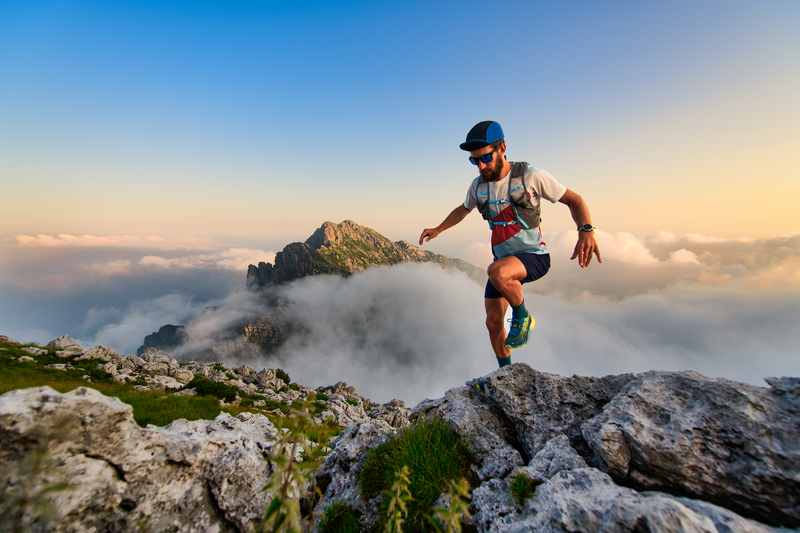
95% of researchers rate our articles as excellent or good
Learn more about the work of our research integrity team to safeguard the quality of each article we publish.
Find out more
OPINION article
Front. Cell Dev. Biol. , 04 May 2022
Sec. Molecular and Cellular Pathology
Volume 10 - 2022 | https://doi.org/10.3389/fcell.2022.893709
This article is part of the Research Topic Tissue-resident Immune Cells in Tumor Immunity and Immunotherapy View all 10 articles
Adenosine, an autacoid nucleoside interacting with P1 receptors, activates four G protein-coupled receptors named A1, A2A, A2B, and A3, crucially regulating several human pathologies (Borea et al., 2018). It affects both neoplastic and immune cells, promoting cancer cell proliferation, neo-angiogenesis, immunoescape, and metastasis (Arab and Hadjati, 2019). Extracellular nucleotides such as ATP, ADP, and UTP also function as cell-to-cell communication signals by binding and activating P2 receptors belonging to the P2X and P2Y subfamilies (Kennedy, 2021). These receptors are further subdivided into different subtypes (Khakh et al., 2021). The differential expressions of P1 and P2 receptors both in immune and tumor cells generate a complex picture. Cancers are able to convert extracellular ATP into immunosuppressive adenosine, through the activation of CD39 ectonucleotidase that hydrolyzes ATP to AMP, and a subsequent CD73 enzyme that transforms AMP into adenosine, with the stimulation of adenosine receptors on immune cells activating numerous immunosuppressive effects (Borea et al., 2018; Boison and Yegutkin 2019). The shift from P2 to P1 activation is important for limiting the inflammatory response, thus preventing tissue damage, but may also deleteriously inhibit immunosurveillance (Antonioli et al., 2013; Allard et al., 2019). Targeting CD39 and CD73 has, therefore, become a new way to fight cancer (Perrot et al., 2019; Moesta et al., 2020; Li et al., 2019). This review conjugates the current knowledge of purinergic signaling in cancer biology with techniques involving nanomaterials to increase anticancer immune responses.
Two hallmarks connecting adenosine to cancer include 1) solid tumors develop hypoxia and increase adenosine from nanomolar to micromolar concentrations and 2) the A2A receptor is an essential brake of immune cells (Sitkovsky M. V., 2020; Hatfild and Sitkovsky, 2020). The hypoxic activation of the master oxygen-sensitive transcriptional regulator HIF-1α upregulates ecto-5′-nucleotidase (CD73), generating adenosine accumulation associated with poor prognosis in many neoplasms (Borea et al., 2017). Adenosine activates cAMP-elevating A2A receptors to inhibit CD8+, CD4+ lymphocytes, and natural killer (NK) cells but stimulates B and T regulatory lymphocytes (Treg), tumor-associated macrophages (TAMs), and myeloid-derived suppressor cells (MDSCs), thus establishing a typically immunosuppressive tumor microenvironment (TME) (Vijayan et al., 2017). This encouraged immunologists to recognize adenosine as a new “immune checkpoint regulator” that stimulated the classic anti-cytotoxic T-like antigen 4 (CTLA4) and anti–programmed death-ligand 1 (PD-L1) to increase immunoescape (Sitkovsky M. V., 2020). Indeed, CTLA4 and PD-L1 inhibitors have been well-tolerated in cancer patients, improving overall morbidity and survival versus standard chemotherapy. However, efficacy may be limited to relatively few patients in some tumor types, reflecting the presence of alternative immunosuppressive factors in TME. Notably, anti-PD1 therapy increased immunosuppressant A2A receptors on CD8+ T cells; moreover, patients resistant to immunotherapy showed CD73 upregulation, suggesting that adenosine machinery counteracted the effects of immune checkpoint inhibitor drugs (Zarek et al., 2008). One improvement strategy has been implemented to inhibit (Kotulová et al., 2021) the hypoxia-HIF-1α-A2A receptor-mediated pathway in the TME through A2A receptor antagonists (Hatfield and Sitkovsky, 2020; Willingham et al., 2020). Accordingly, genetic silencing of the A2A receptor strongly increased inflammation and tumor rejection in mice (Ohta and Sitkovsky, 2001; Ohta et al., 2006; Sitkovsky M. V., 2020). A series of phase I/II clinical trials, evaluating the safety and efficacy of A2A receptor blockers/CD73 inhibitors including oleclumab, CPI-006, BMS-986179, and NZV-930 and A2A receptor antagonists such as ciforadenant, inupadenant, taminadenant, AZD4635, and preladenant alone or coadministered with immune checkpoint inhibitors such as anti-PD1 or anti-PDL1, are under evaluation (Arab and Hadjati, 2019; Arab et al., 2021; Franco et al., 2021; Thompson and Powell, 2021).
Beyond targeting the A2A receptor, anticancer immunotherapy can also be potentiated by inhibiting the A2B receptor, a subtype also capable of stimulating cAMP in T cells. Phase I clinical trials of A2B blockers in patients with advanced cancer are underway (Franco et al., 2021). Arguably, this pharmacological approach might only succeed in patients bearing hypoxic tumors with a sufficient number of tumor-reactive T cells, yet this consideration remains to be resolved (Sitkovsky M. V., 2020; Fong et al., 2020).
The TME is rich in ATP and its metabolites modulating tumor and immune cell biology and responses (Di Virgilio et al., 2018). The contribution of P2 receptors to cancer biology has been intensively investigated (Chiarella et al., 2021). The ATP-activated P2X7 receptor has emerged as a pivotal membrane molecule in tumors as it is expressed by cancer cells and by macrophages, dendritic cells, and lymphocytes infiltrating the tumor mass (De Marchi et al., 2019).
Tumor cell cytotoxicity (apoptosis or necrosis) due to prolonged P2X7 receptor activation and pore formation was a desirable anti-tumor response of this membrane molecule (Feng et al., 2006; Fu et al., 2009; Bian et al., 2013; Avanzato et al., 2016). However, subsequent identification of P2X7 receptor variants, with more precise characterization of the responses and measurement of cancer cell expression levels, indicated this subtype was upregulated in many tumor types (McLarnon, 2017; Di Virgilio et al., 2018, Zhang et al., 2019a; 2019b). More significantly, P2X7 receptor stimulation by low extracellular ATP concentrations was pro-tumorigenic, favoring cancer cell survival, proliferation, motility, and chemoresistance (Adinolfi et al., 2012; Schneider et al., 2015; Arnaud-Sampaio et al., 2020). In addition to the P2X7 receptor subtype, the P2X4, P2X5, P2Y6, and P2Y12 receptors also have involvement in tumor biology (Roger et al., 2015). P2X4 and P2X7 receptor subtype expressions concurred with tumor cell proliferation (He et al., 2020). In contrast, P2X5 receptor mediated an anti-proliferative (Zhang et al., 2020) effect by inducing tumor cell differentiation. Cumulative reports have indicated pro-neoplastic P2Y2 receptor-mediated responses conferring resistance to cell apoptosis, stimulation of tumor replication, and dissemination (Limami et al., 2012; Choi et al., 2013; Schumacher et al., 2013). The lack of expression of the P2X7 receptor in P2X7 KO mice induced a decrease in CD8+ lymphocytes while the number of Treg cells increased (De Marchi et al., 2019).
From a pharmacological and therapeutic perspective, P2 receptors have high potential to complement radiation therapy against resistant, highly malignant cancers. The stimulation of P2X7, P2Y6, and P2Y12 receptors was significant in the DNA damage response induced by γ-irradiation of adenocarcinoma A549 cells (Ide et al., 2014). B16 melanoma cells both in vitro and in vivo responded similarly to P2X7 receptor antagonists (Tanamachi et al., 2017). The use of single P2 receptor subtype inhibitors was often sufficient to block tumor cell growth and dissemination (Drill et al., 2021). The growth of human high-grade gliomas was inhibited by P2X7 subtype antagonists (Kan et al., 2020); receptor inhibitors, such as emodin, and the Uncaria tomentosa extract effectively counteracted the P2X7 receptor-mediated breast cancer spread (Zhu et al., 2021). P2X7 receptor antagonization could also usefully reduce pain in cancer patients with metastases. In particular, the P2X7 receptor antagonists AFC5261 and A-740003 were promising in animal models (Li et al., 2018; De Marchi et al., 2019; Falk et al., 2019). Further identification and characterization of new P2X7 receptor modulators and inhibitors were recommended (Hempel et al., 2013). Also for consideration, the expression of P2X7 and other P2 receptors by immune cells participated in immunosurveillance (Jelassi et al., 2013; Grassi and Conti, 2021). The awareness of the importance of P2-mediated signaling in cancer pathogenesis and progression (Figure 1A) has prompted therapeutic strategies targeting extracellular nucleotides. In this light, nanomaterials may improve anticancer outcomes by modulating the immune and tumor cell purinome.
FIGURE 1. (A) Purinergic-mediated responses occurring in the TME: P2 receptor-induced activities are summarized in the upper part of the figure, while P1 receptor-mediated responses are depicted in the lower figure part. (B) Schematic diagram for tailored nanoparticles targeting the TME and its immunological components to potentiate cancer immune therapy.
Although TME immunosurveillance may be markedly heterogeneous, most anticancer agents rely on the reactivation of homeostatic immune defense mechanisms (Joyce and Fearon, 2015; Terry et al., 2017; Ni et al., 2021). Initially, innovative nanomaterials improved upon conventional treatments yet soon drew criticism when nanoparticles elicited toxic effects from immunological alterations (Lenders et al., 2020). Nonetheless, rationally tailored nanomaterials have renewed interest in penetrant TME modulators (Zhang et al., 2021) that address tumor immune evasion (Guevara et al., 2021) by immunotherapy enhancement to promote immunogenic tumor cell death (Aikins et al., 2020) (Nogrady, 2021). The multiple cell types comprising the TME provide alternative nanomaterial targets, and their involvement in intervention design can be reciprocal (Song et al., 2017). For example, to counteract tumor adenosine accumulation, lipid nanoparticles mediating the knockdown of the corresponding A2A receptor in memory T cells could rescue CD8+ T-cell chemotaxis for infiltration into the TME of head and neck squamous cell carcinomas (Newton et al., 2021). Nanoparticle-based delivery approaches also include cell membrane–camouflaged nanocarriers (Grimaudo, 2021) such as tumor-associated macrophage membrane-coated nanoparticles (Chen et al., 2021). Cell membrane-bioinspired nanoparticles can provide superior immune regulation, nanocapsule drug delivery (Zhang et al., 2019c; Irvine and Dane, 2020), tumor targeting, and biocompatibility (Mu et al., 2021).
Yet diversity among tumorigenic cells and between individuals may still Yet, thwart nano-based delivery systems. The improved knowledge of various chronological stages of TME development remains necessary for more effective nanoplatform implementation (Yang et al., 2021) to target the more persistent subpopulation of cancer stem cells (Duan et al., 2021). The highest immunotherapeutic efficacy occurs when nanoparticles achieve precise and timely delivery, specifically targeting neoplastic cells with minimal harm to healthy cells (Muluh et al., 2021). Addressing TME traits, hypoxia-activated nanoparticles have theranostic applications (Wang et al., 2019). Since TME hypoxia blocked antitumor immunity (Singleton et al., 2021), tumor hypoxia-activated polymeric micelles were used to both activate strong cytotoxicity and stimulate a systemic antitumor immunity that effectively eradicated breast cancer in preclinical murine models (Liu et al., 2021). Hypoxia-modifier nanoparticles (Yuan et al., 2021) targeting the blood–brain barrier, enhanced immunotherapy of glioblastoma (Meng et al., 2021), a particularly aggressive form of cancer involving intracellular purine alterations (Debom et al., 2021; Giuliani et al., 2021). Cancer metastasis treatment remains a highlight of nanomedicine-based immunotherapy (Zhang et al., 2019). Excellent efficacy was observed for TME-activated nanoparticle chemodynamic immunotherapy of melanoma-derived lung metastasis (Zhai et al., 2021).
Compared to the relatively heterogenous tumor-cell population, non-tumorigenic supportive cells within the TME such as tumor-associated fibroblasts (TAFs) may present a more consistent target for nanoparticle intervention (Li et al., 2021), yet some limitations persist. nanomaterial-based TME modulation impinging upon purinergic signaling pathways can serve to additionally recruit the immune system to provide more integrative therapy (Laplane et al., 2019; Shi and Lammers, 2019). Nanomaterials can be adapted to modulate purinergic signaling in a number of ways since nanoparticles can be size-tailored to have diameters that match pore sizes present in leaky TME vasculature, thus establishing size-related penetration and accumulation (Yu et al., 2020). Moreover, nanoparticles can assist with improved delivery of drugs such as A2A antagonists that counteracted immunosuppression (Arruga et al., 2021). It is notable that the purinergic signaling network is subjected to modulation by microRNA (miRNA) (Ferrari et al., 2016), and over 30 miRNAs directly or indirectly modulate P1 and P2 receptors and ectoenzymes, with miR-187 capable of modulating both P2X7 and CD73 (Guo et al., 2022). Notably, miRNA that bind the 3′ untranslated region of the P2X7 receptor can affect the development of breast cancer by influencing the P2X7 receptor expression (Zhu et al., 2021). Nanoparticles are well-suited for precision medicine strategies to deliver purinergic signaling-specific miRNA and silencing RNA (siRNA) therapeutics (Kara et al., 2022). It has already been demonstrated that the nanoparticle delivery of siRNA-CD73 to the central nervous system blocked the CD73 expression in the glioblastoma immune microenvironment, inducing apoptosis to delay tumor growth (Azambuja et al., 2020). Smart nanomaterials can be engineered to exploit TME-specific purinergic pathway anomalies. A hydrogel of alginate conjugated with an ATP-specific aptamer hybridized with immunoadjuvant CpG oligonucleotides enabled the release of immune adjuvants in synchrony with low-dose repeated chemo/radiotherapies. This achieved a remarkable synergistic response; in addition to eliminating tumors, the evoked immune memory rejected re-challenged tumors and inhibited distant tumor metastases when combined with immune checkpoint blockade (Sun et al., 2021).
Innate immune interactions include macrophage responsiveness to damage-associated molecular patterns (DAMPs) originating from the cancer cells. M2-like tumor-associated macrophages (TAMs) can efficiently engulf neighboring apoptotic cells abundant in solid tumors, an early immunosuppressive mechanism preventing a DAMP-mediated immune response. The MER proto-oncogene tyrosine kinase (MerTK) can promote an “eat me” signal on dying cells to enhance efferocytosis (Ou et al., 2021). Consequently, apoptotic cells are eliminated before releasing intracellular ATP and cyclic GMP that would otherwise activate the ATP-gated P2X7 channels of TAMs and also cytosolic nucleic acid sensor pathways, including cyclic GMP-AMP synthase (cGAS) producing cyclic guanosine monophosphate–adenosine monophosphate (cGAMP), a second messenger binding and activating the adapter protein, stimulating interferon gene (STING), expressed in TAMs and other cells of the TME. The production of stress-responsive cytokines would ultimately cause M2 macrophages to be polarized toward an immune-activated M1 phenotype (Zhao et al., 2021). Appropriately, macrophages have become key targets for nanoparticle intervention (Medrano-Bosch et al., 2021). A nanoparticle-incorporating STING activator cGAMP enhanced the antitumor immunity in PD-L1-insensitive models of triple-negative breast cancer (Cheng et al., 2018) and improved the clinical outcome of immunotherapy for melanoma (Shae et al., 2019). Cationic silica nanoparticles induced necrotic cell death and activation of the STING in the TME to enhance antitumor immunity (An et al., 2018). Inhalable nanoparticulate agonists of STING synergized with radiotherapy to provide the long-term control of lung metastases (Liu et al., 2019). Combining nanoparticles with compatible forms of therapy such as radiation therapy (Huang et al., 2021) or photodynamic therapy (Jin et al., 2021) improved antitumor efficacy by promoting immunogenic cell death.
Nanomaterials are also capable of enhancing the trained acquired immune response (Magadán et al., 2021), and they have been rationally designed to enhance T-cell expansion, navigate physical barriers, and modulate the TME to overcome barriers to T-cell-based immunotherapies (Gong et al., 2021). Engineered immunomodulating nano-adapter particle rafts such as trispecific natural killer cell nanoengagers (Au et al., 2020) carry more than one monoclonal antibody (mAb) to bridge effector and tumor cells. More effective responses than simply mixing the parental mAbs with T cells, NK cells, natural killer (NK) cells, or macrophages were observed (Jiang et al., 2021). Nanogels selectively released an interleukin-15 cargo upon T-cell receptor activation and expanded T cells in tumors 16-fold relative to the systemic administration of free cytokines. The higher doses of cytokines could be administered, without toxic side effects, to potentiate human chimeric antigen receptor (CAR)-T cell therapy (Tang et al., 2018). Nanoparticle versatility, exemplified in Table 1 and Figure 1B, has meant that numerous clinical nanomaterials and drugs potentiating immunotherapy are currently under development (Li et al., 2020; Hu and Huang, 2022).
TABLE 1. Examples of immunomodulatory nanoparticle types, tumor microenvironment (TME) interactions and co-involved purinergic pathways.
The TME, heavily conditioned by nucleotide/nucleoside release and hydrolysis, makes purinergic signaling an extremely attractive target for strategic modulation of both cancer and immune cells, but responses to antagonists or agonists are highly context-dependent (Hreich et al., 2021). The inhibitors of specific purinome components have successfully blocked tumor progression and metastasis in animal models and preclinical studies, yet improved specific therapeutic strategies are needed. The recent implementation of nanomaterials has shown that they can be very effective agents, acting on their own, delivering mRNA or improving mAb presentation to disrupt the TME refractoriness to immune therapy.
DF, SG, SM, MN, AT, and JB wrote the manuscript. All authors have read and agreed to the published version of the manuscript.
The authors declare that the research was conducted in the absence of any commercial or financial relationships that could be construed as a potential conflict of interest.
All claims expressed in this article are solely those of the authors and do not necessarily represent those of their affiliated organizations, or those of the publisher, the editors, and the reviewers. Any product that may be evaluated in this article, or claim that may be made by its manufacturer, is not guaranteed or endorsed by the publisher.
Adinolfi, E., Raffaghello, L., Giuliani, A. L., Cavazzini, L., Capece, M., Chiozzi, P., et al. (2012). Expression of P2X7 Receptor IncreasesIn VivoTumor Growth. Cancer Res. 72, 2957–2969. doi:10.1158/0008-5472.can-11-1947
Aikins, M. E., Xu, C., and Moon, J. J. (2020). Engineered Nanoparticles for Cancer Vaccination and Immunotherapy. Acc. Chem. Res. 53, 2094–2105. doi:10.1021/acs.accounts.0c00456
Allard, D., Chrobak, P., Allard, B., Messaoudi, N., and Stagg, J. (2019). Targeting the CD73-Adenosine axis in Immuno-Oncology. Immunol. Lett. 205, 31–39. doi:10.1016/j.imlet.2018.05.001
An, M., Yu, C., Xi, J., Reyes, J., Mao, G., Wei, W.-Z., et al. (2018). Induction of Necrotic Cell Death and Activation of STING in the Tumor Microenvironment via Cationic Silica Nanoparticles Leading to Enhanced Antitumor Immunity. Nanoscale 10, 9311–9319. doi:10.1039/c8nr01376d
Antonioli, L., Pacher, P., Vizi, E. S., and Haskó, G. (2013). CD39 and CD73 in Immunity and Inflammation. Trends Mol. Med. 19, 355–367. doi:10.1016/j.molmed.2013.03.005
Arab, S., and Hadjati, J. (2019). Adenosine Blockage in Tumor Microenvironment and Improvement of Cancer Immunotherapy. Immune Netw. 2719, e23. doi:10.4110/in.2019.19.e23
Arab, S., Alizadeh, A., and Asgharzade, S. (2021). Tumor-resident Adenosine-Producing Mesenchymal Stem Cells as a Potential Target for Cancer Treatment. Clin. Exp. Med. 21, 205–213. doi:10.1007/s10238-020-00674-9
Arnaud-Sampaio, V. F., Rabelo, I. L. A., Ulrich, H., and Lameu, C. (2020). The P2X7 Receptor in the Maintenance of Cancer Stem Cells, Chemoresistance and Metastasis. Stem Cel Rev Rep 16, 288–300. doi:10.1007/s12015-019-09936-w
Arruga, F., Serra, S., Vitale, N., Guerra, G., Papait, A., Baffour Gyau, B., et al. (2021). Targeting of the A2A Adenosine Receptor Counteracts Immunosuppression In Vivo in a Mouse Model of Chronic Lymphocytic Leukemia. Haematologica 106, 1343–1353. doi:10.3324/haematol.2019.242016
Au, K. M., Park, S. I., and Wang, A. Z. (2020). Trispecific Natural Killer Cell Nanoengagers for Targeted Chemoimmunotherapy. Sci. Adv. 6, eaba8564. doi:10.1126/sciadv.aba8564
Avanzato, D., Genova, T., Fiorio Pla, A., Bernardini, M., Bianco, S., Bussolati, B., et al. (2016). Activation of P2X7 and P2Y11 Purinergic Receptors Inhibits Migration and Normalizes Tumor-Derived Endothelial Cells via cAMP Signaling. Sci. Rep. 6, 32602. doi:10.1038/srep32602
Azambuja, J. H., Schuh, R. S., Michels, L. R., Iser, I. C., Beckenkamp, L. R., Roliano, G. G., et al. (2020). Blockade of CD73 Delays Glioblastoma Growth by Modulating the Immune Environment. Cancer Immunol. Immunother. 69, 1801–1812. doi:10.1007/s00262-020-02569-w
Bian, S., Sun, X., Bai, A., Zhang, C., Li, L., Enjyoji, K., et al. (2013). P2X7 Integrates PI3K/AKT and AMPK-PRAS40-mTOR Signaling Pathways to Mediate Tumor Cell Death. PLoS One 8, e60184. doi:10.1371/journal.pone.0060184
Boison, D., and Yegutkin, G. G. (2019). Adenosine Metabolism: Emerging Concepts for Cancer Therapy. Cancer Cell 36, 582–596. doi:10.1016/j.ccell.2019.10.007
Borea, P. A., Gessi, S., Merighi, S., Vincenzi, F., and Varani, K. (2017). Pathological Overproduction: the Bad Side of Adenosine. Br. J. Pharmacol. 174, 1945–1960. doi:10.1111/bph.13763
Borea, P. A., Gessi, S., Merighi, S., Vincenzi, F., and Varani, K. (2018). Pharmacology of Adenosine Receptors: The State of the Art. Physiol. Rev. 98, 1591–1625. doi:10.1152/physrev.00049.2017
Chen, C., Song, M., Du, Y., Yu, Y., Li, C., Han, Y., et al. (2021). Tumor-Associated-Macrophage-Membrane-Coated Nanoparticles for Improved Photodynamic Immunotherapy. Nano Lett. 21, 5522–5531. doi:10.1021/acs.nanolett.1c00818
Cheng, N., Watkins-Schulz, R., Junkins, R. D., David, C. N., Johnson, B. M., Montgomery, S. A., et al. (2018). A Nanoparticle-Incorporated STING Activator Enhances Antitumor Immunity in PD-L1-Insensitive Models of Triple-Negative Breast Cancer. JCI Insight 3, 120638. doi:10.1172/jci.insight.120638
Chiarella, A. M., Ryu, Y. K., Manji, G. A., and Rustgi, A. K. (2021). Extracellular ATP and Adenosine in Cancer Pathogenesis and Treatment. Trends Cancer 7, 731–750. doi:10.1016/j.trecan.2021.04.008
Choi, J. H., Ji, Y. G., and Lee, D. H. (2013). Uridine Triphosphate Increases Proliferation of Human Cancerous Pancreatic Duct Epithelial Cells by Activating P2Y2 Receptor. Pancreas 42, 680–686. doi:10.1097/mpa.0b013e318271bb4b
De Marchi, E., Orioli, E., Pegoraro, A., Sangaletti, S., Portararo, P., Curti, A., et al. (2019). The P2X7 Receptor Modulates Immune Cells Infiltration, Ectonucleotidases Expression and Extracellular ATP Levels in the Tumor Microenvironment. Oncogene 38, 3636–3650. doi:10.1038/s41388-019-0684-y
Debom, G. N., Rubenich, D. S., and Braganhol, E. (2021). Adenosinergic Signaling as a Key Modulator of the Glioma Microenvironment and Reactive Astrocytes. Front. Neurosci. 15, 648476. doi:10.3389/fnins.2021.648476
Di Virgilio, F., Sarti, A. C., Falzoni, S., De Marchi, E., and Adinolfi, E. (2018). Extracellular ATP and P2 Purinergic Signalling in the Tumour Microenvironment. Nat. Rev. Cancer 18, 601–618. doi:10.1038/s41568-018-0037-0
Drill, M., Jones, N. C., Hunn, M., O’Brien, T. J., and Monif, M. (2021). Antagonism of the ATP-Gated P2X7 Receptor: a Potential Therapeutic Strategy for Cancer. Purinergic Signal. 17, 215–227. doi:10.1007/s11302-021-09776-9
Duan, H., Liu, Y., Gao, Z., and Huang, W. (2021). Recent Advances in Drug Delivery Systems for Targeting Cancer Stem Cells. Acta Pharmaceutica Sinica B 11, 55–70. doi:10.1016/j.apsb.2020.09.016
Falk, S., Appel, C. K., Bennedbæk, H. B., Al-Dihaissy, T., Unger, A., Dinkel, K., et al. (2019). Chronic High Dose P2X7 Receptor Inhibition Exacerbates Cancer-Induced Bone Pain. Eur. J. Pharmacol. 845, 48–55. doi:10.1016/j.ejphar.2018.12.032
Feng, Y. H., Li, X., Zeng, R., and Gorodeski, G. I. (2006). Endogenously Expressed Truncated P2X7 Receptor Lacking the C-Terminus Is Preferentially Upregulated in Epithelial Cancer Cells and Fails to Mediate Ligand-Induced Pore Formation and Apoptosis. Nucleosides Nucleotides Nucleic Acids 25, 1271–1276. doi:10.1080/15257770600890921
Ferrari, D., Bianchi, N., Eltzschig, H. K., and Gambari, R. (2016). MicroRNAs Modulate the Purinergic Signaling Network. Trends Mol. Med. 22, 905–918. doi:10.1016/j.molmed.2016.08.006
Fong, L., Hotson, A., Powderly, J. D., Sznol, M., Heist, R. S., Choueiri, T. K., et al. (2020). Adenosine 2A Receptor Blockade as an Immunotherapy for Treatment-Refractory Renal Cell Cancer. Cancer Discov. 10, 40–53. doi:10.1158/2159-8290.cd-19-0980
Franco, R., Rivas-Santisteban, R., Navarro, G., and Reyes-Resina, I. (2021). Adenosine Receptor Antagonists to Combat Cancer and to Boost Anti-cancer Chemotherapy and Immunotherapy. Cells 11, 2831. doi:10.3390/cells10112831
Fu, W., McCormick, T., Qi, X., Luo, L., Zhou, L., Li, X., et al. (2009). Activation of P2X(7)-Mediated Apoptosis Inhibits DMBA/TPA-induced Formation of Skin Papillomas and Cancer in Mice. BMC Cancer 9, 114. doi:10.1186/1471-2407-9-114
Giuliani, P., Carluccio, M., and Ciccarelli, R. (2021). Role of Purinome, A Complex Signaling System, in Glioblastoma Aggressiveness. Front. Pharmacol. 12, 632622. doi:10.3389/fphar.2021.632622
Gong, N., Sheppard, N. C., Billingsley, M. M., June, C. H., and Mitchell, M. J. (2021). Nanomaterials for T-Cell Cancer Immunotherapy. Nat. Nanotechnol 16, 25–36. doi:10.1038/s41565-020-00822-y
Grassi, F., and Conti, B. D. P. (2021). The P2X7 Receptor in Tumor Immunity. Front Cel Dev Biol 9, 694831. doi:10.3389/fcell.2021.694831
Grimaudo, M. A. (2021). Nanotechnology for the Development of Nanovaccines in Cancer Immunotherapy. Adv. Exp. Med. Biol. 1295, 303–315. doi:10.1007/978-3-030-58174-9_13
Guevara, M. L., Persano, F., and Persano, S. (2021). Nano-immunotherapy: Overcoming Tumour Immune Evasion. Semin. Cancer Biol. 69, 238–248. doi:10.1016/j.semcancer.2019.11.010
Guo, J., Yang, P., Li, Y. F., Tang, J. F., He, Z. X., Yu, S. G., et al. (2022). MicroRNA: Crucial Modulator in Purinergic Signalling Involved Diseases. Purinergic Signal. doi:10.1007/s11302-022-09840-y
Hatfield, S. M., and Sitkovsky, M. V. (2020). Antihypoxic Oxygenation Agents with Respiratory Hyperoxia to Improve Cancer Immunotherapy. J. Clin. Invest. 130, 5629–5637. doi:10.1172/jci137554
He, J., Zhou, Y., Arredondo Carrera, H. M., Sprules, A., Neagu, R., Zarkesh, S. A., et al. (2020). Inhibiting the P2X4 Receptor Suppresses Prostate Cancer Growth In Vitro and In Vivo, Suggesting a Potential Clinical Target. Cells 9, 2511. doi:10.3390/cells9112511
Hempel, C., Nörenberg, W., Sobottka, H., Urban, N., Nicke, A., Fischer, W., et al. (2013). The Phenothiazine-Class Antipsychotic Drugs Prochlorperazine and Trifluoperazine Are Potent Allosteric Modulators of the Human P2X7 Receptor. Neuropharmacology 75, 365–379. doi:10.1016/j.neuropharm.2013.07.027
Hreich, S. J. D., Benzaquen, J., Hofman, P., and Vouret-Craviari, V. (2021). To Inhibit or to Boost the ATP/P2RX7 Pathway to Fight Cancer-That Is the Question. Purinergic Signal. 17, 619–631. doi:10.1007/s11302-021-09811-9
Hu, M., and Huang, L. (2022). Strategies Targeting Tumor Immune and Stromal Microenvironment and Their Clinical Relevance. Adv. Drug Deliv. Rev. 183, 114137.
Huang, Z., Wang, Y., Yao, D., Wu, J., Hu, Y., and Yuan, A. (2021). Nanoscale Coordination Polymers Induce Immunogenic Cell Death by Amplifying Radiation Therapy Mediated Oxidative Stress. Nat. Commun. 12, 145. doi:10.1038/s41467-020-20243-8
Ide, S., Nishimaki, N., Tsukimoto, M., and Kojima, S. (2014). Purine Receptor P2Y6 Mediates Cellular Response to γ-ray-induced DNA Damage. J. Toxicol. Sci. 39, 15–23. doi:10.2131/jts.39.15
Irvine, D. J., and Dane, E. L. (2020). Enhancing Cancer Immunotherapy with Nanomedicine. Nat. Rev. Immunol. 20, 321–334. doi:10.1038/s41577-019-0269-6
Jelassi, B., Anchelin, M., Chamouton, J., Cayuela, M. L., Clarysse, L., Li, J., et al. (2013). Anthraquinone Emodin Inhibits Human Cancer Cell Invasiveness by Antagonizing P2X7 Receptors. Carcinogenesis 34, 1487–1496. doi:10.1093/carcin/bgt099
Jiang, C. T., Chen, K. G., Liu, A., Huang, H., Fan, Y. N., Zhao, D. K., et al. (2021). Immunomodulating Nano-Adaptors Potentiate Antibody-Based Cancer Immunotherapy. Nat. Commun. 12, 1359. doi:10.1038/s41467-021-21497-6
Jin, F., Liu, D., Xu, X., Ji, J., and Du, Y. (2021). Nanomaterials-Based Photodynamic Therapy with Combined Treatment Improves Antitumor Efficacy through Boosting Immunogenic Cell Death. Int. J. Nanomedicine 16, 4693–4712. doi:10.2147/ijn.s314506
Joyce, J. A., and Fearon, D. T. (2015). T Cell Exclusion, Immune Privilege, and the Tumor Microenvironment. Science 348, 74–80. doi:10.1126/science.aaa6204
Kan, L. K., Seneviratne, S., Drummond, K. J., Williams, D. A., O'Brien, T. J., and Monif, M. (2020). P2X7 Receptor Antagonism Inhibits Tumour Growth in Human High-Grade Gliomas. Purinergic Signal. 16, 327–336. doi:10.1007/s11302-020-09705-2
Kara, G., Calin, G. A., and Ozpolat, B. (2022). RNAi-based Therapeutics and Tumor Targeted Delivery in Cancer. Adv. Drug Deliv. Rev. 182, 114113. doi:10.1016/j.addr.2022.114113
Kennedy, C. (2021). The P2Y/P2X divide: How it Began. Biochem. Pharmacol. May 187, 114408. Epub 2021 Jan 11. PMID: 33444568. doi:10.1016/j.bcp.2021.114408
Khakh, B. S., Burnstock, G., Kennedy, C., King, B. F., North, R. A., Séguéla, P., et al. (2001). International union of Pharmacology. XXIV. Current Status of the Nomenclature and Properties of P2X Receptors and Their Subunits. Pharmacol. Rev. 53, 107–118.
Kotulová, J., Hajdúch, M., and Džubák, P. (2021). Current Adenosinergic Therapies: What Do Cancer Cells Stand to Gain and Lose? Int. J. Mol. Sci. 22, 12569.
Laplane, L., Duluc, D., Bikfalvi, A., Larmonier, N., and Pradeu, T. (2019). Beyond the Tumour Microenvironment. Int. J. Cancer 145, 2611–2618. doi:10.1002/ijc.32343
Lenders, V., Koutsoumpou, X., Sargsian, A., and Manshian, B. B. (2020). Biomedical Nanomaterials for Immunological Applications: Ongoing Research and Clinical Trials. Nanoscale Adv. 2, 5046–5089. doi:10.1039/d0na00478b
Li, P., Zhang, Q., Xiao, Z., Yu, S., Yan, Y., and Qin, Y. (2018). Activation of the P2X7 Receptor in Midbrain Periaqueductal gray Participates in the Analgesic Effect of Tramadol in Bone Cancer Pain Rats. Mol. Pain 14, 1744806918803039. doi:10.1177/1744806918803039
Li, W., Little, N., Park, J., Foster, C. A., Chen, J., and Lu, J. (2021). Tumor-Associated Fibroblast-Targeting Nanoparticles for Enhancing Solid Tumor Therapy: Progress and Challenges. Mol. Pharm. 18, 2889–2905. doi:10.1021/acs.molpharmaceut.1c00455
Li, W., Peng, A., Wu, H., Quan, Y., Li, Y., Lu, L., et al. (2020). Anti-Cancer Nanomedicines: A Revolution of Tumor Immunotherapy. Front. Immunol. 11, 601497. doi:10.3389/fimmu.2020.601497
Li, X. Y., Moesta, A. K., Xiao, C., Nakamura, K., Casey, M., Zhang, H., et al. (2019). Targeting CD39 in Cancer Reveals an Extracellular ATP- and Inflammasome-Driven Tumor Immunity. Cancer Discov. 9, 1754–1773. doi:10.1158/2159-8290.cd-19-0541
Limami, Y., Pinon, A., Leger, D. Y., Pinault, E., Delage, C., Beneytout, J. L., et al. (2012). The P2Y2/Src/p38/COX-2 Pathway Is Involved in the Resistance to Ursolic Acid-Induced Apoptosis in Colorectal and Prostate Cancer Cells. Biochimie 94, 1754–1763. doi:10.1016/j.biochi.2012.04.006
Liu, J., Ai, X., Cabral, H., Liu, J., Huang, Y., and Mi, P. (2021). Tumor Hypoxia-Activated Combinatorial Nanomedicine Triggers Systemic Antitumor Immunity to Effectively Eradicate Advanced Breast Cancer. Biomaterials 273, 120847. doi:10.1016/j.biomaterials.2021.120847
Liu, Y., Crowe, W. N., Wang, L., Lu, Y., Petty, W. J., Habib, A. A., et al. (2019). An Inhalable Nanoparticulate STING Agonist Synergizes with Radiotherapy to Confer Long-Term Control of Lung Metastases. Nat. Commun. 10, 5108. doi:10.1038/s41467-019-13094-5
Magadán, S., Mikelez-Alonso, I., Borrego, F., and González-Fernández, Á. (2021). Nanoparticles and Trained Immunity: Glimpse into the Future. Adv. Drug Deliv. Rev. 175, 113821. doi:10.1016/j.addr.2021.05.031
McLarnon, J. G. (2017). Roles of Purinergic P2X7 Receptor in Glioma and Microglia in Brain Tumors. Cancer Lett. 28 (402), 93–99. doi:10.1016/j.canlet.2017.05.004
Medrano-Bosch, M., Moreno-Lanceta, A., and Melgar-Lesmes, P. (2021). Nanoparticles to Target and Treat Macrophages: The Ockham’s Concept. Pharmaceutics 13, 1340. doi:10.3390/pharmaceutics13091340
Meng, L., Wang, C., Lu, Y., Sheng, G., Yang, L., Wu, Z., et al. (2021). Targeted Regulation of Blood-Brain Barrier for Enhanced Therapeutic Efficiency of Hypoxia-Modifier Nanoparticles and Immune Checkpoint Blockade Antibodies for Glioblastoma. ACS Appl. Mater. Inter. 13, 11657–11671. doi:10.1021/acsami.1c00347
Moesta, A. K., Li, X. Y., and Smyth, M. J. (2020). Targeting CD39 in Cancer. Nat. Rev. Immunol. 20, 739–755.
Mu, D., He, P., Shi, Y., Jiang, L., and Liu, G. (2021). Bioinspired Membrane-Coated Nanoplatform for Targeted Tumor Immunotherapy. Front. Oncol. 11, 819817. doi:10.3389/fonc.2021.819817
Muluh, T. A., Chen, Z., Li, Y., Xiong, K., Jin, J., Fu, S., et al. (2021). Enhancing Cancer Immunotherapy Treatment Goals by Using Nanoparticle Delivery System. Int. J. Nanomedicine 16, 2389–2404. doi:10.2147/ijn.s295300
Newton, H. S., Chimote, A. A., Arnold, M. J., Wise-Draper, T. M., and Conforti, L. (2021). Targeted Knockdown of the Adenosine A2A Receptor by Lipid NPs Rescues the Chemotaxis of Head and Neck Cancer Memory T Cells. Mol. Ther. Methods Clin. Dev. 21, 133–143. doi:10.1016/j.omtm.2021.03.001
Ni, Y., Zhou, X., Yang, J., Shi, H., Li, H., Zhao, X., et al. (2021). The Role of Tumor-Stroma Interactions in Drug Resistance within Tumor Microenvironment. Front. Cel Dev Biol 9, 637675. doi:10.3389/fcell.2021.637675
Nogrady, B. (2021). How Nanotechnology Can Flick the Immunity Switch. Nature 595, 18–19. doi:10.1038/d41586-021-01790-6
Ohta, A., Gorelik, E., Prasad, S. J., Ronchese, F., Lukashev, D., Wong, M. K., et al. (2006). A2A Adenosine Receptor Protects Tumors from Antitumor T Cells. Proc. Natl. Acad. Sci. USA 103, 13132–13137. doi:10.1073/pnas.0605251103
Ohta, A., and Sitkovsky, M. (2001). Role of G-Protein-Coupled Adenosine Receptors in Downregulation of Inflammation and protection from Tissue Damage. Nature 414, 916–920. doi:10.1038/414916a
Ou, L., Zhang, A., Cheng, Y., and Chen, Y. (2021). The cGAS-STING Pathway: A Promising Immunotherapy Target. Front. Immunol. 12, 795048. doi:10.3389/fimmu.2021.795048
Pei, M., Xu, R., Zhang, C., Wang, X., Li, C., and Hu, Y. (2021). Mannose-functionalized Antigen Nanoparticles for Targeted Dendritic Cells, Accelerated Endosomal Escape and Enhanced MHC-I Antigen Presentation. Colloids Surf. B Biointerfaces 197, 111378. doi:10.1016/j.colsurfb.2020.111378
Perrot, I., Michaud, H. A., Giraudon-Paoli, M., Augier, S., Docquier, A., Gros, L., et al. (2019). Blocking Antibodies Targeting the CD39/CD73 Immunosuppressive Pathway Unleash Immune Responses in Combination Cancer Therapies. Cell Rep 27, 2411–2425. e9. doi:10.1016/j.celrep.2019.04.091
Roger, S., Jelassi, B., Couillin, I., Pelegrin, P., Besson, P., and Jiang, L. H. (2015). Understanding the Roles of the P2X7 Receptor in Solid Tumour Progression and Therapeutic Perspectives. Biochim. Biophys. Acta 10 (Pt B), 2584–2602. doi:10.1016/j.bbamem.2014.10.029
Sacchetti, C., Rapini, N., Magrini, A., Cirelli, E., Bellucci, S., Mattei, M., et al. (2013). In Vivo targeting of Intratumor Regulatory T Cells Using PEG-Modified Single-Walled Carbon Nanotubes. Bioconjug. Chem. 24, 852–858. doi:10.1021/bc400070q
Schneider, G., Glaser, T., Lameu, C., Abdelbaset-Ismail, A., Sellers, Z. P., Moniuszko, M., et al. (2015). Extracellular Nucleotides as Novel, Underappreciated Pro-metastatic Factors that Stimulate Purinergic Signaling in Human Lung Cancer Cells. Mol. Cancer 14, 201. doi:10.1186/s12943-015-0469-z
Schumacher, D., Strilic, B., Sivaraj, K. K., Wettschureck, N., and Offermanns, S. (2013). Platelet-Derived Nucleotides Promote Tumor-Cell Transendothelial Migration and Metastasis via P2Y2 Receptor. Cancer Cell 24, 130–137. doi:10.1016/j.ccr.2013.05.008
Shae, D., Becker, K. W., Christov, P., Yun, D. S., Lytton-Jean, A. K. R., Sevimli, S., et al. (2019). Endosomolytic Polymersomes Increase the Activity of Cyclic Dinucleotide STING Agonists to Enhance Cancer Immunotherapy. Nat. Nanotechnol 14, 269–278. doi:10.1038/s41565-018-0342-5
Shi, Y., and Lammers, T. (2019). Combining Nanomedicine and Immunotherapy. Acc. Chem. Res. 52, 1543–1554. doi:10.1021/acs.accounts.9b00148
Singleton, D. C., Macann, A., and Wilson, W. R. (2021). Therapeutic Targeting of the Hypoxic Tumour Microenvironment. Nat. Rev. Clin. Oncol. 18, 751–772. doi:10.1038/s41571-021-00539-4
Sitkovsky, M. V. (2020b). Lessons from the A2A Adenosine Receptor Antagonist-Enabled Tumor Regression and Survival in Patients with Treatment-Refractory Renal Cell Cancer. Cancer Discov. 10, 16–19. doi:10.1158/2159-8290.cd-19-1280
Sitkovsky, M. V. (2020a). Sufficient Numbers of Anti-tumor T Cells Is a Condition of Maximal Efficacy of Anti- Hypoxia-A2-Adenosinergic Drugs during Cancer Immunotherapy. Curr. Opin. Pharmacol 53, 98–100. doi:10.1016/j.coph.2020.07.011
Smith, T. T., Stephan, S. B., Moffett, H. F., McKnight, L. E., Ji, W., Reiman, D., et al. (2017). In Situ programming of Leukaemia-specific T Cells Using Synthetic DNA Nanocarriers. Nat. Nanotechnol 12, 813–820. doi:10.1038/nnano.2017.57
Song, W., Musetti, S. N., and Huang, L. (2017). Nanomaterials for Cancer Immunotherapy. Biomaterials 148, 16–30. doi:10.1016/j.biomaterials.2017.09.017
Sun, L., Shen, F., Tian, L., Tao, H., Xiong, Z., Xu, J., et al. (2021). ATP-responsive Smart Hydrogel Releasing Immune Adjuvant Synchronized with Repeated Chemotherapy or Radiotherapy to Boost Antitumor Immunity. Adv. Mater. 33, e2007910. doi:10.1002/adma.202007910
Tanamachi, K., Nishino, K., Mori, N., Suzuki, T., Tanuma, S. I., Abe, R., et al. (2017). Radiosensitizing Effect of P2X7 Receptor Antagonist on Melanoma In Vitro and In Vivo. Biol. Pharm. Bull. 40 (6), 878–887. doi:10.1248/bpb.b17-00083
Tang, L., Zheng, Y., Melo, M. B., Mabardi, L., Castaño, A. P., Xie, Y. Q., et al. (2018). Enhancing T Cell Therapy through TCR-Signaling- Responsive Nanoparticle Drug Delivery. Nat. Biotechnol. 36, 707–716. doi:10.1038/nbt.4181
Terry, S., Savagner, P., Ortiz-Cuaran, S., Mahjoubi, L., Saintigny, P., Thiery, J. P., et al. (2017). New Insights into the Role of EMT in Tumor Immune Escape. Mol. Oncol. 11, 824–846. doi:10.1002/1878-0261.12093
Thompson, E. A., and Powell, J. D. (2021). Inhibition of the Adenosine Pathway to Potentiate Cancer Immunotherapy: Potential for Combinatorial Approaches. Annu. Rev. Med. 72, 331–348. doi:10.1146/annurev-med-060619-023155
Vijayan, D., Young, A., Teng, M. W. L., and Smyth, M. J. (2017). Targeting Immunosuppressive Adenosine in Cancer. Nat. Rev. Cancer 17, 709–724. doi:10.1038/nrc.2017.86
Wang, Y., Shang, W., Niu, M., Tian, J., and Xu, K. (2019). Hypoxia-active Nanoparticles Used in Tumor Theranostic. Int. J. Nanomedicine 14, 3705–3722. doi:10.2147/ijn.s196959
Willingham, S. B., Hotson, A. N., and Miller, R. A. (2020). Targeting the A2AR in Cancer; Early Lessons from the Clinic. Curr. Opin. Pharmacol. 53, 126–133. doi:10.1016/j.coph.2020.08.003
Yang, M., Li, J., Gu, P., and Fan, X. (2021). The Application of Nanoparticles in Cancer Immunotherapy: Targeting Tumor Microenvironment. Bioact Mater. 6, 1973–1987. doi:10.1016/j.bioactmat.2020.12.010
Yu, W., Liu, R., Zhou, Y., and Gao, H. (2020). Size-Tunable Strategies for a Tumor Targeted Drug Delivery System. ACS Cent. Sci. 6, 100–116. doi:10.1021/acscentsci.9b01139
Yuan, C. S., Deng, Z. W., Qin, D., Mu, Y. Z., Chen, X. G., and Liu, Y. (2021). Hypoxia-modulatory Nanomaterials to Relieve Tumor Hypoxic Microenvironment and Enhance Immunotherapy: Where Do We Stand. Acta Biomater. 125, 1–28. doi:10.1016/j.actbio.2021.02.030
Zarek, P. E., Huang, C. T., Lutz, E. R., Kowalski, J., Horton, M. R., and Linden, J. (2008). A2A Receptor Signaling Promotes Peripheral Tolerance by Inducing T-Cell Anergy and the Generation of Adaptive Regulatory T Cells. Blood 111, 251–259. doi:10.1182/blood-2007-03-081646
Zhai, T., Zhong, W., Gao, Y., Zhou, H., Zhou, Z., Liu, X., et al. (2021). Tumor Microenvironment-Activated Nanoparticles Loaded with an Iron-Carbonyl Complex for Chemodynamic Immunotherapy of Lung Metastasis of Melanoma In Vivo. ACS Appl. Mater. Inter. 13, 39100–39111. doi:10.1021/acsami.1c11485
Zhang, P., Zhai, Y., Cai, Y., Zhao, Y., and Li, Y. (2019). Nanomedicine-Based Immunotherapy for the Treatment of Cancer Metastasis. Adv. Mater. 31, e1904156. doi:10.1002/adma.201904156
Zhang, W. J., Hu, C. G., Zhu, Z. M., and Luo, H. L. (2020). Effect of P2X7 Receptor on Tumorigenesis and its Pharmacological Properties. Biomed. Pharmacother. 125, 109844. doi:10.1016/j.biopha.2020.109844
Zhang, Y., Bush, X., Yan, B., and Chen, J. A. (2019c). Gemcitabine Nanoparticles Promote Antitumor Immunity against Melanoma. Biomaterials 189, 48–59. doi:10.1016/j.biomaterials.2018.10.022
Zhang, Y., Cheng, H., Li, W., Wu, H., and Yang, Y. (2019a). Highly-expressed P2X7 Receptor Promotes Growth and Metastasis of Human HOS/MNNG Osteosarcoma Cells via PI3K/Akt/GSK3beta/beta-Catenin and mTOR/HIF1alpha/VEGF Signaling. Int. J. Cancer 145, 1068–1082. doi:10.1002/ijc.32207
Zhang, Y., Ding, J., and Wang, L. (2019b). The Role of P2X7 Receptor in Prognosis and Metastasis of Colorectal Cancer. Adv. Med. Sci. 64, 388–394. doi:10.1016/j.advms.2019.05.002
Zhang, Y., Han, X., and Nie, G. (2021). Responsive and Activable Nanomedicines for Remodeling the Tumor Microenvironment. Nat. Protoc. 16, 405–430. doi:10.1038/s41596-020-00421-0
Zhao, R., Cao, J., Yang, X., Zhang, Q., Iqbal, M. Z., Lu, J., et al. (2021). Inorganic Material Based Macrophage Regulation for Cancer Therapy: Basic Concepts and Recent Advances. Biomater. Sci. 9, 4568–4590. doi:10.1039/d1bm00508a
Keywords: cancer immune therapy, nanomaterials, tumor microenvironment (TME), A2A adenosine receptor, P2X7 (purino) receptor
Citation: Ferrari D, Gessi S, Merighi S, Nigro M, Travagli A and Burns JS (2022) Potentiating Cancer Immune Therapy via Nanomaterials and Purinergic Signaling. Front. Cell Dev. Biol. 10:893709. doi: 10.3389/fcell.2022.893709
Received: 10 March 2022; Accepted: 28 March 2022;
Published: 04 May 2022.
Edited by:
Wu Qi, Renmin Hospital of Wuhan University, ChinaReviewed by:
Francisco G. Vázquez-Cuevas, Universidad Nacional Autónoma de México, MexicoCopyright © 2022 Ferrari, Gessi, Merighi, Nigro, Travagli and Burns. This is an open-access article distributed under the terms of the Creative Commons Attribution License (CC BY). The use, distribution or reproduction in other forums is permitted, provided the original author(s) and the copyright owner(s) are credited and that the original publication in this journal is cited, in accordance with accepted academic practice. No use, distribution or reproduction is permitted which does not comply with these terms.
*Correspondence: Stefania Merighi, bWhzQHVuaWZlLml0
†These authors have contributed equally to this work
Disclaimer: All claims expressed in this article are solely those of the authors and do not necessarily represent those of their affiliated organizations, or those of the publisher, the editors and the reviewers. Any product that may be evaluated in this article or claim that may be made by its manufacturer is not guaranteed or endorsed by the publisher.
Research integrity at Frontiers
Learn more about the work of our research integrity team to safeguard the quality of each article we publish.