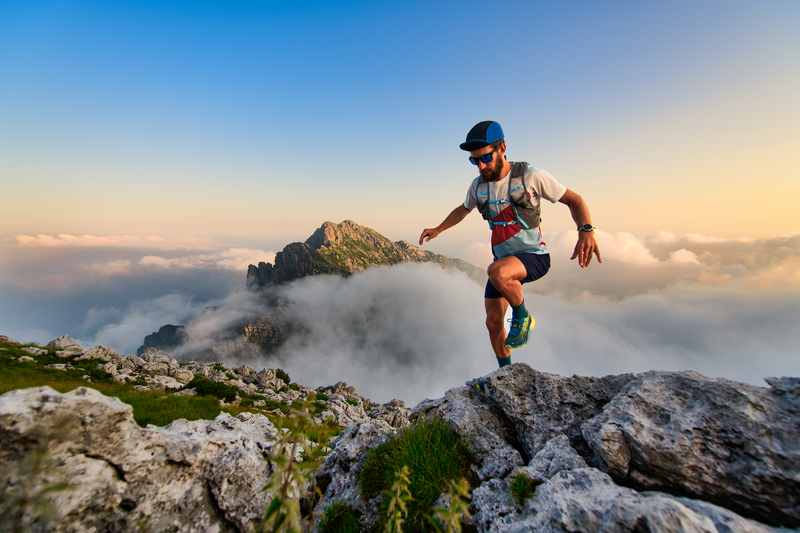
95% of researchers rate our articles as excellent or good
Learn more about the work of our research integrity team to safeguard the quality of each article we publish.
Find out more
REVIEW article
Front. Cell Dev. Biol. , 22 August 2022
Sec. Morphogenesis and Patterning
Volume 10 - 2022 | https://doi.org/10.3389/fcell.2022.943533
This article is part of the Research Topic The Role of Pericytes in Physiology and Pathophysiology View all 6 articles
Here we address the functional importance and role of pericytes in capillary tube network assembly, an essential process that is required for vascularized tissue development, maintenance, and health. Healthy capillaries may be directly capable of suppressing human disease. Considerable advances have occurred in our understanding of the molecular and signaling requirements controlling EC lumen and tube formation in 3D extracellular matrices. A combination of SCF, IL-3, SDF-1α, FGF-2 and insulin (“Factors”) in conjunction with integrin- and MT1-MMP-induced signaling are required for EC sprouting behavior and tube formation under serum-free defined conditions. Pericyte recruitment to the abluminal EC tube surface results in elongated and narrow tube diameters and deposition of the vascular basement membrane. In contrast, EC tubes in the absence of pericytes continue to widen and shorten over time and fail to deposit basement membranes. Pericyte invasion, recruitment and proliferation in 3D matrices requires the presence of ECs. A detailed analysis identified that EC-derived PDGF-BB, PDGF-DD, ET-1, HB-EGF, and TGFβ1 are necessary for pericyte recruitment, proliferation, and basement membrane deposition. Blockade of these individual factors causes significant pericyte inhibition, but combined blockade profoundly interferes with these events, resulting in markedly widened EC tubes without basement membranes, like when pericytes are absent.
A fundamental requirement for the development and maintenance of the vascular system is the interaction between endothelial cells (ECs) and mural cells, which can be pericytes or vascular smooth muscle cells (VSMCs) (Adams and Alitalo, 2007; Armulik et al., 2011; Stratman and Davis, 2012; Bowers et al., 2016; Payne et al., 2020). The vasculature, which is exposed to blood, has an EC lining and on its abluminal side, pericytes or vascular smooth muscle cells are present. In addition, the vascular basement membrane, which has unique components and features compared to epithelial, skeletal muscle or cardiac muscle basement membranes, is found underneath the EC surface and is typically in between the ECs and mural cells (Yurchenco et al., 2004; Davis and Senger, 2005; Stratman et al., 2009a; Senger and Davis, 2011; Yurchenco, 2011; Stratman and Davis, 2012; Davis et al., 2015; Pozzi et al., 2017). Basement membranes can be continuous or discontinuous depending on the tissue location of the vasculature (Eble and Niland, 2009; Augustin and Koh, 2017). Thus, there is an intrinsic polarity to the organization of the vascular wall with the EC layer, an underlying basement membrane, pericytes underlying the basement membrane in capillaries, and then a switch to vascular smooth muscle cells (organized in concentric layers) as arteries and veins increase in size. From a cellular and molecular perspective, how do these vascular assemblies occur and what growth factors, peptides, extracellular matrices (ECM), and signaling events are required for the different steps controlling these processes? Considerable progress has been made to elucidate these key steps, however, many unresolved questions remain. In this review, we will direct our focus to the functional role of pericytes in their ability to control the establishment and maintenance of capillary networks and, also the growth factor and molecular requirements necessary for the assembly of branched arrays of EC-lined tubes, a necessary cellular structural network that interacts with mural cells to generate the different types of blood vessels.
The formation of capillaries requires two cell types: ECs and pericytes. Their interactions are necessary for the capillary assembly process. Capillaries play major roles in tissue development, maturation and maintenance, and deliver signals beyond oxygen and nutrients to tissues to control organismal health. Considerable progress has been made in our understanding of capillary biology. This has occurred in part due to the development of sophisticated in vitro models of human capillary assembly in three-dimensional (3D) matrices (Nakatsu et al., 2003; Koh et al., 2008b; Nakatsu and Hughes, 2008; Stratman et al., 2009a; Stratman et al., 2011). Two key 3D interstitial matrices (i.e. collagen type I and fibrin/fibronectin) are the best matrices (Montesano et al., 1983; Montesano et al., 1987; Davis and Camarillo, 1996; Bayless et al., 2000; Bayless and Davis, 2003; Aplin et al., 2008; Koh et al., 2008b; Nakatsu and Hughes, 2008), to strongly support and drive vascular morphogenesis and, also EC-pericyte tube co-assembly leading to the formation of capillary tube networks. An important fibrin model developed by the laboratory of Christopher Hughes has also been utilized (Nakatsu et al., 2003; Nakatsu and Hughes, 2008). Using a modified design of this system, flow forces can be applied to the EC tube networks in a 3D matrix environment and compared to lack of flow (Wang et al., 2016; Wang et al., 2017). Another significant advance has been the establishment of human EC tube formation, EC sprouting, and EC-pericyte tube co-assembly bioassay systems in both 3D collagen and fibrin matrices under serum-free defined conditions (Stratman et al., 2009a; Stratman et al., 2011; Smith et al., 2013; Bowers et al., 2015; Bowers et al., 2016; Bowers et al., 2020) (Figure 1). These latter models, developed by our laboratory, are allowing us to define the growth factor requirements and signaling events that are necessary for EC tubulogenesis, EC sprouting behavior, pericyte recruitment to EC tubes and pericyte-induced EC tube maturation, which includes vascular basement membrane matrix deposition (Bowers et al., 2016) (Figure 1). A critical point is that the elucidation of the underlying biology of capillary network assembly is essential to understand the pathogenesis of key human disease states, where capillary disassembly or dysfunction is a central issue. Such diseases include diabetes, malignant cancers, vascular malformations, ischemic injuries such as myocardial infarction, stroke, and the progression to chronic kidney disease, hypertension, heart failure, sepsis, and neurodegeneration, including Alzheimer’s disease (Morikawa et al., 2002; Muris et al., 2013; Karaca et al., 2014; Wetzel-Strong et al., 2017; Afsar et al., 2018; Erdener and Dalkara, 2019; Zeng and Chen, 2019; Kida, 2020; Horton and Barrett, 2021; Picoli et al., 2021). In addition, recent studies have strongly implicated capillary loss as an important factor contributing to aging which may accelerate the process (Chen et al., 2021a; Chen et al., 2021b; Grunewald et al., 2021).
FIGURE 1. Key steps controlling capillary tube network assembly in 3D extracellular matrices. In vitro model systems have been developed to mimic the normal process of human capillary network assembly in a 3D matrix environment. Using this defined system, serum-free conditions, and the addition of a combination of five growth factors (“Factors”) consisting of SCF, IL-3, SDF-1α, FGF-2 and insulin allows for human ECs and pericytes to co-assemble into capillary networks over time.
The key steps in capillary tube network assembly occur as follows. During developmental vasculogenesis, where ECs assemble de novo from individual and small clusters of cells, EC interactions with extracellular matrix via integrins leads to cell elongation, intracellular vacuolation and fusion, lumen formation, and multicellular tube assembly over time (Davis et al., 2011) (Figures 1, 2). EC lumen formation is best visualized using histologic cross-sections or by confocal microscopic analysis (Davis and Camarillo, 1996; Koh et al., 2008b; Davis et al., 2011; Stratman et al., 2011; Smith et al., 2013). An important point is that ECs on their own are fully capable of forming lumens and multicellular tube networks in a 3D matrix environment. Suggestions that other cell types such as mural cells are required in conjunction with ECs for this process are not supported by extensive in vitro data. ECs sprout and form lumens and tubes in response to specific growth factor combinations (see later on), and then attract mural cells such as pericytes to create capillary networks. In our model systems under defined serum-free conditions, pericytes on their own show minimal migratory or proliferative behavior in 3D matrices (Stratman et al., 2010; Kemp et al., 2020). However, in the presence of ECs, the pericytes become activated, elongate, migrate, and invade in 3D matrices to reach the EC tube abluminal surface (Stratman et al., 2010; Kemp et al., 2020). They then migrate along this surface in a polarized fashion to form the capillary basement membrane in conjunction with ECs (which deposits abluminally between the two cell types) (Stratman et al., 2009a; Stratman and Davis, 2012; Bowers et al., 2020; Kemp et al., 2020). This pericyte response to the presence of ECs is due to EC production of key pericyte factors that are necessary for pericyte recruitment, proliferation, and pericyte-induced basement membrane deposition (see later on) (Stratman et al., 2010; Kemp et al., 2020).
FIGURE 2. Schematic illustrations showing the fundamental and interconnected steps controlling the assembly of human capillary tube networks. Four key steps are depicted showing the ability of ECs to form lumens, tube networks, and vascular guidance tunnels following by pericyte activation, invasion, and recruitment to these EC-lined tubes in a polarized, abluminal position. This latter step requires the indicated EC-derived factors which consist of PDGF-BB, PDGF-DD, ET-1, TGFβ1, and HB-EGF. EC-pericyte dynamic and polarized interactions within tunnel spaces leads to capillary basement membrane deposition between the two cell types. This figure was prepared on the website, Biorender.com.
In order to form functional capillary networks, EC lumen and tube formation is necessary to allow for connection to the systemic circulation and perfusion of these blood vessels. Further maturation and stabilization of these blood vascular tubes occurs due to this perfusion (Iruela-Arispe and Davis, 2009). For example, EC Notch activation is stimulated downstream of flow forces that induce EC differentiation into the arterial lineage (Lawson et al., 2001), a process which is also accompanied by reduced EC proliferation (Fang et al., 2017). Interestingly, past DNA microarray studies examining differential gene expression during EC tube formation revealed marked downregulation of cell cycle genes associated with proliferation (Bell et al., 2001). Furthermore, recent studies have confirmed this finding by showing that EC tubulogenesis stimulated in vitro using defined growth factors leads to cessation of EC proliferation (Bowers et al., 2020). Interestingly, although EC proliferation is suppressed during tubulogenesis, addition of pericytes leads to pericyte proliferation, a response that depends on ECs and their secretion of key growth factors and peptides, including PDGF-BB, PDGF-DD, HB-EGF, and endothelin-1 (ET-1) during capillary assembly (Stratman et al., 2010; Bowers et al., 2020; Kemp et al., 2020).
Considerable effort has occurred, and great progress has been made toward elucidating the molecular basis for EC lumen and tube formation. EC tube formation is a 3D matrix-specific process that depends on integrin-extracellular matrix (ECM) interactions as well as signaling coordination with specific growth factor-dependent pathways and concurrent MT1-MMP-dependent ECM proteolysis (Stratman et al., 2009b; Sacharidou et al., 2010; Davis et al., 2011; Davis et al., 2015; Bowers et al., 2016). An important finding was the demonstration that EC tubulogenesis requires the creation of vascular guidance tunnels, which are matrix-free spaces that are generated by MT1-MMP-dependent proteolysis of 3D matrices (Stratman et al., 2009b; Sacharidou et al., 2010). Blockade of MT1-MMP, using various chemical or protein MMP inhibitors, completely interferes with vascular guidance tunnel formation. In addition, blockade of EC lumen formation using inhibition of key EC lumen formation regulators, such as protein kinase C epsilon or Src kinases, also completely interferes with tunnel formation (Stratman et al., 2009b). Thus, vascular guidance tunnels are generated during EC tubulogenesis, and interference with their formation completely inhibits the lumen and tube formation process (Stratman et al., 2009b). Once vascular guidance tunnels have been formed, ECs and EC tube networks can migrate and remodel in an MT1-MMP-independent manner in 3D matrices (Stratman et al., 2009b). Another key point is that vascular guidance tunnels are required for the next steps in blood vessel maturation that involve the recruitment of pericytes to the EC tube abluminal surface within the tunnel spaces (Stratman et al., 2009a). The vascular guidance tunnel spaces allow for EC tube remodeling and pericyte motility along the abluminal surface, and these cells together assemble the capillary basement membrane matrix in a polarized manner between the two cell types (Stratman et al., 2009a; Stratman and Davis, 2012) (Figures 1, 2).
Integrin-ECM interactions in 3D matrices are required for the EC tubulogenic process and key integrins that have been shown to stimulate this process are α2β1 and α5β1, which are collagen and fibronectin receptors, respectively (Davis and Camarillo, 1996; Bayless et al., 2000; Bayless and Davis, 2003; Smith et al., 2013). Fibronectin- and collagen type I-rich interstitial matrices appear to be the major ECM stimuli that drives vascular morphogenesis during vascular development and postnatal life (Senger, 1996; Davis and Senger, 2005; Astrof et al., 2007; Hynes, 2007; Astrof and Hynes, 2009; Senger and Davis, 2011). Both α5β1 and α2β1 have been strongly implicated in vivo as well as in vitro as the major integrin stimulators of EC tubulogenesis (Davis and Camarillo, 1996; Bayless et al., 2000; Francis et al., 2002; Senger et al., 2002; Zweers et al., 2007; San Antonio et al., 2009). The αvβ3 integrin, an RGD-binding integrin, has been implicated during tissue injury responses including that occurring during tumor angiogenesis (Brooks et al., 1994). This is due to the fact that it can interact with plasma-derived integrin ligands such as vitronectin and fibronectin (Bayless et al., 2000), injury-induced molecules such as osteopontin (Bayless et al., 1997), or by interacting with denatured collagens (generated during tissue damage) through the exposure of matricryptic RGD sites (Davis, 1992; Davis et al., 2000). Integrins are also important during EC tube maturation responses where ECs interact with basement membrane components (Stratman et al., 2009a). Key EC integrins with affinity for basement membrane matrix proteins include α1β1, α2β1, α3β1, α5β1, α6β1, and αvβ3 (Senger and Davis, 2011; Yurchenco, 2011; Hohenester and Yurchenco, 2013; Davis and Kemp, 2022). Pericytes also express basement membrane binding integrins including α1β1, α2β1, α3β1, α4β1, α5β1, α6β1, and αvβ3 (Stratman et al., 2009a). In contrast to the role of interstitial matrices in stimulating vascular morphogenesis, it appears that basement membrane matrices, have a distinct role which is to affect vascular maturation and stabilization (Stratman et al., 2009a). For example, combined blockade of α3β1 and α6β1, two laminin receptors, inhibited vessel maturation with widened tubes, but did not inhibit the tube formation process itself (Stratman et al., 2009a). More work is needed to investigate the specific functions of these different EC and pericyte integrins during EC tube maturation events, through their interactions with basement membrane matrix components.
Integrin-ECM interactions in conjunction with growth factor receptor activation leads to activation of signal transduction that is necessary for the ability of ECs to form lumens, sprout, and form branched networks of tubes (Somanath et al., 2009; Davis et al., 2011; Bowers et al., 2016). ECs do not proliferate during this morphogenic response and yet signal through the Ras/Map kinase pathway (typically, a mitogenic signaling pathway) to selectively stimulate EC lumen and tube formation and not proliferation. Using defined serum-free conditions, our laboratory discovered a five growth factor combination (i.e. “Factors”) that is required for human ECs to undergo tube morphogenesis and sprouting behavior in either 3D collagen or fibrin matrices (Stratman et al., 2011; Smith et al., 2013; Bowers et al., 2015; Bowers et al., 2016). These growth factors are stem cell factor (SCF), interleukin-3 (IL-3), stromal-derived factor (SDF)-1α, fibroblast growth factor (FGF)-2, and insulin (Stratman et al., 2011; Bowers et al., 2016) (Figures 1–3). We have yet to identify any other combination of growth factors or peptides that stimulate these processes (Bowers et al., 2016). Addition of VEGF alone, or VEGF in combination with FGF-2 fails to stimulate EC tube formation under these defined conditions (Stratman et al., 2011). However, VEGF can act as an upstream priming stimulus, which enhances EC responsiveness to the addition of the downstream “Factors” and, thus, stimulates EC tubulogenesis to a greater extent than the influence of the “Factors” alone (Stratman et al., 2011; Bowers et al., 2020) (see later on). In part, this is due to the ability of VEGF to induce EC expression of c-Kit, IL-3Rα, and CXCR4, which are receptors for three of the “Factors,” SCF, IL-3, and SDF-1α, respectively (Bowers et al., 2020).
FIGURE 3. Defining the growth factors and peptides that are required for both ECs and pericytes to co-assemble into human capillary tube networks. (A) Using a defined serum-free system in 3D collagen or fibrin matrices, the growth factor and peptide requirements for human EC-pericyte tube co-assembly were determined. A five growth factor combination consisting of SCF, IL-3, SDF-1α, FGF-2 and insulin (“Factors”) has been shown to stimulate all aspects of vascular morphogenesis including EC lumen and tube assembly as well as sprouting behavior. Additional work demonstrated a requirement for ECs in stimulating pericytes to recruit to tubes, proliferate, and induce basement membrane deposition. Five EC-derived factors including PDGF-BB, PDGF-DD, ET-1, TGFβ1, and HB-EGF were found to work together to promote these events and their functional roles during this process are indicated.
Coordinated integrin- and “Factor”- mediated signaling in ECs in conjunction with cell surface MT1-MMP-induced ECM proteolysis leads to EC lumen and tube formation as well as sprouting behavior (Davis et al., 2011; Sacharidou et al., 2012; Davis et al., 2015; Bowers et al., 2016). In the former case, multiple small GTPases of the Rho GTPase family have been shown to be critical and activated during EC lumen formation: these include Cdc42, Rac1, Rac2, k-Ras, and Rap1b (Bayless and Davis, 2002; Koh et al., 2008a; Hoang et al., 2011b; a;Barry et al., 2015; Norden et al., 2016). Key effectors (and several binding partners of these effectors) of these GTPases including Pak2, Pak4, IQGAP1, MRCKβ, Rasip1, β-Pix, GIT1, RhoJ, CCM1, and CCM2, are also necessary for the lumen formation process (Koh et al., 2008a; Kleaveland et al., 2009; Koh et al., 2009; Whitehead et al., 2009; Lampugnani et al., 2010; Xu et al., 2011; Yuan et al., 2011; Sacharidou et al., 2012). Other important regulatory or effector kinases that control the EC lumen formation signaling process are protein kinase C epsilon (PKCε), Src family kinases (Src, Fyn, Yes), Raf kinases, Mek kinases and Erk kinase (Alavi et al., 2003; Koh et al., 2009; Sacharidou et al., 2012; Lanahan et al., 2013; Kim et al., 2017). In very recent work, several studies have also implicated Rab GTPases (i.e. Rab27a, Rab8a, Rab11a, Rab3a, Rab3b, Rab35) and Ral (i.e. RalA, RalB) GTPases, which control vesicle trafficking and exocytosis in the EC lumen formation process (Norden et al., 2020; Francis and Kushner, 2021). In total, these molecules and signaling events control the major steps in EC lumen and tube formation. These steps are: 1) to stimulate membrane trafficking events to create and add membrane to the developing apical surface via intracellular vacuole formation, vacuole trafficking and coalescence (Davis and Camarillo, 1996; Bayless and Davis, 2002; Kamei et al., 2006; Kim et al., 2017; Norden et al., 2020); 2) to generate vascular guidance tunnels that are mediated by localized MT1-MMP-dependent matrix proteolysis (Stratman et al., 2009b); and 3) to create cytoskeletal polarization with F-actin distributed basally and post-translationally modified tubulins such as acetylated tubulin distributed in a subapical region which facilitates the trafficking of intracellular vacuoles to the apical membrane surface and then facilitates apical membrane stabilization (Kim et al., 2013; Kim et al., 2017) (Figures 1, 2). Thus, the purpose of the EC tubulogenic signaling cascade is to stimulate the formation of EC-lined tube networks. The establishment of these tubes, then, is coordinated with the recruitment of mural cells, such as pericytes, which are required for capillary maturation and stabilization (Stratman et al., 2009a; Bowers et al., 2020; Kemp et al., 2020).
A central question and issue in the assembly of capillary tube networks is what growth factors, peptides or other molecules control the recruitment of pericytes to the assembling EC tubes. There are different degrees of pericyte recruitment within capillaries depending on the tissue type, which is most accentuated in the brain, where higher percentages of pericytes (relative to ECs) are observed compared to peripheral tissues (Armulik et al., 2011). A series of genetic knockout studies in mice and zebrafish suggest an important role for PDGF-BB, and its receptor, PDGFRβ, which is highly expressed by pericytes (Lindahl et al., 1998; Hellstrom et al., 2001; Nystrom et al., 2006; Stratman et al., 2017) in this process. Other studies using knockout technology have also implicated TGFβ and HB-EGF in EC-mural cell interactions during the development of the vasculature (Oshima et al., 1996; Iivanainen et al., 2003; Carvalho et al., 2004; Lebrin et al., 2005; Ramsauer and D'Amore, 2007; ten Dijke and Arthur, 2007). The use of a defined 3D in vitro model to address these questions is critical because of the complexity of a whole animal. For example, distinguishing biological effects such as mural cell proliferation, survival, or recruitment to EC tubes can be difficult and subject to interpretation in vivo, while these issues can be more readily resolved in vitro.
To address these issues, studies were performed using a serum-free defined EC morphogenesis model using the “Factors,” whereby EC tubulogenesis occurs and pericytes recruit to these tube networks over a 3–5 day period in either 3D collagen or fibrin matrices (Stratman et al., 2009a; Smith et al., 2013; Bowers et al., 2020; Kemp et al., 2020). Pericytes, which were labeled with GFP, to distinguish them from ECs, were seen to become elongated and were also observed to divide during this EC-pericyte tube co-assembly process. In the initial set-up of this assay system, pericytes were seeded at a density of 20% of pericytes relative to ECs, which was optimal. Over time, they were seen to reach the EC tube surface, where 80% or greater of the pericytes were observed to recruit and elongate on the EC tubes by 72 h and beyond. Using these models, immunostaining of gels with antibodies directed to collagen type I or fibrinogen to visualize the ECM, led to the demonstration of vascular guidance tunnel networks. Both the EC-lined tubes and the recruited pericytes were located inside the tunnel spaces (Stratman et al., 2009a; Smith et al., 2013). Real-time movies revealed the dynamic nature of the EC-pericyte tube co-assembly process, and once pericytes had recruited to the EC tubes, they could be observed to be migrating up and down the tube networks, suggesting that they are migrating along the abluminal tube surfaces within the tunnel spaces. Interestingly, occasional pericytes were observed migrating in between tubes and leaving one tube and ending up along another tube surface. Additionally, the presence of pericytes markedly alters the outcome of the EC tube morphogenic process. As EC only tubes progressively get wider and shorter in length over time, pericyte recruitment to tubes leads to maintenance of the initial elongated tube structure with narrow diameters (Stratman et al., 2009a). A key difference between these two conditions, is that EC-pericyte co-cultures markedly deposit vascular basement membrane matrices on the EC abluminal surface, while EC only cultures do not (Stratman et al., 2009a). This conclusion was confirmed using transmission electron microscopy and immunofluorescence microscopy. In the latter case, immunostaining was performed using non-permeabilized (i.e. in the absence of detergent) cultures so that only extracellular deposition of basement membrane components was examined (Stratman et al., 2009a; Smith et al., 2013).
Nuclear GFP-labeled pericytes were utilized to address the role of specific growth factors or peptides affecting pericyte behavior during capillary assembly. These labeled pericytes were seeded in the presence of the EC “Factors” without or with added ECs. Pericytes did not migrate in 3D collagen matrices in the absence of ECs as evaluated by real-time movies imaged under fluorescence where nuclear tracking was assessed over time as a measure of cell motility (Stratman et al., 2010; Kemp et al., 2020). In contrast, when ECs were added with the pericytes, marked motility was observed over time, as they recruit to the EC tube surfaces (Stratman et al., 2010; Kemp et al., 2020). This pericyte motility is actually an invasion response, as an MT-MMP is required for invasion and motility of these cells in 3D matrices (i.e. addition of the MT-MMP inhibitor, GM6001, completely blocks invasion) (Kemp et al., 2020). Thus, EC-derived factors are critical for the pericyte response to EC tubes during capillary formation.
To assess the direct influence of individual EC-derived factors, the ability of 23 different EC-derived factors to affect pericyte invasion of 3D collagen matrices (in the absence of ECs) was examined. Of these, three of them had a strong ability to drive pericyte invasion and they were PDGF-BB, PDGF-DD, and ET-1 (Kemp et al., 2020). When these three factors were combined, even greater stimulation of motility and invasion occurred, and mimicked pericyte invasion of 3D matrices when ECs are present (Kemp et al., 2020). In addition, these factors could stimulate pericyte proliferation along with HB-EGF (which did not directly stimulate pericyte invasion). Interestingly, TGFβ treatment was found to act as an upstream priming stimulus which enhances the ability of PDGFs and ET-1 to stimulate pericyte invasion (Kemp et al., 2020).
An important question was to examine the individual and collective influence of these five EC-derived factors on pericyte interactions with ECs, and the capillary maturation process. Individual blockade of each molecule or its receptor had significant effects on pericyte recruitment in the co-cultures. For example, neutralization of individual factors such as PDGF-BB with blocking antibodies only partially affected pericyte recruitment and proliferation (Kemp et al., 2020). In contrast, neutralization of all five factors in combination resulted in dramatic blockade of pericyte recruitment, such that essentially no recruitment or proliferation of pericytes occurred (Kemp et al., 2020). Also, EC tube formation was not blocked by the addition of these “Pericyte Factor” neutralizing antibodies. Under these conditions, EC tube formation behaved as if pericytes were never added. The EC tubes became markedly widened and shortened in length over time, just like what occurs when ECs are cultured to form tubes by themselves (Kemp et al., 2020). Two different sets of inhibitory cocktails were employed in these studies. One set was pharmacologic agents that were directed at the pericyte receptors, PDGFRβ, endothelin receptor A (EDNRA), EGFR and Alk-5, a TGFβ receptor (i.e. CIGS drug combination). When these agents were added by themselves, a partial inhibitory effect on pericyte recruitment and proliferation were observed, but when combined, there was a marked inhibitory effect (Kemp et al., 2020). The second set of agents utilized neutralizing antibody or receptor trap reagents blocking each of the growth factors. The same conclusions were observed where individual blocking antibodies showed partial effects, but when they were added in combination (along with the EDNRA inhibitor), profound inhibitory effects were observed with no pericyte recruitment or proliferation (Kemp et al., 2020). In addition, both sets of these combined inhibitory agents completely blocked pericyte-induced basement membrane matrix assembly around the EC tube networks (Kemp et al., 2020). This novel study demonstrated that at least five different EC-derived factors (PDGF-BB, PDGF-DD, ET-1, HB-EGF, and TGFβ1) are secreted by ECs during tubulogenesis to drive the pericyte recruitment process, and thus, control capillary tube network assembly and maturation (Kemp et al., 2020) (Figure 3).
There is considerable data in the literature demonstrating a functional role for VEGF and its receptor, VEGFR2, in tissue vascularization responses in vivo in multiple species (Ferrara et al., 1996; Lawson et al., 2002; Lanahan et al., 2013). Of great interest are the findings discussed above showing that VEGF does not directly control EC tube formation or sprouting behavior as a direct acting factor, even when added in combination with FGF-2, using serum-free defined systems in either 3D collagen or fibrin matrices (Stratman et al., 2011; Bowers et al., 2020). In contrast, when VEGF is added to human ECs for 8–16 h as an upstream priming agent, it remarkably stimulates the ability of ECs to respond to the downstream “Factor” combination of SCF, IL-3, SDF-1α, FGF-2 and insulin (Stratman et al., 2011; Bowers et al., 2020). VEGF priming followed by adding VEGF again, FGF-2 alone or their combination does not support EC tubulogenesis. Adding the “Factor” combination as an upstream priming stimulus followed by downstream addition of VEGF alone, FGF-2 alone or VEGF plus FGF-2 fails to support EC tube formation, so reversing the order of addition does not promote this process (Stratman et al., 2011; Bowers et al., 2020). Another important point is that VEGF priming of ECs stimulates the formation of EC tip cells, and accelerates the formation of lumens and tubes, but only following addition of the “Factors” (Bowers et al., 2020). Thus, activities attributed to VEGF in vivo such as driving tip cell sprouting behavior or inducing lumen expansion on its own are not supported by these new insights. Past interesting work demonstrated effects of VEGF on developmental vasculogenesis such as vessel fusion and lumen expansion in response to high levels of VEGF (Drake and Little, 1995, 1999). The new insights on VEGF priming suggest that such biological effects may also involve stimulation of downstream “Factor”-dependent signaling (Bowers et al., 2020). Also, key roles for EC integrin-ECM interactions as well as MT-MMP-dependent proteolysis as requirements for EC tip cell invasion during sprouting behavior are frequently overlooked, but they are crucial regulators of EC tip cell behavior. Finally, there is a marked acceleration of pericyte recruitment, proliferation, and capillary basement membrane deposition following VEGF priming of ECs and addition of the “Factors” during the capillary formative process (Bowers et al., 2020). Thus, VEGF priming accelerates all aspects of EC morphogenesis, while at the same time, stimulates pericyte responsiveness and recruitment to the more rapidly assembling EC tube networks leading to accentuated capillary basement membrane formation (Bowers et al., 2020). All of these events require the downstream presence of the “Factors.”
Why is VEGF able to accelerate vascular morphogenic and maturation events as an upstream primer in response to the downstream “Factors”? Recently, a detailed analysis of the VEGF priming pathway revealed key involvement of VEGFR2, RhoA, PKCα, and PKD2 induced signaling as critical to this priming process (Bowers et al., 2020). In addition, VEGF priming induced the EC expression of the “Factor” receptors, c-Kit, IL-3Rα, and CXCR4, and furthermore, induced the expression of EC-derived factors involved in pericyte recruitment including PDGF-BB, PDGF-DD, and HB-EGF (Bowers et al., 2020). Thus, VEGF priming appears to directly stimulate the ability of ECs to respond to the “Factors” through EC receptor upregulation to drive vascular morphogenesis, but also pericyte recruitment to the EC-lined tubes via greater production of the EC-derived factors that stimulate the pericyte recruitment process (Bowers et al., 2020). Overall, this work suggests that the biological influence of VEGF on vessel assembly, including basement membrane deposition, appears to be primarily mediated by its action as an upstream primer, and that other critical downstream “Factors” are the direct acting ones that stimulate vascular morphogenic and maturation events in conjunction with EC-ECM interactions.
Based on the discussion above, an interesting question concerns the role of VEGF priming and the downstream “Factors” in the development of the arterial system. Of great importance is that VEGF was shown to be required for the development of arteries in the developing zebrafish as well as downstream Notch activation (Notch1 and Notch4 in ECs) (Lawson et al., 2001; Lawson et al., 2002). In developing embryos, the vascular remodeling to create the arterial system is dependent on flow forces (Lucitti et al., 2007; Udan et al., 2013), which also play a role in EC Notch activation, a stimulus that further suppresses EC proliferation (Fang et al., 2017). Data from work in vitro shows that inhibition of Notch activation using γ-secretase inhibitors leads to increase EC sprouting behavior (Sainson et al., 2005; Salvador and Davis, 2018). In our work, this increased EC sprouting response appears to be directly correlated with inhibition of EC lumen formation (Kim et al., 2017; Salvador and Davis, 2018), so Notch activation is important to form and stabilize EC lumens and tubes. In the above description of VEGF priming experiments, it is evident that there is a strong enhancement of pericyte recruitment following this priming stimulus, and thus, VEGF priming, and downstream “Factor” addition leads to rapid assembly of capillary-like structures. Could this assembly process be a developmental precursor to arteriogenesis, perhaps following application of flow forces? Flow forces should enhance Notch activation in ECs and might influence the conversion of pericytes into the vascular smooth muscle lineage. One study, by performing lineage tracing analysis, provided evidence that pericytes are the developmental precursors of vascular smooth muscle cells in the embryonic heart and kidney, a conversion process that required Notch3 (Volz et al., 2015). Interestingly, genetic deletion of Notch3 did not affect the pericyte lineage or their association with developing EC tube networks (Volz et al., 2015). It is important to determine how to create arterial vascular networks in vitro through the association of arterial EC tubes with vascular smooth muscle cells. Are differentiated vascular smooth muscle cells even capable of recruiting to EC tubes or are only pericytes capable of this recruitment? If the latter statement is true, pericytes once recruited to developing arteries would need to differentiate into vascular smooth muscle cells, while pericytes that have recruited to capillaries would remain pericytes. The molecular basis for how such a distinction in differentiation occurs represents an interesting question. An alternative possibility is that vascular smooth muscle cells arise from a different cell type that is capable of recruiting to EC-lined tubes during tissue vascularization. Thus, pericytes, which are functionally designed to recruit to EC tube networks, would be an ideal cell type to be the developmental precursor for VSMCs based on this ability. Future studies need to be performed to further uncover how VSMCs arise and how EC-VSMC interactions regulate the formation and organization of arteries and veins, where VSMCs represent the dominant mural cell type.
It is becoming increasingly clear that capillary dysfunction and regression (i.e. rarefaction) are central pathogenic regulators of major human diseases. Such diseases include hypertension, ischemic tissue injuries including myocardial infarction and stroke, diabetes, heart failure, sepsis, neurodegenerative diseases, malignant cancers, vascular malformations, and aging. Diabetes is a disease whereby abnormalities in EC and pericyte interactions occur and this is a chronic disease state that can become progressively more severe with time. A key pathogenic feature of the disease is pericyte loss (Hammes, 2005), which has been attributed to activation of protein kinase C delta, and the induction of pericyte apoptosis (Geraldes et al., 2009). One of the more severe manifestations of the disease is diabetic retinopathy, with a spectrum of evident retinal injuries including those caused by excessive vascular permeability, and/or hemorrhage (Arboleda-Velasquez et al., 2015). In addition, capillary microaneurysms can manifest, which predispose to retinal hemorrhage (Arboleda-Velasquez et al., 2015). Diabetic capillaries also have abnormal basement membranes as observed by transmission electron microscopy, which are most likely a consequence of abnormal EC-pericyte interactions. Basement membrane degradation may be a pathogenic feature of this disease, which could liberate many growth factors and mediators as discussed earlier, that might allow for continued EC morphogenesis or accelerated regression of vessels. Overall, the capillary vasculature appears to be unstable with elements of formation and regression occurring simultaneously leading to clinically important consequences such as excessive retinal vessel permeability and hemorrhage, both which can contribute to vision loss, for example. The complexity of diabetes and its chronicity in humans, makes it difficult to accurately mimic the human disease using rodent models. This important disease needs to be modeled using the advanced 3D in vitro EC-pericyte model systems of today to add to our molecular understanding of why capillaries are abnormal in diabetes and perhaps develop new therapeutic approaches to treat the disease.
Two other key human disease states with abnormal pericyte associations with EC tubes are malignant cancers and a spectrum of different vascular malformations. In the case of malignant cancers, pericytes appear to become more loosely associated with capillaries, which appears to contribute to the unstable vasculatures within these tumors (Abramsson et al., 2002; Morikawa et al., 2002; Picoli et al., 2021). Like with diabetes, increased vascular permeability and hemorrhage are observed along with focal tumor necrosis, all of which suggest that the capillaries are dysfunctional. Since many malignant tumors produce high levels of VEGF (Dvorak et al., 2011), it has been suggested that VEGF can affect pericyte association with capillaries. Blockade of VEGF or its receptor in these tumor microenvironments does appear to enhance pericyte association with the capillaries and improve perfusion into the tumor, a process that has been termed tumor vessel normalization (Jain, 2005; Goel et al., 2012). These are very interesting observations, and much more work is needed to understand exactly how these abnormalities arise and how they could be potentially therapeutically corrected. As mentioned previously, transient VEGF treatment (i.e. a priming stimulus for 16 h) of ECs leads to upregulation of PDGF-BB, and PDGF-DD, and HB-EGF, which promotes pericyte association with EC tubes, increased pericyte proliferation, and enhanced basement membrane deposition, all steps that drive capillary assembly and eventual stabilization (Bowers et al., 2020). Once capillaries have assembled and stabilized, the activities of PDGF-BB, PDGF-DD, ET-1, HB-EGF and TGFβ1 (which together drive pericyte recruitment, proliferation and basement membrane assembly) may need to be suppressed, so that continued vessel formation does not occur. In contrast, chronic VEGF exposure might enhance the expression and continued release of these EC-derived pericyte activating factors, which induce pericytes to leave capillaries, and invade in between capillaries, creating vessel instability. Such events might also account for pericyte loss in diabetes, where pericytes could be induced to migrate off the capillary abluminal surface. Also, chronic exposure to VEGF (and probable exposure to the EC “Factors”) might further promote EC tube morphogenesis, such as lumen expansion, and basement membrane degradation to further destabilize the capillary walls. More work is needed to elucidate the biology of why capillaries are destabilized in the malignant tumor microenvironment.
Abnormalities in EC-pericyte assembly are also apparent in various vascular malformation syndromes where fewer pericytes/mural cells are observed to be associated with the abnormal vasculature (Cai et al., 2019). In particular, they have been observed in arteriovenous (AVM), venous (VM) and cerebral cavernous malformations (CCM). Lower levels of PDGF-BB have been implicated as a cause of these deficiencies (Redondo et al., 2010). These vascular malformations have in common the presence of activating mutations in key signal transduction molecules including k-Ras with AVM, Tie2 with VM, and PIK3CA with CCM(Limaye et al., 2009; Al-Olabi et al., 2018; Nikolaev et al., 2018; Cai et al., 2019; Ren et al., 2021). CCM also have familial or sporadic mutations in either the CCM1, CCM2 or CCM3 genes (Lampugnani et al., 2017; Wetzel-Strong et al., 2017). Genetic knockouts of each of these CCM genes during vascular development led to EC lumen formation defects (Kleaveland et al., 2009; Whitehead et al., 2009; Lampugnani et al., 2017). VEGF and VEGFR2-dependent signaling have been shown to play a role in previous work on the pathogenesis of a variety of vascular anomalies (Park et al., 2021; Queisser et al., 2021; Snodgrass et al., 2021). An interesting question concerns the potential role of VEGF priming and downstream “Factor”-dependent signaling in the morphogenic manifestations and abnormalities observed in the various vascular anomalies.
In new work from our laboratory, ECs expressing activated k-RasV12 (mimicking ECs within AVM lesions), show markedly accelerated lumen formation mechanisms and form excessively widened tube networks in 3D matrices (Sun et al., 2022). However, these abnormal EC tube networks exhibit strongly reduced pericyte recruitment and basement membrane deposition consistent with findings in vivo in a variety of vascular malformations (Sun et al., 2022). Because multiple EC-derived factors participate in pericyte recruitment to EC-lined tubes during capillary assembly (Kemp et al., 2020), more work needs to be performed to determine the detailed mechanistic reasons why pericytes don’t recruit well to malformed vessels expressing unique sets of genetic mutations. It is probable that the answers here will be complex since EC morphogenesis is abnormal (and unique to the particular malformation syndrome), in combination with deficiencies in the synthesis, secretion (i.e. availability) or stability of the key EC-derived factors necessary for pericyte recruitment, proliferation and pericyte-induced basement membrane deposition (Figure 3). Each case will need to be examined separately in detail because the unique sets of activating mutations or other mutations found in ECs, which underlie these syndromes, will probably regulate these pericyte factors in distinct ways. This information will be necessary to develop novel and effective therapeutic options to treat both the EC morphogenic abnormality as well as the reasons for the EC-mural cell interaction defect.
In this review, we have discussed the fundamental role of pericytes in the assembly, maturation, and stabilization of capillary tube networks. Work over the past several decades using both in vivo and in vitro experimental approaches have led to many key answers regarding the signaling basis for how ECs form tube networks, and how specific sets of growth factors and peptides separately control ECs and pericytes to co-assemble in a polarized manner in 3D extracellular matrix environments. There is remarkable coordination of EC tube network assembly with the recruitment of pericytes along the EC abluminal surface, and then deposition of the capillary basement membrane matrix, a necessary step in the maturation and stabilization of capillaries. Abnormalities and loss of capillaries is a predisposing factor in the development of major human diseases including diabetes, malignant cancers, vascular malformations, and the progression of aging. Despite their critical importance for tissue development and health, not enough emphasis has been placed on comprehensively understanding normal capillary biology as well as capillary dysfunction and regression. For example, capillary regression (i.e. rarefaction) is a fundamental regulator of major human disease states, and only recently, have important advances been made in elucidating key pro-regressive molecules, signal transduction pathways controlling EC tube regression, and pharmacologic approaches to block these processes (Koller et al., 2020). Interestingly, in these studies, the major pro-inflammatory mediators, IL-1β, TNFα, and thrombin, were found to promote EC tube disassembly and regression and EC apoptosis, individually and in combination (Koller et al., 2020). A fascinating finding from this work is that despite the marked loss of EC tubes and individual ECs, these same pro-regressive molecules, did not cause pericyte loss, and instead led to apparent pericyte proliferation and activation (Koller et al., 2020). It is very important to investigate this latter phenomenon in detail to evaluate such pericyte responses resulting from capillary dysfunction and regression which may directly contribute to the underlying pathogenesis of key human diseases.
SK, PL, ZS, MC, KY, MP, and GD all contributed to the writing of the paper. SK and GD prepared the figures.
This work was supported by NIH grants HL136139, HL126518, and HL149748 to GD.
The authors declare that the research was conducted in the absence of any commercial or financial relationships that could be construed as a potential conflict of interest.
All claims expressed in this article are solely those of the authors and do not necessarily represent those of their affiliated organizations, or those of the publisher, the editors and the reviewers. Any product that may be evaluated in this article, or claim that may be made by its manufacturer, is not guaranteed or endorsed by the publisher.
Abramsson, A., Berlin, O., Papayan, H., Paulin, D., Shani, M., and Betsholtz, C. (2002). Analysis of mural cell recruitment to tumor vessels. Circulation 105, 112–117. doi:10.1161/hc0102.101437
Adams, R. H., and Alitalo, K. (2007). Molecular regulation of angiogenesis and lymphangiogenesis. Nat. Rev. Mol. Cell Biol. 8, 464–478. doi:10.1038/nrm2183
Afsar, B., Afsar, R. E., Dagel, T., Kaya, E., Erus, S., Ortiz, A., et al. (2018). Capillary rarefaction from the kidney point of view. Clin. Kidney J. 11, 295–301. doi:10.1093/ckj/sfx133
Al-Olabi, L., Polubothu, S., Dowsett, K., Andrews, K. A., Stadnik, P., Joseph, A. P., et al. (2018). Mosaic RAS/MAPK variants cause sporadic vascular malformations which respond to targeted therapy. J. Clin. Invest. 128, 5185–1508. doi:10.1172/JCI124649
Alavi, A., Hood, J. D., Frausto, R., Stupack, D. G., and Cheresh, D. A. (2003). Role of Raf in vascular protection from distinct apoptotic stimuli. Science 301, 94–96. doi:10.1126/science.1082015
Aplin, A. C., Fogel, E., Zorzi, P., and Nicosia, R. F. (2008). The aortic ring model of angiogenesis. Methods Enzymol. 443, 119–136. doi:10.1016/S0076-6879(08)02007-7
Arboleda-Velasquez, J. F., Valdez, C. N., Marko, C. K., and D'amore, P. A. (2015). From pathobiology to the targeting of pericytes for the treatment of diabetic retinopathy. Curr. Diab. Rep. 15, 573. doi:10.1007/s11892-014-0573-2
Armulik, A., Genove, G., and Betsholtz, C. (2011). Pericytes: Developmental, physiological, and pathological perspectives, problems, and promises. Dev. Cell 21, 193–215. doi:10.1016/j.devcel.2011.07.001
Astrof, S., Crowley, D., and Hynes, R. O. (2007). Multiple cardiovascular defects caused by the absence of alternatively spliced segments of fibronectin. Dev. Biol. 311, 11–24. doi:10.1016/j.ydbio.2007.07.005
Astrof, S., and Hynes, R. O. (2009). Fibronectins in vascular morphogenesis. Angiogenesis 12, 165–175. doi:10.1007/s10456-009-9136-6
Augustin, H. G., and Koh, G. Y. (2017). Organotypic vasculature: From descriptive heterogeneity to functional pathophysiology. Science 357, eaal2379. doi:10.1126/science.aal2379
Barry, D. M., Xu, K., Meadows, S. M., Zheng, Y., Norden, P. R., Davis, G. E., et al. (2015). Cdc42 is required for cytoskeletal support of endothelial cell adhesion during blood vessel formation in mice. Development 142, 3058–3070. doi:10.1242/dev.125260
Bayless, K. J., Davis, G. E., and Meininger, G. A. (1997). Isolation and biological properties of osteopontin from bovine milk. Protein Expr. Purif. 9, 309–314. doi:10.1006/prep.1996.0699
Bayless, K. J., and Davis, G. E. (2003). Sphingosine-1-phosphate markedly induces matrix metalloproteinase and integrin-dependent human endothelial cell invasion and lumen formation in three-dimensional collagen and fibrin matrices. Biochem. Biophys. Res. Commun. 312, 903–913. doi:10.1016/j.bbrc.2003.11.017
Bayless, K. J., and Davis, G. E. (2002). The Cdc42 and Rac1 GTPases are required for capillary lumen formation in three-dimensional extracellular matrices. J. Cell Sci. 115, 1123–1136. doi:10.1242/jcs.115.6.1123
Bayless, K. J., Salazar, R., and Davis, G. E. (2000). RGD-dependent vacuolation and lumen formation observed during endothelial cell morphogenesis in three-dimensional fibrin matrices involves the alpha(v)beta(3) and alpha(5)beta(1) integrins. Am. J. Pathol. 156, 1673–1683. doi:10.1016/s0002-9440(10)65038-9
Bell, S. E., Mavila, A., Salazar, R., Bayless, K. J., Kanagala, S., Maxwell, S. A., et al. (2001). Differential gene expression during capillary morphogenesis in 3D collagen matrices: Regulated expression of genes involved in basement membrane matrix assembly, cell cycle progression, cellular differentiation and G-protein signaling. J. Cell Sci. 114, 2755–2773. doi:10.1242/jcs.114.15.2755
Bowers, S. L. K., Kemp, S. S., Aguera, K. N., Koller, G. M., Forgy, J. C., and Davis, G. E. (2020). Defining an upstream VEGF (vascular endothelial growth factor) priming signature for downstream factor-induced endothelial cell-pericyte tube network coassembly. Arterioscler. Thromb. Vasc. Biol. 40, 2891–2909. doi:10.1161/ATVBAHA.120.314517
Bowers, S. L., Meng, C. X., Davis, M. T., and Davis, G. E. (2015). Investigating human vascular tube morphogenesis and maturation using endothelial cell-pericyte co-cultures and a doxycycline-inducible genetic system in 3D extracellular matrices. Methods Mol. Biol. 1189, 171–189. doi:10.1007/978-1-4939-1164-6_12
Bowers, S. L., Norden, P. R., and Davis, G. E. (2016). Molecular signaling pathways controlling vascular tube morphogenesis and pericyte-induced tube maturation in 3D extracellular matrices. Adv. Pharmacol. 77, 241–280. doi:10.1016/bs.apha.2016.04.005
Brooks, P. C., Clark, R. A., and Cheresh, D. A. (1994). Requirement of vascular integrin alpha v beta 3 for angiogenesis. Science 264, 569–571. doi:10.1126/science.7512751
Cai, Y., Schrenk, S., Goines, J., Davis, G. E., and Boscolo, E. (2019). Constitutive active mutant TIE2 induces enlarged vascular lumen formation with loss of apico-basal polarity and pericyte recruitment. Sci. Rep. 9, 12352. doi:10.1038/s41598-019-48854-2
Carvalho, R. L., Jonker, L., Goumans, M. J., Larsson, J., Bouwman, P., Karlsson, S., et al. (2004). Defective paracrine signalling by TGFbeta in yolk sac vasculature of endoglin mutant mice: A paradigm for hereditary haemorrhagic telangiectasia. Development 131, 6237–6247. doi:10.1242/dev.01529
Chen, J., Lippo, L., Labella, R., Tan, S. L., Marsden, B. D., Dustin, M. L., et al. (2021a). Decreased blood vessel density and endothelial cell subset dynamics during ageing of the endocrine system. EMBO J. 40, e105242. doi:10.15252/embj.2020105242
Chen, J., Sivan, U., Tan, S. L., Lippo, L., De Angelis, J., Labella, R., et al. (2021b). High-resolution 3D imaging uncovers organ-specific vascular control of tissue aging. Sci. Adv. 7, eabd7819. doi:10.1126/sciadv.abd7819
Davis, G. E. (1992). Affinity of integrins for damaged extracellular matrix: Alpha v beta 3 binds to denatured collagen type I through RGD sites. Biochem. Biophys. Res. Commun. 182, 1025–1031. doi:10.1016/0006-291x(92)91834-d
Davis, G. E., Bayless, K. J., Davis, M. J., and Meininger, G. A. (2000). Regulation of tissue injury responses by the exposure of matricryptic sites within extracellular matrix molecules. Am. J. Pathol. 156, 1489–1498. doi:10.1016/S0002-9440(10)65020-1
Davis, G. E., and Camarillo, C. W. (1996). An alpha 2 beta 1 integrin-dependent pinocytic mechanism involving intracellular vacuole formation and coalescence regulates capillary lumen and tube formation in three-dimensional collagen matrix. Exp. Cell Res. 224, 39–51. doi:10.1006/excr.1996.0109
Davis, G. E., and Kemp, S. S. (2022). Extracellular matrix regulation of vascular morphogenesis, maturation, and stabilization. Cold Spring Harb. Perspect. Med. 11, a041156. doi:10.1101/cshperspect.a041156
Davis, G. E., Norden, P. R., and Bowers, S. L. (2015). Molecular control of capillary morphogenesis and maturation by recognition and remodeling of the extracellular matrix: Functional roles of endothelial cells and pericytes in health and disease. Connect. Tissue Res. 56, 392–402. doi:10.3109/03008207.2015.1066781
Davis, G. E., and Senger, D. R. (2005). Endothelial extracellular matrix: Biosynthesis, remodeling, and functions during vascular morphogenesis and neovessel stabilization. Circ. Res. 97, 1093–1107. doi:10.1161/01.RES.0000191547.64391.e3
Davis, G. E., Stratman, A. N., Sacharidou, A., and Koh, W. (2011). Molecular basis for endothelial lumen formation and tubulogenesis during vasculogenesis and angiogenic sprouting. Int. Rev. Cell Mol. Biol. 288, 101–165. doi:10.1016/B978-0-12-386041-5.00003-0
Drake, C. J., and Little, C. D. (1995). Exogenous vascular endothelial growth factor induces malformed and hyperfused vessels during embryonic neovascularization. Proc. Natl. Acad. Sci. U. S. A. 92, 7657–7661. doi:10.1073/pnas.92.17.7657
Drake, C. J., and Little, C. D. (1999). VEGF and vascular fusion: Implications for normal and pathological vessels. J. Histochem. Cytochem. 47, 1351–1356. doi:10.1177/002215549904701101
Dvorak, H. F., Weaver, V. M., Tlsty, T. D., and Bergers, G. (2011). Tumor microenvironment and progression. J. Surg. Oncol. 103, 468–474. doi:10.1002/jso.21709
Eble, J. A., and Niland, S. (2009). The extracellular matrix of blood vessels. Curr. Pharm. Des. 15, 1385–1400. doi:10.2174/138161209787846757
Erdener, S. E., and Dalkara, T. (2019). Small vessels are a big problem in neurodegeneration and neuroprotection. Front. Neurol. 10, 889. doi:10.3389/fneur.2019.00889
Fang, J. S., Coon, B. G., Gillis, N., Chen, Z., Qiu, J., Chittenden, T. W., et al. (2017). Shear-induced Notch-Cx37-p27 axis arrests endothelial cell cycle to enable arterial specification. Nat. Commun. 8, 2149. doi:10.1038/s41467-017-01742-7
Ferrara, N., Carver-Moore, K., Chen, H., Dowd, M., Lu, L., O'shea, K. S., et al. (1996). Heterozygous embryonic lethality induced by targeted inactivation of the VEGF gene. Nature 380, 439–442. doi:10.1038/380439a0
Francis, C. R., and Kushner, E. J. (2021). Capturing membrane trafficking events during 3D angiogenic development in vitro. Microcirculation 20, e12726. doi:10.1111/micc.12726
Francis, S. E., Goh, K. L., Hodivala-Dilke, K., Bader, B. L., Stark, M., Davidson, D., et al. (2002). Central roles of alpha5beta1 integrin and fibronectin in vascular development in mouse embryos and embryoid bodies. Arterioscler. Thromb. Vasc. Biol. 22, 927–933. doi:10.1161/01.atv.0000016045.93313.f2
Geraldes, P., Hiraoka-Yamamoto, J., Matsumoto, M., Clermont, A., Leitges, M., Marette, A., et al. (2009). Activation of PKC-delta and SHP-1 by hyperglycemia causes vascular cell apoptosis and diabetic retinopathy. Nat. Med. 15, 1298–1306. doi:10.1038/nm.2052
Goel, S., Wong, A. H., and Jain, R. K. (2012). Vascular normalization as a therapeutic strategy for malignant and nonmalignant disease. Cold Spring Harb. Perspect. Med. 2, a006486. doi:10.1101/cshperspect.a006486
Grunewald, M., Kumar, S., Sharife, H., Volinsky, E., Gileles-Hillel, A., Licht, T., et al. (2021). Counteracting age-related VEGF signaling insufficiency promotes healthy aging and extends life span. Science 373, eabc8479. doi:10.1126/science.abc8479
Hammes, H. P. (2005). Pericytes and the pathogenesis of diabetic retinopathy. Horm. Metab. Res. 37 (1), 39–43. doi:10.1055/s-2005-861361
Hellstrom, M., Gerhardt, H., Kalen, M., Li, X., Eriksson, U., Wolburg, H., et al. (2001). Lack of pericytes leads to endothelial hyperplasia and abnormal vascular morphogenesis. J. Cell Biol. 153, 543–553. doi:10.1083/jcb.153.3.543
Hoang, M. V., Nagy, J. A., and Senger, D. R. (2011a). Active Rac1 improves pathologic VEGF neovessel architecture and reduces vascular leak: Mechanistic similarities with angiopoietin-1. Blood 117, 1751–1760. doi:10.1182/blood-2010-05-286831
Hoang, M. V., Nagy, J. A., and Senger, D. R. (2011b). Cdc42-mediated inhibition of GSK-3β improves angio-architecture and lumen formation during VEGF-driven pathological angiogenesis. Microvasc. Res. 81, 34–43. doi:10.1016/j.mvr.2010.09.001
Hohenester, E., and Yurchenco, P. D. (2013). Laminins in basement membrane assembly. Cell adh. Migr. 7, 56–63. doi:10.4161/cam.21831
Horton, W. B., and Barrett, E. J. (2021). Microvascular dysfunction in diabetes mellitus and cardiometabolic disease. Endocr. Rev. 42, 29–55. doi:10.1210/endrev/bnaa025
Hynes, R. O. (2007). Cell-matrix adhesion in vascular development. J. Thromb. Haemost. 5 (1), 32–40. doi:10.1111/j.1538-7836.2007.02569.x
Iivanainen, E., Nelimarkka, L., Elenius, V., Heikkinen, S. M., Junttila, T. T., Sihombing, L., et al. (2003). Angiopoietin-regulated recruitment of vascular smooth muscle cells by endothelial-derived heparin binding EGF-like growth factor. FASEB J. 17, 1609–1621. doi:10.1096/fj.02-0939com
Iruela-Arispe, M. L., and Davis, G. E. (2009). Cellular and molecular mechanisms of vascular lumen formation. Dev. Cell 16, 222–231. doi:10.1016/j.devcel.2009.01.013
Jain, R. K. (2005). Normalization of tumor vasculature: An emerging concept in antiangiogenic therapy. Science 307, 58–62. doi:10.1126/science.1104819
Kamei, M., Saunders, W. B., Bayless, K. J., Dye, L., Davis, G. E., and Weinstein, B. M. (2006). Endothelial tubes assemble from intracellular vacuoles in vivo. Nature 442, 453–456. doi:10.1038/nature04923
Karaca, U., Schram, M. T., Houben, A. J., Muris, D. M., and Stehouwer, C. D. (2014). Microvascular dysfunction as a link between obesity, insulin resistance and hypertension. Diabetes Res. Clin. Pract. 103, 382–387. doi:10.1016/j.diabres.2013.12.012
Kemp, S. S., Aguera, K. N., Cha, B., and Davis, G. E. (2020). Defining endothelial cell-derived factors that promote pericyte recruitment and capillary network assembly. Arterioscler. Thromb. Vasc. Biol. 40, 2632–2648. doi:10.1161/ATVBAHA.120.314948
Kida, Y. (2020). Peritubular capillary rarefaction: An underappreciated regulator of CKD progression. Int. J. Mol. Sci. 21, E8255. doi:10.3390/ijms21218255
Kim, D. J., Martinez-Lemus, L. A., and Davis, G. E. (2013). EB1, p150Glued, and Clasp1 control endothelial tubulogenesis through microtubule assembly, acetylation, and apical polarization. Blood 121, 3521–3530. doi:10.1182/blood-2012-11-470179
Kim, D. J., Norden, P. R., Salvador, J., Barry, D. M., Bowers, S. L. K., Cleaver, O., et al. (2017). Src- and Fyn-dependent apical membrane trafficking events control endothelial lumen formation during vascular tube morphogenesis. PLoS One 12, e0184461. doi:10.1371/journal.pone.0184461
Kleaveland, B., Zheng, X., Liu, J. J., Blum, Y., Tung, J. J., Zou, Z., et al. (2009). Regulation of cardiovascular development and integrity by the heart of glass-cerebral cavernous malformation protein pathway. Nat. Med. 15, 169–176. doi:10.1038/nm.1918
Koh, W., Mahan, R. D., and Davis, G. E. (2008a). Cdc42- and Rac1-mediated endothelial lumen formation requires Pak2, Pak4 and Par3, and PKC-dependent signaling. J. Cell Sci. 121, 989–1001. doi:10.1242/jcs.020693
Koh, W., Sachidanandam, K., Stratman, A. N., Sacharidou, A., Mayo, A. M., Murphy, E. A., et al. (2009). Formation of endothelial lumens requires a coordinated PKCepsilon-Src-Pak- and Raf-kinase-dependent signaling cascade downstream of Cdc42 activation. J. Cell Sci. 122, 1812–1822. doi:10.1242/jcs.045799
Koh, W., Stratman, A. N., Sacharidou, A., and Davis, G. E. (2008b). In vitro three dimensional collagen matrix models of endothelial lumen formation during vasculogenesis and angiogenesis. Methods Enzymol. 443, 83–101. doi:10.1016/S0076-6879(08)02005-3
Koller, G. M., Schafer, C., Kemp, S. S., Aguera, K. N., Lin, P. K., Forgy, J. C., et al. (2020). Proinflammatory mediators, IL (Interleukin)-1β, TNF (tumor necrosis factor) α, and thrombin directly induce capillary tube regression. Arterioscler. Thromb. Vasc. Biol. 40, 365–377. doi:10.1161/ATVBAHA.119.313536
Lampugnani, M. G., Malinverno, M., Dejana, E., and Rudini, N. (2017). Endothelial cell disease: Emerging knowledge from cerebral cavernous malformations. Curr. Opin. Hematol. 24, 256–264. doi:10.1097/MOH.0000000000000338
Lampugnani, M. G., Orsenigo, F., Rudini, N., Maddaluno, L., Boulday, G., Chapon, F., et al. (2010). CCM1 regulates vascular-lumen organization by inducing endothelial polarity. J. Cell Sci. 123, 1073–1080. doi:10.1242/jcs.059329
Lanahan, A., Zhang, X., Fantin, A., Zhuang, Z., Rivera-Molina, F., Speichinger, K., et al. (2013). The neuropilin 1 cytoplasmic domain is required for VEGF-A-dependent arteriogenesis. Dev. Cell 25, 156–168. doi:10.1016/j.devcel.2013.03.019
Lawson, N. D., Scheer, N., Pham, V. N., Kim, C. H., Chitnis, A. B., Campos-Ortega, J. A., et al. (2001). Notch signaling is required for arterial-venous differentiation during embryonic vascular development. Development 128, 3675–3683. doi:10.1242/dev.128.19.3675
Lawson, N. D., Vogel, A. M., and Weinstein, B. M. (2002). Sonic hedgehog and vascular endothelial growth factor act upstream of the Notch pathway during arterial endothelial differentiation. Dev. Cell 3, 127–136. doi:10.1016/s1534-5807(02)00198-3
Lebrin, F., Deckers, M., Bertolino, P., and Ten Dijke, P. (2005). TGF-beta receptor function in the endothelium. Cardiovasc. Res. 65, 599–608. doi:10.1016/j.cardiores.2004.10.036
Limaye, N., Wouters, V., Uebelhoer, M., Tuominen, M., Wirkkala, R., Mulliken, J. B., et al. (2009). Somatic mutations in angiopoietin receptor gene TEK cause solitary and multiple sporadic venous malformations. Nat. Genet. 41, 118–124. doi:10.1038/ng.272
Lindahl, P., Hellstrom, M., Kalen, M., Karlsson, L., Pekny, M., Pekna, M., et al. (1998). Paracrine PDGF-B/PDGF-Rbeta signaling controls mesangial cell development in kidney glomeruli. Development 125, 3313–3322. doi:10.1242/dev.125.17.3313
Lucitti, J. L., Jones, E. A., Huang, C., Chen, J., Fraser, S. E., and Dickinson, M. E. (2007). Vascular remodeling of the mouse yolk sac requires hemodynamic force. Development 134, 3317–3326. doi:10.1242/dev.02883
Montesano, R., Orci, L., and Vassalli, P. (1983). In vitro rapid organization of endothelial cells into capillary-like networks is promoted by collagen matrices. J. Cell Biol. 97, 1648–1652. doi:10.1083/jcb.97.5.1648
Montesano, R., Pepper, M. S., Vassalli, J. D., and Orci, L. (1987). Phorbol ester induces cultured endothelial cells to invade a fibrin matrix in the presence of fibrinolytic inhibitors. J. Cell. Physiol. 132, 509–516. doi:10.1002/jcp.1041320313
Morikawa, S., Baluk, P., Kaidoh, T., Haskell, A., Jain, R. K., and Mcdonald, D. M. (2002). Abnormalities in pericytes on blood vessels and endothelial sprouts in tumors. Am. J. Pathol. 160, 985–1000. doi:10.1016/S0002-9440(10)64920-6
Muris, D. M., Houben, A. J., Schram, M. T., and Stehouwer, C. D. (2013). Microvascular dysfunction: An emerging pathway in the pathogenesis of obesity-related insulin resistance. Rev. Endocr. Metab. Disord. 14, 29–38. doi:10.1007/s11154-012-9231-7
Nakatsu, M. N., and Hughes, C. C. (2008). An optimized three-dimensional in vitro model for the analysis of angiogenesis. Methods Enzymol. 443, 65–82. doi:10.1016/S0076-6879(08)02004-1
Nakatsu, M. N., Sainson, R. C., Aoto, J. N., Taylor, K. L., Aitkenhead, M., Perez-Del-Pulgar, S., et al. (2003). Angiogenic sprouting and capillary lumen formation modeled by human umbilical vein endothelial cells (HUVEC) in fibrin gels: The role of fibroblasts and angiopoietin-1. Microvasc. Res. 66, 102–112. doi:10.1016/s0026-2862(03)00045-1
Nikolaev, S. I., Vetiska, S., Bonilla, X., Boudreau, E., Jauhiainen, S., Rezai Jahromi, B., et al. (2018). Somatic activating KRAS mutations in arteriovenous malformations of the brain. N. Engl. J. Med. 378, 1561–1562. doi:10.1056/NEJMc1802190
Norden, P. R., Kim, D. J., Barry, D. M., Cleaver, O. B., and Davis, G. E. (2016). Cdc42 and k-ras control endothelial tubulogenesis through apical membrane and cytoskeletal polarization: Novel stimulatory roles for GTPase effectors, the small GTPases, Rac2 and Rap1b, and inhibitory influence of Arhgap31 and Rasa1. PLoS One 11, e0147758. doi:10.1371/journal.pone.0147758
Norden, P. R., Sun, Z., and Davis, G. E. (2020). Control of endothelial tubulogenesis by Rab and Ral GTPases, and apical targeting of caveolin-1-labeled vacuoles. PLoS One 15, e0235116. doi:10.1371/journal.pone.0235116
Nystrom, H. C., Lindblom, P., Wickman, A., Andersson, I., Norlin, J., Faldt, J., et al. (2006). Platelet-derived growth factor B retention is essential for development of normal structure and function of conduit vessels and capillaries. Cardiovasc. Res. 71, 557–565. doi:10.1016/j.cardiores.2006.05.019
Oshima, M., Oshima, H., and Taketo, M. M. (1996). TGF-beta receptor type II deficiency results in defects of yolk sac hematopoiesis and vasculogenesis. Dev. Biol. 179, 297–302. doi:10.1006/dbio.1996.0259
Park, H., Furtado, J., Poulet, M., Chung, M., Yun, S., Lee, S., et al. (2021). Defective flow-migration coupling causes arteriovenous malformations in hereditary hemorrhagic telangiectasia. Circulation 144, 805–822. doi:10.1161/CIRCULATIONAHA.120.053047
Payne, L. B., Hoque, M., Houk, C., Darden, J., and Chappell, J. C. (2020). Pericytes in vascular development. Curr. Tissue Microenviron. Rep. 1, 143–154. doi:10.1007/s43152-020-00014-9
Picoli, C. C., Goncalves, B. O. P., Santos, G. S. P., Rocha, B. G. S., Costa, A. C., Resende, R. R., et al. (2021). Pericytes cross-talks within the tumor microenvironment. Biochim. Biophys. Acta. Rev. Cancer 1876, 188608. doi:10.1016/j.bbcan.2021.188608
Pozzi, A., Yurchenco, P. D., and Iozzo, R. V. (2017). The nature and biology of basement membranes. Matrix Biol. 57-58, 1–11. doi:10.1016/j.matbio.2016.12.009
Queisser, A., Seront, E., Boon, L. M., and Vikkula, M. (2021). Genetic basis and therapies for vascular anomalies. Circ. Res. 129, 155–173. doi:10.1161/CIRCRESAHA.121.318145
Ramsauer, M., and D'amore, P. A. (2007). Contextual role for angiopoietins and TGFbeta1 in blood vessel stabilization. J. Cell Sci. 120, 1810–1817. doi:10.1242/jcs.003533
Redondo, P., Aguado, L., Marquina, M., Paramo, J. A., Sierra, A., Sanchez-Ibarrola, A., et al. (2010). Angiogenic and prothrombotic markers in extensive slow-flow vascular malformations: Implications for antiangiogenic/antithrombotic strategies. Br. J. Dermatol. 162, 350–356. doi:10.1111/j.1365-2133.2009.09513.x
Ren, A. A., Snellings, D. A., Su, Y. S., Hong, C. C., Castro, M., Tang, A. T., et al. (2021). PIK3CA and CCM mutations fuel cavernomas through a cancer-like mechanism. Nature 594, 271–276. doi:10.1038/s41586-021-03562-8
Sacharidou, A., Koh, W., Stratman, A. N., Mayo, A. M., Fisher, K. E., and Davis, G. E. (2010). Endothelial lumen signaling complexes control 3D matrix-specific tubulogenesis through interdependent Cdc42- and MT1-MMP-mediated events. Blood 115, 5259–5269. doi:10.1182/blood-2009-11-252692
Sacharidou, A., Stratman, A. N., and Davis, G. E. (2012). Molecular mechanisms controlling vascular lumen formation in three-dimensional extracellular matrices. Cells Tissues Organs 195, 122–143. doi:10.1159/000331410
Sainson, R. C., Aoto, J., Nakatsu, M. N., Holderfield, M., Conn, E., Koller, E., et al. (2005). Cell-autonomous notch signaling regulates endothelial cell branching and proliferation during vascular tubulogenesis. Faseb J. 19, 1027–1029. doi:10.1096/fj.04-3172fje
Salvador, J., and Davis, G. E. (2018). Evaluation and characterization of endothelial cell invasion and sprouting behavior. Methods Mol. Biol. 1846, 249–259. doi:10.1007/978-1-4939-8712-2_16
San Antonio, J. D., Zoeller, J. J., Habursky, K., Turner, K., Pimtong, W., Burrows, M., et al. (2009). A key role for the integrin alpha2beta1 in experimental and developmental angiogenesis. Am. J. Pathol. 175, 1338–1347. doi:10.2353/ajpath.2009.090234
Senger, D. R., and Davis, G. E. (2011). Angiogenesis. Cold Spring Harb. Perspect. Biol. 3, a005090. doi:10.1101/cshperspect.a005090
Senger, D. R. (1996). Molecular framework for angiogenesis: A complex web of interactions between extravasated plasma proteins and endothelial cell proteins induced by angiogenic cytokines. Am. J. Pathol. 149, 1–7.
Senger, D. R., Perruzzi, C. A., Streit, M., Koteliansky, V. E., De Fougerolles, A. R., and Detmar, M. (2002). The alpha(1)beta(1) and alpha(2)beta(1) integrins provide critical support for vascular endothelial growth factor signaling, endothelial cell migration, and tumor angiogenesis. Am. J. Pathol. 160, 195–204. doi:10.1016/s0002-9440(10)64363-5
Smith, A. O., Bowers, S. L., Stratman, A. N., and Davis, G. E. (2013). Hematopoietic stem cell cytokines and fibroblast growth factor-2 stimulate human endothelial cell-pericyte tube co-assembly in 3D fibrin matrices under serum-free defined conditions. PLoS One 8, e85147. doi:10.1371/journal.pone.0085147
Snodgrass, R. O., Chico, T. J. A., and Arthur, H. M. (2021). Hereditary haemorrhagic telangiectasia, an inherited vascular disorder in need of improved evidence-based pharmaceutical interventions. Genes (Basel) 12, 174. doi:10.3390/genes12020174
Somanath, P. R., Ciocea, A., and Byzova, T. V. (2009). Integrin and growth factor receptor alliance in angiogenesis. Cell biochem. Biophys. 53, 53–64. doi:10.1007/s12013-008-9040-5
Stratman, A. N., and Davis, G. E. (2012). Endothelial cell-pericyte interactions stimulate basement membrane matrix assembly: Influence on vascular tube remodeling, maturation, and stabilization. Microsc. Microanal. 18, 68–80. doi:10.1017/S1431927611012402
Stratman, A. N., Davis, M. J., and Davis, G. E. (2011). VEGF and FGF prime vascular tube morphogenesis and sprouting directed by hematopoietic stem cell cytokines. Blood 117, 3709–3719. doi:10.1182/blood-2010-11-316752
Stratman, A. N., Malotte, K. M., Mahan, R. D., Davis, M. J., and Davis, G. E. (2009a). Pericyte recruitment during vasculogenic tube assembly stimulates endothelial basement membrane matrix formation. Blood 114, 5091–5101. doi:10.1182/blood-2009-05-222364
Stratman, A. N., Pezoa, S. A., Farrelly, O. M., Castranova, D., Dye, L. E., Butler, M. G., et al. (2017). Interactions between mural cells and endothelial cells stabilize the developing zebrafish dorsal aorta. Development 144, 115–127. doi:10.1242/dev.143131
Stratman, A. N., Saunders, W. B., Sacharidou, A., Koh, W., Fisher, K. E., Zawieja, D. C., et al. (2009b). Endothelial cell lumen and vascular guidance tunnel formation requires MT1-MMP-dependent proteolysis in 3-dimensional collagen matrices. Blood 114, 237–247. doi:10.1182/blood-2008-12-196451
Stratman, A. N., Schwindt, A. E., Malotte, K. M., and Davis, G. E. (2010). Endothelial-derived PDGF-BB and HB-EGF coordinately regulate pericyte recruitment during vasculogenic tube assembly and stabilization. Blood 116, 4720–4730. doi:10.1182/blood-2010-05-286872
Sun, Z., Kemp, S. S., Lin, P. K., Aguera, K. N., and Davis, G. E. (2022). Endothelial k-RasV12 expression induces capillary deficiency attributable to marked tube network expansion coupled to reduced pericytes and basement membranes. Arterioscler. Thromb. Vasc. Biol. 42, 205–222. doi:10.1161/ATVBAHA.121.316798
Ten Dijke, P., and Arthur, H. M. (2007). Extracellular control of TGFbeta signalling in vascular development and disease. Nat. Rev. Mol. Cell Biol. 8, 857–869. doi:10.1038/nrm2262
Udan, R. S., Vadakkan, T. J., and Dickinson, M. E. (2013). Dynamic responses of endothelial cells to changes in blood flow during vascular remodeling of the mouse yolk sac. Development 140, 4041–4050. doi:10.1242/dev.096255
Volz, K. S., Jacobs, A. H., Chen, H. I., Poduri, A., Mckay, A. S., Riordan, D. P., et al. (2015). Pericytes are progenitors for coronary artery smooth muscle. Elife 4, e10036. doi:10.7554/eLife.10036
Wang, X., Phan, D. T., Sobrino, A., George, S. C., Hughes, C. C., and Lee, A. P. (2016). Engineering anastomosis between living capillary networks and endothelial cell-lined microfluidic channels. Lab. Chip 16, 282–290. doi:10.1039/c5lc01050k
Wang, X., Phan, D. T. T., George, S. C., Hughes, C. C. W., and Lee, A. P. (2017). 3D anastomosed microvascular network model with living capillary networks and endothelial cell-lined microfluidic channels. Methods Mol. Biol. 1612, 325–344. doi:10.1007/978-1-4939-7021-6_24
Wetzel-Strong, S. E., Detter, M. R., and Marchuk, D. A. (2017). The pathobiology of vascular malformations: Insights from human and model organism genetics. J. Pathol. 241, 281–293. doi:10.1002/path.4844
Whitehead, K. J., Chan, A. C., Navankasattusas, S., Koh, W., London, N. R., Ling, J., et al. (2009). The cerebral cavernous malformation signaling pathway promotes vascular integrity via Rho GTPases. Nat. Med. 15, 177–184. doi:10.1038/nm.1911
Xu, K., Sacharidou, A., Fu, S., Chong, D. C., Skaug, B., Chen, Z. J., et al. (2011). Blood vessel tubulogenesis requires Rasip1 regulation of GTPase signaling. Dev. Cell 20, 526–539. doi:10.1016/j.devcel.2011.02.010
Yuan, L., Sacharidou, A., Stratman, A. N., Le Bras, A., Zwiers, P. J., Spokes, K., et al. (2011). RhoJ is an endothelial cell-restricted Rho GTPase that mediates vascular morphogenesis and is regulated by the transcription factor ERG. Blood 118, 1145–1153. doi:10.1182/blood-2010-10-315275
Yurchenco, P. D., Amenta, P. S., and Patton, B. L. (2004). Basement membrane assembly, stability and activities observed through a developmental lens. Matrix Biol. 22, 521–538. doi:10.1016/j.matbio.2003.10.006
Yurchenco, P. D. (2011). Basement membranes: Cell scaffoldings and signaling platforms. Cold Spring Harb. Perspect. Biol. 3, a004911. doi:10.1101/cshperspect.a004911
Zeng, H., and Chen, J. X. (2019). Microvascular rarefaction and heart failure with preserved ejection fraction. Front. Cardiovasc. Med. 6, 15. doi:10.3389/fcvm.2019.00015
Keywords: pericytes, endothelial cells, extracellular matrix, capillary assembly, basement membrane deposition
Citation: Kemp SS, Lin PK, Sun Z, Castaño MA, Yrigoin K, Penn MR and Davis GE (2022) Molecular basis for pericyte-induced capillary tube network assembly and maturation. Front. Cell Dev. Biol. 10:943533. doi: 10.3389/fcell.2022.943533
Received: 13 May 2022; Accepted: 25 July 2022;
Published: 22 August 2022.
Edited by:
Kunimasa Ohta, Kyushu University, JapanReviewed by:
Kayoko Hosaka, Karolinska Institutet (KI), SwedenCopyright © 2022 Kemp, Lin, Sun, Castaño, Yrigoin, Penn and Davis. This is an open-access article distributed under the terms of the Creative Commons Attribution License (CC BY). The use, distribution or reproduction in other forums is permitted, provided the original author(s) and the copyright owner(s) are credited and that the original publication in this journal is cited, in accordance with accepted academic practice. No use, distribution or reproduction is permitted which does not comply with these terms.
*Correspondence: George E. Davis, Z2VkYXZpc0B1c2YuZWR1
Disclaimer: All claims expressed in this article are solely those of the authors and do not necessarily represent those of their affiliated organizations, or those of the publisher, the editors and the reviewers. Any product that may be evaluated in this article or claim that may be made by its manufacturer is not guaranteed or endorsed by the publisher.
Research integrity at Frontiers
Learn more about the work of our research integrity team to safeguard the quality of each article we publish.