- 1Department of Obstetrics, Gynecology and Women’s Health, University of Minnesota, Minneapolis, MN, United States
- 2Department of Integrative Biology and Physiology, University of Minnesota Medical School, Minneapolis, MN, United States
- 3Division of Molecular Medicine, Department of Medicine, University of Minnesota, Minneapolis, MN, United States
- 4Department of Biochemistry, Molecular Biology and Biophysics, University of Minnesota, Minneapolis, MN, United States
Metformin is a widely prescribed medication whose mechanism of action is not completely defined and whose role in gestational diabetes management remains controversial. In addition to increasing the risk of fetal growth abnormalities and preeclampsia, gestational diabetes is associated with abnormalities in placental development including impairments in trophoblast differentiation. Given that metformin impacts cellular differentiation events in other systems, we assessed metformin’s impact on trophoblast metabolism and differentiation. Using established cell culture models of trophoblast differentiation, oxygen consumption rates and relative metabolite abundance were determined following 200 µM (therapeutic range) and 2000 µM (supra-therapeutic range) metformin treatment using Seahorse and mass-spectrometry approaches. While no differences in oxygen consumption rates or relative metabolite abundance were detected between vehicle and 200 µM metformin-treated cells, 2000 µM metformin impaired oxidative metabolism and increased the abundance of lactate and TCA cycle intermediates, α-ketoglutarate, succinate, and malate. Examining differentiation, treatment with 2000 μM, but not 200 µM metformin, impaired HCG production and expression of multiple trophoblast differentiation markers. Overall, this work suggests that supra-therapeutic concentrations of metformin impair trophoblast metabolism and differentiation whereas metformin concentrations in the therapeutic range do not strongly impact these processes.
Introduction
Metformin (1,1-dimethylbiguanide) is a first-line treatment for patients with type 2 diabetes given its ability to improve glucose levels, support weight loss, and reduce cardiovascular morbidity (Maruthur et al., 2016). Until recently, metformin was also considered a first-line medication for gestational diabetes mellitus (GDM), improving glucose levels, maternal weight gain, and preeclampsia (Liang et al., 2017; ACOG Practice Bulletin No, 2018; D'Ambrosio et al., 2019; ElSayed et al., 2023). However, metformin is no longer considered a first-line medication for GDM since long-term follow-up has demonstrated increased weight, body mass index, and adiposity at mid-childhood following in utero metformin exposure (Tarry-Adkins et al., 2019). Despite these concerns, there continues to be interest in the use of metformin in pregnancy for potential preeclampsia prevention, reduced maternal weight gain in obese pregnancies, and lower miscarriage rate among pregnancies complicated by polycystic ovarian syndrome (Syngelaki et al., 2016; Løvvik et al., 2019; Adams et al., 2022). However, the mechanisms underlying these potential beneficial outcomes remain unknown.
During pregnancy, the placenta grows to support the health of the developing fetus (Turco and Moffett, 2019). A key cell facilitating nutrient transport to the developing fetus is the syncytiotrophoblast, a unique multi-nucleated cell that supports nutrient transport and gas exchange between maternal and fetal circulation. During the course of pregnancy, cytotrophoblasts divide and fuse to support the development and health of the syncytiotrophoblast (Costa, 2016; Renaud and Jeyarajah, 2022). Trophoblast differentiation is critical for placental development and fetal health. Delayed villous maturation, the most common histopathologic finding in GDM, is associated with poor birth outcomes (Higgins et al., 2011; Treacy et al., 2013; Huynh et al., 2015; Khong et al., 2016; Jaiman et al., 2020). A key finding in delayed villous maturation is an increase in the ratio of cytotrophoblasts to syncytiotrophoblasts, suggestive of potential defects in trophoblast differentiation (Khong et al., 2016). In one limited study, metformin treatment was found to normalize placental histology in pregnancies complicated by GDM (Arshad et al., 2016). However, it is unclear if this is due to improved blood glucose levels or the direct impact of metformin on placental development.
Despite its wide clinical use, metformin’s mechanisms of action are not fully understood, and metformin may have unique impacts within distinct tissues (Rena et al., 2017; Aye et al., 2022). Metformin inhibits complex I of the electron transport chain in a dose-dependent manner leading to changes in nucleotide energy charges: this decrease in ATP to ADP and ATP to AMP ratios leads to the activation of AMPK (LaMoia and Shulman, 2021). However, AMPK-independent mechanisms are also postulated to contribute to improved glycemic profiles (Rena et al., 2017). Impacts of metformin on complex I are demonstrated with supra-therapeutic concentrations, in the 1–5 mM range (LaMoia and Shulman, 2021). In contrast, following oral administration, metformin tissue levels are estimated to achieve levels of 50–100 µM (LaMoia and Shulman, 2021). Recent work suggests that maternal and placental metformin concentrations from GDM patients at the time of delivery may be even lower (Tarry-Adkins et al., 2022a). While the timing of metformin administration relative to blood collection is not clear in this study, maternal serum levels are reported in the 0–5 µM range and are linearly correlated to placental metformin levels. This is significantly lower than the 1–5 mM range thought to directly inhibit complex I, suggesting other mechanisms may underlie metformin’s impact in pregnancy (Aye et al., 2022).
Mitochondria regulate cellular differentiation in multiple systems, and metformin has been found to promote differentiation in some systems and inhibit it in others (Folmes and Terzic, 2016; Lisowski et al., 2018; Jiang and Liu, 2020; Chakrabarty and Chandel, 2021). Specifically, metformin promotes myogenic, neuronal, and osteogenic differentiation through impacts on AMPK (Wang et al., 2012; Dadwal et al., 2015; Senesi et al., 2016). Conversely, metformin inhibits differentiation of cancer stem cells in gastric cancer, osteosarcoma, and breast cancers (Cheung et al., 2019; Tan et al., 2019; Zhao et al., 2019). The impact of metformin on trophoblast differentiation has not been reported.
Given the ongoing interest in metformin for the clinical management of obstetric complications and its known impact on cellular differentiation in other systems, we hypothesized that metformin regulates trophoblast differentiation and cellular metabolism. In this work, we employ cell culture models to test the impact of metformin at therapeutic and supra-therapeutic concentrations on trophoblast metabolism and differentiation. While supra-therapeutic doses of metformin impair cellular metabolism and trophoblast differentiation, no significant impact was seen at therapeutic concentrations.
Materials and methods
Ethics: This study does not meet criteria for human subjects research, confirmed by the University of Minnesota Institutional Review Board (HRD # STUDY00014203).
Cell culture and reagents
BeWo cells were purchased from ATCC and maintained in culture with equal parts F12K (ATCC) (supplemented with 10% Fetal Bovine Serum (R&D Systems), Penicillin-Streptomycin (Gibco)) and DMEM Complete (4.5 g/L glucose, Gibco) (supplemented with Glutamine (Gibco), Sodium Pyruvate (Gibco), 10% Fetal Bovine Serum (Gibco), and Penicillin-Streptomycin (Gibco)). To induce syncytialization, BeWo cells were cultured on tissue-culture treated plates for 24 h. Forskolin (Millipore Sigma) (40 µM) was added for 48 h with DMSO (0.4%) (Sigma-Aldrich) as the vehicle control. Metformin (Cayman) at concentrations of 200 µM or 2000 µM was added for 72 h. PBS (Gibco) was used as a vehicle control for metformin. Media and treatments were replaced every 24 h during syncytialization.
Trophoblast Stem Cells (TSC) (CT29, Acquired from RIKEN Cell Bank) were maintained in a self-renewing state on iMatrix511 (Amsbio) coated plates in TS Complete media. TS Complete media is comprised of DMEM/F12 media (Gibco) containing 100 μg/mL primocin, 0.15% BSA, 1% ITS-X, 1% Knockout Serum Replacement and 0.2 mM ascorbic acid and supplemented with 2.5 µM Y27632, 25 ng/mL EGF, 0.8 mM Valproic Acid, 5 µM A83-01, and 2 µM CHIR99021 (Okae et al., 2018). Cells were cultured at 37°C, 5% CO2. On day 0, cells were plated on a 6-well plate coated with iMatrix511 in TS Complete media and metformin (Cayman) at concentrations of 200 µM or 2000 µM. PBS (Gibco) was used as a vehicle control. On day 1, to induce syncytialization, TS Complete media was replaced with ST Differentiation media containing DMEM/F12 with 100 μg/mL Primocin, 0.1% BSA, 1% ITS-X, 2.5 µM Y27632, 4% KSR, and 2 µM forskolin. Metformin at the respective concentrations was also added during the media change. Self-renewing cells were maintained in TS Complete media with metformin or PBS. On day 4 after plating, RNA was isolated for qPCR.
Protein expression
Cells were washed with ice-cold PBS and lysed with RIPA Buffer (Sigma) supplemented with Protease Inhibitors (Thermo Fisher Scientific), phosphatase inhibitor cocktail (Pierce), and 1 mM DTT (Sigma) in a 6-well plate. Lysate was then centrifuged for 10 min at 4°C at 21,000Xg. Pierce Bicinchoninic Acid Protein Assay Kit (Thermo Scientific) was used to determine protein concentration. 30 μg of protein per well was used to detect protein expression levels. Lysates were run on 4%–12% Bis-Tris gel (Invitrogen), transferred to PVDF, and blocked with 5% milk (Research Products International). Blots were stained overnight at 4°C with total AMPK (Cell Signaling Technology) and pAMPK Thr 172 (Cell Signaling Technology) antibodies in 2% bovine serum albumin (Gemini Bio). Blots were washed with Tris-Buffered Saline and stained with peroxidase-conjugated secondary antibodies (Jackson ImmunoResearch). Blots were developed using SuperSignal West Pico PLUS Chemiluminescent Substrate (Thermo). Total protein loading was assessed by BlotFastStain (G-Biosciences). Blots were imaged using the Bio-Rad ChemiDoc MP imaging system and band density was quantified using ImageJ.
Seahorse assay
30,000 BeWo cells were plated into wells of Cell-Tak-coated XFe96 plates containing 100 µL/well of equal parts F12K (ATCC) and DMEM (Gibco). Cells were plated in the presence of PBS, 200 µM metformin, or 2000 µM metformin, and treated with DMSO or 40 µM forskolin 24 h after plating. 72 h after plating, A Seahorse XF cell Mito stress test was performed to measure ECAR and OCR using the Seahorse Extracellular Flux (XFe96) Analyzer (Seahorse Bioscience Inc. North Billerica, MA). On the day of the assay, the media was switched to 180 µL of Seahorse XF assay media (DMEM) freshly supplemented with 16 mM glucose, 10 mM sodium pyruvate, and 2 mM glutamine (Agilent, Santa Clara, CA). The plate was incubated in a 37°C non-CO2 incubator for 1h prior to measurement. The plate was then transferred to the Seahorse XFe96 Analyzer (Seahorse Bioscience Inc. North Billerica, MA) for analysis. Once in the instrument, cells underwent measurement of basal oxygen consumption and extracellular acidification, followed by successive treatments with oligomycin A (2 µM), FCCP (carbonyl cyanide-ρ-trifluoromethoxyphenylhydrazone) (1 µM), and rotenone and antimycin A (0.5 µM). OCR and ECAR measurements were normalized per well to DNA, measured using the Quant-iT PicoGreen dsDNA Assay kit. For analysis, post-rotenone/antimycin A OCR readings were subtracted from the rest of OCR values to set baseline mitochondrial respiration. Key bioenergetic parameters (basal respiration, ATP-linked respiration, maximal respiration, spare capacity, and proton leak) from the Mito stress test were calculated according to the manufacturer’s protocol (Figure 2B).
Metabolomics
250,000 BeWo cells were plated on 6-well plates, treated with vehicle or metformin, and 24 h after plating, treated with DMSO or 40 µM forskolin as above. A mirror plate was made to facilitate the normalization of metabolite abundance to total protein. 72 h after plating, samples were collected by washing twice with ice-cold PBS, once in ice-cold water, and snap-freezing in liquid nitrogen prior to transferring cells in methanol to a fresh tube. The solvent was evaporated and samples were stored at −80°C until ready for metabolite extraction and analysis.
Relative abundance of glycolytic and TCA cycle intermediates
To determine the relative abundance of selected TCA cycle and glycolytic metabolites, metabolites were extracted as previously described (Ivanisevic et al., 2013; Puchalska et al., 2018; Puchalska et al., 2019), with modifications. Briefly, 1,000 µL of 2:2:1 Acetonitrile (AcN):Water (H20):MeOH (v:v:v) was added to each sample, which underwent three rounds of vortexing, sonication, and snap freezing in liquid nitrogen. The samples were incubated at −20°C for 1–4 h, spun to remove proteins, transferred to fresh tubes, evaporated, and reconstituted in 40 μL of 1:1 AcN:H2O. All analyses were performed on a Thermo Vanquish liquid chromatograph with Thermo Q-Exactive Plus mass spectrometer equipped with a heated ESI (HESI) source.
Samples were analyzed using negative mode on an Atlantis Premier BEH Z-HILIC Column (2.1 mm × 100 mm, 1,7 μm). Separation was completed using gradients of Mobile phase A (15 mM ammonium bicarbonate in water, pH 9.0) and Mobile Phase B (15 mM ammonium bicarbonate pH, 9.0 with 90% AcN). Binary gradients included 10% Mobile Phase A for 5 min, 35% Mobile Phase A for 2 min, and 10% Mobile Phase A for 3 min. Separations were performed at a flow rate of 0.5–1 mL/min and column temperature at 30°C with an injection volume of 2 μL. The mass spectrometer was operated in negative mode using full scan (FS) mode (m/z 68–1,020) with optimized HESI source conditions: auxiliary gas 10, sweep gas 1, sheath gas flow at 30 (arbitrary unit), spray voltage −4 kV, capillary temperature 350°C, S-lens RF 50, and auxiliary gas temperature 350°C. The automatic gain control (AGC) target was set at 3e6 ions with a resolution of 70,000.
Xcalibur’s QuanBrowser from Thermo was used for peak identification and integration. Metabolite profiling data was analyzed using verified peaks and retention times. Metabolite peak identity was confirmed based on retention time, m/z, and compared to authentic standards as described previously (Puchalska et al., 2018; Puchalska et al., 2019). The integrated signal for each metabolite was normalized to total protein determined using Bicinchoninic Acid Assay (BCA) on mirror plates for each condition.
HCG production
After treatment, media was collected and samples were tested for alpha-HCG using ELISA assay (DRG International). DMSO-treated samples were diluted (1:2) with PBS and Forskolin-treated samples were diluted (1:100). HCG expression was detected using the Gen5 program via the Synergy HTX Multimode Reader (BioTek).
Analysis of mRNA expression
Cells were washed with ice-cold PBS. Total RNA was extracted from cells using the Quick RNA Mini-Prep Extraction Kit (Zymo Research). Equal amounts of RNA were used to synthesize cDNA (BioRad). For quantitative real-time PCR, reactions were carried out using SsoAdvanced Universal SYBR Green Supermix (BioRad) on a CFX384 Real-Time System (Bio-Rad). Transcripts were quantified using the 2−ΔΔCT method. and normalized to cyclophilin. See Key Resources Table 1 for specific primers.
Imaging
50,000 BeWo cells were plated on poly-L-lysine pre-treated glass coverslips along with media containing PBS vehicle or 200 µM or 2000 µM metformin. 24 and 48 h after plating, DMSO or 40 µM forskolin was applied. 72 h after plating coverslips were fixed with 4% paraformaldehyde (Fisher Scientific) for 10 min and then permeablized using 0.1% TritonX for 10 min. After washing, coverslips were blocked for 1 h with 5% goat serum in PBS. Coverslips were stained overnight at 4°C with HCG (Abcam) and eCadherin (BD Biosciences) antibodies. The following day, coverslips were washed three times, then incubated at room temperature with mouse (Jackson ImmunoResearch) and rabbit (Jackson ImmunoResearch) secondary antibodies. Coverslips were again washed and mounted on glass slides using mounting media with Prolong Glass Antifade Mountant with NucBlue nuclear Stain (Invitrogen). Images were acquired on an Olympus FluoView BX2 Upright Confocal microscope at the University of Minnesota Imaging Core. Fusion index was quantified as the number of nuclei in syncytia divided by the total number of nuclei using Fiji.
Quantification and statistical analysis
Statistical outliers were excluded following identification by the Grubbs test. ANOVA was used to compare the means of more than two groups. p values less than 0.05 were considered statistically significant. Data were analyzed using GraphPad Prism.
Results
Metformin treatment increases phospho-AMPK signaling in BeWo cells
The BeWo cell line is an established model system where forskolin, an adenylate cyclase activator upstream of protein kinase A, induces both biochemical and morphologic syncytialization (Orendi et al., 2011; Rothbauer et al., 2017). These differentiation parameters are detected through the production of human chorionic gonadotrophin (HCG) and coordinated changes in gene transcription along with the fusion of cell membranes resulting in morphologic syncytia (Costa, 2016; Renaud and Jeyarajah, 2022).
Using the BeWo model system, we first validated metformin activity within undifferentiated cells by assessing AMP-activated protein kinase (AMPK) activity. Metformin increases AMPK activity through phosphorylation of Thr172 in the activation loop of the kinase domain (Musi and Goodyear, 2002). Following treatment of BeWo cells with vehicle (PBS), 200 µM metformin, or 2000 µM metformin for 72 h, phosphorylation of AMP-activated protein kinase (pAMPK at Thr172) relative to total AMPK was detected by Western blotting (Figures 1A, B). Upon treatment with 200 µM metformin, a trending but statistically insignificant increase in pAMPK at Thr172 was observed. However, 2000 µM metformin, increased pAMPK at Thr172 compared to total AMPK. This overall suggests that metformin enters BeWo cells and increases AMPK activity at the 2000 µM concentration.
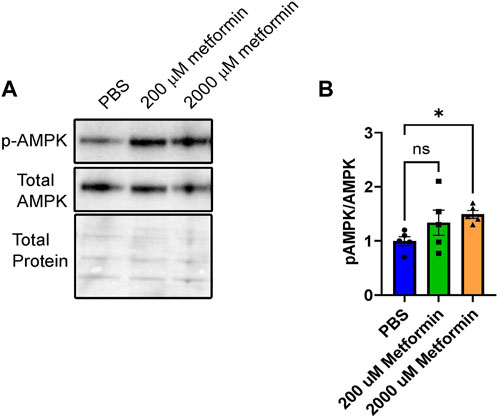
FIGURE 1. Metformin treatment increases pAMPK signaling in BeWo cells. (A) Representative Western blot of HCG, pAMPK, and Total AMPK in BeWo cells treated with two different concentrations of Metformin (200 µM or 2000 µM) compared to vehicle (PBS). (B) Quantification of Western blots (n = 5) demonstrating the relative expression of pAMPK to total AMPK in BeWo cells treated with two different concentrations of Metformin (200 µM or 2000 µM) compared to vehicle. Data are representative of mean ± SEM. *, p < 0.05.
Metformin’s impact on oxidative and glycolytic metabolism
Prior work demonstrates that cytotrophoblasts and syncytiotrophoblasts differ in their glycolytic and oxidative metabolism (Kolahi et al., 2017; Bucher et al., 2021). Given this, we tested the impact of metformin on undifferentiated and differentiated trophoblast metabolism. BeWo cells were plated in the presence of vehicle (PBS), 200 µM or 2000 µM metformin. 48 h after inducing differentiation with forskolin, a Seahorse Mitochondrial Stress Assay was performed to measure how metformin impacts the oxygen consumption rate (OCR) following the sequential addition of inhibitors as depicted in Figures 2A, B. Forskolin treatment decreases basal respiration, ATP-linked respiration, maximum respiration, and spare capacity compared to DMSO-treated control cells (Figures 2C–F). However, no differentiation-dependent change in proton leak or non-mitochondrial respiration was detected. Differentiation in the presence of 200 µM metformin similarly decreased forskolin-dependent basal respiration, ATP-linked respiration, maximum respiration, and spare capacity with no differences detected between vehicle and 200 µM metformin OCR. In contrast, differentiation in the presence of 2000 µM metformin profoundly decreased basal, ATP-linked, maximal, and non-mitochondrial respiration compared to vehicle treatment. Overall, this demonstrates that 2000 µM metformin, not 200 µM metformin, has a broad impact on oxidative metabolism (Figure 2A, C-H).
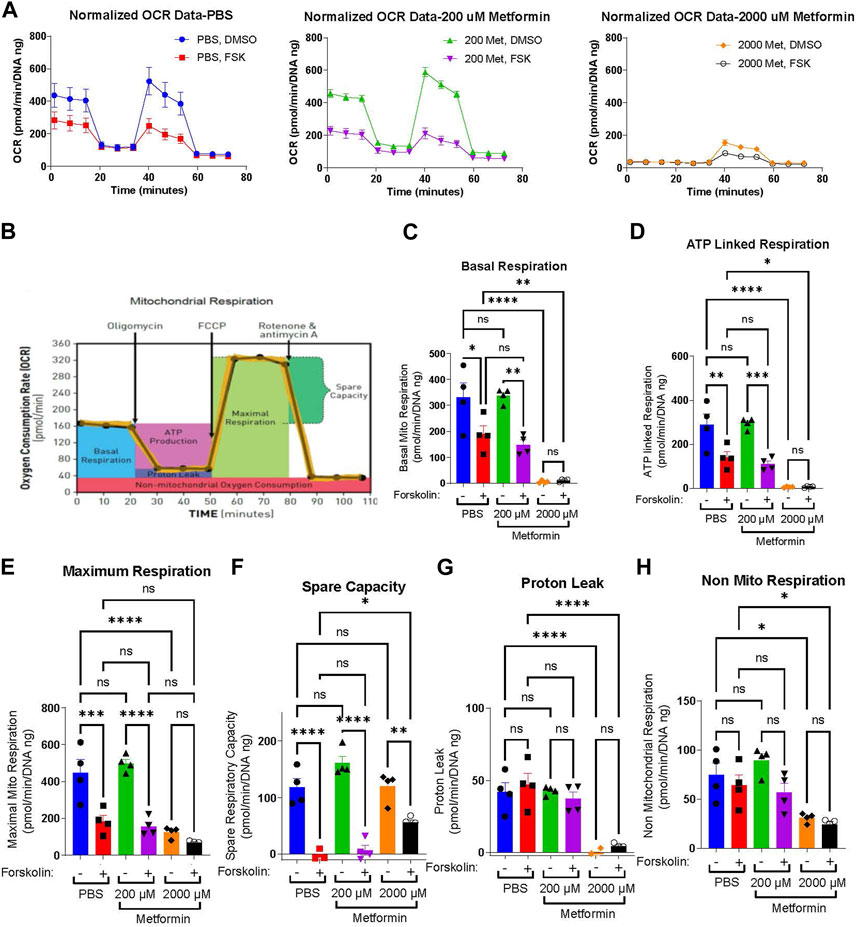
FIGURE 2. Metformin impairs the oxygen consumption rate at high concentrations (A) Normalized Oxygen Consumption Rate (pmol/min/DNA ng) during mitochondrial flux assay for BeWo cells treated with vehicle, 200 µM metformin, or 2000 µM metformin in the presence of DMSO (0.4%, vehicle) or 40 µM forsksolin (FSK) for 48 h. (B) Schematic depicting the impact of sequential inhibitor additions to oxygen consumption rate during Seahorse Mitochondria Stress Test and bio-energetic parameters calculated from the assay. (C–H) Bar graphs representing (C) Basal Mitochondrial Respiration, (D) ATP Linked Respiration, (E) Maximal Respiration, (F) Spare Capacity, (G) Proton Leak and (H) non-mitochondrial respiration (all in (pmol/min/DNA ng)) for vehicle, 200 μM and 2000 µM metformin treated BeWo cells. n = 4 biologic replicates. Data are representative of mean ± SEM. *, p < 0.05; **, p < 0.01; ***, p < 0.001; and ****, p < 0.0001.
Extracellular acidification rate (ECAR) serves as a surrogate measure of glycolysis. Given the profound impacts of 2000 µM metformin on oxidative metabolism, we determined the impact of metformin on basal ECAR. Forskolin treatment slightly increased basal ECAR rates in PBS and 200 µM metformin-treated cells, though no statistically significant differences were detected between groups (Supplemental Figure S1 A, B, D). Following treatment with 2000 µM metformin, ECAR increased significantly in DMSO-treated cells compared to vehicle (Supplemental Figures S1C, D). This is consistent with higher metformin concentrations impairing oxidative phosphorylation and increasing glycolytic rate.
Metformin’s impact on the relative abundance of glycolytic and TCA cycle intermediates
Given the broad differences observed in the OCR with differentiation, we next determined how therapeutic and supra-therapeutic concentrations of metformin impact the relative abundance of cellular metabolites during differentiation. Using a high-resolution liquid chromatography, mass-spectrometry (LC-MS) approach, we determined relative metabolite abundance following treatment of BeWo cells with DMSO or forskolin in the presence of PBS, 200 µM or 2000 µM metformin. Following differentiation, ATP increased in PBS and 200 µM metformin-treated cells (Figure 3A). Surprisingly, 2000 µM metformin maintained ATP levels despite the profound impairments found in oxidative phosphorylation (Figure 2), though no differentiation-dependent increase was found. (Figure 3A). This suggests that upon treatment with 2000 µM metformin, increased glycolytic activity may preserve overall ATP abundance.
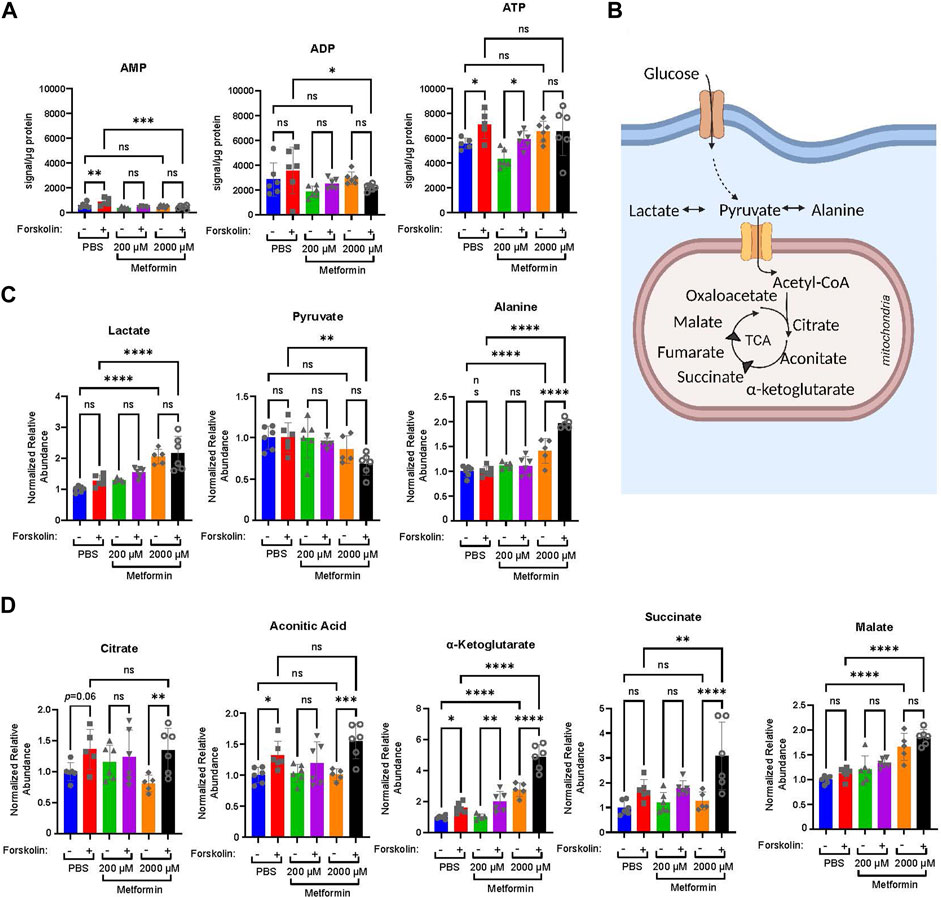
FIGURE 3. Metformin impacts the relative abundance of high energy and TCA cycle metabolites. Forty-eight hours following treatment of BeWo cells with DMSO or Forskolin in the presence of vehicle, Metformin 200 μM, or Metformin 2000 μM, high resolution mass-spectrometry was used to determine the relative abundance of metabolites: (A) Normalized abundance of AMP, ADP, and ATP. Data presented are total metabolite signal normalized to µg protein for each condition. n = 6 biologic replicates. (B) Schematic demonstrating glucose metabolism to pyruvate, lactate, alanine, and TCA cycle metabolites. (C) Normalized relative abundance of lactate, pyruvate, and alanine. Normalized relative abundance is total metabolite signal divided by µg protein for each condition and normalized to DMSO-treated vehicle control. n = 6 biologic replicates. (D) Normalized relative abundance of citrate, aconitate, α-ketoglutarate, succinate, and malate. Data presented are total metabolite signal divided by µg protein for each condition and normalized to DMSO-treated vehicle control. Data are representative of mean ± SEM. n = 6 biologic replicates. *, p < 0.05; **, p < 0.01; ***, p < 0.001; and ****, p < 0.0001.
We next examined the impact of metformin on lactate, pyruvate, alanine, and select TCA cycle intermediates (Figures 3B–D). After glucose enters a cell, it undergoes glycolysis resulting in pyruvate which may be metabolized to lactate, alanine, or enter the TCA cycle. Treatment with 2000 µM metformin increased lactate and alanine abundance compared to PBS and 200 µM metformin treatments (Figure 3C). This is consistent with the observed increase in glycolytic metabolism following 2000 µM metformin treatment.
Differentiation in PBS-treated cells increased citrate, aconitic acid, α-ketoglutarate, and succinate (Figure 3D). This trend persisted in 200 µM treated cells, though a differentiation-dependent increase was only statistically significant for α-ketoglutarate (Figure 3D). In comparison, differentiation following treatment with 2000 µM metformin significantly increased citrate, aconitic acid, α-ketoglutarate, and succinate (Figure 3D). This differentiation-dependent increase in α-ketoglutarate, succinate, and malate with 2000 µM metformin treatment exceeds that observed following vehicle treatment. This suggests that inhibition of oxidative phosphorylation by supra-therapeutic concentrations of metformin may result in the accumulation of TCA intermediate metabolites due to impairments in oxidative metabolism.
Impact of metformin on trophoblast differentiation
Mitochondria regulate cellular differentiation events across multiple systems (Folmes and Terzic, 2016; Lisowski et al., 2018; Chakrabarty and Chandel, 2021). To test the impact of metformin on trophoblast differentiation, we plated BeWo cells in the presence of vehicle, 200 µM or 2000 µM metformin and treated these cells 24 h later with vehicle (DMSO) or forskolin to induce syncytialization. We first assessed the impact of metformin on differentiation by assessing HCG production in media samples. HCG is a peptide hormone made by syncytiotrophoblasts (Fournier et al., 2015). We found significantly lower levels of HCG in media samples obtained from cells treated with 2000 µM metformin compared to those in the vehicle control and 200 µM metformin groups (Figure 4A), suggestive of differentiation defects with supra-therapeutic metformin treatment.
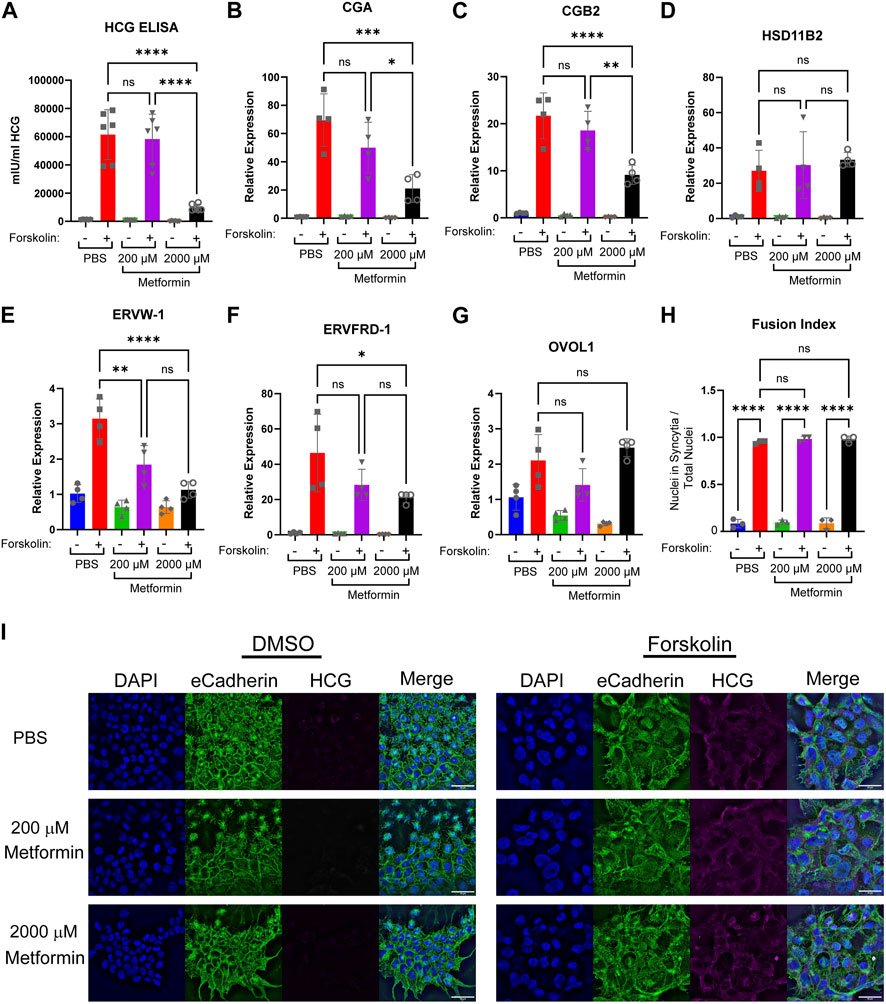
FIGURE 4. Increasing concentrations of metformin impair biochemical syncytialization. Forty-eight hours following treatment of BeWo cells with DMSO (0.4%) or 40 µM forskolin (FSK) in the presence of vehicle, Metformin 200 μM, or Metformin 2000 μM, we assessed: (A) Production of HCG quantified by ELISA. n = 6 biologic replicates. (B–G) Relative gene expression by qPCR: (B) CGA, (C) CGB2 (D) HSD11B2 (E) EFVW-1 (F) ERVFRD-1 (G) OVOL1. n = 4 biological replicates. Data are representative of mean ± SEM. (I) Representative images of nuclear (DAPI, blue), cell membrane (E-cadherin, green), and HCG (magenta) stained BeWo cells treated for 48 h with DMSO or forskolin (FSK) in the presence of vehicle, Metformin 200 μM, or Metformin 2000 µM. Scale bar represents 50 micron. (H) Quantification of fusion index (number of nuclei in syncytia divided by total number of nuclei). n = 6 biologic replicates and 3 fields of view from each replicate. Data are representative of the mean ± SEM. *, p < 0.05; **, p < 0.01; ***, p < 0.001; and ****, p < 0.0001.
Trophoblast differentiation requires upregulation of genes necessary to support transcription, synthetic, and fusogenic machinery during trophoblast differentiation (Costa, 2016; Renaud and Jeyarajah, 2022). To test the impact of metformin on this gene reprogramming, we treated BeWo cells with metformin and induced differentiation. In PBS-treated cells, forskolin increased the expression of CGA and CGB2 (involved in HCG production), HSD11B2 (regulator of glucocorticoid metabolism), ERVW-1 and ERVFRD-1 (fusogenic proteins), and OVOL1 (transcription factor) as expected (Figures 4B–G). Differentiation in the presence of 200 µM metformin similarly increased expression of CGA, CGB2, HSD11B2, ERVFRD-1, and OVOL1 (Figure 4C, D, F, G). However, ERVW-1 expression upon forskolin differentiation was lower (Figure 4E) in the presence of 200 µM of metformin in comparison to PBS. Treatment with 2000 µM metformin impaired forskolin-induced upregulation of CGA, CGB2, ERVW-1, and ERVFRD-1 in comparison to PBS (Figures 4B, C, E, F). However, no difference in OVOL1 or HSD11B2 expression was detected following 2000 µM metformin treatment compared to vehicle (Figures 4D, G). This suggests that 2000 µM metformin may selectively impair transcriptional reprogramming of BeWo cells during differentiation.
To assess morphologic syncytialization, BeWo cells were plated on glass coverslips in the presence of vehicle, 200 or 2000 µM metformin prior to inducing syncytialization. 48 h following differentiation, coverslips were stained with antibodies to HCG, which increases upon differentiation, and E-cadherin, which decreases upon differentiation as membrane fusion occurs (Figure 4I). The degree of fusion was quantified through the fusion index which identifies the number of nuclei found within syncytia divided by the total number of nuclei. We found robust induction of syncytialization upon treatment with forskolin with >90% of nuclei found in syncytia in control cells. Treatment of BeWo cells with 200 and 2000 µM metformin resulted in similar degrees of syncytialization, with no statistically significant differences noted in the fusion index (Figure 4H). Combined with gene expression data, this suggests that supra-therapeutic metformin treatment may de-couple biochemical and morphologic differentiation, which has been previously described (Cheng, 2004; Orendi et al., 2010; Leduc et al., 2012; Omata et al., 2013).
Given that differentiation regulates the expression of nutrient transporters (Karahoda et al., 2022), we assessed changes in nutrient transporter expression in the presence of metformin. This may reflect a mechanism by which metformin could impact fetal growth. SNAT1 (SLC38A1) and SNAT2 (SLC38A2) (Figures 5A, B) are involved in the transport of neutral amino acids, and no difference in expression was detected following treatment with 200 or 2000 µM metformin. LAT1 (SLC7A5) and LAT2 (SLC7A8) (Figure 5 C, D) are involved in the transport of L-amino acids, and following treatment with vehicle, 200 or 2000 µM metformin, a similar forskolin-dependent increase in expression was observed in all investigated groups. Finally, we assessed the expression of FATP6 (SLC27A6) (Figure 5E), which is involved in the transport of fatty acids, and observed increased expression following forskolin treatment in cells treated with 2000 µM metformin compared to vehicle control. This suggests that high doses of metformin may selectively impact nutrient transporter expression.
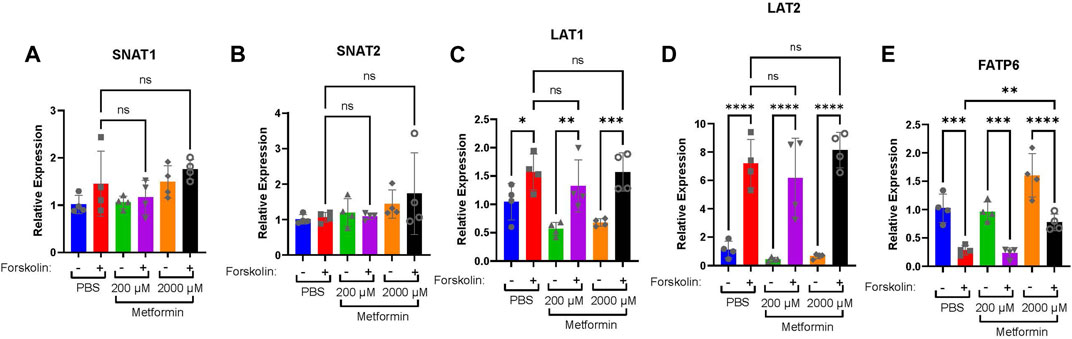
FIGURE 5. High doses of metformin may selectively impact nutrient transporter expression. (A) SNAT1, (B) SNAT2, (C) LAT1, (D) LAT2, and (E) FATP6 gene expression by qPCR in BeWo cells treated with DMSO or Forskolin and 200 µM or 2000 µM metformin compared to vehicle. n = 4 biological replicates. Data are representative of mean ± SEM. *, p < 0.05; **, p < 0.01; ***, p < 0.001; and ****, p < 0.0001.
Impact of metformin on the trophoblast stem cell model of differentiation
While Bewo cells are a widely accepted model of syncytialization, we sought to test the impact of metformin on trophoblast differentiation in another established model system. In the trophoblast stem cell model (Okae et al., 2018), cytotrophoblasts isolated from first-trimester placenta are maintained in culture in the presence of WNT and EGF activators and TFG-B, histone deacetylase, and ROCK inhibitors. Syncytialization is induced by removing these WNT, EGF, and HDAC modulators and adding a low dose of forskolin.
In the trophoblast stem cell model, 2000 µM metformin resulted in cell death in both self-renewing (SR) and differentiation (ST) conditions. However, we did not see evidence of cell death at the 200 µM metformin concentration. Comparing the impact of 200 µM metformin to the vehicle, we did not detect any difference in HCG production by ELISA (Figure 6A). No expression differences were observed in CGA, CGB2, HSD11B2, ERVW-1, ERVFRD-1, or TEAD4 following treatment with 200 µM metformin compared to vehicle (Figure 6B). This again suggests that therapeutic concentrations of metformin do not dysregulate trophoblast differentiation.
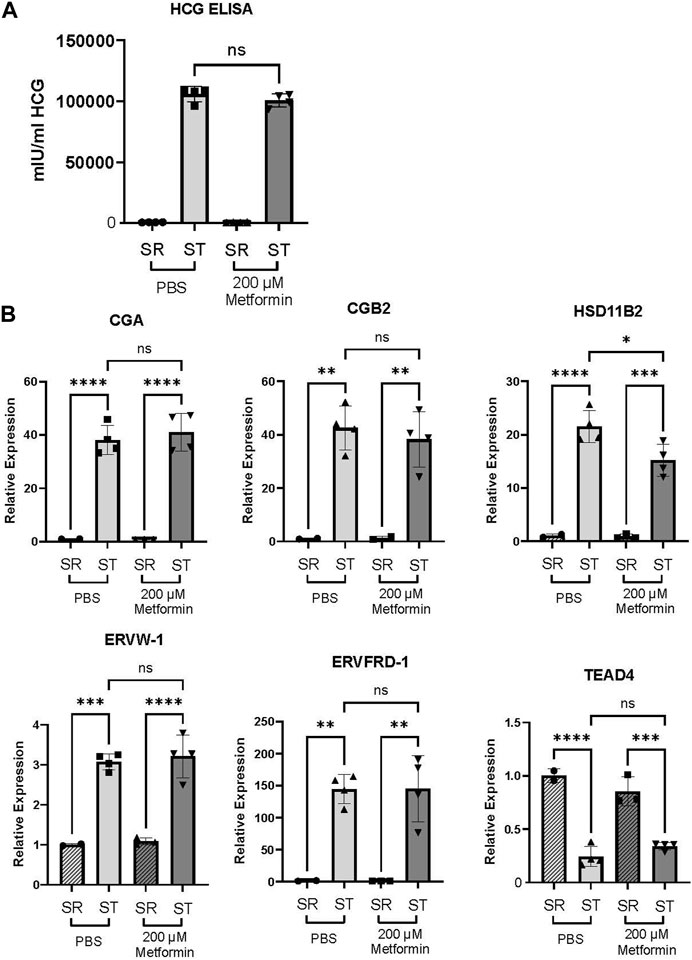
FIGURE 6. Metformin does not impair trophoblast stem cell differentiation. (A) Production of HCG quantified by ELISA in self-renewing (SR) cells or syncytiotrophoblast (ST) Trophoblast stem cells treated with vehicle (PBS) or 200 µM metformin. n = 6 biologic replicates. (B) CGA, CGB, HSD11B2, ERVW, ERVFRD, and TEAD4 gene expression by qPCR in SR or ST Trophoblast stem cells. n = 4 biological replicates. Data are representative of mean ± SEM. *, p < 0.05; **, p < 0.01; ***, p < 0.001; and ****, p < 0.0001.
Discussion
In this work, we evaluated the impact of metformin on trophoblast metabolism and differentiation using established cell culture models of trophoblast differentiation. We found that millimolar doses of metformin inhibited oxidative phosphorylation, resulting in increased glycolysis, lactate, and TCA metabolites. Similar metabolic changes did not occur with 200 µM metformin treatment, consistent with prior reports that therapeutic concentrations of metformin may not function through impairment of Complex I activity. While treatment with 2000 µM of metformin impaired gene expression and HCG production following differentiation, 200 µM of metformin did not result in similar impairments (See Model in Supplemental Figure S2). This overall suggests that therapeutic concentrations of metformin do not impair trophoblast differentiation.
One surprising observation is that supra-physiologic metformin impairs HCG production and broadly impacts the expression of multiple genes involved in trophoblast differentiation: however, we do not detect differences in morphologic syncytialization. Prior studies have identified distinct regulators of biochemical and morphologic syncytialization, including transcription factors and differential activators of protein kinase A, protein kinase C, and mitogen-activated protein kinases (MAPK) pathways (Cheng, 2004; Orendi et al., 2010; Leduc et al., 2012; Omata et al., 2013). We speculate that supra-physiologic concentrations of metformin may impact the expression or activation of specific transcription factors or signaling pathways that distinctly regulate biochemical and morphologic differentiation. Future work will be directed toward delineating this distinction.
Despite its widespread clinical use, metformin’s mechanism of action is not completely defined (LaMoia and Shulman, 2021; Aye et al., 2022). However, millimolar dosages are consistently associated with inhibition of Complex I, which results in the activation of AMPK and its downstream pathways. Recent work examining the impact of 10 and 100 µM metformin on primary trophoblasts demonstrates statistically significant differences in basal respiration, maximal respiration, and ATP production in primary trophoblasts treated with vehicle or 100 µM metformin (Tarry-Adkins et al., 2022a). While this is a different cellular model system, the absolute magnitude of respiration differences remain small and are not normalized to account for variations in cell number. In our assay, we do not detect differences in cellular respiration at the 200 µM concentration, though profound impacts are seen at 2000 µM concentrations. This aligns more closely with recent work demonstrating that metformin does not impact basal respiration, maximal respiration, or ATP-linked respiration at less than 1 mM in healthy syncytiotrophoblasts (Hebert and Myatt, 2023). Additionally, while we find broad changes in the relative metabolite abundance with 2000 µM metformin treatments, we do not see similar changes between vehicle and 200 µM metformin treatments. Overall, this suggests that the potential benefits of metformin in pregnancy may not be attributable to large changes in trophoblast metabolism.
Prior studies have investigated metformin’s impact on osteogenic, neuronal, myogenic, and adipogenic differentiation with varying results (Reviewed in (Jiang and Liu, 2020)). These reports highlight variability in metformin’s impact on differentiation across multiple systems. This may be due to differences in metformin treatments or reflect inherent differences in differentiation across different cell populations. Given that GDM is associated with potential impairments in trophoblast differentiation, we examined metformin’s impact on trophoblast differentiation. While supra-therapeutic levels of metformin impair trophoblast biochemical differentiation in our BeWo cell model, we do not detect impairments in differentiation at therapeutic levels of metformin in either the BeWo or trophoblast stem cell models of differentiation. This suggests that metformin may not be directly contributing to differentiation defects seen in GDM.
The mechanisms underlying 2000 µM metformin’s impact on trophoblast differentiation are not known. Prior reports have implicated AMPK activation as a regulator of trophoblast differentiation with shRNA knock-down of AMPK impairing trophoblast differentiation in the SM10 Trophoblast cell line models (Carey et al., 2014; Waker et al., 2017). It may be that higher doses of metformin modulate AMPK activity and impair differentiation in our system. Mitochondrial metabolism contributes to the epigenetic regulation of gene expression (Dai et al., 2020), and impairments in mitochondrial function at high doses of metformin may dysregulate epigenetic reprogramming during trophoblast differentiation. Human placental explants treated with 7 mM metformin demonstrated increased levels of Histone 3 Lysine 27 acetylation (Jiang et al., 2020). This highlights the potential role for supra-therapeutic concentrations of metformin to epigenetically regulate trophoblast differentiation.
For this work, we primarily relied on the BeWo model of cellular differentiation which may not fully recapitulate all aspects of trophoblast metabolism and differentiation. While this model has been extensively used to study trophoblast differentiation and exhibits many gene and hormone expression changes similar to primary trophoblasts, it may not fully represent all aspects of trophoblast differentiation (Rothbauer et al., 2017; Azar et al., 2018; Pastuschek et al., 2021). For instance, BeWo cells were originally isolated from a choriocarcinoma of male lineage and are aneuploid, which may impact gene regulation and cellular metabolism (Pattillo and Gey, 1968; Weber et al., 2021). Given this limitation, we evaluated the effect of two different concentrations of metformin using a trophoblast stem cell model of differentiation derived from first trimester placenta (Okae et al., 2018). Although supra-therapeutic metformin resulted in cell death, therapeutic concentrations of metformin did not impair trophoblast differentiation, again supporting the observation that metformin is unlikely to impair trophoblast differentiation into syncytiotrophoblasts at therapeutic concentrations. Future work will assess the impact of physiologic concentrations of metformin on primary trophoblast differentiation isolated from term placenta.
Overall, this work suggests that therapeutic concentrations of metformin are not likely to strongly impact trophoblast metabolism or differentiation. Additionally, it highlights the dose-dependent impacts of metformin on cellular metabolism and differentiation events. The potential beneficial impacts of metformin seen clinically in GDM are likely to occur independent of inhibition of complex I and trophoblast differentiation into syncytiotrophoblasts.
Data availability statement
The raw data supporting the conclusion of this article will be made available by the authors, without undue reservation.
Author contributions
SN and SW conceived of the study, designed and completed experiments, analyzed data, prepared figures, and wrote the manuscript. RM performed experiments, analyzed data, and prepared figures. SJ and DS performed experiments. AN, PC, and PP assisted with mass spectrometry analyses and interpretation. SJ and EA performed Seahorse experiments, analyzed, and interpreted data. MG assisted with TSC experiments and data interpretation. All authors reviewed the manuscript. All authors contributed to the article and approved the submitted version.
Funding
SW is supported by the Reproductive Scientist Development Program (RSDP) by the Eunice Kennedy Shriver National Institute of Child Health and Human Development (K12 HD000849) and the American Board of Obstetrics and Gynecology. MG is supported by 5R21HD102770. EA is supported by R56DK131447. PC is supported by R01DK091538 and R01AG069781.
Conflict of interest
PC has served as an external consultant for Pfizer, Inc., Abbott Laboratories, Janssen Research & Development, and Juvenesence.
The remaining authors declare that the research was conducted in the absence of any commercial or financial relationships that could be construed as a potential conflict of interest.
Publisher’s note
All claims expressed in this article are solely those of the authors and do not necessarily represent those of their affiliated organizations, or those of the publisher, the editors and the reviewers. Any product that may be evaluated in this article, or claim that may be made by its manufacturer, is not guaranteed or endorsed by the publisher.
Supplementary material
The Supplementary Material for this article can be found online at: https://www.frontiersin.org/articles/10.3389/fcell.2023.1167097/full#supplementary-material
References
Acog Practice Bulletin No, (2018). ACOG Practice Bulletin No. 190: Gestational diabetes mellitus. Obstet. Gynecol. 131, e49–e64. doi:10.1097/AOG.0000000000002501
Adams, J. H., Poehlmann, J., Racine, J. L., Iruretagoyena, J. I., Eddy, A., Hoppe, K. K., et al. (2022). Weight gain in pregnancy: Can metformin steady the scales? J. Matern. Fetal Neonatal Med. 35, 9504–9510. doi:10.1080/14767058.2022.2044775
Arshad, R., Kanpurwala, M. A., Karim, N., and Hassan, J. A. (2016). Effects of diet and metformin on placental morphology in gestational diabetes mellitus. Pak J. Med. Sci. 32, 1522–1527. doi:10.12669/pjms.326.10872
Aye, I., Aiken, C. E., Charnock-Jones, D. S., and Smith, G. C. S. (2022). Placental energy metabolism in health and disease-significance of development and implications for preeclampsia. Am. J. Obstet. Gynecol. 226, S928–S944. doi:10.1016/j.ajog.2020.11.005
Azar, C., Valentine, M., Trausch-Azar, J., Druley, T., Nelson, D. M., and Schwartz, A. L. (2018). RNA-Seq identifies genes whose proteins are transformative in the differentiation of cytotrophoblast to syncytiotrophoblast, in human primary villous and BeWo trophoblasts. Sci. Rep. 8, 5142. doi:10.1038/s41598-018-23379-2
Bucher, M., Kadam, L., Ahuna, K., and Myatt, L. (2021). Differences in glycolysis and mitochondrial respiration between cytotrophoblast and syncytiotrophoblast in-vitro: Evidence for sexual dimorphism. Int. J. Mol. Sci. 22, 10875. doi:10.3390/ijms221910875
Carey, E. A., Albers, R. E., Doliboa, S. R., Hughes, M., Wyatt, C. N., Natale, D. R., et al. (2014). AMPK knockdown in placental trophoblast cells results in altered morphology and function. Stem Cells Dev. 23, 2921–2930. doi:10.1089/scd.2014.0092
Chakrabarty, R. P., and Chandel, N. S. (2021). Mitochondria as signaling organelles control mammalian stem cell fate. Cell Stem Cell 28, 394–408. doi:10.1016/j.stem.2021.02.011
Cheng, Y. A., Hossain, S., Trapnell, B., Kong, S., and Handwerger, S. (2004). Critical role for transcription factor AP-2alpha in human trophoblast differentiation. Physiol. Genomics 18, 99–107. doi:10.1152/physiolgenomics.00181.2003
Cheung, K. S., Chan, E. W., Wong, A. Y. S., Chen, L., Seto, W. K., Wong, I. C. K., et al. (2019). Metformin use and gastric cancer risk in diabetic patients after Helicobacter pylori eradication. J. Natl. Cancer Inst. 111, 484–489. doi:10.1093/jnci/djy144
Costa, M. A. (2016). Scrutinising the regulators of syncytialization and their expression in pregnancy-related conditions. Mol. Cell Endocrinol. 420, 180–193. doi:10.1016/j.mce.2015.11.010
D'ambrosio, V., Brunelli, R., Vena, F., Di Mascio, D., Marchetti, C., Boccherini, C., et al. (2019). Metformin reduces maternal weight gain in obese pregnant women: A systematic review and meta-analysis of two randomized controlled trials. Diabetes Metab. Res. Rev. 35, e3164. doi:10.1002/dmrr.3164
Dadwal, P., Mahmud, N., Sinai, L., Azimi, A., Fatt, M., Wondisford, F. E., et al. (2015). Activating endogenous neural precursor cells using metformin leads to neural repair and functional recovery in a model of childhood brain injury. Stem Cell Rep. 5, 166–173. doi:10.1016/j.stemcr.2015.06.011
Dai, Z., Ramesh, V., and Locasale, J. W. (2020). The evolving metabolic landscape of chromatin biology and epigenetics. Nat. Rev. Genet. 21, 737–753. doi:10.1038/s41576-020-0270-8
Elsayed, N. A., Aleppo, G., Aroda, V. R., Bannuru, R. R., Brown, F. M., Bruemmer, D., et al. (2023). 9. Pharmacologic approaches to glycemic treatment: Standards of care in diabetes-2023. Diabetes Care 46, S140–S157. doi:10.2337/dc23-S009
Folmes, C. D., and Terzic, A. (2016). Energy metabolism in the acquisition and maintenance of stemness. Semin. Cell Dev. Biol. 52, 68–75. doi:10.1016/j.semcdb.2016.02.010
Fournier, T., Guibourdenche, J., and Evain-Brion, D. (2015). Review: hCGs: Different sources of production, different glycoforms and functions. Placenta 36 (1), S60–S65. doi:10.1016/j.placenta.2015.02.002
Hebert, J. F., and Myatt, L. (2023). Metformin impacts human syncytiotrophoblast mitochondrial function from pregnancies complicated by obesity and gestational diabetes mellitus in a sexually dimorphic manner. Antioxidants (Basel) 12.
Higgins, L., Greenwood, S. L., Wareing, M., Sibley, C. P., and Mills, T. A. (2011). Obesity and the placenta: A consideration of nutrient exchange mechanisms in relation to aberrant fetal growth. Placenta 32, 1–7. doi:10.1016/j.placenta.2010.09.019
Huynh, J., Yamada, J., Beauharnais, C., Wenger, J. B., Thadhani, R. I., Wexler, D., et al. (2015). Type 1, type 2 and gestational diabetes mellitus differentially impact placental pathologic characteristics of uteroplacental malperfusion. Placenta 36, 1161–1166. doi:10.1016/j.placenta.2015.08.004
Ivanisevic, J., Zhu, Z. J., Plate, L., Tautenhahn, R., Chen, S., O'brien, P. J., et al. (2013). Toward 'omic scale metabolite profiling: A dual separation-mass spectrometry approach for coverage of lipid and central carbon metabolism. Anal. Chem. 85, 6876–6884. doi:10.1021/ac401140h
Jaiman, S., Romero, R., Pacora, P., Jung, E., Bhatti, G., Yeo, L., et al. (2020). Disorders of placental villous maturation in fetal death. J. Perinat. Med. 1. doi:10.1515/jpm-2020-0030
Jiang, L. L., and Liu, L. (2020). Effect of metformin on stem cells: Molecular mechanism and clinical prospect. World J. Stem Cells 12, 1455–1473. doi:10.4252/wjsc.v12.i12.1455
Jiang, S., Teague, A. M., Tryggestad, J. B., Jensen, M. E., and Chernausek, S. D. (2020). Role of metformin in epigenetic regulation of placental mitochondrial biogenesis in maternal diabetes. Sci. Rep. 10, 8314. doi:10.1038/s41598-020-65415-0
Karahoda, R., Zaugg, J., Fuenzalida, B., Kallol, S., Moser-Haessig, R., Staud, F., et al. (2022). Trophoblast differentiation affects crucial nutritive functions of placental membrane transporters. Front. Cell Dev. Biol. 10, 820286. doi:10.3389/fcell.2022.820286
Khong, T. Y., Mooney, E. E., Ariel, I., Balmus, N. C., Boyd, T. K., Brundler, M. A., et al. (2016). Sampling and definitions of placental lesions: Amsterdam placental workshop group consensus statement. Arch. Pathol. Lab. Med. 140, 698–713. doi:10.5858/arpa.2015-0225-CC
Kolahi, K. S., Valent, A. M., and Thornburg, K. L. (2017). Cytotrophoblast, not syncytiotrophoblast, dominates glycolysis and oxidative phosphorylation in human term placenta. Sci. Rep. 7, 42941. doi:10.1038/srep42941
Lager, S., and Powell, T. L. (2012). Regulation of nutrient transport across the placenta. J. Pregnancy 2012, 179827. doi:10.1155/2012/179827
Lamoia, T. E., and Shulman, G. I. (2021). Cellular and molecular mechanisms of metformin action. Endocr. Rev. 42, 77–96. doi:10.1210/endrev/bnaa023
Leduc, K., Bourassa, V., Asselin, E., Leclerc, P., Lafond, J., and Reyes-Moreno, C. (2012). Leukemia inhibitory factor regulates differentiation of trophoblastlike BeWo cells through the activation of JAK/STAT and MAPK3/1 MAP kinase-signaling pathways. Biol. Reprod. 86, 54. doi:10.1095/biolreprod.111.094334
Liang, H. L., Ma, S. J., Xiao, Y. N., and Tan, H. Z. (2017). Comparative efficacy and safety of oral antidiabetic drugs and insulin in treating gestational diabetes mellitus: An updated PRISMA-compliant network meta-analysis. Med. Baltim. 96, e7939. doi:10.1097/MD.0000000000007939
Lisowski, P., Kannan, P., Mlody, B., and Prigione, A. (2018). Mitochondria and the dynamic control of stem cell homeostasis. EMBO Rep. 19, e45432. doi:10.15252/embr.201745432
Løvvik, T. S., Carlsen, S. M., Salvesen, Ø., Steffensen, B., Bixo, M., Gómez-Real, F., et al. (2019). Use of metformin to treat pregnant women with polycystic ovary syndrome (PregMet2): A randomised, double-blind, placebo-controlled trial. Lancet Diabetes Endocrinol. 7, 256–266. doi:10.1016/S2213-8587(19)30002-6
Maruthur, N. M., Tseng, E., Hutfless, S., Wilson, L. M., Suarez-Cuervo, C., Berger, Z., et al. (2016). Diabetes medications as monotherapy or metformin-based combination therapy for type 2 diabetes: A systematic review and meta-analysis. Ann. Intern Med. 164, 740–751. doi:10.7326/M15-2650
Musi, N., and Goodyear, L. J. (2002). Targeting the AMP-activated protein kinase for the treatment of type 2 diabetes. Curr. Drug Targets Immune Endocr. Metabol. Disord. 2, 119–127. doi:10.2174/1568005310202020119
Okae, H., Toh, H., Sato, T., Hiura, H., Takahashi, S., Shirane, K., et al. (2018). Derivation of human trophoblast stem cells. Cell Stem Cell 22, 50–63. doi:10.1016/j.stem.2017.11.004
Omata, W., Ackerman, W. E. T., Vandre, D. D., and Robinson, J. M. (2013). Trophoblast cell fusion and differentiation are mediated by both the protein kinase C and a pathways. PLoS One 8, e81003. doi:10.1371/journal.pone.0081003
Orendi, K., Gauster, M., Moser, G., Meiri, H., and Huppertz, B. (2010). The choriocarcinoma cell line BeWo: Syncytial fusion and expression of syncytium-specific proteins. Reproduction 140, 759–766. doi:10.1530/REP-10-0221
Orendi, K., Kivity, V., Sammar, M., Grimpel, Y., Gonen, R., Meiri, H., et al. (2011). Placental and trophoblastic in vitro models to study preventive and therapeutic agents for preeclampsia. Placenta 32, S49–S54. doi:10.1016/j.placenta.2010.11.023
Pastuschek, J., Nonn, O., Gutierrez-Samudio, R. N., Murrieta-Coxca, J. M., Muller, J., Sanft, J., et al. (2021). Molecular characteristics of established trophoblast-derived cell lines. Placenta 108, 122–133. doi:10.1016/j.placenta.2021.02.022
Pattillo, R. A., and Gey, G. O. (1968). The establishment of a cell line of human hormone-synthesizing trophoblastic cells in vitro. Cancer Res. 28, 1231–1236.
Puchalska, P., Huang, X., Martin, S. E., Han, X., Patti, G. J., and Crawford, P. A. (2018). Isotope tracing untargeted metabolomics reveals macrophage polarization-state-specific metabolic coordination across intracellular compartments. iScience 9, 298–313. doi:10.1016/j.isci.2018.10.029
Puchalska, P., Martin, S. E., Huang, X., Lengfeld, J. E., Daniel, B., Graham, M. J., et al. (2019). Hepatocyte-macrophage acetoacetate shuttle protects against tissue fibrosis. Cell Metab. 29, 383–398. doi:10.1016/j.cmet.2018.10.015
Rena, G., Hardie, D. G., and Pearson, E. R. (2017). The mechanisms of action of metformin. Diabetologia 60, 1577–1585. doi:10.1007/s00125-017-4342-z
Renaud, S. J., and Jeyarajah, M. J. (2022). How trophoblasts fuse: An in-depth look into placental syncytiotrophoblast formation. Cell Mol. Life Sci. 79, 433. doi:10.1007/s00018-022-04475-z
Rothbauer, M., Patel, N., Gondola, H., Siwetz, M., Huppertz, B., and Ertl, P. (2017). A comparative study of five physiological key parameters between four different human trophoblast-derived cell lines. Sci. Rep. 7, 5892. doi:10.1038/s41598-017-06364-z
Senesi, P., Montesano, A., Luzi, L., Codella, R., Benedini, S., and Terruzzi, I. (2016). Metformin treatment prevents sedentariness related damages in mice. J. Diabetes Res. 2016, 8274689. doi:10.1155/2016/8274689
Syngelaki, A., Nicolaides, K. H., Balani, J., Hyer, S., Akolekar, R., Kotecha, R., et al. (2016). Metformin versus placebo in obese pregnant women without diabetes mellitus. N. Engl. J. Med. 374, 434–443. doi:10.1056/NEJMoa1509819
Tan, W., Tang, H., Jiang, X., Ye, F., Huang, L., Shi, D., et al. (2019). Metformin mediates induction of miR-708 to inhibit self-renewal and chemoresistance of breast cancer stem cells through targeting CD47. J. Cell Mol. Med. 23, 5994–6004. doi:10.1111/jcmm.14462
Tarry-Adkins, J. L., Aiken, C. E., and Ozanne, S. E. (2019). Neonatal, infant, and childhood growth following metformin versus insulin treatment for gestational diabetes: A systematic review and meta-analysis. PLoS Med. 16, e1002848. doi:10.1371/journal.pmed.1002848
Tarry-Adkins, J. L., Robinson, I. G., Reynolds, R. M., Aye, I., Charnock-Jones, D. S., Jenkins, B., et al. (2022a). Impact of metformin treatment on human placental energy production and oxidative stress. Front. Cell Dev. Biol. 10, 935403. doi:10.3389/fcell.2022.935403
Treacy, A., Higgins, M., Kearney, J. M., Mcauliffe, F., and Mooney, E. E. (2013). Delayed villous maturation of the placenta: Quantitative assessment in different cohorts. Pediatr. Dev. Pathol. 16, 63–66. doi:10.2350/12-06-1218-OA.1
Turco, M. Y., and Moffett, A. (2019). Development of the human placenta. Development 146. doi:10.1242/dev.163428
Waker, C. A., Albers, R. E., Pye, R. L., Doliboa, S. R., Wyatt, C. N., Brown, T. L., et al. (2017). AMPK knockdown in placental labyrinthine progenitor cells results in restriction of critical energy resources and terminal differentiation failure. Stem Cells Dev. 26, 808–817. doi:10.1089/scd.2016.0252
Wang, J., Gallagher, D., Devito, L. M., Cancino, G. I., Tsui, D., He, L., et al. (2012). Metformin activates an atypical PKC-CBP pathway to promote neurogenesis and enhance spatial memory formation. Cell Stem Cell 11, 23–35. doi:10.1016/j.stem.2012.03.016
Weber, M., Weise, A., Vasheghani, F., Gohner, C., Fitzgerald, J. S., Liehr, T., et al. (2021). Cytogenomics of six human trophoblastic cell lines. Placenta 103, 72–75. doi:10.1016/j.placenta.2020.10.011
Keywords: metformin, metabolism, trophoblast stem cell, BeWo, cytotrophoblast, syncytiotrophoblast, differentiation, mitochondria
Citation: Nashif SK, Mahr RM, Jena S, Jo S, Nelson AB, Sadowski D, Crawford PA, Puchalska P, Alejandro EU, Gearhart MD and Wernimont SA (2023) Metformin impairs trophoblast metabolism and differentiation in a dose-dependent manner. Front. Cell Dev. Biol. 11:1167097. doi: 10.3389/fcell.2023.1167097
Received: 15 February 2023; Accepted: 05 May 2023;
Published: 12 May 2023.
Edited by:
Ryan J. Mailloux, McGill University, CanadaReviewed by:
Estela Bevilacqua, University of São Paulo, BrazilSumedha Bagga, Boston University, United States
Copyright © 2023 Nashif, Mahr, Jena, Jo, Nelson, Sadowski, Crawford, Puchalska, Alejandro, Gearhart and Wernimont. This is an open-access article distributed under the terms of the Creative Commons Attribution License (CC BY). The use, distribution or reproduction in other forums is permitted, provided the original author(s) and the copyright owner(s) are credited and that the original publication in this journal is cited, in accordance with accepted academic practice. No use, distribution or reproduction is permitted which does not comply with these terms.
*Correspondence: Sarah A. Wernimont, c3dlcm5pbW9AdW1uLmVkdQ==