- Department of Biology, University of Padova, Padova, Italy
MicroRNAs (miRNAs) are a class of small non-coding RNAs (∼22 nucleotides in length) that negatively regulate protein-coding gene expression post-transcriptionally by targeting mRNAs and triggering either translational repression or RNA degradation. MiRNA genes represent approximately 1% of the genome of different species and it has been estimated that every miRNA can interact with an average of 200 mRNA transcripts, with peaks of 1,500 mRNA targets per miRNA molecule. As a result, miRNAs potentially play a fundamental role in several biological processes including development, metabolism, proliferation, and apoptotic cell death, both in physiological and pathological conditions. Since miRNAs were discovered, Drosophila melanogaster has been used as a model organism to shed light on their functions and their molecular mechanisms in the regulation of many biological and behavioral processes. In this review we focus on the roles of miRNAs in the fruit fly brain, at the level of the visual system that is composed by the compound eyes, each containing ∼800 independent unit eyes called ommatidia, and each ommatidium is composed of eight photoreceptor neurons that project into the optic lobes. We describe the roles of a set of miRNAs in the development and in the proper function of the optic lobes (bantam, miR-7, miR-8, miR-210) and of the compound eyes (bantam, miR-7, miR-9a, miR-210, miR-263a/b, miR-279/996), summarizing also the pleiotropic effects that some miRNAs exert on circadian behavior.
Introduction
microRNAs Biogenesis and Function in Drosophila Melanogaster
MicroRNAs (miRNAs) are a class of small non-coding RNAs (∼22 nucleotides in length) that negatively regulate protein-coding gene expression post-transcriptionally by targeting mRNAs, mostly at the 3′ untranslated region (3′-UTR) and triggering either translational repression or RNA degradation. MiRNA genes represent approximately 1% of the genome of different species and every microRNA has hundreds of targets playing a fundamental role in several biological processes including development, metabolism, proliferation, and apoptotic cell death (He and Hannon, 2004). In the canonical pathway, most miRNA genes are transcribed in the nucleus by RNA polymerase II; the resulting primary miRNA (pri-miRNA) is processed by Drosha and its partner Pasha, which together form the microprocessor complex that is responsible for the cleavage of the pri-miRNA into a 60–70 nucleotide hairpin structure known as precursor miRNA (pre-miRNA) (Wahid et al., 2010). In addition, pre-miRNA-like hairpins can also be generated by the mirtron pathway (Okamura et al., 2007), merging with the canonical pathway when the pre-miRNA is exported into the cytoplasm through EXP5. In the cytoplasm, the pre-miRNA is further processed by Dcr-1 that together with Loqs and AGO1, is responsible for the cleavage of the pre-miRNA hairpin loop and the formation of a miRNA/miRNA* duplex. After cleavage, the passenger strand (miRNA*) is usually degraded, while the guide strand, the mature form of the miRNA, is loaded into AGO1, forming a ribonucleoprotein complex (RISC). Once assembled, the mature miRNA drives RISC toward its mRNA targets which show full or partial sequence complementarity with its seed region and are silenced through mRNA degradation or translation repression (Wahid et al., 2010).
Fly Visual System Development and Anatomy
The fly visual system, composed by the compound eyes and the optic lobes, contains ∼150,000 neurons and glial cells, comprising more than 60% of the neurons of its central nervous system (Nériec and Desplan, 2016).
The Compound Eye Development and Anatomy
The compound eye is derived from an eye-antennal imaginal disc, in which all cells undergo undifferentiated growth until the third larval instar, when a posterior-to-anterior wave of differentiation (morphogenetic furrow) occurs in the eye portion. The retinal progenitor cells start to differentiate because of their position, acquiring specific fates within the ommatidial rudiments by the action of the retinal determination gene network (RDGN) (Kumar, 2010). This developmental process leads to the definition of all the cell types, from the photoreceptor neurons (beginning with R8, followed by R2/R5, R3/R4, R1/R6, and R7 respectively), to the cone cells and the primary, secondary and tertiary pigment cells (Cagan, 2009) (Figure 1A).
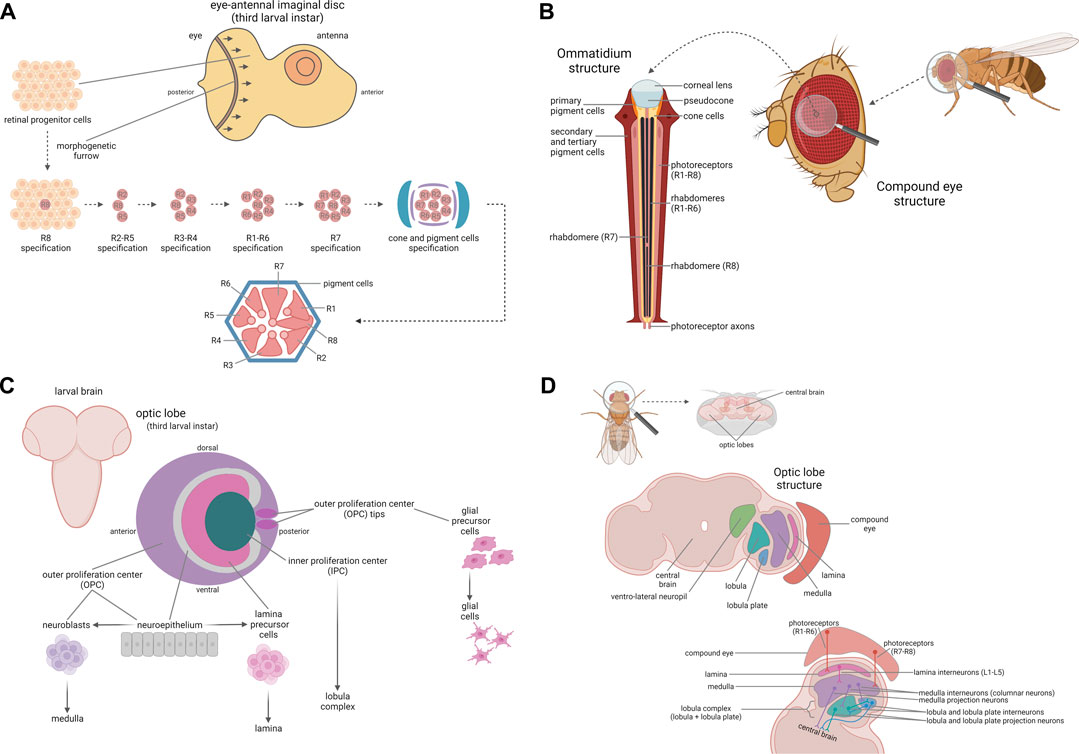
FIGURE 1. Fly visual system. Development and anatomy of the compound eye. (A) The compound eye is derived from the eye-antennal imaginal disc, an epithelium giving rise to both the eye and the antenna. During the third larval instar, a posterior-to-anterior wave of differentiation occurs in the eye portion, leading to the differentiation of the retinal progenitor cells into all the cell types, depending on their positions. The first cells undergoing specification are the photoreceptors, beginning with R8, followed by R2/R5, R3/R4, R1/R6, and R7, respectively, cone cells and pigment cells, which assemble to form the ommatidium final structure, here represented in cross-section. (B) The compound eye is composed of ∼800 units called ommatidia. Each ommatidium is composed of a corneal lens, a pseudocone, as well as cone and pigment cells, which form its backbone and are essential to focus the light on the photoreceptor neurons. In each ommatidium there are eight photoreceptor neurons (R1-R8), six “outer” (R1-R6) and two “inner” (R7-R8) ones, which are responsible for photoreception and for signal transduction from the retina to the optic lobes. Development and anatomy of the optic lobe. (C) The optic lobe is derived from a plate-like structure named optic placode and it is organized into the outer (OPC) and inner proliferation center (IPC). During larval development, the IPC and OPC give rise to the lobula complex and to the lamina, the medulla, and the glial cells respectively. The neuroepithelial cells composing the neuroepithelium of the OPC are converted into the lamina precursor cells in the inner part of the OPC and into the neuroblasts which in turn generate the neurons forming the medulla in the medial part of the OPC. In addition, the tips of the OPC represent the glial precursor cell areas, which will differentiate into the glial cells of the optic lobe. (D) The optic lobe is composed of four neuropils: the lamina, the medulla, the lobula and the lobula plate. The lamina is localized near the retina and is innervated by the outer photoreceptors R1-R6; lamina interneurons L1-L5, targeted by R1-R6 axons, project to the medulla. The medulla is innervated by the inner photoreceptors R7 and R8 and by the lamina interneurons L1-L5; the medulla, similarly to the lamina, is mainly composed of interneurons, and in particular of columnar neurons projecting into the lobula complex. The lobula and the lobula plate, on the other hand, are mainly composed of projection neurons, which transmit the visual information to the ventro-lateral neuropils in the central brain for the last step of visual processing. Created with BioRender.com.
The compound eye is composed of ∼800 units named ommatidia, arranged in an oval shape. Each ommatidium is composed of eight photoreceptor neurons (R1-R8), four cone cells, and two primary pigment cells. In addition, each ommatidium shares six secondary pigment cells and three tertiary pigment cells (also named interommatidial cells) with its surrounding neighbors (Figure 1B). Cone and primary pigment cells are two subsets of non-neuronal cells that secrete the corneal lens and the underlying pseudocone, an extracellular fluid-filled cavity which acts, along with secondary and tertiary pigment cells, to limit light scattering and to focus the light on photoreceptor neurons (Charlton-Perkins and Cook, 2010; Kumar, 2012) that are the specialized neuroepithelial cells responsible for visual phototransduction (Montell, 2012). Among the eight photoreceptors, R1-R6 and R7-R8 are localized in the outer regions and in the central region respectively. Since R7 is localized above R8, only seven photoreceptors are visible in the ommatidium cross-section. The photoreceptor nucleus and organelles reside in the cell body, while photoreception and signal transduction take place in the rhabdomere, a specialized compartment organized in a closely packed stack of ∼50,000 microvilli (Kumar, 2012). Finally, photoreceptor axons project from the retina to the optic lobes for visual information processing (Chang et al., 2018).
The Optic Lobe Development and Anatomy
The optic lobe precursor cells, derived from the neurectoderm of the embryonic head, form a plate-like structure named optic placode, which is then separated into two layers, the outer (OPC) and the inner proliferation center (IPC). After a long period of proliferation until the early pupal stage, the neuroepithelium of the medial part of the OPC is converted into the neuroblasts that generate the neurons forming the medulla, the inner part of the OPC generates the neurons forming the lamina, and the IPC generates the neurons forming the lobula complex (Figure 1C). So, 40 h after puparium formation, the optic lobe reaches its final structure, composed of the four neuropils: lamina, medulla, lobula and lobula plate. The lamina is localized near the retina and is innervated by R1-R6 photoreceptors, responsible for motion detection. Lamina interneurons L1-L5, targeted by R1-R6 photoreceptors axons, project to the medulla. The medulla is organized into distal and proximal medulla and the first one is innervated by the inner photoreceptors R7 and R8, responsible for color vision, and by the lamina interneurons L1-L5, while the proximal medulla receives information from the distal medulla and further computes visual information. Among all the medulla interneurons, only columnar neurons exit from the medulla, projecting into the lobula complex. The lobula complex comprise both the lobula and the lobula plate. In contrast with lamina and medulla, the lobula and the lobula plate are mostly composed of projection neurons, which connect the optic lobe to the central brain. In the lobula plate, involved in motion visual processing, some interneurons (T4 and T5) form connections between lobula plate, lobula and medulla, while the projection neurons transmit visual information to the ventro-lateral neuropils in the central brain, for the last step of visual processing (Nériec and Desplan, 2016; Ngo et al., 2017) (Figure 1D).
Interestingly, some miRNAs play fundamental roles in the development and homeostasis of the fly visual system and also exert a role on its circadian behavior, which is closely related to the capacity of the fly to perceive light and to synchronize to day-night cycles (Rieger et al., 2003).
Drosophila Melanogaster Circadian Clock
Circadian rhythms are a set of physiological and behavioral changes following a cycle of ∼24 h, occurring in most organisms from cyanobacteria to humans and have been extensively studied in D. melanogaster, since the circadian clock influences many of the fruit fly’s behaviors (e.g., locomotor activity). At the molecular level, circadian rhythms are generated by a negative transcriptional feedback loop: the circadian transcription factors CLOCK and CYCLE form a heterodimer that promotes the expression of its own repressors, PERIOD and TIMELESS, which go through various modifications until they are degraded to release CLK/CYC thus starting a new cycle. The stability of these molecules is regulated by a series of post-translational mechanisms, influencing the expression of other clock genes and of hundreds of clock-controlled genes responsible for the rhythmic physiological and behavioral outputs (Tataroglu and Emery, 2015). At the cellular level, the master clock is located in ∼150 neurons, which can be grouped into three major clusters: dorsal neurons (DN1-3), dorsal lateral neurons (LNds), small (s-LNvs) and large ventral lateral neurons (l-LNvs). Notably, a direct communication between the visual system and the circadian clock has been reported, with several photoreceptors targeting different subsets of clock neurons (Schlichting, 2020).
The Role of microRNAs in the Drosophila Melanogaster Visual System
Bantam (ban)
Bantam (ban) is part of several morphogen signaling pathways and specifically of the Hippo pathway, which controls tissue growth. Ban expression is activated by Yorkie (Yki) and is essential for Yki-induced overproliferation. In the uncommitted progenitor cells of the eye imaginal disc, Yki activates the expression of ban to promote cell proliferation and survival; on the other hand, ban expression is downregulated in cells undergoing either cell-cycle arrest or apoptosis, since it represses the translation of the proapoptotic gene head involution defective (hid) (Carthew et al., 2017; Peng et al., 2009) (Figure 2A). Consistently, over-expressing ban in the eye leads to an altered ocular phenotype characterized by bigger eyes (Bejarano and Lai, 2021). In addition, ban is highly expressed in the OPC of the developing optic lobes, where it is required for cell proliferation. In the glial precursor cell areas, ban is important for stem cell maintenance and for glial cell growth, increasing the proliferation of glial precursor and differentiated cells and affecting their distribution, largely through the down-regulation of the transcription factor optomotor-blind (omb) (Figure 2B). Consequently, ban expression affects the photoreceptor axon projection patterns to the optic lobes (Li and Padgett, 2012). Interestingly, ban has been also shown to be implicated in circadian rhythmicity, since it regulates the translation of Clk and ban over-expression induces circadian period lengthening (Li and Liu, 2014).
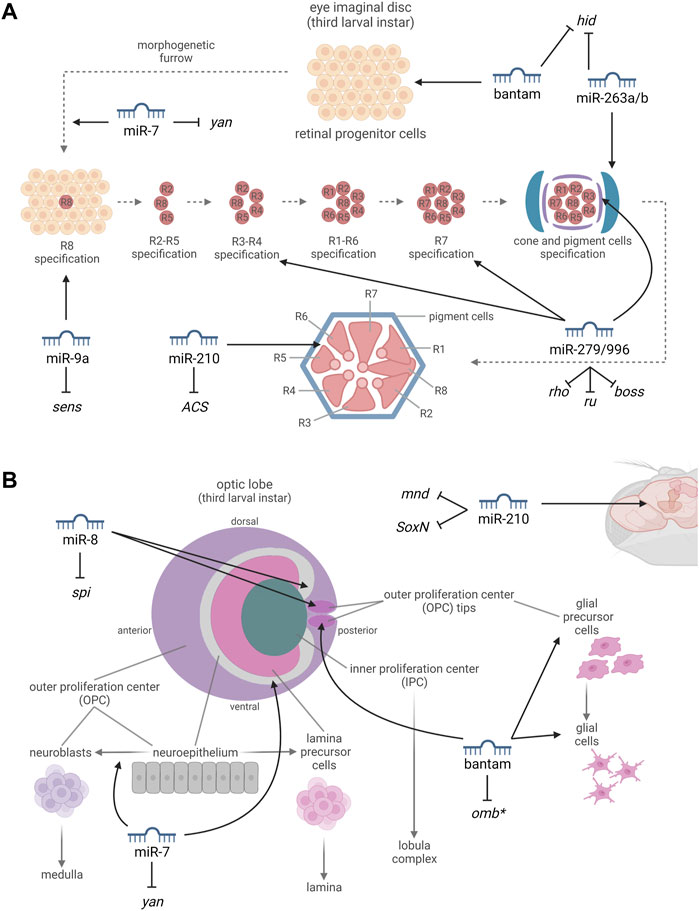
FIGURE 2. miRNAs involved in the development and in the proper functioning of the compound eye (A) and optic lobe (B). (A) By targeting the proapoptotic gene hid, bantam promotes cell proliferation and survival in the progenitor cells of the eye imaginal disc. miR-7, targeting yan, promotes a photoreceptor cell fate. miR-9a, targeting sens, is thought to be involved in the differentiation of the R8 photoreceptor, which occurs by a neuronal selection process such as is observed in the sensory organ precursor cells (SOPs). miR-279/996, targeting rho, ru, and boss, are required for the definition of ommatidial cell fates. miR-263a/b, targeting hid, protect the developing sense organs and interommatidial cells (IOCs), from apoptosis. miR-210, targeting ACS, is essential to prevent progressive retinal degeneration. (B) miR-7, targeting yan, buffers the transition from neuroepithelial cells to neuroblasts. miR-8, expressed in the optic-lobe-associated cortex glia and targeting the growth factor spi, promotes the increase of glial size and is required to limit the growth and neuroblast formation within the neuroepithelium. By leading to the downregulation of omb, bantam is important for stem cell maintenance and for glial cell growth. miR-210, targeting mnd and SoxN, affects neurogenesis. *miRNA-mRNA interactions which have not been experimentally confirmed yet. Created with BioRender.com.
miR-7
miR-7 plays several different roles in flies, and it is involved in the regulation of the Hippo pathway inhibiting yki expression (Yu et al., 2020), establishing an interesting link with the previously discussed ban miRNA. In the eye, miR-7 expression is activated at the beginning of photoreceptor differentiation as part of the EGFR pathway in a reciprocal negative feedback loop mechanism. In the undifferentiated retinal cells, when the transcription factor Yan is degraded, miR-7 expression is activated and it in turn represses Yan activity by binding to its 3′-UTR. In this way, Yan is expressed in progenitor cells and miR-7 is expressed in the developing photoreceptor cells, promoting photoreceptor cell fate at the expense of cone cell fate (Li and Carthew, 2005) (Figure 2A). In addition, in the developing optic lobe, the transition from neuroepithelial cells to neuroblasts, that in turn will generate the neurons forming the medulla, is buffered by miR-7 which is expressed at the level of the neuroepithelial/neuroblast transition zone in response to EGF signaling (Figure 2B). miR-7 limits Notch signaling through the repression of downstream Notch effectors including E(spl)m-γ, causing the switch from the proliferative division of the neuroepithelial cells to the differentiative division of the neuroblasts (Caygill and Brand, 2017).
miR-8
miR-8 is known to be a pleiotropic regulator of fruit fly ontogenesis, controlling physiological processes ranging from body size regulation to neuronal survival (Montigny et al., 2021). In the visual system, miR-8 is expressed from the third larval instar at the level of the optic-lobe-associated cortex glia. It is required to limit the growth and neuroblast formation within the neuroepithelium through the modulation of the growth factor Spitz (spi) which triggers EGFR in order to promote neuroepithelial proliferation and neuroblast formation (Figure 2B). Thus, miR-8 promotes the increase of glial size facilitating the sprouting toward the neuroepithelium and at the same time it negatively regulates Spitz (spi) preventing premature and excessive signaling in the underlying neuroepithelium (Morante et al., 2013).
miR-9a
miR-9a has a key role in the commitment of the sensory organ precursor cells (SOPs) through the interaction with the 3′-UTR of senseless (sens). Sens, encodes for a zinc-finger transcription factor, and acts as a binary switch during SOPs selection, repressing or synergizing with proneural proteins on the basis of its expression levels (Li et al., 2006). In the ontogenesis of the fly eye, the neuronal selection leading to the differentiation of R8 photoreceptors is similar (although not identical) to that described for SOPs. In fact, sens is involved in the regulation of cell survival within the eye imaginal disc and is required for R8 photoreceptor differentiation (Gallicchio et al., 2021; Frankfort et al., 2001) (Figure 2A). Additionally, miR-9a over-expression in the eye results in an altered ocular phenotype characterized by heterogenous eye pigmentation (Bejarano and Lai, 2021), confirming its participation in the development of the fly visual system even if this has not been demonstrated yet.
miR-210
In fruit flies, miR-210 is preferentially expressed in the mushroom bodies, in the antennal lobes and in other olfactory organs, as well as in the main structures of the visual system, from the optic lobes to the compound eyes (Cusumano et al., 2018; Weigelt et al., 2019; Lyu et al., 2021). Weigelt et al. (2019) and Lyu et al. (2021) characterized the eye structure of miR-210 knock-out (KO) flies and reported a progressive retinal degeneration, also accompanied by a reduction in photoreceptor function. In addition, transmission electron microscopy (TEM) analysis revealed the presence of abundant lipid droplet structures in the pigment cells of KO flies, which might represent the cause of the retinal degeneration and suggests the involvement of miR-210 in lipid metabolism. Consistently, Acetyl Coenzyme A synthase (ACS), a homolog of ACSS2, involved in fat storage and utilization, was identified as a direct target of miR-210 (Figure 2A). Nevertheless, additional unidentified targets are most likely yet to be discovered in order to explain the retinal degeneration. Interestingly, the overexpression of miR-210 in clock cells during development led to an altered star shaped morphology of the l-LNvs cell bodies, aberrant arborisations in the optic lobe, and visual defects also affecting circadian rhythmicity. In this context, minidiscs (mnd), which encodes for an amino acid transporter required for imaginal disc growth (Martin et al., 2000), and SoxNeuro (SoxN), encoding a transcription factor that directs embryonic neural development (Ferrero et al., 2014), were identified as direct miR-210 targets (Cusumano et al., 2018) (Figure 2B). Furthermore, miR-210 over- and under-expression within clock neurons modulates circadian outputs such as the time of the morning and evening activity onset (Cusumano et al., 2018; Niu et al., 2019).
miR-263a and miR-263b
miR-263a/b were found to protect the developing sense organs from apoptosis by down-regulating the proapoptotic gene hid and affecting apoptotic pruning, a process occurring to reduce the excess of unspecified progenitor cells during the development of compound eyes (Figure 2A). In this context, considering that 24 h after puparium formation around one out of three interommatidial cells (IOCs) undergo apoptosis, miR-263a/b expression ensure the survival of the remaining cells in order to guarantee the correct arrangement of the ommatidia (Hilgers et al., 2010). Consistently, miR-263b over-expressing flies showed bigger, more curved and very light colored eyes (Bejarano and Lai, 2021), confirming the effects of these miRNAs on IOCs which contain the pigment granules responsible for eye color (Tomlinson, 2012). Furthermore, both miR-263a and miR-263b are implicated in circadian rhythmicity, specifically in the regulation of locomotor activity (Nian et al., 2019; Nian et al., 2020).
miR-279 and miR-996
miR-279 and miR-996 have different sequences but they share similar seed regions and are involved in many functionally redundant cellular activities (Sun et al., 2015). MiR-279/996 exert a prominent role in ommatidial cell fate determination in compound eyes. Indeed, miR-279/996 ectopic expression results in the formation of degenerated R3/R4 photoreceptors with missing or vestigial rhabdomeres, while their deletion leads to the loss of cone cells and to the formation of ectopic R7 photoreceptors. It has been demonstrated that miR-279/996 bind to the 3′-UTRs of multiple positive components of EGFR (rhomboid and roughoid) and Sevenless (bride of sevenless) signaling pathways impairing R7 photoreceptor specification (Duan et al., 2018) (Figure 2A). In addition, miR-279 and miR-996 are implicated in the regulation of fruit fly rest/activity rhythms (Luo and Sehgal, 2012).
Conclusion
The roles of a set of miRNAs involved in the development and proper functioning of the fruit fly visual system was explored. As regards the compound eye, bantam is expressed in the uncommitted progenitor cells of the eye imaginal disc where it promotes cell growth and survival until these cells start to differentiate (Carthew et al., 2017). The differentiation of the several cell types that compose the compound eye is mediated by different miRNAs: miR-263a/b ensure the survival of the proper number of IOCs (Hilgers et al., 2010), miR-7 (Li and Carthew, 2005), miR-9a (Gallicchio et al., 2021), and miR-279/996 (Duan et al., 2018) take part in photoreceptor differentiation, and miR-210 was shown to be essential to photoreceptor maintenance (Lyu et al., 2021) (Figure 2A). As regards the optic lobe, bantam is involved in the proliferation of the OPC (Li and Padgett, 2012) where neuroepithelial cells differentiate into neuroblasts in a process in which both miR-7 (Caygill and Brand, 2017) and miR-8 (Morante et al., 2013) play an important role, while proper expression of miR-210 was shown to be crucial for the correct neurogenesis and arborization of the l-LNvs in the optic lobe (Cusumano et al., 2018) (Figure 2B). In addition, several of these miRNAs (bantam, miR-210, miR-263a/b, miR-279/996) are involved in the regulation of circadian rhythms, further reinforcing the link between the proper functioning of the visual system and physiological circadian rhythmicity. Interestingly, almost all the discussed miRNAs have mammalian homologs and several of them show a highly or even perfectly conserved sequence in Homo sapiens, often sharing similar target genes and also showing similar physiological and pathological effects. The miR-210 seed sequence is 100% identical between flies and humans (Weigelt et al., 2019) and numerous studies have suggested a potential role of miR-210 in eye diseases, since it was found to be elevated in primary open-angle glaucoma patients (Liu et al., 2019) and it is linked to corneal epithelial repair (Kaplan et al., 2022). miR-7 is perfectly conserved from annelids to humans and is highly expressed in the human brain. It participates in the development of the visual system regulating the expression of PAX6 which is an important mediator of ocular formation (Zhao et al., 2020). miR-9 is one of the most highly expressed miRNAs in the vertebrate brain and it is involved in neurogenesis, balancing the proliferation of embryonic neural progenitor cells, also at the level of the retina (Coolen et al., 2013). The miR-200 family, which constitutes the vertebrate homolog of miR-8, has emerged as an important regulator of neurogenesis and gliogenesis (Trümbach and Prakash, 2015) similarly to the role of miR-8 in the Drosophila optic lobes. The miR-183 family, which is the vertebrate homolog of miR-263a and miR-263b, is particularly expressed in the ciliated sensory organs (in mice it is expressed in the bipolar, amacrine, and photoreceptors cells), thus highlighting a conserved main role among different species (Pierce et al., 2008). In particular, the KO of these miRNAs results in retinal degeneration in mice (Lumayag et al., 2013). In conclusion, the architectural similarities between D. melanogaster and vertebrates’ visual system (Sanes and Zipursky, 2010) and the evolutionary conservation of the miRNAs involved in its development and modulation suggest the importance of the fruit fly as a model to further characterize the molecular and neuronal circuits in which miRNAs are involved in the specification and functioning of the visual system of higher organisms including humans. This in turn may pave the way for the identification of promising therapeutic targets for retinal and neurodegenerative diseases.
Author Contributions
DC: Conceptualization, Writing–original draft; CD: Funding acquisition, Supervision, Writing–review & editing.
Funding
This work was supported by the grant PRAT 2014 - University of Padova, N. CPDA142980 to CD.
Conflict of Interest
The authors declare that the research was conducted in the absence of any commercial or financial relationships that could be construed as a potential conflict of interest.
Publisher’s Note
All claims expressed in this article are solely those of the authors and do not necessarily represent those of their affiliated organizations, or those of the publisher, the editors and the reviewers. Any product that may be evaluated in this article, or claim that may be made by its manufacturer, is not guaranteed or endorsed by the publisher.
Acknowledgments
We are grateful to Mauro Zordan (Department of Biology, University of Padova, Italy) for language editing.
References
Bejarano, F., and Lai, E. C. (2021). A Comprehensive Dataset of microRNA Misexpression Phenotypes in the Drosophila Eye. Data in Brief 36, 107037. doi:10.1016/j.dib.2021.107037
Cagan, R. (2009). Chapter 5 Principles of Drosophila Eye Differentiation. Curr. Top. Dev. Biol. 89, 115–135. doi:10.1016/S0070-2153(09)89005-4
Carthew, R. W., Agbu, P., and Giri, R. (2017). MicroRNA Function in Drosophila melanogaster. Semin. Cell Developmental Biol. 65, 29–37. doi:10.1016/j.semcdb.2016.03.015
Caygill, E. E., and Brand, A. H. (2017). miR-7 Buffers Differentiation in the Developing Drosophila Visual System. Cell Rep. 20 (6), 1255–1261. doi:10.1016/j.celrep.2017.07.047
Chang, Y.-C., Tsao, C.-K., and Sun, Y. H. (2018). Temporal and Spatial Order of Photoreceptor and Glia Projections into Optic Lobe in Drosophila. Sci. Rep. 8, 12669. doi:10.1038/s41598-018-30415-8
Charlton-Perkins, M., and Cook, T. A. (2010). Building a Fly Eye. Curr. Top. Dev. Biol. 93, 129–173. doi:10.1016/B978-0-12-385044-7.00005-9
Coolen, M., Katz, S., and Bally-Cuif, L. (2013). miR-9: a Versatile Regulator of Neurogenesis. Front. Cell. Neurosci. 7, 220. doi:10.3389/fncel.2013.00220
Cusumano, P., Biscontin, A., Sandrelli, F., Mazzotta, G. M., Tregnago, C., De Pittà, C., and Costa, R. (2018). Modulation of miR-210 Alters Phasing of Circadian Locomotor Activity and Impairs Projections of PDF Clock Neurons in Drosophila melanogaster. Plos Genet. 14 (7), e1007500. doi:10.1371/journal.pgen.1007500
Duan, H., de Navas, L. F., Hu, F., Sun, K., Mavromatakis, Y. E., Viets, K., et al. (2018). The Mir-279/996 Cluster Represses Receptor Tyrosine Kinase Signaling to Determine Cell Fates in the Drosophila Eye. Development 145 (7), dev159053. doi:10.1242/dev.159053
Ferrero, E., Fischer, B., and Russell, S. (2014). SoxNeuro Orchestrates central Nervous System Specification and Differentiation in Drosophila and Is Only Partially Redundant with Dichaete. Genome Biol. 15 (5), R74. doi:10.1186/gb-2014-15-5-r74
Frankfort, B. J., Nolo, R., Zhang, Z., Bellen, H., and Mardon, G. (2001). Senseless Repression of Rough Is Required for R8 Photoreceptor Differentiation in the Developing Drosophila Eye. Neuron 32 (3), 403–414. doi:10.1016/s0896-6273(01)00480-9
Gallicchio, L., Griffiths-Jones, S., and Ronshaugen, M. (2021). Single-cell Visualization of Mir-9a and Senseless Co-expression during Drosophila melanogaster Embryonic and Larval Peripheral Nervous System Development. G3 (Bethesda) 11 (1), 1–11. doi:10.1093/g3journal/jkaa010
He, L., and Hannon, G. J. (2004). MicroRNAs: Small RNAs with a Big Role in Gene Regulation. Nat. Rev. Genet. 5 (7), 522–531. doi:10.1038/nrg1379
Hilgers, V., Bushati, N., and Cohen, S. M. (2010). Drosophila microRNAs 263a/b Confer Robustness during Development by Protecting Nascent Sense Organs from Apoptosis. Plos Biol. 8 (6), e1000396. doi:10.1371/journal.pbio.1000396
Kaplan, N., Liu, M., Wang, J., Yang, W., Fiolek, E., Peng, H., et al. (2022). Eph Signaling Is Regulated by miRNA-210: Implications for Corneal Epithelial Repair. FASEB J. 36 (1), e22076. doi:10.1096/fj.202101423R
Kumar, J. P. (2012). Building an Ommatidium One Cell at a Time. Dev. Dyn. 241 (1), 136–149. doi:10.1002/dvdy.23707
Kumar, J. P. (2010). Retinal Determination the Beginning of Eye Development. Curr. Top. Dev. Biol. 93, 1–28. doi:10.1016/B978-0-12-385044-7.00001-1
Li, X., and Carthew, R. W. (2005). A microRNA Mediates EGF Receptor Signaling and Promotes Photoreceptor Differentiation in the Drosophila Eye. Cell 123 (7), 1267–1277. doi:10.1016/j.cell.2005.10.040
Li, Y., and Padgett, R. W. (2012). bantam Is Required for Optic Lobe Development and Glial Cell Proliferation. PLoS One 7 (3), e32910. doi:10.1371/journal.pone.0032910
Li, Y., Wang, F., Lee, J. A., and Gao, F. B. (2006). MicroRNA-9a Ensures the Precise Specification of Sensory Organ Precursors in Drosophila. Genes Dev. 20 (20), 2793–2805. doi:10.1101/gad.1466306
Li, Y., and Liu, Z. (2014). Dynamical Analysis of bantam-Regulated Drosophila Circadian Rhythm Model. Int. J. Bifurc. Chaos 24. doi:10.1142/s0218127414501612
Liu, Y., Wang, Y., Chen, Y., Fang, X., Wen, T., Xiao, M., et al. (2019). Discovery and Validation of Circulating Hsa-miR-210-3p as a Potential Biomarker for Primary Open-Angle Glaucoma. Invest. Ophthalmol. Vis. Sci. 60 (8), 2925–2934. doi:10.1167/iovs.19-26663
Lumayag, S., Haldin, C. E., Corbett, N. J., Wahlin, K. J., Cowan, C., Turturro, S., et al. (2013). Inactivation of the microRNA-183/96/182 Cluster Results in Syndromic Retinal Degeneration. Proc. Natl. Acad. Sci. U S A. 110 (6), E507–E516. doi:10.1073/pnas.1212655110
Luo, W., and Sehgal, A. (2012). Regulation of Circadian Behavioral Output via a MicroRNA-JAK/STAT Circuit. Cell 148 (4), 765–779. doi:10.1016/j.cell.2011.12.024
Lyu, J., Chen, Y., Yang, W., Guo, T., Xu, X., Xi, Y., et al. (2021). The Conserved microRNA miR-210 Regulates Lipid Metabolism and Photoreceptor Maintenance in the Drosophila Retina. Cell Death Differ 28 (2), 764–779. doi:10.1038/s41418-020-00622-w
Martin, J. F., Hersperger, E., Simcox, A., and Shearn, A. (2000). Minidiscs Encodes a Putative Amino Acid Transporter Subunit Required Non-autonomously for Imaginal Cell Proliferation. Mech. Dev. 92 (2), 155–167. doi:10.1016/s0925-4773(99)00338-x
Montell, C. (2012). Drosophila Visual Transduction. Trends Neurosci. 35 (6), 356–363. doi:10.1016/j.tins.2012.03.004
Montigny, A., Tavormina, P., Duboe, C., San Clémente, H., Aguilar, M., Valenti, P., et al. (2021). Drosophila Primary microRNA-8 Encodes a microRNA-Encoded Peptide Acting in Parallel of miR-8. Genome Biol. 22 (1), 118. doi:10.1186/s13059-021-02345-8
Morante, J., Vallejo, D. M., Desplan, C., and Dominguez, M. (2013). Conserved miR-8/miR-200 Defines a Glial Niche that Controls Neuroepithelial Expansion and Neuroblast Transition. Dev. Cell 27 (2), 174–187. doi:10.1016/j.devcel.2013.09.018
Nériec, N., and Desplan, C. (2016). From the Eye to the Brain: Development of the Drosophila Visual System. Curr. Top. Dev. Biol. 116, 247–271. doi:10.1016/bs.ctdb.2015.11.032
Ngo, K. T., Andrade, I., and Hartenstein, V. (2017). Spatio-temporal Pattern of Neuronal Differentiation in the Drosophila Visual System: A User's Guide to the Dynamic Morphology of the Developing Optic Lobe. Dev. Biol. 428 (1), 1–24. doi:10.1016/j.ydbio.2017.05.008
Nian, X., Chen, W., Bai, W., and Zhao, Z. (2020). Regulation of Circadian Locomotor Rhythm by miR-263a. Biol. Rhythm Res. 53, 1–11. doi:10.1080/09291016.2020.1726049
Nian, X., Chen, W., Bai, W., Zhao, Z., and Zhang, Y. (2019). miR-263b Controls Circadian Behavior and the Structural Plasticity of Pacemaker Neurons by Regulating the LIM-Only Protein Beadex. Cells 8 (8), 923. doi:10.3390/cells8080923
Niu, Y., Liu, Z., Nian, X., Xu, X., and Zhang, Y. (2019). miR-210 Controls the Evening Phase of Circadian Locomotor Rhythms through Repression of Fasciclin 2. Plos Genet. 15 (7), e1007655. doi:10.1371/journal.pgen.1007655
Okamura, K., Hagen, J. W., Duan, H., Tyler, D. M., and Lai, E. C. (2007). The Mirtron Pathway Generates microRNA-Class Regulatory RNAs in Drosophila. Cell 130 (1), 89–100. doi:10.1016/j.cell.2007.06.028
Peng, H. W., Slattery, M., and Mann, R. S. (2009). Transcription Factor Choice in the Hippo Signaling Pathway: Homothorax and Yorkie Regulation of the microRNA bantam in the Progenitor Domain of the Drosophila Eye Imaginal Disc. Genes Dev. 23 (19), 2307–2319. doi:10.1101/gad.1820009
Pierce, M. L., Weston, M. D., Fritzsch, B., Gabel, H. W., Ruvkun, G., and Soukup, G. A. (2008). MicroRNA-183 Family Conservation and Ciliated Neurosensory Organ Expression. Evol. Dev. 10 (1), 106–113. doi:10.1111/j.1525-142X.2007.00217.x
Rieger, D., Stanewsky, R., and Helfrich-Förster, C. (2003). Cryptochrome, Compound Eyes, Hofbauer-Buchner Eyelets, and Ocelli Play Different Roles in the Entrainment and Masking Pathway of the Locomotor Activity Rhythm in the Fruit Fly Drosophila melanogaster. J. Biol. Rhythms 18 (5), 377–391. doi:10.1177/0748730403256997
Sanes, J. R., and Zipursky, S. L. (2010). Design Principles of Insect and Vertebrate Visual Systems. Neuron 66 (1), 15–36. doi:10.1016/j.neuron.2010.01.018
Schlichting, M. (2020). Entrainment of the Drosophila Clock by the Visual System. Neurosci. Insights 15, 2633105520903708. doi:10.1177/2633105520903708
Sun, K., Jee, D., de Navas, L. F., Duan, H., and Lai, E. C. (2015). Multiple In Vivo Biological Processes Are Mediated by Functionally Redundant Activities of Drosophila Mir-279 and Mir-996. Plos Genet. 11 (6), e1005245. doi:10.1371/journal.pgen.1005245
Tataroglu, O., and Emery, P. (2015). The Molecular Ticks of the Drosophila Circadian Clock. Curr. Opin. Insect Sci. 7, 51–57. doi:10.1016/j.cois.2015.01.002
Tomlinson, A. (2012). The Origin of the Drosophila Subretinal Pigment Layer. J. Comp. Neurol. 520 (12), 2676–2682. doi:10.1002/cne.23063
Trümbach, D., and Prakash, N. (2015). The Conserved miR-8/miR-200 microRNA Family and Their Role in Invertebrate and Vertebrate Neurogenesis. Cell Tissue Res 359 (1), 161–177. doi:10.1007/s00441-014-1911-z
Wahid, F., Shehzad, A., Khan, T., and Kim, Y. Y. (2010). MicroRNAs: Synthesis, Mechanism, Function, and Recent Clinical Trials. Biochim. Biophys. Acta 1803 (11), 1231–1243. doi:10.1016/j.bbamcr.2010.06.013
Weigelt, C. M., Hahn, O., Arlt, K., Gruhn, M., Jahn, A. J., Eßer, J., et al. (2019). Loss of miR-210 Leads to Progressive Retinal Degeneration in Drosophila melanogaster. Life Sci. Alliance 2 (1), e201800149. doi:10.26508/lsa.201800149
Yu, X., Li, M., Cui, M., Sun, B., and Zhou, Z. (2020). Silence of Yki by miR-7 Regulates the Hippo Pathway. Biochem. Biophys. Res. Commun. 532 (3), 446–452. doi:10.1016/j.bbrc.2020.08.069
Keywords: MicroRNAs, Drosophila melanogaster, visual system, optic lobe, compound eye, circadian rhythms
Citation: Colaianni D and De Pittà C (2022) The Role of microRNAs in the Drosophila Melanogaster Visual System. Front. Cell Dev. Biol. 10:889677. doi: 10.3389/fcell.2022.889677
Received: 04 March 2022; Accepted: 21 March 2022;
Published: 04 April 2022.
Edited by:
Lei Pan, Institut Pasteur of Shanghai (CAS), ChinaReviewed by:
Milena Damulewicz, Jagiellonian University, PolandCopyright © 2022 Colaianni and De Pittà. This is an open-access article distributed under the terms of the Creative Commons Attribution License (CC BY). The use, distribution or reproduction in other forums is permitted, provided the original author(s) and the copyright owner(s) are credited and that the original publication in this journal is cited, in accordance with accepted academic practice. No use, distribution or reproduction is permitted which does not comply with these terms.
*Correspondence: Cristiano De Pittà, Y3Jpc3RpYW5vLmRlcGl0dGFAdW5pcGQuaXQ=