- 1Department Biochemistry, Faculty of Medicine and Health Sciences, McGill University, Montreal, QC, Canada
- 2Goodman Cancer Institute, Faculty of Medicine and Health Sciences, McGill University, Montreal, QC, Canada
- 3Department Experimental Medicine, Faculty of Medicine and Health Sciences, McGill University, Montreal, QC, Canada
Breast cancer remains a significant clinical concern affecting millions of women worldwide. Immunotherapy is a rapidly growing drug class that has revolutionized cancer treatment but remains marginally successful in breast cancer. The success of immunotherapy is dependent on the baseline immune responses as well as removing the brakes off pre-existing anti-tumor immunity. In this review, we summarize the different types of immune microenvironment observed in breast cancer as well as provide approaches to target these different immune subtypes. Such approaches have demonstrated pre-clinical success and are currently under clinical evaluation. The impact of combination of these approaches with already approved chemotherapies and immunotherapies may improve patient outcome and survival.
Introduction
Breast cancer remains one of the major causes of death of women, with an estimated 15 Canadian women succumbing to the disease everyday in 2022 (Canadian Cancer Society, 2022). Despite the great progress made in early detection and treatment of breast cancer, it remains the second largest cause of death due to cancer in women (DeSantis et al., 2019; Harbeck et al., 2019). Moreover, almost all cases of death due to breast cancer are caused by metastases to essential organs (Harbeck et al., 2019). Breast cancers are classified according to a combination of molecular and histological subtypes (Harbeck et al., 2019). Tumors are classified as hormone receptor positive (HR+), human epidermal growth factor 2 positive (HER2+) and triple negative breast cancers (TNBCs) (Harbeck et al., 2019). These molecular subtypes display diverse clinical manifestations, disease outcome, treatment options and various immune profiles (Harbeck et al., 2019; Onkar et al., 2023) (Figure 1). It has become clear that the intra- and inter-tumor heterogeneity of breast cancer drives formation of various tumor immune microenvironments (TIMEs), that have become critical in prediction of responses to immunotherapy (Hammerl et al., 2021).
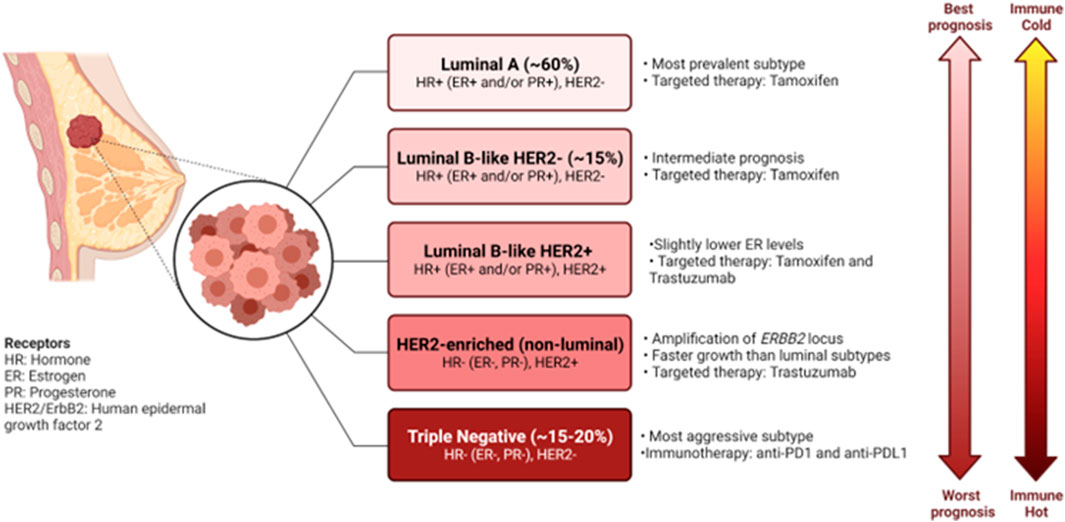
FIGURE 1. Characteristics of the molecular subtypes of breast cancer. Breast cancers can be classified based on gene expression profiles and receptor status. Each of the subtypes exhibit their own clinical manifestations, disease outcome, treatment options and various immune profile. Figure generated with biorender.com.
Immunotherapies have demonstrated strong potential in tumors such as melanoma (Huang and Zappasodi, 2022), however, only remain marginally successful in breast cancers (Schmid et al., 2020), arguing that a better understanding of the diverse immune landscapes of breast cancers and thus tailoring therapies to those tumors is required to improve patient outcome.
In this review, we aim to emphasize how the immune microenvironment may be used for clinical classifications of tumors and how the TIME regulates breast cancer growth, dissemination, and metastasis. These studies highlight immune mediated pathways that are either under clinical evaluation or may provide clinically relevant targets for future therapies (Table 1). We focused exclusively on discoveries made in the context of breast cancer as they represent a unique tumor model arising in mainly females and thus are responsive to estrogens.
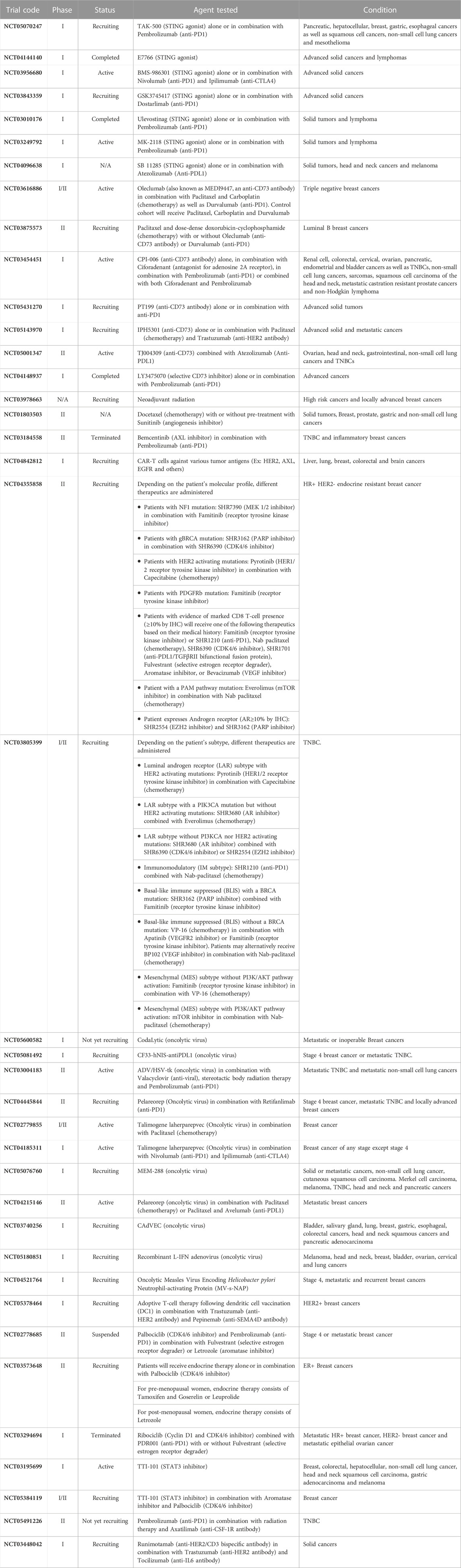
TABLE 1. Summary of the clinical trials mentioned in this paper. Ordered based on appearance in the text.
Immune landscapes of breast cancer
The addition of immunotherapies such as those targeting programed death 1 (PD1) receptor on activated T-cells, programmed death ligand 1 (PDL1) and cytotoxic T-lymphocyte associated 4 (CTLA4) protein has become a standard of care for treatment of melanoma (Huang and Zappasodi, 2022). This is attributed to the highly immunogenic nature of melanoma allowing a permissive environment for immune based interventions (Huang and Zappasodi, 2022). More recently, the anti-PD1 antibody, Pembrolizumab, in combination with chemotherapy has been approved for the treatment of mismatch repair deficient colorectal tumors (Andre et al., 2020) and triple negative breast cancers (TNBCs) (Schmid et al., 2020). With the rise of immunotherapy to treat several solid tumors (Esfahani et al., 2020), a shift towards an immune-based rather than exclusively a histological classification of tumors may allow better segregation of responders and non-responders to the appropriate therapy. In TNBCs, RNA sequencing (seq.) suggested the existence of at least four subtypes within TNBCs including an immunomodulatory subtype, characterized by high T-cell infiltration and upregulation of immune checkpoint inhibitors (Jiang et al., 2019). These findings support that only a subset of TNBCs would respond to immune checkpoint blockade (ICB). Thus, it could be appreciated that classification of breast tumors using the traditional molecular and histological subtypes would not be sufficient to predict responders and non-responders within each breast cancer subtype.
An emerging concept has classified solid tumors into several immune categories. Immune hot (also referred to as immune inflamed), which are classified as being heavily infiltrated by T-cells and express markers of immune cell activation and exhaustion, are robust responders to immunotherapies (Galon and Bruni, 2019; Gruosso et al., 2019; Hammerl et al., 2021). On the other end of the spectrum are immune cold (also referred to as immune desert or ignored) tumors, which exhibit little to no T-cell infiltration and are unresponsive to immunotherapies (Galon and Bruni, 2019; Gruosso et al., 2019; Hammerl et al., 2021). Intermediate immune phenotypes (also referred to as immune altered) where immune cells are restricted to the tumor margin (marginally restricted) or restricted to the stroma (stromal restricted) add to the complexity of the immune microenvironment and are associated with minimal response to immunotherapy (Galon and Bruni, 2019; Gruosso et al., 2019; Hammerl et al., 2021). Moreover, intratumor heterogeneity may result in only a partial response to immunotherapy as well as allow for clonal selection for a resistant population. This is illustrated in the case of hypoxic tumors, with areas being of different immune status depending on their hypoxic status (Pietrobon and Marincola, 2021).
Recent studies using transcriptomic profiling of all subtypes of breast cancer divided tumors into three immune categroies based on immune signature scores: immune high, medium and low (Yao et al., 2021). Immune high tumors were defined as having the highest expression of PDL1 and CD8 to CD4 T-cell ratio (Yao et al., 2021). Interestingly, a higher proportion of TNBCs and HER2+ tumors were classified as immune high tumors whereas estrogen receptor (ER) and progesterone receptor (PR) positive tumors (the HR+ tumors) were mainly immune low (Yao et al., 2021). Analysis from The Cancer Genome Atlas (TCGA) supported this notion by demonstrating TNBCs and HER2+ breast cancers exhibit increased immune metagene expression compared to those of the ER+ subtype (Safonov et al., 2017). This work (Safonov et al., 2017; Yao et al., 2021) argues that ER/PR+ tumors are of lower immunogenicity and thus require tailoring therapeutic regimens to increase their response to immunotherapy.
An increase in number of T-cells infiltrating the tumor is associated with better patient outcome when treated with chemotherapy only (Denkert et al., 2018), chemotherapy with immunotherapies (Li et al., 2021a) as well as in combination with anti-HER2 targeted therapies, such as Trastuzumab (Loi et al., 2014). Therefore, it has become critical to understand the mechanism governing the different TIMEs, which may elucidate better drug targets which ultimately may improve patients clinical progression.
In addition to absolute numbers of T-cells in the tumor being predictive of therapeutic response, studies have demonstrated that the spatial localization of immune cells might be critical in tumor classification and predicted response to therapy (Gruosso et al., 2019; Hammerl et al., 2021) Work by Gruosso et al. (Gruosso et al., 2019) on TNBCs clustered tumors into four subtypes based on the spatial localization of CD8+ (cytotoxic) T-cells (Gruosso et al., 2019) (Figure 2). Immune cold tumors were divided into two subcategories; immune dessert (ignored), which have no CD8+ T-cells cells in the tumor core or margins, and marginally restricted (or excluded), with CD8+ T-cells cells exclusively in the margins. Immune hot tumors were divided into stromal restricted (or excluded) with CD8+ T-cell only in the tumor associated stroma and fully inflamed which have CD8+ T-cells both in the stroma and the tumor nest. Profiling of TNBC tumors exhibiting inflamed, ignored, and excluded (in the margins or stroma) T-cell infiltration phenotypes demonstrated profound transcriptional changes and led to generation of gene signatures that are predictive of T-cell infiltration patterns (Hammerl et al., 2021). Moreover, it was demonstrated that immune inflamed tumors (those with a high immune inflamed gene signature) responded significantly better to anti-PD1 therapy assessed by better overall survival compared those of the immune ignored and excluded categories (Hammerl et al., 2021). These results suggest that transcriptomic profiling of tumors may allow for prediction of the immune landscape and thus response to immunotherapy, allowing therapies to be tailored accordingly. In metastatic HER2+ breast cancer, CD8+ T-cells reaching the tumor core, but not the invasive margin or the stroma, was associated with longer survival with metastasis (Honkanen et al., 2017). Overall, these findings argue that understanding the molecular programs that regulate T-cell spatial localization are critical for improving T-cell activity as well as improved response to immune based therapies.
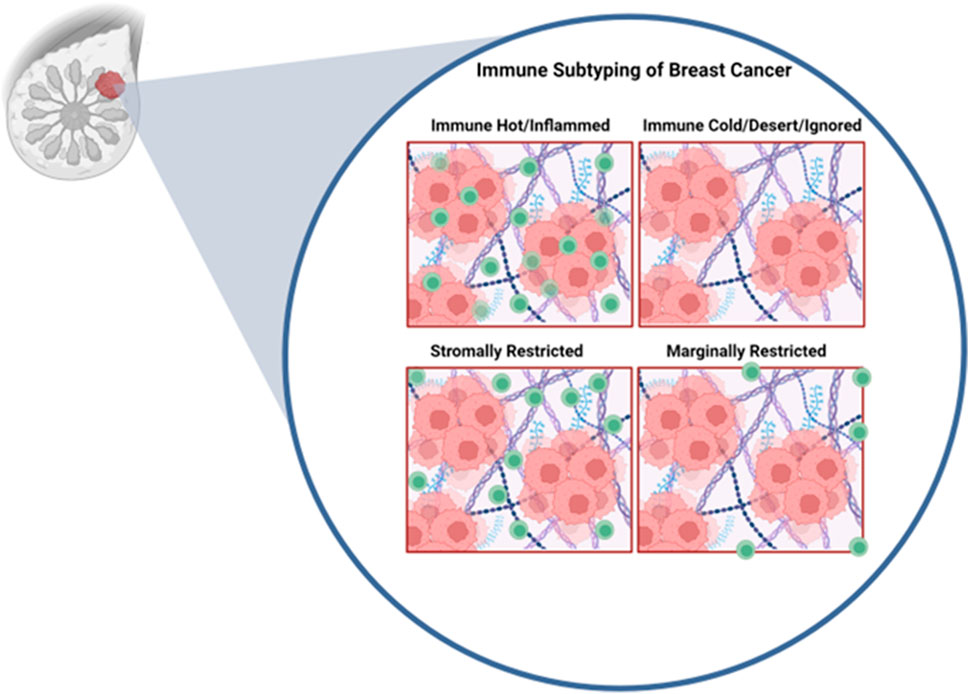
FIGURE 2. Immune profiles of breast cancer. Inspired by Grusso et al. In the immune hot subtype, cytotoxic T-cells are able to fully infiltrate the tumor. Immune cold tumors are fully devoid of cytotoxic T-cells. Stromally restricted tumors are infiltrated by T-cells only in the stroma but not tumor nest. Marginally rrestricted tumors have T-cells limited to the tumor margin and not capable to reaching the tumor nest. Figure generated with biorender.com.
There is a developing consensus that both the number and the spatial localization of T-cells (and potentially other immune cell types) are an important predictor of survival as well as response to approved immunotherapies in breast cancer, particularly in TNBCs (Hammerl et al., 2021). While we described at least three different spatial immune profiles; immune hot, immune cold and excluded (either margin or stroma), most of the studies described in this manuscript rely on the simplistic immune cold (low immune infiltrate in general) and immune hot (inactive/exhausted T-cells). Therefore, for the rest of this manuscript we will follow the nomenclature of the cited work. Additionally, most of this work has been focused on TNBCs. Further research is required to better categorize the TIME in HER2+ and ER+/PR+ tumors and thus improve the prospect of immune based therapies for these breast cancer subtypes, that account for the majority of cases (Harbeck et al., 2019).
Approaches to treat immune cold tumors
Given that increasing numbers of tumor infiltrating CD8+ T-cells cells are associated with better prognosis and response to immunotherapy, work aimed at promoting T-cell infiltration into the tumor (core) could help improve patient outcomes as well as sensitize them to already approved immunotherapies.
Induction of IFN expression through sustaining STING activation
Immune cold tumors arise due to inadequate induction of an innate immune response during early tumor progression (Bonaventura et al., 2019). This is usually due to reduced expression of interferons (IFNs) and interferon stimulated genes (ISGs) (Bonaventura et al., 2019; Galon and Bruni, 2019; Liu and Sun, 2021). Reduced expression of interferons leads to loss of major histocompatibility complex (MHC) class 1 expression which impedes recognition by T-cells (Dhatchinamoorthy et al., 2021). Loss of MHC class 1 is observed in about 60% of TNBCs which may account for the lack of response to immunotherapies in patients (Dusenbery et al., 2021). One of the key regulators of the interferon response is stimulator of interferon genes (STING) (Zhu et al., 2019; Decout et al., 2021). STING is a cytosolic sensor of the cyclic dinucleotide 2′3′-cyclic guanosine monophosphate–adenosine monophosphate (2′3′-cGAMP), which is produced by cyclic GMP-AMP synthase (cGAS) due to cytosolic DNA as a result of cell death or viral infection (Decout et al., 2021). STING activates tank-binding kinase 1 (TBK1), leading to activation of interferon response factor (IRF) three which acts as a transcription factor for ISGs (Decout et al., 2021). Induction of interferons act as a “red flag” for the immune system and results recruitment of anti-tumor immune cells. Blocking this cascade at any stage could result in an immune cold, interferon depleted tumor (Zhu et al., 2019). It is important to note, that 2′3′-cGAMP functions as an immune transmitter between different cancer cells as well as cancer cells and immune cells (such as dendritic cells) whereby one cell can activate STING in a neighbouring cell (Carozza et al., 2020) leading to a positive feedback cascade and increased inflammation. However, excessive inflammation, a hallmark of cancer (Hanahan and Weinberg, 2011), leads to disruption of tissue homeostasis and recruitment of inhibitory immune cells such as regulatory T-cells (Tregs), myeloid derived suppressor cells (MDSCs), macrophages and upregulation of immune checkpoint molecules which sustains an immunosuppressed immune hot TIME.
As a mechanism of stimulating STING in immune cold tumors, either 2′3′-cGAMP or agonists (small molecules that activate STING) have been implemented to stimulate interferon expression and promote an anti-tumor immune response (Deng et al., 2014). Using the 4T1 TNBC model, the STING agonist, cyclic diguanylate, lead to induction of IFNβ expression which was coupled to an increase in activation of STING and its downstream effectors (Yin et al., 2022). Cyclic diguanylate synergized with atezolizumab (anti-PDL1) to reduce tumor growth through activation of cytotoxic T-cells and suppression of Tregs (Yin et al., 2022). Other work on a HER2+ model using the STING activator, ADU-S100, demonstrated that STING activation can induce tumor regression as well as protect against tumor rechallenge in non-tolerized animals (Foote et al., 2017). The effect of ADU-S100 was dependent on both CD8+ and CD4+ T-cells (Foote et al., 2017). Moreover, blockade of immune checkpoints, OX40 receptor and anti-PDL1 lead to a significant increase in ADU-S100 efficacy and complete regression of tumors (Foote et al., 2017).
Several STING agonists are currently in early phases of clinical trials as an adjuvant or in combination with other immunotherapies (NCT05070247, NCT04144140, NCT03956680, NCT03843359, NCT03010176, NCT03249792, NCT04096638). Additionally, already approved poly ADP ribose polymerase (PARP) inhibitor, Olaparib, in a TNBC mouse model demonstrated its capacity to induce CD8+ T-cell infiltration via activation of STING (Pantelidou et al., 2019) as well as promote upregulation of PDL1 in several human derived cell lines (Jiao et al., 2017) suggesting that inducing DNA damage may promote a strong anti-tumor immune response through STING activation. Noteworthy, that increasing the tumor mutational load is associated with favourable immune infiltration and response to immunotherapies (Strickler et al., 2021), potentially due to elevated basal activation of STING. However, only a very small fraction of breast cancers have a high mutational load compared to immunogenic tumors such as lung and melanoma (Thomas et al., 2018; Barroso-Sousa et al., 2020). Additionally. HR + tumors harbour a smaller proportion of tumors with a high mutational burden (Thomas et al., 2018). Mechanisms that increase the mutational load might be clinically beneficial, especially for HR + breast cancer. Indeed, inactivation of the DNA mismatch repair (MMR) by targeting MutL homologue 1 (MLH1) leads to an increased mutational load due to reduced genomic stability, triggering an immune response that sensitizes tumors to anti-PD1 (Germano et al., 2017).
In a cDNA library screen, HER2, which is highly expressed in ∼25% of breast cancers (Harbeck et al., 2019), was found to impede STING activation (Wu et al., 2019). Detailed analysis of this phenomenon demonstrated that the intracellular domain (ICD) of HER2 physically sequesters STING, preventing interaction with TBK1/IRF3 (Wu et al., 2019). Additionally, AKT1 activation downstream HER2, phosphorylates TBK1 at serine 510 which inhibits its downstream activity and association with STING (Wu et al., 2019). This data strongly argues that oncogene mediated signaling can impact the innate immune response and result in an immune cold TIME, which is amenable to receptor tyrosine kinase inhibitors (RTKi) such as lapatinib (Wu et al., 2019). Interestingly, activation of DNA sensing increased the HER2-AKT1 cascade (Wu et al., 2019). We envision that this may behave as a mechanism of resistance in tumors treated with STING activators.
Novel approaches at regulating STING have led to development of small molecules targeting ecto-nucleotide pyrophosphatase/phosphodiesterase 1 (ENPP1) which is an endoplasmic reticulum and cell surface enzyme that degrades natural STING ligand 2′3′-cGAMP as well as ATP/GTP to AMP and GMP (Li et al., 2014; Carozza et al., 2022; Gangar et al., 2022; Goswami et al., 2022). Inhibition of ENPP1 using a small molecule inhibitor was sufficient to induce an active immune response which synergized with radiation therapy (Carozza et al., 2020) potentially due to reduced degradation of 2′3′-cGAMP produced by irradiated cells. Moreover, other studies demonstrated that chromosomally unstable breast tumors suppress an immune response against them via selective upregulation of ENPP1 which degrades 2′3′-cGAMP that may be produced in response to dsDNA released into the cytosol following nuclear envelope ruptures (Li et al., 2021b). Indeed, knockout of ENPP1 in TNBC syngeneic models reduced metastasis to the lung and led to a massive increase in immune cell infiltration at the primary tumor (Li et al., 2021b). Moreover, knockout of ENPP1 synergized with PD1 blockade (Li et al., 2021b). Both patient tumors and mouse models demonstrated that ENPP1 is elevated in relapsed tumors of the TNBC and HER2+ subtypes (Ruiz-Fernandez de Cordoba et al., 2022). ENPP1 promoted neutrophil extracellular trap (NET) formation via tumor cell expression of haptoglobin, supporting relapse and protecting the tumor from radiotherapy (Ruiz-Fernandez de Cordoba et al., 2022). Additionally, both expression of interferons and STING activation are associated with differentiation of the tumor, reduced stem cell capacity and reduced expression of epithelial-mesenchymal transition (EMT) gene signature (Cheng et al., 2020; Cheon et al., 2022; Goswami et al., 2022). Targeting ENPP1 reduced expression of EMT markers and signatures (Goswami et al., 2022). Only one documented case of first-in-human ENPP1 inhibitor in addition to anti-PD1 was performed (Csiki et al., 2022), but no large scale trials have been preformed thus far. We posit that inhibition of ENPP1 may provide strong clinical benefit especially to immune cold TNBCs and HER2+ breast cancers. Combination of 2′3′-cGAMP (Ohkuri et al., 2017) or radiation (Carozza et al., 2020) coupled to ENPP1 inhibition may demonstrate a synergistic effect due to the prolonged accumulation of 2′3′-cGAMP in the extracellular milieu.
AMP produced by ENPP1 is subjected to further hydrolysis by CD73 (Allard et al., 2020). The resultant adenosine may then bind adenosine receptors found on stromal or cancer cells to reinforce an immunosuppressive microenvironment (Allard et al., 2020). CD73 expression was found to be highest in TNBC compared to other breast cancer subtypes and was significantly associated with worse prognosis (Loi et al., 2013). Activity of CD73 in TNBC induced immunosuppression and promoted resistance to anthracyclines (Loi et al., 2013). Targeting CD73 using a monoclonal antibody increased the number of tumor specific CD8+ T-cells and induced INFγ expression (Loi et al., 2013). Combination of anthracycline (doxorubicin) with anti-CD73 delayed death due to metastasis of animals post surgical resection of their primary tumor (Loi et al., 2013). Moreover, anti-CD73 synergized with both anti-PD1 and anti-CTLA4 (Loi et al., 2013). Expression of CD73 has also been correlated with resistance to anti-HER2 targeted therapy, Trastuzumab (Turcotte et al., 2017). Neutralization of CD73 synergized with anti-HER2 monoclonal therapy, eliminated HER2+ tumor growth in syngeneic models as well as reduced pulmonary metastasis (Turcotte et al., 2017). Anti-CD73 targeting antibodies as well as CD73 inhibitors are currently in early phase clinical trials in combination with immune checkpoint blockades and Trastuzumab (NCT03616886, NCT03875573, NCT03454451, NCT05431270, NCT05143970, NCT05001347, NCT04148937).
Use of chemotherapy and radiation
Chemotherapy in combination with anti-PD1 is approved for PDL1+ TNBC (Cortes et al., 2022). While the idea of eventually phasing out chemotherapy and radiation is highly tempting due to their systemic adverse effects; they still remain viable options to prime an immune response and potentially induce upregulation PDL1 and other co-inhibitory molecules (Zitvogel et al., 2008).
DNA damage following radiation induces STING activation which promotes recruitment of dendritic cells which act as antigen presenting cells priming T-cell mediated immunity (Dhatchinamoorthy et al., 2021) (impact is under clinical assessment NCT03978663). Both chemotherapy and radiation can induce cell and DNA damage, generating neoantigens and inducing antigenicity which may sensitize tumors to immunotherapies (Zitvogel et al., 2008; McGranahan et al., 2016; Galon and Bruni, 2019). Additionally, chemotherapy has been demonstrated to selectively deplete MDSCs, Tregs and restores T- and natural (NK) cell functions (Ghiringhelli et al., 2007; Vincent et al., 2010). DNA damage following radiation induces STING activation which promotes recruitment of dendritic cells that act as antigen presenting cells to prime T-cell mediated immunity (Deng et al., 2014). Clinical studies (NCT03978663) are currently underway to correlate radiation treatment with immune cell recruitment and patient outcome.
Resistance to radiation and chemotherapy posses an additional challenge, as tumors need to respond to radiation or chemotherapy to effectively prime an immune response. Resistance to radiation has been linked to hypoxia (briefly discussed in the next section) where lack of molecular oxygen prevents generation of free radicals to react with DNA (Wang et al., 2021). Strategies aimed at promoting tumor oxygenation by vasculature normalization have demonstrated the capacity to sensitize tumors to radiation and improve chemotherapy delivery in multiple tumor models (Telarovic et al., 2021). Tyrosine kinase inhibitor Sunitinib is under clinical investigation in combination with chemotherapy to induce vasculature normalization to enhance chemotherapy delivery (NCT01803503). Blood vessel normalization and perfusion is driven by IFNγ expressing cytotoxic T-cells and is a predictor of response to ICB, anti-PD1 and anti-CTLA4, and the anti-HER2 monoclonal, Trastuzumab (Baker et al., 2018; Zheng et al., 2018). Therefore, strategies at improving vasculature perfusion may permit increased cytotoxic T-cell infiltration, which establishes a positive feedback loop, increasing vasculature perfusion further allowing better delivery of chemotherapy and anti-tumor immune cells. Thus, improved vasculature perfusion may also allow better tumor oxygenation and improved immune responses.
Targeting hypoxia to promote immune cell trafficking
Hypoxia is regarded as a critical phenomenon in breast cancer (Zhang et al., 2021). Indeed, an abundance of work has demonstrated that hypoxia and upregulation of hypoxia inducible factor 1α (HIF1α) in the epithelium regulates breast cancer progression and metastasis via promoting angiogenesis, induction of cancer stem phenotypes and EMT (Muz et al., 2015). Additionally, hypoxia promotes immune suppression either by inhibiting T-cell activity or through exclusion of immune cells by a barrier comprised of low pH, low glucose, and low oxygen conditions (Wang et al., 2021; Zhang et al., 2021). Interestingly, CD73 is under the control of hypoxia and potentially contributes to hypoxia mediated immunosuppression (Allard et al., 2020). Spatial analysis of 4T1 tumors demonstrated that hypoxic areas (HIF1α+) are poorly infiltrated with T-cells as expected (Ma et al., 2022). This work identified that through HIF1α, hypoxia induced silencing of INFγ and tumor necrosis factor (TNF) through histone deacetylase (HDAC) and enhancer of zeste homolog 2 (EZH2) mediated epigenetic silencing in T-cells (Ma et al., 2022). Inhibition or genetic ablation of HIF1α, HDAC or EZH2 sensitized anti-PD1 resistant 4T1 tumors to anti-PD1 (Ma et al., 2022). Knockout of HIF1α in the macrophages of polyoma middle T (PyMT) tumors increased proliferation and cytotoxic activity of T-cells, emphasizing that hypoxia may promote immunosuppression through macrophage function as well (Doedens et al., 2010).
While low oxygen stabilizes HIFs and regulates a hypoxia induced response, receptor tyrosine kinases (RTKs) such as the epidermal growth factor (EGFR) family, which are commonly highly expressed in breast cancers can stabilize HIFs (Lauzier et al., 2007; Lee et al., 2009; Nilsson et al., 2010; Turpin et al., 2016). Interestingly, it was demonstrated that through HIF2α, hypoxia can promote translation of EGFR mRNA (Franovic et al., 2007; Uniacke et al., 2012) implying the presence of a positive feedback loop between HIFs and EGFR. Knockout of AXL RTK in a HER2+ driven mouse model reduced expression of hypoxic markers, reactivated the immune response and improved vasculature perfusion in the primary tumors leading to strong reduction in lung metastasis (Goyette et al., 2021). Inhibition of AXL using R428 synergized with PD1 blockade at blocking metastasis (Goyette et al., 2021). Clinical trials assessing the AXL inhibitor, Bemcentinib, with Pembrolizumab (anti-PD1) on TNBC was attempted on a small cohort of patients (NCT03184558) but none achieved complete response leading to termination of the trial. Newer trials are currently underway to assess impact of chimeric antigen receptor (CAR) T-cells against AXL as well as other RTKs including HER2 and EGFR on advanced tumors (NCT04842812).
Targeting EZH2 to induce inflammatory cytokine expression
EZH2 acts as the catalytic subunit of polycomb repressive complex 2 (PRC2) and places a repressive methyl mark on lysine 27 of histone 3, H3K27me3 (van Mierlo et al., 2019). Progression of PyMT-driven tumors is correlated with an increase in H3K27me3 repressive marks (Cai et al., 2018). EZH2 knockout in PyMT and HER2-driven models demonstrated profound impact on tumor initiation, progression, and metastasis, supporting that EZH2 behaves as on oncogene by suppressing expression of tumor suppressors (Hirukawa et al., 2018; Smith et al., 2019). We have previously demonstrated that inhibition of EZH2 in a HER2+ mouse model induces tumor cell expression of endogenous retroviral elements that promote expression of IFNs and sensitize tumors to anti-HER2 targeted therapy (Hirukawa et al., 2019). Work using ovarian cancer models demonstrated that inhibition of EZH2 induced expression of inflammatory cytokines C-X-C ligand (CXCL) 9 and CXCL10 that promoted recruitment of cytotoxic T-cells into the tumor (Peng et al., 2015). Studies from other tumor models have demonstrated that EZH2 inhibits expression of PDL1 (Xiao et al., 2019) and the MHC class 1 antigen processing pathway (Burr et al., 2019). These studies support that epigenetic inhibition may impact induction of a robust immune response both through the tumor cell proper as well as through direct action on immune cells. The EZH2 inhibitor, SHR2554 is currently in clinical trials in combination with PARP, androgen receptor and cyclin dependent kinases four and 6 (CDK4/6) inhibitors (NCT04355858 and NCT03805399).
Oncolytic viruses to stimulate the immune response
Oncolytic viruses (OVs) are naturally occurring or genetically modified viruses that are capable of selectively infecting and destroying tumor cells (Jin et al., 2021). OVs induce tumor cell death, resulting in release of pathogen-associated molecular patterns (PAMPs) and danger-associated molecular patterns (DAMPs) that can stimulate the anti-tumor immune response (Jin et al., 2021). While OVs are not currently approved, several are currently under pre-clinical and clinical evaluation (Carter et al., 2021; Jin et al., 2021). A herpes simplex virus (HSV)-based OV designated G47Δ, reduced pulmonary metastatic growth (Wang et al., 2012). Addition of IL-12 coding sequence to G47Δ (designated G47Δ-mIL12) killed both murine and human breast cancer cells in vitro and in syngeneic models. It also led to increased infiltration of cytotoxic T-cells and decreased MDSCs infiltration into the tumors (Ghouse et al., 2020). Several OVs are currently in clinical assessment in combination with other immunotherapies such as anti-PDL1 and CAR-T cells (NCT05600582, NCT05081492, NCT03004183, NCT04445844, NCT02779855, NCT04185311, NCT05076760, NCT04215146, NCT03740256, NCT05180851, NCT04521764).
Introduction of genetically engineered anti-tumor immune cells
Given that T-cells are known for their ability to recognize and kill cancer cells, efforts at expanding them ex vivo and reintroducing them back into the patient in a process known as adoptive cell transfer (ACT), has been successful in highly immunogenic tumors (Luo et al., 2022). Recently, ACT of T-cells targeting mutant proteins (SLC3A2, KIAA0368, CADPS2 and CTSB) detected in a patient tumor in combination with interleukin two have been demonstrated to induce tumor regression (Zacharakis et al., 2018). Clinical investigations aimed at expanding anti-HER2 specific T-cells for reintroduction into patients in combination with Trastuzumab (NCT05378464) are currently actively recruiting. Moreover, genetically engineered CAR-T cells have been developed targeting commonly expressed breast cancer antigens such as HER2 (Szoor et al., 2020), EGFR (Liu et al., 2019), EpCAM (Osta et al., 2004), AXL (Zhao et al., 2020a) and c-MET (Tchou et al., 2017). Unfortunately, CAR-T cells are met with the same challenges as regular T-cells where the tumor microenvironment may not be permissive for their survival or activity, presence of inhibitory immune cells as well as lack of homing cues (Li et al., 2020). Studies have addressed this by stimulating STING using 2′3′-cGAMP or DMXAA which improved trafficking and CAR-T cells targeting HER2 in the TIME of breast cancer (Xu et al., 2021). Tumor heterogeneity, whereby some tumor cells may lack the antigen targeted by the CAR or loss of MHC class 1 which is required for recognition of the tumor cell may hinder the impact of CAR-T cells (Li et al., 2020; Luo et al., 2022).
Development of CAR-natural killer (CAR-NK) cells targeting breast cancer tumor antigens (Liu et al., 2020; Portillo et al., 2021) harbours several advantages over CAR-T cells, importantly not requiring MHC matching (Khawar and Sun, 2021). Therefore, CAR-NK need not be from the same patient, have a low risk of graft vs. host disease and do not require MHC to be expressed on the tumor cell (Khawar and Sun, 2021). Additionally, CAR-NKs demonstrate enhanced tumor killing potential compared to CAR-T cells coupled with reduced risk of cytokine release syndrome, which is acute systemic inflammation that could be life threatening (Shimabukuro-Vornhagen et al., 2018; Khawar and Sun, 2021; Portillo et al., 2021).
CAR-macrophages (CAR-Ms) have similar advantages to CAR-NKs with the addition of macrophages having better capacity at infiltrating the tumor (Klichinsky et al., 2020). CAR-M targeting HER2, decreased tumor burden and increased overall survival of tumor bearing mice (Klichinsky et al., 2020). Moreover, they induced expression of inflammatory cytokines capable of converting tumor resident M2 macrophages to the M1 phenotype and boosted anti-tumor T-cell activity (Klichinsky et al., 2020). To our knowledge, no clinical trials are currently underway assessing CAR-NKs or CAR-Ms in breast cancer.
Overall, while immune cold tumors represent a difficult to treat tumor and are associated with poor patient outcome, there is encouraging data suggesting that reactivation of the tumor immunity cycle via STING agonists or targeting ENPP1 or CD73 is sufficient to increase immune cell infiltration into the tumor especially in combination with either chemotherapy or radiotherapy. Targeting hypoxia both directly (through HIF inhibition) and indirectly (through RTKs) may relieve T-cell exclusion therefore, sensitizing patients to traditional therapies and immunotherapies. Epigenetic rewiring of the tumor as well as the immune cells themselves may increase their activation and infiltration into the tumor. Oncolytic virus-based therapies may induce hotness in the tumor due to DAMP and PAMP generation. Finally, to increase the number of anti-tumor immune cells at the tumor generation of genetically engineered T-cells, NKs and macrophages may help reshape the TIME (Figure 3).
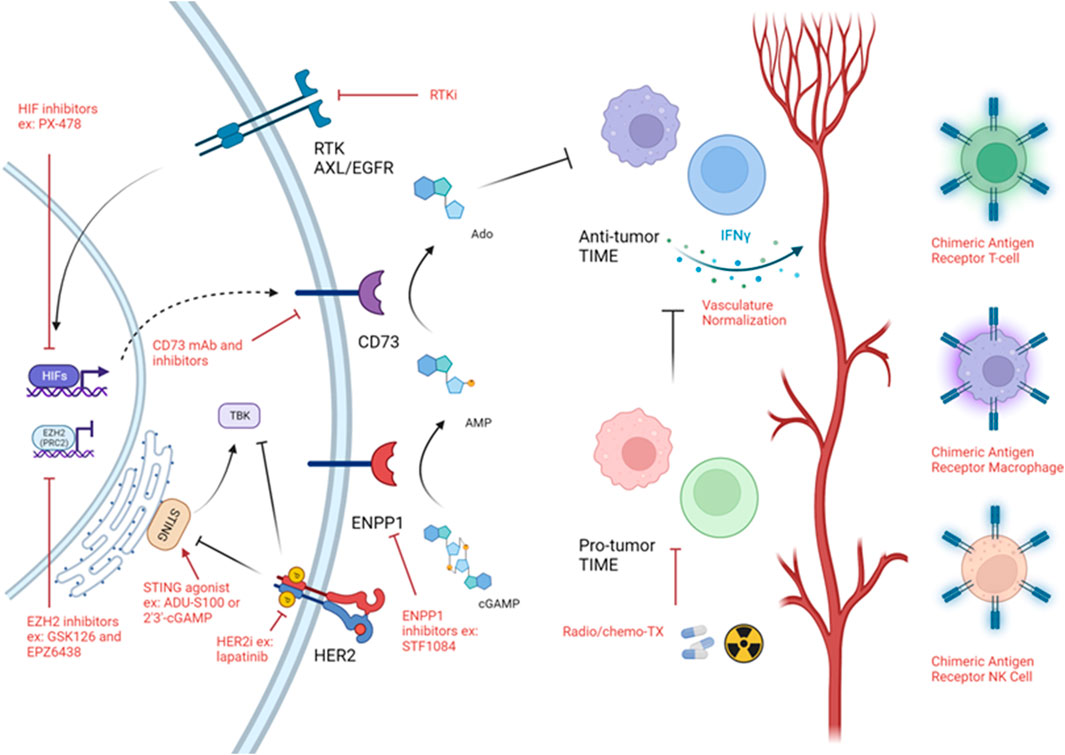
FIGURE 3. Strategies targeting immune cold TIME. Schematic of strategies used to target immune cold timors. Black arrows demonstrates a biologically activating pathway. Black flat head demonstrates a biologically inhibitory pathway. Red arrow implies activating intervention. Red flat head implies inhibitory intervention. Figure generated with biorender.com.
Targeting hormone receptor positive breast cancer—An immune cold subtype
As evident in this review [as well as in others (Goldberg et al., 2021)], significant effort has been focused on understanding the TIME of TNBCs and to a lower extent HER2+ breast cancer given that they represent the most aggressive subtypes. However, 70% of breast cancer diagnoses are ER/PR+ (collectively referred to as hormone receptor positive, HR+), thus understanding the TIME of these tumors is of clinical importance (Goldberg et al., 2021). While endocrine therapy is usually restricted to HR+ breast cancer, the ER is expressed in other cell types, including immune cells (Chakraborty et al., 2023). Moreover, in premenopausal women, significant levels of estrogen may reach the mammary gland and reshape the TIME (Svoronos et al., 2017).
Efforts at understanding the TIME in HR+ breast cancers have been made through use of human breast cancer biopsies and clinical trials. It is now widely accepted that HR+ (particularly HR+/HER2-) status represents immune cold tumors that exhibit reduced T-cell infiltrate (Goldberg et al., 2021). Aforementioned, HR+ breast tumors harbour a lower tumor mutational burden (Thomas et al., 2018; Barroso-Sousa et al., 2020) coupled to significantly lower expression levels of MHC class I and PDL1 compared to other breast cancer subtypes (Zhang et al., 2017; Onkar et al., 2023). Consistent with this notion, activity of the ER is negatively correlated with IFN expression while PR signalling dampens the (signalling transducer and activator of transcription 1 (STAT1)-IFN signaling axis (Lee et al., 2016; Goodman et al., 2019). Additionally, increasing number of stromal T-cells in HR+ breast cancer is a negative prognostic factor (Denkert et al., 2018) and having lower T-cell counts after chemotherapy is associated with improved relapse free survival in HR+ disease (Watanabe et al., 2018). Whether the lower stromal T-cell count is due to increased infiltration into the tumor nest remains to be fully addressed and would explain these discrepancies. Overall, it has become abundantly clear that HR+ tumors are fortified with multiple mechanisms that allow them to support an immune cold TIME.
Estrogen deprivation through inhibition of aromatase by letrozole reduced levels of FOXP3+ Tregs (Generali et al., 2009) with a prominent increase in CD8+/Tregs ratio especially in responders (Chan et al., 2012). Additionally, progesterone supplementation was found to decrease natural killer cell and natural killer T-cell population in a mouse model of PR+ disease (Werner et al., 2021), suggesting that both female hormones may act in concert to generate an immunosuppressive TIME. Tamoxifen is one of the most prescribed breast cancer medications in the adjuvant setting. Studies have demonstrated that tamoxifen treatment induced proliferation of active T-cells (Komi and Lassila, 1998) and increased natural killer cell activity (Richards et al., 2016). Ablation of estrogen activity reduced recruitment of myeloid derived suppressor cells and boosted T-cell activity through its action on the JAK/SRC/STAT3 pathway in the immune cells (Svoronos et al., 2017) indicating that anti-estrogens may have implications in non-ER+ cancers as well. Indeed, estrogen has been demonstrated to induce pro-tumor M2 macrophage polarization (Toniolo et al., 2015) as well as inhibit production of IFNγ and increased expression of indoleamine 2,3-dioxygenase (IDO) which is a T-cell inhibitor, by mature dendritic cells (Liu et al., 2002; Xiao et al., 2004). Attempts have further been made to bolster the anti-tumor immune response generated by tamoxifen treatment through combination with IFNβ and IL2, which prolonged survival of patients harbouring distant metastasis (Nicolini and Carpi, 2005; Nicolini et al., 2005). However, in autoimmune encephalomyelitis, tamoxifen induced upregulation of Th2 cytokine expression (Bebo et al., 2009), as well as in vitro neutrophil NET formation (Corriden et al., 2015). Prolonged treatment of tumors with ER antagonist, fulvestrant, induced upregulation of PDL1 that contributed to immune exhaustion (Huhn et al., 2022). In summary, while tamoxifen and biosimilar agents modulate the TIME positively by increasing immune infiltrate, new accumulating evidence suggests that prolonged treatment with such molecules may establish an immunosuppressive microenvironment. While this immunosuppressive microenvironment may allow for immune escape, it is highly amenable to immunotherapy, further supporting the idea of combination approach.
Activating mutations in the gene encoding the ER (ESR1) have been identified in the context of metastatic breast cancer previously treated with tamoxifen and aromatase inhibitors (Turner et al., 2020; Zundelevich et al., 2020). Mutations in the ER are associated with reduced progression free survival and overall survival (Turner et al., 2020; Zundelevich et al., 2020) as well as resistance to the HR+ breast cancer standard of care (Herzog and Fuqua, 2021; Liang et al., 2022). Interestingly, it was recently demonstrated that tumors harbouring mutations in the ER had elevated levels of inflammatory cytokines S100A8 and S100A9, Tregs and PDL1 positive macrophages in addition to expression of the immunosuppressive cytokine, chitinase-3-like 1 (CHI3L1) (Zhao et al., 2020b; Williams et al., 2021; Li et al., 2022). Neutralizing antibodies against CHI3L1 have demonstrated strong ability to revert immunosuppression and synergized with ICB in other cancer models (Ma et al., 2021) suggesting that targeting CHI3L1in ER mutant breast cancer may be a viable option to alleviate immunosuppression that resulted due to excessive inflammation. Overall, this data contends that while ER mutations promote therapeutic resistance to the traditional standard of care, the switch to an immune hot microenvironment may be associated with immune vulnerabilities. Several clinical trials assessing next-generation, selective estrogen receptor modulators or degraders are currently in progress to identify ones that have a positive clinical impact on patients harbouring mutant ER tumors/metastases [summarized in (Herzog and Fuqua, 2021)].
CDK4/6 inhibition in combination with aromatase inhibitors or ER modulators (such as letrozole and fulvestrant) is currently emerging as the standard of care for HR+ breast cancers due to improved patient survival in clinical trials (Im et al., 2019; Slamon et al., 2020). Emerging clinical and preclinical evidence has suggested that CDK4/6 inhibitors relieve immune suppression (Goel S et al., 2017; Deng et al., 2018; Scirocchi et al., 2022) through increased antigen presentation, expression of endogenous retroviral elements and induction of a Type III IFN response (Goel S et al., 2017). This has warranted the need for clinical trials to address the impact of combined CDK4/6 inhibition, ER modulation and immunotherapy such as anti-PD1 or anti-PDL1 (NCT02778685, NCT03573648 and NCT03294694) (Rampioni Vinciguerra et al., 2022).
Overall, the TIME of HR+ breast cancer is highly complex. Additionally, understanding the TIME to the same degree as TNBC and HER2+ breast cancer is needed to better prognosticate and improve treatment of the various TIME of HR + breast cancer that potentially exists.
Targeting immune hot tumors and metastasis by resolving inflammation
Chronic inflammation is a hallmark of cancer (Hanahan and Weinberg, 2011). Indeed, several benign pathologies marked with chronic inflammation, such as liver cirrhosis or inflammatory bowel disease, are associated with an increased risk of developing cancer (Sangiovanni et al., 2004; Grivennikov et al., 2010; Beaugerie et al., 2013). Oncogenesis is promoted by an unresolved inflammatory response which leads to the accumulation of stromal cells and loss of tissue homeostasis (Hanahan and Weinberg, 2011; Quail and Joyce, 2013). Excessive inflammation and expression of cytokines such as IFNs induce expression of immune checkpoint molecules such as PDL1, which in turn negatively regulate anti-tumor immune cells, leading to immunosuppression that is essential for tumor progression (Hanahan and Weinberg, 2011; Galon and Bruni, 2019). Therefore, strategies treating such tumors attempt to resolve excessive inflammation while making efficient use of immunosuppressive molecule inhibition to counteract tumor-induced T-cell dysfunction.
Tumor-intrinsic immunosuppression through oncogenic pathway induction
Activation of the nuclear factor kappaB (NFΚB) inflammatory pathway is observed predominantly in ER negative tumors through activation of RTKs and/or cellular stress (Biswas et al., 2004; Frasor et al., 2015; Wang et al., 2015). Moreover, active NFKB is correlated with larger, more advanced tumors and correlates with lung and brain metastasis (Wang et al., 2015). NFKB activity is associated with expression of inflammatory cytokines that promote Th2 polarization and maintaining a cancer stem cell population that facilitates oncogenesis (Liu et al., 2010; Wang et al., 2015; Zhang et al., 2022). Inhibition of NFKB delayed tumor onset and abrogated tumor progression in a PyMT driven model of breast cancer (Connelly et al., 2011). Recent work demonstrated that the reticuloendotheliosis viral oncogene homolog A (RELA) subunit of NFKB recruits cat eye syndrome chromosome region candidate 2 (CECR2), an epigenetic factor that induces gene expression of several inflammatory cytokines including colony stimulating factor 1 (CSF1) (Zhang et al., 2022). This interaction was important in recruitment of macrophages as well as their polarization to the M2 state in the lung metastatic niche (Zhang et al., 2022). Targeting CECR2 in mice harbouring orthotopically transplanted 4T1 cells reduced metastasis through reduced M2 macrophage levels and concomitant increase in cytotoxic T-cell activity (Zhang et al., 2022).
NFKB components have been shown to interact with STAT3 and coregulate inflammatory oncogenic pathways in breast cancer (Fan et al., 2013). In addition to STAT3’s ability to regulate the immune microenvironment, STAT3 transcriptional targets regulate anti-apoptotic pathways, EMT and expression of metalloproteinases (MPPs) that act in concert to regulate growth and metastasis (Ma et al., 2020). Interestingly, mammary specific knockout of STAT3 in the PyMT model did not impact nascent tumors, but they were eventually eliminated by an active innate and adaptive immune response (Jones et al., 2016). This finding contends that targeting STAT3 or one of its essential transcriptional targets may be sufficient to induce a robust anti-tumor immune response that leads to complete clearance of tumors. It is noteworthy that our STAT3 knockout was restricted to the mammary epithelium (Jones et al., 2016), future studies using STAT3 inhibitors (Zou et al., 2020) to address the role of STAT3 in other cell types of the TIME are essential for understanding the full role of STAT3 in immunosuppression. To this end, STAT3 activation (assessed using expression of phosphorylated STAT3) in astrocytes of the brain microenvironment supported invasive growth of breast-to-brain metastasis in part through reduced activation of T-cell activity and upregulation of CHI3L1 expression (Priego et al., 2018; Dankner et al., 2022). TTI-101, a STAT3 inhibitor, is currently in early phase clinical trials (NCT03195699 and NCT05384119). Of note, active STAT3 downstream interleukin (IL) six was demonstrated to bind estrogen response elements and promote gene expression independent of the ER or its coactivator forkhead box A1 (FOXA1) promoting metastasis and resistance to anti-estrogen-based therapy (Siersbaek et al., 2020).
Targeting macrophages
Macrophages are the most abundant immune cell type in the TIME, thus representing an attractive therapeutic target. Work from the PyMT mouse model demonstrated that as the tumor progresses, there is a decrease in mammary resident macrophages and an increase in tumor associated macrophages (Attalla et al., 2021). Macrophages are recruited to the tumor site due to expression of inflammatory cytokines, especially CSF1 and chemokine ligand (CCL) 2 (Lin et al., 2001; Franklin et al., 2014; Linde et al., 2018; Neubert et al., 2018). The importance of CSF1 was demonstrated using CSF1 knockout PyMT mice which exhibited delayed disease progression as well as prevented pulmonary metastasis through loss of vascular endothelial growth factor (VEGF) and epidermal growth factor (EGF) expression (Lin et al., 2001). Moreover, macrophages promote tumor cell migration and metastatic dissemination through secretion of EGF and Wnt1 (Wyckoff et al., 2007; Linde et al., 2018). Macrophages, especially those M2 polarized, establish the immunosuppressive microenvironment in immune hot subtypes through expression of cytokines that promote Th2 T-helper cell polarization and inhibition of cytotoxic T-cell function (Liu et al., 2021) (Figure 4A). Moreover, macrophages have been demonstrated to expresses PDL1 to promote exhaustion of T-cells (Ahmed et al., 2020). Inhibition of CSF1 receptor (CSFR) by small molecule (BLZ945) has demonstrated anti-tumor effects in glioma and breast cancer models through loss of M2 polarized macrophages and enhancement of T-cell recruitment to the tumor (Pyonteck et al., 2013; Strachan et al., 2013). Phase II clinical trial for TNBC combining Pembrolizumab (anti-PD1), radiation and Axatilimab (anti-CSFR) is currently ongoing (NCT05491226).
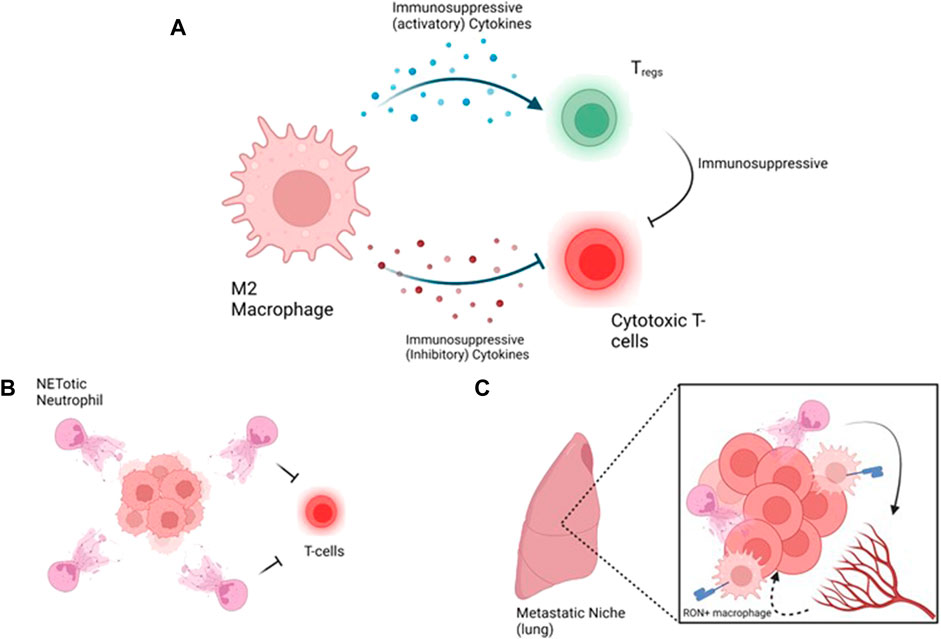
FIGURE 4. Strategies targeting immune hot tumors and metastasis, (A) A loop between macrophages promotes immunosuppression by activating inhibitory T-cells such as Tregs and inhibition of cytotoxic T-cells. Inhibitory T-cells emphasize the suppression of cytotoxic T-cells, (B) At the primary tumor site, NETotic neutrophils protect the tumors from cytotoxic T-cells, (C) At the metastatic site, neutrophils promote vascular permeability to allow extravasyion of the tumor cells and macrophages support the immunosuppressive environment via MSP/RON. Figure generated with biorender.com.
Another macrophage marker that has demonstrated pre-clinical interest is the “do not eat me” signaling axis consisting of signal regulatory protein-alpha (SIRPα) expressed on the macrophage (and other phagocytes) and CD47 expressed on cancer cells (Chen et al., 2022). CD47 is transcriptionally regulated by inflammation, though NFKB and TNF (Betancur et al., 2017) as well as hypoxia, through HIF1α (Zhang et al., 2015). CD47 has also been demonstrated to be an essential breast cancer stem cell marker (Zhang et al., 2015; Kaur et al., 2016; Chen et al., 2022). Engagement of CD47 with SIRPα inhibits the phagocytic capacity of macrophages (Chen et al., 2022). Antibody neutralization of CD47, induced a shift towards M1 macrophages and enhanced antigen presentation (Latour et al., 2001; Zhang et al., 2016; Chen et al., 2022). CD47 blockade also synergized with trastuzumab through enhancing antibody dependent cellular phagocytosis (Tsao et al., 2019). Multiple anti-CD47 antibodies have been developed and are under clinical assessment (Chen et al., 2022).
Targeting neutrophils
Neutrophils are the most common cell type in human blood and have recently gained significant research interest. The neutrophil to lymphocyte ratio in many solid tumors is correlated with patient survival with a higher ratio being associated with worse patient survival (Bartlett et al., 2020; Gago-Dominguez et al., 2020). Neutrophils are recruited to the tumor (site of injury) through secretion of inflammatory cytokines such as tumor growth factor β (TGFβ), CXCL8 (also known as IL-8), CXCL1, CXCL2 as well as IFNs (SenGupta et al., 2021). Furthermore, neutrophils secrete MMP9 (SenGupta et al., 2021) a prominent enzyme that degrades the extracellular matrix, promoting cancer progression and tumor cell dissemination (Owyong et al., 2019). Moreover, expression of chemokine ligand CCL20 by the tumor cell induced expression of PDL1 on neutrophils which reinforces an immunosuppressive microenvironment via exhaustion of T-cells (Kwantwi et al., 2021).
Strikingly, neutrophils have been demonstrated to undergo a process known as NETosis, during which a neutrophil secretes its DNA into a weblike structure (Vorobjeva and Chernyak, 2020). NET formation is promoted by cancer derived factors such as granulocyte-CSF (G-CSF), CXCL5, CXCL6 (Ronchetti et al., 2021). NETosis has been further demonstrated to contribute to cancer progression and metastasis (Ronchetti et al., 2021). Interestingly, NETs have been demonstrated to sequester circulating tumor cells to ensure their successful metastatic colonization (Cools-Lartigue et al., 2013). Depletion of neutrophils reduced metastatic dissemination via reliving inhibition of cytotoxic T-cell activity (Coffelt et al., 2015). Targeting NETosis using DNase I to dissolve the extracellular DNA or inhibiting protein arginine deiminase 4 (PAD4), an enzyme required for NET formation, using small molecules inhibited cancer growth, metastasis and recurrence in models of breast cancer (Park et al., 2016; Ruiz-Fernandez de Cordoba et al., 2022). Moreover, inhibition of PAD4 using GSK484 sensitizes TNBC xenografts to radiotherapy (Wei et al., 2021) and synergizes with anti-PD1/anti-CTLA dual therapy (Teijeira et al., 2020). Interestingly, NETs were demonstrated to encapsulate tumors cells, preventing physical contact between T-cells and tumor cells (Teijeira et al., 2020; Shinde-Jadhav et al., 2021) and thus may promote T-cell exclusion from the tumor (Figure 4B).
Accumulating evidence suggests that targeting pathway associated with inflammation or cells accumulated at the primary tumor site due to the inflammation can act in synergy with other already approved immunotherapies to lead to collapse of the primary tumor or inhibition of metastasis.
Development of bispecific antibodies for treatment of breast cancer
Driven by the idea of combinational therapies, bispecific antibodies (bsAbs) that simultaneously bind multiple tumor specific antigens and/or immune cells leading to an anti-tumor immune response have been generated (Dees et al., 2021). Most bsAbs function as CD3+ T-cell engagers, that direct T-cells to the tumor epithelium to elicit their anti-tumor function (Dees et al., 2021). Therefore, bsAbs that target T-cells and commonly expressed breast cancer cell surface antigens such as HER2 (Sen et al., 2001), EGFR (Stamm et al., 2019), epithelial cell adhesion molecule (EpCAM) (Kubo et al., 2018), trophoblast cell-surface antigen 2 (Trop2) and carcinoembryonic antigen-related cell adhesion molecule 5 (CEACAM5) (Chang et al., 2017) have been developed to specifically target tumors expressing these markers. Prominently, CD3/HER2 targeting bsAb (Runimotamab) is under clinical investigation for HER2+ breast cancer (NCT03448042). In addition to T-cell engagers, novel natural killer (NK) cell redirectors have been generated through the use of CD16 (Fc receptor expressed on natural killers) to target the breast cancer antigen, mesothelin known as MesobsFab (Del Bano et al., 2019). MesobsFab promoted recruitment and penetration of NK cells into spheroids as well as demonstrating anti-tumor effects in humanized mice (Del Bano et al., 2019).
It is important to note that the success of these antibodies is highly dependent on T-cells being capable of fully infiltrating the tumor. A cold TIME or restriction of T-cells to the margin or stroma may dampen the effect of these antibodies and render the tumors resistant. Efforts aimed at improving the immunogenicity of tumors and immune cell trafficking into the tumor (discussed in “Approaches to Treat Immune Cold Tumors”) may enhance the effects of these therapies. Additionally, bsAbs have demonstrated to work additively with anti-PD1 treatment (Chang et al., 2017) especially because some bsAbs have been demonstrated to induce expression of IFNγ (Sen et al., 2001) which may increase expression of PDL1, suggesting that bsAbs monotherapy may not be suffice due to T-cell exhaustion.
Targeting the immune compartment at the metastatic site
Nearly all breast cancer deaths are a result of metastasis to essential organs such as brain, lung and liver (Harbeck et al., 2019). Therefore, efforts at both preventing and more importantly treating metastasis at these sites is crucial for improving patient care. Studies using PyMT mice demonstrated that neutrophils accumulate in the lung even during the pre-metastatic stage and are essential for metastatic colonization from the primary tumor site (Wculek and Malanchi, 2015). Additionally, it was demonstrated that tumor derived exosomes carry RNA to the type II alveolar cells of the lung stimulating expression of CXCL1, CXCL2, CXCL5 and CXCL12, through engagement with the toll like three receptor (TLR3), which recruit neutrophils (Liu et al., 2016). TLR3 knockout was sufficient to reduce pulmonary metastasis, suggesting that targeting TLR3 perhaps by a neutralizing antibody (Duffy et al., 2007) may be a viable option at inhibiting metastasis.
Obesity, which is associated with an increase in systemic inflammation is correlated with an increased incidence of metastasis and disease aggressiveness (Carmichael and Bates, 2004). Mouse modelling demonstrated that obesity due to a high fat diet or using the genetic ob/ob model is associated with lung neutrophilia due to upregulation of granulocyte/macrophage (GM)-CSF and IL5 expression (Quail et al., 2017). Profiling of neutrophils demonstrated that obesity was associated with an increase in markers of mobilization and activation and was sufficient to promote lung colonization of PyMT cells from a tail vein assay (Quail et al., 2017) through neutrophil-mediated vasculature permeability (McDowell et al., 2021). Inhibiting NETosis using GSK484 reduced PyMT breast cancer cell extravasation (McDowell et al., 2021) (Figure 4C). Taken together, these studies strongly demonstrate the connection between obesity and lung colonization by cancer cells through obesity mediated priming of the metastatic niche. However, studies demonstrating whether targeting neutrophils (or other immune cells) in an already established metastatic niche are critical for improving patient care for those with metastatic cancer.
Mice harbouring breast-to-liver, but not breast-to-lung metastasis, had a significant increase in low-density neutrophils (LDNs) which are an “immature subset of neutrophils” recruited due to tumor expression of G-CSF (Hsu et al., 2019). Knockdown of G-CSF in liver metastatic cells reduced LDN recruitment and reduced liver metastatic burden (Hsu et al., 2019). This work emphasizes that neutrophils are a heterogenous population of granulocytes and specific subsets may be required for organotropic metastasis.
Accumulating evidence for neutrophils regulating several stages of cancer progression and therapeutic resistance makes them an interesting clinical target. However, to our best knowledge no clinical trial has been established to assess targeting neutrophils or NETosis in breast cancer.
Macrophages represent another interesting target at both the primary and metastatic site. Pulmonary administration of PLX-3397, a CSFR inhibitor reduced metastatic burden in mice harbouring orthotopic 4T1 tumors (Alhudaithi et al., 2020). This was accompanied by a decrease in M2 macrophages and a concomitant increase in M1 macrophages (Alhudaithi et al., 2020). A series of work has identified the immunosuppressive pathway of macrophage stimulating protein (MSP) to be essential for immunosuppression and metastatic outgrowth (Eyob et al., 2013a; Eyob et al., 2013b). MSP is released from the liver as an inactive precursor which becomes activated once cleaved by matriptase (ST14) found on the surface of cancer cells and macrophages (Eyob et al., 2013a). Active MSP binds its receptor, macrophage stimulating one receptor (MST1R/RON), activating a series of downstream signaling in macrophages which are essential for immunosuppression (Eyob et al., 2013a; Eyob et al., 2013b; Lai et al., 2021). MSP overexpression in the PyMT model promoted more aggressive primary tumor behaviour and promoted osteolytic bone metastasis formation (Welm et al., 2007). Targeting RON through genetic knockout or pharmacological inhibition using BMS-777607/ASLAN002 in the PyMT model prevented the formation of overt lung metastasis through upregulation of a series of anti-tumor cytokines; IL12 and IFNγ and downregulation of pro-tumor cytokines; TNFα and IL10 (Eyob et al., 2013a). Inhibition of RON promoted loss of M2 macrophages and induction of cytotoxic and Th1 T-cell activities resulting in reduced growth of pulmonary metastasis (Eyob et al., 2013b; Lai et al., 2021). Moreover, inhibition of RON synergized with anti-CTLA based therapy to inhibit pulmonary metastasis outgrowth (Ekiz et al., 2018). It is important to emphasize that treatment of established micro-metastasis with BMS-777607/ASLAN002, diminished growth of the lesions (Eyob et al., 2013b; Ekiz et al., 2018). This data indicates that inhibition of RON is a viable option for treatment of metastatic disease.
Conclusions and future directions
It became increasingly clear that novel strategies are required to better classify breast tumors based on a collection of histological, molecular, and immune phenotypes is required to better prognosticate and manage the disease. The breast TIME, at least in the TNBC subtype, is broadly divided into immune hot and immune cold subtypes as well as intermediate subtypes where T-cells are excluded from the tumor nest. While elegant work has been done in understanding the spatial profile of the tumors, functional work identifying pathways to be targeted that lead to abrogation of T-cell exclusion from the tumor nest are still required. Introducing inhibitors that are sufficient to abrogate exclusion of T-cells into a clinical setting may increase the number of responders to T-cell dependent immunotherapy. We are still lacking similar observations to be made in HR+ breast cancer which may allow better stratification of patients and identifying those that respond to immunotherapy versus non-responders. Sophisticated approaches such as single cell sequencing, spatial transcriptomics and multiplex immunohistochemistry and RNA in situ hybridization technologies could be leveraged to better understand the complex immunbiology of breast tumors. This may be a particularly interesting avenue especially in the context of ER mutant disease. There is accumulating pre-clinical evidence suggesting that T-cell infiltration and activation through modulation of the STING/adenosine pathways and epigenetic rewiring of the tumor in combination with chemo/radiotherapy may have a clinically positive impact on patient survival and outcome. Oncolytic viruses are an upcoming avenue which may allow for immune mediated elimination of tumors as well as sensitize tumors to immunotherapies. Clinical evaluation of these interventions may lead to introduction of novel clinical interventions. For immune hot tumors, strategies aimed at resolving inflammation due to oncogene activation as well as eliminating immune cells that accumulate due to the chronic inflammation may synergize with ICB as well as inhibit metastasis. Emerging evidence strongly supports that immunotherapy coupled with other precision oncology agents will eventually become part of standard of care for breast cancer management.
Author contributions
SA, wrote the manuscript and made the figures WM, major edits to the text, reviewing and financial support.
Funding
Canadian Institute of Health Research. SA is supported by the fonds de recherche du Quebec—Sante (FRQS). TT is supporting by the MD/PhD program at McGill university. WJM is supported by the Canada Research Chair of Molecular Oncology.
Acknowledgments
We would like to thank Hailey Proud her critical reading of this work.
Conflict of interest
The authors declare that the research was conducted in the absence of any commercial or financial relationships that could be construed as a potential conflict of interest.
Publisher’s note
All claims expressed in this article are solely those of the authors and do not necessarily represent those of their affiliated organizations, or those of the publisher, the editors and the reviewers. Any product that may be evaluated in this article, or claim that may be made by its manufacturer, is not guaranteed or endorsed by the publisher.
References
Ahmed, F. S., Gaule, P., McGuire, J., Patel, K., Blenman, K., Pusztai, L., et al. (2020). PD-L1 protein expression on both tumor cells and macrophages are associated with response to neoadjuvant durvalumab with chemotherapy in triple-negative breast cancer. Clin. Cancer Res. 26 (20), 5456–5461. doi:10.1158/1078-0432.CCR-20-1303
Alhudaithi, S. S., Almuqbil, R. M., Zhang, H., Bielski, E. R., Du, W., Sunbul, F. S., et al. (2020). Local targeting of lung-tumor-associated macrophages with pulmonary delivery of a CSF-1R inhibitor for the treatment of breast cancer lung metastases. Mol. Pharm. 17 (12), 4691–4703. doi:10.1021/acs.molpharmaceut.0c00983
Allard, B., Allard, D., Buisseret, L., and Stagg, J. (2020). The adenosine pathway in immuno-oncology. Nat. Rev. Clin. Oncol. 17 (10), 611–629. doi:10.1038/s41571-020-0382-2
Andre, T., Shiu, K. K., Kim, T. W., Jensen, B. V., Jensen, L. H., Punt, C., et al. (2020). Pembrolizumab in microsatellite-instability-high advanced colorectal cancer. N. Engl. J. Med. 383 (23), 2207–2218. doi:10.1056/NEJMoa2017699
Attalla, S., Taifour, T., Bui, T., and Muller, W. (2021). Insights from transgenic mouse models of PyMT-induced breast cancer: Recapitulating human breast cancer progression in vivo. Oncogene 40 (3), 475–491. doi:10.1038/s41388-020-01560-0
Baker, J. H. E., Kyle, A. H., Reinsberg, S. A., Moosvi, F., Patrick, H. M., Cran, J., et al. (2018). Heterogeneous distribution of trastuzumab in HER2-positive xenografts and metastases: Role of the tumor microenvironment. Clin. Exp. Metastasis 35 (7), 691–705. doi:10.1007/s10585-018-9929-3
Barroso-Sousa, R., Jain, E., Cohen, O., Kim, D., Buendia-Buendia, J., WinEr, E., et al. (2020). Prevalence and mutational determinants of high tumor mutation burden in breast cancer. Ann. Oncol. 31 (3), 387–394. doi:10.1016/j.annonc.2019.11.010
Bartlett, E. K., Flynn, J. R., Panageas, K. S., Ferraro, R. A., Sta Cruz, J. M., Postow, M. A., et al. (2020). High neutrophil-to-lymphocyte ratio (NLR) is associated with treatment failure and death in patients who have melanoma treated with PD-1 inhibitor monotherapy. Cancer 126 (1), 76–85. doi:10.1002/cncr.32506
Beaugerie, L., Svrcek, M., Seksik, P., Bouvier, A. M., Simon, T., Allez, M., et al. (2013). Risk of colorectal high-grade dysplasia and cancer in a prospective observational cohort of patients with inflammatory bowel disease. Gastroenterology 145 (1), 166–175 e8. doi:10.1053/j.gastro.2013.03.044
Bebo, B. F., Dehghani, B., Foster, S., Kurniawan, A., Lopez, F. J., and Sherman, L. S. (2009). Treatment with selective estrogen receptor modulators regulates myelin specific T-cells and suppresses experimental autoimmune encephalomyelitis. Glia 57 (7), 777–790. doi:10.1002/glia.20805
Betancur, P. A., Abraham, B. J., Yiu, Y. Y., Willingham, S. B., Khameneh, F., Zarnegar, M., et al. (2017). A CD47-associated super-enhancer links pro-inflammatory signalling to CD47 upregulation in breast cancer. Nat. Commun. 8, 14802. doi:10.1038/ncomms14802
Biswas, D. K., Shi, Q., Baily, S., Strickland, I., Ghosh, S., Pardee, A. B., et al. (2004). NF-kappa B activation in human breast cancer specimens and its role in cell proliferation and apoptosis. Proc. Natl. Acad. Sci. U. S. A. 101 (27), 10137–10142. doi:10.1073/pnas.0403621101
Bonaventura, P., Shekarian, T., Alcazer, V., Valladeau-Guilemond, J., Valsesia-Wittmann, S., Amigorena, S., et al. (2019). Cold tumors: A therapeutic challenge for immunotherapy. Front. Immunol. 10, 168. doi:10.3389/fimmu.2019.00168
Burr, M. L., Sparbier, C. E., Chan, K. L., Chan, Y. C., Kersbergen, A., Lam, E. Y. N., et al. (2019). An evolutionarily conserved function of polycomb silences the MHC class I antigen presentation pathway and enables immune evasion in cancer. Cancer Cell. 36 (4), 385–401. doi:10.1016/j.ccell.2019.08.008
Cai, Y., Lin, J. R., Zhang, Q., O'Brien, K., Montagna, C., and Zhang, Z. D. (2018). Epigenetic alterations to Polycomb targets precede malignant transition in a mouse model of breast cancer. Sci. Rep. 8 (1), 5535. doi:10.1038/s41598-018-24005-x
Canadian Cancer Society (2022). New Canadian Cancer Statistics report reveals over 1.5 million people in Canada are living with or beyond cancer. Available from: https://cancer.ca/en/about-us/media-releases/2022/canadian-cancer-statistics-special-report-2022.
Carmichael, A. R., and Bates, T. (2004). Obesity and breast cancer: A review of the literature. Breast 13 (2), 85–92. doi:10.1016/j.breast.2003.03.001
Carozza, J. A., Bohnert, V., Nguyen, K. C., Skariah, G., Shaw, K. E., Brown, J. A., et al. (2020). Extracellular cGAMP is a cancer cell-produced immunotransmitter involved in radiation-induced anti-cancer immunity. Nat. Cancer 1 (2), 184–196. doi:10.1038/s43018-020-0028-4
Carozza, J. A., Cordova, A. F., Brown, J. A., AlSaif, Y., Bohnert, V., Cao, X., et al. (2022). ENPP1's regulation of extracellular cGAMP is a ubiquitous mechanism of attenuating STING signaling. Proc. Natl. Acad. Sci. U. S. A. 119 (21), e2119189119. doi:10.1073/pnas.2119189119
Carter, M. E., Koch, A., Lauer, U. M., and Hartkopf, A. D. (2021). Clinical trials of oncolytic viruses in breast cancer. Front. Oncol. 11, 803050. doi:10.3389/fonc.2021.803050
Chakraborty, B., Byemerwa, J., Krebs, T., Lim, F., Chang, C. Y., and McDonnell, D. P. (2023). Estrogen receptor signaling in the immune system. Endocr. Rev. 44 (1), 117–141. doi:10.1210/endrev/bnac017
Chan, M. S., Wang, L., Felizola, S. J. A., Ueno, T., Toi, M., Loo, W., et al. (2012). Changes of tumor infiltrating lymphocyte subtypes before and after neoadjuvant endocrine therapy in estrogen receptor-positive breast cancer patients--an immunohistochemical study of Cd8+ and Foxp3+ using double immunostaining with correlation to the pathobiological response of the patients. Int. J. Biol. Markers 27 (4), e295–e304. doi:10.5301/JBM.2012.10439
Chang, C. H., Wang, Y., Li, R., Rossi, D. L., Liu, D., Rossi, E. A., et al. (2017). Combination therapy with bispecific antibodies and PD-1 blockade enhances the antitumor potency of T cells. Cancer Res. 77 (19), 5384–5394. doi:10.1158/0008-5472.CAN-16-3431
Chen, C., Wang, R., Chen, X., Hou, Y., and Jiang, J. (2022). Targeting CD47 as a novel immunotherapy for breast cancer. Front. Oncol. 12, 924740. doi:10.3389/fonc.2022.924740
Cheng, H., Xu, Q., Lu, X., Yuan, H., Li, T., Zhang, Y., et al. (2020). Activation of STING by cGAMP regulates MDSCs to suppress tumor metastasis via reversing epithelial-mesenchymal transition. Front. Oncol. 10, 896. doi:10.3389/fonc.2020.00896
Cheon, H., Wang, Y., Wightman, S. M., Jackson, M. W., and Stark, G. R. (2022). How cancer cells make and respond to interferon-I. Trends Cancer 9, 83–92. doi:10.1016/j.trecan.2022.09.003
Coffelt, S. B., Kersten, K., Doornebal, C. W., Weiden, J., Vrijland, K., Hau, C. S., et al. (2015). IL-17-producing γδ T cells and neutrophils conspire to promote breast cancer metastasis. Nature 522 (7556), 345–348. doi:10.1038/nature14282
Connelly, L., Barham, W., Onishko, H. M., Sherrill, T., Chodosh, L. A., Blackwell, T. S., et al. (2011). Inhibition of NF-kappa B activity in mammary epithelium increases tumor latency and decreases tumor burden. Oncogene 30 (12), 1402–1412. doi:10.1038/onc.2010.521
Cools-Lartigue, J., Spicer, J., McDonald, B., Gowing, S., Chow, S., Giannias, B., et al. (2013). Neutrophil extracellular traps sequester circulating tumor cells and promote metastasis. J. Clin. Invest. 123 (8), 3446–3458. doi:10.1172/JCI67484
Corriden, R., Hollands, A., Olson, J., Derieux, J., Lopez, J., Chang, J. T., et al. (2015). Tamoxifen augments the innate immune function of neutrophils through modulation of intracellular ceramide. Nat. Commun. 6, 8369. doi:10.1038/ncomms9369
Cortes, J., Rugo, H. S., Cescon, D. W., Im, S. A., Yusof, M. M., Gallardo, C., et al. (2022). Pembrolizumab plus chemotherapy in advanced triple-negative breast cancer. N. Engl. J. Med. 387 (3), 217–226. doi:10.1056/NEJMoa2202809
Csiki, I., Dong, A., Tuan, B. Y., John, E., O'Toole, L., Seppa, J., et al. (2022). First-in-human experience using RBS2418, an oral ENPP1 inhibitor within an expanded access protocol in combination with pembrolizumab in a patient with metastatic adrenal cancer. J. Clin. Oncol. 40 (16), e14550. doi:10.1200/jco.2022.40.16_suppl.e14550
Dankner, M., Maritan, S. M., Priego, N., Nadaf, J., Nkili, A., Zhuang, R., et al. (2022). Abstract 1569: pSTAT3+ stromal cells drive the invasive growth of brain metastases. Cancer Res. 82 (12), 1569. doi:10.1158/1538-7445.am2022-1569
Decout, A., Katz, J. D., Venkatraman, S., and Ablasser, A. (2021). The cGAS-STING pathway as a therapeutic target in inflammatory diseases. Nat. Rev. Immunol. 21 (9), 548–569. doi:10.1038/s41577-021-00524-z
Dees, S., Ganesan, R., Singh, S., and Grewal, I. S. (2021). Bispecific antibodies for triple negative breast cancer. Trends Cancer 7 (2), 162–173. doi:10.1016/j.trecan.2020.09.004
Del Bano, J., Flores-Flores, R., Josselin, E., Goubard, A., Ganier, L., Castellano, R., et al. (2019). A bispecific antibody-based approach for targeting mesothelin in triple negative breast cancer. Front. Immunol. 10, 1593. doi:10.3389/fimmu.2019.01593
Deng, L., Liang, H., Xu, M., Yang, X., Burnette, B., Arina, A., et al. (2014). STING-dependent cytosolic DNA sensing promotes radiation-induced type I interferon-dependent antitumor immunity in immunogenic tumors. Immunity 41 (5), 843–852. doi:10.1016/j.immuni.2014.10.019
Deng, J., Wang, E. S., Jenkins, R. W., Li, S., Dries, R., Yates, K., et al. (2018). CDK4/6 inhibition augments antitumor immunity by enhancing T-cell activation. Cancer Discov. 8 (2), 216–233. doi:10.1158/2159-8290.CD-17-0915
Denkert, C., von Minckwitz, G., Darb-Esfahani, S., Lederer, B., Heppner, B. I., Weber, K. E., et al. (2018). Tumour-infiltrating lymphocytes and prognosis in different subtypes of breast cancer: A pooled analysis of 3771 patients treated with neoadjuvant therapy. Lancet Oncol. 19 (1), 40–50. doi:10.1016/S1470-2045(17)30904-X
DeSantis, C. E., Ma, J., Gaudet, M. M., Newman, L. A., Miller, K. D., Goding Sauer, A., et al. (2019). Breast cancer statistics, 2019. CA Cancer J. Clin. 69 (6), 438–451. doi:10.3322/caac.21583
Dhatchinamoorthy, K., Colbert, J. D., and Rock, K. L. (2021). Cancer immune evasion through loss of MHC class I antigen presentation. Front. Immunol. 12, 636568. doi:10.3389/fimmu.2021.636568
Doedens, A. L., Stockmann, C., Rubinstein, M. P., Liao, D., Zhang, N., DeNardo, D. G., et al. (2010). Macrophage expression of hypoxia-inducible factor-1 alpha suppresses T-cell function and promotes tumor progression. Cancer Res. 70 (19), 7465–7475. doi:10.1158/0008-5472.CAN-10-1439
Duffy, K. E., Lamb, R. J., San Mateo, L. R., Jordan, J. L., Canziani, G., Brigham-Burke, M., et al. (2007). Down modulation of human TLR3 function by a monoclonal antibody. Cell. Immunol. 248 (2), 103–114. doi:10.1016/j.cellimm.2007.10.002
Dusenbery, A. C., Maniaci, J. L., Hillerson, N. D., Dill, E. A., Bullock, T. N., and Mills, A. M. (2021). MHC class I loss in triple-negative breast cancer: A potential barrier to PD-1/PD-L1 checkpoint inhibitors. Am. J. Surg. Pathol. 45 (5), 701–707. doi:10.1097/PAS.0000000000001653
Ekiz, H. A., Lai, S. C. A., Gundlapalli, H., Haroun, F., Williams, M. A., and Welm, A. L. (2018). Inhibition of RON kinase potentiates anti-CTLA-4 immunotherapy to shrink breast tumors and prevent metastatic outgrowth. Oncoimmunology 7 (9), e1480286. doi:10.1080/2162402X.2018.1480286
Esfahani, K., Roudaia, L., Buhlaiga, N., Del Rincon, S. V., PapNeja, N., and Miller, W. H. (2020). A review of cancer immunotherapy: From the past, to the present, to the future. Curr. Oncol. 27 (2), S87–S97. doi:10.3747/co.27.5223
Eyob, H., Ekiz, H. A., and Welm, A. L. (2013). RON promotes the metastatic spread of breast carcinomas by subverting antitumor immune responses. Oncoimmunology 2 (9), e25670. doi:10.4161/onci.25670
Eyob, H., Ekiz, H. A., Derose, Y. S., Waltz, S. E., Williams, M. A., and Welm, A. L. (2013). Inhibition of ron kinase blocks conversion of micrometastases to overt metastases by boosting antitumor immunity. Cancer Discov. 3 (7), 751–760. doi:10.1158/2159-8290.CD-12-0480
Fan, Y., Mao, R., and Yang, J. (2013). NF-κB and STAT3 signaling pathways collaboratively link inflammation to cancer. Protein Cell. 4 (3), 176–185. doi:10.1007/s13238-013-2084-3
Foote, J. B., Kok, M., Leatherman, J. M., Armstrong, T. D., Marcinkowski, B. C., Ojalvo, L. S., et al. (2017). A STING agonist given with OX40 receptor and PD-L1 modulators primes immunity and reduces tumor growth in tolerized mice. Cancer Immunol. Res. 5 (6), 468–479. doi:10.1158/2326-6066.CIR-16-0284
Franklin, R. A., Liao, W., Sarkar, A., Kim, M. V., Bivona, M. R., Liu, K., et al. (2014). The cellular and molecular origin of tumor-associated macrophages. Science 344 (6186), 921–925. doi:10.1126/science.1252510
Franovic, A., Gunaratnam, L., Smith, K., Robert, I., Patten, D., and Lee, S. (2007). Translational up-regulation of the EGFR by tumor hypoxia provides a nonmutational explanation for its overexpression in human cancer. Proc. Natl. Acad. Sci. U. S. A. 104 (32), 13092–13097. doi:10.1073/pnas.0702387104
Frasor, J., El-Shennawy, L., Stender, J. D., and Kastrati, I. (2015). NFκB affects estrogen receptor expression and activity in breast cancer through multiple mechanisms. Mol. Cell. Endocrinol. 418, 235–239. doi:10.1016/j.mce.2014.09.013
Gago-Dominguez, M., Matabuena, M., Redondo, C. M., Patel, S. P., Carracedo, A., Ponte, S. M., et al. (2020). Neutrophil to lymphocyte ratio and breast cancer risk: Analysis by subtype and potential interactions. Sci. Rep. 10 (1), 13203. doi:10.1038/s41598-020-70077-z
Galon, J., and Bruni, D. (2019). Approaches to treat immune hot, altered and cold tumours with combination immunotherapies. Nat. Rev. Drug Discov. 18 (3), 197–218. doi:10.1038/s41573-018-0007-y
Gangar, M., Goyal, S., Raykar, D., Khurana, P., Martis, A. M., Goswami, A., et al. (2022). Design, synthesis and biological evaluation studies of novel small molecule ENPP1 inhibitors for cancer immunotherapy. Bioorg Chem. 119, 105549. doi:10.1016/j.bioorg.2021.105549
Generali, D., Bates, G., Berruti, A., Brizzi, M. P., Campo, L., Bonardi, S., et al. (2009). Immunomodulation of FOXP3+ regulatory T cells by the aromatase inhibitor letrozole in breast cancer patients. Clin. Cancer Res. 15 (3), 1046–1051. doi:10.1158/1078-0432.CCR-08-1507
Germano, G., Lamba, S., Rospo, G., Barault, L., Magri, A., Maione, F., et al. (2017). Inactivation of DNA repair triggers neoantigen generation and impairs tumour growth. Nature 552 (7683), 116–120. doi:10.1038/nature24673
Ghiringhelli, F., Menard, C., Puig, P. E., Ladoire, S., Roux, S., Martin, F., et al. (2007). Metronomic cyclophosphamide regimen selectively depletes CD4+CD25+ regulatory T cells and restores T and NK effector functions in end stage cancer patients. Cancer Immunol. Immunother. 56 (5), 641–648. doi:10.1007/s00262-006-0225-8
Ghouse, S. M., Nguyen, H. M., Bommareddy, P. K., Guz-Montgomery, K., and Saha, D. (2020). Oncolytic herpes simplex virus encoding IL12 controls triple-negative breast cancer growth and metastasis. Front. Oncol. 10, 384. doi:10.3389/fonc.2020.00384
Goel S, D. M., Watt, A. C., BrinJones, H., Sceneay, J., Li, B. B., Khan, N., et al. (2017). CDK4/6 inhibition triggers anti-tumor immunity. Nature 548, 471–475. doi:10.1038/nature23465
Goldberg, J., Pastorello, R. G., Vallius, T., Davis, J., Cui, Y. X., Agudo, J., et al. (2021). The immunology of hormone receptor positive breast cancer. Front. Immunol. 12, 674192. doi:10.3389/fimmu.2021.674192
Goodman, M. L., Trinca, G. M., Walter, K. R., Papachristou, E. K., D'Santos, C. S., Li, T., et al. (2019). Progesterone receptor attenuates STAT1-mediated IFN signaling in breast cancer. J. Immunol. 202 (10), 3076–3086. doi:10.4049/jimmunol.1801152
Goswami, A., Deb, B., Goyal, S., Gosavi, A., Mali, M., Martis, A. M., et al. (2022). AVA-NP-695 selectively inhibits ENPP1 to activate STING pathway and abrogate tumor metastasis in 4T1 breast cancer syngeneic mouse model. Molecules 27 (19), 6721. doi:10.3390/molecules27196721
Goyette, M. A., Elkholi, I. E., Apcher, C., Kuasne, H., Rothlin, C. V., Muller, W. J., et al. (2021). Targeting Axl favors an antitumorigenic microenvironment that enhances immunotherapy responses by decreasing Hif-1α levels. Proc. Natl. Acad. Sci. U. S. A. 118 (29), e2023868118. doi:10.1073/pnas.2023868118
Grivennikov, S. I., Greten, F. R., and Karin, M. (2010). Immunity, inflammation, and cancer. Cell. 140 (6), 883–899. doi:10.1016/j.cell.2010.01.025
Gruosso, T., Gigoux, M., Manem, V. S. K., Bertos, N., Zuo, D., Perlitch, I., et al. (2019). Spatially distinct tumor immune microenvironments stratify triple-negative breast cancers. J. Clin. Invest. 129 (4), 1785–1800. doi:10.1172/JCI96313
Hammerl, D., Martens, J. W. M., Timmermans, M., Smid, M., Trapman-Jansen, A. M., Foekens, R., et al. (2021). Spatial immunophenotypes predict response to anti-PD1 treatment and capture distinct paths of T cell evasion in triple negative breast cancer. Nat. Commun. 12 (1), 5668. doi:10.1038/s41467-021-25962-0
Hanahan, D., and Weinberg, R. A. (2011). Hallmarks of cancer: The next generation. Cell. 144 (5), 646–674. doi:10.1016/j.cell.2011.02.013
Harbeck, N., Penault-Llorca, F., Cortes, J., Gnant, M., Houssami, N., Poortmans, P., et al. (2019). Breast cancer. Nat. Rev. Dis. Prim. 5 (1), 66. doi:10.1038/s41572-019-0111-2
Herzog, S. K., and Fuqua, S. A. W. (2021). ESR1 mutations and therapeutic resistance in metastatic breast cancer: Progress and remaining challenges. Br. J. Cancer 126 (2), 174–186. doi:10.1038/s41416-021-01564-x
Hirukawa, A., Smith, H. W., Zuo, D., Dufour, C. R., Savage, P., Bertos, N., et al. (2018). Targeting EZH2 reactivates a breast cancer subtype-specific anti-metastatic transcriptional program. Nat. Commun. 9 (1), 2547. doi:10.1038/s41467-018-04864-8
Hirukawa, A., Singh, S., Wang, J., Rennhack, J. P., Swiatnicki, M., Sanguin-Gendreau, V., et al. (2019). Reduction of global H3K27me(3) enhances HER2/ErbB2 targeted therapy. Cell. Rep. 29 (2), 249–257. doi:10.1016/j.celrep.2019.08.105
Honkanen, T. J., Moilanen, T., Karihtala, P., Tiainen, S., Auvinen, P., Vayrynen, J. P., et al. (2017). Prognostic and predictive role of spatially positioned tumour infiltrating lymphocytes in metastatic HER2 positive breast cancer treated with trastuzumab. Sci. Rep. 7 (1), 18027. doi:10.1038/s41598-017-18266-1
Hsu, B. E., Tabaries, S., Johnson, R. M., Andrzejewski, S., Senecal, J., Lehuede, C., et al. (2019). Immature low-density neutrophils exhibit metabolic flexibility that facilitates breast cancer liver metastasis. Cell. Rep. 27 (13), 3902–3915. doi:10.1016/j.celrep.2019.05.091
Huang, A. C., and Zappasodi, R. (2022). A decade of checkpoint blockade immunotherapy in melanoma: Understanding the molecular basis for immune sensitivity and resistance. Nat. Immunol. 23 (5), 660–670. doi:10.1038/s41590-022-01141-1
Huhn, D., Marti-Rodrigo, P., Mouron, S., Hansel, C., Tschapalda, K., Porebski, B., et al. (2022). Prolonged estrogen deprivation triggers a broad immunosuppressive phenotype in breast cancer cells. Mol. Oncol. 16 (1), 148–165. doi:10.1002/1878-0261.13083
Im, S. A., Lu, Y. S., Bardia, A., Harbeck, N., Colleoni, M., Franke, F., et al. (2019). Overall survival with ribociclib plus endocrine therapy in breast cancer. N. Engl. J. Med. 381 (4), 307–316. doi:10.1056/NEJMoa1903765
Jiang, Y. Z., Ma, D., Suo, C., Shi, J., Xue, M., Hu, X., et al. (2019). Genomic and transcriptomic landscape of triple-negative breast cancers: Subtypes and treatment strategies. Cancer Cell. 35 (3), 428–440. doi:10.1016/j.ccell.2019.02.001
Jiao, S., Xia, W., Yamaguchi, H., Wei, Y., Chen, M. K., Hsu, J. M., et al. (2017). PARP inhibitor upregulates PD-L1 expression and enhances cancer-associated immunosuppression. Clin. Cancer Res. 23 (14), 3711–3720. doi:10.1158/1078-0432.CCR-16-3215
Jin, S., Wang, Q., Wu, H., Pang, D., and Xu, S. (2021). Oncolytic viruses for triple negative breast cancer and beyond. Biomark. Res. 9 (1), 71. doi:10.1186/s40364-021-00318-4
Jones, L. M., Broz, M. L., Ranger, J. J., Ozcelik, J., Ahn, R., Zuo, D., et al. (2016). STAT3 establishes an immunosuppressive microenvironment during the early stages of breast carcinogenesis to promote tumor growth and metastasis. Cancer Res. 76 (6), 1416–1428. doi:10.1158/0008-5472.CAN-15-2770
Kaur, S., Elkahloun, A. G., Singh, S. P., Chen, Q. R., Meerzaman, D. M., Song, T., et al. (2016). A function-blocking CD47 antibody suppresses stem cell and EGF signaling in triple-negative breast cancer. Oncotarget 7 (9), 10133–10152. doi:10.18632/oncotarget.7100
Khawar, M. B., and Sun, H. (2021). CAR-NK cells: From natural basis to design for kill. Front. Immunol. 12, 707542. doi:10.3389/fimmu.2021.707542
Klichinsky, M., Ruella, M., Shestova, O., Lu, X. M., Best, A., Zeeman, M., et al. (2020). Human chimeric antigen receptor macrophages for cancer immunotherapy. Nat. Biotechnol. 38 (8), 947–953. doi:10.1038/s41587-020-0462-y
Komi, J., and Lassila, O. (1998). Antioestrogens enhance tumour necrosis factor receptor 2 (TNF-R2) expression and TNF-R2-mediated proliferation in activated T cells. Scand. J. Immunol. 48 (3), 254–260. doi:10.1046/j.1365-3083.1998.00388.x
Kubo, M., Umebayashi, M., Kurata, K., Mori, H., Kai, M., Onishi, H., et al. (2018). Catumaxomab with activated T-cells efficiently lyses chemoresistant EpCAM-positive triple-negative breast cancer cell lines. Anticancer Res. 38 (7), 4273–4279. doi:10.21873/anticanres.12724
Kwantwi, L. B., Wang, S., Zhang, W., Peng, W., Cai, Z., Sheng, Y., et al. (2021). Tumor-associated neutrophils activated by tumor-derived CCL20 (C-C motif chemokine ligand 20) promote T cell immunosuppression via programmed death-ligand 1 (PD-L1) in breast cancer. Bioengineered 12 (1), 6996–7006. doi:10.1080/21655979.2021.1977102
Lai, S. A., Gundlapalli, H., Ekiz, H. A., Jiang, A., Fernandez, E., and Welm, A. L. (2021). Blocking short-form ron eliminates breast cancer metastases through accumulation of stem-like CD4+ T cells that subvert immunosuppression. Cancer Discov. 11 (12), 3178–3197. doi:10.1158/2159-8290.CD-20-1172
Latour, S., Tanaka, H., Demeure, C., Mateo, V., Rubio, M., Brown, E. J., et al. (2001). Bidirectional negative regulation of human T and dendritic cells by CD47 and its cognate receptor signal-regulator protein-alpha: Down-regulation of IL-12 responsiveness and inhibition of dendritic cell activation. J. Immunol. 167 (5), 2547–2554. doi:10.4049/jimmunol.167.5.2547
Lauzier, M. C., Page, E. L., Michaud, M. D., and Richard, D. E. (2007). Differential regulation of hypoxia-inducible factor-1 through receptor tyrosine kinase transactivation in vascular smooth muscle cells. Endocrinology 148 (8), 4023–4031. doi:10.1210/en.2007-0285
Lee, S. H., Koo, K. H., Park, J. W., Kim, H. J., Ye, S. K., Park, J. B., et al. (2009). HIF-1 is induced via EGFR activation and mediates resistance to anoikis-like cell death under lipid rafts/caveolae-disrupting stress. Carcinogenesis 30 (12), 1997–2004. doi:10.1093/carcin/bgp233
Lee, H. J., Song, I. H., Park, I. A., Heo, S. H., Kim, Y. A., Ahn, J. H., et al. (2016). Differential expression of major histocompatibility complex class I in subtypes of breast cancer is associated with estrogen receptor and interferon signaling. Oncotarget 7 (21), 30119–30132. doi:10.18632/oncotarget.8798
Li, L., Yin, Q., Kuss, P., Maliga, Z., Millan, J. L., Wu, H., et al. (2014). Hydrolysis of 2'3'-cGAMP by ENPP1 and design of nonhydrolyzable analogs. Nat. Chem. Biol. 10 (12), 1043–1048. doi:10.1038/nchembio.1661
Li, W., Wu, L., Huang, C., Liu, R., Li, Z., Liu, L., et al. (2020). Challenges and strategies of clinical application of CAR-T therapy in the treatment of tumors-a narrative review. Ann. Transl. Med. 8 (17), 1093. doi:10.21037/atm-20-4502
Li, F., Li, C., Cai, X., Xie, Z., Zhou, L., Cheng, B., et al. (2021). The association between CD8+tumor-infiltrating lymphocytes and the clinical outcome of cancer immunotherapy: A systematic review and meta-analysis. Eclinicalmedicine 41, 101134. doi:10.1016/j.eclinm.2021.101134
Li, J., Duran, M. A., Dhanota, N., Chatila, W. K., Bettigole, S. E., Kwon, J., et al. (2021). Metastasis and immune evasion from extracellular cGAMP hydrolysis. Cancer Discov. 11 (5), 1212–1227. doi:10.1158/2159-8290.CD-20-0387
Li, Z., McGinn, O., Wu, Y., Bahreini, A., Priedigkeit, N. M., Ding, K., et al. (2022). ESR1 mutant breast cancers show elevated basal cytokeratins and immune activation. Nat. Commun. 13 (1), 2011. doi:10.1038/s41467-022-29498-9
Liang, J., Ingalla, E. R., Yao, X., Wang, B. E., Tai, L., Giltnane, J., et al. (2022). Giredestrant reverses progesterone hypersensitivity driven by estrogen receptor mutations in breast cancer. Sci. Transl. Med. 14 (663), eabo5959. doi:10.1126/scitranslmed.abo5959
Lin, E. Y., Nguyen, A. V., Russell, R. G., and Pollard, J. W. (2001). Colony-stimulating factor 1 promotes progression of mammary tumors to malignancy. J. Exp. Med. 193 (6), 727–740. doi:10.1084/jem.193.6.727
Linde, N., Casanova-Acebes, M., Sosa, M. S., Mortha, A., Rahman, A., Farias, E., et al. (2018). Macrophages orchestrate breast cancer early dissemination and metastasis. Nat. Commun. 9 (1), 21. doi:10.1038/s41467-017-02481-5
Liu, Y. T., and Sun, Z. J. (2021). Turning cold tumors into hot tumors by improving T-cell infiltration. Theranostics 11 (11), 5365–5386. doi:10.7150/thno.58390
Liu, H. Y., Buenafe, A. C., Matejuk, A., Ito, A., Zamora, A., Dwyer, J., et al. (2002). Estrogen inhibition of EAE involves effects on dendritic cell function. J. Neurosci. Res. 70 (2), 238–248. doi:10.1002/jnr.10409
Liu, M., Sakamaki, T., Casimiro, M. C., Willmarth, N. E., Quong, A. A., Ju, X., et al. (2010). The canonical NF-kappaB pathway governs mammary tumorigenesis in transgenic mice and tumor stem cell expansion. Cancer Res. 70 (24), 10464–10473. doi:10.1158/0008-5472.CAN-10-0732
Liu, Y., Gu, Y., Han, Y., Zhang, Q., Jiang, Z., Zhang, X., et al. (2016). Tumor exosomal RNAs promote lung pre-metastatic niche formation by activating alveolar epithelial TLR3 to recruit neutrophils. Cancer Cell. 30 (2), 243–256. doi:10.1016/j.ccell.2016.06.021
Liu, Y., Zhou, Y., Huang, K. H., Li, Y., Fang, X., An, L., et al. (2019). EGFR-specific CAR-T cells trigger cell lysis in EGFR-positive TNBC. Aging (Albany NY) 11 (23), 11054–11072. doi:10.18632/aging.102510
Liu, Y., Zhou, Y., Huang, K. H., Fang, X., Li, Y., Wang, F., et al. (2020). Targeting epidermal growth factor-overexpressing triple-negative breast cancer by natural killer cells expressing a specific chimeric antigen receptor. Cell. Prolif. 53 (8), e12858. doi:10.1111/cpr.12858
Liu, J., Geng, X., Hou, J., and Wu, G. (2021). New insights into M1/M2 macrophages: Key modulators in cancer progression. Cancer Cell. Int. 21 (1), 389. doi:10.1186/s12935-021-02089-2
Loi, S., Pommey, S., Haibe-Kains, B., Beavis, P. A., Darcy, P. K., Smyth, M. J., et al. (2013). CD73 promotes anthracycline resistance and poor prognosis in triple negative breast cancer. Proc. Natl. Acad. Sci. U. S. A. 110 (27), 11091–11096. doi:10.1073/pnas.1222251110
Loi, S., MichielS, S., Salgado, R., SirtaiNe, N., Jose, V., Fumagalli, D., et al. (2014). Tumor infiltrating lymphocytes are prognostic in triple negative breast cancer and predictive for trastuzumab benefit in early breast cancer: Results from the FinHER trial. Ann. Oncol. 25 (8), 1544–1550. doi:10.1093/annonc/mdu112
Luo, C., Wang, P., He, S., Zhu, J., Shi, Y., and Wang, J. (2022). Progress and prospect of immunotherapy for triple-negative breast cancer. Front. Oncol. 12, 919072. doi:10.3389/fonc.2022.919072
Ma, J. H., Qin, L., and Li, X. (2020). Role of STAT3 signaling pathway in breast cancer. Cell. Commun. Signal 18 (1), 33. doi:10.1186/s12964-020-0527-z
Ma, B., Akosman, B., Kamle, S., Lee, C. M., He, C. H., Koo, J. S., et al. (2021). CHI3L1 regulates PD-L1 and anti-CHI3L1-PD-1 antibody elicits synergistic antitumor responses. J. Clin. Invest. 131 (21), e137750. doi:10.1172/JCI137750
Ma, S., Zhao, Y., Lee, W. C., Ong, L. T., Lee, P. L., Jiang, Z., et al. (2022). Hypoxia induces HIF1α-dependent epigenetic vulnerability in triple negative breast cancer to confer immune effector dysfunction and resistance to anti-PD-1 immunotherapy. Nat. Commun. 13 (1), 4118. doi:10.1038/s41467-022-31764-9
McDowell, S. A. C., Luo, R. B. E., Arabzadeh, A., Dore, S., Bennett, N. C., Breton, V., et al. (2021). Neutrophil oxidative stress mediates obesity-associated vascular dysfunction and metastatic transmigration. Nat. Cancer 2 (5), 545–562. doi:10.1038/s43018-021-00194-9
McGranahan, N., Furness, A. J. S., Rosenthal, R., Ramskov, S., Lyngaa, R., Saini, S. K., et al. (2016). Clonal neoantigens elicit T cell immunoreactivity and sensitivity to immune checkpoint blockade. Science 351 (6280), 1463–1469. doi:10.1126/science.aaf1490
Muz, B., de la Puente, P., Azab, F., and Azab, A. K. (2015). The role of hypoxia in cancer progression, angiogenesis, metastasis, and resistance to therapy. Hypoxia (Auckl) 3, 83–92. doi:10.2147/HP.S93413
Neubert, N. J., Schmittnaegel, M., Bordry, N., Nassiri, S., Wald, N., Martignier, C., et al. (2018). T cell-induced CSF1 promotes melanoma resistance to PD1 blockade. Sci. Transl. Med. 10 (436), eaan3311. doi:10.1126/scitranslmed.aan3311
Nicolini, A., and Carpi, A. (2005). Beta-interferon and interleukin-2 prolong more than three times the survival of 26 consecutive endocrine dependent breast cancer patients with distant metastases: An exploratory trial. Biomed. Pharmacother. 59 (5), 253–263. doi:10.1016/j.biopha.2004.05.019
Nicolini, A., Carpi, A., and Rossi, G. (2005). An immunotherapy schedule in endocrine-dependent metastatic breast cancer: Correlation between clinical course and immunologic parameters. J. Immunother. 28 (3), 276–279. doi:10.1097/01.cji.0000156827.67572.53
Nilsson, M. B., Zage, P. E., Zeng, L., Xu, L., Cascone, T., Wu, H. K., et al. (2010). Multiple receptor tyrosine kinases regulate HIF-1alpha and HIF-2alpha in normoxia and hypoxia in neuroblastoma: Implications for antiangiogenic mechanisms of multikinase inhibitors. Oncogene 29 (20), 2938–2949. doi:10.1038/onc.2010.60
Ohkuri, T., Kosaka, A., Ishibashi, K., Kumai, T., Hirata, Y., Ohara, K., et al. (2017). Intratumoral administration of cGAMP transiently accumulates potent macrophages for anti-tumor immunity at a mouse tumor site. Cancer Immunol. Immunother. 66 (6), 705–716. doi:10.1007/s00262-017-1975-1
Onkar, S. S., Carleton, N. M., Lucas, P. C., Bruno, T. C., Lee, A. V., Vignali, D. A. A., et al. (2023). The great immune escape: Understanding the divergent immune response in breast cancer subtypes. Cancer Discov. 13 (1), 23–40. doi:10.1158/2159-8290.CD-22-0475
Osta, W. A., Chen, Y., Mikhitarian, K., Mitas, M., Salem, M., Hannun, Y. A., et al. (2004). EpCAM is overexpressed in breast cancer and is a potential target for breast cancer gene therapy. Cancer Res. 64 (16), 5818–5824. doi:10.1158/0008-5472.CAN-04-0754
Owyong, M., Chou, J., van den Bijgaart, R. J., Kong, N., Efe, G., Maynard, C., et al. (2019). MMP9 modulates the metastatic cascade and immune landscape for breast cancer anti-metastatic therapy. Life Sci. Alliance 2 (6), e201800226. doi:10.26508/lsa.201800226
Pantelidou, C., Sonzogni, O., De Oliveria Taveira, M., Mehta, A. K., Kothari, A., Wang, D., et al. (2019). PARP inhibitor efficacy depends on CD8(+) T-cell recruitment via intratumoral STING pathway activation in BRCA-deficient models of triple-negative breast cancer. Cancer Discov. 9 (6), 722–737. doi:10.1158/2159-8290.CD-18-1218
Park, J., Wysocki, R. W., Amoozgar, Z., Maiorino, L., Fein, M. R., Jorns, J., et al. (2016). Cancer cells induce metastasis-supporting neutrophil extracellular DNA traps. Sci. Transl. Med. 8 (361), 361ra138. doi:10.1126/scitranslmed.aag1711
Peng, D., Kryczek, I., Nagarsheth, N., Zhao, L., Wei, S., Wang, W., et al. (2015). Epigenetic silencing of TH1-type chemokines shapes tumour immunity and immunotherapy. Nature 527 (7577), 249–253. doi:10.1038/nature15520
Pietrobon, V., and Marincola, F. M. (2021). Hypoxia and the phenomenon of immune exclusion. J. Transl. Med. 19 (1), 9. doi:10.1186/s12967-020-02667-4
Portillo, A. L., Hogg, R., Poznanski, S. M., Rojas, E. A., Cashell, N. J., Hammill, J. A., et al. (2021). Expanded human NK cells armed with CAR uncouple potent anti-tumor activity from off-tumor toxicity against solid tumors. iScience 24 (6), 102619. doi:10.1016/j.isci.2021.102619
Priego, N., Zhu, L., Monteiro, C., Mulders, M., Wasilewski, D., Bindeman, W., et al. (2018). STAT3 labels a subpopulation of reactive astrocytes required for brain metastasis. Nat. Med. 24 (7), 1024–1035. doi:10.1038/s41591-018-0044-4
Pyonteck, S. M., Akkari, L., Schuhmacher, A. J., Bowman, R. L., Sevenich, L., Quail, D. F., et al. (2013). CSF-1R inhibition alters macrophage polarization and blocks glioma progression. Nat. Med. 19 (10), 1264–1272. doi:10.1038/nm.3337
Quail, D. F., and Joyce, J. A. (2013). Microenvironmental regulation of tumor progression and metastasis. Nat. Med. 19 (11), 1423–1437. doi:10.1038/nm.3394
Quail, D. F., Olson, O. C., Bhardwaj, P., Walsh, L. A., Akkari, L., Quick, M. L., et al. (2017). Obesity alters the lung myeloid cell landscape to enhance breast cancer metastasis through IL5 and GM-CSF. Nat. Cell. Biol. 19 (8), 974–987. doi:10.1038/ncb3578
Rampioni Vinciguerra, G. L., Sonego, M., Segatto, I., Dall'Acqua, A., Vecchione, A., Baldassarre, G., et al. (2022). CDK4/6 inhibitors in combination therapies: Better in company than alone: A mini review. Front. Oncol. 12, 891580. doi:10.3389/fonc.2022.891580
Richards, J. O., Albers, A. J., Smith, T. S., and Tjoe, J. A. (2016). NK cell-mediated antibody-dependent cellular cytotoxicity is enhanced by tamoxifen in HER2/neu non-amplified, but not HER2/neu-amplified, breast cancer cells. Cancer Immunol. Immunother. 65 (11), 1325–1335. doi:10.1007/s00262-016-1885-7
Ronchetti, L., Boubaker, N. S., Barba, M., Vici, P., Gurtner, A., and Piaggio, G. (2021). Neutrophil extracellular traps in cancer: Not only catching microbes. J. Exp. Clin. Cancer Res. 40 (1), 231. doi:10.1186/s13046-021-02036-z
Ruiz-Fernandez de Cordoba, B., Moreno, H., Valencia, K., Perurena, N., Ruedas, P., Walle, T., et al. (2022). Tumor ENPP1(CD203a)/haptoglobin Axis exploits myeloid-derived suppressor cells to promote post-radiotherapy local recurrence in breast cancer. Cancer Discov. 12, 1356–1377. doi:10.1158/2159-8290.cd-21-0932
Safonov, A., Jiang, T., Bianchini, G., Gyorffy, B., Karn, T., Hatzis, C., et al. (2017). Immune gene expression is associated with genomic aberrations in breast cancer. Cancer Res. 77 (12), 3317–3324. doi:10.1158/0008-5472.CAN-16-3478
Sangiovanni, A., Del Ninno, E., Fasani, P., De Fazio, C., Ronchi, G., Romeo, R., et al. (2004). Increased survival of cirrhotic patients with a hepatocellular carcinoma detected during surveillance. Gastroenterology 126 (4), 1005–1014. doi:10.1053/j.gastro.2003.12.049
Schmid, P., Cortes, J., Pusztai, L., McArthur, H., Kummel, S., Bergh, J., et al. (2020). Pembrolizumab for early triple-negative breast cancer. N. Engl. J. Med. 382 (9), 810–821. doi:10.1056/NEJMoa1910549
Scirocchi, F., Scagnoli, S., Botticelli, A., Di Filippo, A., Napoletano, C., Zizzari, I. G., et al. (2022). Immune effects of CDK4/6 inhibitors in patients with HR(+)/HER2(-) metastatic breast cancer: Relief from immunosuppression is associated with clinical response. EBioMedicine 79, 104010. doi:10.1016/j.ebiom.2022.104010
Sen, M., Wankowski, D. M., Garlie, N. K., Siebenlist, R. E., Van Epps, D., LeFever, A. V., et al. (2001). Use of anti-CD3 x anti-HER2/neu bispecific antibody for redirecting cytotoxicity of activated T cells toward HER2/neu+ tumors. J. Hematother Stem Cell. Res. 10 (2), 247–260. doi:10.1089/15258160151134944
SenGupta, S., Hein, L. E., and Parent, C. A. (2021). The recruitment of neutrophils to the tumor microenvironment is regulated by multiple mediators. Front. Immunol. 12, 734188. doi:10.3389/fimmu.2021.734188
Shimabukuro-Vornhagen, A., Godel, P., Subklewe, M., Stemmler, H. J., Schloser, H. A., Schlaak, M., et al. (2018). Cytokine release syndrome. J. Immunother. Cancer 6 (1), 56. doi:10.1186/s40425-018-0343-9
Shinde-Jadhav, S., Mansure, J. J., Rayes, R. F., Marcq, G., Ayoub, M., Skowronski, R., et al. (2021). Role of neutrophil extracellular traps in radiation resistance of invasive bladder cancer. Nat. Commun. 12 (1), 2776. doi:10.1038/s41467-021-23086-z
Siersbaek, R., Scabia, V., Nagarajan, S., Chernukhin, I., Papachristou, E. K., Broome, R., et al. (2020). IL6/STAT3 signaling hijacks estrogen receptor alpha enhancers to drive breast cancer metastasis. Cancer Cell. 38 (3), 412–423. doi:10.1016/j.ccell.2020.06.007
Slamon, D. J., Neven, P., Chia, S., Fasching, P. A., De Laurentiis, M., Im, S. A., et al. (2020). Overall survival with ribociclib plus fulvestrant in advanced breast cancer. N. Engl. J. Med. 382 (6), 514–524. doi:10.1056/NEJMoa1911149
Smith, H. W., Hirukawa, A., Sanguin-Gendreau, V., Nandi, I., Dufour, C. R., Zuo, D., et al. (2019). An ErbB2/c-Src axis links bioenergetics with PRC2 translation to drive epigenetic reprogramming and mammary tumorigenesis. Nat. Commun. 10 (1), 2901. doi:10.1038/s41467-019-10681-4
Stamm, H., Oliveira-Ferrer, L., Grossjohann, E. M., Muschhammer, J., Thaden, V., Brauneck, F., et al. (2019). Targeting the TIGIT-PVR immune checkpoint axis as novel therapeutic option in breast cancer. Oncoimmunology 8 (12), e1674605. doi:10.1080/2162402X.2019.1674605
Strachan, D. C., Ruffell, B., Oei, Y., Bissell, M. J., Coussens, L. M., Pryer, N., et al. (2013). CSF1R inhibition delays cervical and mammary tumor growth in murine models by attenuating the turnover of tumor-associated macrophages and enhancing infiltration by CD8(+) T cells. Oncoimmunology 2 (12), e26968. doi:10.4161/onci.26968
Strickler, J. H., Hanks, B. A., and Khasraw, M. (2021). Tumor mutational burden as a predictor of immunotherapy response: Is more always better? Clin. Cancer Res. 27 (5), 1236–1241. doi:10.1158/1078-0432.CCR-20-3054
Svoronos, N., Perales-Puchalt, A., Allegrezza, M. J., Rutkowski, M. R., Payne, K. K., Tesone, A. J., et al. (2017). Tumor cell-independent estrogen signaling drives disease progression through mobilization of myeloid-derived suppressor cells. Cancer Discov. 7 (1), 72–85. doi:10.1158/2159-8290.CD-16-0502
Szoor, A., Toth, G., Zsebik, B., Szabo, V., Eshhar, Z., Abken, H., et al. (2020). Trastuzumab derived HER2-specific CARs for the treatment of trastuzumab-resistant breast cancer: CAR T cells penetrate and eradicate tumors that are not accessible to antibodies. Cancer Lett. 484, 1–8. doi:10.1016/j.canlet.2020.04.008
Tchou, J., Zhao, Y., Levine, B. L., Zhang, P. J., Davis, M. M., Melenhorst, J. J., et al. (2017). Safety and efficacy of intratumoral injections of chimeric antigen receptor (CAR) T cells in metastatic breast cancer. Cancer Immunol. Res. 5 (12), 1152–1161. doi:10.1158/2326-6066.CIR-17-0189
Teijeira, A., Garasa, S., Gato, M., Alfaro, C., Migueliz, I., Cirella, A., et al. (2020). CXCR1 and CXCR2 chemokine receptor agonists produced by tumors induce neutrophil extracellular traps that interfere with immune cytotoxicity. Immunity 52 (5), 856–871. doi:10.1016/j.immuni.2020.03.001
Telarovic, I., Wenger, R. H., and Pruschy, M. (2021). Interfering with tumor hypoxia for radiotherapy optimization. J. Exp. Clin. Cancer Res. 40 (1), 197. doi:10.1186/s13046-021-02000-x
Thomas, A., Routh, E. D., Pullikuth, A., Jin, G., Su, J., Chou, J. W., et al. (2018). Tumor mutational burden is a determinant of immune-mediated survival in breast cancer. Oncoimmunology 7 (10), e1490854. doi:10.1080/2162402X.2018.1490854
Toniolo, A., Fadini, G. P., Tedesco, S., Cappellari, R., Vegeto, E., Maggi, A., et al. (2015). Alternative activation of human macrophages is rescued by estrogen treatment in vitro and impaired by menopausal status. J. Clin. Endocrinol. Metab. 100 (1), E50–E58. doi:10.1210/jc.2014-2751
Tsao, L. C., Crosby, E. J., Trotter, T. N., Agarwal, P., Hwang, B. J., Acharya, C., et al. (2019). CD47 blockade augmentation of trastuzumab antitumor efficacy dependent on antibody-dependent cellular phagocytosis. JCI Insight 4 (24), e131882. doi:10.1172/jci.insight.131882
Turcotte, M., Allard, D., Mittal, D., Bareche, Y., Buisseret, L., Jose, V., et al. (2017). CD73 promotes resistance to HER2/ErbB2 antibody therapy. Cancer Res. 77 (20), 5652–5663. doi:10.1158/0008-5472.CAN-17-0707
Turner, N. C., Swift, C., Kilburn, L., Fribbens, C., Beaney, M., Garcia-Murillas, I., et al. (2020). ESR1 mutations and overall survival on fulvestrant versus exemestane in advanced hormone receptor-positive breast cancer: A combined analysis of the phase III SoFEA and efect trials. Clin. Cancer Res. 26 (19), 5172–5177. doi:10.1158/1078-0432.CCR-20-0224
Turpin, J., Ling, C., Crosby, E. J., Hartman, Z. C., Simond, A. M., Chodosh, L. A., et al. (2016). The ErbB2ΔEx16 splice variant is a major oncogenic driver in breast cancer that promotes a pro-metastatic tumor microenvironment. Oncogene 35 (47), 6053–6064. doi:10.1038/onc.2016.129
Uniacke, J., Holterman, C. E., Lachance, G., Franovic, A., Jacob, M. D., Fabian, M. R., et al. (2012). An oxygen-regulated switch in the protein synthesis machinery. Nature 486 (7401), 126–129. doi:10.1038/nature11055
van Mierlo, G., Veenstra, G. J. C., Vermeulen, M., and Marks, H. (2019). The complexity of PRC2 subcomplexes. Trends Cell. Biol. 29 (8), 660–671. doi:10.1016/j.tcb.2019.05.004
Vincent, J., Mignot, G., Chalmin, F., Ladoire, S., Bruchard, M., Chevriaux, A., et al. (2010). 5-Fluorouracil selectively kills tumor-associated myeloid-derived suppressor cells resulting in enhanced T cell-dependent antitumor immunity. Cancer Res. 70 (8), 3052–3061. doi:10.1158/0008-5472.CAN-09-3690
Vorobjeva, N. V., and Chernyak, B. V. (2020). NETosis: Molecular mechanisms, role in physiology and pathology. Biochem. (Mosc) 85 (10), 1178–1190. doi:10.1134/S0006297920100065
Wang, J., Hu, P., Zeng, M., Rabkin, S. D., and Liu, R. (2012). Oncolytic herpes simplex virus treatment of metastatic breast cancer. Int. J. Oncol. 40 (3), 757–763. doi:10.3892/ijo.2011.1266
Wang, W., Nag, S. A., and Zhang, R. (2015). Targeting the NFκB signaling pathways for breast cancer prevention and therapy. Curr. Med. Chem. 22 (2), 264–289. doi:10.2174/0929867321666141106124315
Wang, B., Zhao, Q., Zhang, Y., Liu, Z., Zheng, Z., Liu, S., et al. (2021). Targeting hypoxia in the tumor microenvironment: A potential strategy to improve cancer immunotherapy. J. Exp. Clin. Cancer Res. 40 (1), 24. doi:10.1186/s13046-020-01820-7
Watanabe, T., Hida, A. I., Inoue, N., Imamura, M., Fujimoto, Y., Akazawa, K., et al. (2018). Abundant tumor infiltrating lymphocytes after primary systemic chemotherapy predicts poor prognosis in estrogen receptor-positive/HER2-negative breast cancers. Breast Cancer Res. Treat. 168 (1), 135–145. doi:10.1007/s10549-017-4575-z
Wculek, S. K., and Malanchi, I. (2015). Neutrophils support lung colonization of metastasis-initiating breast cancer cells. Nature 528 (7582), 413–417. doi:10.1038/nature16140
Wei, L., Wang, X., Luo, M., Wang, H., Chen, H., and Huang, C. (2021). The PAD4 inhibitor GSK484 enhances the radiosensitivity of triple-negative breast cancer. Hum. Exp. Toxicol. 40 (7), 1074–1083. doi:10.1177/0960327120979028
Welm, A. L., Sneddon, J. B., Taylor, C., Nuyten, D. S. A., van de Vijver, M. J., Hasegawa, B. H., et al. (2007). The macrophage-stimulating protein pathway promotes metastasis in a mouse model for breast cancer and predicts poor prognosis in humans. Proc. Natl. Acad. Sci. U. S. A. 104 (18), 7570–7575. doi:10.1073/pnas.0702095104
Werner, L. R., Gibson, K. A., Goodman, M. L., Helm, D. E., Walter, K. R., Holloran, S. M., et al. (2021). Progesterone promotes immunomodulation and tumor development in the murine mammary gland. J. Immunother. Cancer 9 (5), e001710. doi:10.1136/jitc-2020-001710
Williams, M. M., Spoelstra, N. S., Arnesen, S., O'Neill, K. I., Christenson, J. L., Reese, J., et al. (2021). Steroid hormone receptor and infiltrating immune cell status reveals therapeutic vulnerabilities of ESR1-mutant breast cancer. Cancer Res. 81 (3), 732–746. doi:10.1158/0008-5472.CAN-20-1200
Wu, S., Zhang, Q., Zhang, F., Meng, F., Liu, S., Zhou, R., et al. (2019). HER2 recruits AKT1 to disrupt STING signalling and suppress antiviral defence and antitumour immunity. Nat. Cell. Biol. 21 (8), 1027–1040. doi:10.1038/s41556-019-0352-z
Wyckoff, J. B., Wang, Y., Lin, E. Y., Li, J. F., Goswami, S., Stanley, E. R., et al. (2007). Direct visualization of macrophage-assisted tumor cell intravasation in mammary tumors. Cancer Res. 67 (6), 2649–2656. doi:10.1158/0008-5472.CAN-06-1823
Xiao, B. G., Liu, X., and Link, H. (2004). Antigen-specific T cell functions are suppressed over the estrogen-dendritic cell-indoleamine 2,3-dioxygenase axis. Steroids 69 (10), 653–659. doi:10.1016/j.steroids.2004.05.019
Xiao, G., Jin, L. L., Liu, C. Q., Wang, Y. C., Meng, Y. M., Zhou, Z. G., et al. (2019). EZH2 negatively regulates PD-L1 expression in hepatocellular carcinoma. J. Immunother. Cancer 7 (1), 300. doi:10.1186/s40425-019-0784-9
Xu, N., Palmer, D. C., Robeson, A. C., Shou, P., Bommiasamy, H., Laurie, S. J., et al. (2021). STING agonist promotes CAR T cell trafficking and persistence in breast cancer. J. Exp. Med. 218 (2), e20200844. doi:10.1084/jem.20200844
Yao, J., Li, S., and Wang, X. (2021). Identification of breast cancer immune subtypes by analyzing bulk tumor and single cell transcriptomes. Front. Cell. Dev. Biol. 9, 781848. doi:10.3389/fcell.2021.781848
Yin, M., Hu, J., Yuan, Z., Luo, G., Yao, J., Wang, R., et al. (2022). STING agonist enhances the efficacy of programmed death-ligand 1 monoclonal antibody in breast cancer immunotherapy by activating the interferon-beta signalling pathway. Cell. Cycle 21 (8), 767–779. doi:10.1080/15384101.2022.2029996
Zacharakis, N., Chinnasamy, H., Black, M., Xu, H., Lu, Y. C., Zheng, Z., et al. (2018). Immune recognition of somatic mutations leading to complete durable regression in metastatic breast cancer. Nat. Med. 24 (6), 724–730. doi:10.1038/s41591-018-0040-8
Zhang, H., Lu, H., Xiang, L., Bullen, J. W., Zhang, C., Samanta, D., et al. (2015). HIF-1 regulates CD47 expression in breast cancer cells to promote evasion of phagocytosis and maintenance of cancer stem cells. Proc. Natl. Acad. Sci. U. S. A. 112 (45), E6215–E6223. doi:10.1073/pnas.1520032112
Zhang, M., Hutter, G., Kahn, S. A., Azad, T. D., Gholamin, S., Xu, C. Y., et al. (2016). Anti-CD47 treatment stimulates phagocytosis of glioblastoma by M1 and M2 polarized macrophages and promotes M1 polarized macrophages in vivo. PLoS One 11 (4), e0153550. doi:10.1371/journal.pone.0153550
Zhang, M., Sun, H., Zhao, S., Wang, Y., Pu, H., Wang, Y., et al. (2017). Expression of PD-L1 and prognosis in breast cancer: A meta-analysis. Oncotarget 8 (19), 31347–31354. doi:10.18632/oncotarget.15532
Zhang, Y., Zhang, H., Wang, M., Schmid, T., Xin, Z., Kozhuharova, L., et al. (2021). Hypoxia in breast cancer-scientific translation to therapeutic and diagnostic clinical applications. Front. Oncol. 11, 652266. doi:10.3389/fonc.2021.652266
Zhang, M., Liu, Z. Z., Aoshima, K., Cai, W. L., Sun, H., Xu, T., et al. (2022). CECR2 drives breast cancer metastasis by promoting NF-κB signaling and macrophage-mediated immune suppression. Sci. Transl. Med. 14 (630), eabf5473. doi:10.1126/scitranslmed.abf5473
Zhao, Z., Li, Y., Liu, W., and Li, X. (2020). Engineered IL-7 receptor enhances the therapeutic effect of AXL-CAR-T cells on triple-negative breast cancer. Biomed. Res. Int. 2020, 4795171. doi:10.1155/2020/4795171
Zhao, T., Su, Z., Li, Y., Zhang, X., and You, Q. (2020). Chitinase-3 like-protein-1 function and its role in diseases. Signal Transduct. Target Ther. 5 (1), 201. doi:10.1038/s41392-020-00303-7
Zheng, X., Fang, Z., Liu, X., Deng, S., Zhou, P., Wang, X., et al. (2018). Increased vessel perfusion predicts the efficacy of immune checkpoint blockade. J. Clin. Invest. 128 (5), 2104–2115. doi:10.1172/JCI96582
Zhu, Y., An, X., Zhang, X., Qiao, Y., Zheng, T., and Li, X. (2019). Sting: A master regulator in the cancer-immunity cycle. Mol. Cancer 18 (1), 152. doi:10.1186/s12943-019-1087-y
Zitvogel, L., Apetoh, L., Ghiringhelli, F., and Kroemer, G. (2008). Immunological aspects of cancer chemotherapy. Nat. Rev. Immunol. 8 (1), 59–73. doi:10.1038/nri2216
Zou, S., Tong, Q., Liu, B., Huang, W., Tian, Y., and Fu, X. (2020). Targeting STAT3 in cancer immunotherapy. Mol. Cancer 19 (1), 145. doi:10.1186/s12943-020-01258-7
Zundelevich, A., Dadiani, M., Kahana-Edwin, S., Itay, A., Sella, T., Gadot, M., et al. (2020). ESR1 mutations are frequent in newly diagnosed metastatic and loco-regional recurrence of endocrine-treated breast cancer and carry worse prognosis. Breast Cancer Res. 22 (1), 16. doi:10.1186/s13058-020-1246-5
Keywords: breast cancer, immunology, immune microenvironment, therapies, and clinical trials
Citation: Attalla S, Taifour T and Muller W (2023) Tailoring therapies to counter the divergent immune landscapes of breast cancer. Front. Cell Dev. Biol. 11:1111796. doi: 10.3389/fcell.2023.1111796
Received: 30 November 2022; Accepted: 25 January 2023;
Published: 22 February 2023.
Edited by:
Giusy Tornillo, Cardiff University, United KingdomReviewed by:
Andrea Casazza, Montis Bioscience, BelgiumWenjun Guo, Albert Einstein College of Medicine, United States
Copyright © 2023 Attalla, Taifour and Muller. This is an open-access article distributed under the terms of the Creative Commons Attribution License (CC BY). The use, distribution or reproduction in other forums is permitted, provided the original author(s) and the copyright owner(s) are credited and that the original publication in this journal is cited, in accordance with accepted academic practice. No use, distribution or reproduction is permitted which does not comply with these terms.
*Correspondence: Sherif Attalla, sherif.attalla@mail.mcgill.ca; William Muller, william.muller@mcgill.ca