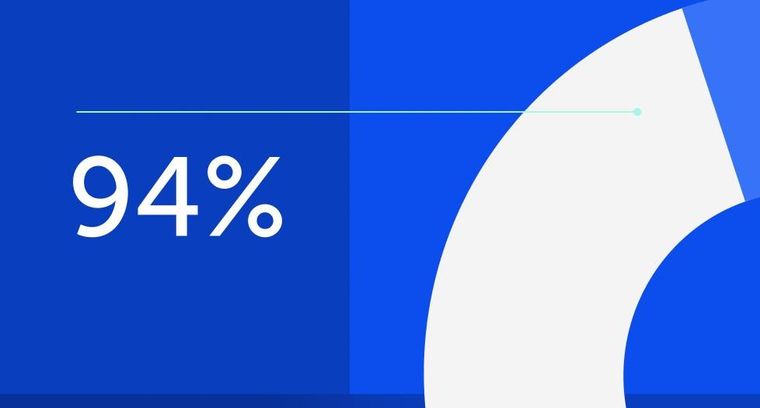
94% of researchers rate our articles as excellent or good
Learn more about the work of our research integrity team to safeguard the quality of each article we publish.
Find out more
REVIEW article
Front. Cell Dev. Biol., 09 August 2023
Sec. Cellular Biochemistry
Volume 11 - 2023 | https://doi.org/10.3389/fcell.2023.1167626
This article is part of the Research TopicMolecular Mechanisms and Potential Therapeutic Intervention of Trace Element-related DiseasesView all 5 articles
Research investigating the correlation between human trace element levels and disease alterations is growing. Epilepsy, a common nervous system disease, has also been found to be closely related to abnormal levels of trace elements. Studies continue to explore mechanisms of various trace elements involved in epileptic seizures through experimental animal models of epilepsy. Thus, we reviewed the research progress on the correlation between trace element levels and epilepsy in recent years and found that the trace elements most closely related to epilepsy are mainly metal ions such as selenium, iron, copper, zinc, and manganese. These results indicate that the changes in some trace elements are closely related to the increase in epilepsy susceptibility. In addition, after treatment with drugs and a ketogenic diet, the concentration of trace elements in the serum of patients with epilepsy changes. In other words, the abnormality of trace element concentrations is of great significance in the occurrence and development of epilepsy. This article is a literature update on the potential role of trace element imbalance in the development of epilepsy, providing new references for the subsequent prevention and treatment of epilepsy.
Epilepsy is a prevalent neurological disorder characterized by persistent susceptibility to seizures, which can lead to various neurobiological, cognitive, psychological, and social ramifications. (Fisher et al., 2005; Fisher et al., 2014). An initial brain injury later results in neuron damage, ion pathway dysfunction, pine fibrosis, glial hyperplasia, synaptic plasticity, inflammatory response, and impairment of brain nerve function, typically resulting in the development of the pathological condition known as epilepsy. In order to prevent and treat epilepsy, it is therefore interesting and crucial to research whether the pathophysiology of epilepsy is related to changes in various trace elements in patients and animal models, leading to fluctuations in susceptibility to epilepsy. According to several pieces of research, the body’s antioxidant defense mechanism relies on the enzymes glutathione peroxidase (GSH-Px) and superoxide dismutase (SOD) to neutralize oxygen free radicals (Yüksel et al., 2000); furthermore, oxidative stress (OS) is the main mechanism underlying epilepsy in patients with epilepsy due to various causes (Zimmer et al., 2021). In particular, iron metabolism and ferroptosis are essential in epilepsy (Chen et al., 2020). Several enzymes, such as tyrosinase and dopamine hydroxylase, require copper as a cofactor. Under normal circumstances, the release of copper during synaptic transmission helps regulate neuronal excitability, while a decrease in serum copper levels can lead to epileptic discharge (Das et al., 2019). Furthermore, modulation of the Zn2+-metal-regulated transcription factor 1 (MTF1)-CaV3.2 cascade reaction also impacts epilepsy (van Loo et al., 2015). Notably, selenium is an antioxidant, and glutathione peroxidase (GSH-px), a selenium-dependent enzyme, reduces organic and hydrogen peroxides in the presence of reduced glutathione (GSH) (Nazıroğlu and Yürekli., 2013). Changes in blood manganese levels in epilepsy-susceptible rats can affect the activity of glutamine synthase (Carl et al., 1993). Moreover, treating epilepsy with drugs and a ketogenic diet (KD) resulted in changes in trace element levels. Thus, trace elements are crucial in the emergence of epilepsy.
Epilepsy has a significant impact on the lives and work of many patients. Despite receiving treatment with antiseizure medications (ASMs), approximately 30% of patients remain drug-resistant. At present, there are many treatment methods for epilepsy, such as the most commonly used drug therapy and surgical treatment; however, the efficacy of these treatments varies among individuals. Ongoing research explores interesting and potentially effective approaches to control epilepsy. Balanced trace element concentrations in people and animal models may aid in the treatment of epilepsy, according to certain clinical evidence or basic research findings. This article reviews and discusses the research on trace elements and epilepsy in recent years, the changes in the levels of trace elements in epilepsy, the possible mechanisms of trace elements involved in epilepsy occurrence, and how to improve epilepsy through these mechanisms.
The interaction of trace elements with the human body has been the subject of extensive research over the last 30 years. During this period, people increasingly recognized the importance of these factors in human diseases, including their contribution to the global disease burden. In recent years, studying the relationship between epilepsy and trace element levels has become a new direction in anti-epilepsy regimens. Research has shown that the levels of micronutrients in children with epilepsy have changed, and further differences have been observed between drug-controlled epilepsy and refractory epilepsy (Verrotti et al., 2002; Ashrafi et al., 2007; Seven et al., 2013; Wojciak et al., 2013; Prasad et al., 2014; Saghazadeh et al., 2015; Dixit et al., 2020; Svetel et al., 2021). This article lists the changes in trace element levels in patients with epilepsy from multiple studies and analyzes the correlation of these levels with epilepsy.
Zinc is essential for maintaining antioxidant activity, metabolic balance, and immune system function (Mocchegiani et al., 2008); furthermore, it has been widely studied because of its contribution to epileptic neurological function. The average serum zinc level in children with refractory epilepsy was found to be significantly lower than that in controls (95.8 ± 29 μg/dl vs. 145 ± 37 μg/dl; p < 0.001) (Saad et al., 2014). Another study similarly showed that the average zinc level in children with epilepsy is significantly lower than that in the controls (60.1 ± 22.6 μg/dl vs. 102.1 ± 18 μg/dl; p < 0.001) (Talat et al., 2015). Patients with epilepsy having serum zinc levels of <70 μg/dl exhibit a 3.56 times greater risk of epilepsy than do those having serum zinc levels of 70–120 μg/dl (Das et al., 2019). However, another study reported that the serum zinc concentration of untreated patients with epilepsy is significantly higher than that of controls (Saghazadeh et al., 2015), and there is no significant difference in zinc levels between the epilepsy and control group (Gündoğdu et al., 2023). In addition, a study on the treatment of refractory epilepsy in children using oral zinc supplementation showed that 31% of children in the intervention group exhibited improvement, while only 4.5% of children in the placebo group did, with a significantly lower frequency of seizures in the intervention group compared to the placebo group (p = 0.02) (Saad et al., 2015). Serum zinc concentrations in patients with epilepsy vary across different research findings. Hence, monitoring the serum zinc concentration of patients is necessary, and supplementing zinc during zinc deficiency may be an effective option during epilepsy treatment.
Hemosiderin, which is stored in the body as iron, is formed in the brain due to hemoglobin breakdown (Zhang et al., 2018). While epilepsy is intimately tied to inflammation, some cytokines (Sanz and Garcia-Gimeno., 2020), such as IL-6 and TNF-α, are associated with iron control and metabolism (Tombini et al., 2013). The presence of iron and its role in regulating inflammation within the epileptic brain can explain the impact of iron on the occurrence and development of epilepsy. One study reported that blood iron levels were substantially lower in the control than the epilepsy group (106 ± 8 μg/dl vs. 88 ± 8 μg/dl; p<0.01) (Ikeda, 2001). Additionally, some studies have discovered that certain seizure types might cause an increase in iron buildup in pyknotic neurons (Zimmer et al., 2021). Further, individuals with numerous metastases may experience iron overload due to medullary aplasias, hemolytic anemias, or hemochromatosis. Therefore, it is important to note that iron excess may be a factor in the second effect, the propensity to produce epilepsy by means of hypoxic damage, OS, and inflammation. Hence, regulating iron metabolism in the brain may provide a new strategy for epilepsy treatment.
Copper can indirectly enhance neurotoxicity by producing oxygen free radicals (Talat et al., 2015) and enhance neural excitability; furthermore, it is closely related to epilepsy. Copper alone inhibits magnesium and sodium-potassium ATPases, leading to changes in sodium and potassium levels, which in turn increases susceptibility to seizures (Donaldson et al., 1971; Garg et al., 2021; Nemati et al., 2021; Sharma et al., 2021). Some studies have shown an increase in serum copper levels in the epilepsy group compared to those of the control group (Wojciak et al., 2013; Gündoğdu et al., 2023), while others have reported no significant difference between the two groups (Sözüer et al., 1995; Chwiej et al., 2008). Another study found that the average copper level is significantly higher in the epilepsy group than in the control group (180.1 ± 32.4 μg/dl vs. 114.5 ± 18.5 μg/dl; p < 0.001) (Talat et al., 2015). Based on the above research results, we believe that controlling serum copper levels may improve the occurrence of epilepsy.
GSH-Px, an antioxidant protein involved in glutamate-mediated excitotoxicity, membrane lipid peroxidation, neurotransmitter turnover, and implicated in the etiology of epilepsy, relies on selenium for its synthesis and functionality (Steinbrenner and Sies., 2013; Murthy et al., 2020; Abokrysha et al., 2021; Kamate et al., 2021). According to research, following rigorous screening, the average blood selenium level of epilepsy patients was 68.88 ± 17.58 ng/ml, whereas the control group exhibited a significantly higher average of 85.93 ± 13.93 ng/ml (p < 0.05) (Ashrafi et al., 2007). The study by Saad et al. (2014) demonstrated that the average serum selenium level of patients with epilepsy is significantly lower than that in the control group (4.2 ± 1.3 μg/dl vs. 8.3 ± 2.3 μg/dl; p < 0.001), which is consistent with the findings of Chakrabarty et al. (2022) (89.7 ± 13.6 μg/dl vs. 95.79 ± 12.66 μg/dl; p = 0.008). In contrast, Gündoğdu et al. (2023) reported that the average level of selenium was significantly higher in the epilepsy group than in the control group (71.95 ± 21.19 μg/l vs. 36.84 ± 9.08 μg/l; p < 0.001). Moreover, another study demonstrated that the serum selenium concentration did differ significantly between untreated patients with epilepsy and individuals in the control group (Das et al., 2019).
In summary, different clinical studies have shown conflicting changes in the levels of trace elements in the serum of patients with epilepsy; this may be due to factors such as insufficient sample size, individual differences, or differences in the environment or drug treatment. Some trace elements with antioxidant effects, such as zinc and selenium, may participate in and regulate some signal pathways and inhibit the occurrence of epilepsy when a stable concentration is maintained. In contrast, copper, iron, and other trace elements can increase the production of oxygen free radicals, enhance neurotoxicity, cause neural necrosis, and potentially increase susceptibility to epilepsy. Therefore, it is essential to monitor the concentration of various trace elements related to epilepsy in the serum for a long time during the anti-epilepsy regimen. If necessary, zinc, selenium, or other trace element supplement should be administered to maintain an adequate concentration of these elements and reduce epilepsy susceptibility. Simultaneously, the concentration of trace elements such as copper and iron that promote epilepsy should be regulated. These bidirectional control conditions may play a positive role in treating epilepsy. To obtain evidence of the correlation between trace element levels and epilepsy, more experimental data and animal model experiments are necessary. These studies would help support the influence of regulating trace element levels on the occurrence of epilepsy and to find countermeasures to reduce the occurrence of epilepsy.
The research on the changes in serum trace element concentrations in patients with epilepsy primarily focuses on quantifying these changes. Clinical studies on the mechanism of action of the trace elements in epilepsy are limited, while more exploration is conducted in experimental animal models.
Zinc can be used as an anticonvulsant while homeostasis is maintained in the body and as a stimulant when homeostasis is disrupted (Prasad et al., 2014). Zinc homeostasis disorders are important in neurodegenerative brain diseases (Pavlica and Gebhardt., 2010). The Zn2+-MTF1-CaV3.2 cascade reaction, which occurs when high amounts of Zn2+ activate MTF-1, which subsequently binds to MRE in the CaV3.2 gene promoter and stimulates transcription of this gene, was discovered in a mouse pilocarpine epilepsy model (van Loo et al., 2015). The expression of CaV3.2 channels is notably increased, leading to elevated ICaT levels due to the upregulation of CaV3.2 mRNA. Furthermore, the hippocampus network becomes more excitable as a result of the CA1 pyramidal cells’ conversion from normal discharge to burst discharge when ICaT is elevated (Sanabria et al., 2001; Yaari et al., 2007). Thus, controlling this cascade reaction to reduce the frequency of recurrent epilepsy is an effective method (van Loo et al., 2015). A low zinc diet in epileptic mice can reduce brain zinc levels and increase susceptibility to epilepsy (Fukahori and Itoh., 1990), whereas a high zinc diet has the opposite effect. Notably, zinc affects brain function during seizures; if its concentration exceeds a certain level (>100 mM), it may cause the death of neural cells (Choi and Koh., 1998). Moreover, zinc can selectively inhibit N-methyl-D-aspartic acid (NMDA) and γ-aminobutyric acid (GABA) receptors, as well as glutamate and GABA transporters, to affect the occurrence of epilepsy (Pavlica and Gebhardt., 2010; Nazıroğlu and Yürekli., 2013; Carver et al., 2016; Das et al., 2019). Zinc can also influence the removal of free radicals since it is a component of the SOD, the most significant antioxidant enzyme (Horning et al., 2000; Yüksel et al., 2000; da Cunha et al., 2003). In summary, low or high levels of zinc in the brain can increase the susceptibility to epilepsy, and zinc seems to have an anti-epileptic effect when its homeostasis is maintained. Therefore, inhibiting zinc release, blocking channels, and modifying zinc transport and buffering in target tissues might all be useful regulatory strategies for treating epilepsy. Additionally, a workable strategy is to moderately supplement with zinc to maintain a balanced zinc level when there is a zinc deficit.
Numerous redox processes use iron molecules, such as DNA biosynthesis, tricarboxylic acid cycle, oxygen transport, and cellular oxidative respiratory chain. Although iron plays a crucial role in certain physiological processes, for the nervous system, iron accumulation caused by iron prolapse may increase susceptibility to epilepsy. Ferroptosis is a newly discovered form of cell death regulation, attributed to severe lipid peroxidation caused by reactive oxygen species (ROS) and iron overload as a result of multiple biological pathways involving ROS, redox GSH, glutathione peroxidase 4 (GPX4), intracellular iron ions (Fe3+ and Fe2+), and lipid peroxidation. In the process of iron ion interaction, the Fenton reaction produces ROS, which is the process of Fe2+ reacting with H2O2 to produce hydroxide and hydroxyl (Mittler, 2017). In addition, there is also a Fenton reaction process in the Haber Weiss cycle, which can generate ROS (Capelletti et al., 2020). ROS are produced more effectively by Fe2+, which also promotes lipid peroxidation and causes ferroptosis (Chen et al., 2020). Long-lasting convulsions in epileptic seizures encourage the generation of too much ROS, which causes oxidative stress and is intimately linked to the development of epileptic activity and neuronal cell death (Freitas, 2009; Eastman et al., 2020). Furthermore, increased ROS levels cause lipid peroxidation, which then results in iron deposition. A significant quantity of Fe2+ from hemoglobin and red blood cells is released into the brain in post-hemorrhagic stroke epilepsy (PHE) and post-traumatic epilepsy (PTE), which may cause ferroptosis (Mori et al., 2004). Ferroptosis can be less frequently seen, and epilepsy development can be delayed with reasonable regulation of ROS levels in the brain.
Additionally, glutamate (Glu), cysteine (Cys), and glycine (Gly) are the key components of the small molecule peptide GSH, which is essential for the body’s ability to eliminate ROS (Xiao and Meierhofer., 2019). GSH serves as GPX4’s substrate, which lowers the frequency of iron poisoning (Ursini and Maiorino, 2020). In epilepsy, GSH levels decrease, and ROS levels increase. Therefore, ferroptosis may be prevented, which is good for treating epilepsy, by controlling the amount of GSH and minimizing harm to neural cells in the epileptic brain.
Manganese is the foundation for the development and function of nerve cells and another fundamental component of many metabolic processes in the brain. The brain’s extracellular fluid may be penetrated by Mn2+ and Mn3+, which can then bind to transferrin released by oligodendrocytes. In a non-binding state, it can be absorbed by choroidal plexus cells and brain parenchyma. Subsequently, neurons obtain ferritin-binding manganese through receptor-mediated endocytosis (Takeda, 2004). Through a transport system akin to neurons, manganese may also enter astrocytes. Once ingested by cells, it will interact with glutamine synthetase (GS) in astrocytes and SOD in neurons (Takeda et al., 1998). The lower capacity of the brain to metabolize glutamate and ammonia may be connected to the reduction in glutamine synthase activity in the brain of epilepsy-prone mice. The buildup of these chemicals may result in a local rise in pH and a local decrease in GABA-dependent chloride ion compression, both of which favor the diffusion of excitement (Carl et al., 1993). This indicates that manganese participates in and inhibits the metabolic pathway of glutamine synthase activity, which may lead to an increase in neural excitability and promote the occurrence of epilepsy. Regulating this pathway may be a new therapeutic target for epilepsy.
Selenium controls or suppresses epileptic seizures caused by excitatory drugs (Nazıroğlu et al., 2008). In particular, the selenium-dependent enzyme GSH-Px is activated by selenium, and its activation allows GSH-Px to control the intracellular amount of hydrogen peroxide and hydroxyl radicals, hence controlling the antioxidant defense mechanism (Naziroğlu et al., 2009). In addition, 11 of the 15 neurodevelopmental disorders related to seizures can be caused by reduced expression of selenoprotein, low selenium status, interference of sodium selenate biosynthesis, or interruption of selenium transport into the brain through sodium selenate (Schweizer et al., 2021). In known animal studies, selenium can prevent the oxidation of nerve cell bodies and actively regulate brain function (Dominiak et al., 2016). However, high doses of selenium cause OS by consuming GSH, which reduces cholinergic signaling pathways’ activation and causes cholinergic neuron degeneration (Lakshmi et al., 2015). Thus, maintaining a selenium balance is important for epilepsy, as it can inhibit neuronal cell death through antioxidant effects and control epilepsy.
Copper is a cofactor of different enzymes. It is a component of copper-zinc-superoxide dismutase (Cu-Zn-SOD) and facilitates a neutralization in the accumulation of free radicals (Puig and Thiele., 2002). Notably, Cu-Zn-SOD not only has anti-epileptic effects by inhibiting cell apoptosis and altering the levels of Nav1.2/NRF2/HO-1 signaling pathway proteins in pilocarpine hydrochloride-induced epileptic rats but also significantly increases the postsynaptic potential latency in epileptic rats (Wen et al., 2022). Nevertheless, Cu’s capacity to accelerate redox processes might mistakenly result in the generation of ROS (Denoyer et al., 2015). In addition, significant brain inflammation brought on by copper is thought to have a role in developing epilepsy (Sozuer et al., 1995; Yilmaz et al., 2009; Płonka-Półtorak et al., 2013). Despite the antioxidant properties of Cu-Zn-SOD, the mechanism of the Cu-catalyzed redox reaction may raise the level of ROS, causing pro-inflammatory responses and raising the risk of neurotoxicity and epilepsy.
In summary, studies on experimental animal models have paid more attention to studying the complex mechanisms of trace elements involved in epilepsy rather than simply measuring the changes in trace element concentrations after epilepsy, which is conducive to discovering the role of various trace elements in epilepsy. Manganese may affect some metabolic and other pathways, reduce the activity of certain enzymes, and promote the occurrence of epilepsy. Further, zinc and selenium can reduce seizures and epilepsy susceptibility through antioxidant, neuroexcitatory, and neuroprotective effects. Although copper is an important component of Cu-Zn-SOD, which scavenges oxygen free radicals, copper itself can increase the erosion of oxygen free radicals on nerve cells, leading to cell death, increasing nerve excitability, and increasing epilepsy susceptibility. Excessive iron accumulation from iron deposition is also recognized as a contributing factor to epilepsy. Furthermore, various trace elements play different roles in epilepsy, and it is possible to determine the mechanisms by which some trace elements damage or protect nerve cells. From the perspective of epilepsy treatment, maintaining a balance of trace element levels in the body may effectively control the frequency and severity of epilepsy. After obtaining more accurate and reliable experimental data in the future, intervening in the pathways associated with these trace elements involved in epilepsy could emerge a novel and effective approach to treating epilepsy.
ASM treatment is the most widely used anti-epileptic regimen, and valproic acid (VPA) and carbamazepine (CBZ) are safe and effective ASMs. Studies from recent years claim that VPA medication can lower serum zinc levels (Graf et al., 1998; Armutcu et al., 2004; Płonka-Półtorak et al., 2011); furthermore, zinc levels have been shown to increase after VPA treatment (Altunbaşak et al., 1997; Hamed et al., 2004). Notably, after receiving VPA, individuals with epilepsy had considerably lower blood copper levels than did the control group (Kaji et al., 1992; Kuzuya et al., 1993; Liu et al., 1997; Armutcu et al., 2004). Specifically, VPA can promote the clearance of copper, selenium, and zinc, reducing the synthesis of free radical scavenging enzymes (Kürekçi et al., 1995). According to other research, there were no variations in the plasma concentrations of copper, zinc, or manganese among individuals receiving sodium VPA or CBZ monotherapy (Gonzalez-Reyes et al., 2007). Some studies also suggest that VPA and CBZ affect the intracellular distribution of zinc (Ilhan et al., 1999).
ASM treatment may affect the homeostasis of trace metal levels in the serum rather than affecting the seizure itself. Furthermore, trace element levels in the body will change while receiving ASM medication, which is difficult to prevent. Nevertheless, through ASM treatment, we can solely regulate the levels of trace elements that contribute to epilepsy, sustain the concentrations of trace elements beneficial for epilepsy, and manage the levels of trace elements that heighten susceptibility to epilepsy. Thus, it is crucial to gather more information through experimental animal studies to further elucidate the mechanisms underlying trace element concentration changes during ASM treatment. This will shed light on the effects of these changes and further enhance the safety of drug therapy for epilepsy.
A highly specialized nutritional diet known as KD is distinguished by its proportional mixing of lipid and non-lipid (protein and carbohydrate) elements. Status epilepticus (SE) may impair intestinal food intake, affecting certain trace elements’ intake and increasing susceptibility to epilepsy. Furthermore, if an individual cannot tolerate a KD, parenteral nutrition can be administered to supplement the necessary trace elements (Dressler et al., 2018). Multiple studies have shown that KD positively affects the treatment of epilepsy, especially refractory epilepsy (Maalouf et al., 2009; Garcia-Penas, 2018; Ułamek-Kozioł et al., 2019). KD may alleviate temporal lobe hippocampal neurodegeneration in rats with epilepsy by activating acid-sensing ion channel 1a (ASIC1a) and mitochondrial-mediated apoptosis pathway (Qiao et al., 2022). While KD is relatively safe for treating epilepsy, some minerals, vitamins, and trace elements, including selenium (Lima et al., 2014; Arslan et al., 2017; El-Rashidy et al., 2020) and zinc (Christodoulides et al., 2012; Hayashi et al., 2013; Frommelt et al., 2014), can decrease in patients with epilepsy, making KD a double-edged sword. Due to the important role of zinc and selenium in the anti-epilepsy process, it is crucial to detect patients’ serum trace element levels during KD treatment and supplement them if necessary. Furthermore, studying the effects of combining KD with various anti-epileptic regimens is a promising area of research.
In conclusion, the levels of trace elements in the patient’s body alter to various degrees following the beginning of epilepsy (Figure 2). Furthermore, the association between epilepsy and trace element levels is further evident in the participation of trace elements in regulating specific signaling pathways involved in epilepsy. For instance, zinc can affect the occurrence of epilepsy by participating in the regulation of pathways involving SOD, MTF1-CaV3.2 cascade reaction, NMDA, and GABA. Selenium is necessary for the synthesis and function of GSH-Px, which contributes to glutamate-mediated excitotoxicity, membrane lipid peroxidation, neurotransmitter turnover, and reduction in the levels of hydrogen oxide and hydroxyl radicals in cells. Although copper is an important component of Cu-Zn-SOD, which can prolong the incubation period of epilepsy, copper can independently inhibit magnesium and sodium-potassium ATPase and produce OS during catalytic redox reactions, causing nerve cell inflammation and promoting epilepsy. Elevated levels of intracellular iron ions (Fe3+ and Fe2+) will produce a large amount of ROS and affect various biological pathways, including those involving GSH, GPX4, and lipid peroxidation, leading to an increased susceptibility to epilepsy. Manganese can enter the brain as ions and combine with SOD and GS in nerve cells, thereby affecting metabolic pathways and leading to epilepsy. The above-mentioned trace elements play a role in the signaling pathways associated with epilepsy and therefore represent new therapeutic targets in treating epilepsy (Figure 1). In patients with epilepsy administered drug therapy, the levels of trace elements change for various reasons compared with those of the control group, and different ASMs may also be involved in these changes. Whether patients with epilepsy are newly diagnosed or have already undergone ASM treatment, long-term monitoring and timely adjustment of serum trace element levels positively affect controlling epilepsy. In addition, KD treatment can change the levels of trace elements in patients with epilepsy. Hence, during KD treatment, it is necessary to supplement trace elements such as zinc and selenium that can positively affect anti-epilepsy effects (Figure 2). Further research exploring the correlation between trace elements and epilepsy is required to understand the close relationship between in vivo trace element levels and epilepsy. This will contribute to a more comprehensive and effective anti-epilepsy regimen.
FIGURE 1. The relationship between epilepsy and trace elements. 1) Zinc is involved in regulating SOD, MTF1-CaV3.2 cascade reaction, NMDA, and GABA to affect the occurrence of epilepsy. 2) Selenium regulates the activity of GSH-Px, which contributes to glutamate-mediated excitotoxicity, membrane lipid peroxidation, and neurotransmitter conversion, thereby reducing the level of intracellular hydrogen oxide and hydroxyl radicalintracellular hydrogen oxide and hydroxyl radical levels. 3) Copper is involved in forming Cu-Zn-SOD, effectively prolonging the latency of epilepsy. However, copper can independently inhibit magnesium and sodium-potassium ATPase, and may produce OS in catalytic redox reactions, causing inflammation of nerve cells and promoting epilepsy. 4) Excessive accumulation of iron ions can produce a large amount of ROS, thereby influencing various biological pathways involving GSH, GPX4, GPX4, and lipid peroxidation. This heightened oxidative stress can subsequently increase vulnerability to epilepsy, affecting various biological pathways involving GSH, GPX4, GPX4, and lipid peroxidation and increasing susceptibility to epilepsy. 5) Manganese enters the nervous system in the form ofas ions, binds to SOD and GS in nerve cells, affects a series of metabolic pathways, and leads to the occurrence of epilepsy.
FIGURE 2. Changes in serum trace element levels in patients with epilepsy. The effect of different anti-epileptic methods on trace elements. 1) The trace element levels in patients with epilepsy vary, including those of zinc and selenium. Copper levels increase or remain unchanged, while iron levels increase. 2) KD reduces serum zinc and selenium levels in patients with epilepsy. 3) The changes in zinc and selenium levels in patients after VPA and CBZ treatment are not uniform.
WL, JX, and LjZ contributed equally to this article. WL, JX, LnZ, FL, LjZ, and ZT designed and wrote the manuscript. JY, HZ, JT, CY, and ZX helped with proofreading and revision. All authors contributed to the article and approved the submitted version.
This work was supported by the grants from the Guizhou epilepsy basic and clinical research scientific and technological innovation talent team project (No: CXTD[2022]013), the Collaborative Innovation Center of the Chinese Ministry of Education (No: 2020 -39), the Guizhou provincial “hundred” level innovative talents funds (No: GCC-2022-038-1), the Guizhou Provincial Science and Technology Foundation (No: ZK 2022-656), and the Zunyi City Science and Technology Foundation (No: 2019-71 and 2021-30).
We would like to express our gratitude for all menbers in valuable assistance and suggestions throughout the collection and analysis of our article. We would also like to acknowledge Editage (www.editage.cn) for their English language editing services.
The authors declare that the research was conducted in the absence of any commercial or financial relationships that could be construed as a potential conflict of interest.
All claims expressed in this article are solely those of the authors and do not necessarily represent those of their affiliated organizations, or those of the publisher, the editors and the reviewers. Any product that may be evaluated in this article, or claim that may be made by its manufacturer, is not guaranteed or endorsed by the publisher.
Abokrysha, N. T., Kishk, N. A., Nawito, A. M., and Mounir, N. (2021). Effect of low-dose naltrexone on Egyptian children with intractable epilepsy: A case series study. Neurol. India 69 (6), 1781–1784. doi:10.4103/0028-3886.333523
Altunbaşak, S., Biatmakoui, F., Baytok, V., Hergüner, O., Burgut, H. R., and Kayrin, L. (1997). Serum and hair zinc levels in epileptic children taking valproic acid. Biol. Trace Elem. Res. 58 (1-2), 117–125. doi:10.1007/BF02910672
Armutcu, F., Ozerol, E., Gurel, A., Kanter, M., Vural, H., Yakinci, C., et al. (2004). Effect of long-term therapy with sodium valproate on nail and serum trace element status in epileptic children. Biol. Trace Elem. Res. 102 (1-3), 1–10. doi:10.1385/BTER:102:1-3:001
Arslan, N., Kose, E., and Guzel, O. (2017). The effect of ketogenic diet on serum selenium levels in patients with intractable epilepsy. Biol. Trace Elem. Res. 178 (1), 1–6. doi:10.1007/s12011-016-0897-7
Ashrafi, M. R., Shabanian, R., Abbaskhanian, A., Nasirian, A., Ghofrani, M., Mohammadi, M., et al. (2007). Selenium and intractable epilepsy: Is there any correlation? Pediatr. Neurol. 36 (1), 25–29. doi:10.1016/j.pediatrneurol.2006.09.001
Capelletti, M. M., Manceau, H., Puy, H., and Peoc'h, K. (2020). Ferroptosis in liver diseases: An overview. Int. J. Mol. Sci. 21 (14), 4908. doi:10.3390/ijms21144908
Carl, G. F., Blackwell, L. K., Barnett, F. C., Thompson, L. A., Rissinger, C. J., Olin, K. L., et al. (1993). Manganese and epilepsy: Brain glutamine synthetase and liver arginase activities in genetically epilepsy prone and chronically seizured rats. Epilepsia 34 (3), 441–446. doi:10.1111/j.1528-1157.1993.tb02584.x
Carver, C. M., Chuang, S-H., and Reddy, D. S. (2016). Zinc selectively blocks neurosteroid-sensitive extrasynaptic δGABAA receptors in the Hippocampus. J. Neurosci. Official J. Soc. Neurosci. 36 (31), 8070–8077. doi:10.1523/JNEUROSCI.3393-15.2016
Chakrabarty, B., Dogra, A. S., Toteja, G. S., Pandey, R. M., Paul, V. K., and Gulati, S. (2022). Serum trace elements in children with well-controlled and drug refractory epilepsy compared to controls: An observational study. Neurol. India 70 (5), 1846–1851. doi:10.4103/0028-3886.359205
Chen, S., Chen, Y., Zhang, Y., Kuang, X., Liu, Y., Guo, M., et al. (2020). Iron metabolism and ferroptosis in epilepsy. Front. Neurosci. 14, 601193. doi:10.3389/fnins.2020.601193
Choi, D. W., and Koh, J. Y. (1998). Zinc and brain injury. Annu. Rev. Neurosci. 21, 347–375. doi:10.1146/annurev.neuro.21.1.347
Christodoulides, S. S., Neal, E. G., Fitzsimmons, G., Chaffe, H. M., Jeanes, Y. M., Aitkenhead, H., et al. (2012). The effect of the classical and medium chain triglyceride ketogenic diet on vitamin and mineral levels. J. Hum. Nutr. Dietetics Official J. Br. Dietetic Assoc. 25 (1), 16–26. doi:10.1111/j.1365-277X.2011.01172.x
Chwiej, J., Winiarski, W., Ciarach, M., Janeczko, K., Lankosz, M., Rickers, K., et al. (2008). The role of trace elements in the pathogenesis and progress of pilocarpine-induced epileptic seizures. J. Biol. Inorg. Chem. JBIC a Publ. Soc. Biol. Inorg. Chem. 13 (8), 1267–1274. doi:10.1007/s00775-008-0411-6
Da-Cunha, S., Filho, F. M. A., Antelo, D. S., and De-Souza, M. M. (2003). Serum sample levels of selenium and copper in healthy volunteers living in Rio de Janeiro city. Sci. Total Environ. 301 (1-3), 51–54. doi:10.1016/s0048-9697(02)00290-5
Das, A., Sarwar, M. S., Hossain, M. S., Karmakar, P., Islam, M. S., Hussain, M. E., et al. (2019). Elevated serum lipid peroxidation and reduced vitamin C and trace element concentrations are correlated with epilepsy. Clin. EEG Neurosci. 50 (1), 63–72. doi:10.1177/1550059418772755
Denoyer, D., Masaldan, S., La-Fontaine, S., and Cater, M. A. (2015). Targeting copper in cancer therapy: 'Copper that cancer. Metallomics Integr. Biometal Sci. 7 (11), 1459–1476. doi:10.1039/c5mt00149h
Dixit, A. B., Srivastava, A., Sharma, D., Tripathi, M., Paul, D., Lalwani, S., et al. (2020). Integrated genome-wide DNA methylation and RNAseq analysis of hippocampal specimens identifies potential candidate genes and aberrant signalling pathways in patients with hippocampal sclerosis. Neurol. India 68 (2), 307–313. doi:10.4103/0028-3886.280649
Dominiak, A., Wilkaniec, A., Wroczyński, P., and Adamczyk, A. (2016). Selenium in the therapy of neurological diseases. Where is it going? Curr. Neuropharmacol. 14 (3), 282–299. doi:10.2174/1570159x14666151223100011
Donaldson, J., St-Pierre, T., Minnich, J., and Barbeau, A. (1971). Seizures in rats associated with divalent cation inhibition of NA+ -K+ -ATP'ase. Can. J. Biochem. 49 (11), 1217–1224. doi:10.1139/o71-175
Dressler, A., Haiden, N., Trimmel-Schwahofer, P., Benninger, F., Samueli, S., Gröppel, G., et al. (2018). Ketogenic parenteral nutrition in 17 pediatric patients with epilepsy. Epilepsia Open 3 (1), 30–39. doi:10.1002/epi4.12084
Eastman, C. L., D'ambrosio, R., and Ganesh, T. (2020). Modulating neuroinflammation and oxidative stress to prevent epilepsy and improve outcomes after traumatic brain injury. Neuropharmacology 172, 107907. doi:10.1016/j.neuropharm.2019.107907
El-Rashidy, O. F., Youssef, M. M., Elgendy, Y. G., Mohsen, M. A., Morsy, S. M., Dawh, S. A., et al. (2020). Selenium and antioxidant levels in children with intractable epilepsy receiving ketogenic diet. Acta Neurol. Belg. 120 (2), 375–380. doi:10.1007/s13760-020-01310-9
Fisher, R. S., Acevedo, C., Arzimanoglou, A., Bogacz, A., Cross, J. H., Elger, C. E., et al. (2014). ILAE official report: A practical clinical definition of epilepsy. Epilepsia 55 (4), 475–482. doi:10.1111/epi.12550
Fisher, R. S., Van-Emde-Boas, W., Blume, W., Elger, C., Genton, P., Lee, P., et al. (2005). Epileptic seizures and epilepsy: Definitions proposed by the international league against epilepsy (ILAE) and the international bureau for epilepsy (IBE). Epilepsia 46 (4), 470–472. doi:10.1111/j.0013-9580.2005.66104.x
Freitas, R. M. (2009). Investigation of oxidative stress involvement in hippocampus in epilepsy model induced by pilocarpine. Neurosci. Lett. 462 (3), 225–229. doi:10.1016/j.neulet.2009.07.037
Frommelt, L., Bielohuby, M., Stoehr, B. J. M., Menhofer, D., Bidlingmaier, M., and Kienzle, E. (2014). Effects of low-carbohydrate, high-fat diets on apparent digestibility of minerals and trace elements in rats. Nutr. (Burbank, Los Angel. Cty. Calif.) 30 (7-8), 869–875. doi:10.1016/j.nut.2013.11.017
Fukahori, M., and Itoh, M. (1990). Effects of dietary zinc status on seizure susceptibility and hippocampal zinc content in the El (epilepsy) mouse. Brain Res. 529 (1-2), 16–22. doi:10.1016/0006-8993(90)90806-m
Garcia-Penas, J. J. (2018). Epilepsy, cognition and ketogenic diet. Rev. Neurol. 66 (01), S71–S75. doi:10.33588/rn.66S01.2017529
Garg, D., Kavitha, M., Kaushik, J. S., and Bala, K. (2021). Favorable response to immunotherapy in a child with hemi-convulsion hemiplegia epilepsy syndrome. Neurol. India 69 (5), 1449–1450. doi:10.4103/0028-3886.329569
Gonzalez-Reyes, R. E., Gutierrez-Alvarez, A. M., and Moreno, C. B. (2007). Manganese and epilepsy: A systematic review of the literature. Brain Res. Rev. 53 (2), 332–336. doi:10.1016/j.brainresrev.2006.10.002
Graf, W. D., Oleinik, O. E., Glauser, T. A., Maertens, P., Eder, D. N., and Pippenger, C. E. (1998). Altered antioxidant enzyme activities in children with a serious adverse experience related to valproic acid therapy. Neuropediatrics 29 (4), 195–201. doi:10.1055/s-2007-973560
Gündoğdu, A., Bolattürk, Ö. F., Aygül, R., and Akyürek, F. (2023). The relationship of fatigue and depression with trace element levels in epileptic patients. Biol. Trace Elem. Res. 201 (3), 1135–1142. doi:10.1007/s12011-022-03258-8
Hamed, S. A., Abdellah, M. M., and El-Melegy, N. (2004). Blood levels of trace elements, electrolytes, and oxidative stress/antioxidant systems in epileptic patients. J. Pharmacol. Sci. 96 (4), 465–473. doi:10.1254/jphs.fpj04032x
Hayashi, A., Kumada, T., Nozaki, F., Hiejima, I., Miyajima, T., and Fujii, T. (2013). Changes in serum levels of selenium, zinc and copper in patients on a ketogenic diet using Ketonformula. No Hattatsu Brain Dev. 45 (4), 288–293.
Horning, M. S., Blakemore, L. J., and Trombley, P. Q. (2000). Endogenous mechanisms of neuroprotection: Role of zinc, copper, and carnosine. Brain Res. 852 (1), 56–61. doi:10.1016/s0006-8993(99)02215-5
Ikeda, M. (2001). Iron overload without the C282Y mutation in patients with epilepsy. J. Neurology, Neurosurg. Psychiatry 70 (4), 551–553. doi:10.1136/jnnp.70.4.551
Ilhan, A., Uz, E., Kali, S., Var, A., and Akyol, O. (1999). Serum and hair trace element levels in patients with epilepsy and healthy subjects: Does the antiepileptic therapy affect the element concentrations of hair? Eur. J. Neurology 6 (6), 705–709. doi:10.1046/j.1468-1331.1999.t01-1-660705.x
Kaji, M., Ito, M., Okuno, T., Momoi, T., Sasaki, H., Yamanaka, C., et al. (1992). Serum copper and zinc levels in epileptic children with valproate treatment. Epilepsia 33 (3), 555–557. doi:10.1111/j.1528-1157.1992.tb01709.x
Kamate, M., Detroja, M., and Hattiholi, V. (2021). Acute leucoencephalopathy with restricted diffusion in children - a case series. Neurol. India 69 (2), 466–469. doi:10.4103/0028-3886.314577
Kürekçi, A. E., Alpay, F., Tanindi, S., Gökçay, E., Ozcan, O., Akin, R., et al. (1995). Plasma trace element, plasma glutathione peroxidase, and superoxide dismutase levels in epileptic children receiving antiepileptic drug therapy. Epilepsia 36 (6), 600–604. doi:10.1111/j.1528-1157.1995.tb02574.x
Kuzuya, T., Hasegawa, T., Shimizu, K., and Nabeshima, T. (1993). Effect of anti-epileptic drugs on serum zinc and copper concentrations in epileptic patients. Int. J. Clin. Pharmacol. Ther. Toxicol. 31 (2), 61–65.
Lakshmi, B. V. S., Sudhakar, M., and Prakash, K. S. (2015). Protective effect of selenium against aluminum chloride-induced alzheimer's disease: Behavioral and biochemical alterations in rats. Biol. Trace Elem. Res. 165 (1), 67–74. doi:10.1007/s12011-015-0229-3
Lima, P. A. D., Sampaio, L. P. D. B., and Damasceno, N. R. T. (2014). Neurobiochemical mechanisms of a ketogenic diet in refractory epilepsy. Clin. (Sao Paulo, Braz. 69 (10), 699–705. doi:10.6061/clinics/2014(10)09
Liu, C. S., Wu, H. M., Kao, S. H., and Wei, Y. H. (1997). Phenytoin-mediated oxidative stress in serum of female epileptics: A possible pathogenesis in the fetal hydantoin syndrome. Hum. Exp. Toxicol. 16 (3), 177–181. doi:10.1177/096032719701600308
Maalouf, M., Rho, J. M., and Mattson, M. P. (2009). The neuroprotective properties of calorie restriction, the ketogenic diet, and ketone bodies. Brain Res. Rev. 59 (2), 293–315. doi:10.1016/j.brainresrev.2008.09.002
Mittler, R. (2017). ROS are good. Trends Plant Sci. 22 (1), 11–19. doi:10.1016/j.tplants.2016.08.002
Mocchegiani, E., Malavolta, M., Muti, E., Costarelli, L., Cipriano, C., Piacenza, F., et al. (2008). Zinc, metallothioneins and longevity: Interrelationships with niacin and selenium. Curr. Pharm. Des. 14 (26), 2719–2732. doi:10.2174/138161208786264188
Mori, A., Yokoi, I., Noda, Y., and Willmore, L. J. (2004). Natural antioxidants may prevent posttraumatic epilepsy: A proposal based on experimental animal studies. Acta Medica Okayama 58 (3), 111–118. doi:10.18926/AMO/32111
Murthy, J. M. K., Jaiswal, S. K., Reddy, M. P., and Srikrishna, S. (2020). Incidence study of epilepsy using the ILAE 2017 classification of epilepsies in a cohort of school children accessing education in government primary schools in south India. Neurol. India 68 (6), 1389–1393. doi:10.4103/0028-3886.304099
Naziroğlu, M., Kutluhan, S., Uğuz, A. C., Celik, O., Bal, R., and Butterworth, P. J. (2009). Topiramate and vitamin e modulate the electroencephalographic records, brain microsomal and blood antioxidant redox system in pentylentetrazol-induced seizure of rats. J. Membr. Biol. 229 (3), 131–140. doi:10.1007/s00232-009-9177-1
Naziroğlu, M., Kutluhan, S., and Yilmaz, M. (2008). Selenium and topiramate modulates brain microsomal oxidative stress values, Ca2+-ATPase activity, and EEG records in pentylentetrazol-induced seizures in rats. J. Membr. Biol. 225 (1-3), 39–49. doi:10.1007/s00232-008-9132-6
Nazıroğlu, M., and Yürekli, V. A. (2013). Effects of antiepileptic drugs on antioxidant and oxidant molecular pathways: Focus on trace elements. Cell. Mol. Neurobiol. 33 (5), 589–599. doi:10.1007/s10571-013-9936-5
Nemati, H., Boroujeni, A. F., Inaloo, S., Katibeh, P., and Shahriari, M. (2021). The cerebrospinal fluid concentration of methyltetrahydrofolate and serum folate in children with developmental delay, regression, and/or refractory epilepsy. Neurol. India 69 (5), 1343–1348. doi:10.4103/0028-3886.329526
Pavlica, S., and Gebhardt, R. (2010). Comparison of uptake and neuroprotective potential of seven zinc-salts. Neurochem. Int. 56 (1), 84–93. doi:10.1016/j.neuint.2009.09.005
Płonka-Półtorak, E., Zagrodzki, P., Chłopicka, J., Bartoń, H., Westermarck, T., Kaipainen, P., et al. (2011). Valproic acid modulates superoxide dismutase, uric acid-independent FRAP and zinc in blood of adult epileptic patients. Biol. Trace Elem. Res. 143 (3), 1424–1434. doi:10.1007/s12011-011-9003-3
Płonka-Półtorak, E., Zagrodzki, P., Nicol, F., Kryczyk, J., Bartoń, H., Westermarck, T., et al. (2013). Antioxidant agents and physiological responses in adult epileptic patients treated with lamotrigine. Pharmacol. Rep. P. R. 65 (1), 99–106. doi:10.1016/s1734-1140(13)70968-x
Prasad, D. K. V., Shaheen, U., Satyanarayana, U., Surya-Prabha, T., Jyothy, A., and Munshi, A. (2014). Association of serum trace elements and minerals with genetic generalized epilepsy and idiopathic intractable epilepsy. Neurochem. Res. 39 (12), 2370–2376. doi:10.1007/s11064-014-1439-3
Puig, S., and Thiele, D. J. (2002). Molecular mechanisms of copper uptake and distribution. Curr. Opin. Chem. Biol. 6 (2), 171–180. doi:10.1016/s1367-5931(02)00298-3
Qiao, Q., Qu, Z., Tian, S., Cao, H., Zhang, Y., Sun, C., et al. (2022). Ketogenic diet alleviates hippocampal neurodegeneration possibly via ASIC1a and the mitochondria-mediated apoptotic pathway in a rat model of temporal lobe epilepsy. Neuropsychiatric Dis. Treat. 18, 2181–2198. doi:10.2147/NDT.S376979
Saad, K., El-Houfey, A. A., Abd El-Hamed, M. A., El-Asheer, O. M., Al-Atram, A. A., and Tawfeek, M. S. K. (2015). A randomized, double-blind, placebo-controlled clinical trial of the efficacy of treatment with zinc in children with intractable epilepsy. Funct. Neurol. 30 (3), 181–185. doi:10.11138/fneur/2015.30.3.181
Saad, K., Hammad, E., Hassan, A. F., and Badry, R. (2014). Trace element, oxidant, and antioxidant enzyme values in blood of children with refractory epilepsy. Int. J. Neurosci. 124 (3), 181–186. doi:10.3109/00207454.2013.831851
Saghazadeh, A., Mahmoudi, M., Meysamie, A., Gharedaghi, M., Zamponi, G. W., and Rezaei, N. (2015). Possible role of trace elements in epilepsy and febrile seizures: A meta-analysis. Nutr. Rev. 73 (11), 760–779. doi:10.1093/nutrit/nuv026
Sanabria, E. R., Su, H., and Yaari, Y. (2001). Initiation of network bursts by Ca2+-dependent intrinsic bursting in the rat pilocarpine model of temporal lobe epilepsy. J. Physiology 532 (1), 205–216. doi:10.1111/j.1469-7793.2001.0205g.x
Sanz, P., and Garcia-Gimeno, M. A. (2020). Reactive glia inflammatory signaling pathways and epilepsy. Int. J. Mol. Sci. 21 (11), 4096. doi:10.3390/ijms21114096
Schweizer, U., Bohleber, S., Zhao, W., and Fradejas-Villar, N. (2021). The neurobiology of selenium: Looking back and to the future. Front. Neurosci. 15, 652099. doi:10.3389/fnins.2021.652099
Seven, M., Basaran, S. Y., Cengiz, M., Unal, S., and Yuksel, A. (2013). Deficiency of selenium and zinc as a causative factor for idiopathic intractable epilepsy. Epilepsy Res. 104 (1-2), 35–39. doi:10.1016/j.eplepsyres.2012.09.013
Sharma, S. R., Sharma, N., Hussain, M., Mobing, H., and Hynniewta, Y. (2021). Levetiracetam use during pregnancy in women with active epilepsy: A hospital-based, retrospective study from a tertiary care hospital in north eastern India. Neurol. India 69 (3), 692–697. doi:10.4103/0028-3886.319234
Sözüer, D. T., Barutçu, U. B., Karakoç, Y., Yalçin, E., and Onen, S. (1995). The effects of antiepileptic drugs on serum zinc and copper levels in children. J. Basic Clin. Physiology Pharmacol. 6 (3-4), 265–269. doi:10.1515/jbcpp.1995.6.3-4.265
Steinbrenner, H., and Sies, H. (2013). Selenium homeostasis and antioxidant selenoproteins in brain: Implications for disorders in the central nervous system. Archives Biochem. Biophysics 536 (2), 152–157. doi:10.1016/j.abb.2013.02.021
Svetel, M., Dragašević, N., Petrović, I., Novaković, I., Tomić, A., Kresojević, N., et al. (2021). NBIA syndromes: A step forward from the previous knowledge. Neurol. India 69 (5), 1380–1388. doi:10.4103/0028-3886.329603
Takeda, A., Devenyi, A., and Connor, J. R. (1998). Evidence for non-transferrin-mediated uptake and release of iron and manganese in glial cell cultures from hypotransferrinemic mice. J. Neurosci. Res. 51 (4), 454–462. doi:10.1002/(SICI)1097-4547(19980215)51:4<454::AID-JNR5>3.0.CO;2-B
Takeda, A. (2004). Essential trace metals and brain function]. Yakugaku Zasshi J. Pharm. Soc. Jpn. 124 (9), 577–585. doi:10.1248/yakushi.124.577
Talat, M. A., Ahmed, A., and Mohammed, L. (2015). Serum levels of zinc and copper in epileptic children during long-term therapy with anticonvulsants. Neurosci. (Riyadh, Saudi Arabia) 20 (4), 341–345. doi:10.17712/nsj.2015.4.20150336
Tombini, M., Squitti, R., Cacciapaglia, F., Ventriglia, M., Assenza, G., Benvenga, A., et al. (2013). Inflammation and iron metabolism in adult patients with epilepsy: Does a link exist? Epilepsy Res. 107 (3), 244–252. doi:10.1016/j.eplepsyres.2013.09.010
Ułamek-Kozioł, M., Czuczwar, S. J., Januszewski, S., and Pluta, R. (2019). Ketogenic diet and epilepsy. Nutrients 11 (10), 2510. doi:10.3390/nu11102510
Ursini, F., and Maiorino, M. (2020). Lipid peroxidation and ferroptosis: the role of GSH and GPx4. Free Radic. Biol. Med. 152, 175–185. doi:10.1016/j.freeradbiomed.2020.02.027
Van-Loo, K. M. J., Schaub, C., Pitsch, J., Kulbida, R., Opitz, T., Ekstein, D., et al. (2015). Zinc regulates a key transcriptional pathway for epileptogenesis via metal-regulatory transcription factor 1. Nat. Commun. 6, 8688. doi:10.1038/ncomms9688
Verrotti, A., Basciani, F., Trotta, D., Pomilio, M. P., Morgese, G., and Chiarelli, F. (2002). Serum copper, zinc, selenium, glutathione peroxidase and superoxide dismutase levels in epileptic children before and after 1 year of sodium valproate and carbamazepine therapy. Epilepsy Res. 48 (1-2), 71–75. doi:10.1016/s0920-1211(01)00322-9
Wen, F., Tan, Z-G., and Xiang, J. (2022). Cu-Zn-SOD suppresses epilepsy in pilocarpine-treated rats and alters SCN2A/Nrf2/HO-1 expression. Epileptic Disord. Int. Epilepsy J. Videotape 24 (4), 647–656. doi:10.1684/epd.2022.1434
Wojciak, R. W., Mojs, E., Stanislawska-Kubiak, M., and Samborski, W. (2013). The serum zinc, copper, iron, and chromium concentrations in epileptic children. Epilepsy Res. 104 (1-2), 40–44. doi:10.1016/j.eplepsyres.2012.09.009
Xiao, Y., and Meierhofer, D. (2019). Glutathione metabolism in renal cell carcinoma progression and implications for therapies. Int. J. Mol. Sci. 20 (15), 3672. doi:10.3390/ijms20153672
Yaari, Y., Yue, C., and Su, H. (2007). Recruitment of apical dendritic T-type Ca2+ channels by backpropagating spikes underlies de novo intrinsic bursting in hippocampal epileptogenesis. J. Physiology 580 (2), 435–450. doi:10.1113/jphysiol.2007.127670
Yilmaz, Y., Tasdemir, H. A., and Paksu, M. S. (2009). The influence of valproic acid treatment on hair and serum zinc levels and serum biotinidase activity. Eur. J. Paediatr. Neurology EJPN Official J. Eur. Paediatr. Neurology Soc. 13 (5), 439–443. doi:10.1016/j.ejpn.2008.08.007
Yüksel, A., Cengiz, M., Seven, M., and Ulutin, T. (2000). Erythrocyte glutathione, glutathione peroxidase, superoxide dismutase and serum lipid peroxidation in epileptic children with valproate and carbamazepine monotherapy. J. Basic Clin. Physiology Pharmacol. 11 (1), 73–81. doi:10.1515/jbcpp.2000.11.1.73
Zhang, L., Zou, X., Zhang, B., Cui, L., Zhang, J., Mao, Y., et al. (2018). Label-free imaging of hemoglobin degradation and hemosiderin formation in brain tissues with femtosecond pump-probe microscopy. Theranostics 8 (15), 4129–4140. doi:10.7150/thno.26946
Keywords: epilepsy, trace element, animal model, treatment, research progress
Citation: Liu W, Xu J, Zhang L, Li F, Zhang L, Tai Z, Yang J, Zhang H, Tuo J, Yu C and Xu Z (2023) Research progress on correlations between trace element levels and epilepsy. Front. Cell Dev. Biol. 11:1167626. doi: 10.3389/fcell.2023.1167626
Received: 16 February 2023; Accepted: 25 July 2023;
Published: 09 August 2023.
Edited by:
Qibing Zeng, Guizhou Medical University, ChinaReviewed by:
Alberto Lazarowski, University of Buenos Aires, ArgentinaCopyright © 2023 Liu, Xu, Zhang, Li, Zhang, Tai, Yang, Zhang, Tuo, Yu and Xu. This is an open-access article distributed under the terms of the Creative Commons Attribution License (CC BY). The use, distribution or reproduction in other forums is permitted, provided the original author(s) and the copyright owner(s) are credited and that the original publication in this journal is cited, in accordance with accepted academic practice. No use, distribution or reproduction is permitted which does not comply with these terms.
*Correspondence: Jinmei Tuo, dGptdGptMTIyN0AxNjMuY29t; Changyin Yu, eXVjaGFuZ3lpbjY4MTJAMTI2LmNvbQ==; Zucai Xu, ZG9jeHpjQDEyNi5jb20=
Disclaimer: All claims expressed in this article are solely those of the authors and do not necessarily represent those of their affiliated organizations, or those of the publisher, the editors and the reviewers. Any product that may be evaluated in this article or claim that may be made by its manufacturer is not guaranteed or endorsed by the publisher.
Research integrity at Frontiers
Learn more about the work of our research integrity team to safeguard the quality of each article we publish.