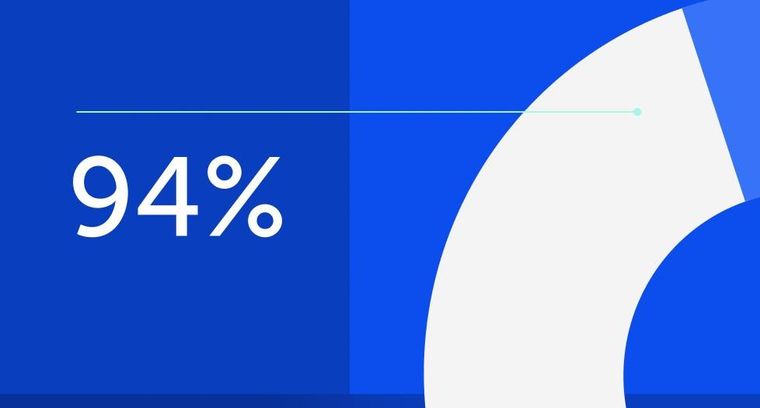
94% of researchers rate our articles as excellent or good
Learn more about the work of our research integrity team to safeguard the quality of each article we publish.
Find out more
REVIEW article
Front. Cell Dev. Biol., 30 January 2023
Sec. Cancer Cell Biology
Volume 11 - 2023 | https://doi.org/10.3389/fcell.2023.1089068
This article is part of the Research TopicThe Role of Cancer Associated Fibroblast (CAF) in EMT/Metastasis in Malignancies of Epithelial TissuesView all 5 articles
Stromal heterogeneity of tumor microenvironment (TME) plays a crucial role in malignancy and therapeutic resistance. Cancer-associated fibroblasts (CAFs) are one of the major players in tumor stroma. The heterogeneous sources of origin and subsequent impacts of crosstalk with breast cancer cells flaunt serious challenges before current therapies to cure triple-negative breast cancer (TNBC) and other cancers. The positive and reciprocal feedback of CAFs to induce cancer cells dictates their mutual synergy in establishing malignancy. Their substantial role in creating a tumor-promoting niche has reduced the efficacy of several anti-cancer treatments, including radiation, chemotherapy, immunotherapy, and endocrine therapy. Over the years, there has been an emphasis on understanding CAF-induced therapeutic resistance in order to enhance cancer therapy results. CAFs, in the majority of cases, employ crosstalk, stromal management, and other strategies to generate resilience in surrounding tumor cells. This emphasizes the significance of developing novel strategies that target particular tumor-promoting CAF subpopulations, which will improve treatment sensitivity and impede tumor growth. In this review, we discuss the current understanding of the origin and heterogeneity of CAFs, their role in tumor progression, and altering the tumor response to therapeutic agents in breast cancer. In addition, we also discuss the potential and possible approaches for CAF-mediated therapies.
Tumors are heterogeneous, which is one of the hallmarks of malignancy. The progression of the tumor depends on the dynamic crosstalk between cancer cells and the other cells in the stromal microenvironment. One of the key players in the tumor microenvironment is cancer-associated fibroblasts (CAFs). CAFs promote tumor progression, extracellular matrix (ECM) remodeling, inflammation, chemoresistance, and immunosuppression (Ohlund et al., 2014; Kalluri, 2016; Czekay et al., 2022). The exact origin of different subtypes of CAFs in breast cancer is not fully understood. Tumor-secreted factors are critical in controlling CAF-precursors’ differentiation into CAFs (Houthuijzen and Jonkers, 2018). Due to the heterogeneity, targeting CAFs remains a significant challenge. In this review, we discuss the current information regarding CAFs biology, origin, function, and how targeting CAFs, is being explored as an opportunity to improve cancer therapies.
Despite being of enormous importance for understanding tumor microenvironment (TME), the lack of precision of the identification markers poses a colossal impediment en route to understanding the source of CAFs. It becomes even more inexplicable when no discernible difference is detectable between the surface markers of normal tissue fibroblasts and CAFs. In order to seal the knowledge gap, several researchers have focused on tracking the fibroblastic properties in the TME at various phases, such as tumorigenic mutation induction and the development of in situ and invasive cancer. These investigations’ findings on human tissue showed that the so-called fibroblastic stroma underwent gradual alterations, and the preliminary step-up in their number eventually paved the way for the induction of malignancies. Noteworthy to mention is that the CAFs continue to encircle the pertinent cancerous lesions as they gradually transform during the early and premalignant episodes. Experimental data suggest circumscribing fibroblasts may have a tumor-suppressive role in the early stages (Rhim et al., 2014). Experimental evidence supporting the increase in the number of stromal fibroblasts alongside the progression of tumorigenesis provides us with a new concept of stromagenesis. This, in turn, prompts the assumption that fibroblasts have a mesenchymal origin and are activated and converted into CAFs during stromagenesis. The new lineage of fibroblasts plays a pro-tumorigenic role, opposite to the initial tumor suppressive role (Sahai et al., 2020a).
Other than differentiating CAFs from normal fibroblasts (NFs) based on inadequately exclusive cell surface markers, the change in shape and size of the NFs was demonstrated to have happened upon treatment with conditioned cell culture medium from human breast cancer cell lines, and this has been associated with conversion into spindle-shaped CAFs (Kumar et al., 2014). However, the entire concept of cellular changes happening in stromal fibroblasts cannot be addressed longitudinally in human biopsy specimens. Although longitudinal sampling is feasible, direct monitoring of stromal fibroblasts is not. Therefore, efforts have concentrated on animal models with properly labeled cells that can track disease development. This has been accomplished by using tissue-specific expression of the “cre-recombinase” in competent laboratory animals with an irreversibly activated reporter gene in cre-positive cells. It might have been easier in the presence of appropriate fibroblast markers (Ping et al., 2021). The “cre-recombinase” technique might have unraveled the potential origin of CAFs to be pericytes, adipocytes, mesenchymal stem cells (MSCs), and endothelial cells. Yeon et al. (2018) found substantial data in favor of the conversion of endothelial cells to CAFs. Data concludes that melanoma-derived exosomes can induce the dedifferentiation of endothelial cells into MSCs (endothelial to mesenchymal transition; EndMT), which are then turned into CAFs. Karnoub et al. (2007) revealed a similar paradigm that explains the transformation of externally administered bone marrow-derived MSCs into CAFs. An interesting study by Nair et al. (2017) demonstrated the effect of conditioned media from T47D and BT549 human breast cancer cells in converting induced pluripotent stem (iPS) cells first into cancer stem cells (CSCs) and then into CAFs.
In contrast, the transition from adipocytes has also been described (Karnoub et al., 2007; Nieman et al., 2011; Bochet et al., 2013). In other circumstances, CAFs are said to prevent adipocyte differentiation. Adipocytes, on the other hand, offer metabolic support regardless of whether they transform into CAFs (Karnoub et al., 2007; Sahai et al., 2020a; Ping et al., 2021). Evidence-based studies showed that Platelet-derived growth factor-BB (PDGF-BB)/Platelet-derived growth factor receptor β (PDGFRβ) signaling system drives pericyte differentiation toward fibroblasts (Hosaka et al., 2016). Further, the epithelial-to-mesenchymal transition (EMT) also feeds the MSCs to CAFs transformation cascade, implying that CAFs have an epithelial origin (Fiori et al., 2019; Mao et al., 2021; Ping et al., 2021). The cytokine generation by the resultant CAFs accounts for tumor cell EMT and promotes cancer spread and invasion (Fiori et al., 2019; Ping et al., 2021).
Information alludes to the idea that both canonical and non-canonical transforming growth factor beta (TGFβ) pathways have a part in fostering the generation of CAFs (Wu et al., 2021). The propagation and differentiation of NFs were correlated with the transmission of TGF and endocytosis by the NFs (Wu et al., 2021). TGFβ and nuclear factor-κB (NF-κB) signaling pathways regulate the conversion of stromal fibroblasts to CAFs in response to a wide range of stimuli, including osteopontin (OPN), interleukin-1 (IL-1), and others that are part of the secretome of the immunological or cancer cells (Fiori et al., 2019). Furthermore, an altered energy metabolism may be vital in converting NFs to CAFs. In the TME, cancer cell-secreted Lysophosphatidic acid (LPA), TGFβ1, or platelet-derived growth factor (PDGF) can potentially trigger the HIF-1α pathway to cause aerobic glycolysis in the fibroblasts (Wu et al., 2021). The transformation of NFs into CAFs is also associated with alterations in how some components express themselves, as shown in several reports (Calvo et al., 2013; Sahai et al., 2020a; Shen et al., 2020). For instance, Yes-associated protein 1 (YAP1), a transcriptional coactivator in NFs, alters the transcription of proto-oncogene c-Src (src) by associating with TEA domain transcription factor-1 (TEAD1) to construct a protein compound, which activates cytoskeletal proteins and leads to the development of CAFs (Calvo et al., 2013; Sahai et al., 2020a).
Physiological and inflammatory stress could also affect NFs to CAFs conversion (Sahai et al., 2020a). IL-1 and IL-6, potent inflammatory cytokines, can predominantly trigger CAF activation via the NF-κB and signal transducers and activators of transcription (STAT) transcription factors (Erez et al., 2010; Sanz-Moreno et al., 2011a). Additionally, crosstalk and positive feedback involving Janus kinase (JAK)-STAT signaling, the contractile cytoskeleton, and histone acetylation all contribute to CAF activation (Albrengues et al., 2014; Albrengues et al., 2015). Heat shock factor-1 (HSF-1) is synthesized in response to physiological stress, and it is thought to enhance stromagenesis and malignancy via transcriptional modification of CAFs (Scherz-Shouval et al., 2014; Sahai et al., 2020a). Other genomic stressors, such as dsDNA break, can initiate an IL-6-mediated response as well as the production of TGFβ family ligand activin A, resulting in the expansion of CAFs or, in some cases, senescent fibroblasts, the removal of which can have severe repercussions for disease relapse (Scherz-Shouval et al., 2014; Ferrari et al., 2019; Sahai et al., 2020a).
Several experimental reports suggest that the nature of CAFs and the TME remains highly volatile and diverse depending upon different signaling pathways and the organ in which they are located. For instance, FGF and TGFβ trigger different regulatory networks through different effector genes to promote carcinogenesis or regulate immune surveillance (Bordignon et al., 2019; Ping et al., 2021). Induction by TGFβ opens up a cascade of events that stimulates tumor cell invasion beyond basal lamina and EMT (Tsubakihara and Moustakas, 2018). As cancer progresses, the alteration of signaling pathways involved in CAF transition from a tumor-suppressive state to a tumor-promoting activated state shows the transcriptomic significance of CAF phenotype plasticity (Ping et al., 2021).
The vast number of activities ascribed to CAFs in myriad experimental models raises the topic of whether a particular kind of CAF accomplishes all of these functions simultaneously or whether CAFs subspecialize or switch between diverse functional states. Enumerable data confirm CAF specialization, which may be similar to the specialization of NFs. The terms “myofibroblast CAFs or myCAFs” and “inflammatory CAFs or iCAFs” are frequently used to refer to different CAFs with the prefixes implying a myofibroblast phenotype and the regulation of inflammatory response, respectively (Öhlund et al., 2017; Biffi et al., 2019). The CAFs nearest in proximity to the cancerous cells in pancreatic cancer, display a myCAF-like phenotype, which is characterized by strong TGFβ-driven αSMA expression and a contractile nature (Öhlund et al., 2017; Sahai et al., 2020a). iCAFs are marked as having elevated IL-6 expression patterns. The TGFβ-mediated regulation of the IL-1 receptor, which is responsible for initiating NF-κB signaling and subsequent IL-6 production, can be used to explain the apparent exclusivity of the two phenotypes (Biffi et al., 2019; Sahai et al., 2020a; Ping et al., 2021).
Bartoschek et al. (2018) categorized breast CAFs into four subpopulations according to vascular development (vCAF), ECM-enriched signaling (mCAF), expression of cycling or proliferative phases (cCAF), and variously expressed differentiation genes (dCAF), via single-cell RNA-sequencing. Another perspective is the assortment of CAF populations based on their origin and location within the TME. vCAFs include angiogenic and vascular regulation functions while predominantly localized near the supposed vasculature. The source of mCAFs was concluded to be of similar marker expression with resident fibroblasts in local mammary tissues. The cCAFs were demonstrated to share the same cluster as vCAFs, being distinguishable only by their proliferating nature. The dCAFs share expression patterns with various stem cells suitable for their putative role in cellular differentiation and tissue morphogenesis (Bartoschek et al., 2018). The demonstrated spatial distribution heterogeneity in these subpopulations elucidates the importance of CAF function concerning their origin and function in inducing a tumor-promoting milieu.
Similarly, Friedman et al. (2020) identified two primary subpopulations of CAFs in breast cancer: pCAFs and sCAFs, which are distinguished by the expression of podoplanin (Pdpn) and S100A4 (also called fibroblast-specific protein one; FSP1), respectively. The makeup and function of these CAFs change as cancer advances and the ratio of different populations give insight into how the patient may respond (Friedman et al., 2020; Asif et al., 2021). The CAF subpopulations classified have distinct phenotypical heterogeneity that distinguishes them from each other. pCAFs were separated into six subgroups based on varying transcriptional differences involving both early and late immune regulation, immune-regulatory signatures, ECM modulation, and inflammation (Friedman et al., 2020). Over time, the transcriptional signatures in breast cancer CAFs transitioned from immune regulatory functions to wound healing and antigen-presentation, as seen with the fraction of pCAFs decreasing over a 4-week period after tumor injection (Friedman et al., 2020). The dynamic nature of CAFs and their ability to transdifferentiate via reprogramming have been further demonstrated through lineage analysis showing the origins of pCAFs from NFs and sCAFs from bone marrow-derived MSCs (Friedman et al., 2020).
Likewise, other diverse types of CAF phenotypes have been identified in breast cancer, with fibroblast activation protein (FAP) being the primary discriminating marker (Costa et al., 2018). Experimental demonstration correlated an elevated expression of FAP in FAP+ fibroblasts with regulatory T-cell (Treg) dependent immunosuppression and poor prognosis. Nonetheless, the FAP+ targeted chimeric antigen receptor (CAR) T-cell therapy unraveled its role in matrix formation besides being considered a potent immunomodulator (Lo et al., 2015). FAP+ fibroblasts have also been identified in PDAC as tumor-promoting CAFs, performing possibly through stromal-derived factor 1 (SDF-1) and C-C motif chemokine ligand 2 (CCL2) dependent manner (Yang et al., 2016a; McAndrews et al., 2022). At the same time, in a genetically engineered mouse model system for PDAC, αSMA+ cells were also identified as a type of myofibroblasts (myCAFs) (Djurec et al., 2018; Sperb et al., 2020), believed to be tumor-restraining in nature. McAndrews et al. (2022) (McAndrews et al., 2022) used a single cell RNA-seq (scRNAsq) analysis that revealed a minimal overlap between FAP+ and αSMA+ type CAFs deciphering them as distinct populations with distinct effects on immune response, chemotherapeutic agents, and chemokine secretion. Costa et al. (2018) (Costa et al., 2018) defined populations of breast CAFs based on the expression of FAP, αSMA, and other various markers such as FSP1, CD29, PDGFR-β, and caveolin-1 (CAV1). CAF-S1 is seen as having a higher expression of all six markers than CAF-S2, which is negative for all. CAF-S3 involved CD29, FSP1, and PDGFR-β expression, and the CAF-S4 subpopulation consisted of high αSMA and CD29 expression along with low to medium expression of FSP1 and PDGFR-β and negative to low expression of CAV1. However, CAF-S1 and CAF-S4 subsets secrete TGFβ and CXCL12 to activate NOTCH signaling and have been associated with TNBC and HER2+ breast cancers, respectively. Along with phenotypical differences, CAF-S1 regulates T-cells, controlling the immune response and suppression in the TME via secretion of IL-6/17/10 and CXCL12 (Costa et al., 2018). Understanding the dynamics of CAF transitions and origins with respect to their differing subpopulations can lead to the precise targeting of tumor-promoting CAF subpopulations (Figure 1).
FIGURE 1. Schematic illustration of heterogeneity in breast CAFs. Broadly, breast CAFs are distinguished under three subclasses based on their heterogeneous function, spatial distribution, and cell surface phenotype. They have been divided into tumor-promoting and tumor-suppressing cell types due to their stage-specific distinct approach toward tumor cells. With the progression of cancer, transcriptional alteration of the tumor-suppressing CAF phenotypes results in the generation of tumor-promoting breast CAFs. Manipulation of the event may prove to be a potent therapeutic tool. Bartoschek et al. (2018) used single-cell RNA data to classify the CAFs according to their spatial distribution. Vascular development, ECM-enriched signaling, expression of proliferative genes, and variously expressed differentiation genes shaped their classification into vCAFs, mCAFs, cCAFs, and dCAFs. Phenotypically breast CAFs could be sub-classified depending upon the i) presence or absence of CD146 (Brechbuhl et al., 2020), ii) expression of αSMA/FAP (Costa et al., 2018), and iii) expression of PDPN/FSP (Friedman et al., 2020). This diagram displays the several subtypes with phenotypical distinctions that are accountable for classified functions at various phases of carcinogenesis. The bottom right graph illustrates the percentage of pCAF/sCAF specific markers over a period of 4 weeks in a breast cancer model.
As documented in the previous section, multiple origins of CAFs account for their equally diverse nature of functions in the TME. They are a significant source of secretory molecules such as growth factors (GFs), cytokines and chemokines, extracellular vesicles (EVs) like exosomes, and extracellular matrices (ECMs). Therefore, the cellular contribution of the CAFs could potentially aid in the initiation and progression of cancer. It also aids in regulating therapeutic resistance through immunomodulation, inflections of local tissue metabolisms, and regulation of the mechanisms establishing hallmarks of cancer, culminating in invasion and metastasis.
However, based on functions, the CAFs may be categorized into two significant subclasses; tumor-promoting CAFs and tumor-suppressing CAFs. Their extraordinary capacity to restructure tumor blood vessels and the extracellular matrix accounts for their reciprocating roles in the TME (Sewell-Loftin et al., 2017). Even though CAFs appear to prevent cancer initially, research findings (Benyahia et al., 2017; Sewell-Loftin et al., 2017; Shen et al., 2017) indicate that they do stimulate cell growth. By forming a “restrictive barrier” encircling a lesion, CAFs effectively prevent the spread of cancer cells by force-mediated ECM-remodeling and through the production of matrix-crosslinking proteins, thus rendering the tumor tissue stiff (Kechagia et al., 2019; Sahai et al., 2020a; Zeltz et al., 2020a; Ping et al., 2021). The altered ECM aids the tumor in developing a therapeutic resistance barrier and a protective canopy to prevent immune cell penetration inside the TME as the disease progresses and the TME evolves (Ping et al., 2021). Apart from promoting metastasis, de novo stimulation of fibroblasts stimulates the release of periostin (POSTN) and tenascin-like molecules, which stimulate Wnt signaling (Chiquet-Ehrismann et al., 2014; Yu et al., 2018a). CAFs thereby accomplish the embodiment of macro-metastasis by regulating stem cell niches through Wnt signaling (Oskarsson et al., 2011; Calon et al., 2012; Malanchi et al., 2012; Dumont et al., 2013a; Madsen et al., 2015; Nabhan et al., 2018). Changes in ECM architecture influence the recruitment of infiltrating leukocytes, which has relevance for tumor immune surveillance (Kaur et al., 2019).
Other secretory functions of CAFs, such as vascular endothelial growth factor (VEGF) synthesis by the stromal cells, can lead to angiogenesis (O'Connell et al., 2011; Fukumura et al., 1998). Various cyto- and chemokines affect the immune response by acting upon CD8+ T-cells, Treg cells, and macrophages (Monteran and Erez, 2019a). Nevertheless, it is widely agreed that the putative function of CAFs is immunosuppressive, with CXC-chemokine ligand 9 (CXCL9) and TGFβ all playing well-established roles in limiting T-cell responses (Fearon, 2014). Antigen cross-presentation by CAFs has been identified more recently (Elyada et al., 2019); this may cause CD4+ T-cell activation and CD8+ T-cell suppression (Lakins et al., 2018a). Further evidence from clinical studies points to an antagonistic relationship between CAFs and CD8+ T-cells (Costa et al., 2018).
Additionally, IL-6 may facilitate immunosuppression by altering metabolism systemically (Flint et al., 2016). In cancer cells, targeting focal adhesion kinase (FAK) reduces stromal fibroblast activation and the emergence of an immunosuppressive milieu. Interfering with the activity of CXCL12 generated by CAFs enhances T-cell-mediated tumor control (Kraman et al., 2010a; Feig et al., 2013; Chen et al., 2019). TNFα can stimulate fibroblast activation in some circumstances, but it is also known to be suppressed by the tumor-promoting immunosuppressive activities of FAP+ fibroblasts (Kraman et al., 2010a; Chaudhry et al., 2013; Lau et al., 2017). Tumor-promoting immunosuppressive activity of FAP+ fibroblasts is connected with the inhibition of TNFα signaling. However, in some circumstances, TNFα can also stimulate fibroblast recruitment, making the situation with tumor necrosis factor (TNFα) generated by CAFs more complicated (Kraman et al., 2010b; Chaudhry et al., 2013; Jiang et al., 2016; Lau et al., 2017). The schematic representation explains the diverse origins and multiple functions of CAFs in Figure 2.
FIGURE 2. Origin and function of CAFs. Schematic representations of possible CAF cell origins have been depicted, as evidenced to date. Different types of cellular differentiation, trans-differentiation, and de-differentiations give rise to the generation of CAFs. CAFs show a plethora of activities in accord with and against cancer cell survival and malignancy, evidently in a stage-specific manner. The only event that results in the suppression of tumors occurs when the CAF-derived secretome includes TGFβ inhibitors. The other roles of CAFs in cancer include the induction of all the hallmarks of cancer via the secretion of various cytokines, chemokines, growth factors, carbohydrate intermediates, and nitrogen sources (amino acids). Their activities include induction of cancer stemness, metastasis, migration, invasion, angiogenesis, ECM remodeling, immunomodulation, metabolic manipulation, and therapeutic resistance (Karnoub et al., 2007; Mani et al., 2008; Erez et al., 2010; Baccelli and Trumpp, 2012; Oskarsson et al., 2014; Albrengues et al., 2015; Yeon et al., 2018; Fiori et al., 2019; Sahai et al., 2020a; Shen et al., 2020; Loh and Ma, 2021; Ping et al., 2021; Wu et al., 2021).
Based on a recent study that revealed matrix-specific hedgehog signaling inhibits tumor growth and invasion by lowering matrix stiffness, scientists have focused on the role of matrix conversion in switching pro-tumor CAFs to anti-tumor CAFs (Yoshida, 2020). In smooth muscle cells and allied CAFs (αSMA+ (αsmooth muscle actin) CAFs), it has been found that an upsurge in hedgehog signaling is correlated with a proportionate suppression of malignant development (Yang et al., 2016b).
The prevalence of CAFs throughout the malignant transformation, from early to late stages, implies that they play an indispensable role in tumorigenesis and progression (Erez et al., 2010). The heterogeneity data demonstrates that the proximal CAFs are empowered with potent juxtacrine TGFβ signaling those aids in transformation into stem cells (Bellomo et al., 2016; Fiori et al., 2019; Nakano et al., 2019; Sahai et al., 2020a). Additionally, the neoplastic cells educate the CAFs to become able to assist through tumor progression and dissemination in other parts of the body (Sahai et al., 2020a; Loh and Ma, 2021; Ping et al., 2021).
Hypothesizing that CAFs foster the niche by enriching the pro-tumor factors, an evidence-based study illustrated that an enhanced expression of TGFβ1 in a CAF-conditioned medium (CAF-CM) remained fundamental to the mesenchymal transformation of the non-CSC bladder cancer cells (Zhuang et al., 2015). Cancer cells induce pro-tumor CAF to establish a pro-favorable niche. TGFβ3 produced from head and neck squamous cell carcinoma (HNSCC) cells can stimulate CAFs to release POSTN, an ECM protein, augmenting the inclination of neoplastic cells towards altered plasticity and metastasizing (Qin et al., 2016; González-González and Periostin, 2018). CSC markers and stemness genes are elevated due to the binding of CAF-derived POSTN to protein tyrosine kinase 7 (PTK7) (Yu et al., 2018a). Likewise, prostate cancer cells turned externally administered bone marrow-derived mesenchymal stem cells (MSCs) into CAFs by TGFβ1 (Barcellos-de-Souza et al., 2016). Interestingly, these stem cell-derived CAFs can change the TME into an M2 (macrophage) phenotype dominant one by transforming residual monocytes. These CAFs can also release cardiotrophin-like cytokine factor (CLCF1) that primarily acts through the JAK-STAT pathway in a positive feedback loop to produce TGFβ and C-X-C motif ligand 6 (CXCL6) (Song et al., 2021). This positive feedback mechanism engages the CAFs to induce stemness by plentiful TGFβ in the TME (Song et al., 2021). In another example citing the role of CSCs in augmenting stemness factors in the TME, a similar paradigm comes into play to employ CAFs by CD24+CD49fhi breast CSCs that trigger the production of components of ECM and pro-tumor paracrine factors in CAFs (Valenti et al., 2017). Valenti et al. (2017) (Valenti et al., 2017) demonstrated that Sonic hedgehog (Shh) derived from breast CSCs were responsible for activating CAFs that, in turn, supplemented the stemness (Valenti et al., 2017; Cazet et al., 2018). Recent research on the origins of chemotherapy resistance found IL-34 and macrophage colony-stimulating factor-1 (M-CSF-1) to be two of those (Baghdadi et al., 2018; Hama et al., 2020). IL-34, with the help of M-CSF-1, acted in a pro-survival way for the tumor cells by helping the monocytes grow alongside converting NFs to CAFs. These CAFs can transform these monocytes into immunosuppressive M2 macrophages, besides secreting stemness-promoting factors like Netrin-1 and FGF2 protein (Suh et al., 2016; Sung et al., 2019). The conversion into the M2 phenotype also inhibits the cytotoxicity of tumor antigen-specific CD8+ T-cells (Baghdadi et al., 2016; Giulianelli et al., 2019; Sung et al., 2019; Blondy et al., 2020). Other examples involve in vitro data, suggesting that the influence of HNSCC cells on the CAFs to produce Wnt3a increases cancer stemness (Le et al., 2019).
CAF-derived factors play critical roles in developing and maintaining CSCs by boosting stemness pathways. Hepatocyte growth factor (HGF), released by stromal cells, like fibroblasts, dramatically enhances stemness and EMT gene expression by increasing the expression of CD44+, CD47+, and CD90+ markers tissue (Yan et al., 2018a; Ding et al., 2018). A rise in the stemness markers and an increase in spheroid formation were demonstrated to be due to the activity of CAF-derived IL-6 through the STAT3 pathway (Xiong et al., 2018; Zhang et al., 2020). Other well-known stemness factors, like NANOG and ALDH, are similarly enhanced by the AKT pathway, which is controlled by the CAF secretome (Li et al., 2018). Furthermore, CAF is a crucial modulator of EMT in cancer cells due to the release of IL-6 (Raskov et al., 2021). A few other molecules causing EMT and stemness are Serglycin (SRGN), Annexin A1 (AnxA1), and Prostaglandin E2 (PGE2), all of which are derived from activated CAFs and act through paracrine signaling on the non-small cell lung cancer (NSCLC) cells, prostate cancer cells, and intestinal cancer cells respectively (Geary et al., 2014; Guo et al., 2017; Roulis et al., 2020). Moreover, exosomal miRNAs such as miR-378c, miR-143, and miR-21, derived from CAFs, play significant roles in EMT and the generation of stem-like cells (Donnarumma et al., 2017; Yang et al., 2017).
All these data allude to the critical role of the CAFs, being an intermediary, following commands from tumor cells to stimulate stemness. Nonetheless limited data in this domain necessitates further efforts to fill knowledge gaps.
The ability of CAFs to induce cancer invasion and metastasis is their most significant distinguishing attribute compared to NFs. Data suggests that CAFs remodel the ECM, leading to cancer progression through invasion and metastasis (Dumont et al., 2013b). Answering the controversy regarding whether the number of CAFs decreases or increases to cause the cancer cells to invade ECM, an exciting study employing a deep learning cell identification algorithm using TCGA suggested an increase in the number of CAFs in the TME (Shapcott et al., 2019). The CAF model differs from typically observed cancer cell metastasis in invadopodia-independent and MMP-independent matrix degradation processes (Cao et al., 2016; Glentis et al., 2017). The heterogeneity and plasticity of CAFs are also critical for invasion and metastasis. The interplay among the microbiota present in the TME portrays itself as the cat’s-paw for the metastatic cells. We have learned about two distinct CAFs, differentiated in terms of heterogeneity of surface markers (CD146+/−). CD146- CAFs have been shown to enrich the TME with the production of proteins responsible for invasiveness, such as tenascin C (TNC), lysyl oxidase (LOX), and fibronectin 1 (FN1) (Brechbuhl et al., 2020). Earlier studies showed that CD146+ cells may represent a more mature pericyte subpopulation than CD146- cells (Hassanpour et al., 2020; Manocha et al., 2022). CD146- is a potent marker for risk analysis for lymph node metastasis, and patients with higher CD146- cells have a poor prognosis (Brechbuhl et al., 2020). In convergence, the accumulated fibronectin (FN) in CAFs accelerates the invasion mainly via integrin-αvβ3. The assembled FN and the expression of αv and β3 integrins are directly proportional to the capacity to breach the basement membrane (BM) (Attieh et al., 2017). However, another integrin α11β1, a stromal cell-specific receptor for fibrillar collagens, indirectly aids in lung metastasis via facilitating trans-differentiation of CAFs (Navab et al., 2016).
It is broadly accepted that the tumor-promoting microenvironment in metastasis lesions emerges prior to the arrival of cancer cells, a process known as premetastatic niche (PMN) development (Fares et al., 2020). Soluble factors such as TGFβ, VEGF, and TNF, and EVs, like exosomes released by CAFs, promote PMN development. These factors then enter the circulatory system and spread to remote targets (Kong et al., 2019; Ping et al., 2021). Exosomes derived from CAFs contain non-coding RNAs (especially miRNAs) that regulate the cancer cells in a paracrine fashion (Yang et al., 2019; Li et al., 2021; Zhan et al., 2021). The differential distribution of these miRNAs in CAFs and NFs draws a thin line between their ability to manipulate the cancer cells to grow and invade distant tissues (Herrera et al., 2018). For example, miR-92a-3p expression was dramatically elevated in colorectal cancer (CRC) cells following phagocytosis of CAFs-derived exosomes (Hu et al., 2019). Again, a decrease in the miR-3188 levels in the CAFs-derived exosomes compared to that of NFs has been stated to have a role in turning on malignant phenotype in head and neck cancer cells (Wang et al., 2019).
POSTN+ CAFs have been demonstrated to enhance lymph node metastasis as well as boost cancer cell proliferation and invasiveness (Hong et al., 2010; Wei et al., 2021). They activated the integrin-FAK/Src-VE-cadherin signaling cascade to penetrate lymphatic endothelial barriers, culminating in metastatic dissemination (Hong et al., 2010). CAFs are also reported to facilitate metastasis depending upon the presence or absence of stanniocalcin-1 (STC1). Pena et al. (2013) (Pena et al., 2013) demonstrated that CRC cells failed to invade and migrate in vitro and in vivo when co-cultured with STC1-deficient CAFs and grown in an orthotopic mouse model, respectively (Pena et al., 2013).
The predominant stromal cells in the TME account for the primary ECM source and thus can easily be attributed to its novel ability to modulate the same. Glentis et al. (2017) demonstrated a crucial role of CAF-containing stroma in paving the matrix-metalloproteinases (MMP)- independent mechanism of invasion by the colon cancer cells (Glentis et al., 2017), in contrast with previous belief that MMP2 and -9 are pre-requisites for metastatic dissemination of cancer cells to distant locations (Taguchi et al., 2014). The activation of CAFs and expression of αSMA rest on the secretome of CAFs. According to Tang et al. (2016), the invasive tendency of breast cancer cells grown in vitro is caused by the downregulation of the miR-200s (miR-200 family), which is consistent with the prometastatic nature of CAFs. These studies also shed insight into the functions of miRNAs in the transdifferentiation of fibroblasts (Tang et al., 2016).
Although molecular aberrations prevail in cancer cells, however, epigenetic alterations and variable miRNA expression are mostly fundamental for the cancer-promoting properties of CAFs. Despite several early reports of p53 mutations in the stromal compartment, it is now widely believed that CAFs do not carry p53 mutations (Hu et al., 2005; Fukino et al., 2007; Bechtel et al., 2010; Zhao et al., 2012). However, an oncogenic gain-of-function is attained inside cancer cells, above and beyond ablating the tumor suppressive function, upon mutation in the TP53 gene (Muller and Vousden, 2013). At the early stage of carcinogenesis, p53 functions as a cell non-autonomous tumor suppressor in the fibroblasts, in part by preventing the production and secretion of several molecules that might promote tumor growth (Kiaris et al., 2005; Moskovits et al., 2006; Addadi et al., 2010; Otomo et al., 2014). However, sustained cross-talk with the cancer cells induces trans-differentiation of their neighboring fibroblasts into CAFs (Kalluri, 2016). In agreement, Arandkar et al. (2018) have demonstrated the role of CAFs in invasion and metastasis correlating to an altered p53 function in the CAFs, suggesting that p53 acted as an essential modulator of the epigenetic machinery of CAFs in comparison with NFs. p53 was shown to have inherent, cell-autonomous, and unique characteristics imparted upon CAFs compared to NFs. A global transcriptome analysis using RNA sequencing data showed that 1,662 genes were expressed differentially in CAFs, supporting cancerous growth, proliferation, invasion, and migration. Cancer cells’ migratory characteristics were attenuated on silencing p53 in the CAFs (Arandkar et al., 2018).
New vasculature connectivity formation is essential because tumor cell proliferation and metastatic spread rely on optimal oxygen intake, supply of nutrients, and waste elimination (Lugano et al., 2020; Notohamiprodjo et al., 2021). The endogenous angiogenic factors responsible for the activation of neovasculature are namely VEGF, bFGF, TGFα, TGFβ, TNFα, angiogenin, PDGF, G-CSF, placental growth factor, IL-8, HGF, and epidermal growth factor (EGF) (Nishida et al., 2006). The role of CAFs in neovasculature to aid hypoxic tumor cells depends broadly on the positive and reciprocal feedback among CAFs and tumor cells. CAFs recruit endothelial cells and stimulate vascularization, triggering angiogenesis for the nutrient supply to cancer cells (Sewell-Loftin et al., 2017). The connective tissue growth factor (CTGF) expression is essential for boosting microvessel density and attracting endothelial cells in experimental xenograft models of CAF-mediated angiogenesis through the expression of SDF-1/CXCL12 (Denton et al., 2018). Apart from that, there is another indirect role of CAFs in triggering angiogenesis. As discussed, CAFs regulate and modify the ECM in favor of the cancer cells to grow and metastasize. Such modifications include the upregulation of MMP9 and MMP13, leading to the secretion of pro-angiogenic growth factors like VEGF (Boire et al., 2005; Vosseler et al., 2009).
Overexpression of SDF-1/CXCL12 by αSMA+ CAFs and simultaneous downregulation of mDia2 protein triggered pro-angiogenic secretome profile in breast cancer (Orimo et al., 2005; Yu et al., 2014; Dvorak et al., 2018). Apart from secreting VEGF, CAFs recruit endothelial progenitor cells (EPCs) in the breast cancer TME resulting in angiogenesis (Orimo et al., 2005; De Francesco et al., 2013). In line, Wang et al. (2014a) showed malignancy and angiogenesis in nasopharyngeal carcinoma (NPC) in a VEGF/CXCL12-CXCR4 dependent manner, and the presence of EPCs was also confirmed with CD133+ cells in the tumor stroma. Two independent research groups reported that the SDF-1/CXCR4 cascade was also employed by αSMA+ CAFs following HIF-1α mediated conversion into CAFs from NFs (Zagzag et al., 2005; Errarte et al., 2020). Recent research unveiled an exciting correlation of αSMA and CD90 of CAFs with neighboring HCC tissue expression of placental growth factor (PGF) (Liu et al., 2020a). PGF causes blunted anti-tumor immunity by M2 polarization and creates a pro-angiogenic environment by triggering the inflammatory markers, NF-κB and COX2 in CAFs (Albonici et al., 2019). With the help of TCGA analysis, Liu et al. (2020a) showed that the co-expression of PGF and CD90 in the tumor milieu could be directly correlated to the angiogenesis markers CD31, CD34, and CD105 leading to poor prognosis of HCC patients.
In a study by Erez et al., 2010, PDGFRα+ CAFs were sorted from a squamous cell carcinoma tumor milieu (SCC) to decipher the role of inflammatory secretome and others in triggering pro-angiogenic signal transduction. Two different pathways could be decoded to reach VEGF expression: directly through OPN and CYR61 expression or secondarily via the expression of inflammatory genes CXCL1/2/5 (Erez et al., 2010).
CAF-secreted exosomes containing miRNAs play a significant part in angiogenesis too. miR-21 was widely accepted to induce VEGF-mediated pro-angiogenic signaling in an autocrine fashion (Asangani et al., 2008; Wu et al., 2017). Nevertheless, the CAF-exosomal miR-21 might also add to the cumulative induction in a paracrine style (Yang et al., 2017). Ten-eleven translocation 2 (TET2) was found to be the target of cancer cell-secreted miR-210 in CAFs, which has been implicated in the pro-angiogenic switch. A recent analysis revealed that miR-210 had the capacity to elevate the expression of some pro-angiogenic factors, including MMP9, FGF2, and VEGFa, in CAFs, by activating the JAK2/STAT3 signaling pathway (Fan et al., 2020). Furthermore, miR-135b-5p upregulation in the CAF-derived exosomes supported the CRC cell migration and angiogenesis (Yin et al., 2021).
The English surgeon Stephen Paget (1855-1926) postulated the “seed and soil” theory a century ago to describe the role of tumor stroma in the growth and dissemination of cancerous cells. The “seed” is the cancer cell, while the stroma or the microenvironment is considered the “soil” for the “seed” (Paget, 1889; Ribatti et al., 2006). Current knowledge suggests that the soil requires positive reciprocal feedback from the seed or that it sometimes needs to be educated about the needs of the seed. For example, we may consider one of the many different means discussed that resulted in a transdifferentiation mesenchymal to mesenchymal transition (MMT) of NFs to CAFs (Sahai et al., 2020a; Ping et al., 2021). p53 non-autonomous expression pattern in the CAFs is believed to be due to the education imparted by the cancer cells. Furthermore, qRT-PCR further confirmed that MMP1, MMP3, and MMP10 mRNA were upregulated in CAFs in a largely p53-dependent manner, precisely opposite to NFs (Arandkar et al., 2018).
CAFs are considered the key ECM modulator in the tumor stroma, influencing tumor development, intravasation, migration, extravasation, and metastasis (Sahai et al., 2020a). Many malignancies are characterized by increased collagen synthesis (Liu et al., 2019a). Increased collagen synthesis and cross-linking, in particular, are linked to increased tumor stiffness and advancement (Xu et al., 2019). A cancer spheroid formation model of ovarian cancer confirmed the role of CAFs in influencing collagen type I, particularly by expressing versican, which promoted cancer invasiveness via the TGFβ pathway (Yeung et al., 2013). In addition, CAF-derived laminin was shown to interact with integrin α6β4 to trigger the migration of cervical cancer cells (Fullar et al., 2015). Following conversion into CAFs, contractile capabilities increase as αSMA and vimentin expression levels rise. That gives rise to its morphological modification by giving it a stellate shape (Kalluri, 2016), and several different soluble and insoluble secretions, including ECM proteins, are observed to be significantly intensified. Fibronectin is deposited first by the CAFs, which produces and intensifies intracellular tensions via increased interaction with actin filaments. In the case of wound healing, a positive feedback loop keeps the fibroblasts activated, and the translocation of yes-associated protein (YAP) into the nucleus triggers overexpression of αSMA (Kollmannsberger et al., 2018). Before producing and conducting tensile strength to the matrix to remodel it, the contractility of the CAFs needs to get amplified. That occurs through the activation of the Rho-ROCK-Myosin II signaling cascade by the signals from newly synthesized ECM and subsequent amalgamation of αSMA with actin-myosin fibers (Gaggioli et al., 2007; Provenzano and Keely, 2011). The CAFs modify the composition by modulating the amount and expression of MMPs, and mechanically, they change the physical properties of the ECM by modifying its organization and stiffness (Jang and Beningo, 2019).
Participation of additional fibroblasts generates a negative feedback loop to reduce the fibronectin/collagen I ratio leading to the relaxation of fibronectin fibers and CAFs entering quiescence. In contrast to the physiological context, fibronectin fibers are not let relaxed to resume quiescence; instead, the fibronectin zones are altered as a preventive measure against relaxation, which, in turn, leads to continuous ECM remodeling in the tumor context (Cox and Erler, 2011; Pickup et al., 2014; Leight et al., 2017; Barbazán and Matic Vignjevic, 2019). These secretions allow the CAFs to stay activated and communicate with the neighboring cells, such as endothelial cells and immune cells, in the stroma. Several trials targeting the alliance between CAFs and ECM modifications in recent years have unraveled that it not only supports the cancer progression, but the efficiency of targeting also depends on the tissue and carcinogenesis stage-specific context (Amakye et al., 2013; Van Cutsem et al., 2020). In some cases (e.g., breast, colon, pancreatic, stomach cancer), the stromal fraction of solid tumors can vary widely, amounting to 60%–90% of the overall tumor mass (Ronnov-Jessen et al., 1996; Dvorak, 1986; Powell et al., 2005; D'Arcangelo et al., 2020). In fact, TME can be very much influenced by the CAFs through the formation of severe desmoplastic reactions creating a desmoplastic TME (Liu et al., 2019a; Zeltz et al., 2020b). Cancer dissemination from PDAC to other organs can be attributed to desmoplasia (Shimosato et al., 1980; Halvorsen and Seim, 1989a; Halvorsen and Seim, 1989b; Hasebe et al., 2002; Iacobuzio-Donahue et al., 2002; Koliopanos et al., 2002; Caporale et al., 2005). However, in the case of CRC, the role of desmoplasia is not as straightforward as PDAC (Hewitt et al., 1993).
In a previous study by Erkan et al., 2008, increased stromal activity (but not stromal density) and diminished collagen deposition were correlated with a worse prognosis. In contrast, lower stromal activity (indicated by low αSMA expression) and increased collagen deposition were synchronized with a better prognosis (Erkan et al., 2008). This came with a contradiction against the dogma that collagen-induced signals highly favor the aggressiveness of cancerous growth (Whatcott et al., 2015; Insua-Rodríguez and Oskarsson, 2016). In another study, Bever et al. (2015) (Bever et al., 2015) illustrated the correlation between stroma density and patient survival and stroma activity using a computer-aided quantitative method in a cohort of 66 PDAC patients under adjuvant medication following a pancreaticoduodenectomy. In line with this, Chen et al. (2021) demonstrated that deletion of type 1 collagen in αSMA+ myofibroblasts exaggerated PDAC, probably due to high stromal activity. More studies in the field of collagen arrangements in the stroma found that rather than the collagen quantity (presence or absence of collagen), it is the collagen quality (thickness and alignment) that is indicative of poor disease prognosis (Drifka et al., 2016; Laklai et al., 2016). That concludes that activation of CAFs and ECM modification is equally essential for cancer progression and metastasis.
The ECM stiffness gives rise to invadopodia formation from actomyosin beams in cancer cells. The rate of invadopodia formation and matrix degradation depends upon the contractility of actomyosin beams (Aung et al., 2014; Jerrell and Parekh, 2014). The invadopodia are enriched with MMPs that form passages for the cancer cells to infiltrate into the ECM by degrading the same (Kessenbrock et al., 2010; Schoumacher et al., 2010). However, recent research reported that CAFs could degrade the ECM in a manner independent of invadopodia. It utilizes the tubular organization, employing MT1-MMP (MMP-14) or MMP-2 protein to remodel the matrix in favor of tumor cell invasion and metastasis (Cao et al., 2016). SPARC, secreted from CAFs, supports the pro-tumor mechanisms in many cancer types. Through the differential nature of executions in a few cancer types, it aids cell invasion by enhancing MMP expression by CAFs as well as by monocytes (Tremble et al., 1993; Neuzillet et al., 2013).
CAFs, educated via TNFα and NF-κB pathway by the p53 mutant cancer cells, in the stroma of metastases have been shown to release significantly high quantities of perlecan in order to attract cancer cells (Pereira et al., 2019). Thus, the cancer cells, CAFs, and immune cells secrete large amounts of VEGF, PDGF, and FGF-β which add to the basement membrane’s (BM) pre-stored growth factors and are finally released after degradation by the MMPS (Kalluri, 2003). The disorders created in the BM enhance tumor cell invasion by helping pro-angiogenic mechanisms.
Moreover, CAFs may indirectly influence inflammatory cell recruitment as well as functioning via ECM deposition and matrix remodeling (Rubin et al., 1995). CAF-directed ECM alteration is important for monocyte and other myeloid cell recruitment. The stiffness of the ECM supports the release of chemoattractants like CCL2 and colony-stimulating factor-1 (CSF-1) from CAFs and tumor cells (Acerbi et al., 2015; Nielsen et al., 2016). The composition, density, and organization of ECM support the passage of immune cells through the stroma. While hyaluronan and fibronectin prevailing stroma can promote T-cell migration through ECM, versican and tenascin can inhibit the same (Loike et al., 2001; Evanko et al., 2012; O'Connor et al., 2012; Bollyky et al., 2011; He and Baum, 2004). On the other hand, the pro-tumorous M2 macrophages can be recruited and blocked by collagen and hyaluronan-rich and SPARC-rich matrices, respectively (Kuang et al., 2007; Kobayashi et al., 2010; Sorokin, 2010; Iijima et al., 2011).
CAFs and immune cells are the most prevailing cell types within the tumor stroma. Among the immune cells, CAF-derived secretome affects many leukocytes, including CD8+ T-cells, Treg cells, and the most abundantly present macrophages (Figure 3). Considering their vast role in TME in tumor progression, invasion, metastasis, and acquired resistance to therapy, the macrophages (henceforth will be designated as ‘tumor-associated macrophages’ or TAMs) will cover a big part of our discussion.
FIGURE 3. Cancer-associated fibroblast (CAF) immunomodulatory mechanisms. CAFs-derived secretome primarily alters the immune microenvironment around the stroma by infusing immunosuppression. Inhibition of NK cells, generation of Treg cells, and M2 polarization of macrophages are the key reasons behind the immunosuppression. Besides, CAFs can block antigen presentation by inhibiting DCs, largely impeding cell-mediated immunity. In addition, CAF-secretome is responsible for N1 to N2 conversion, thereby minimizing the T-cell (CD8+)-mediated cytotoxicity against tumor cells. Moreover, CAFs are known to regress the impact of cancer immunotherapy and favor tumor progression via the reduction of TH1 cells through their conversion into TH2 cells and generation of TH17 cells, respectively (Dikov et al., 2005; Kitamura et al., 2005; Flavell et al., 2010; Allard et al., 2016; Hashimoto et al., 2016; Kitamura et al., 2017; Mantovani et al., 2017; Takahashi et al., 2017; Cheng et al., 2018; Nakamura et al., 2018; Qian et al., 2018; Liu et al., 2019a; Monteran and Erez, 2019a; Masucci et al., 2019; Owusu-Ansah et al., 2019; Zhang et al., 2019; An et al., 2020; Veglia et al., 2021).
Besides TAMs, CAFs have a multifaceted level of interactions with natural killer (NK) cells, dendritic cells (DC), T lymphocytes, myeloid-derived suppressor cells (MDSCs), and tumor-associated neutrophils (TANs), forming a complex tumor-immune interface (An et al., 2020). As discussed in previous sections, the putative impact of the CAF-derived secretome affects immunosurveillance negatively. Exceptions include the interaction of DC cells from hepatocellular carcinoma (HCC), bone marrow cells, and CAFs in a co-culture system generating IL-1β, IL-6, and IL-12p70, which subsequently activates cytotoxic T lymphocytes (TC cells) to release interferon (IFN-) α, and IFN-γ, eliciting an anti-tumor effect (Qian et al., 2018). The interactions with other cells have been discussed in-depth in previous sections detailing the functions and diversity of CAFs (Fearon, 2014; Lakins et al., 2018a; Costa et al., 2018; Elyada et al., 2019). Zhang et al. (2019) have shown that CRC-derived CAFs could upregulate the expression of VCAM1 and secretion of IL-8, thereby recruiting and polarizing macrophages into the M2 phenotype, culminating in diminished NK cell function. Synergism between CAFs and TAMs in crafting an immunosuppressed TME was shown to regulate tumor-infiltrated NK cells (Zhang et al., 2019). Next to NK cells, Cheng et al. (2018) reported a novel mechanism regarding the effect of CAFs on tumor-associated neutrophils (TANs). IL-6 secreted from the CAFs induces STAT3/PD-L1 signaling pathway in TANs triggering chemotaxis and anti-apoptosis mechanism triggering an increase in the number of neutrophils in the TME. A previous report indicated that an N1/N2 phenotypic transition is attributable in the TANs, where N1 is considered to have anti-tumor activity, and N2 is regarded to be associated with immunosuppression, and pro-tumor cellular activities, by DNA instability or by secretion of chemokines and cytokines (Masucci et al., 2019; Lau et al., 2022). The prevalence of the N2 phenotype correlates with poor patient prognosis (Masucci et al., 2019).
Some clues indicate a clear association of CAFs with the polarization of TAMs. CAF-derived secretome includes a plethora of soluble effector molecules, and current studies indicate that CAFs are the regulators of different cell-mediated immune responses (Monteran and Erez, 2019b). In agreement with this, CAFs were able to recruit and differentiate macrophages into TAMs (Ziani et al., 2018; Hegab et al., 2019). CAFs are involved in MCP-1-mediated macrophage infiltration in skin tumors, nourishing chronic inflammation (Zhang et al., 2011). Likewise, podoplanin+ CAFs could recruit and differentiate monocyte into CD204+ TAMs (Sakai et al., 2018). With the key monocyte chemotactic cytokines MCP-1 and SDF-1, the CAFs from invasive human breast cancer were able to transform monocytes into M2-type pro-tumoral macrophages, changing the myeloid lineage structurally as well as functionally (Gok Yavuz et al., 2019). Interestingly, the newly transformed M2-type macrophages (TAMs) can activate CAFs in a positive feedback loop and promote cancer progression (Ueshima et al., 2019). The ephemeral reciprocation in the action of CAFs and TAMs was justified by Hashimoto et al. (2016), where the group demonstrated the advent of CAFs from bone marrow-derived MSCs, their role in increased invasiveness of TAMs, finally culminating in the generation and invasion of more CAFs (Hashimoto et al., 2016; Zheng et al., 2017). In addition to SDF-1, CAFs secrete CXCL14 in prostate cancer, which accelerates the recruitment of monocytes and further leads to their polarization into M2 TAMs (Augsten et al., 2014; Comito et al., 2014). Another mechanism of M2-phenotypic conversion was demonstrated in CRC, in vitro, where CAFs-derived IL-6 and IL-8 were found responsible for the conversion (Kim et al., 2012). ER-/PR-/HER2- triple-negative breast cancer stroma-derived CAFs secrete CXCL16 that attracts monocytes and aids their stromal activation (Allaoui et al., 2016). Adenosine secretion by CAFs warrants great potential for monocyte differentiation and polarization into the M2 phenotype (Regateiro et al., 2012; Della Latta et al., 2013; Allard et al., 2016; de Leve et al., 2019). To describe the TME state that supports the recruitment and conversion of macrophages, Nakamura et al. (2018) deciphered that a hypoxic environment, substantiated by the marker carbonic anhydrase IX, contains both podoplanin+ CAFs as well as CD204+ TAMs (Nakamura et al., 2018). CD204+ TAMs are associated with immune suppression. Evidence-based data support the involvement of FAP+ cells in activating inflammatory STAT3 cascade via the uPAR-FAK-c-Src-JAK2 pathway. Myeloid-derived suppressor cells (MDSCs) are recruited to the tumor stroma to promote immunosuppression. CCL2, derived from FAP+ CAFs, is considered responsible for the CCR2-dependent immunosuppression by the MDSCs (Yang et al., 2016a; Kumar et al., 2017). CD163+ TAMs infiltrated the tumor stroma in triple-negative breast cancer and nasopharyngeal cancers, indicating a close association between CAFs and TAMs (Yu et al., 2018b; Zhou et al., 2018). In addition to direct mechanisms involved in M2 polarization by CAFs, there lies an indirect path as well. The pancreatic stellate cells (PSCs), considered the critical source cells of CAFs in PDAC, could stimulate IL-13-secreting mast-cells, promoting M2 macrophage polarization (Ma et al., 2013; Varricchi et al., 2017).
The crosstalk between CAFs and TAMs (M2-type), along with other cells, and their positive and reciprocating aid towards pro-tumorous, immunosuppressed TME greatly facilitated tumor growth and immune evasion (Hashimoto et al., 2016; Gunaydin, 2021). Further, the CAF-TAM communication was also helpful in advancing cancer with angiogenesis and metastasis (Comito et al., 2014; Doak et al., 2018). CAF/TAM milieu is associated with the generation of perlecan-rich desmoplastic stroma at metastatic sites (Brasil Da Costa et al., 2020). To aid metastasis, CAFs recruit EPCs (Orimo et al., 2005; De Francesco et al., 2013; Wang et al., 2014a) that aid in bringing in epigenetic modifications in the cancer cells in collaboration with the CAFs. The modification leads to a mesenchymal-to-amoeboid transition (MAT), helping in the metastatic spread of tumor cells (Giannoni et al., 2013). Linde et al. (2018) illustrated the role of CAF-educated TAMs (CD206+) in metastasis. The CD206+ M2 macrophages were able to downregulate the E-cadherin in breast cancer cells, promoting EMT (Linde et al., 2018). Moreover, CAFs-secreted IL-33 triggered the M2 TAMs to upregulate the MMP-9 expression, enabling ECM modification (Andersson et al., 2018). There is substantiating evidence indicating the ability of CAFs to induce EMT, invasion, and metastasis (Karagiannis et al., 2012).
Stepping into the roles of CAFs in immune evasion, the primary conception leads us to infer that the M2 phenotypic milieu in the TME is key to immune evasion for the tumor cells. Moreover, CAF-derived secretome includes TGFβ, IL-10, and arginase I to add to the immunosuppressive profile (Takahashi et al., 2017). By secreting adenosine, an immunosuppressive metabolite, the CAFs enhance immunosuppression and myeloid differentiation, proliferation, and invasion (Leone and Emens, 2018). Moreover, the synergism of CAFs and TAMs can hinder adaptive immunity by interrupting antigen presentation. Besides TGFβ and IL-6, CAF-secretome includes tryptophan 2,3-dioxygenase (TDO2), indoleamine-2,3-dioxygenase (IDO), and VEGF that can block DC maturation, a critical antigen-presenting cell (APC). The absence of proper antigen presentation calls for T-cell anergy, a crucial event disrupting the cell-mediated immune response (Gabrilovich et al., 1996; Dikov et al., 2005; Kitamura et al., 2005; Flavell et al., 2010; Kitamura et al., 2017; Ziani et al., 2018; Liu et al., 2019a).
Therefore, the orchestration between the primary stromal cells in association with CAFs promotes the tumor cell via immune evasion and immunosuppression to boost the pro-tumorous TME for enhanced proliferation, growth, invasion, and metastasis of distant tissues, which ultimately leads to disease progression.
Metabolism and metabolic crosstalk are indispensable parts of cancer. The secreted amino acids and other metabolites build a dialogue between the tumor cells and the stromal cells (Kay and Zanivan, 2021). Little is known about these interactions, and it remains open to answering how the CAF metabolism should be targeted for better patient prognosis.
Previous data suggest that CAFs show a characteristic hyper rate of glycolysis, and a proportional rate of autophagy is also typical. This event results in the release of a large amount of lactate, amino acids, and ketone bodies, which are subsequently taken up by tumor cells, helping them grow at a higher rate. The symbiotic dialogue between CAFs and tumor cells is the initial evidence of metabolic crosstalk (Pavlides et al., 2009; Whitaker-Menezes et al., 2011; Guido et al., 2012; Sousa et al., 2016). Aside from lactate, recent reports indicate pyruvate to be the major contribution of CAFs to the metabolome of the stroma as well. Significantly high secretion from breast CAFs and PDAC-CAF conditioned media substantiated the claim for a pyruvate-rich metabolome (Becker et al., 2020; Kerk et al., 2020). Kerk et al. (2020) described that the CAF-secreted extracellular pyruvate maintained redox homeostasis and was beneficial in developing resistance against mitochondrial inhibitors in pancreatic cancer. Strategic aid from pyruvate was again validated by the reports of lymphoma cell survival and ECM remodeling by metastatic breast cancer cells (Sakamoto et al., 2019).
In the case of pancreatic cancer, the quiescent stellate cells get converted into activated fibroblasts (CAFs) and release the stored lipid to assist as a signaling molecule for biomass production. For example, lysophosphatidic acid released from CAFs activates the PI3K/Akt signaling cascade in favor of cancer cell proliferation (Auciello et al., 2019).
The activity of cancer cells-derived-TGFβ and oxidative stress in rewiring CAF metabolism, inspiring glycolysis, and autophagy is widely accepted (Martinez-Outschoorn et al., 2010; Guido et al., 2012). The TGFβ assists the branched-chain keto acid production in CAFs. Branched-chain keto acids are used by cancer cells as carbon and nitrogen sources and were also deciphered from the overexpression of branched-chain amino-acid aminotransferase (BCAT) 1 (Zhu et al., 2020). Autophagy-derived alanine from CAFs is, reportedly, taken up by the PDAC cells to aid the TCA cycle, where the initial starting molecule is derived from pyruvate (Sousa et al., 2016; Auciello et al., 2019; Sanford-Crane et al., 2019). So metabolic convergence between the different sources can be met to justify the proliferation and growth of cancer cells.
Breast cancer-derived exosomes could activate the proto-oncogene MYC in the stromal fibroblasts in a positive and reciprocal feedback loop, as the activated CAFs then secret exosomal factors to be taken up by the cancer cells. This results in cancer cell proliferation via an increase in glucose and glutathione metabolism (Zhao et al., 2016; Yan et al., 2018b). Other metabolic exchanges include glutamine-glutamate exchange that benefits both CAFs and cancer cells. Inhibition in the synthesis of glutamate or glutamine showed reduced tumor growth (Yang et al., 2016c). As mentioned before, if not starvation, nutrient deficiency is a common condition for cancer cells. CAFs have been reported to support prostate cancer cells under glutamine deficiency in an ATF4-dependent manner via pyruvate carboxylase-asparagine synthase overexpression (Linares et al., 2017), where stromal asparagine was used as an alternative source of nitrogen. In stiff ECM, CAFs enhance their rate of glycolysis and oxidative phosphorylation. At this stage, CAF-derived aspartate is taken up by cancer cells, and in convergence, cancer cells-derived glutamate is taken up by the CAFs. Interestingly, this feedback loop stimulating cancer cell growth and invasion offers a common target glutaminase-1 (GLS1) to neutralize both pathways (Bertero et al., 2019).
For CAFs, creating a hypoxic environment is key to nurturing and expediting the growth of cancer cells with more glycolytic enzymes in the stroma. In addition, CAF autophagy also nurtures the metabolic needs of the proliferating cells by delivering lactate and other metabolites (Martinez-Outschoorn et al., 2010; Zhang et al., 2015). An entire epigenetic shift is responsible for adopting the pro-tumorigenic qualities of the TME. It is believed that hypoxia leads to a pro-tumorous gain of characters that include changes in gene expression patterns of CAF markers and ECM components via NNMT (Nicotinamide N-methyltransferase) mediated hypomethylation. Such changes were reported in ovarian CAFs (Eckert et al., 2019). Breast CAFs also supported a similar paradigm by acquiring hypomethylation of promoters of genes responsible for glycolytic enzyme synthesis (Becker et al., 2020). An upregulated NNMT in ovarian, colorectal, and gastric cancers indicates an active role of the enzymes in hypoxia-related hypomethylation (Eckert et al., 2019; Song et al., 2020; Zhang et al., 2021). These suggest that an epigenetic shift occurred, altering the CAFs to be pro-tumorous and remain active even in the absence of the primary inducer.
The function that gets affected by the altered metabolism of CAFs is the immune response against the tumor cells. Netrin G1 (NTNG1) is known for its role in glutamate and glutamine synthesis by CAFs, which is further taken up by cancer cells to expedite their energy needs. NTNG1 is also associated with immunosuppressive cytokine synthesis by CAFs (Kay and Zanivan, 2021). Therefore, the metabolism of CAFs has a multifaceted role inside the stroma, including nutrient supply to cancer cells and pro-tumorigenic immunomodulation.
Studies investigating various types of CAF-induced resistance to chemotherapy, radiotherapy, immunotherapy, and hormone therapy have revealed promising avenues for targeting CAFs and their effect on neighboring cells. CAF-induced resistance includes molecular mechanistic cyclization stimulation in nearby cancer cells, amplification of tumor-promoting secretome, evasion of immune checkpoint regulation, and enhanced paracrine/autocrine signaling or feedback loops that either directly or indirectly affect total tumor resistance. These events lead to tumor development and treatment resistance (Erdogan and Webb, 2017; Hilmi et al., 2020; Linares et al., 2020; Wessolly et al., 2022). Certain populations of CAFs are presumed to mediate the formation of an environment that is tumor-promoting and therapy-resistant (Simon and Salhia, 2022). Given that CAFs are the predominant component of the TME and exert a significant influence on cancer cell sensitivity to anti-cancer therapies, understanding the mechanisms of inhibition and resistance should be a prioritized area of research for sustaining therapy efficacy and overall patient survival.
The main categories of overall chemoresistance induced by CAFs include ECM remodeling, paracrine signaling, induction of stem-like properties in cancer cells, metabolic manipulation, modulation of the immune environment in the TME, and exosomal shuttling between tumor cells and CAFs.
CAF-induced chemotherapy resistance involves multifaceted processes that generate a physical barrier by modifying the extracellular matrix (ECM). This barrier reduces drug accessibility to tumor cells, activates pro-survival signaling pathways, and inhibits apoptotic signaling pathways. ECM remodeling by CAFs may also result in increased EMT and stem-like properties, aggressive cancer cell transitions, epigenetic modulation, and general modulation of the crosstalk between breast tumor cells and stromal components in the TME (Maji et al., 2018). CAFs secrete type 1 collagen, which inhibits chemotherapeutic drug absorption in solid tumors (Luo et al., 2015).
Studies in vitro indicate that stromal-derived paracrine signaling can increase cancer cell survival following chemotherapy (Valkenburg et al., 2018). Chemotherapy-induced DNA damage in CAFs has been observed to increase the expression of various inflammatory, angiogenic, or EMT-inducing signals (HGF) (Vickman et al., 2020). There are cases of chemotherapeutic drugs activating NFs and promoting tumorigenic CAFs that are involved in paracrine signaling. For example, Hedgehog-GLI signaling induces stemness in cancer cells which can result in chemoresistance (Peiris-Pagès et al., 2015). CAF-mediated TGFβ signaling, along with other pro-EMT and tumor-promoting signaling pathways, causes breast tumor cells to resist chemotherapy and become more aggressive. Studies identifying pro-tumorigenic CAF subpopulations have contributed to expanding our scope of identifying paracrine signaling molecules and pathways. Han et al. (2021) investigated the molecular mechanism of paclitaxel resistance, a chemotherapeutic drug, in TNBC and correlated chemotherapy resistance in TNBC PDX models to JAK2 signaling and an enriched cCAF subpopulation (Han et al., 2021a).
The induction of stemness in cancer cells has been a crucial aspect of therapy resistance in all types of cancer. After treatment, the risk of repopulation and relapse is due to the ability of stem-like cancer cells to resist anti-cancer therapies. The evidence that CAFs are also a cause of inducing CSC populations in tumors is essential for comprehending the methodologies of chemotherapy resistance and preventing relapse. Su et al. (2018) demonstrated that the cell membrane proteins CD10⁺ and GPR77⁺ are responsible for the maintenance of stemness in breast CSC populations (Su et al., 2018). These populations are positively correlated with the expression of CD10⁺ GPR77⁺ CAFs, revealing that this subset of CAFs maintains stemness through TME modulation (Su et al., 2018).
Yu et al. (2017) described a stromal GPER-mediated drug resistance and increased mitochondrial activity in the reprogramming of breast cancer energy metabolism. The stromal environment is particularly acidic, but the relationship between lower pH and CAF-secreted lactate can also be associated with chemoresistance through metabolic reprogramming (Tavares-Valente et al., 2013). In agreement with this, the upregulation of Ras signaling in CAFs correlates with an increase in glutamine synthesis, which supports the mitochondrial metabolism of cancer cells and renders them more resistant to chemotherapy, particularly drugs that inhibit androgen signaling (Mishra et al., 2018). Understanding the mechanisms by which CAF influences the metabolism of tumor cells is a promising strategy for addressing issues of therapy resistance and cancer severity.
NFs play an integral role in immune response, and CAFs exploit this function to facilitate cancer cell immune evasion. NK cell abrogation is achieved by exposure to CAF-derived TGFβ through miR-183 mediated DAP12 transcription interruption, thus resisting chemotherapy and aiding cancer cell survival (Powell and Huttenlocher, 2016). The secretion of soluble factors has extensive effects on the EMT, proliferation, migration, and stem-like properties of cancer cells, as well as a prominent influence on immune response and evasion. CXCL secreted by CAFs recruits TAMs, stimulating cancer progression and chemoresistance (Shien et al., 2017). Due to its immunomodulating effects, the clinicopathological significance of CAF PD-L1 expression in TNBC has been identified as a reliable predictor of treatment response and prognosis (Yoshikawa et al., 2021). Interferons, which play a crucial role in immunomodulatory functions, are modulated by CAFs resulting in therapy resistance and tumor progression. Activation of IFN signaling induced by CAFs in claudin-low TNBCs resulted in chemotherapy resistance, as demonstrated by Broad et al. (2021) (Broad et al., 2021). Targeting IFN receptors and other signaling axes involved in CAF-dependent chemoprotection via immunomodulatory functions makes it possible to find more efficacious means of enhancing treatment outcomes. CAF-derived chemokines such as IL-8 or CXCL8 recruit immunosuppressive cells to the TME, consequently enabling effective tumor progression, angiogenesis, and EMT (Han et al., 2021b). Promoting immunosuppression by CAFs in the TME can result in resistance to chemotherapy and other therapies (Hu et al., 2021). CAFs also affect the ratio of immune cells within the TME, notably by increasing the proportion of FOXP3⁺ T-cell Treg to CD8⁺ T lymphocytes, which leads to poor treatment outcomes and a diminished antitumor immune response (Mhaidly and Mechta-Grigoriou, 2021). The mechanisms of CAF immunomodulatory pathways may be crucial to our comprehension of CAF-influenced chemotherapy resistance.
Cancer cells have been seen to have gemcitabine and paclitaxel resistance in pancreatic cancer due to an association between CAFs, TAMs, and stromal-derived insulin-like growth factors (Ireland et al., 2016). Conversely, breast cancer cells increased chemotherapy efficacy by blocking the insulin-like growth factors from stromal components (Ireland et al., 2018). A possible counter to this is to target GPR77 membrane proteins in CAFs to promote chemosensitivity and reduce CSC count (Su et al., 2018). It has also been found that in vitro VCAM-1 knockdown in breast cancer cells reduces the proliferation and migration of IL-6-influenced breast cancer cells, thus increasing chemosensitivity (Wang et al., 2014b). Other methods of countering chemoresistance, such as aspirin treatment, have been reported to be effective as well, although their relationship to CAF-induced stemness still needs to be explored (Saha et al., 2016). More focus needs to be paid to how autophagy influences TME. To combat the chemoresistance brought on by CAFs, small compounds that target the autophagy-related core machinery may be a viable option. In a PDAC animal model, CAF depletion via disruption of the Hh signaling or injection of hyaluronan improved chemotherapy (gemcitabine) delivery (Olive et al., 2009). CAF-targeting strategies have shown progress in our understanding of how to increase chemotherapy efficacy and decrease overall tumor progression.
It has been well established that hormone imbalance, specifically endogenous estrogen and progesterone, can cause breast cancer via receptor-dependent and -independent mechanisms (Trabert et al., 2020). In addition to other secreting growth factors, cytokines, and proteases, estrogen can be secreted from CAFs and may be used to predict the efficacy of endocrine therapy and treatment response (Yu et al., 2017; Ruocco et al., 2018). Adjuvant endocrine therapy is an effective strategy against estrogen receptor-positive and other related types of breast cancer, such as luminal A, luminal B, or HER2⁺ (Schiavon and Smith, 2014; Burstein et al., 2019). In luminal breast cancer, microvesicle-mediated miRNA transfer transforms non-CSCs into CSCs that induce resistance to most therapies, including hormonal therapy. An example of this involves a study confirming the role of CAFs in the presence of hormone therapy resistance by modulating hormonal receptors or activating signaling pathways such as PI3K/AKT and MAPK/ERL1 and ERL2 signaling axis involved in tamoxifen resistance (Martinez-Outschoorn et al., 2011). Anti-estrogen resistance in breast cancer was found to be overcome by inhibiting mitochondrial function in breast cancer cells (Martinez-Outschoorn et al., 2011). Stromal factors influence the TME, and CAF-induced β1 integrin signaling promotes tamoxifen resistance in breast cancer (Pontiggia et al., 2012). Exosomal or vesicular transfer between tumor cells and CAF secretome frequently results in an active feed-forward loop involving CAFs and tumor cells that increases tumor-promoting CAF activation and aggressive features in cancer cells. Specifically, Cosentino et al. (2020) discovered that miR-9-mediated inhibition of EFEMP1 contributed to the upregulation of pro-tumor CAF phenotypes.
Developing methods to prevent the formation of CAF-influenced therapy-resistant tumor cells can increase the clinical efficacy of hormone therapy. Luque et al., 2021 showed that the CAFs, derived from HER2⁺ patients, promoted resistance to trastuzumab and pertuzumab treatment in vitro. However, the correlation with the presence and impact of CAF-S4 was not considered so far. The HER2-amplified breast cancer cells are resistant to tyrosine-kinase inhibitor lapatinib due to CAF manipulation of the ECM rigidity through YAP/TAZ activation (Lin et al., 2015). Soluble factors secreted by CAFs have been explained to cause tumor resistance to anti-HER2 therapies. CAF-derived FGF5 in breast cancer has caused resistance to HER2-targeted therapies through activation of the FGFR2 and c-Src downstream pathways (Fernández-Nogueira et al., 2020). Other secretory growth factors of interest including TGFβ and HGF regulate cancer-related pathways and tumor progression which can be tied to HER2 therapy resistance (Ikushima and Miyazono, 2010; Luraghi et al., 2014). Using a multi-omics approach to identify cytokines, transcription factors, kinases, and miRNAs or other secretory factors that CAFs release can aid in the identification of novel biomarkers that cause therapy resistance in HER2⁺ breast cancer and other forms of cancer.
CAFs can influence the radioresistance of cancer cells with simultaneous activation of alternative mechanisms for their proliferation and progression. Irradiated fibroblasts have been observed to overcome apoptotic signaling and transform into activated phenotypes that promote tumorigenesis (Ansems and Span, 2020). In a recent study, co-cultured CAFs and cancer cells developed resistance to the clastogenic effects of 137Cs gamma rays due to the enhanced capacity of CAFs to repair DNA damage (Domogauer et al., 2021). It is likely that the CAFs that survived radiation therapy modulated the fate of associated cancer cells and caused radiation resistance in the tumor as a whole. Exosomes derived from colon CAFs contribute to radioresistance by promoting cancer stem-like phenotypes (Liu et al., 2020b). With CAF-derived exosomes comes the consequent activation of tumor-promoting signaling pathways and stemness induction, instilling resistance in tumor cells (Liu et al., 2020b). Production of TGFβ by radio-treated CAFs can also promote cancer cell aggressive and invasive properties, acting as a radiotherapy therapeutic escape and resistance (Papadopoulou and Kletsas, 2011; Zhang et al., 2017). Notch signaling in CAFs plays a role in radioresistance, as demonstrated by a recent study that identified single-cell transcriptomic profiles in CAF subpopulations in delta-like canonical Notch ligand 1, Dll1⁺ tumors (Nandi et al., 2022). The connection between Notch signaling and CAF-associated metastasis and radioresistance could be exploited to improve breast cancer patient outcomes by minimizing radioresistance and stemness (Nandi et al., 2022). The increased expression of CXCL12, TGFβ, MMPs, and HGF in CAFs induced by radiation increases the activation of EMT pathways in cancer cells (Ansems and Span, 2020). In the majority of radiotherapies, similar mechanisms involving CAF-derived ECM remodeling and desmoplasia, hypoxia-induced TME, autophagy-induced cancer cell recovery, secretory factors, exosomes, and miRNA cause downstream signaling of cyclization and tumor resistance (Mantoni et al., 2011; Horsman and Overgaard, 2016; Wang et al., 2017). Radiotherapy-induced immunomodulatory mechanisms include CAFs avoiding immune recognition and retaining immunosuppressive properties within the TME (Song et al., 2021). Overall, radiotherapy resistance observed in cancer cells has been linked to CAF activity in the tumor microenvironment; therefore, comprehensive studies on the effects of CAFs on radioresistance can lead to an increase in overall survival and the potential development of successful combination therapies.
Recent research has shown that CAFs play a significant role in immunotherapy resistance by excluding T-cells from tumors. FAP⁺ CAFs can protect tumor cells from tumor necrosis factor and interferon-mediated T-cell necrosis and can express CXC motif chemokine ligands, inhibiting T-cells from accessing tumor cells. ECM remodeling by CAFs also creates a physical barrier preventing immune cell infiltration. The interplay between CAFs and tumor immunosuppressive cells is vital in discovering new ways to promote immunogenic cancer cell death. The degradation, suppression, or reduction of CAFs have been shown to reduce immunosuppressive cells and allow an increased T-cell mediated anti-tumor effect (Antonia et al., 2016). As previously described, the CAF-secretion of IL-6 and IL-8 can promote tumor growth. CAFs aggregate macrophages and activate the NF-κB pathway through the secretion of IL-6 and IL-8, resulting in tumor immunosuppression (Su et al., 2018).
In a scRNA-seq study of a subpopulation of CAFs called CAF-S1 from breast cancer, it was observed that by promoting the attraction, survival, and total quantity of CD4⁺/CD25⁺/FOXP3⁺ regulatory T-cells in the TME, these CAFs promote immunosuppression. More specifically associated with the immunosuppressive environment were the clusters ECM-myCAF and TGFβ-myCAF. The abundance of these clusters correlates with that of PD-1⁺ and CTLA4⁺ CD4⁺ T-cells. The expression of PD-1 and CTLA4 at the surface of FOXP3⁺ T regulatory cells is upregulated by the ECM-myCAFs. Conversely, CD4⁺ CD25⁺ T lymphocytes promote the transformation of ECM-myCAF into TGFβ-myCAF fibroblasts. All these findings allude to the crosstalk between specific CAF clusters and T-lymphocytes that plays a role in immunosuppression and immunotherapy resistance (Kieffer et al., 2020). CAFs also directly contribute to T-cell suppression and deactivation by emulating APCs. In this mechanism, the antigen complexed with MHC-I is processed and presented by these fibroblasts, followed by co-incident antigen-specific upregulation of PD-1/PD-L2 and FAS/FASL on CAFs and T-cells, respectively. The result is enhanced tumor viability due to the dysfunction and death of T-cells (Lakins et al., 2018b). Therefore, the relationship between the TME and breast cancer cells, which is intricately intertwined with the CAF and inflammatory cells, has enormous potential for advancements in the treatment of breast cancer.
A popular approach to targeting CAFs and their tumor-promoting effects includes CAF reprogramming or reversion to a quiescent phenotype. CAF-rich tumors exclude CD8+ T-cells at the tumor margin and upregulate the expression of CTLA-4. A result of this exclusion is the induction of resistance to various immunotherapies, even those that include therapeutic vaccination and αPD1. A study showed that myofibroblastic CAF differentiation is regulated by the enzyme NADPH oxidase-4 (NOX4), and inhibiting this enzyme can transform these myofibroblastic CAFs back to a quiescent, fibroblast-like phenotype (Hanley et al., 2017). Upon further examination using a small-molecule NOX4/1 inhibitor [GKT137831 (setanaxib), genyotex] to target this pathway, it was observed that these drugs could surpass CAF-mediated immunotherapy resistance. These drugs significantly increased CD8+ T-cell infiltration into tumors, efficiently reversed CAF differentiation in vivo, and enhanced the immunotherapy response (Hanley and Thomas, 2020). Other targeting strategies include targeting ECM to allow drug access to tumor cells, TGF-β1 inhibition to prevent tumor progression by facilitating T cell infiltration, and reprogramming CAFs back to a quiescent state by using vitamin A and D agonists to increase the efficacy of chemotherapy (Sherman et al., 2014; Shany et al., 2016; Liu et al., 2019b). Moreover, Hh inhibition remained a significant target in cancer types such as PDAC, where activated CAFs were seen to have elevated Hh signaling and corresponding iCAF production leading to more regulatory T-cells and increased immunosuppression (Steele et al., 2021). Other approaches involve the use of viral vaccines to broaden the cytotoxic range of therapy effects on tumor cells, and CAFs combined (Chen et al., 2015), CAF-directed drug conjugates, inhibition of CTLA-4 and PD-L1 stagnating tumor progression by increasing intratumoral CD8⁺and CD4⁺ T-cells while reducing T regulatory cell count and overall immunosuppressive actions (Fiegle et al., 2019), CXCL12 and CXCR4 inhibition of induced metastasis and invasiveness (Izumi et al., 2016), targeting CAF immune evasion mechanisms, and finally CAF depletion. In many cancers, TAM overexpresses CSF-1R receptors that control macrophages’ production, differentiation, and function. In a murine model, a CSF-1R inhibitor increased T-cell infiltration and CD8⁺ T-cell activity, reduced the recruitment of bone marrow-derived suppressor cells (BMDSC), and suppressed the generation of inflammatory mediators in TAM. As a result of the inhibition of CSF-1R, CAFs neutralized the inhibitor by secreting chemokines and chemokine ligands. The addition of a chemokine receptor antagonist decreased the tumor burden, and the addition of an immune checkpoint inhibitor (anti-PD-1) entirely stopped tumor growth (Kumar et al., 2017). Methods of targeting CAF populations related to immune modulation in the tumor microenvironment can prove to be effective in countering the effects of CAF-induced immunotherapy resistance.
The methods of anti-cancer treatment resistance induced by CAFs can be a gateway to targeting the innate function of CAFs and their effects on tumor progression and therapy efficacy. CAFs are influential in cancer progression and exhibit dichotomous effects of tumor inhibition in early stages and tumor promotion in advanced stages through CAF plasticity, transformation, and secreted growth factors and cytokines such as TGFβ or other secretory factors that induce tumor progression. Targeting specific populations of tumor-promoting CAFs can inhibit tumor progression and improve patient prognosis. Still, clinical studies have been unsuccessful due to the lack of specific biomarkers and their respective ability to transdifferentiate in response to various TME stimuli (Sanz-Moreno et al., 2011b; Özdemir et al., 2014; Sahai et al., 2020b). The different CAF-targeting strategies thus far (Figure 4) include– 1) suppression of CAF transition or formation of the activated state, 2) CAF reprogramming to revert to a NF state, 3) decreasing CAFs in the tumor microenvironment, 4) negating CAF tumor-promoting functions, 5) inhibition of CAF-derived factors, and 6) reducing immunosuppressive functions of CAFs. Using the described mechanisms of targeting CAFs along with immunotherapy, radiotherapy, chemotherapy, hormone therapy, or combined therapy, CAF-induced resistance to therapeutic approaches may be overcome.
FIGURE 4. Various targeting strategies against CAFs1. This illustration includes CAF-targeting approaches, including suppression of CAF transition from inactive to active states (Hanley et al., 2017); CAF reprogramming by targeting Vitamin A&D receptors to revert to the quiescent state (Sherman et al., 2014; Ferrer-Mayorga et al., 2017); decreasing CAFs in TME through CAR-T-cell therapy (Sakemura et al., 2019), vaccination (Chen et al., 2015), monoclonal antibody (Hanley and Thomas, 2020); Negating CAF tumor-promoting functions through inhibition of EMT, stemness, and metastasis (Sakemura et al., 2019); reducing immunosuppressive functions of CAFs to achieve greater T-cell accessibility to tumor cells and increased sensitivity to therapeutic approaches (Hanley and Thomas, 2020); inhibition of CAF-derived chemokines, cytokines, exosomes, miRNA, ECM proteins, and other factors (Izumi et al., 2016).
Through a number of methods, including the exposure of neo-antigens, STING activation, and PD-L1 overexpression, radiation therapy has been demonstrated to synergize with immune modifying therapy (Zhang et al., 2008; Corso et al., 2011; Deng et al., 2014). Tumors can also transform after radiation therapy, making them more sensitive to immunotherapy (Demaria et al., 2005). Therefore, Combination therapy is another promising therapeutic strategy to combat tumor progression. However, the response to the combination of radiotherapy (RT) and immune treatment can vary. The challenge is to determine why some patients respond to RT and immune treatment with a long-lasting response and others with a limited response. CAFs enhance or reduce drug resistance depending on the context. Understanding the connection between the divergent CAF secretome contents and tumor signaling needs may help identify potent combination treatment approaches.
In summary, CAFs play an essential role in the development and progression of breast cancer. In this review, we have summarized recent findings on the various potential origins and diverse functions of CAFs within the tumor microenvironment. These cells have been observed to be both tumor-promotive and tumor-suppressive depending on various factors found within and around the tumor microenvironment and on their heterogeneity. We discuss the molecular and functional heterogeneity of CAFs in order to demonstrate their involvement in different tumor-promoting mechanisms, such as the introduction of stemness, metastasis, migration, invasion, angiogenesis, and therapy resistance, via ECM remodeling, immunomodulation, and altered metabolic activities1. Several studies have been performed to classify different clusters and subsets of CAFs. New methods of classification such as scRNA seq have revealed several unique CAF subtypes and possible markers. A deeper understanding of the molecular and phenotypical differences between CAF populations can lead to addressing issues in therapy resistance and cancer cell targeting. CAF-induced resistance to therapy has been observed to contribute vastly to tumor progression and decreased patient survival. The precise approach of CAF-based treatment is significantly impeded by the fact that the CAFs do not express αSMA or FAP, or any other cell surface marker exclusively. That underscores the need for more knowledge on the numerous strategies CAFs employ to enhance cancer development in the backdrop of a range of therapeutic interventions. This can aid in developing optimal treatment plans and sustained therapy efficacy.
Therefore, from helping in angiogenesis and metastatic invasion to maintaining stemness, CAFs have been established as a potential therapeutic target in breast cancer. Although several exciting and critical findings have been reported in recent years, studies should focus on comparing CAF populations 1) in multiple tumors, 2) in different stages of malignancies, 3) in functional characterization of distinct CAF subtypes, and 4) in development of subtype-specific therapies. Together, this will help to build a more comprehensive picture of the relationship between CAFs, tumor microenvironment, and therapy response.
TS conceived and designed the entire review. MS, TN, EG, ME-S, and OO wrote the paper. TS and BG reviewed and edited the manuscript. All authors read and approved the manuscript.
This publication of this review paper was supported by the Texas A&M University Faculty Development Grant.
The authors declare that the research was conducted in the absence of any commercial or financial relationships that could be construed as a potential conflict of interest.
All claims expressed in this article are solely those of the authors and do not necessarily represent those of their affiliated organizations, or those of the publisher, the editors and the reviewers. Any product that may be evaluated in this article, or claim that may be made by its manufacturer, is not guaranteed or endorsed by the publisher.
1Created with BioRender.com.
Acerbi, I., Cassereau, L., Dean, I., Shi, Q., Au, A., Park, C., et al. (2015). Human breast cancer invasion and aggression correlates with ECM stiffening and immune cell infiltration. Integr. Biol. (Camb). 7 (10), 1120–1134. doi:10.1039/c5ib00040h
Addadi, Y., Moskovits, N., Granot, D., Lozano, G., Carmi, Y., Apte, R. N., et al. (2010). p53 status in stromal fibroblasts modulates tumor growth in an SDF1-dependent manner. Cancer Res. 70 (23), 9650–9658. doi:10.1158/0008-5472.CAN-10-1146
Albonici, L., Giganti, M. G., Modesti, A., Manzari, V., and Bei, R. (2019). Multifaceted role of the placental growth factor (PlGF) in the antitumor immune response and cancer progression. Int. J. Mol. Sci. 20 (12), 2970. doi:10.3390/ijms20122970
Albrengues, J., Bertero, T., Grasset, E., Bonan, S., Maiel, M., Bourget, I., et al. (2015). Epigenetic switch drives the conversion of fibroblasts into proinvasive cancer-associated fibroblasts. Nat. Commun. 6, 10204. doi:10.1038/ncomms10204
Albrengues, J., Bourget, I., Pons, C., Butet, V., Hofman, P., Tartare-Deckert, S., et al. (2014). LIF mediates proinvasive activation of stromal fibroblasts in cancer. Cell. Rep. 7 (5), 1664–1678. doi:10.1016/j.celrep.2014.04.036
Allaoui, R., Bergenfelz, C., Mohlin, S., Hagerling, C., Salari, K., Werb, Z., et al. (2016). Cancer-associated fibroblast-secreted CXCL16 attracts monocytes to promote stroma activation in triple-negative breast cancers. Nat. Commun. 7 (1), 13050. doi:10.1038/ncomms13050
Allard, B., Beavis, P. A., Darcy, P. K., and Stagg, J. (2016). Immunosuppressive activities of adenosine in cancer. Curr. Opin. Pharmacol. 29, 7–16. doi:10.1016/j.coph.2016.04.001
Amakye, D., Jagani, Z., and Dorsch, M. (2013). Unraveling the therapeutic potential of the Hedgehog pathway in cancer. Nat. Med. 19 (11), 1410–1422. doi:10.1038/nm.3389
An, Y., Liu, F., Chen, Y., and Yang, Q. (2020). Crosstalk between cancer-associated fibroblasts and immune cells in cancer. J. Cell. Mol. Med. 24 (1), 13–24. doi:10.1111/jcmm.14745
Andersson, P., Yang, Y., Hosaka, K., Zhang, Y., Fischer, C., Braun, H., et al. (2018). Molecular mechanisms of IL-33–mediated stromal interactions in cancer metastasis. JCI Insight 3 (20), e122375. doi:10.1172/jci.insight.122375
Ansems, M., and Span, P. N. (2020). The tumor microenvironment and radiotherapy response; a central role for cancer-associated fibroblasts. Clin. Transl. Radiat. Oncol. 22, 90–97. doi:10.1016/j.ctro.2020.04.001
Antonia, S. J., Vansteenkiste, J. F., and Moon, E. (2016). Immunotherapy: Beyond anti-PD-1 and anti-PD-L1 therapies. Am. Soc. Clin. Oncol. Educ. Book 35, e450–e458. doi:10.1200/EDBK_158712
Arandkar, S., Furth, N., Elisha, Y., Nataraj, N. B., van der Kuip, H., Yarden, Y., et al. (2018). Altered p53 functionality in cancer-associated fibroblasts contributes to their cancer-supporting features. Proc. Natl. Acad. Sci. U. S. A. 115 (25), 6410–6415. doi:10.1073/pnas.1719076115
Asangani, I. A., Rasheed, S. A., Nikolova, D. A., Leupold, J. H., Colburn, N. H., Post, S., et al. (2008). MicroRNA-21 (miR-21) post-transcriptionally downregulates tumor suppressor Pdcd4 and stimulates invasion, intravasation and metastasis in colorectal cancer. Oncogene 27 (15), 2128–2136. doi:10.1038/sj.onc.1210856
Asif, P. J., Longobardi, C., Hahne, M., and Medema, J. P. (2021). The role of cancer-associated fibroblasts in cancer invasion and metastasis. Cancers (Basel) 13 (18), 4720. doi:10.3390/cancers13184720
Attieh, Y., Clark, A. G., Grass, C., Richon, S., Pocard, M., Mariani, P., et al. (2017). Cancer-associated fibroblasts lead tumor invasion through integrin-β3-dependent fibronectin assembly. J. Cell. Biol. 216 (11), 3509–3520. doi:10.1083/jcb.201702033
Auciello, F. R., Bulusu, V., Oon, C., Tait-Mulder, J., Berry, M., Bhattacharyya, S., et al. (2019). A stromal lysolipid–autotaxin signaling Axis promotes pancreatic tumor progression. Cancer Discov. 9 (5), 617–627. doi:10.1158/2159-8290.CD-18-1212
Augsten, M., Sjöberg, E., Frings, O., Vorrink, S. U., Frijhoff, J., Olsson, E., et al. (2014). Cancer-associated fibroblasts expressing CXCL14 rely upon NOS1-derived nitric oxide signaling for their tumor-supporting properties. Cancer Res. 74 (11), 2999–3010. doi:10.1158/0008-5472.CAN-13-2740
Aung, A., Seo, Y. N., Lu, S., Wang, Y., Jamora, C., del Alamo, J. C., et al. (2014). 3D traction stresses activate protease-dependent invasion of cancer cells. Biophys. J. 107 (11), 2528–2537. doi:10.1016/j.bpj.2014.07.078
Baccelli, I., and Trumpp, A. (2012). The evolving concept of cancer and metastasis stem cells. J. Cell. Biol. 198 (3), 281–293. doi:10.1083/jcb.201202014
Baghdadi, M., Endo, H., Takano, A., Ishikawa, K., Kameda, Y., Wada, H., et al. (2018). High co-expression of IL-34 and M-CSF correlates with tumor progression and poor survival in lung cancers. Sci. Rep. 8 (1), 418. doi:10.1038/s41598-017-18796-8
Baghdadi, M., Wada, H., Nakanishi, S., Abe, H., Han, N., Putra, W. E., et al. (2016). Chemotherapy-induced IL34 enhances immunosuppression by tumor-associated macrophages and mediates survival of chemoresistant lung cancer cells. Cancer Res. 76 (20), 6030–6042. doi:10.1158/0008-5472.CAN-16-1170
Barbazán, J., and Matic Vignjevic, D. (2019). Cancer associated fibroblasts: Is the force the path to the dark side? Curr. Opin. Cell. Biol. 56, 71–79. doi:10.1016/j.ceb.2018.09.002
Barcellos-de-Souza, P., Comito, G., Pons-Segura, C., Taddei, M. L., Gori, V., Becherucci, V., et al. (2016). Mesenchymal stem cells are recruited and activated into carcinoma-associated fibroblasts by prostate cancer microenvironment-derived TGF-β1. Stem Cells 34 (10), 2536–2547. doi:10.1002/stem.2412
Bartoschek, M., Oskolkov, N., Bocci, M., Lovrot, J., Larsson, C., Sommarin, M., et al. (2018). Spatially and functionally distinct subclasses of breast cancer-associated fibroblasts revealed by single cell RNA sequencing. Nat. Commun. 9 (1), 5150. doi:10.1038/s41467-018-07582-3
Bechtel, W., McGoohan, S., Zeisberg, E. M., Muller, G. A., Kalbacher, H., Salant, D. J., et al. (2010). Methylation determines fibroblast activation and fibrogenesis in the kidney. Nat. Med. 16 (5), 544–550. doi:10.1038/nm.2135
Becker, L. M., O’Connell, J. T., Vo, A. P., Cain, M. P., Tampe, D., Bizarro, L., et al. (2020). Epigenetic reprogramming of cancer-associated fibroblasts deregulates glucose metabolism and facilitates progression of breast cancer. Cell. Rep. 31 (9), 107701. doi:10.1016/j.celrep.2020.107701
Bellomo, C., Caja, L., and Moustakas, A. (2016). Transforming growth factor beta as regulator of cancer stemness and metastasis. Br. J. Cancer 115 (7), 761–769. doi:10.1038/bjc.2016.255
Benyahia, Z., Dussault, N., Cayol, M., Sigaud, R., Berenguer-Daizé, C., Delfino, C., et al. (2017). Stromal fibroblasts present in breast carcinomas promote tumor growth and angiogenesis through adrenomedullin secretion. Oncotarget 8 (9), 15744–15762. doi:10.18632/oncotarget.14999
Bertero, T., Oldham, W. M., Grasset, E. M., Bourget, I., Boulter, E., Pisano, S., et al. (2019). Tumor-stroma mechanics coordinate amino acid availability to sustain tumor growth and malignancy. Cell. Metab. 29 (1), 124–140. doi:10.1016/j.cmet.2018.09.012
Bever, K. M., Sugar, E. A., Bigelow, E., Sharma, R., Laheru, D., Wolfgang, C. L., et al. (2015). The prognostic value of stroma in pancreatic cancer in patients receiving adjuvant therapy. HPB Oxf. 17 (4), 292–298. doi:10.1111/hpb.12334
Biffi, G., Oni, T. E., Spielman, B., Hao, Y., Elyada, E., Park, Y., et al. (2019). IL1-Induced JAK/STAT signaling is antagonized by TGFβ to shape CAF heterogeneity in pancreatic ductal adenocarcinoma. Cancer Discov. 9 (2), 282–301. doi:10.1158/2159-8290.CD-18-0710
Blondy, T., d'Almeida, S. M., Briolay, T., Tabiasco, J., Meiller, C., Chene, A. L., et al. (2020). Involvement of the M-CSF/IL-34/CSF-1R pathway in malignant pleural mesothelioma. J. Immunother. Cancer 8 (1), e000182. doi:10.1136/jitc-2019-000182
Bochet, L., Lehuédé, C., Dauvillier, S., Wang, Y. Y., Dirat, B., Laurent, V., et al. (2013). Adipocyte-derived fibroblasts promote tumor progression and contribute to the desmoplastic reaction in breast cancer. Cancer Res. 73 (18), 5657–5668. doi:10.1158/0008-5472.CAN-13-0530
Boire, A., Covic, L., Agarwal, A., Jacques, S., Sherifi, S., and Kuliopulos, A. (2005). PAR1 is a matrix metalloprotease-1 receptor that promotes invasion and tumorigenesis of breast cancer cells. Cell. 120 (3), 303–313. doi:10.1016/j.cell.2004.12.018
Bollyky, P. L., Wu, R. P., Falk, B. A., Lord, J. D., Long, S. A., Preisinger, A., et al. (2011). ECM components guide IL-10 producing regulatory T-cell (TR1) induction from effector memory T-cell precursors. Proc. Natl. Acad. Sci. U. S. A. 108 (19), 7938–7943. doi:10.1073/pnas.1017360108
Bordignon, P., Bottoni, G., Xu, X., Popescu, A. S., Truan, Z., Guenova, E., et al. (2019). Dualism of FGF and TGF-β signaling in heterogeneous cancer-associated fibroblast activation with ETV1 as a critical determinant. Cell. Rep. 28 (9), 2358–2372. doi:10.1016/j.celrep.2019.07.092
Brasil Da Costa, F. H., Lewis, M. S., Truong, A., Carson, D. D., and Farach-Carson, M. C. (2020). SULF1 suppresses Wnt3A-driven growth of bone metastatic prostate cancer in perlecan-modified 3D cancer-stroma-macrophage triculture models. PLOS ONE 15 (5), e0230354. doi:10.1371/journal.pone.0230354
Brechbuhl, H. M., Barrett, A. S., Kopin, E., Hagen, J. C., Han, A. L., Gillen, A. E., et al. (2020). Fibroblast subtypes define a metastatic matrisome in breast cancer. JCI Insight 5 (4), e130751. doi:10.1172/jci.insight.130751
Broad, R. V., Jones, S. J., Teske, M. C., Wastall, L. M., Hanby, A. M., Thorne, J. L., et al. (2021). Inhibition of interferon-signalling halts cancer-associated fibroblast-dependent protection of breast cancer cells from chemotherapy. Br. J. Cancer 124 (6), 1110–1120. doi:10.1038/s41416-020-01226-4
Burstein, H. J., Lacchetti, C., Anderson, H., Buchholz, T. A., Davidson, N. E., Gelmon, K. A., et al. (2019). Adjuvant endocrine therapy for women with hormone receptor–positive breast cancer: ASCO clinical practice guideline focused update. J. Clin. Oncol. 37 (5), 423–438. doi:10.1200/JCO.18.01160
Calon, A., Espinet, E., Palomo-Ponce, S., Tauriello, D. V. F., Iglesias, , Céspedes, M. V., et al. (2012). Dependency of colorectal cancer on a TGF-β-driven program in stromal cells for metastasis initiation. Cancer Cell. 22 (5), 571–584. doi:10.1016/j.ccr.2012.08.013
Calvo, F., Ege, N., Grande-Garcia, A., Hooper, S., Jenkins, R. P., Chaudhry, S. I., et al. (2013). Mechanotransduction and YAP-dependent matrix remodelling is required for the generation and maintenance of cancer-associated fibroblasts. Nat. Cell. Biol. 15 (6), 637–646. doi:10.1038/ncb2756
Cao, H., Eppinga, R. D., Razidlo, G. L., Krueger, E. W., Chen, J., Qiang, L., et al. (2016). Stromal fibroblasts facilitate cancer cell invasion by a novel invadopodia-independent matrix degradation process. Oncogene 35 (9), 1099–1110. doi:10.1038/onc.2015.163
Caporale, A., Vestri, A. R., Benvenuto, E., Mariotti, M., Cosenza, U. M., Scarpini, M., et al. (2005). Is desmoplasia a protective factor for survival in patients with colorectal carcinoma? Clin. Gastroenterology Hepatology 3, 370–375. doi:10.1016/s1542-3565(04)00674-3
Cazet, A. S., Hui, M. N., Elsworth, B. L., Wu, S. Z., Roden, D., Chan, C. L., et al. (2018). Targeting stromal remodeling and cancer stem cell plasticity overcomes chemoresistance in triple negative breast cancer. Nat. Commun. 9 (1), 2897. doi:10.1038/s41467-018-05220-6
Chaudhry, S. I., Hooper, S., Nye, E., Williamson, P., Harrington, K., and Sahai, E. (2013). Autocrine IL-1β-TRAF6 signalling promotes squamous cell carcinoma invasion through paracrine TNFα signalling to carcinoma-associated fibroblasts. Oncogene 32 (6), 747–758. doi:10.1038/onc.2012.91
Chen, I. X., Chauhan, V. P., Posada, J., Ng, M. R., Wu, M. W., Adstamongkonkul, P., et al. (2019). Blocking CXCR4 alleviates desmoplasia, increases T-lymphocyte infiltration, and improves immunotherapy in metastatic breast cancer. Proc. Natl. Acad. Sci. U. S. A. 116 (10), 4558–4566. doi:10.1073/pnas.1815515116
Chen, M., Xiang, R., Wen, Y., Xu, G., Wang, C., Luo, S., et al. (2015). A whole-cell tumor vaccine modified to express fibroblast activation protein induces antitumor immunity against both tumor cells and cancer-associated fibroblasts. Sci. Rep. 5 (1), 14421. doi:10.1038/srep14421
Chen, Y., Kim, J., Yang, S., Wang, H., Wu, C. J., Sugimoto, H., et al. (2021). Type I collagen deletion in αSMA+ myofibroblasts augments immune suppression and accelerates progression of pancreatic cancer. Cancer Cell. 39 (4), 548–565.e6. doi:10.1016/j.ccell.2021.02.007
Cheng, Y., Li, H., Deng, Y., Tai, Y., Zeng, K., Zhang, Y., et al. (2018). Cancer-associated fibroblasts induce PDL1+ neutrophils through the IL6-STAT3 pathway that foster immune suppression in hepatocellular carcinoma. Cell. Death Dis. 9 (4), 422. doi:10.1038/s41419-018-0458-4
Chiquet-Ehrismann, R., Orend, G., Chiquet, M., Tucker, R. P., and Midwood, K. S. (2014). Tenascins in stem cell niches. Matrix Biol. 37, 112–123. doi:10.1016/j.matbio.2014.01.007
Comito, G., Giannoni, E., Segura, C. P., Barcellos-De-Souza, P., Raspollini, M. R., Baroni, G., et al. (2014). Cancer-associated fibroblasts and M2-polarized macrophages synergize during prostate carcinoma progression. Oncogene 33 (19), 2423–2431. doi:10.1038/onc.2013.191
Corso, C. D., Ali, A. N., and Diaz, R. (2011). Radiation-induced tumor neoantigens: Imaging and therapeutic implications. Am. J. Cancer Res. 1 (3), 390–412.
Cosentino, G., Romero-Cordoba, S., Plantamura, I., Cataldo, A., and Iorio, M. V. (2020). miR-9-Mediated inhibition of EFEMP1 contributes to the acquisition of pro-tumoral properties in normal fibroblasts. Cells 9 (9), 2143. doi:10.3390/cells9092143
Costa, A., Kieffer, Y., Scholer-Dahirel, A., Pelon, F., Bourachot, B., Cardon, M., et al. (2018). Fibroblast heterogeneity and immunosuppressive environment in human breast cancer. Cancer Cell. 33 (3), 463–479. doi:10.1016/j.ccell.2018.01.011
Cox, T. R., and Erler, J. T. (2011). Remodeling and homeostasis of the extracellular matrix: Implications for fibrotic diseases and cancer. Dis. Model. Mech. 4 (2), 165–178. doi:10.1242/dmm.004077
Czekay, R. P., Cheon, D. J., Samarakoon, R., Kutz, S. M., and Higgins, P. J. (2022). Cancer-associated fibroblasts: Mechanisms of tumor progression and novel therapeutic targets. Cancers (Basel) 14 (5), 1231. doi:10.3390/cancers14051231
D'Arcangelo, E., Wu, N. C., Cadavid, J. L., and McGuigan, A. P. (2020). The life cycle of cancer-associated fibroblasts within the tumour stroma and its importance in disease outcome. Br. J. Cancer 122 (7), 931–942. doi:10.1038/s41416-019-0705-1
De Francesco, E. M., Lappano, R., Santolla, M. F., Marsico, S., Caruso, A., and Maggiolini, M. (2013). HIF-1α/GPER signaling mediates the expression of VEGF induced by hypoxia in breast cancer associated fibroblasts (CAFs). Breast Cancer Res. 15 (4), R64. doi:10.1186/bcr3458
de Leve, S., Wirsdorfer, F., and Jendrossek, V. (2019). Targeting the immunomodulatory CD73/adenosine system to improve the therapeutic gain of radiotherapy. Front. Immunol. 10, 698. doi:10.3389/fimmu.2019.00698
Della Latta, V., Cabiati, M., Rocchiccioli, S., Del Ry, S., and Morales, M-A. (2013). The role of the adenosinergic system in lung fibrosis. Pharmacol. Res. 76, 182–189. doi:10.1016/j.phrs.2013.08.004
Demaria, S., Kawashima, N., Yang, A. M., Devitt, M. L., Babb, J. S., Allison, J. P., et al. (2005). Immune-mediated inhibition of metastases after treatment with local radiation and CTLA-4 blockade in a mouse model of breast cancer. Clin. Cancer Res. 11 (2), 728–734. doi:10.1158/1078-0432.728.11.2
Deng, L., Liang, H., Xu, M., Yang, X., Burnette, B., Arina, A., et al. (2014). STING-dependent cytosolic DNA sensing promotes radiation-induced type I interferon-dependent antitumor immunity in immunogenic tumors. Immunity 41 (5), 843–852. doi:10.1016/j.immuni.2014.10.019
Denton, A. E., Roberts, E. W., and Fearon, D. T. (2018). “Stromal cells in the tumor microenvironment,” in Stromal immunology. Editors B. M. J. Owens, and M. A. Lakins (Cham: Springer International Publishing), 99–114.
Dikov, M. M., Ohm, J. E., Ray, N., Tchekneva, E. E., Burlison, J., Moghanaki, D., et al. (2005). Differential roles of vascular endothelial growth factor receptors 1 and 2 in dendritic cell differentiation. J. Immunol. 174 (1), 215–222. doi:10.4049/jimmunol.174.1.215
Ding, X., Ji, J., Jiang, J., Cai, Q., Wang, C., Shi, M., et al. (2018). HGF-mediated crosstalk between cancer-associated fibroblasts and MET-unamplified gastric cancer cells activates coordinated tumorigenesis and metastasis. Cell. Death Dis. 9 (9), 867. doi:10.1038/s41419-018-0922-1
Djurec, M., Grana, O., Lee, A., Troule, K., Espinet, E., Cabras, L., et al. (2018). Saa3 is a key mediator of the protumorigenic properties of cancer-associated fibroblasts in pancreatic tumors. Proc. Natl. Acad. Sci. U. S. A. 115 (6), E1147-E1156–E56. doi:10.1073/pnas.1717802115
Doak, G. R., Schwertfeger, K. L., and Wood, D. K. (2018). Distant relations: Macrophage functions in the metastatic niche. Trends Cancer 4 (6), 445–459. doi:10.1016/j.trecan.2018.03.011
Domogauer, J. D., de Toledo, S. M., Howell, R. W., and Azzam, E. I. (2021). Acquired radioresistance in cancer associated fibroblasts is concomitant with enhanced antioxidant potential and DNA repair capacity. Cell. Commun. Signal 19 (1), 30. doi:10.1186/s12964-021-00711-4
Donnarumma, E., Fiore, D., Nappa, M., Roscigno, G., Adamo, A., Iaboni, M., et al. (2017). Cancer-associated fibroblasts release exosomal microRNAs that dictate an aggressive phenotype in breast cancer. Oncotarget 8 (12), 19592–19608. doi:10.18632/oncotarget.14752
Drifka, C. R., Loeffler, A. G., Mathewson, K., Keikhosravi, A., Eickhoff, J. C., Liu, Y., et al. (2016). Highly aligned stromal collagen is a negative prognostic factor following pancreatic ductal adenocarcinoma resection. Oncotarget 7 (46), 76197–76213. doi:10.18632/oncotarget.12772
Dumont, N., Liu, B., DeFilippis, R. A., Chang, H., Rabban, J. T., Karnezis, A. N., et al. (2013). Breast fibroblasts modulate early dissemination, tumorigenesis, and metastasis through alteration of extracellular matrix characteristics. Neoplasia 15 (3), 249–262. doi:10.1593/neo.121950
Dumont, N., Liu, B., Defilippis, R. A., Chang, H., Rabban, J. T., Karnezis, A. N., et al. (2013). Breast fibroblasts modulate early dissemination, tumorigenesis, and metastasis through alteration of extracellular matrix characteristics. Neoplasia 15 (3), 249–262. doi:10.1593/neo.121950
Dvorak, H. F. (1986). Tumors: Wounds that do not heal. Similarities between tumor stroma generation and wound healing. N. Engl. J. Med. 315 (26), 1650–1659. doi:10.1056/NEJM198612253152606
Dvorak, K. M., Pettee, K. M., Rubinic-Minotti, K., Su, R., Nestor-Kalinoski, A., and Eisenmann, K. M. (2018). Carcinoma associated fibroblasts (CAFs) promote breast cancer motility by suppressing mammalian Diaphanous-related formin-2 (mDia2). PLoS One 13 (3), e0195278. doi:10.1371/journal.pone.0195278
Eckert, M. A., Coscia, F., Chryplewicz, A., Chang, J. W., Hernandez, K. M., Pan, S., et al. (2019). Proteomics reveals NNMT as a master metabolic regulator of cancer-associated fibroblasts. Nature 569 (7758), 723–728. doi:10.1038/s41586-019-1173-8
Elyada, E., Bolisetty, M., Laise, P., Flynn, W. F., Courtois, E. T., Burkhart, R. A., et al. (2019). Cross-species single-cell analysis of pancreatic ductal adenocarcinoma reveals antigen-presenting cancer-associated fibroblasts. Cancer Discov. 9 (8), 1102–1123. doi:10.1158/2159-8290.CD-19-0094
Erdogan, B., and Webb, D. J. (2017). Cancer-associated fibroblasts modulate growth factor signaling and extracellular matrix remodeling to regulate tumor metastasis. Biochem. Soc. Trans. 45 (1), 229–236. doi:10.1042/BST20160387
Erez, N., Truitt, M., Olson, P., Arron, S. T., and Hanahan, D. (2010). Cancer-associated fibroblasts are activated in incipient neoplasia to orchestrate tumor-promoting inflammation in an NF-kappaB-Dependent manner. Cancer Cell. 17 (2), 135–147. doi:10.1016/j.ccr.2009.12.041
Erkan, M., Michalski, C. W., Rieder, S., Reiser-Erkan, C., Abiatari, I., Kolb, A., et al. (2008). The activated stroma index is a novel and independent prognostic marker in pancreatic ductal adenocarcinoma. Clin. Gastroenterol. Hepatol. 6 (10), 1155–1161. doi:10.1016/j.cgh.2008.05.006
Errarte, P., Larrinaga, G., and Lopez, J. I. (2020). The role of cancer-associated fibroblasts in renal cell carcinoma. An example of tumor modulation through tumor/non-tumor cell interactions. J. Adv. Res. 21, 103–108. doi:10.1016/j.jare.2019.09.004
Evanko, S. P., Potter-Perigo, S., Bollyky, P. L., Nepom, G. T., and Wight, T. N. (2012). Hyaluronan and versican in the control of human T-lymphocyte adhesion and migration. Matrix Biol. 31 (2), 90–100. doi:10.1016/j.matbio.2011.10.004
Fan, J., Xu, G., Chang, Z., Zhu, L., and Yao, J. (2020). miR-210 transferred by lung cancer cell-derived exosomes may act as proangiogenic factor in cancer-associated fibroblasts by modulating JAK2/STAT3 pathway. Clin. Sci. 134 (7), 807–825. doi:10.1042/CS20200039
Fares, J., Fares, M. Y., Khachfe, H. H., Salhab, H. A., and Fares, Y. (2020). Molecular principles of metastasis: A hallmark of cancer revisited. Signal Transduct. Target Ther. 5 (1), 28. doi:10.1038/s41392-020-0134-x
Fearon, D. T. (2014). The carcinoma-associated fibroblast expressing fibroblast activation protein and escape from immune surveillance. Cancer Immunol. Res. 2 (3), 187–193. doi:10.1158/2326-6066.CIR-14-0002
Feig, C., Jones, J. O., Kraman, M., Wells, R. J., Deonarine, A., Chan, D. S., et al. (2013). Targeting CXCL12 from FAP-expressing carcinoma-associated fibroblasts synergizes with anti-PD-L1 immunotherapy in pancreatic cancer. Proc. Natl. Acad. Sci. U. S. A. 110 (50), 20212–20217. doi:10.1073/pnas.1320318110
Fernández-Nogueira, P., Mancino, M., Fuster, G., López-Plana, A., Jauregui, P., Almendro, V., et al. (2020). Tumor-associated fibroblasts promote HER2-targeted therapy resistance through FGFR2 activation. Clin. Cancer Res. 26 (6), 1432–1448. doi:10.1158/1078-0432.CCR-19-0353
Ferrari, N., Ranftl, R., Chicherova, I., Slaven, N. D., Moeendarbary, E., Farrugia, A. J., et al. (2019). Dickkopf-3 links HSF1 and YAP/TAZ signalling to control aggressive behaviours in cancer-associated fibroblasts. Nat. Commun. 10 (1), 130. doi:10.1038/s41467-018-07987-0
Ferrer-Mayorga, G., Gómez-López, G., Barbáchano, A., Fernández-Barral, A., Peña, C., Pisano, D. G., et al. (2017). Vitamin D receptor expression and associated gene signature in tumour stromal fibroblasts predict clinical outcome in colorectal cancer. Gut 66 (8), 1449–1462. doi:10.1136/gutjnl-2015-310977
Fiegle, E., Doleschel, D., Koletnik, S., Rix, A., Weiskirchen, R., Borkham-Kamphorst, E., et al. (2019). Dual CTLA-4 and PD-L1 blockade inhibits tumor growth and liver metastasis in a highly aggressive orthotopic mouse model of colon cancer. Neoplasia 21 (9), 932–944. doi:10.1016/j.neo.2019.07.006
Fiori, M. E., Di Franco, S., Villanova, L., Bianca, P., Stassi, G., and De Maria, R. (2019). Cancer-associated fibroblasts as abettors of tumor progression at the crossroads of EMT and therapy resistance. Mol. Cancer 18 (1), 70. doi:10.1186/s12943-019-0994-2
Flavell, R. A., Sanjabi, S., Wrzesinski, S. H., and Licona-Limón, P. (2010). The polarization of immune cells in the tumour environment by TGFbeta. Nat. Rev. Immunol. 10 (8), 554–567. doi:10.1038/nri2808
Flint, T. R., Janowitz, T., Connell, C. M., Roberts, E. W., Denton, A. E., Coll, A. P., et al. (2016). Tumor-induced IL-6 reprograms host metabolism to suppress anti-tumor immunity. Cell. Metab. 24 (5), 672–684. doi:10.1016/j.cmet.2016.10.010
Friedman, G., Levi-Galibov, O., David, E., Bornstein, C., Giladi, A., Dadiani, M., et al. (2020). Cancer-associated fibroblast compositions change with breast cancer progression linking the ratio of S100A4+ and PDPN+ CAFs to clinical outcome. Nat. Cancer 1 (7), 692–708. doi:10.1038/s43018-020-0082-y
Fukino, K., Shen, L., Patocs, A., Mutter, G. L., and Eng, C. (2007). Genomic instability within tumor stroma and clinicopathological characteristics of sporadic primary invasive breast carcinoma. JAMA 297 (19), 2103–2111. doi:10.1001/jama.297.19.2103
Fukumura, D., Xavier, R., Sugiura, T., Chen, Y., Park, E-C., Lu, N., et al. (1998). Tumor induction of VEGF promoter activity in stromal cells. Cell. 94 (6), 715–725. doi:10.1016/s0092-8674(00)81731-6
Fullar, A., Dudas, J., Olah, L., Hollosi, P., Papp, Z., Sobel, G., et al. (2015). Remodeling of extracellular matrix by normal and tumor-associated fibroblasts promotes cervical cancer progression. BMC Cancer 15, 256. doi:10.1186/s12885-015-1272-3
Gabrilovich, D. I., Chen, H. L., Girgis, K. R., Cunningham, H. T., Meny, G. M., Nadaf, S., et al. (1996). Production of vascular endothelial growth factor by human tumors inhibits the functional maturation of dendritic cells. Nat. Med. 2 (10), 1096–1103. doi:10.1038/nm1096-1096
Gaggioli, C., Hooper, S., Hidalgo-Carcedo, C., Grosse, R., Marshall, J. F., Harrington, K., et al. (2007). Fibroblast-led collective invasion of carcinoma cells with differing roles for RhoGTPases in leading and following cells. Nat. Cell. Biol. 9 (12), 1392–1400. doi:10.1038/ncb1658
Geary, L. A., Nash, K. A., Adisetiyo, H., Liang, M., Liao, C. P., Jeong, J. H., et al. (2014). CAF-secreted annexin A1 induces prostate cancer cells to gain stem cell-like features. Mol. Cancer Res. 12 (4), 607–621. doi:10.1158/1541-7786.MCR-13-0469
Giannoni, E., Taddei, M. L., Parri, M., Bianchini, F., Santosuosso, M., Grifantini, R., et al. (2013). EphA2-mediated mesenchymal–amoeboid transition induced by endothelial progenitor cells enhances metastatic spread due to cancer-associated fibroblasts. J. Mol. Med. 91 (1), 103–115. doi:10.1007/s00109-012-0941-9
Giulianelli, S., Riggio, M., Guillardoy, T., Perez Pinero, C., Gorostiaga, M. A., Sequeira, G., et al. (2019). FGF2 induces breast cancer growth through ligand-independent activation and recruitment of ERα and PRBΔ4 isoform to MYC regulatory sequences. Int. J. Cancer 145 (7), 1874–1888. doi:10.1002/ijc.32252
Glentis, A., Oertle, P., Mariani, P., Chikina, A., El Marjou, F., Attieh, Y., et al. (2017). Cancer-associated fibroblasts induce metalloprotease-independent cancer cell invasion of the basement membrane. Nat. Commun. 8 (1), 924. doi:10.1038/s41467-017-00985-8
Gok Yavuz, B., Gunaydin, G., Gedik, M. E., Kosemehmetoglu, K., Karakoc, D., Ozgur, F., et al. (2019). Cancer associated fibroblasts sculpt tumour microenvironment by recruiting monocytes and inducing immunosuppressive PD-1(+) TAMs. Sci. Rep. 9 (1), 3172. doi:10.1038/s41598-019-39553-z
González-González, L., and Periostin, Alonso J. (2018). Periostin: A matricellular protein with multiple functions in cancer development and progression. Front. Oncol. 8, 225. doi:10.3389/fonc.2018.00225
Guido, C., Whitaker-Menezes, D., Capparelli, C., Balliet, R., Lin, Z., Pestell, R. G., et al. (2012). Metabolic reprogramming of cancer-associated fibroblasts by TGF-β drives tumor growth: Connecting TGF-β signaling with “Warburg-like” cancer metabolism and L-lactate production. Cell. Cycle 11 (16), 3019–3035. doi:10.4161/cc.21384
Gunaydin, G. (2021). CAFs interacting with TAMs in tumor microenvironment to enhance tumorigenesis and immune evasion. Front. Oncol. 11, 668349. doi:10.3389/fonc.2021.668349
Guo, J. Y., Hsu, H. S., Tyan, S. W., Li, F. Y., Shew, J. Y., Lee, W. H., et al. (2017). Serglycin in tumor microenvironment promotes non-small cell lung cancer aggressiveness in a CD44-dependent manner. Oncogene 36 (17), 2457–2471. doi:10.1038/onc.2016.404
Halvorsen, T. B., and Seim, E. (1989). Association between invasiveness, inflammatory reaction, desmoplasia and survival in colorectal cancer. J. Clin. Pathology 42 (2), 162–166. doi:10.1136/jcp.42.2.162
Halvorsen, T. B., and Seim, E. (1989). Association between invasiveness, inflammatory reaction, desmoplasia and survival in colorectal cancer. J. Clin. Pathology 42 (2), 162–166. doi:10.1136/jcp.42.2.162
Hama, N., Kobayashi, T., Han, N., Kitagawa, F., Kajihara, N., Otsuka, R., et al. (2020). Interleukin-34 limits the therapeutic effects of immune checkpoint blockade. iScience 23 (10), 101584. doi:10.1016/j.isci.2020.101584
Han, J., Yun, J., Quan, M., Kang, W., Jung, J. G., Heo, W., et al. (2021). JAK2 regulates paclitaxel resistance in triple negative breast cancers. J. Mol. Med. Berl. 99 (12), 1783–1795. doi:10.1007/s00109-021-02138-3
Han, Z. J., Li, Y. B., Yang, L. X., Cheng, H. J., Liu, X., and Chen, H. (2021). Roles of the CXCL8-CXCR1/2 Axis in the tumor microenvironment and immunotherapy. Molecules 27 (1), 137. doi:10.3390/molecules27010137
Hanley, C. J., Mellone, M., Ford, K., Thirdborough, S. M., Mellows, T., Frampton, S. J., et al. (2017). Targeting the myofibroblastic cancer-associated fibroblast phenotype through inhibition of NOX4. J. Natl. Cancer Inst. 110 (1), 109–120. doi:10.1093/jnci/djx121
Hanley, C. J., and Thomas, G. J. (2020). T-Cell tumour exclusion and immunotherapy resistance: A role for CAF targeting. Br. J. Cancer 123 (9), 1353–1355. doi:10.1038/s41416-020-1020-6
Hasebe, T., Sasaki, S., Imoto, S., Mukai, K., Yokose, T., and Ochiai, A. (2002). Prognostic significance of fibrotic focus in invasive ductal carcinoma of the breast: A prospective observational study. Mod. Pathol. 15 (5), 502–516. doi:10.1038/modpathol.3880555
Hashimoto, O., Yoshida, M., Koma, Y., Yanai, T., Hasegawa, D., Kosaka, Y., et al. (2016). Collaboration of cancer-associated fibroblasts and tumour-associated macrophages for neuroblastoma development. J. Pathol. 240 (2), 211–223. doi:10.1002/path.4769
Hassanpour, M., Rezaie, J., Darabi, M., Hiradfar, A., Rahbarghazi, R., and Nouri, M. (2020). Autophagy modulation altered differentiation capacity of CD146+ cells toward endothelial cells, pericytes, and cardiomyocytes. Stem Cell. Res. Ther. 11 (1), 139. doi:10.1186/s13287-020-01656-0
He, J., and Baum, L. G. (2004). Presentation of galectin-1 by extracellular matrix triggers T cell death. J. Biol. Chem. 279 (6), 4705–4712. doi:10.1074/jbc.M311183200
Hegab, A. E., Ozaki, M., Kameyama, N., Gao, J., Kagawa, S., Yasuda, H., et al. (2019). Effect of FGF/FGFR pathway blocking on lung adenocarcinoma and its cancer-associated fibroblasts. J. Pathology 249 (2), 193–205. doi:10.1002/path.5290
Herrera, M., Llorens, C., Rodriguez, M., Herrera, A., Ramos, R., Gil, B., et al. (2018). Differential distribution and enrichment of non-coding RNAs in exosomes from normal and Cancer-associated fibroblasts in colorectal cancer. Mol. Cancer 17 (1), 114. doi:10.1186/s12943-018-0863-4
Hewitt, R. E., Powe, D. G., Ian Carter, G., and Turner, D. R. (1993). Desmoplasia and its relevance to colorectal tumour invasion. Int. J. Cancer 53 (1), 62–69. doi:10.1002/ijc.2910530113
Hilmi, M., Nicolle, R., Bousquet, C., and Neuzillet, C. (2020). Cancer-associated fibroblasts: Accomplices in the tumor immune evasion. Cancers (Basel) 12 (10), 2969. doi:10.3390/cancers12102969
Hong, L., Sun, H., Lv, X., Yang, D., Zhang, J., and Shi, Y. (2010). Expression of periostin in the serum of NSCLC and its function on proliferation and migration of human lung adenocarcinoma cell line (A549) in vitro. Mol. Biol. Rep. 37 (5), 2285–2293. doi:10.1007/s11033-009-9721-1
Horsman, M. R., and Overgaard, J. (2016). The impact of hypoxia and its modification of the outcome of radiotherapy. J. Radiat. Res. 57 (1), i90–i98. doi:10.1093/jrr/rrw007
Hosaka, K., Yang, Y., Seki, T., Fischer, C., Dubey, O., Fredlund, E., et al. (2016). Pericyte–fibroblast transition promotes tumor growth and metastasis. Proc. Natl. Acad. Sci. 113 (38), E5618–E5627. doi:10.1073/pnas.1608384113
Houthuijzen, J. M., and Jonkers, J. (2018). Cancer-associated fibroblasts as key regulators of the breast cancer tumor microenvironment. Cancer Metastasis Rev. 37 (4), 577–597. doi:10.1007/s10555-018-9768-3
Hu, J. L., Wang, W., Lan, X. L., Zeng, Z. C., Liang, Y. S., Yan, Y. R., et al. (2019). CAFs secreted exosomes promote metastasis and chemotherapy resistance by enhancing cell stemness and epithelial-mesenchymal transition in colorectal cancer. Mol. Cancer 18 (1), 91. doi:10.1186/s12943-019-1019-x
Hu, M., Yao, J., Cai, L., Bachman, K. E., van den Brule, F., Velculescu, V., et al. (2005). Distinct epigenetic changes in the stromal cells of breast cancers. Nat. Genet. 37 (8), 899–905. doi:10.1038/ng1596
Hu, S., Ma, J., Su, C., Chen, Y., Shu, Y., Qi, Z., et al. (2021). Engineered exosome-like nanovesicles suppress tumor growth by reprogramming tumor microenvironment and promoting tumor ferroptosis. Acta Biomater. 135, 567–581. doi:10.1016/j.actbio.2021.09.003
Iacobuzio-Donahue, C. A., Ryu, B., Hruban, R. H., and Kern, S. E. (2002). Exploring the host desmoplastic response to pancreatic carcinoma: Gene expression of stromal and neoplastic cells at the site of primary invasion. Am. J. Pathology 160 (1), 91–99. doi:10.1016/S0002-9440(10)64353-2
Iijima, J., Konno, K., and Itano, N. (2011). Inflammatory alterations of the extracellular matrix in the tumor microenvironment. Cancers (Basel) 3 (3), 3189–3205. doi:10.3390/cancers3033189
Ikushima, H., and Miyazono, K. (2010). TGFbeta signalling: A complex web in cancer progression. Nat. Rev. Cancer 10 (6), 415–424. doi:10.1038/nrc2853
Insua-Rodríguez, J., and Oskarsson, T. (2016). The extracellular matrix in breast cancer. Adv. Drug Deliv. Rev. 97, 41–55. doi:10.1016/j.addr.2015.12.017
Ireland, L., Santos, A., Ahmed, M. S., Rainer, C., Nielsen, S. R., Quaranta, V., et al. (2016). Chemoresistance in pancreatic cancer is driven by stroma-derived insulin-like growth factors. Cancer Res. 76 (23), 6851–6863. doi:10.1158/0008-5472.CAN-16-1201
Ireland, L., Santos, A., Campbell, F., Figueiredo, C., Hammond, D., Ellies, L. G., et al. (2018). Blockade of insulin-like growth factors increases efficacy of paclitaxel in metastatic breast cancer. Oncogene 37 (15), 2022–2036. doi:10.1038/s41388-017-0115-x
Izumi, D., Ishimoto, T., Miyake, K., Sugihara, H., Eto, K., Sawayama, H., et al. (2016). CXCL12/CXCR4 activation by cancer-associated fibroblasts promotes integrin β1 clustering and invasiveness in gastric cancer. Int. J. Cancer 138 (5), 1207–1219. doi:10.1002/ijc.29864
Jang, I., and Beningo, K. A. (2019). Integrins, CAFs and mechanical forces in the progression of cancer. Cancers (Basel) 11 (5), 721. doi:10.3390/cancers11050721
Jerrell, R. J., and Parekh, A. (2014). Cellular traction stresses mediate extracellular matrix degradation by invadopodia. Acta Biomater. 10 (5), 1886–1896. doi:10.1016/j.actbio.2013.12.058
Jiang, H., Hegde, S., Knolhoff, B. L., Zhu, Y., Herndon, J. M., Meyer, M. A., et al. (2016). Targeting focal adhesion kinase renders pancreatic cancers responsive to checkpoint immunotherapy. Nat. Med. 22 (8), 851–860. doi:10.1038/nm.4123
Kalluri, R. (2003). Basement membranes: Structure, assembly and role in tumour angiogenesis. Nat. Rev. Cancer 3 (6), 422–433. doi:10.1038/nrc1094
Kalluri, R. (2016). The biology and function of fibroblasts in cancer. Nat. Rev. Cancer 16 (9), 582–598. doi:10.1038/nrc.2016.73
Karagiannis, G. S., Poutahidis, T., Erdman, S. E., Kirsch, R., Riddell, R. H., and Diamandis, E. P. (2012). Cancer-associated fibroblasts drive the progression of metastasis through both paracrine and mechanical pressure on cancer tissue. Mol. Cancer Res. 10 (11), 1403–1418. doi:10.1158/1541-7786.MCR-12-0307
Karnoub, A. E., Dash, A. B., Vo, A. P., Sullivan, A., Brooks, M. W., Bell, G. W., et al. (2007). Mesenchymal stem cells within tumour stroma promote breast cancer metastasis. Nature 449 (7162), 557–563. doi:10.1038/nature06188
Kaur, A., Ecker, B. L., Douglass, S. M., Kugel, C. H., Webster, M. R., Almeida, F. V., et al. (2019). Remodeling of the collagen matrix in aging skin promotes melanoma metastasis and affects immune cell motility. Cancer Discov. 9 (1), 64–81. doi:10.1158/2159-8290.CD-18-0193
Kay, E. J., and Zanivan, S. (2021). Metabolic pathways fuelling protumourigenic cancer-associated fibroblast functions. Curr. Opin. Syst. Biol. 28, 100377. doi:10.1016/j.coisb.2021.100377
Kechagia, J. Z., Ivaska, J., and Roca-Cusachs, P. (2019). Integrins as biomechanical sensors of the microenvironment. Nat. Rev. Mol. Cell. Biol. 20 (8), 457–473. doi:10.1038/s41580-019-0134-2
Kerk, S. A., Lin, L., Myers, A. L., Zhang, Y., Jiménez, J. A., Sajjakulnukit, P., et al. (2020). The pancreatic tumor microenvironment compensates for loss of GOT2.
Kessenbrock, K., Plaks, V., and Werb, Z. (2010). Matrix metalloproteinases: Regulators of the tumor microenvironment. Cell. 141 (1), 52–67. doi:10.1016/j.cell.2010.03.015
Kiaris, H., Chatzistamou, I., Trimis, G., Frangou-Plemmenou, M., Pafiti-Kondi, A., and Kalofoutis, A. (2005). Evidence for nonautonomous effect of p53 tumor suppressor in carcinogenesis. Cancer Res. 65 (5), 1627–1630. doi:10.1158/0008-5472.CAN-04-3791
Kieffer, Y., Hocine, H. R., Gentric, G., Pelon, F., Bernard, C., Bourachot, B., et al. (2020). Single-cell analysis reveals fibroblast clusters linked to immunotherapy resistance in cancer. Cancer Discov. 10 (9), 1330–1351. doi:10.1158/2159-8290.CD-19-1384
Kim, J. H., Oh, S-H., Kim, E-J., Park, S. J., Hong, S. P., Cheon, J. H., et al. (2012). The role of myofibroblasts in upregulation of S100A8 and S100A9 and the differentiation of myeloid cells in the colorectal cancer microenvironment. Biochem. Biophysical Res. Commun. 423 (1), 60–66. doi:10.1016/j.bbrc.2012.05.081
Kitamura, H., Kamon, H., Sawa, S-I., Park, S-J., Katunuma, N., Ishihara, K., et al. (2005). IL-6-STAT3 controls intracellular MHC class II alphabeta dimer level through cathepsin S activity in dendritic cells. Immunity 23 (5), 491–502. doi:10.1016/j.immuni.2005.09.010
Kitamura, H., Ohno, Y., Toyoshima, Y., Ohtake, J., Homma, S., Kawamura, H., et al. (2017). Interleukin-6/STAT3 signaling as a promising target to improve the efficacy of cancer immunotherapy. Cancer Sci. 108 (10), 1947–1952. doi:10.1111/cas.13332
Kobayashi, N., Miyoshi, S., Mikami, T., Koyama, H., Kitazawa, M., Takeoka, M., et al. (2010). Hyaluronan deficiency in tumor stroma impairs macrophage trafficking and tumor neovascularization. Cancer Res. 70 (18), 7073–7083. doi:10.1158/0008-5472.CAN-09-4687
Koliopanos, A., Friess, H., di Mola, F. F., Tang, W-H., Kubulus, D., Brigstock, D., et al. (2002). Connective tissue growth factor gene expression alters tumor progression in esophageal cancer. World J. Surg. 26 (4), 420–427. doi:10.1007/s00268-001-0242-x
Kollmannsberger, P., Bidan, C. M., Dunlop, J. W. C., Fratzl, P., and Vogel, V. (2018). Tensile forces drive a reversible fibroblast-to-myofibroblast transition during tissue growth in engineered clefts. Sci. Adv. 4 (1), eaao4881. doi:10.1126/sciadv.aao4881
Kong, J., Tian, H., Zhang, F., Zhang, Z., Li, J., Liu, X., et al. (2019). Extracellular vesicles of carcinoma-associated fibroblasts creates a pre-metastatic niche in the lung through activating fibroblasts. Mol. Cancer 18 (1), 175. doi:10.1186/s12943-019-1101-4
Kraman, M., Bambrough, P. J., Arnold, J. N., Roberts, E. W., Magiera, L., Jones, J. O., et al. (2010). Suppression of antitumor immunity by stromal cells expressing fibroblast activation protein-alpha. Science 330 (6005), 827–830. doi:10.1126/science.1195300
Kraman, M., Bambrough, P. J., Arnold, J. N., Roberts, E. W., Magiera, L., Jones, J. O., et al. (2010). Suppression of antitumor immunity by stromal cells expressing fibroblast activation protein–α. Science 330 (6005), 827–830.
Kuang, D. M., Wu, Y., Chen, N., Cheng, J., Zhuang, S. M., and Zheng, L. (2007). Tumor-derived hyaluronan induces formation of immunosuppressive macrophages through transient early activation of monocytes. Blood 110 (2), 587–595. doi:10.1182/blood-2007-01-068031
Kumar, S., Shabi, T. S., and Goormaghtigh, E. (2014). A FTIR imaging characterization of fibroblasts stimulated by various breast cancer cell lines. PLoS ONE 9 (11), e111137. doi:10.1371/journal.pone.0111137
Kumar, V., Donthireddy, L., Marvel, D., Condamine, T., Wang, F., Lavilla-Alonso, S., et al. (2017). Cancer-associated fibroblasts neutralize the anti-tumor effect of CSF1 receptor blockade by inducing PMN-MDSC infiltration of tumors. Cancer Cell. 32 (5), 654–668. doi:10.1016/j.ccell.2017.10.005
Lakins, M. A., Ghorani, E., Munir, H., Martins, C. P., and Shields, J. D. (2018). Cancer-associated fibroblasts induce antigen-specific deletion of CD8 (+) T Cells to protect tumour cells. Nat. Commun. 9 (1), 948. doi:10.1038/s41467-018-03347-0
Lakins, M. A., Ghorani, E., Munir, H., Martins, C. P., and Shields, J. D. (2018). Cancer-associated fibroblasts induce antigen-specific deletion of CD8+T Cells to protect tumour cells. Nat. Commun. 9 (1), 948. doi:10.1038/s41467-018-03347-0
Laklai, H., Miroshnikova, Y. A., Pickup, M. W., Collisson, E. A., Kim, G. E., Barrett, A. S., et al. (2016). Genotype tunes pancreatic ductal adenocarcinoma tissue tension to induce matricellular fibrosis and tumor progression. Nat. Med. 22 (5), 497–505. doi:10.1038/nm.4082
Lau, D., Lechermann, L. M., and Gallagher, F. A. (2022). Clinical translation of neutrophil imaging and its role in cancer. Mol. Imaging Biol. 24 (2), 221–234. doi:10.1007/s11307-021-01649-2
Lau, T. S., Chan, L. K. Y., Wong, E. C. H., Hui, C. W. C., Sneddon, K., Cheung, T. H., et al. (2017). A loop of cancer-stroma-cancer interaction promotes peritoneal metastasis of ovarian cancer via TNFα-TGFα-EGFR. Oncogene 36 (25), 3576–3587. doi:10.1038/onc.2016.509
Le, P. N., Keysar, S. B., Miller, B., Eagles, J. R., Chimed, T-S., Reisinger, J., et al. (2019). Wnt signaling dynamics in head and neck squamous cell cancer tumor-stroma interactions. Mol. Carcinog. 58 (3), 398–410. doi:10.1002/mc.22937
Leight, J. L., Drain, A. P., and Weaver, V. M. (2017). Extracellular matrix remodeling and stiffening modulate tumor phenotype and treatment response. Annu. Rev. Cancer Biol. 1 (1), 313–334. doi:10.1146/annurev-cancerbio-050216-034431
Leone, R. D., and Emens, L. A. (2018). Targeting adenosine for cancer immunotherapy. J. Immunother. Cancer 6 (1), 57. doi:10.1186/s40425-018-0360-8
Li, C., Teixeira, A. F., Zhu, H. J., and Ten Dijke, P. (2021). Cancer associated-fibroblast-derived exosomes in cancer progression. Mol. Cancer 20 (1), 154. doi:10.1186/s12943-021-01463-y
Li, Q., Wang, C., Wang, Y., Sun, L., Liu, Z., Wang, L., et al. (2018). HSCs-derived COMP drives hepatocellular carcinoma progression by activating MEK/ERK and PI3K/AKT signaling pathways. J. Exp. Clin. Cancer Res. 37 (1), 231. doi:10.1186/s13046-018-0908-y
Lin, C-H., Pelissier, F. A., Zhang, H., Lakins, J., Weaver, V. M., Park, C., et al. (2015). Microenvironment rigidity modulates responses to the HER2 receptor tyrosine kinase inhibitor lapatinib via YAP and TAZ transcription factors. Mol. Biol. Cell. 26 (22), 3946–3953. doi:10.1091/mbc.E15-07-0456
Linares, J., Marín-Jiménez, J. A., Badia-Ramentol, J., and Calon, A. (2020). Determinants and functions of CAFs secretome during cancer progression and therapy. Front. Cell. Dev. Biol. 8, 621070. doi:10.3389/fcell.2020.621070
Linares, J. F., Cordes, T., Duran, A., Reina-Campos, M., Valencia, T., Ahn, C. S., et al. (2017). ATF4-Induced metabolic reprograming is a synthetic vulnerability of the p62-deficient tumor stroma. Cell. Metab. 26 (6), 817–829. doi:10.1016/j.cmet.2017.09.001
Linde, N., Casanova-Acebes, M., Sosa, M. S., Mortha, A., Rahman, A., Farias, E., et al. (2018). Macrophages orchestrate breast cancer early dissemination and metastasis. Nat. Commun. 9 (1), 21. doi:10.1038/s41467-017-02481-5
Liu, L., Zhang, Z., Zhou, L., Hu, L., Yin, C., Qing, D., et al. (2020). Cancer associated fibroblasts-derived exosomes contribute to radioresistance through promoting colorectal cancer stem cells phenotype. Exp. Cell. Res. 391 (2), 111956. doi:10.1016/j.yexcr.2020.111956
Liu, T., Han, C., Wang, S., Fang, P., Ma, Z., Xu, L., et al. (2019). Cancer-associated fibroblasts: An emerging target of anti-cancer immunotherapy. J. Hematol. Oncol. 12 (1), 86. doi:10.1186/s13045-019-0770-1
Liu, T., Han, C., Wang, S., Fang, P., Ma, Z., Xu, L., et al. (2019). Cancer-associated fibroblasts: An emerging target of anti-cancer immunotherapy. J. Hematol. Oncol. 12 (1), 86. doi:10.1186/s13045-019-0770-1
Liu, Z., Chen, M., Zhao, R., Huang, Y., Liu, F., Li, B., et al. (2020). CAF-induced placental growth factor facilitates neoangiogenesis in hepatocellular carcinoma. Acta Biochim. Biophys. Sin. (Shanghai). 52 (1), 18–25. doi:10.1093/abbs/gmz134
Lo, A., Wang, L. S., Scholler, J., Monslow, J., Avery, D., Newick, K., et al. (2015). Tumor-promoting desmoplasia is disrupted by depleting FAP-expressing stromal cells. Cancer Res. 75 (14), 2800–2810. doi:10.1158/0008-5472.CAN-14-3041
Loh, J. J., and Ma, S. (2021). The role of cancer-associated fibroblast as a dynamic player in mediating cancer stemness in the tumor microenvironment. Front. Cell. Dev. Biol. 9, 727640. doi:10.3389/fcell.2021.727640
Loike, J. D., Cao, L., Budhu, S., Hoffman, S., and Silverstein, S. C. (2001). Blockade of alpha 5 beta 1 integrins reverses the inhibitory effect of tenascin on chemotaxis of human monocytes and polymorphonuclear leukocytes through three-dimensional gels of extracellular matrix proteins. J. Immunol. 166 (12), 7534–7542. doi:10.4049/jimmunol.166.12.7534
Lugano, R., Ramachandran, M., and Dimberg, A. (2020). Tumor angiogenesis: Causes, consequences, challenges and opportunities. Cell. Mol. Life Sci. 77 (9), 1745–1770. doi:10.1007/s00018-019-03351-7
Luo, H., Tu, G., Liu, Z., and Liu, M. (2015). Cancer-associated fibroblasts: A multifaceted driver of breast cancer progression. Cancer Lett. 361 (2), 155–163. doi:10.1016/j.canlet.2015.02.018
Luque, M., Sanz-Álvarez, M., Santamaría, A., Zazo, S., Cristóbal, I., de la Fuente, L., et al. (2021). Targeted therapy modulates the secretome of cancer-associated fibroblasts to induce resistance in HER2-positive breast cancer. Int. J. Mol. Sci. 22 (24), 13297. doi:10.3390/ijms222413297
Luraghi, P., Reato, G., Cipriano, E., Sassi, F., Orzan, F., Bigatto, V., et al. (2014). MET signaling in colon cancer stem-like cells blunts the therapeutic response to EGFR inhibitors. Cancer Res. 74 (6), 1857–1869. doi:10.1158/0008-5472.CAN-13-2340-T
Ma, Y., Hwang, R. F., Logsdon, C. D., and Ullrich, S. E. (2013). Dynamic mast cell–stromal cell interactions promote growth of pancreatic cancer. Cancer Res. 73 (13), 3927–3937. doi:10.1158/0008-5472.CAN-12-4479
Madsen, C. D., Pedersen, J. T., Venning, F. A., Singh, L. B., Moeendarbary, E., Charras, G., et al. (2015). Hypoxia and loss of PHD2 inactivate stromal fibroblasts to decrease tumour stiffness and metastasis. EMBO Rep. 16 (10), 1394–1408. doi:10.15252/embr.201540107
Maji, S., Panda, S., Samal, S. K., Shriwas, O., Rath, R., Pellecchia, M., et al. (2018). Bcl-2 antiapoptotic family proteins and chemoresistance in cancer. Adv. Cancer Res. 137, 37–75. doi:10.1016/bs.acr.2017.11.001
Malanchi, I., Santamaria-Martínez, A., Susanto, E., Peng, H., Lehr, H-A., Delaloye, J-F., et al. (2012). Interactions between cancer stem cells and their niche govern metastatic colonization. Nature 481 (7379), 85–89. doi:10.1038/nature10694
Mani, S. A., Guo, W., Liao, M. J., Eaton, E. N., Ayyanan, A., Zhou, A. Y., et al. (2008). The epithelial-mesenchymal transition generates cells with properties of stem cells. Cell. 133 (4), 704–715. doi:10.1016/j.cell.2008.03.027
Manocha, E., Consonni, A., Baggi, F., Ciusani, E., Cocce, V., Paino, F., et al. (2022). CD146+ pericytes subset isolated from human micro-fragmented fat tissue display a strong interaction with endothelial cells: A potential cell target for therapeutic angiogenesis. Int. J. Mol. Sci. 23 (10), 5806. doi:10.3390/ijms23105806
Mantoni, T. S., Lunardi, S., Al-Assar, O., Masamune, A., and Brunner, T. B. (2011). Pancreatic stellate cells radioprotect pancreatic cancer cells through β1-integrin signaling. Cancer Res. 71 (10), 3453–3458. doi:10.1158/0008-5472.CAN-10-1633
Mantovani, A., Marchesi, F., Malesci, A., Laghi, L., and Allavena, P. (2017). Tumour-associated macrophages as treatment targets in oncology. Nat. Rev. Clin. Oncol. 14 (7), 399–416. doi:10.1038/nrclinonc.2016.217
Mao, X., Xu, J., Wang, W., Liang, C., Hua, J., Liu, J., et al. (2021). Crosstalk between cancer-associated fibroblasts and immune cells in the tumor microenvironment: New findings and future perspectives. Mol. Cancer 20 (1), 131. doi:10.1186/s12943-021-01428-1
Martinez-Outschoorn, U. E., Goldberg, A., Lin, Z., Ko, Y. H., Flomenberg, N., Wang, C., et al. (2011). Anti-estrogen resistance in breast cancer is induced by the tumor microenvironment and can be overcome by inhibiting mitochondrial function in epithelial cancer cells. Cancer Biol. Ther. 12 (10), 924–938. doi:10.4161/cbt.12.10.17780
Martinez-Outschoorn, U. E., Trimmer, C., Lin, Z., Whitaker-Menezes, D., Chiavarina, B., Zhou, J., et al. (2010). Autophagy in cancer associated fibroblasts promotes tumor cell survival: Role of hypoxia, HIF1 induction and NFκB activation in the tumor stromal microenvironment. Cell. Cycle 9 (17), 3515–3533. doi:10.4161/cc.9.17.12928
Masucci, M. T., Minopoli, M., and Carriero, M. V. (2019). Tumor associated neutrophils. Their role in tumorigenesis, metastasis, prognosis and therapy. Front. Oncol. 9, 1146. doi:10.3389/fonc.2019.01146
McAndrews, K. M., Chen, Y., Darpolor, J. K., Zheng, X., Yang, S., Carstens, J. L., et al. (2022). Identification of functional heterogeneity of carcinoma-associated fibroblasts with distinct IL6-mediated therapy resistance in pancreatic cancer. Cancer Discov. 12 (6), 1580–1597. doi:10.1158/2159-8290.CD-20-1484
Mhaidly, R., and Mechta-Grigoriou, F. (2021). Role of cancer-associated fibroblast subpopulations in immune infiltration, as a new means of treatment in cancer. Immunol. Rev. 302 (1), 259–272. doi:10.1111/imr.12978
Mishra, R., Haldar, S., Placencio, V., Madhav, A., Rohena-Rivera, K., Agarwal, P., et al. (2018). Stromal epigenetic alterations drive metabolic and neuroendocrine prostate cancer reprogramming. J. Clin. Investig. 128 (10), 4472–4484. doi:10.1172/JCI99397
Monteran, L., and Erez, N. (2019). The dark side of fibroblasts: Cancer-associated fibroblasts as mediators of immunosuppression in the tumor microenvironment. Front. Immunol. 10, 1835. doi:10.3389/fimmu.2019.01835
Monteran, L., and Erez, N. (2019). The dark side of fibroblasts: Cancer-associated fibroblasts as mediators of immunosuppression in the tumor microenvironment. Front. Immunol. 10, 1835. doi:10.3389/fimmu.2019.01835
Moskovits, N., Kalinkovich, A., Bar, J., Lapidot, T., and Oren, M. (2006). p53 Attenuates cancer cell migration and invasion through repression of SDF-1/CXCL12 expression in stromal fibroblasts. Cancer Res. 66 (22), 10671–10676. doi:10.1158/0008-5472.CAN-06-2323
Muller, P. A., and Vousden, K. H. (2013). p53 mutations in cancer. Nat. Cell. Biol. 15 (1), 2–8. doi:10.1038/ncb2641
Nabhan, A. N., Brownfield, D. G., Harbury, P. B., Krasnow, M. A., and Desai, T. J. (2018). Single-cell Wnt signaling niches maintain stemness of alveolar type 2 cells. Science 359 (6380), 1118–1123. doi:10.1126/science.aam6603
Nair, N., Calle, A. S., Zahra, M. H., Prieto-Vila, M., Oo, A. K. K., Hurley, L., et al. (2017). A cancer stem cell model as the point of origin of cancer-associated fibroblasts in tumor microenvironment. Sci. Rep. 7 (1), 6838. doi:10.1038/s41598-017-07144-5
Nakamura, H., Ichikawa, T., Nakasone, S., Miyoshi, T., Sugano, M., Kojima, M., et al. (2018). Abundant tumor promoting stromal cells in lung adenocarcinoma with hypoxic regions. Lung Cancer 115, 56–63. doi:10.1016/j.lungcan.2017.11.013
Nakano, M., Kikushige, Y., Miyawaki, K., Kunisaki, Y., Mizuno, S., Takenaka, K., et al. (2019). Dedifferentiation process driven by TGF-beta signaling enhances stem cell properties in human colorectal cancer. Oncogene 38 (6), 780–793. doi:10.1038/s41388-018-0480-0
Nandi, A., Debnath, R., Nayak, A., To, T. K. J., Thacker, G., Reilly, M., et al. (2022). Dll1-mediated Notch signaling drives tumor cell crosstalk with cancer associated fibroblasts to promote radioresistance in breast cancer. Cambridge, UK: Cancer Research.
Navab, R., Strumpf, D., To, C., Pasko, E., Kim, K. S., Park, C. J., et al. (2016). Integrin α11β1 regulates cancer stromal stiffness and promotes tumorigenicity and metastasis in non-small cell lung cancer. Oncogene 35 (15), 1899–1908. doi:10.1038/onc.2015.254
Neuzillet, C., Tijeras-Raballand, A., Cros, J., Faivre, S., Hammel, P., and Raymond, E. (2013). Stromal expression of SPARC in pancreatic adenocarcinoma. Cancer Metastasis Rev. 32 (3), 585–602. doi:10.1007/s10555-013-9439-3
Nielsen, S. R., Quaranta, V., Linford, A., Emeagi, P., Rainer, C., Santos, A., et al. (2016). Macrophage-secreted granulin supports pancreatic cancer metastasis by inducing liver fibrosis. Nat. Cell. Biol. 18 (5), 549–560. doi:10.1038/ncb3340
Nieman, K. M., Kenny, H. A., Penicka, C. V., Ladanyi, A., Buell-Gutbrod, R., Zillhardt, M. R., et al. (2011). Adipocytes promote ovarian cancer metastasis and provide energy for rapid tumor growth. Nat. Med. 17 (11), 1498–1503. doi:10.1038/nm.2492
Nishida, N., Yano, H., Fau - Nishida, T., Nishida T Fau - Kamura, T., Kamura T Fau - Kojiro, M., and Kojiro, M. (2006). Angiogenesis in cancer. Vasc Health Risk Manag. 2 (3), 213–219. doi:10.2147/vhrm.2006.2.3.213
Notohamiprodjo, S., Varasteh, Z., Beer, A. J., Niu, G., Chen, X., Weber, W., et al. (2021). “Chapter 45 - tumor vasculature,” in Molecular imaging. Editors B. D. Ross, and S. S. Gambhir Second Edition (Academic Press), 831–867.
O'Connell, J. T., Sugimoto, H., Cooke, V. G., MacDonald, B. A., Mehta, A. I., LeBleu, V. S., et al. (2011). VEGF-A and Tenascin-C produced by S100A4+ stromal cells are important for metastatic colonization. Proc. Natl. Acad. Sci. U. S. A. 108 (38), 16002–16007. doi:10.1073/pnas.1109493108
O'Connor, R. S., Hao, X., Shen, K., Bashour, K., Akimova, T., Hancock, W. W., et al. (2012). Substrate rigidity regulates human T cell activation and proliferation. J. Immunol. 189 (3), 1330–1339. doi:10.4049/jimmunol.1102757
Ohlund, D., Elyada, E., and Tuveson, D. (2014). Fibroblast heterogeneity in the cancer wound. J. Exp. Med. 211 (8), 1503–1523. doi:10.1084/jem.20140692
Öhlund, D., Handly-Santana, A., Biffi, G., Elyada, E., Almeida, A. S., Ponz-Sarvise, M., et al. (2017). Distinct populations of inflammatory fibroblasts and myofibroblasts in pancreatic cancer. J. Exp. Med. 214 (3), 579–596. doi:10.1084/jem.20162024
Olive, K. P., Jacobetz, M. A., Davidson, C. J., Gopinathan, A., McIntyre, D., Honess, D., et al. (2009). Inhibition of Hedgehog signaling enhances delivery of chemotherapy in a mouse model of pancreatic cancer. Science 324 (5933), 1457–1461. doi:10.1126/science.1171362
Orimo, A., Gupta, P. B., Sgroi, D. C., Arenzana-Seisdedos, F., Delaunay, T., Naeem, R., et al. (2005). Stromal fibroblasts present in invasive human breast carcinomas promote tumor growth and angiogenesis through elevated SDF-1/CXCL12 secretion. Cell. 121 (3), 335–348. doi:10.1016/j.cell.2005.02.034
Oskarsson, T., Acharyya, S., Zhang, X. H. F., Vanharanta, S., Tavazoie, S. F., Morris, P. G., et al. (2011). Breast cancer cells produce tenascin C as a metastatic niche component to colonize the lungs. Nat. Med. 17 (7), 867–874. doi:10.1038/nm.2379
Oskarsson, T., Batlle, E., and Massague, J. (2014). Metastatic stem cells: Sources, niches, and vital pathways. Cell. Stem Cell. 14 (3), 306–321. doi:10.1016/j.stem.2014.02.002
Otomo, R., Otsubo, C., Matsushima-Hibiya, Y., Miyazaki, M., Tashiro, F., Ichikawa, H., et al. (2014). TSPAN12 is a critical factor for cancer-fibroblast cell contact-mediated cancer invasion. Proc. Natl. Acad. Sci. U. S. A. 111 (52), 18691–18696. doi:10.1073/pnas.1412062112
Owusu-Ansah, K. G., Song, G., Chen, R., Edoo, M. I. A., Li, J., Chen, B., et al. (2019). COL6A1 promotes metastasis and predicts poor prognosis in patients with pancreatic cancer. Int. J. Oncol. 55 (2), 391–404. doi:10.3892/ijo.2019.4825
Özdemir, B. C., Pentcheva-Hoang, T., Carstens, J. L., Zheng, X., Wu, C. C., Simpson, T. R., et al. (2014). Depletion of carcinoma-associated fibroblasts and fibrosis induces immunosuppression and accelerates pancreas cancer with reduced survival. Cancer Cell. 25 (6), 719–734. doi:10.1016/j.ccr.2014.04.005
Paget, S. (1889). The distribution of secondary growths in cancer of the breast. Lancet 133 (3421), 571–573. doi:10.1016/s0140-6736(00)49915-0
Papadopoulou, A., and Kletsas, D. (2011). Human lung fibroblasts prematurely senescent after exposure to ionizing radiation enhance the growth of malignant lung epithelial cells in vitro and in vivo. Int. J. Oncol. 39 (4), 989–999. doi:10.3892/ijo.2011.1132
Pavlides, S., Whitaker-Menezes, D., Castello-Cros, R., Flomenberg, N., Witkiewicz, A. K., Frank, P. G., et al. (2009). The reverse Warburg effect: Aerobic glycolysis in cancer associated fibroblasts and the tumor stroma. Cell. Cycle 8 (23), 3984–4001. doi:10.4161/cc.8.23.10238
Peiris-Pagès, M., Sotgia, F., and Lisanti, M. P. (2015). Chemotherapy induces the cancer-associated fibroblast phenotype, activating paracrine Hedgehog-GLI signalling in breast cancer cells. Oncotarget 6 (13), 10728–10745. doi:10.18632/oncotarget.3828
Pena, C., Cespedes, M. V., Lindh, M. B., Kiflemariam, S., Mezheyeuski, A., Edqvist, P. H., et al. (2013). STC1 expression by cancer-associated fibroblasts drives metastasis of colorectal cancer. Cancer Res. 73 (4), 1287–1297. doi:10.1158/0008-5472.CAN-12-1875
Pereira, B. A., Vennin, C., Papanicolaou, M., Chambers, C. R., Herrmann, D., Morton, J. P., et al. (2019). CAF subpopulations: A new reservoir of stromal targets in pancreatic cancer. Trends Cancer 5 (11), 724–741. doi:10.1016/j.trecan.2019.09.010
Pickup, M. W., Mouw, J. K., and Weaver, V. M. (2014). The extracellular matrix modulates the hallmarks of cancer. EMBO Rep. 15 (12), 1243–1253. doi:10.15252/embr.201439246
Ping, Q., Yan, R., Cheng, X., Wang, W., Zhong, Y., Hou, Z., et al. (2021). Cancer-associated fibroblasts: Overview, progress, challenges, and directions. Cancer Gene Ther. 28 (9), 984–999. doi:10.1038/s41417-021-00318-4
Pontiggia, O., Sampayo, R., Raffo, D., Motter, A., Xu, R., Bissell, M. J., et al. (2012). The tumor microenvironment modulates tamoxifen resistance in breast cancer: A role for soluble stromal factors and fibronectin through β1 integrin. Breast Cancer Res. Treat. 133 (2), 459–471. doi:10.1007/s10549-011-1766-x
Powell, D. R., and Huttenlocher, A. (2016). Neutrophils in the tumor microenvironment. Trends Immunol. 37 (1), 41–52. doi:10.1016/j.it.2015.11.008
Powell, D. W., Adegboyega, P. A., Mari, J. F. D., and Mifflin, R. C. (2005). Epithelial Cells and Their Neighbors I. Role of intestinal myofibroblasts in development, repair, and cancer. Am. J. Physiology-Gastrointestinal Liver Physiology 289 (1), G2–G7. doi:10.1152/ajpgi.00075.2005
Provenzano, P. P., and Keely, P. J. (2011). Mechanical signaling through the cytoskeleton regulates cell proliferation by coordinated focal adhesion and Rho GTPase signaling. J. Cell. Sci. 124 (8), 1195–1205. doi:10.1242/jcs.067009
Qian, L., Tang, Z., Yin, S., Mo, F., Yang, X., Hou, X., et al. (2018). Fusion of dendritic cells and cancer-associated fibroblasts for activation of anti-tumor cytotoxic T lymphocytes. J. Biomed. Nanotechnol. 14 (10), 1826–1835. doi:10.1166/jbn.2018.2616
Qin, X., Yan, M., Zhang, J., Wang, X., Shen, Z., Lv, Z., et al. (2016). TGFβ3-mediated induction of Periostin facilitates head and neck cancer growth and is associated with metastasis. Sci. Rep. 6, 20587. doi:10.1038/srep20587
Raskov, H., Orhan, A., Gaggar, S., and Gogenur, I. (2021). Cancer-associated fibroblasts and tumor-associated macrophages in cancer and cancer immunotherapy. Front. Oncol. 11, 668731. doi:10.3389/fonc.2021.668731
Regateiro, F. S., Cobbold, S. P., and Waldmann, H. (2012). CD73 and adenosine generation in the creation of regulatory microenvironments. Clin. Exp. Immunol. 171 (1), 1–7. doi:10.1111/j.1365-2249.2012.04623.x
Rhim, A. D., Oberstein, P. E., Thomas, D. H., Mirek, E. T., Palermo, C. F., Sastra, S. A., et al. (2014). Stromal elements act to restrain, rather than support, pancreatic ductal adenocarcinoma. Cancer Cell. 25 (6), 735–747. doi:10.1016/j.ccr.2014.04.021
Ribatti, D., Mangialardi, G., and Vacca, A. (2006). Stephen Paget and the 'seed and soil' theory of metastatic dissemination. Clin. Exp. Med. 6 (4), 145–149. doi:10.1007/s10238-006-0117-4
Ronnov-Jessen, L., Petersen, O. W., and Bissell, M. J. (1996). Cellular changes involved in conversion of normal to malignant breast: Importance of the stromal reaction. Physiol. Rev. 76 (1), 69–125. doi:10.1152/physrev.1996.76.1.69
Roulis, M., Kaklamanos, A., Schernthanner, M., Bielecki, P., Zhao, J., Kaffe, E., et al. (2020). Paracrine orchestration of intestinal tumorigenesis by a mesenchymal niche. Nature 580 (7804), 524–529. doi:10.1038/s41586-020-2166-3
Rubin, P., Johnston, Cj, Fau - Williams, J. P., Williams Jp Fau - McDonald, S., McDonald S Fau - Finkelstein, J. N., and Finkelstein, J. N. (1995). A perpetual cascade of cytokines postirradiation leads to pulmonary fibrosis. Int. J. Radiat. Oncol. Biol. Phys. 33 (1), 99–109. doi:10.1016/0360-3016(95)00095-G
Ruocco, R. M., Avagliano, A., Granato, G., Imparato, V., Masone, S., Masullo, M., et al. (2018). Involvement of breast cancer-associated fibroblasts in tumor development, therapy resistance and evaluation of potential therapeutic strategies. Curr. Med. Chem. 25 (29), 3414–3434. doi:10.2174/0929867325666180309120746
Saha, S., Mukherjee, S., Khan, P., Kajal, K., Mazumdar, M., Manna, A., et al. (2016). Aspirin suppresses the acquisition of chemoresistance in breast cancer by disrupting an nf?b-IL6 signaling Axis responsible for the generation of cancer stem cells. Cancer Res. 76 (7), 2000–2012. doi:10.1158/0008-5472.CAN-15-1360
Sahai, E., Astsaturov, I., Cukierman, E., DeNardo, D. G., Egeblad, M., Evans, R. M., et al. (2020). A framework for advancing our understanding of cancer-associated fibroblasts. Nat. Rev. Cancer 20 (3), 174–186. doi:10.1038/s41568-019-0238-1
Sahai, E., Astsaturov, I., Cukierman, E., DeNardo, D. G., Egeblad, M., Evans, R. M., et al. (2020). A framework for advancing our understanding of cancer-associated fibroblasts. Nat. Rev. Cancer 20 (3), 174–186. doi:10.1038/s41568-019-0238-1
Sakai, T., Aokage, K., Neri, S., Nakamura, H., Nomura, S., Tane, K., et al. (2018). Link between tumor-promoting fibrous microenvironment and an immunosuppressive microenvironment in stage I lung adenocarcinoma. Lung Cancer 126, 64–71. doi:10.1016/j.lungcan.2018.10.021
Sakamoto, A., Kunou, S., Shimada, K., Tsunoda, M., Aoki, T., Iriyama, C., et al. (2019). Pyruvate secreted from patient-derived cancer-associated fibroblasts supports survival of primary lymphoma cells. Cancer Sci. 110 (1), 269–278. doi:10.1111/cas.13873
Sakemura, R., Cox, M., Hansen, M., Hefazi, M., Roman, C., Schick, K., et al. (2019). Targeting cancer associated fibroblasts in the bone marrow prevents resistance to chimeric antigen receptor T cell therapy in multiple myeloma. Blood 134, 865. doi:10.1182/blood-2019-123277
Sanford-Crane, H., Abrego, J., and Sherman, M. H. (2019). Fibroblasts as modulators of local and systemic cancer metabolism. Cancers 11 (5), 619. doi:10.3390/cancers11050619
Sanz-Moreno, V., Gaggioli, C., Yeo, M., Albrengues, J., Wallberg, F., Viros, A., et al. (2011). ROCK and JAK1 signaling cooperate to control actomyosin contractility in tumor cells and stroma. Cancer Cell. 20 (2), 229–245. doi:10.1016/j.ccr.2011.06.018
Sanz-Moreno, V., Gaggioli, C., Yeo, M., Albrengues, J., Wallberg, F., Viros, A., et al. (2011). ROCK and JAK1 signaling cooperate to control actomyosin contractility in tumor cells and stroma. Cancer Cell. 20 (2), 229–245. doi:10.1016/j.ccr.2011.06.018
Scherz-Shouval, R., Santagata, S., Mendillo, M. L., Sholl, L. M., Ben-Aharon, I., Beck, A. H., et al. (2014). The reprogramming of tumor stroma by HSF1 is a potent enabler of malignancy. Cell. 158 (3), 564–578. doi:10.1016/j.cell.2014.05.045
Schiavon, G., and Smith, I. E. (2014). Status of adjuvant endocrine therapy for breast cancer. Breast Cancer Res. 16 (2), 206. doi:10.1186/bcr3636
Schoumacher, M., Goldman, R. D., Louvard, D., and Vignjevic, D. M. (2010). Actin, microtubules, and vimentin intermediate filaments cooperate for elongation of invadopodia. J. Cell. Biol. 189 (3), 541–556. doi:10.1083/jcb.200909113
Sewell-Loftin, M. K., Bayer, S. V. H., Crist, E., Hughes, T., Joison, S. M., Longmore, G. D., et al. (2017). Cancer-associated fibroblasts support vascular growth through mechanical force. Sci. Rep. 7 (1), 12574. doi:10.1038/s41598-017-13006-x
Shany, S., Sigal-Batikoff, I., and Lamprecht, S. (2016). Vitamin D and myofibroblasts in fibrosis and cancer: At cross-purposes with TGF-β/SMAD signaling. Anticancer Res. 36 (12), 6225–6234. doi:10.21873/anticanres.11216
Shapcott, M., Hewitt, K. J., and Rajpoot, N. (2019). Deep learning with sampling in colon cancer histology. Front. Bioeng. Biotechnol. 7, 52. doi:10.3389/fbioe.2019.00052
Shen, T., Li, Y., Zhu, S., Yu, J., Zhang, B., Chen, X., et al. (2020). YAP1 plays a key role of the conversion of normal fibroblasts into cancer-associated fibroblasts that contribute to prostate cancer progression. J. Exp. Clin. Cancer Res. 39 (1), 36. doi:10.1186/s13046-020-1542-z
Shen, Z., Qin, X., Yan, M., Li, R., Chen, G., Zhang, J., et al. (2017). Cancer-associated fibroblasts promote cancer cell growth through a miR-7-RASSF2-PAR-4 axis in the tumor microenvironment. Oncotarget 8 (1), 1290–1303. doi:10.18632/oncotarget.13609
Sherman, M. H., Yu, R. T., Engle, D. D., Ding, N., Atkins, A. R., Tiriac, H., et al. (2014). Vitamin D receptor-mediated stromal reprogramming suppresses pancreatitis and enhances pancreatic cancer therapy. Cell. 159 (1), 80–93. doi:10.1016/j.cell.2014.08.007
Shien, K., Papadimitrakopoulou, V. A., Ruder, D., Behrens, C., Shen, L., Kalhor, N., et al. (2017). JAK1/STAT3 activation through a proinflammatory cytokine pathway leads to resistance to molecularly targeted therapy in non-small cell lung cancer. Mol. Cancer Ther. 16 (10), 2234–2245. doi:10.1158/1535-7163.MCT-17-0148
Shimosato, Y., Hashimoto, T., Kodama, T., Kameya, T., Suzuki, A., Nishiwaki, Y., et al. (1980). Prognostic implications of fibrotic focus (scar) in small peripheral lung cancers. Am. J. Surg. Pathology 4 (4), 365–373. doi:10.1097/00000478-198008000-00005
Simon, T., and Salhia, B. (2022). Cancer-associated fibroblast subpopulations with diverse and dynamic roles in the tumor microenvironment. Mol. Cancer Res. 20 (2), 183–192. doi:10.1158/1541-7786.MCR-21-0282
Song, M., He, J., Pan, Q. Z., Yang, J., Zhao, J., Zhang, Y. J., et al. (2021). Cancer-associated fibroblast-mediated cellular crosstalk supports hepatocellular carcinoma progression. Hepatology 73 (5), 1717–1735. doi:10.1002/hep.31792
Song, M., Li, Y., Miao, M., Zhang, F., Yuan, H., Cao, F., et al. (2020). High stromal nicotinamide N-methyltransferase (NNMT) indicates poor prognosis in colorectal cancer. Cancer Med. 9 (6), 2030–2038. doi:10.1002/cam4.2890
Sorokin, L. (2010). The impact of the extracellular matrix on inflammation. Nat. Rev. Immunol. 10 (10), 712–723. doi:10.1038/nri2852
Sousa, C. M., Biancur, D. E., Wang, X., Halbrook, C. J., Sherman, M. H., Zhang, L., et al. (2016). Pancreatic stellate cells support tumour metabolism through autophagic alanine secretion. Nature 536 (7617), 479–483. doi:10.1038/nature19084
Sperb, N., Tsesmelis, M., and Wirth, T. (2020). Crosstalk between tumor and stromal cells in pancreatic ductal adenocarcinoma. Int. J. Mol. Sci. 21 (15), 5486. doi:10.3390/ijms21155486
Steele, N. G., Biffi, G., Kemp, S. B., Zhang, Y., Drouillard, D., Syu, L., et al. (2021). Inhibition of hedgehog signaling alters fibroblast composition in pancreatic cancer. Clin. Cancer Res. 27 (7), 2023–2037. doi:10.1158/1078-0432.CCR-20-3715
Su, S., Chen, J., Yao, H., Liu, J., Yu, S., Lao, L., et al. (2018). CD10+GPR77+ cancer-associated fibroblasts promote cancer formation and chemoresistance by sustaining cancer stemness. Cell. 172 (4), 841–856. doi:10.1016/j.cell.2018.01.009
Suh, J., Kim, D-H., and Surh, Y-J. (2016). Abstract 4096: Fibroblast growth factor-2-derived from cancer-associated fibroblasts stimulates proliferation and migration of human breast cancer cells. Cancer Res. 76 (14), 4096. doi:10.1158/1538-7445.am2016-4096
Sung, P. J., Rama, N., Imbach, J., Fiore, S., Ducarouge, B., Neves, D., et al. (2019). Cancer-associated fibroblasts produce netrin-1 to control cancer cell plasticity. Cancer Res. 79 (14), 3651–3661. doi:10.1158/0008-5472.CAN-18-2952
Taguchi, A., Kawana, K., Tomio, K., Yamashita, A., Isobe, Y., Nagasaka, K., et al. (2014). Matrix metalloproteinase (MMP)-9 in cancer-associated fibroblasts (CAFs) is suppressed by omega-3 polyunsaturated fatty acids in vitro and in vivo. PLoS One 9 (2), e89605. doi:10.1371/journal.pone.0089605
Takahashi, H., Sakakura, K., Kudo, T., Toyoda, M., Kaira, K., Oyama, T., et al. (2017). Cancer-associated fibroblasts promote an immunosuppressive microenvironment through the induction and accumulation of protumoral macrophages. Oncotarget 8 (5), 8633–8647. doi:10.18632/oncotarget.14374
Tang, X., Hou, Y., Yang, G., Wang, X., Tang, S., Du, Y. E., et al. (2016). Stromal miR-200s contribute to breast cancer cell invasion through CAF activation and ECM remodeling. Cell. Death Differ. 23 (1), 132–145. doi:10.1038/cdd.2015.78
Tavares-Valente, D., Baltazar, F., Moreira, R., and Queirós, O. (2013). Cancer cell bioenergetics and pH regulation influence breast cancer cell resistance to paclitaxel and doxorubicin. J. Bioenerg. Biomembr. 45 (5), 467–475. doi:10.1007/s10863-013-9519-7
Trabert, B., Sherman, M. E., Kannan, N., and Stanczyk, F. Z. (2020). Progesterone and breast cancer. Endocr. Rev. 41 (2), 320–344. doi:10.1210/endrev/bnz001
Tremble, P. M., Lane, T. F., Sage, E. H., and Werb, Z. (1993). SPARC, a secreted protein associated with morphogenesis and tissue remodeling, induces expression of metalloproteinases in fibroblasts through a novel extracellular matrix-dependent pathway. J. Cell. Biol. 121 (6), 1433–1444. doi:10.1083/jcb.121.6.1433
Tsubakihara, Y., and Moustakas, A. (2018). Epithelial-mesenchymal transition and metastasis under the control of transforming growth factor β. Int. J. Mol. Sci. 19 (11), 3672. doi:10.3390/ijms19113672
Ueshima, E., Fujimori, M., Kodama, H., Felsen, D., Chen, J., Durack, J. C., et al. (2019). Macrophage-secreted TGF-β1 contributes to fibroblast activation and ureteral stricture after ablation injury. Am. J. Physiology-Renal Physiology 317 (1), F52-F64–F64. doi:10.1152/ajprenal.00260.2018
Valenti, G., Quinn, H. M., Heynen, G., Lan, L., Holland, J. D., Vogel, R., et al. (2017). Cancer stem cells regulate cancer-associated fibroblasts via activation of hedgehog signaling in mammary gland tumors. Cancer Res. 77 (8), 2134–2147. doi:10.1158/0008-5472.CAN-15-3490
Valkenburg, K. C., de Groot, A. E., and Pienta, K. J. (2018). Targeting the tumour stroma to improve cancer therapy. Nat. Rev. Clin. Oncol. 15 (6), 366–381. doi:10.1038/s41571-018-0007-1
Van Cutsem, E., Tempero, M. A., Sigal, D., Oh, D. Y., Fazio, N., Macarulla, T., et al. (2020). Randomized phase III trial of pegvorhyaluronidase alfa with nab-paclitaxel plus gemcitabine for patients with hyaluronan-high metastatic pancreatic adenocarcinoma. J. Clin. Oncol. 38 (27), 3185–3194. doi:10.1200/JCO.20.00590
Varricchi, G., Galdiero, M. R., Loffredo, S., Marone, G., Iannone, R., Marone, G., et al. (2017). Are mast cells MASTers in cancer? Front. Immunol. 8, 424. doi:10.3389/fimmu.2017.00424
Veglia, F., Sanseviero, E., and Gabrilovich, D. I. (2021). Myeloid-derived suppressor cells in the era of increasing myeloid cell diversity. Nat. Rev. Immunol. 21 (8), 485–498. doi:10.1038/s41577-020-00490-y
Vickman, R. E., Faget, D. V., Beachy, P., Beebe, D., Bhowmick, N. A., Cukierman, E., et al. (2020). Deconstructing tumor heterogeneity: The stromal perspective. Oncotarget 11 (40), 3621–3632. doi:10.18632/oncotarget.27736
Vosseler, S., Lederle, W., Airola, K., Obermueller, E., Fusenig, N. E., and Mueller, M. M. (2009). Distinct progression-associated expression of tumor and stromal MMPs in HaCaT skin SCCs correlates with onset of invasion. Int. J. Cancer 125 (10), 2296–2306. doi:10.1002/ijc.24589
Wang, P. C., Weng, C. C., Hou, Y. S., Jian, S. F., Fang, K. T., Hou, M. F., et al. (2014). Activation of VCAM-1 and its associated molecule CD44 leads to increased malignant potential of breast cancer cells. Int. J. Mol. Sci. 15 (3), 3560–3579. doi:10.3390/ijms15033560
Wang, S., Ma, N., Kawanishi, S., Hiraku, Y., Oikawa, S., Xie, Y., et al. (2014). Relationships of alpha-SMA-positive fibroblasts and SDF-1-positive tumor cells with neoangiogenesis in nasopharyngeal carcinoma. Biomed. Res. Int. 2014, 507353. doi:10.1155/2014/507353
Wang, X., Qin, X., Yan, M., Shi, J., Xu, Q., Li, Z., et al. (2019). Loss of exosomal miR-3188 in cancer-associated fibroblasts contributes to HNC progression. J. Exp. Clin. Cancer Res. 38 (1), 151. doi:10.1186/s13046-019-1144-9
Wang, Y., Gan, G., Wang, B., Wu, J., Cao, Y., Zhu, D., et al. (2017). Cancer-associated fibroblasts promote irradiated cancer cell recovery through autophagy. EBioMedicine 17, 45–56. doi:10.1016/j.ebiom.2017.02.019
Wei, W. F., Chen, X. J., Liang, L. J., Yu, L., Wu, X. G., Zhou, C. F., et al. (2021). Periostin(+) cancer-associated fibroblasts promote lymph node metastasis by impairing the lymphatic endothelial barriers in cervical squamous cell carcinoma. Mol. Oncol. 15 (1), 210–227. doi:10.1002/1878-0261.12837
Wessolly, M., Mairinger, E., Borchert, S., Bankfalvi, A., Mach, P., Schmid, K. W., et al. (2022). CAF-associated paracrine signaling worsens outcome and potentially contributes to chemoresistance in epithelial ovarian cancer. Front. Oncol. 12, 798680. doi:10.3389/fonc.2022.798680
Whatcott, C. J., Diep, C. H., Jiang, P., Watanabe, A., LoBello, J., Sima, C., et al. (2015). Desmoplasia in primary tumors and metastatic lesions of pancreatic cancer. Clin. Cancer Res. 21 (15), 3561–3568. doi:10.1158/1078-0432.CCR-14-1051
Whitaker-Menezes, D., Martinez-Outschoorn, U. E., Lin, Z., Ertel, A., Flomenberg, N., Witkiewicz, A. K., et al. (2011). Evidence for a stromal-epithelial "lactate shuttle" in human tumors: MCT4 is a marker of oxidative stress in cancer-associated fibroblasts. Cell. Cycle 10 (11), 1772–1783. doi:10.4161/cc.10.11.15659
Wu, F., Yang, J., Liu, J., Wang, Y., Mu, J., Zeng, Q., et al. (2021). Signaling pathways in cancer-associated fibroblasts and targeted therapy for cancer. Signal Transduct. Target Ther. 6 (1), 218. doi:10.1038/s41392-021-00641-0
Wu, Y., Song, Y., Xiong, Y., Wang, X., Xu, K., Han, B., et al. (2017). MicroRNA-21 (Mir-21) promotes cell growth and invasion by repressing tumor suppressor PTEN in colorectal cancer. Cell. Physiol. Biochem. 43 (3), 945–958. doi:10.1159/000481648
Xiong, S., Wang, R., Chen, Q., Luo, J., Wang, J., Zhao, Z., et al. (2018). Cancer-associated fibroblasts promote stem cell-like properties of hepatocellular carcinoma cells through IL-6/STAT3/Notch signaling. Am. J. cancer Res. 8 (2), 302–316.
Xu, S., Xu, H., Wang, W., Li, S., Li, H., Li, T., et al. (2019). The role of collagen in cancer: From bench to bedside. J. Transl. Med. 17 (1), 309. doi:10.1186/s12967-019-2058-1
Yan, B., Jiang, Z., Cheng, L., Chen, K., Zhou, C., Sun, L., et al. (2018). Paracrine HGF/c-MET enhances the stem cell-like potential and glycolysis of pancreatic cancer cells via activation of YAP/HIF-1α. Exp. Cell. Res. 371 (1), 63–71. doi:10.1016/j.yexcr.2018.07.041
Yan, W., Wu, X., Zhou, W., Fong, M. Y., Cao, M., Liu, J., et al. (2018). Cancer-cell-secreted exosomal miR-105 promotes tumour growth through the MYC-dependent metabolic reprogramming of stromal cells. Nat. Cell. Biol. 20 (5), 597–609. doi:10.1038/s41556-018-0083-6
Yang, F., Ning, Z., Ma, L., Liu, W., Shao, C., Shu, Y., et al. (2017). Exosomal miRNAs and miRNA dysregulation in cancer-associated fibroblasts. Mol. Cancer 16 (1), 148. doi:10.1186/s12943-017-0718-4
Yang, L., Achreja, A., Yeung, T-L., Mangala, L. S., Jiang, D., Han, C., et al. (2016). Targeting stromal glutamine synthetase in tumors disrupts tumor microenvironment-regulated cancer cell growth. Cell. Metab. 24 (5), 685–700. doi:10.1016/j.cmet.2016.10.011
Yang, X., Li, Y., Zou, L., and Zhu, Z. (2019). Role of exosomes in crosstalk between cancer-associated fibroblasts and cancer cells. Front. Oncol. 9, 356. doi:10.3389/fonc.2019.00356
Yang, X., Lin, Y., Shi, Y., Li, B., Liu, W., Yin, W., et al. (2016). FAP promotes immunosuppression by cancer-associated fibroblasts in the tumor microenvironment via STAT3–CCL2 signaling. Cancer Res. 76 (14), 4124–4135. doi:10.1158/0008-5472.CAN-15-2973
Yang, Z., Peng, Y-C., Gopalan, A., Gao, D., Chen, Y., and Joyner, A. L. (2016). Stromal Hedgehog signaling maintains smooth muscle and hampers micro-invasive prostate cancer. Dis. Models Mech. 10 (1), 39–52. doi:10.1242/dmm.027417
Yeon, J. H., Jeong, H. E., Seo, H., Cho, S., Kim, K., Na, D., et al. (2018). Cancer-derived exosomes trigger endothelial to mesenchymal transition followed by the induction of cancer-associated fibroblasts. Acta Biomater. 76, 146–153. doi:10.1016/j.actbio.2018.07.001
Yeung, T. L., Leung, C. S., Wong, K. K., Samimi, G., Thompson, M. S., Liu, J., et al. (2013). TGF-beta modulates ovarian cancer invasion by upregulating CAF-derived versican in the tumor microenvironment. Cancer Res. 73 (16), 5016–5028. doi:10.1158/0008-5472.CAN-13-0023
Yin, H., Yu, S., Xie, Y., Dai, X., Dong, M., Sheng, C., et al. (2021). Cancer-associated fibroblasts-derived exosomes upregulate microRNA-135b-5p to promote colorectal cancer cell growth and angiogenesis by inhibiting thioredoxin-interacting protein. Cell. Signal. 84, 110029. doi:10.1016/j.cellsig.2021.110029
Yoshida, G. J. (2020). Regulation of heterogeneous cancer-associated fibroblasts: The molecular pathology of activated signaling pathways. J. Exp. Clin. Cancer Res. 39 (1), 112. doi:10.1186/s13046-020-01611-0
Yoshikawa, K., Ishida, M., Yanai, H., Tsuta, K., Sekimoto, M., and Sugie, T. (2021). Prognostic significance of PD-L1-positive cancer-associated fibroblasts in patients with triple-negative breast cancer. BMC Cancer 21 (1), 239. doi:10.1186/s12885-021-07970-x
Yu, B., Wu, K., Wang, X., Zhang, J., Wang, L., Jiang, Y., et al. (2018). Periostin secreted by cancer-associated fibroblasts promotes cancer stemness in head and neck cancer by activating protein tyrosine kinase 7. Cell. Death Dis. 9 (11), 1082. doi:10.1038/s41419-018-1116-6
Yu, T., Yang, G., Hou, Y., Tang, X., Wu, C., Wu, X. A., et al. (2017). Cytoplasmic GPER translocation in cancer-associated fibroblasts mediates cAMP/PKA/CREB/glycolytic axis to confer tumor cells with multidrug resistance. Oncogene 36 (15), 2131–2145. doi:10.1038/onc.2016.370
Yu, Y., Ke, L., Lv, X., Ling, Y., Lu, J., Liang, H., et al. (2018). The prognostic significance of carcinoma-associated fibroblasts and tumor-associated macrophages in nasopharyngeal carcinoma. Cancer Manag. Res 10, 1935–1946. doi:10.2147/CMAR.S167071
Yu, Y., Xiao, C. H., Tan, L. D., Wang, Q. S., Li, X. Q., and Feng, Y. M. (2014). Cancer-associated fibroblasts induce epithelial-mesenchymal transition of breast cancer cells through paracrine TGF-beta signalling. Br. J. Cancer 110 (3), 724–732. doi:10.1038/bjc.2013.768
Zagzag, D., Krishnamachary, B., Yee, H., Okuyama, H., Chiriboga, L., Ali, M. A., et al. (2005). Stromal cell-derived factor-1alpha and CXCR4 expression in hemangioblastoma and clear cell-renal cell carcinoma: von Hippel-lindau loss-of-function induces expression of a ligand and its receptor. Cancer Res. 65 (14), 6178–6188. doi:10.1158/0008-5472.CAN-04-4406
Zeltz, C., Primac, I., Erusappan, P., Alam, J., Noel, A., and Gullberg, D. (2020). Cancer-associated fibroblasts in desmoplastic tumors: Emerging role of integrins. Seminars Cancer Biol. 62, 166–181. doi:10.1016/j.semcancer.2019.08.004
Zeltz, C., Primac, I., Erusappan, P., Alam, J., Noel, A., and Gullberg, D. (2020). Cancer-associated fibroblasts in desmoplastic tumors: Emerging role of integrins. Semin. Cancer Biol. 62, 166–181. doi:10.1016/j.semcancer.2019.08.004
Zhan, Y., Du, J., Min, Z., Ma, L., Zhang, W., Zhu, W., et al. (2021). Carcinoma-associated fibroblasts derived exosomes modulate breast cancer cell stemness through exonic circHIF1A by miR-580-5p in hypoxic stress. Cell. Death Discov. 7 (1), 141. doi:10.1038/s41420-021-00506-z
Zhang, D., Wang, Y., Shi, Z., Liu, J., Sun, P., Hou, X., et al. (2015). Metabolic reprogramming of cancer-associated fibroblasts by IDH3α downregulation. Cell. Rep. 10 (8), 1335–1348. doi:10.1016/j.celrep.2015.02.006
Zhang, H., Xie, C., Yue, J., Jiang, Z., Zhou, R., Xie, R., et al. (2017). Cancer-associated fibroblasts mediated chemoresistance by a FOXO1/TGFβ1 signaling loop in esophageal squamous cell carcinoma. Mol. Carcinog. 56 (3), 1150–1163. doi:10.1002/mc.22581
Zhang, J., Chen, L., Xiao, M., Wang, C., and Qin, Z. (2011). FSP1+ fibroblasts promote skin carcinogenesis by maintaining MCP-1-mediated macrophage infiltration and chronic inflammation. Am. J. Pathol. 178 (1), 382–390. doi:10.1016/j.ajpath.2010.11.017
Zhang, L., Song, M., Zhang, F., Yuan, H., Chang, W., Yu, G., et al. (2021). Accumulation of nicotinamide N-methyltransferase (NNMT) in cancer-associated fibroblasts: A potential prognostic and predictive biomarker for gastric carcinoma. J. Histochem. Cytochem. 69 (3), 165–176. doi:10.1369/0022155420976590
Zhang, M., Yang, H., Wan, L., Wang, Z., Wang, H., Ge, C., et al. (2020). Single-cell transcriptomic architecture and intercellular crosstalk of human intrahepatic cholangiocarcinoma. J. Hepatol. 73 (5), 1118–1130. doi:10.1016/j.jhep.2020.05.039
Zhang, P., Su, D. M., Liang, M., and Fu, J. (2008). Chemopreventive agents induce programmed death-1-ligand 1 (PD-L1) surface expression in breast cancer cells and promote PD-L1-mediated T cell apoptosis. Mol. Immunol. 45 (5), 1470–1476. doi:10.1016/j.molimm.2007.08.013
Zhang, R., Qi, F., Zhao, F., Li, G., Shao, S., Zhang, X., et al. (2019). Cancer-associated fibroblasts enhance tumor-associated macrophages enrichment and suppress NK cells function in colorectal cancer. Cell. Death Dis. 10 (4), 273. doi:10.1038/s41419-019-1435-2
Zhao, H., Yang, L., Baddour, J., Achreja, A., Bernard, V., Moss, T., et al. (2016). Tumor microenvironment derived exosomes pleiotropically modulate cancer cell metabolism. eLife 5, e10250. doi:10.7554/eLife.10250
Zhao, L., Sun, Y., Hou, Y., Peng, Q., Wang, L., Luo, H., et al. (2012). MiRNA expression analysis of cancer-associated fibroblasts and normal fibroblasts in breast cancer. Int. J. Biochem. Cell. Biol. 44 (11), 2051–2059. doi:10.1016/j.biocel.2012.08.005
Zheng, X., Turkowski, K., Mora, J., Brüne, B., Seeger, W., Weigert, A., et al. (2017). Redirecting tumor-associated macrophages to become tumoricidal effectors as a novel strategy for cancer therapy. Oncotarget 8 (29), 48436–48452. doi:10.18632/oncotarget.17061
Zhou, J., Wang, X-H., Zhao, Y-X., Chen, C., Xu, X-Y., Sun, Q., et al. (2018). Cancer-associated fibroblasts correlate with tumor-associated macrophages infiltration and lymphatic metastasis in triple negative breast cancer patients. J. Cancer 9 (24), 4635–4641. doi:10.7150/jca.28583
Zhu, Z., Achreja, A., Meurs, N., Animasahun, O., Owen, S., Mittal, A., et al. (2020). Tumour-reprogrammed stromal BCAT1 fuels branched-chain ketoacid dependency in stromal-rich PDAC tumours. Nat. Metab. 2 (8), 775–792. doi:10.1038/s42255-020-0226-5
Zhuang, J., Lu, Q., Shen, B., Huang, X., Shen, L., Zheng, X., et al. (2015). TGFβ1 secreted by cancer-associated fibroblasts induces epithelial-mesenchymal transition of bladder cancer cells through lncRNA-ZEB2NAT. Sci. Rep. 5, 11924. doi:10.1038/srep11924
Keywords: CAF, breast cancer, tumor microenvironment, heterogeneity, targeting
Citation: Sarkar M, Nguyen T, Gundre E, Ogunlusi O, El-Sobky M, Giri B and Sarkar TR (2023) Cancer-associated fibroblasts: The chief architect in the tumor microenvironment. Front. Cell Dev. Biol. 11:1089068. doi: 10.3389/fcell.2023.1089068
Received: 03 November 2022; Accepted: 12 January 2023;
Published: 30 January 2023.
Edited by:
Roberto Piñeiro, Health Research Institute of Santiago de Compostela (IDIS), SpainReviewed by:
Sergey Novitskiy, Vanderbilt University Medical Center, United StatesCopyright © 2023 Sarkar, Nguyen, Gundre, Ogunlusi, El-Sobky, Giri and Sarkar. This is an open-access article distributed under the terms of the Creative Commons Attribution License (CC BY). The use, distribution or reproduction in other forums is permitted, provided the original author(s) and the copyright owner(s) are credited and that the original publication in this journal is cited, in accordance with accepted academic practice. No use, distribution or reproduction is permitted which does not comply with these terms.
*Correspondence: Biplab Giri, YmdpcmkuZW1zY3JsQGdtYWlsLmNvbQ==; Tapasree Roy Sarkar, dHNhcmthckBiaW8udGFtdS5lZHU=
†These authors have contributed equally to this work
Disclaimer: All claims expressed in this article are solely those of the authors and do not necessarily represent those of their affiliated organizations, or those of the publisher, the editors and the reviewers. Any product that may be evaluated in this article or claim that may be made by its manufacturer is not guaranteed or endorsed by the publisher.
Research integrity at Frontiers
Learn more about the work of our research integrity team to safeguard the quality of each article we publish.