- 1Department of Biology, York University, Toronto, ON, Canada
- 2Centre for Research on Biomolecular Interactions, York University, Toronto, ON, Canada
It is now well-established that microRNAs (miRNAs) are important regulators of gene expression. The role of miRNAs in placental development and trophoblast function is constantly expanding. Trophoblast invasion and their ability to remodel uterine spiral arteries are essential for proper placental development and successful pregnancy outcome. Many miRNAs are reported to be dysregulated in pregnancy complications, especially preeclampsia and they exert various regulatory effects on trophoblasts. In this review, we provide a brief overview of miRNA biogenesis and their mechanism of action, as well as of trophoblasts differentiation, invasion and spiral artery remodeling. We then discuss the role of miRNAs in trophoblasts invasion and spiral artery remodeling, focusing on miRNAs that have been thoroughly investigated, especially using multiple model systems. We also discuss the potential role of miRNAs in the pathogenesis of preeclampsia.
1 Introduction
MicroRNAs (miRNAs) are involved in the regulation of gene expression and thereby exert a wide range of biological functions not only within the cells in which they are made but can also be secreted to mediate cell-cell communication (O'Brien et al., 2018). Many studies have shown that miRNAs are important regulators of placental development and trophoblast functions and their abnormal expression/secretion is associated with pregnancy-related disorders, including preeclampsia (PE) (Fu et al., 2013a; Farrokhnia et al., 2014; Mouillet et al., 2014; Zhang M. et al., 2016; Hayder et al., 2018; Skalis et al., 2019; Aplin et al., 2020).
The placenta is a transient organ that supports the development of mammalian embryos (Burton and Fowden, 2015; Maltepe and Fisher, 2015; Turco and Moffett, 2019). It regulates gas, nutrients, and waste exchange between the mother and the fetus, and secrets many pregnancy-associated hormones that are important for fetal growth and the progression of pregnancy (Iliodromiti et al., 2012; Camm et al., 2018). One of the key events during placental development is the invasion of trophoblasts into the decidua and subsequent remodeling of uterine spiral arteries to provide adequate placental perfusion to support the demands of the growing fetus (Lyall et al., 2013; Fisher, 2015). The remodeling of the spiral arteries by trophoblasts starts as early as 8 weeks of gestation (Burton et al., 2009; Whitley and Cartwright, 2010) and insufficient remodeling has been implicated in several pregnancy complications, including PE (Redman, 1991; Opichka et al., 2021; Pankiewicz et al., 2021; Staff et al., 2022). Therefore, knowledge of both spatial and temporal regulation of placental development is critical to our understanding of healthy pregnancy progression and the mechanisms underlying the development of many pregnancy complications and adverse fetal outcomes (Genbacev et al., 1997; Burton et al., 2007; Fisher, 2015; Hayder et al., 2018; Aplin et al., 2020).
Since their discovery, miRNAs have been shown to regulate all stages of normal pregnancy from implantation to labour (Renthal et al., 2013; Hayder et al., 2018). They are also reported to be dysregulated in many pregnancy-related diseases including PE (Fu et al., 2013a; Bidarimath et al., 2014; Mouillet et al., 2015; Munaut et al., 2016; Cai et al., 2017; Carreras-Badosa et al., 2017; Lykoudi et al., 2018; Ali et al., 2021). In particular, secreted miRNAs have been intensely studied as potential predictive and diagnostic markers for many of these conditions as well as possible therapeutic targets (Zou et al., 2018; Hornakova et al., 2020; Ali et al., 2021; Foley et al., 2021). As research into miRNA regulation and functions continues to evolve, our understanding of their role in placental pathophysiology advances.
In this review, we have provided an updated overview of miRNA biology and their role in regulating trophoblast invasion, spiral artery remodeling (SAR) and PE development, focusing on miRNAs that have been extensively investigated and/or those that have been studied using multiple experimental models.
2 Overview of microRNAs
2.1 Biogenesis
miRNAs are small non-coding single-stranded RNAs averaging 22 nucleotides in length (O'Brien et al., 2018). Canonically, a miRNA is transcribed by RNA polymerase II, or less often by RNA polymerase III, into a primary miRNA (pri-mRNA). This can either be from intragenic miRNA genes located mostly within the intron of a host gene, or from intergenic miRNA genes that exist independent of a host gene and are being regulated by their own promotor (Ha and Kim, 2014). Pri-miRNA stem-loop (hairpin) structure is then recognized by the microprocessor complex compromised of an RNA binding protein, DiGeorge syndrome Critical Region 8 (DGCR8) and a ribonuclease III enzyme, Drosha, which leads to the generation of precursor miRNA (pre-miRNA) (Denli et al., 2004; Han et al., 2004). Pre-miRNA is then exported into the cytoplasm by an exportin 5 (XPO5)/RanGTP complex and is further processed by Dicer, an RNase III endonuclease that removes pre-miRNA terminal loop forming a mature miRNA duplex (Zhang et al., 2004; Okada et al., 2009). Several RNA-binding proteins, including transactivation response element binding protein (TRBP), function as cofactors of Dicer to ensure accurate processing of pre-miRNA (Wilson et al., 2015; Fareh et al., 2016). The miRNA duplex unwinds into two single-stranded mature miRNAs, denoted by the -5p and -3p suffix, that can be loaded into the Argonaute (AGO) family of proteins (AGO1-4 in humans) in an ATP-dependent manner (Yoda et al., 2010; Medley et al., 2021) (Figure 1).
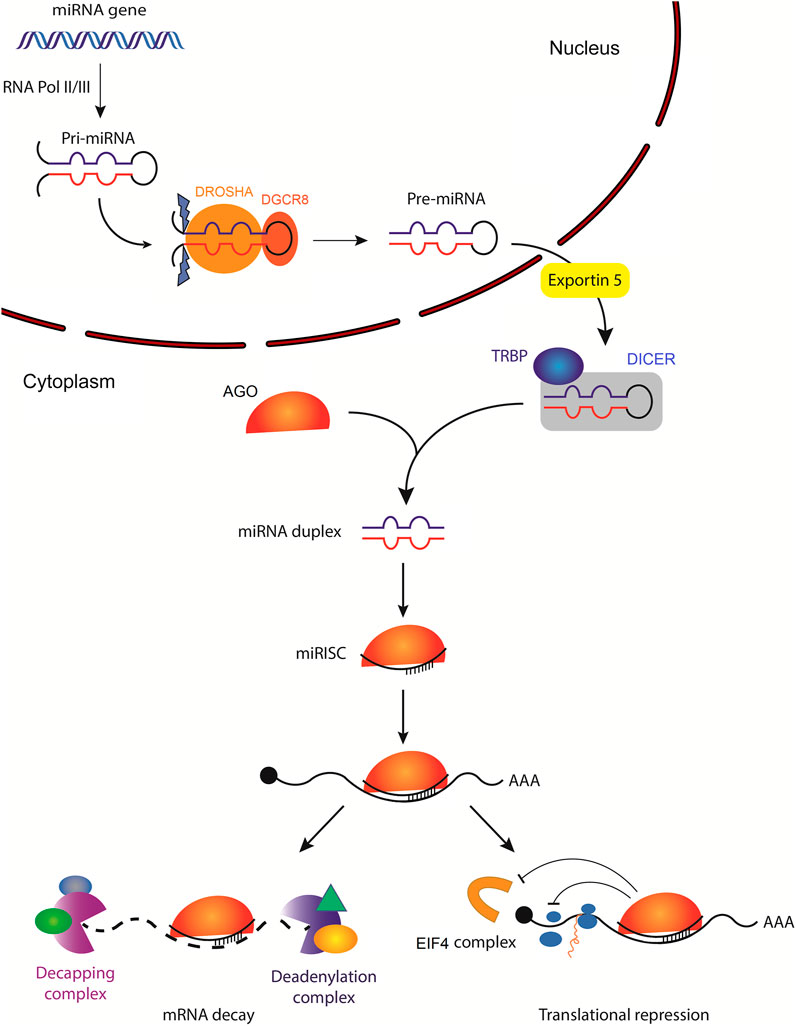
FIGURE 1. Biogenesis of microRNAs and their mechanisms of action. The primary miRNA (pri-miRNA) is transcribed by RNA polymerase II (less often RNA polymerase III) from a miRNA gene. Pri-miRNA is then processed by the DROSHA/DGCR8 complex to generate hairpin loop-containing precursor miRNA (pre-miRNA), which is exported from the nucleus by exportin-5. In the cytoplasm, pre-miRNA is first cleaved by DICER with the help of several RNA-binding proteins, including transactivation response element-binding protein (TRBP), which functions as a cofactor of DICER to ensure efficient processing of pre-miRNA. Subsequently, the mature miRNA duplex is unwound and one of the strands is loaded into the Argonaute (AGO) family of proteins to form a minimal miRNA-induced silencing complex (miRISC). Once miRISC is bound to its target mRNA, it can inhibit the initiation of translation by affecting the eukaryotic translation initiation factor 4 (EIF4) cap recognition and/or by inhibiting the formation of the 80S ribosomal complex. miRISC can also promote poly(A) deadenylation and mRNA decapping resulting in mRNA decay.
Although less common, non-canonical miRNA biogenesis is also observed (Abdelfattah et al., 2014). It can be classified into two groups, the Drosha/DGCR8-independent and the Dicer-independent pathways (Kim et al., 2016; O'Brien et al., 2018). These, for example, include miRNAs that originate from splicing the introns of messenger RNA (mRNA), mirtrons, or those that are processed from endogenous short hairpin RNA (shRNA), respectively (Ruby et al., 2007; Yang and Lai, 2011).
2.2 Mechanisms of action
Regardless of their biogenesis pathway, miRNAs regulate gene expression through the formation of the minimal miRNA-induced silencing complex (miRISC) which consists of a single-stranded mature miRNA guide strand and an AGO family protein (AGO1-4) (Kawamata and Tomari, 2010). The addition of effector complexes to minimal miRISC facilitates its function to negatively (and less frequently, positively) regulate gene expression at both transcriptional and posttranscriptional levels (Benhamed et al., 2012; O'Brien et al., 2018). The most well-studied mechanism of action is dependent on the interaction between miRISC and target mRNA at the 3′ untranslated region (UTR) (Valinezhad Orang et al., 2014; Jo et al., 2015; Salem et al., 2018; Wang N. Y. et al., 2019). This interaction between miRISC and target mRNA is typically stabilized by the 5′ seed region of the loaded miRNA (nucleotides 2–8) (Bartel, 2009; Ellwanger et al., 2011; Xu W. et al., 2014) and its association with a target sequence termed the miRNA response element (MRE) on the target mRNA (Behm-Ansmant et al., 2006; Nair et al., 2020).
An important early step in the regulation of target mRNA by miRISC is the formation of stable interactions between them (Chandradoss et al., 2015). This is dependent on miRISC and target RNA subcellular localization, RNA secondary structure, RNA-binding proteins, miRNA:RNA binding affinity, and miRNA:MRE copy number ratios. Within the cytoplasm, functional MRE are most commonly located within the 3′ UTR of mRNA (Huntzinger and Izaurralde, 2011; Ipsaro and Joshua-Tor, 2015) and to a much lesser extent the 5′ UTR (Zhang et al., 2018) and coding sequence (Fang and Rajewsky, 2011; Gu W. J. et al., 2013). miRNAs have also been detected in the nucleus to regulate gene transcription (Huang and Li, 2012; Liu H. et al., 2018). The affinity between miRNA and MRE itself significantly affects the stability of the interaction (Jo et al., 2015). Mismatches within the seed region, particularly within the first four nucleotides, substantially reduce miRISC interaction stability (Schirle et al., 2014; Chandradoss et al., 2015; Salomon et al., 2015), whereas supplementary interactions between MRE and miRNA within the central and 3’ regions have been shown, in some cases, to increase target specificity and stability (Sheu-Gruttadauria et al., 2019).
The mode of miRISC regulation is highly dependent on the set of proteins in complex with miRISC but in most cases, the formation of miRISC leads to the inhibition of gene expression via two major mechanisms: mRNA destabilization and translational repression (Figure 1). Following miRISC interaction with its cognate MRE within the cytoplasm, a miRISC adaptor protein, trinucleotide repeat-containing adaptor 6A (TNRC6A), interacts with poly(A) binding protein C (PABPC) (Liu et al., 2005; Huntzinger et al., 2013), poly(A)-nuclease deadenylation complex subunit 2 (PAN2/3), and the carbon catabolite repressor protein 4 (CCR4):NOT complex. Together, they localize the target mRNA poly(A) tail to miRISC, promoting efficient poly(A) deadenylation (Chen et al., 2009; Braun et al., 2011; Jonas and Izaurralde, 2015). After poly(A) deadenylation, the target mRNA is decapped by decapping protein 1/2 (DCP1/2) complexes, (Behm-Ansmant et al., 2006). mRNA decapping eventually leads to 5′ to 3’ exoribonuclease degradation by exoribonuclease 1 (XRN1) (Braun et al., 2012). Simultaneously, miRISC inhibits translation initiation by promoting the dissociation of eukaryotic translation initiation factor 4A1 (EIF4A1)/EIF4A2 and/or by inhibiting the formation of the 80S ribosomal complex (Behm-Ansmant et al., 2006; Fabian and Sonenberg, 2012; Meijer et al., 2013; Fukao et al., 2014). Although target mRNA degradation is typically observed following miRISC interaction, in some cases mRNA stability is left unchanged while inhibition of translation initiation results in decreased protein output (Pillai et al., 2005). Taken together, miRNA-bound AGO acts as a mediator of miRISC posttranscriptional regulatory potential in at least two primary ways: the miRNA-dependent localization of miRISC to target mRNA and the AGO-dependent recruitment of effector proteins that modulate target mRNA stability and protein output.
3 Overview of trophoblast differentiation, invasion, spiral artery remodeling, and preeclampsia
The main functions of the placenta are accomplished by trophoblasts, which are specialized cells of the placenta that play an essential role in embryo implantation and interaction with the decidualized maternal uterus (Imakawa and Nakagawa, 2017; Turco and Moffett, 2019). Abnormality in trophoblast differentiation and invasion, as well as spiral artery remodeling, is associated with various pregnancy-related disorders, such as PE.
3.1 Trophoblast differentiation
The human placenta originates from the trophectoderm, the outer layer of the pre-implantation embryo known as the blastocyst (Aplin, 2000). Following implantation, the trophectoderm undergoes proliferation and further differentiates into a branching network of villi that are in direct contact with the maternal circulation while maintaining a barrier between the fetal and maternal blood (Kaufmann et al., 2004; Wooding and Burton, 2008; Schmidt et al., 2015). The villous trees are made up of cytotrophoblasts (CTBs) covering the villous core, and a syncytiotrophoblast (STB) layer covering the villous surface that is in direct contact with the maternal blood. The CTBs are stem-like progenitor cells, which rapidly proliferate and fuse to give rise to the multinucleated STB layer. The STB layer of the placental villus functions as a physical barrier between the fetus and the mother to protect the fetus against vertical transmission of pathogens and immune attacks; it also regulates the exchange of O2, CO2, and nutrients between maternal and fetal blood, as well as secretes growth factors and hormones that are essential for normal pregnancy (Costa, 2016; Carrasco-Wong et al., 2021).
The CTBs also aggregate into cell columns at the tips of the anchoring villi. Cells at the distal region of the CTB column and in contact with the decidualized stroma lose most of their proliferative ability (Xu et al., 2002) and differentiate into invasive extravillous trophoblasts (EVTs). EVTs can be further grouped into interstitial EVTs (iEVTs) that invade the uterine stroma and endovascular EVTs (enEVTs) that invade spiral arteries to replace the endothelial cells (Pollheimer et al., 2018). Two possible origins of enEVTs have been proposed. First, they could be derived from the iEVTs surrounding the spiral arteries (Pijnenborg et al., 2006). Second, the shell CTBs that form the initial spiral artery plugs could differentiate into enEVTs which then start migrating within the spiral arteries in a retrograde manner (Pijnenborg et al., 2006; Burton et al., 2021). Some iEVTs can invade as far as the inner third of the myometrium, where they fuse to form multinucleated placental bed giant cells (Turco and Moffett, 2019). In addition, endoglandular EVTs (egEVTs) have also been identified as a potential subtype of iEVTs (Moser et al., 2010; Moser et al., 2015).
EVTs express a unique profile of adhesion molecules and histocompatibility antigens that facilitate their invasion ability as well as evasion of the maternal immune response. Villous CTBs anchored to the villous basement membrane retain their polarized epithelial state and express integrins ITGA6 and ITGB4 (α6β4) on their surface (Fisher and Damsky, 1993; Ji et al., 2013). As cells at the distal tip of the CTB column differentiate into EVTs, α6β4 is downregulated while integrin α5β1 is upregulated (Damsky et al., 1994). Integrin α1β1 is highly upregulated in EVTs that invade deeper into the decidua (Damsky et al., 1994; Damsky and Fisher, 1998). On the other hand, cadherin 1 (CDH1/E-cadherin) is downregulated in EVTs, resulting in the loss of cell-cell contact while invasive EVTs are characterized by the expression of cadherin 5 (CDH5/VE-cadherin) instead (Bulla et al., 2005; Ji et al., 2013). To avoid activating decidual natural killer (dNK) cells, iEVT express a distinct profile of HLA class I major histocompatibility complex (MHC) antigens that include HLA-C, HLA-E, and HLA-G (McMaster et al., 1995; Ferreira et al., 2017; Hackmon et al., 2017). HLA-E and HLA-G are thought to be vital for maternal tolerance of the semi-allogeneic fetus (Blaschitz et al., 2001). The placenta-specific HLA-G is highly expressed in invasive EVTs and can modulate the activity of not only dNK, but also macrophages and uterine T and B cells (Guillard et al., 2008; Ferreira et al., 2017).
3.2 Trophoblast invasion and spiral artery remodeling
During early pregnancy, EVT invasion is a crucial process for placental development, as the extent of the invasion is a key determinant for the quality of anchorage (Anin et al., 2004). This process is tightly regulated (Zhu et al., 2012) and insufficient invasion of EVTs is a hallmark of abnormal placentation and is associated with severe complications, such as PE, fetal growth restriction (FGR), and miscarriage. On the other hand, excessive invasion may result in placenta accreta and gestational trophoblastic disorders, including choriocarcinoma (Ning et al., 2019).
The precise mechanism underlying trophoblast invasion is not fully understood. Hormones, cytokines, growth factors, and proteinases are reported to be implicated in EVT invasion. Among them, matrix metalloproteinases (MMPs) play a critical role in mediating trophoblast invasion into the decidua by degrading the extracellular matrix (ECM) (Bischof et al., 2000; Anacker et al., 2011). For instance, MMP2 and MMP9 are two of the best-known key enzymes in trophoblast invasion (Huppertz et al., 1997; Staun-Ram et al., 2004; Jovanović et al., 2010).
Starting around 10 weeks of gestation, uterine spiral arteries undergo a remodeling process and are transformed from high-resistance vessels into dilated, low-resistance ones to ensure sufficient oxygen and nutrient supplies for the growing embryo (Anin et al., 2004; Lyall et al., 2013; Burton et al., 2021). This process involves coordinated actions of enEVTs, iEVTs, and decidual cells (Pijnenborg et al., 2006; Harris, 2010). The un-remodeled spiral artery contains endothelial cells surrounded by a layer of ECM and an outer layer of vascular smooth muscle cells (VSMCs). Early in the remodeling process, dNK cells and macrophages “prime” the vessels by disrupting the VSMCs and endothelial cell layers and triggering apoptosis (Smith et al., 2009). In the meantime, iEVTs invade the decidua into the areas surrounding the spiral arteries (Kam et al., 1999; Lyall et al., 2013) to remove VSMCs and replace the ECM with extracellular fibrinoid deposits, which are essential steps for normal spiral artery transformation (Kam et al., 1999; Harris, 2010; Lyall et al., 2013). iEVTs also release cytokines to help initiate the SAR process. For example, iEVTs release interleukin 6 (IL6) and C-X-C motif chemokine ligand 8 (CXCL8) to induce endothelial cells to secrete chemokines CCL14 and CXCL6 (Choudhury et al., 2017; Tao et al., 2019); in turn, the increased levels of CCL14 and CXCL6 recruit dNK cells and macrophages to further promote the remodeling process (Choudhury et al., 2017). Recent studies have also suggested that increased uterine arterial wall shear stress, as a result of pregnancy-associated increase in maternal blood volume and the decrease in vascular resistance, is one of the main physiological stimuli inducing uterine vascular remodeling (Ko et al., 2018; Khankin et al., 2021). The increase in wall sheer stress induces the remodeling, in part, by regulating endothelial cell signaling and the extent of arterial circumferential growth (Ko et al., 2018; Roux et al., 2020; Khankin et al., 2021).
The major function of enEVTs is to replace the endothelial cells lining the maternal uterine vasculature and this process is known as vascular mimicry (Ji et al., 2013). Interestingly, the invasion of enEVTs occurs in the arteries surrounded by perivascular iEVTs, suggesting that iEVT invasion could prepare for the subsequent enEVT invasion (Pijnenborg et al., 1983). Invasion of both iEVTs and enEVTs toward the spiral arteries is regulated in a spatiotemporal manner, as their invasive processes are terminated by 18–20 weeks of gestation and are limited to the inner third of myometrium (Pijnenborg et al., 1981). This remodeling process enhances placental perfusion in order to support the developing fetus.
3.3 Preeclampsia
According to the American College of Obstetricians and Gynecologists (ACOG) (2002) and the International Society for the Study of Hypertension in Pregnancy (ISSHP) (Davey and MacGillivray, 1988), PE is defined by de novo development of hypertension and multiple organ damage after 20 weeks of gestation (Lyall et al., 2013). This pregnancy-related disorder is a major cause of maternal and fetal morbidity and mortality and affects 2–8% of pregnancies worldwide (Huppertz, 2018; Phipps et al., 2019). The ISSHP groups PE into early-onset PE (EOPE), if patients are diagnosed and deliver before 34 weeks of gestation, and late-onset PE (LOPE, ≥ 34 weeks of gestation) (Raymond and Peterson, 2011; Tranquilli et al., 2013; Burton et al., 2019). More recently, detailed transcriptomic analyses of patients’ samples suggest PE can be subclassified based on molecular signatures; these subclasses can better describe the etiologies and the maternal–placental contributions to PE pathology (Leavey et al., 2016). Although the pathophysiology of PE is not completely understood, abnormal placental development is believed to play a central role in PE pathogenesis, particularly the EOPE (Noris et al., 2005; Brosens et al., 2011; Staff, 2019; Aplin et al., 2020).
PE is proposed as a two-stage disorder: the placental dysfunction stage and the maternal clinical syndrome stage (Redman, 1991; Staff, 2019; Carrasco-Wong et al., 2021). In the first stage, abnormal placental development due to defective trophoblast differentiation, shallow EVT invasion into the uterus and subsequent insufficient remodeling of spiral arteries leads to placental malperfusion. This in turn causes progressive oxidative stress of STB, characterized by erosion and damage of the surface of STB, increased shedding of inflammatory factors into the maternal circulation, decreased cell-cell fusion events, and reduced number of nuclei (Hubel, 1999; Cheng and Wang, 2009). Furthermore, some evidence suggests the existence of a switch from the normal apoptotic to the necrotic pathway making the shedded debris more pro-inflammatory compared to normal pregnancies (Paria et al., 2002).
Placental malperfusion also leads to an imbalanced release of various pro-angiogenic, anti-angiogenic, pro-inflammatory, and anti-inflammatory factors into the maternal circulation (Benyo et al., 2001; Redman et al., 2014; Tannetta et al., 2017; Burton et al., 2019; Carrasco-Wong et al., 2021) (Figure 2A). This, in turn, provokes widespread endothelial dysfunction and results in the second stage of PE, the maternal clinical syndrome stage, characterized by the development of maternal hypertension, proteinuria, and/or multi-organ damage (Redman, 1991; Huppertz, 2018; Staff, 2019; Aplin et al., 2020; Carrasco-Wong et al., 2021). Soluble fms-like tyrosine kinase 1 (sFLT1), also known as soluble vascular endothelial growth factor receptor 1 (sVEGFR1), is commonly reported as being elevated in maternal serum in PE (Shibata et al., 2005). The upregulation of sFLT1 correlates with the downregulation of vascular endothelial growth factor A (VEGFA) and placental growth factor (PlGF) in PE, both of which are required for normal trophoblast function (Carty et al., 2008). In addition, decreased VEGFA levels contribute to the inhibition of EVT invasion and EVT-induced spiral artery remodeling (Norwitz, 2007). Studies of CTBs in vivo have also indicated that high level of sFLT1 decreases EVT invasiveness (Wang et al., 2009). The ratio of sFLT1/PlGF has been extensively evaluated as a biomarker for PE (Zeisler et al., 2016; Nikuei et al., 2020; Ohkuchi et al., 2021). Furthermore, many studies have also investigated the possibility of using miRNAs, secreted by trophoblasts via exosomes into the maternal circulation, as predictive markers of PE (Li H. et al., 2020; Matsubara et al., 2021; Palma et al., 2021; Xu et al., 2021).
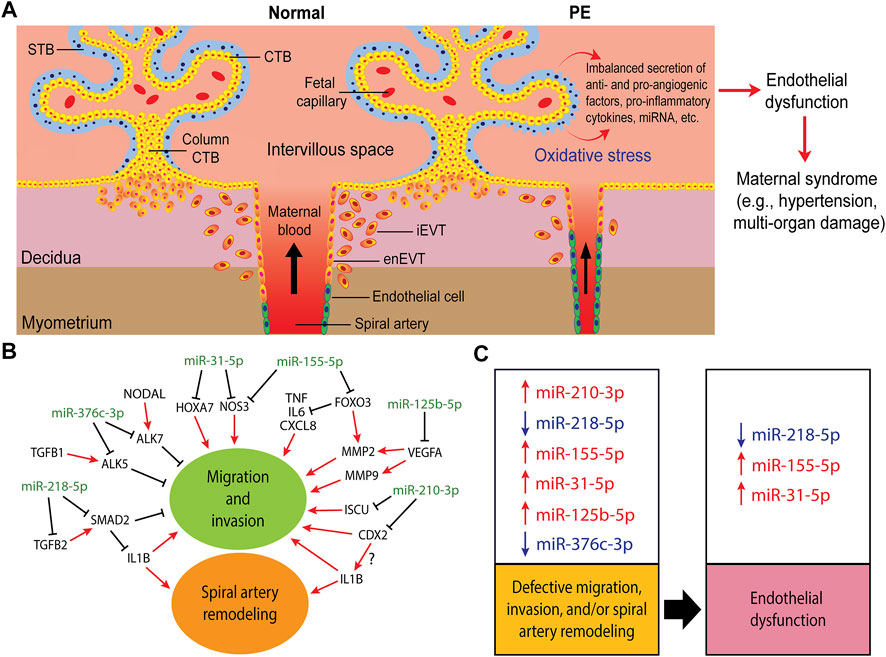
FIGURE 2. Trophoblast invasion, spiral artery remodeling, and their contribution to the pathogenesis of preeclampsia (PE). (A) In normal pregnancy, after implantation, the trophectoderm grows and differentiates into tree-like structures called villi which are the functional units of the placenta. A villous tree is made up of cytotrophoblasts (CTBs) covering the villous core, and a syncytiotrophoblast (STB) layer covering the villous surface that is in direct contact with the maternal blood. The CTBs also aggregate into cell columns at the tips of the anchoring villi. Cells at the distal region of the CTB column in contact with the decidualized uterine wall differentiate into invasive extravillous trophoblasts (EVTs). Interstitial EVTs (iEVTs) invade the decidua as far as the inner third of the myometrium. Some iEVTs move towards the maternal spiral arteries to help in their remodeling while endovascular trophoblasts (enEVTs) replace the endothelial cells lining these vessels. In PE, shallow EVT invasion and insufficient spiral artery remodeling lead to placental malperfusion and cause oxidative stress, resulting in the imbalanced secretion of pro- and anti-angiogenic factors, including miRNAs, into the maternal circulation. These factors lead to widespread endothelial dysfunction which results in the onset of maternal symptoms, such as hypertension, proteinuria, and/or multi-organ dysfunction. (B) A simplified regulatory network of some of the well-investigated miRNAs involved in regulating trophoblasts migration, invasion, and spiral artery remodeling. (C) The dysregulation of these miRNAs in PE (arrows denote up or down in PE) and their potential contribution into the proposed two-stages of PE pathogenesis: impaired placental development stage as a result of defective migration, invasion and spiral artery remodeling, and the maternal clinical syndrome stage due to endothelial dysfunction.
Many studies have investigated changes in the placental transcriptome, including miRNA expression, in pregnancy-related disorders such as PE. Studying PE pregnancies that are also compromised by other pregnancy complications, such as small or large for gestational age babies, gestational diabetes, or FGR, can help to better understand the mechanisms that give rise to these complications and also identify unique molecular signatures as potential biomarkers and therapeutic targets for these disorders (Mayor-Lynn et al., 2011; Sõber et al., 2015; Luo et al., 2017; Zhong et al., 2019). Thus, the identification of reliable PE-specific biomarkers, including miRNA, is one of the main interests of PE research (Lala and Chakraborty, 2003; Carty et al., 2008; Rana et al., 2020; Stepan et al., 2020; Martinez-Fierro and Garza-Veloz, 2021).
4 microRNA regulation of trophoblast invasion, spiral artery remodeling, and preeclampsia development
To date, many miRNAs have been shown to regulate trophoblast invasion (Fu et al., 2013a; Doridot et al., 2013; Ji et al., 2013; Schjenken et al., 2016; Hayder et al., 2018), and cell-cell communication at the maternal-fetal interface (Morales-Prieto et al., 2020) and to be dysregulated in PE (Choi et al., 2013; Parada-Niño et al., 2022). Some miRNAs have also been implicated in SAR (Brkić et al., 2018; Hu and Zhang, 2019; Hayder et al., 2021; Pankiewicz et al., 2021). These studies range from the use of a single cell line, most commonly, HTR8/SVneo (Graham et al., 1993) to ex vivo and in vivo models with contradictory findings are often reported. As the HTR8/SVneo cells in different labs appear to be cultured under different conditions and gain different characteristics (Kilburn et al., 2000; Takao et al., 2011; Weber et al., 2013; Lee et al., 2016; Abou-Kheir et al., 2017; Brkić et al., 2020), it is important to use multiple experimental models when studying trophoblast behaviours.
When investigating the effects of miRNA regulation, there are multiple biological aspects that need be considered. A miRNA can target many genes; conversely, a gene can be regulated directly by many miRNAs. Also, there are numerous ways for miRNA to indirectly regulate genes. For example, a miRNA may target a set of genes, which in turn, regulate the expression of other genes. Therefore, to confirm the direct regulation of a target gene by a miRNA, the following studies should be performed. First, one should determine the direct interaction between the miRNA and the predicted MRE using a reporter assay (e.g., luciferase) and the effect of miRNA overexpression/inhibition on the mRNA and protein levels of the target gene. Second, the function of the target gene should be investigated. Finally, functional rescue experiments should be performed to determine if overexpression of the target gene will reverse the effect of the miRNA. Alternatively, or additionally, some researchers determine if silencing of the target gene can attenuate the effect of the miRNA inhibitor in their rescue experiments.
We searched PubMed for miRNAs that have been reported to regulate trophoblast invasion or SAR and are also dysregulated in PE. Table 1 lists miRNAs that have been reported to be either up- or downregulated in preeclamptic placentas or in exosomes isolated from these placentas and are found to regulate trophoblast migration, invasion, and/or SAR using at least 2 cell lines or 1 cell line with ex vivo or in vivo models. For target genes that have not been comprehensively investigated using expression analyses and functional rescue experiments, we include them in the table but denote them as partially validated. Table 1 is by no means an extensive list and we recommend some recent reviews on miRNAs in placental development and pregnancy complications (Lv et al., 2019; Skalis et al., 2019; Hemmatzadeh et al., 2020; Ali et al., 2021; Parada-Niño et al., 2022). Given the large number of papers published in these areas, we focus the discussion on the role of miRNAs that have been more extensively studied using multiple models in regulating trophoblast invasion and SAR (Figure 2B) and their potential contributions to the pathogenesis of PE (Figure 2C).
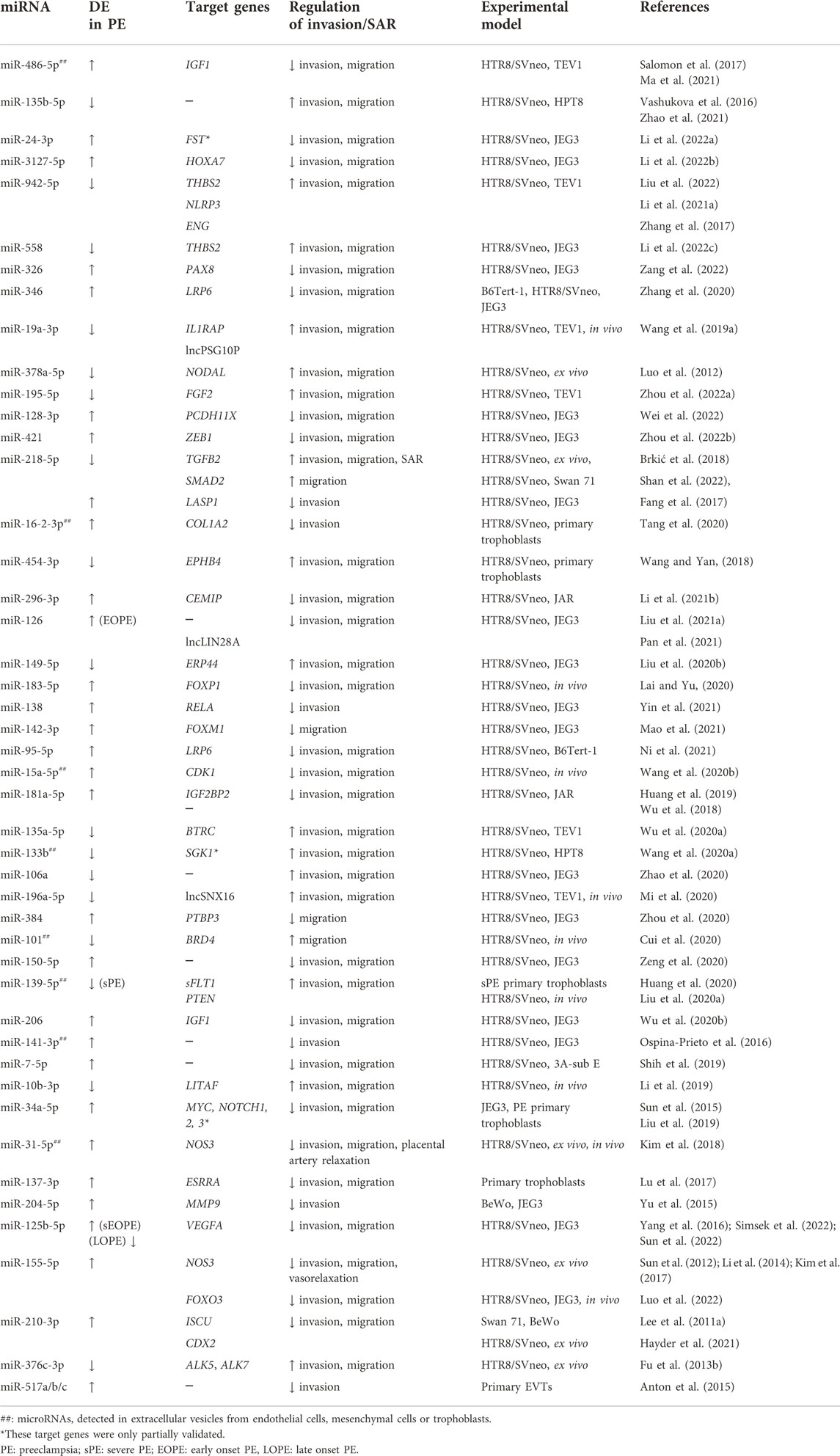
TABLE 1. Differentially expressed (DE) miRNAs in preeclampsia that affect trophoblast migration/invasion and/or spiral artery remodeling.
4.1 miR-210-3p
Perhaps one of the best-known miRNAs involved in trophoblast invasion through targeting of multiple genes is hsa-miR-210-3p. This master hypoxamir (hypoxia-regulated miRNAs) is directly regulated by the binding of the hypoxia-induced transcription factor 1 α (HIF1A) to its promoter region (Chan et al., 2012), and by another hypoxia-regulated transcription factor, nuclear factor κ B subunit 1 (NFKB1) (Zhang et al., 2012). Early placental development normally occurs under low oxygen conditions (2–3% O2); this is important for trophoblast proliferation and to help maintain the trophoblast stem cell population. It is also advantageous in order to reduce reactive oxygen species production, thus limiting the oxidative damage to the zygotic DNA and maintaining pluripotency of embryonic cells through the stabilization of HIF family members (Burton et al., 2021). In one of our studies, we found that miR-210-3p expression was highest in first trimester (weeks 5–12) and lowest in third trimester (week 26–40) placental samples from normal pregnancies (Hayder et al., 2021). This higher first trimester expression level is consistent with the notion that miR-210-3p may play a role in trophoblast adaptation to a hypoxic environment during the early stages of placental development (Zaccagnini et al., 2013; Krawczynski et al., 2016) when trophoblasts have reduced mitochondrial function and rely heavily on glycolysis for ATP production (Kolahi et al., 2017; Burton et al., 2021), processes of which miR-210-3p is involved in regulating (Chen Z. et al., 2010; Muralimanoharan et al., 2012).
Several studies have reported that overexpression of miR-210-3p in different trophoblast cell lines and first trimester placental explants decreases trophoblast invasion by targeting various genes. For example, miR-210-3p targets iron-sulfur cluster assembly enzyme (ISCU), a key protein involved in mitochondrial function, and its downregulation results in decreased trophoblasts invasion (Lee D. C. et al., 2011; Colleoni et al., 2013). We recently reported that caudal-related homeobox transcription factor 2 (CDX2), a key transcription factor in trophectoderm formation, is also a target of miR-210-3p. We showed that overexpressing miR-210-3p or downregulating CDX2 decreased trophoblast migration and invasion, as well as EVT outgrowth into the Matrigel, in first trimester placental explants (Hayder et al., 2021). However, the mechanisms by which ISCU and CDX2 promote trophoblast invasion remain to be investigated.
In primary EVTs, miR-210-3p overexpression decreases cell invasion by activating the ERK/MAPK pathway (Anton et al., 2013), which is known to be activated by both hypoxia and lipopolysaccharide (LPS) treatment used to induce PE-like conditions (Park et al., 2011). We also found that overexpression of miR-210-3p reduced the ability of the HTR8/SVneo cell line to form endothelial-like networks, and decreased the mRNA expression of cytokines, interleukin one beta (IL1B), CXCL8 and CXCL1 (Hayder et al., 2021); which are important in regulating immune cells recruitment to SAR sites. However, using miR-210-3p inhibitor did not improve the network formation ability or cytokine expression levels in trophoblasts (Hayder et al., 2021). These findings suggest that while miR-210-3p may be dispensable for SAR, its excessive production could contribute to the defective SAR observed in PE.
The in vivo function of mir-210 has been investigated in several animal models; however, findings varied among species and environmental conditions. One study showed that neither mir-210 knockout nor placenta-specific overexpression of miR-210-3p in mice displayed changes in fetal and placental weight or morphology when compared to controls (Krawczynski et al., 2016). In another study, however, when mir-210 knockout mice were subjected to maternal hypoxic stress (10.5% O2) mimicking living at high altitude, a decrease in fetal weight and impaired placental development with reduced spongiotrophoblast layer and labyrinth fetal blood vessels were observed (Bian et al., 2021). In contrast, a study on pregnant sheep living at high altitude showed increased miR-210-3p levels in the uterine arteries and impaired uterine arterial adaptation to pregnancy by increasing pressure-dependent vascular resistance of these arteries (Hu et al., 2017). Interestingly, in humans, pregnant women living at high altitude have an increased risk of developing PE and FGR due to a lack of normal vascular adjustments to pregnancy including a lack of change in uterine vascular resistance, but whether miR-210-3p is involved in this was not verified (Palmer et al., 1999; Keyes et al., 2003). Thus, more studies are needed to fully understand the role of miR-210-3p in regulating placental development and maternal adaptation to pregnancy, especially under maternal stress conditions.
Caution should be applied when considering findings obtained from animal models in which PE has been induced. Namely, PE is exclusively a primate disorder and thus needs to be induced surgically or pharmacologically in mice. Also, while there are many similarities in placental structures and developmental processes between rodents and humans, there are still significant differences such as the extent of trophoblast invasion and the fact that the SAR process starts later in rodents compare to humans (Frazier et al., 2020). Moreover, placental malperfusion seen in preeclamptic placentas results in some areas being hyperoxic while others relatively hypoxic rather than the placenta just being hypoxic (Staff, 2019; Burton et al., 2021). This cycle of hypoxia-reoxygenation in PE could lead to differences in cellular responses to oxidative stress (Mukherjee et al., 2021; Pi et al., 2021; Qiu et al., 2021). Regardless, these studies demonstrated the emerging complexities of the role of miR-210-3p in placental development and the need to better understand its function in pregnancy.
The upregulation of miR-210-3p in the placenta and serum of women with PE is well-established (Pineles et al., 2007; Zhu et al., 2009; Liu et al., 2012; Muralimanoharan et al., 2012; Anton et al., 2013; Hayder et al., 2021; Jairajpuri et al., 2021). Studies have also shown that miR-210-3p levels in the serum of women who developed PE later in gestation were higher during the second trimester than in women who did not develop PE (Xu P. et al., 2014; Munaut et al., 2016), suggesting the possibility of using miR-210-3p as a predictive biomarker for PE. However, whether miR-210 upregulation is a consequence or cause of PE is yet to be determined; it is possible that the high miR-210-3p levels in PE can restrict trophoblast invasion and SAR thereby further contributing to PE pathogenesis.
4.2 miR-218-5p
Hsa-miR-218-5p is a highly conserved miRNA in mammalian species. In humans, miR-218-5p is transcribed from mir-218–1 (4p15.31) and mir-218–2 (5q35.1), which are located within the introns of the SLIT2 and SLIT3, respectively (Mott and Mohr, 2015). Two precursors, mir-218–1 and mir-218–2, produce the same mature miR-218-5p and a precursor-specific miR-218-3p, miR-218-1-3p and miR-218-2-3p. In HTR8/SVneo cells stably transfected with mir-218–1, miR-218-5p was strongly upregulated while no significant change in miR-218-1-3p level was detected (Brkić et al., 2018), suggesting that miR-218-5p is the dominant mature miR-218 form. While little is known about the role of SLIT3 in the placenta, SLIT2 and its receptor, roundabout guidance receptor 1 (ROBO1), have been suggested to regulate trophoblast proliferation and differentiation and their aberrant signaling may be associated with various pregnancy-related disorders (Li et al., 2015; Li et al., 2017; Tiensuu et al., 2019). Interestingly, ROBO1, which is a functional target of miR-218-5p (Liu Y. et al., 2021), is upregulated in preeclamptic placentas (Liao et al., 2012).
We have investigated the function of miR-218-5p in trophoblast invasion, differentiation and SAR using various models and experimental methods. In trophoblast cell lines, stable or transient overexpression of miR-218-5p promoted migration, invasion, and the expression of various genes related to enEVT differentiation and SAR, as well as the formation of endothelial-like networks; meanwhile, the inhibition of endogenous miR-218-5p had the opposite effects (Brkić et al., 2018; Shan et al., 2022). In addition, miR-218-5p promoted the outgrowth of EVTs in first trimester placental explants. Finally, using a placental explants-decidua co-culture model, we demonstrated that overexpressing miR-218-5p in the explants increased the depth of trophoblast invasion into the decidua, induced the recruitment of immune cells, and enhanced the loss of smooth muscle cells surrounding the spiral arteries (Brkić et al., 2018). These findings suggest that miR-218-5p promotes enEVT differentiation and accelerates SAR. Mechanistically, we identified transforming growth factor β 2 (TGFB2) (Brkić et al., 2018) and its downstream mediator, SMAD2 (Shan et al., 2022) as direct targets of miR-218-5p. We also showed that TGFB2 and SMAD2 exert inhibitory effects on enEVT differentiation (Brkić et al., 2018; Shan et al., 2022). Interestingly, inhibition of this pathway induced the expression and secretion of IL1B (Shan et al., 2022). We and other groups have shown that physiological concentrations of IL1B promotes trophoblast migration and invasion, and enEVT differentiation (Prutsch et al., 2012; Shan et al., 2022). Thus, one of the mechanisms by which miR-218-5p induces enEVT differentiation and SAR is to suppress TGFB2/SMAD2 signaling and to induce IL1B.
Several studies have reported that miR-218-5p is downregulated in preeclamptic placentas. Our lab has observed that miR-218-5p levels were significantly lower in PE placentas between 35–39 weeks of gestation than in their gestational age-matched controls in two sets of clinical samples collected in China and Canada (Brkić et al., 2018). Similarly, two miRNA profiling studies have reported the downregulation of miR-218-5p in placentas from women with severe PE and delivered at term (Zhu et al., 2009; Xu P. et al., 2014). Moreover, a recent study showed that miR-218-5p was significantly decreased in a rat model of PE while magnesium sulfate, which has been recommended for the treatment of PE (WHO, 2011), increased miR-218-5p levels (Zheng et al., 2022). In contrast, two other studies reported the upregulation of miR-218-5p in the plasma of PE patients at gestational age 20–40 weeks (Akgor et al., 2021) and in preeclamptic placental tissues collected primarily from 34–40 weeks of pregnancies (Fang et al., 2017). The reasons for these inconsistent findings are not clear. However, differences in gestational stages, sample size, clinical characteristics of the study subjects, and cell populations within the placental biopsies used for the miRNA measurement may contribute to such discrepancy.
While in vivo study directly investigating the contribution of miR-218-5p to PE pathogenesis remains to be conducted, based on in vitro and ex vivo studies, it is possible that downregulation of miR-218-5p could impede trophoblast migration, invasion, enEVT differentiation, and SAR, thereby contributing to the first stage of PE development. We also found that transfection of miR-218-5p into first trimester placental explants increased the secretion of pro-invasive and pro-angiogenic cytokines but decreased the secretion of an anti-angiogenic factor, soluble endoglin (sENG) (Brkić et al., 2018), which plays important roles in the second stage of PE development (Perez-Roque et al., 2021; Margioula-Siarkou et al., 2022). These findings, together with the observation that miR-218-5p is downregulated in a rat PE model but induced by magnesium sulfate to reverse the hypertension and proteinuria in these preeclamptic rats, support the notion that insufficient production of miR-218-5p is also linked to the development of maternal symptoms.
4.3 miR-155-5p
Hsa-miR-155-5p is a known pro-inflammatory miRNA encoded by the host gene, MIRHG155, previously known as the B-cell Integration Cluster (BIC) gene. Several transcription factors binding sites have been identified in the promoter region of MIRHG155, including NFKB1, SMAD4, interferon-sensitive response element (ISRE), interferon regulatory factors (IRF) and HIF1A (Mahesh and Biswas, 2019). Overexpressing miR-155-5p in trophoblasts decreased their invasion and migration while inhibiting miR-155-5p resulted in the opposite phenotype (Zhang et al., 2010; Dai et al., 2012; Li et al., 2014; Luo et al., 2022). A recent study reported that the forkhead-box class O transcription factor 3 (FOXO3), is targeted by miR-155-5p. It also showed that FOXO3 promoted trophoblast migration and invasion by decreasing the levels of tumor necrosis factor (TNF), IL6, and CXCL8 and by increasing the levels of MMP2 (Luo et al., 2022). These findings suggest that miR-155-5p can negatively regulate trophoblast invasion. However, direct evidence of the negative role of miR-155-5p in human SAR remains to be investigated.
Nitric oxide (NO) is an important signaling molecule that acts as a vasodilator but has also been shown to regulate trophoblast motility (Costa and Biaggioni, 1998; Harris et al., 2008). NO is produced in endothelial cells by the action of nitric oxide synthase 3 (NOS3), formerly known as endothelial nitric oxide synthase, eNOS. Both NO bioavailability and NOS3 levels are downregulated in PE (Albrecht et al., 2003; Kim et al., 2006; Guerby et al., 2019). It has been shown that miR-155-5p targets NOS3 to inhibit HTR8/SVneo invasion (Li et al., 2014; Kim et al., 2017). Moreover, treatment of human vascular endothelial cells (HUVECs) with exosomes isolated from preeclamptic pregnancies and from miR-155-5p overexpressing BeWo cells suppressed NOS3 expression (Shen et al., 2018) and that miR-155-5p decreased endothelium-dependent vasorelaxation (Sun et al., 2012). Thus, it is possible that by targeting NOS3, miR-155-5p reduces NO production, thereby impeding vasodilation and increasing blood pressure (Cardillo et al., 2000). This notion is supported by in vivo findings from a pregnant hypertension rat model, in which inhibition of miR-155-5p improved in blood pressure levels (Liu D. F. et al., 2018).
TNF is a well-known pro-inflammatory cytokine implicated in PE (Chen L. M. et al., 2010; Bellos et al., 2018). The inflammatory response serves a vital biological function and is finely controlled by many transcription factors, including NFKB1, to protect against tissue damage. However, prolonged and persistent activation of NFKB1 leads to increased production of cytokines, such as TNF and the interleukin family (Kim et al., 2013; Cornelius, 2018). This increase in pro-inflammatory cytokines is associated with impaired trophoblast invasion, endothelial dysfunction, and is considered a risk factor for PE (Vaughan and Walsh, 2012; Kim et al., 2018). TNF treatment has been reported to upregulate miR-155-5p expression, which in turn mediates TNF-induced downregulation of NOS3 (Sun et al., 2012). Interestingly, treatment with aspirin can prevent NOS3 downregulation by blocking both TNF-mediated miR-155-5p biogenesis and NFKB1-mediated MIR155HG expression (Kim et al., 2017). Aspirin is known to reduce hypoxia-induced sFLT1 release in trophoblasts and endothelial cells and to promote trophoblast invasion (Lin et al., 2019; Su et al., 2019). Low doses of aspirin are currently used to reduce the incidence of hypertensive complications during pregnancy (Henderson et al., 2014; Khanabdali et al., 2018) and the onset of EOPE if given daily to high-risk women before 16 weeks of gestation (Roberge et al., 2018; Ducat et al., 2019; Rolnik et al., 2022). In addition, several studies have reported the upregulation of miR-155-5p in preeclamptic placental (Lasabová et al., 2015; Azizi et al., 2017; Wang et al., 2021) and in maternal serum samples (Yang et al., 2017; Shen et al., 2018; Kim et al., 2020). Moreover, there is a strong positive correlation between placental and serum miR-155-5p levels suggesting a placental origin of serum miR-155-5p (Yang et al., 2017). When measuring extracellular miRNAs in maternal serum samples collected between 17 and 28 weeks of gestation, and before the onset of PE symptoms, a study showed that miR-155-5p was one of the best predictive biomarkers of PE (Srinivasan et al., 2020).
Together, these findings strongly suggest that aberrant overexpression of miR-155-5p can contribute to both stages of PE pathogenesis. Regardless of whether elevated miR-155-5p level are an actual cause or a consequence of other abnormal processes, it can further promote placental dysfunction by inhibiting trophoblast invasion. Additionally, higher miR-155-5p levels can induce endothelial dysfunction. Future studies should also explore the possibility of targeting miR-155-5p as a new preventive or therapeutic strategy for PE.
4.4 miR-31-5p
Several interesting studies have investigated the role of hsa-miR-31-5p in placental development. One study reported a negative role of miR-31-5p in HTR8/SVneo cell migration and invasion via targeting homeobox protein A7 (HOXA7), a member of the HOX family that has been shown to promote migration, invasion, autophagy and proliferation and inhibits apoptosis in HTR8/SVneo (Dong et al., 2020). Activated autophagy is an important mechanism for EVT invasion and vascular remodeling. It is thought to provide an “emergency” nutrient and energy supply for invading EVTs (Saito and Nakashima, 2014), especially under stress conditions such as starvation or hypoxia. In HTR8/SVneo and HUVEC co-culture model of vascular remodeling, autophagy-deficient HTR8/SVneo cell lines failed to replace endothelial-like networks formed by HUVEC; suggesting that autophagy is important for HTR8/SVneo to acquire endothelial-like phenotype (Zhang Y. et al., 2016; Nakashima et al., 2020; Li Y. M. et al., 2021; Li Z. J. et al., 2022). Thus, in light of the role of HOXA7 in promoting autophagy in EVTs, it is postulated that miR-31-5p-mediated downregulation of HOXA7 could limit deep invasion of EVTs and contribute to some of the phenotypes observed in preeclamptic placentas.
Treatment of HUVECs and HTR8/SVneo cells with TNF led to the upregulation of miR-31-5p (Kim et al., 2020) and this effect was mediated by NFKB1 (Kim et al., 2018). Interestingly, transfection of HUVECs with miR-31-5p mimic or treatment of HUVECs with TNF resulted in a decreased invasion of HTR8/SVneo co-cultured with these HUVECs. Further analysis revealed that miR-31-5p decreases trophoblast invasion by targeting NOS3 (Kim et al., 2018). In addition to NOS3, miR-31-5p also negatively regulates HUVECs and human mammary epithelial cells (HMECs) proliferation, migration, and network-like formation by targeting endothelin receptor type B (ETBR), a receptor of endothelin-1 (ET1) which upon activation, causes vasodilation (Mazzuca et al., 2014; Li W. et al., 2020). ETBR is downregulated in PE (Li W. et al., 2020) and hypertensive pregnancies (Mazzuca et al., 2014) and abnormal ETBR expression contributes to microvascular dysfunction in postpartum women who have developed PE (Stanhewicz et al., 2017). miR-31-5p is upregulated in PE placentas (Li W. et al., 2020) and in serum of women with PE (Kim et al., 2018; Kim et al., 2020). In an ex vivo cultured model of placental arterial vessels from healthy pregnant women, TNF treatment or miR-31-5p mimic induced endothelial dysfunction and inhibited vasorelaxation (Kim et al., 2018) which are thought to underpin the onset of the clinical syndrome stage of PE (Staff, 2019; Aplin et al., 2020). Hence, by inhibiting trophoblast invasion and inducing endothelial dysfunction, elevated miR-31-5p levels in PE could contribute to both stages of the disorder but this requires further investigation.
4.5 miR-125b-5p
Hsa-miR-125b-5p, previously known as miR-125b, is generated from two different precursors, mir-125b-1 and mir-125b-2. In normal pregnancy, miR-125b-5p is expressed at higher levels in the third trimester than in first trimester placentas (Gu Y. et al., 2013). Also, miR-125b-5p overexpression reduces trophoblast invasion and migration (Li Q. H. et al., 2020; Xueya et al., 2020; Tang et al., 2021; Sun et al., 2022). This is mediated, in part, by targeting VEGFA (Sun et al., 2022), a well-known pro-angiogenic factor and a regulator of trophoblast function and placental angiogenesis (Amirchaghmaghi et al., 2015; Fan et al., 2021). VEGFA is also known to activate ERK1/2 signaling leading to upregulation of MMP2 and MMP9 to promote trophoblast invasion (Su et al., 2017; Wang et al., 2017). Moreover, placenta-specific overexpression of miR-125b-5p in mice decreased placental and fetal weights and increased abortion rate. Further analysis in these mice revealed that the observed phenotypes were due to a significant reduction in branch density of placental vasculature and in trophoblast invasion into the decidua and the spiral arteries (Sun et al., 2022). These findings strongly support an inhibitory role for miR-125b-5p in trophoblast invasion, placental angiogenesis and SAR.
Studies of miR-125b-5p expression in pregnancy complications vary based on the type and onset of these disorders. In preeclamptic placentas, one study reported that miR-125b-5p is upregulated in severe EOPE placentas (Yang et al., 2016) while another one showed downregulated miR-125b-5p in LOPE (Simsek et al., 2022), and a third study reported no difference (Inno et al., 2021). However, miR-125b-5p was consistently shown to be upregulated in umbilical cord blood (Simsek et al., 2022) and in serum of women affected by EOPE (Yang et al., 2016; Tang et al., 2021; Simsek et al., 2022). Interestingly, miR-125b-5p was also shown to be elevated at 12 weeks of gestation in women who developed PE later in their pregnancies, suggesting that miR-125b-5p miRNA could be used as a predicative marker for PE (Licini et al., 2021). The detection of aberrant upregulation of miR-125b-5p in first trimester, together with the findings that placenta-specific overexpression of miR-125-5p in mice resulted in limited trophoblast invasion into the decidua and spiral artery, strongly supports the role of this miRNA in PE development, particularly EOPE, and its potential as a therapeutic target for preventive PE strategy.
4.6 Chromosome 14 miRNA cluster
Chromosome 14 miRNA cluster (C14MC) is conserved among placental mammals. In humans, this cluster contains 54 miRNA genes (Welten et al., 2014; Nayak et al., 2018) with 84 mature miRNAs identified so far (Smith et al., 2021). It is located on chromosome 14q32 at the imprinted DLK1-DIO3 domain (mouse distal chromosome 12 domain cluster) where it can be expressed from the maternally inherited chromosome 14 (Seitz et al., 2004; Glazov et al., 2008; Morales-Prieto et al., 2013; Welten et al., 2014). Although many C14MC members appear to have variable expression patterns in the first trimester, the majority of them are expressed at a higher level in the second trimester than in other gestational stages (Morales-Prieto et al., 2012; Gu Y. et al., 2013; Gonzalez et al., 2021; Inno et al., 2021; Smith et al., 2021). Also, many C14MC miRNAs are detected in both fetal and maternal plasma in normal pregnancy (Paquette et al., 2018). As expected, heterozygous mouse pups with maternal, but not paternal, deletion of this maternally-inherited miRNA cluster exhibited partially penetrant neonatal lethality due to defects in the maintenance of energy homeostasis (Labialle et al., 2014), suggesting an essential role of these miRNAs during pregnancy.
The role of specific members of the C14MC in trophoblasts function and placental development has been investigated. For example, our lab reported that miR-376c-3p inhibits transforming growth factor β 1 (TGFB1) and NODAL signaling by targeting their respective type I receptors, activin receptor-like kinase 5 (ALK5) and ALK7. This, in turn, significantly increases HTR8/SVneo invasion and EVT outgrowth of first trimester placental explants (Fu et al., 2013b). We and others have shown that TGFB1 and NODAL negatively regulate trophoblast invasion and EVT outgrowth of first trimester placental explants (Nadeem et al., 2011; Luo et al., 2012; Shi et al., 2019; Brkić et al., 2020). miR-376c-3p is also downregulated in both placental and maternal plasma samples from PE patients compared to those from normal pregnancies (Fu et al., 2013b; Sandrim et al., 2016). Thus, lower miR-376c-3p levels would possibly lead to insufficient trophoblast invasion and thereby contributes to the development of PE. However, in vivo studies are needed to confirm this possibility.
Another member of C14MC is miR-494-3p; it promotes migration and invasion of trophoblast cell lines by targeting high-temperature requirement A serine protease 1 (HTRA1) (Ou et al., 2020). HTRA1 is a secreted protein that inhibits trophoblast migration and invasion (Ajayi et al., 2008; Fantone et al., 2021). HTRA1 interacts with HTRA3 to antagonize and degrade another HTRA member, HTRA4 that is known to promote trophoblast invasion (Wang et al., 2012; Chen et al., 2014; Fantone et al., 2021). HTRA1 is upregulated in EOPE (Ajayi et al., 2008; Liu C. H. et al., 2018), and surprisingly, so is miR-494-3p (Zhao et al., 2022). However, HtrA1-deficient mice display smaller placentas and exhibit impaired SAR and FGR (Hasan et al., 2015). Thus, identifying other targets of miR-494-3p in human trophoblasts, can shed the light on the precise mechanisms by which miR-494-3p regulate placental development and its implication in PE.
In preeclamptic placentas, some of C14MC miRNAs are upregulated, while others are downregulated (Timofeeva et al., 2018; Devor et al., 2020; Inno et al., 2021); however, a detailed analysis of all miRNAs in this cluster is yet to be done. Due to the lack of studies on the specific role of many C14MC members in trophoblast invasion, it is unclear how these cluster miRNAs may contribute to the development of PE. Therefore, more studies, especially ex vivo and in vivo ones, are needed to further determine how C14MC members regulate placental development, if and how they are dysregulated in PE, and how they may play a role in PE pathogenesis.
4.7 Chromosome 19 miRNA cluster
Chromosome 19 miRNA cluster (C19MC) is a primate-specific miRNA cluster that is expressed almost exclusively in the placenta (Xie et al., 2014). It is located on chromosome 19q13.41 and contains 46 miRNA genes that are processed from RNA polymerase II non-protein coding transcripts (Bortolin-Cavaille et al., 2009). The C19MC is maternally imprinted and expressed exclusively from the paternally inherited chromosome 19 (Noguer-Dance et al., 2010). In primary trophoblasts isolated from normal term placentas, 41 out of the 46 C19MC miRNA genes were expressed and they represented the majority of miRNAs expressed in these cells as well as in the exosomes secreted by them (Luo et al., 2009; Donker et al., 2012; Lee et al., 2016). Temporally, studies have shown that C19MC miRNAs exhibit a dynamic gestational expression profile (Gottlieb et al., 2021); however, there are inconsistencies among different reports. One study showed that 46 mature C19MC miRNAs were higher in third trimester villous trophoblasts compared to first trimester cells (Donker et al., 2012; Morales-Prieto et al., 2012), while another study showed that the expression of some C19MC members were higher in first trimester samples (ranged 6–10 weeks) than in second trimester ones (ranged 11–23 weeks) (Smith et al., 2021). Nevertheless, C19MC miRNAs still constituted 15% of total miRNA transcripts at term placentas (Smith et al., 2021).
Some C19MC members displayed strong expression in first trimester human villous CTBs. Within the CTB column, C19MC expression levels gradually decreased from proximal to distal cells as CTBs differentiated into EVTs (Xie et al., 2014; Mong et al., 2020). Consonantly, many C19MC miRNAs were detected in JEG3, JAR and BeWo choriocarcinoma trophoblast cell lines but not in the EVT-like HTR8/SVneo cell line (Donker et al., 2012). Interestingly, ectopic stable expression of the entire C19MC in HTR8/SVneo reduced cell migration (Xie et al., 2014). Gene ontology analysis using the C19MC overexpressing HTR8/SVneo showed a downregulation of many genes involved in cell motility and adhesion (Xie et al., 2014). These findings suggest that C19MC exerts a negative regulatory role in EVT differentiation and migration. However, the role of individual C19MC members has not been well established. Only few studies have investigated the role of specific C19MC members in trophoblast function and done so using only a single cell line (Anton et al., 2015; Ding et al., 2015; Zhang et al., 2021; Zheng et al., 2021).
Recent studies suggest that C19MC plays important roles in placenta development. In human embryonic stem (hES) cells, C19MC expression is important for the differentiation of hES into human trophoblast stem cells (hTS). Using CRISPR/Cas gene editing tools, the authors further demonstrate that C19MC is critical in hTS maintenance (Kobayashi et al., 2022). The exact mechanism behind C19MC stem cell maintenance is uncertain but it may involve the C19MC-induced upregulation of pluripotent factors octamer-binding transcription factor 4 (OCT4) and fibroblast growth factor 4 (FGF4) (Mong et al., 2020). In addition, transgenic mice expressing the human C19MC miRNAs had also been developed. The C19MC transgenic mice had larger placentas with enhanced trophoblast proliferation and altered trophoblast migration (Mouillet et al., 2020). Compared with the wild type mice, the C19MC transgenic mice express a higher level of Tpbpb, a marker of the junctional zone glycogen cells (similar to human EVTs) and Mmp1a, a gene involved in trophoblast migration and invasion (Soncin et al., 2015; Mouillet et al., 2020). Thus, these findings appear to be at odds with those from the in vitro studies using human trophoblast cell lines. Further studies are required to elucidate the role of this miRNA cluster in trophoblast migration, invasion, and SAR.
In PE, many members of the C19MC were shown to be dysregulated with the majority of them being upregulated (Hromadnikova et al., 2013; Miura et al., 2015; Inno et al., 2021). For example, miR-512-5p, miR-515-5p, miR-517a/b/c, miR-518a/e/f-5p, miR-519a/b/c/e-5p, miR-519days/c-3p, miR-520a/b/c-5p and miR-520a-3p were consistently upregulated in PE (Anton et al., 2015; Ding et al., 2015; Zhang M. et al., 2016; Inno et al., 2021) while miR-524-5p and miR-525-5p were consistently downregulated (Zhang et al., 2021; Zheng et al., 2021). Given the large number of miRNAs within the C19MC cluster, the differential regulation of at least some cluster members and their potentially varying function, it can be expected that individual miRNAs in this cluster have distinct expression profiles throughout gestation. Nevertheless, C19MC appears to be critical for placental development in at least two key areas: the maintenance and proliferation of hTS and their differentiation into EVT. However, following EVT differentiation, C19MC expression may be deleterious by decreasing migration and modulating adhesion potential. Thus, differential expression of C19MC members in PE requires a high spatial resolution, particularly between villous trophoblast populations and EVT populations. It may be that the upregulation of C19MC in PE is compensatory, attempting to increase CTB cell number and STB integrity via STB differentiation. If the PE environment impairs C19MC downregulation following EVT differentiation, this may further exacerbate insufficient EVT invasion and SAR. Consequently, it is important that future research on this cluster obtain detailed analysis of the expression of all C19MC mature miRNAs in PE to be able to determine their involvement in PE pathophysiology and further our understanding of cluster miRNA dynamics.
5 Concluding remarks
Placental development is highly regulated and involves a complex interplay between fetal and maternal cells to ensure a successful pregnancy. To date, many studies have investigated the role of miRNAs in regulating placental development, including trophoblast invasion, using multiple in vitro, ex vivo and in vivo models. Although several studies have suggested that some miRNAs play a role in the differentiation of enEVTs and SAR, our understanding of how miRNAs are involved in trophoblasts-mediated remodeling of uterine spiral arteries is still very limited.
Studies of miRNAs in the human placenta have been mostly carried out in vitro. While some papers reported the use of primary trophoblasts, placental villous explants, and villous explants/decidua co-culture, most published work have been done using established trophoblast cell lines. The use of a single cell line has its limitations considering the differences in marker gene expression and origin of many trophoblast cell lines (Pastuschek et al., 2021). Furthermore, the same cell line (e.g. HTR8/SVneo) in different labs appears to have different characteristics (Brkić et al., 2020). Cell line cross-contamination has also been reported (Nelson-Rees et al., 1981; Capes-Davis et al., 2010; Kniss and Summerfield, 2014; Ye et al., 2015). Additionally, some miRNAs might target different genes in different trophoblast cell lines (Chaiwangyen et al., 2015). Finally, while the use of animal models has advanced our understanding of miRNA functions in healthy and PE pregnancy (Rossant and Cross, 2001; Khalil and Granger, 2002; Carter, 2007; Palei et al., 2013; Rana et al., 2019; Frazier et al., 2020; Gatford et al., 2020; Bakrania et al., 2022), there are challenges in translating the findings to human applications. This is, in part, due to differences in placental structures between humans and non-primate models (Chaouat and Clark, 2015; Schmidt et al., 2015), the need for genetic, surgical or pharmacological manipulations to induce PE-like symptoms in these models (Kumasawa, 2018; Bakrania et al., 2022), and the fact that some miRNAs are not expressed in certain animals (Glazov et al., 2008; Noguer-Dance et al., 2010; Nayak et al., 2018; Schmidt et al., 2018). Therefore, it is important to use multiple systems such as cell lines, primary cells, ex vivo and in vivo models, as well as clinical samples, to study miRNA functions in human placentation and their involvement in the development of pregnancy-related disorders.
Many studies have reported miRNA expression profiles throughout gestation and their dysregulation in preeclamptic placentas and maternal serum; however, inconsistent findings are also often reported. This could be due to differences in the gestational age of the tissues, the composition of cell types in the samples, and clinical characteristics of the patients (Morales-Prieto et al., 2012; Pique-Regi et al., 2019; Smith et al., 2021). Thus, differential expression of miRNAs in healthy and PE placentas requires higher spatial resolution. Advanced techniques, such as single-cell sequencing, are powerful tools that will allow us to investigate miRNA expression and gene networks regulated by miRNAs within a cell population at unprecedented resolution. They will also reveal key sets of molecular events occurring throughout trophoblast differentiation, help elucidate molecular mechanisms underlying PE pathogenesis, and identify early PE markers to provide the means for early prediction and intervention of PE to improve maternal and fetal outcomes.
Author contributions
All authors listed have made a substantial, direct, and intellectual contribution to the work and approved it for publication.
Funding
Work in our laboratory was supported by grants from the Canadian Institutes of Health Research (MOP-81370, CCI-92222, CCI-132565, MOP-89931, and PJT-153146) and the York University Research Chair program to CP.
Conflict of interest
The authors declare that the research was conducted in the absence of any commercial or financial relationships that could be construed as a potential conflict of interest.
Publisher’s note
All claims expressed in this article are solely those of the authors and do not necessarily represent those of their affiliated organizations, or those of the publisher, the editors and the reviewers. Any product that may be evaluated in this article, or claim that may be made by its manufacturer, is not guaranteed or endorsed by the publisher.
References
Abdelfattah, A. M., Park, C., and Choi, M. Y. (2014). Update on non-canonical microRNAs. Biomol. Concepts 5 (4), 275–287. doi:10.1515/bmc-2014-0012
Abou-Kheir, W., Barrak, J., Hadadeh, O., and Daoud, G. (2017). HTR-8/SVneo cell line contains a mixed population of cells. Placenta 50, 1–7. doi:10.1016/j.placenta.2016.12.007
Ajayi, F., Kongoasa, N., Gaffey, T., Asmann, Y. W., Watson, W. J., Baldi, A., et al. (2008). Elevated expression of serine protease HtrA1 in preeclampsia and its role in trophoblast cell migration and invasion. Am. J. Obstet. Gynecol. 199 (5), e1–10557. e510. doi:10.1016/j.ajog.2008.04.046
Akgor, U., Ayaz, L., and Cayan, F. (2021). Expression levels of maternal plasma microRNAs in preeclamptic pregnancies. J. Obstet. Gynaecol. 41 (6), 910–914. doi:10.1080/01443615.2020.1820465
Albrecht, E. W. J. A., Stegeman, C. A., Heeringa, P., Henning, R. H., and van Goor, H. (2003). Protective role of endothelial nitric oxide synthase. J. Pathol. 199 (1), 8–17. doi:10.1002/path.1250
Ali, A., Hadlich, F., Abbas, M. W., Iqbal, M. A., Tesfaye, D., Bouma, G. J., et al. (2021). MicroRNA–mRNA networks in pregnancy complications: A comprehensive downstream analysis of potential biomarkers. Int. J. Mol. Sci. 22 (5), 2313. doi:10.3390/ijms22052313
American College of Obstetricians and Gynecologists (2002). ACOG practice bulletin. Diagnosis and management of preeclampsia and eclampsia. Int. J. Gynaecol. Obstet. 77 (1), 67–75. doi:10.1016/S0020-7292(02)80002-9
Amirchaghmaghi, E., Rezaei, A., Moini, A., Roghaei, M. A., Hafezi, M., and Aflatoonian, R. (2015). Gene expression analysis of VEGF and its receptors and assessment of its serum level in unexplained recurrent spontaneous abortion. Cell J. 16 (4), 538–545. doi:10.22074/cellj.2015.498
Anacker, J., Segerer, S. E., Hagemann, C., Feix, S., Kapp, M., Bausch, R., et al. (2011). Human decidua and invasive trophoblasts are rich sources of nearly all human matrix metalloproteinases. Mol. Hum. Reprod. 17 (10), 637–652. doi:10.1093/molehr/gar033
Anin, S. A., Vince, G., and Quenby, S. (2004). Trophoblast invasion. Hum. Fertil. 7 (3), 169–174. doi:10.1080/14647270400006911
Anton, L., Olarerin-George, A. O., Hogenesch, J. B., and Elovitz, M. A. (2015). Placental expression of miR-517a/b and miR-517c contributes to trophoblast dysfunction and preeclampsia. PLOS ONE 10 (3), e0122707. doi:10.1371/journal.pone.0122707
Anton, L., Olarerin-George, A. O., Schwartz, N., Srinivas, S., Bastek, J., Hogenesch, J. B., et al. (2013). miR-210 inhibits trophoblast invasion and is a serum biomarker for preeclampsia. Am. J. Pathol. 183 (5), 1437–1445. doi:10.1016/j.ajpath.2013.07.021
Aplin, J. D., Myers, J. E., Timms, K., and Westwood, M. (2020). Tracking placental development in health and disease. Nat. Rev. Endocrinol. 16 (9), 479–494. doi:10.1038/s41574-020-0372-6
Aplin, J. D. (2000). The cell biological basis of human implantation. Baillieres Best. Pract. Res. Clin. Obstet. Gynaecol. 14 (5), 757–764. doi:10.1053/beog.2000.0116
Azizi, F., Saleh Gargari, S., Asadi Shahmirzadi, S., Dodange, F., Amiri, V., Mirfakhraie, R., et al. (2017). Evaluation of placental mir-155-5p and long non-coding RNA sONE expression in patients with severe pre-eclampsia. Int. J. Mol. Cell. Med. 6 (1), 22–30.
Bakrania, B. A., George, E. M., and Granger, J. P. (2022). Animal models of preeclampsia: Investigating pathophysiology and therapeutic targets. Am. J. Obstet. Gynecol. 226 (2), S973–S987. doi:10.1016/j.ajog.2020.10.025
Bartel, D. P. (2009). MicroRNAs: Target recognition and regulatory functions. Cell 136 (2), 215–233. doi:10.1016/j.cell.2009.01.002
Behm-Ansmant, I., Rehwinkel, J., Doerks, T., Stark, A., Bork, P., and Izaurralde, E. (2006). MRNA degradation by miRNAs and GW182 requires both CCR4 : NOT deadenylase and DCP1 : DCP2 decapping complexes. Genes Dev. 20 (14), 1885–1898. doi:10.1101/gad.1424106
Bellos, I., Karageorgiou, V., Kapnias, D., Karamanli, K.-E., and Siristatidis, C. (2018). The role of interleukins in preeclampsia: A comprehensive review. Am. J. Reprod. Immunol. 80 (6), e13055. doi:10.1111/aji.13055
Benhamed, M., Herbig, U., Ye, T., Dejean, A., and Bischof, O. (2012). Senescence is an endogenous trigger for microRNA-directed transcriptional gene silencing in human cells. Nat. Cell Biol. 14 (3), 266–275. doi:10.1038/ncb2443
Benyo, D. F., Smarason, A., Redman, C. W. G., Sims, C., and Conrad, K. P. (2001). Expression of inflammatory cytokines in placentas from women with preeclampsia. J. Clin. Endocrinol. Metab. 86 (6), 2505–2512. doi:10.1210/jcem.86.6.7585
Bian, X., Liu, J., Yang, Q., Liu, Y., Jia, W., Zhang, X., et al. (2021). MicroRNA-210 regulates placental adaptation to maternal hypoxic stress during pregnancy. Biol. Reprod. 104 (2), 418–429. doi:10.1093/biolre/ioaa187
Bidarimath, M., Khalaj, K., Wessels, J. M., and Tayade, C. (2014). MicroRNAs, immune cells and pregnancy. Cell. Mol. Immunol. 11 (6), 538–547. doi:10.1038/cmi.2014.45
Bischof, P., Meisser, A., and Campana, A. (2000). Paracrine and autocrine regulators of trophoblast invasion--a review. Placenta 21, S55–S60. doi:10.1053/plac.2000.0521
Blaschitz, A., Hutter, H., and Dohr, G. (2001). HLA Class I protein expression in the human placenta. Early Pregnancy (Cherry Hill) 5 (1), 67–69.
Bortolin-Cavaille, M.-L., Dance, M., Weber, M., and Cavaillé, J. (2009). C19MC microRNAs are processed from introns of large Pol-II, non-protein-coding transcripts. Nucleic Acids Res. 37 (10), 3464–3473. doi:10.1093/nar/gkp205
Braun, J. E., Huntzinger, E., Fauser, M., and Izaurralde, E. (2011). GW182 proteins directly recruit cytoplasmic deadenylase complexes to miRNA targets. Mol. Cell 44 (1), 120–133. doi:10.1016/j.molcel.2011.09.007
Braun, J. E., Truffault, V., Boland, A., Huntzinger, E., Chang, C. T., Haas, G., et al. (2012). A direct interaction between DCP1 and XRN1 couples mRNA decapping to 5' exonucleolytic degradation. Nat. Struct. Mol. Biol. 19 (12), 1324–1331. doi:10.1038/nsmb.2413
Brkić, J., Dunk, C., O'Brien, J., Fu, G., Nadeem, L., Wang, Y. L., et al. (2018). MicroRNA-218-5p promotes endovascular trophoblast differentiation and spiral artery remodeling. Mol. Ther. 26 (9), 2189–2205. doi:10.1016/j.ymthe.2018.07.009
Brkić, J., Dunk, C., Shan, Y., O'Brien, J. A., Lye, P., Qayyum, S., et al. (2020). Differential role of Smad2 and Smad3 in the acquisition of an endovascular trophoblast-like phenotype and preeclampsia. Front. Endocrinol. 11, 436. doi:10.3389/fendo.2020.00436
Brosens, I., Pijnenborg, R., Vercruysse, L., and Romero, R. (2011). The “Great Obstetrical Syndromes” are associated with disorders of deep placentation. Am. J. Obstet. Gynecol. 204 (3), 193–201. doi:10.1016/j.ajog.2010.08.009
Bulla, R., Villa, A., Bossi, F., Cassetti, A., Radillo, O., Spessotto, P., et al. (2005). VE-cadherin is a critical molecule for trophoblast–endothelial cell interaction in decidual spiral arteries. Exp. Cell Res. 303 (1), 101–113. doi:10.1016/j.yexcr.2004.09.015
Burton, G. J., Cindrova-Davies, T., Yung, H. W., and Jauniaux, E. (2021). Hypoxia and reproductive health: Oxygen and development of the human placenta. Reprod. Camb. Engl. 161 (1), F53–F65. doi:10.1530/rep-20-0153
Burton, G. J., and Fowden, A. L. (2015). The placenta: A multifaceted, transient organ. Philos. Trans. R. Soc. Lond. B Biol. Sci. 370 (1663), 20140066. doi:10.1098/rstb.2014.0066
Burton, G. J., Jauniaux, E., and Charnock-Jones, D. S. (2007). Human early placental development: Potential roles of the endometrial glands. Placenta 28, S64–S69. doi:10.1016/j.placenta.2007.01.007
Burton, G. J., Redman, C. W., Roberts, J. M., and Moffett, A. (2019). Pre-eclampsia: Pathophysiology and clinical implications. BMJ 366, l2381. doi:10.1136/bmj.l2381
Burton, G. J., Woods, A. W., Jauniaux, E., and Kingdom, J. C. P. (2009). Rheological and physiological consequences of conversion of the maternal spiral arteries for uteroplacental blood flow during human pregnancy. Placenta 30 (6), 473–482. doi:10.1016/j.placenta.2009.02.009
Cai, M., Kolluru, G. K., and Ahmed, A. (2017). Small molecule, big prospects: MicroRNA in pregnancy and its complications. J. Pregnancy 2017, 6972732. doi:10.1155/2017/6972732
Camm, E. J., Botting, K. J., and Sferruzzi-Perri, A. N. (2018). Near to one's heart: The intimate relationship between the placenta and fetal heart. Front. Physiol. 9, 629. doi:10.3389/fphys.2018.00629
Capes-Davis, A., Theodosopoulos, G., Atkin, I., Drexler, H. G., Kohara, A., MacLeod, R. A. F., et al. (2010). Check your cultures! A list of cross-contaminated or misidentified cell lines. Int. J. Cancer 127 (1), 1–8. doi:10.1002/ijc.25242
Cardillo, C., Kilcoyne, C. M., Cannon, R. O., and Panza, J. A. (2000). Interactions between nitric oxide and endothelin in the regulation of vascular tone of human resistance vessels in vivo. Hypertension 35(6), 1237–1241. doi:10.1161/01.HYP.35.6.1237
Carrasco-Wong, I., Aguilera-Olguin, M., Escalona-Rivano, R., Chiarello, D. I., Barragan-Zuniga, L. J., Sosa-Macias, M., et al. (2021). Syncytiotrophoblast stress in early onset preeclampsia: The issues perpetuating the syndrome. Placenta 113, 57–66. doi:10.1016/j.placenta.2021.05.002
Carreras-Badosa, G., Bonmatí, A., Ortega, F.-J., Mercader, J.-M., Guindo-Martínez, M., Torrents, D., et al. (2017). Dysregulation of placental miRNA in maternal obesity is associated with pre- and postnatal growth. J. Clin. Endocrinol. Metab. 102 (7), 2584–2594. doi:10.1210/jc.2017-00089
Carter, A. M. (2007). Animal models of human placentation--a review. Placenta 28, S41–S47. doi:10.1016/j.placenta.2006.11.002
Carty, D. M., Delles, C., and Dominiczak, A. F. (2008). Novel biomarkers for predicting preeclampsia. Trends cardiovasc. Med. 18 (5), 186–194. doi:10.1016/j.tcm.2008.07.002
Chaiwangyen, W., Ospina-Prieto, S., Photini, S. M., Schleussner, E., Markert, U. R., and Morales-Prieto, D. M. (2015). Dissimilar microRNA-21 functions and targets in trophoblastic cell lines of different origin. Int. J. Biochem. Cell Biol. 68, 187–196. doi:10.1016/j.biocel.2015.08.018
Chan, Y. C., Banerjee, J., Choi, S. Y., and Sen, C. K. (2012). miR-210: The master hypoxamir. Microcirculation 19 (3), 215–223. doi:10.1111/j.1549-8719.2011.00154.x
Chandradoss, S. D., Schirle, N. T., Szczepaniak, M., MacRae, I. J., and Joo, C. (2015). A dynamic search process underlies MicroRNA targeting. Cell 162 (1), 96–107. doi:10.1016/j.cell.2015.06.032
Chaouat, G., and Clark, D. A. (2015). Are animal models useful or confusing in understanding the human feto-maternal relationship? A debate. J. Reprod. Immunol. 108, 56–64. doi:10.1016/j.jri.2014.10.004
Chen, C. Y. A., Zheng, D. H., Xia, Z. F., and Shyu, A. B. (2009). Ago-TNRC6 triggers microRNA-mediated decay by promoting two deadenylation steps. Nat. Struct. Mol. Biol. 16 (11), 1160–U1166. doi:10.1038/nsmb.1709
Chen, L. M., Liu, B., Zhao, H. B., Stone, P., Chen, Q., and Chamley, L. (2010a). IL-6, TNFalpha and TGFbeta promote nonapoptotic trophoblast deportation and subsequently causes endothelial cell activation. Placenta 31 (1), 75–80. doi:10.1016/j.placenta.2009.11.005
Chen, Y.-Y., Chuang, P.-Y., Chen, C.-P., Chiu, Y.-H., Lo, H.-F., Cheong, M.-L., et al. (2014). Functional antagonism between high temperature requirement protein A (HtrA) family members regulates trophoblast invasion. J. Biol. Chem. 289 (33), 22958–22968. doi:10.1074/jbc.M114.576744
Chen, Z., Li, Y., Zhang, H., Huang, P., and Luthra, R. (2010b). Hypoxia-regulated microRNA-210 modulates mitochondrial function and decreases ISCU and COX10 expression. Oncogene 29 (30), 4362–4368. doi:10.1038/onc.2010.193
Cheng, M. H., and Wang, P. H. (2009). Placentation abnormalities in the pathophysiology of preeclampsia. Expert Rev. Mol. diagn. 9 (1), 37–49. doi:10.1586/14737159.9.1.37
Choi, S. Y., Yun, J., Lee, O. J., Han, H. S., Yeo, M. K., Lee, M. A., et al. (2013). MicroRNA expression profiles in placenta with severe preeclampsia using a PNA-based microarray. Placenta 34 (9), 799–804. doi:10.1016/j.placenta.2013.06.006
Choudhury, R. H., Dunk, C. E., Lye, S. J., Aplin, J. D., Harris, L. K., and Jones, R. L. (2017). Extravillous trophoblast and endothelial cell crosstalk mediates leukocyte infiltration to the early remodeling decidual spiral arteriole wall. J. Immunol. 198 (10), 4115–4128. doi:10.4049/jimmunol.1601175
Colleoni, F., Padmanabhan, N., Yung, H. W., Watson, E. D., Cetin, I., Tissot van Patot, M. C., et al. (2013). Suppression of mitochondrial electron transport chain function in the hypoxic human placenta: A role for miRNA-210 and protein synthesis inhibition. PLoS One 8 (1), e55194. doi:10.1371/journal.pone.0055194
Cornelius, D. C. (2018). Preeclampsia: From inflammation to immunoregulation. Clin. Med. Insights. Blood Disord. 11, 1179545X17752325. doi:10.1177/1179545x17752325
Costa, F., and Biaggioni, I. (1998). Role of nitric oxide in adenosine-induced vasodilation in humans. Hypertension 31 (5), 1061–1064. doi:10.1161/01.hyp.31.5.1061
Costa, M. A. (2016). The endocrine function of human placenta: An overview. Reprod. Biomed. Online 32 (1), 14–43. doi:10.1016/j.rbmo.2015.10.005
Cui, J., Chen, X., Lin, S., Li, L., Fan, J., Hou, H., et al. (2020). MiR-101-containing extracellular vesicles bind to BRD4 and enhance proliferation and migration of trophoblasts in preeclampsia. Stem Cell Res. Ther. 11 (1), 231. doi:10.1186/s13287-020-01720-9
Dai, Y., Qiu, Z., Diao, Z., Shen, L., Xue, P., Sun, H., et al. (2012). MicroRNA-155 inhibits proliferation and migration of human extravillous trophoblast derived HTR-8/SVneo cells via down-regulating cyclin D1. Placenta 33 (10), 824–829. doi:10.1016/j.placenta.2012.07.012
Damsky, C. H., and Fisher, S. J. (1998). Trophoblast pseudo-vasculogenesis: Faking it with endothelial adhesion receptors. Curr. Opin. Cell Biol. 10 (5), 660–666. doi:10.1016/S0955-0674(98)80043-4
Damsky, C. H., Librach, C., Lim, K. H., Fitzgerald, M. L., McMaster, M. T., Janatpour, M., et al. (1994). Integrin switching regulates normal trophoblast invasion. Development 120 (12), 3657–3666. doi:10.1242/dev.120.12.3657
Davey, D. A., and MacGillivray, I. (1988). The classification and definition of the hypertensive disorders of pregnancy. Am. J. Obstet. Gynecol. 158 (4), 892–898. doi:10.1016/0002-9378(88)90090-7
Denli, A. M., Tops, B. B., Plasterk, R. H., Ketting, R. F., and Hannon, G. J. (2004). Processing of primary microRNAs by the Microprocessor complex. Nature 432 (7014), 231–235. doi:10.1038/nature03049
Devor, E., Santillan, D., Scroggins, S., Warrier, A., and Santillan, M. (2020). Trimester-specific plasma exosome microRNA expression profiles in preeclampsia. J. Matern. Fetal. Neonatal Med. 33 (18), 3116–3124. doi:10.1080/14767058.2019.1569614
Ding, J., Huang, F., Wu, G., Han, T., Xu, F., Weng, D., et al. (2015). MiR-519d-3p suppresses invasion and migration of trophoblast cells via targeting MMP-2. PLoS One 10 (3), e0120321. doi:10.1371/journal.pone.0120321
Dong, X., Zhang, Y., Chen, X., and Xue, M. (2020). Long noncoding RNA LINC00511 regulates the proliferation, apoptosis, invasion and autophagy of trophoblast cells to mediate pre-eclampsia progression through modulating the miR-31-5p/homeobox protein A7 axis. J. Obstet. Gynaecol. Res. 46 (8), 1298–1309. doi:10.1111/jog.14344
Donker, R. B., Mouillet, J. F., Chu, T., Hubel, C. A., Stolz, D. B., Morelli, A. E., et al. (2012). The expression profile of C19MC microRNAs in primary human trophoblast cells and exosomes. Mol. Hum. Reprod. 18 (8), 417–424. doi:10.1093/molehr/gas013
Doridot, L., Miralles, F., Barbaux, S., and Vaiman, D. (2013). Trophoblasts, invasion, and microRNA. Front. Genet. 4, 248. doi:10.3389/fgene.2013.00248
Ducat, A., Vargas, A., Doridot, L., Bagattin, A., Lerner, J., Vilotte, J. L., et al. (2019). Low-dose aspirin protective effects are correlated with deregulation of HNF factor expression in the preeclamptic placentas from mice and humans. Cell Death Discov. 5, 94. doi:10.1038/s41420-019-0170-x
Ellwanger, D. C., Buttner, F. A., Mewes, H. W., and Stumpflen, V. (2011). The sufficient minimal set of miRNA seed types. Bioinformatics 27 (10), 1346–1350. doi:10.1093/bioinformatics/btr149
Fabian, M. R., and Sonenberg, N. (2012). The mechanics of miRNA-mediated gene silencing: A look under the hood of miRISC. Nat. Struct. Mol. Biol. 19 (6), 586–593. doi:10.1038/nsmb.2296
Fan, X. J., Muruganandan, S., Shallie, P. D., Dhal, S., Petitt, M., and Nayak, N. R. (2021). VEGF maintains maternal vascular space homeostasis in the mouse placenta through modulation of trophoblast giant cell functions. Biomolecules 11 (7), 1062. doi:10.3390/biom11071062
Fang, M., Du, H., Han, B., Xia, G., Shi, X., Zhang, F., et al. (2017). Hypoxia-inducible microRNA-218 inhibits trophoblast invasion by targeting LASP1: Implications for preeclampsia development. Int. J. Biochem. Cell Biol. 87, 95–103. doi:10.1016/j.biocel.2017.04.005
Fang, Z., and Rajewsky, N. (2011). The impact of miRNA target sites in coding sequences and in 3'UTRs. PLoS One 6 (3), e18067. doi:10.1371/journal.pone.0018067
Fantone, S., Giannubilo, S. R., Marzioni, D., and Tossetta, G. (2021). HTRA family proteins in pregnancy outcome. Tissue Cell 72, 101549. doi:10.1016/j.tice.2021.101549
Fareh, M., Yeom, K.-H., Haagsma, A. C., Chauhan, S., Heo, I., and Joo, C. (2016). TRBP ensures efficient Dicer processing of precursor microRNA in RNA-crowded environments. Nat. Commun. 7 (1), 13694. doi:10.1038/ncomms13694
Farrokhnia, F., Aplin, J. D., Westwood, M., and Forbes, K. (2014). MicroRNA regulation of mitogenic signaling networks in the human placenta. J. Biol. Chem. 289 (44), 30404–30416. doi:10.1074/jbc.M114.587295
Ferreira, L. M. R., Meissner, T. B., Tilburgs, T., and Strominger, J. L. (2017). HLA-G: At the interface of maternal–fetal tolerance. Trends Immunol. 38 (4), 272–286. doi:10.1016/j.it.2017.01.009
Fisher, S. J., and Damsky, C. H. (1993). Human cytotrophoblast invasion. Semin. Cell Biol. 4 (3), 183–188. doi:10.1006/scel.1993.1022
Fisher, S. J. (2015). Why is placentation abnormal in preeclampsia? Am. J. Obstet. Gynecol. 213 (4), S115–S122. doi:10.1016/j.ajog.2015.08.042
Foley, H. B., Howe, C. G., Eckel, S. P., Chavez, T., Gevorkian, L., Reyes, E. G., et al. (2021). Extracellular vesicle-enriched miRNA profiles across pregnancy in the MADRES cohort. PLOS ONE 16 (5), e0251259. doi:10.1371/journal.pone.0251259
Frazier, S., McBride, M. W., Mulvana, H., and Graham, D. (2020). From animal models to patients: The role of placental microRNAs, miR-210, miR-126, and miR-148a/152 in preeclampsia. Clin. Sci. 134 (8), 1001–1025. doi:10.1042/cs20200023
Fu, G., Brkic, J., Hayder, H., and Peng, C. (2013a). MicroRNAs in human placental development and pregnancy complications. Int. J. Mol. Sci. 14 (3), 5519–5544. doi:10.3390/ijms14035519
Fu, G., Ye, G., Nadeem, L., Ji, L., Manchanda, T., Wang, Y., et al. (2013b). MicroRNA-376c impairs transforming growth factor-beta and nodal signaling to promote trophoblast cell proliferation and invasion. Hypertension 61 (4), 864–872. doi:10.1161/HYPERTENSIONAHA.111.203489
Fukao, A., Mishima, Y., Takizawa, N., Oka, S., Imataka, H., Pelletier, J., et al. (2014). MicroRNAs trigger dissociation of eIF4AI and eIF4AII from target mRNAs in humans. Mol. Cell 56 (1), 79–89. doi:10.1016/j.molcel.2014.09.005
Gatford, K. L., Andraweera, P. H., Roberts, C. T., and Care, A. S. (2020). Animal models of preeclampsia: Causes, consequences, and interventions. Hypertension 75(6), 1363–1381. doi:10.1161/HYPERTENSIONAHA.119.14598
Genbacev, O., Zhou, Y., Ludlow, J. W., and Fisher, S. J. (1997). Regulation of human placental development by oxygen tension. Science 277 (5332), 1669–1672. doi:10.1126/science.277.5332.1669
Glazov, E. A., McWilliam, S., Barris, W. C., and Dalrymple, B. P. (2008). Origin, evolution, and biological role of miRNA cluster in DLK-DIO3 genomic region in placental mammals. Mol. Biol. Evol. 25 (5), 939–948. doi:10.1093/molbev/msn045
Gonzalez, T. L., Eisman, L. E., Joshi, N. V., Flowers, A. E., Wu, D., Wang, Y., et al. (2021). High-throughput miRNA sequencing of the human placenta: Expression throughout gestation. Epigenomics 13 (13), 995–1012. doi:10.2217/epi-2021-0055
Gottlieb, A., Flor, I., Nimzyk, R., Burchardt, L., Helmke, B., Langenbuch, M., et al. (2021). The expression of miRNA encoded by C19MC and miR-371-3 strongly varies among individual placentas but does not differ between spontaneous and induced abortions. Protoplasma 258 (1), 209–218. doi:10.1007/s00709-020-01548-3
Graham, C. H., Hawley, T. S., Hawley, R. C., MacDougall, J. R., Kerbel, R. S., Khoo, N., et al. (1993). Establishment and characterization of first trimester human trophoblast cells with extended lifespan. Exp. Cell Res. 206 (2), 204–211. doi:10.1006/excr.1993.1139
Gu, W. J., Wang, X. F., Zhai, C. Y., Zhou, T., and Xie, X. Y. (2013a). Biological basis of miRNA action when their targets are located in human protein coding region. Plos One 8 (5), e63403. ARTN e63403. doi:10.1371/journal.pone.0063403
Gu, Y., Sun, J., Groome, L. J., and Wang, Y. (2013b). Differential miRNA expression profiles between the first and third trimester human placentas. Am. J. Physiol. Endocrinol. Metab. 304 (8), E836–E843. doi:10.1152/ajpendo.00660.2012
Guerby, P., Swiader, A., Auge, N., Parant, O., Vayssiere, C., Uchida, K., et al. (2019). High glutathionylation of placental endothelial nitric oxide synthase in preeclampsia. Redox Biol. 22, 101126. doi:10.1016/j.redox.2019.101126
Guillard, C., Zidi, I., Marcou, C., Menier, C., Carosella, E. D., and Moreau, P. (2008). Role of HLA-G in innate immunity through direct activation of NF-kappaB in natural killer cells. Mol. Immunol. 45 (2), 419–427. doi:10.1016/j.molimm.2007.06.160
Ha, M., and Kim, V. N. (2014). Regulation of microRNA biogenesis. Nat. Rev. Mol. Cell Biol. 15 (8), 509–524. doi:10.1038/nrm3838
Hackmon, R., Pinnaduwage, L., Zhang, J., Lye, S. J., Geraghty, D. E., and Dunk, C. E. (2017). Definitive class I human leukocyte antigen expression in gestational placentation: HLA-F, HLA-E, HLA-C, and HLA-G in extravillous trophoblast invasion on placentation, pregnancy, and parturition. Am. J. Reprod. Immunol. 77 (6), e12643. doi:10.1111/aji.12643
Han, J., Lee, Y., Yeom, K. H., Kim, Y. K., Jin, H., and Kim, V. N. (2004). The Drosha-DGCR8 complex in primary microRNA processing. Genes Dev. 18 (24), 3016–3027. doi:10.1101/gad.1262504
Harris, L. K., McCormick, J., Cartwright, J. E., Whitley, G. S. J., and Dash, P. R. (2008). S-nitrosylation of proteins at the leading edge of migrating trophoblasts by inducible nitric oxide synthase promotes trophoblast invasion. Exp. Cell Res. 314 (8), 1765–1776. doi:10.1016/j.yexcr.2008.02.010
Harris, L. K. (2010). Review: Trophoblast-vascular cell interactions in early pregnancy: How to remodel a vessel. Placenta 31, S93–S98. doi:10.1016/j.placenta.2009.12.012
Hasan, M. Z., Ikawati, M., Tocharus, J., Kawaichi, M., and Oka, C. (2015). Abnormal development of placenta in HtrA1-deficient mice. Dev. Biol. 397 (1), 89–102. doi:10.1016/j.ydbio.2014.10.015
Hayder, H., Fu, G., Nadeem, L., O’Brien, J. A., Lye, S. J., and Peng, C. (2021). Overexpression of miR-210-3p impairs extravillous trophoblast functions associated with uterine spiral artery remodeling. Int. J. Mol. Sci. 22 (8), 3961. doi:10.3390/ijms22083961
Hayder, H., O'Brien, J., Nadeem, U., and Peng, C. (2018). MicroRNAs: Crucial regulators of placental development. Reproduction 155 (6), R259–R271. doi:10.1530/rep-17-0603
Hemmatzadeh, M., Shomali, N., Yousefzadeh, Y., Mohammadi, H., Ghasemzadeh, A., and Yousefi, M. (2020). MicroRNAs: Small molecules with a large impact on pre-eclampsia. J. Cell. Physiol. 235 (4), 3235–3248. doi:10.1002/jcp.29286
Henderson, J. T., Whitlock, E. P., O’Connor, E., Senger, C. A., Thompson, J. H., and Rowland, M. G. (2014). Low-dose aspirin for prevention of morbidity and mortality from preeclampsia: A systematic evidence review for the US preventive services task force. Ann. Intern. Med. 160 (10), 695–703. doi:10.7326/M13-2844
Hornakova, A., Kolkova, Z., Holubekova, V., Loderer, D., Lasabova, Z., Biringer, K., et al. (2020). Diagnostic potential of MicroRNAs as biomarkers in the detection of preeclampsia. Genet. Test. Mol. Biomarkers 24 (6), 321–327. doi:10.1089/gtmb.2019.0264
Hromadnikova, I., Kotlabova, K., Ondrackova, M., Kestlerova, A., Novotna, V., Hympanova, L., et al. (2013). Circulating C19MC MicroRNAs in preeclampsia, gestational hypertension, and fetal growth restriction. Mediat. Inflamm. 2013, 186041. doi:10.1155/2013/186041
Hu, X.-Q., Dasgupta, C., Xiao, D., Huang, X., Yang, S., and Zhang, L. (2017). Micro RNA-210 targets TET1 and suppresses pregnancy-mediated adaptation of BKCa channel expression and function in ovine uterine arteries. Hypertens. (Dallas, TexHYPERTENSIONAHA 117, 09864. doi:10.1161/HYPERTENSIONAHA.117.09864
Hu, X. Q., and Zhang, L. (2019). MicroRNAs in uteroplacental vascular dysfunction. Cells 8 (11), E1344. doi:10.3390/cells8111344
Huang, J., Zheng, L., Kong, H., Wang, F., Su, Y., and Xin, H. (2020). miR-139-5p promotes the proliferation and invasion of trophoblast cells by targeting sFlt-1 in preeclampsia. Placenta 92, 37–43. doi:10.1016/j.placenta.2020.02.003
Huang, V., and Li, L. C. (2012). miRNA goes nuclear. RNA Biol. 9 (3), 269–273. doi:10.4161/rna.19354
Huang, X. H., Wu, L., Zhang, G. Y., Tang, R. R., and Zhou, X. (2019). Elevated MicroRNA-181a-5p contributes to trophoblast dysfunction and preeclampsia. Reprod. Sci. 26 (8), 1121–1129. doi:10.1177/1933719118808916
Hubel, C. A. (1999). Oxidative stress in the pathogenesis of preeclampsia. Proc. Soc. Exp. Biol. Med. 222 (3), 222–235. doi:10.1046/j.1525-1373.1999.d01-139.x
Huntzinger, E., and Izaurralde, E. (2011). Gene silencing by microRNAs: Contributions of translational repression and mRNA decay. Nat. Rev. Genet. 12 (2), 99–110. doi:10.1038/nrg2936
Huntzinger, E., Kuzuoglu-Ozturk, D., Braun, J. E., Eulalio, A., Wohlbold, L., and Izaurralde, E. (2013). The interactions of GW182 proteins with PABP and deadenylases are required for both translational repression and degradation of miRNA targets. Nucleic Acids Res. 41 (2), 978–994. doi:10.1093/nar/gks1078
Huppertz, B., Kertschanska, S., Demir, A. Y., Frank, H.-G., and Kaufmann, P. (1997). Immunohistochemistry of matrix metalloproteinases (MMP), their substrates, and their inhibitors (TIMP) during trophoblast invasion in the human placenta. Cell Tissue Res. 291 (1), 133–148. doi:10.1007/s004410050987
Huppertz, B. (2018). The critical role of abnormal trophoblast development in the etiology of preeclampsia. Curr. Pharm. Biotechnol. 19 (10), 771–780. doi:10.2174/1389201019666180427110547
Iliodromiti, Z., Antonakopoulos, N., Sifakis, S., Tsikouras, P., Daniilidis, A., Dafopoulos, K., et al. (2012). Endocrine, paracrine, and autocrine placental mediators in labor. Horm. (Athens) 11 (4), 397–409. doi:10.14310/horm.2002.1371
Imakawa, K., and Nakagawa, S. (2017). The phylogeny of placental evolution through dynamic integrations of retrotransposons. Prog. Mol. Biol. Transl. Sci. 145, 89–109. doi:10.1016/bs.pmbts.2016.12.004
Inno, R., Kikas, T., Lillepea, K., and Laan, M. (2021). Coordinated expressional landscape of the human placental miRNome and transcriptome. Front. Cell Dev. Biol. 9, 697947. doi:10.3389/fcell.2021.697947
Ipsaro, J. J., and Joshua-Tor, L. (2015). From guide to target: Molecular insights into eukaryotic RNA-interference machinery. Nat. Struct. Mol. Biol. 22 (1), 20–28. doi:10.1038/nsmb.2931
Jairajpuri, D. S., Malalla, Z. H., Sarray, S., and Mahmood, N. (2021). Analysis of differential expression of hypoxia-inducible microRNA-210 gene targets in mild and severe preeclamptic patients. Noncoding. RNA Res. 6 (1), 51–57. doi:10.1016/j.ncrna.2021.03.001
Ji, L., Brkic, J., Liu, M., Fu, G., Peng, C., and Wang, Y. L. (2013). Placental trophoblast cell differentiation: Physiological regulation and pathological relevance to preeclampsia. Mol. Asp. Med. 34 (5), 981–1023. doi:10.1016/j.mam.2012.12.008
Jo, M. H., Shin, S., Jung, S. R., Kim, E., Song, J. J., and Hohng, S. (2015). Human Argonaute 2 has diverse reaction pathways on target RNAs. Mol. Cell 59 (1), 117–124. doi:10.1016/j.molcel.2015.04.027
Jonas, S., and Izaurralde, E. (2015). Towards a molecular understanding of microRNA-mediated gene silencing. Nat. Rev. Genet. 16 (7), 421–433. doi:10.1038/nrg3965
Jovanović, M., Stefanoska, I., Radojcić, L., and Vićovac, L. (2010). Interleukin-8 (CXCL8) stimulates trophoblast cell migration and invasion by increasing levels of matrix metalloproteinase (MMP)2 and MMP9 and integrins alpha5 and beta1. Reprod. Camb. Engl. 139 (4), 789–798. doi:10.1530/REP-09-0341
Kam, E. P. Y., Gardner, L., Loke, Y. W., and King, A. (1999). The role of trophoblast in the physiological change in decidual spiral arteries. Hum. Reprod. 14 (8), 2131–2138. doi:10.1093/humrep/14.8.2131
Kaufmann, P., Mayhew, T. M., and Charnock-Jones, D. S. (2004). Aspects of human fetoplacental vasculogenesis and angiogenesis. II. Changes during normal pregnancy. Placenta 25 (2-3), 114–126. doi:10.1016/j.placenta.2003.10.009
Kawamata, T., and Tomari, Y. (2010). Making RISC. Trends biochem. Sci. 35 (7), 368–376. doi:10.1016/j.tibs.2010.03.009
Keyes, L. E., Armaza, F. J., Niermeyer, S., Vargas, E., Young, D. A., and Moore, L. G. (2003)., 54. Dc, 20–25. doi:10.1203/01.PDR.0000069846.64389.DCIntrauterine growth restriction, preeclampsia, and intrauterine mortality at high altitude in BoliviaPediatr. Res.1
Khalil, R. A., and Granger, J. P. (2002). Vascular mechanisms of increased arterial pressure in preeclampsia: Lessons from animal models. Am. J. Physiol. Regul. Integr. Comp. Physiol. 283 (1), R29–R45. doi:10.1152/ajpregu.00762.2001
Khanabdali, R., Shakouri-Motlagh, A., Wilkinson, S., Murthi, P., Georgiou, H. M., Brennecke, S. P., et al. (2018). Low-dose aspirin treatment enhances the adhesion of preeclamptic decidual mesenchymal stem/stromal cells and reduces their production of pro-inflammatory cytokines. J. Mol. Med. 96 (11), 1215–1225. doi:10.1007/s00109-018-1695-9
Khankin, E. V., Ko, N. L., Mandala, M., Karumanchi, S. A., and Osol, G. (2021). Normalization of wall shear stress as a physiological mechanism for regulating maternal uterine artery expansive remodeling during pregnancy. FASEB Bioadv. 3 (9), 702–708. doi:10.1096/fba.2021-00019
Kilburn, B. A., Wang, J., Duniec-Dmuchowski, Z. M., Leach, R. E., Romero, R., Armant, D. R., et al. (2000). Extracellular matrix composition and hypoxia regulate the expression of HLA-G and integrins in a human trophoblast cell line. Biol. Reprod. 62 (3), 739–747. doi:10.1095/biolreprod62.3.739
Kim, J., Lee, K.-S., Kim, J.-H., Lee, D.-K., Park, M., Choi, S., et al. (2017). Aspirin prevents TNF-α-induced endothelial cell dysfunction by regulating the NF-κB-dependent miR-155/eNOS pathway: Role of a miR-155/eNOS axis in preeclampsia. Free Radic. Biol. Med. 104, 185–198. doi:10.1016/j.freeradbiomed.2017.01.010
Kim, S., Lee, K. S., Choi, S., Kim, J., Lee, D. K., Park, M., et al. (2018). NF-κB-responsive miRNA-31-5p elicits endothelial dysfunction associated with preeclampsia via down-regulation of endothelial nitric-oxide synthase. J. Biol. Chem. 293 (49), 18989–19000. doi:10.1074/jbc.RA118.005197
Kim, S., Park, M., Kim, J. Y., Kim, T., Hwang, J. Y., Ha, K. S., et al. (2020). Circulating miRNAs associated with dysregulated vascular and trophoblast function as target-based diagnostic biomarkers for preeclampsia. Cells 9 (9), E2003. doi:10.3390/cells9092003
Kim, Y.-W., West, X. Z., and Byzova, T. V. (2013). Inflammation and oxidative stress in angiogenesis and vascular disease. J. Mol. Med. 91 (3), 323–328. doi:10.1007/s00109-013-1007-3
Kim, Y. J., Park, H. S., Lee, H. Y., Ha, E. H., Suh, S. H., Oh, S. K., et al. (2006). Reduced l-arginine level and decreased placental eNOS activity in preeclampsia. Placenta 27 (4), 438–444. doi:10.1016/j.placenta.2005.04.011
Kim, Y. K., Kim, B., and Kim, V. N. (2016). Re-evaluation of the roles of DROSHA, Export in 5, and DICER in microRNA biogenesis. Proc. Natl. Acad. Sci. U. S. A. 113 (13), E1881–E1889. doi:10.1073/pnas.1602532113
Kniss, D. A., and Summerfield, T. L. (2014). Discovery of HeLa cell contamination in HES cells: Call for cell line authentication in reproductive biology research. Reprod. Sci. 21 (8), 1015–1019. doi:10.1177/1933719114522518
Ko, N. L., Mandala, M., John, L., Gelinne, A., and Osol, G. (2018). Venoarterial communication mediates arterial wall shear stress-induced maternal uterine vascular remodeling during pregnancy. Am. J. Physiol. Heart Circ. Physiol. 315 (3), H709–H717. doi:10.1152/ajpheart.00126.2018
Kobayashi, N., Okae, H., Hiura, H., Kubota, N., Kobayashi, E. H., Shibata, S., et al. (2022). The microRNA cluster C19MC confers differentiation potential into trophoblast lineages upon human pluripotent stem cells. Nat. Commun. 13 (1), 3071. doi:10.1038/s41467-022-30775-w
Kolahi, K. S., Valent, A. M., and Thornburg, K. L. (2017). Cytotrophoblast, not syncytiotrophoblast, dominates glycolysis and oxidative phosphorylation in human term placenta. Sci. Rep. 7 (1), 42941. doi:10.1038/srep42941
Krawczynski, K., Mishima, T., Huang, X., and Sadovsky, Y. (2016). Intact feto-placental growth in microRNA-210 deficient mice. Placenta 47, 113–115. doi:10.1016/j.placenta.2016.09.007
Kumasawa, K. (2018). “Animal models in preeclampsia,” in Preeclampsia: Basic, genomic, and clinical. Editor S. Saito, 141–155.
Labialle, S., Marty, V., Bortolin-Cavaillé, M. L., Hoareau-Osman, M., Pradère, J. P., Valet, P., et al. (2014). The miR-379/miR-410 cluster at the imprinted Dlk1-Dio3 domain controls neonatal metabolic adaptation. Embo J. 33 (19), 2216–2230. doi:10.15252/embj.201387038
Lai, W., and Yu, L. (2020). Elevated MicroRNA 183 impairs trophoblast migration and invasiveness by downregulating FOXP1 expression and elevating GNG7 expression during preeclampsia. Mol. Cell. Biol. 41 (1), e00236–00220. doi:10.1128/MCB.00236-20
Lala, P. K., and Chakraborty, C. (2003). Factors regulating trophoblast migration and invasiveness: Possible derangements contributing to pre-eclampsia and fetal injury. Placenta 24 (6), 575–587. doi:10.1016/s0143-4004(03)00063-8
Lasabová, Z., Vazan, M., Zibolenova, J., and Svecova, I. (2015). Overexpression of miR-21 and miR-122 in preeclamptic placentas. Neuro Endocrinol. Lett. 36 (7), 695–699.
Leavey, K., Benton, S. J., Grynspan, D., Kingdom, J. C., Bainbridge, S. A., and Cox, B. J. (2016). Unsupervised placental gene expression profiling identifies clinically relevant subclasses of human preeclampsia. Hypertension 68(1), 137–147. doi:10.1161/HYPERTENSIONAHA.116.07293
Lee, Cheryl Q. E., Gardner, L., Turco, M., Zhao, N., Murray, Matthew J., Coleman, N., et al. (2016). What is trophoblast? A combination of criteria define human first-trimester trophoblast. Stem Cell Rep. 6 (2), 257–272. doi:10.1016/j.stemcr.2016.01.006
Lee, D.-C., Romero, R., Kim, J.-S., Tarca, A. L., Montenegro, D., Pineles, B. L., et al. (2011a). miR-210 targets iron-sulfur cluster scaffold homologue in human trophoblast cell lines: siderosis of interstitial trophoblasts as a novel pathology of preterm preeclampsia and small-for-gestational-age pregnancies. Am. J. Pathol. 179 (2), 590–602. doi:10.1016/j.ajpath.2011.04.035
Lee, D. C., Romero, R., Kim, J. S., Tarca, A. L., Montenegro, D., Pineles, B. L., et al. (2011b). miR-210 targets iron-sulfur cluster scaffold homologue in human trophoblast cell lines siderosis of interstitial trophoblasts as a novel pathology of preterm preeclampsia and small-for-gestational-age pregnancies. Am. J. Pathol. 179 (2), 590–602. doi:10.1016/j.ajpath.2011.04.035
Li, H., Ouyang, Y. S., Sadovsky, E., Parks, W. T., Chu, T. J., and Sadovsky, Y. (2020a). Unique microRNA signals in plasma exosomes from pregnancies complicated by preeclampsia. Hypertension 75 (3), 762–771. doi:10.1161/hypertensionaha.119.14081
Li, H., Zhou, L., Zhang, C., Xi, Q., Lv, J., Huo, W., et al. (2022a). Follistatin dysregulation impaired trophoblast biological functions by GDF11-Smad2/3 axis in preeclampsia placentas. Placenta 121, 145–154. doi:10.1016/j.placenta.2022.03.015
Li, J., Han, J. Y., Zhao, A. M., and Zhang, G. X. (2022b). CircPAPPA regulates the proliferation, migration, invasion, apoptosis, and cell cycle of trophoblast cells through the miR-3127-5p/HOXA7 Axis. Reprod. Sci. 29 (4), 1215–1225. doi:10.1007/s43032-021-00802-0
Li, P., Peng, H., Lu, W.-H., Shuai, H.-L., Zha, Q.-B., Yeung, C.-K., et al. (2015). Role of Slit2/Robo1 in trophoblast invasion and vascular remodeling during ectopic tubal pregnancy. Placenta 36 (10), 1087–1094. doi:10.1016/j.placenta.2015.08.002
Li, P., Shi, Y., Shuai, H., Cai, Y., Lu, W., Wang, G., et al. (2017). Alterted SLIT2/ROBO1 signalling is linked to impaired placentation of missed and threatened miscarriage in early pregnancy. Histopathology 71 (4), 543–552. doi:10.1111/his.13250
Li, Q. H., Han, Y. Y., Xu, P., Yin, L. X., Si, Y. R., Zhang, C. J., et al. (2020b). Elevated microRNA-125b inhibits cytotrophoblast invasion and impairs endothelial cell function in preeclampsia. Cell Death Discov. 6 (1), 35. doi:10.1038/s41420-020-0269-0
Li, R., Wang, N., Xue, M., Long, W., Cheng, C., Mi, C., et al. (2019). A potential regulatory network among WDR86-AS1, miR-10b-3p, and LITAF is possibly involved in preeclampsia pathogenesis. Cell. Signal. 55, 40–52. doi:10.1016/j.cellsig.2018.12.006
Li, W., Yu, N., Fan, L., Chen, S.-H., and Wu, J.-L. (2020c). Circ_0063517 acts as ceRNA, targeting the miR-31-5p-ETBR axis to regulate angiogenesis of vascular endothelial cells in preeclampsia. Life Sci. 244, 117306. doi:10.1016/j.lfs.2020.117306
Li, X., Li, C., Dong, X., and Gou, W. (2014). MicroRNA-155 inhibits migration of trophoblast cells and contributes to the pathogenesis of severe preeclampsia by regulating endothelial nitric oxide synthase. Mol. Med. Rep. 10 (1), 550–554. doi:10.3892/mmr.2014.2214
Li, X. P., Yang, R., Xu, Y., and Zhang, Y. S. (2021a). Circ_0001438 participates in the pathogenesis of preeclampsia via the circ_0001438/miR-942/NLRP3 regulatory network. Placenta 104, 40–50. doi:10.1016/j.placenta.2020.11.005
Li, X. S., Liang, L., Zhang, J., Tong, M. Y., Xia, C. F., Yang, Q. X., et al. (2021b). MiR-296-3p promotes the development and progression of preeclampsia via targeting the CEMIP. Eur. Rev. Med. Pharmacol. Sci. 25 (11), 3938–3946. doi:10.26355/eurrev_202106_26034
Li, Y., Chen, J., and Song, S. (2022c). Circ-OPHN1 suppresses the proliferation, migration, and invasion of trophoblast cells through mediating miR-558/THBS2 axis. Drug Dev. Res. 83, 1034–1046. doi:10.1002/ddr.21931
Li, Y. M., Zhao, X. Y., He, B. W., Wu, W. B., Zhang, H. J., Yang, X. Y., et al. (2021c). Autophagy activation by hypoxia regulates angiogenesis and apoptosis in oxidized low-density lipoprotein-induced preeclampsia. Front. Mol. Biosci. 8, 709751. doi:10.3389/fmolb.2021.709751
Li, Z. J., Wang, S. S., and Li, L. P. (2022d). Advanced oxidative protein products drive trophoblast cells into senescence by inhibiting the autophagy: The potential implication of preeclampsia. Front. Cell Dev. Biol. 10, 810282. doi:10.3389/fcell.2022.810282
Liao, W. X., Laurent, L. C., Agent, S., Hodges, J., and Chen, D. B. (2012). Human placental expression of SLIT/ROBO signaling cues: Effects of preeclampsia and hypoxia. Biol. Reprod. 86 (4), 111. doi:10.1095/biolreprod.110.088138
Licini, C., Avellini, C., Picchiassi, E., Mensà, E., Fantone, S., Ramini, D., et al. (2021). Pre-eclampsia predictive ability of maternal miR-125b: A clinical and experimental study. Transl. Res. 228, 13–27. doi:10.1016/j.trsl.2020.07.011
Lin, L., Li, G. L., Zhang, W. Y., Wang, Y. L., and Yang, H. (2019). Low-dose aspirin reduces hypoxia-induced sFlt1 release via the JNK/AP-1 pathway in human trophoblast and endothelial cells. J. Cell. Physiol. 234 (10), 18928–18941. doi:10.1002/jcp.28533
Liu, B. B., Liu, L., Cui, S. H., Qi, Y., and Wang, T. T. (2021a). Expression and significance of microRNA-126 and VCAM-1 in placental tissues of women with early-onset preeclampsia. J. Obstet. Gynaecol. Res. 47 (6), 2042–2050. doi:10.1111/jog.14732
Liu, C. H., Xing, F., He, Y. Y., Zong, S. S., Luo, C. F., Li, C. Q., et al. (2018a). Elevated HTRA1 and HTRA4 in severe preeclampsia and their roles in trophoblast functions. Mol. Med. Rep. 18 (3), 2937–2944. doi:10.3892/mmr.2018.9289
Liu, C., Zhou, Y., and Zhang, Z. (2012). MiR-210: An important player in the pathogenesis of preeclampsia? J. Cell. Mol. Med. 16 (4), 943–944. doi:10.1111/j.1582-4934.2011.01370.x
Liu, D. F., Li, S. M., Zhu, Q. X., and Jiang, W. (2018b). The involvement of miR-155 in blood pressure regulation in pregnant hypertension rat via targeting FOXO3a. Eur. Rev. Med. Pharmacol. Sci. 22 (20), 6591–6598. doi:10.26355/eurrev_201810_16133
Liu, H., Lei, C., He, Q., Pan, Z., Xiao, D., and Tao, Y. (2018c). Nuclear functions of mammalian MicroRNAs in gene regulation, immunity and cancer. Mol. Cancer 17 (1), 64. doi:10.1186/s12943-018-0765-5
Liu, H., Wang, F., Zhang, Y., Xing, Y., and Wang, Q. (2020a). Exosomal microRNA-139-5p from mesenchymal stem cells accelerates trophoblast cell invasion and migration by motivation of the ERK/MMP-2 pathway via downregulation of protein tyrosine phosphatase. J. Obstet. Gynaecol. Res. 46 (12), 2561–2572. doi:10.1111/jog.14495
Liu, J. D., Rivas, F. V., Wohlschlegel, J., Yates, J. R., Parker, R., and Hannon, G. J. (2005). A role for the P-body component GW182 in microRNA function. Nat. Cell Biol. 7 (12), 1261–1266. doi:10.1038/ncb1333
Liu, J. J., Zhang, L., Zhang, F. F., Luan, T., Yin, Z. M., Rui, C., et al. (2019). Influence of miR-34a on preeclampsia through the Notch signaling pathway. Eur. Rev. Med. Pharmacol. Sci. 23 (3), 923–931. doi:10.26355/eurrev_201902_16978
Liu, J. Y., Yang, Y., Liu, W. L., and Lan, R. L. (2022). circ_0085296 inhibits the biological functions of trophoblast cells to promote the progression of preeclampsia via the miR-942-5p/THBS2 network. Open Med. 17 (1), 577–588. doi:10.1515/med-2022-0427
Liu, R., Wang, X., and Yan, Q. (2020b). The regulatory network of lncRNA DLX6-AS1/miR-149-5p/ERP44 is possibly related to the progression of preeclampsia. Placenta 93, 34–42. doi:10.1016/j.placenta.2020.02.001
Liu, Y., Wu, Y., Liu, S., and Dai, Y. (2021b). Long non-coding RNA TRIM52-AS1 promotes growth and metastasis via miR-218-5p/ROBO1 in hepatocellular carcinoma. Cancer Manag. Res. 13, 547–558. doi:10.2147/cmar.S286205
Lu, T.-M., Lu, W., and Zhao, L.-J. (2017). MicroRNA-137 affects proliferation and migration of placenta trophoblast cells in preeclampsia by targeting ERRα. Reprod. Sci. 24 (1), 85–96. doi:10.1177/1933719116650754
Luo, L., Ye, G., Nadeem, L., Fu, G., Yang, B. B., Honarparvar, E., et al. (2012). MicroRNA-378a-5p promotes trophoblast cell survival, migration and invasion by targeting Nodal. J. Cell Sci. 125 (13), 3124–3132. doi:10.1242/jcs.096412
Luo, S., Cao, N., Tang, Y., and Gu, W. (2017). Identification of key microRNAs and genes in preeclampsia by bioinformatics analysis. PLOS ONE 12 (6), e0178549. doi:10.1371/journal.pone.0178549
Luo, S. S., Ishibashi, O., Ishikawa, G., Ishikawa, T., Katayama, A., Mishima, T., et al. (2009). Human villous trophoblasts express and secrete placenta-specific microRNAs into maternal circulation via exosomes. Biol. Reprod. 81 (4), 717–729. doi:10.1095/biolreprod.108.075481
Luo, X., Pan, C., Guo, X., Gu, C., Huang, Y., Guo, J., et al. (2022). Methylation mediated silencing of miR-155 suppresses the development of preeclampsia in vitro and in vivo by targeting FOXO3. Mediat. Inflamm. 2022, 4250621. doi:10.1155/2022/4250621
Lv, Y., Lu, C., Ji, X., Miao, Z., Long, W., Ding, H., et al. (2019). Roles of microRNAs in preeclampsia. J. Cell. Physiol. 234 (2), 1052–1061. doi:10.1002/jcp.27291
Lyall, F., Robson, S. C., and Bulmer, J. N. (2013). Spiral artery remodeling and trophoblast invasion in preeclampsia and fetal growth restriction: Relationship to clinical outcome. Hypertension 62 (6), 1046–1054. doi:10.1161/HYPERTENSIONAHA.113.01892
Lykoudi, A., Kolialexi, A., Lambrou, G. I., Braoudaki, M., Siristatidis, C., Papaioanou, G. K., et al. (2018). Dysregulated placental microRNAs in early and late onset preeclampsia. Placenta 61, 24–32. doi:10.1016/j.placenta.2017.11.005
Ma, R. X., Liang, Z. J., Shi, X. M., Xu, L. L., Li, X. W., Wu, J. H., et al. (2021). Exosomal miR-486-5p derived from human placental microvascular endothelial cells regulates proliferation and invasion of trophoblasts via targeting IGF1. Hum. Cell 34 (5), 1310–1323. doi:10.1007/s13577-021-00543-x
Mahesh, G., and Biswas, R. (2019). MicroRNA-155: A master regulator of inflammation. J. Interferon Cytokine Res. 39 (6), 321–330. doi:10.1089/jir.2018.0155
Maltepe, E., and Fisher, S. J. (2015). Placenta: The forgotten organ. Annu. Rev. Cell Dev. Biol. 31, 523–552. doi:10.1146/annurev-cellbio-100814-125620
Mao, Y., Hou, B., Shan, L., Sun, X. T., and Wang, L. (2021). Aberrantly up-regulated miR-142-3p inhibited the proliferation and invasion of trophoblast cells by regulating FOXM1. Placenta 104, 253–260. doi:10.1016/j.placenta.2021.01.002
Margioula-Siarkou, G., Margioula-Siarkou, C., Petousis, S., Margaritis, K., Vavoulidis, E., Gullo, G., et al. (2022). The role of endoglin and its soluble form in pathogenesis of preeclampsia. Mol. Cell. Biochem. 477 (2), 479–491. doi:10.1007/s11010-021-04294-z
Martinez-Fierro, M. L., and Garza-Veloz, I. (2021). Analysis of circulating microRNA signatures and preeclampsia development. Cells 10 (5), 1003. doi:10.3390/cells10051003
Matsubara, K., Matsubara, Y., Uchikura, Y., and Sugiyama, T. (2021). Pathophysiology of preeclampsia: The role of exosomes. Int. J. Mol. Sci. 22 (5), 2572. doi:10.3390/ijms22052572
Mayor-Lynn, K., Toloubeydokhti, T., Cruz, A. C., and Chegini, N. (2011). Expression profile of MicroRNAs and mRNAs in human placentas from pregnancies complicated by preeclampsia and preterm labor. Reprod. Sci. 18 (1), 46–56. doi:10.1177/1933719110374115
Mazzuca, M. Q., Li, W., Reslan, O. M., Yu, P., Mata, K. M., and Khalil, R. A. (2014). Downregulation of microvascular endothelial type B endothelin receptor is a central vascular mechanism in hypertensive pregnancy. Hypertension 64(3), 632–643. doi:10.1161/HYPERTENSIONAHA.114.03315
McMaster, M. T., Librach, C. L., Zhou, Y., Lim, K. H., Janatpour, M. J., DeMars, R., et al. (1995). Human placental HLA-G expression is restricted to differentiated cytotrophoblasts. J. Immunol. 154 (8), 3771–3778.
Medley, J. C., Panzade, G., and Zinovyeva, A. Y. (2021). microRNA strand selection: Unwinding the rules. Wiley Interdiscip. Rev. RNA 12 (3), e1627. doi:10.1002/wrna.1627
Meijer, H. A., Kong, Y. W., Lu, W. T., Wilczynska, A., Spriggs, R. V., Robinson, S. W., et al. (2013). Translational repression and eIF4A2 activity are critical for MicroRNA-mediated gene regulation. Science 340 (6128), 82–85. doi:10.1126/science.1231197
Mi, C., Ye, B., Gao, Z., Du, J., Li, R., and Huang, D. (2020). BHLHE40 plays a pathological role in pre-eclampsia through upregulating SNX16 by transcriptional inhibition of miR-196a-5p. Mol. Hum. Reprod. 26 (7), 532–548. doi:10.1093/molehr/gaaa037
Miura, K., Higashijima, A., Murakami, Y., Tsukamoto, O., Hasegawa, Y., Abe, S., et al. (2015). Circulating chromosome 19 miRNA cluster microRNAs in pregnant women with severe pre-eclampsia. J. Obstet. Gynaecol. Res. 41 (10), 1526–1532. doi:10.1111/jog.12749
Mong, E. F., Yang, Y., Akat, K. M., Canfield, J., VanWye, J., Lockhart, J., et al. (2020). Chromosome 19 microRNA cluster enhances cell reprogramming by inhibiting epithelial-to-mesenchymal transition. Sci. Rep. 10 (1), 3029. doi:10.1038/s41598-020-59812-8
Morales-Prieto, D. M., Chaiwangyen, W., Ospina-Prieto, S., Schneider, U., Herrmann, J., Gruhn, B., et al. (2012). MicroRNA expression profiles of trophoblastic cells. Placenta 33 (9), 725–734. doi:10.1016/j.placenta.2012.05.009
Morales-Prieto, D. M., Favaro, R. R., and Markert, U. R. (2020). Placental miRNAs in feto-maternal communication mediated by extracellular vesicles. Placenta 102, 27–33. doi:10.1016/j.placenta.2020.07.001
Morales-Prieto, D. M., Ospina-Prieto, S., Chaiwangyen, W., Schoenleben, M., and Markert, U. R. (2013). Pregnancy-associated miRNA-clusters. J. Reprod. Immunol. 97 (1), 51–61. doi:10.1016/j.jri.2012.11.001
Moser, G., Gauster, M., Orendi, K., Glasner, A., Theuerkauf, R., and Huppertz, B. (2010). Endoglandular trophoblast, an alternative route of trophoblast invasion? Analysis with novel confrontation co-culture models. Hum. Reprod. 25 (5), 1127–1136. doi:10.1093/humrep/deq035
Moser, G., Weiss, G., Gauster, M., Sundl, M., and Huppertz, B. (2015). Evidence from the very beginning: Endoglandular trophoblasts penetrate and replace uterine glands in situ and in vitro. Hum. Reprod. 30 (12), 2747–2757. doi:10.1093/humrep/dev266
Mott, J. L., and Mohr, A. M. (2015). Overview of MicroRNA biology. Semin. Liver Dis. 35 (1), 3–11. doi:10.1055/s-0034-1397344
Mouillet, J. F., Goff, J., Sadovsky, E., Sun, H. J., Parks, T., Chu, T. J., et al. (2020). Transgenic expression of human C19MC miRNAs impacts placental morphogenesis. Placenta 101, 208–214. doi:10.1016/j.placenta.2020.09.069
Mouillet, J. F., Ouyang, Y., Coyne, C. B., and Sadovsky, Y. (2015). MicroRNAs in placental health and disease. Am. J. Obstet. Gynecol. 213 (4), S163–S172. doi:10.1016/j.ajog.2015.05.057
Mouillet, J. F., Ouyang, Y. S., Bayer, A., Coyne, C. B., and Sadovsky, Y. (2014). The role of trophoblastic microRNAs in placental viral infection. Int. J. Dev. Biol. 58 (2-4), 281–289. doi:10.1387/ijdb.130349ys
Mukherjee, I., Dhar, R., Singh, S., Sharma, J. B., Nag, T. C., Mridha, A. R., et al. (2021). Oxidative stress-induced impairment of trophoblast function causes preeclampsia through the unfolded protein response pathway. Sci. Rep. 11 (1), 18415. doi:10.1038/s41598-021-97799-y
Munaut, C., Tebache, L., Blacher, S., Noël, A., Nisolle, M., and Chantraine, F. (2016). Dysregulated circulating miRNAs in preeclampsia. Biomed. Rep. 5 (6), 686–692. doi:10.3892/br.2016.779
Muralimanoharan, S., Maloyan, A., Mele, J., Guo, C., Myatt, L. G., and Myatt, L. (2012). MIR-210 modulates mitochondrial respiration in placenta with preeclampsia. Placenta 33 (10), 816–823. doi:10.1016/j.placenta.2012.07.002
Nadeem, L., Munir, S., Fu, G. D., Dunk, C., Baczyk, D., Caniggia, I., et al. (2011). Nodal signals through activin receptor-like kinase 7 to inhibit trophoblast migration and invasion implication in the pathogenesis of preeclampsia. Am. J. Pathol. 178 (3), 1177–1189. doi:10.1016/j.ajpath.2010.11.066
Nair, A. A., Tang, X. J., Thompson, K. J., Vedell, P. T., Kalari, K. R., and Subramanian, S. (2020). Frequency of MicroRNA response elements identifies pathologically relevant signaling pathways in triple-negative breast cancer. Iscience 23 (6), 101249. ARTN 101249. doi:10.1016/j.isci.2020.101249
Nakashima, A., Cheng, S. B., Ikawa, M., Yoshimori, T., Huber, W. J., Menon, R., et al. (2020). Evidence for lysosomal biogenesis proteome defect and impaired autophagy in preeclampsia. Autophagy 16 (10), 1771–1785. doi:10.1080/15548627.2019.1707494
Nayak, S., Aich, M., Kumar, A., Sengupta, S., Bajad, P., Dhapola, P., et al. (2018). Novel internal regulators and candidate miRNAs within miR-379/miR-656 miRNA cluster can alter cellular phenotype of human glioblastoma. Sci. Rep. 8 (1), 7673. doi:10.1038/s41598-018-26000-8
Nelson-Rees, W. A., Daniels, D. W., and Flandermeyer, R. R. (1981). Cross-contamination of cells in culture. Science 212 (4493), 446–452. doi:10.1126/science.6451928
Ni, H. J., Wang, X., Qu, H. M., Gao, X. L., and Yu, X. Y. (2021). MiR-95-5p involves in the migration and invasion of trophoblast cells by targeting low density lipoprotein receptor-related protein 6. J. Obstet. Gynaecol. Res. 47 (1), 184–197. doi:10.1111/jog.14451
Nikuei, P., Rajaei, M., Roozbeh, N., Mohseni, F., Poordarvishi, F., Azad, M., et al. (2020). Diagnostic accuracy of sFlt1/PlGF ratio as a marker for preeclampsia. BMC Pregnancy Childbirth 20 (1), 80. doi:10.1186/s12884-020-2744-2
Ning, F., Hou, H., Morse, A. N., and Lash, G. E. (2019). Understanding and management of gestational trophoblastic disease. F1000Res 8. doi:10.12688/f1000research.14953.1
Noguer-Dance, M., Abu-Amero, S., Al-Khtib, M., Lefèvre, A., Coullin, P., Moore, G. E., et al. (2010). The primate-specific microRNA gene cluster (C19MC) is imprinted in the placenta. Hum. Mol. Genet. 19 (18), 3566–3582. doi:10.1093/hmg/ddq272
Noris, M., Perico, N., and Remuzzi, G. (2005). Mechanisms of disease: Pre-eclampsia. Nat. Clin. Pract. Nephrol. 1 (2), 98–114. quiz 120. doi:10.1038/ncpneph0035
Norwitz, E. R. (2007). Defective implantation and placentation: Laying the blueprint for pregnancy complications. Reprod. Biomed. Online 14, 101–109. Spec No 1. doi:10.1016/S1472-6483(10)61464-2
O'Brien, J., Hayder, H., Zayed, Y., and Peng, C. (2018). Overview of MicroRNA biogenesis, mechanisms of actions, and circulation. Front. Endocrinol. 9 (402). doi:10.3389/fendo.2018.00402
Ohkuchi, A., Saito, S., Yamamoto, T., Minakami, H., Masuyama, H., Kumasawa, K., et al. (2021). Short-term prediction of preeclampsia using the sFlt-1/PlGF ratio: A subanalysis of pregnant Japanese women from the PROGNOSIS asia study. Hypertens. Res. 44 (7), 813–821. doi:10.1038/s41440-021-00629-x
Okada, C., Yamashita, E., Lee, S. J., Shibata, S., Katahira, J., Nakagawa, A., et al. (2009). A high-resolution structure of the pre-microRNA nuclear export machinery. Science 326 (5957), 1275–1279. doi:10.1126/science.1178705
Opichka, M. A., Rappelt, M. W., Gutterman, D. D., Grobe, J. L., and McIntosh, J. J. (2021). Vascular dysfunction in preeclampsia. Cells 10 (11), 3055. doi:10.3390/cells10113055
Ospina-Prieto, S., Chaiwangyen, W., Herrmann, J., Groten, T., Schleussner, E., Markert, U. R., et al. (2016). MicroRNA-141 is upregulated in preeclamptic placentae and regulates trophoblast invasion and intercellular communication. Transl. Res. 172, 61–72. doi:10.1016/j.trsl.2016.02.012
Ou, Y., Zhu, L., Wei, X., Bai, S., Chen, M., Chen, H., et al. (2020). Circular RNA circ_0111277 attenuates human trophoblast cell invasion and migration by regulating miR-494/HTRA1/Notch-1 signal pathway in pre-eclampsia. Cell Death Dis. 11 (6), 479. doi:10.1038/s41419-020-2679-6
Palei, A. C., Spradley, F. T., Warrington, J. P., George, E. M., and Granger, J. P. (2013). Pathophysiology of hypertension in pre-eclampsia: A lesson in integrative physiology. Acta Physiol. 208 (3), 224–233. doi:10.1111/apha.12106
Palma, C., Jellins, J., Lai, A., Salas, A., Campos, A., Sharma, S., et al. (2021). Extracellular vesicles and preeclampsia: Current knowledge and future research directions. Subcell. Biochem. 97, 455–482. doi:10.1007/978-3-030-67171-6_18
Palmer, S. K., Moore, L. G., Young, D. A., Cregger, B., Berman, J. C., and Zamudio, S. (1999). Altered blood pressure course during normal pregnancy and increased preeclampsia at high altitude (3100 meters) in Colorado. Am. J. Obstet. Gynecol. 180(5), 1161–1168. doi:10.1016/S0002-9378(99)70611-3
Pan, X. L., Noguchi, S., Ando, M., Nishimura, T., and Tomi, M. (2021). MicroRNA-126 suppresses the invasion of trophoblast-model JEG-3 cells by targeting LIN28A. Biochem. Biophys. Res. Commun. 545, 132–137. doi:10.1016/j.bbrc.2021.01.077
Pankiewicz, K., Fijałkowska, A., Issat, T., and Maciejewski, T. M. (2021). Insight into the key points of preeclampsia pathophysiology: Uterine artery remodeling and the role of MicroRNAs. Int. J. Mol. Sci. 22 (6), 3132. doi:10.3390/ijms22063132
Paquette, A. G., Chu, T. J., Wu, X. G., Wang, K., Price, N. D., and Sadovsky, Y. (2018). Distinct communication patterns of trophoblastic miRNA among the maternal-placental-fetal compartments. Placenta 72-73, 28–35. doi:10.1016/j.placenta.2018.10.004
Parada-Niño, L., Castillo-León, L. F., and Morel, A. (2022). Preeclampsia, natural history, genes, and miRNAs associated with the syndrome. J. Pregnancy 2022, 3851225. doi:10.1155/2022/3851225
Paria, B. C., Reese, J., Das, S. K., and Dey, S. K. (2002). Deciphering the cross-talk of implantation: Advances and challenges. Science 296 (5576), 2185–2188. doi:10.1126/science.1071601
Park, M.-H., Galan, H. L., and Arroyo, J. A. (2011). Effect of hypoxia on endothelial nitric oxide synthase, NO production, intracellular survival signaling (p-ERK1/2 and p-AKT) and apoptosis in human term trophoblast. Am. J. Reprod. Immunol. 65 (4), 407–414. doi:10.1111/j.1600-0897.2010.00886.x
Pastuschek, J., Nonn, O., Gutiérrez-Samudio, R. N., Murrieta-Coxca, J. M., Müller, J., Sanft, J., et al. (2021). Molecular characteristics of established trophoblast-derived cell lines. Placenta 108, 122–133. doi:10.1016/j.placenta.2021.02.022
Perez-Roque, L., Nunez-Gomez, E., Rodriguez-Barbero, A., Bernabeu, C., Lopez-Novoa, J. M., and Pericacho, M. (2021). Pregnancy-induced high plasma levels of soluble endoglin in mice lead to preeclampsia symptoms and placental abnormalities. Int. J. Mol. Sci. 22 (1), E165. doi:10.3390/ijms22010165
Phipps, E. A., Thadhani, R., Benzing, T., and Karumanchi, S. A. (2019). Pre-eclampsia: Pathogenesis, novel diagnostics and therapies. Nat. Rev. Nephrol. 15 (5), 275–289. doi:10.1038/s41581-019-0119-6
Pi, Y. L., Tian, X. Y., Ma, J., Zhang, H. F., and Huang, X. H. (2021). Vitamin D alleviates hypoxia/reoxygenation-induced injury of human trophoblast HTR-8 cells by activating autophagy. Placenta 111, 10–18. doi:10.1016/j.placenta.2021.05.008
Pijnenborg, R., Bland, J. M., Robertson, W. B., and Brosens, I. (1983). Uteroplacental arterial changes related to interstitial trophoblast migration in early human pregnancy. Placenta 4 (4), 397–413. doi:10.1016/S0143-4004(83)80043-5
Pijnenborg, R., Bland, J. M., Robertson, W. B., Dixon, G., and Brosens, I. (1981). The pattern of interstitial trophoblastic invasion of the myometrium in early human pregnancy. Placenta 2 (4), 303–316. doi:10.1016/s0143-4004(81)80027-6
Pijnenborg, R., Vercruysse, L., and Hanssens, M. (2006). The uterine spiral arteries in human pregnancy: Facts and controversies. Placenta 27 (9-10), 939–958. doi:10.1016/j.placenta.2005.12.006
Pillai, R. S., Bhattacharyya, S. N., Artus, C. G., Zoller, T., Cougot, N., Basyuk, E., et al. (2005). Inhibition of translational initiation by Let-7 MicroRNA in human cells. Science 309 (5740), 1573–1576. doi:10.1126/science.1115079
Pineles, B. L., Romero, R., Montenegro, D., Tarca, A. L., Han, Y. M., Kim, Y. M., et al. (2007). Distinct subsets of microRNAs are expressed differentially in the human placentas of patients with preeclampsia. Am. J. Obstet. Gynecol. 196 (3), 261.e261–e6. doi:10.1016/j.ajog.2007.01.008
Pique-Regi, R., Romero, R., Tarca, A. L., Sendler, E. D., Xu, Y., Garcia-Flores, V., et al. (2019). Single cell transcriptional signatures of the human placenta in term and preterm parturition. Elife 8, e52004. doi:10.7554/eLife.52004
Pollheimer, J., Vondra, S., Baltayeva, J., Beristain, A. G., and Knöfler, M. (2018). Regulation of placental extravillous trophoblasts by the maternal uterine environment. Front. Immunol. 9, 2597. doi:10.3389/fimmu.2018.02597
Prutsch, N., Fock, V., Haslinger, P., Haider, S., Fiala, C., Pollheimer, J., et al. (2012). The role of interleukin-1β in human trophoblast motility. Placenta 33 (9), 696–703. doi:10.1016/j.placenta.2012.05.008
Qiu, D. M., Wu, J. F., Li, M., Wang, L., Zhu, X. G., and Chen, Y. G. (2021). Impaction of factors associated with oxidative stress on the pathogenesis of gestational hypertension and preeclampsia A Chinese patients based study. Medicine 100 (11), e23666. doi:10.1097/md.0000000000023666
Rana, S., Burke, S. D., and Karumanchi, S. A. (2020). Imbalances in circulating angiogenic factors in the pathophysiology of preeclampsia and related disorders. Am. J. Obstet. Gynecol. 226, S1019–S1034. doi:10.1016/j.ajog.2020.10.022
Rana, S., Lemoine, E., Granger, J. P., and Karumanchi, S. A. (2019). Preeclampsia: Pathophysiology, challenges, and perspectives. Circ. Res. 124 (7), 1094–1112. doi:10.1161/CIRCRESAHA.118.313276
Raymond, D., and Peterson, E. (2011). A critical review of early-onset and late-onset preeclampsia. Obstet. Gynecol. Surv. 66 (8), 497–506. doi:10.1097/OGX.0b013e3182331028
Redman, C., Sargent, I., and Staff, A. (2014). IFPA senior award lecture: Making sense of pre-eclampsia–two placental causes of preeclampsia? Placenta 35, S20–S25. doi:10.1016/j.placenta.2013.12.008
Redman, C. W. G. (1991). Pre-eclampsia and the placenta. Placenta 12 (4), 301–308. doi:10.1016/0143-4004(91)90339-H
Renthal, N. E., Williams, K. r. C., and Mendelson, C. R. (2013). MicroRNAs—Mediators of myometrial contractility during pregnancy and labour. Nat. Rev. Endocrinol. 9 (7), 391–401. doi:10.1038/nrendo.2013.96
Roberge, S., Bujold, E., and Nicolaides, K. H. (2018). Aspirin for the prevention of preterm and term preeclampsia: Systematic review and metaanalysis. Am. J. Obstet. Gynecol. 218 (3), 287287–287293. doi:10.1016/j.ajog.2017.11.561
Rolnik, D. L., Nicolaides, K. H., and Poon, L. C. (2022). Prevention of preeclampsia with aspirin. Am. J. Obstet. Gynecol. 226 (2), S1108–S1119. doi:10.1016/j.ajog.2020.08.045
Rossant, J., and Cross, J. C. (2001). Placental development: Lessons from mouse mutants. Nat. Rev. Genet. 2 (7), 538–548. doi:10.1038/35080570
Roux, E., Bougaran, P., Dufourcq, P., and Couffinhal, T. (2020). Fluid shear stress sensing by the endothelial layer. Front. Physiol. 11, 861. doi:10.3389/fphys.2020.00861
Ruby, J. G., Jan, C. H., and Bartel, D. P. (2007). Intronic microRNA precursors that bypass Drosha processing. Nature 448 (7149), 83–86. doi:10.1038/nature05983
Saito, S., and Nakashima, A. (2014). A review of the mechanism for poor placentation in early-onset preeclampsia: The role of autophagy in trophoblast invasion and vascular remodeling. J. Reprod. Immunol. 101-102, 80–88. doi:10.1016/j.jri.2013.06.002
Salem, M., O'Brien, J. A., Bernaudo, S., Shawer, H., Ye, G., Brkic, J., et al. (2018). miR-590-3p promotes ovarian cancer growth and metastasis via a novel FOXA2-versican pathway. Cancer Res. 78 (15), 4175–4190. doi:10.1158/0008-5472.Can-17-3014
Salomon, C., Guanzon, D., Scholz-Romero, K., Longo, S., Correa, P., Illanes, S. E., et al. (2017). Placental exosomes as early biomarker of preeclampsia: Potential role of exosomal MicroRNAs across gestation. J. Clin. Endocrinol. Metab. 102 (9), 3182–3194. doi:10.1210/jc.2017-00672
Salomon, W. E., Jolly, S. M., Moore, M. J., Zamore, P. D., and Serebrov, V. (2015). Single-molecule imaging reveals that Argonaute reshapes the binding properties of its nucleic acid guides. Cell 162 (1), 84–95. doi:10.1016/j.cell.2015.06.029
Sandrim, V. C., Luizon, M. R., Palei, A. C., Tanus-Santos, J. E., and Cavalli, R. C. (2016). Circulating microRNA expression profiles in pre-eclampsia: Evidence of increased miR-885-5p levels. Bjog 123 (13), 2120–2128. doi:10.1111/1471-0528.13903
Schirle, N. T., Sheu-Gruttadauria, J., and MacRae, I. J. (2014). Structural basis for microRNA targeting. Science 346 (6209), 608–613. doi:10.1126/science.1258040
Schjenken, J. E., Zhang, B., Chan, H. Y., Sharkey, D. J., Fullston, T., and Robertson, S. A. (2016). miRNA regulation of immune tolerance in early pregnancy. Am. J. Reprod. Immunol. 75 (3), 272–280. doi:10.1111/aji.12490
Schmidt, A., Morales-Prieto, D. M., Pastuschek, J., Frohlich, K., and Markert, U. R. (2015). Only humans have human placentas: Molecular differences between mice and humans. J. Reprod. Immunol. 108, 65–71. doi:10.1016/j.jri.2015.03.001
Schmidt, J. K., Block, L. N., and Golos, T. G. (2018). Defining the rhesus macaque placental miRNAome: Conservation of expression of placental miRNA clusters between the macaque and human. Placenta 65, 55–64. doi:10.1016/j.placenta.2018.04.003
Seitz, H., Royo, H., Bortolin, M. L., Lin, S. P., Ferguson-Smith, A. C., and Cavaille, J. (2004). A large imprinted microRNA gene cluster at the mouse Dlk1-Gtl2 domain. Genome Res. 14 (9), 1741–1748. doi:10.1101/gr.2743304
Shan, Y., Chen, Y., Brkić, J., Fournier, L., Ma, H., and Peng, C. (2022). miR-218-5p induces interleukin-1β and endovascular trophoblast differentiation by targeting the transforming growth factor β-SMAD2 pathway. Front. Endocrinol. 13, 842587. doi:10.3389/fendo.2022.842587
Shen, L., Li, Y., Li, R., Diao, Z., Yany, M., Wu, M., et al. (2018). Placenta-associated serum exosomal miR-155 derived from patients with preeclampsia inhibits eNOS expression in human umbilical vein endothelial cells. Int. J. Mol. Med. 41 (3), 1731–1739. doi:10.3892/ijmm.2018.3367
Sheu-Gruttadauria, J., Xiao, Y., Gebert, L. F. R., and MacRae, I. J. (2019). Beyond the seed: Structural basis for supplementary microRNA targeting by human Argonaute2. Embo J. 38 (13), e101153. ARTN e101153. doi:10.15252/embj.2018101153
Shi, Z. Y., She, K. E., Li, H., Yuan, X. H., Han, X., and Wang, Y. Q. (2019). MicroRNA-454 contributes to sustaining the proliferation and invasion of trophoblast cells through inhibiting Nodal/ALK7 signaling in pre-eclampsia. Chem. Biol. Interact. 298, 8–14. doi:10.1016/j.cbi.2018.10.012
Shibata, E., Rajakumar, A., Powers, R. W., Larkin, R. W., Gilmour, C., Bodnar, L. M., et al. (2005). Soluble fms-like tyrosine kinase 1 is increased in preeclampsia but not in normotensive pregnancies with small-for-gestational-age neonates: Relationship to circulating placental growth factor. J. Clin. Endocrinol. Metab. 90 (8), 4895–4903. doi:10.1210/jc.2004-1955
Shih, J.-C., Lin, H.-H., Hsiao, A.-C., Su, Y.-T., Tsai, S., Chien, C.-L., et al. (2019). Unveiling the role of microRNA-7 in linking TGF-β-Smad-mediated epithelial-mesenchymal transition with negative regulation of trophoblast invasion. FASEB J. 33 (5), 6281–6295. doi:10.1096/fj.201801898RR
Simsek, F., Turunc, E., Keskin-Arslan, E., Erol, H., Acar, S., Atakul, B. K., et al. (2022). Molecular mechanisms involved in pre-eclampsia through expressional regulation of endothelin-1. Placenta 124, 55–61. doi:10.1016/j.placenta.2022.05.012
Skalis, G., Katsi, V., Miliou, A., Georgiopoulos, G., Papazachou, O., Vamvakou, G., et al. (2019). MicroRNAs in preeclampsia. Microrna 8 (1), 28–35. doi:10.2174/2211536607666180813123303
Smith, M. D., Pillman, K., Jankovic-Karasoulos, T., McAninch, D., Wan, Q., Bogias, K. J., et al. (2021). Large-scale transcriptome-wide profiling of microRNAs in human placenta and maternal plasma at early to mid gestation. RNA Biol. 18 (1), 507–520. doi:10.1080/15476286.2021.1963105
Smith, S. D., Dunk, C. E., Aplin, J. D., Harris, L. K., and Jones, R. L. (2009). Evidence for immune cell involvement in decidual spiral arteriole remodeling in early human pregnancy. Am. J. Pathol. 174 (5), 1959–1971. doi:10.2353/ajpath.2009.080995
Sõber, S., Reiman, M., Kikas, T., Rull, K., Inno, R., Vaas, P., et al. (2015). Extensive shift in placental transcriptome profile in preeclampsia and placental origin of adverse pregnancy outcomes. Sci. Rep. 5 (1), 13336. doi:10.1038/srep13336
Soncin, F., Natale, D., and Parast, M. M. (2015). Signaling pathways in mouse and human trophoblast differentiation: A comparative review. Cell. Mol. Life Sci. 72 (7), 1291–1302. doi:10.1007/s00018-014-1794-x
Srinivasan, S., Treacy, R., Herrero, T., Olsen, R., Leonardo, T. R., Zhang, X., et al. (2020). Discovery and verification of extracellular miRNA biomarkers for non-invasive prediction of pre-eclampsia in asymptomatic women. Cell Rep. Med. 1 (2), 100013. doi:10.1016/j.xcrm.2020.100013
Staff, A. C., Fjeldstad, H. E., Fosheim, I. K., Moe, K., Turowski, G., Johnsen, G. M., et al. (2022). Failure of physiological transformation and spiral artery atherosis: Their roles in preeclampsia. Am. J. Obstet. Gynecol. 226 (2), S895–s906. doi:10.1016/j.ajog.2020.09.026
Staff, A. C. (2019). The two-stage placental model of preeclampsia: An update. J. Reprod. Immunol. 134-135, 1–10. doi:10.1016/j.jri.2019.07.004
Stanhewicz, Anna E., Jandu, S., Santhanam, L., and Alexander, Lacy M. (2017). Alterations in endothelin type B receptor contribute to microvascular dysfunction in women who have had preeclampsia. Clin. Sci. 131 (23), 2777–2789. doi:10.1042/cs20171292
Staun-Ram, E., Goldman, S., Gabarin, D., and Shalev, E. (2004). Expression and importance of matrix metalloproteinase 2 and 9 (MMP-2 and -9) in human trophoblast invasion. Reprod. Biol. Endocrinol. 2, 59. doi:10.1186/1477-7827-2-59
Stepan, H., Hund, M., and Andraczek, T. (2020). Combining biomarkers to predict pregnancy complications and redefine preeclampsia: The angiogenic-placental syndrome. Hypertension 75 (4), 918–926. 10.1161/hypertensionaha.119.13763.
Su, M. T., Tsai, P. Y., Tsai, H. L., Chen, Y. C., and Kuo, P. L. (2017). miR-346 and miR-582-3p-regulated EG-VEGF expression and trophoblast invasion via matrix metalloproteinases 2 and 9. Biofactors 43 (2), 210–219. doi:10.1002/biof.1325
Su, M. T., Wang, C. Y., Tsai, P. Y., Chen, T. Y., Tsai, H. L., and Kuo, P. L. (2019). Aspirin enhances trophoblast invasion and represses soluble fms-like tyrosine kinase 1 production: A putative mechanism for preventing preeclampsia. J. Hypertens. 37 (12), 2461–2469. doi:10.1097/hjh.0000000000002185
Sun, F., Cai, H., Tan, L., Qin, D., Zhang, J., Hua, J., et al. (2022). Placenta-specific miR-125b overexpression leads to increased rates of pregnancy loss in mice. Int. J. Mol. Sci. 23 (2), 943. doi:10.3390/ijms23020943
Sun, H.-X., Zeng, D.-Y., Li, R.-T., Pang, R.-P., Yang, H., Hu, Y.-L., et al. (2012). Essential role of MicroRNA-155 in regulating endothelium-dependent vasorelaxation by targeting endothelial nitric oxide synthase. Hypertension 60(6), 1407–1414. doi:10.1161/HYPERTENSIONAHA.112.197301
Sun, M., Chen, H., Liu, J., Tong, C., and Meng, T. (2015). MicroRNA-34a inhibits human trophoblast cell invasion by targeting MYC. BMC Cell Biol. 16, 21. doi:10.1186/s12860-015-0068-2
Takao, T., Asanoma, K., Kato, K., Fukushima, K., Tsunematsu, R., Hirakawa, T., et al. (2011). Isolation and characterization of human trophoblast side-population (SP) cells in primary villous cytotrophoblasts and HTR-8/SVneo cell line. PLOS ONE 6 (7), e21990. doi:10.1371/journal.pone.0021990
Tang, J. N., Wang, D., Lu, J., and Zhou, X. Y. (2021). MiR-125b participates in the occurrence of preeclampsia by regulating the migration and invasion of extravillous trophoblastic cells through STAT3 signaling pathway. J. Recept. Signal Transduct. Res. 41 (2), 202–208. doi:10.1080/10799893.2020.1806318
Tannetta, D., Masliukaite, I., Vatish, M., Redman, C., and Sargent, I. (2017). Update of syncytiotrophoblast derived extracellular vesicles in normal pregnancy and preeclampsia. J. Reprod. Immunol. 119, 98–106. doi:10.1016/j.jri.2016.08.008
Tao, H., Liu, X., Liu, X., Liu, W., Wu, D., Wang, R., et al. (2019). LncRNA MEG3 inhibits trophoblast invasion and trophoblast-mediated VSMC loss in uterine spiral artery remodeling. Mol. Reprod. Dev. 86 (6), 686–695. doi:10.1002/mrd.23147
Teng, L., Liu, P., Song, X., Wang, H., Sun, J., and Yin, Z. (2020). Long non-coding RNA nuclear-enriched abundant transcript 1 (NEAT1) represses proliferation of trophoblast cells in rats with preeclampsia via the MicroRNA-373/FLT1 Axis. Med. Sci. Monit. 26, e927305–927301. doi:10.12659/MSM.927305
Tiensuu, H., Haapalainen, A. M., Karjalainen, M. K., Pasanen, A., Huusko, J. M., Marttila, R., et al. (2019). Risk of spontaneous preterm birth and fetal growth associates with fetal SLIT2. PLoS Genet. 15 (6), e1008107. doi:10.1371/journal.pgen.1008107
Timofeeva, A. V., Gusar, V. A., Kan, N. E., Prozorovskaya, K. N., Karapetyan, A. O., Bayev, O. R., et al. (2018). Identification of potential early biomarkers of preeclampsia. Placenta 61, 61–71. doi:10.1016/j.placenta.2017.11.011
Tranquilli, A. L., Brown, M. A., Zeeman, G. G., Dekker, G., and Sibai, B. M. (2013). The definition of severe and early-onset preeclampsia. Statements from the international society for the study of hypertension in pregnancy (ISSHP). Pregnancy Hypertens. 3 (1), 44–47. doi:10.1016/j.preghy.2012.11.001
Turco, M. Y., and Moffett, A. (2019). Development of the human placenta. Development 146 (22), dev163428. doi:10.1242/dev.163428
Valinezhad Orang, A., Safaralizadeh, R., and Kazemzadeh-Bavili, M. (2014). Mechanisms of miRNA-mediated gene regulation from common downregulation to mRNA-specific upregulation. Int. J. Genomics 2014, 970607. doi:10.1155/2014/970607
Vashukova, E. S., Glotov, A. S., Fedotov, P. V., Efimova, O. A., Pakin, V. S., Mozgovaya, E. V., et al. (2016). Placental microRNA expression in pregnancies complicated by superimposed pre-eclampsia on chronic hypertension. Mol. Med. Rep. 14 (1), 22–32. doi:10.3892/mmr.2016.5268
Vaughan, J. E., and Walsh, S. W. (2012). Activation of NF-κB in placentas of women with preeclampsia. Hypertens. Pregnancy 31 (2), 243–251. doi:10.3109/10641955.2011.642436
Wang, A., Rana, S., and Karumanchi, S. A. (2009). Preeclampsia: The role of angiogenic factors in its pathogenesis. Physiol. (Bethesda) 24, 147–158. doi:10.1152/physiol.00043.2008
Wang, C. Y., Tsai, H. L., Syu, J. S., Chen, T. Y., and Su, M. T. (2017). Primary cilium-regulated EG-VEGF signaling facilitates trophoblast invasion. J. Cell. Physiol. 232 (6), 1467–1477. doi:10.1002/jcp.25649
Wang, D., Na, Q., Song, G. Y., and Wang, L. L. (2020a). Human umbilical cord mesenchymal stem cell-derived exosome-mediated transfer of microRNA-133b boosts trophoblast cell proliferation, migration and invasion in preeclampsia by restricting SGK1. Cell Cycle 19 (15), 1869–1883. doi:10.1080/15384101.2020.1769394
Wang, F. R., and Yan, J. (2018). MicroRNA-454 is involved in regulating trophoblast cell proliferation, apoptosis, and invasion in preeclampsia by modulating the expression of ephrin receptor B4. Biomed. Pharmacother. 107, 746–753. doi:10.1016/j.biopha.2018.08.055
Wang, L. J., Cheong, M. L., Lee, Y. S., Lee, M. T., and Chen, H. (2012). High-temperature requirement protein A4 (HtrA4) suppresses the fusogenic activity of syncytin-1 and promotes trophoblast invasion. Mol. Cell. Biol. 32 (18), 3707–3717. doi:10.1128/mcb.00223-12
Wang, N., Li, R. Z., and Xue, M. (2019a). Potential regulatory network in the PSG10P/miR-19a-3p/IL1RAP pathway is possibly involved in preeclampsia pathogenesis. J. Cell. Mol. Med. 23 (2), 852–864. doi:10.1111/jcmm.13985
Wang, N. Y., Zheng, J., Chen, Z., Liu, Y., Dura, B., Kwak, M., et al. (2019b). Single-cell microRNA-mRNA co-sequencing reveals non-genetic heterogeneity and mechanisms of microRNA regulation. Nat. Commun. 10, 95. ARTN 95. doi:10.1038/s41467-018-07981-6
Wang, Y., Du, X., and Wang, J. (2020b). Transfer of miR-15a-5p by placental exosomes promotes pre-eclampsia progression by regulating PI3K/AKT signaling pathway via CDK1. Mol. Immunol. 128, 277–286. doi:10.1016/j.molimm.2020.10.019
Wang, Z. P., Shan, Y. H., Yang, Y., Wang, T. S., and Guo, Z. H. (2021). MicroRNA-155 is upregulated in the placentas of patients with preeclampsia and affects trophoblast apoptosis by targeting SHH/GLi1/BCL2. Hum. Exp. Toxicol. 40 (3), 439–451. doi:10.1177/0960327120954252
Weber, M., Knoefler, I., Schleussner, E., Markert, U. R., and Fitzgerald, J. S. (2013). HTR8/SVneo cells display trophoblast progenitor cell-like characteristics indicative of self-renewal, repopulation activity, and expression of “stemness-” associated transcription factors. Biomed. Res. Int. 2013, 243649. doi:10.1155/2013/243649
Wei, X., Yuan, Y., and Yang, Q. (2022). SNHG22 promotes migration and invasion of trophoblasts via miR-128-3p/PCDH11X axis and activates PI3K/Akt signaling pathway. Clinics 77, 100055. doi:10.1016/j.clinsp.2022.100055
Welten, S. M. J., Bastiaansen, A. J. N. M., Jong, R. C. M. d., Vries, M. R. d., Peters, E. A. B., Boonstra, M. C., et al. (2014). Inhibition of 14q32 MicroRNAs miR-329, miR-487b, miR-494, and miR-495 increases neovascularization and blood flow recovery after ischemia. Circ. Res. 115(8), 696–708. doi:10.1161/CIRCRESAHA.114.304747
Whitley, G. S., and Cartwright, J. E. (2010). Cellular and molecular regulation of spiral artery remodelling: Lessons from the cardiovascular field. Placenta 31 (6), 465–474. doi:10.1016/j.placenta.2010.03.002
WHO (2011). World Health organization recommendations for prevention and treatment of pre-eclampsia and eclampsia. [Online]. Available at: https://www.ncbi.nlm.nih.gov/books/NBK140561/(Accessed July 14, 2022).
Wilson, R. C., Tambe, A., Kidwell, M. A., Noland, C. L., Schneider, C. P., and Doudna, J. A. (2015). Dicer-TRBP complex formation ensures accurate mammalian microRNA biogenesis. Mol. Cell 57 (3), 397–407. doi:10.1016/j.molcel.2014.11.030
Wooding, F. B. P., and Burton, G. (2008). Comparative placentation : Structures, functions and evolution. Berlin: Springer.
Wu, D., Shi, L., Hong, L., Chen, X., and Cen, H. (2020a). MiR-135a-5p promotes the migration and invasion of trophoblast cells in preeclampsia by targeting β-TrCP. Placenta 99, 63–69. doi:10.1016/j.placenta.2020.07.028
Wu, H.-Y., Wang, X.-H., Liu, K., and Zhang, J.-L. (2020b). LncRNA MALAT1 regulates trophoblast cells migration and invasion via miR-206/IGF-1 axis. Cell CycleGeorget. Tex.) 19 (1), 39–52. doi:10.1080/15384101.2019.1691787
Wu, L., Song, W. Y., Xie, Y., Hu, L. L., Hou, X. M., Wang, R., et al. (2018). miR-181a-5p suppresses invasion and migration of HTR-8/SVneo cells by directly targeting IGF2BP2. Cell Death Dis. 9, 16. doi:10.1038/s41419-017-0045-0
Xie, L., Mouillet, J. F., Chu, T., Parks, W. T., Sadovsky, E., Knöfler, M., et al. (2014). C19MC microRNAs regulate the migration of human trophoblasts. Endocrinology 155 (12), 4975–4985. doi:10.1210/en.2014-1501
Xu, G., Guimond, M.-J. e., Chakraborty, C., and Lala, P. K. (2002). Control of proliferation, migration, and invasiveness of human extravillous trophoblast by decorin, a decidual product. Biol. Reprod. 67 (2), 681–689. doi:10.1095/biolreprod67.2.681
Xu, P., Ma, Y., Wu, H., and Wang, Y. L. (2021). Placenta-derived MicroRNAs in the pathophysiology of human pregnancy. Front. Cell Dev. Biol. 9, 646326. doi:10.3389/fcell.2021.646326
Xu, P., Zhao, Y., Liu, M., Wang, Y., Wang, H., Li, Y. X., et al. (2014a). Variations of microRNAs in human placentas and plasma from preeclamptic pregnancy. Hypertension 63 (6), 1276–1284. doi:10.1161/hypertensionaha.113.02647
Xu, W., San Lucas, A., Wang, Z., and Liu, Y. (2014b). Identifying microRNA targets in different gene regions. BMC Bioinforma. 15, S4. Suppl 7. doi:10.1186/1471-2105-15-S7-S4
Xueya, Z., Yamei, L., Sha, C., Dan, C., Hong, S., Xingyu, Y., et al. (2020). Exosomal encapsulation of miR-125a-5p inhibited trophoblast cell migration and proliferation by regulating the expression of VEGFA in preeclampsia. Biochem. Biophys. Res. Commun. 525 (3), 646–653. doi:10.1016/j.bbrc.2020.02.137
Yang, J. S., and Lai, E. C. (2011). Alternative miRNA biogenesis pathways and the interpretation of core miRNA pathway mutants. Mol. Cell 43 (6), 892–903. doi:10.1016/j.molcel.2011.07.024
Yang, W., Wang, A., Zhao, C., Li, Q., Pan, Z., Han, X., et al. (2016). miR-125b enhances IL-8 production in early-onset severe preeclampsia by targeting sphingosine-1-phosphate lyase 1. PLoS One 11 (12), e0166940. doi:10.1371/journal.pone.0166940
Yang, X., Zhang, J., and Ding, Y. (2017). Association of microRNA-155, interleukin 17A, and proteinuria in preeclampsia. Med. Baltim. 96 (18), e6509. doi:10.1097/md.0000000000006509
Ye, F., Chen, C., Qin, J., Liu, J., and Zheng, C. (2015). Genetic profiling reveals an alarming rate of cross-contamination among human cell lines used in China. Faseb J. 29 (10), 4268–4272. doi:10.1096/fj.14-266718
Yin, A. L., Chen, Q., Zhong, M., and Jia, B. (2021). MicroRNA-138 improves LPS-induced trophoblast dysfunction through targeting RELA and NF-kappa B signaling. Cell Cycle 20 (5-6), 508–521. doi:10.1080/15384101.2021.1877927
Yoda, M., Kawamata, T., Paroo, Z., Ye, X., Iwasaki, S., Liu, Q., et al. (2010). ATP-dependent human RISC assembly pathways. Nat. Struct. Mol. Biol. 17 (1), 17–23. doi:10.1038/nsmb.1733
Yu, Y., Wang, L., Liu, T., and Guan, H. (2015). MicroRNA-204 suppresses trophoblast-like cell invasion by targeting matrix metalloproteinase-9. Biochem. Biophys. Res. Commun. 463 (3), 285–291. doi:10.1016/j.bbrc.2015.05.052
Zaccagnini, G., Maimone, B., Di Stefano, V., Fasanaro, P., Greco, S., Perfetti, A., et al. (2013). Hypoxia-induced miR-210 modulates tissue response to acute peripheral ischemia. Antioxid. Redox Signal. 21 (8), 1177–1188. doi:10.1089/ars.2013.5206
Zang, J. J., Yan, M., Zhang, Y., Peng, W., Zuo, J. X., Zhou, H. S., et al. (2022). MiR-326 inhibits trophoblast growth, migration, and invasion by targeting PAX8 via Hippo pathway. Reprod. Biol. Endocrinol. 20 (1), 38. doi:10.1186/s12958-022-00909-2
Zeisler, H., Llurba, E., Chantraine, F., Vatish, M., Staff, A. C., Sennström, M., et al. (2016). Predictive value of the sFlt-1:PlGF ratio in women with suspected preeclampsia. N. Engl. J. Med. 374 (1), 13–22. doi:10.1056/NEJMoa1414838
Zeng, Y., Wei, L., Lali, M. S., Chen, Y., Yu, J., and Feng, L. (2020). miR-150-5p mediates extravillous trophoblast cell migration and angiogenesis functions by regulating VEGF and MMP9. Placenta 93, 94–100. doi:10.1016/j.placenta.2020.02.019
Zhang, H., Kolb, F. A., Jaskiewicz, L., Westhof, E., and Filipowicz, W. (2004). Single processing center models for human Dicer and bacterial RNase III. Cell 118 (1), 57–68. doi:10.1016/j.cell.2004.06.017
Zhang, J., Zhou, W., Liu, Y., Liu, T., Li, C., and Wang, L. (2018). Oncogenic role of microRNA-532-5p in human colorectal cancer via targeting of the 5'UTR of RUNX3. Oncol. Lett. 15 (5), 7215–7220. doi:10.3892/ol.2018.8217
Zhang, L., Li, H. H., Li, M. B., Zhang, W. X., Yang, Z., and Zhang, S. Q. (2020). LRP6 is involved in the proliferation, migration and invasion of trophoblast cells via miR-346. Int. J. Mol. Med. 46 (1), 211–223. doi:10.3892/ijmm.2020.4570
Zhang, M., Li, P., Mao, X. M., and Zhang, H. Y. (2021). Regulatory mechanism of miR-525-5p in over-invasion of trophoblast. J. Obstet. Gynaecol. Res. 47 (2), 679–688. doi:10.1111/jog.14581
Zhang, M., Muralimanoharan, S., Wortman, A. C., and Mendelson, C. R. (2016a). Primate-specific miR-515 family members inhibit key genes in human trophoblast differentiation and are upregulated in preeclampsia. Proc. Natl. Acad. Sci. U. S. A. 113 (45), E7069–E7076. doi:10.1073/pnas.1607849113
Zhang, Y., Diao, Z., Su, L., Sun, H., Li, R., Cui, H., et al. (2010). MicroRNA-155 contributes to preeclampsia by down-regulating CYR61. Am. J. Obstet. Gynecol. 202 (5), 466.e461–e7. doi:10.1016/j.ajog.2010.01.057
Zhang, Y., Fei, M., Xue, G., Zhou, Q., Jia, Y., Li, L., et al. (2012). Elevated levels of hypoxia-inducible microRNA-210 in pre-eclampsia: New insights into molecular mechanisms for the disease. J. Cell. Mol. Med. 16 (2), 249–259. doi:10.1111/j.1582-4934.2011.01291.x
Zhang, Y., Hu, X., Gao, G., Wang, Y., Chen, P., and Ye, Y. (2016b). Autophagy protects against oxidized low density lipoprotein-mediated inflammation associated with preeclampsia. Placenta 48, 136–143. doi:10.1016/j.placenta.2016.09.015
Zhang, Y., Huang, G., Zhang, Y., Yang, H., Long, Y., Liang, Q., et al. (2017). MiR-942 decreased before 20 weeks gestation in women with preeclampsia and was associated with the pathophysiology of preeclampsia in vitro. Clin. Exp. Hypertens. 39 (2), 108–113. doi:10.1080/10641963.2016.1210619
Zhao, X., Zhang, X., Wu, Z., Mei, J., Li, L., and Wang, Y. (2021). Up-regulation of microRNA-135 or silencing of PCSK6 attenuates inflammatory response in preeclampsia by restricting NLRP3 inflammasome. Mol. Med. 27, 82. doi:10.1186/s10020-021-00335-x
Zhao, Y. H., Liu, Y. L., Fei, K. L., and Li, P. (2020). Long non-coding RNA HOTAIR modulates the progression of preeclampsia through inhibiting miR-106 in an EZH2-dependent manner. Life Sci. 253, 117668. doi:10.1016/j.lfs.2020.117668
Zhao, Y., Zhao, G., and Li, W. (2022). MicroRNA-495 suppresses pre-eclampsia via activation of p53/PUMA axis. Cell Death Discov. 8 (1), 132. doi:10.1038/s41420-022-00874-0
Zheng, J., Tian, M., Liu, L., Jia, X., Sun, M., and Lai, Y. (2022). Magnesium sulfate reduces vascular endothelial cell apoptosis in rats with preeclampsia via the miR-218-5p/HMGB1 pathway. Clin. Exp. Hypertens. 44 (2), 159–166. doi:10.1080/10641963.2021.2013492
Zheng, L. M., Song, J., Tang, R., Chen, X. J., Wang, L., Wu, D. C., et al. (2021). MicroRNA-524-5p regulates the proliferation and invasion of HTR-8/SVneo trophoblasts by targeting NUMB in the Notch signaling pathway. Mol. Med. Rep. 23 (6), 436. doi:10.3892/mmr.2021.12075
Zhong, Y., Zhu, F., and Ding, Y. (2019). Differential microRNA expression profile in the plasma of preeclampsia and normal pregnancies. Exp. Ther. Med. 18 (1), 826–832. doi:10.3892/etm.2019.7637
Zhou, D., Xu, X., Liu, Y., Liu, H., Cheng, X., Gu, Y., et al. (2022a15298). MiR-195-5p facilitates the proliferation, migration, and invasion of human trophoblast cells by targeting FGF2. J. Obstet. Gynaecol. Res. 48, 2122–2133. doi:10.1111/jog.15298
Zhou, F., Liu, H., Zhang, R., and Sun, Y. (2022b). Circ_0007121 facilitates trophoblastic cell proliferation, migration, and invasion via the regulation of the miR-421/ZEB1 Axis in preeclampsia. Reprod. Sci. 29 (1), 100–109. doi:10.1007/s43032-021-00713-0
Zhou, W., She, G., Yang, K., Zhang, B., Liu, J., and Yu, B. (2020). MiR-384 inhibits proliferation and migration of trophoblast cells via targeting PTBP3. Pregnancy Hypertens. 21, 132–138. doi:10.1016/j.preghy.2020.05.017
Zhu, J. Y., Pang, Z. J., and Yu, Y. H. (2012). Regulation of trophoblast invasion: The role of matrix metalloproteinases. Rev. Obstet. Gynecol. 5 (3-4), e137–143.
Zhu, X.-m., Han, T., Sargent, I. L., Yin, G.-w., and Yao, Y.-q. (2009). Differential expression profile of microRNAs in human placentas from preeclamptic pregnancies vs normal pregnancies. Am. J. Obstet. Gynecol. 200 (6), 661.e661–e7. doi:10.1016/j.ajog.2008.12.045
Keywords: microRNA, trophoblasts, invasion, spiral artery remodeling, preeclampsia
Citation: Hayder H, Shan Y, Chen Y, O’Brien JA and Peng C (2022) Role of microRNAs in trophoblast invasion and spiral artery remodeling: Implications for preeclampsia. Front. Cell Dev. Biol. 10:995462. doi: 10.3389/fcell.2022.995462
Received: 15 July 2022; Accepted: 25 August 2022;
Published: 03 October 2022.
Edited by:
Peeyush Lala, Western University, CanadaReviewed by:
Ernawati Ernawati, Airlangga University, IndonesiaNihar Nayak, University of Missouri–Kansas City, United States
Copyright © 2022 Hayder, Shan, Chen, O’Brien and Peng. This is an open-access article distributed under the terms of the Creative Commons Attribution License (CC BY). The use, distribution or reproduction in other forums is permitted, provided the original author(s) and the copyright owner(s) are credited and that the original publication in this journal is cited, in accordance with accepted academic practice. No use, distribution or reproduction is permitted which does not comply with these terms.
*Correspondence: Chun Peng, Y3BlbmdAeW9ya3UuY2E=