- 1Univ. Grenoble Alpes, CNRS, UMR 5525, VetAgro Sup, Grenoble INP, CHU Grenoble Alpes, TIMC, T-RAIG, Grenoble, France
- 2Université Paris-Saclay, CNRS UMR 8000, Institut de Chimie Physique, Orsay, France
Reactive oxygen species (ROS), produced by the phagocyte NADPH oxidase, NOX2, are involved in many leukocyte functions. An excessive or inappropriate ROS production can lead to oxidative stress and tissue damage. On the other hand, an absence of ROS production due to a lack of a functional NADPH oxidase is associated with recurrent infections as well as inflammation disorders. Thus, it is clear that the enzyme NADPH oxidase must be tightly regulated. The NOX2 complex bears both membrane and cytosolic subunits. The membrane subunits constitute the flavocytochrome b558, consisting of gp91phox (Nox2) and p22phox subunits. The cytosolic subunits form a complex in resting cells and are made of three subunits (p47phox, p40phox, p67phox). Upon leukocyte stimulation, the cytosolic subunits and the small GTPase Rac assemble with the flavocytochrome b558 in order to make a functional complex. Depending on the stimulus, the NADPH oxidase can assemble either at the phagosomal membrane or at the plasma membrane. Many studies have explored NOX2 activation; however, how this activation is sustained and regulated is still not completely clear. Here we review the multiple roles of NOX2 in neutrophil functions, with a focus on description of its components and their assembly mechanisms. We then explain the role of energy metabolism and phosphoinositides in regulating NADPH oxidase activity. In particular, we discuss: 1) the link between metabolic pathways and NOX2 activity regulation through neutrophil activation and the level of released ROS, and 2) the role of membrane phosphoinositides in controlling the duration of NOX2 activity.
Introduction: NADPH Oxidase Roles in Neutrophil Functions
The first discovered role of ROS produced by NOX2 was pathogen killing. Upon phagocytosis the NADPH oxidase is activated and produces superoxide anions, O2.-, inside the phagosome as soon as the phagosome has formed (Tlili et al., 2011). Inside the phagosome, O2.- dismutates into hydrogen peroxide, H2O2. In neutrophils, the granules release myeloperoxidase (MPO) which catalyzes the formation of hypochlorous acid (HOCl) from chloride ions and H2O2. Several millimolars of O2.- are produced in the phagosome. Neither the ROS chemistry nor the mechanism of toxicity of this species within the peculiar environment of the phagosome have been well defined (Winterbourn et al., 2016).
Evidence for the involvement of ROS in pathogen killing comes largely from people who lack a functional NADPH oxidase as in the chronic granulomatous disease (CGD). In this genetic disease, people are faced with chronic and persistent infections. Neutrophils isolated from these patients are defective in killing bacteria and fungi (Klebanoff, 2005). Moreover, recent data indicate that CGD neutrophils have an increased cytokine production and secretion of the chemoattractant leukotriene B4 when they are challenged with fungus particles (Song et al., 2020a; Yoo et al., 2021). This increased cytokine and leukotriene B4 production probably contributes to the aberrant inflammation observed in CGD patients. The production of H2O2, thanks to NADPH oxidase activation, modulates the signaling pathways involved in cytokine production although their specific targets have not been identified (Yoo et al., 2021). Among the potential targets, the activity of NF-κB, which is involved in inflammatory mediator transcription, has been shown to undergo a redox regulation (Trevelin et al., 2020).
NADPH oxidase activation is triggered by phagocytosis but also by soluble stimuli. Long-lasting NADPH oxidase activity has been observed in vitro in adherent neutrophils stimulated by cytokines or bacterial chemotactic peptides such as N-formyl-methionyl-leucyl-phenylalanine (fMLP) (Fumagalli et al., 2013; McMillan et al., 2014; Song et al., 2020b). Following stimulus of this type, NOX2 assembles at the plasma membrane and produces ROS in the extracellular medium.
This ROS production then regulates the migration of neutrophils (Trevelin et al., 2020). Kuiper et al. showed that upon stimulation by a fMLP gradient, the ROS produced inhibit the activity of the lipid phosphatase PTEN (Phosphatase and TENsin homolog) at the cell front (Kuiper et al., 2011). The inhibition occurs through oxidation of cysteine 124 in the catalytic site (Lee et al., 2002). PTEN inhibition prevents the phosphatidylinositol 3,4,5-trisphosphate (PIP3) dephosphorylation at the cell front. This PIP3 accumulation is a key event for the activation of signaling molecules involved in neutrophil polarization and chemotaxis (Wang, 2009). Furthermore, ROS induce actin glutathionylation which is necessary for chemotaxis in vitro and for the recruitment of neutrophils to the site of infection in vivo (Sakai et al., 2012).
NADPH oxidase activity has also been shown to be involved in neutrophil apoptosis (Dupre-Crochet et al., 2013) and, more recently, in the formation of neutrophil extracellular traps (NETs) which consist of decondensed chromatin associated with granule proteins such as MPO and neutrophil elastase. The release of NETs can be fatal to neutrophils since they eventually explode to release these NETs. The involvement of ROS in NETs formation seems to depend on the stimulus (Kenny et al., 2017). NETs avert the dissemination of the pathogen; however, produced in excess or in an inappropriate context, NETs may in fact contribute to the disease process (Sollberger et al., 2018). Recently NETs have been described in COVID infections, where they have been shown to contribute to tissue injury and immunothrombosis (Veras et al., 2020; Ackermann et al., 2021).
Moreover, an excessive NADPH oxidase activation can be detrimental for the surrounding tissues. Excess ROS production by neutrophils has been involved in auto-immune diseases (Glennon-Alty et al., 2018) and chronic inflammatory disease states, such as periodontal disease or chronic obstructive pulmonary disease (COPD) (Jasper et al., 2019; Zeng et al., 2019). In COPD, activated neutrophils are recruited to the lungs of the patients. ROS produced by the NADPH oxidase contribute to the oxidative stress, which leads to increased inflammation, cellular senescence, altered organelle functions especially mitochondria functions and DNA damage (Barnes, 2022). These pathophysiological effects of oxidative stress in COPD has been described in recent reviews (Manevski et al., 2020; Barnes, 2022).
Below, we discuss the NADPH oxidase components and their assembly mechanism, and then review the role of energy metabolism and phosphoinositides in regulating the NADPH oxidase activity.
NADPH Oxidase Components and Assembly
The phagocyte NADPH oxidase is made of two membrane subunits, gp91phox/Nox2 and p22phox, three cytosolic subunits, p40phox, p47phox, p67phox, and the small GTPase Rac. The membrane-bound NADPH oxidase subunits are located at the plasma membrane and also in the endocytic compartments in macrophages and neutrophils (Casbon et al., 2009; Joly et al., 2020). In these latter cells however most of the membrane subunits of the NADPH oxidase reside in granules (Borregaard et al., 1983; Lominadze et al., 2005). During phagocytosis, granules and endocytic compartments fuse with the phagosome providing it with membrane subunits as well as lytic enzymes. Nox2 is a 91 kDa glycoprotein responsible for NOX2 catalytic activity. It transfers electrons from cytosolic NADPH to O2 producing superoxide anions (Vermot et al., 2021). The p22phox subunit stabilizes Nox2 at the plasma membrane and enables Nox2 heme acquisition (DeLeo et al., 2000). In its C-terminus, p22phox bears a proline-rich region (PRR), which interacts with p47phox SH3 (SRC Homology 3) domains and may also interact with the same domain in p40phox (Tamura et al., 2007). In the resting state, the cytosolic subunits p47phox, p67phox and p40phox form a heterotrimeric complex. p67phox binds to the PRR domain of p47phox via its SH3 domain. p40phox-p67phox interaction involves the PB1 (Phox and Bem1) domains of both proteins (Vermot et al., 2021).
A 3D model of the complex has recently been proposed based on biophysical studies and structural data: the complex has an elongated shape; the non-structured C-ter of p47phox constitutes a flexible region that may facilitate interaction with the membrane subunits (Ziegler et al., 2019). p47phox and p40phox also contain a PX (PhoX homology) domain that interacts with anionic phospholipids of the membrane (see paragraph 2.3 below) (Ellson et al., 2001; Kanai et al., 2001; Karathanassis et al., 2002). However, in the resting state, p47phox, like p40phox, is in an auto-inhibitory conformation, preventing the formation of the complex (Ago et al., 1999; Karathanassis et al., 2002; Honbou et al., 2007).
NADPH oxidase can be activated by signalling pathways triggered by soluble stimuli such as fMLP or by pathogen phagocytosis. The phagocytosis is promoted by opsonins i.e., immunoglobulins G and complement molecules (C3b and C3bi) that cover the pathogen following antibody production and activation of the complement. Certain agents can potentiate the activation of NOX2. NOX2 is then in a “primed” state and the NOX2 activity is higher after an activation by a second stimulus such as those mentioned above. Such priming agents include some cytokines, chemo-attractants and Toll-like receptor agonists (El-Benna et al., 2016). EL-Benna et al. describe these priming events in detail in the preceding review.
Upon neutrophil stimulation, p47phox specific serines are phosphorylated by different kinases (MAPK, PKC ...) (Belambri et al., 2018) and these phosphorylations unfold p47phox. P47phox can then interact with p22phox and anionic phospholipids at the plasma membrane thus mediating the recruitment of p67phox and p40phox. Concomitantly, Rac2, highly expressed in neutrophils, dissociates from a GDP dissociation inhibitor (GDI) and exchanges its GDP with GTP. The GTP-bound Rac2 associates with the membrane via its polybasic domain and prenyl group, and binds to p67phox (Bokoch and Diebold, 2002). Rac2-p67phox interaction favours the binding of p67phox to Nox2 and may participate together with p67phox, in regulating the electron flow from NADPH to oxygen, leading to superoxide anion production (Nisimoto et al., 1999; Bokoch and Diebold, 2002).
Different studies, including our own, have observed the dynamics of the cytosolic subunits during phagocytosis using time-lapse confocal video-microscopy (Li et al., 2009; Matute et al., 2009; Tlili et al., 2012; Faure et al., 2013; Song et al., 2017). The following model can be proposed: the complex assembly occurs as soon as the phagocytosis starts, then p47phox and Rac2 leave the phagosome within a few minutes, whereas p40phox and p67phox stay until the end of ROS production (Figure 2A, see paragraph 2.3).
Because of their major roles in neutrophil functions, ROS production and NADPH oxidase activity are highly regulated in space and time. This regulation has been extensively studied and depends on the subunit expression (Nunes et al., 2013), the phosphorylation of NOX2 subunits (Belambri et al., 2018), the trafficking of the subunits to the phagosomal or plasma membrane and ion fluxes (Nunes et al., 2013).
In the following section, we will focus on the role of energy metabolism and phosphoinositides in regulating NADPH oxidase activity.
Interconnection of Neutrophil Energy Metabolism and NADPH Oxidase Activity
NADPH oxidase activation is dependent on cytoskeleton modifications (Bengtsson et al., 1991), on phosphorylation of cytosolic factors, especially p47phox, and membrane cytochrome b558 (Nox2 and p22phox) (El Benna et al., 1997; Bouin et al., 1998; Regier et al., 1999; Raad et al., 2009), and on NADPH availability. All these processes require energy.
In physiological conditions, neutrophil cell metabolism depends essentially on glucose and on the glycolytic pathway for ATP production and energy supply (Borregaard and Herlin, 1982; Anderson et al., 1991; Maianski et al., 2004). Treatment of neutrophils with a glycolysis inhibitor completely abolished phorbol myristate acetate (PMA)-induced NOX2 activity (Chacko et al., 2013).
Furthermore, 6-phosphofructo-2-kinase (PFK2), an enzyme involved in glycolysis regulation, has been identified in the active NOX2 complex isolated from neutrophils stimulated with PMA. Inhibition of PFK2 expression leads to a decrease in NOX2 activity, indicating spatial and functional interactions between enzymes involved in energy metabolism and the phagocyte NOX2 complex (Baillet et al., 2017).
However, the story is not so simple! Neutrophils were considered for decades as a homogeneous cell population with a short half-life and a nearly absence of transcriptional activity (Mantovani et al., 2011). However, recent studies reported heterogeneity of neutrophil phenotypes and revealed the highly-developed plasticity of these cells in response to various physiological and pathological conditions (Silvestre-Roig et al., 2019; Yang et al., 2019). This heterogeneity is especially evident in their functions and their capacity to produce reactive oxygen species via NOX2 activation.
When NOX2 is activated, electrons are transferred from the donor, NADPH, to the acceptor, O2 then leading to the release of superoxide anions. This process called “oxidative burst” is extremely fast and requires a large amount of NADPH, the NOX2 cofactor. NADPH availability appears to be a key element in spatial and temporal NOX2 activation. NADPH concentration has been shown to oscillate in a wave-like manner in resting neutrophils. In stimulated neutrophils, the amplitude and/or frequency of NADPH oscillations increase according to the nature of the stimulus (Petty, 2001). Changes in stimulus-induced NADPH oscillations have been correlated with abnormality of NOX2-derived ROS production in neutrophils from patients suffering from chronic inflammatory disorders. This observation suggests a link between NADPH concentration and NOX2 activity (Petty, 2001).
The main source of NADPH in neutrophils is the glucose-dependent pentose phosphate pathway (PPP). Activation of neutrophils with various stimuli leads to an increase in PPP metabolites (Britt et al., 2022). In the PPP oxidative phase, the enzymes glucose-6-phosphate dehydrogenase (G6PD) and 6-phosphogluconate dehydrogenase (6 PGDH) catalyse the two steps leading to NADPH generation (Curi et al., 2020). Activity of G6PD and 6 PGDH is involved in NOX2 activity regulation. Patients with severe G6PD deficiency are more susceptible to infections and present dysfunctions in neutrophil microbicidal mechanisms (Gray et al., 1973). Moreover, G6PD deficiency may result in an absence of ROS production by PMA-stimulated neutrophils (Tsai et al., 1998). At the molecular level, G6PD and 6 PGDH form a supramolecular complex mainly localized at the periphery of neutrophils. This localization facilitates the interaction with the G6PD substrate i.e., glucose-6-phosphate, produced at the plasma membrane, and thus the production of NADPH (Kindzelskii et al., 2004). Interestingly, in neutrophils from pregnant women, the complex G6PD/6 PGDH is relocalized to the microtubule-organizing-center, modifying the site of NADPH release. This difference correlates with the decrease in NOX2-derived ROS production observed in neutrophils from pregnant women (Kindzelskii et al., 2004). Excitingly, proteomic analysis of the constitutively active NOX2 complex isolated from neutrophils has revealed the association of 6 PGDH with the active NOX2 complex and the role of 6 PGDH in the modulation of ROS production via NADPH availability (Baillet et al., 2011).
Cellular micro-compartmentation, coupling energy metabolism to ROS production, provides an additional level of NADPH oxidase activity regulation (Figure 1).
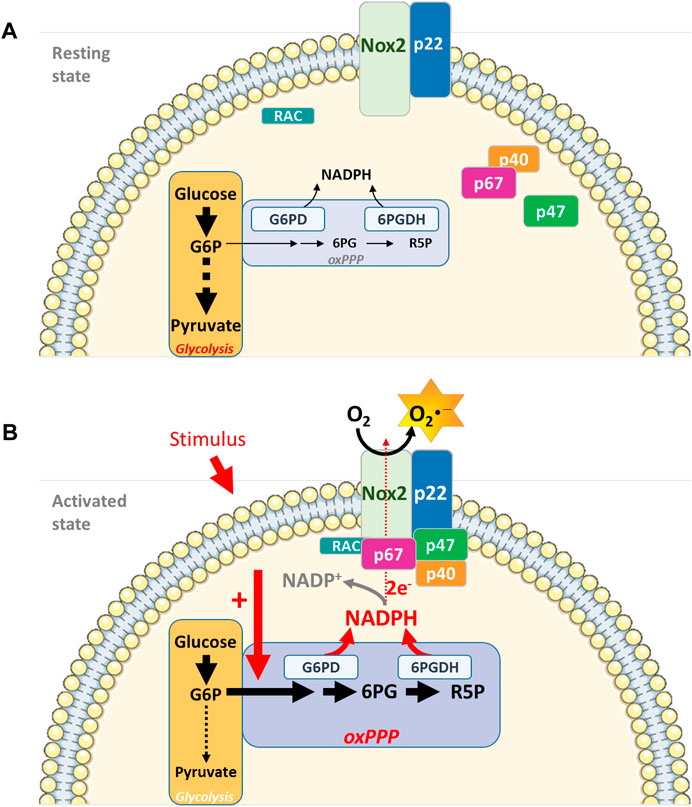
FIGURE 1. Interconnecting cell metabolism and NOX2 activity in neutrophils. (A). In resting neutrophils, energy metabolism depends essentially on glucose and on the glycolytic pathway for ATP production and energy supply. NOX2 is dissociated and inactive. (B). Upon cell stimulation (PMA, opsonized bacteria, fMLP), the oxidative phase of the PPP is activated, leading to an increase in NADPH concentration, a limiting cofactor for NOX2 complex activity. Micro-compartmentation coupling enzymes involved in the energy metabolism (G6PD and 6 PGDH) and NOX2 complex represent an additional level of NADPH oxidase activity regulation (G6P: Glucose-6-Phosphate; 6 PG: 6-PhosphoGluconate; R5P: Ribulose-5-Phosphate)Parts of this figure were drawn by using pictures from Servier Medical Art. Servier Medical Art by Servier is licensed under a Creative Commons Attribution 3.0 Unported License (https://creativecommons.org/licenses/by/3.0/).
Phosphoinositide Dynamics and NADPH Oxidase Regulation
Metabolism contributes to the regulation of NOX2 activity whose assembly is dependent on the subunit interaction. However, not only protein-protein binding is important for NADPH oxidase activity, but also protein-lipid binding. P47phox and p40phox have a PX domain. The PX domain of p47phox has two binding pockets: one prefers phosphatidylinositol 3,4-bisphosphate (PI(3,4)P2) and the other binds phosphatidic acid and phosphatidylserine, whereas the PX domain of p40phox binds PI3P (Ellson et al., 2001; Kanai et al., 2001; Karathanassis et al., 2002; Stahelin et al., 2003). The phosphoinositide composition of the inner leaflet of the membrane during neutrophil activation is crucial to sustain NADPH oxidase activity. The understanding of the importance of the p40phox-PX for ROS production came from the discovery of a CGD patient with a mutation of a critical residue for PI3P binding in the PX domain (Matute et al., 2009). Neutrophils of this patient presented a substantial defect in intracellular ROS production during phagocytosis of Aspergillus fumigatus hyphae or serum opsonized fungus particles but not upon activation of neutrophils by soluble stimuli (Matute et al., 2009; Bagaitkar et al., 2012). Neutrophils of mice bearing a PX mutation in p40phox also had a reduced ROS production upon phagocytosis although this depends on the stimulus (Ellson et al., 2006; Anderson et al., 2010). In p40phox, the PX domain is masked by an intramolecular interaction (Honbou et al., 2007) which is removed in the presence of H2O2 and when p40phox is targeted to the membrane (Ueyama et al., 2011). Thus, it may be only when the p40phox/p67phox/p47phox complex is at the membrane that p40phox can bind to PI3P.
The NADPH oxidase complex assembles at the phagosomal cup. At this time PI (3,4)P2 and PIP3 accumulate in the inner leaflet of the phagosomal cup. This accumulation is transient and followed by the rise of PI3P in the inner leaflet 1 min after the phagosome sealing. PI3P can be generated through dephosphorylation of PI(3,4)P2 and by phosphorylation of phosphatidylinositol by the class III phosphoinositide 3-kinase (PI3K) (Vieira et al., 2001; Valenta et al., 2020; Montaño-Rendón et al., 2021). Using the PX of p40phox tagged with GFP as a PI3P biosensor, we observed that PI3P lasted around 15min at the phagosome of opsonized fungus particles in neutrophil-like PLB-985 cells. Expressing fluorescent protein fusion of p40phox and p67phox in these cells allowed us to prove that the timing of their disappearance at the level of the phagosome correlated with that of PI3P. Moreover, we showed that the protein Rubicon, a negative regulator of Class III PI3K (Sun et al., 2011), and the PI3P phosphatase Myotubular Myopathy 1 (MTM1) (Cao et al., 2008; Amoasii et al., 2013), were present at the phagosome. Knocking down these two proteins increased the time that the PI3P biosensor, p40phox and p67phox remained present at the phagosomal membrane and also ROS production inside the phagosome. In contrast, overexpression of MTM1 at the phagosome prevented the accumulation of PI3P, p40phox and p67phox and ROS production. Thus, the disappearance of PI3P from the phagosomal membrane controls the disassembly of the NADPH oxidase complex and thus the ROS production inside the phagosome (Song et al., 2017) (Figure 2A).
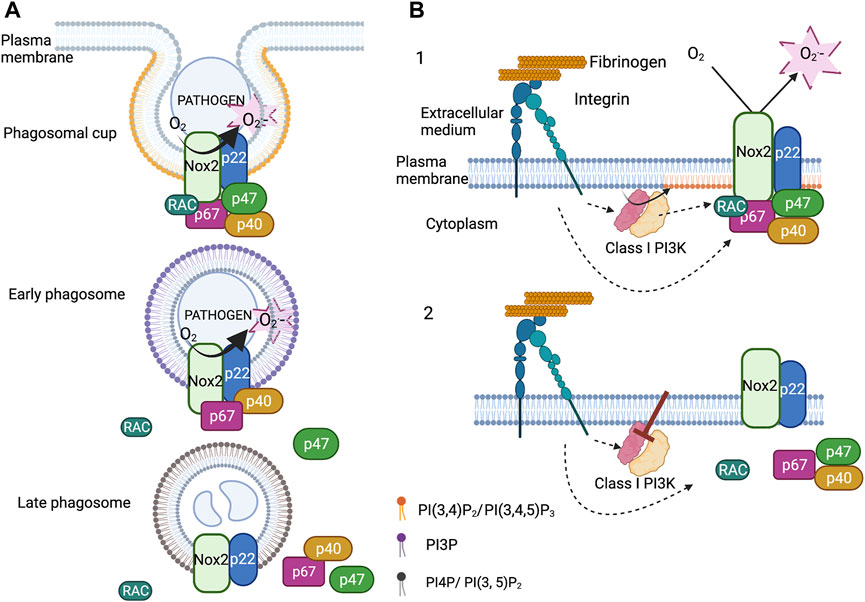
FIGURE 2. Regulation of the NADPH oxidase by phosphoinositides. (A). Upon phagocytosis of serum opsonized fungus particles, PI(3,4)P2 and PIP3 accumulate in the inner leaflet of the phagosomal membrane. At the same time, the cytosolic subunits (p67phox, p47phox and p40phox) and the small GTPases Rac2 associate with the membrane subunits (Nox2 and p22phox). Soon after phagosome closure, p47phox and Rac2 leave the phagosome. The detachment of p47phox is concomitant to the decrease in the level of PI(3,4)P2 and the accumulation of PI3P in the cytosolic leaflet of the early phagosome. The binding of p40phox to PI3P sustains the NADPH oxidase activity. The disappearance of PI3P induces the disassembly of the complex. (B). Integrin dependent adherent neutrophils, stimulated or not by fMLP, produce ROS via NADPH oxidase activation. Class I PI3Ks are also activated and are necessary to sustain NADPH oxidase activation. Inhibition of Class I PI3Ks, especially the β isoform, deactivate the NADPH oxidase by triggering its disassembly. Class I PI3K products maintain the cytosolic subunits at the plasma membrane probably via the PX domain of p47phox (created with “BioRender.com”).
The NADPH oxidase also assembles at the plasma membrane and produces ROS in the extracellular medium. This ROS production is involved in neutrophil migration and may have other physiological consequences. However, this can also be detrimental contributing to thrombus formation (Gutmann et al., 2020). A long-lasting NADPH oxidase activation at the plasma membrane has been observed, in vitro, in β2 integrin dependent adherent neutrophils stimulated or not by fMLP or TNF (Fumagalli et al., 2013; Houslay et al., 2016; Song et al., 2020b). Integrins, in their active conformation, stimulated different intracellular pathways including the production of PI(3,4)P2 and PIP3 by class I PI3K (Mócsai et al., 2015). Class I PI3K works as a heterodimer possessing p110 catalytic subunits and regulatory subunits. Two subclasses, IA and IB, can be distinguished. Subclass IA comprises three types of catalytic subunits (p110α, p110β, p110δ) that share several regulatory subunits: p85, p50 and p55 (Balla, 2013).
The pharmacological inhibition of class I PI3K in adherent neutrophils, and especially p110β, halted integrin mediated ROS production. Expression of tagged p47phox, p40phox and p67phox in neutrophil-like PLB-985 cells allowed us to observe, by total internal reflexion fluorescence video-microscopy and after class I PI3K inhibition, the release of these subunits from the plasma membrane. Our results suggest that this mechanism involves the PX domain of p47phox, which binds PI (3,4)P2 (Figure 2B). This is coherent with the fact that mutation in some critical residues involved in PI(3,4)P2 binding only slightly modified ROS production at the phagosome but drastically affected that at the plasma membrane following fMLP activation (Li et al., 2010; Olsson et al., 2017). Thus, Class I PI3K products may be important for the deactivation of the NADPH oxidase at the plasma membrane whereas PI3P would act on the timing of NADPH oxidase activation at the phagosome (Figure 2).
Concluding Remarks
A fine-tuned regulation of the NADPH oxidase is necessary. Polymorphism in the NADPH oxidase genes leading to low ROS production has recently been associated with autoimmune diseases such as systemic lupus erythematosus (Olsson et al., 2017). In contrast, excess activation of neutrophils and NADPH oxidase dependent ROS production contribute to several chronic inflammatory diseases. Inhibitors of class I PI3K isoforms are currently in clinical testing or approved for drug use (Miller et al., 2019) and thus may be interesting targets in order to reduce the duration of NADPH activity at the plasma membrane. Modulating cellular metabolism by regulating the PPP pathway could be a way to moderate or, on the contrary, to increase NOX2-dependent ROS production. Furthermore, it may be of great interest to selectively target NOX2 at the plasma membrane versus phagosomal membrane.
Author Contributions
All authors listed have made a substantial, direct, and intellectual contribution to the work and approved it for publication.
Funding
SL has benefited from a grant from the French ministry of research. This work is supported by the CNRS, the Université Paris-Saclay and the Université Grenoble Alpes.
Conflict of Interest
The authors declare that the research was conducted in the absence of any commercial or financial relationships that could be construed as a potential conflict of interest.
Publisher’s Note
All claims expressed in this article are solely those of the authors and do not necessarily represent those of their affiliated organizations, or those of the publisher, the editors and the reviewers. Any product that may be evaluated in this article, or claim that may be made by its manufacturer, is not guaranteed or endorsed by the publisher.
Acknowledgments
We thank Kirsty Grant for reading the manuscript and for help with English language corrections.
References
Ackermann, M., Anders, H.-J., Bilyy, R., Bowlin, G. L., Daniel, C., De Lorenzo, R., et al. (2021). Patients with COVID-19: in the Dark-NETs of Neutrophils. Cell Death Differ. 28, 3125–3139. doi:10.1038/s41418-021-00805-z
Ago, T., Nunoi, H., Ito, T., and Sumimoto, H. (1999). Mechanism for Phosphorylation-Induced Activation of the Phagocyte NADPH Oxidase Protein P47. J. Biol. Chem. 274, 33644–33653. doi:10.1074/jbc.274.47.33644
Amoasii, L., Hnia, K., Chicanne, G., Brech, A., Cowling, B. S., Müller, M. M., et al. (2013). Myotubularin and PtdIns3P Remodel the Sarcoplasmic Reticulum in Muscle In Vivo. J. Cell Sci. 126, 1806–1819. doi:10.1242/jcs.118505
Anderson, K. E., Chessa, T. A. M., Davidson, K., Henderson, R. B., Walker, S., Tolmachova, T., et al. (2010). PtdIns3P and Rac Direct the Assembly of the NADPH Oxidase on a Novel, Pre-phagosomal Compartment during FcR-Mediated Phagocytosis in Primary Mouse Neutrophils. Blood 116, 4978–4989. doi:10.1182/blood-2010-03-275602
Anderson, R., Van Rensburg, C. E., Jooné, G. K., and Lessing, A. (1991). Auranofin Inactivates Phosphofructokinase in Human Neutrophils, Leading to Depletion of Intracellular ATP and Inhibition of Superoxide Generation and Locomotion. Mol. Pharmacol. 40, 427–434. Available at: http://www.ncbi.nlm.nih.gov/pubmed/1654515.
Bagaitkar, J., Matute, J. D., Austin, A., Arias, A. A., and Dinauer, M. C. (2012). Activation of Neutrophil Respiratory Burst by Fungal Particles Requires Phosphatidylinositol 3-phosphate Binding to P40(phox) in Humans but Not in Mice. Blood 120, 3385–3387. doi:10.1182/blood-2012-07-445619
Baillet, A., Hograindleur, M. A., El Benna, J., Grichine, A., Berthier, S., Morel, F., et al. (2017). Unexpected Function of the Phagocyte NADPH Oxidase in Supporting Hyperglycolysis in Stimulated Neutrophils: Key Role of 6-Phosphofructo-2-Kinase. FASEB J. 31, 663–673. doi:10.1096/fj.201600720R
Baillet, A., Xu, R., Grichine, A., Berthier, S., Morel, F., and Paclet, M. H. (2011). Coupling of 6-phosphogluconate Dehydrogenase with NADPH Oxidase in Neutrophils: Nox2 Activity Regulation by NADPH Availability. FASEB J. 25, 2333–2343. doi:10.1096/fj.10-173807
Balla, T. (2013). Phosphoinositides: Tiny Lipids with Giant Impact on Cell Regulation. Physiol. Rev. 93, 1019–1137. doi:10.1152/physrev.00028.2012
Barnes, P. J. (2022). Oxidative Stress in Chronic Obstructive Pulmonary Disease. Antioxidants 11, 965. doi:10.3390/antiox11050965
Belambri, S. A., Rolas, L., Raad, H., Hurtado-Nedelec, M., Dang, P. M.-C., and El-Benna, J. (2018). NADPH Oxidase Activation in Neutrophils: Role of the Phosphorylation of its Subunits. Eur. J. Clin. Invest. 48, e12951. doi:10.1111/eci.12951
Bengtsson, T., Dahlgren, C., Stendahl, O., and Andersson, T. (1991). Actin Assembly and Regulation of Neutrophil Function: Effects of Cytochalasin B and Tetracaine on Chemotactic Peptide-Induced O2 - Production and Degranulation. J. Leukoc. Biol. 49, 236–244. doi:10.1002/jlb.49.3.236
Benna, J. E., Dang, P. M.-C., Gaudry, M., Fay, M., Morel, F., Hakim, J., et al. (1997). Phosphorylation of the Respiratory Burst Oxidase Subunit P67(phox) during Human Neutrophil Activation: Regulation by Protein Kinase C-dependent and Independent Pathways. J. Biol. Chem. 272, 17204–17208. doi:10.1074/jbc.272.27.17204
Bokoch, G. M., and Diebold, B. A. (2002). Current Molecular Models for NADPH Oxidase Regulation by Rac GTPase. Blood 100, 2692–2695. doi:10.1182/blood-2002-04-1149
Borregaard, N., Heiple, J. M., Simons, E. R., and Clark, R. A. (1983). Subcellular Localization of the B-Cytochrome Component of the Human Neutrophil Microbicidal Oxidase: Translocation during Activation. J. Cell Biol. 97, 52–61. doi:10.1083/jcb.97.1.52
Borregaard, N., and Herlin, T. (1982). Energy Metabolism of Human Neutrophils during Phagocytosis. J. Clin. Invest. 70, 550–557. doi:10.1172/JCI110647
Bouin, A.-P., Grandvaux, N., Vignais, P. V., and Fuchs, A. (1998). p40 Is Phosphorylated on Threonine 154 and Serine 315 during Activation of the Phagocyte NADPH Oxidase. J. Biol. Chem. 273, 30097–30103. doi:10.1074/jbc.273.46.30097
Britt, E. C., Lika, J., Giese, M. A., Schoen, T. J., Seim, G. L., Huang, Z., et al. (2022). Switching to the Cyclic Pentose Phosphate Pathway Powers the Oxidative Burst in Activated Neutrophils. Nat. Metab. 4, 389–403. doi:10.1038/s42255-022-00550-8
Cao, C., Backer, J. M., Laporte, J., Bedrick, E. J., and Wandinger-Ness, A. (2008). Sequential Actions of Myotubularin Lipid Phosphatases Regulate Endosomal PI(3)P and Growth Factor Receptor Trafficking. MBoC 19, 3334–3346. doi:10.1091/mbc.e08-04-0367
Casbon, A.-J., Allen, L.-A. H., Dunn, K. W., and Dinauer, M. C. (2009). Macrophage NADPH Oxidase FlavocytochromebLocalizes to the Plasma Membrane and Rab11-Positive Recycling Endosomes. J. Immunol. 182, 2325–2339. doi:10.4049/jimmunol.0803476
Chacko, B. K., Kramer, P. A., Ravi, S., Johnson, M. S., Hardy, R. W., Ballinger, S. W., et al. (2013). Methods for Defining Distinct Bioenergetic Profiles in Platelets, Lymphocytes, Monocytes, and Neutrophils, and the Oxidative Burst from Human Blood. Lab. Invest. 93, 690–700. doi:10.1038/labinvest.2013.53
Curi, R., Levada-Pires, A. C., Silva, E. B. da, Poma, S. de O., Zambonatto, R. F., Domenech, P., et al. (2020). The Critical Role of Cell Metabolism for Essential Neutrophil Functions. Cell. Physiol. biochem. 54, 629–647. doi:10.33594/000000245
DeLeo, F. R., Burritt, J. B., Yu, L., Jesaitis, A. J., Dinauer, M. C., and Nauseef, W. M. (2000). Processing and Maturation of Flavocytochromeb 558 Include Incorporation of Heme as a Prerequisite for Heterodimer Assembly. J. Biol. Chem. 275, 13986–13993. doi:10.1074/jbc.275.18.13986
Dupré-Crochet, S., Erard, M., and Nüβe, O. (2013). ROS Production in Phagocytes: Why, when, and where? J. Leukoc. Biol. 94, 657–670. doi:10.1189/jlb.1012544
El-Benna, J., Hurtado-Nedelec, M., Marzaioli, V., Marie, J.-C., Gougerot-Pocidalo, M.-A., and Dang, P. M.-C. (2016). Priming of the Neutrophil Respiratory Burst: Role in Host Defense and Inflammation. Immunol. Rev. 273, 180–193. doi:10.1111/imr.12447
Ellson, C., Davidson, K., Anderson, K., Stephens, L. R., and Hawkins, P. T. (2006). PtdIns3P Binding to the PX Domain of P40phox Is a Physiological Signal in NADPH Oxidase Activation. EMBO J. 25, 4468–4478. doi:10.1038/sj.emboj.7601346
Ellson, C. D., Gobert-Gosse, S., Anderson, K. E., Davidson, K., Erdjument-Bromage, H., Tempst, P., et al. (2001). PtdIns(3)P Regulates the Neutrophil Oxidase Complex by Binding to the PX Domain of P40phox. Nat. Cell Biol. 3, 679–682. doi:10.1038/35083076
Faure, M. C., Sulpice, J.-C., Delattre, M., Lavielle, M., Prigent, M., Cuif, M.-H., et al. (2013). The Recruitment of P47phoxand Rac2G12V at the Phagosome Is Transient and Phosphatidylserine Dependent. Biol. Cell 105, 501–518. doi:10.1111/boc.201300010
Fumagalli, L., Campa, C. C., Germena, G., Lowell, C. A., Hirsch, E., and Berton, G. (2013). Class I Phosphoinositide-3-Kinases and Src Kinases Play a Nonredundant Role in Regulation of Adhesion-independent and -Dependent Neutrophil Reactive Oxygen Species Generation. J. I. 190, 3648–3660. doi:10.4049/jimmunol.1201951
Glennon-Alty, L., Hackett, A. P., Chapman, E. A., and Wright, H. L. (2018). Neutrophils and Redox Stress in the Pathogenesis of Autoimmune Disease. Free Radic. Biol. Med. 125, 25–35. doi:10.1016/j.freeradbiomed.2018.03.049
Gray, G. R., Stamatoyannopoulos, G., Naiman, S. C., Kliman, M. R., Klebanoff, S. J., Austin, T., et al. (1973). Neutrophil Dysfunction, Chronic Granulomatous Disease, and Non-spherocytic Hæmolytic Anæmia Caused by Complete Deficiency of Glucose-6-Phosphate Dehydrogenase. Lancet 302, 530–534. doi:10.1016/S0140-6736(73)92350-7
Gutmann, C., Siow, R., Gwozdz, A. M., Saha, P., and Smith, A. (2020). Reactive Oxygen Species in Venous Thrombosis. Ijms 21, 1918. doi:10.3390/ijms21061918
Honbou, K., Minakami, R., Yuzawa, S., Takeya, R., Suzuki, N. N., Kamakura, S., et al. (2007). Full-length P40phox Structure Suggests a Basis for Regulation Mechanism of its Membrane Binding. EMBO J. 26, 1176–1186. doi:10.1038/sj.emboj.7601561
Houslay, D. M., Anderson, K. E., Chessa, T., Kulkarni, S., Fritsch, R., Downward, J., et al. (2016). Coincident Signals from GPCRs and Receptor Tyrosine Kinases Are Uniquely Transduced by PI3Kβ in Myeloid Cells. Sci. Signal. 9, ra82. doi:10.1126/scisignal.aae0453
Jasper, A. E., McIver, W. J., Sapey, E., and Walton, G. M. (2019). Understanding the Role of Neutrophils in Chronic Inflammatory Airway Disease. F1000Res 8, 557. doi:10.12688/f1000research.18411.1
Joly, J., Hudik, E., Lecart, S., Roos, D., Verkuijlen, P., Wrona, D., et al. (2020). Membrane Dynamics and Organization of the Phagocyte NADPH Oxidase in PLB-985 Cells. Front. Cell Dev. Biol. 8, 1–10. doi:10.3389/fcell.2020.608600
Kanai, F., Liu, H., Field, S. J., Akbary, H., Matsuo, T., Brown, G. E., et al. (2001). The PX Domains of P47phox and P40phox Bind to Lipid Products of PI(3)K. Nat. Cell Biol. 3, 675–678. doi:10.1038/35083070
Karathanassis, D., Stahelin, R. V., Bravo, J., Perisic, O., Pacold, C. M., Cho, W., et al. (2002). Binding of the PX Domain of P47phox to Phosphatidylinositol 3,4-bisphosphate and Phosphatidic Acid Is Masked by an Intramolecular Interaction. EMBO J. 21, 5057–5068. doi:10.1093/emboj/cdf519
Kenny, E. F., Herzig, A., Krüger, R., Muth, A., Mondal, S., Thompson, P. R., et al. (2017). Diverse Stimuli Engage Different Neutrophil Extracellular Trap Pathways. Elife 6. doi:10.7554/eLife.24437
Kindzelskii, A. L., Ueki, T., Michibata, H., Chaiworapongsa, T., Romero, R., and Petty, H. R. (2004). 6-Phosphogluconate Dehydrogenase and Glucose-6-Phosphate Dehydrogenase Form a Supramolecular Complex in Human Neutrophils that Undergoes Retrograde Trafficking during Pregnancy. J. Immunol. 172, 6373–6381. doi:10.4049/jimmunol.172.10.6373
Klebanoff, S. J. (2005). Myeloperoxidase: Friend and Foe. J. Leukoc. Biol. 77, 598–625. doi:10.1189/jlb.1204697
Kuiper, J. W. P., Sun, C., Magalhães, M. A. O., and Glogauer, M. (2011). Rac Regulates PtdInsP3 Signaling and the Chemotactic Compass through a Redox-Mediated Feedback Loop. Blood 118, 6164–6171. doi:10.1182/blood-2010-09-310383
Lee, S.-R., Yang, K.-S., Kwon, J., Lee, C., Jeong, W., and Rhee, S. G. (2002). Reversible Inactivation of the Tumor Suppressor PTEN by H2O2. J. Biol. Chem. 277, 20336–20342. doi:10.1074/jbc.M111899200
Li, X. J., Marchal, C. C., Stull, N. D., Stahelin, R. V., and Dinauer, M. C. (2010). p47 Phox Homology Domain Regulates Plasma Membrane but Not Phagosome Neutrophil NADPH Oxidase Activation. J. Biol. Chem. 285, 35169–35179. doi:10.1074/jbc.M110.164475
Li, X. J., Tian, W., Stull, N. D., Grinstein, S., Atkinson, S., and Dinauer, M. C. (2009). A Fluorescently Tagged C-Terminal Fragment of p47phoxDetects NADPH Oxidase Dynamics during Phagocytosis. MBoC 20, 1520–1532. doi:10.1091/mbc.e08-06-0620
Lominadze, G., Powell, D. W., Luerman, G. C., Link, A. J., Ward, R. A., and McLeish, K. R. (2005). Proteomic Analysis of Human Neutrophil Granules. Mol. Cell. Proteomics 4, 1503–1521. doi:10.1074/mcp.M500143-MCP200
Maianski, N. A., Geissler, J., Srinivasula, S. M., Alnemri, E. S., Roos, D., and Kuijpers, T. W. (2004). Functional Characterization of Mitochondria in Neutrophils: a Role Restricted to Apoptosis. Cell Death Differ. 11, 143–153. doi:10.1038/sj.cdd.4401320
Manevski, M., Muthumalage, T., Devadoss, D., Sundar, I. K., Wang, Q., Singh, K. P., et al. (2020). Cellular Stress Responses and Dysfunctional Mitochondrial-Cellular Senescence, and Therapeutics in Chronic Respiratory Diseases. Redox Biol. 33, 101443. doi:10.1016/j.redox.2020.101443
Mantovani, A., Cassatella, M. A., Costantini, C., and Jaillon, S. (2011). Neutrophils in the Activation and Regulation of Innate and Adaptive Immunity. Nat. Rev. Immunol. 11, 519–531. doi:10.1038/nri3024
Matute, J. D., Arias, A. A., Wright, N. A. M., Wrobel, I., Waterhouse, C. C. M., Li, X. J., et al. (2009). A New Genetic Subgroup of Chronic Granulomatous Disease with Autosomal Recessive Mutations in P40phox and Selective Defects in Neutrophil NADPH Oxidase Activity. Blood 114, 3309–3315. doi:10.1182/blood-2009-07-231498
McMillan, S. J., Sharma, R. S., Richards, H. E., Hegde, V., and Crocker, P. R. (2014). Siglec-E Promotes β2-Integrin-dependent NADPH Oxidase Activation to Suppress Neutrophil Recruitment to the Lung. J. Biol. Chem. 289, 20370–20376. doi:10.1074/jbc.M114.574624
Miller, M., Thompson, P., and Gabelli, S. (2019). Structural Determinants of Isoform Selectivity in PI3K Inhibitors. Biomolecules 9, 82. doi:10.3390/biom9030082
Mócsai, A., Walzog, B., and Lowell, C. A. (2015). Intracellular Signalling during Neutrophil Recruitment. Cardiovasc. Res. 107, 373–385. doi:10.1093/cvr/cvv159
Montaño-Rendón, F., Grinstein, S., and Walpole, G. F. W. (2021). Monitoring Phosphoinositide Fluxes and Effectors during Leukocyte Chemotaxis and Phagocytosis. Front. Cell Dev. Biol. 9. doi:10.3389/fcell.2021.626136
Nisimoto, Y., Motalebi, S., Han, C.-H., and Lambeth, J. D. (1999). The P67 Activation Domain Regulates Electron Flow from NADPH to Flavin in Flavocytochrome B(558). J. Biol. Chem. 274, 22999–23005. doi:10.1074/jbc.274.33.22999
Nunes, P., Demaurex, N., and Dinauer, M. C. (2013). Regulation of the NADPH Oxidase and Associated Ion Fluxes during Phagocytosis. Traffic 14, a–n. doi:10.1111/tra.12115
Olsson, L. M., Johansson, Å. C., Gullstrand, B., Jönsen, A., Saevarsdottir, S., Rönnblom, L., et al. (2017). A Single Nucleotide Polymorphism in theNCF1gene Leading to Reduced Oxidative Burst Is Associated with Systemic Lupus Erythematosus. Ann. Rheum. Dis. 76, 1607–1613. doi:10.1136/annrheumdis-2017-211287
Petty, H. R. (2001). Neutrophil Oscillations: Temporal and Spatiotemporal Aspects of Cell Behavior. Ir 23, 85–94. doi:10.1385/IR:23:1:85
Raad, H., Paciet, M.-H., Boussetta, T., Kroviarski, Y., Morel, F., Quinn, M. T., et al. (2009). Regulation of the Phagocyte NADPH Oxidase Activity: Phosphorylation of gp91phox/NOX2 by Protein Kinase C Enhances its Diaphorase Activity and Binding to Rac2, P67phox, and P47phox. FASEB J. 23, 1011–1022. doi:10.1096/fj.08-114553
Regier, D. S., Waite, K. A., Wallin, R., and McPhail, L. C. (1999). A Phosphatidic Acid-Activated Protein Kinase and Conventional Protein Kinase C Isoforms Phosphorylate P22(phox), an NADPH Oxidase Component. J. Biol. Chem. 274, 36601–36608. doi:10.1074/jbc.274.51.36601
Sakai, J., Li, J., Subramanian, K. K., Mondal, S., Bajrami, B., Hattori, H., et al. (2012). Reactive Oxygen Species-Induced Actin Glutathionylation Controls Actin Dynamics in Neutrophils. Immunity 37, 1037–1049. doi:10.1016/j.immuni.2012.08.017
Silvestre-Roig, C., Fridlender, Z. G., Glogauer, M., and Scapini, P. (2019). Neutrophil Diversity in Health and Disease. Trends Immunol. 40, 565–583. doi:10.1016/j.it.2019.04.012
Sollberger, G., Tilley, D. O., and Zychlinsky, A. (2018). Neutrophil Extracellular Traps: The Biology of Chromatin Externalization. Dev. Cell 44, 542–553. doi:10.1016/j.devcel.2018.01.019
Song, Z., Huang, G., Chiquetto Paracatu, L., Grimes, D., Gu, J., Luke, C. J., et al. (2020a). NADPH Oxidase Controls Pulmonary Neutrophil Infiltration in the Response to Fungal Cell Walls by Limiting LTB4. Blood 135, 891–903. doi:10.1182/blood.2019003525
Song, Z., Hudik, E., Le Bars, R., Roux, B., Dang, P. M.-C., El Benna, J., et al. (2020b). Class I Phosphoinositide 3-kinases Control Sustained NADPH Oxidase Activation in Adherent Neutrophils. Biochem. Pharmacol. 178, 114088. doi:10.1016/j.bcp.2020.114088
Song, Z. M., Bouchab, L., Hudik, E., Le Bars, R., Nüsse, O., and Dupré-Crochet, S. (2017). Phosphoinositol 3-phosphate Acts as a Timer for Reactive Oxygen Species Production in the Phagosome. J. Leukoc. Biol. 101, 1155–1168. doi:10.1189/jlb.1A0716-305R
Stahelin, R. V., Burian, A., Bruzik, K. S., Murray, D., and Cho, W. (2003). Membrane Binding Mechanisms of the PX Domains of NADPH Oxidase P40 and P47. J. Biol. Chem. 278, 14469–14479. doi:10.1074/jbc.M212579200
Sun, Q., Zhang, J., Fan, W., Wong, K. N., Ding, X., Chen, S., et al. (2011). The RUN Domain of Rubicon Is Important for hVps34 Binding, Lipid Kinase Inhibition, and Autophagy Suppression. J. Biol. Chem. 286, 185–191. doi:10.1074/jbc.M110.126425
Tamura, M., Shiozaki, I., Ono, S., Miyano, K., Kunihiro, S., and Sasaki, T. (2007). p40 Phox as an Alternative Organizer to P47 Phox in Nox2 Activation: A New Mechanism Involving an Interaction with P22 Phox. FEBS Lett. 581, 4533–4538. doi:10.1016/j.febslet.2007.08.040
Tlili, A., Dupré-Crochet, S., Erard, M., and Nüße, O. (2011). Kinetic Analysis of Phagosomal Production of Reactive Oxygen Species. Free Radic. Biol. Med. 50, 438–447. doi:10.1016/j.freeradbiomed.2010.11.024
Tlili, A., Erard, M., Faure, M.-C., Baudin, X., Piolot, T., Dupré-Crochet, S., et al. (2012). Stable Accumulation of P67(phox) at the Phagosomal Membrane and ROS Production within the Phagosome. J. Leukoc. Biol. 91, 83–95. doi:10.1189/jlb.1210701
Trevelin, S. C., Shah, A. M., and Lombardi, G. (2020). Beyond Bacterial Killing: NADPH Oxidase 2 Is an Immunomodulator. Immunol. Lett. 221, 39–48. doi:10.1016/j.imlet.2020.02.009
Tsai, K.-J., Hung, I.-J., Chow, C. K., Stern, A., Chao, S. S., and Chiu, D. T.-Y. (1998). Impaired Production of Nitric Oxide, Superoxide, and Hydrogen Peroxide in Glucose 6-Phosphate-Dehydrogenase-Deficient Granulocytes. FEBS Lett. 436, 411–414. doi:10.1016/S0014-5793(98)01174-0
Ueyama, T., Nakakita, J., Nakamura, T., Kobayashi, T., Kobayashi, T., Son, J., et al. (2011). Cooperation of P40 Phox with P47 Phox for Nox2-Based NADPH Oxidase Activation during Fcγ Receptor (FcγR)-Mediated Phagocytosis. J. Biol. Chem. 286, 40693–40705. doi:10.1074/jbc.M111.237289
Valenta, H., Erard, M., Dupré-Crochet, S., and Nüβe, O. (2020). The NADPH Oxidase and the Phagosome. Adv. Exp. Med. Biol. 1246, 153–177. doi:10.1007/978-3-030-40406-2_9
Veras, F., Pontelli, M., Silva, C., Toller-Kawahisa, J., Lima, M. d., Nascimento, D., et al. (2020). SARS-CoV-2 Triggered Neutrophil Extracellular Traps (NETs) Mediate COVID-19 Pathology. J. Exp. Med. 217. doi:10.1101/2020.06.08.20125823
Vermot, A., Petit-Härtlein, I., Smith, S. M. E., and Fieschi, F. (2021). NADPH Oxidases (NOX): An Overview from Discovery, Molecular Mechanisms to Physiology and Pathology. Antioxidants 10, 890. doi:10.3390/antiox10060890
Vieira, O. V., Botelho, R. J., Rameh, L., Brachmann, S. M., Matsuo, T., Davidson, H. W., et al. (2001). Distinct Roles of Class I and Class III Phosphatidylinositol 3-kinases in Phagosome Formation and Maturation. J. Cell Biol. 155, 19–26. doi:10.1083/jcb.200107069
Wang, F. (2009). The Signaling Mechanisms Underlying Cell Polarity and Chemotaxis. Cold Spring Harb. Perspect. Biol. 1, a002980. doi:10.1101/cshperspect.a002980
Winterbourn, C. C., Kettle, A. J., and Hampton, M. B. (2016). Reactive Oxygen Species and Neutrophil Function. Annu. Rev. Biochem. 85, 765–792. doi:10.1146/annurev-biochem-060815-014442
Yang, P., Li, Y., Xie, Y., and Liu, Y. (2019). Different Faces for Different Places: Heterogeneity of Neutrophil Phenotype and Function. J. Immunol. Res. 2019, 1–18. doi:10.1155/2019/8016254
Yoo, D.-g., Paracatu, L. C., Xu, E., Lin, X., and Dinauer, M. C. (2021). NADPH Oxidase Limits Collaborative Pattern-Recognition Receptor Signaling to Regulate Neutrophil Cytokine Production in Response to Fungal Pathogen-Associated Molecular Patterns. J. I. 207, 923–937. doi:10.4049/jimmunol.2001298
Zeng, M. Y., Miralda, I., Armstrong, C. L., Uriarte, S. M., and Bagaitkar, J. (2019). The Roles of NADPH Oxidase in Modulating Neutrophil Effector Responses. Mol. Oral Microbiol. 34, 27–38. doi:10.1111/omi.12252
Keywords: NADPH oxidase (NOX2), phagocytose, phosphoinositides, metabolism, neutrophil
Citation: Paclet M-H, Laurans S and Dupré-Crochet S (2022) Regulation of Neutrophil NADPH Oxidase, NOX2: A Crucial Effector in Neutrophil Phenotype and Function. Front. Cell Dev. Biol. 10:945749. doi: 10.3389/fcell.2022.945749
Received: 16 May 2022; Accepted: 23 June 2022;
Published: 14 July 2022.
Edited by:
Zhichao Fan, UCONN Health, United StatesReviewed by:
Qixin Wang, University of Rochester, United StatesGlenn F. W. Walpole, University of Toronto, Canada
Copyright © 2022 Paclet, Laurans and Dupré-Crochet. This is an open-access article distributed under the terms of the Creative Commons Attribution License (CC BY). The use, distribution or reproduction in other forums is permitted, provided the original author(s) and the copyright owner(s) are credited and that the original publication in this journal is cited, in accordance with accepted academic practice. No use, distribution or reproduction is permitted which does not comply with these terms.
*Correspondence: Sophie Dupré-Crochet, c29waGllLmR1cHJlQHVuaXZlcnNpdGUtcGFyaXMtc2FjbGF5LmZy