- Laboratory of Reproductive Biology, Graduate School of Science, Nagoya University, Nagoya, Japan
Gametogenesis, the production of eggs and sperm, is a fundamental process in sexually reproducing animals. Following gametogenesis commitment and sexual fate decision, germ cells undergo several developmental processes to halve their genomic size and acquire sex-specific characteristics of gametes, including cellular size, motility, and cell polarity. However, it remains unclear how different gametogenesis processes are initially integrated. With the advantages of the teleost fish medaka (Oryzias latipes), in which germline stem cells continuously produce eggs and sperm in mature gonads and a sexual switch gene in germ cells is identified, we found that distinct pathways initiate gametogenesis cooperatively after commitment to gametogenesis. This evokes the concept of functional modules, in which functionally interlocked genes are grouped to yield distinct gamete characteristics. The various combinations of modules may allow us to explain the evolution of diverse reproductive systems, such as parthenogenesis and hermaphroditism.
Introduction
For sexually reproductive organisms, life begins with the fusion of two haploid gametes, an egg and a sperm. Gametes are generated from common germline stem cells through tightly regulated developmental processes. Once germline stem cells undergo gametogenesis commitment and sperm-egg fate decisions, the cells halve their genomic size through meiosis. Germline stem cells go through folliculogenesis or spermatogenesis to acquire sex-specific characteristics such as cellular size, motility, and polarity. Although the mechanisms underlying the development of eggs and sperm have been well studied, the initial integration of meiosis, sexually dimorphic folliculogenesis, and spermatogenesis remains elusive.
In mice, the first sexual difference in germ cell development is indicated by the timing of meiosis initiation. Ovarian germ cells initiate meiosis before birth, whereas testicular germ cells do not embark on meiosis until puberty (Spiller and Bowles, 2019). Several signaling pathways involved in meiotic initiation have been identified. One is retinoic acid (RA) signaling, which activates the downstream transcription factor Stra8 (stimulated by retinoic acid 8) and Meiosin (meiosis initiator) to drive meiotic gene expression (Bowles et al., 2006; Koubova et al., 2006; Ishiguro et al., 2020). Another is BMP (bone morphogenetic protein) signaling, which is essential for meiosis initiation in vivo and in vitro, and ligands are presumably secreted from ovarian somatic cells (Wu et al., 2016; Miyauchi et al., 2017; Nagaoka et al., 2020). The other is WNT/β-catenin signaling, which is required for Stra8 expression in germ cells, although whether this signaling acts on germ cells directly or indirectly remains unclear (Tomizuka et al., 2008; Naillat et al., 2010; Chassot et al., 2011). In contrast, in the testes, RA is degraded by Cyp26b1 (cytochrome P450 family 26 subfamily B member 1) expressed in somatic cells, thereby preventing germ cells from entering meiosis during embryogenesis (Bowles et al., 2006; Koubova et al., 2006). Additionally, male-specific Fgf9 (fibroblast growth factor 9) also contributes to meiosis suppression via the upregulation of Nanos2 (nanos C2HC-type zinc finger 2) in germ cells, thereby making them less responsive to RA (Suzuki and Saga, 2008; Bowles et al., 2010). Instead, germ cells enter mitotic arrest and are specified as spermatogonial cells (Spiller and Bowles, 2019).
Although the molecular mechanisms that promote or suppress meiosis have been investigated intensively in mice, it is difficult to identify germ cell sex determinants hidden among intertwined developmental processes. Meiosis initiation per se is not a female-specific event; thus, it is not equivalent to germline feminization. The absence of mouse ovarian germline stem cells also makes the sexual fate decision of the germline difficult to be clarified (Tanaka, 2016). In male mice, certain genes, including Nanos2, Piwil4 (piwi like RNA-mediated gene silencing 4), and Dnmt3l (DNA methyltransferase 3 like), are specifically induced when germ cells are fated to undergo spermatogenesis (Tsuda et al., 2003; La Salle et al., 2004; Aravin et al., 2008). However, in some teleost species, nanos2 is expressed not only in spermatogonia, but also in oogonia (Aoki et al., 2009; Nakamura et al., 2010; Beer and Draper, 2013), suggesting that a gene(s) induced in male germ cells can be recognized as the acquisition of stemness rather than germline masculinization.
The teleost fish medaka (Oryzias latipes) is one of the ideal vertebrate models, in which the presence of germline stem cells in both ovaries and testes has been proven experimentally (Nakamura et al., 2010). This makes it possible to analyze the initial step of sexual development by comparing the profiles of ovarian and testicular germline stem cells. Consequently, we are beginning to understand the presence of a modular structure in gametogenesis that yields sex- and gamete-specific characteristics. This view may explain the diversity observed among reproductive systems.
Germ Cell Proliferation in Early Gametogenesis
Germline stem cells produce progenitor cells that proliferate mitotically and enter meiosis (Wu et al., 2013). In diverse organisms, germline stem cells undergo two types of mitotic cell division. One is stem-type cell division that produces isolated daughter cells. The other is transit-amplifying cell division, in which several rounds of cell division followed by incomplete cytokinesis form a cluster of cells that are connected via intercellular bridges (Pepling et al., 1999; Spradling et al., 2011).
In medaka, stem-type and transit-amplifying cell divisions are referred to as type-I and type-II cell divisions, respectively (Saito et al., 2007; Nakamura et al., 2010; Sumita et al., 2021). Type I/II cell division can be observed in adult testes and ovaries, supporting the presence of germline stem cells in both sexes (Nakamura et al., 2010; Sumita et al., 2021). Importantly, medaka germ cells transplanted into gonads of the other sex give rise to gametes according to the recipient sex, suggesting that germline stem cells are sexually undifferentiated (Tanaka, 2016). In other words, medaka germline stem cells continuously make sexual fate decisions at the time of their commitment to gametogenesis throughout their reproductive life. This prompted us to explore the germ cell sex determinants acting on gametogenesis-committed germ cell types.
Of note, stra8 has been lost from the medaka genome (Pasquier et al., 2016). In addition, medaka germ cells are not responsive to RA signaling at the time of sex determination (Adolfi et al., 2016; Adolfi et al., 2021). Therefore, factors other than RA signaling components are involved in germline sex determination in medaka.
Role of Foxl3 in Germ Cell Sex Determination in Medaka
By comparing the transcriptomes of medaka germ cells between genetic males (XY) and females (XX), a switch gene for the sexual fate decision of germ cells, foxl3 (forkhead box L3), has been identified (Nishimura et al., 2015). Foxl3 is initially expressed in type I germ cells in both sexes, but its expression is maintained only in female type II germ cells (Nishimura et al., 2015; Nishimura and Tanaka, 2016). A loss-of-function mutant of foxl3 leads to germ cell-specific sex reversal in XX gonads and spermatogenesis in the ovarian environment (Nishimura et al., 2015). In addition, transplantation of foxl3−/− germ cells into wild-type ovaries results in spermatogenesis of foxl3−/− germ cells, showing that Foxl3 functions in a cell-autonomous manner as a germ cell sex determinant in medaka ovaries (Nishimura et al., 2015).
Foxl3 is widely conserved in vertebrate genomes except for placental mammals (Bertho et al., 2016). Although the role of Foxl3 in other vertebrate species remains largely unknown, its expression in germ cells has been reported in some teleost species including Nile tilapia (Oreochromis niloticus) and Japanese eel (Anguilla Japonica) (Wu et al., 2019; Dai et al., 2021). Furthermore, a loss-of-function mutant of tilapia foxl3 leads to production of spermatogenic cells in XX ovaries (Dai et al., 2021), suggesting a conserved role of foxl3 in germ cell feminization.
Dmrt1 may play an important role in germline sexual development by antagonizing foxl3 function. In tilapia, loss of foxl3 leads to dmrt1 expression in XX gonads, whereas dmrt1 mutation causes ectopic foxl3 expression in XY gonads. This suggests that sexual fate of tilapia germline is regulated by antagonistic roles of foxl3 and dmrt1 (Dai et al., 2021). Another important player in germline sexual development is germ cell itself. In medaka, germ cells have an inherent feminizing effect during early gonadal development, which acts independently of developmental stage and sex of germ cells (Kurokawa et al., 2007; Morinaga et al., 2007; Nakamura et al., 2012; Nishimura et al., 2018). The same effect of germ cells has been reported in zebrafish (Slanchev et al., 2005; Tzung et al., 2015), where germ cell apoptosis triggers testis differentiation from juvenile ovaries (Uchida et al., 2002). This could be explained by two juxtacrine signaling pathways. One secreted from germ cells suppresses gonadal masculinization via dmrt1 downregulation, while the dmrt1-downstream signal secreted from somatic cells suppresses germline feminization via foxl3 downregulation in germ cells.
Although a full view of the Foxl3 regulatory network remains elusive, RNA sequencing has revealed genes downstream of foxl3 (Kikuchi et al., 2019). Comparative transcriptome analysis between wild-type and foxl3−/− XX germ cells identified 1,480 differentially expressed genes (DEGs), comprising 600 upregulated and 880 downregulated genes. Gene ontology and pathway enrichment analyses suggest that, in female germ cells, pathways related to the extracellular matrix, oogenesis, and RNA regulation are activated, while the cytoskeletal network and cell cycle pathway are suppressed. These results indicate that Foxl3 may promote germ cell feminization via the transcriptional activation of oogenesis-related genes and reorganization of the microtubule network.
In addition, discovery of the Foxl3-binding motif (5′-DHAAACAA-3′) and in silico searches for the motif within DEG promoter regions suggest that Foxl3 may bind to most DEG promoters and directly regulate their expression (Kikuchi et al., 2019). Foxl3 likely initiates regulatory events as a pioneer transcription factor that determines the sexual fate of germ cells by targeting silent chromatin and enabling states of its competence to be activated or repressed (Zaret and Mango, 2016). Many pioneer factors are involved in cell fate decisions, as exemplified by FoxA, which persists at enhancer elements in chromatin as endoderm is induced to liver fate (Gualdi et al., 1996; Bossard and Zaret, 2000; Lee et al., 2005).
Foxl3 Initiates Distinct Genetic Pathways Regulating Meiosis and Folliculogenesis
The following question arises: Which developmental processes does Foxl3 initiate to yield egg characteristics? Recent studies have revealed two genes, rec8a (REC8 meiotic recombination protein a) and fbxo47 (F-box protein 47) to be direct targets of Foxl3. Similar to foxl3, rec8a and fbxo47 are expressed in female type II germ cells, and loss-of-function mutations cause female-specific sterility and ovarian dysgenesis, suggesting their crucial roles in oogenesis (Kikuchi et al., 2019; Kikuchi et al., 2020).
Medaka rec8a is one of two mammalian Rec8 orthologs originating from a teleost-specific whole-genome duplication event (Braasch et al., 2016). rec8a encodes an α-kleisin subunit of the meiotic cohesin. The cohesin complex forms a ring-like structure that holds sister chromatids during mitosis and meiosis. Meiotic cohesin is required not only for sister chromatid cohesion, but also for chromosomal axis formation, association of homologous chromosomes, meiotic recombination, and accurate chromosome segregation (Ishiguro, 2019). Mouse Rec8-null mutants of both sexes fail to complete meiotic prophase I and become sterile (Bannister et al., 2004; Xu et al., 2005). Similarly, medaka rec8a–/– fish display defects in synapsis between homologous chromosomes during meiotic prophase I, which results in meiotic arrest at the pachytene-like stage. The phenotypes of medaka rec8a–/– are female specific: medaka rec8a–/– males are fertile and develop normal testes containing mature sperm (Kikuchi et al., 2020). It seems likely that the rec8a paralogous gene rec8b contributes to spermatogenesis. Nevertheless, rec8a was the first gene to be identified as a female-specific factor involved in meiosis and may provide a clue to explore the molecular mechanisms regulating sexual dimorphism in meiosis (Cahoon and Libuda, 2019; Sardell and Kirkpatrick, 2020).
Another Foxl3 target, fbxo47, encodes a member of the F-box protein family. The F-box protein is widely conserved in eukaryotes and is involved in various cellular functions (Cardozo and Pagano, 2004). F-box proteins are known to act as components of Skp1/Cullin/F-box-protein (SCF) E3 ubiquitin ligase or independently of the ubiquitin-mediated pathway (Kipreos and Pagano, 2000). The medaka fbxo47−/− mutant mimics the phenotype of foxl3−/−: Precocious spermatogenesis in XX ovaries. Moreover, fbxo47 acts genetically upstream of the folliculogenesis-related transcription factors lhx8b, figla, and nobox (Kikuchi et al., 2020). These results indicate that medaka fbxo47 is involved in the suppression of spermatogenesis and progression of folliculogenesis.
Caenorhabditis elegans FBXO47 (also known as PROM-1) mediates the mitosis-meiosis transition via ubiquitination and degradation of cyclin E1 (CYE-1), and SCFPROM-1 also promotes homologous chromosome pairing as a positive regulator of CHK-2 serine/threonine kinase (Mohammad et al., 2018). In addition, a recent study revealed that mouse Fbxo47 prevents precocious disassembly of the synaptonemal complex independently of SCF E3 ligase (Tanno et al., 2022). Thus, Fbxo47 may have conserved roles in gametogenesis via ubiquitination-dependent and ubiquitination-independent pathways.
Importantly, epistasis analysis revealed that pathways involving rec8a and fbxo47 are genetically independent (Kikuchi et al., 2020). Hence, the studies described above shed light on two genetic pathways acting downstream of foxl3: one promotes female-specific meiosis and the other regulates folliculogenesis and suppression of spermatogenesis (Figure 1). The mechanisms underlying the suppression of spermatogenesis will be an important area for future research.
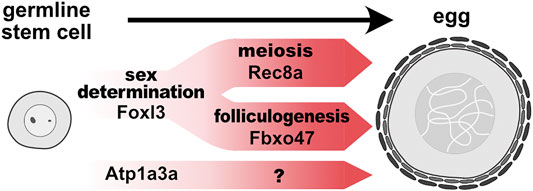
FIGURE 1. Genetically distinct pathways promote germline feminization in medaka. In germline stem cells, foxl3, an intrinsic factor of germ cell sex, directly activates expression of rec8a and fbxo47 to initiate meiosis and folliculogenesis, respectively. Other female-specific pathways (e.g., atp1a3a) may also promote another module independently of foxl3.
Model: Functionally Distinct Modules Integrate Gametogenesis
Accumulating evidence based on genetic analyses indicates that meiosis, folliculogenesis, and spermatogenesis are genetically dissociable. In mice, Stra8-deficient ovarian germ cells develop into oocyte-like cells without undergoing meiosis (Dokshin et al., 2013). Loss-of-function mutants of medaka rec8a also lead to meiotic arrest of ovarian germ cells at the pachytene-like stage, but they still express transcription factors involved in folliculogenesis, including lhx8b, figla, and nobox (Kikuchi et al., 2020). Meanwhile, medaka dmc1−/− testes can produce a small number of motile sperm with the ability to inseminate, although the ploidy is abnormal (Chen et al., 2016). In contrast, meiosis progresses when folliculogenesis or spermatogenesis is disrupted. Lhx8−/− female mice display severe defects in folliculogenesis, with no abnormalities involving meiotic marker expression and chromosomal structures (Choi et al., 2008). The same phenomenon occurs in medaka fbxo47−/− ovaries, where folliculogenesis is severely disrupted, while the meiotic gene rec8a is normally expressed and spermatogenesis proceeds (Kikuchi et al., 2020). Collectively, these phenotypic analyses clearly indicate that although meiosis is dispensable for gametogenesis, processes driving folliculogenesis and spermatogenesis can be activated in the absence of meiosis.
This evokes the concept of ‘functional modules’ where expression of a group of genes are tightly coordinated to act in the same developmental process (Niehrs and Pollet, 1999; Szenker-Ravi et al., 2022), featuring essential gamete characteristics. For example, a module of meiosis contributes to halving the genome, whereas a module of folliculogenesis and spermatogenesis renders gametes feminized or masculinized, respectively.
This raises the interesting view that modules can be referred to as the basis for creating various modes of reproduction during evolution (Figure 2). In apomictic parthenogenesis (development of embryos from unfertilized eggs), bypassing meiotic modules allows gametogenesis to circumvent meiosis, resulting in the production of diploid eggs (Lampert, 2008; Mirzaghaderi and Horandl, 2016). This type of reproduction has been reported in bdelloid rotifers and many arthropods (Simon et al., 2003). Another unisexual mode of reproduction, hybridogenesis, could also be explained by alterations to the meiotic module so that one of the parental genomes is selectively transferred to the gametes, while the other one is lost during meiosis (Lehtonen et al., 2013; Lavanchy and Schwander, 2019). Hybridogenesis has been found in a diverge range of animals, including fishes (Poeciliopsis lucida-monacha), frogs (Pelophylax esculentus), and insects (Bacillus rossius-grandii) (Schultz, 1969; Mantovani and Scali, 1992; Vorburger et al., 2009). Additionally, hermaphrodites (e.g., Caenorhabditis elegans) consecutively produce egg and sperm from germline stem cells by acquiring a mechanism for switching a module of folliculogenesis from that of spermatogenesis (Hubbard and Greenstein, 2000). In addition, sexually dimorphic (anisogamous) gametes seem to have descended from equal-sized (isogamous) gametes, which is common in algae and protists (Kirk, 2006). Thus, during the course of evolution, the folliculogenesis and spermatogenesis modules might have been progressively evoked and selected to develop large eggs and small sperm.
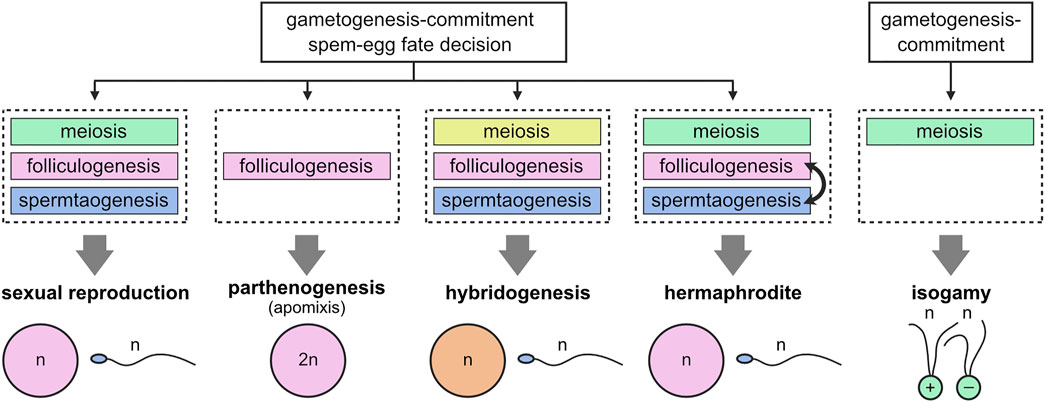
FIGURE 2. Modular structure of gametogenesis. Initial events of gametogenesis-commitment and sperm-egg fate decision trigger distinct developmental processes, corresponding to distinct modules (meiosis, folliculogenesis, and spermatogenesis). Gain, loss, or alteration of modules during evolution can explain adaptive diversification of reproductive systems.
Regarding folliculogenesis, it is likely that this module diverges into other submodules, leading to more specific gametogenic characteristics, such as maternal RNA accumulation and fertilization capability. Additionally, the finding of oogenesis-specific, but foxl3-independent, gene expression suggests that characteristics independent of meiosis and folliculogenesis are also present. Atp1a3a (ATPase Na+/K+ transporting subunit alpha 3a) is one of the genes that we found from our RNA-seq data to be upregulated specifically in female germ cells but independently of foxl3 (Kikuchi et al., 2019) (Figure 1). During oogenesis, this cation-exchanging transporter could be involved in cell enlargement by regulating osmotic pressure.
In conclusion, we propose a modular structure of gametogenesis, in which genetically distinct modules build the development of functional gametes. Modification and/or loss of modules will provide a way to explain the diversification of reproductive systems.
Data Availability Statement
The original contributions presented in the study are included in the article/Supplementary Material, further inquiries can be directed to the corresponding author.
Ethics Statement
The animal study was reviewed and approved by the Center for Animal Research and Education, Nagoya University, approval #S210527-003.
Author Contributions
MK conceived the idea and wrote the manuscript. MT critically reviewed the manuscript and revised the manuscript. All authors contributed to the article and approved the submitted version.
Funding
This work was supported by a Grant-in-Aid for Early-Career Scientists (21K15133: MK) and a Grant-in-Aid for Scientific Research on Innovative Areas (17H06430, MT).
Conflict of Interest
The authors declare that the research was conducted in the absence of any commercial or financial relationships that could be construed as a potential conflict of interest.
Publisher’s Note
All claims expressed in this article are solely those of the authors and do not necessarily represent those of their affiliated organizations, or those of the publisher, the editors and the reviewers. Any product that may be evaluated in this article, or claim that may be made by its manufacturer, is not guaranteed or endorsed by the publisher.
References
Adolfi, M. C., Herpin, A., Martinez-Bengochea, A., Kneitz, S., Regensburger, M., Grunwald, D. J., et al. (2021). Crosstalk between Retinoic Acid and Sex-Related Genes Controls Germ Cell Fate and Gametogenesis in Medaka. Front. Cell. Dev. Biol. 8, 613497. doi:10.3389/fcell.2020.613497
Adolfi, M. C., Herpin, A., Regensburger, M., Sacquegno, J., Waxman, J. S., and Schartl, M. (2016). Retinoic Acid and Meiosis Induction in Adult versus Embryonic Gonads of Medaka. Sci. Rep. 6, 34281. doi:10.1038/srep34281
Aoki, Y., Nakamura, S., Ishikawa, Y., and Tanaka, M. (2009). Expression and Syntenic Analyses of FournanosGenes in Medaka. Zoological Sci. 26, 112–118. doi:10.2108/zsj.26.112
Aravin, A. A., Sachidanandam, R., Bourc'his, D., Schaefer, C., Pezic, D., Toth, K. F., et al. (2008). A piRNA Pathway Primed by Individual Transposons Is Linked to De Novo DNA Methylation in Mice. Mol. Cell. 31, 785–799. doi:10.1016/j.molcel.2008.09.003
Bannister, L. A., Reinholdt, L. G., Munroe, R. J., and Schimenti, J. C. (2004). Positional Cloning and Characterization of Mousemei8, a Disrupted Allele of the Meiotic cohesinRec8. Genesis 40, 184–194. doi:10.1002/gene.20085
Beer, R. L., and Draper, B. W. (2013). nanos3 Maintains Germline Stem Cells and Expression of the Conserved Germline Stem Cell Gene Nanos2 in the Zebrafish Ovary. Dev. Biol. 374, 308–318. doi:10.1016/j.ydbio.2012.12.003
Bertho, S., Pasquier, J., Pan, Q., Le Trionnaire, G., Bobe, J., Postlethwait, J. H., et al. (2016). Foxl2 and its Relatives Are Evolutionary Conserved Players in Gonadal Sex Differentiation. Sex. Dev. 10, 111–129. doi:10.1159/000447611
Bossard, P., and Zaret, K. S. (2000). Repressive and Restrictive Mesodermal Interactions with Gut Endoderm: Possible Relation to Meckel's Diverticulum. Development 127, 4915–4923. doi:10.1242/dev.127.22.4915
Bowles, J., Feng, C.-W., Spiller, C., Davidson, T.-L., Jackson, A., and Koopman, P. (2010). FGF9 Suppresses Meiosis and Promotes Male Germ Cell Fate in Mice. Dev. Cell. 19, 440–449. doi:10.1016/j.devcel.2010.08.010
Bowles, J., Knight, D., Smith, C., Wilhelm, D., Richman, J., Mamiya, S., et al. (2006). Retinoid Signaling Determines Germ Cell Fate in Mice. Science 312, 596–600. doi:10.1126/science.1125691
Braasch, I., Gehrke, A. R., Smith, J. J., Kawasaki, K., Manousaki, T., Pasquier, J., et al. (2016). Erratum: Corrigendum: The Spotted Gar Genome Illuminates Vertebrate Evolution and Facilitates Human-Teleost Comparisons. Nat. Genet. 48, 700. doi:10.1038/ng0616-700c
Cahoon, C. K., and Libuda, D. E. (2019). Leagues of Their Own: Sexually Dimorphic Features of Meiotic Prophase I. Chromosoma 128, 199–214. doi:10.1007/s00412-019-00692-x
Cardozo, T., and Pagano, M. (2004). The SCF Ubiquitin Ligase: Insights into a Molecular Machine. Nat. Rev. Mol. Cell. Biol. 5, 739–751. doi:10.1038/nrm1471
Chassot, A.-A., Gregoire, E. P., Lavery, R., Taketo, M. M., de Rooij, D. G., Adams, I. R., et al. (2011). RSPO1/β-Catenin Signaling Pathway Regulates Oogonia Differentiation and Entry into Meiosis in the Mouse Fetal Ovary. PLoS. One. 6, e25641. doi:10.1371/journal.pone.0025641
Chen, J., Cui, X., Jia, S., Luo, D., Cao, M., Zhang, Y., et al. (2016). Disruption of Dmc1 Produces Abnormal Sperm in Medaka (Oryzias latipes). Sci. Rep. 6, 30912. doi:10.1038/srep30912
Choi, Y., Ballow, D. J., Xin, Y., and Rajkovic, A. (2008). Lim Homeobox Gene, Lhx8, Is Essential for Mouse Oocyte Differentiation and Survival1. Biol. Reprod. 79, 442–449. doi:10.1095/biolreprod.108.069393
Dai, S., Qi, S., Wei, X., Liu, X., Li, Y., Zhou, X., et al. (2021). Germline Sexual Fate Is Determined by the Antagonistic Action of Dmrt1 and Foxl3/foxl2 in Tilapia. Development 148, dev199380. doi:10.1242/dev.199380
Dokshin, G. A., Baltus, A. E., Eppig, J. J., and Page, D. C. (2013). Oocyte Differentiation Is Genetically Dissociable from Meiosis in Mice. Nat. Genet. 45, 877–883. doi:10.1038/ng.2672
Gualdi, R., Bossard, P., Zheng, M., Hamada, Y., Coleman, J. R., and Zaret, K. S. (1996). Hepatic Specification of the Gut Endoderm In Vitro: Cell Signaling and Transcriptional Control. Genes Dev. 10, 1670–1682. doi:10.1101/gad.10.13.1670
Hubbard, E. J. A., and Greenstein, D. (2000). TheCaenorhabditis Elegans Gonad: A Test Tube for Cell and Developmental Biology. Dev. Dyn. 218, 2–22. doi:10.1002/(SICI)1097-017710.1002/(sici)1097-0177(200005)218:1<2:aid-dvdy2>3.0.co;2-w
Ishiguro, K.-I., Matsuura, K., Tani, N., Takeda, N., Usuki, S., Yamane, M., et al. (2020). MEIOSIN Directs the Switch from Mitosis to Meiosis in Mammalian Germ Cells. Dev. Cell. 52, 429–445. doi:10.1016/j.devcel.2020.01.010
Ishiguro, K.-I. (2019). The Cohesin Complex in Mammalian Meiosis. Genes. Cells. 24, 6–30. doi:10.1111/gtc.12652
Kikuchi, M., Nishimura, T., Ishishita, S., Matsuda, Y., and Tanaka, M. (2020). foxl3, a Sexual Switch in Germ Cells, Initiates Two Independent Molecular Pathways for Commitment to Oogenesis in Medaka. Proc. Natl. Acad. Sci. U.S.A. 117, 12174–12181. doi:10.1073/pnas.1918556117
Kikuchi, M., Nishimura, T., Saito, D., Shigenobu, S., Takada, R., Gutierrez-Triana, J. A., et al. (2019). Novel Components of Germline Sex Determination Acting Downstream of Foxl3 in Medaka. Dev. Biol. 445, 80–89. doi:10.1016/j.ydbio.2018.10.019
Kipreos, E. T., and Pagano, M. (2000). The F-Box Protein Family. Genome. Biol. 1, reviews30021. doi:10.1186/gb-2000-1-5-reviews3002
Kirk, D. L. (2006). Oogamy: Inventing the Sexes. Curr. Biol. 16, R1028–R1030. doi:10.1016/j.cub.2006.11.015
Koubova, J., Menke, D. B., Zhou, Q., Capel, B., Griswold, M. D., and Page, D. C. (2006). Retinoic Acid Regulates Sex-specific Timing of Meiotic Initiation in Mice. Proc. Natl. Acad. Sci. U.S.A. 103, 2474–2479. doi:10.1073/pnas.0510813103
Kurokawa, H., Saito, D., Nakamura, S., Katoh-Fukui, Y., Ohta, K., Baba, T., et al. (2007). Germ Cells Are Essential for Sexual Dimorphism in the Medaka Gonad. Proc. Natl. Acad. Sci. U.S.A. 104, 16958–16963. doi:10.1073/pnas.0609932104
La Salle, S., Mertineit, C., Taketo, T., Moens, P. B., Bestor, T. H., and Trasler, J. M. (2004). Windows for Sex-specific Methylation Marked by DNA Methyltransferase Expression Profiles in Mouse Germ Cells. Dev. Biol. 268, 403–415. doi:10.1016/j.ydbio.2003.12.031
Lampert, K. P. (2008). Facultative Parthenogenesis in Vertebrates: Reproductive Error or Chance? Sex. Dev. 2, 290–301. doi:10.1159/000195678
Lavanchy, G., and Schwander, T. (2019). Hybridogenesis. Curr. Biol. 29, R9–R11. doi:10.1016/j.cub.2018.11.046
Lee, C. S., Sund, N. J., Behr, R., Herrera, P. L., and Kaestner, K. H. (2005). Foxa2 Is Required for the Differentiation of Pancreatic α-cells. Dev. Biol. 278, 484–495. doi:10.1016/j.ydbio.2004.10.012
Lehtonen, J., Schmidt, D. J., Heubel, K., and Kokko, H. (2013). Evolutionary and Ecological Implications of Sexual Parasitism. Trends Ecol. Evol. 28, 297–306. doi:10.1016/j.tree.2012.12.006
Mantovani, B., and Scali, V. (1992). Hybridogenesis and Androgenesis in the Stick-Insectbacillus Rossius-Grandii Benazzii(Insecta, Phasmatodea). Evolution 46, 783–796. doi:10.1111/j.1558-5646.1992.tb02084.x
Mirzaghaderi, G., and Hörandl, E. (2016). The Evolution of Meiotic Sex and its Alternatives. Proc. R. Soc. B 283, 20161221. doi:10.1098/rspb.2016.1221
Miyauchi, H., Ohta, H., Nagaoka, S., Nakaki, F., Sasaki, K., Hayashi, K., et al. (2017). Bone Morphogenetic Protein and Retinoic Acid Synergistically Specify Female Germ‐cell Fate in Mice. EMBO. J. 36, 3100–3119. doi:10.15252/embj.201796875
Mohammad, A., Vanden Broek, K., Wang, C., Daryabeigi, A., Jantsch, V., Hansen, D., et al. (2018). Initiation of Meiotic Development Is Controlled by Three Post-Transcriptional Pathways in Caenorhabditis elegans. Genetics 209, 1197–1224. doi:10.1534/genetics.118.300985
Morinaga, C., Saito, D., Nakamura, S., Sasaki, T., Asakawa, S., Shimizu, N., et al. (2007). The Hotei Mutation of Medaka in the Anti-müllerian Hormone Receptor Causes the Dysregulation of Germ Cell and Sexual Development. Proc. Natl. Acad. Sci. U.S.A. 104, 9691–9696. doi:10.1073/pnas.0611379104
Nagaoka, S. I., Nakaki, F., Miyauchi, H., Nosaka, Y., Ohta, H., Yabuta, Y., et al. (2020). ZGLP1 Is a Determinant for the Oogenic Fate in Mice. Science 367, eaaw4115. doi:10.1126/science.aaw4115
Naillat, F., Prunskaite-Hyyryläinen, R., Pietilä, I., Sormunen, R., Jokela, T., Shan, J., et al. (2010). Wnt4/5a Signalling Coordinates Cell Adhesion and Entry into Meiosis during Presumptive Ovarian Follicle Development. Hum. Mol. Genet. 19, 1539–1550. doi:10.1093/hmg/ddq027
Nakamura, S., Kobayashi, K., Nishimura, T., Higashijima, S.-I., and Tanaka, M. (2010). Identification of Germline Stem Cells in the Ovary of the Teleost Medaka. Science 328, 1561–1563. doi:10.1126/science.1185473
Nakamura, S., Watakabe, I., Nishimura, T., Picard, J.-Y., Toyoda, A., Taniguchi, Y., et al. (2012). Hyperproliferation of Mitotically Active Germ Cells Due to Defective Anti-müllerian Hormone Signaling Mediates Sex Reversal in Medaka. Development 139, 2283–2287. doi:10.1242/dev.076307
Niehrs, C., and Pollet, N. (1999). Synexpression Groups in Eukaryotes. Nature 402, 483–487. doi:10.1038/990025
Nishimura, T., Sato, T., Yamamoto, Y., Watakabe, I., Ohkawa, Y., Suyama, M., et al. (2015). foxl3 Is a Germ Cell-Intrinsic Factor Involved in Sperm-Egg Fate Decision in Medaka. Science 349, 328–331. doi:10.1126/science.aaa2657
Nishimura, T., and Tanaka, M. (2016). The Mechanism of Germline Sex Determination in Vertebrates. Biol. Reproduction 95, 30. doi:10.1095/biolreprod.115.138271
Nishimura, T., Yamada, K., Fujimori, C., Kikuchi, M., Kawasaki, T., Siegfried, K. R., et al. (2018). Germ Cells in the Teleost Fish Medaka Have an Inherent Feminizing Effect. PLoS. Genet. 14, e1007259. doi:10.1371/journal.pgen.1007259
Pasquier, J., Cabau, C., Nguyen, T., Jouanno, E., Severac, D., Braasch, I., et al. (2016). Gene Evolution and Gene Expression after Whole Genome Duplication in Fish: The PhyloFish Database. Bmc. Genomics. 17, 368. doi:10.1186/s12864-016-2709-z
Pepling, M. E., de Cuevas, M., and Spradling, A. C. (1999). Germline Cysts: A Conserved Phase of Germ Cell Development? Trends Cell. Biol. 9, 257–262. doi:10.1016/s0962-8924(99)01594-9
Saito, D., Morinaga, C., Aoki, Y., Nakamura, S., Mitani, H., Furutani-Seiki, M., et al. (2007). Proliferation of Germ Cells during Gonadal Sex Differentiation in Medaka: Insights from Germ Cell-Depleted Mutant Zenzai. Dev. Biol. 310, 280–290. doi:10.1016/j.ydbio.2007.07.039
Sardell, J. M., and Kirkpatrick, M. (2020). Sex Differences in the Recombination Landscape. Am. Nat. 195, 361–379. doi:10.1086/704943
Schultz, R. J. (1969). Hybridization, Unisexuality, and Polyploidy in the Teleost Poeciliopsis (Poeciliidae) and Other Vertebrates. Am. Nat. 103, 605–619. doi:10.1086/282629
Simon, J.-C., Delmotte, F., Rispe, C., and Crease, T. (2003). Phylogenetic Relationships between Parthenogens and Their Sexual Relatives: the Possible Routes to Parthenogenesis in Animals. Biol. J. Linn. Soc. 79, 151–163. doi:10.1046/j.1095-8312.2003.00175.x
Slanchev, K., Stebler, J., Cueva-Méndez, G., and Cueva-Méndez, E. (2005). Development without Germ Cells: the Role of the Germ Line in Zebrafish Sex Differentiation. Proc. Natl. Acad. Sci. U.S.A. 102, 4074–4079. doi:10.1073/pnas.0407475102
Spiller, C., and Bowles, J. (2019). Sexually Dimorphic Germ Cell Identity in Mammals. Curr. Top. Dev. Biol. 134, 253–288. doi:10.1016/bs.ctdb.2019.01.011
Spradling, A., Fuller, M. T., Braun, R. E., and Yoshida, S. (2011). Germline Stem Cells. Cold Spring Harb. Perspect. Biol. 3, a002642. doi:10.1101/cshperspect.a002642
Sumita, R., Nishimura, T., and Tanaka, M. (2021). Dynamics of Spermatogenesis and Change in Testicular Morphology under 'Mating' and 'Non-Mating' Conditions in Medaka (Oryzias latipes). Zoological Sci. 38, 436–443. doi:10.2108/zs210025
Suzuki, A., and Saga, Y. (2008). Nanos2 Suppresses Meiosis and Promotes Male Germ Cell Differentiation. Genes Dev. 22, 430–435. doi:10.1101/gad.1612708
Szenker-Ravi, E., Ott, T., Khatoo, M., de Bellaing, A. M., Goh, W. X., Chong, Y. L., et al. (2022). Discovery of a Genetic Module Essential for Assigning Left-Right Asymmetry in Humans and Ancestral Vertebrates. Nat. Genet. 54, 62–72. doi:10.1038/s41588-021-00970-4
Tanaka, M. (2016). Germline Stem Cells Are Critical for Sexual Fate Decision of Germ Cells. Bioessays 38, 1227–1233. doi:10.1002/bies.201600045
Tanno, N., Takemoto, K., Takada-Horisawa, Y., Shimada, R., Fujimura, S., Tani, N., et al. (2022). FBXO47 Is Essential for Preventing the Synaptonemal Complex from Premature Disassembly in Mouse Male Meiosis. iScience 25, 104008. doi:10.1016/j.isci.2022.104008
Tomizuka, K., Horikoshi, K., Kitada, R., Sugawara, Y., Iba, Y., Kojima, A., et al. (2008). R-spondin1 Plays an Essential Role in Ovarian Development through Positively Regulating Wnt-4 Signaling. Hum. Mol. Genet. 17, 1278–1291. doi:10.1093/hmg/ddn036
Tsuda, M., Sasaoka, Y., Kiso, M., Abe, K., Haraguchi, S., Kobayashi, S., et al. (2003). Conserved Role of Nanos Proteins in Germ Cell Development. Science 301, 1239–1241. doi:10.1126/science.1085222
Tzung, K.-W., Goto, R., Saju, J. M., Sreenivasan, R., Saito, T., Arai, K., et al. (2015). Early Depletion of Primordial Germ Cells in Zebrafish Promotes Testis Formation. Stem. Cell. Rep. 4, 61–73. doi:10.1016/j.stemcr.2014.10.011
Uchida, D., Yamashita, M., Kitano, T., and Iguchi, T. (2002). Oocyte Apoptosis during the Transition from Ovary-like Tissue to Testes during Sex Differentiation of Juvenile Zebrafish. J. Exp. Biol. 205, 711–718. doi:10.1242/jeb.205.6.711
Vorburger, C., Schmeller, D. S., Hotz, H., Guex, G.-D., and Reyer, H.-U. (2009). “Masked Damage: Mutational Load in Hemiclonal Water Frogs,” in Masked Damage: Mutational Load in Hemiclonal Water FrogsLost Sex. Editors I. Schön, K. Martens, and P. V. Dijk (Berlin: Springer), 433–446. doi:10.1007/978-90-481-2770-2_20
Wu, G.-C., Jeng, S.-R., Pan, Y.-T., Li, H.-W., Ku, W.-L., Lin, C.-J., et al. (2019). The Germline-specific Expression of Foxl3a and its Paralogous Foxl3b Are Associated with Male Gonadal Differentiation in the Japanese Eel, Anguilla Japonica. General Comp. Endocrinol. 277, 56–65. doi:10.1016/j.ygcen.2019.03.008
Wu, J., Luo, H., and Wang, H. (2013). Germline Stem Cells. Curr. Top. Dev. Biol. 102, 97–126. doi:10.1016/B978-0-12-416024-8.00004-0
Wu, Q., Fukuda, K., Kato, Y., Zhou, Z., Deng, C.-X., and Saga, Y. (2016). Sexual Fate Change of XX Germ Cells Caused by the Deletion of SMAD4 and STRA8 Independent of Somatic Sex Reprogramming. PLoS. Biol. 14, e1002553. doi:10.1371/journal.pbio.1002553
Xu, H., Beasley, M. D., Warren, W. D., van der Horst, G. T. J., and McKay, M. J. (2005). Absence of Mouse REC8 Cohesin Promotes Synapsis of Sister Chromatids in Meiosis. Dev. Cell. 8, 949–961. doi:10.1016/j.devcel.2005.03.018
Keywords: gametogenesis, germ cell, sex, meiosis, folliculogenesis
Citation: Kikuchi M and Tanaka M (2022) Functional Modules in Gametogenesis. Front. Cell Dev. Biol. 10:914570. doi: 10.3389/fcell.2022.914570
Received: 07 April 2022; Accepted: 09 May 2022;
Published: 26 May 2022.
Edited by:
Kei-Ichiro Ishiguro, Kumamoto University, JapanReviewed by:
Sean Burgess, University of California, Davis, United StatesMateus Contar Adolfi, Julius Maximilian University of Würzburg, Germany
Copyright © 2022 Kikuchi and Tanaka. This is an open-access article distributed under the terms of the Creative Commons Attribution License (CC BY). The use, distribution or reproduction in other forums is permitted, provided the original author(s) and the copyright owner(s) are credited and that the original publication in this journal is cited, in accordance with accepted academic practice. No use, distribution or reproduction is permitted which does not comply with these terms.
*Correspondence: Minoru Tanaka, mtanaka@bio.nagoya-u.ac.jp