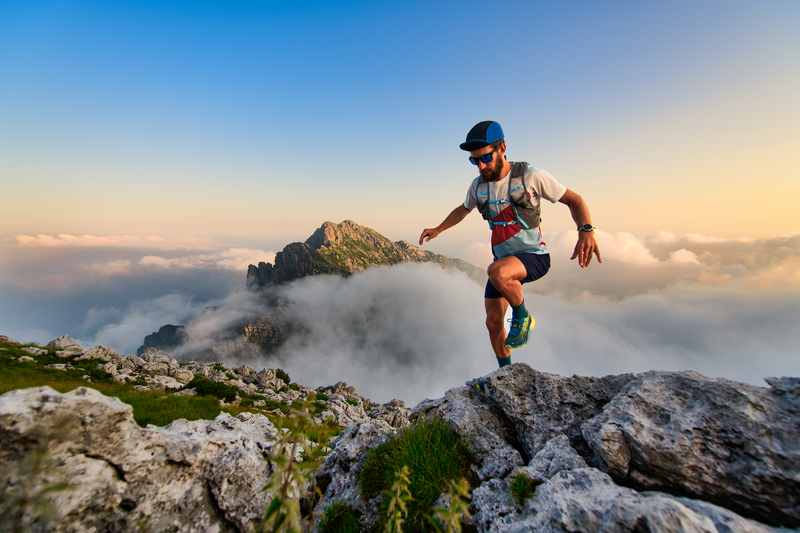
95% of researchers rate our articles as excellent or good
Learn more about the work of our research integrity team to safeguard the quality of each article we publish.
Find out more
HYPOTHESIS AND THEORY article
Front. Cell Dev. Biol. , 08 September 2022
Sec. Cell Adhesion and Migration
Volume 10 - 2022 | https://doi.org/10.3389/fcell.2022.956432
This article is part of the Research Topic Space Mechanobiology and Medicine - Volume II View all 4 articles
Dysbiosis of the human skin microbiome has long been associated with changes to the pH of the skin, dermal immune function and chronic skin conditions. Dermatological issues have been noted as the most prevalent medical presentation in the microgravity environment of space. The change in gravitational forces has been implicated in human immuno-suppression, also impacted by changes in the gastrointestinal-skin axis and its impact on Vitamin D metabolism, altered microbial gene expression in resident flora (leading changes in biofilm formation) and increased virulence factors in potential pathogens. There are also other stressors to the skin microbiome unique to space travel, including increased exposure to radiation, prolonged periods of dry washing technique, air quality and changes in microbe replication and growth parameters. Optimal microbiome health leads to enhanced skin barrier manufacture and maintenance, along with improved skin immune function and healing. In a microgravity environment expected to be experienced during long space flights, disruptions to the skin microbiome, coupled with increased virulence of pathological viruses and bacteria has implications for holistic skin health, astronaut cognitive function and mental health, and is coupled with slowed rates of wound healing. Scenario management for holistic skin health and restoration of microbiome homeostasis on long space flights require consideration.
Our skin microbiome has evolved, and continues to evolve on Earth as a commensal system, vital for the health and life of the individual. The challenges of space flight on human health and physiology is a relatively new area of research, and as humankind’s ambition to move towards space colonization increases, there is increased likelihood of new disease encounters and physiological dysfunction of astronauts skin (Taylor, 1993; Horneck et al., 2003; Horneck and Comet, 2006; Taylor, 2015). Identifying methods to preserve the health of the commensal organisms that make up the human skin microbiome, and thus the overall health of the skin, during long haul space flight is of great clinical importance.
Thus far, human microgravity experience is limited to missions on the International Space Station (ISS) and Salyut, and Skylab stations, with additional information gained from space shuttle data collections and isolated analog experiments. There are numerous factors at play which affect the skin differently to that on Earth, skin microbiome changes are noted and further complicated by differences in microbial growth rates in microgravity (Taylor, 2015). Clinicians have been cognisant of medically important microorganisms populating astronauts early in the history of space flight (Coiletti Louis et al., 1991; Taylor, 1993; Williams, 2003a; Taylor, 2015). Changes to the human immune system, even after short-duration space flights, have been noted (Taylor, 1993). Physiological processes in the skin contribute to wound heating, multi-system health, mental health and cognitive functioning and is a relatively neglected area of space medicine.
For reasons not yet ascertained, microgravity environments affect the human immune response; this effect has been recorded after relatively low exposure (Nefedov et al., 1971; Stowe et al., 2001; Williams, 2003a; Mehta et al., 2014). Some of the catalogued changes to the human immune system range from differences in cellular genetic expression, changes to specific classes of immune cell activation (i.e., monocytes, leukocytes), interruptions of the gastrointestinal-skin axis, changes in cellular response (i.e., monocyte cellular imbalance) to endotoxins (cytokine blunting), as well as changes in the growth and nature of common commensals, pathogenic bacteria and viruses (Canova Sabrina et al., 2005; Kaur et al., 2005; Kaur et al., 2008; Baqai et al., 2009; Guéguinou et al., 2009; McPhee and Charles, 2010; Jennifer et al., 2021; Spatz et al., 2021). It is hypothesised, but the mechanism not fully understood, physiological changes monitored in humans may be directly influenced by the microgravity environment of space. With a resulting change of tertiary and quaternary molecular conformation leading, for example, to molecular conformational mismatch and structural stress, in turn causing cytokine blunting, affecting the inflammatory mediated immune response with dysregulation of signaling and recruitment pathways (Nickerson et al., 2000; Kaur et al., 2005; Kaur et al., 2008; Guéguinou et al., 2009; Picardo and Monica, 2014; Jennifer et al., 2021; Spatz et al., 2021). Consideration should also be given to the formation of lymph and its propulsion within the human lymph system in microgravity environments. Lymph contributes to fluid homeostasis of the interstitial and serosal cavities, but also acts as an “immune cell highway” propelling classes of immune cells to sites of immune responses as part of the immune reaction (Canova Sabrina et al., 2005; Kaur et al., 2005; Kaur et al., 2008; Baqai et al., 2009; Guéguinou et al., 2009; McPhee and Charles, 2010; Jennifer et al., 2021; Solari et al., 2021; Spatz et al., 2021). It is noted ISS astronauts experience gross disturbance of this system (evidenced by pseudo-sinusitis and “puffy faces,” which do not respond to traditional diuretics) in microgravity (Solari et al., 2021).
Recent enclosed analog research has been focussed on the biological translocation of bacteria within closed environments/habitats, potential bacterial-derived toxicity, as well as exploration of tissue-derived toxins (involving the lymph system). However the contributing impact of microgravity on the lymphatic system has not been extensively explored (Zwart et al., 2011; SanMiguel and Grice, 2015; Cabalín et al., 2021; Tang et al., 2021). Solari et.al. (2021) note that there is little data on the modulation of gut lymphatic drainage and movement in disease management, and attention to lymph drainage remains the domain of specific areas of cancer management (i.e., breast cancer lymphoedema) (Smith et al., 1999; Zwart et al., 2011; SanMiguel and Grice, 2015; Lund et al., 2016; Schwager and Detmar, 2019; Cabalín et al., 2021; Solari et al., 2021). Limited research has reviewed the impact of artificially imposed lymph flow perhaps improving the composition of gut microbiome, which may in turn impact or reduce future gut-microbiome imbalance associated illnesses and nutritional deficiencies. A secondary effect may be the improved immunosurveillance and delivery of immune cells. (Smith et al., 2005; Scott et al., 2021; Smith et al., 2021; Solari et al., 2021; Tang et al., 2021).
Nutrient studies demonstrate that lipid transport is associated closely with the gut microenvironment; changes to this environment can affect the absorption of dietary lipids and their associated lipid soluble nutrients. In turn, changed gut microenvironments impact on the effective immunosurveillance between the villi interstitial space and mesenteric lymph nodes (Solari et al., 2021). It is appreciated that there are widespread associations: reduced lipid transport into the blood impacts immunosurveillance and increases risk tissue oedema (an independent risk factor for infection); and reduced dietary lipid transport affects uptake of vital nutrients and mineral co-enzymes ultimately affecting the gastrointestinal-skin axis. (Sonnenfield and Shearer, 2002; Smith et al., 2005; Zwart et al., 2011; Zwart et al., 2013; Carlson, 2014; Shen et al., 2014; SanMiguel and Grice, 2015; Anna et al., 2016; Scott et al., 2021; Smith et al., 2021; Solari et al., 2021; Tang et al., 2021).
More recently noted effects also attributed to microgravity affects include altered T cell activation, decreased sensitivity and activation of molecular signalling pathways (leading to reduced functionality of protein co-activators) by mechanisms yet to be fully elucidated (Nickerson et al., 2000; Sonnenfield and Shearer, 2002; Kaur et al., 2008; Zwart et al., 2011; Zwart et al., 2013; Carlson, 2014; Picardo and Monica, 2014; Shen et al., 2014; SanMiguel and Grice, 2015; Anna et al., 2016) A recent study confirmed the ability of T cells to influence the bacterial microbiome of the skin (Shen et al., 2014). An example, the reactivation of latent viral infections, due in part to the de-activation or down regulation of some parts of the human immune system, has been observed (Stowe et al., 2001; Baqai et al., 2009; Zwart et al., 2013; Carlson, 2014; Taylor, 2015). Further studies with regards to primary immuno-deficiencies show increased microbial permissiveness and growth in opportunistic pathogens (SanMiguel and Grice, 2015).
Vitamin D plays a cardinal role regulating the human skin’s immune response and contributes to the healing potential of the skin. Low Vitamin D status leads to increased risk of topical infections and systemic and multi-organ changes (i.e. osteoporosis, pulmonary fibrosis); also noted is decreased skin immune responses with disordered wound healing (Smith et al., 1999; Zwart et al., 2011; Schwager and Detmar, 2019; Cabalín et al., 2021; Smith et al., 2021; Tang et al., 2021). The immunological mechanisms required for the production of Vitamin D via the 7-DHC pathway can be clinically interrupted in enclosed space environments. The regulation of keratinocyte differentiation is dependent on Vitamin D, creating a nexus between the body’s Vitamin D stocks, regulation and differentiation of keratinocytes and their co-activators (Cabalín et al., 2021; Smith et al., 2021). Which in turn helps with growth regulation of keratinocytes in the human body (Lund et al., 2016; Claudel et al., 2019a). Autoflora sharing in the gastrointestinal tract affects individual gastrointestinal-skin axis, and in turn may indirectly affect the metabolism and availability of vitamins, such as Vitamin D, leading to changes the skin microbiome (Cogoli et al., 1984; Bikle et al., 2003; SanMiguel and Grice, 2015; Zwart and ScottSmith, 2020).
There is mounting evidence that some autoimmune pathologies are associated with low Vitamin D status, including the development of disordered skin growth (vitiligo and scleroderma) as well as heart disease, increased topical and systemic infection and diabetes development (Cogoli et al., 1984; Bikle, 2004; Bikle et al., 2004; DeLuca, 2004; Smith et al., 2009; Matheson et al., 2010; Hu et al., 2014; Zwart and ScottSmith, 2020). On a cellular level, lowered levels can affect murine and human plasmacytoid dendritic cellular function, creating a responsive-suppressive effect on cancer genesis and immune cell behavior (Zwart et al., 2013; Shen et al., 2014; Scott et al., 2021). Nasal colonisation by methicillin-resistant Staphylococcus aureus, MRSA, in individuals with low Vitamin D is recorded, demonstrating that commensals may out-compete other bacteria in a low Vitamin D environment (Penna, 2000; DeLuca, 2004; De Haes et al., 2005; Kostner et al., 2009; Smith et al., 2009; Matheson et al., 2010; Malodobra-Mazur et al., 2012; Karthaus et al., 2014; SanMiguel and Grice, 2015).
Future multi-generational long haul space flights may provide multiple mental health challenges, where mood management, cognitive stability and cooperative individuals will be needed for success. Vitamin D receptors are found in the brain, and a deficiency of Vitamin D can lead to decreases or alterations in cognitive functioning (Penna, 2000; Searing and Leung, 2010; Slominski et al., 2011; Pludowski et al., 2013; Saleh et al., 2013; Thill et al., 2015; Wierzbicka et al., 2015; Smith et al., 2021). Changes in the gastrointestinal-skin axis secondary to astronaut autoflora exchange may in turn impact Vitamin D production and the vitamin’s wider availability to the human immune system (Cogoli et al., 1984; Coiletti Louis et al., 1991; Taylor, 1993; Williams, 2003a; Bikle et al., 2003; Bikle, 2004; Bikle et al., 2004; Horneck and Comet, 2006; Kaur et al., 2008; Guéguinou et al., 2009; Carlson, 2014; Hu et al., 2014; Taylor, 2015; Zwart and ScottSmith, 2020; Spatz et al., 2021). Though supplementation guidelines are readily available, they may not be applicable in microgravity environments due to the acknowledged change in gastrointestinal microbiome and autoflora phenomena, creating dysfunction with the gastrointestinal-skin axis, complicated further by molecular structural stress (Adorini and Penna, 2008; Slominski et al., 2013d; Jennifer et al., 2021; Spatz et al., 2021). Oral supplementation presents itself as an obvious solution, but may also create challenges on longer haul space flights with regards to adequate supply (dosage for the protective and healthy immune response the human body requires) and changed gastrointestinal microbiome may lead to inadequate dosage uptake for individual needs (Taylor, 1974; Peterson et al., 2013; SanMiguel and Grice, 2015; NASA, 2021; Sole and Santamaria, 2021). UV supplementation is another avenue to explore, however Australian studies have shown that UV sun-damaged skin becomes compromised, falling behind on the production of Vitamin D utilized by other physiological systems in the body (Holick, 2004; Eckberg et al., 2005; Eyles et al., 2005; Heath and Elovic, 2006; Hawker et al., 2007; O’Hara and Shanahan, 2007; Garcia et al., 2011; Haussler et al., 2011; Duygu Gezen et al., 2012; Hu et al., 2014).
Extrapolating from bacteria and conformational molecular changes in microgravity, the warping of functional three dimensional (3D) structures (immunomolecular molecular structures) causes molecular structural stress, leading to further down regulation or dysfunctionality of keratinocyte co-activator contribution to kertinocyte growth and differentiation, creating an environment for mircobiome dysbiosis (Nickerson et al., 2000; Kaur et al., 2005; Kaur et al., 2008; Carlson, 2014; Picardo and Monica, 2014; Taylor, 2015; Schwager and Detmar, 2019; Jennifer et al., 2021; Spatz et al., 2021) In effect, along with compromised human immune functioning and wound healing, this can lead to longer and more infectious microorganism profiles (Schwager and Detmar, 2019; Cueto, 2022). Down-regulation of the human immune system in microgravity is competing with a range of pathogenic insults, including risk of encountering novel diseases in space, causing questions to be asked if humans could biologically survive in space (Nefedov et al., 1971; Guéguinou et al., 2009; Schwager and Detmar, 2019). It is becoming evident that general human health and well being is highly dependent on resident Vitamin D status (Smith et al., 1999; Holick, 2004; Wang et al., 2004; Eckberg et al., 2005; Eyles et al., 2005; Heath and Elovic, 2006; Hawker et al., 2007; Holick, 2007; O’Hara and Shanahan, 2007; Kaur et al., 2008; Misra et al., 2008; Garcia et al., 2011; Haussler et al., 2011; Zwart et al., 2011; Bikle, 2012; Duygu Gezen et al., 2012; Slominski et al., 2013d; Hossein-nezhad and Holick, 2013; Zwart et al., 2013; Carlson, 2014; Zwart and ScottSmith, 2020; Scott et al., 2021; Smith et al., 2021; Cueto, 2022).
Many potential pathogens inhabit and colonise the human body, and apart from a possible direct pathogenic threat, gastrointestinal commensal balance can also affect the absorption and activation of much needed vitamins and nutrients to maintain a healthy functioning immune system (Canova Sabrina et al., 2005; Carlson, 2014; Wierzbicka et al., 2014). Space flights have been demonstrated to cause changes to the gastrointestinal and gastrointestinal-skin axis and nasal colonisation with resident respiratory bacterial flora (Taylor, 1993; Stowe et al., 2001; Du et al., 2002; Baqai et al., 2009; Guéguinou et al., 2009; Du et al., 2011; Taylor, 2015; Smith et al., 2021; Sole and Santamaria, 2021; Spatz et al., 2021; Tang et al., 2021). Maintaining a healthy microbiome is challenged by a two-fold antagonistic process: the rapid growth of potential pathological organisms (up to four times Earth-bound growth rates), coupled with the noted lack of medication stability and effectiveness in space (often discussed as increased resistance) (Du et al., 2002; Malodobra-Mazur et al., 2012; Taylor et al., 2012; Wierzbicka et al., 2014; Taylor, 2015; Jennifer et al., 2021; Spatz et al., 2021). Anaerobic and aerobic organisms experience decreased generational replication times, perhaps not allowing uptake of systemic antibiotic agents at the correct replication phase for effectiveness, potentially rendering antibiotic treatments minimally effective.
Additionally, changes in the nature and type of cytoskeleton expression appear to provide some species of bacteria a competitive advantage. Salmonella typhimurium, Staphylococcus epidermis (a normal skin commensal) have demonstrated predatory behavior with these changes, effectively out-completing other commensals (Nefedov et al., 1971; Coiletti Louis et al., 1991; Nickerson et al., 2000; Du et al., 2002; Picardo and Monica, 2014; Taylor, 2015; Lund et al., 2016; Jennifer et al., 2021). Further research is investigating the effects of radiation and microbiological organisms genetic response (Wang et al., 2004). Genetic mutations have been noted in Saccharomyces cerevisiae after longer space flights, and changed Escherichia coli gene expression is noted. These changes, coupled with host human epigenetic ageing (in response to radiation, stress, and microgravity environment) provide a compromised course for the senescence immune response (Sonnenfield and Taylor, 1991; Klaus et al., 1997; Fukuda et al., 2000; Wang et al., 2004; Karthaus et al., 2014; Nickerson et al., 2016; Zea et al., 2017; Nwanaji-Enwerem Jamaji et al., 2020).
Some microorganisms have demonstrated persistent resident populations in microgravity, surviving successive space flights (Aunins Thomas et al., 2018; Singh et al., 2018; More et al., 2019; Mortazavi, 2019). These include those indicated as a biosafety level 2 microorganism: Acinetobacter baumannii, Haemophilus influenzae, Klebsiella pneumoniae, Salmonella enterica, Shigella sonnei, Staphylococcus aureus, Yersinia frederiksenii, and Aspergillus lentulus (Aunins Thomas et al., 2018). Of the persistent space flight bacteria, Staphylococcus aureus and Yersinia frederiksenii are known skin pathogens, and Aspergillus lentulus, Haemophilus influenzae, and Klebsiella pneumoniae are more routinely encountered clinically in the human respiratory system. Whilst Salmonella enterica and Shigella sonnei are mainly considered gastrointestinal pathogens, Acinetobacter baumannii has demonstrated its pathological capabilities, particularly with indwelling urinary catheters in the immunocompromised individuals experiencing prolonged hospitalization (Bijlani et al., 2021). Although the conditions in space habitats and microgravity environments are selective, they do not alter the micro-biological affect on human health and the same disease profile can be expected with a predicted quicker timeline (Singh et al., 2018; More et al., 2019; Mortazavi, 2019).
Resistance per se may not be the correct conceptual terminology to describe the ineffectiveness of antibiotics, for example resistance may be due to faster generational/replication time, affecting antibiotics and antiretrovirals introduction into the organisms at the “right moment”to disrupt their replication pathways. Given the expected change in gut flora due to autoflora colonisation in astronauts, storage and antibiotic resistance concerns, the bio-availability of therapeutics in long space flight will need to be considered (Taylor, 1974; Teize and Putcha, 1994; Du et al., 2002; Canova Sabrina et al., 2005; Du et al., 2011; Putcha et al., 2011; Taylor et al., 2012; Turroni et al., 2020). Medication storage in microgravity and the effect of increased radiation (over periods of time) coupled with other (as yet) unquantifiable factors, may well impact on medication integrity (Nefedov et al., 1971; Coiletti Louis et al., 1991; Smith et al., 1999; Nickerson et al., 2000; Stowe et al., 2001; Du et al., 2002; Williams, 2003a; Canova Sabrina et al., 2005; Kaur et al., 2005; Kaur et al., 2008; Baqai et al., 2009; Guéguinou et al., 2009; McPhee and Charles, 2010; Du et al., 2011; Zwart et al., 2011; Zwart et al., 2013; Carlson, 2014; Mehta et al., 2014; Picardo and Monica, 2014; SanMiguel and Grice, 2015; Schwager and Detmar, 2019; Cabalín et al., 2021; Jennifer et al., 2021; Solari et al., 2021; Spatz et al., 2021). As treatment options become compromised, and antibiotics lose a level of their effectiveness, resistance will inevitably increase, particularly with rapid generational rates (Taylor et al., 2012). It is evident the nature of long haul space flight will create the conditions conducive to dysbiosis of the skin microbiome, autoflora changes will impact Vitamin D production, which in turn affects skin barrier growth and function, encouraging barrier dysfunction (Du et al., 2011).
Each individual provides an ecosystem of variable habitats, with bacterial, viral and fungal commensals demonstrating preferential environments. Colonization and autoflora exchange in closed environments is a known effect of both simulated and actual space environments; individual physiology dictates how a population of flora will inhabit different individuals. Gastrointestinal autoflora exchange has been quite well documented (Zea et al., 2017; Aunins Thomas et al., 2018; Singh et al., 2018; Mortazavi, 2019). Conversely, the mechanisms behind skin autoflora exchange and microbiological persistence in space flight vehicles and analogs is not well understood (Taylor and Sommer, 2005; Maloney et al., 2014; Aunins Thomas et al., 2018; Turroni et al., 2020; Yu et al., 2020). The microbiome of the gut and skin is now understood to be shared between participants who are housed in closed quarters (Williams, 2003b; Kostner et al., 2009; Karthaus et al., 2014; Mann et al., 2019). Skin commensal exchange, even when aseptic techniques and sterility is maintained, is a little more difficult to catalog. One presumes that the hygiene is strong in space vehicles and analog environments (Naik et al., 2012; Voorhies and Lorenzi, 2016; Tan et al., 2017; Ehrhardt, 2018; Rainer Barbara et al., 2020; NASA, 2021). The persistence and increased virulence of some microbiological organisms in space vehicles would suggest a more subtle transference vector (Zea et al., 2017; Aunins Thomas et al., 2018; Surber et al., 2018b; Mann et al., 2019).
As outlined, multivariate factors impact on skin health, and the relatively recent understanding of these factors working together to create a healthy skin environment has highlighted the fragility of the system once outside the Earthly environment (Horneck and Comet, 2006; Peterson et al., 2013). It is recognised that skin immunity is contributed to by resident commensals, and skin health can be affected by a change in the population dynamics of the resident commensals, and a change in the surface pH (NASA, 2021; Sole and Santamaria, 2021). Earth-bound studies have shown that pathological conditions such as rosacea and acne can be communicable, with individuals demonstrating a genetic propensity for these diseases which thrive in an alkaline pH environment [(Naik et al., 2012; Hu et al., 2014; Voorhies and Lorenzi, 2016; Tan et al., 2017; Surber et al., 2018a; Ehrhardt, 2018; Rainer Barbara et al., 2020), 135].
Changes in pH can greatly affect the habitat and composition of skin microbiome (Wheatcroft, 1989; Naik et al., 2012; Angelova-Fischer et al., 2018; Kahraman et al., 2019; Farkas and Farkas, 2021). This in turn, when coupled with human immune system dysfunction, could be expect to lead to disease proliferation, i.e., latent common viral diseases reactivating in microgravity environments, and novel and persistent habitat infiltration (Taylor and Sommer, 2005; Maloney et al., 2014; Turroni et al., 2020; Yu et al., 2020). Novel technical solutions for maintaining or repopulation of a healthy microbiome may include autoflora generalisation (generic autoflora distribution across individuals in a closed environment before long-haul space flight) of gastrointestinal and skin microbiome flora to maximise vitamin and nutrient absorption (Williams, 2003a).
A survey of reported medical issues during space flights has demonstrated that dermatological issues appear as the most regular complaint (Stowe et al., 2001; Horneck and Comet, 2006; Mehta et al., 2014). Skin complaints after space travel have included dryness, eczema, itch, acne and other infections, thinning skin, and erythemous or mastoidcyotic reactions to stimuli, suggesting changes to the barrier functioning of the skin and creation of dybiosis (Wheatcroft, 1989; Naik et al., 2012; Youn et al., 2013; Angelova-Fischer et al., 2018; Surber et al., 2018b; Kahraman et al., 2019; Farkas and Farkas, 2021). These factors can profoundly impact astronaut health, well-being and comfort in microgravity. Skin dysbiosis can act as a medical distraction, and should be a cardinal consideration on long haul space flights (Williams, 2003a). It is further appreciated that both short and long duration space flight have the potential to severely disrupt the skin microbiome, which will also be prone to currently unquantifiable radiation influences (Taylor, 1974; Penna, 2000; DeLuca, 2004; De Haes et al., 2005; O’Hara and Shanahan, 2007; Adorini and Penna, 2008; Kostner et al., 2009; Smith et al., 2009; Matheson et al., 2010; Searing and Leung, 2010; Slominski et al., 2011; Malodobra-Mazur et al., 2012; Slominski et al., 2013d; Peterson et al., 2013; Pludowski et al., 2013; Saleh et al., 2013; Hu et al., 2014; Karthaus et al., 2014; Thill et al., 2015; Wierzbicka et al., 2015; NASA, 2021; Sole and Santamaria, 2021).
Optimal skin health occurs within a range of pH value. Depending on the location/site of the human body, a number of factors affect skin pH: age, anatomical site, genetic and ethic inheritance, sebum, sweat and skin moisture (Wheatcroft, 1989; Naik et al., 2012; Angelova-Fischer et al., 2018; Kahraman et al., 2019; Farkas and Farkas, 2021). As an example of ethic inheritance affecting skin behaviour, with darkly pigmented skin demonstrates a lower pH and far superior barrier integrity than lighter skin (Naik et al., 2012; Farkas and Farkas, 2021). The pH tends to attract and help stabilise the human microbiome ecosystem within the realms of its symbiotic relationship, and the microbiome is greatly affected by pH and changes in pH (Wheatcroft, 1989). Newly hypothesized dysbiosis causative agents, such as Staphylococcus epidermidis, persist in the microgravity environment (Wheatcroft, 1989; Naik et al., 2012; Youn et al., 2013; Surber et al., 2018b; Farkas and Farkas, 2021).
Bacteria that comprise the microbiome contribute growth factors and peptides to the skin, stimulating skin repair. Staphylococcus aureus is one of the mainstays of this contribution, but may be outcompeted by Staphylococcus epidermidis, having an additional deleterious effect on wound healing (Youn et al., 2013; Surber et al., 2018a; Surber et al., 2018b). Common pathogenic bacteria such as Staphylococcus aureus show optimal growth at pH of 7.5, whilst Propionibacterium acnes has optimal growth at pH 6.3, and the newer organism contributing to acne, the bacteria Staphylococcus epidermidis is persistent in space flight, also preferring a more alkaline pH (Jürgen et al., 2011; Voorhies and Lorenzi, 2016; Tan et al., 2017; Surber et al., 2018a; Ehrhardt, 2018; Nanna, 2018; Claudel et al., 2019b; Kahraman et al., 2019; Lynde et al., 2019; Mortazavi, 2019; Cueto, 2022). The hydrophobic character and lipid distribution of the stratum corneum requires the organisation of lipids into a series of lamellar layers, and the synthesis of ceramides greatly improves with a lowered pH (Naik et al., 2012). It has been demonstrated that a lowered pH improves barrier functioning and integrity by creating an environment whereby ceramides can be generated by maximal enzymatic reaction of critical components of the permeability barrier of the skin. Two important enzymes, β-glucocerebrosidase and acidic sphingomyelinase, work at specific pH of 5.6 and 4.5 respectively (Naik et al., 2012; Angelova-Fischer et al., 2018; Kahraman et al., 2019). The change to sebum composition, which occurs under hormonal influences, reduces fatty acids presence and increases squalene and pH.
The basic principles for management of skin microbiome should include correcting pH, a balanced level of fatty acids present (secreted by the resident flora), and avoiding substances that damage or disrupt the microbiome. Treatment of elderly skin, which suffers from much of the same complaints as those astronauts who have experienced space flight, also suggest appropriate lowering of the pH in skincare products (Youn et al., 2013; Claudel et al., 2019b). Impaired wound healing has been documented in the space environment. Factors such as 3D molecular structural stress, changes in pH and barrier function, may lead to compromised functioning and contributing to noted skin atrophy and poor skin health of returning astronauts (Youn et al., 2013; Schreml et al., 2014; Neutelings et al., 2015; Braun et al., 2019; Lynde et al., 2019; Afshinnekoo et al., 2020; Cubo-Mateo and Gelinsky, 2021). Newer techniques, being developed on Earth (stem cell culture, bio-mechanical printing, placental growth factor harvesting, patches to deliver growth factors and progenitor wound healing molecules), may not have the availability or same behavior in the microgravity environment to be of value in space flight (Skardal et al., 2012; Neutelings et al., 2015; Widgerow et al., 2016; Dyer and Miller, 2018; Braun et al., 2019; Tottoli et al., 2020). Medications and treatments for infection, skin health maintenance and pH balance will face manufacturing, medication stability, and storage challenges in the remote and potentially hostile environment of spacecraft designed for long-duration space flight. A lowered skin pH would increase the wound healing capabilities of the skin, and could be as simple as using slightly acidic wipes for washing (without removal), to promote an acidic environment, perhaps three times a week whilst in the microgravity environment.
In the overall concext of space travel, it is becoming obvious that the area of medicine and astronaut health which may become cardinal to ensuring successful missions is maintenance of a healthy skin microbiome within the microgravity environment (Horneck and Comet, 2006; Stewart et al., 2007; Taddeo et al., 2008; Skardal et al., 2012; Saba, 2013; Tottoli et al., 2020; Paul et al., 2021). It is now appreciated that space flight contributes to skin dysbiosis, including, but not limited to, dryness, acne, topical infections, (with recorded increased virulence/reactivation of a herpetic/viral infections) and slowed wound healing.
This admix of poor barrier function, compromised immune response, the impact of changed individual autoflora, and disruptions of the gastrointestinal-skin axis (i.e. impaired Vitamin D metabolism and deficiency), together with poor wound healing, has the potential to lead to skin dysbiosis. The collective storm of decreased/altered human immune response, decreased wound healing, changes to microbiome, coupled with biologically demonstrated increased microbe replication and infective states (with reduced antibiotic and antiretroviral effectiveness), will inevitably lead to increased dysbiosis and risk of infections. Microgravity-induced 3D molecular stress, leading to molecular dysfunctionality of co-enzymes and interruptions of signalling/immune cellular recruitment pathways, cannot be ignored in planning for long-duration space flight. Consideration of innate pharmaceutical failure (packaging, longevity of medications, radiation damage), and ability to manufacture specifically designed medications, coupled with packaging and preservation, it is essential to give good “shelf life” for vital medicinal, food and personal care products during long-duration spaceflights. By definition, in normal use, sealed packaging is designed to prevent microbial growth, and thus is a limiting factor for microbiome-restorative medicaments.
Ensuring optimal functioning of the skin microbiome is paramount, but what are some practical measures to ensure this happens? The sebum consists of fatty acids, which are liberated by the microbiome, and are one of the mechanisms by which the skin remains acidic. It is acknowledged that an acidic pH is vitally important to the microbiome health, and in turn the biomechanics of the underlying human tissue (Naik et al., 2012; Youn et al., 2013; Angelova-Fischer et al., 2018; Kahraman et al., 2019; Lynde et al., 2019). A logical scenario would be to replicate the environment in which we know the microbiome exists optimally, bar the effects of microgravity. Whilst still in its infancy, there is enough data to piece together evidence-based strategies on such a concept. It is now known that in higher or more alkaline pH the microflora of the skin changes its composition and certain bacterial and viral species become more virulent (Jürgen et al., 2011; Nanna, 2018; Kahraman et al., 2019; Rainer Barbara et al., 2020; Farkas and Farkas, 2021). New data suggests that a lower than expected pH would create a healthier environment for skin’s microflora (Stewart et al., 2007; Taddeo et al., 2008; Saba, 2013; Youn et al., 2013; Eshelby, 2021; Paul et al., 2021; Cueto, 2022). One aspect of skin health in the changed immunological environment of microgravity, the pH, could be a simple, but effective, way to increase skin health, decrease topical infection rate, increase skin immune responses, and maintain a healthy biome (Youn et al., 2013; Lynde et al., 2019).
The authors suggest consistently maintaining a lowered skin pH in the microgravity environment as a direct methodology for maintaining astronaut skin barrier health whilst reducing the risk of infection. This approach would result in the maintenance of the skin barrier, reduce dryness, and slow disease development and topical infections (such as acne and rosacea), whilst helping at the same time to maintain the skin’s microbiome (Jürgen et al., 2011; Youn et al., 2013; Nanna, 2018; Lynde et al., 2019). A lowered pH would lead to increased skin wound healing capabilities.
International space agencies are planning for long-duration human spaceflight. However at our current level of technology, they present several medical challenges for human health. Good health and wellbeing for spacefarers has become a complex immunological, nutritional and medical issue, whereby new technology and solutions for expected and novel diseases and infections require careful thought and deployment of resources. Keeping crews fit and healthy (accounting for age-related disease profiles) require consideration of the biological, physiological, and pathogenic changes stemming from both potential pathogens, changes to skin microbiome, gastrointestinal autoflora, and the gastrointestinal-skin axis. It is also important to account for the molecular structural stress experienced in microgravity environment, and the effect of this on the human immune system.
Reducing skin pH, adequate Vitamin D supplementation, maintenance of the gastrointestinal-skin axis, and protection and monitoring of the skin microbiome, may provide a novel prophylactic and treatment course to help maintain a healthy individual skin microbiome. Improving and maintaining skin microbiome quality will reduce the risk of skin dysbiosis and infections during long-haul space flights.
The original contributions presented in the study are included in the article/supplementary material, further inquiries can be directed to the corresponding author.
Ethical review and approval was not required for the study on human participants in accordance with the local legislation and institutional requirements. The patients/participants provided their written informed consent to participate in this study.
All authors listed have made a substantial, direct, and intellectual contribution to the work and approved it for publication.
The authors declare that the research was conducted in the absence of any commercial or financial relationships that could be construed as a potential conflict of interest.
All claims expressed in this article are solely those of the authors and do not necessarily represent those of their affiliated organizations, or those of the publisher, the editors and the reviewers. Any product that may be evaluated in this article, or claim that may be made by its manufacturer, is not guaranteed or endorsed by the publisher.
Adorini, L., and Penna, G. (2008). Control of autoimmune diseases by the vitamin D endocrine system. Nat. Clin. Pract. Rheumatol. 4, 404–412. doi:10.1038/ncprheum0855
Afshinnekoo, E., Scott, R. T., MacKay, M. J., Pariset, E., Cekanaviciute, E., Barker, R., et al. (2020). Fundamental biological features of spaceflight: Advancing the field to enable deep-space exploration. Cell 183, 1162–1184. doi:10.1016/j.cell.2020.10.050
Angelova-Fischer, I., Fischer, T. W., Abels, C., and Zillikens, D. (2018). Accelerated barrier recovery and enhancement of the barrier integrity and properties by topical application of a pH 4 vs. a pH 5·8 water-in-oil emulsion in aged skin. Br. J. Dermatol. 179 (2), 471–477. doi:10.1111/bjd.16591
Anna, P., Wierzbicka, J., and Żmijewski, M. A. (2016). Vitamin D in the skin physiology and pathology. Acta Biochim. Pol. 63, 17–29. doi:10.18388/abp.2015_1104
Aunins Thomas, R., Erickson, K. E., Prasad, N., Levy, S. E., Jones, A., Shrestha, S., et al. (2018). Luis Zea and anushree chatterjee. Spaceflight modifies Escherichia coli gene expression in response to antibiotic exposure and reveals role of oxidative stress response. Front. Microbiol. 9, 310. doi:10.3389/fmicb.2018.00310
Baqai, F. P., Gridley, D. S., Slater, J. M., Luo-Owen, X., Stodieck, L. S., Ferguson, V., et al. (2009). Effects of spaceflight on innate immune function and antioxidant gene expression. J. Appl. Physiol. 106 (6), 1935–1942. doi:10.1152/japplphysiol.91361.2008
Bijlani, S., Stephens, E., Singh, N. K., Venkateswaran, K., and Wang, C. C. C. (2021). Advances in space microbiology. iScience 24 (5), 102395. doi:10.1016/j.isci.2021.102395
Bikle, D. D., Oda, Y., and Xie, Z. (2004). Calcium and 1, 25(OH)2D: Interacting drivers of epidermal differentiation. J. Steroid Biochem. Mol. Biol. 89 (90), 355–360. doi:10.1016/j.jsbmb.2004.03.020
Bikle, D. D., Tu, C. L., Xie, Z., and Oda, Y. (2003). Vitamin D regulated keratinocyte differentiation: role of coactivators. J. Cell. Biochem. 88, 290–295. doi:10.1002/jcb.10339
Bikle, D. D. (2012). Vitamin D and the skin: Physiology and pathophysiology. Rev. Endocr. Metab. Disord. 13, 3–19. doi:10.1007/s11154-011-9194-0
Bikle, D. D. (2004). Vitamin D regulated keratinocyte differentiation. J. Cell. Biochem. 92, 436–444. doi:10.1002/jcb.20095
Braun, N., Binder, S., Grosch, H., Theek, C., Ülker, J., Tronnier, H., et al. (2019). Current data on effects of long-term missions on the international space station on skin physiological parameters. Skin. Pharmacol. Physiol. 32, 43–51. doi:10.1159/000494688
Cabalín, C., Iturriaga, C., Pérez-Mateluna, G., Echeverría, D., Camargo, C. A., and Borzutzky, A. (2021). Vitamin D status and supplementation in Antarctica: a systematic review and meta- analysis. Int. J. Circumpolar Health 80, 1926133. doi:10.1080/22423982.2021.1926133
Canova Sabrina, C., Fiorasi, F., Mognato, M., Grifalconi, M., and Elena, R. (2005). Antonella russo and lucia celotti "modeled microgravity" affects cell response to ionizing radiation and increases genomic damage. Radiat. Res. 163 (2), 191–199. doi:10.1667/RR3304
Carlson, J. (2014). Lymphedema and subclinical lymphostasis (microlymphedema) facilitate cutaneous infection, inflammatory dermatoses, and neoplasia: A locus minoris resistentiae. Clin. Dermatol. 32, 599–615. doi:10.1016/j.clindermatol.2014.04.007
Claudel, J. P., Auffret, N., Leccia, M. T., Poli, F., Corvec, S., and Dréno, B. (2019). Staphylococcus epidermidis: A potential new player in the physiopathology of acne? Dermatology 235 (4), 287–294. doi:10.1159/000499858
Claudel, J. P., Auffret, N., Leccia, M. T., Poli, F., Corvec, S., and Dréno, B. (2019). Staphylococcus epidermidis: A potential new player in the physiopathology of acne? Dermatology 235 (4), 287–294. Epub 2019 May 21. PMID: 31112983. doi:10.1159/000499858
Cogoli, A., Tschopp, A., and Fushs-Bislin, P. (1984). Cell sensitivity to gravity. Science 225 (4658), 228–230. doi:10.1126/science.6729481
Coiletti Louis, A., Peierson, D L., and Mishra, S. K. (1991). Microbioal growth and physiology in space: A ReviewSAE transactions. J. Aerosp. 1002, 1594–1604.
Cubo-Mateo, N., and Gelinsky, M. (2021). Wound and skin healing in space: The 3D bioprinting perspective. Front. Bioeng. Biotechnol. 9, 720217. doi:10.3389/fbioe.2021.720217
Cueto, I. (2022). Acne bacteria, study suggests, thrive when skin oil turns infection-fighting cells into accomplices. Available at: https://www.statnews.com/2022/07/22/how-acne-bacteria-thrives-despite-immune-system/.
De Haes, P., Garmyn, M., Verstuyf, A., De Clercq, P., Vandewalle, M., Degreef, H., et al. (2005). 1, 25-Dihydroxyvitamin D3 and analogues protect primary human keratinocytes against UVB-induced DNA damage. J. Photochem. Photobiol. B 78, 141–148. doi:10.1016/j.jphotobiol.2004.09.010
DeLuca, H. F. (2004). Overview of general physiologic features and functions of vitamin D. Am. J. Clin. Nutr. 80, 1689S–1696S. doi:10.1093/ajcn/80.6.1689S
Du, J., Daniels, V. R., Vaksman, Z., Boyd, J. L., Crady, C., and Putcha, L. (2011). Evaluation of physical and chemical changes in pharmaceuticals flown on space missions. AAPS J, Sci. 13 (2), 299–308. doi:10.1208/s12248-011-9270-0
Du, J., Gatlin, K., Vaksman, Z., Berens, K., and Putcha, L. (2002). Stability of pharmaceuticals during space flight. AAPS Pharm. Sci. 4, T3153. doi:10.2147/IDR.S67275
Duygu Gezen, -Ak, Erdinç, D., Basar, B., Hasmet, H., Turan, E., Hakan, G., et al. (2012). Selma Yilmazer Vitamin D receptor gene haplotype is associated with late-onset Alzheimer's disease. Tohoku J. Exp. Med. 228 (3), 189–196. doi:10.1620/tjem.228.189
Dyer, J. M., and Miller, R. A. (2018). Chronic skin fragility of aging: Current concepts in the pathogenesis, recognition, and management of dermatoporosis. J. Clin. Aesthet. Dermatol. 11, 13–18. PMCID: PMC5788262.
Eckberg, P. B., Bik, E. M., Berstein, C. N., Purdom, E., Dethlefsen, L., Sargent, M., et al. (2005). Diversity of the human intestinal microbial flora. Science 380 (5728), 1635–1638. doi:10.1126/science.1110591
Ehrhardt, Proksch (2018). pH in nature, humans and skin. J. Dermatol. 45, 1044–1052. doi:10.1111/1346-8138.14489
Eyles, D. W., Smith, S., Kinobe, R., Hewison, M., and McGrath, J. J. (2005). Distribution of the vitamin D receptor and 1 alpha-hydroxylase in human brain. J. Chem. Neuroanat. 29, 21–30. doi:10.1016/j.jchemneu.2004.08.006
Farkas, Á., and Farkas, G. (2021). Effects of spaceflight on human skin. Skin. Pharmacol. Physiol. 34, 239–245. doi:10.1159/000515963
Fukuda, T., Fukuda, K., Takahasi, A., Ohnishi, T., Nakano, T., Sato, M., et al. (2000). Analysis of deletion mutation of the rpsL gene in the yeast Saccharomyces cerevisiae detected after lon-term flight on the Russian space station Mir. Mutat. Res. 470, 125–132. doi:10.1016/s1383-5742(00)00054-5
Garcia, L. A., King, K. K., Ferrini, M. G., Norris, K. C., and Artaza, J. N. (2011). 1, 25(OH)2vitamin D3 stimulates myogenic differentiation by inhibiting cell proliferation and modulating the expression of promyogenic growth factors and myostatin in C2C12 skeletal muscle cells. Endocrinology 152, 2976–2986. doi:10.1210/en.2011-0159
Guéguinou, N., Huin-Schohn, C., Bascove, M., Bueb, J. L., Tschirhart, E., Legrand-Frossi, C., et al. (2009). Could spaceflight association immune system; weakening preclude the expansion of human presence beyond earth’s orbit? J. J. Leukoc. Biol. 86 (5), 1027–1038. doi:10.1189/jlb.0309167
Haussler, M. R., Jurutka, P. W., Mizwicki, M., and Norman, A. W. (2011). Vitamin D receptor (VDR)-mediated actions of 1α, 25(OH) vitamin D: Genomic and non-genomic mechanisms. Best. Pract. Res. Clin. Endocrinol. Metab. 25, 543–559. doi:10.1016/j.beem.2011.05.010
Hawker, N. P., Pennypacker, S. D., Chang, S. M., and Bikle, D. D. (2007). Regulation of human epidermal keratinocyte differentiation by the vitamin D receptor and its coactivators DRIP205, SRC2, and SRC3. J. Invest. Dermatol. 127, 874–880. doi:10.1038/sj.jid.5700624
Heath, K. M., and Elovic, E. P. (2006). Vitamin D deficiency: Implications in the rehabilitation setting. Am. J. Phys. Med. Rehabil. 85, 916–923. doi:10.1097/01.phm.0000242622.23195.61
Holick, M. F. (2004). Vitamin D: Importance in the prevention of cancers, type 1 diabetes, heart disease, and osteoporosis. Am. J. Clin. Nutr. 79, 362–371. doi:10.1093/ajcn/79.3.362
Horneck, G., and Comet, B. (2006). General human health issues for Moon and Mars missions: Results from the HUMEX study. Adv. Space Res. 37, 100–108. doi:10.1016/j.asr.2005.06.077
Horneck, G., Facius, R., Reichert, M., Rettberg, P., Seboldt, W., Manzey, D., et al. (2003). HUMEX, a study on the survivability and adaptation of humans to long-duration exploratory missions, part I: Lunar missions. Adv. Space Res. 31, 2389–2401. doi:10.1016/s0273-1177(03)00568-4
Hossein-nezhad, A., and Holick, M. F. (2013). Vitamin D for health: a global perspective. Mayo Clin. Proc. 88, 720–755. doi:10.1016/j.mayocp.2013.05.011
Hu, L., Bikle, D. D., and Oda, Y. (2014). Reciprocal role of vitamin D receptor on β-catenin regulated keratinocyte proliferation and differentiation. J. Steroid Biochem. Mol. Biol. 144, 237–241. doi:10.1016/j.jsbmb.2013.11.002
Jennifer, B., Darker, S. F., Hansmeier, N., Yang, S., Buss, K., Park, J., et al. (2021). Evaluating the effect of spaceflight on the host-pathogen interaction between human intestinal epithelial cells and Salmonella Typhimurium. npj Microgravity 7, 9. doi:10.1038/s41526-02136w
Jürgen, B., Wohlfart, R., and Nanna, Y. (2011). Treatment of aged skin with a pH 4 skin care product normalizes increased skin surface pH and improves barrier function: Results of a pilot study. J. Cosmet. Dermatological Sci. Appl. 1, 50–58. Published Online September 2011. doi:10.4236/jcdsa.2011.13009
Kahraman, E., Melis, K., Bektay, H. Ş., and Sevgi, G. (2019). Recent advances on topical application of ceramides to restore barrier function of skin. Cosmetics 6 (3), 52. doi:10.3390/cosmetics6030052
Karthaus, N., van Spriel, A. B., Looman, M. W., Chen, S., Spilgies, L. M., Lieben, L., et al. (2014). Vitamin d controls murine and human plasmacytoid dendritic cell function. J. Invest. Dermatol. 134, 1255–1264. doi:10.1038/jid.2013.501
Kaur, I., Simons, E. R., Castro, V. A., Ott, C. M., and Pierson, D. L. (2005). Changes in monocyte functions of astronauts. Brain Behav. Immun. 19 (6), 547–554. doi:10.1016/j.bbi.2004.12.006
Kaur, I., Simons, E. R., Kapadia, A. S., Ott, C., and Pierson, D. L. (2008). Effect of spaceflight on ability of monocytes to respond to endotoxins of gram-negative bacteria. Clin. Vaccine Immunol. 15 (10), 1523–1528. doi:10.1128/CVI.00065-08
Klaus, D., Steven, S., Todd, P., and Stodieck, L. (1997). Investigation of space flight effects on Escherichia coli and a proposed model of underlying physical mechanisms. Microbiology 143 (2), 449–455. doi:10.1099/00221287-143-2-449
Kostner, K., Denzer, N., Muller, C. S., Klein, R., Tilgen, W., and Reichrath, J. (2009). The relevance of vitamin D receptor (VDR) gene polymorphisms for cancer: a review of the literature. Anticancer Res. 29, 3511–3536. PMID: 19667145.
Lund, A. W., Medler, T. R., Leachman, S. A., and Coussens, L. M. (2016). Lymphatic vessels, inflammation, and immunity in skin cancer. Cancer Discov. 6 (1), 22–35. Epub 2015 Nov 9. PMID: 26552413PMCID: PMC4707138. doi:10.1158/2159-8290.CD-15-0023
Lynde, C., Tan, J., Andriesse, A., Skotnicki, S., Beecker, J., Claveau, J., et al. (2019). Clinical insights about the role of pH in acne. J. Drugs Dermatol. 18 (12), 221. PMID: 31860223.
Malodobra-Mazur, M., Paduch, A., Lebioda, A., Konopacka, M., Rogolinski, J., Szymczyk, C., et al. (2012). VDR gene single nucleotide polymorphisms and their association with risk of oral cavity carcinoma. Acta Biochim. Pol. 59, 627–630. doi:10.18388/abp.2012_2102
Maloney, R. D., Desbonnet, L., Clarke, G., Dinan, T. G., and Cryan, J. F. (2014). The micro-biome: stress, health and disease. Mamm. Genome 25 (1-2), 49–74. doi:10.1007/s00335-013-9488-5
Mann, V., Sundaresan, A., Mehta, S. K., Crucian, B., Doursout, M. F., and Devakottai, S. (2019). Effects of microgravity and other space stressors in immunosuppression and viral reactivation with potential nervous system involvement. Rev. Neurol. India 67, S198–S203. doi:10.4103/0028-3886.259125
Matheson, E. M., Mainous, A. G., Hueston, W. J., Diaz, V. A., and Everett, C. J. (2010). Vitamin D and methicillin-resistant Staphylococcus aureus nasal carriage. Scand. J. Infect. Dis. 42, 455–460. doi:10.3109/00365541003602049
McPhee, J. C., and Charles, J. B. (2010). Human health and performance risks of space exploration missions evidence reviewed by the NASA Human Research Program. Houston, TX: National Aeronautics and Space Administration. Lyndon B. Johnson Space Center.
Mehta, S. K., Laudenslager, M. L., Stowe, R. P., Crucian, B. E., Sams, C. F., and Pierson, D. L. (2014). Multiple latent viruses reactivate in astronauts during Space Shuttle missions. Brain Behav. Immun. 41, 210–217. doi:10.1016/j.bbi.2014.05.014
Misra, M., Pacaud, D., Petryk, A., Collett-Solberg, P. F., and Kappy, M. (2008). Vitamin D deficiency in children and its management: review of current knowledge and recommendations. Pediatrics 122, 398–417. doi:10.1542/peds.2007-189410.1542/peds.2007-1894
More, M., Wink, L., Kögler, I., Alexander, M., Rettberg, P., Schwendner, P., et al. (2019). Space Station conditions are selective but do not alter microbial characteristics relevant to human health. Nat. Commun. 10, 3990. Article number: 3990. doi:10.1038/s41467-019-11682-z
Mortazavi, S. M. J. (2019). Acquired antibiotic resistance in Escherichia coli exposed to simulated microgravity: Possible role of other space stressors and adaptive responses. ASM Journals mBio 10 (2). doi:10.1128/mBio.00165-19
Naik, S., Bouladoux, N., Wilhelm, C., Molloy, M. J., Salcedo, R., Kastenmuller, W., et al. (2012). Compartmentalized control of skin immunity by resident commensals. Science 337, 1115–1119. doi:10.1126/science.1225152
Nanna, S. (2018). pH and Acne. Curr. Probl. Dermatol 54, 115–122. Epub 2018 Aug 21. doi:10.1159/000489525
NASA (2021). Study of the impact of long-term space travel on the astronauts’ microbiome (Microbiome). Houston: National Aeronautics and Space Administration. Available at: https://www.nasa.gov/mission_pages/station/research/experiments/explorer/Investigation.html?#id=982 (Accessed July 24, 2021).
Nefedov, Y. G., Shilov, V. M., Koustantinova, I. V., and Zaloguev, S. N. (1971). Microbiological and immunological aspects of extended manned space flights. Life Sci. Space Res. 9, 11–16.
Neutelings, T., Nusgens, B. V., Liu, Y., Tavella, S., Ruggiu, A., Cancedda, R., et al. (2015). Skin physiology in microgravity: A 3-month stay aboard ISS induces dermal atrophy and affects cutaneous muscle and hair follicles cycling in mice. NPJ Microgravity 1, 15002. doi:10.1038/npjmgrav.2015.2
Nickerson, C. A., Ott, C. M., Mister, S. J., Morrow, B. J., Burns-KeLiher, L., and Pierson, D. L. (2000). Microgravity as a novel environmental signal affecting Salmonella enterica serovar Typhimurium virulence. Infect. Immun. 68, 3147–3152. doi:10.1128/iai.68.6.3147-3152.2000
Nickerson, C. A., Pelllis, N. R., and Ott, C. M. (2016). Effect of spaceflight and spaceflight analogue culture on human and microbial cells: Novel insights into disease mechanisms. Springer.
Nwanaji-Enwerem Jamaji, C., Nwanaji-Enwerem, U., Van Der Laan, L., Galazka, J. M., Redeker, N. S., and Andres, C. (2020). A longitudinal epigenetic aging and leukocyte analysis of simulated space travel: The mars-500 mission. Cell Rep. Open Access. doi:10.1016/j.celrep.2020.108406
O’Hara, A. M., and Shanahan, F. (2007). Gut microbiota: mining for therapeutic potential. Clin. Gastroenterol. Hepatol. 5 (3), 274–284. doi:10.1016/j.cgh.2006.12.009
Paul, W., and Sharma, C. P. (2021). “Tissue and organ regeneration: An introduction,” in Regenerated organs. Editor C. P. Shrama (Academic Press), 3–9. doi:10.1016/b978-0-12-821085-7.00001-4
Penna, G. (2000). 1 Alpha, 25-dihydroxyvitamin D3 inhibits differentiation, maturation, activation, and survival of dendritic cells leading to impaired alloreactive T cell activation. J. Immunol. 164, 2405–2411. doi:10.4049/jimmunol.164.5.2405
Peterson, A. L., Murchison, C., Zabetian, C., Leverenz, J. B., Watson, G. S., Montine, T., et al. (2013). Memory, mood, and vitamin D in persons with Parkinson’s disease. J. Park. Dis. 3, 547–555. doi:10.3233/JPD-130206
Picardo, M., and Monica, O. (2014). Skin microbiome and skin disease: the example of rosacea. J. Clin. Gastroenterol. 48, S85–S86. Number Supp. 1, November/December 2014. doi:10.1097/MCG.0000000000000241
Pludowski, P., Karczmarewicz, E., Bayer, M., Carter, G., Chlebna-Sokol, D., Czech-Kowalska, J., et al. (2013). Practical guidelines for the supplementation of vitamin D and the treatment of deficits in central Europe — recommended vitamin D intakes in the general population and groups at risk of vitamin D deficiency. Endokrynol. Pol. 64, 319–327. doi:10.5603/ep.2013.0012
Putcha, L., Taylor, P. W., and Boyd, Jl (2011). Biopharmaceutical challenges of therapeutics in space: formulation and packaging considerations. Ther. Deliv. 2 (11), 1373–1376. doi:10.4155/tde.11.115
Rainer Barbara, M., Thompson, K. G., Antonescu, C., Florea, L., Mongodin, E. F., Bui, J., et al. (2020). Characterization and analysis of the skin microbiota in rosacea: A case-control study. Am. J. Clin. Dermatol. 21, 139–147. doi:10.1007/s40257-019-00471-5
Saba, M. (2013). Skin pH: from basic science to basic skin care. Acta Derm. Venereol. 93, 261–267. doi:10.2340/00015555-1531
Saleh, H. M., Abdel Fattah, N. S., and Hamza, H. T. (2013). Evaluation of serum 25-hydroxyvitamin D levels in vitiligo patients with and without autoimmune diseases. Photodermatol. Photoimmunol. Photomed. 29, 34–40. doi:10.1111/phpp.12016
SanMiguel, A., and Grice, E. A. (2015). Interactions between host factors and the skin microbiome. Cell Mol. Life Sci. 72 (8), 1499–1515. Epub 2014 Dec 30. PMID: 25548803; PMCID: PMC4376244. doi:10.1007/s00018-014-1812-z
Schreml, S., Kemper, M., and Abels, C. (2014). Skin pH in the elderly and appropriate skin care. EMJ Dermatol 2, 86–94.
Schwager, S., and Detmar, M. (2019). Inflammation and lymphatic function. Front. Immunol. 10, 308. PMID: 30863410; PMCID: PMC6399417. doi:10.3389/fimmu.2019.00308
Scott, S., Heer, M., and Zwart, S. (2021). Nutrition and human space flight: Evidence from 4–6 Month missions to the international space station. Curr. Dev. Nutr. 5. doi:10.1093/cdn/nzab047_026
Searing, D. A., and Leung, D. Y. (2010). Vitamin D in atopic dermatitis, asthma and allergic diseases. Immunol. Allergy Clin. North Am. 30, 397–409. doi:10.1016/j.iac.2010.05.005
Shen, W., Li, W., Hixon, J. A., Bouladoux, N., Belkaid, Y., Dzutzev, A., et al. (2014). Adaptive immunity to murine skin commensals. Proc. Natl. Acad. Sci. U. S. A. 111 (29), E2977–E2986. doi:10.1073/pnas.1401820111
Singh, N. K., Wood, J. M., Karouia, F., and Venkateswaran, K. (2018). Succession and persistence of microbial communities and antimicrobial resistance genes associated with International Space Station environmental surfaces. Microbiome 6, 204. doi:10.1186/s40168-018-0585-2
Skardal, A., Mack, D., Kapetanovic, E., Atala, A., Jackson, J. D., Yoo, J., et al. (2012). Bioprinted amniotic fluid-derived stem cells accelerate healing of large skin wounds. Stem Cell Transl. Med. 1, 792–802. doi:10.5966/sctm.2012-0088
Slominski, A., Li, W., Bhattacharya, S. K., Smith, R. A., Johnson, P. L., Chen, J., et al. (2011). Novel vitamin D analog 17, 20S(OH)2pD and 17, 20R(OH)2pD are noncalcemic and exhibit antifibrotic activity. J. Invest. Dermatol. 131, 1167–1169. doi:10.1038/jid.2010.425
Slominski, A., Zbytek, B., Nikolakis, G., Manna, P. R., Skobowiat, C., Zmijewski, M., et al. (2013d). Steroidogenesis in the skin: Implications for local immune functions. J. Steroid Biochem. Mol. Biol. 137, 107–123. doi:10.1016/j.jsbmb.2013.02.006
Smith, S. M., Zwart, S. R., Douglas, G. L., and Heer, M. (2021). Human adaptation to space flight: The role of food and nutrition. Second Edition. Houston, TX: National Aeronautics and Space Administration. Available at: https://www.nasa.gov/sites/default/files/atoms/files/human_adaptation_2021_final.pdf.
Smith, S. M., Gardner, K. K., Locke, J., and Zwart, S. R. (2009). Vitamin D supplementation during Antarctic winter. Am. J. Clin. Nutr. 89, 1092–1098. doi:10.3945/ajcn.2008.27189
Smith, S. M., Wastney, M. E., Morukov, B. V., Larina, I. M., Nyquist, L. E., Abrams, S. A., et al. (1999). Calcium metabolism before, during, and after a 3-mo spaceflight: kinetic and biochemical changes. Am. J. Physiol. 277, R1–R10. doi:10.1152/ajpregu.1999.277.1.r1
Smith, S. M., Zwart, S. R., Block, G., Rice, B. L., and Davis-Street, J. E. (2005). The nutritional status of astronauts is altered after long-term space flight aboard the International Space Station. J. Nutr. 135, 437–443. doi:10.1093/jn/135.3.437
Solari, E., Marcozzi, C., Negrini, D., and Moriondo, A. (2021). Interplay between gut lymphatic vessels and microbiota. Cells 10 (10), 2584. doi:10.3390/cells10102584
Sole, P., and Santamaria, P. (2021). Re-programming autoreactive T cells into T-regulatory type 1 cells for the treatment of autoimmunity. Front. Immunol. 12. doi:10.3889/immu.2021.684240
Sonnenfield, G., and Shearer, W. T. (2002). Immune Function during space flight. Nutrition 18 (10), 899–903. doi:10.1016/s0899-9007(02)00903-6
Sonnenfield, G., and Taylor, G. R. (1991). Effects of microgravity on the immune system. SAR transactions. J. Aerosp. 100, 1617–1622. Part 2.
Spatz, J. M., Huges Fulford, M., Tsai, A., Gaudilliere, D., Hedou, J., Ganio, E., et al. (2021). Human immune system adaptations to simulated microgravity revealed by single-cell mass cytometry. Sci. Rep. 11, 11872. Nature. doi:10.1038/s41598-021-90458-2
Stewart, L. H., Trunkey, D., and Regbagliati, G. S. (2007). Emergency medicine in space. J. Emerg. Med. 32 (1), 45–54. doi:10.1016/j.jemermed.2006.05.031
Stowe, R. P., Mehta, S. K., Ferrando, A. A., Feeback, D. L., and Pierson, D. L. (2001). Immune responses and latent herpesvirus reactivation in spaceflight. Aviat. Space Environ. Med. 72 (10), 884–891. PMID: 11601551.
Surber, C., Abels, C., and Maibach, H. (2018). pH of the skin: Issues and challenges, Curr. Probl. Dermatol. 54, 87–94. doi:10.1159/000489522
Surber, C., Humbert, P., Abels, C., and Maibach, H. (2018). “Background and introduction the acid mantle: A myth or an essential part of skin health?,” in pH of the skin: Issues and challenges. Editors C. Surber, C. Abels, and H. Maibach (Basel, Karger: Curr Probl Dermatol), 54, 1–10. doi:10.1159/000489512
Taddeo, T. A., and Armstrong, C. W. (2008). “Spaceflight medical systems,” in Principles of clinical medicine for space flight. Editors M. Barratt, and S. R. Pool (New York: Springer), 69–100. doi:10.1080/09553002.2020.1820603
Tan, J., Almeida, L. M. C., Bewley, A., Cribier, B., Dlova, N. C., Gallo, R., et al. (2017). Updating the diagnosis, classification and assessment of rosacea: recommendations from the global ROSacea COnsensus (ROSCO) panel. Br. J. Dermatol 176 (2), 431–438. doi:10.1111/bjd.15122
Tang, H., Rising, H. H., Majji, M., and Brown, R. D. (2021). Long-Term space nutrition: A scoping review. Nutrients 14 (1), 194. PMID: 35011072; PMCID: PMC8747021. doi:10.3390/nu14010194
Taylor, G. R. (1993). Immune changes during short-duration missions. J. Leukoc. Biol. 54 (3), 202–208. doi:10.1002/jlb.54.3.202
Taylor, G. R. (1974). Recovery of medically important microorganisms from Apollo astronauts. Aerosp. Med. 45 (8), 824–828.
Taylor, P. W. (2015). Impact of space flight on bacterial virulence and antibiotic susceptibility. Infect. Drug Resist. 8, 249–262. doi:10.2147/IDR.S67275
Taylor, P. W., and Putcha, L. (2012). “Stability of medications in space: Therapeutic implications for extended duration space missions,” in Advances in engineering research. Editor V. M. Petrova (Hauppaguge: Nova Science Publishers), 1, 221–239.
Taylor, P. W., and Sommer, A. P. (2005). Towards rational treatment of bacterial infections during extended space travel. Int. J. Antimicrob. Agents 26, 183–187. doi:10.1016/j.ijantimicag.2005.06.002
Teize, K. J., and Putcha, L. (1994). Factors affecting drug availability in space. J. Clin. Pharmacol. 34, 671–676.
Thill, M., Woeste, A., Reichert, K., Fischer, D., Rody, A., Friedrich, M., et al. (2015). Vitamin D inhibits ovarian cancer cell line proliferation in combination with celecoxib and suppresses cyclooxygenase-2 expression. Anticancer Res. 35, 1197–1203. –1203PMID: 25667511.
Tottoli, E. M., Dorati, R., Genta, I., Chiesa, E., Pisani, S., and Conti, B. (2020). Skin wound healing process and new emerging technologies for skin wound care and regeneration. Pharmaceutics 12, 735. doi:10.3390/pharmaceutics12080735
Turroni, S., Magnani, M., Pukar, K. C., Lesnick, P., Vidal, H., and Heer, M. (2020). Gut microbiome and space travelers' health: State of the art and possible pro/prebiotic strategies for long-term space missions. Front. Physiol. 11, 553929. doi:10.3389/fphys.2020.553929
Voorhies, A. A., and Lorenzi, H. A. (2016). The Challenge of maintaining a healthy microbiome during long-duration space missions. Front. Astron. Space Sci. 3. doi:10.3389/fspas.2016.00023
Wang, T. T., Nestel, F. P., Bourdeau, V., Nagai, Y., Wang, Q., Liao, J., et al. (2004). Cutting edge: 1, 25-dihydroxyvitamin D3 is a direct inducer of antimicrobial peptide gene expression. J. Immunol. 173, 2909–2912. doi:10.4049/jimmunol.173.5.2909
Wheatcroft, W. (1989). Effects of simulated Skylab missions on the oral health of astronauts. J. Gr Houst. Dent. Soc. 61, 7. doi:10.14219/jada.archive.1976.0502
Widgerow, A. D., Fabi, S. G., Palestine, R. F., Rivkin, A., Ortiz, A., Bucay, V. W., et al. (2016). Extracellular matrix modulation: Optimizing skin care and rejuvenation procedures. J. Drugs Dermatol. 15 (4), s63–s71.
Wierzbicka, J. M., Binek, A., Ahrends, T., Nowacka, J. D., Szydlowska, A., Turczyk, L., et al. (2015). Differential antitumor effects of vitamin D analogues on colorectal carcinoma in culture. Int. J. Oncol. 47, 1084–1096. doi:10.3892/ijo.2015.3088
Wierzbicka, J., Piotrowska, A., and Żmijewski, M. A. (2014). The renaissance of vitamin D. Acta Biochim. Pol. 61, 679–686. PMID: 25566549. doi:10.18388/abp.2014_1830
Williams, D. R. (2003). The biomedical challenges of space flight. Annu. Rev. Med. 54, 245–256. doi:10.1146/annurev.med.54.101601.152215
Youn, S. H., Choi, C. W., Choi, J. W., and Youn, S. W. (2013). The skin surface pH and its different influence on the development of acne lesion according to gender and age. Skin. Res. Technol. 19 (2), 131–136. doi:10.1111/srt.12023
Yu, S-Y., Guo, L-N., Xiao, M., Zhou, M-L., Yuan, Y., Yao, W., et al. (2020). Clinical and microbiological characterization of invasive pulmonary aspergillosis caused by Aspergillus lentulus in China. Front. Microbiol. 11, 1672. doi:10.3389/fmicb.2020.01672
Zea, L., Larson, M., Estante, F., Qvrtrup, K., Moeller, R., Dias de Oliveira, S., et al. (2017). Phenotypic changes exhibited by E. coli cultured in space. Front. Microbiol. 8, 1598. doi:10.1620/tjem.228.189
Zwart, S. R., and ScottSmith, M. (2020). Vitamin D and COVID-19: Lessons from spaceflight analogs. J. Nutr. 150 (10), 2624–2627. doi:10.1093/jn/nxaa233
Zwart, S. R., Mehta, S. K., Ploutz-Snyder, R., Bourbeau, Y., Locke, J. P., Pierson, D. L., et al. (2011). Response to vitamin D supplementation during Antarctic winter is related to BMI, and supplementation can mitigate Epstein-Barr Virus Reactivation. J. Nutr. 141 (4), 692–697. doi:10.3945/jn.110.134742
Zwart, S. R., Parsons, H., Kimlin, M., Innis, S. M., Locke, J. P., and Smith, S. M. (2013). A 250 µg/week dose of vitamin D was as effective as a 50 µg/d dose in healthy adults, but a regimen of four weekly followed by monthly doses of 1250 µg raised the risk of hypercalciuria. Br. J. Nutr. 110, 1866–1872. doi:10.1017/S000711451300113X
Keywords: space flight, skin pH, microgravity, 3D molecular structure, immuno-suppression, Vitamin D, microbiome, dysbiosis
Citation: Caswell G and Eshelby B (2022) Skin microbiome considerations for long haul space flights. Front. Cell Dev. Biol. 10:956432. doi: 10.3389/fcell.2022.956432
Received: 30 May 2022; Accepted: 19 August 2022;
Published: 08 September 2022.
Edited by:
Rowena Christiansen, The University of Melbourne, AustraliaReviewed by:
Matthew Melin, Fairview Health Services, United StatesCopyright © 2022 Caswell and Eshelby. This is an open-access article distributed under the terms of the Creative Commons Attribution License (CC BY). The use, distribution or reproduction in other forums is permitted, provided the original author(s) and the copyright owner(s) are credited and that the original publication in this journal is cited, in accordance with accepted academic practice. No use, distribution or reproduction is permitted which does not comply with these terms.
*Correspondence: Gabrielle Caswell, ZHJjYXN3ZWxsQHNwYWNlcG9ydGF1c3RyYWxpYS5jb20uYXU=
Disclaimer: All claims expressed in this article are solely those of the authors and do not necessarily represent those of their affiliated organizations, or those of the publisher, the editors and the reviewers. Any product that may be evaluated in this article or claim that may be made by its manufacturer is not guaranteed or endorsed by the publisher.
Research integrity at Frontiers
Learn more about the work of our research integrity team to safeguard the quality of each article we publish.