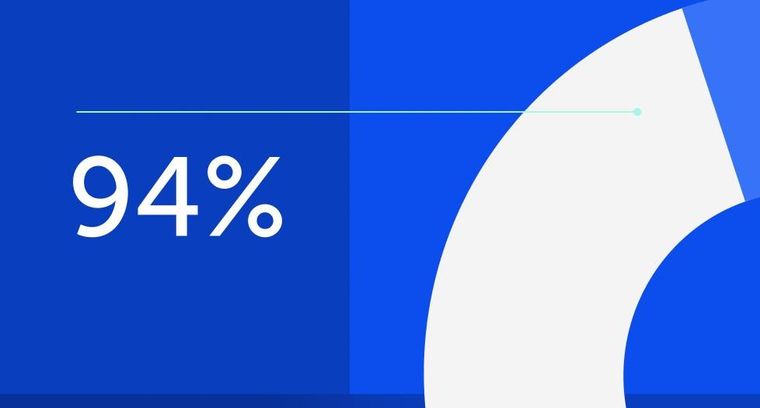
94% of researchers rate our articles as excellent or good
Learn more about the work of our research integrity team to safeguard the quality of each article we publish.
Find out more
REVIEW article
Front. Cell Dev. Biol., 30 August 2022
Sec. Cell Growth and Division
Volume 10 - 2022 | https://doi.org/10.3389/fcell.2022.976736
The skeleton is one of the largest organ systems in the body and is richly innervated by the network of nerves. Peripheral nerves in the skeleton include sensory and sympathetic nerves. Crosstalk between bones and nerves is a hot topic of current research, yet it is not well understood. In this review, we will explore the role of nerves in bone repair and remodeling, as well as summarize the molecular mechanisms by which neurotransmitters regulate osteogenic differentiation. Furthermore, we discuss the skeleton’s role as an endocrine organ that regulates the innervation and function of nerves by secreting bone-derived factors. An understanding of the interactions between nerves and bone can help to prevent and treat bone diseases caused by abnormal innervation or nerve function, develop new strategies for clinical bone regeneration, and improve patient outcomes.
The nerves are spatially connected to the skeleton. The skeleton is extensively innervated by sensory and sympathetic nerves (Togari et al., 2012). However, our understanding of the interrelationship between nerve and bone is still limited, which also hinders the treatment of neuro-skeletal disorders. Neuroskeletal regulation has received more attention in recent years, and some pioneering theories and ideas have been proposed. Skeletal function and regeneration were affected by neurological defects or mental disorders (Kelly et al., 2020; Yang et al., 2020). Furthermore, Ducy et al. found that bone remodeling was regulated by the central nervous system and its derived leptin (Ducy et al., 2000; Takeda et al., 2002), suggesting that nerves have the ability to modulate bone. Since then, researchers have explored the mechanisms by which neurons in the central and peripheral nervous systems communicate with cells in the skeletal microenvironment and have identified the contributions of sensory and sympathetic nerves to bone development, bone homeostasis, and bone remodeling (Grassel, 2014; Chen et al., 2019; Tomlinson et al., 2020; Wang et al., 2020). Moreover, the skeleton makes up approximately 15% of the total body weight and is one of the largest organ systems in the body, supporting the movement and protecting vital organs (Han et al., 2018; Su et al., 2019; Rath and Durairaj, 2022). However, in the last 20 years, it has gradually been discovered that the skeleton also acted as a secretory organ, regulating other tissue functions by secreting different bone-derived factors (Li et al., 2019; Qian et al., 2021). The crosstalk between bone and nerves is a hot topic of research currently. As part of this review, we will examine the role of nerves in bone formation and repair, analyze the regulation of the nervous system by the skeleton, and summarize the mechanisms of mutual regulation between nerves and bone.
Connecting the central nervous system (CNS) to the skeleton is the peripheral nervous system (PNS), which acts as a transmitter of information. In the PNS, neurons are found in sensory and autonomic ganglia, collections of nerve cell bodies with axons that extend to target tissues (Brazill et al., 2019). Long bones and the calvaria are innervated by nerves that originate at different locations. Sensory neurons in the limbs originate in the dorsal root ganglion (DRG) next to the spinal cord and have a pseudo-unipolar morphology (Sorkin et al., 2018). Its axons bifurcate into peripheral projections that innervate the target tissue (Figure 1A) and can sense pain, pressure, heat and other stimuli and transmit them to central processing (Brazill et al., 2019). Nociceptors are the receptors that are sensitive to injurious stimuli (Dubin and Patapoutian, 2010). They can be further subdivided into peptidergic and non-peptidergic primary afferent neurons (Kestell et al., 2015). Nerve growth factor (NGF) is necessary for the survival and recruitment of peptidergic fibers, whereas fibers lacking peptides are dependent on glial-derived neurotrophic factor (GDNF) (Averill et al., 1995). Peptide-rich fibers expressing substance P (SP) and calcitonin gene-related peptide (CGRP) are prevalent in the skeleton of vertebrate species (Hill and Elde, 1991; Brazill et al., 2019). In addition, the collateral axon branches of these neurons can generate efferent signals that target their peripheral target tissues (Brazill et al., 2019). This local release of neuropeptides indicates the trophic nature of sensory nerves and their ability to modulate skeletal activity.
FIGURE 1. Distribution of nerves in limb and skull. (A) Pan-neuronal tubulin beta 3 Class III (TUBB3) whole-mount immunohistochemical staining of the mouse ulnar periosteum. (B, C) Whole-mount images of the mouse calvaria, showing the TUBB3+ nerve fibers in the (B) dura and (C) periosteum. White dashed lines indicate margins of the interfrontal suture, imaged from the endocranial aspect. B, bone; BM, bone marrow; PO, periosteum. White scale bar, 100 μm.
The autonomic nervous system (ANS) is a part of the peripheral efferent nervous system that innervates and regulates the activity of all organs of the body, including sympathetic and parasympathetic nerves (McCorry, 2007). The autonomic nerves in the limbs are mainly sympathetic (Brazill et al., 2019). Through preganglionic neurons in the spinal cord, autonomic nerves synapse with postganglionic neurons in the paravertebral ganglia of the sympathetic chain (Cherruau et al., 2003; Elefteriou, 2018). Sympathetic postganglionic neurons are noradrenergic, and they can project to a wide range of tissues in the body (Elefteriou, 2018). Sympathetic adrenergic neurons, which are abundant in bone, express tyrosine hydroxylase (TH) and promote the synthesis of norepinephrine (NE) (Hill and Elde, 1991; Mach et al., 2002). In addition, cholinergic neurons containing acetylcholine (ACh) and the neuropeptide vasoactive intestinal peptide (VIP) innervate bone (Hohmann et al., 1986; Cherruau et al., 2003).
Reports on nerve innervation in the skull are very limited. The ganglia that innervate the craniofacial skeleton are located in the cranial nerve ganglia (Brazill et al., 2019). Calvarial bone is surrounded by both intracranial (dura) and extracranial (periosteal) sheaths (Figures 1B,C) that contain sensory, sympathetic, and parasympathetic nerve endings (Kosaras et al., 2009). In the mature calvaria, the sutures are thought to serve as the major passageway of innervation between the dura and periosteum. From the dura, the fibers appear to penetrate the suture and emerge into the periosteum (Kosaras et al., 2009). Moreover, our results showed that the sutures were dominated by sensory nerves with a small portion of sympathetic nerves (Meyers et al., 2020). The regulatory mechanisms of neurodevelopment are complex. The influence of nerve formation and distribution on cranial repair remains to be elucidated.
In summary, the skeleton is covered by neural networks. Their location in space determines that they necessarily modulate each other. However, we know very little about the interaction between the skeleton and the nervous system, which has been a hot topic of research in recent years.
Bone is innervated by sensory nerve fibers expressing tropomyosin receptor kinase A (TrkA), which are required for the proliferation of osteochondral progenitors during skeletal development in mammals (Castaneda-Corral et al., 2011; Mantyh, 2014). Besides pain, little is known about the role of sensory innervation in bone regeneration. NGF is the first discovered neurotrophic factor that is primarily involved in the regulation of the growth, survival, as well as regeneration of neurons (Ebendal, 1992). In addition to directly activating TrkA+ sensory neurons to transmit injury signals, NGF determines the amount and type of innervation required for repair and regulates the growth of TrkA+ neurons into the cartilage membrane of long bones during embryogenesis, which is required for progenitor cell differentiation and bone mineralization (Reichardt, 2006; Tomlinson et al., 2016). Primary and secondary ossification centers exhibited delayed vascular invasion when TrkA+ signaling and innervation were inhibited, which further decreased the number of Osterix+ osteoprogenitor cells and reduced the length and volume of femur (Tomlinson et al., 2016). The regeneration of entire appendages by starfish and certain amphibians depends on nerves. We also found that mammalian fracture repair required TrkA signaling from sensory nerves (Li et al., 2019). NGF was significantly upregulated in the early stage of repair after fracture. The bone repair process involves the regulation of inflammation by macrophages and the formation of bone by mesenchymal stem cells (MSCs). In our study, the main sources of NGF production were periosteal MSCs and osteocalcin (OCN)+ bone-lining osteoblasts, followed by macrophages. TrkA+ nerve sprouting was positively correlated with NGF expression. The level of NGF and the number of newly generated nerve fibers peaked on day 3 and then dropped significantly. Moreover, the nerves in the callus were predominantly sensory and peptidergic fibers, with a small proportion of TH+ sympathetic nerve fibers. Inhibition of NGF-TrkA signaling impaired sensory nerve re-growth and vascular remodeling, leading to failure of bone healing. The research suggests that NGF regulates nerve ingrowth at an early stage, which facilitates vascular and bone regeneration. Thus, we reveal the sequence of cellular events that occur during re-innervation of fracture-healed tissue and demonstrate a critical role of TrkA sensory nerves in bone healing.
The flat bones of the skull are densely innervated, which is essential for coordinated bone development and repair (Warren et al., 2003; Doro et al., 2017). Our group next characterized the innervation of the mouse skull and explored their role in cranial bone defect repair (Meyers et al., 2020). Nerve fibers were observed in the uninjured skull. During the early stages of calvarial defect repair, macrophages were activated and expanded rapidly, releasing significant amounts of NGF. Locally increased NGF promoted nerve regeneration and axonal ingrowth. Furthermore, immunohistochemical staining identified the sprouting nerve as primarily CGRP+ peptidergic nociceptors. The nerve regeneration process further promoted vascular remodeling and bone regeneration at the defect site. Like the long bone, TrkA is universally expressed on fibers that innervate the calvarium from the trigeminal ganglia. A mutation in TrkA protein inhibited NGF signaling and subsequently impaired the re-innervation of tubulin beta 3 Class III (TUBB3)+ nerve fibers. These findings suggest that nerve regeneration in the skull is also controlled by the NGF-TrkA signaling pathway. Blocking the NGF-TrkA signaling pathway led to reduced vascularity and decreased osteogenic capacity, which significantly delayed bone defect healing (Meyers et al., 2020). Interestingly, we found that NGF also regulated the migration of MSCs. Locally released NGF recruited the aggregation of MSCs to the defect site via the NGF-p75 signaling pathway, thereby coupling the neuro-skeletal interaction (Figure 2) (Xu et al., 2022). Despite these findings, we have yet to address the downstream molecular mechanisms of neuroskeletal intercommunication. It is particularly important to investigate how nociceptive fibers affect bone repair through secondary messengers.
FIGURE 2. NGF promotes neural regeneration and MSC migration. There are two receptors for nerve growth factor (NGF): tropomyosin receptor kinase A (TrkA) and p75 (also known as CD271). Upon bone injury, NGF produced by macrophages activates the TrkA pathway to promote nerve fiber re-innervation. Furthermore, NGF recruits mesenchymal stem cells via the p75 signaling pathway to migrate toward bone defects and enhance bone formation.
Along with NGF, semaphorin 3A (Sema3A) is also a well-known axon guidance molecule (Tamagnone and Comoglio, 2004; Kruger et al., 2005; Fukuda et al., 2013). Neuron-derived Sema3A is essential for the normal development of peripheral neurons in vivo and contributes to nerve growth and regeneration (Zhang et al., 2018). Fukuda et al. demonstrated that Sema3A produced in neurons regulated bone remodeling by modulating sensory nerve innervation to the bone (Fukuda et al., 2013). Specific deletion of Sema3A in neurons resulted in lower bone mass, whereas mice with a Sema3A deficiency specific to osteoblasts had normal bone mass. Further studies revealed a significant reduction in the number of sensory innervations of trabecular bone in neuron-specific Sema3A-deficient mice, leading to skeletal abnormalities. In contrast, sympathetic nerve fibers, which inhibit the increase in bone mass, were not significantly affected. In combination with our transcriptome sequencing data (Xu et al., 2022), we found high expression of Sema3A locally in bone injury sites. Based on its role in bone remodeling, we hypothesize that Sema3A also plays an important regulatory role in bone repair by stimulating sensory nerve innervation and thus promoting bone regeneration. However, the relative roles of Sema3A and NGF in bone regeneration need to be further explored.
As the skeleton develops and fractures heal, nerve fibers are closely associated with blood vessels (Tomlinson et al., 2016; Li et al., 2019). Our study showed that nerve regeneration occurred before revascularization and contributed to vascular regrowth (Meyers et al., 2020). Angiogenesis is promoted by sensory nerve-released SP and CGRP via neurokinin-1 (NK1) and CGRPR receptors on endothelial cells (Marrella et al., 2018). We previously found that vascular extracellular vesicles (EVs) promoted MSC proliferation, migration, and differentiation and improved bone regenerative capacity (Xu et al., 2019). Thus, nerves can indirectly regulate bone repair through the vasculature in addition to their paracrine role in regulating bone regeneration.
Clear nerve-derived factors mediating bone formation, including neurotransmitters and extracellular vesicles (Figure 3), have been elucidated in several in vitro studies and regenerative contexts. Bone lineage cells express receptors for neurotransmitters or neuropeptides that can be modulated by neurogenic substances. CGRP and SP, which are derived from sensory nerves, stimulate osteogenic differentiation and bone formation. CGRP is a critical and highly expressed sensory signal. It produces in both peripheral and central neurons and can function in the transmission of nociception (Rosenfeld et al., 1983; Crenshaw et al., 1987). CGRP promoted the proliferation of osteoprogenitor cells, reduced their apoptosis and enhanced the expression of osteogenic genes and the activity of osteoblasts via inhibiting NF-κB activation (Mrak et al., 2010; Wang et al., 2010). In addition, CGRP promoted the recruitment and cell proliferation of MSCs and enhanced osteogenic differentiation by activating mitogen-activated protein kinase (MAPK) signaling pathway (Yang et al., 2017; Wang et al., 2020) and upregulating the expression of alkaline phosphatase (ALP) and runt-related transcription factor 2 (Runx2) (Fang et al., 2013; Xu and Jiang, 2014). Following bone injury or fracture, CGRP-containing fibers may trigger perivascular bone regeneration. Local injection of CGRP was effective in accelerating bone formation (Jia et al., 2019). CGRP expression in the peripheral femoral cortex and dorsal root ganglion (DRG) was significantly increased following in vivo implantation of biodegradable magnesium, which promoted new bone formation (Zhang et al., 2016).
FIGURE 3. Bone regeneration is regulated by nerves through paracrine action. Nerve growth factor (NGF) and semaphorin 3A (Sema3A) promote nerve regeneration. Neurotransmitters and small extracellular vesicles (sEVs) are released by neural cells to regulate angiogenesis and osteogenesis. Red sharp arrows (→) indicate stimulation, while blue blunt arrows (┴) indicate inhibition. The role of neuropeptide Y (NPY) in regulating bone formation remains controversial. SP, substance P; CGRP, calcitonin gene-related peptide; NE, norepinephrine; ACh, acetylcholine; VIP, vasoactive intestinal peptide.
SP belongs to the tachykinin family and is a highly conserved 11-amino acid neuropeptide involved in pain perception (Hong et al., 2009; Steinhoff et al., 2014). SP is expressed in the peripheral nerves and is often released together with CGRP (Brazill et al., 2019). Similar to CGRP, SP has been shown to increase the proliferation and osteogenic differentiation of MSCs (Nagao et al., 2014). SP bound to and activated the NK-1 receptor (NK-1R), which was expressed by bone lineage cells (Goto et al., 1998; Goto et al., 2007; Wang et al., 2020). SP directly regulated bone metabolism through NK-1R (Goto et al., 1998). SP stimulated the proliferation of BMSCs in a concentration-dependent manner. Wang et al. found that low concentrations of SP promoted ALP activity and Runx2 expression, and high concentrations of SP contributed to matrix mineralization (Wang et al., 2009). Furthermore, SP enhanced MAPK, Wnt and bone morphogenetic protein 2 (Bmp2) signaling pathways as well as upregulated vascular endothelial growth factor (VEGF) expression, thereby promoting angiogenesis and osteogenic differentiation (Hong et al., 2009; Fu et al., 2014; Wang et al., 2020). SP also increased the mobilization of MSCs to the osteogenic region, accelerating bone remodeling and formation (Imai et al., 1997; Hong et al., 2009; Zhang et al., 2014).
Neuronal cells can also regulate bone regeneration remotely via extracellular vesicles. According to clinical studies, patients with craniocerebral injury and fractures have a faster callus formation and a shorter fracture healing time than patients with fractures alone. Xia et al. demonstrated that damaged neurons released small extracellular vesicles (sEVs) rich in pro-osteogenic microRNAs (miRNAs) after traumatic brain injury (TBI) (Xia et al., 2021). Fibronectin 1 on the membrane surface of sEVs might be a key protein for brain-derived sEVs to target bone tissue. Moreover, miR-328a-3p and miR-150-5p enriched in sEVs accelerated bone healing by regulating the transcription of FOXO4 and CBL proteins, respectively.
Sympathetic signaling reduces bone mass. Sympathetic tone activation upregulated intracellular OPN expression and induced bone loss. Mechanistically, intracellular OPN reduced the cAMP-response element binding (CREB) phosphorylation and osteogenic differentiation via the inhibition of β2-adrenergic receptor (Nagao et al., 2011). NE is the main neurotransmitter of the sympathetic nervous system and is synthesized from the amino acid tyrosine by the action of TH (Elefteriou, 2018). Adrenergic nerves release NE that inhibits bone formation. Its receptors include a- and ß-adrenergic receptors (ARs), such as β1, β2, α1B, α2A, α2B, and α2C (Grassel, 2014; Tank and Lee Wong, 2015; Brazill et al., 2019; Wan et al., 2021). NE participates in the regulation of bone remodeling mainly via its β2 receptor. Sympathetic signaling via β2-adrenergic receptors (β2-AR) present on osteoblasts controlled bone formation. Disruption of the noradrenergic signaling axis or knock of β2-AR increased bone mass (Elefteriou et al., 2005). Mechanistically, sympathetic tone signals in osteoblasts suppressed CREB phosphorylation via β2-AR, thereby reducing osteoblast proliferation (Takeda et al., 2002; Kajimura et al., 2011). Du et al. found that NE inhibited stem cell migration, downregulated Runx2 and OCN expression, and prevented the formation of mineralized bone nodules via β3-adrenergic receptor (Du et al., 2014).
Cholinergic nerve-derived ACh and VIP, as well as adrenergic nerve-produced neuropeptide Y (NPY) also play important roles in bone formation. ACh functions by binding to both muscarinic (mAChRs) and nicotinic acetylcholine receptors (nAChRs) (Jones et al., 2012). These receptors are expressed on bone lineage cells, suggesting that ACh may affect bone formation (Wan et al., 2021). Ma et al. demonstrated the beneficial effect of ACh signaling on bone mass increase, while it also promoted the maintenance of peak bone mass in adult mice (Ma and Elefteriou, 2020). Acetylcholinesterase (AChE) is a classical cholinergic hydrolase that mainly degrades acetylcholine. AChE decelerated bone growth by terminating the action of acetylcholine to balance acetylcholine concentrations at low levels, thereby promoting chondrocyte apoptosis and impeding bone mineralization. Conversely, reducing AChE increased ACh activity and promoted bone formation (Luo et al., 2020). VIP is normally released from cholinergic neurons together with acetylcholine. There are two known receptors for the VIP termed VPAC1 and VPAC2. VIP promoted osteogenic differentiation of MSCs by activating the Wnt/β-catenin signaling pathway. VIP also increased the expression of VEGF in MSCs to stimulate angiogenesis. Additionally, VIP significantly boosted cranial defect repair, bone formation, and angiogenesis in vivo (Shi et al., 2020). PKA activation by VIP resulted in the accumulation of cAMP and affected multiple signaling pathways that regulate osteoblast differentiation (Juhasz et al., 2016). VIP also upregulated ERK 1/2 activation in osteoblasts (Juhasz et al., 2015). NPY is usually accompanied by the release of NE in sympathetic nerves (Wan et al., 2021). NPY acts through five G protein-coupled receptor isoforms, Y1R, Y2R, Y4R, Y5R and Y6R, of which Y1R and Y2R are usually involved in bone mass regulation (Chen and Zhang, 2022). Osteoblast-specific Y1 receptor knockout mice exhibited high bone mass, suggesting that the NPY-Y1R signaling axis regulates bone homeostasis (Yahara et al., 2017). Reduced Runx2 levels and mineralized nodules after NPY-treated MSC osteogenesis confirmed that NPY impaired osteogenic differentiation (Zhang et al., 2021). Blockade of NPY enhanced bone mass (Baldock et al., 2009; Lee et al., 2010; Xie et al., 2020). However, some studies have also reported a positive role for NPY in bone formation and bone repair (Chen and Zhang, 2022). Low doses of NPY promoted osteogenesis and mineralization in MSCs, whereas high concentrations of NPY had the opposite effect (Liu et al., 2016). NPY overexpression significantly upregulated osterix and Runx2 levels and enhanced osteogenic capacity (Zhang et al., 2020). The specific regulatory role of NPY on bone formation needs to be further clarified.
Nevertheless, our understanding of the exact key molecules released from nerve fibers within a bone injury site that regulate bone regeneration in vivo remains unclear and is the subject of continued investigation.
Bone has long been thought to serve only as a supportive and protective organ (Zhu et al., 2020). In the last 20 years, it has slowly become apparent that bone can also act as an endocrine organ by secreting various bone-derived factors that have regulatory effects on the central nervous system, immune system, energy metabolism, etc (Guntur and Rosen, 2012; Moser and van der Eerden, 2018; Du et al., 2021). OCN is a marker gene for osteogenic differentiation and is synthesized solely in osteoblasts (Klingelhoffer et al., 2016; Moser and van der Eerden, 2018). It plays an important role in regulating bone calcium metabolism. OCN is considered as a multifunctional bone-derived hormone that regulates a variety of physiological activities and developmental processes (Karsenty and Olson, 2016; Obri et al., 2018; Qian et al., 2021). OCN regulates the neuronal function and is required for both brain development and brain function in mice (Obri et al., 2018). Khrimian et al. found that the target receptor for OCN in the brain was the G-protein coupled receptor Gpr158, which was expressed in neurons in the CA3 region of the hippocampus (Khrimian et al., 2017). Despite this, Gpr158 was not expressed in all OCN-active brain regions, suggesting that other receptors respond to osteocalcin signaling in the mouse brain. Recently, Qian et al. demonstrated that OCN regulated the transition of oligodendrocyte precursor cells (OPCs) into mature oligodendrocytes (OLs) and was essential for OL myelination and remyelination after demyelination injury (Qian et al., 2021). OCN deficiency led to upregulated myelin-related gene expression and excessive myelin formation in the central nervous system. G Protein-Coupled Receptor 37 (GPR37) was abundantly expressed in mature OLs and was a novel specific receptor for OCN. OCN suppressed myelin gene regulatory factor (Myrf) through GPR37, which was required for OCN to regulate OL differentiation and myelination. This study reveals reciprocal crosstalk between bone secreted-OCN and glial cell function, providing evidence for an important role of OCN in regulating OL myelin formation. Furthermore, osteoblast-derived OCN could cross the blood-brain barrier and bind to neurons in the brainstem, midbrain, and hippocampus, enhancing the production of monoamine neurotransmitters, preventing anxiety and depression, and facilitating learning and memory. In addition to these, before the embryos synthesized OCN, the mother delivered this hormone to the fetus via the placenta to prevent neuronal apoptosis (Oury et al., 2013).
Increased prostaglandin E2 (PGE2) levels in osteoporotic mice were accompanied by a significant reduction in CGRP+ sensory fibers (Chen et al., 2019). PGE2 receptor 4 (EP4) is the main receptor for PGE2 (Hawcroft et al., 2007; Konya et al., 2013), which is expressed in bone sensory nerves. Chen et al. clarified that PGE2 secretion by osteoblasts was elevated under conditions of reduced bone mass, and this signal, transmitted through EP4, regulated osteoblast-mediated bone formation (Chen et al., 2019). The specific mechanism was that PGE2 stimulated hypothalamic CREB signaling through EP4 in sensory nerves to inhibit sympathetic tone, which in turn regulated osteoblast differentiation and promoted bone regeneration (Figure 4).
FIGURE 4. Bone regulates neural function and activity. Osteocalcin (OCN) released from skeleton controls oligodendrocyte (OL) differentiation and neural function through G-protein coupled receptors, affecting cognition and mood. Moreover, bone produces prostaglandin E2 (PGE2), which binds to EP4 receptors on sensory nerves to inhibit sympathetic nerve activity, thereby promoting bone formation. Red arrow indicates an increase, while blue arrow indicates a decrease. Blue blunt arrows (┴) indicate inhibition.
During early embryonic development in mice, NGF produced by osteochondral progenitor cells promoted skeletal nerve innervation and function by activating TrkA signaling, which directed sensory nerve axons to the site of initial ossification (Tomlinson et al., 2016). In a long bone stress fracture model, we found that periosteal MSC- and osteoblast-derived NGF could regulate the reinnervation of nerves, representing the role of skeletal regulation of nerves after injury (Li et al., 2019). In contrast, MSC-derived NGF was not a key factor in regulating nerve regeneration during calvarial defect repair (Meyers et al., 2020), suggesting that the mechanisms regulating nerve ingrowth during regeneration differ depending on the physiological site.
The peripheral nerves in the skeleton include sensory and sympathetic nerves. The mechanisms of skeletal-neural crosstalk are complex, and a rich variety of cells and factors are involved. There are still many unknown regulatory effects. In this review, we explore the role of nerves in the regulation of bone remodeling and repair and parse the effect of bone as an endocrine organ on nerves. In our research, we are more interested in the mechanisms of neural regulation of the skeleton. Innervation of bone occurs almost simultaneously with endochondral ossification during embryonic development (Bidegain et al., 1995; Sisask et al., 2013). The increase in nerve density in bone coincides with bone growth and remodeling, forming a dense network covering the bone (Tomlinson et al., 2020). Bone injury is accompanied by nerve damage, and nerve reinnervation is essential for bone repair. We found that MSCs, osteoblasts and macrophages all secreted NGF. NGF-expressing cells that play a key role in long bone and calvarial bone injuries are differential. The specific roles of skeletal and immune cells in injury repair and neural regeneration remain to be investigated. Moreover, the results from single-cell studies revealed that NGF was highly expressed in perivascular stem cells (especially pericytes) and that the NGF-TrkA signaling pathway promoted angiogenesis and vascular regeneration (Graiani et al., 2004; Vera et al., 2014; Ahluwalia et al., 2016). The fact that EVs of vascular origin could stimulate stem cell activity and regenerate skeletal tissues suggests the diversity of NGF sources and its multiple roles. In this regard, further research is required.
Signals from nerves can promote bone formation and prevent bone degeneration. Targeting pro-neural regenerative pathways or specific signals from peripheral nerves can accelerate bone healing. Dissecting the neuro-skeletal crosstalk could advance our understanding of bone metabolism and the pathogenesis of diseases such as osteoporosis, osteoarthritis, heterotopic ossification and bone tumors, as well as identify potential targets for the mitigation and prevention of these diseases. Related research could also contribute to the development of novel bone repair materials. Materials that target both nerve and bone regeneration could offer new hope and solutions to major clinical challenges, such as large bone defects.
JX and AJ wrote the first draft of the manuscript. ZZ, JZ, and QQ wrote sections of the manuscript. CM and SL collected and contributed the data. All authors contributed to manuscript revision, read, and approved the submitted version.
American Cancer Society (Research Scholar Grant, RSG-18-027-01-CSM), NIH/NIAMS (R01 AR070773 and R01 AR079171), Department of Defense (USAMRAA W81XWH-18-1-0336, W81XWH-18-1-0121, W81XWH-20-1-0795 and W81XWH-20-1-0302), and the Maryland Stem Cell Research Foundation.
Some drawing elements in Figures 2–4 were modified from Servier Medical Art (http://smart.servier.com/), licensed under a Creative Common Attribution 3.0 Generic License (https://creativecommons.org/licenses/by/3.0/).
AJ is a paid consultant for Novadip and Lifesprout LLC. This arrangement has been reviewed and approved by the Johns Hopkins University in accordance with its conflict of interest policies.
The remaining authors declare that the research was conducted in the absence of any commercial or financial relationships that could be construed as a potential conflict of interest.
All claims expressed in this article are solely those of the authors and do not necessarily represent those of their affiliated organizations, or those of the publisher, the editors and the reviewers. Any product that may be evaluated in this article, or claim that may be made by its manufacturer, is not guaranteed or endorsed by the publisher.
Ahluwalia, A., Jones, M. K., Brzozowski, T., and Tarnawski, A. S. (2016). Nerve growth factor is critical requirement for in vitro angiogenesis in gastric endothelial cells. Am. J. Physiol. Gastrointest. Liver Physiol. 311 (5), G981–G987. doi:10.1152/ajpgi.00334.2016
Averill, S., McMahon, S. B., Clary, D. O., Reichardt, L. F., and Priestley, J. V. (1995). Immunocytochemical localization of trkA receptors in chemically identified subgroups of adult rat sensory neurons. Eur. J. Neurosci. 7 (7), 1484–1494. doi:10.1111/j.1460-9568.1995.tb01143.x
Baldock, P. A., Lee, N. J., Driessler, F., Lin, S., Allison, S., Stehrer, B., et al. (2009). Neuropeptide Y knockout mice reveal a central role of NPY in the coordination of bone mass to body weight. PLoS One 4 (12), e8415. doi:10.1371/journal.pone.0008415
Bidegain, M., Roos, B. A., Hill, E. L., Howard, G. A., and Balkan, W. (1995). Calcitonin gene-related peptide (CGRP) in the developing mouse limb. Endocr. Res. 21 (4), 743–755. doi:10.1080/07435809509030488
Brazill, J. M., Beeve, A. T., Craft, C. S., Ivanusic, J. J., and Scheller, E. L. (2019). Nerves in bone: Evolving concepts in pain and anabolism. J. Bone Min. Res. 34 (8), 1393–1406. doi:10.1002/jbmr.3822
Castaneda-Corral, G., Jimenez-Andrade, J. M., Bloom, A. P., Taylor, R. N., Mantyh, W. G., Kaczmarska, M. J., et al. (2011). The majority of myelinated and unmyelinated sensory nerve fibers that innervate bone express the tropomyosin receptor kinase A. Neuroscience 178, 196–207. doi:10.1016/j.neuroscience.2011.01.039
Chen, H., Hu, B., Lv, X., Zhu, S., Zhen, G., Wan, M., et al. (2019). Prostaglandin E2 mediates sensory nerve regulation of bone homeostasis. Nat. Commun. 10 (1), 181. doi:10.1038/s41467-018-08097-7
Chen, Q. C., and Zhang, Y. (2022). The role of NPY in the regulation of bone metabolism. Front. Endocrinol. 13, 833485. doi:10.3389/fendo.2022.833485
Cherruau, M., Morvan, F. O., Schirar, A., and Saffar, J. L. (2003). Chemical sympathectomy-induced changes in TH-VIP-and CGRP-immunoreactive fibers in the rat mandible periosteum: Influence on bone resorption. J. Cell. Physiol. 194 (3), 341–348. doi:10.1002/jcp.10209
Crenshaw, E. B., Russo, A. F., Swanson, L. W., and Rosenfeld, M. G. (1987). Neuron-specific alternative RNA processing in transgenic mice expressing a metallothionein-calcitonin fusion gene. Cell. 49 (3), 389–398. doi:10.1016/0092-8674(87)90291-1
Doro, D. H., Grigoriadis, A. E., and Liu, K. J. (2017). Calvarial suture-derived stem cells and their contribution to cranial bone repair. Front. Physiol. 8, 956. doi:10.3389/fphys.2017.00956
Du, Y., Zhang, L., Wang, Z., Zhao, X., and Zou, J. (2021). Endocrine regulation of extra-skeletal organs by bone-derived secreted protein and the effect of mechanical stimulation. Front. Cell. Dev. Biol. 9, 778015. doi:10.3389/fcell.2021.778015
Du, Z., Wang, L., Zhao, Y., Cao, J., Wang, T., Liu, P., et al. (2014). Sympathetic denervation-induced MSC mobilization in distraction osteogenesis associates with inhibition of MSC migration and osteogenesis by norepinephrine/adrb3. PLoS One 9 (8), e105976. doi:10.1371/journal.pone.0105976
Dubin, A. E., and Patapoutian, A. (2010). Nociceptors: The sensors of the pain pathway. J. Clin. Invest. 120 (11), 3760–3772. doi:10.1172/JCI42843
Ducy, P., Amling, M., Takeda, S., Priemel, M., Schilling, A. F., Beil, F. T., et al. (2000). Leptin inhibits bone formation through a hypothalamic relay: A central control of bone mass. Cell. 100 (2), 197–207. doi:10.1016/s0092-8674(00)81558-5
Ebendal, T. (1992). Function and evolution in the NGF family and its receptors. J. Neurosci. Res. 32 (4), 461–470. doi:10.1002/jnr.490320402
Elefteriou, F., Ahn, J. D., Takeda, S., Starbuck, M., Yang, X., Liu, X., et al. (2005). Leptin regulation of bone resorption by the sympathetic nervous system and CART. Nature 434 (7032), 514–520. doi:10.1038/nature03398
Elefteriou, F. (2018). Impact of the autonomic nervous system on the skeleton. Physiol. Rev. 98 (3), 1083–1112. doi:10.1152/physrev.00014.2017
Fang, Z., Yang, Q., Xiong, W., Li, G. H., Liao, H., Xiao, J., et al. (2013). Effect of CGRP-adenoviral vector transduction on the osteoblastic differentiation of rat adipose-derived stem cells. PLoS One 8 (8), e72738. doi:10.1371/journal.pone.0072738
Fu, S., Mei, G., Wang, Z., Zou, Z. L., Liu, S., Pei, G. X., et al. (2014). Neuropeptide substance P improves osteoblastic and angiogenic differentiation capacity of bone marrow stem cells in vitro. Biomed. Res. Int. 2014, 596023. doi:10.1155/2014/596023
Fukuda, T., Takeda, S., Xu, R., Ochi, H., Sunamura, S., Sato, T., et al. (2013). Sema3A regulates bone-mass accrual through sensory innervations. Nature 497 (7450), 490–493. doi:10.1038/nature12115
Goto, T., Nakao, K., Gunjigake, K. K., Kido, M. A., Kobayashi, S., and Tanaka, T. (2007). Substance P stimulates late-stage rat osteoblastic bone formation through neurokinin-1 receptors. Neuropeptides 41 (1), 25–31. doi:10.1016/j.npep.2006.11.002
Goto, T., Yamaza, T., Kido, M. A., and Tanaka, T. (1998). Light- and electron-microscopic study of the distribution of axons containing substance P and the localization of neurokinin-1 receptor in bone. Cell. Tissue Res. 293 (1), 87–93. doi:10.1007/s004410051100
Graiani, G., Emanueli, C., Desortes, E., Van Linthout, S., Pinna, A., Figueroa, C. D., et al. (2004). Nerve growth factor promotes reparative angiogenesis and inhibits endothelial apoptosis in cutaneous wounds of Type 1 diabetic mice. Diabetologia 47 (6), 1047–1054. doi:10.1007/s00125-004-1414-7
Grassel, S. G. (2014). The role of peripheral nerve fibers and their neurotransmitters in cartilage and bone physiology and pathophysiology. Arthritis Res. Ther. 16 (6), 485. doi:10.1186/s13075-014-0485-1
Guntur, A. R., and Rosen, C. J. (2012). Bone as an endocrine organ. Endocr. Pract. 18 (5), 758–762. doi:10.4158/EP12141.RA
Han, Y., You, X., Xing, W., Zhang, Z., and Zou, W. (2018). Paracrine and endocrine actions of bone-the functions of secretory proteins from osteoblasts, osteocytes, and osteoclasts. Bone Res. 6, 16. doi:10.1038/s41413-018-0019-6
Hawcroft, G., Ko, C. W., and Hull, M. A. (2007). Prostaglandin E2-EP4 receptor signalling promotes tumorigenic behaviour of HT-29 human colorectal cancer cells. Oncogene 26 (21), 3006–3019. doi:10.1038/sj.onc.1210113
Hill, E. L., and Elde, R. (1991). Distribution of CGRP-VIP-D beta H-SP-and NPY-immunoreactive nerves in the periosteum of the rat. Cell. Tissue Res. 264 (3), 469–480. doi:10.1007/BF00319037
Hohmann, E. L., Elde, R. P., Rysavy, J. A., Einzig, S., and Gebhard, R. L. (1986). Innervation of periosteum and bone by sympathetic vasoactive intestinal peptide-containing nerve fibers. Science 232 (4752), 868–871. doi:10.1126/science.3518059
Hong, H. S., Lee, J., Lee, E., Kwon, Y. S., Lee, E., Ahn, W., et al. (2009). A new role of substance P as an injury-inducible messenger for mobilization of CD29(+) stromal-like cells. Nat. Med. 15 (4), 425–435. doi:10.1038/nm.1909
Imai, S., Tokunaga, Y., Maeda, T., Kikkawa, M., and Hukuda, S. (1997). Calcitonin gene-related peptide, substance P, and tyrosine hydroxylase-immunoreactive innervation of rat bone marrows: An immunohistochemical and ultrastructural investigation on possible efferent and afferent mechanisms. J. Orthop. Res. 15 (1), 133–140. doi:10.1002/jor.1100150120
Jia, S., Zhang, S. J., Wang, X. D., Yang, Z. H., Sun, Y. N., Gupta, A., et al. (2019). Calcitonin gene-related peptide enhances osteogenic differentiation and recruitment of bone marrow mesenchymal stem cells in rats. Exp. Ther. Med. 18 (2), 1039–1046. doi:10.3892/etm.2019.7659
Jones, C. K., Byun, N., and Bubser, M. (2012). Muscarinic and nicotinic acetylcholine receptor agonists and allosteric modulators for the treatment of schizophrenia. Neuropsychopharmacology 37 (1), 16–42. doi:10.1038/npp.2011.199
Juhasz, T., Helgadottir, S. L., Tamas, A., Reglodi, D., and Zakany, R. (2015). PACAP and VIP signaling in chondrogenesis and osteogenesis. Peptides 66, 51–57. doi:10.1016/j.peptides.2015.02.001
Juhasz, T., Tamas, A., Zakany, R., and Reglodi, D. (2016). “Role of PACAP and VIP signalling in regulation of chondrogenesis and osteogenesis,” in Pituitary adenylate cyclase activating polypeptide — pacap. Editors D. Reglodi, and A. Tamas (Cham: Springer International Publishing), 337–353.
Kajimura, D., Hinoi, E., Ferron, M., Kode, A., Riley, K. J., Zhou, B., et al. (2011). Genetic determination of the cellular basis of the sympathetic regulation of bone mass accrual. J. Exp. Med. 208 (4), 841–851. doi:10.1084/jem.20102608
Karsenty, G., and Olson, E. N. (2016). Bone and muscle endocrine functions: Unexpected paradigms of inter-organ communication. Cell. 164 (6), 1248–1256. doi:10.1016/j.cell.2016.02.043
Kelly, R. R., Sidles, S. J., and LaRue, A. C. (2020). Effects of neurological disorders on bone health. Front. Psychol. 11, 612366. doi:10.3389/fpsyg.2020.612366
Kestell, G. R., Anderson, R. L., Clarke, J. N., Haberberger, R. V., and Gibbins, I. L. (2015). Primary afferent neurons containing calcitonin gene-related peptide but not substance P in forepaw skin, dorsal root ganglia, and spinal cord of mice. J. Comp. Neurol. 523 (17), 2555–2569. doi:10.1002/cne.23804
Khrimian, L., Obri, A., Ramos-Brossier, M., Rousseaud, A., Moriceau, S., Nicot, A. S., et al. (2017). Gpr158 mediates osteocalcin's regulation of cognition. J. Exp. Med. 214 (10), 2859–2873. doi:10.1084/jem.20171320
Klingelhoffer, C., Reck, A., and Morsczeck, C. (2016). The extracellular concentration of osteocalcin decreased in dental follicle cell cultures during biomineralization. Cytotechnology 68 (5), 2171–2176. doi:10.1007/s10616-016-0012-0
Konya, V., Marsche, G., Schuligoi, R., and Heinemann, A. (2013). E-type prostanoid receptor 4 (EP4) in disease and therapy. Pharmacol. Ther. 138 (3), 485–502. doi:10.1016/j.pharmthera.2013.03.006
Kosaras, B., Jakubowski, M., Kainz, V., and Burstein, R. (2009). Sensory innervation of the calvarial bones of the mouse. J. Comp. Neurol. 515 (3), 331–348. doi:10.1002/cne.22049
Kruger, R. P., Aurandt, J., and Guan, K. L. (2005). Semaphorins command cells to move. Nat. Rev. Mol. Cell. Biol. 6 (10), 789–800. doi:10.1038/nrm1740
Lee, N. J., Doyle, K. L., Sainsbury, A., Enriquez, R. F., Hort, Y. J., Riepler, S. J., et al. (2010). Critical role for Y1 receptors in mesenchymal progenitor cell differentiation and osteoblast activity. J. Bone Min. Res. 25 (8), 1736–1747. doi:10.1002/jbmr.61
Li, Z., Meyers, C. A., Chang, L., Lee, S., Li, Z., Tomlinson, R., et al. (2019). Fracture repair requires TrkA signaling by skeletal sensory nerves. J. Clin. Invest. 129 (12), 5137–5150. doi:10.1172/JCI128428
Liu, S., Jin, D., Wu, J. Q., Xu, Z. Y., Fu, S., Mei, G., et al. (2016). Neuropeptide Y stimulates osteoblastic differentiation and VEGF expression of bone marrow mesenchymal stem cells related to canonical Wnt signaling activating in vitro. Neuropeptides 56, 105–113. doi:10.1016/j.npep.2015.12.008
Luo, X., Lauwers, M., Layer, P. G., and Wen, C. (2020). Non-neuronal role of acetylcholinesterase in bone development and degeneration. Front. Cell. Dev. Biol. 8, 620543. doi:10.3389/fcell.2020.620543
Ma, Y., and Elefteriou, F. (2020). Brain-derived acetylcholine maintains peak bone mass in adult female mice. J. Bone Min. Res. 35 (8), 1562–1571. doi:10.1002/jbmr.4024
Mach, D. B., Rogers, S. D., Sabino, M. C., Luger, N. M., Schwei, M. J., Pomonis, J. D., et al. (2002). Origins of skeletal pain: Sensory and sympathetic innervation of the mouse femur. Neuroscience 113 (1), 155–166. doi:10.1016/s0306-4522(02)00165-3
Mantyh, P. W. (2014). The neurobiology of skeletal pain. Eur. J. Neurosci. 39 (3), 508–519. doi:10.1111/ejn.12462
Marrella, A., Lee, T. Y., Lee, D. H., Karuthedom, S., Syla, D., Chawla, A., et al. (2018). Engineering vascularized and innervated bone biomaterials for improved skeletal tissue regeneration. Mat. Today 21 (4), 362–376. doi:10.1016/j.mattod.2017.10.005
McCorry, L. K. (2007). Physiology of the autonomic nervous system. Am. J. Pharm. Educ. 71 (4), 78. doi:10.5688/aj710478
Meyers, C. A., Lee, S., Sono, T., Xu, J., Negri, S., Tian, Y., et al. (2020). A neurotrophic mechanism directs sensory nerve transit in cranial bone. Cell. Rep. 31 (8), 107696. doi:10.1016/j.celrep.2020.107696
Moser, S. C., and van der Eerden, B. C. J. (2018). Osteocalcin-A versatile bone-derived hormone. Front. Endocrinol. 9, 794. doi:10.3389/fendo.2018.00794
Mrak, E., Guidobono, F., Moro, G., Fraschini, G., Rubinacci, A., and Villa, I. (2010). Calcitonin gene-related peptide (CGRP) inhibits apoptosis in human osteoblasts by beta-catenin stabilization. J. Cell. Physiol. 225 (3), 701–708. doi:10.1002/jcp.22266
Nagao, M., Feinstein, T. N., Ezura, Y., Hayata, T., Notomi, T., Saita, Y., et al. (2011). Sympathetic control of bone mass regulated by osteopontin. Proc. Natl. Acad. Sci. U. S. A. 108 (43), 17767–17772. doi:10.1073/pnas.1109402108
Nagao, S., Goto, T., Kataoka, S., Toyono, T., Joujima, T., Egusa, H., et al. (2014). Expression of neuropeptide receptor mRNA during osteoblastic differentiation of mouse iPS cells. Neuropeptides 48 (6), 399–406. doi:10.1016/j.npep.2014.10.004
Obri, A., Khrimian, L., Karsenty, G., and Oury, F. (2018). Osteocalcin in the brain: From embryonic development to age-related decline in cognition. Nat. Rev. Endocrinol. 14 (3), 174–182. doi:10.1038/nrendo.2017.181
Oury, F., Khrimian, L., Denny, C. A., Gardin, A., Chamouni, A., Goeden, N., et al. (2013). Maternal and offspring pools of osteocalcin influence brain development and functions. Cell. 155 (1), 228–241. doi:10.1016/j.cell.2013.08.042
Qian, Z., Li, H., Yang, H., Yang, Q., Lu, Z., Wang, L., et al. (2021). Osteocalcin attenuates oligodendrocyte differentiation and myelination via GPR37 signaling in the mouse brain. Sci. Adv. 7 (43), eabi5811. doi:10.1126/sciadv.abi5811
Rath, N. C., and Durairaj, V. (2022). “Chapter 22 - avian bone physiology and poultry bone disorders,” in Sturkie's avian physiology. Editors C. G. Scanes, and S. Dridi. Seventh Edition (San Diego: Academic Press), 549–563.
Reichardt, L. F. (2006). Neurotrophin-regulated signalling pathways. Philos. Trans. R. Soc. Lond. B Biol. Sci. 361 (1473), 1545–1564. doi:10.1098/rstb.2006.1894
Rosenfeld, M. G., Mermod, J. J., Amara, S. G., Swanson, L. W., Sawchenko, P. E., Rivier, J., et al. (1983). Production of a novel neuropeptide encoded by the calcitonin gene via tissue-specific RNA processing. Nature 304 (5922), 129–135. doi:10.1038/304129a0
Shi, L., Feng, L., Zhu, M. L., Yang, Z. M., Wu, T. Y., Xu, J., et al. (2020). Vasoactive intestinal peptide stimulates bone marrow-mesenchymal stem cells osteogenesis differentiation by activating wnt/β-catenin signaling pathway and promotes rat skull defect repair. Stem Cells Dev. 29 (10), 655–666. doi:10.1089/scd.2019.0148
Sisask, G., Silfversward, C. J., Bjurholm, A., and Nilsson, O. (2013). Ontogeny of sensory and autonomic nerves in the developing mouse skeleton. Auton. Neurosci. 177 (2), 237–243. doi:10.1016/j.autneu.2013.05.005
Sorkin, L. S., Eddinger, K. A., Woller, S. A., and Yaksh, T. L. (2018). Origins of antidromic activity in sensory afferent fibers and neurogenic inflammation. Semin. Immunopathol. 40 (3), 237–247. doi:10.1007/s00281-017-0669-2
Steinhoff, M. S., von Mentzer, B., Geppetti, P., Pothoulakis, C., and Bunnett, N. W. (2014). Tachykinins and their receptors: Contributions to physiological control and the mechanisms of disease. Physiol. Rev. 94 (1), 265–301. doi:10.1152/physrev.00031.2013
Su, N., Yang, J., Xie, Y., Du, X., Chen, H., Zhou, H., et al. (2019). Bone function, dysfunction and its role in diseases including critical illness. Int. J. Biol. Sci. 15 (4), 776–787. doi:10.7150/ijbs.27063
Takeda, S., Elefteriou, F., Levasseur, R., Liu, X., Zhao, L., Parker, K. L., et al. (2002). Leptin regulates bone formation via the sympathetic nervous system. Cell. 111 (3), 305–317. doi:10.1016/s0092-8674(02)01049-8
Tamagnone, L., and Comoglio, P. M. (2004). To move or not to move? Semaphorin signalling in cell migration. EMBO Rep. 5 (4), 356–361. doi:10.1038/sj.embor.7400114
Tank, A. W., and Lee Wong, D. (2015). Peripheral and central effects of circulating catecholamines. Compr. Physiol. 5 (1), 1–15. doi:10.1002/cphy.c140007
Togari, A., Arai, M., Kondo, H., Kodama, D., and Niwa, Y. (2012). The neuro-osteogenic network: The sympathetic regulation of bone resorption. Jpn. Dent. Sci. Rev. 48 (2), 61–70. doi:10.1016/j.jdsr.2011.12.002
Tomlinson, R. E., Christiansen, B. A., Giannone, A. A., and Genetos, D. C. (2020). The role of nerves in skeletal development, adaptation, and aging. Front. Endocrinol. 11, 646. doi:10.3389/fendo.2020.00646
Tomlinson, R. E., Li, Z., Zhang, Q., Goh, B. C., Li, Z., Thorek, D. L. J., et al. (2016). NGF-TrkA signaling by sensory nerves coordinates the vascularization and ossification of developing endochondral bone. Cell. Rep. 16 (10), 2723–2735. doi:10.1016/j.celrep.2016.08.002
Vera, C., Tapia, V., Vega, M., and Romero, C. (2014). Role of nerve growth factor and its TRKA receptor in normal ovarian and epithelial ovarian cancer angiogenesis. J. Ovarian Res. 7, 82. doi:10.1186/s13048-014-0082-6
Wan, Q. Q., Qin, W. P., Ma, Y. X., Shen, M. J., Li, J., Zhang, Z. B., et al. (2021). Crosstalk between bone and nerves within bone. Adv. Sci. 8 (7), 2003390. doi:10.1002/advs.202003390
Wang, L., Shi, X., Zhao, R., Halloran, B. P., Clark, D. J., Jacobs, C. R., et al. (2010). Calcitonin-gene-related peptide stimulates stromal cell osteogenic differentiation and inhibits RANKL induced NF-kappaB activation, osteoclastogenesis and bone resorption. Bone 46 (5), 1369–1379. doi:10.1016/j.bone.2009.11.029
Wang, L., Zhao, R., Shi, X., Wei, T., Halloran, B. P., Clark, D. J., et al. (2009). Substance P stimulates bone marrow stromal cell osteogenic activity, osteoclast differentiation, and resorption activity in vitro. Bone 45 (2), 309–320. doi:10.1016/j.bone.2009.04.203
Wang, X. D., Li, S. Y., Zhang, S. J., Gupta, A., Zhang, C. P., and Wang, L. (2020). The neural system regulates bone homeostasis via mesenchymal stem cells: A translational approach. Theranostics 10 (11), 4839–4850. doi:10.7150/thno.43771
Warren, S. M., Greenwald, J. A., Nacamuli, R. P., Fong, K. D., Song, H. J., Fang, T. D., et al. (2003). Regional dura mater differentially regulates osteoblast gene expression. J. Craniofac. Surg. 14 (3), 363–370. doi:10.1097/00001665-200305000-00015
Xia, W., Xie, J., Cai, Z., Liu, X., Wen, J., Cui, Z. K., et al. (2021). Damaged brain accelerates bone healing by releasing small extracellular vesicles that target osteoprogenitors. Nat. Commun. 12 (1), 6043. doi:10.1038/s41467-021-26302-y
Xie, W., Han, Y., Li, F., Gu, X., Su, D., Yu, W., et al. (2020). Neuropeptide Y1 receptor antagonist alters gut microbiota and alleviates the ovariectomy-induced osteoporosis in rats. Calcif. Tissue Int. 106 (4), 444–454. doi:10.1007/s00223-019-00647-5
Xu, G., and Jiang, D. (2014). The role and mechanism of exogenous calcitonin gene-related peptide on mesenchymal stem cell proliferation and osteogenetic formation. Cell. biochem. Biophys. 69 (2), 369–378. doi:10.1007/s12013-013-9809-z
Xu, J., Li, Z., Tower, R. J., Negri, S., Wang, Y., Meyers, C. A., et al. (2022). NGF-p75 signaling coordinates skeletal cell migration during bone repair. Sci. Adv. 8 (11), eabl5716. doi:10.1126/sciadv.abl5716
Xu, J., Wang, Y., Hsu, C. Y., Gao, Y., Meyers, C. A., Chang, L., et al. (2019). Human perivascular stem cell-derived extracellular vesicles mediate bone repair. Elife 8, e48191. doi:10.7554/eLife.48191
Yahara, M., Tei, K., and Tamura, M. (2017). Inhibition of neuropeptide Y Y1 receptor induces osteoblast differentiation in MC3T3E1 cells. Mol. Med. Rep. 16 (3), 2779–2784. doi:10.3892/mmr.2017.6866
Yang, F., Liu, Y., Chen, S., Dai, Z., Yang, D., Gao, D., et al. (2020). A GABAergic neural circuit in the ventromedial hypothalamus mediates chronic stress-induced bone loss. J. Clin. Invest. 130 (12), 6539–6554. doi:10.1172/JCI136105
Yang, Z., Wu, B., Jia, S., Zhao, Y., Hou, R., Liu, X., et al. (2017). The mechanically activated p38/MMP-2 signaling pathway promotes bone marrow mesenchymal stem cell migration in rats. Arch. Oral Biol. 76, 55–60. doi:10.1016/j.archoralbio.2017.01.017
Zhang, B., Zhang, X., Xiao, J., Zhou, X., Chen, Y., and Gao, C. (2020). Neuropeptide Y upregulates Runx2 and osterix and enhances osteogenesis in mouse MC3T3E1 cells via an autocrine mechanism. Mol. Med. Rep. 22 (5), 4376–4382. doi:10.3892/mmr.2020.11506
Zhang, M., Zhou, Q., Luo, Y., Nguyen, T., Rosenblatt, M. I., and Guaiquil, V. H. (2018). Semaphorin3A induces nerve regeneration in the adult cornea-a switch from its repulsive role in development. PLoS One 13 (1), e0191962. doi:10.1371/journal.pone.0191962
Zhang, Y. B., Wang, L., Jia, S., Du, Z. J., Zhao, Y. H., Liu, Y. P., et al. (2014). Local injection of substance P increases bony formation during mandibular distraction osteogenesis in rats. Br. J. Oral Maxillofac. Surg. 52 (8), 697–702. doi:10.1016/j.bjoms.2014.07.002
Zhang, Y., Chen, C. Y., Liu, Y. W., Rao, S. S., Tan, Y. J., Qian, Y. X., et al. (2021). Neuronal induction of bone-fat imbalance through osteocyte neuropeptide Y. Adv. Sci. 8 (24), e2100808. doi:10.1002/advs.202100808
Zhang, Y., Xu, J., Ruan, Y. C., Yu, M. K., O'Laughlin, M., Wise, H., et al. (2016). Implant-derived magnesium induces local neuronal production of CGRP to improve bone-fracture healing in rats. Nat. Med. 22 (10), 1160–1169. doi:10.1038/nm.4162
Keywords: nervous system, skeletal system, crosstalk, bone repair and regeneration, nerve innervation
Citation: Xu J, Zhang Z, Zhao J, Meyers CA, Lee S, Qin Q and James AW (2022) Interaction between the nervous and skeletal systems. Front. Cell Dev. Biol. 10:976736. doi: 10.3389/fcell.2022.976736
Received: 23 June 2022; Accepted: 08 August 2022;
Published: 30 August 2022.
Edited by:
Yu Li, Sichuan University, ChinaReviewed by:
Sean Shishu Huang, West China Hospital, Sichuan University, ChinaCopyright © 2022 Xu, Zhang, Zhao, Meyers, Lee, Qin and James. This is an open-access article distributed under the terms of the Creative Commons Attribution License (CC BY). The use, distribution or reproduction in other forums is permitted, provided the original author(s) and the copyright owner(s) are credited and that the original publication in this journal is cited, in accordance with accepted academic practice. No use, distribution or reproduction is permitted which does not comply with these terms.
*Correspondence: Aaron W. James, YXdqYW1lc0BqaG1pLmVkdQ==
†These authors have contributed equally to this work
Disclaimer: All claims expressed in this article are solely those of the authors and do not necessarily represent those of their affiliated organizations, or those of the publisher, the editors and the reviewers. Any product that may be evaluated in this article or claim that may be made by its manufacturer is not guaranteed or endorsed by the publisher.
Research integrity at Frontiers
Learn more about the work of our research integrity team to safeguard the quality of each article we publish.