- 1Department of Biomedical Sciences, University of Padova, Padova, Italy
- 2Department of Biology, University of Padova, Padova, Italy
- 3Department of Chemical Sciences, University of Padova, Padova, Italy
- 4Institute of Neuroscience, National Research Council (CNR), Padova, Italy
- 5Faculty of Health and Medical Sciences, University of Surrey, Guildford, United Kingdom
- 6Department of Neurosciences, University of Padova, Padova, Italy
- 7Veneto Institute of Molecular Medicine, Padova, Italy
The redox activity of cytochrome c oxidase (COX), the terminal oxidase of the mitochondrial respiratory chain (MRC), depends on the incorporation of iron and copper into its catalytic centers. Many mitochondrial proteins have specific roles for the synthesis and delivery of metal-containing cofactors during COX biogenesis. In addition, a large set of different factors possess other molecular functions as chaperones or translocators that are also necessary for the correct maturation of these complexes. Pathological variants in genes encoding structural MRC subunits and these different assembly factors produce respiratory chain deficiency and lead to mitochondrial disease. COX deficiency in Drosophila melanogaster, induced by downregulated expression of three different assembly factors and one structural subunit, resulted in decreased copper content in the mitochondria accompanied by different degrees of increase in the cytosol. The disturbances in metal homeostasis were not limited only to copper, as some changes in the levels of cytosolic and/or mitochondrial iron, manganase and, especially, zinc were observed in several of the COX-deficient groups. The altered copper and zinc handling in the COX defective models resulted in a transcriptional response decreasing the expression of copper transporters and increasing the expression of metallothioneins. We conclude that COX deficiency is generally responsible for an altered mitochondrial and cellular homeostasis of transition metals, with variations depending on the origin of COX assembly defect.
Introduction
The function of the mitochondrial respiratory chain (MRC) depends on a series of redox reactions that transfer electrons from reduced substrates (NADH and FADH2) to the final acceptor, molecular oxygen (O2). The electronic transfer is mediated by MRC complexes I-IV and two mobile electron carriers, coenzyme Q (CoQ) and cytochrome c (cyt c), that donate electrons to complex III and complex IV, respectively. Complex IV or cytochrome c oxidase (COX) is the terminal oxidase, where four electrons are transferred from four cyt c molecules to one molecule of oxygen (O2), reducing it to two molecules of water (H2O). As part of the family of the heme-copper oxidases, COX catalytic centers contain both iron and copper (Pereira et al., 2001). Cyt c donates electrons to the CuA center, which is composed of two closely bound atoms of Cu contained in the core subunit COX2 (MT-CO2). On the other hand, O2 reduction occurs in the catalytic center within the COX1 (MT-CO1) subunit, composed of a low spin heme a and the binuclear center of heme a3 and one Cu atom (CuB). The process of electron transfer is coupled to proton pumping from the mitochondrial matrix to the intermembrane space, contributing to the generation of the proton motive force essential for ATP synthesis (Pereira et al., 2008; Wikstrom et al., 2018). All cytochrome c oxidases possess a third core subunit (COX3; MT-CO3) that does not contain an active center but it is believed to be necessary to preserve the catalytic activity (Sharma et al., 2015). In addition to the three mitochondrial DNA-encoded core subunits, COX in animals, including Drosophila melanogaster (Brischigliaro et al., submitted), contains 11 “supernumerary” subunits with no catalytic role but important for the enzyme stability, function and regulation (Pitceathly and Taanman, 2018; Ramzan et al., 2021).
Human COX is assembled in a modular fashion, where each module is defined by each of the core subunits (Vidoni et al., 2017). Upon translation in the proximity of the mitochondrial inner membrane, MT-CO1 binds to a plethora of chaperones that help stabilize the apoprotein and serve as a platform for its metalation (Timon-Gomez et al., 2018). The assembly factor SURF1 is part of this intermediate complex and it is believed to have a role in heme A delivery to the catalytic center, whereas COX10 and COX15, two enzymes also essential for COX function, synthesize heme A (Timon-Gomez et al., 2018). Copper delivery to the CuB center in MT-CO1 is mediated by COX17 and COX11 (Cobine et al., 2006). The incorporation of the CuA center bound to MT-CO2 requires the participation of several different metallochaperones, namely COX17, COA6, SCO1 and SCO2, that function in the delivery of the two Cu ions to the apoprotein (Jett and Leary, 2018). All of these Cu-delivering proteins are localized in the mitochondrial intermembrane space and contain Cys residues, important for the redox regulation of their import, stability and Cu binding (Geldon et al., 2021). Genetic variants resulting in defects of structural subunits and assembly factors, including those involved in the metalation of COX such as COX10, COX15, SURF1, COA6, SCO1 and SCO2, are the cause of mitochondrial disease associated with COX deficiency in humans (Brischigliaro and Zeviani, 2021). In addition to COX deficiency, mutations in SCO1 and SCO2 produce an overall reduction in the Cu content in cultured cells and in tissues (Leary et al., 2007), related with the loss of the plasma membrane copper transporter CTR1 (Hlynialuk et al., 2015; Baker et al., 2017). Copper transport within the cell and into mitochondria must be accurately controlled due to the toxicity of this metal, which at high levels can determine redox stress and inactivate Fe-containing enzymes (Cobine et al., 2021). Decompensation of cellular Cu levels can lead to various diseases, including neurodegeneration (Cobine et al., 2021; Ruiz et al., 2021). Also, primary Cu deficiencies result in decreased COX activity, most likely contributing to the degenerative clinical course displayed by these patients (Spinazzi et al., 2014). The correlation between altered mitochondrial metal homeostasis and disease is not exclusive for Cu, as dysregulation of Fe is also well known to be associated with neurodegenerative processes (Kozlowski et al., 2009; Dietz et al., 2021; Cheng et al., 2022). In addition, Zn overload can cause neuronal damage principally related with mitochondrial respiratory chain dysfunction (Kozlowski et al., 2009; Liu et al., 2021). This connection might be reciprocal, as complex III deficiency in yeast leads to a depletion of the labile intra-mitochondrial Zn pools (Atkinson et al., 2011).
Drosophila melanogaster models have been useful to understand metal homeostasis in animal cells (Navarro and Schneuwly, 2017), as well as mitochondrial dysfunction (Foriel et al., 2015). In this work we have used well characterized D. melanogaster RNAi strains targeting three COX assembly factors: Coa3/Ccdc56 (Peralta et al., 2012), Scox, which is the single SCO protein homolog present in flies (Porcelli et al., 2010; Nguyen et al., 2014) and Coa8 (Brischigliaro et al., 2019). For this study we have also included a knock-down strain of a COX supernumerary structural subunit cype/COX6C (Szuplewski and Terracol, 2001; Fernandez-Ayala et al., 2009). Using these well-established models of COX deficiency, we have separately analyzed the cytosolic and the mitochondrial content of four of the most biologically-relevant transition metals (Cu, Fe, Mn, and Zn). We conclude that COX deficiency in general produces an imbalance in the compartmentalization of Cu and Zn and, at a lesser extent, of Fe and Mn as well. In addition, these COX-deficient strains showed altered transcript levels of Cu transporters and metallothioneins. We hypothesize that these alterations could be a contributing factor in the pathogenesis of mitochondrial disease associated with COX deficiency.
Materials and Methods
Fly Stocks and Maintenance
Flies were raised on standard cornmeal medium and kept at 23°C, 70% humidity on a 12:12 h light/dark cycle. Fly strains used in this study were obtained from Bloomington Drosophila Stock Center (BDSC) and Vienna Drosophila Resource Center (VDRC). Genotypes used in this study were: w1118 (BDSC 6326), act5c-gal4>CyO.GFP (BDSC 4414), UAS-Coa8-IR (VDRC ID 100605), UAS-cype-IR (VDRC ID 102336), UAS-Scox-IR (VDRC ID 7861), UAS-Ccdc56-IR (VDRC ID 27948). Control individuals were obtained by crossing the act5c-gal4 driver line with flies from the w1118 strain.
Isolation of Mitochondria
Mitochondria from D. melanogaster were prepared by differential centrifugation (Brischigliaro et al., 2022). Briefly, 150 individuals were homogenized in 10 ml of homogenization buffer (225 mM mannitol, 75 mM sucrose, 5 mM HEPES-KOH pH 7.4, 1% fatty-acid free BSA) with 15–20 strokes at 1,000 rpm in a motor driven Teflon-glass Elvehjem potter on ice. Samples were centrifuged at 1,000 X g for 10 min at 4°C and filtered using a 100 μm strainer. Samples were centrifuged at 6,000 X g for 10 min at 4°C. Supernatants (containing the cytosolic, post-mitochondrial fraction) were collected and pellets (containing the mitochondrial fraction) were washed with homogenization buffer and centrifuged at 6,000 X g for 10 min at 4°C. Mitochondrial pellets were washed again with homogenization buffer without BSA, collected by centrifugation at 7,000 X g at 4°C and resuspended in 1 ml of homogenization buffer without BSA.
Mitochondrial Enzyme Activity Measurements
Mitochondria prepared as described above were used for spectrophotometric kinetic measurements of cytochrome c oxidase (COX) and citrate synthase (CS) activity as described (Brischigliaro et al., 2022). COX in gel activity was performed after mitochondrial membrane solubilization with n-dodecyl-β-maltoside (DDM) and blue-native gel electrophoresis as described (Fernandez-Vizarra and Zeviani, 2021a).
Inductively Coupled Plasma Mass Spectrometry
Sample solution (1 g) was digested with 0.7 g of 69% HNO3 (CAS 7697-37-2 Sigma Aldrich). A microwave digestion system CEM EXPLORER SP-D PLUS was used for the acid digestion according to the following protocol: ramp temperature from room to 180°C in 4 min, then 180°C for 6 min, and 300 W power with medium stirring and with a pressure of 300 PSI. Samples were adjusted to 10 g with milliQ water (resistivity 18.2 MΩ cm-1) and 100 μg L−1 of internal standard (IS) which was the mixture Agilent 5183-4681, containing 6Li, 45Sc, 72Ge, 103Rh, 115In, 159Tb, 175Lu and 209Bi.
Four elements (Cu, Mn, Fe, and Zn) were quantified in each sample by using inductively coupled plasma coupled to a mass spectrometer (ICP-MS, Agilent Technologies 7700x. Agilent Technologies International Japan, Ltd., Tokyo, Japan). The operating conditions and data acquisition parameters were the same as reported (Badocco et al., 2015). The multi-element calibration standard used for all calibrations was the IV-ICPMS-71A (Inorganic-Ventures, 100 ml) containing 10 mg/L of Ag, Al, As, B, Ba, Be, Ca, Cd, Ce, Co, Cr, Cs, Cu, Dy, Er, Eu, Fe, Ga, Gd, Ho, K, La, Lu, Mg, Mn, Na, Nd, Ni, P, Pb, Pr, Rb, S, Se, Sm, Sr, Th, Tl, Tm, U, V, Yb and Zn. The multielement standard solutions for calibration were prepared in 5% HNO3 by gravimetric serial dilution at eight different concentrations between 0.5 ng L−1 and 500 ng L−1. All regressions were calculated with a non-parametric approach (Lavagnini et al., 2011).
RNA Isolation, Reverse Transcription and qRT-PCR
Total RNA was extracted from 10 individuals of each genotype using TRIzol (Thermo Fisher Scientific), according to the manufacturer’s protocol. Reverse transcription was performed using GoScript Reverse Transcriptase kit (Promega). qRT-PCRs were performed using GoTaq qPCR SYBR Green (Promega) and a Bio-Rad CFX 96 Touch System (Bio-Rad). The 2-∆∆Ct method was used to calculate the relative expression levels of the targets using Rp49 as the reference gene. The oligonucleotides used in this study are: PF_Ctr1A_qRT (5′-ACCGTGCGCATTTTGTTT-3′), PR_Ctr1A_qRT (5′-TGACGAACTCAACGGAATGT-3′), PF_Ctr1B_qRT(5′-GCCAAGTCCTGCCCTATG-3′), PR_ Ctr1B_qRT(5′-CGAACTCCGTCACAGTGGA-3′), PF_mtnA_ qRT (5′-TGCATCAGTTGTGGTCAG-3′), PR_mtnA_qRT (5′-AAAGGTAGGTATGGGCTATTTAG-3′), PF_MtnD_qRT (5′-GCAAGGCTTGTGGAACAAA-3′), PR_MtnD_qRT (5′-TCCGTTCTAGCAGGAGCACT-3′), PF_Rp49_qRT (5′-ATCGGTTACGGATCGAACAA-3′) and PR_Rp49_qRT (5′-GACAATCTCCTTGCGCTTCT-3′).
Western Blot and Immunodetection Analysis
The proteins separated in 4%–12% SDS-PAGE Bis-Tris pre-cast gels (Invitrogen) were transferred to PVDF membranes using Tris-Glycine buffer (25 mM Tris-HCl, 192 mM Glycine, 20% methanol, 0.025% SDS). PVDF membranes were blocked with 5% skimmed milk in PBS-T (0.1% Tween-20) at RT for 1 h. Primary antibodies were diluted in 3% BSA in PBS-T and incubated overnight at 4°C. HRP- conjugated secondary antibodies were diluted in 1% skimmed milk in PBS-T and incubated for 1 h at room temperature. Chemiluminescent signals were recorded using an Alliance Mini HD9 instrument (UVITEC). The primary antibodies used were: mouse monoclonal anti-Hsp70 (Sigma-Aldrich, H5147, 1:1,000) and mouse monoclonal anti-ATP5A (Abcam, ab14748, 1:1,000).
Results
To investigate the compartmentalization of transition metals in genetic models of COX deficiency, we separated washed mitochondrial and cytosolic (‘post-mitochondrial’ soluble supernatant) fractions from whole homogenates obtained from adults Coa8 RNAi and Coa3 RNAi adults. The analyses of the cype RNAi and Scox RNAi, were performed using third instar larvae as these individuals do not reach the adult stage (Fernandez-Ayala et al., 2009; Nguyen et al., 2014). To determine the extent of the COX defect in these models, we used mitochondrial fractions from the knock-down (KD) flies to perform COX spectrophotometric kinetic enzyme activity measurements (Figure 1A), and COX in-gel-activity assays (Figure 1B). All the KD samples presented COX deficiency to some extent, being the least affected the Coa3 KD adults with a decrease of ∼30% compared with the control, followed by the Coa8 KD adults and cype and Scox larvae, all showing a ∼50% reduction of COX activity, normalized by the activity of citrate synthase (CS). The metal content in both the separated cytosolic and mitochondrial fractions was determined by ICP-MS and normalized by the protein amount in each of the samples (Figures 2, 3).
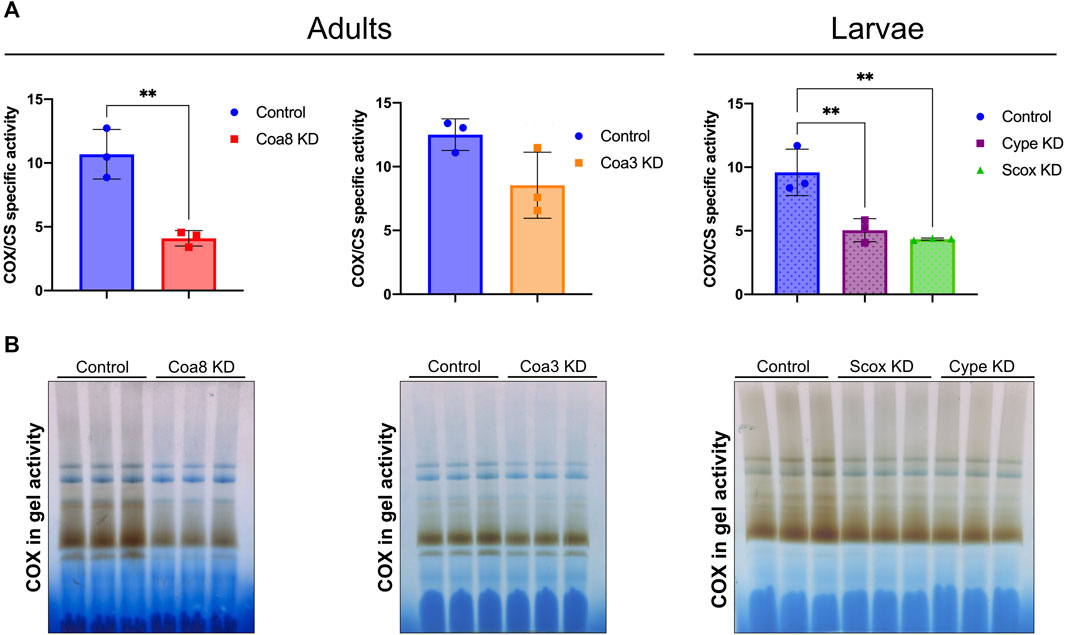
FIGURE 1. Extent of the COX deficiency in D. melanogaster KD models. (A) Spectrophotometric kinetic enzyme activity measurements of COX activity, normalized by the activity of citrate synthase (COX/CS) in mitochondrial fractions from control adults (solid blue bars), Coa8 KD adults (solid red bars), Coa3 KD adults (solid orange bars), control larvae (dotted blue bars), cype KD larvae (dotted purple bars) and Scox KD larvae (dotted green bars). The symbols represent the individual values of each replicate measurement, and the bars represent the mean ± SD. The statistical significance was calculated using Student’s t-test for the adult pairwise comparisons, and one-way ANOVA with Tukey’s multiple comparisons test for the three larvae groups (**p ≤ 0.01). (B) COX in gel activity assays performed in DDM-solubilized mitochondrial samples, separated through blue-native electrophoresis gels, from three independent replicates for each of the indicated experimental group.
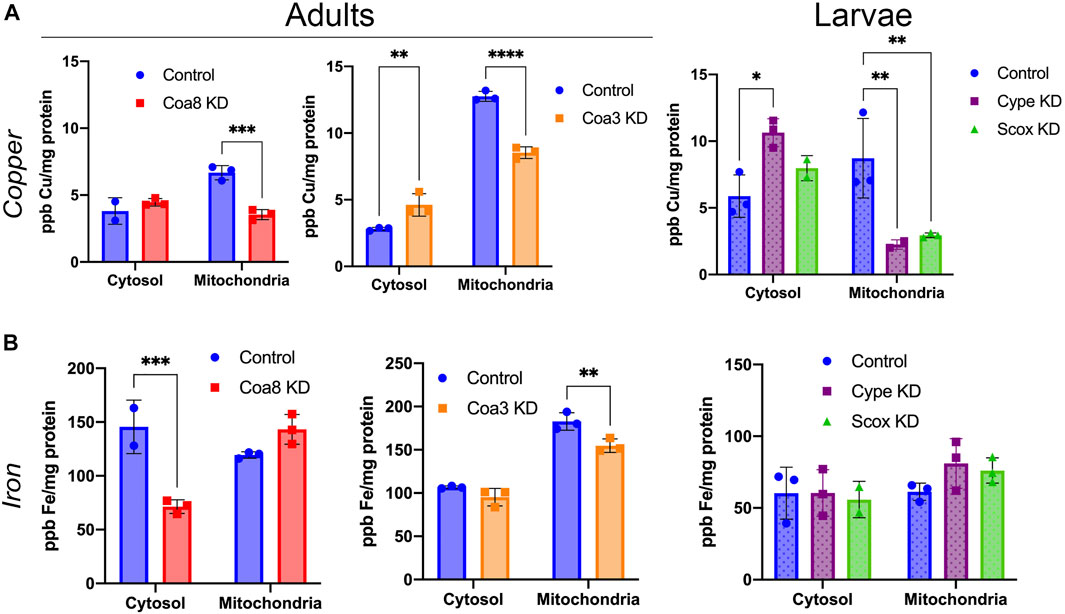
FIGURE 2. Cellular copper and iron compartmentalization in D. melanogaster models of COX deficiency. (A) Copper and (B) iron content in parts per billion (ppb) and normalized by protein content (mg protein) in cytosolic and mitochondrial fractions from control adults (solid blue bars), Coa8 KD adults (solid red bars), Coa3 KD adults (solid orange bars), control larvae (dotted blue bars), cype KD larvae (dotted purple bars) and Scox KD larvae (dotted green bars). The symbols represent the individual values of each replicate measurement, and the bars represent the mean ± SD. The statistical significance was calculated using two-way ANOVA and Sidak’s multiple comparison tests (*p ≤ 0.05, **p ≤ 0.01, ***p ≤ 0.001, ****p ≤ 0.0001).
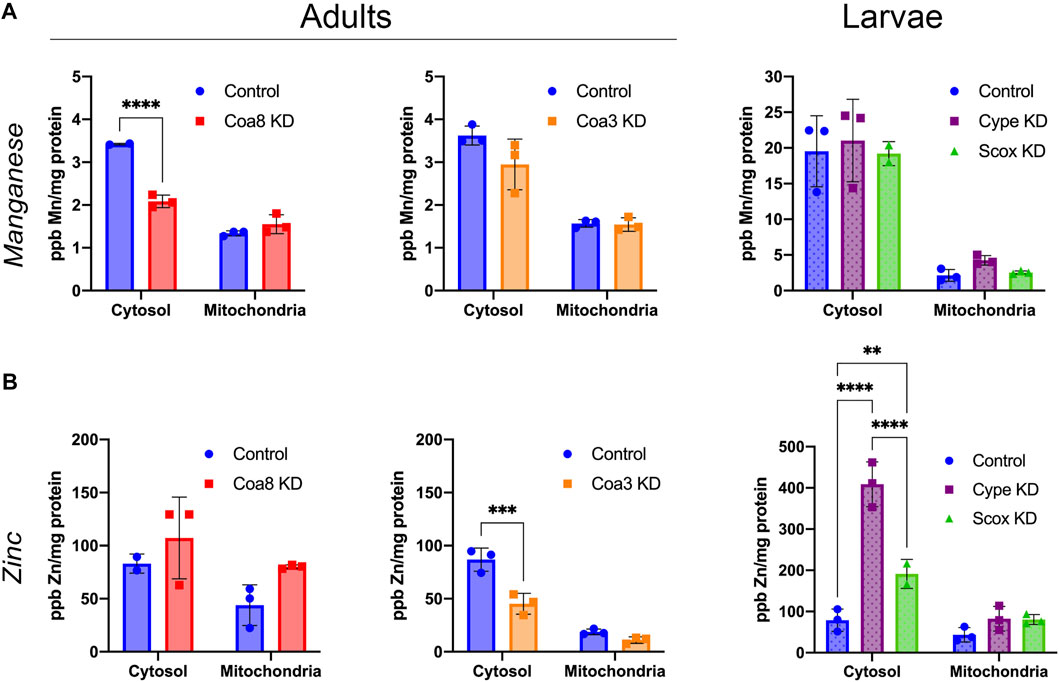
FIGURE 3. Cellular manganese and zinc compartmentalization in D. melanogaster models of COX deficiency. (A) Manganese and (B) zinc content in parts per billion (ppb) and normalized by protein content (mg protein) in cytosolic and mitochondrial fractions from control adults (solid blue bars), Coa8 KD adults (solid red bars), Coa3 KD adults (solid orange bars), control larvae (dotted blue bars), cype KD larvae (dotted purple bars) and Scox KD larvae (dotted green bars). The symbols represent the individual values of each replicate measurement and the bars represent the mean ± SD. The statistical significance was calculated using two-way ANOVA and Sidak’s multiple comparison tests (**p ≤ 0.01, ***p ≤ 0.001, ****p ≤ 0.0001).
COX Deficiency Significantly Impacts Cellular Copper Compartmentalization
As shown in Figure 2A, mitochondrial copper levels were significantly reduced in all the analyzed COX-deficient models with a concomitant increase in cytosolic Cu content, particularly in the Coa3 KD adult flies and the cype KD larvae. Mitochondria from cype and Scox KD individuals showed the biggest differences, being the Cu content reduced by 74 and 67%, respectively, whereas Coa8 and Coa3 KD mitochondria contained 48 and 33% less copper than controls, respectively. On the other hand, cype and Coa3 KD cytosolic fractions had 81 and 64% increase in copper levels, whereas the metal was increased by 33% in Scox KD and, at minor extent (17%), in Coa8 KD, (Figure 2A). Even though Coa8 cytosolic Cu levels were not significantly different than in the controls, the compartmentalization of this metal was clearly altered also in this model, as in normal conditions mitochondrial Cu content is around 2-fold that of the cytosol (Figure 2A).
The effects of COX deficiency on Fe homeostasis did not appear as consistent or severe as on Cu. The most noticeable effects were found in the Coa8 KD model where Fe content in the mitochondria was increased by about 20%, with a significant reduction in the cytosol to about 50% of the control (Figure 2B). No changes were observed in any of the two larval models, whereas the Fe in the mitochondria of the Coa3 KD adults was decreased only by about 15%, without any alterations in the cytosolic amount.
Cellular and Mitochondrial Zn and Mn Homeostasis are Altered in COX-Deficient Flies
Using the same ICP-MS analysis, we focused on other biologically relevant transition metals that are not directly involved in the formation of the COX catalytic centers. In particular, we observed some changes in Mn and especially in Zn levels. Manganese cytosolic levels were approximately 6-fold higher in the larvae than in the control adults, while the amounts within the mitochondria were roughly the same in both stages of development (Figure 3A). However, the only analyzed COX-deficient models showing altered Mn amounts were Coa8 KD adults in which Mn levels were reduced by ca. 40% and cype KD mitochondria with a 2-fold increase in Mn concentration (Figure 3A).
On the other hand, we observed noticeable effects on Zn compartmentalization in most of the COX KD models. cype and Scox KD showed increased intra-mitochondrial zinc levels of 83, 90 and 85%, respectively, compared with the controls (Figure 3B). Furthermore, Coa8 KD mitochondria contained 83% more Zn than control on average, although this increase was not statistically significant according to the applied test (Figure 3B). In addition, the Zn in the cytosol was significantly increased in cype and Scox KD larvae by 5.2- and 2.4-fold, respectively (Figure 3B, right panel). Curiously, downregulation of Coa3, resulted in a reduction of Zn concentration of about half in the mitochondria and the cytosol, being the decrease in the latter statistically significant (Figure 3B, central panel).
Defective COX Assembly Triggers a Cellular Copper Homeostatic Response
Given the strong and consistent alterations observed in copper compartmentalization in all our COX KD models, we aimed to understand the cellular responses consequent to these homeostatic abnormalities. To this end, we determined the expression levels of genes under the control of the metal-responsive transcription factor-1 (MTF-1), which are mainly copper transporters and metallothioneins (Selvaraj et al., 2005; Günther et al., 2012). Firstly, we quantified the expression of genes encoding the plasma membrane copper transporters Ctr1A (human CTR1 homolog) and Ctr1B (human CTR2 homolog). As shown in Figure 4, the expression of Ctr1A was somewhat decreased in the cype and Scox larvae and, especially, in Coa3 KD adults where the mRNA levels were significantly reduced by 65%. Conversely, Ctr1B expression was significantly decreased in all COX deficient samples except for Scox KD individuals, where, interestingly, its expression at the mRNA level was significantly increased 1.5-fold. Furthermore, we assessed the expression levels of MtnA and MtnD, which are Cu-responsive metallothioneins involved in metal ion binding for trafficking and detoxification (Navarro and Schneuwly, 2017). Again, we found changes in the MtnA and MtnD transcript levels in the COX-deficient flies, probably related to the higher Cu content found in the cytoplasm of these individuals. The effect was not the same in all the models as we found that the expression levels of both MtnA and MtnD were significantly increased (2.8–3.5 fold) in the Coa8 and Coa3 KD models, whereas in cype KD and Scox KD, MtnA and D expression was only mildly and not significantly increased (Figure 4).
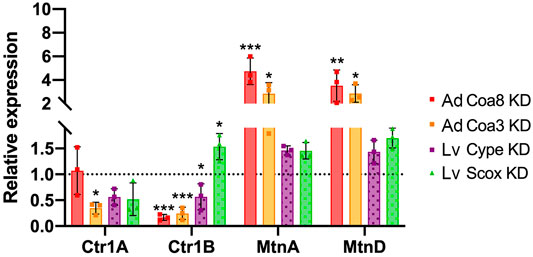
FIGURE 4. COX deficiency alters the expression of metal-responsive transcription factor 1 (MTF-1) target genes. Transcript levels measured by quantitative PCR of the copper transporters Ctr1A, Ctr1B, and the metallothioneins MtnA, MtnD in different genetic models of COX deficiency. Expression levels are normalized to the expression in the control strains (set to 1). Genotypes are: Coa8 KD (solid red bars), Coa3 KD (solid orange bars), cype KD (dotted purple bars) and Scox KD (dotted green bars). The bars represent the mean ± SD of n = 3 biological replicates, each measured in triplicate. One-way ANOVA and Dunett’s multiple comparison test of KD vs. control (*p ≤ 0.05, **p ≤ 0.01 ***p ≤ 0.001). Ad: Adults; Lv: Larvae.
Discussion
Mitochondrial enzymatic complex IV (COX) deficiency is a frequent biochemical hallmark in mitochondrial disease, and it can be originated by genetic defects affecting either COX structural components or assembly factors necessary for the correct assembly and function of the enzyme (Fernandez-Vizarra and Zeviani, 2021b; Brischigliaro and Zeviani, 2021). In this work we have used D. melanogaster models deficient in three different assembly factors with a distinct role in the process of COX assembly. Coa3 is a chaperone and/or stabilizing factor of nascent MT-CO1, playing a role in the early assembly stages (Mick et al., 2010; Mick et al., 2012; Peralta et al., 2012; Clemente et al., 2013). The function of Coa8 is still not clear, but evidence collected so far points out to a redox sensing function necessary to promote the middle assembly stages of COX biogenesis (Brischigliaro et al., 2019; Signes et al., 2019). Scox is the D. melanogaster homologue of the human copper-chaperones SCO1 and SCO2, necessary for Cu delivery to the CuA center in MT-CO2 (Leary et al., 2004; Leary et al., 2009). In addition, we have included in our analysis a KD strain for cype, the fly homolog of mammalian COX6C subunit. Although mutations in COX6C have not been associated with human mitochondrial disease to date, defects in cype produce severe phenotypes in flies (Szuplewski and Terracol, 2001; Fernandez-Ayala et al., 2009).
The consequences of SCO1 and SCO2 mutations on Cu homeostasis are well documented, resulting in a general cellular Cu deficiency in human and mouse cells and tissues (Leary et al., 2007; Hlynialuk et al., 2015; Baker et al., 2017). This is compatible with the observations in the Scox flies, where the levels of Cu in the mitochondria-enriched fractions were significantly lower than in the controls, without a variation in the cytosolic amounts. When the Cu amounts in the two fractions are added together, a lower global Cu amount occurs in the Scox KD flies. This is also similar to what was observed in a Sco2 KO/KI model, mimicking the most frequently found mutation in humans, where the levels of mitochondrial Cu were reduced in several tissues (Yang et al., 2010). Even though in this study the mitochondria were not purified using density gradients and contaminants from other membranous organelles containing metals could have been present, the simple washing of the crude pellets, as it was done in the samples for this study, eliminates a great proportion of microsomes, peroxisomes and lysosomes (Fernandez-Vizarra et al., 2006). Accordingly, mitochondria purified from adult flies and larvae are devoid of cytoplasmic markers (Supplementary Figure S1B). Therefore, mitochondria are the major contents in these fractions (Supplementary Figure S1B), and the mitochondrial yield in the mitochondrial preparations from the COX deficient models is similar or even higher than in the controls (Supplementary Figure S1A). Furthermore, we found dysregulation of Cu compartmentalization in all the analyzed COX deficient models, including Scox KD. This indicates that not only defects in one of the chaperones directly handling Cu for the delivery to COX produce alterations in Cu homeostasis, but also the failure to assemble COX is associated with lower intra-mitochondrial Cu levels. In fact, there appears to be a direct correlation between COX activity and mitochondrial Cu amounts in the adults as both parameters are around 50% of the control in the Coa8 KD and ca. 70% in the Coa3 KD. In the larvae, the Cu deficiency (∼20% in both models) is more severe than the COX defect (∼50% in the two KD strains). However, even though the nature of the defect is different, i.e., a structural defect (cype KD) vs. a Cu chaperone defect (Scox KD), a similar COX deficiency results in comparable Cu depletion in the larvae. This is compatible with the fact that COX is the most abundant Cu-containing enzyme in the cell types in which mitochondria are present (Ruiz et al., 2021). Cu depletion was also observed in human samples from individuals carrying mutations in COX10, COX15 and SURF1, but Cu cellular levels could be recovered without increasing COX activity by overexpressing the Cu-binding proteins SCO1 and SCO2 (Leary et al., 2007). This observation points out to a general effect of COX deficiency determining Cu depletion inside mitochondria and either no changes or an increase in cytoplasmic Cu concentrations, as observed in our D. melanogaster models.
We were also interested in analyzing the amounts of other transition metals in the mitochondrial and cytosolic fractions of the four COX-deficient models. Iron, which is the other metal present in the catalytic centers of COX, does not appear to be generally altered as a consequence of a generic COX deficiency. We only found an alteration in Fe compartmentalization in the Coa8 KD model, and a modest decrease in the mitochondria of Coa3 KD flies. This could reflect the consequences of the specific COX assembly defect in these models, probably relating to an accumulation of metalated Cox1 in the Coa8 KD flies (Signes et al., 2019), and a reduction in the amounts of Cox1 in the Coa3 KD (Peralta et al., 2012; Clemente et al., 2013). The changes in Mn were also model-specific and rather modest compared with the other metals. On the other hand, some changes in Zn homeostasis were observed in the COX-deficient strains, with increases in mitochondria of the Coa8 KD adults and the Scox and cype KD larvae, and with a very significant elevation in the cytosol of the larval models. Conversely, Zn concentration was lower in both cytosol and mitochondria from Coa3 KD adults. It is difficult to explain why Zn levels are dysregulated in the case of COX deficiency, as Zn is not a component of the enzyme. One could speculate this could be related to changes in the redox state of both mitochondria and cytosol associated with the COX enzymatic and assembly defect (Geldon et al., 2021) or, alternatively, to the tight connection between the metabolism of Cu and Zn observed in yeast, humans and D. melanogaster (Cobine et al., 2004; Kozlowski et al., 2009; Spinazzi et al., 2014; Navarro and Schneuwly, 2017; Barber et al., 2021). The reasons why the alteration in Zn concentrations in the Coa3 KD flies goes in the opposite direction than in the other models is difficult to interpret, being that these same flies show a decrease in intra-mitochondrial Cu and an increase in the cytosol, similar to the other three models. However, this again might reflect early assembly defect driven by Coa3 deficiency, compared with the other factors and structural subunit that have a role in later (middle) stages of COX assembly (Brischigliaro and Zeviani, 2021).
Defects in SCO1 in mouse produce the loss of the copper transporter CTR1 from the plasma membrane by internalization and/or proteasomal degradation (Hlynialuk et al., 2015; Baker et al., 2017), consequently decreasing Cu influx. In the Scox KD flies the transcript levels of Ctr1A, the D. melanogaster homolog of CTR1, were significantly reduced and this was also observed to a lesser extent in cype and Coa3 KD individuals. This may reflect species-specific mechanisms but also suggest a conserved response aimed to reduce Cu uptake at the plasma membrane, likely as a consequence of increased Cu levels in the cytoplasm. In fact, Ctr1A mRNA levels were comparable to the control in the Coa8 KD, where Cu cytoplasmic levels were not increased. In addition, the expression of other genes under the control of MTF-1, such as the ‘low affinity’ Cu transporter Ctr1B and metallothioneins MtnA and MtnD (Egli et al., 2003; Selvaraj et al., 2005; Turski and Thiele, 2007; Günther et al., 2012), were also found altered in the COX-deficient flies. Specifically, the expression of Ctr1B (CTR2 in mammals) was reduced in all the strains except for Scox KD. The role of Ctr1B/CTR2 is not as well defined as that of CTR1 but there seems to be an interplay between the two transporters (Wee et al., 2013). This observation can be interpreted as an attempt to up-regulate Cu uptake, possibly to compensate the reduced activity of Scox. In fact, previous studies demonstrated that increasing copper availability can rescue the COX deficiency arising from SCO2 genetic defects in human cell lines (Jaksch et al., 2001; Salviati et al., 2002; Casarin et al., 2012; Soma et al., 2018). Interestingly, we observed increased expression of metallothioneins MtnA and MtnD in Coa8 and Coa3 KD individuals, which is most likely linked to altered Cu and Zn compartmentalization, and would increase metal trafficking and detoxification (Navarro and Schneuwly, 2017). This effect was strong in adults and negligible at the larval stage, possibly reflecting differences in response to metal overload at different developmental stages in D. melanogaster. Another possibility is that the marked upregulation of Zn levels in the cytoplasm of Scox and cype KD larvae might mitigate the Cu-driven responses, consistent with the fact that increased Zn cellular levels are usually correlated with a decrease in Cu amounts (Spinazzi et al., 2014; Barber et al., 2021).
Mitochondrial diseases are highly heterogeneous in their clinical presentation and additional elements other than ATP deficiency must play a role in the pathogenic mechanisms (Nunnari and Suomalainen, 2012; Suomalainen and Battersby, 2018). Altered metal homeostasis is associated with different pathological states, especially with neurodegeneration (Kozlowski et al., 2009). Although not considered as a main clinical feature in most mitochondrial diseases, iron overload has been reported in GRACILE syndrome, caused by a mutation in the complex III assembly factor BCS1L (Visapaa et al., 2002; Lynn et al., 2012; Kasapkara et al., 2014). In addition, complex III deficiency caused by defects in different biogenetical factors, causes a decrease in mitochondrial Zn pools in yeast (Atkinson et al., 2010; Atkinson et al., 2011). Moreover, evidences of the contribution of altered Fe homeostasis to the pathogenetic mechanisms of Leigh syndrome associated with the loss of the Fe-S binding complex I subunit NDUFS4 have been found (Grillo et al., 2021).
In conclusion, we hypothesize that the disturbance of mitochondrial and, consequently, cellular transition metal content, as well as the responses triggered by these alterations, might play a role in the pathogenesis of the neurodegenerative phenotypes observed in some mitochondrial disorders, especially those involving COX deficiency. Future work is warranted to confirm these observations with larger datasets and additional models in order to explore this hypothesis and investigate the role of transition metal homeostasis in mitochondrial disorders.
Data Availability Statement
The original contributions presented in the study are included in the article/Supplementary Material, further inquiries can be directed to the corresponding authors.
Author Contributions
Conceptualization: MB, RC, MZ, and EF-V. Methodology: MB and DB. Formal analysis: MB, DB and EF-V. Investigation: MB and DB. Resources: DB, RC, CV, PP and MZ. Writing—original draft: MB and EF-V. Writing—review and editing: MB, DB, RC, CV, MZ, PP, and EF-V. Supervision: RC, CV, MZ, PP, and EF-V.
Funding
Our work is supported by: Telethon Foundation (GGP19007 to MZ, GGP20013 to CV and GGP15041 to RC), Fondation NRJ pour les Neurosciences—Institute de France Grant (to MZ), Associazione Luigi Comini Onlus (to MZ and CV), AFM—Telethon 23706 (to CV), EU Horizon 2020 under the Marie Sklodowska-Curie grant agreement No. 765937 (CINCHRON) (to RC).
Conflict of Interest
The authors declare that the research was conducted in the absence of any commercial or financial relationships that could be construed as a potential conflict of interest.
Publisher’s Note
All claims expressed in this article are solely those of the authors and do not necessarily represent those of their affiliated organizations, or those of the publisher, the editors and the reviewers. Any product that may be evaluated in this article, or claim that may be made by its manufacturer, is not guaranteed or endorsed by the publisher.
Acknowledgments
We are grateful to Paul A. Cobine (Auburn University, Auburn, AL, United States) and Scot C. Leary (University of Saskatchewan, Saskatoon, Canada) for critical discussion of the data.
Supplementary Material
The Supplementary Material for this article can be found online at: https://www.frontiersin.org/articles/10.3389/fcell.2022.892069/full#supplementary-material
References
Atkinson, A., Khalimonchuk, O., Smith, P., Sabic, H., Eide, D., and Winge, D. R. (2010). Mzm1 Influences a Labile Pool of Mitochondrial Zinc Important for Respiratory Function. J. Biol. Chem. 285 (25), 19450–19459. doi:10.1074/jbc.m110.109793
Atkinson, A., Smith, P., Fox, J. L., Cui, T.-Z., Khalimonchuk, O., and Winge, D. R. (2011). The LYR Protein Mzm1 Functions in the Insertion of the Rieske Fe/S Protein in Yeast Mitochondria. Mol. Cell Biol. 31 (19), 3988–3996. MCB.05673-11 [pii] 10.1128/MCB.05673-11. doi:10.1128/mcb.05673-11
Badocco, D., Lavagnini, I., Mondin, A., Favaro, G., and Pastore, P. (2015). Definition of the Limit of Quantification in the Presence of Instrumental and Non-instrumental Errors. Comparison Among Various Definitions Applied to the Calibration of Zinc by Inductively Coupled Plasma-Mass Spectrometry. Spectrochim. Acta Part B At. Spectrosc. 114, 81–86. doi:10.1016/j.sab.2015.10.004
Baker, Z. N., Jett, K., Boulet, A., Hossain, A., Cobine, P. A., Kim, B.-E., et al. (2017). The Mitochondrial Metallochaperone SCO1 Maintains CTR1 at the Plasma Membrane to Preserve Copper Homeostasis in the Murine Heart. Hum. Mol. Genet. 26 (23), 4617–4628. doi:10.1093/hmg/ddx344
Barber, R. G., Grenier, Z. A., and Burkhead, J. L. (2021). Copper Toxicity Is Not Just Oxidative Damage: Zinc Systems and Insight from Wilson Disease. Biomedicines 9 (3), 316. doi:10.3390/biomedicines9030316
Brischigliaro, M., Corrà, S., Tregnago, C., Fernandez-Vizarra, E., Zeviani, M., Costa, R., et al. (2019). Knockdown of APOPT1/COA8 Causes Cytochrome C Oxidase Deficiency, Neuromuscular Impairment, and Reduced Resistance to Oxidative Stress in Drosophila melanogaster. Front. Physiol. 10, 1143. doi:10.3389/fphys.2019.01143
Brischigliaro, M., Frigo, E., Fernandez-Vizarra, E., Bernardi, P., and Viscomi, C. (2022). Measurement of Mitochondrial Respiratory Chain Enzymatic Activities in Drosophila melanogaster Samples. Star. Protoc. 3 (2), 101322. doi:10.1016/j.xpro.2022.101322
Brischigliaro, M., and Zeviani, M. (2021). Cytochrome C Oxidase Deficiency. Biochimica Biophysica Acta (BBA) - Bioenergetics 1862 (1), 148335. doi:10.1016/j.bbabio.2020.148335
Casarin, A., Giorgi, G., Pertegato, V., Siviero, R., Cerqua, C., Doimo, M., et al. (2012). Copper and Bezafibrate Cooperate to Rescue Cytochrome C Oxidase Deficiency in Cells of Patients with SCO2 Mutations. Orphanet J. Rare Dis. 7, 21. doi:10.1186/1750-1172-7-21
Cheng, R., Dhorajia, V. V., Kim, J., and Kim, Y. (2022). Mitochondrial Iron Metabolism and Neurodegenerative Diseases. Neurotoxicology 88, 88–101. doi:10.1016/j.neuro.2021.11.003
Clemente, P., Peralta, S., Cruz-Bermudez, A., Echevarría, L., Fontanesi, F., Barrientos, A., et al. (2013). hCOA3 Stabilizes Cytochrome C Oxidase 1 (COX1) and Promotes Cytochrome C Oxidase Assembly in Human Mitochondria. J. Biol. Chem. 288 (12), 8321–8331. doi:10.1074/jbc.M112.422220
Cobine, P. A., Moore, S. A., and Leary, S. C. (2021). Getting Out what You Put in: Copper in Mitochondria and its Impacts on Human Disease. Biochimica Biophysica Acta (BBA) - Mol. Cell Res. 1868 (1), 118867. doi:10.1016/j.bbamcr.2020.118867
Cobine, P. A., Ojeda, L. D., Rigby, K. M., and Winge, D. R. (2004). Yeast Contain a Non-proteinaceous Pool of Copper in the Mitochondrial Matrix. J. Biol. Chem. 279 (14), 14447–14455. doi:10.1074/jbc.M312693200
Cobine, P. A., Pierrel, F., and Winge, D. R. (2006). Copper Trafficking to the Mitochondrion and Assembly of Copper Metalloenzymes. Biochimica Biophysica Acta (BBA) - Mol. Cell Res. 1763 (7), 759–772. doi:10.1016/j.bbamcr.2006.03.002
Dietz, J. V., Fox, J. L., and Khalimonchuk, O. (2021). Down the Iron Path: Mitochondrial Iron Homeostasis and beyond. Cells 10 (9), 2198. doi:10.3390/cells10092198
Egli, D., Selvaraj, A., Yepiskoposyan, H., Zhang, B., Hafen, E., Georgiev, O., et al. (2003). Knockout of 'metal-Responsive Transcription Factor' MTF-1 in Drosophila by Homologous Recombination Reveals its Central Role in Heavy Metal Homeostasis. Embo J. 22 (1), 100–108. doi:10.1093/emboj/cdg012
Fernandez‐Vizarra, E., and Zeviani, M. (2021b). Mitochondrial Disorders of the OXPHOS System. FEBS Lett. 595 (8), 1062–1106. doi:10.1002/1873-3468.13995
Fernandez-Ayala, D. J. M., Sanz, A., Vartiainen, S., Kemppainen, K. K., Babusiak, M., Mustalahti, E., et al. (2009). Expression of the Ciona intestinalis Alternative Oxidase (AOX) in Drosophila Complements Defects in Mitochondrial Oxidative Phosphorylation. Cell Metab. 9 (5), 449–460. doi:10.1016/j.cmet.2009.03.004
Fernandez-Vizarra, E., and Zeviani, M. (2021a). “Blue-Native Electrophoresis to Study the OXPHOS Complexes,” in Mitochondrial Gene Expression: Methods and Protocols. Editors M. Minczuk, and J. Rorbach (New York, NY: Springer US), 287–311. doi:10.1007/978-1-0716-0834-0_20
Fernandezvizarra, E., Fernandezsilva, P., and Enriquez, J. (2006). “Isolation of Mitochondria from Mammalian Tissues and Cultured Cells,” in Cell Biology: A Laboratory Handbook. Editor J. E. Celis (Elsevier-Academic Press), 69–77. doi:10.1016/b978-012164730-8/50082-4
Foriel, S., Willems, P., Smeitink, J., Schenck, A., and Beyrath, J. (2015). Mitochondrial Diseases: Drosophila melanogaster as a Model to Evaluate Potential Therapeutics. Int. J. Biochem. Cell Biol. 63, 60–65. doi:10.1016/j.biocel.2015.01.024
Geldon, S., Fernández-Vizarra, E., and Tokatlidis, K. (2021). Redox-Mediated Regulation of Mitochondrial Biogenesis, Dynamics, and Respiratory Chain Assembly in Yeast and Human Cells. Front. Cell Dev. Biol. 9, 720656. doi:10.3389/fcell.2021.720656
Grillo, A. S., Kelly, C., Ha, V. T., Bodart, C. M., Huff, S., Couch, R. K., et al. (2021). Iron Status Influences Mitochondrial Disease Progression in Complex I-Deficient Mice. bioRxiv. 2021.2009.2029.462431. doi:10.1101/2021.09.29.462431
Günther, V., Lindert, U., and Schaffner, W. (2012). The Taste of Heavy Metals: Gene Regulation by MTF-1. Biochimica Biophysica Acta (BBA) - Mol. Cell Res. 1823 (9), 1416–1425. doi:10.1016/j.bbamcr.2012.01.005
Hlynialuk, C. J., Ling, B., Baker, Z. N., Cobine, P. A., Yu, L. D., Boulet, A., et al. (2015). The Mitochondrial Metallochaperone SCO1 Is Required to Sustain Expression of the High-Affinity Copper Transporter CTR1 and Preserve Copper Homeostasis. Cell Rep. 10 (6), 933–943. doi:10.1016/j.celrep.2015.01.019
Jaksch, M., Horvath, R., Horn, N., Auer, D. P., Macmillan, C., Peters, J., et al. (2001). Homozygosity (E140K) in SCO2 Causes Delayed Infantile Onset of Cardiomyopathy and Neuropathy. Neurology 57 (8), 1440–1446. doi:10.1212/wnl.57.8.1440
Jett, K. A., and Leary, S. C. (2018). Building the CuA Site of Cytochrome C Oxidase: A Complicated, Redox-dependent Process Driven by a Surprisingly Large Complement of Accessory Proteins. J. Biol. Chem. 293 (13), 4644–4652. doi:10.1074/jbc.R117.816132
Kasapkara, Ç. S., Tümer, L., Ezgü, F. S., Küçükçongar, A., and Hasanoğlu, A. (2014). BCS1L Gene Mutation Causing GRACILE Syndrome: Case Report. Ren. Fail. 36 (6), 953–954. doi:10.3109/0886022X.2014.900422
Kozlowski, H., Janicka-Klos, A., Brasun, J., Gaggelli, E., Valensin, D., and Valensin, G. (2009). Copper, Iron, and Zinc Ions Homeostasis and Their Role in Neurodegenerative Disorders (Metal Uptake, Transport, Distribution and Regulation). Coord. Chem. Rev. 253 (21), 2665–2685. doi:10.1016/j.ccr.2009.05.011
Lavagnini, I., Badocco, D., Pastore, P., and Magno, F. (2011). Theil-Sen Nonparametric Regression Technique on Univariate Calibration, Inverse Regression and Detection Limits. Talanta 87, 180–188. doi:10.1016/j.talanta.2011.09.059
Leary, S. C., Cobine, P. A., Kaufman, B. A., Guercin, G.-H., Mattman, A., Palaty, J., et al. (2007). The Human Cytochrome C Oxidase Assembly Factors SCO1 and SCO2 Have Regulatory Roles in the Maintenance of Cellular Copper Homeostasis. Cell Metab. 5 (1), 9–20. doi:10.1016/j.cmet.2006.12.001
Leary, S. C., Kaufman, B. A., Pellecchia, G., Guercin, G. H., Mattman, A., Jaksch, M., et al. (2004). Human SCO1 and SCO2 Have Independent, Cooperative Functions in Copper Delivery to Cytochrome C Oxidase. Hum. Mol. Genet. 13 (17), 1839–1848. doi:10.1093/hmg/ddh197
Leary, S. C., Sasarman, F., Nishimura, T., and Shoubridge, E. A. (2009). Human SCO2 Is Required for the Synthesis of CO II and as a Thiol-Disulphide Oxidoreductase for SCO1. Hum. Mol. Genet. 18 (12), 2230–2240. doi:10.1093/hmg/ddp158
Liu, H. Y., Gale, J. R., Reynolds, I. J., Weiss, J. H., and Aizenman, E. (2021). The Multifaceted Roles of Zinc in Neuronal Mitochondrial Dysfunction. Biomedicines 9 (5), 489. doi:10.3390/biomedicines9050489
Lynn, A. M., King, R. I., Mackay, R. J., Florkowski, C. M., and Wilson, C. J. (2012). BCS1L Gene Mutation Presenting with GRACILE-like Syndrome and Complex III Deficiency. Ann. Clin. Biochem. 49 (Pt 2), 201–203. doi:10.1258/acb.2011.011180
Mick, D. U., Dennerlein, S., Wiese, H., Reinhold, R., Pacheu-Grau, D., Lorenzi, I., et al. (2012). MITRAC Links Mitochondrial Protein Translocation to Respiratory-Chain Assembly and Translational Regulation. Cell 151 (7), 1528–1541. doi:10.1016/j.cell.2012.11.053
Mick, D. U., Vukotic, M., Piechura, H., Meyer, H. E., Warscheid, B., Deckers, M., et al. (2010). Coa3 and Cox14 Are Essential for Negative Feedback Regulation of COX1 Translation in Mitochondria. J. Cell Biol. 191 (1), 141–154. doi:10.1083/jcb.201007026
Navarro, J. A., and Schneuwly, S. (2017). Copper and Zinc Homeostasis: Lessons from Drosophila melanogaster. Front. Genet. 8, 223. doi:10.3389/fgene.2017.00223
Nguyen, T. B., Ida, H., Shimamura, M., Kitazawa, D., Akao, S., Yoshida, H., et al. (2014). Role of SCOX in Determination of Drosophila melanogaster Lifespan. Am. J. Cancer Res. 4 (4), 325–336.
Nunnari, J., and Suomalainen, A. (2012). Mitochondria: in Sickness and in Health. Cell 148 (6), 1145–1159. doi:10.1016/j.cell.2012.02.035
Peralta, S., Clemente, P., Sánchez-Martínez, Á., Calleja, M., Hernández-Sierra, R., Matsushima, Y., et al. (2012). Coiled Coil Domain-Containing Protein 56 (CCDC56) Is a Novel Mitochondrial Protein Essential for Cytochrome C Oxidase Function. J. Biol. Chem. 287 (29), 24174–24185. doi:10.1074/jbc.M112.343764
Pereira, M. M., Santana, M., and Teixeira, M. (2001). A Novel Scenario for the Evolution of Haem-Copper Oxygen Reductases. Biochim. Biophys. Acta 1505 (2-3), 185–208. doi:10.1016/s0005-2728(01)00169-4
Pereira, M. M., Sousa, F. L., Veríssimo, A. F., and Teixeira, M. (2008). Looking for the Minimum Common Denominator in Haem-Copper Oxygen Reductases: towards a Unified Catalytic Mechanism. Biochimica Biophysica Acta (BBA) - Bioenergetics 1777 (7-8), 929–934. doi:10.1016/j.bbabio.2008.05.441
Pitceathly, R. D. S., and Taanman, J.-W. (2018). NDUFA4 (Renamed COXFA4) Is a Cytochrome-C Oxidase Subunit. Trends Endocrinol. Metabolism 29 (7), 452–454. doi:10.1016/j.tem.2018.03.009
Porcelli, D., Oliva, M., Duchi, S., Latorre, D., Cavaliere, V., Barsanti, P., et al. (2010). Genetic, Functional and Evolutionary Characterization of Scox, the Drosophila melanogaster Ortholog of the Human SCO1 Gene. Mitochondrion 10 (5), 433–448. doi:10.1016/j.mito.2010.04.002
Ramzan, R., Kadenbach, B., and Vogt, S. (2021). Multiple Mechanisms Regulate Eukaryotic Cytochrome C Oxidase. Cells 10 (3), 514. doi:10.3390/cells10030514
Ruiz, L. M., Libedinsky, A., and Elorza, A. A. (2021). Role of Copper on Mitochondrial Function and Metabolism. Front. Mol. Biosci. 8, 711227. doi:10.3389/fmolb.2021.711227
Salviati, L., Hernandez-Rosa, E., Walker, W. F., Sacconi, S., DiMauro, S., Schon, E. A., et al. (2002). Copper Supplementation Restores Cytochrome C Oxidase Activity in Cultured Cells from Patients with SCO2 Mutations. Biochem. J. 363 (Pt 2), 321–327. doi:10.1042/0264-6021:3630321
Selvaraj, A., Balamurugan, K., Yepiskoposyan, H., Zhou, H., Egli, D., Georgiev, O., et al. (2005). Metal-responsive Transcription Factor (MTF-1) Handles Both Extremes, Copper Load and Copper Starvation, by Activating Different Genes. Genes Dev. 19 (8), 891–896. doi:10.1101/gad.1301805
Sharma, V., Ala-Vannesluoma, P., Vattulainen, I., Wikström, M., and Róg, T. (2015). Role of Subunit III and its Lipids in the Molecular Mechanism of Cytochrome C Oxidase. Biochimica Biophysica Acta (BBA) - Bioenergetics 1847 (8), 690–697. doi:10.1016/j.bbabio.2015.04.007
Signes, A., Cerutti, R., Dickson, A. S., Benincá, C., Hinchy, E. C., Ghezzi, D., et al. (2019). APOPT 1/COA 8 Assists COX Assembly and Is Oppositely Regulated by UPS and ROS. EMBO Mol. Med. 11 (1). doi:10.15252/emmm.201809582
Soma, S., Latimer, A. J., Chun, H., Vicary, A. C., Timbalia, S. A., Boulet, A., et al. (2018). Elesclomol Restores Mitochondrial Function in Genetic Models of Copper Deficiency. Proc. Natl. Acad. Sci. U.S.A. 115 (32), 8161–8166. doi:10.1073/pnas.1806296115
Spinazzi, M., Sghirlanzoni, A., Salviati, L., and Angelini, C. (2014). Impaired Copper and Iron Metabolism in Blood Cells and Muscles of Patients Affected by Copper Deficiency Myeloneuropathy. Neuropathol. Appl. Neurobiol. 40 (7), 888–898. doi:10.1111/nan.12111
Suomalainen, A., and Battersby, B. J. (2018). Mitochondrial Diseases: the Contribution of Organelle Stress Responses to Pathology. Nat. Rev. Mol. Cell Biol. 19 (2), 77–92. doi:10.1038/nrm.2017.66
Szuplewski, S., and Terracol, R. (2001). The Cyclope Gene of Drosophila Encodes a Cytochrome C Oxidase Subunit VIc Homolog. Genetics 158 (4), 1629–1643. doi:10.1093/genetics/158.4.1629
Timón-Gómez, A., Nývltová, E., Abriata, L. A., Vila, A. J., Hosler, J., and Barrientos, A. (2018). Mitochondrial Cytochrome C Oxidase Biogenesis: Recent Developments. Seminars Cell & Dev. Biol. 76, 163–178. doi:10.1016/j.semcdb.2017.08.055
Turski, M. L., and Thiele, D. J. (2007). Drosophila Ctr1A Functions as a Copper Transporter Essential for Development. J. Biol. Chem. 282 (33), 24017–24026. doi:10.1074/jbc.M703792200
Vidoni, S., Harbour, M. E., Guerrero-Castillo, S., Signes, A., Ding, S., Fearnley, I. M., et al. (2017). MR-1S Interacts with PET100 and PET117 in Module-Based Assembly of Human Cytochrome C Oxidase. Cell Rep. 18 (7), 1727–1738. doi:10.1016/j.celrep.2017.01.044
Visapää, I., Fellman, V., Vesa, J., Dasvarma, A., Hutton, J. L., Kumar, V., et al. (2002). GRACILE Syndrome, a Lethal Metabolic Disorder with Iron Overload, Is Caused by a Point Mutation in BCS1L. Am. J. Hum. Genet. 71 (4), 863–876. doi:10.1086/342773
Wee, N. K. Y., Weinstein, D. C., Fraser, S. T., and Assinder, S. J. (2013). The Mammalian Copper Transporters CTR1 and CTR2 and Their Roles in Development and Disease. Int. J. Biochem. Cell Biol. 45 (5), 960–963. doi:10.1016/j.biocel.2013.01.018
Wikström, M., Krab, K., and Sharma, V. (2018). Oxygen Activation and Energy Conservation by Cytochrome C Oxidase. Chem. Rev. 118 (5), 2469–2490. doi:10.1021/acs.chemrev.7b00664
Keywords: mitochondrial respiratory chain, cytochrome c oxidase, copper, iron, zinc, manganese, metal homeostasis
Citation: Brischigliaro M, Badocco D, Costa R, Viscomi C, Zeviani M, Pastore P and Fernández-Vizarra E (2022) Mitochondrial Cytochrome c Oxidase Defects Alter Cellular Homeostasis of Transition Metals. Front. Cell Dev. Biol. 10:892069. doi: 10.3389/fcell.2022.892069
Received: 08 March 2022; Accepted: 28 April 2022;
Published: 19 May 2022.
Edited by:
Oleh Khalimonchuk, University of Nebraska-Lincoln, United StatesReviewed by:
Gavin P. Mcstay, Staffordshire University, United KingdomMarco Spinazzi, Centre Hospitalier Universitaire d'Angers, France
Copyright © 2022 Brischigliaro, Badocco, Costa, Viscomi, Zeviani, Pastore and Fernández-Vizarra. This is an open-access article distributed under the terms of the Creative Commons Attribution License (CC BY). The use, distribution or reproduction in other forums is permitted, provided the original author(s) and the copyright owner(s) are credited and that the original publication in this journal is cited, in accordance with accepted academic practice. No use, distribution or reproduction is permitted which does not comply with these terms.
*Correspondence: Michele Brischigliaro, bWljaGVsZS5icmlzY2hpZ2xpYXJvQHVuaXBkLml0; Erika Fernández-Vizarra, ZXJpa2EuZmVybmFuZGV6dml6YXJyYUB1bmlwZC5pdA==