- 1Center for Molecular and Translational Human Infectious Diseases Research, Houston Methodist Research Institute, Houston, TX, United States
- 2Department of Pathology and Genomic Medicine, Houston Methodist Hospital, Houston, TX, United States
Pathogenic streptococci require manganese for survival in the host. In response to invading pathogens, the host recruits nutritional immune effectors at infection sites to withhold manganese from the pathogens and control bacterial growth. The manganese scarcity impairs several streptococcal processes including oxidative stress defenses, de novo DNA synthesis, bacterial survival, and virulence. Emerging evidence suggests that pathogens also encounter manganese toxicity during infection and manganese excess impacts streptococcal virulence by manganese mismetallation of non-cognate molecular targets involved in bacterial antioxidant defenses and cell division. To counter host-imposed manganese stress, the streptococcal species employ a sophisticated sensory system that tightly coordinates manganese stress-specific molecular strategies to negate host induced manganese stress and proliferate in the host. Here we review the molecular details of host-streptococcal interactions in the battle for manganese during infection and the significance of streptococcal effectors involved to bacterial pathophysiology.
Introduction
Transition metals such as iron (Fe), manganese (Mn), and zinc (Zn) are critical micronutrients required for the proliferation of pathogenic bacteria (Nairz et al., 2010; Brophy & Nolan, 2015; Lopez & Skaar, 2018; Lonergan & Skaar, 2019). Metal ions participate in major cellular processes as enzymatic or structural cofactors for various macromolecular machineries (Andreini et al., 2008). Given the near essentiality of metals for bacterial growth, the eukaryotic host evolved mechanisms to either starve or poison the invading pathogen with metals as a means to control microbial growth (Kehl-Fie & Skaar, 2010; Becker & Skaar, 2014; Makthal & Kumaraswami, 2017). As a counter mechanism, pathogenic bacteria possess molecular strategies to sense different spectrum of metal stress and overcome host nutritional defenses by employing stress-specific adaptive strategies. Thus, the battle for metals during infection is at the crossroads between health and disease and has major implications on the clinical course of infection.
Manganese plays a central role in bacterial pathogenicity due to its contribution to cellular processes that are critical for microbial growth (Bosma et al., 2021). In addition to its well-known role in bacterial antioxidant defenses, Mn also functions as a cofactor for molecules involved in carbon metabolism, nucleotide metabolism, virulence factor production, and protein synthesis (Chander et al., 1998; Boal et al., 2010; Martin & Imlay, 2011; Aguirre & Culotta, 2012; Barnese et al., 2012; Juttukonda & Skaar, 2015). As a result, the host employs intracellular and extracellular immune strategies to target bacterial Mn requirement and controls pathogen proliferation by Mn sequestration (Cellier et al., 2007; Kehl-Fie & Skaar, 2010; Brophy & Nolan, 2015). However, pathogenic bacteria evade host nutritional immune defenses by orchestrating adaptive strategies that promote its survival under Mn stress growth conditions (Kehl-Fie & Skaar, 2010; Diaz-Ochoa et al., 2014). Given the significance of Mn to bacterial pathogenesis and host defenses, elucidation of the roles of various host and bacterial molecules involved in the competition for Mn is likely to uncover new strategies to treat or prevent infections.
In this review, we discuss the molecular strategies used by streptococci to negate Mn stress during infection and their significance to bacterial virulence. Specifically, we will focus on the role of Mn homeostasis in the pathophysiology of major pathogens belong to Streptococcus genus that include human pathogens S. pneumoniae, S. pyogenes (known as group A streptococcus; GAS), S. agalactiae (known as group B streptococcus; GBS) and S. mutans, opportunistic pathogens S. sanguinis and S. parasanguinis, and swine pathogen S. suis.
Manganese-Dependent Streptococcal Processes
Although Mn is speculated to function as a cofactor for various streptococcal proteins, only the activity of superoxide dismutases (SOD) and ribonucleotide reductases (RNR) are characterized to be Mn dependent (Makhlynets et al., 2014; Schatzman & Culotta, 2018; Ruskoski & Boal, 2021). The link between cellular Mn levels and bacterial resistance to oxidative stress is indicative of a critical role for Mn in bacterial antioxidant defenses. Superoxide dismutases are key component of streptococcal antioxidant defenses as SODs enzymatically detoxify superoxide generated by neutrophils during infection. The gene encoding sodA is identified in several streptococci including GAS, S. pneumoniae, S. gordonii, S. agalactiae, S. sanguinis, S. mutans, and S. suis, and is critical for bacterial oxidative stress resistance and virulence (Gaillot et al., 1997; Gerlach et al., 1998; Yesilkaya et al., 2000; Jakubovics et al., 2002; Tang et al., 2012; Fujishima et al., 2013; Crump et al., 2014; Turner et al., 2019). In all the characterized streptococcal SodA, Mn serves as a cofactor and SodA enzymatic activity depends on Mn uptake by Mn importers. In GAS, the activity of purified recombinant SodA is Mn dependent and SodA is inactive in its Fe-metallated or apo form (Gerlach et al., 1998). Consistent with the Mn dependency of SodA, the Mn uptake by MtsABC is critical for the elevated SodA function and the SodA activity in WT GAS increased during oxidative stress (Turner et al., 2019). Similar dependence of SodA activity on Mn uptake systems has also been observed in other streptococcal species including S. sanguinis, S. pneumoniae, and S. gordonii (Jakubovics et al., 2002; Crump et al., 2014; Eijkelkamp et al., 2014).
A second major cellular process affected by Mn limitation is de novo DNA synthesis by ribonucleotide reductases (RNR) (Boal et al., 2010; Ruskoski & Boal, 2021). The RNR catalyzes the conversion of ribonucleotides into deoxy ribonucleotides, the fundamental units required for DNA synthesis, repair, replication, and bacterial survival (Ruskoski & Boal, 2021). Typically, streptococci encode two copies of RNRs, a class III RNR that is responsible for DNA synthesis during anaerobic growth, and a class Ib RNR that catalyzes dNTP synthesis during aerobic growth (Roca et al., 2008; Makhlynets et al., 2014). In S. sanguinis, the catalytic activity of class Ib RNR is Mn-dependent and the Mn-metallated class Ib RNR has higher enzymatic activity than iron-bound RNRIb (Makhlynets et al., 2014). Furthermore, only the Mn-dependent class Ib RNR is required for S. sanguinis virulence in a rabbit model of infective endocarditis (Rhodes et al., 2014), indicating that Mn-dependent DNA synthesis by class Ib RNR is critical for S. sanguinis survival in the host.
Host Mechanisms Withholding Manganese During Infection
The host employs extracellular as well as intracellular Mn withholding strategies to control the growth of pathogenic bacteria. The calprotectin (CP)-mediated Mn sequestration is the only characterized host mechanism that imposes extracellular Mn limitation on bacterial pathogens (Corbin et al., 2008; Kehl-Fie et al., 2011; Damo et al., 2013; Hayden et al., 2013; Diaz-Ochoa et al., 2016; Nakashige et al., 2016). CP derived from neutrophils and other host cells such as monocytes, macrophages, and epithelial cells is present in sub milligram quantities in the infected abscesses (Corbin et al., 2008; Makthal et al., 2017). The extracellular CP binds to calcium in the host tissues and chelates metals from the infection sites (Corbin et al., 2008; Hayden et al., 2013). CP, a heterodimer of S100A8 and S100A9 proteins, binds to Fe, Mn, and Zn with high affinity in calcium-dependent manner (Kehl-Fie et al., 2011; Hayden et al., 2013; Nakashige et al., 2015; Nakashige et al., 2016). The CP heterodimer has two metal binding sites located at the intersubunit interface and metal ligands for each site are provided by both S100A8 and S100A9 subunits. The His3Asp motif of site 1 constitutes the Zn-binding site and is formed by H83 and H87 of S100A8 and H20 and D30 of S100A9. The site 2 is characterized by a His6 motif that is comprised of H17 and H27 of S100A8 and H91 and H95 of S100A9 as well as H103 and H105 of the S100A9 C-terminal tail (Brophy et al., 2013; Damo et al., 2013; Nakashige et al., 2016). The site 2 with the hexa-histidine motif can bind Mn, Fe, and Zn in vitro (Brophy et al., 2013; Nakashige et al., 2015; Nakashige et al., 2016; Zygiel & Nolan, 2018). The effect of Mn sequestration by CP on bacterial pathogenesis has been well characterized in S. aureus and gram-negative bacteria (Kehl-Fie et al., 2011; Kehl-Fie et al., 2013; Diaz-Ochoa et al., 2016). The CP-imposed Mn deficiency impairs the enzymatic detoxification of reactive oxygen species (ROS) by Mn-dependent superoxide dismutase (SOD) in S. aureus, which leads to increased susceptibility to oxidative stress, reduced bacterial survival, and attenuated bacterial virulence (Kehl-Fie et al., 2011; Kehl-Fie et al., 2013) (Figure 1). CP is present in GAS-infected abscesses (Makthal et al., 2017), and CP-mediated Zn sequestration impacts streptococcal growth and pathogenesis (De Filippo et al., 2014; Makthal et al., 2017; Burcham et al., 2020; Makthal et al., 2020). However, the significance of Mn withholding by CP on streptococcal proliferation in vivo and virulence remains unelucidated. Analyses of the growth kinetics of ∆sloC and ∆mntH mutants of S. mutans in the presence of CP in vitro indicated that Mn import by SloC and MntH is critical for S. mutans CP resistance (Kajfasz et al., 2020). Thus, as observed in other pathogens, it is likely that streptococcal pathogens may also engage in competition for Mn with CP during infection.
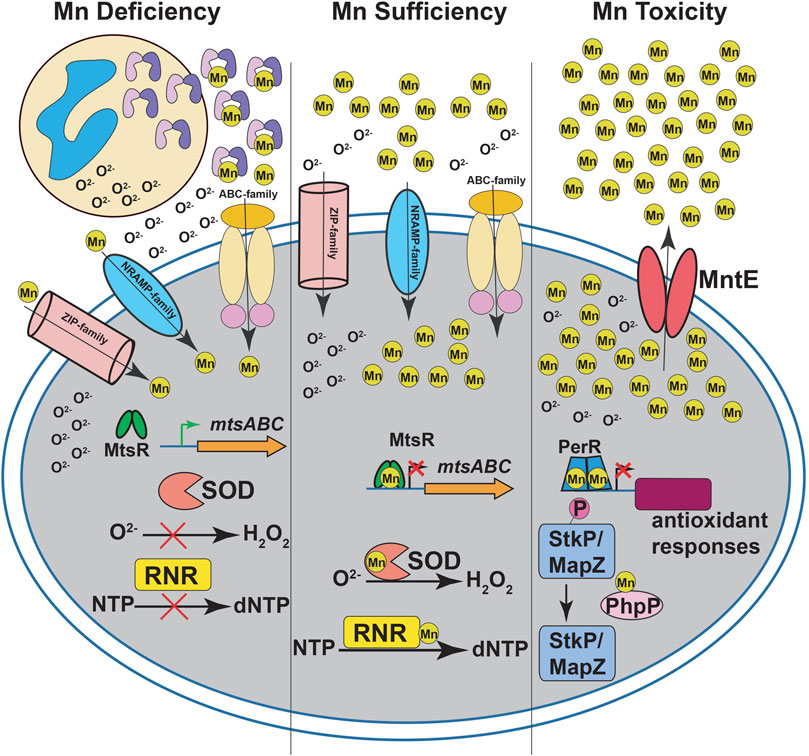
FIGURE 1. Manganese (Mn) homeostasis during streptococcal infections. During infection, neutrophils release calprotectin (CP) at infection sites (left panel). CP sequesters Mn from the invading pathogen to inhibit streptococcal growth. The intracellular Mn deficiency impairs the activity of Mn-dependent superoxide dismutases (SOD) and ribonucleotide reductases (RNR), which leads to defective antioxidant defenses against superoxide (O2−) and de novo DNA synthesis, respectively. The CP-imposed Mn deficiency is sensed by Mn-sensing metalloregulator, MtsR, and the Mn-free MtsR relieves the repression of ABC family Mn importer mtsABC. The Mn uptake during nutrient-limiting growth conditions in streptococci is mediated primarily by ABC transporters. In some streptococcal species, secondary transporters belonging to NRAMP- and ZIP-family transporters are also involved in Mn acquisition. During Mn sufficiency (middle panel), MtsR binds to Mn, interacts with promoters, and represses the expression of target genes including mtsABC. The optimal Mn levels in the cytosol promotes the activity of SOD and RNR. During Mn toxicity (right panel), the Mn efflux pump MntE detoxifies bacterial cytosol of excess Mn and promotes streptococcal survival. In the absence of MntE, high cytosolic Mn levels lead to mismetallation of peroxide sensing regulator (PerR) with Mn and results in defective antioxidant responses. Furthermore, Mn mismetallated PhpP phosphatase dephosphorylates cell division kinases StkP and MapZ, which causes improper cell division and altered bacterial cell morphologies.
In addition to extracellular Mn chelation, the host also limits Mn availability to intracellular bacteria by employing natural resistance-associated macrophage protein 1 (NRAMP1) transporters (Jabado et al., 2000; Zaharik et al., 2004; Peracino et al., 2006; Cellier et al., 2007). The NRAMP1 importers are associated with the lysosomes and proposed to transport Mn and Fe out of the phagolysosomes into host cytosol (Jabado et al., 2000; Cellier et al., 2007). The Mn withdrawal by NRAMP1 from phagolysosomes is suggested to starve the phagocytosed pathogen of Mn and impair bacterial Mn-dependent cellular processes including antioxidant defenses and bacterial survival. Consistent with this, NRAMP1 plays a major role in controlling the pathogenicity of Salmonella enterica in mouse models of infection (Zaharik et al., 2004). Experimental evidence for the role of NRAMP1 in host defenses against streptococcal growth is lacking and additional investigations are required to assess the contribution of NRAMP1 in limiting intracellular streptococcal growth.
Streptococcal Strategies to Acquire Manganese
Despite the presence of host immune mechanisms withholding Mn, streptococcal pathogens colonize the host and establish infection, suggesting that pathogens possess mechanisms to acquire Mn and evade host-imposed Mn sequestration. The streptococcal species deploy three different family of Mn acquisition systems to obtain extracellular Mn. These transporters belong to ATP-binding cassette (ABC)-family, natural resistance-associated macrophage protein (NRAMP) family, and ZRT-, IRT-like protein (ZIP) family of transporters (Figure 1). The ABC-family manganese transporters are universally present in all the characterized streptococcal genomes (Burnette-Curley et al., 1995; Kolenbrander et al., 1998; Kitten et al., 2000; Janulczyk et al., 2003; Paik et al., 2003; Johnston et al., 2004; Schreur et al., 2011; Crump et al., 2014; Kajfasz et al., 2020). Contrary to this, the NRAMP-family Mn transporters are less abundant among streptococcal species (Bray et al., 2009; Shabayek et al., 2016; Kajfasz et al., 2020), whereas a ZIP family Mn importer is characterized only in S. sanguinis (Puccio et al., 2022a).
The ABC family of transporters are the primary streptococcal Mn importers and the best studied. All the characterized ABC-family Mn importers share similar molecular architecture and are composed of three components: a surface-exposed, membrane anchored solute binding protein that binds to extracellular Mn with high affinity, an integral membrane protein that mediates Mn influx, and a cytoplasmic ATPase that facilitates Mn import by ATP hydrolysis (Li et al., 2014; Neville et al., 2021). The well characterized ABC transporters that are involved in streptococcal Mn acquisition include PsaBCA of S. pneumoniae, MtsABC of S. pyogenes, ScaCBA of S. gordonii, SloABC of S. mutans, TroABC of S. suis, SsaACB of S. sanguinis, and FimABC of S. parasanguinis (Burnette-Curley et al., 1995; Berry & Paton, 1996; Kolenbrander et al., 1998; Kitten et al., 2000; Janulczyk et al., 2003; Paik et al., 2003; Johnston et al., 2004; Schreur et al., 2011; Crump et al., 2014; Kajfasz et al., 2020). Since streptococci also use ABC transporters for the import of Fe and Zn (Sun et al., 2010; Makthal et al., 2017; Makthal & Kumaraswami, 2017), it is challenging to assign the cognate ligand for these transporters. A parallel approach that includes the identification of Mn-specific binding motifs in the solute-binding subunit of ABC transporters along with the characterization of importer-inactivated mutants for Mn-specific biological phenotypes is often required to identify the cognate ligand for ABC transporters. Most of the well characterized Mn-binding extracellular subunits of streptococcal ABC transporters such as PsaA from S. pneumoniae, MtsA from GAS, ScaA from S. gordonii, SloC from S. mutans, and FimA from S. parasanguinis contain a His-His-Glu-Asp motif that binds Mn (Sun et al., 2009; Couñago et al., 2014). Contrary to this, the Zn-binding motif in the surface-exposed Zn-binding subunit AdcA of streptococcal Zn importers AdcABC contain a His-His-His-Glu motif (Luo et al., 2021). However, the S. suis Mn importer TroA has a Zn-like His-His-His-Asp binding motif, which underscores the necessity to define the metal-specific biological phenotypes to assign the correct ligand for ABC-family metal importers (Schreur et al., 2011). Some of the biological phenotypes to identify Mn-specific transporters include Mn-specific transcription regulation of the genes encoding transporters, and phenotypes associated with transporter inactivated mutants such as reduced intracellular Mn levels, defective growth in Mn limiting growth conditions, restoration of growth specifically by Mn supplementation, and increased sensitivity to oxidative stress. In accordance with this, inactivation of psaABC from S. pneumoniae, mtsABC from GAS, scaABC from S. gordonii, ssaABC from S. sanguinis, fimABC from S. parasanguinis, and troABC from S. suis resulted in decreased cytosolic Mn levels, impaired growth during Mn limitation, restoration of growth by exogenous provision of Mn, and defective antioxidant defenses (Burnette-Curley et al., 1995; Kolenbrander et al., 1998; Kitten et al., 2000; Janulczyk et al., 2003; Paik et al., 2003; Johnston et al., 2004; Schreur et al., 2011; Crump et al., 2014; Kajfasz et al., 2020).
S. agalactiae and S. mutans employ two Mn transporters to cope with Mn limitation. The Mn uptake in S. mutans is mediated by an ABC-transporter sloABC and a NRAMP-family importer mntH (Kajfasz et al., 2020). The single ∆sloC or ∆mntH mutant had WT-like phenotype in Mn uptake, growth in Mn limiting growth conditions, and oxidative stress tolerance (Kajfasz et al., 2020). However, inactivation of both transporters resulted in defective phenotypes typically associated with Mn deficiency in vitro (Kajfasz et al., 2020), indicating the functional redundancy and complementarity between the two importers for Mn acquisition by S. mutans. Intriguingly, when tested for their resistance against CP in vitro, the ∆sloC mutant was more sensitive to CP, whereas the ∆mntH mutant had WT-like resistance to CP, suggesting that the two transporters may have distinct roles in S. mutans resistance to CP-mediated metal limitation (Kajfasz et al., 2020). Although two different Mn transporters are implicated in Mn uptake by S. agalactiae, their collective roles in Mn acquisition are not fully defined. S. agalactiae encodes an ABC-transporter mtsABC and a NRAMP-family importer mntH (Bray et al., 2009; Shabayek et al., 2016; Zhu et al., 2020). Consistent with a role in Mn acquisition, the mtsA expression is upregulated during Mn limitation (Bray et al., 2009) and mtsABC is critical for oxidative stress resistance and S. agalactiae survival in human blood and plasma (Zhu et al., 2020). Similarly, the mntH is also involved in Mn uptake and antioxidant defenses of S. agalactiae (Shabayek et al., 2016). Further studies are required to delineate the individual as well as collective roles of the two Mn transporters in Mn acquisition, antioxidant defenses, CP resistance, and virulence of S. agalactiae.
S. sanguinis encode three Mn transporters, ABC-transporter SsaACB, NRAMP-transporter MntH, and ZIP family transporter TmpA (Puccio et al., 2022b). Functional characterization of the transporters indicate that SsaACB is the primary Mn transporter, whereas TmpA and MntH are functionally redundant secondary transporters. Inactivation of either tmpA or mntH alone did not cause any Mn-associated defective phenotypes. However, the double mutant ∆ssaACB∆tmpA was more defective than the single ∆ssaABC mutant in Mn acquisition, and survival in Mn limiting growth conditions (Puccio et al., 2022a). The growth phenotype of triple mutant ∆ssaACB∆tmpA∆mntH in Mn limiting growth conditions was similar to the double mutant, suggesting the functional redundancy of secondary transporters (Puccio et al., 2022b).
Manganese Acquisition and Streptococcal Virulence
Consistent with the significant contribution of Mn importers for streptococcal growth in the presence of various stressors in vitro, the Mn uptake systems are critical for streptococcal virulence in several animal models of systemic infection mimicking various forms of streptococcal disease manifestations. The psaABC in S. pneumoniae, and troA in S. suis are critical for bacterial virulence in intraperitoneal or intravenous mouse models of systemic infection (Marra et al., 2002; Johnston et al., 2004; McAllister et al., 2004; Schreur et al., 2011). Similarly, the bacterial virulence of ∆sloC or ∆sloA of S. mutans, ∆fimA of S. parasanguinis, and ∆ssaABC of S. sanguinis was significantly attenuated in animal models of infective endocarditis (Burnette-Curley et al., 1995; Kitten et al., 2000; Paik et al., 2003; Crump et al., 2014). Intriguingly, the deletion of secondary Mn transporters mntH or tmpA alone did not affect the virulence phenotype S. sanguinis in rabbit model of infective endocarditis (Puccio et al., 2022a). However, deletion of either mntH or tmpA in ∆ssaABC mutant strain caused further reduction in bacterial survival in vivo compared to single ∆ssaABC mutant (Puccio et al., 2022a). These results suggest that the ABC-transporter SsaABC is the primary Mn uptake system in vivo, however, MntH or TmpA also has a role, albeit a lesser role, in Mn acquisition during S. sanguinis infection. In GAS, the Mn import by MtsABC contributes significantly to bacterial pathogenesis in a subcutaneous air sac model of invasive infection as inactivation of mtsABC resulted in reduced mortality compared to wild type GAS (Janulczyk et al., 2003). Contrary to the systemic mouse models of infection, the Mn importer mutant strains had a varied virulence phenotype in experimental models simulating localized streptococcal infections. In S. mutans, inactivation of either ABC-transporter sloC or NRAMP-transporter mntH failed to affect bacterial survival ex vivo in human saliva (Kajfasz et al., 2020). However, when both transporters were inactivated, the ∆sloC∆mntH double mutant strain had defective growth in saliva (Kajfasz et al., 2020). Similarly, inactivation of sloC failed to affect S. mutans plaque formation in gnotobiotic rat model of caries (Kitten et al., 2000). The S. sanguinis Mn importer SsaABC is dispensable for bacterial colonization in a mouse model of oral colonization (Puccio et al., 2022b). Contrary to this, the Mn importer PsaABC is critical for of S. pneumoniae colonization in mouse model of intranasal colonization. Inactivation of different components of psaABC system caused decreased nasopharyngeal colonization, reduced bacterial burden in lungs, and decreased mortality (Berry & Paton, 1996; Marra et al., 2002; McAllister et al., 2004). These observations suggest that pathogen-specific and host niche-specific variations exist in the contribution of streptococcal Mn importers to bacterial pathogenesis.
Despite the demonstrated significance of Mn importers to streptococcal virulence, their roles in direct competition with host Mn sequestration systems such as calprotectin in vivo are yet to be demonstrated. Future investigations assessing the contribution of Mn uptake systems to streptococcal pathogenesis in mice lacking CP or NRAMP1 transporters are required to delineate the precise role of Mn importers in streptococcal resistance to host-imposed Mn limitation.
Manganese Toxicity and Streptococcal Pathogenesis
Unlike CP-mediated Mn limitation on the pathogen, evidence for host mechanisms imposing Mn toxicity is lacking. However, the ubiquitous presence of genes encoding Mn exporters in streptococcal genomes and their demonstrated significance to bacterial pathogenesis in animal models of infection suggest that pathogens encounter Mn toxicity in vivo. The Mn efflux pump MntE has been identified and characterized in S. pneumoniae, GAS, S. mutans, and S. suis (Rosch et al., 2009; Turner et al., 2015; Xu et al., 2017; O'Brien et al., 2020) (Figure 1). MntE belongs to cation diffusion facilitator (CDF) family of transporters that couple import of H+ ions with Mn efflux (Kolaj-Robin et al., 2015; Martin & Giedroc, 2016). The MntE exporters exist as a dimer and each subunit is composed of an amino terminal transmembrane domain (TMD) with 6 transmembrane helices and a carboxy terminal cytoplasmic domain (CTD). Both domains are critical for Mn export by MntE (Martin & Giedroc, 2016). Although 3 metal binding sites, sites A-C, are identified, the significance of each site for metal binding and metal export vary among CDF transporters. The metal binding site A located in the transmembrane domain constitutes the active site for metal transport and has the determinants for metal selectivity (Martin & Giedroc, 2016). In MntE and its paralogs, the site A is composed of a Asn-Asp-Asp-Asp (N-D-D-D) motif with contributions from amino acids Asn47 and Asp51 of transmembrane helix TM2 and Asp155 and Asp159 of TM5. Inactivating alanine substitutions at each of the site 1 amino acids of pneumococcal MntE resulted in increased Mn sensitivity, indicating the critical role of site 1 in Mn efflux by MntE (Martin & Giedroc, 2016). Comparison of the amino acid sequence of MntE with Zn-binding CDF exporters identified a H-D-H-D motif in Zn exporters in place of N-D-D-D motif of MntE. Interestingly, swapping studies substituting N-D-D-D motif of MntE with H-D-H-D motif switched the metal specificity and export activities of MntE from Mn to Zn, suggesting that site A of MntE contains the specificity determinant for Mn binding and efflux (Martin & Giedroc, 2016). The site B is located at the interdomain interface between TMD and CTD and involved in metal binding. However, it remains unclear whether metal binding at site B participates in Mn influx directly or indirectly by influencing MntE stability and/or Mn binding at site A (Martin & Giedroc, 2016). The site C is critical for metal efflux by Zn exporters, however, it is dispensable for Mn efflux by MntE (Martin & Giedroc, 2016).
Although the full extent of cellular processes affected by Mn toxicity is yet to be elucidated, few streptococcal pathophysiological mechanisms influenced by Mn excess have been characterized. Invariably, the mismetallation of non-cognate molecular targets with Mn during Mn excess is the basis for the observed defective phenotypes. GAS upregulate mntE expression selectively in response to Mn excess (Turner et al., 2015), suggesting that GAS deploy MntE to negate Mn toxicity. Inactivation of mntE resulted in increased intracellular accumulation of Mn, impaired oxidative stress defenses, and growth defect during GAS growth in vitro in the presence of excess Mn (Turner et al., 2015). Further characterization of MntE suggested that mismetallation of GAS peroxide stress sensor PerR during Mn toxicity is likely the basis for the impaired GAS antioxidant defenses (Figure 1). The Mn- or Fe-metallated PerR represses the transcription of genes involved in GAS oxidative stress defenses. During oxidative stress, only the Fe-bound PerR is responsive to reactive oxygen species and responds by derepressing GAS oxidative stress regulon (Makthal et al., 2013). Contrary to this, the PerR:Mn is recalcitrant to oxidative stress and metallation with Mn during Mn surplus locks PerR in repressor state. Consequently, the failure of Mn mismetallated PerR to derepress oxidative stress regulon in response to ROS likely contributes to impaired GAS antioxidant defenses (Makthal et al., 2013). Consistent with this, unlike the increased sensitivity of ∆mntE mutant to oxidative stress, the ∆perR∆mntE double mutant had WT-like resistance to peroxide stress (Turner et al., 2015). Similarly, the Mn-metallated PerR in ∆mntE mutant was proposed to cause repression of a secreted Dnase sdaI, a major GAS virulence determinant that is critical for the degradation of neutrophil extracellular traps (NETs) (Grifantini et al., 2011; Wang et al., 2013). Consequently, the impaired antioxidant defenses of ∆mntE mutant caused defective GAS survival during growth in the presence of neutrophils (Turner et al., 2015). In pneumococci, intracellular Mn excess caused by the inactivation of mntE resulted in asymmetric cell division and cells with altered morphologies. During Mn toxicity, the ratio of Mn:Zn was altered, which resulted in Mn mismetallation and hyperactivity of a cell division regulator, PhpP phosphatase (Martin et al., 2017). The increased phosphatase activity of PhpP led to dephosphorylation of cell division regulatory kinases, StkP and MapZ, and likely contribute to the dysregulation of pneumococcal cell division (Figure 1) (Martin et al., 2017). Transcriptome analyses of pneumococcal ∆mntE mutant revealed that genes encoding pilus regulator rlrA and pilus subunit rrgB were upregulated during Mn excess (Rosch et al., 2009). However, the downstream consequences of pilus upregulation during Mn intoxication to pneumococcal pathophysiology remain unclear.
The assessment of ∆mntE virulence phenotype in animal models of infection revealed niche-specific roles for Mn exporters in streptococcal pathogenesis. The pneumococcal ∆mntE mutant was attenuated for nasal colonization, dissemination to secondary sites, and bacterial survival in intranasal mouse model of pneumococcal infection (Rosch et al., 2009). Similarly, competition infection studies with S. suis WT and ∆mntE mutant showed that the ∆mntE mutant had reduced survival compared to WT in systemic mouse model of infection (Xu et al., 2017). Contrary to this, inactivation of mntE did not affect GAS virulence in transgenic humanized plasminogen mouse model of invasive infection (Turner et al., 2015). These observations suggest that host-imposed Mn intoxication is an anatomic site-specific nutritional immune mechanism and MntE is critical for streptococcal virulence in specific host niches.
Manganese Sensing and Gene Regulation During Manganese Limitation by Streptococci
Pathogens are tasked with the challenge of sensing host induced alterations in Mn levels and maintaining the balance between acquiring sufficient Mn and not incurring Mn toxicity during infection. Successful pathogens monitor the fluctuations in Mn levels via cytosolic Mn sensing transcription regulators and tightly regulate the Mn stress-specific adaptive responses including Mn uptake (Merchant & Spatafora, 2014; Chandrangsu et al., 2017). During Mn sufficiency, Mn-sensing transcription regulators bind Mn, interact with target promoters with high affinity, repress expression of Mn importers, and reduce Mn uptake (Figure 1). Conversely, under Mn limiting conditions, the metal-free apo transcription regulators dissociate from the promoters, induce gene expression by relieving the repression, and facilitate Mn acquisition via upregulation of Mn importers (Figure 1) (Merchant & Spatafora, 2014; Do et al., 2019). The streptococcal Mn sensors belong to a highly conserved diphtheria toxin repressor (DtxR)-family of transcription regulators that include PsaR from S. pneumoniae, MtsR from GAS, SloR from S. mutans, ScaR from S. gordonii, and TroR from S. suis (Jakubovics et al., 2000; Paik et al., 2003; Johnston et al., 2006; Stoll et al., 2009; Do et al., 2019; Zheng et al., 2021).
Structural studies of MtsR and ScaR dimers revealed that these regulators adopt a typical DtxR family fold with 3 distinct domains per subunit: an amino-terminal DNA-binding domain with a winged helix-turn-helix (wHTH) DNA binding motif, a central dimerization domain, and a carboxy-terminal FeoA-like domain (Stoll et al., 2009; Do et al., 2019). Each MtsR subunit has two metal sensing sites that are located in the central dimerization domain. The Mn sensing site 1 is at the interface between the dimerization and C-terminal FeoA domains, whereas the site 2 is situated between the dimerization and N-terminal DNA-binding domains. The MtsR amino acids His76, Glu80, Cys123, and His125 constitute Mn site 1, whereas the site 2 is made of His32, His95, His161, and Asp163 (Do et al., 2019). Metal occupancy in MtsR promotes high affinity interactions between MtsR and mts motifs in target promoters and mediates Mn-dependent transcription repression by MtsR. Both sites are critical for metalloregulation by MtsR and single alanine substitutions at His76 and E80 of the site 1 as well as His95 and His161 of the site 2 caused reduced Mn binding, decreased affinity of MtsR for mts motifs, defective promoter binding by MtsR, and loss of transcription repression of target genes in the presence of Mn. Although both sites are critical for metal binding and gene regulation by MtsR, the disruption of site 1 had greater effect than site 2 with the site 1 mutants had complete loss of repression, while the site 2 mutants exhibited only partial loss of repression (Do et al., 2019). These observations prompted the speculation that the site 1 is the primary Mn sensing site and the site 2 is the secondary Mn sensing site, and the dissociation of Mn from these sites may allow gradual derepression of target genes in response to the degree of severity of Mn deficiency. The site 1 metal ligands are conserved among all the characterized streptococcal Mn sensing regulators and the site 1 ligands His76, Glu80, Cys123, and His125 in SloR are critical for promoter binding and transcription regulation (Haswell et al., 2013). As observed in MtsR, the site 2 in SloR is also located between the dimerization and N-terminal DNA-binding domains (Haswell et al., 2013). However, the SloR site 2 is distinct from that of MtsR as it varies in the amino acid composition (Do et al., 2019). The site 2 in SloR is composed of Asp7, Glu99, Glu102, and His103 and the site 2 metal ligands are critical for SloR metalloregulation (Haswell et al., 2013). Thus, the metal sensing and gene regulation by site 1 is likely common among all the streptococcal Mn metalloregulators and the composition of site 2 may vary.
Although MtsR exists as a dimer in solution, MtsR oligomerizes upon binding to the promoter. The structural analyses of MtsR uncovered an intermolecular interface between the C-terminal FeoA domains of adjacent MtsR dimers that is involved in MtsR oligomerization (Do et al., 2019). Consistent with this observation, alanine substitutions in the oligomerization interface did not affect MtsR dimerization, however, it disrupted MtsR multimerization on target promoter and caused loss of repression of target gene expression by MtsR (Do et al., 2019). The oligomerization of Mn sensors on promoter sequences has also been observed for SloR from S. mutans and ScaR from S. gordonii (Jakubovics et al., 2000; Spatafora et al., 2015) and the amino acids involved in MtsR oligomerization are conserved among Mn sensing streptococcal transcription regulators. Further characterization of the C-terminal FeoA domain-mediated oligomerization of other streptococcal Mn sensors on the target promoters and its impact on their gene regulation is required to assess whether FeoA domain has a similar role in other Mn-sensing metalloregulators.
Comparative transcriptome analyses of several streptococci with their corresponding Mn-sensing regulator inactivated strains provided critical insights into the streptococcal adaptive responses that aid bacterial growth during Mn scarcity. In most cases, the Mn-sensing regulators primarily control the expression of streptococcal genes encoding ABC-family Mn importers in response to Mn availability. Three independent studies assessing the regulatory influence of PsaR and Mn showed that the core components of S. pneumoniae adaptive responses to Mn limitation include Mn importer psaABC, the choline binding virulence factor pcpA, and secreted serine protease prtA (Johnston et al., 2006; Hendriksen et al., 2009; Ogunniyi et al., 2010). However, strain-specific variations were observed in PsaR regulon as inactivation of psaR in S. pneumoniae strain D39 resulted in 37 differentially regulated genes, whereas the expression of 19 genes were altered in ∆psaR mutant in S. pneumoniae TIGR4 background (Hendriksen et al., 2009). Although a larger GAS MtsR regulon was previously reported (Toukoki et al., 2010), a recent study showed that the core MtsR regulon is much smaller and consists of 26 genes (Do et al., 2019). The MtsR-regulated GAS adaptive responses to Mn limitation include Mn importer mtsABC, sia operon encoding putative iron transport system, iron efflux pump pmtA, and ribonucleotide reductases (Do et al., 2019). Similarly, transcription profiling of ∆troR mutant in S. suis revealed that TroR primarily controls the expression of Mn importer troABCD in response to Mn limitation (Zheng et al., 2021). Contrary to these observations, SloR in S. mutans exerts major regulatory influence with a total of 686 genes are differentially regulated in the ∆sloR mutant compared to WT (O'Rourke et al., 2010). In addition to Mn importer sloABC, some of the characterized SloR-repressed genes include pdhABC genes involved in sugar and amino acid metabolism, citBZC genes encoding citrate synthase, and glgBCDA operon involved in carbohydrate metabolism. Interestingly, SloR also functions as a Mn-dependent activator and induces the expression of genes encoding thiol peroxidase tpx, and bacteriocin transporter complex bta (O'Rourke et al., 2010).
An efficient adaptation to fluctuating Mn levels during infection is likely critical for bacterial virulence. Thus, Mn sensing and regulation of streptococcal adaptive responses by metalloregulators can be critical for bacterial virulence as dysregulation of Mn uptake may cause intracellular Mn stress. Consistent with this, Consistent with this, the Mn sensing, oligomerization, and gene regulation function of MtsR is cricital for GAS virulence in an intramuscular mouse model of invasive infection (Do et al., 2019). Contrary to this, gene regulation by TroR is dispensable for S. suis virulence in an intraperitoneal mouse model of infection (Zheng et al., 2021). Similarly, inactivation of psaR failed to affect S. pneumoniae colonization in a mouse model of nasopharyngeal colonization. However, the ∆psaR mutant had minimal but inconsistent differences in virulence in mouse models of pneumonia compared to their parental strain (Hendriksen et al., 2009). These observations suggest that Mn availability and the requirement for tight regulation of Mn uptake may vary in different host niches.
Summary and Future Perspectives
Growing evidence suggest that Mn has a critical role in various cellular processes associated with the pathophysiology of streptococci during infection. However, with the exception for few examples, the full repertoire of Mn-dependent streptococcal molecular machineries and cellular processes remains unknown. Efforts are required to identify bacterial processes that require Mn as such evidence will not only identify new Mn-dependent bacterial proteins and processes but also uncover additional evasive strategies used by pathogens to overcome host nutritional immune defenses.
It is evident that streptococcal pathogens experience host induced Mn stress during infection. However, little is known about the host factors involved, and the nature and spatiotemporal distribution of Mn based nutritional immune mechanisms employed by the host during streptococcal infections. Given the significant differences in Mn levels among various host tissues (Aschner & Aschner, 2005; Kehl-Fie et al., 2013; Juttukonda et al., 2017; Monteith et al., 2022) and Mn requirements among different pathogens (Chandrangsu et al., 2017), it is critical to determine the nature of host nutritional immune strategies at different host sites and assess their contribution to the prevention of infections caused by various streptococcal pathogens. Elucidation of the host site-specific and streptococcal species-specific molecules involved in the host-pathogen competition for Mn may improve our understanding of bacterial pathogenesis and pave the foundation for developing novel therapeutic strategies.
Author Contributions
SA and MK wrote the manuscript. MK edited the manuscript.
Conflict of Interest
The authors declare that the research was conducted in the absence of any commercial or financial relationships that could be construed as a potential conflict of interest.
Publisher’s Note
All claims expressed in this article are solely those of the authors and do not necessarily represent those of their affiliated organizations, or those of the publisher, the editors and the reviewers. Any product that may be evaluated in this article, or claim that may be made by its manufacturer, is not guaranteed or endorsed by the publisher.
Acknowledgments
This work was supported in part by the National Institutes of Health grants R01 AI146048 and R01AI162748 to MK.
References
Aguirre, J. D., and Culotta, V. C. (2012). Battles with Iron: Manganese in Oxidative Stress Protection. J. Biol. Chem. 287, 13541–13548. doi:10.1074/jbc.r111.312181
Andreini, C., Bertini, I., Cavallaro, G., Holliday, G. L., and Thornton, J. M. (2008). Metal Ions in Biological Catalysis: from Enzyme Databases to General Principles. J. Biol. Inorg. Chem. 13, 1205–1218. doi:10.1007/s00775-008-0404-5
Aschner, J. L., and Aschner, M. (2005). Nutritional Aspects of Manganese Homeostasis. Mol. Aspects Med. 26, 353–362. doi:10.1016/j.mam.2005.07.003
Barnese, K., Gralla, E. B., Valentine, J. S., and Cabelli, D. E. (2012). Biologically Relevant Mechanism for Catalytic Superoxide Removal by Simple Manganese Compounds. Proc. Natl. Acad. Sci. U.S.A. 109, 6892–6897. doi:10.1073/pnas.1203051109
Becker, K. W., and Skaar, E. P. (2014). Metal Limitation and Toxicity at the Interface between Host and Pathogen. FEMS Microbiol. Rev. 38, 1235–1249. doi:10.1111/1574-6976.12087
Berry, A. M., and Paton, J. C. (1996). Sequence Heterogeneity of PsaA, a 37-kilodalton Putative Adhesin Essential for Virulence of Streptococcus Pneumoniae. Infect. Immun. 64, 5255–5262. doi:10.1128/iai.64.12.5255-5262.1996
Boal, A. K., Cotruvo, J. A., Stubbe, J., and Rosenzweig, A. C. (2010). Structural Basis for Activation of Class Ib Ribonucleotide Reductase. Science 329, 1526–1530. doi:10.1126/science.1190187
Bosma, E. F., Rau, M. H., van Gijtenbeek, L. A., and Siedler, S. (2021). Regulation and Distinct Physiological Roles of Manganese in Bacteria. FEMS Microbiol. Rev. 45. doi:10.1093/femsre/fuab028
Bray, B. A., Sutcliffe, I. C., and Harrington, D. J. (2009). Expression of the MtsA Lipoprotein of Streptococcus Agalactiae A909 Is Regulated by Manganese and Iron. Ant. Van Leeuwenhoek 95, 101–109. doi:10.1007/s10482-008-9291-6
Brophy, M. B., and Nolan, E. M. (2015). Manganese and Microbial Pathogenesis: Sequestration by the Mammalian Immune System and Utilization by Microorganisms. ACS Chem. Biol. 10, 641–651. doi:10.1021/cb500792b
Brunjes Brophy, M., Nakashige, T. G., Gaillard, A., and Nolan, E. M. (2013). Contributions of the S100A9 C-Terminal Tail to High-Affinity Mn(II) Chelation by the Host-Defense Protein Human Calprotectin. J. Am. Chem. Soc. 135, 17804–17817. doi:10.1021/ja407147d
Burcham, L. R., Le Breton, Y., Radin, J. N., Spencer, B. L., Deng, L., Hiron, A., et al. (2020). Identification of Zinc-dependent Mechanisms Used by Group B Streptococcus to Overcome Calprotectin-Mediated Stress. mBio 11, e02302–02320. doi:10.1128/mBio.02302-20
Burnette-Curley, D., Wells, V., Viscount, H., Munro, C. L., Fenno, J. C., Fives-Taylor, P., et al. (1995). FimA, a Major Virulence Factor Associated with Streptococcus Parasanguis Endocarditis. Infect. Immun. 63, 4669–4674. doi:10.1128/iai.63.12.4669-4674.1995
Cellier, M. F., Courville, P., and Campion, C. (2007). Nramp1 Phagocyte Intracellular Metal Withdrawal Defense. Microbes Infect. 9, 1662–1670. doi:10.1016/j.micinf.2007.09.006
Chander, M., Setlow, B., and Setlow, P. (1998). The Enzymatic Activity of Phosphoglycerate Mutase from Gram-Positive Endospore-Forming Bacteria Requires Mn2+ and Is pH Sensitive. Can. J. Microbiol. 44, 759–767. doi:10.1139/w98-060
Chandrangsu, P., Rensing, C., and Helmann, J. D. (2017). Metal Homeostasis and Resistance in Bacteria. Nat. Rev. Microbiol. 15, 338–350. doi:10.1038/nrmicro.2017.15
Corbin, B. D., Seeley, E. H., Raab, A., Feldmann, J., Miller, M. R., Torres, V. J., et al. (2008). Metal Chelation and Inhibition of Bacterial Growth in Tissue Abscesses. Science 319, 962–965. doi:10.1126/science.1152449
Couñago, R. M., Ween, M. P., Begg, S. L., Bajaj, M., Zuegg, J., Mara, M. L., et al. (2014). Imperfect Coordination Chemistry Facilitates Metal Ion Release in the Psa Permease. Nat. Chem. Biol. 10, 35–41. doi:10.1038/nchembio.1382
Crump, K. E., Bainbridge, B., Brusko, S., Turner, L. S., Ge, X., Stone, V., et al. (2014). The Relationship of the Lipoprotein SsaB, Manganese and Superoxide Dismutase inStreptococcus Sanguinisvirulence for Endocarditis. Mol. Microbiol. 92, 1243–1259. doi:10.1111/mmi.12625
Damo, S. M., Kehl-Fie, T. E., Sugitani, N., Holt, M. E., Rathi, S., Murphy, W. J., et al. (2013). Molecular Basis for Manganese Sequestration by Calprotectin and Roles in the Innate Immune Response to Invading Bacterial Pathogens. Proc. Natl. Acad. Sci. U.S.A. 110, 3841–3846. doi:10.1073/pnas.1220341110
De Filippo, K., Neill, D. R., Mathies, M., Bangert, M., McNeill, E., Kadioglu, A., et al. (2014). A New Protective Role for S100A9 in Regulation of Neutrophil Recruitment during Invasive Pneumococcal Pneumonia. FASEB J. 28, 3600–3608. doi:10.1096/fj.13-247460
Diaz-Ochoa, V. E., Jellbauer, S., Klaus, S., and Raffatellu, M. (2014). Transition Metal Ions at the Crossroads of Mucosal Immunity and Microbial Pathogenesis. Front. Cell. Infect. Microbiol. 4, 2. doi:10.3389/fcimb.2014.00002
Diaz-Ochoa, V. E., Lam, D., Lee, C. S., Klaus, S., Behnsen, J., Liu, J. Z., et al. (2016). Salmonella Mitigates Oxidative Stress and Thrives in the Inflamed Gut by Evading Calprotectin-Mediated Manganese Sequestration. Cell Host Microbe 19, 814–825. doi:10.1016/j.chom.2016.05.005
Do, H., Makthal, N., Chandrangsu, P., Olsen, R. J., Helmann, J. D., Musser, J. M., et al. (2019). Metal Sensing and Regulation of Adaptive Responses to Manganese Limitation by MtsR Is Critical for Group A streptococcus Virulence. Nucl. Acids Res. 47, 7476–7493. doi:10.1093/nar/gkz524
Eijkelkamp, B. A., Morey, J. R., Ween, M. P., Ong, C.-l. Y., McEwan, A. G., Paton, J. C., et al. (2014). Extracellular Zinc Competitively Inhibits Manganese Uptake and Compromises Oxidative Stress Management in Streptococcus Pneumoniae. PLoS One 9, e89427. doi:10.1371/journal.pone.0089427
Fujishima, K., Kawada-Matsuo, M., Oogai, Y., Tokuda, M., Torii, M., and Komatsuzawa, H. (2013). Dpr and Sod in Streptococcus Mutans Are Involved in Coexistence with S. Sanguinis, and PerR Is Associated with Resistance to H 2 O 2. Appl. Environ. Microbiol. 79, 1436–1443. doi:10.1128/aem.03306-12
Gaillot, O., Poyart, C., Berche, P., and Trieu-Cuot, P. (1997). Molecular Characterization and Expression Analysis of the Superoxide Dismutase Gene from Streptococcus Agalactiae. Gene 204, 213–218. doi:10.1016/s0378-1119(97)00548-9
Gerlach, D., Reichardt, W., and Vettermann, S. (1998). Extracellular Superoxide Dismutase fromStreptococcus Pyogenestype 12 Strain Is Manganese-dependent. FEMS Microbiol. Lett. 160, 217–224. doi:10.1111/j.1574-6968.1998.tb12914.x
Grifantini, R., Toukoki, C., Colaprico, A., and Gryllos, I. (2011). Peroxide Stimulon and Role of PerR in Group A Streptococcus. J. Bacteriol. 193, 6539–6551. doi:10.1128/jb.05924-11
Haswell, J. R., Pruitt, B. W., Cornacchione, L. P., Coe, C. L., Smith, E. G., and Spatafora, G. A. (2013). Characterization of the Functional Domains of the SloR Metalloregulatory Protein in Streptococcus Mutans. J. Bacteriol. 195, 126–134. doi:10.1128/jb.01648-12
Hayden, J. A., Brophy, M. B., Cunden, L. S., and Nolan, E. M. (2013). High-Affinity Manganese Coordination by Human Calprotectin Is Calcium-dependent and Requires the Histidine-Rich Site Formed at the Dimer Interface. J. Am. Chem. Soc. 135, 775–787. doi:10.1021/ja3096416
Hendriksen, W. T., Bootsma, H. J., van Diepen, A., Estevão, S., Kuipers, O. P., de Groot, R., et al. (2009). Strain-specific Impact of PsaR of Streptococcus Pneumoniae on Global Gene Expression and Virulence. Microbiology 155, 1569–1579. doi:10.1099/mic.0.025072-0
Jabado, N., Jankowski, A., Dougaparsad, S., Picard, V., Grinstein, S., and Gros, P. (2000). Natural Resistance to Intracellular Infections. J. Exp. Med. 192, 1237–1248. doi:10.1084/jem.192.9.1237
Jakubovics, N. S., Smith, A. W., and Jenkinson, H. F. (2000). Expression of the Virulence-Related Sca (Mn2+) Permease in Streptococcus Gordonii Is Regulated by a Diphtheria Toxin Metallorepressor-like Protein ScaR. Mol. Microbiol. 38, 140–153. doi:10.1046/j.1365-2958.2000.02122.x
Jakubovics, N. S., Smith, A. W., and Jenkinson, H. F. (2002). Oxidative Stress Tolerance Is Manganese (Mn2+) Regulated in Streptococcus Gordonii. Microbiology 148, 3255–3263. doi:10.1099/00221287-148-10-3255
Janulczyk, R., Ricci, S., and Björck, L. (2003). MtsABC Is Important for Manganese and Iron Transport, Oxidative Stress Resistance, and Virulence of Streptococcus Pyogenes. Infect. Immun. 71, 2656–2664. doi:10.1128/iai.71.5.2656-2664.2003
Johnston, J. W., Briles, D. E., Myers, L. E., and Hollingshead, S. K. (2006). Mn 2+ -Dependent Regulation of Multiple Genes in Streptococcus Pneumoniae through PsaR and the Resultant Impact on Virulence. Infect. Immun. 74, 1171–1180. doi:10.1128/iai.74.2.1171-1180.2006
Johnston, J. W., Myers, L. E., Ochs, M. M., Benjamin, W. H., Briles, D. E., and Hollingshead, S. K. (2004). Lipoprotein PsaA in Virulence of Streptococcus Pneumoniae : Surface Accessibility and Role in Protection from Superoxide. Infect. Immun. 72, 5858–5867. doi:10.1128/iai.72.10.5858-5867.2004
Juttukonda, L. J., Berends, E. T. M., Zackular, J. P., Moore, J. L., Stier, M. T., Zhang, Y., et al. (2017). Dietary Manganese Promotes Staphylococcal Infection of the Heart. Cell Host Microbe 22, 531–542.e8. doi:10.1016/j.chom.2017.08.009
Juttukonda, L. J., and Skaar, E. P. (2015). Manganese Homeostasis and Utilization in Pathogenic Bacteria. Mol. Microbiol. 97, 216–228. doi:10.1111/mmi.13034
Kajfasz, J. K., Katrak, C., Ganguly, T., Vargas, J., Wright, L., Peters, Z. T., et al. (2020). Manganese Uptake, Mediated by SloABC and MntH, Is Essential for the Fitness of Streptococcus Mutans. msphere 5, e00764–00719. doi:10.1128/mSphere.00764-19
Kehl-Fie, T. E., Chitayat, S., Hood, M. I., Damo, S., Restrepo, N., Garcia, C., et al. (2011). Nutrient Metal Sequestration by Calprotectin Inhibits Bacterial Superoxide Defense, Enhancing Neutrophil Killing of Staphylococcus aureus. Cell Host Microbe 10, 158–164. doi:10.1016/j.chom.2011.07.004
Kehl-Fie, T. E., and Skaar, E. P. (2010). Nutritional Immunity beyond Iron: a Role for Manganese and Zinc. Curr. Opin. Chem. Biol. 14, 218–224. doi:10.1016/j.cbpa.2009.11.008
Kehl-Fie, T. E., Zhang, Y., Moore, J. L., Farrand, A. J., Hood, M. I., Rathi, S., et al. (2013). MntABC and MntH Contribute to Systemic Staphylococcus aureus Infection by Competing with Calprotectin for Nutrient Manganese. Infect. Immun. 81, 3395–3405. doi:10.1128/iai.00420-13
Kitten, T., Munro, C. L., Michalek, S. M., and Macrina, F. L. (2000). Genetic Characterization of a Streptococcus Mutans LraI Family Operon and Role in Virulence. Infect. Immun. 68, 4441–4451. doi:10.1128/iai.68.8.4441-4451.2000
Kolaj-Robin, O., Russell, D., Hayes, K. A., Pembroke, J. T., and Soulimane, T. (2015). Cation Diffusion Facilitator Family: Structure and Function. FEBS Lett. 589, 1283–1295. doi:10.1016/j.febslet.2015.04.007
Kolenbrander, P. E., Andersen, R. N., Baker, R. A., and Jenkinson, H. F. (1998). The Adhesion-Associated Sca Operon in Streptococcus Gordonii Encodes an Inducible High-Affinity ABC Transporter for Mn 2+ Uptake. J. Bacteriol. 180, 290–295. doi:10.1128/jb.180.2.290-295.1998
Li, N., Yang, X.-Y., Guo, Z., Zhang, J., Cao, K., Han, J., et al. (2014). Varied Metal-Binding Properties of Lipoprotein PsaA in Streptococcus Pneumoniae. J. Biol. Inorg. Chem. 19, 829–838. doi:10.1007/s00775-014-1114-9
Lonergan, Z. R., and Skaar, E. P. (2019). Nutrient Zinc at the Host-Pathogen Interface. Trends Biochem. Sci. 44, 1041–1056. doi:10.1016/j.tibs.2019.06.010
Lopez, C. A., and Skaar, E. P. (2018). The Impact of Dietary Transition Metals on Host-Bacterial Interactions. Cell Host Microbe 23, 737–748. doi:10.1016/j.chom.2018.05.008
Luo, Z., Morey, J. R., Deplazes, E., Motygullina, A., Tan, A., Ganio, A., et al. (2021). A Trap-Door Mechanism for Zinc Acquisition by Streptococcus Pneumoniae AdcA. mBio 12, e01958–01920. doi:10.1128/mbio.01958-20
Makhlynets, O., Boal, A. K., Rhodes, D. V., Kitten, T., Rosenzweig, A. C., and Stubbe, J. (2014). Streptococcus Sanguinis Class Ib Ribonucleotide Reductase. J. Biol. Chem. 289, 6259–6272. doi:10.1074/jbc.m113.533554
Makthal, N., Do, H., Wendel, B. M., Olsen, R. J., Helmann, J. D., Musser, J. M., et al. (2020). Group A Streptococcus AdcR Regulon Participates in Bacterial Defense against Host-Mediated Zinc Sequestration and Contributes to Virulence. Infect. Immun. 88, e00097–00020. doi:10.1128/IAI.00097-20
Makthal, N., and Kumaraswami, M. (2017). Zinc'ing it Out: Zinc Homeostasis Mechanisms and Their Impact on the Pathogenesis of Human Pathogen Group A streptococcus. Metallomics 9, 1693–1702. doi:10.1039/c7mt00240h
Makthal, N., Nguyen, K., Do, H., Gavagan, M., Chandrangsu, P., Helmann, J. D., et al. (2017). A Critical Role of Zinc Importer AdcABC in Group A Streptococcus-Host Interactions during Infection and its Implications for Vaccine Development. EBioMedicine 21, 131–141. doi:10.1016/j.ebiom.2017.05.030
Makthal, N., Rastegari, S., Sanson, M., Ma, Z., Olsen, R. J., Helmann, J. D., et al. (2013). Crystal Structure of Peroxide Stress Regulator from Streptococcus Pyogenes Provides Functional Insights into the Mechanism of Oxidative Stress Sensing. J. Biol. Chem. 288, 18311–18324. doi:10.1074/jbc.m113.456590
Marra, A., Lawson, S., Asundi, J. S., Brigham, D., and Hromockyj, A. E. (2002). In Vivo characterization of the Psa Genes from Streptococcus Pneumoniae in Multiple Models of Infection. Microbiology 148, 1483–1491. doi:10.1099/00221287-148-5-1483
Martin, J. E., and Giedroc, D. P. (2016). Functional Determinants of Metal Ion Transport and Selectivity in Paralogous Cation Diffusion Facilitator Transporters CzcD and MntE in Streptococcus Pneumoniae. J. Bacteriol. 198, 1066–1076. doi:10.1128/jb.00975-15
Martin, J. E., and Imlay, J. A. (2011). The Alternative Aerobic Ribonucleotide Reductase of Escherichia coli, NrdEF, Is a Manganese-dependent Enzyme that Enables Cell Replication during Periods of Iron Starvation. Mol. Microbiol. 80, 319–334. doi:10.1111/j.1365-2958.2011.07593.x
Martin, J. E., Lisher, J. P., Winkler, M. E., and Giedroc, D. P. (2017). Perturbation of Manganese Metabolism Disrupts Cell Division inStreptococcus Pneumoniae. Mol. Microbiol. 104, 334–348. doi:10.1111/mmi.13630
McAllister, L. J., Tseng, H.-J., Ogunniyi, A. D., Jennings, M. P., McEwan, A. G., and Paton, J. C. (2004). Molecular Analysis of the Psa Permease Complex of Streptococcus Pneumoniae. Mol. Microbiol. 53, 889–901. doi:10.1111/j.1365-2958.2004.04164.x
Merchant, A. T., and Spatafora, G. A. (2014). A Role for the DtxR Family of Metalloregulators in Gram-Positive Pathogenesis. Mol. Oral Microbiol. 29, 1–10. doi:10.1111/omi.12039
Monteith, A. J., Miller, J. M., Beavers, W. N., Juttukonda, L. J., Skaar, E. P., and Torres, V. J. (2022). Increased Dietary Manganese Impairs Neutrophil Extracellular Trap Formation Rendering Neutrophils Ineffective at Combating Staphylococcus aureus. Infect. Immun. 90, e00685–00621. doi:10.1128/iai.00685-21
Nairz, M., Schroll, A., Sonnweber, T., and Weiss, G. (2010). The Struggle for Iron - a Metal at the Host-Pathogen Interface. Cell Microbiol. 12, 1691–1702. doi:10.1111/j.1462-5822.2010.01529.x
Nakashige, T. G., Stephan, J. R., Cunden, L. S., Brophy, M. B., Wommack, A. J., Keegan, B. C., et al. (2016). The Hexahistidine Motif of Host-Defense Protein Human Calprotectin Contributes to Zinc Withholding and its Functional Versatility. J. Am. Chem. Soc. 138, 12243–12251. doi:10.1021/jacs.6b06845
Nakashige, T. G., Zhang, B., Krebs, C., and Nolan, E. M. (2015). Human Calprotectin Is an Iron-Sequestering Host-Defense Protein. Nat. Chem. Biol. 11, 765–771. doi:10.1038/nchembio.1891
Neville, S. L., Sjöhamn, J., Watts, J. A., MacDermott-Opeskin, H., Fairweather, S. J., Ganio, K., et al. (2021). The Structural Basis of Bacterial Manganese Import. Sci. Adv. 7, eabg3980. doi:10.1126/sciadv.abg3980
O'Brien, J., Pastora, A., Stoner, A., and Spatafora, G. (2020). The S. Mutans mntE Gene Encodes a Manganese Efflux Transporter. Mol. Oral Microbiol. 35, 129–140. doi:10.1111/omi.12286
O'Rourke, K. P., Shaw, J. D., Pesesky, M. W., Cook, B. T., Roberts, S. M., Bond, J. P., et al. (2010). Genome-Wide Characterization of the SloR Metalloregulome in Streptococcus Mutans. J. Bacteriol. 192, 1433–1443. doi:10.1128/jb.01161-09
Ogunniyi, A. D., Mahdi, L. K., Jennings, M. P., McEwan, A. G., McDevitt, C. A., Van der Hoek, M. B., et al. (2010). Central Role of Manganese in Regulation of Stress Responses, Physiology, and Metabolism in Streptococcus Pneumoniae. J. Bacteriol. 192, 4489–4497. doi:10.1128/jb.00064-10
Paik, S., Brown, A., Munro, C. L., Cornelissen, C. N., and Kitten, T. (2003). The sloABCR Operon of Streptococcus Mutans Encodes an Mn and Fe Transport System Required for Endocarditis Virulence and its Mn-dependent Repressor. J. Bacteriol. 185, 5967–5975. doi:10.1128/jb.185.20.5967-5975.2003
Peracino, B., Wagner, C., Balest, A., Balbo, A., Pergolizzi, B., Noegel, A. A., et al. (2006). Function and Mechanism of Action of Dictyostelium Nramp1 (Slc11a1) in Bacterial Infection. Traffic 7, 22–38. doi:10.1111/j.1600-0854.2005.00356.x
Puccio, T., An, S. S., Schultz, A. C., Lizarraga, C. A., Bryant, A. S., Culp, D. J., et al. (2022a). Manganese Transport by Streptococcus Sanguinis in Acidic Conditions and its Impact on Growth In Vitro and In Vivo. Mol. Microbiol. 117, 375–393. doi:10.1111/mmi.14854
Puccio, T., Kunka, K. S., An, S. S., and Kitten, T. (2022b). Contribution of a ZIP‐family Protein to Manganese Uptake and Infective Endocarditis Virulence in Streptococcus Sanguinis. Mol. Microbiol. 117, 353–374. doi:10.1111/mmi.14853
Rhodes, D. V., Crump, K. E., Makhlynets, O., Snyder, M., Ge, X., Xu, P., et al. (2014). Genetic Characterization and Role in Virulence of the Ribonucleotide Reductases of Streptococcus Sanguinis. J. Biol. Chem. 289, 6273–6287. doi:10.1074/jbc.m113.533620
Roca, I., Torrents, E., Sahlin, M., Gibert, I., and Sjöberg, B.-M. (2008). NrdI Essentiality for Class Ib Ribonucleotide Reduction in Streptococcus Pyogenes. J. Bacteriol. 190, 4849–4858. doi:10.1128/jb.00185-08
Rosch, J. W., Gao, G., Ridout, G., Wang, Y.-D., and Tuomanen, E. I. (2009). Role of the Manganese Efflux System mntE for Signalling and Pathogenesis in Streptococcus Pneumoniae. Mol. Microbiol. 72, 12–25. doi:10.1111/j.1365-2958.2009.06638.x
Ruskoski, T. B., and Boal, A. K. (2021). The Periodic Table of Ribonucleotide Reductases. J. Biol. Chem. 297, 101137. doi:10.1016/j.jbc.2021.101137
Schatzman, S. S., and Culotta, V. C. (2018). Chemical Warfare at the Microorganismal Level: a Closer Look at the Superoxide Dismutase Enzymes of Pathogens. ACS Infect. Dis. 4, 893–903. doi:10.1021/acsinfecdis.8b00026
Schreur, P. J. W., Rebel, J. M. J., Smits, M. A., van Putten, J. P. M., and Smith, H. E. (2011). TroA of Streptococcus Suis Is Required for Manganese Acquisition and Full Virulence. J. Bacteriol. 193, 5073–5080. doi:10.1128/jb.05305-11
Shabayek, S., Bauer, R., Mauerer, S., Mizaikoff, B., and Spellerberg, B. (2016). A Streptococcal NRAMP Homologue Is Crucial for the Survival ofStreptococcus Agalactiaeunder Low pH Conditions. Mol. Microbiol. 100, 589–606. doi:10.1111/mmi.13335
Spatafora, G., Corbett, J., Cornacchione, L., Daly, W., Galan, D., Wysota, M., et al. (2015). Interactions of the Metalloregulatory Protein SloR from Streptococcus Mutans with its Metal Ion Effectors and DNA Binding Site. J. Bacteriol. 197, 3601–3615. doi:10.1128/jb.00612-15
Stoll, K. E., Draper, W. E., Kliegman, J. I., Golynskiy, M. V., Brew-Appiah, R. A. T., Phillips, R. K., et al. (2009). Characterization and Structure of the Manganese-Responsive Transcriptional Regulator ScaR,. Biochemistry 48, 10308–10320. doi:10.1021/bi900980g
Sun, X., Baker, H. M., Ge, R., Sun, H., He, Q.-Y., and Baker, E. N. (2009). Crystal Structure and Metal Binding Properties of the Lipoprotein MtsA, Responsible for Iron Transport in Streptococcus Pyogenes. Biochemistry 48, 6184–6190. doi:10.1021/bi900552c
Sun, X., Ge, R., Zhang, D., Sun, H., and He, Q.-Y. (2010). Iron-containing Lipoprotein SiaA in SiaABC, the Primary Heme Transporter of Streptococcus Pyogenes. J. Biol. Inorg. Chem. 15, 1265–1273. doi:10.1007/s00775-010-0684-4
Tang, Y., Zhang, X., Wu, W., Lu, Z., and Fang, W. (2012). Inactivation of the sodA Gene of Streptococcus Suis Type 2 Encoding Superoxide Dismutase Leads to Reduced Virulence to Mice. Veterinary Microbiol. 158, 360–366. doi:10.1016/j.vetmic.2012.02.028
Toukoki, C., Gold, K. M., McIver, K. S., and Eichenbaum, Z. (2010). MtsR Is a Dual Regulator that Controls Virulence Genes and Metabolic Functions in Addition to Metal Homeostasis in the Group A streptococcus. Mol. Microbiol. 76, 971–989. doi:10.1111/j.1365-2958.2010.07157.x
Turner, A. G., Ong, C. L., Gillen, C. M., Davies, M. R., West, N. P., McEwan, A. G., et al. (2015). Manganese Homeostasis in Group A Streptococcus Is Critical for Resistance to Oxidative Stress and Virulence. MBio 6, e00278–00215. doi:10.1128/mBio.00278-15
Turner, A. G., Djoko, K. Y., Ong, C.-l. Y., Barnett, T. C., Walker, M. J., and McEwan, A. G. (2019). Group A Streptococcus Co-ordinates Manganese Import and Iron Efflux in Response to Hydrogen Peroxide Stress. Biochem. J. 476, 595–611. doi:10.1042/bcj20180902
Wang, C.-H., Chiang-Ni, C., Kuo, H.-T., Zheng, P.-X., Tsou, C.-C., Wang, S., et al. (2013). Peroxide Responsive Regulator PerR of Group A Streptococcus Is Required for the Expression of Phage-Associated DNase Sda1 under Oxidative Stress. PLoS One 8, e81882. doi:10.1371/journal.pone.0081882
Xu, J., Zheng, C., Cao, M., Zeng, T., Zhao, X., Shi, G., et al. (2017). The Manganese Efflux System MntE Contributes to the Virulence of Streptococcus Suis Serotype 2. Microb. Pathog. 110, 23–30. doi:10.1016/j.micpath.2017.06.022
Yesilkaya, H., Kadioglu, A., Gingles, N., Alexander, J. E., Mitchell, T. J., and Andrew, P. W. (2000). Role of Manganese-Containing Superoxide Dismutase in Oxidative Stress and Virulence of Streptococcus Pneumoniae. Infect. Immun. 68, 2819–2826. doi:10.1128/iai.68.5.2819-2826.2000
Zaharik, M. L., Cullen, V. L., Fung, A. M., Libby, S. J., Kujat Choy, S. L., Coburn, B., et al. (2004). The Salmonella enterica Serovar Typhimurium Divalent Cation Transport Systems MntH and SitABCD Are Essential for Virulence in an Nramp1 G169 Murine Typhoid Model. Infect. Immun. 72, 5522–5525. doi:10.1128/iai.72.9.5522-5525.2004
Zheng, C., Wei, M., Qiu, J., Jia, M., Zhou, X., Jiao, X., et al. (2021). TroR Negatively Regulates the TroABCD System and Is Required for Resistance to Metal Toxicity and Virulence in Streptococcus Suis. Appl. Environ. Microbiol. 87, e01375–01321. doi:10.1128/aem.01375-21
Zhu, L., Yerramilli, P., Pruitt, L., Ojeda Saavedra, M., Cantu, C. C., Olsen, R. J., et al. (2020). Genome-Wide Assessment of Streptococcus Agalactiae Genes Required for Survival in Human Whole Blood and Plasma. Infect. Immun. 88, e00357–00320. doi:10.1128/IAI.00357-20
Keywords: nutritional immunity, bacterial virulence, streptococcal infection, manganese homeostasis, manganese uptake, manganese toxicity
Citation: Aggarwal S and Kumaraswami M (2022) Managing Manganese: The Role of Manganese Homeostasis in Streptococcal Pathogenesis. Front. Cell Dev. Biol. 10:921920. doi: 10.3389/fcell.2022.921920
Received: 16 April 2022; Accepted: 01 June 2022;
Published: 21 June 2022.
Edited by:
Thomas Kehl-Fie, University of Illinois at Urbana-Champaign, United StatesReviewed by:
Jose Lemos, University of Florida, United StatesTodd Kitten, Virginia Commonwealth University, United States
Grace Ann Spatafora, Middlebury College, United States
Copyright © 2022 Aggarwal and Kumaraswami. This is an open-access article distributed under the terms of the Creative Commons Attribution License (CC BY). The use, distribution or reproduction in other forums is permitted, provided the original author(s) and the copyright owner(s) are credited and that the original publication in this journal is cited, in accordance with accepted academic practice. No use, distribution or reproduction is permitted which does not comply with these terms.
*Correspondence: Muthiah Kumaraswami, bWt1bWFyYXN3YW1pQGhvdXN0b25tZXRob2Rpc3Qub3Jn