- 1Departments of Pathology, University of Alabama at Birmingham, Birmingham, AL, United States
- 2Department of Cell Developmental and Integrative Biology, University of Alabama at Birmingham, Birmingham, AL, United States
- 3Department of Ophthalmology and Visual Sciences, University of Alabama at Birmingham, Birmingham, AL, United States
Thrombospondin-1 (TSP-1) is a matricellular extracellular matrix protein. Matricellular proteins are components of the extracellular matrix (ECM) that regulate key cellular functions and impact ECM organization, but which lack direct primary structural roles in the ECM. TSP-1 expression is upregulated in response to injury, hypoxia, growth factor stimulation, inflammation, glucose, and by reactive oxygen species. Relevant to glaucoma, TSP-1 is also a mechanosensitive molecule upregulated by mechanical stretch. TSP-1 expression is increased in ocular remodeling in glaucoma in both the trabecular meshwork and in the optic nerve head. The exact roles of TSP-1 in glaucoma remain to be defined, however. It plays important roles in cell behavior and in ECM remodeling during wound healing, fibrosis, angiogenesis, and in tumorigenesis and metastasis. At the cellular level, TSP-1 can modulate cell adhesion and migration, protease activity, growth factor activity, anoikis resistance, apoptosis, and collagen secretion and matrix assembly and cross-linking. These multiple functions and macromolecular and receptor interactions have been ascribed to specific domains of the TSP-1 molecule. In this review, we will focus on the cell regulatory activities of the TSP-1 N-terminal domain (NTD) sequence that binds to cell surface calreticulin (Calr) and which regulates cell functions via signaling through Calr complexed with LDL receptor related protein 1 (LRP1). We will describe TSP-1 actions mediated through the Calr/LRP1 complex in regulating focal adhesion disassembly and cytoskeletal reorganization, cell motility, anoikis resistance, and induction of collagen secretion and matrix deposition. Finally, we will consider the relevance of these TSP-1 functions to the pathologic remodeling of the ECM in glaucoma.
Introduction
The extracellular matrix (ECM) is a meshwork of proteins and carbohydrate-rich molecules secreted by cells and organized into distinct cell and context-specific structures in the extracellular space. Cellular interactions with ECM regulate and direct key cellular functions, such as differentiation, survival, proliferation, adhesion, and migration/invasion. In turn, cells both determine the expression and secretion of specific ECM components as well as the organization of the ECM network, a concept termed “dynamic reciprocity” (Bornstein and Ash, 1977; Bissell et al., 1982; Bissell and Aggeler, 1987; Bissell and Barcellos-Hoff, 1987). Multi-cellular life depends on ECM. New approaches that enrich ECM components in samples for mass spectrometry/proteomic analyses have increased our understanding of the breadth of molecules contained in the “matrisome” and importantly, enabled comparative studies to assess how the composition of the ECM differs in health and disease (Naba et al., 2012; Naba et al., 2016). These studies have identified over 278 core matrisome components consisting of various collagens, proteoglycans, and modular glycoproteins in addition to matrisome-associated components consisting of proteases, cross-linking enzymes, growth factors, and glycan structures (Naba et al., 2016).
The eye is an ECM-rich organ. For example, the vitreous, cornea, sclera, and lamina cribrosa are collagen rich structures with distinct tissue-specific collagen type expression and organization. Type IV collagen rich basement membranes are key to integrity of the retinal vasculature and the trabecular meshwork. Thus, it is not surprising that aberrant remodeling of the ECM is critical to the pathogenesis of multiple ocular diseases, including myopia, cataract formation and fibrous encapsulation, age-related macular degeneration, and importantly, glaucoma (Hernandez, 1993; Yang et al., 1993; Kantorow et al., 2000; Norose et al., 2000; Sawhney, 2002; Yan et al., 2003; Mansergh et al., 2004; Rada et al., 2006; He et al., 2014; Kuchtey and Kuchtey, 2014; Biasella et al., 2020; Wu et al., 2020; Grillo et al., 2021; Reinhard et al., 2021; Keller and Peters, 2022; Powell et al., 2022).
Extracellular Matrix and Glaucoma
Glaucoma is the second leading cause of irreversible blindness as a result of damage to the optic nerve (Quigley, 2011; Tham et al., 2014). Elevated intraocular pressure (IOP) is the most predominant risk factor for glaucoma, although there are other genetic factors that can cause glaucoma and patients with normal intraocular pressures can also develop glaucoma (Downs et al., 2011; Quigley, 2011). In addition, glucocorticoid treatment can lead to glaucoma (Chan et al., 2019; Roberti et al., 2020). Intraocular pressure is maintained by a balance of aqueous humor production and its outflow primarily through the conventional trabecular meshwork (TM) pathway in the anterior of the eye (Acott et al., 2021). Complex remodeling of diverse ECM components is linked to increased outflow resistance in the TM and elevated IOP (Vranka et al., 2015a; Vranka et al., 2015b; Acott et al., 2021; Keller and Peters, 2022). Chronic IOP can lead to deformation of the collagenous lamina cribrosa (LC) and adjacent sclera at the posterior of the eye: cupping of the LC is associated with axonal damage to the retinal ganglion cells of the optic nerve (Downs et al., 2011). Abnormal elastin fibers, increases in matrix metalloproteinases, and altered collagen organization in the laminar beams and at the posterior laminar boundary are among the ECM changes in the glaucomatous LC (Hernandez et al., 1990; Pena et al., 1998). Remodeling of the ECM affects the structure and function of both the TM and LC and it is accepted that deleterious ECM remodeling is a significant factor in the pathogenesis of glaucoma (Hernandez et al., 1990; Hernandez, 1993; Lutjen-Drecoll, 1999; Tektas and Lutjen-Drecoll, 2009; Vranka et al., 2015b; Wang et al., 2017; Kelly et al., 2021). Matricellular ECM proteins, a distinct type of ECM protein described below, are widely considered to be important in the pathogenesis of glaucoma [reviewed in (Rhee et al., 2009; Chatterjee et al., 2014; Wallace et al., 2014)].
Matricellular Proteins
Distinct from ECM proteins that comprise the primary structure of the ECM and that support cell adhesion, such as collagens, laminins, and fibronectin, there is another group of ECM proteins, matricellular proteins, that instead modify ECM structure and organization and which are primarily de-adhesive (Sage and Bornstein, 1991; Bornstein, 1995; Murphy-Ullrich and Sage, 2014). Common to both matricellular and structural ECM components, matricellular proteins bind to diverse cellular receptors to regulate cellular functions. In addition, they also bind to multiple other ECM components and modulate growth factor and protease activity. In the mid-1990’s when the first knockout mice of matricellular proteins, such as tenascin-C, SPARC, and TSP-1, were generated, there was surprise in the field that these genetic knockouts did not result in embryonic lethality. However, distinct phenotypes became apparent when animals were subjected to stresses, aging, or injury, suggesting important roles for matricellular proteins in adaptive and pathologic responses to injury and stress (Erickson, 1997; Settles et al., 1997; Lawler et al., 1998; Bassuk et al., 1999; Brekken and Sage, 2000; Bradshaw et al., 2002; Bradshaw et al., 2003a; Bradshaw et al., 2003b; Haddadin et al., 2009). These roles of matricellular proteins in adaptive cellular responses have been borne out by subsequent studies from multiple labs and are consistent with the highly regulated patterns of matricellular protein expression during development, following injury, and under conditions of cellular and metabolic stress (Chen et al., 2000; Adams and Lawler, 2011; Stenina-Adognravi, 2014; Giblin and Midwood, 2015; Midwood et al., 2016; Zuliani-Alvarez et al., 2017). The number of ECM proteins that are now considered to be “matricellular” has also expanded since the first insights with TSP-1, tenascin-C, and SPARC (Murphy-Ullrich and Sage, 2014). Myocilin, a protein expressed in glaucoma, has long been considered a matricellular protein because of its de-adhesive effects on TM cells and interactions with multiple ECM proteins (Tamm, 2002; Wentz-Hunter et al., 2004; Shen et al., 2008; Chatterjee et al., 2014).
Thrombospondin-1
Here, we will highlight the matricellular ECM glycoprotein, thrombospondin-1 (TSP-1), encoded by THBS1, and discuss possible roles for TSP-1 signaling of intermediate adhesion and its associated functions in glaucoma. TSP-1 is a homotrimeric, modular ECM glycoprotein that is classified as a matricellular ECM protein. TSP-1 was first identified as a protein released from the α-granules of thrombin-stimulated platelets and subsequently found to be widely expressed by cells in culture. TSP-1 is part of a family of five related proteins, with trimeric TSP-1 and TSP-2, members of the Group A TSP family, being the most similar [reviewed in (Adams and Lawler, 2011)]. Typical of ECM proteins, TSP-1 is a modular glycoprotein composed of multiple repeats of distinct structural domains. TSP-1 interacts with diverse molecules, including other ECM proteins, proteases, and growth factors, and it has multiple receptors, imparting an array of functionalities in different contexts (Adams and Lawler, 2011; Murphy-Ullrich and Sage, 2014; Resovi et al., 2014) (Figure 1). TSP-1 has many biologic functions, including regulation of cell adhesion and motility, platelet aggregation, cell death, protease activity, ECM organization, and growth factor regulation. Given this multiplicity of functions, TSP-1 has diverse roles in regulation of angiogenesis, inhibition of nitric oxide signaling, endothelial cell barrier function, inflammation/immunity, endoplasmic reticulum stress, fibrosis, and latent TGF-β activation (Adams and Lawler, 2004; Sweetwyne and Murphy-Ullrich, 2012; Murphy-Ullrich and Suto, 2018; Murphy-Ullrich, 2019; Forbes et al., 2021; Kale et al., 2021; Kaur et al., 2021; Morandi et al., 2021). The reader is referred to several excellent reviews covering broader roles of TSP-1 and other matricellular proteins in glaucoma and in fibrotic ECM remodeling for a more extensive discussion (Rhee et al., 2009; Chatterjee et al., 2014; Wallace et al., 2014; Murphy-Ullrich and Downs, 2015; Murphy-Ullrich, 2019; Keller and Peters, 2022). In this review, we will focus specifically on the N-terminal domain (NTD) calreticulin (Calr) binding sequence of TSP-1. This sequence, mimicked by the “hep I” peptide, signals induction of the intermediate cell adhesive state, first associated with the de-adhesive activity of TSP-1 (Murphy-Ullrich et al., 1993).
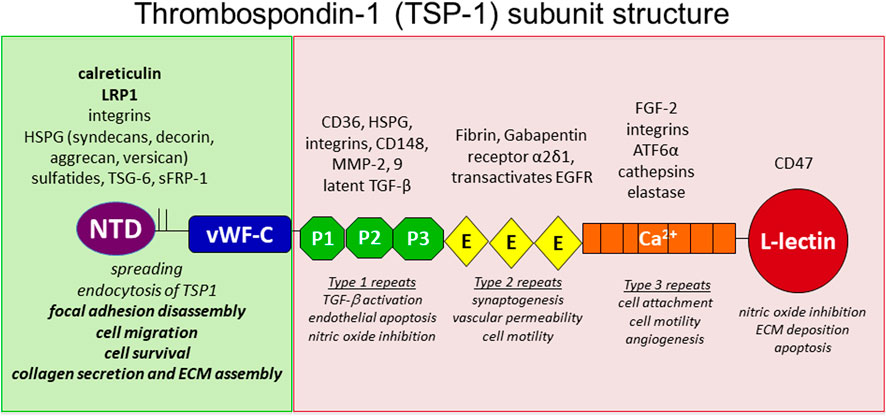
FIGURE 1. Model of Thrombospondin-1. TSP-1 is a homotrimer linked by interchain disulfide bonds in the oligomerization domain between the laminin G like N-terminal domain (NTD) and the vWF-C like domain. It is comprised of 3 properdin-like repeats (also called TSR or Type 1 repeats), followed by 3 EGF-like repeats (Type 2 repeats). This is followed by 13 calcium-binding Type 3 repeats that interact with the C-terminal L-lectin like domain. Various receptors and interacting molecules for each domain are indicated above the structure and functions ascribed to each domain are indicated below the structure.
Thrombospondin-1 Regulation of Intermediate Cell Adhesion
Characterization of the Intermediate Adhesive State: Altered Focal Adhesion and Cytoskeletal Organization
Matricellular proteins are distinct from adhesion supporting ECM proteins such as fibronectin, in that although cells might attach and partially spread on substrates of matricellular proteins, cells do not undergo complete cytoskeletal organization indicative of a fully adhesive cell (Lahav et al., 1987; Tuszynski et al., 1987; Murphy-Ullrich and Hook, 1989; Chiquet-Ehrismann, 1991; Adams and Lawler, 1993). It was originally noted that cells attached to substrates of TSP-1 had limited spreading with the formation of fascin-containing microspikes and also the absence of central focal adhesions and actin stress fiber organization (Murphy-Ullrich and Hook, 1989; Adams, 1995; Adams et al., 2001). In addition, soluble TSP-1 can prevent bovine aortic endothelial cells from forming focal adhesions on fibronectin substrates (Murphy-Ullrich and Hook, 1989). Similarly, tenascin-C can prevent cell adhesion to fibronectin substrates by blocking fibronectin-syndecan-4 binding (Huang et al., 2001; Midwood et al., 2004; Midwood et al., 2006). Moreover, TSP-1 and other matricellular proteins can induce alterations in fully adherent cells containing organized F-actin containing stress fibers and focal adhesions, characterized by disassembly of existing focal adhesions, but not cell detachment, a state called “intermediate cell adhesion” (Greenwood and Murphy-Ullrich, 1998; Murphy-Ullrich, 2001). Intermediate adhesion refers to this altered adhesive state in which cells are poised to respond to stress through altering their cytoskeletal organization and activation of signaling pathways that stimulate cell motility, survival, and ECM organization. These observations were first made in studies with SPARC, tenascin-C, and TSP-1 (Murphy-Ullrich and Hook, 1989; Murphy-Ullrich et al., 1991; Sage and Bornstein, 1991; Murphy-Ullrich et al., 1995). Mesenchymal cells (endothelial cells, fibroblasts) adherent to substrates such as fibronectin or vitronectin when treated with soluble forms of these matricellular proteins undergo cytoskeletal reorganization with loss of vinculin and α-actinin from focal adhesions at the termini of actin stress fibers. Interestingly, cells remain attached and partially spread. Furthermore, integrin remains clustered at these regions, suggesting that matricellular proteins are triggering intracellular signaling events that cause cytoskeletal reorganization, rather than direct physical disruption of integrin-ECM interactions (Murphy-Ullrich, 2001). The upregulation of TSP-1 expression in cell injury and in response to multiple forms of cellular stress, including hypoxia, mechanical stimulation, and high glucose, among others, supports the idea that TSP-1 signaling of intermediate adhesion might be a cell adaptive response (Stenina-Adognravi, 2014).
The Calreticulin/LRP1 Receptor Co-Complex Mediates Thrombospondin-1 Induced Intermediate Adhesion
The N-terminal heparin binding domain of TSP-1, specifically amino acids 17–35, binds to a calcium binding protein, calreticulin (Calr), to mediate focal adhesion disassembly and this function of TSP-1 can be mimicked by a peptide comprising this sequence, hep I (Murphy-Ullrich et al., 1993; Goicoechea et al., 2000). Notably, this sequence and function are conserved in TSP-2, although direct binding of TSP-2 to Calr has not been experimentally confirmed. The TSP binding site in Calr has been mapped to a sequence in the Calr N-domain (aa 19–36) on the face opposite Calr’s lectin binding site (Goicoechea et al., 2002). Calr is a chaperone involved in mediating glycoprotein folding in the endoplasmic reticulum (ER) (Michalak et al., 2009). It also is a calcium binding protein that mediates calcium release from the ER leading to downstream NFAT activation (Michalak et al., 1999; Groenendyk et al., 2004). However, Calr is found in multiple different cellular compartments, including the juxtamembrane cytosol where it interacts with integrin alpha subunits and also at the cell membrane on the cell surface (Dedhar, 1994; Coppolino and Dedhar, 1999; Gold et al., 2010; Liu et al., 2016). It is not clear how Calr is transported and localized to the cell surface, although conditions of cellular stress increase cell surface expression of Calr and there is evidence to suggest that Calr is transported with phosphatidyl serine to the surface of apoptotic cells (Wiersma et al., 2015). Integrin expression also regulates transit of Calr to the cell surface (Liu et al., 2016).
In addition to mediating TSP-1 induced focal adhesion disassembly, migration, anoikis, and collagen matrix assembly (see below), cell surface Calr, together with LDL Receptor Related Protein 1, (LRP1), is an important component of immunogenic cell death, serving as a cell surface signal for phagocytosis, independent of ligation by TSP-1 (Ogden et al., 2001; Gardai et al., 2005; Obeid et al., 2007). Secreted, extracellular Calr (eCRT) also facilitates scarless wound healing primarily via binding the scavenger and signaling receptor, LRP1 (discussed below), which leads to release of TGF-β3 and autocrine signaling to induce expression of ECM proteins (Nanney et al., 2008; Greives et al., 2012; Pandya et al., 2020). Interestingly, the ability of eCRT to induce ECM is dependent on intracellular Calr, consistent with previous observations showing the importance of endoplasmic Calr mediated calcium release and NFAT activation in mediating TGF-β stimulation of ECM (Van Duyn Graham et al., 2010; Zimmerman et al., 2013; Zimmerman et al., 2015; Owusu et al., 2018; Lu et al., 2020). Whether TSP-1 binding to cell surface Calr and complex formation with LRP1 has a role in this process remains unknown. In addition, Calr on the surface of the parasite Trypanisoma cruzi (TcCRT) binds TSP-1 and recombinant NTD, and localizes TSP-1 to the surface of the parasite, which increases parasite infectivity of mammalian cells (Johnson et al., 2012; Nde et al., 2012).
Since Calr is a peripheral membrane protein that lacks a transmembrane domain, Calr must associate with a transmembrane molecule to transmit intracellular signaling resulting from TSP-1 ligation of cell surface Calr. Basu and Srivastava previously showed an association between cell surface Calr and CD91, otherwise known as LRP1 or the α2-macroglobulin receptor (Strickland et al., 1995; Basu and Srivastava, 1999; Basu et al., 2001; Lillis et al., 2008). LRP1 is a large, multi-domain protein that binds an array of ligands. It is comprised of two disulfide-linked proteins, the smaller of which traverses the cell membrane (Strickland et al., 1995). The cytoplasmic domain of LRP-1 contains NPXY-1 sites that are involved in mediating intracellular signal transduction from bound ligand (Lillis et al., 2008). LRP1 mediates TSP-1 stimulated focal adhesion disassembly, cell migration, and anoikis resistance (Orr et al, 2003a; Orr et al, 2003b; Pallero et al., 2008). It was previously shown that TSP-1 binds to LRP1 and that LRP1 is a major mediator of endocytosis of TSP-1, a process enhanced by heparan sulfate proteoglycans (Godyna et al., 1995; Mikhailenko et al., 1995; Mikhailenko et al., 1997; Wang et al., 2004). This LRP1 binding site is localized to the N-terminal domain of TSP-1 (aa 1–90), although this site is distinct from the hep I site (aa 17–35) that binds Calr and mediates focal adhesion disassembly (Goicoechea et al., 2000; Wang et al., 2004). Furthermore, there is no evidence that the Calr-binding site of TSP-1 binds LRP1 (Orr et al, 2003b). LRP1 mediated endocytosis of TSP-1 could be an important factor in limiting TSP-1 action in a localized cellular milieu and there is also evidence suggesting that this endocytic role of LRP1 is involved in clearance of TSP-1/TSP-2 ligands such as MMPs and VEGF [discussed in (Lillis et al., 2008)].
It should be noted that different matricellular proteins signal intermediate adhesion via distinct receptors. For example, the large form of tenascin-C containing the alternatively spliced TNfnA-D domain binds to annexin II to trigger focal adhesion disassembly (Chung et al., 1996), whereas, the active receptor for SPARC-mediated focal adhesion disassembly has not been identified.
Signal Transduction Pathways in Intermediate Adhesion
Greenwood et al. (1998), Greenwood and Murphy-Ullrich (1998) showed that TSP-1 or the hep I peptide stimulated increased PI3K activity and binding of PIP3 to α-actinin, an actin bundling protein, inducing α-actinin dissociation from cytoplasmic focal adhesion complexes. Separate studies using PDGF showed that PIP3 induces α-actinin dissociation from the cytoplasmic tail of the integrin beta subunit and it is presumed that TSP-1 acts similarly (Greenwood et al., 2000). TSP-1 activates FAK, PI3K, and ERK in a manner involving both Gαi2 and Gβγ heterotrimeric G protein subunits (Orr et al., 2002). TSP-1 mediated focal adhesion disassembly requires FAK activation, which induces both PI3K and ERK activity that results in a transient down regulation of Rho activity (Orr et al., 2002; Orr et al., 2004). Furthermore, both TSP-1 and tenascin-C stimulation of focal adhesion disassembly requires Protein Kinase G activity (Murphy-Ullrich et al., 1996). Interestingly, Barker et al. (2004b) showed that the GPI-linked protein, Thy-1 (CD90), present in lipid rafts, is required for src family kinase (SFK) activation and TSP-1/hep I-mediated focal adhesion disassembly: both intact lipid rafts and the GPI-anchor of Thy-1 are required for responsiveness to TSP-1/hep I (Barker et al., 2004a; Barker et al., 2004b; Rege et al., 2006). Further work by Rege et al. (2006) showed that Thy-1 associates with FAK and SFK in lipid rafts following TSP-1/hep I stimulation of fibroblasts to induce cell migration and focal adhesion disassembly. There is no evidence for a direct association between Thy-1 and either LRP1 or Calr (Barker et al., 2004b), although both Calr and LRP1 have been localized to lipid rafts (Boucher et al., 2002; Ghiran et al., 2003). These intriguing results suggest a role for Thy-1 in potentially mediating formation of multi-factor signaling complexes associated with Calr/LRP1 following TSP-1/hep I stimulation (Rege et al., 2006). The signaling pathways activated by TSP-1 binding to the Calr/LRP-1 co-complex are summarized in Figure 2.
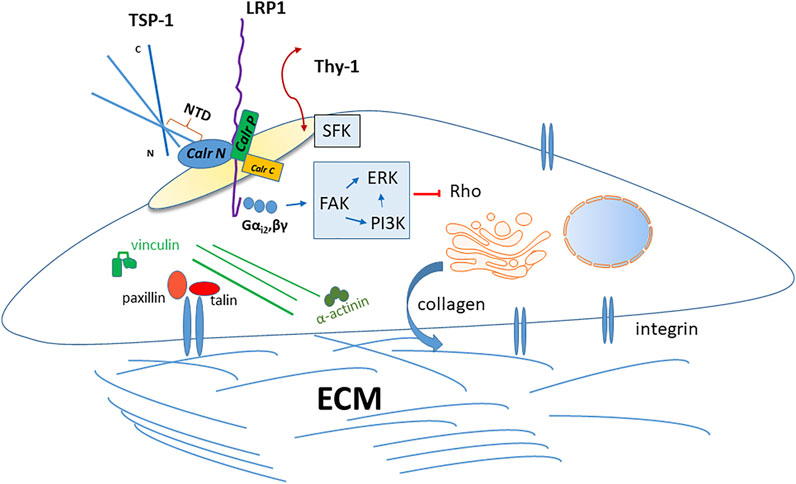
FIGURE 2. Thrombospondin-1 signaling through the caleticulin-LRP1 complex. The sequence represented by the hep I peptide (amino acids 17–35) in the NTD of TSP-1 bind to the N-terminal domain of calreticulin at the cell surface. The TSP-1 binding site has been localized to amino acids 19–26 in calreticulin. Binding of TSP-1 to calreticulin induces altered interactions between the calreticulin N and P domains to expose the putative LRP1 binding site and increase association of calreticulin with LRP1 and activation of downstream signaling. This occurs in lipids rafts. The GPI-linked protein, Thy-1, must also be present in lipid rafts and it activates Src family kinases, which are necessary for TSP-1 signaling through calreticulin/LRP-1, although direct interactions of Thy-1 with this complex have not been demonstrated. TSP-1-calreticulin engagement of LRP1 induces association with the Gαi2 heterotrimeric G protein subunit. This activates downstream FAK that induces PI3K and ERK signaling, culminating in a transient downregulation of Rho kinase activity. In fibroblasts and endothelial cells, TSP-1 signaling through calreticulin/LRP1 induces focal adhesion disassembly with cytoskeletal reorganization accompanied by loss of vinculin and α-actinin from integrin-talin clusters. This state of intermediate adhesion renders cells more motile and resistant to anoikis in a PI3K-dependent manner. In vivo expression of the secreted TSP-1 calreticulin binding sequence in a model of the foreign body response increases pericapsular collagen density and increased collagen matrix assembly in vitro, similarly in a PI3K-dependent manner.
Role of Lipid Rafts in Thrombospondin-1/Calr/LRP1 Signaling
Lipids rafts are important in mediating a number of complex effects of various TSP-1 receptors (Morandi et al., 2021). The importance of lipid rafts in mediating TSP-1 interactions with Calr/LRP1 complexes was shown in a series of elegant studies using molecular dynamics simulation approaches (Wang et al., 2014; Wang et al., 2019; Yan et al., 2010; Yan et al., 2011). In initial studies, Yan et al. (2010) showed that TSP-1 binding to the Calr N-terminal domain amino acids 19–36 induced conformational changes in Calr. TSP-1 binding to Calr induced a more open interaction between the Calr N domain and the P domain loop, potentially exposing the LRP1 binding site in Calr. This is consistent with biochemical observations that treatment of cells with the TSP-1 hep I peptide induced increased co-immunoprecipitation of Calr with LRP1 in endothelial cells (Orr et al, 2003b). Further modeling studies confirmed the importance of lysines 24 and 32 in TSP-1 and amino acid 24–26 and 32–34 in Calr for these interactions, consistent with biochemical observations (Murphy-Ullrich et al., 1993; Goicoechea et al., 2002; Yan et al., 2011). The Song lab then modeled the role of various membrane lipid configurations to explore the role of membrane domains in mediating Calr interactions with the plasma membrane and the effect on TSP-1 binding. A raft-like lipid bilayer (containing cholesterol) stabilized Calr binding to the lipid as compared to a palmitoyl phosphocholine bilayer and increased TSP-1 induced Calr conformational change to a more open configuration (Wang et al., 2014). Sphingomyelin in the cholesterol containing raft enhanced these interactions and TSP-1 binding to Calr further induced redistribution of cholesterol into raft-like aggregates (Wang et al., 2014). Furthermore, modeling of accessible sites in raft-bound opened Calr led to the hypothesis that Calr amino acids 96–150 near the N and P domain junction potentially represent the Calr-LRP1 binding site, although this remains to be confirmed biochemically (Wang et al., 2014). Calr-LRP1 interactions on apoptotic cells trigger phagocytosis (Ogden et al., 2001; Gardai et al., 2005) and play a role in immunogenic cell death (Obeid et al., 2007). This process is independent of TSP-1 or hep I binding to Calr, however (Gardai et al., 2005). Interestingly, modeling of Calr-LRP1 interactions in a phosphatidylserine rich lipid raft, representing apoptotic cells, showed that phosphatidyl serine rafts increased Calr membrane binding and interactions with LRP1 by increasing Calr stability at the apoptotic membrane (Wang et al., 2014; Wang et al., 2019). However, interactions of Calr bound to apoptotic membranes with TSP-1 showed reduced favorability of binding as calculated by free binding energy (Wang et al., 2014; Wang and Song, 2020), perhaps accounting for the TSP-1 independence of Calr-LRP1 mediated clearance of apoptotic cells (Gardai et al., 2005). This finding could also suggest decreased responsiveness to TSP-1 induced cellular migration and anoikis resistance under apoptotic conditions.
Functional Roles of Thrombospondin-1 Signaling Through Calr/LRP1 Complexes
Initial studies regarding TSP-1 signaling through Calr/LRP1 complexes were focused on focal adhesion disassembly and induction of intermediate cell adhesion, a potentially biologically adaptive state between rounded, weakly adherent cells and stationary, non-migratory cells with fully organized stress fibers and focal adhesion complexes (Greenwood and Murphy-Ullrich, 1998; Murphy-Ullrich, 2001). The characteristics of this adhesion modulation were described above. In addition to the direct effects of the TSP-1/Calr/LRP1 pathway on focal adhesion disassembly and cytoskeletal reorganization, this pathway has been shown to impact several biological functions of cells, including cell migration, resistance to anoikis, and enhancement of type 1 collagen secretion and matrix assembly. These functions might be the direct result of focal adhesion disassembly (i.e., migration) or more complex indirect effects of signaling pathways activated through Calr/LRP1 signaling (i.e., anoikis resistance, collagen secretion, and assembly) in the intermediate adhesive state. The impact of TSP-1 signaling through Calr/LRP1 on each of these cellular activities will be discussed.
Cell Migration
The ability of individual cells to migrate requires fine coordination between adhesive, protrusive, and contractile forces (Lauffenburger and Horwitz, 1996; Webb et al., 2002). TSP-1 has long been known to support cell migration, from haptotaxis on TSP-1 substrates through its C-terminal domain (Taraboletti et al., 1987) to stimulation of chemotaxis by the heparin-binding domain of soluble TSP-1 (Taraboletti et al., 1987; Mansfield et al., 1990; Taraboletti et al., 1990; Mansfield and Suchard, 1994). Given the ability of the Calr binding sequence of the heparin-binding domain of TSP-1 to stimulate focal adhesion disassembly (Murphy-Ullrich and Hook, 1989; Murphy-Ullrich et al., 1993), we asked whether TSP-1 and a peptide representing the Calr-binding sequence, hep I, stimulated directed and random cell migration (Orr et al, 2003a). In transwell assays, both TSP-1 and the hep I peptide, but not an inactive modified hep I peptide, stimulated directed (chemotactic) and random (cytokinesis) migration of bovine aortic endothelial cells at concentrations similar to those required to induce focal adhesion disassembly (Orr et al, 2003a). We observed increased directed cell migration with TSP-1 or hep I under chemotactic gradients. Under chemokinetic conditions, TSP-1 or hep I increased the proportion of cell migrating and migration speed. This migratory response to TSP-1 or hep I was absent in either Calr or LRP1 knockout fibroblasts or in wildtype fibroblasts treated with the LRP1 antagonist, RAP. Treatment with TSP-1 or hep I increased cell migration to a gradient of acidic FGF, whereas TSP-1/hep I reduced basic FGF directed migration (Orr et al, 2003a). Interestingly, TSP-1 had minimal chemoattractant effects on TM cells in a modified Boyden chamber assay, although it is not clear whether migrated cells were able to attach to the membranes in the presence of TSP-1 (Hogg et al., 1995). In our transwell assays with bovine aortic endothelial cells, it was necessary to coat membranes with a mix of fibronectin and vitronectin to support cell attachment of migrated cells in the presence of TSP-1 in the lower wells (Orr et al, 2003a). The TSP-1 binding sequence of Calr (aa 19–36) can also stimulate T cell motility through a 3D type 1 collagen ECM and endogenous TSP-1/cell surface Calr induced T cell motility via CD47, a receptor for the C-terminal domain of TSP-1; interestingly, in these studies, the Calr binding sequence of TSP-1 (hep I peptide) blocked T cell motility, presumably by competing with endogenous TSP-1/Calr (Li et al., 2005). These data suggest that signaling through the hep I sequence of TSP-1 binding to Calr in complex with LRP1 potentially impacts tissue remodeling through regulating cell adhesion and migration.
Anoikis Resistance
Non-hematologic cells require interactions with ECM molecules to generate pro-survival, anti-apoptotic signals (Meredith et al., 1993; Frisch and Francis, 1994). Signaling through integrins is a primary mediator of pro-survival signaling, although in some cases, integrin signaling is not sufficient (Zahir et al., 2003). PI3K induced Akt activation and FAK signaling are primary survival pathways regulated by cell-ECM adhesion (Xia et al., 2004; Yamaki et al., 2007). When cells are deprived of these cell adhesion survival signals, they undergo a form of apoptosis termed “anoikis,” derived from the Greek for “homeless wanderer” (Frisch and Francis, 1994). Interestingly, Rho-mediated cytoskeletal tension is an important mediator of anoikis (Yamaki et al., 2007). Anoikis is important in tissue remodeling during development and in response to injury (Frisch and Francis, 1994). Failure of anoikis impedes resolution of wound healing and can lead to fibrosis through fibroblast persistence (Horowitz et al., 2007). Tumor cell adhesion-independence, meaning survival in the absence of cell-matrix interactions, is a form of anoikis resistance and facilitates tumor metastasis (Liotta and Kohn, 2004).
TSP-1 signaling through binding of its type 1 repeats to CD36 and VEGF antagonism, binding of the C-terminal domain to CD47, as well as inhibition of basic FGF signaling via binding of the TSP-1 type 3 repeats, and the involvement of integrins, have been shown to induce endothelial apoptosis and this plays an important role in the anti-angiogenic function of TSP-1 (Good et al., 1990; Taraboletti et al., 1990; Tolsma et al., 1993; Streit et al., 1999; Cursiefen et al., 2004; Isenberg et al., 2009; Sun et al., 2009; Lawler and Lawler, 2012; Rusnati et al., 2019; Morandi et al., 2021). However, the NTD of TSP-1 is known to function differently from its C-terminal regions (Elzie and Murphy-Ullrich, 2004). The NTD heparin binding domain supports endothelial cell proliferation and angiogenesis through both syndecan-4, a transmembrane heparan sulfate proteoglycan, and integrin signaling (Tolsma et al., 1993; Ferrari do Outeiro-Bernstein et al., 2002; Calzada et al., 2003; Nunes et al., 2008). Given that the hep I Calr binding sequence in the TSP-1 NTD activates two pro-survival pathways, PI3K and FAK signaling, and transiently down regulates Rho activity that is necessary for anoikis, we asked whether TSP-1 binding to the Calr-LRP1 co-complex might support cell survival under adhesion-deprived conditions that trigger anoikis (Greenwood et al., 1998; Orr et al., 2004; Pallero et al., 2008). Fibroblasts plated on poly-HEMA coated coverslips, which only weakly supports attachment, undergo apoptosis. Treatment with TSP-1, hep I peptide, or a recombinant form of the NTD trimer (NoC1) increased cell viability and prevented apoptosis with decreased Caspase 3 activity and cleaved PARP1. TSP-1/hep I/NoC1 mediated cell survival is dependent on PI3K activity and Akt activity as the survival effects are blocked by wortmannin. Furthermore, TSP-1 mediated anoikis resistance occurs through Calr/LRP1 signaling as a peptide that blocks TSP-1 binding to Calr (CRT19.36), expression of Calr mutated in the TSP-1 binding site, and knockout of LRP1 expression all prevent TSP-1’s pro-survival signaling during anoikis (Pallero et al., 2008). These findings suggest a possible role for TSP-1/Calr/LRP1 signaling in wound repair with early anoikis resistance potentially supporting initial cell survival during migration into wounds, but possibly detrimental effects during later wound resolution due to fibroblast persistence, potentially contributing to fibrosis.
Collagen Matrix Assembly and the Foreign Body Response
To begin to assess possible roles of TSP-1 signaling through the Calr-LRP1 complex in vivo, we used a model of the foreign body response in which surgical sponges loaded with type 1 collagen and plasmid to express a secreted, EGFP-tagged version of the TSP-1 Calr binding sequence are implanted subcutaneously (Sweetwyne et al., 2010). In this model, cells invade the sponge and ingest the collagen and plasmid, becoming locally transfected to express the secreted TSP-1 Calr binding sequence. Given the known roles of this TSP-1 sequence in supporting cell migration and survival and the observations of delayed granulation tissue in Thbs1 null excisional wounds, we hypothesized that local expression of the TSP-1 Calr binding sequence would augment cell migration and wound healing (DiPietro et al., 1996; Agah et al., 2002; Orr et al, 2003a; Pallero et al., 2008). Surprisingly sponges with local TSP-1 Calr expression, but not control sponges expressing TSP-1 mutated in Calr binding sequences, developed a robust collagenous capsule surrounding the foreign body. This could not be explained by increased cellular or myofibroblast infiltration. Further in vitro studies established that the TSP-1 Calr sequence directly increased intracellular collagen expression, soluble collagen secretion, and collagen deposition into a deoxycholate insoluble matrix (Sweetwyne et al., 2010). The effects of the hep I sequence on fibronectin matrix assembly are not known. This increase in collagen matrix is dependent of Akt signaling, but independent of TGF-β signaling. Although the specific mechanism of TSP-1/Calr signaling increased collagen matrix assembly is not clear yet, it does not involve alterations in type 1 collagen transcript or processing of its N and C-terminal propeptides. We do not think that TSP-1/Calr/LRP-1 signaling affects MMP-mediated tissue remodeling by preventing or inhibiting LRP1-mediated MMP clearance as we failed to observe any differences in MMP activity in the presence of hep I peptide (Bing Su, Mariya Sweetwyne, Joanne Murphy-Ullrich, unpublished data). Although the specific roles of TSP-1 signaling through the Calr-LRP1 axis in normal and pathologic wound repair and tissue remodeling remain to be determined, these data provide support for TSP-1/Calr/LRP1 mediated signaling in regulating tissue repair responses (Sweetwyne and Murphy-Ullrich, 2012). Intriguing studies showed that TSP-1, via an unknown binding domain, can bind to both the KGHR sequence in the mature triple helical domain of collagen and to the C-propeptide domain of intracellular collagen and directly affect collagen fibril formation by preventing BMP-1 activation of prolysyl oxidase (Rosini et al., 2018). Peptides that block the TSP-1/KGHR interaction increase myofibroblasts through TGF-β signaling, suggesting a complex role for TSP-1 in regulating collagen processing and secretion and ECM assembly through multiple mechanisms.
Relevance to Glaucoma
So what role might these functions of the NTD of TSP-1 play in the pathologic changes to ocular tissue structure in glaucoma? Multiple studies suggest that TSP-1 expression is variably increased in the glaucomatous TM and in the (LC of the optic nerve head and sclera at the posterior of the eye (Tripathi et al., 1991; Hiscott et al., 1996; Flugel-Koch et al., 2004; Rhee et al., 2009; Chatterjee et al., 2014; Semba et al., 2021). Furthermore, knockout of the Thbs1 gene in mice reduces intra-ocular pressure (Haddadin et al., 2012). Recently a new gene variant of THBS1 was identified in a family with primary open angle glaucoma with elevated intraocular pressure that encodes the known N700S missense mutation (Wirtz et al., 2022). This variant was first identified as a risk factor for familial premature myocardial infarction and although the N700S mutation occurs in the C-terminal calcium binding wire region, protein stability and heparin binding functions are altered by this mutation, suggesting alterations in functions ascribed to other TSP-1 domains: the impact of the N700S mutation on TSP-1Calr/LRP1 signaling is unknown (Zhou et al., 2004; Stenina et al., 2005; Carlson et al., 2008). Links between increased TSP-1 expression and upregulated TGF-β activity suggest a role for TSP-1 in modulating TGF-β activation and possible MMP activity in glaucoma (Wallace et al., 2014; Murphy-Ullrich and Downs, 2015; Keller and Peters, 2022). TSP-1 can activate TGF-β 1-3 isoforms, whereas integrin-dependent TGF-β activation affects only TGF-β1 and 3 activation: since TGF-β2 is a primary factor in glaucoma, this suggests a significant role for TSP-1 in regulating TGF-β1 and 2 activity in glaucoma (Murphy-Ullrich and Downs, 2015; Murphy-Ullrich and Suto, 2018). Post-surgical levels of TSP-1 and TGF-β2 levels in aqueous humor are major independent predictors of trabeculectomy failure associated with fibrotic responses in primary open angle glaucoma patients (Zhu et al., 2019). Another pilot study confirmed elevated aqueous humor TGF-β2 levels in patients with failed trabeculectomy (Gajda-Derylo et al., 2019). It is not known whether aqueous humor levels of these proteins reflect actual signaling at the cellular level or rather are surrogate markers of underlying disease pathogenesis. Despite the focus on TSP-1 as a regulator of TGF-β activation in glaucoma, in contrast, the possible functions of the TSP-1/Calr/LRP1 complex in glaucoma remain unexplored and unknown.
Factors Involved in Glaucoma Pathogenesis Regulate TSP-1 Expression
Mechanical Forces and Mechanotransduction Pathways
It is well documented that TSP-1 is a mechano-responsive gene with numerous examples in studies with both LC and TM cells, as well as in other tissues, such as lung and skin (Kirwan et al, 2005a; Vittal et al., 2005; Warburton and Kaartinen, 2007; Chen et al., 2011). One mechanism for mechano-regulation of TSP-1 expression might occur via cellular contractility as recent studies showed that a Rho-kinase inhibitor decreased TSP-1 expression by TM cells, which was associated with decreased cell migration and increased outflow (Shan et al., 2021). Interestingly, in these studies, the LSKL peptide, which prevents TSP-1-mediated latent TGF-β activation, also decreased TSP-1 expression in this system, suggesting that TGF-β regulates TSP-1 expression under these conditions and that TM cell motility occurs downstream of Rho-mediated cytoskeletal contractility. It was not shown whether active Rho kinase regulates TSP-1 expression via TGF-β or more directly via Rho. It should be noted that the LSKL peptide only prevents TSP-1 binding to the latent TGF-β complex and does not block the action of the hep I peptide on focal adhesion disassembly and F-actin re-organization (unpublished data). Others showed that TSP-1 regulates TGF-β1 induced cell contractility in systemic sclerosis fibroblasts via MEK/ERK signaling, which can be blocked by the LSKL peptide (Chen et al., 2011). We showed that the TSP-1 Calr binding sequence activates ERK and transiently downregulates Rho kinase activity and stimulates cell migration (Orr et al., 2002; Orr et al, 2003a; Orr et al., 2004). The studies by Shan et al. (2021) showing that Rho kinase inhibition downregulated TSP-1 expression resulting in decreased cell migration would be consistent with a role for the TSP-1 Calr binding site in TM cell migration, although this remains to be shown directly and one cannot rule out effects due to TSP-1 mediated TGF-β activation. Moreover, the interplay between Rho regulated TSP-1 expression and TGF-β activation requires further study and these data suggest that the multiplicity of TSP-1 actions in a given tissue in specific context are likely to operate in the context of highly regulated feedback system.
The YAP-TAZ pathway is a key effector in mechanotransduction pathways and it has recently been shown that YAP is increased in LC cells from donors with glaucoma (Murphy et al., 2022). Culturing normal LC cells on stiff matrices increases YAP, expression of collagen and α-smooth muscle actin, and cell proliferation: YAP also regulates myofibroblast differentiation of scleral fibroblasts (Hu et al., 2021; Murphy et al., 2022). In addition, YAP regulates fibrotic activity of TM cells in response to dexamethasone (Liu et al., 2021). Interestingly, TSP-1 expression is regulated by YAP/TAZ and TSP-1 can also regulate YAP/TAZ signaling (Arun et al., 2020; Yamashiro et al., 2020; Arun et al., 2022). In vascular smooth muscle cells under cyclic mechanical stretch, TSP-1 binds to integrin αvβ1 and induces focal adhesion formation to stimulate nuclear shuttling of YAP, which impacts vascular remodeling in injury models (Yamashiro et al., 2020). In contrast, heart endothelial cells expressing TSP-1 had lower levels of unphosphorylated YAP and decreased nuclear YAP when challenged with the parasite T. cruzi as compared to endothelial cells from TSP-1 knockout mice (Arun et al., 2020). Furthermore, TSP-1 modulated YAP/β-catenin signaling in this system (Arun et al., 2022). As noted previously, infection of mammalian cells with T. cruzi increases TSP-1 expression and TSP-1 binding to parasite Calr on the surface of the trypanosome increases infectivity (Johnson et al., 2012). Interestingly, TSP-1 itself is transcriptionally induced by YAP/TAZ signaling (Shen et al., 2018; Boopathy and Hong, 2019). Exosomes derived from stiff matrices are enriched in TSP-1 and secreted in a YAP-dependent manner, which drives motility and invasiveness of breast cancer cells in a FAK and metalloproteinase manner, although these observations were associated with increased, not decreased, focal adhesions (Patwardhan et al., 2021). Given the importance of mechanotransduction and ECM stiffness in glaucoma (Last et al., 2011; Raghunathan et al., 2013; Powell et al., 2022), the roles of TSP-1 and signaling through its Calr-binding sequence warrant further investigation.
Thrombospondin-1 Regulation by Endoplasmic Reticulum Stress
Elevated TGF-β levels and dexamethasone are known factors in the pathogenesis of glaucoma (Flugel-Koch et al., 2004; Kirwan et al, 2005b). Chronically increased ER stress due to aging, oxidative stress, and glucocorticoid (dexamethasone) treatment also plays a role in glaucoma (Peters et al., 2015). ER stress also increases TSP-1 expression in pulmonary tissues subjected to intermittent hypoxia and TSP-1 plays an important role in cardiac hypertrophy-mediated autophagy through the PERK-ATF4 pathway, where recombinant NTD, and to a lesser extent the CTD, co-immunoprecipitated with PERK (Shi et al., 2020; Vanhoutte et al., 2021). Interestingly, TSP-1 is induced in hypertrophic hearts, as are ER stress proteins including Calr: induction of Calr by hypertrophy is attenuated in Thbs1 -/- mice, suggesting that TSP-1 can regulate expression of Calr, consistent with our unpublished studies (Vanhoutte et al., 2021). Recent studies show that TSP-1 also upregulates ER stress via increasing the ATF6-CHOP axis in renal tubular epithelial cells under high glucose conditions (Yue and Du, 2022): unfortunately, neither the active domain of TSP-1 nor the expression of Calr was investigated in these studies. Nonetheless, possible links between TSP-1 signaling and ER stress in glaucoma would be interesting to investigate.
Thrombospondin-1 Co-Receptors Calr/LRP1 in Glaucoma
Little is known about regulation of the TSP-1 co-receptors, Calr and LRP1, in glaucoma. Calr expression and its localization to the cell surface are increased in ER stress. In a fraction of glaucoma cases in which patients express myocilin with mutations that affect protein folding, aggregation, and retention in the ER [juvenile open-angle, ∼4% of adult onset primary open-angle (Kwon et al., 2009)], mutant myocilin engages pro-glaucoma alterations in cell adhesion and signaling and autophagy (Stone et al., 1997; Liu and Vollrath, 2004; Yan et al., 2020; Sharma and Grover, 2021). The Calr/calnexin chaperones bind to mutant myocilin: chemical chaperones reduce mutant myocilin-Calr binding and reduce ER stress induced cell death (Liu and Vollrath, 2004; Yam et al., 2007; Yan et al., 2020). As dexamethasone can stimulate ER stress in glucocorticoid-induced glaucoma, it is possible that cell surface Calr is also upregulated in this form of glaucoma (Zode et al., 2014). Similarly, chronic conditions associated with glaucoma, such as aging and oxidative stress increase ER stress (Peters et al., 2015). Nonetheless, whether ER stress induces expression of Calr on the cell surface of cells important to TM, scleral, or LC remodeling, where it could mediate signaling via TSP-1 ligation, remains unexplored.
There is evidence of extracellular release of Calr from on cytotrophoblast cells under ER stress: given the role of extracellular Calr in scarless wound repair, it would be interesting to know if Calr is increased in the aqueous fluid from glaucomatous TM (Pandya et al., 2020; Iwahashi et al., 2021). Calr plays a role in mediating fibrotic TGF-β signaling through ER-mediated calcium release and NFAT activation and increased levels of Calr have been detected in the medial layer of atherosclerotic rabbits, implicating ER and secreted Calr in ECM remodeling (Gold et al., 2010; Van Duyn Graham et al., 2010; Zimmerman et al., 2013; Owusu et al., 2018).
Similarly, there is little known about modulation of LRP1 activity (endocytosis, signaling) in glaucoma. As LRP1 is a scavenger receptor that mediates endocytosis of TSP-1 and also MMPs, it could play a role in regulating local levels of these proteins. Furthermore, Calr and LRP1 act in concert to signal clearance of apoptotic cells, independent of TSP-1 binding to Calr, an activity that could mediate cellular clearance of damaged TM cells to potentially increase aqueous outflow (Ogden et al., 2001; Gardai et al., 2005; Obeid et al., 2007). However, these TSP-1 independent roles have not been tested in glaucoma and could be potentially important avenues of investigation.
Localization of the N-Terminal Domain Calr-Binding Sequence in the Glaucomatous Milieu
It is possible that TSP-1 signaling through the NTD Calr-binding sequence plays significant roles in glaucomatous ECM remodeling. The NTD is susceptible to proteolysis by a variety of enzymes, including ADAMTS1, neutrophil elastase, and cathepsin G (Raugi et al., 1984; Rabhi-Sabile et al., 1996; Lee et al., 2006). BMP-1 also cleaves TSP-1 and releases the C-terminal region, which is associated with increased TGF-β activity (Anastasi et al., 2020). Lee et al. (2006) reported the presence of a TSP-1 fragment corresponding to the NTD, both in wound tissue and in wound fluids. The C-terminus determines TSP-1 ECM localization, supporting the idea of released soluble NTD (Adams et al., 2008). This is consistent with our observations of the secreted hep I sequence both in wound fluid and deposited into the wounds of mice with engineered expression of the Calr-binding hep I sequence in the model of the foreign body response described above (Sweetwyne et al., 2010). In a situation of injury, one would expect that the soluble fragment would be the predominant form of TSP-1, although deposition into and release from the provisional or early wound matrix remains a possibility. The influence of “stiff” matrices as found in glaucomatous tissue might also impact deposition and/or release of the TSP-1 NTD.
Thrombospondin-1/Calr/LRP1 Signaling and Cross-Linked Actin Networks Formation
Despite the role of the TSP-1 Calr binding sequence in stimulating focal adhesion disassembly and re-organization of actin-containing stress fibers, a possible role for TSP-1 in either mediating or antagonizing cross-linked actin networks (CLAN) formation in glaucomatous cells is unknown. The αvβ3 integrin is critical for CLAN formation and fibronectin fibrillogenesis, associated with increased cellular contractility, increased intraocular pressure, and increased TGF-β2 signaling (Filla et al., 2006; Filla et al., 2009; Filla et al., 2011; Filla et al., 2021). Interestingly, the TSP-1 Calr binding sequence activates many of the same pathways (PI3K, ERK, FAK) associated with integrin signaling; however, TSP-1 induces dissociation of α-actinin and vinculin from focal adhesions leading to dissociation of actin stress fibers from focal adhesions without dissociation of αvβ3 integrin clusters, paxillin, or talin (Murphy-Ullrich and Hook, 1989; Greenwood and Murphy-Ullrich, 1998). In the glaucomatous TM, stiffer ECMs are associated with increased stress fibers and CLAN formation: although TSP-1 signaling through Calr/LRP1 can increase collagen matrix, potentially leading to a stiffer ECM, this could be counteracted by induction of focal adhesion disassembly and loss of organized stress fibers that would decrease contractility. It is possible that short-term signaling through TSP-1/Calr/LRP1 would decrease CLAN formation due to focal adhesion disassembly, but support long-term increased CLAN formation due to a denser collagen ECM.
Discussion: Gaps and Opportunities
Here we have focused specifically on the roles of the TSP-1/Calr binding sequence and its signaling in concert with LRP1 in regulating focal adhesion disassembly and cell motility, promotion of cell survival under de-adhesive conditions, and collagen matrix assembly. The importance of these TSP-1 functions in TM, LC, and scleral remodeling in glaucoma has been largely unexplored. It is not clear whether these functions might contribute to the pathogenesis of glaucoma through stimulation of cell migration, cell persistence, and collagen deposition (Figure 3). The ability of the TSP-1/Calr/LRP1 axis to promote collagen ECM assembly suggests ECM stiffness might be increased via this pathway, which could increase pathologic remodeling. However, the disruption of focal adhesion connections to actin-containing stress fibers suggests that TSP-1/Calr/LRP1 signaling would decrease cellular contractility and be protective against glaucoma. Increased cell survival and persistence of ECM-producing cells in response to injury is associated with fibrotic remodeling, suggesting that TSP-1 induced anoikis resistance might increase survival of fibrosis promoting cells, especially during migration (Thannickal and Horowitz, 2006). Alternately, TM cell loss is characteristic of glaucoma and TSP-1 promotion of cell survival might be protective. Some think that TM cell loss is partially due to TM cell migration out of the outflow tract to the Schlemm’s canal lumen after injury, which potentially could be exacerbated by TSP-1 signaling through Calr/LRP1 (Calthorpe et al., 1991; Hogg et al., 1995; Zhou et al., 1999; Hogg et al., 2000). On the other hand, TSP-1 signaling could attract stem cells to sites of injury or surgical intervention and enhance repair (Kelley et al., 2009; Yun et al., 2016).
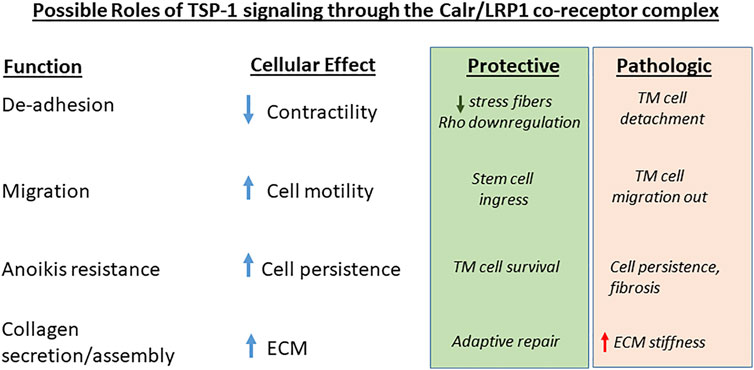
FIGURE 3. Possible roles of TSP-1/Calr/LRP1 signaling in glaucoma. The four major functions ascribed to TSP-1 signaling through the Calr/LRP1 receptor co-complex and their cellular effects are designated. Potential beneficial or pathologic effects of these activities in glaucoma cell responses to injury and ECM remodeling are indicated.
Only direct studies in vitro and in relevant animal models can address these questions. If data suggest that TSP-1 signaling through this pathway is deleterious, one could deliver an antagonist peptide of the Calr TSP-1 binding sequence (CRT19.36) (Sweetwyne et al., 2010). Alternately, the hep I peptide (aa 17–35) could be used to mimic and augment any beneficial TSP-1 effects. Similar approaches using intraocular delivery of collagen mimetic peptides to repair retinal ganglion cells have been shown to have benefit in rodent models (McGrady et al., 2021; Ribeiro et al., 2022).
Author Contributions
JM-U conceptualized and wrote this manuscript. She is accountable for the content of the work.
Funding
JEMU was supported by NIH NEI R01 EY027924-01A1.
Conflict of Interest
The authors declare that the research was conducted in the absence of any commercial or financial relationships that could be construed as a potential conflict of interest.
Publisher’s Note
All claims expressed in this article are solely those of the authors and do not necessarily represent those of their affiliated organizations, or those of the publisher, the editors and the reviewers. Any product that may be evaluated in this article, or claim that may be made by its manufacturer, is not guaranteed or endorsed by the publisher.
Acknowledgments
The author wishes to acknowledge the seminal contributions of past trainees and many collaborators to the original studies reported in this review. This is your work and I hope I have done it justice.
References
Acott, T. S., Vranka, J. A., Keller, K. E., Raghunathan, V., and Kelley, M. J. (2021). Normal and Glaucomatous Outflow Regulation. Prog. Retin. Eye Res. 82, 100897. doi:10.1016/j.preteyeres.2020.100897
Adams, J. C., Bentley, A. A., Kvansakul, M., Hatherley, D., and Hohenester, E. (2008). Extracellular Matrix Retention of Thrombospondin 1 Is Controlled by its Conserved C-Terminal Region. J. Cell Sci. 121 (Pt 6), 784–795. doi:10.1242/jcs.021006
Adams, J. C. (1995). Formation of Stable Microspikes Containing Actin and the 55 kDa Actin Bundling Protein, Fascin, Is a Consequence of Cell Adhesion to Thrombospondin-1: Implications for the Anti-adhesive Activities of Thrombospondin-1. J. Cell Sci. 108 (Pt 5), 1977–1990. doi:10.1242/jcs.108.5.1977
Adams, J. C., and Lawler, J. (1993). Diverse Mechanisms for Cell Attachment to Platelet Thrombospondin. J. Cell Sci. 104 (Pt 4), 1061–1071. doi:10.1242/jcs.104.4.1061
Adams, J. C., Kureishy, N., and Taylor, A. L. (2001). A Role for Syndecan-1 in Coupling Fascin Spike Formation by Thrombospondin-1. J. Cell Biol. 152 (6), 1169–1182. doi:10.1083/jcb.152.6.1169
Adams, J. C., and Lawler, J. (2004). The Thrombospondins. Int. J. Biochem. Cell Biol. 36 (6), 961–968. doi:10.1016/j.biocel.2004.01.004
Adams, J. C., and Lawler, J. (2011). The Thrombospondins. Cold Spring Harb. Perspect. Biol. 3 (10), a009712. doi:10.1101/cshperspect.a009712
Agah, A., Kyriakides, T. R., Lawler, J., and Bornstein, P. (2002). The Lack of Thrombospondin-1 (TSP1) Dictates the Course of Wound Healing in Double-Tsp1/tsp2-Null Mice. Am. J. Pathology 161 (3), 831–839. doi:10.1016/s0002-9440(10)64243-5
Anastasi, C., Rousselle, P., Talantikite, M., Tessier, A., Cluzel, C., Bachmann, A., et al. (2020). BMP-1 Disrupts Cell Adhesion and Enhances TGF-β Activation through Cleavage of the Matricellular Protein Thrombospondin-1. Sci. Signal 13 (639). doi:10.1126/scisignal.aba3880
Arun, A., Rayford, K. J., Cooley, A., Rachakonda, G., Villalta, F., Pratap, S., et al. (2020). Thrombospondin-1 Plays an Essential Role in Yes-Associated Protein Nuclear Translocation during the Early Phase of Trypanosoma Cruzi Infection in Heart Endothelial Cells. Int. J. Mol. Sci. 21 (14). doi:10.3390/ijms21144912
Arun, A., Rayford, K. J., Cooley, A., Rana, T., Rachakonda, G., Villalta, F., et al. (2022). Thrombospondin-1 Expression and Modulation of Wnt and Hippo Signaling Pathways during the Early Phase of Trypanosoma Cruzi Infection of Heart Endothelial Cells. PLoS Negl. Trop. Dis. 16 (1), e0010074. doi:10.1371/journal.pntd.0010074
Barker, T. H., Grenett, H. E., MacEwen, M. W., Tilden, S. G., Fuller, G. M., Settleman, J., et al. (2004a). Thy-1 Regulates Fibroblast Focal Adhesions, Cytoskeletal Organization and Migration through Modulation of P190 RhoGAP and Rho GTPase Activity. Exp. Cell Res. 295 (2), 488–496. doi:10.1016/j.yexcr.2004.01.026
Barker, T. H., Pallero, M. A., MacEwen, M. W., Tilden, S. G., Woods, A., Murphy-Ullrich, J. E., et al. (2004b). Thrombospondin-1-induced Focal Adhesion Disassembly in Fibroblasts Requires Thy-1 Surface Expression, Lipid Raft Integrity, and Src Activation. J. Biol. Chem. 279 (22), 23510–23516. doi:10.1074/jbc.m402169200
Bassuk, J. A., Birkebak, T., Rothmier, J. D., Clark, J. M., Bradshaw, A., Muchowski, P. J., et al. (1999). Disruption of theSparcLocus in Mice Alters the Differentiation of Lenticular Epithelial Cells and Leads to Cataract Formation. Exp. Eye Res. 68 (3), 321–331. doi:10.1006/exer.1998.0608
Basu, S., Binder, R. J., Ramalingam, T., and Srivastava, P. K. (2001). CD91 Is a Common Receptor for Heat Shock Proteins Gp96, Hsp90, Hsp70, and Calreticulin. Immunity 14 (3), 303–313. doi:10.1016/s1074-7613(01)00111-x
Basu, S., and Srivastava, P. K. (1999). Calreticulin, a Peptide-Binding Chaperone of the Endoplasmic Reticulum, Elicits Tumor- and Peptide-specific Immunity. J. Exp. Med. 189 (5), 797–802. doi:10.1084/jem.189.5.797
Biasella, F., Plössl, K., Karl, C., Weber, B. H. F., and Friedrich, U. (2020). Altered Protein Function Caused by AMD-Associated Variant Rs704 Links Vitronectin to Disease Pathology. Invest. Ophthalmol. Vis. Sci. 61 (14), 2. doi:10.1167/iovs.61.14.2
Bissell, M. J., and Aggeler, J. (1987). Dynamic Reciprocity: How Do Extracellular Matrix and Hormones Direct Gene Expression? Prog. Clin. Biol. Res. 249, 251–262.
Bissell, M. J., and Barcellos-Hoff, M. H. (1987). The Influence of Extracellular Matrix on Gene Expression: Is Structure the Message? J. Cell Sci. Suppl. 1987, 327–343. doi:10.1242/jcs.1987.supplement_8.18
Bissell, M. J., Hall, H. G., and Parry, G. (1982). How Does the Extracellular Matrix Direct Gene Expression? J. Theor. Biol. 99 (1), 31–68. doi:10.1016/0022-5193(82)90388-5
Boopathy, G. T. K., and Hong, W. (2019). Role of Hippo Pathway-YAP/TAZ Signaling in Angiogenesis. Front. Cell Dev. Biol. 7, 49. doi:10.3389/fcell.2019.00049
Bornstein, P., and Ash, J. F. (1977). Cell Surface-Associated Structural Proteins in Connective Tissue Cells. Proc. Natl. Acad. Sci. U.S.A. 74 (6), 2480–2484. doi:10.1073/pnas.74.6.2480
Bornstein, P. (1995). Diversity of Function Is Inherent in Matricellular Proteins: an Appraisal of Thrombospondin 1. J. Cell Biol. 130 (3), 503–506. doi:10.1083/jcb.130.3.503
Boucher, P., Liu, P., Gotthardt, M., Hiesberger, T., Anderson, R. G. W., and Herz, J. (2002). Platelet-derived Growth Factor Mediates Tyrosine Phosphorylation of the Cytoplasmic Domain of the Low Density Lipoprotein Receptor-Related Protein in Caveolae. J. Biol. Chem. 277 (18), 15507–15513. doi:10.1074/jbc.m200428200
Bradshaw, A. D., Graves, D. C., Motamed, K., and Sage, E. H. (2003a). SPARC-null Mice Exhibit Increased Adiposity without Significant Differences in Overall Body Weight. Proc. Natl. Acad. Sci. U.S.A. 100 (10), 6045–6050. doi:10.1073/pnas.1030790100
Bradshaw, A. D., Puolakkainen, P., Wight, T. N., Helene Sage, E., Dasgupta, J., and Davidson, J. M. (2003b). SPARC-null Mice Display Abnormalities in the Dermis Characterized by Decreased Collagen Fibril Diameter and Reduced Tensile Strength. J. Investigative Dermatology 120 (6), 949–955. doi:10.1046/j.1523-1747.2003.12241.x
Bradshaw, A. D., Reed, M. J., and Sage, E. H. (2002). SPARC-null Mice Exhibit Accelerated Cutaneous Wound Closure. J. Histochem Cytochem. 50 (1), 1–10. doi:10.1177/002215540205000101
Brekken, R. A., and Sage, E. H. (2000). SPARC, a Matricellular Protein: at the Crossroads of Cell-Matrix. Matrix Biol. 19 (7), 569–580. doi:10.1016/s0945-053x(00)00105-0
Calthorpe, C. M., Grierson, I., and Hitchings, R. A. (1991). Chemoattractants Produced by Ocular Cells Induce Trabecular Meshwork Cell Migration. Int. Ophthalmol. 15 (3), 185–191. doi:10.1007/bf00153926
Calzada, M. J., Sipes, J. M., Krutzsch, H. C., Yurchenco, P. D., Annis, D. S., Mosher, D. F., et al. (2003). Recognition of the N-Terminal Modules of Thrombospondin-1 and Thrombospondin-2 by α6β1 Integrin. J. Biol. Chem. 278 (42), 40679–40687. doi:10.1074/jbc.m302014200
Carlson, C. B., Liu, Y., Keck, J. L., and Mosher, D. F. (2008). Influences of the N700S Thrombospondin-1 Polymorphism on Protein Structure and Stability. J. Biol. Chem. 283 (29), 20069–20076. doi:10.1074/jbc.m800223200
Chan, W., Wiggs, J. L., and Sobrin, L. (2019). The Genetic Influence on Corticosteroid-Induced Ocular Hypertension: A Field Positioned for Discovery. Am. J. Ophthalmol. 202, 1–5. doi:10.1016/j.ajo.2019.02.001
Chatterjee, A., Villarreal, G., and Rhee, D. J. (2014). Matricellular Proteins in the Trabecular Meshwork: Review and Update. J. Ocular Pharmacol. Ther. 30 (6), 447–463. doi:10.1089/jop.2014.0013
Chen, H., Herndon, M. E., and Lawler, J. (2000). The Cell Biology of Thrombospondin-1. Matrix Biol. 19 (7), 597–614. doi:10.1016/s0945-053x(00)00107-4
Chen, Y., Leask, A., Abraham, D. J., Kennedy, L., Shi-Wen, X., Denton, C. P., et al. (2011). Thrombospondin 1 Is a Key Mediator of Transforming Growth Factor β-mediated Cell Contractility in Systemic Sclerosis via a Mitogen-Activated Protein Kinase Kinase (MEK)/extracellular Signal-Regulated Kinase (ERK)-dependent Mechanism. Fibrogenes. Tissue Repair 4 (1), 9. doi:10.1186/1755-1536-4-9
Chiquet-Ehrismann, R. (1991). Anti-adhesive Molecules of the Extracellular Matrix. Curr. Opin. Cell Biol. 3 (5), 800–804. doi:10.1016/0955-0674(91)90053-2
Chung, C. Y., Murphy-Ullrich, J. E., and Erickson, H. P. (1996). Mitogenesis, Cell Migration, and Loss of Focal Adhesions Induced by Tenascin-C Interacting with its Cell Surface Receptor, Annexin II. MBoC 7 (6), 883–892. doi:10.1091/mbc.7.6.883
Coppolino, M. G., and Dedhar, S. (1999). Ligand-specific, Transient Interaction between Integrins and Calreticulin during Cell Adhesion to Extracellular Matrix Proteins Is Dependent upon Phosphorylation/dephosphorylation Events. Biochem. J. 340 (Pt 1), 41–50. doi:10.1042/bj3400041
Cursiefen, C., Masli, S., Ng, T. F., Dana, M. R., Bornstein, P., Lawler, J., et al. (2004). Roles of Thrombospondin-1 and -2 in Regulating Corneal and Iris Angiogenesis. Invest. Ophthalmol. Vis. Sci. 45 (4), 1117–1124. doi:10.1167/iovs.03-0940
Dedhar, S. (1994). Novel Functions for Calreticulin: Interaction with Integrins and Modulation of Gene Expression? Trends Biochem. Sci. 19 (7), 269–271. doi:10.1016/0968-0004(94)90001-9
DiPietro, L. A., Nissen, N. N., Gamelli, R. L., Koch, A. E., Pyle, J. M., and Polverini, P. J. (1996). Thrombospondin 1 Synthesis and Function in Wound Repair. Am. J. Pathol. 148 (6), 1851–1860.
Downs, J. C., Roberts, M. D., and Sigal, I. A. (2011). Glaucomatous Cupping of the Lamina Cribrosa: a Review of the Evidence for Active Progressive Remodeling as a Mechanism. Exp. Eye Res. 93 (2), 133–140. doi:10.1016/j.exer.2010.08.004
Elzie, C. A., and Murphy-Ullrich, J. E. (2004). The N-Terminus of Thrombospondin: the Domain Stands Apart. Int. J. Biochem. Cell Biol. 36 (6), 1090–1101. doi:10.1016/j.biocel.2003.12.012
Erickson, H. P. (1997). A Tenascin Knockout with a Phenotype. Nat. Genet. 17 (1), 5–7. doi:10.1038/ng0997-5
Ferrari do Outeiro-Bernstein, M. A., Nunes, S. S., Andrade, A. C. M., Alves, T. R., Legrand, C., and Morandi, V. (2002). A Recombinant NH2-terminal Heparin-Binding Domain of the Adhesive Glycoprotein, Thrombospondin-1, Promotes Endothelial Tube Formation and Cell Survival: a Possible Role for Syndecan-4 Proteoglycan. Matrix Biol. 21 (4), 311–324. doi:10.1016/s0945-053x(02)00010-0
Filla, M. S., Meyer, K. K., Faralli, J. A., and Peters, D. M. (2021). Overexpression and Activation of Alphavbeta3 Integrin Differentially Affects TGFbeta2 Signaling in Human Trabecular Meshwork Cells. Cells 10 (8). doi:10.3390/cells10081923
Filla, M. S., Schwinn, M. K., Nosie, A. K., Clark, R. W., and Peters, D. M. (2011). Dexamethasone-Associated Cross-Linked Actin Network Formation in Human Trabecular Meshwork Cells Involves β3 Integrin Signaling. Invest. Ophthalmol. Vis. Sci. 52 (6), 2952–2959. doi:10.1167/iovs.10-6618
Filla, M. S., Schwinn, M. K., Sheibani, N., Kaufman, P. L., and Peters, D. M. (2009). Regulation of Cross-Linked Actin Network (CLAN) Formation in Human Trabecular Meshwork (HTM) Cells by Convergence of Distinct β1 and β3 Integrin Pathways. Invest. Ophthalmol. Vis. Sci. 50 (12), 5723–5731. doi:10.1167/iovs.08-3215
Filla, M. S., Woods, A., Kaufman, P. L., and Peters, D. M. (2006). β1 and β3 Integrins Cooperate to Induce Syndecan-4-Containing Cross-Linked Actin Networks in Human Trabecular Meshwork Cells. Invest. Ophthalmol. Vis. Sci. 47 (5), 1956–1967. doi:10.1167/iovs.05-0626
Flügel-Koch, C., Ohlmann, A., Fuchshofer, R., Welge-Lüssen, U., and Tamm, E. R. (2004). Thrombospondin-1 in the Trabecular Meshwork: Localization in Normal and Glaucomatous Eyes, and Induction by TGF-Β1 and Dexamethasone In Vitro. Exp. Eye Res. 79 (5), 649–663. doi:10.1016/j.exer.2004.07.005
Forbes, T., Pauza, A. G., and Adams, J. C. (2021). In the Balance: How Do Thrombospondins Contribute to the Cellular Pathophysiology of Cardiovascular Disease? Am. J. Physiology-Cell Physiology 321 (5), C826–C845. doi:10.1152/ajpcell.00251.2021
Frisch, S., and Francis, H. (1994). Disruption of Epithelial Cell-Matrix Interactions Induces Apoptosis. J. Cell Biol. 124 (4), 619–626. doi:10.1083/jcb.124.4.619
Gajda-Deryło, B., Stahnke, T., Struckmann, S., Warsow, G., Birke, K., Birke, M. T., et al. (2019). Comparison of Cytokine/chemokine Levels in Aqueous Humor of Primary Open-Angle Glaucoma Patients with Positive or Negative Outcome Following Trabeculectomy. Biosci. Rep. 39 (5). doi:10.1042/BSR20181894
Gardai, S. J., McPhillips, K. A., Frasch, S. C., Janssen, W. J., Starefeldt, A., Murphy-Ullrich, J. E., et al. (2005). Cell-surface Calreticulin Initiates Clearance of Viable or Apoptotic Cells through Trans-activation of LRP on the Phagocyte. Cell 123 (2), 321–334. doi:10.1016/j.cell.2005.08.032
Ghiran, I., Klickstein, L. B., and Nicholson-Weller, A. (2003). Calreticulin Is at the Surface of Circulating Neutrophils and Uses CD59 as an Adaptor Molecule. J. Biol. Chem. 278 (23), 21024–21031. doi:10.1074/jbc.m302306200
Giblin, S. P., and Midwood, K. S. (2015). Tenascin-C: Form versus Function. Cell Adh Migr. 9 (1-2), 48–82. doi:10.4161/19336918.2014.987587
Godyna, S., Liau, G., Popa, I., Stefansson, S., and Argraves, W. S. (1995). Identification of the Low Density Lipoprotein Receptor-Related Protein (LRP) as an Endocytic Receptor for Thrombospondin-1. J. Cell Biol. 129 (5), 1403–1410. doi:10.1083/jcb.129.5.1403
Goicoechea, S., Orr, A. W., Pallero, M. A., Eggleton, P., and Murphy-Ullrich, J. E. (2000). Thrombospondin Mediates Focal Adhesion Disassembly through Interactions with Cell Surface Calreticulin. J. Biol. Chem. 275 (46), 36358–36368. doi:10.1074/jbc.m005951200
Goicoechea, S., Pallero, M. A., Eggleton, P., Michalak, M., and Murphy-Ullrich, J. E. (2002). The Anti-adhesive Activity of Thrombospondin Is Mediated by the N-Terminal Domain of Cell Surface Calreticulin. J. Biol. Chem. 277 (40), 37219–37228. doi:10.1074/jbc.m202200200
Gold, L. I., Eggleton, P., Sweetwyne, M. T., Van Duyn, L. B., Greives, M. R., Naylor, S. M., et al. (2010). Calreticulin: Non‐endoplasmic Reticulum Functions in Physiology and Disease. FASEB J. 24 (3), 665–683. doi:10.1096/fj.09-145482
Good, D. J., Polverini, P. J., Rastinejad, F., Le Beau, M. M., Lemons, R. S., Frazier, W. A., et al. (1990). A Tumor Suppressor-dependent Inhibitor of Angiogenesis Is Immunologically and Functionally Indistinguishable from a Fragment of Thrombospondin. Proc. Natl. Acad. Sci. U.S.A. 87 (17), 6624–6628. doi:10.1073/pnas.87.17.6624
Greenwood, J. A., and Murphy-Ullrich, J. E. (1998). Signaling of De-adhesion in Cellular Regulation and Motility. Microsc. Res. Tech. 43 (5), 420–432. doi:10.1002/(sici)1097-0029(19981201)43:5<420::aid-jemt8>3.0.co;2-b
Greenwood, J. A., Pallero, M. A., Theibert, A. B., and Murphy-Ullrich, J. E. (1998). Thrombospondin Signaling of Focal Adhesion Disassembly Requires Activation of Phosphoinositide 3-kinase. J. Biol. Chem. 273 (3), 1755–1763. doi:10.1074/jbc.273.3.1755
Greenwood, J. A., Theibert, A. B., Prestwich, G. D., and Murphy-Ullrich, J. E. (2000). Restructuring of Focal Adhesion Plaques by Pi 3-Kinase. J. Cell Biol. 150 (3), 627–642. doi:10.1083/jcb.150.3.627
Greives, M. R., Samra, F., Pavlides, S. C., Blechman, K. M., Naylor, S. M., Woodrell, C. D., et al. (2012). Exogenous Calreticulin Improves Diabetic Wound Healing. Wound Repair Regen. 20 (5), 715–730. doi:10.1111/j.1524-475X.2012.00822.x
Grillo, S. L., Etzel, J. D., Weber, S. R., Ondeck, C., Wang, W., Zhao, Y., et al. (2021). Descriptive Analysis of Fibulin-3 and the Extracellular Vesicle Marker, Alix, in Drusen from a Small Cohort of Postmortem Human Eyes. Exp. Eye Res. 203, 108422. doi:10.1016/j.exer.2020.108422
Groenendyk, J., Lynch, J., and Michalak, M. (2004). Calreticulin, Ca2+, and Calcineurin - Signaling from the Endoplasmic Reticulum. Mol. Cells 17 (3), 383–389.
Haddadin, R. I., Oh, D.-J., Kang, M. H., Filippopoulos, T., Gupta, M., Hart, L., et al. (2009). SPARC-null Mice Exhibit Lower Intraocular Pressures. Invest. Ophthalmol. Vis. Sci. 50 (8), 3771–3777. doi:10.1167/iovs.08-2489
Haddadin, R. I., Oh, D.-J., Kang, M. H., Villarreal, G., Kang, J.-H., Jin, R., et al. (2012). Thrombospondin-1 (TSP1)-Null and TSP2-Null Mice Exhibit Lower Intraocular Pressures. Invest. Ophthalmol. Vis. Sci. 53 (10), 6708–6717. doi:10.1167/iovs.11-9013
He, L., Frost, M. R., Siegwart, J. T., and Norton, T. T. (2014). Gene Expression Signatures in Tree Shrew Choroid during Lens-Induced Myopia and Recovery. Exp. Eye Res. 123, 56–71. doi:10.1016/j.exer.2014.04.005
Hernandez, M. R., Andrzejewska, W. M., and Neufeld, A. H. (1990). Changes in the Extracellular Matrix of the Human Optic Nerve Head in Primary Open-Angle Glaucoma. Am. J. Ophthalmol. 109 (2), 180–188. doi:10.1016/s0002-9394(14)75984-7
Hernandez, M. R. (1993). Extracellular Matrix Macromolecules of the Lamina Cribrosa. J. Glaucoma 2 (1), 50–57. doi:10.1097/00061198-199300210-00014
Hiscott, P., Schlötzer-Schrehardt, U., and Naumann, G. O. H. (1996). Unexpected Expression of Thrombospondin 1 by Corneal and Iris Fibroblasts in the Pseudoexfoliation Syndrome. Hum. Pathol. 27 (12), 1255–1258. doi:10.1016/s0046-8177(96)90333-0
Hogg, P., Calthorpe, M., Batterbury, M., and Grierson, I. (2000). Aqueous Humor Stimulates the Migration of Human Trabecular Meshwork Cells In Vitro. Invest Ophthalmol. Vis. Sci. 41 (5), 1091–1098.
Hogg, P., Calthorpe, M., Ward, S., and Grierson, I. (1995). Migration of Cultured Bovine Trabecular Meshwork Cells to Aqueous Humor and Constituents. Invest Ophthalmol. Vis. Sci. 36 (12), 2449–2460.
Horowitz, J. C., Rogers, D. S., Sharma, V., Vittal, R., White, E. S., Cui, Z., et al. (2007). Combinatorial Activation of FAK and AKT by Transforming Growth Factor-Β1 Confers an Anoikis-Resistant Phenotype to Myofibroblasts. Cell. Signal. 19 (4), 761–771. doi:10.1016/j.cellsig.2006.10.001
Hu, D., Jiang, J., Ding, B., Xue, K., Sun, X., and Qian, S. (2021). Mechanical Strain Regulates Myofibroblast Differentiation of Human Scleral Fibroblasts by YAP. Front. Physiol. 12, 712509. doi:10.3389/fphys.2021.712509
Huang, W., Chiquet-Ehrismann, R., Moyano, J. V., Garcia-Pardo, A., and Orend, G. (2001). Interference of Tenascin-C with Syndecan-4 Binding to Fibronectin Blocks Cell Adhesion and Stimulates Tumor Cell Proliferation. Cancer Res. 61 (23), 8586–8594.
Isenberg, J. S., Shiva, S., and Gladwin, M. (2009). Thrombospondin-1-CD47 Blockade and Exogenous Nitrite Enhance Ischemic Tissue Survival, Blood Flow and Angiogenesis via Coupled NO-cGMP Pathway Activation. Nitric Oxide 21 (1), 52–62. doi:10.1016/j.niox.2009.05.005
Iwahashi, N., Ikezaki, M., Nishitsuji, K., Yamamoto, M., Matsuzaki, I., Kato, N., et al. (2021). Extracellularly Released Calreticulin Induced by Endoplasmic Reticulum Stress Impairs Syncytialization of Cytotrophoblast Model BeWo Cells. Cells 10 (6). doi:10.3390/cells10061305
Johnson, C. A., Kleshchenko, Y. Y., Ikejiani, A. O., Udoko, A. N., Cardenas, T. C., Pratap, S., et al. (2012). Thrombospondin-1 Interacts with Trypanosoma Cruzi Surface Calreticulin to Enhance Cellular Infection. PLoS One 7 (7), e40614. doi:10.1371/journal.pone.0040614
Kale, A., Rogers, N. M., and Ghimire, K. (2021). Thrombospondin-1 CD47 Signalling: From Mechanisms to Medicine. Int. J. Mol. Sci. 22 (8). doi:10.3390/ijms22084062
Kantorow, M., Huang, Q., Yang, X. J., Sage, E. H., Magabo, K. S., Miller, K. M., et al. (2000). Increased Expression of Osteonectin/SPARC mRNA and Protein in Age-Related Human Cataracts and Spatial Expression in the Normal Human Lens. Mol. Vis. 6, 24–29.
Kaur, S., Bronson, S. M., Pal-Nath, D., Miller, T. W., Soto-Pantoja, D. R., and Roberts, D. D. (2021). Functions of Thrombospondin-1 in the Tumor Microenvironment. Int. J. Mol. Sci. 22 (9). doi:10.3390/ijms22094570
Keller, K. E., and Peters, D. M. (2022). Pathogenesis of Glaucoma: Extracellular Matrix Dysfunction in the Trabecular Meshwork-A Review. Clin. Exp. Ophthalmol.
Kelley, M. J., Rose, A. Y., Keller, K. E., Hessle, H., Samples, J. R., and Acott, T. S. (2009). Stem Cells in the Trabecular Meshwork: Present and Future Promises. Exp. Eye Res. 88 (4), 747–751. doi:10.1016/j.exer.2008.10.024
Kelly, R. A., Perkumas, K. M., Campbell, M., Farrar, G. J., Stamer, W. D., Humphries, P., et al. (2021). Fibrotic Changes to Schlemm's Canal Endothelial Cells in Glaucoma. Int. J. Mol. Sci. 22 (17). doi:10.3390/ijms22179446
Kirwan, R. P., Fenerty, C. H., Crean, J., Wordinger, R. J., Clark, A. F., and O'Brien, C. J. (2005a). Influence of Cyclical Mechanical Strain on Extracellular Matrix Gene Expression in Human Lamina Cribrosa Cells In Vitro. Mol. Vis. 11, 798–810.
Kirwan, R. P., Leonard, M. O., Murphy, M., Clark, A. F., and O'Brien, C. J. (2005b). Transforming Growth Factor-β-Regulated Gene Transcription and Protein Expression in Human GFAP-Negative Lamina Cribrosa Cells. Glia 52 (4), 309–324. doi:10.1002/glia.20247
Kuchtey, J., and Kuchtey, R. W. (2014). The Microfibril Hypothesis of Glaucoma: Implications for Treatment of Elevated Intraocular Pressure. J. Ocul. Pharmacol. Ther. 30 (2-3), 170–180. doi:10.1089/jop.2013.0184
Kwon, Y. H., Fingert, J. H., Kuehn, M. H., and Alward, W. L. M. (2009). Primary Open-Angle Glaucoma. N. Engl. J. Med. 360 (11), 1113–1124. doi:10.1056/nejmra0804630
Lahav, J., Dardik, R., and Stein, O. (1987). Endothelial Cell Thrombospondin and its Possible Role in Cell Adhesion. Semin. Thromb. Hemost. 13 (3), 352–360. doi:10.1055/s-2007-1003511
Last, J. A., Pan, T., Ding, Y., Reilly, C. M., Keller, K., Acott, T. S., et al. (2011). Elastic Modulus Determination of Normal and Glaucomatous Human Trabecular Meshwork. Invest. Ophthalmol. Vis. Sci. 52 (5), 2147–2152. doi:10.1167/iovs.10-6342
Lauffenburger, D. A., and Horwitz, A. F. (1996). Cell Migration: a Physically Integrated Molecular Process. Cell 84 (3), 359–369. doi:10.1016/s0092-8674(00)81280-5
Lawler, J., Sunday, M., Thibert, V., Duquette, M., George, E. L., Rayburn, H., et al. (1998). Thrombospondin-1 Is Required for Normal Murine Pulmonary Homeostasis and its Absence Causes Pneumonia. J. Clin. Invest. 101 (5), 982–992. doi:10.1172/jci1684
Lawler, P. R., and Lawler, J. (2012). Molecular Basis for the Regulation of Angiogenesis by Thrombospondin-1 and -2. Cold Spring Harb. Perspect. Med. 2 (5), a006627. doi:10.1101/cshperspect.a006627
Lee, N. V., Sato, M., Annis, D. S., Loo, J. A., Wu, L., Mosher, D. F., et al. (2006). ADAMTS1 Mediates the Release of Antiangiogenic Polypeptides from TSP1 and 2. EMBO J. 25 (22), 5270–5283. doi:10.1038/sj.emboj.7601400
Li, S. S., Forslöw, A., and Sundqvist, K.-G. (2005). Autocrine Regulation of T Cell Motility by Calreticulin-Thrombospondin-1 Interaction. J. Immunol. 174 (2), 654–661. doi:10.4049/jimmunol.174.2.654
Lillis, A. P., Van Duyn, L. B., Murphy-Ullrich, J. E., and Strickland, D. K. (2008). LDL Receptor-Related Protein 1: Unique Tissue-specific Functions Revealed by Selective Gene Knockout Studies. Physiol. Rev. 88 (3), 887–918. doi:10.1152/physrev.00033.2007
Liotta, L. A., and Kohn, E. (2004). Cancer and the Homeless Cell. Nature 430 (7003), 973–974. doi:10.1038/430973a
Liu, C. C., Leclair, P., Monajemi, M., Sly, L. M., Reid, G. S., and Lim, C. J. (2016). α-Integrin Expression and Function Modulates Presentation of Cell Surface Calreticulin. Cell Death Dis. 7, e2268. doi:10.1038/cddis.2016.176
Liu, Y., and Vollrath, D. (2004). Reversal of Mutant Myocilin Non-secretion and Cell Killing: Implications for Glaucoma. Hum. Mol. Genet. 13 (11), 1193–1204. doi:10.1093/hmg/ddh128
Liu, Z., Li, S., Qian, X., Li, L., Zhang, H., and Liu, Z. (2021). RhoA/ROCK-YAP/TAZ Axis Regulates the Fibrotic Activity in Dexamethasone-Treated Human Trabecular Meshwork Cells. Front. Mol. Biosci. 8, 728932. doi:10.3389/fmolb.2021.728932
Lu, A., Pallero, M. A., Owusu, B. Y., Borovjagin, A. V., Lei, W., Sanders, P. W., et al. (2020). Calreticulin Is Important for the Development of Renal Fibrosis and Dysfunction in Diabetic Nephropathy. Matrix Biol. Plus 8, 100034. doi:10.1016/j.mbplus.2020.100034
Lütjen-Drecoll, E. (1999). Functional Morphology of the Trabecular Meshwork in Primate Eyes. Prog. Retin. Eye Res. 18 (1), 91–119. doi:10.1016/s1350-9462(98)00011-1
Mansergh, F. C., Wride, M. A., Walker, V. E., Adams, S., Hunter, S. M., and Evans, M. J. (2004). Gene Expression Changes during Cataract Progression in Sparc Null Mice: Differential Regulation of Mouse Globins in the Lens. Mol. Vis. 10, 490–511.
Mansfield, P. J., Boxer, L. A., and Suchard, S. J. (1990). Thrombospondin Stimulates Motility of Human Neutrophils. J. Cell Biol. 111 (6 Pt 2), 3077–3086. doi:10.1083/jcb.111.6.3077
Mansfield, P. J., and Suchard, S. J. (1994). Thrombospondin Promotes Chemotaxis and Haptotaxis of Human Peripheral Blood Monocytes. J. Immunol. 153 (9), 4219–4229.
McGrady, N. R., Pasini, S., Baratta, R. O., Del Buono, B. J., Schlumpf, E., and Calkins, D. J. (2021). Restoring the Extracellular Matrix: A Neuroprotective Role for Collagen Mimetic Peptides in Experimental Glaucoma. Front. Pharmacol. 12, 764709. doi:10.3389/fphar.2021.764709
Meredith, J. E., Fazeli, B., and Schwartz, M. A. (1993). The Extracellular Matrix as a Cell Survival Factor. MBoC 4 (9), 953–961. doi:10.1091/mbc.4.9.953
Michalak, M., Corbett, E. F., Mesaeli, N., Nakamura, K., and Opas, M. (1999). Calreticulin: One Protein, One Gene, Many Functions. Biochem. J. 344, 281–292. doi:10.1042/bj3440281
Michalak, M., Groenendyk, J., Szabo, E., Gold, L. I., and Opas, M. (2009). Calreticulin, a Multi-Process Calcium-Buffering Chaperone of the Endoplasmic Reticulum. Biochem. J. 417 (3), 651–666. doi:10.1042/bj20081847
Midwood, K. S., Chiquet, M., Tucker, R. P., and Orend, G. (2016). Tenascin-C at a Glance. J. Cell Sci. 129 (23), 4321–4327. doi:10.1242/jcs.190546
Midwood, K. S., Mao, Y., Hsia, H. C., Valenick, L. V., and Schwarzbauer, J. E. (2006). Modulation of Cell-Fibronectin Matrix Interactions during Tissue Repair. J. Investigative Dermatology Symposium Proc. 11 (1), 73–78. doi:10.1038/sj.jidsymp.5650005
Midwood, K. S., Valenick, L. V., Hsia, H. C., and Schwarzbauer, J. E. (2004). Coregulation of Fibronectin Signaling and Matrix Contraction by Tenascin-C and Syndecan-4. MBoC 15 (12), 5670–5677. doi:10.1091/mbc.e04-08-0759
Mikhailenko, I., Kounnas, M. Z., and Strickland, D. K. (1995). Low Density Lipoprotein Receptor-Related Protein/α2-Macroglobulin Receptor Mediates the Cellular Internalization and Degradation of Thrombospondin. J. Biol. Chem. 270 (16), 9543–9549. doi:10.1074/jbc.270.16.9543
Mikhailenko, I., Krylov, D., Argraves, K. M., Roberts, D. D., Liau, G., and Strickland, D. K. (1997). Cellular Internalization and Degradation of Thrombospondin-1 Is Mediated by the Amino-Terminal Heparin Binding Domain (HBD). J. Biol. Chem. 272 (10), 6784–6791. doi:10.1074/jbc.272.10.6784
Morandi, V., Petrik, J., and Lawler, J. (2021). Endothelial Cell Behavior Is Determined by Receptor Clustering Induced by Thrombospondin-1. Front. Cell Dev. Biol. 9, 664696. doi:10.3389/fcell.2021.664696
Murphy, R., Irnaten, M., Hopkins, A., O'Callaghan, J., Stamer, W. D., Clark, A. F., et al. (2022). Matrix Mechanotransduction via Yes-Associated Protein in Human Lamina Cribrosa Cells in Glaucoma. Invest. Ophthalmol. Vis. Sci. 63 (1), 16. doi:10.1167/iovs.63.1.16
Murphy-Ullrich, J. E., Pallero, M. A., Boerth, N., Greenwood, J. A., Lincoln, T. M., and Cornwell, T. L. (1996). Cyclic GMP-dependent Protein Kinase Is Required for Thrombospondin and Tenascin Mediated Focal Adhesion Disassembly. J. Cell Sci. 109 (Pt 10), 2499–2508. doi:10.1242/jcs.109.10.2499
Murphy-Ullrich, J. E., and Downs, J. C. (2015). The Thrombospondin1-TGF-β Pathway and Glaucoma. J. Ocular Pharmacol. Ther. 31 (7), 371–375. doi:10.1089/jop.2015.0016
Murphy-Ullrich, J. E., Gurusiddappa, S., Frazier, W. A., and Höök, M. (1993). Heparin-binding Peptides from Thrombospondins 1 and 2 Contain Focal Adhesion-Labilizing Activity. J. Biol. Chem. 268 (35), 26784–26789. doi:10.1016/s0021-9258(19)74381-6
Murphy-Ullrich, J. E., and Höök, M. (1989). Thrombospondin Modulates Focal Adhesions in Endothelial Cells. J. Cell Biol. 109 (3), 1309–1319. doi:10.1083/jcb.109.3.1309
Murphy-Ullrich, J. E., Lane, T. F., Pallero, M. A., and Sage, E. H. (1995). SPARC Mediates Focal Adhesion Disassembly in Endothelial Cells through a Follistatin-like Region and the Ca2+-Binding EF-Hand. J. Cell. Biochem. 57 (2), 341–350. doi:10.1002/jcb.240570218
Murphy-Ullrich, J. E., Lightner, V. A., Aukhil, I., Yan, Y. Z., Erickson, H. P., and Hook, M. (1991). Focal Adhesion Integrity Is Downregulated by the Alternatively Spliced Domain of Human Tenascin [published Erratum Appears in J Cell Biol 1992 Feb;116(3):833]. J. Cell Biol. 115 (4), 1127–1136. doi:10.1083/jcb.115.4.1127
Murphy-Ullrich, J. E., and Sage, E. H. (2014). Revisiting the Matricellular Concept. Matrix Biol. 37, 1–14. doi:10.1016/j.matbio.2014.07.005
Murphy-Ullrich, J. E., and Suto, M. J. (2018). Thrombospondin-1 Regulation of Latent TGF-β Activation: A Therapeutic Target for Fibrotic Disease. Matrix Biol. 68-69, 28–43. doi:10.1016/j.matbio.2017.12.009
Murphy-Ullrich, J. E. (2001). The De-adhesive Activity of Matricellular Proteins: Is Intermediate Cell Adhesion an Adaptive State? J. Clin. Invest. 107 (7), 785–790. doi:10.1172/jci12609
Murphy-Ullrich, J. E. (2019). Thrombospondin 1 and its Diverse Roles as a Regulator of Extracellular Matrix in Fibrotic Disease. J. Histochem Cytochem. 67 (9), 683–699. doi:10.1369/0022155419851103
Naba, A., Clauser, K. R., Hoersch, S., Liu, H., Carr, S. A., and Hynes, R. O. (2012). The Matrisome: In Silico Definition and In Vivo Characterization by Proteomics of Normal and Tumor Extracellular Matrices. Mol. Cell Proteomics 11 (4), M111–M014647. doi:10.1074/mcp.M111.014647
Naba, A., Clauser, K. R., Ding, H., Whittaker, C. A., Carr, S. A., and Hynes, R. O. (2016). The Extracellular Matrix: Tools and Insights for the "omics" Era. Matrix Biol. 49, 10–24. doi:10.1016/j.matbio.2015.06.003
Nanney, L. B., Woodrell, C. D., Greives, M. R., Cardwell, N. L., Pollins, A. C., Bancroft, T. A., et al. (2008). Calreticulin Enhances Porcine Wound Repair by Diverse Biological Effects. Am. J. Pathology 173 (3), 610–630. doi:10.2353/ajpath.2008.071027
Nde, P. N., Lima, M. F., Johnson, C. A., Pratap, S., and Villalta, F. (2012). Regulation and Use of the Extracellular Matrix by Trypanosoma Cruzi during Early Infection. Front. Immun. 3, 337. doi:10.3389/fimmu.2012.00337
Norose, K., Lo, W.-K., Clark, J. I., Sage, E. H., and Howe, C. C. (2000). Lenses of SPARC-Null Mice Exhibit an Abnormal Cell Surface-Basement Membrane Interface. Exp. Eye Res. 71 (3), 295–307. doi:10.1006/exer.2000.0884
Nunes, S. S., Outeiro-Bernstein, M. A. F. d., Juliano, L., Vardiero, F., Nader, H. B., Woods, A., et al. (2008). Syndecan-4 Contributes to Endothelial Tubulogenesis through Interactions with Two Motifs inside the Pro-angiogenic N-Terminal Domain of Thrombospondin-1. J. Cell. Physiol. 214 (3), 828–837. doi:10.1002/jcp.21281
Obeid, M., Tesniere, A., Ghiringhelli, F., Fimia, G. M., Apetoh, L., Perfettini, J.-L., et al. (2007). Calreticulin Exposure Dictates the Immunogenicity of Cancer Cell Death. Nat. Med. 13 (1), 54–61. doi:10.1038/nm1523
Ogden, C. A., deCathelineau, A., Hoffmann, P. R., Bratton, D., Ghebrehiwet, B., Fadok, V. A., et al. (2001). C1q and Mannose Binding Lectin Engagement of Cell Surface Calreticulin and CD91 Initiates Macropinocytosis and Uptake of Apoptotic Cells. J. Exp. Med. 194 (6), 781–796. doi:10.1084/jem.194.6.781
Orr, A. W., Elzie, C. A., Kucik, D. F., and Murphy-Ullrich, J. E. (2003a). Thrombospondin Signaling through the Calreticulin/LDL Receptor-Related Protein Co-complex Stimulates Random and Directed Cell Migration. J. Cell Sci. 116 (Pt 14), 2917–2927. doi:10.1242/jcs.00600
Orr, A. W., Pallero, M. A., and Murphy-Ullrich, J. E. (2002). Thrombospondin Stimulates Focal Adhesion Disassembly through Gi- and Phosphoinositide 3-kinase-dependent ERK Activation. J. Biol. Chem. 277 (23), 20453–20460. doi:10.1074/jbc.m112091200
Orr, A. W., Pallero, M. A., Xiong, W.-C., and Murphy-Ullrich, J. E. (2004). Thrombospondin Induces RhoA Inactivation through FAK-dependent Signaling to Stimulate Focal Adhesion Disassembly. J. Biol. Chem. 279 (47), 48983–48992. doi:10.1074/jbc.m404881200
Orr, A. W., Pedraza, C. E., Pallero, M. A., Elzie, C. A., Goicoechea, S., Strickland, D. K., et al. (2003b). Low Density Lipoprotein Receptor-Related Protein Is a Calreticulin Coreceptor that Signals Focal Adhesion Disassembly. J. Cell Biol. 161 (6), 1179–1189. doi:10.1083/jcb.200302069
Owusu, B. Y., Zimmerman, K. A., and Murphy-Ullrich, J. E. (2018). The Role of the Endoplasmic Reticulum Protein Calreticulin in Mediating TGF-β-Stimulated Extracellular Matrix Production in Fibrotic Disease. J. Cell Commun. Signal. 12 (1), 289–299. doi:10.1007/s12079-017-0426-2
Pallero, M. A., Elzie, C. A., Chen, J., Mosher, D. F., and Murphy‐Ullrich, J. E. (2008). Thrombospondin 1 Binding to calreticulin‐LRP1 Signals Resistance to Anoikis. FASEB J. 22 (11), 3968–3979. doi:10.1096/fj.07-104802
Pandya, U. M., Manzanares, M. A., Tellechea, A., Egbuta, C., Daubriac, J., Jimenez‐Jaramillo, C., et al. (2020). Calreticulin Exploits TGF‐β for Extracellular Matrix Induction Engineering a Tissue Regenerative Process. FASEB J. 34 (12), 15849–15874. doi:10.1096/fj.202001161r
Patwardhan, S., Mahadik, P., Shetty, O., and Sen, S. (2021). ECM Stiffness-Tuned Exosomes Drive Breast Cancer Motility through Thrombospondin-1. Biomaterials 279, 121185. doi:10.1016/j.biomaterials.2021.121185
Pena, J. D. O., Netland, P. A., Vidal, I., Dorr, D. A., Rasky, A., and Hernandez, M. R. (1998). Elastosis of the Lamina Cribrosa in Glaucomatous Optic Neuropathy. Exp. Eye Res. 67 (5), 517–524. doi:10.1006/exer.1998.0539
Peters, J. C., Bhattacharya, S., Clark, A. F., and Zode, G. S. (2015). Increased Endoplasmic Reticulum Stress in Human Glaucomatous Trabecular Meshwork Cells and Tissues. Invest. Ophthalmol. Vis. Sci. 56 (6), 3860–3868. doi:10.1167/iovs.14-16220
Powell, S., Irnaten, M., and O’Brien, C. (2022). Glaucoma - 'A Stiff Eye in a Stiff Body'. Curr. Eye Res., 1–9. doi:10.1080/02713683.2022.2039204
Rabhi-Sabile, S., Pidard, D., Lawler, J., Renesto, P., Chignard, M., and Legrand, C. (1996). Proteolysis of Thrombospondin during Cathepsin-G-Induced Platelet Aggregation: Functional Role of the 165-kDa Carboxy-Terminal Fragment. FEBS Lett. 386 (1), 82–86. doi:10.1016/0014-5793(96)00408-5
Raghunathan, V. K., Morgan, J. T., Dreier, B., Reilly, C. M., Thomasy, S. M., Wood, J. A., et al. (2013). Role of Substratum Stiffness in Modulating Genes Associated with Extracellular Matrix and Mechanotransducers YAP and TAZ. Invest. Ophthalmol. Vis. Sci. 54 (1), 378–386. doi:10.1167/iovs.12-11007
Raugi, G. J., Mumby, S. M., Ready, C. A., and Bornstein, P. (1984). Location and Partial Characterization of the Heparin-Binding Fragment of Platelet Thrombospondin. Thrombosis Res. 36 (2), 165–175. doi:10.1016/0049-3848(84)90338-4
Rege, T. A., Pallero, M. A., Gomez, C., Grenett, H. E., Murphy-Ullrich, J. E., and Hagood, J. S. (2006). Thy-1, via its GPI Anchor, Modulates Src Family Kinase and Focal Adhesion Kinase Phosphorylation and Subcellular Localization, and Fibroblast Migration, in Response to Thrombospondin-1/hep I. Exp. Cell Res. 312 (19), 3752–3767. doi:10.1016/j.yexcr.2006.07.029
Reinhard, J., Wiemann, S., Hildebrandt, S., and Faissner, A. (2021). Extracellular Matrix Remodeling in the Retina and Optic Nerve of a Novel Glaucoma Mouse Model. Biol. (Basel) 10 (3). doi:10.3390/biology10030169
Resovi, A., Pinessi, D., Chiorino, G., and Taraboletti, G. (2014). Current Understanding of the Thrombospondin-1 Interactome. Matrix Biol. 37, 83–91. doi:10.1016/j.matbio.2014.01.012
Rhee, D. J., Haddadin, R. I., Kang, M. H., and Oh, D.-J. (2009). Matricellular Proteins in the Trabecular Meshwork. Exp. Eye Res. 88 (4), 694–703. doi:10.1016/j.exer.2008.11.032
Ribeiro, M., McGrady, N. R., Baratta, R. O., Del Buono, B. J., Schlumpf, E., and Calkins, D. J. (2022). Intraocular Delivery of a Collagen Mimetic Peptide Repairs Retinal Ganglion Cell Axons in Chronic and Acute Injury Models. Int. J. Mol. Sci. 23 (6). doi:10.3390/ijms23062911
Roberti, G., Oddone, F., Agnifili, L., Katsanos, A., Michelessi, M., Mastropasqua, L., et al. (2020). Steroid-induced Glaucoma: Epidemiology, Pathophysiology, and Clinical Management. Surv. Ophthalmol. 65 (4), 458–472. doi:10.1016/j.survophthal.2020.01.002
Rosini, S., Pugh, N., Bonna, A. M., Hulmes, D. J. S., Farndale, R. W., and Adams, J. C. (2018). Thrombospondin-1 Promotes Matrix Homeostasis by Interacting with Collagen and Lysyl Oxidase Precursors and Collagen Cross-Linking Sites. Sci. Signal 11 (532). doi:10.1126/scisignal.aar2566
Rusnati, M., Borsotti, P., Moroni, E., Foglieni, C., Chiodelli, P., Carminati, L., et al. (2019). The Calcium-Binding Type III Repeats Domain of Thrombospondin-2 Binds to Fibroblast Growth Factor 2 (FGF2). Angiogenesis 22 (1), 133–144. doi:10.1007/s10456-018-9644-3
Sage, E. H., and Bornstein, P. (1991). Extracellular Proteins that Modulate Cell-Matrix Interactions. SPARC, Tenascin, and Thrombospondin. J. Biol. Chem. 266 (23), 14831–14834. doi:10.1016/s0021-9258(18)98545-5
Sawhney, R. (2002). Expression and Regulation of SPARC, Fibronectin, and Collagen IV by Dexamethasone in Lens Epithelial Cells. Cell Biol. Int. 26 (11), 971–983. doi:10.1006/cbir.2002.0955
Semba, R. D., Zhang, P., Dufresne, C., Gao, T., Al-Jadaan, I., Craven, E. R., et al. (2021). Primary Angle Closure Glaucoma Is Characterized by Altered Extracellular Matrix Homeostasis in the Iris. Proteomics Clin. Appl. 15 (6), e2000094. doi:10.1002/prca.202000094
Settles, D. L., Kusakabe, M., Steindler, D. A., Fillmore, H., and Erickson, H. P. (1997). Tenascin-C Knockout Mouse Has No Detectable Tenascin-C Protein. J. Neurosci. Res. 47 (1), 109–117. doi:10.1002/(sici)1097-4547(19970101)47:1<109::aid-jnr12>3.0.co;2-0
Shan, S. W., Do, C. W., Lam, T. C., Li, H. L., Stamer, W. D., and To, C. H. (2021). Thrombospondin‐1 Mediates Rho‐kinase Inhibitor‐induced Increase in Outflow‐facility. J. Cell Physiol. 236 (12), 8226–8238. doi:10.1002/jcp.30492
Sharma, R., and Grover, A. (2021). Myocilin-associated Glaucoma: A Historical Perspective and Recent Research Progress. Mol. Vis. 27, 480–493.
Shen, J., Cao, B., Wang, Y., Ma, C., Zeng, Z., Liu, L., et al. (2018). Hippo Component YAP Promotes Focal Adhesion and Tumour Aggressiveness via Transcriptionally Activating THBS1/FAK Signalling in Breast Cancer. J. Exp. Clin. Cancer Res. 37 (1), 175. doi:10.1186/s13046-018-0850-z
Shen, X., Koga, T., Park, B.-C., SundarRaj, N., and Yue, B. Y. J. T. (2008). Rho GTPase and cAMP/protein Kinase A Signaling Mediates Myocilin-Induced Alterations in Cultured Human Trabecular Meshwork Cells. J. Biol. Chem. 283 (1), 603–612. doi:10.1074/jbc.m708250200
Shi, Z., Xu, L., Xie, H., Ouyang, R., Ke, Y., Zhou, R., et al. (2020). Attenuation of Intermittent Hypoxia-Induced Apoptosis and Fibrosis in Pulmonary Tissues via Suppression of ER Stress Activation. BMC Pulm. Med. 20 (1), 92. doi:10.1186/s12890-020-1123-0
Stenina, O. I., Ustinov, V., Krukovets, I., Marinic, T., Topol, E. J., and Plow, E. F. (2005). Polymorphisms A387P in Thrombospondin‐4 and N700S in Thrombospondin‐1 Perturb Calcium Binding Sites. FASEB J. 19 (13), 1893–1895. doi:10.1096/fj.05-3712fje
Stenina-Adognravi, O. (2014). Invoking the Power of Thrombospondins: Regulation of Thrombospondins Expression. Matrix Biol. 37, 69–82. doi:10.1016/j.matbio.2014.02.001
Stone, E. M., Fingert, J. H., Alward, W. L. M., Nguyen, T. D., Polansky, J. R., Sunden, S. L. F., et al. (1997). Identification of a Gene that Causes Primary Open Angle Glaucoma. Science 275 (5300), 668–670. doi:10.1126/science.275.5300.668
Streit, M., Velasco, P., Brown, L. F., Skobe, M., Richard, L., Riccardi, L., et al. (1999). Overexpression of Thrombospondin-1 Decreases Angiogenesis and Inhibits the Growth of Human Cutaneous Squamous Cell Carcinomas. Am. J. Pathology 155 (2), 441–452. doi:10.1016/s0002-9440(10)65140-1
Strickland, D. K., Kounnas, M. Z., and Argraves, W. S. (1995). LDL Receptor‐related Protein: a Multiligand Receptor for Lipoprotein and Proteinase Catabolism. FASEB J. 9 (10), 890–898. doi:10.1096/fasebj.9.10.7615159
Summers Rada, J. A., Shelton, S., and Norton, T. T. (2006). The Sclera and Myopia. Exp. Eye Res. 82 (2), 185–200. doi:10.1016/j.exer.2005.08.009
Sun, J., Hopkins, B. D., Tsujikawa, K., Perruzzi, C., Adini, I., Swerlick, R., et al. (2009). Thrombospondin-1 Modulates VEGF-A-Mediated Akt Signaling and Capillary Survival in the Developing Retina. Am. J. Physiology-Heart Circulatory Physiology 296 (5), H1344–H1351. doi:10.1152/ajpheart.01246.2008
Sweetwyne, M. T., and Murphy-Ullrich, J. E. (2012). Thrombospondin1 in Tissue Repair and Fibrosis: TGF-β-dependent and Independent Mechanisms. Matrix Biol. 31 (3), 178–186. doi:10.1016/j.matbio.2012.01.006
Sweetwyne, M. T., Pallero, M. A., Lu, A., Van Duyn Graham, L., and Murphy-Ullrich, J. E. (2010). The Calreticulin-Binding Sequence of Thrombospondin 1 Regulates Collagen Expression and Organization during Tissue Remodeling. Am. J. pathology 177 (4), 1710–1724. doi:10.2353/ajpath.2010.090903
Tamm, E. R. (2002). Myocilin and Glaucoma: Facts and Ideas. Prog. Retin. Eye Res. 21 (4), 395–428. doi:10.1016/s1350-9462(02)00010-1
Taraboletti, G., Roberts, D. D., and Liotta, L. A. (1987). Thrombospondin-induced Tumor Cell Migration: Haptotaxis and Chemotaxis Are Mediated by Different Molecular Domains. J. Cell Biol. 105 (5), 2409–2415. doi:10.1083/jcb.105.5.2409
Taraboletti, G., Roberts, D., Liotta, L. A., and Giavazzi, R. (1990). Platelet Thrombospondin Modulates Endothelial Cell Adhesion, Motility, and Growth: a Potential Angiogenesis Regulatory Factor. J. cell Biol. 111 (2), 765–772. doi:10.1083/jcb.111.2.765
Tektas, O.-Y., and Lütjen-Drecoll, E. (2009). Structural Changes of the Trabecular Meshwork in Different Kinds of Glaucoma. Exp. Eye Res. 88 (4), 769–775. doi:10.1016/j.exer.2008.11.025
Tham, Y.-C., Li, X., Wong, T. Y., Quigley, H. A., Aung, T., and Cheng, C.-Y. (2014). Global Prevalence of Glaucoma and Projections of Glaucoma Burden through 2040. Ophthalmology 121 (11), 2081–2090. doi:10.1016/j.ophtha.2014.05.013
Thannickal, V. J., and Horowitz, J. C. (2006). Evolving Concepts of Apoptosis in Idiopathic Pulmonary Fibrosis. Proc. Am. Thorac. Soc. 3 (4), 350–356. doi:10.1513/pats.200601-001tk
Tolsma, S., Volpert, O., Good, D., Frazier, W., Polverini, P., and Bouck, N. (1993). Peptides Derived from Two Separate Domains of the Matrix Protein Thrombospondin-1 Have Anti-angiogenic Activity. J. Cell Biol. 122 (2), 497–511. doi:10.1083/jcb.122.2.497
Tripathi, B. J., Tripathi, R. C., Yang, C., Millard, C. B., and Dixit, V. M. (1991). Synthesis of a Thrombospondin-like Cytoadhesion Molecule by Cells of the Trabecular Meshwork. Invest Ophthalmol. Vis. Sci. 32 (1), 181–188.
Tuszynski, G. P., Rothman, V., Murphy, A., Siegler, K., Smith, L., Smith, S., et al. (1987). Thrombospondin Promotes Cell-Substratum Adhesion. Science 236 (4808), 1570–1573. doi:10.1126/science.2438772
Van Duyn Graham, L., Sweetwyne, M. T., Pallero, M. A., and Murphy-Ullrich, J. E. (2010). Intracellular Calreticulin Regulates Multiple Steps in Fibrillar Collagen Expression, Trafficking, and Processing into the Extracellular Matrix. J. Biol. Chem. 285 (10), 7067–7078. doi:10.1074/jbc.m109.006841
Vanhoutte, D., Schips, T. G., Vo, A., Grimes, K. M., Baldwin, T. A., Brody, M. J., et al. (2021). Thbs1 Induces Lethal Cardiac Atrophy through PERK-ATF4 Regulated Autophagy. Nat. Commun. 12 (1), 3928. doi:10.1038/s41467-021-24215-4
Vittal, V., Rose, A., Gregory, K. E., Kelley, M. J., and Acott, T. S. (2005). Changes in Gene Expression by Trabecular Meshwork Cells in Response to Mechanical Stretching. Invest. Ophthalmol. Vis. Sci. 46 (8), 2857–2868. doi:10.1167/iovs.05-0075
Vranka, J. A., Bradley, J. M., Yang, Y.-F., Keller, K. E., and Acott, T. S. (2015a). Mapping Molecular Differences and Extracellular Matrix Gene Expression in Segmental Outflow Pathways of the Human Ocular Trabecular Meshwork. PLoS One 10 (3), e0122483. doi:10.1371/journal.pone.0122483
Vranka, J. A., Kelley, M. J., Acott, T. S., and Keller, K. E. (2015b). Extracellular Matrix in the Trabecular Meshwork: Intraocular Pressure Regulation and Dysregulation in Glaucoma. Exp. Eye Res. 133, 112–125. doi:10.1016/j.exer.2014.07.014
Wallace, D. M., Murphy-Ullrich, J. E., Downs, J. C., and O'Brien, C. J. (2014). The Role of Matricellular Proteins in Glaucoma. Matrix Biol. 37, 174–182. doi:10.1016/j.matbio.2014.03.007
Wang, K., Read, A. T., Sulchek, T., and Ethier, C. R. (2017). Trabecular Meshwork Stiffness in Glaucoma. Exp. Eye Res. 158, 3–12. doi:10.1016/j.exer.2016.07.011
Wang, L., Murphy-Ullrich, J. E., and Song, Y. (2014). Molecular Insight into the Effect of Lipid Bilayer Environments on Thrombospondin-1 and Calreticulin Interactions. Biochemistry 53 (40), 6309–6322. doi:10.1021/bi500662v
Wang, L., Murphy-Ullrich, J. E., and Song, Y. (2019). Multiscale Simulation of the Interaction of Calreticulin-Thrombospondin-1 Complex with a Model Membrane Microdomain. J. Biomol. Struct. Dyn. 37 (3), 811–822. doi:10.1080/07391102.2018.1433065
Wang, L., and Song, Y. (2020). Molecular Insights into the Effect of an Apoptotic Raft-like Bilayer on the Conformation and Dynamics of Calreticulin. Biochimica Biophysica Acta (BBA) - Biomembr. 1862 (2), 183146. doi:10.1016/j.bbamem.2019.183146
Wang, S., Herndon, M. E., Ranganathan, S., Godyna, S., Lawler, J., Argraves, W. S., et al. (2004). Internalization but Not Binding of Thrombospondin-1 to Low Density Lipoprotein Receptor-Related Protein-1 Requires Heparan Sulfate Proteoglycans. J. Cell. Biochem. 91 (4), 766–776. doi:10.1002/jcb.10781
Warburton, D., and Kaartinen, V. (2007). When the Lung Is Stretched, Could it Be Thrombospondin via TGFbeta1 Peptide Activation? J. Physiol. 584 (Pt 2), 365. doi:10.1113/jphysiol.2007.144394
Webb, D. J., Parsons, J. T., and Horwitz, A. F. (2002). Adhesion Assembly, Disassembly and Turnover in Migrating Cells - over and over and over Again. Nat. Cell Biol. 4 (4), E97–E100. doi:10.1038/ncb0402-e97
Wentz-Hunter, K., Shen, X., Okazaki, K., Tanihara, H., and Yue, B. Y. J. T. (2004). Overexpression of Myocilin in Cultured Human Trabecular Meshwork Cells. Exp. Cell Res. 297 (1), 39–48. doi:10.1016/j.yexcr.2004.02.024
Wiersma, V. R., Michalak, M., Abdullah, T. M., Bremer, E., and Eggleton, P. (2015). Mechanisms of Translocation of ER Chaperones to the Cell Surface and Immunomodulatory Roles in Cancer and Autoimmunity. Front. Oncol. 5, 7. doi:10.3389/fonc.2015.00007
Wirtz, M. K., Sykes, R., Samples, J., Edmunds, B., Choi, D., Keene, D. R., et al. (2022). Identification of Missense Extracellular Matrix Gene Variants in a Large Glaucoma Pedigree and Investigation of the N700S Thrombospondin-1 Variant in Normal and Glaucomatous Trabecular Meshwork Cells. Curr. Eye Res. 47 (1), 79–90. doi:10.1080/02713683.2021.1945109
Wu, H.-J., Kuchtey, J., and Kuchtey, R. W. (2020). Increased Susceptibility to Glaucomatous Damage in Microfibril Deficient Mice. Invest. Ophthalmol. Vis. Sci. 61 (10), 28. doi:10.1167/iovs.61.10.28
Xia, H., Nho, R. S., Kahm, J., Kleidon, J., and Henke, C. A. (2004). Focal Adhesion Kinase Is Upstream of Phosphatidylinositol 3-Kinase/Akt in Regulating Fibroblast Survival in Response to Contraction of Type I Collagen Matrices via a β1 Integrin Viability Signaling Pathway. J. Biol. Chem. 279 (31), 33024–33034. doi:10.1074/jbc.m313265200
Yam, G. H.-F., Gaplovska-Kysela, K., Zuber, C., and Roth, J. r. (2007). Sodium 4-phenylbutyrate Acts as a Chemical Chaperone on Misfolded Myocilin to Rescue Cells from Endoplasmic Reticulum Stress and Apoptosis. Invest. Ophthalmol. Vis. Sci. 48 (4), 1683–1690. doi:10.1167/iovs.06-0943
Yamaki, N., Negishi, M., and Katoh, H. (2007). RhoG Regulates Anoikis through a Phosphatidylinositol 3-kinase-dependent Mechanism. Exp. Cell Res. 313 (13), 2821–2832. doi:10.1016/j.yexcr.2007.05.010
Yamashiro, Y., Thang, B. Q., Ramirez, K., Shin, S. J., Kohata, T., Ohata, S., et al. (2020). Matrix Mechanotransduction Mediated by Thrombospondin-1/integrin/YAP in the Vascular Remodeling. Proc. Natl. Acad. Sci. U.S.A. 117 (18), 9896–9905. doi:10.1073/pnas.1919702117
Yan, Q., Blake, D., Clark, J. I., and Sage, E. H. (2003). Expression of the Matricellular Protein SPARC in Murine Lens: SPARC Is Necessary for the Structural Integrity of the Capsular Basement Membrane. J. Histochem Cytochem. 51 (4), 503–511. doi:10.1177/002215540305100412
Yan, Q., Murphy-Ullrich, J. E., and Song, Y. (2011). Molecular and Structural Insight into the Role of Key Residues of Thrombospondin-1 and Calreticulin in Thrombospondin-1−Calreticulin Binding. Biochemistry 50 (4), 566–573. doi:10.1021/bi101639y
Yan, Q., Murphy-Ullrich, J. E., and Song, Y. (2010). Structural Insight into the Role of Thrombospondin-1 Binding to Calreticulin in Calreticulin-Induced Focal Adhesion Disassembly. Biochemistry 49 (17), 3685–3694. doi:10.1021/bi902067f
Yan, X., Wu, S., Liu, Q., Li, Y., Zhu, W., and Zhang, J. (2020). Accumulation of Asn450Tyr Mutant Myocilin in ER Promotes Apoptosis of Human Trabecular Meshwork Cells. Mol. Vis. 26, 563–573.
Yang, J. L., Neufeld, A. H., Zorn, M. B., and Hernandez, M. R. (1993). Collagen Type I mRNA Levels in Cultured Human Lamina Cribrosa Cells: Effects of Elevated Hydrostatic Pressure. Exp. Eye Res. 56 (5), 567–574. doi:10.1006/exer.1993.1070
Yue, L. L., and Du, X. (2022). Thrombospondin 1 Promotes Endoplasmic Reticulum Stress and Apoptosis in HK-2 Cells by Upregulating ATF6-CHOP. Curr. Med. Sci. doi:10.1007/s11596-022-2513-8
Yun, H., Zhou, Y., Wills, A., and Du, Y. (2016). Stem Cells in the Trabecular Meshwork for Regulating Intraocular Pressure. J. Ocular Pharmacol. Ther. 32 (5), 253–260. doi:10.1089/jop.2016.0005
Zahir, N., Lakins, J. N., Russell, A., Ming, W., Chatterjee, C., Rozenberg, G. I., et al. (2003). Autocrine Laminin-5 Ligates α6β4 Integrin and Activates RAC and NFκB to Mediate Anchorage-independent Survival of Mammary Tumors. J. Cell Biol. 163 (6), 1397–1407. doi:10.1083/jcb.200302023
Zhou, L., Li, Y., and Yue, B. Y. J. T. (1999). Alteration of Cytoskeletal Structure, Integrin Distribution, and Migratory Activity by Phagocytic Challenge in Cells from an Ocular Tissue-The Trabecular Meshwork. Vitro Cell.Dev.Biol.-Animal 35 (3), 144–149. doi:10.1007/s11626-999-0016-6
Zhou, X., Huang, J., Chen, J., Zhao, J., Ge, D., Yang, W., et al. (2004). Genetic Association Analysis of Myocardial Infarction with Thrombospondin-1 N700S Variant in a Chinese Population. Thromb. Res. 113 (3-4), 181–186. doi:10.1016/j.thromres.2004.02.016
Zhu, J. D., Xie, L. L., Li, Z. Y., and Lu, X. H. (2019). The Prognosis of Trabeculectomy in Primary Angle-Closure Glaucoma Patients. Int. J. Ophthalmol. 12 (1), 66–72. doi:10.18240/ijo.2019.01.10
Zimmerman, K. A., Graham, L. V., Pallero, M. A., and Murphy-Ullrich, J. E. (2013). Calreticulin Regulates Transforming Growth Factor-β-Stimulated Extracellular Matrix Production. J. Biol. Chem. 288 (20), 14584–14598. doi:10.1074/jbc.m112.447243
Zimmerman, K. A., Xing, D., Pallero, M. A., Lu, A., Ikawa, M., Black, L., et al. (2015). Calreticulin Regulates Neointima Formation and Collagen Deposition Following Carotid Artery Ligation. J. Vasc. Res. 52 (5), 306–320. doi:10.1159/000443884
Zode, G. S., Sharma, A. B., Lin, X., Searby, C. C., Bugge, K., Kim, G. H., et al. (2014). Ocular-specific ER Stress Reduction Rescues Glaucoma in Murine Glucocorticoid-Induced Glaucoma. J. Clin. Invest. 124 (5), 1956–1965. doi:10.1172/jci69774
Keywords: thrombospondin-1, calreticulin, LRP1, glaucoma, focal adhesions, anoikis, matricellular, cell migration
Citation: Murphy-Ullrich JE (2022) Thrombospondin-1 Signaling Through the Calreticulin/LDL Receptor Related Protein 1 Axis: Functions and Possible Roles in Glaucoma. Front. Cell Dev. Biol. 10:898772. doi: 10.3389/fcell.2022.898772
Received: 17 March 2022; Accepted: 10 May 2022;
Published: 27 May 2022.
Edited by:
Donna Peters, University of Wisconsin-Madison, United StatesReviewed by:
Ted Acott, Oregon Health and Science University, United StatesWeiming Mao, Indiana University, United States
Paloma Liton, Duke University, United States
Copyright © 2022 Murphy-Ullrich. This is an open-access article distributed under the terms of the Creative Commons Attribution License (CC BY). The use, distribution or reproduction in other forums is permitted, provided the original author(s) and the copyright owner(s) are credited and that the original publication in this journal is cited, in accordance with accepted academic practice. No use, distribution or reproduction is permitted which does not comply with these terms.
*Correspondence: Joanne E. Murphy-Ullrich, am11cnBoeUB1YWJtYy5lZHU=