- Universite Bordeaux, CNRS, IMN, UMR 5293, F-33000 Bordeaux, France
Macroautophagy, an evolutionary conserved catabolic process in the eukaryotic cell, regulates cellular homeostasis and plays a decisive role in self-engulfing proteins, protein aggregates, dysfunctional or damaged organelles, and invading pathogens. Growing evidence from in vivo and in vitro models shows that autophagy dysfunction plays decisive role in the pathogenesis of various neurodegenerative diseases, including Parkinson’s disease (PD). PD is an incurable and second most common neurodegenerative disease characterised by neurological and motor dysfunction accompanied of non-motor symptoms that can also reduce the life quality of patients. Despite the investment in research, the aetiology of the disease is still unknown and the therapies available are aimed mostly at ameliorating motor symptoms. Hence, therapeutics regulating the autophagy pathway might play an important role controlling the disease progression, reducing neuronal loss and even ameliorating non-motor symptoms. In this review, we highlight potential therapeutic opportunities involved in different targeting options like an initiation of autophagy, Leucine-rich repeat kinase 2 (LRRK2) inhibition, mitophagy, lysosomes, lipid metabolism, immune system, gene expression, biomarkers, and also non-pharmacological interventions. Thus, strategies to identify therapeutics targeting the pathways modulating autophagy might hold a future for therapy development against PD.
Introduction
Macroautophagy (hereafter called autophagy) is an evolutionarily conserved catabolic process that regulates cellular homeostasis by recycling cytoplasmic components such as proteins, aggregates, damaged organelles, and pathogens. Autophagy consists of several steps (Figure 1A) for the engulfment of the cytosplasmic components into the autophagosome that in turn fuses to the lysosome, giving raise to the autolysosome where the lysosomal hydrolases degrade the target material (De Duve, 1963; Mari et al., 2011). Several studies show that alterations of autophagy are associated with neurodegeneration and various neurodegenerative diseases such as Alzheimer’s disease (AD), Amyotrophic lateral sclerosis (ALS), Huntington’s disease (HD) and Parkinson’s disease (PD) (Komatsu et al., 2006; Liang et al., 2010; Friedman et al., 2012) (Aman et al., 2021).
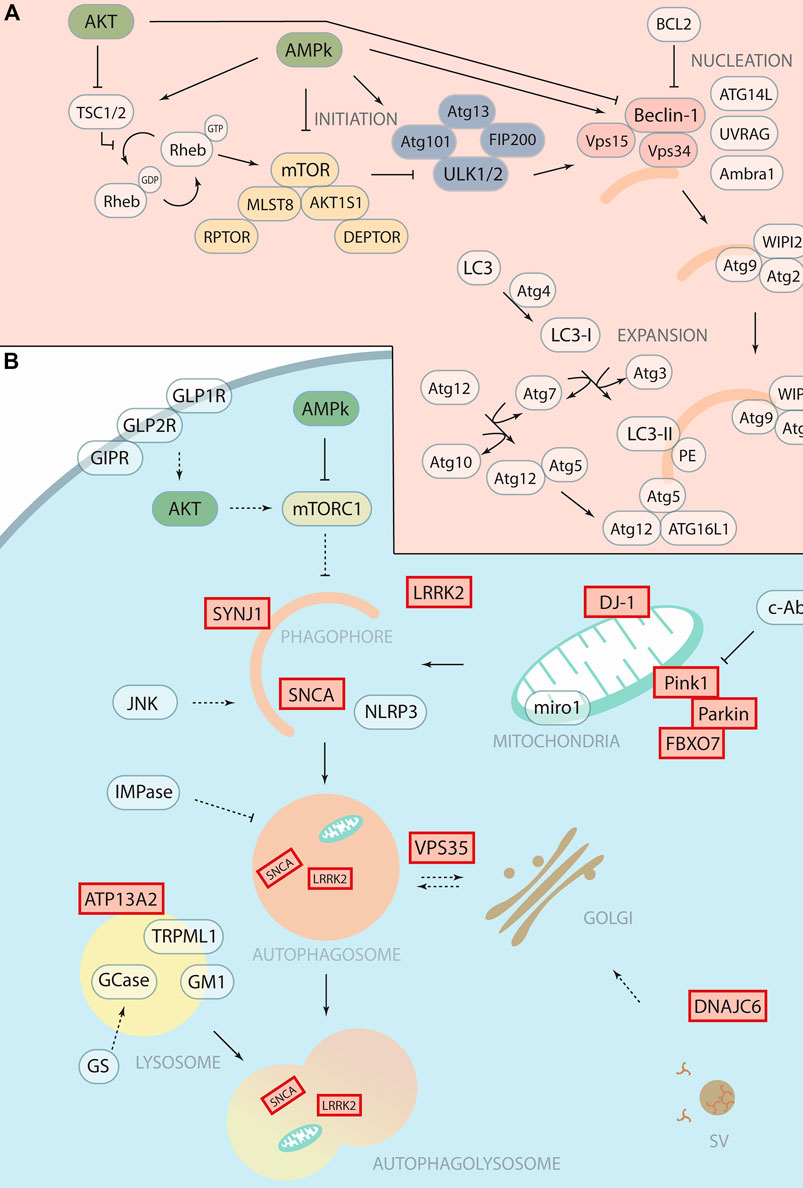
FIGURE 1. Therapeutic targeting of autophagy with potential application in Parkinson’s Disease. (A) Schematic representation of autophagosomal formation with autophagic core proteins involved in the different steps such as initiation, nucleation and expansion. We highlight in yellow the mTOR complex 1, in blue the ULK1 complex and in soft red the PI3K Class III complex. (B) Schematic representation of the autophagy pathway shows the forming phagophore that matures to the autophagosome and then fuses with the lysosome for degradation. Proteins encoded by “Parkinson’s disease genes,” labelled with red boxes, function in the autophagy pathway and related processes at different steps. The therapeutic targets or drugable nodes within the autophagy pathway that are targeted by compounds discussed in this minireview are marked in blue.
PD is the second most common neurodegenerative disorder, after AD, that affects mainly the dopaminergic (DA) neurons in the substantia nigra pars compacta (SNc). PD is characterised by three cardinal motor symptoms, akinesia/bradykinesia, tremor at rest and rigidity, in combination with other non-motor symptoms. Currently, there is no known cure or treatment to stop the progression of the disease. PD is often diagnosed when motor symptoms appear, and at this stage around 30%–70% of the DA neurons in the SNc (Fearnley and Lees, 1991; Cheng et al., 2010; Giguère et al., 2018) has already degenerated. Interestingly, various PD causative proteins have a direct role in autophagy, such as LRRK2, Synaptojanin1, Pink1, and Parkin (Soukup et al., 2016; Vanhauwaert et al., 2017) (Figure 1B). Another PD hallmark often associated with the loss of DA neurons is SNCA (α-Synuclein) aggregation (Soukup et al., 2018) that results from either mutations in SNCA or an overabundance of the protein. Although the resulting aggregates can be degraded by autophagy (Vogiatzi et al., 2008) SNCA can also impair autophagic protein degradation in PD while autophagy inhibition seems to exacerbate SNCA accumulation (Lei et al., 2019) (Song et al., 2014) (Klucken et al., 2012). Genome Wide Association Studies (GWAS) and post-mortem analysis linked various autophagy associated genes as risk factors in PD (Anglade et al., 1997; Chen et al., 2013; Mamais et al., 2018).
This review recapitulates the molecular mechanisms of macroautophagy in PD, focusing on recent clinical studies seeking to exploit autophagy to halt the progression of PD. Finally, we discuss the current gap and future perspective of pharmacological and non-pharmaceutical approaches that modulate autophagy as a therapeutic avenue to treat PD.
Targeting the Initiation of Autophagy
Amino acid starvation ultimately leads to mTOR phosphorylation and inactivation, promoting autophagy. Similarly, changes in cellular energy levels (AMP/ATP ratio) or glucose starvation can activate AMP-activated protein kinase (PRKAA1/AMPK), leading to the inactivation of mTOR, activation of ULK1, and initiation of autophagy. Conversely, the AKT pathway can negatively regulate autophagy and is often deregulated in neurodegenerative disorders (Long et al., 2021). Thus, therapeutical strategies could exploit metabolic pathways like AMPK, AKT, or mTOR to control autophagy. However, targeting these proteins produce widespread off-target effects (Xiang et al., 2020). For example, the phosphodiesterase inhibitor ibudilast inhibits mTOR complex 1 and promotes the nuclear translocation of transcription factor EB resulting in higher transcription of autophagy genes that induces lysosomal and autophagy biogenesis (Chen et al., 2020). The flavonoid Icariin restore the levels of autophagy-related proteins (LC3-II, beclin1, p62, and mTOR) and has shown to be neuroprotective, in rotenone induced rat PD model and PC12 cells (Zeng et al., 2019; Wang S. et al., 2021). Further, combinations of drugs like the mTOR allosteric-inhibitor sirolimus (also known as rapamycin) with RTB101 (ATP-competitor that also inhibits mTOR) that crosses the blood-brain barrier (BBB) in concentrations capable of inducing autophagy, may provide novel potential approaches in PD therapy (GlobeNewsWire, 2020). However, compounds such as Trehalose that promote autophagy via mTOR-independent pathway are also emerging as a promising therapeutic candidates for treating PD (Khalifeh et al., 2019).
Currently, symptomatic PD treatment relies on a dopamine replacement therapy consisting of levodopa (L-Dopa) administration. Unfortunately, this treatment causes several problems including motor fluctuations and abnormal involuntary movements named dyskinesia; although the mechanism is still unclear, a recent study reported that the administration of rapamycin effectively reduced this L-dopa-induced dyskinesia (Feyder et al., 2021). Glucagon-like peptide 1 receptor (GLP1R) that indirectly targets AKT might help as an adjuvant therapy. For example, the Glucagon-like peptide 1 analogue Liraglutide reduces the adverse side effects of L-DOPA administration (Badawi et al., 2019) and also enhances mitophagy flux (Lin et al., 2021). New approaches to restore autophagy, such as dual formulation with GLPR agonists (DA-CH5) (Zhang et al., 2020) or GLP2R analogues (Su Y. et al., 2021), are being explored.
Drugs modulating the neuroprotective AMPK pathway are also being tested. For example, the anti-diabetic drug Metformin triggers autophagy by activating AMPK and increases the tyrosine hydroxylase-stained neurons in the SN in rotenone induced mouse PD model (El-Ghaiesh et al., 2020; Katila et al., 2020). Unfortunately, this drug was associated with an increased PD development risk (Ping et al., 2020; Qin et al., 2021). The polyphenol Resveratrol can induce autophagy by mTOR inhibition and shows neuroprotection in MPTP (Su C.-F. et al., 2021), rotenone, 6-OHDA, paraquat, maneb induced and transgenic mouse PD model (Liu et al., 2019) but whether this drug acts via AMPK or PI3K/AKT is still unclear (Arbo et al., 2020). Non-pharmacological therapeutic approaches targeting energy metabolism, such as the ketone diet, improved motor (Norwitz et al., 2020) and cognitive (Krikorian et al., 2019) performances in PD patients and the ketone βHB can act through HCAR2, activating AMPK and therefore autophagy (Kovács et al., 2021). Overall, exercise seems to demonstrate neuroprotection in PD patients (Yang et al., 2021a), and restore autophagy markers (Ferreira et al., 2021) but additional research is necessary to explore the cellular circuits and molecular mechanisms underlying the intersection between autophagy and exercise leading to neuroprotection.
Leucine-Rich Repeat Kinase 2 Inhibition
LRRK2 is a multifunctional protein with threonine and serine kinase domains that genetically contributes to PD (Paisán-Ruı́z et al., 2004). Various studies reported a context-dependent function of LRRK2 in several cellular processes such as autophagy and lysosomal degradation depending on the specific LRRK2 mutation, cell type or even in the step of the autophagy (Madureira et al., 2020) (Wallings et al., 2015). At the presynaptic terminal, LRRK2 phosphorylates Endophilin-A to attract autophagic proteins like Atg3, thus initiating autophagy induction (Soukup et al., 2016). The Endophilin-A LRRK2 interaction seems to function upstream of the autophagic protein Endophilin-B in autophagy induction (Hernandez-Diaz et al., 2022). This is particularly interesting since Histone deacetylase 2 (HDAC2) regulates neuronal Endophilin-B1 expression (Wang D. B. et al., 2019) and could be a promising therapeutic target to restore impaired autophagy in PD patients (Mazzocchi et al., 2020). Indeed, LRRK2 kinase gain-of-function mutations, such as G2019S, are the most common cause of familial PD and activation of LRRK2 has also been reported in sporadic PD (Maio et al., 2018). Therefore, the inhibition of LRRK2 kinase activity is being explored as a potential therapy (Ding and Ren, 2020) and reducing LRRK2 activity in animal models of PD is reported to minimise SNCA aggregation, neuroinflammation, and dopaminergic neuron loss (Daher et al., 2014) (Daher et al., 2015). An example of this strategy is the inhibitor DNL151, proceeding into later clinical phases due to its dosage flexibility and ability to cross BBB (Denali Therapeutics Inc, 2020). Antisense oligonucleotides constitute an alternative approach to target LRRK2. Intracerebral injection of antisense oligonucleotides in a PD mouse model reduced LRRK2 protein levels as well as SNCA aggregation and dopaminergic neurodegeneration (Zhao et al., 2017). Indeed, intrathecal injection of the drug BIIB094, an antisense oligonucleotide that targets LRRK2 mRNA for degradation, is currently under clinical phase 1 (Supplementary Table S1).
Mitophagy
Mitochondria plays an important role in the pathogenesis of PD (González-Rodríguez et al., 2021) (Sanchez-mirasierra et al., 2021) (Cen et al., 2021). Mitophagy is the selective autophagy mechanism that targets mitochondria to the autophagy pathway (Lemasters, 2005) via specific mitophagy receptors such as optineurin (OPTN) or nuclear dot protein 52 kDa (NDP52) (Lazarou et al., 2015), (Heo et al., 2015). There are Pink1/Parkin dependent and independent mechanisms of mitophagy. PINK1 and PRKN act sequentially in mitophagy to target damaged mitochondria for degradation (Narendra et al., 2008) (Narendra et al., 2010). While Parkin/Pink1 may play additional roles in mitochondrial fission and fussion events (Deng et al., 2008), loss-of-function mutation in Parkin and PINK1 are linked to early-onset PD [(Kitada et al., 1998) (Valente et al., 2001)]. Targeting PARKIN activity regulating posttranscriptional modifications, such as ULK1 phosphorilation of PARKIN (Hung et al., 2021) or directly controlling mitochondrial fission and fusion (Deng et al., 2008) emerge as therapeutic opportunities in PD. However, most of the studies involving the role of PINK1 and PARKIN in mitophagy have been done in vitro. Experimental in vivo evidence provides a more complex picture showing that the role of PARKIN-dependent mitophagy is restricted to the cell soma and not to axonal or other distal compartments (Sung et al., 2016). Moreover, several in vivo studies report tissue-specific differences in the levels of basal mitophagy (Sun et al., 2015) (McWilliams et al., 2016) or even showing that basal mitophagy can occur independently of PINK1 (McWilliams et al., 2018). This evidence demonstrates the importance of context and cell specificity in mitophagy and should be taken into account for therapeutic approaches. In PINK1-PARKIN-independent mitophagy, mitochondrial proteins interact directly with the autophagic LC3/GABARAP family proteins (Antón et al., 2016). For example, genetic and pharmacological induction of the mitochondrial receptor NIX restores mitophagy independently of PINK1/PARKIN in PD patients cells (Koentjoro et al., 2017). Unfortunately, the molecular mechanisms of mitochondrial receptors underlying mitophagy are not well understood in vivo. Nevertheless, therapeutics targeting mitophagy are a promising approach. For instance, celastrol promotes DA neuron survival in mice PD models (Zhang C. et al., 2021) (Lin et al., 2019) and suppresses PARKIN recruitment to the mitochondria by inactivating PINK1 (Zhang et al., 2019). An alternative therapeutic target is the protein Miro1, a mitochondrial protein, that is, a risk factor (Grossmann et al., 2020) and a biomarker of PD (Hsieh et al., 2019) even in the prodromal phase (Nguyen et al., 2021). The accumulation of this protein delays mitophagy (Shaltouki et al., 2018) and the R272Q mutation in the Miro1 protein blocks autophagy flux (Berenguer-escuder et al., 2020). However, evidence also suggests that complete loss of Miro1 disrupts mitophagy and induces a stress response (Lopez-Domenech et al., 2021).
Targeting Lysosomes
At the latest step of autophagy, the autophagosome fuses with the acidic lysosome raising the autolysosome, where degradation occurs. Mutations in ATP13A2 gene, which encodes a lysosomal P5-type ATPase, leads to parkinsonism (Ramirez et al., 2006) and impaired lysosomal acidification, thus decreasing lysosome mediated clearance of autophagosomes (Dehay et al., 2012). Therefore, enhancing the degradation step of autophagy emerged as a therapeutic strategy. The drugs like rifampicin (Liang et al., 2020) and clioquinol (Wallings et al., 2019) (Shi et al., 2020) are promising candidates for restoring lysosomal acidification in mammalian models of PD. The compound ML-SA1 reduces SNCA accumulation in DA neurons from PD patients by increasing lysosomal biogenesis and function (Tedeschi et al., 2019; Tsunemi et al., 2019). Targeting lysosomal function currently has an ample clinical pipeline: the drug Ambroxol is currently approved for the treatment of respiratory diseases, but preclinical and clinical data support a therapeutic potential for PD patients (Silveira et al., 2019; Mullin et al., 2020; Istaiti et al., 2021). These findings strongly support the hypothesis that dysfunction of the lysosome has a pivotal role in the etiology of PD. Hence, drugs targeting the lysosomal pathway might prevent neuronal death and neuronal function, thereby halting PD progression.
Other pharmacological alternatives focus on the inhibition of c-Abl, a kinase that functions in the late stage of the autophagy pathway by regulating lysosome maturation via the lysosomal enzymes cathepsin D and cathepsin L (Yogalingam and Pendergast, 2008). Activation of this protein causes autophagy deficits and accumulation of SNCA, resulting in DA neurodegeneration (Karim et al., 2020). The c-Abl modulator, Nilotinib, has a safety profile but showed no improvement in motor scores and whether this candidate will further proceed to phase 3 in clinical trials is unclear (Pagan et al., 2020; Simuni et al., 2021).
Targeting Lipid Metabolism
Emerging evidence shows that deregulation of lipid metabolism can ultimately play a role in the onset and progression of PD (Hernandez-Diaz and Soukup, 2020). Several lipid families (fatty acyls, glycerolipids, glycerophospholipids, sphingolipids, and sterols) have been so far identified as regulators of the PD aetiological processes such as SNCA aggregation, oxidative- and endoplasmic reticulum-stress, endosomal-lysosomal dysfunction, and immune response (Xicoy et al., 2019b). Moreover, several enzymes involved in lipid metabolism can contribute directly or indirectly to the mechanism of PD progression (Alecu and Bennett, 2019). Therefore, molecules targeting those enzymes, lipid species and specific lipid pathways might interfere with the disease progression. For instance, GWAS indicates that the gene Glucocerebrosidase1 (GBA1) is a risk factor for PD. Mutations in GBA1 are present in about 10% of all patients with sporadic PD worldwide (Avenali et al., 2020). GBA1 encodes for the lysosomal enzyme glucocerebrosidase (GCase), which catalyses the hydrolysis of glucosylceramide to ceramide and glucose. GCase mutations can decrease GCase function, impairing Ceramide (Cer) production, synthesis and recycling of sphingolipids (Wigger et al., 2019) and also promoting the accumulation of the GCase substrate glucosylceramide. Changes in Cer, glucosylceramide, cholesterol and different sphingolipid species can modulate lipid membrane morphology (Akiyama et al., 2013) (García-Sanz et al., 2021), autophagy at different levels (Hernandez-Diaz and Soukup, 2020) and even the levels of lysosomal cholesterol could contribute to change in lipid rafts that are necessary for synaptic function and integrity (García-Sanz et al., 2021). Mutations in GBA1 and glucosylceramide accumulation can impair lysosomal function, mitochondrial alterations, endoplasmic reticulum stress, and abnormal accumulation of SNCA (Avenali et al., 2020) (Farfel-Becker et al., 2014). Indeed, drugs targeting mutant GBA1 could reduce the progression of PD by halting the activity of glucocerebrosidase and increasing activity in cellular and mice models along with a reduction in SNCA accumulation (Fernandes et al., 2016; Parnetti et al., 2017). Currently, several compounds targeting GBA (PR001, Ambroxol, venglustat GZ/SAR402671) are in various phases of clinical trials (Mullin et al., 2020) (Supplementary Table S1).
Gangliosides are glycosphingolipids that contain a sialic acid group. These oligosaccharides are important components of the neuronal plasma membranes. Accumulating evidence supports the role of gangliosides in the onset and progression of PD (Alecu and Bennett, 2019). In particular, experiments in animal PD models have demonstrated that gangliosides (GM1 and GM2) partially restore depleted dopamine levels, rescue motor symptoms, restore neurotransmitter levels, reduce SNCA aggregation and promote neuronal recovery (Schneider et al., 1995; Chiricozzi et al., 2019). In line with those findings, gangliosides or Ganglioside analogues (LIGA-20) intake promotes neuronal and motor function recovery (Alecu and Bennett, 2019). Thus, understanding ganglioside metabolism and neuroprotective roles seem essential to design novel therapeutic approaches. A recent study reported that PD patients exhibit lower serum levels of total cholesterol (TC), low density lipoprotein-cholesterol (LDL-C), and triglycerides (TG) compared to healthy individuals (Saedi et al., 2021). Further in vitro studies have demonstrated that change in the lipid profile is associated with PD causative agents (i.e., oxidative stress, endosomal-lysosomal function, endoplasmic reticulum stress, and immune response), and thus in PD etiology (Alecu and Bennett, 2019). Taken together these results highlights a strong correlation between abnormalities in lipid metabolism and PD. How these lipid alterations impact the lipid composition of the autophagosome structures is still unknown (Hernandez-Diaz and Soukup, 2020). Moreover, there is limited knowledge regarding the lipid profiles of the autophagic structures (Laczkó-Dobos et al., 2021) and a precise characterisation of the lipid composition of autophagosomal membranes in brain cells is currently missing. Taken together, the development of specific and safe drugs targeting lipid metabolism might open new avenues for treatments in PD.
Immune System
The immune system may be overactive in PD and preclinical studies have further enlightened that neuroinflammation is associated with T cell infiltration, Lewy body formation, and microglia activation, which intensify the neuronal dysfunction by generating a detrimental immune response (Kannarkat et al., 2013; von Euler Chelpin and Vorup-Jensen, 2017). Further, studies reported that inflammation causes loss of DA neurons and how altered immune response could influence the onset and progression of neurodegenerative diseases, particularly PD (Tansey and Goldberg, 2010; Kannarkat et al., 2013; Chao et al., 2014). Currently several studies provide evidence supporting a correlation between blood brain barrier (BBB) disruption, DA neuronal cell death and PD (Rite et al., 2007) (Al-Bachari et al., 2020) Positron emission tomography (PET) and histological studies in patients with PD demonstrated dysfunction of the BBB system, alteration of the blood vessels and neurodegeneration in the SNc (Kortekaas et al., 2005) (Gray and Woulfe, 2015). In summary, these studies indicate that neuroinflammatory changes affect BBB dysfunction by altering transport systems, enhancing immune cell entry thereby enhancing PD pathogenesis and progression. There are some connections between inflammation, autophagy and DA neuronal loss. For example, in mice, loss of the autophagic core protein Atg5 leads to the activation of the inflammasome in microglia, leading to the protection of DA neurons and the recapitulation of some PD motor symptoms (Wang X. et al., 2019) Following cellular damage or infection, the inflammasome, a multimeric protein complex, is recruited by NOD-like receptor protein 3 (NLRP3) and SNCA oligomers mediate the activation of the NLRP3-inflammasome in PD by upregulating the expression of the autophagy-related protein ATG5 (Wang X. et al., 2019). Indeed, pharmacological inhibition of NLRP3 in mice lacking ATG5 in microglia rescues DA neuron loss (Cheng et al., 2020).
LRRK2 protein is expressed in the nervous system and immune cells, especially in response to inflammatory signals [reviewed in (Wallings and Tansey, 2019)]. Strikingly, growing evidence supports the role of LRRK2 in inflammation and the onset of inflammatory diseases such as Crohn’s disease, an inflammatory Bowel disease (IBD) (Hui et al., 2018) and patients with IBD are more likely to develop PD (Villumsen et al., 2019). However, further research is needed to understand how LRRK2 regulates immune pathways and whether current and new drugs targeting LRRK2 may also target the abnormal inflammation seen in PD patients.
Several studies have shown that active (boosting self-generate antibodies) or passive (administration of antibodies externally) immunotherapies could halt PD progression. For instance, using specific antibodies against toxic variants of SNCA can slow the progression of the disease (Lee and Lee, 2016; Oliveira et al., 2021). For instance, the injection of immunomodulatory growth factors like Sargramostim (GM-CSF Leukine) may protect DA neurons, resulting in the reduction of motor symptoms (Gendelman et al., 2017). However, the interactions between neuronal dysfunction, innate immune activation and neuroinflammation are still not well understood. Further research in this area is necessary to improve therapeutic approaches to alleviate motor and cognitive behaviours in PD.
Targeting Gene Expression
Intensive research based on GWAS has identified that 5%–10% of individuals suffering from PD show dominant or recessive mutations in specific autophagy-related genes such as LRRK2, VPS35, DNAJC6; PARKIN, ATP13A2/Park9 or PINK1/Park6 (Satake et al., 2009; Simón-Sánchez et al., 2009; Klein and Westenberger, 2012). Hence, efforts to treat PD using gene therapy could restore pathophysiological pathways, halting neurodegeneration. This approach could compensate for the DA signalling in the basal ganglia, thereby providing neuroprotective effects and rescuing motor symptoms (Axelsen and Woldbye, 2018).
Recent in vivo and in vitro studies have shown that drugs targeting transcription factors (TFs) that regulate autophagy and lysosomal functions can be a promising strategy to prevent neurodegeneration. Transcription factor EB (TFEB) is a master regulator of lysosomal genes (Sardiello et al., 2009) and modulating TFEB activity is proposed as a therapeutic approach to treat PD pathogenesis (Decressac et al., 2013) and consequently there are a number of TFEB agonists being tested in preclinical trials (Chen et al., 2021). An additional therapeutic candidate is the TFs family FoxO, which transactivate genes that control autophagosome biogenesis and the formation of the autolysosome. Regulation of FoxO activity constitute a promising therapeutic strategy in the fields of senescence and age-related diseases (Calissi et al., 2021) which could potentially benefit the treatment of PD For instance, targeting the expression of the transcription factor FoxO6 (Desai et al., 2020) and FoxO3 (Pino et al., 2014) emerges as a promising alternative to regulate autophagy and prevent neurodegeneration by promoting the removal of misfolded, aggregated, toxic proteins (Dumitriu et al., 2012; Santo and Paik, 2018). Overall, based on the preclinical studies, it is clear that approaches targeting gene expression can effectively treat PD. However, further studies are essential to understand the pathways and signals playing a pivotal role in the onset and progression of PD.
Biomarkers
One of the major hindrances to the development of a therapy for PD is the long latency period between the onset of degeneration of DA neurons and the manifestation of clinical symptoms (Schrag et al., 2017; Li and Le, 2020; Santaella et al., 2020). Biomarkers can accurately make risk predictions, prognostic, staging, theragnostic, and response evaluation of the disease years before the manifestation of major clinical features (Xicoy et al., 2019a; Shao and Le, 2019; Lee et al., 2021). Hence, identifying early predictive and prognostic PD biomarkers is essential for monitoring disease progression and evaluating of the positive response of the therapeutic intervention. Dysregulation of autophagy occurs in PD and biomarkers monitoring autophagy or autophagy-associated proteins will be valuable in managing PD (Zhang K. et al., 2021). For instance, a study has shown that the autophagy protein LC3B in cerebrospinal fluid can be a potential biomarker for early diagnosis of PD (Youn et al., 2018). In recent years, microRNAs (miRNAs) and long non-coding RNAs (lncRNAs) have emerged as effective biomarkers for the onset and progression of the disease. Several miRNAs (e.g., MiR124, MiR7, MiR153, miR253, and miR223) are under evaluation as diagnostic or prognostic biomarkers for cancer, heart disorders and also neurodegenerative diseases as PD (Ma et al., 2018; Angelopoulou et al., 2019; Akkoc and Gozuacik, 2020; Yang et al., 2021b). Moreover, biomarkers for the activity of autophagy could also provide information concerning the autophagy-related endosomal, lysosomal and secretary pathways; synucleinopathy processes, axonal damage biomarkers and even inherited PD-related mutations (DJ-1, LRRK2) (Youn et al.; Pan et al., 2008; Xicoy et al., 2019a).
Hence, identifying potential biomarkers associated with autophagy for the early diagnosis, prognosis and tracking the severity of disease could be essential for developing effective neuroprotective treatment strategies.
Discussion
Currently available treatments of PD are based on symptomatic relief but neither restore lost neurons nor prevent or stop neurodegeneration. A rapid way to implement new therapies is through drug repurposing, which allows previously approved drugs to go directly into phase 2, shortening the length and cost of clinical trials as they already have a safety track record. In the pipeline of drug candidates to treat PD, at least 16 compounds have that characteristic (Stott et al., 2021), including drugs targeting proteins linked to autophagy, such as quetiapine, an antipsychotic that targets GCase (Burbulla et al., 2021). However, drug repurposing can be challenging (Keerie et al., 2021) and the identification of novel autophagy targets [such as ubiquitin (Schmidt et al., 2021)] and new drugs targeting this process is of utmost importance to use autophagy as a therapeutic tool in PD (Stacchiotti and Corsetti, 2020; Wang Z.-Y. et al., 2021; Li et al., 2021). Supplementary Table S1 enlists the pharmaceutical modulators of autophagy discussed in this minireview.
Medical chemistry can also help find novel treatments by optimising formulations to improve some characteristics of the existing compounds. For example, this approach has been used to enhance the anti-parkinsonian efficacy of resveratrol (Xiong et al., 2020), to increase the neuroprotective effect of metformin by the upregulation of autophagy (Agostini et al., 2022) and to improve mitochondrial function (Schlichtmann et al., 2021). Targeting the brain is still a challenge for any pharmacological approach. Thus, further investigations of new ways of encapsulation and administration would be essential for valid treatments (Ribeiro et al., 2020; Cunha et al., 2021). A promising way of delivery is through exosomes (Luo et al., 2021). However, the interplay between autophagy and exosome release and uptake needs to be further investigated at a cellular level, especially in the context of PD.
PD is currently considered a multifactorial and heterogeneous disease and novel classifications using PD subtypes are emerging to improve treatments and design better clinical trials. Indeed, this heterogeneity can impact drug treatment. For instance, the different mutations identified in LRRK2 gene produce neuropathological variations and the outcome of using LRRK2 inhibitors, which will differ in binding the open or the close conformation to produce different phosphorylated substrates making one mutation resistant to one type of inhibitor but not the other (Tasegian et al., 2021). Moreover, among the mutations involving GBA1, there are also null mutations that will require a replacement therapy and will not benefit from an activator (von Linstow et al., 2020). A precision medicine approach (using current knowledge of genetic risk in combination with genetic information and biomarkers from individual patients) will help to solve some of the current challenges in the design of clinical trials and therapies for PD patients (Schneider et al., 2020).
Another challenge to design trials to evaluate neuroprotection is how to select the patients in the prodromal phase (before developing motor symptoms) of PD. For example, accumulation of Miro1 occurs in PD patients (sporadic or associated with mutations) whereas, in healthy conditions, this protein is rapidly cleared in response to mitochondria damage, allowing the initiation of mitophagy. Thus, the clearance of Miro1 is proposed as a test to improve the success of clinical trials (Bharat and Wang, 2020). Another criterion that has been used with a metformin trial is to check its neuroprotection property in patients with idiopathic rapid eye movement behaviour disorder (iRBD). Patients with iRBD might develop PD, dementia with Lewy bodies or multiple system atrophy in 80% or more cases (Sportelli et al., 2020). To act effectively, we need markers for the onset and the progression of the disease. For example, macrophages from GBA-PD were successfully used to assess ambroxol therapy (Kopytova et al., 2021). Herein, Figure 1B we have discussed the drugable nodes as well as the known-genes implicated in the autophagic process and PD that has been discussed in this minireview.
Further investigations are needed to delimit autophagy therapeutical potential because of the dual face of autophagy mediating survival and cell death (Lamb, 2020). Hence understanding how autophagy proteins participate in other pathways is necessary to anticipate and minimise noxious off-target effects upon targeting these proteins (Xiang et al., 2020). Pharmacological efforts need to be made to improve organ, cell (neuron, glia) or even compartment specificity (soma, axon, synapse) of the drugs, as the effects of autophagy activation and inhibition can be context specific. Besides, non-pharmacological approaches to modulate autophagy should be explored, such as exercise or diet. In conclusion, autophagy represents a great therapeutic opportunity to treat PD; yet the details of the molecular mechanisms governing autophagy in different brain cells and compounds targeting it, as well as the crosstalk between autophagy and other pathways need to be elucidated, to unlock the therapeutic potential to fight against PD in the near future.
Author Contributions
IS-M, SG, SH-D, and S-FS conceptualised the work and wrote the manuscript.
Funding
Research in the laboratory is supported by the Initiative d'Excellence de l'Université de Bordeaux (IDEX Neurocampus Chair), GPR BRAIN_2030 and the Region Nouvelle-Aquitaine.
Conflict of Interest
The authors declare that the research was conducted in the absence of any commercial or financial relationships that could be construed as a potential conflict of interest.
Publisher’s Note
All claims expressed in this article are solely those of the authors and do not necessarily represent those of their affiliated organizations, or those of the publisher, the editors and the reviewers. Any product that may be evaluated in this article, or claim that may be made by its manufacturer, is not guaranteed or endorsed by the publisher.
Acknowledgments
We thank Dr. Abdelhamid Benazzouz (IMN/CNRS/University Bordeaux) for critical reading of the manuscript.
Supplementary Material
The Supplementary Material for this article can be found online at: https://www.frontiersin.org/articles/10.3389/fcell.2022.921314/full#supplementary-material
References
Agostini, F., Masato, A., and Bubacco, L. (2022). Metformin Repurposing for Parkinson Disease Therapy : Opportunities and Challenges. Int. J. Mol. Sci. 23 (1), 398. doi:10.3390/ijms23010398
Akiyama, H., Kobayashi, S., Hirabayashi, Y., and Murakami-Murofushi, K. (2013). Cholesterol Glucosylation Is Catalyzed by Transglucosylation Reaction of β-glucosidase 1. Biochem. Biophysical Res. Commun. 441, 838–843. doi:10.1016/j.bbrc.2013.10.145
Akkoc, Y., and Gozuacik, D. (2020). MicroRNAs as Major Regulators of the Autophagy Pathway. Biochimica Biophysica Acta (BBA) - Mol. Cell Res. 1867, 118662. doi:10.1016/J.BBAMCR.2020.118662
Al-Bachari, S., Naish, J. H., Parker, G. J. M., Emsley, H. C. A., and Parkes, L. M. (2020). Blood-Brain Barrier Leakage Is Increased in Parkinson's Disease. Front. Physiol. 11, 593026. doi:10.3389/fphys.2020.593026
Alecu, I., and Bennett, S. A. L. (2019). Dysregulated Lipid Metabolism and its Role in α-Synucleinopathy in Parkinson's Disease. Front. Neurosci. 13, 328. doi:10.3389/FNINS.2019.00328/BIBTEX
Aman, Y., Schmauck-Medina, T., Hansen, M., Morimoto, R. I., Simon, A. K., Bjedov, I., et al. (2021). Autophagy in Healthy Aging and Disease. Nat. Aging 1, 634–650. doi:10.1038/s43587-021-00098-4
Angelopoulou, E., Paudel, Y. N., and Piperi, C. (2019). miR-124 and Parkinson's Disease: A Biomarker with Therapeutic Potential. Pharmacol. Res. 150, 104515. doi:10.1016/J.PHRS.2019.104515
Anglade, P., Vyas, S., Herrero, M. T., Michel, P. P., and Marquez, J. (1997). Apoptosis and Autophagy in Nigral Neurons of Patients with Parkinson ’ S Disease. Histol. Histopathol. 12, 25–31.
Antón, Z., Landajuela, A., Hervás, J. H., Montes, L. R., Hernández-Tiedra, S., Velasco, G., et al. (2016). Human Atg8-Cardiolipin Interactions in Mitophagy: Specific Properties of LC3B, GABARAPL2 and GABARAP. Autophagy 12, 2386–2403. doi:10.1080/15548627.2016.1240856
Arbo, B. D., André-Miral, C., Nasre-Nasser, R. G., Schimith, L. E., Santos, M. G., Costa-Silva, D., et al. (2020). Resveratrol Derivatives as Potential Treatments for Alzheimer's and Parkinson's Disease. Front. Aging Neurosci. 12, 1–15. doi:10.3389/fnagi.2020.00103
Avenali, M., Blandini, F., and Cerri, S. (2020). Glucocerebrosidase Defects as a Major Risk Factor for Parkinson's Disease. Front. Aging Neurosci. 12, 1–14. doi:10.3389/fnagi.2020.00097
Axelsen, T. M., and Woldbye, D. P. D. (2018). Gene Therapy for Parkinson's Disease, an Update. Jpd 8, 195–215. doi:10.3233/JPD-181331
Badawi, G. A., Abd El Fattah, M. A., Zaki, H. F., and El Sayed, M. I. (2019). Sitagliptin and Liraglutide Modulate L-Dopa Effect and Attenuate Dyskinetic Movements in Rotenone-Lesioned Rats. Neurotox. Res. 35, 635–653. doi:10.1007/s12640-019-9998-3
Berenguer-escuder, C., Grossmann, D., Antony, P., Arena, G., Wasner, K., Massart, F., et al. (2020). Impaired Mitochondrial-Endoplasmic Reticulum Interaction and Mitophagy in Miro1-Mutant Neurons in Parkinson's Disease. Hum. Mol. Genet. 29, 1353–1364. doi:10.1093/hmg/ddaa066
Bharat, V., and Wang, X. (2020). Precision Neurology for Parkinson's Disease: Coupling Miro1‐Based Diagnosis with Drug Discovery. Mov. Disord. 35, 1502–1508. doi:10.1002/mds.28194
Burbulla, L. F., Zheng, J., Song, P., Jiang, W., Johnson, M. E., Brundin, P., et al. (2021). Direct Targeting of Wild-type Glucocerebrosidase by Antipsychotic Quetiapine Improves Pathogenic Phenotypes in Parkinson's Disease Models. JCI Insight 6, 1–8. doi:10.1172/jci.insight.148649
Calissi, G., Lam, E. W.-F., and Link, W. (2021). Therapeutic Strategies Targeting FOXO Transcription Factors. Nat. Rev. Drug Discov. 20, 21–38. doi:10.1038/s41573-020-0088-2
Cen, X., Zhang, M., Zhou, M., Ye, L., and Xia, H. (2021). Mitophagy Regulates Neurodegenerative Diseases. Cells 10, 1876. doi:10.3390/cells10081876
Chao, Y., Wong, S. C., and Tan, E. K. (2014). Evidence of Inflammatory System Involvement in Parkinson's Disease. BioMed Res. Int. 2014, 1–9. doi:10.1155/2014/308654
Chen, D., Pang, S., Feng, X., Huang, W., Hawley, R. G., and Yan, B. (2013). Genetic Analysis of the ATG7 Gene Promoter in Sporadic Parkinson's Disease. Neurosci. Lett. 534, 193–198. doi:10.1016/j.neulet.2012.12.039
Chen, M., Dai, Y., Liu, S., Fan, Y., Ding, Z., and Li, D. (2021). TFEB Biology and Agonists at a Glance. Cells 10, 333. doi:10.3390/cells10020333
Chen, Y., Wang, H., Ying, Z., and Gao, Q. (2020). Ibudilast Enhances the Clearance of SOD1 and TDP-43 Aggregates through TFEB-Mediated Autophagy and Lysosomal Biogenesis: The New Molecular Mechanism of Ibudilast and its Implication for Neuroprotective Therapy. Biochem. Biophysical Res. Commun. 526, 231–238. doi:10.1016/j.bbrc.2020.03.051
Cheng, H.-C., Ulane, C. M., and Burke, R. E. (2010). Clinical Progression in Parkinson Disease and the Neurobiology of Axons. Ann. Neurol. 67, 715–725. doi:10.1002/ana.21995
Cheng, J., Liao, Y., Dong, Y., Hu, H., Yang, N., Kong, X., et al. (2020). Microglial Autophagy Defect Causes Parkinson Disease-like Symptoms by Accelerating Inflammasome Activation in Mice. Autophagy 16, 2193–2205. doi:10.1080/15548627.2020.1719723
Chiricozzi, E., Mauri, L., Lunghi, G., Di Biase, E., Fazzari, M., Maggioni, M., et al. (2019). Parkinson's Disease Recovery by GM1 Oligosaccharide Treatment in the B4galnt1+/− Mouse Model. Sci. Rep. 9, 19330. doi:10.1038/s41598-019-55885-2
Cunha, A., Gaubert, A., Latxague, L., and Dehay, B. (2021). PLGA-based Nanoparticles for Neuroprotective Drug Delivery in Neurodegenerative Diseases. Pharmaceutics 13, 1042–1124. doi:10.3390/pharmaceutics13071042
Daher, J. P. L., Abdelmotilib, H. A., Hu, X., Volpicelli-Daley, L. A., Moehle, M. S., Fraser, K. B., et al. (2015). Leucine-rich Repeat Kinase 2 (LRRK2) Pharmacological Inhibition Abates α-Synuclein Gene-Induced Neurodegeneration. J. Biol. Chem. 290, 19433–19444. doi:10.1074/jbc.M115.660001
Daher, J. P. L., Volpicelli-Daley, L. A., Blackburn, J. P., Moehle, M. S., and West, A. B. (2014). Abrogation of α-synuclein-mediated Dopaminergic Neurodegeneration in LRRK2-Deficient Rats. Proc. Natl. Acad. Sci. U.S.A. 111, 9289–9294. doi:10.1073/pnas.1403215111
de Oliveira Junior, E. R., Truzzi, E., Ferraro, L., Fogagnolo, M., Pavan, B., Beggiato, S., et al. (2020). Nasal Administration of Nanoencapsulated Geraniol/ursodeoxycholic Acid Conjugate: Towards a New Approach for the Management of Parkinson's Disease. J. Control. Release 321, 540–552. doi:10.1016/j.jconrel.2020.02.033
Decressac, M., Mattsson, B., Weikop, P., Lundblad, M., Jakobsson, J., and Björklund, A. (2013). TFEB-mediated Autophagy Rescues Midbrain Dopamine Neurons from α-synuclein Toxicity. Proc. Natl. Acad. Sci. U.S.A. 110, E1817–E1826. doi:10.1073/pnas.1305623110
Dehay, B., Ramirez, A., Martinez-Vicente, M., Perier, C., Canron, M.-H., Doudnikoff, E., et al. (2012). Loss of P-type ATPase ATP13A2/PARK9 Function Induces General Lysosomal Deficiency and Leads to Parkinson Disease Neurodegeneration. Proc. Natl. Acad. Sci. U.S.A. 109, 9611–9616. doi:10.1073/pnas.1112368109
Denali Therapeutics Inc (2020). Denali Therapeutics Announces Decision to Advance DNL151 into Late Stage Clinical Studies in Parkinson’s Patients. California, United States: GlobeNewswire. Available at: https://www.denalitherapeutics.com/investors/press-release?id=7661l (Accessed October 31, 2021).
Deng, H., Dodson, M. W., Huang, H., and Guo, M. (2008). The Parkinson's Disease Genes Pink1 and Parkin Promote Mitochondrial Fission And/or Inhibit Fusion in Drosophila. Proc. Natl. Acad. Sci. U.S.A. 105, 14503–14508. doi:10.1073/pnas.0803998105
Desai, S., Pansare, P., Sainani, S., Doke, R., Bhalchim, V., and Rode, K. (2020). Foxo6 - A Novel Target for Parkinson's Disease. Biomed. Pharmacol. J. 13, 367–381. doi:10.13005/BPJ/1897
Ding, X., and Ren, F. (2020). Leucine-rich Repeat Kinase 2 Inhibitors: a Patent Review (2014-present). Expert Opin. Ther. Pat. 30, 275–286. doi:10.1080/13543776.2020.1729354
Dumitriu, A., Latourelle, J. C., Hadzi, T. C., Pankratz, N., Garza, D., Miller, J. P., et al. (2012). Gene Expression Profiles in Parkinson Disease Prefrontal Cortex Implicate FOXO1 and Genes under its Transcriptional Regulation. PLOS Genet. 8, e1002794. doi:10.1371/JOURNAL.PGEN.1002794
El-Ghaiesh, S. H., Bahr, H. I., Ibrahiem, A. T., Ghorab, D., Alomar, S. Y., Farag, N. E., et al. (2020). Metformin Protects from Rotenone-Induced Nigrostriatal Neuronal Death in Adult Mice by Activating AMPK-FOXO3 Signaling and Mitigation of Angiogenesis. Front. Mol. Neurosci. 13, 1–14. doi:10.3389/fnmol.2020.00084
Farfel-Becker, T., Vitner, E. B., Kelly, S. L., Bame, J. R., Duan, J., Shinder, V., et al. (2014). Neuronal Accumulation of Glucosylceramide in a Mouse Model of Neuronopathic Gaucher Disease Leads to Neurodegeneration. Hum. Mol. Genet. 23, 843–854. doi:10.1093/hmg/ddt468
Fearnley, J. M., and Lees, A. J. (1991). Ageing and Parkinson's Disease: Substantia Nigra Regional Selectivity. Brain 114 (Pt 5), 2283–2301. doi:10.1093/brain/114.5.2283
Fernandes, H. J. R., Hartfield, E. M., Christian, H. C., Emmanoulidou, E., Zheng, Y., Booth, H., et al. (2016). ER Stress and Autophagic Perturbations Lead to Elevated Extracellular α-Synuclein in GBA-N370s Parkinson's iPSC-Derived Dopamine Neurons. Stem Cell Rep. 6, 342–356. doi:10.1016/j.stemcr.2016.01.013
Ferreira, A. F. F., Binda, K. H., and Real, C. C. (2021). The Effects of Treadmill Exercise in Animal Models of Parkinson's Disease: A Systematic Review. Neurosci. Biobehav. Rev. 131, 1056–1075. doi:10.1016/j.neubiorev.2021.10.019
Feyder, M., Plewnia, C., Lieberman, O. J., Spigolon, G., Piccin, A., Urbina, L., et al. (2021). Involvement of Autophagy in Levodopa‐Induced Dyskinesia. Mov. Disord. 36, 1137–1146. doi:10.1002/mds.28480
Friedman, L. G., Lachenmayer, M. L., Wang, J., He, L., Poulose, S. M., Komatsu, M., et al. (2012). Disrupted Autophagy Leads to Dopaminergic Axon and Dendrite Degeneration and Promotes Presynaptic Accumulation of -Synuclein and LRRK2 in the Brain. J. Neurosci. 32, 7585–7593. doi:10.1523/JNEUROSCI.5809-11.2012
García‐Sanz, P., Aerts, J., and Moratalla, R. (2021). The Role of Cholesterol in α‐Synuclein and Lewy Body Pathology in GBA1 Parkinson's Disease. Mov. Disord. 36, 1070–1085. doi:10.1002/mds.28396
Gendelman, H. E., Zhang, Y., Santamaria, P., Olson, K. E., Schutt, C. R., Bhatti, D., et al. (2017). Evaluation of the Safety and Immunomodulatory Effects of Sargramostim in a Randomized, Double-Blind Phase 1 Clinical Parkinson's Disease Trial. npj Parkinson's Dis. 3. doi:10.1038/S41531-017-0013-5
Giguère, N., Burke Nanni, S., and Trudeau, L.-E. (2018). On Cell Loss and Selective Vulnerability of Neuronal Populations in Parkinson's Disease. Front. Neurol. 9, 455. doi:10.3389/fneur.2018.00455
GlobeNewsWire (2020). resTORbio Announces Interim Results for Phase 1b/2a Trial of RTB101 in Patients with Parkinson’s Disease and Provides Corporate UpdateNo Title. Available at: https://www.globenewswire.com/en/news-release/2020/02/19/1986962/0/en/resTORbio-Announces-Interim-Results-for-Phase-1b-2a-Trial-of-RTB101-in-Patients-with-Parkinson-s-Disease-and-Provides-Corporate-Update.html [Accessed October 21, 2021].
González-Rodríguez, P., Zampese, E., Stout, K. A., Guzman, J. N., Ilijic, E., Yang, B., et al. (2021). Disruption of Mitochondrial Complex I Induces Progressive Parkinsonism. Nature 599, 650–656. doi:10.1038/s41586-021-04059-0
Gray, M. T., and Woulfe, J. M. (2015). Striatal Blood-Brain Barrier Permeability in Parkinson'S Disease. J. Cereb. Blood Flow. Metab. 35, 747–750. doi:10.1038/jcbfm.2015.32
Grossmann, D., Berenguer-Escuder, C., Chemla, A., Arena, G., and Krüger, R. (2020). The Emerging Role of RHOT1/Miro1 in the Pathogenesis of Parkinson'S Disease. Front. Neurol. 11, 1–20. doi:10.3389/fneur.2020.00587
Heo, J.-M., Ordureau, A., Paulo, J. A., Rinehart, J., and Harper, J. W. (2015). The PINK1-PARKIN Mitochondrial Ubiquitylation Pathway Drives a Program of OPTN/NDP52 Recruitment and TBK1 Activation to Promote Mitophagy. Mol. Cell 60, 7–20. doi:10.1016/j.molcel.2015.08.016
Hernandez-Diaz, S., Ghimire, S., Sanchez-Mirasierra, I., Montecinos-Oliva, C., Swerts, J., Kuenen, S., et al. (2022). Endophilin-B Regulates Autophagy during Synapse Development and Neurodegeneration. Neurobiol. Dis. 163, 105595. doi:10.1016/j.nbd.2021.105595
Hernandez-Diaz, S., and Soukup, S.-F. (2020). The Role of Lipids in Autophagy and its Implication in Neurodegeneration. Cst 4, 167–186. doi:10.15698/cst2020.07.225
Hsieh, C.-H., Li, L., Vanhauwaert, R., Nguyen, K. T., Davis, M. D., Bu, G., et al. (2019). Miro1 Marks Parkinson's Disease Subset and Miro1 Reducer Rescues Neuron Loss in Parkinson's Models. Cell Metab. 30, 1131–1140.e7. doi:10.1016/j.cmet.2019.08.023
Hui, K. Y., Fernandez-Hernandez, H., Hu, J., Schaffner, A., Pankratz, N., Hsu, N.-Y., et al. (2018). Functional Variants in the LRRK2 Gene Confer Shared Effects on Risk for Crohn's Disease and Parkinson's Disease. Sci. Transl. Med. 10, eaai7795. doi:10.1126/scitranslmed.aai7795
Hung, C.-M., Lombardo, P. S., Malik, N., Brun, S. N., Hellberg, K., Van Nostrand, J. L., et al. (2021). AMPK/ULK1-mediated Phosphorylation of Parkin ACT Domain Mediates an Early Step in Mitophagy. Sci. Adv. 7, 1–14. doi:10.1126/SCIADV.ABG4544
Istaiti, M., Revel‐Vilk, S., Becker‐Cohen, M., Dinur, T., Ramaswami, U., Castillo‐Garcia, D., et al. (2021). Upgrading the Evidence for the Use of Ambroxol in Gaucher Disease and GBA Related Parkinson: Investigator Initiated Registry Based on Real Life Data. Am. J Hematol 96, 545–551. doi:10.1002/ajh.26131
Kannarkat, G. T., Boss, J. M., and Tansey, M. G. (2013). The Role of Innate and Adaptive Immunity in Parkinson's Disease. J. Park. Dis. 3, 493–514. doi:10.3233/JPD-130250
Karim, M. R., Liao, E. E., Kim, J., Meints, J., Martinez, H. M., Pletnikova, O., et al. (2020). α-Synucleinopathy Associated C-Abl Activation Causes P53-dependent Autophagy Impairment. Mol. Neurodegener. 15, 1–18. doi:10.1186/s13024-020-00364-w
Katila, N., Bhurtel, S., Park, P.-H., Hong, J. T., and Choi, D.-Y. (2020). Activation of AMPK/aPKCζ/CREB Pathway by Metformin Is Associated with Upregulation of GDNF and Dopamine. Biochem. Pharmacol. 180, 114193. doi:10.1016/j.bcp.2020.114193
Keerie, A., Brown-Wright, H., Kirkland, I., Grierson, A., Alix, J. J. P., Holscher, C., et al. (2021). The GLP-1 Receptor Agonist, Liraglutide, Fails to Slow Disease Progression in SOD1G93A and TDP-43Q331K Transgenic Mouse Models of ALS. Sci. Rep. 11, 1–10. doi:10.1038/s41598-021-96418-0
Khalifeh, M., Barreto, G. E., and Sahebkar, A. (2019). Trehalose as a Promising Therapeutic Candidate for the Treatment of Parkinson's Disease. Br. J. Pharmacol. 176, 1173–1189. doi:10.1111/bph.14623
Kitada, T., Asakawa, S., Hattori, N., Matsumine, H., Yamamura, Y., Minoshima, S., et al. (1998). Mutations in the Parkin Gene Cause Autosomal Recessive Juvenile Parkinsonism. Nature 392, 605–608. Available at: https://www.nature.com/articles/33416.pdf. doi:10.1038/33416
Klein, C., and Westenberger, A. (2012). Genetics of Parkinson's Disease. Cold Spring Harb. Perspect. Med. 2, a008888. doi:10.1101/CSHPERSPECT.A008888
Klucken, J., Poehler, A.-M., Ebrahimi-Fakhari, D., Schneider, J., Nuber, S., Rockenstein, E., et al. (2012). Alpha-synuclein Aggregation Involves a Bafilomycin A1-Sensitive Autophagy Pathway. Autophagy 8, 754–766. doi:10.4161/auto.19371
Koentjoro, B., Park, J.-S., and Sue, C. M. (2017). Nix Restores Mitophagy and Mitochondrial Function to Protect against PINK1/Parkin-Related Parkinson's Disease. Sci. Rep. 7, 1–11. doi:10.1038/srep44373
Komatsu, M., Waguri, S., Chiba, T., Murata, S., Iwata, J.-i., Tanida, I., et al. (2006). Loss of Autophagy in the Central Nervous System Causes Neurodegeneration in Mice. Nature 441, 880–884. doi:10.1038/nature04723
Kopytova, A. E., Rychkov, G. N., Nikolaev, M. A., Baydakova, G. V., Cheblokov, A. A., Senkevich, K. A., et al. (2021). Ambroxol Increases Glucocerebrosidase (GCase) Activity and Restores GCase Translocation in Primary Patient-Derived Macrophages in Gaucher Disease and Parkinsonism. Park. Relat. Disord. 84, 112–121. doi:10.1016/j.parkreldis.2021.02.003
Kortekaas, R., Leenders, K. L., van Oostrom, J. C. H., Vaalburg, W., Bart, J., Willemsen, A. T. M., et al. (2005). Blood-brain Barrier Dysfunction in Parkinsonian Midbrain In Vivo. Ann. Neurol. 57, 176–179. doi:10.1002/ana.20369
Kovács, Z., Brunner, B., and Ari, C. (2021). Beneficial Effects of Exogenous Ketogenic Supplements on Aging Processes and Age-Related Neurodegenerative Diseases. Nutrients 13, 2197–2235. doi:10.3390/nu13072197
Krikorian, R., Shidler, M. D., Summer, S. S., Sullivan, P. G., Duker, A. P., Isaacson, R. S., et al. (2019). Nutritional Ketosis for Mild Cognitive Impairment in Parkinson's Disease: A Controlled Pilot Trial. Clin. Park. Relat. Disord. 1, 41–47. doi:10.1016/j.prdoa.2019.07.006
Laczkó-Dobos, H., Maddali, A. K., Jipa, A., Bhattacharjee, A., Végh, A. G., and Juhász, G. (2021). Lipid Profiles of Autophagic Structures Isolated from Wild Type and Atg2 Mutant Drosophila. Biochimica Biophysica Acta (BBA) - Mol. Cell Biol. Lipids 1866, 158868. doi:10.1016/j.bbalip.2020.158868
Lamb, H. M. (2020). Double Agents of Cell Death: Novel Emerging Functions of Apoptotic Regulators. Febs J. 287, 2647–2663. doi:10.1111/febs.15308
Lazarou, M., Sliter, D. A., Kane, L. A., Sarraf, S. A., Wang, C., Burman, J. L., et al. (2015). The Ubiquitin Kinase PINK1 Recruits Autophagy Receptors to Induce Mitophagy. Nature 524, 309–314. doi:10.1038/nature14893
Lee, J. S., and Lee, S.-J. (2016). Mechanism of Anti-α-synuclein Immunotherapy. Jmd 9, 14–19. doi:10.14802/JMD.15059
Lee, S. Y. H., Yates, N. J., and Tye, S. J. (2021). Inflammatory Mechanisms in Parkinson's Disease: From Pathogenesis to Targeted Therapies. The Neuroscientist. doi:10.1177/1073858421992265
Lei, Z., Cao, G., and Wei, G. (2019). A30P Mutant α-synuclein Impairs Autophagic Flux by Inactivating JNK Signaling to Enhance ZKSCAN3 Activity in Midbrain Dopaminergic Neurons. Cell Death Dis. 10, 133. doi:10.1038/s41419-019-1364-0
Lemasters, J. J. (2005). Selective Mitochondrial Autophagy, or Mitophagy, as a Targeted Defense against Oxidative Stress, Mitochondrial Dysfunction, and Aging. Rejuvenation Res. 8, 3–5. doi:10.1089/rej.2005.8.3
Li, S., Sun, X., Bi, L., Tong, Y., and Liu, X. (2021). Research Progress on Natural Product Ingredients' Therapeutic Effects on Parkinson's Disease by Regulating Autophagy. Evidence-Based Complementary Altern. Med. 2021, 1–11. doi:10.1155/2021/5538200
Li, T., and Le, W. (2020). Biomarkers for Parkinson's Disease: How Good Are They? Neurosci. Bull. 36, 183–194. doi:10.1007/S12264-019-00433-1/TABLES/2
Liang, C.-C., Wang, C., Peng, X., Gan, B., and Guan, J.-L. (2010). Neural-specific Deletion of FIP200 Leads to Cerebellar Degeneration Caused by Increased Neuronal Death and Axon Degeneration. J. Biol. Chem. 285, 3499–3509. doi:10.1074/jbc.M109.072389
Liang, Y., Zheng, D., Peng, S., Lin, D., Jing, X., Zeng, Z., et al. (2020). Rifampicin Attenuates Rotenone-Treated Microglia Inflammation via Improving Lysosomal Function. Toxicol. Vitro 63, 104690. doi:10.1016/j.tiv.2019.104690
Lin, M.-W., Lin, C. C., Chen, Y.-H., Yang, H.-B., and Hung, S.-Y. (2019). Celastrol Inhibits Dopaminergic Neuronal Death of Parkinson's Disease through Activating Mitophagy. Antioxidants 9, 37. doi:10.3390/antiox9010037
Lin, T.-K., Lin, K.-J., Lin, H.-Y., Lin, K.-L., Lan, M.-Y., Wang, P.-W., et al. (2021). Glucagon-Like Peptide-1 Receptor Agonist Ameliorates 1-Methyl-4-Phenyl-1,2,3,6-Tetrahydropyridine (MPTP) Neurotoxicity through Enhancing Mitophagy Flux and Reducing α-Synuclein and Oxidative Stress. Front. Mol. Neurosci. 14, 1–17. doi:10.3389/fnmol.2021.697440
Liu, Q., Zhu, D., Jiang, P., Tang, X., Lang, Q., Yu, Q., et al. (2019). Resveratrol Synergizes with Low Doses of L-DOPA to Improve MPTP-Induced Parkinson Disease in Mice. Behav. Brain Res. 367, 10–18. doi:10.1016/j.bbr.2019.03.043
Long, H.-Z., Cheng, Y., Zhou, Z.-W., Luo, H.-Y., Wen, D.-D., and Gao, L.-C. (2021). PI3K/AKT Signal Pathway: A Target of Natural Products in the Prevention and Treatment of Alzheimer's Disease and Parkinson's Disease. Front. Pharmacol. 12, 1–20. doi:10.3389/fphar.2021.648636
López‐Doménech, G., Howden, J. H., Covill‐Cooke, C., Morfill, C., Patel, J. V., Bürli, R., et al. (2021). Loss of Neuronal Miro1 Disrupts Mitophagy and Induces Hyperactivation of the Integrated Stress Response. Embo J. 40, 1–20. doi:10.15252/embj.2018100715
Luo, S., Sun, X., Huang, M., Ma, Q., Du, L., and Cui, Y. (2021). Enhanced Neuroprotective Effects of Epicatechin Gallate Encapsulated by Bovine Milk-Derived Exosomes against Parkinson's Disease through Antiapoptosis and Antimitophagy. J. Agric. Food Chem. 69, 5134–5143. doi:10.1021/acs.jafc.0c07658
Ma, J., Weng, L., Wang, Z., Jia, Y., Liu, B., Wu, S., et al. (2018). MiR-124 Induces Autophagy-Related Cell Death in Cholangiocarcinoma Cells through Direct Targeting of the EZH2-STAT3 Signaling axis. Exp. Cell Res. 366, 103–113. doi:10.1016/J.YEXCR.2018.02.037
Madureira, M., Connor-Robson, N., and Wade-Martins, R. (2020). LRRK2: Autophagy and Lysosomal Activity. Front. Neurosci. 14, 498. doi:10.3389/fnins.2020.00498
Maio, R. Di, Hoffman, E. K., Rocha, E. M., Keeney, M. T., Laurie, H., Miranda, B. R. De, et al. (2018). A Central Role for LRRK2 in Idiopathic Parkinson Disease. Sci. Transl. Med. 10, eaar5429. doi:10.1126/scitranslmed.aar5429.A
Mamais, A., Manzoni, C., Nazish, I., Arber, C., Sonustun, B., Wray, S., et al. (2018). Analysis of Macroautophagy Related Proteins in G2019S LRRK2 Parkinson's Disease Brains with Lewy Body Pathology. Brain Res. 1701, 75–84. doi:10.1016/j.brainres.2018.07.023
Mari, M., Tooze, S. A., and Reggiori, F. (2011). The Puzzling Origin of the Autophagosomal Membrane. F1000 Biol. Rep. 3, 25. doi:10.3410/B3-25
Mazzocchi, M., Collins, L. M., Sullivan, A. M., and O'Keeffe, G. W. (2020). The Class II Histone Deacetylases as Therapeutic Targets for Parkinson's Disease. Neuronal Signal 4, NS20200001. doi:10.1042/NS20200001
McWilliams, T. G., Prescott, A. R., Allen, G. F. G., Tamjar, J., Munson, M. J., Thomson, C., et al. (2016). Mito-QC Illuminates Mitophagy and Mitochondrial Architecture In Vivo. J. Cell Biol. 214, 333–345. doi:10.1083/jcb.201603039
McWilliams, T. G., Prescott, A. R., Montava-Garriga, L., Ball, G., Singh, F., Barini, E., et al. (2018). Basal Mitophagy Occurs Independently of PINK1 in Mouse Tissues of High Metabolic Demand. Cell Metab. 27, 439–449.e5. doi:10.1016/j.cmet.2017.12.008
Mullin, S., Smith, L., Lee, K., D’Souza, G., Woodgate, P., Elflein, J., et al. (2020). Ambroxol for the Treatment of Patients with Parkinson Disease with and without Glucocerebrosidase Gene Mutations. JAMA Neurol. 77, 427–434. doi:10.1001/JAMANEUROL.2019.4611
Narendra, D. P., Jin, S. M., Tanaka, A., Suen, D.-F., Gautier, C. A., Shen, J., et al. (2010). PINK1 Is Selectively Stabilized on Impaired Mitochondria to Activate Parkin. PLoS Biol. 8, e1000298. doi:10.1371/journal.pbio.1000298
Narendra, D., Tanaka, A., Suen, D.-F., and Youle, R. J. (2008). Parkin Is Recruited Selectively to Impaired Mitochondria and Promotes Their Autophagy. J. Cell Biol. 183, 795–803. doi:10.1083/jcb.200809125
Norwitz, N. G., Dearlove, D. J., Lu, M., Clarke, K., Dawes, H., and Hu, M. T. (2020). A Ketone Ester Drink Enhances Endurance Exercise Performance in Parkinson's Disease. Front. Neurosci. 14, 1–11. doi:10.3389/fnins.2020.584130
Oliveira, L. M. A., Gasser, T., Edwards, R., Zweckstetter, M., Melki, R., Stefanis, L., et al. (2021). Alpha-synuclein Research: Defining Strategic Moves in the Battle against Parkinson's Disease. npj Park. Dis. 7 (1), 65. doi:10.1038/s41531-021-00203-9
Pagan, F. L., Hebron, M. L., Wilmarth, B., Torres-Yaghi, Y., Lawler, A., Mundel, E. E., et al. (2020). Nilotinib Effects on Safety, Tolerability, and Potential Biomarkers in Parkinson Disease. JAMA Neurol. 77, 309–317. doi:10.1001/jamaneurol.2019.4200
Paisán-Ruı́z, C., Jain, S., Evans, E. W., Gilks, W. P., Simón, J., van der Brug, M., et al. (2004). Cloning of the Gene Containing Mutations that Cause PARK8-Linked Parkinson's Disease. Neuron 44, 595–600. doi:10.1016/j.neuron.2004.10.023
Pan, T., Kondo, S., Le, W., and Jankovic, J. (2008). The Role of Autophagy-Lysosome Pathway in Neurodegeneration Associated with Parkinson's Disease. Brain 131, 1969–1978. doi:10.1093/BRAIN/AWM318
Parnetti, L., Paciotti, S., Eusebi, P., Dardis, A., Zampieri, S., Chiasserini, D., et al. (2017). Cerebrospinal Fluid β-glucocerebrosidase Activity Is Reduced in Parkinson's Disease Patients. Mov. Disord. 32, 1423–1431. doi:10.1002/MDS.27136
Ping, F., Jiang, N., and Li, Y. (2020). Association between Metformin and Neurodegenerative Diseases of Observational Studies: Systematic Review and Meta-Analysis. BMJ Open Diab Res. Care 8, e001370. doi:10.1136/bmjdrc-2020-001370
Pino, E., Amamoto, R., Zheng, L., Cacquevel, M., Sarria, J.-C., Knott, G. W., et al. (2014). FOXO3 Determines the Accumulation of α-synuclein and Controls the Fate of Dopaminergic Neurons in the Substantia Nigra. Hum. Mol. Genet. 23, 1435–1452. doi:10.1093/hmg/ddt530
Qin, X., Zhang, X., Li, P., Wang, M., Yan, L., Bao, Z., et al. (2021). Association between Diabetes Medications and the Risk of Parkinson's Disease: A Systematic Review and Meta-Analysis. Front. Neurol. 12, 1–10. doi:10.3389/fneur.2021.678649
Ramirez, A., Heimbach, A., Gründemann, J., Stiller, B., Hampshire, D., Cid, L. P., et al. (2006). Hereditary Parkinsonism with Dementia Is Caused by Mutations in ATP13A2, Encoding a Lysosomal Type 5 P-type ATPase. Nat. Genet. 38, 1184–1191. doi:10.1038/ng1884
Saedi, S., Hemmati-Dinarvand, M., Barmaki, H., Mokhtari, Z., Musavi, H., Valilo, M., et al. (2021). Serum Lipid Profile of Parkinson’s Disease Patients: A Study from the Northwest of Iran. Casp. J. Intern. Med. 12, 155–161. doi:10.22088/cjim.12.2.155
Sanchez-mirasierra, I., Hernandez-diaz, S., Ghimire, S., Main, P., and Soukup, S. (2021). “Altered Autophagy on the Path to Parkinson’s Disease,” in Autophagy in Health and Disease. Editors B. A. Rothermel, and D. Abhinav (Netherlands: Elsevier), 271–286.
Santaella, A., Kuiperij, H. B., Van Rumund, A., Esselink, R. A. J., Van Gool, A. J., Bloem, B. R., et al. (2020). Inflammation Biomarker Discovery in Parkinson's Disease and Atypical Parkinsonisms. BMC Neurol. 20, 26–28. doi:10.1186/S12883-020-1608-8/FIGURES/2
Santo, E. E., and Paik, J. (2018). FOXO in Neural Cells and Diseases of the Nervous System. Curr. Top. Dev. Biol. 127, 105–118. doi:10.1016/BS.CTDB.2017.10.002
Sardiello, M., Palmieri, M., di Ronza, A., Medina, D. L., Valenza, M., Gennarino, V. A., et al. (2009). A Gene Network Regulating Lysosomal Biogenesis and Function. Science 325, 473–477. doi:10.1126/science.1174447
Satake, W., Nakabayashi, Y., Mizuta, I., Hirota, Y., Ito, C., Kubo, M., et al. (2009). Genome-wide Association Study Identifies Common Variants at Four Loci as Genetic Risk Factors for Parkinson's Disease. Nat. Genet. 41, 1303–1307. doi:10.1038/ng.485
Schlichtmann, B. W., Kalyanaraman, B., Schlichtmann, R. L., Panthani, M. G., Anantharam, V., Kanthasamy, A. G., et al. (2021). Functionalized Polyanhydride Nanoparticles for Improved Treatment of Mitochondrial Dysfunction. J. Biomed. Mater Res. 110, 450–459. doi:10.1002/jbm.b.34922
Schmidt, M. F., Gan, Z. Y., Komander, D., and Dewson, G. (2021). Ubiquitin Signalling in Neurodegeneration: Mechanisms and Therapeutic Opportunities. Cell Death Differ. 28, 570–590. doi:10.1038/s41418-020-00706-7
Schneider, J. S., Roeltgen, D. P., Rothblat, D. S., Chapas-Crilly, J., Seraydarian, L., and Rao, J. (1995). GM1 Ganglioside Treatment of Parkinson's Disease: An Open Pilot Study of Safety and Efficacy. Neurology 45, 1149–1154. doi:10.1212/WNL.45.6.1149
Schneider, S. A., Hizli, B., and Alcalay, R. N. (2020). Emerging Targeted Therapeutics for Genetic Subtypes of Parkinsonism. Neurotherapeutics 17, 1378–1392. doi:10.1007/s13311-020-00920-8
Schrag, A., Siddiqui, U. F., Anastasiou, Z., Weintraub, D., and Schott, J. M. (2017). Clinical Variables and Biomarkers in Prediction of Cognitive Impairment in Patients with Newly Diagnosed Parkinson's Disease: a Cohort Study. Lancet Neurology 16, 66–75. doi:10.1016/S1474-4422(16)30328-3
Shaltouki, A., Hsieh, C.-H., Kim, M. J., and Wang, X. (2018). Alpha-synuclein Delays Mitophagy and Targeting Miro Rescues Neuron Loss in Parkinson's Models. Acta Neuropathol. 136, 607–620. doi:10.1007/s00401-018-1873-4
Shao, Y., and Le, W. (2019). Recent Advances and Perspectives of Metabolomics-Based Investigations in Parkinson's Disease. Mol. Neurodegener. 14, 3. doi:10.1186/s13024-018-0304-2
Shi, L., Huang, C., Luo, Q., Xia, Y., Liu, W., Zeng, W., et al. (2020). Clioquinol Improves Motor and Non-motor Deficits in MPTP-Induced Monkey Model of Parkinson's Disease through AKT/mTOR Pathway. Aging 12, 9515–9533. doi:10.18632/aging.103225
Silveira, C. R. A., MacKinley, J., Coleman, K., Li, Z., Finger, E., Bartha, R., et al. (2019). Ambroxol as a Novel Disease-Modifying Treatment for Parkinson's Disease Dementia: Protocol for a Single-Centre, Randomized, Double-Blind, Placebo-Controlled Trial. BMC Neurol. 19, 1–10. doi:10.1186/s12883-019-1252-3
Simón-Sánchez, J., Schulte, C., Bras, J. M., Sharma, M., Gibbs, J. R., Berg, D., et al. (2009). Genome-wide Association Study Reveals Genetic Risk Underlying Parkinson's Disease. Nat. Genet. 41, 1308–1312. doi:10.1038/ng.487
Simuni, T., Fiske, B., Merchant, K., Coffey, C. S., Klingner, E., Caspell-garcia, C., et al. (2021). Efficacy of Nilotinib in Patients with Moderately Advanced Parkinson Disease. JAMA Neurol. 78, 312–320. doi:10.1001/jamaneurol.2020.4725
Song, J.-X., Lu, J.-H., Liu, L.-F., Chen, L.-L., Durairajan, S. S. K., Yue, Z., et al. (2014). HMGB1 Is Involved in Autophagy Inhibition Caused by SNCA/α-synuclein Overexpression. Autophagy 10, 144–154. doi:10.4161/auto.26751
Soukup, S.-F., Kuenen, S., Vanhauwaert, R., Manetsberger, J., Hernández-Díaz, S., Swerts, J., et al. (2016). A LRRK2-Dependent EndophilinA Phosphoswitch Is Critical for Macroautophagy at Presynaptic Terminals. Neuron 92, 829–844. doi:10.1016/j.neuron.2016.09.037
Soukup, S. F., Vanhauwaert, R., and Verstreken, P. (2018). Parkinson's Disease: Convergence on Synaptic Homeostasis. EMBO J. 37, 1–16. doi:10.15252/embj.201898960
Sportelli, C., Urso, D., Jenner, P., and Chaudhuri, K. R. (2020). Metformin as a Potential Neuroprotective Agent in Prodromal Parkinson's Disease-Viewpoint. Front. Neurol. 11, 1–10. doi:10.3389/fneur.2020.00556
Stacchiotti, A., and Corsetti, G. (2020). Natural Compounds and Autophagy: Allies against Neurodegeneration. Front. Cell Dev. Biol. 8, 1–16. doi:10.3389/fcell.2020.555409
Stott, S. R. W., Wyse, R. K., and Brundin, P. (2021). Drug Repurposing for Parkinson's Disease: The International Linked Clinical Trials Experience. Front. Neurosci. 15, 1–9. doi:10.3389/fnins.2021.653377
Su, C.-F., Jiang, L., Zhang, X.-W., Iyaswamy, A., and Li, M. (2021a). Resveratrol in Rodent Models of Parkinson's Disease: A Systematic Review of Experimental Studies. Front. Pharmacol. 12, 1–10. doi:10.3389/fphar.2021.644219
Su, Y., Zhang, Z., Li, H., Ma, J., Sun, L., Shao, S., et al. (2021b). A GLP-2 Analogue Protects SH-SY5Y and Neuro-2a Cells against Mitochondrial Damage, Autophagy Impairments and Apoptosis in a Parkinson Model. Drug Res. (Stuttg) 71, 43–50. doi:10.1055/a-1266-3263
Sun, N., Yun, J., Liu, J., Malide, D., Liu, C., Rovira, I. I., et al. (2015). Measuring In Vivo Mitophagy. Mol. Cell 60, 685–696. doi:10.1016/j.molcel.2015.10.009
Sung, H., Tandarich, L. C., Nguyen, K., and Hollenbeck, P. J. (2016). Compartmentalized Regulation of Parkin-Mediated Mitochondrial Quality Control in the Drosophila Nervous System In Vivo. J. Neurosci. 36, 7375–7391. doi:10.1523/JNEUROSCI.0633-16.2016
Tansey, M. G., and Goldberg, M. S. (2010). Neuroinflammation in Parkinson's Disease: Its Role in Neuronal Death and Implications for Therapeutic Intervention. Neurobiol. Dis. 37, 510–518. doi:10.1016/j.nbd.2009.11.004
Tasegian, A., Singh, F., Ganley, I. G., Reith, A. D., and Alessi, D. R. (2021). Impact of Type II LRRK2 Inhibitors on Signaling and Mitophagy. Biochem. J. 478, 3555–3573. doi:10.1042/bcj20210375
Tedeschi, V., Petrozziello, T., Sisalli, M. J., Boscia, F., Canzoniero, L. M. T., and Secondo, A. (2019). The Activation of Mucolipin TRP Channel 1 (TRPML1) Protects Motor Neurons from L-BMAA Neurotoxicity by Promoting Autophagic Clearance. Sci. Rep. 9, 1–11. doi:10.1038/s41598-019-46708-5
Tsunemi, T., Perez-Rosello, T., Ishiguro, Y., Yoroisaka, A., Jeon, S., Hamada, K., et al. (2019). Increased Lysosomal Exocytosis Induced by Lysosomal Ca2+ Channel Agonists Protects Human Dopaminergic Neurons from α-Synuclein Toxicity. J. Neurosci. 39, 5760–5772. doi:10.1523/JNEUROSCI.3085-18.2019
Valente, E. M., Bentivoglio, A. R., Dixon, P. H., Ferraris, A., Ialongo, T., Frontali, M., et al. (2001). Localization of a Novel Locus for Autosomal Recessive Early-Onset Parkinsonism, PARK6, on Human Chromosome 1p35-P36. Am. J. Hum. Genet. 68, 895–900. doi:10.1086/319522
Vanhauwaert, R., Kuenen, S., Masius, R., Bademosi, A., Manetsberger, J., Schoovaerts, N., et al. (2017). The SAC 1 Domain in Synaptojanin Is Required for Autophagosome Maturation at Presynaptic Terminals. EMBO J. 36, 1392–1411. doi:10.15252/embj.201695773
Villumsen, M., Aznar, S., Pakkenberg, B., Jess, T., and Brudek, T. (2019). Inflammatory Bowel Disease Increases the Risk of Parkinson's Disease: a Danish Nationwide Cohort Study 1977-2014. Gut 68, 18–24. doi:10.1136/GUTJNL-2017-315666
Vogiatzi, T., Xilouri, M., Vekrellis, K., and Stefanis, L. (2008). Wild Type α-Synuclein Is Degraded by Chaperone-Mediated Autophagy and Macroautophagy in Neuronal Cells. J. Biol. Chem. 283, 23542–23556. doi:10.1074/jbc.M801992200
von Euler Chelpin, M., and Vorup-Jensen, T. (2017). Targets and Mechanisms in Prevention of Parkinson's Disease through Immunomodulatory Treatments. Scand. J. Immunol. 85, 321–330. doi:10.1111/SJI.12542
von Linstow, C. U., Gan-Or, Z., and Brundin, P. (2020). Precision Medicine in Parkinson's Disease Patients with LRRK2 and GBA Risk Variants - Let's Get Even More Personal. Transl. Neurodegener. 9, 1–10. doi:10.1186/s40035-020-00218-x
Wallings, R., Connor-Robson, N., and Wade-Martins, R. (2019). LRRK2 Interacts with the Vacuolar-type H+-ATPase Pump A1 Subunit to Regulate Lysosomal Function. Hum. Mol. Genet. 28, 2696–2710. doi:10.1093/hmg/ddz088
Wallings, R. L., and Tansey, M. G. (2019). LRRK2 Regulation of Immune-Pathways and Inflammatory Disease. Biochem. Soc. Trans. 47, 1581–1595. doi:10.1042/BST20180463
Wallings, R., Manzoni, C., and Bandopadhyay, R. (2015). Cellular Processes Associated with LRRK2 Function and Dysfunction. FEBS J. 282, 2806–2826. doi:10.1111/febs.13305
Wang, D. B., Kinoshita, C., Kinoshita, Y., Sopher, B. L., Uo, T., Lee, R. J., et al. (2019a). Neuronal Susceptibility to Beta-Amyloid Toxicity and Ischemic Injury Involves Histone Deacetylase-2 Regulation of Endophilin-B1. Brain Pathol. 29, 164–175. doi:10.1111/bpa.12647
Wang, S., Ma, J., Zeng, Y., Zhou, G., Wang, Y., Zhou, W., et al. (2021a). Icariin, an Up-And-Coming Bioactive Compound against Neurological Diseases: Network Pharmacology-Based Study and Literature Review. Dddt 15, 3619–3641. doi:10.2147/DDDT.S310686
Wang, X., Chi, J., Huang, D., Ding, L., Zhao, X., Jiang, L., et al. (2019b). α-Synuclein Promotes Progression of Parkinson's Disease by Upregulating Autophagy Signaling Pathway to Activate NLRP3 Inflammasome. Exp. Ther. Med. 19, 931–938. doi:10.3892/etm.2019.8297
Wang, Z.-Y., Liu, J., Zhu, Z., Su, C.-F., Sreenivasmurthy, S. G., Iyaswamy, A., et al. (2021b). Traditional Chinese Medicine Compounds Regulate Autophagy for Treating Neurodegenerative Disease: A Mechanism Review. Biomed. Pharmacother. 133, 110968. doi:10.1016/j.biopha.2020.110968
Wigger, D., Gulbins, E., Kleuser, B., and Schumacher, F. (2019). Monitoring the Sphingolipid De Novo Synthesis by Stable-Isotope Labeling and Liquid Chromatography-Mass Spectrometry. Front. Cell Dev. Biol. 7, 210. doi:10.3389/FCELL.2019.00210/BIBTEX
Xiang, H., Zhang, J., Lin, C., Zhang, L., Liu, B., and Ouyang, L. (2020). Targeting Autophagy-Related Protein Kinases for Potential Therapeutic Purpose. Acta Pharm. Sin. B 10, 569–581. doi:10.1016/j.apsb.2019.10.003
Xicoy, H., Peñuelas, N., Vila, M., and Laguna, A. (2019a). Autophagic- and Lysosomal-Related Biomarkers for Parkinson's Disease: Lights and Shadows. Cells 8, 1317. doi:10.3390/CELLS8111317
Xicoy, H., Wieringa, B., and Martens, G. J. M. (2019b). The Role of Lipids in Parkinson's Disease. Cells 8, 27. doi:10.3390/CELLS8010027
Xiong, S., Liu, W., Zhou, Y., Mo, Y., Liu, Y., Chen, X., et al. (2020). Enhancement of Oral Bioavailability and Anti-Parkinsonian Efficacy of Resveratrol through a Nanocrystal Formulation. Asian J. Pharm. Sci. 15, 518–528. doi:10.1016/j.ajps.2019.04.003
Yang, Y., Chen, L., Yao, J., Wang, N., Liu, D., Wang, Y., et al. (2021a). Early Implementation of Intended Exercise Improves Quality of Life in Parkinson's Disease Patients. Neurol. Sci. 43, 1761–1767. doi:10.1007/s10072-021-05530-6
Yang, Y., Li, Y., Yang, H., Guo, J., and Li, N. (2021b). Circulating MicroRNAs and Long Non-coding RNAs as Potential Diagnostic Biomarkers for Parkinson's Disease. Front. Mol. Neurosci. 14, 631553. doi:10.3389/FNMOL.2021.631553
Yogalingam, G., and Pendergast, A. M. (2008). Abl Kinases Regulate Autophagy by Promoting the Trafficking and Function of Lysosomal Components. J. Biol. Chem. 283, 35941–35953. doi:10.1074/jbc.M804543200
Youn, J., Lee, S.-B., Lee, H. S., Yang, H. O., Park, J., Kim, J. S., et al. (2018). Cerebrospinal Fluid Levels of Autophagy-Related Proteins Represent Potentially Novel Biomarkers of Early-Stage Parkinson's Disease. Sci. Rep. 8, 16866. doi:10.1038/s41598-018-35376-6
Zeng, R., Zhou, Q., Zhang, W., Fu, X., Wu, Q., Lu, Y., et al. (2019). Icariin-mediated Activation of Autophagy Confers Protective Effect on Rotenone Induced Neurotoxicity In Vivo and In Vitro. Toxicol. Rep. 6, 637–644. doi:10.1016/j.toxrep.2019.06.014
Zhang, C., Wang, R., Liu, Z., Bunker, E., Lee, S., Giuntini, M., et al. (2019). The Plant Triterpenoid Celastrol Blocks PINK1-dependent Mitophagy by Disrupting PINK1's Association with the Mitochondrial Protein TOM20. J. Biol. Chem. 294, 7472–7487. doi:10.1074/jbc.RA118.006506
Zhang, C., Zhao, M., Wang, B., Su, Z., Guo, B., Qin, L., et al. (2021a). The Nrf2-NLRP3-Caspase-1 axis Mediates the Neuroprotective Effects of Celastrol in Parkinson's Disease. Redox Biol. 47, 102134. doi:10.1016/j.redox.2021.102134
Zhang, K., Zhu, S., Li, J., Jiang, T., Feng, L., Pei, J., et al. (2021b). Targeting Autophagy Using Small-Molecule Compounds to Improve Potential Therapy of Parkinson's Disease. Acta Pharm. Sin. B 11, 3015–3034. doi:10.1016/J.APSB.2021.02.016
Zhang, L., Zhang, L., Li, Y., Li, L., Melchiorsen, J. U., Rosenkilde, M., et al. (2020). The Novel Dual GLP-1/GIP Receptor Agonist DA-CH5 Is Superior to Single GLP-1 Receptor Agonists in the MPTP Model of Parkinson's Disease. Jpd 10, 523–542. doi:10.3233/JPD-191768
Zhao, H. T., John, N., Delic, V., Ikeda-Lee, K., Kim, A., Weihofen, A., et al. (2017). LRRK2 Antisense Oligonucleotides Ameliorate α-Synuclein Inclusion Formation in a Parkinson's Disease Mouse Model. Mol. Ther. - Nucleic Acids 8, 508–519. doi:10.1016/j.omtn.2017.08.002
Keywords: autophagy, Parkinson’s disease, autophagy modulators, PD causative proteins, potential therapeutic avenues
Citation: Sanchez-Mirasierra I, Ghimire S, Hernandez-Diaz S and Soukup S-F (2022) Targeting Macroautophagy as a Therapeutic Opportunity to Treat Parkinson’s Disease. Front. Cell Dev. Biol. 10:921314. doi: 10.3389/fcell.2022.921314
Received: 15 April 2022; Accepted: 13 June 2022;
Published: 06 July 2022.
Edited by:
Patrice X. Petit, Centre National de la Recherche Scientifique (CNRS), FranceReviewed by:
Dan Lindholm, University of Helsinki, FinlandCopyright © 2022 Sanchez-Mirasierra, Ghimire, Hernandez-Diaz and Soukup. This is an open-access article distributed under the terms of the Creative Commons Attribution License (CC BY). The use, distribution or reproduction in other forums is permitted, provided the original author(s) and the copyright owner(s) are credited and that the original publication in this journal is cited, in accordance with accepted academic practice. No use, distribution or reproduction is permitted which does not comply with these terms.
*Correspondence: Sandra-Fausia Soukup, c2FuZHJhLnNvdWt1cEB1LWJvcmRlYXV4LmZy
†These authors have contributed equally to this work