- 1Alliance for Research on the Mediterranean and North Africa (ARENA), University of Tsukuba, Tsukuba, Japan
- 2Faculty of Life and Environmental Sciences, University of Tsukuba, Tsukuba, Japan
- 3AIST-University of Tsukuba Open Innovation Laboratory for Food and Medicinal Resource Engineering (FoodMed-OIL), AIST, University of Tsukuba, Tsukuba, Japan
- 4R&D Center for Tailor-made QOL, University of Tsukuba, Tsukuba, Japan
Stem cells isolated from perinatal tissue sources possess tremendous potential for biomedical and clinical applications. On the other hand, emerging data have demonstrated that bioactive natural compounds regulate numerous cellular and biochemical functions in stem cells and promote cell migration, proliferation, and attachment, resulting in maintaining stem cell proliferation or inducing controlled differentiation. In our previous studies, we have reported for the first time that various natural compounds could induce targeted differentiation of hAESCs in a lineage-specific manner by modulating early biological and molecular events and enhance the therapeutic potential of hAESCs through modulating molecular signaling. In this perspective, we will discuss the advantages of using naturally occurring active compounds in hAESCs and their potential implications for biological research and clinical applications.
Introduction
The term placenta is considered as an exploitable source of a number of pluripotent stem cells including, human amniotic epithelial stem cells (hAESCs), human amniotic mesenchymal stromal cells (hAMSCs), and human umbilical cord mesenchymal stromal cells (hUMSCs) (Miki and Strom, 2006; Ilancheran et al., 2007; Toda et al., 2007; Hu et al., 2009; Antoniadou and David, 2016; De Coppi and Atala, 2019). As derived from the biological waste product placenta, these perinatal stem cells are readily available, have an abundant supply, require no invasive harvesting procedures as well as have minimal ethical constraints. However, hAESCs possess unique biological characteristics compared to other perinatal pluripotent cells because of their developmental origin from the epiblast at around eight days after fertilization (Miki et al., 2005). They are derived from the innermost single layer of epithelial cells of the amnion that contacts the amniotic fluid directly. Isolated hAESCs express octamer-binding transcription factor-4 (OCT-4), a key transcription factor that maintain pluripotency and self-renewal in embryonic stem cells (ESCs) and induced pluripotent stem cells (iPSCs). hAESCs also express other pluripotent stem cell markers, such as Nanog homeobox (NANOG), SRY-Box transcription factor 2 (SOX2), stage-specific embryonic antigen (SSEA)3 and SSEA4, and tumor rejection antigen (TRA)1-60 and TRA 1-80 (Miki et al., 2005; Miki et al., 2010; Murphy et al., 2010; Gaggi et al., 2020). hAESCs lack telomerase activity and have short telomeres, which limit their proliferation efficiency (Gaggi et al., 2020). However, because of their limited proliferation capacity, hAESCs do not pose the risk of tumor or teratoma formation like ESCs (Miki et al., 2005). Moreover, under appropriate differentiation protocol, hAESCs can be differentiated into cells from all three germ layers, such as cells from the endodermal origin-liver, pancreas and lung epithelium, neural cells from the ectodermal origin, and bone and fat cells from mesodermal origin (Sakuragawa et al., 1996; Cai et al., 2005; Miki et al., 2005; Pan et al., 2006; Toda et al., 2007; Miki et al., 2010; Niknejad et al., 2010; Serra et al., 2018; Furuya et al., 2019). Notably, hAESCs have distinct expression profiles of human leukocyte antigens (HLAs). hAESCs show low expression of classical HLA-I: HLA-A, B, and C and no expression of HLA-II: HLA-DP, DQ, and DR, which contribute to immune recognition and rejection of PSCs after transplantation. hAESCs also express non-classical HLA-I: HLA-E, F, and G, specifically HLA-G, which have inhibitory effects on immune cells (Akle et al., 1981; Li et al., 2005). Thus, hAESCs are regarded as a promising source of stem cells in biological research and regenerative medicine.
On the other hand, natural resource-derived biologically active compounds, such as polyphenols, flavonoids, tannins, terpenoids, and fatty acids, have long been investigated for promoting cell division, and differentiation of pluripotent and adult stem cells (PSCs) under standard culture conditions (Udalamaththa et al., 2016; Udagama and Udalamaththa, 2018). Effects of plant extracts and their bioactive compounds on the proliferation and differentiation of mesenchymal stem cells (MSCs) have been extensively studied (Kornicka et al., 2017; Saud et al., 2019; Maeda, 2020). However, in spite of the fact that hAESCs were discovered nearly two decades ago, only a few studies have attempted to investigate the effects of natural compounds in hAESCs. As part of our continual effort to explore the bioactivities and functionalities of natural compounds of plant origin, we have been investigating their effects on modulating the early biological events in hAESCs (Ferdousi et al., 2019; Aonuma et al., 2020; Ferdousi et al., 2020; Uchida et al., 2020; Bejaoui et al., 2021; Ferdousi et al., 2021; Takahashi et al., 2021). In this perspective, we will discuss the multidirectional research opportunities through integrating natural bioactive compounds with the existing hAESCs research platforms.
Natural Compound-Treated hAESCs: Potential Research Opportunities
Natural Bioactive Compounds as Promising Differentiation Inducers of hAESCs
As hAESCs are derived from the pluripotent epiblast, these cells exert a high level of differentiation plasticity. A series of studies demonstrated successful induction of hAESCs into hepatocyte-like cells (Marongiu et al., 2011; Maymó et al., 2018; Furuya et al., 2019), hepatic sinusoidal endothelial cells (Serra et al., 2018), insulin-producing pancreatic β cells (Szukiewicz et al., 2010) through a combined approach using growth factors, cytokines, extracellular matrix proteins, or cocultured with mouse hepatocytes. Similarly, following treatment with noggin, serum, basic fibroblast growth factor (bFGF), and retinoic acid, hAESCs are able to differentiate into neural cells (Ishii et al., 1999; Okawa et al., 2001; Niknejad et al., 2010). Additionally, proper culture condition also induces mesodermal-lineage cells, including adipocytes, osteocytes, chondrocytes, and cardiomyocytes (Miki and Strom, 2006; Fang et al., 2012). Therefore, hAESCs provide an excellent cell source for cell therapy and regenerative medicine. However, hAESCs consist of a heterogeneous cell population according to different stem cell markers profiling (Centurione et al., 2018), which hinders the large-scale clinical transformation of hAESCs.
Additionally, the recombinant growth factors, synthetic and semi-biological cytokines, and proteins used for maintaining proliferation and inducing differentiation of stem cells, are reported to have toxic effects and possible risk of rejection. Also, these reagents are rapidly degradable and are required to replace continuously, making the whole procedure highly expensive, hence limiting their use in therapeutic tissue engineering (Marion and Mao, 2006; Raghavan et al., 2013). In this regard, exploring new biological approaches to facilitate hAESCs differentiation potential is highly needed.
In recent years, a new research stream has been developing to use naturally occurring bioactive compounds as stimulants of stem cells because of their high availability, low toxicity, and minimum side effects. Certain phytochemicals have been extensively studied for adult stem cell proliferation and inhibition of cancer cell proliferation (Udalamaththa et al., 2016). Those plant-derived pharmacologically active substances are reported to increase the rate of cell division and differentiation through modulating complex signal pathways and to facilitate tissue regeneration and immunomodulation. However, in hAESCs, the effects of natural compounds have not been explored widely. In our previous studies, we have reported for the first time that several natural compounds could regulate early biological events in hAESCs suitable for controlled differentiation of hAESCs. A caffeic acid ester, rosmarinic acid (RA), showed the potential of enhanced neural cell differentiation in hAESCs through downregulating the gene expressions related to canonical WNT pathway, BMP/TGF-b pathway, and notch signaling pathway (Ferdousi et al., 2019). RA also upregulated the expression of nemo like kinase (NLK), the positive effector of non-canonical WNT pathway. A caffeoylquinic acid derivative, 3,4,5-Tri-O-Caffeoylquinic acid (TCQA), enhanced the expressions of catenin beta 1 (CTNNB1), bone morphogenetic protein 5 (BMP5), versican (VCAN), melanocortin 1 receptor (MC1R), and dermokine (DMKN) in hAESCs, which are known to be involved in neural and pigment cell differentiation (Bejaoui et al., 2021). A flavonol aglycone isorhamnetin could induce the expression of several hepatic progenitor markers, like delta-like non-canonical Notch ligand 1 (DLK1), epithelial cell adhesion molecule (EPCAM), and albumin (ALB). Isorhamnetin-treated hAESCs also showed several mature hepatocyte functions, including ICG uptake, glycogen storage, and urea production, and weak hepatic cytochrome P450 (CYP) enzyme activity (Uchida et al., 2020). An anthocyanin, cyanidin 3-glucoside (CY3G), upregulated the expression of meteorin like glial cell differentiation regulator (METRNL) in hAESCs, which is an adipomyokine with pleiotropic activities in adipose tissue (Takahashi et al., 2021). These findings in hAESCs are supported by previous studies on these compounds in different in vitro and in vivo settings. For example, RA has been reported to exert neuroprotective effects in neuroinflammatory and neurodegenerative diseases (Takeda et al., 2002; Ito et al., 2008; Sasaki et al., 2013; Kondo et al., 2015; Makhathini et al., 2018), which has been attributed to RA’s capacity to induce neural differentiation and neurotransmitter release. Similarly, TCQA has been reported to improve cognitive function in aging model mice through inducing adult neurogenesis (Sasaki et al., 2019a). TCQA has also been reported to promote hair regrowth and pigmentation in vitro and in vivo (Bejaoui et al., 2019; 2020). Isorhamnetin has been widely reported to alleviate hepatic fibrosis in a number of in vivo models (Lee et al., 2008; Ganbold et al., 2019; Liu et al., 2019), while CY3G is known for its anti-obesity and anti-diabetic effects through modulating adipocyte differentiation (Matsukawa et al., 2015; Olivas-Aguirre et al., 2016; Saulite et al., 2019). In Figure 1, we have shown the enriched cell types by differentially expressed genes in different compound-treated hAESCs. Detailed experimental and analysis procedures are available in our previously published paper (Ferdousi et al., 2019). In the future, establishing the optimal hAESCs culture procedure by utilizing appropriate preconditioning with natural compounds is worth further investigation.
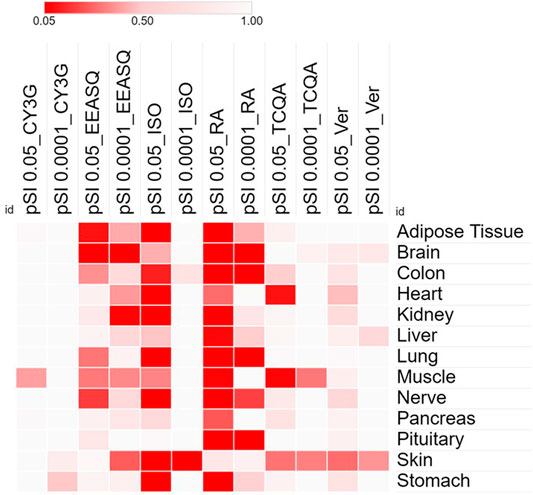
FIGURE 1. Heat map showing the significance and specificity of the tissue expressions by the differentially expressed genes of different compounds in hAESCs. Cells were treated with compounds for 7–10 days and RNAs were isolated from the control and treated hAESCs for microarray experiments using the Affymetrix’s GeneAtlas® System (Affymetrix Inc., Santa Clara, CA, USA, human genome array strips; HG-U219). Genes with a linear fold change >1.1 (verbenalin), 1.2 (RA, TCQA and EEASQ), and 2 (ISO, CY3G) and a p-value < 0.05 (one-way between-subjects ANOVA) were considered as differentially expressed genes. Enrichment analysis was conducted using the Tissue Specific Expression Analysis (TSEA) tool (http://genetics.wustl.edu/jdlab/tsea//). Heat map was generated on Morpheus tool (https://software.broadinstitute.org/morpheus//). Significance of tissue enrichment were identified by Fisher’s Exact test. pSI, Specificity Index thresholds; pSI 0.05, significantly enriched all transcripts; pSI 0.0001, most specific subset of significantly enriched transcripts; CY3G, cyanidin 3-glucoside; EEASQ, ethanol extract of Aurantiochytrium-derived squalene; ISO, isorhamnetin; RA, rosmarinic acid; TCQA, 3,4,5-tri-O-caffeoylquinic acid; Ver, verbenalin.
Natural Bioactive Compounds to Enhance Therapeutic Potential of hAESCs
The distinct immunomodulatory properties of hAESC make it the most promising candidate for cell-based therapy (Miki, 2011). Specifically, hAESCs have very low immunogenicity, thus are suitable for allotransplantation. Indeed, mounting studies have revealed the beneficial outcomes of hAESCs-based therapy for wound healing (Zhao et al., 2018; Zheng et al., 2018), skin graft (Li et al., 2012), injury repair (Kamiya et al., 2005; Parmar et al., 2006; Bai et al., 2020), pulmonary and liver fibrosis (Manuelpillai et al., 2012; Tan et al., 2014; Miki, 2016; Tan et al., 2017; Cargnoni et al., 2018), and importantly in neurological diseases (Di Germanio et al., 2016; Sanluis-Verdes et al., 2017), including spinal cord injury (Gao et al., 2014), Parkinson’s disease (Yang et al., 2010), Alzheimer’s disease (AD) (Xue et al., 2012; Kim et al., 2020), and multiple sclerosis (McDonald et al., 2011; Liu et al., 2012). However, successful clinical outcomes of hAESC transplantation depend on its immunomodulating functions. A previous study showed that expansion of hAESCs in serum-free culture media leads to significantly different expressions of stem cell markers, increased differentiation capacity and immunosuppression (Yang et al., 2018). Another study reported that prolonged exposure of hAESCs to the inflammatory cytokines, namely interleukin (IL)-1β and interferon (INF)-γ, resulted in enhanced secretion of immunomodulatory molecules (Kolanko et al., 2019). However, while current studies focus on the safety and efficacy of translating hAESC-based therapy into clinical practices, using natural compounds for priming approaches to improve the therapeutic efficacy of hAESCs has not been explored.
Our previous studies showed that treatment with natural compounds increases anti-inflammatory potential of hAESCs (Bejaoui et al., 2021; Ferdousi et al., 2021; Takahashi et al., 2021). We have also reported that isorhamnetin may have the potential to improve anti-fibrotic effects of hAESCs (Aonuma et al., 2020). Additionally, we showed that an iridoid glucoside verbenalin may enhance therapeutic potential of hAESCs for AD through targeting multiple pathologies simultaneously, including lysosomal dysfunction, pathological angiogenesis, neurometabolic aging, pathological protein aggregation, and circadian rhythms (Ferdousi et al., 2020). A recent interesting study reported that a combination of oral administration of lycopene, a carotenoid hydrocarbon found in bright red fruits and vegetables, and hAESCs transplantation could significantly ameliorate cognitive function in an in vivo AD model compared to a single treatment of lycopene and hAESC (Xu et al., 2021). Additionally, combination treatment of lycopene and hAESC also improved immunosuppressive activities in chroid plexus of AD rats. In Table 1, biological functions of different compounds in hAESCs are listed. We envision the emerging combination of naturally occurring compounds and hAESCs will offer additional opportunities for successful clinical translation of hAESC.
hAESCs as a Drug Screening Tool for Natural Compounds
Human PSCs, including both ESCs and iPSCs, have been used extensively as physiologically relevant in vitro human models in high-throughput drug screening, from target identification to preclinical compound evaluation. Stem cell-based methods reduce the timelines and attrition rate of new therapeutics (McNeish, 2004; Ebert and Svendsen, 2010; Laustriat et al., 2010; Grskovic et al., 2011; Rubin and Haston, 2011; Engle and Puppala, 2013). However, limited cell resources, invasive extraction procedures, expensive cell reprogramming and maintenance procedures, and ethical constraints are the main challenges for the large-scale use of ESCs and iPSCs for drug screening and toxicity testing.
On the other hand, a huge number of small molecules derived from or based on natural compounds become available for drug screening and biological investigations each year. However, despite substantial technological advances, the rate of new medicine discovery is exceptionally low. Indeed, drug discovery is greatly hampered by the gap between the validation of the compound and its successful clinical application. The unpredictability of the currently used in vitro cellular models, where the crucial elements of drug-biology interaction are lost, and the complexity of the in vivo microenvironment are behind the translational inefficiency of new target compounds.
In this regard, hAESCs and other perinatal stem cells, which are derived from biological waste products, may offer promising cell sources in drug screening and toxicity testing efforts. In Figure 2, we have shown important biological functions of different natural compounds observed in hAESCs (please refer to Supplementary Figure file for details). In hAESCs, isorhamnetin showed anti-fibrotic potential, which was then validated in the cardiac fibrosis in vivo model (Aonuma et al., 2020). The observed neuroprotective potential of microalgae-derived squalene (Ferdousi et al., 2021) has also been validated in aging model mice (Sasaki et al., 2019b; Sasaki et al., 2020). Similarly, the chemical synaptic transmission activity of RA was observed in depression model mice (Sasaki et al., 2013; Kondo et al., 2015), and the neurogenesis-regulating effect of TCQA was confirmed in aging mice (Sasaki et al., 2019a). Our observations strongly suggest that hAESCs would provide a promising platform to perform initial functionality screening of natural compounds.
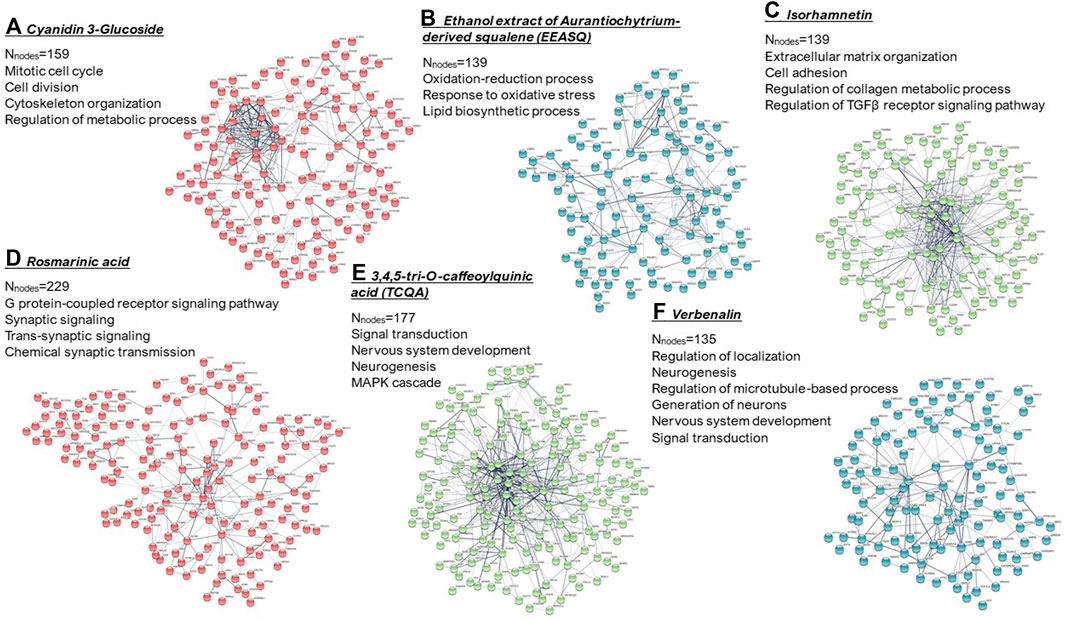
FIGURE 2. k-means clustering and PPI network of the differentially expressed genes (computed using STRING; https://string-db.org/). Significantly enriched gene ontologies (biological processes) in each network are presented. Nnodes = number of nodes. (A) Cyanidin 3-glucoside; (B) ethanol extract of Aurantiochytrium-derived squalene (EEASQ); (C) Isorhamnetin; (D) Rosmarinic acid; (E) 3,4,5-tri-O-caffeoylquinic acid (TCQA); (F) Verbenalin. Cells were treated with compounds for 7–10 days and RNAs were isolated from the control and treated hAESCs for microarray experiments using the Affymetrix’s GeneAtlas® System (Affymetrix Inc., Santa Clara, CA, USA, human genome array strips; HG-U219). Genes with a linear fold change >1.1 (verbenalin), 1.2 (RA, TCQA and EEASQ), and 2 (ISO, CY3G) and a p-value < 0.05 (one-way between-subjects ANOVA) were considered as differentially expressed genes.
Discussion
Biologically active compounds have been incorporated into stem cell research to maintain stem cell proliferation or to facilitate controlled differentiation into more defined tissues (Udalamaththa et al., 2016; Udagama and Udalamaththa, 2018; Saud et al., 2019). Our previous studies have suggested the potential of natural compounds in optimizing the microenvironment and regulating the early biological events to induce directed differentiation of hAESCs. Although hAESCs have already been studied extensively for their therapeutic potential (Toda et al., 2007), we anticipate that the emerging combination of natural compounds and hAESCs would lead to a stable molecular signature, enhanced proliferation capacity, and improved therapeutic efficacy.
One of the major challenges in hAESCs research is the heterogeneity in primary amnion-derived epithelial cell populations based on their cell surface profiling (Centurione et al., 2018; Ghamari et al., 2020). For example, studies showed that NANOG is expressed in only 1–3% of hAESCs, about 50% of term hAESCs express SSEA-4, and co-expression of SSEA-4, TRA1-60, and TRA1-81 is found in 4% of amniotic epithelial cells (Miki et al., 2005; Miki and Strom, 2006; Miki et al., 2007; Bryzek et al., 2013). Additionally, hAESCs derived from different areas of amniotic membrane exhibited different pluripotent surface markers expression and proliferative ability (Centurione et al., 2018). However, several studies have proposed better controllable approaches for generating hAESCs homogeneous enough for biological and clinical application (Miki et al., 2010; Murphy et al., 2010; Zhou et al., 2013; Gramignoli et al., 2016; Gottipamula and Sridhar, 2018; Yang et al., 2018). Another study showed that expansion of hAESCs in 3D culture system and subsequent isolation from the adherent subpopulations may enhance the stemness properties of hAESCs (Furuya et al., 2019).
From one human term amniotic membrane, nearly 200 million hAESCs can be harvested, allowing sufficient cell supply for large-scale use in academic research, pharmaceutical industry, and clinical application. For our studies on natural compound-treated hAESCs, we received the cells from ‘The Tsukuba Human Tissue Biobank Center (THB)’ established at the University of Tsukuba Hospital in 2013 (Takeuchi et al., 2016). The hAESCs were isolated from the mothers’ donated placenta who underwent cesarean section. Biobanking of perinatal stem cells began over three decades ago with the establishment of umbilical cord blood biobank. However, as the field of perinatal cells and regenerative medicine is progressing rapidly, biobanking of other types of perinatal stem cells, including hAESCs, will be an integral part of successful cell-based therapy.
Recent advances in genome-wide expression profiling, single-cell multi-omics analysis followed by machine learning-based analyses permit systematic approaches to the biological discovery of regulatory mechanisms and biochemical pathways (Chavan et al., 2006; Kumar et al., 2012). They have indeed provided certain unique opportunities for widening the application of hAESC research platform.
In the future, integrating natural compounds to hAESCs to establish an optimal culture condition, to achieve appropriate preconditioning for enhancing the therapeutic potential would be new opportunities for further investigation.
Data Availability Statement
Publicly available datasets were analyzed in this study. This data can be found here: Microarray data are deposited in the Gene Expression Omnibus (GEO) under accession numbers GSE148776 (CY3G), GSE188411 (EEASQ), GSE148777 (Isorhamnetin), GSE133277 (Rosmarinic acid), GSE153617 (TCQA), and GSE137061 (Verbenalin).
Author Contributions
FF: conceptualization, data curation, formal analysis, visualization, writing—original draft; HI: conceptualization, funding acquisition, project administration, supervision and writing—review and editing. Both authors made substantial contributions to this article and approved the final article.
Funding
This research was supported by Japan Science and Technology Agency (JST)-Science and Technology Research Partnership for Sustainable Development (SATREPS, Grant No. JPMJSA1506).
Conflict of Interest
The authors declare that the research was conducted in the absence of any commercial or financial relationships that could be construed as a potential conflict of interest.
Publisher’s Note
All claims expressed in this article are solely those of the authors and do not necessarily represent those of their affiliated organizations, or those of the publisher, the editors and the reviewers. Any product that may be evaluated in this article, or claim that may be made by its manufacturer, is not guaranteed or endorsed by the publisher.
Acknowledgments
We thankfully acknowledge Professor Nobuhiro Ohkohchi, Professor Yun-Wen Zheng, Dr. Kazunori Sasaki and Dr. Kinji Furuya for their active contribution to this project.
Supplementary Material
The Supplementary Material for this article can be found online at: https://www.frontiersin.org/articles/10.3389/fcell.2022.865810/full#supplementary-material
References
Akle, C. A., Welsh, K. I., Adinolfi, M., Leibowitz, S., and McColl, I. (1981). Immunogenicity of Human Amniotic Epithelial Cells after Transplantation into Volunteers. The Lancet 318 (8254), 1003–1005. doi:10.1016/s0140-6736(81)91212-5
Antoniadou, E., and David, A. L. (2016). Placental Stem Cells. Best Pract. Res. Clin. Obstet. Gynaecol. 31, 13–29. doi:10.1016/j.bpobgyn.2015.08.014
Aonuma, K., Ferdousi, F., Xu, D., Tominaga, K., and Isoda, H. (2020). Effects of Isorhamnetin in Human Amniotic Epithelial Stem Cells In Vitro and its Cardioprotective Effects In Vivo. Front Cell Dev Biol 8, 578197. doi:10.3389/fcell.2020.578197
Bai, X., Liu, J., Yuan, W., Liu, Y., Li, W., Cao, S., et al. (2020). Therapeutic Effect of Human Amniotic Epithelial Cells in Rat Models of Intrauterine Adhesions. Cell Transpl. 29, 963689720908495. doi:10.1177/0963689720908495
Bejaoui, M., Ferdousi, F., Zheng, Y.-W., Oda, T., and Isoda, H. (2021). Regulating Cell Fate of Human Amnion Epithelial Cells Using Natural Compounds: an Example of Enhanced Neural and Pigment Differentiation by 3,4,5-Tri-O-Caffeoylquinic Acid. Cell Commun Signal 19 (1), 26. doi:10.1186/s12964-020-00697-5
Bejaoui, M., Villareal, M. O., and Isoda, H. (2020). 3,4,5-Tri-O-Caffeoylquinic Acid Promoted Hair Pigmentation through β-Catenin and its Target Genes. Front. Cell Dev. Biol. 8, 175. doi:10.3389/fcell.2020.00175
Bejaoui, M., Villareal, M. O., and Isoda, H. (2019). β-catenin-mediated Hair Growth Induction Effect of 3,4,5-Tri-O-Caffeoylquinic Acid. Aging 11 (12), 4216–4237. doi:10.18632/aging.102048
Bryzek, A., Czekaj, P., Plewka, D., Komarska, H., Tomsia, M., Lesiak, M., et al. (2013). Expression and Co-expression of Surface Markers of Pluripotency on Human Amniotic Cells Cultured in Different Growth media. Ginekol Pol. 84 (12), 1012–1024. doi:10.17772/gp/1673
Cai, Z., Pan, L., and Shu, J. (2005). Specific Proteins of Neural Stem Cell Expressed by Human Amnion Cells. Chin. J. Rehabil. Theor. Pract. 11 (12), 965–967.
Cargnoni, A., Farigu, S., Cotti Piccinelli, E., Bonassi Signoroni, P., Romele, P., Vanosi, G., et al. (2018). Effect of Human Amniotic Epithelial Cells on Pro-fibrogenic Resident Hepatic Cells in a Rat Model of Liver Fibrosis. J. Cell Mol Med 22 (2), 1202–1213. doi:10.1111/jcmm.13396
Centurione, L., Passaretta, F., Centurione, M. A., De Munari, S., Vertua, E., Silini, A., et al. (2018). Mapping of the Human Placenta. Cell Transpl. 27 (1), 12–22. doi:10.1177/0963689717725078
Chavan, P., Joshi, K., and Patwardhan, B. (2006). DNA Microarrays in Herbal Drug Research. Evidence-Based Complement. Altern. Med. 3 (4), 447–457. doi:10.1093/ecam/nel075
De Coppi, P., and Atala, A. (2019). “Stem Cells from the Amnion,” in Principles of Regenerative Medicine (Cambridge, Massachusetts: Academic Press, Elsevier), 133–148. doi:10.1016/b978-0-12-809880-6.00009-6
Di Germanio, C., Bernier, M., de Cabo, R., and Barboni, B. (2016). Amniotic Epithelial Cells: A New Tool to Combat Aging and Age-Related Diseases? Front. Cell Dev. Biol. 4, 135. doi:10.3389/fcell.2016.00135
Ebert, A. D., and Svendsen, C. N. (2010). Human Stem Cells and Drug Screening: Opportunities and Challenges. Nat. Rev. Drug Discov. 9 (5), 367–372. doi:10.1038/nrd3000
Engle, S. J., and Puppala, D. (2013). Integrating Human Pluripotent Stem Cells into Drug Development. Cell stem cell 12 (6), 669–677. doi:10.1016/j.stem.2013.05.011
Fang, C.-H., Jin, J., Joe, J.-H., Song, Y.-S., So, B.-I., Lim, S. M., et al. (2012). In Vivo differentiation of Human Amniotic Epithelial Cells into Cardiomyocyte-like Cells and Cell Transplantation Effect on Myocardial Infarction in Rats: Comparison with Cord Blood and Adipose Tissue-Derived Mesenchymal Stem Cells. Cell Transpl. 21 (8), 1687–1696. doi:10.3727/096368912x653039
Ferdousi, F., Furuya, K., Sasaki, K., Zheng, Y.-W., Oda, T., and Isoda, H. (2021). DNA Microarray-Based Global Gene Expression Profiling in Human Amniotic Epithelial Cells Predicts the Potential of Microalgae-Derived Squalene for the Nervous System and Metabolic Health. Biomedicines 10 (1), 48. doi:10.3390/biomedicines10010048
Ferdousi, F., Kondo, S., Sasaki, K., Uchida, Y., Ohkohchi, N., Zheng, Y.-W., et al. (2020). Microarray Analysis of Verbenalin-Treated Human Amniotic Epithelial Cells Reveals Therapeutic Potential for Alzheimer's Disease. Aging 12 (6), 5516–5538. doi:10.18632/aging.102985
Ferdousi, F., Sasaki, K., Uchida, Y., Ohkohchi, N., Zheng, Y.-W., and Isoda, H. (2019). Exploring the Potential Role of Rosmarinic Acid in Neuronal Differentiation of Human Amnion Epithelial Cells by Microarray Gene Expression Profiling. Front. Neurosci. 13, 779. doi:10.3389/fnins.2019.00779
Furuya, K., Zheng, Y.-W., Sako, D., Iwasaki, K., Zheng, D.-X., Ge, J.-Y., et al. (2019). Enhanced Hepatic Differentiation in the Subpopulation of Human Amniotic Stem Cells under 3D Multicellular Microenvironment. Wjsc 11 (9), 705–721. doi:10.4252/wjsc.v11.i9.705
Gaggi, G., Di Credico, A., Izzicupo, P., Antonucci, I., Crescioli, C., Di Giacomo, V., et al. (2020). Epigenetic Features of Human Perinatal Stem Cells Redefine Their Stemness Potential. Cells 9 (5), 1304. doi:10.3390/cells9051304
Ganbold, M., Owada, Y., Ozawa, Y., Shimamoto, Y., Ferdousi, F., Tominaga, K., et al. (2019). Isorhamnetin Alleviates Steatosis and Fibrosis in Mice with Nonalcoholic Steatohepatitis. Sci. Rep. 9 (1), 16210–16211. doi:10.1038/s41598-019-52736-y
Gao, S., Ding, J., Xiao, H.-J., Li, Z.-Q., Chen, Y., Zhou, X.-S., et al. (2014). Anti-inflammatory and Anti-apoptotic Effect of Combined Treatment with Methylprednisolone and Amniotic Membrane Mesenchymal Stem Cells after Spinal Cord Injury in Rats. Neurochem. Res. 39 (8), 1544–1552. doi:10.1007/s11064-014-1344-9
Ghamari, S.-H., Abbasi-Kangevari, M., Tayebi, T., Bahrami, S., and Niknejad, H. (2020). The Bottlenecks in Translating Placenta-Derived Amniotic Epithelial and Mesenchymal Stromal Cells into the Clinic: Current Discrepancies in Marker Reports. Front. Bioeng. Biotechnol. 8, 180. doi:10.3389/fbioe.2020.00180
Gottipamula, S., and Sridhar, K. N. (2018). Large-scale Isolation, Expansion and Characterization of Human Amniotic Epithelial Cells. Ijsc 11 (1), 87–95. doi:10.15283/ijsc18001
Gramignoli, R., Srinivasan, R. C., Kannisto, K., and Strom, S. C. (2016). Isolation of Human Amnion Epithelial Cells According to Current Good Manufacturing Procedures. Curr. Protoc. Stem Cell Biol 37 (1), 1E–13E. doi:10.1310.1002/cpsc.2
Grskovic, M., Javaherian, A., Strulovici, B., and Daley, G. Q. (2011). Induced Pluripotent Stem Cells - Opportunities for Disease Modelling and Drug Discovery. Nat. Rev. Drug Discov. 10 (12), 915–929. doi:10.1038/nrd3577
Hu, J., Cai, Z., and Zhou, Z. (2009). Progress in Studies on the Characteristics of Human Amnion Mesenchymal Cells. Prog. Nat. Sci. 19 (9), 1047–1052. doi:10.1016/j.pnsc.2008.12.005
Ilancheran, S., Michalska, A., Peh, G., Wallace, E. M., Pera, M., and Manuelpillai, U. (2007). Stem Cells Derived from Human Fetal Membranes Display Multilineage Differentiation Potential. Biol. Reprod. 77 (3), 577–588. doi:10.1095/biolreprod.106.055244
Ishii, T., Ohsugi, K., Nakamura, S., Sato, K., Hashimoto, M., Mikoshiba, K., et al. (1999). Gene Expression of Oligodendrocyte Markers in Human Amniotic Epithelial Cells Using Neural Cell-type-specific Expression System. Neurosci. Lett. 268 (3), 131–134. doi:10.1016/s0304-3940(99)00297-9
Ito, N., Yabe, T., Gamo, Y., Nagai, T., Oikawa, T., Yamada, H., et al. (2008). Rosmarinic Acid from Perillae Herba Produces an Antidepressant-like Effect in Mice through Cell Proliferation in the hippocampus. Biol. Pharm. Bull. 31 (7), 1376–1380. doi:10.1248/bpb.31.1376
Kamiya, K., Wang, M., Uchida, S., Amano, S., Oshika, T., Sakuragawa, N., et al. (2005). Topical Application of Culture Supernatant from Human Amniotic Epithelial Cells Suppresses Inflammatory Reactions in Cornea. Exp. Eye Res. 80 (5), 671–679. doi:10.1016/j.exer.2004.11.018
Kim, K., Suh, Y.-H., and Chang, K.-A. (2020). Therapeutic Effects of Human Amniotic Epithelial Stem Cells in a Transgenic Mouse Model of Alzheimer's Disease. Ijms 21 (7), 2658. doi:10.3390/ijms21072658
Kolanko, E., Kopaczka, K., Koryciak-Komarska, H., Czech, E., Szmytkowska, P., Gramignoli, R., et al. (2019). Increased Immunomodulatory Capacity of Human Amniotic Cells after Activation by Pro-inflammatory Chemokines. Eur. J. Pharmacol. 859, 172545. doi:10.1016/j.ejphar.2019.172545
Kondo, S., El Omri, A., Han, J., and Isoda, H. (2015). Antidepressant-like Effects of Rosmarinic Acid through Mitogen-Activated Protein Kinase Phosphatase-1 and Brain-Derived Neurotrophic Factor Modulation. J. Funct. Foods 14, 758–766. doi:10.1016/j.jff.2015.03.001
Kornicka, K., Kocherova, I., and Marycz, K. (2017). The Effects of Chosen Plant Extracts and Compounds on Mesenchymal Stem Cells-A Bridge between Molecular Nutrition and Regenerative Medicine- Concise Review. Phytother. Res. 31 (7), 947–958. doi:10.1002/ptr.5812
Kumar, A., Asthana, M., Sharma, S., Roy, P., Amdekar, S., Singh, V., et al. (2012). Importance of Using DNA Microarray in Studying Medicinal Plant.
Laustriat, D., Gide, J., and Peschanski, M. (2010). Human Pluripotent Stem Cells in Drug Discovery and Predictive Toxicology. Biochem. Soc. Trans. 38 (4), 1051–1057.
Lee, M.-K., Yang, H.-K., Ha, N.-R., Sung, S.-H., and Kim, Y.-C. (2008). Isorhamnetin from Oenanthe Javanica Attenuates Fibrosis in Rat Hepatic Stellate Cells via Inhibition of ERK Signaling Pathway. Nat. Product. Sci. 14 (2), 81–85.
Li, H., Chu, Y., Zhang, Z., Zhang, G., Jiang, L., Wu, H., et al. (2012). Construction of Bilayered Tissue-Engineered Skin with Human Amniotic Mesenchymal Cells and Human Amniotic Epithelial Cells. Artif. organs 36 (10), 911–919. doi:10.1111/j.1525-1594.2012.01461.x
Li, H., Niederkorn, J. Y., Neelam, S., Mayhew, E., Word, R. A., McCulley, J. P., et al. (2005). Immunosuppressive Factors Secreted by Human Amniotic Epithelial Cells. Invest. Ophthalmol. Vis. Sci. 46 (3), 900–907. doi:10.1167/iovs.04-0495
Liu, N., Feng, J., Lu, X., Yao, Z., Liu, Q., Lv, Y., et al. (2019). Isorhamnetin Inhibits Liver Fibrosis by Reducing Autophagy and Inhibiting Extracellular Matrix Formation via the TGF-β1/Smad3 and TGF-Β1/p38 MAPK Pathways. Mediators Inflamm. 2019. doi:10.1155/2019/6175091
Liu, Y. H., Vaghjiani, V., Tee, J. Y., To, K., Cui, P., Oh, D. Y., et al. (2012). Amniotic Epithelial Cells from the Human Placenta Potently Suppress a Mouse Model of Multiple Sclerosis. PloS one 7 (4), e35758. doi:10.1371/journal.pone.0035758
Maeda, A. (2020). Recruitment of Mesenchymal Stem Cells to Damaged Sites by Plant-Derived Components. Front. Cell Dev. Biol. 8, 437. doi:10.3389/fcell.2020.00437
Makhathini, K. B., Mabandla, M. V., and Daniels, W. M. U. (2018). Rosmarinic Acid Reverses the Deleterious Effects of Repetitive Stress and Tat Protein. Behav. Brain Res. 353, 203–209. doi:10.1016/j.bbr.2018.07.010
Manuelpillai, U., Lourensz, D., Vaghjiani, V., Tchongue, J., Lacey, D., Tee, J.-Y., et al. (2012). Human Amniotic Epithelial Cell Transplantation Induces Markers of Alternative Macrophage Activation and Reduces Established Hepatic Fibrosis. PloS one 7 (6), e38631. doi:10.1371/journal.pone.0038631
Marion, N. W., and Mao, J. J. (2006). Mesenchymal Stem Cells and Tissue Engineering. Methods Enzymol. 420, 339–361. doi:10.1016/s0076-6879(06)20016-8
Marongiu, F., Gramignoli, R., Dorko, K., Miki, T., Ranade, A. R., Paola Serra, M., et al. (2011). Hepatic Differentiation of Amniotic Epithelial Cells. Hepatology 53 (5), 1719–1729. doi:10.1002/hep.24255
Matsukawa, T., Inaguma, T., Han, J., Villareal, M. O., and Isoda, H. (2015). Cyanidin-3-glucoside Derived from Black Soybeans Ameliorate Type 2 Diabetes through the Induction of Differentiation of Preadipocytes into Smaller and Insulin-Sensitive Adipocytes. J. Nutr. Biochem. 26 (8), 860–867. doi:10.1016/j.jnutbio.2015.03.006
Maymó, J. L., Riedel, R., Pérez-Pérez, A., Magatti, M., Maskin, B., Dueñas, J. L., et al. (2018). Proliferation and Survival of Human Amniotic Epithelial Cells during Their Hepatic Differentiation. PloS one 13 (1), e0191489.
McDonald, C., Siatskas, C., and C.A. Bernard, C. (2011). The Emergence of Amnion Epithelial Stem Cells for the Treatment of Multiple Sclerosis. Inflamm. Regen. 31 (3), 256–271. doi:10.2492/inflammregen.31.256
McNeish, J. (2004). Embryonic Stem Cells in Drug Discovery. Nat. Rev. Drug Discov. 3 (1), 70–80. doi:10.1038/nrd1281
Miki, T., Marongiu, F., Dorko, K., Ellis, E. C., and Strom, S. C. (2010). Isolation of Amniotic Epithelial Stem Cells. Curr. Protoc. Stem Cell Biol. 12, 1E.3.1–1E.3.10. doi:10.1002/9780470151808.sc01e03s12
Miki, T. (2016). A Rational Strategy for the Use of Amniotic Epithelial Stem Cell Therapy for Liver Diseases. Stem Cell translational Med. 5 (4), 405–409. doi:10.5966/sctm.2015-0304
Miki, T. (2011). Amnion-derived Stem Cells: in Quest of Clinical Applications. Stem Cell Res Ther 2 (3), 25. doi:10.1186/scrt66
Miki, T., Lehmann, T., Cai, H., Stolz, D. B., and Strom, S. C. (2005). Stem Cell Characteristics of Amniotic Epithelial Cells. Stem cells 23 (10), 1549–1559. doi:10.1634/stemcells.2004-0357
Miki, T., Mitamura, K., Ross, M. A., Stolz, D. B., and Strom, S. C. (2007). Identification of Stem Cell Marker-Positive Cells by Immunofluorescence in Term Human Amnion. J. Reprod. Immunol. 75 (2), 91–96. doi:10.1016/j.jri.2007.03.017
Miki, T., and Strom, S. C. (2006). Amnion-derived Pluripotent/multipotent Stem Cells. Stem Cell Rev 2 (2), 133–141. doi:10.1007/s12015-006-0020-0
Murphy, S., Rosli, S., Acharya, R., Mathias, L., Lim, R., Wallace, E., et al. (2010). Amnion Epithelial Cell Isolation and Characterization for Clinical Use. Curr. Protoc. Stem Cell Biol 13, 1E.6.1–1E.6.25. doi:10.1002/9780470151808.sc01e06s13
Niknejad, H., Peirovi, H., Peirovi, H., Ahmadiani, A., Ghanavi, J., and Jorjani, M. (2010). Differentiation Factors that Influence Neuronal Markers Expression In Vitro from Human Amniotic Epithelial Cells. eCM 19, 22–29. doi:10.22203/ecm.v019a03
Okawa, H., Okuda, O., Arai, H., Sakuragawa, N., and Sato, K. (2001). Amniotic Epithelial Cells Transform into Neuron-like Cells in the Ischemic Brain. Neuroreport 12 (18), 4003–4007. doi:10.1097/00001756-200112210-00030
Olivas-Aguirre, F., Rodrigo-García, J., Martínez-Ruiz, N., Cárdenas-Robles, A., Mendoza-Díaz, S., Álvarez-Parrilla, E., et al. (2016). Cyanidin-3-O-glucoside: Physical-Chemistry, Foodomics and Health Effects. Molecules 21 (9), 1264. doi:10.3390/molecules21091264
Pan, L., Shu, J., and Cai, Z. (2006). The Morphologic Study of the Characteristics of Neurobiology of the Amniotic Membrane. Chin. J. Rehabil. Med. 21 (1), 46–49.
Parmar, D. N., Alizadeh, H., Awwad, S. T., Li, H., Neelam, S., Bowman, R. W., et al. (2006). Ocular Surface Restoration Using Non-surgical Transplantation of Tissue-Cultured Human Amniotic Epithelial Cells. Am. J. Ophthalmol. 141 (2), 299–307. doi:10.1016/j.ajo.2005.09.008
Raghavan, R. N., Somanathan, N., and Sastry, T. P. (2013). Evaluation of Phytochemical-Incorporated Porous Polymeric Sponges for Bone Tissue Engineering: a Novel Perspective. Proc. Inst. Mech. Eng. H 227 (8), 859–865. doi:10.1177/0954411913489804
Rubin, L. L., and Haston, K. M. (2011). Stem Cell Biology and Drug Discovery. BMC Biol. 9 (1), 42–11. doi:10.1186/1741-7007-9-42
Sakuragawa, N., Thangavel, R., Mizuguchi, M., Hirasawa, M., and Kamo, I. (1996). Expression of Markers for Both Neuronal and Glial Cells in Human Amniotic Epithelial Cells. Neurosci. Lett. 209 (1), 9–12. doi:10.1016/0304-3940(96)12599-4
Sanluis-Verdes, A., Sanluis-Verdes, N., Manso-Revilla, M. J., Castro-Castro, A. M., Pombo-Otero, J., Fraga-Mariño, M., et al. (2017). Tissue Engineering for Neurodegenerative Diseases Using Human Amniotic Membrane and Umbilical Cord. Cell Tissue Bank 18 (1), 1–15. doi:10.1007/s10561-016-9595-0
Sasaki, K., Davies, J., Doldán, N. G., Arao, S., Ferdousi, F., Szele, F. G., et al. (2019a). 3,4,5-Tricaffeoylquinic Acid Induces Adult Neurogenesis and Improves Deficit of Learning and Memory in Aging Model Senescence-Accelerated Prone 8 Mice. Aging 11 (2), 401–422. doi:10.18632/aging.101748
Sasaki, K., El Omri, A., Kondo, S., Han, J., and Isoda, H. (2013). Rosmarinus Officinalis Polyphenols Produce Anti-depressant like Effect through Monoaminergic and Cholinergic Functions Modulation. Behav. Brain Res. 238, 86–94. doi:10.1016/j.bbr.2012.10.010
Sasaki, K., Geribaldi-Doldan, N., Wu, Q., Davies, J., Szele, F. G., and Isoda, H. (2020). The Microalgae Aurantiochytrium Sp. Increases Neurogenesis and Improves Spatial Learning and Memory in Senescence-Accelerated Prone 8 Mice. Front. Cell Developmental Biol. 8, 1877.
Sasaki, K., Othman, M. B., Ferdousi, F., Yoshida, M., Watanabe, M., Tominaga, K., et al. (2019b). Modulation of the Neurotransmitter Systems through the Anti-inflammatory and Antidepressant-like Effects of Squalene from Aurantiochytrium Sp. Plos one 14 (6), e0218923. doi:10.1371/journal.pone.0218923
Saud, B., Malla, R., and Shrestha, K. (2019). A Review on the Effect of Plant Extract on Mesenchymal Stem Cell Proliferation and Differentiation. Stem Cell Int. 2019. doi:10.1155/2019/7513404
Saulite, L., Jekabsons, K., Klavins, M., Muceniece, R., and Riekstina, U. (2019). Effects of Malvidin, Cyanidin and Delphinidin on Human Adipose Mesenchymal Stem Cell Differentiation into Adipocytes, Chondrocytes and Osteocytes. Phytomedicine 53, 86–95. doi:10.1016/j.phymed.2018.09.029
Serra, M., Marongiu, M., Contini, A., Miki, T., Cadoni, E., Laconi, E., et al. (2018). Evidence of Amniotic Epithelial Cell Differentiation toward Hepatic Sinusoidal Endothelial Cells. Cell Transpl. 27 (1), 23–30. doi:10.1177/0963689717727541
Szukiewicz, D., Pyzlak, M., Stangret, A., Rongies, W., and Maslinska, D. (2010). Decrease in Expression of Histamine H2 Receptors by Human Amniotic Epithelial Cells during Differentiation into Pancreatic Beta-like Cells. Inflamm. Res. 59 (2), S205–S207. doi:10.1007/s00011-009-0131-6
Takahashi, S., Ferdousi, F., Zheng, Y.-W., Oda, T., and Isoda, H. (2021). Human Amniotic Epithelial Cells as a Tool to Investigate the Effects of Cyanidin 3-O-Glucoside on Cell Differentiation. Ijms 22 (7), 3768. doi:10.3390/ijms22073768
Takeda, H., Tsuji, M., Miyamoto, J., and Matsumiya, T. (2002). Rosmarinic Acid and Caffeic Acid Reduce the Defensive Freezing Behavior of Mice Exposed to Conditioned Fear Stress. Psychopharmacology 164 (2), 233–235. doi:10.1007/s00213-002-1253-5
Takeuchi, T., Noguchi, M., Kawakami, Y., and Ohkohchi, N. (2016). Use of Human Biospecimen Resources for Drug Discovery —Approach of Tsukuba Human Tissue Biobank Center—. Regul. Sci. Med. Prod. 6 (1), 57–63. doi:10.14982/rsmp.6.57
Tan, J. L., Chan, S. T., Wallace, E. M., and Lim, R. (2014). Human Amnion Epithelial Cells Mediate Lung Repair by Directly Modulating Macrophage Recruitment and Polarization. Cell Transpl. 23 (3), 319–328. doi:10.3727/096368912x661409
Tan, J. L., Tan, Y. Z., Muljadi, R., Chan, S. T., Lau, S. N., Mockler, J. C., et al. (2017). Amnion Epithelial Cells Promote Lung Repair via Lipoxin A4. Stem Cell translational Med. 6 (4), 1085–1095. doi:10.5966/sctm.2016-0077
Toda, A., Okabe, M., Yoshida, T., and Nikaido, T. (2007). The Potential of Amniotic Membrane/amnion-Derived Cells for Regeneration of Various Tissues. J. Pharmacol. Sci. 105 (3), 215–228. doi:10.1254/jphs.cr0070034
Uchida, Y., Ferdousi, F., Zheng, Y.-W., Oda, T., and Isoda, H. (2020). Global Gene Expression Profiling Reveals Isorhamnetin Induces Hepatic-Lineage Specific Differentiation in Human Amniotic Epithelial Cells. Front. Cell Dev. Biol. 8 (1260). doi:10.3389/fcell.2020.578036
Udalamaththa, V. L., Jayasinghe, C. D., and Udagama, P. V. (2016). Potential Role of Herbal Remedies in Stem Cell Therapy: Proliferation and Differentiation of Human Mesenchymal Stromal Cells. Stem Cell Res Ther 7 (1), 110. doi:10.1186/s13287-016-0366-4
Vidya Udagama, P., and Udalamaththa, V. (2018). “Application of Herbal Medicine as Proliferation and Differentiation Effectors of Human Stem Cells,” in Herbal Medicine. IntechOpen (London: IntechOpen Limited). doi:10.5772/intechopen.72711
Xu, Z., Liu, C., Wang, R., Gao, X., Hao, C., and Liu, C. (2021). A Combination of Lycopene and Human Amniotic Epithelial Cells Can Ameliorate Cognitive Deficits and Suppress Neuroinflammatory Signaling by Choroid Plexus in Alzheimer's Disease Rat. J. Nutr. Biochem. 88, 108558. doi:10.1016/j.jnutbio.2020.108558
Xue, S., Chen, C., Dong, W., Hui, G., Liu, T., and Guo, L. (2012). Therapeutic Effects of Human Amniotic Epithelial Cell Transplantation on Double-Transgenic Mice Co-expressing APPswe and PS1ΔE9-Deleted Genes. Sci. China Life Sci. 55 (2), 132–140. doi:10.1007/s11427-012-4283-1
Yang, P.-j., Yuan, W.-x., Liu, J., Li, J.-y., Tan, B., Qiu, C., et al. (2018). Biological Characterization of Human Amniotic Epithelial Cells in a Serum-free System and Their Safety Evaluation. Acta Pharmacol. Sin 39 (8), 1305–1316. doi:10.1038/aps.2018.22
Yang, X., Song, L., Wu, N., Liu, Z., Xue, S., and Hui, G. (2010). An Experimental Study on Intracerebroventricular Transplantation of Human Amniotic Epithelial Cells in a Rat Model of Parkinson's Disease. Neurol. Res. 32 (10), 1054–1059. doi:10.1179/016164110x12681290831207
Zhao, B., Li, X., Shi, X., Shi, X., Zhang, W., Wu, G., et al. (2018). Exosomal microRNAs Derived from Human Amniotic Epithelial Cells Accelerate Wound Healing by Promoting the Proliferation and Migration of Fibroblasts. Stem Cell Int. 2018. doi:10.1155/2018/5420463
Zheng, Y., Zheng, S., Fan, X., Li, L., Xiao, Y., Luo, P., et al. (2018). Amniotic Epithelial Cells Accelerate Diabetic Wound Healing by Modulating Inflammation and Promoting Neovascularization. Stem Cell Int 2018, 1082076. doi:10.1155/2018/1082076
Keywords: human amniotic epithelial cells, natural compound, differentiation inducer, drug screening, cell priming, functional foods, biobank
Citation: Ferdousi F and Isoda H (2022) Regulating Early Biological Events in Human Amniotic Epithelial Stem Cells Using Natural Bioactive Compounds: Extendable Multidirectional Research Avenues. Front. Cell Dev. Biol. 10:865810. doi: 10.3389/fcell.2022.865810
Received: 30 January 2022; Accepted: 07 March 2022;
Published: 01 April 2022.
Edited by:
Valerie Kouskoff, The University of Manchester, United KingdomReviewed by:
Toshio Miki, Nihon University, JapanAngela Di Baldassarre, University of Studies G. d'Annunzio Chieti and Pescara, Italy
Copyright © 2022 Ferdousi and Isoda. This is an open-access article distributed under the terms of the Creative Commons Attribution License (CC BY). The use, distribution or reproduction in other forums is permitted, provided the original author(s) and the copyright owner(s) are credited and that the original publication in this journal is cited, in accordance with accepted academic practice. No use, distribution or reproduction is permitted which does not comply with these terms.
*Correspondence: Hiroko Isoda, aXNvZGEuaGlyb2tvLmdhQHUudHN1a3ViYS5hYy5qcA==