- Helmholtz Institute for Metabolic, Obesity and Vascular Research (HI-MAG) of the Helmholtz Zentrum München at the University of Leipzig and University Hospital Leipzig, Leipzig, Germany
In humans, various dietary and social factors led to the development of increased brain sizes alongside large adipose tissue stores. Complex reciprocal signaling mechanisms allow for a fine-tuned interaction between the two organs to regulate energy homeostasis of the organism. As an endocrine organ, adipose tissue secretes various hormones, cytokines, and metabolites that signal energy availability to the central nervous system (CNS). Vice versa, the CNS is a critical regulator of adipose tissue function through neural networks that integrate information from the periphery and regulate sympathetic nerve outflow. This review discusses the various reciprocal signaling mechanisms in the CNS and adipose tissue to maintain organismal energy homeostasis. We are focusing on the integration of afferent signals from the periphery in neuronal populations of the mediobasal hypothalamus as well as the efferent signals from the CNS to adipose tissue and its implications for adipose tissue function. Furthermore, we are discussing central mechanisms that fine-tune the immune system in adipose tissue depots and contribute to organ homeostasis. Elucidating this complex signaling network that integrates peripheral signals to generate physiological outputs to maintain the optimal energy balance of the organism is crucial for understanding the pathophysiology of obesity and metabolic diseases such as type 2 diabetes.
Introduction
In most mammalian species, the size of the brain and adipose depots are inversely correlated, indicating compensatory buffering strategies to protect against starvation (Navarrete et al., 2011). However, in humans, dietary and social factors have led to increased brain sizes alongside large adipose tissue stores despite substantial energetic costs (Navarrete et al., 2011; Heldstab et al., 2016). Adipose depots make up a remarkable proportion of human body mass, allowing physiological buffering and efficient partitioning of unutilized calories (Ofenheimer et al., 2020; Liu et al., 2021). The size of the human brain allows for increased cognitive flexibility, representing an additional mechanism to keep the energy intake constant and to maintain the body’s energy requirements (Heldstab et al., 2016). Therefore, a fine-tuned crosstalk between the two organs orchestrates the regulation of feeding, energy storage, and energy expenditure. Adipose depots constitute a dynamic endocrine organ secreting multiple adipokines into circulation that signal energy availability to the brain. Furthermore, sensory innervation of adipose depots allows for the detection of locally released free fatty acids and adipokines and therefore represents an additional afferent route from adipose tissue to the central nervous system (CNS) (Murphy et al., 2013; Garretson et al., 2016). The CNS integrates these peripheral signals to generate physiological responses to maintain the optimal energy balance of the organism.
This review highlights the coordinated reciprocal signaling between the CNS and white adipose tissue. We are discussing the integration of afferent signals from the periphery in neuronal populations of the mediobasal hypothalamus as well as the efferent signals from the CNS to adipose tissue and its implications for adipose tissue function. Furthermore, we are focusing on central mechanisms that regulate resident immune cell function in adipose tissue depots and subsequently contribute to organ homeostasis.
Efferent signals from the central nervous system are crucial regulators of white adipose tissue function
The brain interacts with white adipose tissue depots through distinct efferent sympathetic nerves, releasing the catecholamine norepinephrine (NE) from their nerve terminals. In white adipose tissue (WAT), sympathetic nerve terminals are located adjacent to >90% of adipocytes, forming a dense network of sympathetic arborizations (Jiang et al., 2017). Importantly, sympathetic outflow to the adipose tissue is the principal initiator of adipose tissue lipolysis (Fredholm and Karlsson, 1970; Youngstrom and Bartness, 1995; Dodt et al., 2003; Bartness et al., 2010). Electrical stimulation of sympathetic nerve fibres in rat epididymal adipose tissue explants resulted in the rapid release of fatty acids and glycerol into the incubation medium, which provided some of the first evidence for this regulation (Correll, 1963). The necessity of WAT sympathetic nervous system (SNS) innervation for lipolysis was elegantly demonstrated by direct optogenetic activation of sympathetic inputs to adipose tissue, which was sufficient to promote a local lipolytic response and fat mass reduction (Zeng et al., 2015). It is worth noting that the extent of sympathetic innervation and outflow differs between the different depots of WAT (Youngstrom and Bartness, 1995; Brito et al., 2007; Brito et al., 2008). Furthermore, the rate of extracellular NE clearance also influences the sympathetic tone. Most NE is sequestered from the synapse through the solute carrier family six member 2 (SLC6A2) monoamine transporter expressed on sympathetic neurons. Pirzgalska et al. (2017) reported a macrophage subtype capable of dampening the sympathetic tone in adipose tissue by lowering noradrenaline bioavailability. These specialized sympathetic neuron-associated macrophages (SAMs) are able to scavenge noradrenaline through the transporter Slc6a2 and degrade it using the enzyme monoamine oxidase A (MAOa) (Camell et al., 2017). Treating aged mice with an MAOA inhibitor increased adipose tissue concentrations of norepinephrine (NE) and restored the aging-related fasting-induced lipolysis defect (Camell et al., 2017). In addition, genetic ablation of Slc6a2 was shown to be sufficient to increase NE levels in serum, which results in improved brown adipose tissue (BAT) performance and browning of WAT (Pirzgalska et al., 2017). Of note, adipocytes express the organic cation transporter 3 (Oct3; Slc22a3), allowing the clearance of NE (Ayala-Lopez et al., 2015; Song et al., 2019). This indicates that several cell types are involved in the regulation of NE bioavailability specifically in white adipose microenvironments. Collectively, the bioavailability of catecholamines, such as NE, constitutes a specific regulatory mechanism in adipose tissue homeostasis.
Stimulation of lipolysis requires the activation of G-protein–coupled α- and β-adrenoceptors (α- and β-ARs) on adipocytes (Barbe et al., 1996). The extent of the lipolytic activity depends on a balance between lipolysis stimulation by β-ARs (β1–3-AR) and lipolysis inhibition by α2-ARs [for review see (Evans et al., 2019; Collins, 2022)]. Activating β-adrenergic receptors leads to dissociation of the receptor-coupled Gs protein and activation of adenylate cyclase (AC), which increases intracellular cAMP levels. High cAMP levels activate protein kinase A (PKA), which phosphorylates hormone-sensitive lipase (HSL) and perilipin-A (PLIN1). This initiates a signaling cascade that leads to the activation of lipases, such as adipose triglyceride lipase (ATGL) and monoglyceride lipase (MGL) or α/β hydrolase-domain 6 (ABHD6), allowing triglycerides to be hydrolyzed sequentially into fatty acids (FA) and glycerol (Figure 1) (Grabner et al., 2021).
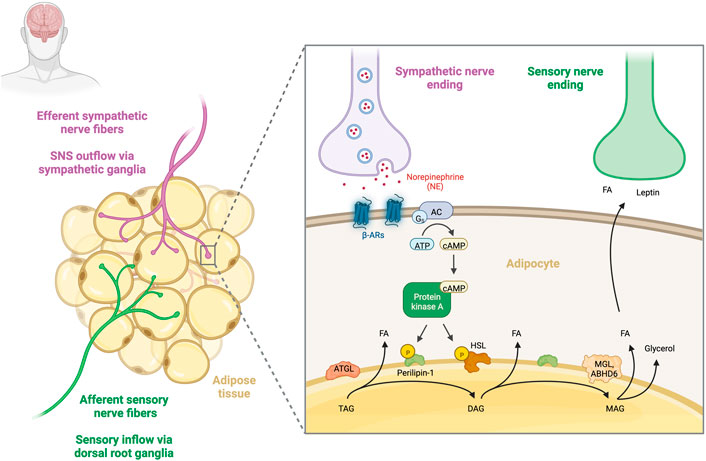
FIGURE 1. Sympathetic regulation of adipose tissue lipolysis. Sympathetic and sensory innervation of human adipose tissue. Sympathetic nerve fibres that travel from the CNS to innervate adipose tissue (purple) and sensory nerve fibres that relay information from adipose tissue to the CNS (green) are shown. Release of noradrenaline (NE) from efferent sympathetic fibres leads to the activation of β-adrenergic receptors and the subsequent dissociation of the receptor-coupled Gs protein leads to activation of adenylate cyclase (AC), which increases intracellular cAMP levels. High cAMP levels activate protein kinase A (PKA), phosphorylating hormone-sensitive lipase (HSL) and perilipin-A (PLIN1). This initiates the activation of a set of lipases, such as adipose triglyceride lipase (ATGL) and monoglyceride lipase (MGL) or α/β hydrolase-domain 6 (ABHD6), allowing for consecutive hydrolysis of TGs into fatty acids (FA) and glycerol. Increased lipolysis in turn activates WAT afferent sensory nerve endings, which are able to sense local FA and leptin concentrations (Garretson et al., 2016). Created with BioRender.com.
In order to fine-tune adipose lipolytic activity, neuropeptide Y (NPY), which is co-stored with NE, is released from sympathetic nerve terminals and inhibits lipolysis by binding to its receptor NPYR1 (Lundberg et al., 1990; Labelle et al., 1997; Bradley et al., 2005). NPY receptors are highly expressed on human adipocytes, and most abundant in subcutaneous adipose depots (Castan et al., 1993; Serradeil-Le Gal et al., 2000; Kos et al., 2007). The release of NPY on the other hand has a considerable proliferative effect on adipocyte precursors and stimulates adipogenesis (Kuo et al., 2007; Yang et al., 2008). In this context, NPY promotes the accumulation and storage of energy.
Lipolysis increases the availability of free fatty acids which in turn activate local WAT afferents that mediate acute induction of thermogenesis in distant BAT depots (Garretson et al., 2016; Nguyen et al., 2017). This data is strong indication of WAT and BAT crosstalk via afferent sensory feedback in order to maintain thermoregulation.
Efferent signals from the central nervous system regulate adipose tissue expansion
In addition to its role as principal regulator of adipose tissue lipolysis, the SNS is also involved in the regulation of adipose tissue mass and plasticity. Here, the local release of NE constitutes an important negative regulator of adipogenesis (Jones et al., 1992). Surgical local denervation or chemical sympathectomy of WAT increased adipocyte precursor cell proliferation and accelerated preadipocyte differentiation (Cousin et al., 1993; Bowers et al., 2004; Foster and Bartness, 2006). However, surgical denervation of nerve bundles results in a mixed denervation of sensory and sympathetic nerve fibres that impacts the interpretation of these findings. Furthermore, transgenic mice with a deficiency of the neuronal transcription factor Nscl-2 displayed reduced nerve density in white adipose depots and this reduction in innervation was accompanied by an increase in numbers of preadipocytes (Ruschke et al., 2009). Conversely, sensory denervation (through local microinjections of capsaicin) did not affect preadipocyte proliferation and differentiation (Foster and Bartness, 2006). The inhibitory effect of NE on the proliferation of WAT adipose progenitor cell populations is likely mediated through β1-adrenergic receptors (β1-ARs). Schneider et al. (2018) demonstrated that the selective β1-AR agonist dobutamine diminished preadipocyte proliferation. Interestingly, the activation of the parasympathetic nervous system (PSNS) may play an opposing role to that of the SNS. Here, reduced melanocortin signaling due to increased vagal activity within the splanchnic compartment actively facilitates adipose tissue expansion (Holland et al., 2019). Importantly, a lack of parasympathetic innervation in the WAT has been reported and therefore further research is needed to determine how the brain-melanocortin-vagus efferent axis regulates fat mass gain (Giordano et al., 2006).
Central integration of adipose tissue signals
Retrograde tracing experiments have revealed numerous brain areas that are polysynaptically connected to adipose tissue. This includes several nuclei in the mediobasal hypothalamus, such as the arcuate nucleus (ARC), the ventromedial hypothalamus (VMH), the dorsomedial hypothalamus (DMH), the lateral hypothalamus (LH) and the paraventricular nucleus of the hypothalamus (PVH) (Bamshad et al., 1998; Ryu and Bartness, 2014). These nuclei contain several functionally distinct neuronal populations that adapt integrative physiology to the organismal energy state (Aponte et al., 2011; Brandt et al., 2018; Chen et al., 2015; Sutton and Krashes, 2020; van den Top et al., 2004; Zhan et al., 2013). These neurons express high levels of receptors that allow for the integration of peripheral metabolic feedback signals within the CNS in order to generate physiological output (Jais and Bruning, 2022). This output is conveyed as sympathetic outflow to adipose tissue (and other peripheral organs) (Guilherme et al., 2019).
The most well-defined neurocircuit in the context of integrative physiology is the melanocortin system, which consists of the functionally antagonistic anorexigenic proopiomelanocortin (POMC)-expressing neurons and the orexigenic agouti-related peptide (AgRP)-expressing neurons in the arcuate nucleus (ARC) of the mediobasal hypothalamus (Gautron et al., 2015). POMC neurons are activated by energy surplus and inhibit food intake after prolonged periods of feeding (Aponte et al., 2011). These neurons release the melanocortin α-melanocyte-stimulating hormone (α-MSH) into the paraventricular nucleus (PVH) where it binds to the melanocortin receptor 4 (MC4R), resulting in reduced food intake and increased energy expenditure (Cone, 2006; Zhan et al., 2013; Krashes et al., 2016). AgRP neurons are located adjacent to the third ventricle in close proximity to the median eminence (ME), allowing them to sense peripheral metabolic signals. A negative energy balance increases AgRP neuronal excitability, which is rapidly suppressed upon the initiation of feeding (Hahn et al., 1998; Aponte et al., 2011; Betley et al., 2015). Mechanistically, AgRP acts as an inverse agonist for the melanocortin receptor 4 (MC4R) that competes with α-MSH released from POMC neurons for binding sites on the MC4R (Haskell-Luevano and Monck, 2001). AgRP neurons also release neuropeptide Y (NPY) as well as the inhibitory neurotransmitter gamma-aminobutyric acid (GABA), and the promotion of feeding depends on both NPY and GABA release from these cells (Tong et al., 2008; Krashes et al., 2013).
Importantly, both AgRP and POMC neurons express receptors for leptin, insulin and other energy-state communicating hormones and are therefore subject to feedback regulation (Belgardt and Bruning, 2010; Varela and Horvath, 2012; Vogt and Bruning, 2013; Biglari et al., 2021; Deem et al., 2022).
Leptin signaling in the mediobasal hypothalamus
The discovery of leptin revealed a central mechanism of how adipose tissue communicates with the CNS (Zhang et al., 1994; Halaas et al., 1995; Montague et al., 1997). Leptin is a hormone primarily released by WAT proportional to the size of fat stores and central leptin signaling is an essential regulator of lipid storage (Frederich et al., 1995; Klein et al., 1996; Fruhbeck et al., 1998). The lipolytic effects of leptin are mediated by neuronal pathways as evidenced by the fact that selective denervation within WAT depots prevents the lipolysis-increasing effects of leptin (Buettner et al., 2008; Zeng et al., 2015). Several studies have identified POMC neurons in the ARC as potential regulators of lipolysis (Kaushik et al., 2012; Shin et al., 2017; Gomez-Valades et al., 2021). For example, the loss of autophagy in POMC neurons decreases α-melanocyte-stimulating hormone (MSH) levels, which in turn leads to impaired lipolysis (Kaushik et al., 2012). Leptin directly activates POMC neurons through the leptin receptor (LEPR) (Cowley et al., 2001; Balthasar et al., 2004). In addition, leptin reduces the inhibitory tone of presynaptic GABAergic neurons to postsynaptic POMC neurons (Vong et al., 2011). Mechanistically, leptin signaling in the mediobasal hypothalamus increases adipose tissue lipolysis by post-translational regulation of hormone-sensitive lipase (HSL) in adipocytes (Buettner et al., 2008). In this regard, several nuclei of the mediobasal hypothalamus, such as the ARC, VMH, DMH, LH, and PVH are mediating leptin action on SNS activity (Rahmouni and Morgan, 2007; Simonds et al., 2012; Harlan and Rahmouni, 2013; Shi et al., 2020). Moreover, leptin-stimulated central PI3K signaling regulates energy expenditure through activation of SNS activity to WAT leading to browning of adipocytes and increased energy expenditure (Plum et al., 2007). Sympathetic nerve fibers in white adipose tissue establish neuro-adipose junctions, thereby allowing for the local regulation of lipolysis (Zeng et al., 2015). Leptin also regulates the plasticity of the sympathetic innervation of adipose tissue. Here, a population of BDNF-expressing neurons in the paraventricular nucleus of the hypothalamus (BDNFPVH) dynamically regulates the sympathetic innervation downstream of leptin-sensitive AGRP and POMC neurons in the ARC (Wang et al., 2020).
De novo lipogenesis, the process of converting carbohydrates into fatty acids, is regulated by hypothalamic leptin signaling as demonstrated by acute infusion of leptin into the mediobasal hypothalamus, which potently suppresses key de novo lipogenic enzymes (Buettner et al., 2008). Interestingly, de novo lipogenesis in adipocytes might provide regulatory feedback for sympathetic neuronal activity as the suppression of the key lipogenic enzyme fatty acid synthase (FASN) in white adipocytes enhances sympathetic activity (Guilherme et al., 2017). Furthermore, leptin regulates its own expression in adipocytes through a SNS-dependent mechanism that requires NE. Acute treatment of mice with β3-adrenoceptor agonists suppresses leptin secretion from adipocytes through a β3-adrenergic receptor (β3-AR)–cAMP-dependent mechanism (Trayhurn et al., 1996). Conversely, systemic inhibition of catecholamine synthesis in rats increased plasma leptin levels by 15-fold (Sivitz et al., 1999).
Insulin signaling in the mediobasal hypothalamus
Another important signaling molecule in the CNS-adipose crosstalk involving melanocortin neurons is insulin. Insulin is an anabolic peptide hormone secreted by β-cells of the pancreas and insulin signaling in the mediobasal hypothalamus dampens sympathetic nerve activity to adipose tissue, suppresses lipolysis and allows for increased adipose tissue retention of fatty acids (Scherer et al., 2011; Shin et al., 2017). Furthermore, this reduction in lipolysis reduces hepatic glucose production by limiting the flux of energy substrates necessary for gluconeogenesis (Scherer et al., 2011). Importantly, genetic disruption of the insulin receptor (IR) on POMC neurons resulted in impaired suppression of adipose tissue lipolysis (Shin et al., 2017). However, POMC neurons are a heterogeneous cell population (Dodd et al., 2018; Biglari et al., 2021). The phosphatase TCPTP mediates insulins effects on POMC neurons as elevated expression of the phosphatase TCPTP in POMC neurons represses insulin signaling. Conversely, a decreased expression of TCPTP enhances insulin signaling and therefore the proportion of POMC neurons activated by insulin (Dodd et al., 2018). However, the effects of POMC-specific TCPTP expression on the regulation of lipolysis are still unknown.
Sensing of free fatty acids in the mediobasal hypothalamus
Various cell types in the mediobasal hypothalamus are capable of sensing circulating fatty acids. Tanycytes, specialized ependymal glia cells that line the wall of the third ventricle near the ARC, sense free fatty acids and subsequently regulate WAT lipolysis through hypothalamic FGF21 signaling (Geller et al., 2019). Importantly, long-chain fatty acids (LCFA) such as oleic acid (OA) are able to excite a subset of POMC neurons directly through inhibition of ATP-activated potassium (KATP) channels (Jo et al., 2009). Moreover, central administration of a MC4R antagonist abolished the (anorexigenic) actions of OA (Schwinkendorf et al., 2011).
These findings indicate that the melanocortin system acts as a signaling hub for regulating WAT lipolysis. Indeed, SNS outflow neurons to WAT express melanocortin-4 receptor (MC4R) mRNA (Song et al., 2005). Pharmacological activation of MC3/4R in the CNS stimulates lipolysis independent of food intake (Nogueiras et al., 2007). Furthermore, central infusion with MC3/4R agonists provokes differential sympathetic drives to various adipose tissue depots (Brito et al., 2007).
It is worth noting that peripheral signals are relayed not only via the circulation, but also through sensory innervation of adipose depots. Sensory nerve endings allow for the detection of local leptin levels directly in the adipose tissue depot (Fishman and Dark, 1987; Niijima, 1998, 1999). Therefore, the integration of information from leptin reaching the brain via the circulation and information about individual adipose tissue depots leptin levels via sensory fibers might help to adjust the sympathetic tone from the CNS in an adipose tissue depot-specific manner.
In conclusion, these data clearly highlight the importance of the melanocortin system in the CNS-adipose crosstalk. Further efforts are needed however to understand the deregulation of these signaling networks during the development of leptin and insulin resistance.
Resident immune cells contribute to adipose tissue homeostasis through multiple mechanisms
Immune mechanisms in the adipose tissue have been widely studied within the onset of obesity and insulin resistance (Pekala et al., 1983; Wellen and Hotamisligil, 2003; Lumeng et al., 2007a; Lumeng et al., 2007b). Nevertheless, adipose tissue contains resident immune cells that maintain organ homeostasis. Adipose tissue macrophages (ATMs) are the most abundant immune cell type, which occupy up to 10% of stromal cells under a steady-state and are usually uniformly distributed (Weisberg et al., 2003). ATMs help maintain adipose tissue homeostasis by controlling key signaling pathways involved in adipogenesis, lipogenesis, lipolysis, and lipid uptake (Kosteli et al., 2010; Bilkovski et al., 2011; Nguyen et al., 2011; Brunner et al., 2020; Chen et al., 2021). In addition, ATMs play a critical role in the vascular homeostasis of the organ as an adequate blood flow is essential for adipose tissue expansion and metabolic functions. This was demonstrated by co-culturing macrophages and adipocytes, which lead to increased expression of VEGFA and other pro-angiogenic factors (Yadav et al., 2020). Interestingly, VEGFA exerts potent neurotrophic and synaptotrophic effects as well (Sondell et al., 1999; Pelletier et al., 2015; Calvo et al., 2018). However, the role of VEGFA in regulating adipose tissue innervation has not been explored to date. In addition, the comparison of adipose tissue obtained from wildtype and ATM-depleted mice revealed that ATMs play a pivotal role in suppressing the expression of pro-inflammatory cytokines (Chen et al., 2021).
Bi-directional crosstalk between adipose tissue-resident immune cells and nerve fibres
Extensive crosstalk between the SNS and resident ATMs regulates adipose tissue homeostasis and the activation of β-adrenergic signaling is a powerful regulator of adipose tissue function. Adrenergic receptors are expressed in macrophages, particularly β2-adrenergic receptors (β2-AR) (Pavlov et al., 2018; Petkevicius et al., 2021). ATMs from mice treated with CL316,243 (a β-adrenergic agonist) increased beige adipogenesis significantly (Lee et al., 2016). This process entails triggering the death of unilocular white adipocytes, clearance of dead cells, and the recruitment of UCP1+ adipocyte progenitors and further differentiation (Lee et al., 2016). Moreover, β2-AR stimulation was also shown to be essential for maintaining low TNFα expression levels in ATMs in lean mice and for M2 macrophage polarization (Grailer et al., 2014; Tang et al., 2015). Interestingly, Petkevicius et al. (2021) have recently shown that macrophage β2-AR activation is dispensable for the development of metabolic inflammation. However, nerve-associated macrophages can be found in several tissues throughout the body, where they are involved in regulation of metabolic homeostasis potentially utilizing different signaling mechanisms [for review see (Kolter et al., 2020)]. Therefore, further studies on the function of nerve-associated macrophages in metabolic homeostasis are required.
Furthermore, β2-AR stimulation in macrophages promotes thermogenesis via the production and secretion of acetylcholine, which acts on adipocytes via acetylcholine receptors, stimulating the PKA pathway and subsequently inducing thermogenic gene expression (Figure 2A) (Knights et al., 2021; Meng et al., 2021).
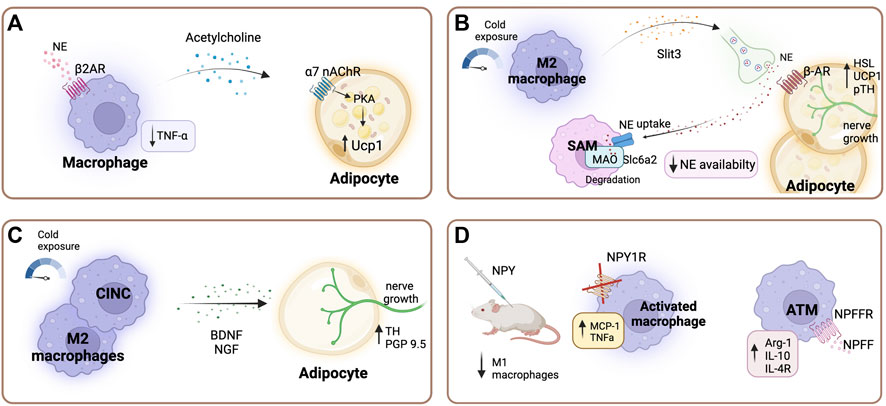
FIGURE 2. Sympathetic regulation of adipose tissue macrophages. (A) Beta-2 adrenergic receptor (β2-AR) stimulation in macrophages promotes the production and secretion of acetylcholine, which acts on adipocytes via acetylcholine receptors, stimulating thermogenesis through the PKA pathway and consequently inducing thermogenic gene expression (Knights et al., 2021; Meng et al., 2021). Additionally, β2-AR stimulation is essential for maintaining low tumor necrosis factor -alpha (TNFα) levels (Wang et al., 2003). (B) After cold stimulation M2 macrophages secrete cytokine Slit3, which promotes sympathetic nerve growth pathway, and stimulates the synthesis and release of norepinephrine (NE), subsequently inducing thermogenesis (Wang et al., 2021). Specialized sympathetic neuron-associated macrophages (SAMs) scavenge noradrenaline through the transporter Slc6a2 and degrade it using the enzyme monoamine oxidase A (MAOa). Thereby regulating local adipose tissue availability of NE (Camell et al., 2017; Pirzgalska et al., 2017). (C) A subset of macrophages belonging to the cold-induced neuroimmune cells (CINCs) and M2 macrophages secrete neurotrophic factors such as brain-derived neurotrophic factor (BDNF) upon cold exposure, promoting adipocyte nerve growth (Blaszkiewicz et al., 2022; Xie et al., 2022). (D) Neuropeptide Y (NPY) modulates inflammatory response in macrophages. NPY supplementation in lean mice leads to a decreased number of M1 adipose tissue macrophages (ATMs) (Singer et al., 2013). Deficiency of NPY1 receptor increases the secretion of TNFα and monocyte chemoattractant protein-1 (MCP-1) under inflammatory conditions (Macia et al., 2012). Neuropeptide FF receptor 2 (NPFFR2) is predominantly expressed in ATMs compared to other macrophage populations. In ATMs neuropeptide FF (NPFF) increases arginase 1, interleukin (Il-) 10, and Il-4 receptor expression (Waqas et al., 2017). Created with BioRender.com.
In addition, cholinergic signaling in macrophages through the alpha-7 nicotinic acetylcholine receptor plays an anti-inflammatory role by inhibiting TNFα release (Wang et al., 2003). TNFα has long been implicated in development of insulin resistance (Hotamisligil et al., 1993), and reduced TNFα activity improves systemic insulin resistance (Togashi et al., 2002). Exposure to low-dose TNFα impairs central insulin and leptin signaling (Romanatto et al., 2007). Furthermore, TNFα induces the expression of PTP1B in the ARC through NF-κB activation (Zabolotny et al., 2008). This represses insulin signaling and therefore lowers the proportion of POMC neurons activated by insulin.
Furthermore, through the secretion of the cytokine Slit3 upon cold exposure ATMs exert a modulatory function in the sympathetic innervation of adipose tissue. Slit3 promotes sympathetic nerve growth via the Slit-ROBO pathway, and additionally stimulates the synthesis and release of NE, which in turn promotes thermogenesis (Figure 2B) (Wang et al., 2021). A subset of macrophages, cold-induced neuroimmune cells (CINCs), are recruited to the tissue in response to cold stimulation and have shown to coordinate gene expression involved in nerve survival and plasticity (Blaszkiewicz et al., 2022). These cells secrete neurotrophic factors such as brain-derived neurotrophic factor (BDNF), which has been shown to exert an important role in WAT innervation (Blaszkiewicz et al., 2020; Wang et al., 2020) (Figure 2C). For instance, the knockout of BNDF in myeloid lineage cells in mice leads to decreased innervation of inguinal subcutaneous WAT depots (Blaszkiewicz et al., 2020). In addition, the TrkB receptor (a receptor with high binding affinity for BDNF) was shown to be expressed on sensory and sympathetic nerve fibers in subcutaneous WAT (Blaszkiewicz et al., 2022). Moreover, hypothalamic BDNF overexpression in DIO and lean mice lead to an up-regulation of β-ARs and UCP-1 in the WAT (Cao et al., 2009; Cao et al., 2011). Whereas heterozygous BDNF knockout mice (mice with approximately 40% less BDNF protein than wild type control animals) showed a selective suppression of β-ARs in WAT (Cao et al., 2011).
Neuropeptide Y regulates the function of ATMs
NPY potently influences metabolic function in peripheral tissue and has been shown to play a role in the inflammatory response modulation of ATMs (Ruohonen et al., 2008; Singer et al., 2013). ATMs express Y1, Y2, and Y5 receptors and the in vitro blockade of these receptors enhances the expression of pro-inflammatory genes (Singer et al., 2013). The Y1 receptor expression in immune cells of adipose tissue depots was critical in controlling inflammation and insulin resistance in obesity (Figure 2D) (Macia et al., 2012). In this study, the authors investigated periovarian adipose depots from female mice. Moreover, in vivo NPY supplementation decreased M1 proinflammatory macrophages in lean mice (Singer et al., 2013). Park et al. (2021) demonstrated that NPY secreted by macrophages also upregulates adipogenic and lipogenic gene expression profiles. Nevertheless, obese mice show higher levels of circulating NPY, and increased expression of NPY and NPY 2-receptor (NPY2R) mRNA in subcutaneous adipose tissue, indicating that circulating NPY originates from adipose tissue (Kuo et al., 2007). Additionally, Kuo et al. reported that stress in mice leads to the release of NPY from sympathetic nerve fibres in WAT and activation of NPY2R, which stimulates macrophage infiltration and a metabolic syndrome-like condition (Kuo et al., 2007). NPY function is believed to be determined by site-specific NPY and NPY receptor expression. In lean animals, NPY is expressed in non-ATMs, such as adipocytes, as well as ATMs. However, during obesity NPY expression is significantly induced in ATMs (Schwarz et al., 1994; Kos et al., 2007; Singer et al., 2013). However, the contribution of NPY release from sympathetic nerve endings to the total adipose tissue NPY levels has not been investigated to date. Hence, further studies are needed to clarify the exact role of adipose tissue-specific NPY in obesity and insulin resistance development.
Neuropeptide FF effects on adipose tissue
Furthermore, neuropeptide FF (NPFF) has been shown to decrease food intake in mice and inhibit adipocyte development (Murase et al., 1996; Herrera-Herrera and Salazar-Olivo, 2008; Ruohonen et al., 2008). NPFF is potentially released from nerve endings in adipose tissue, although this has not been demonstrated so far (van Harmelen et al., 2010). This neuropeptide shows potent effects in the regulation of ATM function. In isolated ATMs, NPFF treatment increased arginase 1, IL-10, and IL-4R expression and in mice NPFF treatment improved glucose tolerance and insulin sensitivity (Figure 2D) (Waqas et al., 2017). Neuropeptide FF receptor 2 (NPFFR2) is predominantly expressed in ATMs compared to other macrophage populations (Waqas et al., 2017). Moreover, sustained exposure to NPFF can increase ATMs numbers more effectively than IL-4, a cytokine known to induce M2 activation (Waqas et al., 2017). Interestingly, hypothalamic NPFF signaling through the NPFFR2 receptor plays a key role in mediating diet-induced adaptative thermogenesis as evidenced by an impaired BAT response in Npffr2 knockout mice (Zhang et al., 2018).
Melanocortins also play an important anti-inflammatory role through the activation of melanocortin receptors (MCRs) expressed in adipose tissue resident immune cells (Wang et al., 2019). Several in vivo and in vitro studies have shown anti-inflammatory effects mediated by melanocortin agonists acting on macrophages (Wang et al., 2019). For example, Getting et al. (1999) reported that MC3/4R activation in peritoneal macrophages reduces the release of pro-inflammatory cytokines. In addition, α-melanocyte-stimulating hormone (α-MSH) was shown to inhibit the production of nitric oxide and NF-κB nuclear translocation in cultured macrophages (Star et al., 1995; Rajora et al., 1996; Mandrika et al., 2001). Activation of POMC neurons in the ARC and the anterior pituitary leads to the release of α-MSH into the circulation and subsequent activation of MCRs in the adipose tissue (Elias et al., 2000; Cowley et al., 2001). However, the POMC gene is expressed in various immune cells (residing within the adipose tissue compartment) as well (Lyons and Blalock, 1997; Blalock, 1999). These local effects of adipose tissue-derived peptide products of the POMC gene need further investigation. Of note, in both mouse and human preadipocytes α-MSH inhibits proliferation and in adipocytes it decreases the expression and secretion of leptin (Smith et al., 2003; Hoggard et al., 2004).
It is clear that ATMs impact the innervation of adipose tissue in numerous ways and vice versa the adipose tissue innervation directly impacts ATM functions via neurotransmitter release.
Furthermore, several other adipose tissue immune cell types are subject to sympathetic regulation. For instance, group 2 innate lymphoid cells (ILC2) were recently shown to be indirectly regulated by the SNS (Figure 3A). Activation of β2-ARs on mesenchymal stromal cells (MSC) leads to the secretion of glial-derived neurotrophic factor (GDNF) from these cells (Cardoso et al., 2021). Subsequently, GDNF acts on ILC2 cells via the neuroregulatory receptor RET, which ultimately leads to an increased cytokine secretion [such as interleukin (IL-)5, IL-13 and Met-enkephalin], thereby regulating adipocyte function and energy expenditure (Cardoso et al., 2021). Mice with RET receptor gain-of-function display improved glucose tolerance, decreased adipocyte size and increased UCP1 expression (Cardoso et al., 2021). Notably, sympathetic tone is required for ILC2 cells, since sympathetic denervation results in significantly suppressed ILC2 function (Ding et al., 2016).
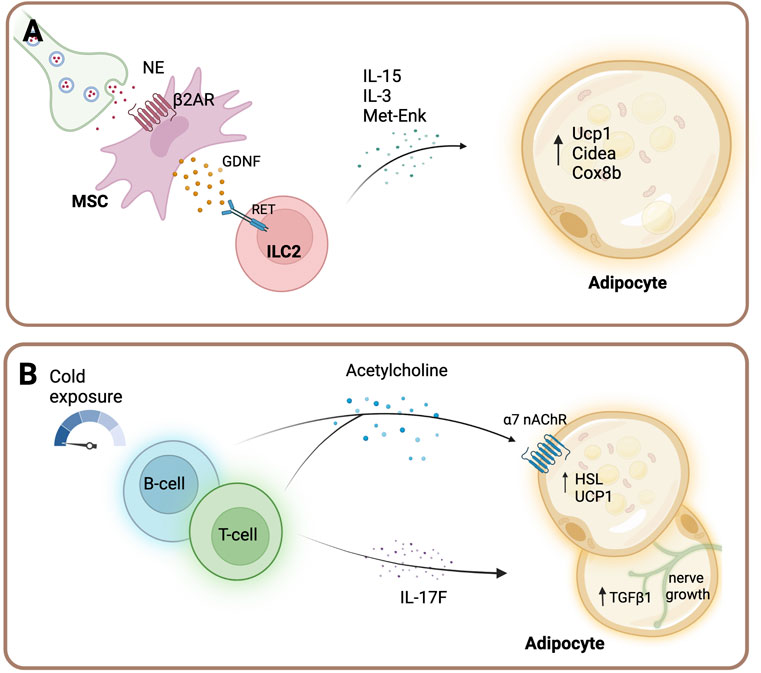
FIGURE 3. Sympathetic regulation of immune cells in adipose tissue. (A) Sympathetic outflow acts on β2-AR of mesenchymal cells (MSC), which release glial-derived neurotrophic factor (GDNF). GDNF in turn activates group 2 innate lymphoid cells (ILC2) cells via the receptor RET. Activated ILC2 cells secrete interleukin (IL-)5, IL-13 cytokines and Met-enkephalin (Met-enk) and subsequently regulate adipocyte function and energy expenditure (Cardoso et al., 2021). (B) B cells and T cells release acetylcholine upon acute cold exposure in inguinal white adipose tissue (Jun et al., 2018). Furthermore, Gamma delta (yδ) T cells maintain sympathetic innervation of adipose tissue by driving the expression of transforming growth factor beta-1 (TGFβ1) in adipocytes via the IL-17F effector cytokine (Hu et al., 2020). Created with BioRender.com.
The neurotransmitter acetylcholine (ACh) is an important contributor to immune cell function [for review see (Fujii et al., 2017) and (Cox et al., 2020)] and B cells and T cells are important (non-neuronal) acetylcholine-producing cells upon acute cold exposure in the inguinal WAT (Figure 3B) (Jun et al., 2018). Mice with hematopoietic ablation of the gene encoding for choline acetyltransferase (the rate-limiting enzyme that mediates the biosynthesis of acetylcholine) show thermogenic defects. Furthermore, the expression of the beige-fat-specific cholinergic receptor alpha two subunit (Chrna2) correlated with the local acetylcholine production and its activation was shown to be mediated in a paracrine manner (Jun et al., 2018). Furthermore, T cells, specifically yδ T cells, play a key role in the maintenance of sympathetic innervation of adipose tissue by driving the expression of TGFβ1 in adipocytes via the IL-17F effector cytokine (Hu et al., 2020). TGFβ1 possesses neurotrophic activity and promotes sympathetic innervation (Hu et al., 2020). Collectively, these data indicate that immune cells in adipose tissue closely associate with the SNS to maintain tissue homeostasis.
Concluding remarks
Understanding the complex signaling networks that integrate energy availability signals from adipose tissue in the CNS to generate physiological outputs is crucial for understanding the pathophysiology of obesity and metabolic diseases such as type 2 diabetes. The findings discussed in this review clearly highlight the importance of the melanocortin system in the CNS-adipose crosstalk. However, specific neuronal populations in the mediobasal hypothalamus modify the activity of melanocortin neurons. Defining the exact molecular nature of these regulatory neurons has proven challenging. Owing to their structural and functional diversity, our current understanding of the neurocircuits involved in the control of adipose tissue is still limited. Recent technical advances in neuroscience have led to the possibility of identifying and characterizing the neurocircuits involved in the control of adipose tissue homeostasis. Identifying druggable targets on these specific neuronal populations is a prerequisite for developing novel interventions and therapeutic approaches for obesity and associated metabolic diseases.
Author contributions
All authors listed have made a substantial, direct, and intellectual contribution to the work and approved it for publication.
Funding
This work was supported by the Helmholtz Center Munich and the federal government of Saxony, Germany.
Acknowledgments
The authors apologize to all authors not cited who have made important contributions.
Conflict of interest
The authors declare that the research was conducted in the absence of any commercial or financial relationships that could be construed as a potential conflict of interest.
The handling editor SU declared a shared affiliation with the authors at the time of review.
Publisher’s note
All claims expressed in this article are solely those of the authors and do not necessarily represent those of their affiliated organizations, or those of the publisher, the editors and the reviewers. Any product that may be evaluated in this article, or claim that may be made by its manufacturer, is not guaranteed or endorsed by the publisher.
References
Aponte, Y., Atasoy, D., and Sternson, S. M. (2011). AGRP neurons are sufficient to orchestrate feeding behavior rapidly and without training. Nat. Neurosci. 14, 351–355. doi:10.1038/nn.2739
Ayala-Lopez, N., Jackson, W. F., Burnett, R., Wilson, J. N., Thompson, J. M., and Watts, S. W. (2015). Organic cation transporter 3 contributes to norepinephrine uptake into perivascular adipose tissue. Am. J. Physiol. Heart Circ. Physiol. 309, H1904–H1914. doi:10.1152/ajpheart.00308.2015
Balthasar, N., Coppari, R., McMinn, J., Liu, S. M., Lee, C. E., Tang, V., et al. (2004). Leptin receptor signaling in POMC neurons is required for normal body weight homeostasis. Neuron 42, 983–991. doi:10.1016/j.neuron.2004.06.004
Bamshad, M., Aoki, V. T., Adkison, M. G., Warren, W. S., and Bartness, T. J. (1998). Central nervous system origins of the sympathetic nervous system outflow to white adipose tissue. Am. J. Physiol. 275, R291–R299. doi:10.1152/ajpregu.1998.275.1.R291
Barbe, P., Millet, L., Galitzky, J., Lafontan, M., and Berlan, M. (1996). In situ assessment of the role of the beta 1-beta 2- and beta 3-adrenoceptors in the control of lipolysis and nutritive blood flow in human subcutaneous adipose tissue. Br. J. Pharmacol. 117, 907–913. doi:10.1111/j.1476-5381.1996.tb15279.x
Bartness, T. J., Shrestha, Y. B., Vaughan, C. H., Schwartz, G. J., and Song, C. K. (2010). Sensory and sympathetic nervous system control of white adipose tissue lipolysis. Mol. Cell. Endocrinol. 318, 34–43. doi:10.1016/j.mce.2009.08.031
Belgardt, B. F., and Bruning, J. C. (2010). CNS leptin and insulin action in the control of energy homeostasis. Ann. N. Y. Acad. Sci. 1212, 97–113. doi:10.1111/j.1749-6632.2010.05799.x
Betley, J. N., Xu, S., Cao, Z. F. H., Gong, R., Magnus, C. J., Yu, Y., et al. (2015). Neurons for hunger and thirst transmit a negative-valence teaching signal. Nature 521, 180–185. doi:10.1038/nature14416
Biglari, N., Gaziano, I., Schumacher, J., Radermacher, J., Paeger, L., Klemm, P., et al. (2021). Functionally distinct POMC-expressing neuron subpopulations in hypothalamus revealed by intersectional targeting. Nat. Neurosci. 24, 913–929. doi:10.1038/s41593-021-00854-0
Bilkovski, R., Schulte, D. M., Oberhauser, F., Mauer, J., Hampel, B., Gutschow, C., et al. (2011). Adipose tissue macrophages inhibit adipogenesis of mesenchymal precursor cells via wnt-5a in humans. Int. J. Obes. 35, 1450–1454. doi:10.1038/ijo.2011.6
Blalock, J. E. (1999). Proopiomelanocortin and the immune-neuroendocrine connection. Ann. N. Y. Acad. Sci. 885, 161–172. doi:10.1111/j.1749-6632.1999.tb08673.x
Blaszkiewicz, M., Gunsch, G., Willows, J. W., Gardner, M. L., Sepeda, J. A., Sas, A. R., et al. (2022). Adipose tissue myeloid-lineage neuroimmune cells express genes important for neural plasticity and regulate adipose innervation. Front. Endocrinol. 13, 864925. doi:10.3389/fendo.2022.864925
Blaszkiewicz, M., Wood, E., Koizar, S., Willows, J., Anderson, R., Tseng, Y. H., et al. (2020). The involvement of neuroimmune cells in adipose innervation. Mol. Med. 26, 126. doi:10.1186/s10020-020-00254-3
Bowers, R. R., Festuccia, W. T., Song, C. K., Shi, H., Migliorini, R. H., and Bartness, T. J. (2004). Sympathetic innervation of white adipose tissue and its regulation of fat cell number. Am. J. Physiol. Regul. Integr. Comp. Physiol. 286, R1167–R1175. doi:10.1152/ajpregu.00558.2003
Bradley, R. L., Mansfield, J. P., and Maratos-Flier, E. (2005). Neuropeptides, including neuropeptide Y and melanocortins, mediate lipolysis in murine adipocytes. Obes. Res. 13, 653–661. doi:10.1038/oby.2005.73
Brandt, C., Nolte, H., Henschke, S., Engstrom Ruud, L., Awazawa, M., Morgan, D. A., et al. (2018). Food perception primes hepatic ER homeostasis via melanocortin-dependent control of mTOR activation. Cell 175, 1321–1335. doi:10.1016/j.cell.2018.10.015
Brito, M. N., Brito, N. A., Baro, D. J., Song, C. K., and Bartness, T. J. (2007). Differential activation of the sympathetic innervation of adipose tissues by melanocortin receptor stimulation. Endocrinology 148, 5339–5347. doi:10.1210/en.2007-0621
Brito, N. A., Brito, M. N., and Bartness, T. J. (2008). Differential sympathetic drive to adipose tissues after food deprivation, cold exposure or glucoprivation. Am. J. Physiol. Regul. Integr. Comp. Physiol. 294, R1445–R1452. doi:10.1152/ajpregu.00068.2008
Brunner, J. S., Vogel, A., Lercher, A., Caldera, M., Korosec, A., Puhringer, M., et al. (2020). The PI3K pathway preserves metabolic health through MARCO-dependent lipid uptake by adipose tissue macrophages. Nat. Metab. 2, 1427–1442. doi:10.1038/s42255-020-00311-5
Buettner, C., Muse, E. D., Cheng, A., Chen, L., Scherer, T., Pocai, A., et al. (2008). Leptin controls adipose tissue lipogenesis via central, STAT3-independent mechanisms. Nat. Med. 14, 667–675. doi:10.1038/nm1775
Calvo, P. M., de la Cruz, R. R., and Pastor, A. M. (2018). Synaptic loss and firing alterations in Axotomized Motoneurons are restored by vascular endothelial growth factor (VEGF) and VEGF-B. Exp. Neurol. 304, 67–81. doi:10.1016/j.expneurol.2018.03.004
Camell, C. D., Sander, J., Spadaro, O., Lee, A., Nguyen, K. Y., Wing, A., et al. (2017). Inflammasome-driven catecholamine catabolism in macrophages blunts lipolysis during ageing. Nature 550, 119–123. doi:10.1038/nature24022
Cao, L., Choi, E. Y., Liu, X., Martin, A., Wang, C., Xu, X., et al. (2011). White to Brown fat phenotypic switch induced by genetic and environmental activation of a hypothalamic-adipocyte axis. Cell Metab. 14, 324–338. doi:10.1016/j.cmet.2011.06.020
Cao, L., Lin, E. J., Cahill, M. C., Wang, C., Liu, X., and During, M. J. (2009). Molecular therapy of obesity and diabetes by a physiological autoregulatory approach. Nat. Med. 15, 447–454. doi:10.1038/nm.1933
Cardoso, F., Klein Wolterink, R. G. J., Godinho-Silva, C., Domingues, R. G., Ribeiro, H., da Silva, J. A., et al. (2021). Neuro-mesenchymal units control ILC2 and obesity via a brain-adipose circuit. Nature 597, 410–414. doi:10.1038/s41586-021-03830-7
Castan, I., Valet, P., Larrouy, D., Voisin, T., Remaury, A., Daviaud, D., et al. (1993). Distribution of PYY receptors in human fat cells: An antilipolytic system alongside the alpha 2-adrenergic system. Am. J. Physiol. 265, E74–E80. doi:10.1152/ajpendo.1993.265.1.E74
Chen, Q., Lai, S. M., Xu, S., Tan, Y., Leong, K., Liu, D., et al. (2021). Resident macrophages restrain pathological adipose tissue remodeling and protect vascular integrity in obese mice. EMBO Rep. 22, e52835. doi:10.15252/embr.202152835
Chen, Y., Lin, Y. C., Kuo, T. W., and Knight, Z. A. (2015). Sensory detection of food rapidly modulates arcuate feeding circuits. Cell 160, 829–841. doi:10.1016/j.cell.2015.01.033
Collins, S. (2022). β-Adrenergic receptors and adipose tissue metabolism: Evolution of an old story. Annu. Rev. Physiol. 84, 1–16. doi:10.1146/annurev-physiol-060721-092939
Cone, R. D. (2006). Studies on the physiological functions of the melanocortin system. Endocr. Rev. 27, 736–749. doi:10.1210/er.2006-0034
Correll, J. W. (1963). Adipose tissue: Ability to respond to nerve stimulation in vitro. Science 140, 387–388. doi:10.1126/science.140.3565.387
Cousin, B., Casteilla, L., Lafontan, M., Ambid, L., Langin, D., Berthault, M. F., et al. (1993). Local sympathetic denervation of white adipose tissue in rats induces preadipocyte proliferation without noticeable changes in metabolism. Endocrinology 133, 2255–2262. doi:10.1210/endo.133.5.8404678
Cowley, M. A., Smart, J. L., Rubinstein, M., Cerdan, M. G., Diano, S., Horvath, T. L., et al. (2001). Leptin activates anorexigenic POMC neurons through a neural network in the arcuate nucleus. Nature 411, 480–484. doi:10.1038/35078085
Cox, M. A., Bassi, C., Saunders, M. E., Nechanitzky, R., Morgado-Palacin, I., Zheng, C., et al. (2020). Beyond neurotransmission: Acetylcholine in immunity and inflammation. J. Intern. Med. 287, 120–133. doi:10.1111/joim.13006
Deem, J. D., Faber, C. L., and Morton, G. J. (2022). AgRP neurons: Regulators of feeding, energy expenditure, and behavior. FEBS J. 289, 2362–2381. doi:10.1111/febs.16176
Ding, X., Luo, Y., Zhang, X., Zheng, H., Yang, X., Yang, X., et al. (2016). IL-33-driven ILC2/eosinophil axis in fat is induced by sympathetic tone and suppressed by obesity. J. Endocrinol. 231, 35–48. doi:10.1530/JOE-16-0229
Dodd, G. T., Michael, N. J., Lee-Young, R. S., Mangiafico, S. P., Pryor, J. T., Munder, A. C., et al. (2018). Insulin regulates POMC neuronal plasticity to control glucose metabolism. Elife 7, e38704. doi:10.7554/eLife.38704
Dodt, C., Lonnroth, P., Wellhoner, J. P., Fehm, H. L., and Elam, M. (2003). Sympathetic control of white adipose tissue in lean and obese humans. Acta Physiol. Scand. 177, 351–357. doi:10.1046/j.1365-201X.2003.01077.x
Elias, C. F., Kelly, J. F., Lee, C. E., Ahima, R. S., Drucker, D. J., Saper, C. B., et al. (2000). Chemical characterization of leptin-activated neurons in the rat brain. J. Comp. Neurol. 423, 261–281. doi:10.1002/1096-9861(20000724)423:2<261::aid-cne6>3.0.co;2-6
Evans, B. A., Merlin, J., Bengtsson, T., and Hutchinson, D. S. (2019). Adrenoceptors in white, Brown, and brite adipocytes. Br. J. Pharmacol. 176, 2416–2432. doi:10.1111/bph.14631
Fishman, R. B., and Dark, J. (1987). Sensory innervation of white adipose tissue. Am. J. Physiol. 253, R942–R944. doi:10.1152/ajpregu.1987.253.6.R942
Foster, M. T., and Bartness, T. J. (2006). Sympathetic but not sensory denervation stimulates white adipocyte proliferation. Am. J. Physiol. Regul. Integr. Comp. Physiol. 291, R1630–R1637. doi:10.1152/ajpregu.00197.2006
Frederich, R. C., Hamann, A., Anderson, S., Lollmann, B., Lowell, B. B., and Flier, J. S. (1995). Leptin levels reflect body lipid content in mice: Evidence for diet-induced resistance to leptin action. Nat. Med. 1, 1311–1314. doi:10.1038/nm1295-1311
Fredholm, B. B., and Karlsson, J. (1970). Metabolic effects of prolonged sympathetic nerve stimulation in canine subcutaneous adipose tissue. Acta Physiol. Scand. 80, 567–576. doi:10.1111/j.1748-1716.1970.tb04824.x
Fruhbeck, G., Aguado, M., Gomez-Ambrosi, J., and Martinez, J. A. (1998). Lipolytic effect of in vivo leptin administration on adipocytes of lean and ob/ob mice, but not db/db mice. Biochem. Biophys. Res. Commun. 250, 99–102. doi:10.1006/bbrc.1998.9277
Fujii, T., Mashimo, M., Moriwaki, Y., Misawa, H., Ono, S., Horiguchi, K., et al. (2017). Expression and function of the cholinergic system in immune cells. Front. Immunol. 8, 1085. doi:10.3389/fimmu.2017.01085
Garretson, J. T., Szymanski, L. A., Schwartz, G. J., Xue, B., Ryu, V., and Bartness, T. J. (2016). Lipolysis sensation by white fat afferent nerves triggers Brown fat thermogenesis. Mol. Metab. 5, 626–634. doi:10.1016/j.molmet.2016.06.013
Gautron, L., Elmquist, J. K., and Williams, K. W. (2015). Neural control of energy balance: Translating circuits to therapies. Cell 161, 133–145. doi:10.1016/j.cell.2015.02.023
Geller, S., Arribat, Y., Netzahualcoyotzi, C., Lagarrigue, S., Carneiro, L., Zhang, L., et al. (2019). Tanycytes regulate lipid homeostasis by sensing free fatty acids and signaling to key hypothalamic neuronal populations via FGF21 secretion. Cell Metab. 30, 833–844. doi:10.1016/j.cmet.2019.08.004
Getting, S. J., Gibbs, L., Clark, A. J., Flower, R. J., and Perretti, M. (1999). POMC gene-derived peptides activate melanocortin type 3 receptor on murine macrophages, suppress cytokine release, and inhibit neutrophil migration in acute experimental inflammation. J. Immunol. 162, 7446–7453.
Giordano, A., Song, C. K., Bowers, R. R., Ehlen, J. C., Frontini, A., Cinti, S., et al. (2006). White adipose tissue lacks significant vagal innervation and immunohistochemical evidence of parasympathetic innervation. Am. J. Physiol. Regul. Integr. Comp. Physiol. 291, R1243–R1255. doi:10.1152/ajpregu.00679.2005
Gomez-Valades, A. G., Pozo, M., Varela, L., Boudjadja, M. B., Ramirez, S., Chivite, I., et al. (2021). Mitochondrial cristae-remodeling protein OPA1 in POMC neurons couples Ca(2+) homeostasis with adipose tissue lipolysis. Cell Metab. 33, 1820–1835.e9. doi:10.1016/j.cmet.2021.07.008
Grabner, G. F., Xie, H., Schweiger, M., and Zechner, R. (2021). Lipolysis: Cellular mechanisms for lipid mobilization from fat stores. Nat. Metab. 3, 1445–1465. doi:10.1038/s42255-021-00493-6
Grailer, J. J., Haggadone, M. D., Sarma, J. V., Zetoune, F. S., and Ward, P. A. (2014). Induction of M2 regulatory macrophages through the β2-adrenergic receptor with protection during endotoxemia and acute lung injury. J. Innate Immun. 6, 607–618. doi:10.1159/000358524
Guilherme, A., Henriques, F., Bedard, A. H., and Czech, M. P. (2019). Molecular pathways linking adipose innervation to insulin action in obesity and diabetes mellitus. Nat. Rev. Endocrinol. 15, 207–225. doi:10.1038/s41574-019-0165-y
Guilherme, A., Pedersen, D. J., Henchey, E., Henriques, F. S., Danai, L. V., Shen, Y., et al. (2017). Adipocyte lipid synthesis coupled to neuronal control of thermogenic programming. Mol. Metab. 6, 781–796. doi:10.1016/j.molmet.2017.05.012
Hahn, T. M., Breininger, J. F., Baskin, D. G., and Schwartz, M. W. (1998). Coexpression of Agrp and NPY in fasting-activated hypothalamic neurons. Nat. Neurosci. 1, 271–272. doi:10.1038/1082
Halaas, J. L., Gajiwala, K. S., Maffei, M., Cohen, S. L., Chait, B. T., Rabinowitz, D., et al. (1995). Weight-reducing effects of the plasma protein encoded by the obese gene. Science 269, 543–546. doi:10.1126/science.7624777
Harlan, S. M., and Rahmouni, K. (2013). Neuroanatomical determinants of the sympathetic nerve responses evoked by leptin. Clin. Auton. Res. 23, 1–7. doi:10.1007/s10286-012-0168-4
Haskell-Luevano, C., and Monck, E. K. (2001). Agouti-related protein functions as an inverse agonist at a constitutively active brain melanocortin-4 receptor. Regul. Pept. 99, 1–7. doi:10.1016/s0167-0115(01)00234-8
Heldstab, S. A., van Schaik, C. P., and Isler, K. (2016). Being fat and smart: A comparative analysis of the fat-brain trade-off in mammals. J. Hum. Evol. 100, 25–34. doi:10.1016/j.jhevol.2016.09.001
Herrera-Herrera, M. L., and Salazar-Olivo, L. A. (2008). RFamide neuropeptides inhibit murine and human adipose differentiation. Biochem. Biophys. Res. Commun. 377, 29–34. doi:10.1016/j.bbrc.2008.09.071
Hoggard, N., Hunter, L., Duncan, J. S., and Rayner, D. V. (2004). Regulation of adipose tissue leptin secretion by alpha-melanocyte-stimulating hormone and agouti-related protein: Further evidence of an interaction between leptin and the melanocortin signalling system. J. Mol. Endocrinol. 32, 145–153. doi:10.1677/jme.0.0320145
Holland, J., Sorrell, J., Yates, E., Smith, K., Arbabi, S., Arnold, M., et al. (2019). A brain-melanocortin-vagus Axis mediates adipose tissue expansion independently of energy intake. Cell Rep. 27, 2399–2410. e2396. doi:10.1016/j.celrep.2019.04.089
Hotamisligil, G. S., Shargill, N. S., and Spiegelman, B. M. (1993). Adipose expression of tumor necrosis factor-alpha: Direct role in obesity-linked insulin resistance. Science 259, 87–91. doi:10.1126/science.7678183
Hu, B., Jin, C., Zeng, X., Resch, J. M., Jedrychowski, M. P., Yang, Z., et al. (2020). γδ T cells and adipocyte IL-17RC control fat innervation and thermogenesis. Nature 578, 610–614. doi:10.1038/s41586-020-2028-z
Jais, A., and Bruning, J. C. (2022). Arcuate nucleus-dependent regulation of metabolism-pathways to obesity and diabetes mellitus. Endocr. Rev. 43, 314–328. doi:10.1210/endrev/bnab025
Jiang, H., Ding, X., Cao, Y., Wang, H., and Zeng, W. (2017). Dense intra-adipose sympathetic arborizations are essential for cold-induced beiging of mouse white adipose tissue. Cell Metab. 26, 686–692. doi:10.1016/j.cmet.2017.08.016
Jo, Y. H., Su, Y., Gutierrez-Juarez, R., and Chua, S. (2009). Oleic acid directly regulates POMC neuron excitability in the hypothalamus. J. Neurophysiol. 101, 2305–2316. doi:10.1152/jn.91294.2008
Jones, D. D., Ramsay, T. G., Hausman, G. J., and Martin, R. J. (1992). Norepinephrine inhibits rat pre-adipocyte proliferation. Int. J. Obes. Relat. Metab. Disord. 16, 349–354.
Jun, H., Yu, H., Gong, J., Jiang, J., Qiao, X., Perkey, E., et al. (2018). An immune-beige adipocyte communication via nicotinic acetylcholine receptor signaling. Nat. Med. 24, 814–822. doi:10.1038/s41591-018-0032-8
Kaushik, S., Arias, E., Kwon, H., Lopez, N. M., Athonvarangkul, D., Sahu, S., et al. (2012). Loss of autophagy in hypothalamic POMC neurons impairs lipolysis. EMBO Rep. 13, 258–265. doi:10.1038/embor.2011.260
Klein, S., Coppack, S. W., Mohamed-Ali, V., and Landt, M. (1996). Adipose tissue leptin production and plasma leptin kinetics in humans. Diabetes 45, 984–987. doi:10.2337/diab.45.7.984
Knights, A. J., Liu, S., Ma, Y., Nudell, V. S., Perkey, E., Sorensen, M. J., et al. (2021). Acetylcholine-synthesizing macrophages in subcutaneous fat are regulated by β2 -adrenergic signaling. EMBO J. 40, e106061. doi:10.15252/embj.2020106061
Kolter, J., Kierdorf, K., and Henneke, P. (2020). Origin and differentiation of nerve-associated macrophages. J. Immunol. 204, 271–279. doi:10.4049/jimmunol.1901077
Kos, K., Harte, A. L., James, S., Snead, D. R., O'Hare, J. P., McTernan, P. G., et al. (2007). Secretion of neuropeptide Y in human adipose tissue and its role in maintenance of adipose tissue mass. Am. J. Physiol. Endocrinol. Metab. 293, E1335–E1340. doi:10.1152/ajpendo.00333.2007
Kosteli, A., Sugaru, E., Haemmerle, G., Martin, J. F., Lei, J., Zechner, R., et al. (2010). Weight loss and lipolysis promote a dynamic immune response in murine adipose tissue. J. Clin. Invest. 120, 3466–3479. doi:10.1172/JCI42845
Krashes, M. J., Lowell, B. B., and Garfield, A. S. (2016). Melanocortin-4 receptor-regulated energy homeostasis. Nat. Neurosci. 19, 206–219. doi:10.1038/nn.4202
Krashes, M. J., Shah, B. P., Koda, S., and Lowell, B. B. (2013). Rapid versus delayed stimulation of feeding by the endogenously released AgRP neuron mediators GABA, NPY, and AgRP. Cell Metab. 18, 588–595. doi:10.1016/j.cmet.2013.09.009
Kuo, L. E., Kitlinska, J. B., Tilan, J. U., Li, L., Baker, S. B., Johnson, M. D., et al. (2007). Neuropeptide Y acts directly in the periphery on fat tissue and mediates stress-induced obesity and metabolic syndrome. Nat. Med. 13, 803–811. doi:10.1038/nm1611
Labelle, M., Boulanger, Y., Fournier, A., St Pierre, S., and Savard, R. (1997). Tissue-specific regulation of fat cell lipolysis by NPY in 6-OHDA-treated rats. Peptides 18, 801–808. doi:10.1016/s0196-9781(97)00028-4
Lee, Y. H., Kim, S. N., Kwon, H. J., Maddipati, K. R., and Granneman, J. G. (2016). Adipogenic role of alternatively activated macrophages in beta-adrenergic remodeling of white adipose tissue. Am. J. Physiol. Regul. Integr. Comp. Physiol. 310, R55–R65. doi:10.1152/ajpregu.00355.2015
Liu, B., Du, Y., Wu, Y., Snetselaar, L. G., Wallace, R. B., and Bao, W. (2021). Trends in obesity and adiposity measures by race or ethnicity among adults in the United States 2011-18: Population based study. BMJ 372, n365. doi:10.1136/bmj.n365
Lumeng, C. N., Bodzin, J. L., and Saltiel, A. R. (2007a). Obesity induces a phenotypic switch in adipose tissue macrophage polarization. J. Clin. Invest. 117, 175–184. doi:10.1172/JCI29881
Lumeng, C. N., Deyoung, S. M., Bodzin, J. L., and Saltiel, A. R. (2007b). Increased inflammatory properties of adipose tissue macrophages recruited during diet-induced obesity. Diabetes 56, 16–23. doi:10.2337/db06-1076
Lundberg, J. M., Franco-Cereceda, A., Hemsen, A., Lacroix, J. S., and Pernow, J. (1990). Pharmacology of noradrenaline and neuropeptide tyrosine (NPY)-mediated sympathetic cotransmission. Fundam. Clin. Pharmacol. 4, 373–391. doi:10.1111/j.1472-8206.1990.tb00692.x
Lyons, P. D., and Blalock, J. E. (1997). Pro-opiomelanocortin gene expression and protein processing in rat mononuclear leukocytes. J. Neuroimmunol. 78, 47–56. doi:10.1016/s0165-5728(97)00081-7
Macia, L., Yulyaningsih, E., Pangon, L., Nguyen, A. D., Lin, S., Shi, Y. C., et al. (2012). Neuropeptide Y1 receptor in immune cells regulates inflammation and insulin resistance associated with diet-induced obesity. Diabetes 61, 3228–3238. doi:10.2337/db12-0156
Mandrika, I., Muceniece, R., and Wikberg, J. E. (2001). Effects of melanocortin peptides on lipopolysaccharide/interferon-gamma-induced NF-kappaB DNA binding and nitric oxide production in macrophage-like RAW 264.7 cells: Evidence for dual mechanisms of action. Biochem. Pharmacol. 61, 613–621. doi:10.1016/s0006-2952(00)00583-9
Meng, W., Xiao, T., Liang, X., Wen, J., Peng, X., Wang, J., et al. (2021). The miR-182-5p/FGF21/acetylcholine axis mediates the crosstalk between adipocytes and macrophages to promote beige fat thermogenesis. JCI Insight 6, 150249. doi:10.1172/jci.insight.150249
Montague, C. T., Farooqi, I. S., Whitehead, J. P., Soos, M. A., Rau, H., Wareham, N. J., et al. (1997). Congenital leptin deficiency is associated with severe early-onset obesity in humans. Nature 387, 903–908. doi:10.1038/43185
Murase, T., Arima, H., Kondo, K., and Oiso, Y. (1996). Neuropeptide FF reduces food intake in rats. Peptides 17, 353–354. doi:10.1016/0196-9781(95)02137-x
Murphy, K. T., Schwartz, G. J., Nguyen, N. L., Mendez, J. M., Ryu, V., and Bartness, T. J. (2013). Leptin-sensitive sensory nerves innervate white fat. Am. J. Physiol. Endocrinol. Metab. 304, E1338–E1347. doi:10.1152/ajpendo.00021.2013
Navarrete, A., van Schaik, C. P., and Isler, K. (2011). Energetics and the evolution of human brain size. Nature 480, 91–93. doi:10.1038/nature10629
Nguyen, K. D., Qiu, Y., Cui, X., Goh, Y. P., Mwangi, J., David, T., et al. (2011). Alternatively activated macrophages produce catecholamines to sustain adaptive thermogenesis. Nature 480, 104–108. doi:10.1038/nature10653
Nguyen, N. L., Barr, C. L., Ryu, V., Cao, Q., Xue, B., and Bartness, T. J. (2017). Separate and shared sympathetic outflow to white and Brown fat coordinately regulates thermoregulation and beige adipocyte recruitment. Am. J. Physiol. Regul. Integr. Comp. Physiol. 312, R132–R145. doi:10.1152/ajpregu.00344.2016
Niijima, A. (1998). Afferent signals from leptin sensors in the white adipose tissue of the epididymis, and their reflex effect in the rat. J. Auton. Nerv. Syst. 73, 19–25. doi:10.1016/s0165-1838(98)00109-x
Niijima, A. (1999). Reflex effects from leptin sensors in the white adipose tissue of the epididymis to the efferent activity of the sympathetic and vagus nerve in the rat. Neurosci. Lett. 262, 125–128. doi:10.1016/s0304-3940(99)00054-3
Nogueiras, R., Wiedmer, P., Perez-Tilve, D., Veyrat-Durebex, C., Keogh, J. M., Sutton, G. M., et al. (2007). The central melanocortin system directly controls peripheral lipid metabolism. J. Clin. Invest. 117, 3475–3488. doi:10.1172/JCI31743
Ofenheimer, A., Breyer-Kohansal, R., Hartl, S., Burghuber, O. C., Krach, F., Schrott, A., et al. (2020). Reference values of body composition parameters and visceral adipose tissue (VAT) by DXA in adults aged 18-81 years-results from the LEAD cohort. Eur. J. Clin. Nutr. 74, 1181–1191. doi:10.1038/s41430-020-0596-5
Park, S., Komatsu, T., Hayashi, H., Mori, R., and Shimokawa, I. (2021). The role of neuropeptide Y in adipocyte-macrophage crosstalk during high fat diet-induced adipose inflammation and liver steatosis. Biomedicines 9, 1739. doi:10.3390/biomedicines9111739
Pavlov, V. A., Chavan, S. S., and Tracey, K. J. (2018). Molecular and functional neuroscience in immunity. Annu. Rev. Immunol. 36, 783–812. doi:10.1146/annurev-immunol-042617-053158
Pekala, P., Kawakami, M., Vine, W., Lane, M. D., and Cerami, A. (1983). Studies of insulin resistance in adipocytes induced by macrophage mediator. J. Exp. Med. 157, 1360–1365. doi:10.1084/jem.157.4.1360
Pelletier, J., Roudier, E., Abraham, P., Fromy, B., Saumet, J. L., Birot, O., et al. (2015). VEGF-A promotes both pro-angiogenic and neurotrophic capacities for nerve recovery after compressive neuropathy in rats. Mol. Neurobiol. 51, 240–251. doi:10.1007/s12035-014-8754-1
Petkevicius, K., Bidault, G., Virtue, S., Newland, S. A., Dale, M., Dugourd, A., et al. (2021). Macrophage beta2-adrenergic receptor is dispensable for the adipose tissue inflammation and function. Mol. Metab. 48, 101220. doi:10.1016/j.molmet.2021.101220
Pirzgalska, R. M., Seixas, E., Seidman, J. S., Link, V. M., Sanchez, N. M., Mahu, I., et al. (2017). Sympathetic neuron-associated macrophages contribute to obesity by importing and metabolizing norepinephrine. Nat. Med. 23, 1309–1318. doi:10.1038/nm.4422
Plum, L., Rother, E., Munzberg, H., Wunderlich, F. T., Morgan, D. A., Hampel, B., et al. (2007). Enhanced leptin-stimulated Pi3k activation in the CNS promotes white adipose tissue transdifferentiation. Cell Metab. 6, 431–445. doi:10.1016/j.cmet.2007.10.012
Rahmouni, K., and Morgan, D. A. (2007). Hypothalamic arcuate nucleus mediates the sympathetic and arterial pressure responses to leptin. Hypertension 49, 647–652. doi:10.1161/01.HYP.0000254827.59792.b2
Rajora, N., Ceriani, G., Catania, A., Star, R. A., Murphy, M. T., and Lipton, J. M. (1996). alpha-MSH production, receptors, and influence on neopterin in a human monocyte/macrophage cell line. J. Leukoc. Biol. 59, 248–253. doi:10.1002/jlb.59.2.248
Romanatto, T., Cesquini, M., Amaral, M. E., Roman, E. A., Moraes, J. C., Torsoni, M. A., et al. (2007). TNF-alpha acts in the hypothalamus inhibiting food intake and increasing the respiratory quotient-effects on leptin and insulin signaling pathways. Peptides 28, 1050–1058. doi:10.1016/j.peptides.2007.03.006
Ruohonen, S. T., Pesonen, U., Moritz, N., Kaipio, K., Roytta, M., Koulu, M., et al. (2008). Transgenic mice overexpressing neuropeptide Y in noradrenergic neurons: A novel model of increased adiposity and impaired glucose tolerance. Diabetes 57, 1517–1525. doi:10.2337/db07-0722
Ruschke, K., Ebelt, H., Kloting, N., Boettger, T., Raum, K., Bluher, M., et al. (2009). Defective peripheral nerve development is linked to abnormal architecture and metabolic activity of adipose tissue in Nscl-2 mutant mice. PLoS One 4, e5516. doi:10.1371/journal.pone.0005516
Ryu, V., and Bartness, T. J. (2014). Short and long sympathetic-sensory feedback loops in white fat. Am. J. Physiol. Regul. Integr. Comp. Physiol. 306, R886–R900. doi:10.1152/ajpregu.00060.2014
Scherer, T., O'Hare, J., Diggs-Andrews, K., Schweiger, M., Cheng, B., Lindtner, C., et al. (2011). Brain insulin controls adipose tissue lipolysis and lipogenesis. Cell Metab. 13, 183–194. doi:10.1016/j.cmet.2011.01.008
Schneider, M. K., Xue, B., and Shi, H. (2018). Activation of the sympathetic nervous system suppresses mouse white adipose tissue hyperplasia through the β1 adrenergic receptor. Physiol. Rep. 6, e13645. doi:10.14814/phy2.13645
Schwarz, H., Villiger, P. M., von Kempis, J., and Lotz, M. (1994). Neuropeptide Y is an inducible gene in the human immune system. J. Neuroimmunol. 51, 53–61. doi:10.1016/0165-5728(94)90128-7
Schwinkendorf, D. R., Tsatsos, N. G., Gosnell, B. A., and Mashek, D. G. (2011). Effects of central administration of distinct fatty acids on hypothalamic neuropeptide expression and energy metabolism. Int. J. Obes. 35, 336–344. doi:10.1038/ijo.2010.159
Serradeil-Le Gal, C., Lafontan, M., Raufaste, D., Marchand, J., Pouzet, B., Casellas, P., et al. (2000). Characterization of NPY receptors controlling lipolysis and leptin secretion in human adipocytes. FEBS Lett. 475, 150–156. doi:10.1016/s0014-5793(00)01649-5
Shi, Z., Pelletier, N. E., Wong, J., Li, B., Sdrulla, A. D., Madden, C. J., et al. (2020). Leptin increases sympathetic nerve activity via induction of its own receptor in the paraventricular nucleus. Elife 9, e55357. doi:10.7554/eLife.55357
Shin, A. C., Filatova, N., Lindtner, C., Chi, T., Degann, S., Oberlin, D., et al. (2017). Insulin receptor signaling in POMC, but not AgRP, neurons controls adipose tissue insulin action. Diabetes 66, 1560–1571. doi:10.2337/db16-1238
Simonds, S. E., Cowley, M. A., and Enriori, P. J. (2012). Leptin increasing sympathetic nerve outflow in obesity: A cure for obesity or a potential contributor to metabolic syndrome? Adipocyte 1, 177–181. doi:10.4161/adip.20690
Singer, K., Morris, D. L., Oatmen, K. E., Wang, T., DelProposto, J., Mergian, T., et al. (2013). Neuropeptide Y is produced by adipose tissue macrophages and regulates obesity-induced inflammation. PLoS One 8, e57929. doi:10.1371/journal.pone.0057929
Sivitz, W. I., Fink, B. D., Morgan, D. A., Fox, J. M., Donohoue, P. A., and Haynes, W. G. (1999). Sympathetic inhibition, leptin, and uncoupling protein subtype expression in normal fasting rats. Am. J. Physiol. 277, E668–E677. doi:10.1152/ajpendo.1999.277.4.E668
Smith, S. R., Gawronska-Kozak, B., Janderova, L., Nguyen, T., Murrell, A., Stephens, J. M., et al. (2003). Agouti expression in human adipose tissue: Functional consequences and increased expression in type 2 diabetes. Diabetes 52, 2914–2922. doi:10.2337/diabetes.52.12.2914
Sondell, M., Lundborg, G., and Kanje, M. (1999). Vascular endothelial growth factor has neurotrophic activity and stimulates axonal outgrowth, enhancing cell survival and Schwann cell proliferation in the peripheral nervous system. J. Neurosci. 19, 5731–5740. doi:10.1523/jneurosci.19-14-05731.1999
Song, C. K., Jackson, R. M., Harris, R. B., Richard, D., and Bartness, T. J. (2005). Melanocortin-4 receptor mRNA is expressed in sympathetic nervous system outflow neurons to white adipose tissue. Am. J. Physiol. Regul. Integr. Comp. Physiol. 289, R1467–R1476. doi:10.1152/ajpregu.00348.2005
Song, W., Luo, Q., Zhang, Y., Zhou, L., Liu, Y., Ma, Z., et al. (2019). Organic cation transporter 3 (Oct3) is a distinct catecholamines clearance route in adipocytes mediating the beiging of white adipose tissue. PLoS Biol. 17, e2006571. doi:10.1371/journal.pbio.2006571
Star, R. A., Rajora, N., Huang, J., Stock, R. C., Catania, A., and Lipton, J. M. (1995). Evidence of autocrine modulation of macrophage nitric oxide synthase by alpha-melanocyte-stimulating hormone. Proc. Natl. Acad. Sci. U. S. A. 92, 8016–8020. doi:10.1073/pnas.92.17.8016
Sutton, A. K., and Krashes, M. J. (2020). Integrating hunger with rival motivations. Trends Endocrinol. Metab. 31, 495–507. doi:10.1016/j.tem.2020.04.006
Tang, L., Okamoto, S., Shiuchi, T., Toda, C., Takagi, K., Sato, T., et al. (2015). Sympathetic nerve activity maintains an anti-inflammatory state in adipose tissue in male mice by inhibiting TNF-alpha gene expression in macrophages. Endocrinology 156, 3680–3694. doi:10.1210/EN.2015-1096
Togashi, N., Ura, N., Higashiura, K., Murakami, H., and Shimamoto, K. (2002). Effect of TNF-alpha-converting enzyme inhibitor on insulin resistance in fructose-fed rats. Hypertension 39, 578–580. doi:10.1161/hy0202.103290
Tong, Q., Ye, C. P., Jones, J. E., Elmquist, J. K., and Lowell, B. B. (2008). Synaptic release of GABA by AgRP neurons is required for normal regulation of energy balance. Nat. Neurosci. 11, 998–1000. doi:10.1038/nn.2167
Trayhurn, P., Duncan, J. S., Rayner, D. V., and Hardie, L. J. (1996). Rapid inhibition of ob gene expression and circulating leptin levels in lean mice by the beta 3-adrenoceptor agonists BRL 35135A and ZD2079. Biochem. Biophys. Res. Commun. 228, 605–610. doi:10.1006/bbrc.1996.1704
van den Top, M., Lee, K., Whyment, A. D., Blanks, A. M., and Spanswick, D. (2004). Orexigen-sensitive NPY/AgRP pacemaker neurons in the hypothalamic arcuate nucleus. Nat. Neurosci. 7, 493–494. doi:10.1038/nn1226
van Harmelen, V., Dicker, A., Sjolin, E., Blomqvist, L., Wiren, M., Hoffstedt, J., et al. (2010). Effects of pain controlling neuropeptides on human fat cell lipolysis. Int. J. Obes. 34, 1333–1340. doi:10.1038/ijo.2010.46
Varela, L., and Horvath, T. L. (2012). Leptin and insulin pathways in POMC and AgRP neurons that modulate energy balance and glucose homeostasis. EMBO Rep. 13, 1079–1086. doi:10.1038/embor.2012.174
Vogt, M. C., and Bruning, J. C. (2013). CNS insulin signaling in the control of energy homeostasis and glucose metabolism - from embryo to old age. Trends Endocrinol. Metab. 24, 76–84. doi:10.1016/j.tem.2012.11.004
Vong, L., Ye, C., Yang, Z., Choi, B., Chua, S., and Lowell, B. B. (2011). Leptin action on GABAergic neurons prevents obesity and reduces inhibitory tone to POMC neurons. Neuron 71, 142–154. doi:10.1016/j.neuron.2011.05.028
Wang, H., Yu, M., Ochani, M., Amella, C. A., Tanovic, M., Susarla, S., et al. (2003). Nicotinic acetylcholine receptor alpha7 subunit is an essential regulator of inflammation. Nature 421, 384–388. doi:10.1038/nature01339
Wang, P., Loh, K. H., Wu, M., Morgan, D. A., Schneeberger, M., Yu, X., et al. (2020). A leptin-BDNF pathway regulating sympathetic innervation of adipose tissue. Nature 583, 839–844. doi:10.1038/s41586-020-2527-y
Wang, W., Guo, D. Y., Lin, Y. J., and Tao, Y. X. (2019). Melanocortin regulation of inflammation. Front. Endocrinol. 10, 683. doi:10.3389/fendo.2019.00683
Wang, Y. N., Tang, Y., He, Z., Ma, H., Wang, L., Liu, Y., et al. (2021). Slit3 secreted from M2-like macrophages increases sympathetic activity and thermogenesis in adipose tissue. Nat. Metab. 3, 1536–1551. doi:10.1038/s42255-021-00482-9
Waqas, S. F. H., Hoang, A. C., Lin, Y. T., Ampem, G., Azegrouz, H., Balogh, L., et al. (2017). Neuropeptide FF increases M2 activation and self-renewal of adipose tissue macrophages. J. Clin. Invest. 127, 3559. doi:10.1172/JCI95841
Weisberg, S. P., McCann, D., Desai, M., Rosenbaum, M., Leibel, R. L., and Ferrante, A. W. (2003). Obesity is associated with macrophage accumulation in adipose tissue. J. Clin. Invest. 112, 1796–1808. doi:10.1172/JCI19246
Wellen, K. E., and Hotamisligil, G. S. (2003). Obesity-induced inflammatory changes in adipose tissue. J. Clin. Invest. 112, 1785–1788. doi:10.1172/JCI20514
Xie, H., Heier, C., Meng, X., Bakiri, L., Pototschnig, I., Tang, Z., et al. (2022). An immune-sympathetic neuron communication axis guides adipose tissue browning in cancer-associated cachexia. Proc. Natl. Acad. Sci. U. S. A. 119, e2112840119. doi:10.1073/pnas.2112840119
Yadav, N. V. S., Barcikowski, A., Uehana, Y., Jacobs, A. T., and Connelly, L. (2020). Breast adipocyte Co-culture increases the expression of pro-angiogenic factors in macrophages. Front. Oncol. 10, 454. doi:10.3389/fonc.2020.00454
Yang, K., Guan, H., Arany, E., Hill, D. J., and Cao, X. (2008). Neuropeptide Y is produced in visceral adipose tissue and promotes proliferation of adipocyte precursor cells via the Y1 receptor. FASEB J. 22, 2452–2464. doi:10.1096/fj.07-100735
Youngstrom, T. G., and Bartness, T. J. (1995). Catecholaminergic innervation of white adipose tissue in Siberian hamsters. Am. J. Physiol. 268, R744–R751. doi:10.1152/ajpregu.1995.268.3.R744
Zabolotny, J. M., Kim, Y. B., Welsh, L. A., Kershaw, E. E., Neel, B. G., and Kahn, B. B. (2008). Protein-tyrosine phosphatase 1B expression is induced by inflammation in vivo. J. Biol. Chem. 283, 14230–14241. doi:10.1074/jbc.M800061200
Zeng, W., Pirzgalska, R. M., Pereira, M. M., Kubasova, N., Barateiro, A., Seixas, E., et al. (2015). Sympathetic neuro-adipose connections mediate leptin-driven lipolysis. Cell 163, 84–94. doi:10.1016/j.cell.2015.08.055
Zhan, C., Zhou, J., Feng, Q., Zhang, J. E., Lin, S., Bao, J., et al. (2013). Acute and long-term suppression of feeding behavior by POMC neurons in the brainstem and hypothalamus, respectively. J. Neurosci. 33, 3624–3632. doi:10.1523/JNEUROSCI.2742-12.2013
Zhang, L., Ip, C. K., Lee, I. J., Qi, Y., Reed, F., Karl, T., et al. (2018). Diet-induced adaptive thermogenesis requires neuropeptide FF receptor-2 signalling. Nat. Commun. 9, 4722. doi:10.1038/s41467-018-06462-0
Keywords: central nervous system, hypothalamus, adipose tissue, sympathetic regulation, lipolysis, adipogenesis, adipose tissue macrophage, resident immune cells
Citation: Puente-Ruiz SC and Jais A (2022) Reciprocal signaling between adipose tissue depots and the central nervous system. Front. Cell Dev. Biol. 10:979251. doi: 10.3389/fcell.2022.979251
Received: 27 June 2022; Accepted: 24 August 2022;
Published: 16 September 2022.
Edited by:
Siegfried Ussar, Helmholtz Association of German Research Centres (HZ), GermanyReviewed by:
Kristy L. Townsend, The Ohio State University Wexner Medical CenterPetra Kotzbeck, Medical University of Graz, Austria
Copyright © 2022 Puente-Ruiz and Jais. This is an open-access article distributed under the terms of the Creative Commons Attribution License (CC BY). The use, distribution or reproduction in other forums is permitted, provided the original author(s) and the copyright owner(s) are credited and that the original publication in this journal is cited, in accordance with accepted academic practice. No use, distribution or reproduction is permitted which does not comply with these terms.
*Correspondence: Alexander Jais, YWxleGFuZGVyLmphaXNAaGVsbWhvbHR6LW11ZW5jaGVuLmRl