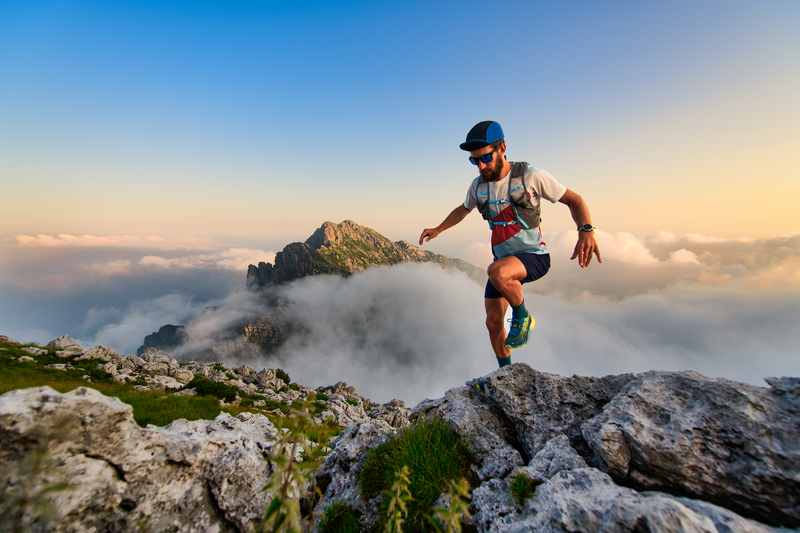
95% of researchers rate our articles as excellent or good
Learn more about the work of our research integrity team to safeguard the quality of each article we publish.
Find out more
REVIEW article
Front. Cell Dev. Biol. , 19 May 2022
Sec. Stem Cell Research
Volume 10 - 2022 | https://doi.org/10.3389/fcell.2022.903904
This article is part of the Research Topic Inflammation, Stem Cells and Wound Healing in Skin Aging View all 15 articles
Skin is the largest organ in human body, harboring a plethora of cell types and serving as the organismal barrier. Skin aging such as wrinkling and hair graying is graphically pronounced, and the molecular mechanisms behind these phenotypic manifestations are beginning to unfold. As in many other organs and tissues, epigenetic and metabolic deregulations have emerged as key aging drivers. Particularly in the context of the skin epithelium, the epigenome and metabolome coordinately shape lineage plasticity and orchestrate stem cell function during aging. Our review discusses recent studies that proposed molecular mechanisms that drive the degeneration of hair follicles, a major appendage of the skin. By focusing on skin while comparing it to model organisms and adult stem cells of other tissues, we summarize literature on genotoxic stress, nutritional sensing, metabolic rewiring, mitochondrial activity, and epigenetic regulations of stem cell plasticity. Finally, we speculate about the rejuvenation potential of rate-limiting upstream signals during aging and the dominant role of the tissue microenvironment in dictating aged epithelial stem cell function.
As the body’s largest organ, skin harbors a cadre of cell types. First and foremost, epithelial cells serve as the fundamental units of our barrier; they reside in the interfollicular epidermis and pilosebaceous unit, the latter including the sebaceous gland and the hair follicle (Hardy, 1992; Fuchs, 1995; Paus and Cotsarelis, 1999; Watt, 2001; Lopez-Pajares et al., 2013). Fibroblasts in the dermis secrete bulk extracellular matrix in the tissue for not only structural support but also mediate signaling (Gurtner et al., 2008; Sennett and Rendl, 2012; Heitman et al., 2019; Plikus et al., 2021; McAndrews et al., 2022). Subcutaneous adipocytes and dermal pre-adipocytes exhibit remarkable lineage plasticity to mediate metabolic and signaling regulations (Horsley and Watt, 2017). Immune cells including those of the innate and adaptive systems provide tissue surveillance (Allison and Havran, 1991; Merad et al., 2008; Heath and Carbone, 2013), support repair and regeneration (DeStefano and Christiano, 2014; Ali et al., 2017; Eming et al., 2017), and communicate with the microbiome (Nakatsuji and Gallo, 2012; Byrd et al., 2018; Kobayashi et al., 2019). Oxygen and nutrients are exchanged via the endothelial and lymphatic vasculature, sensations are conducted through the intertwining neuronal network, ultraviolet radiation protection is afforded by melanocytes, and hair follicles are erected by arrector pili muscles; all of these cell types exert their respective functions while maintaining crosstalk with juxtaposed epithelial stem cells (Nishimura et al., 2005; Brownell et al., 2011; Fujiwara et al., 2011; Chang et al., 2013; Gur-Cohen et al., 2019; Shwartz et al., 2020; Zhang et al., 2020).
The skin epithelium is maintained by its resident stem cells, harboring the capacity for long-term self-renewal and multi-lineage differentiation. The hair follicle as a major skin appendage is maintained by hair follicle stem cells (HFSCs) residing in the bulge, an anatomic location beneath the sebaceous gland and isthmus of the pilosebaceous unit (Cotsarelis et al., 1990) (Figure 1A). HFSCs fuel the cyclic regeneration of hair follicles during homeostatic hair growth (Morris et al., 2004; Tumbar et al., 2004; Greco et al., 2009; Hsu et al., 2011). They contribute to both follicular and epidermal regeneration during transplantation and wound repair (Taylor et al., 2000; Blanpain et al., 2004; Claudinot et al., 2005; Ito et al., 2005; Levy et al., 2005), and they initiate skin squamous cell carcinomas upon oncogenic transformation (Lapouge et al., 2011). Likewise, exhibiting remarkable plasticity, several HFSC populations adjacent to the bulge contribute to wound repair and tumorigenesis (Nijhof et al., 2006; Jaks et al., 2008; Jensen et al., 2009; Snippert et al., 2010; Page et al., 2013; Donati et al., 2017; Ge et al., 2017; Ge and Fuchs, 2018) (Figure 1B).
FIGURE 1. Molecular mechanisms underlying hair follicle miniaturization in aging (A) The hair follicle as a major skin appendage is maintained by hair follicle stem cells (HFSCs) residing in the bulge, an anatomic location beneath the sebaceous gland and isthmus of the pilosebaceous unit. HFSCs fuel the cyclic regeneration of hair follicles during homeostatic hair growth, whereas epidermal stem cells (EpdSC) and junctional zone stem cells (JzSCs) maintain the epidermis and junctional zone, respectively (B) HFSCs drive both follicular and epidermal regeneration during wound repair. Depending on the wounding depth, different populations of stem cells are induced to contribute to wound repair (C–E) Several cellular and molecular mechanisms have been recently proposed to explain the fate of aging HFSCs in murine models, including epidermal transdifferentiation (C), mechanical compression in situ (D), and escape into dermis (E).
Aging in the skin is graphically pronounced and includes a wrinkly surface due to dermal extracellular matrix atrophy and gray hair owing to a loss of melanocytes. Accompanying these overtly notable signs, the old skin manifests many hallmarks of aging (Lopez-Otin et al., 2013) such as stem cell exhaustion, genotoxic stress, metabolic deregulation, and epigenetic erosion. In our current essay, we focus on skin aging, especially in the context of stem cell function and the origin and consequence of lineage deregulation. Whenever applicable, we compare mammalian skin to model organisms and adult stem cell of other tissues and organs. Overall, we entertain the idea that skin is an ideal system to understand and tackle organismal aging.
Stem cells decline over time in number and/or in activity across organs and tissues. Curiously, this functional decline is often accompanied by skewed lineage output. For example, aging in the hematopoietic system is signified by pronounced myeloid lineage expansion at the expense of lymphoid cells (Sudo et al., 2000) (Cho et al., 2008; Florian et al., 2013). Aged skeletal stem cells have decreased bone- and cartilage-forming potential but produce more stromal lineages, leading to not only bone fragility but also hematopoietic skewing (Ambrosi et al., 2021). Secretory cell types dominate the aged intestinal stem cell output (Nalapareddy et al., 2017). The question of the fate of HFSCs during aging is a particularly intriguing one. Consistent with the lineage-skewing phenomenon at the organ level, hair follicles undergo miniaturization as HFSCs are diminished during aging, resulting in macroscopically sparse hair. In contrast, the epidermis and sebaceous gland undergo hyperplasia. Several cellular and molecular mechanisms have been recently proposed to explain the fate of aging HFSCs in murine models (Figures 1C–E).
In the skin, stem cell lineage infidelity where HFSCs expand their fate to regenerate the epidermis is observed during wound repair (Ge et al., 2017) (Figure 1B) and could be recapitulated upon the ablation of several key HFSC fate transcription factors in adult skin, including SOX9 (Kadaja et al., 2014) and NFIB/NFIX (Adam et al., 2020), suggesting a default HFSC response of epidermal differentiation under injury. In a more extreme scenario, epidermal cysts form in Notch signaling–deficient skin, suggesting blocked hair follicle differentiation (Yamamoto et al., 2003; Pan et al., 2004; Vauclair et al., 2005; Estrach et al., 2006; Demehri and Kopan, 2009). During aging, stress signals including DNA damage and a high-fat diet have been shown to drive epidermal conversion of HFSCs by compromising basement membrane integrity (Matsumura et al., 2016), altering stem cell symmetric divisions (Matsumura and Nishimura, 2021), and inducing oxidative stress (Morinaga et al., 2021), all of which contribute to the epidermal conversion of HFSCs and hair follicle miniaturization (Figure 1C).
On the other hand, deficiency of several HFSC quiescence regulators, such as LHX2 (Folgueras et al., 2013) or beta-catenin (Lien et al., 2014), appears to push HFSCs into the sebaceous gland lineage. In this regard, loss of BMP (Guha et al., 2004; Plikus et al., 2004; Qiu et al., 2011) or aberrant activation of LEF1 (Merrill et al., 2001; Niemann et al., 2002; Petersson et al., 2011), NOTCH1 (Estrach et al., 2006), and GLI2 (Allen et al., 2003; Gu and Coulombe, 2008) also manifests as ectopic sebaceous glands, although in some of these scenarios, it remains unclear whether sebaceous glands were mis-specified from HFSCs at the expense of hair follicles. Since sebaceous gland hyperplasia, like that of the epidermis, is a prominent signature of skin aging, it would be tempting to speculate that aged HFSCs also skew toward sebaceous gland differentiation, resulting in hair follicle miniaturization (Figures 1B,C).
An alternative model for the loss of aged HFSCs was recently proposed (Figure 1D): mechanical compression induces Piezo channel activation, calcium influx, and subsequently HFSC apoptosis (Xie et al., 2022). While the loss of inhibitory K6+ niche cells is known to cause HFSC activation (Hsu et al., 2011), in this context, mechanical cues play a dominant function, since the reinsertion of the hair shaft alone without the K6+ cells returned bulge HFSCs to quiescence (Xie et al., 2022). Corroborating this model, repetitive hair depilation induces hair follicle aging (Keyes et al., 2013; Lay et al., 2016), likely evoking mechanical compressions and the Piezo1-calcium-TNFα axis (Xie et al., 2022). Therefore, mechanical compressions induced HFSC apoptosis is likely a major contribution of HFSC exhaustion and hair follicle miniaturization during aging.
Remarkably, via intravital imaging, aged HFSCs have been shown to escape into the dermis (Zhang et al., 2021) (Figure 1E). This escape could be recapitulated by depletion of HFSC quiescence regulators FOXC1 (Lay et al., 2016; Wang et al., 2016) and NFATC1 (Keyes et al., 2013) in the hair follicles and is caused by the aging-associated loss of extracellular matrix proteins (Ge et al., 2020; Zhang et al., 2021). On the other hand, deregulation of the aging extracellular matrix due to niche stiffening underlies nuclear cytoskeletal remodeling and subsequently epigenome remodeling in the aging HFSCs (Koester et al., 2021), providing an important molecular link as to how the extracellular matrix niche determines aged HFSC fate (Ge et al., 2020; Koester et al., 2021).
Telomeres shorten each time the genome duplicates, serving as a major driver for replicative aging. In mammals, telomerase expression is present and often abundant in adult stem cells (Sharpless and DePinho, 2004), including those of the skin (Figure 2). Among the defects in many highly proliferative organs, a signature phenotype of telomerase-deficient mice is alopecia and hair graying (Lee et al., 1998; Rudolph et al., 1999; Flores et al., 2005; Sarin et al., 2005). Likewise, in humans, mutations in the gene encoding for dyskerin, critical for telomere stability, lead to dyskeratosis congenita, a progressive bone-marrow failure syndrome characterized by abnormal skin pigmentation (Mitchell et al., 1999; Vulliamy et al., 2001).
FIGURE 2. The impact of genotoxic stress on skin aging. Numerous causes of genotoxic stresses including telomere attrition, DNA damage, circadian reprograming, and loss of quiescence could lead to stem cell functional decline in aging. The outcomes include increased oxidative stress, induction of P16 and cellular senescence, loss of cell adhesion, and activated innate immune pathways, among others.
Similar to telomere attrition, DNA damage–induced genotoxic stress due to replication error, mutagens, and reactive oxygen species (ROS) is well documented to accelerate aging (Figure 2). Among DNA repair pathways, it has been shown that non-homologous end joining (NHEJ) is specifically enhanced in quiescent hematopoietic stem cells (HSCs), whereas committed progenitors preferentially use homologous recombination as their repair mechanism (Mohrin et al., 2010). Similarly, quiescent bulge HFSCs are relatively protected against ionizing radiation, likely because of their elevated anti-apoptotic programs and enhanced NHEJ capacity (Sotiropoulou et al., 2010). Deficiencies in various NHEJ components (Difilippantonio et al., 2000; Gao et al., 2000) (Baker et al., 2004; Nijnik et al., 2007), spindle assembly checkpoint proteins (Baker et al., 2004), and DNA damage response pathways (Ito et al., 2004; Ruzankina et al., 2007) collectively contribute to premature aging of the hair follicles.
Disruption of the circadian clock results in pre-mature aging (Janich et al., 2011; Kondratov et al., 2006; Yu et al., 2021), and its associated molecular mechanisms are only beginning to emerge (Figure 2). It has been suggested the transit-amplifying cells of the hair follicles exhibit elevated sensitivity toward DNA damage during the day when their mitotic activities peak (Plikus et al., 2013), whereas the epidermis preferentially proliferates at night to avoid the high level of oxidative phosphorylation (with its byproduct ROS) during the day, presumably as a protective mechanism against genotoxicity (Stringari et al., 2015). In aged epidermal stem cells, arrhythmic and prolonged DNA replication combined with otherwise-normal oscillatory oxidative phosphorylation programs may therefore underlie the increased oxidative damage (Solanas et al., 2017). Nevertheless, complete disruption of the clock did not recapitulate selective rhythmic deregulation in a physiological aging setting, nor did circadian rewiring induced by a high-fat diet, suggesting the direct cause of aging-associated circadian reprogramming remains unclear (Solanas et al., 2017).
Furthermore, chronic inflammatory signals also contribute to DNA damage and genotoxicity. For example, IFNα stimulate HSCs to exit quiescence via IFNAR and STAT1 signaling, elevate mitochondrial ROS levels, and lead to DNA damage (Essers et al., 2009; Sato et al., 2009; Walter et al., 2015) (Figure 2). ROS may act upstream of DNA damage, evidenced by the observation that antioxidant treatment is able to suppress DNA damage and thus delay HSC aging (Essers et al., 2009; Walter et al., 2015). Genotoxic stress could further exacerbate ROS deregulation and subsequently activate p38MAPK and upregulate the expression of P16, resulting in HSC exhaustion (Ito et al., 2004) (Ito et al., 2006). In the case of HFSCs, DNA damage induces proteolysis of collagen XVII, a critical cell junction collagen, and induces stem cell differentiation (Matsumura et al., 2016). Likewise, ionizing radiation–induced DNA damage leads to melanocyte stem cell exhaustion and hair graying (Inomata et al., 2009). Strikingly, hair graying is completely suppressed in mice that are deficient in the double-stranded DNA-sensing cGAS/STING pathway (Dou et al., 2017), suggesting that the innate immunity pathway serves as a key mediator of radiation-induced aging.
Pioneering work has established several nutrient-sensing and energy-sensing pathways to be critically involved in lifespan extension across model organisms (Figure 3A), including insulin/insulin-like growth factor (IIS) (Kenyon, 2010), AMP-activated protein kinase (AMPK) (Herzig and Shaw, 2018), mammalian or mechanistic target of rapamycin (mTOR) (Wullschleger et al., 2006; Laplante and Sabatini, 2012), and sirtuin (Haigis and Guarente, 2006; Longo and Kennedy, 2006; Houtkooper et al., 2012) pathways. While mechanistic studies of these pathways in skin aging are rapidly emerging, in this section, we will review the historical aspects of these molecular mechanisms in various model organisms and adult stem cells in order to provide a broader context for skin aging.
FIGURE 3. Nutritional and metabolic regulation of skin aging (A) IIS (insulin/insulin-like growth factor) and AMPK/mTOR are two major pathways in regulating nutrients and metabolism that have been described in contributing to organismal aging (red circle) and longevity (green circle). Asterix indicates components of these pathways that have been demonstrated to contribute to skin aging (B) Mitochondrial biology is closely intertwined with aging biology. Highlighted in red are several key genes that have been shown to regulate skin stem cell aging in vivo. LDH, lactate dehydrogenase; PDH, pyruvate dehydrogenase; MPC, mitochondrial pyruvate carrier; GLS, glutaminase; IDH, isocitrate dehydrogenase (converting isocitrate into alpha-ketoglutarate). Asterix indicates components of these pathways that have been demonstrated to contribute to skin aging.
In the IIS pathway, the selective ablation of signaling cascade components led to activation of downstream transcription factors FOXO (Lee et al., 2003; Murphy et al., 2003) and NRF (Sykiotis and Bohmann, 2008; Tullet et al., 2008), eliciting cellular protective programs against oxidative stress and promoting longevity (Tothova et al., 2007; Blackwell et al., 2015). AMPK senses cellular energy decline and activates a plethora of catabolic pathways while suppressing anabolic processes, promoting lifespan extension (Mair et al., 2011; Herzig and Shaw, 2018). The antidiabetic antineoplastic drug metformin exerts both AMPK-dependent (Ma et al., 2022) and AMPK-independent activities (Foretz et al., 2014) and, when used at a high dose, inhibits mitochondrial electron transport chain complex I (described further below), all of which may contribute to its utility in anti-aging (Zhou et al., 2001). Both IIS and AMPK pathways converge on mTOR. Nutrient and amino acid starvation inhibits mTOR activity, resulting in suppressed protein translation and enhanced autophagy via key mTOR targets S6K1 (ribosome protein S6 kinase B1), 4EBP1 (eukaryotic translation initiation factor 4E-binding protein 1), and ULK1 (unc-51 like autophagy activating kinase 1) (Wullschleger et al., 2006; Laplante and Sabatini, 2012). Sirtuins are NAD+ (nicotinamide adenine dinucleotide)–dependent deacetylases that act on both histone substrates to silence heterochromatin (Oberdoerffer et al., 2008; Dang et al., 2009) and on non-histone substrates such as P53 (Luo et al., 2001; Vaziri et al., 2001), HSF1 (heat shock factor 1), FOXO (Brunet et al., 2004), and PGC1 (Rodgers et al., 2005), among others, to regulate aging.
Among these regulators, mTOR function has been genetically tackled in several adult stem cell, aging, and malignancy contexts. Hyperactivation of mTOR by deletion of Pten or TSC1 exhausted neural stem cells (Bonaguidi et al., 2011) and HSCs (Yilmaz et al., 2006; Zhang et al., 2006; Gan et al., 2008; Chen et al., 2009), and the HSCs were transformed to become precursors of myeloproliferative disorder. On the other hand, loss of mTOR function via ablation of the mTORC1 component Raptor (regulatory-associated protein of mTOR) in mouse HSCs leads to non-lethal pancytopenia, splenomegaly, and accumulation of monocytoid cells. Raptor conditional knockout also compromised HSC regeneration and inhibited leukemogenesis evoked by Pten deficiency (Kalaitzidis et al., 2012). p53 and p16 mediate HSC exhaustion and serve as a roadblock to leukemic transformation upon Pten deletion (Lee J. Y. et al., 2010b). In adult but not childhood leukemia, deletion of the mTORC2 component Rictor blocked leukemogenesis and HSC depletion (Magee et al., 2012). Mechanistically, it has been shown that in Drosophila larvae, chronic stimulation of TOR (via constitutively active insulin receptor expression) induces ROS and activates JNK and FOXO, resulting in accumulation of Sestrin (a family of stress-sensing proteins) and activation of AMPK, facilitating autophagic clearance of damaged mitochondria, protein aggregates, or lipids (Lee J. H. et al., 2010a). Loss of Sestrin resulted in age-associated pathologies including triglyceride accumulation, mitochondrial dysfunction, muscle degeneration, and cardiac malfunction, which could be reversed by AMPK activation or TOR inhibition. In the skin, deletion of either Mtor itself or its complex components Raptor of mTORC1 or Rictor of mTORC2 led to skin barrier defects during development (Ding et al., 2016). Treatment with rapamycin (preferentially targeting mTORC1) reversed HFSC exhaustion induced by WNT1 overactivation (Castilho et al., 2009), whereas mTORC2 has been suggested to mediate glutaminase suppression in returning HFSCs to quiescence (Kim et al., 2020).
Calorie restriction or dietary restriction is widely known to ameliorate aging-associated decline of stem cells, such as HSCs (Chen et al., 2003; Warr et al., 2013; Tang et al., 2016), germline stem cells (Mair et al., 2010), and stem cells of the intestine (Regan et al., 2016; Akagi et al., 2018; Mihaylova et al., 2018), skeletal muscle (Cerletti et al., 2012), and skin (Forni et al., 2017; Solanas et al., 2017). Mechanistically, in the context of intestinal stem cells, calorie restriction enhances stem cell activity through a niche-dependent paracrine signal (Yilmaz et al., 2012). In HSCs, calorie restriction cell-autonomously preserves the functional autophagy-high and oxidative phosphorylation–low populations during aging (Warr et al., 2013; Ho et al., 2017). Similar to basal autophagy, chaperon mediated autophagy is also required for maintaining HSC function during aging (Dong et al., 2021). In aged neural stem cells, calorie restriction induced lysosome activation clears aggregates and restores stem cell activation (Leeman et al., 2018), and likewise, chaperon mediated autophagy is essential for preventing proteome collapse and neurodegenerations (Bourdenx et al., 2021). Calorie restriction also restores the transcriptional circadian rhythm of aged epidermal and muscle stem cells to their youthful level, such as replication and autophagy, respectively (Solanas et al., 2017).
By sensing energy demand and nutrient supply, stem cells adaptively tune the level of glycolysis (breakdown of glucose into pyruvate under aerobic conditions or lactate under anaerobic conditions) and oxidative phosphorylation pathways (including oxidation of pyruvate, glutamine, or fatty acid in mitochondria, TCA cycle, electron transport chain activity) (Figure 3B). It is commonly believed that long-term self-renewing stem cells reside in a hypoxic niche and downregulate mitochondrial activity (Mazumdar et al., 2010; Simsek et al., 2010; Takubo et al., 2010). Blocking the influx of glycolytic metabolites into mitochondria by overexpression of pyruvate dehydrogenase kinase (which inhibits pyruvate dehydrogenase and hence pyruvate oxidation) increases the long-term self-renewal capacity of HSCs (Simsek et al., 2010; Takubo et al., 2013), while deletion of mitochondrial pyruvate carrier (which transports pyruvate into mitochondria for oxidation) enhances the organoid-forming potential of intestinal stem cells (Schell et al., 2017). Likewise, in the skin, depletion of mitochondrial pyruvate carrier induced HFSC activation, whereas ablation of lactate dehydrogenase (which converts pyruvate to lactate during anaerobic glycolysis) blocked HFSC activation (Flores et al., 2017). Interestingly, glutamine oxidation is highly upregulated in HFSC progenies compared to HFSCs in organoid culture, and it has been proposed to promote HFSCs returning to quiescence at the end of the hair cycle (Kim et al., 2020). While oxidative phosphorylation and mitochondrial activity are unequivocally induced upon stem cell activation, whether glucose or glutamine influx contributes to the increased oxidation is challenging to directly determine in vivo. Tissue-specific depletion of glutaminase (which converts glutamine to glutamate), similar to the experiments performed on mitochondrial pyruvate carrier and lactate dehydrogenase, will likely provide further insights into this interesting question.
Mitochondrial biology has long been intertwined with aging biology (Beckman and Ames, 1998). Mice with mutated mitochondrial DNA polymerase Polg age prematurely (Trifunovic et al., 2004; Kujoth et al., 2005), inducing a differentiation block in tissues with high turnover rate including skin (Norddahl et al., 2011; Ahlqvist et al., 2012). Mice with epidermis-specific loss of TFAM (mitochondrial transcription factor A), required for the transcription of mitochondrial genes encoding electron transport chain subunits, showed impaired epidermal differentiation and hair follicle growth (Hamanaka et al., 2013; Kloepper et al., 2015). Indeed, electron transport chain activity declines in aging, and enhancing mitochondrial function by overexpression of PGC1 (Rera et al., 2011; Dillon et al., 2012) or supply of NAD+ (Gomes et al., 2013) delays aging. Consistently, during aging, telomere dysfunction compromises mitochondrial function via p53-mediated inhibition of PGC1 levels (Sahin and Depinho, 2010). In an interesting twist, it has been shown that mild mitochondrial stress prolongs lifespan in worms (Durieux et al., 2011) and yeast (Pan et al., 2011). The specific context under which these pathways operate and the cell types responding to these various manipulations likely play a major role in explaining these seemingly complex observations. It would be of future interest to examine how restoring ETC activity or inducing mild mitochondrial stress would affect skin ageing.
A hallmark of aging-associated stem cell degeneration is transcriptional noise and epigenetic erosion, in which heterochromatin silencing and transcriptional fidelity appear crucial. Pioneering genetic and biochemical experiments have mapped out the central pathway for heterochromatin regulation (Jenuwein and Allis, 2001; Zhang and Reinberg, 2001; Grewal and Moazed, 2003; Jaenisch and Bird, 2003; Roeder, 2005; Workman, 2006; Kouzarides, 2007; Zaratiegui et al., 2007; Law and Jacobsen, 2010; Piunti and Shilatifard, 2016; Schuettengruber et al., 2017), a critical vulnerability during organismal aging. Epigenetic deregulations in aging have been extensively characterized in model organisms, where several parallels could be drawn into the mammalian systems including skin (Figure 4).
FIGURE 4. Transcriptional and epigenetic noise in aging stem cells. Several proposed origins of the transcriptional and epigenetic noise in aged stem cells, including genome-wide hypomethylation and derepression of transposable elements, spurious transcription from the gene body, and deregulated transcription from ribosomal DNA (rDNA) at nucleoli. Global hypomethylation is accompanied by regional hypermethylation at gene promoters as well as formation of senescence-associated heterochromatin foci (SAHF). Some of the enzymes and post-translational histone modifications have been shown to regulate aging and longevity in a transgenerational fashion.
A key gene-silencing mechanism in mammals, DNA methylation is not conserved or has a very limited role in yeast, flies, and worms. Rather, histone modifications are heavily involved in transcriptional silencing in the latter. For example, during yeast replicative aging, decline of the deacetylase Sir2 results in acetylated histone 4 lysine 16 (H4K16ac) and increased transcription at subtelomeric regions (Dang et al., 2009), as well as enhanced ribosomal DNA (rDNA) transcription by RNA polymerase I and formation of extrachromosomal rDNA circles (Sinclair and Guarente, 1997) (H4K16ac and RNA polymerase I transcription factors are Sir2 substrates). Interestingly, aged HSCs exhibit global DNA hypomethylation that includes the rDNA regions (Sun et al., 2014), and they accumulate non-canonical gH2AX and ATR at nucleoli where rDNA transcription occurs, disrupting ribosome biogenesis (Flach et al., 2014). Notably, SIRT1 (the mammalian homolog of yeast Sir2) appears to contribute to repeat silencing and is involved in the DNA damage response in mammalian cells (Oberdoerffer et al., 2008), although its role in mammalian rDNA regulation is unknown. Since, unlike yeast, mammals use both DNA and histone methylation to mediate transcriptional repression, it will be interesting to see which of these pathways are involved in rDNA silencing during skin aging. Such effort will now be greatly facilitated by the recently completed draft of gapless human genome covering highly repetitive regions including rDNAs (Nurk et al., 2022).
Besides repetitive rDNA, another type of highly abundant repeats in the mammalian genome is transposons (Figure 4). The aging epigenome is shaped by global DNA hypomethylation, typically at gene-poor and late-replicating regions, accompanied by focal hypermethylation at genic regions, bearing strong resemblance to the cancer genome (Cruickshanks et al., 2013; Yuan et al., 2015). In yeast, decline of histone chaperones and global loss of histones during aging lead to transposon derepression and genome instability (Feser et al., 2010; Hu et al., 2014; O'Sullivan et al., 2010). Deficiencies in histone deacetylase SIRT6 in mice (Simon et al., 2019), exonuclease TREX1 in human cells (Thomas et al., 2017), and endonuclease AGO2 in flies (Li et al., 2013) have been shown to induce transposons in aging and inflammatory diseases. In a unique group of premature aging diseases, progeria and laminopathies, lamin-associated domains become disintegrated (Kudlow et al., 2007), accompanied by global loss of H3K9me3 and structural changes in the heterochromatin (Scaffidi and Misteli, 2006; Zhang et al., 2015) and subsequently nuclear autophagy and cytosolic chromatin fragment–induced inflammation (Dou et al., 2015). Indeed, in keratinocytes, it has been shown that mechanical strain leads to H3K9me3 heterochromatin loss at the nuclear lamina and disruption of lineage gene expression during aging (Le et al., 2016; Koester et al., 2021), suggesting a viable hypothesis that deregulation of heterochromatin drives skin aging. Among H3K9me3 methyltransferases, G9a has been functionally examined in the mammalian epithelium. While G9a is minimally involved in homeostasis potentially because of functional redundancy, it is critical for tumorigenesis (Avgustinova et al., 2018; Avgustinova and Benitah, 2021).
Another source of spurious transcription in aging could be emanated from gene bodies (Figure 4). H3K36me3 methyltransferases are among the first discovered epigenetic regulators of longevity: Set2 in yeast (Carrozza et al., 2005; Sen et al., 2015) and Met-1 in worms (Hamilton et al., 2005; Pu et al., 2015). In these model systems, it has been shown that RNA polymerase II–associated methyltransferase deposits H3K36me3 along the gene body, recruiting histone deacetylase to suppress spurious transcription initiated from the gene body, and this transcriptional fidelity becomes compromised in aging. A similar mechanism has recently been described in mammalian cells (Neri et al., 2017; McCauley et al., 2021), in which DNA methyltransferase is likely recruited by H3K36me3 along the gene body to mediate the suppression of cryptic transcription (Dhayalan et al., 2010). The mammalian H3K36me3 is maintained by multiple methyltransferases, Setd2 and Nsd1/2/3, which exhibit both overlapping and distinct functions (Barral et al., 2022).
Both H3K9me3 and H3K36me3 are closely associated with DNA methylation and frequently exhibit interdependency. Epidermis-specific deletion of the de novo DNA methyltransferase Dnmt1 led to hair follicle miniaturization along with epidermal and sebaceous gland hyperplasia, a signature of premature aging in the skin (Li et al., 2012). Consistently, in the epidermis, Dnmt1 along with its foreshadowing E3 ubiquitin ligase Uhrf1 are required to maintain stem cell self-renewal (Sen et al., 2010; Mulder et al., 2012), loss of which results in autoinflammatory conditions in the skin (Beck et al., 2021). A related enzyme, Tet2, that catalyzes 5-hydroxymethylcytosine (5hmC) and promotes DNA demethylation, is essential to maintain epidermal differentiation (Boudra et al., 2021). Furthermore, deletion of the maintenance DNA methyltransferases Dnmt3a/b from epithelial cells largely spared skin homeostasis but contributed to malignant transformation (Rinaldi et al., 2016; Rinaldi et al., 2017). Interesting, deletion of mediator complex component Med1 (Nakajima et al., 2013) or the SWI/SNF-like BAF complex catalytic subunit Brg1 (Xiong et al., 2013) also elicits HFSC exhaustion and alopecia phenotype, although in the case of BAF complex, its function is context dependent and regulates either stem cell expansion or differentiation based on which lineage is examined (Bao et al., 2013; Mardaryev et al., 2014).
Another repressive histone post-translational modification proposed to regulate aging is the inactive or poised chromatin marker H3K27me3, which declines due to increased demethylase UTX-1 during aging (Jin et al., 2011; Maures et al., 2011). In this case, the impact of H3K27me3 loss on aging has been attributed to its specific regulation of the IIS (insulin/insulin-like growth factor) genes. In contrast, the excessive levels of the active chromatin marker H3K4me3 and its responsible methyltransferase, trithorax, has been shown to be detrimental to longevity (Greer et al., 2010; Greer et al., 2011). On the other hand, H3K4me3 demethylase promotes longevity in worms, with a remarkable transgenerational memory effect observed in this context (Greer et al., 2014). Mild mitochondrial stress-induced lifespan extension is associated with epigenetic remodeling via histone methyltransferases (Tian et al., 2016) and demethylases (Merkwirth et al., 2016). Remarkably, genome-wide mapping of 5mC and 5hmC in HSCs revealed extended regions of low-methylation canyons that are distinct from CpG islands and shores (Jeong et al., 2014). These canyons harbor either H3K27me3 or poised H3K27me3/H3K4me3 histone markers, whose borders are demarcated by 5hmC, and are deregulated upon Dnmt3a/Tet1 deletion or in aging (Jeong et al., 2014; Sun et al., 2014; Dixon et al., 2021).
In skin, histone methyltransferases of H3K27me3 (polycomb group) and H3K4me3 (trithorax group) regulate epidermal stem cell self-renewal and differentiation, respectively, during development and regeneration (Millar, 2011; Frye and Benitah, 2012; Perdigoto et al., 2014; Guan et al., 2020). For example, in PRC2 Ezh2 conditional knockout, P16 is derepressed, resulting in cell cycle arrest and epithelium hypoplasia (Ezhkova et al., 2009), similar to PRC1 deficiency (Cordisco et al., 2010; Luis et al., 2011). In contrast, the H3K27me3 demethylase promotes epidermal differentiation (Sen et al., 2008). Likewise, histone deacetylases HDAC1/2 (LeBoeuf et al., 2010) (Hughes et al., 2014) and HDAC3 (Szigety et al., 2020) have opposing functions in stem cell proliferation versus differentiation. On the other hand, the histone methyltransferase MLL4 (KMT2D) that catalyzes H3K4me1 is required to maintain epidermal homeostasis, whose loss resulted in the disruption of skin stratification and lipid metabolism (Lin-Shiao et al., 2018; Egolf et al., 2021). Interestingly, lack of Bmi1, a component of PRC1, leads to impaired mitochondrial function and to increased ROS and DNA damage, which could be reversed by antioxidant treatment or genetic disruption of Chk2 (Liu et al., 2009). These findings suggest that global chromatin disruption may override defects from specific gene deregulations, that it is not an individual gene or a few genes per se that elicit the phenotype. These molecular and cellular mechanisms are potentially extendable to aging.
Compared to global hypomethylation, equally noteworthy is the accompanying focal hypermethylation in aging chromatin (Figure 4). A prominent feature in cellular senescence is the formation of the senescence-associated secretory phenotype (SASP) on one hand (Coppé et al., 2010) and senescence-associated heterochromatin foci (SAHF) on the other (Narita et al., 2003; Zhang et al., 2005; Di Micco et al., 2011; Rai et al., 2014; Ricketts et al., 2015). Remarkably, a sparse hair coat in aged mice is recovered upon FOXO4 peptide treatment, which blocks p53 nuclear localization and induces the apoptosis of senescent cells (Baar et al., 2017), supporting the functional significance of senescence in skin aging. SAHF has been suggested as a survival mechanism to “plug the hole” that assembles heterochromatin onto euchromatin regions, given the compromised nuclear lamina that otherwise is necessary to maintain the physiological heterochromatin. Alternatively, one could speculate these foci may titrate away rate-limiting factors (H3.3, methyltransferase, or metabolites required for transcriptional repression; see Discussion) from transposon repeats and heterochromatic regions, exacerbating the already-weakened epigenetic regulation during aging. Although this model has not been formally tested in aging, a similar titration- or competition-based inhibitory mechanism from H3K27M and H3K36M oncohistones has been shown in cancer (Lewis et al., 2013; Lu et al., 2016), leaving the field with the intriguing question of how the lineage specificity and gene selectivity is achieved in the context of global epigenetic deregulations.
While the identity of upstream signals that lead to defective epigenetic machinery in aging remains unclear, it appears safe to assume these factors would be rate-limiting. As mentioned, nutrients and metabolites, in addition to their impact on cell signaling transductions and mitochondrial biology, are appealing candidates that appear to dictate the aging transcriptome and epigenome. Many epigenetic enzymes that regulate methylation or acetylation exhibit KM in the range of observed substrate concentrations in cells, suggesting rates of these reactions are highly sensitive to the cellular fluctuations of the corresponding metabolites, such as S-adenosyl methionine, acetyl-CoA, alpha-ketoglutarate, NAD+, and beta-hydroxybutyrate (Schvartzman et al., 2018), raising the intriguing question of the extent to which cellular metabolite deregulation shapes the aging epigenome. Indeed, it has been shown that in autophagy-deficient HSCs, DNA methylation and lineage gene expression are regulated by S-adenosyl methionine and alpha-ketoglutarate levels (Ho et al., 2017). Ascorbate and its downstream target TET2 dictate HSCs (Agathocleous et al., 2017) as well as (Boudra et al., 2021) epidermal function and tumorigenesis. NAD+ being the rate-limiting factor, competition between PARP-1 (also NAD+ dependent) and SIRT1 dictates cellular response in DNA damage and epigenome regulation (Bai et al., 2011) (Scheibye-Knudsen et al., 2014). RNA sequencing (RNA-seq) and assay for transposase-accessible chromatin with sequencing (ATAC-seq) at single cell level, and together with spatial transcriptomics provided unprecedented throughput and resolution of cell type, lineage trajectory, and tissue-level crosstalk in many biological contexts, and are likely to be powerful technologies in aging research. The current challenge lies in the metabolomics at a sub-cellular level and within the intact organism in vivo, both of which are critical to understand molecular determinants of aging.
Local tissue microenvironment plays a dominant role in dictating stem cell function, including aging. Aged dermal fibroblasts maintain their positional identity (Marsh et al., 2018) while manifesting adipogenic and inflammatory traits (Salzer et al., 2018; Mahmoudi et al., 2019). Secreted factors of BMP and WNT pathways enriched in adipocytes (Keyes et al., 2013; Chen et al., 2014) and pre-adipocytes (Festa et al., 2011) known to dictate hair follicle regeneration are disrupted in aged dermis (Chen et al., 2014; Chen et al., 2015). Skin-resident immune cells are drastically remodeled during aging (Giangreco et al., 2008; Doles et al., 2012), including regulatory T cells that are known to support hair follicle regeneration (Ali et al., 2017) and decline significantly in aged dermis (Ge et al., 2020). So are the arrector pili muscles and nerves (Brownell et al., 2011; Fujiwara et al., 2011; Shwartz et al., 2020) that provide niche input to HFSCs, both of which become dislodged in aged skin (Ge et al., 2020). Of significance, aged HFSCs can be rejuvenated by resident cell types and niche components of the young skin (Chen et al., 2014; Ge et al., 2020; Koester et al., 2021), suggesting that the tissue microenvironment drives stem cell function during aging. Future work will need to exploit the molecular identity, regulatory signals, and therapeutic potential of such rejuvenation factors.
YL and YG conceived the concept and wrote the manuscript.
YG is supported by grants from the NIH (1K01AR072132), CPRIT (FP00006955), UT Rising STARs program, Cancer Center Support Grant new faculty award, Andrew Sabin Family Award and MD Anderson Cancer Center startup funding. This manuscript was edited by Sarah Bronson, ELS, of the Research Medical Library at The University of Texas MD Anderson Cancer Center.
The authors declare that the research was conducted in the absence of any commercial or financial relationships that could be construed as a potential conflict of interest.
All claims expressed in this article are solely those of the authors and do not necessarily represent those of their affiliated organizations, or those of the publisher, the editors and the reviewers. Any product that may be evaluated in this article, or claim that may be made by its manufacturer, is not guaranteed or endorsed by the publisher.
Adam, R. C., Yang, H., Ge, Y., Infarinato, N. R., Gur-Cohen, S., Miao, Y., et al. (2020). NFI Transcription Factors Provide Chromatin Access to Maintain Stem Cell Identity while Preventing Unintended Lineage Fate Choices. Nat. Cell Biol. 22, 640–650. doi:10.1038/s41556-020-0513-0
Agathocleous, M., Meacham, C. E., Burgess, R. J., Piskounova, E., Zhao, Z., Crane, G. M., et al. (2017). Ascorbate Regulates Haematopoietic Stem Cell Function and Leukaemogenesis. Nature 549, 476–481. doi:10.1038/nature23876
Ahlqvist, K. J., Hämäläinen, R. H., Yatsuga, S., Uutela, M., Terzioglu, M., Götz, A., et al. (2012). Somatic Progenitor Cell Vulnerability to Mitochondrial DNA Mutagenesis Underlies Progeroid Phenotypes in Polg Mutator Mice. Cell metab. 15, 100–109. doi:10.1016/j.cmet.2011.11.012
Akagi, K., Wilson, K. A., Katewa, S. D., Ortega, M., Simons, J., Hilsabeck, T. A., et al. (2018). Dietary Restriction Improves Intestinal Cellular Fitness to Enhance Gut Barrier Function and Lifespan in D. melanogaster. PLoS Genet. 14, e1007777. doi:10.1371/journal.pgen.1007777
Ali, N., Zirak, B., Rodriguez, R. S., Pauli, M. L., Truong, H.-A., Lai, K., et al. (2017). Regulatory T Cells in Skin Facilitate Epithelial Stem Cell Differentiation. Cell 169, 1119–1129. e1111. doi:10.1016/j.cell.2017.05.002
Allen, M., Grachtchouk, M., Sheng, H., Grachtchouk, V., Wang, A., Wei, L., et al. (2003). Hedgehog Signaling Regulates Sebaceous Gland Development. Am. J. pathology 163, 2173–2178. doi:10.1016/s0002-9440(10)63574-2
Allison, J. P., and Havran, W. L. (1991). The Immunobiology of T Cells with Invariant Gammadelta Antigen Receptors. Annu. Rev. Immunol. 9, 679–705. doi:10.1146/annurev.iy.09.040191.003335
Ambrosi, T. H., Marecic, O., McArdle, A., Sinha, R., Gulati, G. S., Tong, X., et al. (2021). Aged Skeletal Stem Cells Generate an Inflammatory Degenerative Niche. Nature 597 (7875), 256–262. doi:10.1038/s41586-021-03795-7
Avgustinova, A., and Benitah, S. A. (2021). Repression of Endogenous Retroviruses Prevents Antiviral Immune Response and Is Required for Mammary Gland Development. Cell Stem Cell 28 (10), 1790–1804. e8. doi:10.1016/j.stem.2021.04.030
Avgustinova, A., Symeonidi, A., Castellanos, A., Urdiroz-Urricelqui, U., Sole-Boldo, L., Martin, M., et al. (2018). Loss of G9a Preserves Mutation Patterns but Increases Chromatin Accessibility, Genomic Instability and Aggressiveness in Skin Tumours. Nat. Cell Biol. 20 (12), 1400–1409. doi:10.1038/s41556-018-0233-x
Baar, M. P., Brandt, R. M. C., Putavet, D. A., Klein, J. D. D., Derks, K. W. J., Bourgeois, B. R. M., et al. (2017). Targeted Apoptosis of Senescent Cells Restores Tissue Homeostasis in Response to Chemotoxicity and Aging. Cell 169, 132–147. e116. doi:10.1016/j.cell.2017.02.031
Bai, P., Cantó, C., Oudart, H., Brunyánszki, A., Cen, Y., Thomas, C., et al. (2011). PARP-1 Inhibition Increases Mitochondrial Metabolism through SIRT1 Activation. Cell metab. 13, 461–468. doi:10.1016/j.cmet.2011.03.004
Baker, D. J., Jeganathan, K. B., Cameron, J. D., Thompson, M., Juneja, S., Kopecka, A., et al. (2004). BubR1 Insufficiency Causes Early Onset of Aging-Associated Phenotypes and Infertility in Mice. Nat. Genet. 36, 744–749. doi:10.1038/ng1382
Bao, X., Tang, J., Lopez-Pajares, V., Tao, S., Qu, K., Crabtree, G. R., et al. (2013). ACTL6a Enforces the Epidermal Progenitor State by Suppressing SWI/SNF-dependent Induction of KLF4. Cell Stem Cell 12, 193–203. doi:10.1016/j.stem.2012.12.014
Barral, A., Pozo, G., Ducrot, L., Papadopoulos, G. L., Sauzet, S., Oldfield, A. J., et al. (2022). SETDB1/NSD-dependent H3K9me3/H3K36me3 Dual Heterochromatin Maintains Gene Expression Profiles by Bookmarking Poised Enhancers. Mol. Cell 82, 816–832. e12. doi:10.1016/j.molcel.2021.12.037
Beck, M. A., Fischer, H., Grabner, L. M., Groffics, T., Winter, M., Tangermann, S., et al. (2021). DNA Hypomethylation Leads to cGAS-Induced Autoinflammation in the Epidermis. Embo J. 40, e108234. doi:10.15252/embj.2021108234
Beckman, K. B., and Ames, B. N. (1998). The Free Radical Theory of Aging Matures. Physiol. Rev. 78, 547–581. doi:10.1152/physrev.1998.78.2.547
Blackwell, T. K., Steinbaugh, M. J., Hourihan, J. M., Ewald, C. Y., and Isik, M. (2015). SKN-1/Nrf, Stress Responses, and Aging in Caenorhabditis elegans. Free Radic. Biol. Med. 88, 290–301. doi:10.1016/j.freeradbiomed.2015.06.008
Blanpain, C., Lowry, W. E., Geoghegan, A., Polak, L., and Fuchs, E. (2004). Self-renewal, Multipotency, and the Existence of Two Cell Populations within an Epithelial Stem Cell Niche. Cell 118, 635–648. doi:10.1016/j.cell.2004.08.012
Bonaguidi, M. A., Wheeler, M. A., Shapiro, J. S., Stadel, R. P., Sun, G. J., Ming, G.-l., et al. (2011). In Vivo clonal Analysis Reveals Self-Renewing and Multipotent Adult Neural Stem Cell Characteristics. Cell 145, 1142–1155. doi:10.1016/j.cell.2011.05.024
Boudra, R., Woappi, Y., Wang, D., Xu, S., Wells, M., Schmults, C. D., et al. (2021). Regulation of 5-Hydroxymethylcytosine by TET2 Contributes to Squamous Cell Carcinoma Tumorigenesis. J. investigative dermatology 142 (21), 1270–1279. doi:10.1016/j.jid.2021.09.026
Bourdenx, M., Martín-Segura, A., Scrivo, A., Rodriguez-Navarro, J. A., Kaushik, S., Tasset, I., et al. (2021). Chaperone-mediated Autophagy Prevents Collapse of the Neuronal Metastable Proteome. Cell 184, 2696–2714. e2625. doi:10.1016/j.cell.2021.03.048
Brownell, I., Guevara, E., Bai, C. B., Loomis, C. A., and Joyner, A. L. (2011). Nerve-derived Sonic Hedgehog Defines a Niche for Hair Follicle Stem Cells Capable of Becoming Epidermal Stem Cells. Cell Stem Cell 8, 552–565. doi:10.1016/j.stem.2011.02.021
Brunet, A., Sweeney, L. B., Sturgill, J. F., Chua, K. F., Greer, P. L., Lin, Y., et al. (2004). Stress-dependent Regulation of FOXO Transcription Factors by the SIRT1 Deacetylase. Science 303, 2011–2015. doi:10.1126/science.1094637
Byrd, A. L., Belkaid, Y., and Segre, J. A. (2018). The Human Skin Microbiome. Nat. Rev. Microbiol. 16, 143–155. doi:10.1038/nrmicro.2017.157
Carrozza, M. J., Li, B., Florens, L., Suganuma, T., Swanson, S. K., Lee, K. K., et al. (2005). Histone H3 Methylation by Set2 Directs Deacetylation of Coding Regions by Rpd3S to Suppress Spurious Intragenic Transcription. Cell 123, 581–592. doi:10.1016/j.cell.2005.10.023
Castilho, R. M., Squarize, C. H., Chodosh, L. A., Williams, B. O., and Gutkind, J. S. (2009). mTOR Mediates Wnt-Induced Epidermal Stem Cell Exhaustion and Aging. Cell Stem Cell 5, 279–289. doi:10.1016/j.stem.2009.06.017
Cerletti, M., Jang, Y. C., Finley, L. W. S., Haigis, M. C., and Wagers, A. J. (2012). Short-term Calorie Restriction Enhances Skeletal Muscle Stem Cell Function. Cell Stem Cell 10, 515–519. doi:10.1016/j.stem.2012.04.002
Chang, C. Y., Pasolli, H. A., Giannopoulou, E. G., Guasch, G., Gronostajski, R. M., Elemento, O., et al. (2013). NFIB Is a Governor of Epithelial-Melanocyte Stem Cell Behaviour in a Shared Niche. Nature 495, 98–102. doi:10.1038/nature11847
Chen, C., Liu, Y., Liu, Y., and Zheng, P. (2009). mTOR Regulation and Therapeutic Rejuvenation of Aging Hematopoietic Stem Cells. Sci. Signal 2, ra75. doi:10.1126/scisignal.2000559
Chen, C.-C., Murray, P. J., Jiang, T. X., Plikus, M. V., Chang, Y.-T., Lee, O. K., et al. (2014). Regenerative Hair Waves in Aging Mice and Extra-follicular Modulators Follistatin, Dkk1, and Sfrp4. J. Investigative Dermatology 134, 2086–2096. doi:10.1038/jid.2014.139
Chen, C.-C., Wang, L., Plikus, M. V., Jiang, T. X., Murray, P. J., Ramos, R., et al. (2015). Organ-level Quorum Sensing Directs Regeneration in Hair Stem Cell Populations. Cell 161, 277–290. doi:10.1016/j.cell.2015.02.016
Chen, J., Astle, C., and Harrison, D. (2003). Hematopoietic Senescence Is Postponed and Hematopoietic Stem Cell Function Is Enhanced by Dietary Restriction*1. Exp. Hematol. 31, 1097–1103. doi:10.1016/s0301-472x(03)00238-8
Cho, R. H., Sieburg, H. B., and Muller-Sieburg, C. E. (2008). A New Mechanism for the Aging of Hematopoietic Stem Cells: Aging Changes the Clonal Composition of the Stem Cell Compartment but Not Individual Stem Cells. Blood 111, 5553–5561. doi:10.1182/blood-2007-11-123547
Claudinot, S., Nicolas, M., Oshima, H., Rochat, A., and Barrandon, Y. (2005). Long-term Renewal of Hair Follicles from Clonogenic Multipotent Stem Cells. Proc. Natl. Acad. Sci. U.S.A. 102, 14677–14682. doi:10.1073/pnas.0507250102
Coppé, J. P., Desprez, P. Y., Krtolica, A., and Campisi, J. (2010). The Senescence-Associated Secretory Phenotype: the Dark Side of Tumor Suppression. Annu. Rev. Pathol. 5, 99–118. doi:10.1146/annurev-pathol-121808-102144
Cordisco, S., Maurelli, R., Bondanza, S., Stefanini, M., Zambruno, G., Guerra, L., et al. (2010). Bmi-1 Reduction Plays a Key Role in Physiological and Premature Aging of Primary Human Keratinocytes. J. Investigative Dermatology 130, 1048–1062. doi:10.1038/jid.2009.355
Cotsarelis, G., Sun, T.-T., and Lavker, R. M. (1990). Label-retaining Cells Reside in the Bulge Area of Pilosebaceous Unit: Implications for Follicular Stem Cells, Hair Cycle, and Skin Carcinogenesis. Cell 61, 1329–1337. doi:10.1016/0092-8674(90)90696-c
Cruickshanks, H. A., McBryan, T., Nelson, D. M., Vanderkraats, N. D., Shah, P. P., van Tuyn, J., et al. (2013). Senescent Cells Harbour Features of the Cancer Epigenome. Nat. Cell Biol. 15, 1495–1506. doi:10.1038/ncb2879
Dang, W., Steffen, K. K., Perry, R., Dorsey, J. A., Johnson, F. B., Shilatifard, A., et al. (2009). Histone H4 Lysine 16 Acetylation Regulates Cellular Lifespan. Nature 459, 802–807. doi:10.1038/nature08085
Daniel Ricketts, M., Frederick, B., Hoff, H., Tang, Y., Schultz, D. C., Singh Rai, T., et al. (2015). Ubinuclein-1 Confers Histone H3.3-Specific-Binding by the HIRA Histone Chaperone Complex. Nat. Commun. 6, 7711. doi:10.1038/ncomms8711
Demehri, S., and Kopan, R. (2009). Notch Signaling in Bulge Stem Cells Is Not Required for Selection of Hair Follicle Fate. Development 136, 891–896. doi:10.1242/dev.030700
DeStefano, G. M., and Christiano, A. M. (2014). The Genetics of Human Skin Disease. Cold Spring Harb. Perspect. Med. 4, a015172. doi:10.1101/cshperspect.a015172
Dhayalan, A., Rajavelu, A., Rathert, P., Tamas, R., Jurkowska, R. Z., Ragozin, S., et al. (2010). The Dnmt3a PWWP Domain Reads Histone 3 Lysine 36 Trimethylation and Guides DNA Methylation. J. Biol. Chem. 285, 26114–26120. doi:10.1074/jbc.m109.089433
Di Micco, R., Sulli, G., Dobreva, M., Liontos, M., Botrugno, O. A., Gargiulo, G., et al. (2011). Interplay between Oncogene-Induced DNA Damage Response and Heterochromatin in Senescence and Cancer. Nat. Cell Biol. 13, 292–302. doi:10.1038/ncb2170
Difilippantonio, M. J., Zhu, J., Chen, H. T., Meffre, E., Nussenzweig, M. C., Max, E. E., et al. (2000). DNA Repair Protein Ku80 Suppresses Chromosomal Aberrations and Malignant Transformation. Nature 404, 510–514. doi:10.1038/35006670
Dillon, L. M., Williams, S. L., Hida, A., Peacock, J. D., Prolla, T. A., Lincoln, J., et al. (2012). Increased Mitochondrial Biogenesis in Muscle Improves Aging Phenotypes in the mtDNA Mutator Mouse. Hum. Mol. Genet. 21, 2288–2297. doi:10.1093/hmg/dds049
Ding, X., Bloch, W., Iden, S., Rüegg, M. A., Hall, M. N., Leptin, M., et al. (2016). mTORC1 and mTORC2 Regulate Skin Morphogenesis and Epidermal Barrier Formation. Nat. Commun. 7, 13226. doi:10.1038/ncomms13226
Dixon, G., Pan, H., Yang, D., Rosen, B. P., Jashari, T., Verma, N., et al. (2021). QSER1 Protects DNA Methylation Valleys from De Novo Methylation. Science 372, eabd0875. doi:10.1126/science.abd0875
Doles, J., Storer, M., Cozzuto, L., Roma, G., and Keyes, W. M. (2012). Age-associated Inflammation Inhibits Epidermal Stem Cell Function. Genes Dev. 26, 2144–2153. doi:10.1101/gad.192294.112
Donati, G., Rognoni, E., Hiratsuka, T., Liakath-Ali, K., Hoste, E., Kar, G., et al. (2017). Wounding Induces Dedifferentiation of Epidermal Gata6+ Cells and Acquisition of Stem Cell Properties. Nat. Cell Biol. 19 (6), 603–613. doi:10.1038/ncb3532
Dong, S., Wang, Q., Kao, Y.-R., Diaz, A., Tasset, I., Kaushik, S., et al. (2021). Chaperone-mediated Autophagy Sustains Haematopoietic Stem-Cell Function. Nature 591, 117–123. doi:10.1038/s41586-020-03129-z
Dou, Z., Ghosh, K., Vizioli, M. G., Zhu, J., Sen, P., Wangensteen, K. J., et al. (2017). Cytoplasmic Chromatin Triggers Inflammation in Senescence and Cancer. Nature 550, 402–406. doi:10.1038/nature24050
Dou, Z., Xu, C., Donahue, G., Shimi, T., Pan, J.-A., Zhu, J., et al. (2015). Autophagy Mediates Degradation of Nuclear Lamina. Nature 527, 105–109. doi:10.1038/nature15548
Durieux, J., Wolff, S., and Dillin, A. (2011). The Cell-Non-Autonomous Nature of Electron Transport Chain-Mediated Longevity. Cell 144, 79–91. doi:10.1016/j.cell.2010.12.016
Egolf, S., Zou, J., Anderson, A., Simpson, C. L., Aubert, Y., Prouty, S., et al. (2021). MLL4 Mediates Differentiation and Tumor Suppression through Ferroptosis. Sci. Adv. 7, eabj9141. doi:10.1126/sciadv.abj9141
Eming, S. A., Wynn, T. A., and Martin, P. (2017). Inflammation and Metabolism in Tissue Repair and Regeneration. Science 356, 1026–1030. doi:10.1126/science.aam7928
Essers, M. A. G., Offner, S., Blanco-Bose, W. E., Waibler, Z., Kalinke, U., Duchosal, M. A., et al. (2009). IFNα Activates Dormant Haematopoietic Stem Cells In Vivo. Nature 458, 904–908. doi:10.1038/nature07815
Estrach, S., Ambler, C. A., Lo Celso, C. L., Hozumi, K., and Watt, F. M. (2006). Jagged 1 Is a β-catenin Target Gene Required for Ectopic Hair Follicle Formation in Adult Epidermis. Development 133, 4427–4438. doi:10.1242/dev.02644
Ezhkova, E., Pasolli, H. A., Parker, J. S., Stokes, N., Su, I.-h., Hannon, G., et al. (2009). Ezh2 Orchestrates Gene Expression for the Stepwise Differentiation of Tissue-specific Stem Cells. Cell 136, 1122–1135. doi:10.1016/j.cell.2008.12.043
Feser, J., Truong, D., Das, C., Carson, J. J., Kieft, J., Harkness, T., et al. (2010). Elevated Histone Expression Promotes Life Span Extension. Mol. Cell 39, 724–735. doi:10.1016/j.molcel.2010.08.015
Festa, E., Fretz, J., Berry, R., Schmidt, B., Rodeheffer, M., Horowitz, M., et al. (2011). Adipocyte Lineage Cells Contribute to the Skin Stem Cell Niche to Drive Hair Cycling. Cell 146, 761–771. doi:10.1016/j.cell.2011.07.019
Flach, J., Bakker, S. T., Mohrin, M., Conroy, P. C., Pietras, E. M., Reynaud, D., et al. (2014). Replication Stress Is a Potent Driver of Functional Decline in Ageing Haematopoietic Stem Cells. Nature 512, 198–202. doi:10.1038/nature13619
Flores, A., Schell, J., Krall, A. S., Jelinek, D., Miranda, M., Grigorian, M., et al. (2017). Lactate Dehydrogenase Activity Drives Hair Follicle Stem Cell Activation. Nat. Cell Biol. 19, 1017–1026. doi:10.1038/ncb3575
Flores, I., Cayuela, M. L., and Blasco, M. A. (2005). Effects of Telomerase and Telomere Length on Epidermal Stem Cell Behavior. Science 309, 1253–1256. doi:10.1126/science.1115025
Florian, M. C., Nattamai, K. J., Dörr, K., Marka, G., Überle, B., Vas, V., et al. (2013). A Canonical to Non-canonical Wnt Signalling Switch in Haematopoietic Stem-Cell Ageing. Nature 503, 392–396. doi:10.1038/nature12631
Folgueras, A. R., Guo, X., Pasolli, H. A., Stokes, N., Polak, L., Zheng, D., et al. (2013). Architectural Niche Organization by LHX2 Is Linked to Hair Follicle Stem Cell Function. Cell Stem Cell 13, 314–327. doi:10.1016/j.stem.2013.06.018
Foretz, M., Guigas, B., Bertrand, L., Pollak, M., and Viollet, B. (2014). Metformin: from Mechanisms of Action to Therapies. Cell metab. 20, 953–966. doi:10.1016/j.cmet.2014.09.018
Forni, M. F., Peloggia, J., Braga, T. T., Chinchilla, J. E. O., Shinohara, J., Navas, C. A., et al. (2017). Caloric Restriction Promotes Structural and Metabolic Changes in the Skin. Cell Rep. 20, 2678–2692. doi:10.1016/j.celrep.2017.08.052
Frye, M., and Benitah, S. A. (2012). Chromatin Regulators in Mammalian Epidermis. Seminars Cell & Dev. Biol. 23, 897–905. doi:10.1016/j.semcdb.2012.08.009
Fuchs, E. (1995). Keratins and the Skin. Annu. Rev. Cell Dev. Biol. 11, 123–154. doi:10.1146/annurev.cb.11.110195.001011
Fujiwara, H., Ferreira, M., Donati, G., Marciano, D. K., Linton, J. M., Sato, Y., et al. (2011). The Basement Membrane of Hair Follicle Stem Cells Is a Muscle Cell Niche. Cell 144, 577–589. doi:10.1016/j.cell.2011.01.014
Gan, B., Sahin, E., Jiang, S., Sanchez-Aguilera, A., Scott, K. L., Chin, L., et al. (2008). mTORC1-dependent and -independent Regulation of Stem Cell Renewal, Differentiation, and Mobilization. Proc. Natl. Acad. Sci. U.S.A. 105, 19384–19389. doi:10.1073/pnas.0810584105
Gao, Y., Ferguson, D. O., Xie, W., Manis, J. P., Sekiguchi, J., Frank, K. M., et al. (2000). Interplay of P53 and DNA-Repair Protein XRCC4 in Tumorigenesis, Genomic Stability and Development. Nature 404, 897–900. doi:10.1038/35009138
Ge, Y., and Fuchs, E. (2018). Stretching the Limits: from Homeostasis to Stem Cell Plasticity in Wound Healing and Cancer. Nat. Rev. Genet. 19, 311–325. doi:10.1038/nrg.2018.9
Ge, Y., Gomez, N. C., Adam, R. C., Nikolova, M., Yang, H., Verma, A., et al. (2017). Stem Cell Lineage Infidelity Drives Wound Repair and Cancer. Cell 169, 636–650. doi:10.1016/j.cell.2017.03.042
Ge, Y., Miao, Y., Gur-Cohen, S., Gomez, N., Yang, H., Nikolova, M., et al. (2020). The Aging Skin Microenvironment Dictates Stem Cell Behavior. Proc. Natl. Acad. Sci. U.S.A. 117, 5339–5350. doi:10.1073/pnas.1901720117
Giangreco, A., Qin, M., Pintar, J. E., and Watt, F. M. (2008). Epidermal Stem Cells Are Retained In Vivo throughout Skin Aging. Aging Cell 7, 250–259. doi:10.1111/j.1474-9726.2008.00372.x
Gomes, A. P., Price, N. L., Ling, A. J. Y., Moslehi, J. J., Montgomery, M. K., Rajman, L., et al. (2013). Declining NAD+ Induces a Pseudohypoxic State Disrupting Nuclear-Mitochondrial Communication during Aging. Cell 155, 1624–1638. doi:10.1016/j.cell.2013.11.037
Greco, V., Chen, T., Rendl, M., Schober, M., Pasolli, H. A., Stokes, N., et al. (2009). A Two-step Mechanism for Stem Cell Activation during Hair Regeneration. Cell Stem Cell 4, 155–169. doi:10.1016/j.stem.2008.12.009
Greer, E. L., Beese-Sims, S. E., Brookes, E., Spadafora, R., Zhu, Y., Rothbart, S. B., et al. (2014). A Histone Methylation Network Regulates Transgenerational Epigenetic Memory in C. elegans. Cell Rep. 7, 113–126. doi:10.1016/j.celrep.2014.02.044
Greer, E. L., Maures, T. J., Hauswirth, A. G., Green, E. M., Leeman, D. S., Maro, G. S., et al. (2010). Members of the H3K4 Trimethylation Complex Regulate Lifespan in a Germline-dependent Manner in C. elegans. Nature 466, 383–387. doi:10.1038/nature09195
Greer, E. L., Maures, T. J., Ucar, D., Hauswirth, A. G., Mancini, E., Lim, J. P., et al. (2011). Transgenerational Epigenetic Inheritance of Longevity in Caenorhabditis elegans. Nature 2011, 1. doi:10.1038/nature10572
Grewal, S. I. S., and Moazed, D. (2003). Heterochromatin and Epigenetic Control of Gene Expression. Science 301, 798–802. doi:10.1126/science.1086887
Gu, L.-H., and Coulombe, P. A. (2008). Hedgehog Signaling, Keratin 6 Induction, and Sebaceous Gland Morphogenesis. Am. J. pathology 173, 752–761. doi:10.2353/ajpath.2008.071089
Guan, Y., Yang, Y. J., Nagarajan, P., and Ge, Y. (2020). Transcriptional and Signalling Regulation of Skin Epithelial Stem Cells in Homeostasis, Wounds and Cancer. Exp. Dermatol. 30 (4), 529–545. doi:10.1111/exd.14247
Guha, U., Mecklenburg, L., Cowin, P., Kan, L., O'Guin, W. M., D'Vizio, D., et al. (2004). Bone Morphogenetic Protein Signaling Regulates Postnatal Hair Follicle Differentiation and Cycling. Am. J. pathology 165, 729–740. doi:10.1016/s0002-9440(10)63336-6
Gur-Cohen, S., Yang, H., Baksh, S. C., Miao, Y., Levorse, J., Kataru, R. P., et al. (2019). Stem Cell-Driven Lymphatic Remodeling Coordinates Tissue Regeneration. Science 366, 1218–1225. doi:10.1126/science.aay4509
Gurtner, G. C., Werner, S., Barrandon, Y., and Longaker, M. T. (2008). Wound Repair and Regeneration. Nature 453, 314–321. doi:10.1038/nature07039
Haigis, M. C., and Guarente, L. P. (2006). Mammalian Sirtuins-Emerging Roles in Physiology, Aging, and Calorie Restriction. Genes Dev. 20, 2913–2921. doi:10.1101/gad.1467506
Hamanaka, R. B., Glasauer, A., Hoover, P., Yang, S., Blatt, H., Mullen, A. R., et al. (2013). Mitochondrial Reactive Oxygen Species Promote Epidermal Differentiation and Hair Follicle Development. Sci. Signal 6, ra8. doi:10.1126/scisignal.2003638
Hamilton, B., Dong, Y., Shindo, M., Liu, W., Odell, I., Ruvkun, G., et al. (2005). A Systematic RNAi Screen for Longevity Genes in C. elegans. Genes Dev. 19, 1544–1555. doi:10.1101/gad.1308205
Hardy, M. (1992). The Secret Life of the Hair Follicle. Trends Genet. 8, 55–61. doi:10.1016/0168-9525(92)90044-5
Heath, W. R., and Carbone, F. R. (2013). The Skin-Resident and Migratory Immune System in Steady State and Memory: Innate Lymphocytes, Dendritic Cells and T Cells. Nat. Immunol. 14, 978–985. doi:10.1038/ni.2680
Heitman, N., Sennett, R., Mok, K. W., Saxena, N., Srivastava, D., Martino, P., et al. (2019). Dermal Sheath Contraction Powers Stem Cell Niche Relocation during Hair Cycle Regression. Science 367 (6474), 161–166. doi:10.1126/science.aax9131
Herzig, S., and Shaw, R. J. (2018). AMPK: Guardian of Metabolism and Mitochondrial Homeostasis. Nat. Rev. Mol. Cell Biol. 19, 121–135. doi:10.1038/nrm.2017.95
Ho, T. T., Warr, M. R., Adelman, E. R., Lansinger, O. M., Flach, J., Verovskaya, E. V., et al. (2017). Autophagy Maintains the Metabolism and Function of Young and Old Stem Cells. Nature 543, 205–210. doi:10.1038/nature21388
Horsley, V., and Watt, F. (2017). Repeal and Replace: Adipocyte Regeneration in Wound Repair. Cell Stem Cell 20, 424–426. doi:10.1016/j.stem.2017.03.015
Houtkooper, R. H., Pirinen, E., and Auwerx, J. (2012). Sirtuins as Regulators of Metabolism and Healthspan. Nat. Rev. Mol. Cell Biol. 13, 225–238. doi:10.1038/nrm3293
Hsu, Y.-C., Pasolli, H. A., and Fuchs, E. (2011). Dynamics between Stem Cells, Niche, and Progeny in the Hair Follicle. Cell 144, 92–105. doi:10.1016/j.cell.2010.11.049
Hu, Z., Chen, K., Xia, Z., Chavez, M., Pal, S., Seol, J.-H., et al. (2014). Nucleosome Loss Leads to Global Transcriptional Up-Regulation and Genomic Instability during Yeast Aging. Genes Dev. 28, 396–408. doi:10.1101/gad.233221.113
Hughes, M. W., Jiang, T.-X., Lin, S.-J., Leung, Y., Kobielak, K., Widelitz, R. B., et al. (2014). Disrupted Ectodermal Organ Morphogenesis in Mice with a Conditional Histone Deacetylase 1, 2 Deletion in the Epidermis. J. Investigative Dermatology 134, 24–32. doi:10.1038/jid.2013.283
Inomata, K., Aoto, T., Binh, N. T., Okamoto, N., Tanimura, S., Wakayama, T., et al. (2009). Genotoxic Stress Abrogates Renewal of Melanocyte Stem Cells by Triggering Their Differentiation. Cell 137, 1088–1099.
Ito, K., Hirao, A., Arai, F., Matsuoka, S., Takubo, K., Hamaguchi, I., et al. (2004). Regulation of Oxidative Stress by ATM Is Required for Self-Renewal of Haematopoietic Stem Cells. Nature 431, 997–1002. doi:10.1038/nature02989
Ito, K., Hirao, A., Arai, F., Takubo, K., Matsuoka, S., Miyamoto, K., et al. (2006). Reactive Oxygen Species Act through P38 MAPK to Limit the Lifespan of Hematopoietic Stem Cells. Nat. Med. 12, 446–451. doi:10.1038/nm1388
Ito, M., Liu, Y., Yang, Z., Nguyen, J., Liang, F., Morris, R. J., et al. (2005). Stem Cells in the Hair Follicle Bulge Contribute to Wound Repair but Not to Homeostasis of the Epidermis. Nat. Med. 11, 1351–1354. doi:10.1038/nm1328
Jaenisch, R., and Bird, A. (2003). Epigenetic Regulation of Gene Expression: How the Genome Integrates Intrinsic and Environmental Signals. Nat. Genet. 33, 245–254. doi:10.1038/ng1089
Jaks, V., Barker, N., Kasper, M., van Es, J. H., Snippert, H. J., Clevers, H., et al. (2008). Lgr5 Marks Cycling, yet Long-Lived, Hair Follicle Stem Cells. Nat. Genet. 40, 1291–1299. doi:10.1038/ng.239
Janich, P., Pascual, G., Merlos-Suárez, A., Batlle, E., Ripperger, J., Albrecht, U., et al. (2011). The Circadian Molecular Clock Creates Epidermal Stem Cell Heterogeneity. Nature 480, 209–214. doi:10.1038/nature10649
Jensen, K. B., Collins, C. A., Nascimento, E., Tan, D. W., Frye, M., Itami, S., et al. (2009). Lrig1 Expression Defines a Distinct Multipotent Stem Cell Population in Mammalian Epidermis. Cell Stem Cell 4, 427–439. doi:10.1016/j.stem.2009.04.014
Jenuwein, T., and Allis, C. D. (2001). Translating the Histone Code. Science 293, 1074–1080. doi:10.1126/science.1063127
Jeong, M., Sun, D., Luo, M., Huang, Y., Challen, G. A., Rodriguez, B., et al. (2014). Large Conserved Domains of Low DNA Methylation Maintained by Dnmt3a. Nat. Genet. 46, 17–23. doi:10.1038/ng.2836
Jin, C., Li, J., Green, C. D., Yu, X., Tang, X., Han, D., et al. (2011). Histone Demethylase UTX-1 Regulates C. elegans Life Span by Targeting the insulin/IGF-1 Signaling Pathway. Cell Metab. 14, 161–172. doi:10.1016/j.cmet.2011.07.001
Kadaja, M., Keyes, B. E., Lin, M., Pasolli, H. A., Genander, M., Polak, L., et al. (2014). SOX9: a Stem Cell Transcriptional Regulator of Secreted Niche Signaling Factors. Genes Dev. 28, 328–341. doi:10.1101/gad.233247.113
Kalaitzidis, D., Sykes, S. M., Wang, Z., Punt, N., Tang, Y., Ragu, C., et al. (2012). mTOR Complex 1 Plays Critical Roles in Hematopoiesis and Pten-Loss-Evoked Leukemogenesis. Cell Stem Cell 11, 429–439. doi:10.1016/j.stem.2012.06.009
Keyes, B. E., Segal, J. P., Heller, E., Lien, W. H., Chang, C. Y., Guo, X., et al. (2013). Nfatc1 Orchestrates Aging in Hair Follicle Stem Cells. Proc. Natl. Acad. Sci. U. S. A. 110, E4950–E4959. doi:10.1073/pnas.1320301110
Kim, C. S., Ding, X., Allmeroth, K., Biggs, L. C., Kolenc, O. I., L’Hoest, N., et al. (2020). Glutamine Metabolism Controls Stem Cell Fate Reversibility and Long-Term Maintenance in the Hair Follicle. Cell metab. 32, 629–642. e628. doi:10.1016/j.cmet.2020.08.011
Kloepper, J. E., Baris, O. R., Reuter, K., Kobayashi, K., Weiland, D., Vidali, S., et al. (2015). Mitochondrial Function in Murine Skin Epithelium Is Crucial for Hair Follicle Morphogenesis and Epithelial-Mesenchymal Interactions. J. Investigative Dermatology 135, 679–689. doi:10.1038/jid.2014.475
Kobayashi, T., Voisin, B., Kim, D. Y., Kennedy, E. A., Jo, J.-H., Shih, H.-Y., et al. (2019). Homeostatic Control of Sebaceous Glands by Innate Lymphoid Cells Regulates Commensal Bacteria Equilibrium. Cell 176, 982–997. e916. doi:10.1016/j.cell.2018.12.031
Koester, J., Miroshnikova, Y. A., Ghatak, S., Chacón-Martínez, C. A., Morgner, J., Li, X., et al. (2021). Niche Stiffening Compromises Hair Follicle Stem Cell Potential during Ageing by Reducing Bivalent Promoter Accessibility. Nat. Cell Biol. 23 (7), 771–781. doi:10.1038/s41556-021-00705-x
Kondratov, R. V., Kondratova, A. A., Gorbacheva, V. Y., Vykhovanets, O. V., and Antoch, M. P. (2006). Early Aging and Age-Related Pathologies in Mice Deficient in BMAL1, the Core Componentof the Circadian Clock. Genes Dev. 20, 1868–1873. doi:10.1101/gad.1432206
Kouzarides, T. (2007). Chromatin Modifications and Their Function. Cell 128, 693–705. doi:10.1016/j.cell.2007.02.005
Kudlow, B. A., Kennedy, B. K., and Monnat, R. J. (2007). Werner and Hutchinson-Gilford Progeria Syndromes: Mechanistic Basis of Human Progeroid Diseases. Nat. Rev. Mol. Cell Biol. 8, 394–404. doi:10.1038/nrm2161
Kujoth, G. C., Hiona, A., Pugh, T. D., Someya, S., Panzer, K., Wohlgemuth, S. E., et al. (2005). Mitochondrial DNA Mutations, Oxidative Stress, and Apoptosis in Mammalian Aging. Science 309, 481–484. doi:10.1126/science.1112125
Laplante, M., and Sabatini, D. M. (2012). mTOR Signaling in Growth Control and Disease. Cell 149, 274–293. doi:10.1016/j.cell.2012.03.017
Lapouge, G., Youssef, K. K., Vokaer, B., Achouri, Y., Michaux, C., Sotiropoulou, P. A., et al. (2011). Identifying the Cellular Origin of Squamous Skin Tumors. Proc. Natl. Acad. Sci. U.S.A. 108, 7431–7436. doi:10.1073/pnas.1012720108
Law, J. A., and Jacobsen, S. E. (2010). Establishing, Maintaining and Modifying DNA Methylation Patterns in Plants and Animals. Nat. Rev. Genet. 11, 204–220. doi:10.1038/nrg2719
Lay, K., Kume, T., and Fuchs, E. (2016). FOXC1 Maintains the Hair Follicle Stem Cell Niche and Governs Stem Cell Quiescence to Preserve Long-Term Tissue-Regenerating Potential. Proc. Natl. Acad. Sci. U. S. A. 113, E1506–E1515. doi:10.1073/pnas.1601569113
Le, H. Q., Ghatak, S., Yeung, C.-Y. C., Tellkamp, F., Günschmann, C., Dieterich, C., et al. (2016). Mechanical Regulation of Transcription Controls Polycomb-Mediated Gene Silencing during Lineage Commitment. Nat. Cell Biol. 18, 864–875. doi:10.1038/ncb3387
LeBoeuf, M., Terrell, A., Trivedi, S., Sinha, S., Epstein, J. A., Olson, E. N., et al. (2010). Hdac1 and Hdac2 Act Redundantly to Control P63 and P53 Functions in Epidermal Progenitor Cells. Dev. Cell 19, 807–818. doi:10.1016/j.devcel.2010.10.015
Lee, H.-W., Blasco, M. A., Gottlieb, G. J., Horner, J. W., Greider, C. W., and DePinho, R. A. (1998). Essential Role of Mouse Telomerase in Highly Proliferative Organs. Nature 392, 569–574. doi:10.1038/33345
Lee, J. H., Budanov, A. V., Park, E. J., Birse, R., Kim, T. E., Perkins, G. A., et al. (2010a). Sestrin as a Feedback Inhibitor of TOR that Prevents Age-Related Pathologies. Science 327, 1223–1228. doi:10.1126/science.1182228
Lee, J. Y., Nakada, D., Yilmaz, O. H., Tothova, Z., Joseph, N. M., Lim, M. S., et al. (2010b). mTOR Activation Induces Tumor Suppressors that Inhibit Leukemogenesis and Deplete Hematopoietic Stem Cells after Pten Deletion. Cell Stem Cell 7, 593–605. doi:10.1016/j.stem.2010.09.015
Lee, S. S., Kennedy, S., Tolonen, A. C., and Ruvkun, G. (2003). DAF-16 Target Genes that Control C. elegans Life-Span and Metabolism. Science 300, 644–647. doi:10.1126/science.1083614
Leeman, D. S., Passegue, E., Rando, T. A., Frydman, J., and Brunet, A. (2018). Lysosome Activation Clears Aggregates and Enhances Quiescent Neural Stem Cell Activation during Aging. Science 359 (6381), 1277–1283. doi:10.1126/science.aag3048
Levy, V., Lindon, C., Harfe, B. D., and Morgan, B. A. (2005). Distinct Stem Cell Populations Regenerate the Follicle and Interfollicular Epidermis. Dev. Cell 9, 855–861. doi:10.1016/j.devcel.2005.11.003
Lewis, P. W., Müller, M. M., Koletsky, M. S., Cordero, F., Lin, S., Banaszynski, L. A., et al. (2013). Inhibition of PRC2 Activity by a Gain-Of-Function H3 Mutation Found in Pediatric Glioblastoma. Science 340, 857–861. doi:10.1126/science.1232245
Li, J., Jiang, T.-X., Hughes, M. W., Wu, P., Widelitz, R. B., Fan, G., et al. (2012). Progressive Alopecia Reveals Decreasing Stem Cell Activation Probability during Aging of Mice with Epidermal Deletion of DNA Methyltransferase 1. J. Investigative Dermatology 132, 2681–2690. doi:10.1038/jid.2012.206
Li, W., Prazak, L., Chatterjee, N., Grüninger, S., Krug, L., Theodorou, D., et al. (2013). Activation of Transposable Elements during Aging and Neuronal Decline in Drosophila. Nat. Neurosci. 16, 529–531. doi:10.1038/nn.3368
Lien, W.-H., Polak, L., Lin, M., Lay, K., Zheng, D., and Fuchs, E. (2014). In Vivo transcriptional Governance of Hair Follicle Stem Cells by Canonical Wnt Regulators. Nat. Cell Biol. 16, 179–190. doi:10.1038/ncb2903
Lin-Shiao, E., Lan, Y., Coradin, M., Anderson, A., Donahue, G., Simpson, C. L., et al. (2018). KMT2D Regulates P63 Target Enhancers to Coordinate Epithelial Homeostasis. Genes Dev. 32, 181–193. doi:10.1101/gad.306241.117
Liu, J., Cao, L., Chen, J., Song, S., Lee, I. H., Quijano, C., et al. (2009). Bmi1 Regulates Mitochondrial Function and the DNA Damage Response Pathway. Nature 459, 387–392. doi:10.1038/nature08040
Longo, V. D., and Kennedy, B. K. (2006). Sirtuins in Aging and Age-Related Disease. Cell 126, 257–268. doi:10.1016/j.cell.2006.07.002
López-Otín, C., Blasco, M. A., Partridge, L., Serrano, M., and Kroemer, G. (2013). The Hallmarks of Aging. Cell 153, 1194–1217. doi:10.1016/j.cell.2013.05.039
Lopez-Pajares, V., Yan, K., Zarnegar, B. J., Jameson, K. L., and Khavari, P. A. (2013). Genetic Pathways in Disorders of Epidermal Differentiation. Trends Genet. 29, 31–40. doi:10.1016/j.tig.2012.10.005
Lu, C., Jain, S. U., Hoelper, D., Bechet, D., Molden, R. C., Ran, L., et al. (2016). Histone H3K36 Mutations Promote Sarcomagenesis through Altered Histone Methylation Landscape. Science 352, 844–849. doi:10.1126/science.aac7272
Luis, N. M., Morey, L., Mejetta, S., Pascual, G., Janich, P., Kuebler, B., et al. (2011). Regulation of Human Epidermal Stem Cell Proliferation and Senescence Requires Polycomb- Dependent and -independent Functions of Cbx4. Cell Stem Cell 9, 233–246. doi:10.1016/j.stem.2011.07.013
Luo, J., Nikolaev, A. Y., Imai, S.-i., Chen, D., Su, F., Shiloh, A., et al. (2001). Negative Control of P53 by Sir2α Promotes Cell Survival under Stress. Cell 107, 137–148. doi:10.1016/s0092-8674(01)00524-4
Ma, T., Tian, X., Zhang, B., Li, M., Wang, Y., Yang, C., et al. (2022). Low-dose Metformin Targets the Lysosomal AMPK Pathway through PEN2. Nature 603 (7899), 159–165. doi:10.1038/s41586-022-04431-8
Magee, J. A., Ikenoue, T., Nakada, D., Lee, J. Y., Guan, K.-L., and Morrison, S. J. (2012). Temporal Changes in PTEN and mTORC2 Regulation of Hematopoietic Stem Cell Self-Renewal and Leukemia Suppression. Cell Stem Cell 11, 415–428. doi:10.1016/j.stem.2012.05.026
Mahmoudi, S., Mancini, E., Xu, L., Moore, A., Jahanbani, F., Hebestreit, K., et al. (2019). Heterogeneity in Old Fibroblasts Is Linked to Variability in Reprogramming and Wound Healing. Nature 574, 553–558. doi:10.1038/s41586-019-1658-5
Mair, W., McLeod, C. J., Wang, L., and Jones, D. L. (2010). Dietary Restriction Enhances Germline Stem Cell Maintenance. Aging Cell 9, 916–918. doi:10.1111/j.1474-9726.2010.00602.x
Mair, W., Morantte, I., Rodrigues, A. P. C., Manning, G., Montminy, M., Shaw, R. J., et al. (2011). Lifespan Extension Induced by AMPK and Calcineurin Is Mediated by CRTC-1 and CREB. Nature 470, 404–408. doi:10.1038/nature09706
Mardaryev, A. N., Gdula, M. R., Yarker, J. L., Emelianov, V. N., Poterlowicz, K., Sharov, A. A., et al. (2014). p63 and Brg1 Control Developmentally Regulated Higher-Order Chromatin Remodelling at the Epidermal Differentiation Complex Locus in Epidermal Progenitor Cells. Dev. Camb. Engl. 141, 101–111. doi:10.1242/dev.103200
Marsh, E., Gonzalez, D. G., Lathrop, E. A., Boucher, J., and Greco, V. (2018). Positional Stability and Membrane Occupancy Define Skin Fibroblast Homeostasis In Vivo. Cell 175, 1620–1633. doi:10.1016/j.cell.2018.10.013
Matsumura, H., Mohri, Y., Binh, N. T., Morinaga, H., Fukuda, M., Ito, M., et al. (2016). Hair Follicle Aging Is Driven by Transepidermal Elimination of Stem Cells via COL17A1 Proteolysis. Science 351, aad4395. doi:10.1126/science.aad4395
Matsumura, H., Liu, N., Nanba, D., Ichinose, S., Takada, A., Kurata, S., et al. (2021). Distinct Types of Stem Cell Divisions Determine Organ Regeneration and Aging in Hair Follicles. Nat. Aging 1, 190–204. doi:10.1038/s43587-021-00033-7
Maures, T. J., Greer, E. L., Hauswirth, A. G., and Brunet, A. (2011). The H3K27 Demethylase UTX-1 Regulates C. elegans Lifespan in a Germline-independent, Insulin-dependent Manner. Aging Cell 10, 980–990. doi:10.1111/j.1474-9726.2011.00738.x
Mazumdar, J., O'Brien, W. T., Johnson, R. S., LaManna, J. C., Chavez, J. C., Klein, P. S., et al. (2010). O2 Regulates Stem Cells through Wnt/β-Catenin Signalling. Nat. Cell Biol. 12, 1007–1013. doi:10.1038/ncb2102
McAndrews, K. M., Miyake, T., Ehsanipour, E. A., Kelly, P. J., Becker, L. M., McGrail, D. J., et al. (2022). Dermal αSMA(+) Myofibroblasts Orchestrate Skin Wound Repair via β1 Integrin and Independent of Type I Collagen Production. Embo J. 41, e109470. doi:10.15252/embj.2021109470
McCauley, B. S., Sun, L., and Dang, W. (2021). Altered Chromatin States Drive Cryptic Transcription in Aging Mammalian Stem Cells. Nat. Aging. 1 (8), 684–697. doi:10.1038/s43587-021-00091-x
Merad, M., Ginhoux, F., and Collin, M. (2008). Origin, Homeostasis and Function of Langerhans Cells and Other Langerin-Expressing Dendritic Cells. Nat. Rev. Immunol. 8, 935–947. doi:10.1038/nri2455
Merkwirth, C., Jovaisaite, V., Durieux, J., Matilainen, O., Jordan, S. D., Quiros, P. M., et al. (2016). Two Conserved Histone Demethylases Regulate Mitochondrial Stress-Induced Longevity. Cell 165 (5), 1209–1223. doi:10.1016/j.cell.2016.04.012
Merrill, B. J., Gat, U., DasGupta, R., and Fuchs, E. (2001). Tcf3 and Lef1 Regulate Lineage Differentiation of Multipotent Stem Cells in Skin. Genes Dev. 15, 1688–1705. doi:10.1101/gad.891401
Mihaylova, M. M., Cheng, C.-W., Cao, A. Q., Tripathi, S., Mana, M. D., Bauer-Rowe, K. E., et al. (2018). Fasting Activates Fatty Acid Oxidation to Enhance Intestinal Stem Cell Function during Homeostasis and Aging. Cell Stem Cell 22, 769–778. e764. doi:10.1016/j.stem.2018.04.001
Millar, S. E. (2011). Committing to a Hairy Fate: Epigenetic Regulation of Hair Follicle Stem Cells. Cell Stem Cell 9, 183–184. doi:10.1016/j.stem.2011.08.009
Mitchell, J. R., Wood, E., and Collins, K. (1999). A Telomerase Component Is Defective in the Human Disease Dyskeratosis Congenita. Nature 402, 551–555. doi:10.1038/990141
Mohrin, M., Bourke, E., Alexander, D., Warr, M. R., Barry-Holson, K., Le Beau, M. M., et al. (2010). Hematopoietic Stem Cell Quiescence Promotes Error-Prone DNA Repair and Mutagenesis. Cell Stem Cell 7, 174–185. doi:10.1016/j.stem.2010.06.014
Morinaga, H., Mohri, Y., Grachtchouk, M., Asakawa, K., Matsumura, H., Oshima, M., et al. (2021). Obesity Accelerates Hair Thinning by Stem Cell-Centric Converging Mechanisms. Nature 595 (7866), 266–271. doi:10.1038/s41586-021-03624-x
Morris, R. J., Liu, Y., Marles, L., Yang, Z., Trempus, C., Li, S., et al. (2004). Capturing and Profiling Adult Hair Follicle Stem Cells. Nat. Biotechnol. 22, 411–417. doi:10.1038/nbt950
Mulder, K. W., Wang, X., Escriu, C., Ito, Y., Schwarz, R. F., Gillis, J., et al. (2012). Diverse Epigenetic Strategies Interact to Control Epidermal Differentiation. Nat. Cell Biol. 14, 753–763. doi:10.1038/ncb2520
Murphy, C. T., McCarroll, S. A., Bargmann, C. I., Fraser, A., Kamath, R. S., Ahringer, J., et al. (2003). Genes that Act Downstream of DAF-16 to Influence the Lifespan of Caenorhabditis elegans. Nature 424, 277–283. doi:10.1038/nature01789
Nakajima, T., Inui, S., Fushimi, T., Noguchi, F., Kitagawa, Y., Reddy, J. K., et al. (2013). Roles of MED1 in Quiescence of Hair Follicle Stem Cells and Maintenance of Normal Hair Cycling. J. Investigative Dermatology 133, 354–360. doi:10.1038/jid.2012.293
Nakatsuji, T., and Gallo, R. L. (2012). Antimicrobial Peptides: Old Molecules with New Ideas. J. Investigative Dermatology 132, 887–895. doi:10.1038/jid.2011.387
Nalapareddy, K., Nattamai, K. J., Kumar, R. S., Karns, R., Wikenheiser-Brokamp, K. A., Sampson, L. L., et al. (2017). Canonical Wnt Signaling Ameliorates Aging of Intestinal Stem Cells. Cell Rep. 18, 2608–2621.
Narita, M., Nuñez, S., Heard, E., Narita, M., Lin, A. W., Hearn, S. A., et al. (2003). Rb-mediated Heterochromatin Formation and Silencing of E2F Target Genes during Cellular Senescence. Cell 113, 703–716. doi:10.1016/s0092-8674(03)00401-x
Neri, F., Rapelli, S., Krepelova, A., Incarnato, D., Parlato, C., Basile, G., et al. (2017). Intragenic DNA Methylation Prevents Spurious Transcription Initiation. Nature 543, 72–77. doi:10.1038/nature21373
Niemann, C., Owens, D. M., Hülsken, J., Birchmeier, W., and Watt, F. M. (2002). Expression of ΔNLef1 in Mouse Epidermis Results in Differentiation of Hair Follicles into Squamous Epidermal Cysts and Formation of Skin Tumours. Development 129, 95–109. doi:10.1242/dev.129.1.95
Nijhof, J. G. W., Braun, K. M., Giangreco, A., van Pelt, C., Kawamoto, H., Boyd, R. L., et al. (2006). The Cell-Surface Marker MTS24 Identifies a Novel Population of Follicular Keratinocytes with Characteristics of Progenitor Cells. Development 133, 3027–3037. doi:10.1242/dev.02443
Nijnik, A., Woodbine, L., Marchetti, C., Dawson, S., Lambe, T., Liu, C., et al. (2007). DNA Repair Is Limiting for Haematopoietic Stem Cells during Ageing. Nature 447, 686–690. doi:10.1038/nature05875
Nishimura, E. K., Granter, S. R., and Fisher, D. E. (2005). Mechanisms of Hair Graying: Incomplete Melanocyte Stem Cell Maintenance in the Niche. Science 307, 720–724. doi:10.1126/science.1099593
Norddahl, G. L., Pronk, C. J., Wahlestedt, M., Sten, G., Nygren, J. M., Ugale, A., et al. (2011). Accumulating Mitochondrial DNA Mutations Drive Premature Hematopoietic Aging Phenotypes Distinct from Physiological Stem Cell Aging. Cell Stem Cell 8, 499–510. doi:10.1016/j.stem.2011.03.009
Nurk, S., Koren, S., Rhie, A., Rautiainen, M., Bzikadze, A. V., Mikheenko, A., et al. (2022). The Complete Sequence of a Human Genome. Science 376, 44–53. doi:10.1126/science.abj6987
O'Sullivan, R. J., Kubicek, S., Schreiber, S. L., and Karlseder, J. (2010). Reduced Histone Biosynthesis and Chromatin Changes Arising from a Damage Signal at Telomeres. Nat. Struct. Mol. Biol. 17, 1218–1225. doi:10.1038/nsmb.1897
Oberdoerffer, P., Michan, S., McVay, M., Mostoslavsky, R., Vann, J., Park, S.-K., et al. (2008). SIRT1 Redistribution on Chromatin Promotes Genomic Stability but Alters Gene Expression during Aging. Cell 135, 907–918. doi:10.1016/j.cell.2008.10.025
Page, M. E., Lombard, P., Ng, F., Göttgens, B., and Jensen, K. B. (2013). The Epidermis Comprises Autonomous Compartments Maintained by Distinct Stem Cell Populations. Cell Stem Cell 13, 471–482. doi:10.1016/j.stem.2013.07.010
Pan, Y., Lin, M.-H., Tian, X., Cheng, H.-T., Gridley, T., Shen, J., et al. (2004). γ-Secretase Functions through Notch Signaling to Maintain Skin Appendages but Is Not Required for Their Patterning or Initial Morphogenesis. Dev. Cell 7, 731–743. doi:10.1016/j.devcel.2004.09.014
Pan, Y., Schroeder, E. A., Ocampo, A., Barrientos, A., and Shadel, G. S. (2011). Regulation of Yeast Chronological Life Span by TORC1 via Adaptive Mitochondrial ROS Signaling. Cell Metab. 13, 668–678. doi:10.1016/j.cmet.2011.03.018
Paus, R., and Cotsarelis, G. (1999). The Biology of Hair Follicles. N. Engl. J. Med. 341, 491–497. doi:10.1056/nejm199908123410706
Perdigoto, C. N., Valdes, V. J., Bardot, E. S., and Ezhkova, E. (2014). Epigenetic Regulation of Epidermal Differentiation. Cold Spring Harb. Perspect. Med. 4, a015263. doi:10.1101/cshperspect.a015263
Petersson, M., Brylka, H., Kraus, A., John, S., Rappl, G., Schettina, P., et al. (2011). TCF/Lef1 Activity Controls Establishment of Diverse Stem and Progenitor Cell Compartments in Mouse Epidermis. Embo J. 30, 3004–3018. doi:10.1038/emboj.2011.199
Piunti, A., and Shilatifard, A. (2016). Epigenetic Balance of Gene Expression by Polycomb and COMPASS Families. Science 352, aad9780. doi:10.1126/science.aad9780
Plikus, M. V., Vollmers, C., de la Cruz, D., Chaix, A., Ramos, R., Panda, S., et al. (2013). Local Circadian Clock Gates Cell Cycle Progression of Transient Amplifying Cells during Regenerative Hair Cycling. Proc. Natl. Acad. Sci. U. S. A. 110, E2106–E2115. doi:10.1073/pnas.1215935110
Plikus, M. V., Wang, X., Sinha, S., Forte, E., Thompson, S. M., Herzog, E. L., et al. (2021). Fibroblasts: Origins, Definitions, and Functions in Health and Disease. Cell 184, 3852–3872. doi:10.1016/j.cell.2021.06.024
Plikus, M., Wang, W. P., Liu, J., Wang, X., Jiang, T.-X., and Chuong, C.-M. (2004). Morpho-Regulation of Ectodermal Organs. Am. J. pathology 164, 1099–1114. doi:10.1016/s0002-9440(10)63197-5
Pu, M., Ni, Z., Wang, M., Wang, X., Wood, J. G., Helfand, S. L., et al. (2015). Trimethylation of Lys36 on H3 Restricts Gene Expression Change during Aging and Impacts Life Span. Genes Dev. 29, 718–731. doi:10.1101/gad.254144.114
Qiu, W., Li, X., Tang, H., Huang, A. S., Panteleyev, A. A., Owens, D. M., et al. (2011). Conditional Activin Receptor Type 1B (Acvr1b) Knockout Mice Reveal Hair Loss Abnormality. J. Investigative Dermatology 131, 1067–1076. doi:10.1038/jid.2010.400
Rai, T. S., Cole, J. J., Nelson, D. M., Dikovskaya, D., Faller, W. J., Vizioli, M. G., et al. (2014). HIRA Orchestrates a Dynamic Chromatin Landscape in Senescence and Is Required for Suppression of Neoplasia. Genes Dev. 28, 2712–2725. doi:10.1101/gad.247528.114
Regan, J. C., Khericha, M., Dobson, A. J., Bolukbasi, E., Rattanavirotkul, N., and Partridge, L. (2016). Sex Difference in Pathology of the Ageing Gut Mediates the Greater Response of Female Lifespan to Dietary Restriction. eLife 5, e10956. doi:10.7554/eLife.10956
Rera, M., Bahadorani, S., Cho, J., Koehler, C. L., Ulgherait, M., Hur, J. H., et al. (2011). Modulation of Longevity and Tissue Homeostasis by the Drosophila PGC-1 Homolog. Cell metab. 14, 623–634. doi:10.1016/j.cmet.2011.09.013
Rinaldi, L., Avgustinova, A., Martín, M., Datta, D., Solanas, G., Prats, N., et al. (2017). Loss of Dnmt3a and Dnmt3b Does Not Affect Epidermal Homeostasis but Promotes Squamous Transformation through PPAR-γ. eLife 6, e21697. doi:10.7554/eLife.21697
Rinaldi, L., Datta, D., Serrat, J., Morey, L., Solanas, G., Avgustinova, A., et al. (2016). Dnmt3a and Dnmt3b Associate with Enhancers to Regulate Human Epidermal Stem Cell Homeostasis. Cell Stem Cell 19 (4), 491–501. doi:10.1016/j.stem.2016.06.020
Rodgers, J. T., Lerin, C., Haas, W., Gygi, S. P., Spiegelman, B. M., and Puigserver, P. (2005). Nutrient Control of Glucose Homeostasis through a Complex of PGC-1α and SIRT1. Nature 434, 113–118. doi:10.1038/nature03354
Roeder, R. G. (2005). Transcriptional Regulation and the Role of Diverse Coactivators in Animal Cells. FEBS Lett. 579, 909–915. doi:10.1016/j.febslet.2004.12.007
Rudolph, K. L., Chang, S., Lee, H.-W., Blasco, M., Gottlieb, G. J., Greider, C., et al. (1999). Longevity, Stress Response, and Cancer in Aging Telomerase-Deficient Mice. Cell 96, 701–712. doi:10.1016/s0092-8674(00)80580-2
Ruzankina, Y., Pinzon-Guzman, C., Asare, A., Ong, T., Pontano, L., Cotsarelis, G., et al. (2007). Deletion of the Developmentally Essential Gene ATR in Adult Mice Leads to Age-Related Phenotypes and Stem Cell Loss. Cell Stem Cell 1, 113–126. doi:10.1016/j.stem.2007.03.002
Sahin, E., and Depinho, R. A. (2010). Linking Functional Decline of Telomeres, Mitochondria and Stem Cells during Ageing. Nature 464, 520–528. doi:10.1038/nature08982
Salzer, M. C., Lafzi, A., Berenguer-Llergo, A., Youssif, C., Castellanos, A., Solanas, G., et al. (2018). Identity Noise and Adipogenic Traits Characterize Dermal Fibroblast Aging. Cell 175, 1575–1590. doi:10.1016/j.cell.2018.10.012
Sarin, K. Y., Cheung, P., Gilison, D., Lee, E., Tennen, R. I., Wang, E., et al. (2005). Conditional Telomerase Induction Causes Proliferation of Hair Follicle Stem Cells. Nature 436, 1048–1052. doi:10.1038/nature03836
Sato, T., Onai, N., Yoshihara, H., Arai, F., Suda, T., and Ohteki, T. (2009). Interferon Regulatory Factor-2 Protects Quiescent Hematopoietic Stem Cells from Type I Interferon-dependent Exhaustion. Nat. Med. 15, 696–700. doi:10.1038/nm.1973
Scaffidi, P., and Misteli, T. (2006). Lamin A-dependent Nuclear Defects in Human Aging. Science 312, 1059–1063. doi:10.1126/science.1127168
Scheibye-Knudsen, M., Mitchell, S. J., Fang, E. F., Iyama, T., Ward, T., Wang, J., et al. (2014). A High-Fat Diet and NAD + Activate Sirt1 to Rescue Premature Aging in Cockayne Syndrome. Cell metab. 20, 840–855. doi:10.1016/j.cmet.2014.10.005
Schell, J. C., Wisidagama, D. R., Bensard, C., Zhao, H., Wei, P., Tanner, J., et al. (2017). Control of Intestinal Stem Cell Function and Proliferation by Mitochondrial Pyruvate Metabolism. Nat. Cell Biol. 19, 1027–1036. doi:10.1038/ncb3593
Schuettengruber, B., Bourbon, H.-M., Di Croce, L., and Cavalli, G. (2017). Genome Regulation by Polycomb and Trithorax: 70 Years and Counting. Cell 171, 34–57. doi:10.1016/j.cell.2017.08.002
Schvartzman, J. M., Thompson, C. B., and Finley, L. W. S. (2018). Metabolic Regulation of Chromatin Modifications and Gene Expression. J. Cell Biol. 217, 2247–2259. doi:10.1083/jcb.201803061
Sen, G. L., Reuter, J. A., Webster, D. E., Zhu, L., and Khavari, P. A. (2010). DNMT1 Maintains Progenitor Function in Self-Renewing Somatic Tissue. Nature 463, 563–567. doi:10.1038/nature08683
Sen, G. L., Webster, D. E., Barragan, D. I., Chang, H. Y., and Khavari, P. A. (2008). Control of Differentiation in a Self-Renewing Mammalian Tissue by the Histone Demethylase JMJD3. Genes Dev. 22, 1865–1870. doi:10.1101/gad.1673508
Sen, P., Dang, W., Donahue, G., Dai, J., Dorsey, J., Cao, X., et al. (2015). H3K36 Methylation Promotes Longevity by Enhancing Transcriptional Fidelity. Genes Dev. 29, 1362–1376. doi:10.1101/gad.263707.115
Sennett, R., and Rendl, M. (2012). Mesenchymal-epithelial Interactions during Hair Follicle Morphogenesis and Cycling. Seminars Cell & Dev. Biol. 23, 917–927. doi:10.1016/j.semcdb.2012.08.011
Sharpless, N. E., and DePinho, R. A. (2004). Telomeres, Stem Cells, Senescence, and Cancer. J. Clin. Invest. 113, 160–168. doi:10.1172/jci20761
Shwartz, Y., Gonzalez-Celeiro, M., Chen, C.-L., Pasolli, H. A., Sheu, S.-H., Fan, S. M.-Y., et al. (2020). Cell Types Promoting Goosebumps Form a Niche to Regulate Hair Follicle Stem Cells. Cell 182, 578–593. doi:10.1016/j.cell.2020.06.031
Simon, M., Van Meter, M., Ablaeva, J., Ke, Z., Gonzalez, R. S., Taguchi, T., et al. (2019). LINE1 Derepression in Aged Wild-type and SIRT6-Deficient Mice Drives Inflammation. Cell metab. 29, 871–885. e875. doi:10.1016/j.cmet.2019.02.014
Simsek, T., Kocabas, F., Zheng, J., Deberardinis, R. J., Mahmoud, A. I., Olson, E. N., et al. (2010). The Distinct Metabolic Profile of Hematopoietic Stem Cells Reflects Their Location in a Hypoxic Niche. Cell Stem Cell 7, 380–390. doi:10.1016/j.stem.2010.07.011
Sinclair, D. A., and Guarente, L. (1997). Extrachromosomal rDNA Circles- A Cause of Aging in Yeast. Cell 91, 1033–1042. doi:10.1016/s0092-8674(00)80493-6
Snippert, H. J., Haegebarth, A., Kasper, M., Jaks, V., van Es, J. H., Barker, N., et al. (2010). Lgr6 Marks Stem Cells in the Hair Follicle that Generate All Cell Lineages of the Skin. Science 327, 1385–1389. doi:10.1126/science.1184733
Solanas, G., Peixoto, F. O., Perdiguero, E., Jardí, M., Ruiz-Bonilla, V., Datta, D., et al. (2017). Aged Stem Cells Reprogram Their Daily Rhythmic Functions to Adapt to Stress. Cell 170, 678–692. e620. doi:10.1016/j.cell.2017.07.035
Sotiropoulou, P. A., Candi, A., Mascré, G., De Clercq, S., Youssef, K. K., Lapouge, G., et al. (2010). Bcl-2 and Accelerated DNA Repair Mediates Resistance of Hair Follicle Bulge Stem Cells to DNA-Damage-Induced Cell Death. Nat. Cell Biol. 12, 572–582. doi:10.1038/ncb2059
Stringari, C., Wang, H., Geyfman, M., Crosignani, V., Kumar, V., Takahashi, J. S., et al. (2015). In Vivo single-cell Detection of Metabolic Oscillations in Stem Cells. Cell Rep. 10, 1–7. doi:10.1016/j.celrep.2014.12.007
Sudo, K., Ema, H., Morita, Y., and Nakauchi, H. (2000). Age-Associated Characteristics of Murine Hematopoietic Stem Cells. J. Exp. Med. 192, 1273–1280.
Sun, D., Luo, M., Jeong, M., Rodriguez, B., Xia, Z., Hannah, R., et al. (2014). Epigenomic Profiling of Young and Aged HSCs Reveals Concerted Changes during Aging that Reinforce Self-Renewal. Cell Stem Cell 14, 673–688. doi:10.1016/j.stem.2014.03.002
Sykiotis, G. P., and Bohmann, D. (2008). Keap1/Nrf2 Signaling Regulates Oxidative Stress Tolerance and Lifespan in Drosophila. Dev. Cell 14, 76–85. doi:10.1016/j.devcel.2007.12.002
Szigety, K. M., Liu, F., Yuan, C. Y., Moran, D. J., Horrell, J., Gochnauer, H. R., et al. (2020). HDAC3 Ensures Stepwise Epidermal Stratification via NCoR/SMRT-Reliant Mechanisms Independent of its Histone Deacetylase Activity. Genes Dev. 34, 973–988. doi:10.1101/gad.333674.119
Takubo, K., Goda, N., Yamada, W., Iriuchishima, H., Ikeda, E., Kubota, Y., et al. (2010). Regulation of the HIF-1α Level Is Essential for Hematopoietic Stem Cells. Cell Stem Cell 7, 391–402. doi:10.1016/j.stem.2010.06.020
Takubo, K., Nagamatsu, G., Kobayashi, C. I., Nakamura-Ishizu, A., Kobayashi, H., Ikeda, E., et al. (2013). Regulation of Glycolysis by Pdk Functions as a Metabolic Checkpoint for Cell Cycle Quiescence in Hematopoietic Stem Cells. Cell Stem Cell 12, 49–61. doi:10.1016/j.stem.2012.10.011
Tang, D., Tao, S., Chen, Z., Koliesnik, I. O., Calmes, P. G., Hoerr, V., et al. (2016). Dietary Restriction Improves Repopulation but Impairs Lymphoid Differentiation Capacity of Hematopoietic Stem Cells in Early Aging. J. Exp. Med. 213, 535–553. doi:10.1084/jem.20151100
Taylor, G., Lehrer, M. S., Jensen, P. J., Sun, T.-T., and Lavker, R. M. (2000). Involvement of Follicular Stem Cells in Forming Not Only the Follicle but Also the Epidermis. Cell 102, 451–461. doi:10.1016/s0092-8674(00)00050-7
Thomas, C. A., Tejwani, L., Trujillo, C. A., Negraes, P. D., Herai, R. H., Mesci, P., et al. (2017). Modeling of TREX1-dependent Autoimmune Disease Using Human Stem Cells Highlights L1 Accumulation as a Source of Neuroinflammation. Cell Stem Cell 21, 319–331. e318. doi:10.1016/j.stem.2017.07.009
Tian, Y., Garcia, G., Bian, Q., Steffen, K. K., Joe, L., Wolff, S., et al. (2016). Mitochondrial Stress Induces Chromatin Reorganization to Promote Longevity and UPR Mt. Cell 165, 1197–1208. doi:10.1016/j.cell.2016.04.011
Tothova, Z., Kollipara, R., Huntly, B. J., Lee, B. H., Castrillon, D. H., Cullen, D. E., et al. (2007). FoxOs Are Critical Mediators of Hematopoietic Stem Cell Resistance to Physiologic Oxidative Stress. Cell 128, 325–339. doi:10.1016/j.cell.2007.01.003
Trifunovic, A., Wredenberg, A., Falkenberg, M., Spelbrink, J. N., Rovio, A. T., Bruder, C. E., et al. (2004). Premature Ageing in Mice Expressing Defective Mitochondrial DNA Polymerase. Nature 429, 417–423. doi:10.1038/nature02517
Tullet, J. M. A., Hertweck, M., An, J. H., Baker, J., Hwang, J. Y., Liu, S., et al. (2008). Direct Inhibition of the Longevity-Promoting Factor SKN-1 by Insulin-like Signaling in C. elegans. Cell 132, 1025–1038. doi:10.1016/j.cell.2008.01.030
Tumbar, T., Guasch, G., Greco, V., Blanpain, C., Lowry, W. E., Rendl, M., et al. (2004). Defining the Epithelial Stem Cell Niche in Skin. Science 303, 359–363. doi:10.1126/science.1092436
Vauclair, S., Nicolas, M., Barrandon, Y., and Radtke, F. (2005). Notch1 Is Essential for Postnatal Hair Follicle Development and Homeostasis. Dev. Biol. 284, 184–193. doi:10.1016/j.ydbio.2005.05.018
Vaziri, H., Dessain, S. K., Eaton, E. N., Imai, S.-I., Frye, R. A., Pandita, T. K., et al. (2001). hSIR2SIRT1 Functions as an NAD-dependent P53 Deacetylase. Cell 107, 149–159. doi:10.1016/s0092-8674(01)00527-x
Vulliamy, T., Marrone, A., Goldman, F., Dearlove, A., Bessler, M., Mason, P. J., et al. (2001). The RNA Component of Telomerase Is Mutated in Autosomal Dominant Dyskeratosis Congenita. Nature 413, 432–435. doi:10.1038/35096585
Walter, D., Lier, A., Geiselhart, A., Thalheimer, F. B., Huntscha, S., Sobotta, M. C., et al. (2015). Exit from Dormancy Provokes DNA-Damage-Induced Attrition in Haematopoietic Stem Cells. Nature 520, 549–552. doi:10.1038/nature14131
Wang, L., Siegenthaler, J. A., Dowell, R. D., and Yi, R. (2016). Foxc1 Reinforces Quiescence in Self-Renewing Hair Follicle Stem Cells. Science 351, 613–617. doi:10.1126/science.aad5440
Warr, M. R., Binnewies, M., Flach, J., Reynaud, D., Garg, T., Malhotra, R., et al. (2013). FOXO3A Directs a Protective Autophagy Program in Haematopoietic Stem Cells. Nature 494, 323–327. doi:10.1038/nature11895
Watt, F. M. (2001). Stem Cell Fate and Patterning in Mammalian Epidermis. Curr. Opin. Genet. Dev. 11, 410–417. doi:10.1016/s0959-437x(00)00211-2
Workman, J. L. (2006). Nucleosome Displacement in Transcription: Figure 1. Genes Dev. 20, 2009–2017. doi:10.1101/gad.1435706
Wullschleger, S., Loewith, R., and Hall, M. N. (2006). TOR Signaling in Growth and Metabolism. Cell 124, 471–484. doi:10.1016/j.cell.2006.01.016
Xie, Y., Chen, D., Jiang, K., Song, L., Qian, N., Du, Y., et al. (2022). Hair Shaft Miniaturization Causes Stem Cell Depletion through Mechanosensory Signals Mediated by a Piezo1-Calcium-TNF-α axis. Cell Stem Cell 29, 70–85. e76. doi:10.1016/j.stem.2021.09.009
Xiong, Y., Li, W., Shang, C., Chen, R. M., Han, P., Yang, J., et al. (2013). Brg1 Governs a Positive Feedback Circuit in the Hair Follicle for Tissue Regeneration and Repair. Dev. Cell 25, 169–181. doi:10.1016/j.devcel.2013.03.015
Yamamoto, N., Tanigaki, K., Han, H., Hiai, H., and Honjo, T. (2003). Notch/RBP-J Signaling Regulates Epidermis/hair Fate Determination of Hair Follicular Stem Cells. Curr. Biol. 13, 333–338. doi:10.1016/s0960-9822(03)00081-2
Yilmaz, Ö. H., Katajisto, P., Lamming, D. W., Gültekin, Y., Bauer-Rowe, K. E., Sengupta, S., et al. (2012). mTORC1 in the Paneth Cell Niche Couples Intestinal Stem-Cell Function to Calorie Intake. Nature 486, 490–495. doi:10.1038/nature11163
Yilmaz, Ö. H., Valdez, R., Theisen, B. K., Guo, W., Ferguson, D. O., Wu, H., et al. (2006). Pten Dependence Distinguishes Haematopoietic Stem Cells from Leukaemia-Initiating Cells. Nature 441, 475–482. doi:10.1038/nature04703
Yu, W., Liu, C., Li, Q., and Zhang, L. (2021). A Stress-Induced miR-31–CLOCK–ERK Pathway Is a Key Driver and Therapeutic Target for Skin Aging. Nat. Aging 1, 1. doi:10.1038/s43587-021-00094-8
Yuan, T., Jiao, Y., de Jong, S., Ophoff, R. A., Beck, S., and Teschendorff, A. E. (2015). An Integrative Multi-Scale Analysis of the Dynamic DNA Methylation Landscape in Aging. PLoS Genet. 11, e1004996. doi:10.1371/journal.pgen.1004996
Zaratiegui, M., Irvine, D. V., and Martienssen, R. A. (2007). Noncoding RNAs and Gene Silencing. Cell 128, 763–776. doi:10.1016/j.cell.2007.02.016
Zhang, B., Ma, S., Rachmin, I., He, M., Baral, P., Choi, S., et al. (2020). Hyperactivation of Sympathetic Nerves Drives Depletion of Melanocyte Stem Cells. Nature 577, 676–681. doi:10.1038/s41586-020-1935-3
Zhang, C., Wang, D., and Yi, R. (2021). Escape of Hair Follicle Stem Cells Causes Stem Cell Exhaustion during Aging. Nat. Aging 1, 1. doi:10.1038/s43587-021-00103-w
Zhang, J., Grindley, J. C., Yin, T., Jayasinghe, S., He, X. C., Ross, J. T., et al. (2006). PTEN Maintains Haematopoietic Stem Cells and Acts in Lineage Choice and Leukaemia Prevention. Nature 441, 518–522. doi:10.1038/nature04747
Zhang, R., Poustovoitov, M. V., Ye, X., Santos, H. A., Chen, W., Daganzo, S. M., et al. (2005). Formation of MacroH2A-Containing Senescence-Associated Heterochromatin Foci and Senescence Driven by ASF1a and HIRA. Dev. Cell 8, 19–30. doi:10.1016/j.devcel.2004.10.019
Zhang, W., Li, J., Suzuki, K., Qu, J., Wang, P., Zhou, J., et al. (2015). A Werner Syndrome Stem Cell Model Unveils Heterochromatin Alterations as a Driver of Human Aging. Science 348, 1160–1163. doi:10.1126/science.aaa1356
Zhang, Y., and Reinberg, D. (2001). Transcription Regulation by Histone Methylation: Interplay between Different Covalent Modifications of the Core Histone Tails. Genes Dev. 15, 2343–2360. doi:10.1101/gad.927301
Keywords: skin aging, stem cell lineage plasticity, inflammaging, epigenetics, metabolism
Citation: Lyu Y and Ge Y (2022) Toward Elucidating Epigenetic and Metabolic Regulation of Stem Cell Lineage Plasticity in Skin Aging. Front. Cell Dev. Biol. 10:903904. doi: 10.3389/fcell.2022.903904
Received: 24 March 2022; Accepted: 21 April 2022;
Published: 19 May 2022.
Edited by:
Wen-Hui Lien, Catholic University of Louvain, BelgiumReviewed by:
Brian C. Capell, University of Pennsylvania, United StatesCopyright © 2022 Lyu and Ge. This is an open-access article distributed under the terms of the Creative Commons Attribution License (CC BY). The use, distribution or reproduction in other forums is permitted, provided the original author(s) and the copyright owner(s) are credited and that the original publication in this journal is cited, in accordance with accepted academic practice. No use, distribution or reproduction is permitted which does not comply with these terms.
*Correspondence: Yejing Ge, WUdlMUBtZGFuZGVyc29uLm9yZw==
Disclaimer: All claims expressed in this article are solely those of the authors and do not necessarily represent those of their affiliated organizations, or those of the publisher, the editors and the reviewers. Any product that may be evaluated in this article or claim that may be made by its manufacturer is not guaranteed or endorsed by the publisher.
Research integrity at Frontiers
Learn more about the work of our research integrity team to safeguard the quality of each article we publish.