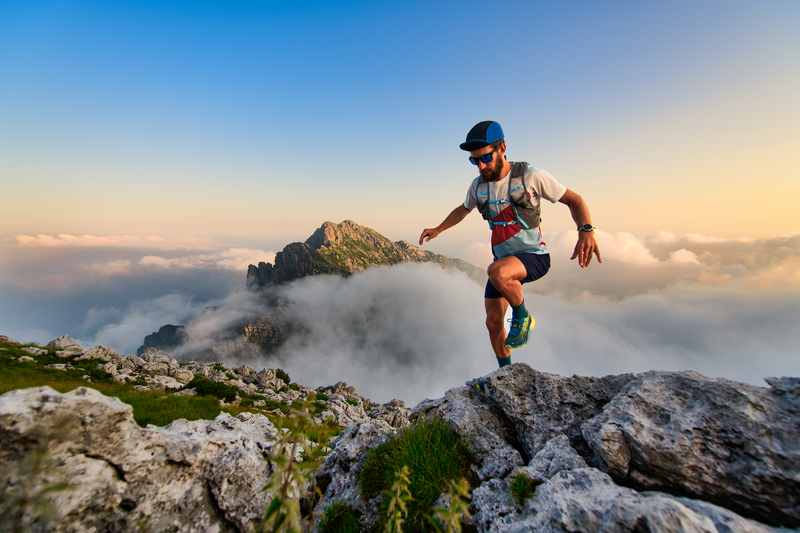
95% of researchers rate our articles as excellent or good
Learn more about the work of our research integrity team to safeguard the quality of each article we publish.
Find out more
REVIEW article
Front. Cell Dev. Biol. , 15 May 2023
Sec. Molecular and Cellular Reproduction
Volume 11 - 2023 | https://doi.org/10.3389/fcell.2023.1166232
This article is part of the Research Topic Editors' Showcase 2022: Insights in Molecular and Cellular Reproduction View all 15 articles
Sperm are terminally differentiated cells that lack most of the membranous organelles, resulting in a high abundance of ether glycerolipids found across different species. Ether lipids include plasmalogens, platelet activating factor, GPI-anchors and seminolipid. These lipids play important roles in sperm function and performance, and thus are of special interest as potential fertility markers and therapeutic targets. In the present article, we first review the existing knowledge on the relevance of the different types of ether lipids for sperm production, maturation and function. To further understand ether-lipid metabolism in sperm, we then query available proteomic data from highly purified sperm, and produce a map of metabolic steps retained in these cells. Our analysis pinpoints the presence of a truncated ether lipid biosynthetic pathway that would be competent for the production of precursors through the initial peroxisomal core steps, but devoid of subsequent microsomal enzymes responsible for the final synthesis of all complex ether-lipids. Despite the widely accepted notion that sperm lack peroxisomes, the thorough analysis of published data conducted herein identifies nearly 70% of all known peroxisomal resident proteins as part of the sperm proteome. In view of this, we highlight open questions related to lipid metabolism and possible peroxisomal functions in sperm. We propose a repurposed role for the truncated peroxisomal ether-lipid pathway in detoxification of products from oxidative stress, which is known to critically influence sperm function. The likely presence of a peroxisomal-derived remnant compartment that could act as a sink for toxic fatty alcohols and fatty aldehydes generated by mitochondrial activity is discussed. With this perspective, our review provides a comprehensive metabolic map associated with ether-lipids and peroxisomal-related functions in sperm and offers new insights into potentially relevant antioxidant mechanisms that warrant further research.
Sperm are terminally differentiated haploid cells with a unique structure necessary for the different stages of fertilization and early embryonic development. To achieve fertilization, sperm leaving the testis must first undergo a series of physiological changes in the epididymis and the female tract, known as maturation and capacitation, respectively (Yanagimachi, 1994). As a consequence of these processes, sperm become able to undergo the acrosome reaction, an exocytotic event that takes place in their head, and to develop a specific flagellar movement termed hyperactivation. These physiological changes enable sperm to cross the cumulus oophorus that surrounds the egg, then bind to and penetrate the zona pellucida, and finally fuse with the egg’s plasma membrane (Figure 1). In this regard, it is important to mention that, since sperm are transcriptionally and translationally silent cells, signal transduction cascades and metabolic pathways, among others, are essential for the regulation of the changes conducive to fertilization. Moreover, sperm function is critically influenced by reactive oxygen species (ROS) mainly derived from aerobic metabolism in the mitochondria. Small quantities of ROS are needed for normal sperm function, stimulating both sperm capacitation and fertilization reviewed by O’Flaherty and Scarlata (2022). However, high levels of ROS induce an oxidative stress state that has a detrimental effect on sperm motility, capacitation, acrosome reaction and DNA integrity, leading to male infertility reviewed by Aitken et al., (2022). As terminally differentiated cells, sperm are particularly vulnerable to ROS attack. Since the late 70’s, evidence has been accumulating showing the critical role of lipid peroxidation in both combating and propagating this damage (Jones and Mann, 1976; Aitken et al., 1989; Gomez et al., 1998; Aitken and Baker, 2006). Therefore, a delicate balance between ROS production and antioxidant mechanisms should exist in normal sperm to ensure sperm survival for successful fertilization.
FIGURE 1. Schematic representation of the different steps involved in sperm production, maturation, and fertilization. The described roles for the different types of ether lipids in these steps are included in the table. Abbreviations: AR, acrosomal reaction; PLs, phospholipids; PAF, platelet activating factor; SGG, sulfogalactosylglycerolipid; GPI, glycosylphosphatidylinositol.
As a result of several studies focusing on the evaluation of sperm lipid composition, increased attention has been given to the relationship between sperm lipid composition and fertility (Rivera-Egea et al., 2018; Lopalco et al., 2019). Thus, lipids are of special interest as potential fertility markers and therapeutic targets not only because of their structural functions in sperm, but also because they are sensitive to external and environmental signals, many of which can be recreated in vitro. Ether lipids are a particularly highly abundant subclass of glycerophospholipids found in sperm of different species, such as humans, boars, stallions, bulls, and lions (Parks and Lynch, 1992; Leßig et al., 2004; Oresti et al., 2011; Jakop et al., 2022), suggesting they play a critical role in sperm physiology. In other cell types, ether lipids are key components of cell membranes, providing unique structural attributes with effects on membrane dynamics, including membrane fluidity and membrane fusion, facilitating signaling processes, regulating cell differentiation, and protecting membranes from oxidation by acting as potential endogenous antioxidants reviewed by Dean and Lodhi, (2018), Jiménez-Rojo and Riezman, (2019). Structurally, ether-phospholipids are characterized by the presence of an ether bond at the sn-1 position of the glycerol backbone (Figure 2). Lipids with distinctive ether linkages may be categorized into a variety of subspecies that include: a) plasmanyl and plasmenyl (plasmalogens) glycerophospholipids that compose approximately 20% of the mammalian total phospholipid pool (Dean and Lodhi, 2018), b) platelet-activating factor (PAF) that mediates inflammatory responses (Kelesidis et al., 2015), c) glycosylphosphatidylinositol (GPI) anchors, a lipid anchor for many cell-surface proteins added via posttranslational modifications (Kinoshita, 2016), and d) sulfogalactosylglycerolipid (SGG), also known as seminolipid, which is a testis-specific sulfoglycolipid essential for germ cell function in spermatogenesis (Zhang et al., 2005) (Figure 2). It is not clear when and where this array of ether lipids present in sperm is synthesized. The enzymes responsible for the initial steps in the synthesis of ether lipids are localized to the peroxisome where the alkyl precursor, 1-alkyl-glycerol-3-phosphate (1-alkyl-G3P), is made, and then is transported to the endoplasmic reticulum (ER) and Golgi, where it is consumed to produce complex ether lipids. It is currently accepted that sperm are devoid of these organelles, as these are lost with the residual body and cytoplasmic droplet during spermiogenesis and epididymal maturation, along with other membranous organelles such as lysosomes (Aveldano et al., 1992; Lüers et al., 2006), precluding the idea that they can synthesize ether lipids for themselves. As such, the source of ether lipids in sperm may be linked to early stages of the spermatogenesis or to acquisition during epididymal transit, particularly since the incorporation of exogenous phospholipids is possible through extracellular vesicles such as epididymosomes (Sullivan and Saez, 2013). Intriguingly, reports on sperm proteomes from different species have consistently identified peroxisomal resident proteins, including the enzymes involved in the production of ether lipids (Chauvin et al., 2012; Amaral et al., 2013; 2014; Castillo et al., 2018; Martín-Cano et al., 2020; Greither et al., 2023). Therefore, sperm seem to preserve selected peroxisomal pathways relevant for lipid metabolism. Here, we first summarize existing knowledge on the biological significance of ether lipid species plasmanyl and plasmenyl glycerophospholipids, PAF, SGG and GPI, particularly regarding sperm maturation and fertilization and their impact on male fertility and sperm performance. Using published data from highly purified human and mice sperm, we then embark in a thorough analysis of the sperm proteome identifying peroxisomal resident proteins and ether-lipid metabolic enzymes. This analysis unveils the presence of a truncated biosynthetic pathway where peroxisomal steps capable of producing ether lipid precursors are present, while late ER/Golgi steps are missing. Lastly, we discuss open questions related to lipid metabolism and peroxisomal functions in sperm, which require further research to be answered.
FIGURE 2. Chemical structures of ether and diacyl glycerophospholipids and their precursors. The acyl precursor of diacyl phospholipids, 1-acyl-G3P (also known as lysophosphatidic acid) is shown for comparison. This precursor is mainly made in the endoplasmic reticulum. Blue box points to the ester bond at the sn-1 position of the glycerol backbone (A). The precursor of all ether lipids is 1-alkyl-glycerol-3-phosphate (1-alkyl-G3P) which is synthesized in peroxisomes. Red box points to ether linkage at the sn-1 position of the glycerol backbone (B). Structures of representative ether lipid species discussed in this review are shown with the ether linkage boxed in red (C). Note that in the case of plasmenyl glycerophospholipids a vinyl ether bond is found at the sn-1 position.
The vast majority of reports on ether lipids in the male reproductive system and sperm date back from two decades ago. The relatively recent identification of genes coding for all the enzymes involved in the biosynthesis of ether lipids (Dean and Lodhi, 2018), combined with powerful techniques using proteomics and lipidomics of sperm samples has revamped research in this field, providing unique opportunities to advance in our knowledge on the role of these relegated lipids highly abundant in sperm.
Examples of the known roles of ether lipids in the different stages of sperm production and function are included in Figure 1.
a) Plasmanyl and plasmenyl phospholipids
During epididymal maturation, the number of phospholipids per cell decreases by around 50% in ram, bull, and rat sperm (Scott et al., 1967; Poulos et al., 1973; Aveldano et al., 1992) due to the loss of the cytoplasmic droplet. Interestingly, this reduction of phospholipids during epididymal transit is selective for diacyl phospholipids whereas plasmalogen levels do not significantly change, resulting in an increase in its proportion. In fact, early lipid analyses have revealed that both plasmanyl (O-) and plasmenyl (P-) forms of phosphatidylcholine and phosphatidylethanolamine are the major phospholipid classes in mature porcine and bovine sperm (Evans et al., 1980; Selivonchick et al., 1980). More recent lipidomics analysis of sperm from humans and other species have confirmed the prevalence of these ether lipids (Wood et al., 2016; Rivera-Egea et al., 2018; Ramal-Sanchez et al., 2020). Although the precise biological function of plasmanyl and plasmenyl glycerophospholipids in the male reproductive system has yet to be elucidated, it is hypothesized that the high proportion of these lipids in mature sperm protects these cells against oxidative damage due to their known antioxidant properties (Shan et al., 2021). Based on their chemical structure, plasmalogens have been considered to act as endogenous antioxidants. Due to their vinyl-ether linkage, plasmalogens are prone to oxidation serving as scavengers for radical species while protecting membrane lipids (Skaff et al., 2008; Broniec et al., 2011). It has been proposed that the susceptibility of plasmalogens to oxidative damage is due to a low hydrogen bond dissociation energy at the carbon adjacent to the vinyl ether bond (Murphy, 2001). Furthermore, the vinyl ether bond might be more exposed to the oxidative agents due to its proximity to the water-lipid interface, making them the first targets of ROS (Lankalapalli et al., 2009). In this regard, oxidation of plasmalogen by interaction with singlet oxygen/ROS occurs significantly faster than their diacyl counterparts (Broniec et al., 2011). However, the formation of further toxic molecules like fatty aldehydes generated from plasmalogens oxidation has also been reported, questioning their role towards a pro-oxidative effect (Stadelmann-Ingrand et al., 2004). This is relevant for sperm, considering the critical effect of ROS, oxidative stress and lipid peroxidation on sperm function, and their high content of polyunsaturated fatty acids incorporated to plasmalogens reviewed by Gibb et al., (2020), Gautier and Aurich, (2022).
Further challenging the idea that all plasmanyl/plasmenyl species play similar roles, is the fact that specific ether lipid species have been linked to opposite phenotypes in sperm performance. For example, 40:4 plasmanyl-PC [(O-40:4)] and 40:5 plasmenyl-PC [(P-40:5)] were identified as potential motility markers in canine spermatozoa (Lucio et al., 2017) while PC (O-42:4) and PE (P-34:2) were found to be significantly higher in sperm from patients without pregnancy success after intracytoplasmic sperm injection (Rivera-Egea et al., 2018). In addition, lack of plasmenyl species did not seem to affect sperm viability (Werner et al., 2020).
In summary, it is possible that ether lipid species with specific length and degree of unsaturation of their fatty acid tails may differentially affect the physical and functional properties of sperm. Indeed, it is known that sperm lipid composition can be influenced by factors such as the intake of dietary lipids (Saez and Drevet, 2019). As such, future investigations may be directed towards establishing a link between ether lipid saturation and alkyl/acyl chain length, the reproductive performance of sperm and its dependence on diet.
b) Platelet activating factor (PAF).
Studies in different species, including humans, have drawn a positive relationship between PAF content and sperm motility (Minhas et al., 1991; 1993; Roudebush et al., 2002), fertilization rates (Toledo et al., 2003) and clinical pregnancy after assisted reproduction treatments (Roudebush and Purnell, 2000). PAF acts through binding to its receptor, which has been found to be concentrated in the proximal head and midpiece of sperm from different species (Reinhardt et al., 1999; Levine et al., 2002). Human sperm samples with less than 50% forward motility possess low PAF receptor levels (Roudebush et al., 2000), leading to the hypothesis that this ether lipid is relevant for sperm function. This has been further supported by the presence of enzymes responsible for PAF catabolism and remodeling in human sperm and seminal plasma (Gujrati et al., 1987).
The association between PAF and sperm function has been studied using experiments where exogenous PAF was added during capacitation. Incubation of mouse sperm in a medium containing PAF resulted in an increase in fertilization rate, while incubation with a PAF receptor antagonist significantly decreased motility and fertilization rate, which was reversed with the addition of PAF (Sengoku et al., 1992). In this regards, PAF-acetylhydrolase, the primary enzyme responsible for inactivating PAF, has been proposed as a decapacitation factor (Letendre et al., 1992; Roudebush and Purnell, 2000; Zhu et al., 2006). More recently, PAF was found to dose-dependently induce the acrosome reaction in capacitated human spermatozoa and has been proposed to be associated with ERK-signaling pathways (Wu et al., 2020).
c) Sulfogalactosylglycerolipid (SGG)
SGG (also known as seminolipid) is an anionic glycolipid found selectively on the outer leaflet of mammalian primary spermatocytes membranes (Ishizuka, 1997; Tanphaichitr et al., 2003). SGG levels reach a maximum in round spermatids and remain constant in sperm (Iwamori et al., 2020; Kongmanas et al., 2021). Saturated C16:0-alkyl-C16:0-acyl is the primary species, with SGG containing other alkyl/acyl chain lengths comprising less than 10% of the total SGG content (Goto-Inoue et al., 2009).
SGG is essential in sperm production as spermatogenesis is arrested in animals lacking SGG synthesis, leading to infertility (Fujimoto et al., 2000; Honke et al., 2002). Moreover, male animals defective in SGG turnover are subfertile (Xu et al., 2011). Altogether, these results support the notion that SGG homeostasis in the testis is critical for normal spermatogenesis.
In addition, SGG involvement in capacitation and fertilization has also been proposed. Several studies have shown that during capacitation, SGG migrates to the equatorial region of the sperm head (Flesch and Gadella, 2000), likely to allow the acrosome reaction to occur. Moreover, most SGG is localized in low-density detergent resistant membranes in capacitated sperm cells, reflecting the ability of SGG to form lipid rafts (Khalil et al., 2006). A role for SGG in sperm-ZP interaction has also been proposed (White et al., 2000; Weerachatyanukul et al., 2001).
In humans, the ratio of cholesterol to SGG is found to be a potential biomarker for semen quality. Oligoasthenozoospermic (i.e., low sperm number and motility) patients were found to have a five-fold higher cholesterol to SGG ratio than individuals with normal sperm motility values, although this ratio has not been implicated to affect fertility (Lopalco et al., 2019).
d) Glycosylphosphatidylinositol (GPI)
GPI is a lipid anchor added post-translationally to many cell-surface proteins (Figure 2). The GPI anchors are assembled on a phosphatidylinositol (PI) lipid in the ER, and then covalently attached to the carboxyl terminus of proteins possessing a GPI-attachment signal peptide (Kinoshita, 2016). In mammals, despite the fact that most free cellular phosphatidylinositols contain unsaturated diacyl glycerol forms, GPI anchors have mainly 1-alkyl-2-acyl phosphatidylinositol with saturated fatty acid tails that make them raft-compatible lipid structures (Kanzawa et al., 2009). GPI anchored proteins (GPI-AP) are typically associated with membrane microdomains or lipid rafts (Kinoshita, 2020).
GPI-APs have been described to play different roles in sperm function. Their origin on the sperm surface is primarily from de novo synthesis by spermatogenic cells and/or by transfer from epididymal exosomes (epididymosomes) (Martin-DeLeon, 2015; Yoshitake and Araki, 2020). Database analysis showed more than 25 GPI-APs from testicular origin, and many of them are present in sperm (Yoshitake and Araki, 2020). Among them, TEX101 and Ly6K are essential for male fertility as sperm from knockout mice are unable to reach the fertilization site in the oviduct (Fujihara et al., 2014). Other GPI-APs have been implicated in different steps of gamete interaction (i.e., GLIPR1-like protein 1, SPAM1, SPACA4) (Phelps et al., 1988; Lin et al., 1994; Shetty et al., 2003; Gibbs et al., 2010).
The key steps in the synthesis of GPI anchors are summarized in Figure 3 (Kinoshita, 2020). GPI-AP synthesis is initiated with a phosphatidylinositol precursor that is converted to a 1-alkyl 2- acyl “three-footed” PI moiety where the inositol ring is acylated in addition to the two alkyl/acyl tails of PI (Kinoshita, 2020). Incorporation of the alkyl-based lipid moiety in the GPI anchor occurs through a remodeling pathway. Although the exact enzymatic steps of this remodeling are unclear, it is known that the peroxisomal alkyl-phospholipid biosynthetic pathway is required for biosynthesis of mammalian GPI anchors (Kanzawa et al., 2009). The inositol ring in the GPI precursor first gets deacylated by the GPI inositol-deacylase Pgap1. Interestingly, Pgap1 has been detected in the proteome of mouse sperm (Chauvin et al., 2012) and PGAP1 knockout mice showed male infertility (Ueda et al., 2007). Therefore, lack of deacylation of the GPI anchor may abolish the subsequent fatty acid remodeling to incorporate the ether lipid moiety, which has been shown to be critical for raft association of GPI-APs (Maeda et al., 2007). This highlights the relevance of alkyl-GPI anchors in male fertility.
FIGURE 3. Key steps in the synthesis of GPI-APs. The lipid precursor is phosphatidylinositol (PI, green) containing two acyl tails with mostly unsaturated fatty acids in sn-2. 1) A diacyl to alkyl/acyl occurs through lipid remodelling involving a peroxisomal precursor. In addition the inositol ring gets palmitoylated. 2) The deacylase PGAP1 removes the acyl tail. 3) Further acyl tail remodeling occurs to introduce a saturated fatty acid in position sn-2. Once mature, the GPI-AP can be delivered to the plasma membrane. Modified from (Kinoshita, 2020).
The synthesis of all ether lipids is initiated by a core pathway that operates in peroxisomes and is conserved along evolution (Figure 4). In the first step of this pathway, dihydroxyacetone phosphate (DHAP) is acylated at the sn-1 position by the enzyme DHAP acyltransferase (GNPAT/DHAPAT) encoded by the mammalian GNPAT gene (Dean and Lodhi, 2018). The acyl group on 1-acyl-DHAP is subsequently exchanged for a fatty alcohol via alkyl-DHAP synthase (AGPS/ADHAPS) encoded by the AGPS gene. Therefore, this second step is responsible for the characteristic ether bond at the sn-1 position, generating the intermediate 1-alkyl-DHAP (Dean and Lodhi, 2018). Studies involving patients deficient in either GNPAT or AGPS revealed a dependence on the presence of the catalytically competent AGPS for the stability and maximal activity of GNPAT (Itzkovitz et al., 2012), leading to the hypothesis that the two enzymes form a functional complex in order to channel 1-acyl-DHAP from GNPAT to AGPS. Both enzymes are localized on the luminal side of the peroxisomal membrane (de Vet E. C. et al., 1997; Thai et al., 1997; Braverman and Moser, 2012) and may exist as a heterotrimeric complex in a 2:1 ratio of GNPAT to AGPS (Biermann et al., 1999; Piano et al., 2016).
FIGURE 4. Peroxisomal core ether lipid biosynthetic pathway is present in sperm. In somatic cells, ether lipid biosynthesis is initiated in peroxisomes (A) and is finalized in the endoplasmic reticulum (B) where enzymes may be able to use both acyl and alkyl precursors (question mark). Boxed enzymes and pathways labelled in gold are found in sperm according to Chauvin et al., 2012; Amaral et al., 2013, 2014; Castillo et al., 2018; Martín-Cano et al., 2020 and Greither et al., 2023. Note peroxisome and ER compartments are believed to be missing in sperm. DHAP, Dihydroxyacetone phosphate; G3P, glycerol 3-phosphate; PA, phosphatidic acid; DAG, diacylglycerol; GNPAT, DHAP acyltransferase; AGPS, alkyl-DHAP synthase; DHRS7B, acyl/alkyl DHAP reductase; FAR; fatty acyl-CoA reductase; FALDH fatty aldehyde dehydrogenase; GPAT, G3-P acyltransferase; AGPAT, 1-acyl G3-P acyltransferase; LPIN, lipin PA phosphatase.
The fatty alcohol (AGPS substrate), typically restricted to C16:0, C18:0, and C18:1 species, is generated from a peroxisomal membrane-associated fatty acyl-CoA reductase (FAR), which catalyzes the reduction of acyl-CoA (Cheng and Russell, 2004). Two isoforms, FAR1 and FAR2, with a 58% sequence identity are ubiquitously expressed, albeit at varying levels in different tissues (Cheng and Russell, 2004). FAR1 activity is regulated by the cellular levels of ether lipids as its degradation has been associated with high levels of plasmenyl-PE (Honsho et al., 2010). Therefore, FAR1 is strongly implicated to be the rate-limiting step in ether lipid biosynthesis (Honsho et al., 2013). Another source of fatty alcohols is derived from the interconversion with fatty aldehydes. Evidence supporting the presence of this FAR-independent pathway comes from patients with the inherited metabolic disease Sjögren–Larsson Syndrome (SLS) (Weustenfeld et al., 2019), characterized by a deficiency in the enzyme fatty aldehyde dehydrogenase (FALDH) which oxidizes fatty aldehydes to fatty acids (Keller et al., 2014; Weustenfeld et al., 2019). FALDH deficiency results in an increase of fatty alcohols inducing ether lipid synthesis, reflected by the accumulation of ether lipids in the brain of SLS patients (Staps et al., 2020; Koch et al., 2022).
The last step in the core peroxisomal pathway for ether lipid biosynthesis involves the conversion of 1-alkyl-DHAP to 1-alkyl-glycerol-3 phosphate (1-alkyl-G3P), mediated by an acyl/alkyl DHAP reductase (DHRS7B/ADHAPR) encoded by the DHRS7B gene (Lodhi et al., 2012; Honsho et al., 2020). This reductase has the capacity to also reduce 1-acyl-DHAP to 1-acyl-G3P, which is a precursor for the synthesis of phosphatidic acid and all derived acyl-glycerophospholipids (Figure 4). Although the expression of enzymes involved in the previous steps in the pathway are non-tissue specific, DHRS7B expression is high in thyroid, muscle, testis, epididymis, and seminal vesicle (Sjöstedt et al., 2020; Human Protein Atlas, 2022). 1-alkyl-G3P is exported from the peroxisome for further acylation and dephosphorylation in the ER, giving rise to the different species of ether lipids discussed above (Honsho et al., 2020).
Peroxisomal disorders associated with ether lipid deficiency typically result in severe clinical phenotypes, highlighting its significance in human pathophysiology. The deficiency of ether lipids could be due to mutations in the biosynthetic peroxisomal proteins (Figure 4) or defects in peroxisomal import complexes. Patients afflicted with Zellweger spectrum disorder lack the ability to assemble functional peroxisomes due to pathogenic variants of PEX genes, which encode proteins known as peroxins (Kim and Hettema, 2015). Among these genes are PEX5 and PEX7, coding for cytosolic receptors that recognize Peroxisomal Targeting Signals, PTS1 or PTS2, respectively, which are necessary for the import of matrix proteins to the peroxisome (Hasan et al., 2013). The core enzymes for the biosynthesis of ether lipids display peroxisomal import signals. Whereas GNPAT contains a PTS1 in its C-end, AGPS presents a PTS2 in its N-end (de Vet E. C. J. M. et al., 1997; Thai et al., 1997; Ofman et al., 1998). A second disorder, rhizomelic chondrodysplasia punctata (RCDP), corresponds specifically to defects in the core ether lipid biosynthesis pathway. Five types of RCDP have been identified; RCDP1 and RCDP5 are associated with defects in the PEX7 and PEX5, respectively, with their abnormal function preventing the import of GNPAT and AGPS to the peroxisomal matrix. Mutations leading to deficiencies in GNPAT, AGPS, and FAR1 are respectively referred to as RCDP2, RCDP3, and RCDP4 (Barøy et al., 2015). Clinical characteristics of RCDP include the shortening of limbs (rhizomelia), premature calcifications of the epiphyseal cartilage, microcephaly, facial dysmorphism, congenital cataracts, and psychomotor retardation (Dean and Lodhi, 2018). Approximately 50% of affected patients succumb by age six due to the severity of these phenotypes, and most do not survive past adolescence (Braverman and Moser, 2012). Studies to elucidate the underlying mechanisms that result in these phenotypes were primarily conducted in knockout mouse models. Mice deficient in GNPAT displayed symptoms observed in RCDP patients, such as dwarfism, cataract formation, and abnormalities in myelination (Rodemer et al., 2003; Teigler et al., 2009). Another symptom of ether lipid deficiency displayed in the mouse models includes male sterility, a characteristic not studied in humans due to the premature mortality associated with the disorder. A targeted disruption of the GNPAT gene results in abnormalities of the male reproductive system, including testicular atrophy, decreased seminiferous tubule diameter and absence of sperm in the epididymis (Rodemer et al., 2003). Based on the presence of apoptotic, multinucleated cells within the seminiferous epithelium and a lack of mature sperm or elongating spermatids, a spermatogenic arrest is hypothesized to occur between the pachytene spermatocytes and round spermatid stages (Rodemer et al., 2003). Blind-sterile2 (bs2) mice which express aberrantly spliced AGPS transcripts exhibited similar phenotypes (Liegel et al., 2011). These observations indicate an essential role of ether lipids in the male reproductive system, raising the question of whether they can serve as valid fertility markers.
As stated before, it is currently accepted that sperm are devoid of peroxisomes, but reports on the proteome of these cells from different species have consistently identified peroxisomal proteins (Chauvin et al., 2012; Amaral et al., 2013; 2014; Castillo et al., 2018; Martín-Cano et al., 2020; Greither et al., 2023). Based on available data on peroxisomal residents at the time, Amaral and others highlighted the presence of fifteen proteins with exclusive peroxisomal localization in the human sperm tail proteome. Furthermore, the expression of peroxisomal membrane protein 11 (PEX11) and peroxisomal 3-ketoacyl-CoA thiolase (ACAA1) was confirmed via immunocytochemistry (PEX11 and ACAA1) and Western blot (ACAA1) in highly purified sperm samples (Amaral et al., 2013). Consistent with MS/MS results using sperm tails, both Pex11 and Acaa1 proteins localized to sperm midpiece and in the case of Acaa1, the full protein was detected by Western blot, indicating it is potentially functional. In order to better define the extent of the peroxisomal presence in the sperm proteome we conducted a meticulous analysis of published proteomic data from human and mouse sperm (Chauvin et al., 2012; Amaral et al., 2013; 2014; Castillo et al., 2018; Greither et al., 2023) and compared it to the known mammalian peroxisomal resident list of proteins (Yifrach et al., 2018). Out of 195 peroxisomal residents, 138 proteins (∼71%) were detected at least once in sperm from humans or mice, representing a much larger incidence than previously anticipated (Supplementary Table S1). It is worth noting that 12 peroxins are present in sperm, with only 4 remaining undetected. Interestingly, two of the missing peroxins (Pex2 and Pex10) belong to the Really Interesting Genes (RING) finger E3 ligases family, and have been recently shown to act as sensors of intracellular FAs, regulating lipolysis in response to ROS levels (Ding et al., 2021). Therefore sperm are equipped with a large set of peroxisomal proteins, with its most abundant matrix proteins (e.g.,.ACOX1, HSD17B4, CAT, THIKA, EPHX2), membrane proteins (ABCD1, ABCD3, ACBD5) and biogenesis associated peroxins in addition to the enzymes from the ether lipid pathway. This probably reflects the presence of a previously undetected peroxisomal remnant compartment which based on previous immunocytochemistry (PEX11 and ACAA1) may be localized to the midpiece in close proximity to mitochondria. Consistently among all sperm proteomes analyzed was the absence of the peroxisomal enzymes FAR1 and FAR2. As highlighted in Figure 4, the core enzymes GNPAT, AGPS and DHRS7B have all been detected in sperm proteomic studies, but the pathway seems to be FAR-independent, suggesting a detrimental role for fatty alcohols in sperm. Our analysis also points to a truncated ether-lipid biosynthetic pathway, with few extra peroxisomal steps present in sperm. A large set of LysoPA acyltransferases (AGPAT1, 2, 3, and 5) and a PA phosphohydrolase (LPIN1), which are the putative enzymes responsible for the production of diacyl- and 1-alkyl-2 acyl- PA and DAG respectively, are also present in sperm (Figure 4). After these steps, the enzymes of the Kennedy pathway known to consume the acyl- and alkyl-DAG intermediates in the ER/Golgi, as well as PEDS, the desaturase that introduces the vinyl bond for the synthesis of plasmalogens (Werner et al., 2020), all seem to be missing from the sperm proteome.
The presence of this truncated ether-lipid biosynthetic pathway suggests a divergent role for the peroxisomal core pathway in sperm. Given the pathway is FAR-independent it must consume an alternative source of fatty-alcohols at the AGPS step. The production of both fatty-aldehydes and fatty-alcohols from the catabolism of membrane lipids in sperm has been documented (Evans et al., 2021). Interestingly, mitochondrial cytochrome c has been found to act as a plasmalogenase that cleaves plasmenylcholine and plasmenylethanolamine at the sn-1 vinyl ether linkage, releasing fatty aldehydes in response to oxidative stress (Jenkins et al., 2018). The presence of peroxisomal FALDH in sperm also supports a role of a putative peroxisomal-like compartment in the detoxification of fatty aldehydes.
We therefore propose that sperm contain a repurposed FAR-independent ether lipid core pathway, probably localized to a peroxisome-remnant compartment intimately connected to mitochondria in order to act as a sink for toxic fatty alcohols and fatty aldehydes produced from lipid catabolism (Figure 5). Although somehow limited, evidence on the midpiece localization of the peroxisomal residents PEX11 and ACAA1 supports the close proximity of such compartment to mitochondria (Amaral et al., 2013).
FIGURE 5. Metabolic lipid pathways present in sperm. Proposed localization of the core peroxisomal pathway to a remnant peroxisomal compartment in close contact with mitochondria, for the synthesis ether lipid precursors with a main fatty alcohol detoxifying role. Abbreviations: Cyt c, cytochrome c; FFA, free fatty acid; FA, fatty acid; TCA, tri-carboxylic acids; CoA, coenzyme A. Created with BioRender.com.
In summary, sperm ether lipids, produced in early stages of spermatogenesis and/or provided by the epididymis, play a variety of roles in sperm function included in this review, and probably others to be elucidated in future studies. In addition, this review puts forward the concept that an enhanced collaboration between mitochondria and peroxisomal-like remnant structures may exist in sperm, in order to deal with the oxidative stress burden generated during sperm performance by the high mitochondrial activity (Balbach et al., 2020; Ferreira et al., 2021; Giaccagli et al., 2021).
Ether lipids are particularly abundant in sperm. As we have described in the first sections of this review, their diversity and relevance for sperm production, maturation, and function have been addressed by different laboratories over time. Many outstanding questions remain regarding the regulation of their synthesis and metabolism during the various steps preparing sperm for fertilization. The analysis of available sperm proteomic data carried out in this review identified key lipid metabolic pathways, opening new avenues in this regard. It is well known that sperm damage generated by oxidative stress as a consequence of a systemic inflammation produced by exogenous insults is one of the main causes of male infertility (Agarwal et al., 2018; Su et al., 2022). Therefore, the potential involvement of peroxisomal enzymes in a new antioxidant mechanism to help sperm deal with this damage contributes critical information that has the potential of significantly improving the diagnosis and treatment of male infertility. Future research is needed to challenge the proposed hypothesis and to further investigate the localization of the large peroxisomal subproteome present in sperm.
We performed this study by searching for keywords from PubMed and Web of Science on all articles in English published prior to April 2023. Search terms were based on the following keywords: Ether lipids, Plasmanyl, Plasmenyl, Plasmalogen, Platelet-activating factor, Sulfogalactosylglycerolipid, Seminolipid, Glycosylphosphatidylinositol, GNPAT/DHAPAT, AGPS, DHRS7B, fatty alcohols, oxidative stress, proteomics, and peroxisome combined to male reproductive tract, sperm and fertilization. In all cases, references cited in the analyzed articles were also considered.
DC and VZ: conceptualization, data curation, funding acquisition, investigation, writing—original draft, review and editing. MH, WL, LG, and VL: data curation, investigation, and writing—original draft. VD: data curation, funding acquisition and writing—review and editing. All authors contributed to the article and approved the submitted version.
This study was partially supported by National Agency for Scientific and Technological Promotion (ANPCyT) Grant PICT 2019-1508 to DC, and the National Research Council (PIP 2022-2024) of Argentina to DC and VD and by a Discovery Grant and a Discovery Accelerator Supplement from the Natural Sciences and Engineering Research Council (NSERC) PIN303585-2016 to VZ.
The authors would like to thank Alina Bertolesi for insightful comments about this manuscript.
The authors declare that the research was conducted in the absence of any commercial or financial relationships that could be construed as a potential conflict of interest.
All claims expressed in this article are solely those of the authors and do not necessarily represent those of their affiliated organizations, or those of the publisher, the editors and the reviewers. Any product that may be evaluated in this article, or claim that may be made by its manufacturer, is not guaranteed or endorsed by the publisher.
The Supplementary Material for this article can be found online at: https://www.frontiersin.org/articles/10.3389/fcell.2023.1166232/full#supplementary-material
Agarwal, A., Rana, M., Qiu, E., AlBunni, H., Bui, A. D., and Henkel, R. (2018). Role of oxidative stress, infection and inflammation in male infertility. Andrologia 50, 13126. doi:10.1111/and.13126
Aitken, R. J., and Baker, M. A. (2006). Oxidative stress, sperm survival and fertility control. Mol. Cell. Endocrinol. 250, 66–69. doi:10.1016/j.mce.2005.12.026
Aitken, R. J., Clarkson, J. S., and Fishel, S. (1989). Generation of reactive oxygen species, lipid peroxidation, and human sperm function. Biol. Reprod. 41, 183–197. doi:10.1095/biolreprod41.1.183
Aitken, R. J., Drevet, J. R., Moazamian, A., and Gharagozloo, P. (2022). Male infertility and oxidative stress: A focus on the underlying mechanisms. Antioxidants 11, 306. doi:10.3390/antiox11020306
Amaral, A., Castillo, J., Estanyol, J. M., Ballesca, J. L., Ramalho-Santos, J., and Oliva, R. (2013). Human sperm tail proteome suggests new endogenous metabolic pathways. Mol. Cell. Proteomics 12, 330–342. doi:10.1074/mcp.M112.020552
Amaral, A., Castillo, J., Ramalho-Santos, J., and Oliva, R. (2014). The combined human sperm proteome: Cellular pathways and implications for basic and clinical science. Hum. Reprod. Update 20, 40–62. doi:10.1093/humupd/dmt046
Aveldano, M. I., Rotstein, N. P., and Vermouth, N. T. (1992). Lipid remodelling during epididymal maturation of rat spermatozoa. Enrichment in plasmenylcholines containing long-chain polyenoic fatty acids of the n-9 series. Biochem. J. 283, 235–241. doi:10.1042/bj2830235
Baba, D., Kashiwabara, S., Honda, A., Yamagata, K., Wu, Q., Ikawa, M., et al. (2002). Mouse sperm lacking cell surface hyaluronidase PH-20 can pass through the layer of cumulus cells and fertilize the egg. J. Biol. Chem. 277, 30310–30314. doi:10.1074/jbc.M204596200
Balbach, M., Gracia Gervasi, M., Hidalgo, D. M., Visconti, P. E., Levin, L. R., and Buck, J. (2020). Metabolic changes in mouse sperm during capacitation. Biol. Reprod. 103, 791–801. doi:10.1093/biolre/ioaa114
Barøy, T., Koster, J., Strømme, P., Ebberink, M. S., Misceo, D., Ferdinandusse, S., et al. (2015). A novel type of rhizomelic chondrodysplasia punctata, RCDP5, is caused by loss of the PEX5 long isoform. Hum. Mol. Genet. 24, 5845–5854. doi:10.1093/hmg/ddv305
Biermann, J., Just, W. W., Wanders, R. J. A., and Van Den Bosch, H. (1999). Alkyl-dihydroxyacetone phosphate synthase and dihydroxyacetone phosphate acyltransferase form a protein complex in peroxisomes. Eur. J. Biochem. 261, 492–499. doi:10.1046/j.1432-1327.1999.00295.x
Braverman, N. E., and Moser, A. B. (2012). Functions of plasmalogen lipids in health and disease. Biochim. Biophys. Acta - Mol. Basis Dis. 1822, 1442–1452. doi:10.1016/j.bbadis.2012.05.008
Broniec, A., Klosinski, R., Pawlak, A., Wrona-Krol, M., Thompson, D., and Sarna, T. (2011). Interactions of plasmalogens and their diacyl analogs with singlet oxygen in selected model systems. Free Radic. Biol. Med. 50, 892–898. doi:10.1016/j.freeradbiomed.2011.01.002
Castillo, J., Jodar, M., and Oliva, R. (2018). The contribution of human sperm proteins to the development and epigenome of the preimplantation embryo. Hum. Reprod. Update 24, 535–555. doi:10.1093/humupd/dmy017
Chauvin, T., Xie, F., Liu, T., Nicora, C. D., Yang, F., Camp II, D. G., et al. (2012). A systematic analysis of a deep mouse epididymal sperm proteome. Biol. Reprod. 87, 141. doi:10.1095/biolreprod.112.104208
Cheng, J. B., and Russell, D. W. (2004). Mammalian wax biosynthesis: I. Identification of two fatty acyl-coenzyme A reductases with different substrate specificities and tissue distributions. J. Biol. Chem. 279, 37789–37797. doi:10.1074/jbc.M406225200
de Vet, E. C., Biermann, J., and van den Bosch, H. (1997a). Immunological localization and tissue distribution of alkyldihydroxyacetonephosphate synthase and deficiency of the enzyme in peroxisomal disorders. Eur. J. Biochem. 247, 511–517. doi:10.1111/j.1432-1033.1997.00511.x
de Vet, E. C. J. M., van den Broek, B. T. E., and van den Bosch, H. (1997b). Nucleotide sequence of human alkyl-dihydroxyacetonephosphate synthase cDNA reveals the presence of a peroxisomal targeting signal 2. Biochim. Biophys. Acta - Lipids Lipid Metab. 1346, 25–29. doi:10.1016/S0005-2760(97)00014-3
Dean, J. M., and Lodhi, I. J. (2018). Structural and functional roles of ether lipids. Protein Cell 9, 196–206. doi:10.1007/s13238-017-0423-5
Ding, L., Sun, W., Balaz, M., He, A., Klug, M., Wieland, S., et al. (2021). Peroxisomal β-oxidation acts as a sensor for intracellular fatty acids and regulates lipolysis. Nat. Metab. 3, 1648–1661. doi:10.1038/s42255-021-00489-2
Evans, H. C., Dinh, T. T. N., Hardcastle, M. L., Gilmore, A. A., Ugur, M. R., Hitit, M., et al. (2021). Advancing semen evaluation using lipidomics. Front. Vet. Sci. 8, 601794. doi:10.3389/fvets.2021.601794
Evans, R. W., Weaver, D. E., and Clegg, E. D. (1980). Diacyl, alkenyl, and alkyl ether phospholipids in ejaculated, in utero-and in vitro-incubated porcine spermatozoa. J. Lipid Res. 21, 223–228. doi:10.1016/s0022-2275(20)39828-x
Ferreira, J. J., Cassina, A., Irigoyen, P., Ford, M., Pietroroia, S., Peramsetty, N., et al. (2021). Increased mitochondrial activity upon CatSper channel activation is required for mouse sperm capacitation. Redox Biol. 48, 102176. doi:10.1016/j.redox.2021.102176
Flesch, F. M., and Gadella, B. M. (2000). Dynamics of the mammalian sperm plasma membrane in the process of fertilization. Biochim. Biophys. Acta 1469, 197–235. doi:10.1016/s0304-4157(00)00018-6
Fujihara, Y., Okabe, M., and Ikawa, M. (2014). GPI-anchored protein complex, LY6K/TEX101, is required for sperm migration into the oviduct and male fertility in mice. Biol. Reprod. 90, 60. doi:10.1095/biolreprod.113.112888
Fujihara, Y., Tokuhiro, K., Muro, Y., Kondoh, G., Araki, Y., Ikawa, M., et al. (2013). Expression of TEX101, regulated by ACE, is essential for the production of fertile mouse spermatozoa. Proc. Natl. Acad. Sci. 110, 8111–8116. doi:10.1073/pnas.1222166110
Fujimoto, H., Tadano-Aritomi, K., Tokumasu, A., Ito, K., Hikita, T., Suzuki, K., et al. (2000). Requirement of seminolipid in spermatogenesis revealed by UDP-galactose:ceramide galactosyltransferase-deficient mice. J. Biol. Chem. 275, 22623–22626. doi:10.1074/jbc.C000200200
Gautier, C., and Aurich, C. (2022). Fine feathers make fine birds – the mammalian sperm plasma membrane lipid composition and effects on assisted reproduction. Anim. Reprod. Sci. 246, 106884. doi:10.1016/j.anireprosci.2021.106884
Giaccagli, M. M., Gómez-Elías, M. D., Herzfeld, J. D., Marín-Briggiler, C. I., Cuasnicú, P. S., Cohen, D. J., et al. (2021). Capacitation-Induced mitochondrial activity is required for sperm fertilizing ability in mice by modulating hyperactivation. Front. Cell Dev. Biol. 9, 767161. doi:10.3389/fcell.2021.767161
Gibb, Z., Griffin, R. A., Aitken, R. J., and De Iuliis, G. N. (2020). Functions and effects of reactive oxygen species in male fertility. Anim. Reprod. Sci. 220, 106456. doi:10.1016/j.anireprosci.2020.106456
Gibbs, G. M., Lo, J. C. Y., Nixon, B., Jamsai, D., O’Connor, A. E., Rijal, S., et al. (2010). Glioma pathogenesis-related 1-like 1 is testis enriched, dynamically modified, and redistributed during male germ cell maturation and has a potential role in sperm-oocyte binding. Endocrinology 151, 2331–2342. doi:10.1210/en.2009-1255
Gomez, E., Irvine, D. S., and Aitken, R. J. (1998). Evaluation of a spectrophotometric assay for the measurement of malondialdehyde and 4-hydroxyalkenals in human spermatozoa: Relationships with semen quality and sperm function. Int. J. Androl. 21, 81–94. doi:10.1046/j.1365-2605.1998.00106.x
Goto-Inoue, N., Hayasaka, T., Zaima, N., and Setou, M. (2009). The specific localization of seminolipid molecular species on mouse testis during testicular maturation revealed by imaging mass spectrometry. Glycobiology 19, 950–957. doi:10.1093/glycob/cwp089
Greither, T., Dejung, M., Behre, H. M., Butter, F., and Herlyn, H. (2023). The human sperm proteome—toward a panel for male fertility testing. Androl. n/a. doi:10.1111/andr.13431
Gujrati, V. R., Naukam, R. J., and Sastry, B. V. R. R. (1987). Enzymatic deacetylation and acetylation of ether phospholipids related to platelet-activating factor in human semen with short and long liquefaction times. Ann. N. Y. Acad. Sci. 513, 583–585. doi:10.1111/j.1749-6632.1987.tb25114.x
Hasan, S., Platta, H. W., and Erdmann, R. (2013). Import of proteins into the peroxisomal matrix. Front. Physiol. 4, 261. doi:10.3389/fphys.2013.00261
Honke, K., Hirahara, Y., Dupree, J., Suzuki, K., Popko, B., Fukushima, K., et al. (2002). Paranodal junction formation and spermatogenesis require sulfoglycolipids. Proc. Natl. Acad. Sci. U. S. A. 99, 4227–4232. doi:10.1073/pnas.032068299
Honsho, M., Asaoku, S., and Fujiki, Y. (2010). Posttranslational regulation of fatty acyl-CoA reductase 1, Far1, controls ether glycerophospholipid synthesis. J. Biol. Chem. 285, 8537–8542. doi:10.1074/jbc.M109.083311
Honsho, M., Asaoku, S., Fukumoto, K., and Fujiki, Y. (2013). Topogenesis and homeostasis of fatty Acyl-CoA reductase 1. J. Biol. Chem. 288, 34588–34598. doi:10.1074/jbc.M113.498345
Honsho, M., Tanaka, M., Zoeller, R. A., and Fujiki, Y. (2020). Distinct functions of acyl/alkyl dihydroxyacetonephosphate reductase in peroxisomes and endoplasmic reticulum. Front. Cell Dev. Biol. 8, 855. doi:10.3389/fcell.2020.00855
Human Protein Atlas (2022). Hum. Protein atlas. Available at: https://www.proteinatlas.org/.
Hunnicutt, G. R., Primakoff, P., and Myles, D. G. (1996). Sperm surface protein PH-20 is bifunctional: One activity is a hyaluronidase and a second, distinct activity is required in secondary sperm-zona binding. Biol. Reprod. 55, 80–86. doi:10.1095/biolreprod55.1.80
Ishizuka, I. (1997). Chemistry and functional distribution of sulfoglycolipids. Prog. Lipid Res. 36, 245–319. doi:10.1016/S0163-7827(97)00011-8
Itzkovitz, B., Jiralerspong, S., Nimmo, G., Loscalzo, M., Horovitz, D. D. G., Snowden, A., et al. (2012). Functional characterization of novel mutations in GNPAT and AGPS, causing rhizomelic chondrodysplasia punctata (RCDP) types 2 and 3. Hum. Mutat. 33, 189–197. doi:10.1002/humu.21623
Iwamori, M., Adachi, S., Lin, B., Tanaka, K., Aoki, D., and Nomura, T. (2020). Spermatogenesis-associated changes of fucosylated glycolipids in murine testis. Hum. Cell 33, 23–28. doi:10.1007/s13577-019-00304-x
Jakop, U., Müller, K., Müller, P., Neuhauser, S., Rodríguez, I. C., Grunewald, S., et al. (2022). Seminal lipid profiling and antioxidant capacity: A species comparison. PLoS One 17 (3), e0264675. doi:10.1371/journal.pone.0264675
Jenkins, C. M., Yang, K., Liu, G., Moon, S. H., Dilthey, B. G., and Gross, R. W. (2018). Cytochrome c is an oxidative stress-activated plasmalogenase that cleaves plasmenylcholine and plasmenylethanolamine at the sn-1 vinyl ether linkage. J. Biol. Chem. 293, 8693–8709. doi:10.1074/jbc.RA117.001629
Jiménez-Rojo, N., and Riezman, H. (2019). On the road to unraveling the molecular functions of ether lipids. FEBS Lett. 593, 2378–2389. doi:10.1002/1873-3468.13465
Jones, R., and Mann, T. (1976). Lipid peroxides in spermatozoa; formation, role of plasmalogen, and physiological significance. Proc. R. Soc. Lond. - Biol. Sci. 193, 317–333. doi:10.1098/rspb.1976.0050
Kanzawa, N., Maeda, Y., Ogiso, H., Murakami, Y., Taguchi, R., and Kinoshita, T. (2009). Peroxisome dependency of alkyl-containing GPI-anchor biosynthesis in the endoplasmic reticulum. Proc. Natl. Acad. Sci. U. S. A. 106, 17711–17716. doi:10.1073/pnas.0904762106
Kelesidis, T., Papakonstantinou, V., Detopoulou, P., Fragopoulou, E., Chini, M., Lazanas, M. C., et al. (2015). The role of platelet-activating factor in chronic inflammation, immune activation, and comorbidities associated with HIV infection. AIDS Rev. 17, 191–201.
Keller, M. A., Zander, U., Fuchs, J. E., Kreutz, C., Watschinger, K., Mueller, T., et al. (2014). A gatekeeper helix determines the substrate specificity of Sjögren–Larsson Syndrome enzyme fatty aldehyde dehydrogenase. Nat. Commun. 5, 4439. doi:10.1038/ncomms5439
Khalil, M. B., Chakrabandhu, K., Xu, H., Weerachatyanukul, W., Buhr, M., Berger, T., et al. (2006). Sperm capacitation induces an increase in lipid rafts having zona pellucida binding ability and containing sulfogalactosylglycerolipid. Dev. Biol. 290, 220–235. doi:10.1016/j.ydbio.2005.11.030
Kim, P. K., and Hettema, E. H. (2015). Multiple pathways for protein transport to peroxisomes. J. Mol. Biol. 427, 1176–1190. doi:10.1016/j.jmb.2015.02.005
Kinoshita, T. (2020). Biosynthesis and biology of mammalian GPI-anchored proteins. Open Biol. 10, 190290. doi:10.1098/rsob.190290
Kinoshita, T. (2016). Glycosylphosphatidylinositol (GPI) anchors: Biochemistry and cell biology: Introduction to a thematic review series. J. Lipid Res. 57, 4–5. doi:10.1194/jlr.E065417
Koch, J., Watschinger, K., Werner, E. R., and Keller, M. A. (2022). Tricky isomers—the evolution of analytical strategies to characterize plasmalogens and plasmanyl ether lipids. Front. Cell Dev. Biol. 10, 864716. doi:10.3389/fcell.2022.864716
Kongmanas, K., Saewu, A., Kiattiburut, W., Baker, M. A., Faull, K. F., Burger, D., et al. (2021). Accumulation of seminolipid in sertoli cells is associated with increased levels of reactive oxygen species and male subfertility: Studies in aging arsa null male mice. Antioxidants 10, 912. doi:10.3390/antiox10060912
Lankalapalli, R. S., Eckelkamp, J. T., Sircar, D., Ford, D. A., Subbaiah, P. V., and Bittman, R. (2009). Synthesis and antioxidant properties of an unnatural plasmalogen analogue bearing a trans O-vinyl ether linkage. Org. Lett. 11, 2784–2787. doi:10.1021/ol9009078
Letendre, E. D., Miron, P., Roberts, K. D., and Langlais, J. (1992). Platelet-activating factor acetylhydrolase in human seminal plasma. Fertil. Steril. 57, 193–198. doi:10.1016/S0015-0282(16)54800-6
Levine, A. S., Kort, H. I., Toledo, A. A., and Roudebush, W. E. (2002). A review of the effect of platelet-activating factor on male reproduction and sperm function. J. Androl. 23, 471–476. doi:10.1002/j.1939-4640.2002.tb02262.x
Leßig, J., Gey, C., Süß, R., Schiller, J., Glander, H.-J., and Arnhold, J. (2004). Analysis of the lipid composition of human and boar spermatozoa by MALDI-TOF mass spectrometry, thin layer chromatography and 31P NMR spectroscopy. Comp. Biochem. Physiol. Part B Biochem. Mol. Biol. 137, 265–277. doi:10.1016/j.cbpc.2003.12.001
Liegel, R., Chang, B., Dubielzig, R., and Sidjanin, D. J. (2011). Blind sterile 2 (bs2), a hypomorphic mutation in Agps, results in cataracts and male sterility in mice. Mol. Genet. Metab. 103, 51–59. doi:10.1016/j.ymgme.2011.02.002
Lin, Y., Mahan, K., Lathrop, W. F., Myles, D. G., and Primakoff, P. (1994). A hyaluronidase activity of the sperm plasma membrane protein PH-20 enables sperm to penetrate the cumulus cell layer surrounding the egg. J. Cell Biol. 125, 1157–1163. doi:10.1083/jcb.125.5.1157
Lodhi, I. J., Yin, L., Jensen-Urstad, A. P. L., Funai, K., Coleman, T., Baird, J. H., et al. (2012). Inhibiting adipose tissue lipogenesis reprograms thermogenesis and PPARγ activation to decrease diet-induced obesity. Cell Metab. 16, 189–201. doi:10.1016/j.cmet.2012.06.013
Lopalco, P., Vitale, R., Cho, Y. S., Totaro, P., Corcelli, A., and Lobasso, S. (2019). Alteration of cholesterol sulfate/seminolipid ratio in semen lipid profile of men with oligoasthenozoospermia. Front. Physiol. 10, 1344. doi:10.3389/fphys.2019.01344
Lucio, C. F., Brito, M. M., Angrimani, D. S. R., Belaz, K. R. A., Morais, D., Zampieri, D., et al. (2017). Lipid composition of the canine sperm plasma membrane as markers of sperm motility. Reprod. Domest. Anim. 52, 208–213. doi:10.1111/rda.12860
Lüers, G. H., Thiele, S., Schad, A., Völkl, A., Yokota, S., and Seitz, J. (2006). Peroxisomes are present in murine spermatogonia and disappear during the course of spermatogenesis. Histochem. Cell Biol. 125, 693–703. doi:10.1007/s00418-005-0114-9
Maeda, Y., Tashima, Y., Houjou, T., Fujita, M., Yoko-o, T., Jigami, Y., et al. (2007). Fatty acid remodeling of GPI-anchored proteins is required for their raft association. Mol. Biol. Cell 18, 1497–1506. doi:10.1091/mbc.e06-10-0885
Martín-Cano, F. E., Gaitskell-Phillips, G., Ortiz-Rodríguez, J. M., Silva-Rodríguez, A., Román, Á., Rojo-Domínguez, P., et al. (2020). Proteomic profiling of stallion spermatozoa suggests changes in sperm metabolism and compromised redox regulation after cryopreservation. J. Proteomics 221, 103765. doi:10.1016/j.jprot.2020.103765
Martin-DeLeon, P. (2015). Epididymosomes: Transfer of fertility-modulating proteins to the sperm surface. Asian J. Androl. 17, 720–725. doi:10.4103/1008-682X.155538
Minhas, B. S., Kim, H. N., Zhu, Y. P., Ripps, B. A., and Buster, J. E. (1993). Platelet activating factor treatment of spermatozoa enhances fertilization rates of rabbit oocytes utilizing subzonal insertion of sperm. Theriogenology 39, 269. doi:10.1016/0093-691x(93)90124-n
Minhas, B. S., Kumar, R., Ricker, D. D., Robertson, J. L., and Dodson, M. G. (1991). The presence of platelet-activating factor-like activity in human spermatozoa. Fertil. Steril. 55, 372–376. doi:10.1016/s0015-0282(16)54132-6
Minhas, B. S. (1993). Platelet-activating factor treatment of human spermatozoa enhances fertilization potential. Am. J. Obstet. Gynecol. 168, 1314–1317. doi:10.1016/0002-9378(93)90387-x
Murphy, R. C. (2001). Free-radical-Induced oxidation of arachidonoyl plasmalogen phospholipids: Antioxidant mechanism and precursor pathway for bioactive eicosanoids. Chem. Res. Toxicol. 14, 463–472. doi:10.1021/tx000250t
Nakamura, Y., Yoshida, M., Tanigawa, K., Harada, A., Kihara-Negishi, F., Maruyama, K., et al. (2021). Deficiency of type I platelet-activating factor-acetylhydrolase catalytic subunits causes an increase in body weight. Biol. Pharm. Bull. 44, 920–925. doi:10.1248/bpb.b20-00936
Nikolopoulou, M., Soucek, D. A., and Vary, J. C. (1985). Changes in the lipid content of boar sperm plasma membranes during epididymal maturation. Biochim. Biophys. Acta 815, 486–498. doi:10.1016/0005-2736(85)90377-3
O’Flaherty, C., and Scarlata, E. (2022). Oxidative stress and reproductive function: The protection of mammalian spermatozoa against oxidative stress. Reproduction 164, F67–F78. doi:10.1530/REP-22-0200
Ofman, R., Hettema, E. H., Hogenhout, E. M., Caruso, U., Muijsers, A. O., and Wanders, R. J. A. (1998). Acyl-CoA:Dihydroxyacetonephosphate acyltransferase: Cloning of the human cDNA and resolution of the molecular basis in rhizomelic chondrodysplasia punctata type 2. Hum. Mol. Genet. 7, 847–853. doi:10.1093/hmg/7.5.847
Oresti, G. M., Luquez, J. M., Furland, N. E., and Aveldaño, M. I. (2011). Uneven distribution of ceramides, sphingomyelins and glycerophospholipids between heads and tails of rat spermatozoa. Lipids 46, 1081–1090. doi:10.1007/s11745-011-3601-x
Parks, J. E., and Lynch, D. V. (1992). Lipid composition and thermotropic phase behavior of boar, bull, stallion, and rooster sperm membranes. Cryobiology 29, 255–266. doi:10.1016/0011-2240(92)90024-v
Phelps, B. M., Primakoff, P., Koppel, D. E., Low, M. G., and Myles, D. G. (1988). Restricted lateral diffusion of PH-20, a PI-anchored sperm membrane protein. Sci. (80-. ) 240, 1780–1782. doi:10.1126/science.3381102
Piano, V., Nenci, S., Magnani, F., Aliverti, A., and Mattevi, A. (2016). Recombinant human dihydroxyacetonephosphate acyl-transferase characterization as an integral monotopic membrane protein. Biochem. Biophys. Res. Commun. 481, 51–58. doi:10.1016/j.bbrc.2016.11.019
Poulos, A., Voglmayr, J. K., and White, I. G. (1973). Phospholipid changes in spermatozoa during passage through the genital tract of the bull. Biochim. Biophys. Acta (BBA)-Lipids Lipid Metab. 306, 194–202. doi:10.1016/0005-2760(73)90225-7
Ramal-Sanchez, M., Bernabo, N., Tsikis, G., Blache, M.-C. C., Labas, V., Druart, X., et al. (2020). Progesterone induces sperm release from oviductal epithelial cells by modifying sperm proteomics, lipidomics and membrane fluidity. Mol. Cell. Endocrinol. 504, 110723. doi:10.1016/j.mce.2020.110723
Reinhardt, J. C., Cui, X., and Roudebush, W. E. (1999). Immunofluorescent evidence of the platelet-activating factor receptor on human spermatozoa. Fertil. Steril. 71, 941–942. doi:10.1016/S0015-0282(99)00096-5
Rivera-Egea, R., Garrido, N., Sota, N., Meseguer, M., Remohí, J., and Dominguez, F. (2018). Sperm lipidic profiles differ significantly between ejaculates resulting in pregnancy or not following intracytoplasmic sperm injection. J. Assist. Reprod. Genet. 35, 1973–1985. doi:10.1007/s10815-018-1284-4
Rodemer, C., Thai, T. P., Brugger, B., Kaercher, T., Werner, H., Nave, K. A., et al. (2003). Inactivation of ether lipid biosynthesis causes male infertility, defects in eye development and optic nerve hypoplasia in mice. Hum. Mol. Genet. 12, 1881–1895. doi:10.1093/hmg/ddg191
Roudebush, W. E., Gerald, M. S., Cano, J. A., Lussier, I. D., Westergaard, G., and Higley, J. D. (2002). Relationship between platelet-activating factor concentration in rhesus monkey (Macaca mulatta) spermatozoa and sperm motility. Am. J. Primatol. 56, 1–7. doi:10.1002/ajp.1059
Roudebush, W. E., and Purnell, E. T. (2000). Platelet-activating factor content in human spermatozoa and pregnancy outcome. Fertil. Steril. 74, 257–260. doi:10.1016/S0015-0282(00)00646-4
Roudebush, W. E., Wild, M. D., and Maguire, E. H. (2000). Expression of the platelet-activating factor receptor in human spermatozoa: Differences in messenger ribonucleic acid content and protein distribution between normal and abnormal spermatozoa. Fertil. Steril. 73, 967–971. doi:10.1016/S0015-0282(00)00485-4
Saez, F., and Drevet, J. R. (2019). Dietary cholesterol and lipid overload: Impact on male fertility. Oxid. Med. Cell. Longev. 2019, 4521786. doi:10.1155/2019/4521786
Scott, T. W., Voglmayr, J. K., and Setchell, B. P. (1967). Lipid composition and metabolism in testicular and ejaculated ram spermatozoa. Biochem. J. 102, 456–461. doi:10.1042/bj1020456
Selivonchick, D. P., Schmid, P. C., Natarajan, V., and Schmid, H. H. O. (1980). Structure and metabolism of phospholipids in bovine epididymal spermatozoa. Biochim. Biophys. Acta (BBA)/Lipids Lipid Metab. 618, 242–254. doi:10.1016/0005-2760(80)90030-2
Sengoku, K., Ishikawa, M., Tamate, K., and Shimizu, T. (1992). Effects of platelet activating factor on mouse sperm function. J. Assist. Reprod. Genet. 9, 447–453. doi:10.1007/BF01204050
Shan, S., Xu, F., Hirschfeld, M., and Brenig, B. (2021). Sperm lipid markers of male fertility in mammals. Int. J. Mol. Sci. 22, 8767. doi:10.3390/ijms22168767
Shetty, J., Wolkowicz, M. J., Digilio, L. C., Klotz, K. L., Jayes, F. L., Diekman, A. B., et al. (2003). SAMP14, a novel, acrosomal membrane-associated, glycosylphosphatidylinositol-anchored member of the Ly-6/urokinase-type plasminogen activator receptor superfamily with a role in sperm-egg interaction. J. Biol. Chem. 278, 30506–30515. doi:10.1074/jbc.M301713200
Sjöstedt, E., Zhong, W., Fagerberg, L., Karlsson, M., Mitsios, N., Adori, C., et al. (2020). An atlas of the protein-coding genes in the human, pig, and mouse brain. Science 367, 5947. doi:10.1126/science.aay5947
Skaff, O., Pattison, D. I., and Davies, M. J. (2008). The vinyl ether linkages of plasmalogens are favored targets for myeloperoxidase-derived oxidants: A kinetic study. Biochemistry 47, 8237–8245. doi:10.1021/bi800786q
Stadelmann-Ingrand, S., Pontcharraud, R., and Fauconneau, B. (2004). Evidence for the reactivity of fatty aldehydes released from oxidized plasmalogens with phosphatidylethanolamine to form Schiff base adducts in rat brain homogenates. Chem. Phys. Lipids 131, 93–105. doi:10.1016/j.chemphyslip.2004.04.008
Staps, P., Rizzo, W. B., Vaz, F. M., Bugiani, M., Giera, M., Heijs, B., et al. (2020). Disturbed brain ether lipid metabolism and histology in Sjögren-Larsson syndrome. J. Inherit. Metab. Dis. 43, 1265–1278. doi:10.1002/jimd.12275
Su, Y., Liu, Z., Xie, K., Ren, Y., Li, C., and Chen, W. (2022). Ferroptosis: A novel type of cell death in male reproduction. Genes (Basel) 14, 43. doi:10.3390/genes14010043
Sullivan, R., and Saez, F. (2013). Epididymosomes, prostasomes, and liposomes: Their roles in mammalian male reproductive physiology. Reproduction 146, R21–R35. doi:10.1530/REP-13-0058
Tanphaichitr, N., Khalil, M. B., Weerachatyanukul, W., Kates, M., Xu, H., Carmona, E., et al. (2003). Physiological and biophysical properties of male germ cell sulfogalactosylglycerolipid. Male Fertil. lipid Metab., 125–148.
Teigler, A., Komljenovic, D., Draguhn, A., Gorgas, K., and Just, W. W. (2009). Defects in myelination, paranode organization and Purkinje cell innervation in the ether lipid-deficient mouse cerebellum. Hum. Mol. Genet. 18, 1897–1908. doi:10.1093/hmg/ddp110
Thai, T. P., Heid, H., Rackwitz, H. R., Hunziker, A., Gorgas, K., and Just, W. W. (1997). Ether lipid biosynthesis: Isolation and molecular characterization of human dihydroxyacetonephosphate acyltransferase. FEBS Lett. 420, 205–211. doi:10.1016/S0014-5793(97)01495-6
Toledo, A. A., Mitchell-Leef, D., Elsner, C. W., Slayden, S. M., and Roudebush, W. E. (2003). Fertilization potential of human sperm is correlated with endogenous platelet-activating factor content. J. Assist. Reprod. Genet. 20, 192–195. doi:10.1023/A:1023622126870
Ueda, Y., Yamaguchi, R., Ikawa, M., Okabe, M., Morii, E., Maeda, Y., et al. (2007). PGAP1 knock-out mice show otocephaly and male infertility. J. Biol. Chem. 282, 30373–30380. doi:10.1074/jbc.M705601200
Weerachatyanukul, W., Rattanachaiyanont, M., Carmona, E., Furimsky, A., Mai, A., Shoushtarian, A., et al. (2001). Sulfogalactosylglycerolipid is involved in human gamete interaction. Mol. Reprod. Dev. 60, 569–578. doi:10.1002/mrd.1122
Werner, E. R., Keller, M. A., Sailer, S., Lackner, K., Koch, J., Hermann, M., et al. (2020). The TMEM189 gene encodes plasmanylethanolamine desaturase which introduces the characteristic vinyl ether double bond into plasmalogens. Proc. Natl. Acad. Sci. U. S. A. 117, 7792–7798. doi:10.1073/pnas.1917461117
Weustenfeld, M., Eidelpes, R., Schmuth, M., Rizzo, W. B., Zschocke, J., and Keller, M. A. (2019). Genotype and phenotype variability in Sjögren-Larsson syndrome. Hum. Mutat. 40, 177–186. doi:10.1002/humu.23679
White, D., Weerachatyanukul, W., Gadella, B., Kamolvarin, N., Attar, M., and Tanphaichitr, N. (2000). Role of sperm sulfogalactosylglycerolipid in mouse sperm-zona pellucida binding. Biol. Reprod. 63, 147–155. doi:10.1095/biolreprod63.1.147
Wood, P. L., Scoggin, K., Ball, B. A., Troedsson, M. H., and Squires, E. L. (2016). Lipidomics of equine sperm and seminal plasma: Identification of amphiphilic (O-acyl)-ω-hydroxy-fatty acids. Theriogenology 86, 1212–1221. doi:10.1016/j.theriogenology.2016.04.012
Wu, H., Gao, J., Wang, X., Leung, T. Y., Duan, Y. G., and Chiu, P. C. N. (2020). Platelet-activating factor induces acrosome reaction via the activation of extracellular signal-regulated kinase in human spermatozoa. Andrologia 52, e13565–e13567. doi:10.1111/and.13565
Xu, H., Kongmanas, K., Kadunganattil, S., Smith, C. E., Rupar, T., Goto-Inoue, N., et al. (2011). Arylsulfatase A deficiency causes seminolipid accumulation and a lysosomal storage disorder in Sertoli cells. J. Lipid Res. 52, 2187–2197. doi:10.1194/jlr.M019661
Yamano, S., Yamazaki, J., Irahara, M., Tokumura, A., Nakagawa, K., and Saito, H. (2004). Human spermatozoa capacitated with progesterone or a long incubation show accelerated internalization by an alkyl ether lysophospholipid. Fertil. Steril. 81, 605–610. doi:10.1016/j.fertnstert.2003.07.036
Yifrach, E., Fischer, S., Oeljeklaus, S., Schuldiner, M., Zalckvar, E., and Warscheid, B. (2018). Defining the mammalian peroxisomal proteome. Subcell. Biochem. 89, 47–66. doi:10.1007/978-981-13-2233-4_2
Yoshitake, H., and Araki, Y. (2020). Role of the glycosylphosphatidylinositol-anchored protein TEX101 and its related molecules in spermatogenesis. Int. J. Mol. Sci. 21, 6628. doi:10.3390/ijms21186628
Zhang, Y., Hayashi, Y., Cheng, X., Watanabe, T., Wang, X., Taniguchi, N., et al. (2005). Testis-specific sulfoglycolipid, seminolipid, is essential for germ cell function in spermatogenesis. Glycobiology 15, 649–654. doi:10.1093/glycob/cwi043
Keywords: sperm, ether lipid, peroxisome, metabolism, oxidative stress, fertility
Citation: Horta Remedios M, Liang W, González LN, Li V, Da Ros VG, Cohen DJ and Zaremberg V (2023) Ether lipids and a peroxisomal riddle in sperm. Front. Cell Dev. Biol. 11:1166232. doi: 10.3389/fcell.2023.1166232
Received: 15 February 2023; Accepted: 26 May 2023;
Published: 15 May 2023.
Edited by:
Fredrick Rosario-Joseph, University of Colorado Denver, United StatesReviewed by:
Mariana Camargo, Federal University of São Paulo, BrazilCopyright © 2023 Horta Remedios, Liang, González, Li, Da Ros, Cohen and Zaremberg. This is an open-access article distributed under the terms of the Creative Commons Attribution License (CC BY). The use, distribution or reproduction in other forums is permitted, provided the original author(s) and the copyright owner(s) are credited and that the original publication in this journal is cited, in accordance with accepted academic practice. No use, distribution or reproduction is permitted which does not comply with these terms.
*Correspondence: Débora J. Cohen, ZGNvaGVuQGRuYS51YmEuYXI=; Vanina Zaremberg, dnphcmVtYmVAdWNhbGdhcnkuY2E=
Disclaimer: All claims expressed in this article are solely those of the authors and do not necessarily represent those of their affiliated organizations, or those of the publisher, the editors and the reviewers. Any product that may be evaluated in this article or claim that may be made by its manufacturer is not guaranteed or endorsed by the publisher.
Research integrity at Frontiers
Learn more about the work of our research integrity team to safeguard the quality of each article we publish.