- 1Centre Hospitalier Universitaire de Québec, Centre de Recherche Du Centre Hospitalier de L’Université Laval (CHUQ-CHUL), Axe Neurosciences, Université Laval, Quebec City, QC, Canada
- 2Département de Réadaptation, Faculté de Médecine, Université Laval, Quebec City, QC, Canada
Although their physiology and functions are very different, bones, skeletal and smooth muscles, as well as the heart have the same embryonic origin. Skeletal muscles and bones interact with each other to enable breathing, kinesis, and the maintenance of posture. Often, muscle and bone tissues degenerate synchronously under various conditions such as cancers, space travel, aging, prolonged bed rest, and neuromuscular diseases. In addition, bone tissue, skeletal and smooth muscles, and the heart share common signaling pathways. The RANK/RANKL/OPG pathway, which is essential for bone homeostasis, is also implicated in various physiological processes such as sarcopenia, atherosclerosis, and cardiovascular diseases. Several studies have reported bone-skeletal muscle crosstalk through the RANK/RANKL/OPG pathway. This review will summarize the current evidence indicating that the RANK/RANKL/OPG pathway is involved in muscle function. First, we will briefly discuss the role this pathway plays in bone homeostasis. Then, we will present results from various sources indicating that it plays a physiopathological role in skeletal, smooth muscle, and cardiac functions. Understanding how the RANK/RANKL/OPG pathway interferes in several physiological disorders may lead to new therapeutic approaches aimed at protecting bones and other tissues with a single treatment.
Introduction
Muscles and bones share a common embryonic origin, the mesoderm. Both tissues develop together, and their proximity and interaction enable, among other things, kinesis, stability, and support for the body. Bones and muscles are constantly adapting to varying mechanical, physiological, and biochemical demands. Bone formation and resorption depend largely on the mechanical load resulting from gravity and muscle contractions (Brotto and Bonewald, 2015). Bone density increases rapidly during the teenage years and continues to increase into the 30s (Greenlund and Nair, 2003) in synchrony with muscle mass, which follows the same trajectory. In the elderly, physical activity and anabolic hormones such as testosterone and estrogen decrease, inducing a progressive loss of bone density and muscle mass, an important component of frailty syndrome. Cast immobilization, prolonged bed rest, microgravity, critical illness, and neuromuscular diseases can markedly accelerate the osteoporotic and sarcopenic processes (Hamrick et al., 2006; McKay and Smith, 2008; Russo, 2009; Ness and Apkon, 2014; Owen et al., 2015). Bone and skeletal muscles are thus very dynamic tissues that undergo several modifications throughout life.
Several reports over the past 10–15 years have demonstrated that the synchronicity between bones and skeletal muscle extends beyond solely mechanical loading and that osteokines and myokines released by these two tissues can potentially influence bone and skeletal muscle mass (Brotto and Bonewald, 2015; Boulanger Piette et al., 2018). We hypothesized over a decade ago that the triad composed of receptor activator of nuclear factor kappa B (RANK), its ligand (RANKL), and osteoprotegerin (OPG), an inhibitor of RANKL that plays a central role in bone remodeling and homeostasis (Yasuda, 2021), is also involved in the regulation of skeletal muscle function (Dutka et al., 2021; Yasuda, 2021). In this review, we take a closer look at the role of RANK/RANKL/OPG (RRO) in muscle function. We examine current evidence implicating the triad in cardiac, skeletal, and smooth muscle function in health and disease.
RANK/RANKL/OPG in Bone Homeostasis and Disease
RANKL is a type II transmembrane protein expressed by the osteoblasts, osteocytes, and immune cells making up bone tissue. Structurally, RANKL has a short N-terminal intracellular tail and a larger C-terminal extracellular region (Nelson et al., 2012). Its ectodomain is cleaved to generate soluble RANKL, which is released into the extracellular milieu (Ono et al., 2020). Precursor messenger RNA (pre-mRNA) alternative splicing generates soluble and circulating RANKL (Ikeda et al., 2001). Three isomers of RANKL have been identified, including RANKL 3, a soluble protein devoid of intracellular or transmembrane domains (Ono et al., 2020; Ikeda et al., 2001). In solution, RANKL forms a homotrimer that interacts with its receptor RANK and the decoy receptor OPG (Nelson et al., 2012). RANK is a type I transmembrane protein located on osteoclast progenitors, mature osteoclasts, and immune cells (Wada et al., 2006). Osteoblast membrane-bound RANKL binds to RANK at the surface of osteoclast progenitor cells to stimulate osteoclastogenesis, bone remodeling, and calcium homeostasis (Li et al., 2000). The cytoplasmic region of RANK has no intrinsic kinase activity and requires adapter molecules for downstream signaling. Upon activation by RANKL, RANK receptors trimerize and recruit the tumor necrosis factor (TNF) associated with factor-6 (TRAF-6), an E3 ubiquitin ligase required for osteoclast differentiation (Armstrong et al., 2002). While TRAF- 2, 5, and 6 all have the ability to bind the cytoplasmic domain of RANK (Hsu et al., 1999), only TRAF-6 mutations lead to osteopetrosis, or overly dense bones, resulting from the loss of osteoclast function (Darnay et al., 1998; Lomaga et al., 1999; Kobayashi et al., 2001). RANKL/RANK binding activates downstream intracellular signaling pathways through TRAF-6, including MAPK, NF-κB, and PI3K, resulting in an increase in the expression of NFATc1, which promotes osteoclastogenesis and bone resorption (Boyce et al., 2015) (Figure 1). OPG belongs to the TNF receptor superfamily. It is secreted by osteoblasts and acts as a soluble decoy receptor of RANKL. The OPG/RANKL interaction prevents osteoclast formation and bone resorption in vivo (Yasuda et al., 1998). The RANKL/OPG ratio is an indicator of bone health and reflects the balance between bone formation and resorption. Unsurprisingly, RANKL/RANK expression and bone remodeling are also regulated by other cytokines, hormones, and environmental factors. For example, cytokines like interleukin-1 (IL-1 β), IL-11, and TNF-α, and hormones like 1α,25-dihydroxyvitamin D3, parathyroid hormone (PTH), and prostaglandin (PG) can all stimulate bone resorption by inducing the membrane expression of RANKL (Hofbauer et al., 2000; Udagawa et al., 2000). Adding to this complexity, the RANKL/RANK interaction is also implicated in bone formation. A study has reported that vesicular RANK, which is secreted from maturing osteoclasts, promotes bone formation by triggering RANKL reverse signaling and the activation of Runt-related transcription factor 2 (Runx2). This reverse signaling occurs via the proline-rich motif of the RANKL cytoplasmic domain (Ikebuchi et al., 2018) (Figure 1). The disruption of components of the RRO triad causes bone dysfunction and results in a pathological condition. RANKL-deficient mice exhibit a significant increase in bone mass, causing severe osteopetrosis with a lack of marrow spaces, dental eruption, and a total absence of osteoclasts. These mice show signs of growth retardation affecting several bones of the limbs, skull, and vertebrae (Kim et al., 2000). Similarly, RANK-deficient mice are resistant to bone resorption induced by TNF-α, IL-1β, calcitriol, and parathyroid hormone-related protein (PTHrP) (Li et al., 2000). In addition, osteoclast differentiation is blocked, resulting in significant osteopetrosis (Dougall et al., 1999) (Figure 2, left box). On the other hand, OPG-deficient mice develop early onset osteoporosis (Bucay et al., 1998) (Figure 2, left box). In humans, genetic mutations affecting the RANK, RANKL, and OPG genes are associated with familial forms of bone abnormalities. Mutations in the signal peptide region of RANK have been linked to familial Paget disease (Hughes et al., 2000) and to forms of singular anomalies such as expansile skeletal hyperphosphatasia. This anomaly, which has been observed in a daughter and her mother, is caused by a 15-base pair duplication in the RANK gene and is characterized by accelerated bone remodeling that results in bone, dental, and metabolic dysfunctions (Whyte and Hughes, 2002). Mutations in the gene encoding OPG cause idiopathic hyperphosphatasia, an autosomal recessive bone disease characterized by deformities of long bones, kyphosis, and acetabular protrusion (Cundy et al., 2002). The severity of the condition increases during adolescence. Polymorphisms in the OPG gene are also associated with osteoporotic fractures (Langdahl et al., 2002). A recent study showed that RANKL is involved in the pathophysiology of fibrous dysplasia of bone (FD), a genetic disease affecting the skeleton where postnatal skeletal stem cells acquire a fibroblastic phenotype and proliferate, replacing bone marrow resident cells and causing bone demineralization, an increase in osteoclast density, and, consequently, the dysregulation of osteoclastogenesis (de Castro et al., 2019). Patients with FD exhibit a 12-fold increase in serum RANKL/OPG ratios, which is significantly correlated with the FD burden (de Castro et al., 2019). The RANKL/RANK signaling pathway is also implicated in osteoporosis associated with estrogen deficiency in early postmenopausal women. Increased cell surface expression of RANKL by bone marrow cells has been observed, which directly correlates with increased osteoclast formation and bone resorption in aging women (Eghbali-Fatourechi et al., 2003). Targeting the RRO signaling pathway remains a promising therapeutic strategy to reduce bone resorption and ultimately bone loss. Denosumab, a human monoclonal antibody targeting RANKL, has received FDA approval for treating osteoporosis in men and postmenopausal women and minimizing bone loss associated with metastases in patients with advanced solid tumors (Cummings et al., 2009; Lacey et al., 2012). Denosumab has recently been shown to be more effective in treating glucocorticoid (GC)-induced osteoporosis than bisphosphonates (Yanbeiy and Hansen, 2019). RRO has also been reported to be involved in osteoclast formation and bone resorption in rheumatoid arthritis (RA), a systemic autoimmune disease (Tanaka and Tanaka, 2021). A recent case-controlled study and meta-analysis has indicated that the RANKL gene polymorphism increases the risk for RA. However, RANK and OPG gene locus polymorphisms are not associated risk factors (Yang et al., 2019). A recent study has shown that RANKL serum concentrations are highest in RA associated with periodontal disease (PD) and that a denosumab treatment suppresses the progression of RA in a randomized controlled trial (Panezai et al., 2018; Tanaka and Tanaka, 2021). Lastly, numerous studies have shown that the RRO pathway is involved in bone metastases (Ono et al., 2020; Okamoto, 2021) and that the neutralization of RANKL appears to have a beneficial effect on bone health. Clinical trials are currently investigating whether denosumab can be used to treat different cancers (Raje et al., 2018). However, a recent international randomized controlled trial with denosumab failed to improve disease-related outcomes for women with high-risk early breast cancer (Coleman et al., 2020). The RRO pathway is thus essential for the regulation of bone homeostasis, while its deregulation may be involved in bone, autoimmune, and cancerous diseases.
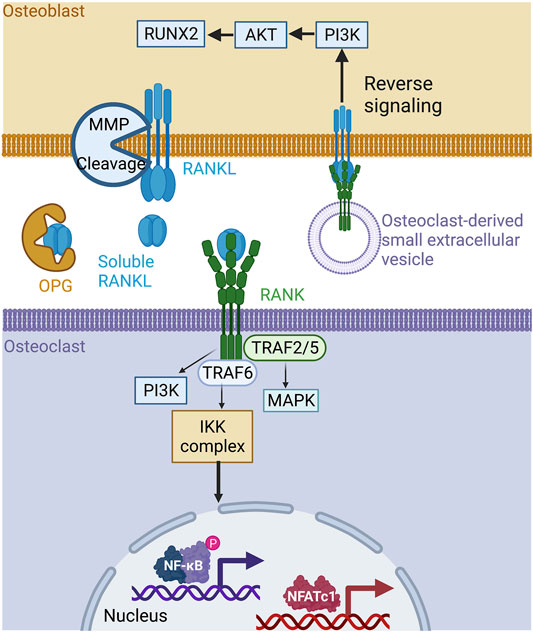
FIGURE 1. The RANK/RANKL/OPG signaling pathway in bone. RANKL is produced by osteoblasts in membrane form and is cleaved by metalloproteinases to its soluble form. The soluble form of RANKL is neutralized by circulating OPG or is bound to RANK at the osteoclast membrane, inducing a signaling cascade involving TRAF−2, −5, −6, PI3K, and MAPK, leading to the activation of the transcription factors NFATC1 and NF-κB, which are essential for bone resorption. Mature osteoclasts can also produce small extracellular RANK vesicles on their surface. These vesicles bind to the membrane form of RANKL on osteoblasts, inducing reverse signaling and promoting osteoblast differentiation. Created with BioRender.com.
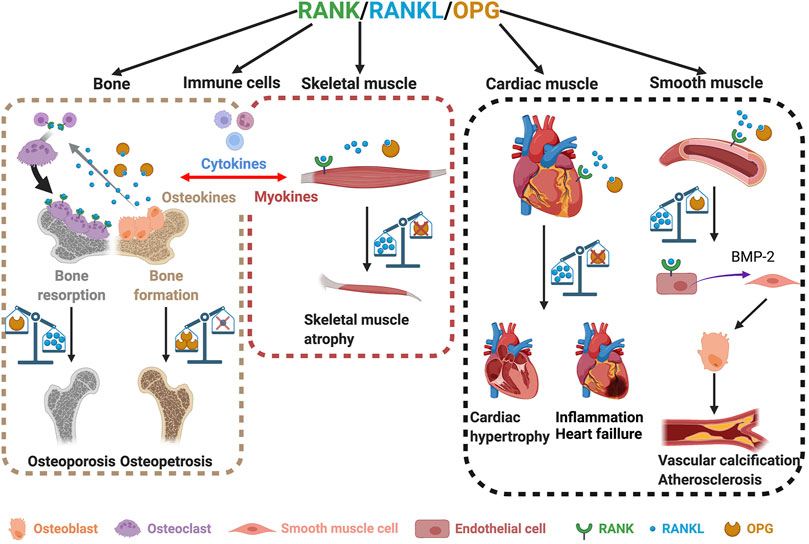
FIGURE 2. The roles of the RANK/RANKL/OPG triad in bone and skeletal, cardiac, and smooth muscles. Involvement of RANK/RANKL/OPG in bone homeostasis (left box). An imbalance between RANKL and OPG leads to either osteoporosis or osteopetrosis (left box). Skeletal muscles also express RANK, RANKL, and OPG (middle box). The overexpression of RANKL and/or the absence of OPG lead to muscle atrophy (middle box). An imbalance in the RANKL/OPG ratio also affects heart and smooth muscles and induces cardiac hypertrophy, heart failure, and vascular calcification (right box). Created with BioRender.com.
RANK/RANKL/OPG in Cardiac and Smooth Muscles
It is now well established that the role of the triad RRO goes beyond bone homeostasis, mammary gland development, and immunity. Interestingly, studies of heart failure in rodents have shown a high and persistent expression of the RANK, RANKL, and OPG genes in the ischemic and nonischemic areas of the heart (Ueland et al., 2005; Slavic et al., 2018). Conversely, the selective inhibition of RANKL in hematopoietic cells is sufficient to reduce the expression of pro-inflammatory cytokine IL1-ß and maintain post-ischemic cardiac function (Slavic et al., 2018). Furthermore, intravenous post-infarction anti-RANKL treatments in C57BL/6 mice reduce infarct size and cardiac neutrophil infiltration (Carbone et al., 2016). Likewise, OPG-deficient mice exhibit cardiac hypertrophy and myocardial contractile dysfunction at as early as 2.5 months of age (Hao et al., 2016). These morphological changes are accompanied by an increase in the number of apoptotic cells and the activation of TNF-related apoptosis-inducing ligand (TRAIL). A 28-day treatment with exogenous OPG partially rescued left ventricular structure and function in OPG-deficient mice (Hao et al., 2016). The inhibition of TRAIL decreases myocardial infarction by preventing cardiac cell death and inflammation in rats, pigs, and monkeys (Wang et al., 2020). Mechanistically, TRAIL induces the death of recruited leukocytes and activated cardiomyocytes, causing cardiac injury (Wang et al., 2020). In a model of pressure overload, a marked increase in RANKL expression has been observed in hypertrophying myocardium while in vitro RANKL stimulates the expression of TNFα, IL-1α, and IL-1β via the TRAF6-NF-κB signaling pathway in neonatal cardiomyocytes (Ock et al., 2012). These results from animal models have been confirmed in patients with heart failure who also exhibit increased RANK, RANKL, and OPG protein concentrations, suggesting that they are involved in the development of heart failure (Ueland et al., 2005). As such, the RRO pathway is thought to be involved in cardiac remodeling following immunoinflammatory myocardial diseases or during chronic heart failure (Liu et al., 2008) (Figure 2, right box).
Smooth muscles form the dense layers of many tissues, including blood vessels and hollow organs. Unlike skeletal and cardiac muscles, smooth muscles do not have transverse striations. Vascular smooth muscle cells (VSMCs) are involved in vascular tone, and their contractile function is not under voluntary control (Iyemere et al., 2006). VSMCs participate in vascular and valve calcification and share common characteristics with bone remodeling and metabolism (Kawakami et al., 2016). Many bone proteins are expressed in calcified vessel plaques, including bone morphogenetic protein-2 (BMP-2), an osteogenic differentiation factor capable of differentiating VSMCs into osteoblast-like cells (Kawakami et al., 2016). Under basal conditions human aortic smooth muscle cells (HASMCs) produce OPG whose expression increases with inflammatory stimuli (Davenport et al., 2018). OPG expression is inhibited when exogenous RANKL is added to the microenvironment of HASMCs (Davenport et al., 2018). RANKL acts also on human aortic endothelial cells (HAECs), increasing the release of BMP-2 from HAECs, which, in turn, can potentially stimulate osteoblast-like cell activity in HASMCs (Davenport et al., 2016). OPG is naturally present in blood vessels. The presence of RANKL inhibits OPG secretion by HASMCs and disrupts the RANKL/OPG ratio, promoting vascular calcification (Davenport et al., 2016; Davenport et al., 2018). Like OPG, matrix Gla protein (MGP) is an inhibitor of calcification that prevents the precipitation of calcium salts. Estrogen inhibits RANKL-mediated BMP-2 release and increases MGP expression (Osako et al., 2010). This supports the observation that aging women with estrogen deficiency are more at risk of both cardiovascular diseases and osteoporosis after menopause (Lampropoulos et al., 2012; Sprini et al., 2014). The RRO pathway thus plays an active role in angiogenesis, pathological inflammation, cell survival, and VSMC calcification (Rochette et al., 2019), pointing to potential cross-talk between blood vessels and bones (Figure 2, right box).
A recent review presented current knowledge on the roles of the different elements of the RRO triad in heart failure and cardiovascular diseases (Dutka et al., 2021). OPG plays an important role in the cardiovascular system through its interactions with endothelial and smooth muscle cells and its ligands, TRAIL and RANKL. Circulating OPG concentrations have been proposed as a biochemical marker for assessing the risk of cardiovascular complications in osteoporotic patients (Barbu et al., 2017). Elevated OPG concentrations in serum may play a protective role in the early stages of cardiovascular pathology. However, the maintenance of high OPG concentrations may have deleterious effects on the vascular system by participating in atherogenesis and vascular injury (Dutka et al., 2021). On the other hand, elevated serum RANKL concentrations may increase the risk of cardiovascular diseases (Kiechl et al., 2007). To go further in highlighting the crosstalk between bones and vessels, few studies have also reported the interaction between the RRO triad and blood coagulation factors. An in vitro model showed that differentiated osteoclasts would release RANKL, activating the extrinsic coagulation pathway and the conversion of prothrombin to thrombin (Karlström et al., 2010). Another study showed that thrombin receptor deficiency leads to a decrease in RANKL/OPG ratio which was associated with a high bone density phenotype (Tudpor et al., 2015). Conversely, a significant increase in the RANKL/OPG ratio and signs of osteoporosis were noticed in a mouse model of hemophilia (Yen et al., 2022). Moreover, OPG may play a role in regulating thrombus formation by binding to Von Willebrand factor (VWF), an essential factor for platelet adhesion (Wohner et al., 2022). Although significant progress has been made in the field, the molecular mechanisms by which RANK, RANKL, and OPG modulate cardiovascular diseases, heart failure and to some extent hemostasis remain unclear and need further investigation.
The RANK/RANKL/OPG Pathway in Dystrophic Skeletal Muscle, Inflammation, and Repair
Like bone cells and cardiac and smooth muscles, skeletal muscles also express the RRO triad (Baud’huin et al., 2013; Dufresne et al., 2018; Dufresne et al., 2016). A study on healthy human volunteers has shown that intense exercise-induced muscle damage increases serum OPG and decreases RANKL concentrations, suggesting that they are involved in muscle inflammation and repair processes in response to damage (Philippou et al., 2009). Changes in circulating OPG and RANKL concentrations are correlated with the distance traveled by runners, implying that the positive effects of long distance running on skeletal mass may, in part, be mediated by OPG/RANKL (Ziegler et al., 2005). Previous work from our laboratory has also shown that muscle-specific RANK deletion modulates the regulation of Ca2+ storage and sarco-endoplasmic reticulum Ca2+-ATPase (SERCA) activity in skeletal muscle (Dufresne et al., 2016). In the context of muscle disease, dystrophic mdx muscles exhibit significantly higher concentrations of RANK than wild-type muscles, suggesting that RANK is involved in muscular dystrophy (Dufresne et al., 2018; Guiraud et al., 2017). We have shown that daily injections of OPG-Fc, restore the function of the extensor digitorum longus (EDL) muscle in young dystrophic mdx mice (Dufresne et al., 2015). The inhibition of the RANKL/RANK interaction with an antibody specifically targeting RANKL also improves muscle strength in mdx mice (Hamoudi et al., 2019). We used a severe myotoxic agent to induce skeletal muscle injury and showed that the daily administration of the recombinant full-length OPG-Fc (FL-OPG-Fc) protein improves muscle strength, regeneration, and repair (Bouredji et al., 2021). On the other hand, genetic deletion of OPG in mice results in osteoporosis at as early as 3 months of age, with progressive muscle atrophy by 5 months (Hamoudi et al., 2020) (Figure 2, middle box). The concentration of circulating RANKL increases 20-fold in these OPG-deficient mice (Hamoudi et al., 2020). We showed that a 2-month treatment with anti-RANKL significantly improves muscle strength and reduces osteoporosis in OPG-deficient mice (Hamoudi et al., 2020). Conversely, mice that overexpress RANKL or that lack the myogenic factor peroxisome proliferator-activated receptor beta (Pparb) exhibit lower maximal strength and velocity and reduced muscle mass (Bonnet et al., 2019) (Figure 2, middle box). Treatments with truncated OPG-Fc (TR-OPG-Fc) or denosumab increase the muscle mass and strength of these two mouse models (Bonnet et al., 2019). They used a translational experimental approach to show that postmenopausal women with osteoporosis who were treated with denosumab for 3 years exhibit improved lean appendicular mass and grip strength while bisphosphonates have no effect (Bonnet et al., 2019). Moreover, other conditions such as chronic cigarette usage or chronic obstructive pulmonary disease (COPD) correlate with high serum RANKL concentrations (Bai et al., 2011; Nogueira and Breen, 2021) and low BMD and muscle dysfunction (Nogueira and Breen, 2021). A 6-month chronic exposure to cigarette smoking increases RANK and RANKL concentrations in skeletal muscle fibers (Xiong et al., 2021). Interestingly, the neutralization of RANKL restores muscle strength and function in mice exposed to tobacco smoke particles (Xiong et al., 2021). Furthermore, the exposure of muscle cells to tobacco smoke particles in vitro causes an increase in the expression of RANKL/RANK, which is responsible for the inflammation and atrophy of muscle fibers (Xiong et al., 2021). Lastly, mice with non-metastatic ovarian cancer exhibit cachexia associated with high RANKL concentrations, while an anti-RANKL treatment reduces bone loss and improves muscle function (Pin et al., 2021). Taken together, this evidence shows that RANK and RANKL are involved in skeletal muscle dysfunction while their inhibitors, anti-RANKL, TR-OPG-Fc, and FL-OPG-Fc, provide beneficial effects and may serve as a potential therapeutic approach in the future.
Crosstalk Between Bone and Skeletal Muscle Through the RANK/RANKL/OPG Pathway
Physical activity contributes to cytokine release and bone development and remodeling (Tobeiha et al., 2020). For example, circulating concentrations of OPG and RANKL increase significantly immediately following high intensity aerobic exercise, suggesting that bone remodeling mediators have been activated (Mezil et al., 2015). Furthermore, a one-year training program significantly increases OPG concentrations. This has been associated with a significant reduction in bone loss in postmenopausal women compared to sedentary controls (Bergström et al., 2012). Conversely, mechanical unloading and estrogen deficiency reduce serum OPG concentrations compared with menstruating women who exercise, which provides support for the positive osteogenic effect of OPG and exercise (West et al., 2009). Recent discoveries have revealed the importance of mutual cross-talk between muscle-bone through the release of myokines and osteokines. For instance, transforming growth factor (TGF-β), which is mainly produced by osteocytes and is stored in the bone matrix (Xu et al., 2018), plays a direct role in skeletal muscle weakness in pathological conditions. The increase in circulating TGF-β following metastasis-induced bone destruction increases the oxidation of muscle proteins and Ca2+ receptors through the upregulation of NADPH oxidase 4 (Nox4) and contributes to muscle weakness (Waning et al., 2015). It has been shown that circulating IL-6, the first identified myokine, increases 100-fold during exercise (Pedersen and Febbraio, 2008), sending a signal to osteoblasts to favor osteoclast differentiation and the release of bioactive osteocalcin, an anabolic hormone that plays an essential role in bone and muscle mass and muscle performance (Mera et al., 2016; Chowdhury et al., 2020). IL-6 also increases the expression of RANKL, which is important for the release of bioactive osteocalcin (Chowdhury et al., 2020). Additionally, IL-6 has been reported to induce the expression of IL-10, another anti-inflammatory myokine (Steensberg et al., 2003; Santos et al., 2020). The deletion of IL-10 in mice accelerates bone resorption and has an impact on bone homeostasis (Dresner-Pollak et al., 2004). The stimulation of bone cells in vitro with IL-10 increase the expression of OPG and decreases the expression of RANKL (Liu et al., 2006) while the deletion of IL-10 in mice accelerates bone resorption, suggesting that IL-10 myokine may influence bone by modulating the RRO pathway. In the same vein, the administration of irisin, a myokine released from skeletal muscles after physical exercise, attenuates the negative effect of unloading on OPG, thus maintaining the equilibrium of RANKL/OPG ratio in unloaded mice (Colaianni et al., 2017). Myostatin, a negative regulator of muscle mass is another myokine that promotes the expression of several bone regulators, including RANKL, in osteolytic cell cultures (Qin et al., 2017). Mounting evidence has thus confirmed the existence of bidirectional and mutual molecular crosstalk between bone and skeletal muscle that, to some extent, directly or indirectly impacts the expression of RRO (Figure 2, left and middle boxes).
Concluding Remarks and Future Directions
This mini review focuses on the multifaceted aspects of the RRO pathway. Although the RRO triad is the most important regulator of bone homeostasis, cumulative evidence has elegantly demonstrated that it also plays a role in skeletal, smooth, and cardiac muscles. Targeting similar pathways that regulate different tissues is undoubtedly a valuable strategy for addressing pathological and aging conditions with multiple comorbidities. While the RRO signaling cascade is well defined in bone cells, the cellular and molecular mechanisms by which RRO regulates muscle cell function is far from being well understood. RANKL is clearly associated with the activation of the inflammatory-atrophic and inflammatory-hypertrophic pathways in skeletal muscle and the heart, respectively. Future research directions should focus on investigating the clinical relevance of neutralizing RANKL in muscular and cardiac disorders such as dystrophic diseases, aging, and cancer-related cachexia. As for OPG, a clear understanding of the involvement of each of its three domains, that is, the RANKL-, TRAIL- and heparin-binding domains, is a prerequisite for deciphering the contribution of RRO in skeletal, smooth, and cardiac muscles.
Author Contributions
All authors listed have made a substantial, direct, and intellectual contribution to the work and approved it for publication.
Funding
This work was supported by the grant to JF from the Natural Sciences and Engineering Research Council of Canada (RGPIN-2016-05845).
Conflict of Interest
The authors declare that the research was conducted in the absence of any commercial or financial relationships that could be construed as a potential conflict of interest.
Publisher’s Note
All claims expressed in this article are solely those of the authors and do not necessarily represent those of their affiliated organizations, or those of the publisher, the editors and the reviewers. Any product that may be evaluated in this article, or claim that may be made by its manufacturer, is not guaranteed or endorsed by the publisher.
References
Armstrong, A. P., Tometsko, M. E., Glaccum, M., Sutherland, C. L., Cosman, D., and Dougall, W. C. (2002). A RANK/TRAF6-dependent Signal Transduction Pathway Is Essential for Osteoclast Cytoskeletal Organization and Resorptive Function. J. Biol. Chem. 277 (46), 44347–44356. doi:10.1074/jbc.M202009200
Bai, P., Sun, Y., Jin, J., Hou, J., Li, R., Zhang, Q., et al. (2011). Disturbance of the OPG/RANK/RANKL Pathway and Systemic Inflammation in COPD Patients with Emphysema and Osteoporosis. Respir. Res. 12, 1–8. doi:10.1186/1465-9921-12-157
Barbu, C. G., Arsene, A. L., Florea, S., Albu, A., Sirbu, A., Martin, S., et al. (2017). Cardiovascular Risk Assessment in Osteoporotic Patients Using Osteoprotegerin as a Reliable Predictive Biochemical Marker. Mol. Med. Rep. 16 (5), 6059–6067. doi:10.3892/mmr.2017.7376
Baud’huin, M., Duplomb, L., Teletchea, S., Lamoureux, F., Ruiz-Velasco, C., Maillasson, M., et al. (2013). Osteoprotegerin: Multiple Partners for Multiple Functions. Cytokine & Growth Factor Rev. 24, 401–409. Published online. doi:10.1016/j.cytogfr.2013.06.001
Bergström, I., Parini, P., Gustafsson, S. A., Andersson, G., and Brinck, J. (2012). Physical Training Increases Osteoprotegerin in Postmenopausal Women. J. Bone Min. Metab. 30 (2), 202–207. doi:10.1007/s00774-011-0304-6
Bonnet, N., Bourgoin, L., Biver, E., Douni, E., and Ferrari, S. (2019). RANKL Inhibition Improves Muscle Strength and Insulin Sensitivity and Restores Bone Mass. J. Clin. Invest. 129 (8), 3214–3223. doi:10.1172/JCI125915
Boulanger Piette, A., Hamoudi, D., Marcadet, L., Morin, F., Argaw, A., Ward, L., et al. (2018). Targeting the Muscle-Bone Unit: Filling Two Needs with One Deed in the Treatment of Duchenne Muscular Dystrophy. Curr. Osteoporos. Rep. 16 (5), 541–553. doi:10.1007/s11914-018-0468-2
Bouredji, Z., Hamoudi, D., Marcadet, L., Argaw, A., and Frenette, J. (2021). Testing the Efficacy of a Human Full-Length OPG-Fc Analog in a Severe Model of Cardiotoxin-Induced Skeletal Muscle Injury and Repair. Mol. Ther. - Methods & Clin. Dev. 21, 559–573. doi:10.1016/j.omtm.2021.03.022
Boyce, B. F., Xiu, Y., Li, J., Xing, L., and Yao, Z. (2015). NF-κB-Mediated Regulation of Osteoclastogenesis. Endocrinol. Metab. 30 (1), 35–44. doi:10.3803/EnM.2015.30.1.35
Brotto, M., and Bonewald, L. (2015). Bone and Muscle: Interactions beyond Mechanical. Bone 80, 109–114. doi:10.1016/j.bone.2015.02.010
Bucay, N., Sarosi, I., Dunstan, C. R., Morony, S., Tarpley, J., Capparelli, C., et al. (1998). Osteoprotegerin-deficient Mice Develop Early Onset Osteoporosis and Arterial Calcification. Genes & Dev. 12 (9), 1260–1268. doi:10.1101/gad.12.9.1260
Carbone, F., Crowe, L. A., Roth, A., Burger, F., Lenglet, S., Braunersreuther, V., et al. (2016). Treatment with Anti-RANKL Antibody Reduces Infarct Size and Attenuates Dysfunction Impacting on Neutrophil-Mediated Injury. J. Mol. Cell. Cardiol. 94, 82–94. doi:10.1016/j.yjmcc.2016.03.013
Chowdhury, S., Schulz, L., Palmisano, B., Singh, P., Berger, J. M., Yadav, V. K., et al. (2020). Muscle-derived Interleukin 6 Increases Exercise Capacity by Signaling in Osteoblasts. J. Clin. Invest. 130 (6), 2888–2902. doi:10.1172/JCI133572
Colaianni, G., Mongelli, T., Cuscito, C., Pignataro, P., Lippo, L., Spiro, G., et al. (2017). Irisin Prevents and Restores Bone Loss and Muscle Atrophy in Hind-Limb Suspended Mice. Sci. Rep. 7 (1), 1–16. doi:10.1038/s41598-017-02557-8
Coleman, R., Finkelstein, D. M., Barrios, C., Martin, M., Iwata, H., Hegg, R., et al. (2020). Adjuvant Denosumab in Early Breast Cancer (D-CARE): an International, Multicentre, Randomised, Controlled, Phase 3 Trial. Lancet Oncol. 21 (1), 60–72. doi:10.1016/S1470-2045(19)30687-4
Cummings, S. R., Martin, J. S., McClung, M. R., Siris, E. S., Eastell, R., Reid, I. R., et al. (2009). Denosumab for Prevention of Fractures in Postmenopausal Women with Osteoporosis. N. Engl. J. Med. 361 (8), 756–765. doi:10.1056/NEJMoa0809493
Cundy, T., Hegde, M., Naot, D., Chong, B., King, A., Wallace, R., et al. (2002). A Mutation in the Gene TNFRSF11B Encoding Osteoprotegerin Causes an Idiopathic Hyperphosphatasia Phenotype. Hum. Mol. Genet. 11 (18), 2119–2127. doi:10.1093/hmg/11.18.2119
Darnay, B. G., Haridas, V., Ni, J., Moore, P. A., and Aggarwal, B. B. (1998). Characterization of the Intracellular Domain of Receptor Activator of NF-Κb (RANK). J. Biol. Chem. 273 (32), 20551–20555. doi:10.1074/jbc.273.32.20551
Davenport, C., Harper, E., Forde, H., Rochfort, K. D., Murphy, R. P., Smith, D., et al. (2016). RANKL Promotes Osteoblastic Activity in Vascular Smooth Muscle Cells by Upregulating Endothelial BMP-2 Release. Int. J. Biochem. Cell Biol. 77, 171–180. doi:10.1016/j.biocel.2016.06.009
Davenport, C., Harper, E., Rochfort, K. D., Forde, H., Smith, D., and Cummins, P. M. (2018). RANKL Inhibits the Production of Osteoprotegerin from Smooth Muscle Cells under Basal Conditions and Following Exposure to Cyclic Strain. J. Vasc. Res. 55 (2), 111–123. doi:10.1159/000486787
de Castro, L. F., Burke, A. B., Wang, H. D., Tsai, J., Florenzano, P., Pan, K. S., et al. (2019). Activation of RANK/RANKL/OPG Pathway Is Involved in the Pathophysiology of Fibrous Dysplasia and Associated with Disease Burden. J. Bone Min. Res. 34 (2), 290–294. doi:10.1002/jbmr.3602
Dougall, W. C., Glaccum, M., Charrier, K., Rohrbach, K., Brasel, K., De Smedt, T., et al. (1999). RANK Is Essential for Osteoclast and Lymph Node Development. Genes & Dev. 13 (18), 2412–2424. doi:10.1101/gad.13.18.2412
Dresner-Pollak, R., Gelb, N., Rachmilewitz, D., Karmeli, F., and Weinreb, M. (2004). Interleukin 10-deficient Mice Develop Osteopenia, Decreased Bone Formation, and Mechanical Fragility of Long Bones. Gastroenterology 127 (3), 792–801. doi:10.1053/j.gastro.2004.06.013
Dufresne, S. S., Boulanger-Piette, A., Bossé, S., Argaw, A., Hamoudi, D., Marcadet, L., et al. (2018). Genetic Deletion of Muscle RANK or Selective Inhibition of RANKL Is Not as Effective as Full-Length OPG-Fc in Mitigating Muscular Dystrophy. Acta Neuropathol. Commun. 6 (1), 31. doi:10.1186/s40478-018-0533-1
Dufresne, S. S., Dumont, N. A., Bouchard, P., Lavergne, É., Penninger, J. M., and Frenette, J. (2015). Osteoprotegerin Protects against Muscular Dystrophy. Am. J. Pathology 185 (4), 920–926. doi:10.1016/j.ajpath.2015.01.006
Dufresne, S. S., Dumont, N. A., Boulanger-Piette, A., Fajardo, V. A., Gamu, D., Kake-Guena, S.-A., et al. (2016). Muscle RANK Is a Key Regulator of Ca2+ Storage, SERCA Activity, and Function of Fast-Twitch Skeletal Muscles. Am. J. Physiology-Cell Physiology 310 (8), C663–C672. doi:10.1152/ajpcell.00285.2015
Dutka, M., Bobiński, R., Wojakowski, W., Francuz, T., Pająk, C., and Zimmer, K. (2021). Osteoprotegerin and RANKL-RANK-OPG-TRAIL Signalling axis in Heart Failure and Other Cardiovascular Diseases. Heart Fail Rev. doi:10.1007/s10741-021-10153-2
Eghbali-Fatourechi, G., Khosla, S., Sanyal, A., Boyle, W. J., Lacey, D. L., and Riggs, B. L. (2003). Role of RANK Ligand in Mediating Increased Bone Resorption in Early Postmenopausal Women. J. Clin. Invest. 111 (8), 1221–1230. doi:10.1172/JCI200317215
Greenlund, L., and Nair, K. S. (2003). Sarcopenia-consequences, Mechanisms, and Potential Therapies. Mech. Ageing Dev. 124 (3), 287–299. doi:10.1016/S0047-6374(02)00196-3
Guiraud, S., Edwards, B., Squire, S. E., Babbs, A., Shah, N., Berg, A., et al. (2017). Identification of Serum Protein Biomarkers for Utrophin Based DMD Therapy. Sci. Rep. 7, 43697. doi:10.1038/srep43697
Hamoudi, D., Bouredji, Z., Marcadet, L., Yagita, H., Landry, L.-B., Argaw, A., et al. (2020). Muscle Weakness and Selective Muscle Atrophy in Osteoprotegerin-Deficient Mice. Hum. Mol. Genet. 29 (3), 483–494. doi:10.1093/hmg/ddz312
Hamoudi, D., Marcadet, L., Piette Boulanger, A., Yagita, H., Bouredji, Z., Argaw, A., et al. (2019). An Anti-RANKL Treatment Reduces Muscle Inflammation and Dysfunction and Strengthens Bone in Dystrophic Mice. Hum. Mol. Genet. 28 (18), 3101–3112. doi:10.1093/hmg/ddz124
Hamrick, M. W., Ding, K.-H., Pennington, C., Chao, Y. J., Wu, Y.-D., Howard, B., et al. (2006). Age-related Loss of Muscle Mass and Bone Strength in Mice Is Associated with a Decline in Physical Activity and Serum Leptin. Bone 39 (4), 845–853. doi:10.1016/j.bone.2006.04.011
Hao, Y., Tsuruda, T., Sekita-Hatakeyama, Y., Kurogi, S., Kubo, K., Sakamoto, S., et al. (2016). Cardiac Hypertrophy Is Exacerbated in Aged Mice Lacking the Osteoprotegerin Gene. Cardiovasc Res. 110 (1), 62–72. doi:10.1093/cvr/cvw025
Hofbauer, L. C., Khosla, S., Dunstan, C. R., Lacey, D. L., Boyle, W. J., and Riggs, B. L. (2000). The Roles of Osteoprotegerin and Osteoprotegerin Ligand in the Paracrine Regulation of Bone Resorption. J. Bone Min. Res. 15 (1), 2–12. doi:10.1359/jbmr.2000.15.1.2
Hsu, H., Lacey, D. L., Dunstan, C. R., Solovyev, I., Colombero, A., Timms, E., et al. (1999). Tumor Necrosis Factor Receptor Family Member RANK Mediates Osteoclast Differentiation and Activation Induced by Osteoprotegerin Ligand. Proc. Natl. Acad. Sci. U.S.A. 96 (7), 3540–3545. doi:10.1073/pnas.96.7.3540
Hughes, A. E., Ralston, S. H., Marken, J., Bell, C., MacPherson, H., Wallace, R. G. H., et al. (2000). Mutations in TNFRSF11A, Affecting the Signal Peptide of RANK, Cause Familial Expansile Osteolysis. Nat. Genet. 24 (1), 45–48. doi:10.1038/71667
Ikebuchi, Y., Aoki, S., Honma, M., Hayashi, M., Sugamori, Y., Khan, M., et al. (2018). Coupling of Bone Resorption and Formation by RANKL Reverse Signalling. Nature 561 (7722), 195–200. doi:10.1038/s41586-018-0482-7
Ikeda, T., Kasai, M., Utsuyama, M., and Hirokawa, K. (2001). Determination of Three Isoforms of the Receptor Activator of Nuclear Factor-Κβ Ligand and Their Differential Expression in Bone and Thymus*. Endocrinology 142 (4), 1419–1426. doi:10.1210/endo.142.4.8070
Iyemere, V. P., Proudfoot, D., Weissberg, P. L., and Shanahan, C. M. (2006). Vascular Smooth Muscle Cell Phenotypic Plasticity and the Regulation of Vascular Calcification. J. Intern Med. 260 (3), 192–210. doi:10.1111/j.1365-2796.2006.01692.x
Karlström, E., Ek-Rylander, B., Wendel, M., and Andersson, G. (2010). RANKL Induces Components of the Extrinsic Coagulation Pathway in Osteoclasts. Biochem. Biophysical Res. Commun. 394 (3), 593–599. doi:10.1016/j.bbrc.2010.03.025
Kawakami, R., Nakagami, H., Noma, T., Ohmori, K., Kohno, M., and Morishita, R. (2016). RANKL System in Vascular and Valve Calcification with Aging. Inflamm. Regen. 36 (1), 4–9. doi:10.1186/s41232-016-0016-3
Kiechl, S., Schett, G., Schwaiger, J., Seppi, K., Eder, P., Egger, G., et al. (2007). Soluble Receptor Activator of Nuclear Factor-Κb Ligand and Risk for Cardiovascular Disease. Circulation 116 (4), 385–391. doi:10.1161/CIRCULATIONAHA.106.686774
Kim, N., Odgren, P. R., Kim, D.-K., Marks, S. C., and Choi, Y. (2000). Diverse Roles of the Tumor Necrosis Factor Family Member TRANCE in Skeletal Physiology Revealed by TRANCE Deficiency and Partial Rescue by a Lymphocyte-Expressed TRANCE Transgene. Proc. Natl. Acad. Sci. U.S.A. 97 (20), 10905–10910. doi:10.1073/pnas.200294797
Kobayashi, N., Kadono, Y., Naito, A., Matsumoto, K., Yamamoto, T., Tanaka, S., et al. (2001). Segregation of TRAF6-Mediated Signaling Pathways Clarifies its Role in Osteoclastogenesis. EMBO J. 20 (6), 1271–1280. doi:10.1093/emboj/20.6.1271
Lacey, D. L., Boyle, W. J., Simonet, W. S., Kostenuik, P. J., Dougall, W. C., Sullivan, J. K., et al. (2012). Bench to Bedside: Elucidation of the OPG-RANK-RANKL Pathway and the Development of Denosumab. Nat. Rev. Drug Discov. 11 (5), 401–419. doi:10.1038/nrd3705
Lampropoulos, C. E., Papaioannou, I., and D'Cruz, D. P. (2012). Osteoporosis-a Risk Factor for Cardiovascular Disease? Nat. Rev. Rheumatol. 8 (10), 587–598. doi:10.1038/nrrheum.2012.120
Langdahl, B. L., Carstens, M., Stenkjaer, L., and Eriksen, E. F. (2002). Polymorphisms in the Osteoprotegerin Gene Are Associated with Osteoporotic Fractures. J. Bone Min. Res. 17 (7), 1245–1255. doi:10.1359/jbmr.2002.17.7.1245
Li, J., Sarosi, I., Yan, X.-Q., Morony, S., Capparelli, C., Tan, H.-L., et al. (2000). RANK Is the Intrinsic Hematopoietic Cell Surface Receptor that Controls Osteoclastogenesis and Regulation of Bone Mass and Calcium Metabolism. Proc. Natl. Acad. Sci. U.S.A. 97 (4), 1566–1571. doi:10.1073/pnas.97.4.1566
Liu, D., Yao, S., and Wise, G. E. (2006). Effect of Interleukin-10 on Gene Expression of Osteoclastogenic Regulatory Molecules in the Rat Dental Follicle. Eur. J. Oral Sci. 114 (1), 42–49. doi:10.1111/j.1600-0722.2006.00283.x
Liu, W., Feng, W., Wang, F., Li, W., Gao, C., Zhou, B., et al. (2008). Osteoprotegerin/RANK/RANKL axis in Cardiac Remodeling Due to Immuno-Inflammatory Myocardial Disease. Exp. Mol. Pathology 84, 213–217. doi:10.1016/j.yexmp.2008.02.004
Lomaga, M. A., Yeh, W.-C., Sarosi, I., Duncan, G. S., Furlonger, C., Ho, A., et al. (1999). TRAF6 Deficiency Results in Osteopetrosis and Defective Interleukin-1, CD40, and LPS Signaling. Genes & Dev. 13 (8), 1015–1024. doi:10.1101/gad.13.8.1015
McKay, H., and Smith, E. (2008). Winning the Battle against Childhood Physical Inactivity: The Key to Bone Strength? J. Bone Min. Res. 23 (7), 980–985. doi:10.1359/jbmr.080306
Mera, P., Laue, K., Ferron, M., Confavreux, C., Wei, J., Galán-Díez, M., et al. (2016). Osteocalcin Signaling in Myofibers Is Necessary and Sufficient for Optimum Adaptation to Exercise. Cell Metab. 23 (6), 1078–1092. doi:10.1016/j.cmet.2016.05.004
Mezil, Y. A., Allison, D., Kish, K., Ditor, D., Ward, W. E., Tsiani, E., et al. (2015). Response of Bone Turnover Markers and Cytokines to High-Intensity Low-Impact Exercise. Med. Sci. Sports Exerc 47 (7), 1495–1502. doi:10.1249/MSS.0000000000000555
Nelson, C. A., Warren, J. T., Wang, M. W.-H., Teitelbaum, S. L., and Fremont, D. H. (2012). RANKL Employs Distinct Binding Modes to Engage RANK and the Osteoprotegerin Decoy Receptor. Structure 20 (11), 1971–1982. doi:10.1016/j.str.2012.08.030
Ness, K., and Apkon, S. D. (2014). Bone Health in Children with Neuromuscular Disorders. J. Pediatr. Rehabil. Med. 7, 133–142. doi:10.3233/PRM-140282
Nogueira, L., and Breen, E. C. (2021). Cigarettes Make You Weak: RANKL/RANK Link Changes in Muscle and Bone. Am. J. Respir. Cell Mol. Biol. 64 (5), 533–535. doi:10.1165/rcmb.2021-0098ED
Ock, S., Ahn, J., Lee, S. H., Park, H., Son, J. W., Oh, J. G., et al. (2012). Receptor Activator of Nuclear Factor-Κb Ligand Is a Novel Inducer of Myocardial Inflammation. Cardiovasc Res. 94 (1), 105–114. doi:10.1093/cvr/cvs078
Okamoto, K. (2021). Role of RANKL in Cancer Development and Metastasis. J. Bone Min. Metab. 39 (1), 71–81. doi:10.1007/s00774-020-01182-2
Ono, T., Hayashi, M., Sasaki, F., and Nakashima, T. (2020). RANKL Biology: Bone Metabolism, the Immune System, and beyond. Inflamm. Regen. 40 (1), 1–16. doi:10.1186/s41232-019-0111-3
Osako, M. K., Nakagami, H., Koibuchi, N., Shimizu, H., Nakagami, F., Koriyama, H., et al. (2010). Estrogen Inhibits Vascular Calcification via Vascular RANKL System. Circ. Res. 107 (4), 466–475. doi:10.1161/CIRCRESAHA.110.216846
Owen, H. C., Vanhees, I., Gunst, J., Van Cromphaut, S., and Van den Berghe, G. (2015). Critical Illness-Induced Bone Loss Is Related to Deficient Autophagy and Histone Hypomethylation. ICMx 3 (1), 1–16. doi:10.1186/s40635-015-0052-3
Panezai, J., Ghaffar, A., Altamash, M., Engström, P.-E., and Larsson, A. (2018). Periodontal Disease Influences Osteoclastogenic Bone Markers in Subjects with and without Rheumatoid Arthritis. PLoS One 13 (6), e0197235–15. doi:10.1371/journal.pone.0197235
Pedersen, B. K., and Febbraio, M. A. (2008). Muscle as an Endocrine Organ: Focus on Muscle-Derived Interleukin-6. Physiol. Rev. 88 (4), 1379–1406. doi:10.1152/physrev.90100.2007
Philippou, A., Bogdanis, G., Maridaki, M., Halapas, A., Sourla, A., and Koutsilieris, M. (2009). Systemic Cytokine Response Following Exercise-Induced Muscle Damage in Humans. Clin. Chem. Lab. Med. 47 (6), 777–782. doi:10.1515/CCLM.2009.163
Pin, F., Jones, A. J., Huot, J. R., Narasimhan, A., Zimmers, T. A., Bonewald, L. F., et al. (2021). RANKL Blockade Reduces Cachexia and Bone Loss Induced by Non‐Metastatic Ovarian Cancer in Mice. J. Bone Min. Res. 37 (3), 381–396. doi:10.1002/jbmr.4480
Qin, Y., Peng, Y., Zhao, W., Pan, J., Ksiezak-Reding, H., Cardozo, C., et al. (2017). Myostatin Inhibits Osteoblastic Differentiation by Suppressing Osteocyte-Derived Exosomal microRNA-218: A Novel Mechanism in Muscle-Bone Communication. J. Biol. Chem. 292 (26), 11021–11033. doi:10.1074/jbc.M116.770941
Raje, N., Terpos, E., Willenbacher, W., Shimizu, K., García-Sanz, R., Durie, B., et al. (2018). Denosumab versus Zoledronic Acid in Bone Disease Treatment of Newly Diagnosed Multiple Myeloma: an International, Double-Blind, Double-Dummy, Randomised, Controlled, Phase 3 Study. Lancet Oncol. 19 (3), 370–381. doi:10.1016/S1470-2045(18)30072-X
Rochette, L., Meloux, A., Rigal, E., Zeller, M., Cottin, Y., and Vergely, C. (2019). The Role of Osteoprotegerin and its Ligands in Vascular Function. Ijms 20 (3), 705. doi:10.3390/ijms20030705
Russo, C. R. (2009). The Effects of Exercise on Bone. Basic Concepts and Implications for the Prevention of Fractures. Clin. Cases Min. Bone Metab. 6 (3), 223–228.
Santos, J. d. M. B. d., Bachi, A. L. L., Luna Junior, L. A., Foster, R., Sierra, A. P. R., Benetti, M., et al. (2020). The Relationship of IL-8 and IL-10 Myokines and Performance in Male Marathon Runners Presenting Exercise-Induced Bronchoconstriction. Ijerph 17 (8), 2622. doi:10.3390/ijerph17082622
Slavic, S., Andrukhova, O., Ford, K., Handschuh, S., Latic, N., Reichart, U., et al. (2018). Selective Inhibition of Receptor Activator of NF-Κb Ligand (RANKL) in Hematopoietic Cells Improves Outcome after Experimental Myocardial Infarction. J. Mol. Med. 96 (6), 559–573. doi:10.1007/s00109-018-1641-x
Sprini, D., Rini, G. B., Di Stefano, L., Cianferotti, L., and Napoli, N. (2014). Correlation between Osteoporosis and Cardiovascular Disease. ccmbm 11 (2), 117–119. doi:10.11138/ccmbm/2014.11.2.117
Steensberg, A., Fischer, C. P., Keller, C., Møller, K., and Pedersen, B. K. (2003). IL-6 Enhances Plasma IL-1ra, IL-10, and Cortisol in Humans. Am. J. Physiology-Endocrinology Metabolism 285 (2 48-2), E433–E437. doi:10.1152/ajpendo.00074.2003
Tanaka, S., and Tanaka, Y. (2021). RANKL as a Therapeutic Target of Rheumatoid Arthritis. J. Bone Min. Metab. 39 (1), 106–112. doi:10.1007/s00774-020-01159-1
Tobeiha, M., Moghadasian, M. H., Amin, N., and Jafarnejad, S. (2020). RANKL/RANK/OPG Pathway: A Mechanism Involved in Exercise-Induced Bone Remodeling. BioMed Res. Int. 2020, 1–11. doi:10.1155/2020/6910312
Tudpor, K., van der Eerden, B. C. J., Jongwattanapisan, P., Roelofs, J. J. T. H., van Leeuwen, J. P. T. M., Bindels, R. J. M., et al. (2015). Thrombin Receptor Deficiency Leads to a High Bone Mass Phenotype by Decreasing the RANKL/OPG Ratio. Bone 72, 14–22. doi:10.1016/j.bone.2014.11.004
Udagawa, N., Takahashi, N., Yasuda, H., Mizuno, A., Itoh, K., Ueno, Y., et al. (2000). Osteoprotegerin Produced by Osteoblasts Is an Important Regulator in Osteoclast Development and Function*. Endocrinology 141 (9), 3478–3484. doi:10.1210/endo.141.9.7634
Ueland, T., Yndestad, A., Øie, E., Florholmen, G., Halvorsen, B., Frøland, S. S., et al. (2005). Dysregulated Osteoprotegerin/RANK Ligand/RANK axis in Clinical and Experimental Heart Failure. Circulation 111 (19), 2461–2468. doi:10.1161/01.CIR.0000165119.62099.14
Wada, T., Nakashima, T., Hiroshi, N., and Penninger, J. M. (2006). RANKL-RANK Signaling in Osteoclastogenesis and Bone Disease. Trends Mol. Med. 12 (1), 17–25. doi:10.1016/j.molmed.2005.11.007
Wang, Y., Zhang, H., Wang, Z., Wei, Y., Wang, M., Liu, M., et al. (2020). Blocking the Death Checkpoint Protein TRAIL Improves Cardiac Function after Myocardial Infarction in Monkeys, Pigs, and Rats. Sci. Transl. Med. 12 (540), eaaw3172. doi:10.1126/scitranslmed.aaw3172
Waning, D. L., Mohammad, K. S., Reiken, S., Xie, W., Andersson, D. C., John, S., et al. (2015). Excess TGF-β Mediates Muscle Weakness Associated with Bone Metastases in Mice. Nat. Med. 21 (11), 1262–1271. doi:10.1038/nm.3961
West, S. L., Scheid, J. L., and De Souza, M. J. (2009). The Effect of Exercise and Estrogen on Osteoprotegerin in Premenopausal Women. Bone 44 (1), 137–144. doi:10.1016/j.bone.2008.09.008
Whyte, M. P., and Hughes, A. E. (2002). Expansile Skeletal Hyperphosphatasia Is Caused by a 15-base Pair Tandem Duplication in TNFRSF11A Encoding RANK and Is Allelic to Familial Expansile Osteolysis. J. Bone Min. Res. 17 (1), 26–29. doi:10.1359/jbmr.2002.17.1.26
Wohner, N., Sebastian, S., Muczynski, V., Huskens, D., de Laat, B., de Groot, P. G., et al. (2022). Osteoprotegerin modulates platelet adhesion to von Willebrand factor during release from endothelial cells. J Thrombosis Haemost 20 (3), 755–766. doi:10.1111/jth.15598
Xiong, J., Le, Y., Rao, Y., Zhou, L., Hu, Y., Guo, S., et al. (2021). RANKL Mediates Muscle Atrophy and Dysfunction in a Cigarette Smoke-Induced Model of Chronic Obstructive Pulmonary Disease. Am. J. Respir. Cell Mol. Biol. 64 (5), 617–628. doi:10.1165/rcmb.2020-0449OC
Xu, X., Zheng, L., Yuan, Q., Zhen, G., Crane, J. L., Zhou, X., et al. (2018). Transforming Growth Factor-β in Stem Cells and Tissue Homeostasis. Bone. Res. 6 (1), 2. doi:10.1038/s41413-017-0005-4
Yanbeiy, Z. A., and Hansen, K. E. (2019). Denosumab in the Treatment of Glucocorticoid-Induced Osteoporosis: a Systematic Review and Meta-Analysis. Dddt 13, 2843–2852. doi:10.2147/DDDT.S148654
Yang, H., Liu, W., Zhou, X., Rui, H., Zhang, H., and Liu, R. (2019). The Association between RANK, RANKL and OPG Gene Polymorphisms and the Risk of Rheumatoid Arthritis: A Case-Controlled Study and Meta-Analysis. Biosci. Rep. 39 (6), 1–11. doi:10.1042/BSR20182356
Yasuda, H. (2021). Discovery of the RANKL/RANK/OPG System. J. Bone Min. Metab. 39 (1), 2–11. doi:10.1007/s00774-020-01175-1
Yasuda, H., Shima, N., Nakagawa, N., Mochizuki, S.-I., Yano, K., Fujise, N., et al. (1998). Identity of Osteoclastogenesis Inhibitory Factor (OCIF) and Osteoprotegerin (OPG): A Mechanism by Which OPG/OCIF Inhibits Osteoclastogenesisin Vitro1. Endocrinology 139 (3), 1329–1337. doi:10.1210/endo.139.3.5837
Yen, C.-C., Liu, Y.-W., Chang, G. R.-L., Lan, Y.-W., Kao, Y.-T., Cheng, S.-N., et al. (2022). Therapeutic Effects of Kefir Peptides on Hemophilia-Induced Osteoporosis in Mice with Deficient Coagulation Factor VIII. Front. Cell Dev. Biol. 10 (February), 1–13. doi:10.3389/fcell.2022.794198
Keywords: RANK, RANKL, OPG, myokines, osteokines, heart, skeletal muscle, smooth muscle
Citation: Marcadet L, Bouredji Z, Argaw A and Frenette J (2022) The Roles of RANK/RANKL/OPG in Cardiac, Skeletal, and Smooth Muscles in Health and Disease. Front. Cell Dev. Biol. 10:903657. doi: 10.3389/fcell.2022.903657
Received: 24 March 2022; Accepted: 09 May 2022;
Published: 26 May 2022.
Edited by:
Marielle Saclier, Institut Pasteur, FranceReviewed by:
Giulia Battafarano, Bambino Gesù Children’s Hospital (IRCCS), ItalyCopyright © 2022 Marcadet, Bouredji, Argaw and Frenette. This is an open-access article distributed under the terms of the Creative Commons Attribution License (CC BY). The use, distribution or reproduction in other forums is permitted, provided the original author(s) and the copyright owner(s) are credited and that the original publication in this journal is cited, in accordance with accepted academic practice. No use, distribution or reproduction is permitted which does not comply with these terms.
*Correspondence: Jérôme Frenette, jerome.frenette@crchudequebec.ulaval.ca
†These authors have contributed equally to this work and share first authorship