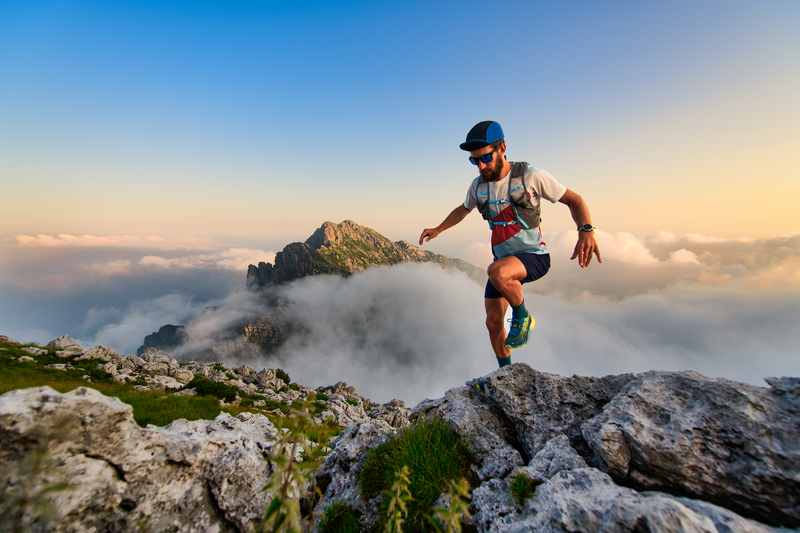
95% of researchers rate our articles as excellent or good
Learn more about the work of our research integrity team to safeguard the quality of each article we publish.
Find out more
ORIGINAL RESEARCH article
Front. Cell Dev. Biol. , 01 June 2023
Sec. Developmental Epigenetics
Volume 11 - 2023 | https://doi.org/10.3389/fcell.2023.1151108
This article is part of the Research Topic Editors' Showcase 2021: Insights in Developmental Epigenetics View all 4 articles
Cadmium (Cd) is a toxic heavy metal found throughout the environment and one of the top ten toxicants of major public health concern identified by the World Health Organization. In utero Cd exposure causes fetal growth restriction, malformation, and spontaneous abortion; however, the mechanisms by which Cd impacts these outcomes are poorly understood. Cd accumulates in the placenta, suggesting that these negative outcomes may be a consequence of disrupted placental function and placental insufficiency. To understand the impact of Cd on gene expression within the placenta, we developed a mouse model of Cd-induced fetal growth restriction through maternal consumption of CdCl2 and performed RNA-seq on control and CdCl2 exposed placentae. The top differentially expressed transcript was the Tcl1 Upstream Neuron-Associated (Tuna) long non-coding RNA, which was upregulated over 25-fold in CdCl2 exposed placentae. Tuna has been shown to be critical for neural stem cell differentiation. However, within the placenta, there is no evidence that Tuna is normally expressed or functional at any developmental stage. To determine the spatial expression of Cd-activated Tuna within the placenta, we used in situ hybridization as well as placental layer-specific RNA isolation and analysis. Both methods confirmed the absence of Tuna expression in control samples and determined that Cd-induced Tuna expression is specific to the junctional zone. Since many lncRNAs regulate gene expression, we hypothesized that Tuna forms part of the mechanism of Cd-induced transcriptomic changes. To test this, we over-expressed Tuna in cultured choriocarcinoma cells and compared gene expression profiles to those of control and CdCl2 exposed cells. We demonstrate significant overlap between genes activated by Tuna overexpression and genes activated by CdCl2 exposure, with enrichment in the NRF2-mediated oxidative stress response. Herein we analyze the NRF2 pathway and show that Tuna increases NRF2/NRF2 both at the transcript and protein levels. Tuna drives increased NRF2 target gene expression, a result that is abrogated with the use of an NRF2 inhibitor, confirming that Tuna activates oxidative stress response genes through this pathway. This work identifies the lncRNA Tuna as a potential novel player in Cd-induced placental insufficiency.
Declared as one of the top ten environmental toxicants of public health concern by the World Health Organization, Cd is toxic to almost all forms of life (Rahimzadeh et al., 2017). Cd occurs naturally in the Earth’s crust at low levels; however, anthropogenic and natural activity release it into the atmosphere where it can reach toxic concentrations in air, water, and soil (Rahimzadeh et al., 2017). Health effects of chronic Cd exposure include the development of cancer, kidney disease, rapid bone demineralization, reproductive dysfunction, diabetes, and osteoporosis, as well as other metabolic, cardiovascular, and behavioral disorders (Satarug et al., 2010). With a biological half-life of up to 30 years, Cd can accumulate in the kidneys and liver (Gerhardsson et al., 2012). Negative outcomes associated with in utero Cd exposure include fetal growth restriction (FGR), malformation, and spontaneous abortion (Kippler et al., 2010). FGR, affecting 3%–7% of pregnancies, is defined by failure to reach growth potential due to placental dysfunction resulting in low micronutrient and gas transport to the fetus (Burton and Jauniaux, 2018). During pregnancy Cd accumulates in the placenta and is inefficiently transferred to the fetus, indicating that its impacts on fetal development may be a result of placental impairment (Osman et al., 2000). While the effects of Cd on development have long been established, the mechanisms underlying Cd-induced placental dysfunction are poorly understood.
The most common cause for FGR is placental insufficiency (Salavati et al., 2019). The placenta is a crucial interface for providing gas and nutrients to the growing fetus from the maternal blood supply (Burton and Jauniaux, 2015), and placental insufficiency is described as when improper formation or damage negatively impacts this function (Gagnon, 2003). In mice, the placenta is composed of three layers: decidua, junctional zone (JZ), and labyrinth (Lab) (Watson and Cross, 2005). The decidua is composed of fetal extravillous trophoblasts that have invaded the maternal endometrium to restructure maternal spiral arteries, allowing for placental access to maternal blood supplies (Simmons et al., 2007; Silva and Serakides, 2016). The JZ is primarily composed of glycogen trophoblasts (GlyTs), which serve as energy storage, and spongiotrophoblasts (SpTs), which synthesize and secrete endocrine hormones for the placenta and fetus (Eaton et al., 2020). The Lab is composed of syncytiotrophoblasts (SynT) that line the highly vascularized basal layer enabling gas and nutrient exchange to the fetus (Simmons and Cross, 2005). Impairment to placentation and placental development are one of the main causes of stillbirths, preterm births, preeclampsia, and FGR (Gibbins et al., 2020). In this study we examine the transcriptional profiles of placentae associated with Cd-induced FGR.
Long non-coding RNAs (lncRNAs) are >200 bp long transcripts with no protein coding capabilities. They can interact with mRNA, DNA, protein, and miRNA to regulate epigenetic, transcriptional, post-transcriptional, translational, and post-translational pathways (Zhang et al., 2019). At a cellular level, lncRNAs have been shown to influence trophoblast migration, proliferation, and invasion (Muys et al., 2016; Pengjie et al., 2018; Feng et al., 2021). In addition, several lncRNAs have been associated with conditions such as pre-eclampsia and intrauterine growth restriction (Gremlich et al., 2014; Zhang et al., 2015). In humans, relationships have been found between Cd exposure, lncRNA transcript abundance in the placenta, and low birthweights (Hussey et al., 2020).
In a previous study, we established a mouse model of developmental Cd exposure which caused FGR and morphological changes to the placenta (Simmers et al., 2022). Briefly, both male and female embryos exposed to 50 ppm CdCl2 through maternal drinking water from 5 weeks prior to mating until collection at embryonic day 18.5 (e18.5) displayed significantly decreased weights. Placental weights expressed as a proportion of total embryo weights were significantly decreased in female embryos indicating placental insufficiency. Both male and female CdCl2 exposed placentae showed a significantly increased ratio of Lab to JZ signifying a major disruption to placental morphology. Herein we examine the differentially expressed genes (DEGs) between CdCl2 exposed and control placentae through RNA-seq. We identify the lncRNA Tuna as the most differentially expressed gene and use an in vitro overexpression model to demonstrate that it drives the nuclear factor erythroid 2-related factor 2 (NRF2) oxidative stress response pathway, suggesting that Tuna may be a key part of the cellular response to Cd. Our findings have implications for understanding the mechanisms through which Cd causes placental dysfunction and FGR.
Mice were maintained on a 14-h/10-h light/dark cycle at 30%–70% humidity, 22°C ± 4°C. Mating, exposure, and collection of whole placentae and layer-specific tissue were performed as previously described (Simmers et al., 2022). In short, 5-week-old female C57BL/6J mice were exposed for 5 weeks to 0 or 50 ppm CdCl2 through drinking water (Sigma-Aldrich, 202908). In this model, 5 weeks of exposure to 50 ppm CdCl2 results in blood Cd levels in dams of 5.23 ± 0.99 μg/L (mean ± standard error) (Hudson et al., 2019), similar to the blood Cd levels of ∼6 μg/L found in people living in a contaminated community in Japan (Sasaki et al., 2019). At 10 weeks of age, mice were mated with unexposed CAST/EiJ males. The use of divergent parental strains facilitated the analysis of imprinted genes in our previous study (Simmers et al., 2022). CdCl2 exposure continued throughout mating and gestation. Placentae and fetuses were isolated at e18.5; collected tissues were weighed, snap-frozen immediately upon dissection and stored at −80°C or prepared for histological analysis as described below.
Eight female placental samples representing at least four litters from independent dams were selected from each treatment group for nucleic acid isolation. Tissues were homogenized in lysis buffer and RNA was isolated via AllPrep DNA/RNA/miRNA kit (Qiagen). RNA from layer-specific tissues was isolated via AllPrep DNA/RNA Micro kit (Qiagen). RNA from Jeg-3 cells was isolated via NucleoSpin RNA kit (Macherey-Nagel). Nucleic acids were quantified on a Nanodrop 2000 and RNA integrity was confirmed by electrophoresis.
500 ng of total RNA from whole placenta, 100 ng of layer-specific total RNA, or 500 ng of total RNA from Jeg-3 cells was used to synthesize first strand cDNA according to the manufacturer’s protocol (M-MLV RT enzyme, Promega) (Simmers et al., 2022). qRT-PCR was performed in triplicate on 96-well plates with a QuantStudio 3 Real-Time PCR system (Applied Biosystems) using SsoAdvanced Universal SYBR Green Supermix (Bio-Rad). The cycling conditions were as follows: 95°C for 30 s; 40 cycles of 95°C for 15 s, 60°C for 30 s; dissociation curve of 60.0°C–95.0°C. The primer sequences are provided in Supplementary Table S1. Mouse Polr2a or human GAPDH were used as reference genes and were not significantly differentially expressed between treatment groups (data not shown). Gene expression was quantified using the ΔΔCt method (Livak and Schmittgen, 2001). Tuna/TUNA expression was quantified using the ΔCt method to determine its expression as a percentage of the housekeeper (Polr2a/GAPDH).
Jeg-3 cells were purchased from the American Type Culture Collection (Manassas, VA) and maintained in minimum essential media (Corning Life Sciences) supplemented with 10% FBS (Genesee Scientific) and 100 μg/mL Streptomycin, 100 U/mL Penicillin (HyClone), and humidified in 5% CO2 at 37°C.
Cells were seeded in 6-well plates for 24 h at 4 × 105 cells/well until reaching ∼80% confluency. Cells were starved with serum free media for 24 h at which point serum free media was aspirated, cells were washed in PBS, and exposed to 0, 0.5, 1.0, 2.5, 5.0, and 10.0 µM concentrations of CdCl2, PbAc, or NaAsO2 in serum free media. Each exposure was done in triplicate, cells were collected after 24 h of exposure, and each experiment was repeated 3 times. Viability was determined using CytoTox-Glo (Promega) according to the manufacturer’s instructions.
Cells were seeded in 6-well plates for 24 h at 4 × 105 cells/well until reaching ∼80% confluency. Expression of GFP (control) or Tuna was achieved by transfecting cells with 3.5 μg of pcopGFP or pcopGFP-mTuna using Lipofectamine 3000 according to the manufacturer’s protocol (ThermoFisher). PcopGFP-mTuna was created by inserting the mouse Tuna sequence into the multiple cloning site (MCS) of pCDH-CMV-MCS-EF1α-GreenPuro Cloning and Expression Lentivector (System Biosciences). To remove the Tuna sequence and generate the pcopGFP control vector, the pcopGFP-mTuna construct was cut at the EcoRI and BamHI restriction sites, blunted with T4 DNA polymerase and ligated with T4 DNA ligase (New England Biolabs). Each condition was done in triplicate, transfected cells were collected 24, 48, or 72 h post transfection, and all experiments were repeated 3 times.
Fresh Frozen–In a cryostat, fresh frozen e18.5 placentae samples were partially embedded in OCT compound (Tissue-Tek), sectioned (20 µm thickness) and fixed in 10% formalin.
Formalin-Fixed Paraffin-Embedded (FFPE)—Placentae were collected at e18.5 and fixed in 4% formaldehyde for 24 h. Within 24 h of fixation, placentae were delivered in 70% ethanol to the NC State Histology Laboratory for dehydration and paraffin embedding. Samples were then sectioned to 20 µm and pretreated according to the Formalin-Fixed Paraffin-Embedded (FFPE) Sample Preparation and Pretreatment protocol by Advanced Cell Diagnostics.
All samples were then dehydrated, dried, treated, and probed using the RNAscope 2.5 HD assay according to the manufacturer’s protocol (ACD) using Mm-Tunar-O1 (REF#579711) or Mm-Polr2a (REF#312471) probes. The protocol was modified to limit protease exposure to 15 min, and a 30 s eosin counterstain step was added directly after the hematoxylin stain and ammonia water rinse.
Cell viability and proliferation assays for transfected cells were conducted using the trypan blue exclusion test (Strober, 2015). Cells were grown and exposed or transfected as described above. All conditions were done in triplicate, viability was checked after 24, 48, and 72 h post transfection, and each experiment was repeated 3 times. At each time point the ratio of living cells to total cells was used to calculate percent viability and total cell count was used for proliferation.
Levels of H2O2 were determined via ROS-Glo H2O2 Kit (Promega). Cells were seeded in 96-well plates at 1 × 104 cells/well and cultured overnight until reaching ∼80% confluency. Expression of GFP (control) or Tuna was achieved as described above. Cells were exposed to 50 µM menadione for 2 h to induce oxidative stress as a positive control. Each condition was done in triplicate. Transfected, control, and menadione treated cells were analyzed at 24 h, and all experiments were repeated 3 times.
RNA was isolated from e18.5 female mice or Jeg-3 cells as described above. Integrity, purity, and concentration were determined via the Agilent 2100 Bioanalyzer with an RNA 6000 Nano Chip (Agilent Technologies, United States). All samples had an RNA integrity number (RIN) > 9.0. Samples were submitted to the NC State University Genomic Sciences Laboratory for Illumina RNA library construction and sequencing. cDNA libraries for Illumina sequencing were constructed via the NEBNext Ultra Directional RNA Library Prep Kit (NEB) and NEBNext Multiplex Oligos for Illumina (NEB) according to the manufacturer’s protocol. Samples were sequenced on the Illumina NovaSeq S4, utilizing a 150 × 2 bp paired end S4 sequencing reagent kit (Illumina).
Data analysis was performed in consultation with the Bioinformatics Core at NC State University’s Center for Human Health and the Environment. An average of ∼61 million paired-end raw RNA-seq reads were generated for each replicate. The quality of sequenced data was assessed using the fastqc application, and 12 poor-quality bases were trimmed from the 5’-end. The remaining good-quality reads were aligned to the mouse or human reference genome (mm10 version 87 or GRCh38 respectively) databases using the STAR aligner (Dobin et al., 2013). To validate single nucleotide polymorphisms (SNPs) in the hybrid mouse model, the following WASP options were passed to the STAR aligner (--varVCFfile C57BL_6NJ.mgp.v5.snps.dbSNP142.vcf--waspOutputMode SAMtag). Per-gene counts of uniquely mapped reads for each replicate were calculated using the htseq-count script from the HTSeq (Anders et al., 2015) Python package. The count matrix was imported to R statistical computing environment for further analysis. Initially, genes that had no count in greater than half of the replicate samples were discarded. The remaining count data were normalized for sequencing depth and distortion, and dispersion was estimated using DESeq2 (Love et al., 2014) Bioconductor package. We fitted a linear model using the treatment levels, and differentially expressed genes (DEGs) were identified after applying multiple testing corrections using the Benjamini–Hochberg procedure (Benjamini and Hochberg, 1995). The final list of significant genes was generated using an adjusted p-value <0.05. RNA-seq data are deposited in the Gene Expression Omnibus (accession number GSE233282).
For gene set enrichment analysis, the common DEGs between the 0 vs. 5 µM CdCl2 exposed Jeg-3 cells and pcopGFP vs. pcopGFP-mTuna transfected cells detected through RNA-seq were analyzed via hypergeometric calculation as described previously (Baptissart et al., 2022).
Ensembl IDs of significant DEGs were input for core analysis using Ingenuity Pathway Analysis (IPA) software (Qiagen). For all analyses, the ‘Upstream regulators’ were filtered to only consider the molecule type ‘Genes, RNAs and proteins’.
Cells were exposed or transfected as previously described and washed with PBS and lysed in ice-cold RIPA buffer (ThermoFisher) with protease inhibitor cocktail (Sigma-Aldrich). Protein concentration was determined with the BCA Protein Assay Kit (Pierce) and samples were boiled with 4X Laemmli buffer for 5 min. Equal amounts of protein were resolved in 4%–15% gradient SDS-PAGE gels (BioRad) and transferred to polyvinylidene difluoride membranes (Merck Millipore). Membranes were cut according to target molecular weight and probed with anti-NRF2 (Abcam, #137550), TXNRD1 (Abcam, #124954), or GAPDH (Abcam, #245355) overnight on a shaker at 4°C. Antibody dilutions are presented in Supplementary Table S2. Membranes were washed 3X in 5% non-fat milk for 15 min each before incubating for 1 h on a shaker at room temperature in secondary antibody (Abcam, #7090). Finally, membranes were washed 3X in TBST for 15 min each, incubated in enhanced chemiluminescence reagents (ThermoFisher), and imaged with the AI600 (GE). Band density analysis was performed using Photoshop (Adobe).
All statistical analyses unless otherwise specified were performed on values determined through median absolute deviation (MAD) analysis using a one-way analysis of variance (ANOVA) or Student’s t-test, two-tailed. Data are presented as the mean ± standard error of the mean. *p < 0.05, **p < 0.01, ***p < 0.001, ****p < 0.0001. The numbers of animals, litters and samples used in each experiment are presented in Supplementary Table S2.
To begin to understand how Cd could impact placental function thereby modulating fetal growth, we performed RNA-seq on female control and 50 ppm CdCl2 exposed placentae collected from our mouse model of Cd-induced FGR (Simmers et al., 2022). Females were selected because CdCl2 exposure induces FGR in females without impacting placental weight; in contrast, CdCl2 exposed male placentae show significantly reduced weight at e16.5 although this difference does not persist at e18.5. RNA-seq revealed a total of 783 significant DEGs (adjusted p-value <0.05), consisting of 433 downregulated (55.3%) and 350 upregulated (44.7%) genes. Of those, Tcl1 Upstream Neuron-Associated (Tuna) lncRNA was the top differentially expressed transcript with a ∼25-fold increase in expression in CdCl2 exposed placentae compared to controls (Figure 1A; Supplementary Table S3). Despite being conserved in all vertebrates with a sequenced genome, Tuna has not been widely studied. It has been described as a crucial factor for neural stem cell differentiation, a tumor suppressor involved in breast cancer progression, an oncogene in glioma cells, and a differentiation and implantation factor in the endometrium (Lin et al., 2014; Li et al., 2017; Wang et al., 2020). However, a role for Tuna in the placenta or in the cellular response to an environmental stressor has not been described.
FIGURE 1. Visualization and quantification of Tuna transcript abundance through RNA-seq and qRT-PCR. (A) RNA-seq reads mapped to Tuna in the mm10 genome build visualized through UCSC Genome Browser. Sample names are listed on the left (four 0 ppm and four 50 ppm CdCl2 exposed placentae, each from unique litters). Peaks are based on a proportion of 125 maximum reads. (B) Tuna transcript abundance in e18.5 female whole placenta determined through qRT-PCR. (C) Tuna transcript abundance in e18.5 female placental Lab and JZ. Data are presented as a percentage of Polr2a. Data are presented as means ± SE (n = 8 per group). Whole placenta qRT-PCR results were analyzed by Student’s t-test, two-tailed test comparing 0 ppm and 50 ppm exposures. **p < 0.01. ND = not detected.
To validate the finding from RNA-seq, the abundance of placental Tuna transcripts was analyzed through qRT-PCR. Using this approach, CdCl2 exposure was associated with a 7-fold increase in placental Tuna transcripts compared to controls (Figure 1B). Together, our results from RNA-seq and qRT-PCR confirm Tuna to be poorly expressed in control placentae; however, in response to CdCl2 exposure, Tuna transcript abundance is significantly increased.
Given the different functions performed by the layers of the placenta, we aimed to determine where in the e18.5 placenta Tuna is expressed in response to CdCl2 exposure. Using layer-specific micropunches of control and 50 ppm CdCl2 exposed placentae obtained and validated in our previous study, we analyzed the spatial expression of Tuna through qRT-PCR (Simmers et al., 2022). Tuna was only detectable within the JZ of the 50 ppm CdCl2 samples; both the JZ and Lab fractions of control placentae as well as the Lab fraction of 50 ppm CdCl2 exposed placentae demonstrated undetectable levels of Tuna transcripts (Figure 1C).
To further confirm this layer specificity and identify the cell type responsible for the increase in Tuna transcripts, we performed in situ hybridization on e18.5 placental sections. Positive (Polr2a) and negative controls demonstrated the expected results, validating the efficacy of the protocol (Figures 2A, B). In fresh frozen placental sections, Tuna transcripts were undetectable in the Lab of both control and 50 ppm CdCl2 exposed samples as well as control JZ samples (Figures 2C–E). In support of our qRT-PCR data, Tuna transcripts were detectable exclusively in the JZ of the 50 ppm CdCl2 exposed samples (Figure 2F). Analysis of the Lab/JZ intersection showed the dispersed signal of Tuna transcripts present with the JZ (Figure 2G, top right) and absence of signal in the Lab (bottom left). To obtain better cell morphology and inform on the spatial distribution of Tuna transcripts within the JZ, we performed in situ hybridization on formalin fixed paraffin embedded tissue. Consistent with our prior results, no Tuna signal was found in 50 ppm CdCl2 exposed Lab sections (Supplementary Figure S1) but was present within the JZ of 50 ppm CdCl2 exposed placentae including SpTs (Figure 2H).
FIGURE 2. Distribution of Tuna transcripts in female e18.5 mouse placental sections. (A) Detection of positive control Polr2a in H&E counter-stained fresh frozen e18.5 placenta; brown signal represents at least a single Polr2a mRNA molecule. (B) Negative control. (C) Tuna in 0 ppm Lab. (D) Tuna in 50 ppm Lab. (E) Tuna in 0 ppm JZ. (F) Tuna in 50 ppm JZ. (G) Tuna at the intersection of Lab and JZ in 50 ppm CdCl2 sections. (H) Tuna in 50 ppm JZ formalin fixed paraffin embedded sections. Arrows indicate positive Tuna signal. In situ hybridization was performed on n = 4 per group; representative images are shown.
We developed an in vitro cell model using the human choriocarcinoma cell line Jeg-3 to determine if TUNA is activated by Cd in a cell autonomous manner. Jeg-3 cells were exposed to 0, 0.5, 1.0, 2.5, 5.0, and 10.0 µM concentrations of CdCl2 for 24 h. No significant changes to cell viability were observed (Figure 3A). TUNA transcript abundance significantly increased in a CdCl2 dose dependent manner across all exposure concentrations (Figure 3B). Next, we explored whether this response was specific to CdCl2 or could be induced by other toxic metals. To test this, Jeg-3 cells were exposed to 0, 0.5, 1.0, 2.5, 5.0, and 10.0 µM concentrations of lead acetate (PbAc) or sodium arsenite (NaAsO2) for 24 h. No significant changes to viability were detected with the exception of 10.0 µM NaAsO2 (Figure 3A). As a control for the uptake of CdCl2, PbAc and NaAsO2, we compared the transcript abundance of the oxidative stress marker Cu/Zn Superoxide Dismutase 1 (SOD1). SOD1 transcript abundance is a known marker of oxidative stress as it encodes an enzyme responsible for the conversion of superoxide radicals into molecular oxygen and hydrogen peroxide (Banks and Andersen, 2019). CdCl2, PbAc and NaAsO2 exposures all resulted in significant increases in SOD1 expression (Figure 3C).
FIGURE 3. Viability and TUNA expression in Jeg-3 cells after 24 h of toxic metal exposure. (A) Viability of Jeg-3 cells exposed to 0, 0.5, 1.0, 2.5, 5.0, and 10.0 µM concentrations of CdCl2, PbAc, and NaAsO2 for 24 h, detected by CytoTox-Glo. (B) TUNA transcript abundance in Jeg-3 cells in response to 0, 0.5, 1.0, 2.5, 5.0, and 10.0 µM concentrations of CdCl2, PbAc, and NaAsO2 analyzed by qRT-PCR. (C) SOD1 transcript abundance in unexposed control cells and in 5 μM CdCl2, 5 μM PbAc, 5 μM NaAsO2 exposed Jeg-3 cells at 24 h. Data are presented as mean of mean ± SE from three technical replicates within each of three independent experiments. Analyzed by one-way ANOVA with Tukey’s post-hoc test on values determined through MAD analysis. *p < 0.05, **p < 0.01, ****p < 0.0001.
Neither PbAc or NaAsO2 significantly increased TUNA transcript abundance at any concentration confirming that the increase in TUNA expression is specific to CdCl2 exposure (Figure 3B).
To begin to study the functions of Cd-induced Tuna in the placenta, we created an expression construct (pcopGFP-mTuna) using the spliced mouse Tuna sequence detected within the placentae of our FGR model. An empty vector (pcopGFP) served as a control (Figure 4A). Constructs were transfected into Jeg-3 cells and successful transfection was determined by visualizing fluorescence from the GFP protein. GFP transcript abundance was measured by qRT-PCR to ensure no significant differences were detected between pcopGFP and pcopGFP-mTuna transfections within experiments (data not shown). Tuna expression was confirmed in cells transfected with the pcopGFP-mTuna construct (Figure 4B).
FIGURE 4. Tuna expression construct, viability, and proliferation assays of control vs. Tuna overexpressing Jeg-3 cells at 24, 48, and 72 h after transfection. (A) Structure of empty control vector (pcopGFP) and Tuna expression vector (pcopGFP-mTuna). (B) Tuna transcript abundance in Jeg-3 cells 24 h after transfection determined by qRT-PCR. (C) Viability of Jeg-3 cells at 24, 48, and 72 h post transfection, represented as a percent of total pcopGFP cells at 0 h. (D) Proliferation of Jeg-3 cells at 24, 48, and 72 h post transfection, data points represent total cell number at each time point. Experiments were performed three times, each with three technical replicates. Analyzed by Student’s t-test, two-tailed, comparing pcopGFP and pcopGFP-mTuna across the experimental replicates determined through MAD analysis. *p < 0.05.
To test if Tuna plays a role in the response of cells to environmental exposures capable of inducing oxidative stress, we compared both viability and proliferation between pcopGFP and pcopGFP-mTuna transfected cells at 24, 48, and 72 h after transfection. There were no significant differences in viability at any time point between the two groups of cells (Figure 4C). However, proliferation was significantly decreased at 24 and 72 h in the pcopGFP-mTuna transfected cells compared to controls (Figure 4D). This suggests that Cd-induced increases in Tuna transcript abundance may contribute to the anti-proliferative effects associated with Cd toxicity (Erboga and Kanter, 2016).
To understand the role Tuna might play in the cellular response to CdCl2, we aimed to compare RNA-seq results from CdCl2 exposure and Tuna overexpression experiments for enrichment of DEGs. RNA isolated from pcopGFP and pcopGFP-mTuna transfected Jeg-3 cells was submitted for RNA-seq alongside RNA from control (0 μM) and 5 μM CdCl2 exposed cells (Supplementary Tables S4, S5). RNA-seq of pcopGFP versus pcopGFP-mTuna transfected cells revealed a total of 1221 DEGs, of which 455 were downregulated (37.3%) and 766 upregulated (62.7%). RNA-seq of 0 versus 5 μM CdCl2 exposed cells revealed a total of 8357 DEGs, 4124 of which were downregulated (49.4%) and 4233 upregulated (50.6%). A total of 759 DEGs were shared between the two datasets, representing a statistically significant enrichment (enrichment x1.15; p-value = 7.53e−10; hypergeometric test) (Figure 5; Supplementary Table S6). This finding provides support for Tuna playing a role in the response to Cd exposure in the placenta. We analyzed the shared DEGs from the two datasets using Ingenuity Pathway Analysis (IPA) to identify common pathways. Oxidative phosphorylation, mitochondrial dysfunction, and NRF2-mediated oxidative stress response were the three most significantly enriched pathways (Supplementary Table S7). The NRF2-mediated oxidative stress response pathway showed the highest activation z-score indicating the pathway with the most likely activation of biological function.
FIGURE 5. RNA-seq analysis on Tuna overexpressing and CdCl2 exposed Jeg-3 cells at 24 h. Venn diagram represents the number of CdCl2 DEGs and their intersection with Tuna overexpressing DEGs in Jeg-3 cells at 24 h exposure or post transfection. RNA-seq was performed on 4 replicates per group.
Nuclear factor erythroid (NF-E2)-related factor 2 (NRF2) is a transcription factor responsible for coordinating and regulating the xenobiotic and oxidative stress response. NRF2 binds antioxidant response elements (AREs) to promote the expression of over 200 genes (Rojo de la Vega et al., 2018; He et al., 2020). In addition to its role in cellular defense, NRF2 has been associated with metabolism, inflammation, autophagy, proteostasis, mitochondrial physiology, and immune system functions (Tonelli et al., 2018; Song et al., 2021).
To further investigate the potential role of Tuna in the NRF2-mediated oxidative stress response pathway, we analyzed NRF2/NRF2 transcript and protein levels, the transcript abundance of NRF2 targets (TXNRD1, PRDX1, GSR, and FTH1), and TXNRD1 protein abundance in Jeg-3 cells exposed to CdCl2 and cells overexpressing Tuna. We first analyzed the pathway in response to CdCl2. 5 μM CdCl2 induced significant increases of NRF2 transcript and protein abundance compared to controls (Figures 6A, B). Similarly, the NRF2 targets TXNRD1, PRDX1, and FTH1 were significantly increased, while GSR showed no significant changes (Figure 6C). Lastly, TXNRD1 protein levels were significantly increased in response to CdCl2 (Figure 6D). Next, we analyzed the same pathway in the context of Tuna overexpression. Similar to CdCl2 exposure, Tuna overexpression caused a significant increase in both NRF2 transcript and protein abundance when compared to pcopGFP controls (Figures 6E, F). In addition, TXNRD1 and GSR showed increased transcript abundance while PRDX1 and FTH1 showed no significant changes (Figure 6G). The increased abundance of TXNRD1 transcripts was not reflected by an increase in TXNRD1 protein abundance (Figure 6H). These results suggest that in addition to driving NRF2 expression, Tuna at least partially activates the NRF2-mediated oxidative stress response pathway.
FIGURE 6. NRF2 Oxidative Stress Response Pathway transcript and protein abundance in Jeg-3 cells in response to CdCl2 exposure and Tuna overexpression. (A) NRF2 transcript abundance in Jeg-3 cells in response to 0 and 5 μM CdCl2 at 24 h. (B) NRF2 protein abundance in response to 0 and 5 µM CdCl2 at 24 h. (C) TXNRD1, PRDX1, GSR, and FTH1 transcript abundance in response to 0 and 5 µM CdCl2 at 24 h. (D) TXNRD1 protein abundance in response to 0 and 5 µM CdCl2 at 24 h. (E) NRF2 transcript abundance in Jeg-3 cells in response to pcopGFP and pcopGFP-mTuna at 24 h. (F) NRF2 protein abundance in response to pcopGFP and pcopGFP-mTuna at 24 h. (G) TXNRD1, PRDX1, GSR, and FTH1 transcript abundance in response to pcopGFP and pcopGFP-mTuna at 24 h. (H) TXNRD1 protein abundance in response to pcopGFP and pcopGFP-mTuna at 24 h. Analyzed by Student’s t-test, two-tailed, on values determined through MAD analysis from three independent experiments, each with three technical replicates. *p < 0.05, ***p < 0.001. Representative westerns blots are shown in panels (B), (D), (F), and (H). Membranes were cut and probed independently for NRF2, TXNRD1, and GAPDH therefore GAPDH images are duplicated between (B) and (D), and between (F) and (H).
The capability of lncRNAs to interact with DNA, RNA, and proteins allows them to regulate gene transcription and signaling pathways through a variety of mechanisms (Statello et al., 2021). While it is possible that Tuna-induced activation of selected NRF2 target genes occurs through direct binding of Tuna transcripts to the promoters of these genes, our observation that Tuna upregulates NRF2/NRF2 transcript and protein levels suggests that Tuna might drive the expression of these targets through the NRF2 pathway. To test this hypothesis, we compared NRF2 target transcript abundance between cells transfected with pcopGFP, pcopGFP-mTuna, and pcopGFP-mTuna in the presence of the NRF2 inhibitor ML385. As before, the expression of TXNRD1 and GSR was significantly increased in cells overexpressing Tuna compared to GFP overexpressing controls, but this did not occur in the presence of ML385 (Figure 7). These data show that Tuna is acting through NRF2 to drive the NRF2-mediated oxidative stress response.
FIGURE 7. NRF2 target gene expression in response to GFP control, Tuna overexpression, and Tuna overexpression + ML385 in Jeg-3 cells 24 h post transfection. Data are presented as mean of means ± SE from three technical replicates within each of three independent experiments. Analyzed by one-way ANOVA with Tukey’s post-hoc test on values determined through MAD analysis. *p < 0.05, **p < 0.01.
To determine if Tuna drives the generation of reactive oxygen species (ROS) and thereby activates the oxidative stress response, we analyzed the H2O2 levels present in Jeg-3 cells overexpressing Tuna at 24 h post transfection. As a positive control for ROS, cells were treated with menadione. As expected, cells exposed to menadione displayed a significant increase in ROS compared to all other conditions (Figure 8). Neither GFP overexpressing controls nor Tuna overexpressing cells produced elevated levels of ROS. In addition, there was no significant difference between the ROS of GFP overexpressing controls and Tuna overexpressing cells, indicating that the activation of the NRF2-mediated oxidative stress response pathway in Tuna over-expressing cells cannot be explained by increased ROS. These results suggest that Tuna overexpression is driving the NRF2-mediated oxidative stress response independent of ROS.
FIGURE 8. ROS levels in vehicle (control) and menadione exposed Jeg-3 cells compared to GFP control and Tuna overexpressing cells at 24 h post transfection. Data are presented as mean of means ± SE from three technical replicates within each of three independent experiments. Analyzed by one-way ANOVA with Tukey’s post-hoc test on values determined through MAD analysis. ****p < 0.0001.
FGR is a condition in which the fetus fails to reach its growth potential (Burton and Jauniaux, 2018). Affecting up to 7% of pregnancies, FGR is not only the leading cause of stillbirths and prenatal mortality but is one of the most characterized risk factors for cardiovascular disease in adult life, the leading cause of premature mortality globally (Barker, 1995; Audette and Kingdom, 2018; Crispi et al., 2018; Francula-Zaninovic and Nola, 2018; Woods et al., 2018). Placental insufficiency is one of the most common causes of FGR, and both placental dysfunction as well as FGR have been correlated with Cd exposure (Taylor et al., 2016; Burton and Jauniaux, 2018; Geng and Wang, 2019). While the mechanisms behind Cd-induced FGR and placental insufficiency are not fully understood, prior research has revealed altered expression of placental gene networks present in those with metal exposure-associated FGR (Deyssenroth et al., 2017). We hypothesized that aberrant transcript expression in the placenta caused by Cd exposure drives improper placental development leading to placental insufficiency and negative birth outcomes.
We previously established a mouse model of Cd-induced FGR wherein 50 ppm CdCl2 administered through drinking water before and throughout pregnancy caused significant FGR and placental insufficiency at embryonic day 18.5 (e18.5) (Simmers et al., 2022). In the current study, whole placenta RNA was isolated from control and 50 ppm exposed samples for transcriptomic analysis. Through RNA-seq we identified 783 DEGs between control and 50 ppm CdCl2 exposed samples. The top DEG, Tuna, was upregulated ∼25 fold in the placentae of offspring exposed to CdCl2 versus controls. qRT-PCR confirmed the increase in Tuna transcript abundance; however, the increase was ∼7 fold. The discrepancy between fold increases is likely due to differences in the sensitivity of the two techniques.
Tuna, first described in 2011 and also called ‘megamind’, is a ∼3200 bp lncRNA with high evolutionary conservation across vertebrates (Lin et al., 2014; Bouckenheimer et al., 2018). While it has been detected within the endometrium of the uterine wall potentially indicating a critical role in decidualization, no studies have identified it within the placenta at any stage of development (Wang et al., 2020). In mice, Tuna has been described as a crucial factor for both neural stem cell differentiation and maintenance of pluripotency through the formation of an RNA/protein complex that targets promoters of Nanog, Sox2, and Fgf4 to induce their expression (Lin et al., 2014). TUNA was found to be increased in human breast cancer tissues, and in vitro experiments on human breast cancer cell lines suggest that TUNA promotes oncogenic activity through the expression of SOX2 and promotion of epithelial-to-mesenchymal transition (Li et al., 2017). More recently, it has been revealed that Tuna transcripts previously thought to have no protein coding capability may encode microproteins (proteins smaller than 100 amino acids), responsible for reduced differentiation potential in mouse embryonic stem cells (Senís et al., 2021). The capability of Tuna to form multiple complexes and possible microproteins dependent upon developmental stage and tissue make it a potential regulatory molecule through which environmental exposures could modulate transcription. Differentially expressed lncRNAs have been associated with low birth weights and in addition Cd exposure has been shown to disrupt the expression of lncRNAs critical to fetal growth (Hussey et al., 2020).
Through the isolation of RNA from specific layers of the placenta, we determined that Tuna was expressed exclusively in the JZ in response to CdCl2 exposure, an area composed of SpTs, GlyTs and a layer of trophoblast giant cells directly bordering the decidua (Simmons et al., 2007). Identifying the specific cell type in fresh frozen sections was not possible due to the loss of clear cell morphology. However, in FFPE samples, which better preserve morphological features with the exception of GlyTs that were digested in the fixation process, we observed both cytoplasmic and nuclear signals throughout all cell types present within the JZ. This indicated that SpTs were the most likely cell type responsible for the highest Tuna expression. Outside of the fact that the JZ acts as an endocrine compartment maintaining progesterone secretion, little is known about the full purpose and function of SpTs within the JZ (Tanaka et al., 1997; Coan et al., 2006).
To further study the effect of CdCl2 on Tuna expression we switched to an in vitro model using the human choriocarcinoma cell line Jeg-3. Induction of TUNA expression by CdCl2 exposure was cell autonomous and was specific to CdCl2 rather than being a general response to heavy metal exposure, as no detectable changes in TUNA transcript were detected in response to PbAc and NaAsO2.
To further understand the role of Tuna in CdCl2 exposed Jeg-3 cells, we created a Tuna expression construct. While we used the mouse Tuna sequence in these studies, the sequence shows high evolutionary conservation with the human ortholog. Overexpression of Tuna activated the NRF2-mediated oxidative stress response pathway shown through increased NRF2/NRF2 transcript and protein abundance, as well as increased transcript abundance of several of its targets including TXNRD1 and GSR as illustrated in the proposed model (Figure 9). Not all NRF2 targets analyzed displayed differential expression in response to Tuna overexpression. It is possible that Tuna is only partially activating the NRF2-mediated stress response pathway and that other cofactors only present in oxidative stress conditions lead to the increased expression of other gene targets. Alternatively, only a subset of targets may appear to be activated due to the time point analyzed. This might also account for the differences in TXNRD1 protein detection between CdCl2 exposure and Tuna overexpression.
Further, use of the NRF2 protein inhibitor ML385 showed that Tuna drives NRF2 target gene expression by activating the NRF2 pathway rather than independently driving the expression of NRF2 target genes. NRF2 is a critical transcription factor in the protective response to oxidative and electrophilic stress by controlling the regulation of detoxifying enzymes and antioxidant proteins (Baird and Yamamoto, 2020). NRF2 is negatively regulated by Kelch-like ECH-associated protein 1 (KEAP1), an adapter protein for ubiquitin ligase Cullin3 (CUL3)-containing E3 ubiquitin ligase complex, which retains NRF2 in the cytoplasm and enables constant ubiquitination and degradation by the ubiquitin proteasome system (UPS), maintaining low basal levels of NRF2 (Zhang et al., 2005; Villeneuve et al., 2010). Under conditions of oxidative stress NRF2 dissociates from the CUL3-KEAP1-E3 complex, translocating to the nucleus where it forms heterodimers with small musculoaponeurotic fibrosarcomas (sMAFs), binding the antioxidant response element (ARE), leading to expression of NRF2 target genes (Kaspar et al., 2000; Nam and Keum, 2019). NRF2 targets include over 200 transcripts that range from xenobiotic response, oxidative stress response, and Phase II metabolizing enzymes (Gao et al., 2021).
Our results show that both NRF2 transcript and protein levels increase in response to Tuna overexpression. However, it is unknown whether the activation of the NRF2 pathway is a result of Tuna driving NRF2 expression to levels outcompeting NRF2 sequestration or if Tuna modulates the CUL3-KEAP1-E3 complex. Further experiments are required to understand the mechanisms through which Tuna controls NRF2 protein levels.
Studies in rat cells have shown that CdCl2 activates Nrf2, protecting against Cd-induced apoptosis (Chen and Shaikh, 2009). However, our viability and proliferation assays of Tuna overexpression did not show any changes in viability and actually showed decreased proliferation. This may indicate that there is a protective function of Tuna that is acting outside of NRF2. LncRNAs have been shown to act as scaffolding for protein complexes capable of targeting enhancer and promoter regions of the genome. Utilizing an RNA/chromatin interaction assay such as chromatin isolation by RNA purification (ChIRP) would help to identify potential genomic locations to which Tuna binds and may reveal potential novel roles of Tuna in controlling the cellular response to Cd.
Tuna was recently shown to affect decidualization and differentiation of endometrial stromal cells (Wang et al., 2020). Patients with recurrent implantation failure (RIF), described as three failed in vitro fertilization attempts, showed significantly increased Tuna transcript levels in the endometrium compared to controls, potentially indicating a role in implantation and decidualization success (Bashiri et al., 2018). Decidualization involves the invasion of extravillous trophoblasts into the endometrium allowing for the restructuring of spiral arteries. Tuna may therefore play distinct roles in the endometrium and the JZ of the placenta.
These data show evidence of a novel role for Tuna within the placenta and present the first study to analyze the function of Tuna within a placental cell model. Further studies of the molecular mechanisms underlying Cd-induced Tuna activation and its relevance to placental insufficiency could identify new pathways underlying FGR.
The datasets presented in this study can be found in online repositories. The names of the repository/repositories and accession number(s) can be found in the article/Supplementary Material.
Animal work was approved by the North Carolina State University Institutional Animal Care and Use Committee, under protocols 16-045-B and 19-049-B. Experiments were completed in accordance with the Guiding Principles in the Use of Animals in Toxicology.
MS and MC conceived the project, designed the study, conducted experiments, analyzed and interpreted the data, and wrote the manuscript. DJ performed bioinformatics analyses. YT contributed to experimental design and data interpretation.
This work was funded by the National Institutes of Health under K22ES027510, P30ES025128 and T32ES007046.
We thank members of the Cowley Lab for helpful discussion of the work and the manuscript.
The authors declare that the research was conducted in the absence of any commercial or financial relationships that could be construed as a potential conflict of interest.
All claims expressed in this article are solely those of the authors and do not necessarily represent those of their affiliated organizations, or those of the publisher, the editors and the reviewers. Any product that may be evaluated in this article, or claim that may be made by its manufacturer, is not guaranteed or endorsed by the publisher.
The Supplementary Material for this article can be found online at: https://www.frontiersin.org/articles/10.3389/fcell.2023.1151108/full#supplementary-material
Anders, S., Pyl, P. T., and Huber, W. (2015). HTSeq - a Python framework to work with high-throughput sequencing data. Bioinformatics 31, 166–169. doi:10.1093/bioinformatics/btu638
Audette, M. C., and Kingdom, J. C. (2018). Screening for fetal growth restriction and placental insufficiency. Semin. Fetal Neonatal Med. 23, 119–125. doi:10.1016/j.siny.2017.11.004
Baird, L., and Yamamoto, M. (2020). The molecular mechanisms regulating the KEAP1-NRF2 pathway. Mol. Cell. Biol. 40, 000999-20–e123. doi:10.1128/MCB.00099-20
Banks, C. J., and Andersen, J. L. (2019). Mechanisms of SOD1 regulation by post-translational modifications. Redox Biol. 26, 101270. doi:10.1016/j.redox.2019.101270
Baptissart, M., Bradish, C. M., Jones, B. S., Walsh, E., Tehrani, J., Marrero-Colon, V., et al. (2022). Zac1 and the Imprinted Gene Network program juvenile NAFLD in response to maternal metabolic syndrome. Hepatology 76, 1090–1104. doi:10.1002/hep.32363
Barker, D. J. P. (1995). Fetal origins of coronary heart disease. BMJ 311, 171–174. doi:10.1136/bmj.311.6998.171
Bashiri, A., Halper, K. I., and Orvieto, R. (2018). Recurrent Implantation Failure-update overview on etiology, diagnosis, treatment and future directions. Reprod. Biol. Endocrinol. 16, 121–218. doi:10.1186/s12958-018-0414-2
Benjamini, Y., and Hochberg, Y. (1995). Controlling the false discovery rate: A practical and powerful approach to multiple testing. J. R. Stat. Soc. 57, 289–300. doi:10.1111/j.2517-6161.1995.tb02031.x
Bouckenheimer, J., Fauque, P., Lecellier, C. H., Bruno, C., Commes, T., Lemaître, J. M., et al. (2018). Differential long non-coding RNA expression profiles in human oocytes and cumulus cells. Sci. Rep. 8, 2202–2213. doi:10.1038/s41598-018-20727-0
Burton, G. J., and Jauniaux, E. (2018). Pathophysiology of placental-derived fetal growth restriction. Am. J. Obstet. Gynecol. 218, S745–S761. doi:10.1016/j.ajog.2017.11.577
Burton, G. J., and Jauniaux, E. (2015). What is the placenta? Am. J. Obstet. Gynecol. 213, S6.e1–S6.e4. doi:10.1016/j.ajog.2015.07.050
Chen, J., and Shaikh, Z. A. (2009). Activation of Nrf2 by cadmium and its role in protection against cadmium-induced apoptosis in rat kidney cells. Toxicol. Appl. Pharmacol. 241, 81–89. doi:10.1016/j.taap.2009.07.038
Coan, P. M., Conroy, N., Burton, G. J., and Ferguson-Smith, A. C. (2006). Origin and characteristics of glycogen cells in the developing murine placenta. Dev. Dyn. 235, 3280–3294. doi:10.1002/dvdy.20981
Crispi, F., Miranda, J., and Gratacós, E. (2018). Long-term cardiovascular consequences of fetal growth restriction: Biology, clinical implications, and opportunities for prevention of adult disease. Am. J. Obstet. Gynecol. 218, S869–S879. doi:10.1016/j.ajog.2017.12.012
Deyssenroth, M. A., Gennings, C., Liu, S., and Peng, S. (2017). Intrauterine multi-metal exposure is associated with reduced fetal growth through modulation of the placental gene network. Physiol. Behav. 176, 139–148.
Dobin, A., Davis, C. A., Schlesinger, F., Drenkow, J., Zaleski, C., Jha, S., et al. (2013). Star: Ultrafast universal RNA-seq aligner. Bioinformatics 29, 15–21. doi:10.1093/bioinformatics/bts635
Eaton, M., Davies, A. H., Devine, J., Zhao, X., Simmons, D. G., Maríusdóttir, E., et al. (2020). Complex patterns of cell growth in the placenta in normal pregnancy and as adaptations to maternal diet restriction. PLoS One 15, 02267355–e226827. doi:10.1371/journal.pone.0226735
Erboga, M., and Kanter, M. (2016). Effect of cadmium on trophoblast cell proliferation and apoptosis in different gestation periods of rat placenta. Biol. Trace Elem. Res. 169, 285–293. doi:10.1007/s12011-015-0439-8
Feng, C., Cheng, L., Jin, J., Liu, X., and Wang, F. (2021). Long non-coding RNA MALAT1 regulates trophoblast functions through VEGF/VEGFR1 signaling pathway. Arch. Gynecol. Obstet. 304, 873–882. doi:10.1007/s00404-021-05987-y
Francula-Zaninovic, S., and Nola, I. A. (2018). Management of measurable variable cardiovascular disease’ risk factors. Curr. Cardiol. Rev. 14, 153–163. doi:10.2174/1573403X14666180222102312
Gagnon, R. (2003). Placental insufficiency and its consequences. Eur. J. Obstet. Gynecol. Reprod. Biol. 110, 99–107. doi:10.1016/s0301-2115(03)00179-9
Gao, L., Kumar, V., Vellichirammal, N. N., Park, S. Y., Rudebush, T. L., Yu, L., et al. (2021). Functional, proteomic, and bioinformatic analyses of Nrf2-and Keap1- null skeletal muscle. J. Physiol. 598, 5427–5451. doi:10.1113/JP280176
Geng, H. X., and Wang, L. (2019). Cadmium: Toxic effects on placental and embryonic development. Environ. Toxicol. Pharmacol. 67, 102–107. doi:10.1016/j.etap.2019.02.006
Gerhardsson, L., Englyst, V., Lundstrom, N.-G., Sandberg, S., and Nordberg, G. (2012). Cadmium, copper and zinc in tissues of deceased copper smelter workers. J. Allergy Clin. Immunol. 130, 556.
Gibbins, K. J., Pinar, H., Reddy, U. M., Saade, G. R., Goldenberg, R. L., Dudley, D. J., et al. (2020). Findings in stillbirths associated with placental disease. Am. J. Perinatol. 37, 708–715. doi:10.1055/s-0039-1688472
Gremlich, S., Damnon, F., Reymondin, D., Braissant, O., Schittny, J. C., Baud, D., et al. (2014). The long non-coding RNA NEAT1 is increased in IUGR placentas, leading to potential new hypotheses of IUGR origin/development. Placenta 35, 44–49. doi:10.1016/j.placenta.2013.11.003
He, F., Ru, X., and Wen, T. (2020). NRF2, a transcription factor for stress response and beyond. Int. J. Mol. Sci. 21, 4777–4823. doi:10.3390/ijms21134777
Hudson, K. M., Belcher, S. M., and Cowley, M. (2019). Maternal cadmium exposure in the mouse leads to increased heart weight at birth and programs susceptibility to hypertension in adulthood. Sci. Rep. 9, 13553. doi:10.1038/s41598-019-49807-5
Hussey, M. R., Burt, A., Deyssenroth, M. A., Jackson, B. P., Hao, K., Peng, S., et al. (2020). Placental lncRNA expression associated with placental cadmium concentrations and birth weight. Environ. Epigenetics 6, dvaa003–10. doi:10.1093/eep/dvaa003
Kaspar, J., Niture, S., and Jaiswal, A. (2000). Nrf2:INrf2(Keap1) signaling in oxidative stress james. Child. Dev. 73, 543–562.
Kippler, M., Hoque, A. M. W., Raqib, R., Ohrvik, H., Ekström, E. C., and Vahter, M. (2010). Accumulation of cadmium in human placenta interacts with the transport of micronutrients to the fetus. Toxicol. Lett. 192, 162–168. doi:10.1016/j.toxlet.2009.10.018
Li, H., Zhu, L., Xu, L., Qin, K., Liu, C., Yu, Y., et al. (2017). Long noncoding RNA linc00617 exhibits oncogenic activity in breast cancer. Mol. Carcinog. 56, 3–17. doi:10.1002/mc.22338
Lin, N., Chang, K. Y., Li, Z., Gates, K., Rana, Z. A., Dang, J., et al. (2014). An evolutionarily conserved long noncoding RNA TUNA controls pluripotency and neural lineage commitment. Mol. Cell 53, 1005–1019. doi:10.1016/j.molcel.2014.01.021
Livak, K. J., and Schmittgen, T. D. (2001). Analysis of relative gene expression data using real-time quantitative PCR and the 2(-Delta Delta C(T)) Method. Methods 25, 402–408. doi:10.1006/meth.2001.1262
Love, M. I., Huber, W., and Anders, S. (2014). Moderated estimation of fold change and dispersion for RNA-seq data with DESeq2. Genome Biol. 15, 550–621. doi:10.1186/s13059-014-0550-8
Muys, B. R., Lorenzi, J. C. C., Zanette, D. L., Lima e Bueno, R. d. B., de Araújo, L. F., Dinarte-Santos, A. R., et al. (2016). Placenta-enriched LincRNAs MIR503HG and LINC00629 decrease migration and invasion potential of JEG-3 cell line. PLoS One 11, 01515600–e151617. doi:10.1371/journal.pone.0151560
Nam, L. B., and Keum, Y. S. (2019). Binding partners of NRF2: Functions and regulatory mechanisms. Arch. Biochem. Biophys. 678, 108184. doi:10.1016/j.abb.2019.108184
Osman, K., Akesson, A., Berglund, M., Bremme, K., Schütz, A., Ask, K., et al. (2000). Toxic and essential elements in placentas of Swedish women. Clin. Biochem. 33, 131–138. doi:10.1016/s0009-9120(00)00052-7
Pengjie, Z., Xionghui, C., Yueming, Z., Ting, X., Na, L., Jianying, T., et al. (2018). LncRNA uc003fir promotes CCL5 expression and negatively affects proliferation and migration of trophoblast cells in preeclampsia. Pregnancy Hypertens. 14, 90–96. doi:10.1016/j.preghy.2018.08.449
Rahimzadeh, M. R., Rahimzadeh, M. R., Kazemi, S., and Moghadamnia, A. A. (2017). Cadmium toxicity and treatment: An update. Casp. J. Intern. Med. 8, 135–145. doi:10.22088/cjim.8.3.135
Rojo de la Vega, M., Chapman, E., and Zhang, D. D. (2018). NRF2 and the hallmarks of cancer. Cancer Cell 34, 21–43. doi:10.1016/j.ccell.2018.03.022
Salavati, N., Smies, M., Ganzevoort, W., Charles, A. K., Erwich, J. J., Plösch, T., et al. (2019). The possible role of placental morphometry in the detection of fetal growth restriction. Front. Physiol. 10. doi:10.3389/fphys.2018.01884
Sasaki, T., Horiguchi, H., Arakawa, A., Oguma, E., Komatsuda, A., Sawada, K., et al. (2019). Hospital-based screening to detect patients with cadmium nephropathy in cadmium-polluted areas in Japan. Environ. Health. Prev. Med. 24, 8. doi:10.1186/s12199-019-0762-3
Satarug, S., Garrett, S. H., Sens, M. A., and Sens, D. A. (2010). Cadmium, environmental exposure, and health outcomes. Environ. Health Perspect. 118, 182–190. doi:10.1289/ehp.0901234
Senís, E., Esgleas, M., Najas, S., Jiménez-Sábado, V., Bertani, C., Giménez-Alejandre, M., et al. (2021). TUNAR lncRNA encodes a microprotein that regulates neural differentiation and neurite formation by modulating calcium dynamics. Front. Cell Dev. Biol. 9, 747667–747714. doi:10.3389/fcell.2021.747667
Silva, J. F., and Serakides, R. (2016). Intrauterine trophoblast migration: A comparative view of humans and rodents. Cell Adhes. Migr. 10, 88–110. doi:10.1080/19336918.2015.1120397
Simmers, M. D., Hudson, K. M., Baptissart, M., and Cowley, M. (2022). Epigenetic control of the imprinted growth regulator Cdkn1c in cadmium-induced placental dysfunction. Epigenetics. In press.
Simmons, D. G., and Cross, J. C. (2005). Determinants of trophoblast lineage and cell subtype specification in the mouse placenta. Dev. Biol. 284, 12–24. doi:10.1016/j.ydbio.2005.05.010
Simmons, D. G., Fortier, A. L., and Cross, J. C. (2007). Diverse subtypes and developmental origins of trophoblast giant cells in the mouse placenta. Dev. Biol. 304, 567–578. doi:10.1016/j.ydbio.2007.01.009
Song, M. Y., Lee, D. Y., Chun, K. S., and Kim, E. H. (2021). The role of nrf2/keap1 signaling pathway in cancer metabolism. Int. J. Mol. Sci. 22, 4376–4416. doi:10.3390/ijms22094376
Statello, L., Guo, C. J., Chen, L. L., and Huarte, M. (2021). Gene regulation by long non-coding RNAs and its biological functions. Nat. Rev. Mol. Cell Biol. 22, 96–118. doi:10.1038/s41580-020-00315-9
Strober, W. (2015). Trypan blue exclusion test of cell viability. Curr. Protoc. Immunol. 111, A3–A3.B.3. doi:10.1002/0471142735.ima03bs111
Tanaka, M., Gertsenstein, M., Rossant, J., and Nagy, A. (1997). Mash2 acts cell autonomously in mouse spongiotrophoblast development. Dev. Biol. 190, 55–65. doi:10.1006/dbio.1997.8685
Taylor, C. M., Golding, J., and Emond, A. M. (2016). Moderate prenatal cadmium exposure and adverse birth outcomes: A role for sex-specific differences? Paediatr. Perinat. Epidemiol. 30, 603–611. doi:10.1111/ppe.12318
Tonelli, C., Chio, I. I. C., and Tuveson, D. A. (2018). Transcriptional regulation by Nrf2. Antioxidants Redox Signal 29, 1727–1745. doi:10.1089/ars.2017.7342
Villeneuve, N. F., Lau, A., and Zhang, D. D. (2010). Regulation of the Nrf2-keap1 antioxidant response by the ubiquitin proteasome system: An insight into cullin-ring ubiquitin ligases. Antioxidants Redox Signal 13, 1699–1712. doi:10.1089/ars.2010.3211
Wang, Y., Hu, S., Yao, G., Zhu, Q., He, Y., Lu, Y., et al. (2020). A novel molecule in human cyclic endometrium: LncRNA TUNAR is involved in embryo implantation. Front. Physiol. 11, 587448–587511. doi:10.3389/fphys.2020.587448
Watson, E. D., and Cross, J. C. (2005). Development of structures and transport functions in the mouse placenta. Physiology 20, 180–193. doi:10.1152/physiol.00001.2005
Woods, L., Perez-Garcia, V., and Hemberger, M. (2018). Regulation of placental development and its impact on fetal growth—new insights from mouse models. Front. Endocrinol. (Lausanne). 9, 570–618. doi:10.3389/fendo.2018.00570
Zhang, D. D., Lo, S. C., Sun, Z., Habib, G. M., Lieberman, M. W., and Hannink, M. (2005). Ubiquitination of Keap1, a BTB-Kelch substrate adaptor protein for Cul3, targets Keap1 for degradation by a proteasome-independent pathway. J. Biol. Chem. 280, 30091–30099. doi:10.1074/jbc.M501279200
Zhang, X., Wang, W., Zhu, W., Dong, J., Cheng, Y., Yin, Z., et al. (2019). Mechanisms and functions of long non-coding RNAs at multiple regulatory levels. Int. J. Mol. Sci. 20, 5573. doi:10.3390/ijms20225573
Keywords: lncRNA, Tuna, placental insufficiency, fetal growth restriction (FGR), Cadmium, Nrf2, oxidative stress
Citation: Simmers MD, Jima DD, Tsuji Y and Cowley M (2023) LncRNA Tuna is activated in cadmium-induced placental insufficiency and drives the NRF2-mediated oxidative stress response. Front. Cell Dev. Biol. 11:1151108. doi: 10.3389/fcell.2023.1151108
Received: 25 January 2023; Accepted: 24 April 2023;
Published: 01 June 2023.
Edited by:
Jianrong Lu, University of Florida, United StatesReviewed by:
Jamie L. McCall, West Virginia University, United StatesCopyright © 2023 Simmers, Jima, Tsuji and Cowley. This is an open-access article distributed under the terms of the Creative Commons Attribution License (CC BY). The use, distribution or reproduction in other forums is permitted, provided the original author(s) and the copyright owner(s) are credited and that the original publication in this journal is cited, in accordance with accepted academic practice. No use, distribution or reproduction is permitted which does not comply with these terms.
*Correspondence: Michael Cowley, bWFjb3dsZXlAbmNzdS5lZHU=
Disclaimer: All claims expressed in this article are solely those of the authors and do not necessarily represent those of their affiliated organizations, or those of the publisher, the editors and the reviewers. Any product that may be evaluated in this article or claim that may be made by its manufacturer is not guaranteed or endorsed by the publisher.
Research integrity at Frontiers
Learn more about the work of our research integrity team to safeguard the quality of each article we publish.