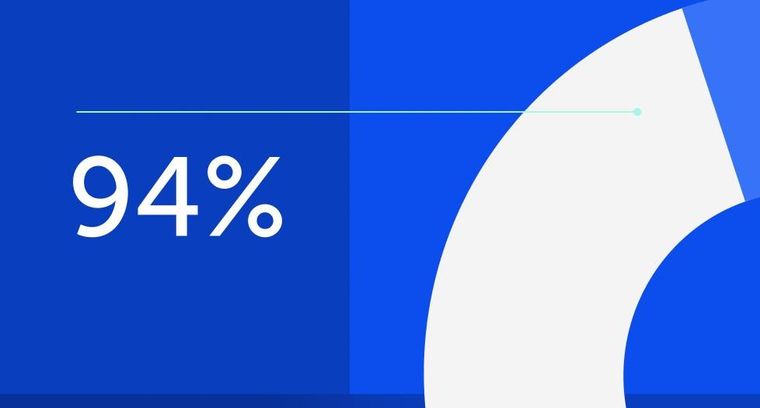
94% of researchers rate our articles as excellent or good
Learn more about the work of our research integrity team to safeguard the quality of each article we publish.
Find out more
REVIEW article
Front. Cell Dev. Biol., 14 February 2023
Sec. Stem Cell Research
Volume 11 - 2023 | https://doi.org/10.3389/fcell.2023.1089028
This article is part of the Research TopicPlasticity and Adaptability of the Gastrointestinal EpitheliumView all 4 articles
Metaplasia, dysplasia, and cancer arise from normal epithelia via a plastic cellular transformation, typically in the setting of chronic inflammation. Such transformations are the focus of numerous studies that strive to identify the changes in RNA/Protein expression that drive such plasticity along with the contributions from the mesenchyme and immune cells. However, despite being widely utilized clinically as biomarkers for such transitions, the role of glycosylation epitopes is understudied in this context. Here, we explore 3′-Sulfo-Lewis A/C, a clinically validated biomarker for high-risk metaplasia and cancer throughout the gastrointestinal foregut: esophagus, stomach, and pancreas. We discuss the clinical correlation of sulfomucin expression with metaplastic and oncogenic transformation, as well as its synthesis, intracellular and extracellular receptors and suggest potential roles for 3′-Sulfo-Lewis A/C in contributing to and maintaining these malignant cellular transformations.
Cellular plasticity can be defined as a cell undergoing a phenotypic change resulting in a different histological appearance and RNA/Protein expression profile. This process occurs at homeostasis in tissues constitutively propagated by stem cells; however, more recently it has been shown to be a necessary aspect of tissue repair after injury. In the setting of prolonged/chronic injury and presumed mutational burden, plasticity allows tissue to transform into metaplasia as well as dysplasia, and cancer.
Evidence for cellular plasticity of the GI foregut in injury and cancer largely derive from lineage tracing experiments using either Cre-reporters (Kopp et al., 2012; Han et al., 2019; Caldwell et al., 2022; Min et al., 2022) or pulse-chase experiments (Burclaff et al., 2020). Recently single-cell RNAseq (scRNAseq) trajectory/velocity analysis is also supportive of such transitions (Bockerstett et al., 2020a; Bockerstett et al., 2020b; Ma et al., 2022). Our knowledge of these metaplastic and oncologic tissue transitions derive primarily from in vivo murine studies due to several limitations of in vitro systems, namely: 1) the inability of cultured cancer cells or organoids from metaplastic tissue or cancer to reliably differentiate into homeostatic tissue with loss of cancer-associated transcription factors and glycosylation epitopes, (e.g. Figure 1) 2) the effects of growth conditions on organoids fueling constitutive proliferation reminiscent of dysplasia or cancer (Brown et al., 2022), 3) the lack of mesenchymal and immune interactions critical for metaplastic transition, (Petersen et al., 2014; Meyer et al., 2020; De Salvo et al., 2021), and 4) the lack of a deep mutational burden acquired over prolonged periods of inflammation (Baslan et al., 2022; Redston et al., 2022).
FIGURE 1. Anti-3′-Sulfo-LeA/C (mAb Das-1) immunohistochemistry of Barrett’s esophageal organoids. Organoids were grown from endoscopic biopsies of individuals with Barrett’s esophagus as we have previously reported. (Jin et al., 2021). Immunohistochemistry of paraffin embedded organoids were stained as we have described in Brown et al., 2021a. Hematoxylin (blue) was used as a nuclear counterstain. Orange Asterix denotes the organoid lumen, which is bordered by the apical domain of the metaplastic cells through which the sulfomucins are being secreted.
Clinically, these high-risk plastic cellular transformations are a hallmark of gastrointestinal pathology in practice. Indeed, while metaplastic transitions are prevalent in the setting of chronic inflammation in the form of Barrett’s esophagus, gastric intestinal metaplasia, and pancreatic intraepithelial neoplasia for example, they form the basis for dysplastic transformation into esophageal, gastric, and pancreatic adenocarcinomas, which have been recapitulated in murine models. Identification of patients at an early stage of metaplastic transition may allow for reversal (i.e. eradication of H. pylori infection in the stomach) or slowing (i.e. proton pump inhibitor therapy for Barrett’s esophagus). Similarly, by successfully identifying patients early in the metaplasia-to-dysplasia transition, minimally invasive endoscopic therapies such as endoscopic submucosal dissection of dysplastic gastric lesions or radiofrequency ablation of Barrett’s esophagus may be undertaken to potentially abrogate this process. The clinical challenge lies in identification of patients with fairly common metaplastic changes and subsequent risk stratification of patients with metaplasia who have undergone early stage dysplasia that requires intervention. For example, Barrett’s esophagus, prevalent in 1%–2% of the general population (Ronkainen et al., 2005) is readily identifiable with expensive, invasive, endoscopy; however, even once low-grade dysplasia has emerged the risk of cancer formation is <1% per year (Wani et al., 2011). While ever improving endoscopic and surgical approaches to dysplasia have emerged (Shaheen et al., 2022) and non-invasive means to assess the esophagus have been invented (Fitzgerald et al., 2020) the incidence of esophageal cancer has not significantly changed in the same period. Additionally unique challenges emerge for pancreatic intraepithelial neoplasia (PanIN) which is not readily detectable on radiographic modalities (Das and Early, 2017) or metaplasia of the stomach which may be endoscopically or histopathologically subtle to subtype.
Clearly, in the hopes of developing less invasive means of assaying for these high-risk plastic transitions, numerous groups are trying to identify biomarkers. Interestingly, nearly all commercially available serologic tests for metaplasia and cancer depend on assays for neoglycosylation epitopes and secreted glycoproteins (Table 1). However, there is a paucity of knowledge and understanding as to why cells begin to express and secrete these aberrant glycosylation epitopes and glycoproteins as they undergo plastic transitions to metaplasia and cancer. Further, there is a disconnect between the data produced from murine models of metaplasia, which primarily focuses on RNA/protein expression as well as the contributions of tumor microenvironment and clinically utilized assays for glycosylation epitopes and glycosylated proteins.
Here, we take a Top-Down approach: we start from a clinically validated biomarker, 3′-Sulfo-LeA/C, which has recently been recognized as the epitope of the antibody Das-1 (Brown et al., 2021a), for metaplasia and cancer throughout the GI foregut and review the literature in order to gain insight into how and why metaplasia and cancers might stereotypically express this antigen. With glycosylation epitopes, this approach is necessary because, as will be discussed, the cellular glycome cannot be readily predicted from cellular RNA/protein expression.
Aberrantly sulfated glycosylation epitopes have long been recognized as a feature of high-risk metaplasia of the esophagus and stomach (Hakkinen et al., 1968; Bodger et al., 2003; Shah et al., 2020) as well as with dysplasia and cancer (Montero and Segura, 1980; Torrado et al., 1992; Fitzgerald et al., 2002). In the stomach, sulfation appears to be correlated with intestinal-type rather than diffuse-type of gastric cancer (Driessen et al., 1998).
Despite correlation with these plastic cellular transformations, sulfation remains poorly understood in part due to the paucity of commercial antibodies and lectins that are reactive towards it (McKitrick et al., 2021). Traditionally, histological identification of sulfated epitopes relies on high-iron diamine (HID) staining and specific Alcian blue stains at pH 1 (AB-1) (Reid et al., 1989; Padra and Linden, 2022). In addition to being agnostic to the moiety carrying the sulfate, these chemical stains are not compatible with more sophisticated imaging technologies like confocal or live cell imaging. 35S radiolabeling can be used to assay for sulfation; however, it has no spatial resolution within the tissue and again is agnostic to the moiety carrying the sulfate. Lastly, standard glycomic MS/MS analysis like collision-induced dissociation struggles to detect sulfate groups due to experimental loss with this technique (Kailemia et al., 2012).
Despite a lack of commercially available tools to detect sulfated glycans, historically, at least five groups have generated antibodies against 3′-Sulfated type I Lewis antigens (Table 2) and demonstrated that their reactivity phenocopies high-iron diamine staining in metaplasias and cancer of the gastrointestinal foregut (Das et al., 1987; Yamori et al., 1989; Rathman et al., 1990; Mitsuoka et al., 1998; Loveless et al., 2001). This suggests that at least a portion of the sulfated groups identified by HID/AB-1 staining represent 3′-Sulfo-LeA/C. Immunohistochemical surveys using these antibodies have demonstrated a consistent tissue distribution of 3′-Sulfo-LeA/C in both normal fetal and adult tissue as well as during progression to metaplasia and cancer, cross-validating the specificity of these “homegrown” antibodies in tissue (Table 3). Three of these antibodies are specifically reactive towards 3′-Sulfo-LeA/C [Das-1, F2, and 91.9H] and an additional two antibodies have affinity towards both 3′-Sulfo-LeA/C and 3′-Sulfo-LeX [SU59, MIN/3/60] (e.g. these latter reagents are unable to discriminate between Type I v Type II LacNAc; Figure 2 and Table 2).
TABLE 2. List of antibodies that are specifically reactive towards 3’-Sulfated galactose containing epitopes.
FIGURE 2. Synthesis of Type I Lewis epitopes. (A). Schematic representation of type 1 v. type II Lewis antigens. (B). Diagrams of sulfated and sialylated type I Lewis antigens. (C). Presumed synthetic pathway for 3′-Sulfated LeA/C.
Sulfatides (3-O galactosylceramide, e.g. SM4) are a class of sulfolipid that express a terminal 3′-Sulfo-Galactose, similar to 3′-Sulfated Lewis epitopes. Despite sharing the terminal 3′-Sulfo-Gal, antibodies recognizing sulfatides and 3′-Sulfo-LeA/C have mutually exclusive tissue distributions. Anti-sulfatide antibodies are reactive towards kidney, pancreatic islets, and nervous tissue (Fredman et al., 1988; Miyake et al., 1992; Buschard et al., 1993a; Buschard et al., 1993b; Buschard et al., 1994; Colsch et al., 2008), which is distinct from the distribution for 3′-Sulfo-LeA/C (Table 3). Thus although, the antibodies reactive to 3′-Sulfo-LeA/C require a 3′-Sulfate on the terminal galactose they also require the adjacent glycans.
Unlike protein expression, which usually correlates with RNA levels, protein glycosylation cannot be simply predicted from RNA or protein expression due to numerous competing factors. Following the initial glycosylation event in either the endoplasmic reticulum (for N-Linked glycans) or Golgi apparatus (for O-linked glycans), numerous enzymes compete with one another to add additional sugars (glycosyltransferases), remove sugars (glycosidases), and/or create branch points as the receptive moiety moves through the endoplasmic reticulum to the Golgi apparatus and beyond. These enzymes are not evenly distributed within each organelle, but instead are sorted to different regions and thus enzyme activity within the endoplasmic reticulum or cis-Golgi will affect the ability of more distally located enzymes in the trans-Golgi to act by changing the available glycosylation epitopes. In addition to differential expression of the proteins accepting these glycosylation epitopes, the speed, route of transport of the glycan acceptor, and availability of nucleotide-sugar donors will also differentially affect the end-product. Further, it is beginning to be appreciated that the activity of these glycosylation enzymes is also regulated (Li et al., 2022). Lastly, these glycans are modified not only by sulfotransferases (discussed here), but also by acetyl- and methyl transferases creating even greater diversity. Thus, even if the activity and donor specificity of each relevant glycosylation enzyme is known, it is essentially impossible to accurately predict a cellular glycome and this results in the production of heterogeneous products in most situations.
With all this complexity, it might be surprising that specific glycosylation epitopes are stereotypically expressed during specific plastic cellular transitions. However, the utility of such epitopes is clearly demonstrated by their clinical use as biomarkers to 1) diagnose cancer, 2) stage cancer, 3) monitor therapeutic response and/or 4) evaluate for recurrence (Table 1). While the synthesis, cellular effects, and tissue/organismal effects of 3′-Sulfo-LeA/C is discussed here, much research is required to understand how and why such epitopes are stereotypically produced and secreted during these cellular transformations across many divergent organs.
LeA/C are type 1 Lewis antigens: they contain an N-Acetyl Glucosamine (GlcNAc) bound to a galactose via a β1-3 linkage (Figure 2). Lewis A differs from Lewis C in that it also has a fucose attached to GlcNAc via a one to four linkage. Type 1 Lewis antigens have been reported on proteins by both N- and O-Linked glycans as well as on glycosphingolipids.
In humans, there are five enzymes (β3GALT-1,-2,-4,-5,-6) that attach a galactose via a β3-link. Sequence-based phylogenetic analysis suggests that these enzymes segregate into three separate groups 1) β3GALT-1,-2, and,-5, 2) β3GALT-4, and 3) β3GALT-6, which parallel the preferred acceptor glycan (Togayachi et al., 2006) (Supplementary Image S1). β3GALT-4 is responsible for transferring galactose to glycosphingolipids (Daniotti et al., 1999) as well as glycoylphosphatidylinositol (GPI) (Wang et al., 2020). β3GALT-6 adds galactose to xylose in glycosylaminoglycans (Bai et al., 2001). β3GALT-1,-2, and -5 are capable of producing type I Lewis antigens on N-linked glycans; however, only β3GALT5 (Zhou et al., 1999) appears capable of adding this to O-linked glycans (Holgersson and Lofling, 2006). Since, the expression of O-Linked sulfated mucins have been best described in plastic cellular transformations of the GI foregut and β3GALT5 is generally believed to be responsible for the production of type I Lewis antigens in GI cancers (Isshiki et al., 1999; Salvini et al., 2001) we will focus on this enzyme. Further, overexpression β3GALT5 (in conjunction with FUT3) was able to generate Sialyl-LeA (CA19-9) in a doxycycline inducible murine model that resulted in pancreatitis and augmented the development of tumors in the KrasLSL-G12D background (Engle et al., 2019).
The expression of β3GALT5 is complex, being promoted by CDX1 and CDX2 and potentially HNF1α/β that acts on a retroviral LTR promoter present only in old world monkeys and humans (absent in mice and new world monkeys); however, it is unclear whether this later transcriptional promoter is relevant (Isshiki et al., 2003). In addition to transcriptional factors, epigenetic methylation appears to play an important role in expression of β3GALT5 (Aronica et al., 2017).
Unlike β3GALT5, which specifically synthesizes type 1 Lewis antigens, the remaining two enzymes (FUT3 and GAL3ST2) that are necessary to synthesize 3′-Sulfo-Lewis A act on both type 1 [Galβ(1–3)GlcNAc] and type 2 [Galβ(1–4)GlcNAc] backbones (Figure 2). FUT3 is unique among the numerous fucosyltransferases in that it is the only one capable of adding an α1,4-linked fucose to GlcNAc for the creation of Lewis A. Interestingly in mice, Fut3 is a non-functional pseudogene due to multiple frameshift and non-sense mutations (Gersten et al., 1995) and as such it is believed that mice are only able synthesize LeC and not LeA. Expression of Fut3 is under the control of at least NFκB (Norden et al., 2017) and c-Myc (Sakuma et al., 2012).
3′-Phosphoadenosine 5′-phosphosulfate (PAPS) is a universal sulfate donor for all sulfation reactions: those on glycans and those on aglycone moieties like tyrosine. This high-energy donor is generated from ATP via two, bifunctional homologous enzymes, PAPSS1 and PAPSS2 (Venkatachalam, 2003). PAPSS1 is reported to be located in the nucleus (Besset et al., 2000), while PAPSS2 is predominantly cytoplasmic (Schroder et al., 2012). The relative contribution of cellular PAPS from these enzymes is unclear and may vary by cell type. Since PAPS is produced in the nucleocytoplasm and sulfation of glycans occurs within vesicular compartments, there are two PAPS transporters (PAPST1 and PAPST2) on the Golgi membrane (Kamiyama et al., 2003; Kamiyama et al., 2006). Knockdown of PAPST1 and PAPST2 in DLD-1 cells (a cell line known to express 3′-Sulfo-LeA/C (Hassan et al., 1995)) was shown to decrease sulfate incorporation not only in glycoproteins, but also chondroitin sulfate and heparan sulfate (Kamiyama et al., 2011) (Figure 3).
FIGURE 3. Schematic representation of the synthesis and transport of PAPS into the Golgi cisternae, where it is utilized by GAL3ST2 to sulfate Type I Lewis epitopes. PAPS is synthesized by the bifunctional enzymes PAPSS1 and PAPSS2. These paralogous proteins initially utilize the ATP sulfurylase domain to produce APS (Adenosine-5′-phosphosulfate). The APS intermediate is then acted upon by the APS kinase domain to generate PAPS. PAPS is subsequently transported from the cytosol into the Golgi lumen by two transporters PAPST1 and PAPST2. In the Golgi, PAPS is utilized by multiple different enzymes, one of which is GAL3ST2 that transfers the sulfate from PAPS to the 3′-site of galactose. These proteins were drawn in the medial Golgi with artistic license, as it is unclear where within the Golgi they reside. All structures were generated from the atomic coordinates utilizing the program chimera (Pettersen et al., 2004). The coordinates of PAPSS2 where obtained from PDB 7FHA (Zhang et al., 2022), while those for PAPSS1 and GAL3ST2 were predicted by the program AlphaFold (Jumper et al., 2021) and downloaded from the AlphaFold webserver (Varadi et al., 2022).
In both humans and mice, there are four distinct sulfotranferases capable of transferring a sulfate from PAPS to the 3′-site of a terminal galactose (GAL3ST1 (Honke et al., 1997), GAL3ST2 (Honke et al., 2001), GAL3ST3 (El-Fasakhany et al., 2001; Suzuki et al., 2001), and GAL3ST4 (Seko et al., 2001)). Like the galactosyltransferases, these enzymes have different tissue expression profiles and preferred glycan acceptors. It should also be noted that, in the 1990s when these genes were being cloned, the nomenclature was not standardized: GAL3ST3 had been briefly called GAL3ST2 (El-Fasakhany et al., 2001) and likewise GAL3ST2 was called GP3ST (Honke et al., 2001; Ikeda et al., 2001), and GAL3ST1 referred to as CST in several publications (cerebroside 3′-sulfotransferase). Each of these sulfotransferases have a unique acceptor specificity with GAL3ST2 being the only enzyme with strong activity toward Type I Lewis antigens (Honke et al., 2001; Suzuki et al., 2001; Seko et al., 2002). There has been a report of weak activity of GAL3ST3 toward Type 1 Lewis antigens; however, this might be due to cross-reactivity of the F2 antibody used (El-Fasakhany et al., 2001) as others were not able to detect activity when measuring transfer to pure substrates (Suzuki et al., 2001). GAL3ST1 is reportedly exclusively active toward ceramides (Honke et al., 1997), while GAL3ST4 has no activity against Galβ(1–3)GlcNAc (Seko et al., 2001). Thus GAL3ST2 appears to be the 3′-Sulfotransferase responsible for generating 3′-Sulfo-LewisA/C. This enzyme is agnostic to the glycan carrier, being able to sulfate O-Linked, N-Linked, and glycolipids (Sun et al., 2022).
In mice, Gal3st2 underwent a triplication into Gal3st2, Gal3st2b, and Gal3st2c, which exist in rapid succession over ∼110 kbp. Both the RNA and protein sequences are over 97% identical (Supplementary Image S2), which makes it virtually impossible to determine which of the three genes are translated or expressed by qPCR or other techniques. The transcriptional regulation of GAL3ST2 is likewise unknown and some have argued that activity does not correlate with RNA/Protein levels and thus may be regulated by other means (Castro et al., 2012). Despite a relatively compact predicted structure by alpha fold (Supplementary Image S3), no high-resolution structural data exists for either of these enzymes or homologous proteins. Thus the molecular mechanism and critical residues by which they function remain to be determined as well as the possible regulation.
Immediately following the GAL3ST2 gene in humans, mice, chicken, etc., is NEU4, which removes a 2,3-linked sialic acid from Lewis A epitopes (Shiozaki et al., 2011). Databases like String (Szklarczyk et al., 2019) report that GAL3ST2 and NEU4 are coexpressed (Supplementary Image S4) potentially in cis on the same piece of mRNA (e.g. chimera) as this fusion transcripts has been reported in patent applications (https://patentimages.storage.googleapis.com/13/65/1e/27c585e9570bc4/US20160078168A1.pdf). as well as in cancer (Liu et al., 2021). Neu4 has been reported to be present in the endoplasmic reticulum and mitochondria (long isoform) (Yamaguchi et al., 2005; Bigi et al., 2010) as well as the lysosome (Seyrantepe et al., 2004). The lysosomal distribution is best supported by in vitro data as the enzymatic activity is negatively correlated with pH being most active at ∼3.2 (Monti et al., 2004), the pH of the lysosome. Mice lacking Neu4 develop lysosomal storage pathology (Seyrantepe et al., 2008).
This location is interesting as autophagy has been demonstrated to be an essential aspect of plastic cellular transformation, specifically in the process of paligenosis (Willet et al., 2018; Brown et al., 2022). Potentially, sialylated proteins (e.g. those with 3′-Sialyl-LeA e.g. CA19-9) could be shuttled to the lysosome where sialic acid is removed by the neuraminidase activity of Neu4, making the glycan amenable to sulfation. Despite being purely conjecture as the dependency of NEU4 on GAL3ST2 activity has never been reported, indirect evidence from tissue surveys of these plastic cellular transitions suggests a transition from sialylated to sulfated mucins as pathology progresses towards cancer. For example, the sole discriminating feature differentiating Type II intestinal metaplasia of the stomach from Type III is that the former expresses sialyated mucins and type III expresses sulfated mucins (Shah et al., 2020). Analogous to the stomach, acinar-to-ductal metaplasia and Pan-IN2 express 3′-Sialyl-LeA (e.g. CA19-9) (Shi et al., 2014), which is in contrast to 3′-Sulfo-LeA/C which is restricted to high-grade lesions (Pan-IN3 and PDAC) (Das et al., 2021). Thus, in multiple tissues the transition from sialylated to sulfated mucins (esp. involving LeA/C) correlates with the transition to higher risk metaplasia and cancer. Two potential explanations for the transition from sialylated-to-sulfated mucins are 1) increased expression of NEU4 and GAL3ST2 in cis or 2) altered vesicular transport routing mucins towards these enzymes. It remains to be determined whether either of these processes are required for the transition to sulfated mucins that correlate with greater incidence of having or developing foregut cancers.
While several groups have described the distribution of 3′-Sulfo-LeA/C in mature adult tissue, a widespread survey of human fetal expression has only been described using the antibody Das-1 (formerly 7E12H12). In fetal tissue (analyzed at 7–22 weeks human gestational age), 3′-Sulfo-LeA/C expression is widespread, but in many cases is restricted to a subset of cells in these organ systems (Table 3) (Das et al., 1987; Das et al., 1990; Das et al., 1992; Badve et al., 2000). In adult tissue at homeostasis, the 3′-Sulfo-LeA/C epitope is not restricted to the germ layer and found in the colon (both enterocyte and goblet), extrahepatic biliary tract, MG1/MUC5B producing salivary glands, (Veerman et al., 2003), keratinocytes, and Hassall’s corpuscles of the thymus (Veerman et al., 1997b; Badve et al., 2000). In addition, low-grade patchy expression has been reported in the uterine cervix and submucosal glands of the lung (Veerman et al., 1997b).
Despite the absence of expression in the mature, adult esophagus, small intestine, pancreas, bladder, and lung, 3′-Sulfo-LeA/C is re-expressed during the plastic cellular transformation into premalignant metaplasia and cancer (Tables 3, 4). As such, 3′-Sulfo-LeA/C is a true oncofetal antigen in that expression is lost during maturation but reappears as cells transition into metaplasia and cancer. Re-expresssion of this antigen has been best studied in GI foregut: esophagus (Barrett’s esophagus and adenocarcinoma) (Das et al., 1994; Endo et al., 1998; Glickman et al., 2001; DeMeester et al., 2002; Bodger et al., 2003; Piazuelo et al., 2004; Su et al., 2004; Hahn et al., 2009; Moriichi et al., 2009), stomach (type III incomplete intestinal metaplasia and gastric adenocarcinoma) (Ohe et al., 1994; Veerman et al., 1997b; DeMeester et al., 2002; Bodger et al., 2003; Mirza et al., 2003; Piazuelo et al., 2004; O'Connell et al., 2005; Sun et al., 2006; Watari et al., 2008; Watari et al., 2012; Feng et al., 2013), and more recently by us in the exocrine pancreas [Pancreatic Intraepithelial Neoplasia (Pan-IN3), High-grade intraductal papillary mucinous neoplasm (IPMN), and pancreatic ductal adenocarcinoma (PDAC)] (Das et al., 2014; Das et al., 2019; Das et al., 2021).
TABLE 4. mAb Das-1 reactivity in gastrointestinal precancerous epithelial lesions and cancers derived therefrom.
As previously discussed, expression of 3′-Sulfo-LeA/C appears to be restricted to “higher-risk” histology relative to sialylated glycans. For example, sulfated mucins like 3′-Sulfo-LeA/C are observed in type III intestinal metaplasia of the stomach as well as gastric adenocarcinoma (GC) (Bodger et al., 2003; Mirza et al., 2003), and by definition are not present in type I or II intestinal metaplasia of the stomach (Shah et al., 2020). Similarly, 3′-Sulfo-LeA/C is restricted to Pan-IN3 and PDAC and not present in reversible lesions like acinar-to-ductal metaplasia or Pan-IN1 & Pan-IN2 (Das et al., 2021).
The corresponding sialic analog of 3′-Sulfo-LeA is CA19-9 (3′-Sialyl-LeA) a widely used, commercially available biomarker. Although CA19-9 is a sensitive biomarker for the recurrence of pancreatic cancer after surgical resection or in response to chemotherapeutic treatment failure/non-response (Humphris et al., 2012), it can be non-specifically elevated in patients with and without pancreaticobiliary disease (Kim et al., 2020). In serum, elevated CA19-9 was observed in a minority of patients up to 2 years prior to the diagnosis of pancreatic ductal adenocarcinoma; however, this approached 60% sensitivity in 6 months preceding the diagnosis (Fahrmann et al., 2021). In patients with pancreatic cysts suspected of possible malignancy, serum CA19-9 has a sensitivity and specificity of 34.2% and 92.4%, respectively (Kim et al., 2015), which has been confirmed in larger studies and meta-analyses (Tanaka et al., 2019; Ciprani et al., 2020). Likewise, CA19-9 levels in cyst fluid perform poorly at differentiating benign IPMN from those harboring malignancy, with many studies demonstrating non-significant differences in the levels between these two cohorts (Nagashio et al., 2014; Kurita et al., 2019). In contrast, assaying for 3′-Sulfo-LeA/C is 88% sensitive and 100% specific for identifying those cysts that harbor high-grade dysplasia and cancer (Das et al., 2014; Das et al., 2019). Thus, akin to the difference between Type II (Sialylated mucins) and Type III (Sulfated mucins) intestinal metaplasia of the stomach (Shah et al., 2020), in the pancreas sulfated mucins (3′-Sulfo-LeA/C) may be more sensitive and specific to high-grade dysplasia and cancer than the sialylated analogs (e.g. CA19-9).
One notable difference between the expression of 3′-Sulfo-LeA/C in normal columnar cells compared to cells that express 3′-Sulfo-LeA/C only after plastic transition to metaplasia/dysplasia and cancer is the subcellular distribution. In normal columnar epithelial cells of the biliary tract and colon 3′-Sulfo-LeA/C is primarily found on the apical extracellular membrane, presumably on the outer leaflet (Das et al., 1987; Das et al., 1992). (Figures 4A,B) In contrast, in metaplasia and cancer, the 3′-Sulfo-LeA/C is expressed intracellularly and secreted being largely absent from extracellular membrane (Brown et al., 2021a) (Figure 1; Figures 4C–E). This secretion explains how we and others are able to detect 3′-Sulfo-LeA in the serum and extracellular fluid upon plastic cellular transformation to high-grade dysplasia and cancer (Zheng et al., 2009; Das et al., 2014; Tanaka-Okamoto et al., 2017; Das et al., 2019). The reason for this different cellular distribution is unknown but almost certainly involves different vesicular trafficking pathways invoked during these plastic cellular transformations. Studying these divergent vesicular trafficking trajectories is an area of active investigation in our laboratory.
FIGURE 4. Subcellular expression patterns of 3′-Sulfo-LeA/C in tissues at homeostasis and in metaplasia and cancer. (A). Immunohistochemistry of human gallbladder mucosa demonstrating expression of 3′-Sulfo-LeA/C on the apical membrane of the biliary epithelium. (B). Expression of 3′-Sulfo-LeA/C on the outer membrane of the colonic enterocytes (Inset), but intracellular in the goblet cells. In contrast, in metaplasia and cancer 3′-Sulfo-LeA/C is absent from the apical membrane, being present intracellularly and is secreted as demonstrated in (C). Barrett’s Esophagus, (D). Gastric Cancer, and (E). Pancreatic cancer. Secretion denoted by yellow arrow arrowhead. (Panel A was reproduced with permission from Mandal et al., 1994; Panels C–E were reproduced from Brown et al., 2021a).
It is also interesting to note that expression of 3′-Sulfo-LeA/C in human metaplasias often exhibits a variegated cell-by-cell expression where clonally-related neighboring cells may differentially express or not express the sulfomucins (Brown et al., 2021a; Das et al., 2021) (Figure 4C). Using synchronous mouse models, we believe that this occurs because of coordinated cellular process of expression and expulsion (Brown, 2021b; Brown, 2021c). Although secretion of sulfated mucins still occurs when cells progress from metaplasia to cancer, the variegated appearance is largely absent and all cells express similar quantities of the sulfated mucins (Brown et al., 2021a; Das et al., 2021) (Figure 4).
Precise epitope mapping suggests that at least two antibodies (F2 and Das-1) (Veerman et al., 1997b; Brown, 2021b) recognize the 3′-Sulfo-Galβ(1–3)GlcNAc (3′-Sulfo-LeC) chain and the fucose moiety of LeA is not absolutely required; however, in some cases it may increase affinity. The independence of the α(1–4)fucose in LeA becomes important when using mouse models to study the expression of this epitope because the sole alpha-4-fucosyltransferase (Fut3, Fut3) ortholog in mice is a non-functional pseudogene (Gersten et al., 1995). Thus, the consequences of 3′-Sulfation of Galβ1-3GlcNAc (Lewis C; LeC) in cells and at the tissue level can still be studied using the antibody Das-1 or the historically produced antibody F2 (Veerman et al., 1997b). In contrast, analysis of the epitope recognized by the historically generated 91.9H presumably requires both the fucose, as well as additional proximal sugars in the tetra- and pentasaccharide (Loveless et al., 1998). Using the KrasLSL-G12D; TP53; Pdx1-cre mouse, we have demonstrated that the expression of 3′-Sulfo-LeC in mice (Brown et al., 2021a) parallels expression in humans (Das et al., 2021), being restricted to high-grade Pan-INs and pancreatic ductal adenocarcinoma. As such, genetic murine models appear to be a plausible tool to molecularly dissect how and why these sulfated mucins are expressed during the plastic transition from normal, homeostatic tissue into metaplasia, dysplasia, and cancer.
Galactose containing epitopes are generally recognized by a family of proteins called galectins (galactose binding lectins). At least three of the numerous galectins bind galactose containing epitopes that have a 3′-sulfate with greater or equal affinity: Galectin-3 (Ideo et al., 2002; Stowell et al., 2008; Xiao et al., 2018), Galectin-4 (Ideo et al., 2002; Ideo et al., 2005; Bum-Erdene et al., 2016; Xiao et al., 2018), and Galectin-8 (Ideo et al., 2003; Carlsson et al., 2007a; Carlsson et al., 2007b; Ideo et al., 2011; Xiao et al., 2018). It should be noted that in experiments the effect of 3′-Sulfation was tested compared to non-sulfated glycans (frequently with an adjacent GlcNAc), however, in none of these experiments was a fucose present in Lewis A. Thus, although these galactose binding motifs may prefer 3′-Sulfated galactose, it is unclear whether they bind 3′-Sulfo-LeA, or potentially just the afucosylalated 3′-Sulfo-LeC. The residues that are critical to recognizing the 3′-sulfate have begun to be investigated using the crystal structures of Galectins in complex with these sugars (Ideo et al., 2011; Bum-Erdene et al., 2015; 2016).
Two of the galectins that preferentially bind 3′-Sulfated galactose, Galectin-3 and -4, are among the 20 most upregulated proteins in gastric cancer relative to adjacent non-cancerous tissue (Zhang et al., 2021). The simultaneous expression of sulfomucins and their cognate galectins, suggests an intracellular function. In cell lines, when Galectin-3 and Galectin-8 recognize their glycans, they route the associated vesicular compartments to the lysosome for degradation (Thurston et al., 2012; Jia et al., 2020a; b). In addition to its role in stimulating autophagy, Galectin-8 has also been shown to modulate mTORC1 activity through the ragulator complex (Jia et al., 2018). Both autophagy and mTORC are essential components that are reciprocally regulated in paligenosis, a conserved process by which normal, homeostatic cells transform into metaplasia (Willet et al., 2018; Brown et al., 2022).
In the extracellular milieu, 3′-Sulfo-LeA/C has been shown to be a potent ligand for selectins. E.g.: E-Selectin (Yuen et al., 1992; Yuen et al., 1994; Wang et al., 2022), L-Selectin (Green et al., 1992; Galustian et al., 1997; Galustian et al., 1999; Galustian et al., 2002), and P-selectin (Galustian et al., 2002; Appeldoorn et al., 2005). Selectins are a group of proteins expressed on numerous immune cells (E− & L-Selectins), as well as activated endothelial cells and platelets (P-selectins). These proteins assist in homing of cells to proper locations as an initial step in chemotaxis.
In addition to being potent ligands for selectins, 3′-Sulfo-LeA has also been shown to be a preferred ligand for another receptor on macrophages: the cysteine-rich domain of the macrophages mannose receptor (Leteux et al., 2000), as well as on dendritic cells: dendritic cell immunoreceptor (DCIR) (Bloem et al., 2014). Thus, the expression and secretion of 3′-Sulfo-LeA/C likely is involved in shaping the response to pathogen associated molecular patterns (PAMPs) and danger associated molecular patterns (DAMPs) through association and activation of these receptors.
In this context, it is interesting to speculate that tissues which express 3′-Sulfo-LeA/C on their surface at homeostasis (e.g. biliary tract and colon; Figures 4A,B) might reduce expression upon oncogenic transformation to evade the immune system. While metaplastic, dysplastic tissues and cancer that lack the epitope on their surface (Figures 4C–E), might secrete these sulfomucins to potentially release decoys to evade the immune recognition or modulate the tumor microenvironment.
In the stomach, H. pylori is the predominant cause of metaplastic transformation and gastric cancer. Salivary Muc5B containing 3′-Sulfo-LeA/C has been demonstrated to be a ligand for H. pylori (Veerman et al., 1997a). The interaction between these sulfated mucins and H. pylori has been demonstrated to be sensitive to pH such that binding is increased in the acidic milieu of the stomach. The H. pylori protein recognizing 3′-Sulfo-LeA/C is Neutrophil Activating Protein (HP-NapA) (Teneberg et al., 1997; Namavar et al., 1998), which is a 17 kDa iron binding protein that oligomerizes into a dodecameric quaternary structure (Tonello et al., 1999). HP-NapA appears to bind the terminal 3′-Sulfo-Gal as affinity towards this truncated epitope is similar to that of 3′-Sulfo-LeA (Veerman et al., 1997a; Namavar et al., 1998). Although levels may vary, HP-NapA is ubiquitously expressed among H. pylori stains (Evans et al., 1995) and appears to be located both in the cytosol (Namavar et al., 1998) and associated with the outer membrane vesicles after autolysis (Phadnis et al., 1996) analogous to virulence factors like Urease. Evidence for this mechanism comes from 1) HP-NapA has the exact same epitope specificity as was determined for H. pylori (3′-galactose and 3′-Sulfo-LeA) (Veerman et al., 1997a; Namavar et al., 1998) 2) vaccination of mice with HP-NapA protected the mice from subsequent challenge with H. pylori demonstrating that the protein was exposed to extracellular surface and an essential virulence factor (Satin et al., 2000). These data suggest that salivary sulfated Muc5B may associate with and potentially coat H. pylori and its associated OMV virulence factors limiting this pathogen’s ability to establish a niche in the stomach. The salivary Muc5B acting as a constitutively produced, prophylactic source of sulfated mucins, while induced expression and secretion during injury/metaplastic transformation is a secondary, reactionary source.
Distal to the GI foregut, secreted sulfated mucins have also been shown to be important in modulating commensal and pathogenic bacteria. Recently Xu and others have demonstrated that when synthesis of sulfated mucins are significantly reduced by intestinal deletion of Papss2, mice developed greater inflammation in murine models of colitis as well as a greater predilection to develop tumors (Xu et al., 2021). Others have also recently reported that bacteria have developed specific sulfatases towards 3′-Sulfo-LeA (and other sulfated glycosylation epitopes) that are important in bacterial tropism within the colon (Luis et al., 2021).
Overexpression of GAL3ST2, the enzyme that adds the terminal 3′-Sulfate group to Galβ(1–3)GlcNAc, in 3T3L1 preadipocytes resulted in failure to differentiate as judged by accumulation of triglycerides and C/EBPβ expression (Guerra et al., 2015). These data suggest that 3′-Sulfo-LeA/C may play a direct role in regulating cellular differentiation with expression favoring a dedifferentated phenotype, analogous to the transition from normal differentiated cells into metaplasia. Much more work needs to be done to elucidate the cellular phenotype elicited by the expression of sulfated mucins.
The stereotyped expression and secretion of 3′-Sulfated LeA/C in high-risk metaplasia and cancer throughout the GI foregut (Table 4) has allowed several groups, including our own, to use it as both a histologic tool as well as biomarker for diagnosing these malignant cellular transformations. The utility of assaying for these sulfomucins is most important proximally as the human esophagus, stomach, and pancreas do not express this antigen under homeostatic conditions. This is in contrast to the colon and biliary tract, which express the epitope at homeostasis on their apical membrane, but reduce expression upon oncogenic transformation.
Despite the strong correlation of 3′-Sulfated LeA/C with high-risk metaplasia and cancer in the gastrointestinal foregut, it is unknown why this epitope is stereotypically expressed. Although decreased expression of the enzymes necessary to synthesize 3′-Sulfo-LeA/C correlates with the decreased expression in colon cancer, the contrapositive is not true as β3GALT5, FUT3, or GAL3ST2 do not appear to be significantly upregulated in all foregut cancers. As such, the expression of 3′-Sulfo-LeA/C is potentially a consequence of altered vesicular trafficking or induction of autophagy, which are important aspects of these plastic cellular transitions. Alternatively, increased expression or differential expression of the mucins that accept these glycans as cells change their phenotype could also explain the different levels of these oncofetal epitopes. Even metabolic changes that occur in cancer could alter the availability of sulfate and/or activated sugars to assemble these epitopes.
Although it is known that the secreted sulfated mucins bind H. pylori, it is not known whether this increases the ability of H. pylori to penetrate deeper into the gland or is a protective mechanism e.g. coats the bacteria preventing them from adhering to the mucosa. The work in the colon might suggest that the later is likely the case as inhibition of sulfated mucins via intestinal specific knockout of Papss2 results in greater injury and appears to augment carcinogenesis (Xu et al., 2021); however, these are different pathogens and colonic bacteria have even evolved sulfatases that function as colonization factors (Luis et al., 2021).
What also is not known is whether the expression of these sulfated epitopes are necessary for the plastic transition for metaplasia-to-dysplasia or cancer. A single study in cell lines suggested that the expression of sulfated mucins prevented differentiation, albeit in a non-epithelial lineage (Guerra et al., 2015). However, this in conjunction with the knowledge that the Galectins overexpressed in cancer (e.g. Galectin-3) and which bind this epitope induce autophagy an essential aspect of cellular plasticity, suggests that the expressions of these sulfated glycosylation epitopes might play a role in the cellular transformation and maintenance of the metaplastic, dysplastic, and oncogenic state. Further, the secreted sulfated mucins by way of immune receptors may play important roles in the tumor microenvironment. Overall, much work is needed to understand how and why specific glycosylation epitopes like 3′-Sulfo-LeA/C are stereotypically expressed during transition into metaplasia, dysplasia, and cancer.
KD and JB conceived of and wrote the manuscript.
JB is supported by K08 DK132496, the Department of Defense, through the PRCRP program under Award No. W81XWH-20-1-0630, NIH T32 DK007130-42, NIH R21 AI156236, the Doris Duke Fund to Retain Clinical Scientist (Doris Duke Charitable Foundation), the Digestive Disease Research Core Centers Pilot and Feasibility Grant as part of P30 DK052574, and the American Gastroenterological Association AGA2021-5101. Development of mAb Das-1 was supported in part by research grants NIDDK, R01 DK47673 and R01 DK63618.
We thank Ramon Jin and Jason Mills for kindly providing slides of Barrett’s organoids, Matt Ciorba for slides of human colon, as well as Stuart Kornfeld for critical reading and helpful discussion during the preparation of the manuscript.
KD has been granted a patent for the use of Das-1 in the detection of cancerous pancreatic lesions (patent# US9575073B2; https://patentimages.storage.googleapis.com/de/90/97/d650045c1ed674/US9575073.pdf).
The remaining author declares that the research was conducted in the absence of any commercial or financial relationships that could be construed as a potential conflict of interest.
All claims expressed in this article are solely those of the authors and do not necessarily represent those of their affiliated organizations, or those of the publisher, the editors and the reviewers. Any product that may be evaluated in this article, or claim that may be made by its manufacturer, is not guaranteed or endorsed by the publisher.
The Supplementary Material for this article can be found online at: https://www.frontiersin.org/articles/10.3389/fcell.2023.1089028/full#supplementary-material
Supplementary image S1 | (A) Phylogenetic tree of the five human B3GALTs computed using clustal omega software. (B) Uncurated multisequence alignment of the five human B3GALTs.
Supplementary image S2 | Multisequence alignment of the murine triplicated Gal3st2 genes. Uncurated alignment computed with clustal omega (https://www.ebi.ac.uk/Tools/msa/clustalo/).
Supplementary image S3 | Alpha fold prediction of human GAL3ST2. (A,B) Two views rotated 90 degrees about the Y-Axis. (C) The alpha fold confidence matrix computed for GAL3ST2 suggests that the transmembrane alpha helix and the globular domains were predicted with high confidence; however, the orientation (quaternary structure) of these two domains relative to one another is less certain.
Supplementary image S4 | Reciprocal String-DB analysis of (A) GAL3ST2 and (B) NEU4 suggest that these genes are co-expressed.
HID, High-Iron Diamine; AB-1, Alcian Blue at pH 1; LeA, Lewis A; LeC, Lewis C; LeX, Lewis X, GIM, gastric intestinal metaplasia; PDAC, pancreatic adenocarcinoma; PAN-IN, pancreatic intraepithelial neoplasia; GC, gastric cancer.
Appeldoorn, C. C., Bonnefoy, A., Lutters, B. C., Daenens, K., Van Berkel, T. J., Hoylaerts, M. F., et al. (2005). Gallic acid antagonizes P-selectin-mediated platelet-leukocyte interactions: Implications for the French paradox. Circulation 111, 106–112. doi:10.1161/01.CIR.0000151307.10576.02
Aronica, A., Avagliano, L., Caretti, A., Tosi, D., Bulfamante, G. P., and Trinchera, M. (2017). Unexpected distribution of CA19.9 and other type 1 chain Lewis antigens in normal and cancer tissues of colon and pancreas: Importance of the detection method and role of glycosyltransferase regulation. Biochim. Biophys. Acta Gen. Subj. 1861, 3210–3220. doi:10.1016/j.bbagen.2016.08.005
Badve, S., Logdberg, L., Sokhi, R., Sigal, S. H., Botros, N., Chae, S., et al. (2000). An antigen reacting with das-1 monoclonal antibody is ontogenically regulated in diverse organs including liver and indicates sharing of developmental mechanisms among cell lineages. Pathobiology 68, 76–86. doi:10.1159/000028117
Bai, X., Zhou, D., Brown, J. R., Crawford, B. E., Hennet, T., and Esko, J. D. (2001). Biosynthesis of the linkage region of glycosaminoglycans: Cloning and activity of galactosyltransferase II, the sixth member of the beta 1, 3-galactosyltransferase family (beta 3GalT6). J. Biol. Chem. 276, 48189–48195. doi:10.1074/jbc.M107339200
Baslan, T., Morris, J. P. T., Zhao, Z., Reyes, J., Ho, Y. J., Tsanov, K. M., et al. (2022). Ordered and deterministic cancer genome evolution after p53 loss. Nature 608, 795–802. doi:10.1038/s41586-022-05082-5
Besset, S., Vincourt, J. B., Amalric, F., and Girard, J. P. (2000). Nuclear localization of PAPS synthetase 1: A sulfate activation pathway in the nucleus of eukaryotic cells. FASEB J. 14, 345–354. doi:10.1096/fasebj.14.2.345
Bigi, A., Morosi, L., Pozzi, C., Forcella, M., Tettamanti, G., Venerando, B., et al. (2010). Human sialidase NEU4 long and short are extrinsic proteins bound to outer mitochondrial membrane and the endoplasmic reticulum, respectively. Glycobiology 20, 148–157. doi:10.1093/glycob/cwp156
Bloem, K., Vuist, I. M., Van Den Berk, M., Klaver, E. J., Van Die, I., Knippels, L. M., et al. (2014). DCIR interacts with ligands from both endogenous and pathogenic origin. Immunol. Lett. 158, 33–41. doi:10.1016/j.imlet.2013.11.007
Bockerstett, K. A., Lewis, S. A., Noto, C. N., Ford, E. L., Saenz, J. B., Jackson, N. M., et al. (2020a). Single-cell transcriptional analyses identify lineage-specific epithelial responses to inflammation and metaplastic development in the gastric corpus. Gastroenterology 159, 2116–2129. doi:10.1053/j.gastro.2020.08.027
Bockerstett, K. A., Lewis, S. A., Wolf, K. J., Noto, C. N., Jackson, N. M., Ford, E. L., et al. (2020b). Single-cell transcriptional analyses of spasmolytic polypeptide-expressing metaplasia arising from acute drug injury and chronic inflammation in the stomach. Gut 69, 1027–1038. doi:10.1136/gutjnl-2019-318930
Bodger, K., Campbell, F., and Rhodes, J. M. (2003). Detection of sulfated glycoproteins in intestinal metaplasia: A comparison of traditional mucin staining with immunohistochemistry for the sulfo-Lewis(a) carbohydrate epitope. J. Clin. Pathol. 56, 703–708. doi:10.1136/jcp.56.9.703
Brown, J. W., Cho, C. J., and Mills, J. C. (2022). Paligenosis: Cellular remodeling during tissue repair. Annu. Rev. Physiol. 84, 461–483. doi:10.1146/annurev-physiol-061121-035954
Brown, J. W., Das, K. K., Kalas, V., Das, K. M., and Mills, J. C. (2021b). 3’-Sulfated Lewis A is a biomarker for metaplastic and carcinomatous transformation of several gastrointestinal epithelia. Gastroenterology 160, S71.
Brown, J. W., Das, K. K., Kalas, V., Das, K. M., and Mills, J. C. (2021c). 3’-Sulfo-LeA/C annotates cathartocytosis, A novel subcellular process used by cells to efficiently dedifferentiate during metaplastic and oncogenic transformation. Glycobiology 31, 1684–1685.
Brown, J. W., Das, K. K., Kalas, V., Das, K. M., and Mills, J. C. (2021a). mAb Das-1 recognizes 3'-Sulfated Lewis A/C, which is aberrantly expressed during metaplastic and oncogenic transformation of several gastrointestinal Epithelia. PLoS One 16 16, e0261082. doi:10.1371/journal.pone.0261082
Bum-Erdene, K., Leffler, H., Nilsson, U. J., and Blanchard, H. (2016). Structural characterisation of human galectin-4 N-terminal carbohydrate recognition domain in complex with glycerol, lactose, 3'-sulfo-lactose, and 2'-fucosyllactose. Sci. Rep. 6, 20289. doi:10.1038/srep20289
Bum-Erdene, K., Leffler, H., Nilsson, U. J., and Blanchard, H. (2015). Structural characterization of human galectin-4 C-terminal domain: Elucidating the molecular basis for recognition of glycosphingolipids, sulfated saccharides and blood group antigens. FEBS J. 282, 3348–3367. doi:10.1111/febs.13348
Burclaff, J., Willet, S. G., Saenz, J. B., and Mills, J. C. (2020). Proliferation and differentiation of gastric mucous neck and chief cells during homeostasis and injury-induced metaplasia. Gastroenterology 158, 598–609. doi:10.1053/j.gastro.2019.09.037
Buschard, K., Josefsen, K., Hansen, S. V., Horn, T., Marshall, M. O., Persson, H., et al. (1994). Sulphatide in islets of langerhans and in organs affected in diabetic late complications: A study in human and animal tissue. Diabetologia 37, 1000–1006. doi:10.1007/BF00400463
Buschard, K., Josefsen, K., Horn, T., and Fredman, P. (1993a). Sulphatide and sulphatide antibodies in insulin-dependent diabetes mellitus. Lancet 342, 840. doi:10.1016/0140-6736(93)92697-r
Buschard, K., Josefsen, K., Horn, T., Larsen, S., and Fredman, P. (1993b). Sulphatide antigen in islets of Langerhans and in diabetic glomeruli, and anti-sulphatide antibodies in type 1 diabetes mellitus. APMIS 101, 963–970. doi:10.1111/j.1699-0463.1993.tb00208.x
Caldwell, B., Meyer, A. R., Weis, J. A., Engevik, A. C., and Choi, E. (2022). Chief cell plasticity is the origin of metaplasia following acute injury in the stomach mucosa. Gut 71, 1068–1077. doi:10.1136/gutjnl-2021-325310
Carlsson, S., Carlsson, M. C., and Leffler, H. (2007a). Intracellular sorting of galectin-8 based on carbohydrate fine specificity. Glycobiology 17, 906–912. doi:10.1093/glycob/cwm059
Carlsson, S., Oberg, C. T., Carlsson, M. C., Sundin, A., Nilsson, U. J., Smith, D., et al. (2007b). Affinity of galectin-8 and its carbohydrate recognition domains for ligands in solution and at the cell surface. Glycobiology 17, 663–676. doi:10.1093/glycob/cwm026
Castro, I., Aguilera, S., Brockhausen, I., Alliende, C., Quest, A. F., Molina, C., et al. (2012). Decreased salivary sulphotransferase activity correlated with inflammation and autoimmunity parameters in Sjogren's syndrome patients. Rheumatol. Oxf. 51, 482–490. doi:10.1093/rheumatology/ker351
Ciprani, D., Morales-Oyarvide, V., Qadan, M., Hank, T., Weniger, M., Harrison, J. M., et al. (2020). An elevated CA 19-9 is associated with invasive cancer and worse survival in IPMN. Pancreatology 20, 729–735. doi:10.1016/j.pan.2020.04.002
Colsch, B., Baumann, N., and Ghandour, M. S. (2008). Generation and characterization of the binding epitope of a novel monoclonal antibody to sulfatide (sulfogalactosylceramide) OL-2: Applications of antigen immunodetections in brain tissues and urinary samples. J. Neuroimmunol. 193, 52–58. doi:10.1016/j.jneuroim.2007.10.008
Daniotti, J. L., Martina, J. A., Zurita, A. R., and Maccioni, H. J. (1999). Mouse beta 1, 3-galactosyltransferase (GA1/GM1/GD1b synthase): Protein characterization, tissue expression, and developmental regulation in neural retina. J. Neurosci. Res. 58, 318–327. doi:10.1002/(sici)1097-4547(19991015)58:2<318::aid-jnr12>3.0.co;2-u
Das, K. K., Brown, J. W., Fernandez Del-Castillo, C., Huynh, T., Mills, J. C., Matsuda, Y., et al. (2021). mAb das-1 identifies pancreatic ductal adenocarcinoma and high-grade pancreatic intraepithelial neoplasia with high accuracy. Hum. Pathol. 111, 36–44. doi:10.1016/j.humpath.2021.01.003
Das, K. K., and Early, D. (2017). Pancreatic cancer screening. Curr. Treat. Options Gastroenterol. 15, 562–575. doi:10.1007/s11938-017-0149-8
Das, K. K., Geng, X., Brown, J. W., Morales-Oyarvide, V., Huynh, T., Pergolini, I., et al. (2019). Cross validation of the monoclonal antibody das-1 in identification of high-risk mucinous pancreatic cystic lesions. Gastroenterology 157, 720–730. doi:10.1053/j.gastro.2019.05.014
Das, K. K., Xiao, H., Geng, X., Fernandez-Del-Castillo, C., Morales-Oyarvide, V., Daglilar, E., et al. (2014). mAb Das-1 is specific for high-risk and malignant intraductal papillary mucinous neoplasm (IPMN). Gut 63, 1626–1634. doi:10.1136/gutjnl-2013-306219
Das, K. M., Prasad, I., Garla, S., and Amenta, P. S. (1994). Detection of a shared colon epithelial epitope on Barrett epithelium by a novel monoclonal antibody. Ann. Intern Med. 120, 753–756. doi:10.7326/0003-4819-120-9-199405010-00006
Das, K. M., Sakamaki, S., Vecchi, M., and Diamond, B. (1987). The production and characterization of monoclonal antibodies to a human colonic antigen associated with ulcerative colitis: Cellular localization of the antigen by using the monoclonal antibody. J. Immunol. 139, 77–84. doi:10.4049/jimmunol.139.1.77
Das, K. M., Squillante, L., Chitayet, D., and Kalousek, D. K. (1992). Simultaneous appearance of a unique common epitope in fetal colon, skin, and biliary epithelial cells. A possible link for extracolonic manifestations in ulcerative colitis. J. Clin. Gastroenterol. 15, 311–316. doi:10.1097/00004836-199212000-00009
Das, K. M., Vecchi, M., and Sakamaki, S. (1990). A shared and unique epitope(s) on human colon, skin, and biliary epithelium detected by a monoclonal antibody. Gastroenterology 98, 464–469. doi:10.1016/0016-5085(90)90839-s
De Salvo, C., Pastorelli, L., Petersen, C. P., Butto, L. F., Buela, K. A., Omenetti, S., et al. (2021). Interleukin 33 triggers early eosinophil-dependent events leading to metaplasia in a chronic model of gastritis-prone mice. Gastroenterology 160, 302–316. doi:10.1053/j.gastro.2020.09.040
Demeester, S. R., Wickramasinghe, K. S., Lord, R. V., Friedman, A., Balaji, N. S., Chandrasoma, P. T., et al. (2002). Cytokeratin and DAS-1 immunostaining reveal similarities among cardiac mucosa, CIM, and Barrett's esophagus. Am. J. Gastroenterol. 97, 2514–2523. doi:10.1111/j.1572-0241.2002.06033.x
Driessen, A., Ectors, N., Creemers, J., Filez, L., Penninckx, F., Van Cutsem, E., et al. (1998). Intestinal metaplasia in gastric malignancy: A comparison between carcinoma and lymphoma. Eur. J. Gastroenterol. Hepatol. 10, 595–600. doi:10.1097/00042737-199807000-00013
El-Fasakhany, F. M., Uchimura, K., Kannagi, R., and Muramatsu, T. (2001). A novel human gal-3-O-sulfotransferase: Molecular cloning, characterization, and its implications in biosynthesis of (SO(4)-3)Galbeta1-4(fucalpha1-3)GlcNAc. J. Biol. Chem. 276, 26988–26994. doi:10.1074/jbc.M100348200
Endo, T., Tamaki, K., Arimura, Y., Itoh, F., Hinoda, Y., Hareyama, M., et al. (1998). Expression of sulfated carbohydrate chain and core peptides of mucin detected by monoclonal antibodies in Barrett's esophagus and esophageal adenocarcinoma. J. Gastroenterol. 33, 811–815. doi:10.1007/s005350050180
Engle, D. D., Tiriac, H., Rivera, K. D., Pommier, A., Whalen, S., Oni, T. E., et al. (2019). The glycan CA19-9 promotes pancreatitis and pancreatic cancer in mice. Science 364, 1156–1162. doi:10.1126/science.aaw3145
Evans, D. J., Evans, D. G., Takemura, T., Nakano, H., Lampert, H. C., Graham, D. Y., et al. (1995). Characterization of a Helicobacter pylori neutrophil-activating protein. Infect. Immun. 63, 2213–2220. doi:10.1128/IAI.63.6.2213-2220.1995
Fahrmann, J. F., Schmidt, C. M., Mao, X., Irajizad, E., Loftus, M., Zhang, J., et al. (2021). Lead-time trajectory of CA19-9 as an anchor marker for pancreatic cancer early detection. Gastroenterology 160, 1373–1383. doi:10.1053/j.gastro.2020.11.052
Feng, X. S., Wang, Y. F., Hao, S. G., Ru, Y., Gao, S. G., and Wang, L. D. (2013). Expression of Das-1, Ki67 and sulfuric proteins in gastric cardia adenocarcinoma and intestinal metaplasia lesions. Exp. Ther. Med. 5, 1555–1558. doi:10.3892/etm.2013.1038
Fitzgerald, R. C., Abdalla, S., Onwuegbusi, B. A., Sirieix, P., Saeed, I. T., Burnham, W. R., et al. (2002). Inflammatory gradient in barrett's oesophagus: Implications for disease complications. Gut 51, 316–322. doi:10.1136/gut.51.3.316
Fitzgerald, R. C., Di Pietro, M., O'donovan, M., Maroni, R., Muldrew, B., Debiram-Beecham, I., et al. (2020). Cytosponge-trefoil factor 3 versus usual care to identify barrett's oesophagus in a primary care setting: A multicentre, pragmatic, randomised controlled trial. Lancet 396, 333–344. doi:10.1016/S0140-6736(20)31099-0
Fredman, P., Mattsson, L., Andersson, K., Davidsson, P., Ishizuka, I., Jeansson, S., et al. (1988). Characterization of the binding epitope of a monoclonal antibody to sulphatide. Biochem. J. 251, 17–22. doi:10.1042/bj2510017
Galustian, C., Childs, R. A., Stoll, M., Ishida, H., Kiso, M., and Feizi, T. (2002). Synergistic interactions of the two classes of ligand, sialyl-Lewis(a/x) fuco-oligosaccharides and short sulpho-motifs, with the P- and L-selectins: Implications for therapeutic inhibitor designs. Immunology 105, 350–359. doi:10.1046/j.1365-2567.2002.01369.x
Galustian, C., Childs, R. A., Yuen, C. T., Hasegawa, A., Kiso, M., Lubineau, A., et al. (1997). Valency dependent patterns of binding of human L-selectin toward sialyl and sulfated oligosaccharides of le(a) and le(x) types: Relevance to anti-adhesion therapeutics. Biochemistry 36, 5260–5266. doi:10.1021/bi962887a
Galustian, C., Lubineau, A., Le Narvor, C., Kiso, M., Brown, G., and Feizi, T. (1999). L-selectin interactions with novel mono- and multisulfated Lewisx sequences in comparison with the potent ligand 3'-sulfated Lewisa. J. Biol. Chem. 274, 18213–18217. doi:10.1074/jbc.274.26.18213
Gersten, K. M., Natsuka, S., Trinchera, M., Petryniak, B., Kelly, R. J., Hiraiwa, N., et al. (1995). Molecular cloning, expression, chromosomal assignment, and tissue-specific expression of a murine alpha-(1, 3)-fucosyltransferase locus corresponding to the human ELAM-1 ligand fucosyl transferase. J. Biol. Chem. 270, 25047–25056. doi:10.1074/jbc.270.42.25047
Glickman, J. N., Wang, H., Das, K. M., Goyal, R. K., Spechler, S. J., Antonioli, D., et al. (2001). Phenotype of barrett's esophagus and intestinal metaplasia of the distal esophagus and gastroesophageal junction: An immunohistochemical study of cytokeratins 7 and 20, das-1 and 45 MI. Am. J. Surg. Pathol. 25, 87–94. doi:10.1097/00000478-200101000-00010
Green, P. J., Tamatani, T., Watanabe, T., Miyasaka, M., Hasegawa, A., Kiso, M., et al. (1992). High affinity binding of the leucocyte adhesion molecule L-selectin to 3'-sulphated-Le(a) and -Le(x) oligosaccharides and the predominance of sulphate in this interaction demonstrated by binding studies with a series of lipid-linked oligosaccharides. Biochem. Biophys. Res. Commun. 188, 244–251. doi:10.1016/0006-291x(92)92376-9
Guerra, L. N., Suarez, C., Soto, D., Schiappacasse, A., Sapochnik, D., Sacca, P., et al. (2015). GAL3ST2 from mammary gland epithelial cells affects differentiation of 3T3-L1 preadipocytes. Clin. Transl. Oncol. 17, 511–520. doi:10.1007/s12094-014-1267-6
Hahn, H. P., Blount, P. L., Ayub, K., Das, K. M., Souza, R., Spechler, S., et al. (2009). Intestinal differentiation in metaplastic, nongoblet columnar epithelium in the esophagus. Am. J. Surg. Pathol. 33, 1006–1015. doi:10.1097/PAS.0b013e31819f57e9
Hakkinen, I., Jarvi, O., and Gronroos, J. (1968). Sulphoglycoprotein antigens in the human alimentary canal and gastric cancer. An immunohistological study. Int. J. Cancer 3, 572–581. doi:10.1002/ijc.2910030506
Halstensen, T. S., Das, K. M., and Brandtzaeg, P. (1993). Epithelial deposits of immunoglobulin G1 and activated complement colocalise with the M(r) 40 kD putative autoantigen in ulcerative colitis. Gut 34, 650–657. doi:10.1136/gut.34.5.650
Han, S., Fink, J., Jorg, D. J., Lee, E., Yum, M. K., Chatzeli, L., et al. (2019). Defining the identity and dynamics of adult gastric isthmus stem cells. Cell Stem Cell 25, 342–356. doi:10.1016/j.stem.2019.07.008
Hassan, T., Kanisawa, Y., Meyers, S., Dasgupta, A., and Das, K. M. (1995). Expression of a unique protein on colon cancer cells that reacts with a novel monoclonal antibody and ulcerative colitis serum. Clin. Exp. Immunol. 100, 457–462. doi:10.1111/j.1365-2249.1995.tb03722.x
Holgersson, J., and Lofling, J. (2006). Glycosyltransferases involved in type 1 chain and Lewis antigen biosynthesis exhibit glycan and core chain specificity. Glycobiology 16, 584–593. doi:10.1093/glycob/cwj090
Honke, K., Tsuda, M., Hirahara, Y., Ishii, A., Makita, A., and Wada, Y. (1997). Molecular cloning and expression of cDNA encoding human 3'-phosphoadenylylsulfate:galactosylceramide 3'-sulfotransferase. J. Biol. Chem. 272, 4864–4868. doi:10.1074/jbc.272.8.4864
Honke, K., Tsuda, M., Koyota, S., Wada, Y., Iida-Tanaka, N., Ishizuka, I., et al. (2001). Molecular cloning and characterization of a human beta-Gal-3'-sulfotransferase that acts on both type 1 and type 2 (Gal beta 1-3/1-4GlcNAc-R) oligosaccharides. J. Biol. Chem. 276, 267–274. doi:10.1074/jbc.M005666200
Humphris, J. L., Chang, D. K., Johns, A. L., Scarlett, C. J., Pajic, M., Jones, M. D., et al. (2012). The prognostic and predictive value of serum CA19.9 in pancreatic cancer. Ann. Oncol. 23, 1713–1722. doi:10.1093/annonc/mdr561
Ideo, H., Matsuzaka, T., Nonaka, T., Seko, A., and Yamashita, K. (2011). Galectin-8-N-domain recognition mechanism for sialylated and sulfated glycans. J. Biol. Chem. 286, 11346–11355. doi:10.1074/jbc.M110.195925
Ideo, H., Seko, A., Ishizuka, I., and Yamashita, K. (2003). The N-terminal carbohydrate recognition domain of galectin-8 recognizes specific glycosphingolipids with high affinity. Glycobiology 13, 713–723. doi:10.1093/glycob/cwg094
Ideo, H., Seko, A., Ohkura, T., Matta, K. L., and Yamashita, K. (2002). High-affinity binding of recombinant human galectin-4 to SO(3)(-)-->3Galbeta1-->3GalNAc pyranoside. Glycobiology 12, 199–208. doi:10.1093/glycob/12.3.199
Ideo, H., Seko, A., and Yamashita, K. (2005). Galectin-4 binds to sulfated glycosphingolipids and carcinoembryonic antigen in patches on the cell surface of human colon adenocarcinoma cells. J. Biol. Chem. 280, 4730–4737. doi:10.1074/jbc.M410362200
Ikeda, N., Eguchi, H., Nishihara, S., Narimatsu, H., Kannagi, R., Irimura, T., et al. (2001). A remodeling system of the 3'-sulfo-Lewis a and 3'-sulfo-Lewis x epitopes. J. Biol. Chem. 276, 38588–38594. doi:10.1074/jbc.M107390200
Irimura, T., Wynn, D. M., Hager, L. G., Cleary, K. R., and Ota, D. M. (1991). Human colonic sulfomucin identified by a specific monoclonal antibody. Cancer Res. 51, 5728–5735.
Isshiki, S., Kudo, T., Nishihara, S., Ikehara, Y., Togayachi, A., Furuya, A., et al. (2003). Lewis type 1 antigen synthase (beta3Gal-T5) is transcriptionally regulated by homeoproteins. J. Biol. Chem. 278, 36611–36620. doi:10.1074/jbc.M302681200
Isshiki, S., Togayachi, A., Kudo, T., Nishihara, S., Watanabe, M., Kubota, T., et al. (1999). Cloning, expression, and characterization of a novel UDP-galactose:beta-N-acetylglucosamine beta1, 3-galactosyltransferase (beta3Gal-T5) responsible for synthesis of type 1 chain in colorectal and pancreatic epithelia and tumor cells derived therefrom. J. Biol. Chem. 274, 12499–12507. doi:10.1074/jbc.274.18.12499
Jia, J., Abudu, Y. P., Claude-Taupin, A., Gu, Y., Kumar, S., Choi, S. W., et al. (2018). Galectins control mTOR in response to endomembrane damage. Mol. Cell 70, 120–135. doi:10.1016/j.molcel.2018.03.009
Jia, J., Claude-Taupin, A., Gu, Y., Choi, S. W., Peters, R., Bissa, B., et al. (2020a). Galectin-3 coordinates a cellular system for lysosomal repair and removal. Dev. Cell 52, 69–87. doi:10.1016/j.devcel.2019.10.025
Jia, J., Claude-Taupin, A., Gu, Y., Choi, S. W., Peters, R., Bissa, B., et al. (2020b). MERIT, a cellular system coordinating lysosomal repair, removal and replacement. Autophagy 16, 1539–1541. doi:10.1080/15548627.2020.1779451
Jin, R. U., Brown, J. W., Li, Q. K., Bayguinov, P. O., Wang, J. S., and Mills, J. C. (2021). Tropism of severe acute respiratory syndrome coronavirus 2 for barrett's esophagus may increase susceptibility to developing coronavirus disease 2019. Gastroenterology 160, 2165–2168.e4. doi:10.1053/j.gastro.2021.01.024
Jumper, J., Evans, R., Pritzel, A., Green, T., Figurnov, M., Ronneberger, O., et al. (2021). Highly accurate protein structure prediction with AlphaFold. Nature 596, 583–589. doi:10.1038/s41586-021-03819-2
Kailemia, M. J., Li, L., Ly, M., Linhardt, R. J., and Amster, I. J. (2012). Complete mass spectral characterization of a synthetic ultralow-molecular-weight heparin using collision-induced dissociation. Anal. Chem. 84, 5475–5478. doi:10.1021/ac3015824
Kamiyama, S., Ichimiya, T., Ikehara, Y., Takase, T., Fujimoto, I., Suda, T., et al. (2011). Expression and the role of 3'-phosphoadenosine 5'-phosphosulfate transporters in human colorectal carcinoma. Glycobiology 21, 235–246. doi:10.1093/glycob/cwq154
Kamiyama, S., Sasaki, N., Goda, E., Ui-Tei, K., Saigo, K., Narimatsu, H., et al. (2006). Molecular cloning and characterization of a novel 3'-phosphoadenosine 5'-phosphosulfate transporter, PAPST2. J. Biol. Chem. 281, 10945–10953. doi:10.1074/jbc.M508991200
Kamiyama, S., Suda, T., Ueda, R., Suzuki, M., Okubo, R., Kikuchi, N., et al. (2003). Molecular cloning and identification of 3'-phosphoadenosine 5'-phosphosulfate transporter. J. Biol. Chem. 278, 25958–25963. doi:10.1074/jbc.M302439200
Kim, J. R., Jang, J. Y., Kang, M. J., Park, T., Lee, S. Y., Jung, W., et al. (2015). Clinical implication of serum carcinoembryonic antigen and carbohydrate antigen 19-9 for the prediction of malignancy in intraductal papillary mucinous neoplasm of pancreas. J. Hepatobiliary Pancreat. Sci. 22, 699–707. doi:10.1002/jhbp.275
Kim, S., Park, B. K., Seo, J. H., Choi, J., Choi, J. W., Lee, C. K., et al. (2020). Carbohydrate antigen 19-9 elevation without evidence of malignant or pancreatobiliary diseases. Sci. Rep. 10, 8820. doi:10.1038/s41598-020-65720-8
Kopp, J. L., Von Figura, G., Mayes, E., Liu, F. F., Dubois, C. L., Morris, J. P. T., et al. (2012). Identification of Sox9-dependent acinar-to-ductal reprogramming as the principal mechanism for initiation of pancreatic ductal adenocarcinoma. Cancer Cell 22, 737–750. doi:10.1016/j.ccr.2012.10.025
Kurita, Y., Kuwahara, T., Hara, K., Mizuno, N., Okuno, N., Matsumoto, S., et al. (2019). Diagnostic ability of artificial intelligence using deep learning analysis of cyst fluid in differentiating malignant from benign pancreatic cystic lesions. Sci. Rep. 9, 6893. doi:10.1038/s41598-019-43314-3
Leteux, C., Chai, W., Loveless, R. W., Yuen, C. T., Uhlin-Hansen, L., Combarnous, Y., et al. (2000). The cysteine-rich domain of the macrophage mannose receptor is a multispecific lectin that recognizes chondroitin sulfates A and B and sulfated oligosaccharides of blood group Lewis(a) and Lewis(x) types in addition to the sulfated N-glycans of lutropin. J. Exp. Med. 191, 1117–1126. doi:10.1084/jem.191.7.1117
Li, H., Lee, W. S., Feng, X., Bai, L., Jennings, B. C., Liu, L., et al. (2022). Structure of the human GlcNAc-1-phosphotransferase αβ subunits reveals regulatory mechanism for lysosomal enzyme glycan phosphorylation. Nat. Struct. Mol. Biol. 29, 348–356. doi:10.1038/s41594-022-00748-0
Liu, C. C., Veeraraghavan, J., Tan, Y., Kim, J. A., Wang, X., Loo, S. K., et al. (2021). A novel neoplastic fusion transcript, rad51ap1-DYRK4, confers sensitivity to the MEK inhibitor trametinib in aggressive breast cancers. Clin. Cancer Res. 27, 785–798. doi:10.1158/1078-0432.CCR-20-2769
Loveless, R. W., Yuen, C. T., Tsuiji, H., Irimura, T., and Feizi, T. (1998). Monoclonal antibody 91.9H raised against sulfated mucins is specific for the 3'-sulfated Lewisa tetrasaccharide sequence. Glycobiology 8, 1237–1242. doi:10.1093/glycob/8.12.1237
Loveless, W., Feizi, T., Valeri, M., Day, R., and Bay, S. (2001). A monoclonal antibody, MIN/3/60, that recognizes the sulpho-Lewis(x) and sulpho-Lewis(a) sequences detects a sub-population of epithelial glycans in the crypts of human colonic epithelium. Hybridoma 20, 223–229. doi:10.1089/027245701753179794
Luis, A. S., Jin, C., Pereira, G. V., Glowacki, R. W. P., Gugel, S. R., Singh, S., et al. (2021). A single sulfatase is required to access colonic mucin by a gut bacterium. Nature 598, 332–337. doi:10.1038/s41586-021-03967-5
Ma, Z., Lytle, N. K., Chen, B., Jyotsana, N., Novak, S. W., Cho, C. J., et al. (2022). Single-cell transcriptomics reveals a conserved metaplasia program in pancreatic injury. Gastroenterology 162, 604–620. doi:10.1053/j.gastro.2021.10.027
Mandal, A., Dasgupta, A., Jeffers, L., Squillante, L., Hyder, S., Reddy, R., et al. (1994). Autoantibodies in sclerosing cholangitis against a shared peptide in biliary and colon epithelium. Gastroenterology 106, 185–192. doi:10.1016/s0016-5085(94)95271-x
Mckitrick, T. R., Bernard, S. M., Noll, A. J., Collins, B. C., Goth, C. K., Mcquillan, A. M., et al. (2021). Novel lamprey antibody recognizes terminal sulfated galactose epitopes on mammalian glycoproteins. Commun. Biol. 4, 674. doi:10.1038/s42003-021-02199-7
Meyer, A. R., Engevik, A. C., Madorsky, T., Belmont, E., Stier, M. T., Norlander, A. E., et al. (2020). Group 2 innate lymphoid cells coordinate damage response in the stomach. Gastroenterology 159, 2077–2091. doi:10.1053/j.gastro.2020.08.051
Min, J., Zhang, C., Bliton, R. J., Caldwell, B., Caplan, L., Presentation, K. S., et al. (2022). Dysplastic stem cell plasticity functions as a driving force for neoplastic transformation of precancerous gastric mucosa. Gastroenterology 163, 875–890. doi:10.1053/j.gastro.2022.06.021
Mirza, Z. K., Das, K. K., Slate, J., Mapitigama, R. N., Amenta, P. S., Griffel, L. H., et al. (2003). Gastric intestinal metaplasia as detected by a monoclonal antibody is highly associated with gastric adenocarcinoma. Gut 52, 807–812. doi:10.1136/gut.52.6.807
Mitsuoka, C., Sawada-Kasugai, M., Ando-Furui, K., Izawa, M., Nakanishi, H., Nakamura, S., et al. (1998). Identification of a major carbohydrate capping group of the L-selectin ligand on high endothelial venules in human lymph nodes as 6-sulfo sialyl Lewis X. J. Biol. Chem. 273, 11225–11233. doi:10.1074/jbc.273.18.11225
Miyake, M., Taki, T., Kannagi, R., and Hitomi, S. (1992). First establishment of a human monoclonal antibody directed to sulfated glycosphingolipids SM4s-Gal and SM4g, from a patient with lung cancer. Cancer Res. 52, 2292–2297.
Montero, C., and Segura, D. I. (1980). Retrospective histochemical study of mucosubstances in adenocarcinomas of the gastrointestinal tract. Histopathology 4, 281–291. doi:10.1111/j.1365-2559.1980.tb02922.x
Monti, E., Bassi, M. T., Bresciani, R., Civini, S., Croci, G. L., Papini, N., et al. (2004). Molecular cloning and characterization of NEU4, the fourth member of the human sialidase gene family. Genomics 83, 445–453. doi:10.1016/j.ygeno.2003.08.019
Moriichi, K., Watari, J., Das, K. M., Tanabe, H., Fujiya, M., Ashida, T., et al. (2009). Effects of Helicobacter pylori infection on genetic instability, the aberrant CpG island methylation status and the cellular phenotype in Barrett's esophagus in a Japanese population. Int. J. Cancer 124, 1263–1269. doi:10.1002/ijc.24092
Murphy, D. P., Pantuck, A. J., Amenta, P. S., Das, K. M., Cummings, K. B., Keeney, G. L., et al. (1999). Female urethral adenocarcinoma: Immunohistochemical evidence of more than 1 tissue of origin. J. Urol. 161, 1881–1884. doi:10.1016/s0022-5347(05)68833-7
Nagashio, Y., Hijioka, S., Mizuno, N., Hara, K., Imaoka, H., Bhatia, V., et al. (2014). Combination of cyst fluid CEA and CA 125 is an accurate diagnostic tool for differentiating mucinous cystic neoplasms from intraductal papillary mucinous neoplasms. Pancreatology 14, 503–509. doi:10.1016/j.pan.2014.09.011
Namavar, F., Sparrius, M., Veerman, E. C., Appelmelk, B. J., and Vandenbroucke-Grauls, C. M. (1998). Neutrophil-activating protein mediates adhesion of Helicobacter pylori to sulfated carbohydrates on high-molecular-weight salivary mucin. Infect. Immun. 66, 444–447. doi:10.1128/IAI.66.2.444-447.1998
Norden, R., Samuelsson, E., and Nystrom, K. (2017). NFκB-mediated activation of the cellular FUT3, 5 and 6 gene cluster by herpes simplex virus type 1. Glycobiology 27, 999–1005. doi:10.1093/glycob/cwx079
O'connell, F. P., Wang, H. H., and Odze, R. D. (2005). Utility of immunohistochemistry in distinguishing primary adenocarcinomas from metastatic breast carcinomas in the gastrointestinal tract. Arch. Pathol. Lab. Med. 129, 338–347. doi:10.1043/1543-2165(2005)129<338:UOIIDP>2.0.CO;2
Ohe, Y., Hinoda, Y., Irimura, T., Imai, K., and Yachi, A. (1994). Expression of sulfated carbohydrate chains detected by monoclonal antibody 91.9H in human gastric cancer tissues. Jpn. J. Cancer Res. 85, 400–408. doi:10.1111/j.1349-7006.1994.tb02373.x
Onuma, E. K., Amenta, P. S., Jukkola, A. F., Mohan, V., Borra, S., and Das, K. M. (2001). A phenotypic change of small intestinal epithelium to colonocytes in small intestinal adenomas and adenocarcinomas. Am. J. Gastroenterol. 96, 2480–2485. doi:10.1111/j.1572-0241.2001.04056.x
Padra, J. T., and Linden, S. K. (2022). Optimization of Alcian blue pH 1.0 histo-staining protocols to match mass spectrometric quantification of sulfomucins and circumvent false positive results due to sialomucins. Glycobiology 32, 6–10. doi:10.1093/glycob/cwab091
Petersen, C. P., Weis, V. G., Nam, K. T., Sousa, J. F., Fingleton, B., and Goldenring, J. R. (2014). Macrophages promote progression of spasmolytic polypeptide-expressing metaplasia after acute loss of parietal cells. Gastroenterology 146, 1727–1738. doi:10.1053/j.gastro.2014.02.007
Pettersen, E. F., Goddard, T. D., Huang, C. C., Couch, G. S., Greenblatt, D. M., Meng, E. C., et al. (2004). UCSF Chimera–a visualization system for exploratory research and analysis. J. Comput. Chem. 25, 1605–1612. doi:10.1002/jcc.20084
Phadnis, S. H., Parlow, M. H., Levy, M., Ilver, D., Caulkins, C. M., Connors, J. B., et al. (1996). Surface localization of Helicobacter pylori urease and a heat shock protein homolog requires bacterial autolysis. Infect. Immun. 64, 905–912. doi:10.1128/IAI.64.3.905-912.1996
Piazuelo, M. B., Haque, S., Delgado, A., Du, J. X., Rodriguez, F., and Correa, P. (2004). Phenotypic differences between esophageal and gastric intestinal metaplasia. Mod. Pathol. 17, 62–74. doi:10.1038/sj.modpathol.3800016
Rathman, W. M., Van Den Keybus, P. A., Van Zeyl, M. J., Dopp, E. A., Veerman, E. C., and Nieuw Amerongen, A. V. (1990). Characterization of monoclonal antibodies to human salivary (glyco) proteins. Cellular localization of mucin, cystatin-like 14 kD protein and 20 kD glycoprotein in the human submandibular gland. J. Biol. Buccale 18, 19–27.
Redston, M., Noffsinger, A., Kim, A., Akarca, F. G., Rara, M., Stapleton, D., et al. (2022). Abnormal TP53 predicts risk of progression in patients with barrett's esophagus regardless of a diagnosis of dysplasia. Gastroenterology 162, 468–481. doi:10.1053/j.gastro.2021.10.038
Reid, P. E., Owen, D. A., Fletcher, K., Rowan, R. E., Reimer, C. L., Rouse, G. J., et al. (1989). The histochemical specificity of high iron diamine-alcian blue. Histochem J. 21, 501–507. doi:10.1007/BF01845800
Ronkainen, J., Aro, P., Storskrubb, T., Johansson, S. E., Lind, T., Bolling-Sternevald, E., et al. (2005). Prevalence of barrett's esophagus in the general population: An endoscopic study. Gastroenterology 129, 1825–1831. doi:10.1053/j.gastro.2005.08.053
Sakuma, K., Aoki, M., and Kannagi, R. (2012). Transcription factors c-Myc and CDX2 mediate E-selectin ligand expression in colon cancer cells undergoing EGF/bFGF-induced epithelial-mesenchymal transition. Proc. Natl. Acad. Sci. U. S. A. 109, 7776–7781. doi:10.1073/pnas.1111135109
Salvini, R., Bardoni, A., Valli, M., and Trinchera, M. (2001). Beta 1, 3-Galactosyltransferase beta 3Gal-T5 acts on the GlcNAcbeta 1-->3Galbeta 1-->4GlcNAcbeta 1-->R sugar chains of carcinoembryonic antigen and other N-linked glycoproteins and is down-regulated in colon adenocarcinomas. J. Biol. Chem. 276, 3564–3573. doi:10.1074/jbc.M006662200
Satin, B., Del Giudice, G., Della Bianca, V., Dusi, S., Laudanna, C., Tonello, F., et al. (2000). The neutrophil-activating protein (HP-NAP) of Helicobacter pylori is a protective antigen and a major virulence factor. J. Exp. Med. 191, 1467–1476. doi:10.1084/jem.191.9.1467
Schroder, E., Gebel, L., Eremeev, A. A., Morgner, J., Grum, D., Knauer, S. K., et al. (2012). Human PAPS synthase isoforms are dynamically regulated enzymes with access to nucleus and cytoplasm. PLoS One 7, e29559. doi:10.1371/journal.pone.0029559
Seko, A., Hara-Kuge, S., and Yamashita, K. (2001). Molecular cloning and characterization of a novel human galactose 3-O-sulfotransferase that transfers sulfate to gal beta 1-->3galNAc residue in O-glycans. J. Biol. Chem. 276, 25697–25704. doi:10.1074/jbc.M101558200
Seko, A., Nagata, K., Yonezawa, S., and Yamashita, K. (2002). Down-regulation of Gal 3-O-sulfotransferase-2 (Gal3ST-2) expression in human colonic non-mucinous adenocarcinoma. Jpn. J. Cancer Res. 93, 507–515. doi:10.1111/j.1349-7006.2002.tb01285.x
Seyrantepe, V., Canuel, M., Carpentier, S., Landry, K., Durand, S., Liang, F., et al. (2008). Mice deficient in Neu4 sialidase exhibit abnormal ganglioside catabolism and lysosomal storage. Hum. Mol. Genet. 17, 1556–1568. doi:10.1093/hmg/ddn043
Seyrantepe, V., Landry, K., Trudel, S., Hassan, J. A., Morales, C. R., and Pshezhetsky, A. V. (2004). Neu4, a novel human lysosomal lumen sialidase, confers normal phenotype to sialidosis and galactosialidosis cells. J. Biol. Chem. 279, 37021–37029. doi:10.1074/jbc.M404531200
Shah, S. C., Gawron, A. J., Mustafa, R. A., and Piazuelo, M. B. (2020). Histologic subtyping of gastric intestinal metaplasia: Overview and considerations for clinical practice. Gastroenterology 158, 745–750. doi:10.1053/j.gastro.2019.12.004
Shaheen, N. J., Falk, G. W., Iyer, P. G., Souza, R. F., Yadlapati, R. H., Sauer, B. G., et al. (2022). Diagnosis and management of barrett's esophagus: An updated ACG guideline. Am. J. Gastroenterol. 117, 559–587. doi:10.14309/ajg.0000000000001680
Shi, C., Merchant, N., Newsome, G., Goldenberg, D. M., and Gold, D. V. (2014). Differentiation of pancreatic ductal adenocarcinoma from chronic pancreatitis by PAM4 immunohistochemistry. Arch. Pathol. Lab. Med. 138, 220–228. doi:10.5858/arpa.2013-0056-OA
Shiozaki, K., Yamaguchi, K., Takahashi, K., Moriya, S., and Miyagi, T. (2011). Regulation of sialyl Lewis antigen expression in colon cancer cells by sialidase NEU4. J. Biol. Chem. 286, 21052–21061. doi:10.1074/jbc.M111.231191
Stowell, S. R., Arthur, C. M., Mehta, P., Slanina, K. A., Blixt, O., Leffler, H., et al. (2008). Galectin-1, -2, and -3 exhibit differential recognition of sialylated glycans and blood group antigens. J. Biol. Chem. 283, 10109–10123. doi:10.1074/jbc.M709545200
Su, Y., Chen, X., Klein, M., Fang, M., Wang, S., Yang, C. S., et al. (2004). Phenotype of columnar-lined esophagus in rats with esophagogastroduodenal anastomosis: Similarity to human barrett's esophagus. Lab. Invest 84, 753–765. doi:10.1038/labinvest.3700079
Sun, J. H., Das, K. K., Amenta, P. S., Yokota, K., Watari, J., Sato, T., et al. (2006). Preferential expression of cyclooxygenase-2 in colonic-phenotype of gastric intestinal metaplasia: Association with helicobacter pylori and gastric carcinoma. J. Clin. Gastroenterol. 40, 122–128. doi:10.1097/01.mcg.0000196461.15186.0d
Sun, L., Konstantinidi, A., Ye, Z., Nason, R., Zhang, Y., Bull, C., et al. (2022). Installation of O-glycan sulfation capacities in human HEK293 cells for display of sulfated mucins. J. Biol. Chem. 298, 101382. doi:10.1016/j.jbc.2021.101382
Suzuki, A., Hiraoka, N., Suzuki, M., Angata, K., Misra, A. K., Mcauliffe, J., et al. (2001). Molecular cloning and expression of a novel human beta-Gal-3-O-sulfotransferase that acts preferentially on N-acetyllactosamine in N- and O-glycans. J. Biol. Chem. 276, 24388–24395. doi:10.1074/jbc.M103135200
Szklarczyk, D., Gable, A. L., Lyon, D., Junge, A., Wyder, S., Huerta-Cepas, J., et al. (2019). STRING v11: Protein-protein association networks with increased coverage, supporting functional discovery in genome-wide experimental datasets. Nucleic Acids Res. 47, D607–D613. doi:10.1093/nar/gky1131
Tanaka, M., Heckler, M., Liu, B., Heger, U., Hackert, T., and Michalski, C. W. (2019). Cytologic analysis of pancreatic juice increases specificity of detection of malignant IPMN-A systematic review. Clin. Gastroenterol. Hepatol. 17, 2199–2211. doi:10.1016/j.cgh.2018.12.034
Tanaka-Okamoto, M., Mukai, M., Takahashi, H., Fujiwara, Y., Ohue, M., and Miyamoto, Y. (2017). Various sulfated carbohydrate tumor marker candidates identified by focused glycomic analyses. Glycobiology 27, 400–415. doi:10.1093/glycob/cww133
Teneberg, S., Miller-Podraza, H., Lampert, H. C., Evans, D. J., Evans, D. G., Danielsson, D., et al. (1997). Carbohydrate binding specificity of the neutrophil-activating protein of Helicobacter pylori. J. Biol. Chem. 272, 19067–19071. doi:10.1074/jbc.272.30.19067
Thurston, T. L., Wandel, M. P., Von Muhlinen, N., Foeglein, A., and Randow, F. (2012). Galectin 8 targets damaged vesicles for autophagy to defend cells against bacterial invasion. Nature 482, 414–418. doi:10.1038/nature10744
Togayachi, A., Sato, T., and Narimatsu, H. (2006). Comprehensive enzymatic characterization of glycosyltransferases with a beta3GT or beta4GT motif. Methods Enzymol. 416, 91–102. doi:10.1016/S0076-6879(06)16006-1
Tonello, F., Dundon, W. G., Satin, B., Molinari, M., Tognon, G., Grandi, G., et al. (1999). The Helicobacter pylori neutrophil-activating protein is an iron-binding protein with dodecameric structure. Mol. Microbiol. 34, 238–246. doi:10.1046/j.1365-2958.1999.01584.x
Torrado, J., Correa, P., Ruiz, B., Bernardi, P., Zavala, D., and Bara, J. (1992). Lewis antigen alterations in gastric cancer precursors. Gastroenterology 102, 424–430. doi:10.1016/0016-5085(92)90086-e
Tsuiji, H., Hayashi, M., Wynn, D. M., and Irimura, T. (1998a). Expression of mucin-associated sulfo-Lea carbohydrate epitopes on human colon carcinoma cells. Jpn. J. Cancer Res. 89, 1267–1275. doi:10.1111/j.1349-7006.1998.tb00523.x
Tsuiji, H., Hong, J. C., Kim, Y. S., Ikehara, Y., Narimatsu, H., and Irimura, T. (1998b). Novel carbohydrate specificity of monoclonal antibody 91.9H prepared against human colonic sulfomucin: Recognition of sulfo-Lewis(a) structure. Biochem. Biophys. Res. Commun. 253, 374–381. doi:10.1006/bbrc.1998.9726
Varadi, M., Anyango, S., Deshpande, M., Nair, S., Natassia, C., Yordanova, G., et al. (2022). AlphaFold protein structure database: Massively expanding the structural coverage of protein-sequence space with high-accuracy models. Nucleic Acids Res. 50, D439–D444. doi:10.1093/nar/gkab1061
Veerman, E. C., Bank, C. M., Namavar, F., Appelmelk, B. J., Bolscher, J. G., and Nieuw Amerongen, A. V. (1997a). Sulfated glycans on oral mucin as receptors for Helicobacter pylori. Glycobiology 7, 737–743. doi:10.1093/glycob/7.6.737
Veerman, E. C., Bolscher, J. G., Appelmelk, B. J., Bloemena, E., Van Den Berg, T. K., and Nieuw Amerongen, A. V. (1997b). A monoclonal antibody directed against high M(r) salivary mucins recognizes the SO3-3gal beta 1-3GlcNAc moiety of sulfo-Lewis(a): A histochemical survey of human and rat tissue. Glycobiology 7, 37–43. doi:10.1093/glycob/7.1.37
Veerman, E. C., Van Den Keijbus, P. A., Nazmi, K., Vos, W., Van Der Wal, J. E., Bloemena, E., et al. (2003). Distinct localization of MUC5B glycoforms in the human salivary glands. Glycobiology 13, 363–366. doi:10.1093/glycob/cwg037
Venkatachalam, K. V. (2003). Human 3'-phosphoadenosine 5'-phosphosulfate (PAPS) synthase: Biochemistry, molecular biology and genetic deficiency. IUBMB Life 55, 1–11. doi:10.1080/1521654031000072148
Wang, X., Hanes, M. S., Cummings, R. D., and Woods, R. J. (2022). Computationally guided conversion of the specificity of E-selectin to mimic that of Siglec-8. Proc. Natl. Acad. Sci. U. S. A. 119, e2117743119. doi:10.1073/pnas.2117743119
Wang, Y., Maeda, Y., Liu, Y. S., Takada, Y., Ninomiya, A., Hirata, T., et al. (2020). Cross-talks of glycosylphosphatidylinositol biosynthesis with glycosphingolipid biosynthesis and ER-associated degradation. Nat. Commun. 11, 860. doi:10.1038/s41467-020-14678-2
Wani, S., Falk, G., Hall, M., Gaddam, S., Wang, A., Gupta, N., et al. (2011). Patients with nondysplastic Barrett's esophagus have low risks for developing dysplasia or esophageal adenocarcinoma. Clin. Gastroenterol. Hepatol. 9, 220–227. doi:10.1016/j.cgh.2010.11.008
Watari, J., Das, K. K., Amenta, P. S., Tanabe, H., Tanaka, A., Geng, X., et al. (2008). Effect of eradication of Helicobacter pylori on the histology and cellular phenotype of gastric intestinal metaplasia. Clin. Gastroenterol. Hepatol. 6, 409–417. doi:10.1016/j.cgh.2007.12.044
Watari, J., Moriichi, K., Tanabe, H., Kashima, S., Nomura, Y., Fujiya, M., et al. (2012). Biomarkers predicting development of metachronous gastric cancer after endoscopic resection: An analysis of molecular pathology of Helicobacter pylori eradication. Int. J. Cancer 130, 2349–2358. doi:10.1002/ijc.26275
Willet, S. G., Lewis, M. A., Miao, Z. F., Liu, D., Radyk, M. D., Cunningham, R. L., et al. (2018). Regenerative proliferation of differentiated cells by mTORC1-dependent paligenosis. EMBO J. 37, e98311. doi:10.15252/embj.201798311
Xiao, Q., Ludwig, A. K., Romano, C., Buzzacchera, I., Sherman, S. E., Vetro, M., et al. (2018). Exploring functional pairing between surface glycoconjugates and human galectins using programmable glycodendrimersomes. Proc. Natl. Acad. Sci. U. S. A. 115, E2509–E2518. doi:10.1073/pnas.1720055115
Xu, P., Xi, Y., Zhu, J., Zhang, M., Luka, Z., Stolz, D. B., et al. (2021). Intestinal sulfation is essential to protect against colitis and colonic carcinogenesis. Gastroenterology 161, 271–286. doi:10.1053/j.gastro.2021.03.048
Yamachika, T., Nakanishi, H., Inada, K., Kitoh, K., Kato, T., Irimura, T., et al. (1997). Reciprocal control of colon-specific sulfomucin and sialosyl-Tn antigen expression in human colorectal neoplasia. Virchows Arch. 431, 25–30. doi:10.1007/s004280050065
Yamaguchi, K., Hata, K., Koseki, K., Shiozaki, K., Akita, H., Wada, T., et al. (2005). Evidence for mitochondrial localization of a novel human sialidase (NEU4). Biochem. J. 390, 85–93. doi:10.1042/BJ20050017
Yamori, T., Ota, D. M., Cleary, K. R., Hoff, S., Hager, L. G., and Irimura, T. (1989). Monoclonal antibody against human colonic sulfomucin: Immunochemical detection of its binding sites in colonic mucosa, colorectal primary carcinoma, and metastases. Cancer Res. 49, 887–894.
Yuen, C. T., Bezouska, K., O'brien, J., Stoll, M., Lemoine, R., Lubineau, A., et al. (1994). Sulfated blood group Lewis(a). A superior oligosaccharide ligand for human E-selectin. J. Biol. Chem. 269, 1595–1598.
Yuen, C. T., Lawson, A. M., Chai, W., Larkin, M., Stoll, M. S., Stuart, A. C., et al. (1992). Novel sulfated ligands for the cell adhesion molecule E-selectin revealed by the neoglycolipid technology among O-linked oligosaccharides on an ovarian cystadenoma glycoprotein. Biochemistry 31, 9126–9131. doi:10.1021/bi00153a003
Zhang, M., Hu, S., Min, M., Ni, Y., Lu, Z., Sun, X., et al. (2021). Dissecting transcriptional heterogeneity in primary gastric adenocarcinoma by single cell RNA sequencing. Gut 70, 464–475. doi:10.1136/gutjnl-2019-320368
Zhang, P., Zhang, L., Hou, Z., Lin, H., Gao, H., and Zhang, L. (2022). Structural basis for the substrate recognition mechanism of ATP-sulfurylase domain of human PAPS synthase 2. Biochem. Biophys. Res. Commun. 586, 1–7. doi:10.1016/j.bbrc.2021.11.062
Zheng, J., Bao, W. Q., Sheng, W. Q., Guo, L., Zhang, H. L., Wu, L. H., et al. (2009). Serum 3'-sulfo-Lea indication of gastric cancer metastasis. Clin. Chim. Acta 405, 119–126. doi:10.1016/j.cca.2009.04.017
Keywords: sulfation, glycosylation, barrett’s esophagus, gastric metaplasia, pancreatic intraepithelial neoplasia, pancreatic cancer, intraductal papillary mucinous neoplasm
Citation: Das KK and Brown JW (2023) 3′-sulfated LewisA/C: An oncofetal epitope associated with metaplastic and oncogenic plasticity of the gastrointestinal foregut. Front. Cell Dev. Biol. 11:1089028. doi: 10.3389/fcell.2023.1089028
Received: 03 November 2022; Accepted: 10 January 2023;
Published: 14 February 2023.
Edited by:
Atsushi Suzuki, Kyushu University, JapanReviewed by:
Anurag Kumar Singh, Martin Luther University of Halle-Wittenberg, GermanyCopyright © 2023 Das and Brown. This is an open-access article distributed under the terms of the Creative Commons Attribution License (CC BY). The use, distribution or reproduction in other forums is permitted, provided the original author(s) and the copyright owner(s) are credited and that the original publication in this journal is cited, in accordance with accepted academic practice. No use, distribution or reproduction is permitted which does not comply with these terms.
*Correspondence: Koushik K. Das, Sy5EYXNAd3VzdGwuZWR1; Jeffrey W. Brown, YnJvd25qd0B3dXN0bC5lZHU=
Disclaimer: All claims expressed in this article are solely those of the authors and do not necessarily represent those of their affiliated organizations, or those of the publisher, the editors and the reviewers. Any product that may be evaluated in this article or claim that may be made by its manufacturer is not guaranteed or endorsed by the publisher.
Research integrity at Frontiers
Learn more about the work of our research integrity team to safeguard the quality of each article we publish.