- School of Human Sciences, The University of Western Australia, Perth, WA, Australia
Breast cancer remains a significant burden with 1 in 8 women affected and metastasis posing a significant challenge for patient survival. Disease progression involves remodeling of the extracellular matrix (ECM). In breast cancer, tissue stiffness increases owing to an increase in collagen production by recruited cancer-associated fibroblasts (CAFs). These stromal modifications are notable during primary tumor growth and have a dualistic action by creating a hard capsule to prevent penetration of anti-cancer therapies and forming a favorable environment for tumor progression. Remodeling of the tumor microenvironment immediately presented to cells can include changes in protein composition, concentration and structural arrangement and provides the first mechanical stimuli in the metastatic cascade. Not surprisingly, metastatic cancer cells possess the ability to mechanically adapt, and their adaptability ensures not only survival but successful invasion within altered environments. In the past decade, the importance of the microenvironment and its regulatory role in diseases have gained traction and this is evident in the shift from plastic culture to the development of novel biomaterials that mimic in vivo tissue. With these advances, elucidations can be made into how ECM remodeling and more specifically, altered cell-ECM adhesions, regulate tumor growth and cancer cell plasticity. Such enabling tools in mechanobiology will identify fundamental mechanisms in cancer progression that eventually help develop preventative and therapeutic treatment from a clinical perspective. This review will focus on current platforms engineered to mimic the micro and nano-properties of the tumor microenvironment and subsequent understanding of mechanically regulated pathways in cancer.
1 Introduction
As cancer, particularly metastasis, remains a leading cause of death globally (WHO, 2021), there is a call to action for researchers to develop novel approaches to enable new treatments. While the biochemical and genetic drivers of metastasis have been extensively studied, (Chatterjee et al., 2018; De Francesco et al., 2018; Siegel et al., 2018), there is a growing appreciation for the regulatory roles that biophysical cues play in tumor progression and the onset of metastasis. Changes to mechanical inputs such as stiffness (Sherman-Baust et al., 2003; Paszek and Weaver, 2004; Lu et al., 2011; Gierach et al., 2012) and ligand presentation (Allinen et al., 2004; Larsen et al., 2006; Oskarsson, 2013) are transduced to the cell nucleus via a process coined mechanotransduction. The microenvironment is composed of micro-and nanoscale features which undergo extensive remodeling during tumor development (Leiss et al., 2008; Wang et al., 2017). While these aberrant mechanical stimuli are acknowledged in tumor progression, the contribution and specific mechanism for this is still largely unknown. Understanding how mechanical stimuli regulate cancer cell fate largely relies on enabling biomimetic and tuneable materials that precisely recapitulate specific properties of the tumor microenvironment (TME) and more specifically the ECM. In this review, we discuss the functional consequences that alterations of the ECM, at the nano- and micro-scale, have on cancer cell growth and invasion and the platforms allowing the study of this complex disease.
2 What is the Tumor Microenvironment (TME)
TME refers to the cellular (fibroblasts, immune cells, endothelial cells, and adipocytes, etc) and non-cellular (proteins that make up the ECM) components that form the microenvironment of a tumour. In this review, we focus on the non-cellular component, the ECM, present in all tissues and providing the physical scaffolding and biomechanical cues required for tissue morphogenesis, differentiation and homeostasis. The ECM consists of a multitude of proteins (e.g. collagen, laminin, and fibronectin) and glycosaminoglycans/proteoglycan (e.g. hyaluronic acid, heparin). Utilising certain cell membrane receptors (e.g., mainly integrins) cells can adhere to specific ligands expressed on the ECM. In this way, cells and the microenvironment maintain a dynamic dialogue, whereby healthy tissues remain in a state of homeostasis. This crosstalk is disrupted in tumorigenesis, where extensive remodification to the TME results in altered cell behaviour (Lu et al., 2011). The ECM is remodelled by ECM modifying factors (MMP and LOX) which alter cross-linking of the matrix or by paracrine signalling to cancer-associated fibroblasts (CAFs) resulting in increased deposition of ECM proteins (Özdemir et al., 2014; Rhim et al., 2014; Sahai et al., 2020; Lee and Chaudhuri, 2021). Changes in ECM composition by prolonged cross-linking/degradation will present altered mechanical properties (e.g., stiffness), cell-ECM ligand specificity (e.g., fibronectin dominant to collagen dominant), and altered spacing between ligands (e.g., nano-spacing of GFOGER, peptide in collagen that binds integrins α1β1 and α2β1) (Figure 1). As physical properties of the ECM influence cell behaviour via mechanotransduction and these properties are altered in cancer, acknowledging the cancer ECM and its regulatory role in controlling cell behaviour are critical for understanding cancer progression. (Young et al., 2016).
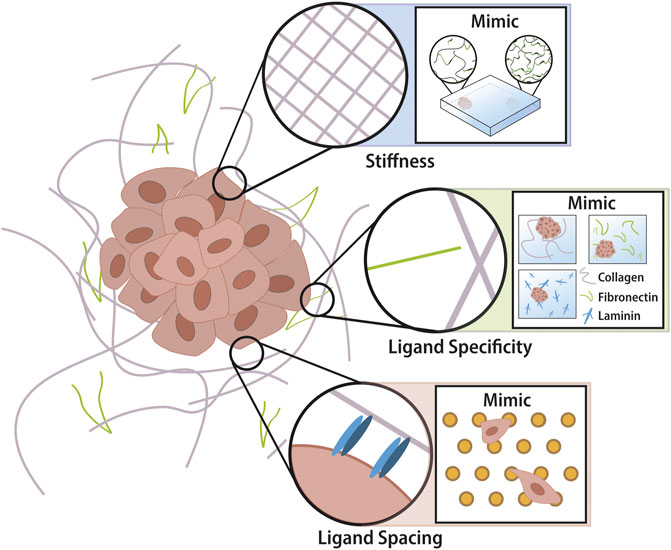
FIGURE 1. Tumor extracellular matrix in nano- and micro-scale. Properties of tumor ECM include microscale stiffness and ligand chemistry changes as well as nanoscale ligand spacing changes. The intelligent design of biomaterials allows recapitulation of these properties into tuneable devices for the study of cancer phenotypes. Hydrogels utilise cross-linking technology which investigators can control spatially across one gel by altering UV penetration. There is a multitude of biomaterials each utilising different backbones and employing different ligands for cells to adhere to (i.e. collagen = GFOGER and GelMA = RGD). Novel advancements in nanotechnology have enabled the production of a platform whereby the nano-spacing of ligands can be altered according to the micelle-nano-array of gold-nanoparticles that have attached peptides.
2.1 ECM Stiffness Activates Mechanotransduction Pathways to Regulate Cancer Fate
Changes to tissue stiffness following tumor development is one of the most well-established characteristics of the TME and this knowledge is exploited during cancer screening (e.g., palpation) (Khaled et al., 2004). Tumor stiffening is a downstream effect of increased deposition of ECM proteins (such as collagen) by the recruitment and activation of CAFs (Tlsty and Coussens, 2006). Collagen is increasingly more crosslinked by lysyl oxidase (LOX) which is implicated with increasing tension between cytoskeletal components and integrins and mediating cell-matrix focal adhesions (FA) (Choquet et al., 1997; Cox et al., 2013). Establishing a mechanical connection between cell and ECM, via FA, will result in the activation of various signalling cascades and the generation of intracellular force through actin-myosin. This process, coined mechanotransduction, begins at the cell-ECM interface whereby specific integrins bind to the ECM proteins to recruit and form actin stress fibers which maintain the cytoskeletal tension (Bershadsky et al., 2003). Filamentous Actin binds to Lamin-A within the nuclear membrane via adaptor proteins such as Nesprin and SUN (Wang et al., 2009), translating mechanical input to the nucleus of the cell. Tension applied to nuclear lamina (Lamin-A is one of the key building blocks) leads to translocation of mechanosensitive proteins such as transcriptional coactivator with PDZ-binding motif (TAZ) and yes-associated protein (YAP). This mechanotransduction pathway utilises biochemical and biomechanical signals to alter cancer cell growth, modes of invasion and eventually govern the fate of cells in the TME. Increased ECM stiffness enhances YAP and TAZ activity which evidence suggests can drive tumour-initiating cells (cancer stem cells (CSCs) to maintain self-renewal and tumor-initiation capacities of breast cancer cells and induce EMT (Cordenonsi et al., 2011; Dupont et al., 2011; Maugeri-Saccà et al., 2015). Alongside integrin-mediated mechanotransduction, increasing traction force not only regulates Rho-associated protein kinase (ROCK), a protein largely responsible for actin cytoskeleton dynamics and regulating breast cancer epithelial cell differentiation (Wozniak et al., 2003), but also activates mitogen-activated protein kinase (MAPK), which is responsible for many diverse cellular programs including cell proliferation, differentiation, motility and survival (Provenzano et al., 2009; Cargnello and Roux, 2011). Overall, a stiff ECM activates mechanotransduction which induces a gene expression signature in cancer cells that is associated with a greater risk for invasive breast carcinoma (Habel et al., 2004; Provenzano et al., 2009).
2.2 TME Stiffness is Dynamic at the Nanoscale
Previous investigations into the effects of stiffening on cancer phenotypes arose from the common consensus that tumors are stiffer than their healthy counterpart. However, the TME, and more specifically the ECM, has displayed levels of complexity not previously appreciated. A recent investigation into the nanoscale stiffness of breast explants revealed non-metastatic tumors with this characteristic increase in stiffness, but metastatic tumors with a “softer” more “heterogenous” stiffness profile with 3 distinct mechanical properties (Plodinec et al., 2012). These nanoscale measurements translate to clinical measurements, with high mammographic density associated with a greater risk of developing breast cancer, whilst low density is linked to an increased risk of cancer invasion (Ye et al., 2014). An examination of transgenic mice showed initial stiffening following tumor growth (spatially heterogeneous) followed by tissue softening at the onset of tumor dissemination as a result of ECM digestion (Sameni et al., 2000). These studies highlight the complexity of the TME and more importantly the dynamic nature of cancer ECM at the nanoscale.
2.3 Tumour Progression Involves Altered ECM Composition
2.3.1 Protein Composition Changes and Integrin Specificity
In diseases where ECM is misregulated, changes to ECM composition results in changes to the ligand presentation (e.g., density (Sherman-Baust et al., 2003; Paszek and Weaver, 2004; Gierach et al., 2012) chemistry and geometry (Allinen et al., 2004; Larsen et al., 2006; Oskarsson, 2013)) which mediates integrin binding. Integrins are a family of transmembrane glycoproteins receptors consisting of 18 α and 8 β units which form heterodimers that mediate cell-matrix interactions (Ruoslahti, 1991; Hynes, 1992). As receptors, integrins mediate recognition of ECM constituents (e.g., cells that express α1β1 and α2β1 bind to GFOGER), recruiting focal adhesion complexes to establish traction force generation and actin filament assembly (Massia and Hubbell, 1991; Arnold et al., 2004). For this reason, integrin-mediated mechanotransduction has gained traction as a highly specific process that regulates cytoskeletal tension, intracellular signalling and gene expression related to proliferation, migration and survival. In breast cancer, an aberrant ECM can destabilise integrin-mediated adhesion resulting in enhanced metastatic potential and drug resistance (Young et al., 2020).
In the majority of cancers, significant accumulation of collagen has been associated with metastatic recurrence, aggressive behaviour, and chemoresistance (Lochter and Bissell, 1995; Ramaswamy et al., 2003; Oskarsson, 2013; Holle et al., 2016; Ondeck et al., 2019). Integrins α1β1 and α2β1 are known to be primarily collagen receptors and their expression has shown to play a role in melanoma cell migration in 3D collagen matrices (Koistinen and Heino, 2013). Increased expression of these two heterodimers is noted in melanoma metastatic cells when compared to cells in the primary tumor (Klein et al., 1991). Interestingly, studies utilising mammary epithelium and aorta-derived smooth muscles have shown collagen to activate distinct signalling pathways and alter cell behaviour independent of mechanical stimuli (stiffness) (Giancotti and Ruoslahti, 1999; Engler et al., 2004; Brownfield et al., 2013). Accompanying increased collagen deposition during a fibrotic response is the deposition of fibronectin. Fibronectin is a glycoprotein known to enhance the growth of breast epithelial cells (McIntosh et al., 2010), increase breast cancer invasion (via STAT3 and MAPK pathways) (Balanis et al., 2013) and is upregulated in circulating tumor cells (Raimondi et al., 2011). Cells adhere to RGD motifs expressed on fibronectin via the α5β1 receptor and αVβ3 which have emerged as essential mediators in many human carcinomas and are largely implicated in tumour proliferation and metastasis, with αVβ3 identified as a melanoma tumour progression marker (Hsu et al., 1998; Koistinen and Heino, 2013; Hou et al., 2020).
2.3.2 Glycosaminoglycan Expression During Tumor Progression
Aside from ECM proteins, glycosaminoglycans (GAGs) have also proven essential in cancer progression. Hyaluronan is a GAG that is highly expressed in breast cancer (Karousou et al., 2014). Hyaluronan can interact with cancer cells via cell surface receptors CD44 and RHAMM (Toole, 2004). CD44 is a cell surface adhesion receptor (not an integrin) and is largely recognized as a cancer stem cell (CSC) marker expressed by almost every tumour cell (Jaggupilli and Elkord, 2012; Lin and Ding, 2017; Wang et al., 2018). Employing alternative signaling cascades then integrin-driven adhesion, CD44 has shown regulatory roles in the Hippo pathway (Wang et al., 2014) and interacts with RHAMM and ERK (Hamilton et al., 2007) as well as Rho-ROCK (Chellaiah et al., 2003; Ohata et al., 2012) to mediate breast cancer cell motility and enhance stemness of colon cancer-initiating cells. This offers an alternative perspective on the regulatory roles of GAGs and less understood cell-ECM interactions. Overall, these studies emphasize the specificity of cell-ECM adhesions which promote sustained altered signaling cascades and govern particular cell fates.
2.3.3 Altered Nano-Spacing of Ligands in TME
Compositional remodeling due to increased deposition of ECM proteins, enhanced cross-linking by LOX and localized degradation by secreted MMP factors, has an implication in altering the spacing between individual ligands (e.g. GFOGER). Cells sense the variations in the spacing of ECM proteins through either single integrin proteins or recruitment of larger integrin-containing adhesion complexes (Oria et al., 2017). This spatial sensing has shown to play a role in physiological and pathological states (Daley et al., 2008). Oria et al., 2017 demonstrated that human breast myoepithelial integrin clustering and subsequent recruitment of focal adhesion is inhibited when cell-ECM interactions are separated by more than a few tens of nanometers. These nanometer-scale alterations have regulatory roles in cellular migration, morphology, focal adhesion assembly, cell adhesion and traction force generation (Arnold et al., 2004; Cavalcanti-Adam et al., 2006; Cavalcanti-Adam et al., 2007; Selhuber-Unkel et al., 2010; Oria et al., 2017). Interestingly, breast cancer cell survival, in response to chemotherapeutic treatment, is highly dependent on the nanoscale ligand spacing (Young et al., 2020).
Collagen fibres interact with each other at defined spacing intervals termed periodicity (Gelse et al., 2003; Young et al., 2016). This periodicity has shown intervals ranging from 63 to 72 nm and is the interval at which cells interact with the protein (Wallace et al., 2010; Young et al., 2016). In vitro studies have shown when periodicity is increased above 73 nm melanocytes and osteoclasts were inhibited in their ability to cluster integrins (Arnold et al., 2004). Fibronectin, also implicated in cancer, has shown a regular fibril arrangement of 42 nm in thick fibres and 84 nm in thin fibres. The fibrils at 84 nm will be unfolded and at a state of extension whilst the thick fibres are a result of staggered fibronectin dimers (Dzamba and Peters, 1991). It is hypothesized that the latter could be exacerbated in cancer due to the highly dense protein structures in the TME (Young et al., 2016). As the spatial organization of the available ligand binding partner changes, this mediates integrin clustering affecting force-mediated contractility of the cell and governing cell fate (Li et al., 2015). To confirm this theory, cartilage cells were placed on ligands of discreet spacing; the result was significantly greater cell area on the smaller nano-spaced ligands, inferring aberrant integrin-clustering on the larger nano-spacing (Li et al., 2015). In breast cancer cells, altering the nano-spacing of ligands showed altered cellular properties including morphology, focal adhesion formation, migration and chemoprotection (smaller ligand spacing hinders survival against chemotherapeutic drugs) (Young et al., 2020).
3 Tools for Recapitulating the Tumor Microenvironment
3.1 Stiffness Tunable Hydrogels
The TME offers a complex assortment of biochemical and biomechanical inputs that dynamically change following cancer progression and onset of metastasis. To better understand the functional consequences of ECM mechanics on cell phenotype, investigators have employed a combination of 2D and 3D hydrogel-based platforms. Being highly water-based and tunable, hydrogels offer superior recapitulation of specific characteristics of the ECM including stiffness, composition, and ligand spacing (Supplementary Table S1).
As mentioned previously, one of the most well-established characteristics of the TME is changes in tissue stiffness which is largely correlated with the risk of breast cancer development (Habel et al., 2004; Provenzano et al., 2008). Because of this, the production of biomaterials was largely centred around hydrogels whose chemistry would allow easy manipulation of stiffness. Synthetic gels such as polyacrylamide can produce discrete, static 2D gels of different stiffnesses and spatial gradients which offer greater biomimicry of the spatial heterogeneity seen in vivo (Engler et al., 2006; Insua-Rodríguez and Oskarsson, 2016; Hadden et al., 2017; Chin et al., 2021). Seeding metastatic breast cancer cells on uniform stiffness hydrogels which varied from 2.4–10.6 kPa demonstrated stiffness-dependent differences in traction forces, strain energies, and morphologies (Massalha and Weihs, 2017). As robust as PA is, the presence of free radicals during polymerization inhibits cell encapsulation and limits translatability from a 3D perspective.
Appreciation for the 3D architecture and its regulatory role on cell phenotype has geared the development of novel 3D-capable hydrogels. Collagen, being the primary constituent and major structural component in many tissues is very attractive for cell-ECM studies. Formed mainly from fibrous protein collagen type I, fabrication involves manipulating collagen concentration and raising the solution temperature until gelation occurs. Their soft mesh-like matrix makes collagen hydrogels ideal for invasion assays, with the most recent investigation showing collective cancer cell migration inhibited in the stiffest microenvironment (2.5 mg/ml) (Raghuraman et al., 2022). The drawback of this platform includes limited stiffness ranges (up to ∼5 kPa) and temporal tuneability, with reduced long-term stability and high batch-batch variability.
For these reasons, semi-synthetic materials such as gelatin methacryloyl (GelMA), synthesized from denatured collagen, has gained significant traction (Bertlein et al., 2017). Containing cross-linkable methacrylate groups, GelMA, will undergo polymerization upon the addition of a photoinitiator (Irgacure 2,959) and exposure to UV light. In this way, investigators gain greater tuneability over GelMA whilst still maintaining biological relevance (contains RGD). GelMA can also employ a gradient stiffness model which allows for a more holistic understanding of mechanically driven cell phenotypes (Major et al., 2019; Kim et al., 2020).
The use of these hydrogels allows for high throughput fabrication of readily adaptable and physiologically relevant environments, making it appealing for the study of ECM for cancer proliferation and invasion. Other approaches to mimicking the 3D microenvironment include 3D bioprinting which is beneficial in creating an exact replication of target tissue with consideration for cellular components, ECM and 3D spatial components (for reviews see; (Charbe et al., 2017; Ro et al., 2022)). Alternatively, the decellularization of tumors also offers a new Frontier in tissue engineering that maintains not only the mechanical components of the ECM but also the composition and structure (for review see; (García-Gareta et al., 2022)).
Stiffness is just one ECM characteristic supplying biophysical input to the cell. Tumor progression also proceeds extensive modification to ECM composition (i.e., going from fibronectin-laminin rich matrix to primarily collagen dominated) which alters the integrin profile and at the nanoscale alters the spacing at which cells are interacting with ECM proteins (Sherman-Baust et al., 2003; Allinen et al., 2004; Paszek and Weaver, 2004; Larsen et al., 2006; Gierach et al., 2012; Oskarsson, 2013).
3.2 Tools to Control ECM Composition
Polyacrylamide is inert in nature and requires conjugation with proteins to enable cell attachment. Using a bifunctional cross-linker sulfo-SANPAH, proteins can be covalently bound to the PA and enable cell attachment (Caliari and Burdick, 2016). Because of this, PA is an attractive choice when investigating the effects of specific adhesive ligands, as cell-ECM interactions can be tightly controlled (Tse and Engler, 2010). For 3D investigation different biomaterials which utilize different ligands can be employed. Collagen as mentioned previously expresses abundant GFOGER which will activate integrins α1β1 and α2β1. GelMA, adapted from native gelatin, contains RGD binding motifs (Bertlein et al., 2017) which will recruit integrins α5β1 and αVβ3. Alginate and polyethylene glycol diacrylate (PEGDA), do not express native ligands, similar to PA, and offers a “blank canvas” allowing preferential modification with adhesive ligands within a 3D context (Caliari and Burdick, 2016).
Hyaluronic acid (HA) as a hydrogel has several important advantages including its biological relevance and chemical tunability. HA functional groups can be modified to enable a wide range of cross-linking chemistries, useful for cellular mechanotransduction investigations (Fraser et al., 1997). Unlike collagen and GelMA, HA lacks integrin-mediated cell adhesion but presents cell surface markers including CD44 which can be beneficial when studying alternative cell-ECM adhesions and indirect pathways interacting with integrin-mediated mechanotransduction.
3.3 Tools for Investigating Ligand Nano-Spacing
Recapitulating and controlling for the nanoscale properties of the microenvironment remains a great challenge in biomaterials. Photolithography and optical lithography are the two most commonly used techniques in nanofabrication (Lohmüller et al., 2011). However, these two methods rely on light and achieving structural dimensions below 100 nm is hardly feasible (Lohmüller et al., 2011). Alternative methods, such as block copolymer micelle lithography (BCMN), rely on the self-organization of molecules to generate structural materials and can reach these sub-nanometer resolutions (Gates et al., 2004). BCMN involves the formation of spontaneous microphase-separated morphologies from amphiphilic block copolymers (Lohmüller et al., 2011). The distribution of nanoparticles due to block copolymer micelle self-assembly can be manipulated by changing micelle size, the concentration of polymer solution, the amount of metal precursor and the retraction speed from the substrate. Once the micro-arrays are established, peptides can be adhered to the nanoparticles enabling precise control over ligand spacing. Seeding cancer cells atop this platform has shown to influence key cellular properties such as morphology, focal adhesion formation, migration as well as drug sensitivity (Young et al., 2020).
4 Conclusion and Future Perspectives
The phenomenon responsible for the successful spread of primary cancer cells from their tumor microenvironment to secondary sites is still largely uncharacterized at the molecular level. Universally, the metastatic cascade employs mechanical challenges in which cancer cells will morphologically adapt to survive in these altered environments (Amos and Choi, 2021). Numerous in vivo and in vitro studies have examined the initial stages of tumor growth and invasion and begun to outline some of the nano- and micro-scale changes occurring in the TME–including changes to ECM stiffness, ligand chemistry and ligand nanospacing. Micro-scale changes, such as stiffness and ligand chemistry, have shown greater advancements in biomaterials than nano-scale properties where the primary obstacle has been developing tools in vivo to examine these changes and establishing platforms in vitro that allow for tuneability at this level. Overall, intelligent design of synthetic and biological biomaterials should incorporate these properties so that investigators can focus on the crucial differences between these properties and appreciate the role of the ECM and its regulation of cell fate even at the smallest, and perhaps most crucial, of metrics.
Author Contributions
DV and YC drafted manuscript.
Funding
This work was supported by Heart Foundation Future Leader Fellowship 101173 (to YC) and Australian Government Research Training Program (to DV)
Conflict of Interest
The authors declare that the research was conducted in the absence of any commercial or financial relationships that could be construed as a potential conflict of interest.
Publisher’s Note
All claims expressed in this article are solely those of the authors and do not necessarily represent those of their affiliated organizations, or those of the publisher, the editors and the reviewers. Any product that may be evaluated in this article, or claim that may be made by its manufacturer, is not guaranteed or endorsed by the publisher.
Supplementary Material
The Supplementary Material for this article can be found online at: https://www.frontiersin.org/articles/10.3389/fcell.2022.908799/full#supplementary-material
References
Allinen, M., Beroukhim, R., Cai, L., Brennan, C., Lahti-Domenici, J., Huang, H., et al. (2004). Molecular Characterization of the Tumor Microenvironment in Breast Cancer. Cancer Cell 6, 17–32. doi:10.1016/j.ccr.2004.06.010
Amos, S. E., Choi, Y. S., and Glass, R. (2021). The Cancer Microenvironment: Mechanical Challenges of the Metastatic Cascade. Front. Bioeng. Biotechnol. 9, 56.
Arnold, M., Cavalcanti-Adam, E. A., Glass, R., Blümmel, J., Eck, W., Kantlehner, M., et al. (2004). Activation of Integrin Function by Nanopatterned Adhesive Interfaces. Chem. Phys. Chem. 5, 383–388. doi:10.1002/cphc.200301014
Balanis, N., Wendt, M. K., Schiemann, B. J., Wang, Z., Schiemann, W. P., and Carlin, C. R. (2013). Epithelial to Mesenchymal Transition Promotes Breast Cancer Progression via a Fibronectin-dependent STAT3 Signaling Pathway. J. of Biol. Chem. 288, 17954–17967. doi:10.1074/jbc.m113.475277
Bershadsky, A. D., Balaban, N. Q., and Geiger, B. (2003). Adhesion-dependent Cell Mechanosensitivity. Annu. Rev. Cell. Dev. Biol. 19, 677–695. doi:10.1146/annurev.cellbio.19.111301.153011
Bertlein, S., Brown, G., Lim, K. S., Jungst, T., Boeck, T., Blunk, T., et al. (2017). Thiol-Ene Clickable Gelatin: A Platform Bioink for Multiple 3D Biofabrication Technologies. Adv. Mat. 29, 1703404. doi:10.1002/adma.201703404
Brownfield, D. G., Venugopalan, G., Lo, A., Mori, H., Tanner, K., Fletcher, D. A., et al. (2013). Patterned Collagen Fibers Orient Branching Mammary Epithelium through Distinct Signaling Modules. Curr. Biol. 23, 703–709. doi:10.1016/j.cub.2013.03.032
Caliari, S. R., and Burdick, J. A. (2016). A Practical Guide to Hydrogels for Cell Culture. Nat. Methods 13, 405–414. doi:10.1038/nmeth.3839
Cargnello, M., and Roux, P. P. (2011). Activation and Function of the MAPKs and Their Substrates, the MAPK-Activated Protein Kinases. Microbiol. Mol. Biol. Rev. 75, 50–83. doi:10.1128/mmbr.00031-10
Cavalcanti-Adam, E. A., Micoulet, A., Blümmel, J., Auernheimer, J., Kessler, H., and Spatz, J. P. (2006). Lateral Spacing of Integrin Ligands Influences Cell Spreading and Focal Adhesion Assembly. Eur. J. of Cell Biol. 85, 219–224. doi:10.1016/j.ejcb.2005.09.011
Cavalcanti-Adam, E. A., Volberg, T., Micoulet, A., Kessler, H., Geiger, B., and Spatz, J. P. (2007). Cell Spreading and Focal Adhesion Dynamics Are Regulated by Spacing of Integrin Ligands. Biophysical J. 92, 2964–2974. doi:10.1529/biophysj.106.089730
Charbe, N., McCarron, P. A., and Tambuwala, M. M. (2017). Three-dimensional Bio-Printing: A New Frontier in Oncology Research. Wjco 8, 21. doi:10.5306/wjco.v8.i1.21
Chatterjee, A., Rodger, E. J., and Eccles, M. R. (2018). “Epigenetic Drivers of Tumourigenesis and Cancer Metastasis,” in Seminars in Cancer Biology (Netherlands: Elsevier), 51, 149–159. doi:10.1016/j.semcancer.2017.08.004
Chellaiah, M. A., Biswas, R. S., Rittling, S. R., Denhardt, D. T., and Hruska, K. A. (2003). Rho-dependent Rho Kinase Activation Increases CD44 Surface Expression and Bone Resorption in Osteoclasts. J. of Biol. Chem. 278, 29086–29097. doi:10.1074/jbc.m211074200
Chin, I. L., Hool, L., and Choi, Y. S. (2021). Interrogating Cardiac Muscle Cell Mechanobiology on Stiffness Gradient Hydrogels. Biomater. Sci. 9, 6795–6806. doi:10.1039/d1bm01061a
Choquet, D., Felsenfeld, D. P., and Sheetz, M. P. (1997). Extracellular Matrix Rigidity Causes Strengthening of Integrin-Cytoskeleton Linkages. Cell. 88, 39–48. doi:10.1016/s0092-8674(00)81856-5
Cordenonsi, M., Zanconato, F., Azzolin, L., Forcato, M., Rosato, A., Frasson, C., et al. (2011). The Hippo Transducer TAZ Confers Cancer Stem Cell-Related Traits on Breast Cancer Cells. Cell. 147, 759–772. doi:10.1016/j.cell.2011.09.048
Cox, T. R., Bird, D., Baker, A.-M., Barker, H. E., Ho, M. W.-Y., Lang, G., et al. (2013). LOX-mediated Collagen Crosslinking Is Responsible for Fibrosis-Enhanced Metastasis. Cancer Res. 73, 1721–1732. doi:10.1158/0008-5472.can-12-2233
Daley, W. P., Peters, S. B., and Larsen, M. (2008). Extracellular Matrix Dynamics in Development and Regenerative Medicine. J. of Cell Sci. 121, 255–264. doi:10.1242/jcs.006064
De Francesco, E. M., Sotgia, F., and Lisanti, M. P. (2018). Cancer Stem Cells (CSCs): Metabolic Strategies for Their Identification and Eradication. Biochem. J. 475, 1611–1634. doi:10.1042/bcj20170164
Dupont, S., Morsut, L., Aragona, M., Enzo, E., Giulitti, S., Cordenonsi, M., et al. (2011). Role of YAP/TAZ in Mechanotransduction. Nature 474, 179–183. doi:10.1038/nature10137
Dzamba, B. J., and Peters, D. M. (1991). Arrangement of Cellular Fibronectin in Noncollagenous Fibrils in Human Fibroblast Cultures. J. of Cell Sci. 100, 605–612. doi:10.1242/jcs.100.3.605
Engler, A., Bacakova, L., Newman, C., Hategan, A., Griffin, M., and Discher, D. (2004). Substrate Compliance versus Ligand Density in Cell on Gel Responses. Biophysical J. 86, 617–628. doi:10.1016/s0006-3495(04)74140-5
Engler, A. J., Sen, S., Sweeney, H. L., and Discher, D. E. (2006). Matrix Elasticity Directs Stem Cell Lineage Specification. Cell. 126, 677–689. doi:10.1016/j.cell.2006.06.044
Fraser, J. R. E., Laurent, T. C., and Laurent, U. B. G. (1997). Hyaluronan: its Nature, Distribution, Functions and Turnover. J. of Intern. Med. 242, 27–33. doi:10.1046/j.1365-2796.1997.00170.x
García-Gareta, E., Pérez, M. Á., and García-Aznar, J. M. (2022). Decellularization of Tumours: A New Frontier in Tissue Engineering. J. of Tissue Eng. 13, 20417314221091682. doi:10.1177/20417314221091682
Gates, B. D., Xu, Q., Love, J. C., Wolfe, D. B., and Whitesides, G. M. (2004). Unconventional Nanofabrication. Annu. Rev. Mat. Res. 34, 339–372. doi:10.1146/annurev.matsci.34.052803.091100
Gelse, K., Pöschl, E., and Aigner, T. (2003). Collagens-structure, Function, and Biosynthesis. Adv. drug Deliv. Rev. 55, 1531–1546. doi:10.1016/j.addr.2003.08.002
Giancotti, F. G., and Ruoslahti, E. (1999). Integrin Signaling. science 285, 1028–1033. doi:10.1126/science.285.5430.1028
Gierach, G. L., Ichikawa, L., Kerlikowske, K., Brinton, L. A., Farhat, G. N., Vacek, P. M., et al. (2012). Relationship between Mammographic Density and Breast Cancer Death in the Breast Cancer Surveillance Consortium. J. of Natl. Cancer Inst. 104, 1218–1227. doi:10.1093/jnci/djs327
Habel, L. A., Dignam, J. J., Land, S. R., Salane, M., Capra, A. M., and Julian, T. B. (2004). Mammographic Density and Breast Cancer after Ductal Carcinoma In Situ. JNCI J. of Natl. Cancer Inst. 96, 1467–1472. doi:10.1093/jnci/djh260
Hadden, W. J., Young, J. L., Holle, A. W., McFetridge, M. L., Kim, D. Y., Wijesinghe, P., et al. (2017). Stem Cell Migration and Mechanotransduction on Linear Stiffness Gradient Hydrogels. Proc. Natl. Acad. Sci. U.S.A. 114, 5647–5652. doi:10.1073/pnas.1618239114
Hamilton, S. R., Fard, S. F., Paiwand, F. F., Tolg, C., Veiseh, M., Wang, C., et al. (2007). The Hyaluronan Receptors CD44 and Rhamm (CD168) Form Complexes with ERK1,2 that Sustain High Basal Motility in Breast Cancer Cells. J. of Biol. Chem. 282, 16667–16680. doi:10.1074/jbc.m702078200
Holle, A. W., Young, J. L., and Spatz, J. P. (2016). In Vitro cancer Cell-ECM Interactions Inform In Vivo Cancer Treatment. Adv. drug Deliv. Rev. 97, 270–279. doi:10.1016/j.addr.2015.10.007
Hou, J., Yan, D., Liu, Y., Huang, P., and Cui, H. (2020). The Roles of Integrin α5β1 in Human Cancer. Ott 13, 13329–13344. doi:10.2147/ott.s273803
Hsu, M.-Y., Shih, D.-T., Meier, F. E., Van Belle, P., Hsu, J.-Y., Elder, D. E., et al. (1998). Adenoviral Gene Transfer of β3 Integrin Subunit Induces Conversion from Radial to Vertical Growth Phase in Primary Human Melanoma. The Am. J. of pathology 153, 1435–1442. doi:10.1016/s0002-9440(10)65730-6
Hynes, R. O. (1992). Integrins: Versatility, Modulation, and Signaling in Cell Adhesion. Cell. 69, 11–25. doi:10.1016/0092-8674(92)90115-s
Insua-Rodríguez, J., and Oskarsson, T. (2016). The Extracellular Matrix in Breast Cancer. Adv. drug Deliv. Rev. 97, 41–55. doi:10.1016/j.addr.2015.12.017
Jaggupilli, A., and Elkord, E. (2012). Significance of CD44 and CD24 as Cancer Stem Cell Markers: an Enduring Ambiguity. Clin. Dev. Immunol. 2012, 708036. doi:10.1155/2012/708036
Karousou, E., D'Angelo, M. L., Kouvidi, K., Vigetti, D., Viola, M., Nikitovic, D., et al. (2014). Collagen VI and Hyaluronan: the Common Role in Breast Cancer. BioMed Res. Int. 2014, 606458. doi:10.1155/2014/606458
Khaled, W., Reichling, S., Bruhns, O., Boese, H., Baumann, M., Monkman, G., et al. (2004). “Palpation Imaging Using a Haptic System for Virtual Reality Applications in Medicine,” in Perspective in Image-Guided Surgery (Singapore: World Scientific), 407–414. doi:10.1142/9789812702678_0055
Kim, C., Young, J. L., Holle, A. W., Jeong, K., Major, L. G., Jeong, J. H., et al. (2020). Stem Cell Mechanosensation on Gelatin Methacryloyl (GelMA) Stiffness Gradient Hydrogels. Ann. Biomed. Eng. 48, 893–902. doi:10.1007/s10439-019-02428-5
Klein, C. E., Dressel, D., Steinmayer, T., Mauch, C., Eckes, B., Krieg, T., et al. (1991). Integrin Alpha 2 Beta 1 Is Upregulated in Fibroblasts and Highly Aggressive Melanoma Cells in Three-Dimensional Collagen Lattices and Mediates the Reorganization of Collagen I Fibrils. The J. of Cell Biol. 115, 1427–1436. doi:10.1083/jcb.115.5.1427
Koistinen, P., and Heino, J. (2013). “Integrins in Cancer Cell Invasion,” in Madame Curie Bioscience Database (Texas(United States): Landes Bioscience). Internet.
Larsen, M., Artym, V. V., Green, J. A., and Yamada, K. M. (2006). The Matrix Reorganized: Extracellular Matrix Remodeling and Integrin Signaling. Curr. Opin. Cell Biol. 18, 463–471. doi:10.1016/j.ceb.2006.08.009
Lee, J. Y., and Chaudhuri, O. (2021). Modeling the Tumor Immune Microenvironment for Drug Discovery Using 3D Culture. Apl. Bioeng. 5, 010903. doi:10.1063/5.0030693
Leiss, M., Beckmann, K., Girós, A., Costell, M., and Fässler, R. (2008). The Role of Integrin Binding Sites in Fibronectin Matrix Assembly In Vivo. Curr. Opin. Cell Biol. 20, 502–507. doi:10.1016/j.ceb.2008.06.001
Li, S., Wang, X., Cao, B., Ye, K., Li, Z., and Ding, J. (2015). Effects of Nanoscale Spatial Arrangement of Arginine-Glycine-Aspartate Peptides on Dedifferentiation of Chondrocytes. Nano Lett. 15, 7755–7765. doi:10.1021/acs.nanolett.5b04043
Lin, J., and Ding, D. (2017). The Prognostic Role of the Cancer Stem Cell Marker CD44 in Ovarian Cancer: a Meta-Analysis. Cancer Cell. Int. 17, 8–11. doi:10.1186/s12935-016-0376-4
Lochter, A., and Bissell, M. J. (1995). “Involvement of Extracellular Matrix Constituents in Breast Cancer,” in Seminars in Cancer Biology (Berkeley, CA (United States): Lawrence Berkeley National Lab.), 6. doi:10.1006/scbi.1995.0017
Lohmüller, T., Aydin, D., Schwieder, M., Morhard, C., Louban, I., Pacholski, C., et al. (2011). Nanopatterning by Block Copolymer Micelle Nanolithography and Bioinspired Applications. Biointerphases 6, MR1–MR12. doi:10.1116/1.3536839
Lu, P., Takai, K., Weaver, V. M., and Werb, Z. (2011). Extracellular Matrix Degradation and Remodeling in Development and Disease. Cold Spring Harb. Perspect. Biol. 3, a005058. doi:10.1101/cshperspect.a005058
Major, L. G., Holle, A. W., Young, J. L., Hepburn, M. S., Jeong, K., Chin, I. L., et al. (2019). Volume Adaptation Controls Stem Cell Mechanotransduction. ACS Appl. Mat. Interfaces 11, 45520–45530. doi:10.1021/acsami.9b19770
Massalha, S., and Weihs, D. (2017). Metastatic Breast Cancer Cells Adhere Strongly on Varying Stiffness Substrates, Initially without Adjusting Their Morphology. Biomech. Model. Mechanobiol. 16, 961–970. doi:10.1007/s10237-016-0864-4
Massia, S. P., and Hubbell, J. A. (1991). An RGD Spacing of 440 Nm Is Sufficient for Integrin Alpha V Beta 3-mediated Fibroblast Spreading and 140 Nm for Focal Contact and Stress Fiber Formation. The J. of Cell Biol. 114, 1089–1100. doi:10.1083/jcb.114.5.1089
Maugeri-Saccà, M., Barba, M., Pizzuti, L., Vici, P., Di Lauro, L., Dattilo, R., et al. (2015). The Hippo Transducers TAZ and YAP in Breast Cancer: Oncogenic Activities and Clinical Implications. Expert Rev. Mol. Med. 17, e14. doi:10.1017/erm.2015.12
McIntosh, J., Dennison, G., Holly, J. M. P., Jarrett, C., Frankow, A., Foulstone, E. J., et al. (2010). IGFBP-3 Can Either Inhibit or Enhance EGF-Mediated Growth of Breast Epithelial Cells Dependent upon the Presence of Fibronectin. J. of Biol. Chem. 285, 38788–38800. doi:10.1074/jbc.m110.177311
Ohata, H., Ishiguro, T., Aihara, Y., Sato, A., Sakai, H., Sekine, S., et al. (2012). Induction of the Stem-like Cell Regulator CD44 by Rho Kinase Inhibition Contributes to the Maintenance of Colon Cancer-Initiating Cells. Cancer Res. 72, 5101–5110. doi:10.1158/0008-5472.can-11-3812
Ondeck, M. G., Kumar, A., Placone, J. K., Plunkett, C. M., Matte, B. F., Wong, K. C., et al. (2019). Dynamically Stiffened Matrix Promotes Malignant Transformation of Mammary Epithelial Cells via Collective Mechanical Signaling. Proc. Natl. Acad. Sci. U.S.A. 116, 3502–3507. doi:10.1073/pnas.1814204116
Oria, R., Wiegand, T., Escribano, J., Elosegui-Artola, A., Uriarte, J. J., Moreno-Pulido, C., et al. (2017). Force Loading Explains Spatial Sensing of Ligands by Cells. Nature 552, 219–224. doi:10.1038/nature24662
Oskarsson, T. (2013). Extracellular Matrix Components in Breast Cancer Progression and Metastasis. The Breast 22, S66–S72. doi:10.1016/j.breast.2013.07.012
Özdemir, B. C., Pentcheva-Hoang, T., Carstens, J. L., Zheng, X., Wu, C.-C., Simpson, T. R., et al. (2014). Depletion of Carcinoma-Associated Fibroblasts and Fibrosis Induces Immunosuppression and Accelerates Pancreas Cancer with Reduced Survival. Cancer Cell 25, 719–734. doi:10.1016/j.ccr.2014.04.005
Paszek, M. J., and Weaver, V. M. (2004). The Tension Mounts: Mechanics Meets Morphogenesis and Malignancy. J. Mammary Gland. Biol. Neoplasia 9, 325–342. doi:10.1007/s10911-004-1404-x
Plodinec, M., Loparic, M., Monnier, C. A., Obermann, E. C., Zanetti-Dallenbach, R., Oertle, P., et al. (2012). The Nanomechanical Signature of Breast Cancer. Nat. Nanotech 7, 757–765. doi:10.1038/nnano.2012.167
Provenzano, P. P., Inman, D. R., Eliceiri, K. W., and Keely, P. J. (2009). Matrix Density-Induced Mechanoregulation of Breast Cell Phenotype, Signaling and Gene Expression through a FAK-ERK Linkage. Oncogene 28, 4326–4343. doi:10.1038/onc.2009.299
Provenzano, P. P., Inman, D. R., Eliceiri, K. W., Trier, S. M., and Keely, P. J. (2008). Contact Guidance Mediated Three-Dimensional Cell Migration Is Regulated by Rho/ROCK-dependent Matrix Reorganization. Biophysical J. 95, 5374–5384. doi:10.1529/biophysj.108.133116
Raghuraman, S., Schubert, A. S., Bröker, S., Jurado, A., Müller, A., Brandt, M., et al. (2022). Pressure Drives Rapid Burst‐Like Coordinated Cellular Motion from 3D Cancer Aggregates. Adv. Sci. 9, 2104808. doi:10.1002/advs.202104808
Raimondi, C., Gradilone, A., Naso, G., Vincenzi, B., Petracca, A., Nicolazzo, C., et al. (2011). Epithelial-mesenchymal Transition and Stemness Features in Circulating Tumor Cells from Breast Cancer Patients. Breast Cancer Res. Treat. 130, 449–455. doi:10.1007/s10549-011-1373-x
Ramaswamy, S., Ross, K. N., Lander, E. S., and Golub, T. R. (2003). A Molecular Signature of Metastasis in Primary Solid Tumors. Nat. Genet. 33, 49–54. doi:10.1038/ng1060
Rhim, A. D., Oberstein, P. E., Thomas, D. H., Mirek, E. T., Palermo, C. F., Sastra, S. A., et al. (2014). Stromal Elements Act to Restrain, rather Than Support, Pancreatic Ductal Adenocarcinoma. Cancer Cell 25, 735–747. doi:10.1016/j.ccr.2014.04.021
Ro, J., Kim, J., and Cho, Y.-K. (2022). Recent Advances in Spheroid-Based Microfluidic Models to Mimic Tumour Microenvironment. Analyst 147, 2023–2034. doi:10.1039/D2AN00172A
Sahai, E., Astsaturov, I., Cukierman, E., DeNardo, D. G., Egeblad, M., Evans, R. M., et al. (2020). A Framework for Advancing Our Understanding of Cancer-Associated Fibroblasts. Nat. Rev. Cancer 20, 174–186. doi:10.1038/s41568-019-0238-1
Sameni, M., Moin, K., and Sloane, B. F. (2000). Imaging Proteolysis by Living Human Breast Cancer Cells. Neoplasia 2, 496–504. doi:10.1038/sj.neo.7900116
Selhuber-Unkel, C., Erdmann, T., López-García, M., Kessler, H., Schwarz, U. S., and Spatz, J. P. (2010). Cell Adhesion Strength Is Controlled by Intermolecular Spacing of Adhesion Receptors. Biophysical J. 98, 543–551. doi:10.1016/j.bpj.2009.11.001
Sherman-Baust, C. A., Weeraratna, A. T., Rangel, L. B. A., Pizer, E. S., Cho, K. R., Schwartz, D. R., et al. (2003). Remodeling of the Extracellular Matrix through Overexpression of Collagen VI Contributes to Cisplatin Resistance in Ovarian Cancer Cells. Cancer Cell 3, 377–386. doi:10.1016/s1535-6108(03)00058-8
Siegel, M. B., He, X., Hoadley, K. A., Hoyle, A., Pearce, J. B., Garrett, A. L., et al. (2018). Integrated RNA and DNA Sequencing Reveals Early Drivers of Metastatic Breast Cancer. The J. of Clin. investigation 128, 1371–1383. doi:10.1172/jci96153
Tlsty, T. D., and Coussens, L. M. (2006). Tumor Stroma and Regulation of Cancer Development. Annu. Rev. Pathol. Mech. Dis. 1, 119–150. doi:10.1146/annurev.pathol.1.110304.100224
Toole, B. P. (2004). Hyaluronan: from Extracellular Glue to Pericellular Cue. Nat. Rev. Cancer 4, 528–539. doi:10.1038/nrc1391
Tse, J. R., and Engler, A. J. (2010). Preparation of Hydrogel Substrates with Tunable Mechanical Properties. Curr. Protoc. Cell. Biol. 10. Unit 10.16. doi:10.1002/0471143030.cb1016s47
Wallace, J. M., Chen, Q., Fang, M., Erickson, B., Orr, B. G., and Banaszak Holl, M. M. (2010). Type I Collagen Exists as a Distribution of Nanoscale Morphologies in Teeth, Bones, and Tendons. Langmuir 26, 7349–7354. doi:10.1021/la100006a
Wang, K., Wu, F., Seo, B. R., Fischbach, C., Chen, W., Hsu, L., et al. (2017). Breast Cancer Cells Alter the Dynamics of Stromal Fibronectin-Collagen Interactions. Matrix Biol. 60-61, 86–95. doi:10.1016/j.matbio.2016.08.001
Wang, L., Zuo, X., Xie, K., and Wei, D. (2018). “The Role of CD44 and Cancer Stem Cells,” in Cancer Stem Cells (Berlin(Germany): Springer), 31–42. doi:10.1007/978-1-4939-7401-6_3
Wang, N., Tytell, J. D., and Ingber, D. E. (2009). Mechanotransduction at a Distance: Mechanically Coupling the Extracellular Matrix with the Nucleus. Nat. Rev. Mol. Cell. Biol. 10, 75–82. doi:10.1038/nrm2594
Wang, Z., Wu, Y., Wang, H., Zhang, Y., Mei, L., Fang, X., et al. (2014). Interplay of Mevalonate and Hippo Pathways Regulates RHAMM Transcription via YAP to Modulate Breast Cancer Cell Motility. Proc. Natl. Acad. Sci. U. S. A. 111, E89–E98. doi:10.1073/pnas.1319190110
Wozniak, M. A., Desai, R., Solski, P. A., Der, C. J., and Keely, P. J. (2003). ROCK-generated Contractility Regulates Breast Epithelial Cell Differentiation in Response to the Physical Properties of a Three-Dimensional Collagen Matrix. The J. of Cell Biol. 163, 583–595. doi:10.1083/jcb.200305010
Ye, J., Wu, D., Wu, P., Chen, Z., and Huang, J. (2014). The Cancer Stem Cell Niche: Cross Talk between Cancer Stem Cells and Their Microenvironment. Tumor Biol. 35, 3945–3951. doi:10.1007/s13277-013-1561-x
Young, J. L., Holle, A. W., and Spatz, J. P. (2016). Nanoscale and Mechanical Properties of the Physiological Cell-ECM Microenvironment. Exp. Cell. Res. 343, 3–6. doi:10.1016/j.yexcr.2015.10.037
Keywords: extracellular matrix, mechanobiology, invasion, hydrogel, stiffness, adhesion
Citation: Vahala D and Choi YS (2022) Modelling the Tumor Microenvironment: Recapitulating Nano- and Micro-Scale Properties that Regulate Tumor Progression. Front. Cell Dev. Biol. 10:908799. doi: 10.3389/fcell.2022.908799
Received: 31 March 2022; Accepted: 20 May 2022;
Published: 14 June 2022.
Edited by:
Samantha Jane Stehbens, The University of Queensland, AustraliaReviewed by:
Sarah Boyle, Centre for Cancer Biology (CCB), AustraliaCopyright © 2022 Vahala and Choi. This is an open-access article distributed under the terms of the Creative Commons Attribution License (CC BY). The use, distribution or reproduction in other forums is permitted, provided the original author(s) and the copyright owner(s) are credited and that the original publication in this journal is cited, in accordance with accepted academic practice. No use, distribution or reproduction is permitted which does not comply with these terms.
*Correspondence: Yu Suk Choi, eXVzdWsuY2hvaUB1d2EuZWR1LmF1