- Department of Immunology, Faculty of Medicine, Jagiellonian University Medical College, Krakow, Poland
At present, extracellular vesicles (EVs) are considered key candidates for cell-free therapies, including treatment of allergic and autoimmune diseases. However, their therapeutic effectiveness, dependent on proper targeting to the desired cells, is significantly limited due to the reduced bioavailability resulting from their rapid clearance by the cells of the mononuclear phagocyte system (MPS). Thus, developing strategies to avoid EV elimination is essential when applying them in clinical practice. On the other hand, malfunctioning MPS contributes to various immune-related pathologies. Therapeutic reversal of these effects with EVs would be beneficial and could be achieved, for example, by modulating the macrophage phenotype or regulating antigen presentation by dendritic cells. Additionally, intended targeting of EVs to MPS macrophages for replication and repackaging of their molecules into new vesicle subtype can allow for their specific targeting to appropriate populations of acceptor cells. Herein, we briefly discuss the under-explored aspects of the MPS-EV interactions that undoubtedly require further research in order to accelerate the therapeutic use of EVs.
1 Therapeutic extracellular vesicles
Extracellular vesicles (EVs), usually divided into exosomes, microvesicles, and less studied apoptotic bodies, are released by all types of human cells and present in all body fluids (Wiklander et al., 2019). However, EV’s isolation, characterization, and classification especially, poses many difficulties, constituting a challenge limiting the practical use of these membranous structures (Théry et al., 2019). In addition, they are isolated from other eukaryotic cells, including fungi (Rizzo et al., 2020) and plants (Urzì et al., 2021), and can also be released by bacteria (Sartorio et al., 2021). The therapeutic potential of these lipid membrane-enclosed vesicles and thus the future development of a new class of EV-based therapeutics has been clearly emphasized in recent years (Conlan et al., 2017; Bernardi and Balbi, 2020; Jahromi and Fuhrmann, 2021; Cheng and Hill, 2022). EVs derived from immune cells, such as T cells, dendritic cells (DCs) or macrophages, as well as from other sources, such as mesenchymal stem cells (MSCs), have a clear immunomodulatory capacity (Zhang et al., 2014; Zhou et al., 2020; Hazrati et al., 2022) due to the expression of costimulatory molecules, antigen presenting activity and transfer of specific cargos, which makes them useful tools in the propagation of anti-tumor response or autoimmune suppression (Marar et al., 2021). New opportunities for EVs’ engineering are proposed for the treatment of neurological, bone, cardiac and metabolic diseases, as well as cancers (Nazimek and Bryniarski, 2020b; Liu et al., 2022; Sun et al., 2023), and in regenerative medicine (Lelek and Zuba-Surma, 2020; Lee and Kim, 2021; Karnas et al., 2023). Moreover, modified EVs are described as promising vehicles for targeted drug delivery, especially in cancer therapy (Chen J. et al., 2022; Sun et al., 2022, 2023; Tan et al., 2022).
2 Biodistribution of EVs in the context of their therapeutic efficacy and clearance
In vivo biodistribution studies are one of the necessary steps towards the translational application of EVs (De Sousa et al., 2023). Biodistribution of EVs depends on various parameters, including the route of administration, source of parental cells, target cells, as well as the size of vesicles (Wiklander et al., 2015; Murphy et al., 2019). Obviously, the appropriate dose of administered EVs is equally important for their future fate in the organism (Gupta et al., 2021). EVs with their cargos are able to reach different distant organs following various routes of administration, and the most commonly described targeted organs are those enriched in cells of the mononuclear phagocyte system (MPS), and include liver, spleen, kidneys, lungs, intestines, heart and brain (Wen et al., 2016; Manca et al., 2018; Kang et al., 2021; Samuel et al., 2021; Verweij et al., 2021; Driedonks et al., 2022; López de las Hazas et al., 2022; Lorca et al., 2022). These findings suggest the crucial role of MPS cells in the uptake and clearance of exogenously-delivered EVs. Due to the technical difficulties encountered, the issue of MPS uptake of endogenous EVs remains open. However, it can be concluded that the vast majority of vesicles secreted by body cells are naturally removed from the extracellular space by this route. Similarly to other new therapeutics, EVs’ biodistribution studies focus on validation of pharmacokinetic parameters, including half-lives of distribution and elimination phases (Kang et al., 2021). The rapid clearance of therapeutic EVs, resulting in their short half-life in circulation, is one of the main difficulties when adapting them to therapy (Esmaeili et al., 2022; Lu et al., 2022). Accordingly, some researchers point to the relatively short half-life of EV in various tissues, estimated at 30 min or less (Lai et al., 2014; Ronquist, 2019), while others note the time-dependent changes in the circulation and biodistribution of administered EVs, as recently analyzed by Kang et al. (2021).
Under physiological and disease-associated conditions, excretion of EVs into urine or even exhaled air makes them promising biomarkers, but accelerates their removal from circulation (Lai et al., 2014; Lucchetti et al., 2021). However, the crucial role in EVs’ rapid clearance has been attributed to mononuclear phagocyte system.
3 Mononuclear phagocyte system
There have been many milestones along the way to the current definition of the mononuclear phagocyte system (MPS), formerly known as the reticuloendothelial system (RES) (Yona and Gordon, 2015). Gordon and Plüddemann (2019) define it as a dispersed organ (Figure 1), due to different tissue residence of MPS cells that include monocytes, macrophages and DCs (Chow et al., 2011), but some authors prefer to focus only on monocytes and macrophages (Hume et al., 2019). MPS cells inhabit all tissues of the body where they can acquire specific, tissue-oriented functions, as in the case of microglia and osteoclasts (Ngo et al., 2022). Conversely, DCs are more motile than macrophages and therefore more likely to migrate to local lymphoid tissues to present antigens during the induction phase of an immune response, while macrophages rather induce an effector phase at the site of inflammation (Hull et al., 2014).
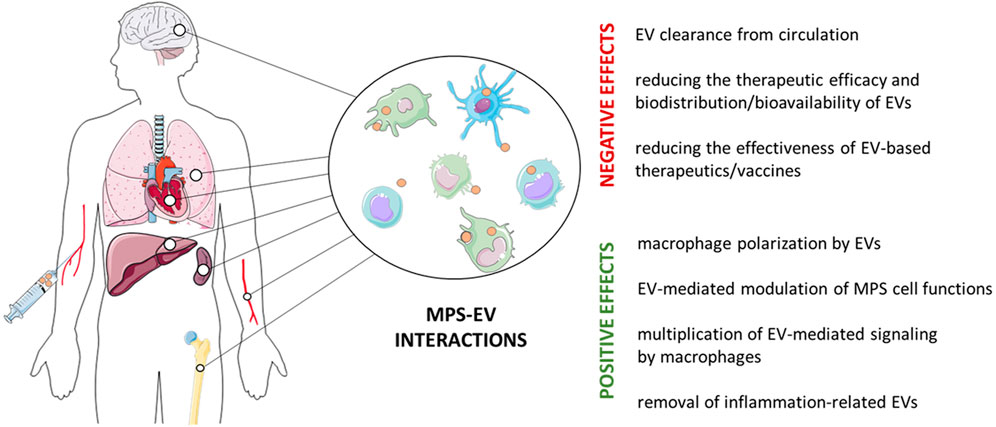
FIGURE 1. Positive and negative effects of the interaction of the mononuclear phagocyte system (MPS) and extracellular vesicles (EVs). MPS cells, such as monocytes, macrophages and dendritic cells, are found in virtually all body tissues, where they play an important role in tissue homeostasis and in immune defense. However, MPS cells remove both intrinsically-released and therapeutically-administered EVs, which limits their bioavailability. On the other hand, EVs targeting MPS cells can restore their impaired functions to induce the expected biological/clinical effect.
This system is essential for maintaining homeostasis as a major part of the first line of defense against pathogens. Physiologically, MPS is mainly responsible for phagocytosis of self- and foreign antigens, as well as antigen processing and presentation to T cells. Therefore, MPS is considered to link the innate and adaptive immunity (Pahari et al., 2018; Uribe-Querol and Rosales, 2020), and to play a critical role in tissue repair (Viola et al., 2019) as well as in the clearance of damaged, senescent, dying and apoptotic cells (Gordon and Plüddemann, 2018). However, MPS also contributes to immune-related pathologies, especially in infections and chronic inflammation (Hume et al., 2021).
After systemic administration, accumulation of EVs in MPS cell-enriched organs, such as spleen and liver, causes their rapid clearance and inhibit their delivery to distant target organs (Charoenviriyakul et al., 2017; Mentkowski et al., 2018; Tian et al., 2018). Additionally, this may significantly affect the effectiveness of EV-based vaccines, e.g., in anti-tumor immunotherapies (Chen W. et al., 2022) (Figure 1). The lack or significant decline of EVs’ elimination from circulation in macrophage-depleted mice confirms the essential role of MPS in this process (Imai et al., 2015; Matsumoto et al., 2020; Warashina et al., 2022). It is worth to note, however, that EVs released by MPS cells, such as monocytes, appear to be less extensively phagocytosed (You et al., 2022).
MPS cells are also considered the major biological barrier limiting the efficacy of systemically administered therapeutic nanomaterials or synthetic nanoparticles (Cong et al., 2022; Mills et al., 2022; Ruan et al., 2022; Lu et al., 2023), especially due to their accumulation in liver macrophages (Ngo et al., 2022), which promotes research into strategies to avoid MPS phagocytosis.
4 Evasion from clearance by MPS
EVs’ escape from phagocytosis has been described as one of the strategies delaying their clearance and improving uptake by targeted cells (Esmaeili et al., 2022). Different camouflage approaches are proposed to avoid reducing EVs amount as a result of MPS action after systemic administration (Parada et al., 2021). The “don’t eat me” signal transmitted by CD47 on tumor cells contributes to the inhibition of their phagocytosis by interacting with signal regulatory protein-alpha (SIRPα) displayed by macrophages (Liu Y. et al., 2023). Also, the expression of CD47 or CD24 molecules protecting against phagocytosis on tumor cell-derived EVs has been reported (Altevogt et al., 2020). A similar strategy is also suggested for therapeutic EVs (Belhadj et al., 2020). Kamerkar et al. demonstrated that CD47 expression on fibroblasts-derived EVs limits their clearance by circulating monocytes (Kamerkar et al., 2017). Additionally, Li Y. et al. (2022) showed that overexpression of this molecule on EVs, unlike cells, does not transmit cell death signals. Zhang et al. (2019) constructed artificial chimeric exosomes by integrating membrane proteins from red blood cells (containing surface CD47) and cancer cells into a synthetic phospholipid bilayer, that have anti-tumor activity and the ability to resist phagocytosis. Similarly, Du et al. (2021) showed that CD47-overexpressing EVs loaded with ferroptosis inducer and photosensitizer effectively evade MPS phagocytosis, which improved their bioavailability and delivery to targeted tumor. Moreover, CD47-containing EVs may competitively interact with macrophage-expressed SIRPα to disturb “don’t eat me” signaling, thereby promoting tumor cell phagocytosis (Cheng et al., 2021). Other molecules that could be expressed by EVs to avoid their phagocytosis and extend the half-life are CD31, CD44, or β2-microglobulin (Parada et al., 2021).
Rapid clearance after intravenous administration disturbs targeted EVs delivery to injured heart tissue (Chen et al., 2021), while therapeutic, miRNA-loaded EVs derived from CD47-overexpressing MSCs were present in serum longer than unmodified EVs and preferentially accumulated in the heart of mice with myocardial infarction reperfusion injury (Wei et al., 2021). A two-step strategy of successful EVs’ delivery to myocardium has also been described recently. First, blocking the macrophage-expressed endocytosis gene CLTC for the clathrin heavy chain with EV-delivered siRNA was used to impair the phagocytic activity of hepatic and splenic macrophages. Secondly, therapeutic, miR-21a-containing EVs were injected to significantly improve the cardiac function (Wan et al., 2020).
Another method to reduce the clearance of intravenously administered EVs is based on their conjugation with micelles containing polyethylene glycol (PEG) (Kooijmans et al., 2016). In addition, combination of PEG and CD47 expression on engineered lipid nanoparticles greatly increased their anti-HIV activity by escaping from MPS phagocytosis (Zhang et al., 2023). Modern research approaches propose the use of PEGylation to protect EVs from phagocytosis by MPS cells, which may also support the targeted cargo delivery by constructing “smart exosome platforms” (Guo et al., 2021). Moreover, reduction of the amount of negatively charged phosphatidylserine-derived groups on the EVs’ membranes may also suppress their uptake by macrophages (Matsumoto et al., 2017; Esmaeili et al., 2022).
Various strategies are proposed to solve similar problems with the therapeutic administration of synthetic nanoparticles, especially that Wilhelm et al. (2016) estimated the level of their delivery to solid tumors at only 0.7% of the administered dose. These approaches involve either manipulation of nanomaterials by surface coating with protective factors or changing their shape, or inhibiting and depleting MPS cells (Liu et al., 2017; Ai et al., 2018; Xia et al., 2019; Mills et al., 2022; Lu et al., 2023), and should be combined to increase the biological efficacy. It might be a good idea to use bacteria as an example, as they develop different mechanisms to escape phagocytosis, allowing them to expand and weaken the host’s immune system (Leseigneur et al., 2020; Pidwill et al., 2023).
5 Phagocytosis of EVs as a desirable process
Therapeutic functions of EVs depend on the suitable targeting of acceptor cells by direct interaction with extracellular receptors or fusion with cell membrane (Gurung et al., 2021). They are then captured by target cell through different pathways, including caveola-, clathrin- or receptor-mediated and lipid raft-dependent endocytosis as well as macro- and micropinocytosis (Kwok et al., 2021; Pedrioli and Paganetti, 2021; Hazrati et al., 2022). Moreover, internalization of EVs by phagocytosis is also considered (Tkach and Théry, 2016; Jadli et al., 2020). Some studies described phagocytosis as the most efficient mechanism of internalization of cancer and leukemic cell-derived EVs (Feng et al., 2010; Emam et al., 2018). However, EV phagocytosis appears to be a very complex process. Accordingly, observations by Montecalvo et al. (2012) on EV-shuttled miRNA transfer between DCs suggest that EVs release their content to targeted cell cytosol by the complete fusion with the phagosome membrane. This can be preceded by EV hemifusion with the cell membrane followed by endocytosis and/or by internalization as free vesicles.
Moreover, resident alveolar macrophages internalize most of the microvesicles released into the alveoli under the homeostatic conditions (Soni et al., 2022), and their phagocytosis results in alleviation of inflammation during acute lung injury in mice (Mohning et al., 2018), while impaired EV phagocytosis in cystic fibrosis significantly reduces antibacterial immune defenses (Koeppen et al., 2021). The diversity of surface receptors on phagocytic cells allows for the binding of a large number of ligands on the EV surface, which makes phagocytes almost ideal recipient cells (Gonda et al., 2020). Thus, under certain circumstances, it can be assumed that targeting EVs to phagocytes is a desirable process (Figure 1).
6 MPS cells as the target of EVs
Under certain circumstances, MPS cells contribute to immune-related pathologies. Thus, targeting them by EVs becomes an interesting immunotherapeutic approach. While maturation, migration, and antigen-presentation processes are the primary targets of DC-directed immunomodulatory EVs (Liu X. et al., 2023), switching and balancing the activation/polarization status appears to be most effective in targeting macrophages (Hu et al., 2021).
As recently reviewed, MSC-derived EVs rather downregulate the antigen-presenting capabilities of DCs (Liu X. et al., 2023), while EVs from other cell sources, including engineered CAR-T lymphocytes, can stimulate the presentation of antigens by DCs, e.g., in cancer (Buzas, 2023).
However, tissue-resident macrophages seem to attract more research attention. Activated microglia are involved in neuroinflammation and related disorders, including neurodegenerations such as Alzheimer’s and Parkinson’s diseases (Muzio et al., 2021). Thus, microglia as MPS cell population can be considered as an interesting target for therapeutic EVs (Xin et al., 2021). Recent studies indicate the possibility of modulating microglial cells by administering EVs isolated from human induced pluripotent stem cell-derived neural stem cells. Following EV administration, a dose-dependent decrease in the secretion of tumor necrosis factor-α (TNF-α) and interleukin 1β (IL-1β) was observed, mediating anti-inflammatory effects of EVs on proinflammatory microglia (Upadhya et al., 2022). Furthermore, the latest findings demonstrated a therapeutic effect of intravenously injected Schwann cell-derived EVs on spinal cord injury by suppressing M1- and stimulating M2-polarization of infiltrating macrophages and microglia (Ren et al., 2023). The latter suggest that EV-mediated MPS cell phenotype switching may produce therapeutic effects.
Accordingly, the contribution of EVs to macrophage polarization and induction of regulatory phenotype is emphasized (Hyvärinen et al., 2018; Li et al., 2021; Gharavi et al., 2022). For instance, MSC-derived EVs reduced IL-23 and IL-22 secretion by CD80low/intermediate, CD86+, CD163low, and CD206low regulatory macrophages, enhancing their anti-inflammatory and tolerance-promoting phenotype (Hyvärinen et al., 2018). Moreover, MSC-derived EVs may polarize human macrophages into radioprotective cells that exhibit high phagocytic activity and have an ability to improve hematopoiesis in mice with lethal acute radiation syndrome (Kink et al., 2019).
In addition, MSC-derived EVs were shown to attenuate myocardial ischemia-reperfusion injury by promoting macrophage polarization towards M2 phenotype (Zhao et al., 2019; Li Q. et al., 2022). Interestingly, fusion of MSC-derived EVs with platelet membrane fragments promoted their trafficking to the ischemic myocardium due to the binding to circulating monocytes (Li Q. et al., 2022). Similar therapeutic effect was observed in myocardial infarction under the activity of miRNA-transferring, DC-derived EVs that activated M2 macrophages in a Treg cell-dependent manner (Zhang et al., 2021).
After local administration, MSC-derived EVs containing therapeutic miRNAs may promote M2 macrophage-mediated angiogenesis and tendon regeneration after its rapture (Xu et al., 2023). Additionally, EVs isolated from adipose tissue-derived MSCs ameliorated tendinopathy by promoting phagocytosis and M2 polarization of macrophages (Wu et al., 2023). Furthermore, stimulating M2 macrophage phenotype by MSC-derived EVs may also improve ligament healing (Chamberlain et al., 2021). Adipose tissue macrophages from lean mice release EVs that modulate macrophage polarization via contained miRNAs to promote wound healing in diabetic mice (Xia et al., 2023), whereas human serum-derived EVs encouraged angiogenesis and osteogenesis by reducing the expression of M1-related genes in macrophages (Xiang et al., 2023). Therapeutic EVs may also diminish the activity of M1 macrophages to alleviate periodontitis (Luo et al., 2023).
M1 macrophages exert anti-tumor activity in cancer environment, and could be induced by miRNA-33- and miRNA-130-overexpressing EVs (Moradi-Chaleshtori et al., 2021) as well as by macrophage-derived EVs expressing human glycyl-tRNA synthetase-1 that trigger cancer cell death (Park et al., 2022). Interestingly, EVs isolated from plasma of post-irradiated patients with cervical cancer promoted the M1 phenotype switch in tumor-associated macrophages (Ren et al., 2022). Similar reprogramming could be induced by tumor cell-derived microparticles loaded with chemotherapeutic drugs (Wei et al., 2023). Furthermore, engineered hybrid cell membrane nanovesicles containing M2-to-M1 repolarization signals and expressing SIRPα prevented both local cancer recurrence and distant metastasis, through triggering an anti-tumor immune response (Rao et al., 2020).
Macrophage activation status in bacterial-host communication may be modulated by EVs. MCS-derived, miRNA-466-containing EVs may participate in the host immune response to multidrug-resistant bacteria by promoting macrophage phagocytosis (Shi et al., 2021). However, internalization of bacterial EVs by macrophages modifies their antimicrobial activity against Escherichia coli (Guangzhang et al., 2023). Moreover, both bacterial EVs and EVs derived from infected macrophages may alter macrophage polarization during infection (Qu et al., 2022). Interestingly, microvesicles released by host cells and carrying bacterial pore-forming toxins can be delivered to macrophages, which induces their polarization into the CD14+MHCIIlowCD86low cells that exhibit an enhanced response to Gram-positive bacterial ligands (Köffel et al., 2018). Recently, the mechanism of inflammasome activation or silencing in monocytes by EVs isolated from amniotic fluid during pregnancy has been described (Nunzi et al., 2023), suggesting that monocyte activation status may also be modulated by EVs.
Efficient clearance of dying and apoptotic cells by MPS allows for the maintenance of immune homeostasis and peripheral tolerance (Trahtemberg and Mevorach, 2017; Gordon and Plüddemann, 2018). Thus, EV-mediated strategies to restore and/or increase the phagocytosis of apoptotic cells by MPS may induce therapeutic effects in autoimmune and inflammatory diseases. Recently, significantly enhanced efferocytosis of apoptotic cardiomyocytes by macrophages was observed after treatment with EVs secreted by cardiosphere-derived cells to induce the cardioprotective effects (de Couto et al., 2019). Moreover, opsonization of apoptotic cardiomyocytes with MSC-derived EVs significantly increased their phagocytosis by macrophages, which augmented cardiac repair and function (Patil et al., 2021). On the other hand, Chen et al. (2019) showed that apoptotic cell-derived EVs increased macrophage production of transforming growth factor (TGF)-β, which in turn enhanced the clearance of dead cells, which led to the alleviation of colitis.
Our recent findings demonstrated that macrophages can multiply the EV-mediated immunoregulatory signaling (Nazimek et al., 2021). After selective engulfment of suppressor T cell-derived, miRNA-150-carrying EVs that depends on the interaction of antibody light chains with antigenic determinants complexed with MHC class II (Bryniarski et al., 2013; Nazimek et al., 2015, 2018, 2019, 2020), macrophages appear to synthesize additional miRNA-150 molecules and then package them into antigen/MHC-expressing EVs, which enables specific targeting of acceptor T cells (Nazimek et al., 2021). Thus, one can speculate that MPS cells can replicate and repackage immunoregulatory and therapeutic molecules derived from primary EVs to then allow the signal to specifically reach the desired target cell via secondary EV transmission.
7 Conclusion
EVs are considered key candidates for cell-free therapies, including allergy and autoimmunity treatment (Nazimek and Bryniarski, 2020a; Nazimek and Bryniarski, 2021). However, EV therapeutic efficacy is affected by limited bioavailability due to their rapid clearance by MPS cells. Thus, strategies to avoid vesicle removal by MPS are considered essential to circumvent the limitations associated with their clinical use. On the other hand, dysregulated MPS cell functions contribute to various immune-related pathologies. Thus, restoring MPS cell activity to normal by EV treatment would be beneficial. Finally, the bystander effect of EV removal by MPS cells can be turned positive by considering macrophages as a multiplier of signaling contained in EVs. Hence, all the aspects discussed briefly in this summary (Figure 1), which have not been sufficiently researched so far, are undoubtedly an interesting direction worth further research in order to accelerate the use of EVs in therapy.
However, future research needs to be directed towards standardization of processes for the production and isolation of therapeutic EVs along with the development of strategies allowing EVs to specifically target the desired cells when administered at established doses, routes and schedules.
Author contributions
MC drafted the manuscript, KB revised the manuscript, and KN conceptualized and revised the manuscript. All authors contributed to the article and approved the submitted version.
Funding
This work was supported by grant awarded by the Polish Ministry of Education and Science through the Jagiellonian University Medical College, project number N41/DBS/001026 to KN.
Conflict of interest
The authors declare that the research was conducted in the absence of any commercial or financial relationships that could be construed as a potential conflict of interest.
Publisher’s note
All claims expressed in this article are solely those of the authors and do not necessarily represent those of their affiliated organizations, or those of the publisher, the editors and the reviewers. Any product that may be evaluated in this article, or claim that may be made by its manufacturer, is not guaranteed or endorsed by the publisher.
References
Ai, X., Hu, M., Wang, Z., Zhang, W., Li, J., Yang, H., et al. (2018). Recent advances of membrane-cloaked nanoplatforms for biomedical applications. Bioconjug Chem. 29, 838–851. doi:10.1021/acs.bioconjchem.8b00103
Altevogt, P., Sammar, M., Hüser, L., Umansky, V., and Utikal, J. (2020). Perspective – escape from destruction: How cancer-derived EVs are protected from phagocytosis. TEV 2, 60–64. doi:10.47184/tev.2020.01.08
Belhadj, Z., He, B., Deng, H., Song, S., Zhang, H., Wang, X., et al. (2020). A combined “eat me/don’t eat me” strategy based on extracellular vesicles for anticancer nanomedicine. J. Extracell. Vesicles 9, 1806444. doi:10.1080/20013078.2020.1806444
Bernardi, S., and Balbi, C. (2020). Extracellular vesicles: From biomarkers to therapeutic tools. Biol. (Basel) 9, 258. doi:10.3390/biology9090258
Bryniarski, K., Ptak, W., Jayakumar, A., Püllmann, K., Caplan, M. J., Chairoungdua, A., et al. (2013). Antigen-specific, antibody-coated, exosome-like nanovesicles deliver suppressor T-cell microRNA-150 to effector T cells to inhibit contact sensitivity. J. Allergy Clin. Immunol. 132, 170–181. doi:10.1016/j.jaci.2013.04.048
Buzas, E. I. (2023). The roles of extracellular vesicles in the immune system. Nat. Rev. Immunol. 23, 236–250. doi:10.1038/s41577-022-00763-8
Chamberlain, C. S., Kink, J. A., Wildenauer, L. A., McCaughey, M., Henry, K., Spiker, A. M., et al. (2021). Exosome-educated macrophages and exosomes differentially improve ligament healing. Stem Cells 39, 55–61. doi:10.1002/stem.3291
Charoenviriyakul, C., Takahashi, Y., Morishita, M., Matsumoto, A., Nishikawa, M., and Takakura, Y. (2017). Cell type-specific and common characteristics of exosomes derived from mouse cell lines: Yield, physicochemical properties, and pharmacokinetics. Eur. J. Pharm. Sci. 96, 316–322. doi:10.1016/j.ejps.2016.10.009
Chen, H., Kasagi, S., Chia, C., Zhang, D., Tu, E., Wu, R., et al. (2019). Extracellular vesicles from apoptotic cells promote TGFβ production in macrophages and suppress experimental colitis. Sci. Rep. 9, 5875. doi:10.1038/s41598-019-42063-7
Chen, J., Tan, Q., Yang, Z., and Jin, Y. (2022a). Engineered extracellular vesicles: Potentials in cancer combination therapy. J. Nanobiotechnol 20, 132. doi:10.1186/s12951-022-01330-y
Chen, P., Wang, L., Fan, X., Ning, X., Yu, B., Ou, C., et al. (2021). Targeted delivery of extracellular vesicles in heart injury. Theranostics 11, 2263–2277. doi:10.7150/thno.51571
Chen, W., Wu, Y., Deng, J., Yang, Z., Chen, J., Tan, Q., et al. (2022b). Phospholipid-membrane-based nanovesicles acting as vaccines for tumor immunotherapy: Classification, mechanisms and applications. Pharmaceutics 14, 2446. doi:10.3390/pharmaceutics14112446
Cheng, L., and Hill, A. F. (2022). Therapeutically harnessing extracellular vesicles. Nat. Rev. Drug Discov. 21, 379–399. doi:10.1038/s41573-022-00410-w
Cheng, L., Zhang, X., Tang, J., Lv, Q., and Liu, J. (2021). Gene-engineered exosomes-thermosensitive liposomes hybrid nanovesicles by the blockade of CD47 signal for combined photothermal therapy and cancer immunotherapy. Biomaterials 275, 120964. doi:10.1016/j.biomaterials.2021.120964
Chow, A., Brown, B. D., and Merad, M. (2011). Studying the mononuclear phagocyte system in the molecular age. Nat. Rev. Immunol. 11, 788–798. doi:10.1038/nri3087
Cong, Y., Baimanov, D., Zhou, Y., Chen, C., and Wang, L. (2022). Penetration and translocation of functional inorganic nanomaterials into biological barriers. Adv. Drug Deliv. Rev. 191, 114615. doi:10.1016/j.addr.2022.114615
Conlan, R. S., Pisano, S., Oliveira, M. I., Ferrari, M., and Mendes Pinto, I. (2017). Exosomes as reconfigurable therapeutic systems. Trends Mol. Med. 23, 636–650. doi:10.1016/j.molmed.2017.05.003
de Couto, G., Jaghatspanyan, E., DeBerge, M., Liu, W., Luther, K., Wang, Y., et al. (2019). Mechanism of enhanced MerTK-dependent macrophage efferocytosis by extracellular vesicles. Arterioscler. Thromb. Vasc. Biol. 39, 2082–2096. doi:10.1161/ATVBAHA.119.313115
De Sousa, K. P., Rossi, I., Abdullahi, M., Ramirez, M. I., Stratton, D., and Inal, J. M. (2023). Isolation and characterization of extracellular vesicles and future directions in diagnosis and therapy. WIREs Nanomed Nanobiotechnol 15, e1835. doi:10.1002/wnan.1835
Driedonks, T., Jiang, L., Carlson, B., Han, Z., Liu, G., Queen, S. E., et al. (2022). Pharmacokinetics and biodistribution of extracellular vesicles administered intravenously and intranasally to Macaca nemestrina. J Extracell. Bio 1, e59. doi:10.1002/jex2.59
Du, J., Wan, Z., Wang, C., Lu, F., Wei, M., Wang, D., et al. (2021). Designer exosomes for targeted and efficient ferroptosis induction in cancer via chemo-photodynamic therapy. Theranostics 11, 8185–8196. doi:10.7150/thno.59121
Emam, S. E., Ando, H., Lila, A. S. A., Shimizu, T., Okuhira, K., Ishima, Y., et al. (2018). Liposome co-incubation with cancer cells secreted exosomes (extracellular vesicles) with different proteins expressions and different uptake pathways. Sci. Rep. 8, 14493. doi:10.1038/s41598-018-32861-w
Esmaeili, A., Alini, M., Baghaban Eslaminejad, M., and Hosseini, S. (2022). Engineering strategies for customizing extracellular vesicle uptake in a therapeutic context. Stem Cell Res. Ther. 13, 129. doi:10.1186/s13287-022-02806-2
Feng, D., Zhao, W.-L., Ye, Y.-Y., Bai, X.-C., Liu, R.-Q., Chang, L.-F., et al. (2010). Cellular internalization of exosomes occurs through phagocytosis. Traffic 11, 675–687. doi:10.1111/j.1600-0854.2010.01041.x
Gharavi, A. T., Hanjani, N. A., Movahed, E., and Doroudian, M. (2022). The role of macrophage subtypes and exosomes in immunomodulation. Cell Mol. Biol. Lett. 27, 83. doi:10.1186/s11658-022-00384-y
Gonda, A., Moyron, R., Kabagwira, J., Vallejos, A. P., and Wall, R. N. (2020). “Cellular-defined microenvironmental internalization of exosomes,” in Extracellular vesicles and their importance in human health. Editors A. Gil De Bona, and J. Antonio Reales Calderon (London: IntechOpen). doi:10.5772/intechopen.86020
Gordon, S., and Plüddemann, A. (2018). Macrophage clearance of apoptotic cells: A critical assessment. Front. Immunol. 9, 127. doi:10.3389/fimmu.2018.00127
Gordon, S., and Plüddemann, A. (2019). The mononuclear phagocytic system. Generation of diversity. Front. Immunol. 10, 1893. doi:10.3389/fimmu.2019.01893
Guangzhang, C., Fangfang, F., Siqian, D., Xinyi, X., Xiaochuan, B., Yihan, R., et al. (2023). Outer membrane vesicles from Escherichia coli are efficiently internalized by macrophage cells and alter their inflammatory response. Microb. Pathog. 175, 105965. doi:10.1016/j.micpath.2022.105965
Guo, Y., Wan, Z., Zhao, P., Wei, M., Liu, Y., Bu, T., et al. (2021). Ultrasound triggered topical delivery of Bmp7 mRNA for white fat browning induction via engineered smart exosomes. J. Nanobiotechnology 19, 402. doi:10.1186/s12951-021-01145-3
Gupta, D., Zickler, A. M., and El Andaloussi, S. (2021). Dosing extracellular vesicles. Adv. Drug Deliv. Rev. 178, 113961. doi:10.1016/j.addr.2021.113961
Gurung, S., Perocheau, D., Touramanidou, L., and Baruteau, J. (2021). The exosome journey: From biogenesis to uptake and intracellular signalling. Cell Commun. Signal 19, 47. doi:10.1186/s12964-021-00730-1
Hazrati, A., Soudi, S., Malekpour, K., Mahmoudi, M., Rahimi, A., Hashemi, S. M., et al. (2022). Immune cells-derived exosomes function as a double-edged sword: Role in disease progression and their therapeutic applications. Biomark. Res. 10, 30. doi:10.1186/s40364-022-00374-4
Hu, Q., Lyon, C. J., Fletcher, J. K., Tang, W., Wan, M., and Hu, T. Y. (2021). Extracellular vesicle activities regulating macrophage- and tissue-mediated injury and repair responses. Acta Pharm. Sin. B 11, 1493–1512. doi:10.1016/j.apsb.2020.12.014
Hull, T. D., Agarwal, A., and George, J. F. (2014). The mononuclear phagocyte system in homeostasis and disease: A role for heme oxygenase-1. Antioxid. Redox Signal 20, 1770–1788. doi:10.1089/ars.2013.5673
Hume, D. A., Caruso, M., Keshvari, S., Patkar, O. L., Sehgal, A., Bush, S. J., et al. (2021). The mononuclear phagocyte system of the rat. J. Immunol. 206, 2251–2263. doi:10.4049/jimmunol.2100136
Hume, D. A., Irvine, K. M., and Pridans, C. (2019). The mononuclear phagocyte system: The relationship between monocytes and macrophages. Trends Immunol. 40, 98–112. doi:10.1016/j.it.2018.11.007
Hyvärinen, K., Holopainen, M., Skirdenko, V., Ruhanen, H., Lehenkari, P., Korhonen, M., et al. (2018). Mesenchymal stromal cells and their extracellular vesicles enhance the anti-inflammatory phenotype of regulatory macrophages by downregulating the production of interleukin (IL)-23 and IL-22. Front. Immunol. 9, 771. doi:10.3389/fimmu.2018.00771
Imai, T., Takahashi, Y., Nishikawa, M., Kato, K., Morishita, M., Yamashita, T., et al. (2015). Macrophage-dependent clearance of systemically administered B16BL6-derived exosomes from the blood circulation in mice. J. Extracell. Vesicles 4, 26238. doi:10.3402/jev.v4.26238
Jadli, A. S., Ballasy, N., Edalat, P., and Patel, V. B. (2020). Inside(sight) of tiny communicator: Exosome biogenesis, secretion, and uptake. Mol. Cell Biochem. 467, 77–94. doi:10.1007/s11010-020-03703-z
Jahromi, L. P., and Fuhrmann, G. (2021). Bacterial extracellular vesicles: Understanding biology promotes applications as nanopharmaceuticals. Adv. Drug Deliv. Rev. 173, 125–140. doi:10.1016/j.addr.2021.03.012
Kamerkar, S., LeBleu, V. S., Sugimoto, H., Yang, S., Ruivo, C. F., Melo, S. A., et al. (2017). Exosomes facilitate therapeutic targeting of oncogenic KRAS in pancreatic cancer. Nature 546, 498–503. doi:10.1038/nature22341
Kang, M., Jordan, V., Blenkiron, C., and Chamley, L. W. (2021). Biodistribution of extracellular vesicles following administration into animals: A systematic review. J. Extracell. Vesicles 10, e12085. doi:10.1002/jev2.12085
Karnas, E., Dudek, P., and Zuba-Surma, E. K. (2023). Stem cell-derived extracellular vesicles as new tools in regenerative medicine - immunomodulatory role and future perspectives. Front. Immunol. 14, 1120175. doi:10.3389/fimmu.2023.1120175
Kink, J. A., Forsberg, M. H., Reshetylo, S., Besharat, S., Childs, C. J., Pederson, J. D., et al. (2019). Macrophages educated with exosomes from primed mesenchymal stem cells treat acute radiation syndrome by promoting hematopoietic recovery. Biol. Blood Marrow Transpl. 25, 2124–2133. doi:10.1016/j.bbmt.2019.07.026
Koeppen, K., Nymon, A., Barnaby, R., Li, Z., Hampton, T. H., Ashare, A., et al. (2021). CF monocyte-derived macrophages have an attenuated response to extracellular vesicles secreted by airway epithelial cells. Am. J. Physiol. Lung Cell Mol. Physiol. 320, L530–L544. doi:10.1152/ajplung.00621.2020
Köffel, R., Wolfmeier, H., Larpin, Y., Besançon, H., Schoenauer, R., Babiychuk, V. S., et al. (2018). Host-derived microvesicles carrying bacterial pore-forming toxins deliver signals to macrophages: A novel mechanism of shaping immune responses. Front. Immunol. 9, 1688. doi:10.3389/fimmu.2018.01688
Kooijmans, S. A. A., Fliervoet, L. A. L., van der Meel, R., Fens, M. H. A. M., Heijnen, H. F. G., van Bergen en Henegouwen, P. M. P., et al. (2016). PEGylated and targeted extracellular vesicles display enhanced cell specificity and circulation time. J. Control. Release 224, 77–85. doi:10.1016/j.jconrel.2016.01.009
Kwok, Z. H., Wang, C., and Jin, Y. (2021). Extracellular vesicle transportation and uptake by recipient cells: A critical process to regulate human diseases. Processes 9, 273. doi:10.3390/pr9020273
Lai, C. P., Mardini, O., Ericsson, M., Prabhakar, S., Maguire, C., Chen, J. W., et al. (2014). Dynamic biodistribution of extracellular vesicles in vivo using a multimodal imaging reporter. ACS Nano 8, 483–494. doi:10.1021/nn404945r
Lee, J. Y., and Kim, H.-S. (2021). Extracellular vesicles in regenerative medicine: Potentials and challenges. Tissue Eng. Regen. Med. 18, 479–484. doi:10.1007/s13770-021-00365-w
Lelek, J., and Zuba-Surma, E. K. (2020). Perspectives for future use of extracellular vesicles from umbilical cord- and adipose tissue-derived mesenchymal stem/stromal cells in regenerative therapies—synthetic review. IJMS 21, 799. doi:10.3390/ijms21030799
Leseigneur, C., Lê-Bury, P., Pizarro-Cerdá, J., and Dussurget, O. (2020). Emerging evasion mechanisms of macrophage defenses by pathogenic bacteria. Front. Cell. Infect. Microbiol. 10, 577559. doi:10.3389/fcimb.2020.577559
Li, Q., Huang, Z., Wang, Q., Gao, J., Chen, J., Tan, H., et al. (2022a). Targeted immunomodulation therapy for cardiac repair by platelet membrane engineering extracellular vesicles via hitching peripheral monocytes. Biomaterials 284, 121529. doi:10.1016/j.biomaterials.2022.121529
Li, Y., Tan, J., Miao, Y., and Zhang, Q. (2021). MicroRNA in extracellular vesicles regulates inflammation through macrophages under hypoxia. Cell Death Discov. 7, 285. doi:10.1038/s41420-021-00670-2
Li, Y., Wu, Y., Federzoni, E. A., Wang, X., Dharmawan, A., Hu, X., et al. (2022b). CD47 cross-dressing by extracellular vesicles expressing CD47 inhibits phagocytosis without transmitting cell death signals. Elife 11, e73677. doi:10.7554/eLife.73677
Liu, G., Wu, J., Chen, G., and Shang, A. (2022). The potential therapeutic value and application prospect of engineered exosomes in human diseases. Front. Cell Dev. Biol. 10, 1051380. doi:10.3389/fcell.2022.1051380
Liu, X., Wei, Q., Lu, L., Cui, S., Ma, K., Zhang, W., et al. (2023a). Immunomodulatory potential of mesenchymal stem cell-derived extracellular vesicles: Targeting immune cells. Front. Immunol. 14, 1094685. doi:10.3389/fimmu.2023.1094685
Liu, Y., Wang, Y., Yang, Y., Weng, L., Wu, Q., Zhang, J., et al. (2023b). Emerging phagocytosis checkpoints in cancer immunotherapy. Sig Transduct. Target Ther. 8, 104. doi:10.1038/s41392-023-01365-z
Liu, Y., Wang, Z., Liu, Y., Zhu, G., Jacobson, O., Fu, X., et al. (2017). Suppressing nanoparticle-mononuclear phagocyte system interactions of two-dimensional gold nanorings for improved tumor accumulation and photothermal ablation of tumors. ACS Nano 11, 10539–10548. doi:10.1021/acsnano.7b05908
López de las Hazas, M.-C., del Pozo-Acebo, L., Hansen, M. S., Gil-Zamorano, J., Mantilla-Escalante, D. C., Gómez-Coronado, D., et al. (2022). Dietary bovine milk miRNAs transported in extracellular vesicles are partially stable during GI digestion, are bioavailable and reach target tissues but need a minimum dose to impact on gene expression. Eur. J. Nutr. 61, 1043–1056. doi:10.1007/s00394-021-02720-y
Lorca, C., Laparra, M., Céspedes, M. V., Casaní, L., Florit, S., Jové, M., et al. (2022). Industrial by-products as a novel circular source of biocompatible extracellular vesicles. Adv. Funct. Mater. 32, 2202700. doi:10.1002/adfm.202202700
Lu, J., Gao, X., Wang, S., He, Y., Ma, X., Zhang, T., et al. (2023). Advanced strategies to evade the mononuclear phagocyte system clearance of nanomaterials. Exploration 3, 20220045. doi:10.1002/EXP.20220045
Lu, S., Wang, R., Fu, W., and Si, Y. (2022). Applications of extracellular vesicles in abdominal aortic aneurysm. Front. Cardiovasc Med. 9, 927542. doi:10.3389/fcvm.2022.927542
Lucchetti, D., Santini, G., Perelli, L., Ricciardi-Tenore, C., Colella, F., Mores, N., et al. (2021). Detection and characterisation of extracellular vesicles in exhaled breath condensate and sputum of COPD and severe asthma patients. Eur. Respir. J. 58, 2003024. doi:10.1183/13993003.03024-2020
Luo, H., Chen, D., Li, R., Li, R., Teng, Y., Cao, Y., et al. (2023). Genetically engineered CXCR4-modified exosomes for delivery of miR-126 mimics to macrophages alleviate periodontitis. J. Nanobiotechnol 21, 116. doi:10.1186/s12951-023-01863-w
Manca, S., Upadhyaya, B., Mutai, E., Desaulniers, A. T., Cederberg, R. A., White, B. R., et al. (2018). Milk exosomes are bioavailable and distinct microRNA cargos have unique tissue distribution patterns. Sci. Rep. 8, 11321. doi:10.1038/s41598-018-29780-1
Marar, C., Starich, B., and Wirtz, D. (2021). Extracellular vesicles in immunomodulation and tumor progression. Nat. Immunol. 22, 560–570. doi:10.1038/s41590-021-00899-0
Matsumoto, A., Takahashi, Y., Chang, H.-Y., Wu, Y.-W., Yamamoto, A., Ishihama, Y., et al. (2020). Blood concentrations of small extracellular vesicles are determined by a balance between abundant secretion and rapid clearance. J. Extracell. Vesicles 9, 1696517. doi:10.1080/20013078.2019.1696517
Matsumoto, A., Takahashi, Y., Nishikawa, M., Sano, K., Morishita, M., Charoenviriyakul, C., et al. (2017). Role of phosphatidylserine-derived negative surface charges in the recognition and uptake of intravenously injected B16bl6-derived exosomes by macrophages. J. Pharm. Sci. 106, 168–175. doi:10.1016/j.xphs.2016.07.022
Mentkowski, K. I., Snitzer, J. D., Rusnak, S., and Lang, J. K. (2018). Therapeutic potential of engineered extracellular vesicles. AAPS J. 20, 50. doi:10.1208/s12248-018-0211-z
Mills, J. A., Liu, F., Jarrett, T. R., Fletcher, N. L., and Thurecht, K. J. (2022). Nanoparticle based medicines: Approaches for evading and manipulating the mononuclear phagocyte system and potential for clinical translation. Biomater. Sci. 10, 3029–3053. doi:10.1039/d2bm00181k
Mohning, M. P., Thomas, S. M., Barthel, L., Mould, K. J., McCubbrey, A. L., Frasch, S. C., et al. (2018). Phagocytosis of microparticles by alveolar macrophages during acute lung injury requires MerTK. Am. J. Physiol. Lung Cell Mol. Physiol. 314, L69–L82. doi:10.1152/ajplung.00058.2017
Montecalvo, A., Larregina, A. T., Shufesky, W. J., Beer Stolz, D., Sullivan, M. L. G., Karlsson, J. M., et al. (2012). Mechanism of transfer of functional microRNAs between mouse dendritic cells via exosomes. Blood 119, 756–766. doi:10.1182/blood-2011-02-338004
Moradi-Chaleshtori, M., Bandehpour, M., Soudi, S., Mohammadi-Yeganeh, S., and Hashemi, S. M. (2021). In vitro and in vivo evaluation of anti-tumoral effect of M1 phenotype induction in macrophages by miR-130 and miR-33 containing exosomes. Cancer Immunol. Immunother. 70, 1323–1339. doi:10.1007/s00262-020-02762-x
Murphy, D. E., de Jong, O. G., Brouwer, M., Wood, M. J., Lavieu, G., Schiffelers, R. M., et al. (2019). Extracellular vesicle-based therapeutics: Natural versus engineered targeting and trafficking. Exp. Mol. Med. 51, 1–12. doi:10.1038/s12276-019-0223-5
Muzio, L., Viotti, A., and Martino, G. (2021). Microglia in neuroinflammation and neurodegeneration: From understanding to therapy. Front. Neurosci. 15, 742065. doi:10.3389/fnins.2021.742065
Nazimek, K., Askenase, P. W., and Bryniarski, K. (2018). Antibody light chains dictate the specificity of contact hypersensitivity effector cell suppression mediated by exosomes. Int. J. Mol. Sci. 19, E2656. doi:10.3390/ijms19092656
Nazimek, K., and Bryniarski, K. (2020a). Approaches to inducing antigen-specific immune tolerance in allergy and autoimmunity: Focus on antigen-presenting cells and extracellular vesicles. Scand. J. Immunol. 91, e12881. doi:10.1111/sji.12881
Nazimek, K., and Bryniarski, K. (2021). Increasing the therapeutic efficacy of extracellular vesicles from the antigen-specific antibody and light chain perspective. Front. Cell Dev. Biol. 9, 790722. doi:10.3389/fcell.2021.790722
Nazimek, K., and Bryniarski, K. (2020b). Perspectives in manipulating EVs for therapeutic applications: Focus on cancer treatment. Int. J. Mol. Sci. 21, E4623. doi:10.3390/ijms21134623
Nazimek, K., Bryniarski, K., Ptak, W., Groot Kormelink, T., and Askenase, P. W. (2020). Orally administered exosomes suppress mouse delayed-type hypersensitivity by delivering miRNA-150 to antigen-primed macrophage APC targeted by exosome-surface anti-peptide antibody light chains. Int. J. Mol. Sci. 21, E5540. doi:10.3390/ijms21155540
Nazimek, K., Bustos-Morán, E., Blas-Rus, N., Nowak, B., Ptak, W., Askenase, P. W., et al. (2019). Syngeneic red blood cell-induced extracellular vesicles suppress delayed-type hypersensitivity to self-antigens in mice. Clin. Exp. Allergy 49, 1487–1499. doi:10.1111/cea.13475
Nazimek, K., Bustos-Morán, E., Blas-Rus, N., Nowak, B., Totoń-Żurańska, J., Seweryn, M. T., et al. (2021). Antibodies enhance the suppressive activity of extracellular vesicles in mouse delayed-type hypersensitivity. Pharm. (Basel) 14, 734. doi:10.3390/ph14080734
Nazimek, K., Ptak, W., Nowak, B., Ptak, M., Askenase, P. W., and Bryniarski, K. (2015). Macrophages play an essential role in antigen-specific immune suppression mediated by T CD8+ cell-derived exosomes. Immunology 146, 23–32. doi:10.1111/imm.12466
Ngo, W., Ahmed, S., Blackadar, C., Bussin, B., Ji, Q., Mladjenovic, S. M., et al. (2022). Why nanoparticles prefer liver macrophage cell uptake in vivo. Adv. Drug Deliv. Rev. 185, 114238. doi:10.1016/j.addr.2022.114238
Nunzi, E., Mezzasoma, L., Bellezza, I., Zelante, T., Orvietani, P., Coata, G., et al. (2023). Microbiota-associated HAF-EVs regulate monocytes by triggering or inhibiting inflammasome activation. Int. J. Mol. Sci. 24, 2527. doi:10.3390/ijms24032527
Pahari, S., Kaur, G., Negi, S., Aqdas, M., Das, D. K., Bashir, H., et al. (2018). Reinforcing the functionality of mononuclear phagocyte system to control tuberculosis. Front. Immunol. 9, 193. doi:10.3389/fimmu.2018.00193
Parada, N., Romero-Trujillo, A., Georges, N., and Alcayaga-Miranda, F. (2021). Camouflage strategies for therapeutic exosomes evasion from phagocytosis. J. Adv. Res. 31, 61–74. doi:10.1016/j.jare.2021.01.001
Park, M. C., Goughnour, P. C., Jun, S., Cho, S., Song, E., Kim, S. B., et al. (2022). Two distinct receptor-binding domains of human glycyl-tRNA synthetase 1 displayed on extracellular vesicles activate M1 polarization and phagocytic bridging of macrophages to cancer cells. Cancer Lett. 539, 215698. doi:10.1016/j.canlet.2022.215698
Patil, M., Saheera, S., Dubey, P. K., Kahn-Krell, A., Kumar Govindappa, P., Singh, S., et al. (2021). Novel mechanisms of exosome-mediated phagocytosis of dead cells in injured heart. Circ. Res. 129, 1006–1020. doi:10.1161/CIRCRESAHA.120.317900
Pedrioli, G., and Paganetti, P. (2021). Hijacking endocytosis and autophagy in extracellular vesicle communication: Where the inside meets the outside. Front. Cell Dev. Biol. 8, 595515. doi:10.3389/fcell.2020.595515
Pidwill, G. R., Pyrah, J. F., Sutton, J. A. F., Best, A., Renshaw, S. A., and Foster, S. J. (2023). Clonal population expansion of Staphylococcus aureus occurs due to escape from a finite number of intraphagocyte niches. Sci. Rep. 13, 1188. doi:10.1038/s41598-023-27928-2
Qu, M., Zhu, H., and Zhang, X. (2022). Extracellular vesicle-mediated regulation of macrophage polarization in bacterial infections. Front. Microbiol. 13, 1039040. doi:10.3389/fmicb.2022.1039040
Rao, L., Wu, L., Liu, Z., Tian, R., Yu, G., Zhou, Z., et al. (2020). Hybrid cellular membrane nanovesicles amplify macrophage immune responses against cancer recurrence and metastasis. Nat. Commun. 11, 4909. doi:10.1038/s41467-020-18626-y
Ren, J., Li, L., Yu, B., Xu, E., Sun, N., Li, X., et al. (2022). Extracellular vesicles mediated proinflammatory macrophage phenotype induced by radiotherapy in cervical cancer. BMC Cancer 22, 88. doi:10.1186/s12885-022-09194-z
Ren, J., Zhu, B., Gu, G., Zhang, W., Li, J., Wang, H., et al. (2023). Schwann cell-derived exosomes containing MFG-E8 modify macrophage/microglial polarization for attenuating inflammation via the SOCS3/STAT3 pathway after spinal cord injury. Cell Death Dis. 14, 70. doi:10.1038/s41419-023-05607-4
Rizzo, J., Rodrigues, M. L., and Janbon, G. (2020). Extracellular vesicles in fungi: Past, present, and future perspectives. Front. Cell. Infect. Microbiol. 10, 346. doi:10.3389/fcimb.2020.00346
Ronquist, G. K. (2019). Extracellular vesicles and energy metabolism. Clin. Chim. Acta 488, 116–121. doi:10.1016/j.cca.2018.10.044
Ruan, S., Greenberg, Z., Pan, X., Zhuang, P., Erwin, N., and He, M. (2022). Extracellular vesicles as an advanced delivery biomaterial for precision cancer immunotherapy. Adv. Healthc. Mater. 11, 2100650. doi:10.1002/adhm.202100650
Samuel, M., Fonseka, P., Sanwlani, R., Gangoda, L., Chee, S. H., Keerthikumar, S., et al. (2021). Oral administration of bovine milk-derived extracellular vesicles induces senescence in the primary tumor but accelerates cancer metastasis. Nat. Commun. 12, 3950. doi:10.1038/s41467-021-24273-8
Sartorio, M. G., Pardue, E. J., Feldman, M. F., and Haurat, M. F. (2021). Bacterial outer membrane vesicles: From discovery to applications. Annu. Rev. Microbiol. 75, 609–630. doi:10.1146/annurev-micro-052821-031444
Shi, M.-M., Zhu, Y.-G., Yan, J.-Y., Rouby, J.-J., Summah, H., Monsel, A., et al. (2021). Role of miR-466 in mesenchymal stromal cell derived extracellular vesicles treating inoculation pneumonia caused by multidrug-resistant Pseudomonas aeruginosa. Clin. Transl. Med. 11, e287. doi:10.1002/ctm2.287
Soni, S., O’Dea, K. P., Abe, E., Khamdan, M., Shah, S. V., Sarathchandra, P., et al. (2022). Microvesicle-mediated communication within the alveolar space: Mechanisms of uptake by epithelial cells and alveolar macrophages. Front. Immunol. 13, 853769. doi:10.3389/fimmu.2022.853769
Sun, K., Zheng, X., Jin, H., Yu, F., and Zhao, W. (2022). Exosomes as CNS drug delivery tools and their applications. Pharmaceutics 14, 2252. doi:10.3390/pharmaceutics14102252
Sun, Y., Sun, F., Xu, W., and Qian, H. (2023). Engineered extracellular vesicles as a targeted delivery platform for precision therapy. Tissue Eng. Regen. Med. 20, 157–175. doi:10.1007/s13770-022-00503-y
Tan, Z.-L., Li, J.-F., Luo, H.-M., Liu, Y.-Y., and Jin, Y. (2022). Plant extracellular vesicles: A novel bioactive nanoparticle for tumor therapy. Front. Pharmacol. 13, 1006299. doi:10.3389/fphar.2022.1006299
Théry, C., Witwer, K. W., Aikawa, E., Alcaraz, M. J., Anderson, J. D., Andriantsitohaina, R., et al. (2019). Minimal information for studies of extracellular vesicles 2018 (MISEV2018): A position statement of the international society for extracellular vesicles and update of the MISEV2014 guidelines. J. Extracell. Vesicles 7, 1535750. doi:10.1080/20013078.2018.1535750
Tian, T., Zhang, H.-X., He, C.-P., Fan, S., Zhu, Y.-L., Qi, C., et al. (2018). Surface functionalized exosomes as targeted drug delivery vehicles for cerebral ischemia therapy. Biomaterials 150, 137–149. doi:10.1016/j.biomaterials.2017.10.012
Tkach, M., and Théry, C. (2016). Communication by extracellular vesicles: Where we are and where we need to go. Cell 164, 1226–1232. doi:10.1016/j.cell.2016.01.043
Trahtemberg, U., and Mevorach, D. (2017). Apoptotic cells induced signaling for immune homeostasis in macrophages and dendritic cells. Front. Immunol. 8, 1356. doi:10.3389/fimmu.2017.01356
Upadhya, R., Madhu, L. N., Rao, S., and Shetty, A. K. (2022). Proficiency of extracellular vesicles from hiPSC-derived neural stem cells in modulating proinflammatory human microglia: Role of pentraxin-3 and miRNA-21-5p. Front. Mol. Neurosci. 15, 845542. doi:10.3389/fnmol.2022.845542
Uribe-Querol, E., and Rosales, C. (2020). Phagocytosis: Our current understanding of a universal biological process. Front. Immunol. 11, 1066. doi:10.3389/fimmu.2020.01066
Urzì, O., Raimondo, S., and Alessandro, R. (2021). Extracellular vesicles from plants: Current knowledge and open questions. Int. J. Mol. Sci. 22, 5366. doi:10.3390/ijms22105366
Verweij, F. J., Balaj, L., Boulanger, C. M., Carter, D. R. F., Compeer, E. B., D’Angelo, G., et al. (2021). The power of imaging to understand extracellular vesicle biology in vivo. Nat. Methods 18, 1013–1026. doi:10.1038/s41592-021-01206-3
Viola, A., Munari, F., Sánchez-Rodríguez, R., Scolaro, T., and Castegna, A. (2019). The metabolic signature of macrophage responses. Front. Immunol. 10, 1462. doi:10.3389/fimmu.2019.01462
Wan, Z., Zhao, L., Lu, F., Gao, X., Dong, Y., Zhao, Y., et al. (2020). Mononuclear phagocyte system blockade improves therapeutic exosome delivery to the myocardium. Theranostics 10, 218–230. doi:10.7150/thno.38198
Warashina, S., Zouda, M., Mohri, K., Wada, Y., Maeda, K., Watanabe, Y., et al. (2022). 64Cu-labeling of small extracellular vesicle surfaces via a cross-bridged macrocyclic chelator for pharmacokinetic study by positron emission tomography imaging. Int. J. Pharm. 624, 121968. doi:10.1016/j.ijpharm.2022.121968
Wei, K., Zhang, H., Yang, S., Cui, Y., Zhang, B., Liu, J., et al. (2023). Chemo-drugs in cell microparticles reset antitumor activity of macrophages by activating lysosomal P450 and nuclear hnRNPA2B1. Signal Transduct. Target Ther. 8, 22. doi:10.1038/s41392-022-01212-7
Wei, Z., Chen, Z., Zhao, Y., Fan, F., Xiong, W., Song, S., et al. (2021). Mononuclear phagocyte system blockade using extracellular vesicles modified with CD47 on membrane surface for myocardial infarction reperfusion injury treatment. Biomaterials 275, 121000. doi:10.1016/j.biomaterials.2021.121000
Wen, S. W., Sceneay, J., Lima, L. G., Wong, C. S. F., Becker, M., Krumeich, S., et al. (2016). The biodistribution and immune suppressive effects of breast cancer–derived exosomes. Cancer Res. 76, 6816–6827. doi:10.1158/0008-5472.CAN-16-0868
Wiklander, O. P. B., Brennan, M. Á., Lötvall, J., Breakefield, X. O., and El Andaloussi, S. (2019). Advances in therapeutic applications of extracellular vesicles. Sci. Transl. Med. 11, eaav8521. doi:10.1126/scitranslmed.aav8521
Wiklander, O. P. B., Nordin, J. Z., O’Loughlin, A., Gustafsson, Y., Corso, G., Mäger, I., et al. (2015). Extracellular vesicle in vivo biodistribution is determined by cell source, route of administration and targeting. J. Extracell. Vesicles 4, 26316. doi:10.3402/jev.v4.26316
Wilhelm, S., Tavares, A. J., Dai, Q., Ohta, S., Audet, J., Dvorak, H. F., et al. (2016). Analysis of nanoparticle delivery to tumours. Nat. Rev. Mater 1, 16014. doi:10.1038/natrevmats.2016.14
Wu, G., Su, Q., Li, J., Xue, C., Zhu, J., Cai, Q., et al. (2023). NAMPT encapsulated by extracellular vesicles from young adipose-derived mesenchymal stem cells treated tendinopathy in a “One-Stone-Two-Birds” manner. J. Nanobiotechnology 21, 7. doi:10.1186/s12951-022-01763-5
Xia, Q., Zhang, Y., Li, Z., Hou, X., and Feng, N. (2019). Red blood cell membrane-camouflaged nanoparticles: A novel drug delivery system for antitumor application. Acta Pharm. Sin. B 9, 675–689. doi:10.1016/j.apsb.2019.01.011
Xia, W., Liu, Y., Jiang, X., Li, M., Zheng, S., Zhang, Z., et al. (2023). Lean adipose tissue macrophage derived exosome confers immunoregulation to improve wound healing in diabetes. J. Nanobiotechnol 21, 128. doi:10.1186/s12951-023-01869-4
Xiang, X., Pathak, J. L., Wu, W., Li, J., Huang, W., Wu, Q., et al. (2023). Human serum-derived exosomes modulate macrophage inflammation to promote VCAM1 -mediated angiogenesis and bone regeneration. J. Cell. Mol. Medi 27, 1131–1143. doi:10.1111/jcmm.17727
Xin, D., Li, T., Chu, X., Ke, H., Liu, D., and Wang, Z. (2021). MSCs-extracellular vesicles attenuated neuroinflammation, synapse damage and microglial phagocytosis after hypoxia-ischemia injury by preventing osteopontin expression. Pharmacol. Res. 164, 105322. doi:10.1016/j.phrs.2020.105322
Xu, H., Zhu, Y., Hsiao, A. W.-T., Xu, J., Tong, W., Chang, L., et al. (2023). Bioactive glass-elicited stem cell-derived extracellular vesicles regulate M2 macrophage polarization and angiogenesis to improve tendon regeneration and functional recovery. Biomaterials 294, 121998. doi:10.1016/j.biomaterials.2023.121998
Yona, S., and Gordon, S. (2015). From the reticuloendothelial to mononuclear phagocyte system – the unaccounted years. Front. Immunol. 6, 328. doi:10.3389/fimmu.2015.00328
You, B., Yang, Y., Zhou, Z., Yan, Y., Zhang, L., Jin, J., et al. (2022). Extracellular vesicles: A new frontier for cardiac repair. Pharmaceutics 14, 1848. doi:10.3390/pharmaceutics14091848
Zhang, B., Yin, Y., Lai, R. C., and Lim, S. K. (2014). Immunotherapeutic potential of extracellular vesicles. Front. Immunol. 5, 518. doi:10.3389/fimmu.2014.00518
Zhang, J., Han, J., Li, H., Li, Z., Zou, P., Li, J., et al. (2023). Lymphocyte membrane- and 12p1-dual-functionalized nanoparticles for free HIV-1 trapping and precise siRNA delivery into HIV-1-Infected cells. Adv. Sci. (Weinh) 10, e2300282. doi:10.1002/advs.202300282
Zhang, K.-L., Wang, Y.-J., Sun, J., Zhou, J., Xing, C., Huang, G., et al. (2019). Artificial chimeric exosomes for anti-phagocytosis and targeted cancer therapy. Chem. Sci. 10, 1555–1561. doi:10.1039/C8SC03224F
Zhang, Y., Cai, Z., Shen, Y., Lu, Q., Gao, W., Zhong, X., et al. (2021). Hydrogel-load exosomes derived from dendritic cells improve cardiac function via Treg cells and the polarization of macrophages following myocardial infarction. J. Nanobiotechnol 19, 271. doi:10.1186/s12951-021-01016-x
Zhao, J., Li, X., Hu, J., Chen, F., Qiao, S., Sun, X., et al. (2019). Mesenchymal stromal cell-derived exosomes attenuate myocardial ischaemia-reperfusion injury through miR-182-regulated macrophage polarization. Cardiovasc. Res. 115, 1205–1216. doi:10.1093/cvr/cvz040
Keywords: cell-free therapeutics, dendritic cells, exosomes, extracellular vesicles, immunotherapy, macrophages, monocytes, mononuclear phagocyte system
Citation: Cieślik M, Bryniarski K and Nazimek K (2023) Biodelivery of therapeutic extracellular vesicles: should mononuclear phagocytes always be feared?. Front. Cell Dev. Biol. 11:1211833. doi: 10.3389/fcell.2023.1211833
Received: 25 April 2023; Accepted: 26 June 2023;
Published: 05 July 2023.
Edited by:
Hinrich Peter Hansen, University of Cologne, GermanyReviewed by:
Alexander N. Kapustin, AstraZeneca, United KingdomKatrin S. Reiners, University Hospital Bonn, Germany
Copyright © 2023 Cieślik, Bryniarski and Nazimek. This is an open-access article distributed under the terms of the Creative Commons Attribution License (CC BY). The use, distribution or reproduction in other forums is permitted, provided the original author(s) and the copyright owner(s) are credited and that the original publication in this journal is cited, in accordance with accepted academic practice. No use, distribution or reproduction is permitted which does not comply with these terms.
*Correspondence: Katarzyna Nazimek, a2F0YXJ6eW5hLm5hemltZWtAdWouZWR1LnBs