- 1Institute of Molecular and Cellular Anatomy, RWTH Aachen University, Aachen, Germany
- 2Department of Biosciences, University of Durham, Upper Mountjoy Science Site, Durham, United Kingdom
- 3Biophysical Sciences Institute, University of Durham, Durham, United Kingdom
- 4Department of Biological Structure, University of Washington, Seattle, WA, United States
Editorial on the Research Topic
3D architecture of intermediate filaments in tissue mechanics and function
In this special issue there is a collection of articles that highlight the mechano-biological signalling of and the integration of intermediate filaments within the cytoskeletal machinery. The individual and collective contribution of the individual cytoskeletal elements have been well documented (Ge et al., 2020; Serres et al., 2020; Lois-Bermejo et al., 2022; Nunes Vicente et al., 2022; Ridge et al., 2022; Sivagurunathan et al., 2022; Wu et al., 2022). Indeed the importance of the cytoskeleton as an integrated unit is accepted fully in the literature (Pegoraro et al., 2017; Hohmann and Dehghani, 2019). Intermediate filaments interconnect all subcellular compartments and they are the one cytoskeletal element where cross-β-interactions form intracellular hydrogels (Kato and McKnight, 2018) by virtue of their N- and C-terminal intrinsically disordered domains ((IDDs) (Kornreich et al., 2015))—or plainly put—assist their assembly and their associated phase separation events e.g., (Li et al., 2020). It is no coincidence that previously noted “zones of exclusion” observed by conventional transmission electron microscopy (Blose and Chacko, 1976; Borenfreund et al., 1980) should now be interpreted as evidence of their hydrogel potential e.g., nuclear pores (Fiserova et al., 2014) and cytoplasmic intermediate filament networks (Kornreich et al., 2015). The importance of these IDDs to cell behaviour and to their emergent properties (Ridge et al., 2022) is a hot Research Topic in current debate. This has given rise to exciting hypotheses to explain complex cell behaviours such as motility (see the contributions by Infante and Etienne-Manneville; Kim et al., in this research topic issue) cell polarisation (ibid Despin-Guitard et al.,) epithelial-mesenchymal transitions and inflammatory responses (Ridge et al., 2022).
One such hypothesis is the “wetware” concept (Bray, 2009) as a way to conceptualise cellular and tissue decision-making at the level of individual components and processes (Kulkarni et al., 2022). Cell Biology compartmentalises systems and structures, but each function within the context of the cell and the tissue require integration and localised responses of metabolic, structural and cellular pathways. The cytoskeleton collectively provides the architecture (Figure 1) that is needed to sense, communicate and respond to the legion of stimuli received at any one time by each individual cell. It, and its associated biomolecules, can deliver the processing logic for the cell because it provides the required connections (Bray, 2009). In this respect, the intermediate filament cytoskeleton is part and parcel of the stress response (Welch et al., 1985; Quinlan et al., 2002; Landsbury et al., 2010; Toivola et al., 2010) and to the transcriptional (Shimi and Goldman, 2014; Nazer, 2022) and to translational regulation (Magin et al., 2007; Kim and Coulombe, 2010; Mohanasundaram et al., 2022), to chaperone mediated autophagy (Bandyopadhyay et al., 2010), to respiratory efficiency (Diokmetzidou et al., 2016) and to cell division (Matsuyama et al., 2013). This identifies intermediate filaments as key interconnectors for subcellular interaction networks (Kulkarni et al., 2022). Indeed, the intermediate filament provides a surface to facilitate biomolecular folding, biomolecular complex assembly and complex organisation. Intermediate filaments as a collective provide a scale-free network across diverse length scales especially as a result of the inter-cellular organisation they afford within a tissue via their connection to cell-cell junctions such as the desmosome (see Green et al., in this research topic issue). Their integrative role in mechano-signalling (Infante and Etienne-Manneville; this research topic issue) is well founded and super-resolution microscopy demonstrates that stretching filaments will reveal new, and quite possibly novel, functional nanodomains (Massou et al., 2020; Nunes Vicente et al., 2022) as also shown for lipo-oxidative stress (Lois-Bermejo et al., 2022). Stress reveals the importance of the C-terminal IDDs to the biophysical properties of intermediate filaments (Aufderhorst-Roberts and Koenderink, 2019) as well as to their assembly and ultimately therefore also to cell morphology (Zhou et al., 2021).
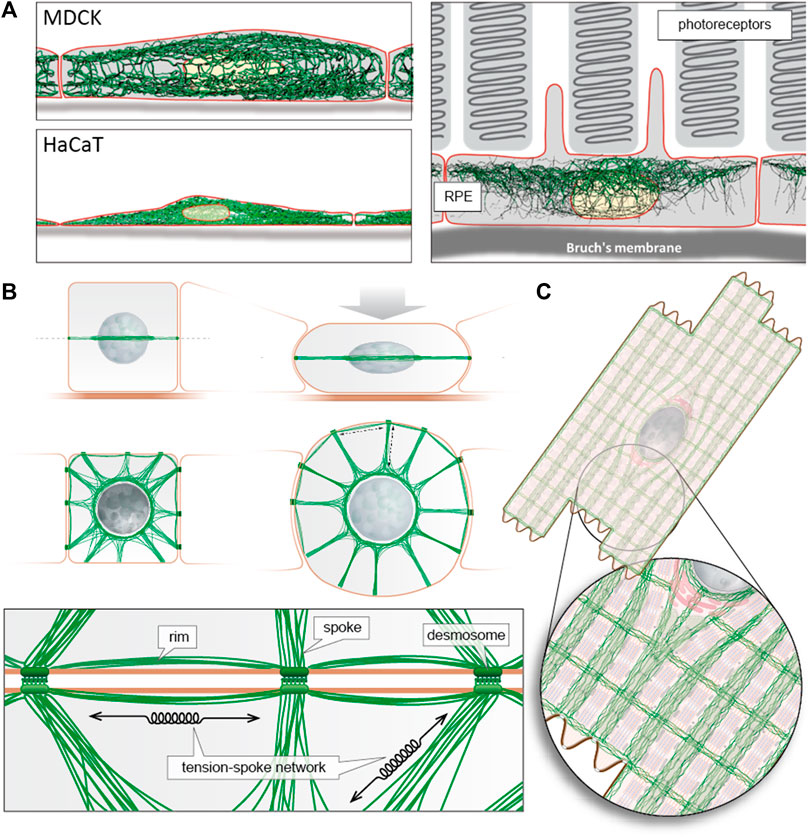
FIGURE 1. Intermediate filament architecture is cell type specific to support tissue mechanics and function. (A) The digital reconstructions of intermediate filament networks that are superimposed on schematic drawings of the corresponding cells are taken from Windoffer et al. (2022). They were derived from 3D recordings of fluorescently labelled keratins in polarized canine MDCK cells, human immortalized epidermal HaCaT keratinocytes and murine retinal pigment epithelial cells in situ. Note the different network distributions ranging from apical enrichment (RPE) to apical and basal enrichment (MDCK) and pan cytoplasmic (HaCaT). (B) The modified scheme from Quinlan et al. (2017) highlights the circumferential rim and radial spokes arrangement of keratin intermediate filaments connecting the network to the perinuclear cage and adjacent cells through desmosomes. The resulting transcellular tension-spoke system provides mechanical resilience. (C) Schematic representation of desmin intermediate filament architecture in cardiomyocytes providing defined subcellular spaces for the ordered contractile apparatus, mitochondria, the nucleus and attachment sites to neighbouring cardiomyocytes and the extracellular matrix (adapted from (Behrendt, 1977; Tokuyasu et al. 1983; Wang and Ramirez-Mitchell, 1983)).
The Research Topic highlights aspects of epithelial keratin network organization. Using a Krt8:YFP reporter mouse Desprin-Guitard and colleagues (see Despin-Guitard et al., in this research topic issue) study the keratin intermediate filament network in the developing mouse embryo revealing a kaleidoscope of temporally and spatially determined expression profiles in embryonic and extraembryonic tissues which are interpreted as plastic adaptations of cell mechanics to growth and morphological changes. The review by Green and colleagues in this research topic issue focuses on the epidermal desmosome-keratin system as an integrator of mechanically-determined signalling. In concert with other junctions, desmosomes dictate epidermal polarization and differentiation forming a barrier by stratum-specific junctional and cytoskeletal arrangements. The authors suggest that these arrangements counteract inflammation. The paper by Yoon and colleagues in this research topic issue presents technical advancements for multidimensional and multimodal monitoring of keratin filament architecture and function. High resolution microscopy of fluorescent keratins is enabled on defined matrices and combined with traction force microscopy. In this way, the interrelationship between extracellular matrix cues with global 3D cytoskeletal network properties at the keratin filament/keratin bundle level and local forces is quantified by refined image analysis. It is further illustrated that these tools can be used for monitoring the consequences of local keratin network perturbations and ECM composition on cell mechanics in the context of transcellular network arrangement.
Infante and Etienne-Manneville in this research topic issue summarize current knowledge about the spatial arrangement and integration of cytoplasmic and nuclear intermediate filaments and their interaction with other cytoplasmic filament systems during cell migration. They emphasize the different properties of the different intermediate filament types as a basis of cell type- and function-related cellular mechanics. They further highlight the cooperativity between intermediate filaments with the other cytoskeletal systems determining motile properties of single cells and cell collectives. Direct experimental assessment of vimentin’s function during metastatic invasion is finally provided by Kim and colleagues in this research topic issue. Using a novel vimentin-stabilizing drug they report on altered vimentin network morphology with consequences on adhesion and contractility resulting in cell shape changes, increased tractions forces and perturbed migration.
Figure 1 presents examples of intermediate filament network organization to illustrate their function both as organizers of the subcellular space (Schwarz and Leube, 2016) and as transcellular integrators to facilitate and support coordinated mechanical and biochemical functions in the context of tissues rather than individual cells (Hatzfeld et al., 2017). It is this framework upon which the contributions in this Research Topic have been made.
Author contributions
All authors listed have made a substantial, direct, and intellectual contribution to the work and approved it for publication.
Acknowledgments
We dedicate this editorial to Professor Werner Franke, whose passion for Intermediate Filaments and cell-cell junctions inspired many generations of cell biologists. We thank our respective academic institutions (Universities of Aachen, Durham and Washington). REL thanks the German Research Council for continued support (LE566/18-2; GRK2415/363055819). RAQ and REL thank the Biophysical Sciences Institute and the Institute of Advanced Study (Durham University) for financial support.
Conflict of interest
The authors declare that the research was conducted in the absence of any commercial or financial relationships that could be construed as a potential conflict of interest.
Publisher’s note
All claims expressed in this article are solely those of the authors and do not necessarily represent those of their affiliated organizations, or those of the publisher, the editors and the reviewers. Any product that may be evaluated in this article, or claim that may be made by its manufacturer, is not guaranteed or endorsed by the publisher.
References
Aufderhorst-Roberts, A., and Koenderink, G. H. (2019). Stiffening and inelastic fluidization in vimentin intermediate filament networks. Soft Matter 15 (36), 7127–7136. doi:10.1039/c9sm00590k
Bandyopadhyay, U., Sridhar, S., Kaushik, S., Kiffin, R., and Cuervo, A. M. (2010). Identification of regulators of chaperone-mediated autophagy. Mol. Cell 39 (4), 535–547. doi:10.1016/j.molcel.2010.08.004
Behrendt, H. (1977). Effect of anabolic steroids on rat heart muscle cells. I. Intermediate filaments. Cell Tissue Res. 180 (3), 303–315. doi:10.1007/BF00227598
Blose, S. H., and Chacko, S. (1976). Rings of intermediate (100 A) filament bundles in the perinuclear region of vascular endothelial cells. Their mobilization by colcemid and mitosis. J. Cell Biol. 70 (21), 459–466. doi:10.1083/jcb.70.2.459
Borenfreund, E., Schmid, E., Bendich, A., and Franke, W. W. (1980). Constitutive aggregates of intermediate-sized filaments of the vimentin and cytokeratin type in cultured hepatoma cells and their dispersal by butyrate. Exp. Cell Res. 127, 215–235. doi:10.1016/0014-4827(80)90428-0
Despin-Guitard, E., Quenec'Hdu, R., Nahaboo, W., Schwarz, N., Leube, R. E., Chazaud, C., et al. (2022). Regionally specific levels and patterns of keratin 8 expression in the mouse embryo visceral endoderm emerge upon anterior-posterior axis determination. Front. Cell Dev. Biol. 2022 Dec 1; 10:1037041. doi:10.3389/fcell.2022.1037041
Diokmetzidou, A., Soumaka, E., Kloukina, I., Tsikitis, M., Makridakis, M., Varela, A., et al. (2016). Desmin and αB-crystallin interplay in the maintenance of mitochondrial homeostasis and cardiomyocyte survival. J. Cell Sci. 129 (20), 3705–3720. doi:10.1242/jcs.192203
Fiserova, J., Spink, M., Richards, S. A., Saunter, C., and Goldberg, M. W. (2014). Entry into the nuclear pore complex is controlled by a cytoplasmic exclusion zone containing dynamic GLFG-repeat nucleoporin domains. J. Cell Sci. 127 (1), 124–136. doi:10.1242/jcs.133272
Ge, X., Zhang, T., Yu, X., Muwonge, A. N., Anandakrishnan, N., Wong, N. J., et al. (2020). LIM-nebulette reinforces podocyte structural integrity by linking actin and vimentin filaments. J. Am. Soc. Nephrol. 31 (10), 2372–2391. doi:10.1681/ASN.2019121261
Green, K. J., Niessen, C. M., Rübsam, M., Perez White, B. E., and Broussard, J. A. (2022). The Desmosome-Keratin Scaffold Integrates ErbB Family and Mechanical Signaling to Polarize Epidermal Structure and Function. Front. Cell Dev. Biol. 10, 903696. doi:10.3389/fcell.2022.903696
Hatzfeld, M., Keil, R., and Magin, T. M. (2017). Desmosomes and intermediate filaments: Their consequences for tissue mechanics. Cold Spring Harb. Perspect. Biol. 9 (6), a029157. doi:10.1101/cshperspect.a029157
Hohmann, T., and Dehghani, F. (2019). The cytoskeleton-A complex interacting meshwork. Cells 8 (4), 362. doi:10.3390/cells8040362
Kato, M., and McKnight, S. L. (2018). A solid-state conceptualization of information transfer from gene to message to protein. Annu. Rev. Biochem. 87, 351–390. doi:10.1146/annurev-biochem-061516-044700
Kim, HR., Warrington, SJ., López-Guajardo, A., Al Hennawi, K., Cook, SL., Griffith,, Z. D. J., et al. (2022). ALD-R491 regulates vimentin filament stability and solubility, cell contractile force, cell migration speed and directionality. Front. Cell Dev. Biol. 10, 926283. doi:10.3389/fcell.2022.926283
Kim, S., and Coulombe, P. A. (2010). Emerging role for the cytoskeleton as an organizer and regulator of translation. Nat. Rev. Mol. Cell Biol. 11 (1), 75–81. doi:10.1038/nrm2818
Kornreich, M., Avinery, R., Malka-Gibor, E., Laser-Azogui, A., and Beck, R. (2015). Order and disorder in intermediate filament proteins. FEBS Lett. 589 (19), 2464–2476. doi:10.1016/j.febslet.2015.07.024
Kulkarni, P., Bhattacharya, S., Achuthan, S., Behal, A., Jolly, M. K., Kotnala, S., et al. (2022). Intrinsically disordered proteins: Critical components of the wetware. Chem. Rev. 122 (6), 6614–6633. doi:10.1021/acs.chemrev.1c00848
Landsbury, A. (2010). “Functional symbiosis between the intermediate filament cytoskeleton and small heat shock proteins,” in Small stress proteins and human diseases. Editors S. Simon, and A. P. Arrigo, 55–87.
Li, Y., Liu, X., Xia, C. H., FitzGerald, P. G., Li, R., Wang, J., et al. (2020). CP49 and filensin intermediate filaments are essential for formation of cold cataract. Mol. Vis. 26, 603–612.
Lois-Bermejo, I., Gonzalez-Jimenez, P., Duarte, S., Pajares, M. A., and Perez-Sala, D. (2022). Vimentin tail segments are differentially exposed at distinct cellular locations and in response to stress. Front. Cell Dev. Biol. 10, 908263. doi:10.3389/fcell.2022.908263
Magin, T. M., Vijayaraj, P., and Leube, R. E. (2007). Structural and regulatory functions of keratins. Exp. Cell Res. 313 (10), 2021–2032. doi:10.1016/j.yexcr.2007.03.005
Massou, S., Nunes Vicente, F., Wetzel, F., Mehidi, A., Strehle, D., Leduc, C., et al. (2020). Cell stretching is amplified by active actin remodelling to deform and recruit proteins in mechanosensitive structures. Nat. Cell Biol. 22 (8), 1011–1023. doi:10.1038/s41556-020-0548-2
Matsuyama, M., Tanaka, H., Inoko, A., Goto, H., Yonemura, S., Kobori, K., et al. (2013). Defect of mitotic vimentin phosphorylation causes microophthalmia and cataract via aneuploidy and senescence in lens epithelial cells. J. Biol. Chem. 288 (50), 35626–35635. doi:10.1074/jbc.M113.514737
Mohanasundaram, P., Coelho-Rato, L. S., Modi, M. K., Urbanska, M., Lautenschlager, F., Cheng, F., et al. (2022). Cytoskeletal vimentin regulates cell size and autophagy through mTORC1 signaling. PLoS Biol. 20 (9), e3001737. doi:10.1371/journal.pbio.3001737
Nazer, E. (2022). To be or not be (in the LAD): Emerging roles of lamin proteins in transcriptional regulation. Biochem. Soc. Trans. 50 (2), 1035–1044. doi:10.1042/BST20210858
Nunes Vicente, F., Lelek, M., Tinevez, J. Y., Tran, Q. D., Pehau-Arnaudet, G., Zimmer, C., et al. (2022). Molecular organization and mechanics of single vimentin filaments revealed by super-resolution imaging. Sci. Adv. 8 (8), eabm2696. doi:10.1126/sciadv.abm2696
Pegoraro, A. F., Janmey, P., and Weitz, D. A. (2017). Mechanical properties of the cytoskeleton and cells. Cold Spring Harb. Perspect. Biol. 9 (11), a022038. doi:10.1101/cshperspect.a022038
Quinlan, R. A. (2002). “Cytoskeletal competence requires protein chaperones,” in Progress in molecular and subcellular Biology. Small stress proteins. Editors A.-P. Arrigo, and W. E. G. Muller, 28, 219–233.
Quinlan, R. A., Schwarz, N., Windoffer, R., Richardson, C., Hawkins, T., Broussard, J. A., et al. (2017). A rim-and-spoke hypothesis to explain the biomechanical roles for cytoplasmic intermediate filament networks. J. Cell Sci. 130 (20), 3437–3445. doi:10.1242/jcs.202168
Ridge, K. M., Eriksson, J. E., Pekny, M., and Goldman, R. D. (2022). Roles of vimentin in health and disease. Genes Dev. 36 (7-8), 391–407. doi:10.1101/gad.349358.122
Schwarz, N., and Leube, R. E. (2016). Intermediate filaments as organizers of cellular space: How they affect mitochondrial structure and function. Cells 5 (3), 30. doi:10.3390/cells5030030
Serres, M. P., Samwer, M., Truong Quang, B. A., Lavoie, G., Perera, U., Gorlich, D., et al. (2020). F-actin interactome reveals vimentin as a key regulator of actin organization and cell mechanics in mitosis. Dev. Cell 52 (2), 210–222. doi:10.1016/j.devcel.2019.12.011
Shimi, T., and Goldman, R. D. (2014). Nuclear lamins and oxidative stress in cell proliferation and longevity. Adv. Exp. Med. Biol. 773, 415–430. doi:10.1007/978-1-4899-8032-8_19
Sivagurunathan, S., Vahabikashi, A., Yang, H., Zhang, J., Vazquez, K., Rajasundaram, D., et al. (2022). Expression of vimentin alters cell mechanics, cell-cell adhesion, and gene expression profiles suggesting the induction of a hybrid EMT in human mammary epithelial cells. Front. Cell Dev. Biol. 10, 929495. doi:10.3389/fcell.2022.929495
Sun, H., Wang, A., and He, S. (2022). Temporal and spatial analysis of alzheimer's disease based on an improved convolutional neural network and a resting-state FMRI brain functional network. Int. J. Environ. Res. Public Health 19 (8), 4508. doi:10.3390/ijerph19084508
Tokuyasu, K. T., Dutton, A. H., and Singer, S. J. (1983). Immunoelectron microscopic studies of desmin (skeletin) localization and intermediate filament organization in chicken cardiac muscle. J. Cell Biol. 96 (6), 1736–1742. doi:10.1083/jcb.96.6.1736
Toivola, D. M., Strnad, P., Habtezion, A., and Omary, M. B. (2010). Intermediate filaments take the heat as stress proteins. Trends Cell Biol. 20 (2), 79–91. doi:10.1016/j.tcb.2009.11.004
Wang, K., and Ramirez-Mitchell, R. (1983). A network of transverse and longitudinal intermediate filaments is associated with sarcomeres of adult vertebrate skeletal muscle. J. Cell Biol. 96 (2), 562–570. doi:10.1083/jcb.96.2.562
Welch, W. J., Feramisco, J. R., and Blose, S. H. (1985). The mammalian stress response and the cytoskeleton: Alterations in intermediate filaments. Ann. N. Y. Acad. Sci. 455 (57), 57–67. doi:10.1111/j.1749-6632.1985.tb50403.x
Windoffer, R., Schwarz, N., Yoon, S., Piskova, T., Scholkemper, M., Stegmaier, J., et al. (2022). Quantitative mapping of keratin networks in 3D. Elife 11, e75894. doi:10.7554/eLife.75894
Wu, H., Shen, Y., Sivagurunathan, S., Weber, M. S., Adam, S. A., Shin, J. H., et al. (2022). Vimentin intermediate filaments and filamentous actin form unexpected interpenetrating networks that redefine the cell cortex. Proc. Natl. Acad. Sci. U. S. A. 119 (10), e2115217119. doi:10.1073/pnas.2115217119
Yoon, S., Windoffer, R., Kozyrina, A. N., Piskova, T., Di Russo, J., and Leube, R. E. (2022). Combining image restoration and traction force microscopy to study extracellular matrix-dependent keratin filament network plasticity. plasticity. Front. Cell Dev. Biol. 10, 901038. doi:10.3389/fcell.2022.901038
Keywords: cytoskeleton, intermediate filament, cell junctions, cell shape, cell polarity and migration, wetware
Citation: Leube RE and Quinlan RA (2023) Editorial: The wetware credentials of intermediate filaments involves coordinating, organising and networking in cells and tissues. Front. Cell Dev. Biol. 11:1146618. doi: 10.3389/fcell.2023.1146618
Received: 17 January 2023; Accepted: 23 January 2023;
Published: 13 February 2023.
Edited and reviewed by:
Claudia Tanja Mierke, Leipzig University, GermanyCopyright © 2023 Leube and Quinlan. This is an open-access article distributed under the terms of the Creative Commons Attribution License (CC BY). The use, distribution or reproduction in other forums is permitted, provided the original author(s) and the copyright owner(s) are credited and that the original publication in this journal is cited, in accordance with accepted academic practice. No use, distribution or reproduction is permitted which does not comply with these terms.
*Correspondence: Rudolf E. Leube, cmxldWJlQHVrYWFjaGVuLmRl; Roy A. Quinlan, ci5hLnF1aW5sYW5AZHVyaGFtLmFjLnVr