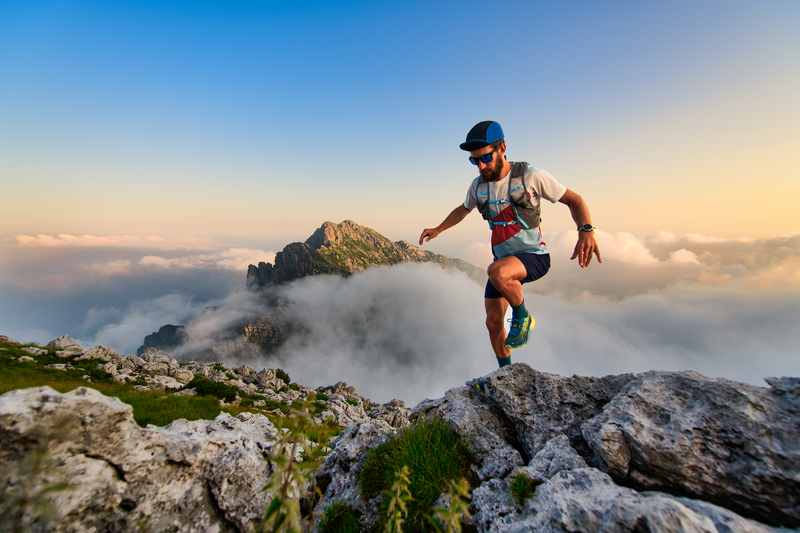
95% of researchers rate our articles as excellent or good
Learn more about the work of our research integrity team to safeguard the quality of each article we publish.
Find out more
REVIEW article
Front. Cell Dev. Biol. , 01 April 2022
Sec. Cell Growth and Division
Volume 10 - 2022 | https://doi.org/10.3389/fcell.2022.856261
This article is part of the Research Topic Growth and Tissue Regeneration with Focus on Proteoglycans View all 5 articles
This review highlights the multifunctional properties of perlecan (HSPG2) and its potential roles in repair biology. Perlecan is ubiquitous, occurring in vascular, cartilaginous, adipose, lymphoreticular, bone and bone marrow stroma and in neural tissues. Perlecan has roles in angiogenesis, tissue development and extracellular matrix stabilization in mature weight bearing and tensional tissues. Perlecan contributes to mechanosensory properties in cartilage through pericellular interactions with fibrillin-1, type IV, V, VI and XI collagen and elastin. Perlecan domain I - FGF, PDGF, VEGF and BMP interactions promote embryonic cellular proliferation, differentiation, and tissue development. Perlecan domain II, an LDLR-like domain interacts with lipids, Wnt and Hedgehog morphogens. Perlecan domain III binds FGF-7 and 18 and has roles in the secretion of perlecan. Perlecan domain IV, an immunoglobulin repeat domain, has cell attachment and matrix stabilizing properties. Perlecan domain V promotes tissue repair through interactions with VEGF, VEGF-R2 and α2β1 integrin. Perlecan domain-V LG1-LG2 and LG3 fragments antagonize these interactions. Perlecan domain V promotes reconstitution of the blood brain barrier damaged by ischemic stroke and is neurogenic and neuroprotective. Perlecan-VEGF-VEGFR2, perlecan-FGF-2 and perlecan-PDGF interactions promote angiogenesis and wound healing. Perlecan domain I, III and V interactions with platelet factor-4 and megakaryocyte and platelet inhibitory receptor promote adhesion of cells to implants and scaffolds in vascular repair. Perlecan localizes acetylcholinesterase in the neuromuscular junction and is of functional significance in neuromuscular control. Perlecan mutation leads to Schwartz-Jampel Syndrome, functional impairment of the biomechanical properties of the intervertebral disc, variable levels of chondroplasia and myotonia. A greater understanding of the functional working of the neuromuscular junction may be insightful in therapeutic approaches in the treatment of neuromuscular disorders. Tissue engineering of salivary glands has been undertaken using bioactive peptides (TWSKV) derived from perlecan domain IV. Perlecan TWSKV peptide induces differentiation of salivary gland cells into self-assembling acini-like structures that express salivary gland biomarkers and secrete α-amylase. Perlecan also promotes chondroprogenitor stem cell maturation and development of pluripotent migratory stem cell lineages, which participate in diarthrodial joint formation, and early cartilage development. Recent studies have also shown that perlecan is prominently expressed during repair of adult human articular cartilage. Perlecan also has roles in endochondral ossification and bone development. Perlecan domain I hydrogels been used in tissue engineering to establish heparin binding growth factor gradients that promote cell migration and cartilage repair. Perlecan domain I collagen I fibril scaffolds have also been used as an FGF-2 delivery system for tissue repair. With the availability of recombinant perlecan domains, the development of other tissue repair strategies should emerge in the near future. Perlecan co-localization with vascular elastin in the intima, acts as a blood shear-flow endothelial sensor that regulates blood volume and pressure and has a similar role to perlecan in canalicular fluid, regulating bone development and remodeling. This complements perlecan’s roles in growth plate cartilage and in endochondral ossification to form the appendicular and axial skeleton. Perlecan is thus a ubiquitous, multifunctional, and pleomorphic molecule of considerable biological importance. A greater understanding of its diverse biological roles and functional repertoires during tissue development, growth and disease will yield valuable insights into how this impressive proteoglycan could be utilized successfully in repair biology.
This review highlights the interactive properties of perlecan (HSPG2) and its potential applications in repair biology. Perlecan is often referred to as a large heparan sulfate proteoglycan (HS-PG) but exists as a HS/chondroitin sulfate (CS) hybrid form in most tissues, endothelial cells however synthesize a monosubstituted HS glycoform (Melrose et al., 2008). Keratinocytes produce a form of perlecan substituted with keratan sulfate (KS), HS and CS side chains and it is one of only a few PGs, which are found with such a glycosaminoglycan (GAG) substitution pattern (Knox et al., 2005). Perlecan, domain I contains three 70–100 kDa HS or CS chains attached to serine 65, 71, and 76, mouse perlecan also contain a HS or CS substitution site on Ser 3593 or Serine 3250 respectively on domain V (Tapanadechopone et al., 1999). Perlecan has a ubiquitous distribution and occurs in vascular, poorly vascularized cartilaginous, fibro-cartilaginous, adipose, lymphoreticular systems, neural, bone and bone marrow stromal tissues (Melrose et al., 2008). Perlecan is a major basement membrane component (Figure 1) as are type IV collagen, laminin and nidogen. PRELP (proline/arginine-rich end leucine-rich repeat protein), a HS binding SLRP (small leucine rich proteoglycan) and type IV collagen both interact and anchor perlecan in the vascular basement membrane (Bengtsson et al., 2002). The basement membrane is a widely distributed, specialized, thin, dense, sheet-like structure tailored to the needs of specific tissues and organs with roles as a cellular scaffold and cell signaling platform (Timpl, 1996; Pozzi et al., 2017). While cartilaginous tissues are devoid of sheet-like basement membrane structures it has been suggested that the pericellular matrix (PCM) surrounding each chondrocyte serves a similar role (Kvist et al., 2008). Von Willebrand factor A‐domain‐related protein (WARP) also interacts with perlecan, as a bridging structure to type VI collagen in the chondrocyte PCM (Allen et al., 2006; Fitzgerald, 2020), and stabilizes the basement membrane of basal structures in peripheral nerves (Allen et al., 2008; Allen et al., 2009). WARP is found in a distinct sub-set of nerve basement membrane and neural vasculature (Allen et al., 2008; Fitzgerald, 2020).
FIGURE 1. Immunofluorescent colocalisation of perlecan and elastin in transverse sections of blood vessels (A–D) and immunolocalisation of elastin (E,F) and perlecan (G,H) in venules (E,G) and capillaries (F,H). The underlying schematic depicts the major extracellular matrix components of basement membranes highlighting perlecan’s interactive role in maintaining integrity of this structure (I). (A–H) reproduced from (Hayes et al., 2011a) with permission.
Perlecan colocalizes with elastin in blood vessels and this contributes to their visco-elastic properties in vasculogenesis during establishment of early vascular networks and tissue development (Hayes et al., 2011a) and is also a key component of basement membranes (Figure 1). Perlecan occurs in specialized neuroprogenitor stem cell niches termed fractones in the sub-ventricular and sub-granular dentate gyrus of the hippocampus where it promotes neural cell survival and proliferation through sequestered FGF-2 (Kerever et al., 2014; Kerever et al., 2007). Perlecan is also associated with chondroprogenitor cell niches in cartilage rudiments where it promotes the attainment of stem cell pluripotency and migratory stem cell lineages that participate in joint cavitation, cartilage development and the expansion of cartilaginous rudiments (Hayes et al., 2016a; Melrose and Melrose, 2016) (Figure 2). Perlecan promotes chondrocyte proliferation and differentiation and ECM production and the assembly of a transient developmental cartilaginous scaffold (Melrose et al., 2016). Perlecan is up-regulated in hypertrophic chondrocytes that establish the primary and secondary ossification centers in the rudiments, these will give rise to the long bone cartilage growth plates (Melrose et al., 2004; Smith et al., 2010). Perlecan also promotes the establishment of primitive vascular networks in the stromal tissues surrounding fetal rudiments that provide nutrition to the rapidly expanding cell numbers within the mesenchymal condensations and cartilage rudiments (Melrose et al., 2003; Shu et al., 2013). Perlecan provides mechanical stability to the developing ECM through interactions with a range of HS-binding structural matrix components. Pericellular type VI and XI collagen interactions with perlecan aids in the stabilization and functional properties of mature cartilaginous ECMs (Hayes et al., 2016b; Smith and Melrose, 2019; Guilak et al., 2021). Interactions of perlecan with a wide range of HS interactive structural matrix proteins and cell adhesive glycoproteins, ensure efficient cell-matrix communication with weight bearing and tensional stresses providing mechanotransductive signals to chondrocytes to maintain tissue homeostasis (Knox and Whitelock, 2006; Melrose et al., 2008; Melrose, 2020). Perlecan contributes to mechano-sensory regulatory properties in cartilaginous tissues mediated by pericellular interactions with type VI and XI collagen, fibrillin-1 and elastin (Hayes et al., 2013; Hayes et al., 2016b; Smith and Melrose, 2019; Melrose, 2020; Guilak et al., 2021; Hayes et al., 2021).
FIGURE 2. Immunolocalisation of perlecan in 14-week-old human foetal knee joint (A–D), newborn ovine cartilaginous tissues (E–H) and mature human adipocytes (I). Perlecan has a diffuse distribution in cartilage rudiments in the femur and tibia of a foetal knee joint (A). The boxed areas in (A) are depicted at higher magnification in (B,C). These show strong localization of perlecan in putative stem cell niches in the surface region of the cartilage rudiments (B,C) and diffuse extracellular staining in the rudiment. Perlecan localization in putative stem cell niches within the surface regions of a hip rudiment (D). The boxed area in (D) is depicted at higher magnification in the inset. Scale bars in (A–D), 50 μm. Macroscopic immunolocalisation of perlecan in a newborn ovine medial meniscus. Perlecan is concentrated predominantly within the inner cartilage-like meniscal zone (E). Pericellular immunolocalisation (arrows) of perlecan within neonatal ovine femoral head articular cartilage (F) and resting zone tibial growth plate chondrocytes. Double headed arrow indicates extracellular gradient of perlecan immunolabel extending from the resting zone through to the columnar proliferating and hypertrophic growth plate chondrocytes (G). Polarised pericellular immunolocalisation of perlecan in strings of cells in the newborn ovine ACL (H). Pericellular immunolocalization of perlecan around human adipocytes (I). (A–D) modified from (Melrose and Melrose, 2016) with permission, images © Melrose 2016, (E–H) modified from (Smith et al., 2010), (I) reproduced from (Yamashita et al., 2018) with permission.
Perlecan is a modular multifunctional PG with five distinct domains. Domain I is unique to perlecan and its GAG chains bind fibroblast growth factors (FGFs), platelet-derived growth factor (PDGF), vascular endothelial growth factor (VEGF) and bone morphogenetic proteins (BMPs) promoting cellular proliferation, differentiation and tissue development (Melrose et al., 2008; Knox and Whitelock, 2006; Melrose, 2020; Whitelock et al., 2008) (Figure 3). Perlecan-HS mediated interactions with ECM components stabilize tissues (Arikawa-Hirasawa et al., 1999; Costell et al., 1999; Nicole et al., 2000; Gomes et al., 2002; Mongiat et al., 2003; Knox and Whitelock, 2006; Melrose et al., 2006; Farach-Carson and Carson, 2007; Rodgers et al., 2007; Kvist et al., 2008; Melrose et al., 2008; Smith et al., 2010; Hayes et al., 2011a; Hayes et al., 2011b; Singhal and Martin, 2011; Thompson et al., 2011; Hayes et al., 2013; Shu et al., 2013; Hayes et al., 2014; Wilusz et al., 2014; Shu et al., 2016a; Hayes et al., 2016b; Sadatsuki et al., 2017; Smith and Melrose, 2019; Melrose, 2020; Ocken et al., 2020). Domain II bears homology with low-density lipoprotein (LDL) receptor and has roles in the clearance of LDL and very low-density lipoprotein (VLDL) from the bloodstream. Domain II binds the poorly soluble Wnt and Hedgehog morphogens and acts as a transport PG, establishing gradients of these morphogens in tissues. Domain III of perlecan binds FGF-7 and 18 (Mongiat et al., 2000; Smith et al., 2007) and domain IV, an immunoglobulin (Ig) repeat domain has cell attachment properties, bears homology with the cell membrane Ig receptor family and neural cell adhesion molecule (NCAM) and also has roles as a scaffolding material (Noonan et al., 1991; Murdoch et al., 1992; Hopf et al., 1999; Farach-Carson et al., 2008; Martinez et al., 2018).
FIGURE 3. Schematic of the modular structure of perlecan and the interactive and cell instructive properties of each of its domains.
The carboxyl terminal domain V of perlecan contains three laminin-type G domains (LG) and four EGF-like repeats (Noonan et al., 1991; Murdoch et al., 1992). These LG domains are homologous with the α chain globular domains of laminin and facilitate cell-ECM interactions (Timpl, 1996) and unique divergent roles in angiogenesis, vascular cell interactions, wound healing and autophagy (Gubbiotti et al., 2017). Missense mutations, alternatively spliced, truncated or ablated sequences in perlecan domain V are evident in an autosomal recessive disorder called Schwartz Jampel Syndrome (SJS) characterized by neuromuscular deficits and myotonia (Gubbiotti et al., 2017). Domain V interacts with α2β1 integrin on endothelial cells in the assembly of angiogenic capillary tubes (Lord and Whitelock, 2013). Recombinant perlecan domain V has been expressed using human, mouse and Drosophila (domain V homologue unc-52) DNA sequences in bacterial and mammalian expression systems (Lord and Whitelock, 2013). Human domain V sequence from Glu3687 to Ser4391 when expressed in HEK-293 cells resulted in the synthesis of a GAG-free perlecan domain V, this was termed endorepellin. However when mouse perlecan domain V (Brown et al., 1997; Friedrich et al., 1999; Tapanadechopone et al., 1999), or human domain V encompassing 37 amino acids of the C-terminal region of perlecan domain IV from Leu3626 to Ser439 was expressed in HEK-293 cells a perlecan domain V containing HS and CS chains was produced. rhPerlecan domain V is a fully functional vascular PG in its own right supporting endothelial cell interactions as effectively as full-length perlecan thus it has considerable promise in repair biology (Lin et al., 2020).
Endogenously produced perlecan domain V released by MMPs from full-length perlecan also promotes tissue repair through angiogenic interactions with VEGF, VEGF-R2, α2β1 integrin, ECM-1 and progranulin (Brown et al., 1997; Bix, 2013; Bix et al., 2013). LG1-LG2 and LG3 modules of perlecan domain V antagonize these interactions and inhibit tube formation and in-growth of new blood vessels. Perlecan domain V promotes repair of the disrupted blood brain barrier that occurs after ischemic stroke (Bix, 2013; Bix et al., 2013). Interactions between perlecan domain V and pro-angiogenic glycoproteins such as extracellular matrix protein 1 (ECM1) (Mongiat et al., 2003) and progranulin (Gonzalez et al., 2003) promote angiogenesis and tissue repair. Perlecan complexes with dystroglycan and acetylcholinesterase occur in the neuromuscular junction (NMJ) and are of functional significance with essential roles in the assembly and function of this structure. Perlecan domain V α5β1 integrin interactions promote pericyte migration, enhance PDGF-BB-induced phosphorylation of platelet-derived growth factor receptor β (PDGFRβ), SHP-2 (Src homology region 2 domain-containing phosphatase-2), and focal adhesion kinase (FAK) (Nakamura et al., 2019). This supports the maintenance of the normal blood brain barrier and repair processes of this structure following ischemic stroke (Nakamura et al., 2015a; Nakamura et al., 2019). Perlecan domain V and its LG1LG2 and LG3 modules differentially modulate interactions with VEGF-1, 2; FGF-7; PDGF promoting or inhibiting angiogenesis. Perlecan domain V is neuroprotective, and has anti-inflammatory properties leading to the proposal of domain-V for the treatment of Alzheimer’s disease (AD), stroke patients (Lee et al., 2011; Clarke et al., 2012; Kahle et al., 2012; Bix, 2013; Bix et al., 2013; Guell and Bix, 2014; Marcelo and Bix, 2014; Edwards and Bix, 2019a; Edwards and Bix, 2019b), vascular dementia (Marcelo and Bix, 2015) and brain trauma (Badaut and Bix, 2014). Perlecan and VEGF incorporated into bio-scaffolds promote angiogenesis and tissue repair (Rnjak-Kovacina et al., 2016; Lord et al., 2017). Recombinant perlecan domain V supports angiogenesis, vascular cell interactions and wound healing (Lee et al., 2011; Clarke et al., 2012; Kahle et al., 2012; Marcelo and Bix, 2014; Marcelo and Bix, 2015; Poluzzi et al., 2016; Rnjak-Kovacina et al., 2017). Interactions between perlecan PDGF BB, FGF-2, and TGF-β1 promote fibroblast migration and collagenous repair of the corneal stroma and other connective tissues to regulate tissue fibrosis. Perlecan-VEGF-VEGF-R2 interactions promote angiogenesis and wound healing (Lord et al., 2014). Perlecan domain III and V interactions with platelet factor 4 and domain I of perlecan with megakaryocyte and platelet inhibitory receptor G6b-R promote adhesion of cells to implants and scaffolds in vascular repair applications (Rnjak-Kovacina et al., 2017). With the availability of recombinant perlecan, applications are now emerging for perlecan in repair biology. Perlecan expression by adipocytes and smooth muscle cells mechano-regulate lipid and glucose catabolism and oxidative muscle fiber composition and the systemic metabolism of adipose tissue and skeletal muscle (Yamashita et al., 2018). Perlecan thus has physiological roles in obesity, the onset of metabolic syndrome and in lipoprotein retention in diabetic atherosclerosis (Tannock and King, 2008; Ng et al., 2021). Polarised M2 macrophages retain LDL in atherosclerotic plaques through interactions with perlecan domain I HS chains and the LDL receptor of perlecan domain II. Perlecan as a basement membrane component of blood vessels, blood brain barrier and nerve basal structures in moto-neuron synapses of the NMJ is thus a ubiquitous pleomorphic molecule involved in the regulation of many physiological processes in tissues of varied form and function. With a full appreciation of perlecan’s biology in tissues this insightful information would greatly aid in future tissue repair strategies involving this remarkable PG.
Perlecan is a large multi-domain ECM HSPG detectable in four cell blastocysts during embryogenesis, its expression changes spatiotemporally during implantation of the embryo and in the placentation stage of embryonic development (Smith et al., 1997). Perlecan is expressed by many embryonic cell types and tissues (Roediger et al., 2009) including the trophoblast and tropho-ectoderm, the basal lamina that underlies the uterine epithelia and endothelia, and developing decidua (Smith et al., 1997). Perlecan promotes many biological processes in the embryo during cell adhesion, growth factor binding, and modulation of apoptotic events (Girós et al., 2007; Soulintzi and Zagris, 2007). Perlecan expression and activity is controlled at the transcriptional level, through alternative splicing, and proteolytic processing of perlecan in the extracellular environment (Gomes et al., 2002). Full length perlecan acts as an extracellular scaffold supporting cell attachment, growth factor and morphogen sequestration that promoting cell proliferation and differentiation, matrix production and tissue expansion during development (Farach-Carson and Carson, 2007). Perlecan also stabilises the ECM through multi-component interactions mediated by its core protein and its GAG chain substitution in domain I. Perlecan is a ubiquitous modular instructive multifunctional extracellular and pericellular PG that regulates cellular migration, differentiation and proliferation (McGrath et al., 2005; Whitelock et al., 2008; Lord et al., 2014; Nakamura et al., 2015b). Perlecan in cartilaginous tissues promotes proliferation and differentiation of chondrogenic cell types, stimulates matrix synthesis and contributes to tissue expansion and skeletogenesis. It also helps stabilize the extracellular matrix (ECM) and promotes various tissue repair processes (Costell et al., 1999; French et al., 2002; Gomes et al., 2004; Sadatsuki et al., 2017; Gao et al., 2021; Garcia et al., 2021). In vascular tissues, perlecan has different effects; for example, it promotes angiogenic repair of skin wounds through FGF-2 sequestered by its domain I HS chains (Zhou et al., 2004) but inhibits smooth muscle cell (SMC) proliferation and migration (Koyama et al., 1998; Gotha et al., 2014) (Figures 3, 4).
FIGURE 4. Immunolocalisation of perlecan in a 14-week gestational age human hallux (big toe). Top left panel shows a macroscopic view with boxed regions depicted at higher magnification in subjacent panels (A,B). Right-hand panel shows periodic acid-schiff (PAS) staining of the foetal hallux showing selected regions of its anterior and posterior surfaces. The strong reaction (purple staining) indicates the presence of perlecan HS and other matrix polysaccharides (e.g., hyaluronic acid, HA) within the basement membrane (BM) and epidermis (C,D) of the digit. Images modified from (Smith and Melrose, 2015) with permission. Images © the authors 2015.
In a carotid artery injury model using MΔ3/Δ3 mice lacking perlecan HS chains the medial thickness, medial area/lumen ratio, and macrophage infiltration were significantly increased (Gotha et al., 2014). Perlecan lacking HS side chains has a reduced ability to inhibit SMC proliferation in vitro. The HS side chains of perlecan have critical roles to play in the vessel wall, as an interleukin (IL)-2 receptor for vascular SMCs (Arumugam et al., 2019). IL-2, a 15 kDa immunoregulatory cytokine secreted by T cells, promotes peripheral immune cell growth and development initiating a defensive immune response as an initial response in the wound repair process. While SMC proliferation is inhibited by HS-PGs, digestion of HS chains from perlecan reverses this effect. SMC perlecan is a hybrid HS/CS PG while endothelial perlecan is mono-substituted with HS (Whitelock et al., 2008; Lord et al., 2014; Melrose, 2020). SMCs bind to perlecan core protein only when perlecan’s GAG side chains have been removed. This involves a novel binding site in perlecan domain III, perlecan domain V and α2β1 integrin. Endothelial cells, however, adhere to perlecan core protein containing intact GAG substitution (Whitelock et al., 1999; Lord et al., 2014).
SMC perlecan binds FGF-1 and FGF-2 via HS side chain interactions promoting FGF-2, but not FGF-1 signaling (Lord et al., 2014). Endothelial cell perlecan also binds both FGF isoforms through HS but, in this case, promotes signaling through both FGF-1 and FGF-2. Perlecan differentially regulates cellular proliferation and cell signaling promoted by growth factors to regulate tissue repair processes (Segev et al., 2004; McGrath et al., 2005; Hultgårdh-Nilsson and Durbeej, 2007). The form of perlecan present in tissues can vary with different cell populations assembling variable GAG side chain components in domain I. Perlecan is also subject to post-translational modifications including truncations, mis-sense and point mutations in its core protein and proteolytic modifications leading to the generation of perlecan fragments (Melrose, 2020). Domain IV of full-length perlecan is particularly susceptible to cleavage by matrix metalloproteinases (MMPs) leading to the generation of bioactive domain I and V matricryptic fragments.
Attachment of perlecan to the endothelial cell surface as a dynamic flow sensor at the endothelium-blood interface (Siegel et al., 2014) can also modulate charge density at the endothelial cell surface affecting membrane polarization (Siegel et al., 2014). Shear stress signaling to endothelial cells regulates vascular ECM remodeling and induction of angiogenesis (Russo et al., 2020). Membrane polarization regulates cell proliferation, cell signaling, cytoskeletal organization and gene expression (Gradilla et al., 2018). Cell polarization facilitates cell-cell signalling and is interfaced with stimulatory biophysical forces at the cell surface that regulate cell differentiation and tissue development (Saha et al., 2018). Calcium signalling initiated through transient receptor potential (TRP) endothelial cell channels (Thakore and Earley, 2019) drives vasculogenesis and controls the contractile properties of SMCs, vasodilation and blood pressure (Thompson et al., 2011). Perlecan domain II is a LDL receptor that facilitates lipid clearance from the circulation (Ebara et al., 2000). Membrane de-polymerization resulting from binding of LDL to perlecan leads to vasoconstriction, lowering of cyclic guanosine monophosphate (cGMP) SMC levels and deleteriously contributes to atherosclerosis. Lipid binding to perlecan in bone may also modulate its flow sensory properties and the regulatory properties it conveys to osteocytes (Thompson et al., 2011; Wang, 2018).
Perlecan is localized in the periphery of stem cell niches in foetal cartilage rudiments (Melrose and Melrose, 2016) (Figures 2B–D). Perlecan regulates the attainment of stem cell pluripotency and the development of migratory chondroprogenitor stem cell lineages with roles in diarthrodial joint development, expansion of the cartilage rudiments and development of primary and secondary ossification center precursors to the cartilage growth plate cartilages. These are important features of relevance in potential repair applications that might be developed using perlecan in repair biology.
While perlecan is a component of basement membranes in vascularised tissues it also has a wide distribution in tensional and weight bearing cartilages such as the meniscus, tendon, ligament and intervertebral disc (IVD). These are predominantly avascular tissues devoid of a basement membrane, however the PCM of chondrocytes has been suggested to represent an intrinsic basement membrane around each cell (Kvist et al., 2008). Atomic force microscopy (AFM) studies demonstrate that perlecan imparts compliancy to the PCM and is cytoprotective (Guilak et al., 2021). Cell-ECM interconnections in cartilages and perlecan as a biosensor, facilitates cell-matrix communication allowing cells to perceive and respond to perturbations in their biomechanical microenvironments and to orchestrate tissue homeostasis. Perlecan also monitors the flow of cannalicular fluid in the osteocyte PCM and acts as a mechanosensor that regulates bone development (Thompson et al., 2011; Wang et al., 2014; Wijeratne et al., 2016).
Perlecan has critical roles in basement membrane in the blood brain barrier and important roles in NMJ assembly and function (Figures 5, 6). Perlecan-FGF-2 interactions in the neural stem cell niche (fractone) regulate the survival, proliferation and differentiation of neuroprogenitor stem cell populations in the sub-ventricular and dentate gyrus of the hippocampus (Kerever et al., 2007; Douet et al., 2013; Kerever et al., 2014; Mercier, 2016; Kerever and Arikawa-Hirasawa, 2021; Kerever et al., 2021; Kim et al., 2021).
FIGURE 5. Schematic depiction of perlecan’s role as an integral structural component involved in the assembly and function of the neuromuscular junction (NMJ) showing its interactions with cell surface integrins, type IV, VI, XVIII, ColQ collagens, MuSK (Muscle-Specific Kinase) and dystroglycan (DG) and localization of catalytically active acetylcholinesterase sub-units (A). Other structural components of the NMJ include extracellular components such as matriglycan, neurexin, laminin, type XVIII collagen and agrin and the cell membrane components MuSK, sarcoglycan, dystrophin and sarcospan (B). This schematic is a simplified interpretation of data from the many publications that have outlined the very complex structure and function of the NMJ (Geppert et al., 1992; Crosbie et al., 1999; Arikawa-Hirasawa et al., 2002b; Steen and Froehner, 2003; Rotundo et al., 2008; Sigoillot et al., 2010; Knight et al., 2011; Singhal and Martin, 2011; Ohno et al., 2013; Arredondo et al., 2014; Yoshida-Moriguchi and Campbell, 2015; Banerjee et al., 2017; Cescon et al., 2018; Belhasan and Akaaboune, 2020; Legay and Dobbertin, 2020) and illustrates how mutations in perlecan evident in SJS that result in severely diminished tissue levels of perlecan compromise the functional properties of the NMJ manifesting in the neurological and muscular deficits evident in SJS. A better understanding of the functional basis of the NMJ is also of relevance to synaptic functions in musculoskeletal disorders in general. Perlecan has key roles to play in the assembly, function and regulation of the NMJ (Aldunate et al., 2004; Cartaud et al., 2004; Kimbell et al., 2004; Rotundo et al., 2005; Rudenko, 2017; Südhof, 2018; Noborn and Sterky, 2021). Perlecan has central roles in the clustering of acetylcholinesterase (Ache) at the synaptic basal membrane through formation of a ternary complex with MuSK, DG and ColQ (C). The collagen-tailed form of AChE is localized at the NMJ through interaction with the transmembrane DG complex by binding to perlecan (Kimbell et al., 2004). HS binding domains in ColQ anchor it to the synaptic basal lamina. ColQ-AChE/perlecan complex co-localizes in the NMJ with dystroglycan, rapsyn, laminin and MuSK (Rotundo et al., 2005). MuSK is a receptor tyrosine kinase with important roles to play in the clustering of active AChE sub units at the NMJ in a ternary complex with ColQ and perlecan (Cartaud et al., 2004) of functional importance (Aldunate et al., 2004). Neurexin HS chains also recruit HS-binding proteins required for synaptic assembly and the maintenance of synaptic plasticity (Südhof, 2018; Noborn and Sterky, 2021). A large collection of synaptic adhesion/organizing molecules (SAMs) exist in the mammalian brain with roles in synapse development and maintenance. SAMs, include neurexins, neuroligins, cadherins, and contactins implicated in neuropsychiatric and neurodevelopmental diseases, including autism, schizophrenia, and bipolar disorder (Rudenko, 2017). A greater understanding of the process of synaptic assembly, function and regulation at the molecular level may further the development of novel synaptic therapeutics. A recent publication proposes that HS-PGs are key players in Alzheimer’s disease (AD). In a unifying hypothesis HS-PGs are considered central to all aspects of AD neuropathology, i.e., plaque/tangle development amyloid deposition, neuroinflammation and apolipeprotein E (ApoE) accumulation (Snow et al., 2021).
FIGURE 6. Histochemical localization of a motor neuron attaching to muscle fibre showing the myelinated nerve bundle (MNB), motor endplates (MEP) and striated muscle fibres (SMF) (A). and perlecan-positive striated muscle fibres stained with MAb A7L6 to perlecan domain IV in murine extensor digitorum longus muscle (B). A lower power image depicting perlecan-positive features in a foetal human elbow joint (C). 1) flattened venule, 2) capillary with entrapped red blood cells, 3) small nerve -bundle in cross-section, 4) muscle fibres, 5) longitudinal nerve fibre bundles in ulnar nerve of the elbow. Schematic of the major features of the motor end plate (C). Figure segment a, stock image 2AD3P00 from Alamy Science Photolibrary reproduced under license. (B,C) modified from (Shu et al., 2019) Open Access under CC BY NC-ND License to Publish. (D). A wave of membrane depolarization emanating from the nerve soma produces an action potential that travels down the nerve axon resulting in activation of voltage gated Ca2+ channels in the nerve synapse and a resultant influx of Ca2+. This results in mobilization of synaptic vesicles in the nerve to the post synaptic membrane. These merge with the post-synaptic membrane releasing their neurotransmitter contents which include acetylcholine into the synaptic gap. Acetylcholine is captured by acetylcholine receptors on the muscle synaptic membrane which results in an influx of Na+ ions through Na+ channels into the muscle causing muscular contraction. Acetylcholinesterase released by the nerve mops up any excess of acetylcholine. Acetylcholinesterase is one of nature’s most efficient enzymes and hydrolyses acetylcholine regulating muscular relaxation (Vigny et al., 1978). The myotonia found in SJS is due to a breakdown in this mechanism due to a deficiency of perlecan at the NMJ and a deficiency of AChE that is normally clustered in synapses by perlecan (Silman and Sussman, 2008).
The importance of perlecan to the functional weight bearing and tensile properties of cartilaginous tissues is well illustrated in SJS (Schwartz-Jampel syndrome; chondrodystrophic myotonia) (Arikawa-Hirasawa et al., 2002a) and Dyssegmental dysplasia Silverman-Handmaker type (DDSH) (Arikawa-Hirasawa et al., 2001a; Arikawa-Hirasawa et al., 2001b). The former is a relatively mild skeletal condition characterized by reduced perlecan levels in tissues; however, DDSH is a very severe condition where perlecan levels in tissues are severely depleted or totally absent. SJS is an autosomal recessive disease caused by mutation in the HSPG2 gene resulting in skeletal dysplasia and neuromuscular hyperactivity (Nicole et al., 2000; Bauché et al., 2013). In this condition mutant fibroblasts secrete reduced levels of perlecan and display impaired migratory properties but normal proliferative rates (Arikawa-Hirasawa et al., 2002a). DDSH (MIM 224410) in contrast is a very severe but extremely rare condition caused by functional null mutations in the perlecan HSPG2 gene (Arikawa-Hirasawa et al., 2001a). Less than forty DDSH cases have been reported in the literature, and only four of these were detected prenatally.
Perlecan knockout is a lethal condition. Conventional perlecan knockout (i.e., Hspg2−/−, KO) mice die just after birth mainly due to respiratory failure (Arikawa-Hirasawa et al., 1999; Costell et al., 1999). The few mice that survive to birth display macroscopic abnormalities in cephalic development and have short squat frames with severely distorted axial and appendicular skeletal development. Furthermore, internal examination shows major abnormalities in the development of the major outflow tracts from the heart in these mice. Perlecan-null mice, form normal basement membranes but these soon deteriorate at areas of increased mechanical stress e.g., areas of myocardial contraction and brain vesicle expansion. Perlecan-null mice die around E10–12, due to heart, lung, and brain defects. Weakened embryonic heart basements membranes and “leaky” cardiomyocyte-endothelial cell interfaces result in cardiac arrest due to blood leakage into the pericardial space. Major defects in lung development also contribute to the lethality of perlecan deficiency. Abnormalities in cephalic development, distortion in normal brain laminar architecture and development of holes in the fore- and midbrain also occur. Perlecan-null mice experience severe bleeding in the lung, skin, and brain, due to weakened blood vessels. Perlecan null mice also display distorted growth plate architecture and a disturbed chondrocyte organization with a loss of normal columnar chondrocyte spatial organization and expansion and distortion of the resting, proliferative and hypertrophic zones consistent with the massive disruptions seen macroscopically in skeletal development in this mouse model. Perlecan knock-out mice are not suitable for the examination of perlecan’s roles in postnatal tissue development, but starkly demonstrate the importance of perlecan in the development of pre-natal vascular and non-vascular tissues.
In order to avoid the lethality of perlecan KO mice, conditional perlecan-deficient (Hspg2−/−, TG) mice were developed that express the perlecan transgene only in cartilage using the Col2a1promoter and enhancer (Xu et al., 2010; Xu et al., 2016). These perlecan TG mice develop a phenotype similar to DDSH but also share features of SJS (Arikawa-Hirasawa et al., 2001a; Arikawa-Hirasawa et al., 2002a). Perlecan knockdown impacts on cartilage development and skeletogenesis and impairs the normal weight bearing properties of cartilaginous tissues and functional properties of the pericellular matrix of chondrocytes (Xu et al., 2016; Ocken et al., 2020).
In Hspg2−/−-Tg (Hspg2−/−; Col2a1-Hspg2Tg/−) mice, perlecan is only expressed in cartilage but not the synovium. This results in less development of osteophytic spurs in the tibial and femoral joint margins (Arikawa-Hirasawa et al., 1999; Xu et al., 2010; Ishijima et al., 2012; Kaneko et al., 2013). A perlecan exon 3 null mouse model has also been developed. Perlecan domain-I encoded by exon 3 contains its GAG attachment points thus perlecan exon 3 null mice produce perlecan deficient in HS. A 20 kDa drop in the size of the perlecan core protein is also evident. This model has yielded important information on the role of perlecan HS in the regulation of chondrocyte behavior and in tissue homeostasis and perlecan HS mediated interactions with ECM components (Whitelock et al., 2008; Smith et al., 2010; Hayes et al., 2011a; Hayes et al., 2011b; Hayes et al., 2013; Shu et al., 2013; Hayes et al., 2014; Shu et al., 2016a; Hayes et al., 2016b; Shu et al., 2018; Shu et al., 2019; Smith and Melrose, 2019). This exon 3 null GAG free form of perlecan does not participate in growth factor and morphogen sequestration and cell signaling like the full length GAG substituted form of perlecan resulting in a loss in its ability to act as a co-receptor for the presentation of these growth factors to their cognate receptors (Zhou et al., 2004; Whitelock et al., 2008). However, there are GAG-free regions of the perlecan core protein that can also bind certain growth factors. For example, perlecan domain III can bind FGF-7 and FGF-18, however it is not known to what extent this interaction can provide the same cell proliferative and differentiative properties provided by growth factors that bind to the GAG chains of perlecan domain I. Perlecan exon 3 null HS-deficient mice do not store TGF-β1 in skin tissues like the full-length perlecan does (Shu et al., 2016a). Participation in the wound healing response, previously provided by FGF-2 and VEGF perlecan interactions and the angiogenic responses they provide, is also lost in perlecan exon 3 null mice (Zhou et al., 2004). As previously discussed, full length HS substituted perlecan has major roles in tissue and organ development and wound healing orchestrated by the binding and signaling of mitogens and morphogens with cells in a temporal and dynamic fashion. FGF-7, −18 and PDGF can also bind to perlecan domains III, IV and V, such interactions may also mediate wound healing and cell signaling responses. Binding of PDGF-BB has been mapped to domain III-2 (Kd = 8 nM), lower binding affinities are evident for domains I, IV-1 and V (Kd = 34–64 nM). PDGF-AA binds to domain III-2 (Göhring et al., 1998). Perlecan HS deficiency impairs pulmonary vascular development (Chang et al., 2015). Perlecan HS chains also recruit pericytes to pulmonary vessels. HS deficiency in perlecan attenuates hypoxia-induced pulmonary hypertension involving impaired FGF-2/FGFR1 interactions (Chang et al., 2015). Perlecan exon 3 null mice display reduced healing responses due to impaired FGF-2 and VEGF signaling (Zhou et al., 2004). The chondroprotection evident in perlecan exon 3 null mice in a post traumatic OA model may be attributable to the preservation of FGFR-3-FGF-18 signaling and perlecan domain III-mediated interactions (Shu et al., 2016b), contributing to significantly reduced joint margin osteophytosis, synovial perlecan is required for osteophyte formation in knee OA (Kaneko et al., 2013). Hspg2 exon 3 null murine chondrocytes display increased hypertrophic maturational changes and chondrocyte proliferative rates in vitro and in vivo, accelerated growth plate maturation, elevated GAG deposition, and exostosis formation in the IVD (Shu et al., 2019). Perlecan HS may thus exert repressive control over chondrocytes in mature cartilage explaining why cartilage has such a poor healing response (Garcia et al., 2021).
A model of SJS in which a 4595G to A point mutation occurs in the perlecan gene displays reduced perlecan secretion and incorporation into the PCM similar to the clinical features of human SJS (Rodgers et al., 2007; Stum et al., 2008). Mice homozygous for Hspg2C1532Y-Neo (Neo/Neo) have short stature, impaired mineralization, misshapen bones, OA-like joint dysplasias and myotonia (Rodgers et al., 2007; Stum et al., 2008).
A model of SJS has also been developed that displays congenital peripheral nerve hyper-excitability, neuromyotonia, demyelination and peripheral neuropathies (Bangratz et al., 2012). This model revealed roles for perlecan in the regulation of longitudinal elongation of myelin by Schwann cells (Echaniz-Laguna et al., 2009; Bangratz et al., 2012). Perlecan-deficient mice displaying shorter internodes, had increased levels of impaired functional voltage-gated K (+) channels (Echaniz-Laguna et al., 2009). Electrophysiological studies have demonstrated muscle fiber hyper-excitability arising from such alterations in peripheral nerve organization, muscle hypertrophy and compositional changes (Xu et al., 2010).
Cerebral artery occlusion stroke models in Yucatan miniature pigs, dogs and mice (Platt et al., 2014; Vasquez et al., 2019; Llovera et al., 2021) have facilitated examination of perlecan’s roles in the repair of the blood brain barrier following stroke. Perlecan domain V has neurogenic and neuroprotective properties and promotes angiogenic repair of the blood brain barrier (Lee et al., 2011; Bix, 2013; Marcelo and Bix, 2014). Perlecan transgenic mice have demonstrated important roles for perlecan domain V in pericyte recruitment in the promotion of BBB repair processes (Nakamura et al., 2019). IL-1 is also neuroprotective and has neuron restoring properties in experimental ischemic stroke studies (Salmeron et al., 2019). Recombinant perlecan domain V is now available for blood brain barrier repair strategies (Rnjak-Kovacina et al., 2017). Recombinant perlecan domain V decorated with HS and CS chains is a vascular PG in its own right and supports endothelial cell interactions as well as full-length perlecan (Rnjak-Kovacina et al., 2017). Repair of the blood brain barrier involves pericyte recruitment, triggered by an up-regulation in PDGFRβ (Arimura et al., 2012; Makihara et al., 2015; Shibahara et al., 2020), this drives pericyte migration required for pericyte endothelial tube repair interactions in the neurovascular unit (Hellstrom et al., 1999). Perlecan binds PDGF and promotes pericyte migration and integrin α5β1 and α2β1 mediated interactions in endothelial tube formation (Hellstrom et al., 1999; Arimura et al., 2012; Shen et al., 2012; Makihara et al., 2015; Nakamura et al., 2019).
While perlecan promotes the proliferation and differentiation of endothelial cells, as well as many other cell types, it inhibits SMC proliferation through the tumor repressor PTEN (tumor suppressor phosphatase and tensin homolog), including upregulation of FRNK (focal adhesion kinase–related non-kinase) and down regulation of FAK signalling (Walker et al., 2003; Garl et al., 2004).
HS’s interactions with FGF-2, PDGF, VEGF, HGF, BMP2, GM-CSF, angiopoietin-3, and activin A illustrate the potential of perlecan domain I as a co-receptor for growth factor delivery and receptor activation. The ITIM megakaryocyte-platelet receptor (G6b-B-R) is an additional ligand for the HS chains of perlecan domain I (Vögtle et al., 2019). G6b-B-R is highly expressed in mature megakaryocytes (MKs) that regulates platelet activation (Lord et al., 2018; Vögtle et al., 2019). Binding of G6b-B-R to perlecan HS chains mediates functional responses in MKs and platelets, negatively regulating platelet adhesion to fibrinogen and collagen. It also modulates platelet adhesion to vascular graft materials and explains the varied roles of perlecan in fibrosis (Lord et al., 2009; Lord et al., 2018).
Perlecan attached to endothelial cells in the lumen of blood vessels acts as a shear flow sensor that interacts with Ca2+ or Na+ regulating charge density at the endothelial cell surface. This also regulates membrane polarization in endothelial cells and SMCs, ion transport regulates vasoconstriction and relaxation in blood vessels (Siegel et al., 2014) and is crucial for cell-cell signalling coupled with stimulatory biophysical forces that promote cell differentiation and tissue development (Saha et al., 2018). Calcium signalling through endothelial cell TRP channels (Thakore and Earley, 2019) drives vasculogenesis (Moccia et al., 2019) and SMC contractility which in turn regulates vasodilation and blood pressure (Ishai-Michaeli et al., 1990).
While the HS chains of perlecan mediate growth factor interactions in skeletogenesis, degradation of HS in situ has been shown to improve wound healing through the re-mobilization of sequestered growth factors locally at sites of tissue repair (Ishai-Michaeli et al., 1990; Zcharia et al., 2005; Nasser, 2008). Heparanase expression in osteoblastic cells also promotes bone formation and tissue remodeling at the osteochondral interface during endochondral ossification (Kram et al., 2006).
Given the crucial role that perlecan plays in multiple biological processes, it is unsurprising that perlecan, or its components, have been utilized in multiple therapeutic applications. Perlecan’s known ability to bind, sequester, and deliver a myriad of growth factors and other bioactive molecules is a key feature that has been harnessed to translate this molecule into potential therapeutics for multiple applications, from angiogenesis to the regeneration of cartilage (Gao et al., 2021; Garcia et al., 2021). Perlecan’s ability to modulate processes in cardiovascular applications has been explored through an immune-purified form of perlecan from human coronary arterial endothelial cells. This immunopurified perlecan was used to coat expanded polytetrafluoroethylene (ePTFE) vascular grafts. Implantation of the vascular grafts into the carotid arteries of an ovine model demonstrated that the perlecan-coated grafts reduced platelet adhesion and enhanced endothelial cell growth along the implanted graft when compared with the uncoated vascular graft (Lord et al., 2009). While this study demonstrated the ability for perlecan to improve vascular graft patency, isolation of perlecan from tissues or purified from conditioned medium, can be cost-prohibitive due to the small amounts available and the quantities required.
An alternative option that has been explored is through the use of recombinant fragments of perlecan. The protein core of perlecan contains five domains, many with bioactive properties, though use of recombinant perlecan fragments has focused on the use of domain-I and -V due to these domains containing GAG attachment sites. The protein component of perlecan domain I contains three GAG attachment sites. The GAGs that decorate the perlecan domain I core protein are predominantly HS, though it may also be decorated with CS. Recombinant perlecan domain I decorated with HS has been incorporated into 3D structures or scaffolds for multiple therapeutic applications. The incorporation of perlecan domain-I into 3D structures has resulted in increased retention of FGF-2 (Yang et al., 2005), as well as BMP2 (Jha et al., 2009; Srinivasan et al., 2012; Chiu et al., 2016) for cartilage repair and regeneration. More recently, advances in fabrication and microfluidics has enabled the ability to produce growth factor gradients (Hubka et al., 2019), an approach that has been utilized to generate chemotactic gradients with FGF-2R The ability to create gradients using perlecan, perlecan fragments and other bioactive components of the ECM, has significant potential in tissue repair and regeneration, including the development of smart biomaterials and constructs. It will also greatly improve the understanding of many developmental and disease processes, e.g., in cancer biology.
As mentioned above, perlecan domain V, like domain I, contains a GAG attachment site. Recombinant perlecan has been explored in several applications due to its growth factor interactions. Recombinant perlecan domain V is substituted with HS and CS chains and promotes angiogenesis by enhancing growth factor signaling (Lin et al., 2020). When perlecan or perlecan DNA is immobilized on silk or chitosan scaffolds (Lord et al., 2017) increased vascular ingrowth and integration in vivo underlies the importance of perlecan in angiogenesis/vasculogenesis.
The complexity and nuances of perlecan’s ability to modulate biological processes has been explored by immobilization technique, and the presence of GAG chains plays a key role in the orientation of this PG (Rnjak-Kovacina et al., 2016). Immobilization of perlecan domain V by physisorption or covalently, in addition with immobilization of the protein core of perlecan domain V only, or protein core decorated with GAG chains, modulated the interaction and attachment of both endothelial cells and platelets. The ability to control the interaction of different cell types holds tremendous importance to tissue repair, for example in cardiac and vascular repair procedures where tissue grafts and implants, require cues to facilitate migration and re-endothelization of the repair tissue, whist minimizing platelet adhesion. The potential of perlecan domain V to modulate platelet adhesion has been explored by incorporating this domain onto different polymeric surfaces including poly (vinyl chloride) (Chandrasekar et al., 2021), silk (Lau et al., 2021) and chitosan (Lord et al., 2017). Incorporation of perlecan domain I plasmid DNA in conjunction with VEGF189 in a rodent wound model demonstrated increased re-epithelialization of the wound, formation of sub-endothelial tissue and neo-angiogenesis within the wound bed (Lord et al., 2017).
Peptides derived from perlecan domain IV (TWSKV), laminin-111 (YIGSR, IKVAV), and fibronectin (RGDSP) have been incorporated into HA scaffolds with RGDSP and TWSKV peptide HA scaffolds significantly accelerating cell proliferation (Fowler et al., 2021). Perlecan peptide TWSKV triggers differentiation of salivary gland cells into self-assembling acini-like structures expressing salivary gland biomarkers that secrete α-amylase (Pradhan et al., 2009; Pradhan et al., 2010). Purified ECM-derived peptides have been suggested as stimulatory molecules that direct the proliferation and differentiation of progenitor cell populations. These are of potential application in tissue repair processes using human embryonic stem cells (hESCs) and induced pluripotent stem cells (iPSCs) (Rowland et al., 2013). Laminin-111 peptide fibrin hydrogels restore salivary gland function (Nam et al., 2017). An extensive range of laminin-111-derived peptides conjugated to chitosan scaffolds also show promise in tissue engineering applications designed to promote tissue regeneration (Hozumi et al., 2012). Laminin-111 peptide-HA hydrogels have also been shown to act as a synthetic basement membrane (Yamada et al., 2013). Conjugation of RGDSP peptide to HA gels improves cell viability, accelerates formation of epithelial spheroids, and promotes the expansion of 3D progenitor cell populations, representing the first step toward the development of an engineered salivary gland (Fowler et al., 2021). Primary salivary human progenitor stem cells undergo acinar-like differentiation in HA hydrogel cultures and incorporation of basement membrane peptides derived from perlecan and laminin-111 further directs the development of 3D salivary gland-like spheroids (Srinivasan et al., 2017). Full-length perlecan also has directive roles over the 3D development of submandibular salivary glands. Heparanase colocalizes with perlecan in submandibular gland basement membrane. Cleavage of perlecan HS side chains by heparanase regulates salivary gland branching morphogenesis by modulating FGF-10 mediated cell signaling. Heparanase releases FGF-10 from perlecan HS in the basement membrane. This increases MAPK signaling, epithelial clefting, and lateral branching thus increasing submandibular gland branching morphogenesis (Patel et al., 2007). The size and sulfation patterns of the perlecan HS side chains regulate FGF-10-mediated interactions during proliferation, salivary gland duct elongation, bud expansion, and differentiation. The spatio-temporal localization of specific HS structures in salivary tissues provides a mechanistic insight as to how salivary gland developmental processes mediated by FGF10 occur in vivo (Patel et al., 2008). FGF-10 is a multi-functional paracrine growth factor that mediates mesenchymal-epithelial signaling during tissue development, growth and disease and has significant relevance to regenerative medicine (Itoh and Ohta, 2014; Itoh, 2016).
Once damaged, articular cartilage has a notoriously poor ability to repair itself (Armiento et al., 2019). Perlecan’s important well-established roles in chondrogenesis and cartilage development (Smith et al., 2010; Melrose et al., 2012) imply potential roles in cartilage repair by recapitulating developmental roles in damaged/diseased tissues (Gao et al., 2021; Garcia et al., 2021). Perlecan promotes chondroprogenitor stem cell maturation and development of pluripotent migratory stem cell lineages with roles in diarthrodial joint formation and early cartilage development (Hayes et al., 2016a; Melrose and Melrose, 2016). Perlecan’s cartilage stabilizing properties through interactions with ECM components also establish its potential in cartilage repair (SundarRaj et al., 1995; Gomes et al., 2002; Melrose et al., 2006; Melrose et al., 2008). Perlecan domain I hydrogels have been used to establish heparin binding growth factor gradients that promote cell migration of potential use to promote cartilage repair (Hubka et al., 2019). Perlecan domain I BMP-2 hydrogels have also been shown to promote chondrogenesis and cartilage repair in a murine early OA model (Yang et al., 2006; Jha et al., 2009; Srinivasan et al., 2012). BMP-2 and BMP-9 can also promote chondrogenic differentiation of human multipotential mesenchymal stem cells (Majumdar et al., 2001; Shestovskaya et al., 2021). Perlecan domain I collagen I fibril scaffolds have been used as an FGF-2 delivery system that could also be useful in cartilage repair strategies (Yang et al., 2005). FGF-2 directs non-committed mesenchymal stem cells to a chondrogenic phenotype (Shu et al., 2016c).
The duration of synaptic transmission of impulses at the NMJ is chiefly controlled by acetylcholinesterase (AChE), an enzyme which hydrolyzes acetylcholine (ACh) to choline and acetate (Katz and Miledi, 1973; Rosenberry, 1979). Perlecan, nidogens, glycoproteins and collagen fill the synaptic cleft and are relevant for signal transduction as well as the maintenance of mechanical strength in the NMJ. At the NMJ, the most abundant form of AChE is the AChET which is anchored to the synaptic basal lamina by non-fibrillar collagen Q (ColQ) (Krejci et al., 1991; Krejci et al., 1997). ColQ, a triple helical structure, binds to perlecan domain 1 via two heparan sulfate PG binding motifs and to muscle specific kinase (MuSK) respectively (Rotundo et al., 1997; Casanueva et al., 1998; Deprez et al., 2003; Cartaud et al., 2004; Nakata et al., 2013).
Experimental reports demonstrate the functional relationship between perlecan and AChE (Peng et al., 1999; Arikawa-Hirasawa et al., 2002b; Massoulié and Millard, 2009; Maselli et al., 2012) and the cellular co-localization of perlecan and AChE at the synaptic basal lamina in vitro and in pre-clinical models of the NMJ (Peng et al., 1999; Arikawa-Hirasawa et al., 2002b; Massoulié and Millard, 2009; Maselli et al., 2012). Perlecan null mice lack AChE clusters at the NMJ (Peng et al., 1999; Martinez-Pena y Valenzuela et al., 2007).
Ischemic stroke is a leading cause of permanent disability and mortality and there is an urgent need for adjunctive therapies for concurrent administration with existing clot-busting (tissue plasminogen activator, tPA) and endovascular clot removal (thrombectomy) treatments. Whilst these primary treatments remove the underlying cause of the stroke, they do little to reverse or prevent subsequent brain injury. Perlecan domain V is potentially one such therapy whose efficacy has been experimentally demonstrated in both young and aged rodent models of ischemic stroke, where it has been reported to have neuroprotective, neuroreparative and functionally restorative effects (Lee et al., 2011; Bix et al., 2013; Trout et al., 2021). These benefits of perlecan domain V have been linked to its maintenance of the BBB through α5β1 integrin modulation and increased VEGF secretion (Clarke et al., 2012; Nakamura et al., 2019). Bix and colleagues showed that brain expression of perlecan is substantially and chronically increased following experimental and clinical ischemic stroke (Lee et al., 2011; Bix et al., 2013; Trout et al., 2021). This observation has led to the hypothesis that perlecan plays a significant role in brain repair following ischemic insult. Accordingly, larger infarct volumes and severe motor-sensory deficits in transgenic mice with only 10% of total perlecan bioavailability (perlecan hypomorphs), when compared to age-matched wild type controls, have been independently reported and corroborated (Lee et al., 2011; Nakamura et al., 2019). This suggests that physiologic levels of perlecan are important for the brain to repair itself after stroke, and that diminished perlecan levels are detrimental for stroke outcomes. It follows that exogenously administered perlecan domain V would enhance the brain’s ability in self-repair by increasing the availability of perlecan for BBB maintenance, thereby improving brain injury and functional deficits following ischemic stroke.
Perlecan promotes blood brain barrier repair following acute brain injury by modulating the survival and migration of PDGFRβ-positive pericytes to the ischemic brain region (Arimura et al., 2012; Shen et al., 2012; Nakamura et al., 2016; Hoffmann et al., 2018; Nakamura et al., 2019). Perlecan domain V binds to PDGFRβ and α5β1 in order to maintain blood brain integrity in the injured brain area (Nakamura et al., 2019) Also, following ischemic brain injury, α5β1 integrin expression increases and the exogenously administered perlecan domain V binds to this integrin on endothelial cells to bring about the increased secretion of VEGF (Clarke et al., 2012). The elevated levels of VEGF enables angiogenesis via the downstream activation of PI3K-Akt, MEK-ERK and HIF-1α (Milner et al., 2008; Clarke et al., 2012). Surprisingly, the increased VEGF does not worsen the BBB disruption following perlecan domain V administration after ischemic stroke (Lee et al., 2011). The intraperitoneal administration of perlecan domain V decreases infarct volume as well as immuno-expression of markers of apoptosis (TUNEL and Caspase 3 cleavage) (Lee et al., 2011). More so, perlecan domain V modulates the activation and proliferation of astrocytes and ameliorates astrogliosis in vitro and in vivo. This indicates that perlecan domain V rescues neuronal death and may actively dampen scar formation after ischemic stroke suggesting it has instructive regulatory properties in tissue fibrosis (Lord et al., 2018). Matricryptin peptides derived from ECM components also have roles in tissue remodeling (Amruta et al., 2020; de Castro Brás and Frangogiannis, 2020). The in vivo benefits of perlecan domain V have been evaluated in rodents using various models of ischemic using middle cerebral artery occlusion which includes tandem ipsilateral common carotid and middle cerebral arteries occlusion (CCA/MCAO) model that induces mild cortical lesion (Trout et al., 2021), endothelin (ET)-1 injection (mild to moderate brain lesion) (Lee et al., 2011), photothrombotic (Bix et al., 2013) and intraluminal filament MCAO model -severe brain injury.
The therapeutic potential of exogenously administered perlecan domain V in a severe pre-clinical ischemic stroke model (photothrombotic and intraluminal MCAO) is essentially dependent on how quickly the first dose of perlecan domain V is administered after ischemic stroke induction. A recent report by Nakamura et al. (Nakamura et al., 2019) found mild effects of exogenously administered recombinant perlecan domain V (5 mg/kg) on infarct volume after intraluminal MCAO induction. Perlecan domain V was administered 24 h after reperfusion and daily for three to four consecutive days. However, Bix and colleagues had previously reported that the timely administration of perlecan domain V (2 mg/kg) after severe ischemic stroke (photothrombotic model) is vital for neuroprotective benefits (Clarke et al., 2012). Indeed, the specific report indicates that when perlecan domain V is administered 3 hours after stroke induction, infarct volume is smaller when compared to perlecan domain V administration when commenced six, twelve and 24 hours post-stroke induction. Importantly, infarct volume increases as the start of perlecan domain V treatment after stroke induction period increases. Additionally, recent findings show a significant decrease in infarct volume when perlecan domain V was co-administered during reperfusion in a mouse model of intraluminal MCAO (Biose et al., 2021).
Early reports have shown that human recombinant LG3, the C-terminal-most portion of perlecan domain V, may confer the neuroprotective efficacy of full length perlecan domain V (Saini and Bix, 2012; Bix, 2013). Like domain V, LG3 is increased in the compromised brain hemisphere after 24 hours of transient MCAO and it persistently remains elevated for 3 days after stroke in rats.3 Similarly, 2 hours of oxygen-glucose deprivation (OGD) in astrocytes and neuron cultures substantially increases LG3 levels. LG3 is anti-apoptotic as it decreases Caspase 3 after OGD-reperfusion (Lee et al., 2011; Saini and Bix, 2012). Also, LG3 preserved the integrity of fetal cortical neurons by reducing Caspase 3 immunohistochemistry after in vitro OGD when compared to control dishes (Saini and Bix, 2012). Investigations are ongoing to determine the effect of LG3 on infarct volume and motor-sensory functions in various preclinical models of ischemic stroke (Biose et al., 2021).
In summary, perlecan is actively processed for brain repair after ischemic stroke and is neuroprotective, improves angiogenesis and motor-sensory functions and modulates astrocyte activity when administered in a timely manner following stroke. Furthermore, LG3 which confers the biological activity of perlecan domain V in infarct volume reduction may have potential adjunctive stroke therapeutic value in preventing mortality among clinical stroke patients (Biose et al., 2021).
Perlecan is a ubiquitous, pleomorphic, and morphologically important PG with roles in many physiological processes in tissues of varied form and function. A greater understanding of perlecan’s tissue specific roles during growth, development and disease will be insightful as to how this multifunctional PG might be utilized to maximal effect in repair biology. The current biological understanding of perlecan demonstrates its enormous capacity as a “fix-it” molecule with many beneficial attributes (e.g., matrix stabilization, cell guidance and general tissue development) that could be potentially harnessed in repair biology. Emerging improvements in implant design, therapeutic blood brain barrier repair strategies following stroke, modeling of artificial neural stem cell niches and neuromuscular synaptic interface tissues may find application in the treatment of neurodegenerative disorders (Wang et al., 2013; Gill et al., 2015; Buzanska et al., 2018). Acetylcholine is a cholinergic agonist and chief neurotransmitter of the parasympathetic autonomic nervous system that contracts smooth muscle. Efficient regulation of acetylcholine is essential and achieved by acetyl cholinesterase located in the NMJ allowing muscle to undergo relaxation following contraction. Dysregulation of this process can have far-reaching and potentially fatal consequences with a cessation of activity of muscle groups that control breathing. As shown in this review perlecan has key roles in the assembly of the NMJ through interactions with type VI collagen (Cescon et al., 2018), Coll-Q, MuSK and dystroglycan (Peng et al., 1999) responsible for localization of acetylchinesterase in the NMJ (Rotundo et al., 2005; Rotundo et al., 2008; Rudenko, 2017). Acetylcholinesterase is one of the most rapidly acting enzymes in the human body with extremely high catalytic efficiency. Mutations in the perlecan gene (HSPG2) have been identified in two classes of musculoskeletal conditions in the relatively mild Schwartz-Jampel syndrome and more severe but relatively rare neonatal lethal dyssegmental dysplasia, Silverman-Handmaker type (Arikawa-Hirasawa et al., 2001a; Arikawa-Hirasawa et al., 2001b; Arikawa-Hirasawa et al., 2002a). A unique mutation in perlecan in Schwartz-Jampel syndrome compromises the biomechanical competence of the intervertebral disc and is also associated with myotonia and various degrees of chondroplasia (Lin et al., 2021). We have also shown perlecan’s essential roles in the development of cartilaginous components with essential roles in endochondral ossification and development of the axial and appendicular skeleton. A greater understanding of the regulation of acetylcholinesterase in the NMJ is of relevance to research into how muscular dystrophy, muscular sclerosis and the erratic muscular movements of Parkinson’a disease occur (Jacobson et al., 2001; Aldunate et al., 2004). Changes in the basement membrane have also been observed in these and related medical conditions (Jayadev and Sherwood, 2017; Pozzi et al., 2017; Xu et al., 2018; Johnson, 2019; Punga et al., 2022). Perlecan is a functional component of basement membranes and its application in the repair of basal structures in the blood brain barrier following ischemic stroke continue to improve the recovery of this important structure (Bix, 2013; Bix et al., 2013). Synaptic HS-PGs have also now been identified with important roles in the regulation of neural responses. Incorrect assembly or distribution of these synaptic HS-PGs occurs in a number of neurological diseases showing their relevance to disease modification (Knight et al., 2011; Banerjee et al., 2017; Noborn and Sterky, 2021). Interest in the electro-conductive properties of GAGs in the design of dopant films in artificial synaptic membranes along with significant improvements in organic microelectronics and micro fabrication have led to improvement in functional artificial synapses capable of regulating neural responses (Wang et al., 2013; Gill et al., 2015; Buzanska et al., 2018). Artificial synapses that mimic biological synapse neuron-driven interactions have been proposed as next-generation memristor information systems driven by low energy requirements, high energy efficiency and fast computing speed (Liu et al., 2020; Zeng et al., 2021). These offer exciting possibilities in functional neural repair following trauma or disease. Basic studies on the biology of perlecan are thus warranted to optimally utilise recombinant perlecan domains for growth factor delivery and matrix stabilisation and in the regulation of cellular activity in many therapeutic procedures. Studies of this sort are likely to discover properties of perlecan of potential benefit to a diverse range of applications in repair biology. It is worthwhile noting that perlecan encodes significant biological information both in its core protein and attached HS side chains. A cursory examination of the HS interactome and advances in GAG analysis methodology amply demonstrate the biodiversity and interactive capability of HS interactions at the cell surface and their potential to control cell behaviour (Gómez Toledo et al., 2021; Szekeres et al., 2021; Vallet et al., 2021). Much still needs to be learned of the complex information conveyed by the GAG glycocode and how this information translates to the cellular mechanisms that drive tissue processes during development, growth, and disease. However, elucidation of the first draft of the GAG-protein interactome (Vallet et al., 2021) shows that this system has significant potential in the area of tissue repair processes and these useful properties are slowly becoming understood (Melrose, 2016; Hayes and Melrose, 2020). For example, perlecan domain I has been used as a FGF-2 and BMP-2 delivery vehicle in tissue repair strategies (Jha et al., 2009; Srinivasan et al., 2012). Recombinant perlecan domain V, a HS and CS substituted PG in its own right with equivalent stimulatory properties to full length perlecan (Lord and Whitelock, 2013), also shows promise in the repair of vascularized and cartilaginous tissues (Lord and Whitelock, 2013; Lord et al., 2014; Lin et al., 2020; Lord et al., 2020). Perlecan domains I and V thus offer exciting possibilities in repair biology that will continue to emerge and are eagerly anticipated.
JM conceptualised the review, organised and reviewed contributions from all the authors and finalised the final version of the manuscript. BF contributed information on perlecan biology and its application in vascular repair and edited drafts. IF and GB contributed information on neural repair and edited all drafts of the manuscript. AH had input to the writing of all sections of the manuscript and edited all versions of the manuscript. All authors agreed to the final version of the manuscript.
This work was supported by funding to JM from NHMRC Australia (NHMRC Project Grant 512167), Arthritis Foundation of Australia (Win Dunne Fund), Arthritis Australia (Jack Bloomfield Fund) and University of NSW Engineering Faculty Grant support.
Author JM has received consultancy fees from ARTHOPHARM and SYLVAN Pharmaceuticals, Bondi, NSW, Australia.
The remaining authors declare that the research was conducted in the absence of any commercial or financial relationships that could be construed as a potential conflict of interest.
All claims expressed in this article are solely those of the authors and do not necessarily represent those of their affiliated organizations, or those of the publisher, the editors and the reviewers. Any product that may be evaluated in this article, or claim that may be made by its manufacturer, is not guaranteed or endorsed by the publisher.
Aldunate, R., Casar, J. C., Brandan, E., and Inestrosa, N. C. (2004). Structural and Functional Organization of Synaptic Acetylcholinesterase. Brain Res. Brain Res. Rev. 47 (1-3), 96–104. doi:10.1016/j.brainresrev.2004.07.019
Allen, J., Brachvogel, B., Farlie, P., Fitzgerald, J., and Bateman, J. (2008). The Extracellular Matrix Protein WARP Is a Novel Component of a Distinct Subset of Basement Membranes. Matrix Biol. 27 (4), 295–305. doi:10.1016/j.matbio.2008.01.005
Allen, J. M., Bateman, J. F., Hansen, U., Wilson, R., Bruckner, P., Owens, R. T., et al. (2006). WARP Is a Novel Multimeric Component of the Chondrocyte Pericellular Matrix that Interacts with Perlecan. J. Biol. Chem. 281 (11), 7341–7349. doi:10.1074/jbc.m513746200
Allen, J. M., Zamurs, L., Brachvogel, B., Schlötzer-Schrehardt, U., Hansen, U., Lamandé, S. R., et al. (2009). Mice Lacking the Extracellular Matrix Protein WARP Develop Normally but Have Compromised Peripheral Nerve Structure and Function. J. Biol. Chem. 284 (18), 12020–12030. doi:10.1074/jbc.m806968200
Amruta, N., Rahman, A. A., Pinteaux, E., and Bix, G. (2020). Neuroinflammation and Fibrosis in Stroke: The Good, the Bad and the Ugly. J. Neuroimmunology 346, 577318. doi:10.1016/j.jneuroim.2020.577318
Arikawa-Hirasawa, E., Le, A. H., Nishino, I., Nonaka, I., Ho, N. C., Francomano, C. A., et al. (2002). Structural and Functional Mutations of the Perlecan Gene Cause Schwartz-Jampel Syndrome, with Myotonic Myopathy and Chondrodysplasia. Am. J. Hum. Genet. 70 (5), 1368–1375. doi:10.1086/340390
Arikawa-Hirasawa, E., Rossi, S. G., Rotundo, R. L., and Yamada, Y. (2002). Absence of Acetylcholinesterase at the Neuromuscular Junctions of Perlecan-Null Mice. Nat. Neurosci. 5 (2), 119–123. doi:10.1038/nn801
Arikawa-Hirasawa, E., Watanabe, H., Takami, H., Hassell, J. R., and Yamada, Y. (1999). Perlecan Is Essential for Cartilage and Cephalic Development. Nat. Genet. 23 (3), 354–358. doi:10.1038/15537
Arikawa-Hirasawa, E., Wilcox, W. R., Le, A. H., Silverman, N., Govindraj, P., Hassell, J. R., et al. (2001). Dyssegmental Dysplasia, Silverman-Handmaker Type, Is Caused by Functional Null Mutations of the Perlecan Gene. Nat. Genet. 27 (4), 431–434. doi:10.1038/86941
Arikawa-Hirasawa, E., Wilcox, W. R., and Yamada, Y. (2001). Dyssegmental Dysplasia, Silverman-Handmaker Type: Unexpected Role of Perlecan in Cartilage Development. Am. J. Med. Genet. 106 (4), 254–257. doi:10.1002/ajmg.10229
Arimura, K., Ago, T., Kamouchi, M., Nakamura, K., Ishitsuka, K., Kuroda, J., et al. (2012). PDGF Receptor β Signaling in Pericytes Following Ischemic Brain Injury. Cnr 9, 1–9. doi:10.2174/156720212799297100
Armiento, A. R., Alini, M., and Stoddart, M. J. (2019). Articular Fibrocartilage - Why Does Hyaline Cartilage Fail to Repair? Adv. Drug Deliv. Rev. 146, 289–305. doi:10.1016/j.addr.2018.12.015
Arredondo, J., Lara, M., Ng, F., Gochez, D. A., Lee, D. C., Logia, S. P., et al. (2014). COOH-terminal Collagen Q (COLQ) Mutants Causing Human Deficiency of Endplate Acetylcholinesterase Impair the Interaction of ColQ with Proteins of the Basal Lamina. Hum. Genet. 133 (5), 599–616. doi:10.1007/s00439-013-1391-3
Arumugam, P., Carroll, K. L., Berceli, S. A., Barnhill, S., and Wrenshall, L. E. (2019). Expression of a Functional IL-2 Receptor in Vascular Smooth Muscle Cells. J.I. 202 (3), 694–703. doi:10.4049/jimmunol.1701151
Badaut, J., and Bix, G. J. (2014). Vascular Neural Network Phenotypic Transformation after Traumatic Injury: Potential Role in Long-Term Sequelae. Transl. Stroke Res. 5, 394–406. doi:10.1007/s12975-013-0304-z
Banerjee, S., Venkatesan, A., and Bhat, M. A. (2017). Neurexin, Neuroligin and Wishful Thinking Coordinate Synaptic Cytoarchitecture and Growth at Neuromuscular Junctions. Mol. Cell Neurosci. 78, 9–24. doi:10.1016/j.mcn.2016.11.004
Bangratz, M., Sarrazin, N., Devaux, J., Zambroni, D., Echaniz-Laguna, A., René, F., et al. (2012). A Mouse Model of Schwartz-Jampel Syndrome Reveals Myelinating Schwann Cell Dysfunction with Persistent Axonal Depolarization In Vitro and Distal Peripheral Nerve Hyperexcitability when Perlecan Is Lacking. Am. J. Pathol. 180 (5), 2040–2055. doi:10.1016/j.ajpath.2012.01.035
Bauché, S., Boerio, D., Davoine, C. S., Bernard, V., Stum, M., Bureau, C., et al. (2013). Peripheral Nerve Hyperexcitability with Preterminal Nerve and Neuromuscular junction Remodeling Is a Hallmark of Schwartz-Jampel Syndrome. Neuromuscul. Disord. 23 (12), 998–1009. doi:10.1016/j.nmd.2013.07.005
Belhasan, D. C., and Akaaboune, M. (2020). The Role of the Dystrophin Glycoprotein Complex on the Neuromuscular System. Neurosci. Lett. 722, 134833. doi:10.1016/j.neulet.2020.134833
Bengtsson, E., Mörgelin, M., Sasaki, T., Timpl, R., Heinegård, D., and Aspberg, A. (2002). The Leucine-Rich Repeat Protein PRELP Binds Perlecan and Collagens and May Function as a Basement Membrane Anchor. J. Biol. Chem. 277 (17), 15061–15068. doi:10.1074/jbc.m108285200
Biose, I. J., Rutkai, I., Adkisson, D., and Clossen, B. (2021). Perlecan LG3 Is Neuroprotective and Functionally Restorative in Experimental Ischemic Stroke. Stroke 52, AP755. doi:10.1161/str.52.suppl_1.p755
Bix, G. J., Gowing, E. K., and Clarkson, A. N. (2013). Perlecan Domain V Is Neuroprotective and Affords Functional Improvement in a Photothrombotic Stroke Model in Young and Aged Mice. Transl. Stroke Res. 4 (5), 515–523. doi:10.1007/s12975-013-0266-1
Bix, G. J. (2013). Perlecan Domain V Therapy for Stroke: a beacon of hope? ACS Chem. Neurosci. 4 (3), 370–374. doi:10.1021/cn300197y
Brown, J. C., Sasaki, T., Gohring, W., Yamada, Y., and Timpl, R. (1997). The C-Terminal Domain V of Perlecan Promotes Beta1 Integrin-Mediated Cell Adhesion, Binds Heparin, Nidogen and Fibulin-2 and Can Be Modified by Glycosaminoglycans. Eur. J. Biochem. 250, 39–46. doi:10.1111/j.1432-1033.1997.t01-1-00039.x
Buzanska, L., Zychowicz, M., and Kinsner-Ovaskainen, A. (2018). Bioengineering of the Human Neural Stem Cell Niche: A Regulatory Environment for Cell Fate and Potential Target for Neurotoxicity. Results Probl. Cel Differ 66, 207–230. doi:10.1007/978-3-319-93485-3_9
Cartaud, A., Strochlic, L., Guerra, M., Blanchard, B., Lambergeon, M., Krejci, E., et al. (2004). MuSK Is Required for Anchoring Acetylcholinesterase at the Neuromuscular junction. J. Cel Biol 165 (4), 505–515. doi:10.1083/jcb.200307164
Casanueva, O. I., Deprez, P., Garcı́a-Huidobro, T., and Inestrosa, N. C. (1998). At Least Two Receptors of Asymmetric Acetylcholinesterase Are Present at the Synaptic Basal Lamina ofTorpedoElectric Organ. Biochem. Biophysical Res. Commun. 250 (2), 312–317. doi:10.1006/bbrc.1998.9303
Cescon, M., Gregorio, I., Eiber, N., Borgia, D., Fusto, A., Sabatelli, P., et al. (2018). Collagen VI Is Required for the Structural and Functional Integrity of the Neuromuscular junction. Acta Neuropathol. 136 (3), 483–499. doi:10.1007/s00401-018-1860-9
Chandrasekar, K., Farrugia, B. L., Johnson, L., Marks, D., Irving, D., Elgundi, Z., et al. (2021). Effect of Recombinant Human Perlecan Domain V Tethering Method on Protein Orientation and Blood Contacting Activity on Polyvinyl Chloride. Adv. Healthc. Mater. 10 (14), 2100388. doi:10.1002/adhm.202100388
Chang, Y.-T., Tseng, C.-N., Tannenberg, P., Eriksson, L., Yuan, K., de Jesus Perez, V. A., et al. (2015). Perlecan Heparan Sulfate Deficiency Impairs Pulmonary Vascular Development and Attenuates Hypoxic Pulmonary Hypertension. Cardiovasc. Res. 107 (1), 20–31. doi:10.1093/cvr/cvv143
Chiu, Y.-C., Fong, E. L., Grindel, B. J., Kasper, F. K., Harrington, D. A., and Farach-Carson, M. C. (2016). Sustained Delivery of Recombinant Human Bone Morphogenetic Protein-2 from Perlecan Domain I - Functionalized Electrospun Poly (ε-Caprolactone) Scaffolds for Bone Regeneration. J. Exp. Ortop 3 (1), 25. doi:10.1186/s40634-016-0057-1
Clarke, D. N., Al Ahmad, A., Lee, B., Parham, C., Auckland, L., Fertala, A., et al. (2012). Perlecan Domain V Induces VEGf Secretion in Brain Endothelial Cells through Integrin α5β1 and ERK-dependent Signaling Pathways. PLoS One 7, e45257. doi:10.1371/journal.pone.0045257
Costell, M., Gustafsson, E., Aszódi, A., Mörgelin, M., Bloch, W., Hunziker, E., et al. (1999). Perlecan Maintains the Integrity of Cartilage and Some Basement Membranes. J. Cel Biol 147 (5), 1109–1122. doi:10.1083/jcb.147.5.1109
Crosbie, R. H., Lebakken, C. S., Holt, K. H., Venzke, D. P., Straub, V., Lee, J. C., et al. (1999). Membrane Targeting and Stabilization of Sarcospan Is Mediated by the Sarcoglycan Subcomplex. J. Cel Biol 145 (1), 153–165. doi:10.1083/jcb.145.1.153
de Castro Brás, L., and Frangogiannis, N. G. (2020). Extracellular Matrix-Derived Peptides in Tissue Remodeling and Fibrosis. Matrix Biol. 91-92, 176–187. doi:10.1016/j.matbio.2020.04.006
Deprez, P., Inestrosa, N. C., and Krejci, E. (2003). Two Different Heparin-Binding Domains in the Triple-Helical Domain of ColQ, the Collagen Tail Subunit of Synaptic Acetylcholinesterase. J. Biol. Chem. 278, 23233–23242. doi:10.1074/jbc.m301384200
Douet, V., Kerever, A., Arikawa‐Hirasawa, E., and Mercier, F. (2013). Fractone‐heparan Sulphates Mediate FGF‐2 Stimulation of Cell Proliferation in the Adult Subventricular Zone. Cell Prolif. 46 (2), 137–145. doi:10.1111/cpr.12023
Ebara, T., Conde, K., Kako, Y., Liu, Y., Xu, Y., Ramakrishnan, R., et al. (2000). Delayed Catabolism of apoB-48 Lipoproteins Due to Decreased Heparan Sulfate Proteoglycan Production in Diabetic Mice. J. Clin. Invest. 105, 1807–1818. doi:10.1172/jci8283
Echaniz-Laguna, A., Rene, F., Marcel, C., Bangratz, M., Fontaine, B., Loeffler, J.-P., et al. (2009). Electrophysiological Studies in a Mouse Model of Schwartz-Jampel Syndrome Demonstrate Muscle Fiber Hyperactivity of Peripheral Nerve Origin. Muscle Nerve 40 (1), 55–61. doi:10.1002/mus.21253
Edwards, D. N., and Bix, G. J. (2019). Roles of Blood-Brain Barrier Integrins and Extracellular Matrix in Stroke. Am. J. Physiology-Cell Physiol. 316, C252–C263. doi:10.1152/ajpcell.00151.2018
Edwards, D. N., and Bix, G. J. (2019). The Inflammatory Response after Ischemic Stroke: Targeting β2 and β1 Integrins. Front. Neurosci. 13, 540. doi:10.3389/fnins.2019.00540
Farach-Carson, M. C., Brown, A. J., Lynam, M., Safran, J. B., and Carson, D. D. (2008). A Novel Peptide Sequence in Perlecan Domain IV Supports Cell Adhesion, Spreading and FAK Activation. Matrix Biol. 27 (2), 150–160. doi:10.1016/j.matbio.2007.09.007
Farach-Carson, M. C., and Carson, D. D. (2007). Perlecan a Multifunctional Extracellular Proteoglycan Scaffold. Glycobiology 17 (9), 897–905. doi:10.1093/glycob/cwm043
Fitzgerald, J. (2020). WARP: A Unique Extracellular Matrix Component of Cartilage, Muscle, and Endothelial Cell Basement Membranes. Anat. Rec. 303 (6), 1619–1623. doi:10.1002/ar.24087
Fowler, E. W., Ravikrishnan, A., Witt, R. L., Pradhan-Bhatt, S., and Jia, X. (2021). RGDSP-decorated Hyaluronate Hydrogels Facilitate Rapid 3D Expansion of Amylase-Expressing Salivary Gland Progenitor Cells. ACS Biomater. Sci. Eng. 7 (12), 5749–5761. doi:10.1021/acsbiomaterials.1c00745
French, M. M., Gomes, R. R., Timpl, R., Höök, M., Czymmek, K., Farach-Carson, M. C., et al. (2002). Chondrogenic Activity of the Heparan Sulfate Proteoglycan Perlecan Maps to the N-Terminal Domain I. J. Bone Miner Res. 17 (1), 48–55. doi:10.1359/jbmr.2002.17.1.48
Friedrich, M. V. K., Göhring, W., Mörgelin, M., Brancaccio, A., David, G., and Timpl, R. (1999). Structural Basis of Glycosaminoglycan Modification and of Heterotypic Interactions of Perlecan Domain V 1 1Edited by I. B. Holland. J. Mol. Biol. 294 (1), 259–270. doi:10.1006/jmbi.1999.3259
Gao, G., Chen, S., Pei, Y. A., and Pei, M. (2021). Impact of Perlecan, a Core Component of Basement Membrane, on Regeneration of Cartilaginous Tissues. Acta Biomater. 135 (2121), 13–26. doi:10.1016/j.actbio.2021.08.032
Garcia, J., McCarthy, H. S., Kuiper, J. H., Melrose, J., and Roberts, S. (2021). Perlecan in the Natural and Cell Therapy Repair of Human Adult Articular Cartilage: Can Modifications in This Proteoglycan Be a Novel Therapeutic Approach? Biomolecules 11 (1), 92. doi:10.3390/biom11010092
Garl, P. J., Wenzlau, J. M., Walker, H. A., Whitelock, J. M., Costell, M., and Weiser-Evans, M. C. M. (2004). Perlecan-induced Suppression of Smooth Muscle Cell Proliferation Is Mediated through Increased Activity of the Tumor Suppressor PTEN. Circ. Res. 94, 175–183. doi:10.1161/01.res.0000109791.69181.b6
Geppert, M., Ushkaryov, Y. A., Hata, Y., Davletov, B., Petrenko, A. G., and Sudhof, T. C. (1992). Neurexins. Cold Spring Harbor Symposia Quantitative Biol. 57, 483–490. doi:10.1101/sqb.1992.057.01.053
Gill, A. A., Ortega, Í., Kelly, S., and Claeyssens, F. (2015). Towards the Fabrication of Artificial 3D Microdevices for Neural Cell Networks. Biomed. Microdevices 17 (2), 27. doi:10.1007/s10544-015-9929-x
Girós, A., Morante, J., Gil-Sanz, C., Fairén, A., and Costell, M. (2007). Erlecan Controls Neurogenesis in the Developing Telencephalon. BMC Dev. Biol. 7, 29. doi:10.1186/1471-213X-7-29
Göhring, W., Sasak, i. T., Heldin, C. H., and Timpl, R. (1998). Mapping of the Binding of Platelet-Derived Growth Factor to Distinct Domains of the Basement Membrane Proteins BM-40 and Perlecan and Distinction from the BM-40 Collagen-Binding Epitope. Eur. J. Biochem. 255 (1), 60–66.
Gomes, R., Kirn-Safran, C., Farach-Carson, M. C., and Carson, D. D. (2002). Perlecan: an Important Component of the Cartilage Pericellular Matrix. J. Musculoskelet. Neuronal Interact 2 (6), 511–516.
Gomes, R. R., Farach-Carson, M. C., and Carson, D. D. (2004). Perlecan Functions in Chondrogenesis: Insights from In Vitro and In Vivo Models. Cells Tissues Organs 176 (1-3), 79–86. doi:10.1159/000075029
Gómez Toledo, A., Sorrentino, J. T., Sandoval, D. R., Malmström, J., Lewis, N. E., and Esko, J. D. (2021). A Systems View of the Heparan Sulfate Interactome. J. Histochem. Cytochem. 69 (2), 105–119.
Gonzalez, E. M., Mongiat, M., Slater, S. J., Baffa, R., and Iozzo, R. V. (2003). A Novel Interaction between Perlecan Protein Core and Progranulin. J. Biol. Chem. 278 (40), 38113–38116. doi:10.1074/jbc.c300310200
Gotha, L., Lim, S. Y., Osherov, A. B., Wolff, R., Qiang, B., Erlich, I., et al. (2014). Heparan Sulfate Side Chains Have a Critical Role in the Inhibitory Effects of Perlecan on Vascular Smooth Muscle Cell Response to Arterial Injury. Am. J. Physiology-Heart Circulatory Physiol. 307 (3), H337–H345. doi:10.1152/ajpheart.00654.2013
Gradilla, A.-C., Sanchez-Hernandez, D., Brunt, L., and Scholpp, S. (2018). From Top to Bottom: Cell Polarity in Hedgehog and Wnt Trafficking. BMC Biol. 16, 37. doi:10.1186/s12915-018-0511-x
Gubbiotti, M. A., Neill, T., and Iozzo, R. V. (2017). A Current View of Perlecan in Physiology and Pathology: A Mosaic of Functions. Matrix Biol. 57-58, 285–298. doi:10.1016/j.matbio.2016.09.003
Guell, K., and Bix, G. J. (2014). Brain Endothelial Cell Specific Integrins and Ischemic Stroke. Expert Rev. Neurotherapeutics 14, 1287–1292. doi:10.1586/14737175.2014.964210
Guilak, F., Hayes, A. J., and Melrose, J. (2021). Perlecan in Pericellular Mechanosensory Cell-Matrix Communication, Extracellular Matrix Stabilisation and Mechanoregulation of Load-Bearing Connective Tissues. Int. J. Mol. Sci. 22 (5), 2716. doi:10.3390/ijms22052716
Hayes, A. J., Smith, S. M., Gibson, M. A., and Melrose, J. (2011). Comparative Immunolocalization of the Elastin Fiber-Associated Proteins Fibrillin-1, LTBP-2, and MAGP-1 with Components of the Collagenous and Proteoglycan Matrix of the Fetal Human Intervertebral Disc. Spine (Phila Pa 1976) 36 (21), E1365–E1372. doi:10.1097/BRS.0b013e31821fd23e
Hayes, A. J., Gibson, M. A., Shu, C., and Melrose, J. (2014). Confocal Microscopy Demonstrates Association of LTBP-2 in Fibrillin-1 Microfibrils and Colocalisation with Perlecan in the Disc Cell Pericellular Matrix. Tissue and Cell 46 (3), 185–197. doi:10.1016/j.tice.2014.04.002
Hayes, A. J., Hughes, C. E., Smith, S. M., Caterson, B., Little, C. B., and Melrose, J. (2016). The CS Sulfation Motifs 4C3, 7D4, 3B3[−]; and Perlecan Identify Stem Cell Populations and Their Niches, Activated Progenitor Cells and Transitional Areas of Tissue Development in the Fetal Human Elbow. Stem Cell Development 25 (11), 836–847. doi:10.1089/scd.2016.0054
Hayes, A. J., Lord, M. S., Smith, S. M., Smith, M. M., Whitelock, J. M., Weiss, A. S., et al. (2011). Colocalization In Vivo and Association In Vitro of Perlecan and Elastin. Histochem. Cel Biol 136 (4), 437–454. doi:10.1007/s00418-011-0854-7
Hayes, A. J., and Melrose, J. (2020). Electro‐Stimulation, a Promising Therapeutic Treatment Modality for Tissue Repair: Emerging Roles of Sulfated Glycosaminoglycans as Electro‐Regulatory Mediators of Intrinsic Repair Processes. Adv. Therap. 3, 2000151. doi:10.1002/adtp.202000151
Hayes, A. J., Smith, S. M., and Melrose, J. (2013). Comparative Immunolocalisation of Fibrillin-1 and Perlecan in the Human Foetal, and HS-Deficient Hspg2 Exon 3 Null Mutant Mouse Intervertebral Disc. Histochem. Cel Biol 139 (1), 1–11. doi:10.1007/s00418-012-1041-1
Hayes, A., Melrose, J., and Melrose, J. (2021). 3D Distribution of Perlecan within Intervertebral Disc Chondrons Suggests Novel Regulatory Roles for This Multifunctional Modular Heparan Sulphate Proteoglycan. eCM 41, 73–89. doi:10.22203/ecm.v041a06
Hayes, A., Shu, C. C., Shu, C., Lord, M., Little, C., Whitelock, J., et al. (2016). Pericellular Colocalisation and Interactive Properties of Type VI Collagen and Perlecan in the Intervertebral Disc. eCM 32, 40–57. doi:10.22203/ecm.v032a03
Hellstrom, M., Kal n, M., Lindahl, P., Abramsson, A., and Betsholtz, C. (1999). Role of PDGF-B and PDGFR-Beta in Recruitment of Vascular Smooth Muscle Cells and Pericytes during Embryonic Blood Vessel Formation in the Mouse. Development 126, 3047–3055. doi:10.1242/dev.126.14.3047
Hoffmann, A., Dege, T., Kunze, R., Ernst, A.-S., Lorenz, H., Böhler, L.-I., et al. (2018). Early Blood-Brain Barrier Disruption in Ischemic Stroke Initiates Multifocally Around Capillaries/Venules. Stroke 49 (6), 1479–1487. doi:10.1161/strokeaha.118.020927
Hopf, M., Göhring, W., Kohfeldt, E., Yamada, Y., and Timpl, R. (1999). Recombinant Domain IV of Perlecan Binds to Nidogens, Laminin-Nidogen Complex, Fibronectin, Fibulin-2 and Heparin. Eur. J. Biochem. 259 (3), 917–925. doi:10.1046/j.1432-1327.1999.00127.x
Hozumi, K., Sasaki, A., Yamada, Y., Otagiri, D., Kobayashi, K., Fujimori, C., et al. (2012). Reconstitution of Laminin-111 Biological Activity Using Multiple Peptide Coupled to Chitosan Scaffolds. Biomaterials 33 (17), 4241–4250. doi:10.1016/j.biomaterials.2012.02.055
Hubka, K. M., Carson, D. D., Harrington, D. A., and Farach-Carson, M. C. (2019). Perlecan Domain I Gradients Establish Stable Biomimetic Heparin Binding Growth Factor Gradients for Cell Migration in Hydrogels. Acta Biomater. 97, 385–398. doi:10.1016/j.actbio.2019.07.040
Hultgårdh-Nilsson, A., and Durbeej, M. (2007). Role of the Extracellular Matrix and its Receptors in Smooth Muscle Cell Function: Implications in Vascular Development and Disease. Curr. Opin. Lipidol. 18 (5), 540–545.
Ishai-Michaeli, R., Eldor, A., and Vlodavsky, I. (1990). Heparanase Activity Expressed by Platelets, Neutrophils, and Lymphoma Cells Releases Active Fibroblast Growth Factor from Extracellular Matrix. Cell Regul 1, 833–842. doi:10.1091/mbc.1.11.833
Ishijima, M., Suzuki, N., Hozumi, K., Matsunobu, T., Kosaki, K., Kaneko, H., et al. (2012). Perlecan Modulates VEGF Signaling and Is Essential for Vascularization in Endochondral Bone Formation. Matrix Biol. 31, 234–245. doi:10.1016/j.matbio.2012.02.006
Itoh, N. (2016). FGF10: A Multifunctional Mesenchymal-Epithelial Signaling Growth Factor in Development, Health, and Disease. Cytokine Growth Factor. Rev. 28, 63–69. doi:10.1016/j.cytogfr.2015.10.001
Itoh, N., and Ohta, H. (2014). Fgf10: a Paracrine-Signaling Molecule in Development, Disease, and Regenerative Medicine. Cmm 14 (4), 504–509. doi:10.2174/1566524014666140414204829
Jacobson, C., Côté, P. D., Rossi, S. G., Rotundo, R. L., and Carbonetto, S. (2001). The Dystroglycan Complex Is Necessary for Stabilization of Acetylcholine Receptor Clusters at Neuromuscular Junctions and Formation of the Synaptic Basement Membrane. J. Cel Biol 152 (3), 435–450. doi:10.1083/jcb.152.3.435
Jayadev, R., and Sherwood, D. R. (2017). Basement Membranes. Curr. Biol. 27 (6), R207–R211. doi:10.1016/j.cub.2017.02.006
Jha, A. K., Yang, W., Kirn-Safran, C. B., Farach-Carson, M. C., and Jia, X. (2009). Perlecan Domain I-Conjugated, Hyaluronic Acid-Based Hydrogel Particles for Enhanced Chondrogenic Differentiation via BMP-2 Release. Biomaterials 30 (36), 6964–6975. doi:10.1016/j.biomaterials.2009.09.009
Johnson, N. E. (2019). Myotonic Muscular Dystrophies. CONTINUUM: Lifelong Learn. Neurol. 25 (6), 1682–1695. doi:10.1212/con.0000000000000793
Kahle, M. P., Lee, B., Pourmohamad, T., Cunningham, A., Su, H., Kim, H., et al. (2012). Perlecan Domain V Is Upregulated in Human Brain Arteriovenous Malformation and Could Mediate the Vascular Endothelial Growth Factor Effect in Lesional Tissue. Neuroreport 23, 627–630. doi:10.1097/00001756-201207110-00010
Kaneko, H., Ishijima, M., Futami, I., Tomikawa-Ichikawa, N., Kosaki, K., Sadatsuki, R., et al. (2013). Synovial Perlecan Is Required for Osteophyte Formation in Knee Osteoarthritis. Matrix Biol. 32 (3-4), 178–187. doi:10.1016/j.matbio.2013.01.004
Katz, B., and Miledi, R. (1973). The Binding of Acetylcholine to Receptors and its Removal from the Synaptic Cleft. J. Physiol. (Lond). 231, 549–574. doi:10.1113/jphysiol.1973.sp010248
Kerever, A., Nagahara, F., Keino-Masu, K., Masu, M., van Kuppevelt, T. H., Vivès, R. R., et al. (2021). Regulation of Fractone Heparan Sulfate Composition in Young and Aged Subventricular Zone Neurogenic Niches. Glycobiology 1, cwab081. doi:10.1093/glycob/cwab081
Kerever, A., and Arikawa-Hirasawa, E. (2021). Optimal Extracellular Matrix Niches for Neurogenesis: Identifying Glycosaminoglycan Chain Composition in the Subventricular Neurogenic Zone. Front. Neuroanat. 15, 764458. doi:10.3389/fnana.2021.764458
Kerever, A., Mercier, F., Nonaka, R., de Vega, S., Oda, Y., Zalc, B., et al. (2014). Perlecan Is Required for FGF-2 Signaling in the Neural Stem Cell Niche. Stem Cel Res. 12 (2), 492–505. doi:10.1016/j.scr.2013.12.009
Kerever, A., Schnack, J., Vellinga, D., Ichikawa, N., Moon, C., Arikawa-Hirasawa, E., et al. (2007). Novel Extracellular Matrix Structures in the Neural Stem Cell Niche Capture the Neurogenic Factor Fibroblast Growth Factor 2 from the Extracellular Milieu. Stem Cells 25 (9), 2146–2157. doi:10.1634/stemcells.2007-0082
Kim, H. J., Lee, E., Nam, M., Chung, J. K., Joo, S., Nam, Y., et al. (2021). Contribution of Extracellular Matrix Component Landscapes in the Adult Subventricular Zone to the Positioning of Neural Stem/Progenitor Cells. Exp. Neurobiol. 30 (4), 275–284. doi:10.5607/en21012
Kimbell, L. M., Ohno, K., Engel, A. G., and Rotundo, R. L. (2004). C-terminal and Heparin-Binding Domains of Collagenic Tail Subunit Are Both Essential for Anchoring Acetylcholinesterase at the Synapse. J. Biol. Chem. 279 (12), 10997–11005. doi:10.1074/jbc.m305462200
Knight, D., Xie, W., and Boulianne, G. L. (2011). Neurexins and Neuroligins: Recent Insights from Invertebrates. Mol. Neurobiol. 44 (3), 426–440. doi:10.1007/s12035-011-8213-1
Knox, S., Fosang, A. J., Last, K., Melrose, J., and Whitelock, J. (2005). Perlecan from Human Epithelial Cells Is a Hybrid Heparan/chondroitin/keratan Sulfate Proteoglycan. FEBS Lett. 579 (22), 5019–5023. doi:10.1016/j.febslet.2005.07.090
Knox, S. M., and Whitelock, J. M. (2006). Perlecan: How Does One Molecule Do So many Things? Cell. Mol. Life Sci. 63 (21), 2435–2445. doi:10.1007/s00018-006-6162-z
Koyama, N., Kinsella, M. G., Wight, T. N., Hedin, U., and Clowes, A. W. (1998). Heparan Sulfate Proteoglycans Mediate a Potent Inhibitory Signal for Migration of Vascular Smooth Muscle Cells. Circ. Res. 83 (3), 305–313. doi:10.1161/01.res.83.3.305
Kram, V., Zcharia, E., Yacoby-Zeevi, O., Metzger, S., Chajek-Shaul, T., Gabet, Y., et al. (2006). Heparanase Is Expressed in Osteoblastic Cells and Stimulates Bone Formation and Bone Mass. J. Cel. Physiol. 207, 784–792. doi:10.1002/jcp.20625
Krejci, E., Coussen, F., Duval, N., Chatel, J. M., Legay, C., Puype, M., et al. (1991). Primary Structure of a Collagenic Tail Peptide of Torpedo Acetylcholinesterase: Co-expression with Catalytic Subunit Induces the Production of Collagen-Tailed Forms in Transfected Cells. EMBO J. 10, 1285–1293. doi:10.1002/j.1460-2075.1991.tb08070.x
Krejci, E., Thomine, S., Boschetti, N., Legay, C., Sketelj, J., and Massoulié, J. (1997). The Mammalian Gene of Acetylcholinesterase-Associated Collagen. J. Biol. Chem. 272, 22840–22847. doi:10.1074/jbc.272.36.22840
Kvist, A. J., Nyström, A., Hultenby, K., Sasaki, T., Talts, J. F., and Aspberg, A. (2008). The Major Basement Membrane Components Localize to the Chondrocyte Pericellular Matrix - A Cartilage Basement Membrane Equivalent? Matrix Biol. 27 (1), 22–33. doi:10.1016/j.matbio.2007.07.007
Lau, K., Waterhouse, A., Akhavan, B., Gao, L., Kim, H. N., Tang, F., et al. (2021). Biomimetic Silk Biomaterials: Perlecan-Functionalized Silk Fibroin for Use in Blood-Contacting Devices. Acta Biomater. 132, 162–175. doi:10.1016/j.actbio.2021.02.014
Lee, B., Clarke, D., Al Ahmad, A., Kahle, M., Parham, C., Auckland, L., et al. (2011). Perlecan Domain V Is Neuroprotective and Proangiogenic Following Ischemic Stroke in Rodents. J. Clin. Invest. 121 (8), 3005–3023. doi:10.1172/jci46358
Legay, C., and Dobbertin, A. (2020). Collagens at the Vertebrate Neuromuscular junction, from Structure to Pathologies. Neurosci. Lett. 735, 135155. doi:10.1016/j.neulet.2020.135155
Lin, P.-Y., Hung, J.-H., Hsu, C.-K., Chang, Y.-T., and Sun, Y.-T. (2021). A Novel Pathogenic HSPG2 Mutation in Schwartz-Jampel Syndrome. Front. Neurol. 12, 632336. doi:10.3389/fneur.2021.632336
Lin, X., Tang, F., Jiang, S., Khamis, H., Bongers, A., Whitelock, J. M., et al. (2020). A Biomimetic Approach toward Enhancing Angiogenesis: Recombinantly Expressed Domain V of Human Perlecan Is a Bioactive Molecule that Promotes Angiogenesis and Vascularization of Implanted Biomaterials. Adv. Sci. 7 (17), 2000900. doi:10.1002/advs.202000900
Liu, Z., Tang, J., Gao, B., Yao, P., Li, X., Liu, D., et al. (2020). Neural Signal Analysis with Memristor Arrays towards High-Efficiency Brain-Machine Interfaces. Nat. Commun. 11 (1), 4234. doi:10.1038/s41467-020-18105-4
Llovera, G., Simats, A., and Liesz, A. (2021). Modeling Stroke in Mice: Transient Middle Cerebral Artery Occlusion via the External Carotid Artery. J. Vis. Exp. 1, 171. doi:10.3791/62573
Lord, M. S., Chuang, C. Y., Melrose, J., Davies, M. J., Iozzo, R. V., and Whitelock, J. M. (2014). The Role of Vascular-Derived Perlecan in Modulating Cell Adhesion, Proliferation and Growth Factor Signaling. Matrix Biol. 35, 112–122. doi:10.1016/j.matbio.2014.01.016
Lord, M. S., Ellis, A. L., Farrugia, B. L., Whitelock, J. M., Grenett, H., Li, C., et al. (2017). Perlecan and Vascular Endothelial Growth Factor-Encoding DNA-Loaded Chitosan Scaffolds Promote Angiogenesis and Wound Healing. J. Controlled Release 250, 48–61. doi:10.1016/j.jconrel.2017.02.009
Lord, M. S., Tang, F., Rnjak-Kovacina, J., Smith, J. G. W., Melrose, J., and Whitelock, J. M. (2018). The Multifaceted Roles of Perlecan in Fibrosis. Matrix Biol. 68-69, 150–166. doi:10.1016/j.matbio.2018.02.013
Lord, M. S., and Whitelock, J. M. (2013). Recombinant Production of Proteoglycans and Their Bioactive Domains. FEBS J. 280, 2490–2510. doi:10.1111/febs.12197
Lord, M. S., Yu, W., Cheng, B., Simmons, A., Poole-Warren, L., and Whitelock, J. M. (2009). The Modulation of Platelet and Endothelial Cell Adhesion to Vascular Graft Materials by Perlecan. Biomaterials 30 (28), 4898–4906. doi:10.1016/j.biomaterials.2009.05.063
Lord, M., Whitelock, J., and Turnbull, J. E. (2020). Better Growth-Factor Binding Aids Tissue Repair. Nat. Biomed. Eng. 4, 368–369. doi:10.1038/s41551-020-0548-3
Majumdar, M. K., Wang, E., and Morris, E. A. (2001). BMP-2 and BMP-9 Promotes Chondrogenic Differentiation of Human Multipotential Mesenchymal Cells and Overcomes the Inhibitory Effect of IL-1. J. Cel. Physiol. 189 (3), 275–284. doi:10.1002/jcp.10025
Makihara, N., Arimura, K., Ago, T., Tachibana, M., Nishimura, A., Nakamura, K., et al. (2015). Involvement of Platelet-Derived Growth Factor Receptor β in Fibrosis through Extracellular Matrix Protein Production after Ischemic Stroke. Exp. Neurol. 264, 127–134. doi:10.1016/j.expneurol.2014.12.007
Marcelo, A., and Bix, G. (2014). Investigating the Role of Perlecan Domain V in post-ischemic Cerebral Angiogenesis. Methods Mol. Biol. 1135, 331–341. doi:10.1007/978-1-4939-0320-7_27
Marcelo, A., and Bix, G. (2015). The Potential Role of Perlecan Domain V as Novel Therapy in Vascular Dementia. Metab. Brain Dis. 30, 1–5. doi:10.1007/s11011-014-9576-6
Martinez, J. R., Grindel, B. J., Hubka, K. M., Dodge, G. R., and Farach‐Carson, M. C. (2018). Perlecan/HSPG2: Signaling Role of Domain IV in Chondrocyte Clustering with Implications for Schwartz‐Jampel Syndrome. J. Cel Biochem 120, 2138–2150. doi:10.1002/jcb.27521
Martinez-Pena y Valenzuela, I., Akaaboune, M., and Akaaboune, M. (2007). Acetylcholinesterase Mobility and Stability at the Neuromuscular junction of Living Mice. MBoC 18, 2904–2911. doi:10.1091/mbc.e07-02-0093
Maselli, R. A., Arredondo, J., Ferns, M. J., and Wollmann, R. L. (2012). Synaptic Basal Lamina-Associated Congenital Myasthenic Syndromes. Ann. N. Y Acad. Sci. 1275, 36–48. doi:10.1111/j.1749-6632.2012.06807.x
Massoulié, J., and Millard, C. B. (2009). Cholinesterases and the Basal Lamina at Vertebrate Neuromuscular Junctions. Curr. Opin. Pharmacol. 9, 316–325. doi:10.1016/j.coph.2009.04.004
McGrath, J. C., Deighan, C., Briones, A. M., Shafaroudi, M. M., McBride, M., Adler, J., et al. (2005). New Aspects of Vascular Remodelling: the Involvement of All Vascular Cell Types. Exp. Physiol. 90 (4), 469–475. doi:10.1113/expphysiol.2005.030130
Melrose, J. (2020). Perlecan, a Modular Instructive Proteoglycan with Diverse Functional Properties. Int. J. Biochem. Cel Biol 128, 105849. doi:10.1016/j.biocel.2020.105849
Melrose, J. (2016). Glycosaminoglycans in Wound Healing. Bone Tissue Regen. Insights 7, BTRI.S38670. doi:10.4137/BTRI.S38670
Melrose, J., Hayes, A. J., Whitelock, J. M., and Little, C. B. (2008). Perlecan, the "jack of All Trades" Proteoglycan of Cartilaginous Weight-Bearing Connective Tissues. Bioessays 30 (5), 457–469. doi:10.1002/bies.20748
Melrose, J., Isaacs, M. D., Smith, S. M., Hughes, C. E., Little, C. B., Caterson, B., et al. (2012). Chondroitin Sulphate and Heparan Sulphate Sulphation Motifs and Their Proteoglycans Are Involved in Articular Cartilage Formation during Human Foetal Knee Joint Development. Histochem. Cel Biol 138 (3), 461–475. doi:10.1007/s00418-012-0968-6
Melrose, J., and Melrose, J. (2016). Perlecan Delineates Stem Cell Niches in Human Foetal Hip, Knee and Elbow Cartilage Rudiments and Has Potential Roles in the Regulation of Stem Cell Differentiation. Srdt 3, 1–7. doi:10.24966/SRDT-2060/100009
Melrose, J., Roughley, P., Knox, S., Smith, S., Lord, M., and Whitelock, J. (2006). The Structure, Location, and Function of Perlecan, a Prominent Pericellular Proteoglycan of Fetal, Postnatal, and Mature Hyaline Cartilages. J. Biol. Chem. 281 (48), 36905–36914. doi:10.1074/jbc.m608462200
Melrose, J., Shu, C., Whitelock, J. M., and Lord, M. S. (2016). The Cartilage Extracellular Matrix as a Transient Developmental Scaffold for Growth Plate Maturation. Matrix Biol. 52-54, 363–383. doi:10.1016/j.matbio.2016.01.008
Melrose, J., Smith, S., Ghosh, P., and Whitelock, J. (2003). Perlecan, the Multidomain Heparan Sulfate Proteoglycan of Basement Membranes, Is Also a Prominent Component of the Cartilaginous Primordia in the Developing Human Fetal Spine. J. Histochem. Cytochem. 51 (10), 1331–1341. doi:10.1177/002215540305101010
Melrose, J., Smith, S., and Whitelock, J. (2004). Perlecan Immunolocalizes to Perichondrial Vessels and Canals in Human Fetal Cartilaginous Primordia in Early Vascular and Matrix Remodeling Events Associated with Diarthrodial Joint Development. J. Histochem. Cytochem. 52 (11), 1405–1413. doi:10.1369/jhc.4a6261.2004
Mercier, F. (2016). Fractones: Extracellular Matrix Niche Controlling Stem Cell Fate and Growth Factor Activity in the Brain in Health and Disease. Cel. Mol. Life Sci. 73 (24), 4661–4674. doi:10.1007/s00018-016-2314-y
Milner, R., Hung, S., Erokwu, B., Dore-Duffy, P., Lamanna, J. C., and Del Zoppo, G. J., (2008). Increased Expression of Fibronectin and the α5β1 Integrin in Angiogenic Cerebral Blood Vessels of Mice Subject to Hypobaric Hypoxia. Mol. Cell Neurosci. 38, 43–52. doi:10.1016/j.mcn.2008.01.013
Moccia, F., Negri, S., Shekha, M., Faris, P., and Guerra, G. (2019). Endothelial Ca2+ Signaling, Angiogenesis and Vasculogenesis: Just what it Takes to Make a Blood Vessel. Int. J. Mol. Sci. 20. doi:10.3390/ijms20163962
Mongiat, M., Fu, J., Oldershaw, R., Greenhalgh, R., Gown, A. M., and Iozzo, R. V. (2003). Perlecan Protein Core Interacts with Extracellular Matrix Protein 1 (ECM1), a Glycoprotein Involved in Bone Formation and Angiogenesis. J. Biol. Chem. 278 (19), 17491–17499. doi:10.1074/jbc.m210529200
Mongiat, M., Taylor, K., Otto, J., Aho, S., Uitto, J., Whitelock, J. M., et al. (2000). The Protein Core of the Proteoglycan Perlecan Binds Specifically to Fibroblast Growth Factor-7. J. Biol. Chem. 275 (10), 7095–7100. doi:10.1074/jbc.275.10.7095
Murdoch, A. D., Dodge, G. R., Cohen, I., Tuan, R. S., and Iozzo, R. V. (1992). Primary Structure of the Human Heparan Sulfate Proteoglycan from Basement Membrane (HSPG2/perlecan). A Chimeric Molecule with Multiple Domains Homologous to the Low Density Lipoprotein Receptor, Laminin, Neural Cell Adhesion Molecules, and Epidermal Growth Factor. J. Biol. Chem. 267 (12), 8544–8557. doi:10.1016/s0021-9258(18)42478-7
Nakamura, K., Arimura, K., Nishimura, A., Tachibana, M., Yoshikawa, Y., Makihara, N., et al. (2016). Possible Involvement of Basic FGF in the Upregulation of PDGFRβ in Pericytes after Ischemic Stroke. Brain Res. 1630, 98–108. doi:10.1016/j.brainres.2015.11.003
Nakamura, K., Ikeuchi, T., Nara, K., Rhodes, C. S., Zhang, P., Chiba, Y., et al. (2019). Perlecan Regulates Pericyte Dynamics in the Maintenance and Repair of the Blood-Brain Barrier. J. Cel Biol 218 (10), 3506–3525. doi:10.1083/jcb.201807178
Nakamura, R., Nakamura, F., and Fukunaga, S. (2015). Diverse Functions of Perlecan in central Nervous System Cellsin Vitro. Anim. Sci. J. 86, 904–911. doi:10.1111/asj.12376
Nakamura, R., Nakamura, F., and Fukunaga, S. (2015). Perlecan Diversely Regulates the Migration and Proliferation of Distinct Cell Types In Vitro. Cells Tissues Organs 200 (6), 374–393. doi:10.1159/000440950
Nakata, T., Ito, M., Azuma, Y., Otsuka, K., Noguchi, Y., Komaki, H., et al. (2013). Mutations in the C-Terminal Domain of ColQ in Endplate Acetylcholinesterase Deficiency Compromise ColQ MuSK Interaction. Hum. Mutat. 34 (7), 997–1004. doi:10.1002/humu.22325
Nam, K., Maruyama, C. L., Wang, C.-S., Trump, B. G., Lei, P., Andreadis, S. T., et al. (2017). Laminin-111-derived Peptide Conjugated Fibrin Hydrogel Restores Salivary Gland Function. PLoS One 12 (11), e0187069. doi:10.1371/journal.pone.0187069
Nasser, N. J. (2008). Heparanase Involvement in Physiology and Disease. Cel. Mol. Life Sci. 65, 1706–1715. doi:10.1007/s00018-008-7584-6
Ng, C.-y., Whitelock, J. M., Williams, H., Kim, H. N., Medbury, H. J., and Lord, M. S. (2021). Macrophages Bind LDL Using Heparan Sulfate and the Perlecan Protein Core. J. Biol. Chem. 296, 100520. doi:10.1016/j.jbc.2021.100520
Nicole, S., Davoine, C.-S., Topaloglu, H., Cattolico, L., Barral, D., Beighton, P., et al. (2000). Perlecan, the Major Proteoglycan of Basement Membranes, Is Altered in Patients with Schwartz-Jampel Syndrome (Chondrodystrophic Myotonia). Nat. Genet. 26 (4), 480–483. doi:10.1038/82638
Noborn, F., and Sterky, F. H. (2021). Role of Neurexin Heparan Sulfate in the Molecular Assembly of Synapses - Expanding the Neurexin Code? FEBS J. 1, 1. doi:10.1111/febs.16251
Noonan, D. M., Fulle, A., Valente, P., Cai, S., Horigan, E., Sasaki, M., et al. (1991). The Complete Sequence of Perlecan, a Basement Membrane Heparan Sulfate Proteoglycan, Reveals Extensive Similarity with Laminin A Chain, Low Density Lipoprotein-Receptor, and the Neural Cell Adhesion Molecule. J. Biol. Chem. 266 (34), 22939–22947. doi:10.1016/s0021-9258(18)54445-8
Ocken, A. R., Ku, M. M., Kinzer-Ursem, T. L., and Calve, S. (2020). Perlecan Knockdown Significantly Alters Extracellular Matrix Composition and Organization during Cartilage Development. Mol. Cell Proteomics 19 (7), 1220–1235. doi:10.1074/mcp.ra120.001998
Ohno, K., Ito, M., Kawakami, Y., Krejci, E., and Engel, A. G. (2013). Specific Binding of Collagen Q to the Neuromuscular junction Is Exploited to Cure Congenital Myasthenia and to Explore Bases of Myasthenia Gravis. Chemico-Biological Interactions 203 (1), 335–340. doi:10.1016/j.cbi.2012.08.020
Patel, V. N., Knox, S. M., Likar, K. M., Lathrop, C. A., Hossain, R., Eftekhari, S., et al. (2007). Heparanase Cleavage of Perlecan Heparan Sulfate Modulates FGF10 Activity during Ex Vivo Submandibular Gland Branching Morphogenesis. Development 134 (23), 4177–4186. doi:10.1242/dev.011171
Patel, V. N., Likar, K. M., Zisman-Rozen, S., Cowherd, S. N., Lassiter, K. S., Sher, I., et al. (2008). Specific Heparan Sulfate Structures Modulate FGF10-Mediated Submandibular Gland Epithelial Morphogenesis and Differentiation. J. Biol. Chem. 283 (14), 9308–9317. doi:10.1074/jbc.m709995200
Peng, H. B., Xie, H., Rossi, S. G., and Rotundo, R. L. (1999). Acetylcholinesterase Clustering at the Neuromuscular junction Involves Perlecan and Dystroglycan. J. Cel Biol 145, 911–921. doi:10.1083/jcb.145.4.911
Platt, S. R., Holmes, S. P., Howerth, E. W., Duberstein, K. J. J., Dove, C. R., Kinder, H. A., et al. (2014). Development and Characterization of a Yucatan Miniature Biomedical Pig Permanent Middle Cerebral Artery Occlusion Stroke Model. Exp. Trans. Stroke Med. 6 (1), 5. doi:10.1186/2040-7378-6-5
Poluzzi, C., Iozzo, R. V., and Schaefer, L. (2016). Endostatin and Endorepellin: A Common Route of Action for Similar Angiostatic Cancer Avengers. Adv. Drug Deliv. Rev. 97, 156–173. doi:10.1016/j.addr.2015.10.012
Pozzi, A., Yurchenco, P. D., and Iozzo, R. V. (2017). The Nature and Biology of Basement Membranes. Matrix Biol. 57-58, 1–11. doi:10.1016/j.matbio.2016.12.009
Pradhan, S., Liu, C., Zhang, C., Jia, X., Farach-Carson, M. C., and Witt, R. L. (2010). Lumen Formation in Three-Dimensional Cultures of Salivary Acinar Cells. Otolaryngol. Head Neck Surg. 142 (2), 191–195. doi:10.1016/j.otohns.2009.10.039
Pradhan, S., Zhang, C., Jia, X., Carson, D. D., Witt, R., and Farach-Carson, M. C. (2009). Perlecan Domain IV Peptide Stimulates Salivary Gland Cell AssemblyIn Vitro. Tissue Eng. A 15 (11), 3309–3320. doi:10.1089/ten.tea.2008.0669
Punga, A. R., Maddison, P., Heckmann, J. M., Guptill, J. T., and Evoli, A. (2022). Epidemiology, Diagnostics, and Biomarkers of Autoimmune Neuromuscular junction Disorders. Lancet Neurol. 21 (2), 176–188. doi:10.1016/s1474-4422(21)00297-0
Rnjak-Kovacina, J., Tang, F., Lin, X., Whitelock, J. M., and Lord, M. S. (2017). Recombinant Domain V of Human Perlecan Is a Bioactive Vascular Proteoglycan. Biotechnol. J. 12, 12. doi:10.1002/biot.201700196
Rnjak-Kovacina, J., Tang, F., Whitelock, J. M., and Lord, M. S. (2016). Silk Biomaterials Functionalized with Recombinant Domain V of Human Perlecan Modulate Endothelial Cell and Platelet Interactions for Vascular Applications. Colloids Surf. B: Biointerfaces 148, 130–138. doi:10.1016/j.colsurfb.2016.08.039
Rodgers, K. D., Sasaki, T., Aszodi, A., and Jacenko, O. (2007). Reduced Perlecan in Mice Results in Chondrodysplasia Resembling Schwartz-Jampel Syndrome. Hum. Mol. Genet. 16, 515–528. doi:10.1093/hmg/ddl484
Roediger, M., Kruegel, J., Miosge, N., and Gersdorff, N. (2009). Tissue Distribution of Perlecan Domains III and V during Embryonic and Fetal Human Development. Histol. Histopathol 24 (7), 859–868. doi:10.14670/HH-24.859
Rosenberry, T. L. (1979). Quantitative Simulation of Endplate Currents at Neuromuscular Junctions Based on the Reaction of Acetylcholine with Acetylcholine Receptor and Acetylcholinesterase. Biophysical J. 26, 263–289. doi:10.1016/s0006-3495(79)85249-2
Rotundo, R. L., Ruiz, C. A., Marrero, E., Kimbell, L. M., Rossi, S. G., Rosenberry, T., et al. (2008). Assembly and Regulation of Acetylcholinesterase at the Vertebrate Neuromuscular junction. Chem. Biol. Interact 175 (1-3), 26–29. doi:10.1016/j.cbi.2008.05.025
Rotundo, R. L., Rossi, S. G., and Anglister, L. (1997). Transplantation of Quail Collagen-Tailed Acetylcholinesterase Molecules onto the Frog Neuromuscular Synapse. J. Cel Biol136, 367–374. doi:10.1083/jcb.136.2.367
Rotundo, R. L., Rossi, S. G., Kimbell, L. M., Ruiz, C., and Marrero, E. (2005). Targeting Acetylcholinesterase to the Neuromuscular Synapse. Chemico-Biological Interactions 157-158, 15–21. doi:10.1016/j.cbi.2005.10.007
Rowland, T. J., Blaschke, A. J., Buchholz, D. E., Hikita, S. T., Johnson, L. V., and Clegg, D. O. (2013). Differentiation of Human Pluripotent Stem Cells to Retinal Pigmented Epithelium in Defined Conditions Using Purified Extracellular Matrix Proteins. J. Tissue Eng. Regen. Med. 7 (8), 642–653. doi:10.1002/term.1458
Rudenko, G. (2017). Dynamic Control of Synaptic Adhesion and Organizing Molecules in Synaptic Plasticity. Neural Plast. 2017, 6526151. doi:10.1155/2017/6526151
Russo, T. A., Banuth, A. M. M., Nader, H. B., and Dreyfuss, J. L. (2020). Altered Shear Stress on Endothelial Cells Leads to Remodeling of Extracellular Matrix and Induction of Angiogenesis. PLoS One 15 (11), e0241040. doi:10.1371/journal.pone.0241040
Sadatsuki, R., Kaneko, H., Kinoshita, M., Futami, I., Nonaka, R., Culley, K. L., et al. (2017). Perlecan Is Required for the Chondrogenic Differentiation of Synovial Mesenchymal Cells through Regulation of Sox9 Gene Expression. J. Orthop. Res. 35 (4), 837–846. doi:10.1002/jor.23318
Saha, S., Nagy, T. L., and Weiner, O. D. (2018). Joining Forces: Crosstalk between Biochemical Signalling and Physical Forces Orchestrates Cellular Polarity and Dynamics. Philos. Trans. R. Soc. Lond. B Biol. Sci. 373, 20170145. doi:10.1098/rstb.2017.0145
Saini, M. G., and Bix, G. J. (2012). Oxygen-glucose Deprivation (OGD) and Interleukin-1 (IL-1) Differentially Modulate Cathepsin B/L Mediated Generation of Neuroprotective Perlecan LG3 by Neurons. Brain Res. 1438, 65–74. doi:10.1016/j.brainres.2011.12.027
Salmeron, K. E., Maniskas, M. E., Edwards, D. N., Wong, R., Rajkovic, I., Trout, A., et al. (2019). Interleukin 1 Alpha Administration Is Neuroprotective and Neuro-Restorative Following Experimental Ischemic Stroke. J. Neuroinflammation 16 (1), 222. doi:10.1186/s12974-019-1599-9
Segev, A., Nili, N., and Strauss, B. H. (2004). The Role of Perlecan in Arterial Injury and Angiogenesis. Cardiovasc. Res. 63 (4), 603–610. doi:10.1016/j.cardiores.2004.03.028
Shen, J., Ishii, Y., Xu, G., Dang, T. C., Hamashima, T., Matsushima, T., et al. (2012). PDGFR-β as a Positive Regulator of Tissue Repair in a Mouse Model of Focal Cerebral Ischemia. J. Cereb. Blood Flow Metab. 32, 353–367. doi:10.1038/jcbfm.2011.136
Shestovskaya, M. V., Bozhkova, S. A., Sopova, J. V., Khotin, M. G., and Bozhokin, M. S. (2021). Methods of Modification of Mesenchymal Stem Cells and Conditions of Their Culturing for Hyaline Cartilage Tissue Engineering. Biomedicines 9 (11), 1666. doi:10.3390/biomedicines9111666
Shibahara, T., Ago, T., Nakamura, K., Tachibana, M., Yoshikawa, Y., Komori, M., et al. (2020). Pericyte-Mediated Tissue Repair through PDGFRβ Promotes Peri-Infarct Astrogliosis, Oligodendrogenesis, and Functional Recovery after Acute Ischemic Stroke. eNeuro 7 (2), 1. doi:10.1523/ENEURO.0474-19.2020
Shu, C. C., Jackson, M. T., Smith, M. M., Smith, S. M., Penm, S., Lord, M. S., et al. (2016). Ablation of Perlecan Domain 1 Heparan Sulfate Reduces Progressive Cartilage Degradation, Synovitis, and Osteophyte Size in a Preclinical Model of Posttraumatic Osteoarthritis. Arthritis Rheumatol. 68 (4), 868–879. doi:10.1002/art.39529
Shu, C. C., Smith, M. M., Appleyard, R. C., Little, C. B., and Melrose, J. (2018). Achilles and Tail Tendons of Perlecan Exon 3 Null Heparan Sulphate Deficient Mice Display Surprising Improvement in Tendon Tensile Properties and Altered Collagen Fibril Organisation Compared to C57BL/6 Wild Type Mice. PeerJ 6, e5120. doi:10.7717/peerj.5120
Shu, C. C., Smith, S. M., Little, C. B., and Melrose, J. (2019). Elevated Hypertrophy, Growth Plate Maturation, Glycosaminoglycan Deposition, and Exostosis Formation in the Hspg2 Exon 3 Null Mouse Intervertebral Disc. Biochem. J. 476 (2), 225–243. doi:10.1042/bcj20180695
Shu, C., Smith, S. M., Little, C. B., and Melrose, J. (2016). Use of FGF-2 and FGF-18 to Direct Bone Marrow Stromal Stem Cells to Chondrogenic and Osteogenic Lineages. Future Sci. OA 2 (4), FSO142. doi:10.4155/fsoa-2016-0034
Shu, C., Smith, S. M., and Melrose, J. (2016). The Heparan Sulphate Deficient Hspg2 Exon 3 Null Mouse Displays Reduced Deposition of TGF-Β1 in Skin Compared to C57BL/6 Wild Type Mice. J. Mol. Hist. 47 (3), 365–374. doi:10.1007/s10735-016-9677-0
Shu, C., Smith, S. S., Little, C. B., and Melrose, J. (2013). Comparative Immunolocalisation of Perlecan, Heparan Sulphate, Fibroblast Growth Factor-18, and Fibroblast Growth Factor Receptor-3 and Their Prospective Roles in Chondrogenic and Osteogenic Development of the Human Foetal Spine. Eur. Spine J. 22 (8), 1774–1784. doi:10.1007/s00586-013-2680-1
Siegel, G., Malmsten, M., and Ermilov, E. (2014). Anionic Biopolyelectrolytes of the Syndecan/perlecan Superfamily: Physicochemical Properties and Medical Significance. Adv. Colloid Interf. Sci. 205, 275–318. doi:10.1016/j.cis.2014.01.009
Sigoillot, S. M., Bourgeois, F., Lambergeon, M., Strochlic, L., and Legay, C. (2010). ColQ Controls Postsynaptic Differentiation at the Neuromuscular junction. J. Neurosci. 30 (1), 13–23. doi:10.1523/jneurosci.4374-09.2010
Silman, I., and Sussman, J. L. (2008). Acetylcholinesterase: How Is Structure Related to Function? Chem. Biol. Interact 175 (1-3), 3–10. doi:10.1016/j.cbi.2008.05.035
Singhal, N., and Martin, P. T. (2011). Role of Extracellular Matrix Proteins and Their Receptors in the Development of the Vertebrate Neuromuscular junction. Devel Neurobio 71 (11), 982–1005. doi:10.1002/dneu.20953
Smith, M. M., and Melrose, J. (2015). Proteoglycans in Normal and Healing Skin. Adv. Wound Care 4 (3), 152–173. doi:10.1089/wound.2013.0464
Smith, S. E., French, M. M., Julian, J., Paria, B. C., Dey, S. K., and Carson, D. D. (1997). Expression of Heparan Sulfate Proteoglycan (Perlecan) in the Mouse Blastocyst Is Regulated during normal and Delayed Implantation. Developmental Biol. 184 (1), 38–47. doi:10.1006/dbio.1997.8521
Smith, S. M.-L., West, L. A., and Hassell, J. R. (2007). The Core Protein of Growth Plate Perlecan Binds FGF-18 and Alters its Mitogenic Effect on Chondrocytes. Arch. Biochem. Biophys. 468 (2), 244–251. doi:10.1016/j.abb.2007.10.006
Smith, S. M., and Melrose, J. (2019). Type XI Collagen-Perlecan-HS Interactions Stabilise the Pericellular Matrix of Annulus Fibrosus Cells and Chondrocytes Providing Matrix Stabilisation and Homeostasis. J. Mol. Hist. 50 (3), 285–294. doi:10.1007/s10735-019-09823-1
Smith, S. M., Shu, C., and Melrose, J. (2010). Comparative Immunolocalisation of Perlecan with Collagen II and Aggrecan in Human Foetal, Newborn and Adult Ovine Joint Tissues Demonstrates Perlecan as an Early Developmental Chondrogenic Marker. Histochem. Cel Biol 134 (3), 251–263. doi:10.1007/s00418-010-0730-x
Snow, A. D., Cummings, J. A., and Lake, T. (2021). The Unifying Hypothesis of Alzheimer's Disease: Heparan Sulfate Proteoglycans/Glycosaminoglycans Are Key as First Hypothesized over 30 Years Ago. Front. Aging Neurosci. 13, 710683. doi:10.3389/fnagi.2021.710683
Soulintzi, N., and Zagris, N. (2007). Spatial and Temporal Expression of Perlecan in the Early Chick Embryo. Cells Tissues Organs 186 (4), 243–256. doi:10.1159/000107948
Srinivasan, P. P., McCoy, S. Y., Jha, A. K., Yang, W., Jia, X., Farach-Carson, M. C., et al. (2012). Injectable Perlecan Domain 1-hyaluronan Microgels Potentiate the Cartilage Repair Effect of BMP2 in a Murine Model of Early Osteoarthritis. Biomed. Mater. 7 (2), 024109. doi:10.1088/1748-6041/7/2/024109
Srinivasan, P. P., Patel, V. N., Liu, S., Harrington, D. A., Hoffman, M. P., Jia, X., et al. (2017). Primary Salivary Human Stem/Progenitor Cells Undergo Microenvironment-Driven Acinar-like Differentiation in Hyaluronate Hydrogel Culture. Stem Cell Transl Med 6 (1), 110–120. doi:10.5966/sctm.2016-0083
Steen, M. S., and Froehner, S. C. (2003). PerleCan Fix Your Muscle AChEs. Trends Neurosciences 26 (5), 241–242. doi:10.1016/s0166-2236(03)00077-8
Stum, M., Girard, E., Bangratz, M., Bernard, V., Herbin, M., Vignaud, A., et al. (2008). Evidence of a Dosage Effect and a Physiological Endplate Acetylcholinesterase Deficiency in the First Mouse Models Mimicking Schwartz-Jampel Syndrome Neuromyotonia. Hum. Mol. Genet. 17 (20), 3166–3179. doi:10.1093/hmg/ddn213
Südhof, T. (2018). Towards an Understanding of Synapse Formation. Neuron 100, 276–293. doi:10.1016/j.neuron.2018.09.040
SundarRaj, N., Fite, D., Ledbetter, S., Chakravarti, S., and Hassell, J. R. (1995). Perlecan Is a Component of Cartilage Matrix and Promotes Chondrocyte Attachment. J. Cel Sci. 108 (Pt 7), 2663–2672. doi:10.1242/jcs.108.7.2663
Szekeres, G., Pagel, K., and Heiner, Z. (2021). Analytical Challenges of Glycosaminoglycans at Biological Interfaces. Anal. Bioanal. Chem. 1, 1–9. doi:10.1007/s00216-021-03705-w
Tannock, L. R., and King, V. L. (2008). Proteoglycan Mediated Lipoprotein Retention: a Mechanism of Diabetic Atherosclerosis. Rev. Endocr. Metab. Disord. 9 (4), 289–300. doi:10.1007/s11154-008-9078-0
Tapanadechopone, P., Hassell, J. R., Rigatti, B., and Couchman, J. R. (1999). Localization of Glycosaminoglycan Substitution Sites on Domain V of Mouse Perlecan. Biochem. Biophysical Res. Commun. 265 (3), 680–690. doi:10.1006/bbrc.1999.1714
Thakore, P., and Earley, S. (2019). Transient Receptor Potential Channels and Endothelial Cell Calcium Signaling. Compr. Physiol. 9, 1249–1277. doi:10.1002/cphy.c180034
Thompson, W. R., Modla, S., Grindel, B. J., Czymmek, K. J., Kirn-Safran, C. B., Wang, L., et al. (2011). Perlecan/Hspg2 Deficiency Alters the Pericellular Space of the Lacunocanalicular System Surrounding Osteocytic Processes in Cortical Bone. J. Bone Miner Res. 26 (3), 618–629. doi:10.1002/jbmr.236
Timpl, R. (1996). Macromolecular Organization of Basement Membranes. Curr. Opin. Cel Biol. 8, 618–624. doi:10.1016/s0955-0674(96)80102-5
Trout, A. L., Kahle, M. P., Roberts, J. M., Marcelo, A., de Hoog, L., Boychuk, J. A., et al. (2021). Perlecan Domain-V Enhances Neurogenic Brain Repair after Stroke in Mice. Transl. Stroke Res. 12, 72–86. doi:10.1007/s12975-020-00800-5
Vallet, S. D., Clerc, O., and Ricard-Blum, S. (2021). Glycosaminoglycan-Protein Interactions: The First Draft of the Glycosaminoglycan Interactome. J. Histochem. Cytochem. 69 (2), 93–104. doi:10.1369/0022155420946403
Vasquez, C. A., Moen, S. L., Juliano, M. J., Jagadeesan, B. D., Pluhar, G. E., Chen, C. C., et al. (2019). Development of a Novel Canine Model of Ischemic Stroke: Skull Base Approach with Transient Middle Cerebral Artery Occlusion. World Neurosurg. 127, e251–e260. doi:10.1016/j.wneu.2019.03.082
Vigny, M., Bon, S., Massoulie, J., and Leterrier, F. (1978). Active-site Catalytic Efficiency of Acetylcholinesterase Molecular Forms in Electrophorus, torpedo, Rat and Chicken. Eur. J. Biochem. 85 (2), 317–323. doi:10.1111/j.1432-1033.1978.tb12241.x
Vögtle, T., Sharma, S., Mori, J., Nagy, Z., Semeniak, D., Scandola, C., et al. (2019). Heparan Sulfates Are Critical Regulators of the Inhibitory Megakaryocyte-Platelet Receptor G6b-B. Elife 8. doi:10.7554/eLife.46840
Walker, H. A., Whitelock, J. M., Garl, P. J., Nemenoff, R. A., Stenmark, K. R., and Weiser-Evans, M. C. M. (2003). Perlecan Up-Regulation of FRNK Suppresses Smooth Muscle Cell Proliferation via Inhibition of FAK Signaling. MBoC 14, 1941–1952. doi:10.1091/mbc.e02-08-0508
Wang, B., Lai, X., Price, C., Thompson, W. R., Li, W., Quabili, T. R., et al. (2014). Perlecan-containing Pericellular Matrix Regulates Solute Transport and Mechanosensing within the Osteocyte Lacunar-Canalicular System. J. Bone Miner Res. 29 (4), 878–891. doi:10.1002/jbmr.2105
Wang, L. (2018). Solute Transport in the Bone Lacunar-Canalicular System (LCS). Curr. Osteoporos. Rep. 16, 32–41. doi:10.1007/s11914-018-0414-3
Wang, Y., Cooke, M. J., Sachewsky, N., Morshead, C. M., and Shoichet, M. S. (2013). Bioengineered Sequential Growth Factor Delivery Stimulates Brain Tissue Regeneration after Stroke. J. Controlled Release 172 (1), 1–11. doi:10.1016/j.jconrel.2013.07.032
Whitelock, J. M., Graham, L. D., Melrose, J., Murdoch, A. D., Iozzo, R. V., and Anne Underwood, P. (1999). Human Perlecan Immunopurified from Different Endothelial Cell Sources Has Different Adhesive Properties for Vascular Cells. Matrix Biol. 18 (2), 163–178. doi:10.1016/s0945-053x(99)00014-1
Whitelock, J. M., Melrose, J., and Iozzo, R. V. (2008). Diverse Cell Signaling Events Modulated by Perlecan. Biochemistry 47 (43), 11174–11183. doi:10.1021/bi8013938
Wijeratne, S. S., Martinez, J. R., Grindel, B. J., Frey, E. W., Li, J., Wang, L., et al. (2016). Single Molecule Force Measurements of perlecan/HSPG2: A Key Component of the Osteocyte Pericellular Matrix. Matrix Biol. 50, 27–38. doi:10.1016/j.matbio.2015.11.001
Wilusz, R. E., Sanchez-Adams, J., and Guilak, F. (2014). The Structure and Function of the Pericellular Matrix of Articular Cartilage. Matrix Biol. 39, 25–32. doi:10.1016/j.matbio.2014.08.009
Xu, L., Nirwane, A., and Yao, Y. (2018). Basement Membrane and Blood-Brain Barrier. Stroke Vasc. Neurol. 4 (2), 78–82. doi:10.1136/svn-2018-000198
Xu, X., Li, Z., Leng, Y., Neu, C. P., and Calve, S. (2016). Knockdown of the Pericellular Matrix Molecule Perlecan Lowers In Situ Cell and Matrix Stiffness in Developing Cartilage. Developmental Biol. 418 (2), 242–247. doi:10.1016/j.ydbio.2016.08.029
Xu, Z., Ichikawa, N., Kosaki, K., Yamada, Y., Sasaki, T., Sakai, L. Y., et al. (2010). Perlecan Deficiency Causes Muscle Hypertrophy, a Decrease in Myostatin Expression, and Changes in Muscle Fiber Composition. Matrix Biol. 29 (6), 461–470. doi:10.1016/j.matbio.2010.06.001
Yamada, Y., Hozumi, K., Katagiri, F., Kikkawa, Y., and Nomizu, M. (2013). Laminin-111-derived Peptide-Hyaluronate Hydrogels as a Synthetic Basement Membrane. Biomaterials 34 (28), 6539–6547. doi:10.1016/j.biomaterials.2013.05.044
Yamashita, Y., Nakada, S., Yoshihara, T., Nara, T., Furuya, N., Miida, T., et al. (2018). Perlecan, a Heparan Sulfate Proteoglycan, Regulates Systemic Metabolism with Dynamic Changes in Adipose Tissue and Skeletal Muscle. Sci. Rep. 8 (1), 7766. doi:10.1038/s41598-018-25635-x
Yang, W. D., Gomes, R. R., Alicknavitch, M., Farach-Carson, M. C., and Carson, D. D. (2005). Perlecan Domain I Promotes Fibroblast Growth Factor 2 Delivery in Collagen I Fibril Scaffolds. Tissue Eng. 11 (1-2), 76–89. doi:10.1089/ten.2005.11.76
Yang, W., Gomes, R. R., Brown, A. J., Burdett, A. R., Alicknavitch, M., Farach-Carson, M. C., et al. (2006). Chondrogenic Differentiation on Perlecan Domain I, Collagen II, and Bone Morphogenetic Protein-2-Based Matrices. Tissue Eng. 12 (7), 2009–2024. doi:10.1089/ten.2006.12.2009
Yoshida-Moriguchi, T., and Campbell, K. P. (2015). Matriglycan: a Novel Polysaccharide that Links Dystroglycan to the Basement Membrane. Glycobiology 25 (7), 702–713. doi:10.1093/glycob/cwv021
Zcharia, E., Zilka, R., Yaar, A., Yacoby-Zeevi, O., Zetser, A., Metzger, S., et al. (2005). Heparanase Accelerates Wound Angiogenesis and Wound Healing in Mouse and Rat Models. FASEB j. 19, 211–221. doi:10.1096/fj.04-1970com
Zeng, X., Huang, S., Ye, Q., Rajagopalan, P., Li, W., Kuang, H., et al. (2021). Controllable High-Performance Memristors Based on 2D Fe2GeTe3oxide for Biological Synapse Imitation. Nanotechnology 32, 32. doi:10.1088/1361-6528/abfd58
Zhou, Z., Wang, J., Cao, R., Morita, H., Soininen, R., Chan, K. M., et al. (2004). Impaired Angiogenesis, Delayed Wound Healing and Retarded Tumor Growth in Perlecan Heparan Sulfate-Deficient Mice. Cancer Res. 64 (14), 4699–4702. doi:10.1158/0008-5472.can-04-0810
AChE Acetylcholinesterase
Ach Acetylcholine
ACL Anterior cruciate ligament
AD Alzheimer’s disease
AFM Atomic force microscopy
ApoE Apolipoprotein E
BBB Blood brain barrier
BM Basement membrane
BMP Bone morphogenetic protein
CCA Common carotid artery
cGMP Cyclic guanosine monophosphate
Col Collagen
CS Chondroitin sulphate
CTGF Connective tissue growth factor
DDSH Dyssegmental dysplasia Silverman-Handmaker type
DG Dystroglycan
DMOAD Disease modifying OA drug
Dok7 Docking protein 7
ECM Extracellular matrix
EGF Epidermal growth factor
ERK Extracellular signal-regulated kinase
ET Endothelin
ePTFE Expanded polytetrafluoroethylene
FAK Focal adhesion kinase
FGF Fibroblast growth factor
FGFR Fibroblast growth factor receptor
FRNK Focal adhesion kinase–related non-kinase
GAG Glycosaminoglycan
GM-CSF Granulocyte macrophage-colony stimulating factor
G6b-B-R Megakaryocyte-platelet receptor
HA Hyaluronic acid
HEK Human embryonic kidney
hESC Human embryonic stem cell
HIF Hypoxia-inducible factor
HGF Hepatocyte growth factor
HS Heparan Sulphate
HS-PG Heparan Sulphate Proteoglycan
HSPG2 Heparan sulphate proteoglycan 2/perlecan
Ig Immunoglobulin
IL Interleukin
iPSC Induced pluripotent stem cell
ITIM Immunoreceptor tyrosine-based inhibition motif
IVD Intervertebral disc
KO Knockout
KS Keratan sulphate
LDL Low-density lipoprotein
LDLR Low-density lipoprotein receptor
LG Laminin type G domain
LRP4 Low-density lipoprotein receptor-related protein 4
Mab Monoclonal antibody
MAPK Mitogen-activated protein kinase
MCAO Middle cerebral artery occlusion
MEK Mitogen-activated protein kinase kinase
MEP Motor end plate
MK Megakaryocyte
MMP Matrix metalloproteinase
MNB Myelinated nerve bundle
MuSK Muscle-Specific Kinase
NCAM Neural cell adhesion molecule
NMJ Neuromuscular junction
OA Osteoarthritis
OGD Oxygen-glucose deprivation
PCM Pericellular matrix
PDGF Platelet derived growth factor
PDGFRβ Platelet derived growth factor receptor beta
PG Proteoglycan
PRELP Proline/arginine-rich end leucine-rich repeat protein
PTEN Tumor suppressor phosphatase and tensin homolog
SAM Synaptic adhesion/organizing molecules
SHP-2 Src homology region 2 domain-containing phosphatase-2
SJS Schwartz Jampel Syndrome
SLRP Small leucine rich proteoglycan
SMC Smooth muscle cell
SMF Striated muscle fibre
TGF-β Transforming growth factor-β
TRP Transient receptor potential
TUNEL Terminal deoxynucleotidyl transferase
dUTP nick end labeling
VEGF Vascular endothelial growth factor
VEGF-R2 Vascular endothelial growth factor receptor 2
VLDL Very low-density lipoprotein
WARP Von Willebrand factor A‐domain‐related protein.
Keywords: perlecan, repair biology, vascular repair, cartilage repair, repair of blood brain barrier, perlecan domain-I, perlecan domain-V, growth factor delivery
Citation: Hayes AJ, Farrugia BL, Biose IJ, Bix GJ and Melrose J (2022) Perlecan, A Multi-Functional, Cell-Instructive, Matrix-Stabilizing Proteoglycan With Roles in Tissue Development Has Relevance to Connective Tissue Repair and Regeneration. Front. Cell Dev. Biol. 10:856261. doi: 10.3389/fcell.2022.856261
Received: 17 January 2022; Accepted: 28 February 2022;
Published: 01 April 2022.
Edited by:
Sissel Beate Rønning, Norwegian Institute of Food, Fisheries and Aquaculture Research (Nofima), NorwayReviewed by:
Linda Hiebert, University of Saskatchewan, CanadaCopyright © 2022 Hayes, Farrugia, Biose, Bix and Melrose. This is an open-access article distributed under the terms of the Creative Commons Attribution License (CC BY). The use, distribution or reproduction in other forums is permitted, provided the original author(s) and the copyright owner(s) are credited and that the original publication in this journal is cited, in accordance with accepted academic practice. No use, distribution or reproduction is permitted which does not comply with these terms.
*Correspondence: James Melrose, amFtZXMubWVscm9zZUBzeWRuZXkuZWR1LmF1
Disclaimer: All claims expressed in this article are solely those of the authors and do not necessarily represent those of their affiliated organizations, or those of the publisher, the editors and the reviewers. Any product that may be evaluated in this article or claim that may be made by its manufacturer is not guaranteed or endorsed by the publisher.
Research integrity at Frontiers
Learn more about the work of our research integrity team to safeguard the quality of each article we publish.