- 1Research Department, Mediterranean Institute for Transplantation and Advanced Specialized Therapies (IRCCS ISMETT), Palermo, Italy
- 2Ri.MED Foundation, Palermo, Italy
- 3Department of Pharmacy, School of Medicine, University of Naples Federico II, Naples, Italy
Cancer immunotherapy has led to impressive advances in cancer treatment. Unfortunately, in a high percentage of patients is difficult to consistently restore immune responses to eradicate established tumors. It is well accepted that adaptive immune cells, such as B lymphocytes, CD4+ helper T lymphocytes, and CD8+ cytotoxic T-lymphocytes (CTLs), are the most effective cells able to eliminate tumors. However, it has been recently reported that innate immune cells, including natural killer cells (NK), dendritic cells (DC), macrophages, myeloid-derived suppressor cells (MDSCs), and innate lymphoid cells (ILCs), represent important contributors to modulating the tumor microenvironment and shaping the adaptive tumor response. In fact, their role as a bridge to adaptive immunity, make them an attractive therapeutic target for cancer treatment. Here, we provide a comprehensive overview of the pleiotropic role of tissue-resident innate immune cells in different tumor contexts. In addition, we discuss how current and future therapeutic approaches targeting innate immune cells sustain the adaptive immune system in order to improve the efficacy of current tumor immunotherapies.
1 Introduction
Cancer is considered a major public health concern worldwide and is characterized by an uncontrolled division of altered cells. The human immune system recognizes tumor cells and induces a protective response to eliminate those cells. However, sustained tumors may protect themselves by developing immune escape mechanisms through multiple soluble and cellular mediators. In the last decades, the deep knowledge of tumorigenesis and the study of the complex interaction between the host and the immune system has been the goal for significant advances in anticancer therapy. Conventional anticancer therapy, such as surgical resection, radiotherapy, and cytotoxic drugs, involves multiple targeting of tumor cells. Though, the tumor tissue microenvironment can present a dysregulated, or weakened immune response which, in turn, uncovers pro-tumor activities favouring tumor expansion and progression (Dhara et al., 2022). Recently, new potential targets have been identified based on immunomodulatory therapies, with the aim to re-establish the host anti-tumoral immune response. Since the effect of cancer immunotherapy is largely dependent on the status of the immune system in the tumor microenvironment, the choice of therapy and the development of new therapies based on the immune status in the tumor microenvironment would be predicted to be effective (Sambi et al., 2019). Tissue-resident innate immune cells could be found in different human tissues, performing a strategic role at all stages of the immune response, from maintaining homeostasis to responding to infectious challenges to the resolution of inflammation to tissue repair and finally, to initiating antitumor response (Goff and Danforth, 2021). In humans studying immune cells and responses in tissues is challenging, due to the difficult accessibility of tissue-resident innate immune cells, the biggest pieces of knowledge concerning their responses in tissues have been obtained using murine models or studying immune cells drawn from blood (Segura et al., 2010; Gray and Farber, 2022). In recent years thanks to new knowledge obtained from these studies it has emerged that not only adaptive immune cells are the only effective cells able to eliminate tumors, but also innate cells are able to do it. Indeed, it emerged that natural killer cells (NK), dendritic cells (DC), macrophages, Myeloid-derived suppressor cells (MDSC) and innate lymphoid cells (ILCs), represent important contributors to modulating the tumor microenvironment and shaping the adaptive tumor response (Wang et al., 2019b). This review provides an overview of the different types of tissue-resident innate immune cells involved in the suppressor activity of anti-tumor immunity. The deep knowledge of the mechanisms underlying these processes could significantly improve the clinical utility of tissue-resident innate immune cells in cancer and eventually can support the identification of biomarkers for cancer prognosis and the development of novel therapeutic approaches for cancer treatment.
1.1 Tissue-Resident Dendritic Cells in Tumor Immunity
Dendritic cells (DCs) represent a heterogeneous family of immune cells, consisting of various subgroups of specialized antigen-presenting cells, mainly involved in initiating and regulating innate and adaptive immune responses (Wculek et al., 2020). Together with macrophages and B cells, they are considered the three major professional antigen-presenting cells (APCs). DCs play a critical role in promoting immunity by providing immunomodulatory signals, such as the secretion of cytokines and growth factors, but can also promote tolerance by presenting antigens to T cells (Patente et al., 2018; Wculek et al., 2020). They are a sort of sentinel able to collect a broad spectrum of environmental signals or stimuli such as bacterial and viral PAMPs and/or DAMPs, processing an extensive spectrum of specific tissue responses and influencing the immunological outcome, one of the most important, promoting T cell-mediated immunity (Levings et al., 2005; Steinman, 2006). DCs originate in bone marrow from unique precursor CD34+ that can differentiate into myeloid (MP) and lymphoid (LP) precursors. The first type of precursor gives rise to monocytes and DC precursors (MDP), which are further differentiated in common DC precursors (CDP), from which finally arise preclassical DC (pre-cDC) and plasmacytoid DC (pDC). In the last step of differentiation, pre-cDC will give rise to the most represented cDC subpopulations, named cDC1 and cDC2. Regarding the second differentiation way, or else LP, the ontogenic pathway is not completely elucidated, so nowadays we only know that it can give rise to pDC (Geissmann et al., 2010). DCs can be found in practically all tissue, they are a very plastic and dynamic cell population that can change its phenotype based on the tissue microenvironment in which is located. DCs represent the link between innate and adaptive immune responses, without inflammatory stimuli they are in an immature or tolerogenic state contributing to immune tolerance. Immature DCs express low levels of costimulatory molecules such as CD40, CD80, and CD86, besides they can infiltrate the tumor micro-environment performing a preponderant role in beginning antitumor immune response (Ganguly et al., 2013). The biggest knowledge concerning DC subpopulations have been obtained from the studies on murine DCs, mostly due to the wide range of tissue accessibility; unfortunately, the same type of characterization cannot be done for human DCs (Segura et al., 2010). Indeed, almost all studies on human DCs were performed mainly on peripheral blood where, among others, DCs constitute a rare cell population. Initially, DCs have been simply divided according to the cell localization, dividing them into resident lymphoid tissue DCs and migratory non-lymphoid tissue DCs (Haniffa et al., 2013). Nowadays, the development of new technologies, especially single-cell RNAseq, allowed to bring light new characteristics of this very heterogeneous cell population providing more information usable for classification criteria, including phenotypical, functional, and developmental criteria (Heath and Carbone, 2013).
Currently, DCs are divided into at least four wide groups using either functional or phenotypical characteristics. From a phenotypical point of view, all human DCs show a high expression of major histocompatibility complex (MHC) class II molecules (MHC-II) and of CD11c, which are expressed also on other cells, and many other molecules which allow their classification into various subtypes. Conversely, they lack key markers of T cells, B cells, natural killer (NK) cells, granulocytes, and monocytes. DCs subset can be classified into Conventional DC Type 1 (cDC1), Conventional DC Type 2, Plasmacytoid DC (pDC), and Monocyte-derived DCs (moDC), and each subset plays a different role within tumors and during their therapy (Kim et al., 2021).
The cDC1 are characterized by the presence of specific markers surface including thrombin receptor THRM (CD141), the chemokine receptor XCR1, C-type lectin CLEC9A and the cell adhesion molecule CADM1 (homologous of CD8α/CD103/XCR1 in mice) (Reynolds and Haniffa, 2015). The two mainly transcription factors involved in their generation are BATF3, a basic leucine Zipper ATF-Like Transcription Factor 3 and the IFN-regulatory factor 8 (IRF8). cDC1 are localized especially in peripheral blood and in lymphoid and non-lymphoid tissue, where they are specialized in cross-presentation, realizing the priming of CD8+ T cells against extracellular antigens such as bacteria and viruses (Haniffa et al., 2012). Recently, using different tumor murine models emerged that cDC1 also play a critical role in the induction of the cancer-immune cycle, exercised through the transport of antigens from tumor towards draining lymph nodes, inducing a robust activation/proliferation of CD8+ T cells or transfer of antigen to resident myeloid cells (Roberts et al., 2016; Salmon et al., 2016; Gardner et al., 2020). The antitumor immune responses mediated by cDC1s are critical in the mechanism of tumor rejection and responses to immunotherapies, like the immune-checkpoint blockade and adoptive T cell therapy (Kim et al., 2021). In addition, the presence of cDC1 within human melanoma tumors correlated with improved response to anti-PD-1 therapy (Barry et al., 2018) as well as with higher CD8+ T cell infiltration into tumors (Binnewies et al., 2019) which is associated with a positive prognosis across multiple tumor types (Fridman et al., 2012). In addition to PD-1/PD-L1 expression, it was also observed clustered expression of TIM-3 on cDC cells within tumors, particularly CD103+ cDCs. de Mingo Pulido et al. (2018), have shown that the use of αTIM-3 antibody-induced an increase in cell death within tumors and an improvement in response to chemotherapy, suggesting a key role of TIM-3 as a target for therapy. Recently, Roberts et al. (2016) have shown that anti-tumour activity of migratory cDC1s subtype can be done through the expression of chemokine receptor CCR7; in fact, in mice with cDC1 defective for CCR7, it was observed a loss priming of T cell in lymph nodes area and a lack of antigen hand-off to resident myeloid cells, which led to a failure of immune control with consequential increased tumor growth. Moreover, an analysis of tumour-infiltrating cell populations, isolated by human melanoma biopsies, showed that only CD141+ DC expressed a detectable CCR7 on the surface, demonstrating that CCR7 is particularly prominent on CD141+ DC in human tumors. In addition, it has been demonstrated that cross-presentation activity by cDC1 is improved by I interferon (IFN) signaling, and cells that lack IFNAR1 (IFN-α/β receptor 1) are unable to perform tumor-specific T cell priming and tumor elimination (Diamond et al., 2011; Fuertes et al., 2011).
The cDC2 are a heterogeneous subset of cells that co-express high levels of CD1c and SIRPα (CD172a) (homologous of CD11b and CD172a in mice) and a range of other markers that are tissue-specific (Shortman and Liu, 2002; Reynolds and Haniffa, 2015; Wculek et al., 2020). The transcriptional factors involved in cDC2 maturation and differentiation are mainly three, ID2 (Inhibitor of DNA binding 2), IRF8 (Interferon Regulatory Factor 8) and IRF4 (Interferon Regulatory Factor 4), which seems to have a preponderant role in CD8(+) dendritic cell differentiation (Rees et al., 1990; Li et al., 2016; Wculek et al., 2020). Using single-cell RNA-seq analysis, Villani and collaborators described two novel cDC1 subpopulations, namely cDC2 and cDC3 that show both the expression of CD11c+ but diverged for the expression of other molecular markers, including CD163/CD36 and CD32B (Villani et al., 2017). cDC2 are the dominant DC subset in blood but they are also localized in lymphoid and non-lymphoid tissue, which are involved in the induction of Th1, Th2, and Th17 responses (Haniffa et al., 2013; Haniffa et al., 2012; Segura et al., 2012). Moreover, recent studies in the literature suggest that cDC2 may be involved in presenting tumor-derived antigens to CD4+ T cells, which assist and support CD8+ T cells in their antitumor activity. Despite their role in tumorigenesis is still not well known, it emerged that they are effective stimulators of naïve T cell proliferation, required to mount an anti-tumor response (Villani et al., 2017). Besides, Binnewies and collaborators observed, in a murine model of melanoma, that the depletion of regulatory T cells into the tumoral site induced a cDC2 increase activity in eliciting intra-tumoral CD4+ T cell responses with subsequent tumor growth control (Villani et al., 2017). Similarly to the mouse model, it was observed an increase of CD4+ T in patients that show cDC2 abundance to the detriment of Treg suggests that the combination of high levels of cDC2 and low levels of Treg correlate with better tumor prognosis and with clinical responsiveness to immunotherapy (including anti-PD-1 therapy), though the increase of the levels of CD4+ T cell infiltration (Quezada et al., 2006; Balachandran et al., 2011; Wallin et al., 2016). It will be interesting to understand how the Treg may control cDC2 function and influence the anti-tumor CD4+ T cells response, both in melanoma and in other tumors (Sato et al., 2005; Binnewies et al., 2019).
Plasmacytoid DC (pDC) are characterized by the absence of CD11c and the expression of CD123 (IL-3R), CD303 (CLEC4C), and CD304 (neuropilin) markers (homologues to CD11cint CD11b− B220+ SiglecH+ CD317+ in mouse) (Dzionek et al., 2000; Collin et al., 2013). They arise in two different ways, directly from LP precursors and indirectly by CDP precursors, through the MP precursor’s line. The transcriptional factors that are essential for pDC development belong to the family of E2.2 expressed in both humans and mice (Liu, 2005; Cisse et al., 2008; Cheng et al., 2015). pDCs are a subset of DC specialized in response to viral RNA and DNA infection thus, for this reason, they express very high levels of TLR7 and TLR9, the two toll-like receptors specialized in signal transduction of viral and self-nucleic acids (Lande et al., 2007; Gilliet et al., 2008). The ligation of viral antigens to their TLR7 and TLR9 induces a very strong release of type I interferon (IFN-I) together with other inflammatory cytokines, including IL6 and TNFα (Patente et al., 2018). The role of pDCs in human tumors is less known compared to the other DCs subset and the data in the literature sometimes results controversial. The state of the art of pDCs asserts that they have an inert role in anti-tumor immune responses, but it also emerged that most cancers, including breast cancer (Sisirak et al., 2012), melanoma (Gerlini et al., 2007), and ovarian carcinoma (Labidi-Galy et al., 2011) are highly infiltrated by pDCs (Le Mercier et al., 2013; Kranz et al., 2016). Le Mercier et al. (2013) reported that pDCs infiltrating human primary tumors, represent an important prognostic factor associated with poor outcomes (Treilleux et al., 2004). Other studies reported that pDCs are able to limit tumoral progression, probably through IFN-α secretion (Kranz et al., 2016). Additionally, in tumor sites, it has been identified tumor-associated pDCs (TApDC) that, compared to normal pDCS, express a partially mature phenotype and an altered IFN-α, ΤΝF-α, and IL-6 production, able to induce an increase in Treg expansion, are associated with tumor progression with a poor overall prognosis (Hartmann et al., 2003; Treilleux et al., 2004; Labidi-Galy et al., 2011). Conversely, it was observed, in vivo, that an intra-tumoral injection of a TLR7 ligand led to TApDC activation displaying a potent curative effect, suggesting that TApDC could become an efficient therapeutic target (Kranz et al., 2016). Thus, several therapeutic protocols have been developed in cancers to stimulate pDC production of IFN-α. Among these, Imiquimod, a TLR7 agonist, has notably been used in cancer therapy because of its antitumoral action associated with the activation of NF-κB, which leads to the induction of proinflammatory cytokines such as IFN-α (Schon and Schon, 2008).
Monocyte-derived DCs (moDC) are a particular subset of DCs that differentiate separately from CD14hi monocytes in humans (homologous to Ly6C+ in mice), in response to inflammation conditions (Jordan et al., 2020; Wculek et al., 2020). The knowledge acquired in the last years on human DCs was obtained using differentiated monocytes by in vitro culture with GM-CSF and IL4 (Sallusto and Lanzavecchia, 2018). The main surface marker of MoDCs overlaps with those expressed in macrophages, cDC2s and other immune cells, including CD1c, CD11b, CD14, CD209, CD172a CD1a, and CCR2 (Guilliams et al., 2014; Wculek et al., 2020). Recently, the evaluation of the Fc receptors FcγRI and FcεRI expression on moDCs allowed a better distinction between subsets, as moDCs express high levels of activating Fc receptors for IgG (FcγRs). Even if the physiological relevance of MoDC is unclear, it was noted that moDCs are produced in response to inflammation-inducing IFN-γ by CD4+ T cells promoting Th1 immune response. Phenotypical and functional alterations in moDCs have been identified in patients with different types of cancer, including breast cancer (Ramos et al., 2012) chronic lymphocytic leukemia (Toniolo et al., 2016), chronic myeloid leukemia (Brown et al., 2014), colorectal cancer (Orsini et al., 2013) and cervical neoplasia (Lopes et al., 2017). The most observed phenotypical alteration was reduced levels of specific markers involved in antigen presentation and lymphocytes activation, including HLA-DR, CD80, CD86, and CD83. Besides, phenotypical alterations were related to loss of function in inducing proliferation of both CD4+ and CD8+ T cells (Toniolo et al., 2016). The peculiarity of the mo-DCs to present the antigens in both MHC class I and class II molecules have been extensively used in the clinic, mostly as vaccines to induce anti-tumor immune responses in cancer patients. In the last decade, the use of DCs is considered a hopeful adjuvant for inducing immunity to cancer and their manipulation could represent a great potential for cancer immunotherapy (Thurner et al., 1999). A series of clinical trials on cancer therapy aimed to promote DCs activation, and consequently T cell priming against tumor antigen through the administration of specific cytokines and or adjuvant, such as FLT3L, GM-CSF and/or agents blocking a series of soluble factors released by cancer cells or specific signaling pathways that contrast DCs maturation (Saito et al., 2008; Merad et al., 2013; Johnson et al., 2018; Kerdidani et al., 2019).
1.2 Tissue-Resident Macrophages in Tumor Immunity
Tissue resident macrophages (TRMs) represent an important cell component of the innate immune system, with a wide distribution in every tissue throughout the body (Epelman et al., 2014b). Despite macrophage’s origin being thought to be derived from circulating blood monocytes infiltrating the tissue and differentiating into macrophages, recent literature has demonstrated that macrophage ontology is not that simple (Locati et al., 2020). Several recent pieces of evidence showed that TRMs can have embryonic progenitors, such as liver resident Kupffer cells (KCs), lung alveolar, microglia, splenic, and peritoneal macrophages (Hashimoto et al., 2013; Yona et al., 2013). Interestingly, these cells are fully differentiated before birth and self-renew in a monocytes-independent manner. However, some classes of TRMs, such as adult cardiac and skeletal muscle, derive from yolk-sac, and foetal monocytes progenitors, thus they can be substituted by blood monocytes (Epelman et al., 2014a; Wang et al., 2020). TRMs have a key role in innate immunity, as they represent the first line of defence that our body put in place upon infection with pathogens or microbes. In addition, they function by presenting antigens to T cells thus stimulating T cell response in a different types of disease conditions (Greenhalgh et al., 2018). Furthermore, macrophages maintain tissue homeostasis, by specifically contributing to the clearance of cellular debris (Herzog et al., 2019), tissue repair and remodelling (Bosurgi et al., 2017). In order to exert their functions, macrophages get activated by different stimuli coming from the tissue microenvironment in vivo and in vitro by a specific cocktail of cytokines. For a better comprehension of macrophages phenotype, Mills and collaborators classified them as classically activated TRMs (M1) and alternatively activated ones (M2) (Mills et al., 2000). While M1 macrophages release proinflammatory cytokines (TNF-α, IL-1β, IL-6, among others) and produce reactive oxygen species (ROS) to promote inflammation and defend against external pathogens, M2 ones stimulate the secretion of IL-10 and TGF-β to inhibit inflammation and to promote tissue repair and angiogenesis. However, a defined difference between these two classes of macrophages cannot be done, as their polarization can be switched according to the tissue conditions, whether it is in a steady-state or a pathological state. Thus, despite the efforts recently done to characterize M1 and M2 macrophages, a real definition of how TRMs work in physiological conditions is far from being achieved. Given their role within organs, TRMs have an important role during tumorigenesis, as they interact directly with tumor cells during progression and metastasis. Together with monocytes-derived macrophages, they represent the most abundant cells within a tumor, representing an important component of the tumor microenvironment (TME). It has been proposed that almost 50% of the tumor mass is generally represented by tumour-associated macrophages (TAMs) (Poh and Ernst, 2018). Thus, TAMs can have then different origins, although they all exert similar functions even if with different molecular mechanisms. For instance, it has been shown in transgenic mouse models of lung adenocarcinoma that while monocyte-derived macrophages stimulate tumor dissemination, TRMs are sufficient to induce tumor growth (Loyher et al., 2018). Similar results were obtained in TRMs-depleted transgenic mouse model (Csf1op/op), showing that alveolar macrophages depletion did not affect the dissemination of mammary tumor cells (Qian et al., 2009). However, this is not the case of pancreatic ductal adenocarcinoma (PDAC), where the pro-tumoral role of TRMs and monocyte-derived macrophages seems to be the exact opposite (Zhu et al., 2017c). These observations clearly suggest that the contribution of each macrophage population to tumor progression is strictly correlated with the tissue they reside. On the same lines of evidence, even the response to chemotherapy is different between TRMs and monocyte-derived macrophages, as it might change according to the type of tumor. Indeed, Loyher et al. (2018) elegantly demonstrated that monocyte-derived macrophages recovered faster from cyclophosphamide treatment when compared with TRMs in a transgenic mouse model of lung cancer. TAMs, either monocyte-derived macrophages or TRMs, are associated with tumor progression and poor prognosis, because of their role in controlling tumor survival and resistance to conventional therapies. In particular, different studies support the hypothesis that tumor-promoting macrophages have a M2-like phenotype (Mantovani et al., 2002; Boutilier and Elsawa, 2021), while the M1-like one is associated with anti-tumor properties (Noy and Pollard, 2014; Ubil et al., 2018; Wanderley et al., 2018). However, as discussed above for macrophages during homeostasis, macrophage polarization is influenced by cues and stimuli by the microenvironment (Sica and Mantovani, 2012; Najafi et al., 2019; Gunassekaran et al., 2021). For instance, different studies showed that TGF-β increased in hepatocellular carcinomas the expression of TIM-3, an immune checkpoint blockade inhibitor, thus leading to 1) M1 to M2 macrophages polarization, and 2) increased tumor progression and metastatization (Yan et al., 2015). This is a very important aspect, as the induction of an M1-like polarization could be an important therapeutic strategy to generate tumor-suppressive macrophages. TAMs have a pivotal role in defining therapeutic efficiency (Zitvogel et al., 2008; Hughes et al., 2015). For instance, they inhibit T cell response via inhibition of cytotoxic CD8+ T cells, either by expressing inhibitory immune checkpoint molecules (PD-L1 and PD-L2), blocking antigen presentation or by the secretion of immunosuppressive proteins, such as IL-10, TGF-β, and prostaglandin E2 (Blumenthal et al., 2001; Munn and Mellor, 2003; Matlack et al., 2006). In addition, they modulate T cell exclusion from the tumor by the activation of MMPs and cathepsins, and by stimulation of fibrotic mechanisms (Nielsen et al., 2016; Zhu et al., 2017c; Quaranta et al., 2018). Interestingly, it has been shown that when TAMs are depleted, cytotoxic CD8+ T cells increased their presence in the tumor context, thus improving the therapeutic outcome of the treatments (Denardo et al., 2009; Andreu et al., 2010). Along with inhibition of T cell response, TAMs are also responsible for chemotherapy and radiotherapy resistance and tumor relapse. Recent works demonstrated that standard care of treatment for several tumors determine a release of bioactive factors, such as VCAM1 and CCL2, that are involved in the increased macrophages infiltration in the tumor microenvironment (Kalbasi et al., 2017; Takahashi et al., 2020). In addition, in vivo studies on the prostate cancer model clearly showed that macrophages depletion further improve the docetaxel chemotherapy response by reducing tumor progression (Guan et al., 2019). Furthermore, macrophages induce resistance to chemotherapy by suppressing cancer cell apoptosis via release of soluble factors (colon and ovarian cancer model) (Feng et al., 2011; Au Yeung et al., 2016), or by exosomal delivery of miRNA-21 (gastric cancer cells) (Zheng et al., 2017). Finally, TAMs reduce immune checkpoint blockade therapy (ICB) via the expression of inhibitory immune checkpoint molecules (PD-L1, PD-L2, and TIM-3), thus blocking T cell response (Thommen et al., 2015; Anfray et al., 2019). For all these reasons, TAMs have been considered an important target in tumor immunity, although preclinical studies, as well as clinical trials, did not define in the past a proper clear-cut on possible therapies aiming at TAMs eradication. Thus, different approaches are currently understudies to target macrophages for anti-cancer therapies. Among others, therapies to deplete macrophages, inhibit monocyte-derived macrophages recruitment, and stimulate TAMs repolarization towards an M1 phenotype are currently under investigation in a preclinical stage, as well as in clinical trials. Macrophages depletion via CSF-1R blockade as monotherapy to affect tumor growth, despite the encouraging preliminary data in preclinical studies (Strachan et al., 2013; Quail et al., 2016), did not provide substantial benefits for the treatment of established solid tumors (O'Brien et al., 2021). On the contrary, a combination of tumor resection followed by macrophage depletion did provide a valuable reduction of melanoma recurrence and metastasis (Tham et al., 2015). Thus, combined therapies, along with the identification of the right timing of the treatment itself, might be the path to contrast tumor progression. Different approaches have been described to deplete macrophages, either in normal or tumoral tissues. For instance, liposomes loaded with clodronate have been shown to reduce tumor growth in mouse models of mammary cancer. In addition, its combination with protein kinase inhibitors, such as sorafenib, was able to drastically diminish tumor angiogenesis and metastasis in a hepatocellular carcinoma model (Zhang et al., 2010). Interestingly, different groups successfully attempted to eradicate TAMs using trabectedin, a chemotherapic used for the treatment of ovarian cancer and sarcomas. Trabectedin acts by stimulating macrophages apoptosis via activation of TRAIL-R2, a death receptor specifically expressed in macrophages (Allavena et al., 2005; Germano et al., 2013; Gordon et al., 2016). Thus, trabectedin is currently under analysis in combined therapies in several clinical trials. TAMs depletion can be obtained also by inhibiting CSF1/CSF-1R signaling axis, via monoclonal antibodies or small molecule inhibitors. In addition, targeting macrophages’ surface receptors (CD52, CD206, FR-β, among others) is another approach that has been recently taken for the same purpose. In all cases, these attempts result quite encouraging in the preclinical set, and some of them are now in clinical trials in combined chemotherapies for the treatment of lymphomas and chronic lymphocytic leukemia (NCT00069238, NCT01361711, and NCT01030900). Blocking monocyte-derived macrophages recruitment in the tumor microenvironment is another approach that has been tried to target TAMs. This type of therapy mostly relies on monoclonal antibodies aiming at the inhibition of the interaction between monocyte chemokines and their specific receptors. The most studied signaling axis in this context has been the CCL2/CCR2, as CCR2 is highly expressed in tumors and have been shown to stimulate macrophages recruitment in the tumor microenvironment (Lim et al., 2016). Also, in this case though, while the pre-clinical studies were encouraging, the clinic ones did not provide important results for the treatment of some type of prostate cancers (Pienta et al., 2013). However, the combination with anti-PD-1 immune checkpoint blockade therapy demonstrated quite efficiency for metastasis inhibition of mouse models of bladder and lung cancer (Tu et al., 2020).
Finally, TAMs repolarization towards an M1-phenotype is another valid strategy that has been developed in recent years. Macrophage re-polarization has been obtained with different methods, including treatments with TLR agonists (van Dalen et al., 2018), CSF-1R (Pyonteck et al., 2013) and PI3Kγ (Kaneda et al., 2016) inhibition. TLR agonists, including LPS and several lipoproteins, determine the activation of the NFkB signaling pathway, thus stimulating the production of pro-inflammatory cytokines typical of the M1 phenotype. For instance, Poly:IC, a synthetic molecule mimicking viral dsRNA, binds to TLR3 and induces macrophages polarization and colon cancer arrest. However, TLR agonists result cytotoxic for use in anti-cancer therapies, thus stimulating alternative methods for their in-situ delivery. Interestingly, TLR7/8 agonists loaded into nanoparticles induced in vitro and in vivo polarization of M1-like macrophages in different models of solid tumors, including lung and colon adenocarcinoma (Rodell et al., 2018). CSF-1/CSF-1R axis inhibition, despite being firstly considered a valid strategy for TAM depletion, is now a well-accepted method to repolarize macrophages. An elegant work published in 2013 clearly demonstrated in a glioblastoma multiforme (GBM) tumor model that CSF-1 blockade did not eradicate TAMs, but instead “re-educate” them within the tumor microenvironment by decreasing M2 macrophage gene signature, and at the same time promoting overall survival in patients with GBM (Pyonteck et al., 2013). Furthermore, CSF-1 blockade was found to stimulate TAMs polarization and improved animal survival in mouse models of hepatocellular carcinoma and PDAC (Zhu et al., 2014; Ao et al., 2017). Finally, PI3Kγ selective small molecules inhibitors nicely demonstrated their effectiveness in polarizing TAMs in mouse models of PDAC, thus promoting CD8+ T cell infiltration and tumor arrest.
1.3 Tissue-Resident Myeloid-Derived Suppressor Cells in Tumor Immunity
Myeloid-derived suppressor cells (MDSCs) are a heterogeneous group of immature myeloid cells, derived from the bone marrow hematopoietic precursor cells, which constitute one of the main suppressive cell populations of the innate immune system (Veglia et al., 2018). In physiological conditions, immature myeloid cells differentiate into the different innate immune cells, such as macrophages, granulocytes, and dendritic cells, and migrate to the corresponding tissues, exerting their normal immune functions (Groth et al., 2019). In pathological conditions, such as infectious diseases, cancers or autoimmune disorders, deregulation on myeloid differentiation occurs, which, combined with a persistent stimulation of myelopoiesis, results in the expansion of MDSCs (Consonni et al., 2019; Lim et al., 2020). This deregulated generation and expansion of immunosuppressive MDSCs is promoted by a series of cytokines, such as GM-CSF, VEGF, IL-1β, IL-6, and IL-10 (Cheng et al., 2021). Significantly increased immature myeloid cells have been observed in the bone marrow and peripheral blood of patients with cancer (Cheng et al., 2021), and the presence of enriched MDSCs have been related to poor prognosis for multiple types of cancer (Jiang et al., 2015; Tian et al., 2015; De Cicco et al., 2020). In fact, throughout the entire pathological process that leads to tumor formation, MDSCs increase up to10-fold and migrate to the periphery, exerting their suppressor activity interfering with the normal functions of circulating T and other immune cells involved in the anti-tumor immunity (Safarzadeh et al., 2019; Nakamura and Smyth, 2020; Ma et al., 2022). Unlike mice’s MDSCs, where these cells have been well characterized, human MDSCs are less clearly defined. Typically, they are described as lineage cells that co-express high levels of CD33 and CD11b surface markers but lack HLA-DR. Human CD33+CD11b+HLA-DR− MDSCs can also be subdivided in three distinct populations of CD14+CD15− monocytic-MDSCs (M-MDSCs), CD15+CD66b+CD14− granulocytic-MDSCs (G-MDSCs) and CD14−CD15− early-MDSCs (E-MDSCs), which comprised more immature progenitors (Gabrilovich and Nagaraj, 2009; Mandruzzato et al., 2016; Ma et al., 2022) myeloid markers, such as PD-L1, CD40, CD49d, CD80, CD115, and CD124, which all mediate immunosuppression, has also been discovered to describe specific patterns of MDSCs (Gabrilovich and Nagaraj, 2009; Mandruzzato et al., 2016; Ma et al., 2022). Two functional proteins, such as CCAAT/enhancer-binding protein (c/EBPβ) and STAT3, which promote generation, differentiation, and expansion of MDSCs, despite are not surface markers, could help to define two different MDSCs subgroups (CD11b+HLA-DR−c/EBPβ+ and CD33+HLA-DR-STAT3+) and could provide new diagnostic and therapeutic tools for cancer immunotherapy (Lechner et al., 2011; Wu et al., 2011; Wang et al., 2019a). MDSCs have a key role in accelerating the progression of cancer, by producing a broad range of suppressive factors that prevent immune cells’ anti-tumor reactivity (Ma et al., 2022). The main mechanisms by which MDSCs act as immunosuppressive cells are oxidative stress, amino acid consumption, cytokines secretion, cell-cell interaction and exosomes release. MDSCs mediate immunosuppressive effects under oxidative stress, producing reactive oxygen species (ROS), nitric oxide (NO), and reactive nitrogen species (RNS). Increased ROS production induces damages on adaptive immune response by interfering with TCR CD3ζ expression, which acts on IFN-γ expression, hampering activation, viability and proliferation of T cells (Belikov et al., 2015; Ohl and Tenbrock, 2018; Cheng et al., 2021). MDSCs express nitrogen-oxygen synthase 2 (iNOS). The upregulated expression of iNOS leads to NO production that suppresses T cell’s function by inhibiting JAK3/STAT5 activation (Bingisser et al., 1998) and decreasing MHC class II expression (Harari and Liao, 2004). NO also induce T and NK cell apoptosis in tumor microenvironment through different mechanisms, such as impaired expression of the Bcl-2 family proteins, increased expression of the p53 tumor suppressor protein, damage of mitochondrial functions, DNA fragmentation, and activation of the caspase cascade (Umansky and Schirrmacher, 2001). Additionally, in tumor cell aggregation sites enriched with MDSCs, NO reacts with superoxide forming the RNS, namely peroxynitrite, a strong nitrifying agent. It can nitrate tyrosine residues in T cell receptor, inducing reduced IL-2 production with a consequent impaired T cell activation and proliferation (Bentz et al., 2000; Cobbs et al., 2003; Szabo et al., 2007; Gabrilovich et al., 2012; Cheng et al., 2021) (Feng et al., 2018). Besides, peroxynitrite can modify TCR conformational flexibility affecting its interaction with MHC class I molecules, causing the decreased response of cytotoxic CD8 T cells to antigen-specific stimulation (Nagaraj et al., 2007). Additionally, peroxynitrite prevents antitumor infiltration of antigen-specific CD8 T cells by nitration of CCL2 chemokine (Molon et al., 2011). The second MDSCs’ immunosuppressive mechanism consists in the exhaustion of some amino acids with a key role in T cell functioning. The high production of arginase-1 (Arg-1) by MDSCs causes L-arginine deficiency in the tumor microenvironment, which either provokes the cell cycle arrest to the G0–G1 phase of T cells (Rodriguez et al., 2007) and the downregulation of TCR expression, inducing T cell dysfunction and tumor escape in vivo (Rodriguez et al., 2002). Another essential amino acid for T cell activation is cysteine (Levring et al., 2015). Dendritic cells (DC) and macrophages can import extracellular cysteine and export it in the tumor microenvironment, making this amino acid available for T cell utilization. MDSCs competitively import extracellular cysteine, but, contrary to DC and macrophages, MDSCs are not able to export cysteine, due to the lacked expression of cysteine transporters, preventing T cell activation (Srivastava et al., 2010). The MDSCs overexpression of indoleamine 2, 3-dioxygenase (IDO), an enzyme that, metabolizing tryptophan, has been referred to support immunosuppressive properties of these cells. Tryptophan depletion causes T cell proliferation arrest (Srivastava et al., 2010) and antigen presentation impairment (Fallarino et al., 2006). Besides, highly IDO levels produced by MDSCs promotes the differentiation and expansion of T regulatory (Treg) cells, exacerbating the inhibition of anti-tumor T cells function (Curti et al., 2007). In response to tumor microenvironment, MDSCs acquire the ability to produce multiple immunosuppressive molecules, such as cytokines, chemokines, and growth factors (Lechner et al., 2010). The tumor microenvironment is characterized by high levels of IL-10 and MDSCs are the principal producers of this cytokine (Cheng et al., 2021) IL-10, in turn, strengthens the immunosuppressive ability of MDSCs in a vicious cycle, by upregulating the expression of different immunosuppressive molecules (Xiu et al., 2015; Lamichhane et al., 2017). IL-10 produced by MDSCs induce increased expression of lymphocyte activation gene 3 (LAG3) and the consequent decreased IL-2, IL-12, and IFNγ secretion by T cells, which hampered their proliferation and anti-tumor activity (Vuk-Pavlovic et al., 2010; Li et al., 2015). As IL-10, increased TGFβ production by MDSCs has been reported in various tumor types. TGFβ is a potent immune regulator cytokine that can inhibit proliferation, activation, differentiation, and cytotoxic activity of effector T cells (Cheng et al., 2021). This cytokine blocked Th1 differentiation and activation by silencing the expression of TBET and STAT4, which are key transcription factors for the formation of this important subset of anti-tumor T cells (Gorelik et al., 2002). Moreover, acting on Smad3 signaling, TGFβ could decrease IL-2 production (McKarns et al., 2004) and downregulated the expression of granzyme B and IFNγ (Zhang and Bevan, 2012). Highly production of IL-10 and TGFβ by MDSCs can also lead to the differentiation and expansion of Treg cells, by inducing FoxP3 and CD25 expression on naïve CD4 T cells (Fu et al., 2004; Heo et al., 2010). Besides, MDSCs can produce high levels of some chemokines, such as CCL3, CCL4, and CCL5, which drive CCR5-expressing Treg cells through the tumor microenvironment, supporting the tumor growth (Schlecker et al., 2012). MDSCs could also impair T cell trafficking in tumor-bearing hosts. ADAM17 (a disintegrin and metalloproteinase domain 17) expressed on MDSCs directly cleaves the ectodomain of L-Selectin on T cells to inhibit their homing to tumor sites and peripheral lymph nodes (Li et al., 2021). Other than suppressing anti-tumor immunity, MDSCs can directly promote tumor progression and metastasis by inducing stemness of tumor cells, angiogenesis, and degradation of extracellular matrix (ECM). Many immunosuppressive factors, such as IL-10, TGFβ, and IL-6 produced by MDSCs, are able to induce stem cell properties in various tumor cells (Schlegel et al., 2015; Zhu et al., 2017a; Yang et al., 2019). MDSCs produce high levels of VEGF (Shojaei et al., 2009), the most important cytokine involved in angiogenesis, which binding its receptor (VEGFR) on epithelial cells, promoting neo-angiogenesis by activating JAK2/STAT3 pathway. MDSCs also express high levels of VEGFR2, which, activated by VEGF secreted either by tumor cells or by themselves, lead to a vicious cycle that contributes to maintaining MDSCs angiogenic activity (Min et al., 2017). Besides, MDSCs produce matrix metalloproteinases (MMPs) that, degrading the ECM, contribute to tumor metastasis (Zhang et al., 2020). Another mechanism provided by MDSCs to suppress immune response is through cell-to-cell contact. MDSCs constitutively express on their surface molecules involved in the suppression of immune cells. Among these molecules, Fas ligand (Fas-L) is highly expressed by tumor-infiltrating MDSCs and can induce apoptosis of CD8 cytotoxic T cells by activating Fas-Fas-L axis, with a consequent local immune suppression as demonstrated in mice models (Zhu et al., 2017b; Rashid et al., 2021). Besides, MDSCs in tumor microenvironment bear high levels of ligands of negative immune checkpoint regulators, such as PD-L1 and Galectin-9, which respectively binding PD-1 and TIM3, inducing T cell anergy (Cheng et al., 2021). Moreover, it has been reported that MDSCs are able to induce decreased cytotoxicity, reduced IFNγ production and downregulated expression of NKG2D of NK cells, due to membrane-bound TGFβ in a cell-cell contact mode (Li et al., 2009). Additionally, through the cell-to-cell transfer of the metabolite methylglyoxal, MDSCs could paralyze T cells, reducing their anti-tumor activity (Baumann et al., 2020). Finally, MDSCs can also exert their immunosuppressive function by releasing exosomes. Similarly, to parental MDSCs, exosomes secreted from MDSCs contain pro-tumorigenic factors and can play a crucial role in immunosuppression, tumor growth, angiogenesis, invasion, and metastasis by distributing their contents into the tumor milieu. It has been demonstrated that MDSCs-derived exosomes contain matrix metalloproteinases (MMPs) and different cytokines, chemokines, and growth factors (CSF, VEGF, MCP, SDF1α, TNFα, and IFNγ), which establish a pro-metastatic microenvironment that allows the metastatic progression of tumor cells (Umansky et al., 2016). Moreover, MDSCs-derived exosomes can induce exhaustion and apoptosis of CD8 T cells by either increasing ROS production or inducing the activation of Fas/Fas-ligand pathway (Rashid et al., 2021). Additionally, MDSCs-derived exosomes bearing the membrane-bound PD-L1, could induce the transformation of naïve B cells into B regulatory cells, recently identified as an immunosuppressive cell population (Rosser and Mauri, 2015), thus inhibiting antitumor immune response (Lee-Chang et al., 2019). Finally, some microRNA contained in MDSCs-derived exosomes, such as miR-126a and miR9, promotes tumor angiogenesis by reprogramming endothelial cells (Baroni et al., 2016; Deng et al., 2017). The deep knowledge of the mechanisms by which MDSCs exert their powerful immunosuppressive functions and pro-tumoral activity could help to develop new effective immunotherapeutic strategies for the treatment of tumors or could intensify the effectiveness of tumor treatments already used. For these reasons, several clinical trials targeting MDSCs and their products are ongoing. Among these, the use of monoclonal antibodies (mAb) against immune checkpoints inhibitors seems to improve cancer patients’ outcomes, in combination with other anti-cancer therapies. Ipilimumab is a fully humanized mAb that acts blocking CTLA-4. Ipilimumab, alone or in combination with other anti-tumoral treatments, could potentiate the anti-tumor T cell response and could lower the frequency of MDSCs in tumor microenvironment, ameliorating the outcome of patients with different kinds of solid tumors (Hodi et al., 2010; Sade-Feldman et al., 2016; Tobin et al., 2018). Pembrolizumab, a PD-1 blocking mAb, alone or in combination with BL-8040, a CXCR4 antagonist, was approved to treat unresectable or metastatic solid tumors, due to its ability to reduce MDSCs number and increase effector T cell tumor infiltration (Redman et al., 2016; Vachhani and Chen, 2016; Syn et al., 2017; Bockorny et al., 2020). Another target of immunotherapies is the blockade of MDSCs’ recruitment into the tumor microenvironment, by the antagonist of some chemokine receptors highly expressed on these cells. A CCR2 antagonist, namely 747, displayed anti-cancer properties and potentiate the efficacy of sorafenib in a model of hepatocellular carcinoma (Yao et al., 2017). Other chemokine receptors antagonists, such as reparixin (anti-CXCR1/2), LY2510924 and ulocuplumab (anti-CXCR4), in association with chemotherapics agents, have shown significant results in the treatment of different solid tumors (Galsky et al., 2014; Schott et al., 2017; Ghobrial et al., 2020). Another important strategy for cancer treatment is the inhibition of MDSC activation, by inducing the transition of immature MDSCs in mature myeloid cells. All-trans retinoic acid (ATRA) and the active form of vitamin D have been recognized as ideal inducers of MDSCs differentiation and have been used for different types of both hematopoietic and solid tumors (Iclozan et al., 2013; Tobin et al., 2018; Fleet et al., 2020; Makitie et al., 2020). More recently, the use of CSF-1R inhibitors, such as GW-2580, Imatinib, and pexidartinib, due to their ability to inhibit the expansion of MDSCs, have revealed important results in different solid and hematopoietic cancer treatments (Giallongo et al., 2018; Edwards et al., 2019; Wesolowski et al., 2019). Finally, Arg-1 and iNOS, critical factors in MDSC-mediated immunosuppression, are the targets of inhibitor agents (INCB001158 for Arg-1 and L-NMMA for iNOS), which, in combination with immunotherapy or chemotherapy, have shown good results in some solid tumors treatment (Cheng et al., 2021; Chung et al., 2021).
1.4 Tissue-Resident Natural Killer and Natural Killer T Cells in Tumor Immunity
Natural Killer (NK) and Natural Killer T (NKT) cells are lymphocytes of the innate immune system playing pivotal roles in immune surveillance and response against virus-infected and tumor cells. NK and NKT cells share some common phenotypes and function such as the secretion of interleukin-2 (IL-2), interferon-γ (IFN-γ) and tumor necrosis factor α (TNFα) upon interaction with the ligand or antigen (Shimizu et al., 2020). However, they express distinct lineage development, tissue distribution, antigen recognition and regulatory mechanisms in health and cancer (Vivier et al., 2012).
1.4.1 Natural Killer Cells
Human NK cells are commonly divided into two subsets: immune-regulatory cytokine-responsive CD56brightCD16− and cytotoxic CD56dimCD16+ with potent IFN-γ, TNF-α, and GM-CSF secretion activity upon stimulation (Cooper et al., 2001). The diatribe of whether CD56dim are a mature form of CD56bright (Romagnani et al., 2007) or a distinct subpopulation originating from a separate lineage is still a matter of debate (Cichocki et al., 2019).
NK cells infiltrate has been found in several types of cancers (Mamessier et al., 2011; Ali et al., 2014). Interestingly CD56bright more efficiently traffic to the TME as they respond to the chemokines produced within the tumor bed. For example, IFN-γ stimulates tumor-infiltrating immune cells to release CXCL9-11, which is known to recruit CD56bright NK cells (Wendel et al., 2008). By contrast, highly cytotoxic CD56dim usually express receptors for chemokines produced at low levels and their traffic within the tumor site is often insufficient (Berahovich et al., 2006). NK cells vary expression of chemokine receptors following cytokine stimulation and therefore the composition of the NK cells in the TME changes accordingly (Pachynski et al., 2012). For example, CD56bright NK cells respond to IL-15 by upregulating the expression of the chemokine receptor CCR5 and induce migration to the TME. Conversely, the same cytokine inhibits infiltration of cytotoxic CD56dim cells by dampening expression of CXCR4 and CX3CR1 receptors (Sechler et al., 2004). In patients with advanced melanoma, an inverse correlation between the abundance of circulating CD56bright NK cells and patients’ survival was found, pointing out a role of these cells in the modulation of cancer response (de Jonge et al., 2019). Likewise, the proportion of CD56bright cells and production of IFNγ have been reported to be significantly lower in patients with prostate cancer than in controls (Koo et al., 2013).
Recently, by single-cell profiling a population of CD56brightCD127+CD160−CD52+ cells, NK0 was identified from human bone marrow and represent the precursors of conventional NK2/CD56brightCD160+CD52− cells and NK1/CD56dimPerforinhigh cells (Crinier et al., 2021). In patients with acute myeloid leukemia, transcriptomic analysis of the bone marrow revealed inhibition of the NK cell effector function and, importantly, patients with a good prognosis exhibited increased levels in the population of CD160+ NK cells (Crinier et al., 2021). Differently to T cells, NK cells recognize their target with a mechanism known as “missing self,” where the specificity for a given antigen is dispensable. NK cell function is modulated by a dynamic balance of activating and inhibitory signals and adhesion receptors in response to “altered self” cells such as tumor cells. When the inhibitory receptors do not recognize their target via the interaction with “self-identifier” (HLA) molecules, NK cells kill their target (Elliott and Yokoyama, 2011). NK inhibitory receptors are killer cell Ig-like receptors (KIRs) which engage with HLA types A, B or C (Harel-Bellan et al., 1986; Marsh et al., 2003), and NKG2A which binds to the highly conserved HLA-E (Sivori et al., 1996; Islam et al., 2021). More lately, other inhibitory molecules have been added to the list such as CD161, KLRG1, SIGLEC7, SIGLEC9, PD-1, TIGIT, LAG3, and TIM3 (Cozar et al., 2021). Conversely, activation receptors include CD16, NKp30, NKp44, NKp46, NKG2D, NKG2C, and activating KIRs. NK-cell activity is also enhanced by co-receptors like DNAX accessory molecule 1 (DNAM1) (Sanchez-Correa et al., 2012), NKp80 and 2B4 (Freud et al., 2017). For many years, it has been believed that NK cells uniformly recirculate. However, alongside conventional NK (cNK) cells, NK cells resident in the peripheral tissues, termed tissue-resident NK (trNK) cells have been reported in liver, kidney, skin uterus, salivary glands, and adipose tissue (Sojka et al., 2014; Fan and Rudensky, 2016; Sun et al., 2019). These tissue-resident lymphocytes do not recirculate in the blood or lymphatic system and have a distinct phenotype, like for example, the tissue-resident EOMES−T-bet+CD49a+ NK cells in the human liver (Sun et al., 2019). NK cells present immune-regulatory functions as they promote the activation of other innate and adaptive immune cells both by releasing cytokines and chemokines, or through direct cell–cell contact (Vivier et al., 2011). NK cells shape adaptive immune responses through the cross-talk with other cells such as T cells, B cells, and dendritic cells (DCs) (Malmberg et al., 2017). By inducing maturation of DC, NK cells trigger T cells mediated response; by producing IFN-γ, NK cells promote Th1 polarization (Moretta et al., 2008). Additionally, it has been proposed that NK cells can increase the function of cytotoxic CD8+ T cells by suppressing their state of exhaustion (Zheng et al., 2016) and, importantly, NK cell play a fundamental role in checkpoint blockade therapy by exacerbating antitumor or antiviral function of CD8+ T cells (Zhang et al., 2018). NK cells rapidly kill newly arising tumors or metastases, but their anti-tumor potency is less efficient against established solid formations. This is the result of the many strategies developed by tumors to escape immune surveillance, accompanied by a scarce capacity of NK cell to infiltrate the tumor site, as they tend to accumulate at the margins (Platonova et al., 2011). The tumor microenvironment (TME) is hostile to immune cells. NK cell effector function is limited by mechanisms of defense such as hypoxia, where tumor cells release abundant H2O2, thus limiting infiltration of CD56bright NK cells (Izawa et al., 2011; Terrén et al., 2019). Additionally, excessive production of the metabolic enzyme Indoleamine 2, 3-dioxygenase 1 (IDO1) causes immunosuppression and NK and T-cell (Pietra et al., 2012). Likewise, Prostaglandin-E2 (PGE2) produced by cancer cells decreases NK-cell cytotoxicity (Galland et al., 2017). Additionally, tumor cells directly inhibit the expression of NK cell markers such as NKp30, NKp44, NKp46, and NKG2D by releasing soluble factors such as TGFβ (Lee et al., 2004; Sconocchia et al., 2012; Close et al., 2020). NK cells show impaired cytotoxic function hampered by cancer cells and upregulation of inhibitory receptors like NKG2A (Mamessier et al., 2011). The TME is also populated by different cell types that orchestrate suppression of the anti-tumor immune response. These include stromal cells, regulatory T cells (Treg), fibroblasts and myeloid-derived suppressor cells (MDSC). Inhibition of CD8+ T and NK cells is exerted by cell-to-cell contact or via release of TGFβ and IL10 or production of nitric oxide (Ghiringhelli et al., 2005). The TME negatively influences NK cell immune response. The phenotype, metabolism and function of intra-tumor NK cells dynamically change during the different stages of tumor occurrence and progression (Liu et al., 2021). In the early stages of breast cancer development, NK cells have cytotoxic functions, which are lost at a later phase, thus promoting tumor progression. NK cells have impaired functions and are exhausted in advanced cancers and are characterized by increased glucose and lipid metabolism (Liu et al., 2021). Downregulation of cytotoxicity genes was reported in biopsies of chemotherapy-resistant breast cancer samples by microarray expression assay (Garcia-Chagollan et al., 2018). In gastric cancer, the overall phenotype of NK cells did not differ between the population of circulating and infiltrating NK cells. What was reported, however, was impaired function in tumor infiltrating NK cells, with reduced IFNγ, TNFα, and proliferation (Peng et al., 2017). Likewise, in HCC tissues, a population of tumor infiltrating CD11b−CD27− double negative NK cells was found to be characterized by poor effector functions and cytokine release (Zhang et al., 2017). In the TME, prolonged exposure to NKG2D ligands is associated with a reduction NKG2D expression, which causes NK cell function impairment and evasion of immune surveillance (Thompson et al., 2017). For example, in ovarian cancer, a reduction of the membrane-bound MICA/B proteins has been underpinned as an immune escape strategy adopted by tumor cells. MICA/B molecules bind to the receptor NKG2D which is expressed by NK cells, but also γδ+ and CD8+ T cells (Xie et al., 2014). NKG2D engagement corresponds to the inhibition of NK cell cytotoxic function and tumor progression (Xie et al., 2014). The metalloproteinases ADAM 10 and 17 are expressed by tumor cells and are implicated in immune surveillance escape. These mediate the shedding of B7-H6, ligand of NKp30. Increases of the B7-H6 in its soluble form determine drop in NKp30 expression and loss of NK effector function in different cancers (Schlecker et al., 2014; Pesce et al., 2015; Semeraro et al., 2015; Mantovani et al., 2019). PD-1 is an exhaustion marker on both T cells and NK cells (Liu et al., 2017b; Pesce et al., 2017). Importantly, NK mediated tumor response can be restored by blocking the immune checkpoint PD-1/PD-L1 axes (Liu et al., 2017b). Additionally to PD1, co-expression of a second exhaustion marker like TIM-3 has been associated to impaired NK cell function in many cancers, with reduced release of Granzyme B and IFN-γ and inhibited cytotoxicity (Seo et al., 2017). An interesting immune checkpoint is represented by the inhibitory receptor NKG2A. Its ligand, HLA-E is expressed by many different tumor cells (Zhen et al., 2013; Sun et al., 2017). Blockade of NKG2A/HLA-E axes with the antibody Monalizumab, for example, has proofed to unlock both NK cell and T cells function and corroborate anti-tumor immunity (André et al., 2018). Several studies have tried to unravel the role of NK cells in tumor immunity highlighting discrepancies mainly based on the type of tumor (Rezaeifard et al., 2021). Divergent results on the rate of NK cells infiltration in solid tumors have highlighted the urge to find reliable markers to address this question. The NK Cells Receptor (NCR) NKp46 has been recently identified as a robust biomarker to quantify tumor-infiltrating NK cells (Cózar et al., 2021). Although NK cells seem to reach the tumor bed to a lesser extent than other lymphocytes such as CD4+ and CD8+ T cells, their presence within the tumor correlates with a higher survival rate, as reported in head and neck squamous cell carcinoma (Weil et al., 2017; Concha-Benavente et al., 2018), colorectal cancer (Sconocchia et al., 2014), prostate tumor (Izawa et al., 2011) in gastric and esophageal cancers (Lorenzo-Herrero et al., 2019) and metastatic melanoma (Cursons et al., 2019b). Patients with metastatic skin melanoma showed better survival rates if infiltrating NK cells were detected in the tumor biopsies (Cursons et al., 2019a). Moreover, increased numbers of NK cells were correlated with a better response to anti–PD-1 immunotherapy and with an accumulation of pro-active DCs with a protective anti-cancer role at the tumor site (Barry et al., 2018). By contrast, the opposite has also been reported with inverse correlation between the advance stages of cancer and NK cells infiltrating the tumor (Vgenopoulou et al., 2003) or accumulating into the lymph nodes draining the tumor (Rezaeifard et al., 2019). Recently, a subset of CD49a+Eomes+ NK cells with known proangiogenic function has been described to accumulate at the site of liver tumor. This population has impaired cytotoxic function and reduced TNF-α release, strongly suggesting a pro-oncogenic activity in HCC (Zecca et al., 2021). NK cell infiltrate has been described in metastases, solid tumors and lymph nodes draining the tumor (Gulubova et al., 2009; Ali et al., 2014; Carrega et al., 2014). However, the extent of NK cell infiltration in the tumor greatly depends on the nature of the tumor and its localization. Some organs are more easily reached by NK cells such as the lungs, liver and kidney, while the intestinal tract appear to be less permissive (Remark et al., 2013). That said, high infiltration rate does not solely correlate with good prognostic factors. Expression of specific activating markers on NK cell surface represent a biomarker for more accurate prognosis (Schleypen et al., 2006), as described for the HLA-E receptor NKG2A and the activating marker NKG2C in endometrial cancer (Versluis et al., 2017). Tumor infiltrating NK and T cells represent a therapeutic target to tackle tumor. NK cells express the marker CD161, regulated by the gene KLRB1. CD161 expression orchestrates NK cell cytotoxic function in several cancers (Cheng et al., 2022). A good prognosis rate is observed in those cancer patients where KLRB1 is highly upregulated as infiltration of immune cells at tumor site and sensitivity to chemotherapy is KLRB1-dependent in many cancer types (Cheng et al., 2022). Nectar Therapeutics is developing an engineered IL-2 cytokine for the treatment of solid tumors. NKTR-214 is designed to sustain growth and survival of specific cancer-killing T cells and NK ells that specifically recognize a tumor target by targeting tumor infiltrating cells lymphocytes by binding to the CD122 receptor expressed by effector CD8+ T cells and NK cells (Bentebibel et al., 2019). However, it has also been reported that this effect is still partial, since a portion of the patients enrolled in the study displayed a proliferation of Treg cells (Sullivan, 2019).
The efficacy of this cytokine has been tested in clinical trial also in combination with checkpoint inhibitor anti-PD1 demonstrating efficacy and safety in the treatment of solid tumor like melanoma, renal cell carcinoma, and non-small cell lung cancer (Diab et al., 2020). In Soft Tissue Sarcoma (STS), compared to circulating cells, intra-tumoral NK and T cells have upregulated TIGIT, a marker of exhaustion. TIGIT+ lymphocytes are considered prognostic in STS and recently it has been proposed that TIGIT blockade may be a promising clinical strategy in STS (Judge et al., 2020). Similarly, in colon tumor biopsies, tumor-infiltrating NK cells have increased levels of TIGIT expression than circulating NK, suggesting an exhausted phenotype (Zhang et al., 2018). Finally, CD155 is the ligand of both activating receptor DNAM1 and inhibitory ligand TIGIT and is expressed in many types of cancer. DNAM1 engagement with CD155 is associated to inhibition of NK cell function (Nakai et al., 2010; Li et al., 2020). NK cells participate to response to tumors and represent potential targets for cancer immunotherapy. However, the TME negatively affects NK cell function, phenotype, survival, and rate of infiltration as a mechanism of escape. This inactivation can be however reverted by using immune checkpoints inhibitors specific, for instance, for NKG2A and TIGIT, already used in clinical trials (Galot et al., 2021). Alternatively, some studies have addressed mechanisms to increase NK cell infiltration at the tumor bed by neutralizing soluble factors that suppress NK cells function such as TGFβ, already entered in clinical trials also in combination with anti-PD1 (Dodagatta-Marri et al., 2019).
1.4.2 Natural Killer T Cells
Unlike conventional T cells, NKT cells recognize lipid antigens in a CD1d-dependent or independent manner (Vivier et al., 2012). CD1d+ NKT cells are divided into Type I or Type II NKT cells. Type I NKT cells are also known as invariant-NKT (iNKT) cells and express the invariant Vα14Jα18 TCR in mouse or Vα24Jα18 in human. This TCR recognize α-Galactosydcerimide (α-GalCer) lipid antigen (Terabe and Berzofsky, 2008). Human iNKT cells develop within the thymus and can be subdivided into functional subsets based on their expression of CD4 and CD8 into CD4+ iNKT cells, CD8+ iNKT cells, and DN iNKT cells (Lee et al., 2002). The DN and CD8+ iNKT cells have increased IFN-γ secretion and cytotoxic function upon activation while CD4+ iNKT cells have a pronounced helper function with release of type 2 (Th2) cytokines, such as IL-4 and IL-13 (Lee et al., 2002). In common with NK cells, human NKT cells express markers such as 2B4, NKG2D, DNAM-1, CD94, and NKG2A (Shimizu et al., 2020).
Frequency and function of intratumor or circulating iNKT cells have been assumed to correlate with overall survival in several types of cancers (Yanagisawa et al., 2002; Fujii et al., 2003; Tachibana et al., 2005; Schneiders et al., 2012), thus implying a role for iNKT cells in tumor immune surveillance. In details, reduced iNKT-cell numbers correlated with poor overall survival in head and neck squamous cell carcinoma (Molling et al., 2007) and acute myeloid leukemia (Najera Chuc et al., 2012). Conversely, increased numbers of intratumor or circulating iNKT cells have been associated with improved prognosis in colon cancer, prostate cancer, hematologic malignancies, and neuroblastoma (Metelitsa et al., 2004; Tachibana et al., 2005; Shaulov et al., 2008).
Human iNKT cytotoxicity cells against target cells may occur via TCR-dependent or independent signaling. During immune-surveillance, activation of iNKT cells can occur indirectly by cross-presentation of tumor lipids by APCs (Wu et al., 2003). Sialylated glycolipids on tumor cell membranes may become targets for iNKT cells and during tumor progression might be modified, representing a mechanism of immune surveillance escape. Several types of tumors, such as melanoma, small-cell lung cancer (SCLC), sarcoma, and neuroblastoma, highly express some gangliosides in comparison with corresponding normal tissue (Lo et al., 2010). These gangliosides might activate both CD4+CD8− iNKT and CD4−CD8− iNKT cells to produce IL-4 (Wu et al., 2003) and, acting as NKT cell ligands, might be related to prognosis in some cancers.
Activation of iNKT cells can also occur directly, via presentation of self-lipids by CD1d-positive tumors. CD1d expression has been found on solid tumors, such as prostate cancer (Tahir et al., 2001; Nowak et al., 2010), renal cell carcinoma (Chong et al., 2015), breast cancer (Hix et al., 2011), and tumors of the nervous system (Dhodapkar et al., 2004; Liu et al., 2013). For instance, in myeloma patients the frequency of iNKT cells in PBMCs are inversely correlated with disease progression (Dhodapkar et al., 2003; Jiang et al., 2018). This is due to the fact that primary myeloma cells express CD1d (Dhodapkar et al., 2003); however, its expression decreases in advanced stage cancer cells (Spanoudakis et al., 2009). Furthermore, human iNKT cells have been reported to kill CD1d+ osteosarcoma cells, but not CD1d− osteoblasts, confirming the CD1d restriction of iNKT cell–dependent cytotoxicity (Fallarini et al., 2012).
Interestingly, iNKT cells from cancer patients are not impaired; rather they are in an anergic state as they can be expanded and activated by stimulation with DCs loaded with α-GalCer, thus pointing out iNKT cells as potential target for anti-cancer therapy (Iyoda et al., 2018). In myeloid leukemia patients, for instance, purified PBMCs-derived iNKT cells are responsive to stimulation with α-GalCer/CD1d-tetramer with production of IFN-γ, TNF-α, IL-2, and IL-4 and cytotoxic against autologous leukemic cells, that are CD1d+ (Metelitsa et al., 2003).
iNKT cells have the capacity to alter the immune tumor microenvironment thus influencing the ability of the host to limit growth of cancer cells. iNKT cells thus represent a population to be harnessed for the development of anticancer clinical therapeutics. Indeed, the identification of strong cell agonists, such as α-GalCer and its analogues, has led to the production of synthetic lipids that have shown potential in cancer vaccination and treatment (McEwen-Smith et al., 2015). Some approaches include using nanovectors/nanoparticle-based delivery systems (Ghinnagow et al., 2017) or α-GalCer loaded exosomes (Liu et al., 2017a). Moreover, vaccination with DCs pulsed with α-Gal-Cer with the aim to expand and activate iNKT cells in human cancer patients is also being evaluated (Richter et al., 2013; Shimizu et al., 2013).
To conclude, successful therapeutic treatments should aim at increasing the rate of tumor infiltrating NK and iNKT cells and rescuing their effector function.
1.5 Tissue-Resident Innate Lymphoid Cells in Tumor Immunity
Although NK cells are defined as the prototypical innate lymphocyte population, over the past decade, innate lymphoid cells (ILCs) have expanded the definition of innate immune cells. ILCs are mainly located in barrier tissues including skin, intestine and lung and are involved in multiple physiological and pathophysiologic processes (Mjosberg and Spits, 2016). Human ILCs are identified as Lineage−, CD127+ cells since they lack the expression of classical lymphocyte surface markers, the recombination activating gene (RAG)-rearranged antigen receptors and other surface molecules whilst express the CD127 (also known as IL-7 receptor). The lineage markers for ILCs identification in human include: CD3, CD4, CD8, CD14, CD15, CD16, CD19, CD20, CD33, CD34, CD203c, and FcERI in order to exclude macrophages, dendritic cells, red blood cells, T, B, and NK cells (Trabanelli et al., 2018). Likewise, mouse ILCs are identified as Lin−, CD127+, CD90+ where the lineage mix consists of: CD3ε, CD5, CD8α, CD11c, CD11b, CD19, B220, FCRεI, TCRαβ, TCRγδ, DX5, and Ter119) (Gomez-Cadena et al., 2020). ILCs are defined as the innate counterpart of T lymphocytes subpopulations. In fact, similarly to Th1, Th2, and Th17 cells, ILCs are classified in ILC1, ILC2, and ILC3 cells mirroring the expression of transcription factors and the cytokine secretion of their adaptive counterpart (Ercolano et al., 2020b). In particular, ILC1s express T-bet and produce IFN-γ and TNF-α, ILC2s express GATA-3 and secrete IL-4, IL-5, and IL-13 and ILC3s express RORγt and secreting IL- 17A and/or IL-22. In the human peripheral circulation, ILC3s also comprise a population of progenitor cells (referred as ILCPs) able to differentiate into all ILC subsets and natural killer (NK) cells (Lim et al., 2017). In addition, has been recently reported that ILCPs, thanks to the expression of CD62L are able to migrate to the lymph node (Bar-Ephraim et al., 2019). As tissue-resident cells, ILCs establish close interaction with other cells in the tissues contributing to the first-line defense against different threats shaping both innate and adaptive immune response by producing their prototypic, subset specific cytokine sets. The impact of ILCs in different diseases including allergy, asthma, rheumatoid arthritis, and inflammatory bowel disease, has been widely described during the last years (Pasha et al., 2019; Wu and Shen, 2020; Ercolano et al., 2022; Trabanelli et al., 2022). Nevertheless, our group and others reported a controversial role of ILCs in cancer showing both pro and anti-tumor effects according to the tumor type and the consequent cytokines and other cells that constitute the tumor microenvironment (TME) (Ercolano et al., 2019; Ghaedi and Ohashi, 2020; Jacquelot et al., 2022). Given their ability to secrete IFN-γ and TNF-α and the expression of the natural cytotoxicity receptor (NCR) NKp46, ILC1s are often teamed to NK cells and difficult to discriminate in both human and mouse (Zhang and Huang, 2017). However, at steady state these two subsets can be distinguished according to the expression of the transcription factors Tbet and Eomes (Daussy et al., 2014; Klose et al., 2014). In addition, unlike NK cells, ILC1s show a weak cytotoxic capacity due to their poor ability to secrete granzyme B and perforin (Vivier et al., 2018). Nevertheless, recent findings based on single-cell RNA sequencing and flow cytometric analysis identified an ILC1 subset with cytotoxic ability in the liver (Di Censo et al., 2021; Friedrich et al., 2021; Chen et al., 2022). Different data demonstrated that these two populations differ in both their development and distribution. In fact, while ILC1s are predominantly tissue-resident cells, NK cells are found mostly in the bloodstream and within secondary lymphoid tissues (Gao et al., 2017). Nevertheless, has been demonstrated that, under pathological conditions, including tumor development and metastasis, NK cells can acquire an ILC1-like phenotype. In particular, in a murine model of fibrosarcoma and melanoma, has been shown that NK cells were converted in ILC1s limiting the NK cell–mediated tumor immunosurveillance (Gao et al., 2017). TGF-β resulted to be the principal mediator involved in this switching of NK phenotype suggesting that the use of antibodies targeting TGF-β and its receptors may offer a promising strategy for cancer immunotherapy inhibiting the conversion of NK cells into ILC1s. In line with these findings, Salome et al. (2019) described an uncommon population of ILC1-like cells population with cytotoxic properties which were impaired in human acute myeloid leukemia (AML) by TGF-β and AhR ligands. Together with TGF-β, different cytokines present in the TME can modulate both the pro- and anti-tumoral effect of ILC1s. For instance, an IL-15 rich environment promotes the generation of a tissue-resident ILC1-like cells, characterized by a powerful cytotoxic activity towards cancer cells as demonstrated by using a MMTV-PyMT (PyMT) mammary tumor model (Dadi et al., 2016). Likewise, high levels of IL-15 have been observed in human colorectal cancer (CRC) biopsies, as well as high expression of T-bet and IFN-γ supporting the anti-tumoral role of ILC1s in this context (Mlecnik et al., 2014). In human melanoma, our own group reported an increase in the frequency of ILC1s in both peripheral blood mononuclear cells (PBMC) and tumor-infiltrated lymph nodes (TILN) of melanoma patients compared to healthy donors. Nevertheless, ILC1s from melanoma patients were functionally impaired as demonstrated by the reduced secretion of IFN-γ. By dissecting the different mediators present in the TME, we focused on adenosine and indoleamine. In fact, it is widely known that these two mediators are highly expressed in cancer and play a key role in the progression of melanoma and other types of cancer (Vijayan et al., 2017; Jennings et al., 2021). In particular, we observed that these two mediators are involved in ILC1 exhaustion leading to a reduction in type-1 cytokine secretion (Ercolano et al., 2020a). This effect was reverted by using an adenosine receptors inhibitor showing new evidence to sustain blocking these immunosuppressive pathways in melanoma patients by targeting the innate lymphoid cells arm.
ILC2s are assigned as a primarily pro-tumorigenic subset given their ability to produce type-2 cytokines, such as IL-13 and IL-5, and other immunosuppressive mediators. Different studies reported a higher frequency of tumor-infiltrating ILC2 in gastric, breast and prostate cancer. Particularly, in a first work published by Jovanovic et al. (2014), has been reported an increase of ILC2-derived IL-13 in vivo by using the 4T1 syngeneic murine model. Next, Salimi et al. (2018), observed an increase of ILC2s and investigated on the expression of different activatory and inhibitory receptors in tumor-infiltrating ILCs in both human breast and gastric cancer. Similarly, Trabanelli et al. (2017), found an enrichment of ILC2s in prostate cancer patients which was correlated with tumor stage and myeloid derived suppressor cells (MDSCs) frequency. Increased numbers of ILC2 have been showed also in the tumors of gastric cancer patients infected with Helicobacter pylori (Li et al., 2017). Furthermore, very recently, has been reported a link between ILC2 and tuft cells (a rare population of epithelial cells present at the gastrointestinal and respiratory tract). In particular, the tuft cells/ILC2 axis seems to be involved in the development of gastric cancer as demonstrated by using a murine model of intestinal metaplasia. This result was also confirmed in tumor microarrays of intestinal-type gastric cancer patients showing a unique correlation for tuft cells, ILC2s and survival in intestinal-type gastric cancer. IL-25 and IL-13 play a key role in dictating the crosstalk between tuft cells and ILC2s, in fact, the treatment with either α-IL13 or α-IL25 neutralizing antibodies significantly restrained tumor growth which coincided with a reduced frequency of both tuft cells and ILC2s in mice (O’Keefe et al., 2022). The pro- and anti-tumoral role of ILC2s is frequently associated with the overexpression of various cytokines involved in their activation including IL-33, IL-25, and TSLP commonly defined as “alarmins” (Roan et al., 2019). Our group recently demonstrated that IL-33 is highly expressed in tissues as well as in the serum of CRC patients. The high presence of IL-33 didn’t affect the frequency of ILC2s but increased their activity in term of IL-13 and IL-5 production which in turn sustain CRC progression through the modulation of the epithelial-to-mesenchymal transition (EMT) phenomenon (Ercolano et al., 2021). Conversely, it has been recently reported that in pancreatic adenocarcinoma, IL-33 induces ILC2 expansion that was accompanied by enhanced intratumoral CD8+ T cells pushing tissue-specific tumor immunity (Moral et al., 2020). Interestingly, they also reported that tumor-infiltrated ILC2s express the inhibitory checkpoint receptor PD-1 hypothesizing that PD-1 blockade could further boost ILC2 activation to enhance anti-tumor efficacy. In line with these findings, (Jacquelot et al., 2021a) demonstrated that PD-1 blockade increased ILC2 and eosinophil recruitment and enhanced anti-tumor responses in melanoma context (Jacquelot et al., 2021b). These findings suggest the need to further dissect investigation on the broad array of checkpoint inhibitors as target for ILC2 in cancer immunotherapy.
The role of ILC3s in cancer is emerging as complex and highly dependent on the tumor type as also described for their adaptive counterpart (Bruchard and Ghiringhelli, 2019). In fact, similarly to Th17 cells, ILC3s exert both pro- and anti-tumor functions showing different phenotypes according to the tissue microenvironment. Although ILC3s are generally dependent on the transcription factor RORγt, there are more complex subsets that further subdivide ILC3s in NCR+ ILC3s and NCR− ILC3s depending on the expression of the natural cytotoxicity receptors (NCRs) NKp46 and NKp44 in both human and mice (Melo-Gonzalez and Hepworth, 2017). In addition, lymphoid-tissue inducer (LTi) cells are a further subclass of ILC3 closely related to NCR- ILC3s, involved in lymph nodes development (Spits et al., 2013). ILC3s have been associated with the pathogenesis and progression of different type of cancer. For instance, a profound reduction of ILC3 NCR+ cells were observed also in acute myeloid leukemia (AML) patients which was completely recovered after two cycles of treatment with standard chemotherapy (represented by daunorubicin/cytarabine) (Trabanelli et al., 2015). Conversely, Liu et al. (2019) demonstrated that NCR− ILC3s were the tissue-resident cells mainly present in the liver of hepatocellular carcinoma-bearing mice. In particular, NCR- ILC3s supported the early phase of tumour development by promoting the establishment of an IL-17-rich tumor microenvironment in response to IL-23 sustaining the differentiation of other IL-17-producing cells. These findings suggest that NCR- ILC3s, as well as IL-23, could be an interesting target to exploit for the prevention of hepatocellular carcinoma progression. Given the essential role of LTi cells in generating secondary lymphoid tissues during embryogenesis, it is possible to hypothesize an involvement of these cells in cancer context. In fact, has been demonstrated that an IL-12 enriched environment promotes the recruitment and proliferation of NKp46+ LTi cells, able to inhibit tumor growth and thwart the development of lung metastases in a murine model of cutaneous melanoma (Eisenring et al., 2010). Importantly, (Jacquelot et al., 2021b) recently reviewed that LTi cells are implicated in the development of tertiary lymphoid structures that are formed in inflamed tissues (including tumor) and are composed by different immune cells driving the immune response against tumor development and progression and improving the clinical outcome (Jacquelot et al., 2021a). Moreover, in subcutaneous melanoma, it has been showed that LTi cells exert a tumoricidal effect by increasing the expression levels of adhesion molecules in the tumor which in turn promote the recruitment of adaptive immune cells and the subsequent tumor control (Eisenring et al., 2010). The ability of ILCs to foster the recruitment of other immune cells has been recently described in bladder cancer settings. In particular, Vanoni et al. (2021) demonstrated that ILCPs (the ILC3 progenitor subset present in the peripheral blood) interact with endothelial cells inducing, on one hand, the expression of adhesion molecules on the surface of endothelial cells and acquiring and, on the other, an ILC3-like phenotype sharing some phenotypical markers with LTi cells. However, in human high-grade bladder carcinoma samples, ILCPs are barely detected and their ability to upregulate adhesion molecule expression on endothelial cells was impaired (Vanoni et al., 2021). These findings could describe one of the mechanisms through which tumour cells block the infiltration of immune cells into the tumour site highlighting a new attractive target to regulate the immune infiltration and establish an antitumor immune response.
2 Concluding Remarks
Immune system evasion is a distinctive hallmark of cancer (Hanahan, 2022). In the last decades, cancer immunotherapy has experienced important clinical progresses in the treatment of different types of cancer. For instance, immune checkpoint inhibitors such as ipilimumab, nivolumab, and pembrolizumab, whose primary purpose is to unleash effector T cells response, have recast the treatment of aggressive forms of tumor including melanoma, non-small cell lung carcinoma and colorectal cancer (Bagchi et al., 2021). However, cancer relapse and recurrence occur for most patients increasing the need to find new therapeutic targets to improve cancer immunotherapy. The tumor milieu is a complex assortment of immune cells, blood vessels, connective tissue cells and extracellular matrix molecules, which all exert a remarkable influence on the cancerous cells they surround. The better understanding of this microenvironment, with particular attention focused on the function of immune cells within this region is today of particular interest. In this context, tissue-resident innate immune cells exert both positive and negative immune regulatory functions (Figure 1), representing important contributors in modulating the tumor microenvironment and shaping the adaptive tumor response (Wang et al., 2019b). Thus, investigate on the innate-adaptive lymphocyte crosstalk in cancer, could represent an interesting approach in order to increase the efficacy of the current immunotherapies.
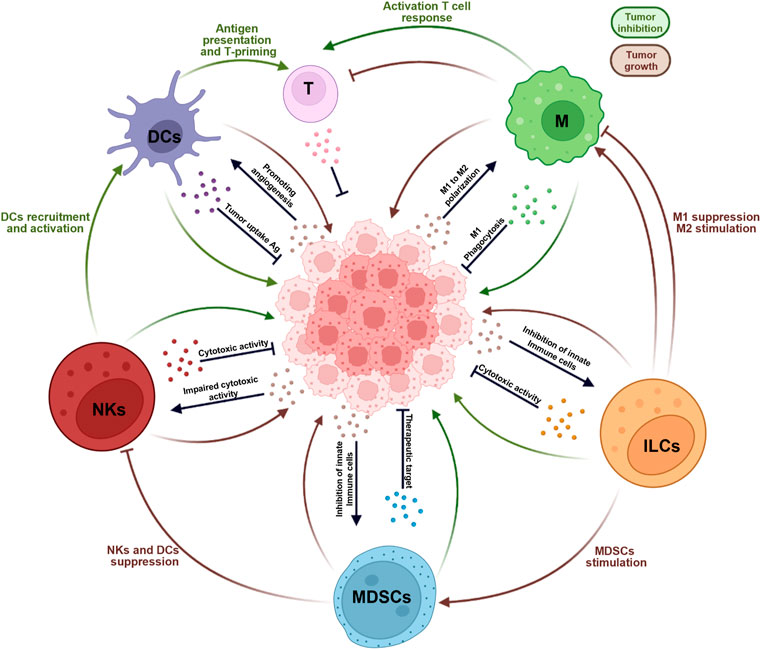
FIGURE 1. Orchestration of cancer immune control. Within the tumor each tissue-resident innate immune cells exert both positive (tumor inhibition, in green) and negative (tumor growth, in brown) immune regulatory functions. The tissue-resident innate immune cells are important contributors in modulating the tumor microenvironment though the secretion of growth factors, chemokines, and cytokines. As show in the figure, the mainly subpopulation involved are dendritic cells (DCs), macrophages, myeloid-derived suppressor cells (MDSCs), natural killer cells (NKs), and innate lymphoid cells (ILCs).
Author Contributions
RB, MB, EB, GZ, DM, and PC did the bibliographic research and wrote the manuscript. GE and AI critically revised the article for intellectual content. All authors contributed to the article and approved the submitted version.
Funding
This work was supported by Associazione Italiana per la Ricerca sul Cancro (AIRC) (MFAG No. 26002 to GE), and by the Italian Government grants (PRIN 2017 No. 2017BA9LM5 to AI).
Conflict of Interest
The authors declare that the research was conducted in the absence of any commercial or financial relationships that could be construed as a potential conflict of interest.
Publisher’s Note
All claims expressed in this article are solely those of the authors and do not necessarily represent those of their affiliated organizations, or those of the publisher, the editors and the reviewers. Any product that may be evaluated in this article, or claim that may be made by its manufacturer, is not guaranteed or endorsed by the publisher.
References
Ali, T. H., Pisanti, S., Ciaglia, E., Mortarini, R., Anichini, A., Garofalo, C., et al. (2014). Enrichment of CD56dimKIR+CD57+ Highly Cytotoxic NK Cells in Tumour-Infiltrated Lymph Nodes of Melanoma Patients. Nat. Commun. 5, 5639. doi:10.1038/ncomms6639
Allavena, P., Signorelli, M., Chieppa, M., Erba, E., Bianchi, G., Marchesi, F., et al. (2005). Anti-inflammatory Properties of the Novel Antitumor Agent Yondelis (Trabectedin): Inhibition of Macrophage Differentiation and Cytokine Production. Cancer Res. 65, 2964–2971. doi:10.1158/0008-5472.can-04-4037
André, P., Denis, C., Soulas, C., Bourbon-Caillet, C., Lopez, J., Arnoux, T., et al. (2018). Anti-NKG2A mAb Is a Checkpoint Inhibitor that Promotes Anti-tumor Immunity by Unleashing Both T and NK Cells. Cell 175, 1731–e13. e1713. doi:10.1016/j.cell.2018.10.014
Andreu, P., Johansson, M., Affara, N. I., Pucci, F., Tan, T., Junankar, S., et al. (2010). FcRγ Activation Regulates Inflammation-Associated Squamous Carcinogenesis. Cancer Cell 17, 121–134. doi:10.1016/j.ccr.2009.12.019
Anfray, C., Ummarino, A., Andon, F. T., and Allavena, P. (2019). Current Strategies to Target Tumor-Associated-Macrophages to Improve Anti-tumor Immune Responses. Cells 9, 46. doi:10.3390/cells9010046
Ao, J.-Y., Zhu, X.-D., Chai, Z.-T., Cai, H., Zhang, Y.-Y., Zhang, K.-Z., et al. (2017). Colony-stimulating Factor 1 Receptor Blockade Inhibits Tumor Growth by Altering the Polarization of Tumor-Associated Macrophages in Hepatocellular Carcinoma. Mol. Cancer Ther. 16, 1544–1554. doi:10.1158/1535-7163.mct-16-0866
Au Yeung, C. L., Co, N.-N., Tsuruga, T., Yeung, T.-L., Kwan, S.-Y., Leung, C. S., et al. (2016). Exosomal Transfer of Stroma-Derived miR21 Confers Paclitaxel Resistance in Ovarian Cancer Cells through Targeting APAF1. Nat. Commun. 7, 11150. doi:10.1038/ncomms11150
Bagchi, S., Yuan, R., and Engleman, E. G. (2021). Immune Checkpoint Inhibitors for the Treatment of Cancer: Clinical Impact and Mechanisms of Response and Resistance. Annu. Rev. Pathol. Mech. Dis. 16, 223–249. doi:10.1146/annurev-pathol-042020-042741
Balachandran, V. P., Cavnar, M. J., Zeng, S., Bamboat, Z. M., Ocuin, L. M., Obaid, H., et al. (2011). Imatinib Potentiates Antitumor T Cell Responses in Gastrointestinal Stromal Tumor through the Inhibition of Ido. Nat. Med. 17, 1094–1100. doi:10.1038/nm.2438
Bar-Ephraim, Y. E., Koning, J. J., Burniol Ruiz, E., Konijn, T., Mourits, V. P., Lakeman, K. A., et al. (2019). CD62L Is a Functional and Phenotypic Marker for Circulating Innate Lymphoid Cell Precursors. J. I. 202, 171–182. doi:10.4049/jimmunol.1701153
Baroni, S., Romero-Cordoba, S., Plantamura, I., Dugo, M., D’Ippolito, E., Cataldo, A., et al. (2016). Exosome-mediated Delivery of miR-9 Induces Cancer-Associated Fibroblast-like Properties in Human Breast Fibroblasts. Cell Death Dis. 7–e2312. doi:10.1038/cddis.2016.224
Barry, K. C., Hsu, J., Broz, M. L., Cueto, F. J., Binnewies, M., Combes, A. J., et al. (2018). A Natural Killer-Dendritic Cell axis Defines Checkpoint Therapy-Responsive Tumor Microenvironments. Nat. Med. 24, 1178–1191. doi:10.1038/s41591-018-0085-8
Baumann, T., Dunkel, A., Schmid, C., Schmitt, S., Hiltensperger, M., Lohr, K., et al. (2020). Regulatory Myeloid Cells Paralyze T Cells through Cell-Cell Transfer of the Metabolite Methylglyoxal. Nat. Immunol. 21, 555–566. doi:10.1038/s41590-020-0666-9
Belikov, A. V., Schraven, B., and Simeoni, L. (2015). T Cells and Reactive Oxygen Species. J. Biomed. Sci. 22, 85. doi:10.1186/s12929-015-0194-3
Bentebibel, S.-E., Hurwitz, M. E., Bernatchez, C., Haymaker, C., Hudgens, C. W., Kluger, H. M., et al. (2019). A First-In-Human Study and Biomarker Analysis of NKTR-214, a Novel IL2Rβγ-Biased Cytokine, in Patients with Advanced or Metastatic Solid Tumors. Cancer Discov. 9, 711–721. doi:10.1158/2159-8290.cd-18-1495
Bentz, B. G., Haines, G. K., and Radosevich, J. A. (2000). Increased Protein Nitrosylation in Head and Neck Squamous Cell Carcinogenesis. Head. Neck 22, 64–70. doi:10.1002/(sici)1097-0347(200001)22:1<64::aid-hed10>3.0.co;2-j
Berahovich, R. D., Lai, N. L., Wei, Z., Lanier, L. L., and Schall, T. J. (2006). Evidence for NK Cell Subsets Based on Chemokine Receptor Expression. J. Immunol. 177, 7833–7840. doi:10.4049/jimmunol.177.11.7833
Bingisser, R. M., Tilbrook, P. A., Holt, P. G., and Kees, U. R. (1998). Macrophage-derived Nitric Oxide Regulates T Cell Activation via Reversible Disruption of the Jak3/STAT5 Signaling Pathway. J. Immunol. 160, 5729–5734.
Binnewies, M., Mujal, A. M., Pollack, J. L., Combes, A. J., Hardison, E. A., Barry, K. C., et al. (2019). Unleashing Type-2 Dendritic Cells to Drive Protective Antitumor CD4+ T Cell Immunity. Cell 177, 556–571. doi:10.1016/j.cell.2019.02.005
Blumenthal, R. L., Campbell, D. E., Hwang, P., Dekruyff, R. H., Frankel, L. R., and Umetsu, D. T. (2001). Human Alveolar Macrophages Induce Functional Inactivation in Antigen-specific CD4 T Cells. J. Allergy Clin. Immunol. 107, 258–264. doi:10.1067/mai.2001.112845
Bockorny, B., Semenisty, V., Macarulla, T., Borazanci, E., Wolpin, B. M., Stemmer, S. M., et al. (2020). BL-8040, a CXCR4 Antagonist, in Combination with Pembrolizumab and Chemotherapy for Pancreatic Cancer: the COMBAT Trial. Nat. Med. 26, 878–885. doi:10.1038/s41591-020-0880-x
Bosurgi, L., Cao, Y. G., Cabeza-Cabrerizo, M., Tucci, A., Hughes, L. D., Kong, Y., et al. (2017). Macrophage Function in Tissue Repair and Remodeling Requires IL-4 or IL-13 with Apoptotic Cells. Science 356, 1072–1076. doi:10.1126/science.aai8132
Boutilier, A. J., and Elsawa, S. F. (2021). Macrophage Polarization States in the Tumor Microenvironment. Int. J. Mol. Sci. 22. doi:10.3390/ijms22136995
Brown, S., Hutchinson, C. V., Aspinall-O'dea, M., Whetton, A. D., Johnson, S. M., Rees-Unwin, K., et al. (2014). Monocyte-derived Dendritic Cells from Chronic Myeloid Leukaemia Have Abnormal Maturation and Cytoskeletal Function that Is Associated with Defective Localisation and Signalling by Normal ABL1 Protein. Eur. J. Haematol. 93, 96–102. doi:10.1111/ejh.12306
Bruchard, M., and Ghiringhelli, F. (2019). Deciphering the Roles of Innate Lymphoid Cells in Cancer. Front. Immunol. 10, 656. doi:10.3389/fimmu.2019.00656
Carrega, P., Bonaccorsi, I., Di Carlo, E., Morandi, B., Paul, P., Rizzello, V., et al. (2014). CD56brightPerforinlow Noncytotoxic Human NK Cells Are Abundant in Both Healthy and Neoplastic Solid Tissues and Recirculate to Secondary Lymphoid Organs via Afferent Lymph. J. I. 192, 3805–3815. doi:10.4049/jimmunol.1301889
Chen, Y., Wang, X., Hao, X., Li, B., Tao, W., Zhu, S., et al. (2022). Ly49E Separates Liver ILC1s into Embryo-Derived and Postnatal Subsets with Different Functions. J. Exp. Med. 219. doi:10.1084/jem.20211805
Cheng, J.-N., Yuan, Y.-X., Zhu, B., and Jia, Q. (2021). Myeloid-Derived Suppressor Cells: A Multifaceted Accomplice in Tumor Progression. Front. Cell Dev. Biol. 9, 740827. doi:10.3389/fcell.2021.740827
Cheng, M., Zhang, X., Yu, H., Du, P., Plumas, J., Chaperot, L., et al. (2015). Characterization of Species-specific Genes Regulated by E2-2 in Human Plasmacytoid Dendritic Cells. Sci. Rep. 5, 10752. doi:10.1038/srep10752
Cheng, X., Cao, Y., Wang, X., Cheng, L., Liu, Y., Lei, J., et al. (2022). Systematic Pan-Cancer Analysis of KLRB1 with Prognostic Value and Immunological Activity across Human Tumors. J. Immunol. Res. 2022, 5254911. doi:10.1155/2022/5254911
Chong, T. W., Goh, F. Y., Sim, M. Y., Huang, H. H., Thike, D. A. A., Lim, W. K., et al. (2015). CD1d Expression in Renal Cell Carcinoma Is Associated with Higher Relapse Rates, Poorer Cancer-specific and Overall Survival. J. Clin. Pathol. 68, 200–205. doi:10.1136/jclinpath-2014-202735
Chung, A. W., Anand, K., Anselme, A. C., Chan, A. A., Gupta, N., Venta, L. A., et al. (2021). A Phase 1/2 Clinical Trial of the Nitric Oxide Synthase Inhibitor L-NMMA and Taxane for Treating Chemoresistant Triple-Negative Breast Cancer. Sci. Transl. Med. 13, eabj5070. doi:10.1126/scitranslmed.abj5070
Cichocki, F., Grzywacz, B., and Miller, J. S. (2019). Human NK Cell Development: One Road or Many? Front. Immunol. 10, 2078. doi:10.3389/fimmu.2019.02078
Cisse, B., Caton, M. L., Lehner, M., Maeda, T., Scheu, S., Locksley, R., et al. (2008). Transcription Factor E2-2 Is an Essential and Specific Regulator of Plasmacytoid Dendritic Cell Development. Cell 135, 37–48. doi:10.1016/j.cell.2008.09.016
Close, H. J., Stead, L. F., Nsengimana, J., Reilly, K. A., Droop, A., Wurdak, H., et al. (2020). Expression Profiling of Single Cells and Patient Cohorts Identifies Multiple Immunosuppressive Pathways and an Altered NK Cell Phenotype in Glioblastoma. Clin. Exp. Immunol. 200, 33–44. doi:10.1111/cei.13403
Cobbs, C. S., Whisenhunt, T. R., Wesemann, D. R., Harkins, L. E., Van Meir, E. G., and Samanta, M. (2003). Inactivation of Wild-type P53 Protein Function by Reactive Oxygen and Nitrogen Species in Malignant Glioma Cells. Cancer Res. 63, 8670–8673.
Collin, M., Mcgovern, N., and Haniffa, M. (2013). Human Dendritic Cell Subsets. Immunology 140, 22–30. doi:10.1111/imm.12117
Concha-Benavente, F., Kansy, B., Moskovitz, J., Moy, J., Chandran, U., and Ferris, R. L. (2018). PD-L1 Mediates Dysfunction in Activated PD-1+ NK Cells in Head and Neck Cancer Patients. Cancer Immunol. Res. 6, 1548–1560. doi:10.1158/2326-6066.cir-18-0062
Consonni, F. M., Porta, C., Marino, A., Pandolfo, C., Mola, S., Bleve, A., et al. (2019). Myeloid-Derived Suppressor Cells: Ductile Targets in Disease. Front. Immunol. 10, 949. doi:10.3389/fimmu.2019.00949
Cooper, M. A., Fehniger, T. A., Turner, S. C., Chen, K. S., Ghaheri, B. A., Ghayur, T., et al. (2001). Human Natural Killer Cells: a Unique Innate Immunoregulatory Role for the CD56bright Subset. Blood, J. Am. Soc. Hematol. 97, 3146–3151. doi:10.1182/blood.v97.10.3146
Cózar, B., Greppi, M., Carpentier, S., Narni-Mancinelli, E., Chiossone, L., and Vivier, E. (2021). Tumor-Infiltrating Natural Killer Cells. Cancer Discov. 11, 34–44. doi:10.1158/2159-8290.cd-20-0655
Cózar, B., Greppi, M., Carpentier, S., Narni-Mancinelli, E., Chiossone, L., and Vivier, E. (2021). Tumor-infiltrating Natural Killer Cells. Cancer Discov. 11, 34–44.
Crinier, A., Dumas, P.-Y., Escalière, B., Piperoglou, C., Gil, L., Villacreces, A., et al. (2021). Single-cell Profiling Reveals the Trajectories of Natural Killer Cell Differentiation in Bone Marrow and a Stress Signature Induced by Acute Myeloid Leukemia. Cell Mol. Immunol. 18, 1290–1304. doi:10.1038/s41423-020-00574-8
Cursons, J., Souza-Fonseca-Guimaraes, F., Foroutan, M., Anderson, A., Hollande, F., Hediyeh-Zadeh, S., et al. (2019a). A Gene Signature Predicting Natural Killer Cell Infiltration and Improved Survival in Melanoma Patients. Cancer Immunol. Res. 7, 1162–1174. doi:10.1158/2326-6066.cir-18-0500
Cursons, J., Souza-Fonseca-Guimaraes, F., Foroutan, M., Anderson, A., Hollande, F., Hediyeh-Zadeh, S., et al. (2019b). A Gene Signature Predicting Natural Killer Cell Infiltration and Improved Survival in Melanoma Patients. Cancer Immunol. Res. 7, 1162–1174. doi:10.1158/2326-6066.cir-18-0500
Curti, A., Pandolfi, S., Valzasina, B., Aluigi, M., Isidori, A., Ferri, E., et al. (2007). Modulation of Tryptophan Catabolism by Human Leukemic Cells Results in the Conversion of CD25− into CD25+ T Regulatory Cells. Blood 109, 2871–2877. doi:10.1182/blood-2006-07-036863
Dadi, S., Chhangawala, S., Whitlock, B. M., Franklin, R. A., Luo, C. T., Oh, S. A., et al. (2016). Cancer Immunosurveillance by Tissue-Resident Innate Lymphoid Cells and Innate-like T Cells. Cell 164, 365–377. doi:10.1016/j.cell.2016.01.002
Daussy, C., Faure, F., Mayol, K., Viel, S., Gasteiger, G., Charrier, E., et al. (2014). T-bet and Eomes Instruct the Development of Two Distinct Natural Killer Cell Lineages in the Liver and in the Bone Marrow. J. Exp. Med. 211, 563–577. doi:10.1084/jem.20131560
De Cicco, P., Ercolano, G., and Ianaro, A. (2020). The New Era of Cancer Immunotherapy: Targeting Myeloid-Derived Suppressor Cells to Overcome Immune Evasion. Front. Immunol. 11, 1680. doi:10.3389/fimmu.2020.01680
De Jonge, K., Ebering, A., Nassiri, S., Maby-El Hajjami, H., Ouertatani-Sakouhi, H., Baumgaertner, P., et al. (2019). Circulating CD56bright NK Cells Inversely Correlate with Survival of Melanoma Patients. Sci. Rep. 9, 4487. doi:10.1038/s41598-019-40933-8
De Mingo Pulido, Á., Gardner, A., Hiebler, S., Soliman, H., Rugo, H. S., Krummel, M. F., et al. (2018). TIM-3 Regulates CD103+ Dendritic Cell Function and Response to Chemotherapy in Breast Cancer. Cancer Cell 33, 60–74. doi:10.1016/j.ccell.2017.11.019
Denardo, D. G., Barreto, J. B., Andreu, P., Vasquez, L., Tawfik, D., Kolhatkar, N., et al. (2009). CD4+ T Cells Regulate Pulmonary Metastasis of Mammary Carcinomas by Enhancing Protumor Properties of Macrophages. Cancer Cell 16, 91–102. doi:10.1016/j.ccr.2009.06.018
Deng, Z., Rong, Y., Teng, Y., Zhuang, X., Samykutty, A., Mu, J., et al. (2017). Exosomes miR-126a Released from MDSC Induced by DOX Treatment Promotes Lung Metastasis. Oncogene 36, 639–651. doi:10.1038/onc.2016.229
Dhara, V., Shetty, S. S., De Arruda, J. A. A., Silva, T. A., Russo, R. C., Shetty, N. J., et al. (2022). Decoding the Influence of the Immune System and Immunotherapy Targets on Carcinomas: A Hidden Prism in Oral Cancer Therapy. Disease-a-Month 2022, 101353. doi:10.1016/j.disamonth.2022.101353
Dhodapkar, K. M., Cirignano, B., Chamian, F., Zagzag, D., Miller, D. C., Finlay, J. L., et al. (2004). Invariant Natural Killer T Cells Are Preserved in Patients with Glioma and Exhibit Antitumor Lytic Activity Following Dendritic Cell-Mediated Expansion. Int. J. Cancer 109, 893–899. doi:10.1002/ijc.20050
Dhodapkar, M. V., Geller, M. D., Chang, D. H., Shimizu, K., Fujii, S.-I., Dhodapkar, K. M., et al. (2003). A Reversible Defect in Natural Killer T Cell Function Characterizes the Progression of Premalignant to Malignant Multiple Myeloma. J. Exp. Med. 197, 1667–1676. doi:10.1084/jem.20021650
Di Censo, C., Marotel, M., Mattiola, I., Müller, L., Scarno, G., Pietropaolo, G., et al. (2021). Granzyme A and CD160 Expression Delineates ILC1 with Graded Functions in the Mouse Liver. Eur. J. Immunol. 51, 2568–2575. doi:10.1002/eji.202149209
Diab, A., Tannir, N. M., Bentebibel, S.-E., Hwu, P., Papadimitrakopoulou, V., Haymaker, C., et al. (2020). Bempegaldesleukin (NKTR-214) Plus Nivolumab in Patients with Advanced Solid Tumors: Phase I Dose-Escalation Study of Safety, Efficacy, and Immune Activation (PIVOT-02). Cancer Discov. 10, 1158–1173. doi:10.1158/2159-8290.cd-19-1510
Diamond, M. S., Kinder, M., Matsushita, H., Mashayekhi, M., Dunn, G. P., Archambault, J. M., et al. (2011). Type I Interferon Is Selectively Required by Dendritic Cells for Immune Rejection of Tumors. J. Exp. Med. 208, 1989–2003. doi:10.1084/jem.20101158
Dodagatta-Marri, E., Meyer, D., Reeves, M., Paniagua, R., To, M., Binnewies, M., et al. (2019). alpha-PD-1 Therapy Elevates Treg/Th Balance and Increases Tumor Cell pSmad3 that Are Both Targeted By-TGF Antibody to Promote Durable Rejection and Immunity in Squamous Cell Carcinomas. J. Immunother. CANCER 7. doi:10.1186/s40425-018-0493-9
Dzionek, A., Fuchs, A., Schmidt, P., Cremer, S., Zysk, M., Miltenyi, S., et al. (2000). BDCA-2, BDCA-3, and BDCA-4: Three Markers for Distinct Subsets of Dendritic Cells in Human Peripheral Blood. J. Immunol. 165, 6037–6046. doi:10.4049/jimmunol.165.11.6037
Edwards, D. K., Watanabe-Smith, K., Rofelty, A., Damnernsawad, A., Laderas, T., Lamble, A., et al. (2019). CSF1R Inhibitors Exhibit Antitumor Activity in Acute Myeloid Leukemia by Blocking Paracrine Signals from Support Cells. Blood 133, 588–599. doi:10.1182/blood-2018-03-838946
Eisenring, M., Vom Berg, J., Kristiansen, G., Saller, E., and Becher, B. (2010). IL-12 Initiates Tumor Rejection via Lymphoid Tissue-Inducer Cells Bearing the Natural Cytotoxicity Receptor NKp46. Nat. Immunol. 11, 1030–1038. doi:10.1038/ni.1947
Elliott, J. M., and Yokoyama, W. M. (2011). Unifying Concepts of MHC-dependent Natural Killer Cell Education. Trends Immunol. 32, 364–372. doi:10.1016/j.it.2011.06.001
Epelman, S., Lavine, K. J., Beaudin, A. E., Sojka, D. K., Carrero, J. A., Calderon, B., et al. (2014a). Embryonic and Adult-Derived Resident Cardiac Macrophages Are Maintained through Distinct Mechanisms at Steady State and during Inflammation. Immunity 40, 91–104. doi:10.1016/j.immuni.2013.11.019
Epelman, S., Lavine, K. J., and Randolph, G. J. (2014b). Origin and Functions of Tissue Macrophages. Immunity 41, 21–35. doi:10.1016/j.immuni.2014.06.013
Ercolano, G., Falquet, M., Vanoni, G., Trabanelli, S., and Jandus, C. (2019). ILC2s: New Actors in Tumor Immunity. Front. Immunol. 10, 2801. doi:10.3389/fimmu.2019.02801
Ercolano, G., Garcia-Garijo, A., Salomé, B., Gomez-Cadena, A., Vanoni, G., Mastelic-Gavillet, B., et al. (2020a). Immunosuppressive Mediators Impair Proinflammatory Innate Lymphoid Cell Function in Human Malignant Melanoma. Cancer Immunol. Res. 8, 556–564. doi:10.1158/2326-6066.cir-19-0504
Ercolano, G., Gomez-Cadena, A., Dumauthioz, N., Vanoni, G., Kreutzfeldt, M., Wyss, T., et al. (2021). PPARɣ Drives IL-33-dependent ILC2 Pro-tumoral Functions. Nat. Commun. 12, 2538. doi:10.1038/s41467-021-22764-2
Ercolano, G., Trabanelli, S., Jandus, C., and Jandus, P. (2022). IL-9R Loss in Innate Lymphoid Cell Type 2 (ILC2) Reflects Treg Impairment in Mastocytosis Patients. J. Investig. Allergol. Clin. Immunol. 0. doi:10.18176/jiaci.0815
Ercolano, G., Wyss, T., Salomé, B., Romero, P., Trabanelli, S., and Jandus, C. (2020b). Distinct and Shared Gene Expression for Human Innate versus Adaptive Helper Lymphoid Cells. J. Leukoc. Biol. 108, 723–737. doi:10.1002/jlb.5ma0120-209r
Fallarini, S., Paoletti, T., Orsi Battaglini, N., and Lombardi, G. (2012). Invariant NKT Cells Increase Drug-Induced Osteosarcoma Cell Death. Br. J. Pharmacol. 167, 1533–1549. doi:10.1111/j.1476-5381.2012.02108.x
Fallarino, F., Grohmann, U., You, S., Mcgrath, B. C., Cavener, D. R., Vacca, C., et al. (2006). The Combined Effects of Tryptophan Starvation and Tryptophan Catabolites Down-Regulate T Cell Receptor ζ-Chain and Induce a Regulatory Phenotype in Naive T Cells. J. Immunol. 176, 6752–6761. doi:10.4049/jimmunol.176.11.6752
Fan, X., and Rudensky, A. Y. (2016). Hallmarks of Tissue-Resident Lymphocytes. Cell 164, 1198–1211. doi:10.1016/j.cell.2016.02.048
Feng, S., Cheng, X., Zhang, L., Lu, X., Chaudhary, S., Teng, R., et al. (2018). Myeloid-derived Suppressor Cells Inhibit T Cell Activation through Nitrating LCK in Mouse Cancers. Proc. Natl. Acad. Sci. U.S.A. 115, 10094–10099. doi:10.1073/pnas.1800695115
Feng, Y.-H., Wu, C.-L., Tsao, C.-J., Chang, J.-G., Lu, P.-J., Yeh, K.-T., et al. (2011). Deregulated Expression of Sprouty2 and microRNA-21 in Human Colon Cancer: Correlation with the Clinical Stage of the Disease. Cancer Biol. Ther. 11, 111–121. doi:10.4161/cbt.11.1.13965
Fleet, J. C., Burcham, G. N., Calvert, R. D., Elzey, B. D., and Ratliff, T. L. (2020). 1α, 25 Dihydroxyvitamin D (1,25(OH)2D) Inhibits the T Cell Suppressive Function of Myeloid Derived Suppressor Cells (MDSC). J. Steroid Biochem. Mol. Biol. 198, 105557. doi:10.1016/j.jsbmb.2019.105557
Freud, A. G., Mundy-Bosse, B. L., Yu, J., and Caligiuri, M. A. (2017). The Broad Spectrum of Human Natural Killer Cell Diversity. Immunity 47, 820–833. doi:10.1016/j.immuni.2017.10.008
Fridman, W. H., Pagès, F., Sautès-Fridman, C., and Galon, J. (2012). The Immune Contexture in Human Tumours: Impact on Clinical Outcome. Nat. Rev. Cancer 12, 298–306. doi:10.1038/nrc3245
Friedrich, C., Taggenbrock, R. L. R. E., Doucet-Ladevèze, R., Golda, G., Moenius, R., Arampatzi, P., et al. (2021). Effector Differentiation Downstream of Lineage Commitment in ILC1s Is Driven by Hobit across Tissues. Nat. Immunol. 22, 1256–1267. doi:10.1038/s41590-021-01013-0
Fu, S., Zhang, N., Yopp, A. C., Chen, D., Mao, M., Chen, D., et al. (2004). TGF-beta Induces Foxp3 + T-Regulatory Cells from CD4 + CD25 - Precursors. Am. J. Transpl. 4, 1614–1627. doi:10.1111/j.1600-6143.2004.00566.x
Fuertes, M. B., Kacha, A. K., Kline, J., Woo, S.-R., Kranz, D. M., Murphy, K. M., et al. (2011). Host Type I IFN Signals Are Required for Antitumor CD8+ T Cell Responses through CD8α+ Dendritic Cells. J. Exp. Med. 208, 2005–2016. doi:10.1084/jem.20101159
Fujii, S.-i., Shimizu, K., Klimek, V., Geller, M. D., Nimer, S. D., and V. Dhodapkar, M. (2003). Severe and Selective Deficiency of Interferon-γ-Producing Invariant Natural Killer T Cells in Patients with Myelodysplastic Syndromes. Br. J. Haematol. 122, 617–622. doi:10.1046/j.1365-2141.2003.04465.x
Gabrilovich, D. I., and Nagaraj, S. (2009). Myeloid-derived Suppressor Cells as Regulators of the Immune System. Nat. Rev. Immunol. 9, 162–174. doi:10.1038/nri2506
Gabrilovich, D. I., Ostrand-Rosenberg, S., and Bronte, V. (2012). Coordinated Regulation of Myeloid Cells by Tumours. Nat. Rev. Immunol. 12, 253–268. doi:10.1038/nri3175
Galland, S., Vuille, J., Martin, P., Letovanec, I., Caignard, A., Fregni, G., et al. (2017). Tumor-derived Mesenchymal Stem Cells Use Distinct Mechanisms to Block the Activity of Natural Killer Cell Subsets. Cell Rep. 20, 2891–2905. doi:10.1016/j.celrep.2017.08.089
Galot, R., Le Tourneau, C., Saada-Bouzid, E., Daste, A., Even, C., Debruyne, P., et al. (2021). A Phase II Study of Monalizumab in Patients with Recurrent/metastatic Squamous Cell Carcinoma of the Head and Neck: The I1 Cohort of the EORTC-HNCG-1559 UPSTREAM Trial. Eur. J. Cancer 158, 17–26. doi:10.1016/j.ejca.2021.09.003
Galsky, M. D., Vogelzang, N. J., Conkling, P., Raddad, E., Polzer, J., Roberson, S., et al. (2014). A Phase I Trial of LY2510924, a CXCR4 Peptide Antagonist, in Patients with Advanced Cancer. Clin. Cancer Res. 20, 3581–3588. doi:10.1158/1078-0432.ccr-13-2686
Ganguly, D., Haak, S., Sisirak, V., and Reizis, B. (2013). The Role of Dendritic Cells in Autoimmunity. Nat. Rev. Immunol. 13, 566–577. doi:10.1038/nri3477
Gao, Y., Souza-Fonseca-Guimaraes, F., Bald, T., Ng, S. S., Young, A., Ngiow, S. F., et al. (2017). Tumor Immunoevasion by the Conversion of Effector NK Cells into Type 1 Innate Lymphoid Cells. Nat. Immunol. 18, 1004–1015. doi:10.1038/ni.3800
Garcia-Chagollan, M., Carranza-Torres, I. E., Carranza-Rosales, P., Guzmán-Delgado, N. E., Ramírez-Montoya, H., Martínez-Silva, M. G., et al. (2018). Expression of NK Cell Surface Receptors in Breast Cancer Tissue as Predictors of Resistance to Antineoplastic Treatment. Technol. cancer Res. Treat. 17, 1533033818764499. doi:10.1177/1533033818764499
Gardner, A., De Mingo Pulido, Á., and Ruffell, B. (2020). Dendritic Cells and Their Role in Immunotherapy. Front. Immunol. 11, 924. doi:10.3389/fimmu.2020.00924
Geissmann, F., Manz, M. G., Jung, S., Sieweke, M. H., Merad, M., and Ley, K. (2010). Development of Monocytes, Macrophages, and Dendritic Cells. Science 327, 656–661. doi:10.1126/science.1178331
Gerlini, G., Urso, C., Mariotti, G., Di Gennaro, P., Palli, D., Brandani, P., et al. (2007). Plasmacytoid Dendritic Cells Represent a Major Dendritic Cell Subset in Sentinel Lymph Nodes of Melanoma Patients and Accumulate in Metastatic Nodes. Clin. Immunol. 125, 184–193. doi:10.1016/j.clim.2007.07.018
Germano, G., Frapolli, R., Belgiovine, C., Anselmo, A., Pesce, S., Liguori, M., et al. (2013). Role of Macrophage Targeting in the Antitumor Activity of Trabectedin. Cancer Cell 23, 249–262. doi:10.1016/j.ccr.2013.01.008
Ghaedi, M., and Ohashi, P. S. (2020). ILC Transdifferentiation: Roles in Cancer Progression. Cell Res. 30, 562–563. doi:10.1038/s41422-020-0326-5
Ghinnagow, R., Cruz, L. J., Macho-Fernandez, E., Faveeuw, C., and Trottein, F. (2017). Enhancement of Adjuvant Functions of Natural Killer T Cells Using Nanovector Delivery Systems: Application in Anticancer Immune Therapy. Front. Immunol. 8, 879. doi:10.3389/fimmu.2017.00879
Ghiringhelli, F., Ménard, C., Terme, M., Flament, C., Taieb, J., Chaput, N., et al. (2005). CD4+CD25+ Regulatory T Cells Inhibit Natural Killer Cell Functions in a Transforming Growth Factor-β-dependent Manner. J. Exp. Med. 202, 1075–1085. doi:10.1084/jem.20051511
Ghobrial, I. M., Liu, C.-J., Redd, R. A., Perez, R. P., Baz, R., Zavidij, O., et al. (2020). A Phase Ib/II Trial of the First-In-Class Anti-CXCR4 Antibody Ulocuplumab in Combination with Lenalidomide or Bortezomib Plus Dexamethasone in Relapsed Multiple Myeloma. Clin. Cancer Res. 26, 344–353. doi:10.1158/1078-0432.ccr-19-0647
Giallongo, C., Parrinello, N. L., La Cava, P., Camiolo, G., Romano, A., Scalia, M., et al. (2018). Monocytic Myeloid-Derived Suppressor Cells as Prognostic Factor in Chronic Myeloid Leukaemia Patients Treated with Dasatinib. J. Cell Mol. Med. 22, 1070–1080. doi:10.1111/jcmm.13326
Gilliet, M., Cao, W., and Liu, Y.-J. (2008). Plasmacytoid Dendritic Cells: Sensing Nucleic Acids in Viral Infection and Autoimmune Diseases. Nat. Rev. Immunol. 8, 594–606. doi:10.1038/nri2358
Goff, S. L., and Danforth, D. N. (2021). The Role of Immune Cells in Breast Tissue and Immunotherapy for the Treatment of Breast Cancer. Clin. Breast Cancer 21, e63–e73. doi:10.1016/j.clbc.2020.06.011
Gomez-Cadena, A., Romero, P., Trabanelli, S., and Jandus, C. (2020). Detecting and Analyzing Murine Innate Lymphoid Cells. Methods Enzymol. 631, 329–342. doi:10.1016/bs.mie.2019.10.022
Gordon, E. M., Sankhala, K. K., Chawla, N., and Chawla, S. P. (2016). Trabectedin for Soft Tissue Sarcoma: Current Status and Future Perspectives. Adv. Ther. 33, 1055–1071. doi:10.1007/s12325-016-0344-3
Gorelik, L., Constant, S., and Flavell, R. A. (2002). Mechanism of Transforming Growth Factor β-induced Inhibition of T Helper Type 1 Differentiation. J. Exp. Med. 195, 1499–1505. doi:10.1084/jem.20012076
Gray, J. I., and Farber, D. L. (2022). Tissue-Resident Immune Cells in Humans. Annu. Rev. Immunol. 40, 195–220. doi:10.1146/annurev-immunol-093019-112809
Greenhalgh, A. D., Zarruk, J. G., Healy, L. M., Baskar Jesudasan, S. J., Jhelum, P., Salmon, C. K., et al. (2018). Peripherally Derived Macrophages Modulate Microglial Function to Reduce Inflammation after CNS Injury. PLoS Biol. 16, e2005264. doi:10.1371/journal.pbio.2005264
Groth, C., Hu, X., Weber, R., Fleming, V., Altevogt, P., Utikal, J., et al. (2019). Immunosuppression Mediated by Myeloid-Derived Suppressor Cells (MDSCs) during Tumour Progression. Br. J. Cancer 120, 16–25. doi:10.1038/s41416-018-0333-1
Guan, W., Hu, J., Yang, L., Tan, P., Tang, Z., West, B. L., et al. (2019). Inhibition of TAMs Improves the Response to Docetaxel in Castration-Resistant Prostate Cancer. Endocr. Relat. Cancer 26, 131–140. doi:10.1530/erc-18-0284
Guilliams, M., Bruhns, P., Saeys, Y., Hammad, H., and Lambrecht, B. N. (2014). The Function of Fcγ Receptors in Dendritic Cells and Macrophages. Nat. Rev. Immunol. 14, 94–108. doi:10.1038/nri3582
Gulubova, M., Manolova, I., Kyurkchiev, D., Julianov, A., and Altunkova, I. (2009). Decrease in Intrahepatic CD56+ Lymphocytes in Gastric and Colorectal Cancer Patients with Liver Metastases. Apmis 117, 870–879. doi:10.1111/j.1600-0463.2009.02547.x
Gunassekaran, G. R., Poongkavithai Vadevoo, S. M., Baek, M.-C., and Lee, B. (2021). M1 Macrophage Exosomes Engineered to Foster M1 Polarization and Target the IL-4 Receptor Inhibit Tumor Growth by Reprogramming Tumor-Associated Macrophages into M1-like Macrophages. Biomaterials 278, 121137. doi:10.1016/j.biomaterials.2021.121137
Hanahan, D. (2022). Hallmarks of Cancer: New Dimensions. Cancer Discov. 12, 31–46. doi:10.1158/2159-8290.cd-21-1059
Haniffa, M., Collin, M., and Ginhoux, F. (2013). Ontogeny and Functional Specialization of Dendritic Cells in Human and Mouse. Adv. Immunol. 120, 1–49. doi:10.1016/b978-0-12-417028-5.00001-6
Haniffa, M., Shin, A., Bigley, V., Mcgovern, N., Teo, P., See, P., et al. (2012). Human Tissues Contain CD141hi Cross-Presenting Dendritic Cells with Functional Homology to Mouse CD103+ Nonlymphoid Dendritic Cells. Immunity 37, 60–73. doi:10.1016/j.immuni.2012.04.012
Harari, O., and Liao, J. (2004). Inhibition of MHC II Gene Transcription by Nitric Oxide and Antioxidants. Cpd 10, 893–898. doi:10.2174/1381612043452893
Harel-Bellan, A., Quillet, A., Marchiol, C., Demars, R., Tursz, T., and Fradelizi, D. (1986). Natural Killer Susceptibility of Human Cells May Be Regulated by Genes in the HLA Region on Chromosome 6. Proc. Natl. Acad. Sci. U.S.A. 83, 5688–5692. doi:10.1073/pnas.83.15.5688
Hartmann, E., Wollenberg, B., Rothenfusser, S., Wagner, M., Wellisch, D., Mack, B., et al. (2003). Identification and Functional Analysis of Tumor-Infiltrating Plasmacytoid Dendritic Cells in Head and Neck Cancer. Cancer Res. 63, 6478–6487.
Hashimoto, D., Chow, A., Noizat, C., Teo, P., Beasley, M. B., Leboeuf, M., et al. (2013). Tissue-resident Macrophages Self-Maintain Locally throughout Adult Life with Minimal Contribution from Circulating Monocytes. Immunity 38, 792–804. doi:10.1016/j.immuni.2013.04.004
Heath, W. R., and Carbone, F. R. (2013). The Skin-Resident and Migratory Immune System in Steady State and Memory: Innate Lymphocytes, Dendritic Cells and T Cells. Nat. Immunol. 14, 978–985. doi:10.1038/ni.2680
Heo, Y.-J., Joo, Y.-B., Oh, H.-J., Park, M.-K., Heo, Y.-M., Cho, M.-L., et al. (2010). IL-10 Suppresses Th17 Cells and Promotes Regulatory T Cells in the CD4+ T Cell Population of Rheumatoid Arthritis Patients. Immunol. Lett. 127, 150–156. doi:10.1016/j.imlet.2009.10.006
Herzog, C., Pons Garcia, L., Keatinge, M., Greenald, D., Moritz, C., Peri, F., et al. (2019). Rapid Clearance of Cellular Debris by Microglia Limits Secondary Neuronal Cell Death after Brain Injury In Vivo. Development 146, dev174698. doi:10.1242/dev.174698
Hix, L. M., Shi, Y. H., Brutkiewicz, R. R., Stein, P. L., Wang, C.-R., and Zhang, M. (2011). CD1d-expressing Breast Cancer Cells Modulate NKT Cell-Mediated Antitumor Immunity in a Murine Model of Breast Cancer Metastasis. PLoS One 6, e20702. doi:10.1371/journal.pone.0020702
Hodi, F. S., O'day, S. J., Mcdermott, D. F., Weber, R. W., Sosman, J. A., Haanen, J. B., et al. (2010). Improved Survival with Ipilimumab in Patients with Metastatic Melanoma. N. Engl. J. Med. 363, 711–723. doi:10.1056/nejmoa1003466
Hughes, R., Qian, B.-Z., Rowan, C., Muthana, M., Keklikoglou, I., Olson, O. C., et al. (2015). Perivascular M2 Macrophages Stimulate Tumor Relapse after Chemotherapy. Cancer Res. 75, 3479–3491. doi:10.1158/0008-5472.can-14-3587
Iclozan, C., Antonia, S., Chiappori, A., Chen, D.-T., and Gabrilovich, D. (2013). Therapeutic Regulation of Myeloid-Derived Suppressor Cells and Immune Response to Cancer Vaccine in Patients with Extensive Stage Small Cell Lung Cancer. Cancer Immunol. Immunother. 62, 909–918. doi:10.1007/s00262-013-1396-8
Islam, R., Pupovac, A., Evtimov, V., Boyd, N., Shu, R., Boyd, R., et al. (2021). Enhancing a Natural Killer: Modification of NK Cells for Cancer Immunotherapy. Cells 10. doi:10.3390/cells10051058
Iyoda, T., Yamasaki, S., Hidaka, M., Kawano, F., Abe, Y., Suzuki, K., et al. (2018). Amelioration of NK Cell Function Driven by Vα24 + Invariant NKT Cell Activation in Multiple Myeloma. Clin. Immunol. 187, 76–84. doi:10.1016/j.clim.2017.10.007
Izawa, S., Kono, K., Mimura, K., Kawaguchi, Y., Watanabe, M., Maruyama, T., et al. (2011). H2O2 Production within Tumor Microenvironment Inversely Correlated with Infiltration of CD56dim NK Cells in Gastric and Esophageal Cancer: Possible Mechanisms of NK Cell Dysfunction. Cancer Immunol. Immunother. 60, 1801–1810. doi:10.1007/s00262-011-1082-7
Jacquelot, N., Seillet, C., Vivier, E., and Belz, G. T. (2022). Innate Lymphoid Cells and Cancer. Nat. Immunol. 23, 371–379. doi:10.1038/s41590-022-01127-z
Jacquelot, N., Seillet, C., Wang, M., Pizzolla, A., Liao, Y., Hediyeh-Zadeh, S., et al. (2021b). Blockade of the Co-inhibitory Molecule PD-1 Unleashes ILC2-dependent Antitumor Immunity in Melanoma. Nat. Immunol. 22, 851–864. doi:10.1038/s41590-021-00943-z
Jacquelot, N., Tellier, J., Nutt, S. I., and Belz, G. T. (2021a). Tertiary Lymphoid Structures and B Lymphocytes in Cancer Prognosis and Response to Immunotherapies. Oncoimmunology 10, 1900508. doi:10.1080/2162402x.2021.1900508
Jennings, M. R., Munn, D., and Blazeck, J. (2021). Immunosuppressive Metabolites in Tumoral Immune Evasion: Redundancies, Clinical Efforts, and Pathways Forward. J. Immunother. Cancer 9. doi:10.1136/jitc-2021-003013
Jiang, F., Liu, H., Liu, Z., Yan, S., Chen, J., Shao, Q., et al. (2018). Deficient Invariant Natural Killer T Cells Had Impaired Regulation on Osteoclastogenesis in Myeloma Bone Disease. J. Cell Mol. Med. 22, 2706–2716. doi:10.1111/jcmm.13554
Jiang, H., Gebhardt, C., Umansky, L., Beckhove, P., Schulze, T. J., Utikal, J., et al. (2015). Elevated Chronic Inflammatory Factors and Myeloid-Derived Suppressor Cells Indicate Poor Prognosis in Advanced Melanoma Patients. Int. J. Cancer 136, 2352–2360. doi:10.1002/ijc.29297
Johnson, D. E., O'keefe, R. A., and Grandis, J. R. (2018). Targeting the IL-6/JAK/STAT3 Signalling axis in Cancer. Nat. Rev. Clin. Oncol. 15, 234–248. doi:10.1038/nrclinonc.2018.8
Jordan, V. A., Lunos, S., Sieger, G., Horvath, K. J., Cohen, S., and Misono, S. (2020). Association of Voice and Mental Health Diagnoses with Differences in Voice‐related Care Utilization. Laryngoscope 130, 1496–1502. doi:10.1002/lary.28277
Jovanovic, I. P., Pejnovic, N. N., Radosavljevic, G. D., Pantic, J. M., Milovanovic, M. Z., Arsenijevic, N. N., et al. (2014). Interleukin-33/ST2 axis Promotes Breast Cancer Growth and Metastases by Facilitating Intratumoral Accumulation of Immunosuppressive and Innate Lymphoid Cells. Int. J. Cancer 134, 1669–1682. doi:10.1002/ijc.28481
Judge, S. J., Darrow, M. A., Thorpe, S. W., Gingrich, A. A., O'donnell, E. F., Bellini, A. R., et al. (2020). Analysis of Tumor-Infiltrating NK and T Cells Highlights IL-15 Stimulation and TIGIT Blockade as a Combination Immunotherapy Strategy for Soft Tissue Sarcomas. J. Immunother. Cancer 8. doi:10.1136/jitc-2020-001355
Kalbasi, A., Komar, C., Tooker, G. M., Liu, M., Lee, J. W., Gladney, W. L., et al. (2017). Tumor-Derived CCL2 Mediates Resistance to Radiotherapy in Pancreatic Ductal Adenocarcinoma. Clin. Cancer Res. 23, 137–148. doi:10.1158/1078-0432.ccr-16-0870
Kaneda, M. M., Cappello, P., Nguyen, A. V., Ralainirina, N., Hardamon, C. R., Foubert, P., et al. (2016). Macrophage PI3Kγ Drives Pancreatic Ductal Adenocarcinoma Progression. Cancer Discov. 6, 870–885. doi:10.1158/2159-8290.cd-15-1346
Kerdidani, D., Chouvardas, P., Arjo, A. R., Giopanou, I., Ntaliarda, G., Guo, Y. A., et al. (2019). Wnt1 Silences Chemokine Genes in Dendritic Cells and Induces Adaptive Immune Resistance in Lung Adenocarcinoma. Nat. Commun. 10, 1405. doi:10.1038/s41467-019-09370-z
Kim, C. W., Kim, K.-D., and Lee, H. K. (2021). The Role of Dendritic Cells in Tumor Microenvironments and Their Uses as Therapeutic Targets. BMB Rep. 54, 31–43. doi:10.5483/bmbrep.2021.54.1.224
Klose, C. S. N., Flach, M., Möhle, L., Rogell, L., Hoyler, T., Ebert, K., et al. (2014). Differentiation of Type 1 ILCs from a Common Progenitor to All Helper-like Innate Lymphoid Cell Lineages. Cell 157, 340–356. doi:10.1016/j.cell.2014.03.030
Koo, K. C., Shim, D. H., Yang, C. M., Lee, S.-B., Kim, S. M., Shin, T. Y., et al. (2013). Reduction of the CD16−CD56bright NK Cell Subset Precedes NK Cell Dysfunction in Prostate Cancer. PLoS One 8, e78049. doi:10.1371/journal.pone.0078049
Kranz, L. M., Diken, M., Haas, H., Kreiter, S., Loquai, C., Reuter, K. C., et al. (2016). Systemic RNA Delivery to Dendritic Cells Exploits Antiviral Defence for Cancer Immunotherapy. Nature 534, 396–401. doi:10.1038/nature18300
Labidi-Galy, S. I., Sisirak, V., Meeus, P., Gobert, M., Treilleux, I., Bajard, A., et al. (2011). Quantitative and Functional Alterations of Plasmacytoid Dendritic Cells Contribute to Immune Tolerance in Ovarian Cancer. Cancer Res. 71, 5423–5434. doi:10.1158/0008-5472.can-11-0367
Lamichhane, P., Karyampudi, L., Shreeder, B., Krempski, J., Bahr, D., Daum, J., et al. (2017). IL10 Release upon PD-1 Blockade Sustains Immunosuppression in Ovarian Cancer. Cancer Res. 77, 6667–6678. doi:10.1158/0008-5472.can-17-0740
Lande, R., Gregorio, J., Facchinetti, V., Chatterjee, B., Wang, Y.-H., Homey, B., et al. (2007). Plasmacytoid Dendritic Cells Sense Self-DNA Coupled with Antimicrobial Peptide. Nature 449, 564–569. doi:10.1038/nature06116
Le Mercier, I., Poujol, D., Sanlaville, A., Sisirak, V., Gobert, M., Durand, I., et al. (2013). Tumor Promotion by Intratumoral Plasmacytoid Dendritic Cells Is Reversed by TLR7 Ligand Treatment. Cancer Res. 73, 4629–4640. doi:10.1158/0008-5472.can-12-3058
Lechner, M. G., Liebertz, D. J., and Epstein, A. L. (2010). Characterization of Cytokine-Induced Myeloid-Derived Suppressor Cells from Normal Human Peripheral Blood Mononuclear Cells. J. I. 185, 2273–2284. doi:10.4049/jimmunol.1000901
Lechner, M. G., Megiel, C., Russell, S. M., Bingham, B., Arger, N., Woo, T., et al. (2011). Functional Characterization of Human Cd33+ and Cd11b+ Myeloid-Derived Suppressor Cell Subsets Induced from Peripheral Blood Mononuclear Cells Co-cultured with a Diverse Set of Human Tumor Cell Lines. J. Transl. Med. 9, 90. doi:10.1186/1479-5876-9-90
Lee, J.-C., Lee, K.-M., Kim, D.-W., and Heo, D. S. (2004). Elevated TGF-Β1 Secretion and Down-Modulation of NKG2D Underlies Impaired NK Cytotoxicity in Cancer Patients. J. Immunol. 172, 7335–7340. doi:10.4049/jimmunol.172.12.7335
Lee, P. T., Benlagha, K., Teyton, L., and Bendelac, A. (2002). Distinct Functional Lineages of Human Vα24 Natural Killer T Cells. J. Exp. Med. 195, 637–641. doi:10.1084/jem.20011908
Lee-Chang, C., Rashidi, A., Miska, J., Zhang, P., Pituch, K. C., Hou, D., et al. (2019). Myeloid-Derived Suppressive Cells Promote B Cell-Mediated Immunosuppression via Transfer of PD-L1 in Glioblastoma. Cancer Immunol. Res. 7, 1928–1943. doi:10.1158/2326-6066.cir-19-0240
Levings, M. K., Gregori, S., Tresoldi, E., Cazzaniga, S., Bonini, C., and Roncarolo, M. G. (2005). Differentiation of Tr1 Cells by Immature Dendritic Cells Requires IL-10 but Not CD25+CD4+ Tr Cells. Blood 105, 1162–1169. doi:10.1182/blood-2004-03-1211
Levring, T. B., Kongsbak, M., Rode, A. K. O., Woetmann, A., Ødum, N., Bonefeld, C. M., et al. (2015). Human CD4+ T Cells Require Exogenous Cystine for Glutathione and DNA Synthesis. Oncotarget 6, 21853–21864. doi:10.18632/oncotarget.5213
Li, G., Wu, K., Tao, K., Lu, X., Ma, J., Mao, Z., et al. (2015). Vasoactive Intestinal Peptide Induces CD14+HLA-DR−/low Myeloid-Derived Suppressor Cells in Gastric Cancer. Mol. Med. Rep. 12, 760–768. doi:10.3892/mmr.2015.3374
Li, H., Han, Y., Guo, Q., Zhang, M., and Cao, X. (2009). Cancer-Expanded Myeloid-Derived Suppressor Cells Induce Anergy of NK Cells through Membrane-Bound TGF-Β1. J. Immunol. 182, 240–249. doi:10.4049/jimmunol.182.1.240
Li, H. S., Liu, C., Xiao, Y., Chu, F., Liang, X., Peng, W., et al. (2016). Bypassing STAT3-Mediated Inhibition of the Transcriptional Regulator ID2 Improves the Antitumor Efficacy of Dendritic Cells. Sci. Signal 9, ra94. doi:10.1126/scisignal.aaf3957
Li, K., Shi, H., Zhang, B., Ou, X., Ma, Q., Chen, Y., et al. (2021). Myeloid-derived Suppressor Cells as Immunosuppressive Regulators and Therapeutic Targets in Cancer. Sig Transduct. Target Ther. 6, 362. doi:10.1038/s41392-021-00670-9
Li, R., Jiang, X. X., Zhang, L. F., Liu, X. M., Hu, T. Z., Xia, X. J., et al. (2017). Group 2 Innate Lymphoid Cells Are Involved in Skewed Type 2 Immunity of Gastric Diseases Induced by Helicobacter pylori Infection. Mediat. Inflamm. 2017, 4927964. doi:10.1155/2017/4927964
Li, Y.-C., Zhou, Q., Song, Q.-K., Wang, R.-B., Lyu, S., Guan, X., et al. (2020). Overexpression of an Immune Checkpoint (CD155) in Breast Cancer Associated with Prognostic Significance and Exhausted Tumor-Infiltrating Lymphocytes: a Cohort Study. J. Immunol. Res. 2020, 3948928. doi:10.1155/2020/3948928
Lim, A. I., Li, Y., Lopez-Lastra, S., Stadhouders, R., Paul, F., Casrouge, A., et al. (2017). Systemic Human ILC Precursors Provide a Substrate for Tissue ILC Differentiation. Cell 168, 1086–1100. doi:10.1016/j.cell.2017.02.021
Lim, H. X., Kim, T. S., and Poh, C. L. (2020). Understanding the Differentiation, Expansion, Recruitment and Suppressive Activities of Myeloid-Derived Suppressor Cells in Cancers. Int. J. Mol. Sci. 21. doi:10.3390/ijms21103599
Lim, S. Y., Yuzhalin, A. E., Gordon-Weeks, A. N., and Muschel, R. J. (2016). Targeting the CCL2-CCR2 Signaling axis in Cancer Metastasis. Oncotarget 7, 28697–28710. doi:10.18632/oncotarget.7376
Liu, D., Song, L., Brawley, V. S., Robison, N., Wei, J., Gao, X., et al. (2013). Medulloblastoma Expresses CD1d and Can Be Targeted for Immunotherapy with NKT Cells. Clin. Immunol. 149, 55–64. doi:10.1016/j.clim.2013.06.005
Liu, H., Chen, L., Liu, J., Meng, H., Zhang, R., Ma, L., et al. (2017a). Co-delivery of Tumor-Derived Exosomes with Alpha-Galactosylceramide on Dendritic Cell-Based Immunotherapy for Glioblastoma. Cancer Lett. 411, 182–190. doi:10.1016/j.canlet.2017.09.022
Liu, X., Li, L., Si, F., Huang, L., Zhao, Y., Zhang, C., et al. (2021). NK and NKT Cells Have Distinct Properties and Functions in Cancer. Oncogene 40, 4521–4537. doi:10.1038/s41388-021-01880-9
Liu, Y.-J. (2005). IPC: Professional Type 1 Interferon-Producing Cells and Plasmacytoid Dendritic Cell Precursors. Annu. Rev. Immunol. 23, 275–306. doi:10.1146/annurev.immunol.23.021704.115633
Liu, Y., Cheng, Y., Xu, Y., Wang, Z., Du, X., Li, C., et al. (2017b). Increased Expression of Programmed Cell Death Protein 1 on NK Cells Inhibits NK-Cell-Mediated Anti-tumor Function and Indicates Poor Prognosis in Digestive Cancers. Oncogene 36, 6143–6153. doi:10.1038/onc.2017.209
Liu, Y., Song, Y., Lin, D., Lei, L., Mei, Y., Jin, Z., et al. (2019). NCR− Group 3 Innate Lymphoid Cells Orchestrate IL-23/IL-17 axis to Promote Hepatocellular Carcinoma Development. EBioMedicine 41, 333–344. doi:10.1016/j.ebiom.2019.02.050
Lo, A. S. Y., Ma, Q., Liu, D. L., and Junghans, R. P. (2010). Anti-GD3 Chimeric sFv-Cd28/t-Cell Receptor ζ Designer T Cells for Treatment of Metastatic Melanoma and Other Neuroectodermal Tumors. Clin. Cancer Res. 16, 2769–2780. doi:10.1158/1078-0432.ccr-10-0043
Locati, M., Curtale, G., and Mantovani, A. (2020). Diversity, Mechanisms, and Significance of Macrophage Plasticity. Annu. Rev. Pathol. Mech. Dis. 15, 123–147. doi:10.1146/annurev-pathmechdis-012418-012718
Lopes, A. M. M., Michelin, M. A., and Murta, E. F. C. (2017). Monocyte-derived Dendritic Cells from Patients with Cervical Intraepithelial Lesions. Oncol. Lett. 13, 1456–1462. doi:10.3892/ol.2017.5595
Lorenzo-Herrero, S., López-Soto, A., Sordo-Bahamonde, C., Gonzalez-Rodriguez, A. P., Vitale, M., and Gonzalez, S. (2019). NK Cell-Based Immunotherapy in Cancer Metastasis. Cancers 11, 29.
Loyher, P.-L., Hamon, P., Laviron, M., Meghraoui-Kheddar, A., Goncalves, E., Deng, Z., et al. (2018). Macrophages of Distinct Origins Contribute to Tumor Development in the Lung. J. Exp. Med. 215, 2536–2553. doi:10.1084/jem.20180534
Ma, T., Renz, B. W., Ilmer, M., Koch, D., Yang, Y., Werner, J., et al. (2022). Myeloid-Derived Suppressor Cells in Solid Tumors. Cells 11. doi:10.3390/cells11020310
Mäkitie, A., Tuokkola, I., Laurell, G., Mäkitie, O., Olsen, K., Takes, R. P., et al. (2020). Vitamin D in Head and Neck Cancer: a Systematic Review. Curr. Oncol. Rep. 23, 5. doi:10.1007/s11912-020-00996-7
Malmberg, K.-J., Carlsten, M., Björklund, A., Sohlberg, E., Bryceson, Y. T., and Ljunggren, H.-G. (2017). Natural Killer Cell-Mediated Immunosurveillance of Human Cancer. Semin. Immunol. 2017, 20–29. doi:10.1016/j.smim.2017.08.002
Mamessier, E., Sylvain, A., Thibult, M.-L., Houvenaeghel, G., Jacquemier, J., Castellano, R., et al. (2011). Human Breast Cancer Cells Enhance Self Tolerance by Promoting Evasion from NK Cell Antitumor Immunity. J. Clin. Invest. 121, 3609–3622. doi:10.1172/jci45816
Mandruzzato, S., Brandau, S., Britten, C. M., Bronte, V., Damuzzo, V., Gouttefangeas, C., et al. (2016). Toward Harmonized Phenotyping of Human Myeloid-Derived Suppressor Cells by Flow Cytometry: Results from an Interim Study. Cancer Immunol. Immunother. 65, 161–169. doi:10.1007/s00262-015-1782-5
Mantovani, A., Sozzani, S., Locati, M., Allavena, P., and Sica, A. (2002). Macrophage Polarization: Tumor-Associated Macrophages as a Paradigm for Polarized M2 Mononuclear Phagocytes. Trends Immunol. 23, 549–555. doi:10.1016/s1471-4906(02)02302-5
Mantovani, S., Oliviero, B., Lombardi, A., Varchetta, S., Mele, D., Sangiovanni, A., et al. (2019). Deficient Natural Killer Cell NKp30-Mediated Function and Altered NCR3 Splice Variants in Hepatocellular Carcinoma. Hepatology 69, 1165–1179. doi:10.1002/hep.30235
Marsh, S. G. E., Parham, P., Dupont, B., Geraghty, D. E., Trowsdale, J., Middleton, D., et al. (2003). Killer-cell Immunoglobulin-like Receptor (KIR) Nomenclature Report, 2002. Immunogenetics 55, 220–226. doi:10.1007/s00251-003-0571-z
Matlack, R., Yeh, K., Rosini, L., Gonzalez, D., Taylor, J., Silberman, D., et al. (2006). Peritoneal Macrophages Suppress T-Cell Activation by Amino Acid Catabolism. Immunology 117, 386–395. doi:10.1111/j.1365-2567.2005.02312.x
Mcewen-Smith, R. M., Salio, M., and Cerundolo, V. (2015). The Regulatory Role of Invariant NKT Cells in Tumor Immunity. Cancer Immunol. Res. 3, 425–435. doi:10.1158/2326-6066.cir-15-0062
Mckarns, S. C., Schwartz, R. H., and Kaminski, N. E. (2004). Smad3 Is Essential for TGF-Β1 to Suppress IL-2 Production and TCR-Induced Proliferation, but Not IL-2-Induced Proliferation. J. Immunol. 172, 4275–4284. doi:10.4049/jimmunol.172.7.4275
Melo-Gonzalez, F., and Hepworth, M. R. (2017). Functional and Phenotypic Heterogeneity of Group 3 Innate Lymphoid Cells. Immunology 150, 265–275. doi:10.1111/imm.12697
Merad, M., Sathe, P., Helft, J., Miller, J., and Mortha, A. (2013). The Dendritic Cell Lineage: Ontogeny and Function of Dendritic Cells and Their Subsets in the Steady State and the Inflamed Setting. Annu. Rev. Immunol. 31, 563–604. doi:10.1146/annurev-immunol-020711-074950
Metelitsa, L. S., Weinberg, K. I., Emanuel, P. D., and Seeger, R. C. (2003). Expression of CD1d by Myelomonocytic Leukemias Provides a Target for Cytotoxic NKT Cells. Leukemia 17, 1068–1077. doi:10.1038/sj.leu.2402943
Metelitsa, L. S., Wu, H.-W., Wang, H., Yang, Y., Warsi, Z., Asgharzadeh, S., et al. (2004). Natural Killer T Cells Infiltrate Neuroblastomas Expressing the Chemokine CCL2. J. Exp. Med. 199, 1213–1221. doi:10.1084/jem.20031462
Mills, C. D., Kincaid, K., Alt, J. M., Heilman, M. J., and Hill, A. M. (2000). M-1/M-2 Macrophages and the Th1/Th2 Paradigm. J. Immunol. 164, 6166–6173. doi:10.4049/jimmunol.164.12.6166
Min, Y., Li, J., Qu, P., and Lin, P. C. (2017). C/EBP-δ Positively Regulates MDSC Expansion and Endothelial VEGFR2 Expression in Tumor Development. Oncotarget 8, 50582–50593. doi:10.18632/oncotarget.16410
Mjösberg, J., and Spits, H. (2016). Human Innate Lymphoid Cells. J. Allergy Clin. Immunol. 138, 1265–1276. doi:10.1016/j.jaci.2016.09.009
Mlecnik, B., Bindea, G., Angell, H. K., Sasso, M. S., Obenauf, A. C., Fredriksen, T., et al. (2014). Functional Network Pipeline Reveals Genetic Determinants Associated with In Situ Lymphocyte Proliferation and Survival of Cancer Patients. Sci. Transl. Med. 6, 228ra37. doi:10.1126/scitranslmed.3007240
Molling, J. W., Langius, J. A. E., Langendijk, J. A., Leemans, C. R., Bontkes, H. J., Van Der Vliet, H. J. J., et al. (2007). Low Levels of Circulating Invariant Natural Killer T Cells Predict Poor Clinical Outcome in Patients with Head and Neck Squamous Cell Carcinoma. Jco 25, 862–868. doi:10.1200/jco.2006.08.5787
Molon, B., Ugel, S., Del Pozzo, F., Soldani, C., Zilio, S., Avella, D., et al. (2011). Chemokine Nitration Prevents Intratumoral Infiltration of Antigen-specific T Cells. J. Exp. Med. 208, 1949–1962. doi:10.1084/jem.20101956
Moral, J. A., Leung, J., Rojas, L. A., Ruan, J., Zhao, J., Sethna, Z., et al. (2020). ILC2s Amplify PD-1 Blockade by Activating Tissue-specific Cancer Immunity. Nature 579, 130–135. doi:10.1038/s41586-020-2015-4
Moretta, A., Marcenaro, E., Parolini, S., Ferlazzo, G., and Moretta, L. (2008). NK Cells at the Interface between Innate and Adaptive Immunity. Cell Death Differ. 15, 226–233. doi:10.1038/sj.cdd.4402170
Muhammad Ali Tahir, S., Cheng, O., Shaulov, A., Koezuka, Y., Bubley, G. J., Wilson, S. B., et al. (2001). Loss of IFN-γ Production by Invariant NK T Cells in Advanced Cancer. J. Immunol. 167, 4046–4050. doi:10.4049/jimmunol.167.7.4046
Munn, D., and Mellor, A. (2003). Macrophages and the Regulation of Self-Reactive T Cells. Cpd 9, 257–264. doi:10.2174/1381612033392026
Nagaraj, S., Gupta, K., Pisarev, V., Kinarsky, L., Sherman, S., Kang, L., et al. (2007). Altered Recognition of Antigen Is a Mechanism of CD8+ T Cell Tolerance in Cancer. Nat. Med. 13, 828–835. doi:10.1038/nm1609
Najafi, M., Hashemi Goradel, N., Farhood, B., Salehi, E., Nashtaei, M. S., Khanlarkhani, N., et al. (2019). Macrophage Polarity in Cancer: A Review. J. Cell Biochem. 120, 2756–2765. doi:10.1002/jcb.27646
Najera Chuc, A. E., Cervantes, L. A. M., Retiguin, F. P., Ojeda, J. V., and Maldonado, E. R. (2012). Low Number of Invariant NKT Cells Is Associated with Poor Survival in Acute Myeloid Leukemia. J. Cancer Res. Clin. Oncol. 138, 1427–1432. doi:10.1007/s00432-012-1251-x
Nakai, R., Maniwa, Y., Tanaka, Y., Nishio, W., Yoshimura, M., Okita, Y., et al. (2010). Overexpression of Necl-5 Correlates with Unfavorable Prognosis in Patients with Lung Adenocarcinoma. Cancer Sci. 101, 1326–1330. doi:10.1111/j.1349-7006.2010.01530.x
Nakamura, K., and Smyth, M. J. (2020). Myeloid Immunosuppression and Immune Checkpoints in the Tumor Microenvironment. Cell Mol. Immunol. 17, 1–12. doi:10.1038/s41423-019-0306-1
Nielsen, S. R., Quaranta, V., Linford, A., Emeagi, P., Rainer, C., Santos, A., et al. (2016). Macrophage-secreted Granulin Supports Pancreatic Cancer Metastasis by Inducing Liver Fibrosis. Nat. Cell Biol. 18, 549–560. doi:10.1038/ncb3340
Nowak, M., Arredouani, M. S., Tun-Kyi, A., Schmidt-Wolf, I., Sanda, M. G., Balk, S. P., et al. (2010). Defective NKT Cell Activation by CD1d+ TRAMP Prostate Tumor Cells Is Corrected by Interleukin-12 with Alpha-Galactosylceramide. PLoS One 5, e11311. doi:10.1371/journal.pone.0011311
Noy, R., and Pollard, J. W. (2014). Tumor-associated Macrophages: from Mechanisms to Therapy. Immunity 41, 49–61. doi:10.1016/j.immuni.2014.06.010
O'brien, S. A., Orf, J., Skrzypczynska, K. M., Tan, H., Kim, J., Devoss, J., et al. (2021). Activity of Tumor-Associated Macrophage Depletion by CSF1R Blockade Is Highly Dependent on the Tumor Model and Timing of Treatment. Cancer Immunol. Immunother. 70, 2401–2410. doi:10.1007/s00262-021-02861-3
Ohl, K., and Tenbrock, K. (2018). Reactive Oxygen Species as Regulators of MDSC-Mediated Immune Suppression. Front. Immunol. 9, 2499. doi:10.3389/fimmu.2018.02499
O’keefe, R. N., Carli, A. L. E., Baloyan, D., Afshar-Sterle, S., Eissmann, M. F., Poh, A. R., et al. (2022). Inhibition of the Tuft cell/ILC2 axis Reduces Gastric Tumor Development in Mice.
Orsini, G., Legitimo, A., Failli, A., Ferrari, P., Nicolini, A., Spisni, R., et al. (2013). Defective Generation and Maturation of Dendritic Cells from Monocytes in Colorectal Cancer Patients during the Course of Disease. Ijms 14, 22022–22041. doi:10.3390/ijms141122022
Pachynski, R. K., Zabel, B. A., Kohrt, H. E., Tejeda, N. M., Monnier, J., Swanson, C. D., et al. (2012). The Chemoattractant Chemerin Suppresses Melanoma by Recruiting Natural Killer Cell Antitumor Defenses. J. Exp. Med. 209, 1427–1435. doi:10.1084/jem.20112124
Pasha, M. A., Patel, G., Hopp, R., and Yang, Q. (2019). Role of Innate Lymphoid Cells in Allergic Diseases. Allergy Asthma Proc. 40, 138–145. doi:10.2500/aap.2019.40.4217
Patente, T. A., Pinho, M. P., Oliveira, A. A., Evangelista, G. C. M., Bergami-Santos, P. C., and Barbuto, J. A. M. (2018). Human Dendritic Cells: Their Heterogeneity and Clinical Application Potential in Cancer Immunotherapy. Front. Immunol. 9, 3176. doi:10.3389/fimmu.2018.03176
Peng, L.-S., Zhang, J.-Y., Teng, Y.-S., Zhao, Y.-L., Wang, T.-T., Mao, F.-Y., et al. (2017). Tumor-Associated Monocytes/Macrophages Impair NK-Cell Function via TGFβ1 in Human Gastric Cancer. Cancer Immunol. Res. 5, 248–256. doi:10.1158/2326-6066.cir-16-0152
Pesce, S., Greppi, M., Tabellini, G., Rampinelli, F., Parolini, S., Olive, D., et al. (2017). Identification of a Subset of Human Natural Killer Cells Expressing High Levels of Programmed Death 1: A Phenotypic and Functional Characterization. J. Allergy Clin. Immunol. 139, 335–346. e333. doi:10.1016/j.jaci.2016.04.025
Pesce, S., Tabellini, G., Cantoni, C., Patrizi, O., Coltrini, D., Rampinelli, F., et al. (2015). B7-H6-mediated Downregulation of NKp30 in NK Cells Contributes to Ovarian Carcinoma Immune Escape. Oncoimmunology 4, e1001224. doi:10.1080/2162402x.2014.1001224
Pienta, K. J., Machiels, J.-P., Schrijvers, D., Alekseev, B., Shkolnik, M., Crabb, S. J., et al. (2013). Phase 2 Study of Carlumab (CNTO 888), a Human Monoclonal Antibody against CC-Chemokine Ligand 2 (CCL2), in Metastatic Castration-Resistant Prostate Cancer. Invest New Drugs 31, 760–768. doi:10.1007/s10637-012-9869-8
Pietra, G., Vitale, M., Moretta, L., and Mingari, M. C. (2012). How Melanoma Cells Inactivate NK Cells. Oncoimmunology 1, 974–975. doi:10.4161/onci.20405
Platonova, S., Cherfils-Vicini, J., Damotte, D., Crozet, L., Vieillard, V., Validire, P., et al. (2011). Profound Coordinated Alterations of Intratumoral NK Cell Phenotype and Function in Lung Carcinoma. Cancer Res. 71, 5412–5422. doi:10.1158/0008-5472.can-10-4179
Poh, A. R., and Ernst, M. (2018). Targeting Macrophages in Cancer: From Bench to Bedside. Front. Oncol. 8, 49. doi:10.3389/fonc.2018.00049
Pyonteck, S. M., Akkari, L., Schuhmacher, A. J., Bowman, R. L., Sevenich, L., Quail, D. F., et al. (2013). CSF-1R Inhibition Alters Macrophage Polarization and Blocks Glioma Progression. Nat. Med. 19, 1264–1272. doi:10.1038/nm.3337
Qian, B., Deng, Y., Im, J. H., Muschel, R. J., Zou, Y., Li, J., et al. (2009). A Distinct Macrophage Population Mediates Metastatic Breast Cancer Cell Extravasation, Establishment and Growth. PLoS One 4, e6562. doi:10.1371/journal.pone.0006562
Quail, D. F., Bowman, R. L., Akkari, L., Quick, M. L., Schuhmacher, A. J., Huse, J. T., et al. (2016). The Tumor Microenvironment Underlies Acquired Resistance to CSF-1R Inhibition in Gliomas. Science 352, aad3018. doi:10.1126/science.aad3018
Quaranta, V., Rainer, C., Nielsen, S. R., Raymant, M. L., Ahmed, M. S., Engle, D. D., et al. (2018). Macrophage-Derived Granulin Drives Resistance to Immune Checkpoint Inhibition in Metastatic Pancreatic Cancer. Cancer Res. 78, 4253–4269. doi:10.1158/0008-5472.can-17-3876
Quezada, S. A., Peggs, K. S., Curran, M. A., and Allison, J. P. (2006). CTLA4 Blockade and GM-CSF Combination Immunotherapy Alters the Intratumor Balance of Effector and Regulatory T Cells. J. Clin. Investigation 116, 1935–1945. doi:10.1172/jci27745
Ramos, R. N., Chin, L. S., Dos Santos, A. P. S. A., Bergami-Santos, P. C., Laginha, F., and Barbuto, J. A. M. (2012). Monocyte-derived Dendritic Cells from Breast Cancer Patients Are Biased to Induce CD4+CD25+Foxp3+ Regulatory T Cells. J. Leukoc. Biol. 92, 673–682. doi:10.1189/jlb.0112048
Rashid, M., Borin, T., Ara, R., Piranlioglu, R., Achyut, B., Korkaya, H., et al. (2021). Critical Immunosuppressive Effect of MDSC-derived E-xosomes in the T-umor M-icroenvironment. Oncol. Rep. 45, 1171–1181. doi:10.3892/or.2021.7936
Redman, J. M., Gibney, G. T., and Atkins, M. B. (2016). Advances in Immunotherapy for Melanoma. BMC Med. 14, 20. doi:10.1186/s12916-016-0571-0
Rees, B., Bilwes, A., Samama, J. P., and Moras, D. (1990). CardiotoxinV4II fromNaja Mossambica Mossambica. J. Mol. Biol. 214, 281–297. doi:10.1016/0022-2836(90)90161-e
Remark, R., Alifano, M., Cremer, I., Lupo, A., Dieu-Nosjean, M.-C., Riquet, M., et al. (2013). Characteristics and Clinical Impacts of the Immune Environments in Colorectal and Renal Cell Carcinoma Lung Metastases: Influence of Tumor Origin. Clin. Cancer Res. 19, 4079–4091. doi:10.1158/1078-0432.ccr-12-3847
Reynolds, G., and Haniffa, M. (2015). Human and Mouse Mononuclear Phagocyte Networks: A Tale of Two Species? Front. Immunol. 6, 330. doi:10.3389/fimmu.2015.00330
Rezaeifard, S., Safaei, A., Talei, A., Faghih, Z., and Erfani, N. (2019). NK, NKT and Invariant-NKT Cells in Tumor Draining Lymph Nodes of Patients with Breast Cancer. Iran. J. Immunol. 16, 291–298. doi:10.22034/IJI.2019.80280
Rezaeifard, S., Talei, A., Shariat, M., and Erfani, N. (2021). Tumor Infiltrating NK Cell (TINK) Subsets and Functional Molecules in Patients with Breast Cancer. Mol. Immunol. 136, 161–167. doi:10.1016/j.molimm.2021.03.003
Richter, J., Neparidze, N., Zhang, L., Nair, S., Monesmith, T., Sundaram, R., et al. (2013). Clinical Regressions and Broad Immune Activation Following Combination Therapy Targeting Human NKT Cells in Myeloma. Blood 121, 423–430. doi:10.1182/blood-2012-06-435503
Roan, F., Obata-Ninomiya, K., and Ziegler, S. F. (2019). Epithelial Cell-Derived Cytokines: More Than Just Signaling the Alarm. J. Clin. Invest 129, 1441–1451. doi:10.1172/jci124606
Roberts, E. W., Broz, M. L., Binnewies, M., Headley, M. B., Nelson, A. E., Wolf, D. M., et al. (2016). Critical Role for CD103+/CD141+ Dendritic Cells Bearing CCR7 for Tumor Antigen Trafficking and Priming of T Cell Immunity in Melanoma. Cancer Cell 30, 324–336. doi:10.1016/j.ccell.2016.06.003
Rodell, C. B., Arlauckas, S. P., Cuccarese, M. F., Garris, C. S., Li, R., Ahmed, M. S., et al. (2018). TLR7/8-agonist-loaded Nanoparticles Promote the Polarization of Tumour-Associated Macrophages to Enhance Cancer Immunotherapy. Nat. Biomed. Eng. 2, 578–588. doi:10.1038/s41551-018-0236-8
Rodriguez, P. C., Quiceno, D. G., and Ochoa, A. C. (2007). L-arginine Availability Regulates T-Lymphocyte Cell-Cycle Progression. Blood 109, 1568–1573. doi:10.1182/blood-2006-06-031856
Rodriguez, P. C., Zea, A. H., Culotta, K. S., Zabaleta, J., Ochoa, J. B., and Ochoa, A. C. (2002). Regulation of T Cell Receptor CD3ζ Chain Expression Byl-Arginine. J. Biol. Chem. 277, 21123–21129. doi:10.1074/jbc.m110675200
Romagnani, C., Juelke, K., Falco, M., Morandi, B., D’Agostino, A., Costa, R., et al. (2007). CD56brightCD16− Killer Ig-like Receptor− NK Cells Display Longer Telomeres and Acquire Features of CD56dim NK Cells upon Activation. J. Immunol. 178, 4947–4955. doi:10.4049/jimmunol.178.8.4947
Rosser, E. C., and Mauri, C. (2015). Regulatory B Cells: Origin, Phenotype, and Function. Immunity 42, 607–612. doi:10.1016/j.immuni.2015.04.005
Sade-Feldman, M., Kanterman, J., Klieger, Y., Ish-Shalom, E., Olga, M., Saragovi, A., et al. (2016). Clinical Significance of Circulating CD33+CD11b+HLA-DR− Myeloid Cells in Patients with Stage IV Melanoma Treated with Ipilimumab. Clin. Cancer Res. 22, 5661–5672. doi:10.1158/1078-0432.ccr-15-3104
Safarzadeh, E., Hashemzadeh, S., Duijf, P. H. G., Mansoori, B., Khaze, V., Mohammadi, A., et al. (2019). Circulating Myeloid‐derived Suppressor Cells: An Independent Prognostic Factor in Patients with Breast Cancer. J. Cell. Physiology 234, 3515–3525. doi:10.1002/jcp.26896
Saito, T., Takayama, T., Osaki, T., Nagai, S., Suzuki, T., Sato, M., et al. (2008). Combined Mobilization and Stimulation of Tumor-Infiltrating Dendritic Cells and Natural Killer Cells with Flt3 Ligand and IL-18 In Vivo Induces Systemic Antitumor Immunity. Cancer Sci. 99, 2028–2036. doi:10.1111/j.1349-7006.2008.00907.x
Salimi, M., Wang, R., Yao, X., Li, X., Wang, X., Hu, Y., et al. (2018). Activated Innate Lymphoid Cell Populations Accumulate in Human Tumour Tissues. BMC Cancer 18, 341. doi:10.1186/s12885-018-4262-4
Sallusto, F., and Lanzavecchia, A. (2018). Pillars Article: Efficient Presentation of Soluble Antigen by Cultured Human Dendritic Cells Is Maintained by Granulocyte/macrophage Colony-Stimulating Factor Plus Interleukin 4 and Downregulated by Tumor Necrosis Factor α. J. Exp. Med. 1994. 179:1109-1118. J. Exp. MedJ Immunol. 200, 887–896.
Salmon, H., Idoyaga, J., Rahman, A., Leboeuf, M., Remark, R., Jordan, S., et al. (2016). Expansion and Activation of CD103 + Dendritic Cell Progenitors at the Tumor Site Enhances Tumor Responses to Therapeutic PD-L1 and BRAF Inhibition. Immunity 44, 924–938. doi:10.1016/j.immuni.2016.03.012
Salomé, B., Gomez-Cadena, A., Loyon, R., Suffiotti, M., Salvestrini, V., Wyss, T., et al. (2019). CD56 as a Marker of an ILC1-like Population with NK Cell Properties that Is Functionally Impaired in AML. Blood Adv. 3, 3674–3687. doi:10.1182/bloodadvances.2018030478
Sambi, M., Bagheri, L., and Szewczuk, M. R. (2019). Current Challenges in Cancer Immunotherapy: Multimodal Approaches to Improve Efficacy and Patient Response Rates. J. Oncol. 2019, 4508794. doi:10.1155/2019/4508794
Sanchez-Correa, B., Gayoso, I., Bergua, J. M., Casado, J. G., Morgado, S., Solana, R., et al. (2012). Decreased Expression of DNAM-1 on NK Cells from Acute Myeloid Leukemia Patients. Immunol. Cell Biol. 90, 109–115. doi:10.1038/icb.2011.15
Sato, E., Olson, S. H., Ahn, J., Bundy, B., Nishikawa, H., Qian, F., et al. (2005). Intraepithelial CD8 + Tumor-Infiltrating Lymphocytes and a High CD8 +/regulatory T Cell Ratio Are Associated with Favorable Prognosis in Ovarian Cancer. Proc. Natl. Acad. Sci. U.S.A. 102, 18538–18543. doi:10.1073/pnas.0509182102
Schlecker, E., Fiegler, N., Arnold, A., Altevogt, P., Rose-John, S., Moldenhauer, G., et al. (2014). Metalloprotease-Mediated Tumor Cell Shedding of B7-H6, the Ligand of the Natural Killer Cell-Activating Receptor NKp30. Cancer Res. 74, 3429–3440. doi:10.1158/0008-5472.can-13-3017
Schlecker, E., Stojanovic, A., Eisen, C., Quack, C., Falk, C. S., Umansky, V., et al. (2012). Tumor-infiltrating Monocytic Myeloid-Derived Suppressor Cells Mediate CCR5-dependent Recruitment of Regulatory T Cells Favoring Tumor Growth. J. I. 189, 5602–5611. doi:10.4049/jimmunol.1201018
Schlegel, N. C., Von Planta, A., Widmer, D. S., Dummer, R., and Christofori, G. (2015). PI3K Signalling Is Required for a TGFβ-Induced Epithelial-mesenchymal-like Transition (EMT-like) in Human Melanoma Cells. Exp. Dermatol 24, 22–28. doi:10.1111/exd.12580
Schleypen, J. S., Baur, N., Kammerer, R., Nelson, P. J., Rohrmann, K., Gröne, E. F., et al. (2006). Cytotoxic Markers and Frequency Predict Functional Capacity of Natural Killer Cells Infiltrating Renal Cell Carcinoma. Clin. Cancer Res. 12, 718–725. doi:10.1158/1078-0432.ccr-05-0857
Schneiders, F. L., De Bruin, R. C. G., Van Den Eertwegh, A. J. M., Scheper, R. J., Leemans, C. R., Brakenhoff, R. H., et al. (2012). Circulating Invariant Natural Killer T-Cell Numbers Predict Outcome in Head and Neck Squamous Cell Carcinoma: Updated Analysis with 10-year Follow-Up. Jco 30, 567–570. doi:10.1200/jco.2011.38.8819
Schön, M. P., and Schön, M. (2008). TLR7 and TLR8 as Targets in Cancer Therapy. Oncogene 27, 190–199. doi:10.1038/sj.onc.1210913
Schott, A. F., Goldstein, L. J., Cristofanilli, M., Ruffini, P. A., Mccanna, S., Reuben, J. M., et al. (2017). Phase Ib Pilot Study to Evaluate Reparixin in Combination with Weekly Paclitaxel in Patients with HER-2-Negative Metastatic Breast Cancer. Clin. Cancer Res. 23, 5358–5365. doi:10.1158/1078-0432.ccr-16-2748
Sconocchia, G., Arriga, R., Tornillo, L., Terracciano, L., Ferrone, S., and Spagnoli, G. C. (2012). Melanoma Cells Inhibit NK Cell Functions-Letter. Cancer Res. 72, 5428–5429. doi:10.1158/0008-5472.can-12-1181
Sconocchia, G., Eppenberger, S., Spagnoli, G. C., Tornillo, L., Droeser, R., Caratelli, S., et al. (2014). NK Cells and T Cells Cooperate during the Clinical Course of Colorectal Cancer. Oncoimmunology 3, e952197. doi:10.4161/21624011.2014.952197
Sechler, J. M., Barlic, J., Grivel, J.-C., and Murphy, P. M. (2004). IL-15 Alters Expression and Function of the Chemokine Receptor CX3CR1 in Human NK Cells. Cell. Immunol. 230, 99–108. doi:10.1016/j.cellimm.2004.10.001
Segura, E., Kapp, E., Gupta, N., Wong, J., Lim, J., Ji, H., et al. (2010). Differential Expression of Pathogen-Recognition Molecules between Dendritic Cell Subsets Revealed by Plasma Membrane Proteomic Analysis. Mol. Immunol. 47, 1765–1773. doi:10.1016/j.molimm.2010.02.028
Segura, E., Valladeau-Guilemond, J., Donnadieu, M.-H., Sastre-Garau, X., Soumelis, V., and Amigorena, S. (2012). Characterization of Resident and Migratory Dendritic Cells in Human Lymph Nodes. J. Exp. Med. 209, 653–660. doi:10.1084/jem.20111457
Semeraro, M., Rusakiewicz, S., Minard-Colin, V., Delahaye, N. F., Enot, D., Vély, F., et al. (2015). Clinical Impact of the NKp30/B7-H6 axis in High-Risk Neuroblastoma Patients. Sci. Transl. Med. 7, 283ra55. doi:10.1126/scitranslmed.aaa2327
Seo, H., Jeon, I., Kim, B. S., Park, M., Bae, E. A., Song, B., et al. (2017). IL-21-mediated Reversal of NK Cell Exhaustion Facilitates Anti-tumour Immunity in MHC Class I-Deficient Tumours. Nat. Commun. 8, 15776–15814. doi:10.1038/ncomms15776
Shaulov, A., Yue, S., Wang, R., Joyce, R. M., Balk, S. P., Kim, H. T., et al. (2008). Peripheral Blood Progenitor Cell Product Contains Th1-Biased Noninvariant CD1d-Reactive Natural Killer T Cells: Implications for Posttransplant Survival. Exp. Hematol. 36, 464–472. doi:10.1016/j.exphem.2007.12.010
Shimizu, K., Iyoda, T., Yamasaki, S., Kadowaki, N., Tojo, A., and Fujii, A. S. (2020). NK and NKT Cell-Mediated Immune Surveillance against Hematological Malignancies. Cancers (Basel) 12. doi:10.3390/cancers12040817
Shimizu, K., Mizuno, T., Shinga, J., Asakura, M., Kakimi, K., Ishii, Y., et al. (2013). Vaccination with Antigen-Transfected, NKT Cell Ligand-Loaded, Human Cells Elicits Robust In Situ Immune Responses by Dendritic Cells. Cancer Res. 73, 62–73. doi:10.1158/0008-5472.can-12-0759
Shojaei, F., Wu, X., Qu, X., Kowanetz, M., Yu, L., Tan, M., et al. (2009). G-CSF-initiated Myeloid Cell Mobilization and Angiogenesis Mediate Tumor Refractoriness to Anti-VEGF Therapy in Mouse Models. Proc. Natl. Acad. Sci. U.S.A. 106, 6742–6747. doi:10.1073/pnas.0902280106
Shortman, K., and Liu, Y.-J. (2002). Mouse and Human Dendritic Cell Subtypes. Nat. Rev. Immunol. 2, 151–161. doi:10.1038/nri746
Sica, A., and Mantovani, A. (2012). Macrophage Plasticity and Polarization: In Vivo Veritas. J. Clin. Invest. 122, 787–795. doi:10.1172/jci59643
Sisirak, V., Faget, J., Gobert, M., Goutagny, N., Vey, N., Treilleux, I., et al. (2012). Impaired IFN-α Production by Plasmacytoid Dendritic Cells Favors Regulatory T-Cell Expansion that May Contribute to Breast Cancer Progression. Cancer Res. 72, 5188–5197. doi:10.1158/0008-5472.can-11-3468
Sivori, S., Vitale, M., Bottino, C., Marcenaro, E., Sanseverino, L., Parolini, S., et al. (1996). CD94 Functions as a Natural Killer Cell Inhibitory Receptor for Different HLA Class I Alleles: Identification of the Inhibitory Form of CD94 by the Use of Novel Monoclonal Antibodies. Eur. J. Immunol. 26, 2487–2492. doi:10.1002/eji.1830261032
Sojka, D. K., Tian, Z., and Yokoyama, W. M. (2014). Tissue-resident Natural Killer Cells and Their Potential Diversity. Seminars Immunol. 26, 127–131. doi:10.1016/j.smim.2014.01.010
Spanoudakis, E., Hu, M., Naresh, K., Terpos, E., Melo, V., Reid, A., et al. (2009). Regulation of Multiple Myeloma Survival and Progression by CD1d. Blood 113, 2498–2507. doi:10.1182/blood-2008-06-161281
Spits, H., Artis, D., Colonna, M., Diefenbach, A., Di Santo, J. P., Eberl, G., et al. (2013). Innate Lymphoid Cells - a Proposal for Uniform Nomenclature. Nat. Rev. Immunol. 13, 145–149. doi:10.1038/nri3365
Srivastava, M. K., Sinha, P., Clements, V. K., Rodriguez, P., and Ostrand-Rosenberg, S. (2010). Myeloid-derived Suppressor Cells Inhibit T-Cell Activation by Depleting Cystine and Cysteine. Cancer Res. 70, 68–77. doi:10.1158/0008-5472.can-09-2587
Steinman, R. M. (2006). Linking Innate to Adaptive Immunity through Dendritic Cells. Novartis Found. Symp. 279, 216–109.
Strachan, D. C., Ruffell, B., Oei, Y., Bissell, M. J., Coussens, L. M., Pryer, N., et al. (2013). CSF1R Inhibition Delays Cervical and Mammary Tumor Growth in Murine Models by Attenuating the Turnover of Tumor-Associated Macrophages and Enhancing Infiltration by CD8+T Cells. Oncoimmunology 2, e26968. doi:10.4161/onci.26968
Sullivan, R. J. (2019). Back to the Future: Rethinking and Retooling IL2 in the Immune Checkpoint Inhibitor Era. Cancer Discov. 9, 694–695. doi:10.1158/2159-8290.cd-19-0412
Sun, C., Xu, J., Huang, Q., Huang, M., Wen, H., Zhang, C., et al. (2017). High NKG2A Expression Contributes to NK Cell Exhaustion and Predicts a Poor Prognosis of Patients with Liver Cancer. Oncoimmunology 6, e1264562. doi:10.1080/2162402x.2016.1264562
Sun, H., Liu, L., Huang, Q., Liu, H., Huang, M., Wang, J., et al. (2019). Accumulation of Tumor-Infiltrating CD49a+ NK Cells Correlates with Poor Prognosis for Human Hepatocellular Carcinoma. Cancer Immunol. Res. 7, 1535–1546. doi:10.1158/2326-6066.cir-18-0757
Syn, N. L., Teng, M. W. L., Mok, T. S. K., and Soo, R. A. (2017). De-novo and Acquired Resistance to Immune Checkpoint Targeting. Lancet Oncol. 18, e731–e741. doi:10.1016/s1470-2045(17)30607-1
Szabó, C., Ischiropoulos, H., and Radi, R. (2007). Peroxynitrite: Biochemistry, Pathophysiology and Development of Therapeutics. Nat. Rev. Drug Discov. 6, 662–680. doi:10.1038/nrd2222
Tachibana, T., Onodera, H., Tsuruyama, T., Mori, A., Nagayama, S., Hiai, H., et al. (2005). Increased Intratumor Vα24-Positive Natural Killer T Cells: A Prognostic Factor for Primary Colorectal Carcinomas. Clin. Cancer Res. 11, 7322–7327. doi:10.1158/1078-0432.ccr-05-0877
Takahashi, R., Ijichi, H., Sano, M., Miyabayashi, K., Mohri, D., Kim, J., et al. (2020). Soluble VCAM-1 Promotes Gemcitabine Resistance via Macrophage Infiltration and Predicts Therapeutic Response in Pancreatic Cancer. Sci. Rep. 10, 21194. doi:10.1038/s41598-020-78320-3
Terabe, M., and Berzofsky, J. A. (2008). Chapter 8 the Role of NKT Cells in Tumor Immunity. Adv. Cancer Res. 101, 277–348. doi:10.1016/s0065-230x(08)00408-9
Terrén, I., Orrantia, A., Vitallé, J., Zenarruzabeitia, O., and Borrego, F. (2019). NK Cell Metabolism and Tumor Microenvironment. Front. Immunol. 10, 2278. doi:10.3389/fimmu.2019.02278
Tham, M., Khoo, K., Yeo, K. P., Kato, M., Prevost-Blondel, A., Angeli, V., et al. (2015). Macrophage Depletion Reduces Postsurgical Tumor Recurrence and Metastatic Growth in a Spontaneous Murine Model of Melanoma. Oncotarget 6, 22857–22868. doi:10.18632/oncotarget.3127
Thommen, D. S., Schreiner, J., Müller, P., Herzig, P., Roller, A., Belousov, A., et al. (2015). Progression of Lung Cancer Is Associated with Increased Dysfunction of T Cells Defined by Coexpression of Multiple Inhibitory Receptors. Cancer Immunol. Res. 3, 1344–1355. doi:10.1158/2326-6066.cir-15-0097
Thompson, T. W., Kim, A. B., Li, P. J., Wang, J., Jackson, B. T., Huang, K. T. H., et al. (2017). Endothelial Cells Express NKG2D Ligands and Desensitize Antitumor NK Responses. Elife 6, e30881. doi:10.7554/eLife.30881
Thurner, B., Haendle, I., Röder, C., Dieckmann, D., Keikavoussi, P., Jonuleit, H., et al. (1999). Vaccination with mage-3A1 Peptide-Pulsed Mature, Monocyte-Derived Dendritic Cells Expands Specific Cytotoxic T Cells and Induces Regression of Some Metastases in Advanced Stage IV Melanoma. J. Exp. Med. 190, 1669–1678. doi:10.1084/jem.190.11.1669
Tian, T., Gu, X., Zhang, B., Liu, Y., Yuan, C., Shao, L., et al. (2015). Increased Circulating CD14(+)HLA-DR-/low Myeloid-Derived Suppressor Cells Are Associated with Poor Prognosis in Patients with Small-Cell Lung Cancer. Cbm 15, 425–432. doi:10.3233/cbm-150473
Tobin, R. P., Jordan, K. R., Robinson, W. A., Davis, D., Borges, V. F., Gonzalez, R., et al. (2018). Targeting Myeloid-Derived Suppressor Cells Using All-Trans Retinoic Acid in Melanoma Patients Treated with Ipilimumab. Int. Immunopharmacol. 63, 282–291. doi:10.1016/j.intimp.2018.08.007
Toniolo, P. A., Liu, S., Yeh, J. E., Ye, D. Q., Barbuto, J. A. M., and Frank, D. A. (2016). Deregulation of SOCS5 Suppresses Dendritic Cell Function in Chronic Lymphocytic Leukemia. Oncotarget 7, 46301–46314. doi:10.18632/oncotarget.10093
Trabanelli, S., Chevalier, M. F., Martinez-Usatorre, A., Gomez-Cadena, A., Salomé, B., Lecciso, M., et al. (2017). Tumour-derived PGD2 and NKp30-B7h6 Engagement Drives an Immunosuppressive ILC2-MDSC axis. Nat. Commun. 8, 593. doi:10.1038/s41467-017-00678-2
Trabanelli, S., Curti, A., Lecciso, M., Salome, B., Riether, C., Ochsenbein, A., et al. (2015). CD127+ Innate Lymphoid Cells Are Dysregulated in Treatment Naive Acute Myeloid Leukemia Patients at Diagnosis. Haematologica 100, e257–e260. doi:10.3324/haematol.2014.119602
Trabanelli, S., Ercolano, G., Wyss, T., Gomez-Cadena, A., Falquet, M., Cropp, D., et al. (2022). c-Maf Enforces Cytokine Production and Promotes Memory-like Responses in Mouse and Human Type 2 Innate Lymphoid Cells. EMBO J. 2022, e109300. doi:10.15252/embj.2021109300
Trabanelli, S., Gomez-Cadena, A., Salomé, B., Michaud, K., Mavilio, D., Landis, B. N., et al. (2018). Human Innate Lymphoid Cells (ILCs): Toward a Uniform Immune-Phenotyping. Cytometry 94, 392–399. doi:10.1002/cyto.b.21614
Treilleux, I., Blay, J.-Y., Bendriss-Vermare, N., Ray-Coquard, I., Bachelot, T., Guastalla, J.-P., et al. (2004). Dendritic Cell Infiltration and Prognosis of Early Stage Breast Cancer. Clin. Cancer Res. 10, 7466–7474. doi:10.1158/1078-0432.ccr-04-0684
Tu, M. M., Abdel-Hafiz, H. A., Jones, R. T., Jean, A., Hoff, K. J., Duex, J. E., et al. (2020). Inhibition of the CCL2 Receptor, CCR2, Enhances Tumor Response to Immune Checkpoint Therapy. Commun. Biol. 3, 720. doi:10.1038/s42003-020-01441-y
Ubil, E., Caskey, L., Holtzhausen, A., Hunter, D., Story, C., and Earp, H. S. (2018). Tumor-secreted Pros1 Inhibits Macrophage M1 Polarization to Reduce Antitumor Immune Response. J. Clin. Invest 128, 2356–2369. doi:10.1172/jci97354
Umansky, V., Blattner, C., Gebhardt, C., and Utikal, J. (2016). The Role of Myeloid-Derived Suppressor Cells (MDSC) in Cancer Progression. Vaccines (Basel) 4, 36. doi:10.3390/vaccines4040036
Umansky, V., and Schirrmacher, V. (2001). Nitric Oxide-Induced Apoptosis in Tumor Cells. Adv. Cancer Res. 82, 107–131. doi:10.1016/s0065-230x(01)82004-2
Vachhani, P., and Chen, H. (2016). Spotlight on Pembrolizumab in Non-small Cell Lung Cancer: the Evidence to Date. Onco Targets Ther. 9, 5855–5866. doi:10.2147/OTT.S97746
Van Dalen, F. J., Van Stevendaal, M. H. M. E., Fennemann, F. L., Verdoes, M., and Ilina, O. (2018). Molecular Repolarisation of Tumour-Associated Macrophages. Molecules 24. doi:10.3390/molecules24010009
Vanoni, G., Ercolano, G., Candiani, S., Rutigliani, M., Lanata, M., Derré, L., et al. (2021). Human Primed ILCPs Support Endothelial Activation through NF-Κb Signaling. Elife 10. doi:10.7554/eLife.58838
Veglia, F., Perego, M., and Gabrilovich, D. (2018). Myeloid-derived Suppressor Cells Coming of Age. Nat. Immunol. 19, 108–119. doi:10.1038/s41590-017-0022-x
Versluis, M. A. C., Marchal, S., Plat, A., De Bock, G. H., Van Hall, T., De Bruyn, M., et al. (2017). The Prognostic Benefit of Tumour-Infiltrating Natural Killer Cells in Endometrial Cancer Is Dependent on Concurrent Overexpression of Human Leucocyte Antigen-E in the Tumour Microenvironment. Eur. J. Cancer 86, 285–295. doi:10.1016/j.ejca.2017.09.008
Vgenopoulou, S., Lazaris, A. C., Markopoulos, C., Boltetsou, E., Kyriakou, V., Kavantzas, N., et al. (2003). Immunohistochemical Evaluation of Immune Response in Invasive Ductal Breast Cancer of Not-otherwise-specified Type. Breast 12, 172–178. doi:10.1016/s0960-9776(03)00004-3
Vijayan, D., Young, A., Teng, M. W. L., and Smyth, M. J. (2017). Targeting Immunosuppressive Adenosine in Cancer. Nat. Rev. Cancer 17, 709–724. doi:10.1038/nrc.2017.86
Villani, A. C., Satija, R., Reynolds, G., Sarkizova, S., Shekhar, K., Fletcher, J., et al. (2017). Single-cell RNA-Seq Reveals New Types of Human Blood Dendritic Cells, Monocytes, and Progenitors. Science 356. doi:10.1126/science.aah4573
Vivier, E., Artis, D., Colonna, M., Diefenbach, A., Di Santo, J. P., Eberl, G., et al. (2018). Innate Lymphoid Cells: 10 Years on. Cell 174, 1054–1066. doi:10.1016/j.cell.2018.07.017
Vivier, E., Raulet, D. H., Moretta, A., Caligiuri, M. A., Zitvogel, L., Lanier, L. L., et al. (2011). Innate or Adaptive Immunity? the Example of Natural Killer Cells. Science 331, 44–49. doi:10.1126/science.1198687
Vivier, E., Ugolini, S., Blaise, D., Chabannon, C., and Brossay, L. (2012). Targeting Natural Killer Cells and Natural Killer T Cells in Cancer. Nat. Rev. Immunol. 12, 239–252. doi:10.1038/nri3174
Vuk-Pavlović, S., Bulur, P. A., Lin, Y., Qin, R., Szumlanski, C. L., Zhao, X., et al. (2010). Immunosuppressive CD14+HLA-DRlow/- Monocytes in Prostate Cancer. Prostate 70, 443–455. doi:10.1002/pros.21078
Wallin, J. J., Bendell, J. C., Funke, R., Sznol, M., Korski, K., Jones, S., et al. (2016). Atezolizumab in Combination with Bevacizumab Enhances Antigen-specific T-Cell Migration in Metastatic Renal Cell Carcinoma. Nat. Commun. 7, 12624. doi:10.1038/ncomms12624
Wanderley, C. W., Colón, D. F., Luiz, J. P. M., Oliveira, F. F., Viacava, P. R., Leite, C. A., et al. (2018). Paclitaxel Reduces Tumor Growth by Reprogramming Tumor-Associated Macrophages to an M1 Profile in a TLR4-dependent Manner. Cancer Res. 78, 5891–5900. doi:10.1158/0008-5472.CAN-17-3480
Wang, W., Xia, X., Mao, L., and Wang, S. (2019a). The CCAAT/Enhancer-Binding Protein Family: Its Roles in MDSC Expansion and Function. Front. Immunol. 10, 1804. doi:10.3389/fimmu.2019.01804
Wang, X., Sathe, A. A., Smith, G. R., Ruf-Zamojski, F., Nair, V., Lavine, K. J., et al. (2020). Heterogeneous Origins and Functions of Mouse Skeletal Muscle-Resident Macrophages. Proc. Natl. Acad. Sci. U.S.A. 117, 20729–20740. doi:10.1073/pnas.1915950117
Wang, Z., Wang, Z., Li, B., Wang, S., Chen, T., and Ye, Z. (2019b). Innate Immune Cells: A Potential and Promising Cell Population for Treating Osteosarcoma. Front. Immunol. 10, 1114. doi:10.3389/fimmu.2019.01114
Wculek, S. K., Cueto, F. J., Mujal, A. M., Melero, I., Krummel, M. F., and Sancho, D. (2020). Dendritic Cells in Cancer Immunology and Immunotherapy. Nat. Rev. Immunol. 20, 7–24. doi:10.1038/s41577-019-0210-z
Weil, S., Memmer, S., Lechner, A., Huppert, V., Giannattasio, A., Becker, T., et al. (2017). Natural Killer Group 2D Ligand Depletion Reconstitutes Natural Killer Cell Immunosurveillance of Head and Neck Squamous Cell Carcinoma. Front. Immunol. 8, 387. doi:10.3389/fimmu.2017.00387
Wendel, M., Galani, I. E., Suri-Payer, E., and Cerwenka, A. (2008). Natural Killer Cell Accumulation in Tumors Is Dependent on IFN-γ and CXCR3 Ligands. Cancer Res. 68, 8437–8445. doi:10.1158/0008-5472.can-08-1440
Wesolowski, R., Sharma, N., Reebel, L., Rodal, M. B., Peck, A., West, B. L., et al. (2019). Phase Ib Study of the Combination of Pexidartinib (PLX3397), a CSF-1R Inhibitor, and Paclitaxel in Patients with Advanced Solid Tumors. Ther. Adv. Med. Oncol. 11, 1758835919854238. doi:10.1177/1758835919854238
Wu, D. Y., Segal, N. H., Sidobre, S., Kronenberg, M., and Chapman, P. B. (2003). Cross-presentation of Disialoganglioside GD3 to Natural Killer T Cells. J. Exp. Med. 198, 173–181. doi:10.1084/jem.20030446
Wu, L., Du, H., Li, Y., Qu, P., and Yan, C. (2011). Signal Transducer and Activator of Transcription 3 (Stat3C) Promotes Myeloid-Derived Suppressor Cell Expansion and Immune Suppression during Lung Tumorigenesis. Am. J. Pathology 179, 2131–2141. doi:10.1016/j.ajpath.2011.06.028
Wu, Y., and Shen, J. (2020). Innate Lymphoid Cells in Crohn's Disease. Front. Immunol. 11, 554880. doi:10.3389/fimmu.2020.554880
Xie, J., Liu, M., Li, Y., Nie, Y., Mi, Q., and Zhao, S. (2014). Ovarian Tumor-Associated microRNA-20a Decreases Natural Killer Cell Cytotoxicity by Downregulating MICA/B Expression. Cell Mol. Immunol. 11, 495–502. doi:10.1038/cmi.2014.30
Xiu, B., Lin, Y., Grote, D. M., Ziesmer, S. C., Gustafson, M. P., Maas, M. L., et al. (2015). IL-10 Induces the Development of Immunosuppressive CD14+HLA-DRlow/− Monocytes in B-Cell Non-hodgkin Lymphoma. Blood Cancer J. 5, e328. doi:10.1038/bcj.2015.56
Yan, W., Liu, X., Ma, H., Zhang, H., Song, X., Gao, L., et al. (2015). Tim-3 Fosters HCC Development by Enhancing TGF-β-Mediated Alternative Activation of Macrophages. Gut 64, 1593–1604. doi:10.1136/gutjnl-2014-307671
Yanagisawa, K., Seino, K.-i., Ishikawa, Y., Nozue, M., Todoroki, T., and Fukao, K. (2002). Impaired Proliferative Response of Vα24 NKT Cells from Cancer Patients against α-Galactosylceramide. J. Immunol. 168, 6494–6499. doi:10.4049/jimmunol.168.12.6494
Yang, L., Dong, Y., Li, Y., Wang, D., Liu, S., Wang, D., et al. (2019). IL‐10 Derived from M2 Macrophage Promotes Cancer Stemness via JAK1/STAT1/NF‐κB/Notch1 Pathway in Non‐small Cell Lung Cancer. Int. J. Cancer 145, 1099–1110. doi:10.1002/ijc.32151
Yao, W., Ba, Q., Li, X., Li, H., Zhang, S., Yuan, Y., et al. (2017). A Natural CCR2 Antagonist Relieves Tumor-Associated Macrophage-Mediated Immunosuppression to Produce a Therapeutic Effect for Liver Cancer. EBioMedicine 22, 58–67. doi:10.1016/j.ebiom.2017.07.014
Yona, S., Kim, K.-W., Wolf, Y., Mildner, A., Varol, D., Breker, M., et al. (2013). Fate Mapping Reveals Origins and Dynamics of Monocytes and Tissue Macrophages under Homeostasis. Immunity 38, 79–91. doi:10.1016/j.immuni.2012.12.001
Zecca, A., Barili, V., Rizzo, D., Olivani, A., Biasini, E., Laccabue, D., et al. (2021). Intratumor Regulatory Noncytotoxic NK Cells in Patients with Hepatocellular Carcinoma. Cells. doi:10.3390/cells10030614
Zhang, J., Han, X., Shi, H., Gao, Y., Qiao, X., Li, H., et al. (2020). Lung Resided Monocytic Myeloid-Derived Suppressor Cells Contribute to Premetastatic Niche Formation by Enhancing MMP-9 Expression. Mol. Cell. Probes 50, 101498. doi:10.1016/j.mcp.2019.101498
Zhang, N., and Bevan, M. J. (2012). TGF-β Signaling to T Cells Inhibits Autoimmunity during Lymphopenia-Driven Proliferation. Nat. Immunol. 13, 667–673. doi:10.1038/ni.2319
Zhang, Q.-F., Yin, W.-W., Xia, Y., Yi, Y.-Y., He, Q.-F., Wang, X., et al. (2017). Liver-infiltrating CD11b−CD27− NK Subsets Account for NK-Cell Dysfunction in Patients with Hepatocellular Carcinoma and Are Associated with Tumor Progression. Cell Mol. Immunol. 14, 819–829. doi:10.1038/cmi.2016.28
Zhang, Q., Bi, J., Zheng, X., Chen, Y., Wang, H., Wu, W., et al. (2018). Blockade of the Checkpoint Receptor TIGIT Prevents NK Cell Exhaustion and Elicits Potent Anti-tumor Immunity. Nat. Immunol. 19, 723–732. doi:10.1038/s41590-018-0132-0
Zhang, W., Zhu, X.-D., Sun, H.-C., Xiong, Y.-Q., Zhuang, P.-Y., Xu, H.-X., et al. (2010). Depletion of Tumor-Associated Macrophages Enhances the Effect of Sorafenib in Metastatic Liver Cancer Models by Antimetastatic and Antiangiogenic Effects. Clin. Cancer Res. 16, 3420–3430. doi:10.1158/1078-0432.ccr-09-2904
Zhang, Y., and Huang, B. (2017). The Development and Diversity of ILCs, NK Cells and Their Relevance in Health and Diseases. Adv. Exp. Med. Biol. 1024, 225–244. doi:10.1007/978-981-10-5987-2_11
Zhen, Z. J., Ling, J. Y., Cai, Y., Luo, W. B., and He, Y. J. (2013). Impact of HLA-E Gene Polymorphism on HLA-E Expression in Tumor Cells and Prognosis in Patients with Stage III Colorectal Cancer. Med. Oncol. 30, 482–489. doi:10.1007/s12032-013-0482-2
Zheng, M., Sun, R., Wei, H., and Tian, Z. (2016). NK Cells Help Induce Anti-hepatitis B Virus CD8+ T Cell Immunity in Mice. J. I. 196, 4122–4131. doi:10.4049/jimmunol.1500846
Zheng, P., Chen, L., Yuan, X., Luo, Q., Liu, Y., Xie, G., et al. (2017). Exosomal Transfer of Tumor-Associated Macrophage-Derived miR-21 Confers Cisplatin Resistance in Gastric Cancer Cells. J. Exp. Clin. Cancer Res. 36, 53. doi:10.1186/s13046-017-0528-y
Zhu, H., Gu, Y., Xue, Y., Yuan, M., Cao, X., and Liu, Q. (2017a). CXCR2+ MDSCs Promote Breast Cancer Progression by Inducing EMT and Activated T Cell Exhaustion. Oncotarget 8, 114554–114567. doi:10.18632/oncotarget.23020
Zhu, J., Powis De Tenbossche, C. G., Cané, S., Colau, D., Van Baren, N., Lurquin, C., et al. (2017b). Resistance to Cancer Immunotherapy Mediated by Apoptosis of Tumor-Infiltrating Lymphocytes. Nat. Commun. 8, 1404. doi:10.1038/s41467-017-00784-1
Zhu, Y., Herndon, J. M., Sojka, D. K., Kim, K.-W., Knolhoff, B. L., Zuo, C., et al. (2017c). Tissue-Resident Macrophages in Pancreatic Ductal Adenocarcinoma Originate from Embryonic Hematopoiesis and Promote Tumor Progression. Immunity 47, 323–338. doi:10.1016/j.immuni.2017.07.014
Zhu, Y., Knolhoff, B. L., Meyer, M. A., Nywening, T. M., West, B. L., Luo, J., et al. (2014). CSF1/CSF1R Blockade Reprograms Tumor-Infiltrating Macrophages and Improves Response to T-Cell Checkpoint Immunotherapy in Pancreatic Cancer Models. Cancer Res. 74, 5057–5069. doi:10.1158/0008-5472.can-13-3723
Keywords: innate immune cells, macrophages, innate lymphoid cells (ILC), NK cells, tissue-resident immune cells, cancer, MDSC (myeloid-derived suppressor cell)
Citation: Busà R, Bulati M, Badami E, Zito G, Maresca DC, Conaldi PG, Ercolano G and Ianaro A (2022) Tissue-Resident Innate Immune Cell-Based Therapy: A Cornerstone of Immunotherapy Strategies for Cancer Treatment. Front. Cell Dev. Biol. 10:907572. doi: 10.3389/fcell.2022.907572
Received: 29 March 2022; Accepted: 03 May 2022;
Published: 26 May 2022.
Edited by:
Peng Liu, Institut National de la Santé et de la Recherche Médicale (INSERM), FranceReviewed by:
Gianluca Scarno, Sapienza University of Rome, ItalyAlvaro De Mingo Pulido, Moffitt Cancer Center, United States
Copyright © 2022 Busà, Bulati, Badami, Zito, Maresca, Conaldi, Ercolano and Ianaro. This is an open-access article distributed under the terms of the Creative Commons Attribution License (CC BY). The use, distribution or reproduction in other forums is permitted, provided the original author(s) and the copyright owner(s) are credited and that the original publication in this journal is cited, in accordance with accepted academic practice. No use, distribution or reproduction is permitted which does not comply with these terms.
*Correspondence: Giuseppe Ercolano, R2l1c2VwcGUuZXJjb2xhbm9AdW5pbmEuaXQ=
†These authors have contributed equally to this work