- Guangdong Key Laboratory of Non-human Primate Research, Guangdong-Hongkong-Macau Institute of CNS Regeneration, Jinan University, Guangzhou, China
The foundation for investigating the mechanisms of human diseases is the establishment of animal models, which are also widely used in agricultural industry, pharmaceutical applications, and clinical research. However, small animals such as rodents, which have been extensively used to create disease models, do not often fully mimic the key pathological changes and/or important symptoms of human disease. As a result, there is an emerging need to establish suitable large animal models that can recapitulate important phenotypes of human diseases for investigating pathogenesis and developing effective therapeutics. However, traditional genetic modification technologies used in establishing small animal models are difficultly applied for generating large animal models of human diseases. This difficulty has been overcome to a great extent by the recent development of gene editing technology, especially the clustered regularly interspaced short palindromic repeats (CRISPR)/CRISPR-associated protein 9 (Cas9). In this review, we focus on the applications of CRISPR/Cas9 system to establishment of large animal models, including nonhuman primates, pigs, sheep, goats and dogs, for investigating disease pathogenesis and treatment. We also discuss the limitations of large animal models and possible solutions according to our current knowledge. Finally, we sum up the applications of the novel genome editing tool Base Editors (BEs) and its great potential for gene editing in large animals.
Introduction
Animal models play an important role in scientific research, including the study of disease mechanisms, medicinal development and the production of agricultural products (McGonigle and Ruggeri, 2014). To create ideal animal models, researchers often genetically modify animals to achieve desirable traits. Gene-modified small rodent models, especially mice and rats, provide a large amount of experimental data and play an important role in the study of disease mechanisms as well as important biology function (Ribitsch et al., 2020). However, these small animal models also have some shortcomings. First, because of considerable differences between small animals and humans in physiological, anatomical, and genomic structures, small animal models are often unable to mimic the disease characteristics of humans, leading to the inability of researchers to fully understand the pathogenesis of diseases. This has also led to the failure of many drugs screened from small animal models in clinical trials (Prabhakar, 2012; Zhao et al., 2019). In addition, small animal models play less roles in agricultural activities, such as the production of animal by-products.
To overcome these limitations, scientists are increasingly focusing on large animal models (including non-human primates (NHPs), pigs, dogs, goats, and sheep). Large animals represented by NHPs are more ideal animal models for human diseases due to their similarities in genetics, physiology, developmental biology, social behavior and cognition (Shen, 2013). For example, the brain mass of different species, which are depicted in Figure 1, are apparently very different. However, many factors, including the difficulties in genome editing, have limited the establishment of gene modified large animal models.
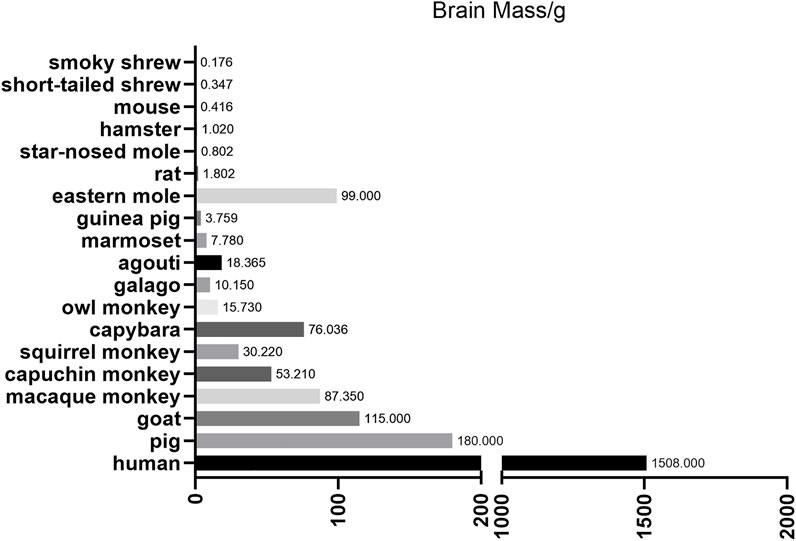
FIGURE 1. Brain mass for different animals. This figure mainly depicts the brain mass of different large animals and small animals. Notice that large animals, such as pigs and nonhuman primates, are much closer to human beings compared with small animals to some extent.
With the development of gene editing technology in recent twodecades, like zinc finger nucleases (ZFNs), transcription activator-like effector nucleases (TALENs) and CRISPR/Cas9, this difficulty has been overcome greatly. The ZFNs system consists of two components, including DNA-binding zinc-finger protein (ZFP) domain at the amino terminus and the Fok I nuclease cleavage domain at the carboxyl terminus. Each Fok I monomer link with a ZFP to form a ZFN that recognizes a specific site. Under certain conditions, two ZFNs can perform enzyme function of cleavage, leading to double-strand breaks (DSBs), thus mediating DNA site-specific cleavage (Durai et al., 2005; Carroll, 2011). ZFNs have been used in a variety of species, including plants, animal and mammalian cells (Carroll, 2011). However, due to the differences between large animals and small animals, it is still difficult to establish a large animal model using ZFNs(Chen et al., 2016). TALENs are gene-editing tools similar to ZFNs(Christian et al., 2010; Mussolino and Cathomen, 2012; Joung and Sander, 2013). They are also made by two structures: a transcription activator-like (TAL) effector DNA-binding domain and a DNA cleavage domain (a nuclease which cuts DNA strands). Thus, TALENs can also be designed to induce site-specific DSBs to target specific gene sequences (Yoshimi and Mashimo, 2018). Compared with ZFNs, the synthesis and design of TALENs components are simpler, so some large animal models are successfully established by TALENs strategy. For example, in 2014, researchers created methyl CpG binding protein 2 (MECP2) Mutant Rhesus and Cynomolgus monkeys using TALENs(Liu et al., 2014).
Although ZFNs and TALENs have greatly improved the efficiency of establishing gene editing animal models, CRISPR/Cas9 is the most popular and effective gene editing method at present. The CRISPR/Cas9 system also consists of a recognition component, small RNAs called single-guide RNAs (sgRNAs), and a cleavage component, Cas9 nuclease. CRISPR/Cas9 can target almost any loosened (non-condensed) part of the genomes through base pairing between sgRNAs and DNA as well as the recognition of protospacer adjacent motif (PAM) sequences (Jinek et al., 2012). Cas9 then cleaves the double-strand DNA at the target site to form DSBs. Subsequently, cells repair DSBs sites by non-homologous end joining (NHEJ) or homology-directed repair (HDR). The repair process can go wrong, resulting in mutations at specific genetic loci. Since the discovery of CRISPR/Cas9, researchers have rapidly implemented a series of optimizations to the system, and applied it in the establishment of gene-editing models of small animal, including mice (Wang et al., 2013), rats (Ma et al., 2014) and zebrafishes (Kim and Kim, 2014). As a result, the CRISPR/Cas9 system has greatly accelerated the research of gene editing large animal models, which is what we mainly discuss in this article.
The Application of CRISPR/Cas9 in Nonhuman Primate Models
Undoubtedly, the nonhuman primate is the most representative animal that is capable of mimicking the human disease for the similarities in terms of genetics, physiology, developmental biology, social behaviors and cognition. However, it is really difficult to create a nonhuman primate transgenic model compared with small animals, such as the mouse because of various factors including the long breeding cycle and ethical factors. The first transgenic mouse model was built as early as 1974 (Jaenisch and Mintz, 1974), but the first genetically modified monkey model did not appear until 2001 (Chan et al., 2001). Afterwards, in 2008, Yang et al. developed a transgenic model of Huntington’s disease (HD) in a rhesus macaque that expressed polyglutamine (polyQ)-expanded huntingtin protein (HTT) by injecting lentiviral vector into mature rhesus oocytes followed by fertilization through intracytoplasmic sperm injection and embryo transplantation (Yang et al., 2008). This is the first transgenic nonhuman primate disease model, which could already show some features similar to those found in HD patients, including chorea, dystonia as well as nuclear inclusion and neuropil aggregates in the brains. In the later experiment, they used the same transgenic strategy to introduce mutant huntingtin (HTT) genes into monkey oocytes, which also expressed exon1 of HTT with a 147Q tract (previous models containing a 65–88Q tract and dying soon) in transgenic monkeys (Wang et al., 2008). These HD monkeys display degeneration of axons and neuronal processes, suggesting that the disruption in axons or dendrites can lead to the neuronal degeneration in HD.
The success of transgenic monkey models of human disease and the advances in gene editing technology inspired scientists to establish genome editing monkey models. The generation of a gene-modified monkey via CRISPR/Cas9 was first reported in 2014 (Niu et al., 2014). By coinjection of Cas9 mRNA and sgRNAs into one-cell-stage embryos, researchers successfully achieved precise gene targeting in cynomolgus monkeys. They also showed that this system enabled simultaneous disruption of two target genes (peroxisome proliferator-activated receptor gamma (PPARG) and recombination activating gene 1 (RAG1) in one step without detectable off-target effects. They also demonstrated that germline transmission could happen in the Cas9-manipulated monkeys by examining gene targeting in gonads and germ cells (Chen et al., 2015a). However, the resulting transgenic monkey exhibited mosaic mutations accompanied by the presence of wild type allele in different tissues, which left an issue of whether the mosaic mutations could influence the function study. Then, Chen et al. used Cas9 to disrupt the dystrophin gene (DMD) in rhesus monkeys, which exhibited markedly depleted dystrophin and muscle degeneration seen in early Duchenne muscular dystrophy (DMD) (Chen et al., 2015b), indicating that CRISPR/Cas9 can efficiently generate monkey models of human diseases regardless of inheritance patterns.
Next, some experiments were conducted aimed at eliminating the mosaic mutations. Researchers showed that biallelic gene mutation can be efficiently generated in monkeys by zygote injection with an optimized Cas9/sgRNA combination in one-step (Wan et al., 2015). After optimization and innovation of the approach, Zuo et al. also showed that a single gene or multiple genes can be completely knocked out in monkey embryos by zygotic injection of Cas9 mRNA and multiple adjacent sgRNAs without mosaicism (Zuo et al., 2017). Apart from that, another research indicated that shortening the half-life of Cas9 in fertilized zygotes reduced mosaic mutations and increased its ability to modify genomes in monkey embryos (Tu et al., 2017). Following this approach, they used the Cas9/sgRNA method to disrupt SH3 and ankyrin repeat domains 3 gene (SHANK3) in cynomolgus monkeys, which showed altered neurogenesis and disrupted expression of synaptic proteins in the prefrontal cortex, which was not found in the mouse model (Zhao et al., 2017).
Apart from knockout animals, researchers have also attempted to obtain knock-in nonhuman primates in recent years. Yao et al. first established knock-in monkeys by homology-mediated end joining (HMEJ)-based method. However, monkeys generated by this approach showed mosaicism. Therefore, further serial crossbreeding is required to generate complete gene knock-in monkeys (Yao et al., 2018). A similar work to achieve precise OCT4-hrGFP (octamer-binding transcription factor 4-humanized recombinant green fluorescent protein) knock-in in cynomolgus monkey model was also tried via CRISPR/Cas9-assisted HR (Cui et al., 2018).
However, a genetically modified nonhuman primate is expensive to maintain and requires the facility that is only available to a small number of laboratories. Thus, researchers have tried to establish the modified embryonic stem cells (ESCs) of rhesus monkey using the CRISPR/Cas9 system, which can undergo unlimited self-renewal while maintaining the potential to give rise to all cell types (Zhu et al., 2015; Kobayashi et al., 2019). Using a dual-guide gene targeting approach, another group achieved biallelic deletions in the CCR5 gene (C-C motif chemokine receptor 5) of cynomolgus macaque embryos (23–37%) (Schmidt et al., 2020).
Although CRISPR/Cas9 system is the most widely used strategy in the creation of large animal models nowadays, there were also nonhuman primate models using different approaches like TALENs(Liu et al., 2014; Liu et al., 2016; Chen et al., 2017). An obvious issue is the safety of the gene-editing in nonhuman primates due to potential off-target mutations. Using whole-genome sequencing to comprehensively assess on- and off-target mutations in previously produced CRISPR/Cas9 editing monkeys (Chen et al., 2015b), researchers found that CRISPR/Cas9-based gene editing is active on the expected genomic sites without producing off-target modifications in other functional regions of the genome, suggesting that the CRISPR/Cas9 technique could be relatively safe and effective in modeling genetic disease in nonhuman primates (Wang S. et al., 2018; Luo et al., 2019).
CRISPR/Cas9 editing monkeys have already shown better phenotypes in human diseases than mouse models despite a limited number of successfully established models (Seita et al., 2020; Yang et al., 2021; Yin et al., 2022). Yang et al. generated PTEN-induced kinase 1 (PINK1, whose mutations cause early-onset Parkinson’s disease (PD) mutant monkeys by targeting two exons in the PINK1 gene (Yang et al., 2019a; Yang et al., 2019b), which showed remarkable neuronal loss in the cortex, substantia nigra and striatum. However, neuronal loss was not reported in Pink1 KO mice (Kitada et al., 2007; Dawson et al., 2010) or pigs (Zhou et al., 2015; Wang et al., 2016b). Similar results were confirmed in acute monkey models created by CRISPR/Cas9 system (Li et al., 2021; Sun et al., 2022; Yang et al., 2022). In another example, Kang et al. achieved dosage-sensitive sex reversal, adrenal hypoplasia critical region, on chromosome X, gene 1 (DAX1) knockout in the monkey, which could recapitulate the phenotypes of human adrenal hypoplasia congenita (AHC) and hypogonadotropic hypogonadism (HH).
Nonhuman primate models of human diseases were also used for developing therapies. Tu et al. found that abnormal behaviors and brain activities of autism spectrum disorder (ASD) in the previously established monkey models (Zhao et al., 2017) were alleviated by the antidepressant fluoxetine treatment (Tu et al., 2019). This finding demonstrated that the genetically modified non-human primate can be used for translational research of therapeutics for ASD, pointing out that the nonhuman primate models of human disease have a great potential for clinical research like drug tests.
The Application of CRISPR/Cas9 in Pig Models
The pig is also an important animal with several unique features that make it a promising alternative animal model (Prather et al., 2003). Pigs are model animals that are also close to humans (Meurens et al., 2012). Pigs and humans are extremely similar in terms of anatomy, physiology and biochemical metabolism (Roura et al., 2016). Pigs have the advantages of early sexual maturity, short reproductive cycle, high number of offspring per litter (Zou et al., 2019). Moreover, the recent development of somatic cell nuclear transfer (SCNT) technology and genome editing technology has made it possible to generate genetically modified large animals efficiently (Yang et al., 2014). The first genome editing pigs were generated in 1985 using pronuclear DNA microinjection in zygotes (Hammer et al., 1985). With the development of CRISPR/Cas9 technology, the speed of building genome-edited pig models has been greatly accelerated.
Hai et al. first showed that zygotes microinjection of the CRISPR/Cas9 system can efficiently generate genome-modified pigs in one step (Hai et al., 2014), and the mutations can be transmitted into the germline efficiently. Different researchers then reported that single- or double-gene targeted pigs can be effectively achieved by using the CRISPR/Cas9 system combined with SCNT, which avoids mosaic mutation and detectable off-target effects (Whitworth et al., 2014; Zhou et al., 2015). Also, the modification of multiple genes like triple gene-targeted pigs was feasible to be generated (Wang et al., 2016b).
Apart from knockout pigs, CRISPR/Cas system is also used to establish knock-in models that mimic human diseases. Yan et al. used CRISPR/Cas9 to insert a large CAG repeat (150 CAGs) into the endogenous pig HTT gene in fibroblast cells and employed the SCNT to generate a HD KI pig model expressing full-length mutant HTT at the endogenous level (Yan et al., 2018), whose brains presented severe and preferential neurodegeneration in the medium spiny neurons like HD patients. Other examples include genes or large fragment knock-in pig models (Ruan et al., 2015; Li G. et al., 2020).
Based on these successful trials in establishing knockout or knock-in animals using CRISPR/Cas9 system, a number of pig models that could recapture the features of human diseases have been created, such as 5-hydroxytryptamine (5-HT) deficiency (Li et al., 2017b), complement protein deficiency (Zhang W. et al., 2017), cardiovascular disease (Huang et al., 2017), cancer (Kang et al., 2016), type II collagenopathy (Zhang et al., 2020), and HD (Yan et al., 2018).
On the other hand, the pig is one of the most important livestock in the agriculture industry. Genetically modified pigs may offer distinct features, such as increased mass of muscle and resistance to the pathogen. As a result, researchers have made great efforts to improve the mass of muscle by using CRISPR/Cas9 system to disrupt genes that hinder the hypertrophy of muscle (Wang K. et al., 2015; Wang et al., 2017; Zou et al., 2018; Liu et al., 2019; Li R. et al., 2020) and improve the resistance to virus (Xie et al., 2020).
Apart from this, the pig is considered to be an important resource of donor organs for transplantation because of the growing demand in the xenotransplantation. A key problem after xenotransplantation is the pig-to-human immunological compatibility. Therefore, a great deal of genome editing pigs has been established to eliminate antigens leading to immunological rejection in human (Petersen et al., 2016; Chuang et al., 2017; Gao et al., 2017; Wu et al., 2017; Joanna et al., 2018). Another problem is the risk of cross-species transmission of porcine endogenous retroviruses (PERVs). Therefore, researchers inactivated all of the PERVs in a porcine primary cell line and generated PERV-inactivated pigs via SCNT and CRISPR/Cas9 system (Yang et al., 2015; Niu D. et al., 2017; Yue et al., 2021). These efforts were aimed to make the clinical usage of pig organs safer. Recently, a series of stunning reports have provided the first results showing the feasibility of transplanting organs from transgenic pigs into humans, including the kidney and the heart (2022; Porrett et al., 2022), which marked a great breakthrough in the clinical application.
The Application of CRISPR/Cas9 in Sheep and Goat Models
Sheep and goats have also become important model animals in biomedical research due to their suitable size and short gestation period. Like pigs, sheep and goats also play an important role in agricultural and pharmaceutical field for their meat, milk, fiber, and other by-products. Han et al. reported the successful one-step generation of gene knockout sheep using a one-step zygote injection of the CRISPR/Cas9 system by targeting the myostatin (MSTN) gene (Zhengxing et al., 2014), which demonstrated the feasibility of gene targeting in sheep using the CRISPR/Cas9 system at the first time. At the same year, Ni et al. showed for the first time that the CRISPR/Cas9 mediated genome editing can be efficiently accomplished in goats (Ni et al., 2014) and the single-gene knockout fibroblasts were successfully used for SCNT and resulted in live-born goats harboring biallelic mutations.
Apart from the knockout strategy based on the aberrant DNA repair to generate frameshifting insertion-deletion mutations (indels), whether this genomic engineering technique involving HR can be used to introduce defined point mutations is another question. Subsequently, Niu et al. reported a G→A point mutation in the growth differentiation factor 9 (GDF9) gene that has a large effect on the litter size of cashmere goats successfully (Niu et al., 2018). Moreover, Wu et al. succeeded in integrating an exogenous tGFP (turboGFP) gene into targeted genes in frame with high efficiency (Wu et al., 2016), which was the first gene knock-in sheep via CRISPR/Cas9 system. Another research specifically inserted the thymosin beta 4 (Tβ4) gene into the goat CCR5 locus (Li X. et al., 2019), which also provided an example for the establishment of knock-in goats models.
Sheep and goats have been used as interesting models in biomedical research. Compared to experimental rodents, sheep and goats offer the advantage of being more suitable in mimicking human diseases due to their similar size and anatomy. There have already been some examples of genome editing sheep or goats by CRISPR/Cas9 system to mimic human disease. Fan et al. created the first sheep model of human disease of cystic fibrosis (CF) generated by CRISPR/Cas9 mediated disruption of the cystic fibrosis transmembrane conductance regulator (CFTR) gene (Fan et al., 2018). The newborn CFTR−/- sheep developed severe disease phenotypes consistent with CF pathology in humans, like pancreatic fibrosis, intestinal obstruction, and substantial liver and gallbladder disease reflecting CF liver disease that is evident in humans. Another study reported for the first time the generation of otoferlin (OTOF) gene disrupted sheep, which provided a model allowing better understanding and development of new therapies for human deafness related to genetic disorders (Menchaca et al., 2020). Additionally, Williams et al. have also reported an interesting sheep model, recapitulating human hypophosphatasia (HPP, a rare metabolic bone disease) by applying CRISPR/Cas9(Williams et al., 2018). In this study, a single point mutation in the tissue-nonspecific alkaline phosphatase gene (ALPL) was introduced. Thus, the generated gene-edited lambs accurately phenocopied human HPP, providing a useful large animal model for the study of rare human bone diseases. The results of these reports corroborate the great potential of the CRISPR/Cas9 system to generate gene-edited sheep or goats that recapitulate human diseases (Kalds et al., 2019).
Similar to pigs, the sheep or the goat is also one of the most important livestock in agriculture industry, which urges scientists to reform its traits via genome editing from different aspects according to physical demand. Sheep and goats could provide us with donor organs for xenotransplantation by serving as the host for the growth of human organs. As a result, Vilarino et al. created PDX1−/− (pancreatic and duodenal homeobox protein 1) fetus lacking a pancreas, which provided the basis for the production of gene-edited sheep as a host for interspecies organ generation (Vilarino et al., 2017). BMPR-IB (bone morphogenetic protein receptor type IB, also known as FecB) is a key candidate gene for the genetic control of sheep reproductive performance. Researchers created loss-of-function mutations in the sheep BMPR-IB by using the CRISPR/Cas9 system, leading to an increase in ovulation rate and consequently larger litter size (Zhang et al., 2017c). Ma et al. established an AANAT/ASMT (aralkylamine N-acetyltransferase/acetylserotonin O-methyltransferase) transgenic animal model constructed with CRISPR/Cas9 system, which served as the mammary gland bioreactor to produce melatonin-enriched milk in the sheep (Ma et al., 2017) or in goats (Zhou et al., 2017; Tian et al., 2018). Another research showed that CRISPR/Cas9-mediated loss of fibroblast growth factor 5 (FGF5) activity could promote the wool growth and, consequently, increase the wool length and yield in sheep (Li et al., 2017a; Hu et al., 2017) or in goats (Wang et al., 2016a), and similar research was performed in order to change the coat color of sheep and goats (Zhang et al., 2017b).
To meet the growing demand for the meat product of sheep and goats, many experiments are aimed at improving the yield and quality of the meat production of sheep or goats, most of which were conducted by hindering the muscle producing genes in sheep (Crispo et al., 2015; Niu Y. et al., 2017; Zhang Y. et al., 2018) or in goats (Wang X. et al., 2015; Guo et al., 2016; Zhang J. et al., 2018; Wang X. et al., 2018; He et al., 2018), like MSTN. This strategy was also used in the pig.
The Application of CRISPR/Cas9 in Dog Models
The dog is also a typical species used in scientific research, though there are not many labs focusing on the topic of CRISPR/Cas9 system editing dogs. Zou et al. demonstrated for the first time that a single injection of Cas9 mRNA and sgRNA corresponding to a specific gene into zygotes, combined with an auto-embryo transfer strategy, can efficiently generate site-specific genome-modified dogs (Zou et al., 2015). Their team also generated the apolipoprotein E (APOE) deficient dogs via similar strategy 3 years later (Feng et al., 2018).
The most exciting breakthrough about gene edited dogs related to CRISPR/Cas9 system happened in the year of 2018. Researchers of Olson lab working with dogs successfully fixed a genetic glitch that causes DMD by further damaging the DNA. They used adeno-associated viruses (AAV) to deliver CRISPR/Cas9 gene editing components to four dogs, which allowed the mutated gene to again make a key muscle protein and greatly alleviated the disease (Amoasii et al., 2018). The feat-achieved for the first time in a large animal-raised hope that such genetic surgery could 1 day prevent or treat this crippling and deadly disease in people, which created a great interest in the field (Cohen, 2018; Duan, 2018; Wasala et al., 2019; Mata Lopez et al., 2020).
Discussion
Limitations of CRISPR/Cas9 System in Large Animal Models
Above all, the progress of genome editing large animal models is accelerated tremendously by CRISPR/Cas9 system because of many aspects of advantages. First, this system is nearly able to target any locus of the genome in animals theoretically as long as there are PAM sequences near the location (Mali et al., 2013), which really expanded the range of editing genes compared with previous strategy. Next, because the targeting strategy relies on 23 base pair matches, CRISPR/Cas9 can target to virtually any genes in a sequence-dependent manner. As a result, CRISPR/Cas9 can target two alleles to cause a null mutation in the founder animals, which would avoid the procedure of mating heterozygous mutant animals to generate homozygous mutants. This advantage is really critical to large animals because the outcrossing process may take much longer time than mouse models, even several years in nonhuman primates. Third, the CRISPR/Cas9 system can simultaneously manipulate several genes by the co-injection of several sgRNAs with Cas9 into fertilized eggs at the one-cell stage, which makes the establishment of animal models of multigenic disease possible, especially the complex neurodegenerative diseases, such as PD and Alzheimer’s disease (AD). As a result, these models may mimic the genetic mutations and the symptoms in patients in a better way.
Although the CRISPR/Cas9 system has brought great hope for the use of large animal models for studying human diseases, several challenges remain. The first one is the off-targeting effect. When the approximate 23 base pairs that enable the specific cutting of Cas9 match other areas of the genomic DNA, nonspecific editing may happen and cause off-targeting mutations. Although the off-target events can be diluted over generations in small animals with short breeding times, this strategy is infeasible for large animals like monkeys because their sexual maturation usually requires 4–5 years (Niu et al., 2014). To eliminate or reduce the side effects of off-target mutations, truncated guide RNAs in the CRISPR/Cas9 system can be used to improve the specificity of Cas9 nucleases or paired nickases (Fu et al., 2013; Sakuma et al., 2014). On the other hand, the use of bioinformatic screening to search for unique genomic targets and the use of paired Cas9 nickases can also reduce off-targets (Tu et al., 2015).
The second problem is the mosaicism in CRISPR/Cas9-mediated genome editing, which means the presence of more than one genotype in one individual. The mechanisms of the mosaicism are still unclear, and there may be several causes. It is possible that the translation of Cas9 mRNA to produce an active enzymatic form is delayed until after the first cell division, and this delay may play a major role in genetic mosaicism. The mosaicism problem may also result from the prolonged expression of Cas9 mRNA. Alternatively, differential DNA repair and non-homozygous recombination activities in zygotes and divided embryonic cells can also influence genetic mutation rates and mosaicism (Tu et al., 2015). In a word, the CRISPR/Cas9 system can continuously target and cleave genes at different stages of embryonic development in different ways, as a result, leading to mosaicism of the introduced mutations. Although the mosaicism is an undesired result in genome editing animals, there may be several advantages, which include enabling animals to survive beyond the lethal phase when manipulated genes are essential to animals. In addition, mosaic animals help us better understand dosage effects of genes on developmental defects, especially those which may mimic human congenital disorders (Zhong et al., 2015). However, the mosaicism caused by CRISPR/Cas9 is undesirable in most cases. The biggest problem of chimeric large animals is that the mutation may be difficult to be transmitted into the offspring (Oliver et al., 2015) because of the long breeding cycle of large animals especially nonhuman primates. There are several possible strategies to reduce the mosaicism. The first way is to speed up the editing process by the introduction of CRISPR/Cas9 components in an appropriate format [Cas9/sgRNA ribonucleoprotein (RNP)] and concentration into very early pronuclear stage zygotes by using electroporation (Mehravar et al., 2019), so the CRISPR/Cas9 system will work at the earliest stage of zygotes. Secondly, as described above, shortening the longevity of Cas9 in combination with embryo splitting to eliminate the delayed function of Cas9 also makes a difference (Tu et al., 2017). The third strategy is to use germline modification. In this way, genetically-modified somatic cells can be used as nuclear donors for SCNT into enucleated germ cells. In another way, generally targeted gene edited spermatogonial stem cells (SSCs) can be used as donors for transplantation into testis directly.
The last problem is the efficiency of the CRISPR/Cas9 system. According to the previous study, the efficiency of gene targeting with CRISPR in large animals (like nonhuman primates) is more variable and lower than that in mice (Chen et al., 2016). Therefore, precise gene editing technologies need to be further improved, to increase the efficiency of gene targeting and the rate of homozygous mutation by using new Cas protein [like Cpf1 (Cas12a)] and new systems (like base editing system).
Base Editing in Large Animals and Prime Editing
Although the CRISPR/Cas9 system has been used to establish gene editing models in multiple species, it is more likely to induce random indels through error-prone NHEJ rather than the error-free HDR during gene editing (Kim and Kim, 2014), which makes indels more likely to occur at the editing site than single-nucleotide substitutions. In addition, DNA sequencing results show that point mutations, not indels, cause the vast majority of human genetic diseases (Yuan et al., 2020), which suggests the importance of the newly developed gene-editing tool base editing in establishing animal models of human disease. Base editing is a gene editing tool developed in recent years, which can lead to gene mutations through changes in a single base pair (Komor et al., 2016; Nishida et al., 2016; Gaudelli et al., 2017). At the genome level, BEs can achieve all four kinds of single base transition, including C to T, G to A, A to G, and T to C (adenine (A), cytosine (C), guanine (G), and thymine (T)). There are several basic base editors, include cytosine base editors (CBEs), adenine base editors (ABEs) and RNA base editors (RBEs). CBEs is capable of converting the base pair C-G to into T-A, while ABEs can achieve the transition from A-T into G-C. RBEs are able to achieve the conversion of A to Inosine (I) in the level of RNA (Molla and Yang, 2019) (the structure of these BEs are depicted in Figure 2).
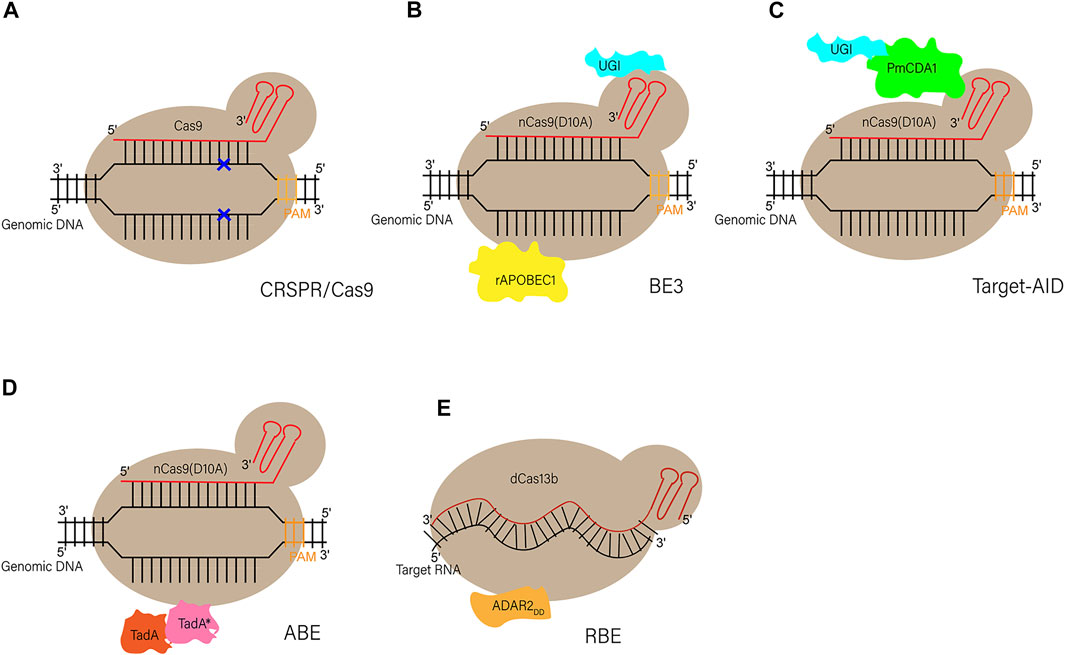
FIGURE 2. Schematic diagram of CRISPR/Cas9 and different BEs (A) Schematic diagram of CRISPR/Cas9. Through base pairing between sgRNAs and DNA as well as the PAM sequence, Cas9 can recognize and cleave the double-strand DNA at the target site to form DSBs (B,C) Schematic diagram of two original base editors (CBEs). BE3: a SpCas9 nickase (D10A) is linked to a rat cytidine deaminase (rAPOBEC1) through the N terminus, and to a uracil glycosylase inhibitor (UGI) at the C terminus. Target-AID: the C terminus of SpCas9 nickase (D10A) is linked to both cytidine deaminase from Petromyzon marinus (PmCDA1) and UGI (D) Schematic diagram of adenine base editor (ABEs). ABEs: fusion of artificially evolved DNA adenine deaminase (TadA*-TadA) with SpCas9 nickase (D10A) generates ABEs (TadA, wildtype Escherichia coil tRNA adenosine deaminase; TadA*, mutated TadA) (E) Schematic diagram of RNA base editor (RBEs). RBEs: catalytically dead Prevotella sp. Cas13 (dCas13b) is tethered with deaminase domain of human“adenosine deaminase acting on RNA” (ADAR2DD) to form RBEs.
Although there are only several years after the invention of base editing, scientists have used it to achieve gene editing successfully in many small animals and plants, such as mouse (Ryu et al., 2018), rat (Ma et al., 2018), rabbit (Lee et al., 2018; Liu et al., 2018), zebrafish (Zhang Y. et al., 2017), rice (Hua et al., 2018) and wheat (Li C. et al., 2018).
Apart from these, there are also some examples of gene editing large animal models created by BEs. In the 2018, a group demonstrated the BE3 (one kind of CBEs)-mediated base editing can induce nonsense mutations in the goat FGF5 gene. They further characterized the phenotypic and genetic changes to investigate the consequence of base pair editing, and provided strong supporting evidence that the BE3 induced off-target mutations were rare at genome-wide level (Li G. et al., 2019). These successful attempts in goats opened up unlimited possibilities of genome engineering by base editing in large animals. Inspired by this, researchers also created BE3-mediated sheep by co-injection of a BE3 mRNA and guide RNA aiming at the SOCS2 (suppressor of cytokine signaling 2) gene in the next year (Zhou et al., 2019).
By using BE3 to target the TWIST2 (twist-related protein 2) gene [responsible for the ablepharon macrostomia syndrome (AMS) in human)] and the tyrosinase (TYR) gene [the causal gene for oculocutaneous albinism type 1 (OCA1)], researchers created a gene editing pig model successfully in the same year of the first base editing goats (Li Z. et al., 2018), which mimicked the phenotypic characteristics of human diseases well. The group of Liangxue Lai also achieved efficient base editing for several genes in pigs by combining CBEs with SCNT in the next year, including DMD, RAG1 (recombination activating gene 1), RAG2 (recombination activating gene 1) and IL2RG (interleukin 2 receptor subunit gamma) (Xie et al., 2019). Another group also achieved precise base conversion in three genes {[GGTA1 (glycoprotein alpha-galactosyltransferase 1), B4GALNT2 (beta-1,4-N-acetyl-galactosaminyltransferase 2), and CMAH (cytidine monophospho-N-acetylneuraminic acid hydroxylasegenes)]} in pig genome (Yuan et al., 2020). As for NHPs, researchers generated the first Hutchinson-Gilford progeria syndrome (HGPS) monkey model by delivering a BE mRNA and guide RNA (gRNA) targeting the LMNA (lamin A/C) gene via microinjection into monkey zygotes, and the typical HGPS phenotypes including growth retardation, bone alterations, and vascular abnormalities confirmed the reliability of this model (Wang et al., 2020). Since the appearance of BEs, this tool has been applied in some species of large animals, like NHPs, goats, sheep and pigs. It is really convenient to establish disease models or improve the traits in large animals by base editing for its ability of precise single base pair editing.
Although base editing has played a great role in precise genome editing, it is still unable to achieve all base conversions. To solve this problem, researchers created the prime editing. This system consists of a Cas9 nickase (H840A mutation) fused to a reverse transcriptase domain and a modified sgRNA, named prime editing guide RNA (pegRNA). The basic principle is to use nCas9 to nick the non-target strand at the target location, and then use reverse transcriptase to generate the required sequence using the template RNA. Then the FEN1 endonuclease is able to excise the sequence called flap during this process and contributes to the genome repairing (Anzalone et al., 2019; Caso and Davies, 2022). Although this technique has only been reported in the establishment of small animal models (Liu et al., 2020), it is of great significance for the study of diseases caused by various base mutations in large animal models because it can mediate almost all types of base conversions.
Prospects and Challenges
Large animal models can be used in many areas including disease pathogenesis investigation and pre-clinical research. Due to the lack of ESCs from large animals, it has been difficult to use traditional gene targeting technology to establish large animal models of human diseases. Nowadays, the development of precise genome editing tools especially the CRISPR/Cas9 system has greatly advanced this field. Many genome-edited large animals have been created including knockout or knock-in that cover almost all types of currently used experimental animals, such as nonhuman primates, pigs, sheep, goats and dogs. Even so, there are still many limitations in the establishment of large animal models, which may involve the inadequate gene targeting efficiency, mosaicism, and off-targeting. Many strategies and optimized components of the system have been brought up to reduce these drawbacks or to improve efficiency.
The BEs recently provide us with a new tool in the establishment of large animal models with the rapid development and optimization of this new system. Although there are only few successful examples in large animals, the BEs greatly expanded the scope of this area promisingly because the vast majority of human genetic diseases are induced by point mutations. On the other hand, all kinds of other technologies, like somatic cell nuclear transfer, genome editing of SSCs, and tetraploid complementation, have played more and more important roles in the establishment of rodents or even large animal models nowadays. Therefore, the combination of the BEs or optimized CRISPR/Cas9 components with other platforms previously described will make precise genome modification in large animals more efficient and easier (the procedure of gene modified large animal models is depicted in Figure 3).
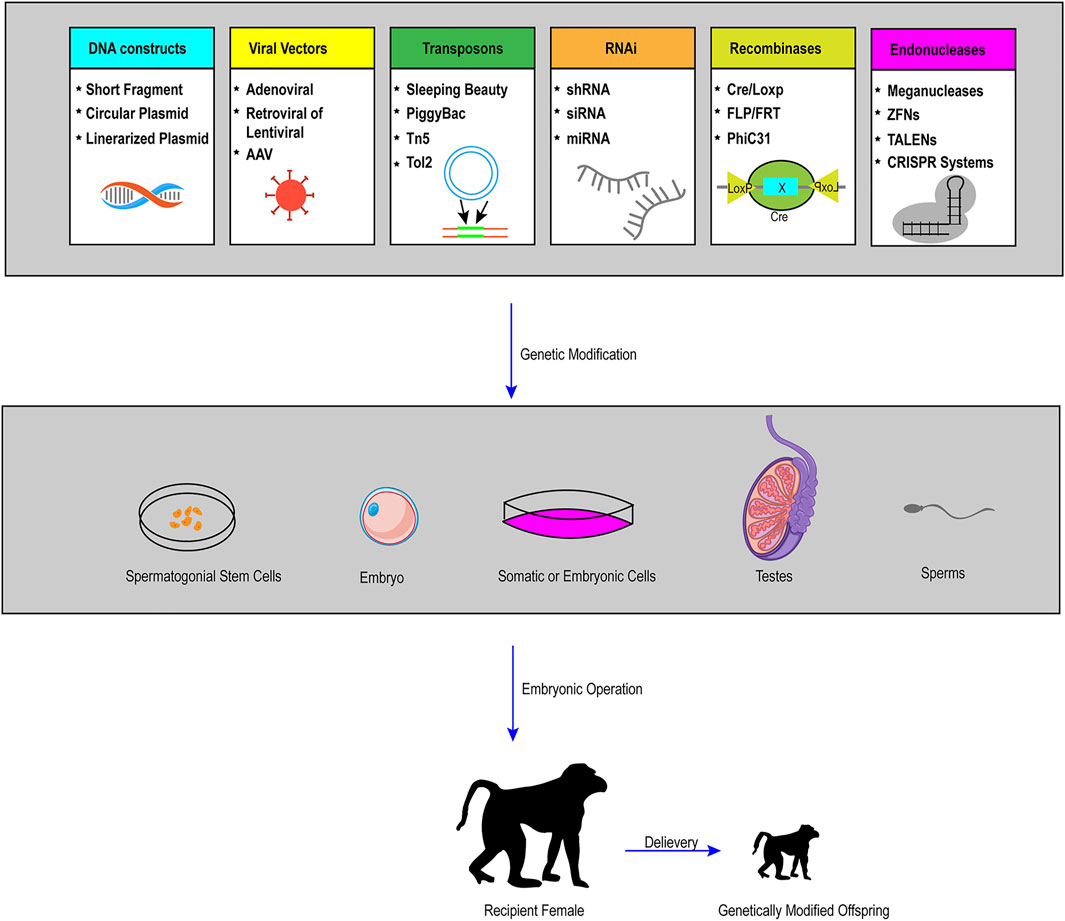
FIGURE 3. Schematic representation of practical and possible pathways of genetic modification in large animals. To achieve the generation of live founders with desired genetic modifications (pronuclear injection and nuclear transfer are the two primary procedures), the first step is to conduct gene manipulations in a variety of cells or organs, including somatic cells, embryonic cells, embryos, spermatozoa, SSCs and other targeted organs by the use of many tools, such as viral vectors, recombinases, transposons, RNA interference (RNAi), and endonucleases. Through a series of embryonic operations, such as nuclear transfer, genetically modified cells or embryos can produce genetically modified offspring.
In clinical research, it is a promising direction to use gene therapy to treat inherited human diseases. The FDA has approved some gene therapy products for patients, such as patients with B-cell precursor acute lymphoblastic leukemia (ALL). Before applying the strategy on patients, evaluating the efficacy and safety using genetically modified animal models that can mimic the characteristics of human disease is necessary, which further underscores the importance of the establishment of genome editing large animal models. Xenotransplantation is another promising direction, as the shortage of human organs is a common problem, and model animals can provide a sufficient supply of organ products. Genetic modification of donor animals can eliminate many problems, especially the immunological compatibility, and increase the probability of success during xenotransplantation. The recent success of transplanting organs from transgenic pigs into the humans is a stunning breakthrough in this field, which will in turn lead to the development of gene-editing large animal models.
In summary, with the development and application of precise genome editing tools represented by CRISPR/Ca9 system, a great number of large animal models will be established (the examples of genome-edited large animals described in this article is listed in Table 1). The improvement will create ideal animal models that are more similar to the human, benefit the study of the mechanisms of human diseases, and make important contribution to the clinical application.
Author Contributions
Designed the paper: YL, ZT, SY. Wrote the paper: YL, JL. Collected literatures: YL, CL. Edited the paper: SY, SL, X-JL
Funding
This work was supported by National Key Research and Development Program of China (2021YFA0805300), The National Natural Science Foundation of China (81922026,82171244), and Guangzhou Key Research Program on Brain Science (202007030008).
Conflict of Interest
The authors declare that the research was conducted in the absence of any commercial or financial relationships that could be construed as a potential conflict of interest.
Publisher’s Note
All claims expressed in this article are solely those of the authors and do not necessarily represent those of their affiliated organizations, or those of the publisher, the editors and the reviewers. Any product that may be evaluated in this article, or claim that may be made by its manufacturer, is not guaranteed or endorsed by the publisher.
References
Amoasii, L., Hildyard, J. C. W., Li, H., Sanchez-Ortiz, E., Mireault, A., Caballero, D., et al. (2018). Gene Editing Restores Dystrophin Expression in a Canine Model of Duchenne Muscular Dystrophy. Science 362 (6410), 86–91. doi:10.1126/science.aau1549
Anzalone, A. V., Randolph, P. B., Davis, J. R., Sousa, A. A., Koblan, L. W., Levy, J. M., et al. (2019). Search-and-replace Genome Editing without Double-Strand Breaks or Donor DNA. Nature 576 (7785), 149–157. doi:10.1038/s41586-019-1711-4
Carroll, D. (2011). Genome Engineering with Zinc-Finger Nucleases. Genetics 188 (4), 773–782. doi:10.1534/genetics.111.131433
Caso, F., and Davies, B. (2022). Base Editing and Prime Editing in Laboratory Animals. Lab. Anim. 56 (1), 35–49. doi:10.1177/0023677221993895
Chan, A. W. S., Chong, K. Y., Martinovich, C., Simerly, C., and Schatten, G. (2001). Transgenic Monkeys Produced by Retroviral Gene Transfer into Mature Oocytes. Science 291 (5502), 309–312. doi:10.1126/science.291.5502.309
Chen, Y., Cui, Y., Shen, B., Niu, Y., Zhao, X., Wang, L., et al. (2015a). Germline Acquisition of Cas9/RNA-Mediated Gene Modifications in Monkeys. Cell Res. 25 (2), 262–265. doi:10.1038/cr.2014.167
Chen, Y., Zheng, Y., Kang, Y., Yang, W., Niu, Y., Guo, X., et al. (2015b). Functional Disruption of the Dystrophin Gene in Rhesus Monkey Using CRISPR/Cas9. Hum. Mol. Genet. 24 (13), 3764–3774. doi:10.1093/hmg/ddv120
Chen, Y., Niu, Y., and Ji, W. (2016). Genome Editing in Nonhuman Primates: Approach to Generating Human Disease Models. J. Intern Med. 280 (3), 246–251. doi:10.1111/joim.12469
Chen, Y., Yu, J., Niu, Y., Qin, D., Liu, H., Li, G., et al. (2017). Modeling Rett Syndrome Using TALEN-Edited MECP2 Mutant Cynomolgus Monkeys. Cell 169 (5), 945–955. e910. doi:10.1016/j.cell.2017.04.035
Christian, M., Cermak, T., Doyle, E. L., Schmidt, C., Zhang, F., Hummel, A., et al. (2010). Targeting DNA Double-Strand Breaks with TAL Effector Nucleases. Genetics 186 (2), 757–761. doi:10.1534/genetics.110.120717
Chuang, C.-K., Chen, C.-H., Huang, C.-L., Su, Y.-H., Peng, S.-H., Lin, T.-Y., et al. (2017). Generation of GGTA1 Mutant Pigs by Direct Pronuclear Microinjection of CRISPR/Cas9 Plasmid Vectors. Anim. Biotechnol. 28 (3), 174–181. doi:10.1080/10495398.2016.1246453
Cohen, J. (2018). In Dogs, CRISPR Fixes a Muscular Dystrophy. Science 361 (6405), 835. doi:10.1126/science.361.6405.835
Crispo, M., Mulet, A. P., Tesson, L., Barrera, N., Cuadro, F., dos Santos-Neto, P. C., et al. (2015). Efficient Generation of Myostatin Knock-Out Sheep Using CRISPR/Cas9 Technology and Microinjection into Zygotes. PLoS One 10 (8), e0136690. doi:10.1371/journal.pone.0136690
Cui, Y., Niu, Y., Zhou, J., Chen, Y., Cheng, Y., Li, S., et al. (2018). Generation of a Precise Oct4-hrGFP Knockin Cynomolgus Monkey Model via CRISPR/Cas9-assisted Homologous Recombination. Cell Res. 28 (3), 383–386. doi:10.1038/cr.2018.10
Dawson, T. M., Ko, H. S., and Dawson, V. L. (2010). Genetic Animal Models of Parkinson's Disease. Neuron 66 (5), 646–661. doi:10.1016/j.neuron.2010.04.034
Duan, D. (2018). CRISPR Alleviates Muscular Dystrophy in Dogs. Nat. Biomed. Eng. 2 (11), 795–796. doi:10.1038/s41551-018-0320-0
Durai, S., Mani, M., Kandavelou, K., Wu, J., Porteus, M. H., and Chandrasegaran, S. (2005). Zinc Finger Nucleases: Custom-Designed Molecular Scissors for Genome Engineering of Plant and Mammalian Cells. Nucleic Acids Res. 33 (18), 5978–5990. doi:10.1093/nar/gki912
Fan, Z., Perisse, I. V., Cotton, C. U., Regouski, M., Meng, Q., Domb, C., et al. (2018). A Sheep Model of Cystic Fibrosis Generated by CRISPR/Cas9 Disruption of the CFTR Gene. JCI Insight 3 (19), e123529. doi:10.1172/jci.insight.123529
Feng, C., Wang, X., Shi, H., Yan, Q., Zheng, M., Li, J., et al. (2018). Generation of ApoE Deficient Dogs via Combination of Embryo Injection of CRISPR/Cas9 with Somatic Cell Nuclear Transfer. J. Genet. Genomics 45 (1), 47–50. doi:10.1016/j.jgg.2017.11.003
Fu, Y., Foden, J. A., Khayter, C., Maeder, M. L., Reyon, D., Joung, J. K., et al. (2013). High-frequency Off-Target Mutagenesis Induced by CRISPR-Cas Nucleases in Human Cells. Nat. Biotechnol. 31 (9), 822–826. doi:10.1038/nbt.2623
Gao, H., Zhao, C., Xiang, X., Li, Y., Zhao, Y., Li, Z., et al. (2017). Production of α1,3-galactosyltransferase and Cytidine Monophosphate-N-Acetylneuraminic Acid Hydroxylase Gene Double-Deficient Pigs by CRISPR/Cas9 and Handmade Cloning. J. Reproduction Dev. 63 (1), 17–26. doi:10.1262/jrd.2016-079
Gaudelli, N. M., Komor, A. C., Rees, H. A., Packer, M. S., Badran, A. H., Bryson, D. I., et al. (2017). Programmable Base Editing of at to GC in Genomic DNA without DNA Cleavage. Nature 551 (7681), 464–471. doi:10.1038/nature24644
Guo, R., Wan, Y., Xu, D., Cui, L., Deng, M., Zhang, G., et al. (2016). Generation and Evaluation of Myostatin Knock-Out Rabbits and Goats Using CRISPR/Cas9 System. Sci. Rep. 6, 29855. doi:10.1038/srep29855
Hai, T., Teng, F., Guo, R., Li, W., and Zhou, Q. (2014). One-step Generation of Knockout Pigs by Zygote Injection of CRISPR/Cas System. Cell Res. 24 (3), 372–375. doi:10.1038/cr.2014.11
Hammer, R. E., Pursel, V. G., Rexroad, C. E., Wall, R. J., Bolt, D. J., Ebert, K. M., et al. (1985). Production of Transgenic Rabbits, Sheep and Pigs by Microinjection. Nature 315 (6021), 680–683. doi:10.1038/315680a0
He, Z., Zhang, T., Jiang, L., Zhou, M., Wu, D., Mei, J., et al. (2018). Use of CRISPR/Cas9 Technology Efficiently Targetted Goat Myostatin through Zygotes Microinjection Resulting in Double-Muscled Phenotype in Goats. Biosci. Rep. 38 (6), BSR20180742. doi:10.1042/BSR20180742
Hu, R., Fan, Z. Y., Wang, B. Y., Deng, S. L., Zhang, X. S., Zhang, J. L., et al. (2017). RAPID COMMUNICATION: Generation of FGF5 Knockout Sheep via the CRISPR/Cas9 System12. J. Anim. Sci. 95 (5), 2019–2024. doi:10.2527/jas.2017.1503
Hua, K., Tao, X., Yuan, F., Wang, D., and Zhu, J.-K. (2018). Precise A·T to G·C Base Editing in the Rice Genome. Mol. Plant 11 (4), 627–630. doi:10.1016/j.molp.2018.02.007
Huang, L., Hua, Z., Xiao, H., Cheng, Y., Xu, K., Gao, Q., et al. (2017). CRISPR/Cas9-mediated ApoE-/- and LDLR-/- Double Gene Knockout in Pigs Elevates Serum LDL-C and TC Levels. Oncotarget 8 (23), 37751–37760. doi:10.18632/oncotarget.17154
Jaenisch, R., and Mintz, B. (1974). Simian Virus 40 DNA Sequences in DNA of Healthy Adult Mice Derived from Preimplantation Blastocysts Injected with Viral DNA. Proc. Natl. Acad. Sci. U.S.A. 71 (4), 1250–1254. doi:10.1073/pnas.71.4.1250
Jiahuan Chen, J., Beiying An, B., Biao Yu, B., Xiaohuan Peng, X., Hongming Yuan, H., and Qiangbing Yang, Q. (2021). CRISPR/Cas9-mediated Knockin of Human Factor IX into Swine Factor IX Locus Effectively Alleviates Bleeding in Hemophilia B Pigs. haematol 106 (3), 829–837. doi:10.3324/haematol.2019.224063
Jinek, M., Chylinski, K., Fonfara, I., Hauer, M., Doudna, J. A., and Charpentier, E. (2012). A Programmable Dual-RNA-Guided DNA Endonuclease in Adaptive Bacterial Immunity. Science 337 (6096), 816–821. doi:10.1126/science.1225829
Joanna, Z., Magdalena, H., Agnieszka, N.-T., Jacek, J., Ryszard, S., Zdzisław, S., et al. (2018). The Production of UL16-Binding Protein 1 Targeted Pigs Using CRISPR Technology. 3 Biotech. 8 (1), 70. doi:10.1007/s13205-018-1107-4
Joung, J. K., and Sander, J. D. (2013). TALENs: a Widely Applicable Technology for Targeted Genome Editing. Nat. Rev. Mol. Cell Biol. 14 (1), 49–55. doi:10.1038/nrm3486
Kalds, P., Zhou, S., Cai, B., Liu, J., Wang, Y., Petersen, B., et al. (2019). Sheep and Goat Genome Engineering: From Random Transgenesis to the CRISPR Era. Front. Genet. 10, 750. doi:10.3389/fgene.2019.00750
Kang, J.-T., Ryu, J., Cho, B., Lee, E.-J., Yun, Y.-J., Ahn, S., et al. (2016). Generation ofRUNX3knockout Pigs Using CRISPR/Cas9-mediated Gene Targeting. Reprod. Dom. Anim. 51 (6), 970–978. doi:10.1111/rda.12775
Kim, H., and Kim, J.-S. (2014). A Guide to Genome Engineering with Programmable Nucleases. Nat. Rev. Genet. 15 (5), 321–334. doi:10.1038/nrg3686
Kitada, T., Pisani, A., Porter, D. R., Yamaguchi, H., Tscherter, A., Martella, G., et al. (2007). Impaired Dopamine Release and Synaptic Plasticity in the Striatum of PINK1 -deficient Mice. Proc. Natl. Acad. Sci. U.S.A. 104 (27), 11441–11446. doi:10.1073/pnas.0702717104
Kobayashi, K., Tsukiyama, T., Nakaya, M., Kageyama, S., Tomita, K., Murai, R., et al. (2019). Generation of an OCT3/4 Reporter Cynomolgus Monkey ES Cell Line Using CRISPR/Cas9. Stem Cell Res. 37, 101439. doi:10.1016/j.scr.2019.101439
Komor, A. C., Kim, Y. B., Packer, M. S., Zuris, J. A., and Liu, D. R. (2016). Programmable Editing of a Target Base in Genomic DNA without Double-Stranded DNA Cleavage. Nature 533 (7603), 420–424. doi:10.1038/nature17946
Lee, H. K., Willi, M., Miller, S. M., Kim, S., Liu, C., Liu, D. R., et al. (2018). Targeting Fidelity of Adenine and Cytosine Base Editors in Mouse Embryos. Nat. Commun. 9 (1), 4804. doi:10.1038/s41467-018-07322-7
Li, W.-R., Liu, C.-X., Zhang, X.-M., Chen, L., Peng, X.-R., He, S.-G., et al. (2017a). CRISPR/Cas9-mediated Loss of FGF5 Function Increases Wool Staple Length in Sheep. FEBS J. 284 (17), 2764–2773. doi:10.1111/febs.14144
Li, C., Zong, Y., Wang, Y., Jin, S., Zhang, D., Song, Q., et al. (2018a). Expanded Base Editing in Rice and Wheat Using a Cas9-Adenosine Deaminase Fusion. Genome Biol. 19 (1), 59. doi:10.1186/s13059-018-1443-z
Li, Z., Duan, X., An, X., Feng, T., Li, P., Li, L., et al. (2018b). Efficient RNA-Guided Base Editing for Disease Modeling in Pigs. Cell Discov. 4, 64. doi:10.1038/s41421-018-0065-7
Li, G., Zhou, S., Li, C., Cai, B., Yu, H., Ma, B., et al. (2019a). Base Pair Editing in Goat: Nonsense Codon Introgression into FGF 5 Results in Longer Hair. FEBS J. 286 (23), 4675–4692. doi:10.1111/febs.14983
Li, X., Hao, F., Hu, X., Wang, H., Dai, B., Wang, X., et al. (2019b). Generation of Tβ4 Knock-In Cashmere Goat Using CRISPR/Cas9. Int. J. Biol. Sci. 15 (8), 1743–1754. doi:10.7150/ijbs.34820
Li, G., Zhang, X., Wang, H., Mo, J., Zhong, C., Shi, J., et al. (2020a). CRISPR/Cas9-Mediated Integration of Large Transgene into Pig CEP112 Locus. G3 (Bethesda) 10 (2), 467–473. doi:10.1534/g3.119.400810
Li, R., Zeng, W., Ma, M., Wei, Z., Liu, H., Liu, X., et al. (2020b). Precise Editing of Myostatin Signal Peptide by CRISPR/Cas9 Increases the Muscle Mass of Liang Guang Small Spotted Pigs. Transgenic Res. 29 (1), 149–163. doi:10.1007/s11248-020-00188-w
Li, H., Wu, S., Ma, X., Li, X., Cheng, T., Chen, Z., et al. (2021). Co-editing PINK1 and DJ-1 Genes via Adeno-Associated Virus-Delivered CRISPR/Cas9 System in Adult Monkey Brain Elicits Classical Parkinsonian Phenotype. Neurosci. Bull. 37 (9), 1271–1288. doi:10.1007/s12264-021-00732-6
Liu, H., Chen, Y., Niu, Y., Zhang, K., Kang, Y., Ge, W., et al. (2014). TALEN-mediated Gene Mutagenesis in Rhesus and Cynomolgus Monkeys. Cell Stem Cell 14 (3), 323–328. doi:10.1016/j.stem.2014.01.018
Liu, Z., Li, X., Zhang, J.-T., Cai, Y.-J., Cheng, T.-L., Cheng, C., et al. (2016). Autism-like Behaviours and Germline Transmission in Transgenic Monkeys Overexpressing MeCP2. Nature 530 (7588), 98–102. doi:10.1038/nature16533
Liu, Z., Chen, M., Chen, S., Deng, J., Song, Y., Lai, L., et al. (2018). Highly Efficient RNA-Guided Base Editing in Rabbit. Nat. Commun. 9 (1), 2717. doi:10.1038/s41467-018-05232-2
Liu, X., Liu, H., Wang, M., Li, R., Zeng, J., Mo, D., et al. (2019). Disruption of the ZBED6 Binding Site in Intron 3 of IGF2 by CRISPR/Cas9 Leads to Enhanced Muscle Development in Liang Guang Small Spotted Pigs. Transgenic Res. 28 (1), 141–150. doi:10.1007/s11248-018-0107-9
Liu, Y., Li, X., He, S., Huang, S., Li, C., Chen, Y., et al. (2020). Efficient Generation of Mouse Models with the Prime Editing System. Cell Discov. 6 (1), 27. doi:10.1038/s41421-020-0165-z
Luo, X., He, Y., Zhang, C., He, X., Yan, L., Li, M., et al. (2019). Trio Deep-Sequencing Does Not Reveal Unexpected Off-Target and On-Target Mutations in Cas9-Edited Rhesus Monkeys. Nat. Commun. 10 (1), 5525. doi:10.1038/s41467-019-13481-y
Ma, Y., Zhang, X., Shen, B., Lu, Y., Chen, W., Ma, J., et al. (2014). Generating Rats with Conditional Alleles Using CRISPR/Cas9. Cell Res. 24 (1), 122–125. doi:10.1038/cr.2013.157
Ma, T., Tao, J., Yang, M., He, C., Tian, X., Zhang, X., et al. (2017). AnAANAT/ASMTtransgenic Animal Model Constructed with CRISPR/Cas9 System Serving as the Mammary Gland Bioreactor to Produce Melatonin-Enriched Milk in Sheep. J. Pineal Res. 63 (1), e12406. doi:10.1111/jpi.12406
Ma, Y., Yu, L., Zhang, X., Xin, C., Huang, S., Bai, L., et al. (2018). Highly Efficient and Precise Base Editing by Engineered dCas9-Guide tRNA Adenosine Deaminase in Rats. Cell Discov. 4, 39. doi:10.1038/s41421-018-0047-9
Mali, P., Esvelt, K. M., and Church, G. M. (2013). Cas9 as a Versatile Tool for Engineering Biology. Nat. Methods 10 (10), 957–963. doi:10.1038/nmeth.2649
Mata López, S., Balog-Alvarez, C., Vitha, S., Bettis, A. K., Canessa, E. H., Kornegay, J. N., et al. (2020). Challenges Associated with Homologous Directed Repair Using CRISPR-Cas9 and TALEN to Edit the DMD Genetic Mutation in Canine Duchenne Muscular Dystrophy. PLoS One 15 (1), e0228072. doi:10.1371/journal.pone.0228072
McGonigle, P., and Ruggeri, B. (2014). Animal Models of Human Disease: Challenges in Enabling Translation. Biochem. Pharmacol. 87 (1), 162–171. doi:10.1016/j.bcp.2013.08.006
Mehravar, M., Shirazi, A., Nazari, M., and Banan, M. (2019). Mosaicism in CRISPR/Cas9-mediated Genome Editing. Dev. Biol. 445 (2), 156–162. doi:10.1016/j.ydbio.2018.10.008
Menchaca, A., Dos Santos-Neto, P. C., Souza-Neves, M., Cuadro, F., Mulet, A. P., Tesson, L., et al. (2020). Otoferlin Gene Editing in Sheep via CRISPR-Assisted ssODN-Mediated Homology Directed Repair. Sci. Rep. 10 (1), 5995. doi:10.1038/s41598-020-62879-y
Meurens, F., Summerfield, A., Nauwynck, H., Saif, L., and Gerdts, V. (2012). The Pig: a Model for Human Infectious Diseases. Trends Microbiol. 20 (1), 50–57. doi:10.1016/j.tim.2011.11.002
Molla, K. A., and Yang, Y. (2019). CRISPR/Cas-Mediated Base Editing: Technical Considerations and Practical Applications. Trends Biotechnol. 37 (10), 1121–1142. doi:10.1016/j.tibtech.2019.03.008
Mussolino, C., and Cathomen, T. (2012). TALE Nucleases: Tailored Genome Engineering Made Easy. Curr. Opin. Biotechnol. 23 (5), 644–650. doi:10.1016/j.copbio.2012.01.013
Ni, W., Qiao, J., Hu, S., Zhao, X., Regouski, M., Yang, M., et al. (2014). Efficient Gene Knockout in Goats Using CRISPR/Cas9 System. PLoS One 9 (9), e106718. doi:10.1371/journal.pone.0106718
Nishida, K., Arazoe, T., Yachie, N., Banno, S., Kakimoto, M., Tabata, M., et al. (2016). Targeted Nucleotide Editing Using Hybrid Prokaryotic and Vertebrate Adaptive Immune Systems. Science 353 (6305), aaf8729. doi:10.1126/science.aaf8729
Niu, Y., Shen, B., Cui, Y., Chen, Y., Wang, J., Wang, L., et al. (2014). Generation of Gene-Modified Cynomolgus Monkey via Cas9/RNA-Mediated Gene Targeting in One-Cell Embryos. Cell 156 (4), 836–843. doi:10.1016/j.cell.2014.01.027
Niu, D., Wei, H.-J., Lin, L., George, H., Wang, T., Lee, I.-H., et al. (2017a). Inactivation of Porcine Endogenous Retrovirus in Pigs Using CRISPR-Cas9. Science 357 (6357), 1303–1307. doi:10.1126/science.aan4187
Niu, Y., Jin, M., Li, Y., Li, P., Zhou, J., Wang, X., et al. (2017b). Biallelicβ-carotene Oxygenase 2knockout Results in Yellow Fat in Sheep via CRISPR/Cas9. Anim. Genet. 48 (2), 242–244. doi:10.1111/age.12515
Niu, Y., Zhao, X., Zhou, J., Li, Y., Huang, Y., Cai, B., et al. (2018). Efficient Generation of Goats with Defined Point Mutation (I397V) in GDF9 through CRISPR/Cas9. Reprod. Fertil. Dev. 30 (2), 307–312. doi:10.1071/RD17068
Oliver, D., Yuan, S., McSwiggin, H., and Yan, W. (2015). Pervasive Genotypic Mosaicism in Founder Mice Derived from Genome Editing through Pronuclear Injection. PLoS One 10 (6), e0129457. doi:10.1371/journal.pone.0129457
Petersen, B., Frenzel, A., Lucas-Hahn, A., Herrmann, D., Hassel, P., Klein, S., et al. (2016). Efficient Production of biallelicGGTA1knockout Pigsbycytoplasmic Microinjection of CRISPR/Cas9 into Zygotes. Xenotransplantation 23 (5), 338–346. doi:10.1111/xen.12258
Porrett, P. M., Orandi, B. J., Kumar, V., Houp, J., Anderson, D., Cozette Killian, A., et al. (2022). First Clinical‐grade Porcine Kidney Xenotransplant Using a Human Decedent Model. Am. J Transplant. 22, 1037–1053. doi:10.1111/ajt.16930
Prabhakar, S. (2012). Translational Research Challenges. J. Investig. Med. 60 (8), 1141–1146. doi:10.2310/JIM.0b013e318271fb3b
Prather, R. S., Hawley, R. J., Carter, D. B., Lai, L., and Greenstein, J. L. (2003). Transgenic Swine for Biomedicine and Agriculture. Theriogenology 59 (1), 115–123. doi:10.1016/s0093-691x(02)01263-3
Rao, S., Fujimura, T., Matsunari, H., Sakuma, T., Nakano, K., Watanabe, M., et al. (2016). Efficient Modification of the Myostatin Gene in Porcine Somatic Cells and Generation of Knockout Piglets. Mol. Reprod. Dev. 83 (1), 61–70. doi:10.1002/mrd.22591
Ribitsch, I., Baptista, P. M., Lange-Consiglio, A., Melotti, L., Patruno, M., Jenner, F., et al. (2020). Large Animal Models in Regenerative Medicine and Tissue Engineering: To Do or Not to Do. Front. Bioeng. Biotechnol. 8, 972. doi:10.3389/fbioe.2020.00972
Roura, E., Koopmans, S.-J., Lallès, J.-P., Le Huerou-Luron, I., de Jager, N., Schuurman, T., et al. (2016). Critical Review Evaluating the Pig as a Model for Human Nutritional Physiology. Nutr. Res. Rev. 29 (1), 60–90. doi:10.1017/S0954422416000020
Ruan, J., Li, H., Xu, K., Wu, T., Wei, J., Zhou, R., et al. (2015). Highly Efficient CRISPR/Cas9-mediated Transgene Knockin at the H11 Locus in Pigs. Sci. Rep. 5, 14253. doi:10.1038/srep14253
Ryu, S.-M., Koo, T., Kim, K., Lim, K., Baek, G., Kim, S.-T., et al. (2018). Adenine Base Editing in Mouse Embryos and an Adult Mouse Model of Duchenne Muscular Dystrophy. Nat. Biotechnol. 36 (6), 536–539. doi:10.1038/nbt.4148
Sakuma, T., Nishikawa, A., Kume, S., Chayama, K., and Yamamoto, T. (2014). Multiplex Genome Engineering in Human Cells Using All-In-One CRISPR/Cas9 Vector System. Sci. Rep. 4, 5400. doi:10.1038/srep05400
Schmidt, J. K., Strelchenko, N., Park, M. A., Kim, Y. H., Mean, K. D., Schotzko, M. L., et al. (2020). Genome Editing of CCR5 by CRISPR-Cas9 in Mauritian Cynomolgus Macaque Embryos. Sci. Rep. 10 (1), 18457. doi:10.1038/s41598-020-75295-z
Seita, Y., Morimura, T., Watanabe, N., Iwatani, C., Tsuchiya, H., Nakamura, S., et al. (2020). Generation of Transgenic Cynomolgus Monkeys Overexpressing the Gene for Amyloid-β Precursor Protein. Jad 75 (1), 45–60. doi:10.3233/JAD-191081
Shen, H. (2013). Precision Gene Editing Paves Way for Transgenic Monkeys. Nature 503 (7474), 14–15. doi:10.1038/503014a
Sun, Z., Ye, J., and Yuan, J. (2022). PINK1 Mediates Neuronal Survival in Monkey. Protein Cell 13 (1), 4–5. doi:10.1007/s13238-021-00889-w
Tian, H., Luo, J., Zhang, Z., Wu, J., Zhang, T., Busato, S., et al. (2018). CRISPR/Cas9-mediated Stearoyl-CoA Desaturase 1 (SCD1) Deficiency Affects Fatty Acid Metabolism in Goat Mammary Epithelial Cells. J. Agric. Food Chem. 66 (38), 10041–10052. doi:10.1021/acs.jafc.8b03545
Tu, Z., Yang, W., Yan, S., Guo, X., and Li, X.-J. (2015). CRISPR/Cas9: a Powerful Genetic Engineering Tool for Establishing Large Animal Models of Neurodegenerative Diseases. Mol. Neurodegener. 10, 35. doi:10.1186/s13024-015-0031-x
Tu, Z., Yang, W., Yan, S., Yin, A., Gao, J., Liu, X., et al. (2017). Promoting Cas9 Degradation Reduces Mosaic Mutations in Non-human Primate Embryos. Sci. Rep. 7, 42081. doi:10.1038/srep42081
Tu, Z., Zhao, H., Li, B., Yan, S., Wang, L., Tang, Y., et al. (2019). CRISPR/Cas9-mediated Disruption of SHANK3 in Monkey Leads to Drug-Treatable Autism-like Symptoms. Hum. Mol. Genet. 28 (4), 561–571. doi:10.1093/hmg/ddy367
Vilarino, M., Rashid, S. T., Suchy, F. P., McNabb, B. R., van der Meulen, T., Fine, E. J., et al. (2017). CRISPR/Cas9 Microinjection in Oocytes Disables Pancreas Development in Sheep. Sci. Rep. 7 (1), 17472. doi:10.1038/s41598-017-17805-0
Wan, H., Feng, C., Teng, F., Yang, S., Hu, B., Niu, Y., et al. (2015). One-step Generation of P53 Gene Biallelic Mutant Cynomolgus Monkey via the CRISPR/Cas System. Cell Res. 25 (2), 258–261. doi:10.1038/cr.2014.158
Wang, C.-E., Tydlacka, S., Orr, A. L., Yang, S.-H., Graham, R. K., Hayden, M. R., et al. (2008). Accumulation of N-Terminal Mutant Huntingtin in Mouse and Monkey Models Implicated as a Pathogenic Mechanism in Huntington's Disease. Hum. Mol. Genet. 17 (17), 2738–2751. doi:10.1093/hmg/ddn175
Wang, H., Yang, H., Shivalila, C. S., Dawlaty, M. M., Cheng, A. W., Zhang, F., et al. (2013). One-step Generation of Mice Carrying Mutations in Multiple Genes by CRISPR/Cas-mediated Genome Engineering. Cell 153 (4), 910–918. doi:10.1016/j.cell.2013.04.025
Wang, K., Ouyang, H., Xie, Z., Yao, C., Guo, N., Li, M., et al. (2015a). Efficient Generation of Myostatin Mutations in Pigs Using the CRISPR/Cas9 System. Sci. Rep. 5, 16623. doi:10.1038/srep16623
Wang, X., Yu, H., Lei, A., Zhou, J., Zeng, W., Zhu, H., et al. (2015b). Generation of Gene-Modified Goats Targeting MSTN and FGF5 via Zygote Injection of CRISPR/Cas9 System. Sci. Rep. 5, 13878. doi:10.1038/srep13878
Wang, X., Cai, B., Zhou, J., Zhu, H., Niu, Y., Ma, B., et al. (2016a). Disruption of FGF5 in Cashmere Goats Using CRISPR/Cas9 Results in More Secondary Hair Follicles and Longer Fibers. PLoS One 11 (10), e0164640. doi:10.1371/journal.pone.0164640
Wang, X., Cao, C., Huang, J., Yao, J., Hai, T., Zheng, Q., et al. (2016b). One-step Generation of Triple Gene-Targeted Pigs Using CRISPR/Cas9 System. Sci. Rep. 6, 20620. doi:10.1038/srep20620
Wang, K., Tang, X., Xie, Z., Zou, X., Li, M., Yuan, H., et al. (2017). CRISPR/Cas9-mediated Knockout of Myostatin in Chinese Indigenous Erhualian Pigs. Transgenic Res. 26 (6), 799–805. doi:10.1007/s11248-017-0044-z
Wang, S., Ren, S., Bai, R., Xiao, P., Zhou, Q., Zhou, Y., et al. (2018a). No Off-Target Mutations in Functional Genome Regions of a CRISPR/Cas9-generated Monkey Model of Muscular Dystrophy. J. Biol. Chem. 293 (30), 11654–11658. doi:10.1074/jbc.AC118.004404
Wang, X., Niu, Y., Zhou, J., Zhu, H., Ma, B., Yu, H., et al. (2018b). CRISPR/Cas9-mediatedMSTNdisruption and Heritable Mutagenesis in Goats Causes Increased Body Mass. Anim. Genet. 49 (1), 43–51. doi:10.1111/age.12626
Wang, F., Zhang, W., Yang, Q., Kang, Y., Fan, Y., Wei, J., et al. (2020). Generation of a Hutchinson-Gilford Progeria Syndrome Monkey Model by Base Editing. Protein Cell 11 (11), 809–824. doi:10.1007/s13238-020-00740-8
Wasala, N. B., Hakim, C. H., Chen, S.-J., Yang, N. N., and Duan, D. (2019). Questions Answered and Unanswered by the First CRISPR Editing Study in a Canine Model of Duchenne Muscular Dystrophy. Hum. Gene Ther. 30 (5), 535–543. doi:10.1089/hum.2018.243
Whitworth, K. M., Lee, K., Benne, J. A., Beaton, B. P., Spate, L. D., Murphy, S. L., et al. (2014). Use of the CRISPR/Cas9 System to Produce Genetically Engineered Pigs from In Vitro-Derived Oocytes and Embryos1. Biol. Reprod. 91 (3), 78. doi:10.1095/biolreprod.114.121723
Williams, D. K., Pinzón, C., Huggins, S., Pryor, J. H., Falck, A., Herman, F., et al. (2018). Genetic Engineering a Large Animal Model of Human Hypophosphatasia in Sheep. Sci. Rep. 8 (1), 16945. doi:10.1038/s41598-018-35079-y
Wu, M., Wei, C., Lian, Z., Liu, R., Zhu, C., Wang, H., et al. (2016). Rosa26-targeted Sheep Gene Knock-In via CRISPR-Cas9 System. Sci. Rep. 6, 24360. doi:10.1038/srep24360
Wu, J., Vilarino, M., Suzuki, K., Okamura, D., Bogliotti, Y. S., Park, I., et al. (2017). CRISPR-Cas9 Mediated One-step Disabling of Pancreatogenesis in Pigs. Sci. Rep. 7 (1), 10487. doi:10.1038/s41598-017-08596-5
Xie, J., Ge, W., Li, N., Liu, Q., Chen, F., Yang, X., et al. (2019). Efficient Base Editing for Multiple Genes and Loci in Pigs Using Base Editors. Nat. Commun. 10 (1), 2852. doi:10.1038/s41467-019-10421-8
Xie, Z., Jiao, H., Xiao, H., Jiang, Y., Liu, Z., Qi, C., et al. (2020). Generation of pRSAD2 Gene Knock-In Pig via CRISPR/Cas9 Technology. Antivir. Res. 174, 104696. doi:10.1016/j.antiviral.2019.104696
Yan, S., Tu, Z., Liu, Z., Fan, N., Yang, H., Yang, S., et al. (2018). A Huntingtin Knockin Pig Model Recapitulates Features of Selective Neurodegeneration in Huntington's Disease. Cell 173 (4), 989–1002. e1013. doi:10.1016/j.cell.2018.03.005
Yang, S.-H., Cheng, P.-H., Banta, H., Piotrowska-Nitsche, K., Yang, J.-J., Cheng, E. C. H., et al. (2008). Towards a Transgenic Model of Huntington's Disease in a Non-human Primate. Nature 453 (7197), 921–924. doi:10.1038/nature06975
Yang, H., Wang, G., Sun, H., Shu, R., Liu, T., Wang, C.-E., et al. (2014). Species-dependent Neuropathology in Transgenic SOD1 Pigs. Cell Res. 24 (4), 464–481. doi:10.1038/cr.2014.25
Yang, L., Guell, M., Niu, D., George, H., Lesha, E., Grishin, D., et al. (2015). Genome-wide Inactivation of Porcine Endogenous Retroviruses (PERVs). Science 350 (6264), 1101–1104. doi:10.1126/science.aad1191
Yang, W., Li, S., and Li, X.-J. (2019a). A CRISPR Monkey Model Unravels a Unique Function of PINK1 in Primate Brains. Mol. Neurodegener. 14 (1), 17. doi:10.1186/s13024-019-0321-9
Yang, W., Liu, Y., Tu, Z., Xiao, C., Yan, S., Ma, X., et al. (2019b). CRISPR/Cas9-mediated PINK1 Deletion Leads to Neurodegeneration in Rhesus Monkeys. Cell Res. 29 (4), 334–336. doi:10.1038/s41422-019-0142-y
Yang, W., Chen, X., Li, S., and Li, X.-J. (2021). Genetically Modified Large Animal Models for Investigating Neurodegenerative Diseases. Cell Biosci. 11 (1), 218. doi:10.1186/s13578-021-00729-8
Yang, W., Guo, X., Tu, Z., Chen, X., Han, R., Liu, Y., et al. (2022). PINK1 Kinase Dysfunction Triggers Neurodegeneration in the Primate Brain without Impacting Mitochondrial Homeostasis. Protein Cell 13 (1), 26–46. doi:10.1007/s13238-021-00888-x
Yao, X., Liu, Z., Wang, X., Wang, Y., Nie, Y.-H., Lai, L., et al. (2018). Generation of Knock-In Cynomolgus Monkey via CRISPR/Cas9 Editing. Cell Res. 28 (3), 379–382. doi:10.1038/cr.2018.9
Yin, P., Li, S., Li, X.-J., and Yang, W. (2022). New Pathogenic Insights from Large Animal Models of Neurodegenerative Diseases. Protein Cell 1, 1. doi:10.1007/s13238-022-00912-8
Yoshimi, K., and Mashimo, T. (2018). Application of Genome Editing Technologies in Rats for Human Disease Models. J. Hum. Genet. 63 (2), 115–123. doi:10.1038/s10038-017-0346-2
Yuan, H., Yu, T., Wang, L., Yang, L., Zhang, Y., Liu, H., et al. (2020). Efficient Base Editing by RNA-Guided Cytidine Base Editors (CBEs) in Pigs. Cell. Mol. Life Sci. 77 (4), 719–733. doi:10.1007/s00018-019-03205-2
Yue, Y., Xu, W., Kan, Y., Zhao, H.-Y., Zhou, Y., Song, X., et al. (2021). Extensive Germline Genome Engineering in Pigs. Nat. Biomed. Eng. 5 (2), 134–143. doi:10.1038/s41551-020-00613-9
Ze, L., Hai-Yuan, Y., Ying, W., Man-Ling, Z., Xiao-Rui, L., Qiang, X., et al. (2017b). Generation of Tryptophan Hydroxylase 2 Gene Knockout Pigs by CRISPR/Cas9-mediated Gene Targeting. J. Biomed. Res. 31 (5), 445–452. doi:10.7555/JBR.31.20170026
Zhang, W., Wang, G., Wang, Y., Jin, Y., Zhao, L., Xiong, Q., et al. (2017a). Generation of Complement Protein C3 Deficient Pigs by CRISPR/Cas9-mediated Gene Targeting. Sci. Rep. 7 (1), 5009. doi:10.1038/s41598-017-05400-2
Zhang, X., Li, W., Liu, C., Peng, X., Lin, J., He, S., et al. (2017b). Alteration of Sheep Coat Color Pattern by Disruption of ASIP Gene via CRISPR Cas9. Sci. Rep. 7 (1), 8149. doi:10.1038/s41598-017-08636-0
Zhang, X., Li, W., Wu, Y., Peng, X., Lou, B., Wang, L., et al. (2017c). Disruption of the Sheep BMPR-IB Gene by CRISPR/Cas9 in In Vitro -produced Embryos. Theriogenology 91, 163–172. doi:10.1016/j.theriogenology.2016.10.025
Zhang, Y., Qin, W., Lu, X., Xu, J., Huang, H., Bai, H., et al. (2017d). Programmable Base Editing of Zebrafish Genome Using a Modified CRISPR-Cas9 System. Nat. Commun. 8 (1), 118. doi:10.1038/s41467-017-00175-6
Zhang, J., Cui, M. L., Nie, Y. W., Dai, B., Li, F. R., Liu, D. J., et al. (2018a). CRISPR /Cas9‐mediated Specific Integration of Fat‐1 at the Goat MSTN Locus. FEBS J. 285 (15), 2828–2839. doi:10.1111/febs.14520
Zhang, Y., Wang, Y., Yulin, B., Tang, B., Wang, M., Zhang, C., et al. (2018b). CRISPR/Cas9‐mediated Sheep MSTN Gene Knockout and Promote sSMSCs Differentiation. J. Cell Biochem. 120, 1794–1806. doi:10.1002/jcb.27474
Zhang, B., Wang, C., Zhang, Y., Jiang, Y., Qin, Y., Pang, D., et al. (2020). A CRISPR-Engineered Swine Model of COL2A1 Deficiency Recapitulates Altered Early Skeletal Developmental Defects in Humans. Bone 137, 115450. doi:10.1016/j.bone.2020.115450
Zhao, H., Tu, Z., Xu, H., Yan, S., Yan, H., Zheng, Y., et al. (2017). Altered Neurogenesis and Disrupted Expression of Synaptic Proteins in Prefrontal Cortex of SHANK3-Deficient Non-human Primate. Cell Res. 27 (10), 1293–1297. doi:10.1038/cr.2017.95
Zhao, J., Lai, L., Ji, W., and Zhou, Q. (2019). Genome Editing in Large Animals: Current Status and Future Prospects. Natl. Sci. Rev. 6 (3), 402–420. doi:10.1093/nsr/nwz013
Hongbing, H., Yonghe, M., Tao, W., Ling, L., Xiuzhi, T., Rui, H., et al. (2014). One-step Generation of Myostatin Gene Knockout Sheep via the CRISPR/Cas9 System. Front. Agr. Sci. Eng. 1 (1), 2. doi:10.15302/j-fase-2014007
Zhong, H., Chen, Y., Li, Y., Chen, R., and Mardon, G. (2015). CRISPR-engineered Mosaicism Rapidly Reveals that Loss of Kcnj13 Function in Mice Mimics Human Disease Phenotypes. Sci. Rep. 5, 8366. doi:10.1038/srep08366
Zhou, X., Xin, J., Fan, N., Zou, Q., Huang, J., Ouyang, Z., et al. (2015). Generation of CRISPR/Cas9-mediated Gene-Targeted Pigs via Somatic Cell Nuclear Transfer. Cell. Mol. Life Sci. 72 (6), 1175–1184. doi:10.1007/s00018-014-1744-7
Zhou, W., Wan, Y., Guo, R., Deng, M., Deng, K., Wang, Z., et al. (2017). Generation of Beta-Lactoglobulin Knock-Out Goats Using CRISPR/Cas9. PLoS One 12 (10), e0186056. doi:10.1371/journal.pone.0186056
Zhou, S., Cai, B., He, C., Wang, Y., Ding, Q., Liu, J., et al. (2019). Programmable Base Editing of the Sheep Genome Revealed No Genome-wide Off-Target Mutations. Front. Genet. 10, 215. doi:10.3389/fgene.2019.00215
Zhu, S., Rong, Z., Lu, X., Xu, Y., and Fu, X. (2015). Gene Targeting through Homologous Recombination in Monkey Embryonic Stem Cells Using CRISPR/Cas9 System. Stem Cells Dev. 24 (10), 1147–1149. doi:10.1089/scd.2014.0507
Zou, Q., Wang, X., Liu, Y., Ouyang, Z., Long, H., Wei, S., et al. (2015). Generation of Gene-Target Dogs Using CRISPR/Cas9 System. J. Mol. Cell Biol. 7 (6), 580–583. doi:10.1093/jmcb/mjv061
Zou, Y., Li, Z., Zou, Y., Hao, H., Li, N., and Li, Q. (2018). An FBXO40 Knockout Generated by CRISPR/Cas9 Causes Muscle Hypertrophy in Pigs without Detectable Pathological Effects. Biochem. Biophysical Res. Commun. 498 (4), 940–945. doi:10.1016/j.bbrc.2018.03.085
Zou, X., Ouyang, H., Yu, T., Chen, X., Pang, D., Tang, X., et al. (2019). Preparation of a New Type 2 Diabetic Miniature Pig Model via the CRISPR/Cas9 System. Cell Death Dis. 10 (11), 823. doi:10.1038/s41419-019-2056-5
Keywords: Large animals, CRISPR/Cas9, Off-target, Mosaicism, Base editing
Citation: Lin Y, Li J, Li C, Tu Z, Li S, Li X-J and Yan S (2022) Application of CRISPR/Cas9 System in Establishing Large Animal Models. Front. Cell Dev. Biol. 10:919155. doi: 10.3389/fcell.2022.919155
Received: 13 April 2022; Accepted: 02 May 2022;
Published: 17 May 2022.
Edited by:
Yongye Huang, Northeastern University, ChinaReviewed by:
Simon Sretenovic, University of Maryland, United StatesMing Lei, Sun Yat-Sen Memorial Hospital, China
Desh Deepak Singh, Amity University Rajasthan, India
Copyright © 2022 Lin, Li, Li, Tu, Li, Li and Yan. This is an open-access article distributed under the terms of the Creative Commons Attribution License (CC BY). The use, distribution or reproduction in other forums is permitted, provided the original author(s) and the copyright owner(s) are credited and that the original publication in this journal is cited, in accordance with accepted academic practice. No use, distribution or reproduction is permitted which does not comply with these terms.
*Correspondence: Sen Yan, MjMxeWFuc2VuQDE2My5jb20=