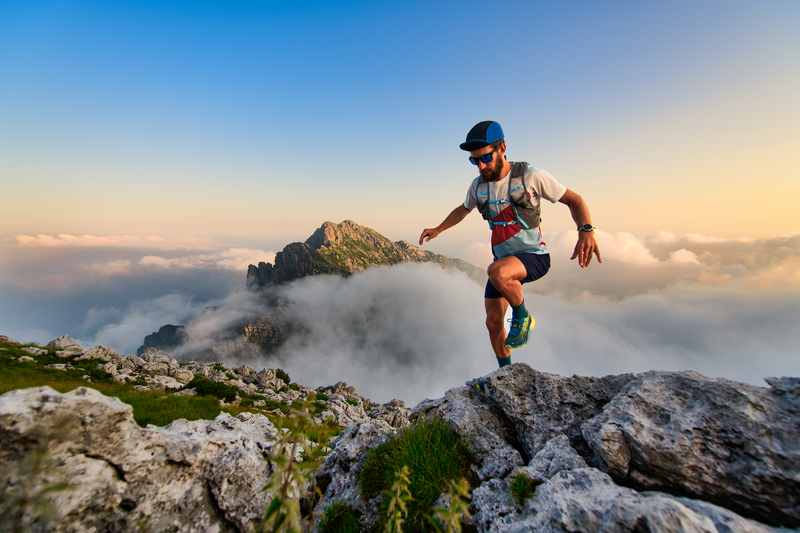
95% of researchers rate our articles as excellent or good
Learn more about the work of our research integrity team to safeguard the quality of each article we publish.
Find out more
REVIEW article
Front. Cell Dev. Biol. , 25 August 2023
Sec. Cell Death and Survival
Volume 11 - 2023 | https://doi.org/10.3389/fcell.2023.1218553
This article is part of the Research Topic The Role and Mechanism of Oxidative Stress, Cells Death and Inflammatory Response in Musculoskeletal Diseases View all articles
Spinal cord injury (SCI) is a sudden onset of disruption to the spinal neural tissue, leading to loss of motor control and sensory function of the body. Oxidative stress is considered a hallmark in SCI followed by a series of events, including inflammation and cellular apoptosis. Melatonin was originally discovered as a hormone produced by the pineal gland. The subcellular localization of melatonin has been identified in mitochondria, exhibiting specific onsite protection to excess mitochondrial reactive oxygen species and working as an antioxidant in diseases. The recent discovery regarding the molecular basis of ligand selectivity for melatonin receptors and the constant efforts on finding synthetic melatonin alternatives have drawn researchers’ attention back to melatonin. This review outlines the application of melatonin in SCI, including 1) the relationship between the melatonin rhythm and SCI in clinic; 2) the neuroprotective role of melatonin in experimental traumatic and ischemia/reperfusion SCI, i.e., exhibiting anti-oxidative, anti-inflammatory, and anti-apoptosis effects, facilitating the integrity of the blood–spinal cord barrier, ameliorating edema, preventing neural death, reducing scar formation, and promoting axon regeneration and neuroplasticity; 3) protecting gut microbiota and peripheral organs; 4) synergizing with drugs, rehabilitation training, stem cell therapy, and biomedical material engineering; and 5) the potential side effects. This comprehensive review provides new insights on melatonin as a natural antioxidant therapy in facilitating rehabilitation in SCI.
Spinal cord injury (SCI) is a sudden onset of disruption to the spinal neural tissue, e.g., spinal cord compression caused by accidents or tumors (Baptiste and Fehlings, 2006), leading to paraplegia, quadriplegia, pain hypersensitivity, and abnormal temperature sensation (Khorasanizadeh et al., 2019; Anjum et al., 2020; Eli et al., 2021). Moreover, a series of complications caused by SCI, such as neurogenic bladder, gastrointestinal dysfunction, and sexual dysfunction, decrease the quality of life of SCI patients, which brings a huge burden to society (Popa et al., 2010; Tollefsen and Fondenes, 2012; Sezer et al., 2015; Tate et al., 2020). Though the initial trauma in SCI is irretrievable, preventing adverse events brought by the secondary injury cascade over the subsequent weeks is achievable.
Oxidative stress is a hallmark of secondary injury. It exists from the acute to chronic phases after traumatic and ischemia/reperfusion SCI (Erten et al., 2003; Song et al., 2013). Briefly, the balance between pro-oxidants and antioxidants is disrupted, excessive reactive oxygen species (ROS) are generated, and lipid peroxidation happens, which results in oxidative stress (McDonald and Sadowsky, 2002). The neurons and glial cells in the spinal cord cannot resist the oxidative stress, and cell death consequently happens followed by large amounts of debris (Bains and Hall, 2012). The deteriorated microenvironment initiates secondary injuries, e.g., neuroinflammation and cellular apoptosis (Wang H. et al., 2022).
Primary sleep disorders, like circadian rhythm sleep–wake disorders and insomnia, are commonly encountered in SCI patients, especially in patients with quadriplegia (Zeitzer et al., 2000). Attributing to the role of melatonin (N-acetyl-5-methoxy tryptamine) in regulating the circadian rhythm sleep–wake cycle (Vasey et al., 2021), Zeitzer et al. (2000) first reported that plasma melatonin concentration failed to increase at night in three lower cervical SCI patients in the year 2000 (Zeitzer et al., 2000). Afterward, the temporal dynamics of melatonin has been measured in SCI patients with various injury degrees at different segments (Table 1). Notably, melatonin secretion decreases and rhythm loss occurs in the tetraplegic patients with complete cervical SCI, while they are not affected in complete thoracic SCI (Zeitzer et al., 2000; Scheer et al., 2006; Verheggen et al., 2012; Thøfner Hultén et al., 2018). A melatonin replacement therapy was proposed by Scheer et al. (2006) to improve sleep quality in tetraplegic patients (Scheer et al., 2006). Paralleled with the clinical reports, a similar pattern of changes in the melatonin rhythm has been revealed in the rat SCI model (Table 1) (Gezici et al., 2010). It has been reported that the rhythmic secretion of melatonin from the pineal gland is regulated by the suprachiasmatic nucleus (SCN) in the anterior hypothalamus (Cardinali and Pévet, 1998). The efferent nerve fibers from the SCN to the pineal gland cross the upper part of the cervical medulla and are connected to the preganglionic cells of the cervical sympathetic ganglion (Møller and Baeres, 2002), which hints the causality of patients with SCI at or above C8 with inadequate melatonin secretion, disrupted melatonin rhythm, and poor sleep quality (Verheggen et al., 2012). However, patients with injuries below the T4 spinal cord maintain their melatonin rhythm (Whelan et al., 2020).
TABLE 1. Relationship between the temporal dynamics of melatonin and spinal cord injury in patients and animal models.
Melatonin is an amphiphilic molecule first isolated by Lerner et al. (1958). Melatonin is synthesized in the pineal gland in the central nervous system (CNS) and peripheral organs, including the retina, intestine, bone marrow, pancreas, and kidney (Reiter, 1991; Ren et al., 2017; Zhang Y. et al., 2018). The subcellular localization of melatonin has been identified in mitochondria due to that the melatonin synthetic enzyme serotonin N-acetyltransferase (SNAT) was found in the matrix and intermembrane space of mitochondria (Reiter and Tan, 2019). Mitochondria are the major source of ROS, the birth place and battle ground of melatonin, and also the site of melatonin metabolism (Reiter and Tan, 2019). Melatonin exhibits specific on-site protection against mitochondrial ROS, which is underlying melatonin’s anti-oxidative mechanism. Reiter and Tan (2019) proposed that melatonin exhibits its major protective effects on neurons at the level of mitochondria (Reiter and Tan, 2019). Melatonin receptor (MT1) is located on the mitochondrial membrane, and it regulates mitochondrial physiology through the MT1-mediated sirtuin 1 (SIRT1)– peroxisome proliferator-activated receptor-gamma coactivator-1 alpha (PGC-1α)–nuclear factor erythroid 2–related factor 2 (NRF2)– peroxisome proliferator-activated receptor-gamma (PPAR-γ) signaling transduction through the MT1-mediated sirtuin 1 (SIRT1)– peroxisome proliferator-activated receptor-gamma coactivator-1 alpha (PGC-1α)–nuclear factor erythroid 2–related factor 2 (NRF2)– peroxisome proliferator-activated receptor-gamma (PPAR-γ) signaling transduction (Guo et al., 2014). It has been reported that melatonin maintains mitochondrial homeostasis by regulating mitochondrial fission, mitophagy, and mitochondrial permeability transition pore (Zheng et al., 2021). Specifically, mitochondrial ROS in cancer amplifies the tumorigenic phenotype, accelerates the accumulation of additional mutations, and leads to metastatic behaviors (Sabharwal and Schumacker, 2014); the cytotoxicity of melatonin to Caco-2 cells (an immortalized cell line of human colorectal adenocarcinoma cells) and Jurkat cells (an immortalized cell line of human T lymphocyte cells), in turn, evidences the effectiveness of melatonin in regulating the mitochondrial metabolism (Wölfler et al., 2001).
Plasma melatonin concentration peaks at night in mammals (Zhang et al., 2022). It has been suggested by experts that the exposure to Sun during the day and avoidance of light at night benefit human wellbeing by improving melatonin production (Tan et al., 2023). In clinic, melatonin has been used to treat primary insomnia (Rondanelli et al., 2011). Moreover, the effectiveness of melatonin in combating oxidative stress, inflammation, and cellular apoptosis has been studied for decades in mood disorders and cognitive dysfunctions (Agahi et al., 2018), stroke and other neurodegenerative diseases (Hosseinzadeh et al., 2023), tumors, and cancers (Rohilla et al., 2023) Recently, the cryogenic electron microscopy (cryo-EM) structures of the active melatonin receptors (MTs) have been revealed (Wang Q. et al., 2022), which is going to take melatonin research forward.
Melatonin regulates sleep and the circadian rhythm by activating two high-affinity G protein–coupled receptors, i.e., MT1 and MT2 (Cecon et al., 2018). In the brain, MT1 is expressed in the locus coeruleus (LC) and perifornical lateral hypothalamus (PFH), regulating the rapid eye movement (REM), while MT2 is found in the thalamic reticular nucleus (TRN) in charge of the non-REM (NREM) activity (Lacoste et al., 2015; Waly and Hallworth, 2015). Initially, melatonin is extracted from the pineal gland and urine; currently, all melatonin used in clinic is obtained from chemical methods, such as the prolonged melatonin preparation (Circadin®) and the synthesized ligand agonist to both MT1 and MT2 (agomelatine, ramelteon, and tasimelteon) (Wang Q. et al., 2022). The technological breakthrough, obtaining biological melatonin from microbes, algae, and plants, has been achieved a few years ago (Arnao et al., 2023). Notably, the microorganisms, including the genetically modified Saccharomyces and Escherichia coli and microbial fermentation Lactobacillus sp., Bifidobacterium sp., Enterococcus sp., and Streptococcus thermophilus, haven been employed to produce melatonin industrially in bioreactors (Arnao et al., 2023). Despite the development of the chemical and biological melatonergic ligands, the in vivo stability and subtype selectivity, especially the MT1 selective drug, are yet to be solved (Boutin et al., 2020). From the pharmacological standpoint, a full characterization of the melatonin receptor subtypes is advocated. Wang H. et al. (2022) revealed that the cryo-EM structures of active MTs with heterotrimeric Gi protein engaged in the receptor core (Wang Q. et al., 2022). Though MT1 and MT2 possess a high similarity in the orthosteric ligand-binding pockets, they display distinctive features in the cryo-EM, i.e., the distinctive structure of the N4.60-Y5.38-H5.46 motif and the “longitudinal channel” in MT1 and the larger sub-pocket in MT2 (Wang Q. et al., 2022).
Attributing to the relationship between the melatonin rhythm and SCI in clinic (Table 1), efforts have been paid to bond the causality between rehabilitation and melatonin in SCI. To provide a thorough summary of melatonin and its effect associated with SCI, literature searches were conducted on the databases of PubMed, Web of Science, and Google Scholar from inception to March 2023 with the keywords combining “spinal cord injury” or “SCI” with “melatonin” or “N-acetyl-5-methoxy tryptamine.” The eligibility criteria of the selected studies are 1) relevant studies with full text published in English and assessable online; 2) outcomes obtained from animal models (mouse, rat, and rabbit); 3) reporting information including model type, treatment, dosage/duration of melatonin, and outcome assessment; and 4) modeling with contusion, compression, radiation, photo thrombosis, and spinal cord ischemia–reperfusion injury (SCIR).
The neuroprotective effects and underlying mechanisms of melatonin were explored in experimental SCI (Table 2).
TABLE 2. Non-comprehensive review of the neuroprotective effects of melatonin in experimental spinal cord injury.
The blood–spinal cord barrier (BSCB) consists of vascular endothelial cells, astrocytes, pericytes, and basement membrane. It regulates molecular exchange between blood and the spinal cord and plays an important role in maintaining the fluid microenvironment of the spinal cord (Haddadi et al., 2013). The compromised integrity of the BSCB increases its permeability to plasma components, e.g., neutrophil infiltration happens at the site of injury following traumatic SCI, leading to vasogenic spinal cord edema (Wu et al., 2014). In traumatic SCI, the BSCB permeability is increased quickly within 48 h post SCI; the melatonin treatment maintains the integrity of the BSCB via regulating the tight junction proteins and protecting endothelial cells and pericytes (Wu et al., 2014). Jing et al. (2016) showed that melatonin attenuates edema by decreasing the expression of aquaporin 4 (AQP4) and intercellular cell adhesion molecule-1 (ICAM1), which restrains microvessel loss and sustains microcirculation (Wu et al., 2014). In the irradiated spinal cord tissue, the mRNA expression of the vascular endothelial growth factor (VEGF), which is critical in BSCB destruction (Haddadi et al., 2013), is increased at 20th and 22nd week after irradiation (Haddadi et al., 2013). Melatonin preconditioning decreases the expression of VEGF and significantly reduces vasodilation, congestion, and cavitation in the white matter, which promotes functional recovery in the irradiated animals (Haddadi et al., 2013).
Neurons are the structural and functional units of the nervous system, while glial cells support neurons. Neurons communicate to one another based on a synaptic structure. Oligodendrocytes form the myelin sheath around axons, which promises an efficient long-distance impulse transmission from neuronal soma to the presynaptic membrane. In SCI, the deteriorated microenvironment after SCI jeopardizes neuronal vitality and causes neuronal death. Wang Q. et al. (2022) found that melatonin inhibits neuronal death at the acute phases of SCI and promotes rehabilitation afterward by attenuating oxidative stress and preventing mitochondrial dysfunction (Wang H. et al., 2022). Moreover, intrathecal melatonin administration significantly preserves the myelinated areas and increases the number of sensory neurons in the white matter after SCI (Fakhri et al., 2021). In a word, melatonin administration prevents axonal degeneration and demyelination (Majidpoor et al., 2020a; Majidpoor et al., 2020b). Parallelly, the endogenous melatonin content in the rostrocaudal region of the spinal cord is increased by a 12-h light/12-h dark cycle, promoting oligodendrogenesis and excitatory synaptic formation (Hong et al., 2019). Neuroplasticity drives functional restoration in SCI. Melatonin facilitates neuronal remodeling by increasing the expression of the brain-derived neurotrophic factor (BDNF) and growth-associated protein-43 (GAP-43) (Jing et al., 2017). Bi et al. (2021) found that melatonin administration after SCI significantly increases the expression of GAP-43, synaptic protein 1 (SYN1), neurofilament 200 (NF200), and postsynaptic density protein 95 (PSD-95) (Bi et al., 2021).
Reactive astrogliosis forms the core of fibrotic scar at the SCI lesion site. The glial scar, a dense limiting border outside the injury site, is the primary cause of the failure in axonal regeneration, and reducing glial scar formation after SCI contributes to the regrowth of transected axons (Krityakiarana et al., 2016). It has been reported that melatonin suppresses the proliferation and accumulation of astrocytes (Yang et al., 2020). Krityakiarana et al. (2016) reported that melatonin reduces inflammatory response and scar formation, which promotes axonal outgrowth (Krityakiarana et al., 2016). Moreover, Hong et al. (2019) reported that the melatonin precursor N-acetylserotonin (NAS) activates the tropomyosin receptor kinase B (TRKB)-induced protein kinase B (AKT)–extracellular-regulated kinase (ERK) signaling cascade, which facilitates axonal growth after SCI (Hong et al., 2019).
Melatonin is an effective free-radical scavenger, sustaining the balance between reduced glutathione (GSH) and oxidized glutathione disulfide (GSSG) and preventing excess lipid peroxidation in the aged mouse brain (Carretero et al., 2009). In traumatic and ischemia/reperfusion SCI, melatonin corrects the level of malondialdehyde (MDA), GSH, superoxide dismutase (SOD), nitrite oxide (NO), xanthine oxidase (XO), thiobarbituric acid reactive substance (TBARS), inducible nitric oxide synthase (iNOS), and myeloperoxidase (MPO), which benefits rehabilitation (Fujimoto et al., 2000; Kaptanoglu et al., 2000; Taskiran et al., 2000; Erten et al., 2003; Liu et al., 2004; Song et al., 2013). Moreover, Gül et al. (2005) revealed a dose-dependent effect of exogenous melatonin on protecting the axons and myelin in white matter at 24 h after SCI, but not on preventing early lipid peroxidation (Gül et al., 2005). Interestingly, Park et al. (2012) reported a loss of efficacy of melatonin treatment in decreasing the mRNA expression of iNOS and accelerating motor function recovery when exposing SCI rats to 24-h constant light, which suggests the significance of endogenous melatonin and melatonin rhythm in functional recovery in SCI (Park et al., 2012).
ROS functions as a critical second messenger that modulates inflammation through redox-sensitive mechanisms. Melatonin works as an antioxidant, attenuating neuroinflammation by altering the oxidative stress-induced gene expression profiles in SCI, such as peroxisome proliferator-activated receptors α (PPAR-α), toll-like receptor-4 (TLR4), nuclear factor kappa B (NF-κB), and NRF2. Specifically, melatonin exhibits anti-inflammatory effects against the secondary injury in wild-type mice by binding to PPAR-α, indicated by a loss of response to melatonin in the PPAR-α knockout (KO) mice (Paterniti et al., 2017). Moreover, melatonin administration during the acute phase after SCI suppresses inflammation through inhibiting the TLR4/NF-κB signaling pathway (Majidpoor et al., 2020a). Recently, Wang Q. et al. (2022) reported that melatonin reduces the generation of nucleotide-binding domain-like receptor protein 3 (NLRP3) inflammasomes via activating NRF2 and downstream antioxidant responsive element (ARE) signaling transduction, which reduces mitochondrial dysfunction and accelerates neurological functional recovery (Wang H. et al., 2022).
M1 microglia (pro-inflammatory) is a main source of tumor necrosis factor alpha (TNF-α), interferon-γ (INF-γ), and interleukin-1β (IL-1β) in CNS. Haddadi and Fardid (2015) found that the TNF-α expression increases within hours in the radiation-injured spinal cord tissue, while oral melatonin administration during the acute phase inhibits TNF-α and aids in SCI (Haddadi and Fardid, 2015). The effectiveness of melatonin in diminishing pro-inflammatory cytokines has been evidenced, such as INF-γ and IL-1β (Xu et al., 2018; Jing et al., 2019; Yang et al., 2020). Moreover, it has been reported that melatonin promotes microglia M2 polarization (anti-inflammatory type) rather than M1 (Zhang et al., 2019).
Oxidative stress is a potent apoptotic inducer, which disrupts Ca2+ homeostasis, causes mitochondrial dysfunction, e.g., mtDNA damage and cytochrome c release, and induces typical apoptotic proteins, e.g., the increased expression of cleaved caspase-3 (CASP3) and B-cell lymphoma 2 (BCL2) and the decrease in the bcl-2-like protein 4 (BAX) content (Aydemir et al., 2016). Aydemir et al. (2016) found that melatonin deficiency impedes sensory and motor function recovery in the ischemic spinal cord injured rats, while pretreatment with melatonin alleviates neuronal apoptosis and accelerates functional recovery by activating the phosphoinositide 3-kinase (PI3K)–akt serine/threonine kinase 1 (AKT1) signaling pathway (Aydemir et al., 2016). Parallelly, the administration of melatonin during the first 3 days after traumatic SCI ameliorates neuronal apoptosis via provoking the wingless–int (Wnt)/β-catenin signaling transduction (Shen et al., 2017).
Oxidative stress damages cellular constituents, e.g., proteins, DNA, and lipids, which turns on the autophagic process (Li et al., 2019). The mechanistic target of rapamycin (mTOR) is a pharmacologic target for autophagy regulation. It has been found that melatonin enhances autophagy and inhibits neuronal apoptosis after SCI through activating the PI3K/AKT/mTOR signaling pathway (Li et al., 2019). In addition, mTOR, 5′AMP-activated protein kinase (AMPK), and NAD-dependent deacetylase sirtuin-1 (SIRT1) interplay in regulating metabolic stress. Gao T. et al. (2020) indicated that melatonin exerts neuroprotection via activating autophagy through the SIRT1/AMPK signaling pathway (Gao K. et al., 2020).
SCI causes functional disorders in the peripheral organs, including the gastrointestinal system (Zhang C. et al., 2018), kidney (Akakin et al., 2013), bladder (Erşahin et al., 2012), testes (Yuan et al., 2016), and corpus cavernosum (Tavukçu et al., 2014), which is attributed to the damaged autonomic innervation by the sympathetic and parasympathetic fibers derived from the spinal cord (Table 3). For example, the damaged autonomic nervous system loses gastrointestinal sympathetic control, leading to insufficient intestinal motility and deficient mucosal secretions (Zhang C. et al., 2018). The gut microbiota works as a virtual endocrine organ, regulating mood and behaviors via the microbiota–gut–brain axis and immunity via the intestinal neuro-immune axis (Jacobson et al., 2021; Huang et al., 2022). Hence, SCI affects the behavior of the gastrointestinal system, alters the constitution of gut microbial community, and causes systemic disorders. The alteration in gut microbiota is associated with the metabolite profiles in SCI (Kang et al., 2023). Gut dysbiosis impairs rehabilitation in SCI (Agahi et al., 2018). The gut microbiota–brain axis mediates the psychological stress after SCI (Musleh-Vega et al., 2022). Fecal microbiota transplantation exerts neuroprotective effects on SCI mice via modulating the microenvironment at the lesion site (Jing et al., 2022). Furthermore, melatonin mitigates the microbiota dysbiosis in the segment of the jejunum in the short-term sleep-deprived mice (Gao T. et al., 2020). Jing et al. (2019) showed that melatonin treatment reduces the relative abundance of Clostridium perfringens and increases that of Lactobacillus, which attenuates SCI-induced dysbiosis (Jing et al., 2019). Moreover, melatonin treatment improves the function of bladder (Erşahin et al., 2012), decreases the SCI-increased permeability of capillaries, and increases the SCI-reduced testicular blood flow (Yuan et al., 2016) by coping with oxidative stress.
TABLE 3. Non-comprehensive review of melatonin protecting peripheral organs in experimental spinal cord injury.
Based on the bulk reports regarding melatonin’s protective roles in SCI, the synergistic effects of melatonin are explored (Table 4), including interacting other medications, functioning as a supplement in rehabilitation training, and working as a preconditioning stimulus in stem cell therapy. Moreover, melatonin has been employed by biomedical material engineering as an antioxidant agent in treating SCI and a differentiation signal to activate the endogenous neural cells.
Methylprednisolone (MP) is a corticosteroid medication applied during the acute phase in SCI (Cayli et al., 2004). Cayli et al. (2004) reported that combining melatonin with MP results in a better neurological recovery during the subacute phase after SCI by inhibiting the accumulation of lipid peroxidation (Cayli et al., 2004). Moreover, melatonin administrated with the corticosteroid dexamethasone (DEX) significantly reduces tissue damage and promotes motor function recovery via reducing apoptosis and polymorphonuclear leukocyte infiltration indicated by the downregulated protein expressions of TNF-α and iNOS in the spinal cord (Genovese et al., 2007). The combined administration elevates the curative effect of DEX, i.e., reducing to a 10th of the original effective concentration, and avoids its side effects, such as infection, osteonecrosis/osteoporosis, and depression (Genovese et al., 2007). Moreover, the combination therapy of melatonin and tadalafil (a medicine for erection problems) is more effective in restoring erectile function via attenuating the oxidative damage to cavernosum in SCI compared to the individual drugs (Tavukçu et al., 2014).
SCI disrupts the signaling transmission between the brain and body, and SCI rehabilitation exercise helps restore brain–body communication via facilitating neuronal regeneration in the injured spinal cord. Melatonin administration combined with motor-driven treadmill exercise suppresses neuroinflammatory response, reduces neuronal deformation, increases endogenous neural stem/progenitor cells, enhances neuronal differentiation, and improves the recovery of motor function after SCI (Park et al., 2010). In line with the previous report, Lee et al. (2014) found that the synergistic effect of melatonin combined with treadmill exercise. It creates a healing niche which facilitates proliferation and differentiation of endogenous neural stem/progenitor cells, promotes neuronal regeneration, and improves neurological function in SCI rats (Lee et al., 2014).
The melatonin precondition provides a promising extracellular vesicle-based or stem cell-based approach for treating SCI. It has been reported in mesenchymal stem cells (MSCs) that melatonin reduces methyltransferase-like 3 (METTL3) expression and global N6-methyladenosine (m6A) modification, which stabilizes the mRNA of ubiquitin specific peptidase 29 (USP29) (Liu et al., 2021). Liu et al. (2021) harvested USP29-contained extracellular vesicles from the melatonin-pretreated MSCs (MEVs) (Liu et al., 2021). The USP29-contained MEVs transfer USP29 to microglia and macrophages in the injured site, which stabilizes NRF2, facilitates M2-like polarization, and consequently, promotes functional behavioral recovery in the SCI mice (Liu et al., 2021). Moreover, melatonin, working as an antioxidant, facilitates the vitality of the adipose-derived stem cells (ADSCs/adMSCs) in vitro before stem cell transplantation (Naeimi et al., 2022). The melatonin-pretreated ADSC/adMSC positively affects the engraftment and neuronal differentiation in SCI (Naeimi et al., 2022). Similarly, melatonin administrated with wnt family member 4 (WNT4) promotes neural cell differentiation of the bovine amniotic epithelial cells, and the neural cell transplantation has been proven to accelerate the motor function recovery in SCI (Gao et al., 2016).
Melatonin is employed in biomedical material engineering to act directly on the endogenous stem cells. Two new sustained-release melatonin delivery systems to prolong melatonin release and enhance melatonin’s role in facilitating M2 polarization are developed in consideration of the complicated conditions and significant inter-individual differences in SCI patients (Zhang et al., 2021). For in situ injection, an injectable Lap/MS@Melatonin micro-gel compound, i.e., poly (lactic-co-glycolic acid) (PLGA) sustained-release microspheres (MSs) loaded with melatonin mixed with Laponite hydrogels, has been designed (Zhang et al., 2021). To sustain and for precision-targeted delivery of melatonin intravenously, a nano-PM compound, namely, PM/MS@Melatonin, i.e., nanospheres coated with a platelet membrane (PM) loaded with melatonin, is designed (Zhang et al., 2021). Moreover, a 3D-printed scaffold for a controlled release of melatonin has been developed for curing long-range nerve defects (Qian et al., 2018). It has been found that this melatonin/polycaprolactone nerve guide conduit reduces oxidative stress, neuroinflammation, and nerve cell apoptosis; provides energy for nerves; facilitates nerve debris clearance; and stimulates neural proliferation (Qian et al., 2018).
Adverse events have been reported in melatonin treating sleep disorders, including excessive sedation (Panda et al., 2022), cognitive disorders (Besag et al., 2019), nocturnal hypotension (Grossman et al., 2011), and impaired glucose tolerance (Rubio-Sastre et al., 2014). The suggested dose of melatonin in clinic is 0.3–1.0 mg/day internationally. However, a clinical trial indicated no significant difference between melatonin and placebo arms in the rate of adverse events, even at a dose as high as 1,600 mg/day (Foley and Steel, 2019). Moreover, systemic reviews indicate no adverse events of life-threatening or of major clinical significance (Grossman et al., 2011; Besag et al., 2019; Panda et al., 2022). The bioavailability of exogenous melatonin is highly variable due to the first-pass metabolism and poor absorption, ranging from 1% to 37% (Andersen et al., 2016). Especially, in cirrhotic patients, the clearance of melatonin is slowed down (Chojnacki et al., 2013). Hence, caution should be exercised when administering melatonin with other drugs (Foster et al., 2015). For example, taking melatonin with sedatives might cause sleepiness and slow breathing (Panda et al., 2022). Furthermore, a case report indicated that a good initial response to melatonin was found at the beginning in treating intellectual disability and sleep disorders; the patients lost response to melatonin afterward potentially due to the delayed melatonin clearance (Braam et al., 2010).
To avoid the adverse effects, lowering effective concentration, increasing the effectiveness of melatonin treatment, and seeking melatonin analogs are considered. For example, the effect of β-methyl-6-chloromelatonin, which exerts a direct soporific effect in treating insomnia without changing in body temperature, heart rate, or blood pressure compared to melatonin (Zemlan et al., 2005), has been studied in SCI though no statistical significant outcome was found (Fee et al., 2010). Other novel synthetic analogs, mainly the melatonin derivatives, are under study, such as the 2-phenylindole derivatives (Suzen et al., 2006), 5-bromoindole derivatives (Gürkök et al., 2009), 2-indole aldehyde derivatives (Suzen et al., 2013), and 5-chloroindole derivatives (Yılmaz et al., 2012).
In this review, the relationship between the melatonin rhythm and SCI has been unveiled in clinic, and the feasibility of melatonin treatment in facilitating rehabilitation under the circumstances of various SCI degrees and segments has been verified in experimental SCI models. Oxidative stress is a primary cause in cytotoxicity and disease. Melatonin administrated independently or synergized with other medications, rehabilitation training, stem cell transplantation, and biomedical material engineering, as a natural antioxidant therapy, aids in SCI. Generally, melatonin copes with oxidative stress and mitigates subsequent neuroinflammation and cellular apoptosis in SCI, resulting in reduced neural death and debris, restored BSCB, attenuated edema, decreased scar formation, a better neuronal regeneration, and neuroplasticity and microcirculation in the injured spinal cord (Figure 1). Moreover, melatonin attenuates oxidative damage, prevents functional disorders in the peripheral organs, and has a beneficial influence on systemic recovery in SCI (Figure 1). Though the melatonin’s protective roles in preventing secondary injuries in SCI have been studied for decades and many signaling pathways are reported involved in melatonin facilitating functional recovery and attenuating tissue damage, the receptor-dependent mechanism is rarely mentioned, e.g., MT1 or MT2. Due to the potential rise of long-term melatonin exposure and the high dose of melatonin used in SCI [orally: 5 mg/kg, intraperitoneal injection: 5–100 mg/kg (reported in experimental SCI research, Table 2); compared to clinical dosage: physiological dose (0.3–1.0 mg/qd) and supraphysiological dose for occasional use (≥3 mg/qd), seeking melatonin analogs to prevent drug resistance and designing selective compound for an effective melatonergic therapy in SCI are advocated.
FIGURE 1. Melatonin facilitates systemic recovery by preventing spinal cord dysfunctions and peripheral organ disorders.
Conceptualization: LX and HW; writing—original draft preparation: LX; writing—review and editing: LX, HW, XH, and TY; and supervision: XH and TY. All authors contributed to the article and approved the submitted version.
This research was supported by the Shandong Province Major Scientific and Technical Innovation Project (2021SFGC0502 to TY) and Qingdao Science and Technology Benefit People Demonstration Guide Special Project (22-3-7-smjk-5-nsh to TY).
The authors declare that the research was conducted in the absence of any commercial or financial relationships that could be construed as a potential conflict of interest.
All claims expressed in this article are solely those of the authors and do not necessarily represent those of their affiliated organizations, or those of the publisher, the editors, and the reviewers. Any product that may be evaluated in this article, or claim that may be made by its manufacturer, is not guaranteed or endorsed by the publisher.
Agahi, M., Akasheh, N., Ahmadvand, A., Akbari, H., and Izadpanah, F. (2018). Effect of melatonin in reducing second-generation antipsychotic metabolic effects: A double blind controlled clinical trial. Diabetes Metab. Syndr. 12, 9–15. doi:10.1016/j.dsx.2017.08.004
Akakin, D., Kiran, D., Ozkan, N., Erşahin, M., Ozdemir-Kumral, Z. N., Yeğen, B., et al. (2013). Protective effects of melatonin against spinal cord injury induced oxidative damage in rat kidney: A morphological and biochemical study. Acta histochem. 115, 827–834. doi:10.1016/j.acthis.2013.04.005
Andersen, L. P., Werner, M. U., Rosenkilde, M. M., Harpsoe, N. G., Fuglsang, H., Rosenberg, J., et al. (2016). Pharmacokinetics of oral and intravenous melatonin in healthy volunteers. BMC Pharmacol. Toxicol. 17, 8. doi:10.1186/s40360-016-0052-2
Anjum, A., Yazid, M. D., Fauzi Daud, M., Idris, J., Ng, A. M. H., Selvi Naicker, A., et al. (2020). Spinal cord injury: pathophysiology, multimolecular interactions, and underlying recovery mechanisms. Int. J. Mol. Sci. 21, 7533. doi:10.3390/ijms21207533
Arnao, M. B., Giraldo-Acosta, M., Castejón-Castillejo, A., Losada-Lorán, M., Sánchez-Herrerías, P., El Mihyaoui, A., et al. (2023). Melatonin from microorganisms, algae, and plants as possible alternatives to synthetic melatonin. Metabolites 13, 72. doi:10.3390/metabo13010072
Ates, O., Cayli, S., Gurses, I., Yucel, N., Altinoz, E., Iraz, M., et al. (2007). Does pinealectomy affect the recovery rate after spinal cord injury? Neurol. Res. 29, 533–539. doi:10.1179/016164107X172121
Aydemir, S., Dogan, D., Kocak, A., and Dilsiz, N. (2016). The effect of melatonin on spinal cord after ischemia in rats. Spinal Cord. 54, 360–363. doi:10.1038/sc.2015.204
Bains, M., and Hall, E. D. (2012). Antioxidant therapies in traumatic brain and spinal cord injury. Biochim. Biophys. Acta 1822, 675–684. doi:10.1016/j.bbadis.2011.10.017
Baptiste, D. C., and Fehlings, M. G. (2006). Pharmacological approaches to repair the injured spinal cord. J. Neurotrauma 23, 318–334. doi:10.1089/neu.2006.23.318
Besag, F. M. C., Vasey, M. J., Lao, K. S. J., and Wong, I. C. K. (2019). Adverse events associated with melatonin for the treatment of primary or secondary sleep disorders: A systematic review. CNS Drugs 33, 1167–1186. doi:10.1007/s40263-019-00680-w
Bi, J., Shen, J., Chen, C., Li, Z., Tan, H., Sun, P., et al. (2021). Role of melatonin in the dynamics of acute spinal cord injury in rats. J. Cell Mol. Med. 25, 2909–2917. doi:10.1111/jcmm.16325
Bi, J., Sun, P., Feng, E., Shen, J., Chen, C., Tan, H., et al. (2022). Melatonin synergizes with methylprednisolone to ameliorate acute spinal cord injury. Front. Pharmacol. 12, 723913. doi:10.3389/fphar.2021.723913
Boutin, J. A., Witt-Enderby, P. A., Sotriffer, C., and Zlotos, D. P. (2020). Melatonin receptor ligands: A pharmaco-chemical perspective. J. Pineal Res. 69, e12672. doi:10.1111/jpi.12672
Braam, W., Van Geijlswijk, I., Keijzer, H., Smits, M. G., Didden, R., and Curfs, L. M. (2010). Loss of response to melatonin treatment is associated with slow melatonin metabolism. J. Intellect. Disabil. Res. 54, 547–555. doi:10.1111/j.1365-2788.2010.01283.x
Cardinali, D. P., and Pévet, P. (1998). Basic aspects of melatonin action. Sleep. Med. Rev. 2, 175–190. doi:10.1016/s1087-0792(98)90020-x
Carretero, M., Escames, G., Lopez, L. C., Venegas, C., Dayoub, J. C., Garcia, L., et al. (2009). Long-term melatonin administration protects brain mitochondria from aging. J. Pineal Res. 47, 192–200. doi:10.1111/j.1600-079X.2009.00700.x
Cayli, S. R., Kocak, A., Yilmaz, U., Tekiner, A., Erbil, M., Ozturk, C., et al. (2004). Effect of combined treatment with melatonin and methylprednisolone on neurological recovery after experimental spinal cord injury. Eur. Spine J. 13, 724–732. doi:10.1007/s00586-003-0550-y
Cecon, E., Oishi, A., and Jockers, R. (2018). Melatonin receptors: molecular pharmacology and signalling in the context of system bias. Br. J. Pharmacol. 175, 3263–3280. doi:10.1111/bph.13950
Chojnacki, C., Wachowska-Kelly, P., Blasiak, J., Reiter, R. J., and Chojnacki, J. (2013). Melatonin secretion and metabolism in patients with hepatic encephalopathy. J. Gastroenterol. Hepatol. 28, 342–347. doi:10.1111/jgh.12055
Eli, I., Lerner, D. P., and Ghogawala, Z. (2021). Acute traumatic spinal cord injury. Neurol. Clin. 39, 471–488. doi:10.1016/j.ncl.2021.02.004
Erol, F. S., Kaplan, M., Tiftikci, M., Yakar, H., Ozercan, I., Ilhan, N., et al. (2008). Comparison of the effects of octreotide and melatonin in preventing nerve injury in rats with experimental spinal cord injury. J. Clin. Neurosci. 15, 784–790. doi:10.1016/j.jocn.2007.06.009
Erşahin, M., Özdemir, Z., Özsavcı, D., Akakın, D., Yeğen, B., Reiter, R. J., et al. (2012). Melatonin treatment protects against spinal cord injury induced functional and biochemical changes in rat urinary bladder. J. Pineal Res. 52, 340–348. doi:10.1111/j.1600-079X.2011.00948.x
Erten, S. F., Kocak, A., Ozdemir, I., Aydemir, S., Colak, A., and Reeder, B. S. (2003). Protective effect of melatonin on experimental spinal cord ischemia. Spinal Cord. 41, 533–538. doi:10.1038/sj.sc.3101508
Esposito, E., Genovese, T., Caminiti, R., Bramanti, P., Meli, R., and Cuzzocrea, S. (2009). Melatonin reduces stress-activated/mitogen-activated protein kinases in spinal cord injury. J. Pineal Res. 46, 79–86. doi:10.1111/j.1600-079X.2008.00633.x
Esposito, E., Genovese, T., Caminiti, R., Bramanti, P., Meli, R., and Cuzzocrea, S. (2008). Melatonin regulates matrix metalloproteinases after traumatic experimental spinal cord injury. J. Pineal Res. 45, 149–156. doi:10.1111/j.1600-079X.2008.00569.x
Fakhri, S., Kiani, A., Jalili, C., Abbaszadeh, F., Piri, S., Farzaei, M. H., et al. (2021). Intrathecal administration of melatonin ameliorates the neuroinflammation- mediated sensory and motor dysfunction in A rat model of compression spinal cord injury. Curr. Mol. Pharmacol. 14, 646–657. doi:10.2174/1874467213666201230101811
Fee, D. B., Swartz, K. R., Scheff, N., Roberts, K., Gabbita, P., and Scheff, S. (2010). Melatonin-analog, beta-methyl-6-chloromelatonin, supplementation in spinal cord injury. Brain Res. 1340, 81–85. doi:10.1016/j.brainres.2010.04.047
Foley, H. M., and Steel, A. E. (2019). Adverse events associated with oral administration of melatonin: A critical systematic review of clinical evidence. Complement. Ther. Med. 42, 65–81. doi:10.1016/j.ctim.2018.11.003
Foster, B. C., Cvijovic, K., Boon, H. S., Tam, T. W., Liu, R., Murty, M., et al. (2015). Melatonin interaction resulting in severe sedation. J. Pharm. Pharm. Sci. 18, 124–131. doi:10.18433/j3ss35
Fujimoto, T., Nakamura, T., Ikeda, T., and Takagi, K. (2000). Potent protective effects of melatonin on experimental spinal cord injury. Spine (Phila Pa 1976) 25, 769–775. doi:10.1097/00007632-200004010-00003
Gao, K., Niu, J., and Dang, X. (2020a). Neuroprotection of melatonin on spinal cord injury by activating autophagy and inhibiting apoptosis via SIRT1/AMPK signaling pathway. Biotechnol. Lett. 42, 2059–2069. doi:10.1007/s10529-020-02939-5
Gao, T., Wang, Z., Cao, J., Dong, Y., and Chen, Y. (2020b). Melatonin attenuates microbiota dysbiosis of jejunum in short-term sleep deprived mice. J. Microbiol. 58, 588–597. doi:10.1007/s12275-020-0094-4
Gao, Y., Bai, C., Zheng, D., Li, C., Zhang, W., Li, M., et al. (2016). Combination of melatonin and Wnt-4 promotes neural cell differentiation in bovine amniotic epithelial cells and recovery from spinal cord injury. J. Pineal Res. 60, 303–312. doi:10.1111/jpi.12311
Genovese, T., Mazzon, E., Crisafulli, C., Esposito, E., Di Paola, R., Muià, C., et al. (2007). Effects of combination of melatonin and dexamethasone on secondary injury in an experimental mice model of spinal cord trauma. J. Pineal Res. 43, 140–153. doi:10.1111/j.1600-079X.2007.00454.x
Genovese, T., Mazzon, E., Muià, C., Bramanti, P., De Sarro, A., and Cuzzocrea, S. (2005). Attenuation in the evolution of experimental spinal cord trauma by treatment with melatonin. J. Pineal Res. 38, 198–208. doi:10.1111/j.1600-079X.2004.00194.x
Gezici, A. R., Karakaş, A., Ergün, R., and Gündüz, B. (2010). Rhythms of serum melatonin in rats with acute spinal cord injury at the cervical and thoracic regions. Spinal Cord. 48, 10–14. doi:10.1038/sc.2009.73
Grossman, E., Laudon, M., and Zisapel, N. (2011). Effect of melatonin on nocturnal blood pressure: meta-analysis of randomized controlled trials. Vasc. Health Risk Manag. 7, 577–584. doi:10.2147/VHRM.S24603
Gül, S., Celik, S. E., Kalayci, M., Taşyürekli, M., Cokar, N., and Bilge, T. (2005). Dose-dependent neuroprotective effects of melatonin on experimental spinal cord injury in rats. Surg. Neurol. 64, 355–361. doi:10.1016/j.surneu.2005.03.036
Guo, P., Pi, H., Xu, S., Zhang, L., Li, Y., Li, M., et al. (2014). Melatonin Improves mitochondrial function by promoting MT1/SIRT1/PGC-1 alpha-dependent mitochondrial biogenesis in cadmium-induced hepatotoxicity in vitro. Toxicol. Sci. 142, 182–195. doi:10.1093/toxsci/kfu164
Guo, Y., Zhang, P., Zhao, H., Xu, C., Lin, S., Mei, X., et al. (2023). Melatonin promotes microglia toward anti-inflammatory phenotype after spinal cord injury. Int. Immunopharmacol. 114, 109599. doi:10.1016/j.intimp.2022.109599
Gürkök, G., Coban, T., and Suzen, S. (2009). Melatonin analogue new indole hydrazide/hydrazone derivatives with antioxidant behavior: synthesis and structure-activity relationships. J. Enzyme Inhib. Med. Chem. 24, 506–515. doi:10.1080/14756360802218516
Haddadi, G. H., and Fardid, R. (2015). Oral administration of melatonin modulates the expression of tumor necrosis factor-α (TNF-α) gene in irradiated rat cervical spinal cord. Rep. Pract. Oncol. Radiother. 20, 123–127. doi:10.1016/j.rpor.2014.11.003
Haddadi, G., Shirazi, A., Sepehrizadeh, Z., Mahdavi, S. R., and Haddadi, M. (2013). Radioprotective effect of melatonin on the cervical spinal cord in irradiated rats. Cell J. 14, 246–253.
Hong, Y., Jin, Y., Park, K., Choi, J., Kang, H., Lee, S. R., et al. (2019). Elevated serum melatonin under constant darkness enhances neural repair in spinal cord injury through regulation of circadian clock proteins expression. J. Clin. Med. 8, 135. doi:10.3390/jcm8020135
Hosseinzadeh, A., Changizi-Ashtiyani, S., Koosha, F., Amiri, S., Karimi-Behnagh, A., and Mehrzadi, S. (2023). Melatonin: therapeutic potential for stroke and other neurodegenerative diseases. Melat. Res. 6, 192–134. doi:10.32794/mr112500144
Huang, X., Hu, J., Peng, H., and Cheng, H.-W. (2022). Embryonic exposure to tryptophan yields bullying victimization via reprogramming the microbiota-gut-brain axis in a chicken model. Nutrients 14, 661. doi:10.3390/nu14030661
Jacobson, A., Yang, D., Vella, M., and Chiu, I. M. (2021). The intestinal neuro-immune axis: crosstalk between neurons, immune cells, and microbes. Mucosal Immunol. 14, 555–565. doi:10.1038/s41385-020-00368-1
Jing, Y., Bai, F., Chen, H., and Dong, H. (2017). Melatonin prevents blood vessel loss and neurological impairment induced by spinal cord injury in rats. J. Spinal Cord. Med. 40, 222–229. doi:10.1080/10790268.2016.1227912
Jing, Y., Bai, F., Chen, H., and Dong, H. (2016). Meliorating microcirculatory with melatonin in rat model of spinal cord injury using laser Doppler flowmetry. Neuroreport 27, 1248–1255. doi:10.1097/WNR.0000000000000686
Jing, Y., Bai, F., Wang, L., Yang, D., Yan, Y., Wang, Q., et al. (2022). Fecal microbiota transplantation exerts neuroprotective effects in a mouse spinal cord injury model by modulating the microenvironment at the lesion site. Microbiol. Spectr. 10, e0017722. doi:10.1128/spectrum.00177-22
Jing, Y., Yang, D., Bai, F., Zhang, C., Qin, C., Li, D., et al. (2019). Melatonin treatment alleviates spinal cord injury-induced gut dysbiosis in mice. J. Neurotrauma 36, 2646–2664. doi:10.1089/neu.2018.6012
Kalkan, E., Ciçek, O., Unlü, A., Abuşoglu, S., Kalkan, S. S., Avunduk, M. C., et al. (2007). The effects of prophylactic zinc and melatonin application on experimental spinal cord ischemia-reperfusion injury in rabbits: experimental study. Spinal Cord. 45, 722–730. doi:10.1038/sj.sc.3102035
Kang, J.-N., Sun, Z.-F., Li, X.-Y., Zhang, X.-D., Jin, Z.-X., Zhang, C., et al. (2023). Alterations in gut microbiota are related to metabolite profiles in spinal cord injury. Neural Regen. Res. 18, 1076–1083. doi:10.4103/1673-5374.355769
Kaptanoglu, E., Tuncel, M., Palaoglu, S., Konan, A., Demirpençe, E., and Kilinç, K. (2000). Comparison of the effects of melatonin and methylprednisolone in experimental spinal cord injury. J. Neurosurg. 93, 77–84. doi:10.3171/spi.2000.93.1.0077
Khorasanizadeh, M., Yousefifard, M., Eskian, M., Lu, Y., Chalangari, M., Harrop, J. S., et al. (2019). Neurological recovery following traumatic spinal cord injury: A systematic review and meta-analysis. J. Neurosurg. Spine 30, 683–699. doi:10.3171/2018.10.SPINE18802
Korkmaz, A., Oyar, E. O., Kardeş, O., and Omeroğlu, S. (2008). Effects of melatonin on ischemic spinal cord injury caused by aortic cross clamping in rabbits. Curr. Neurovasc Res. 5, 46–51. doi:10.2174/156720208783565681
Krityakiarana, W., Sompup, K., Jongkamonwiwat, N., Mukda, S., Pinilla, F. G., Govitrapong, P., et al. (2016). Effects of melatonin on severe crush spinal cord injury-induced reactive astrocyte and scar formation. J. Neurosci. Res. 94, 1451–1459. doi:10.1002/jnr.23930
Lacoste, B., Angeloni, D., Dominguez-Lopez, S., Calderoni, S., Mauro, A., Fraschini, F., et al. (2015). Anatomical and cellular localization of melatonin MT1 and MT2 receptors in the adult rat brain. J. Pineal Res. 58, 397–417. doi:10.1111/jpi.12224
Lee, Y., Lee, S., Lee, S. R., Park, K., Hong, Y., Lee, M., et al. (2014). Beneficial effects of melatonin combined with exercise on endogenous neural stem/progenitor cells proliferation after spinal cord injury. Int. J. Mol. Sci. 15, 2207–2222. doi:10.3390/ijms15022207
Lerner, A. B., Case, J. D., Takahashi, Y., Lee, T. H., and Mori, W. (1958). Isolation of melatonin, the pineal gland factor that lightens melanocytes1. J. Am. Chem. Soc. 80, 2587. doi:10.1021/ja01543a060
Li, C., Chen, X., Qiao, S., Liu, X., Liu, C., Zhu, D., et al. (2014). Melatonin lowers edema after spinal cord injury. Neural Regen. Res. 9, 2205–2210. doi:10.4103/1673-5374.147954
Li, Y., Guo, Y., Fan, Y., Tian, H., Li, K., and Mei, X. (2019). Melatonin enhances autophagy and reduces apoptosis to promote locomotor recovery in spinal cord injury via the PI3K/AKT/mTOR signaling pathway. Neurochem. Res. 44, 2007–2019. doi:10.1007/s11064-019-02838-w
Liu, J. B., Tang, T. S., Yang, H. L., and Xiao, D. S. (2004). Antioxidation of melatonin against spinal cord injury in rats. Chin. Med. J. Engl. 117, 571–575.
Liu, W., Tang, P., Wang, J., Ye, W., Ge, X., Rong, Y., et al. (2021). Extracellular vesicles derived from melatonin-preconditioned mesenchymal stem cells containing USP29 repair traumatic spinal cord injury by stabilizing NRF2. J. Pineal Res. 71, e12769. doi:10.1111/jpi.12769
Liu, X., Wang, Y., Yang, J., Liu, Y., Zhou, D., Hou, M., et al. (2015). Anti-edema effect of melatonin on spinal cord injury in rats. Biomed. Pap. Med. Fac. Univ. Palacky. Olomouc Czech Repub. 159, 220–226. doi:10.5507/bp.2015.012
Majidpoor, J., Khezri, Z., Rostamzadeh, P., Mortezaee, K., Rezaie, M. J., Fathi, F., et al. (2020a). The expressions of NLRP1, NLRP3, and AIM2 inflammasome complexes in the contusive spinal cord injury rat model and their responses to hormonal therapy. Cell Tissue Res. 381, 397–410. doi:10.1007/s00441-020-03250-5
Majidpoor, J., Mortezaee, K., Khezri, Z., Fathi, F., Zali, A., Derakhshan, H. B., et al. (2020b). The effect of the "segment" of spinal cord injury on the activity of the nucleotide-binding domain-like receptor protein 3 inflammasome and response to hormonal therapy. Cell Biochem. Funct. 39, 267–276. doi:10.1002/cbf.3574
Mcdonald, J. W., and Sadowsky, C. (2002). Spinal-cord injury. Lancet 359, 417–425. doi:10.1016/S0140-6736(02)07603-1
Møller, M., and Baeres, F. M. (2002). The anatomy and innervation of the mammalian pineal gland. Cell Tissue Res. 309, 139–150. doi:10.1007/s00441-002-0580-5
Musleh-Vega, S., Ojeda, J., and Vidal, P. M. (2022). Gut microbiota–brain Axis as a potential modulator of psychological stress after spinal cord injury. Biomedicines 10, 847. doi:10.3390/biomedicines10040847
Naeimi, A., Zaminy, A., Amini, N., Balabandi, R., and Golipoor, Z. (2022). Effects of melatonin-pretreated adipose-derived mesenchymal stem cells (MSC) in an animal model of spinal cord injury. BMC Neurosci. 23, 65. doi:10.1186/s12868-022-00752-6
Nesic, O., Lee, J., Unabia, G. C., Johnson, K., Ye, Z., Vergara, L., et al. (2008). Aquaporin 1 - a novel player in spinal cord injury. J. Neurochem. 105, 628–640. doi:10.1111/j.1471-4159.2007.05177.x
Panda, P. K., Panda, P., Dawman, L., and Sharawat, I. K. (2022). Systematic review and meta-analysis of efficacy and safety of melatonin and triclofos for inducing adequate sedation for sleep electroencephalogram in children. J. Neurosci. Rural Pract. 13, 003–011. doi:10.1055/s-0041-1736511
Park, K., Lee, Y., Park, S., Lee, S., Hong, Y., Kil Lee, S., et al. (2010). Synergistic effect of melatonin on exercise-induced neuronal reconstruction and functional recovery in a spinal cord injury animal model. J. Pineal Res. 48, 270–281. doi:10.1111/j.1600-079X.2010.00751.x
Park, S., Lee, S. K., Park, K., Lee, Y., Hong, Y., Lee, S., et al. (2012). Beneficial effects of endogenous and exogenous melatonin on neural reconstruction and functional recovery in an animal model of spinal cord injury. J. Pineal Res. 52, 107–119. doi:10.1111/j.1600-079X.2011.00925.x
Paterniti, I., Campolo, M., Cordaro, M., Impellizzeri, D., Siracusa, R., Crupi, R., et al. (2017). PPAR-Α modulates the anti-inflammatory effect of melatonin in the secondary events of spinal cord injury. Mol. Neurobiol. 54, 5973–5987. doi:10.1007/s12035-016-0131-9
Piao, M. S., Lee, J. K., Jang, J. W., Hur, H., Lee, S. S., Xiao, L., et al. (2014). Melatonin improves functional outcome via inhibition of matrix metalloproteinases-9 after photothrombotic spinal cord injury in rats. Acta Neurochir. (Wien) 156, 2173–2182. doi:10.1007/s00701-014-2119-4
Popa, C., Popa, F., Grigorean, V. T., Onose, G., Sandu, A. M., Popescu, M., et al. (2010). Vascular dysfunctions following spinal cord injury. J. Med. Life 3, 275–285.
Qian, Y., Han, Q., Zhao, X., Song, J., Cheng, Y., Fang, Z., et al. (2018). 3D melatonin nerve scaffold reduces oxidative stress and inflammation and increases autophagy in peripheral nerve regeneration. J. pineal Res. 65, e12516. doi:10.1111/jpi.12516
Reiter, R. J. (1991). Pineal melatonin: cell biology of its synthesis and of its physiological interactions. Endocr. Rev. 12, 151–180. doi:10.1210/edrv-12-2-151
Reiter, R. J., and Tan, D.-X. (2019). Mitochondria: the birth place, battle ground and the site of melatonin metabolism in cells. Melat. Res. 2, 44–66. doi:10.32794/nr11250011
Ren, W., Liu, G., Chen, S., Yin, J., Wang, J., Tan, B., et al. (2017). Melatonin signaling in T cells: functions and applications. J. Pineal Res. 62, e12394. doi:10.1111/jpi.12394
Rohilla, S., Singh, M., Priya, S., Almalki, W. H., Haniffa, S. M., Subramaniyan, V., et al. (2023). Exploring the mechanical perspective of a new anti-tumor agent: melatonin. J. Environ. Pathology, Toxicol. Oncol. 42, 1–16. doi:10.1615/JEnvironPatholToxicolOncol.2022042088
Rondanelli, M., Opizzi, A., Monteferrario, F., Antoniello, N., Manni, R., and Klersy, C. (2011). The effect of melatonin, magnesium, and zinc on primary insomnia in long-term care facility residents in Italy: A double-blind, placebo-controlled clinical trial. J. Am. Geriatr. Soc. 59, 82–90. doi:10.1111/j.1532-5415.2010.03232.x
Rubio-Sastre, P., Scheer, F. A., Gomez-Abellan, P., Madrid, J. A., and Garaulet, M. (2014). Acute melatonin administration in humans impairs glucose tolerance in both the morning and evening. Sleep 37, 1715–1719. doi:10.5665/sleep.4088
Sabharwal, S. S., and Schumacker, P. T. (2014). Mitochondrial ROS in cancer: initiators, amplifiers or an achilles' heel? Nat. Rev. Cancer 14, 709–721. doi:10.1038/nrc3803
Samantaray, S., Sribnick, E. A., Das, A., Knaryan, V. H., Matzelle, D. D., Yallapragada, A. V., et al. (2008). Melatonin attenuates calpain upregulation, axonal damage and neuronal death in spinal cord injury in rats. J. Pineal Res. 44, 348–357. doi:10.1111/j.1600-079X.2007.00534.x
Scheer, F. A., Zeitzer, J. M., Ayas, N. T., Brown, R., Czeisler, C. A., and Shea, S. A. (2006). Reduced sleep efficiency in cervical spinal cord injury; association with abolished night time melatonin secretion. Spinal Cord. 44, 78–81. doi:10.1038/sj.sc.3101784
Schiaveto-De-Souza, A., Da-Silva, C. A., Defino, H. L., and Del Bel, E. A. (2013). Effect of melatonin on the functional recovery from experimental traumatic compression of the spinal cord. Braz J. Med. Biol. Res. 46, 348–358. doi:10.1590/1414-431x20132322
Sezer, N., Akkus, S., and Ugurlu, F. G. (2015). Chronic complications of spinal cord injury. World J. Orthop. 6, 24–33. doi:10.5312/wjo.v6.i1.24
Shen, Z., Zhou, Z., Gao, S., Guo, Y., Gao, K., Wang, H., et al. (2017). Melatonin inhibits neural cell apoptosis and promotes locomotor recovery via activation of the wnt/β-catenin signaling pathway after spinal cord injury. Neurochem. Res. 42, 2336–2343. doi:10.1007/s11064-017-2251-7
Song, Y., Liu, J., Zhang, F., Zhang, J., Shi, T., and Zeng, Z. (2013). Antioxidant effect of quercetin against acute spinal cord injury in rats and its correlation with the p38MAPK/iNOS signaling pathway. Life Sci. 92, 1215–1221. doi:10.1016/j.lfs.2013.05.007
Suzen, S., Bozkaya, P., Coban, T., and Nebioğu, D. (2006). Investigation of the in vitro antioxidant behaviour of some 2-phenylindole derivatives: discussion on possible antioxidant mechanisms and comparison with melatonin. J. Enzyme Inhib. Med. Chem. 21, 405–411. doi:10.1080/14756360500381210
Suzen, S., Tekiner-Gulbas, B., Shirinzadeh, H., Uslu, D., Gurer-Orhan, H., Gumustas, M., et al. (2013). Antioxidant activity of indole-based melatonin analogues in erythrocytes and their voltammetric characterization. J. Enzyme Inhib. Med. Chem. 28, 1143–1155. doi:10.3109/14756366.2012.717223
Tan, D. X., Reiter, R. J., Zimmerman, S., and Hardeland, R. (2023). Melatonin: both a messenger of darkness and a participant in the cellular actions of non-visible solar radiation of near infrared light. Biol. (Basel) 12, 89. doi:10.3390/biology12010089
Taskiran, D., Tanyalcin, T., Sozmen, E. Y., Peker, G. O., Gulmen, V., Cagli, S., et al. (2000). The effects of melatonin on the antioxidant systems in experimental spinal injury. Int. J. Neurosci. 104, 63–73. doi:10.3109/00207450009035009
Tate, D. G., Wheeler, T., Lane, G. I., Forchheimer, M., Anderson, K. D., Biering-Sorensen, F., et al. (2020). Recommendations for evaluation of neurogenic bladder and bowel dysfunction after spinal cord injury and/or disease. J. Spinal Cord. Med. 43, 141–164. doi:10.1080/10790268.2019.1706033
Tavukçu, H. H., Sener, T. E., Tinay, I., Akbal, C., Erşahin, M., Cevik, O., et al. (2014). Melatonin and tadalafil treatment improves erectile dysfunction after spinal cord injury in rats. Clin. Exp. Pharmacol. Physiol. 41, 309–316. doi:10.1111/1440-1681.12216
Thøfner Hultén, V. D., Biering-Sørensen, F., Jørgensen, N. R., and Jennum, P. J. (2018). Melatonin and cortisol in individuals with spinal cord injury. Sleep. Med. 51, 92–98. doi:10.1016/j.sleep.2018.07.008
Tollefsen, E., and Fondenes, O. (2012). Respiratory complications associated with spinal cord injury. Tidsskr. Nor. Laegeforen 132, 1111–1114. doi:10.4045/tidsskr.10.0922
Topsakal, C., Kilic, N., Ozveren, F., Akdemir, I., Kaplan, M., Tiftikci, M., et al. (2003). Effects of prostaglandin E1, melatonin, and oxytetracycline on lipid peroxidation, antioxidant defense system, paraoxonase (PON1) activities, and homocysteine levels in an animal model of spinal cord injury. Spine (Phila Pa 1976) 28, 1643–1652. doi:10.1097/01.BRS.0000083163.03910.B1
Vasey, C., Mcbride, J., and Penta, K. (2021). Circadian rhythm dysregulation and restoration: the role of melatonin. Nutrients 13, 3480. doi:10.3390/nu13103480
Verheggen, R. J., Jones, H., Nyakayiru, J., Thompson, A., Groothuis, J. T., Atkinson, G., et al. (2012). Complete absence of evening melatonin increase in tetraplegics. Faseb J. 26, 3059–3064. doi:10.1096/fj.12-205401
Waly, N. E., and Hallworth, R. (2015). Circadian pattern of melatonin MT1 and MT2 receptor localization in the rat suprachiasmatic nucleus. J. Circadian Rhythms 13, 1. doi:10.5334/jcr.ab
Wang, H., Wang, H., Huang, H., Qu, Z., Ma, D., Dang, X., et al. (2022a). Melatonin attenuates spinal cord injury in mice by activating the Nrf2/ARE signaling pathway to inhibit the NLRP3 inflammasome. Cells 11, 2809. doi:10.3390/cells11182809
Wang, Q., Lu, Q., Guo, Q., Teng, M., Gong, Q., Li, X., et al. (2022b). Structural basis of the ligand binding and signaling mechanism of melatonin receptors. Nat. Commun. 13, 454. doi:10.1038/s41467-022-28111-3
Whelan, A., Halpine, M., Christie, S. D., and Mcveigh, S. A. (2020). Systematic review of melatonin levels in individuals with complete cervical spinal cord injury. J. Spinal Cord. Med. 43, 565–578. doi:10.1080/10790268.2018.1505312
Wölfler, A., Caluba, H.-C., Abuja, P. M., Dohr, G., Schauenstein, K., and Liebmann, P. M. (2001). Prooxidant activity of melatonin promotes fas-induced cell death in human leukemic Jurkat cells. FEBS Lett. 502, 127–131. doi:10.1016/s0014-5793(01)02680-1
Wu, Q., Jing, Y., Yuan, X., Zhang, X., Li, B., Liu, M., et al. (2014). Melatonin treatment protects against acute spinal cord injury-induced disruption of blood spinal cord barrier in mice. J. Mol. Neurosci. 54, 714–722. doi:10.1007/s12031-014-0430-4
Xu, G., Shi, D., Zhi, Z., Ao, R., and Yu, B. (2018). Melatonin ameliorates spinal cord injury by suppressing the activation of inflammasomes in rats. J. Cell Biochem. 120, 5183–5192. doi:10.1002/jcb.27794
Yang, Z., Bao, Y., Chen, W., and He, Y. (2020). Melatonin exerts neuroprotective effects by attenuating astro- and microgliosis and suppressing inflammatory response following spinal cord injury. Neuropeptides 79, 102002. doi:10.1016/j.npep.2019.102002
Yılmaz, A. D., Coban, T., and Suzen, S. (2012). Synthesis and antioxidant activity evaluations of melatonin-based analogue indole-hydrazide/hydrazone derivatives. J. Enzyme Inhib. Med. Chem. 27, 428–436. doi:10.3109/14756366.2011.594048
Yuan, X. C., Wang, P., Li, H. W., Wu, Q. B., Zhang, X. Y., Li, B. W., et al. (2016). Effects of melatonin on spinal cord injury-induced oxidative damage in mice testis. Andrologia 49, e12692. doi:10.1111/and.12692
Zeitzer, J. M., Ayas, N. T., Shea, S. A., Brown, R., and Czeisler, C. A. (2000). Absence of detectable melatonin and preservation of cortisol and thyrotropin rhythms in tetraplegia. J. Clin. Endocrinol. Metab. 85, 2189–2196. doi:10.1210/jcem.85.6.6647
Zemlan, F. P., Mulchahey, J. J., Scharf, M. B., Mayleben, D. W., Rosenberg, R., and Lankford, A. (2005). The efficacy and safety of the melatonin agonist beta-methyl-6-chloromelatonin in primary insomnia: A randomized, placebo-controlled, crossover clinical trial. J. Clin. Psychiatry 66, 384–390. doi:10.4088/jcp.v66n0316
Zhang, C., Zhang, W., Zhang, J., Jing, Y., Yang, M., Du, L., et al. (2018a). Gut microbiota dysbiosis in male patients with chronic traumatic complete spinal cord injury. J. Transl. Med. 16, 353. doi:10.1186/s12967-018-1735-9
Zhang, M., Bai, Y., Xu, C., Lin, J., Jin, J., Xu, A., et al. (2021). Novel optimized drug delivery systems for enhancing spinal cord injury repair in rats. Drug Deliv. 28, 2548–2561. doi:10.1080/10717544.2021.2009937
Zhang, Y., Lang, R., Guo, S., Luo, X., Li, H., Liu, C., et al. (2022). Intestinal microbiota and melatonin in the treatment of secondary injury and complications after spinal cord injury. Front. Neurosci. 16, 981772. doi:10.3389/fnins.2022.981772
Zhang, Y., Liu, Z., Zhang, W., Wu, Q., Zhang, Y., Liu, Y., et al. (2019). Melatonin improves functional recovery in female rats after acute spinal cord injury by modulating polarization of spinal microglial/macrophages. J. Neurosci. Res. 97, 733–743. doi:10.1002/jnr.24409
Zhang, Y., Zhang, W. X., Zhang, Y. J., Liu, Y. D., Liu, Z. J., Wu, Q. C., et al. (2018b). Melatonin for the treatment of spinal cord injury. Neural Regen. Res. 13, 1685–1692. doi:10.4103/1673-5374.238603
Keywords: melatonin, spinal cord injury, oxidative stress, melatonin receptor, secondary injury inflammatory response, cell death
Citation: Xie L, Wu H, Huang X and Yu T (2023) Melatonin, a natural antioxidant therapy in spinal cord injury. Front. Cell Dev. Biol. 11:1218553. doi: 10.3389/fcell.2023.1218553
Received: 07 May 2023; Accepted: 11 August 2023;
Published: 25 August 2023.
Edited by:
Bo Gao, Air Force Military Medical University, ChinaReviewed by:
Chen Chen, Frontage Laboratories, Inc., United StatesCopyright © 2023 Xie, Wu, Huang and Yu. This is an open-access article distributed under the terms of the Creative Commons Attribution License (CC BY). The use, distribution or reproduction in other forums is permitted, provided the original author(s) and the copyright owner(s) are credited and that the original publication in this journal is cited, in accordance with accepted academic practice. No use, distribution or reproduction is permitted which does not comply with these terms.
*Correspondence: Xiaohong Huang, eXNycmVkQGdtYWlsLmNvbQ==; Tengbo Yu, dGVuZ2JvLnl1QHFkdS5lZHUuY24=
†These authors have contributed equally to this work
Disclaimer: All claims expressed in this article are solely those of the authors and do not necessarily represent those of their affiliated organizations, or those of the publisher, the editors and the reviewers. Any product that may be evaluated in this article or claim that may be made by its manufacturer is not guaranteed or endorsed by the publisher.
Research integrity at Frontiers
Learn more about the work of our research integrity team to safeguard the quality of each article we publish.