- 1Department of Medicine, University of California San Diego, San Diego, CA, United States
- 2Institut de Pharmacologie et Biologie Structurale, Université de Toulouse, CNRS, Université Paul Sabatier, Toulouse, France
Integrins regulate the adhesion and migration of blood cells to ensure the proper positioning of these cells in the environment. Integrins detect physical and chemical stimuli in the extracellular matrix and regulate signaling pathways in blood cells that mediate their functions. Integrins are usually in a resting state in blood cells until agonist stimulation results in a high-affinity conformation (“integrin activation”), which is central to integrins’ contribution to blood cells’ trafficking and functions. In this review, we summarize the mechanisms of integrin activation in blood cells with a focus on recent advances understanding of mechanisms whereby Rap1 regulates talin1-integrin interaction to trigger integrin activation in lymphocytes, platelets, and neutrophils.
Introduction
Integrin receptors are heterodimeric α and β cell adhesion molecules that play essential roles in cell-to-cell, cell-to-extracellular matrix, and cell-to-pathogen interactions (Hynes, 2002; Hogg et al., 2011). Integrins regulate signaling pathways in blood cells to mediate cell adhesion, cell migration, cell proliferation and cell differentiation (Schwartz et al., 1995; Hynes, 2002). Blood cells, including leukocytes and platelets, dynamically increase the affinity of integrins for their ligands (activation). This is a central event for many cellular functions (Tadokoro et al., 2003; Shattil et al., 2010). Leukocyte integrins β1, β2, and β7 are essential for innate and adaptive immune responses (Hynes, 2002; Luo et al., 2007; Hogg et al., 2011; Vestweber, 2015); and also contribute to many cardiovascular diseases, including atherosclerosis, thrombosis, stroke and peripheral arterial disease (Clemetson and Clemetson, 1998; Galkina and Ley, 2007; Lal et al., 2007; Lu et al., 2008). When rolling leukocytes are stimulated by chemokines or cytokines, the integrins thus activated interact with their ligands on endothelial cells and induce cell arrest and then extravasation during inflammatory responses (Hynes, 2002; Ley et al., 2007). Likewise, binding of integrin αIIbβ3 to its ligand in a high affinity state is a critical step to enable both stable adhesion to the vessel wall and platelet aggregation for effective hemostasis (Bennett and Vilaire, 1979).
Rap1 GTPases act as a molecular switch that controls talin1-dependent activation (Bos et al., 2001; Bos et al., 2003) of β1 (Tadokoro et al., 2003; Shattil et al., 2010), β2 (Katagiri et al., 2003; Simonson et al., 2006), β3 (Petrich et al., 2007a; Petrich et al., 2007b; Nieswandt et al., 2007; Haling et al., 2011), and β7 (Sun et al., 2014; Sun et al., 2018) and integrins. The Rap1-interacting adaptor molecule (RIAM) is an effector of Rap1 that mediating this function in leukocytes (Klapproth et al., 2015; Su et al., 2015; Lagarrigue et al., 2017; Sun et al., 2021). Recent work indicates that direct Rap1 binding to talin1 plays a vital role in integrin activation in platelets (Bromberger et al., 2018; Lagarrigue et al., 2018; Lagarrigue et al., 2020) and also contributes to integrin activation in leukocytes (Lagarrigue et al., 2020; Bromberger et al., 2021; Lagarrigue et al., 2022).
In this review, we discuss recent insights into the mechanisms of talin1 recruitment to integrins and their subsequent activation in blood cells, focusing on the function of the Rap1-talin1 axis in different cell types and classes of integrins.
Integrin Activation
Integrin α and β subunits are composed of a large ectodomain, a transmembrane domain (TMD) and a cytoplasmic tail (CT) (Hynes, 1987). The integrin β subunit head contains a cation-dependent ligand binding site (Loftus et al., 1990) that is part of an A domain (sometimes called “I domain’ meaning inserted domain) (Xiong et al., 2001). Four β1 integrins and all four β2 integrins of leukocytes contain a second A domain in the α subunit which serves as the primary ligand binding site (Lee et al., 1995). Conformational changes in the extracellular domain increased the affinity of integrins for monomeric ligands and this results from the destabilization of the association of transmembrane and cytoplasmic α and β domains (Hughes et al., 1996; Kim et al., 2003; Mould et al., 2003; Luo et al., 2004; Partridge et al., 2005). Integrins exhibit at least three major conformational states (Takagi et al., 2002; Luo et al., 2007; Chen et al., 2010; Springer and Dustin, 2012): inactive (low affinity, bent-closed, resting state), intermediate (low affinity, extended-closed), and active (high affinity, extended-open). The extended-high affinity state is induced by a conformational rearrangement in the A domain that follows the displacement of the α7 helix in this structure (Emsley et al., 2000). A current model proposes that talin1 associates with the cytoplasmic tail of the β subunit and disrupts the stabilization of the inner membrane clasp (Anthis et al., 2009; Lau et al., 2009). In addition, the binding of talin1 to membrane lipids is also essential for activation (Anthis et al., 2009) because it enables a change in the topology of the β transmembrane domain that disrupts an outer membrane clasp (Kim et al., 2011a) thus breaking the two constraints that stabilize the αβ TMD association. Recent studies with β7 integrin have confirmed the importance of transmission of β TMD topology that disrupts the outer membrane clasp (Sun et al., 2018) in vivo in the development of gut-associated lymphoid tissue. Furthermore, studies of β2 integrins have shown that a mutation that reduces transmission changes in TMD topology blocks integrin extension (Sun et al., 2020) but not the high-affinity conformation.
Integrin Adaptor Proteins
The inside-out signaling of integrins is precisely controlled by the binding of various intracellular adapter proteins to integrins (Abram and Lowell, 2009). A well-described pathway of integrin activation in leukocytes and platelets involves production of Ca2+ and diacylglycerol, protein kinase C (PKC) and activation of Rap1 GTPase. Then, Rap1 interacts with its effector Rap1-GTP-interacting-adaptor molecule (RIAM, product of the APBB1IP gene), which in turn recruits talin1 to the plasma membrane to facilitate its association with integrin (Han et al., 2006; Lee et al., 2009; Lagarrigue et al., 2016). Kindlin3 also participates in this process. Kindlins play an important role in integrin-mediated functions (Tu et al., 2003; Kloeker et al., 2004; Moser et al., 2008; Moser et al., 2009); however, a major role of kindlins is also to enable clustering of integrins leading to avidity modulation and resultant outside-in signaling (Ye et al., 2013).
Rap1 is a small GTPase essential for inside-out signaling of the integrin (Franke et al., 1997; Jeon et al., 2007). It is activated downstream of many agonists by the action of guanine nucleotide-exchange factors (GEFs), which trigger the switch from GDP-bound Rap1 to GTP-bound Rap1, resulting in activation of integrins in leukocytes or platelets. Several Rap1 GEFs such as diacylglycerol-regulated GEF1 (CalDAG-GEF1) and Ca2+ have been identified and are activated in response to a diverse set of upstream stimuli, indicating that multiple pathways may converge on Rap1 GTPases (Abram and Lowell, 2009). RapGEF1, RapGEF3 and RapGEF6 activate Rap1 and promote integrin activation in leukocytes (Abram and Lowell, 2009), while CalDAG-GEF1 is important for Rap1 activation in platelets (Eto et al., 2002; Crittenden et al., 2004; Canault et al., 2014; Lozano et al., 2016). GTP binding causes a conformational change in Rap1 that enables it to engage other proteins that serve as effectors of Rap1-regulated functions.
The ability of intracellular signaling pathways to induce integrin activation depends on the binding of talin1 to the integrin β tail (Tadokoro et al., 2003; Ye et al., 2010; Kim et al., 2011b; Lefort et al., 2012). Talin1 is a major cytoskeletal protein that links integrins and the actin cytoskeleton through its head domain (N-terminal) binding to the integrin β CT domains, and its rod domain (C-terminal) binding to F-actin (Critchley and Gingras, 2008). In mammals, the Tln1 and Tln2 genes encode talin1 and talin2 respectively, but only talin1 is expressed in blood cells and endothelium. The talin1 head domain (THD) comprises an atypical FERM (band 4.1, ezrin, radixin, and moesin) domain grouping four subdomains: F1, F2, F3, and an F0 subdomain derived from the F1 duplication (Calderwood et al., 2013). Structural studies have shown that the F3 subdomain interacts with the conserved proximal NPXY motif in the CT domain of β integrin, leading to the conformational change of integrin and triggering integrin activation (Hemmings et al., 1996; Garcia-Alvarez et al., 2003; Wegener et al., 2007; Shattil et al., 2010; Kim et al., 2011b). The rod domain has 13 subdomains (R1-R13), which include binding sites for RIAM, integrins, vinculin and F-actin (Hemmings et al., 1996; Goult et al., 2013). The mechanism of talin1 activation is still controversial. Binding to PIP5Kγ facilitates the recruitment of talin1 to the plasma membrane, where the talin1-F2 and -F3 subdomains interact with phosphatidylinositol-4,5-bisphosphate (PI(4,5)P2). This binding disrupts the association between the talin1-head domain with the tail and exposes integrin binding sites (Di Paolo et al., 2002; Ling et al., 2002). Germline deletion of talin1 results in embryonic lethality (Monkley et al., 2000), The consequences of the loss of talin1 in mouse blood cells by conditional inactivation have explicitly shown the key role of talin1 in the activation of integrins in leukocytes and platelets (Petrich et al., 2007b; Nieswandt et al., 2007; Manevich-Mendelson et al., 2010; Lefort et al., 2012; Klann et al., 2017). Although the mechanism by which talin1 activates integrins has been well described, there is a need to understand precisely the details of signal transduction events from cell stimulation to the recruitment of talin1 to integrins.
The Rap1-RIAM-talin1 Axis vs. the Rap1-Talin1 Axis
Rap1 is an important signaling hub for the integration of adhesive signals. Rap1 exists in two isoforms, Rap1a and Rap1b, which are encoded by two different genes. RIAM is an effector of Rap1 that binds talin1 to allow its interaction with the cytoplasmic tails of integrins (Lafuente et al., 2004; Lee et al., 2009; Chen et al., 2017). RIAM belongs to the family of Mig-10/RIAM/Lamellipodin (MRL) adapter proteins which are characterized by a structural module comprising a Ras association domain (RA) and a pleckstrin homology domain (PH). RIAM forms an autoinhibitory conformation by an intramolecular interaction between the inhibitory (IN) segment and the RA domain at its binding site to Rap1 and on which the packing of two RIAM molecules by the intermolecular interaction of the PH domain masks the binding to phosphoinositides (Chang et al., 2019; Cho et al., 2021). Phosphorylation of the IN segment and PH domain by focal adhesion kinase (FAK) and proto-oncogene tyrosine-protein kinase (Src), respectively, releases these inhibitory conformations and contribute to RIAM activation thus facilitating its interaction with Rap1 and PI(4,5)P2 to promote talin1-dependent integrin activation (Chang et al., 2019; Cho et al., 2021).
RIAM is crucial for Rap1-dependent integrin activation in leukocytes (Klapproth et al., 2015; Su et al., 2015; Lagarrigue et al., 2017; Sun et al., 2021). RIAM is mainly expressed in leukocytes where it regulates the function of β2 integrins to support their adhesion and migration (Klapproth et al., 2015; Su et al., 2015). In contrast, RIAM is dispensable for α4β1 integrin activation and functions (Klapproth et al., 2015; Su et al., 2015). RIAM depletion in CD4+ T cells inhibits antigen-dependent autoimmunity by interfering with the interaction between effector T cells and antigen presenting cells (Lagarrigue et al., 2017). Interestingly, RIAM has been shown to interact with kindlin-3 even before it binds talin1 (Kliche et al., 2012), but whether RIAM directly interacts with kindlin-3 is unknown. Recently, we showed that RIAM is required for integrin activation in conventional T (Tconv) cells but is dispensable in regulatory T (Treg) cells, although Rap1 is necessary for Treg cell function and RIAM is expressed in Treg cells (Sun et al., 2021).
Lamellipodin (Lpd) (Krause et al., 2004) is a paralogue of RIAM (Lafuente et al., 2004) that compensates for the deficiency of RIAM in the activation of integrins in Treg cells (Sun et al., 2021). Similar to RIAM, Lpd contains talin1 binding sites and triggers integrin activation (Watanabe et al., 2008; Lee et al., 2009). In addition to regulating talin1-dependent integrin activation, RIAM also contributes to β2 integrin-mediated outside-in signaling in neutrophils and macrophages (Klapproth et al., 2015; Torres-Gomez et al., 2020). On the other hand, the role of Lpd in the outside-in signaling of integrins is poorly understood and deserves to be explored.
In vivo studies in mice have revealed that loss of RIAM inhibits integrin activation in a cell type-specific manner. RIAM loss can ameliorate autoimmune diseases such as experimental type I diabetes (Lagarrigue et al., 2017) or inflammatory bowel disease (Sun et al., 2021) by suppressing integrin-mediated activation of Tconv cells while retaining Treg cell function. Platelets express little RIAM or Lpd (Klapproth et al., 2015). Mice deficient in Lpd and RIAM exhibit intact platelet integrin activation (Klapproth et al., 2015; Lagarrigue et al., 2015; Stritt et al., 2015; Su et al., 2015; Sun et al., 2021) and normal hemostasis. Moreover, the fact that RIAM deficiency affects leukocyte integrin β2 function less than talin1 deficiency suggests the existence of alternative RIAM-independent pathways that regulate the recruitment of talin1 to integrins (Klapproth et al., 2015; Sun et al., 2021).
NMR studies indicated a direct interaction between Rap1b and the F0 subdomain on talin1 with low affinity (Goult et al., 2010), suggesting that it is a mechanism of recruitment of talin1 to integrins (Zhu et al., 2017). The direct interaction between TalinB and Rap1 is required for adhesion of Dictyostelium cells (Plak et al., 2016). A mutation that blocks the binding of Rap1 to the F0 subdomain of talin1 is an embryonic lethal mutation in Drosophila (Camp et al., 2018). A quantitative study of the proteome in murine platelets revealed that Rap1 and talin1 are highly expressed in murine platelets at an equimolar ratio (Zeiler et al., 2014). In the absence of other known effectors of Rap1 in platelets, it became clear that the direct interaction of Rap1 with talin1 F0 could play a vital role in integrin activation in platelets. However, a talin1 (R35E) mutation in the F0 subdomain, which blocks direct binding of Rap1, has a modest effect on talin1 induced αIIbβ3 activation (Lagarrigue et al., 2018). In parallel, Moser’s group generated a Tln13mut mouse carrying the K15A, R30A, R35A mutations in the F0 subdomain of talin1 and showed a weak contribution of the F0-Rap1 interaction in the activation of αIIbβ3. Talin1 (R35E) and Tln13mut mice are apparently healthy, fertile, and viable, and showed bleeding times similar to wild-type littermates, indicating mild defects in platelet aggregation and hemostasis (Bromberger et al., 2018; Lagarrigue et al., 2018). Thus, Rap1-talin1 F0 binding has a minor effect on platelet integrin activation despite a critical role of Rap1 and talin1 for αIIbβ3 activation and platelet function (Petrich et al., 2007b; Nieswandt et al., 2007; Stefanini et al., 2018). We recently found a new Rap1 binding site in the talin1 F1 domain (Gingras et al., 2019). The talin1 (R118E) mutation in the F1 domain profoundly reduces the ability of F1 to bind Rap1 and significantly disrupts the ability of Rap1 to mediate talin1-induced integrin activation in platelets (Gingras et al., 2019; Lagarrigue et al., 2020). The talin1 F1 subdomain plays a distinct and much more central role than the F0 domain in Rap1-mediated integrin activation in mammalian cells. Moreover, loss of both Rap1 binding sites in talin1 (R35E,R118E) mutant mice causes a much greater defect in platelet integrin activation, similar to that caused by knockout of both Rap1 a and b isoforms (Stefanini et al., 2018; Lagarrigue et al., 2020). These results suggest that Rap1 binding to the F0 and F1 subdomains of talin1 induces talin1-dependent integrin activation in platelets and plays a fundamental role in hemostasis and thrombosis.
We now have a more complete picture of how the talin1 head domain interacts with the membrane. Positively charged patches in the talin1 F2 and F3 areas bind to membranes to stabilize the weak interaction of talin1 F3 with the cytoplasmic β integrin domain to explain the membrane dependency of talin1-induced activation (Ye et al., 2010; Chinthalapudi et al., 2018; Bromberger et al., 2019). Additionally, the Rap1 binding site in the F1 subdomain of talin1 functions in conjunction with a unique inserted loop that interacts with membrane lipids to enable Rap1 to contribute significantly to the membrane targeting of talin1 (Goult et al., 2010; Gingras et al., 2019). The proximity of the putative membrane-binding helix of the F1 loop to the F1-linked geranyl-geranyl fragment of Rap1 provides a compelling model to explain the complementary role of these two membrane-binding sites in the activation of the integrin. Furthermore, while all studies have focused only on the binding of Rap1b to talin1 (Goult et al., 2010; Bromberger et al., 2018; Lagarrigue et al., 2018; Gingras et al., 2019), we can anticipate that Rap1a may also interact directly with talin1 given that Rap1a and Rap1b share 95% sequence identity. Moreover, the main positively charged residues in talin1 for Rap1 binding are conserved in talin2, in particular K15 and R35 in talin1 F0 and R98 and R118 in talin1 F1, suggesting that Rap1 could also interact directly with talin2. Future studies are needed to determine if direct binding can be extended to each of the Rap1 and talin isoforms.
Cell-Type Specific Connections Between Rap1 and Talin1
The mechanism of recruitment of talin1 by Rap1 has been the subject of several recent studies (Zhu et al., 2017; Bromberger et al., 2018; Bromberger et al., 2019; Gingras et al., 2019; Lagarrigue et al., 2020; Bromberger et al., 2021; Lagarrigue et al., 2022). Interestingly, this dialogue varies by cell type and integrin class. In platelets, lacking endogenous RIAM, talin1 (R35E, R118E) mutations profoundly inhibit integrin αIIbβ3 activation and platelet aggregation, revealing that talin1 is the sole effector of Rap1 (Lagarrigue et al., 2020) (Figure 1).
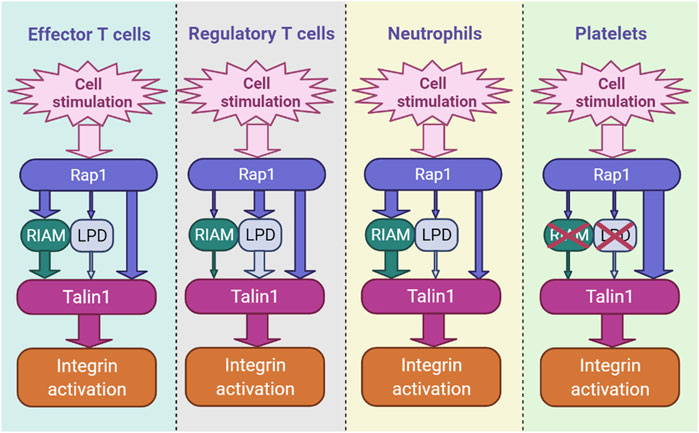
FIGURE 1. The hierarchy of integrin activation pathways differs by cell type. RIAM- and LPD-dependent pathways and the direct binding of Rap1 to talin1 coexist and contribute in parallel in blood cells, including immune cells. The thickness of the arrow indicates the relative importance of each channel.
We have also recently shown that direct binding of Rap1 to talin1 regulates integrin activation in Tconv and Treg cells (Lagarrigue et al., 2022). Inhibition of Rap1 binding to talin1 (R35E,R118E) induces a significant decrease in the activation of integrins α4β1, α4β7 and αLβ2 in Tconv and Treg cells, resulting in defects in T cell homing and functions. However, unlike platelets, RIAM is required for optimal integrin activation in T cells (Klapproth et al., 2015; Su et al., 2015; Lagarrigue et al., 2017; Sun et al., 2021) (Figure 1). While the loss of RIAM strongly impacts the activation of integrins β2 and β3, the effect is minimal on integrin α4β1. In contrast, T cells expressing talin1 (R35E,R118E) show only partial loss of integrin activation (Lagarrigue et al., 2022). RIAM-deficient T cells expressing talin1 (R35E,R118E) show a much deeper impairment in αLβ2, α4β1 and α4β7 activation. RIAM and Lpd depletion combined with talin1 (R35E,R118E) further significantly abrogates integrin activation in Treg cells. RIAM overexpression circumvents the integrin activation deficit in talin1 (R35E,R118E)-expressing T cells, suggesting that RIAM may compensate for Rap1 binding to talin1 during integrin activation (Lagarrigue et al., 2022). Altogether, these results indicate the importance of the interaction between Rap1 and talin1 which promote the activation of integrins αLβ2, α4β1 and α4β7 in T cells (Figure 1).
The two axes Rap1-talin1 and Rap1-RIAM-talin1 act synergistically to regulate integrin β2 activation in leukocytes (Bromberger et al., 2021; Lagarrigue et al., 2022). On the other hand, what distinguishes these two paths is not so clear. The involvement of RIAM adds a higher level of regulation since RIAM must be phosphorylated by Fak and Src to be activated (Chang et al., 2019; Cho et al., 2021). Moreover, RIAM, but also Lpd, couple talin1-mediated integrin activation to the regulation of actin dynamics by forming a molecular complex that results in cellular protrusions at the tips of migrating cells (Lagarrigue et al., 2015). It is therefore tempting to see in the expression of MRL proteins in leukocytes a reflection of their migratory capacity which requires fine tuning of the activation of integrins and the regulation of the actin cytoskeleton. In contrast, one might speculate that in platelets, which do not express RIAM and are much less motile, the Rap1-talin1 pathway occurs more rapidly due to the extra time required for RIAM or Lpd to be phosphorylated and released from the autoinhibition in leukocytes. Further research is needed to determine whether direct binding of Rap1 to talin1, without the use of MRL proteins as an intermediary, reduces the time required for integrin activation in platelets to specifically mediate their binding to the vascular wall under a strong shear force.
Neutrophils are generally considered to be the first immune cells to defend against pathogens or infections. Aberrant neutrophil infiltration is seen in patients with autoimmune disease (Caradonna et al., 2000; Smith et al., 2005). Loss of Rap1 can markedly suppress neutrophil functions largely because it inhibits integrin activation (Bromberger et al., 2018). The Tln13mut mouse (Bromberger et al., 2018), like the talin1 (R35E) mutant mouse (Lagarrigue et al., 2018), does not show leukocytosis, and the neutrophils of the Tln13mut mice show a slight adhesion defect and a reduced extravasation. However, Tln13mut mutation combined with the loss of RIAM clearly suppresses neutrophil integrin functions (Bromberger et al., 2021). In contrast, Tln1R118E/R118E mice exhibit leukocytosis (Lagarrigue et al., 2020), which affects both neutrophils and lymphocytes, suggesting that the F1-Rap1 interaction has a greater functional impact than the F0-Rap1 interaction. The role of Rap1-talin1 binding in neutrophil adhesion and arrest deserves further investigation.
Conclusion
Binding of talin1 to integrin is a terminal event in the inside-out signaling of integrins. Rap1 serves as a convergence point for many adhesive signals. The link between Rap1 and talin1 in integrin activation has long remained a significant gap in our understanding of this event. However, recent advances, partly accelerated by the RIAM and Lpd knockout mice, developed respectively by the laboratories of F.B. Gertler (Klapproth et al., 2015; Su et al., 2015) and M. Krause (Law et al., 2013), have enabled dissections of the role of MRL proteins in different cells. In particular, RIAM appears to play the most important role in most lymphocytes and neutrophils. In Treg cells, however, Lpd is clearly more important. The third connection, via direct binding to Rap1 to two binding sites in the head domain of talin1 (Gingras et al., 2019), is important in all blood cells. In platelets, which express negligible amounts of the MRL proteins, direct binding of Rap1 to talin1 accounts for Rap1-dependent integrin activation (Lagarrigue et al., 2020). Thus, these three pathways act in parallel to connect Rap1 to talin1. The relative contribution of each pathway varies by cell type and integrin type and this variation offers the potential to selectively manipulate integrin activation in a cell type-specific manner.
Author Contributions
HS drafted the manuscript and prepared figures. HS, FL, and MG edited and revised the manuscript. HS approved the final version of the manuscript. All authors contributed to the article and approved the submitted version.
Funding
This work was supported by grants HL 139947 and HL 151433 to MHG, the Crohn’s and Colitis Foundation Career Development Award 902590 to HS, the ATIP-Avenir group leader program (CNRS), ARC Foundation and Foundation de France to FL.
Conflict of Interest
The authors declare that the research was conducted in the absence of any commercial or financial relationships that could be construed as a potential conflict of interest.
Publisher’s Note
All claims expressed in this article are solely those of the authors and do not necessarily represent those of their affiliated organizations, or those of the publisher, the editors and the reviewers. Any product that may be evaluated in this article, or claim that may be made by its manufacturer, is not guaranteed or endorsed by the publisher.
References
Abram, C. L., and Lowell, C. A. (2009). The Ins and Outs of Leukocyte Integrin Signaling. Annu. Rev. Immunol. 27, 339–362. doi:10.1146/annurev.immunol.021908.132554
Anthis, N. J., Wegener, K. L., Ye, F., Kim, C., Goult, B. T., Lowe, E. D., et al. (2009). The Structure of an Integrin/talin Complex Reveals the Basis of Inside-Out Signal Transduction. EMBO J. 28, 3623–3632. doi:10.1038/emboj.2009.287
Bennett, J. S., and Vilaire, G. (1979). Exposure of Platelet Fibrinogen Receptors by ADP and Epinephrine. J. Clin. Invest. 64, 1393–1401. doi:10.1172/jci109597
Bos, J. L., De Bruyn, K., Enserink, J., Kuiperij, B., Rangarajan, S., Rehmann, H., et al. (2003). The Role of Rap1 in Integrin-Mediated Cell Adhesion. Biochem. Soc. Trans. 31, 83–86. doi:10.1042/bst0310083
Bos, J. L., De Rooij, J., and Reedquist, K. A. (2001). Rap1 Signalling: Adhering to New Models. Nat. Rev. Mol. Cell Biol. 2, 369–377. doi:10.1038/35073073
Bromberger, T., Zhu, L., Klapproth, S., Qin, J., and Moser, M. (2019). Rap1 and Membrane Lipids Cooperatively Recruit Talin to Trigger Integrin Activation. J. Cell Sci. 132, jcs235531. doi:10.1242/jcs.235531
Bromberger, T., Klapproth, S., Rohwedder, I., Weber, J., Pick, R., Mittmann, L., et al. (2021). Binding of Rap1 and Riam to Talin1 Fine-Tune β2 Integrin Activity during Leukocyte Trafficking. Front. Immunol. 12, 702345. doi:10.3389/fimmu.2021.702345
Bromberger, T., Klapproth, S., Rohwedder, I., Zhu, L., Mittmann, L., Reichel, C. A., et al. (2018). Direct Rap1/Talin1 Interaction Regulates Platelet and Neutrophil Integrin Activity in Mice. Blood 132, 2754–2762. doi:10.1182/blood-2018-04-846766
Calderwood, D. A., Campbell, I. D., and Critchley, D. R. (2013). Talins and Kindlins: Partners in Integrin-Mediated Adhesion. Nat. Rev. Mol. Cell Biol. 14, 503–517. doi:10.1038/nrm3624
Camp, D., Haage, A., Solianova, V., Castle, W. M., Xu, Q. A., Lostchuck, E., et al. (2018). Direct Binding of Talin to Rap1 Is Required for Cell-ECM Adhesion in Drosophila. J. Cell Sci. 131, jcs225144. doi:10.1242/jcs.225144
Canault, M., Ghalloussi, D., Grosdidier, C., Guinier, M., Perret, C., Chelghoum, N., et al. (2014). Human CalDAG-GEFI Gene (RASGRP2) Mutation Affects Platelet Function and Causes Severe Bleeding. J. Exp. Med. 211, 1349–1362. doi:10.1084/jem.20130477
Caradonna, L., Amati, L., Lella, P., Jirillo, E., and Caccavo, D. (2000). Phagocytosis, Killing, Lymphocyte-Mediated Antibacterial Activity, Serum Autoantibodies, and Plasma Endotoxins in Inflammatory Bowel Disease. Am. J. Gastroenterol. 95, 1495–1502. doi:10.1111/j.1572-0241.2000.02085.x
Chang, Y.-C., Su, W., Cho, E.-a., Zhang, H., Huang, Q., Philips, M. R., et al. (2019). Molecular Basis for Autoinhibition of RIAM Regulated by FAK in Integrin Activation. Proc. Natl. Acad. Sci. U.S.A. 116, 3524–3529. doi:10.1073/pnas.1818880116
Chen, X., Xie, C., Nishida, N., Li, Z., Walz, T., and Springer, T. A. (2010). Requirement of Open Headpiece Conformation for Activation of Leukocyte Integrin α X β 2. Proc. Natl. Acad. Sci. U.S.A. 107, 14727–14732. doi:10.1073/pnas.1008663107
Chen, Y., Ju, L., Rushdi, M., Ge, C., and Zhu, C. (2017). Receptor-mediated Cell Mechanosensing. MBoC 28, 3134–3155. doi:10.1091/mbc.e17-04-0228
Chinthalapudi, K., Rangarajan, E. S., and Izard, T. (2018). The Interaction of Talin with the Cell Membrane Is Essential for Integrin Activation and Focal Adhesion Formation. Proc. Natl. Acad. Sci. U.S.A. 115, 10339–10344. doi:10.1073/pnas.1806275115
Cho, E.-A., Zhang, P., Kumar, V., Kavalchuk, M., Zhang, H., Huang, Q., et al. (2021). Phosphorylation of RIAM by Src Promotes Integrin Activation by Unmasking the PH Domain of RIAM. Structure 29, 320–329. doi:10.1016/j.str.2020.11.011
Clemetson, K. J., and Clemetson, J. M. (1998). Integrins and Cardiovascular Disease. Cell. Mol. Life Sci. (CMLS) 54, 502–513. doi:10.1007/s000180050179
Critchley, D. R., and Gingras, A. R. (2008). Talin at a Glance. J. Cell Sci. 121, 1345–1347. doi:10.1242/jcs.018085
Crittenden, J. R., Bergmeier, W., Zhang, Y., Piffath, C. L., Liang, Y., Wagner, D. D., et al. (2004). CalDAG-GEFI Integrates Signaling for Platelet Aggregation and Thrombus Formation. Nat. Med. 10, 982–986. doi:10.1038/nm1098
Di Paolo, G., Pellegrini, L., Letinic, K., Cestra, G., Zoncu, R., Voronov, S., et al. (2002). Recruitment and Regulation of Phosphatidylinositol Phosphate Kinase Type 1γ by the FERM Domain of Talin. Nature 420, 85–89. doi:10.1038/nature01147
Emsley, J., Knight, C. G., Farndale, R. W., Barnes, M. J., and Liddington, R. C. (2000). Structural Basis of Collagen Recognition by Integrin α2β1. Cell 101, 47–56. doi:10.1016/s0092-8674(00)80622-4
Eto, K., Murphy, R., Kerrigan, S. W., Bertoni, A., Stuhlmann, H., Nakano, T., et al. (2002). Megakaryocytes Derived from Embryonic Stem Cells Implicate CalDAG-GEFI in Integrin Signaling. Proc. Natl. Acad. Sci. U.S.A. 99, 12819–12824. doi:10.1073/pnas.202380099
Franke, B., Akkerman, J. W., and Bos, J. L. (1997). Rapid Ca2+-Mediated Activation of Rap1 in Human Platelets. EMBO J. 16, 252–259. doi:10.1093/emboj/16.2.252
Galkina, E., and Ley, K. (2007). Vascular Adhesion Molecules in Atherosclerosis. Atvb 27, 2292–2301. doi:10.1161/atvbaha.107.149179
García-Alvarez, B., De Pereda, J. M., Calderwood, D. A., Ulmer, T. S., Critchley, D., Campbell, I. D., et al. (2003). Structural Determinants of Integrin Recognition by Talin. Mol. Cell 11, 49–58. doi:10.1016/s1097-2765(02)00823-7
Gingras, A. R., Lagarrigue, F., Cuevas, M. N., Valadez, A. J., Zorovich, M., Mclaughlin, W., et al. (2019). Rap1 Binding and a Lipid-dependent Helix in Talin F1 Domain Promote Integrin Activation in Tandem. J. Cell Biol. 218, 1799–1809. doi:10.1083/jcb.201810061
Goult, B. T., Bouaouina, M., Elliott, P. R., Bate, N., Patel, B., Gingras, A. R., et al. (2010). Structure of a Double Ubiquitin-like Domain in the Talin Head: a Role in Integrin Activation. EMBO J. 29, 1069–1080. doi:10.1038/emboj.2010.4
Goult, B. T., Zacharchenko, T., Bate, N., Tsang, R., Hey, F., Gingras, A. R., et al. (2013). RIAM and Vinculin Binding to Talin Are Mutually Exclusive and Regulate Adhesion Assembly and Turnover. J. Biol. Chem. 288, 8238–8249. doi:10.1074/jbc.m112.438119
Haling, J. R., Monkley, S. J., Critchley, D. R., and Petrich, B. G. (2011). Talin-dependent Integrin Activation Is Required for Fibrin Clot Retraction by Platelets. Blood 117, 1719–1722. doi:10.1182/blood-2010-09-305433
Han, J., Lim, C. J., Watanabe, N., Soriani, A., Ratnikov, B., Calderwood, D. A., et al. (2006). Reconstructing and Deconstructing Agonist-Induced Activation of Integrin αIIbβ3. Curr. Biol. 16, 1796–1806. doi:10.1016/j.cub.2006.08.035
Hemmings, L., Rees, D. J., Ohanian, V., Bolton, S. J., Gilmore, A. P., Patel, B., et al. (1996). Talin Contains Three Actin-Binding Sites Each of Which Is Adjacent to a Vinculin-Binding Site. J. Cell Sci. 109 (Pt 11), 2715–2726. doi:10.1242/jcs.109.11.2715
Hogg, N., Patzak, I., and Willenbrock, F. (2011). The Insider's Guide to Leukocyte Integrin Signalling and Function. Nat. Rev. Immunol. 11, 416–426. doi:10.1038/nri2986
Hughes, P. E., Diaz-Gonzalez, F., Leong, L., Wu, C., Mcdonald, J. A., Shattil, S. J., et al. (1996). Breaking the Integrin Hinge. J. Biol. Chem. 271, 6571–6574. doi:10.1074/jbc.271.12.6571
Hynes, R. (1987). Integrins: a Family of Cell Surface Receptors. Cell 48, 549–554. doi:10.1016/0092-8674(87)90233-9
Jeon, T. J., Lee, D.-J., Lee, S., Weeks, G., and Firtel, R. A. (2007). Regulation of Rap1 Activity by RapGAP1 Controls Cell Adhesion at the Front of Chemotaxing Cells. J. Cell Biol. 179, 833–843. doi:10.1083/jcb.200705068
Katagiri, K., Maeda, A., Shimonaka, M., and Kinashi, T. (2003). RAPL, a Rap1-Binding Molecule that Mediates Rap1-Induced Adhesion through Spatial Regulation of LFA-1. Nat. Immunol. 4, 741–748. doi:10.1038/ni950
Kim, C., Schmidt, T., Cho, E.-G., Ye, F., Ulmer, T. S., and Ginsberg, M. H. (2011a). Basic Amino-Acid Side Chains Regulate Transmembrane Integrin Signalling. Nature 481, 209–213. doi:10.1038/nature10697
Kim, C., Ye, F., and Ginsberg, M. H. (2011b). Regulation of Integrin Activation. Annu. Rev. Cell Dev. Biol. 27, 321–345. doi:10.1146/annurev-cellbio-100109-104104
Kim, M., Carman, C. V., and Springer, T. A. (2003). Bidirectional Transmembrane Signaling by Cytoplasmic Domain Separation in Integrins. Science 301, 1720–1725. doi:10.1126/science.1084174
Klann, J. E., Remedios, K. A., Kim, S. H., Metz, P. J., Lopez, J., Mack, L. A., et al. (2017). Talin Plays a Critical Role in the Maintenance of the Regulatory T Cell Pool. J. I. 198, 4639–4651. doi:10.4049/jimmunol.1601165
Klapproth, S., Sperandio, M., Pinheiro, E. M., Prünster, M., Soehnlein, O., Gertler, F. B., et al. (2015). Loss of the Rap1 Effector RIAM Results in Leukocyte Adhesion Deficiency Due to Impaired β2 Integrin Function in Mice. Blood 126, 2704–2712. doi:10.1182/blood-2015-05-647453
Kliche, S., Worbs, T., Wang, X., Degen, J., Patzak, I., Meineke, B., et al. (2012). CCR7-mediated LFA-1 Functions in T Cells Are Regulated by 2 Independent ADAP/SKAP55 Modules. Blood 119, 777–785. doi:10.1182/blood-2011-06-362269
Kloeker, S., Major, M. B., Calderwood, D. A., Ginsberg, M. H., Jones, D. A., and Beckerle, M. C. (2004). The Kindler Syndrome Protein Is Regulated by Transforming Growth Factor-β and Involved in Integrin-Mediated Adhesion. J. Biol. Chem. 279, 6824–6833. doi:10.1074/jbc.m307978200
Krause, M., Leslie, J. D., Stewart, M., Lafuente, E. M., Valderrama, F., Jagannathan, R., et al. (2004). Lamellipodin, an Ena/VASP Ligand, Is Implicated in the Regulation of Lamellipodial Dynamics. Dev. Cell 7, 571–583. doi:10.1016/j.devcel.2004.07.024
Lafuente, E. M., Van Puijenbroek, A. A. F. L., Krause, M., Carman, C. V., Freeman, G. J., Berezovskaya, A., et al. (2004). RIAM, an Ena/VASP and Profilin Ligand, Interacts with Rap1-GTP and Mediates Rap1-Induced Adhesion. Dev. Cell 7, 585–595. doi:10.1016/j.devcel.2004.07.021
Lagarrigue, F., Gertler, F. B., Ginsberg, M. H., and Cantor, J. M. (2017). Cutting Edge: Loss of T Cell RIAM Precludes Conjugate Formation with APC and Prevents Immune-Mediated Diabetes. J. I. 198, 3410–3415. doi:10.4049/jimmunol.1601743
Lagarrigue, F., Gingras, A. R., Paul, D. S., Valadez, A. J., Cuevas, M. N., Sun, H., et al. (2018). Rap1 Binding to the Talin 1 F0 Domain Makes a Minimal Contribution to Murine Platelet GPIIb-IIIa Activation. Blood Adv. 2, 2358–2368. doi:10.1182/bloodadvances.2018020487
Lagarrigue, F., Kim, C., and Ginsberg, M. H. (2016). The Rap1-RIAM-Talin axis of Integrin Activation and Blood Cell Function. Blood 128, 479–487. doi:10.1182/blood-2015-12-638700
Lagarrigue, F., Paul, D. S., Gingras, A. R., Valadez, A. J., Sun, H., Lin, J., et al. (2020). Talin-1 Is the Principal Platelet Rap1 Effector of Integrin Activation. Blood 136, 1180–1190. doi:10.1182/blood.2020005348
Lagarrigue, F., Tan, B., Du, Q., Fan, Z., Lopez-Ramirez, M. A., Gingras, A. R., et al. (2022). Direct Binding of Rap1 to Talin1 and to MRL Proteins Promotes Integrin Activation in CD4+ T Cells. J. I. 208, 1378–1388. doi:10.4049/jimmunol.2100843
Lagarrigue, F., Vikas Anekal, P., Lee, H.-S., Bachir, A. I., Ablack, J. N., Horwitz, A. F., et al. (2015). A RIAM/lamellipodin-talin-integrin Complex Forms the Tip of Sticky Fingers that Guide Cell Migration. Nat. Commun. 6, 8492. doi:10.1038/ncomms9492
Lal, H., Guleria, R., Foster, D., Lu, G., Watson, L., Sanghi, S., et al. (2007). Integrins: Novel Therapeutic Targets for Cardiovascular Diseases. Chamc 5, 109–132. doi:10.2174/187152507780363223
Lau, T.-L., Kim, C., Ginsberg, M. H., and Ulmer, T. S. (2009). The Structure of the Integrin αIIbβ3 Transmembrane Complex Explains Integrin Transmembrane Signalling. EMBO J. 28, 1351–1361. doi:10.1038/emboj.2009.63
Law, A.-L., Vehlow, A., Kotini, M., Dodgson, L., Soong, D., Theveneau, E., et al. (2013). Lamellipodin and the Scar/WAVE Complex Cooperate to Promote Cell Migration In Vivo. J. Cell Biol. 203, 673–689. doi:10.1083/jcb.201304051
Lee, H.-S., Lim, C. J., Puzon-Mclaughlin, W., Shattil, S. J., and Ginsberg, M. H. (2009). RIAM Activates Integrins by Linking Talin to Ras GTPase Membrane-Targeting Sequences. J. Biol. Chem. 284, 5119–5127. doi:10.1074/jbc.m807117200
Lee, J.-O., Rieu, P., Arnaout, M. A., and Liddington, R. (1995). Crystal Structure of the A Domain from the a Subunit of Integrin CR3 (CD11 b/CD18). Cell 80, 631–638. doi:10.1016/0092-8674(95)90517-0
Lefort, C. T., Rossaint, J., Moser, M., Petrich, B. G., Zarbock, A., Monkley, S. J., et al. (2012). Distinct Roles for Talin-1 and Kindlin-3 in LFA-1 Extension and Affinity Regulation. Blood 119, 4275–4282. doi:10.1182/blood-2011-08-373118
Ley, K., Laudanna, C., Cybulsky, M. I., and Nourshargh, S. (2007). Getting to the Site of Inflammation: the Leukocyte Adhesion Cascade Updated. Nat. Rev. Immunol. 7, 678–689. doi:10.1038/nri2156
Ling, K., Doughman, R. L., Firestone, A. J., Bunce, M. W., and Anderson, R. A. (2002). Type Iγ Phosphatidylinositol Phosphate Kinase Targets and Regulates Focal Adhesions. Nature 420, 89–93. doi:10.1038/nature01082
Loftus, J. C., O'toole, T. E., Plow, E. F., Glass, A., Frelinger, A. L., and Ginsberg, M. H. (1990). A β 3 Integrin Mutation Abolishes Ligand Binding and Alters Divalent Cation-dependent Conformation. Science 249, 915–918. doi:10.1126/science.2392682
Lozano, M. L., Cook, A., Bastida, J. M., Paul, D. S., Iruin, G., Cid, A. R., et al. (2016). Novel Mutations in RASGRP2, Which Encodes CalDAG-GEFI, Abrogate Rap1 Activation, Causing Platelet Dysfunction. Blood 128, 1282–1289. doi:10.1182/blood-2015-11-683102
Lu, X., Lu, D., Scully, M., and Kakkar, V. (2008). The Role of Integrin-Mediated Cell Adhesion in Atherosclerosis: Pathophysiology and Clinical Opportunities. Cpd 14, 2140–2158. doi:10.2174/138161208785740199
Luo, B.-H., Carman, C. V., and Springer, T. A. (2007). Structural Basis of Integrin Regulation and Signaling. Annu. Rev. Immunol. 25, 619–647. doi:10.1146/annurev.immunol.25.022106.141618
Luo, B.-H., Springer, T. A., and Takagi, J. (2004). A Specific Interface between Integrin Transmembrane Helices and Affinity for Ligand. PLoS Biol. 2, e153. doi:10.1371/journal.pbio.0020153
Manevich-Mendelson, E., Grabovsky, V., Feigelson, S. W., Cinamon, G., Gore, Y., Goverse, G., et al. (2010). Talin1 Is Required for Integrin-dependent B Lymphocyte Homing to Lymph Nodes and the Bone Marrow but Not for Follicular B-Cell Maturation in the Spleen. Blood 116, 5907–5918. doi:10.1182/blood-2010-06-293506
Monkley, S. J., Zhou, X.-H., Kinston, S. J., Giblett, S. M., Hemmings, L., Priddle, H., et al. (2000). Disruption of Thetalin Gene Arrests Mouse Development at the Gastrulation Stage. Dev. Dyn. 219, 560–574. doi:10.1002/1097-0177(2000)9999:9999<::aid-dvdy1079>3.0.co;2-y
Moser, M., Bauer, M., Schmid, S., Ruppert, R., Schmidt, S., Sixt, M., et al. (2009). Kindlin-3 Is Required for β2 Integrin-Mediated Leukocyte Adhesion to Endothelial Cells. Nat. Med. 15, 300–305. doi:10.1038/nm.1921
Moser, M., Nieswandt, B., Ussar, S., Pozgajova, M., and Fässler, R. (2008). Kindlin-3 Is Essential for Integrin Activation and Platelet Aggregation. Nat. Med. 14, 325–330. doi:10.1038/nm1722
Mould, A. P., Barton, S. J., Askari, J. A., Mcewan, P. A., Buckley, P. A., Craig, S. E., et al. (2003). Conformational Changes in the Integrin βA Domain Provide a Mechanism for Signal Transduction via Hybrid Domain Movement. J. Biol. Chem. 278, 17028–17035. doi:10.1074/jbc.m213139200
Nieswandt, B., Moser, M., Pleines, I., Varga-Szabo, D., Monkley, S., Critchley, D., et al. (2007). Loss of Talin1 in Platelets Abrogates Integrin Activation, Platelet Aggregation, and Thrombus Formation In Vitro and In Vivo. J. Exp. Med. 204, 3113–3118. doi:10.1084/jem.20071827
Partridge, A. W., Liu, S., Kim, S., Bowie, J. U., and Ginsberg, M. H. (2005). Transmembrane Domain Helix Packing Stabilizes Integrin αIIbβ3 in the Low Affinity State. J. Biol. Chem. 280, 7294–7300. doi:10.1074/jbc.m412701200
Petrich, B. G., Fogelstrand, P., Partridge, A. W., Yousefi, N., Ablooglu, A. J., Shattil, S. J., et al. (2007a). The Antithrombotic Potential of Selective Blockade of Talin-dependent Integrin αIIbβ3 (Platelet GPIIb-IIIa) Activation. J. Clin. Invest. 117, 2250–2259. doi:10.1172/jci31024
Petrich, B. G., Marchese, P., Ruggeri, Z. M., Spiess, S., Weichert, R. A. M., Ye, F., et al. (2007b). Talin Is Required for Integrin-Mediated Platelet Function in Hemostasis and Thrombosis. J. Exp. Med. 204, 3103–3111. doi:10.1084/jem.20071800
Plak, K., Pots, H., Van Haastert, P. J. M., and Kortholt, A. (2016). Direct Interaction between TalinB and Rap1 Is Necessary for Adhesion of Dictyostelium Cells. BMC Cell Biol. 17, 1. doi:10.1186/s12860-015-0078-0
Schwartz, M. A., Schaller, M. D., and Ginsberg, M. H. (1995). Integrins: Emerging Paradigms of Signal Transduction. Annu. Rev. Cell Dev. Biol. 11, 549–599. doi:10.1146/annurev.cb.11.110195.003001
Shattil, S. J., Kim, C., and Ginsberg, M. H. (2010). The Final Steps of Integrin Activation: the End Game. Nat. Rev. Mol. Cell Biol. 11, 288–300. doi:10.1038/nrm2871
Simonson, W. T. N., Franco, S. J., and Huttenlocher, A. (2006). Talin1 Regulates TCR-Mediated LFA-1 Function. J. Immunol. 177, 7707–7714. doi:10.4049/jimmunol.177.11.7707
Smith, P. D., Ochsenbauer-Jambor, C., and Smythies, L. E. (2005). Intestinal Macrophages: Unique Effector Cells of the Innate Immune System. Immunol. Rev. 206, 149–159. doi:10.1111/j.0105-2896.2005.00288.x
Springer, T. A., and Dustin, M. L. (2012). Integrin Inside-Out Signaling and the Immunological Synapse. Curr. Opin. Cell Biol. 24, 107–115. doi:10.1016/j.ceb.2011.10.004
Stefanini, L., Lee, R. H., Paul, D. S., O'shaughnessy, E. C., Ghalloussi, D., Jones, C. I., et al. (2018). Functional Redundancy between RAP1 Isoforms in Murine Platelet Production and Function. Blood 132 (18), 1951–1962. doi:10.1182/blood-2018-03-838714
Stritt, S., Wolf, K., Lorenz, V., Vögtle, T., Gupta, S., Bösl, M. R., et al. (2015). Rap1-GTP-interacting Adaptor Molecule (RIAM) Is Dispensable for Platelet Integrin Activation and Function in Mice. Blood 125, 219–222. doi:10.1182/blood-2014-08-597542
Su, W., Wynne, J., Pinheiro, E. M., Strazza, M., Mor, A., Montenont, E., et al. (2015). Rap1 and its Effector RIAM Are Required for Lymphocyte Trafficking. Blood 126, 2695–2703. doi:10.1182/blood-2015-05-644104
Sun, H., Lagarrigue, F., Wang, H., Fan, Z., Lopez-Ramirez, M. A., Chang, J. T., et al. (2021). Distinct Integrin Activation Pathways for Effector and Regulatory T Cell Trafficking and Function. J. Exp. Med. 218, e20201524. doi:10.1084/jem.20201524
Sun, H., Fan, Z., Gingras, A. R., Lopez‐Ramirez, M. A., Ginsberg, M. H., and Ley, K. (2020). Frontline Science: A Flexible Kink in the Transmembrane Domain Impairs β2 Integrin Extension and Cell Arrest from Rolling. J. Leukoc. Biol. 107, 175–183. doi:10.1002/jlb.1hi0219-073rr
Sun, H., Lagarrigue, F., Gingras, A. R., Fan, Z., Ley, K., and Ginsberg, M. H. (2018). Transmission of Integrin Beta7 Transmembrane Domain Topology Enables Gut Lymphoid Tissue Development. J. Cell Biol. 217 (4), 1453–1465. doi:10.1083/jcb.201707055
Sun, H., Liu, J., Zheng, Y., Pan, Y., Zhang, K., and Chen, J. (2014). Distinct Chemokine Signaling Regulates Integrin Ligand Specificity to Dictate Tissue-specific Lymphocyte Homing. Dev. Cell 30, 61–70. doi:10.1016/j.devcel.2014.05.002
Tadokoro, S., Shattil, S. J., Eto, K., Tai, V., Liddington, R. C., De Pereda, J. M., et al. (2003). Talin Binding to Integrin SS Tails: A Final Common Step in Integrin Activation. Science 302, 103–106. doi:10.1126/science.1086652
Takagi, J., Petre, B. M., Walz, T., and Springer, T. A. (2002). Global Conformational Rearrangements in Integrin Extracellular Domains in Outside-In and Inside-Out Signaling. Cell 110, 599–611. doi:10.1016/s0092-8674(02)00935-2
Torres-Gomez, A., Sanchez-Trincado, J. L., Toribio, V., Torres-Ruiz, R., Rodriguez-Perales, S., Yanez-Mo, M., et al. (2020). RIAM-VASP Module Relays Integrin Complement Receptors in Outside-In Signaling Driving Particle Engulfment. Cells 9, 1166. doi:10.3390/cells9051166
Tu, Y., Wu, S., Shi, X., Chen, K., and Wu, C. (2003). Migfilin and Mig-2 Link Focal Adhesions to Filamin and the Actin Cytoskeleton and Function in Cell Shape Modulation. Cell 113, 37–47. doi:10.1016/s0092-8674(03)00163-6
Vestweber, D. (2015). How Leukocytes Cross the Vascular Endothelium. Nat. Rev. Immunol. 15, 692–704. doi:10.1038/nri3908
Watanabe, N., Bodin, L., Pandey, M., Krause, M., Coughlin, S., Boussiotis, V. A., et al. (2008). Mechanisms and Consequences of Agonist-Induced Talin Recruitment to Platelet Integrin αIIbβ3. J. Cell Biol. 181, 1211–1222. doi:10.1083/jcb.200803094
Wegener, K. L., Partridge, A. W., Han, J., Pickford, A. R., Liddington, R. C., Ginsberg, M. H., et al. (2007). Structural Basis of Integrin Activation by Talin. Cell 128, 171–182. doi:10.1016/j.cell.2006.10.048
Xiong, J.-P., Stehle, T., Diefenbach, B., Zhang, R., Dunker, R., Scott, D. L., et al. (2001). Crystal Structure of the Extracellular Segment of Integrin αVβ3. Science 294, 339–345. doi:10.1126/science.1064535
Ye, F., Hu, G., Taylor, D., Ratnikov, B., Bobkov, A. A., Mclean, M. A., et al. (2010). Recreation of the Terminal Events in Physiological Integrin Activation. J. Cell Biol. 188, 157–173. doi:10.1083/jcb.200908045
Ye, F., Petrich, B. G., Anekal, P., Lefort, C. T., Kasirer-Friede, A., Shattil, S. J., et al. (2013). The Mechanism of Kindlin-Mediated Activation of Integrin αIIbβ3. Curr. Biol. 23, 2288–2295. doi:10.1016/j.cub.2013.09.050
Zeiler, M., Moser, M., and Mann, M. (2014). Copy Number Analysis of the Murine Platelet Proteome Spanning the Complete Abundance Range. Mol. Cell. Proteomics 13, 3435–3445. doi:10.1074/mcp.m114.038513
Keywords: integrin activation, cell adhesion, cell migration, signaling, blood cells
Citation: Sun H, Lagarrigue F and Ginsberg MH (2022) The Connection Between Rap1 and Talin1 in the Activation of Integrins in Blood Cells. Front. Cell Dev. Biol. 10:908622. doi: 10.3389/fcell.2022.908622
Received: 30 March 2022; Accepted: 25 April 2022;
Published: 01 June 2022.
Edited by:
Ben Goult, University of Kent, United KingdomReviewed by:
Esther M. Lafuente, Complutense University of Madrid, SpainLucia Stefanini, Sapienza University of Rome, Italy
Copyright © 2022 Sun, Lagarrigue and Ginsberg. This is an open-access article distributed under the terms of the Creative Commons Attribution License (CC BY). The use, distribution or reproduction in other forums is permitted, provided the original author(s) and the copyright owner(s) are credited and that the original publication in this journal is cited, in accordance with accepted academic practice. No use, distribution or reproduction is permitted which does not comply with these terms.
*Correspondence: Hao Sun, aGFzMDczQHVjc2QuZWR1