- 1Department of Dermatology, Xiangya Hospital, Central South University, Changsha, China
- 2Hunan Key Laboratory of Aging Biology, Xiangya Hospital, Central South University, Changsha, China
- 3National Clinical Research Center for Geriatric Disorders, Xiangya Hospital, Central South University, Changsha, China
Since the embryo, the nervous system and immune system have been interacting to regulate each other’s development and working together to resist harmful stimuli. However, oversensitive neural response and uncontrolled immune attack are major causes of various diseases, especially in barrier organs, while neural-immune interaction makes it worse. As the first defense line, the barrier organs give a guarantee to maintain homeostasis in external environment. And the dense nerve innervation and abundant immune cell population in barrier organs facilitate the neuroimmune interaction, which is the physiological basis of multiple neuroimmune-related diseases. Neuroimmune-related diseases often have complex mechanisms and require a combination of drugs, posing challenges in finding etiology and treatment. Therefore, it is of great significance to illustrate the specific mechanism and exact way of neuro-immune interaction. In this review, we first described the mutual regulation of the two principal systems and then focused on neuro-immune interaction in the barrier organs, including intestinal tract, lungs and skin, to clarify the mechanisms and provide ideas for clinical etiology exploration and treatment.
1 Introduction
Adapting to environmental changes and maintaining homeostasis requires the involvement of both the nervous system and immune system (Veiga-Fernandes and Pachnis, 2017; Zhang et al., 2021). The nervous system dominates tissues or organs mainly through a variety of neurotransmitters, while the immune system resists pathogens by phagocytosis and immune-active substances. According to research in recent years, the two systems can be linked together as a whole through certain agents, such as neurotransmitters, cytokines, hormones, etc (Chavan et al., 2017; Reardon et al., 2018). Neurotransmitter receptors are distributed on some immune cells, which are the foundation of neuromodulation of the immune system. And there are also cytokine receptors on nerve endings, which are the essential pathway for immunomodulation of the nervous system. The nervous system speeds up the immune response, and the immune system correspondingly helps transfer information to the nervous system. Neuro-immune interaction enriches the body’s response to the environment. However, under certain circumstances, neuro-immune interactions are the driving factors for the onset and progression of numerous diseases (Kabata and Artis, 2019; Tanaka and Okusa, 2019; Scheiblich et al., 2020).
For the past few years, there is increasing evidence about the mutual regulation of nerve and immune systems, which reveals that nerves regulate the generation, maturation, and release of immune cells (Elenkov et al., 2000), and immunity is involved in neural development (Cowan and Petri, 2018; Vainchtein et al., 2018). As the first line of defense, the gut-skin-lung barrier plays an important role in clearing harmful substances, resisting pathogen invasion, and maintaining homeostasis, and its dysfunction leads to the occurrence and progression of diseases. What’s more, there are dense nerve innervation and resident immune cell groups on the barrier, and the neuro-immune interaction occupies a more significant position in the pathogenesis of diseases. For example, neuropeptides secreted by sensory nerves trigger neurogenic inflammation and lead to allergic diseases (Sousa-Valente and Brain, 2018), while sympathetic and parasympathetic nerves produce neurotransmitters binding to different receptors on immune cells for immune regulation (Kenney and Ganta, 2014). Cytokine receptors on nerve endings collect information of immune status and transmit feedback signs.
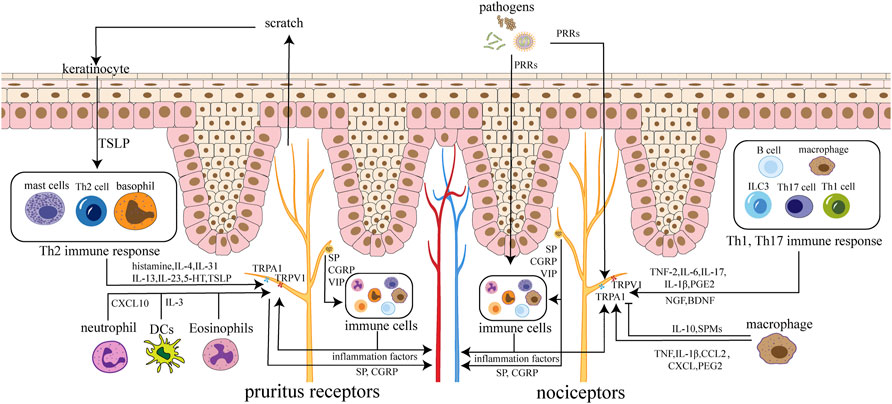
FIGURE 1. Neuroimmune circuits in skin. Immune cells activate receptors and channels on sensory neurons in the skin by secreting cytokines, chemokines, and lipids, leading to itching and pain. Nerve endings can also regulate immune cells by releasing SP, CGRP, VIP and other neuropeptides. Neuropeptides and factors can act on the skin and blood vessels, causing inflammation and edema. Pathogens can stimulate nociceptors directly or by inflammatory cytokines from immune cells. Pruritus receptors’ activation cause scratch, which leads to the destruction of keratinocytes with TSLP released, triggering amplification effect. Nociceptors are mainly involved in Th1 and Th17 immune responses, while pruritus receptors are mainly involved in Th2 immune responses.
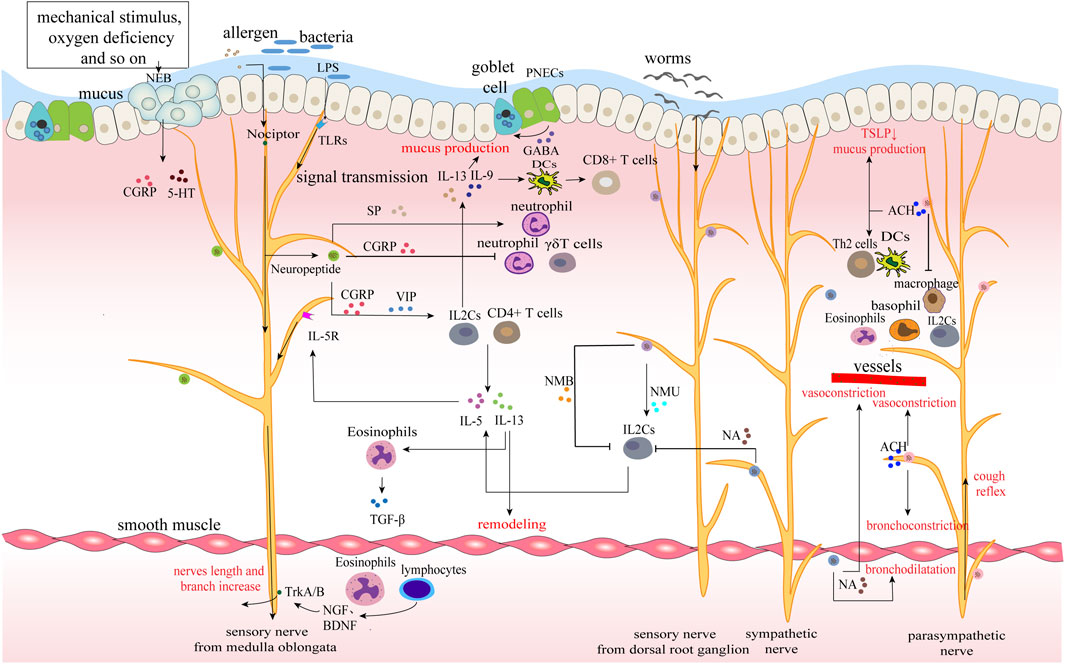
FIGURE 2. Neuroimmune circuits in lungs. The epithelium, nerves and immunity of the lung together constitute the airway barrier. Various allergens, bacteria and parasites stimulate and interact with different nerves to upload signals and regulate immunity. For example, LPS of bacteria combines with TLRs of sensory nerves to promote the release of neuropeptides, while worms act on sympathetic nerves to secrete neurotransmitters and mainly regulate ILC2s. Immune cells transmit immune signals to the nerve by releasing cytokines, forming a closed-loop of nerve and immunity. Moreover, immune cells synthesize nerve growth factor to promote nerve growth and increase nerve exposure in the airway.
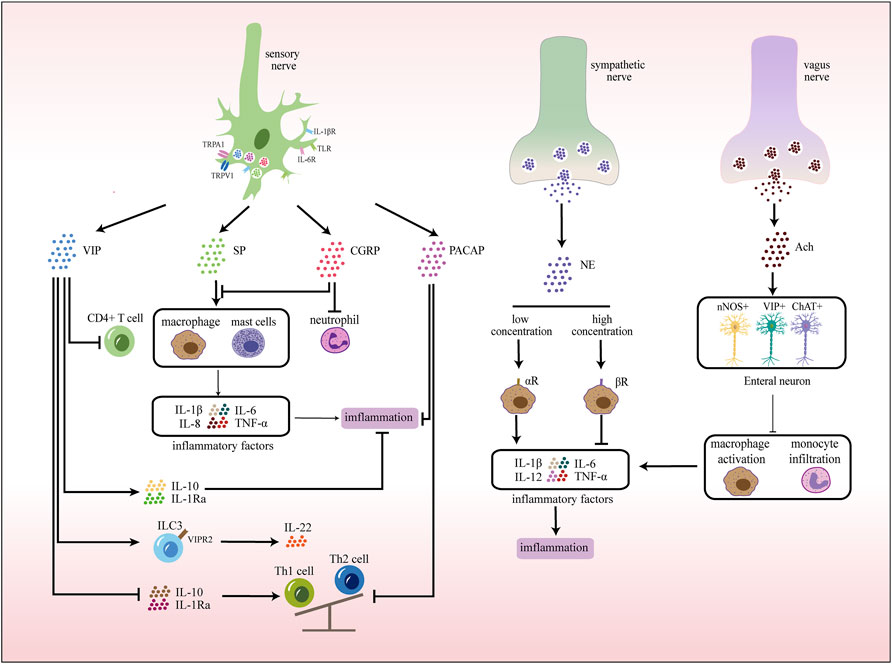
FIGURE 3. Neuroimmune communication in the intestinal tract. In the gut, sensory nerves express receptors that recognize various stimuli, pathogens, and cytokines. Activated sensory nerves release different neurotransmitters that interact with immune cells. In addition, exogenous sympathetic and vagus nerves also participate in inflammatory responses by releasing corresponding neurotransmitters.
At present, the mechanism of neuro-immune interactions remains unclear, and individualized treatment is hard to practice. Further basic research and clinical trials are needed to find out the pattern of neuroimmune-related disease and provide a more rational therapeutic regimen.
2 The Mutual Regulation of Nervous System and Immune System
From the embryonic period, the development of the immune and nervous systems is regulated by each other. Immune cells are almost regulated by nerves from differentiation to function execution. And nerves perform hormone-mediated immune regulation through the hypothalamic-pituitary-adrenal (HPA) axis. The immune system also influences nerve growth and regulates the HPA axis through various immunoactive substances.
2.1 Neural Regulation of Immune Cell Production
Immune cells are originated from hematopoietic stem cells (HSCs) in the bone marrow, differentiate to the lymphoid stem cell stage which differentiate into pro-B and pro-T cells, and finally become a variety of immune cells in the bone marrow and thymus. However, studies have found that the nervous system regulates the generation, migration, and differentiation of HSCs directly or through substances such as neurotransmitters and neuropeptides (Agarwala and Tamplin, 2018).
The formation of HSCs occurs at the embryonic day 27–40, which refers to endothelial-to-hematopoietic transition (EHT) of hematopoietic endothelial cells of the embryonic dorsal aorta. Studies have found that hypoxic stress induces central neural crest cells to produce 5-hydroxytryptamine (5-HT) neurotransmitter signals in the embryonic zebrafish. 5-HT further affects the formation of distant embryonic hematopoietic stem and progenitor cells (HSPCs) via the HPA axis and local glucocorticoid (Kwan et al., 2016). Similarly, central cholinergic signals increase the secretion of catecholamines (CAs) and γ-aminobutyric acid (GABA) through the hypothalamic-pituitary-adrenal (HPA) axis and peripheral neurons, promoting the proliferation and mobilization of immature CD34+ HSCs (Spiegel et al., 2007; Pierce et al., 2017; Shao et al., 2021). In addition, peripheral neurons secrete CAs and calcitonin gene-related peptide (CGRP) to drive granulocyte colony-stimulating factor (G-CSF)-induced mobilization of HSCs (Katayama et al., 2006; Maryanovich et al., 2018; Gao et al., 2021).
2.2 Neural Regulation of Immune Cell Maturation and Release
The nervous system communicates with lymphatic tissues or organs through sensory and autonomic nerves. Among them, the thymus, as a central immune organ, receives dual innervation of sympathetic and parasympathetic nerves. Immunofluorescent staining of mouse thymus shows the inside nerve fibers distribution and indicates that T cell maturation is regulated by nerves (Al-Shalan et al., 2019). Lymph nodes are mainly supplied by sensory and sympathetic nerves. There are extensive and close contacts between nerve fibers and various dendritic cells in mouse lymph nodes (Hu et al., 2019). Sensory neurons detect the immune state of peripheral lymph nodes through nociceptors and undergo remodeling induced by inflammation, playing a vital role in inflammatory signal transduction and immune regulation (Huang et al., 2021). In the spleen, sensory nerves release neuropeptides to regulate the maturation, distribution, and function of immune cells (Felten et al., 1987; Jung et al., 2017), and denervation of the mouse spleen impairs plasma cell formation during T-cell-dependent immune responses (Zhang et al., 2020). The sympathetic nerve regulates the production of CXCL13 to organize the aggregation and distribution of immune cells in the spleen’s white pulp (Murray et al., 2017). Spleen cells of α7-nAChR knockout (α7-KO) mice produce more TNF-α and IL-6 (Fujii et al., 2017), suggesting that vagal nerve stimulation relieves systemic inflammation and prevents organ damage by inhibiting the production of cytokines in the spleen by acetylcholine (Ach) (Bassi et al., 2020).
2.3 Effect of the Immune System on Nerve
In bidirectional neuroimmune communication, immunogenic signals also act on neurons. As an important signal transduction factor of the immune system, cytokines not only coordinate the immune response but also mediate the signal exchange between the immune system and the nervous system, affecting the normal development of the nervous system and the activity of the neuroendocrine axis.
The early life inflammatory cytokine environment including maternal immune activation in utero, perinatal systemic inflammation, and childhood infection, represented by IL-1β, IL-6, TNF-α and IFN-γ, can affect the normal development of the nervous system (Jiang et al., 2018). This is probably due to the interaction of cytokines and major histocompatibility complex I (MHC-I) on neurons, and thus, negatively regulating synaptic plasticity and synaptic pruning (Paolicelli et al., 2011; Schafer et al., 2012). In addition, ciliary neurotrophic factor (CNTF), leukemia inhibitory factor (LIF), cardiotrophin-1 (CT-1), and IL-6 constitute a group of structurally related cytokines that is linked to neuronal nutrition and neuronal development through the NF-kb dependent pathway (Middleton et al., 2000).
Various cytokines have been shown to affect HPA axis activity in vivo. IL-1 increases adrenocorticotropic hormone (ACTH) and Glucocorticoid (GC) levels by influencing the secretory activity of the hypothalamus (Dunn, 2000). Moreover, various hematopoietic cytokines, such as TNF-α, IL-6, LIF, oncostatin M (OM), CNTF, interleukin-1 (IL-11), CT-1, etc., synergically enhance IL-1 mediated ACTH and corticosterone secretion to a certain extent (Turnbull and Rivier, 1999).
3 Neuroimmune Crosstalk in Skin, Lung, and Intestine
Skin, lung, and intestine share common anatomical features, including dense innervation and abundant resident immune cells. Nerve endings express cytokine receptors, and receptors for neuropeptides and neurotransmitters are also widely distributed on immune cells. Nerves and immune cells are close to each other in space. These points laid a physiological foundation for local neuro-immune interaction.
3.1 Neuroimmune Crosstalk in the Skin
The skin consists of epidermis, dermis, and subcutaneous tissue. Various inflammatory factors, neurotransmitters, neuropeptides, and receptors mediate neuroimmune communication between the epidermis, dermis, and appendages to regulate local skin homeostasis (Kobayashi et al., 2019) (Figure 1). The neuroimmune crosstalk of the skin plays a vital role in skin diseases such as psoriasis, atopic dermatitis, allergic dermatitis (Trier et al., 2019; Wang and Kim, 2020).
The nerves in the skin include the sensory, motor and autonomic nerves. Sensory nerves are divided into Aβ-fibers, Aδ-fibers, and C-fibers according to diameter and incoming velocity. C-fibers, including nociceptors and itch receptors, terminate in the epidermis and communicate most closely with neuroimmune (Lumpkin and Caterina, 2007). C-fibers are divided into peptide-energetic neurons and non-peptide-energetic neurons. The peptide-energetic neurons are marked by TRPV1 channels and can secrete neuropeptides, while the NP2 subpopulation of non-peptide-energetic neurons can also express TRPV1. The autonomic nerves of the skin are distributed only in the dermis, and most of them come from cholinergic sympathetic nerve fibers, which are involved in the blood and lymphatic circulation of the skin and the regulation of skin accessory organs. The autonomic nerves of the skin can secrete and release neurotransmitters such as acetylcholine, neuropeptides such as NPY, galanin, CGRP, and VIP, and neuromodulators such as tyrosine hydroxylase (Roosterman et al., 2006).
When sensing external noxious stimuli, skin sensory nerves regulate immunity by releasing neuropeptides, neurotransmitters, and other substances (Table 1) (Gouin et al., 2017). Immune cells also modulate sensory nerve activity by releasing inflammatory factors. Skin sensory nerves are divided into peptidergic neurons and non-peptidergic neurons. Peptidergic neurons marked by the transient receptor potential vanilloid1 (TRPV1) channel release a variety of neuropeptides, mainly Substance P (SP) and CGRP, occupying a leading position in skin neuroimmune (Roosterman et al., 2006). Mas-related G-protein-coupled receptor D-expressing (MrgprD) neurons in non-peptidergic neurons also inhibit mast cells (MCs) activation by releasing glutamate, thereby maintaining skin homeostasis (Zhang et al., 2021). The autonomic nerves of the skin are distributed only in the dermis, and they mainly consist of cholinergic sympathetic nerve fibers. The autonomic nerve participate in the blood and lymph circulation of the skin and the regulation of skin accessory organs by releasing neurotransmitters such as acetylcholine and neuropeptides (Roosterman et al., 2006).
Immune cells such as MCs, macrophages, neutrophils, basophils, T cells, and keratinocytes of the skin also act on neurons by releasing various active substances (Table 2).
3.1.1 Immune Crosstalk of Pruritus Receptors
Nerve endings that transmit itch and pain communicate most closely with immune cells and are highly associated with inflammation (Wang and Kim, 2020).
Most pruritus receptors are non-peptidergic (NP) neurons, consisting of three subgroups: NP1, NP2, and NP3. Each subgroup expresses different receptors and channels. Itch-inducing cytokines are often associated with type 2 cell responses. Keratinocytes release cytokines such as transient receptor potential ankyrin 1 (TSLP), which activate downstream type 2 immunity (Tamari et al., 2021). Type 2 immune cells release histamine, cytokines (IL-4, IL-31, IL-13, IL-33, 5-HT, TSLP), 5-nitro-2-(3-phenylpropylamino)-benzoic acid (NPPB), and other itch factors to activate TRPV1 and transient receptor potential ankyrin 1 (TRPA1), which in turn activate Voltage-gated Na (+) (NaV) channels. Thus, action potentials are generated, and the signals are sent to the brain to generate itch. Itch causes scratching behavior, which make keratinocytes damaged to release TSLP, activating downstream pathways and forming a vicious circle (Wilson et al., 2013a; Mack and Kim, 2018).
Itch is closely related to MCs. In pathological conditions such as psoriasis and epidermal hyperplasia, MCs are increased and located near the primary afferent nerve endings that transmit itch and pain sensations, and there is bidirectional solid crosstalk between them (Voss et al., 2021). On the one hand, MCs release various mediators to activate pruritus receptors through direct or indirect pathways; on the other hand, MCs also generate nerve growth factor (NGF) to promote the growth of sensory nerve fibers (Leon et al., 1994). Furthermore, innervation is also necessary for MCs function. Passive cutaneous anaphylaxis (PCA)-induced degranulation of MCs is significantly reduced under denervation of sensory neurons (Siebenhaar et al., 2008).
Other immune cells are also involved in the generating of itch. Neutrophils activate C-X-C motif chemokine receptor 3 (CXCR3) by upregulating the expression of C-X-C Motif Chemokine Ligand 10 (CXCL10), leading to sensory nerve activation (Walsh et al., 2019). In wounds, dendritic cells, especially type 2 conventional dendritic cells (cDC2s), are the primary source of IL-31. IL-31 induces the expression of TRPV1 and Interleukin 31 Receptor A (IL-31RA) in neurons through the Jak1-Stat3 pathway, thereby increasing the sensitivity of itch sensory neurons (Xu et al., 2020a). Eosinophils, which rise rapidly after skin exposure to toxicants, mediate inflammation and are also able to alter local innervation and promote itch by increasing SP expression (Lee et al., 2015).
3.1.2 Immune Crosstalk of Painful Nerve Endings
Immune cells release cytokines (such as TNF-α, IL-6, IL-17A, IL-1β, NGF, prostaglandin E2), lipids, and other factors. These factors directly activate neurons and lead to the production of pain. And they also indirectly cause pain sensation by causing sensitization of central or peripheral neurons (Cook et al., 2018). Cytokines involved in pain are often associated with type 1 or 17 cell responses.
Macrophages are closely connected with pain perception. After tissue injury or infection, macrophages release various chemokines and lipid mediators. These factors bind to receptors on nociceptors, leading to increased activity of TRPA1/TRPV1 and voltage-gated sodium channels of nociceptors through activation of the downstream mitogen-activated protein kinases (MAPK) pathway and protein kinase A (PKA), resulting in increased pain sensitivity. The activation of nociceptors lead to the release of SP, which binds to macrophage neurokinin-1 receptor (NK-1R), leading to the infiltration of macrophage cells, forming positive feedback (Chen et al., 2020a). In addition, monocytes and macrophages also play an analgesic effect by releasing anti-inflammatory factors such as IL-10 and specialized pro-resolving mediators (SPMs) (Ji et al., 2016). Macrophages also express neurotrophic factors such as NGF and brain derived neurotrophic factor (BDNF), which maintain neuronal growth and survival (Blake et al., 2019a).
Keratinocytes are also closely related to pain receptors. Under physiological conditions, keratinocytes play analgesic and rewarding roles by being able to release β-endorphin, a proopiomelanocortin (POMC)-derived peptide (Fell et al., 2014). However, when keratinocytes are damaged, it leads to hyperalgesia. Pain neurons also release neuropeptides, leading to further activation of keratinocytes, which promotes the development of inflammation (Talagas et al., 2020).
Pain neurons modulate immune cell activity by releasing mediators such as neuropeptides and even miRNA-rich exosomes (McMahon et al., 2015; Simeoli et al., 2017; Baral et al., 2019; Huang et al., 2021). Activation of nociceptors also initiates or enhances neurogenic inflammation (Michoud et al., 2021). During infection, pathogens activate pain receptors through pattern recognition receptors (PRRs), resulting in the release of neuropeptides and the activation of immune cells. And they also directly activate the immune system to activate pain neurons through inflammatory factors. The nervous system and the immune system activate each other and work together to strengthen the defenses (Chiu, 2018).
Pain also leads to neuron-mediated immunosuppression. Nav1.8 is a sodium channel, mainly expressed in C-fibers of sensory neurons in the dorsal root ganglia. During HSV-1 infection, Nav1.8 + nociceptors inhibit skin neutrophil infiltration, limit tissue damage, control dendritic cells (DCs) responses to antigen presentation, and induce the initiation of CD8+ T cells (Filtjens et al., 2021).
3.1.3 Neuroimmune Crosstalk in Cutaneous Host Defense
Skin nerves play an integral role in regulating host defenses. Pathogen molecules such as endotoxin and flagellin directly activate receptors on sensory neurons to induce pain or itch to modulate immune responses (Chiu et al., 2013a; Chiu et al., 2013b). In response to nociceptive stimuli, inflammatory factors, and pathogen molecules, TRPV1+ neurons specifically activate the type 17 immune response by releasing CGRP to stimulate DCs to produce IL-23. IL-23 prompts γδT cells to produce IL-17 and IL-22 cytokines, which in turn recruit neutrophils and monocytes to enhance host defense (Cohen et al., 2019). This means that nerve fibers in the inflamed skin can activate the immune defenses of the uninfected skin, enabling it to fight against the potential threat of infection (Kashem et al., 2015a; Cohen et al., 2019). This pathway enhances the skin’s defense against C. Albicans (Kashem et al., 2015b) and leads to psoriatic dermatitis (Riol-Blanco et al., 2014). During HSV-1 infection, Nav1.8 + nociceptors are activated to inhibit skin neutrophil infiltration, limit tissue damage, and control DC responses to affect antigen presentation and induce the initiation of CD8+ T cells (Filtjens et al., 2021).
Nevertheless, it is worth noting that neuroimmune does not just boost defenses. Pathogens also promote self-transmission by suppressing pain perception through neuroimmune pathways or activating pain to suppress the immune system (Baral et al., 2019). In necrotizing fasciitis, Streptococcus pyogenes secrete streptolysin S (SLS) to activate neurons to generate pain and cause pain neurons to release CGRP. CGRP inhibits the recruitment of neutrophils (Chiu et al., 2013a) and the release of TNF-α from macrophages, which reduces immune bactericidal effect (Chiu, 2018).
3.1.4 Neuroimmune Crosstalk in Skin Inflammation
Skin neurogenic inflammation is caused by the neuropeptides released from sensory nerve endings, manifested as vasodilation, plasma extravasation, edema, cell infiltration, and enhanced nociception (Peters et al., 2005; Sorkin et al., 2018). Neurogenic inflammation promotes skin healing and immune defense but may increase pathological immune responses such as anaphylaxis.
The neuroimmune interaction between peripheral nerve endings and MCs is critical in neurogenic inflammation. After peripheral nerve stimulation, various factors are produced to drive MCs degranulation, which in turn recruits immune cells such as monocytes, eosinophils, and neutrophils (Kulka et al., 2008). SP activates immune cells, causing the release of leukotrienes through the lipoxygenase pathway. Leukotrienes cause plasma extravasation, leading to neurogenic edema (Le Filliatre et al., 2001). SP and CGRP can also act on vascular endothelial cells and smooth muscle cells, increasing vascular permeability and increasing plasma extravasation (Saria, 1984; Brain and Williams, 1989).
Neuropeptides such as SP and CGRP released from nerve endings activate MCs to release mediators such as histamine, leading to the aggregation of inflammatory cells. In turn, MCs and immune cell product activate neurons (Siebenhaar et al., 2008; Steinhoff et al., 2018). Neural and immune promote each other, which forms a vicious circle leading to the generation of neurogenic inflammation (Rosa and Fantozzi, 2013). Interactions between neuropeptides, neuropeptide receptors, and neuropeptide-degrading enzymes are key for the progression of neurogenic inflammation (Cattaruzza et al., 2009).
3.1.5 Neuroimmune Crosstalk in Skin Allergic Diseases
In allergic skin reactions, allergens directly activate TRPV1+ sensory neurons, and results in itch and pain (Perner et al., 2020). TRPV1+ nerve fibers colocalize closely with MRGPRB+ (MAS Related GPR Family Member D) MCs in the skin. During allergy, activated TRPV1+ nerve fibers activate MRGPRB2 on MCs through SP, leading to the release of inflammatory factors (Serhan et al., 2019a; Serhan et al., 2019b). SP also activate MRGPRA1(Mas-related G-protein coupled receptor member A1) on CD301b + DCs, causing their migration to dLN to initiate Th2 cell differentiation and induce skin type 2 inflammation (Perner et al., 2020). The recruited inflammatory cells produce a large number of cytokines, which further activate neurons to generate itch and pain with releasing neuropeptides. Allergens can also directly activate basophils to produce leukotrienes, especially Leukotriene C4 (LTC4) (Chen et al., 2020b). Leukotrienes lead to acute pruritus in atopic dermatitis (AD) through CysLTR2 (Cysteinyl Leukotriene Receptor 2) receptors on a subset of NP3 sensory neurons (Wang et al., 2021). Itch induces scratching behavior, which leads to the release of TSLP from damaged skin keratinocytes. TSLP leads to the aggravation of type 2 inflammation, thus forming a vicious circle (Wilson et al., 2013b; Buhl et al., 2020). Keratinocytes are also capable of secreting NGF and glial cell-line-derived neurotrophic factor (GDNF) to induce neurite outgrowth and increase CGRP + sensory fibers, increasing nerve fiber density in the epidermis of patients with atopic eczema (Roggenkamp et al., 2012).
3.2 Neuroimmune Crosstalk in the Lungs
In the lungs, sensory nerves are distributed in every layer of the airway, especially in the mucosal layer of the airway. Their nerve endings form some special structures, such as nociceptors and neuroepithelial cell body (NEB) (Gelb and Nadel, 2016; Mazzone and Undem, 2016). Sympathetic and parasympathetic nerves enter the lung with blood vessels and bronchus and are distributed around smooth muscle, glands and vessels (Figure 2). Dense nerve distribution facilitates the regulation of airway tension, mucus secretion, and cough reflex, and resident immune cells make a timely immune response to external stimuli. Epithelial cells, neurons, and immune cells interact to maintain the relative homeostasis of the lungs.
Sensory innervation of the respiratory tract mainly comes from the vagus branch from nodose/jugular ganglia and the somatosensory afferent nerves comes from neurons in the dorsal root ganglion (Camp et al., 2021). Pulmonary sensory nerve endings express Toll-like receptors (TLRs, a type of pattern-dependent recognition receptor) that recognize pathogen-associated molecular patterns (PAMPs), such as lipopolysaccharides (LPS, a component of the cell wall of gram-negative bacteria), and transmit signals to the central nervous system (CNS). The CNS makes a comprehensive response and sends signals through the vagus nerve to increase the expression of acetylcholine (Ach), promote cough reflex and regulate immune defense (Chen et al., 2020b; Yamada and Ichinose, 2018a; Jung et al., 2018). Also, pulmonary sensory nerve fibers constitute special nociceptors, which, under the stimulation of allergens and pathogens, on the one hand, cause cough, pain, and bronchoconstrictive reflex for initial resistance, on the other hand, induce neurogenic inflammation through calcium-mediated neuropeptide release (Table 3) (Chen et al., 2020b; Baral et al., 2018). This process is clinically common in allergic diseases, asthma, chronic obstructive pulmonary diseases (COPD), etc., Meanwhile, cytokine receptors such as IL-5R are also expressed in the sensory nerve endings, receiving cytokine signals in the inflammatory response, producing positive feedback and enhancing immunity (Huh and Veiga-Fernandes, 2020). In addition, another sensor, the NEB, is also distributed in the airway. They are compact structures within the mucous membrane of the airway and are innervated by vagal afferent fibers. The apical surface of NEB is exposed to the airway, responding to a series of stimuli such as hypoxia, hypercapnia, mechanical stretching, norepinephrine, etc., and producing several bioactive mediators, including bell bombesin, CGRP, and 5-HT (Table 3) (De Virgiliis and Di Giovanni, 2020; Mahmoud et al., 2021). Sensory nerves from the dorsal root ganglion can not only release neuromedin U (NMU) to promote the proliferation of ILC2 and type 2 inflammatory response (Cardoso et al., 2017; Klose et al., 2017; Wallrapp et al., 2017), but also secrete neuromedin B (NMB) to inhibit ILC2 response, eosinophilia, and mucus production in the stage of allergic inflammation and worm infection, forming a bidirectional regulation (Chen et al., 2020b; Inclan-Rico et al., 2020).
Cholinergic parasympathetic nerves originate from the vagus nucleus of the medulla oblongata and function through the release of ACh (Huang et al., 2019). Binding to M1/M3 receptors, Ach causes a pro-inflammatory effect, promoting bronchoconstriction, mucus secretion and vasodilation, and participating in the cough reflex, which is closely related to asthma, and atopic diseases (Cazzola et al., 2021). However, the effect of Ach on the N receptor is mostly inflammation-resisting. Immune cells express α7 nicotinic acetylcholine receptor (α7nAChR), which binds to Ach to suppress the release of inflammatory cytokines. This specific pathway is known as the cholinergic anti-inflammatory pathway (Yamada and Ichinose, 2018a). For smokers, nicotine, the main component of cigarettes, is the ligand of nicotinic acetylcholine receptor (nACHR), therefore possesses anti-inflammatory properties (Bosmans et al., 2017).
Sympathetic innervation of the lungs originates from the sympathetic ganglion generated from the upper thoracic segment of the spinal cord, and acts on bronchial vessels and submucosal glands through norepinephrine (NE), mainly regulating bronchodilation and mucus secretion under pressure (De Virgiliis and Di Giovanni, 2020). NE inhibits the release of cytokines through β2 adrenergic receptors (β2AR) of immune cells and plays an anti-inflammatory role.
In addition, there are several special cells in the lungs, which are significant in neuroimmune, leading to many pathological states. Pulmonary neuroendocrine cells (PNECs) are endodermal-derived airway epithelial cells innervated by sensory nerves, which are enriched in airway branch points and collect external environmental information from the air (Xu et al., 2020b). PNECs release neuropeptides and neurotransmitters under hypoxia, hypercapnia and nicotine stimulation to regulate inflammatory response, and mucus secretion of airways (Garg et al., 2019). Neuroairway associated macrophage (NAM) is a newly discovered special mesenchymal tissue-resident macrophage, which is closely related to neuronal projection in the airway. It is found that NAM reduces inflammatory damage in influenza virus infection, but the specific mechanism remains unclarified (Ural et al., 2020). Whether NAM participates in the resistance of Severe Acute Respiratory Syndrome Coronavirus 2 (SARS-CoV-2) infection and its possible clinical application is currently a research hotspot (De Virgiliis and Di Giovanni, 2020). Eosinophils and lymphocytes in the lungs synthesize neurotrophic factors (NGF and BDNF) that bind to receptors (TrkA/B) to increase nerve length and branch points, exposing nerve endings to lumen for storage and release of neurotransmitters and neuropeptides from these TRPV1 + fibers. It increases mucus secretion, collagen deposition, and smooth muscle hyperplasia (Pavón-Romero et al., 2021).
3.2.1 Neuroimmune Crosstalk in the Pulmonary Immune Defense Response
It has been found that sensory nerves play a major role in pulmonary infectious diseases (releasing neuropeptides to initiate immune defense (Table 3)). In the case of Staphylococcus aureus, SP stimulates bacterial virulence and promotes the migration, adhesion, and phagocytic activity of neutrophils. TRPV1+ afferent nerve in nociceptor modulates protective immunity by releasing cGRP, inhibiting neutrophil recruitment and γδT cell-mediated defense (Baral et al., 2018; Camp et al., 2021). In helminth infection, NEB can release NMU and NMB to bidirectionally regulate the immune response. NMU activates the second group of innate lymphocytes (ILC2)-mediated type 2 inflammatory response and aggravates inflammatory damage (Cardoso et al., 2017; Xu et al., 2020b). NMB inhibits ILC2 proliferation and reduces inflammation (Inclan-Rico et al., 2020).
Many studies on SARS-CoV-2 suggested that its approach to human host cells is mainly mediated by transmembrane protein angiotensin-converting enzyme 2 (ACE2) and transmembrane protease serine 2 (TMPRSS2), and these receptors are highly expressed in airway epithelium and pulmonary parenchyma (De Virgiliis and Di Giovanni, 2020). Patients are often accompanied by cerebral injury symptoms, such as headache, nausea and vomiting, confusion of consciousness, epilepsy, etc., (Acharya et al., 2020). Studies have revealed that SARS-CoV-2 spreads to the choroid plexus through blood transmission and the breakthrough blood-brain barrier to infect the CNS (Matsuda et al., 2004; Desforges et al., 2019; Fagre et al., 2021). In Corona Virus Disease 2019 (COVID-19), upregulated inflammatory mediators interact with receptors expressed on sensory neurons to activate sensory neurons and release neuropeptides, leading to vasodilation, immune cell recruitment, and neurogenic inflammation (Song et al., 2021). In addition, the newly discovered NAM is proved to relieve inflammation in influenza and has the possibility of fighting the inflammatory storm in severe cases of COVID-19 (Schneider et al., 2014).
3.2.2 Neuroimmune Crosstalk in Pulmonary Inflammation
COPD is a classic type of pulmonary airway inflammation characterized by persistent respiratory symptoms and airflow restriction. We take COPD as an example to illustrate the neuroimmune crosstalk common in pulmonary inflammation.
COPD is developed from chronic bronchitis and emphysema to the stage of continuous airflow limitation in pulmonary function examination, and its incidence and prevalence remain high in China. Increased activity of the cholinergic system in COPD results in airway smooth muscle contraction leading to airflow restriction (Yamada and Ichinose, 2018b). The development of COPD goes through a long inflammatory progression stage, during which the time for Ach release is prolonged, the expression of M1R and M3R in airway structure is increased, and the pro-inflammatory effect of Ach through the M receptor is enhanced (Blake et al., 2019b). While α7nAChR inhibits the release of inflammatory cytokines by inflammatory cells (including macrophages and dendritic cells) and has a pro-inflammatory effect during the inflammatory stage (Hollenhorst and Krasteva-Christ, 2021). During acute inflammation, neuropeptides released by sensory nerve endings modulate the progression of inflammation. SP increases adherent aggregation and phagocytic activity of neutrophils. VIP reduces the contraction response of airway smooth muscle and reduces the degree of airflow restriction (Table 3) (Camp et al., 2021).
3.2.3 Neuroimmune Crosstalk in Asthma
Asthma is a recurrent disease characterized by chronic airway inflammation and airway hyperresponsiveness. Allergens enter the airway and activate T cells through antigen presentation. Activated helper Th2 cells secrete cytokines such as interleukin to directly activate MCs and eosinophils, leading to chronic airway inflammation. B cells can also be activated to produce specific IgE, which binds to MCs and eosinophils to cause smooth muscle contraction. Several neuroimmune processes are involved in the development of asthma.
IL2C is important in allergic diseases. It is regulated by a variety of neuropeptides and neurotransmitters and produces massive cytokines to regulate the immune response. Nociceptors, NEB, and PNECs innervated by sensory nerves receive allergen stimulation and immediately induce the secretion of neuropeptides (VIP, CGRP, SP, etc.,) to cause neurogenic inflammation (Table 3) (Mou et al., 2021). VIP and CGRP enhance the activity of ILC2, TH2, and other cells (Nassenstein et al., 2018), and promote the production of inflammatory factors. SP promotes the degranulation of MCs, release of pro-inflammatory cytokines, and immune migration, increasing the adhesion of neutrophils to bronchial epithelial cells and neutrophils phagocytic activity. In addition, CGRP directly activates ILC2s at a close spatial distance under allergen stimulation, not only triggering downstream type 2 immune response but also promoting ILC2s to produce IL-5, recruiting eosinophils, and aggravating allergic reactions (Camp et al., 2021). PNECs amplify inflammation by releasing CGRP and the neurotransmitter GABA. Among them, GABA induces the hyperplasia of airway epithelial goblet cells and causes excessive secretion of airway mucus (Sui et al., 2018).
The parasympathetic nerve mainly mediates smooth muscle contraction and cough reflex through Ach, exacerbating dyspnea in asthmatic patients (Gosens and Gross, 2018). Ach binds to muscarinic acetylcholine receptor (mAChR) on dendritic cells to promote the polarization of TH2 cells and binds to MCs to increase their activity and aggravate airway edema and exudation (Bosmans et al., 2017). Ach acts on N receptors of immune cells to reduce the production of TSLP, thereby inhibiting the activity of ILC2s, promoting the immunosuppression of Treg cells, reducing the degranulation and cytokines of MCs, and cutting down the release of histamine and migration of eosinophils from basophils. In addition, a7nAChR inhibits the production and release of inflammatory factors on immune cells to cause a cholinergic anti-inflammatory effect, which relieves asthma airway to a certain extent. For example, Ach combined with a7nAChR on ILC2s reduces the proliferation of ILC2, suppresses the production of IL-5 and IL-13, and aggravates ILC2-mediated airway hyperresponsiveness (AHR) (Yamada and Ichinose, 2018a). Macrophage a7nAChR inhibits the inflammatory mediators, such as TNF-α and IL-1β, to produce and reduce inflammatory damage by binding Ach. Anticholinergic and muscarinic receptor antagonists are applied to relieve bronchoconstriction in asthma (Bosmans et al., 2017).
Under stress, NE produced by sympathetic nerve activation binds to β2AR can not only relax smooth muscle but also inhibit ILC2 mediated type 2 inflammation and mucus production, alleviating asthma symptoms. Studies have found that β2AR agonists effectively relax smooth muscle relaxation, inhibit immune cell recruitment, and cytokine release (Voisin et al., 2017).
3.3 Neuroimmune Crosstalk in the Intestinal Tract
Neurons in the intestinal tract are divided into the extraintestinal nerves and the enteric nervous system (ENS) by their location (Furness, 2012). Extraintestinal innervation includes the sympathetic nerve, vagus nerve, dorsal root nerve and nociceptive nerve. Neurons of ENS are mainly located in the submucosa and muscular layer, and together with glial cells form a ganglion network surrounding the intestinal tube, which is spatially divided into two layers: the intramuscular Austenian plexus and the submucosal plexus (SMP) (Furness, 2008; Schemann et al., 2020). ENS forms reflex circuits with the external sympathetic and parasympathetic nervous systems that innervate all intestinal layers, mediating intestinal motility, epithelial secretion, and vascular regulation (Benarroch, 2007; Schneider et al., 2019). Similarly, the intestinal tract is also rich in resident immune cells, which are mostly clustered in the lamina propria and muscularis of the mucosal area, including innate immune cells (macrophages, MCs, NK cells, innate lymphocytes, γδT cells, etc.) and adaptive immune cells (T cells, B cells). This anatomical intimacy between neurons and immune cells provides the basis for neuro-immune interaction (Figure 3).
Gut sensory nerves receive nutrients, mechanical stretching, lumen pressure, and immune stimulation to carry gut sensory signals from the gut to the brain stem and spinal cord (Spencer and Hu, 2020). They recognize harmful and inflammatory stimuli in three ways:
1) PRRs, such as TLRs, to recognize PAMPs produced by invading bacteria or viruses during infection (Barajon et al., 2009; Burgueño et al., 2016);
2) Cytokine receptors, to recognize factors secreted by immune cells (IL-1β、IL-6、IL-10 and TNF-α, etc.,) (Hughes et al., 2013);
3) Danger signal receptors, especially fast electrical channels (TRPV1, TRPV4, TRPA1, and TRPM8), to recognize oxidative stress products (ATP, uric acid, hydroxynonenal, etc.,) during harmful stimuli (heat, acid, and chemicals, etc.,), inflammation and tissue damage (Reinshagen et al., 1994).
Activated sensory nerves, on the one hand, produce reverse axonal reflex. It promotes the release of neuropeptides (SP, CGRP, VIP, etc.,) from nerve terminals on resident immune cells, leading to chemotaxis and activation of neutrophils, macrophages, MCs and lymphocytes (Table 4). At the same time, these neuropeptides can directly act on vascular endothelial cells, thereby promoting vascular dilation and increasing capillary permeability, resulting in plasma extravasation and edema. On the other hand, the activated nervous system produces cytokines to participate in immune activities (Kermarrec et al., 2019; Jarret et al., 2020).
The immune system also acts as a counterforce to the intestinal nervous system. Cytokines are the main signaling molecules, which regulate the intestinal nerve density and function. For example, TNF-α and IL-1β promote neurite growth by increasing glial secretion of GDNF (INVALID CITATIONa), while IL-1β and IL-6 modulate synaptic sensitivity and transmission efficiency (Xia et al., 1999).
3.3.1 Neuroimmune Crosstalk in Intestinal Host Defense
As mentioned above, sensory nerves densely distributed in the gut sense PAMPs and products and actively participate in host defense by releasing neuropeptides (SP, CGRP, VIP, etc.,) (Lai et al., 2017; Mao et al., 2013). On the one hand, these neuropeptides interact with immune cells to mediate neurogenic inflammation, intestinal contraction and mucus secretion, and is important for pathogen clearance (Table 5). On the other hand, due to the similar structure to antibacterial peptides (AMP), neuropeptides act on the negatively charged cell membrane of bacteria, change the membrane structure through depolarization, produce physical pores, and play an antibacterial role (Ganz, 2003; Hansen et al., 2006).
In addition, the intestinal nervous system can produce cytokines directly involved in host defense. Intestinal intermuscular plexus neurons produce IL-18 and stimulate goblet cells to secrete AMP and mucus, thereby enhancing the protective effect of intestinal mucus barrier and mediating protection against bacterial infection (Salmonella) (Jarret et al., 2020).
However, neuro-immune bi-directional communication during host defense is a double-edged sword. Persistent low-grade inflammation of the intestinal tract after infection leads to sensitization and loss of intestinal neurons, which is an important pathogenic factor of intestinal dysfunction after infection. There is an increased risk of irritable bowel syndrome after intestinal infection (Halvorson et al., 2006; Spiller and Garsed, 2009). Many patients have long-term abdominal pain after infection, which may be related to chronical sensitization of TRPV1+ neurons in the intestinal tract (Balemans et al., 2017). In addition, the non-classical inflammasome NlrP6 and caspase-11 (Casp11) -dependent pathway mediates rapid and sustained loss of intestinal neurons in the persistent hypoinflammatory state of postinfection, which is associated with intestinal motility disorders (Matheis et al., 2020).
In contrast, neuroimmunity in the gut also mediates anti-inflammatory responses and tissue damage repair during homeostasis. Tyrosine hydroxylase+ (TH+) neurons signal to myoclastic macrophages (MM) via β2AR to polarize MM into a tissue-protective phenotype (Gabanyi et al., 2016). During the intestinal pathogen infection, these macrophages protect intestinal neurons from Casp11-dependent death by expressing arginase and protective polyamines, which are important tissue-protective effects during postinfection and homeostasis (Matheis et al., 2020; Ahrends et al., 2021).
3.3.2 Neuroimmune Crosstalk in Inflammatory Bowel Disease
During intestinal inflammation, activated neurons release neurotransmitters and neuropeptides that play a pro-inflammatory or anti-inflammatory role and regulate inflammatory and immune states.
The effect of adrenergic neurons on inflammation depends on receptor binding ability. At low concentrations, NE binds a -adrenergic receptors, while at higher concentrations, NE primarily interacts with β receptors (Straub et al., 2006; Willemze et al., 2019). a receptors have pro-inflammatory effects and can promote the production of downstream pro-inflammatory cytokines, which play a significant role in intestinal defense function (Green et al., 2003). Blocking α2-adrenergic receptors down-regulate TNF and IL-1β and improve 2,4,6-trinitrobenzenesulfonic acid (TNB)-induced colitis (Bai et al., 2009). In contrast, several experiments have shown that exogenous NE can act on β receptors and exert dose-dependent anti-inflammatory effects.
They can inhibit the expression of macrophage inflammatory cytokines IL-1β, IL-6, IL-12, TNF-α in the inflammatory state, thereby alleviating colon inflammation and edema symptoms (Willemze et al., 2019; Mallesh et al., 2021). In the inflammatory state of the intestinal mucosa, semaphorin 3C (SEMA3C) and other sympathetic rejection factors are highly expressed, the intestinal sympathetic nerve fibers are lost (Straub et al., 2008), and the synthesis and storage of NE, DA and 5-HT are blocked (Magro et al., 2002). Besides, the density of a receptors is increased and of β receptors is decreased (Martinolle et al., 1993; Blandizzi et al., 2003). All this evidence suggests that imbalance of sympathetic energy transmission may be a part of IBD.
The vagus nerve has an important protective role in IBD, including acute recurrence of experimental colitis and chronic colitis (Ghia et al., 2006; Ghia et al., 2007; Liu et al., 2020). A nationwide cohort study in Sweden showed that patients undergoing vagotomy had an increased risk of developing IBD and that vagotomy was positively associated with later IBD (Liu et al., 2020). In the intestinal tract, the vagus nerve inhibits monocyte infiltration and macrophage activation, and reduces the release of related inflammatory factors (IL-1β, TNF-α, INF-γ, IL-18, etc.,), thereby inhibiting early acute inflammatory response and improving mucosal epithelial integrity (Ghia et al., 2006; Ghia et al., 2007). In addition, sacral nerve stimulation has been shown to improve colonic inflammation and enhance colonic barrier function by enhancing parasympathetic nerve activity and modulating ENS and immune system activity in mice (Tu et al., 2020). Conversely, vagal inhibition and decreased acetylcholine levels increase susceptibility to intestinal inflammation (Ghia et al., 2008). The activity of NF- kB in colonic mucosa and the expression of inflammatory factors such as IL-1β and TNF-α in colonic macrophages of mice with vagal nerve resection were significantly increased (Ghia et al., 2006; O'Mahony et al., 2009).
Sensory neuro-immune communication is also important in the development and progression of IBD (Kihara et al., 2003; Kimball et al., 2004; Engel et al., 2011; Engel et al., 2012a). Multiple pieces of evidence have confirmed the pro-inflammatory role of TRPV1+ and TRPA1+ neurons in experimental colitis. TLR4 co-localization with TRPV1 has been observed in a variety of primary afferent neurons, including DRG. In the course of colitis, the expression of TLR4 in DRG is increased, which upregulates TRPV1 expression and TRPV1 current density, mediates inflammatory pain, and regulates inflammatory state (Shen et al., 2017; Wu et al., 2019; Esquerre et al., 2020). Furthermore, the severity of colitis was positively correlated with the gradient of TRPV1 positive neurons in the colon (Engel et al., 2012a). This may be due to the activation of sensory neurons by inflammatory mediators that sensitize TRPV1 and TRPA1 channels, promote Ca2+ channel opening, and release pro-inflammatory neuropeptide SP, leading to pro-inflammatory events (Engel et al., 2011; Engel et al., 2012a; Bertin et al., 2014). TRPM8+ neurons play a protective role in acute colitis (innate immune cell-mediated) (Ramachandran et al., 2013; de Jong et al., 2015). TRPM8 is located in mucosal epithelial cells and high-threshold colonic sensory neurons (Austroy’s plexus) (Ramachandran et al., 2013), which may co-express TRPV1 and have the ability to cross-desensitize TRPV1 (Brain and Williams, 1989). Activation of TRPM8 blocks TRPV1-mediated calcium signaling, thereby inhibiting inflammation (Ramachandran et al., 2013).
3.3.3 Neuroimmune Crosstalk in Food Allergy
Food allergy is mainly manifested as hypersensitivity reaction (type I) caused by the interaction between submucosal sensory neurons and MCs. It is characterized by increased mucosal secretion and intense contraction of muscle tissue, leading to abdominal pain and diarrhea symptoms (Schemann and Camilleri, 2013; Yashiro et al., 2021).
In allergic reactions, specific IgE binding to FCεR1 on the MC surface recognizes the allergen, degranulates after activation, and releases mixed mediators (histamine, prostaglandin, leukotriene, 5-HT, cytokines, complement, etc.,) (Liu et al., 2003; Van Nassauw et al., 2007). Histamine is an important component of mixed media. On the one hand, it acts on H2 receptors in the postsynaptic membrane of intrinsic primary afferent neurons, resulting in a long-lasting and slow excitatory postsynaptic potential effect, which enhances the synchronous prolongation of the intestinal wall and secretory activity of mucosal epithelial cells (Nemeth et al., 1984). On the other hand, histamine and prostaglandin can inhibit sympathetic nerve activity, which forms inhibitory synapses with submucosal nerves, and increase the excitability of submucosal secreted motor neurons. After that, submucosal motor neurons release VIP and Ach to promote the secretion of water, electrolytes and mucus in the mucosa (Blandizzi et al., 2000; Liu et al., 2003).
In addition, intramuscular gut neurons themselves also express FcepsilonR1s (FcεRIs) (van der Kleij et al., 2010), which are directly activated by IgE during allergic reactions, leading to muscle contraction and diarrhea symptoms, and the production of histamine to further activate MCs and aggravate food allergic reactions (Yashiro et al., 2021).
4 Conclusion
The immune system and nervous system can sense various stimuli inside and outside the body and communicate with each other, helping to maintain host health and homeostasis. Skin, lung, and intestine are the barrier organs of the nervous system and immune cells densely distributed. There are more and more evidences of the existence of neuroimmune units and the close interaction between neuroimmune cells. Elucidating the mechanisms of neuroimmune interaction may have important implications for maintaining and reconstructing tissue homeostasis and discovering new therapeutic targets for barrier organ infectious diseases, inflammatory diseases and allergic diseases.
It is important to note that most of current research methods in the study of neuroimmune drugs promote, physical injury, chemical stimulation, electrical stimulation, etc., while providing preliminary evidence of neuroimmune signal can influence each other, but neuroimmune interaction of group autonomy mechanism is still unclear, and on the study of the neuroimmune cells of the organ is still insufficient. In the future, combined advanced technologies such as tissue-specific optogenetics, chemical genetics and intercellular labeling systems are expected to further explore the cellular intrinsic mechanisms of neuro-immune interactions.
Author Contributions
YZ, SD, and MW were the main authors of the review, and completed the collection and analysis of relevant literature and materials, as well as the writing of the first draft of the paper. ZD and JL were the architects and directors of the project and directed the writing of the paper. All authors have read and agreed to the final text.
Conflict of Interest
The authors declare that the research was conducted in the absence of any commercial or financial relationships that could be construed as a potential conflict of interest.
Publisher’s Note
All claims expressed in this article are solely those of the authors and do not necessarily represent those of their affiliated organizations, or those of the publisher, the editors and the reviewers. Any product that may be evaluated in this article, or claim that may be made by its manufacturer, is not guaranteed or endorsed by the publisher.
References
Acharya, A., Kevadiya, B. D., Gendelman, H. E., and Byrareddy, S. N. (2020). SARS-CoV-2 Infection Leads to Neurological Dysfunction. J. Neuroimmune Pharmacol. 15 (2), 167–173. doi:10.1007/s11481-020-09924-9
Agarwala, S., and Tamplin, O. J. (2018). Neural Crossroads in the Hematopoietic Stem Cell Niche. Trends Cell. Biol. 28 (12), 987–998. doi:10.1016/j.tcb.2018.05.003
Ahrends, T., Aydin, B., Matheis, F., Classon, C. H., Marchildon, F., Furtado, G. C., et al. (2021). Enteric Pathogens Induce Tissue Tolerance and Prevent Neuronal Loss from Subsequent Infections. Cell. 184 (23), 5715–5727. doi:10.1016/j.cell.2021.10.004
Al-Shalan, H. A. M., Hu, D., Nicholls, P. K., Greene, W. K., and Ma, B. (2019). Immunofluorescent Characterization of Innervation and Nerve-Immune Cell Neighborhood in Mouse Thymus. Cell. Tissue Res. 378 (2), 239–254. doi:10.1007/s00441-019-03052-4
Arranz, A., Abad, C., Juarranz, Y., Torroba, M., Rosignoli, F., Leceta, J., et al. (2006). Effect of VIP on TLR2 and TLR4 Expression in Lymph Node Immune Cells during TNBS-Induced Colitis. Ann. N. Y. Acad. Sci. 1070, 129–134. doi:10.1196/annals.1317.001
Asadi, S., Alysandratos, K.-D., Angelidou, A., Miniati, A., Sismanopoulos, N., Vasiadi, M., et al. (2012). Substance P (SP) Induces Expression of Functional Corticotropin-Releasing Hormone Receptor-1 (CRHR-1) in Human Mast Cells. J. Investigative Dermatology 132 (2), 324–329. doi:10.1038/jid.2011.334
Azuma, Y.-T., Hagi, K., Shintani, N., Kuwamura, M., Nakajima, H., Hashimoto, H., et al. (2008). PACAP Provides Colonic Protection against Dextran Sodium Sulfate Induced Colitis. J. Cell. Physiol. 216 (1), 111–119. doi:10.1002/jcp.21381
Bai, A., Lu, N., Guo, Y., Chen, J., and Liu, Z. (2009). Modulation of Inflammatory Response via α2-adrenoceptor Blockade in Acute Murine Colitis. Clin. Exp. Immunol. 156 (2), 353–362. doi:10.1111/j.1365-2249.2009.03894.x
Balemans, D., Mondelaers, S. U., Cibert-Goton, V., Stakenborg, N., Aguilera-Lizarraga, J., Dooley, J., et al. (2017). Evidence for Long-Term Sensitization of the Bowel in Patients with Post-infectious-IBS. Sci. Rep. 7 (1), 13606. doi:10.1038/s41598-017-12618-7
Barajon, I., Serrao, G., Arnaboldi, F., Opizzi, E., Ripamonti, G., Balsari, A., et al. (2009). Toll-like Receptors 3, 4, and 7 Are Expressed in the Enteric Nervous System and Dorsal Root Ganglia. J. Histochem Cytochem. 57 (11), 1013–1023. doi:10.1369/jhc.2009.953539
Baral, P., Udit, S., and Chiu, I. M. (2019). Pain and Immunity: Implications for Host Defence. Nat. Rev. Immunol. 19 (7), 433–447. doi:10.1038/s41577-019-0147-2
Baral, P., Umans, B. D., Li, L., Wallrapp, A., Bist, M., Kirschbaum, T., et al. (2018). Nociceptor Sensory Neurons Suppress Neutrophil and γδ T Cell Responses in Bacterial Lung Infections and Lethal Pneumonia. Nat. Med. 24 (4), 417–426. doi:10.1038/nm.4501
Bassi, G. S., Kanashiro, A., Coimbra, N. C., Terrando, N., Maixner, W., and Ulloa, L. (2020). Anatomical and Clinical Implications of Vagal Modulation of the Spleen. Neurosci. Biobehav. Rev. 112, 363–373. doi:10.1016/j.neubiorev.2020.02.011
Benarroch, E. E. (2007). Enteric Nervous System: Functional Organization and Neurologic Implications. Neurology 69 (20), 1953–1957. doi:10.1212/01.wnl.0000281999.56102.b5
Bertin, S., Aoki-Nonaka, Y., de Jong, P. R., Nohara, L. L., Xu, H., Stanwood, S. R., et al. (2014). The Ion Channel TRPV1 Regulates the Activation and Proinflammatory Properties of CD4+ T Cells. Nat. Immunol. 15 (11), 1055–1063. doi:10.1038/ni.3009
Blake, K. J., Jiang, X. R., and Chiu, I. M. (2019). Neuronal Regulation of Immunity in the Skin and Lungs. Trends Neurosci. 42 (8), 537–551. doi:10.1016/j.tins.2019.05.005
Blake, K. J., Jiang, X. R., and Chiu, I. M. (2019). Neuronal Regulation of Immunity in the Skin and Lungs. Trends Neurosci. 42 (8), 537–551. doi:10.1016/j.tins.2019.05.005
Blandizzi, C., Fornai, M., Colucci, R., Baschiera, F., Barbara, G., Giorgio, R. D., et al. (2003). Altered Prejunctional Modulation of Intestinal Cholinergic and Noradrenergic Pathways by α 2 -adrenoceptors in the Presence of Experimental Colitis. Br. J. Pharmacol. 139 (2), 309–320. doi:10.1038/sj.bjp.0705249
Blandizzi, C., Tognetti, M., Colucci, R., and Tacca, M. D. (2000). Histamine H3receptors Mediate Inhibition of Noradrenaline Release from Intestinal Sympathetic Nerves. Br. J. Pharmacol. 129 (7), 1387–1396. doi:10.1038/sj.bjp.0703194
Bosmans, G., Shimizu Bassi, G., Florens, M., Gonzalez-Dominguez, E., Matteoli, G., and Boeckxstaens, G. E. (2017). Cholinergic Modulation of Type 2 Immune Responses. Front. Immunol. 8, 1873. doi:10.3389/fimmu.2017.01873
Brain, S. D., and Williams, T. J. (1989). Interactions between the Tachykinins and Calcitonin Gene-Related Peptide Lead to the Modulation of Oedema Formation and Blood Flow in Rat Skin. Br. J. Pharmacol. 97 (1), 77–82. doi:10.1111/j.1476-5381.1989.tb11926.x
Buhl, T., Ikoma, A., Kempkes, C., Cevikbas, F., Sulk, M., Buddenkotte, J., et al. (2020). Protease-Activated Receptor-2 Regulates Neuro-Epidermal Communication in Atopic Dermatitis. Front. Immunol. 11, 1740. doi:10.3389/fimmu.2020.01740
Burgueño, J. F., Barba, A., Eyre, E., Romero, C., Neunlist, M., and Fernández, E. (2016). TLR2 and TLR9 Modulate Enteric Nervous System Inflammatory Responses to Lipopolysaccharide. J. Neuroinflammation 13 (1), 187. doi:10.1186/s12974-016-0653-0
Camp, B., Stegemann-Koniszewski, S., and Schreiber, J. (2021). Infection-Associated Mechanisms of Neuro-Inflammation and Neuro-Immune Crosstalk in Chronic Respiratory Diseases. Int. J. Mol. Sci. 22 (11). doi:10.3390/ijms22115699
Cardoso, V., Chesné, J., Ribeiro, H., García-Cassani, B., Carvalho, T., Bouchery, T., et al. (2017). Neuronal Regulation of Type 2 Innate Lymphoid Cells via Neuromedin U. Nature 549 (7671), 277–281. doi:10.1038/nature23469
Cattaruzza, F., Cottrell, G., Vaksman, N., and Bunnett, N. (2009). Endothelin-converting Enzyme 1 Promotes Re-sensitization of Neurokinin 1 Receptor-dependent Neurogenic Inflammation. Br. J. Pharmacol. 156 (5), 730–739. doi:10.1111/j.1476-5381.2008.00039.x
Cazzola, M., Calzetta, L., and Matera, M. G. (2021). Long‐acting Muscarinic Antagonists and Small Airways in Asthma: Which Link? Allergy 76 (7), 1990–2001. doi:10.1111/all.14766
Cevikbas, F., Wang, X., Akiyama, T., Kempkes, C., Savinko, T., Antal, A., et al. (2014). A Sensory Neuron-Expressed IL-31 Receptor Mediates T Helper Cell-dependent Itch: Involvement of TRPV1 and TRPA1. J. Allergy Clin. Immunol. 133 (2), 448–460. doi:10.1016/j.jaci.2013.10.048
Chavan, S. S., Pavlov, V. A., and Tracey, K. J. (2017). Mechanisms and Therapeutic Relevance of Neuro-Immune Communication. Immunity 46 (6), 927–942. doi:10.1016/j.immuni.2017.06.008
Chen, O., Donnelly, C. R., and Ji, R.-R. (2020). Regulation of Pain by Neuro-Immune Interactions between Macrophages and Nociceptor Sensory Neurons. Curr. Opin. Neurobiol. 62, 17–25. doi:10.1016/j.conb.2019.11.006
Chen, W., Shu, Q., and Fan, J. (2020). Neural Regulation of Interactions between Group 2 Innate Lymphoid Cells and Pulmonary Immune Cells. Front. Immunol. 11, 576929. doi:10.3389/fimmu.2020.576929
Chiu, I. M., Heesters, B. A., Ghasemlou, N., Von Hehn, C. A., Zhao, F., Tran, J., et al. (2013). Bacteria Activate Sensory Neurons that Modulate Pain and Inflammation. Nature 501 (7465), 52–57. doi:10.1038/nature12479
Chiu, I. M., Heesters, B. A., Ghasemlou, N., Von Hehn, C. A., Zhao, F., Tran, J., et al. (2013). Bacteria Activate Sensory Neurons that Modulate Pain and Inflammation. Nature 501 (7465), 52–57. doi:10.1038/nature12479
Chiu, I. M. (2018). Infection, Pain, and Itch. Neurosci. Bull. 34 (1), 109–119. doi:10.1007/s12264-017-0098-1
Cohen, J. A., Edwards, T. N., Liu, A. W., Hirai, T., Jones, M. R., Wu, J., et al. (2019). Cutaneous TRPV1+ Neurons Trigger Protective Innate Type 17 Anticipatory Immunity. Cell. 178 (4), 919–e14. doi:10.1016/j.cell.2019.06.022
Cook, A. D., Christensen, A. D., Tewari, D., McMahon, S. B., and Hamilton, J. A. (2018). Immune Cytokines and Their Receptors in Inflammatory Pain. Trends Immunol. 39 (3), 240–255. doi:10.1016/j.it.2017.12.003
Cowan, M., and Petri, W. A. (2018). Microglia: Immune Regulators of Neurodevelopment. Front. Immunol. 9, 2576. doi:10.3389/fimmu.2018.02576
de Jong, P. R., Takahashi, N., Peiris, M., Bertin, S., Lee, J., Gareau, M. G., et al. (2015). TRPM8 on Mucosal Sensory Nerves Regulates Colitogenic Responses by Innate Immune Cells via CGRP. Mucosal Immunol. 8 (3), 491–504. doi:10.1038/mi.2014.82
De Virgiliis, F., and Di Giovanni, S. (2020). Lung Innervation in the Eye of a Cytokine Storm: Neuroimmune Interactions and COVID-19. Nat. Rev. Neurol. 16 (11), 645–652. doi:10.1038/s41582-020-0402-y
Delgado, M., Gonzalez‐Rey, E., and Ganea, D. (2004). VIP/PACAP Preferentially Attract Th2 Effectors through Differential Regulation of Chemokine Production by Dendritic Cells. FASEB J. 18 (12), 1453–1455. doi:10.1096/fj.04-1548fje
Delgado, M., Pozo, D., and Ganea, D. (2004). The Significance of Vasoactive Intestinal Peptide in Immunomodulation. Pharmacol. Rev. 56 (2), 249–290. doi:10.1124/pr.56.2.7
Desforges, M., Le Coupanec, A., Dubeau, P., Bourgouin, A., Lajoie, L., Dubé, M., et al. (2019). Human Coronaviruses and Other Respiratory Viruses: Underestimated Opportunistic Pathogens of the Central Nervous System? Viruses 12 (1). doi:10.3390/v12010014
Ding, W., Manni, M., Stohl, L. L., Zhou, X. K., Wagner, J. A., and Granstein, R. D. (2012). Pituitary Adenylate Cyclase-Activating Peptide and Vasoactive Intestinal Polypeptide Bias Langerhans Cell Ag Presentation toward Th17 Cells. Eur. J. Immunol. 42 (4), 901–911. doi:10.1002/eji.201141958
Dunn, A. J. (2000). Cytokine Activation of the HPA axis. Ann. N. Y. Acad. Sci. 917, 608–617. doi:10.1111/j.1749-6632.2000.tb05426.x
Elenkov, I. J., Wilder, R. L., Chrousos, G. P., and Vizi, E. S. (2000). The Sympathetic Nerve-Aan Integrative Interface between Two Supersystems: the Brain and the Immune System. Pharmacol. Rev. 52 (4), 595–638.
Engel, M. A., Khalil, M., Mueller-Tribbensee, S. M., Becker, C., Neuhuber, W. L., Neurath, M. F., et al. (2012). The Proximodistal Aggravation of Colitis Depends on Substance P Released from TRPV1-Expressing Sensory Neurons. J. Gastroenterol. 47 (3), 256–265. doi:10.1007/s00535-011-0495-6
Engel, M. A., Khalil, M., Siklosi, N., Mueller-Tribbensee, S. M., Neuhuber, W. L., Neurath, M. F., et al. (2012). Opposite Effects of Substance P and Calcitonin Gene-Related Peptide in Oxazolone Colitis. Dig. Liver Dis. 44 (1), 24–29. doi:10.1016/j.dld.2011.08.030
Engel, M. A., Leffler, A., Niedermirtl, F., Babes, A., Zimmermann, K., Filipović, M. R., et al. (2011). TRPA1 and Substance P Mediate Colitis in Mice. Gastroenterology 141 (4), 1346–1358. doi:10.1053/j.gastro.2011.07.002
Esquerre, N., Basso, L., Defaye, M., Vicentini, F. A., Cluny, N., Bihan, D., et al. (2020). Colitis-Induced Microbial Perturbation Promotes Postinflammatory Visceral Hypersensitivity. Cell. Mol. Gastroenterology Hepatology 10 (2), 225–244. doi:10.1016/j.jcmgh.2020.04.003
Fagre, A., Lewis, J., Eckley, M., Zhan, S., Rocha, S. M., Sexton, N. R., et al. (2021). SARS-CoV-2 Infection, Neuropathogenesis and Transmission Among Deer Mice: Implications for Spillback to New World Rodents. PLoS Pathog. 17 (5), e1009585. doi:10.1371/journal.ppat.1009585
Fell, G. L., Robinson, K. C., Mao, J., Woolf, C. J., and Fisher, D. E. (2014). Skin β-Endorphin Mediates Addiction to UV Light. Cell. 157 (7), 1527–1534. doi:10.1016/j.cell.2014.04.032
Felten, D. L., Ackerman, K. D., Wiegand, S. J., and Felten, S. Y. (1987). Noradrenergic Sympathetic Innervation of the Spleen: I. Nerve Fibers Associate with Lymphocytes and Macrophages in Specific Compartments of the Splenic White Pulp. J. Neurosci. Res. 18 (1), 28–36, 118-21. doi:10.1002/jnr.490180107
Filtjens, J., Roger, A., Quatrini, L., Wieduwild, E., Gouilly, J., Hoeffel, G., et al. (2021). Nociceptive Sensory Neurons Promote CD8 T Cell Responses to HSV-1 Infection. Nat. Commun. 12 (1), 2936. doi:10.1038/s41467-021-22841-6
Franke, K., Wang, Z., Zuberbier, T., and Babina, M. (2021). Cytokines Stimulated by IL-33 in Human Skin Mast Cells: Involvement of NF-Κb and P38 at Distinct Levels and Potent Co-operation with FcεRI and MRGPRX2. Int. J. Mol. Sci. 22 (7). doi:10.3390/ijms22073580
Fujii, T., Mashimo, M., Moriwaki, Y., Misawa, H., Ono, S., Horiguchi, K., et al. (2017). Expression and Function of the Cholinergic System in Immune Cells. Front. Immunol. 8, 1085. doi:10.3389/fimmu.2017.01085
Furness, J. B. (2012). The Enteric Nervous System and Neurogastroenterology. Nat. Rev. Gastroenterol. Hepatol. 9 (5), 286–294. doi:10.1038/nrgastro.2012.32
Gabanyi, I., Muller, P. A., Feighery, L., Oliveira, T. Y., Costa-Pinto, F. A., and Mucida, D. (2016). Neuro-immune Interactions Drive Tissue Programming in Intestinal Macrophages. Cell. 164 (3), 378–391. doi:10.1016/j.cell.2015.12.023
Ganz, T. (2003). Defensins: Antimicrobial Peptides of Innate Immunity. Nat. Rev. Immunol. 3 (9), 710–720. doi:10.1038/nri1180
Gao, X., Zhang, D., Xu, C., Li, H., Caron, K. M., and Frenette, P. S. (2021). Nociceptive Nerves Regulate Haematopoietic Stem Cell Mobilization. Nature 589 (7843), 591–596. doi:10.1038/s41586-020-03057-y
Garg, A., Sui, P., Verheyden, J. M., Young, L. R., and Sun, X. (2019). Consider the Lung as a Sensory Organ: A Tip from Pulmonary Neuroendocrine Cells. Curr. Top. Dev. Biol. 132, 67–89. doi:10.1016/bs.ctdb.2018.12.002
Gelb, A. F., and Nadel, J. A. (2016). Affirmation of the Adoration of the Vagi and Role of Tiotropium in Asthmatic Patients. J. Allergy Clin. Immunol. 138 (4), 1011–1013. doi:10.1016/j.jaci.2016.06.024
Ghia, J.-E., Blennerhassett, P., El-Sharkawy, R. T., and Collins, S. M. (2007). The Protective Effect of the Vagus Nerve in a Murine Model of Chronic Relapsing Colitis. Am. J. Physiology-Gastrointestinal Liver Physiology 293 (4), G711–G718. doi:10.1152/ajpgi.00240.2007
Ghia, J. E., Blennerhassett, P., and Collins, S. M. (2008). Impaired Parasympathetic Function Increases Susceptibility to Inflammatory Bowel Disease in a Mouse Model of Depression. J. Clin. Investig. 118 (6), 2209–2218. doi:10.1172/JCI32849
Ghia, J. E., Blennerhassett, P., Kumar–Ondiveeran, H., Verdu, E. F., and Collins, S. M. (2006). The Vagus Nerve: a Tonic Inhibitory Influence Associated with Inflammatory Bowel Disease in a Murine Model. Gastroenterology 131 (4), 1122–1130. doi:10.1053/j.gastro.2006.08.016
Gosens, R., and Gross, N. (2018). The Mode of Action of Anticholinergics in Asthma. Eur. Respir. J. 52 (4). doi:10.1183/13993003.01247-2017
Gouin, O., L'Herondelle, K., Lebonvallet, N., Le Gall-Ianotto, C., Sakka, M., Buhé, V., et al. (2017). TRPV1 and TRPA1 in Cutaneous Neurogenic and Chronic Inflammation: Pro-inflammatory Response Induced by Their Activation and Their Sensitization. Protein Cell. 8 (9), 644–661. doi:10.1007/s13238-017-0395-5
Green, B. T., Lyte, M., Kulkarni-Narla, A., and Brown, D. R. (2003). Neuromodulation of Enteropathogen Internalization in Peyer's Patches from Porcine Jejunum. J. Neuroimmunol. 141 (1-2), 74–82. doi:10.1016/s0165-5728(03)00225-x
Halvorson, H. A., Schlett, C. D., and Riddle, M. S. (2006). Postinfectious Irritable Bowel Syndrome-A Meta-Analysis. Am. J. Gastroenterol. 101 (8), 1894–1899. doi:10.1111/j.1572-0241.2006.00654.x
Hansen, C. J., Burnell, K. K., and Brogden, K. A. (2006). Antimicrobial Activity of Substance P and Neuropeptide Y against Laboratory Strains of Bacteria and Oral Microorganisms. J. Neuroimmunol. 177 (1-2), 215–218. doi:10.1016/j.jneuroim.2006.05.011
Helyes, Z., Kun, J., Dobrosi, N., Sándor, K., Németh, J., Perkecz, A., et al. (2015). Pituitary Adenylate Cyclase-Activating Polypeptide Is Upregulated in Murine Skin Inflammation and Mediates Transient Receptor Potential Vanilloid-1-Induced Neurogenic Edema. J. Investigative Dermatology 135 (9), 2209–2218. doi:10.1038/jid.2015.156
Hoeffel, G., Debroas, G., Roger, A., Rossignol, R., Gouilly, J., Laprie, C., et al. (2021). Sensory Neuron-Derived TAFA4 Promotes Macrophage Tissue Repair Functions. Nature 594 (7861), 94–99. doi:10.1038/s41586-021-03563-7
Hollenhorst, M. I., and Krasteva-Christ, G. (2021). Nicotinic Acetylcholine Receptors in the Respiratory Tract. Molecules 26 (20). doi:10.3390/molecules26206097
Hu, D., Nicholls, P. K., Claus, M., Wu, Y., Shi, Z., Greene, W. K., et al. (2019). Immunofluorescence Characterization of Innervation and Nerve-Immune Cell Interactions in Mouse Lymph Nodes. Eur. J. Histochem 63 (4). doi:10.4081/ejh.2019.3059
Huang, S., Ziegler, C. G. K., Austin, J., Mannoun, N., Vukovic, M., Ordovas-Montanes, J., et al. (2021). Lymph Nodes Are Innervated by a Unique Population of Sensory Neurons with Immunomodulatory Potential. Cell. 184 (2), 441–459. e25. doi:10.1016/j.cell.2020.11.028
Huang, Y., Zhao, C., and Su, X. (2019). Neuroimmune Regulation of Lung Infection and Inflammation. Qjm 112 (7), 483–487. doi:10.1093/qjmed/hcy154
Hughes, P. A., Harrington, A. M., Castro, J., Liebregts, T., Adam, B., Grasby, D. J., et al. (2013). Sensory Neuro-Immune Interactions Differ between Irritable Bowel Syndrome Subtypes. Gut 62 (10), 1456–1465. doi:10.1136/gutjnl-2011-301856
Huh, J. R., and Veiga-Fernandes, H. (2020). Neuroimmune Circuits in Inter-organ Communication. Nat. Rev. Immunol. 20 (4), 217–228. doi:10.1038/s41577-019-0247-z
Inclan-Rico, J. M., Ponessa, J. J., Valero-Pacheco, N., Hernandez, C. M., Sy, C. B., Lemenze, A. D., et al. (2020). Basophils Prime Group 2 Innate Lymphoid Cells for Neuropeptide-Mediated Inhibition. Nat. Immunol. 21 (10), 1181–1193. doi:10.1038/s41590-020-0753-y
Jarret, A., Jackson, R., Duizer, C., Healy, M. E., Zhao, J., Rone, J. M., et al. (2020). Enteric Nervous System-Derived IL-18 Orchestrates Mucosal Barrier Immunity. Cell. 180 (1), 50–63. e12. doi:10.1016/j.cell.2019.12.016
Ji, R.-R., Chamessian, A., and Zhang, Y.-Q. (2016). Pain Regulation by Non-neuronal Cells and Inflammation. Science 354 (6312), 572–577. doi:10.1126/science.aaf8924
Jiang, N. M., Cowan, M., Moonah, S. N., and Petri, W. A. (2018). The Impact of Systemic Inflammation on Neurodevelopment. Trends Mol. Med. 24 (9), 794–804. doi:10.1016/j.molmed.2018.06.008
Joachim, R. A., Kuhlmei, A., Dinh, Q. T., Handjiski, B., Fischer, T., Peters, E. M. J., et al. (2007). Neuronal Plasticity of the "Brain-Skin Connection": Stress-Triggered Up-Regulation of Neuropeptides in Dorsal Root Ganglia and Skin via Nerve Growth Factor-dependent Pathways. J. Mol. Med. 85 (12), 1369–1378. doi:10.1007/s00109-007-0236-8
Jung, W.-C., Levesque, J.-P., and Ruitenberg, M. J. (2017). It Takes Nerve to Fight Back: The Significance of Neural Innervation of the Bone Marrow and Spleen for Immune Function. Seminars Cell. & Dev. Biol. 61, 60–70. doi:10.1016/j.semcdb.2016.08.010
Jung, W. J., Lee, S. Y., Choi, S. I., Kim, B.-K., Lee, E. J., In, K. H., et al. (2018). Toll-like Receptor Expression in Pulmonary Sensory Neurons in the Bleomycin-Induced Fibrosis Model. PLoS One 13 (3), e0193117. doi:10.1371/journal.pone.0193117
Kabata, H., and Artis, D. (2019). Neuro-immune Crosstalk and Allergic Inflammation. J. Clin. Investig. 129 (4), 1475–1482. doi:10.1172/jci124609
Kashem, S. W., Riedl, M. S., Yao, C., Honda, C. N., Vulchanova, L., and Kaplan, D. H. (2015). Nociceptive Sensory Fibers Drive Interleukin-23 Production from CD301b+ Dermal Dendritic Cells and Drive Protective Cutaneous Immunity. Immunity 43 (3), 515–526. doi:10.1016/j.immuni.2015.08.016
Kashem, S. W., Riedl, M. S., Yao, C., Honda, C. N., Vulchanova, L., and Kaplan, D. H. (2015). Nociceptive Sensory Fibers Drive Interleukin-23 Production from CD301b+ Dermal Dendritic Cells and Drive Protective Cutaneous Immunity. Immunity 43 (3), 515–526. doi:10.1016/j.immuni.2015.08.016
Katayama, Y., Battista, M., Kao, W.-M., Hidalgo, A., Peired, A. J., Thomas, S. A., et al. (2006). Signals from the Sympathetic Nervous System Regulate Hematopoietic Stem Cell Egress from Bone Marrow. Cell. 124 (2), 407–421. doi:10.1016/j.cell.2005.10.041
Kenney, M. J., and Ganta, C. K. (2014). Autonomic Nervous System and Immune System Interactions. Compr. Physiol. 4 (3), 1177–1200. doi:10.1002/cphy.c130051
Kermarrec, L., Durand, T., Gonzales, J., Pabois, J., Hulin, P., Neunlist, M., et al. (2019). Rat Enteric Glial Cells Express Novel Isoforms of Interleukine‐7 Regulated during Inflammation. Neurogastroenterol. Motil. 31 (1), e13467. doi:10.1111/nmo.13467
Kihara, N., de la Fuente, S. G., Fujino, K., Takahashi, T., Pappas, T. N., and Mantyh, C. R. (2003). Vanilloid Receptor-1 Containing Primary Sensory Neurones Mediate Dextran Sulphate Sodium Induced Colitis in Rats. Gut 52 (5), 713–719. doi:10.1136/gut.52.5.713
Kimball, E. S., Wallace, N. H., Schneider, C. R., D'Andrea, M. R., and Hornby, P. J. (2004). Vanilloid Receptor 1 Antagonists Attenuate Disease Severity in Dextran Sulphate Sodium-Induced Colitis in Mice. Neurogastroenterol. Motil. 16 (6), 811–818. doi:10.1111/j.1365-2982.2004.00549.x
Kincy-Cain, T., and Bost, K. L. (1996). Increased Susceptibility of Mice to Salmonella Infection Following In Vivo Treatment with the Substance P Antagonist, Spantide II. J. Immunol. 157 (1), 255–264.
Kincy-Cain, T., and Bost, K. L. (1997). Substance P-Induced IL-12 Production by Murine Macrophages. J. Immunol. 158 (5), 2334–2339.
Klose, C. S. N., Mahlakõiv, T., Moeller, J. B., Rankin, L. C., Flamar, A.-L., Kabata, H., et al. (2017). The Neuropeptide Neuromedin U Stimulates Innate Lymphoid Cells and Type 2 Inflammation. Nature 549 (7671), 282–286. doi:10.1038/nature23676
Kobayashi, T., Naik, S., and Nagao, K. (2019). Choreographing Immunity in the Skin Epithelial Barrier. Immunity 50 (3), 552–565. doi:10.1016/j.immuni.2019.02.023
Krämer, H. H., Schmidt, K., Leis, S., Schmelz, M., Sommer, C., and Birklein, F. (2005). Inhibition of Neutral Endopeptidase (NEP) Facilitates Neurogenic Inflammation. Exp. Neurol. 195 (1), 179–184. doi:10.1016/j.expneurol.2005.04.015
Kulka, M., Sheen, C. H., Tancowny, B. P., Grammer, L. C., and Schleimer, R. P. (2008). Neuropeptides Activate Human Mast Cell Degranulation and Chemokine Production. Immunology 123 (3), 398–410. doi:10.1111/j.1365-2567.2007.02705.x
Kwan, W., Cortes, M., Frost, I., Esain, V., Theodore, L. N., Liu, S. Y., et al. (2016). The Central Nervous System Regulates Embryonic HSPC Production via Stress-Responsive Glucocorticoid Receptor Signaling. Cell. stem Cell. 19 (3), 370–382. doi:10.1016/j.stem.2016.06.004
Lai, N. Y., Mills, K., and Chiu, I. M. (2017). Sensory Neuron Regulation of Gastrointestinal Inflammation and Bacterial Host Defence. J. Intern Med. 282 (1), 5–23. doi:10.1111/joim.12591
Lai, N. Y., Musser, M. A., Pinho-Ribeiro, F. A., Baral, P., Jacobson, A., Ma, P., et al. (2020). Gut-Innervating Nociceptor Neurons Regulate Peyer's Patch Microfold Cells and SFB Levels to Mediate Salmonella Host Defense. Cell. 180 (1), 33–49. doi:10.1016/j.cell.2019.11.014
Le Filliatre, G., Sayah, S., Latournerie, V., Renaud, J. F., Finet, M., and Hanf, R. (2001). Cyclo-oxygenase and Lipoxygenase Pathways in Mast Cell Dependent-Neurogenic Inflammation Induced by Electrical Stimulation of the Rat Saphenous Nerve. Br. J. Pharmacol. 132 (7), 1581–1589. doi:10.1038/sj.bjp.0703950
Lee, J. J., Protheroe, C. A., Luo, H., Ochkur, S. I., Scott, G. D., Zellner, K. R., et al. (2015). Eosinophil-dependent Skin Innervation and Itching Following Contact Toxicant Exposure in Mice. J. Allergy Clin. Immunol. 135 (2), 477–487. doi:10.1016/j.jaci.2014.07.003
Leon, A., Buriani, A., Dal Toso, R., Fabris, M., Romanello, S., Aloe, L., et al. (1994). Mast Cells Synthesize, Store, and Release Nerve Growth Factor. Proc. Natl. Acad. Sci. U.S.A. 91 (9), 3739–3743. doi:10.1073/pnas.91.9.3739
Lim, J. E., Chung, E., and Son, Y. (2017). A Neuropeptide, Substance-P, Directly Induces Tissue-Repairing M2 like Macrophages by Activating the PI3K/Akt/mTOR Pathway Even in the Presence of IFNγ. Sci. Rep. 7 (1), 9417. doi:10.1038/s41598-017-09639-7
Liu, B., Wanders, A., Wirdefeldt, K., Sjölander, A., Sachs, M. C., Eberhardson, M., et al. (2020). Vagotomy and Subsequent Risk of Inflammatory Bowel Disease: a Nationwide Register-Based Matched Cohort Study. Aliment. Pharmacol. Ther. 51 (11), 1022–1030. doi:10.1111/apt.15715
Liu, S., Hu, H.-Z., Gao, N., Gao, C., Wang, G., Wang, X., et al. (2003). Neuroimmune Interactions in guinea Pig Stomach and Small Intestine. Am. J. Physiology-Gastrointestinal Liver Physiology 284 (1), G154–G164. doi:10.1152/ajpgi.00241.2002
Lo, C. C. W., Moosavi, S. M., and Bubb, K. J. (2018). The Regulation of Pulmonary Vascular Tone by Neuropeptides and the Implications for Pulmonary Hypertension. Front. Physiol. 9, 1167. doi:10.3389/fphys.2018.01167
Lumpkin, E. A., and Caterina, M. J. (2007). Mechanisms of Sensory Transduction in the Skin. Nature 445 (7130), 858–865. doi:10.1038/nature05662
Mack, M. R., and Kim, B. S. (2018). The Itch-Scratch Cycle: A Neuroimmune Perspective. Trends Immunol. 39 (12), 980–991. doi:10.1016/j.it.2018.10.001
Magro, F., Vieira-Coelho, M. A., Fraga, S., Serrão, M. P., Veloso, F. T., Ribeiro, T., et al. (2002). Impaired Synthesis or Cellular Storage of Norepinephrine, Dopamine, and 5-hydroxytryptamine in Human Inflammatory Bowel Disease. Dig. Dis. Sci. 47 (1), 216–224. doi:10.1023/a:1013256629600
Mahmoud, W., Perniss, A., Poharkar, K., Soultanova, A., Pfeil, U., Hoek, A., et al. (2021). CXCL13 Is Expressed in a Subpopulation of Neuroendocrine Cells in the Murine Trachea and Lung. Giessen: Cell Tissue Res.
Mallesh, S., Schneider, R., Schneiker, B., Lysson, M., Efferz, P., Lin, E., et al. (2021). Sympathetic Denervation Alters the Inflammatory Response of Resident Muscularis Macrophages upon Surgical Trauma and Ameliorates Postoperative Ileus in Mice. Int. J. Mol. Sci. 22 (13). doi:10.3390/ijms22136872
Mao, Y.-K., Kasper, D. L., Wang, B., Forsythe, P., Bienenstock, J., and Kunze, W. A. (2013). Bacteroides Fragilis Polysaccharide A Is Necessary and Sufficient for Acute Activation of Intestinal Sensory Neurons. Nat. Commun. 4, 1465. doi:10.1038/ncomms2478
Martinolle, J. P., Moré, J., Dubech, N., and Garcia-Villar, R. (1993). Inverse Regulation of α- and β-adrenoceptors during Trinitrobenzenesulfonic Acid (TNB)-induced Inflammation in guinea-pig Small Intestine. Life Sci. 52 (18), 1499–1508. doi:10.1016/0024-3205(93)90112-g
Maryanovich, M., Zahalka, A. H., Pierce, H., Pinho, S., Nakahara, F., Asada, N., et al. (2018). Adrenergic Nerve Degeneration in Bone Marrow Drives Aging of the Hematopoietic Stem Cell Niche. Nat. Med. 24 (6), 782–791. doi:10.1038/s41591-018-0030-x
Mashaghi, A., Marmalidou, A., Tehrani, M., Grace, P. M., Pothoulakis, C., and Dana, R. (2016). Neuropeptide Substance P and the Immune Response. Cell. Mol. Life Sci. 73 (22), 4249–4264. doi:10.1007/s00018-016-2293-z
Matheis, F., Muller, P. A., Graves, C. L., Gabanyi, I., Kerner, Z. J., Costa-Borges, D., et al. (2020). Adrenergic Signaling in Muscularis Macrophages Limits Infection-Induced Neuronal Loss. Cell. 180 (1), 64–78. doi:10.1016/j.cell.2019.12.002
Matsuda, K., Park, C. H., Sunden, Y., Kimura, T., Ochiai, K., Kida, H., et al. (2004). The Vagus Nerve Is One Route of Transneural Invasion for Intranasally Inoculated Influenza a Virus in Mice. Vet. Pathol. 41 (2), 101–107. doi:10.1354/vp.41-2-101
Mazzone, S. B., and Undem, B. J. (2016). Vagal Afferent Innervation of the Airways in Health and Disease. Physiol. Rev. 96 (3), 975–1024. doi:10.1152/physrev.00039.2015
McMahon, D. B., Carey, R. M., Kohanski, M. A., Tong, C. C. L., Papagiannopoulos, P., Adappa, N. D., et al. (2020). Neuropeptide Regulation of Secretion and Inflammation in Human Airway Gland Serous Cells. Eur. Respir. J. 55 (4). doi:10.1183/13993003.01386-2019
McMahon, S. B., Russa, F. L., and Bennett, D. L. H. (2015). Crosstalk between the Nociceptive and Immune Systems in Host Defence and Disease. Nat. Rev. Neurosci. 16 (7), 389–402. doi:10.1038/nrn3946
Meng, J., Moriyama, M., Feld, M., Buddenkotte, J., Buhl, T., Szöllösi, A., et al. (2018). New Mechanism Underlying IL-31-induced Atopic Dermatitis. J. Allergy Clin. Immunol. 141 (5), 1677–e8. doi:10.1016/j.jaci.2017.12.1002
Michoud, F., Seehus, C., Schönle, P., Brun, N., Taub, D., Zhang, Z., et al. (2021). Epineural Optogenetic Activation of Nociceptors Initiates and Amplifies Inflammation. Nat. Biotechnol. 39 (2), 179–185. doi:10.1038/s41587-020-0673-2
Middleton, G., Hamanoue, M., Enokido, Y., Wyatt, S., Pennica, D., Jaffray, E., et al. (2000). Cytokine-induced Nuclear Factor Kappa B Activation Promotes the Survival of Developing Neurons. J. Cell. Biol. 148 (2), 325–332. doi:10.1083/jcb.148.2.325
Morita, T., McClain, S. P., Batia, L. M., Pellegrino, M., Wilson, S. R., Kienzler, M. A., et al. (2015). HTR7 Mediates Serotonergic Acute and Chronic Itch. Neuron 87 (1), 124–138. doi:10.1016/j.neuron.2015.05.044
Mou, H., Yang, Y., Riehs, M. A., Barrios, J., Shivaraju, M., Haber, A. L., et al. (2021). Airway Basal Stem Cells Generate Distinct Subpopulations of PNECs. Cell. Rep. 35 (3), 109011. doi:10.1016/j.celrep.2021.109011
Murray, K., Godinez, D. R., Brust-Mascher, I., Miller, E. N., Gareau, M. G., and Reardon, C. (2017). Neuroanatomy of the Spleen: Mapping the Relationship between Sympathetic Neurons and Lymphocytes. PLoS One 12 (7), e0182416. doi:10.1371/journal.pone.0182416
Nassenstein, C., Krasteva-Christ, G., and Renz, H. (2018). New Aspects of Neuroinflammation and Neuroimmune Crosstalk in the Airways. J. Allergy Clin. Immunol. 142 (5), 1415–1422. doi:10.1016/j.jaci.2018.09.011
Nemeth, P. R., Ort, C. A., and Wood, J. D. (1984). Intracellular Study of Effects of Histamine on Electrical Behaviour of Myenteric Neurones in guinea-pig Small Intestine. J. Physiol. 355, 411–425. doi:10.1113/jphysiol.1984.sp015427
O'Mahony, C., van der Kleij, H., Bienenstock, J., Shanahan, F., and O'Mahony, L. (2009). Loss of Vagal Anti-inflammatory Effect: In Vivo Visualization and Adoptive Transfer. Am. J. Physiology-Regulatory, Integr. Comp. Physiology 297 (4), R1118–R1126. doi:10.1152/ajpregu.90904.2008
Oetjen, L. K., Mack, M. R., Feng, J., Whelan, T. M., Niu, H., Guo, C. J., et al. (2017). Sensory Neurons Co-opt Classical Immune Signaling Pathways to Mediate Chronic Itch. Cell. 171 (1), 217–e13. doi:10.1016/j.cell.2017.08.006
Okajima, K., and Harada, N. (2006). Regulation of Inflammatory Responses by Sensory Neurons: Molecular Mechanism(s) and Possible Therapeutic Applications. Curr. Med. Chem. 13 (19), 2241–2251. doi:10.2174/092986706777935131
Paolicelli, R. C., Bolasco, G., Pagani, F., Maggi, L., Scianni, M., Panzanelli, P., et al. (2011). Synaptic Pruning by Microglia Is Necessary for Normal Brain Development. Science 333 (6048), 1456–1458. doi:10.1126/science.1202529
Paus, R., Theoharides, T. C., and Arck, P. C. (2006). Neuroimmunoendocrine Circuitry of the 'brain-Skin Connection'. Trends Immunol. 27 (1), 32–39. doi:10.1016/j.it.2005.10.002
Pavón-Romero, G. F., Serrano-Pérez, N. H., García-Sánchez, L., Ramírez-Jiménez, F., and Terán, L. M. (2021). Neuroimmune Pathophysiology in Asthma. Front. Cell. Dev. Biol. 9, 663535. doi:10.3389/fcell.2021.663535
Perner, C., Flayer, C. H., Zhu, X., Aderhold, P. A., Dewan, Z. N. A., Voisin, T., et al. (2020). Substance P Release by Sensory Neurons Triggers Dendritic Cell Migration and Initiates the Type-2 Immune Response to Allergens. Immunity 53 (5), 1063–e7. doi:10.1016/j.immuni.2020.10.001
Peters, E. M. J., Handjiski, B., Kuhlmei, A., Hagen, E., Bielas, H., Braun, A., et al. (2004). Neurogenic Inflammation in Stress-Induced Termination of Murine Hair Growth Is Promoted by Nerve Growth Factor. Am. J. pathology 165 (1), 259–271. doi:10.1016/s0002-9440(10)63294-4
Peters, E. M. J., Kuhlmei, A., Tobin, D. J., Müller-Röver, S., Klapp, B. F., and Arck, P. C. (2005). Stress Exposure Modulates Peptidergic Innervation and Degranulates Mast Cells in Murine Skin. Brain, Behav. Immun. 19 (3), 252–262. doi:10.1016/j.bbi.2004.08.005
Pierce, H., Zhang, D., Magnon, C., Lucas, D., Christin, J. R., Huggins, M., et al. (2017). Cholinergic Signals from the CNS Regulate G-CSF-Mediated HSC Mobilization from Bone Marrow via a Glucocorticoid Signaling Relay. Cell. stem Cell. 20 (5), 648–e4. doi:10.1016/j.stem.2017.01.002
Ramachandran, R., Hyun, E., Zhao, L., Lapointe, T. K., Chapman, K., Hirota, C. L., et al. (2013). TRPM8 Activation Attenuates Inflammatory Responses in Mouse Models of Colitis. Proc. Natl. Acad. Sci. U.S.A. 110 (18), 7476–7481. doi:10.1073/pnas.1217431110
Reardon, C., Murray, K., and Lomax, A. E. (2018). Neuroimmune Communication in Health and Disease. Physiol. Rev. 98 (4), 2287–2316. doi:10.1152/physrev.00035.2017
Reinshagen, M., Patel, A., Sottili, M., Nast, C., Davis, W., Mueller, K., et al. (1994). Protective Function of Extrinsic Sensory Neurons in Acute Rabbit Experimental Colitis. Gastroenterology 106 (5), 1208–1214. doi:10.1016/0016-5085(94)90011-6
Riol-Blanco, L., Ordovas-Montanes, J., Perro, M., Naval, E., Thiriot, A., Alvarez, D., et al. (2014). Nociceptive Sensory Neurons Drive Interleukin-23-Mediated Psoriasiform Skin Inflammation. Nature 510 (7503), 157–161. doi:10.1038/nature13199
Roggenkamp, D., Falkner, S., Stäb, F., Petersen, M., Schmelz, M., and Neufang, G. (2012). Atopic Keratinocytes Induce Increased Neurite Outgrowth in a Coculture Model of Porcine Dorsal Root Ganglia Neurons and Human Skin Cells. J. Investigative Dermatology 132 (7), 1892–1900. doi:10.1038/jid.2012.44
Roosterman, D., Goerge, T., Schneider, S. W., Bunnett, N. W., and Steinhoff, M. (2006). Neuronal Control of Skin Function: the Skin as a Neuroimmunoendocrine Organ. Physiol. Rev. 86 (4), 1309–1379. doi:10.1152/physrev.00026.2005
Rosa, A. C., and Fantozzi, R. (2013). The Role of Histamine in Neurogenic Inflammation. Br. J. Pharmacol. 170 (1), 38–45. doi:10.1111/bph.12266
Rosas-Ballina, M., Olofsson, P. S., Ochani, M., Valdés-Ferrer, S. I., Levine, Y. A., Reardon, C., et al. (2011)., 334. New York, N.Y.), 98–101. doi:10.1126/science.1209985Acetylcholine-synthesizing T Cells Relay Neural Signals in a Vagus Nerve CircuitScience6052
Sakata, D., Uruno, T., Matsubara, K., Andoh, T., Yamamura, K., Magoshi, Y., et al. (2019). Selective Role of Neurokinin B in IL-31-induced Itch Response in Mice. J. Allergy Clin. Immunol. 144 (4), 1130–e8. doi:10.1016/j.jaci.2019.06.031
Saria, A. (1984). Substance P in Sensory Nerve Fibres Contributes to the Development of Oedema in the Rat Hind Paw after Thermal Injury. Br. J. Pharmacol. 82 (1), 217–222. doi:10.1111/j.1476-5381.1984.tb16461.x
Schafer, D. P., Lehrman, E. K., Kautzman, A. G., Koyama, R., Mardinly, A. R., Yamasaki, R., et al. (2012). Microglia Sculpt Postnatal Neural Circuits in an Activity and Complement-dependent Manner. Neuron 74 (4), 691–705. doi:10.1016/j.neuron.2012.03.026
Scheiblich, H., Trombly, M., Ramirez, A., and Heneka, M. T. (2020). Neuroimmune Connections in Aging and Neurodegenerative Diseases. Trends Immunol. 41 (4), 300–312. doi:10.1016/j.it.2020.02.002
Schemann, M., Frieling, T., and Enck, P. (2020). To Learn, to Remember, to Forget-How Smart Is the Gut? Acta Physiol. (Oxf) 228 (1), e13296. doi:10.1111/apha.13296
Schemann, M., and Camilleri, M. (2013). Functions and Imaging of Mast Cell and Neural axis of the Gut. Gastroenterology 144 (4), 698–704. doi:10.1053/j.gastro.2013.01.040
Schneider, C., Nobs, S. P., Heer, A. K., Kurrer, M., Klinke, G., van Rooijen, N., et al. (2014). Alveolar Macrophages Are Essential for Protection from Respiratory Failure and Associated Morbidity Following Influenza Virus Infection. PLoS Pathog. 10 (4), e1004053. doi:10.1371/journal.ppat.1004053
Schneider, S., Wright, C. M., and Heuckeroth, R. O. (2019). Unexpected Roles for the Second Brain: Enteric Nervous System as Master Regulator of Bowel Function. Annu. Rev. Physiol. 81, 235–259. doi:10.1146/annurev-physiol-021317-121515
Segond von Banchet, G., Boettger, M. K., König, C., Iwakura, Y., Bräuer, R., and Schaible, H.-G. (2013). Neuronal IL-17 Receptor Upregulates TRPV4 but Not TRPV1 Receptors in DRG Neurons and Mediates Mechanical but Not Thermal Hyperalgesia. Mol. Cell. Neurosci. 52, 152–160. doi:10.1016/j.mcn.2012.11.006
Seillet, C., Luong, K., Tellier, J., Jacquelot, N., Shen, R. D., Hickey, P., et al. (2020). The Neuropeptide VIP Confers Anticipatory Mucosal Immunity by Regulating ILC3 Activity. Nat. Immunol. 21 (2), 168–177. doi:10.1038/s41590-019-0567-y
Serhan, N., Basso, L., Sibilano, R., Petitfils, C., Meixiong, J., Bonnart, C., et al. (2019). House Dust Mites Activate Nociceptor-Mast Cell Clusters to Drive Type 2 Skin Inflammation. Nat. Immunol. 20 (11), 1435–1443. doi:10.1038/s41590-019-0493-z
Serhan, N., Basso, L., Sibilano, R., Petitfils, C., Meixiong, J., Bonnart, C., et al. (2019). House Dust Mites Activate Nociceptor-Mast Cell Clusters to Drive Type 2 Skin Inflammation. Nat. Immunol. 20 (11), 1435–1443. doi:10.1038/s41590-019-0493-z
Shao, L., Elujoba-Bridenstine, A., Zink, K. E., Sanchez, L. M., Cox, B. J., Pollok, K. E., et al. (2021). The Neurotransmitter Receptor Gabbr1 Regulates Proliferation and Function of Hematopoietic Stem and Progenitor Cells. Blood 137 (6), 775–787. doi:10.1182/blood.2019004415
Shen, S., Al-Thumairy, H. W., Hashmi, F., and Qiao, L.-Y. (2017). Regulation of Transient Receptor Potential Cation Channel Subfamily V1 Protein Synthesis by the Phosphoinositide 3-kinase/Akt Pathway in Colonic Hypersensitivity. Exp. Neurol. 295, 104–115. doi:10.1016/j.expneurol.2017.06.007
Shimizu, Y., Matsuyama, H., Shiina, T., Takewaki, T., and Furness, J. B. (2008). Tachykinins and Their Functions in the Gastrointestinal Tract. Cell. Mol. Life Sci. 65 (2), 295–311. doi:10.1007/s00018-007-7148-1
Siebenhaar, F., Magerl, M., Peters, E. M. J., Hendrix, S., Metz, M., and Maurer, M. (2008). Mast Cell-Driven Skin Inflammation Is Impaired in the Absence of Sensory Nerves. J. Allergy Clin. Immunol. 121 (4), 955–961. doi:10.1016/j.jaci.2007.11.013
Simeoli, R., Montague, K., Jones, H. R., Castaldi, L., Chambers, D., Kelleher, J. H., et al. (2017). Exosomal Cargo Including microRNA Regulates Sensory Neuron to Macrophage Communication after Nerve Trauma. Nat. Commun. 8 (1), 1778. doi:10.1038/s41467-017-01841-5
Skaper, S. D. (2017). Nerve Growth Factor: a Neuroimmune Crosstalk Mediator for All Seasons. Immunology 151 (1), 1–15. doi:10.1111/imm.12717
Song, W.-J., Hui, C. K. M., Hull, J. H., Birring, S. S., McGarvey, L., Mazzone, S. B., et al. (2021). Confronting COVID-19-Associated Cough and the Post-COVID Syndrome: Role of Viral Neurotropism, Neuroinflammation, and Neuroimmune Responses. Lancet Respir. Med. 9 (5), 533–544. doi:10.1016/s2213-2600(21)00125-9
Sorkin, L. S., Eddinger, K. A., Woller, S. A., and Yaksh, T. L. (2018). Origins of Antidromic Activity in Sensory Afferent Fibers and Neurogenic Inflammation. Semin. Immunopathol. 40 (3), 237–247. doi:10.1007/s00281-017-0669-2
Sousa-Valente, J., and Brain, S. D. (2018). A Historical Perspective on the Role of Sensory Nerves in Neurogenic Inflammation. Semin. Immunopathol. 40 (3), 229–236. doi:10.1007/s00281-018-0673-1
Spencer, N. J., and Hu, H. (2020). Enteric Nervous System: Sensory Transduction, Neural Circuits and Gastrointestinal Motility. Nat. Rev. Gastroenterol. Hepatol. 17 (6), 338–351. doi:10.1038/s41575-020-0271-2
Spiegel, A., Shivtiel, S., Kalinkovich, A., Ludin, A., Netzer, N., Goichberg, P., et al. (2007). Catecholaminergic Neurotransmitters Regulate Migration and Repopulation of Immature Human CD34+ Cells through Wnt Signaling. Nat. Immunol. 8 (10), 1123–1131. doi:10.1038/ni1509
Spiller, R., and Garsed, K. (2009). Postinfectious Irritable Bowel Syndrome. Gastroenterology 136 (6), 1979–1988. doi:10.1053/j.gastro.2009.02.074
Steinhoff, M., Buddenkotte, J., and Lerner, E. A. (2018). Role of Mast Cells and Basophils in Pruritus. Immunol. Rev. 282 (1), 248–264. doi:10.1111/imr.12635
Straub, R. H., Grum, F., Strauch, U., Capellino, S., Bataille, F., Bleich, A., et al. (2008). Anti-inflammatory Role of Sympathetic Nerves in Chronic Intestinal Inflammation. Gut 57 (7), 911–921. doi:10.1136/gut.2007.125401
Straub, R. H., Wiest, R., Strauch, U. G., Harle, P., and Scholmerich, J. (2006). The Role of the Sympathetic Nervous System in Intestinal Inflammation. Gut 55 (11), 1640–1649. doi:10.1136/gut.2006.091322
Subramanian, H., Gupta, K., and Ali, H. (2016). Roles of Mas-Related G Protein-Coupled Receptor X2 on Mast Cell-Mediated Host Defense, Pseudoallergic Drug Reactions, and Chronic Inflammatory Diseases. J. Allergy Clin. Immunol. 138 (3), 700–710. doi:10.1016/j.jaci.2016.04.051
Sui, P., Wiesner, D. L., Xu, J., Zhang, Y., Lee, J., Van Dyken, S., et al. (2018). Pulmonary Neuroendocrine Cells Amplify Allergic Asthma Responses. Science 360 (6393). doi:10.1126/science.aan8546
Szema, A. M., Forsyth, E., Ying, B., Hamidi, S. A., Chen, J. J., Hwang, S., et al. (2017). NFATc3 and VIP in Idiopathic Pulmonary Fibrosis and Chronic Obstructive Pulmonary Disease. PLoS One 12 (1), e0170606. doi:10.1371/journal.pone.0170606
Talagas, M., Lebonvallet, N., Berthod, F., and Misery, L. (2020). Lifting the Veil on the Keratinocyte Contribution to Cutaneous Nociception. Protein Cell. 11 (4), 239–250. doi:10.1007/s13238-019-00683-9
Talbot, S., Doyle, B., Huang, J., Wang, J.-C., Ahmadi, M., Roberson, D. P., et al. (2020). Vagal Sensory Neurons Drive Mucous Cell Metaplasia. J. Allergy Clin. Immunol. 145 (6), 1693–1696. e4. doi:10.1016/j.jaci.2020.01.003
Tamari, M., Ver Heul, A. M., and Kim, B. S. (2021). Immunosensation: Neuroimmune Cross Talk in the Skin. Annu. Rev. Immunol. 39, 369–393. doi:10.1146/annurev-immunol-101719-113805
Tanaka, S., and Okusa, M. D. (2019). AKI and the Neuroimmune Axis. Seminars Nephrol. 39 (1), 85–95. doi:10.1016/j.semnephrol.2018.10.008
Taracanova, A., Alevizos, M., Karagkouni, A., Weng, Z., Norwitz, E., Conti, P., et al. (2017). SP and IL-33 Together Markedly Enhance TNF Synthesis and Secretion from Human Mast Cells Mediated by the Interaction of Their Receptors. Proc. Natl. Acad. Sci. U. S. A. 114 (20), E4002–E4009. doi:10.1073/pnas.1524845114
Thapaliya, M., Chompunud Na Ayudhya, C., Amponnawarat, A., Roy, S., and Ali, H. (2021). Mast Cell-specific MRGPRX2: a Key Modulator of Neuro-Immune Interaction in Allergic Diseases. Curr. Allergy Asthma Rep. 21 (1), 3. doi:10.1007/s11882-020-00979-5
Theoharides, T. C., Zhang, B., Kempuraj, D., Tagen, M., Vasiadi, M., Angelidou, A., et al. (2010). IL-33 Augments Substance P-Induced VEGF Secretion from Human Mast Cells and Is Increased in Psoriatic Skin. Proc. Natl. Acad. Sci. U.S.A. 107 (9), 4448–4453. doi:10.1073/pnas.1000803107
Trier, A. M., Mack, M. R., and Kim, B. S. (2019). The Neuroimmune Axis in Skin Sensation, Inflammation, and Immunity. J. I. 202 (10), 2829–2835. doi:10.4049/jimmunol.1801473
Tu, L., Gharibani, P., Yin, J., and Chen, J. D. Z. (2020). Sacral Nerve Stimulation Ameliorates Colonic Barrier Functions in a Rodent Model of Colitis. Neurogastroenterol. Motil. 32 (10), e13916. doi:10.1111/nmo.13916
Turnbull, A. V., and Rivier, C. L. (1999). Regulation of the Hypothalamic-Pituitary-Adrenal axis by Cytokines: Actions and Mechanisms of Action. Physiol. Rev. 79 (1), 1–71. doi:10.1152/physrev.1999.79.1.1
Ural, B. B., Yeung, S. T., Damani-Yokota, P., Devlin, J. C., de Vries, M., Vera-Licona, P., et al. (2020). Identification of a Nerve-Associated, Lung-Resident Interstitial Macrophage Subset with Distinct Localization and Immunoregulatory Properties. Sci. Immunol. 5 (45). doi:10.1126/sciimmunol.aax8756
Vainchtein, I. D., Chin, G., Cho, F. S., Kelley, K. W., Miller, J. G., Chien, E. C., et al. (2018). Astrocyte-derived Interleukin-33 Promotes Microglial Synapse Engulfment and Neural Circuit Development. Science 359 (6381), 1269–1273. doi:10.1126/science.aal3589
van der Kleij, H., Charles, N., Karimi, K., Mao, Y.-K., Foster, J., Janssen, L., et al. (2010). Evidence for Neuronal Expression of Functional Fc (ε and γ) Receptors. J. Allergy Clin. Immunol. 125 (3), 757–760. doi:10.1016/j.jaci.2009.10.054
Van Nassauw, L., Adriaensen, D., and Timmermans, J.-P. (2007). The Bidirectional Communication between Neurons and Mast Cells within the Gastrointestinal Tract. Aut. Neurosci. 133 (1), 91–103. doi:10.1016/j.autneu.2006.10.003
Veiga-Fernandes, H., and Pachnis, V. (2017). Neuroimmune Regulation during Intestinal Development and Homeostasis. Nat. Immunol. 18 (2), 116–122. doi:10.1038/ni.3634
Voisin, T., Bouvier, A., and Chiu, I. M. (2017). Neuro-immune Interactions in Allergic Diseases: Novel Targets for Therapeutics. Int. Immunol. 29 (6), 247–261. doi:10.1093/intimm/dxx040
Voss, M., Kotrba, J., Gaffal, E., Katsoulis-Dimitriou, K., and Dudeck, A. (2021). Mast Cells in the Skin: Defenders of Integrity or Offenders in Inflammation? Int. J. Mol. Sci. 22 (9). doi:10.3390/ijms22094589
Wallrapp, A., Riesenfeld, S. J., Burkett, P. R., Abdulnour, R.-E. E., Nyman, J., Dionne, D., et al. (2017). The Neuropeptide NMU Amplifies ILC2-Driven Allergic Lung Inflammation. Nature 549 (7672), 351–356. doi:10.1038/nature24029
Walsh, C. M., Hill, R. Z., Schwendinger-Schreck, J., Deguine, J., Brock, E. C., Kucirek, N., et al. (2019). Neutrophils Promote CXCR3-dependent Itch in the Development of Atopic Dermatitis, 8. Berkeley: eLife. doi:10.7554/elife.48448
Walters, N., Trunkle, T., Sura, M., and Pascual, D. W. (2005). Enhanced Immunoglobulin A Response and Protection against Salmonella enterica Serovar Typhimurium in the Absence of the Substance P Receptor. Infect. Immun. 73 (1), 317–324. doi:10.1128/iai.73.1.317-324.2005
Wang, F., Trier, A. M., Li, F., Kim, S., Chen, Z., Chai, J. N., et al. (2021). A Basophil-Neuronal axis Promotes Itch. Cell. 184 (2). doi:10.1016/j.cell.2020.12.033
Wang, F., and Kim, B. S. (2020). Itch: A Paradigm of Neuroimmune Crosstalk. Immunity 52 (5), 753–766. doi:10.1016/j.immuni.2020.04.008
Wang, N., Wang, J., Zhang, Y., Hu, S., Zhang, T., Wu, Y., et al. (2022). Substance P‐induced Lung Inflammation in Mice Is Mast Cell Dependent. Clin. Exp. Allergy 52 (1), 46–58. doi:10.1111/cea.13902
Willemze, R. A., Welting, O., van Hamersveld, P., Verseijden, C., Nijhuis, L. E., Hilbers, F. W., et al. (2019). Loss of Intestinal Sympathetic Innervation Elicits an Innate Immune Driven Colitis. Mol. Med. 25 (1), 1. doi:10.1186/s10020-018-0068-8
Wilson, S. R., Thé, L., Batia, L. M., Beattie, K., Katibah, G. E., McClain, S. P., et al. (2013). The Epithelial Cell-Derived Atopic Dermatitis Cytokine TSLP Activates Neurons to Induce Itch. Cell. 155 (2), 285–295. doi:10.1016/j.cell.2013.08.057
Wilson, S. R., Thé, L., Batia, L. M., Beattie, K., Katibah, G. E., McClain, S. P., et al. (2013). The Epithelial Cell-Derived Atopic Dermatitis Cytokine TSLP Activates Neurons to Induce Itch. Cell. 155 (2), 285–295. doi:10.1016/j.cell.2013.08.057
Wu, Y., Wang, Y., Wang, J., Fan, Q., Zhu, J., Yang, L., et al. (2019). TLR4 Mediates Upregulation and Sensitization of TRPV1 in Primary Afferent Neurons in 2,4,6-trinitrobenzene Sulfate-Induced Colitis. Mol. Pain 15, 1744806919830018. doi:10.1177/1744806919830018
Xia, Y., Hu, H.-Z., Liu, S., Ren, J., Zafirov, D. H., and Wood, J. D. (1999). IL-1β and IL-6 Excite Neurons and Suppress Nicotinic and Noradrenergic Neurotransmission in guinea Pig Enteric Nervous System. J. Clin. Investig. 103 (9), 1309–1316. doi:10.1172/jci5823
Xu, J., Yu, H., and Sun, X. (2020). Less Is More: Rare Pulmonary Neuroendocrine Cells Function as Critical Sensors in Lung. Dev. Cell. 55 (2), 123–132. doi:10.1016/j.devcel.2020.09.024
Xu, J., Zanvit, P., Hu, L., Tseng, P.-Y., Liu, N., Wang, F., et al. (2020). The Cytokine TGF-β Induces Interleukin-31 Expression from Dermal Dendritic Cells to Activate Sensory Neurons and Stimulate Wound Itching. Immunity 53 (2), 371–383. doi:10.1016/j.immuni.2020.06.023
Yamada, M., and Ichinose, M. (2018). The Cholinergic Anti-inflammatory Pathway: an Innovative Treatment Strategy for Respiratory Diseases and Their Comorbidities. Curr. Opin. Pharmacol. 40, 18–25. doi:10.1016/j.coph.2017.12.003
Yamada, M., and Ichinose, M. (2018). The Cholinergic Pathways in Inflammation: A Potential Pharmacotherapeutic Target for COPD. Front. Pharmacol. 9, 1426. doi:10.3389/fphar.2018.01426
Yashiro, T., Ogata, H., Zaidi, S. F., Lee, J., Hayashi, S., Yamamoto, T., et al. (2021). Pathophysiological Roles of Neuro-Immune Interactions between Enteric Neurons and Mucosal Mast Cells in the Gut of Food Allergy Mice. Cells 10 (7). doi:10.3390/cells10071586
Yu, H. B., Yang, H., Allaire, J. M., Ma, C., Graef, F. A., Mortha, A., et al. (2021). Vasoactive Intestinal Peptide Promotes Host Defense against Enteric Pathogens by Modulating the Recruitment of Group 3 Innate Lymphoid Cells. Proc. Natl. Acad. Sci. U. S. A. 118 (41). doi:10.1073/pnas.2106634118
Zhang, S., Edwards, T. N., Chaudhri, V. K., Wu, J., Cohen, J. A., Hirai, T., et al. (2021). Nonpeptidergic Neurons Suppress Mast Cells via Glutamate to Maintain Skin Homeostasis. Cell. 184 (8), 2151–2166. doi:10.1016/j.cell.2021.03.002
Keywords: neuroimmune crosstalk, nerve, immune, barrier organ, neuropeptide, neurotransmitter
Citation: Zhu Y, Duan S, Wang M, Deng Z and Li J (2022) Neuroimmune Interaction: A Widespread Mutual Regulation and the Weapons for Barrier Organs. Front. Cell Dev. Biol. 10:906755. doi: 10.3389/fcell.2022.906755
Received: 29 March 2022; Accepted: 26 April 2022;
Published: 11 May 2022.
Edited by:
Mingxing Lei, Chongqing University, ChinaReviewed by:
Bing Zhang, Westlake University, ChinaJinwei Zhang, Chongqing Hospital of Traditional Chinese Medicine, China
Copyright © 2022 Zhu, Duan, Wang, Deng and Li. This is an open-access article distributed under the terms of the Creative Commons Attribution License (CC BY). The use, distribution or reproduction in other forums is permitted, provided the original author(s) and the copyright owner(s) are credited and that the original publication in this journal is cited, in accordance with accepted academic practice. No use, distribution or reproduction is permitted which does not comply with these terms.
*Correspondence: Zhili Deng, ZGVuZ3poaWxpQGNzdS5lZHUuY24=; Ji Li, bGlqaV94eUBjc3UuZWR1LmNu
†These authors have contributed equally to this work and share first authorship