- 1School of Biomedical Engineering, The Eye Hospital of Wenzhou Medical University, Wenzhou Medical University, Wenzhou, China
- 2Department of Surgery, Hsinchu Cathay General Hospital, Hsinchu, Taiwan
- 3Graduate Institute of Translational and Interdisciplinary Medicine, National Central University, Taoyuan, Taiwan
- 4Department of Reproduction, National Center for Child Health and Development, Tokyo, Japan
- 5Department of Chemistry, College of Sciences, King Saud University, Riyadh, Saudi Arabia
- 6Center of Regenerative Medicine, Renmin Hospital of Wuhan University, Wuhan, China
- 7School and Hospital of Stomatology, Wenzhou Medical University, Wenzhou, China
- 8Department of Chemical and Materials Engineering, National Central University, Taoyuan, Taiwan
- 9Department of Chemical Engineering and R&D Center for Membrane Technology, Chung Yuan Christian University, Taoyuan, Taiwan
Stem cells serve as an ideal source of tissue regeneration therapy because of their high stemness properties and regenerative activities. Mesenchymal stem cells (MSCs) are considered an excellent source of stem cell therapy because MSCs can be easily obtained without ethical concern and can differentiate into most types of cells in the human body. We prepared cell culture materials combined with synthetic polymeric materials of poly-N-isopropylacrylamide-co-butyl acrylate (PN) and extracellular matrix proteins to investigate the effect of cell culture biomaterials on the differentiation of dental pulp stem cells (DPSCs) into neuronal cells. The DPSCs cultured on poly-L-ornithine (PLO)-coated (TPS-PLO) plates and PLO and PN-coated (TPS-PLO-PN) plates showed excellent neuronal marker (βIII-tubulin and nestin) expression and the highest expansion rate among the culture plates investigated in this study. This result suggests that the TPS-PLO and TPS-PN-PLO plates maintained stable DPSCs proliferation and had good capabilities of differentiating into neuronal cells. TPS-PLO and TPS-PN-PLO plates may have high potentials as cell culture biomaterials for the differentiation of MSCs into several neural cells, such as cells in the central nervous system, retinal cells, retinal organoids and oligodendrocytes, which will expand the sources of cells for stem cell therapies in the future.
Introduction
Stem cells are considered as an ideal source of tissue regeneration therapy because of their stemness properties and regenerative abilities. Comparing embryonic stem cells (ESCs) and induced pluripotent stem cells (iPSCs), mesenchymal stem cells (MSCs) are an attractive source of stem cell therapy that does not involve ethical concerns or tumorigenesis possibilities. MSCs, which are typically isolated from bone marrow and fat tissues, have a good ability to differentiate into adipocytes, osteoblasts, and chondrocytes (Belka et al., 2019; Higuchi et al., 2019; Tsai et al., 2019; Albashari et al., 2020; Pan et al., 2020; Tan et al., 2020; Xing et al., 2020; Yang J. et al., 2020; El-Rashidy et al., 2021; Mohammed et al., 2021; Rozila et al., 2021) and show an ability to differentiate into not only these three types of cells but also ectoderm- or endoderm-derived cells (Georgiou et al., 2015; Andrzejewska et al., 2019; Han et al., 2021; He et al., 2020; Luo et al., 2021a; Luo et al., 2021b; Van Rensburg et al., 2021; Wu et al., 2021; Zhu et al., 2021a). For example, 5-azacytidine in cell culture medium induces MSC differentiation into cardiomyocytes and myoblasts (Xu et al., 2004). The induction of MSCs into hepatocytes can be achieved by stimulation with basic fibroblast growth factor (bFGF), epidermal growth factor (EGF) and nicotinamide, followed by additions of oncostatin M, dexamethasone, insulin, transferrin and selenium (Lee et al., 2004). MSCs can differentiate into neuronal cells by using β-mercaptoethanol, insulin, retinoic acid, bFGF, EGF, valproic acid, and hydrocortisol (Anghileri et al., 2008; Pavlova et al., 2012). Most previous studies focused on the development of differentiation medium to induce MSCs into cells derived from three different germ layers (Lee et al., 2004; Xu et al., 2004; Anghileri et al., 2008; Pavlova et al., 2012; Andrzejewska et al., 2019; Higuchi et al., 2019), and these studies demonstrated that MSCs had great potential to differentiate into cells derived from all three germ layers (Lee et al., 2004; Xu et al., 2004; Anghileri et al., 2008; Pavlova et al., 2012; Andrzejewska et al., 2019; Higuchi et al., 2019); in some ways, these characteristics are comparable to hPSCs (Higuchi et al., 2015; Andrzejewska et al., 2019). Currently, only a few studies have investigated the effect of cell culture biomaterials on MSC differentiation into specific lineages of cells, especially neural cell lineages (Prabhakaran et al., 2009; Zhang et al., 2016; Ghorbani et al., 2018; Luo et al., 2018a; Luo et al., 2018b; Hsiao et al., 2020; Luo et al., 2020; Albashari et al., 2021; Zhu et al., 2021b).
Prabhakaran et al. investigated hMSC differentiation into neurons on poly (L-lactic acid)-co-poly (3-caprolactone)/collagen (PLCL/Coll) nanofibrous scaffolds fabricated by using an electron spinning method (Prabhakaran et al., 2009). They found that the cell proliferation ratio of hMSCs cultured on PLCL/Coll nanofibrous scaffolds was the highest among the cell culture biomaterials examined in this study. An 80% higher cell proliferation was observed for the cells on the PLCL/Coll nanofibrous scaffolds than the cells cultured on PLCL nanofibrous scaffolds only (Prabhakaran et al., 2009). Although the cell proliferation on the PLCL/Coll nanofibrous scaffolds was slightly higher than that cultured on tissue culture polystyrene (TPS) plates, it is suggested that the combination of synthetic material (PLCL) and extracellular matrix (ECM) protein (Coll) can provide a balance with a stable cell proliferation rate and efficient differentiation abilities.
Ghorbani et al. investigated human Wharton jelly-derived mesenchymal stem cells (WJ-hMSCs) cultured on polylactic acid (PLA) scaffolds by using a wet-electrospinning method and by subsequently coating with alginate and gelatin, and the WJ-hMSCs were differentiated into neurons (Ghorbani et al., 2018). WJ-hMSCs cultured on the PLA scaffolds were more favored to differentiate into neuronal cells than those cultured on TPS plates, which was validated by immunostaining and qPCR assays of nestin, microtubule-associated protein 2 (MAP2), and neuron-specific enolase (NSE) expression (Ghorbani et al., 2018).
In our previous study (Sung et al., 2021a), human adipose-derived stem cells (hADSCs) were cultivated sequentially in a two-dimensional (2D) biomaterial surface and in a three-dimensional (3D) culturing condition. hADSCs cultured in 3D culture conditions showed higher expression of differentiation markers of osteogenic cells and chondrogenic cells than that of hADSCs cultured in 2D culture conditions when hADSCs were differentiated into osteogenic cells and chondrogenic cells (Sung et al., 2021a). However, the pluripotency and differentiation ability of hADSCs were extremely decreased when they were transferred from 3D culture conditions to 2D culture conditions and vice versa, which suggested that hADSCs have reversible characteristics in terms of their pluripotent characteristics and differentiation potential that depend on their environmental niche in 3D and 2D cultures.
In another study (Gao et al., 2019), hADSCs were cultured on several ECM-coated plates in xeno-free medium with human platelet lysate (hPL). Matrigel-coated surfaces suppressed hADSC differentiation into chondrocytes and facilitated hADSC induction into osteoblasts in media supplemented with 10% hPL (Gao et al., 2019). Fibronectin-coated and recombinant vitronectin-coated surfaces extensively facilitated hADSC induction into chondrocytes and osteoblasts in media supplemented with 5 and 10% hPL (Gao et al., 2019). hPL facilitated hADSC induction into chondrogenic and osteogenic differentiation compared to that of fetal bovine serum (FBS) on all tested ECM-immobilized surfaces.
In this study, we investigated the effect of cell cultivation biomaterials on the differentiation of dental pulp stem cells (DPSCs) into neuronal cells. DPSCs are categorized as one type of MSC. The isolation of DPSCs from a patient is a simple and easy process that does not involve pain or complex surgery. DPSCs can also be obtained from an infant’s tooth as the tooth changes into a permanent tooth. DPSCs show strong MSC markers of CD44, CD73, CD90 and CD105 on their surfaces (Machado et al., 2016; Luzuriaga et al., 2019; Noda et al., 2019). DPSCs and MSCs also share several characteristics. DPSCs belong to the neuronal crest; therefore, DPSCs have a greater tendency to secrete nerve growth factors (NGFs) and to differentiate into neuronal cells than that of bone marrow stem cells. Therefore, DPSCs should be an ideal cell source for neuronal repair in tissue regeneration therapy (d'Aquino et al., 2009; Pisciotta et al., 2015; Bonaventura et al., 2020; Pisciotta et al., 2020).
Suitable polymeric scaffolds can efficiently deliver therapeutic cells to the target site of injuries. Therefore, Feng et al. developed small 3D porous chitosan scaffolds using a freeze-drying process, and they demonstrated that the chitosan scaffolds can promote DPSCs differentiation into neuronal cells in vitro (Feng et al., 2014). They found that the DPSCs in the chitosan scaffolds expressed high expression of nestin, which was reduced sharply following differentiation (Feng et al., 2014). This investigation showed that granular 3D chitosan scaffolds provided a conducive and appropriate microenvironment for cell attachment, proliferation, and neuronal differentiation of DPSCs.
Hsiao et al. reported that DPSCs could successfully adhere to 3D-printed polylactic acid scaffolds (3DP-PLAS) modified by poly-L-lysine and demonstrated morphological changes and related protein expression in DPSCs (Hsiao et al., 2020). They also found that cellular orientations were more easily induced with DPSCs cultured on 3DP-PLAS with 150 μm gaps than with DPSCs cultured on 200 μm gaps. This study suggested that DPSCs cultured on 3DP-PLASs with narrow gaps in width showed better efficiency to differentiate into neurons (Hsiao et al., 2020).
Zhang et al. reported that DPSCs differentiated into neurons on chitosan scaffolds with an average pore diameter of 270 μm and showed the highest neuron marker expression (Zhang et al., 2016). The secretion of NT-3, b-NGF, GDNF and BDNF was significantly increased in the DPSCs/chitosan-scaffold group by 2.3-fold, 4.1-fold, 1.5-fold and 2.2-fold compared with the control group (DPSCs culture on TPS dishes), respectively. In a spinal cord injury (SCI) mouse model, the transplantation of DPSCs-derived neurons on chitosan scaffolds into mice significantly inhibited active caspase-3 (decrease in apoptotic cells) compared with that in the control groups (Zhang et al., 2016). DPSCs cultured on the scaffolds showed not only increased DPSCs-derived neuronal differentiation ability but also elevated efficacy in inhibiting SCI neuronal cell apoptosis after transplantation.
Zheng et al. performed DPSCs culture on chitosan scaffolds, which induced differentiation into neuronal cells for 7 days (Zheng et al., 2021). Among the comparison of DPSCs cultured on the control and DPSCs/chitosan-scaffold groups, the expression of glial fibrillary acidic protein (GFAP), S100β (astrocyte marker) and βⅢ-tubulin (neuronal marker) was extensively enhanced in the DPSCs/chitosan-scaffold group. Based on their findings (Zheng et al., 2021), chitosan scaffolds, which were not cytotoxic to the survival of DPSCs, were more favored for DPSCs neural differentiation.
MSCs typically reside in and are surrounded by extracellular matrix (ECM) proteins. ECM proteins surrounded by DPSCs in vivo typically contain collagen type IV, collagen type III and fibronectin with small amounts of collagen type I (Linde, 1985; Malara et al., 2014). Several glycoproteins, such as proteoglycans, hyaluronic acids and glycosaminoglycans, are also included in the DPSCs niche (Linde, 1985; Malara et al., 2014). These compositions are significantly different depending on the MSCs (Laudani et al., 2020). Therefore, there should be an optimal ECM protein that is suitable for DPSCs differentiation into neuronal cells. However, there is no research to investigate the effect of several ECM proteins as cell culture biomaterials on the differentiation of human DPSCs into neural cells from our database studies, although there are several studies (Arthur et al., 2008; Ebrahimi et al., 2011; Zainal Ariffin et al., 2013; Chang et al., 2014; Feng et al., 2014; Kanafi et al., 2014; Al-Zer et al., 2015; Mattei et al., 2015; Cho et al., 2016; Young et al., 2016; Zhang et al., 2016; Geng et al., 2017; Sanen et al., 2017; Ullah et al., 2017; Zhang et al., 2017; Ganapathy et al., 2019; Hsiao et al., 2020; Rafiee et al., 2020; Solis-Castro et al., 2020; Arimura et al., 2021; Darvishi et al., 2021; Zheng et al., 2021) that address human DPSCs differentiation into neural cells.
In this study, we screened ECM proteins together with synthetic polymeric materials of poly-N-isopropylacrylamide-butyl acrylate (PN), which has high potential to induce DPSCs into neuronal cells. We first evaluated DPSCs growth on PN-coated plates with and without ECM protein coating to achieve the best growth rate of DPSCs. Subsequently, we evaluated DPSCs differentiation into neuronal cells based on the neural marker expression of nestin (neural stem/progenitor cell marker) and βIII-tubulin on PN-coated plates with and without ECM protein coating (Higuchi et al., 2019). We investigated the best composition of the PN and ECM proteins coating materials for DPSCs culture and differentiation into neuronal cells. This research has the potential to help develop optimal conditions for the differentiation of DPSCs into neuronal cells, which may be applied for future regenerative medicine for several neural diseases, such as age-related macular degeneration, spinal cord injury or Parkinson’s disease.
Material and Methods
Materials
The proteins, chemicals and biomaterials used in this research are summarized in Table 1. The other chemicals utilized in this project were received from Sigma-Aldrich (St. Louis, MO, United States).
Dental Pulp Stem Cell Extraction
Dental pulp was isolated from teeth after tooth extraction surgery with informed patient consent (10 patients, 5–18 years old). These informed consents were also acquired the agreement from a parent and/or legal guardian for this study. A schematic of DPSCs extraction from dental pulp is illustrated in Figure 1. The tooth surface was sterilized with 2% streptomycin-penicillin (SP) for 5 min. Teeth were cut and the inner dental pulp was exposed by using a surgical drill, scissors and knives. Dental pulp tissue was washed and suspended in Dulbecco’s modified Eagle’s medium (DMEM) supplemented with 10% FBS and 1% SP. Subsequently, the dental pulp tissue was seeded on TPS plates and incubated at 37°C under a 5% CO2 atmosphere. After the cells (DPSCs) reached 78–82% confluence, DPSCs were detached using a 0.25% trypsin-ethylenediaminetetraacetic acid (EDTA) solution by digestion, centrifuged and passaged into new TPS plates according to a conventional passage procedure. DPSCs at passages 5-7 were used for the following experiments.
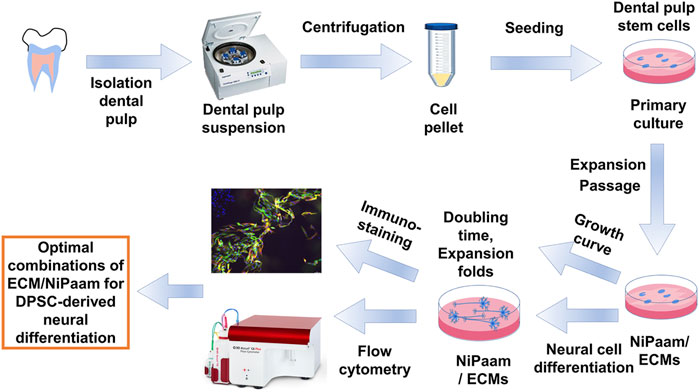
FIGURE 1. Experimental scheme of DPSCs extraction, culture, characterization and differentiation into neuronal cells.
Preparation of Cell Culture Biomaterials
TPS-PN plates (poly-N-isopropylacrylamide-co-butyl acrylate-coated TPS plates) were prepared as follows. Poly-N-isopropylacrylamide-co-butyl acrylate, PN, which has a lower critical solution temperature (LCST) of approximately 30°C with a molecular weight of 88,000, was dissolved to 1.8 mg/ml in 99.5% ethanol. The PN solution was inserted into TPS 6-well plates (1.5 ml per well, surface area of 9.6 cm2) and incubated at 37°C for 2 h. Then, the plates were rinsed using 1 ml of 99.5% ethanol per well four times, and then the plates were rinsed with 1 ml of ultrapure water per well four times. Subsequently, the plates were rinsed with 1 ml of 5, 3 and 1% antibiotic-antimycotic solution per well sequentially.
Laminin-521 (LN), poly-L-ornithine (PLO), and recombinant vitronectin (rVT) were prepared as 5 μg/ml solutions utilizing phosphate buffered solution (PBS). LN, PLO, or rVT solution was inserted into 6-well plates in which PN was either coated (TPS-PN) in advance or not coated (TPS), and the plates were incubated at 37°C for 2 h. Then, the LN, PLO, or rVT solution was removed from 6-well plates, and the plates were utilized for subsequent DPSCs cultivation and differentiation experiments. TPS-LN, TPS-rVT and TPS-PLO plates indicate LN-coated TPS, rVT-coated TPS and PLO-coated TPS plates, respectively. TPS-PN-LN, TPS-PN-rVT and TPS-PN-PLO indicate LN-coated TPS-PN, rVT-coated TPS-PN and PLO-coated TPS-PN plates, respectively.
Characterization of the Cell Culturing Plates
Chemical analysis (N1s and C1s) of TPS and the TPS-PN, TPS-ECM (ECM = rVT, LN or PLO) and TPS-PN-ECM plates were performed using XPS (X-ray photoelectron spectroscopy, Thermal Scientific, Inc., Amarillo, TX, United States) as previously described (Sung et al., 2021b). The binding energy scale adjustment was set from the peak maximum at 284.6 eV in the C1s spectrum.
DPSCs Characterization
Doubling time of DPSCs was calculated from following formula:
where NX indicates cell number at X day and NO indicates the initial cell number.
CD34 (negative marker, hematopoietic stem cell and endothelial progenitor marker), CD73 (MSC marker), and CD105 (MSC marker) expression in DPSCs was evaluated using flow cytometry (BD Accuri™ C6, BD Biosciences, Franklin Lakes, NJ, United States) (Figure 1). The cells were incubated with anti-CD markers (1:500 dilution) or isotype antibodies (1:500 dilution) for 45 min, and the solution was centrifuged at 450 × g for 8 min. The cells were transferred into a phosphate-buffered saline solution and evaluated utilizing flow cytometry as previously described (Sung et al., 2021a).
Inducing the Differentiation of DPSCs Into Neuronal Cell
The procedure of inducing the differentiation of DPSCs into neuronal cells was followed by the method reported by Wang et al. with some modifications [12]. On day −5, DPSCs were inoculated into ECM- and/or PN-coated plates and cultured in DPSCs medium (DMEM supplemented with 10% FBS and 1% SP) until day 0 by exchanging the DPSCs medium every other day. On day 0, DPSCs displayed approximately 85–90% confluence. The DPSCs cultivation medium was discarded, and neurobasal-A medium supplemented with 2 wt% B27, 20 ng/ml EGF and 20 ng/ml bFGF was added to the plates. The media were changed every 3 days. The cells were cultivated until day 15. We investigated whether the purity of DPSCs-derived neuronal cells was facilitated by different cell culture surfaces. These cells were analyzed for the expression levels of βIII-tubulin and nestin using flow cytometry and immunostaining.
Statistical Analysis
Experimental results were analyzed from four replicates. The data are shown as the mean ± standard deviation (SD). Statistical analyses were processed using One-way ANOVA in Excel (Microsoft Corporation) with post-hoc t-test. Probability values (p) less than 0.05 were considered statistically significant.
Results
XPS Assay of PN-Coated and PN Plus ECM-Coated Plates
We developed PN-coated (TPS-PN) and PN plus ECM-coated plates (TPS-PN-ECM) as well as ECM-coated (TPS-ECM) plates for DPSCs culture and differentiation. Although cells can extensively attach to PN-coated plates more than to TPS plates, neuronal cells need to attach to the plates via specific integrin binding. Therefore, not only PN-coated plates but also PLO or ECM (rVT and LN) were further coated on PN-coated plates (TPS-PN-PLO, TPS-PN-rVT and TPS-PN-LN) in this study (Figure 1).
These plates were analyzed to investigate the existence of PN and/or ECM on the plate surface utilizing XPS before the cultivation and differentiation of DPSCs into neuronal cells, where ECM-coated plates (TPS-rVT, TPS-LN, TPS-PLO, TPS-PN-rVT, TPS-PN-LN and TPS-PN-PLO) as well as TPS and TPS-PN plates were characterized. Figure 2 displays the high-resolution XPS spectra of the C1s (Figure 2A) and N1s (Figure 2B) peaks of the (a) TPS surface (negative control), (b) TPS-PN (PN-coated TPS) surface, (c) TPS-rVT (rVT-coated TPS) surface, (d) TPS-PN-rVT (rVT-coated TPS-PN) surface, (e) TPS-LN (LN-coated TPS) surface, (f) TPS-PN-LN (LN-coated TPS-PN) surface, (g) TPS-PLO (PLO-coated TPS) surface and (h) TPS-PN-PLO (PLO-coated TPS-PN) surface.
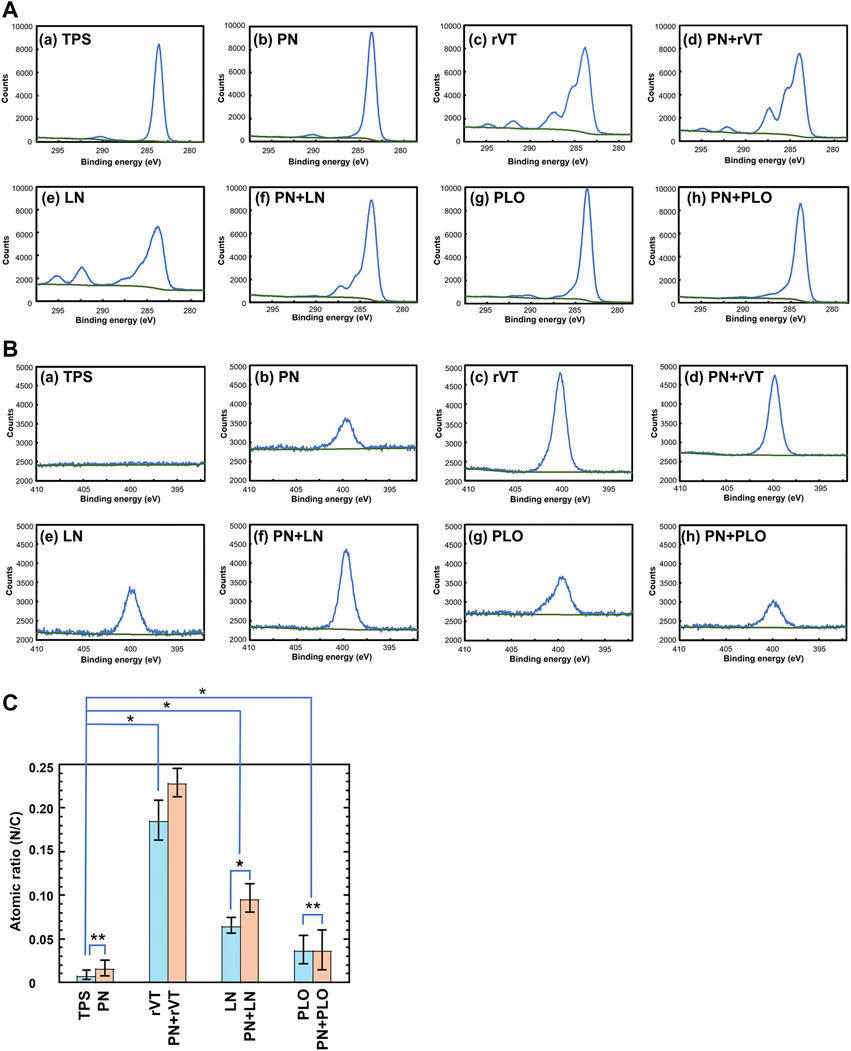
FIGURE 2. Characterization of TPS, TPS-PN, TPS-ECM, TPS-PLO, TPS-PN-ECM and TPS-PN-PLO plates. (A) High-resolution XPS spectra of the C1s peaks on the surface of TPS (a), TPS-PN (b), TPS-rVT (c), TPS-PN-rVT (d), TPS-LN (e), TPS-PN-LN (f), TPS-PLO (g) and TPS-PN-PLO (h) plates. (B) High-resolution XPS spectra of the N1s peaks on the surface of TPS (a), TPS-PN (b), TPS-rVT (c), TPS-PN-rVT (d), TPS-LN (e), TPS-PN-LN (f), TPS-PLO (g) and TPS-PN-PLO (h) plates. (C) The nitrogen to carbon (N/C) atomic ratios on the surface of TPS, TPS-PN, TPS-ECM, TPS-PLO, TPS-PN-ECM and TPS-PN-PLO plates. *p < 0.05. **p > 0.05.
The C1s peak at approximately 285 eV was observed on any surface investigated in this study (Figure 2A). A relatively broad C1s peak was detected on the ECM-coated surface (TPS-rVT, TPS-PN-rVT, TPS-LN and TPS-PN-LN plates) compared to that on the TPS, TPS-PN, TPS-PLO and TPS-PN-PLO plates, which indirectly suggested the existence of ECM on ECM-coated plates.
The N1s peak at 400 eV was extensively observed on TPS-rVT, TPS-PN-rVT, TPS-LN, TPS-PN-LN, TPS-PLO, and TPS-PN- PLO plates, whereas no distinct N1s peak was found on the TPS surface, and a weak N1s peak was observed on the TPS-PN surface (Figure 2B). These findings were directly related to the atomic ratio of N/C described in Figure 2C and suggested the existence of ECM and PLO on ECM-coated and PLO-coated plates.
DPSCs Extraction and Characterization
Dental pulp stem cells (DPSCs) were isolated from discarded teeth after tooth removal surgery. The process of DPSCs preparation is schematically described in Figure 1. The dental pulp was isolated from teeth, the surface of the dental pulp was sterilized with 2% SP solution, and the dental pulp was incised to expose the inner pulp. The inner pulp was suspended in medium and subsequently cut into 1–2 mm2 square small pieces of inner pulp tissue by using sterilized surgery scissors. Then, the pulp tissue was centrifuged to obtain inner pulp tissue pellets. Subsequently, the inner pulp tissues (dental pulp cells) were inserted into (a) TPS plates, (b) PN-coated (TPS-PN) plates, (c) ECM (rVT and LN)-coated (TPS-rVT and TPS-LN) plates, (d) PLO-coated (TPS-PLO) plates and (e) PN-coated plates where ECM (rVT and LN) or PLO was subsequently coated (TPS-PN-rVT, TPS-PN-LN and TPS-PN-PLO) plates. Subsequently, the cells were cultured on these plates for 10 passages in DMEM supplemented with 10% FBS (Figure 3A). After 1 week of cell cultivation, the cells migrated and extended from the pulp, which could be observed under microscopic observation. These cells were considered to be primary DPSCs (passage 0, P0) (Figure 3B). When DPSCs became confluent on the plates, DPSCs at P0 were detached by using a 0.25% trypsin-EDTA digestion for the passage process. After performing a passage and subsequent cell culture for 14 days, the cells started to show more spindle morphologies, which are characteristics of MSCs (DPSCs) (Figure 3B). These primary cells were cultured for 5 passages (P5).
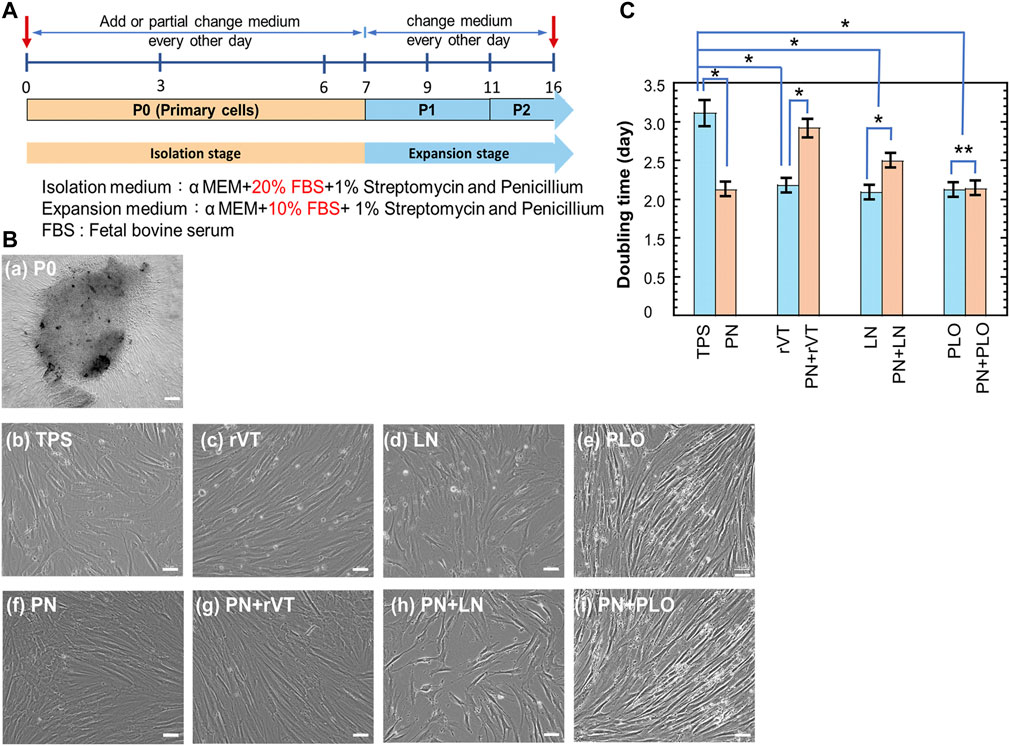
FIGURE 3. Cultivation of DPSCs on TPS, TPS-PN, TPS-ECM, TPS-PLO, TPS-PN-ECM and TPS-PN-PLO plates for five passages. (A) The timeline of primary DPSCs culturing and expansion. (B) The morphologies of primary DPSCs (day 5 at P0) (a) and day 5 at P5 (b–i) on the TPS (b), TPS-rVT (c), TPS-LN (d), TPS-PLO (e), TPS-PN (f), TPS-PN-rVT (g), TPS-PN-LN (h) and TPS-PN-PLO (i) plates. (C) The doubling time of DPSCs on TPS, TPS-PN, TPS-ECM, TPS-PLO, TPS-PN-ECM and TPS-PN-PLO plates at passages 3-5. The bar indicates 200 μm (a) and 50 μm (b–i). *p < 0.05. **p > 0.05.
We evaluated the doubling time of the cells, which were cultured on different cell culture biomaterials, during passages 3-5 and the results are shown in Figure 3C. The fastest DPSC doubling time was 2.1 days, in which DPSCs were cultured on TPS-PN, TPS-rVT, TPS-LN, TPS-PLO and TPS-PN-PLO plates. Compared with the DPSCs on TPS plates (doubling time = 3.1 days), DPSCs on TPS-PN, TPS-rVT, TPS-LN, TPS-PLO and TPS-PN-PLO plates (doubling time = 2.1 days) had a doubling time that was faster by 1 day (p < 0.05). In the other ECM-coated plate group, the doubling times of DPSCs on TPS-PN-rVT and TPS-PN-LN plates were 2.9 and 2.5 days, respectively, which were 0.2 and 0.6 days faster than the cells cultured on TPS plates, respectively (p < 0.05 for TPS vs. TPS-PN-LN). This indicates that PN coating with ECMs causes a longer doubling time for DPSCs cultured on PN and ECM coating plates compared to that of the cells on the same ECM-coated plates without PN coatings (p < 0.05). However, the doubling time of DPSCs was not significantly different when the cells were cultured on TPS-PLO and TPS-PN-PLO plates (p > 0.05), in which the doubling time of DPSCs on these plates was the shortest (2.1 days) in this study. This result indicated that the TPS-PLO and TPS-PN-PLO plates were the most stable culturing materials for DPSCs cultivation and expansion.
MSC Marker Expression of DPSCs Cultured on ECM and PN-Coated Plates
We evaluated the MSC marker expression of CD 73 and CD 105 and hematopoietic marker expression of CD 34 on DPSCs cultured on (a) TPS plates, (b) PN-coated (TPS-PN) plates, (c) ECM-coated (TPS-rVT and TPS-LN) plates, (d) PLO-coated (TPS-PLO) plates and (e) PN-coated plates where ECM (TPS-PN-rVT and TPS-PN-LN) or PLO was subsequently coated (TPS-PN-PLO) were examined, and the results are shown in Figure 4. In general, all MSC markers of DPSCs cultured on different materials showed expressions of more than 90% except for DPSCs on TPS-rVT and TPS-PN-rVT plates, whereas CD34 was not expressed in the DPSCs cultured on any plates investigated in this study. DPSCs showed the lowest CD 73 and CD105 marker expression on TPS-rVT plates at 79.5 and 80.2%, respectively. The highest CD73 and CD105 expression was found for DPSCs cultured on TPS-PN-PLO plates, which was 97 and 92.8%, respectively. The difference among DPSCs cultured on ECM- or PLO-coated plates with or without PN coating were less distinct. These results suggested that the MSC marker expression of DPSCs was relatively high on any cell culture biomaterial investigated in this study. However, it should be noted that DPSCs cultured on TPS-PN-PLO plates showed the highest MSC surface marker expression, which may allow DPSCs to maintain their proliferation ability during the culturing process.
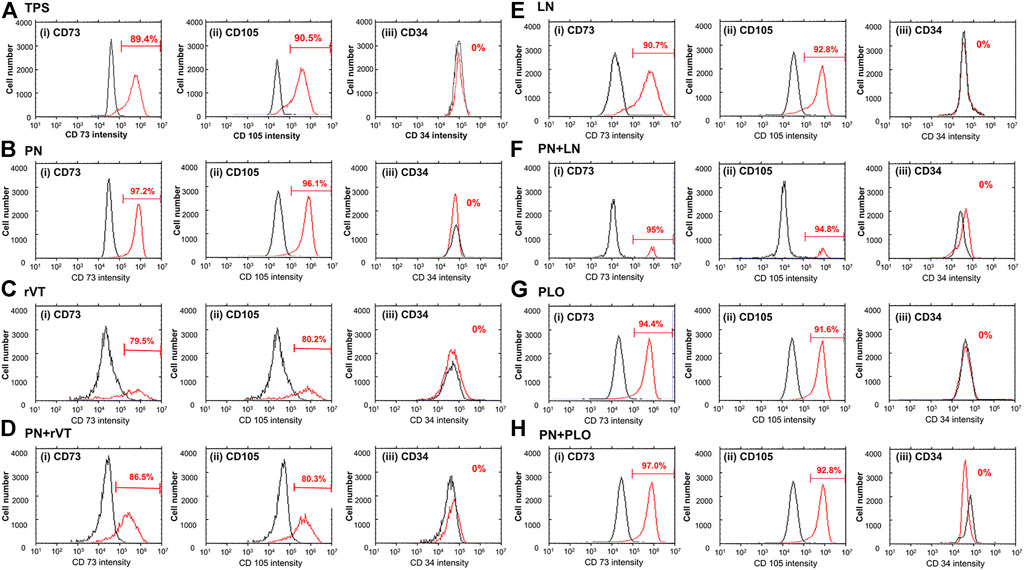
FIGURE 4. Expression of MSC markers [CD73 (i) and CD105 (ii)] and hematopoietic marker [CD34 (iii)] in DPSCs cultured on TPS (A), TPS-PN (B), TPS-rVT (C), TPS-PN-rVT (D), TPS-LN (E), TPS-PN-LN (F), TPS-PLO (G) and TPS-PN-PLO plates (H).
Neuronal Cell Differentiation of DPSCs on ECM- and PN-Coated Plates
DPSCs were induced into neuronal cell differentiation using neuron basal medium supplemented with B27, EGF and bFGF (Figure 5A), which were cultured on ECM-coated (TPS-LN and TPS-rVT) plates, PLO-coated (TPS-PLO) plates and PN-coated (TPS-PN, TPS-PN-rVT, TPS-PN-LN and TPS-PN-PLO) plates as well as noncoated TPS plates. Supplementary Figure S1 displays the sequential morphological changes in DPSCs induced into neuronal cells on ECM- and/or PN-coated plates. Extended long cell colonies began to gradually appear with increasing time after day 4. Several colonies detached from the edge as time passed, especially from the surface of ECM-coated plates, and then, the colonies of the dead cells peeled off from the plates. After day 8, we observed that the cells on each plate surface had neuronal fiber-like morphologies. The neuronal cell marker assay was further performed in the following experiments.
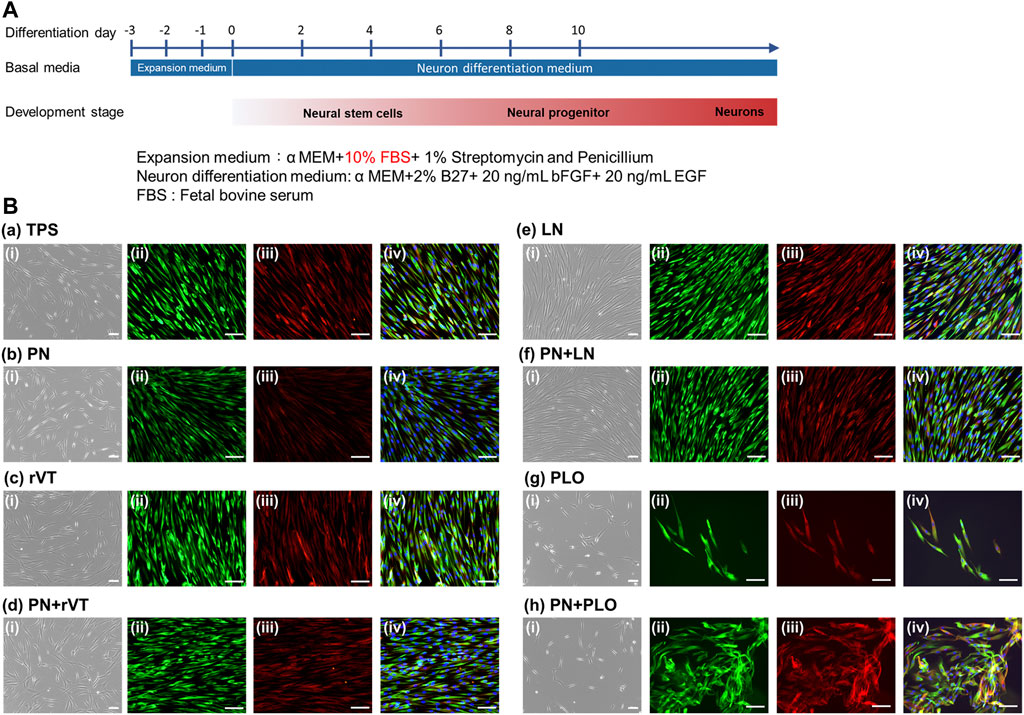
FIGURE 5. Differentiation of DPSCs into neuronal cells after five passages of cultivation on TPS, TPS-PN, TPS-ECM, TPS-PLO, TPS-PN-ECM and TPS-PN-PLO plates. (A) The timeline of the induction of DPSCs differentiation into neuronal cells. (B) The morphologies of DPSCs-derived neuronal cells (i) and the immunohistochemical assay of βIII-tubulin [(ii), green] and nestin [(iii), red)] expression on the TPS (a), TPS-PN (b), TPS-rVT (c), TPS-PN-rVT (d), TPS-LN (e), TPS-PN-LN (f), TPS-PLO (g) and TPS-PN-PLO (h) plates. The photo (iv) was the merged photos of (ii) and (iii). The scale bar indicates 100 μm.
Characterization of DPSCs-Derived Neuronal Cells on ECM and PN-Coated Plates
The expression of the neuronal cell marker proteins βIII-tubulin and nestin on DPSCs-derived neuronal cells, which were cultured on PN-coated plates (TPS-PN, TPS-PN-rVT, TPS-PN-LN and TPS-PN-PLO), ECM-coated plates (TPS-rVT and TPS-LN), PLO-coated plates (TPS-PLO) and TPS plates, was evaluated using an immunofluorescence staining assay at day 15 of differentiation, and the results are shown in Figure 5B. DPSCs-derived neuronal cells, which could induce differentiation on all of the plates in this study, showed extensive expression of βIII-tubulin and nestin. There was no significant difference in the expression of βIII-tubulin and nestin in the cells cultivated on the TPS, TPS-LN and TPS-PN-LN plates or on the TPS-PN, TPS-rVT and TPS-PN-rVT plates. However, we found that the DPSCs differentiated on TPS-PN-PLO plates had the highest expression of βIII-tubulin and nestin in the immunostaining assay in this study (Figure 5B).
We also quantitatively evaluated whether the differentiation rate of DPSCs-derived neuronal cells was enhanced on the PN surface. After 15 days of differentiation, the neuronal marker (βIII-tubulin and nestin) expression of DPSCs-derived neuronal cells was evaluated using flow cytometry. The flow cytometry charts of DPSCs-derived neuronal cells, which were cultivated on different culturing materials (rVT-, LN-, PLO-coated TPS dishes with or without PN coating surface as well as TPS and TPS-PN surface), are shown in Figure 6A for nestin expression and Supplementary Figure S2 for βIII-tubulin expression. The averaged nestin and βIII-tubulin expression of these cells is described in Figures 6B,C, respectively. DPSCs-derived neuronal cells cultured on TPS-PLO and TPS-PN-PLO plates showed more than 90% nestin expression and more than 85% βIII-tubulin expression, which were comparable to that of the cells cultured on TPS-PN or TPS plates. Although DPSCs-derived neuronal cells exhibited high expression of βIII-tubulin and nestin on the TPS plates, the expansion fold of DPSCs on TPS plates was significantly less (the doubling time was high) than that of the cells cultured on TPS-PLO and TPS-PN-PLO plates (Figure 6D).
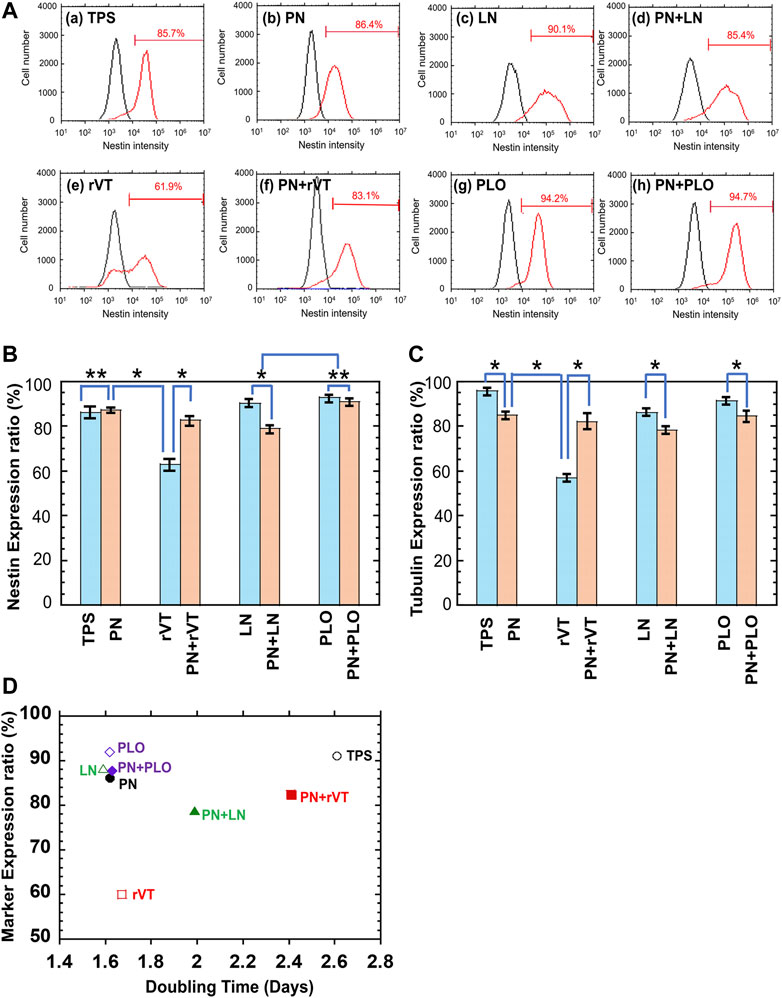
FIGURE 6. Characterization of DPSCs-derived neuronal cells after five passages of DPSCs cultivation on TPS, TPS-PN, TPS-ECM, TPS-PLO, TPS-PN-ECM and TPS-PN-PLO plates. (A) Flow cytometry spectra of nestin expression on DPSCs-derived neuronal cells cultivated on TPS (a), TPS-PN (b), TPS-LN (c), TPS-PN-LN (d), TPS-rVT (e), TPS-PN-rVT (f), TPS-PLO (g) and TPS-PN-PLO (h) plates. (B) Nestin expression ratio cultured on DPSCs-derived neuronal cells, which were cultivated on TPS, TPS-PN, TPS-ECM, TPS-PLO, TPS-PN-ECM and TPS-PN-PLO plates. *p < 0.05. **p > 0.05. (C) βIII tubulin expression ratio cultured on DPSCs-derived neuronal cells, which were cultivated on TPS, TPS-PN, TPS-ECM, TPS-PLO, TPS-PN-ECM and TPS-PN-PLO plates. *p < 0.05. (D) Dependence of the averaged neuronal expression of DPSC-derived neuronal cells on the doubling time of DPSCs cultured on TPS, TPS-PN, TPS-ECM, TPS-PLO, TPS-PN-ECM and TPS-PN-PLO plates.
The relationship between the neuronal marker expression rate (average rate of βIII-tubulin and nestin expression) on DPSCs-derived neuronal cells and the fold expansion of DPSCs was investigated and plotted in Figure 6D. This plot indicated that the TPS-PN, TPS-PLO, TPS-PN-PLO and TPS-LN plates were the most suitable cell culture biomaterials for DPSC expansion and differentiation into neuronal cells.
Discussion
DPSCs and DPSCs-derived neuronal cells were successfully cultured and differentiated on rVT-, LN-, and PLO-coated plates where PN was coated or not coated on the plates in advance. These ECM-coated and PLO-coated plates, which were combined with or without PN coating, could be easily prepared by the coating method. In our previous study (Peng et al., 2016; Yang L. et al., 2020), poly (N-isopropylacrylamide-co-styrene), PN, was used as a coating material for hESC and hiPSC cultivation. The current study indicated that PN-coated plates were not favorable for DPSCs culture and differentiation except for the TPS-PN and TPS-PN-PLO plates (Figure 6D). Neuronal cells derived from DPSCs on TPS-rVT plates showed less βIII-tubulin and nestin expression than that of cells cultured on other the plates investigated in this study, such as the TPS-PN, TPS-PN-rVT, TPS-LN, TPS-PN-LN, TPS-PLO and TPS-PN-PLO plates. By the combination coating of rVT and PN on the plates, the cells on TPS-PN-rVT plates showed similar expression of βIII-tubulin and nestin compared to that of the cells on TPS-PLO and TPS-PN-PLO plates. Therefore, PN coating on the plates is important for rVT-coated plates to enhance neuronal differentiation and the expansion of DPSCs. The cells on the TPS-PN-PLO plates showed similar expressions of βIII-tubulin and nestin and similar expansion folds compared to that of the cells on PLO-TPS plates. The cells on TPS-LN plates also showed high expressions of βIII-tubulin and nestin and had relatively good expansion folds. It should be noted that the cells cultured on TPS-PLO or TPS-PN-PLO plates showed a more than 20-fold expansion rate, which is the highest expansion rate in this study and 2-fold higher than that of the cells on TPS or TPS-PN plates. These results suggest that the DPSCs cultured on TPS-PLO and TPS-PN-PLO plates maintained their stable proliferation and good ability to differentiate to neuronal cells (Figures 3, 6). We expect our culture plates, such as the TPS-PLO and TPS-PN-PLO plates, to have high potential for being applied as cell culture biomaterials for the differentiation of MSCs into other neural cells, such as neural cells in the central nervous system, retinal cells (Smith et al., 2019; Michelet et al., 2020), retinal organoids (Cowan et al., 2020; O'Hara-Wright and Gonzalez-Cordero, 2020) and oligodendrocytes (Higuchi et al., 2019), to extend the sources of cells for stem cell therapies in the future.
Data Availability Statement
The raw data supporting the conclusion of this article will be made available by the authors, without undue reservation.
Ethics Statement
The studies involving human participants were reviewed and approved by the Wenzhou Medical University (2020-135-K-120-01, 01 November 2020). Written informed consent to participate in this study was provided by the participants’ legal guardian/next of kin.
Author Contributions
Conceptualization, AH; methodology, T-CS and AH; software, YG and ZT; validation, YG and ZT; formal analysis, QL and TW; investigation, YG, ZT, QL, and TW; resources, HH-CL, T-CS, and AH; data curation, L-KB, YG and ZT; writing—original draft preparation, T-CS and AH; writing—review and editing, AH and QY; visualization, T-CS, AH, NA, and AA; supervision, HC, AU, QY, and RK.; project administration, AH; funding acquisition, AH, HC, L-KB, HH-CL, and RK. All authors have read and agreed to the published version of the manuscript.
Funding
This research was partially supported by the State Key Laboratory of Ophthalmology, Optometry and Visual Science of China, The Eye Hospital of Wenzhou Medical University under Grant Numbers 4214821001G. The project was also supported by Researchers Supporting Project number (RSP-2021/143), King Saud University, Riyadh, Saudi Arabia. This research was also funded by the Cathay General Hospital Project (MR-A11029 and MR-A11030).
Conflict of Interest
The authors declare that the research was conducted in the absence of any commercial or financial relationships that could be construed as a potential conflict of interest.
Publisher’s Note
All claims expressed in this article are solely those of the authors and do not necessarily represent those of their affiliated organizations, or those of the publisher, the editors and the reviewers. Any product that may be evaluated in this article, or claim that may be made by its manufacturer, is not guaranteed or endorsed by the publisher.
Supplementary Material
The Supplementary Material for this article can be found online at: https://www.frontiersin.org/articles/10.3389/fcell.2022.893241/full#supplementary-material
References
Al-Zer, H., Apel, C., Heiland, M., Friedrich, R. E., Jung, O., Kroeger, N., et al. (2015). Enrichment and Schwann Cell Differentiation of Neural Crest-Derived Dental Pulp Stem Cells. In Vivo 29 (3), 319–326.
Albashari, A. A., He, Y., Albaadani, M. A., Xiang, Y., Ali, J., Hu, F., et al. (2021). Titanium Nanotube Modified with Silver Cross-Linked Basic Fibroblast Growth Factor Improves Osteoblastic Activities of Dental Pulp Stem Cells and Antibacterial Effect. Front. Cell Dev. Biol. 9 (665), 654654. doi:10.3389/fcell.2021.654654
Albashari, A., He, Y., Zhang, Y., Ali, J., Lin, F., Zheng, Z., et al. (2020). Thermosensitive bFGF-Modified Hydrogel with Dental Pulp Stem Cells on Neuroinflammation of Spinal Cord Injury. ACS Omega 5 (26), 16064–16075. doi:10.1021/acsomega.0c01379
Andrzejewska, A., Lukomska, B., and Janowski, M. (2019). Concise Review: Mesenchymal Stem Cells: From Roots to Boost. Stem Cells 37 (7), 855–864. doi:10.1002/stem.3016
Anghileri, E., Marconi, S., Pignatelli, A., Cifelli, P., Galié, M., Sbarbati, A., et al. (2008). Neuronal Differentiation Potential of Human Adipose-Derived Mesenchymal Stem Cells. Stem Cells Dev. 17 (5), 909–916. doi:10.1089/scd.2007.0197
Arimura, Y., Shindo, Y., Yamanaka, R., Mochizuki, M., Hotta, K., Nakahara, T., et al. (2021). Peripheral-Neuron-Like Properties of Differentiated Human Dental Pulp Stem Cells (hDPSCs). PLoS One 16 (5), e0251356. doi:10.1371/journal.pone.0251356
Arthur, A., Rychkov, G., Shi, S., Koblar, S. A., and Gronthos, S. (2008). Adult Human Dental Pulp Stem Cells Differentiate toward Functionally Active Neurons under Appropriate Environmental Cues. Stem Cells 26 (7), 1787–1795. doi:10.1634/stemcells.2007-0979
Belka, J., Nickel, J., and Kurth, D. G. (2019). Growth on Metallo-Supramolecular Coordination Polyelectrolyte (MEPE) Stimulates Osteogenic Differentiation of Human Osteosarcoma Cells (MG63) and Human Bone Marrow Derived Mesenchymal Stem Cells. Polymers 11 (7), 1090. doi:10.3390/polym11071090
Bonaventura, G., Incontro, S., Iemmolo, R., La Cognata, V., Barbagallo, I., Costanzo, E., et al. (2020). Dental Mesenchymal Stem Cells and Neuro-Regeneration: A Focus on Spinal Cord Injury. Cell Tissue Res. 379 (3), 421–428. doi:10.1007/s00441-019-03109-4
Chang, C.-C., Chang, K.-C., Tsai, S.-J., Chang, H.-H., and Lin, C.-P. (2014). Neurogenic Differentiation of Dental Pulp Stem Cells to Neuron-Like Cells in Dopaminergic and Motor Neuronal Inductive Media. J. Formos. Med. Assoc. 113 (12), 956–965. doi:10.1016/j.jfma.2014.09.003
Cho, Y.-A., Kim, D.-S., Song, M., Bae, W.-J., Lee, S., and Kim, E.-C. (2016). Protein Interacting with Never in Mitosis A-1 Induces Glutamatergic and GABAergic Neuronal Differentiation in Human Dental Pulp Stem Cells. J. Endod. 42 (7), 1055–1061. doi:10.1016/j.joen.2016.04.004
Cowan, C. S., Renner, M., De Gennaro, M., Gross-Scherf, B., Goldblum, D., Hou, Y., et al. (2020). Cell Types of the Human Retina and its Organoids at Single-Cell Resolution. Cell 182 (6), 1623–1640. e1634. doi:10.1016/j.cell.2020.08.013
d'Aquino, R., De Rosa, A., Laino, G., Caruso, F., Guida, L., Rullo, R., et al. (2009). Human Dental Pulp Stem Cells: From Biology to Clinical Applications. J. Exp. Zool. 312B, 408–415. doi:10.1002/jez.b.21263
Darvishi, M., Hamidabadi, H. G., Bojnordi, M. N., saeednia, S., Zahiri, M., Niapour, A., et al. (2021). Differentiation of Human Dental Pulp Stem Cells into Functional Motor Neuron: In Vitro and Ex Vivo Study. Tissue Cell 72, 101542. doi:10.1016/j.tice.2021.101542
Ebrahimi, B., Yaghoobi, M., Kamal-abadi, A., and Raoof, M. (2011). Human Dental Pulp Stem Cells Express Many Pluripotency Regulators and Differentiate into Neuronal Cells. Neural Regen. Res. 6 (34), 2666–2672. doi:10.3969/j.issn.1673-5374.2011.34.004
El-Rashidy, A. A., El Moshy, S., Radwan, I. A., Rady, D., Abbass, M. M. S., Dörfer, C. E., et al. (2021). Effect of Polymeric Matrix Stiffness on Osteogenic Differentiation of Mesenchymal Stem/Progenitor Cells: Concise Review. Polymers 13 (17), 2950. doi:10.3390/polym13172950
Feng, X., Lu, X., Huang, D., Xing, J., Feng, G., Jin, G., et al. (2014). 3D Porous Chitosan Scaffolds Suit Survival and Neural Differentiation of Dental Pulp Stem Cells. Cell Mol. Neurobiol. 34 (6), 859–870. doi:10.1007/s10571-014-0063-8
Ganapathy, K., Datta, I., and Bhonde, R. (2019). Astrocyte-Like Cells Differentiated from Dental Pulp Stem Cells Protect Dopaminergic Neurons against 6-Hydroxydopamine Toxicity. Mol. Neurobiol. 56 (6), 4395–4413. doi:10.1007/s12035-018-1367-3
Gao, Y., Ku, N.-J., Sung, T.-C., Higuchi, A., Hung, C.-S., Lee, H. H.-C., et al. (2019). The Effect of Human Platelet Lysate on the Differentiation Ability of Human Adipose-Derived Stem Cells Cultured on ECM-Coated Surfaces. J. Mat. Chem. B 7 (45), 7110–7119. doi:10.1039/C9TB01764J
Geng, Y.-W., Zhang, Z., Liu, M.-Y., and Hu, W.-P. (2017). Differentiation of Human Dental Pulp Stem Cells into Neuronal by Resveratrol. Cell Biol. Int. 41 (12), 1391–1398. doi:10.1002/cbin.10835
Georgiou, M., Golding, J. P., Loughlin, A. J., Kingham, P. J., and Phillips, J. B. (2015). Engineered Neural Tissue with Aligned, Differentiated Adipose-Derived Stem Cells Promotes Peripheral Nerve Regeneration across a Critical Sized Defect in Rat Sciatic Nerve. Biomaterials 37, 242–251. doi:10.1016/j.biomaterials.2014.10.009
Ghorbani, S., Tiraihi, T., and Soleimani, M. (2018). Differentiation of Mesenchymal Stem Cells into Neuron-Like Cells Using Composite 3D Scaffold Combined with Valproic Acid Induction. J. Biomater. Appl. 32 (6), 702–715. doi:10.1177/0885328217741903
He, Y., Cao, Y., Xiang, Y., Hu, F., Tang, F., Zhang, Y., et al. (2020). An Evaluation of Norspermidine on Anti-Fungal Effect on Mature Candida Albicans Biofilms and Angiogenesis Potential of Dental Pulp Stem Cells. Front. Bioeng. Biotechnol. 8, 948. doi:10.3389/fbioe.2020.00948
Higuchi, A., Ling, Q.-D., Kumar, S. S., Chang, Y., Alarfaj, A. A., Munusamy, M. A., et al. (2015). Physical Cues of Cell Culture Materials Lead the Direction of Differentiation Lineages of Pluripotent Stem Cells. J. Mat. Chem. B 3 (41), 8032–8058. doi:10.1039/C5TB01276G
Higuchi, A., Suresh Kumar, S., Benelli, G., Ling, Q.-D., Li, H.-F., Alarfaj, A. A., et al. (2019). Biomaterials Used in Stem Cell Therapy for Spinal Cord Injury. Prog. Mater. Sci. 103, 374–424. doi:10.1016/j.pmatsci.2019.02.002
Hsiao, D., Hsu, S.-H., Chen, R.-S., and Chen, M.-H. (2020). Characterization of Designed Directional Polylactic Acid 3D Scaffolds for Neural Differentiation of Human Dental Pulp Stem Cells. J. Formos. Med. Assoc. 119 (1, Part 2), 268–275. doi:10.1016/j.jfma.2019.05.011
Kanafi, M., Majumdar, D., Bhonde, R., Gupta, P., and Datta, I. (2014). Midbrain Cues Dictate Differentiation of Human Dental Pulp Stem Cells towards Functional Dopaminergic Neurons. J. Cell. Physiol. 229 (10), 1369–1377. doi:10.1002/jcp.24570
Laudani, S., La Cognata, V., Iemmolo, R., Bonaventura, G., Villaggio, G., Saccone, S., et al. (2020). Effect of a Bone Marrow-Derived Extracellular Matrix on Cell Adhesion and Neural Induction of Dental Pulp Stem Cells. Front. Cell Dev. Biol. 8, 100. doi:10.3389/fcell.2020.00100
Lee, K.-D., Kuo, T. K.-C., Whang-Peng, J., Chung, Y.-F., Lin, C.-T., Chou, S.-H., et al. (2004). In Vitro Hepatic Differentiation of Human Mesenchymal Stem Cells. Hepatology 40 (6), 1275–1284. doi:10.1002/hep.20469
Linde, A. (1985). The Extracellular Matrix of the Dental Pulp and Dentin. J. Dent. Res. 64, 523–529. doi:10.1177/002203458506400405
Liu, J., Han, C., Wang, Y.-J., Wang, Y.-C., Guan, X., Wang, L., et al. (2021). Caveolin-1 Downregulation Promotes the Dopaminergic Neuron-Like Differentiation of Human Adipose-Derived Mesenchymal Stem Cells. Neural Regen. Res. 16 (4), 714–720. doi:10.4103/1673-5374.295342
Luo, L., Albashari, A. A., Wang, X., Jin, L., Zhang, Y., Zheng, L., et al. (2018a). Effects of Transplanted Heparin-Poloxamer Hydrogel Combining Dental Pulp Stem Cells and bFGF on Spinal Cord Injury Repair. Stem Cells Int. 2018, 2398521. doi:10.1155/2018/2398521
Luo, L., He, Y., Jin, L., Zhang, Y., Guastaldi, F. P., Albashari, A. A., et al. (2021a). Application of Bioactive Hydrogels Combined with Dental Pulp Stem Cells for the Repair of Large Gap Peripheral Nerve Injuries. Bioact. Mater. 6 (3), 638–654. doi:10.1016/j.bioactmat.2020.08.028
Luo, L., He, Y., Wang, X., Key, B., Lee, B. H., Li, H., et al. (2018b). Potential Roles of Dental Pulp Stem Cells in Neural Regeneration and Repair. Stem Cells Int. 2018, 1731289. doi:10.1155/2018/1731289
Luo, L., Wang, X., Zhang, Y., Wu, Y., Hu, F., Xing, Z., et al. (2020). Biological Behavioral Alterations of the Post-neural Differentiated Dental Pulp Stem Cells through an In Situ Microenvironment. Front. Cell Dev. Biol. 8, 625151. doi:10.3389/fcell.2020.625151
Luo, L., Zhang, Y., Chen, H., Hu, F., Wang, X., Xing, Z., et al. (2021b). Effects and Mechanisms of Basic Fibroblast Growth Factor on the Proliferation and Regenerative Profiles of Cryopreserved Dental Pulp Stem Cells. Cell Prolif. 54 (2), e12969. doi:10.1111/cpr.12969
Luzuriaga, J., Pineda, J. R., Irastorza, I., Uribe-Etxebarria, V., Garcia-Gallastegui, P., Encinas, J. M., et al. (2019). BDNF and NT3 Reprogram Human Ectomesenchymal Dental Pulp Stem Cells to Neurogenic and Gliogenic Neural Crest Progenitors Cultured in Serum-Free Medium. Cell Physiol. Biochem. 52, 1361–1380. doi:10.33594/000000096
Machado, C. V., Passos, S. T., Campos, T. M. C., Bernardi, L., Vilas-Bôas, D. S., Nör, J. E., et al. (2016). The Dental Pulp Stem Cell Niche Based on Aldehyde Dehydrogenase 1 Expression. Int. Endod. J. 49 (8), 755–763. doi:10.1111/iej.12511
Malara, A., Currao, M., Gruppi, C., Celesti, G., Viarengo, G., Buracchi, C., et al. (2014). Megakaryocytes Contribute to the Bone Marrow-Matrix Environment by Expressing Fibronectin, Type IV Collagen, and Laminin. Stem Cells 32 (4), 926–937. doi:10.1002/stem.1626
Mattei, V., Santacroce, C., Tasciotti, V., Martellucci, S., Santilli, F., Manganelli, V., et al. (2015). Role of Lipid Rafts in Neuronal Differentiation of Dental Pulp-Derived Stem Cells. Exp. Cell Res. 339 (2), 231–240. doi:10.1016/j.yexcr.2015.11.012
Michelet, F., Balasankar, A., Teo, N., Stanton, L. W., and Singhal, S. (2020). Rapid Generation of Purified Human RPE from Pluripotent Stem Cells Using 2D Cultures and Lipoprotein Uptake-Based Sorting. Stem Cell Res. Ther. 11 (1), 47. doi:10.1186/s13287-020-1568-3
Mohammed, M., Lai, T.-S., and Lin, H.-C. (2021). Substrate Stiffness and Sequence Dependent Bioactive Peptide Hydrogels Influence the Chondrogenic Differentiation of Human Mesenchymal Stem Cells. J. Mat. Chem. B 9 (6), 1676–1685. doi:10.1039/D0TB02008G
Noda, S., Kawashima, N., Yamamoto, M., Hashimoto, K., Nara, K., Sekiya, I., et al. (2019). Effect of Cell Culture Density on Dental Pulp-Derived Mesenchymal Stem Cells with Reference to Osteogenic Differentiation. Sci. Rep. 9 (1), 5430. doi:10.1038/s41598-019-41741-w
O'Hara-Wright, M., and Gonzalez-Cordero, A. (2020). Retinal Organoids: A Window into Human Retinal Development. Development 147 (24), dev189746. doi:10.1242/dev.189746
Pan, J., Lee, Y.-C., Lee, H. H.-C., Sung, T.-C., Jen, S. H., Ban, L.-K., et al. (2020). Culture and Differentiation of Purified Human Adipose-Derived Stem Cells by Membrane Filtration via Nylon Mesh Filters. J. Mat. Chem. B 8 (24), 5204–5214. doi:10.1039/D0TB00947D
Pavlova, G., Lopatina, T., Kalinina, N., Rybalkina, E., Parfyonova, Y., Tkachuk, V., et al. (2012). In Vitro Neuronal Induction of Adipose-Derived Stem Cells and Their Fate after Transplantation into Injured Mouse Brain. Curr. Med. Chem. 19 (30), 5170–5177. doi:10.2174/092986712803530557
Peng, I.-C., Yeh, C.-C., Lu, Y.-T., Muduli, S., Ling, Q.-D., Alarfaj, A. A., et al. (2016). Continuous Harvest of Stem Cells via Partial Detachment from Thermoresponsive Nanobrush Surfaces. Biomaterials 76, 76–86. doi:10.1016/j.biomaterials.2015.10.039
Pisciotta, A., Bertoni, L., Vallarola, A., Bertani, G., Mecugni, D., and Carnevale, G. (2020). Neural Crest Derived Stem Cells from Dental Pulp and Tooth-Associated Stem Cells for Peripheral Nerve Regeneration. Neural Regen. Res. 15, 373–381. doi:10.4103/1673-5374.266043
Pisciotta, A., Carnevale, G., Meloni, S., Riccio, M., De Biasi, S., Gibellini, L., et al. (2015). Human Dental Pulp Stem Cells (hDPSCs): Isolation, Enrichment and Comparative Differentiation of Two Sub-Populations. BMC Dev. Biol. 15, 14. doi:10.1186/s12861-015-0065-x
Prabhakaran, M. P., Venugopal, J. R., and Ramakrishna, S. (2009). Mesenchymal Stem Cell Differentiation to Neuronal Cells on Electrospun Nanofibrous Substrates for Nerve Tissue Engineering. Biomaterials 30 (28), 4996–5003. doi:10.1016/j.biomaterials.2009.05.057
Rafiee, F., Pourteymourfard-Tabrizi, Z., Mahmoudian-Sani, M.-R., Mehri-Ghahfarrokhi, A., Soltani, A., Hashemzadeh-Chaleshtori, M., et al. (2020). Differentiation of Dental Pulp Stem Cells into Neuron-Like Cells. Int. J. Neurosci. 130 (2), 107–116. doi:10.1080/00207454.2019.1664518
Rozila, I., Azari, P., Munirah, S. b., Safwani, W. K. Z. W., Pingguan-Murphy, B., and Chua, K. H. (2021). Polycaprolactone-Based Scaffolds Facilitates Osteogenic Differentiation of Human Adipose-Derived Stem Cells in a Co-Culture System. Polymers 13 (4), 597. doi:10.3390/polym13040597
Sanen, K., Martens, W., Georgiou, M., Ameloot, M., Lambrichts, I., and Phillips, J. (2017). Engineered Neural Tissue with Schwann Cell Differentiated Human Dental Pulp Stem Cells: Potential for Peripheral Nerve Repair? J. Tissue Eng. Regen. Med. 11 (12), 3362–3372. doi:10.1002/term.2249
Smith, E. N., D'Antonio-Chronowska, A., Greenwald, W. W., Borja, V., Aguiar, L. R., Pogue, R., et al. (2019). Human iPSC-Derived Retinal Pigment Epithelium: A Model System for Prioritizing and Functionally Characterizing Causal Variants at AMD Risk Loci. Stem Cell Rep. 12 (6), 1342–1353. doi:10.1016/j.stemcr.2019.04.012
Solis-Castro, O. O., Boissonade, F. M., and Rivolta, M. N. (2020). Establishment and Neural Differentiation of Neural Crest-Derived Stem Cells from Human Dental Pulp in Serum-Free Conditions. Stem Cells Transl. Med. 9 (11), 1462–1476. doi:10.1002/sctm.20-0037
Sung, T.-C., Heish, C.-W., Lee, H. H.-C., Hsu, J.-Y., Wang, C.-K., Wang, J.-H., et al. (2021a). 3D Culturing of Human Adipose-Derived Stem Cells Enhances Their Pluripotency and Differentiation Abilities. J. Mater. Sci. Technol. 63, 9–17. doi:10.1016/j.jmst.2020.05.003
Sung, T.-C., Lu, M.-W., Tian, Z., Lee, H. H.-C., Pan, J., Ling, Q.-D., et al. (2021b). Poly(vinyl Alcohol-Co-Itaconic Acid) Hydrogels Grafted with Several Designed Peptides for Human Pluripotent Stem Cell Culture and Differentiation into Cardiomyocytes. J. Mat. Chem. B 9 (37), 7662–7673. doi:10.1039/D1TB01555A
Tan, L., Hu, Y., Hou, Y., Chen, M., Xue, C., Chen, M., et al. (2020). Osteogenic Differentiation of Mesenchymal Stem Cells by Silica/Calcium Micro-Galvanic Effects on the Titanium Surface. J. Mat. Chem. B 8 (11), 2286–2295. doi:10.1039/D0TB00054J
Tsai, C.-C., Hong, Y.-J., Lee, R. J., Cheng, N.-C., and Yu, J. (2019). Enhancement of Human Adipose-Derived Stem Cell Spheroid Differentiation in an In Situ Enzyme-Crosslinked Gelatin Hydrogel. J. Mat. Chem. B 7 (7), 1064–1075. doi:10.1039/C8TB02835D
Ullah, I., Park, J.-M., Kang, Y.-H., Byun, J.-H., Kim, D.-G., Kim, J.-H., et al. (2017). Transplantation of Human Dental Pulp-Derived Stem Cells or Differentiated Neuronal Cells from Human Dental Pulp-Derived Stem Cells Identically Enhances Regeneration of the Injured Peripheral Nerve. Stem Cells Dev. 26 (17), 1247–1257. doi:10.1089/scd.2017.0068
Van Rensburg, M. J., Crous, A., and Abrahamse, H. (2021). Potential of Photobiomodulation to Induce Differentiation of Adipose- Derived Mesenchymal Stem Cells into Neural Cells. Curr. Stem Cell Res. Ther. 16 (3), 307–322. doi:10.2174/1574888X15999200918095834
Wu, S.-H., Liao, Y.-T., Hsueh, K.-K., Huang, H.-K., Chen, T.-M., Chiang, E.-R., et al. (2021). Adipose-Derived Mesenchymal Stem Cells From a Hypoxic Culture Improve Neuronal Differentiation and Nerve Repair. Front. Cell Dev. Biol. 9, 658099. doi:10.3389/fcell.2021.658099
Xing, Z., Cai, J., Sun, Y., Cao, M., Li, Y., Xue, Y., et al. (2020). Altered Surface Hydrophilicity on Copolymer Scaffolds Stimulate the Osteogenic Differentiation of Human Mesenchymal Stem Cells. Polymers 12 (7), 1453. doi:10.3390/polym12071453
Xu, W., Zhang, X., Qian, H., Zhu, W., Sun, X., Hu, J., et al. (2004). Mesenchymal Stern Cells from Adult Human Bone Marrow Differentiate into a Cardiomyocyte Phenotype In Vitro. Exp. Biol. Med. (Maywood) 229 (7), 623–631. doi:10.1177/153537020422900706
Yang, J., Xiao, Y., Tang, Z., Luo, Z., Li, D., Wang, Q., et al. (2020a). The Negatively Charged Microenvironment of Collagen Hydrogels Regulates the Chondrogenic Differentiation of Bone Marrow Mesenchymal Stem Cells In Vitro and In Vivo. J. Mat. Chem. B 8 (21), 4680–4693. doi:10.1039/D0TB00172D
Yang, L., Fan, X., Zhang, J., and Ju, J. (2020b). Preparation and Characterization of Thermoresponsive Poly(N-Isopropylacrylamide) for Cell Culture Applications. Polymers 12 (2), 389. doi:10.3390/polym12020389
Young, F. I., Telezhkin, V., Youde, S. J., Langley, M. S., Stack, M., Kemp, P. J., et al. (2016). Clonal Heterogeneity in the Neuronal and Glial Differentiation of Dental Pulp Stem/Progenitor Cells. Stem Cells Int. 2016, 1290561. doi:10.1155/2016/1290561
Zainal Ariffin, S. H., Kermani, S., Zainol Abidin, I. Z., Megat Abdul Wahab, R., Yamamoto, Z., Senafi, S., et al. (2013). Differentiation of Dental Pulp Stem Cells into Neuron-Like Cells in Serum-Free Medium. Stem Cells Int. 2013, 250740. doi:10.1155/2013/250740
Zhang, J., Lian, M., Cao, P., Bao, G., Xu, G., Sun, Y., et al. (2017). Effects of Nerve Growth Factor and Basic Fibroblast Growth Factor Promote Human Dental Pulp Stem Cells to Neural Differentiation. Neurochem. Res. 42 (4), 1015–1025. doi:10.1007/s11064-016-2134-3
Zhang, J., Lu, X., Feng, G., Gu, Z., Sun, Y., Bao, G., et al. (2016). Chitosan Scaffolds Induce Human Dental Pulp Stem Cells to Neural Differentiation: Potential Roles for Spinal Cord Injury Therapy. Cell Tissue Res. 366 (1), 129–142. doi:10.1007/s00441-016-2402-1
Zheng, K., Feng, G., Zhang, J., Xing, J., Huang, D., Lian, M., et al. (2021). Basic Fibroblast Growth Factor Promotes Human Dental Pulp Stem Cells Cultured in 3D Porous Chitosan Scaffolds to Neural Differentiation. Int. J. Neurosci. 131 (7), 625–633. doi:10.1080/00207454.2020.1744592
Zhu, S., Ying, Y., He, Y., Zhong, X., Ye, J., Huang, Z., et al. (2021a). Hypoxia Response Element-Directed Expression of bFGF in Dental Pulp Stem Cells Improve the Hypoxic Environment by Targeting Pericytes in SCI Rats. Bioact. Mater. 6 (8), 2452–2466. doi:10.1016/j.bioactmat.2021.01.024
Keywords: dental pulp stem cells, synthetic cell culture polymer, neuronal cell differentiation, poly-L-ornithine, poly-N-isopropylacrylamide-butyl acrylate, cell therapy
Citation: Gao Y, Tian Z, Liu Q, Wang T, Ban L-K, Lee HH-C, Umezawa A, Almansour AI, Arumugam N, Kumar RS, Ye Q, Higuchi A, Chen H and Sung T-C (2022) Neuronal Cell Differentiation of Human Dental Pulp Stem Cells on Synthetic Polymeric Surfaces Coated With ECM Proteins. Front. Cell Dev. Biol. 10:893241. doi: 10.3389/fcell.2022.893241
Received: 10 March 2022; Accepted: 01 April 2022;
Published: 14 June 2022.
Edited by:
Peter Mei, University of Otago, New ZealandReviewed by:
Alessandra Pisciotta, University of Modena and Reggio Emilia, ItalyPrasad Pethe, Symbiosis International University, India
Copyright © 2022 Gao, Tian, Liu, Wang, Ban, Lee, Umezawa, Almansour, Arumugam, Kumar, Ye, Higuchi, Chen and Sung. This is an open-access article distributed under the terms of the Creative Commons Attribution License (CC BY). The use, distribution or reproduction in other forums is permitted, provided the original author(s) and the copyright owner(s) are credited and that the original publication in this journal is cited, in accordance with accepted academic practice. No use, distribution or reproduction is permitted which does not comply with these terms.
*Correspondence: Qingsong Ye, qingsongye@whu.edu.cn; Akon Higuchi, higuchi@ncu.edu.tw; Hao Chen, chenhao@mail.eye.ac.cn; Tzu-Cheng Sung, stc321@yahoo.com.tw
†These authors have contributed equally to this work