- 1Immunotherapy Program, Lineberger Comprehensive Cancer Center, University of North Carolina, Chapel Hill, NC, United States
- 2Department of Cell Biology and Physiology, School of Medicine, University of North Carolina, Chapel Hill, NC, United States
- 3Hollings Cancer Center, Charleston, SC, United States
- 4Department of Orthopedics and Physical Medicine, Medical University of South Carolina, Charleston, SC, United States
- 5Amherst College, Amherst, MA, United States
The endoplasmic reticulum (ER) is a large continuous membranous organelle that plays a central role as the hub of protein and lipid synthesis while the mitochondria is the principal location for energy production. T cells are an immune subset exhibiting robust dependence on ER and mitochondrial function based on the need for protein synthesis and secretion and metabolic dexterity associated with foreign antigen recognition and cytotoxic effector response. Intimate connections exist at mitochondrial-ER contact sites (MERCs) that serve as the structural and biochemical platforms for cellular metabolic homeostasis through regulation of fission and fusion as well as glucose, Ca2+, and lipid exchange. Work in the tumor immunotherapy field indicates that the complex interplay of nutrient deprivation and tumor antigen stimulation in the tumor microenvironment places stress on the ER and mitochondria, causing dysfunction in organellar structure and loss of metabolic homeostasis. Here, we assess prior literature that establishes how the structural interface of these two organelles is impacted by the stress of solid tumors along with recent advances in the manipulation of organelle homeostasis at MERCs in T cells. These findings provide strong evidence for increased tumor immunity using unique therapeutic avenues that recharge cellular metabolic homeostasis in T cells.
Primer: Immunotherapy and T Cell Biology
The adaptive immune system plays a substantial role in antitumor response with tumor infiltrating T lymphocytes (TILs) serving as the foundation for targeted cancer immunotherapies as they are responsible for penetrating tumors, recognizing tumor antigen, and directly killing malignant cells. High concentrations of CD8+ TILs are correlated with increased survival in multiple solid tumor types (Nakano et al., 2001; Sato et al., 2005; Sharma et al., 2007; Nguyen et al., 2016; Vihervuori et al., 2019), highlighting the critical role T cells play in cancer control. Due to diminished toxicity and robust response rates, development of immunotherapies utilizing TILs has swept the cancer care field. Initial immunotherapy efforts aimed to expand patient TILs ex vivo with high dose cytokines in order to infuse the cell product back to a donor, leading to increased tumor control in comparison to systemic treatment with IL-2 (Rosenberg et al., 1988). Since these initial studies, efforts in adoptive cellular therapy (ACT) have aimed to improve durability of the cellular product. Chimeric antigen receptor (CAR) T cell therapy improved upon traditional ACT by imbuing patient-derived T cells with specific, engineered antigen receptors that bypass the need for major histocompatiblity complex (MHC) presentation and coactivation while targeting molecules expressed on malignant cells, allowing T cells to more efficiently attack tumor (Mohanty et al., 2019).
Upon encounter with tumor antigen, both infused and endogenous tumor-specific T cells quickly exhibit exhaustion and undergo apoptosis, leading to loss of tumor control (Long et al., 2015; Davoodzadeh Gholami et al., 2017). Exhaustion is a phenotype defined by reduced proliferation and expression of inhibitory or checkpoint molecules marked by compromised cytotoxic cytokine production (Wherry and Kurachi, 2015). Programmed cell death protein 1 (PD1) is a specific checkpoint molecule enriched on antigen-specific T cells (Petrovas et al., 2006) that binds programmed cell death ligand 1 (PDL1) on tumor cells (Keir et al., 2008). Tumor cells expressing PDL1 evade the immune system through PD1-PDL1 ligation that leads to silenced effector cell function (Freeman et al., 2000) of the antigen-enriched TIL pool. Impairing PD1-PDL1 ligation through deletion of PD1 promotes clearance of tumors expressing PDL1 (Chen and Han, 2015) and monoclonal antibodies that target the interaction to overturn PDL1-mediated inhibition of TILs have revolutionized cancer care.
Naïve CD8+ T cells are exposed to tumor antigen via major histocompatibility complex I (MHCI) located on antigen-presenting cells (APCs) and subsequent costimulatory signals induce activation and clonal expansion, resulting in a pool of antigen-specific cells (van Stipdonk et al., 2001). Within the CD8+ TIL pool, subsets of effector and memory cells are capable of development, differing in terms of longevity of antigen response, cytotoxicity, and proliferative ability (Gerlach et al., 2010). The effector subset is highly cytotoxic with a short half-life and low capacity for long-term persistence, defined by interferon-γ (IFN-γ), granzyme B, and perforin secretion. Effector CD8+ T cells have high proliferative capability due in part to autocrine secretion of interleukin-2 (IL-2), but are short-lived upon re-exposure to antigen and subsequent cytolysis, dying through activation-induced cell death (Oshima et al., 1976; Smith et al., 1989; Schultz-Cherry et al., 2001; Joshi et al., 2007). From the initial phase of clonal expansion and contraction, a small pool of T cells survives – undergoing molecular changes to generate immunological memory, providing long-lived antigen recognition and rapid recall upon rechallenge (Opferman et al., 1999; Veiga-Fernandes et al., 2000; Kaech and Ahmed, 2001; Kaech et al., 2002).
A seminal discovery for the immunotherapy field was that central memory cells exhibit robust and durable tumor control, often promoting tumor clearance in comparison to effectors (Klebanoff et al., 2005). Similarly, infusion of memory T cells has proven beneficial for CAR T cell therapy, resulting in increased proliferation in vivo and vigorous tumor control (Gattinoni et al., 2005; Berger et al., 2008; Wang et al., 2012a; Sommermeyer et al., 2016). Effector and memory T cells are notably defined by their divergent metabolic programs. Prior to activation, naïve T cells exhibit low energy demand marked by high oxidative phosphorylation (OXPHOS) and fatty acid oxidation (FAO) (Pearce et al., 2013; Bantug et al., 2018b). Upon antigen exposure, differentiation into the effector state requires ATP for expansion, forcing a shift toward aerobic glycolysis (Wieman et al., 2007). In this state of metabolic activation, glucose and amino acid transporters are upregulated, glutaminolysis pathways engage, and FAO is suppressed by upregulation of transcription factor c-Myc (Frauwirth et al., 2002; Wang et al., 2011a; Sinclair et al., 2013). The memory cell metabolic profile opposes that of effectors in displaying sustained OXPHOS and FAO, with the majority of ATP content derived from mitochondrial metabolism (Pearce et al., 2009). Other studies indicate that a source of glucose for memory T cells is stored glycogen produced by an enhanced gluconeogenic cycle. Glycogen catabolism reinforces memory T cell antioxidant capability through generation of reduced glutathione equivalents that prolong survival while imbuing a cell intrinsic source of glucose (Ma et al., 2018).
The distinct metabolic programs of effector and memory cells are the likely cause for their differential antitumor efficacy. The tumor microenvironment (TME) presents a metabolic assault on CD8+ TILs including pH imbalance dysregulating metabolites, amino acid and glucose deprivation (Gajewski et al., 2013), and hypoxia (Petrova et al., 2018), all of which severely undermine the antitumor capability of effector TILs. For example, amino acid availability is crucial for T cell survival and metabolic fitness. Arginine deficiency in the TME compromises T cell tumor control, and L-arginine supplementation in T cells prompts a metabolic shift toward OXPHOS, resulting in therapeutic T cells (Geiger et al., 2016). Glucose deprivation in the TME similarly impairs gene expression events and cytotoxic functions in effector cells (Qiu et al., 2019), but metabolic remodeling of T cells away from glucose dependence drives memory formation and enhances T cell tumor control (Sukumar and Gattinoni, 2014). Also, a picture is emerging that effector cells are disproportionately affected by hypoxia as low oxygen drives abnormal proliferation, forcing increased glucose dependence (Xu et al., 2016) and enhancing terminal exhaustion in the endogenous CD8+ TIL pool (Yu et al., 2020). In contrast, the presence of memory T cells in the tumor bed is often linked to positive immunotherapy outcomes. For example, in the context of checkpoint therapies, memory markers on the TIL pool predict efficacy of treatment (Vanmeerbeek et al., 2021). In order to design successful novel immunotherapies, it is imperative to gain an understanding of the mechanisms through which memory TILs sustain function in the stress of the TME, resisting death, promoting long-lived tumor control.
Tumor Stress Undermines Endoplasmic Reticulum Homeostasis in T Cells
The exquisite persistence of memory T cells in solid tumors is indicative of an intrinsic resistance to the metabolic stress presented by the TME. Perturbations to metabolic homeostasis such as amino acid deprivation, glucose starvation, and hypoxia promote the induction of the unfolded protein response (UPR) (Pan et al., 2003; Bi et al., 2005; Qiu et al., 2010; Bobak et al., 2016; Zanetti et al., 2022) in the endoplasmic reticulum (ER) of cells. As the hub of protein translation, the ER is equipped with three stress sensors, protein kinase RNA-like endoplasmic reticulum kinase (PERK), inositol-requiring enzyme 1 (IRE1α), and activating transcription factor 6 (ATF6) poised to sense a burden of unfolded/misfolded proteins in the ER lumen (ER stress) or other challenges to ER function such as lipid dysregulation (Hetz, 2012). The stress sensors initiate signaling pathways collectively referred to as the UPR that promote translational and transcriptional remodeling aimed to restore accurate protein synthesis and folding for re-establishment of cell homeostasis. Where ER stress pervades, pro-apoptotic signaling is enacted through the UPR, linking cell fate to duration and extent of ER stress (Cunha et al., 2012). Effector CD8+ TILs in mouse and human tumors experience ER stress mediated by PERK (Hurst et al., 2019a) and IRE1α (Song et al., 2018; Yu et al., 2020) and T cell-specific deletion of stress sensors contributes to improved tumor immunity. We have focused on the stress sensor PERK, demonstrating that memory T cells harbor diminished PERK protein relative to effectors (Hurst et al., 2019a) replete with robust reduction of the chronic PERK axis (Hurst et al., 2019a) characterized by transcription factors Atf4, Ddit3 (C/EBP homologous protein (CHOP)) and their downstream transcriptional target Ero1l (Han and Kaufman, 2017). Similarly, the IRE1α-regulated transcription factor X-box-binding protein 1 (XBP1s) was found to contribute to terminal differentiation of antigen-specific T cells, reducing the formation of memory cells (Kamimura and Bevan, 2008). Together, the studies indicate that stress sensors may be differentially regulated among T cell lineages.
Diminished expression of stress sensors could serve to protect memory TILs from metabolic stress in the TME that engage the ER stress response, undermining CD8+ TIL antitumor function. In the TME, CD8+ TILs encounter glucose deprivation, a stress that immediately engages the UPR in glucose-dependent cells (Wengrod et al., 2015). In this context, PERK arrests global cap-dependent translation through phosphorylation of eIF2 alpha subunit (p-eIF2α) in an effort to restore cellular proteostasis (Harding et al., 1999). Our data indicate that translation attenuation in effector CD8+ TILs directed by robust upregulation of p-eIF2α undermines T cell ability to control tumor growth. In contrast, memory-like T cells express diminished p-eIF2α upon culture in TME conditions, sustaining protein translation and tumor control (Hurst et al., 2020; Riesenberg et al., 2022). In solid tumors, CD8+ TILs encounter lipid stress mediated by exposure to high levels of exogenous tumor-derived cholesterol. Exposure to cholesterol was found to induce terminal exhaustion in CD8+ TILs in a process linked to IRE1α activation. Inhibition of XBP1s diminished T cell exhaustion and augmented tumor control (Ma et al., 2019). In ovarian cancers, ascites fluid from ovarian cancer patients prohibited glucose uptake by T cells, ultimately leading to IRE1α-XBP1 activation that repressed mitochondrial respiration. IRE1α-XBP1 activation was found to prohibit the compensatory mechanism of glutamine uptake needed to maintain mitochondrial respiration in glucose deprived states. The study elegantly demonstrated that XBP1-deficient T cells enriched effector function in mouse models of ovarian cancer, providing evidence for the IRE1α axis as an immunotherapeutic target in cancer (Song et al., 2018). Together the data suggest that T cells endowed with mechanisms to support intrinsic resistance to TME metabolic stress exhibit heightened tumor killing capacity.
Tumor Stress Impairs Mitochondrial Function in T Cells
Mitochondrial regulation has largely been the focus of CD8+ TIL immunometabolic study as the intratumoral subset exhibits severe alterations in mitochondrial morphology (Yu et al., 2020). T cells in solid tumors experience diminished mitochondrial biogenesis marked by reduced mitochondrial mass and hypopolarization. Mitochondrial biogenesis, growth, and replication of mitochondria increases mitochondrial mass and metabolic capacities that are crucial for T cell activation, proliferation, and ATP production (Desdin-Mico et al., 2018). Increased biogenesis through overexpression of PPAR-gamma coactivator 1α (PGC1α) enriches mitochondrial mass and supports T cell tumor control (Scharping et al., 2016). In line with these data, it was discovered that memory T cells have enriched mitochondrial mass relative to effectors, illustrating an intrinsic advantage for stress resistance in the TME (Buck et al., 2016). PD-1 (+) (exhausted) TILs display a reduction and shortening of mitochondrial cristae (Ogando et al., 2019) with disrupted membranes and low mitochondrial activity per unit of mitochondrial mass. Hypoxia was identified as a TME condition that represses PGC1α expression in PD-1 TILs as hypoxic exposure coupled with persistent antigen stimulation in the TME drove mitochondrial dysfunction marked by excessive mitochondrial reactive oxygen species (ROS), exacerbating the terminal exhaustion state (Yu et al., 2020). Thus, stimulation of mitochondria through ROS signaling or PGC1a activators augmented the efficacy of T cell response to a-PDL1 therapy (Chamoto et al., 2017). Together, these data illustrate gross dysregulation of mitochondrial biology in CD8+ T cells in tumors.
Endoplasmic Reticulum-Mitochondria Interface Under Pressure
Potential Impacts of Endoplasmic Reticulum-Mitochondrial Interaction in T Cells
The role of the ER in the paradigm of mitochondrial dysfunction in TILs has not been explored. However, studies are emerging that associate CD8+ TIL dysfunction with distress between the two organelles. RNA-seq indicated that exhausted CD8+ TILs experience heightened ER stress relative to other CD8+ TIL subsets, indicative of a profound misfolded protein burden in this T cell pool. Along with exacerbated ER stress, this TIL pool exhibits elevated alterations in mitochondrial morphology characterized by ineffective elimination of damaged mitochondria (mitophagy), abnormal mitochondrial membrane structure, and fewer, shorter cristae compared to autologous splenic T cells (Yu et al., 2020). The data corroborate our previous finding that severe (chronic) ER stress mediated by the PERK axis disproportionately affects exhausted CD8+ TILs, replete with mitochondria dysfunction marked by excessive ROS generation (Hurst et al., 2019a). Together, a picture is emerging that links ER stress to impaired TIL mitochondrial homeostasis, bringing into question the interaction between the two organelles in tumor immunity.
Stress-Mediated Fission and Fusion at Mitochondrial-Endoplasmic Reticulum Contact Sites
ER stress is associated with mitochondrial dysfunction in multiple disease pathologies (Giacomello and Pellegrini, 2016) and physical interactions between the ER and mitochondria often preclude organellar dysfunction. The ER and mitochondria are physically tethered at close distances of ∼10–25 nM (Giacomello and Pellegrini, 2016). These mitochondrial-ER contact sites (MERCs) are unique structures responsible for maintaining physical proximity and biomolecular signals between the two organelles. To this point, over 100 proteins have been identified at these sites as mediators of architecture or biochemical exchange, regulating fission, fusion, calcium (Ca2+), glucose, and lipid metabolism. MERCs may comprise up to 20% of the outer mitochondrial membrane (OMM) (Rizzuto et al., 1998). Though MERCs have not been studied in depth in CD8+TILs, transmission electron microscopy has been used to demonstrate that memory T cells display enhanced MERCs relative to effectors (Buck et al., 2016) (Figures 1A,B). These findings align with a study conducted on effector memory T cells where, upon activation, effector memory cells displayed increased MERCs compared to naïve counterparts, relying on contact sites to modulate mitochondrial function and rapid generation of IFN-γ (Bantug et al., 2018a).
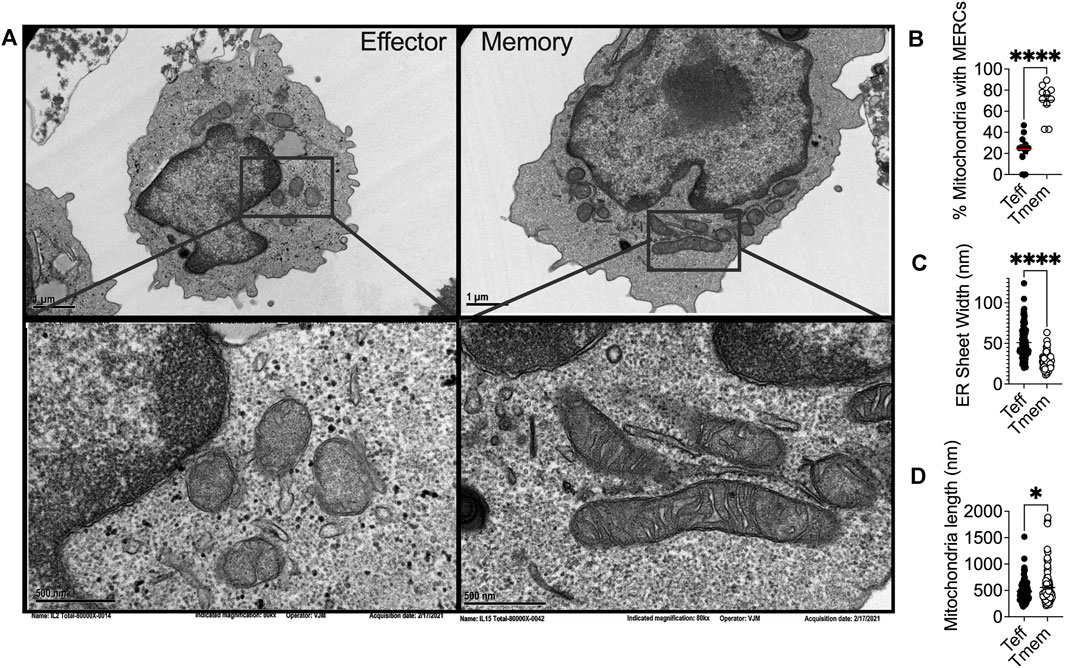
FIGURE 1. (A) Representative transmission electron microscopy of IL2 and IL15-derived effector and memory-like T cells, respectively, illustrating differential ER and mitochondrial biology in T cell subsets in alignment with previous reports that elucidated the phenomenon. Ex vivo expanded OT-1 T cells activated and expanded for 3 days with OVA peptide were conditioned for four more days in IL2 (200U) or IL15 (50 ng/ml) and (B) percentage of mitochondria possessing MERCs (C) ER luminal width and (D) mitochondrial length were quantified.
Endoplasmic Reticulum-Mediated Mitochondrial Fission
MERCs have been identified to play a role in regulating the dynamics of both the ER and mitochondria (Friedman et al., 2010; Friedman et al., 2011). Mitochondria undergo frequent fusion and fission to maintain organellar quality and cell homeostasis (Westermann, 2010) and the process is perceived to be regulated at MERCs. Mitochondrial fission is driven by the dynamin-related GTPase dynamin related protein 1 (Drp1), the fission protein which is recruited to the mitochondrial OMM and forms homo-oligomers around the mitochondria, constricting until one mitochondrion is severed in two (Macdonald et al., 2016). Live-cell imaging demonstrated that ER tubules mediate constriction of mitochondrial membranes, inducing fission in yeast and mammalian cells (Friedman et al., 2011). ER tubules physically wrap around mitochondria, forming MERCs, as the ER protein inverted formin 2 (INF2) recruits Drp1 from the cytosol to initiate fission. These data implicate MERCs and ER morphology in regulation of fission (Korobova et al., 2013) (Lee et al., 2016). Moreover, INF2 impacts ER-localized actin polymerization, enhancing MERCs formation, inter-organelle Ca2+ trafficking, and constriction of the inner mitochondrial membrane (IMM) (Chakrabarti et al., 2018). If MERCs are disrupted by ER tubule reorganization, mitochondrial fission and apoptotic signaling are similarly disturbed (Yedida et al., 2019). This regulation at MERCs may be reciprocal in nature, with disruption of Drp1 leading to disturbed ER morphology as well (Pitts et al., 1999; Westermann, 2011), as Drp1 overexpression can lead to both structural ER changes and mitochondrial fragmentation (Wikstrom et al., 2013; Kobayashi et al., 2020).
In yeast treated with ER stressors, both the ER and the mitochondria displayed expanded membranes prompting ER-mitochondria encounter structures and fission (Kojima et al., 2019). Upon induction of ER stress, the fission protein Drp1 is increased, resulting in mitochondrial fission and fragmentation (Li et al., 2015). ER stress increases MERCs formation, allowing for excessive Ca2+ transfer from the ER to mitochondria (Wanget al., 2021b), leading to mitochondrial fission and impaired bioenergetics (Kaddour-Djebbar et al., 2010; Glancy and Balaban, 2012). These data indicate the interconnectivity between chronic ER stress and mitochondrial fragmentation. ER stress can also induce apoptosis through the mitochondria, specifically through remodeling of cristae junctions for cytochrome c release (Zhang et al., 2008; Pinkaew et al., 2017). In response to ROS-induced ER stress, PERK stabilizes MERCs that relay ROS signals between the organelles, and sustains CHOP that induces mitochondrial apoptosis (Verfaillie et al., 2012). The data illuminate chronic ER stress as a driver of mitochondrial morphological changes, fission dynamics, and apoptotic signaling and could explain our previous finding that effector T cells with fragmented mitochondria express dramatically elevated PERK, failing to possess the resiliency and antitumor capabilities of their memory counterparts, expressing fused mitochondria (Figures 1A,D) (Buck et al., 2016) and diminished PERK (Hurst et al., 2019a).
Endoplasmic Reticulum-Associated Mitochondrial Fusion
Differential mitochondrial biogenesis patterns impact T cell differentiation and immunotherapeutic efficacy. The major fusion proteins of mammalian mitochondria are the OMM GTPases mitofusin 1 and 2 (Mfn1/2) and the IMM GTPase optic atrophy 1 (OPA1) (Gao and Hu, 2021). These proteins serve to facilitate the two sequential steps of mitochondrial fusion at the OMM and IMM, respectfully. Mfn2 has been associated with MERCs formation and stabilization. Mfn2 deletion leads to decreased association between the ER and mitochondria, altering organellar morphology, reducing mitochondrial Ca2+ uptake (de Brito and Scorrano, 2008; Rizzuto et al., 2012). Similar to what has been observed in fission dynamics, the position at which mitochondria fusion occurs is marked by ER tubules (Abrisch et al., 2020), once again indicating that ER-mitochondrial interface acts on mitochondrial fission/fusion dynamics. Of note, memory T cells display fused mitochondrial networks (Figures 1A,D) with greater expression of Mfn2 and OPA1 (Buck et al., 2016). In contrast, effector cells require Drp1 for differentiation, with Drp1−/− T cells displaying memory-like phenotype (Simula et al., 2018). Treatment of effector cells with fusion promoter M1 or Drp-1 inhibitor Mdivi-1 enforced fusion, leading to memory T cell development replete with heightened tumor immunity to murine lymphoma (Buck et al., 2016). Increased fusion is associated with organized cristae morphology (Varanita et al., 2015), enhanced oxidative phosphorylation (Rossignol et al., 2004; Simula et al., 2017), and increased mitochondrial mass (Buck et al., 2016), factors that contribute to enhanced persistence and tumor rejection by CD8+ TILs.
In contrast to chronic stress, acute stress in the ER followed by resolution of the unfolded protein burden promotes fusion of mitochondrial membranes. The PERK arm of the UPR is multifaceted in that it attenuates translation to resolve protein stress (Walter and Ron, 2011) and acutely serves to protect mitochondria homeostasis (Rainbolt et al., 2014). PERK localization to MERCs is required for maintenance of MERCs structure, with PERK−/− mouse embryonic fibroblasts (MEFs) displaying decreased association between ER and mitochondrial proteins, fragmented ER morphology (Verfaillie et al., 2012), and fissed mitochondria (Lebeau et al., 2018). These data indicate that PERK plays a role in modulating mitochondrial fusion and morphology. PERK is critical to production of phosphatidic acid (PA) (Bobrovnikova-Marjon et al., 2012), a lipid responsible for facilitating fusion (Baba et al., 2014), potentially linking resolution of ER stress to mitochondria fusion dynamics through PERK. A direct correlation between PERK activation and mitochondrial dynamics was shown, with PERK-driven phosphorylation of eIF2α being required for mitochondrial hyperfusion in MEFs treated acutely (6–12 h) with ER stress enhancer Thapsigargin (Tg). However, longer treatments with Tg led to increased mitochondrial fragmentation, supporting the concept that chronic ER stress drives fission (Lebeau et al., 2018). Therefore, it is possible that among T cells, memory cells possess the ability to cope with and resolve ER stress, suggesting a cell intrinsic stress resistance that could explain sustained function in the TME.
Stress-Induced Disruption of Endoplasmic Reticulum Dynamics
Disruption of ER structural homeostasis has been shown to directly impact mitochondrial morphology. The peripheral ER is a dynamic structure that interacts with and regulates numerous organelles including mitochondria and must be continuously remodeled to accommodate various demands such as protein production and folding. T cells are highly secretory and the rough ER acts as the primary site where secreted proteins are translated. Of similar importance in T cells, the smooth ER is the primary site of lipid synthesis (Higgins, 1974) and is the principal storage site for cellular Ca2+ ions (Schwarz and Blower, 2016). As a result of the diverse functions that the rough and smooth ER carry out, the ER is comprised of different sub-structures of sheets and tubules, respectively, that branch out to form a complex network consisting of the luminal compartment (Westrate et al., 2015). The distinct domains remain dynamic and undergo rapid changes (West et al., 2011) with the dense network of ER tubules extending to far edges of the cell enabling the ER to interact with other organelles including mitochondria.
In response to the stress of a protein burden in the ER lumen, the ER expands through proliferation of ER sheets to create increased volume for protein folding demand (Bernales et al., 2006). Cytoskeleton-linking membrane protein (Climp63) is the luminal protein that orchestrates ER expansion. Acting as a spacing protein, Climp63 determines the luminal width of ER sheets with depletion and overexpression resulting in decreased and increased luminal width, respectively (Voeltz et al., 2006; Shibata et al., 2010). In response to ER stress, Climp63 expression is upregulated coincident with UPR signaling (Schuck et al., 2009). Overexpression of Climp63 leads to impaired mitochondrial replication and reduced segregation of mtDNA during fission (Lewis et al., 2016). The impact of Climp63 on the mitochondria solidifies a connection between ER morphological proteins and mitochondrial dynamics and function. ER morphology has not been studied in T cell subsets, nor in the context of immunotherapy. Using transmission electron microscopy, we found that the ER of effector T cells displays expansion in comparison to that of memory cells, quantified by ER luminal width (Figures 1A,C). The data identify for the first time the possibility of differential ER morphology between T cell lineages and raise intrigue surrounding the potential impact of ER structure as a feature of T cell tumor immunity.
Biochemical Exchange at Mitochondrial-Endoplasmic Reticulum Contact Sites: Metabolic Hubs
Ca2+, A Matter of Life and Death
MERCs not only serve to shape structural outcomes between the ER and mitochondria, but are conduits for biochemical exchange, serving to facilitate cellular metabolic programming. Ca2+ is a key second messenger regulating apoptosis, exocytosis, and gene transcription (Clapham, 2007), whose exchange is centralized at MERCs. Ca2+ metabolism is paramount in T cells given that T cell receptor (TCR) engagement by antigen results in robust release of ER Ca2+ to tune the process of T cell activation and lineage fate. When the MHC-antigen complex interacts with the TCR, phospholipase C-gamma is phosphorylated leading to cleavage of phosphatidyl inositol, yielding diacylglycerol (DAG) and inositol 1,4,5-triphosphate (IP3) (Putney, 1986). Upon cleavage, IP3 binds IP3Rs, causing Ca2+ release from the ER lumen to cytosol, inducing nuclear factor of activated T-cells (NFAT) transcription (Fenninger and Jefferies, 2019; Hunt et al., 2020; Xiang et al., 2020), followed by IL2 production, T cell proliferation, and disease control (Klein-Hessling et al., 2017). The ER serves as the largest intracellular Ca2+ ion store, relying on Ca2+ signaling for normal protein folding and other homeostatic processes (Groenendyk et al., 2021). The ER Ca2+ gradient is dependent on import of Ca2+ from the cytosol into the ER lumen via sarcoendoplasmic reticulum Ca2+ ATPase pump (SERCA). In T cells, prolonged inhibition of SERCA through overexpression of the enzyme phosphoenolpyruvate carboxykinase 1 (Pck1) resulted in increased cytosolic Ca2+ that extended NFAT signaling, promoting effector function in tumors (Ho et al., 2015).
Specific microdomains known as “hotspots” have been identified where Ca2+ is concentrated around IP3R and mitochondria in close contact with the ER (Rizzuto et al., 1993), introducing the concept that transmission of Ca2+ between the ER and mitochondria occurs at MERCs. It was later demonstrated that interactions between the ER and mitochondria are regulated by the formation of a molecular bridge comprised of IP3R on the ER membrane, voltage dependent anion channels (VDACs) embedded in the OMM, and the chaperone 75-kDa Glucose Regulated Protein (GRP75), which facilitates the physical interaction between the two channels (Marchi et al., 2018). Upon IP3 binding, Ca2+ is released from IP3R at the ER and travels through the OMM into the mitochondrial intermembrane space by way of VDAC Ca2+ transfer. This interaction and subsequent Ca2+ transfer between IP3R and VDAC is mediated by appropriate MERCs formation (Honrath et al., 2017; Wang et al., 2021c). As Ca2+ enters the mitochondria and accumulates in the intermembrane space it passes through the mitochondrial Ca2+ uniporter (MCU) on the IMM to enter the matrix (Raffaello et al., 2016). There, Ca2+ activates dehydrogenases crucial for NADH production via the tricarboxylic acid (TCA) cycle (Figure 2), spurring mitochondrial respiration and ATP production (Vinnakota et al., 2016).
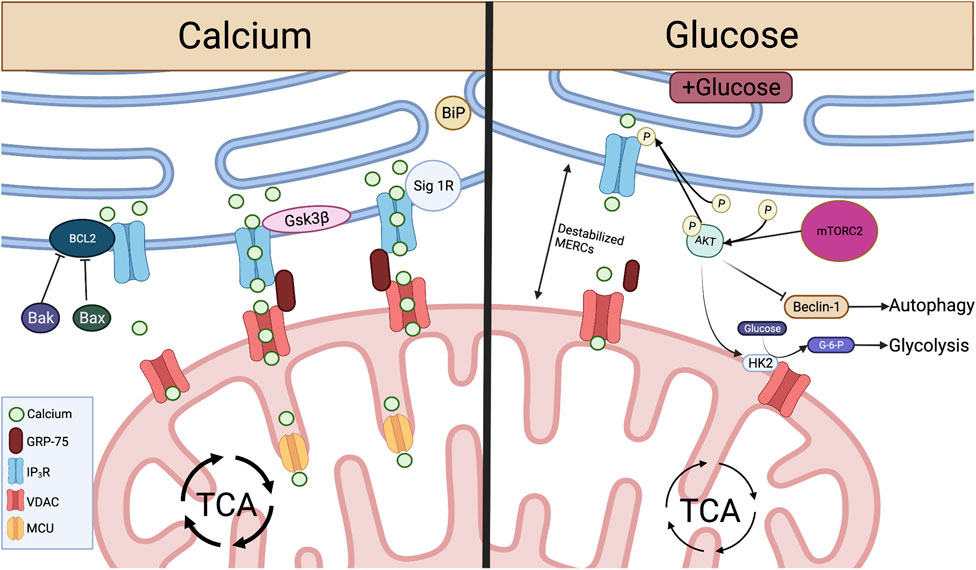
FIGURE 2. Crucial metabolite flow and nutrient sensing occurs at MERCs and is mediated by proteins acting on the IP3R-GRP75-VDAC complex. Left: At MERCs, Ca2+ is transferred from the ER lumen, through the IP3R-GRP75-VDAC complex, into the mitochondrial intermembrane space and through MCU to the mitochondrial matrix. This Ca2+ flux is promoted by Sig-1R dissociation from BiP and the protein Gsk3β interaction with IP3R. Ca2+ flux and IP3R-GRP75-VDAC association is inhibited by Bcl2, and this interaction is inhibited by pro-apoptotic proteins Bax and Bak. Right: When glucose is available, mTORC2 phosphorylation of AKT leads to reduced ER Ca2+ release and disruption of MERCs through inhibition of IP3R. pAKT also inhibits Beclin-1, a promoter of autophagy at MERCs. pAKT activation of HK2 leads to conversion of glucose to G-6-P, spurring glycolysis. Created with BioRender.com.
MERCs-facilitated Ca2+ flux from ER to mitochondria can be enhanced through direct protein-protein interactions with IP3R. Glycogen synthase kinase-3β (Gsk3β) is responsible for direct phosphorylation of IP3R, leading to increased Ca2+ transfer through the IP3R/GRP75/VDAC complex (Figure 2). However, over enrichment of this interaction drives cell susceptibility to apoptotic Ca2+ signaling (Gomez et al., 2016). In T cells targeting tumor, overactivity of Gsk3β has been linked to poor tumor control. Initial studies demonstrated that Gsk3β inhibition arrested T cell products prepared for adoptive cell therapy of melanomas in a stem-like state, enabling prolonged self-renewal and impairing differentiation (Gattinoni et al., 2009). The concept translated to the CAR T cell therapy field as Gsk3β inhibition diminished exhaustion hallmarks in CAR T cells transferred to glioblastomas, resulting in prolonged tumor control (Sengupta et al., 2018). Both studies identified a memory T cell phenotype in the T cell populations characterized by Bcl2high expression, identifying a Ca2+ buffering effect by the antiapoptotic protein.
In contrast to enhanced Ca2+ flow from the ER to the mitochondria, the Bcl2 protein protects against mitochondria-mediated cell death by stabilizing Ca2+ flow through interactions with IP3R. Bcl2 is responsible for inhibition of excessive IP3R-mediated Ca2+ release, protecting cells from apoptotic Ca2+ signaling (Rong et al., 2008). Bcl2 also serves to protect cells from excessive ER Ca2+ buildup through enhancing IP3R sensitivity to low levels of IP3, leading to appropriate Ca2+ flow and increased mitochondrial bioenergetics (Eckenrode et al., 2010; White et al., 2005). Conversely, pro-apoptotic proteins Bax and Bak inhibit Bcl2-mediated IP3R stabilization, leading to reduced IP3R sensitivity and buildup of ER Ca2+, enabling robust Ca2+ release to the mitochondria, initiating programmed cell death (Oakes et al., 2005) (Figure 2).
The Bcl2 family of proteins are well-known for control of T cell antitumor efficacy. During development, Bcl2 expression is low in immature thymocytes. As T cells mature Bcl2 is increased as it is required for thymocytes to survive, protecting cells from programmed cell death (Shanmuganad et al., 2022). Across different T cell subsets, expression of Bcl2 family members differs, with naïve and memory CD8+ T cells expressing elevated Bcl2 in comparison to effectors (Grayson et al., 2000). Moreover, memory differentiation relies on Bcl2 expression as formation is impaired upon Bcl2 inhibition (Kurtulus et al., 2011). The data suggest that Bcl2 is a factor in long-term maintenance of immunity. Overexpression of Bcl2 in tumor-specific T cells promoted cell survival and therapeutic efficacy of adoptively transferred T cells infiltrating mouse melanomas (Charo et al., 2005; Kalbasi et al., 2010). Other studies of constitutive expression of Bcl2 in CAR-T cells resulted in prolonged T cell survival and tumor control in murine lymphomas (Wang et al., 2021a). Together, these data illustrate the importance of mediated Ca2+ flux by Bcl2 in T cell differentiation, longevity, and tumor control.
In addition to protein folding functions, ER chaperone proteins also play a role in control of Ca2+ flux and cell survival at MERCs. In a homeostatic state, the ER chaperone protein Sigma-1 Receptor (Sig-1R) localizes to MERCs and complexes with another chaperone, BiP (Grp78). ER Ca2+ depletion results in Sig-1R dissociation from BiP and subsequent interaction with IP3Rs, enabling prolonged influx of Ca2+ to the mitochondria. Therefore, overexpression of Sig-1R protects from cellular apoptosis, while depletion enhances cell death (Hayashi and Su, 2007) (Figure 2). These studies illustrate the role MERCs play as Ca2+ signaling hubs. Though knockout of IP3Rs leads to diminished ATP and loss of ER-mitochondrial contact maintenance (Cardenas et al., 2010; Bartok et al., 2019), we found that inhibition of IP3R in antigen-stimulated T cells promotes viability and memory formation. The resultant T cell pool showed enriched mitochondrial ATP reserves and robust capability to control murine melanomas (Thaxton et al., 2017), indicating that modulation of Ca2+ flux through IP3R tuning is intimately tied to T cell metabolism and tumor control.
Glucose, Competing for Control
Ca2+ homeostasis is tightly connected to programming of glucose metabolism. Glucose is an essential nutrient for all mammalian cells, serving as a precursor for fatty acids, amino acids, and nucleotides (Navale and Paranjape, 2016). To sustain the energy demands of effector cells, CD8+ T cells shift metabolism from oxidative phosphorylation (OXPHOS) to aerobic glycolysis (Frauwirth et al., 2002; Jones and Thompson, 2007) and this is marked by an increase in glycolytic enzymes, lactate production, and glucose consumption (MacIver et al., 2013; Buck et al., 2015). Glucose is taken up directly by glucose transporters (GLUTS) that reside in the plasma membrane (Navale and Paranjape, 2016) and is phosphorylated by hexokinase 2 (HK2) to form glucose-6-phosphate (G-6-P) which is further broken down via glycolysis to pyruvate (Hay, 2016). In the cytosol, pyruvate can be converted to lactate or imported to the mitochondria through VDAC to fuel the Krebs cycle and electron transport chain. Mammalian target of rapamycin complex 1 (mTORC1) is the energy sensing complex that stimulates glycolysis, supporting protein synthesis and cell growth (Mao and Zhang, 2018). In T cells, deletion of negative mTORC1 regulator tuberous sclerosis complex 2 (TSC2) yielded highly differentiated hyper proliferative effector T cells with enhanced glycolytic dependence. T cells with TSC2 deletion resulted in a pool of robust antitumor T cells, but memory formation was impaired in vivo (Pollizzi et al., 2015). This study illustrated the importance of mTORC1 to produce the initial wave of effector response for tumor immunity, and the significance of mTORC1 to hinder memory cell metabolism and development of cellular longevity.
In response to glucose limitation, cells induce autophagy, a process by which cell components are catabolized to sustain cell function and viability (Yang and Klionsky, 2010; Dall'Armi et al., 2013). Autophagy has been shown to be critical for memory T cell formation (Xu et al., 2014). Active mTORC1 and glycolysis limit autophagy through disruption of a phosphorylation event carried out by energy deprivation sensor AMP-activated protein kinase (AMPK) (Kim et al., 2011), providing a mechanism to impede cellular degradation in nutrient replete settings. Upon induction of autophagy, a vesicular sac is formed enclosing cytoplasmic contents, resulting in a double membranous autophagosome that fuses with a lysosome to degrade contents (Lamb et al., 2013). Amino acids degraded from the process can be used to sustain translation and energy generation (Onodera and Ohsumi, 2005; Guo et al., 2016). A set of autophagy-related (Atg) proteins carries out the autophagic process and the protein light chain 3 (LC3) is widely observed on autophagosome membranes, thus this often serves as a ubiquitous marker for the process (Kabeya et al., 2000; Mizushima et al., 2004; Maruyama et al., 2014; Schaaf et al., 2016). During viral infection, autophagy was rapidly induced at the peak of effector T cell response, with virus-specific T cells exhibiting robust upregulation of LC3 proteins. T cell-specific gene deletion of the Atg7 or Atg5 proteins resulted in defective autophagy at the peak of effector response and impaired memory cell survival leading to faulty virus control (Xu et al., 2014). These data suggest that T cells with excessive mTORC1 activity and inability to form memory responses are likely defective in the initiation of autophagy due to abundant glycolysis.
MERCs play a dominant role in glucose sensing for cells to reprogram metabolism during fluctuations in nutrient availability (Tubbs et al., 2014; Theurey et al., 2016). MERCs were first implicated in nutrient-dependent autophagy by Hailey et al. (2010), who found that starvation-induced autophagy was inhibited after disruption of MERCs by Mfn2 deletion. Accumulation of autophagic proteins Atg14L and Atg5 and subsequent autophagosome formation occurred at MERCs in a starvation-dependent manner (Hamasaki et al., 2013). The process was found to be controlled by mammalian target of rapamycin complex 2 (mTORC2), a protein implicated in cell growth and metabolic function. Specifically, mTORC2-driven activation of the protein kinase AKT inhibits autophagy-promoting protein Beclin-1 (Wang et al., 2012b), resulting in suppression of autophagy. In nutrient deprivation, activation of AKT is inhibited (Betz et al., 2013), leading to induction of autophagy at MERCs through recruitment of Beclin-1 (Gelmetti et al., 2017).
MERCs were further linked to nutrient sensing when it was shown that hepatocytes of fasted mice display increased MERC lengths relative to fed counterparts (Sood et al., 2014). In contrast, hepatocytes from fed mice showed increased mitochondrial fragmentation as a result of decreased MERCs association through disrupted IP3R/VDAC1 interaction (Theurey et al., 2016). With increased pAKT and mTORC2 found at MERCs of fed mice (Betz et al., 2013; Tubbs et al., 2014), it has been hypothesized that this MERC disruption is a result of mTORC2 activation of AKT, resulting in inhibition of IP3R-VDAC interactions at MERCs (Szado et al., 2008; Betz et al., 2013). Glucose serves as the major determinant of these nutrient-dependent changes, with exposure to glucose disrupting MERC interaction both in vitro and in vivo, leading to increased mitochondrial fission and impaired respiration (Theurey et al., 2016). This can be attributed to MTORC2-AKT signaling, which promotes glucose uptake through activation of glucose transporters (Beg et al., 2017) and stimulates HK2-VDAC interactions, enabling conversion of glucose to G-6-P and therefore glycolysis (Stiles, 2009; Betz et al., 2013) (Figure 2). This AKT-dependent glycolytic switch is particularly important in memory T cells and has been shown to promote rapid effector function upon restimulation with antigen.
Two critical studies have offered insight to the necessity of autophagy in antitumor T cells. The studies draw divergent conclusions, highlighting the need for further study. DeVorkin et al. (2019) showed that T cell autophagy was disadvantageous to tumor control as mice deficient in autophagy genes Atg5, Atg14, and Atg16L1 displayed rejection of prostate, breast, and colorectal tumors. The effect was found to be immune-mediated based on diminished tumor growth in wild type mice reconstituted with Atg5−/− bone marrow, and adoptively transferred tumor antigen-specific Atg5−/− T cells demonstrated impaired antitumor immunity. Mechanistically, autophagy was responsible for metabolic dependence on OXPHOS; thus, limiting autophagy increased glycolysis and supported effector function—promoting tumor control (DeVorkin et al., 2019). This contrasts with a study conducted by Vodnala et al. (2019) T cell exposure to extracellular [K+], a cation enriched in the TME, limited T cell nutrient uptake and induced autophagy, diminishing acetylation of effector genes, promoting proliferation and self-renewal, indicative of stem-like T cells. K+-treated T cells were autophagy enriched and represented a pool of T cells with potent cytotoxicity and persistence in the TME that produced tumor clearance (Vodnala et al., 2019). However, the direct contribution of T cell-specific autophagy as a necessity or hindrance for rejection of solid tumors was not addressed. These studies illustrate the need to investigate the role of autophagy in CD8+ TIL metabolism and phenotypic modulation, as autophagy could serve as an avenue to enhance T cell-based immunotherapies.
\The balance between glucose dependence and autophagy that facilitates memory T cell development is an intriguing concept for understanding how to program effective T cell tumor immunity. On one hand hyperglycolytic T cells are powerful effectors for tumor control. On the other hand, T cells experience glucose deprivation in the TME that undermines their ability to persist as effectors (Ho et al., 2015). Interestingly, chronic low glucose conditioning (Klein Geltink et al., 2020) or long-term inhibition of glycolysis (Sukumar and Gattinoni, 2014) reprogrammed T cells toward a memory phenotype, likely as a result of autophagy induction. These data are intriguing in light of our recent discovery that acute glucose deprivation in T cells in the TME induces acute translation arrest through activation of the ER stress response (PERK-p-eIF2α), undermining sustained tumor control. We found that metabolic reprogramming of cells toward a memory lineage alleviated the stress response, abrogating p-eIF2α expression and restoring translation and tumor control to T cells in nutrient deprived environments (Riesenberg et al., 2022). The data raise the possibility that memory cells could use selective autophagy to eliminate stressed ER as a protective measure.
Endoplasmic Reticulum-Associated Signaling and Structures in Autophagy
The ER is a crucial organelle in the autophagic process. ER stress induces autophagy as treatment of cells with nutrient deprivation or ER stressor Tg forced expression of LC3 proteins and formation of autophagosomes. The process appears to be IRE1α-dependent as deletion of IREα leads to diminished autophagosome formation and impaired cell survival in response to ER stress (Ogata et al., 2006). This ER stress-mediated regulation of autophagy has not been well-studied in T cells, but IRE1α signaling has generally been found to be detrimental to T cell development and control of tumor growth. While ER stress instigates autophagy, structural entities derived in the ER are crucial components to orchestrate the autophagic response. Both omegasomes, a subdomain of the ER containing a lipid bilayer enriched for phosphatidylinositol 3-phosphate [PI(3)P], and ER-generated lipid droplets (LDs), are integral to initiation of autophagy. Premature phagophores that eventually mature into autophagosomes initiate from the omegasome and their formation accelerates with starvation (Lamb et al., 2013). LDs are organelles originating from ER membranes encapsulated by a phospholipid monolayer, comprised of a neutral lipid core containing triacylglycerols (TAGs) and cholesterol esters (Walther and Farese, 2012; Hashemi and Goodman, 2015; Olzmann and Carvalho, 2019). In this way, LDs are a stored resource of fatty acids (FAs), providing a lipid rich nutrient source that can be released to the mitochondria upon nutrient limitation (Yan et al., 2019). In response to autophagy induction, LD formation is accelerated in the ER and LDs can be found in close proximity to the mitochondria, acting as a buffer from chronic ER stress (Velazquez et al., 2016) and supporting FAO-mediated OXPHOS through MERCs (Saito et al., 2019) (Figure 3). In acute myeloid leukemia cells, disruption of MERCs through VDAC1 or Mfn2 depletion diminished lipolysis, autophagosome development, and OXPHOS, highlighting the importance of MERCs to regulate lipid metabolism to support autophagy and survival in cells adapting to stress.
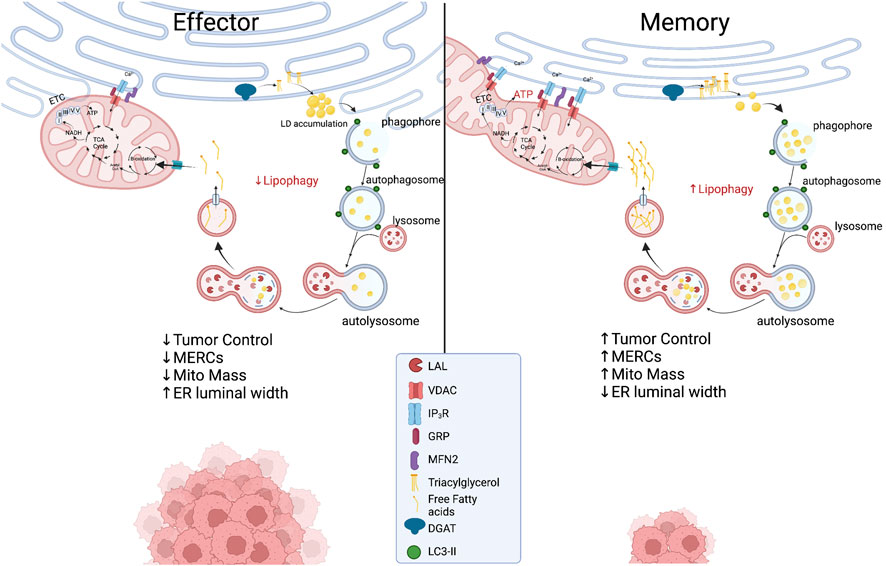
FIGURE 3. Proposed metabolic model at the ER-mitochondria interface in which effector T cells with expanded ER morphology experience chronic ER stress while displaying limited MERCs with punctate and fragmented mitochondria. In memory T cells, ER enzyme DGAT1 synthesizes triacylglycerides (TAGs) stored in lipid droplets that are concurrently degraded through lipophagy by LAL. The process could be accelerated in the nutrient stress of the tumor microenvironment, providing liberated free fatty acids to fused mitochondria to support energy generation in TILs. Created with BioRender.com.
Endoplasmic Reticulum-Associated Signaling and Structures in Lipophagy
As previously discussed, MERCs and autophagy appear to be enriched in memory T cells. A link between ER and mitochondria lipolysis to support autophagy in T cells in tumor stress has not been investigated; however, using pharmacological inhibition, it was discovered that ER-resident diacylglycerol acyltransferases 1/2 (DGAT1/2) that catalyze TAG synthesis sustained FAO and OXPHOS in memory T cells (O'Sullivan et al., 2018). Moreover, overexpression of DGAT1 rescued immunological memory in T cells deficient in TAG synthesis (Cui et al., 2015). Given that LDs were not detected in memory cells, it was discovered that FAs were directly liberated from lysosomes, as the lysosomal acid lipase (LAL) was elevated in memory T cells relative to effectors, proving necessary for cell survival in vivo (O'Sullivan et al., 2018). Thus, it is likely that memory cells use lipophagy to access internal lipid stores and this aligns with their superior function and persistence in nutrient deplete settings. Together, the questions remain: Do memory T cells access ER-derived lipid stores to fuel FAO in the stress of solid tumors? If so, is the process dependent on lipophagy and can the process be facilitated through increased interactions at MERCs (Figure 3)? Understanding lipid dynamics could allow for successful design of potent immunotherapies.
Endoplasmic Reticulum-Phagy
A final intriguing concept for tumor immunotherapy is that of ER-phagy, the orchestrated removal of damaged ER. In solid tumors mitophagy, the selective degradation of marred mitochondria, is impaired and results in persistence of dysmorphic mitochondria in CD8+ TILs. Similarly, cell stress conditions promote ER-stress induced autophagy as well as the process of selective ER-phagy. ER turnover is enabled by recruitment of autophagic machinery to sections of the ER exposed to extreme ER stress. Retreg1 (Fam134b) is among the most researched ER-phagy receptors and binds the LC3/GABARAP protein complex on the autophagosomal membrane mediated by a LC3-containing region (LIR) domain. In mice, disruption of Fam134b led to ER expansion, loss of ER turnover, and diminished ER stress resistance resulting in cell death (Khaminets et al., 2015). Nutrient deprivation induces ER degradation (Hamasaki et al., 2005) and in Fam134b competent MEFs, starvation induced degradation of Retreg1 was accompanied by loss of Climp63 expression. In contrast, in Fam134b−/− MEFs Climp63 expression remained elevated during nutrient starvation (Khaminets et al., 2015), indicating the paramount role of ER-phagy to promote regulation of ER dynamics in nutrient stress. These findings are intriguing, as they highlight the crucial process of maintaining ER homeostasis in cells through membrane remodeling, but this process has yet to be studied in the context of TILs or ER-mitochondrial interactions.
Concluding Remarks
MERCs have emerged as regulatory hubs for maintenance of cellular function and survival. The contacts serve as regulatory points in eukaryotic cells, facilitating metabolite exchange and organelle morphology in order to promote cell survival, differentiation, and proliferation. The importance of MERCs is widely recognized in the fields of metabolism and cell biology, but the profound effects of ER-mitochondrial signaling in T cell differentiation and immune function in the suppressive TME are understudied. Our observations indicate that T cell subsets likely display altered ER morphology that could lead to differential MERC formation and mitochondrial morphology. The variability in MERCs likely impacts metabolic signaling, stress response, and autophagic patterns in T cells, ultimately facilitating the efficacy of an immune response in the TME. Now, we emphasize the need for more comprehensive studies on this topic to elucidate the role of ER-mitochondria interactions responding to cell stress, defining organelle structure, and function in nutrient deprivation in order to help bolster future T cell-based cancer immunotherapies.
Author Contributions
Idea and conceptualization by JT and EH. Figures by EH. Writing and original draft preparation by EH, AA, SL, and JT. Visualization and supervision by JT.
Funding
1R01CA248359-01 (JET), 1R01CA244361-01A1 (JET), T32 CA 193201 (AMA).
Conflict of Interest
The authors declare that the research was conducted in the absence of any commercial or financial relationships that could be construed as a potential conflict of interest.
Publisher’s Note
All claims expressed in this article are solely those of the authors and do not necessarily represent those of their affiliated organizations, or those of the publisher, the editors and the reviewers. Any product that may be evaluated in this article, or claim that may be made by its manufacturer, is not guaranteed or endorsed by the publisher.
Acknowledgments
We are grateful to R. Luke Wiseman, PhD for careful critique and review of the manuscript.
References
Abrisch, R. G., Gumbin, S. C., Wisniewski, B. T., Lackner, L. L., and Voeltz, G. K. (2020). Fission and Fusion Machineries Converge at Er Contact Sites to Regulate Mitochondrial Morphology. J. Cell Biol 219. doi:10.1083/jcb.201911122
Adams, J. M., and Cory, S. (2018). The Bcl-2 Arbiters of Apoptosis and Their Growing Role as Cancer Targets. Cell Death Differ 25, 27–36. doi:10.1038/cdd.2017.161
Baba, T., Kashiwagi, Y., Arimitsu, N., Kogure, T., Edo, A., Maruyama, T., et al. (2014). Phosphatidic Acid (Pa)-Preferring Phospholipase A1 Regulates Mitochondrial Dynamics. J. Biol. Chem. 289, 11497–11511. doi:10.1074/jbc.m113.531921
Bantug, G. R., Fischer, M., Grählert, J., Balmer, M. L., Unterstab, G., Develioglu, L., et al. (2018a). Mitochondria-Endoplasmic Reticulum Contact Sites Function as Immunometabolic Hubs that Orchestrate the Rapid Recall Response of Memory CD8+ T Cells. Immunity 48, 542–555. doi:10.1016/j.immuni.2018.02.012
Bantug, G. R., Galluzzi, L., Kroemer, G., and Hess, C. (2018b). The Spectrum of T Cell Metabolism in Health and Disease. Nat. Rev. Immunol. 18, 19–34. doi:10.1038/nri.2017.99
Bartok, A., Weaver, D., Golenár, T., Nichtova, Z., Katona, M., Bánsághi, S., et al. (2019). Ip3 Receptor Isoforms Differently Regulate Er-Mitochondrial Contacts and Local Calcium Transfer. Nat. Commun. 10, 3726. doi:10.1038/s41467-019-11646-3
Beg, M., Abdullah, N., Thowfeik, F. S., Altorki, N. K., and Mcgraw, T. E. (2017). Distinct Akt Phosphorylation States Are Required for Insulin Regulated Glut4 and Glut1-Mediated Glucose Uptake. Elife 6. doi:10.7554/eLife.26896
Bental, M., and Deutsch, C. (1993). Metabolic Changes in Activated T Cells: An Nmr Study of Human Peripheral Blood Lymphocytes. Magn. Reson. Med. 29, 317–326. doi:10.1002/mrm.1910290307
Berger, C., Jensen, M. C., Lansdorp, P. M., Gough, M., Elliott, C., and Riddell, S. R. (2008). Adoptive Transfer of Effector Cd8+ T Cells Derived from Central Memory Cells Establishes Persistent T Cell Memory in Primates. J. Clin. Invest. 118, 294–305. doi:10.1172/jci32103
Bernales, S., Mcdonald, K. L., and Walter, P. (2006). Autophagy Counterbalances Endoplasmic Reticulum Expansion during the Unfolded Protein Response. Plos Biol. 4, E423. doi:10.1371/journal.pbio.0040423
Betz, C., Stracka, D., Prescianotto-Baschong, C., Frieden, M., Demaurex, N., and Hall, M. N. (2013). mTOR Complex 2-Akt Signaling at Mitochondria-Associated Endoplasmic Reticulum Membranes (MAM) Regulates Mitochondrial Physiology. Proc. Natl. Acad. Sci. U.S.A. 110, 12526–12534. doi:10.1073/pnas.1302455110
Bi, M., Naczki, C., Koritzinsky, M., Fels, D., Blais, J., Hu, N., et al. (2005). Er Stress-Regulated Translation Increases Tolerance to Extreme Hypoxia and Promotes Tumor Growth. Embo J. 24, 3470–3481. doi:10.1038/sj.emboj.7600777
Bobak, Y., Kurlishchuk, Y., Vynnytska-Myronovska, B., Grydzuk, O., Shuvayeva, G., Redowicz, M. J., et al. (2016). Arginine Deprivation Induces Endoplasmic Reticulum Stress in Human Solid Cancer Cells. Int. J. Biochem. Cell Biol. 70, 29–38. doi:10.1016/j.biocel.2015.10.027
Bobrovnikova-Marjon, E., Pytel, D., Riese, M. J., Vaites, L. P., Singh, N., Koretzky, G. A., et al. (2012). Perk Utilizes Intrinsic Lipid Kinase Activity to Generate Phosphatidic Acid, Mediate Akt Activation, and Promote Adipocyte Differentiation. Mol. Cell Biol 32, 2268–2278. doi:10.1128/mcb.00063-12
Brunelle, J. K., and Letai, A. (2009). Control of Mitochondrial Apoptosis by the Bcl-2 Family. J. Cell Sci 122, 437–441. doi:10.1242/jcs.031682
Buck, M. D., O’Sullivan, D., Klein Geltink, R. I., Curtis, J. D., Chang, C.-H., Sanin, D. E., et al. (2016). Mitochondrial Dynamics Controls T Cell Fate through Metabolic Programming. Cell 166, 63–76. doi:10.1016/j.cell.2016.05.035
Buck, M. D., O’Sullivan, D., and Pearce, E. L. (2015). T Cell Metabolism Drives Immunity. J. Exp. Med. 212, 1345–1360. doi:10.1084/jem.20151159
Cárdenas, C., Miller, R. A., Smith, I., Bui, T., Molgó, J., Müller, M., et al. (2010). Essential Regulation of Cell Bioenergetics by Constitutive Insp3 Receptor Ca2+ Transfer to Mitochondria. Cell 142, 270–283. doi:10.1016/j.cell.2010.06.007
Carreras-Sureda, A., Pihán, P., and Hetz, C. (2018). Calcium Signaling at the Endoplasmic Reticulum: Fine-Tuning Stress Responses. Cell Calcium 70, 24–31. doi:10.1016/j.ceca.2017.08.004
Chakrabarti, R., Ji, W.-K., Stan, R. V., De Juan Sanz, J., Ryan, T. A., and Higgs, H. N. (2018). Inf2-Mediated Actin Polymerization at the Er Stimulates Mitochondrial Calcium Uptake, Inner Membrane Constriction, and Division. J. Cell Biol 217, 251–268. doi:10.1083/jcb.201709111
Cham, C. M., Driessens, G., O'keefe, J. P., and Gajewski, T. F. (2008). Glucose Deprivation Inhibits Multiple Key Gene Expression Events and Effector Functions in Cd8+ T Cells. Eur. J. Immunol. 38, 2438–2450. doi:10.1002/eji.200838289
Cham, C. M., and Gajewski, T. F. (2005). Glucose Availability Regulates IFN-γ Production and p70S6 Kinase Activation in CD8+ Effector T Cells. J. Immunol. 174, 4670–4677. doi:10.4049/jimmunol.174.8.4670
Chami, M., Oulès, B., Szabadkai, G., Tacine, R., Rizzuto, R., and Paterlini-Bréchot, P. (2008). Role of Serca1 Truncated Isoform in the Proapoptotic Calcium Transfer from Er to Mitochondria during Er Stress. Mol. Cell 32, 641–651. doi:10.1016/j.molcel.2008.11.014
Chamoto, K., Chowdhury, P. S., Kumar, A., Sonomura, K., Matsuda, F., Fagarasan, S., et al. (2017). Mitochondrial Activation Chemicals Synergize with Surface Receptor Pd-1 Blockade for T Cell-dependent Antitumor Activity. Proc. Natl. Acad. Sci. U S A. 114, E761–E770. doi:10.1073/pnas.1620433114
Chang, C.-H., Qiu, J., O’Sullivan, D., Buck, M. D., Noguchi, T., Curtis, J. D., et al. (2015). Metabolic Competition in the Tumor Microenvironment Is A Driver of Cancer Progression. Cell 162, 1229–1241. doi:10.1016/j.cell.2015.08.016
Charo, J., Finkelstein, S. E., Grewal, N., Restifo, N. P., Robbins, P. F., and Rosenberg, S. A. (2005). Bcl-2 Overexpression Enhances Tumor-specific T-Cell Survival. Cancer Res. 65, 2001–2008. doi:10.1158/0008-5472.can-04-2006
Chen, L., and Han, X. (2015). Anti-Pd-1/Pd-L1 Therapy of Human Cancer: Past, Present, and Future. J. Clin. Invest. 125, 3384–3391. doi:10.1172/jci80011
Cho, B. K., Wang, C., Sugawa, S., Eisen, H. N., and Chen, J. (1999). Functional Differences between Memory and Naive Cd8 T Cells. Proc. Natl. Acad. Sci. U.S.A. 96, 2976–2981. doi:10.1073/pnas.96.6.2976
Chonghaile, T. N., Gupta, S., John, M., Szegezdi, E., Logue, S. E., and Samali, A. (2015). Bcl-2 Modulates the Unfolded Protein Response by Enhancing Splicing of X-Box Binding Protein-1. Biochem. Biophysical Res. Commun. 466, 40–45. doi:10.1016/j.bbrc.2015.08.100
Cousens, L. P., Orange, J. S., and Biron, C. A. (1995). Endogenous Il-2 Contributes to T Cell Expansion and Ifn-Gamma Production during Lymphocytic Choriomeningitis Virus Infection. J. Immunol. 155, 5690–5699.
Csordás, G., Renken, C., Várnai, P., Walter, L., Weaver, D., Buttle, K. F., et al. (2006). Structural and Functional Features and Significance of the Physical Linkage between Er and Mitochondria. J. Cell Biol 174, 915–921. doi:10.1083/jcb.200604016
Cui, G., Staron, M. M., Gray, S. M., Ho, P.-C., Amezquita, R. A., Wu, J., et al. (2015). Il-7-Induced Glycerol Transport and Tag Synthesis Promotes Memory Cd8+ T Cell Longevity. Cell 161, 750–761. doi:10.1016/j.cell.2015.03.021
Cunha, D. A., Igoillo-Esteve, M., Gurzov, E. N., Germano, C. M., Naamane, N., Marhfour, I., et al. (2012). Death Protein 5 and P53-Upregulated Modulator of Apoptosis Mediate the Endoplasmic Reticulum Stress-Mitochondrial Dialog Triggering Lipotoxic Rodent and Human β-Cell Apoptosis. Diabetes 61, 2763–2775. doi:10.2337/db12-0123
Dall'Armi, C., Devereaux, K. A., and Di Paolo, G. (2013). The Role of Lipids in the Control of Autophagy. Curr. Biol. 23, R33–R45. doi:10.1016/j.cub.2012.10.041
Davoodzadeh Gholami, M., Kardar, G. A., Saeedi, Y., Heydari, S., Garssen, J., and Falak, R. (2017). Exhaustion of T Lymphocytes in the Tumor Microenvironment: Significance and Effective Mechanisms. Cell Immunol. 322, 1–14. doi:10.1016/j.cellimm.2017.10.002
de Brito, O. M., and Scorrano, L. (2008). Mitofusin 2 Tethers Endoplasmic Reticulum to Mitochondria. Nature 456, 605–610. doi:10.1038/nature07534
Degenhardt, K., Sundararajan, R., Lindsten, T., Thompson, C., and White, E. (2002). Bax and Bak Independently Promote Cytochrome cRelease from Mitochondria. J. Biol. Chem. 277, 14127–14134. doi:10.1074/jbc.m109939200
Desdín-Micó, G., Soto-Heredero, G., and Mittelbrunn, M. (2018). Mitochondrial Activity in T Cells. Mitochondrion 41, 51–57. doi:10.1016/j.mito.2017.10.006
DeVorkin, L., Pavey, N., Carleton, G., Comber, A., Ho, C., Lim, J., et al. (2019). Autophagy Regulation of Metabolism Is Required for CD8+ T Cell Anti-tumor Immunity. Cell Rep. 27, 502–513. doi:10.1016/j.celrep.2019.03.037
Eckenrode, E. F., Yang, J., Velmurugan, G. V., Foskett, J. K., and White, C. (2010). Apoptosis Protection by Mcl-1 and Bcl-2 Modulation of Inositol 1,4,5-Trisphosphate Receptor-dependent Ca2+ Signaling. J. Biol. Chem. 285, 13678–13684. doi:10.1074/jbc.m109.096040
Fanger, C. M., Hoth, M., Crabtree, G. R., and Lewis, R. S. (1995). Characterization of T Cell Mutants with Defects in Capacitative Calcium Entry: Genetic Evidence for the Physiological Roles of Crac Channels. J. Cell Biol 131, 655–667. doi:10.1083/jcb.131.3.655
Fenninger, F., and Jefferies, W. A. (2019). What's Bred in the Bone: Calcium Channels in Lymphocytes. J.I. 202, 1021–1030. doi:10.4049/jimmunol.1800837
Frauwirth, K. A., Riley, J. L., Harris, M. H., Parry, R. V., Rathmell, J. C., Plas, D. R., et al. (2002). The Cd28 Signaling Pathway Regulates Glucose Metabolism. Immunity 16, 769–777. doi:10.1016/s1074-7613(02)00323-0
Freeman, G. J., Long, A. J., Iwai, Y., Bourque, K., Chernova, T., Nishimura, H., et al. (2000). Engagement of the Pd-1 Immunoinhibitory Receptor by A Novel B7 Family Member Leads to Negative Regulation of Lymphocyte Activation. J. Exp. Med. 192, 1027–1034. doi:10.1084/jem.192.7.1027
Fridman, W. H., Pagès, F., Sautès-Fridman, C., and Galon, J. (2012). The Immune Contexture in Human Tumours: Impact on Clinical Outcome. Nat. Rev. Cancer 12, 298–306. doi:10.1038/nrc3245
Friedman, J. R., Lackner, L. L., West, M., Dibenedetto, J. R., Nunnari, J., and Voeltz, G. K. (2011). Er Tubules Mark Sites of Mitochondrial Division. Science 334, 358–362. doi:10.1126/science.1207385
Friedman, J. R., Webster, B. M., Mastronarde, D. N., Verhey, K. J., and Voeltz, G. K. (2010). Er Sliding Dynamics and Er-Mitochondrial Contacts Occur on Acetylated Microtubules. J. Cell Biol 190, 363–375. doi:10.1083/jcb.200911024
Gajewski, T. F., Schreiber, H., and Fu, Y.-X. (2013). Innate and Adaptive Immune Cells in the Tumor Microenvironment. Nat. Immunol. 14, 1014–1022. doi:10.1038/ni.2703
Gao, S., and Hu, J. (2021). Mitochondrial Fusion: The Machineries in and Out. Trends Cell Biol. 31, 62–74. doi:10.1016/j.tcb.2020.09.008
Gattinoni, L., Klebanoff, C. A., Palmer, D. C., Wrzesinski, C., Kerstann, K., Yu, Z., et al. (2005). Acquisition of Full Effector Function In Vitro Paradoxically Impairs the In Vivo Antitumor Efficacy of Adoptively Transferred Cd8+ T Cells. J. Clin. Invest. 115, 1616–1626. doi:10.1172/jci24480
Gattinoni, L., Zhong, X.-S., Palmer, D. C., Ji, Y., Hinrichs, C. S., Yu, Z., et al. (2009). Wnt Signaling Arrests Effector T Cell Differentiation and Generates Cd8+ Memory Stem Cells. Nat. Med. 15, 808–813. doi:10.1038/nm.1982
Geiger, R., Rieckmann, J. C., Wolf, T., Basso, C., Feng, Y., Fuhrer, T., et al. (2016). L-arginine Modulates T Cell Metabolism and Enhances Survival and Anti-tumor Activity. Cell 167, 829–842. doi:10.1016/j.cell.2016.09.031
Gelmetti, V., De Rosa, P., Torosantucci, L., Marini, E. S., Romagnoli, A., Di Rienzo, M., et al. (2017). Pink1 and Becn1 Relocalize at Mitochondria-Associated Membranes during Mitophagy and Promote Er-Mitochondria Tethering and Autophagosome Formation. Autophagy 13, 654–669. doi:10.1080/15548627.2016.1277309
Gerlach, C., Van Heijst, J. W. J., Swart, E., Sie, D., Armstrong, N., Kerkhoven, R. M., et al. (2010). One Naive T Cell, Multiple Fates in Cd8+ T Cell Differentiation. J. Exp. Med. 207, 1235–1246. doi:10.1084/jem.20091175
Giacomello, M., and Pellegrini, L. (2016). The Coming of Age of the Mitochondria-Er Contact: A Matter of Thickness. Cell Death Differ 23, 1417–1427. doi:10.1038/cdd.2016.52
Glancy, B., and Balaban, R. S. (2012). Role of Mitochondrial Ca2+in the Regulation of Cellular Energetics. Biochemistry 51, 2959–2973. doi:10.1021/bi2018909
Gomez, L., Thiebaut, P.-A., Paillard, M., Ducreux, S., Abrial, M., Crola Da Silva, C., et al. (2016). The SR/ER-mitochondria Calcium Crosstalk Is Regulated by GSK3β during Reperfusion Injury. Cell Death Differ 23, 313–322. doi:10.1038/cdd.2015.101
Gratiot-Deans, J., Merino, R., Nuñez, G., and Turka, L. A. (1994). Bcl-2 Expression during T-Cell Development: Early Loss and Late Return Occur at Specific Stages of Commitment to Differentiation and Survival. Proc. Natl. Acad. Sci. U.S.A. 91, 10685–10689. doi:10.1073/pnas.91.22.10685
Grayson, J. M., Zajac, A. J., Altman, J. D., and Ahmed, R. (2000). Cutting Edge: Increased Expression of Bcl-2 in Antigen-specific Memory Cd8+ T Cells. J. Immunol. 164, 3950–3954. doi:10.4049/jimmunol.164.8.3950
Groenendyk, J., Agellon, L. B., and Michalak, M. (2021). Calcium Signaling and Endoplasmic Reticulum Stress. Int. Rev. Cell Mol Biol 363, 1–20. doi:10.1016/bs.ircmb.2021.03.003
Guo, J. Y., Teng, X., Laddha, S. V., Ma, S., Van Nostrand, S. C., Yang, Y., et al. (2016). Autophagy Provides Metabolic Substrates to Maintain Energy Charge and Nucleotide Pools in Ras-Driven Lung Cancer Cells. Genes Dev. 30, 1704–1717. doi:10.1101/gad.283416.116
Hailey, D. W., Rambold, A. S., Satpute-Krishnan, P., Mitra, K., Sougrat, R., Kim, P. K., et al. (2010). Mitochondria Supply Membranes for Autophagosome Biogenesis during Starvation. Cell 141, 656–667. doi:10.1016/j.cell.2010.04.009
Hales, C. N., Luzio, J. P., Chandler, J. A., and Herman, L. (1974). Localization of Calcium in the Smooth Endoplasmic Reticulum of Rat Isolated Fat Cells. J. Cell Sci 15, 1–15. doi:10.1242/jcs.15.1.1
Hamasaki, M., Furuta, N., Matsuda, A., Nezu, A., Yamamoto, A., Fujita, N., et al. (2013). Autophagosomes Form at Er-Mitochondria Contact Sites. Nature 495, 389–393. doi:10.1038/nature11910
Hamasaki, M., Noda, T., Baba, M., and Ohsumi, Y. (2005). Starvation Triggers the Delivery of the Endoplasmic Reticulum to the Vacuole via Autophagy in Yeast. Traffic 6, 56–65. doi:10.1111/j.1600-0854.2004.00245.x
Han, J., and Kaufman, R. J. (2017). Physiological/Pathological Ramifications of Transcription Factors in the Unfolded Protein Response. Genes Dev. 31, 1417–1438. doi:10.1101/gad.297374.117
Harding, H. P., Zhang, Y., and Ron, D. (1999). Protein Translation and Folding Are Coupled by an Endoplasmic-Reticulum-Resident Kinase. Nature 397, 271–274. doi:10.1038/16729
Hashemi, H. F., and Goodman, J. M. (2015). The Life Cycle of Lipid Droplets. Curr. Opin. Cell Biol. 33, 119–124. doi:10.1016/j.ceb.2015.02.002
Hay, N. (2016). Reprogramming Glucose Metabolism in Cancer: Can it Be Exploited for Cancer Therapy? Nat. Rev. Cancer 16, 635–649. doi:10.1038/nrc.2016.77
Hayashi, T., and Su, T.-P. (2007). Sigma-1 Receptor Chaperones at the ER- Mitochondrion Interface Regulate Ca2+ Signaling and Cell Survival. Cell 131, 596–610. doi:10.1016/j.cell.2007.08.036
Hetz, C. (2012). The Unfolded Protein Response: Controlling Cell Fate Decisions under Er Stress and beyond. Nat. Rev. Mol. Cell Biol 13, 89–102. doi:10.1038/nrm3270
Higgins, J. A. (1974). Studies on the Biogenesis of Smooth Endoplasmic Reticulum Membranes in Hepatocytes of Phenobarbital-Treated Rats. J. Cell Biol 62, 635–646. doi:10.1083/jcb.62.3.635
Ho, P.-C., Bihuniak, J. D., Macintyre, A. N., Staron, M., Liu, X., Amezquita, R., et al. (2015). Phosphoenolpyruvate Is A Metabolic Checkpoint of Anti-tumor T Cell Responses. Cell 162, 1217–1228. doi:10.1016/j.cell.2015.08.012
Honrath, B., Metz, I., Bendridi, N., Rieusset, J., Culmsee, C., and Dolga, A. M. (2017). Glucose-Regulated Protein 75 Determines Er-Mitochondrial Coupling and Sensitivity to Oxidative Stress in Neuronal Cells. Cell Death Discov. 3, 17076. doi:10.1038/cddiscovery.2017.76
Hsieh, W.-C., Sutter, B. M., Ruess, H., Barnes, S. D., Malladi, V. S., and Tu, B. P. (2022). Glucose Starvation Induces A Switch in the Histone Acetylome for Activation of Gluconeogenic and Fat Metabolism Genes. Mol. Cell 82, 60–74. doi:10.1016/j.molcel.2021.12.015
Hunt, H., Tilūnaitė, A., Bass, G., Soeller, C., Roderick, H. L., Rajagopal, V., et al. (2020). Ca2+ Release via IP3 Receptors Shapes the Cardiac Ca2+ Transient for Hypertrophic Signaling. Biophysical J. 119, 1178–1192. doi:10.1016/j.bpj.2020.08.001
Hurst, K. E., Lawrence, K. A., Reyes Angeles, L., Ye, Z., Zhang, J., Townsend, D. M., et al. (2019b). Endoplasmic Reticulum Protein Disulfide Isomerase Shapes T Cell Efficacy for Adoptive Cellular Therapy of Tumors. Cells 8. doi:10.3390/cells8121514
Hurst, K. E., Lawrence, K. A., Essman, M. T., Walton, Z. J., Leddy, L. R., and Thaxton, J. E. (2019a). Endoplasmic Reticulum Stress Contributes to Mitochondrial Exhaustion of CD8+ T Cells. Cancer Immunol. Res. 7, 476–486. doi:10.1158/2326-6066.cir-18-0182
Hurst, K. E., Lawrence, K. A., Robino, R. A., Ball, L. E., Chung, D., and Thaxton, J. E. (2020). Remodeling Translation Primes CD8+ T-Cell Antitumor Immunity. Cancer Immunol. Res. 8, 587–595. doi:10.1158/2326-6066.cir-19-0516
Ingerman, E., Perkins, E. M., Marino, M., Mears, J. A., Mccaffery, J. M., Hinshaw, J. E., et al. (2005). Dnm1 Forms Spirals that Are Structurally Tailored to Fit Mitochondria. J. Cell Biol 170, 1021–1027. doi:10.1083/jcb.200506078
Ishihara, N., Fujita, Y., Oka, T., and Mihara, K. (2006). Regulation of Mitochondrial Morphology through Proteolytic Cleavage of Opa1. Embo J. 25, 2966–2977. doi:10.1038/sj.emboj.7601184
Iwai, Y., Ishida, M., Tanaka, Y., Okazaki, T., Honjo, T., and Minato, N. (2002). Involvement of Pd-L1 on Tumor Cells in the Escape from Host Immune System and Tumor Immunotherapy by Pd-L1 Blockade. Proc. Natl. Acad. Sci. U.S.A. 99, 12293–12297. doi:10.1073/pnas.192461099
Janikiewicz, J., Szymański, J., Malinska, D., Patalas-Krawczyk, P., Michalska, B., Duszyński, J., et al. (2018). Mitochondria-Associated Membranes in Aging and Senescence: Structure, Function, and Dynamics. Cell Death Dis 9, 332. doi:10.1038/s41419-017-0105-5
Jones, R. G., and Thompson, C. B. (2007). Revving the Engine: Signal Transduction Fuels T Cell Activation. Immunity 27, 173–178. doi:10.1016/j.immuni.2007.07.008
Joshi, N. S., Cui, W., Chandele, A., Lee, H. K., Urso, D. R., Hagman, J., et al. (2007). Inflammation Directs Memory Precursor and Short-Lived Effector CD8+ T Cell Fates via the Graded Expression of T-Bet Transcription Factor. Immunity 27, 281–295. doi:10.1016/j.immuni.2007.07.010
Kabeya, Y., Mizushima, N., Ueno, T., Yamamoto, A., Kirisako, T., Noda, T., et al. (2000). Lc3, A Mammalian Homologue of Yeast Apg8p, Is Localized in Autophagosome Membranes after Processing. Embo J. 19, 5720–5728. doi:10.1093/emboj/19.21.5720
Kaddour-Djebbar, I., Choudhary, V., Brooks, C., Ghazaly, T., Lakshmikanthan, V., Dong, Z., et al. (2010). Specific Mitochondrial Calcium Overload Induces Mitochondrial Fission in Prostate Cancer Cells. Int. J. Oncol. 36, 1437–1444. doi:10.3892/ijo_00000629
Kaech, S. M., and Ahmed, R. (2001). Memory CD8+ T Cell Differentiation: Initial Antigen Encounter Triggers a Developmental Program in Naïve Cells. Nat. Immunol. 2, 415–422. doi:10.1038/87720
Kaech, S. M., Hemby, S., Kersh, E., and Ahmed, R. (2002). Molecular and Functional Profiling of Memory Cd8 T Cell Differentiation. Cell 111, 837–851. doi:10.1016/s0092-8674(02)01139-x
Kalbasi, A., Shrimali, R. K., Chinnasamy, D., and Rosenberg, S. A. (2010). Prevention of Interleukin-2 Withdrawal-Induced Apoptosis in Lymphocytes Retrovirally Cotransduced with Genes Encoding an Antitumor T-Cell Receptor and an Antiapoptotic Protein. J. Immunother. 33, 672–683. doi:10.1097/cji.0b013e3181e475cd
Kamimura, D., and Bevan, M. J. (2008). Endoplasmic Reticulum Stress Regulator Xbp-1 Contributes to Effector Cd8+ T Cell Differentiation during Acute Infection. J. Immunol. 181, 5433–5441. doi:10.4049/jimmunol.181.8.5433
Keir, M. E., Butte, M. J., Freeman, G. J., and Sharpe, A. H. (2008). Pd-1 and its Ligands in Tolerance and Immunity. Annu. Rev. Immunol. 26, 677–704. doi:10.1146/annurev.immunol.26.021607.090331
Khaminets, A., Heinrich, T., Mari, M., Grumati, P., Huebner, A. K., Akutsu, M., et al. (2015). Regulation of Endoplasmic Reticulum Turnover by Selective Autophagy. Nature 522, 354–358. doi:10.1038/nature14498
Kim, J., Kundu, M., Viollet, B., and Guan, K.-L. (2011). Ampk and Mtor Regulate Autophagy through Direct Phosphorylation of Ulk1. Nat. Cell Biol 13, 132–141. doi:10.1038/ncb2152
Klebanoff, C. A., Gattinoni, L., Torabi-Parizi, P., Kerstann, K., Cardones, A. R., Finkelstein, S. E., et al. (2005). Central Memory Self/tumor-Reactive CD8 + T Cells Confer superior Antitumor Immunity Compared with Effector Memory T Cells. Proc. Natl. Acad. Sci. U.S.A. 102, 9571–9576. doi:10.1073/pnas.0503726102
Klein Geltink, R. I., Edwards-Hicks, J., Apostolova, P., O’Sullivan, D., Sanin, D. E., Patterson, A. E., et al. (2020). Metabolic Conditioning of CD8+ Effector T Cells for Adoptive Cell Therapy. Nat. Metab. 2, 703–716. doi:10.1038/s42255-020-0256-z
Klein-Hessling, S., Muhammad, K., Klein, M., Pusch, T., Rudolf, R., Flöter, J., et al. (2017). NFATc1 Controls the Cytotoxicity of CD8+ T Cells. Nat. Commun. 8, 511. doi:10.1038/s41467-017-00612-6
Kobayashi, S., Zhao, F., Zhang, Z., Kobayashi, T., Huang, Y., Shi, B., et al. (2020). Mitochondrial Fission and Mitophagy Coordinately Restrict High Glucose Toxicity in Cardiomyocytes. Front. Physiol. 11, 604069. doi:10.3389/fphys.2020.604069
Kojima, R., Kakimoto, Y., Shinmyo, M., Kurokawa, K., Nakano, A., Endo, T., et al. (2019). A Non-canonical Unfolded Protein Response Pathway and Mitochondrial Dynamics Control the Number of Er-Mitochondria Contact Sites. Cold Spring Harbor, NY: Biorxiv, 684753.
Korobova, F., Ramabhadran, V., and Higgs, H. N. (2013). An Actin-dependent Step in Mitochondrial Fission Mediated by the Er-Associated Formin Inf2. Science 339, 464–467. doi:10.1126/science.1228360
Kurtulus, S., Tripathi, P., Moreno-Fernandez, M. E., Sholl, A., Katz, J. D., Grimes, H. L., et al. (2011). Bcl-2 Allows Effector and Memory Cd8+ T Cells to Tolerate Higher Expression of Bim. J.I. 186, 5729–5737. doi:10.4049/jimmunol.1100102
Lamb, C. A., Yoshimori, T., and Tooze, S. A. (2013). The Autophagosome: Origins Unknown, Biogenesis Complex. Nat. Rev. Mol. Cell Biol 14, 759–774. doi:10.1038/nrm3696
Lebeau, J., Saunders, J. M., Moraes, V. W. R., Madhavan, A., Madrazo, N., Anthony, M. C., et al. (2018). The Perk Arm of the Unfolded Protein Response Regulates Mitochondrial Morphology during Acute Endoplasmic Reticulum Stress. Cell Rep. 22, 2827–2836. doi:10.1016/j.celrep.2018.02.055
Lee, J. E., Westrate, L. M., Wu, H., Page, C., and Voeltz, G. K. (2016). Multiple Dynamin Family Members Collaborate to Drive Mitochondrial Division. Nature 540, 139–143. doi:10.1038/nature20555
Levine, B., Sinha, S. C., and Kroemer, G. (2008). Bcl-2 Family Members: Dual Regulators of Apoptosis and Autophagy. Autophagy 4, 600–606. doi:10.4161/auto.6260
Lewis, S. C., Uchiyama, L. F., and Nunnari, J. (2016). Er-Mitochondria Contacts Couple Mtdna Synthesis with Mitochondrial Division in Human Cells. Science 353, Aaf5549. doi:10.1126/science.aaf5549
Li, J., Wang, Y., Wang, Y., Wen, X., Ma, X.-N., Chen, W., et al. (2015). Pharmacological Activation of Ampk Prevents Drp1-Mediated Mitochondrial Fission and Alleviates Endoplasmic Reticulum Stress-Associated Endothelial Dysfunction. J. Mol. Cell Cardiol. 86, 62–74. doi:10.1016/j.yjmcc.2015.07.010
Loh, C., Shaw, K. T.-Y., Carew, J., Viola, J. P. B., Luo, C., Perrino, B. A., et al. (1996). Calcineurin Binds the Transcription Factor Nfat1 and Reversibly Regulates its Activity. J. Biol. Chem. 271, 10884–10891. doi:10.1074/jbc.271.18.10884
Long, A. H., Haso, W. M., Shern, J. F., Wanhainen, K. M., Murgai, M., Ingaramo, M., et al. (2015). 4-1bb Costimulation Ameliorates T Cell Exhaustion Induced by Tonic Signaling of Chimeric Antigen Receptors. Nat. Med. 21, 581–590. doi:10.1038/nm.3838
Ma, R., Ji, T., Zhang, H., Dong, W., Chen, X., Xu, P., et al. (2018). A Pck1-Directed Glycogen Metabolic Program Regulates Formation and Maintenance of Memory CD8+ T Cells. Nat. Cell Biol 20, 21–27. doi:10.1038/s41556-017-0002-2
Ma, X., Bi, E., Lu, Y., Su, P., Huang, C., Liu, L., et al. (2019). Cholesterol Induces CD8+ T Cell Exhaustion in the Tumor Microenvironment. Cell Metab. 30, 143–156. doi:10.1016/j.cmet.2019.04.002
Ma, Y., and Hendershot, L. M. (2003). Delineation of A Negative Feedback Regulatory Loop that Controls Protein Translation during Endoplasmic Reticulum Stress. J. Biol. Chem. 278, 34864–34873. doi:10.1074/jbc.m301107200
Macdonald, P. J., Francy, C. A., Stepanyants, N., Lehman, L., Baglio, A., Mears, J. A., et al. (2016). Distinct Splice Variants of Dynamin-Related Protein 1 Differentially Utilize Mitochondrial Fission Factor as an Effector of Cooperative Gtpase Activity. J. Biol. Chem. 291, 493–507. doi:10.1074/jbc.m115.680181
MacIver, N. J., Michalek, R. D., and Rathmell, J. C. (2013). Metabolic Regulation of T Lymphocytes. Annu. Rev. Immunol. 31, 259–283. doi:10.1146/annurev-immunol-032712-095956
Mao, Z., and Zhang, W. (2018). Role of Mtor in Glucose and Lipid Metabolism. Int. J. Mol. Sci. 19. doi:10.3390/ijms19072043
Marchi, S., Patergnani, S., Missiroli, S., Morciano, G., Rimessi, A., Wieckowski, M. R., et al. (2018). Mitochondrial and Endoplasmic Reticulum Calcium Homeostasis and Cell Death. Cell Calcium 69, 62–72. doi:10.1016/j.ceca.2017.05.003
Maruyama, Y., Sou, Y.-S., Kageyama, S., Takahashi, T., Ueno, T., Tanaka, K., et al. (2014). Lc3b Is Indispensable for Selective Autophagy of P62 but Not Basal Autophagy. Biochem. Biophysical Res. Commun. 446, 309–315. doi:10.1016/j.bbrc.2014.02.093
Mizushima, N., Yamamoto, A., Matsui, M., Yoshimori, T., and Ohsumi, Y. (2004). In Vivo Analysis of Autophagy in Response to Nutrient Starvation Using Transgenic Mice Expressing A Fluorescent Autophagosome Marker. MBoC 15, 1101–1111. doi:10.1091/mbc.e03-09-0704
Mohanty, R., Chowdhury, C. R., Arega, S., Sen, P., Ganguly, P., and Ganguly, N. (2019). Car T Cell Therapy: A New Era for Cancer Treatment (Review). Oncol. Rep. 42, 2183–2195. doi:10.3892/or.2019.7335
Morris, J. L., Gillet, G., Prudent, J., and Popgeorgiev, N. (2021). Bcl-2 Family of Proteins in the Control of Mitochondrial Calcium Signalling: An Old Chap with New Roles. Int. J. Mol. Sci. 22. doi:10.3390/ijms22073730
Nakano, O., Sato, M., Naito, Y., Suzuki, K., Orikasa, S., Aizawa, M., et al. (2001). Proliferative Activity of Intratumoral Cd8(+) T-Lymphocytes as A Prognostic Factor in Human Renal Cell Carcinoma: Clinicopathologic Demonstration of Antitumor Immunity. Cancer Res. 61, 5132–5136.
Navale, A. M., and Paranjape, A. N. (2016). Glucose Transporters: Physiological and Pathological Roles. Biophys. Rev. 8, 5–9. doi:10.1007/s12551-015-0186-2
Nguyen, H. H., Kim, T., Song, S. Y., Park, S., Cho, H. H., Jung, S.-H., et al. (2016). Naïve CD8+ T Cell Derived Tumor-specific Cytotoxic Effectors as a Potential Remedy for Overcoming TGF-β Immunosuppression in the Tumor Microenvironment. Sci. Rep. 6, 28208. doi:10.1038/srep28208
Nichols, B. A., Setzer, P. Y., and Bainton, D. F. (1984). Glucose-6-Phosphatase as A Cytochemical Marker of Endoplasmic Reticulum in Human Leukocytes and Platelets. J. Histochem. Cytochem. 32, 165–171. doi:10.1177/32.2.6319482
O'Sullivan, D., Van Der Windt, G. J. W., Huang, S. C., Curtis, J. D., Chang, C. H., Buck, M. D., et al. (2018). Memory CD8+ T Cells Use Cell-Intrinsic Lipolysis to Support the Metabolic Programming Necessary for Development. Immunity 49, 375–376. doi:10.1016/j.immuni.2018.07.018
Oakes, S. A., Scorrano, L., Opferman, J. T., Bassik, M. C., Nishino, M., Pozzan, T., et al. (2005). Proapoptotic Bax and Bak Regulate the Type 1 Inositol Trisphosphate Receptor and Calcium Leak from the Endoplasmic Reticulum. Proc. Natl. Acad. Sci. U.S.A. 102, 105–110. doi:10.1073/pnas.0408352102
Ogando, J., Sáez, M. E., Santos, J., Nuevo-Tapioles, C., Gut, M., Esteve-Codina, A., et al. (2019). PD-1 Signaling Affects Cristae Morphology and Leads to Mitochondrial Dysfunction in Human CD8+ T Lymphocytes. J. Immunotherapy Cancer 7, 151. doi:10.1186/s40425-019-0628-7
Ogata, M., Hino, S.-i., Saito, A., Morikawa, K., Kondo, S., Kanemoto, S., et al. (2006). Autophagy Is Activated for Cell Survival after Endoplasmic ReticulumStress. Mol. Cell Biol 26, 9220–9231. doi:10.1128/mcb.01453-06
Olzmann, J. A., and Carvalho, P. (2019). Dynamics and Functions of Lipid Droplets. Nat. Rev. Mol. Cell Biol 20, 137–155. doi:10.1038/s41580-018-0085-z
Onodera, J., and Ohsumi, Y. (2005). Autophagy Is Required for Maintenance of Amino Acid Levels and Protein Synthesis under Nitrogen Starvation. J. Biol. Chem. 280, 31582–31586. doi:10.1074/jbc.m506736200
Opferman, J. T., Ober, B. T., and Ashton-Rickardt, P. G. (1999). Linear Differentiation of Cytotoxic Effectors into Memory T Lymphocytes. Science 283, 1745–1748. doi:10.1126/science.283.5408.1745
Oshima, T., Uenishi, N., and Imahori, K. (1976). Simple Assay Methods for Ribonuclease T 1 , T 2 , and Nuclease P 1. Anal. Biochem. 71, 632–634. doi:10.1016/s0003-2697(76)80039-5
Owen, O. E., Reichard, G. A., Patel, M. S., and Boden, G. (1979). Energy Metabolism in Feasting and Fasting. Adv. Exp. Med. Biol. 111, 169–188. doi:10.1007/978-1-4757-0734-2_8
Pan, Y., Chen, H., Siu, F., and Kilberg, M. S. (2003). Amino Acid Deprivation and Endoplasmic Reticulum Stress Induce Expression of Multiple Activating Transcription Factor-3 Mrna Species that, when Overexpressed in Hepg2 Cells, Modulate Transcription by the Human Asparagine Synthetase Promoter. J. Biol. Chem. 278, 38402–38412. doi:10.1074/jbc.m304574200
Pearce, E. L., Poffenberger, M. C., Chang, C.-H., and Jones, R. G. (2013). Fueling Immunity: Insights into Metabolism and Lymphocyte Function. Science 342, 1242454. doi:10.1126/science.1242454
Pearce, E. L., Walsh, M. C., Cejas, P. J., Harms, G. M., Shen, H., Wang, L.-S., et al. (2009). Enhancing Cd8 T-Cell Memory by Modulating Fatty Acid Metabolism. Nature 460, 103–107. doi:10.1038/nature08097
Petrova, V., Annicchiarico-Petruzzelli, M., Melino, G., and Amelio, I. (2018). The Hypoxic Tumour Microenvironment. Oncogenesis 7, 10. doi:10.1038/s41389-017-0011-9
Petrovas, C., Casazza, J. P., Brenchley, J. M., Price, D. A., Gostick, E., Adams, W. C., et al. (2006). Pd-1 Is A Regulator of Virus-specific Cd8+ T Cell Survival in Hiv Infection. J. Exp. Med. 203, 2281–2292. doi:10.1084/jem.20061496
Pinkaew, D., Chattopadhyay, A., King, M. D., Chunhacha, P., Liu, Z., Stevenson, H. L., et al. (2017). Fortilin Binds IRE1α and Prevents ER Stress from Signaling Apoptotic Cell Death. Nat. Commun. 8, 18. doi:10.1038/s41467-017-00029-1
Pitts, K. R., Yoon, Y., Krueger, E. W., and Mcniven, M. A. (1999). The Dynamin-like Protein Dlp1 Is Essential for Normal Distribution and Morphology of the Endoplasmic Reticulum and Mitochondria in Mammalian Cells. MBoC 10, 4403–4417. doi:10.1091/mbc.10.12.4403
Pollizzi, K. N., Patel, C. H., Sun, I.-H., Oh, M.-H., Waickman, A. T., Wen, J., et al. (2015). mTORC1 and mTORC2 Selectively Regulate CD8+ T Cell Differentiation. J. Clin. Invest. 125, 2090–2108. doi:10.1172/jci77746
Putney, J. W. (1986). A Model for Receptor-Regulated Calcium Entry. Cell Calcium 7, 1–12. doi:10.1016/0143-4160(86)90026-6
Qiu, J., Villa, M., Sanin, D. E., Buck, M. D., O’Sullivan, D., Ching, R., et al. (2019). Acetate Promotes T Cell Effector Function during Glucose Restriction. Cell Rep. 27, 2063–2074. doi:10.1016/j.celrep.2019.04.022
Qiu, Y., Mao, T., Zhang, Y., Shao, M., You, J., Ding, Q., et al. (2010). A Crucial Role for Rack1 in the Regulation of Glucose-Stimulated Ire1alpha Activation in Pancreatic Beta Cells. Sci. Signal. 3, Ra7. doi:10.1126/scisignal.2000514
Raffaello, A., Mammucari, C., Gherardi, G., and Rizzuto, R. (2016). Calcium at the Center of Cell Signaling: Interplay between Endoplasmic Reticulum, Mitochondria, and Lysosomes. Trends Biochem. Sci. 41, 1035–1049. doi:10.1016/j.tibs.2016.09.001
Rainbolt, T. K., Saunders, J. M., and Wiseman, R. L. (2014). Stress-Responsive Regulation of Mitochondria through the Er Unfolded Protein Response. Trends Endocrinol. Metab. 25, 528–537. doi:10.1016/j.tem.2014.06.007
Riesenberg, B. P., Hunt, E. G., Tennant, M. D., Hurst, K. E., Andrews, A. M., Leddy, L. R., et al. (2022). Stress-Mediated Attenuation of Translation Undermines T Cell Tumor Control. Cold Spring Harbor, NY: Biorxiv.
Rines, A. K., Sharabi, K., Tavares, C. D. J., and Puigserver, P. (2016). Targeting Hepatic Glucose Metabolism in the Treatment of Type 2 Diabetes. Nat. Rev. Drug Discov. 15, 786–804. doi:10.1038/nrd.2016.151
Rizzuto, R., Brini, M., Murgia, M., and Pozzan, T. (1993). Microdomains with High Ca 2+ Close to IP 3 -Sensitive Channels that Are Sensed by Neighboring Mitochondria. Science 262, 744–747. doi:10.1126/science.8235595
Rizzuto, R., De Stefani, D., Raffaello, A., and Mammucari, C. (2012). Mitochondria as Sensors and Regulators of Calcium Signalling. Nat. Rev. Mol. Cell Biol 13, 566–578. doi:10.1038/nrm3412
Rizzuto, R., Pinton, P., Carrington, W., Fay, F. S., Fogarty, K. E., Lifshitz, L. M., et al. (1998). Close Contacts with the Endoplasmic Reticulum as Determinants of Mitochondrial Ca 2+ Responses. Science 280, 1763–1766. doi:10.1126/science.280.5370.1763
Robey, R. B., and Hay, N. (2006). Mitochondrial Hexokinases, Novel Mediators of the Antiapoptotic Effects of Growth Factors and Akt. Oncogene 25, 4683–4696. doi:10.1038/sj.onc.1209595
Rong, Y.-P., Aromolaran, A. S., Bultynck, G., Zhong, F., Li, X., Mccoll, K., et al. (2008). Targeting Bcl-2-Ip3 Receptor Interaction to Reverse Bcl-2's Inhibition of Apoptotic Calcium Signals. Mol. Cell 31, 255–265. doi:10.1016/j.molcel.2008.06.014
Rosenberg, S. A., Packard, B. S., Aebersold, P. M., Solomon, D., Topalian, S. L., Toy, S. T., et al. (1988). Use of Tumor-Infiltrating Lymphocytes and Interleukin-2 in the Immunotherapy of Patients with Metastatic Melanoma. N. Engl. J. Med. 319, 1676–1680. doi:10.1056/nejm198812223192527
Rossignol, R., Gilkerson, R., Aggeler, R., Yamagata, K., Remington, S. J., and Capaldi, R. A. (2004). Energy Substrate Modulates Mitochondrial Structure and Oxidative Capacity in Cancer Cells. Cancer Res. 64, 985–993. doi:10.1158/0008-5472.can-03-1101
Saito, T., Kuma, A., Sugiura, Y., Ichimura, Y., Obata, M., Kitamura, H., et al. (2019). Autophagy Regulates Lipid Metabolism through Selective Turnover of Ncor1. Nat. Commun. 10, 1567. doi:10.1038/s41467-019-08829-3
Santel, A., and Fuller, M. T. (2001). Control of Mitochondrial Morphology by A Human Mitofusin. J. Cell Sci 114, 867–874. doi:10.1242/jcs.114.5.867
Sato, E., Olson, S. H., Ahn, J., Bundy, B., Nishikawa, H., Qian, F., et al. (2005). Intraepithelial CD8 + Tumor-Infiltrating Lymphocytes and a High CD8 +/regulatory T Cell Ratio Are Associated with Favorable Prognosis in Ovarian Cancer. Proc. Natl. Acad. Sci. U.S.A. 102, 18538–18543. doi:10.1073/pnas.0509182102
Satoh, T., Ross, C. A., Villa, A., Supattapone, S., Pozzan, T., Snyder, S. H., et al. (1990). The Inositol 1,4,5,-Trisphosphate Receptor in Cerebellar Purkinje Cells: Quantitative Immunogold Labeling Reveals Concentration in an Er Subcompartment. J. Cell Biol 111, 615–624. doi:10.1083/jcb.111.2.615
Schaaf, M. B. E., Keulers, T. G., Vooijs, M. A., and Rouschop, K. M. A. (2016). LC3/GABARAP Family Proteins: Autophagy‐(un)related Functions. FASEB j. 30, 3961–3978. doi:10.1096/fj.201600698r
Scharping, N. E., Menk, A. V., Moreci, R. S., Whetstone, R. D., Dadey, R. E., Watkins, S. C., et al. (2016). The Tumor Microenvironment Represses T Cell Mitochondrial Biogenesis to Drive Intratumoral T Cell Metabolic Insufficiency and Dysfunction. Immunity 45, 374–388. doi:10.1016/j.immuni.2016.07.009
Schluns, K. S., Kieper, W. C., Jameson, S. C., and Lefrançois, L. (2000). Interleukin-7 Mediates the Homeostasis of Naïve and Memory CD8 T Cells In Vivo. Nat. Immunol. 1, 426–432. doi:10.1038/80868
Schluns, K. S., Williams, K., Ma, A., Zheng, X. X., and Lefrançois, L. (2002). Cutting Edge: Requirement for Il-15 in the Generation of Primary and Memory Antigen-specific Cd8 T Cells. J. Immunol. 168, 4827–4831. doi:10.4049/jimmunol.168.10.4827
Schuck, S., Prinz, W. A., Thorn, K. S., Voss, C., and Walter, P. (2009). Membrane Expansion Alleviates Endoplasmic Reticulum Stress Independently of the Unfolded Protein Response. J. Cell Biol 187, 525–536. doi:10.1083/jcb.200907074
Schultz-Cherry, S., Dybdahl-Sissoko, N., Neumann, G., Kawaoka, Y., and Hinshaw, V. S. (2001). Influenza Virus Ns1 Protein Induces Apoptosis in Cultured Cells. J. Virol. 75, 7875–7881. doi:10.1128/jvi.75.17.7875-7881.2001
Schwarz, D. S., and Blower, M. D. (2016). The Endoplasmic Reticulum: Structure, Function and Response to Cellular Signaling. Cell. Mol. Life Sci. 73, 79–94. doi:10.1007/s00018-015-2052-6
Sengupta, S., Katz, S. C., Sengupta, S., and Sampath, P. (2018). Glycogen Synthase Kinase 3 Inhibition Lowers Pd-1 Expression, Promotes Long-Term Survival and Memory Generation in Antigen-specific Car-T Cells. Cancer Lett. 433, 131–139. doi:10.1016/j.canlet.2018.06.035
Shamas-Din, A., Brahmbhatt, H., Leber, B., and Andrews, D. W. (2011). Bh3-Only Proteins: Orchestrators of Apoptosis. Biochim. Biophys. Acta (Bba) - Mol. Cell Res. 1813, 508–520. doi:10.1016/j.bbamcr.2010.11.024
Shanmuganad, S., Hummel, S. A., Varghese, V., and Hildeman, D. A. (2022). Bcl-2 Is Necessary to Counteract Bim and Promote Survival of TCRαβ+CD8αα+ Intraepithelial Lymphocyte Precursors in the Thymus. J.I. 208, 651–659. doi:10.4049/jimmunol.2100975
Sharma, P., Shen, Y., Wen, S., Yamada, S., Jungbluth, A. A., Gnjatic, S., et al. (2007). Cd8 Tumor-Infiltrating Lymphocytes Are Predictive of Survival in Muscle-Invasive Urothelial Carcinoma. Proc. Natl. Acad. Sci. U.S.A. 104, 3967–3972. doi:10.1073/pnas.0611618104
Shaw, J., Utz, P., Durand, D., Toole, J., Emmel, E., and Crabtree, G. (1988). Identification of A Putative Regulator of Early T Cell Activation Genes. Science 241, 202–205. doi:10.1126/science.3260404
Shibata, Y., Shemesh, T., Prinz, W. A., Palazzo, A. F., Kozlov, M. M., and Rapoport, T. A. (2010). Mechanisms Determining the Morphology of the Peripheral Er. Cell 143, 774–788. doi:10.1016/j.cell.2010.11.007
Simula, L., Nazio, F., and Campello, S. (2017). The Mitochondrial Dynamics in Cancer and Immune-Surveillance. Semin. Cancer Biol. 47, 29–42. doi:10.1016/j.semcancer.2017.06.007
Simula, L., Pacella, I., Colamatteo, A., Procaccini, C., Cancila, V., Bordi, M., et al. (2018). Drp1 Controls Effective T Cell Immune-Surveillance by Regulating T Cell Migration, Proliferation, and Cmyc-dependent Metabolic Reprogramming. Cell Rep. 25, 3059–3073. doi:10.1016/j.celrep.2018.11.018
Sinclair, L. V., Rolf, J., Emslie, E., Shi, Y.-B., Taylor, P. M., and Cantrell, D. A. (2013). Control of Amino-Acid Transport by Antigen Receptors Coordinates the Metabolic Reprogramming Essential for T Cell Differentiation. Nat. Immunol. 14, 500–508. doi:10.1038/ni.2556
Smith, C. A., Williams, G. T., Kingston, R., Jenkinson, E. J., and Owen, J. J. T. (1989). Antibodies to Cd3/T-Cell Receptor Complex Induce Death by Apoptosis in Immature T Cells in Thymic Cultures. Nature 337, 181–184. doi:10.1038/337181a0
Sommermeyer, D., Hudecek, M., Kosasih, P. L., Gogishvili, T., Maloney, D. G., Turtle, C. J., et al. (2016). Chimeric Antigen Receptor-Modified T Cells Derived from Defined Cd8+ and Cd4+ Subsets Confer Superior Antitumor Reactivity In Vivo. Leukemia 30, 492–500. doi:10.1038/leu.2015.247
Song, M., Sandoval, T. A., Chae, C.-S., Chopra, S., Tan, C., Rutkowski, M. R., et al. (2018). IRE1α-XBP1 Controls T Cell Function in Ovarian Cancer by Regulating Mitochondrial Activity. Nature 562, 423–428. doi:10.1038/s41586-018-0597-x
Sood, A., Jeyaraju, D. V., Prudent, J., Caron, A., Lemieux, P., Mcbride, H. M., et al. (2014). A Mitofusin-2-dependent Inactivating Cleavage of Opa1 Links Changes in Mitochondria Cristae and Er Contacts in the Postprandial Liver. Proc. Natl. Acad. Sci. U.S.A. 111, 16017–16022. doi:10.1073/pnas.1408061111
Stiles, B. L. (2009). PI-3-K and AKT: Onto the Mitochondria☆. Adv. Drug Deliv. Rev. 61, 1276–1282. doi:10.1016/j.addr.2009.07.017
Streb, H., Irvine, R. F., Berridge, M. J., and Schulz, I. (1983). Release of Ca2+ from A Nonmitochondrial Intracellular Store in Pancreatic Acinar Cells by Inositol-1,4,5-Trisphosphate. Nature 306, 67–69. doi:10.1038/306067a0
Su, E. W., Moore, C. J., Suriano, S., Johnson, C. B., Songalia, N., Patterson, A., et al. (2015). IL-2Rα Mediates Temporal Regulation of IL-2 Signaling and Enhances Immunotherapy. Sci. Transl Med. 7, 311ra170. doi:10.1126/scitranslmed.aac8155
Sukumar, M., and Gattinoni, L. (2014). The Short and Sweet of T-Cell Therapy: Restraining Glycolysis Enhances the Formation of Immunological Memory and Antitumor Immune Responses. Oncoimmunology 3, E27573. doi:10.4161/onci.27573
Szabadkai, G., Bianchi, K., Várnai, P., De Stefani, D., Wieckowski, M. R., Cavagna, D., et al. (2006). Chaperone-Mediated Coupling of Endoplasmic Reticulum and Mitochondrial Ca2+ Channels. J. Cell Biol 175, 901–911. doi:10.1083/jcb.200608073
Szado, T., Vanderheyden, V., Parys, J. B., De Smedt, H., Rietdorf, K., Kotelevets, L., et al. (2008). Phosphorylation of Inositol 1,4,5-trisphosphate Receptors by Protein Kinase B/Akt Inhibits Ca 2+ Release and Apoptosis. Proc. Natl. Acad. Sci. U.S.A. 105, 2427–2432. doi:10.1073/pnas.0711324105
Thaxton, J. E., Wallace, C., Riesenberg, B., Zhang, Y., Paulos, C. M., Beeson, C. C., et al. (2017). Modulation of Endoplasmic Reticulum Stress Controls CD4+ T-Cell Activation and Antitumor Function. Cancer Immunol. Res. 5, 666–675. doi:10.1158/2326-6066.cir-17-0081
Theurey, P., Tubbs, E., Vial, G., Jacquemetton, J., Bendridi, N., Chauvin, M.-A., et al. (2016). Mitochondria-Associated Endoplasmic Reticulum Membranes Allow Adaptation of Mitochondrial Metabolism to Glucose Availability in the Liver. J. Mol. Cell Biol 8, 129–143. doi:10.1093/jmcb/mjw004
Tubbs, E., Theurey, P., Vial, G., Bendridi, N., Bravard, A., Chauvin, M.-A., et al. (2014). Mitochondria-Associated Endoplasmic Reticulum Membrane (Mam) Integrity Is Required for Insulin Signaling and Is Implicated in Hepatic Insulin Resistance. Diabetes 63, 3279–3294. doi:10.2337/db13-1751
van Stipdonk, M. J. B., Lemmens, E. E., and Schoenberger, S. P. (2001). Naïve CTLs Require a Single Brief Period of Antigenic Stimulation for Clonal Expansion and Differentiation. Nat. Immunol. 2, 423–429. doi:10.1038/87730
Vanmeerbeek, I., Borras, D. M., Sprooten, J., Bechter, O., Tejpar, S., and Garg, A. D. (2021). Early Memory Differentiation and Cell Death Resistance in T Cells Predicts Melanoma Response to Sequential Anti-ctla4 and Anti-pd1 Immunotherapy. Genes Immun. 22, 108–119. doi:10.1038/s41435-021-00138-4
Varanita, T., Soriano, M. E., Romanello, V., Zaglia, T., Quintana-Cabrera, R., Semenzato, M., et al. (2015). The Opa1-dependent Mitochondrial Cristae Remodeling Pathway Controls Atrophic, Apoptotic, and Ischemic Tissue Damage. Cell Metab. 21, 834–844. doi:10.1016/j.cmet.2015.05.007
Veiga-Fernandes, H., Walter, U., Bourgeois, C., Mclean, A., and Rocha, B. (2000). Response of Naïve and Memory CD8+ T Cells to Antigen Stimulation In Vivo. Nat. Immunol. 1, 47–53. doi:10.1038/76907
Velázquez, A. P., Tatsuta, T., Ghillebert, R., Drescher, I., and Graef, M. (2016). Lipid Droplet-Mediated Er Homeostasis Regulates Autophagy and Cell Survival during Starvation. J. Cell Biol 212, 621–631. doi:10.1083/jcb.201508102
Verfaillie, T., Rubio, N., Garg, A. D., Bultynck, G., Rizzuto, R., Decuypere, J.-P., et al. (2012). Perk Is Required at the Er-Mitochondrial Contact Sites to Convey Apoptosis after Ros-Based Er Stress. Cell Death Differ 19, 1880–1891. doi:10.1038/cdd.2012.74
Vihervuori, H., Autere, T. A., Repo, H., Kurki, S., Kallio, L., Lintunen, M. M., et al. (2019). Tumor-infiltrating Lymphocytes and CD8+ T Cells Predict Survival of Triple-Negative Breast Cancer. J. Cancer Res. Clin. Oncol. 145, 3105–3114. doi:10.1007/s00432-019-03036-5
Vinnakota, K. C., Singhal, A., Van den Bergh, F., Bagher-Oskouei, M., Wiseman, R. W., and Beard, D. A. (2016). Open-Loop Control of Oxidative Phosphorylation in Skeletal and Cardiac Muscle Mitochondria by Ca2+. Biophysical J. 110, 954–961. doi:10.1016/j.bpj.2015.12.018
Vodnala, S. K., Eil, R., Kishton, R. J., Sukumar, M., Yamamoto, T. N., Ha, N. H., et al. (2019). T Cell Stemness and Dysfunction in Tumors Are Triggered by A Common Mechanism. Science 363. doi:10.1126/science.aau0135
Voeltz, G. K., Prinz, W. A., Shibata, Y., Rist, J. M., and Rapoport, T. A. (2006). A Class of Membrane Proteins Shaping the Tubular Endoplasmic Reticulum. Cell 124, 573–586. doi:10.1016/j.cell.2005.11.047
Walter, P., and Ron, D. (2011). The Unfolded Protein Response: From Stress Pathway to Homeostatic Regulation. Science 334, 1081–1086. doi:10.1126/science.1209038
Walther, T. C., and Farese, R. V. (2012). Lipid Droplets and Cellular Lipid Metabolism. Annu. Rev. Biochem. 81, 687–714. doi:10.1146/annurev-biochem-061009-102430
Wang, A., Chandran, S., Shah, S. A., Chiu, Y., Paria, B. C., Aghamolla, T., et al. (2012a). The Stoichiometric Production of IL-2 and IFN-γ mRNA Defines Memory T Cells that Can Self-Renew after Adoptive Transfer in Humans. Sci. Transl Med. 4, 149ra120. doi:10.1126/scitranslmed.3004306
Wang, H., Han, P., Qi, X., Li, F., Li, M., Fan, L., et al. (2021a). Bcl-2 Enhances Chimeric Antigen Receptor T Cell Persistence by Reducing Activation-Induced Apoptosis. Cancers (Basel) 13. doi:10.3390/cancers13020197
Wang, N., Wang, C., Zhao, H., He, Y., Lan, B., Sun, L., et al. (2021b). The Mams Structure and its Role in Cell Death. Cells 10, 10. doi:10.3390/cells10030657
Wang, R. C., Wei, Y., An, Z., Zou, Z., Xiao, G., Bhagat, G., et al. (2012b). Akt-Mediated Regulation of Autophagy and Tumorigenesis through Beclin 1 Phosphorylation. Science 338, 956–959. doi:10.1126/science.1225967
Wang, R., Dillon, C. P., Shi, L. Z., Milasta, S., Carter, R., Finkelstein, D., et al. (2011a). The Transcription Factor Myc Controls Metabolic Reprogramming upon T Lymphocyte Activation. Immunity 35, 871–882. doi:10.1016/j.immuni.2011.09.021
Wang, S. F., Fouquet, S., Chapon, M., Salmon, H., Regnier, F., Labroquère, K., et al. (2011b). Early T Cell Signalling Is Reversibly Altered in Pd-1+ T Lymphocytes Infiltrating Human Tumors. Plos One 6, E17621. doi:10.1371/journal.pone.0017621
Wang, Y., Zhang, X., Wen, Y., Li, S., Lu, X., Xu, R., et al. (2021c). Endoplasmic Reticulum-Mitochondria Contacts: A Potential Therapy Target for Cardiovascular Remodeling-Associated Diseases. Front. Cell Dev. Biol. 9, 774989. doi:10.3389/fcell.2021.774989
Wei, F., Zhong, S., Ma, Z., Kong, H., Medvec, A., Ahmed, R., et al. (2013). Strength of Pd-1 Signaling Differentially Affects T-Cell Effector Functions. Proc. Natl. Acad. Sci. U S A. 110, E2480–E2489. doi:10.1073/pnas.1305394110
Wengrod, J., Wang, D., Weiss, S., Zhong, H., Osman, I., and Gardner, L. B. (2015). Phosphorylation of eIF2α Triggered by mTORC1 Inhibition and PP6C Activation Is Required for Autophagy and Is Aberrant in PP6C-Mutated Melanoma. Sci. Signal. 8, Ra27. doi:10.1126/scisignal.aaa0899
Wentworth, L., Meyers, J. V., Alam, S., Russ, A. J., Suresh, M., and Cho, C. S. (2013). Memory T Cells Are Uniquely Resistant to Melanoma-Induced Suppression. Cancer Immunol. Immunother. 62, 149–159. doi:10.1007/s00262-012-1326-1
Wesselborg, S., Fruman, D. A., Sagoo, J. K., Bierer, B. E., and Burakoff, S. J. (1996). Identification of A Physical Interaction between Calcineurin and Nuclear Factor of Activated T Cells (Nfatp). J. Biol. Chem. 271, 1274–1277. doi:10.1074/jbc.271.3.1274
West, M., Zurek, N., Hoenger, A., and Voeltz, G. K. (2011). A 3d Analysis of Yeast Er Structure Reveals How Er Domains Are Organized by Membrane Curvature. J. Cell Biol 193, 333–346. doi:10.1083/jcb.201011039
Westermann, B. (2010). Mitochondrial Fusion and Fission in Cell Life and Death. Nat. Rev. Mol. Cell Biol 11, 872–884. doi:10.1038/nrm3013
Westermann, B. (2011). Organelle Dynamics: Er Embraces Mitochondria for Fission. Curr. Biol. 21, R922–R924. doi:10.1016/j.cub.2011.10.010
Westrate, L. M., Lee, J. E., Prinz, W. A., and Voeltz, G. K. (2015). Form Follows Function: The Importance of Endoplasmic Reticulum Shape. Annu. Rev. Biochem. 84, 791–811. doi:10.1146/annurev-biochem-072711-163501
Wherry, E. J., and Kurachi, M. (2015). Molecular and Cellular Insights into T Cell Exhaustion. Nat. Rev. Immunol. 15, 486–499. doi:10.1038/nri3862
White, C., Li, C., Yang, J., Petrenko, N. B., Madesh, M., Thompson, C. B., et al. (2005). The Endoplasmic Reticulum Gateway to Apoptosis by Bcl-XL Modulation of the InsP3R. Nat. Cell Biol 7, 1021–1028. doi:10.1038/ncb1302
Wieman, H. L., Wofford, J. A., and Rathmell, J. C. (2007). Cytokine Stimulation Promotes Glucose Uptake via Phosphatidylinositol-3 Kinase/Akt Regulation of Glut1 Activity and Trafficking. MBoC 18, 1437–1446. doi:10.1091/mbc.e06-07-0593
Wikstrom, J. D., Israeli, T., Bachar-Wikstrom, E., Swisa, A., Ariav, Y., Waiss, M., et al. (2013). AMPK Regulates ER Morphology and Function in Stressed Pancreatic β-Cells via Phosphorylation of DRP1. Mol. Endocrinol. 27, 1706–1723. doi:10.1210/me.2013-1109
Xiang, X., Cao, N., Chen, F., Qian, L., Wang, Y., Huang, Y., et al. (2020). Polysaccharide of Atractylodes Macrocephala Koidz (PAMK) Alleviates Cyclophosphamide-Induced Immunosuppression in Mice by Upregulating CD28/IP3R/PLCγ-1/AP-1/NFAT Signal Pathway. Front. Pharmacol. 11, 529657. doi:10.3389/fphar.2020.529657
Xu, X., Araki, K., Li, S., Han, J.-H., Ye, L., Tan, W. G., et al. (2014). Autophagy Is Essential for Effector CD8+ T Cell Survival and Memory Formation. Nat. Immunol. 15, 1152–1161. doi:10.1038/ni.3025
Xu, Y., Chaudhury, A., Zhang, M., Savoldo, B., Metelitsa, L. S., Rodgers, J., et al. (2016). Glycolysis Determines Dichotomous Regulation of T Cell Subsets in Hypoxia. J. Clin. Invest. 126, 2678–2688. doi:10.1172/jci85834
Yan, Y., Wang, H., Wei, C., Xiang, Y., Liang, X., Phang, C.-W., et al. (2019). Hdac6 Regulates Lipid Droplet Turnover in Response to Nutrient Deprivation via P62-Mediated Selective Autophagy. J. Genet. Genomics 46, 221–229. doi:10.1016/j.jgg.2019.03.008
Yang, Z., and Klionsky, D. J. (2010). Eaten Alive: A History of Macroautophagy. Nat. Cell Biol 12, 814–822. doi:10.1038/ncb0910-814
Yedida, G., Milani, M., Cohen, G. M., and Varadarajan, S. (2019). Apogossypol-Mediated Reorganisation of the Endoplasmic Reticulum Antagonises Mitochondrial Fission and Apoptosis. Cell Death Dis 10, 521. doi:10.1038/s41419-019-1759-y
Yu, Y.-R., Imrichova, H., Wang, H., Chao, T., Xiao, Z., Gao, M., et al. (2020). Disturbed Mitochondrial Dynamics in CD8+ TILs Reinforce T Cell Exhaustion. Nat. Immunol. 21, 1540–1551. doi:10.1038/s41590-020-0793-3
Zanetti, M., Xian, S., Dosset, M., and Carter, H. (2022). The Unfolded Protein Response at the Tumor-Immune Interface. Front. Immunol. 13, 823157. doi:10.3389/fimmu.2022.823157
Keywords: endoplasmic recticulum (ER), ER stress, metabolism, cancer immunotherapy, T cell, tumor microenvironment, MERCs
Citation: Hunt EG, Andrews AM, Larsen SR and Thaxton JE (2022) The ER-Mitochondria Interface as a Dynamic Hub for T Cell Efficacy in Solid Tumors. Front. Cell Dev. Biol. 10:867341. doi: 10.3389/fcell.2022.867341
Received: 01 February 2022; Accepted: 28 March 2022;
Published: 27 April 2022.
Edited by:
Yongye Huang, Northeastern University, ChinaReviewed by:
Patrick Legembre, University of Limoges, FranceUlises Ahumada-Castro, University of Chile, Chile
Roberto Bravo-Sagua, University of Chile, Chile
Copyright © 2022 Hunt, Andrews, Larsen and Thaxton. This is an open-access article distributed under the terms of the Creative Commons Attribution License (CC BY). The use, distribution or reproduction in other forums is permitted, provided the original author(s) and the copyright owner(s) are credited and that the original publication in this journal is cited, in accordance with accepted academic practice. No use, distribution or reproduction is permitted which does not comply with these terms.
*Correspondence: Jessica E. Thaxton, amVzc190aGF4dG9uQG1lZC51bmMuZWR1