- Department of Bionanoscience, Kavli Institute of Nanoscience Delft, Delft University of Technology, Delft, Netherlands
The mammalian cytoskeleton forms a mechanical continuum that spans across the cell, connecting the cell surface to the nucleus via transmembrane protein complexes in the plasma and nuclear membranes. It transmits extracellular forces to the cell interior, providing mechanical cues that influence cellular decisions, but also actively generates intracellular forces, enabling the cell to probe and remodel its tissue microenvironment. Cells adapt their gene expression profile and morphology to external cues provided by the matrix and adjacent cells as well as to cell-intrinsic changes in cytoplasmic and nuclear volume. The cytoskeleton is a complex filamentous network of three interpenetrating structural proteins: actin, microtubules, and intermediate filaments. Traditionally the actin cytoskeleton is considered the main contributor to mechanosensitivity. This view is now shifting owing to the mounting evidence that the three cytoskeletal filaments have interdependent functions due to cytoskeletal crosstalk, with intermediate filaments taking a central role. In this Mini Review we discuss how cytoskeletal crosstalk confers mechanosensitivity to cells and tissues, with a particular focus on the role of intermediate filaments. We propose a view of the cytoskeleton as a composite structure, in which cytoskeletal crosstalk regulates the local stability and organization of all three filament families at the sub-cellular scale, cytoskeletal mechanics at the cellular scale, and cell adaptation to external cues at the tissue scale.
Introduction
The cytoskeleton is a fascinating cellular machinery that performs multiple, to some extent contradictory, functions. It acts as a stable structural scaffold providing cells with a specific functional shape and protecting against external forces. Accordingly, genetic defects in cytoskeletal proteins are associated with mechanical defects in cells and tissues, which for instance result in kidney scarring (Feng et al., 2018), skin fragility (Haines and Lane 2012), and muscle failure (Adil et al., 2021). On the other hand, the cytoskeletal structures are also dynamic enough to enable cell migration, division and mechanosensitive response to the environment (Chaudhuri et al., 2020).
Although the cytoskeleton is highly dynamic at the subcellular (nm) scale, it nevertheless maintains structural integrity at the cell scale (microns) and at the tissue scale (up to millimeters). This disparity is most likely due to the composite nature of the cytoskeleton, based around three protein filament networks with distinct structural, mechanical and biochemical properties: actin filaments, microtubules, and intermediate filaments (Figures 1A,B). All three filaments are reversible polymers that self-assemble from weakly interacting subunits whose local availability is a critical determinant of local cytoskeletal dynamics (Plastino and Blanchoin 2018; Ohi et al., 2021). Both actin filaments and microtubules are structurally polar filaments, respectively composed of actin monomers that hydrolyze ATP, and tubulin dimers that hydrolyze GTP. They both exhibit fast ( ∼ seconds to minutes) turnover rates fueled by ATP/GTP hydrolysis (Theriot and Mitchison 1991; McNally 1996). By contrast, intermediate filaments are non-polar filaments that lack intrinsic enzymatic activity (Li et al., 2006; Robert et al., 2015). Their remodeling occurs by slow ( ∼ hours) exchange of filamentous tetramers (Çolakoğlu and Brown 2009; Nöding et al., 2014; Robert et al., 2015). It has been proposed that this long-lived intermediate filament network mechanically integrates the cytoskeleton and provides structural memory that helps maintain cell polarity (Gan et al., 2016).
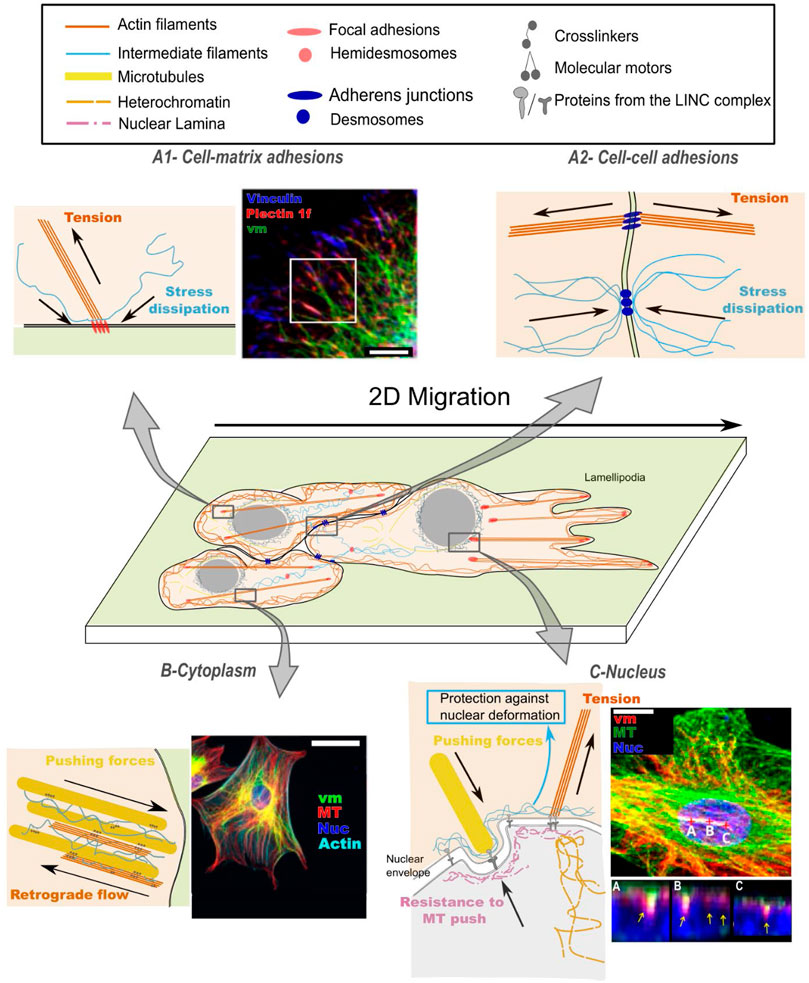
FIGURE 1. Cytoskeletal crosstalk between intermediate filaments (IFs), actin filaments (AFs) and microtubules (MTs) contributes to mechanosensing: (A) at the cell surface: mechanical signals emerging from the matrix (A1) or from neighboring cells (A2); (B) in the propagation of mechanical signals across the cell cytoplasm and (C) up to the cell nucleus. (A1) At cell-matrix adhesions, actin/vimentin crosstalk regulates focal adhesion turnover, which results in dissipation of local stresses. Fluorescence image from (Gregor et al., 2014) shows the intimate spatial relation between vimentin (vm), plectin and vinculin, orchestrating focal adhesion turnover; scale bar is 10 µm. (A2) At cell-cell adhesions, actin/intermediate filament crosstalk is activated upon tensile (pulling) forces and participates in the regulation of cellular prestress. (B) In the dense cytoplasm, mechanical forces are transmitted through all three cytoskeletal networks. The intermediate filament network affects the (de)polymerization rates of the other two networks, and the three networks co-align. Fluorescence image from (Vohnoutka et al., 2019) demonstrates the dense organization of vimentin, microtubules (MT) and actin. Vimentin spans from membrane sites to the nucleus (DAPI) and forms a cage surrounding the nucleus. Scale bar is 50 µm. (C) At the nucleus, forces are transmitted between the cytoskeleton and chromatin through LINC complexes, affecting nuclear shape and heterochromatin density, while intermediate filaments protect the nucleus from large deformations. Fluorescence image from (Feliksiak et al., 2020) shows microtubules and vimentin around the nucleus (DAPI); scale bar = 15 µm. Areas marked by A-B-C demonstrate the tight association of vimentin with nuclear grooves.
Each of the three cytoskeletal networks has its own set of dedicated regulatory proteins that control their structure, dynamics and mechanics with high spatial and temporal precision (Plastino and Blanchoin 2018; Dutour-Provenzano and Etienne-Manneville 2021; Gudimchuk and McIntosh 2021). Cells are thus able to form different specialized cytoskeletal arrays, such as the branched actin networks at the leading edge of migrating cells, the microtubule spindle in dividing cells, and an intermediate filament cage-like structure that protects the nucleus of cells during confined migration. The posttranslational addition of various chemical groups further enhances the complexity of the cytoskeletal proteome, hence the ability of the cell to fine-tune cytoskeletal functions (MacTaggart and Kashina 2021).
It is increasingly recognized that the functions of the three structural systems are tightly coupled via crosslinkers, motors, adhesion complexes and shared signaling factors. Recently, our understanding of the molecular mechanisms of cytoskeletal crosstalk and its consequences for cell shape, mechanics and fundamental processes such as directional migration, has grown (Huber et al., 2015; van Bodegraven and Etienne-Manneville 2020). Still, the role of cytoskeletal crosstalk in cellular mechanosensitivity remains poorly understood. Traditionally actin filaments are considered as the main contributor to mechanosensitivity, since they actively apply contractile (traction) forces to the extracellular matrix and to adjacent cells via specialized adhesion complexes and transmit mechanical signals to the nucleus, for instance via LINC complexes (Sun et al., 2016; Yap et al., 2018). Microtubules are thought to play mainly a regulatory role through their interactions with the actin cytoskeleton and with cell-matrix and cell-cell adhesions (Dogterom and Koenderink 2019; Rafiq et al., 2019; Pimm and Henty-Ridilla 2021). Intermediate filaments are usually considered as passive cytoskeletal elements that maintain cell/tissue integrity. However, there is a growing appreciation that intermediate filaments play a central role in cellular mechanosensitivity, forming a mechanically strong yet responsive network that links to the actin and microtubule cytoskeleton, cell adhesions, and nuclear complexes (Chang and Goldman 2004). This is especially intriguing because intermediate filaments exhibit much more tissue diversity than the other cytoskeletal subsystems. The actin and microtubule cytoskeleton are differently organized in different cell types, but their molecular composition is relatively conserved, with a limited number of isoforms. By contrast, there are ∼ 70 intermediate filament genes in humans, with further diversity arising from alternative splicing. For instance, different types of epithelial cells express different sets of keratins, mesenchymal cells express vimentin, muscle cells express desmin, and neurons express neurofilaments. Intermediate filaments are hence widely used as markers for differentiation (Redmond and Coulombe 2021; Sjöqvist et al., 2021). Herein, we review evidence pointing to the importance of cytoskeletal crosstalk in cellular mechanosensitivity, with a particular focus on the role of intermediate filaments. We demonstrate how intermediate filaments span from cell-cell and cell-matrix adhesion sites, through the cytoplasm and up to the nucleus (see Figure 1), thereby orchestrating long-range mechano-chemical crosstalk between the cytoskeleton, cell adhesions, and internal processes.
Contributions of Cytoskeletal Crosstalk to Mechanosensing at the Cell Membrane
Mechanosensing at Cell/Extracellular Matrix Contacts
Cell-matrix contacts are key players in mechanotransduction as they enable cells to apply forces on the extracellular matrix, in response to its mechanical properties (Sun et al., 2016). These matrix contacts are essential for determining cell polarity, directional migration and the cell’s ability to remodel the extracellular matrix (Brabletz et al., 2021). The role of actin-based transmembrane adhesion complexes, including but not limited to focal adhesions, in mechanosensitivity has been extensively reviewed (Kechagia et al., 2019). The role of the other two cytoskeletal filament families in mechanosensitivity of cell-matrix contacts is recently gaining more attention (Leube et al., 2015; Sanghvi-Shah and Weber 2017; van Bodegraven and Etienne-Manneville 2020).
Focal adhesions are mostly identified with actin-based structures; however, multiple intermediate filament proteins have also been identified at focal adhesions (Figure 1A1). In epithelial cells, keratin filaments are nucleated at focal adhesions and transported inwards assisted by the actin cytoskeleton (Windoffer et al., 2006; Leube et al., 2015). In fibroblasts and endothelial cells, vimentin filaments are anchored to focal adhesions and regulate their size and adhesion strength (Bhattacharya et al., 2009; Kim et al., 2010; Gregor et al., 2014; Kim et al., 2016; Terriac et al., 2017; Vohnoutka et al., 2019). This involves direct force transmission from cell surface integrins to the cell interior (Maniotis et al., 1997), regulating actin stress fibers (Jiu et al., 2015; Jiu et al., 2017) and the associated mechanosensing machinery (Gregor et al., 2014). Focal adhesion anchoring enables vimentin to promote integrin-mediated activation of the major mechanosensor focal adhesion kinase (FAK) and its downstream tension-dependent signaling cascade (Gregor et al., 2014). Actomyosin-dependent rigidity sensing controls microtubule acetylation, which in turn tunes the mechanosensitivity of focal adhesions (Seetharaman et al., 2021). Since microtubule acetylation also affects the association of intermediate filaments with actin bundles at focal adhesions, this points to a complex three-way feedback mechanism that still remains to be disentangled. Cell adhesion is tightly connected to migration; Vimentin tunes cell migration through collagen and fibronectin matrices (Ding et al., 2020; Ostrowska-Podhorodecka et al., 2021, Ostrowska-Podhorodecka, Ding et al. 2021). Persistent collective migration of astrocytes was dependent on the size and turnover of focal adhesions, in a cell-specific (leader vs. follower) manner. The regulation of focal adhesions and cell-cell contacts requires the intermediate filament network, which is composed mainly of glial fibrillar acidic protein (GFAP), vimentin, and nestin (Moeton et al., 2016; De Pascalis et al., 2018). Intermediate filaments also modulate traction forces; in migrating fibroblasts, the vimentin cytoskeleton was shown to slow down actin retrograde flows, while promoting orientation of actin stress fibers and traction forces (Costigliola et al., 2017). Intermediate filaments modulate also forces oriented perpendicularly to the substrate, through invadopodia (Schoumacher et al., 2010), possibly via interacting with actin capping proteins (Lanier et al., 2015).
Intermediate filaments are further anchored to the cell surface by proteins of the plakin family, specifically plectin in hemidesmosomes and desmoplakin in desmosomes (Dowling et al., 1996; Mohammed et al., 2020). Plectin is a major crosslinker connecting the three cytoskeletal filament families (Svitkina et al., 1996; Wiche et al., 2015). Molecular dynamic simulations suggested that plakins act as mechanosensors: pulling forces resulted in plectin and desmoplakin unfolding and exposure of the SH3 domain, which may potentially trigger downstream signaling cascades (Daday et al., 2017). Experimentally, activated plectins were shown to promote microtubule destabilization through their interaction with MAP2, which antagonizes the MAP2-mediated stabilization of MTs (Valencia et al., 2013). Recent studies indicate that FAs and hemidesmosomes are mechanically coupled (Nardone et al., 2017; Wang et al., 2020).
Mechanosignalling at Cell-Cell Contacts
Cell-cell interactions play a crucial role in physiological mechanosensitive processes such as tissue morphogenesis, but also in pathological processes such as inflammatory bowel diseases (Adil et al., 2021). Cells interact through cadherin-based adherens junctions that connect the actin networks of neighboring cells in epithelia and endothelia, and desmosomes that connect the intermediate filaments of neighboring cells and reinforce tissues that experience high mechanical stress such as the epidermis (Rübsam et al., 2018; Broussard et al., 2020) (Figure 1A2). Besides providing mechanical coherence, both adhesions are involved in cell and tissue adaptation to mechanical cues (Charras and Yap 2018; Angulo-Urarte et al., 2020; Zuidema et al., 2020). The mechanosensitivity of adherens junctions was shown to rely on force-sensitive conformational changes of α-catenin and vinculin (Yao et al., 2014; Seddiki et al., 2018). Desmosomes are sites of local assembly/reorganization of keratin filaments (Kim et al., 2021), similar to hemidesmosomes. Desmoplakin, one of the core proteins of desmosomes that binds keratin filaments (Bornslaeger et al., 1996), was recently shown to experiences forces in the pN range in stretched epithelial monolayers, suggesting its load-bearing function (Price et al., 2018).
Although previous studies examining adherens junctions (Engl et al., 2014) and desmosomes (Price et al., 2018) considered the different cytoskeletal networks separately, there is evidence that the three cytoskeletal elements interdependently modulate the dynamical properties of cell-cell junctions. Microtubules promote actin recruitment at adherens junctions and intercellular transmission of the contractile forces generated by the actomyosin network (Ko et al., 2019). In migrating astrocytes, intermediate filaments influence actin-driven retrograde flow of adherens junctions (De Pascalis et al., 2018). In migrating epithelial cells, desmosome dynamics was shown to depend on both intermediate filaments and actin (Roberts et al., 2011). Intermediate filaments also appear to be involved in vascular permeability (Bayir and Sendemir 2021) by helping to organize continuous adherens junctions and the underlying actin network via plectin crosslinking (Osmanagic-Myers et al., 2015). In epithelia, plectin mechanically couples cortical keratin and actin networks and ensures a uniform distribution of actomyosin-generated forces (Prechova et al., 2022). Finally, growing evidence points to collaboration between intermediate filament-desmosome and actin-adherens junction networks during mechanosensing and force generation (reviewed in (Zuidema et al., 2020)).
Contributions of Cytoskeletal Crosstalk to Force Transmission Through the Cytoplasm
Physical interactions between intermediate filaments, actin, and microtubules influence the mechanical properties of the cytoskeleton as a whole, and hence force transmission from the cell surface to the nucleus (Figure 1B). The three cytoskeletal filaments strongly differ in their bending rigidity, as quantified by the persistence length, lp. Intermediate filaments are most flexible, with lp ≈ 0.5–2 μm, microtubules are most rigid, with lp ≈ 1–10 mm, and actin filaments are intermediate with lp ≈ 8 μm (Huber et al., 2015). The filaments also strongly differ in their rupture strain: actin filaments and microtubules only support small tensile strains whereas intermediate filaments support large elongations because their subunits can slide and unfold (Block et al., 2017). Understanding how these single-filament properties translate in cell-scale mechanics is challenging given the molecular and structural complexity of the cytoskeleton. Cell-free reconstitution experiments are hence essential to elucidate the individual and collaborative roles of the different cytoskeletal filaments in cytoskeletal mechanics.
Reconstituted networks of purified actin and intermediate filaments (vimentin or keratin) strain-stiffen when exposed to shear or tensile stresses. These filaments are semiflexible, with a persistence length that is of the same order as the contour length. Experiments and theoretical modelling demonstrated that strain-stiffening occurs because the thermally undulating filaments are straightened out by tensile strains, which reduces the conformational entropy of the fluctuating polymer segments between adjacent crosslinks, and hence opposes further deformation (Gardel et al., 2004; Broedersz and MacKintosh 2014). Depending on the time scale of the imposed mechanical load, reconstituted vimentin networks can additionally dissipate mechanical stress because crosslinks between filaments can remodel and the filaments themselves lengthen by subunit unfolding and sliding elongations (Aufderhorst-Roberts and Koenderink 2019; Forsting et al., 2019). Combining the different cytoskeletal polymers in composite networks demonstrates intriguing co-dependent mechanical properties. Actin/vimentin and actin/microtubule mixtures were shown to exhibit enhanced stiffness and compressibility compared to the single-component networks (Esue et al., 2006; Pelletier et al., 2009; Lin et al., 2011). Furthermore, microtubules were shown to counteract myosin motor-driven contraction of actin networks through their ability to bear large compressive loads (Lee et al., 2021a). Physical interactions also introduce co-dependent polymerization dynamics of the three cytoskeletal polymers. Branched actin networks reduce the growth rate of microtubules and trigger their depolymerization (Colin et al., 2018), while vimentin filaments bind to microtubules and stabilize them against depolymerization (Schaedel et al., 2021) and also bind to actin filaments (Esue et al., 2006). In the presence of crosslinkers and motors, the three filament systems can additionally co-align and (re-)direct each other’s polymerization direction (Preciado López et al., 2014; Gan et al., 2016; Leduc and Etienne-Manneville 2017).
These physical effects identified in simplified reconstituted systems likely contribute to mechanical co-dependencies observed in cells, such as toughening by stress dissipation in the vimentin network (Hu et al., 2019), protection against compressive forces by the vimentin network (Mendez et al., 2014), and vimentin-dependent modulation of actin-myosin contractility (De Pascalis et al., 2018). Raman imaging recently showed that actomyosin forces are transmitted to the intermediate filament cytoskeleton: cells on rigid substrates, where myosin contractility is high (Gupta et al., 2019), contained more unfolded vimentin than on soft substrates, where tension is low (Fleissner et al., 2020). In epithelial monolayers a similar mechanical interplay between the actin and intermediate filament networks was found (Latorre et al., 2018), where cell stretching dilutes the actin cortex and hence decreases tension, while keratin filaments that bear tension re-stiffen the cells. There is evidence that microtubules also contribute to the overall mechanical balance; epithelial folding was for instance shown to emerge from the balance between myosin contractile forces and microtubule-generated pushing forces (Takeda et al., 2018). It would be interesting to evaluate more systematically how mechanical co-dependencies among the three cytoskeletal filament families respond to modified substrate stiffness.
Contributions of Cytoskeletal Crosstalk to Mechanosensitivity at the Nucleus
The nucleus plays a key role in mechanotransduction and mechanosensing (reviewed in (Kirby and Lammerding 2018; Janota et al., 2020)). Nuclear chromatin and the cytoskeleton are physically linked through the LINC (Linker of Nucleoskeleton and Cytoskeleton) complex (Bouzid et al., 2019), which is associated with chromatin and nuclear lamins, members of the intermediate filament family (Figure 1C). Actin filaments are anchored to the nucleus via nesprin-1 and nesprin-2, intermediate filaments via nesprin-3, and microtubules via nesprin-4 (Zhen et al., 2002; Warren et al., 2005; Wilhelmsen et al., 2005; Roux et al., 2009). Considering the role of intermediate filaments in mechanical stabilization of the nucleus, as shown for vimentin (Patteson et al., 2019) and keratin (Almeida et al., 2015), we propose that future work should focus further on resolving the interactions between the three cytoskeletal components at the nuclear envelope in response to changes in substrate rigidity, for instance by superresolution microscopy and molecular tension sensors (Arsenovic et al., 2016; Leduc and Etienne-Manneville 2017).
The physical links between the nuclear lamins and the cytoskeleton provide continuous feedback between the mechanical properties of the nucleus of the cell and its environment (Buxboim et al., 2014; Lomakin et al., 2020; Venturini et al., 2020). Soft substrates promote phosphorylation and turnover of lamin A/C, resulting in softer and less spread nuclei (Buxboim et al., 2014). Pushing forces on the nuclear envelope exerted by microtubules are balanced by the laminA network for the maintenance of a round nuclear shape (Ramdas and Shivashankar 2015; Tariq et al., 2017). In differentiating Hematopoietic Stem and Progenitor (HSPC) cells, local nuclear invaginations associated with centrosomes and microtubule bundles depend on the laminB density and the activity of dynein (Biedzinski et al., 2020). Such local interactions at the nucleus possibly depend on environmental cues. In MEFs plated on micropatterned substrates with independent control over the overall cell shape and the focal adhesion size, the cell-ECM contact size was shown to have more impact than cell shape on overall cell polarization, in a LaminA dependent manner (Lee et al., 2021b). These results strengthen the notion that cytoskeletal crosstalk affects mechanoresponsiveness all the way from the cell surface to the nucleus.
Discussion
In this mini-review, we gathered recent evidence demonstrating the contribution of cytoskeletal crosstalk in transferring mechanical signals from contact points at the plasma membrane to the nucleus. Intermediate filaments play a central role in this crosstalk, by interacting with the actin and microtubule cytoskeleton, cell-cell and cell-matrix adhesions, and nuclear complexes. We propose to shift focus in cytoskeletal and mechanobiological research towards a more holistic view of the cytoskeleton as a composite structure, examining the responses of all three structural families to mechanical cues. The central role of intermediate filaments in mechanosensitivity may render cell/tissue-specific mechanosensitivity. Moreover, during development, aging or pathology, the composition of the intermediate filament cytoskeleton undergoes major changes (Redmond and Coulombe 2021; Sjöqvist et al., 2021). This raises the hypothesis that there may be an “intermediate filament code” that confers cell type-specific mechanosensitive functions.
Elucidating the mechanisms by which intermediate filaments contribute to mechanosensing and mechanotransduction is far from trivial given the molecular complexity of the cytoskeletal proteome together with its cell/tissue specificity. Connecting the manifold molecular-scale interactions to the emergent mechano-biological functions at the cellular level is also challenging. To delineate the functions of different intermediate filament proteins across scales, we believe that it is vital to combine studies in cell culture models and model organisms, where cells can be studied in their native context, with studies of “clean” reconstituted systems, where cytoskeletal crosstalk can be studied under controlled conditions to facilitate combinations with predictive models.
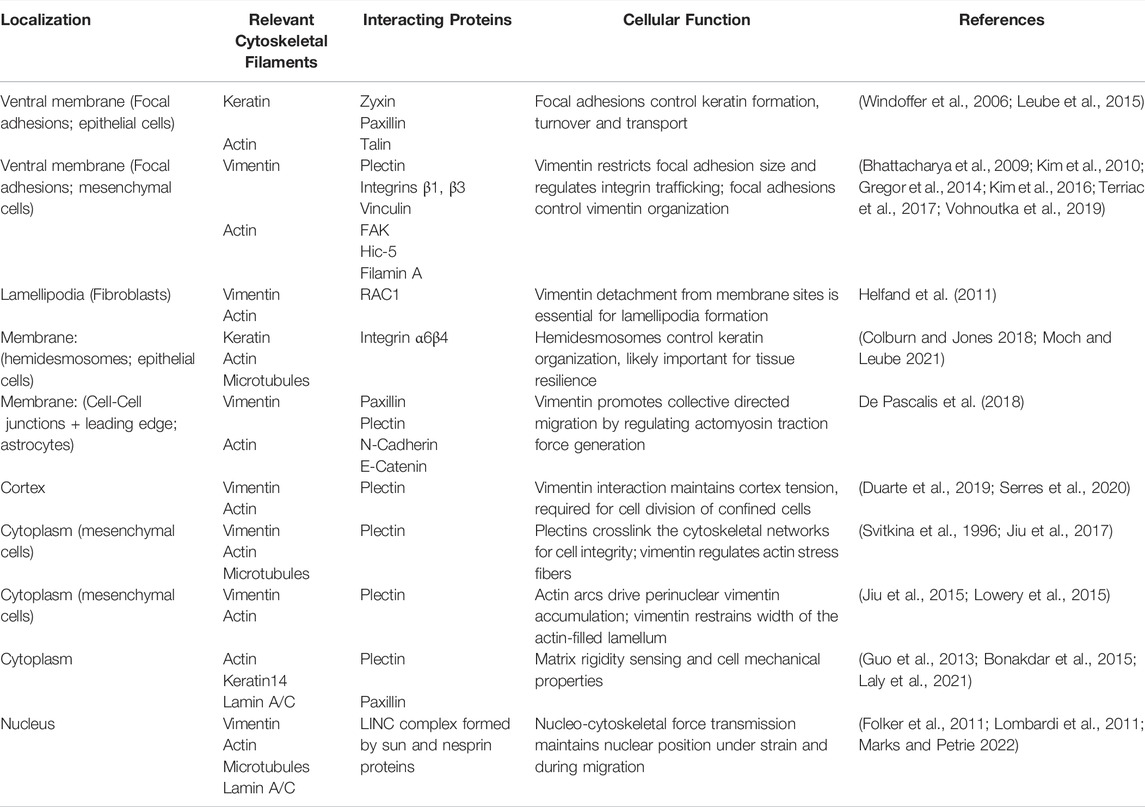
TABLE 1. Selected examples of known cytoskeletal crosstalk interactions relevant for environmental mechanosensing that involve intermediate filaments. Interactions are sorted by subcellular localization, noting the structural and crosslinker proteins known to be involved in the crosstalk, as well as the major cellular function.
Author Contributions
All authors listed have made a substantial, direct, and intellectual contribution to the work and approved it for publication.
Funding
This publication is part of the project “How cytoskeletal teamwork makes cells strong” with project number VI.C.182.004 of the NWO Talent Programme which is financed by the Dutch Research Council (NWO).
Conflict of Interest
The authors declare that the research was conducted in the absence of any commercial or financial relationships that could be construed as a potential conflict of interest.
Publisher’s Note
All claims expressed in this article are solely those of the authors and do not necessarily represent those of their affiliated organizations, or those of the publisher, the editors and the reviewers. Any product that may be evaluated in this article, or claim that may be made by its manufacturer, is not guaranteed or endorsed by the publisher.
Acknowledgments
We thank Pradeep Das for his useful comments on an early version of the manuscipt.
References
Adil, M. S., Narayanan, S. P., and Somanath, P. R. (2021). Cell-cell Junctions: Structure and Regulation in Physiology and Pathology. Tissue Barriers 9 (1), 1848212. doi:10.1080/21688370.2020.1848212
Almeida, F. V., Walko, G., McMillan, J. R., McGrath, J. A., Wiche, G., Barber, A. H., et al. (2015). The Cytolinker Plectin Regulates Nuclear Mechanotransduction in Keratinocytes. J. Cel Sci 128 (24), 4475–4486. doi:10.1242/jcs.173435
Angulo-Urarte, A., van der Wal, T., and Huveneers, S. (2020). Cell-cell Junctions as Sensors and Transducers of Mechanical Forces. Biochim. Biophys. Acta (Bba) - Biomembranes 1862 (9), 183316. doi:10.1016/j.bbamem.2020.183316
Arsenovic, P. T., Ramachandran, I., Bathula, K., Zhu, R., Narang, J. D., Noll, N. A., et al. (2016). Nesprin-2G, a Component of the Nuclear LINC Complex, Is Subject to Myosin-dependent Tension. Biophysical J. 110 (1), 34–43. doi:10.1016/j.bpj.2015.11.014
Aufderhorst-Roberts, A., and Koenderink, G. H. (2019). Stiffening and Inelastic Fluidization in Vimentin Intermediate Filament Networks. Soft Matter 15 (36), 7127–7136. doi:10.1039/c9sm00590k
Bayir, E., and Sendemir, A. (2021). Role of Intermediate Filaments in Blood-Brain Barrier in Health and Disease. Cells 10 (6), 1400. doi:10.3390/cells10061400
Bhattacharya, R., Gonzalez, A. M., Debiase, P. J., Trejo, H. E., Goldman, R. D., Flitney, F. W., et al. (2009). Recruitment of Vimentin to the Cell Surface by Beta3 Integrin and Plectin Mediates Adhesion Strength. J. Cel Sci 122 (Pt 9), 1390–1400. doi:10.1242/jcs.043042
Biedzinski, S., Agsu, G., Vianay, B., Delord, M., Blanchoin, L., Larghero, J., et al. (2020). Microtubules Control Nuclear Shape and Gene Expression during Early Stages of Hematopoietic Differentiation. EMBO J. 39 (23), e103957. doi:10.15252/embj.2019103957
Block, J., Witt, H., Candelli, A., Peterman, E. J., Wuite, G. J., Janshoff, A., et al. (2017). Nonlinear Loading-rate-dependent Force Response of Individual Vimentin Intermediate Filaments to Applied Strain. Phys. Rev. Lett. 118 (4), 048101. doi:10.1103/PhysRevLett.118.048101
Bonakdar, N., Schilling, A., Spörrer, M., Lennert, P., Mainka, A., Winter, L., et al. (2015). Determining the Mechanical Properties of Plectin in Mouse Myoblasts and Keratinocytes. Exp. Cel Res. 331 (2), 331–337. doi:10.1016/j.yexcr.2014.10.001
Bornslaeger, E. A., Corcoran, C. M., Stappenbeck, T. S., and Green, K. J. (1996). Breaking the Connection: Displacement of the Desmosomal Plaque Protein Desmoplakin from Cell-Cell Interfaces Disrupts anchorage of Intermediate Filament Bundles and Alters Intercellular junction Assembly. J. Cel Biol. 134 (4), 985–1001. doi:10.1083/jcb.134.4.985
Bouzid, T., Kim, E., Riehl, B. D., Esfahani, A. M., Rosenbohm, J., Yang, R., et al. (2019). The LINC Complex, Mechanotransduction, and Mesenchymal Stem Cell Function and Fate. J. Biol. Eng. 13 (1), 68. doi:10.1186/s13036-019-0197-9
Brabletz, S., Schuhwerk, H., Brabletz, T., and Stemmler, M. P. (2021). Dynamic EMT: a Multi-Tool for Tumor Progression. EMBO J. 40 (18), e108647. doi:10.15252/embj.2021108647
Broedersz, C. P., and MacKintosh, F. C. (2014). Modeling Semiflexible Polymer Networks. Rev. Mod. Phys. 86 (3), 995–1036. doi:10.1103/revmodphys.86.995
Broussard, J. A., Jaiganesh, A., Zarkoob, H., Conway, D. E., Dunn, A. R., Espinosa, H. D., et al. (2020). Scaling up Single-Cell Mechanics to Multicellular Tissues - the Role of the Intermediate Filament-Desmosome Network. J. Cel Sci 133 (6), 228031. doi:10.1242/jcs.228031
Buxboim, A., Swift, J., Irianto, J., Spinler, K. R., Dingal, P. C. D. P., Athirasala, A., et al. (2014). Matrix Elasticity Regulates Lamin-A,C Phosphorylation and Turnover with Feedback to Actomyosin. Curr. Biol. 24 (16), 1909–1917. doi:10.1016/j.cub.2014.07.001
Chang, L., and Goldman, R. D. (2004). Intermediate Filaments Mediate Cytoskeletal Crosstalk. Nat. Rev. Mol. Cel Biol 5 (8), 601–613. doi:10.1038/nrm1438
Charras, G., and Yap, A. S. (2018). Tensile Forces and Mechanotransduction at Cell-Cell Junctions. Curr. Biol. 28 (8), R445–R457. doi:10.1016/j.cub.2018.02.003
Chaudhuri, O., Cooper-White, J., Janmey, P. A., Mooney, D. J., and Shenoy, V. B. (2020). Effects of Extracellular Matrix Viscoelasticity on Cellular Behaviour. Nature 584 (7822), 535–546. doi:10.1038/s41586-020-2612-2
Çolakoğlu, G., and Brown, A. (2009). Intermediate Filaments Exchange Subunits along Their Length and Elongate by End-To-End Annealing. J. Cel Biol. 185 (5), 769–777. doi:10.1083/jcb.200809166
Colburn, Z. T., and Jones, J. C. R. (2018). Complexes of α6β4 Integrin and Vimentin Act as Signaling Hubs to Regulate Epithelial Cell Migration. J. Cel Sci 131 (14), jcs214593. doi:10.1242/jcs.214593
Colin, A., Singaravelu, P., Théry, M., Blanchoin, L., and Gueroui, Z. (2018). Actin-Network Architecture Regulates Microtubule Dynamics. Curr. Biol. 28 (16), 2647–2656. e2644. doi:10.1016/j.cub.2018.06.028
Costigliola, N., Ding, L., Burckhardt, C. J., Han, S. J., Gutierrez, E., Mota, A., et al. (2017). Vimentin Fibers orient Traction Stress. Proc. Natl. Acad. Sci. U.S.A. 114 (20), 5195–5200. doi:10.1073/pnas.1614610114
Daday, C., Kolšek, K., and Gräter, F. (2017). The Mechano-Sensing Role of the Unique SH3 Insertion in Plakin Domains Revealed by Molecular Dynamics Simulations. Sci. Rep. 7 (1), 11669. doi:10.1038/s41598-017-11017-2
De Pascalis, C., Pérez-González, C., Seetharaman, S., Boëda, B., Vianay, B., Burute, M., et al. (2018). Intermediate Filaments Control Collective Migration by Restricting Traction Forces and Sustaining Cell-Cell Contacts. J. Cel Biol. 217 (9), 3031–3044. doi:10.1083/jcb.201801162
Ding, I., Ostrowska-Podhorodecka, Z., Lee, W., Liu, R. S. C., Carneiro, K., Janmey, P. A., et al. (2020). Cooperative Roles of PAK1 and Filamin A in Regulation of Vimentin Assembly and Cell Extension Formation. Biochim. Biophys. Acta (Bba) - Mol. Cel Res. 1867 (9), 118739. doi:10.1016/j.bbamcr.2020.118739
Dogterom, M., and Koenderink, G. H. (2019). Actin-microtubule Crosstalk in Cell Biology. Nat. Rev. Mol. Cel Biol 20 (1), 38–54. doi:10.1038/s41580-018-0067-1
Dowling, J., Yu, Q. C., and Fuchs, E. (1996). Beta4 Integrin Is Required for Hemidesmosome Formation, Cell Adhesion and Cell Survival. J. Cel Biol. 134 (2), 559–572. doi:10.1083/jcb.134.2.559
Duarte, S., Viedma-Poyatos, Á., Navarro-Carrasco, E., Martínez, A. E., Pajares, M. A., and Pérez-Sala, D. (2019). Vimentin Filaments Interact with the Actin Cortex in Mitosis Allowing normal Cell Division. Nat. Commun. 10 (1), 4200. doi:10.1038/s41467-019-12029-4
Dutour-Provenzano, G., and Etienne-Manneville, S. (2021). Intermediate Filaments. Curr. Biol. 31 (10), R522–r529. doi:10.1016/j.cub.2021.04.011
Engl, W., Arasi, B., Yap, L. L., Thiery, J. P., and Viasnoff, V. (2014). Actin Dynamics Modulate Mechanosensitive Immobilization of E-Cadherin at Adherens Junctions. Nat. Cel Biol 16 (6), 584–591. doi:10.1038/ncb2973
Esue, O., Carson, A. A., Tseng, Y., and Wirtz, D. (2006). A Direct Interaction between Actin and Vimentin Filaments Mediated by the Tail Domain of Vimentin. J. Biol. Chem. 281 (41), 30393–30399. doi:10.1074/jbc.m605452200
Feliksiak, K., Witko, T., Solarz, D., Guzik, M., and Rajfur, Z. (2020). Vimentin Association with Nuclear Grooves in Normal MEF 3T3 Cells. Int. J. Mol. Sci. 21 (20), 7478. doi:10.3390/ijms21207478
Feng, D., DuMontier, C., and Pollak, M. R. (2018). Mechanical Challenges and Cytoskeletal Impairments in Focal Segmental Glomerulosclerosis. Am. J. Physiology-Renal Physiol. 314 (5), F921–f925. doi:10.1152/ajprenal.00641.2017
Fleissner, F., Kumar, S., Klein, N., Wirth, D., Dhiman, R., Schneider, D., et al. (2020). Tension Causes Unfolding of Intracellular Vimentin Intermediate Filaments. Adv. Biosyst. 4 (11), e2000111. doi:10.1002/adbi.202000111
Folker, E. S., Östlund, C., Luxton, G. W. G., Worman, H. J., and Gundersen, G. G. (2011). Lamin A Variants that Cause Striated Muscle Disease Are Defective in Anchoring Transmembrane Actin-Associated Nuclear Lines for Nuclear Movement. Proc. Natl. Acad. Sci. U.S.A. 108 (1), 131–136. doi:10.1073/pnas.1000824108
Forsting, J., Kraxner, J., Witt, H., Janshoff, A., and Köster, S. (2019). Vimentin Intermediate Filaments Undergo Irreversible Conformational Changes during Cyclic Loading. Nano Lett. 19 (10), 7349–7356. doi:10.1021/acs.nanolett.9b02972
Gan, Z., Ding, L., Burckhardt, C. J., Lowery, J., Zaritsky, A., Sitterley, K., et al. (2016). Vimentin Intermediate Filaments Template Microtubule Networks to Enhance Persistence in Cell Polarity and Directed Migration. Cel Syst. 3 (5), 500–501. doi:10.1016/j.cels.2016.11.011
Gardel, M. L., Shin, J. H., MacKintosh, F. C., Mahadevan, L., Matsudaira, P., and Weitz, D. A. (2004). Elastic Behavior of Cross-Linked and Bundled Actin Networks. Science 304 (5675), 1301–1305. doi:10.1126/science.1095087
Gregor, M., Osmanagic‐Myers, S., Burgstaller, G., Wolfram, M., Fischer, I., Walko, G., et al. (2014). Mechanosensing through Focal Adhesion‐anchored Intermediate Filaments. FASEB J. 28 (2), 715–729. doi:10.1096/fj.13-231829
Gudimchuk, N. B., and McIntosh, J. R. (2021). Regulation of Microtubule Dynamics, Mechanics and Function through the Growing Tip. Nat. Rev. Mol. Cel Biol 22 (12), 777–795. doi:10.1038/s41580-021-00399-x
Guo, M., Ehrlicher, A. J., Mahammad, S., Fabich, H., Jensen, M. H., Moore, J. R., et al. (2013). The Role of Vimentin Intermediate Filaments in Cortical and Cytoplasmic Mechanics. Biophysical J. 105 (7), 1562–1568. doi:10.1016/j.bpj.2013.08.037
Gupta, M., Doss, B. L., Kocgozlu, L., Pan, M., Mège, R. M., Callan-Jones, A., et al. (2019). Cell Shape and Substrate Stiffness Drive Actin-Based Cell Polarity. Phys. Rev. E 99 (1-1), 012412. doi:10.1103/PhysRevE.99.012412
Haines, R. L., and Lane, E. B. (2012). Keratins and Disease at a Glance. J. Cel Sci 125 (Pt 17), 3923–3928. doi:10.1242/jcs.099655
Helfand, B. T., Mendez, M. G., Murthy, S. N. P., Shumaker, D. K., Grin, B., Mahammad, S., et al. (2011). Vimentin Organization Modulates the Formation of Lamellipodia. Mol. Biol. Cel 22 (8), 1274–1289. doi:10.1091/mbc.e10-08-0699
Hu, J., Li, Y., Hao, Y., Zheng, T., Gupta, S. K., Parada, G. A., et al. (2019). High Stretchability, Strength, and Toughness of Living Cells Enabled by Hyperelastic Vimentin Intermediate Filaments. Proc. Natl. Acad. Sci. U.S.A. 116 (35), 17175–17180. doi:10.1073/pnas.1903890116
Huber, F., Boire, A., López, M. P., and Koenderink, G. H. (2015). Cytoskeletal Crosstalk: when Three Different Personalities Team up. Curr. Opin. Cel Biol. 32, 39–47. doi:10.1016/j.ceb.2014.10.005
Janota, C. S., Calero-Cuenca, F. J., and Gomes, E. R. (2020). The Role of the Cell Nucleus in Mechanotransduction. Curr. Opin. Cel Biol. 63, 204–211. doi:10.1016/j.ceb.2020.03.001
Jiu, Y., Peränen, J., Schaible, N., Cheng, F., Eriksson, J. E., Krishnan, R., et al. (2017). Vimentin Intermediate Filaments Control Actin Stress Fiber Assembly through GEF-H1 and RhoA. J. Cel Sci 130 (5), 892–902. doi:10.1242/jcs.196881
Jiu, Y., Lehtimäki, J., Tojkander, S., Cheng, F., Jäälinoja, H., Liu, X., et al. (2015). Bidirectional Interplay between Vimentin Intermediate Filaments and Contractile Actin Stress Fibers. Cel Rep. 11 (10), 1511–1518. doi:10.1016/j.celrep.2015.05.008
Kechagia, J. Z., Ivaska, J., and Roca-Cusachs, P. (2019). Integrins as Biomechanical Sensors of the Microenvironment. Nat. Rev. Mol. Cel Biol 20 (8), 457–473. doi:10.1038/s41580-019-0134-2
Kim, H., Nakamura, F., Lee, W., Shifrin, Y., Arora, P., and McCulloch, C. A. (2010). Filamin A Is Required for Vimentin-Mediated Cell Adhesion and Spreading. Am. J. Physiology-Cell Physiol. 298 (2), C221–C236. doi:10.1152/ajpcell.00323.2009
Kim, J., Yang, C., Kim, E. J., Jang, J., Kim, S. J., Kang, S. M., et al. (2016). Vimentin Filaments Regulate Integrin-Ligand Interactions by Binding to the Cytoplasmic Tail of Integrin β3. J. Cel Sci 129 (10), 2030–2042. doi:10.1242/jcs.180315
Kim, Y.-B., Hlavaty, D., Maycock, J., and Lechler, T. (2021). Roles for Ndel1 in Keratin Organization and Desmosome Function. Mol. Biol. Cel 32 (20), ar2. doi:10.1091/mbc.e21-02-0087
Kirby, T. J., and Lammerding, J. (2018). Emerging Views of the Nucleus as a Cellular Mechanosensor. Nat. Cel Biol 20 (4), 373–381. doi:10.1038/s41556-018-0038-y
Ko, C. S., Tserunyan, V., and Martin, A. C. (2019). Microtubules Promote Intercellular Contractile Force Transmission during Tissue Folding. J. Cel Biol. 218 (8), 2726–2742. doi:10.1083/jcb.201902011
Laly, A. C., Sliogeryte, K., Pundel, O. J., Ross, R., Keeling, M. C., Avisetti, D., et al. (2021). The Keratin Network of Intermediate Filaments Regulates Keratinocyte Rigidity Sensing and Nuclear Mechanotransduction. Sci. Adv. 7 (5), 1–12. doi:10.1126/sciadv.abd6187
Lanier, M. H., Kim, T., and Cooper, J. A. (2015). CARMIL2 Is a Novel Molecular Connection between Vimentin and Actin Essential for Cell Migration and Invadopodia Formation. Mol. Biol. Cel 26 (25), 4577–4588. doi:10.1091/mbc.e15-08-0552
Latorre, E., Kale, S., Casares, L., Gómez-González, M., Uroz, M., Valon, L., et al. (2018). Active Superelasticity in Three-Dimensional Epithelia of Controlled Shape. Nature 563 (7730), 203–208. doi:10.1038/s41586-018-0671-4
Leduc, C., and Etienne-Manneville, S. (2017). Regulation of Microtubule-Associated Motors Drives Intermediate Filament Network Polarization. J. Cel Biol 216 (6), 1689–1703. doi:10.1083/jcb.201607045
Lee, G., Han, S.-B., and Kim, D.-H. (2021a). Cell-ECM Contact-Guided Intracellular Polarization Is Mediated via Lamin A/C Dependent Nucleus-Cytoskeletal Connection. Biomaterials 268, 120548. doi:10.1016/j.biomaterials.2020.120548
Lee, G., Leech, G., Rust, M. J., Das, M., McGorty, R. J., Ross, J. L., et al. (2021b). Myosin-driven Actin-Microtubule Networks Exhibit Self-Organized Contractile Dynamics. Sci. Adv. 7 (6), eabe4334. doi:10.1126/sciadv.abe4334
Leube, R. E., Moch, M., and Windoffer, R. (2015). Intermediate Filaments and the Regulation of Focal Adhesion. Curr. Opin. Cel Biol. 32, 13–20. doi:10.1016/j.ceb.2014.09.011
Li, Q.-F., Spinelli, A. M., Wang, R., Anfinogenova, Y., Singer, H. A., and Tang, D. D. (2006). Critical Role of Vimentin Phosphorylation at Ser-56 by P21-Activated Kinase in Vimentin Cytoskeleton Signaling. J. Biol. Chem. 281 (45), 34716–34724. doi:10.1074/jbc.m607715200
Lin, Y.-C., Koenderink, G. H., MacKintosh, F. C., and Weitz, D. A. (2011). Control of Non-linear Elasticity in F-Actin Networks with Microtubules. Soft Matter 7 (3), 902–906. doi:10.1039/c0sm00478b
Lomakin, A. J., Cattin, C. J., Cuvelier, D., Alraies, Z., Molina, M., Nader, G. P. F., et al. (2020). The Nucleus Acts as a Ruler Tailoring Cell Responses to Spatial Constraints. Science 370 (6514), eaba2894. doi:10.1126/science.aba2894
Lombardi, M. L., Jaalouk, D. E., Shanahan, C. M., Burke, B., Roux, K. J., and Lammerding, J. (2011). The Interaction between Nesprins and Sun Proteins at the Nuclear Envelope Is Critical for Force Transmission between the Nucleus and Cytoskeleton. J. Biol. Chem. 286 (30), 26743–26753. doi:10.1074/jbc.m111.233700
López, M. P., Huber, F., Grigoriev, I., Steinmetz, M. O., Akhmanova, A., Koenderink, G. H., et al. (2014). Actin-microtubule Coordination at Growing Microtubule Ends. Nat. Commun. 5, 4778. doi:10.1038/ncomms5778
Lowery, J., Kuczmarski, E. R., Herrmann, H., and Goldman, R. D. (2015). Intermediate Filaments Play a Pivotal Role in Regulating Cell Architecture and Function. J. Biol. Chem. 290 (28), 17145–17153. doi:10.1074/jbc.r115.640359
MacTaggart, B., and Kashina, A. (2021). Posttranslational Modifications of the Cytoskeleton. Cytoskeleton 78 (4), 142–173. doi:10.1002/cm.21679
Maniotis, A. J., Chen, C. S., and Ingber, D. E. (1997). Demonstration of Mechanical Connections between Integrins, Cytoskeletal Filaments, and Nucleoplasm that Stabilize Nuclear Structure. Proc. Natl. Acad. Sci. U.S.A. 94 (3), 849–854. doi:10.1073/pnas.94.3.849
Marks, P. C., and Petrie, R. J. (2022). Push or Pull: How Cytoskeletal Crosstalk Facilitates Nuclear Movement through 3D Environments. Phys. Biol. 19 (2). doi:10.1088/1478-3975/ac45e3
McNally, F. J. (1996). Modulation of Microtubule Dynamics during the Cell Cycle. Curr. Opin. Cel Biol. 8 (1), 23–29. doi:10.1016/s0955-0674(96)80044-5
Mendez, M. G., Restle, D., and Janmey, P. A. (2014). Vimentin Enhances Cell Elastic Behavior and Protects against Compressive Stress. Biophysical J. 107 (2), 314–323. doi:10.1016/j.bpj.2014.04.050
Moch, M., and Leube, R. E. (2021). Hemidesmosome-Related Keratin Filament Bundling and Nucleation. Int. J. Mol. Sci. 22 (4), 2130. doi:10.3390/ijms22042130
Moeton, M., Stassen, O. M. J. A., Sluijs, J. A., van der Meer, V. W. N., Kluivers, L. J., van Hoorn, H., et al. (2016). GFAP Isoforms Control Intermediate Filament Network Dynamics, Cell Morphology, and Focal Adhesions. Cell. Mol. Life Sci. 73 (21), 4101–4120. doi:10.1007/s00018-016-2239-5
Mohammed, F., Trieber, C., Overduin, M., and Chidgey, M. (2020). Molecular Mechanism of Intermediate Filament Recognition by Plakin Proteins. Biochim. Biophys. Acta (Bba) - Mol. Cel Res. 1867 (11), 118801. doi:10.1016/j.bbamcr.2020.118801
Nardone, G., Oliver-De La Cruz, J., Vrbsky, J., Martini, C., Pribyl, J., Skládal, P., et al. (2017). YAP Regulates Cell Mechanics by Controlling Focal Adhesion Assembly. Nat. Commun. 8 (1), 15321. doi:10.1038/ncomms15321
Nöding, B., Herrmann, H., and Köster, S. (2014). Direct Observation of Subunit Exchange along Mature Vimentin Intermediate Filaments. Biophysical J. 107 (12), 2923–2931. doi:10.1016/j.bpj.2014.09.050
Ohi, R., Strothman, C., and Zanic, M. (2021). Impact of the 'tubulin Economy' on the Formation and Function of the Microtubule Cytoskeleton. Curr. Opin. Cel Biol. 68, 81–89. doi:10.1016/j.ceb.2020.09.005
Osmanagic-Myers, S., Rus, S., Wolfram, M., Brunner, D., Goldmann, W. H., Bonakdar, N., et al. (2015). Plectin Reinforces Vascular Integrity by Mediating Crosstalk between the Vimentin and the Actin Networks. J. Cel Sci 128 (22), 4138–4150. doi:10.1242/jcs.172056
Ostrowska-Podhorodecka, Z., Ding, I., Lee, W., Tanic, J., Abbasi, S., Arora, P. D., et al. (2021). Vimentin Tunes Cell Migration on Collagen by Controlling β1 Integrin Activation and Clustering. J. Cel Sci 134 (6), jcs254359. doi:10.1242/jcs.254359
Patteson, A. E., Vahabikashi, A., Pogoda, K., Adam, S. A., Mandal, K., Kittisopikul, M., et al. (2019). Vimentin Protects Cells against Nuclear Rupture and DNA Damage during Migration. J. Cel Biol. 218 (12), 4079–4092. doi:10.1083/jcb.201902046
Pelletier, V., Gal, N., Fournier, P., and Kilfoil, M. L. (2009). Microrheology of Microtubule Solutions and Actin-Microtubule Composite Networks. Phys. Rev. Lett. 102 (18), 188303. doi:10.1103/physrevlett.102.188303
Pimm, M. L., and Henty-Ridilla, J. L. (2021). New Twists in Actin-Microtubule Interactions. Mol. Biol. Cel 32 (3), 211–217. doi:10.1091/mbc.e19-09-0491
Plastino, J., and Blanchoin, L. (2018). Dynamic Stability of the Actin Ecosystem. J. Cel Sci 132 (4), jcs219832. doi:10.1242/jcs.219832
Prechova, M., Adamova, Z., Schweizer, A. L., Maninova, M., Bauer, A., Kah, D., et al. (2022). Plectin-mediated Cytoskeletal Crosstalk Controls Cell Tension and Cohesion in Epithelial Sheets. J. Cel Biol 221 (3), e202105146. doi:10.1083/jcb.202105146
Price, A. J., Cost, A.-L., Ungewiß, H., Waschke, J., Dunn, A. R., and Grashoff, C. (2018). Mechanical Loading of Desmosomes Depends on the Magnitude and Orientation of External Stress. Nat. Commun. 9 (1), 5284. doi:10.1038/s41467-018-07523-0
Rafiq, N. B. M., Nishimura, Y., Plotnikov, S. V., Thiagarajan, V., Zhang, Z., Shi, S., et al. (2019). A Mechano-Signalling Network Linking Microtubules, Myosin IIA Filaments and Integrin-Based Adhesions. Nat. Mater. 18 (6), 638–649. doi:10.1038/s41563-019-0371-y
Ramdas, N. M., and Shivashankar, G. V. (2015). Cytoskeletal Control of Nuclear Morphology and Chromatin Organization. J. Mol. Biol. 427 (3), 695–706. doi:10.1016/j.jmb.2014.09.008
Redmond, C. J., and Coulombe, P. A. (2021). Intermediate Filaments as Effectors of Differentiation. Curr. Opin. Cel Biol. 68, 155–162. doi:10.1016/j.ceb.2020.10.009
Robert, A., Rossow, M. J., Hookway, C., Adam, S. A., and Gelfand, V. I. (2015). Vimentin Filament Precursors Exchange Subunits in an ATP-dependent Manner. Proc. Natl. Acad. Sci. U S A. 112 (27), E3505–E3514. doi:10.1073/pnas.1505303112
Roberts, B. J., Pashaj, A., Johnson, K. R., and Wahl, J. K. (2011). Desmosome Dynamics in Migrating Epithelial Cells Requires the Actin Cytoskeleton. Exp. Cel Res. 317 (20), 2814–2822. doi:10.1016/j.yexcr.2011.09.003
Roux, K. J., Crisp, M. L., Liu, Q., Kim, D., Kozlov, S., Stewart, C. L., et al. (2009). Nesprin 4 Is an Outer Nuclear Membrane Protein that Can Induce Kinesin-Mediated Cell Polarization. Proc. Natl. Acad. Sci. U.S.A. 106 (7), 2194–2199. doi:10.1073/pnas.0808602106
Rübsam, M., Broussard, J. A., Wickström, S. A., Nekrasova, O., Green, K. J., and Niessen, C. M. (2018). Adherens Junctions and Desmosomes Coordinate Mechanics and Signaling to Orchestrate Tissue Morphogenesis and Function: An Evolutionary Perspective. Cold Spring Harb Perspect. Biol. 10 (11), a029207. doi:10.1101/cshperspect.a029207
Sanghvi-Shah, R., and Weber, G. F. (2017). Intermediate Filaments at the Junction of Mechanotransduction, Migration, and Development. Front. Cel Dev. Biol. 5, 1–19. doi:10.3389/fcell.2017.00081
Schaedel, L., Lorenz, C., Schepers, A. V., Klumpp, S., and Köster, S. (2021). Vimentin Intermediate Filaments Stabilize Dynamic Microtubules by Direct Interactions. Nat. Commun. 12 (1), 3799. doi:10.1038/s41467-021-23523-z
Schoumacher, M., Goldman, R. D., Louvard, D., and Vignjevic, D. M. (2010). Actin, Microtubules, and Vimentin Intermediate Filaments Cooperate for Elongation of Invadopodia. J. Cel Biol. 189 (3), 541–556. doi:10.1083/jcb.200909113
Seddiki, R., Narayana, G. H. N. S., Strale, P.-O., Balcioglu, H. E., Peyret, G., Yao, M., et al. (2018). Force-dependent Binding of Vinculin to α-catenin Regulates Cell-Cell Contact Stability and Collective Cell Behavior. Mol. Biol. Cel 29 (4), 380–388. doi:10.1091/mbc.e17-04-0231
Seetharaman, S., Vianay, B., Roca, V., Farrugia, A. J., De Pascalis, C., Boëda, B., et al. (2021). Microtubules Tune Mechanosensitive Cell Responses. Nat. Mater. 21, 366–377. doi:10.1038/s41563-021-01108-x
Serres, M. P., Samwer, M., Truong Quang, B. A., Lavoie, G., Perera, U., Görlich, D., et al. (2020). F-actin Interactome Reveals Vimentin as a Key Regulator of Actin Organization and Cell Mechanics in Mitosis. Dev. Cel 52 (2), 210–222. e217. doi:10.1016/j.devcel.2019.12.011
Sjöqvist, M., Antfolk, D., Suarez-Rodriguez, F., and Sahlgren, C. (2021). From Structural Resilience to Cell Specification - Intermediate Filaments as Regulators of Cell Fate. Faseb J. 35 (1), e21182. doi:10.1096/fj.202001627r
Sun, Z., Guo, S. S., and Fässler, R. (2016). Integrin-mediated Mechanotransduction. J. Cel Biol. 215 (4), 445–456. doi:10.1083/jcb.201609037
Svitkina, T. M., Verkhovsky, A. B., and Borisy, G. G. (1996). Plectin Sidearms Mediate Interaction of Intermediate Filaments with Microtubules and Other Components of the Cytoskeleton. J. Cel Biol. 135 (4), 991–1007. doi:10.1083/jcb.135.4.991
Takeda, M., Sami, M. M., and Wang, Y.-C. (2018). A Homeostatic Apical Microtubule Network Shortens Cells for Epithelial Folding via a Basal Polarity Shift. Nat. Cel Biol 20 (1), 36–45. doi:10.1038/s41556-017-0001-3
Tariq, Z., Zhang, H., Chia-Liu, A., Shen, Y., Gete, Y., Xiong, Z.-M., et al. (2017). Lamin A and Microtubules Collaborate to Maintain Nuclear Morphology. Nucleus 8 (4), 433–446. doi:10.1080/19491034.2017.1320460
Terriac, E., Coceano, G., Mavajian, Z., Hageman, T. A., Christ, A. F., Testa, I., et al. (2017). Vimentin Levels and Serine 71 Phosphorylation in the Control of Cell-Matrix Adhesions, Migration Speed, and Shape of Transformed Human Fibroblasts. Cells 6 (1), 2. doi:10.3390/cells6010002
Theriot, J. A., and Mitchison, T. J. (1991). Actin Microfilament Dynamics in Locomoting Cells. Nature 352 (6331), 126–131. doi:10.1038/352126a0
Valencia, R. G., Walko, G., Janda, L., Novacek, J., Mihailovska, E., Reipert, S., et al. (2013). Intermediate Filament-Associated Cytolinker Plectin 1c Destabilizes Microtubules in Keratinocytes. Mol. Biol. Cel 24 (6), 768–784. doi:10.1091/mbc.e12-06-0488
van Bodegraven, E. J., and Etienne-Manneville, S. (2020). Intermediate Filaments against Actomyosin: the David and Goliath of Cell Migration. Curr. Opin. Cel Biol. 66, 79–88. doi:10.1016/j.ceb.2020.05.006
Venturini, V., Pezzano, F., Català Castro, F., Häkkinen, H.-M., Jiménez-Delgado, S., Colomer-Rosell, M., et al. (2020). The Nucleus Measures Shape Changes for Cellular Proprioception to Control Dynamic Cell Behavior. Science 370 (6514), eaba2644. doi:10.1126/science.aba2644
Vohnoutka, R. B., Gulvady, A. C., Goreczny, G., Alpha, K., Handelman, S. K., Sexton, J. Z., et al. (2019). The Focal Adhesion Scaffold Protein Hic-5 Regulates Vimentin Organization in Fibroblasts. Mol. Biol. Cel 30 (25), 3037–3056. doi:10.1091/mbc.e19-08-0442
Wang, W., Zuidema, A., te Molder, L., Nahidiazar, L., Hoekman, L., Schmidt, T., et al. (2020). Hemidesmosomes Modulate Force Generation via Focal Adhesions. J. Cel Biol 219 (2), e201904137. doi:10.1083/jcb.201904137
Warren, D. T., Zhang, Q., Weissberg, P. L., and Shanahan, C. M. (2005). Nesprins: Intracellular Scaffolds that Maintain Cell Architecture and Coordinate Cell Function? Expert Rev. Mol. Med. 7 (11), 1–15. doi:10.1017/s1462399405009294
Wiche, G., Osmanagic-Myers, S., and Castañón, M. J. (2015). Networking and Anchoring through Plectin: a Key to IF Functionality and Mechanotransduction. Curr. Opin. Cel Biol. 32, 21–29. doi:10.1016/j.ceb.2014.10.002
Wilhelmsen, K., Litjens, S. H. M., Kuikman, I., Tshimbalanga, N., Janssen, H., van den Bout, I., et al. (2005). Nesprin-3, a Novel Outer Nuclear Membrane Protein, Associates with the Cytoskeletal Linker Protein Plectin. J. Cel Biol 171 (5), 799–810. doi:10.1083/jcb.200506083
Windoffer, R., Kölsch, A., Wöll, S., and Leube, R. E. (2006). Focal Adhesions Are Hotspots for Keratin Filament Precursor Formation. J. Cel Biol. 173 (3), 341–348. doi:10.1083/jcb.200511124
Yao, M., Qiu, W., Liu, R., Efremov, A. K., Cong, P., Seddiki, R., et al. (2014). Force-dependent Conformational Switch of α-catenin Controls Vinculin Binding. Nat. Commun. 5 (1), 4525. doi:10.1038/ncomms5525
Yap, A. S., Duszyc, K., and Viasnoff, V. (2018). Mechanosensing and Mechanotransduction at Cell-Cell Junctions. Cold Spring Harb Perspect. Biol. 10 (8), 1–16. doi:10.1101/cshperspect.a028761
Zhen, Y. Y., Libotte, T., Munck, M., Noegel, A. A., and Korenbaum, E. (2002). NUANCE, a Giant Protein Connecting the Nucleus and Actin Cytoskeleton. J. Cel Sci 115 (Pt 15), 3207–3222. doi:10.1242/jcs.115.15.3207
Keywords: mechanobiology, migration, cytoskeleton, vimentin, keratin, actin, microtubules
Citation: Ndiaye A-B, Koenderink GH and Shemesh M (2022) Intermediate Filaments in Cellular Mechanoresponsiveness: Mediating Cytoskeletal Crosstalk From Membrane to Nucleus and Back. Front. Cell Dev. Biol. 10:882037. doi: 10.3389/fcell.2022.882037
Received: 23 February 2022; Accepted: 24 March 2022;
Published: 11 April 2022.
Edited by:
Ming Guo, Massachusetts Institute of Technology, United StatesReviewed by:
Haiqian Yang, Massachusetts Institute of Technology, United StatesSatish Kumar Gupta, Massachusetts Institute of Technology, United States
Copyright © 2022 Ndiaye, Koenderink and Shemesh. This is an open-access article distributed under the terms of the Creative Commons Attribution License (CC BY). The use, distribution or reproduction in other forums is permitted, provided the original author(s) and the copyright owner(s) are credited and that the original publication in this journal is cited, in accordance with accepted academic practice. No use, distribution or reproduction is permitted which does not comply with these terms.
*Correspondence: Michal Shemesh, bS5zaGVtZXNoQHR1ZGVsZnQubmw=; Gijsje H. Koenderink, Zy5oLmtvZW5kZXJpbmtAdHVkZWxmdC5ubA==
†These authors have contributed equally to this work