- Department of Genetics, Development, and Cell Biology, Iowa State University, Ames, IA, United States
Accurate chromosome segregation is vital for cell and organismal viability. The mitotic spindle, a bipolar macromolecular machine composed largely of dynamic microtubules, is responsible for chromosome segregation during each cell replication cycle. Prior to anaphase, a bipolar metaphase spindle must be formed in which each pair of chromatids is attached to microtubules from opposite spindle poles. In this bipolar configuration pulling forces from the dynamic microtubules can generate tension across the sister kinetochores. The tension status acts as a signal that can destabilize aberrant kinetochore-microtubule attachments and reinforces correct, bipolar connections. Historically it has been challenging to isolate the specific role of tension in mitotic processes due to the interdependency of attachment and tension status at kinetochores. Recent technical and experimental advances have revealed new insights into how tension functions during mitosis. Here we summarize the evidence that tension serves as a biophysical signal that unifies multiple aspects of kinetochore and centromere function to ensure accurate chromosome segregation.
Introduction
The mitotic spindle, a highly organized yet morphologically dynamic macromolecular machine composed largely of microtubules and associated proteins, is essential to successfully segregate chromosomes during each round of mitosis. The metaphase spindle has a conserved steady-state structure, which is inherently stable in a bipolar configuration that focuses the microtubules into two poles, crosslinks interpolar microtubules to maintain pole separation, and attaches sister chromatids to kinetochore microtubules from opposite poles (Figure 1).
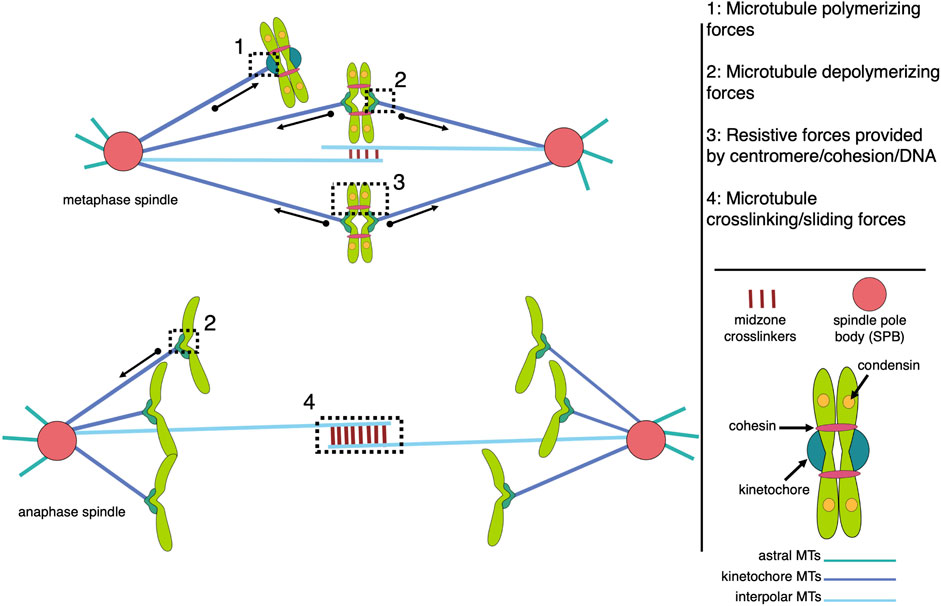
FIGURE 1. Models of microtubule-associated forces in metaphase and anaphase mitotic spindles. The spindle is composed of three major classes of microtubules (interpolar, kinetochore, and astral), each with unique functions that contribute to the forces generated within the bipolar structure. The forces of note include pushing and pulling forces resulting from microtubule polymerization and depolymerization, respectively, which are largely responsible for chromosome movement (1 and 2), and resistive forces, or tension, generated across pairs of sister kinetochores and centromeres, which are coupled by centromere-associated condensin and cohesin protein complexes (3). MAPs (microtubule associated proteins) crosslink antiparallel interpolar microtubules to create a stable midzone that allows kinesin motor proteins to generate sliding forces that push the spindle poles apart (4). While all four types of forces are active in metaphase spindles, tension across sisters is terminated by cohesion cleavage at the metaphase-to-anaphase transition while anaphase chromosome movement is dominated by microtubule-generated pulling forces.
Microtubules are inherently dynamic polymers composed of tubulin protein, a heterodimer of alpha- and beta-tubulin subunits. These dynamic microtubules are organized and coordinated by the actions of many conserved microtubule associated proteins (MAPs). A key aspect of spindle function is that chromatid pairs will be segregated to opposite poles, and thus into different daughter cells, via depolymerizing microtubules, or kinetochore-fibers, by attaching the kinetochores of sister chromatids to microtubules emanating from opposite poles. The kinetochore is a proteinaceous complex that forms on the single centromere of each chromosome. It serves as a physical linkage between the chromosomal centromere and the attached microtubule. When a dynamic microtubule becomes attached to, or captured, by a kinetochore, it can generate a pulling force that creates tension across the sister kinetochores if they are attached to opposite spindle poles. Both the microtubule attachment status and the tension across bipolar attached sister kinetochores serve to ensure chromosomes are accurately segregated during anaphase.
Several decades of work have helped elucidate what proteins mediate the kinetochore-microtubule attachment, how unattached kinetochores act as a signal to delay anaphase onset, and how the phosphorylation of kinetochore proteins regulates the strength of the kinetochore-microtubule attachment. Efforts to discern the mechanisms that sense and respond to microtubule-generated tension at kinetochores have been comparably more difficult due to the interdependency of tension and attachment. Here we provide a brief overview of our understanding of the forces in the bipolar mitotic spindle and how those forces allow dynamic microtubule-kinetochore attachments to generate tension across sister chromatids. We summarize how tension acts as a unifying force that alters kinetochore and centromere structure, mediates Aurora B activity, corrects erroneous attachments, and regulates mitotic progression.
Forces in the mitotic spindle and associated kinetochore-microtubule tension
The forces acting on and within the mitotic spindle must be balanced to facilitate a stable metaphase configuration with bipolar chromatid attachments (Figure 1). Forces in the spindle can be passive, such as friction or structural elasticity, or active, which requires energy input and can result in mechanical output, such as rearrangements or movement (For a review of all spindle forces, see Nazockdast and Redemann (2020); Dumont and Mitchison (2009). In addition to motor proteins, e.g., kinesins, microtubule polymerization (elongation) and depolymerization (shortening) in the spindle can generate these active forces. Microtubule polymerization and depolymerization are both thermodynamically favorable reactions, relying ultimately on the energy of GTP binding to free tubulin and subsequent hydrolysis within the microtubule polymer (Mitchison and Kirschner, 1984).
The forces generated by a single polymerizing microtubule have been measured to be up to 3–4 pN (Dogterom and Yurke, 1997); (Janson et al., 2003). This force is limited in longer microtubules due to an increase in propensity for buckling (Dogterom and Yurke, 1997), yet microtubule bundling by MAPs can increase overall rigidity while additively increasing their combined force-generating potential (Laan et al., 2008). The so-called polar ejection forces are a well-characterized example of microtubule polymerization-driven forces in the mitotic spindle. These forces, which push chromosomes from near the poles toward the central region of the spindle to aid in chromosome congression, are generated by a combination of polymerizing microtubules and kinesins interacting with chromosome arms (Brouhard and Hunt, 2005); (Ke et al., 2009). In Drosophila, polar ejection forces are generated by microtubule polymerization, while NOD, a kinesin-10 motor, couples growing microtubule tips with chromosome arms supporting a polymer ratchet mechanism (Cochran et al., 2009). NOD also has its own plus-end directed motility and plus-end tracking ability via EB1 interaction (Ye et al., 2018). Thus, NOD has two force generating activities that contribute to polar ejection forces (Ye et al., 2018). In HeLa cells, the kinesin-10, Kid, links polymerizing microtubule tips with chromosome arms, while a second motor, the kinesin-4 Kif4A, regulates microtubule growth (Stumpff et al., 2012). Another function of microtubule polymerization-derived force is to position microtubule organizing centers within the cell. For example, microtubules pushing against opposite sides of the cell cortex work to center the nucleus in fission yeast (Tran et al., 2001). More recently, microtubules pushing against the cell cortex were shown to maintain metaphase spindle positioning at the cell center in C. elegans embryos (Garzon-Coral et al., 2016).
The forces associated with microtubule depolymerization are significantly larger than those resulting from polymer growth. In a pioneering study, the wave of curling protofilaments that accompanies depolymerizing microtubules tips was measured to produce 0.5 pN on a bead positioned on one side of the depolymerizing polymer (Grishchuk et al., 2005). In an updated adaptation of this “wave assay”, these forces were measured to be between 8–16 pN (Driver et al., 2017). The total pulling force generated by all protofilaments of a depolymerizing microtubule remains to be directly measured, but has been extrapolated to be 30–65 pN (Grishchuk et al., 2005). Poleward-directed movement of chromosomes on the metaphase plate and during anaphase is driven by the depolymerization of microtubules attached to their kinetochores (Koshland et al., 1988); (Grishchuk and McIntosh, 2006). Indeed, purified budding yeast kinetochores attached to a single microtubule in an end-on manner can withstand load-bearing forces reaching up to 11 pN (Akiyoshi et al., 2010). When a microtubule, attached end-on to a kinetochore, depolymerizes, it generates a pulling force on that kinetochore and its associated chromosome (Figure 2). The kinetochore proteins can oppose this force if there is sufficient resistance to chromosome movement. In the case where the kinetochores of sister chromatids are attached to microtubules from opposite poles, this resistance is mediated by the cohesin and condensin protein complexes linking the chromatids and the elasticity of the pericentromeric chromatin. In this bipolar configuration, the pulling forces generated at one or both microtubule-kinetochore attachments result in a tension force across the coupled sister kinetochores. This force is analogous to the tension transmitted through a rope pulled from opposite ends. When evaluating potential mechanisms involved in sensing and responding to this tension status, it is relevant to consider that the force is transmitted across the entire linkage, including kinetochore components, centromeric DNA/proteins, pericentromeric chromatin, as well as condensin and cohesin complexes. Thus, any component in this linkage could be involved in sensing and responding to the general tension status of the sister kinetochores.
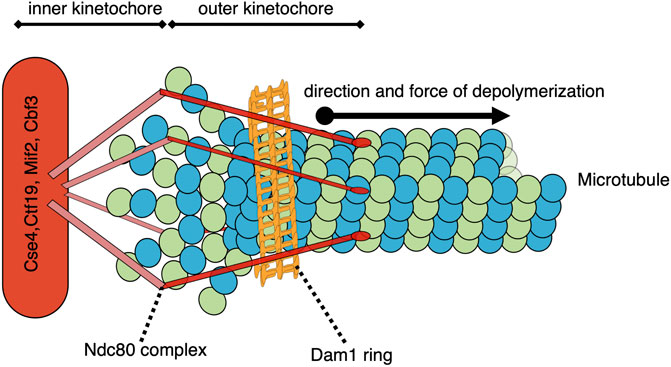
FIGURE 2. Model of a simplified yeast kinetochore-microtubule attachment with a catch bond-like connection. While many proteins comprise the kinetochore and/or participate in the microtubule-kinetochore attachment, two structures of note are the Ndc80 complex and the Dam1/DASH complex ring. As the end-on microtubule depolymerizes into tubulin heterodimers, the 13 protofilaments each curve outward. These protofilaments are constrained within the collar formed by the ring of Dam1/DASH complexes, and their bending drives the collar further onto the depolymerizing microtubule. The Dam1/DASH ring then pulls the associated centromere/kinetochore via the Ndc80-mediated coupling. This pulling force could potentially be sensed by the Dam1/DASH complex, Ndc80, other outer or inner kinetochore proteins, centromere-associated proteins, DNA, or proteins involved in the coupling between the sister centromeres.
The role of tension in accurate chromosome segregation has been a fundamental question since the pioneering experiments in the 1960s with the micromanipulation of chromosomes during meiotic divisions in grasshopper spermatocytes (Nicklas and Koch, 1969). Evidence for tension serving as a prominent force within the spindle comes from many studies. For example, analysis of kinetochores on oscillating metaphase chromosomes revealed sites of active and passive force generation (Dumont and Desai, 2012). In fission yeast, severing the microtubules on one side of the metaphase spindle causes the sister chromatids to move toward the spindle pole on the intact side (Klemm et al., 2018). Overall, evidence demonstrates that microtubule depolymerization generates a pulling force on the attached kinetochore, which, in the case of sister chromatids attached to opposite spindle poles, results in a tension force transmitted across the sister kinetochore linkage.
How microtubule-dependent forces and kinetochore structure lead to chromosome movement
Forces generated by depolymerizing microtubules attached to the kinetochore are vital for creating the pulling forces responsible for chromosome movement during anaphase (Koshland et al., 1988); (Grishchuk and McIntosh, 2006). In addition to depolymerization at the microtubule ‘plus-end’, which is attached to the kinetochore, in most organisms the kinetochore microtubules also undergo a process called flux. This flux is driven by simultaneous polymerization of kinetochore-associated plus-end and depolymerization of the ‘minus-end’ at the spindle pole (Mitchison, 1989). If the depolymerization rate at the pole exceeds polymerization at the kinetochore, it will result in a pulling force toward that pole (Vukušić et al., 2019). The relative contribution of plus-end depolymerization or flux to the overall force experienced at kinetochores varies by organism (Maddox et al., 2000); (Mallavarapu et al., 1999); (Maddox et al., 2002); (Ganem et al., 2005). Overall, microtubule depolymerization at the kinetochore and the poles works to shorten the distance between the spindle pole and the attached kinetochore, thus generating the main forces responsible for poleward chromosome movements and for increasing the tension across bipolar attached sister kinetochores (Inoue, 1953); (Inoué and Ritter Jr, 1975); (Salmon et al., 1976); (Asbury, 2017).
The forces that move chromosomes are significantly larger than the thermally driven background forces in cells and are estimated at 4–5 pN in budding yeast and upwards of hundreds of piconewtons in Drosophila cells (Chacón et al., 2014); (Ye et al., 2016). The microtubule tip must be connected perpendicularly to proteins on the outer face of the kinetochore, forming an end-on attachment to withstand such high forces (Asbury, 2017); (Gudimchuk et al., 2020). The KMN network is a group of kinetochore proteins that is essential for forming end-on attachments, and when impaired, results in chromosome segregation defects (Cheeseman et al., 2006); (DeLuca et al., 2005); (DeLuca et al., 2006); (Kim and Yu, 2015); (McCleland et al., 2003) (for review of KMN network see Varma and Salmon (2012). The conserved protein Ndc80, one of the outermost in the KMN network, is needed for microtubule-dependent force production at kinetochores and specifically needed for generating end-on attachments (Wimbish et al., 2020); (Huis in ’t Veld et al., 2019); (Cheeseman and Desai, 2008); (Alushin et al., 2010); (Cheeseman et al., 2006); (Tooley and Stukenberg, 2011); (Suzuki et al., 2016). Much study has focused on the role of the unstructured tail region of Ndc80 in forming attachments, although results indicate this may vary among organisms. While the tail appears dispensable for generating end-on attachments in S. cerevisiae and C. elegans (Demirel et al., 2012); (Cheerambathur et al., 2013), recent studies using human Ndc80 produced conflicting results (Wimbish et al., 2020); (Huis in ’t Veld et al., 2019). While the exact role of the Ndc80 tail in forming end-on attachment in some organisms remains in question, Ndc80 itself is essential for proper load bearing at kinetochore-microtubule attachments across eukaryotes.
Microtubule associated proteins strengthen load-bearing capacity of kinetochore-microtubule attachments
In addition to central kinetochore components like Ndc80, several less-conserved MAPs are also essential or aid in forming force-generating microtubule attachments at kinetochores. Many of these are recruited to kinetochores via Ndc80 and are important for regulating the attachment to dynamic microtubules (Amin et al., 2019). Highlighted below are MAPs or protein complexes that have been well characterized in their roles to support robust kinetochore-microtubule attachment. For a comprehensive review of MAPs involved in the metaphase spindle and kinetochore-microtubule attachment, see Amin et al. (2019).
In budding yeast, the Dam1/DASH complex is essential for microtubule-kinetochore attachments and dependent on Ndc80 for its kinetochore localization. Although Dam1 appears to be the main microtubule-binding protein in the complex, the nine other DASH subunits are also essential (Westermann et al., 2006); (Asbury et al., 2006); (Grishchuk et al., 2008); (Janke et al., 2001). Mutations in any of the DASH subunits lead to weakened microtubule-kinetochore attachments (Cheeseman et al., 2001). The heterodecameric Dam1/DASH complex oligomerizes to form a ring around the microtubule that is required for persistent kinetochore attachment to dynamic microtubules (Lampert et al., 2010); (Westermann et al., 2005); (Asbury et al., 2006); (Westermann et al., 2006); (Tien et al., 2010); (Tanaka et al., 2007); (Grishchuk et al., 2008). The ring is proposed to function as a collar that can harness the forces produced by depolymerizing microtubules (Figure 2) (Lampert et al., 2010); (Westermann et al., 2005); (Asbury et al., 2006); (Westermann et al., 2006); (Tien et al., 2010); (Tanaka et al., 2007); (Grishchuk et al., 2008); (Kiermaier et al., 2009); (Lacefield et al., 2009); (Lampert et al., 2013); (Miranda et al., 2005); (Umbreit et al., 2014). The rigid, collar-like structure of the ring maintains contact with the microtubule lattice and Ndc80 via flexible C-terminal extensions of the Dam1/DASH complex (Jenni and Harrison, 2018). This flexible connection could potentially accommodate different kinetochore-microtubule configurations, such as lateral versus end-on attachments during the cell cycle. In addition to mediating forces, the Dam1/DASH complex also regulates microtubule-kinetochore attachments via its phosphorylation status. Phosphorylation of the DASH subunit Ask1, by Cdk1, promotes robust microtubule-kinetochore attachment, likely by promoting Dam1/DASH complex oligomerization (Gutierrez et al., 2020). When microtubule-kinetochore attachments are not under sufficient tension, the budding yeast Aurora B homolog, Ipl1, phosphorylates Dam1/DASH complex components to weaken interactions with the microtubule and promote detachment (discussed below) (Keating et al., 2009); (Cheeseman et al., 2002).
Two budding yeast MAPs conserved across eukaryotes also function at force-generating microtubule-kinetochore attachments. The first MAP is Stu2, a well-characterized member of the XMAP215 family that has orthologs in many organisms, with human (chTOG), fission yeast (Dis1), worm (Zyg9), and frog (XMAP215) being among the best described (Amin et al., 2019). XMAP215 family members play prominent roles in controlling the dynamic behavior of microtubules in many cellular processes, including at kinetochores (Amin et al., 2019). Work in budding yeast has shown that Stu2 localizes to kinetochores, where it interacts with Ndc80 (Miller et al., 2016). Disrupted Stu2 kinetochore localization results in microtubule attachment defects, with data indicating Stu2 functions in the establishment of bipolar attachments and stabilizes kinetochore-microtubule attachments under tension (Miller et al., 2019). The second MAP is the homotetrameric kinesin-5 motor protein, Cin8. Kinesin-5 motors are well known for their role in crosslinking and sliding interpolar microtubules in the spindle. Cin8 also localizes to the region of kinetochore-microtubule attachment in an Ndc80-dependent manner (Suzuki et al., 2018). In the absence of Cin8, kinetochores experience less tension as measured by a tension-sensitive FRET module placed within Ndc80. Additional data suggest that Cin8 may promote tension by delivering Protein Phosphatase 1 (PP1) to kinetochores, where it dephosphorylates Ndc80, thus increasing the strength of microtubule attachments (Suzuki et al., 2018).
In metazoan cells, the Ska complex (SKA1, SKA2, and SKA3/Rama1) significantly strengthens microtubule-kinetochore attachments (Auckland et al., 2017); (Gaitanos et al., 2009); (Theis et al., 2009); (Welburn et al., 2009); (Ohta et al., 2011). The Ska complex is proposed to be a functional ortholog of the Dam1/DASH complex in fungi (Welburn et al., 2009); (Van Hooff et al., 2017). When Ska complex localization to kinetochores is prevented in vivo via Ska3 depletion, cells experience a large increase in mitotic duration, indicating difficulty in establishing robust, tension-generating attachments (Zhang et al., 2017). In vitro work has shown that the Ska complex increases the load-bearing capacity of Ndc80-based attachments by binding to both Ndc80 and the microtubule. Using Ndc80 with mutations in the tail region, which lowers affinity for the microtubule, the Ska complex was able to enhance the attachment strength by as much as five-fold (Helgeson et al., 2018); (Huis in ’t Veld et al., 2019). The Ska complex can produce robust attachment, independent of Ndc80 tail phosphorylation status, suggesting it can compensate for the tail-mediated regulation of microtubule binding and perhaps antagonize Aurora B, which phosphorylates the Ndc80 tail to promote the release of tensionless kinetochore attachments (discussed below) (Helgeson et al., 2018); (Wimbish et al., 2020).
Another factor implicated in kinetochore-microtubule attachments in human cells is the Astrin-SKAP complex. Knockdown of Astrin results in disrupted spindle organization and mitotic delay, indicating a vital role(s) in mitotic spindle formation (Gruber et al., 2002). The Astrin-SKAP complex binds microtubules throughout the cell cycle (Kern et al., 2016) yet only localizes to kinetochores once they achieve bipolar attachments in late metaphase (Fang et al., 2009); (Schmidt et al., 2010); (Friese et al., 2016); (Mack and Compton, 2001); (Manning et al., 2010). Astrin-SKAP localization is important for chromosome alignment and maintenance of sister chromatid cohesion (Thein et al., 2007); (Manning et al., 2010); (Dunsch et al., 2011). The localization and function of Astrin-SKAP are inversely related to Aurora B activity, suggesting Astrin-SKAP may antagonize the attachment-destabilizing activity of Aurora B (Fang et al., 2009); (Schmidt et al., 2010). Along those lines, Astrin-SKAP has been shown to facilitate the conversion of kinetochores associated with the lateral side of a microtubule to the end-on configuration (Shrestha et al., 2017). However, the conserved tail of Astrin has been shown to direct PP1 to kinetochores, where PP1 stabilizes microtubule-kinetochore attachments via a mechanism that appears independent of Aurora B activity (Conti et al., 2019). Astrin also interacts with Polo-like Kinase 1 (Plk1), whose phosphorylation of Astrin promotes its kinetochore localization and attachment stabilizing activity (Geraghty et al., 2021). Imaging of mitotic cells indicates that the microtubule attachments of sister kinetochore pairs are under higher tension in cells lacking SKAP (Rosas-Salvans et al., 2022). Moreover, kinetochores move slower on polymerizing and depolymerizing microtubules, and more force is needed to convert shortening kinetochore-attached microtubules back to growth. These observations suggest that Astrin-SKAP works to preserve bipolar attachments by reducing friction or effectively ‘lubricating’ their kinetochore-microtubule connections (Rosas-Salvans et al., 2022). Altogether these findings demonstrate that there are likely multiple layers of tension-regulating mechanisms at the kinetochore, which help to produce more robust attachments in combination.
The roles of tension in promoting accurate chromosome segregation
When unable to generate robust tension across sister kinetochore attachments, cells face prolonged mitotic duration and increased risk of chromosome missegregation, leading to aneuploidy or death. Dynamic microtubules generate robust tension with end-on attachments, whose coupling and regulation requires the function of specialized kinetochore components and MAPs, prominent examples of which are described above. Below we summarize recent advances in understanding how tension can act as a unifying factor, connecting events from the pericentromeric region to the outer kinetochore. Tension can alter kinetochore and centromere structure, regulate attachment strength, act as a modulator of Aurora B activity, and, in conjunction with the Spindle Assembly Checkpoint (SAC) promote timely anaphase onset (Figure 3). Tension across sister kinetochores is a fundamental quality of bipolar attachment that plays a key role in multiple mechanisms and thus unifies their individual functions to achieve accurate chromosome segregation.
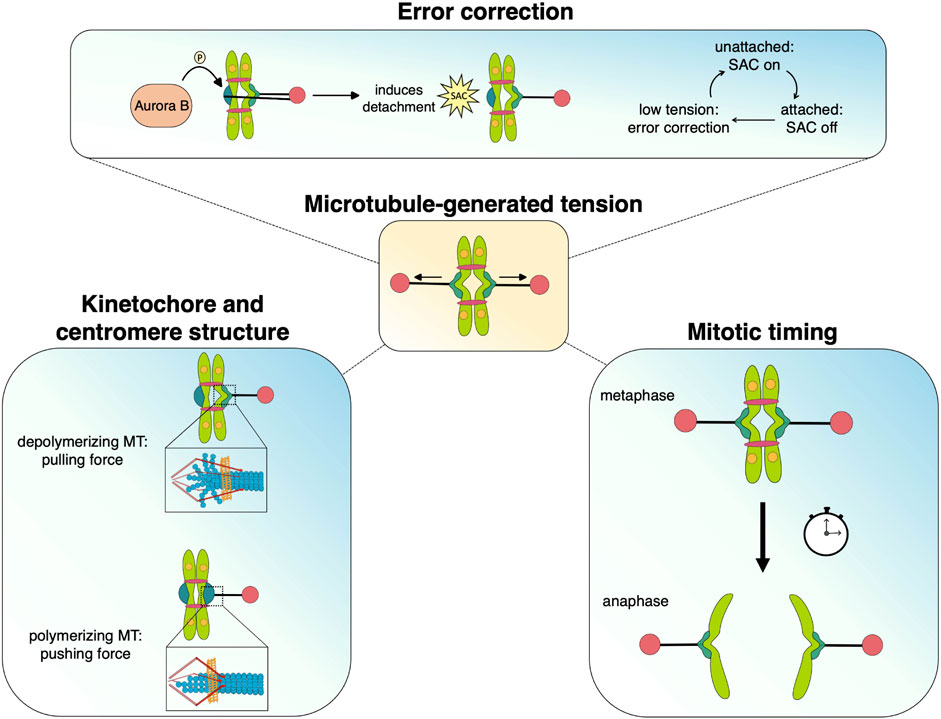
FIGURE 3. Microtubule-generated tension serves as a unifying force that facilitates the processes that promote chromosome segregation during mitosis. Dynamic microtubules generate pushing and pulling forces that move chromosomes as well as alter kinetochore/centromere structure, which may stabilize attachments and silence spindle assembly checkpoint signaling. The tension status at kinetochores also mediates Aurora B-dependent error correction and regulates the timing of anaphase onset.
Tension directly influences kinetochore and centromere structure
The tension produced across sister kinetochores during metaphase is a physiologically relevant force generated within the mitotic spindle (Chacón et al., 2014); (Ye et al., 2016). It has been a long-standing endeavour to elucidate how this tension status is sensed at kinetochores and centromeres. Notably, the structures of both kinetochores and centromeres are influenced by tension. Centromeres are the singular regions of DNA where kinetochore proteins assemble and they serve as a key connection point between replicated sister chromatids (for a review of centromere structure, see Lawrimore and Bloom (2022). It has long been thought that cohesion between sister chromatid DNA strands, provided by the cohesin complex, functions as the major mechanism for resistance to outward forces on their centromeres. One apparent limitation is that cohesin complexes can move relative to the associated DNA and, thus, allow centromeres to be pulled apart with relatively little resistance due to such DNA sliding. This challenge is met by ‘trapping’ the cohesin complexes between a pair of convergently oriented genes on either side of the centromere, thus limiting further DNA sliding and defining the boundaries of the pericentromeric region (Paldi et al., 2020). A notable property of DNA is that it is relatively floppy and easily extended, which does not generate much tension until it is largely extended (Bloom, 2008). Although alternative models have been proposed for the physical arrangement of the pericentromeric DNA between sister centromeres (Paldi et al., 2020), considering the length of DNA within the pericentromere, the bottlebrush model perhaps best accounts for this extensible property of DNA (Lawrimore et al., 2015). Briefly, the bottlebrush model posits that the pericentromeric DNA is organized by condensin and cohesin complexes into a looped loop structure with a central backbone and extending loops resembling the bottlebrushes used to clean test tubes (reviewed in Lawrimore and Bloom (2022). Notably, the bottlebrush model provides a mechanism by which the pericentromeric DNA adopts a stiff structure that allows for microtubule-dependent kinetochore movements to result in relatively greater tension. Centromeric stiffness increases during mitotic progression, with the greatest stiffness during metaphase (Harasymiw et al., 2019). This increased centromeric stiffness in metaphase, when tension is a vital signal for achieving bipolar attachments, passively increases tension in response to active microtubule-depolymerization forces, relative to centromeres with more extendable DNA. This centromeric stiffness creates a more sensitive mechanism to distinguish different kinetochore-microtubule configurations and ensure bipolar attachments are formed (Harasymiw et al., 2019). Altogether, the centromere and associated proteins are critical for proper tension generation and for enhancing the tension-responsive signaling that ensures bipolar kinetochore-microtubule attachments are established.
The kinetochore itself is a mechanically rigid structure compared to DNA. It has been proposed that specific proteins, such as Ndc80, are physically extended by microtubule pulling forces and thus provide a potential mechanism to report the kinetochore tension status (Suzuki et al., 2016). A related idea is that multiple kinetochore proteins or complexes change conformation or position relative to others in response to pulling forces, again serving as a physical indicator of tension (Joglekar et al., 2009); (Maresca and Salmon, 2009); (Uchida et al., 2009); (Wan et al., 2009). Indeed, a tension-dependent change in the shape of inner kinetochore proteins, particularly CENP-T, which undergoes elongation, has been observed in chicken DT40 cells (Suzuki et al., 2011). A FRET-based study using fluorescently labelled Ndc80 and kinetochore microtubules in human U2OS cells found that the number of Ndc80 molecules bound to kinetochore microtubules increased with tension (Yoo et al., 2018). A similar approach demonstrated that the Ndc80 clustering to individual microtubules is comparable at human and yeast kinetochores (Kukreja et al., 2020). Moreover, a distinct structural response has been observed between kinetochores that have lost tension versus those that lost attachment (Roscioli et al., 2020). KNL1 is shown to unravel at the loss of tension, while NDC80 jackknives due to microtubule detachment (Roscioli et al., 2020). This is unique to the other outer kinetochore proteins, which have high nematic order and do not undergo significant structural change in response to loss of tension or attachment (Roscioli et al., 2020). Altogether this evidence supports the idea that physical changes at the kinetochore serve as mechanical cues for tension-dependent processes in mitosis.
Tension regulates Aurora B and strengthens bipolar attachments
Aurora B (Ipl1) is needed to respond to tensionless kinetochores and is the major kinase involved in the associated error correction process (Chan and Botstein, 1993); (Biggins et al., 1999); (Cheeseman et al., 2002); (Tanaka et al., 2002). Error correction is the mechanism by which kinetochore-microtubule attachments experiencing insufficient tension are selectively destabilized, thus granting another chance to establish force-generating bipolar connections. It is mediated by Aurora B via phosphorylation of KMN proteins, such as the tail of Ndc80 (Biggins et al., 1999); (DeLuca et al., 2006); (DeLuca et al., 2011); (Welburn et al., 2010), as well as components of the Dam1/DASH complex (Tien et al., 2010); (Cheeseman et al., 2002). While Aurora B phosphorylates other spindle proteins, such as MAPs associated with the spindle midzone, the modification of kinetochore proteins facilitates error correction. Phosphorylation of the kinetochore components decreases their affinity for the microtubule, weakening the kinetochore-microtubule linkage and promoting detachment (Welburn et al., 2010); (Sarangapani et al., 2013). Conversely, the presence of sufficient tension avoids triggering error correction and, thus, selectively stabilizes bipolar microtubule-kinetochore attachments (McVey et al., 2021). In addition to avoiding Aurora B-mediated error correction, the kinetochore-microtubule linkage behaves like a catch bond in that its binding strength is enhanced under pulling forces (Akiyoshi et al., 2010). As a result, tension-generating bipolar attachments are inherently stabilized.
The mechanism by which Aurora B specifically targets tensionless kinetochore-microtubule attachments, yet avoids those under tension, remains elusive. There are several proposed models for Aurora B activity in response to insufficient tension at kinetochore attachments (for a review of models of Aurora B activity, see McVey et al. (2021). Two well-established classes of models are the spatial dependent (Liu et al., 2009) and the tension-sensitive activation models (Sandall et al., 2006); (Adams et al., 2000); (Bishop et al., 2005). In the spatial dependent models, once Aurora B is localized to the inner centromere, it is constitutively active and will phosphorylate any kinetochore substrate that comes within range. Thus, the components of sister kinetochores that lack bipolar pulling forces and the resultant tension to be sufficiently displaced away from the centralized Aurora B, will be modified, triggering detachment. Conversely, if microtubule-generated forces can pull sister kinetochores far enough apart, concomitantly producing tension, their components will be physically displaced out of the zone of Aurora B phosphorylation, which indirectly stabilizes the attachments. In the activation models, Aurora B activity is modulated by the tension status rather than the proximity of kinetochore substrates. Although Aurora B may encounter its kinetochore substrates, its kinase activity would be inhibited by higher tension or stimulated by low tension. In budding yeast, tension sensing and error correction by Aurora B can occur in the absence of its centromere localization, supporting the hypothesis that Aurora B activation is triggered by tension status (Campbell and Desai, 2013). Further work consistent with the activation model using purified yeast kinetochores suggests that tension-generating attachments can directly regulate Aurora B activity or oppose its outcome (de Regt et al., 2022). Another emerging model, supported by recent findings, is that Aurora B localization to the kinetochore itself, as opposed to the inner centromere, functions to phosphorylate substrates on low-tension kinetochores, while the kinase is evicted from kinetochores that establish tension (Reviewed in (Broad and DeLuca, 2020). This mechanism bears similarity to the activation model in some respects, particularly if Aurora B would be recruited back to kinetochores that subsequently lose tension. In addition to canonical models where Aurora B activity mainly promotes detachment, a recent study provides evidence that the tension status influences the downstream effect of Aurora B phosphorylation. Namely, under low tension Aurora B caused kinetochore microtubules to depolymerize without detachment, but under high tension, the microtubules detach, a difference which the authors propose may be relevant to correcting distinct attachment errors (Chen et al., 2021).
Tension and the spindle assembly checkpoint work together to promote timely metaphase-anaphase transition
Much work has been done to understand how microtubule-generated tension at kinetochores and the SAC work together or independently to promote accurate chromosome segregation. These questions have been difficult to approach experimentally due to the challenge of isolating the effect(s) of the tension status from kinetochore attachment. Very briefly, conditions that reduce tension can also inhibit attachment or induce error correction-mediated detachment, while those preventing attachment also preclude tension. In the presence of unattached kinetochore(s), the SAC will delay anaphase onset (for a full review of the SAC, see (Lara-Gonzalez et al., 2021). Phosphorylation of the kinetochore protein Spc105/KNL1 at unattached kinetochores by Mps1 promotes localization of the Bub (Bub1, Bub3) and Mad (Mad1, Mad2, and BubR1/Mad3) proteins (London et al., 2012); (London and Biggins, 2014). This kinetochore localization leads to catalytic formation of the Mitotic Checkpoint Complex (MCC), which inhibits the Anaphase Promoting Complex (APC/C) by sequestering Cdc20, a required activator of the APC (Lara-Gonzalez et al., 2021).
SAC signalling is necessary to provide sufficient time to establish proper kinetochore-microtubule attachments yet also allows for timely mitotic progression. Along these lines, experiments in budding yeast suggest Bub1 works with the well-established tension-sensitive protein, Sgo1, and the phosphatase PP2A to prevent premature SAC silencing (Jin et al., 2017). On the other hand, recent data indicates that Bub1 and Aurora B work cooperatively to maintain SAC signalling once initiated, even after Mps1 activity at kinetochores has diminished (Roy et al., 2022).
One outstanding mystery, owing to the fact that unattached kinetochores are inherently tensionless, is whether the tension status plays any role in the canonical SAC mechanism at unattached kinetochores. It has been postulated that tension plays a key role in signalling the establishment of proper attachments, and thus silencing the SAC. Although significant work has been directed at understanding how SAC signalling is extinguished once appropriate conditions are met, the mechanistic basis remains largely obscure.
An important step in SAC activation is Mps1-mediated phosphorylation, and thus, silencing the SAC could entail preventing Mps1 phosphorylation of Spc105/KNL1. One potential mechanism is that microtubules and Mps1 competitively bind Ndc80, with microtubule binding displacing Mps1, thus silencing the SAC (Hiruma et al., 2015); (Ji et al., 2015). This competitive binding model has been challenged by work done in Drosophila demonstrating that as microtubule attachments are established, Mps1 localization at kinetochores decreases, except for a small fraction that remains on kinetochores until anaphase onset (Moura et al., 2017). Follow up work revealed that Mps1 localization to syntelic, end-on attached kinetochores proceeded their detachment (Hayward et al., 2022). Mps1 transiently localizes to these kinetochores, which have high levels of Aurora B phosphorylation, to promote timely error correction (Hayward et al., 2022).
Another potential mechanism is that the SAC is silenced by tension-dependent kinetochore stretching, and the sustained deformation of kinetochore components at the attachment interface (Maresca and Salmon, 2009); (Uchida et al., 2009). Other work in budding yeast has posited a mechanical switch in the kinetochore upon end-on attachment that prevents SAC signaling from persisting, where by Mps1 is prevented from phosphorylating Spc105 (Aravamudhan et al., 2015). In this model, Dam1 acts as a barrier preventing Mps1 access to Spc105 (Aravamudhan et al., 2015).
In general, it is thought that extinguishing SAC signaling, regardless of mechanism, entails either eviction of Mps1 from the kinetochore or a decrease in Mps1 activity. In Drosophila, Mps1 activation is regulated by PP1-87B phosphatase activity that antagonizes the increased Mps1 activity resulting from T-loop autophosphorylation (Moura et al., 2017). In human cells, this T-loop autophosphorylation, as well as phosphorylation by Aurora B that impacts Mps1 kinetochore localization and activation, is antagonized by PP2A-B56 (Hayward et al., 2019). In both cases, a decrease in phosphatase activity leading to persistent Mps1 T-loop phosphorylation and Mps1 activity resulted in prolonged mitotic arrest (Moura et al., 2017); (Hayward et al., 2019). Altogether, phosphoregulation of Mps1, resulting in its activation or kinetochore localization, is mediated via PP1-87B and PP2A-B56 to promote SAC silencing and timely anaphase onset.
Recent work has generated conflicting evidence for whether tension is needed to silence the SAC or if microtubule attachment alone is sufficient. PP1 is a phosphatase that antagonizes Mps1 activity at the kinetochore via dephosphorylation of kinetochore substrates. In HeLa cells, intra-kinetochore stretching is diminished in monopolar spindles, leading to decreased PP1 recruitment to kinetochores (Uchida et al., 2021). At these PP1 deficient kinetochores, downstream SAC proteins remained, leading to delayed anaphase onset, irrespective of Mps1 localization (Uchida et al., 2021). This work suggests that tension is needed for kinetochore stretching to silence the SAC. On the other hand, evidence indicates that a lack of tension can, in certain cases, activate the SAC. In HAP1 cells with deficient Kif18A, a kinesin-8 motor, the resulting lack of tension activates the SAC (Janssen et al., 2018). At Mad1 positive kinetochores in these cells, tubulin/microtubule signal was equivalent to that in control cells, demonstrating that kinetochores were fully attached even though the SAC was active (Janssen et al., 2018). These findings indicate that insufficient tension can activate the SAC, regardless of attachment status (Janssen et al., 2018). In budding yeast, deletion of the conserved kinesin-5, Cin8, results in a delay in anaphase onset due to a lack of tension via Ndc80 (Suzuki et al., 2018). This reduced tension is associated with sustained error correction-mediated phosphorylation of Ndc80 due to disrupted kinetochore recruitment of PP1 to antagonize Ipl1 activity, resulting in detachment and SAC activation (Suzuki et al., 2018). Together these studies reveal that the tension generated by end-on attachments is a signal that potentially facilitates multiple mechanisms that activate or silence the SAC.
Contrary to tension being a central signal, other evidence suggests that attachment is the major signal needed to satisfy or silence the SAC. Cells expressing a non-phosphorylatable Ndc80 tail mutant (Hec1-9A) can establish stable end-on attachments. Notably, when these cells are induced to form monopolar spindles, they are unable to generate the normal tension associated with bipolar attachments yet still establish stable end-on connections (Etemad et al., 2015). Although the SAC appears functional in these cells, the low-tension attachments can sufficiently satisfy the checkpoint (Etemad et al., 2015). In another study, Hec1-9A and wild type cells were treated with low dose nocodazole to deplete poleward pulling forces (Tauchman et al., 2015). While nocodazole treatment arrested wild type cells, the Hec1-9A cells satisfied the SAC and entered anaphase, which was corroborated by a decrease in Mad1 positive kinetochores (Tauchman et al., 2015). Additional evidence in human cells show that low kinetochore-microtubule occupancy, or fewer microtubules bound to a kinetochore compared to the typical number at those “fully attached”, does not impact SAC silencing (Etemad et al., 2019). SAC proteins were almost undetectable at kinetochores, despite only about half the total possible microtubules bound to kinetochores (Etemad et al., 2019). This body of work suggests that microtubule-kinetochore attachment is sufficient to silence the SAC, regardless of maximum kinetochore occupancy or tension-generating status. These experiments represent significant technical advances that continue to take us closer to understanding how tension contributes to signalling at kinetochores. They also highlight the intricate relationship between attachment and tension at kinetochores, and the challenges in elucidating the role of each in SAC activation or silencing.
While it remains unclear how or if tension contributes to SAC signalling, the tension status can impact anaphase onset independently of SAC silencing. Work in budding yeast shows Bub1 and Bub3 function outside of their canonical SAC role to delay anaphase onset in response to low-tension but attached kinetochores (Proudfoot et al., 2019). Thus, a tension-sensitive mechanism may provide extra time for kinetochores to come under tension prior to anaphase or to trigger error correction to sample for a bipolar attachment (Proudfoot et al., 2019). Altering the level of tension generated in the spindle by deleting specific MAPs (Cin8, Kip1, Ase1) has been shown to produce a graded response, where detachment mediated by kinetochore protein phosphorylation via Aurora B is enhanced by decreasing centromeric tension (Mukherjee et al., 2019). This work reveals that tension-sensitive signalling mechanisms can be sensitive to the magnitude of forces experienced.
While phosphorylation has been a widely investigated post-translational modification that mediates signalling at centromeres and kinetochores, SUMOylation of proteins has recently been found to also play a role. The SUMOylation status of Sgo1, a well-established tension-sensitive protein, influences the timing of anaphase onset (Su et al., 2021). Sgo1 is recruited to the pericentromeric region during metaphase by phosphorylation of S121 on histone H2A (Fernius and Hardwick, 2007); (Yamagishi et al., 2010). This positioning allows Sgo1 to promote chromosome biorientation by facilitating Chromosome Passenger Complex (CPC) localization to the centromere and recruiting cohesin and condensin (Nerusheva et al., 2014); (Verzijlbergen et al., 2014); (Peplowska et al., 2014). Sgo1 SUMOylation is needed to keep sister chromatids in a stable bioriented state. Metaphase arrested cells harbouring a mutation in the coiled-coil region of Sgo1 (sgo1-4R) that reduces SUMOylation display cycles of biorientation, loss of tension, detachment, then reattachment to become bioriented again (Su et al., 2021). Even with multiple rounds of unnecessary error correction, these cells have significantly lower chromosome missegregation levels than those lacking Sgo1. Together the results suggest that SUMOylation of Sgo1 and the CPC component Bir1 work to decrease Sgo1 localization, and thus dampen Aurora B (Ipl1)-mediated error correction to selectively stabilize bioriented kinetochore-microtubule attachments and promote timely anaphase onset (Su et al., 2021). More work is needed to understand how the tension status is transmitted to these downstream effectors.
Concluding remarks
While multiple classes of microtubule- and motor-dependent forces contribute to formation of the bipolar metaphase spindle, microtubule depolymerizing forces at end-on kinetochore attachments are vital for ensuring accurate chromosome segregation. The tension generated across bipolar attachments serves as a unifying factor linking events from the centromere to the outer kinetochore, including 1) modulating centromere and kinetochore structure, 2) mediating Aurora B activity, and 3) working with the SAC to regulate the timing of anaphase onset. While we have made significant advances in understanding the role of tension in chromosome segregation, there are many questions that remain to be answered. For example, what is the mechanism by which tension status is converted to cellular signalling? How does the tension status initiate, sustain, and/or silence the SAC? To what extent are the mechanisms that sense attachment and tension shared or independent? Despite over 50 years since the importance of tension in chromosome segregation first captivated biologists, elucidating its role(s), both mechanistically and at the molecular level remains challenging. Further advances in genetic and experimental approaches will likely be needed to clearly discriminate the roles of tension and attachment at the kinetochore.
Author contributions
AB and MG contributed to planning and researching, AB wrote, and AB and MG edited the manuscript. AB designed and constructed the figures.
Funding
This work was supported by National Science Foundation grant number MCB-1846262 to MG.
Acknowledgments
We apologize those whose work was omitted due to space and/or scope limitations. We would like to thank members of the lab for their helpful discussions and feedback.
Conflict of interest
The authors declare that the research was conducted in the absence of any commercial or financial relationships that could be construed as a potential conflict of interest.
Publisher’s note
All claims expressed in this article are solely those of the authors and do not necessarily represent those of their affiliated organizations, or those of the publisher, the editors and the reviewers. Any product that may be evaluated in this article, or claim that may be made by its manufacturer, is not guaranteed or endorsed by the publisher.
References
Adams, R., Wheatleya, S., Gouldsworthy, A., Kandels-Lewis, S., Carmena, M., Smythe, C., et al. (2000). Incenp binds the aurora-related kinase airk2 and is required to target it to chromosomes, the central spindle and cleavage furrow. Curr. Biol. 10, 1075–1078. doi:10.1016/s0960-9822(00)00673-4
Akiyoshi, B., Sarangapani, K. K., Powers, A. F., Nelson, C. R., Reichow, S. L., Arellano-Santoyo, H., et al. (2010). Tension directly stabilizes reconstituted kinetochore-microtubule attachments. Nature 468, 576–579. doi:10.1038/nature09594
Alushin, G. M., Ramey, V. H., Pasqualato, S., Ball, D. A., Grigorieff, N., Musacchio, A., et al. (2010). The ndc80 kinetochore complex forms oligomeric arrays along microtubules. Nature 467, 805–810. doi:10.1038/nature09423
Amin, M. A., Agarwal, S., and Varma, D. (2019). Mapping the kinetochore map functions required for stabilizing microtubule attachments to chromosomes during metaphase. Cytoskeleton 76, 398–412. doi:10.1002/cm.21559
Aravamudhan, P., Goldfarb, A. A., and Joglekar, A. P. (2015). The kinetochore encodes a mechanical switch to disrupt spindle assembly checkpoint signalling. Nat. Cell. Biol. 17, 868–879. doi:10.1038/ncb3179
Asbury, C. L. (2017). Anaphase a: Disassembling microtubules move chromosomes toward spindle poles. Biology 6, 15. doi:10.3390/biology6010015
Asbury, C. L., Gestaut, D. R., Powers, A. F., Franck, A. D., and Davis, T. N. (2006). The dam1 kinetochore complex harnesses microtubule dynamics to produce force and movement. Proc. Natl. Acad. Sci. 103, 9873–9878. doi:10.1073/pnas.0602249103
Auckland, P., Clarke, N. I., Royle, S. J., and McAinsh, A. D. (2017). Congressing kinetochores progressively load ska complexes to prevent force-dependent detachment. J. Cell. Biol. 216, 1623–1639. doi:10.1083/jcb.201607096
Biggins, S., Severin, F. F., Bhalla, N., Sassoon, I., Hyman, A. A., and Murray, A. W. (1999). The conserved protein kinase ipl1 regulates microtubule binding to kinetochores in budding yeast. Genes. & Dev. 13, 532–544. doi:10.1101/gad.13.5.532
Bishop, J. D., Han, Z., and Schumacher, J. M. (2005). The caenorhabditis elegans aurora b kinase air-2 phosphorylates and is required for the localization of a bimc kinesin to meiotic and mitotic spindles. Mol. Biol. Cell. 16, 742–756. doi:10.1091/mbc.e04-08-0682
Bloom, K. S. (2008). Beyond the code: The mechanical properties of dna as they relate to mitosis. Chromosoma 117, 103–110. doi:10.1007/s00412-007-0138-0
Broad, A. J., and DeLuca, J. G. (2020). The right place at the right time: Aurora b kinase localization to centromeres and kinetochores. Essays Biochem. 64, 299–311. doi:10.1042/EBC20190081
Brouhard, G. J., and Hunt, A. J. (2005). Microtubule movements on the arms of mitotic chromosomes: Polar ejection forces quantified in vitro. Proc. Natl. Acad. Sci. 102, 13903–13908. doi:10.1073/pnas.0506017102
Campbell, C. S., and Desai, A. (2013). Tension sensing by aurora b kinase is independent of survivin-based centromere localization. Nature 497, 118–121. doi:10.1038/nature12057
Chacón, J. M., Mukherjee, S., Schuster, B. M., Clarke, D. J., and Gardner, M. K. (2014). Pericentromere tension is self-regulated by spindle structure in metaphase. J. Cell. Biol. 205, 313–324. doi:10.1083/jcb.201312024
Chan, C. S., and Botstein, D. (1993). Isolation and characterization of chromosome-gain and increase-in-ploidy mutants in yeast. Genetics 135, 677–691. doi:10.1093/genetics/135.3.677
Cheerambathur, D. K., Gassmann, R., Cook, B., Oegema, K., and Desai, A. (2013). Crosstalk between microtubule attachment complexes ensures accurate chromosome segregation. Science 342, 1239–1242. doi:10.1126/science.1246232
Cheeseman, I. M., Anderson, S., Jwa, M., Green, E. M., Kang, J.-s., Yates, J. R., et al. (2002). Phospho-regulation of kinetochore-microtubule attachments by the aurora kinase ipl1p. Cell. 111, 163–172. doi:10.1016/s0092-8674(02)00973-x
Cheeseman, I. M., Chappie, J. S., Wilson-Kubalek, E. M., and Desai, A. (2006). The conserved kmn network constitutes the core microtubule-binding site of the kinetochore. Cell. 127, 983–997. doi:10.1016/j.cell.2006.09.039
Cheeseman, I. M., and Desai, A. (2008). Molecular architecture of the kinetochore–microtubule interface. Nat. Rev. Mol. Cell. Biol. 9, 33–46. doi:10.1038/nrm2310
Cheeseman, I. M., Enquist-Newman, M., Müller-Reichert, T., Drubin, D. G., and Barnes, G. (2001). Mitotic spindle integrity and kinetochore function linked by the duo1p/dam1p complex. J. Cell. Biol. 152, 197–212. doi:10.1083/jcb.152.1.197
Chen, G.-Y., Renda, F., Zhang, H., Gokden, A., Wu, D. Z., Chenoweth, D. M., et al. (2021). Tension promotes kinetochore–microtubule release by aurora b kinase. J. Cell. Biol. 220, e202007030. doi:10.1083/jcb.202007030
Cochran, J. C., Sindelar, C. V., Mulko, N. K., Collins, K. A., Kong, S. E., Hawley, R. S., et al. (2009). Atpase cycle of the nonmotile kinesin nod allows microtubule end tracking and drives chromosome movement. Cell. 136, 110–122. doi:10.1016/j.cell.2008.11.048
Conti, D., Gul, P., Islam, A., Martín-Durán, J. M., Pickersgill, R. W., and Draviam, V. M. (2019). Kinetochores attached to microtubule-ends are stabilised by astrin bound pp1 to ensure proper chromosome segregation. Elife 8, e49325. doi:10.7554/eLife.49325
de Regt, A. K., Clark, C. J., Asbury, C. L., and Biggins, S. (2022). Tension can directly suppress aurora b kinase-triggered release of kinetochore-microtubule attachments. Nat. Commun. 13, 2152. doi:10.1038/s41467-022-29542-8
DeLuca, J. G., Dong, Y., Hergert, P., Strauss, J., Hickey, J. M., Salmon, E., et al. (2005). Hec1 and nuf2 are core components of the kinetochore outer plate essential for organizing microtubule attachment sites. Mol. Biol. Cell. 16, 519–531. doi:10.1091/mbc.e04-09-0852
DeLuca, J. G., Gall, W. E., Ciferri, C., Cimini, D., Musacchio, A., and Salmon, E. (2006). Kinetochore microtubule dynamics and attachment stability are regulated by hec1. Cell. 127, 969–982. doi:10.1016/j.cell.2006.09.047
DeLuca, K. F., Lens, S. M., and DeLuca, J. G. (2011). Temporal changes in hec1 phosphorylation control kinetochore–microtubule attachment stability during mitosis. J. Cell. Sci. 124, 622–634. doi:10.1242/jcs.072629
Demirel, P. B., Keyes, B. E., Chatterjee, M., Remington, C. E., and Burke, D. J. (2012). A redundant function for the n-terminal tail of ndc80 in kinetochore–microtubule interaction in saccharomyces cerevisiae. Genetics 192, 753–756. doi:10.1534/genetics.112.143818
Dogterom, M., and Yurke, B. (1997). Measurement of the force-velocity relation for growing microtubules. Science 278, 856–860. doi:10.1126/science.278.5339.856
Driver, J. W., Geyer, E. A., Bailey, M. E., Rice, L. M., and Asbury, C. L. (2017). Direct measurement of conformational strain energy in protofilaments curling outward from disassembling microtubule tips. Elife 6, e28433. doi:10.7554/eLife.28433
Dumont, J., and Desai, A. (2012). Acentrosomal spindle assembly and chromosome segregation during oocyte meiosis. Trends Cell. Biol. 22, 241–249. doi:10.1016/j.tcb.2012.02.007
Dumont, S., and Mitchison, T. J. (2009). Force and length in the mitotic spindle. Curr. Biol. 19, R749–R761. doi:10.1016/j.cub.2009.07.028
Dunsch, A. K., Linnane, E., Barr, F. A., and Gruneberg, U. (2011). The astrin–kinastrin/skap complex localizes to microtubule plus ends and facilitates chromosome alignment. J. Cell. Biol. 192, 959–968. doi:10.1083/jcb.201008023
Etemad, B., Kuijt, T. E., and Kops, G. J. (2015). Kinetochore–microtubule attachment is sufficient to satisfy the human spindle assembly checkpoint. Nat. Commun. 6, 8987. doi:10.1038/ncomms9987
Etemad, B., Vertesy, A., Kuijt, T. E., Sacristan, C., van Oudenaarden, A., and Kops, G. J. (2019). Spindle checkpoint silencing at kinetochores with submaximal microtubule occupancy. J. Cell. Sci. 132, jcs231589. doi:10.1242/jcs.231589
Fang, L., Seki, A., and Fang, G. (2009). Skap associates with kinetochores and promotes the metaphase-to-anaphase transition. Cell. cycle 8, 2819–2827. doi:10.4161/cc.8.17.9514
Fernius, J., and Hardwick, K. G. (2007). Bub1 kinase targets sgo1 to ensure efficient chromosome biorientation in budding yeast mitosis. PLoS Genet. 3, e213. doi:10.1371/journal.pgen.0030213
Friese, A., Faesen, A. C., Huis in‘t Veld, P. J., Fischböck, J., Prumbaum, D., Petrovic, A., et al. (2016). Molecular requirements for the inter-subunit interaction and kinetochore recruitment of skap and astrin. Nat. Commun. 7, 11407. doi:10.1038/ncomms11407
Gaitanos, T. N., Santamaria, A., Jeyaprakash, A. A., Wang, B., Conti, E., and Nigg, E. A. (2009). Stable kinetochore–microtubule interactions depend on the ska complex and its new component ska3/c13orf3. EMBO J. 28, 1442–1452. doi:10.1038/emboj.2009.96
Ganem, N. J., Upton, K., and Compton, D. A. (2005). Efficient mitosis in human cells lacking poleward microtubule flux. Curr. Biol. 15, 1827–1832. doi:10.1016/j.cub.2005.08.065
Garzon-Coral, C., Fantana, H. A., and Howard, J. (2016). A force-generating machinery maintains the spindle at the cell center during mitosis. Science 352, 1124–1127. doi:10.1126/science.aad9745
Geraghty, Z., Barnard, C., Uluocak, P., and Gruneberg, U. (2021). The association of plk1 with the astrin–kinastrin complex promotes formation and maintenance of a metaphase plate. J. Cell. Sci. 134, jcs251025. doi:10.1242/jcs.251025
Grishchuk, E. L., and McIntosh, J. R. (2006). Microtubule depolymerization can drive poleward chromosome motion in fission yeast. EMBO J. 25, 4888–4896. doi:10.1038/sj.emboj.7601353
Grishchuk, E. L., Molodtsov, M. I., Ataullakhanov, F. I., and McIntosh, J. R. (2005). Force production by disassembling microtubules. Nature 438, 384–388. doi:10.1038/nature04132
Grishchuk, E., Spiridonov, I., Volkov, V., Efremov, A., Westermann, S., Drubin, D., et al. (2008). Different assemblies of the dam1 complex follow shortening microtubules by distinct mechanisms. Proc. Natl. Acad. Sci. 105, 6918–6923. doi:10.1073/pnas.0801811105
Gruber, J., Harborth, J., Schnabel, J., Weber, K., and Hatzfeld, M. (2002). The mitotic-spindle-associated protein astrin is essential for progression through mitosis. J. Cell. Sci. 115, 4053–4059. doi:10.1242/jcs.00088
Gudimchuk, N. B., Ulyanov, E. V., O’Toole, E., Page, C. L., Vinogradov, D. S., Morgan, G., et al. (2020). Mechanisms of microtubule dynamics and force generation examined with computational modeling and electron cryotomography. Nat. Commun. 11, 3765. doi:10.1038/s41467-020-17553-2
Gutierrez, A., ook Kim, J., Umbreit, N. T., Asbury, C. L., Davis, T. N., Miller, M. P., et al. (2020). Cdk1 phosphorylation of the dam1 complex strengthens kinetochore-microtubule attachments. Curr. Biol. 30, 4491–4499. doi:10.1016/j.cub.2020.08.054
Harasymiw, L. A., Tank, D., McClellan, M., Panigrahy, N., and Gardner, M. K. (2019). Centromere mechanical maturation during mammalian cell mitosis. Nat. Commun. 10, 3188–3199. doi:10.1038/s41467-019-09578-z
Hayward, D., Bancroft, J., Mangat, D., Alfonso-Pérez, T., Dugdale, S., McCarthy, J., et al. (2019). Checkpoint signaling and error correction require regulation of the mps1 t-loop by pp2a-b56. J. Cell. Biol. 218, 3188–3199. doi:10.1083/jcb.201905026
Hayward, D., Roberts, E., and Gruneberg, U. (2022). Mps1 localizes to end-on microtubule-attached kinetochores to promote microtubule release. Curr. Biol. 32, 5200–5208.e8. doi:10.1016/j.cub.2022.10.047
Helgeson, L. A., Zelter, A., Riffle, M., MacCoss, M. J., Asbury, C. L., and Davis, T. N. (2018). Human ska complex and ndc80 complex interact to form a load-bearing assembly that strengthens kinetochore–microtubule attachments. Proc. Natl. Acad. Sci. 115, 2740–2745. doi:10.1073/pnas.1718553115
Hiruma, Y., Sacristan, C., Pachis, S. T., Adamopoulos, A., Kuijt, T., Ubbink, M., et al. (2015). CELL DIVISION CYCLE. Competition between MPS1 and microtubules at kinetochores regulates spindle checkpoint signaling. Science 348, 1264–1267. doi:10.1126/science.aaa4055
Inoue, S. (1953). Polarization optical studies of the mitotic spindle. I. The demonstration of spindle fibers in living cells. Chromosoma 5, 487–500. doi:10.1007/BF01271498
Inoué, S., and Ritter, H. (1975). Dynamics of mitotic spindle organization and function. Soc. General Physiologists Ser. 30, 3–30.
Janke, C., Ortiz, J., Lechner, J., Shevchenko, A., Shevchenko, A., Magiera, M. M., et al. (2001). The budding yeast proteins spc24p and spc25p interact with ndc80p and nuf2p at the kinetochore and are important for kinetochore clustering and checkpoint control. EMBO J. 20, 777–791. doi:10.1093/emboj/20.4.777
Janson, M. E., de Dood, M. E., and Dogterom, M. (2003). Dynamic instability of microtubules is regulated by force. J. Cell. Biol. 161, 1029–1034. doi:10.1083/jcb.200301147
Janssen, L. M., Averink, T. V., Blomen, V. A., Brummelkamp, T. R., Medema, R. H., and Raaijmakers, J. A. (2018). Loss of kif18a results in spindle assembly checkpoint activation at microtubule-attached kinetochores. Curr. Biol. 28, 2685–2696. doi:10.1016/j.cub.2018.06.026
Jenni, S., and Harrison, S. C. (2018). Structure of the dash/dam1 complex shows its role at the yeast kinetochore-microtubule interface. Science 360, 552–558. doi:10.1126/science.aar6436
Ji, Z., Gao, H., and Yu, H. (2015). CELL DIVISION CYCLE. Kinetochore attachment sensed by competitive Mps1 and microtubule binding to Ndc80C. Science 348, 1260–1264. doi:10.1126/science.aaa4029
Jin, F., Bokros, M., and Wang, Y. (2017). Premature silencing of the spindle assembly checkpoint is prevented by the bub1-h2a-sgo1-pp2a axis in saccharomyces cerevisiae. Genetics 205, 1169–1178. doi:10.1534/genetics.116.195727
Joglekar, A. P., Bloom, K., and Salmon, E. (2009). In vivo protein architecture of the eukaryotic kinetochore with nanometer scale accuracy. Curr. Biol. 19, 694–699. doi:10.1016/j.cub.2009.02.056
Ke, K., Cheng, J., and Hunt, A. J. (2009). The distribution of polar ejection forces determines the amplitude of chromosome directional instability. Curr. Biol. 19, 807–815. doi:10.1016/j.cub.2009.04.036
Keating, P., Rachidi, N., Tanaka, T. U., and Stark, M. J. (2009). Ipl1-dependent phosphorylation of dam1 is reduced by tension applied on kinetochores. J. Cell. Sci. 122, 4375–4382. doi:10.1242/jcs.055566
Kern, D. M., Nicholls, P. K., Page, D. C., and Cheeseman, I. M. (2016). A mitotic skap isoform regulates spindle positioning at astral microtubule plus ends. J. Cell. Biol. 213, 315–328. doi:10.1083/jcb.201510117
Kiermaier, E., Woehrer, S., Peng, Y., Mechtler, K., and Westermann, S. (2009). A dam1-based artificial kinetochore is sufficient to promote chromosome segregation in budding yeast. Nat. Cell. Biol. 11, 1109–1115. doi:10.1038/ncb1924
Kim, S., and Yu, H. (2015). Multiple assembly mechanisms anchor the kmn spindle checkpoint platform at human mitotic kinetochores. J. Cell. Biol. 208, 181–196. doi:10.1083/jcb.201407074
Klemm, A. H., Bosilj, A., Gluncic, M., Pavin, N., and Tolic, I. M. (2018). Metaphase kinetochore movements are regulated by kinesin-8 motors and microtubule dynamic instability. Mol. Biol. Cell. 29, 1332–1345. doi:10.1091/mbc.E17-11-0667
Koshland, D. E., Mitchison, T., and Kirschner, M. W. (1988). Polewards chromosome movement driven by microtubule depolymerization in vitro. Nature 331, 499–504. doi:10.1038/331499a0
Kukreja, A. A., Kavuri, S., and Joglekar, A. P. (2020). Microtubule attachment and centromeric tension shape the protein architecture of the human kinetochore. Curr. Biol. 30, 4869–4881.e5. doi:10.1016/j.cub.2020.09.038
Laan, L., Husson, J., Munteanu, E. L., Kerssemakers, J. W., and Dogterom, M. (2008). Force-generation and dynamic instability of microtubule bundles. Proc. Natl. Acad. Sci. 105, 8920–8925. doi:10.1073/pnas.0710311105
Lacefield, S., Lau, D. T., and Murray, A. W. (2009). Recruiting a microtubule-binding complex to dna directs chromosome segregation in budding yeast. Nat. Cell. Biol. 11, 1116–1120. doi:10.1038/ncb1925
Lampert, F., Hornung, P., and Westermann, S. (2010). The dam1 complex confers microtubule plus end–tracking activity to the ndc80 kinetochore complex. J. Cell. Biol. 189, 641–649. doi:10.1083/jcb.200912021
Lampert, F., Mieck, C., Alushin, G. M., Nogales, E., and Westermann, S. (2013). Molecular requirements for the formation of a kinetochore–microtubule interface by dam1 and ndc80 complexes. J. Cell. Biol. 200, 21–30. doi:10.1083/jcb.201210091
Lara-Gonzalez, P., Pines, J., and Desai, A. (2021)., 117. Elsevier, 86–98.Spindle assembly checkpoint activation and silencing at kinetochoresSeminars Cell. & Dev. Biol.
Lawrimore, J., and Bloom, K. (2022). Shaping centromeres to resist mitotic spindle forces. J. Cell. Sci. 135, jcs259532. doi:10.1242/jcs.259532
Lawrimore, J., Vasquez, P. A., Falvo, M. R., Taylor, R. M., Vicci, L., Yeh, E., et al. (2015). Dna loops generate intracentromere tension in mitosis. J. Cell. Biol. 210, 553–564. doi:10.1083/jcb.201502046
Liu, D., Vader, G., Vromans, M. J., Lampson, M. A., and Lens, S. M. (2009). Sensing chromosome bi-orientation by spatial separation of aurora b kinase from kinetochore substrates. Science 323, 1350–1353. doi:10.1126/science.1167000
London, N., and Biggins, S. (2014). Mad1 kinetochore recruitment by mps1-mediated phosphorylation of bub1 signals the spindle checkpoint. Genes. & Dev. 28, 140–152. doi:10.1101/gad.233700.113
London, N., Ceto, S., Ranish, J. A., and Biggins, S. (2012). Phosphoregulation of spc105 by mps1 and pp1 regulates bub1 localization to kinetochores. Curr. Biol. 22, 900–906. doi:10.1016/j.cub.2012.03.052
Mack, G. J., and Compton, D. A. (2001). Analysis of mitotic microtubule-associated proteins using mass spectrometry identifies astrin, a spindle-associated protein. Proc. Natl. Acad. Sci. 98, 14434–14439. doi:10.1073/pnas.261371298
Maddox, P., Desai, A., Oegema, K., Mitchison, T. J., and Salmon, E. (2002). Poleward microtubule flux is a major component of spindle dynamics and anaphase a in mitotic drosophila embryos. Curr. Biol. 12, 1670–1674. doi:10.1016/s0960-9822(02)01183-1
Maddox, P. S., Bloom, K. S., and Salmon, E. (2000). The polarity and dynamics of microtubule assembly in the budding yeast saccharomyces cerevisiae. Nat. Cell. Biol. 2, 36–41. doi:10.1038/71357
Mallavarapu, A., Sawin, K., and Mitchison, T. (1999). A switch in microtubule dynamics at the onset of anaphase b in the mitotic spindle of schizosaccharomyces pombe. Curr. Biol. 9, 1423–1426. doi:10.1016/s0960-9822(00)80090-1
Manning, A. L., Bakhoum, S. F., Maffini, S., Correia-Melo, C., Maiato, H., and Compton, D. A. (2010). Clasp1, astrin and kif2b form a molecular switch that regulates kinetochore-microtubule dynamics to promote mitotic progression and fidelity. EMBO J. 29, 3531–3543. doi:10.1038/emboj.2010.230
Maresca, T. J., and Salmon, E. D. (2009). Intrakinetochore stretch is associated with changes in kinetochore phosphorylation and spindle assembly checkpoint activity. J. Cell. Biol. 184, 373–381. doi:10.1083/jcb.200808130
McCleland, M. L., Gardner, R. D., Kallio, M. J., Daum, J. R., Gorbsky, G. J., Burke, D. J., et al. (2003). The highly conserved ndc80 complex is required for kinetochore assembly, chromosome congression, and spindle checkpoint activity. Genes. & Dev. 17, 101–114. doi:10.1101/gad.1040903
McVey, S. L., Cosby, J. K., and Nannas, N. J. (2021). Aurora b tension sensing mechanisms in the kinetochore ensure accurate chromosome segregation. Int. J. Mol. Sci. 22, 8818. doi:10.3390/ijms22168818
Miller, M. P., Asbury, C. L., and Biggins, S. (2016). A tog protein confers tension sensitivity to kinetochore-microtubule attachments. Cell. 165, 1428–1439. doi:10.1016/j.cell.2016.04.030
Miller, M. P., Evans, R. K., Zelter, A., Geyer, E. A., MacCoss, M. J., Rice, L. M., et al. (2019). Kinetochore-associated stu2 promotes chromosome biorientation in vivo. PLoS Genet. 15, e1008423. doi:10.1371/journal.pgen.1008423
Miranda, J., Wulf, P. D., Sorger, P. K., and Harrison, S. C. (2005). The yeast dash complex forms closed rings on microtubules. Nat. Struct. Mol. Biol. 12, 138–143. doi:10.1038/nsmb896
Mitchison, T., and Kirschner, M. (1984). Dynamic instability of microtubule growth. nature 312, 237–242. doi:10.1038/312237a0
Mitchison, T. (1989). Polewards microtubule flux in the mitotic spindle: Evidence from photoactivation of fluorescence. J. Cell. Biol. 109, 637–652. doi:10.1083/jcb.109.2.637
Moura, M., Osswald, M., Leça, N., Barbosa, J., Pereira, A. J., Maiato, H., et al. (2017). Protein phosphatase 1 inactivates mps1 to ensure efficient spindle assembly checkpoint silencing. Elife 6, e25366. doi:10.7554/eLife.25366
Mukherjee, S., Sandri, B. J., Tank, D., McClellan, M., Harasymiw, L. A., Yang, Q., et al. (2019). A gradient in metaphase tension leads to a scaled cellular response in mitosis. Dev. Cell. 49, 63–76. doi:10.1016/j.devcel.2019.01.018
Nazockdast, E., and Redemann, S. (2020)., 107. Elsevier, 91–102.Mechanics of the spindle apparatusSeminars Cell. & Dev. Biol.
Nerusheva, O. O., Galander, S., Fernius, J., Kelly, D., and Marston, A. L. (2014). Tension-dependent removal of pericentromeric shugoshin is an indicator of sister chromosome biorientation. Genes. & Dev. 28, 1291–1309. doi:10.1101/gad.240291.114
Nicklas, R. B., and Koch, C. A. (1969). Chromosome micromanipulation: Iii. spindle fiber tension and the reorientation of mal-oriented chromosomes. J. Cell. Biol. 43, 40–50. doi:10.1083/jcb.43.1.40
Ohta, S., Bukowski-Wills, J.-C., Wood, L., de Lima Alves, F., Chen, Z., Rappsilber, J., et al. (2011). “Proteomics of isolated mitotic chromosomes identifies the kinetochore protein ska3/rama1,” in Cold spring harbor symposia on quantitative Biology (Cold Spring Harbor, NY: Cold Spring Harbor Laboratory Press). sqb–2010.
Paldi, F., Alver, B., Robertson, D., Schalbetter, S. A., Kerr, A., Kelly, D. A., et al. (2020). Convergent genes shape budding yeast pericentromeres. Nature 582, 119–123. doi:10.1038/s41586-020-2244-6
Peplowska, K., Wallek, A. U., and Storchova, Z. (2014). Sgo1 regulates both condensin and ipl1/aurora b to promote chromosome biorientation. PLoS Genet. 10, e1004411. doi:10.1371/journal.pgen.1004411
Proudfoot, K. G., Anderson, S. J., Dave, S., Bunning, A. R., Roy, P. S., Bera, A., et al. (2019). Checkpoint proteins bub1 and bub3 delay anaphase onset in response to low tension independent of microtubule-kinetochore detachment. Cell. Rep. 27, 416–428. doi:10.1016/j.celrep.2019.03.027
Rosas-Salvans, M., Sutanto, R., Suresh, P., and Dumont, S. (2022). The astrin-skap complex reduces friction at the kinetochore-microtubule interface. Curr. Biol. 32, 2621–2631.e3. doi:10.1016/j.cub.2022.04.061
Roscioli, E., Germanova, T. E., Smith, C. A., Embacher, P. A., Erent, M., Thompson, A. I., et al. (2020). Ensemble-level organization of human kinetochores and evidence for distinct tension and attachment sensors. Cell. Rep. 31, 107535. doi:10.1016/j.celrep.2020.107535
Roy, B., Han, S. J., Fontan, A. N., Jema, S., and Joglekar, A. P. (2022). Aurora b phosphorylates bub1 to promote spindle assembly checkpoint signaling. Curr. Biol. 32, 237–247.e6. doi:10.1016/j.cub.2021.10.049
Salmon, E., Goode, D., Maugel, T., and Bonar, D. B. (1976). Pressure-induced depolymerization of spindle microtubules. iii. differential stability in hela cells. J. Cell. Biol. 69, 443–454. doi:10.1083/jcb.69.2.443
Sandall, S., Severin, F., McLeod, I. X., Yates, J. R., Oegema, K., Hyman, A., et al. (2006). A bir1-sli15 complex connects centromeres to microtubules and is required to sense kinetochore tension. Cell. 127, 1179–1191. doi:10.1016/j.cell.2006.09.049
Sarangapani, K. K., Akiyoshi, B., Duggan, N. M., Biggins, S., and Asbury, C. L. (2013). Phosphoregulation promotes release of kinetochores from dynamic microtubules via multiple mechanisms. Proc. Natl. Acad. Sci. 110, 7282–7287. doi:10.1073/pnas.1220700110
Schmidt, J. C., Kiyomitsu, T., Hori, T., Backer, C. B., Fukagawa, T., and Cheeseman, I. M. (2010). Aurora b kinase controls the targeting of the astrin–skap complex to bioriented kinetochores. J. Cell. Biol. 191, 269–280. doi:10.1083/jcb.201006129
Shrestha, R. L., Conti, D., Tamura, N., Braun, D., Ramalingam, R. A., Cieslinski, K., et al. (2017). Aurora-b kinase pathway controls the lateral to end-on conversion of kinetochore-microtubule attachments in human cells. Nat. Commun. 8, 150. doi:10.1038/s41467-017-00209-z
Stumpff, J., Wagenbach, M., Franck, A., Asbury, C. L., and Wordeman, L. (2012). Kif18a and chromokinesins confine centromere movements via microtubule growth suppression and spatial control of kinetochore tension. Dev. Cell. 22, 1017–1029. doi:10.1016/j.devcel.2012.02.013
Su, X. B., Wang, M., Schaffner, C., Nerusheva, O. O., Clift, D., Spanos, C., et al. (2021). Sumoylation stabilizes sister kinetochore biorientation to allow timely anaphase. J. Cell. Biol. 220, e202005130. doi:10.1083/jcb.202005130
Suzuki, A., Badger, B. L., Haase, J., Ohashi, T., Erickson, H. P., Salmon, E. D., et al. (2016). How the kinetochore couples microtubule force and centromere stretch to move chromosomes. Nat. Cell. Biol. 18, 382–392. doi:10.1038/ncb3323
Suzuki, A., Gupta, A., Long, S. K., Evans, R., Badger, B. L., Salmon, E. D., et al. (2018). A kinesin-5, cin8, recruits protein phosphatase 1 to kinetochores and regulates chromosome segregation. Curr. Biol. 28, 2697–2704. doi:10.1016/j.cub.2018.08.038
Suzuki, A., Hori, T., Nishino, T., Usukura, J., Miyagi, A., Morikawa, K., et al. (2011). Spindle microtubules generate tension-dependent changes in the distribution of inner kinetochore proteins. J. Cell. Biol. 193, 125–140. doi:10.1083/jcb.201012050
Tanaka, K., Kitamura, E., Kitamura, Y., and Tanaka, T. U. (2007). Molecular mechanisms of microtubule-dependent kinetochore transport toward spindle poles. J. Cell. Biol. 178, 269–281. doi:10.1083/jcb.200702141
Tanaka, T. U., Rachidi, N., Janke, C., Pereira, G., Galova, M., Schiebel, E., et al. (2002). Evidence that the ipl1-sli15 (aurora kinase-incenp) complex promotes chromosome bi-orientation by altering kinetochore-spindle pole connections. Cell. 108, 317–329. doi:10.1016/s0092-8674(02)00633-5
Tauchman, E. C., Boehm, F. J., and DeLuca, J. G. (2015). Stable kinetochore–microtubule attachment is sufficient to silence the spindle assembly checkpoint in human cells. Nat. Commun. 6, 10036. doi:10.1038/ncomms10036
Thein, K. H., Kleylein-Sohn, J., Nigg, E. A., and Gruneberg, U. (2007). Astrin is required for the maintenance of sister chromatid cohesion and centrosome integrity. J. Cell. Biol. 178, 345–354. doi:10.1083/jcb.200701163
Theis, M., Slabicki, M., Junqueira, M., Paszkowski-Rogacz, M., Sontheimer, J., Kittler, R., et al. (2009). Comparative profiling identifies c13orf3 as a component of the ska complex required for mammalian cell division. EMBO J. 28, 1453–1465. doi:10.1038/emboj.2009.114
Tien, J. F., Umbreit, N. T., Gestaut, D. R., Franck, A. D., Cooper, J., Wordeman, L., et al. (2010). Cooperation of the dam1 and ndc80 kinetochore complexes enhances microtubule coupling and is regulated by aurora b. J. Cell. Biol. 189, 713–723. doi:10.1083/jcb.200910142
Tooley, J., and Stukenberg, P. T. (2011). The ndc80 complex: Integrating the kinetochore’s many movements. Chromosome Res. 19, 377–391. doi:10.1007/s10577-010-9180-5
Tran, P., Marsh, L., Doye, V., Inoue, S., and Chang, F. (2001). A mechanism for nuclear positioning in fission yeast based on microtubule pushing. J. Cell. Biol. 153, 397–411. doi:10.1083/jcb.153.2.397
Uchida, K. S., Jo, M., Nagasaka, K., Takahashi, M., Shindo, N., Shibata, K., et al. (2021). Kinetochore stretching-mediated rapid silencing of the spindle-assembly checkpoint required for failsafe chromosome segregation. Curr. Biol. 31, 1581–1591.e3. doi:10.1016/j.cub.2021.01.062
Uchida, K. S., Takagaki, K., Kumada, K., Hirayama, Y., Noda, T., and Hirota, T. (2009). Kinetochore stretching inactivates the spindle assembly checkpoint. J. Cell. Biol. 184, 383–390. doi:10.1083/jcb.200811028
Umbreit, N. T., Miller, M. P., Tien, J. F., Cattin Ortolá, J., Gui, L., Lee, K. K., et al. (2014). Kinetochores require oligomerization of dam1 complex to maintain microtubule attachments against tension and promote biorientation. Nat. Commun. 5, 4951. doi:10.1038/ncomms5951
Van Hooff, J. J., Snel, B., and Kops, G. J. (2017). Unique phylogenetic distributions of the ska and dam1 complexes support functional analogy and suggest multiple parallel displacements of ska by dam1. Genome Biol. Evol. 9, 1295–1303. doi:10.1093/gbe/evx088
Varma, D., and Salmon, E. (2012). The kmn protein network–chief conductors of the kinetochore orchestra. J. Cell. Sci. 125, 5927–5936. doi:10.1242/jcs.093724
Verzijlbergen, K. F., Nerusheva, O. O., Kelly, D., Kerr, A., Clift, D., de Lima Alves, F., et al. (2014). Shugoshin biases chromosomes for biorientation through condensin recruitment to the pericentromere. Elife 3, e01374. doi:10.7554/eLife.01374
Huis in ’t Veld, P. J., Volkov, V. A., Stender, I. D., Musacchio, A., and Dogterom, M. (2019). Molecular determinants of the ska-ndc80 interaction and their influence on microtubule tracking and force-coupling. Elife 8, e49539. doi:10.7554/eLife.49539
Vukušić, K., Bua, R., and Tolić, I. M. (2019). Force-generating mechanisms of anaphase in human cells. J. Cell. Sci. 132, jcs231985. doi:10.1242/jcs.231985
Wan, X., O’Quinn, R. P., Pierce, H. L., Joglekar, A. P., Gall, W. E., DeLuca, J. G., et al. (2009). Protein architecture of the human kinetochore microtubule attachment site. Cell. 137, 672–684. doi:10.1016/j.cell.2009.03.035
Welburn, J. P., Grishchuk, E. L., Backer, C. B., Wilson-Kubalek, E. M., Yates, J. R., and Cheeseman, I. M. (2009). The human kinetochore ska1 complex facilitates microtubule depolymerization-coupled motility. Dev. Cell. 16, 374–385. doi:10.1016/j.devcel.2009.01.011
Welburn, J. P., Vleugel, M., Liu, D., Yates, J. R., Lampson, M. A., Fukagawa, T., et al. (2010). Aurora b phosphorylates spatially distinct targets to differentially regulate the kinetochore-microtubule interface. Mol. Cell. 38, 383–392. doi:10.1016/j.molcel.2010.02.034
Westermann, S., Avila-Sakar, A., Wang, H.-W., Niederstrasser, H., Wong, J., Drubin, D. G., et al. (2005). Formation of a dynamic kinetochore-microtubule interface through assembly of the dam1 ring complex. Mol. Cell. 17, 277–290. doi:10.1016/j.molcel.2004.12.019
Westermann, S., Wang, H.-W., Avila-Sakar, A., Drubin, D. G., Nogales, E., and Barnes, G. (2006). The dam1 kinetochore ring complex moves processively on depolymerizing microtubule ends. Nature 440, 565–569. doi:10.1038/nature04409
Wimbish, R. T., DeLuca, K. F., Mick, J. E., Himes, J., Jiménez-Sánchez, I., Jeyaprakash, A. A., et al. (2020). The hec1/ndc80 tail domain is required for force generation at kinetochores, but is dispensable for kinetochore–microtubule attachment formation and ska complex recruitment. Mol. Biol. Cell. 31, 1453–1473. doi:10.1091/mbc.E20-05-0286
Yamagishi, Y., Honda, T., Tanno, Y., and Watanabe, Y. (2010). Two histone marks establish the inner centromere and chromosome bi-orientation. Science 330, 239–243. doi:10.1126/science.1194498
Ye, A. A., Cane, S., and Maresca, T. J. (2016). Chromosome biorientation produces hundreds of piconewtons at a metazoan kinetochore. Nat. Commun. 7, 13221. doi:10.1038/ncomms13221
Ye, A. A., Verma, V., and Maresca, T. J. (2018). Nod is a plus end–directed motor that binds eb1 via a new microtubule tip localization sequence. J. Cell. Biol. 217, 3007–3017. doi:10.1083/jcb.201708109
Yoo, T. Y., Choi, J.-M., Conway, W., Yu, C.-H., Pappu, R. V., and Needleman, D. J. (2018). Measuring ndc80 binding reveals the molecular basis of tension-dependent kinetochore-microtubule attachments. Elife 7, e36392. doi:10.7554/eLife.36392
Keywords: spindle checkpoint, tension, chromosome segregation, microtubule, kinetochore
Citation: Bunning AR and Gupta Jr. ML (2023) The importance of microtubule-dependent tension in accurate chromosome segregation. Front. Cell Dev. Biol. 11:1096333. doi: 10.3389/fcell.2023.1096333
Received: 12 November 2022; Accepted: 11 January 2023;
Published: 23 January 2023.
Edited by:
A. Arockia Jeyaprakash, University of Edinburgh, United KingdomReviewed by:
Carlos Conde, Universidade do Porto, PortugalChip Asbury, University of Washington, United States
Copyright © 2023 Bunning and Gupta Jr.. This is an open-access article distributed under the terms of the Creative Commons Attribution License (CC BY). The use, distribution or reproduction in other forums is permitted, provided the original author(s) and the copyright owner(s) are credited and that the original publication in this journal is cited, in accordance with accepted academic practice. No use, distribution or reproduction is permitted which does not comply with these terms.
*Correspondence: Mohan L. Gupta Jr., bWd1cHRhQGlhc3RhdGUuZWR1