- 1Institute for the Advanced Study of Human Biology (ASHBi), Kyoto University, Kyoto, Japan
- 2Laboratory of Developmental Systems, Institute for Life and Medical Sciences, Kyoto University, Kyoto, Japan
Building limb morphogenesis in vitro would substantially open up avenues for research and applications of appendage development. Recently, advances in stem cell engineering to differentiate desired cell types and produce multicellular structures in vitro have enabled the derivation of limb-like tissues from pluripotent stem cells. However, in vitro recapitulation of limb morphogenesis is yet to be achieved. To formulate a method of building limbs in vitro, it is critically important to understand developmental mechanisms, especially the modularity and the dependency of limb development on the external tissues, as those would help us to postulate what can be self-organized and what needs to be externally manipulated when reconstructing limb development in vitro. Although limbs are formed on the designated limb field on the flank of embryo in the normal developmental context, limbs can also be regenerated on the amputated stump in some animals and experimentally induced at ectopic locations, which highlights the modular aspects of limb morphogenesis. The forelimb-hindlimb identity and the dorsal-ventral, proximal-distal, and anterior-posterior axes are initially instructed by the body axis of the embryo, and maintained in the limb domain once established. In contrast, the aspects of dependency on the external tissues are especially underscored by the contribution of incoming tissues, such as muscles, blood vessels, and peripheral nerves, to developing limbs. Together, those developmental mechanisms explain how limb-like tissues could be derived from pluripotent stem cells. Prospectively, the higher complexity of limb morphologies is expected to be recapitulated by introducing the morphogen gradient and the incoming tissues in the culture environment. Those technological developments would dramatically enhance experimental accessibility and manipulability for elucidating the mechanisms of limb morphogenesis and interspecies differences. Furthermore, if human limb development can be modeled, drug development would be benefited by in vitro assessment of prenatal toxicity on congenital limb deficiencies. Ultimately, we might even create a future in which the lost appendage would be recovered by transplanting artificially grown human limbs.
1 Introduction
The current advances in stem cell biology have enabled the differentiation of the limb bud mesenchyme from pluripotent stem cells (PSCs) such as embryonic stem cells (ESCs) or induced pluripotent stem cells (iPSCs) (Chen et al., 2017; Mori et al., 2019; Yamada et al., 2021). Those findings have provided us with hopes of engineering limb morphogenesis in vitro and utilizing regenerative medicine to rescue damaged limbs. Engineering limb morphogenesis should require recapitulating the developmental environment of the limbs in vitro. Importantly, the modularity of limb development, namely, independence from the development of other organs, might potentially facilitate the self-organization of patterns and structures without external guidance in culture (Schlosser and Wagner, 2004; Sasai, 2013). Therefore, understanding the modular aspects and the dependency on the external tissues in limb development would help to postulate what can be self-organized and what needs to be manipulated in vitro, and to formulate the method for engineering limbs. In this article, we will review the current knowledge on the modularity of limb bud morphogenesis in embryonic development and regeneration as well as its dependence on the externalenvironment of the limb. Based on knowledge of in vivo limb morphogenesis, we will discuss the feasibility of in vitro engineering of the limb.
Limb development initiates as epithelial-mesenchymal transition of the lateral plate triggered by FGF-signaling from ectoderm (Gros and Tabin, 2014). The mesenchymal cells migrate towards the ectoderm at the lateral trunk region, and the outlining ectoderm then differentiates into a signaling center called the apical ectodermal ridge (AER). Signaling interactions between AER and the limb bud mesenchyme regulate massive cell proliferation and patterning of the limb bud.
Once the limb bud is established, the morphogenesis of the limb proceeds in a semi-independent manner, as shown by the fact that limb bud morphogenesis could happen in an unusual part of the body in experimental and regenerative situations. In 1995, Cohn et al. showed that when a bead soaked with FGF or WNT is implanted into the flank region between the forelimb and hindlimb, an ectopic limb bud is induced and the established bud then independently develops into a complete additional limb (Cohn et al., 1995; Kawakami et al., 2001). These studies suggest that once a limb bud is established, the limb morphogenesis requires little, if any, specificity of the tissues proximal to the limb. Thus, the ability of ectopic limb development is one of the striking examples showing the modularity of limb development.
Regeneration of the limbs in urodele amphibians and larval anurans is another example showing the modularity of limb formation, as it can be interpreted as ectopic and heterochronic limb development. When the limb is amputated in those animals, the stump is immediately covered by the wound epidermis and the wound epidermis then serves as the signaling center called apical ectodermal cap (AEC), which functionally corresponds to AER of the developing limb bud (Stoick-Cooper et al., 2007). Underneath the AEC, the cells in the remaining tissues of the limb, including dermis, cartilage, muscles, Schwann cells, and other connective tissues undergo dedifferentiation (Kragl et al., 2009), and the tissue stem cells such as muscle satellite cells are activated (Sandoval-Guzmán et al., 2014). The dedifferentiated cells and the activated tissue stem cells undergo extensive proliferation forming a blastema of mesenchyme, which essentially resembles the developmental limb bud mesenchyme.
Interestingly, Endo et al. (2004) discovered that, in regenerative urodele amphibians, after one peels off a piece of the skin on the lateral side of the limb, reroutes the nerve to the skin wound, and grafts a piece of skin from the side of the limb contralateral to the wound site, a blastema is formed ectopically on the lateral side of the limb. As a result of these manipulations, called the “accessory limb model,” the ectopic blastema continues to grow and forms an additional limb, demonstrating the minimum sufficient conditions to initiate limb regeneration. It was later found that when the same operation is conducted on the lateral trunk, ectopic formation of a blastema and limb morphogenesis can be induced even on the trunk (Hirata et al., 2013). These examples in highly regenerative animals suggested that the proper limb morphogenesis could happen not only in the designated limb field on the flank of the embryo, but also on the completely mature tissues of the adult limb and even the trunk if only the minimal conditions to trigger limb morphogenesis are satisfied.
Those instances highlight the autonomous and modular nature of limb morphogenesis. However, that does not mean limb morphogenesis could occur independently of the proximal tissues. Indeed, the ex vivo culture of chick limb bud fails to develop properly (Strangeways et al., 1926). What kinds of interactions between external tissues are important for limb development and how much independence does the developing limb have? To explore these questions, in the next section, we will review the molecular mechanisms of modularity, namely, how polarities and positional identities are established and maintained and their relationship with the basal tissues.
2 How polarities and positional identities are established?
The identity of limbs as forelimbs and hindlimbs is determined by the position along the rostrocaudal axis of the lateral plate where limb bud mesenchyme is derived (Isaac et al., 1998; Logan et al., 1998; Ohuchi et al., 1998). The positional identity of the lateral plate is governed by the antagonistic gradient of rostral retinoic acid (RA) and caudal FGF signaling (Ribes et al., 2009; Zhao and Duester, 2009). RA is required for the expression of Tbx5 in the presumptive forelimb field of the lateral plate (Zhao et al., 2009). On the other hand, the hindlimb field in the caudal lateral plate is determined by GDF11 secreted from the paraxial mesoderm. GDF11 activates the expression of the hindlimb field and hindlimb bud-specific genes such as Isl1, Pitx1, and Tbx4 in the lateral plate, and thus the initiation of Gdf11 expression controls the position of the hindlimb along the body (McPherron et al., 1999; Jurberg et al., 2013; Matsubara et al., 2017). The anatomical features specific to the forelimb and hindlimb are specified by the expression of Tbx5 in the forelimb and Tbx4/Pitx1 in the hindlimb (Logan et al., 1998; Logan and Tabin, 1999; Rodriguez-Esteban et al., 1999; Takeuchi et al., 1999).
At the later stage of limb development, the same antagonistic gradient of RA and FGF controls the proximodistal identity of the limb bud, namely, stylopod, zeugopod, and autopod, from proximal to distal. This was first suggested in amphibian limb regeneration. When the limb is amputated, the lost limb element will be regenerated depending on the position of the amputation along the proximodistal axis. However, when a limb is treated with RA, the regenerated structure is proximalized relative to the position of amputation in a dose-dependent manner, resulting in a serial proximodistal duplication such that the stylopod is regenerated onto the remaining zeugopod (Niazi and Saxena, 1978; Maden, 1982; Maden, 1983). On the other hand, when blastema of a salamander formed on autopod is transplanted onto stylopod, zeugopod is regenerated in between (Iten and Bryant, 1975; Stocum, 1975; Maden, 1980; McCusker and Gardiner, 2013). This process is referred to as “intercalation”: when the adjacent cells have discontinuous positional information, the intermediate cells are regenerated to fill the gap in the positional identities (French et al., 1976; Agata et al., 2007). The mature autopod tissue is not capable of inducing intercalary regeneration between itself and the stylopod, but FGF treatment of the autopod endows the competency to induce the intercalary formation of zeugopod, suggesting that FGF might be the distal factor (Satoh et al., 2010).
It was later shown in chicken limb development that the transplanted limb bud mesenchyme after exposure to RA exhibits expansion of expression of the proximal marker, Meis1, and develops the proximalized structure. In contrast, exposure to FGF and Wnt, which is secreted from AER in normal development, expands the expression of the distal Hox gene Hoxa13, and distalizes the limb (Cooper et al., 2011; Roselló-Díez et al., 2011). Thus, even after the limb bud is initiated, proximal RA signaling from the trunk is supposedly required for the establishment of proper proximo-distal polarity, although there is controversy about this since in Rdh10 mutant mouse, which lacks RA synthesis, proximal-distal patterning of the hindlimb was not affected (Cunningham et al., 2013).
The key player in establishing the anteroposterior axis of the limb is SHH. The classical experiments using chick embryos strikingly showed that after transplantation of the tissue from the posterior margin of the limb bud, which is known as the zone of polarizing activity (ZPA), to the anterior margin of another limb bud, the mirror-duplicated pattern of three digits is induced along the anteroposterior axis (Saunders and Gasseling, 1968). It was later identified that SHH is the molecular entity of ZPA activity (Riddle et al., 1993). SHH, which is secreted from the posterior margin of the limb bud, specify anteroposterior identity of the digits in its early time window of the expression, and also promotes the expansion of the limb in its broader expression duration (Towers et al., 2008; 2011; Zhu et al., 2008; Zhu et al., 2022).
How is the posterior-biased expression of Shh established and how does the anteroposterior axis of limbs correspond to that of the body? The axial expression of Hox9 paralogous group genes is restricted to the posterior portion of the forelimb-forming region and Hox9 genes activate the expression of Hand2 in the posterior forelimb, which then activates Shh in ZPA (Xu and Wellik, 2011). In contrast, the Hox5 paralogous group genes repress the Shh expression in the anterior forelimb (Xu et al., 2013). Although these regulations by Hox5 and 9 genes only apply to the forelimb, the expression domains of Shh in forelimb and hindlimb buds are regulated by the common downstream effectors such as the anterior repressor, Gli3, and the posterior activator, Hand2 (Büscher et al., 1997; Galli et al., 2010). In hindlimb, the anterior expression of Gli3 is regulated by Sall4, and the posterior expression of Hand2 is regulated by Isl1 (Itou et al., 2012; Akiyama et al., 2015). Interestingly, an additional limb induced in the trunk shows the inverted anteroposterior identity. This might be explained as occurring because the interlimb region posterior to the forelimb field, which would be the anterior portion of the additional limb, has stronger potency of polarizing activity (Tickle, 2015).
In amphibians, the interaction between anterior and posterior tissues at the stump is essential to initiate limb regeneration. If an anterior half of one limb is transplanted on an anterior half of another limb so that the stump has only either anterior identity, the limb fails to be regenerated (Bryant and Baca, 1978; Stocum, 1978). Conversely, if a left limb blastema is grafted onto a right stump so that the anterior tissues of the graft are adjacent to the posterior tissues of the stump, two supernumerary limbs are induced due to intercalation along the anteroposterior axis (Iten and Bryant, 1975). The accessory limb model, mentioned above, also requires a skin graft from a posterior limb onto an anteriorly created wound to initiate intercalation on the stump (Endo et al., 2004). The posterior skin can be substituted by RA or its downstream target, Shh (McCusker et al., 2014; Nacu et al., 2016; Iwata et al., 2020).
Limbs develop on the dorsoventral border of the trunk and AER is induced at the interface of dorsal and ventral ectoderm (Altabef et al., 1997). Dorsal ectoderm expresses Wnt7a (Parr and McMahon, 1995), whereas ventral ectoderm expresses Bmp (Pizette et al., 2001). Recombinant experiments performed by placing ectoderm in dorsoventrally reversed orientation with respect to the mesoderm showed that the signals from ectoderm specify the dorsoventral identity of the limb mesenchyme (MacCabe et al., 1974; Akita, 1996). Like their induction along the anteroposterior axis, supernumerary limbs can be induced by intercalation along the dorsoventral axis (Iten and Bryant, 1975).
How are positional identities interpreted into the anatomical complexity of limbs? Recent single-cell RNA-seq and lineage tracing experiments have shown that Msx-positive naïve limb progenitor cells differentiate into proximal or autopodial progenitors, while the naïve progenitors remain in the distal-most region beneath the AER. Three axes of positional identities affect the differentiation timing of proximal and autopodial progenitors in a complex manner seemingly underlying the mechanisms by which the positional identities are interpreted into the anatomical complexity of limb skeletons (Markman et al., 2023). A comprehensive understanding of this question requires an understanding of the differences in cellular behaviors in three-dimensional space in response to positional identities, as well as physical and mathematical approach to integrate collective cell behaviors into tissue-level morphogenesis. Limb organoids would be powerful model systems for the integral understanding of morphogenesis because simple aspects of three-dimensional morphogenesis can be extracted on a smaller scale in a more experimentally accessible environment.
To summarize this section, forelimb-hindlimb identity is determined by the positional identity of the lateral plate along the rostrocaudal axis. The proximo-distal axis is established through interactions between the trunk, or proximal limb, and distal AER. The anteroposterior axis of the limb is induced by the axial rostrocaudal identity. The dorsoventral axis is instructed by the dorsoventral identity of the ectoderm (Figure 1).Importantly, the fate map and transplantation experiments show that once the limb polarity is established, the positional identity can be maintained in a cell-autonomous manner without reference to the rest of the embryo. (reviewed in (Tickle, 2015)). However, the source of the limb tissues is not just the limb bud mesenchyme and the overlying ectoderm, but also incoming tissues from the trunk after the limb bud is initiated. In the next section, we will review how those incoming tissues acquire morphological information and how those tissues affect the morphogenesis of the other tissues.
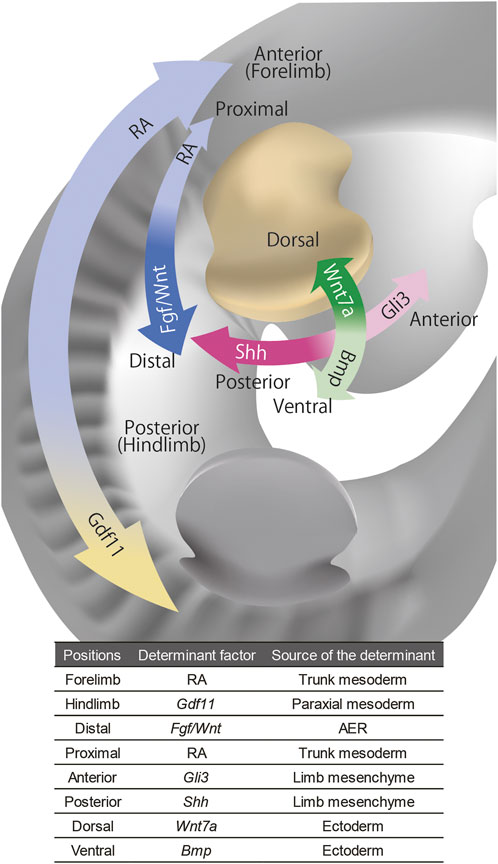
FIGURE 1. The signaling molecules that govern positional information of the limb bud. The distal-proximal axis of the limb bud is governed by FGF/WNT from AER and RA from trunk, the anterior-posterior axis is governed by Shh and Gli3 from limb mesenchyme, and the dorsal-ventral axis is governed by Wnt7a and Bmp from the ectoderm. The anterior-posterior axis of the whole body that governs the forelimb and hindlimb identities is regulated by RA and Gdf11 in axial mesoderm.
3 The contribution of incoming tissues on limb morphogenesis
Muscle cells in the limb are derived from ventral–lateral somites and migrate into the nascent limb bud (Chevallier et al., 1977; Christ et al., 1977; Hayashi and Ozawa, 1991). The migration of muscle precursor cells is induced by Hgf expressed in limb mesenchyme (Bladt et al., 1995; Brand-Saberi et al., 1996; Scaal et al., 1999). Once they have migrated into the limb bud, the muscle precursors cluster into dorsal and ventral muscle masses (Hayashi and Ozawa, 1991). Subsequently, these muscle masses are subdivided into individual, anatomically distinct muscles by muscle connective tissues, which are derived from limb bud mesenchyme (Kardon, 1998; Kardon et al., 2003).
The specific patterns of individual muscles are governed by muscle connective tissues, and their surrounding blood vessels (Grim, 1991; Tozer et al., 2007; Mathew et al., 2011). Thus, muscle patterning defects occur due to mutation of the genes expressed in limb mesenchyme, such as Tbx3, 4, and 5 (Hasson et al., 2010; Colasanto et al., 2016). Tendons are also derived from limb bud mesenchyme and are reciprocally dependent on muscles for proper patterning and maintenance (Christ et al., 1977; Kieny and Chevallier, 1979; Kardon, 1998; Schweitzer et al., 2001; Huang et al., 2015).
Although the initial patterning of bones and cartilage is not dependent on muscles, once the muscles are shaped into a functional unit together with muscle connective tissues and tendons, the mechanical forces applied to skeletons are responsible for proper bone morphogenesis, including longitudinal growth, circumferential growth, and formation of eminence (reviewed in (Felsenthal and Zelzer, 2017)). Strikingly, most of the limb joints fail to be matured and become fused (Pai, 1965; Rot-Nikcevic et al., 2006; Kahn et al., 2009; Nowlan et al., 2010).
Blood vessels are the incoming tissues that substantially affect the morphogenesis of the limb skeleton. Vascularization of the limb bud is initiated as angiogenesis from the dorsal aorta (Seichert and Rychter, 1972a; Seichert and Rychter, 1972b). Concomitantly, somite-derived angioblasts migrate into the limb bud and participate in vasculogenesis (Ambler et al., 2001). The importance of vasculature in limb skeletal morphogenesis is well demonstrated in avascular culture on chorioallantoic membrane (CAM). Chick limb bud graft could develop recognizable limb elements after being transplanted onto CAM, where diffusible nutrients, growth factors, and blood vessels are supplied from the host (Murray and Patrick, 1926). However, if the graft and CAM are separated by an intervening porous filter so that vascular invasion is prevented, the grafts will develop into very small and grossly distorted limbs (Searls, 1968).
The failure of proper cartilage morphogenesis in avascular culture does not seem to be simply due to the lack of oxygen and nutrient supply. Cartilage morphogenesis is associated with vascular remodeling since cartilage requires a hypoxic environment and hypoxic gene regulator, Hif1a, for its development and maintenance (Schipani et al., 2001; Amarilio et al., 2007; Provot et al., 2007; Bentovim et al., 2012). While blood vessels are distributed throughout the limb bud before the emergence of cartilage anlagen, localized vascular regression avascularizes the cartilage-forming area (Feinberg et al., 1986). Although this vascular remodeling is initiated by VEGF secreted from condensed mesenchyme (Eshkar-Oren et al., 2009), vasculatures reciprocally regulate skeletal morphogenesis (Yin and Pacifici, 2001). Furthermore, interdigital apoptosis requires reactive oxygen species produced in the highly vascularized area between digits (Eshkar-Oren et al., 2015).
Unlike cartilage, bone requires vascularization. Limb bone formation occurs via endochondral ossification, in which cartilage anlagen is replaced by bone minerals. At the center of the cartilage anlagen of long bones, the chondrocyte becomes hypertrophic chondrocyte and secretes VEGF to attract invasion of blood vessels (Gerber et al., 1999; Zelzer et al., 2002). Bone minerals are deposited by osteoblast precursors migrated from perichondrium/periosteum along with blood vessels (Maes et al., 2010), although recent evidences show trans-differentiation from hypertrophic chondrocytes is another source of osteoblasts (Yang et al., 2014a; Yang et al., 2014b; Zhou et al., 2014; Park et al., 2015). The vasculature is not only important for longitudinal bone growth, but it also serves as a guiding template for circumferential bone growth (Shoham et al., 2016).
Peripheral nerves are critically important for limb regeneration. It has long been known that if a limb of urodele amphibians is denervated, the amputated limb will fail to regenerate (Todd, 1823). Conversely, the existence of nerves at the wound site is one of the sufficient conditions for inducing ectopic limb regeneration in the accessory limb model (Endo et al., 2004). Rescue experiments in a denervated limb or accessory limb model without nerve rerouting showed that nAG, Fgf, Bmp, and Nrg1 are considered to be “nerve factors” which are secreted from peripheral nerves and induce blastema formation at a stump (Kumar et al., 2007; Makanae et al., 2014; Farkas et al., 2016). However, the nerve dependence seems to be specific to limb regeneration, and not to apply to limb development. When a chick limb bud graft is made such that the graft is not innervated from the host, musculoskeletal development of the grafts is grossly unaffected (Murray and Patrick, 1926; Hunt, 1932; Hamburger, 1939). Furthermore, even in limb regeneration, if a limb bud has never experienced innervation, the limb is competent for regeneration in the absence of nerves (Yntema, 1959). Thus, the requirement for peripheral nerves in limb formation might be contingent on the cellular context of limb progenitors.
In this section, we argued that besides limb bud mesenchyme, tissues such as blood vessels, muscles, and peripheral nerves come into the limb bud and contribute to limb morphogenesis (Figure 2). Musculoskeletal morphologies are fine-tuned based on physical and biochemical interactions in multiple tissues, including muscles and peripheral nerves, which reinforce developmental robustness and malleability of limbs (reviewed in (Tsutsumi et al., 2017)). The invasion of blood vessels plays critical roles in initial skeletal patterning. Thus, among the incoming tissues, blood vessels are seemingly especially important for regulating the morphogenesis of limb mesenchyme.
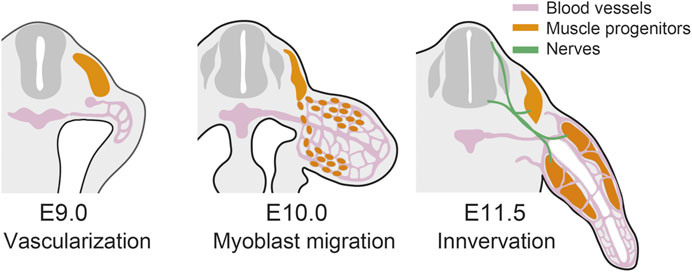
FIGURE 2. Contribution of the incoming tissues in limb development. In mouse forelimb, blood vessels invade the limb bud at E9.0 (Walls et al., 2008), muscle progenitors migrate into the limb at E10.0 (Houzelstein et al., 1999), and the limb bud is innervated at E11.5 (Hurren et al., 2015).
In the context of engineering limb morphogenesis in vitro, is it necessary to direct the morphogenesis of those incoming tissues separately? Since limb morphogenesis is dependent on the interaction of multiple different tissues in the limb, it would be important to establish methods for co-culturing and integrating multiple cell lineages, including limb mesenchyme and the incoming tissues. However, the modular aspect of limb development and regeneration suggests that the incoming tissues as well as limb mesenchyme do not rely on continuous input of morphogenetic information from the external environment. Therefore, in the next section, we will discuss how to achieve the derivation of limb progenitor cells, introduce the positional identities of limb mesenchyme, and integrate them with the incoming tissues.
4 How might we build limbs in vitro?
Recently, Chen et al. reported that three-dimensional culture of mouse iPSCs successfully induced the differentiation of cells with limb bud identity in vitro (Chen et al., 2017) (Figure 3A). Due to the high adhesiveness of PSCs, the cells in suspension culture are self-aggregated into embryoid bodies (Desbaillets et al., 2000). Chen et al. cultured the embryoid body with a WNT/β-catenin signaling agonist, CHIR99021, and FGF8 with a fibrin matrix for 6 days, and subsequently cultured it with SHH agonist Purmorphamine and a TGFβ type I receptor inhibitor, SB431542, together with CHIR99021 and FGF8. Both WNT signaling and FGF8 are present in the primitive streak and play important roles in the formation of mesoderm in the mouse embryo (Takada et al., 1994; Yoshikawa et al., 1997; Liu et al., 1999; Sun et al., 1999; Huelsken et al., 2000). Furthermore, treatment with the WNT/β-catenin signaling agonist induces mesoderm differentiation in the embryoid body (Berge et al., 2008; Brink et al., 2014). Thus, the first 6 days of treatment with the WNT/β-catenin signaling agonist and FGF8 seemed to drive mesodermal differentiation. While SHH has a critical role in the growth of limb bud mesenchyme, the TGFβ type I receptor inhibitor suppresses cartilage differentiation and expands undifferentiated limb bud mesenchyme (Parada et al., 2022).
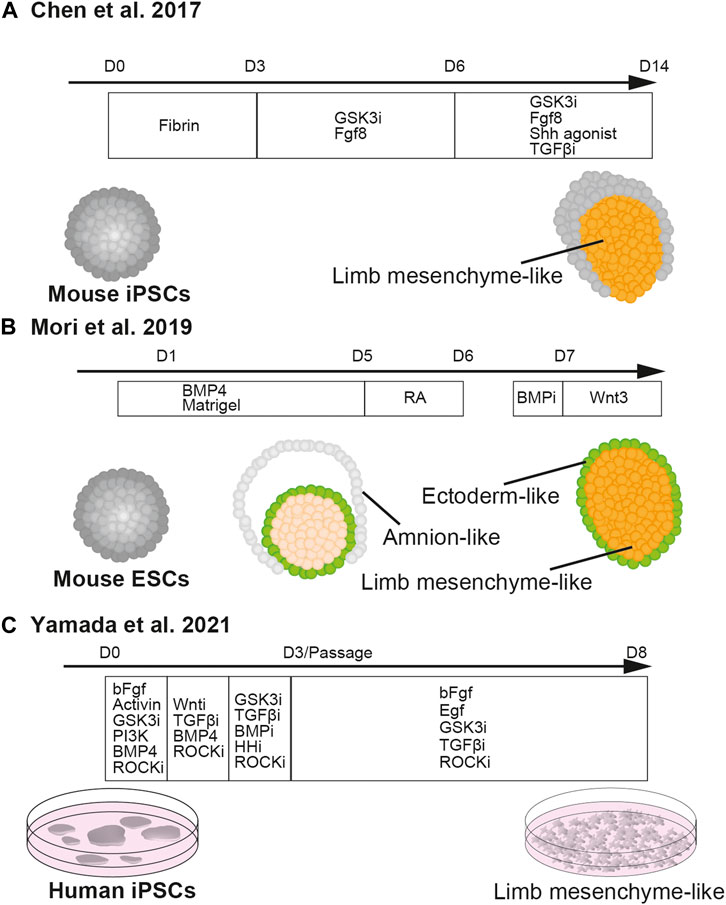
FIGURE 3. In vitro induction of limb mesenchyme from pluripotent stem cells.Chen et al., 2017 described the method of differentiating cell aggregates of mouse iPSCs into limb mesenchyme (A). Mori et al., 2019 reported the differentiation of cell aggregates of mouse ESCs into limb mesenchyme wrapped by the ectoderm (B). Yamada et al., 2021 established the method of differentiating human iPSCs into limb mesenchyme in two-dimensional culture (C).
Strikingly, the limb bud-like aggregates stimulated the regeneration of a mouse digit. Chen et al. transplanted the limb bud-like aggregates derived from iPSCs together with a cocktail of growth factors, FGF8, WNT3a, Thymosin β4, and BMP2 onto the amputated second phalange of an adult mouse digit. While the adult mouse is unable to regenerate the digit after amputation without any manipulation, the grafted digit showed the outgrowth of distal phalange tissues which were composed of both graft-derived and host-derived cells (Chen et al., 2017).
Later, it was reported that another three-dimensional culture method of mouse ESCs successfully recapitulated the differentiation of early limb bud-like structure in vitro, in which Hand2-positive mesenchyme is covered by E-cadherin-positive ectoderm (Mori et al., 2019) (Figure 3B). Essentially, in this study, mouse ESCs were differentiated using the serum-free floating culture of embryoid body-like aggregate with quick reaggregation (SFEBq) method with a high dose of BMP4. In SFEBq, ESCs are seeded in ultra-low adhesive 96-well plates to make embryoid bodies and differentiated in serum-free culture conditions (Eiraku et al., 2008). While without BMP, the embryoid body would spontaneously differentiate into epiblasts and subsequently neural ectoderm (Kamiya et al., 2011; Takata et al., 2016), a high dose of BMP might have contributed to fate decisions towards limb bud mesenchyme at multiple steps of lineage segregation. In the gastrulating mouse embryo, BMP4 secreted from extra-embryonic ectoderm regulates the formation of primitive streak from the epiblasts (Mishina et al., 1995; Winnier et al., 1995; Coucouvanis and Martin, 1999; Beppu et al., 2000). Thus, the first fate decision directed by BMP was to drive differentiation of ESCs towards mesoendoderm, instead of ectoderm (Johansson and Wiles, 1995; Finley et al., 1999; Ying et al., 2003; Ng et al., 2005).
After the primitive streak is established, mesodermal cells from the posterior part of the primitive streak migrate laterally more robustly than those from the anterior part, and thereby the anteroposterior identities of the primitive streak are converted into the mediolateral axis of mesoderm at the later embryonic stage. At this stage, BMP4 that is secreted from the lateral plate and NOGGIN, a BMP antagonist secreted from the notochord, constitute a BMP gradient along the mediolateral axis, specifying the subtypes of mesoderm (Pourquié et al., 1996; Tonegawa et al., 1997; Tonegawa and Takahashi, 1998). Thus, in the culture in Mori et al. (2019) the second fate decision directed by the high dose of BMP was to instruct differentiation of lateral plate mesoderm.
Although it is not very clear how the aggregate with lateral plate identity is differentiated into limb bud mesenchyme in the culture of Mori et al., 2019 the overlying ectoderm might induce epithelial-mesenchymal transition and differentiation of limb bud mesenchyme, as happens in the embryo (Gros and Tabin, 2014). As discussed above, the dorsoventral identity of the limb bud is specified by dorsal Wnt7a and ventral Bmp. Hence, the third fate decision by BMP was to grant ventral identity to the limb bud-like aggregates. Indeed, local inhibition of BMP signaling created a portion with dorsal identity in the aggregate and induced the formation of AER-like structure at the dorsoventral border.
Yamada et al. (2021) reported the derivation of limb bud-like cells from human iPSCs (Figure 3C). In their study, iPSCs were differentiated in a step-wise manner from the mid-primitive streak, lateral plate, and limb bud progenitors by daily changes of medium with composition modified from that described in Loh et al. (2016) so as to maximize the expressions of PRRX1 and other limb bud markers. The differentiated limb bud-like cells could be expanded by activation of EGF, FGF, and canonical WNT signaling, as well as suppression of TGF-β signaling. The limb bud-like cells, which have the potential to produce cartilage, have proven to be useful for drug screening of therapeutic candidates for treating type II collagenopathy (Yamada et al., 2021).
Chen et al. (2017); Mori et al. (2019), and Yamada et al. (2021) showed that the PSC-derived limb bud-like cells are capable of forming skeletal tissues after being transplanted in mouse or rat (Table. 1). However, their ability to undergo cartilage morphogenesis in culture has not been extensively explored. It should be noted that in the study of Mori et al., the anteroposterior and proximo-distal identities in the limb bud-like cells within the aggregate were disorganized. Though the positional identities of the cells in the aggregate were not addressed in Chen et al., given that among the four growth factors in the cocktail, FGF and WNT specify the distal identity of the limb bud, the interaction between the distally specified limb bud-like aggregate and the host tissues might have established the proximo-distal axis, which might have then enabled the organized outgrowth of the skeletal tissues. Furthermore, it can be speculated that blood vessels might have invaded the graft and stimulated the growth and morphogenesis of the regenerating distal digit.
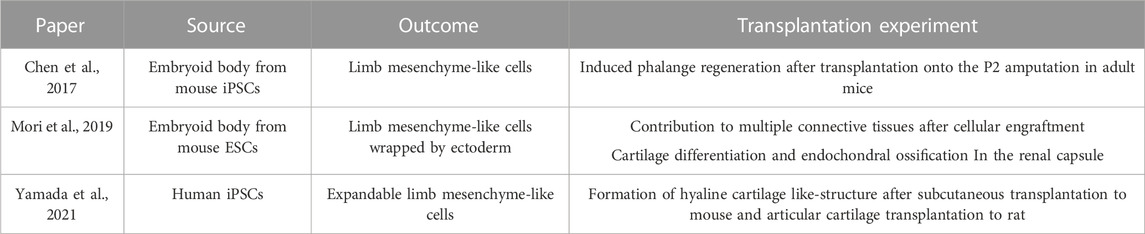
TABLE 1. In vitro inductions of limb mesenchyme and their potency after transplantation experiments.
Is it possible to recapitulate more complex limb morphogenesis with cultures from PSCs? To achieve this goal, it seems essential to induce proper organization of the proximo-distal, dorso-ventral, and anteroposterior axis and to induce vascularization into the limb bud organoid. In fact, these are two of the current major challenges in the field of organoids in general: to provide morphogen gradients that are not self-organized in the aggregate and to induce vascularization.
To establish morphogen gradient in the culture environment of organoids, methods are selected based on the scale and duration of the gradient to be maintained. One commonly used method relies on diffusion from two reservoir chambers. Organoids are embedded in hydrogels and placed between the two chambers (Attayek et al., 2016; Tabata and Lutolf, 2017; Koh and Hagiwara, 2023). Using this method, a chemical gradient spanning 1.5-6 folds in a 1 mm scale can be achieved. When the media in two chambers are kept replenished or the chambers have a large enough volume, the gradient can be maintained for 5–10 days. An orthogonal gradient to create two axes (Such as dorsal-ventral and anterior-posterior) can be established by four microfluidic channels, where two parallel flows are placed perpendicular to each other (Demers et al., 2016).
When a smaller scale of the gradient is required, local administration of signaling molecules can be utilized. As mentioned above, Mori et al. (2019) injected a BMP inhibitor onto a limb bud-like aggregate through a glass capillary and successfully induced a dorsalized hemisphere within the aggregate. In this case, since the aggregate was globally ventralized by BMP signaling prior to the injection, local inhibition of BMP signaling could sufficiently establish a morphogen gradient. Embedding morphogen-secreting cells can generate a gradient with 500 μm scale (Cederquist et al., 2019). By using light-inducible expression of morphogen, more precise special control might be achieved (De Santis et al., 2021).
When a larger scale of the gradient is desired, a 4 mm scale of the gradient can be achieved by embedding organoids on the confluent of two parallel streams (Park et al., 2009). An even larger scale (−20 mm) of the gradient can be achieved by the perpendicular sequential diffusive mixing of two inlet media (Rifes et al., 2020)
Alternatively, the organoid assembly approach might be utilized. In this approach, two organoids with two different positional information are differentiated separately and combined into one “assembloid” (Bagley et al., 2017; Birey et al., 2017; Koike et al., 2019). This approach requires to the identification of the timing at which the positional identities of the two organoids are fixed while two organoids are still capable of fusing, but it is possible that this approach might recapitulate the situation of intercalary and supernumerary regeneration and might powerfully promote limb morphogenesis.
To vascularize organoids, one of the approaches is co-culturing with endothelial progenitor cells. For example, in a brain organoid, human iPS-derived endothelial cells formed a vascular network and improved the survivability and maturation of the organoid (Pham et al., 2018; Cakir et al., 2019). Similarly, vascularized organoids were also generated by co-culturing human PSC-derived cells and human umbilical vein endothelial cells (HUVECs), which are primary endothelial cells with high potential for blood vessel formation in vitro (Takebe et al., 2013; Isshiki et al., 2020; Shi et al., 2020). However, it is still a challenge to achieve functional perfusion of the vascularized organoids in vitro. This goal will likely be achieved by identifying culture environments which allow integration of a microfluidic device with a vascular bed and vascularized organoids (Zhang et al., 2021).
5 Conclusion
It is becoming technically feasible to introduce appropriate morphogen gradients exogenously and to introduce blood vessels and other tissues to limb bud organoids. If engineering a more complex limb bud could be achieved, it would substantially open up avenues for research and applications of appendage development. If we can create in vitro models of three-dimensional limb development, manipulability to elucidate the mechanisms of morphogenesis will be dramatically enhanced. Furthermore, since iPSCs have been established in many non-traditional model organisms, molecular and genetic mechanisms of mammalian limb diversities, including specific features of human limbs, are expected to be studied using organoids from PS cells. For instance, the extensive growth of the proximal hindlimb is one of the remarkable differences between the bodies of modern humans and any other extant animals. It has enabled our upright bipedalism and presumably allowed us to have a heavy brain and has released our hands from their roles for walking. Thus, it would be exciting to have an opportunity to unravel the genetic and developmental mechanisms of the evolution of our limb proportions. Finally, models of human limb development would bring about medical benefits, such as drug screening to assess prenatal toxicity causing congenital limb defects and ultimately rescuing lost appendages by transplanting in vitro-derived human limb bud tissues.
Author contributions
RT conceived, wrote the manuscript, and conceptualized and drew illustrations, upon discussion with ME. All authors listed have made a substantial, direct, and intellectual contribution to the work and approved it for publication.
Funding
JSPS Grant-in-Aid for Young Scientists to RT(22K15122). JST, CREST to ME (JPMJCR1922).
Acknowledgments
We would thank Elizabeth Nakajima for rigorous English and scientific proof reading.
Conflict of interest
The authors declare that the research was conducted in the absence of any commercial or financial relationships that could be construed as a potential conflict of interest.
Publisher’s note
All claims expressed in this article are solely those of the authors and do not necessarily represent those of their affiliated organizations, or those of the publisher, the editors and the reviewers. Any product that may be evaluated in this article, or claim that may be made by its manufacturer, is not guaranteed or endorsed by the publisher.
References
Agata, K., Saito, Y., and Nakajima, E. (2007). Unifying principles of regeneration I: Epimorphosis versus morphallaxis. Dev. Growth & Differ. 49, 73–78. doi:10.1111/j.1440-169X.2007.00919.x
Akita, K. (1996). The effect of the ectoderm on the dorsoventral pattern of epidermis, muscles and joints in the developing chick leg: A new model. Anat. Embryol. 193, 377–386. doi:10.1007/BF00186694
Akiyama, R., Kawakami, H., Wong, J., Oishi, I., Nishinakamura, R., and Kawakami, Y. (2015). Sall4-Gli3 system in early limb progenitors is essential for the development of limb skeletal elements. PNAS 112, 5075–5080. doi:10.1073/pnas.1421949112
Altabef, M., Clarke, J. D., and Tickle, C. (1997). Dorso-ventral ectodermal compartments and origin of apical ectodermal ridge in developing chick limb. Development 124, 4547–4556. doi:10.1242/dev.124.22.4547
Amarilio, R., Viukov, S. V., Sharir, A., Eshkar-Oren, I., Johnson, R. S., and Zelzer, E. (2007). HIF1alpha regulation of Sox9 is necessary to maintain differentiation of hypoxic prechondrogenic cells during early skeletogenesis. Development 134, 3917–3928. doi:10.1242/dev.008441
Ambler, C. A., Nowicki, J. L., Burke, A. C., and Bautch, V. L. (2001). Assembly of trunk and limb blood vessels involves extensive migration and vasculogenesis of somite-derived angioblasts. Dev. Biol. 234, 352–364. doi:10.1006/dbio.2001.0267
Attayek, P. J., Ahmad, A. A., Wang, Y., Williamson, I., Sims, C. E., Magness, S. T., et al. (2016). In vitro polarization of colonoids to create an intestinal stem cell compartment. PLOS ONE 11, e0153795. doi:10.1371/journal.pone.0153795
Bagley, J. A., Reumann, D., Bian, S., Lévi-Strauss, J., and Knoblich, J. A. (2017). Fused cerebral organoids model interactions between brain regions. Nat. Methods 14, 743–751. doi:10.1038/nmeth.4304
Bentovim, L., Amarilio, R., and Zelzer, E. (2012). HIF1α is a central regulator of collagen hydroxylation and secretion under hypoxia during bone development. Development 139, 4473–4483. doi:10.1242/dev.083881
Beppu, H., Kawabata, M., Hamamoto, T., Chytil, A., Minowa, O., Noda, T., et al. (2000). BMP type II receptor is required for gastrulation and early development of mouse embryos. Dev. Biol. 221, 249–258. doi:10.1006/dbio.2000.9670
Berge, D., Koole, W., Fuerer, C., Fish, M., Eroglu, E., and Nusse, R. (2008). Wnt signaling mediates self-organization and Axis formation in embryoid bodies. Cell Stem Cell 3, 508–518. doi:10.1016/j.stem.2008.09.013
Birey, F., Andersen, J., Makinson, C. D., Islam, S., Wei, W., Huber, N., et al. (2017). Assembly of functionally integrated human forebrain spheroids. Nature 545, 54–59. doi:10.1038/nature22330
Bladt, F., Riethmacher, D., Isenmann, S., Aguzzi, A., and Birchmeier, C. (1995). Essential role for the c-met receptor in the migration of myogenic precursor cells into the limb bud. Nature 376, 768–771. doi:10.1038/376768a0
Brand-Saberi, B., Müller, T. S., Wilting, J., Christ, B., and Birchmeier, C. (1996). Scatter factor/hepatocyte growth factor (SF/HGF) induces emigration of myogenic cells at interlimb levelin vivo. Dev. Biol. 179, 303–308. doi:10.1006/dbio.1996.0260
Brink, S. C., Baillie-Johnson, P., Balayo, T., Hadjantonakis, A.-K., Nowotschin, S., Turner, D. A., et al. (2014). Symmetry breaking, germ layer specification and axial organisation in aggregates of mouse embryonic stem cells. Development 141, 4231–4242. doi:10.1242/dev.113001
Bryant, S. V., and Baca, B. A. (1978). Regenerative ability of double-half and half upper arms in the newt, Notophthalmus viridescens. J. Exp. Zoology 204, 307–323. doi:10.1002/jez.1402040302
Büscher, D., Bosse, B., Heymer, J., and Rüther, U. (1997). Evidence for genetic control of Sonic hedgehog by Gli3 in mouse limb development. Mech. Dev. 62, 175–182. doi:10.1016/S0925-4773(97)00656-4
Cakir, B., Xiang, Y., Tanaka, Y., Kural, M. H., Parent, M., Kang, Y.-J., et al. (2019). Engineering of human brain organoids with a functional vascular-like system. Nat. Methods 16, 1169–1175. doi:10.1038/s41592-019-0586-5
Cederquist, G. Y., Asciolla, J. J., Tchieu, J., Walsh, R. M., Cornacchia, D., Resh, M. D., et al. (2019). Specification of positional identity in forebrain organoids. Nat. Biotechnol. 37, 436–444. doi:10.1038/s41587-019-0085-3
Chen, Y., Xu, H., and Lin, G. (2017). Generation of iPSC-derived limb progenitor-like cells for stimulating phalange regeneration in the adult mouse. Cell Discov. 3, 17046. doi:10.1038/celldisc.2017.46
Chevallier, A., Kieny, M., and Mauger, A. (1977). Limb-somite relationship: Origin of the limb musculature. Development 41, 245–258. doi:10.1242/dev.41.1.245
Christ, B., Jacob, H. J., and Jacob, M. (1977). Experimental analysis of the origin of the wing musculature in avian embryos. Anat. Embryol. 150, 171–186. doi:10.1007/BF00316649
Cohn, M. J., Izpisúa-Belmonte, J. C., Abud, H., Heath, J. K., and Tickle, C. (1995). Fibroblast growth factors induce additional limb development from the flank of chick embryos. Cell 80, 739–746. doi:10.1016/0092-8674(95)90352-6
Colasanto, M. P., Eyal, S., Mohassel, P., Bamshad, M., Bonnemann, C. G., Zelzer, E., et al. (2016). Development of a subset of forelimb muscles and their attachment sites requires the ulnar-mammary syndrome gene Tbx3. Dis. Models Mech. 9, 1257–1269. doi:10.1242/dmm.025874
Cooper, K. L., Hu, J. K.-H., Berge, D., Ros, M. A., and Tabin, C. J. (2011). Initiation of proximal-distal patterning in the vertebrate limb by signals and growth. Science 332, 1083–1086. doi:10.1126/science.1199499
Coucouvanis, E., and Martin, G. R. (1999). BMP signaling plays a role in visceral endoderm differentiation and cavitation in the early mouse embryo. Development 126, 535–546. doi:10.1242/dev.126.3.535
Cunningham, T. J., Zhao, X., Sandell, L. L., Evans, S. M., Trainor, P. A., and Duester, G. (2013). Antagonism between retinoic acid and fibroblast growth factor signaling during limb development. Cell Rep. 3, 1503–1511. doi:10.1016/j.celrep.2013.03.036
De Santis, R., Etoc, F., Rosado-Olivieri, E. A., and Brivanlou, A. H. (2021). Self-organization of human dorsal-ventral forebrain structures by light induced SHH. Nat. Commun. 12, 6768. doi:10.1038/s41467-021-26881-w
Demers, C. J., Soundararajan, P., Chennampally, P., Cox, G. A., Briscoe, J., Collins, S. D., et al. (2016). Development-on-chip: In vitro neural tube patterning with a microfluidic device. Development 143, 1884–1892. doi:10.1242/dev.126847
Desbaillets, I., Ziegler, U., Groscurth, P., and Gassmann, M. (2000). Embryoid bodies: An in vitro model of mouse embryogenesis. Exp. Physiol. 85, 645–651. doi:10.1111/j.1469-445X.2000.02104.x
Eiraku, M., Watanabe, K., Matsuo-Takasaki, M., Kawada, M., Yonemura, S., Matsumura, M., et al. (2008). Self-organized formation of polarized cortical tissues from ESCs and its active manipulation by extrinsic signals. Cell Stem Cell 3, 519–532. doi:10.1016/j.stem.2008.09.002
Endo, T., Bryant, S. V., and Gardiner, D. M. (2004). A stepwise model system for limb regeneration. Dev. Biol. 270, 135–145. doi:10.1016/j.ydbio.2004.02.016
Eshkar-Oren, I., Krief, S., Ferrara, N., Elliott, A. M., and Zelzer, E. (2015). Vascular patterning regulates interdigital cell death by a ROS-mediated mechanism. Development 142, 672–680. doi:10.1242/dev.120279
Eshkar-Oren, I., Viukov, S. V., Salameh, S., Krief, S., Oh, C., Akiyama, H., et al. (2009). The forming limb skeleton serves as a signaling center for limb vasculature patterning via regulation of Vegf. Development 136, 1263–1272. doi:10.1242/dev.034199
Farkas, J. E., Freitas, P. D., Bryant, D. M., Whited, J. L., and Monaghan, J. R. (2016). Neuregulin-1 signaling is essential for nerve-dependent axolotl limb regeneration. Development 143, 2724–2731. doi:10.1242/dev.133363
Feinberg, R. N., Latker, C. H., and Beebe, D. C. (1986). Localized vascular regression during limb morphogenesis in the chicken embryo. I. Spatial and temporal changes in the vascular pattern. Anat. Rec. 214, 405–409. doi:10.1002/ar.1092140411
Felsenthal, N., and Zelzer, E. (2017). Mechanical regulation of musculoskeletal system development. Development 144, 4271–4283. doi:10.1242/dev.151266
Finley, M. F. A., Devata, S., and Huettner, J. E. (1999). BMP-4 inhibits neural differentiation of murine embryonic stem cells. J. Neurobiol. 40, 271–287. doi:10.1002/(SICI)1097-4695(19990905)40:3<271::AID-NEU1>3.0.CO;2-C
French, V., Bryant, P. J., and Bryant, S. V. (1976). Pattern regulation in epimorphic fields. Science 193, 969–981. doi:10.1126/science.948762
Galli, A., Robay, D., Osterwalder, M., Bao, X., Bénazet, J.-D., Tariq, M., et al. (2010). Distinct roles of Hand2 in initiating polarity and posterior Shh expression during the onset of mouse limb bud development. PLOS Genet. 6, e1000901. doi:10.1371/journal.pgen.1000901
Gerber, H.-P., Vu, T. H., Ryan, A. M., Kowalski, J., Werb, Z., and Ferrara, N. (1999). VEGF couples hypertrophic cartilage remodeling, ossification and angiogenesis during endochondral bone formation. Nat. Med. 5, 623–628. doi:10.1038/9467
Grim, M. (1991). “Control of muscle morphogenesis and endplate pattern in limb muscles of avian chimeras,” in Developmental Patterning of the vertebrate limb NATO ASI series (Boston, MA: Springer), 293–297. doi:10.1007/978-1-4615-3310-8_39
Gros, J., and Tabin, C. J. (2014). Vertebrate limb bud formation is initiated by localized epithelial-to-mesenchymal transition. Science 343, 1253–1256. doi:10.1126/science.1248228
Hamburger, V. (1939). The development and innervation of transplanted limb primordia of chick embryos. J. Exp. Zoology 80, 347–389. doi:10.1002/jez.1400800302
Hasson, P., DeLaurier, A., Bennett, M., Grigorieva, E., Naiche, L. A., Papaioannou, V. E., et al. (2010). Tbx4 and Tbx5 acting in connective tissue are required for limb muscle and tendon patterning. Dev. Cell 18, 148–156. doi:10.1016/j.devcel.2009.11.013
Hayashi, K., and Ozawa, E. (1991). Vital labelling of somite-derived myogenic cells in the chicken limb bud. Roux’s Arch. Dev. Biol. 200, 188–192. doi:10.1007/BF00361336
Hirata, A., Makanae, A., and Satoh, A. (2013). Accessory limb induction on flank region and its muscle regulation in axolotl. Dev. Dyn. 242, 932–940. doi:10.1002/dvdy.23984
Houzelstein, D., Auda-Boucher, G., Chéraud, Y., Rouaud, T., Blanc, I., Tajbakhsh, S., et al. (1999). The homeobox gene Msx1 is expressed in a subset of somites, and in muscle progenitor cells migrating into the forelimb. Development 126, 2689–2701. doi:10.1242/dev.126.12.2689
Huang, A. H., Riordan, T. J., Pryce, B., Weibel, J. L., Watson, S. S., Long, F., et al. (2015). Musculoskeletal integration at the wrist underlies the modular development of limb tendons. Development 142, 2431–2441. doi:10.1242/dev.122374
Huelsken, J., Vogel, R., Brinkmann, V., Erdmann, B., Birchmeier, C., and Birchmeier, W. (2000). Requirement for β-catenin in anterior-posterior Axis formation in mice. J. Cell Biol. 148, 567–578. doi:10.1083/jcb.148.3.567
Hunt, E. A. (1932). The differentiation of chick limb buds in chorio-allantoic grafts, with special reference to the muscles. J. Exp. Zoology 62, 57–91. doi:10.1002/jez.1400620103
Hurren, B., Collins, J. J. P., Duxson, M. J., and Deries, M. (2015). First neuromuscular contact correlates with onset of primary myogenesis in rat and mouse limb muscles. PLOS ONE 10, e0133811. doi:10.1371/journal.pone.0133811
Isaac, A., Rodriguez-Esteban, C., Ryan, A., Altabef, M., Tsukui, T., Patel, K., et al. (1998). Tbx genes and limb identity in chick embryo development. Development 125, 1867–1875. doi:10.1242/dev.125.10.1867
Isshiki, Y., Kaneko, T., Tamada, A., Muguruma, K., and Yokokawa, R. (2020). “Co-culture of a brain organoid derived from human iPSCs and vasculature on a chip,” in 2020 IEEE 33rd International Conference on Micro Electro Mechanical Systems (MEMS), Vancouver, BC, Canada, 18-22 January 2020 (IEEE), 1024–1027. doi:10.1109/MEMS46641.2020.9056422
Iten, L. E., and Bryant, S. V. (1975). The interaction between the blastema and stump in the establishment of the anterior-posterior and proximal-distal organization of the limb regenerate. Dev. Biol. 44, 119–147. doi:10.1016/0012-1606(75)90381-4
Itou, J., Kawakami, H., Quach, T., Osterwalder, M., Evans, S. M., Zeller, R., et al. (2012). Islet1 regulates establishment of the posterior hindlimb field upstream of the Hand2-Shh morphoregulatory gene network in mouse embryos. Development 139, 1620–1629. doi:10.1242/dev.073056
Iwata, R., Makanae, A., and Satoh, A. (2020). Stability and plasticity of positional memory during limb regeneration in Ambystoma mexicanum. Dev. Dyn. 249, 342–353. doi:10.1002/dvdy.96
Johansson, B. M., and Wiles, M. V. (1995). Evidence for involvement of activin A and bone morphogenetic protein 4 in mammalian mesoderm and hematopoietic development. Mol. Cell. Biol. 15, 141–151. doi:10.1128/MCB.15.1.141
Jurberg, A. D., Aires, R., Varela-Lasheras, I., Nóvoa, A., and Mallo, M. (2013). Switching axial progenitors from producing trunk to tail tissues in vertebrate embryos. Dev. Cell 25, 451–462. doi:10.1016/j.devcel.2013.05.009
Kahn, J., Shwartz, Y., Blitz, E., Krief, S., Sharir, A., Breitel, D. A., et al. (2009). Muscle contraction is necessary to maintain joint progenitor cell fate. Dev. Cell 16, 734–743. doi:10.1016/j.devcel.2009.04.013
Kamiya, D., Banno, S., Sasai, N., Ohgushi, M., Inomata, H., Watanabe, K., et al. (2011). Intrinsic transition of embryonic stem-cell differentiation into neural progenitors. Nature 470, 503–509. doi:10.1038/nature09726
Kardon, G., Harfe, B. D., and Tabin, C. J. (2003). A tcf4-positive mesodermal population provides a prepattern for vertebrate limb muscle patterning. Dev. Cell 5, 937–944. doi:10.1016/S1534-5807(03)00360-5
Kardon, G. (1998). Muscle and tendon morphogenesis in the avian hind limb. Development 125, 4019–4032. doi:10.1242/dev.125.20.4019
Kawakami, Y., Capdevila, J., Büscher, D., Itoh, T., Esteban, C. R., and Belmonte, J. C. I. (2001). WNT signals control FGF-dependent limb initiation and AER induction in the chick embryo. Cell 104, 891–900. doi:10.1016/S0092-8674(01)00285-9
Kieny, M., and Chevallier, A. (1979). Autonomy of tendon development in the embryonic chick wing. Development 49, 153–165. doi:10.1242/dev.49.1.153
Koh, I., and Hagiwara, M. (2023). Gradient to sectioning CUBE workflow for the generation and imaging of organoids with localized differentiation. Commun. Biol. 6, 299–310. doi:10.1038/s42003-023-04694-5
Koike, H., Iwasawa, K., Ouchi, R., Maezawa, M., Giesbrecht, K., Saiki, N., et al. (2019). Modelling human hepato-biliary-pancreatic organogenesis from the foregut–midgut boundary. Nature 574, 112–116. doi:10.1038/s41586-019-1598-0
Kragl, M., Knapp, D., Nacu, E., Khattak, S., Maden, M., Epperlein, H. H., et al. (2009). Cells keep a memory of their tissue origin during axolotl limb regeneration. Nature 460, 60–65. doi:10.1038/nature08152
Kumar, A., Godwin, J. W., Gates, P. B., Garza-Garcia, A. A., and Brockes, J. P. (2007). Molecular basis for the nerve dependence of limb regeneration in an adult vertebrate. Science 318, 772–777. doi:10.1126/science.1147710
Liu, P., Wakamiya, M., Shea, M. J., Albrecht, U., Behringer, R. R., and Bradley, A. (1999). Requirement for Wnt3 in vertebrate axis formation. Nat. Genet. 22, 361–365. doi:10.1038/11932
Logan, M., Simon, H. G., and Tabin, C. (1998). Differential regulation of T-box and homeobox transcription factors suggests roles in controlling chick limb-type identity. Development 125, 2825–2835. doi:10.1242/dev.125.15.2825
Logan, M., and Tabin, C. J. (1999). Role of Pitx1 upstream of Tbx4 in specification of hindlimb identity. Science 283, 1736–1739. doi:10.1126/science.283.5408.1736
Loh, K. M., Chen, A., Koh, P. W., Deng, T. Z., Sinha, R., Tsai, J. M., et al. (2016). Mapping the pairwise choices leading from pluripotency to human bone, heart, and other mesoderm cell types. Cell 166, 451–467. doi:10.1016/j.cell.2016.06.011
MacCabe, J. A., Errick, J., and Saunders, J. W. (1974). Ectodermal control of the dorsoventral axis in the leg bud of the chick embryo. Dev. Biol. 39, 69–82. doi:10.1016/S0012-1606(74)80009-6
Maden, M. (1980). Intercalary regeneration in the amphibian limb and the rule of distal transformation. Development 56, 201–209. doi:10.1242/dev.56.1.201
Maden, M. (1983). The effect of vitamin A on the regenerating axolotl limb. Development 77, 273–295. doi:10.1242/dev.77.1.273
Maden, M. (1982). Vitamin A and pattern formation in the regenerating limb. Nature 295, 672–675. doi:10.1038/295672a0
Maes, C., Kobayashi, T., Selig, M. K., Torrekens, S., Roth, S. I., Mackem, S., et al. (2010). Osteoblast precursors, but not mature osteoblasts, move into developing and fractured bones along with invading blood vessels. Dev. Cell 19, 329–344. doi:10.1016/j.devcel.2010.07.010
Makanae, A., Mitogawa, K., and Satoh, A. (2014). Co-operative Bmp- and Fgf-signaling inputs convert skin wound healing to limb formation in urodele amphibians. Dev. Biol. 396, 57–66. doi:10.1016/j.ydbio.2014.09.021
Markman, S., Zada, M., David, E., Giladi, A., Amit, I., and Zelzer, E. (2023). A single-cell census of mouse limb development identifies complex spatiotemporal dynamics of skeleton formation. Dev. Cell 58, 565–581.e4. doi:10.1016/j.devcel.2023.02.013
Mathew, S. J., Hansen, J. M., Merrell, A. J., Murphy, M. M., Lawson, J. A., Hutcheson, D. A., et al. (2011). Connective tissue fibroblasts and Tcf4 regulate myogenesis. Development 138, 371–384. doi:10.1242/dev.057463
Matsubara, Y., Hirasawa, T., Egawa, S., Hattori, A., Suganuma, T., Kohara, Y., et al. (2017). Anatomical integration of the sacral–hindlimb unit coordinated by GDF11 underlies variation in hindlimb positioning in tetrapods. Nat. Ecol. Evol. 1, 1392–1399. doi:10.1038/s41559-017-0247-y
McCusker, C. D., and Gardiner, D. M. (2013). Positional information is reprogrammed in blastema cells of the regenerating limb of the axolotl (Ambystoma mexicanum). PLOS ONE 8, e77064. doi:10.1371/journal.pone.0077064
McCusker, C., Lehrberg, J., and Gardiner, D. (2014). Position-specific induction of ectopic limbs in non-regenerating blastemas on axolotl forelimbs. Regeneration 1, 27–34. doi:10.1002/reg2.10
McPherron, A. C., Lawler, A. M., and Lee, S.-J. (1999). Regulation of anterior/posterior patterning of the axial skeleton by growth/differentiation factor 11. Nat. Genet. 22, 260–264. doi:10.1038/10320
Mishina, Y., Suzuki, A., Ueno, N., and Behringer, R. R. (1995). Bmpr encodes a type I bone morphogenetic protein receptor that is essential for gastrulation during mouse embryogenesis. Genes Dev. 9, 3027–3037. doi:10.1101/gad.9.24.3027
Mori, S., Sakakura, E., Tsunekawa, Y., Hagiwara, M., Suzuki, T., and Eiraku, M. (2019). Self-organized formation of developing appendages from murine pluripotent stem cells. Nat. Commun. 10, 3802–3813. doi:10.1038/s41467-019-11702-y
Murray, P. D. F., and Patrick, D. F. (1926). An experimental study of the development the limbs of the chick. Germany: Universitätsbibliothek Johann Christian Senckenberg.
Nacu, E., Gromberg, E., Oliveira, C. R., Drechsel, D., and Tanaka, E. M. (2016). FGF8 and SHH substitute for anterior–posterior tissue interactions to induce limb regeneration. Nature 533, 407–410. doi:10.1038/nature17972
Ng, E. S., Azzola, L., Sourris, K., Robb, L., Stanley, E. G., and Elefanty, A. G. (2005). The primitive streak gene Mixl1 is required for efficient haematopoiesis and BMP4-induced ventral mesoderm patterning in differentiating ES cells. Development 132, 873–884. doi:10.1242/dev.01657
Niazi, I. A., and Saxena, S. (1978). Abnormal hind limb regeneration in tadpoles of the toad, Bufo andersoni, exposed to excess vitamin A. Folia Biol. 26, 3–8.
Nowlan, N. C., Bourdon, C., Dumas, G., Tajbakhsh, S., Prendergast, P. J., and Murphy, P. (2010). Developing bones are differentially affected by compromised skeletal muscle formation. Bone 46, 1275–1285. doi:10.1016/j.bone.2009.11.026
Ohuchi, H., Takeuchi, J., Yoshioka, H., Ishimaru, Y., Ogura, K., Takahashi, N., et al. (1998). Correlation of wing-leg identity in ectopic FGF-induced chimeric limbs with the differential expression of chick Tbx5 and Tbx4. Development 125, 51–60. doi:10.1242/dev.125.1.51
Pai, A. C. (1965). Developmental genetics of a lethal mutation, muscular dysgenesis (mdg), in the mouse. Dev. Biol. 11, 82–92. doi:10.1016/0012-1606(65)90038-2
Parada, C., Banavar, S. P., Khalilian, P., Rigaud, S., Michaut, A., Liu, Y., et al. (2022). Mechanical feedback defines organizing centers to drive digit emergence. Dev. Cell 57, 854–866.e6. doi:10.1016/j.devcel.2022.03.004
Park, J., Gebhardt, M., Golovchenko, S., Perez-Branguli, F., Hattori, T., Hartmann, C., et al. (2015). Dual pathways to endochondral osteoblasts: A novel chondrocyte-derived osteoprogenitor cell identified in hypertrophic cartilage. Biol. Open 4, 608–621. doi:10.1242/bio.201411031
Park, J. Y., Kim, S.-K., Woo, D.-H., Lee, E.-J., Kim, J.-H., and Lee, S.-H. (2009). Differentiation of neural progenitor cells in a microfluidic chip-generated cytokine gradient. STEM CELLS 27, 2646–2654. doi:10.1002/stem.202
Parr, B. A., and McMahon, A. P. (1995). Dorsalizing signal Wnt-7a required for normal polarity of D–V and A–P axes of mouse limb. Nature 374, 350–353. doi:10.1038/374350a0
Pham, M. T., Pollock, K. M., Rose, M. D., Cary, W. A., Stewart, H. R., Zhou, P., et al. (2018). Generation of human vascularized brain organoids. NeuroReport 29, 588–593. doi:10.1097/WNR.0000000000001014
Pizette, S., Abate-Shen, C., and Niswander, L. (2001). BMP controls proximodistal outgrowth, via induction of the apical ectodermal ridge, and dorsoventral patterning in the vertebrate limb. Development 128, 4463–4474. doi:10.1242/dev.128.22.4463
Pourquié, O., Fan, C.-M., Coltey, M., Hirsinger, E., Watanabe, Y., Bréant, C., et al. (1996). Lateral and axial signals involved in avian somite patterning: A role for BMP4. Cell 84, 461–471. doi:10.1016/S0092-8674(00)81291-X
Provot, S., Zinyk, D., Gunes, Y., Kathri, R., Le, Q., Kronenberg, H. M., et al. (2007). Hif-1alpha regulates differentiation of limb bud mesenchyme and joint development. J. Cell Biol. 177, 451–464. doi:10.1083/jcb.200612023
Ribes, V., Roux, I. L., Rhinn, M., Schuhbaur, B., and Dollé, P. (2009). Early mouse caudal development relies on crosstalk between retinoic acid, Shh and Fgf signalling pathways. Development 136, 665–676. doi:10.1242/dev.016204
Riddle, R. D., Johnson, R. L., Laufer, E., and Tabin, C. (1993). Sonic hedgehog mediates the polarizing activity of the ZPA. Cell 75, 1401–1416. doi:10.1016/0092-8674(93)90626-2
Rifes, P., Isaksson, M., Rathore, G. S., Aldrin-Kirk, P., Møller, O. K., Barzaghi, G., et al. (2020). Modeling neural tube development by differentiation of human embryonic stem cells in a microfluidic WNT gradient. Nat. Biotechnol. 38, 1265–1273. doi:10.1038/s41587-020-0525-0
Rodriguez-Esteban, C., Tsukui, T., Yonei, S., Magallon, J., Tamura, K., and Belmonte, J. C. I. (1999). The T-box genes Tbx4 and Tbx5 regulate limb outgrowth and identity. Nature 398, 814–818. doi:10.1038/19769
Roselló-Díez, A., Ros, M. A., and Torres, M. (2011). Diffusible signals, not autonomous mechanisms, determine the main proximodistal limb subdivision. Science 332, 1086–1088. doi:10.1126/science.1199489
Rot-Nikcevic, I., Reddy, T., Downing, K. J., Belliveau, A. C., Hallgrímsson, B., Hall, B. K., et al. (2006). Myf5−/−:MyoD−/− amyogenic fetuses reveal the importance of early contraction and static loading by striated muscle in mouse skeletogenesis. Dev. Genes Evol. 216, 1–9. doi:10.1007/s00427-005-0024-9
Sandoval-Guzmán, T., Wang, H., Khattak, S., Schuez, M., Roensch, K., Nacu, E., et al. (2014). Fundamental differences in dedifferentiation and stem cell recruitment during skeletal muscle regeneration in two salamander species. Cell Stem Cell 14, 174–187. doi:10.1016/j.stem.2013.11.007
Sasai, Y. (2013). Cytosystems dynamics in self-organization of tissue architecture. Nature 493, 318–326. doi:10.1038/nature11859
Satoh, A., Cummings, G. M. C., Bryant, S. V., and Gardiner, D. M. (2010). Regulation of proximal-distal intercalation during limb regeneration in the axolotl (Ambystoma mexicanum). Dev. Growth & Differ. 52, 785–798. doi:10.1111/j.1440-169X.2010.01214.x
Saunders, J. W. J., and Gasseling, M. T. (1968). “Ectodermal-mesenchymal interactions in the origin of limb symmetry,” in Epithelial-mesenchymal interactions (United States: Williams & Wilkins), 78–97. Available at: https://ci.nii.ac.jp/naid/10019237905/(Accessed October 8, 2020).
Scaal, M., Bonafede, A., Dathe, V., Sachs, M., Cann, G., Christ, B., et al. (1999). SF/HGF is a mediator between limb patterning and muscle development. Development 126, 4885–4893. doi:10.1242/dev.126.21.4885
Schipani, E., Ryan, H. E., Didrickson, S., Kobayashi, T., Knight, M., and Johnson, R. S. (2001). Hypoxia in cartilage: HIF-1alpha is essential for chondrocyte growth arrest and survival. Genes Dev. 15, 2865–2876. doi:10.1101/gad.934301
Schlosser, G., and Wagner, G. P. (2004). Modularity in development and evolution. Chicago, IL: University of Chicago Press. Available at: https://press.uchicago.edu/ucp/books/book/chicago/M/bo3636976.html (Accessed August 19, 2022).
Schweitzer, R., Chyung, J. H., Murtaugh, L. C., Brent, A. E., Rosen, V., Olson, E. N., et al. (2001). Analysis of the tendon cell fate using Scleraxis, a specific marker for tendons and ligaments. Development 128, 3855–3866. doi:10.1242/dev.128.19.3855
Searls, R. L. (1968). Development of the embryonic chick limb bud in avascular culture. Dev. Biol. 17, 382–399. doi:10.1016/0012-1606(68)90071-7
Seichert, V., and Rychter, Z. (1972a). Vascularization of developing anterior lamb of the chick embryo. II. Differentiation of vascular bed and its significance for the location of morphogenetic processes inside the limb bud. Folia Morphol. (Praha) 20, 352–361.
Seichert, V., and Rychter, Z. (1972b). Vascularization of the developing anterior limb of the chick embryo. 3. Developmental changes in the perimetacarpal capillary network. Folia Morphol. (Praha) 20, 397–405.
Shi, Y., Sun, L., Wang, M., Liu, J., Zhong, S., Li, R., et al. (2020). Vascularized human cortical organoids (vOrganoids) model cortical development in vivo. PLOS Biol. 18, e3000705. doi:10.1371/journal.pbio.3000705
Shoham, A. B., Rot, C., Stern, T., Krief, S., Akiva, A., Dadosh, T., et al. (2016). Deposition of collagen type I onto skeletal endothelium reveals a new role for blood vessels in regulating bone morphology. Development 143, 3993–3943. doi:10.1242/dev.139253
Stocum, D. L. (1978). Regeneration of symmetrical hindlimbs in larval salamanders. Science 200, 790–793. doi:10.1126/science.644323
Stocum, D. L. (1975). Regulation after proximal or distal transposition of limb regeneration blastemas and determination of the proximal boundary of the regenerate. Dev. Biol. 45, 112–136. doi:10.1016/0012-1606(75)90246-8
Stoick-Cooper, C. L., Moon, R. T., and Weidinger, G. (2007). Advances in signaling in vertebrate regeneration as a prelude to regenerative medicine. Genes Dev. 21, 1292–1315. doi:10.1101/gad.1540507
Strangeways, T. S. P., Fell, H. B., and Hardy, W. B. (1926). Experimental studies on the differentiation of embryonic tissues growing in vivo and in vitro—I, the development of the undifferentiated limb-bud (a) when subcutaneously grafted into the post-embryonic chick and (b) when cultivated in vitro. Proc. R. Soc. Lond. 99, 340–366. doi:10.1098/rspb.1926.0017
Sun, X., Meyers, E. N., Lewandoski, M., and Martin, G. R. (1999). Targeted disruption of Fgf8 causes failure of cell migration in the gastrulating mouse embryo. Genes Dev. 13, 1834–1846. doi:10.1101/gad.13.14.1834
Tabata, Y., and Lutolf, M. P. (2017). Multiscale microenvironmental perturbation of pluripotent stem cell fate and self-organization. Sci. Rep. 7, 44711. doi:10.1038/srep44711
Takada, S., Stark, K. L., Shea, M. J., Vassileva, G., McMahon, J. A., and McMahon, A. P. (1994). Wnt-3a regulates somite and tailbud formation in the mouse embryo. Genes Dev. 8, 174–189. doi:10.1101/gad.8.2.174
Takata, N., Sakakura, E., Kasukawa, T., Sakuma, T., Yamamoto, T., and Sasai, Y. (2016). Establishment of functional genomics pipeline in mouse epiblast-like tissue by combining transcriptomic analysis and gene knockdown/knockin/knockout, using RNA interference and CRISPR/Cas9. Hum. Gene Ther. 27, 436–450. doi:10.1089/hum.2015.148
Takebe, T., Sekine, K., Enomura, M., Koike, H., Kimura, M., Ogaeri, T., et al. (2013). Vascularized and functional human liver from an iPSC-derived organ bud transplant. Nature 499, 481–484. doi:10.1038/nature12271
Takeuchi, J. K., Koshiba-Takeuchi, K., Matsumoto, K., Vogel-Höpker, A., Naitoh-Matsuo, M., Ogura, K., et al. (1999). Tbx5 and Tbx4 genes determine the wing/leg identity of limb buds. Nature 398, 810–814. doi:10.1038/19762
Tickle, C. (2015). How the embryo makes a limb: Determination, polarity and identity. J. Anat. 227, 418–430. doi:10.1111/joa.12361
Todd, T. (1823). On the process of reproduction of the members of the aquatic salamander. Quart. J. Sci. Arts Lib. 16, 84–86.
Tonegawa, A., Funayama, N., Ueno, N., and Takahashi, Y. (1997). Mesodermal subdivision along the mediolateral axis in chicken controlled by different concentrations of BMP-4. Development 124, 1975–1984. doi:10.1242/dev.124.10.1975
Tonegawa, A., and Takahashi, Y. (1998). Somitogenesis controlled by noggin. Dev. Biol. 202, 172–182. doi:10.1006/dbio.1998.8895
Towers, M., Mahood, R., Yin, Y., and Tickle, C. (2008). Integration of growth and specification in chick wing digit-patterning. Nature 452, 882–886. doi:10.1038/nature06718
Towers, M., Signolet, J., Sherman, A., Sang, H., and Tickle, C. (2011). Insights into bird wing evolution and digit specification from polarizing region fate maps. Nat. Commun. 2, 426. doi:10.1038/ncomms1437
Tozer, S., Bonnin, M.-A., Relaix, F., Savino, S. D., García-Villalba, P., Coumailleau, P., et al. (2007). Involvement of vessels and PDGFB in muscle splitting during chick limb development. Development 134, 2579–2591. doi:10.1242/dev.02867
Tsutsumi, R., Tran, M. P., and Cooper, K. L. (2017). Changing while staying the same: Preservation of structural continuity during limb evolution by developmental integration. Integr. Comp. Biol. 57, 1269–1280. doi:10.1093/icb/icx092
Walls, J. R., Coultas, L., Rossant, J., and Henkelman, R. M. (2008). Three-dimensional analysis of vascular development in the mouse embryo. PLOS ONE 3, e2853. doi:10.1371/journal.pone.0002853
Winnier, G., Blessing, M., Labosky, P. A., and Hogan, B. L. (1995). Bone morphogenetic protein-4 is required for mesoderm formation and patterning in the mouse. Genes Dev. 9, 2105–2116. doi:10.1101/gad.9.17.2105
Xu, B., Hrycaj, S. M., McIntyre, D. C., Baker, N. C., Takeuchi, J. K., Jeannotte, L., et al. (2013). Hox5 interacts with Plzf to restrict Shh expression in the developing forelimb. PNAS 110, 19438–19443. doi:10.1073/pnas.1315075110
Xu, B., and Wellik, D. M. (2011). Axial Hox9 activity establishes the posterior field in the developing forelimb. PNAS 108, 4888–4891. doi:10.1073/pnas.1018161108
Yamada, D., Nakamura, M., Takao, T., Takihira, S., Yoshida, A., Kawai, S., et al. (2021). Induction and expansion of human PRRX1+ limb-bud-like mesenchymal cells from pluripotent stem cells. Nat. Biomed. Eng. 5, 926–940. doi:10.1038/s41551-021-00778-x
Yang, G., Tsang, K. Y., Tang, H. C., Chan, D., and Cheah, K. S. E. (2014b). Hypertrophic chondrocytes can become osteoblasts and osteocytes in endochondral bone formation. PNAS 111, 12097–12102. doi:10.1073/pnas.1302703111
Yang, G., Zhu, L., Hou, N., Lan, Y., Wu, X.-M., Zhou, B., et al. (2014a). Osteogenic fate of hypertrophic chondrocytes. Cell Res. 24, 1266–1269. doi:10.1038/cr.2014.111
Yin, M., and Pacifici, M. (2001). Vascular regression is required for mesenchymal condensation and chondrogenesis in the developing limb. Dev. Dyn. 222, 522–533. doi:10.1002/dvdy.1212
Ying, Q.-L., Stavridis, M., Griffiths, D., Li, M., and Smith, A. (2003). Conversion of embryonic stem cells into neuroectodermal precursors in adherent monoculture. Nat. Biotechnol. 21, 183–186. doi:10.1038/nbt780
Yntema, C. L. (1959). Regeneration in sparsely innervated and aneurogenic forelimbs of Amblystoma larvae. J. Exp. Zoology 140, 101–123. doi:10.1002/jez.1401400106
Yoshikawa, Y., Fujimori, T., McMahon, A. P., and Takada, S. (1997). Evidence that absence ofWnt-3aSignaling promotes neuralization instead of paraxial mesoderm development in the mouse. Dev. Biol. 183, 234–242. doi:10.1006/dbio.1997.8502
Zelzer, E., McLean, W., Ng, Y.-S., Fukai, N., Reginato, A. M., Lovejoy, S., et al. (2002). Skeletal defects in VEGF120/120 mice reveal multiple roles for VEGF in skeletogenesis. Development 129, 1893–1904. doi:10.1242/dev.129.8.1893
Zhang, S., Wan, Z., and Kamm, D. R. (2021). Vascularized organoids on a chip: Strategies for engineering organoids with functional vasculature. Lab a Chip 21, 473–488. doi:10.1039/D0LC01186J
Zhao, X., and Duester, G. (2009). Effect of retinoic acid signaling on Wnt/beta-catenin and FGF signaling during body axis extension. Gene Expr. Patterns 9, 430–435. doi:10.1016/j.gep.2009.06.003
Zhao, X., Sirbu, I. O., Mic, F. A., Molotkova, N., Molotkov, A., Kumar, S., et al. (2009). Retinoic acid promotes limb induction through effects on body Axis extension but is unnecessary for limb patterning. Curr. Biol. 19, 1050–1057. doi:10.1016/j.cub.2009.04.059
Zhou, X., Mark, K., Henry, S., Norton, W., Adams, H., and Crombrugghe, B. (2014). Chondrocytes transdifferentiate into osteoblasts in endochondral bone during development, postnatal growth and fracture healing in mice. PLOS Genet. 10, e1004820. doi:10.1371/journal.pgen.1004820
Zhu, J., Nakamura, E., Nguyen, M.-T., Bao, X., Akiyama, H., and Mackem, S. (2008). Uncoupling sonic hedgehog control of pattern and expansion of the developing limb bud. Dev. Cell 14, 624–632. doi:10.1016/j.devcel.2008.01.008
Keywords: limb organoid, stem cell engineering, limb development, pluripotent stem cells, morphogenesis
Citation: Tsutsumi R and Eiraku M (2023) How might we build limbs in vitro informed by the modular aspects and tissue-dependency in limb development?. Front. Cell Dev. Biol. 11:1135784. doi: 10.3389/fcell.2023.1135784
Received: 01 January 2023; Accepted: 10 May 2023;
Published: 22 May 2023.
Edited by:
Yuji Atsuta, Kyushu University, JapanReviewed by:
Gufa Lin, Tongji University, ChinaJesus Chimal-Monroy, National Autonomous University of Mexico, Mexico
Copyright © 2023 Tsutsumi and Eiraku. This is an open-access article distributed under the terms of the Creative Commons Attribution License (CC BY). The use, distribution or reproduction in other forums is permitted, provided the original author(s) and the copyright owner(s) are credited and that the original publication in this journal is cited, in accordance with accepted academic practice. No use, distribution or reproduction is permitted which does not comply with these terms.
*Correspondence: Rio Tsutsumi, dHN1dHN1bWkucmlvLjh4QGt5b3RvLXUuYWMuanA=; Mototsugu Eiraku, ZWlyYWt1Lm1vdG90c3VndS4ydUBreW90by11LmFjLmpw