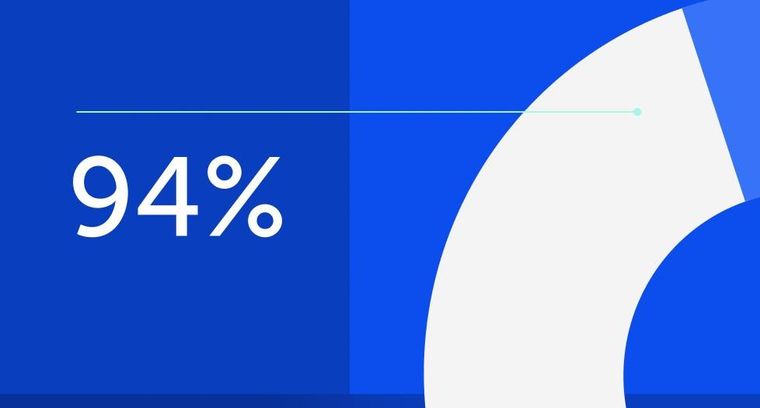
94% of researchers rate our articles as excellent or good
Learn more about the work of our research integrity team to safeguard the quality of each article we publish.
Find out more
ORIGINAL RESEARCH article
Front. Cell Dev. Biol., 16 August 2023
Sec. Morphogenesis and Patterning
Volume 11 - 2023 | https://doi.org/10.3389/fcell.2023.1141893
This article is part of the Research TopicAdvances in Morphogenesis and Patterning: Zebrafish as a Model OrganismView all 5 articles
Craniofacial development is a complex and tightly regulated process and disruptions can lead to structural birth defects, the most common being nonsyndromic cleft lip and palate (NSCLP). Previously, we identified FOS as a candidate regulator of NSCLP through family-based association studies, yet its specific contributions to oral and palatal formation are poorly understood. This study investigated the role of fos during zebrafish craniofacial development through genetic disruption and knockdown approaches. Fos was expressed in the periderm, olfactory epithelium and other cell populations in the head. Genetic perturbation of fos produced an abnormal craniofacial phenotype with a hypoplastic oral cavity that showed significant changes in midface dimensions by quantitative facial morphometric analysis. Loss and knockdown of fos caused increased cell apoptosis in the head, followed by a significant reduction in cranial neural crest cells (CNCCs) populating the upper and lower jaws. These changes resulted in abnormalities of cartilage, bone and pharyngeal teeth formation. Periderm cells surrounding the oral cavity showed altered morphology and a subset of cells in the upper and lower lip showed disrupted Wnt/β-catenin activation, consistent with modified inductive interactions between mesenchymal and epithelial cells. Taken together, these findings demonstrate that perturbation of fos has detrimental effects on oral epithelial and CNCC-derived tissues suggesting that it plays a critical role in zebrafish craniofacial development and a potential role in NSCLP.
Vertebrate craniofacial development relies on a complex and tightly regulated series of tissue growth and fusion, and even minor disruptions to this intricate process can lead to birth defects, the most common of which is nonsyndromic cleft lip and palate (NSCLP) (Cordero et al., 2011; Gorlin et al., 2011). The cranial neural crest cells (CNCCs) are a multipotent population of cells that give rise to the various tissues comprising the craniofacial structures, including cartilage and bone, muscles, sensory neurons, and pigment cells (Cordero et al., 2011; Mork and Crump, 2015; Dash and Trainor, 2020). In human embryonic development, CNCCs give rise to the facial prominences during the fourth week of gestation, which over the subsequent 6–8 weeks, grow, make contact and fuse to form complete facial structures (Marazita and Mooney, 2004). Additionally, epithelial cells in the facial prominences provide important signals to CNCCs, directing their proliferation and patterning to further regulate craniofacial growth and morphogenesis (Chai and Maxson, 2006; Jiang et al., 2006). These interactions involve multiple molecular signaling pathways, including, but not limited to, wingless-type MMTV integration site family (WNT), fibroblast growth factor (FGF), bone morphogenetic protein (BMP), hedgehog (Hh) and ectodysplasin A (EDA) (Chai and Maxson, 2006; Reynolds et al., 2019).
Deficiencies in cell migration, changes in mitotic activity or apoptosis, and interruptions to epithelial-mesenchymal interactions can affect the shape and size of the facial prominences and increase the likelihood of an orofacial cleft (Jiang et al., 2006; Dixon et al., 2011; Ji et al., 2020). Quantitative studies of facial form using geometric morphometrics (GMM) have provided important information for understanding processes disrupted during craniofacial development by identifying phenotypic changes in facial prominences that contribute to orofacial clefts (Young et al., 2007).
In previous studies, we reported an association of CRISPLD2 with NSCLP that was independently replicated in different populations (Chiquet et al., 2007; Letra et al., 2010; Shen et al., 2011; Mijiti et al., 2015; Ge et al., 2018). Further, we showed that knockdown and loss of crispld2 in zebrafish caused severe craniofacial defects resulting from altered migration of CNCCs (Yuan et al., 2012; Swindell et al., 2015; Chiquet et al., 2018). RNA-seq analysis of crispld2 wild type (WT) and morphant embryos revealed that fosab (zebrafish homologue of FOS) was differentially expressed. This finding, and a positive association between FOS/rs1046117 and NSCLP, identified FOS as a novel candidate NSCLP gene (Chiquet et al., 2018).
Fos is part of the activating protein-1 (AP-1) transcription factor complex and FOS has roles in oncogenic processes such as tumor growth and progression, as well as biological processes like proliferation, differentiation, epithelial-to-mesenchymal transition and apoptosis (Wagner, 2002; Milde-Langosch, 2005; Durchdewald et al., 2009; Velazquez et al., 2015; Chen et al., 2017; Rodriguez-Berdini et al., 2020). Fos is also an activator in the lipid synthesis pathway in the endoplasmic reticulum (Wagner, 2002; Durchdewald et al., 2009; Rodriguez-Berdini et al., 2020). Mice lacking Fos display severe osteopetrosis, impaired gametogenesis, abnormal hematopoiesis and behavioral changes (Grigoriadis et al., 1995). Craniofacial abnormalities such as a domed skull with a shorter snout, absence of tooth eruption, as well as a reduced neocortex are also common features in Fos null mice (Johnson et al., 1992; Wagner, 2002; Alfaqeeh et al., 2015; Velazquez et al., 2015). Importantly, reporter expression is found in orofacial tissues, including the medial edge epithelium (MEE) of the palate, the dental papilla mesenchyme, the periderm, and cells at the midline of the nasal septum of fos-lacZ mice (Smeyne et al., 1993a; Smeyne et al., 1993b). Fos protein is also detected in the rat MEE just before the elevation of the palatal shelves, the facial epidermis, Meckel’s cartilage and the mesenchymal condensations that precede bone and muscle formation (Yano et al., 1996). These studies provide strong evidence that FOS plays a role during craniofacial development. However, its contributions to mouth and palate formation remain to be elucidated.
Zebrafish is a commonly used animal model for craniofacial studies of embryonic development and offers many advantages that make them ideal for detailed studies, including the ability to obtain large numbers of transparent, externally developing embryos (Yelick and Schilling, 2002; Mork and Crump, 2015; Duncan et al., 2017; Raterman et al., 2020). Additionally, zebrafish craniofacial development has been well characterized and is comparable to mammalian craniofacial development (Schilling and Le Pabic, 2009; Eames et al., 2013; Mork and Crump, 2015). The ethmoid plate in zebrafish, for example, is thought to be functionally analogous to palatal development in mammals, however, there are also differences (Swartz et al., 2011; Dougherty et al., 2013; Cusack et al.,2017; Raterman et al., 2020). This study investigated the role of fos during craniofacial development in zebrafish using geometric morphometrics combined with tissue and cell analysis to determine its potential involvement in craniofacial morphogenesis and potential contributions to NSCLP.
Fluorescent in situ hybridization using hybridization chain reaction (HCR) (Choi et al., 2018) was used to identify fosab (referred to as fos) mRNA expression during 1–5 days post fertilization (dpf) of zebrafish development. Overall, low levels of fos mRNA were observed in the embryonic head at 1 dpf, however, expression was detected in a subset of tissues around the eyes (Figure 1A, A’, B, B’; Supplementary Figure S1A). Increased fos expression was observed at 2 dpf as well as around the nares/olfactory placodes, midfacial region, eyes, oral cavity and brain (Figure 1B, 1B’ 1C, 1C’). By 5dpf, strong expression was observed in the areas surrounding the nares/olfactory pits, perioral tissues (upper and lower lips), and more diffusely in the lower jaw (Figure 1D, 1D’, 1E, 1E’). Expression was also observed in the otic vesicle and lens (Figure 1D, 1D’). Examination of individual z-slices at 5 dpf showed fluorescent signal in the brain and olfactory epithelium, and the outer epithelial layer (Supplementary Figure S2). Quantitative real-time PCR of fos transcripts at these developmental time points were normalized to beta actin (actb1) and supported the in situ results, with lower fos expression observed at 1 dpf and highest at 5 dpf (Figure 1H). These findings support the expression of fos in craniofacial tissues throughout early development stages in zebrafish.
FIGURE 1. Fos is expressed in craniofacial tissues during zebrafish development. (A) Hybridization chain reaction (HCR) in situ with zebrafish fosab RNA probe set showed localized expression around the developing brain and olfactory placodes at 1 day post fertilization (dpf) (4 animals imaged), shown in lateral view and denoted by arrowheads (A, A’, B, B’), increased expression in the midface and oral cavity at 2 dpf (7 animals imaged)shown in lateral and ventral views (C, C’, D, D’, E, E’) and 5 dpf show in lateral and rostral views (5 animals imaged) (F, F’, G, G’). (B) Quantitative RT-PCR showing relatively lower mRNA expression at 1 dpf and higher expression at 2 dpf and 5 dpf (3 separate batches of 5 embryos each in each group).
To define the role of fos in craniofacial development, zebrafish F0 mutants (referred to as crispants (Burger et al., 2016)) were generated using CRISPR/Cas9 with two single guide RNAs (sgRNAs) targeting exons 1 and 4 of the fos gene. Genotyping revealed efficient mutagenesis with 89% of embryos showing a deletion of approximately 1,500 base pairs (bp) of the fos coding region (Figure 2A). F0 embryos (84%) displayed an abnormal phenotype at 5 dpf, with smaller head and eyes, cardiac edema, abnormal yolk extension, missing swim bladder and curved body axis compared to uninjected control embryos (UIC) and tyrosinase sgRNA-injected F0 embryos (0%) (Figures 2B,C; Supplementary Figure S4). Abnormal mouth shape, displaced and merged neuromasts (arrowheads), and anomalies in lower jaw tissues were observed (Figures 2D,E). In situ hybridization showed reduced fos mRNA expression in the head at all developmental time points examined (Supplementary Figure S1). A small number of embryos (16%) appeared normal, likely reflecting mosaicism resulting from F0 CRISPR mutagenesis. Stable fos mutants (F2 generation) with the same deletion as F0 crispants had significantly reduced fos mRNA levels and a normal phenotype. Genetic compensation studies of closely related genes showed that fosb was significantly upregulated in the mutants at both 2 and 3 dpf (p = 0.03 and 0.006 respectively) (Supplementary Figure S3). Moreover, providing additional evidence for fos excision in these animals, injection of the fos sgRNAs into the stable F2 mutants did not cause an abnormal craniofacial phenotype (Supplementary Figure S4A).
FIGURE 2. Fos mutants and morphants have distinctive keyhole-shaped mouth and abnormal craniofacies. (A) Schematic of CRISPR guides targeting exons 1 and 4 of the fosab gene and resulting in an almost complete deletion of the coding region with a 1.523 kb deletion confirmed by DNA sequencing. (B–L) Representative brightfield images of 5dpf zebrafish showing normal phenotype (B) and abnormalities in fos F0 mutants (15/24 embryos, 63%) and morphants (102/132 embryos, 77%) (C and F) compared to UIC and MM controls (110/122 embryos, normal phenotype, 90%) (B, G). (D–M) Confocal images of rostrally mounted DAPI-stained samples showed a distinctively abnormal facial and mouth shape in both fos mutant (103 embryos) (H) and morphant (138 embryos) (I) embryos compared to UIC (93 embryos) (D) and MM (47 embryos) controls (I). Arrowheads indicate merged or displaced neuromasts. Co-injection of human FOS mRNA rescued the morphant phenotype (J and K) while overexpression alone caused other abnormalities including cyclopia and an elongated lower jaw (L, M). UIC, uninjected control; F0, crispant; MO, morpholino; MM, mismatch; Hu-FOS, human FOS mRNA.
To further evaluate the consequences of reduced fos on craniofacial development, a translation-blocking morpholino (MO) against fos was used. Fos morphant embryos displayed similar abnormalities to crispants at 5 dpf (Figures 2F,H), while mismatch (MM) control injected embryos were similar to the UICs (Figures 2G, I). Co-injection with a morpholino directed to p53 did not improve the phenotype, supporting that the abnormal morphant phenotype was independent of non-specific cell death (Boer et al., 2016) (Supplementary Figure S4B). Additionally, fos MO was injected in stable fos F3 mutants and did not lead to an abnormal phenotype, supporting the specificity of the fos MO. To further test the specificity of fos knockdown, human FOS (Hu-FOS) mRNA, which is not targeted by zebrafish fos MO, was co-injected and rescued the morphant abnormalities including the size of the head and eyes, as well as oral cavity shape (Figures 2J, K). While 82% of fos morphants had an abnormal mouth and face, only 45% had an abnormal phenotype after human FOS mRNA co-injection (Supplementary Figure S5A). Interestingly, overexpression of human-FOS RNA, as well as zebrafish fos mRNA, caused severe facial abnormalities, including an extended lower jaw and cyclopia in more than 50% of surviving injected embryos (Figures 2L, M). Pharmacological perturbation of AP-1 transcription factor complex activity mediated by fos using SR 11302 resulted in craniofacial abnormalities, similar to those observed in fos crispant and morphant embryos, including a narrow face, smaller mouth, and altered olfactory pits (Supplementary Figure S6). As expected, this resulting phenotype was more severe because of the wider range of disruption by the AP-1 inhibitor, which targets the AP-1 dimer complex composed of different members of the fos and jun protein families (fos, fosb, fra-1, fra-2, jun, junB, junD) (Wagner, 2002). AP-1 inhibitor treatment led to a more severe mouth phenotype in stable F3 fos mutants compared to wild type and DMSO treated groups, suggesting that while the stable mutants have a normal phenotype, they are more sensitive to AP-1 perturbations (Supplementary Figure S6). Taken together, these results suggest a key role for fos in regulating craniofacial development in zebrafish embryos.
To identify the orofacial features altered by genetic perturbation of fos at 5 dpf, we used zFACE, a geometric morphometric tool for quantitative deep phenotyping of the craniofacial region in zebrafish embryos (Maili et al., 2023). This tool uses 26 anatomical landmarks, comparable to those used in human geometric morphometric (GMM) studies (Weinberg et al., 2008), to calculate 39 facial measurements, including linear distances, areas and angles (Maili et al., 2023). As shown in Table 1, significant alterations were observed for 16 facial features in both fos crispants and morphants compared to UIC and MM controls (ANOVA with post hoc Tukey’s test, p < 0.0001). These included differences in oral cavity dimensions and angles, olfactory placode positioning, as well as area measurements in the lower half of the face. Similar phenotypic results were observed at 6 dpf, indicating that the facial anomalies did not improve with time and were not the result of a delay in development (Supplementary Figure S7; Supplementary Table S1). Nine of the 16 altered dimensions (2 olfactory to mouth angles, mouth height, and 6 oral cavity angles) were fully rescued by Hu-FOS mRNA injection (Table 1; Figure 3A). Olfactory distance, neuromast width and mouth perimeter were partially rescued; they were different compared to the controls and to the morphants, representing an intermediate phenotype (Table 1; Figure 3B). Four facial features, chin width, a neuromast angle, area bottom and area combined, were not rescued (Table 1; Figure 3C). Morphometric analysis could not be performed in the overexpression group due to the severity of the phenotype and many anatomical landmarks missing.
TABLE 1. Results of morphometric analysis Fos crispants (F0 mutants), morphants and MO + mRNA co-injected (rescue) embryos were compared using morphometric analysis of the facial region.
FIGURE 3. Quantitative analysis confirms facial abnormalities in fos crispants and morphants. (A) ZFACE morphometrics identified significant measurement differences in 16 individual craniofacial parameters for fos mutants (n = 103) and morphants (n = 138) compared to MM (n = 47)/UIC (n = 93) controls. Nine of these parameters were completely rescued (only the labiale superius left, an oral cavity angle, is shown as an example). (B) Three parameters were partially rescued (olfactory distance shown as an example) while (C) 4 were not rescued (area of the bottom half of the face shown). (D) Principal component analysis of Procrustes transformed landmarks showed an overlap of fos crispant and morphant groups, as well as separation from the UIC and MM groups, while the rescue group plotted closer to the controls. (E) Summary of overall shape differences between control and experimental groups, highlighting the similarities between UIC and MM controls, crispants and morphants, and the differences between UIC/MM controls and crispants/morphants. Additionally, rescue with fos mRNA showed a more normal average mouth and face shape. UIC, uninjected control; F0, crispant; MO, morpholino; MM, mismatch; rescue, human FOS mRNA injected morphant.
Unbiased multivariate analysis using principal component analysis (PCA) was next performed after Procrustes transformation to determine overall shape changes after fos disruption. The first two components (PC1, PC2) were responsible for 63% of the variance in the dataset. Landmarks in the lower part of the oral cavity and midface had the largest vectors of change across PC1, while landmarks outlining the lower jaw, location of the pupils and upper lip had the largest vectors of change across PC2 (Supplementary Figure S8). A component plot for PC1 and PC2 showed overlap of fos crispant and MO groups, as well as separation of the crispant and MO groups from the UIC and MM controls (Figure 3D). A small subset (n = 5, 10%) of crispants clustered close to the UIC and MM control groups, likely due to mosaicism. The rescue group mapped closer to the control groups, with some individuals in the intermediate area of the plot, likely reflecting the partial rescue observed in the previous analysis (Figure 3D).
Shape analysis was also performed using discriminant function analysis (DFA) to determine face shape differences between experimental and control groups (Klingenberg, 2011). As expected, face shape in UIC and MM controls was similar (Procrustes distance = 0.03, p = 0.06). Although a significant difference was found between fos crispants and morphants, comparison of wireframe representations revealed only minor alterations in face shape (Procrustes distance = 0.06, p < 0.001) (Figure 3E). Comparisons between both crispants to UICs (Procrustes distance = 0.18, p < 0.001) and morphants to MM controls (Procrustes distance = 0.17, p < 0.001) showed significant shape differences involving the oral cavity, midface and lower jaw in both comparisons (Figure 3E). DFA also highlighted the improvement in the rescue group when compared to UICs, specifically in the upper lip and upper face regions, however the lower lip and jaw landmarks remained abnormal (Figure 3E). Together, these quantitative analyses demonstrated that perturbation of fos affected oral cavity and midface dimensions and overall facial shape.
Given that the shape of the underlying skeletal tissues is a major contributing factor to facial shape, the bone and cartilage based structures that comprise craniofacial tissues were examined (Murillo-Rincon and Kaucka, 2020). Skeletal staining showed reduced and abnormally shaped jaw cartilages, including smaller ethmoid plate, trabeculae and parachordal cartilages, reduced Meckel’s and palatoquadrate cartilages and missing basibranchial cartilages in both fos crispants and morphants (Figures 4B, B’, C, C’) compared to UIC and fos MM controls (Figures 4A, A’, D, D’). The parasphenoid bone, branchiostegal rays and fifth ceratobranchial arches (CB5) showed impaired ossification and smaller or missing pharyngeal teeth (Figures 4 B-C, asterisks). Fused otoliths and asymmetric neurocranial structures were also observed (Figure 4C’). Morphants generally displayed a more severe disruption of the craniofacial skeleton. These cranioskeletal abnormalities were dose-dependent, ranging from mild (0.5ng/uL MO injection) to very severe (2ng/uL MO injection) (Supplementary Figure S9).
FIGURE 4. Perturbation of fos causes bone, cartilage, and tooth abnormalities. (A–D) Fos crispants (16/19) and morphants (61/62) show abnormally shaped jaw cartilages, including a smaller ethmoid plate, Meckel’s and palatoquadrate cartilages, missing basibranchial cartilages (B–C’) red arrows) and missing pharyngeal teeth (red asterisks). (E–H) Only 24% of fos crispant and morphant zebrafish showed mineralization at the fifth ceratobranchial arch (F, G), white arrows) compared to 100% of the UIC/MM (E and H). Tooth number was reduced, and tooth shape/size was altered in fos crispants and morphants compared to UIC/MM (E–H), white asterisks). UIC, uninjected control; F0, crispant; MO, morpholino; MM, mismatch.
Abnormal pharyngeal/branchial arch formation was suggested based on the multiple bone and cartilage anomalies. Fos knockdown in the Tg (-4.9sox10:GFP) zebrafish line was used to visualize the developing pharyngeal arches (PAs) (Carney et al., 2006). At 5dpf, PAs 1 and 2 were abnormal, with crispants/morphants showing cellular disorganization, vertical and horizontal constrictions and arch asymmetry (Supplementary Figure S10). Approximately 20% of crispant, and 37% of morphant larvae showed merged PA1 and PA2; while all crispants/morphants had abnormally-shaped but intact PAs 3–7 (Figure 4G-H; Supplementary Figure S10). Additionally, mineralization of the fifth ceratobranchial (CB5/PA 7), visualized by alizarin red staining, was observed in 100% of the UIC and MM embryos, compared to only 19% of fos crispants and 24% of morphants (Figures 4E–H arrow; Supplementary Figure S11; Supplementary Table S2). Decreased mineralization persisted during subsequent developmental stages, with only 68% of morphants showing evidence of mineralization by 8dpf (Supplementary Figure S11D). Smaller cleithrums and opercles were also observed, indicating abnormal ossification in craniofacial dermal bones (Figure 4E–H).
Development of zebrafish pharyngeal dentition was also examined in fos crispants and morphants. At 5dpf, 3 bilateral rows of pharyngeal teeth (4V1, 3V1 and 5V1), were observed in both UIC and MM controls, with 4V1 ankylosed/attached to the perichondral bone of CB5, as expected (Figures 4E,H; Supplementary Figure S11C). In comparison, crispant and morphant larvae had only 1 tooth (4V1) on each side of the arch, which was smaller and of abnormal morphology compared to the controls. In mild phenotypic crispants/morphants, 4V1 had attached/deposited bone around the non-mineralized CB5 cartilage, while in crispants/morphants showing severe phenotypes only 4V1 buds were visible (Figures 4F,G; Supplementary Figure S11C). Defects in tooth attachment, morphogenesis, and in CB5 mineralization persisted to 8dpf (Supplementary Figure S11D; Supplementary Table S2). These abnormalities in bone, cartilage, pharyngeal arch and tooth formation in both fos crispants and morphants are consistent with perturbation of a common developmental program.
Cell populations important for craniofacial development in fos crispants and morphants were next examined to understand the abnormal phenotype resulting from fos perturbation. Since expression of fos mRNA was detected in the outer epithelial layer, epithelial cell number and morphology were analyzed using a keratin-4 transgenic reporter line Tg(krt4:gfp) (Gong et al., 2002). Fos crispants and morphants showed abnormal cellular arrangement, particularly around the oral cavity and nares/olfactory pits compared to UIC and MM controls at 3 and 5 dpf (Figures 5A–D). Visualization of the borders and cell-cell junctions of epithelial cells also further showed the smaller oral cavities and olfactory pits in crispants/morphants (Figures 5E–H). In addition to altered arrangement of epithelial cells around the oral cavity and lower jaw, cells in the perioral region of both crispants and morphants had a smaller area compared to controls at both 3 dpf (p < 0.0001, Student’s t-test) and 5 dpf (p < 0.0001) (Figure 5 I, J; see Supplementary Figure S12 for a description of facial region analyses). These results suggested a role for fos in regulating epithelial cell size and arrangement around the oral cavity.
FIGURE 5. Perturbation of fos causes epithelial abnormalities. (A–H) Examination of Keratin-4 expressing cells showed abnormal arrangements of peridermal epithelial cells around the oral cavity in both crispants (B and F) and morphants (C and G) compared to controls at both 3dpf (A and D) and 5dpf (E and H). Periderm cell size was quantified and both crispants and morphants (minimum of n = 60 cells per condition; n≥5 embryos at each timepoint) statistical test showed reduced perioral cell area at 3dpf (I) and 5dpf (J) compared to controls. UIC, uninjected control; F0, crispant; MO, morpholino; MM, mismatch.
The transgenic line Tg(-4.9sox10:EGFP) (Carney et al., 2006) that labels pre-migratory and migrating CNCCs was used to examine these cells because of their large contributions to craniofacial bone and cartilage. Early in development (1dpf, Figure 6A) CNCC numbers and migration were unaffected in the crispants and morphants when compared to controls (p = 0.27). However, by 2dpf, significant differences were observed in the number and migration pattern of CNCCs in fos crispants and morphants (p < 0.0001) (Figures 6A,B). At 3dpf, crispants and morphants continued to show reduced CNCC derivatives compared to both UICs and MMs and these differences were more pronounced at 5dpf, with significant decrease of GFP fluorescence in crispants/morphants compared to UIC controls (p < 0.0001 for both, Figures 6A,B). Immunostaining for activated caspase-3 showed a dramatic increase in the number of positively stained cells around the eyes and in the midline in both crispants and morphants suggesting high, localized cell death between 1dpf and 2dpf (Figure 6C). While MOs are known to cause an increase in cell death (Boer et al., 2016), very similar results were also observed in the F0 crispant larvae, suggesting this precocious death is promoted by a lack of fos. Together, these results demonstrated that absence/knockdown of fos compromised the survival of critical subpopulations of CNCCs and contributed to the phenotypic anomalies observed later in development.
FIGURE 6. Craniofacial cell populations are significantly affected in fos crispant and morphant zebrafish embryos. (A) Time course evaluation of CNCCs shown at 1dpf, where sox10 fluorescence was similar in all 4 groups, but at 2dpf significant differences in NCC populations and migration were observed in the fos crispants and morphants and these differences persisted to 5dpf in NCC derived mesenchyme as observed in rostral views of embryos. (B) Quantification of average fluorescence showed significant reductions at both 2dpf and 5dpf for crispants and morphants (statistical test numbers quantified in each group were: 2dpf: UIC = 23, F0 = 11, MO = 41, MM = 13; 5dpf: UIC = 28, F0 = 10, MO = 39, MM = 15). (C) Fos crispants/morphants showed increased apoptosis in the head region at 1dpf, shown by activated caspase 3 immunolabeling, where affected CNCC populations around the eye and optic stalk will give rise to the ethmoid plate and oral ectoderm. (D) The increase caspase 3 positive cells in the head region was significantly increased in crispants and morphants compared to controls (The number of embryos quantified for each group was UIC = 6, F0 = 10, MO = 5 and MM = 2.). (E) Mutation and knockdown of fos in a Wnt reporter line showed a reduction in β-catenin signaling starting at 1 dpf (triangles), when a group of cells observed in controls was largely missing in the mutants/morphants and persisting to 3 dpf, when reporter expression in cells of the upper and lower lip was severely reduced in fos mutants and morphants (white asterisks) (F) 3D quantification of the oral cavity region supported the significant reduction in Wnt-responsive cell number in these structures. The number of embryos quantified was UIC = 10, F0 = 11, MO = 6 and MM = 3. UIC, uninjected control; F0, crispant; MO, morphant; MM, mismatch control morpholino, CNCC, cranial neural crest cells, *p < 0.05, **p < 0.01, ***p < 0.001, ****p < 0.0001.
Interactions between the mesenchyme and epithelial cells are known to induce Wnt signaling centers that control cell fates and behaviors in the surrounding tissues (Jussila and Thesleff, 2012). Based on the changes observed in CNCC derivatives and epithelial cells, a reporter zebrafish line that serves as a biosensor for Wnt signaling (Tg(7xTCF-Xla.Siam:GFP)) was evaluated for changes in β-catenin mediated Wnt activity (Moro et al., 2012). A general reduction in WNT-responsive cells, in both fos crispants and morphants, was identified in the head starting at 1dpf and 2dpf (p < 0.01, Figure 6; Supplementary Figure S13). At 3dpf, we observed a group of WNT responsive cells that were restricted to the upper and lower lip. Intriguingly, a dramatic reduction of these Wnt responsive cells was observed in both fos crispants and morphants (Figure 6, asterisks). Quantification of these cells in 3D analysis using Imaris supported the significantly reduced number of reporter positive cells in the oral cavity region (p = 4.08E-12 and p = 0.007, crispants compared to UIC and morphants compared to MM controls, respectively). By 5dpf, β-catenin active cells had recovered around the oral cavity in crispants/morphants, however the organization of the cells followed the abnormal mouth shape. Together, these results suggested that Wnt responsive cells were reduced at early developmental time points and severely disrupted around the mouth at 3dpf, implicating that absence or reduction of fos impacted the ability of these cells to properly pattern the oral cavity.
Fos plays a role in embryonic and craniofacial development (Wagner, 2002; Durchdewald et al., 2009; Alfaqeeh et al., 2015; Velazquez et al., 2015) and was previously identified in a network of differentially expressed genes in a zebrafish model of orofacial clefts and confirmed in a human family-based association study (Chiquet et al., 2018). In this study, we sought to characterize the role of Fos in orofacial morphogenesis and oral cavity formation. Using both fos crispant and morphant embryos, we showed that absence or reduction of fos in developing zebrafish led to an abnormal craniofacial morphology with a distinctive abnormal ovoid oral cavity shape, suggesting a key role in orofacial morphogenesis. Deep phenotyping using geometric morphometric analyses showed that midface dimensions and oral cavity shape were the facial features most affected by fos perturbation. Further, perioral epithelial cells had altered morphologies and cranial neural crest cells (CNCCs) were reduced in number. These changes corresponded to a reduction in Wnt-responsive cells around the oral cavity, suggesting that fos plays an important regulatory role in guiding the interactions between the epithelial and mesenchymal cell populations that induce WNT signaling during oral cavity development. Together, our findings provide a better understanding of the contributions of fos in vertebrate craniofacial development and suggest that variation in FOS expression can alter facial dimensions during development and potentially play an etiologic role in orofacial clefting.
Cell migration and movements during craniofacial development facilitate interactions between different cell and tissue types, and their environment (Murillo-Rincon and Kaucka, 2020). These interactions are critical to create spatially defined sources of morphogenetic signals (e.g., WNTs, FGFs, BMP, Hedgehog), commonly referred to as signaling centers or organizers (Perrimon et al., 2012; Martinez Arias and Steventon, 2018; Murillo-Rincon and Kaucka, 2020). Both the initiation of the signaling centers in the developing head and the expression levels of inductive signals can be modified by several mechanisms, such as 1) changes in gene expression that perturb survival or migration of specific cell types and 2) the timing of the cell/tissue interactions (Perrimon et al., 2012).
Although CNCC death can be associated with morpholino oligonucleotide use (Boer et al., 2016), the marked upregulation of apoptosis of CNCC in the head and around the eyes was also identified in the crispants, but not in mismatch morpholino injected embryos. These results suggest that the observed apoptosis is not solely due to morpholino-related toxicity. Importantly, areas in which increased caspase-3 was detected at 1dpf also showed specific regional fos mRNA expression. The CNCC in these regions have been shown to migrate rostrally and caudally to reach the oral ectoderm and ethmoid plate (Eberhart et al., 2008) and the observed aberrant apoptosis likely led to the regional reduction of CNCCs, resulting in abnormal arches and anomalous jaw skeletal elements. Indeed, fos knockdown has been shown to cause increased apoptosis in osteosarcoma cells (Wang et al., 2017), supporting the relationship between reduced levels of fos and increased apoptosis observed in our crispant and morphant zebrafish models. In C. elegans, fos is also a cell autonomous regulator of cell invasion, a process critical for many cell types and also NCCs, as they need to invade through extracellular matrix, mesoderm and migrating endothelial cells to reach their destination, making their motility and invasive ability crucial (Sherwood et al., 2005; McLennan et al., 2020). Overall, perturbation of zebrafish fos expression affected the survival and migration of a specific subset of NCCs that populate the oral ectoderm and ethmoid plate, suggesting that one of the mechanisms responsible for the abnormal orofacial phenotype is the early disruption of NCCs.
Epithelial-mesenchymal interactions play a crucial role during the development of several organs and tissues and are mediated by cell-cell contact, or the diffusion of soluble factors such as Wnts (Ribatti and Santoiemma, 2014). During tooth development, regional signals from the ectoderm induce molecular changes in the cranial neural crest-derived ectomesenchyme (Jussila an Thesleff, 2012). The activation of β-catenin signaling is required in the oral epithelium for the induction of placodes and formation of enamel knots; this signaling is also necessary in the odontogenic mesenchyme beyond the bud stage (Chen et al., 2009; Jussila and Thesleff, 2012). Both fos crispants and morphants showed a dramatic reduction of Wnt-responsive cells around the oral cavity at 3dpf, whereas the brain was less affected, indicating that Wnt signaling was perturbed in this region. Consistent with disrupted inductive signaling, fos crispants/morphants showed abnormal tooth development, with marked delays in tooth formation and anomalies in morphology and number of teeth. Even though the process of tooth eruption in mice is different from pharyngeal tooth attachment to CB5 in zebrafish, these results are consistent with observations of smaller teeth in Fos null mice that fail to erupt and form roots (Van der Heyden and Huysseune, 2000; Alfaqeeh et al., 2015).
The periderm serves important functions in craniofacial structures that fuse during development, such as the oral cavity and the palate (Hammond et al., 2019). In mammalian models, abnormal periderm development leads to epithelial adhesions and orofacial clefts, while failure of periderm removal from fusing palatal shelves can contribute to cleft palate (Richardson et al., 2014; Hammond et al., 2019). Periderm anomalies were observed starting at 3dpf in the perioral region, suggesting that loss of fos could also be contributing to peridermal abnormalities linked to abnormal orofacial development. Future experiments will identify how fos regulates the molecular mechanisms in periderm cells. Interestingly, our results showed that at 3 dpf, CNCC derivatives were greatly reduced in the upper and lower jaws, at the same time that oral periderm cells showed altered morphologies and arrangement, indicating that perturbation of fos interrupted both epithelial and mesenchymal cell populations around the developing oral cavity. This specific effect on cells around the oral cavity corresponds with the mouth and midface shape anomalies detected at 5dpf, suggesting a disruption to critical inductive signals that regulate orofacial morphology. This could result from either missing β-catenin active cells or inability of these cells to activate β-catenin signaling and warrants further studies. These findings suggest that fos plays an important inductive role in regulating interactions between epithelial and mesenchymal cell populations and its absence affects both cells around the oral cavity and midface and mineralized tissue like bones and teeth.
Additionally, Wnt/β-catenin signaling delineates areas of rapid growth in the facial prominences in murine models, and both genetic and pharmacologic perturbation of this signaling pathway results in altered craniofacial dimensions during embryonic development (Brugmann et al., 2007; He and Chen, 2012). The use of geometric morphometrics allowed identification of regional alterations to dimensions such as mouth height, olfactory to mouth angles and olfactory distance, and identified significant shape changes to the mouth and midface, strongly supporting the role of fos in morphogenesis of these structures (Maili et al., 2023). Together with the reduced number of Wnt responsive cells in the perioral region, these findings suggest insufficient growth in these tissues when fos is absent or reduced. Interestingly, a correlation between the dimensions of the maxillae and nasal pits and susceptibility for developing cleft lip and palate has been reported in mice (Young et al., 2007; Parsons et al., 2008; Green et al., 2015). Additionally, human morphometric studies have shown that there are facial differences in the upper lip, philtrum and nasolabial angles in individuals predisposed to orofacial clefts as well as their unaffected relatives (Fraser and Pashayan, 1970; Weinberg et al., 2006a; Weinberg et al., 2006b; Weinberg et al., 2009; El Sergani et al., 2020). Together with the findings that variation in FOS is associated with NSCLP, these results suggest that altered fos expression affects the growth and shape of the oral cavity and midface regions and potentially plays an etiologic role in the development of an orofacial cleft.
Crispants, morphants and pharmacologic inhibition of the AP-1 transcription factor complex produced similar orofacial anomalies, supporting our findings. In the majority of surviving larvae, fos mRNA overexpression also caused a cyclopia craniofacial phenotype suggesting possible connections between dysregulated fos expression and ciliopathies and/or other developmental signaling pathways such as Nodal or Hedgehog. Stable fos mutants (F1, F2, F3 generations) had no phenotype even though they had 1.5 kb deletions and absent fos mRNA expression and thus were not useful for these studies. Similar results from multiple studies suggest that this is likely related to genetic compensation unique to Crispr/Cas9 mutagenesis methods (Rossi et al., 2015; Buglo et al., 2020). Increased expression of fosl1a, fosb and fosl2 was observed in the fosab mutants (Supplementary Figure S3). Additionally, injection of the guide RNAs in fosab mutant embryos did not produce a phenotype, however, these stable mutants were more sensitive to pharmacological AP-1 disruption (Supplementary Figure S4, S6). These data support the idea that compensatory mechanisms by similar gene family members may be responsible for buffering the phenotype.
In conclusion, this study demonstrates that fos is critical for zebrafish craniofacial development and fos perturbation affects multiple craniofacial tissues resulting in abnormal orofacial structures. Loss of fos has a profound effect on facial progenitor cells, suggesting that it is important for epithelial and mesenchymal cell populations and regulates interactions between them that are crucial for proper oral cavity morphogenesis. Although cleft lip was not seen in our zebrafish embryos, severe orofacial anomalies were observed. This result may be related to species-specific differences in mouth development (Soukup et al., 2013). Based on the current work as well as results suggesting a role for FOS in NSCLP (Chiquet et al., 2018), future investigations in zebrafish and humans should target identification of other genes and pathways by which fos regulates craniofacial development.
Zebrafish (Danio rerio) were housed and maintained at 28°C as described previously (Westerfield, 1993). All work involving the use of animals was performed with approval of the UTHealth Animal Welfare Committee (AWC-20–0052).
Zebrafish fos antisense morpholinos (Fos MO: GCGTTAAGGCTGGTAAACATCATCC) targeting the ATG start site in exon 1 and a mismatched control (Fos MM: GaGTTAAcGCTcGTAAAaATCATaC, mismatch in small caps) were designed by GeneTools (Philomath, OR). Morpholinos were suspended in MilliQ water to a stock concentration of 16.67 mg/mL or 2 mM. Injections of morpholinos were diluted to 0.5 ng/uL to 3 ng/nL in Danieu buffer. A plasmid containing the full-length human FOS cDNA (NM_005252.4) was purchased from Addgene (Plasmid #59140). The full-length FOS cDNA was cloned into the pCS2 vector and FOS mRNA was generated using the mMessage mMachine Sp6 kit (Ambion, Austin, TX). mRNA was resuspended in nuclease-free water to a stock concentration of 2 ng/nL and diluted to 0.5 ng/nL in 0.1 M KCl for injections. Fos F0 crispants were created using IDT Alt-R™ CRISPR-Cas9 System (Coralville, IA). Two Crispr RNAs (crRNAs) specific to Fos gene (fos crRNA1: CGAGCAAGGAAATACAAGAC and fos crRNA2: GGTTGGGGAATTCAAGGAGT) were hybridized separately with trans activating crispr RNA (tracrRNA) to form a functional gRNA complex. 60uM of each gRNA was incubated with 5ug/ul of Cas9 protein (Alt-R® S.p. Cas9 Nuclease, IDT) for 10 min at 37°C to generate the ribonucleoprotein (RNP) complex. Equimolar amounts of the two RNPs were mixed and injected into the zebrafish embryos. For all zebrafish injections, one-cell embryos were injected with 1 nL of MO, mRNA or RNPs.
Embryos were fixed in 4% paraformaldehyde (PFA) (Sigma) in 1X phosphate buffered saline with Triton X-100 (PBST) at room temperature for 4 h and stained with 0.2 mg/L DAPI (Life Technologies) for 30 min at room temperature. Embryos were mounted rostrally in 1% low-melt agarose (Research Products International, Mount Prospect, IL) and imaged with Zeiss LSM 800 Confocal Microscope (Thornwood, NJ). Twenty-six anatomic landmarks including eyes, olfactory pits, neuromasts and mouth were identified from the confocal images and measurements between these landmarks were calculated to extract phenotypic features and understand which anatomical structures were altered as a result of fos knockdown. ANOVA and Tukey’s test for multiple comparisons was applied or each measurement and Bonferroni correction for 39 measurements was applied to determine statistical significance. GraphPad Prism 9.0.0 was used to plot and visualize the data.
Dimensionality reduction was performed using Principal Component Analysis (PCA) in StataIC 14 (StataCorp. 2015). Components with an eigenvalue of greater than or equal to 1 (following the Kaiser-Guttman method) were retained for analysis. Promax rotation, which accounts for correlations between the different factors (zFACE measurements) was used because a high correlation was observed/expected between features calculated using shared landmarks. Principal component (PC) scores were predicted and logistic regression models were utilized to regress morphant/crispant status by PC scores.
Additionally, to focus on facial shape and remove variation due to size, position, or rotation, the 2D landmark data points from the 26 zFACE coordinates were uploaded into MorphoJ version 1.07 A and principal axes Procrustes superimposition was performed (Klingenberg, 2011). After Procrustes transformation, PCA was used to examine general shape variation in the combined groups. Additionally, discriminant function analysis (DFA), which maximizes the between-group variance relative to within-group variance, was used to pinpoint shape in fos morphants/crispants compared to controls. Mean Procrustes distance with 10,000 permutations was used to statistically test shape differences between control and experimental groups.
Alcian blue (Anatech LTD.) and alizarin red (Sigma-Aldrich) staining was performed using standard techniques (Kimmel and Trammell 1981) to visualize the bone and cartilage structures. Briefly, 5-8dpf embryos were collected and fixed in 2% PFA/1X PBXT for 1 h at room temperature and stored in methanol over night at −20 °C. After removing methanol, embryos were incubated in 0.04% alcian blue solution (100 mmol/L Tris, pH 7.5, 10 mmol/L MgCl2, 64% ethanol) overnight at room temperature. They were destained in 3% H2O2/0.5% KOH for 10 min at room temperature and then stained in 0.02% alizarin red solution (100 mmol/L Tris, pH 7.5, 25% glycerol) for 30 min at room temperature. Embryos were then destained in 50% glycerol/0.1% KOH for 30 min and stored in 50% glycerol. Imaging was performed using the LAS Montage Module (Leica). For visualizing teeth, a 0.5% Alizarin Red solution was used to stain fixed sox10.gfp reporter embryos for 1 h.
Periderm cells were measured in Zen software (Zeiss, Thornwood, NJ) using the contour graphics tool. Midface and perioral cells were analyzed separately due to already existing differences in their size. In each individual embryo, 10 cells were measured from each region and at least 10 individuals were analyzed from each group.
Average fluorescence signal was measured in ImageJ (Schneider et al., 2012). Wnt-responsive cells were counted using the Spots detection tool and automatic detection settings in Imaris software (Bitplane, Oxford Instruments) utilizing default settings with at least 3 animals analyzed for each condition.
Embryos were fixed at 1dpf in 2% PFA/1XPBS overnight at 4 °C. After washing, they were incubated with block solution (1% DMSO, 2 mg/ml BSA, 0.5% Triton X-100, 10% inactivated goat serum in PBS) for 2 h at room temperature, washed and then incubated with 1:700 dilution of Rabbit Activated Caspase 3 (BD Biosciences, Franklin Lakes, NJ) in block solution overnight at 4 °C. 1:150 dilution of Donkey Anti-Rabbit 647 (Molecular Probes, Eugene, OR) was used for secondary antibody staining. Washed embryos were mounted in 1% low-melt agarose for confocal imaging.
HCR assay was performed using Molecular Instruments Multiplexed v3.0 protocol and reagents (Molecular Instruments, Los Angeles, CA). Briefly, embryos were fixed at different stages in 2% PFA/1XPBS over night at 4 °C. They were pre-hybridized with probe hybridization buffer for 30 min at 37°C, then incubated with 4 nM of the probe (fosab HCR probe set B2 Alexa Fluor 546) in probe hybridization buffer overnight at 37 °C. Embryos were washed with 5X SSCT and incubated with amplification buffer for 30 min at room temperature. 30 pmol of hairpins h1 and h2 corresponding to the probe were snap cooled (heated at 95C for 90 s and cooled to room temperature in a dark drawer), diluted in 500 uL of amplification buffer and added to the embryos for overnight incubation in the dark at room temperature. Washed embryos were mounted in 1% low-melt agarose for confocal imaging.
Total RNA was extracted from a group of 5 embryos using Trizol (Invitrogen) as described previously (Chiquet et al., 2018). Further purification was done using RNeasy Mini Kit (Qiagen). The purified RNA was reverse transcribed to cDNA using QuantitectTM Reverse Transcription Kit (Qiagen). Gene expression of different fos family genes in zebrafish was analyzed using QuantitectTM Primer Assays (Qiagen) for each individual gene–fosaa, fosab, fosb, fosl1a, fosl1b and fosl2 – and data was normalized using beta-actin (actb1) as an endogenous control. All samples were run in triplicate and compared using Student’s t-tests.
AP-1 inhibitor SR 11302 (Tocris Bio-Techne, Minneapolis, MN; Catalog No.2476, Batch No.7, Minimum Purity>98%) was dissolved in DMSO to prepare a stock solution. Varying concentrations of drug (1, 5, and 10 uM) were added to embryo dishes starting at either 1 or 2 dpf with daily media changes until collection at 5 dpf. The controls included treatment with the same volume of DMSO and untreated dishes.
Perturbation of fos, a candidate gene associated with nonsyndromic cleft lip and palate in humans, causes a distinctive orofacial phenotype in zebrafish as a result of abnormal development of craniofacial tissues. Disruption of fos in the oral epithelial, cranial neural crest and Wnt responsive cell populations around the oral cavity causes anomalies that suggest a potential role in the etiology of NSCLP.
The original contributions presented in the study are included in the article/Supplementary Material, further inquiries can be directed to the corresponding author.
The animal study was reviewed and approved by UTHealth Animal Welfare Committee.
LM, BT, AL, GE and JH conceptualized the project and methodology. LM, BT, QY, FC and SM performed all the experiments and subsequent analyses. SH provided assistance with statistical analysis. LM and BT wrote the manuscript and AL, GE and JH edited the manuscript. All authors contributed to the article and approved the submitted version.
This study was supported by NIH grants R01-DE11931 (to JH) and F31-DE28187 (to LM); the Gulf Coast Consortium Collaborative Research Award to JH and GE; and the Cancer Prevention Research Institute of Texas, RR140077; the National Institute of General Medical Sciences, National Institutes of Health, R01GM124043; and the Mark and Linda Quick Basic Science Award to GE.
We thank Christian Urbina for morphometric data analysis assistance.
The authors declare that the research was conducted in the absence of any commercial or financial relationships that could be construed as a potential conflict of interest.
All claims expressed in this article are solely those of the authors and do not necessarily represent those of their affiliated organizations, or those of the publisher, the editors and the reviewers. Any product that may be evaluated in this article, or claim that may be made by its manufacturer, is not guaranteed or endorsed by the publisher.
The Supplementary Material for this article can be found online at: https://www.frontiersin.org/articles/10.3389/fcell.2023.1141893/full#supplementary-material
Alfaqeeh, S., Oralova, V., Foxworthy, M., Matalova, E., Grigoriadis, A. E., and Tucker, A. S. (2015). Root and eruption defects in c-fos mice are driven by loss of osteoclasts. J. Dent. Res. 94 (12), 1724–1731. doi:10.1177/0022034515608828
Boer, E. F., Jette, C. A., and Stewart, R. A. (2016). Neural crest migration and survival are susceptible to morpholino-induced artifacts. PLoS One 11 (12), e0167278. doi:10.1371/journal.pone.0167278
Brugmann, S. A., Goodnough, L. H., Gregorieff, A., Leucht, P., ten Berge, D., Fuerer, C., et al. (2007). Wnt signaling mediates regional specification in the vertebrate face. Development 134 (18), 3283–3295. doi:10.1242/dev.005132
Buglo, E., Sarmiento, E., Martuscelli, N. B., Sant, D. W., Danzi, M. C., Abrams, A. J., et al. (2020). Genetic compensation in a stable slc25a46 mutant zebrafish: A case for using F0 crispr mutagenesis to study phenotypes caused by inherited disease. PLoS One 15 (3), e0230566. doi:10.1371/journal.pone.0230566
Burger, A., Lindsay, H., Felker, A., Hess, C., Anders, C., Chiavacci, E., et al. (2016). Maximizing mutagenesis with solubilized CRISPR-Cas9 ribonucleoprotein complexes. Development 143 (11), 2025–2037. doi:10.1242/dev.134809
Carney, T. J., Dutton, K. A., Greenhill, E., Delfino-Machin, M., Dufourcq, P., Blader, P., et al. (2006). A direct role for Sox10 in specification of neural crest-derived sensory neurons. Development 133 (23), 4619–4630. doi:10.1242/dev.02668
Chai, Y., and Maxson, R. E. (2006). Recent advances in craniofacial morphogenesis. Dev. Dyn. 235 (9), 2353–2375. doi:10.1002/dvdy.20833
Chen, J., Lan, Y., Baek, J. A., Gao, Y., and Jiang, R. (2009). Wnt/beta-catenin signaling plays an essential role in activation of odontogenic mesenchyme during early tooth development. Dev. Biol. 334 (1), 174–185. doi:10.1016/j.ydbio.2009.07.015
Chen, J., Zhang, E., Zhang, W., Liu, Z., Lu, P., Zhu, T., et al. (2017). Fos promotes early stage teno-lineage differentiation of tendon stem/progenitor cells in tendon. Stem Cells Transl. Med. 6 (11), 2009–2019. doi:10.1002/sctm.15-0146
Chiquet, B. T., Lidral, A. C., Stal, S., Mulliken, J. B., Moreno, L. M., Arcos-Burgos, M., et al. (2007). CRISPLD2: A novel NSCLP candidate gene. Hum. Mol. Genet. 16 (18), 2241–2248. doi:10.1093/hmg/ddm176
Chiquet, B. T., Yuan, Q., Swindell, E. C., Maili, L., Plant, R., Dyke, J., et al. (2018). Knockdown of Crispld2 in zebrafish identifies a novel network for nonsyndromic cleft lip with or without cleft palate candidate genes. Eur. J. Hum. Genet. 26 (10), 1441–1450. doi:10.1038/s41431-018-0192-5
Choi, H. M. T., Schwarzkopf, M., Fornace, M. E., Acharya, A., Artavanis, G., Stegmaier, J., et al. (2018). Third-generation in situ hybridization chain reaction: multiplexed, quantitative, sensitive, versatile, robust. Development 145 (12), dev165753. doi:10.1242/dev.165753
Cordero, D. R., Brugmann, S., Chu, Y., Bajpai, R., Jame, M., and Helms, J. A. (2011). Cranial neural crest cells on the move: their roles in craniofacial development. Am. J. Med. Genet. A 155A (2), 270–279. doi:10.1002/ajmg.a.33702
Cusack, B. J., Parsons, T. E., Weinberg, S. M., Vieira, A. R., and Szabo-Rogers, H. L. (2017). Growth factor signaling alters the morphology of the zebrafish ethmoid plate. J. Anat. 230 (5), 701–709. doi:10.1111/joa.12592
Dash, S., and Trainor, P. A. (2020). The development, patterning and evolution of neural crest cell differentiation into cartilage and bone. Bone 137, 115409. doi:10.1016/j.bone.2020.115409
Dixon, M. J., Marazita, M. L., Beaty, T. H., and Murray, J. C. (2011). Cleft lip and palate: understanding genetic and environmental influences. Nat. Rev. Genet. 12 (3), 167–178. doi:10.1038/nrg2933
Dougherty, M., Kamel, G., Grimaldi, M., Gfrerer, L., Shubinets, V., Ethier, R., et al. (2013). Distinct requirements for wnt9a and irf6 in extension and integration mechanisms during zebrafish palate morphogenesis. Development 140 (1), 76–81. doi:10.1242/dev.080473
Duncan, K. M., Mukherjee, K., Cornell, R. A., and Liao, E. C. (2017). Zebrafish models of orofacial clefts. Dev. Dyn. 246 (11), 897–914. doi:10.1002/dvdy.24566
Durchdewald, M., Angel, P., and Hess, J. (2009). The transcription factor fos: A janus-type regulator in health and disease. Histol. Histopathol. 24 (11), 1451–1461. doi:10.14670/HH-24.1451
Eames, B. F., DeLaurier, A., Ullmann, B., Huycke, T. R., Nichols, J. T., Dowd, J., et al. (2013). FishFace: interactive atlas of zebrafish craniofacial development at cellular resolution. BMC Dev. Biol. 13, 23. doi:10.1186/1471-213X-13-23
Eberhart, J. K., He, X., Swartz, M. E., Yan, Y. L., Song, H., Boling, T. C., et al. (2008). MicroRNA Mirn140 modulates Pdgf signaling during palatogenesis. Nat. Genet. 40 (3), 290–298. doi:10.1038/ng.82
El Sergani, A. M., Brandebura, S., Padilla, C., Butali, A., Adeyemo, W. L., Valencia-Ramirez, C., et al. (2020). Parents of children with nonsyndromic orofacial clefting show altered palate shape. Cleft Palate Craniofac J. 1055665620967235, 847–853. doi:10.1177/1055665620967235
Fraser, F. C., and Pashayan, H. (1970). Relation of face shape to susceptibility to congenital cleft lip. A preliminary report. J. Med. Genet. 7 (2), 112–117. doi:10.1136/jmg.7.2.112
Ge, X., Shi, Q. M., Ding, Z., Ju, Q., Wang, H., Wang, Q., et al. (2018). Association between CRISPLD2 polymorphisms and the risk of nonsyndromic clefts of the lip and/or palate: A meta-analysis. Cleft Palate Craniofac J. 55 (3), 328–334. doi:10.1177/1055665617738995
Gong, Z., Ju, B., Wang, X., He, J., Wan, H., Sudha, P. M., et al. (2002). Green fluorescent protein expression in germ-line transmitted transgenic zebrafish under a stratified epithelial promoter from keratin8. Dev. Dyn. 223 (2), 204–215. doi:10.1002/dvdy.10051
Gorlin, R. J., Cohen, M. M., and Hennekam, R. C. M. (2011). Syndromes of the head and neck. Fourth Edition ed. New York: Oxford University Press.
Green, R. M., Feng, W., Phang, T., Fish, J. L., Li, H., Spritz, R. A., et al. (2015). Tfap2a-dependent changes in mouse facial morphology result in clefting that can be ameliorated by a reduction in Fgf8 gene dosage. Dis. Model Mech. 8 (1), 31–43. doi:10.1242/dmm.017616
Grigoriadis, A. E., Wang, Z. Q., and Wagner, E. F. (1995). Fos and bone cell development: lessons from a nuclear oncogene. Trends Genet. 11 (11), 436–441. doi:10.1016/s0168-9525(00)89142-8
Hammond, N. L., Dixon, J., and Dixon, M. J. (2019). Periderm: life-cycle and function during orofacial and epidermal development. Semin. Cell Dev. Biol. 91, 75–83. doi:10.1016/j.semcdb.2017.08.021
He, F., and Chen, Y. (2012). Wnt signaling in lip and palate development. Front. Oral Biol. 16, 81–90. doi:10.1159/000337619
Ji, Y., Garland, M. A., Sun, B., Zhang, S., Reynolds, K., McMahon, M., et al. (2020). Cellular and developmental basis of orofacial clefts. Birth Defects Res. 112 (19), 1558–1587. doi:10.1002/bdr2.1768
Jiang, R., Bush, J. O., and Lidral, A. C. (2006). Development of the upper lip: morphogenetic and molecular mechanisms. Dev. Dyn. 235 (5), 1152–1166. doi:10.1002/dvdy.20646
Johnson, R. S., Spiegelman, B. M., and Papaioannou, V. (1992). Pleiotropic effects of a null mutation in the c-fos proto-oncogene. Cell 71 (4), 577–586. doi:10.1016/0092-8674(92)90592-z
Jussila, M., and Thesleff, I. (2012). Signaling networks regulating tooth organogenesis and regeneration, and the specification of dental mesenchymal and epithelial cell lineages. Cold Spring Harb. Perspect. Biol. 4 (4), a008425. doi:10.1101/cshperspect.a008425
Klingenberg, C. P. (2011). MorphoJ: an integrated software package for geometric morphometrics. Mol. Ecol. Resour. 11 (2), 353–357. doi:10.1111/j.1755-0998.2010.02924.x
Letra, A., Menezes, R., Cooper, M., Fonseca, R., Tropp, S., Govil, M., et al. (2010). Crispld2 variants including a C471t silent mutation may contribute to nonsyndromic cleft lip with or without cleft palate. Cleft Palate Craniofac J. 48, 363–370. doi:10.1597/09-227
Maili, L., Ruiz, O. E., Kahan, P. H., Chiu, F., Larson, S. T., Hashmi, S., et al. (2023). Facial analytics based on a coordinate extrapolation system (zFACE) for morphometric phenotyping of developing zebrafish. Dis Model Mech 16 (6), dmm049868. doi:10.1242/dmm.049868
Marazita, M. L., and Mooney, M. P. (2004). Current concepts in the embryology and genetics of cleft lip and cleft palate. Clin. Plast. Surg. 31 (2), 125–140. doi:10.1016/S0094-1298(03)00138-X
Martinez Arias, A., and Steventon, B. (2018). On the nature and function of organizers. Development 145 (5), dev159525. doi:10.1242/dev.159525
McLennan, R., McKinney, M. C., Teddy, J. M., Morrison, J. A., Kasemeier-Kulesa, J. C., Ridenour, D. A., et al. (2020). Neural crest cells bulldoze through the microenvironment using Aquaporin 1 to stabilize filopodia. Development 147 (1), dev185231. doi:10.1242/dev.185231
Mijiti, A., Ling, W., Maimaiti, A., Tuerdi, M., Tuerxun, J., and Moming, A. (2015). Preliminary evidence of an interaction between the CRISPLD2 gene and non-syndromic cleft lip with or without cleft palate (nsCL/P) in Xinjiang Uyghur population, China. Int. J. Pediatr. Otorhinolaryngol. 79 (2), 94–100. doi:10.1016/j.ijporl.2014.10.043
Milde-Langosch, K. (2005). The Fos family of transcription factors and their role in tumourigenesis. Eur. J. Cancer 41, 2449–2461. doi:10.1016/j.ejca.2005.08.008
Mork, L., and Crump, G. (2015). Zebrafish craniofacial development: A window into early patterning. Curr. Top. Dev. Biol. 115, 235–269. doi:10.1016/bs.ctdb.2015.07.001
Moro, E., Ozhan-Kizil, G., Mongera, A., Beis, D., Wierzbicki, C., Young, R. M., et al. (2012). In vivo Wnt signaling tracing through a transgenic biosensor fish reveals novel activity domains. Dev. Biol. 366 (2), 327–340. doi:10.1016/j.ydbio.2012.03.023
Murillo-Rincon, A. P., and Kaucka, M. (2020). Insights into the complexity of craniofacial development from a cellular perspective. Front. Cell Dev. Biol. 8, 620735. doi:10.3389/fcell.2020.620735
Parsons, T. E., Kristensen, E., Hornung, L., Diewert, V. M., Boyd, S. K., German, R. Z., et al. (2008). Phenotypic variability and craniofacial dysmorphology: increased shape variance in a mouse model for cleft lip. J. Anat. 212 (2), 135–143. doi:10.1111/j.1469-7580.2007.00845.x
Perrimon, N., Pitsouli, C., and Shilo, B. Z. (2012). Signaling mechanisms controlling cell fate and embryonic patterning. Cold Spring Harb. Perspect. Biol. 4 (8), a005975. doi:10.1101/cshperspect.a005975
Raterman, S. T., Metz, J. R., Wagener, F., and Von den Hoff, J. W. (2020). Zebrafish models of craniofacial malformations: interactions of environmental factors. Front. Cell Dev. Biol. 8, 600926. doi:10.3389/fcell.2020.600926
Reynolds, K., Kumari, P., Sepulveda Rincon, L., Gu, R., Ji, Y., Kumar, S., et al. (2019). Wnt signaling in orofacial clefts: crosstalk, pathogenesis and models. Dis. Model Mech. 12 (2), dmm037051. doi:10.1242/dmm.037051
Ribatti, D., and Santoiemma, M. (2014). Epithelial-mesenchymal interactions: A fundamental developmental Biology mechanism. Int. J. Dev. Biol. 58 (5), 303–306. doi:10.1387/ijdb.140143dr
Richardson, R. J., Hammond, N. L., Coulombe, P. A., Saloranta, C., Nousiainen, H. O., Salonen, R., et al. (2014). Periderm prevents pathological epithelial adhesions during embryogenesis. J. Clin. Invest. 124 (9), 3891–3900. doi:10.1172/JCI71946
Rodriguez-Berdini, L., Ferrero, G. O., Bustos Plonka, F., Cardozo Gizzi, A. M., Prucca, C. G., Quiroga, S., et al. (2020). The moonlighting protein c-Fos activates lipid synthesis in neurons, an activity that is critical for cellular differentiation and cortical development. J. Biol. Chem. 295 (26), 8808–8818. doi:10.1074/jbc.RA119.010129
Rossi, A., Kontarakis, Z., Gerri, C., Nolte, H., Holper, S., Kruger, M., et al. (2015). Genetic compensation induced by deleterious mutations but not gene knockdowns. Nature 524 (7564), 230–233. doi:10.1038/nature14580
Schilling, T. F., and Le Pabic, P. (2009). Fishing for the signals that pattern the face. J. Biol. 8 (11), 101. doi:10.1186/jbiol205
Schneider, C. A., Rasband, W. S., and Eliceiri, K. W. (2012). NIH image to ImageJ: 25 years of image analysis. Nat. Methods 9 (7), 671–675. doi:10.1038/nmeth.2089
Shen, X., Liu, R. M., Yang, L., Wu, H., Li, P. Q., Liang, Y. L., et al. (2011). The CRISPLD2 gene is involved in cleft lip and/or cleft palate in a Chinese population. Birth defects Res. Part A, Clin. Mol. Teratol. 91 (10), 918–924. doi:10.1002/bdra.20840
Sherwood, D. R., Butler, J. A., Kramer, J. M., and Sternberg, P. W. (2005). FOS-1 promotes basement-membrane removal during anchor-cell invasion in C. elegans. Cell 121 (6), 951–962. doi:10.1016/j.cell.2005.03.031
Smeyne, R. J., Schilling, K., Oberdick, J., Robertson, L., Luk, D., Curran, T., et al. (1993a). A fos-lac Z transgenic mouse that can be used for neuroanatomic mapping. Adv. Neurol. 59, 285–291. Retrieved from http://www.ncbi.nlm.nih.gov/pubmed/8420113.
Smeyne, R. J., Vendrell, M., Hayward, M., Baker, S. J., Miao, G. G., Schilling, K., et al. (1993b). Continuous c-fos expression precedes programmed cell death in vivo. Nature 363 (6425), 166–169. doi:10.1038/363166a0
Soukup, V., Horacek, I., and Cerny, R. (2013). Development and evolution of the vertebrate primary mouth. J. Anat. 222 (1), 79–99. doi:10.1111/j.1469-7580.2012.01540.x
Swartz, M. E., Sheehan-Rooney, K., Dixon, M. J., and Eberhart, J. K. (2011). Examination of a palatogenic gene program in zebrafish. Dev. Dyn. 240 (9), 2204–2220. doi:10.1002/dvdy.22713
Swindell, E. C., Yuan, Q., Maili, L. E., Tandon, B., Wagner, D. S., and Hecht, J. T. (2015). Crispld2 is required for neural crest cell migration and cell viability during zebrafish craniofacial development. Genesis 53 (10), 660–667. doi:10.1002/dvg.22897
Van der Heyden, C., and Huysseune, A. (2000). Dynamics of tooth formation and replacement in the zebrafish (Danio rerio) (Teleostei, Cyprinidae). Dev. Dyn. 219 (4), 486–496. doi:10.1002/1097-0177(2000)9999:9999<::AID-DVDY1069>3.0.CO;2-Z
Velazquez, F. N., Prucca, C. G., Etienne, O., D'Astolfo, D. S., Silvestre, D. C., Boussin, F. D., et al. (2015). Brain development is impaired in c-fos -/- mice. Oncotarget 6 (19), 16883–16901. doi:10.18632/oncotarget.4527
Wagner, E. F. (2002). Functions of AP1 (Fos/Jun) in bone development. Ann. Rheum. Dis. 61, ii40–42. doi:10.1136/ard.61.suppl_2.ii40
Wang, Q., Liu, H., Wang, Q., Zhou, F., Liu, Y., Zhang, Y., et al. (2017). Involvement of c-Fos in cell proliferation, migration, and invasion in osteosarcoma cells accompanied by altered expression of Wnt2 and Fzd9. PLoS One 12 (6), e0180558. doi:10.1371/journal.pone.0180558
Weinberg, S. M., Maher, B. S., and Marazita, M. L. (2006a). Parental craniofacial morphology in cleft lip with or without cleft palate as determined by cephalometry: A meta-analysis. Orthod. Craniofac Res. 9 (1), 18–30. doi:10.1111/j.1601-6343.2006.00339.x
Weinberg, S. M., Naidoo, S. D., Bardi, K. M., Brandon, C. A., Neiswanger, K., Resick, J. M., et al. (2009). Face shape of unaffected parents with cleft affected offspring: combining three-dimensional surface imaging and geometric morphometrics. Orthod. Craniofac Res. 12 (4), 271–281. doi:10.1111/j.1601-6343.2009.01462.x
Weinberg, S. M., Neiswanger, K., Martin, R. A., Mooney, M. P., Kane, A. A., Wenger, S. L., et al. (2006b). The pittsburgh oral-facial cleft study: expanding the cleft phenotype. Background and justification. Cleft Palate Craniofac J. 43 (1), 7–20. doi:10.1597/04-122r1.1
Weinberg, S. M., Neiswanger, K., Richtsmeier, J. T., Maher, B. S., Mooney, M. P., Siegel, M. I., et al. (2008). Three-dimensional morphometric analysis of craniofacial shape in the unaffected relatives of individuals with nonsyndromic orofacial clefts: A possible marker for genetic susceptibility. Am. J. Med. Genet. A 146A (4), 409–420. doi:10.1002/ajmg.a.32177
M. Westerfield (Editor) (1993). The zebrafish book: A guide for the laboratory use of zebrafish (brachydanio rerio) (Eugene, OR: M. Westerfield).
Yano, H., Ohtsuru, A., Ito, M., Fujii, T., and Yamashita, S. (1996). Involvement of c-Fos proto-oncogene during palatal fusion and interdigital space formation in the rat. Dev. Growth Differ. 38 (4), 351–357. doi:10.1046/j.1440-169X.1996.t01-3-00003.x
Yelick, P. C., and Schilling, T. F. (2002). Molecular dissection of craniofacial development using zebrafish. Crit. Rev. Oral Biol. Med. 13 (4), 308–322. doi:10.1177/154411130201300402
Young, N. M., Wat, S., Diewert, V. M., Browder, L. W., and Hallgrimsson, B. (2007). Comparative morphometrics of embryonic facial morphogenesis: implications for cleft-lip etiology. Anat. Rec. Hob. N.J. 2007) 290 (1), 123–139. doi:10.1002/ar.20415
Keywords: fos, morphogenesis, craniofacial development, orofacial cleft, geometric morphometrics
Citation: Maili L, Tandon B, Yuan Q, Menezes S, Chiu F, Hashmi SS, Letra A, Eisenhoffer GT and Hecht JT (2023) Disruption of fos causes craniofacial anomalies in developing zebrafish. Front. Cell Dev. Biol. 11:1141893. doi: 10.3389/fcell.2023.1141893
Received: 10 January 2023; Accepted: 21 June 2023;
Published: 16 August 2023.
Edited by:
Maria Iribarne, University of Notre Dame, United StatesReviewed by:
Yongchu Pan, Nanjing Medical University, ChinaCopyright © 2023 Maili, Tandon, Yuan, Menezes, Chiu, Hashmi, Letra, Eisenhoffer and Hecht. This is an open-access article distributed under the terms of the Creative Commons Attribution License (CC BY). The use, distribution or reproduction in other forums is permitted, provided the original author(s) and the copyright owner(s) are credited and that the original publication in this journal is cited, in accordance with accepted academic practice. No use, distribution or reproduction is permitted which does not comply with these terms.
*Correspondence: Jacqueline T. Hecht, amFjcXVlbGluZS50LmhlY2h0QHV0aC50bWMuZWR1
†ORCID: George T. Eisenhoffer, https://orcid.org/0000-0003-0188-6782; Jacqueline T. Hecht, https://orcid.org/0000-0002-9087-3379
Disclaimer: All claims expressed in this article are solely those of the authors and do not necessarily represent those of their affiliated organizations, or those of the publisher, the editors and the reviewers. Any product that may be evaluated in this article or claim that may be made by its manufacturer is not guaranteed or endorsed by the publisher.
Research integrity at Frontiers
Learn more about the work of our research integrity team to safeguard the quality of each article we publish.