- Plant Molecular Biology Department, Instituto de Biotecnología, Universidad Nacional Autónoma de México (UNAM), Cuernavaca, Mexico
Plants are the source of our understanding of several fundamental biological principles. It is well known that Gregor Mendel discovered the laws of Genetics in peas and that maize was used for the discovery of transposons by Barbara McClintock. Plant models are still useful for the understanding of general key biological concepts. In this article, we will focus on discussing the recent plant studies that have shed new light on the mysterious mechanisms of meiotic crossover (CO) interference, heterochiasmy, obligatory CO, and CO homeostasis. Obligatory CO is necessary for the equilibrated segregation of homologous chromosomes during meiosis. The tight control of the different male and female CO rates (heterochiasmy) enables both the maximization and minimization of genome shuffling. An integrative model can now predict these observed aspects of CO patterning in plants. The mechanism proposed considers the Synaptonemal Complex as a canalizing structure that allows the diffusion of a class I CO limiting factor linearly on synapsed bivalents. The coarsening of this limiting factor along the SC explains the interfering spacing between COs. The model explains the observed coordinated processes between synapsis, CO interference, CO insurance, and CO homeostasis. It also easily explains heterochiasmy just considering the different male and female SC lengths. This mechanism is expected to be conserved in other species.
1 Introduction
1.1 Crossing over interference: a short historical perspective
In sexually reproducing organisms, parental alleles are distributed in the offspring following the laws of segregation defined by Gregor Mendel more than 150 years ago (Mendel, 1866; Birchler, 2015). The chromosomal theory of heredity proposed by Thomas H. Morgan (1926) explains how new chromosomic (genomic) combinations are formed during the meiotic and fertilization processes. During meiosis, new chromosomes are formed using recombination between parental DNA molecules. The junctions between parental chromosomes named crossing-overs correspond to the cytologically observed chiasma defined by Frans A. Janssens (Janssens, 1909) (translated into English by Koszul and Zickler, 2012). Using Drosophila, Alfred Sturtevant genetically defined that one CO in a genetic interval reduces the probability of observing another CO in the adjacent interval (Sturtevant, 1913; Sturtevant, 1965), a phenomenon Hermann J. Muller coined ‘CO interference’ in 1916 (Muller, 1916). John B.S. Haldane explained the genetic mechanism of interference by the statistical non-Poisson distribution of chiasmata observed in bivalents of various Angiosperms (Haldane, 1931). The correspondence between genetic crossing-overs (CO) and cytological chiasmata was elegantly demonstrated in maize (Creighton and McClintock, 1931; Coe and Kass, 2005). Various models of CO formation have been developed (reviewed in Haber, 2008; Gray and Cohen, 2016; Chuang and Smith, 2023), including various models trying to explain CO interference, ever since Haldane (Berchowitz and Copenhaver, 2010; Sun et al., 2017; Otto and Payseur, 2019; Saito and Colaiácovo, 2019; Chuang and Smith, 2023).
In this minireview, we will discuss what we have recently learned from plants regarding the integrated mechanisms regulating synapsis, CO interference, CO insurance, CO homeostasis, and heterochiasmy.
1.2 An integrated molecular model for CO formation in plants
The meiotic division leads to the reduction by half of the chromosome number in order to balance the fertilization process (Zickler and Kleckner, 2015). Meiosis is one of the most dynamic plant cellular processes (Ronceret and Pawlowski, 2010). It allows, after one step of replication, the equilibrated segregation of homologous chromosomes (meiosis I) followed by the segregation of sister chromatids (meiosis II) to produce four haploid spores (Mercier et al., 2015; Wang and Copenhaver, 2018; Gutierrez-Pinzón et al., 2021; Lloyd, 2023). During meiotic prophase I, homologous chromosomes undergo a genetically regulated process of recombination to create unique new chromosomes from two parental ones. The molecular models for meiotic CO formation of the last 40 years are based on the DNA Double-Strand Breaks (DSBs) Repair model of meiotic recombination proposed by Resnick, 1976 and refined by Szostak et al., 1983. This model explains how two DNA molecules can form a new recombined one using the error-prone repair mechanism of homologous recombination (HR). The basic mechanism of meiotic homologous recombination involves the formation of hundreds of programmed DSBs inflicted to the genome at the beginning of the leptotene stage (de Massy, 2013; Mercier et al., 2015; Yadav and Claeys Bouuaert, 2021). The number of DSBs varies from species to species: 150 to 250 in Arabidopsis (Vignard et al., 2007), 450 to 550 in maize (Pawlowski et al., 2003), and around 400 to 1,500 in hexaploid wheat (Benyahya et al., 2020). These various DSBs are formed in plants by the SPO11 complex containing SPO11-1 (Grelon et al., 2001; Shingu et al., 2012; Sprink and Hartung, 2014; Da Ines et al., 2020; Ku et al., 2020), SPO11-2 (Stacey et al., 2006; Benyahya et al., 2020; Fayos et al., 2020; Sprink and Hartung, 2021; Li et al., 2022; Hyde et al., 2023), MTOPVIB (Fu et al., 2016; Vrielynck et al., 2016; Xue et al., 2019; Jing et al., 2020; Steckenborn et al., 2023), PRD1 (de Muyt et al., 2007; Shi et al., 2021; Wang et al., 2022), PRD2 (de Muyt et al., 2009; Wang C. et al., 2023), PRD3 (de Muyt et al., 2009; Lambing et al., 2022; Wang et al., 2022), and DFO (Zhang et al., 2012). All these DSB factors form a complex where SPO11-1/2 (TOPVIA-like subunit) presents the catalytic transesterase (endonuclease) activity associated with MTOPVIB (TOPVIB-like subunit), underlining that the SPO11 complex conserve the structure of a DNA topoisomerase (Vrielynck et al., 2016; Vrielynck et al., 2021; Wang Y. et al., 2023). In this model, all original DSBs are not necessarily transformed into COs, but all COs are designed from a subset of DSBs (Figure 1). In plants, only a small subset of DSB sites is converted into COs (Mercier et al., 2015). Though in most species studied, the global genomic position of DSBs is randomly distributed along the length of chromosomes (He et al., 2017; Choi et al., 2018), it is known that at smaller scale, there are hotspots of DSBs in open chromatin regions near transcriptional start sites with low nucleosome density in Arabidopsis (He et al., 2017; Choi et al., 2018). It was recently shown that these DSB sites are also silenced to avoid recombination on transcribed genes (Wang et al., 2022). The number of DSBs can finally affect the genomic distribution of CO (Xue et al., 2018). The SPO11 complex is associated with axial elements (AE) of the synaptonemal complex (SC) including the HORMA domain ASY1/PAIR2 (Armstrong et al., 2002; Nonomura et al., 2006; Sanchez-Moran et al., 2007; Cuacos et al., 2021; Wang C. et al., 2023) as well as the ASY3/PAIR3/DSY2 (Wang et al., 2011; Ferdous et al., 2012; Lee et al., 2015) and ASY4 coiled-coil proteins (Chambon et al., 2018), supposably forming the basis of chromatin loops (Vrielynck et al., 2021; Lambing et al., 2022) (Figure 1 Leptotene stage).
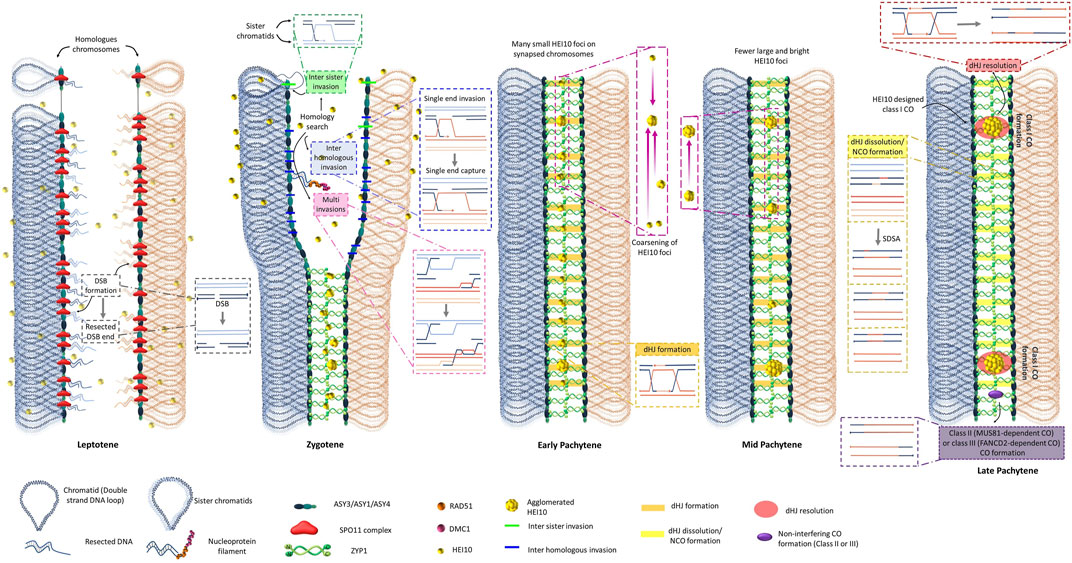
FIGURE 1. An integrated model explaining CO interference. Schematic representation of the formation of CO in just a pair of homologous chromosomes. To simplify, the global arrangement of the chromosome is depicted with two different colors for the homologs (red and blue) and two different tones for sister chromatids (light red and light blue). At this scale, each double-stranded DNA molecule is depicted as a simplifying line. The molecular steps necessary to understand the molecular mechanism of CO formation are depicted in magnified insets, where the 5′ ends of each DNA strand are represented by line with extremities with dots. In leptotene, the replicated chromosomes are represented with their important features for meiotic recombination. The axial elements (AE) including ASY1, ASY3, and ASY4 are assembled at the base of the loop of two sister chromatids attached by meiotic cohesins including REC8. Establishment of the proteinaceous axial element is essential to form the Synaptonemal Complex (SC). The formation of tens of DNA double-strand breaks (DSBs) for each of the only two homologous chromosomes pictured is processed by the evolutionarily conserved SPO11 complex. DSB ends are resected at either 5′ end to produce 3′ overhang, which are recognized by Rad51 and Dmc1 to generate inter-homologue strand-invasion. The 3′ end can invade either a homolog or a sister chromatid. In addition, multi-invasion of the 3′ tails on a homolog and/or sister chromatid is also possible to find an intact homologous template for HR repair. 3′ tail invasion in the homologous intact molecule generates a displacement loop (D-loop) through single end invasion (SEI). In zygotene, multiple SEI are used for homologous chromosome recognition/pairing. The transverse filaments ZYP1 of the synaptonemal complex (SC) start to polymerize at the center of the tripartite SC between two lateral elements. In early pachytene, the homologous chromosomes are fully synapsed from telomere to telomere. The SC allows the diffusion in only one dimension of the limiting CO factor HEI10 that can form coarsening recombination nodules explaining interference between CO class I (adapted from Morgan et al., 2021; Durand et al., 2022). In mid pachytene, various recombinant intermediates can be associated with HEI10 foci that progressively form larger agglomerated foci and eliminate the possibility of close CO design, except in the case of non-interfering COs that are not HEI10-dependant. At the end of pachytene, most of the chromosomal positions with SEI have not been transformed in CO but are resolved as NCO. At least one and generally few interferent (spaced out) COs are present on each bivalent, except where non-interfering (class II or class III) COs are formed.
The process of meiotic recombination is realized in a context of replicated chromosomes. Each DSB can therefore be repaired by homologous recombination using different undamaged homologous sequences as a repair template. The sites where the identical sister chromatid is used as the repair template form non-CO events (Figure 1). The sites where the DSB repair is processed using either one of the two polymorphic DNA sequences of the homologous chromosomes as the repair template can enter the pathway to form COs (Figure 1). During meiosis, a specific cohesin complex is installed between sister chromatids and contains REC8 instead of SCC1 (Chelysheva et al., 2005; Golubovskaya et al., 2006; Shao et al., 2011) as well as SMC1/3 (Lam et al., 2005; Bolaños-Villegas, 2021), SCC2 (Wang et al., 2020), SCC3 (Chelysheva et al., 2005), CTF7 (Bolaños-Villegas et al., 2013), and PDS5 (Pradillo et al., 2015). The putative helicase MCM8 (Crismani et al., 2013) and the cohesion-like SMC5/6 complex that favors sister chromatin HR repair in somatic cells are also tightly controlled during meiosis to allow the activity of interhomolog HR (Watanabe et al., 2009; Liu et al., 2014; Knoll et al., 2014; Chen et al., 2021; Zhu et al., 2021; Jiang et al., 2022). These DSBs are readily resected 5′ to 3′ by the exonuclease activity of MRE11 to form single-strand overhang 3′ extremities (Puizina et al., 2004; Ji et al., 2013) and allow the liberation of SPO11-bound oligonucleotides (Choi et al., 2018). MRE11 forms a complex with RAD50 (Bleuyard et al., 2004), NBS1 (Akutsu et al., 2007; Waterworth et al., 2007), and COM1 (Uanschou et al., 2007; Ji et al., 2013; Wang et al., 2018). These 3’ free single-strand DNA extremities are protected by various RPAs (Chang et al., 2009; Osman et al., 2009; Li et al., 2018; Aklilu et al., 2020), replaced by recA type recombinases RAD51 (Bleuyard et al., 2005; Da Ines et al., 2022; Li et al., 2022), RAD51C (Bleuyard et al., 2005; Jing et al., 2019), XRCC3 (Bleuyard and White, 2004; Zhang et al., 2015), and DMC1 (Couteau et al., 1999; Deng and Wang, 2007; Kurzbauer et al., 2012; Wang et al., 2016; Colas et al., 2019; Szurman-Zubrzycka et al., 2019). BRCA2 directly interacts with RAD51 and DMC1 and could facilitate their loading on the resected end of DSBs (Dray et al., 2006; Fu et al., 2020). These recombinases have the property to form nucleoprotein filaments that can bring together the single-strand DNA extremities with its specific homologous double-strand DNA molecules in a process known as single-end invasions (SEIs) (review in Emmenecker et al., 2022). Different recombinase subcomplexes exist and have differential functions between inter-sister SEI and interhomolog SEI (Kurzbauer et al., 2012; Pradillo et al., 2012; Su et al., 2017). The main biochemical difference between the Arabidopsis RAD51 and DMC1 recombinases tested in vitro is that RAD51 pairing activity seem not to be influenced by Ca2+ while DMC1 pairing activity is greatly enhanced in the presence of Ca2+ (Kobayashi et al., 2019). The most recently supported model proposes a symmetrical loading of RAD51 and DMC1 homotypic filaments on both DSB ends of a break (Da Ines et al., 2022). Interestingly, the role of the meiotic specific DMC1 seems to attenuate the strand exchange activity of the mitotic and meiotic RAD51 recombinase (Da Ines et al., 2022) (Figure 1 Zygotene stage). Interhomolog SEI is also promoted during meiosis by axial proteins such as ASY1 (Sanchez-Moran et al., 2007; De Muyt et al., 2009). Other recombinase accessory proteins modulate SEI (review in Emmenecker et al., 2022). For example, RAD54 assists the RAD51 interhomolog repair of meiotic DSB (Hernandez Sanchez-Rebato et al., 2021), while the meiotic specific HOP2 Vignard et al., 2007; Uanschou et al., 2013; Shi et al., 2019, Emmenecker et al., 2022) and MND1 (Kerzendorfer et al., 2006; Lu et al., 2020) form a complex that could assist DMC1 strand invasion (Kang et al., 2015). HOP2 could also prevent illegitimate recombination between non homologous chromosome regions (Farahani-Tafreshi et al., 2022) (Figure 1 Zygotene stages). In rice, HOP2 can directly interact with the ZEP1 SC central component (Shi et al., 2019).
Hundreds of DSB sites allow the formation of the corresponding hundreds of SEI sites along the zygotene homologous chromosomes (Figure 1 Zygotene stage). These multiple sites of SEI connections (known as joint molecules JMs), involving base pair DNA sequence homology recognition, are usually proposed to explain the mechanism used for the correct association (also known as pairing process) between the homologous chromosomes (Zickler and Kleckner, 1999; Pawlowski et al., 2003; Higgins et al., 2004). Most of these numerous SEIs will not form CO but are proposed to allow the accurate alignment between the right partners not only on a local scale but along the whole chromosome length. The excess of SEIs compared to the restricted final number of COs could therefore explain the accuracy of the pairing process between chromosome bivalents concomitant to synapsis (Gutierrez-Pinzón et al., 2021) (Figure 1 Zygotene stage). The recognized paired interaction zones are stabilized by the zipper-like proteinaceous structure known as the synaptonemal complex (SC). ZYP1 (ZEP1 in rice) is the central element forming a ladder between the two axial elements of the SC and connecting homologs from telomere to telomere (Higgins et al., 2005; Wang et al., 2010; Barakate et al., 2014; Capilla-Pérez et al., 2021; France et al., 2021) (Figure 1 Zygotene stage). The various SEI sites form regions of DNA triple helices known as Displacement-loops (D-loops). Most D-loops do not enter a CO pathway and several anti-recombinase pathways (described later) can dissociate the invading strand from the D-loop and re-anneal with the other DSB end, leading to small patches of hybrid DNA known as non-Crossover (NCO). The dissolved D-loops are proposed to be repaired via the Synthesis-Dependent Strand Annealing (SDSA) model during meiosis (Allers and Lichten, 2001; Puchta, 2005; Vu et al., 2017; Wang and Copenhaver, 2018) (Figure 1 Zygotene and pachytene stages).
For the few D-loops that enter a CO pathway, the invading strand is extended by DNA polymerases that realize a local DNA synthesis using the undamaged strand to copy it (Figure 1 Zygotene stage). The displaced strand of the undamaged DNA molecule hybridizes with the other 3’ end of the DSB in a process known as second end capture (Figure 1 Zygotene stage). These joint molecules can interchange part of their homologous strands extending the hybridization zone in a process known as branch migration that leads to the formation of double Holliday junctions (dHJs) (Figure 1 Pachytene stages). The asymmetric resolution of these dHJs can lead to the formation of COs that covalently link new portions of two homologous chromosomes (Szostak et al., 1983).
Two principal classes of CO pathways, one sensitive to interference (class I) and the other insensitive to interference (class II), have been defined in S. cerevisiae, animals, and plants (Mercier et al., 2005; Holloway et al., 2008; Gray and Cohen, 2016).
The major pathway (accounting for around 85% of COs in Arabidopsis) is called class I and is subject to CO interference. The formation of the class I CO involves members of the ZMM pathway composed of ZIP4 (Chelysheva et al., 2007; Shen et al., 2012), MER3 (Mercier et al., 2005; Wang et al., 2009), HEI10 (Chelysheva et al., 2012; Wang K. et al., 2012), PTD (Wijeratne et al., 2006; Ren et al., 2019), SHOC1/ZIP2 (Macaisne et al., 2008; Ren et al., 2019), mutS-like MSH4 (Higgins et al., 2004; Zhang et al., 2014b), mutS-like MSH5 (Higgins et al., 2008a; Luo et al., 2013), mutL-like MLH1 (Dion et al., 2007; Liu et al., 2022), and mutL-like MLH3 (Jackson et al., 2006; Colas et al., 2016; Mao et al., 2021). The mut-Lγ resolvase (formed by the MLH1-MLH3 heterodimer of the ZMM complex) can potentially stabilize D-loop structures and mature them as CO (review in Ziolkowski, 2023). This interfering pathway also requires the leading strand DNA polymerase epsilon (Ronceret et al., 2005; Huang et al., 2015; Wang et al., 2022a; Wang et al., 2022b) as well as the potential lagging strand DNA polymerase POLD1 (Wang et al., 2019) and RFC1 factor (Wang Y. et al., 2012) probably necessary for the synthesis steps of HR. This interfering pathway is limited by the HCR1 encoding a Protein Phosphatase X1 that can interact with HEI10, PTD, MSH5, and MLH1 (Nageswaran et al., 2021). In rice, a new plant specific ZMM member named HEIP1 interacting with HEI10, ZIP4, and MSH5 was also identified (Li et al., 2018). In tetraploid wheat, MSH4/MSH5 mutants also demonstrate an 85%–15% proportion of the two CO pathways in wheat species (Desjardins et al., 2020). In hexaploid wheat, the classical Ph1 locus controlling pairing and recombination between homeologous chromosomes, which is very important for breeding strategies, corresponds to a ZIP4 homolog (Rey et al., 2017).
The second minor non-interfering pathway (accounting for 15% of COs in Arabidopsis, around 10% of COs in rice) is called class II and involves the MUS81 protein (Berchowitz et al., 2007; Higgins et al., 2008b; Geuting et al., 2009). The MUS81 complex has a highly controlled endonuclease activity acting on selective DNA structures, such as D-loop and HJ, and can act on several replication and recombination intermediates during mitosis and meiosis depending on its interacting partners and phosphorylation status (Pfander and Matos, 2017). In rice, GEN1, the homolog of the Holliday junction resolvase is necessary for class II CO formation (Wang et al., 2017) while it is not in Arabidopsis (Bauknecht and Kobbe, 2014; Olivier et al., 2016), suggesting that the MUS81 pathways have diverged between dicots and monocots. Compared to Arabidopsis, the MUS81 pathway contributes even less to CO designation in rice (Wang et al., 2017; Mu et al., 2022), suggesting that the weight of the different pathways described in this review probably depend on each species.
In Arabidopsis, a second non-interfering pathway of CO, depending on FANCD2 and parallel to the MUS81 non-interfering CO pathway, contributes to the formation of some type II COs (Kurzbauer et al., 2018). The FANCD2 pathway, but not the MUS81 pathway, affects the distribution of class I CO (Li et al., 2021). In order to avoid confusion, this distinct FANCD2 pathway could be designed as the non-interfering class III CO pathway (Gutierrez-Pinzón et al., 2021).
Various meiotic interhomolog intermediate dissolution pathways (or anti-recombinase pathways) have been identified in Arabidopsis using meiotic mutant suppressor screens restoring fertility. Double mutant and cytological marker analysis have shown that they all reduce specifically the MUS81 dependent non-interfering class II CO pathway. These anti-recombinase pathways act using parallel mechanisms since mutants combining the different pathways lead to additive massive meiotic non-interfering CO formation (Crismani et al., 2012; Girard et al., 2015; Séguéla-Arnaud et al., 2015; Serra et al., 2018; Singh et al., 2023).
The first anti CO pathway requires the RECQ4A and RECQ4B helicase activity homolog to mammal BLM and yeast SGS1 (Hartung et al., 2007; Higgins et al., 2011; Séguéla-Arnaud et al., 2015; Serra et al., 2018). Combine with TOP3α (Hartung et al., 2008) and RMI/BLAP75 (Chelysheva et al., 2008), this complex is known as the RTR complex in plants and is similar to the ‘dissolvosome’ BTR complex in mammals and the yeast STR complex (Emmenecker et al., 2022). In Arabidopsis, recq4A mutant suppresses CO dependent on MUS81 activity (Séguéla-Arnaud et al., 2015). The RECQ4A protein is found on recombination intermediates of telomeres during meiosis and could particularly restrict CO class II on these chromosomal regions (Higgins et al., 2011). Recq4 mutants increase by around three times the number of COs in rice, tomato, and pea (Mieulet et al., 2018). It seems that this RTR complex has functionally diverged between Arabidopsis and tomato (Whitbread et al., 2021).
The second anti CO class II pathway requires FIDGETIN-like AAA-ATPase (FIGL1) (Girard et al., 2015) associated with FLIP (Fernandes et al., 2018). This FIGL1 is an antagonist to the BRAC2 recombinase (Kumar et al., 2019). In rice, the FLIP-like MEICA protein can interact with the TOP3α and MSH7 (Hu et al., 2017), suggesting coordination between the first anti CO pathway at least in rice. MEICA and FIGNL1 both have an anti CO activity affecting class II CO (Zhang et al., 2017; Yang C. et al., 2022). These pathways could also have different strengths in male versus female meiosis as observed by the male specific effect of the FIGL mutation in rice (Zhang et al., 2017).
The third anti CO pathway requires the Fanconi Anaemia (FA) pathway comprising the FANCM helicase (Crismani et al., 2012); its cofactors MHF1 and MHF2 (Girard et al., 2015); and the FANCC, FANCE, and FANCF subcomplex (Singh et al., 2023).
It is important to clarify that any of these three parallel anti-recombinase pathways, which substantially increase the number of class II CO, have been shown to abolish CO interference measured genetically. The interpretation of these confounding results is that the large number of class II COs, by decreasing the space between COs, mask the class I spacing mechanism expected to remain unaffected (Crismani et al., 2012; Girard et al., 2015; Séguéla-Arnaud et al., 2017; Fernandes et al., 2018; Li et al., 2021).
Interestingly, in Arabidopsis, FANCD2, FANCM, FIGL1, and RMI1 not only suppress non-interfering CO but also have a role in regulating the distribution of class I CO among chromosomes to insure at least one CO per bivalent (Li et al., 2021). In wheat, it was shown that FANCM not only suppresses class II non-interfering COs but also promotes class I interfering COs and insures at least one CO by bivalent (Desjardins et al., 2022).
It has been proposed that these dissolution pathways leading to NCO could be especially important for multi-invasion complexes formed between more than two DNA molecules that would otherwise lead to aberrant recombination intermediates (Emmenecker et al., 2022; Mu et al., 2022). Whether or not these pathways could be involved in a putative chromatid interference phenomenon (Zhao et al., 1995; Sarens et al., 2021) is still unknown.
In general, the combination of these different pro- and anti- CO pathways leads to the formation of only one or two COs per chromosome arm in plants, fungi, and animals (Otto and Payseur, 2019; Saito and Colaiácovo, 2019; Pazhayam et al., 2021; Tock et al., 2021; Lloyd, 2023).
However, despite the understanding of the molecular factors involved in CO formation in various model species, the mechanism by which COs tend to space out from each other has been debated for more than a century. The other mechanisms of the obligatory CO, CO homeostasis (where the variation of the initial number of recombination intermediate does not affect the final number of COs), and heterochiasmy (where CO rate is different between male and female meiosis) have also gained various insights from recent analysis in plant meiotic models.
1.3 A model of CO interference based on HEI10 coarsening along the SC compartment
Recently, several lines of evidence converged to pinpoint the fundamental role of the localization dynamics of the specific pro-crossover ZMM HEI10 factor on meiotic chromosomes to explain the CO interference phenomenon. HEI10 is a member of a family of RING-finger domain E3 ubiquitin ligase (De Muyt et al., 2014) including Zip3 in S. cerevisiae (Agarwal and Roeder, 2000), HEI10 and RNF212 in mammals (Ward et al., 2007; Kong et al., 2008), Vilya in Drosophila (Lake et al., 2015) and ZHP-3 in C. elegans (Bhalla et al., 2008; Zhang et al., 2018). In Arabidopsis, the expression of HEI10 responds similarly to its overlapping adjacent gene MRD1 to pathogen inoculations via the OZF1 transcription factor (Singh et al., 2022). AtHEI10 is also transcriptionally repressed by a Heat Shock Binding Protein (HSBP) that contributes to the adaptative CO formation in response to change in temperature (Kim et al., 2022) suggesting that HEI10 expression is responding to various environmental factors. In immunolocalization experiments, HEI10 foci show a peculiar pattern with a foci number progressively decreasing from late zygotene to diakinesis with late signal associated with other members of the class I CO such as MLH1 and MLH3 in Arabidopsis and rice (Chelysheva et al., 2012; Wang K. et al., 2012). It was noticed that HEI10 can show either small and faint numerous foci at the beginning of the zygotene stage or large and bright signals at the end of the pachytene stage in rice, Arabidopsis, and wheat meiocytes (Wang Y. et al., 2012; Duroc et al., 2014; Desjardins et al., 2020; Osman et al., 2021). Natural variation in this gene is associated with variation in meiotic recombination rate observed between Arabidopsis ecotypes (Ziolkowski et al., 2017). The dosage of HEI10, reduced in hei10 heterozygotes or increased in HEI10 overexpression line is a determinant for CO number and the strength of CO interference (Ziolkowski et al., 2017; Serra et al., 2018; Morgan et al., 2021). Using super-resolution SIM track of the HEI10 immunolocalization signal on fully synapsed pachytene bivalents, it was observed that the intensity of HEI10 signal per foci depends on the total number of observed foci per bivalent, with brighter signal when unique foci are present on bivalents (Morgan et al., 2021). During early zygotene, faint HEI10 signals are uniformly distributed along the bivalents (Figure 1. Zygotene stage), the number of HEI10 foci progressively decreases during zygotene and pachytene stages with an aggregation of brighter and larger HEI10 foci at the expense of the fainter and smaller adjacent foci (Figure 1 Zygotene and Pachytene stages). Ultimately by late pachytene, few large bright foci have been spaced out by the mechanism of HEI10 coarsening that has depleted the surrounding HEI10 sites on bivalents (Figure Fig1. Late Pachytene stage). However, by which mechanism HEI10 can coarsen and how it is related to other ubiquitination processes during meiosis require further investigations (Orr et al., 2021; Ren et al., 2021). It is worth underlining that the analysis of pachytene bivalents using electron microscopy had already noticed different sizes and shapes of early (poorly interfering) versus late interfering ‘recombination nodules’ (RN) localize along the synaptonemal complex (Anderson and Stack, 2005). Morgan and colleagues proposed a mathematical ‘diffusion mediated HEI10 coarsening model’ where the SC is seen as a linear structure allowing the diffusion of the HEI10 signal in only one dimension (Morgan et al., 2021). This model convincingly predicts differently tested situations in WT and hei10 mutant or HEI10oe overexpression line (Morgan et al., 2021) (Figure 2). The model explains very well how the HEI10 recombination nodules become progressively distant from each other. This model also implicitly explains how the mechanism of interference is not affected by the formation of either class II MUS81 dependent CO, nor class III FANCD2 dependent CO, though either of the three pathways can use the same SEI initial recombination intermediates. It also suggests that the remaining SEI sites without HEI10 bright foci are transformed into NCO or non-interfering COs (Figure 1 Pachytene stages).
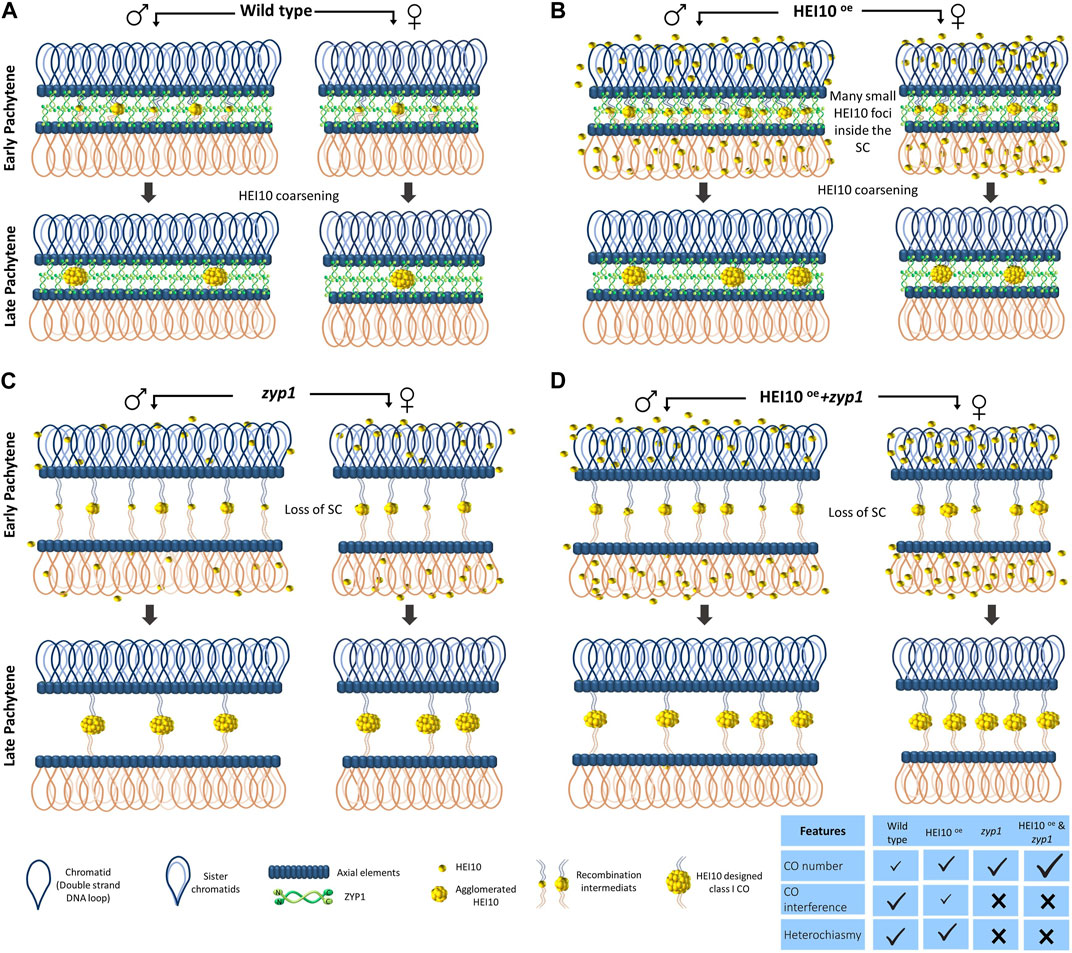
FIGURE 2. Many mechanisms of crossover interference can be explained by the HEI10 coarsening model along the ZYP1 synaptonemal complex structure. Schematic representation of the HEI10 localization at pachytene in Arabidopsis thaliana wild type (A), zyp1 mutant (B), HEI10oe overexpressed line (C), and zyp1+ HEI10oe (D) adapted from Durand et al., 2022. In wild type, HEI10 proteins are localized into foci. Many small HEI10 foci on synapsed chromosomes appear along the SC. With the progression of the pachytene stages, the number of HEI10 foci gradually decreases and few of them coarsen by agglomerating the smaller foci into the larger ones. Fewer foci are generated in females due to the shorter SC and leads to heterochiasmy (A). In HEI10 OE, the coarsening process occurs as well as wild type and by keeping the heterochiasmy state, CO numbers in both sexes increase while CO interference decreases (B). In the absence of SC, zyp1, unlike the presence of SC ones, free diffusion of HEI10 occurs in the nucleoplasm and abolish the competition among foci to absorb the HEI10 and all initial foci grow continuously by capturing the HEI10. Hence, this results in a loss of crossover interference and heterochiasmy but an increase in the CO number (C). As it is expected, the combination HEI10 (OE) and zyp1 provides larger increases in CO number than zyp1 and HEI10 OE alone, and CO interference and heterochiasmy are abolished (D).
The coarsening model was initially proposed in C. elegans, whose HEI10 homolog ZHP-3 has a similar behavior (Bhalla et al., 2008; Zhang et al., 2018; Zhang et al., 2021). The SC also seems to have peculiar liquid-crystal properties that can facilitate long range signal transduction (Rog et al., 2017). Whether or not similar properties of the plant SC could help the progressive coarsening of HEI10 remains to be determined in plants.
The situation in mammals could be similar since the HEI10 accumulates at designed CO sites (Qiao et al., 2014). However, in mammals, the interference mechanism might not involve the HEI10 protein itself but rather its antagonist RNF212 SUMO ligase (Qiao et al., 2014; Rao et al., 2017). Indeed, in mammals, RNF212 seems to be the limiting factor for CO class I design since it has sequence variants explaining the recombination rate in human populations (Kong et al., 2008) and shows a sensitive dosage role during mouse meiotic recombination (Reynolds et al., 2013).
1.4 The SC/HEI10 coarsening could also explain the mechanisms of obligatory CO and CO homeostasis
The mechanism by which at least one CO is formed on each bivalent was recognized as essential to segregate the full set of homologous chromosomes during the meiotic reductional division (Darlington and Dark, 1932). This obligate CO was defined by Owen in 1949 (Owen, 1949). This ability to ensure CO (also known as CO insurance) on each bivalent can easily be integrated into the HEI10 coarsening model along the SC. The HEI10 coarsening model assumes that if enough HEI10 is deposited on a bivalent, it will produce at least one CO per bivalent explaining CO insurance.
The mechanism of CO homeostasis was defined by Martini et al., 2006 to explain a situation observed in budding yeast where the variation of the number of DSB (i.e., 80% reduction) does not significantly affect the final number of COs. In Arabidopsis, a 30%–40% reduction in DSB number leads to a proportionate though smaller reduction of CO number, affecting their distribution and redirecting them toward the telomeres (Xue et al., 2018). In maize though, it appears that CO homeostasis is somehow limited (Sidhu et al., 2015). Anyway, the coarsening HEI10 model could also account for CO homeostasis if we imagine that a DSB-independent limiting amount of expressed HEI10 per meiocyte limit the final number of the major class I CO. The large excess of DSB and SEI intermediates compared to the final CO number could explain how the bright and large HEI foci designing class I COs might not be affected by a change in the number of DSB and earlier SEI intermediates, as long as the number of these intermediates is at least the same as or exceeds the number of final COs.
1.5 Role of synapsis in interference, obligate CO and heterochiasmy
In species with different CO rates between male and female meiosis (heterochiasmy), the length of SC is particularly different as well. SC length also depends on chromosome size and the SC length is proportional to CO rate (Zickler and Kleckner, 2015).
As already mentioned, the ZYP1 is the central element of the SC. In contrast to the S cerevisiae, Zip1 homolog is considered a member of the ZMM pathway that is indispensable for synapsis and CO formation (Sym et al., 1993), the mutation of zyp1 in Arabidopsis and rice disprupts synapsis but allows the formation of a higher number of COs per meiosis (Wang et al., 2010; Capilla-Pérez et al., 2021; France et al., 2021). It appears that this uncoupling of SC and CO class I formation in plants fortunately allowed the role of the SC in the interference mechanism to be refined. In addition, plants do not have a bone fine pachytene checkpoint leading to apoptosis when bivalents cannot form (De Jaeger-Braet et al., 2022), allowing easier observations of the consequences of the zyp1 mutation than in animals or budding yeast. In the absence of zyp1, the number of COs increases, but interference, obligatory CO, and heterochiasmy are lost (Capilla-Pérez et al., 2021; France et al., 2021). In zyp1 mutants, the HEI10 foci are still formed despite the loss of the SC and the formation of bright HEI10 signals (Capilla-Pérez et al., 2021), suggesting that the HEI10 diffusion still occurs but in three dimensions in the nucleoplasm, instead of being canalyzed by the SC on each bivalent (Figure 2C). So when synapsis is lost, heterochiasmy is also lost. This data is interpreted as the fact that heterochiasmy does not rely on peculiar regulation of the RH machinery itself except the one imposed by the SC length. It is predicted that all the factors affecting longer SC length will increase CO rate; the one decreasing SC length will decrease CO rate.
The HEI10 coarsening model proposed by Morgan et al., 2021 also reliably accounts for these observed facts in male and female meiosis of Arabidopsis (Durand et al., 2022). The longer male SC can integrate more HEI10 and have a longer distance to space them out, leading to both a higher recombination rate and higher interference (Figure 2B). Finally the HEI10 coarsening model also predicted as well the combination of zyp1 with HEI10oe situations (Durand et al., 2022; Fozard et al., 2023) (Figure 2D). The situation is also observed in a new separation of function zyp1 allele (Yang S. et al., 2022). ASY1 also mediates CO insurance and interference (Lambing et al., 2020; Pochon et al., 2022). The effect of ASY1, one of the axial elements of the SC, on CO and interference might also be partly due to the absence of ZYP1 installation in this mutant.
A previous model known as the beam-film model has been extensively proposed to explain the interference mechanism by a redistribution of the mechanical stress that dissipates at the site of CO designation, impeding the formation of another CO nearby (Kleckner et al., 2004; Zhang et al., 2014a; Otto and Payseur, 2019). The beam-film model and the HEI coarsening model are possibly not exclusive and the mechanisms of HEI10 diffusion or coarsening could be influenced by a mechanical stress potentially perceived through SC remodeling (Lambing et al., 2020; Yang C. et al., 2022).
Most of the meiotic studies are still made in male meiocytes but immunolocalization techniques used to analyze female meiocytes have now been developed (Escobar-Guzmán et al., 2015; Gordillo et al., 2020) in order to better understand heterochiasmy and identify the basis of the regulation of the male and female SC length difference.
2 Conclusion
Forty years after the DSB repair model (Szostak et al., 1983), the molecular pathways and factors leading to the formation of CO have been described in various fungi, animal, and plant models. An integrated model for understanding the CO patterning at the whole genome level remained more difficult to establish. The model reviewed here requires the observed excess of DBS sites for correct pairing between homologs and synapsis. The excess SEI sites are also required for interference and the progressive design of COs along bivalents that lead to the restricted number of final COs. The SEI sites unused to form CO are still repaired and explain the presence of NCO.
In the model reviewed here, which was initially proposed in C. elegans by Zhang et al., 2021 as well as in Arabidopsis by Morgan et al., 2021 and refined by Durand et al., 2022 and Fozard et al., 2023, the role of the synaptonemal complex SC, an evolutionary well conserved structure only present during meiosis, is clearly assigned: it allows the HR machinery to work on the bivalent as a unit separated from the rest of the nucleoplasm. This explains how SC is tightly interconnected with the homologous recombination process during meiosis. The SC can therefore restrict the diffusion of important recombination regulators such as HEI10 on a linear basis and explain the observed coordination of mechanisms controlling CO insurance, homeostasis, and interference. In this model, heterochiasmy relies on different SC lengths, as observed cytologically in various species (Zickler and Kleckner, 1999).
Author contributions
NR wrote a draft of the manuscript and realized the figures. AR wrote the manuscript.
Funding
Relevant research in the AR lab was supported by a grant from the Mexican SEP-CONACYT. Ciencia Básica A1-S-8496 to AR. NR received a postdoctoral UNAM-DGAPA fellowship.
Acknowledgments
We acknowledge Patricia Rueda for their technical support and the plant meiotic community for their stimulating discussions. We thank the reviewer for their careful and insightful suggestions, which helped us to improve our manuscript.
Conflict of interest
The authors declare that the research was conducted in the absence of any commercial or financial relationships that could be construed as a potential conflict of interest.
Publisher’s note
All claims expressed in this article are solely those of the authors and do not necessarily represent those of their affiliated organizations, or those of the publisher, the editors and the reviewers. Any product that may be evaluated in this article, or claim that may be made by its manufacturer, is not guaranteed or endorsed by the publisher.
References
Agarwal, S., and Roeder, G. S. (2000). Zip3 provides a link between recombination enzymes and synaptonemal complex proteins. Cell 102 (2), 245–255. PMID: 10943844. doi:10.1016/s0092-8674(00)00029-5
Aklilu, B. B., Peurois, F., Saintomé, C., Culligan, K. M., Kobbe, D., Leasure, C., et al. (2020). Functional diversification of replication protein A paralogs and telomere length maintenance in Arabidopsis. Genetics 215 (4), 989–1002. Epub 2020 Jun 12. PMID: 32532801; PMCID: PMC7404240. doi:10.1534/genetics.120.303222
Akutsu, N., Iijima, K., Hinata, T., and Tauchi, H. (2007). Characterization of the plant homolog of Nijmegen breakage syndrome 1: Involvement in DNA repair and recombination. Biochem. Biophys. Res. Commun. 353 (2), 394–398. Epub 2006 Dec 13. PMID: 17182003. doi:10.1016/j.bbrc.2006.12.030
Allers, T., and Lichten, M. (2001). Differential timing and control of noncrossover and crossover recombination during meiosis. Cell 106 (1), 47–57. PMID: 11461701. doi:10.1016/s0092-8674(01)00416-0
Anderson, L. K., and Stack, S. M. (2005). Recombination nodules in plants. Cytogenet Genome Res. 109 (1-3), 198–204. doi:10.1159/000082400
Armstrong, S. J., Caryl, A. P., Jones, G. H., and Franklin, F. C. (2002). Asy1, a protein required for meiotic chromosome synapsis, localizes to axis-associated chromatin in Arabidopsis and Brassica. J. Cell Sci. 115 (18), 3645–3655. PMID: 12186950. doi:10.1242/jcs.00048
Barakate, A., Higgins, J. D., Vivera, S., Stephens, J., Perry, R. M., Ramsay, L., et al. (2014). The synaptonemal complex protein ZYP1 is required for imposition of meiotic crossovers in barley. Plant Cell 26 (2), 729–740. Epub 2014 Feb 21. PMID: 24563202; PMCID: PMC3967036. doi:10.1105/tpc.113.121269
Bauknecht, M., and Kobbe, D. (2014). AtGEN1 and AtSEND1, two paralogs in Arabidopsis, possess holliday junction resolvase activity. Plant Physiol. 166 (1), 202–216. Epub 2014 Jul 18. PMID: 25037209; PMCID: PMC4149707. doi:10.1104/pp.114.237834
Benyahya, F., Nadaud, I., Da Ines, O., Rimbert, H., White, C., and Sourdille, P. (2020). SPO11.2 is essential for programmed double-strand break formation during meiosis in bread wheat (Triticum aestivum L). Plant J. 104 (1), 30–43. doi:10.1111/tpj.14903
Berchowitz, L. E., and Copenhaver, G. P. (2010). Genetic interference: don't stand so close to me. Curr. Genomics 11 (2), 91–102. PMID: 20885817; PMCID: PMC2874225. doi:10.2174/138920210790886835
Berchowitz, L. E., Francis, K. E., Bey, A. L., and Copenhaver, G. P. (2007). The role of AtMUS81 in interference-insensitive crossovers in A. thaliana. PLoS Genet. 3 (8), e132. Epub 2007 Jun 26. PMID: 17696612; PMCID: PMC1941751. doi:10.1371/journal.pgen.0030132
Bhalla, N., Wynne, D. J., Jantsch, V., and Dernburg, A. F. (2008). ZHP-3 acts at crossovers to couple meiotic recombination with synaptonemal complex disassembly and bivalent formation in C. elegans. PLoS Genet. 4 (10), e1000235. doi:10.1371/journal.pgen.1000235
Birchler, J. A. (2015). Mendel, mechanism, models, marketing, and more. Cell 163 (1), 9–11. doi:10.1016/j.cell.2015.09.008
Bleuyard, J. Y., Gallego, M. E., Savigny, F., and White, C. I. (2005). Differing requirements for the Arabidopsis Rad51 paralogs in meiosis and DNA repair. Plant J. 41 (4), 533–545. PMID: 15686518. doi:10.1111/j.1365-313X.2004.02318.x
Bleuyard, J. Y., Gallego, M. E., and White, C. I. (2004). Meiotic defects in the Arabidopsis rad50 mutant point to conservation of the MRX complex function in early stages of meiotic recombination. Chromosoma 113 (4), 197–203. Epub 2004 Aug 10. PMID: 15309561. doi:10.1007/s00412-004-0309-1
Bleuyard, J. Y., and White, C. I. (2004). The Arabidopsis homologue of Xrcc3 plays an essential role in meiosis. EMBO J. 23 (2), 439–449. Epub 2004 Jan 15. PMID: 14726957; PMCID: PMC1271761. doi:10.1038/sj.emboj.7600055
Bolaños-Villegas, P., Yang, X., Wang, H. J., Juan, C. T., Chuang, M. H., Makaroff, C. A., et al. (2013). Arabidopsis CHROMOSOME TRANSMISSION FIDELITY 7 (AtCTF7/ECO1) is required for DNA repair, mitosis and meiosis. Plant J. 75 (6), 927–940. Epub 2013 Jul 20. PMID: 23750584; PMCID: PMC3824207. doi:10.1111/tpj.12261
Bolaños-Villegas, P. (2021). The role of structural maintenance of chromosomes complexes in meiosis and genome maintenance: Translating biomedical and model plant research into crop breeding opportunities. Front. Plant Sci. 12, 659558. PMID: 33868354; PMCID: PMC8044525. doi:10.3389/fpls.2021.659558
Capilla-Pérez, L., Durand, S., Hurel, A., Lian, Q., Chambon, A., Taochy, C., et al. (2021). The synaptonemal complex imposes crossover interference and heterochiasmy in Arabidopsis. Proc. Natl. Acad. Sci. U. S. A. 118 (12), e2023613118. doi:10.1073/pnas.2023613118
Chambon, A., West, A., Vezon, D., Horlow, C., De Muyt, A., Chelysheva, L., et al. (2018). Identification of ASYNAPTIC4, a component of the meiotic chromosome Axis. Plant Physiol. 178 (1), 233–246. Epub 2018 Jul 12. PMID: 30002256; PMCID: PMC6130017. doi:10.1104/pp.17.01725
Chang, Y., Gong, L., Yuan, W., Li, X., Chen, G., Li, X., et al. (2009). Replication protein A (RPA1a) is required for meiotic and somatic DNA repair but is dispensable for DNA replication and homologous recombination in rice. Plant Physiol. 151 (4), 2162–2173. Epub 2009 Oct 7. PMID: 19812186; PMCID: PMC2785997. doi:10.1104/pp.109.142877
Chelysheva, L., Diallo, S., Vezon, D., Gendrot, G., Vrielynck, N., Belcram, K., et al. (2005). AtREC8 and AtSCC3 are essential to the monopolar orientation of the kinetochores during meiosis. J. Cell Sci. 118 (20), 4621–4632. Epub 2005 Sep 21. PMID: 16176934. doi:10.1242/jcs.02583
Chelysheva, L., Gendrot, G., Vezon, D., Doutriaux, M. P., Mercier, R., and Grelon, M. (2007). Zip4/Spo22 is required for class I CO formation but not for synapsis completion in Arabidopsis thaliana. PLoS Genet. 3 (5), e83. PMID: 17530928; PMCID: PMC1877879. doi:10.1371/journal.pgen.0030083
Chelysheva, L., Vezon, D., Belcram, K., Gendrot, G., and Grelon, M. (2008). The Arabidopsis BLAP75/Rmi1 homologue plays crucial roles in meiotic double-strand break repair. PLoS Genet. 4 (12), e1000309. doi:10.1371/journal.pgen.1000309
Chelysheva, L., Vezon, D., Chambon, A., Gendrot, G., Pereira, L., Lemhemdi, A., et al. (2012). The Arabidopsis HEI10 is a new ZMM protein related to Zip3. PLoS Genet. 8 (7), e1002799. Epub 2012 Jul 26. PMID: 22844245; PMCID: PMC3405992. doi:10.1371/journal.pgen.1002799
Chen, H., He, C., Wang, C., Wang, X., Ruan, F., Yan, J., et al. (2021). RAD51 supports DMC1 by inhibiting the SMC5/6 complex during meiosis. Plant Cell 33 (8), 2869–2882. PMID: 34009315; PMCID: PMC8408460. doi:10.1093/plcell/koab136
Choi, K., Zhao, X., Tock, A. J., Lambing, C., Underwood, C. J., Hardcastle, T. J., et al. (2018). Nucleosomes and DNA methylation shape meiotic DSB frequency in Arabidopsis thaliana transposons and gene regulatory regions. Genome Res. 28 (4), 532–546. doi:10.1101/gr.225599.117
Chuang, Y. C., and Smith, G. R. (2023). Meiotic crossover interference: Methods of analysis and mechanisms of action. Curr. Top. Dev. Biol. 151, 217–244. Epub 2022 Aug 24. PMID: 36681471. doi:10.1016/bs.ctdb.2022.04.006
Coe, E., and Kass, L. B. (2005). Proof of physical exchange of genes on the chromosomes. Proc. Natl. Acad. Sci. U. S. A. 102 (19), 6641–6646. Epub 2005 May 2. PMID: 15867161; PMCID: PMC1100733. doi:10.1073/pnas.0407340102
Colas, I., Barakate, A., Macaulay, M., Schreiber, M., Stephens, J., Vivera, S., et al. (2019). desynaptic5 carries a spontaneous semi-dominant mutation affecting Disrupted Meiotic cDNA 1 in barley. J. Exp. Bot. 70 (10), 2683–2698. PMID: 31028386; PMCID: PMC6509107. doi:10.1093/jxb/erz080
Colas, I., Macaulay, M., Higgins, J. D., Phillips, D., Barakate, A., Posch, M., et al. (2016). A spontaneous mutation in MutL-Homolog 3 (HvMLH3) affects synapsis and crossover resolution in the barley desynaptic mutant des10. New Phytol. 212 (3), 693–707. doi:10.1111/nph.14061
Couteau, F., Belzile, F., Horlow, C., Grandjean, O., Vezon, D., and Doutriaux, M. P. (1999). Random chromosome segregation without meiotic arrest in both male and female meiocytes of a dmc1 mutant of Arabidopsis. Plant Cell 11 (9), 1623–1634. PMID: 10488231; PMCID: PMC144309. doi:10.1105/tpc.11.9.1623
Creighton, H. B., and McClintock, B. A. (1931). A correlation of cytological and genetical crossing-over in zea mays. Proc. Natl. Acad. Sci. U. S. A. 17 (8), 492–497. PMID: 16587654; PMCID: PMC1076098. doi:10.1073/pnas.17.8.492
Crismani, W., Girard, C., Froger, N., Pradillo, M., Santos, J. L., Chelysheva, L., et al. (2012). FANCM limits meiotic crossovers. Science 336 (6088), 1588–1590. PMID: 22723424. doi:10.1126/science.1220381
Crismani, W., Portemer, V., Froger, N., Chelysheva, L., Horlow, C., Vrielynck, N., et al. (2013). MCM8 is required for a pathway of meiotic double-strand break repair independent of DMC1 in Arabidopsis thaliana. PLoS Genet. 9 (1), e1003165. Epub 2013 Jan 3. PMID: 23300481; PMCID: PMC3536722. doi:10.1371/journal.pgen.1003165
Cuacos, M., Lambing, C., Pachon-Penalba, M., Osman, K., Armstrong, S. J., Henderson, I. R., et al. (2021). Meiotic chromosome axis remodelling is critical for meiotic recombination in Brassica rapa. J. Exp. Bot. 72 (8), 3012–3027. PMID: 33502451; PMCID: PMC8023211. doi:10.1093/jxb/erab035
Da Ines, O., Bazile, J., Gallego, M. E., and White, C. I. (2022). DMC1 attenuates RAD51-mediated recombination in Arabidopsis. PLoS Genet. 18 (8), e1010322. PMID: 36007010; PMCID: PMC9451096. doi:10.1371/journal.pgen.1010322
Da Ines, O., Michard, R., Fayos, I., Bastianelli, G., Nicolas, A., Guiderdoni, E., et al. (2020). Bread wheat TaSPO11-1 exhibits evolutionarily conserved function in meiotic recombination across distant plant species. Plant J. 103 (6), 2052–2068. Epub 2020 Jul 10. PMID: 32559326. doi:10.1111/tpj.14882
Darlington, C. D., and Dark, S. O. (1932). The origin and behaviour of chiasmata II. Stenobothrus parallelus. Cytol. (tokyo) 63, 169–185. doi:10.1508/cytologia.3.169
De Jaeger-Braet, J., Krause, L., Buchholz, A., and Schnittger, A. (2022). Heat stress reveals a specialized variant of the pachytene checkpoint in meiosis of Arabidopsis thaliana. Plant Cell 34 (1), 433–454. PMID: 34718750; PMCID: PMC8846176. doi:10.1093/plcell/koab257
de Massy, B. (2013). Initiation of meiotic recombination: How and where? Conservation and specificities among eukaryotes. Annu. Rev. Genet. 47, 563–599. PMID: 24050176. doi:10.1146/annurev-genet-110711-155423
De Muyt, A., Pereira, L., Vezon, D., Chelysheva, L., Gendrot, G., Chambon, A., et al. (2009). A high throughput genetic screen identifies new early meiotic recombination functions in Arabidopsis thaliana. PLoS Genet. 5 (9), e1000654. Epub 2009 Sep 18. PMID: 19763177; PMCID: PMC2735182. doi:10.1371/journal.pgen.1000654
De Muyt, A., Vezon, D., Gendrot, G., Gallois, J. L., Stevens, R., and Grelon, M. (2007). AtPRD1 is required for meiotic double strand break formation in Arabidopsis thaliana. EMBO J. 26 (18), 4126–4137. Epub 2007 Aug 30. PMID: 17762870; PMCID: PMC2230667. doi:10.1038/sj.emboj.7601815
De Muyt, A., Zhang, L., Piolot, T., Kleckner, N., Espagne, E., and Zickler, D. (2014). E3 ligase Hei10: A multifaceted structure-based signaling molecule with roles within and beyond meiosis. Genes Dev. 28 (10), 1111–1123. PMID: 24831702; PMCID: PMC4035539. doi:10.1101/gad.240408.114
Deng, Z. Y., and Wang, T. (2007). OsDMC1 is required for homologous pairing in Oryza sativa. Plant Mol. Biol. 65 (1-2), 31–42. Epub 2007 Jun 12. PMID: 17562186. doi:10.1007/s11103-007-9195-2
Desjardins, S. D., Ogle, D. E., Ayoub, M. A., Heckmann, S., Henderson, I. R., Edwards, K. J., et al. (2020). MutS homologue 4 and MutS homologue 5 maintain the obligate crossover in wheat despite stepwise gene loss following polyploidization. Plant Physiol. 183 (4), 1545–1558. Epub 2020 Jun 11. PMID: 32527734; PMCID: PMC7401138. doi:10.1104/pp.20.00534
Desjardins, S. D., Simmonds, J., Guterman, I., Kanyuka, K., Burridge, A. J., Tock, A. J., et al. (2022). FANCM promotes class I interfering crossovers and suppresses class II non-interfering crossovers in wheat meiosis. Nat. Commun. 13 (1), 3644. PMID: 35752733; PMCID: PMC9233680. doi:10.1038/s41467-022-31438-6
Dion, E., Li, L., Jean, M., and Belzile, F. (2007). An Arabidopsis MLH1 mutant exhibits reproductive defects and reveals a dual role for this gene in mitotic recombination. Plant J. 51 (3), 431–440. PMID: 17559505. doi:10.1111/j.1365-313X.2007.03145.x
Dray, E., Siaud, N., Dubois, E., and Doutriaux, M. P. (2006). Interaction between Arabidopsis Brca2 and its partners Rad51, Dmc1, and Dss1. Plant Physiol. 140 (3), 1059–1069. Epub 2006 Jan 13. PMID: 16415210; PMCID: PMC1400560. doi:10.1104/pp.105.075838
Durand, S., Lian, Q., Jing, J., Ernst, M., Grelon, M., Zwicker, D., et al. (2022). Joint control of meiotic crossover patterning by the synaptonemal complex and HEI10 dosage. Nat. Commun. 13 (1), 5999. PMID: 36224180; PMCID: PMC9556546. doi:10.1038/s41467-022-33472-w
Duroc, Y., Lemhemdi, A., Larchevêque, C., Hurel, A., Cuacos, M., Cromer, L., et al. (2014). The kinesin AtPSS1 promotes synapsis and is required for proper crossover distribution in meiosis. PLoS Genet. 10 (10), e1004674. PMID: 25330379; PMCID: PMC4199493. doi:10.1371/journal.pgen.1004674
Emmenecker, C., Mézard, C., and Kumar, R. (2022). Repair of DNA double-strand breaks in plant meiosis: Role of eukaryotic RecA recombinases and their modulators. Plant Reprod. 36, 17–41. Epub ahead of print. PMID: 35641832. doi:10.1007/s00497-022-00443-6
Escobar-Guzmán, R., Rodríguez-Leal, D., Vielle-Calzada, J. P., and Ronceret, A. (2015). Whole-mount immunolocalization to study female meiosis in Arabidopsis. Nat. Protoc. 10 (10), 1535–1542. Epub 2015 Sep 10. PMID: 26357009. doi:10.1038/nprot.2015.098
Farahani-Tafreshi, Y., Wei, C., Gan, P., Daradur, J., Riggs, C. D., and Hasenkampf, C. A. (2022). The Arabidopsis HOP2 gene has a role in preventing illegitimate connections between nonhomologous chromosome regions. Chromosome Res. 30 (1), 59–75. Epub 2022 Jan 22. PMID: 35064347. doi:10.1007/s10577-021-09681-2
Fayos, I., Meunier, A. C., Vernet, A., Navarro-Sanz, S., Portefaix, M., Lartaud, M., et al. (2020). Assessment of the roles of SPO11-2 and SPO11-4 in meiosis in rice using CRISPR/Cas9 mutagenesis. J. Exp. Bot. 71 (22), 7046–7058. PMID: 32842152. doi:10.1093/jxb/eraa391
Ferdous, M., Higgins, J. D., Osman, K., Lambing, C., Roitinger, E., Mechtler, K., et al. (2012). Inter-homolog crossing-over and synapsis in Arabidopsis meiosis are dependent on the chromosome axis protein AtASY3. PLoS Genet. 8 (2), e1002507. Epub 2012 Feb 2. PMID: 22319460; PMCID: PMC3271061. doi:10.1371/journal.pgen.1002507
Fernandes, J. B., Duhamel, M., Seguéla-Arnaud, M., Froger, N., Girard, C., Choinard, S., et al. (2018). FIGL1 and its novel partner FLIP form a conserved complex that regulates homologous recombination. PLoS Genet. 14 (4), e1007317. PMID: 29608566; PMCID: PMC5897033. doi:10.1371/journal.pgen.1007317
Fozard, J. A., Morgan, C., and Howard, M. (2023). Coarsening dynamics can explain meiotic crossover patterning in both the presence and absence of the synaptonemal complex. eLife 12, e79408. doi:10.7554/eLife.79408
France, M. G., Enderle, J., Röhrig, S., Puchta, H., Franklin, F. C. H., and Higgins, J. D. (2021). ZYP1 is required for obligate cross-over formation and cross-over interference in Arabidopsis. Proc. Natl. Acad. Sci. U. S. A. 118 (14), e2021671118. PMID: 33782125; PMCID: PMC8040812. doi:10.1073/pnas.2021671118
Fu, M., Wang, C., Xue, F., Higgins, J., Chen, M., Zhang, D., et al. (2016). The DNA topoisomerase VI-B subunit OsMTOPVIB is essential for meiotic recombination initiation in rice. Mol. Plant 9 (11), 1539–1541. Epub 2016 Jul 28. PMID: 27477684. doi:10.1016/j.molp.2016.07.006
Fu, R., Wang, C., Shen, H., Zhang, J., Higgins, J. D., and Liang, W. (2020). Rice OsBRCA2 is required for DNA double-strand break repair in meiotic cells. Front. Plant Sci. 11, 600820. PMID: 33304374; PMCID: PMC7701097. doi:10.3389/fpls.2020.600820
Geuting, V., Kobbe, D., Hartung, F., Dürr, J., Focke, M., and Puchta, H. (2009). Two distinct MUS81-EME1 complexes from Arabidopsis process Holliday junctions. Plant Physiol. 150 (2), 1062–1071. Epub 2009 Apr 1. PMID: 19339504; PMCID: PMC2689967. doi:10.1104/pp.109.136846
Girard, C., Chelysheva, L., Choinard, S., Froger, N., Macaisne, N., Lemhemdi, A., et al. (2015). AAA-ATPase FIDGETIN-LIKE 1 and helicase FANCM antagonize meiotic crossovers by distinct mechanisms. PLoS Genet. 11 (7), e1005369. doi:10.1371/journal.pgen.1005369
Golubovskaya, I. N., Hamant, O., Timofejeva, L., Wang, C. J., Braun, D., Meeley, R., et al. (2006). Alleles of afd1 dissect REC8 functions during meiotic prophase I. J. Cell Sci. 119 (16), 3306–3315. Epub 2006 Jul 25. PMID: 16868028. doi:10.1242/jcs.03054
Gordillo, S. V. G., Escobar-Guzman, R., Rodriguez-Leal, D., Vielle-Calzada, J. P., and Ronceret, A. (2020). Whole-Mount immunolocalization procedure for plant female meiocytes. Methods Mol. Biol. 2061, 13–24. PMID: 31583649. doi:10.1007/978-1-4939-9818-0_2
Gray, S., and Cohen, P. E. (2016). Control of meiotic crossovers: From double-strand break formation to designation. Annu. Rev. Genet. 23 (50), 175–210. Epub 2016 Sep 14. PMID: 27648641; PMCID: PMC5319444. doi:10.1146/annurev-genet-120215-035111
Grelon, M., Vezon, D., Gendrot, G., and Pelletier, G. (2001). AtSPO11-1 is necessary for efficient meiotic recombination in plants. EMBO J. 20 (3), 589–600. PMID: 11157765; PMCID: PMC133473. doi:10.1093/emboj/20.3.589
Gutiérrez Pinzón, Y., González Kise, J. K., Rueda, P., and Ronceret, A. (2021). The Formation of bivalents and the control of plant meiotic recombination. Front. Plant Sci. 12, 717423. PMID: 34557215; PMCID: PMC8453087. doi:10.3389/fpls.2021.717423
Haber, J. (2008). “Evolution of models of homologous recombination,” in Recombination and meiosis. Editors R. Egel, and D.-H. Lankenau (Berlin Heidelberg: Springer-Verlag), 1–64. Genome Dyn Stab (3)Published online: 22 December 2007. doi:10.1007/7050_2007_037/
Haldane, J. B. S. (1931). The cytological basis of genetical interference. Cytologia 3 (1), 54–65. doi:10.1508/cytologia.3.54
Hartung, F., Suer, S., and Puchta, H. (2007). Two closely related RecQ helicases have antagonistic roles in homologous recombination and DNA repair in Arabidopsis thaliana. Proc. Natl. Acad. Sci. U. S. A. 104 (47), 18836–18841. doi:10.1073/pnas.0705998104
Hartung, F., Suer, S., Knoll, A., Wurz-Wildersinn, R., and Puchta, H. (2008). Topoisomerase 3alpha and RMI1 suppress somatic crossovers and are essential for resolution of meiotic recombination intermediates in Arabidopsis thaliana. PLoS Genet. 4 (12), e1000285. doi:10.1371/journal.pgen.1000285
He, Y., Wang, M., Dukowic-Schulze, S., Zhou, A., Tiang, C. L., Shilo, S., et al. (2017). Genomic features shaping the landscape of meiotic double-strand-break hotspots in maize. Proc. Natl. Acad. Sci. U. S. A. 114 (46), 12231–12236. Epub 2017 Oct 30. PMID: 29087335; PMCID: PMC5699076. doi:10.1073/pnas.1713225114
Hernandez Sanchez-Rebato, M., Bouatta, A. M., Gallego, M. E., White, C. I., and Da Ines, O. (2021). RAD54 is essential for RAD51-mediated repair of meiotic DSB in Arabidopsis. PLoS Genet. 17 (5), e1008919. PMID: 34003859; PMCID: PMC8162660. doi:10.1371/journal.pgen.1008919
Higgins, J. D., Armstrong, S. J., Franklin, F. C., and Jones, G. H. (2004). The Arabidopsis MutS homolog AtMSH4 functions at an early step in recombination: Evidence for two classes of recombination in Arabidopsis. Genes Dev. 18 (20), 2557–2570. PMID: 15489296; PMCID: PMC529542. doi:10.1101/gad.317504
Higgins, J. D., Buckling, E. F., Franklin, F. C., and Jones, G. H. (2008a). Expression and functional analysis of AtMUS81 in Arabidopsis meiosis reveals a role in the second pathway of crossing-over. Plant J. 54 (1), 152–162. PMID: 18182028. doi:10.1111/j.1365-313X.2008.03403.x
Higgins, J. D., Ferdous, M., Osman, K., and Franklin, F. C. (2011). The RecQ helicase AtRECQ4A is required to remove inter-chromosomal telomeric connections that arise during meiotic recombination in Arabidopsis. Plant J. 65 (3), 492–502. Epub 2010 Dec 30. PMID: 21265901. doi:10.1111/j.1365-313X.2010.04438.x
Higgins, J. D., Sanchez-Moran, E., Armstrong, S. J., Jones, G. H., and Franklin, F. C. (2005). The Arabidopsis synaptonemal complex protein ZYP1 is required for chromosome synapsis and normal fidelity of crossing over. Genes Dev. 19 (20), 2488–2500. PMID: 16230536; PMCID: PMC1257403. doi:10.1101/gad.354705
Higgins, J. D., Vignard, J., Mercier, R., Pugh, A. G., Franklin, F. C., and Jones, G. H. (2008b). AtMSH5 partners AtMSH4 in the class I meiotic crossover pathway in Arabidopsis thaliana, but is not required for synapsis. Plant J. 55 (1), 28–39. doi:10.1111/j.1365-313X.2008.03470.x
Holloway, J. K., Booth, J., Edelmann, W., McGowan, C. H., and Cohen, P. E. (2008). MUS81 generates a subset of MLH1-MLH3-independent crossovers in mammalian meiosis. PLoS Genet. 4 (9), :e1000186. PMID: 18787696; PMCID: PMC2525838. doi:10.1371/journal.pgen.1000186
Hu, Q., Li, Y., Wang, H., Shen, Y., Zhang, C., Du, G., et al. (2017). Meiotic chromosome association 1 interacts with TOP3α and regulates meiotic recombination in rice. Plant Cell 29 (7), 1697–1708. Epub 2017 Jul 10. PMID: 28696221; PMCID: PMC5559755. doi:10.1105/tpc.17.00241
Huang, J., Cheng, Z., Wang, C., Hong, Y., Su, H., Wang, J., et al. (2015). Formation of interference-sensitive meiotic cross-overs requires sufficient DNA leading-strand elongation. Proc. Natl. Acad. Sci. U. S. A. 112 (40), 12534–12539. Epub 2015 Sep 21. PMID: 26392549; PMCID: PMC4603498. doi:10.1073/pnas.1507165112
Hyde, L., Osman, K., Winfield, M., Sanchez-Moran, E., Higgins, J. D., Henderson, I. R., et al. (2023). Identification, characterization, and rescue of CRISPR/Cas9 generated wheat SPO11-1 mutants. Plant Biotechnol. J. 21 (2), 405–418. Epub 2022 Dec 10. PMID: 36373224; PMCID: PMC9884015. doi:10.1111/pbi.13961
Jackson, N., Sanchez-Moran, E., Buckling, E., Armstrong, S. J., Jones, G. H., and Franklin, F. C. (2006). Reduced meiotic crossovers and delayed prophase I progression in AtMLH3-deficient Arabidopsis. EMBO J. 25 (6), 1315–1323. Epub 2006 Feb 9. PMID: 16467846; PMCID: PMC1422170. doi:10.1038/sj.emboj.7600992
Janssens, F. A. (1909). La Thorie de la Chiasmatypie. Nouvelle interprétation des cinèses de maturation. La Cell. 25, 389–411.
Ji, J., Tang, D., Wang, M., Li, Y., Zhang, L., Wang, K., et al. (2013). MRE11 is required for homologous synapsis and DSB processing in rice meiosis. Chromosoma 122 (5), 363–376. Epub 2013 Jun 22. PMID: 23793712. doi:10.1007/s00412-013-0421-1
Jiang, J., Ou, X., Han, D., He, Z., Liu, S., Mao, N., et al. (2022). A diRNA-protein scaffold module mediates SMC5/6 recruitment in plant DNA repair. Plant Cell. 27;34 (10), 3899–3914. doi:10.1093/plcell/koac191
Jing, J., Zhang, T., Wang, Y., Cui, Z., and He, Y. (2019). ZmRAD51C is essential for double-strand break repair and homologous recombination in maize meiosis. Int. J. Mol. Sci. 20 (21), 5513. PMID: 31694261; PMCID: PMC6861927. doi:10.3390/ijms20215513
Jing, J. L., Zhang, T., Kao, Y. H., Huang, T. H., Wang, C. R., and He, Y. (2020). ZmMTOPVIB enables DNA double-strand break formation and bipolar spindle assembly during maize meiosis. Plant Physiol. 184 (4), 1811–1822. Epub 2020 Oct 19. PMID: 33077613; PMCID: PMC7723106. doi:10.1104/pp.20.00933
Kang, H. A., Shin, H. C., Kalantzi, A. S., Toseland, C. P., Kim, H. M., Gruber, S., et al. (2015). Crystal structure of Hop2-Mnd1 and mechanistic insights into its role in meiotic recombination. Nucleic Acids Res. 43 (7), 3841–3856. Epub 2015 Mar 3. PMID: 25740648; PMCID: PMC4402518. doi:10.1093/nar/gkv172
Kerzendorfer, C., Vignard, J., Pedrosa-Harand, A., Siwiec, T., Akimcheva, S., Jolivet, S., et al. (2006). The Arabidopsis thaliana MND1 homologue plays a key role in meiotic homologous pairing, synapsis and recombination. J. Cell Sci. 119 (12), 2486–2496. PMID: 16763194. doi:10.1242/jcs.02967
Kim, J., Park, J., Kim, H., Son, N., Kim, E. J., Kim, J., et al. (2022). Arabidopsis HEAT SHOCK FACTOR BINDING PROTEIN is required to limit meiotic crossovers and HEI10 transcription. EMBO J. 41 (14), e109958. Epub 2022 Jun 7. PMID: 35670129; PMCID: PMC9289711. doi:10.15252/embj.2021109958
Kleckner, N., Zickler, D., Jones, G. H., Dekker, J., Padmore, R., Henle, J., et al. (2004). A mechanical basis for chromosome function. Proc. Natl. Acad. Sci. U. S. A. 101 (34), 12592–12597. Epub 2004 Aug 6. PMID: 15299144; PMCID: PMC515102. doi:10.1073/pnas.0402724101
Knoll, A., Fauser, F., and Puchta, H. (2014). DNA recombination in somatic plant cells: Mechanisms and evolutionary consequences. Chromosome Res. 22 (2), 191–201. PMID: 24788060. doi:10.1007/s10577-014-9415-y
Kobayashi, W., Liu, E., Ishii, H., Matsunaga, S., Schlögelhofer, P., and Kurumizaka, H. (2019). Homologous pairing activities of Arabidopsis thaliana RAD51 and DMC1. J. Biochem. 165 (3), 289–295. PMID: 30517709. doi:10.1093/jb/mvy105
Kong, A., Thorleifsson, G., Stefansson, H., Masson, G., Helgason, A., Gudbjartsson, D. F., et al. (2008). Sequence variants in the RNF212 gene associate with genome-wide recombination rate. Science 319 (5868), 1398–1401. Epub 2008 Jan 31. PMID: 18239089. doi:10.1126/science.1152422
Koszul, R., and Zickler, D. (2012). La theorie de la Chiasmatypie 1909. Genetics 191 (2), 319–346. doi:10.1534/genetics.112.139725
Ku, J. C., Ronceret, A., Golubovskaya, I., Lee, D. H., Wang, C., Timofejeva, L., et al. (2020). Dynamic localization of SPO11-1 and conformational changes of meiotic axial elements during recombination initiation of maize meiosis. PLoS Genet. 16 (4), e1007881. PMID: 32310948; PMCID: PMC7192515. doi:10.1371/journal.pgen.1007881
Kumar, R., Duhamel, M., Coutant, E., Ben-Nahia, E., and Mercier, R. (2019). Antagonism between BRCA2 and FIGL1 regulates homologous recombination. Nucleic Acids Res. 47 (10), 5170–5180. doi:10.1093/nar/gkz225
Kurzbauer, M. T., Pradillo, M., Kerzendorfer, C., Sims, J., Ladurner, R., Oliver, C., et al. (2018). Arabidopsis thaliana FANCD2 promotes meiotic crossover formation. Plant Cell 30 (2), 415–428. Epub 2018 Jan 19. PMID: 29352063; PMCID: PMC5868695. doi:10.1105/tpc.17.00745
Kurzbauer, M. T., Uanschou, C., Chen, D., and Schlögelhofer, P. (2012). The recombinases DMC1 and RAD51 are functionally and spatially separated during meiosis in Arabidopsis. Plant Cell 24 (5), 2058–2070. Epub 2012 May 15. PMID: 22589466; PMCID: PMC3442587. doi:10.1105/tpc.112.098459
Lake, C. M., Nielsen, R. J., Guo, F., Unruh, J. R., Slaughter, B. D., Hawley, R. S., et al. (2015). Vilya, a component of the recombination nodule, is required for meiotic double-strand break formation in Drosophila. Elife. 4, e08287. doi:10.7554/eLife.08287
Lam, W. S., Yang, X., and Makaroff, C. A. (2005). Characterization of Arabidopsis thaliana SMC1 and SMC3: Evidence that AtSMC3 may function beyond chromosome cohesion. J. Cell Sci. 118 (14), 3037–3048. Epub 2005 Jun 21. PMID: 15972315. doi:10.1242/jcs.02443
Lambing, C., Kuo, P., Kim, J., Osman, K., Whitbread, A. L., Yang, J., et al. (2022). Differentiated function and localisation of SPO11-1 and PRD3 on the chromosome axis during meiotic DSB formation in Arabidopsis thaliana. PLoS Genet. 18 (7), e1010298. PMID: 35857772; PMCID: PMC9342770. doi:10.1371/journal.pgen.1010298
Lambing, C., Kuo, P. C., Tock, A. J., Topp, S. D., and Henderson, I. R. (2020). ASY1 acts as a dosage-dependent antagonist of telomere-led recombination and mediates crossover interference in Arabidopsis. Proc. Natl. Acad. Sci. U. S. A. 117 (24), 13647–13658. Epub 2020 Jun 4. PMID: 32499315; PMCID: PMC7306779. doi:10.1073/pnas.1921055117
Lee, D. H., Kao, Y. H., Ku, J. C., Lin, C. Y., Meeley, R., Jan, Y. S., et al. (2015). The axial element protein DESYNAPTIC2 mediates meiotic double-strand break formation and synaptonemal complex assembly in maize. Plant Cell 27 (9), 2516–2529. Epub 2015 Aug 21. PMID: 26296964; PMCID: PMC4815100. doi:10.1105/tpc.15.00434
Li, M., Li, S., He, Y., Wang, Y., Zhang, T., Li, P., et al. (2022). ZmSPO11-2 is critical for meiotic recombination in maize. Chromosome Res. 30 (4), 415–428. Epub 2022 Jun 8. PMID: 35674907. doi:10.1007/s10577-022-09694-5
Li, X., Zhang, J., Huang, J., Xu, J., Chen, Z., Copenhaver, G. P., et al. (2021). Regulation of interference-sensitive crossover distribution ensures crossover assurance in Arabidopsis. Proc. Natl. Acad. Sci. U. S. A. 118 (47), e2107543118. PMID: 34795056; PMCID: PMC8617516. doi:10.1073/pnas.2107543118
Li, Y., Qin, B., Shen, Y., Zhang, F., Liu, C., You, H., et al. (2018). HEIP1 regulates crossover formation during meiosis in rice. Proc. Natl. Acad. Sci. U. S. A. 115 (42), 10810–10815. Epub 2018 Oct 1. PMID: 30275327; PMCID: PMC6196533. doi:10.1073/pnas.1807871115
Liu, K., Chen, E., Gu, Z., Dai, B., Wang, A., Zhu, Z., et al. (2022). A retrotransposon insertion in MUTL-HOMOLOG 1 affects wild rice seed set and cultivated rice crossover rate. Plant Physiol. 190 (3), 1747–1762. PMID: 35976143; PMCID: PMC9614510. doi:10.1093/plphys/kiac378
Liu, M., Shi, S., Zhang, S., Xu, P., Lai, J., Liu, Y., et al. (2014). SUMO E3 ligase AtMMS21 is required for normal meiosis and gametophyte development in Arabidopsis. BMC Plant Biol. 14, 153. PMID: 24893774; PMCID: PMC4189105. doi:10.1186/1471-2229-14-153
Liu, X., Cao, Y., Du, G., Zhang, C., Xu, M., Cheng, Z., et al. (2022). OsRAD51 plays a vital role in promoting homologous recombination in rice meiosis. Int. J. Mol. Sci. 23 (17), 9906. PMID: 36077304; PMCID: PMC9456343. doi:10.3390/ijms23179906
Lloyd, A. (2023). Crossover patterning in plants. Plant Reprod. 36 (1), 55–72. Epub 2022 Jul 14. PMID: 35834006; PMCID: PMC9957876. doi:10.1007/s00497-022-00445-4
Lu, J., Wang, C., Wang, H., Zheng, H., Bai, W., Lei, D., et al. (2020). OsMFS1/OsHOP2 complex participates in rice male and female development. Front. Plant Sci. 11, 518. PMID: 32499797; PMCID: PMC7243175. doi:10.3389/fpls.2020.00518
Luo, Q., Tang, D., Wang, M., Luo, W., Zhang, L., Qin, B., et al. (2013). The role of OsMSH5 in crossover formation during rice meiosis. Mol. Plant 6 (3), 729–742. Epub 2012 Dec 8. PMID: 23220939. doi:10.1093/mp/sss145
Macaisne, N., Novatchkova, M., Peirera, L., Vezon, D., Jolivet, S., Froger, N., et al. (2008). SHOC1, an XPF endonuclease-related protein, is essential for the formation of class I meiotic crossovers. Curr. Biol. 18 (18), 1432–1437. doi:10.1016/j.cub.2008.08.041
Mao, B., Zheng, W., Huang, Z., Peng, Y., Shao, Y., Liu, C., et al. (2021). Rice MutLγ, the MLH1-MLH3 heterodimer, participates in the formation of type I crossovers and regulation of embryo sac fertility. Plant Biotechnol. J. 19 (7), 1443–1455. Epub 2021 Feb 22. PMID: 33544956; PMCID: PMC8313138. doi:10.1111/pbi.13563
Martini, E., Diaz, R. L., Hunter, N., and Keeney, S. (2006). Crossover homeostasis in yeast meiosis. Cell 126 (2), 285–295. PMID: 16873061; PMCID: PMC1949389. doi:10.1016/j.cell.2006.05.044
Mendel, G. (1866). “Experiments in plant hybridization,” in Proceedings of the natural history society of brunn. (Translated by william bateson and corrected by roger blumberg). Available at: http://www.mendelweb.org/Mendel.html.
Mercier, R., Jolivet, S., Vezon, D., Huppe, E., Chelysheva, L., Giovanni, M., et al. (2005). Two meiotic crossover classes cohabit in Arabidopsis: One is dependent on MER3,whereas the other one is not. Curr. Biol. 15 (8), 692–701. doi:10.1016/j.cub.2005.02.056
Mercier, R., Mézard, C., Jenczewski, E., Macaisne, N., and Grelon, M. (2015). The molecular biology of meiosis in plants. Annu. Rev. Plant Biol. 66, 297–327. Epub 2014 Dec 1. PMID: 25494464. doi:10.1146/annurev-arplant-050213-035923
Mieulet, D., Aubert, G., Bres, C., Klein, A., Droc, G., Vieille, E., et al. (2018). Unleashing meiotic crossovers in crops. Nat. Plants 4 (12), 1010–1016. Epub 2018 Nov 26. PMID: 30478361. doi:10.1038/s41477-018-0311-x
Morgan, C., Fozard, J. A., Hartley, M., Henderson, I. R., Bomblies, K., and Howard, M. (2021). Diffusion-mediated HEI10 coarsening can explain meiotic crossover positioning in Arabidopsis. Nat. Commun. 12 (1), 4674. PMID: 34344879; PMCID: PMC8333306. doi:10.1038/s41467-021-24827-w
Morgan (1926). The theory of the gene. Yale University Press. Available at: http://www.esp.org/books/morgan/theory/facsimile/.
Mu, N., Li, Y., Li, S., Shi, W., Shen, Y., Yang, H., et al. (2022). MUS81 is required for atypical recombination intermediate resolution but not crossover designation in rice. New Phytol. 237, 2422–2434. Epub ahead of print. PMID: 36495065. doi:10.1111/nph.18668
Muller, H. J. (1916). The mechanism of crossing-over. II. IV. The manner of occurrence of crossing-over. Am. Nat. 50, 284–305. doi:10.1086/279541
Nageswaran, D. C., Kim, J., Lambing, C., Kim, J., Park, J., Kim, E. J., et al. (2021). HIGH CROSSOVER RATE1 encodes PROTEIN PHOSPHATASE X1 and restricts meiotic crossovers in Arabidopsis. Nat. Plants 7 (4), 452–467. Epub 2021 Apr 12. PMID: 33846593; PMCID: PMC7610654. doi:10.1038/s41477-021-00889-y
Nonomura, K., Nakano, M., Eiguchi, M., Suzuki, T., and Kurata, N. (2006). PAIR2 is essential for homologous chromosome synapsis in rice meiosis I. J. Cell Sci. 119 (2), 217–225. PMID: 16410547. doi:10.1242/jcs.02736
Olivier, M., Da Ines, O., Amiard, S., Serra, H., Goubely, C., White, C. I., et al. (2016). The structure-specific endonucleases MUS81 and SEND1 are essential for telomere stability in Arabidopsis. Plant Cell 28 (1), 74–86. Epub 2015 Dec 24. PMID: 26704385; PMCID: PMC4746687. doi:10.1105/tpc.15.00898
Orr, J. N., Waugh, R., and Colas, I. (2021). Ubiquitination in plant meiosis: Recent advances and high throughput methods. Front. Plant Sci. 12, 667314. PMID: 33897750; PMCID: PMC8058418. doi:10.3389/fpls.2021.667314
Osman, K., Algopishi, U., Higgins, J. D., Henderson, I. R., Edwards, K. J., Franklin, F. C. H., et al. (2021). Distal bias of meiotic crossovers in hexaploid bread wheat reflects spatio-temporal asymmetry of the meiotic program. Front. Plant Sci. 12, 631323. PMID: 33679846; PMCID: PMC7928317. doi:10.3389/fpls.2021.631323
Osman, K., Sanchez-Moran, E., Mann, S. C., Jones, G. H., and Franklin, F. C. (2009). Replication protein A (AtRPA1a) is required for class I crossover formation but is dispensable for meiotic DNA break repair. EMBO J. 28 (4), 394–404. Epub 2009 Jan 15. PMID: 19153602; PMCID: PMC2646153. doi:10.1038/emboj.2008.295
Otto, S. P., and Payseur, B. A. (2019). Crossover interference: Shedding light on the evolution of recombination. Annu. Rev. Genet. 53, 19–44. Epub 2019 Aug 20. PMID: 31430178; PMCID: PMC8715713. doi:10.1146/annurev-genet-040119-093957
Owen, A. R. G. (1949). A possible interpretation of the apparent interference across the centromere found by callan and montalenti in culex pipiens. Hered. (Edinb) 3, 357–367. doi:10.1038/hdy.1949.26
Pawlowski, W. P., Golubovskaya, I. N., and Cande, W. Z. (2003). Altered nuclear distribution of recombination protein RAD51 in maize mutants suggests the involvement of RAD51 in meiotic homology recognition. Plant Cell 15 (8), 1807–1816. PMID: 12897254; PMCID: PMC167171. doi:10.1105/tpc.012898
Pazhayam, N. M., Turcotte, C. A., and Sekelsky, J. (2021). Meiotic crossover patterning. Front. Cell Dev. Biol. 9, 681123. PMID: 34368131; PMCID: PMC8344875. doi:10.3389/fcell.2021.681123
Pfander, B., and Matos, J. (2017). Control of Mus81 nuclease during the cell cycle. FEBS Lett. 591 (14), 2048–2056. Epub 2017 Jul 10. PMID: 28640495. doi:10.1002/1873-3468.12727
Pochon, G., Henry, I. M., Yang, C., Lory, N., Fernández-Jiménez, N., Böwer, F., et al. (2022). The Arabidopsis Hop1 homolog ASY1 mediates cross-over assurance and interference. PNAS Nexus 2022, pgac302. doi:10.1093/pnasnexus/pgac302
Pradillo, M., Knoll, A., Oliver, C., Varas, J., Corredor, E., Puchta, H., et al. (2015). Involvement of the cohesin cofactor PDS5 (SPO76) during meiosis and DNA repair in Arabidopsis thaliana. Front. Plant Sci. 1 (6), 1034. PMID: 26648949; PMCID: PMC4664637. doi:10.3389/fpls.2015.01034
Pradillo, M., López, E., Linacero, R., Romero, C., Cuñado, N., Sánchez-Morán, E., et al. (2012). Together yes, but not coupled: New insights into the roles of RAD51 and DMC1 in plant meiotic recombination. Plant J. 69 (6), 921–933. Epub 2011 Dec 15. PMID: 22066484. doi:10.1111/j.1365-313X.2011.04845.x
Puchta, H. (2005). The repair of double-strand breaks in plants: Mechanisms and consequences for genome evolution. J. Exp. Bot. 56 (409), 1–14. Epub 2004 Nov 22. PMID: 15557293. doi:10.1093/jxb/eri025
Puizina, J., Siroky, J., Mokros, P., Schweizer, D., and Riha, K. (2004). Mre11 deficiency in Arabidopsis is associated with chromosomal instability in somatic cells and Spo11-dependent genome fragmentation during meiosis. Plant Cell 16 (8), 1968–1978. Epub 2004 Jul 16. PMID: 15258261; PMCID: PMC519189. doi:10.1105/tpc.104.022749
Qiao, H., Prasada Rao, H. B., Yang, Y., Fong, J. H., Cloutier, J. M., Deacon, D. C., et al. (2014). Antagonistic roles of ubiquitin ligase HEI10 and SUMO ligase RNF212 regulate meiotic recombination. Nat. Genet. 46 (2), 194–199. Epub 2014 Jan 5. PMID: 24390283; PMCID: PMC4356240. doi:10.1038/ng.2858
Rao, H. B., Qiao, H., Bhatt, S. K., Bailey, L. R., Tran, H. D., Bourne, S. L., et al. (2017). A SUMO-ubiquitin relay recruits proteasomes to chromosome axes to regulate meiotic recombination. Science. 355 (6323), 403–407. Epub 2017 Jan 5. PMID: 28059716; PMCID: PMC5569317. doi:10.1126/science.aaf6407
Ren, L., Zhao, T., Zhao, Y., Du, G., Yang, S., Mu, N., et al. (2021). The E3 ubiquitin ligase DESYNAPSIS1 regulates synapsis and recombination in rice meiosis. Cell Rep. 37 (5), 109941. doi:10.1016/j.celrep.2021.109941
Ren, Y., Chen, D., Li, W., Zhou, D., Luo, T., Yuan, G., et al. (2019). OsSHOC1 and OsPTD1 are essential for crossover formation during rice meiosis. Plant J. 98 (2), 315–328. Epub 2019 Feb 12. PMID: 30589140. doi:10.1111/tpj.14214
Resnick, M. A. (1976). The repair of double-strand breaks in DNA; a model involving recombination. J. Theor. Biol. 59 (1), 97–106. doi:10.1016/s0022-5193(76)80025-2
Rey, M. D., Martín, A. C., Higgins, J., Swarbreck, D., Uauy, C., Shaw, P., et al. (2017). Exploiting the ZIP4 homologue within the wheat Ph1 locus has identified two lines exhibiting homoeologous crossover in wheat-wild relative hybrids. Mol. Breed. 37 (8), 95. Epub 2017 Jul 18. PMID: 28781573; PMCID: PMC5515957. doi:10.1007/s11032-017-0700-2
Reynolds, A., Qiao, H., Yang, Y., Chen, J. K., Jackson, N., Biswas, K., et al. (2013). RNF212 is a dosage-sensitive regulator of crossing-over during mammalian meiosis. Nat. Genet. 45 (3), 269–278. Epub 2013 Feb 10. PMID: 23396135; PMCID: PMC4245152. doi:10.1038/ng.2541
Rog, O., Köhler, S., and Dernburg, A. F. (2017). The synaptonemal complex has liquid crystalline properties and spatially regulates meiotic recombination factors. Elife 6, e21455. doi:10.7554/eLife.21455
Ronceret, A., Guilleminot, J., Lincker, F., Gadea-Vacas, J., Delorme, V., Bechtold, N., et al. (2005). Genetic analysis of two Arabidopsis DNA polymerase epsilon subunits during early embryogenesis. Plant J. 44 (2), 223–236. doi:10.1111/j.1365-313X.2005.02521.x
Ronceret, A., and Pawlowski, W. P. (2010). Chromosome dynamics in meiotic prophase I in plants. Cytogenet Genome Res. 129 (1-3), 173–183. Epub 2010 Jun 10. PMID: 20551605. doi:10.1159/000313656
Saito, T. T., and Colaiácovo, M. P. (2019). Regulation of crossover frequency and distribution during meiotic recombination. Cold Spring Harb. Symp. Quant. Biol. 82, 223–234. doi:10.1101/sqb.2017.82.034132
Sanchez-Moran, E., Santos, J. L., Jones, G. H., and Franklin, F. C. (2007). ASY1 mediates AtDMC1-dependent interhomolog recombination during meiosis in Arabidopsis. Genes Dev. 21 (17), 2220–2233. PMID: 17785529; PMCID: PMC1950860. doi:10.1101/gad.439007
Sarens, M., Copenhaver, G. P., and De Storme, N. (2021). The role of chromatid interference in determining meiotic crossover patterns. Front. Plant Sci. 12, 656691. PMID: 33767725; PMCID: PMC7985435. doi:10.3389/fpls.2021.656691
Séguéla-Arnaud, M., Crismani, W., Larchevêque, C., Mazel, J., Froger, N., Choinard, S., et al. (2015). Multiple mechanisms limit meiotic crossovers: TOP3α and two BLM homologs antagonize crossovers in parallel to FANCM. Proc. Natl. Acad. Sci. U. S. A. 112 (15), 4713–4718. Epub 2015 Mar 30. PMID: 25825745; PMCID: PMC4403193. doi:10.1073/pnas.1423107112
Séguéla-Arnaud, M., Choinard, S., Larchevêque, C., Girard, C., Froger, N., Crismani, W., et al. (2017). RMI1 and TOP3α limit meiotic CO formation through their C-terminal domains. Nucleic Acids Res. 45 (4), 1860–1871. doi:10.1093/nar/gkw1210
Serra, H., Lambing, C., Griffin, C. H., Topp, S. D., Nageswaran, D. C., Underwood, C. J., et al. (2018). Massive crossover elevation via combination of HEI10 and recq4a recq4b during Arabidopsis meiosis. Proc. Natl. Acad. Sci. U. S. A. 115 (10), 2437–2442. Epub 2018 Feb 20. PMID: 29463699; PMCID: PMC5877939. doi:10.1073/pnas.1713071115
Shao, T., Tang, D., Wang, K., Wang, M., Che, L., Qin, B., et al. (2011). OsREC8 is essential for chromatid cohesion and metaphase I monopolar orientation in rice meiosis. Plant Physiol. 156 (3), 1386–1396. Epub 2011 May 23. PMID: 21606318; PMCID: PMC3135945. doi:10.1104/pp.111.177428
Shen, Y., Tang, D., Wang, K., Wang, M., Huang, J., Luo, W., et al. (2012). ZIP4 in homologous chromosome synapsis and crossover formation in rice meiosis. J. Cell Sci. 125 (11), 2581–2591. Epub 2012 Mar 5. PMID: 22393242. doi:10.1242/jcs.090993
Shi, W., Ji, J., Xue, Z., Zhang, F., Miao, Y., Yang, H., et al. (2021). PRD1, a homologous recombination initiation factor, is involved in spindle assembly in rice meiosis. New Phytol. 230 (2), 585–600. Epub 2021 Feb 10. PMID: 33421144. doi:10.1111/nph.17178
Shi, W., Tang, D., Shen, Y., Xue, Z., Zhang, F., Zhang, C., et al. (2019). OsHOP2 regulates the maturation of crossovers by promoting homologous pairing and synapsis in rice meiosis. New Phytol. 222 (2), 805–819. Epub 2019 Jan 25. PMID: 30584664. doi:10.1111/nph.15664
Shingu, Y., Tokai, T., Agawa, Y., Toyota, K., Ahamed, S., Kawagishi-Kobayashi, M., et al. (2012). The double-stranded break-forming activity of plant SPO11s and a novel rice SPO11 revealed by a Drosophila bioassay. BMC Mol. Biol. 13, 1. PMID: 22248237; PMCID: PMC3273433. doi:10.1186/1471-2199-13-1
Sidhu, G. K., Fang, C., Olson, M. A., Falque, M., Martin, O. C., and Pawlowski, W. P. (2015). Recombination patterns in maize reveal limits to crossover homeostasis. Proc. Natl. Acad. Sci. U. S. A. 112 (52), 15982–15987. Epub 2015 Dec 14. PMID: 26668366; PMCID: PMC4703008. doi:10.1073/pnas.1514265112
Singh, A., Sharma, A., Singh, N., and Nandi, A. K. (2022). MTO1-RESPONDING DOWN 1 (MRD1) is a transcriptional target of OZF1 for promoting salicylic acid-mediated defense in Arabidopsis. Plant Cell Rep. 41 (5), 1319–1328. Epub 2022 Mar 24. PMID: 35325291. doi:10.1007/s00299-022-02861-2
Singh, D. K., Gamboa, R. S., Singh, A. K., Walkemeier, B., Van Leene, J., De Jaeger, G., et al. (2023). The FANCC-FANCE-FANCF complex is evolutionarily conserved and regulates meiotic recombination. Nucleic Acids Res. 51, 2516–2528. Epub ahead of print. PMID: 36652992. doi:10.1093/nar/gkac1244
Sprink, T., and Hartung, F. (2021). Heterologous complementation of SPO11-1 and -2 depends on the splicing pattern. Int. J. Mol. Sci. 22 (17), 9346. PMID: 34502253; PMCID: PMC8430568. doi:10.3390/ijms22179346
Sprink, T., and Hartung, F. (2014). The splicing fate of plant SPO11 genes. Front. Plant Sci. 5, 214. PMID: 25018755; PMCID: PMC4071758. doi:10.3389/fpls.2014.00214
Stacey, N. J., Kuromori, T., Azumi, Y., Roberts, G., Breuer, C., Wada, T., et al. (2006). Arabidopsis SPO11-2 functions with SPO11-1 in meiotic recombination. Plant J. 48 (2), 206–216. doi:10.1111/j.1365-313X.2006.02867.x
Steckenborn, S., Cuacos, M., Ayoub, M. A., Feng, C., Schubert, V., Hoffie, I., et al. (2023). The meiotic topoisomerase VI B subunit (MTOPVIB) is essential for meiotic DNA double-strand break formation in barley (Hordeum vulgare L). Plant Reprod. 36 (1), 1–15. doi:10.1007/s00497-022-00444-5
Sturtevant (1965). A history of genetics. Cold Spring Harbor Laboratory Press. Available at: www.esp.org/books/sturt/history.
Sturtevant, A. H. (1913). The linear arrangement of six sex-linked factors in Drosophila, as shown by their mode of association. J. Exp. Zool. 14, 43–59. doi:10.1002/jez.1400140104
Su, H., Cheng, Z., Huang, J., Lin, J., Copenhaver, G. P., Ma, H., et al. (2017). Arabidopsis RAD51, RAD51C and XRCC3 proteins form a complex and facilitate RAD51 localization on chromosomes for meiotic recombination. PLoS Genet. 13 (5), e1006827. PMID: 28562599; PMCID: PMC5470734. doi:10.1371/journal.pgen.1006827
Sun, L., Wang, J., Sang, M., Jiang, L., Zhao, B., Cheng, T., et al. (2017). Landscaping crossover interference across a genome. Trends Plant Sci. 22 (10), 894–907. Epub 2017 Aug 16. PMID: 28822625. doi:10.1016/j.tplants.2017.06.008
Sym, M., Engebrecht, J. A., and Roeder, G. S. (1993). ZIP1 is a synaptonemal complex protein required for meiotic chromosome synapsis. Cell 72 (3), 365–378. PMID: 7916652. doi:10.1016/0092-8674(93)90114-6
Szostak, J. W., Orr-Weaver, T. L., Rothstein, R. J., and Stahl, F. W. (1983). The double-strand-break repair model for recombination. Cell 33 (1), 25–35. PMID:6380756. doi:10.1016/0092-8674(83)90331-8
Szurman-Zubrzycka, M., Baran, B., Stolarek-Januszkiewicz, M., Kwaśniewska, J., Szarejko, I., and Gruszka, D. (2019). The dmc1 mutant allows an insight into the DNA double-strand break repair during meiosis in barley (Hordeum vulgare L). Front. Plant Sci. 10, 761. doi:10.3389/fpls.2019.00761
Tock, A. J., Holland, D. M., Jiang, W., Osman, K., Sanchez-Moran, E., Higgins, J. D., et al. (2021). Crossover-active regions of the wheat genome are distinguished by DMC1, the chromosome axis, H3K27me3, and signatures of adaptation. Genome Res. 31 (9), 1614–1628. Epub 2021 Aug 23. PMID: 34426514; PMCID: PMC8415368. doi:10.1101/gr.273672.120
Uanschou, C., Ronceret, A., Von Harder, M., De Muyt, A., Vezon, D., Pereira, L., et al. (2013). Sufficient amounts of functional HOP2/MND1 complex promote interhomolog DNA repair but are dispensable for intersister DNA repair during meiosis in Arabidopsis. Plant Cell 25 (12), 4924–4940. Epub 2013 Dec 20. PMID: 24363313; PMCID: PMC3903996. doi:10.1105/tpc.113.118521
Uanschou, C., Siwiec, T., Pedrosa-Harand, A., Kerzendorfer, C., Sanchez-Moran, E., Novatchkova, M., et al. (2007). A novel plant gene essential for meiosis is related to the human CtIP and the yeast COM1/SAE2 gene. EMBO J. 26 (24), 5061–5070. Epub 2007 Nov 15. PMID: 18007598; PMCID: PMC2140101. doi:10.1038/sj.emboj.7601913
Vignard, J., Siwiec, T., Chelysheva, L., Vrielynck, N., Gonord, F., Armstrong, S. J., et al. (2007). The interplay of RecA-related proteins and the MND1-HOP2 complex during meiosis in Arabidopsis thaliana. PLoS Genet. 3 (10), 1894–1906. Epub 2007 Aug 30. PMID: 17937504; PMCID: PMC2014788. doi:10.1371/journal.pgen.0030176
Vrielynck, N., Chambon, A., Vezon, D., Pereira, L., Chelysheva, L., De Muyt, A., et al. (2016). A DNA topoisomerase VI-like complex initiates meiotic recombination. Science 351 (6276), 939–943. PMID: 26917763. doi:10.1126/science.aad5196
Vrielynck, N., Schneider, K., Rodriguez, M., Sims, J., Chambon, A., Hurel, A., et al. (2021). Conservation and divergence of meiotic DNA double strand break forming mechanisms in Arabidopsis thaliana. Nucleic Acids Res. 49 (17), 9821–9835. PMID: 34458909; PMCID: PMC8464057. doi:10.1093/nar/gkab715
Vu, G. T. H., Cao, H. X., Fauser, F., Reiss, B., Puchta, H., and Schubert, I. (2017). Endogenous sequence patterns predispose the repair modes of CRISPR/Cas9-induced DNA double-stranded breaks in Arabidopsis thaliana. Plant J. 92 (1), 57–67. Epub 2017 Aug 14. PMID: 28696528. doi:10.1111/tpj.13634
Wang, C., Higgins, J. D., He, Y., Lu, P., Zhang, D., and Liang, W. (2017). Resolvase OsGEN1 mediates DNA repair by homologous recombination. Plant Physiol. 173 (2), 1316–1329. Epub 2017 Jan 3. PMID: 28049740; PMCID: PMC5291025. doi:10.1104/pp.16.01726
Wang, C., Huang, J., Li, Y., Zhang, J., He, C., Li, T., et al. (2022b). DNA polymerase epsilon binds histone H3.1-H4 and recruits MORC1 to mediate meiotic heterochromatin condensation. Proc. Natl. Acad. Sci. U. S. A. 119 (43), e2213540119. Epub 2022 Oct 19. PMID: 36260743; PMCID: PMC9618065. doi:10.1073/pnas.2213540119
Wang, C., Huang, J., Zhang, J., Wang, H., Han, Y., Copenhaver, G. P., et al. (2019). The largest subunit of DNA polymerase delta is required for normal formation of meiotic type I crossovers. Plant Physiol. 179 (2), 446–459. Epub 2018 Nov 20. PMID: 30459265; PMCID: PMC6426404. doi:10.1104/pp.18.00861
Wang, C., Huang, J., Zhang, J., Yu, Y., Copenhaver, G. P., You, C., et al. (2022a). DNA polymerase epsilon interacts with SUVH2/9 to repress the expression of genes associated with meiotic DSB hotspot in Arabidopsis. Proc. Natl. Acad. Sci. U. S. A. 119 (41), e2208441119. Epub 2022 Oct 3. PMID: 36191225; PMCID: PMC9564942. doi:10.1073/pnas.2208441119
Wang, C., Qu, S., Zhang, J., Fu, M., Chen, X., and Liang, W. (2023). OsPRD2 is essential for double-strand break formation, but not spindle assembly during rice meiosis. Front. Plant Sci. 13, 1122202. PMID: 36714725; PMCID: PMC9880466. doi:10.3389/fpls.2022.1122202
Wang, H., Hu, Q., Tang, D., Liu, X., Du, G., Shen, Y., et al. (2016). OsDMC1 is not required for homologous pairing in rice meiosis. Plant Physiol. 171 (1), 230–241. Epub 2016 Mar 9. PMID: 26960731; PMCID: PMC4854709. doi:10.1104/pp.16.00167
Wang, H., Xu, W., Sun, Y., Lian, Q., Wang, C., Yu, C., et al. (2020). The cohesin loader SCC2 contains a PHD finger that is required for meiosis in land plants. PLoS Genet. 16 (6), e1008849. PMID: 32516352; PMCID: PMC7304647. doi:10.1371/journal.pgen.1008849
Wang, K., Tang, D., Wang, M., Lu, J., Yu, H., Liu, J., et al. (2009). MER3 is required for normal meiotic crossover formation, but not for presynaptic alignment in rice. J. Cell Sci. 122 (12), 2055–2063. Epub 2009 May 26. PMID: 19470578. doi:10.1242/jcs.049080
Wang, K., Wang, M., Tang, D., Shen, Y., Miao, C., Hu, Q., et al. (2012). The role of rice HEI10 in the formation of meiotic crossovers. PLoS Genet. 8 (7), e1002809. Epub 2012 Jul 5. PMID: 22792078; PMCID: PMC3390396. doi:10.1371/journal.pgen.1002809
Wang, K., Wang, M., Tang, D., Shen, Y., Qin, B., Li, M., et al. (2011). PAIR3, an axis-associated protein, is essential for the recruitment of recombination elements onto meiotic chromosomes in rice. Mol. Biol. Cell 22 (1), 12–19. Epub 2010 Nov 30. PMID: 21119003; PMCID: PMC3016970. doi:10.1091/mbc.E10-08-0667
Wang, M., Wang, K., Tang, D., Wei, C., Li, M., Shen, Y., et al. (2010). The central element protein ZEP1 of the synaptonemal complex regulates the number of crossovers during meiosis in rice. Plant Cell 22 (2), 417–430. Epub 2010 Feb 12. PMID: 20154151; PMCID: PMC2845403. doi:10.1105/tpc.109.070789
Wang, Y., Cheng, Z., Huang, J., Shi, Q., Hong, Y., Copenhaver, G. P., et al. (2012). The DNA replication factor RFC1 is required for interference-sensitive meiotic crossovers in Arabidopsis thaliana. PLoS Genet. 8 (11), e1003039. Epub 2012 Nov 8. PMID: 23144629; PMCID: PMC3493451. doi:10.1371/journal.pgen.1003039
Wang, Y., and Copenhaver, G. P. (2018). Meiotic recombination: Mixing it up in plants. Annu. Rev. Plant Biol. 69, 577–609. Epub 2018 Feb 28. PMID: 29489392. doi:10.1146/annurev-arplant-042817-040431
Wang, Y., Jiang, L., Zhang, T., Jing, J., and He, Y. (2018). ZmCom1 is required for both mitotic and meiotic recombination in maize. Front. Plant Sci. 9, 1005. PMID: 30061907; PMCID: PMC6055016. doi:10.3389/fpls.2018.01005
Wang, Y., Li, S. Y., Wang, Y. Z., and He, Y. (2023). ZmASY1 interacts with ZmPRD3 and is crucial for meiotic double-strand break formation in maize. New Phytol. 237 (2), 454–470. 18528. Epub 2022 Nov 30. PMID: 36221195. doi:10.1111/nph.18528
Wang, Y., Wang, Y., Zang, J., Chen, H., and He, Y. (2022). ZmPRD1 is essential for double-strand break formation, but is not required for bipolar spindle assembly during maize meiosis. J. Exp. Bot. 73 (11), 3386–3400. doi:10.1093/jxb/erac075
Ward, J. O., Reinholdt, L. G., Motley, W. W., Niswander, L. M., Deacon, D. C., Griffin, L. B., et al. (2007). Mutation in mouse hei10, an e3 ubiquitin ligase, disrupts meiotic crossing over. PLoS Genet. 3 (8), e139. Epub 2007 Jul 6. PMID: 17784788; PMCID: PMC1959360. doi:10.1371/journal.pgen.0030139
Watanabe, K., Pacher, M., Dukowic, S., Schubert, V., Puchta, H., and Schubert, I. (2009). The STRUCTURAL MAINTENANCE OF CHROMOSOMES 5/6 complex promotes sister chromatid alignment and homologous recombination after DNA damage in Arabidopsis thaliana. Plant Cell 21 (9), 2688–2699. Epub 2009 Sep 8. PMID: 19737979; PMCID: PMC2768936. doi:10.1105/tpc.108.060525
Waterworth, W. M., Altun, C., Armstrong, S. J., Roberts, N., Dean, P. J., Young, K., et al. (2007). NBS1 is involved in DNA repair and plays a synergistic role with ATM in mediating meiotic homologous recombination in plants. Plant J. 52 (1), 41–52. Epub 2007 Aug 2. PMID: 17672843. doi:10.1111/j.1365-313X.2007.03220.x
Whitbread, A. L., Dorn, A., Röhrig, S., and Puchta, H. (2021). Different functional roles of RTR complex factors in DNA repair and meiosis in Arabidopsis and tomato. Plant J. 106 (4), 965–977. Epub 2021 Mar 25. PMID: 33619799. doi:10.1111/tpj.15211
Wijeratne, A. J., Chen, C., Zhang, W., Timofejeva, L., and Ma, H. (2006). The Arabidopsis thaliana PARTING DANCERS gene encoding a novel protein is required for normal meiotic homologous recombination. Mol. Biol. Cell 17 (3), 1331–1343. Epub 2006 Jan 4. PMID: 16394097; PMCID: PMC1382321. doi:10.1091/mbc.e05-09-0902
Xue, M., Wang, J., Jiang, L., Wang, M., Wolfe, S., Pawlowski, W. P., et al. (2018). The number of meiotic double-strand breaks influences crossover distribution in Arabidopsis. Plant Cell 30 (10), 2628–2638. Epub 2018 Oct 3. PMID: 30282794; PMCID: PMC6241269. doi:10.1105/tpc.18.00531
Xue, Z., Liu, C., Shi, W., Miao, Y., Shen, Y., Tang, D., et al. (2019). OsMTOPVIB is required for meiotic bipolar spindle assembly. Proc. Natl. Acad. Sci. U. S. A. 116 (32), 15967–15972. Epub 2019 Jul 24. PMID: 31341087; PMCID: PMC6689953. doi:10.1073/pnas.1821315116
Yadav, V. K., and Claeys Bouuaert, C. (2021). Mechanism and control of meiotic DNA double-strand break formation in S. cerevisiae. Front. Cell Dev. Biol. 9, 642737. PMID: 33748134; PMCID: PMC7968521. doi:10.3389/fcell.2021.642737
Yang, C., Sofroni, K., Hamamura, Y., Hu, B., Elbasi, H. T., Balboni, M., et al. (2022). ZYP1-mediated recruitment of PCH2 to the synaptonemal complex remodels the chromosome axis leading to crossover restriction. Nucleic Acids Res. 50 (22), 12924–12937. PMID: 36504011; PMCID: PMC9825157. doi:10.1093/nar/gkac1160
Yang, S., Zhang, C., Cao, Y., Du, G., Tang, D., Li, Y., et al. (2022). FIGNL1 inhibits non-homologous chromosome association and crossover formation. Front. Plant Sci. 11 (13), 945893. PMID: 35898226; PMCID: PMC9310568. doi:10.3389/fpls.2022.945893
Zhang, B., Wang, M., Tang, D., Li, Y., Xu, M., Gu, M., et al. (2015). XRCC3 is essential for proper double-strand break repair and homologous recombination in rice meiosis. J. Exp. Bot. 66 (19), 5713–5725. Epub 2015 Jun 1. PMID: 26034131. doi:10.1093/jxb/erv253
Zhang, C., Song, Y., Cheng, Z. H., Wang, Y. X., Zhu, J., Ma, H., et al. (2012). The Arabidopsis thaliana DSB formation (AtDFO) gene is required for meiotic double-strand break formation. Plant J. 72 (2), 271–281. Epub 2012 Jul 26. PMID: 22694475. doi:10.1111/j.1365-313X.2012.05075.x
Zhang, L., Köhler, S., Rillo-Bohn, R., and Dernburg, A. F. (2018). A compartmentalized signaling network mediates crossover control in meiosis. Elife 7, e30789. PMID: 29521627; PMCID: PMC5906097. doi:10.7554/eLife.30789
Zhang, L., Liang, Z., Hutchinson, J., and Kleckner, N. (2014a). Crossover patterning by the beam-film model: Analysis and implications. PLoS Genet. 10 (1), e1004042. PMID: 24497834; PMCID: PMC3907302. doi:10.1371/journal.pgen.1004042
Zhang, L., Stauffer, W., Zwicker, D., and Dernburg, A. F. (2021). Crossover patterning through kinase-regulated condensation and coarsening of recombination nodules. bioRxiv 2021.08.26.457865.
Zhang, L., Tang, D., Luo, Q., Chen, X., Wang, H., Li, Y., et al. (2014b). Crossover formation during rice meiosis relies on interaction of OsMSH4 and OsMSH5. Genetics 198 (4), 1447–1456. Epub 2014 Oct 2. PMID: 25278554; PMCID: PMC4256764. doi:10.1534/genetics.114.168732
Zhang, P., Zhang, Y., Sun, L., Sinumporn, S., Yang, Z., Sun, B., et al. (2017). The rice AAA-ATPase OsFIGNL1 is essential for male meiosis. Front. Plant Sci. 8, 1639. PMID: 29021797; PMCID: PMC5624289. doi:10.3389/fpls.2017.01639
Zhao, H., McPeek, M. S., and Speed, T. P. (1995). Statistical analysis of chromatid interference. Genetics 139 (2), 1057–1065. PMID: 7713408; PMCID: PMC1206356. doi:10.1093/genetics/139.2.1057
Zhu, L., Fernández-Jiménez, N., Szymanska-Lejman, M., Pelé, A., Underwood, C. J., Serra, H., et al. (2021). Natural variation identifies SNI1, the SMC5/6 component, as a modifier of meiotic crossover in Arabidopsis. Proc. Natl. Acad. Sci. U. S. A. 118 (33), e2021970118. PMID: 34385313; PMCID: PMC8379953. doi:10.1073/pnas.2021970118
Zickler, D., and Kleckner, N. (1999). Meiotic chromosomes: Integrating structure and function. Annu. Rev. Genet. 33, 603–754. PMID: 10690419. doi:10.1146/annurev.genet.33.1.603
Zickler, D., and Kleckner, N. (2015). Recombination, pairing, and synapsis of homologs during meiosis. Cold Spring Harb. Perspect. Biol. 7 (6), a016626. PMID: 25986558; PMCID: PMC4448610. doi:10.1101/cshperspect.a016626
Ziolkowski, P. A., Underwood, C. J., Lambing, C., Martinez-Garcia, M., Lawrence, E. J., Ziolkowska, L., et al. (2017). Natural variation and dosage of the HEI10 meiotic E3 ligase control Arabidopsis crossover recombination. Genes Dev. 31 (3), 306–317. Epub 2017 Feb 21. PMID: 28223312; PMCID: PMC5358726. doi:10.1101/gad.295501.116
Keywords: meiotic recombination, crossing-over, interference, synapsis, heterochiasmy, CO insurance, CO homeostasis, HEI10 coarsening model
Citation: Rafiei N and Ronceret A (2023) Crossover interference mechanism: New lessons from plants. Front. Cell Dev. Biol. 11:1156766. doi: 10.3389/fcell.2023.1156766
Received: 01 February 2023; Accepted: 17 April 2023;
Published: 19 May 2023.
Edited by:
Pedro A San-Segundo, CSIC-University of Salamanca, SpainReviewed by:
Eugenio Sanchez Moran, University of Birmingham, United KingdomCopyright © 2023 Rafiei and Ronceret. This is an open-access article distributed under the terms of the Creative Commons Attribution License (CC BY). The use, distribution or reproduction in other forums is permitted, provided the original author(s) and the copyright owner(s) are credited and that the original publication in this journal is cited, in accordance with accepted academic practice. No use, distribution or reproduction is permitted which does not comply with these terms.
*Correspondence: Arnaud Ronceret, YXJuYXVkLnJvbmNlcmV0QGlidC51bmFtLm14