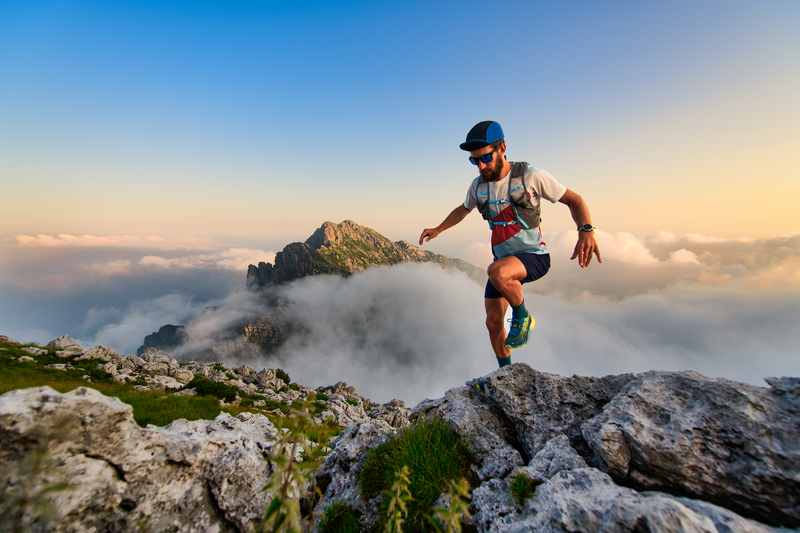
94% of researchers rate our articles as excellent or good
Learn more about the work of our research integrity team to safeguard the quality of each article we publish.
Find out more
PERSPECTIVE article
Front. Cell Dev. Biol. , 26 April 2022
Sec. Stem Cell Research
Volume 10 - 2022 | https://doi.org/10.3389/fcell.2022.854604
This article is part of the Research Topic Developmental Models 2.0 View all 16 articles
Generation of three-dimensional (3D)-structured functional human islets is expected to be an alternative cell source for cadaveric human islet transplantation for the treatment of insulin-dependent diabetes. Human pluripotent stem cells (hPSCs), such as human embryonic stem cells (hESCs) and human induced pluripotent stem cells (hiPSCs), offer infinite resources for newly synthesized human islets. Recent advancements in hPSCs technology have enabled direct differentiation to human islet-like clusters, which can sense glucose and secrete insulin, and those islet clusters can ameliorate diabetes when transplanted into rodents or non-human primates (NHPs). However, the generated hPSC-derived human islet-like clusters are functionally immature compared with primary human islets. There remains a challenge to establish a technology to create fully functional human islets in vitro, which are functionally and transcriptionally indistinguishable from cadaveric human islets. Understanding the complex differentiation and maturation pathway is necessary to generate fully functional human islets for a tremendous supply of high-quality human islets with less batch-to-batch difference for millions of patients. In this review, I summarized the current progress in the generation of 3D-structured human islets from pluripotent stem cells and discussed the importance of adapting physiology for in vitro functional human islet organogenesis and possible improvements with environmental cues.
Diabetes is a complicated chronic disease that affects more than 450 million people worldwide (Cho et al., 2018; Huang et al., 2018; Rogers et al., 2020; Rogers and Kim, 2020; Shah et al., 2020; Smalls et al., 2020; Tan et al., 2020). Microvasculature defects caused by diabetes lead to stroke, blindness, and kidney failure in some patients (Creager et al., 2003; Luscher et al., 2003; Beckman et al., 2013; Paneni et al., 2013; Cayabyab et al., 2021). Although the discovery of insulin a century ago made significant improvements in diabetic therapeutics, from lethal to treatable, even modern insulin therapy cannot be an alternative to endogenous functional insulin-producing β cells. Insulin treatment requires manual glucose monitoring and adjustment of insulin injection in both amount and timing every day, which also means the high maintenance and economic impact for the patients and expenditure on healthcare in all countries. Continuous, rapid fine-tuning of glucose or other nutrition-sensing insulin secretion is necessary for functional cure of diabetes. Cadaveric islet transplantation provides a functional cure for insulin-dependent type 1 diabetes (T1D) with a success rate of more than 90%, and its efficacy has been sustained for over 20 years in some cases (Lemos et al., 2021). The short supply and requirement of immunosuppressants, which increase the risk of adverse effects, limit the islet transplantation for the treatment of diabetes. Owing to their pluripotency and self-renewal function, human pluripotent stem cells (hPSCs), such as human induced pluripotent stem cells (hiPSCs) (Takahashi et al., 2007) and human embryonic stem cells (hESCs) (Thomson et al., 1998), offer an alternative cell resource for human cadaveric islets. Step-by-step treatment of small chemicals and recombinant proteins, which induce differentiation from hPSCs, was developed by integrating the knowledge of specific signaling pathways that contribute to the pancreatic lineage specification and islet differentiation. While recent advances in this approach have made it possible to reproducibly generate functional hPSC-derived insulin-producing β-like cells, which are capable of sensing glucose concentration and secreting insulin, there are several major limitations to our knowledge in generating fully functional human β cells or human islets. First, we have not yet clarified the pathway to induce each endocrine cell type separately. Although there are several reports on the production of more biasedly α cells rather than β cells from hPSCs (Rezania et al., 2011; Peterson et al., 2020), these established protocols do not generate a single-cell type but rather contain other cell types together with differentiation. Second, we have not completely understood the pathway of postnatal functional islet maturation, which typically occurs a few years after birth in humans. hPSC-derived insulin-producing cells are generated either by β-like cells (hPSC-derived β-like cells; monolayer or isolated insulin-producing cells) or human islet-like organoid clusters (hPSC-derived islets; α, β, γ-cells containing 3D-structured cell cluster). Therefore, the current protocol of generating fully functional hPSC-derived β-like cells or islets remains a challenge. Identifying such a pathway is necessary to improve the immaturity of generated islets and reduce the risk of batch-to-batch differences. In this review, I aimed to outline the current progress in generating functional human pancreatic islets from the perspective of 1) pancreatic lineage specification, 2) postnatal functional maturation, 3) differences between primary human islets and hPSC-derived islets, 4) missing factors, and 5) further adaptation of the environmental physiological responses (Figure 1). Additionally, I aimed to discuss future perspectives to improve the current protocol for generating functional human islets from hPSCs.
Since the establishment of hESCs (Thomson et al., 1998), insulin-producing β cells have been identified in spontaneously differentiated cells from hESCs (Lumelsky et al., 2001; Assady et al., 2001). In addition with the efforts to identify the essential pathways for pancreatic lineage specification, efficient endodermal differentiation (D'Amour et al., 2005) and pancreatic progenitor (PP) differentiation (D'Amour et al., 2006) protocols have been developed. hESC- or hiPSC-derived PPs are capable to differentiate into mature functional β cells in vivo within a couple of months of transplantation (Kroon et al., 2008), suggesting that these protocols represent the right track for generating functional β cells in vitro. The key features of stage-specific differentiation are controlled by stage-specific transcriptional factors (TFs) and key identity genes (Tahbaz and Yoshihara, 2021). hPSCs undergo definitive endodermal (DE) differentiation by targeting the Activin A pathway and canonical Wnt (cWnt) pathway, Wnt3A. SRY-box transcription factor 17 (SOX17) and Forkhead box A2tbox2 (FOXA2) are prominent DE markers. Fibroblast growth factor 7 (FGF7/KGF) induces a primitive gut tube (PGT). Hepatocyte nuclear factor 1β (HNF1β) and hepatocyte nuclear factor-4α (HNF4α) are well-known PGT markers. Retinoic acid (RA) signaling activation, combined with sonic hedgehog (SSH) inhibition, bone morphogenetic protein (BMP) signal inhibition, and protein kinase C (PKC) activation, leads to PPs. PPs are pancreatic and duodenal homeobox 1 (PDX-1) and NK6 homeobox 1 (NKX6-1) double-positive cells, which can further generate mono-hormonal β cells, α cells (glucagon producing), and δ cells (somatostatin producing). RAs activation, SSH inhibition, BMP signal inhibition, transforming growth factor β (TGFβ) signal inhibition, and thyroid hormone signaling activation (THR) lead to endocrine progenitor (EP) generation. NEUROG3 (NGN3) is the key transcriptional factor of EP. It has been recently observed that targeting the cytoskeleton by inhibiting actin polymerization enhances NGN3 expression in the endocrine stage (Mamidi et al., 2018; Hogrebe et al., 2020). γ-secretase inhibition, BMP signal inhibition, TGFβ signal inhibition, and THR activation lead to the generation of endocrine hormone-producing cells (α, β, and γ cells). Pre-matured glucose-response β-like cells are generated (Pagliuca et al., 2014; Rezania et al., 2014; Russ et al., 2015). Although these previous studies established reproducible GSIS, impaired glucose-stimulated Ca2+ flux, reduced production of insulin, and many transcriptional differences have been observed in hPSC-derived β-like cells compared to those of primary β cells or islets (Veres et al., 2019; Augsornworawat et al., 2020; Yoshihara et al., 2020; Balboa et al., 2022). These results suggested that hPSC-derived β-like cells may remain a feature of the juvenile/neonatal stage of β cells (Hrvatin et al., 2014).
Increasing evidence suggests that multiple functional maturation pathways play an important role in the postnatal acquisition of robust GSIS function (Alvarez-Dominguez and Melton, 2021). Therefore, the current focus on improving the protocol for generating functional islets relies on the identification of the new pathways for functional maturation. Islet functional maturation is accelerated after birth to adapt to oxygen- and nutrition-rich environments. There are a number of transcriptional factors and key β-cell marker gene expressions such as INSULIN (INS), MAF bZIP transcription factor A (MAFA), SIX homeobox 2 (SIX2), glucose-6-phosphatase catalytic subunit 2 (G6PC2), and urocortin 3 (UCN3), which are lower in hPSC-derived β-like cells generated in vitro (Balboa et al., 2022). Chromatin remodeling and miRNA expression have been suggested to control the gene expression for functional β-cell maturation (Xie et al., 2013; Dhawan et al., 2015; Jacovetti et al., 2015). MAFA expression, regulated by T3 and other unknown signals, is important for INS and G6PC2 transcription (Martin et al., 2008; Artner et al., 2010; Aguayo-Mazzucato et al., 2011; Hang and Stein, 2011). G6PC2 expression suppresses low-glucose concentration-stimulated insulin secretion, leading to amplification of the GSIS function. MAF BZIP transcription factor B (MAFB) alternatively regulates INS gene expression in human β cells (Russell et al., 2020). Metabolic shift triggered by weaning enhances GSIS (Jacovetti et al., 2015; Stolovich-Rain et al., 2015). SIX2 is important for sustaining key β-cell lineage and metabolic genes (Arda et al., 2016; Velazco-Cruz et al., 2020a; Bevacqua et al., 2021). ATP and other nutritions, including glucose, have been reported to enhance the maturity of juvenile/neonate β cells in rodents (Rorsman et al., 1989; Hellerstrom and Swenne, 1991). We have previously demonstrated that estrogen-related receptor-γ (ERRγ) and related metabolic genes are upregulated during postnatal development in mouse islets (Yoshihara et al., 2016). ERRγ directly regulates gene clusters related to mitochondrial pyruvate metabolism, tricarboxylic acid (TCA) cycle, and oxidative phosphorylation (OxPhos), causing enhanced glucose-stimulated ATP production and insulin secretion (Yoshihara et al., 2016; Ahmadian et al., 2018; Fan et al., 2018). These metabolic reprogramming may be postnatally coordinated by the non-canonical WNT pathway (ncWNT4) (Bader et al., 2016; Yoshihara et al., 2020). Flattop (Fltp), a receptor of Wnt4, is upregulated postnatally in murine β cells, and Wnt4 enhances the maturation of β cells in mice and humans. We demonstrated that Wnt4 expression is significantly lower in murine neonatal islets or hPSC-derived β-like cells than that in murine adult β cells or primary adult human islets (Bader et al., 2016; Yoshihara et al., 2016; Yoshihara et al., 2020). Additionally, Wnt4 treatment enhances oxidative features and GSIS function in hPSC-derived 3D-structured human islet-like organoids (HILOs). These studies indicate that not only lineage commitment but also metabolic adaptation is important for enhancing the functional maturation of hPSC-derived β-like cells or islets. Other lineage-dependent transcriptional factors (LDTFs) and signal-dependent transcriptional factors (SDTFs), which collaboratively modulate functional maturation, have been discussed elsewhere (Wortham and Sander, 2021). The β-cell function is enhanced through aging in rodents, and p16 plays a key role in reducing cell proliferation and enhancing GSIS function. Circadian rhythms acquired during development also play an important role in β-cell maturation (Alvarez-Dominguez et al., 2020). Recent advances in single-cell technologies have enabled recapturing of the gene clusters involved in the differentiation and in vivo maturation of hPSC-derived β-like cells (Veres et al., 2019; Augsornworawat et al., 2021; Balboa et al., 2022). Several key β-cell genes in hPSC-derived β-like cells, including INS, MAFA, G6PC2, and UCN3, have been observed to be expressed in equivalent amounts only after in vivo maturation (Veres et al., 2019; Augsornworawat et al., 2021; Balboa et al., 2022). From these studies, the key changes during functional β-cell maturation seem to be advanced lineage commitment, metabolic reprograming to a more oxidative status with efficient glucose utilization, cell cycle reduction, and adaptation to environmental physiological changes in vivo.
Although there has been a better understanding of the pancreatic differentiation, islets maturation, and the technologies mimicking human organogenesis, there are many differences between the primary human islets supplied by the human donors and the generated hPSC-derived islets. Generally, hPSC-derived islets exhibit lower insulin production and GSIS fold changes than adult human primary islets (Pagliuca et al., 2014; Rezania et al., 2014; Velazco-Cruz et al., 2020b; Yoshihara et al., 2020). These functional differences may be insufficiently represented since the primary adult islets functionally differ among individuals, and the donor islets were typically shipped at 4 C overnight or a few days, which makes the condition of primary human islets unideal. This suggests that the functional differences between hPSC-derived islets and primary adult islets maybe even bigger than the ones described or thought of previously. In 2014, Hrvatin et al. (2014) revealed that the hPSC-derived β-like cells resemble human fetal β cells more transcriptionally rather than adult β cells. Remarkable technological advances have been made to create more glucose-responsive hPSC-derived β-like cells, which are capable of secreting insulin in response to high glucose, to produce mono-hormonal insulin-producing cells rather than poly-hormonal insulin-producing cells, and for improved differentiation efficacy to create insulin-producing cells to about ∼70%. The insulin production levels are significantly higher than those in previous protocols (Pagliuca et al., 2014; Rezania et al., 2014; Russ et al., 2015). Although considerable progress has been made in creating functional islets from hPSCs, there are still significant differences between hPSC-derived β-like cells and human primary β cells. Ca2+ influx, which is triggered by the closure of the KATP channel in response to ATP amplification typically induced by glucose metabolism in the mitochondria of hPSC-derived β-like cells, is much slower and abnormal compared with that in the primary human islets 23,24,4. This is linked to the slower and fewer insulin secretion responses in hPSC-derived β-like cells (Pagliuca et al., 2014; Rezania et al., 2014; Nair et al., 2019). Patch-clamp recordings revealed heterogeneity and aberrant ion current such as Na+ (Balboa et al., 2022). Aberrant metabolic features in hPSC-derived β-like cells or islets, such as glycolysis, TCA cycle, and OxPhos, were observed and demonstrated as the target pathways to improve GSIS (Yoshihara et al., 2016; Nair et al., 2019; Davis et al., 2020; Balboa et al., 2022). It has been observed that longer culturing time of hPSC-derived islets at the maturation stage in vitro improves some maturation marker gene expression and GSIS; however, it has been identified that in vivo engraftment for 6 months is necessary to promote further maturation (Veres et al., 2019; Augsornworawat et al., 2020; Balboa et al., 2022). There remain several missing factors to understand how pancreatic islets adapt to the physiological environment during functional maturation. These factors should be considered to further improve the generation of functional human islets from hPSCs (Figure 1).
Blood glucose levels are elevated postnatally triggered by weaning owing to the alternation of nutritional sources from fat-rich breast milk to a carbohydrate-rich chow diet (Stolovich-Rain et al., 2015). Glucose has been identified as a maturation factor in rodent β cells (Hellerstrom and Swenne, 1991). Therefore, the current protocol for in vitro β-cell generation is intended to increase glucose concentration during differentiation from the PP stage to the β-cell maturation stage (Yoshihara et al., 2020). The bottleneck of glycolysis (Davis et al., 2020) or mitochondrial metabolism (Yoshihara et al., 2016) in hPSC-derived β-like cells may limit the glucose-induced maturation efficacy. Improving β-cell metabolism has been highlighted as the target for improving maturation. We have previously demonstrated that forced expression of estrogen-related receptor γ (ERRγ), a master regulator of mitochondrial gene expression, including pyruvate metabolism, the TCA cycle, and OxPhos improves GSIS in hPSC-derived β-like cells (Yoshihara et al., 2016). Reaggregation (Nair et al., 2019) or the ncWnt4/planar cell polarity (PCP) pathway (Bader et al., 2016; Yoshihara et al., 2020) enhances mitochondrial metabolism, which enhances GSIS function in hPSC-derived β-like cells. ncWnt/PCP effector Flattop (Fltp) expression increases during postnatal β cell maturation and is heterogeneously expressed in mature β cells. Fltp-positive (Fltp+) β cells are characterized by higher mitochondrial gene expression and activity (Bader et al., 2016). Wnt4, a component of ncWnt pathway increases during postnatal β cell maturation, and Wnt4 expression is lower in hPSC-derived β-like cells than that in the primary human β cells. Wnt4 stimulation enhances expression in mitochondrial gene expression and activity and GSIS function in hPSC-derived human islet-like organoids (HILOs). We have reported that ERRγ is a downstream factor of Wnt4 signaling to regulate mitochondrial gene expression and activity (Yoshihara et al., 2020). Declining mammalian target of rapamycin complex 1 (mTORC1) signaling from neonate to adult maturation has been observed (Ni et al., 2017; Jaafar et al., 2019; Helman et al., 2020). The constitutively active mTORC pathway in mouse β cells increases β-cell proliferation while reducing β cells’ maturation gene expressions such as Ins1, Ucn3, Mafa, and G6pc2 and mitochondrial metabolic genes (Jaafar et al., 2019). mTORC1 enhances the responses of amino acid-stimulated insulin secretion, which increases insulin secretion at low glucose concentrations and reduces GSIS thresholds (Helman et al., 2020). In addition to mitochondria metabolism, glycolysis is impaired in hPSC-derived β-like cells, which limits the pyruvate and related fuel mitochondrial metabolite production. The enzymatic activity, but not the expression of glyceraldehyde 3-phosphate dehydrogenase (GAPDH) and phosphoglycerate kinase 1 (PGK1), which are important for the enzymatic conversion of glyceraldehyde 3-phosphate to 3-phosphoglycerate in glycolysis in hPSC-derived β-like cells, is defective (Davis et al., 2020). This bottleneck of enzymatic activity slows down the glycolysis activity, limiting the production of phosphoenolpyruvate to fuel ATP generation through the mitochondria. Cell-permeable intermediate metabolites such as methyl-succinate and methyl-pyruvates partially rescue the GSIS defect in hPSC-derived β-like cells. Since most of the hPSC-derived β-like cells generated in vitro present significantly lower maximum respiration of the mitochondria, efficient supply of the mitochondria and improvement of the mitochondria are some of the key features of immature hPSC-derived β-like cells.
Dynamic continuous nutritional exchange is the natural condition for endogenous functional β-like cells; however, it has not been replicated for in vitro-cultured hPSC-derived β-like cells. The current protocol relies on feeding nutrition-rich media during β-like cell differentiation every day or every other day. In this setting, glucose is utilized without refill for more than 24–48 h and waste such as lactate from the cells continues to accumulate in the culture condition. Lactate and pyruvate transporters, such as monocarboxylate transporter 1 (MCT1), are disallowance genes in functional β cells, and their expression has been observed in neonates or hPSC-derived β-like cells (Yoshihara et al., 2016). This notion raises the concern that accumulated lactate not only oxidizes the media but is also re-absorbed in the hPSC-derived β-like cells to interrupt the proper metabolic activity and differentiation in β cells. In addition, a recent study found that hPSC-derived islets are hyper-sensitive to pyruvate-induced insulin secretion, which typically is not observed in primary adult islets (Balboa et al., 2022). Aberrant pyruvate transporter expression may contribute to this abnormal phenotype of hPSC-derived islets. In vivo-mimicking continuous nutritional exchange by techniques such as microfluidic perfusion (Jun et al., 2019) may aid in improving the metabolic disorder in hPSC-derived β-like cells.
In addition to nutrition, oxygen is a critical factor responsible for the functionality of β cells. Mature islets are surrounded by microvessels, which provide circulating oxygen tension (approximately 100 mmHg) (Jansson and Hellerstrom, 1983). It has been observed that in vitro-cultured human islet viability, especially hypoxia-induced necrosis in the center of islets, is improved by hyperoxia (approximately 350 mmHg) (Komatsu et al., 2016; Komatsu et al., 2017). Since there is no oxygen supply from microvessel in vitro-cultured hPSC-derived islets, current typical cell culture conditions, 5% CO2, 20% O2 (approximately 160 mmHg), may not be ideal for differentiation and maintenance. The inner cell mass of blastocyst is likely to maintain low O2 condition (approximately 5% O2) (Rodesch et al., 1992; Clark et al., 2006; Harvey, 2007; Lees et al., 2019). This is reasonable since hPSC metabolism is characterized by being highly dependent on glycolysis and up to 70% of glucose is converted to lactate (Lees et al., 2019). During differentiation and maturation, this metabolism is shifted to a more oxidative mitochondria-dependent process (Varum et al., 2011). High O2 levels (approximately 35% O2) promote the growth of ex vivo-cultured mouse pancreatic buds (Fraker et al., 2007). Similarly, rat pancreata cultured with high O2 (60–80% O2) promotes β-cell differentiation (Heinis et al., 2010). Mechanistically, hypoxia activates hypoxia-inducible factor 1α (HIF1α), which in turn activates the hairy and enhancer of split (HES1) to prevent β-cell differentiation (Heinis et al., 2010). High O2 (60% O2) exposure between the endoderm and EP stage facilitated differentiation of EPs by activating cWnt signaling and suppressing HIF1α and HES1 expression, which leads to the promotion of NGN3 expression in the insulin-producing cell differentiation model of mouse and human ES cells (Cechin et al., 2014; Hakim et al., 2014). In contrast, an excessive amount of O2 may increase the reactive oxygen species (ROS) and cause oxidative stress which triggers β-cell dysfunction (Robertson et al., 2004; Gerber and Rutter, 2017). Both hypoxia and oxidative stress/ROS induce thioredoxin-interacting protein (Txnip), a major cause of β-cell dysfunction in diabetes (Yoshihara et al., 2010a; Yoshihara et al., 2010b; Yoshihara et al., 2014; Yoshihara, 2020). Therefore, rather than a continuous supply of excessive O2, more dynamic regulation of O2 supply should be considered to improve the current protocol for the differentiation of the β cells. Monitoring the dynamic O2 supply will benefit the better differentiation and function of hPSC-derived β cells.
Islet microenvironments consist of pancreatic fibroblasts, acinar cells, vasculature cells, and immune cells such as macrophages. Increasing evidence suggests that exocrine acinar cells play a critical role in maintaining islet function and defects in exocrine link to the pathogenesis of some types of diabetes (Rickels et al., 2020; Chiou et al., 2021). The pancreatic organ-matched mesenchyme promotes self-renewal of the hPSC-derived PPs without further direct differentiation (Sneddon et al., 2012). These studies suggest that the islet microenvironment created by non-endocrine cells contributes to islet development and functional regulation. Further investigation of the spatiotemporal interaction between the endocrine cells and non-endocrine cells in islets is required to identify the key pathway that facilitates islet differentiation and physiological activity during fetal and postnatal development.
It has been widely recognized that central and peripheral neurons play an important role in regulating the exocrine and endocrine pancreas (Buijs et al., 2001; Chandra and Liddle, 2013). Neural projections from the dorsal motor neuron (dMN) in the brain stem innervate the pancreas and regulate enzymatic and hormonal secretions in the exocrine and endocrine systems, respectively. Thyrosine hydroxylase (TH)-positive sympathetic nerves have been detected in the fetal pancreas starting from the early stages, such as 8 weeks post-conception (w.p.c) (Krivova et al., 2021). The sympathetic nerve innervation of mouse islets is reported to be a critical factor for proper islet architecture and function in a β-adrenergic dependent manner (Borden et al., 2013). Although how the innervation of peripheral nerves in human islets is important for organizing islet architecture, development, and function is still controversial or remains unknown, many receptors for neurotransmitters are expressed in human islets and regulate hormone secretion (Ahren, 2000; Burns et al., 2015; Rosario et al., 2016; Lin et al., 2021).
Islets are multicellular mini-organs consisting of differential hormone-expressing cells with paracrine hormone regulation (Huising, 2020). Α cells secrete glucagon and glucagon-like peptide-1 (GLP-1), which enhance insulin secretion in β cells. δ cells secrete somatostatin, which suppresses insulin secretion in β cells and glucagon secretion from α cells (Strowski et al., 2000). Insulin inhibits glucagon secretion by stimulating somatostatin secretion by sodium-glucose co-transporter-2 (SGLT2) (Vergari et al., 2019). UCN3, co-secreted with insulin, also stimulates somatostatin secretion from δ cells to enhance paracrine feedback regulation of β cells (van der Meulen et al., 2015). Although this paracrine hormone regulation plays an important role in proper insulin secretion in islets, the effect of paracrine signaling on islet differentiation and maturation has not yet been elucidated.
Islets are embedded in the exocrine pancreas while through the nervous innervation and vasculature network receiving the signals from outside the pancreas. The intestine plays a central role in regulating β-cell function through the enteroendocrine hormone secretion directly or via the vagus nerve–brain–pancreas axis. Adipokines and hepatokines also play an important role in β-cell function (El Ouaamari et al., 2013; Prentice et al., 2021). These signals from outside the pancreas influence the differentiation and maturation of islets remain poorly understood. Since the organs are simultaneously differentiated and maturate during fetal and postnatal development, it is possible that organ–organ interactions also play an important role in islet differentiation and maturation.
Adapting to physiological environmental stimuli is a hallmark of mature adult organs, which are broadly regulated by nuclear hormone receptor (NR) signaling (Evans and Mangelsdorf, 2014). NR, a superfamily of 48 transcriptional factors in humans, regulates gene expression in response to various hormones, lipids, and metabolites and plays a master role in development and physiology (Evans and Mangelsdorf, 2014). Many NRs are expressed in rodent and human islets and are related to the β-cell physiology in response to lipid metabolism (Chuang et al., 2008) (Table 1). Free fatty acids, such as palmitate, stimulate insulin secretion for the short term, whereas chronic exposure to high concentrations of free fatty acids induces compensatory β-cell expansion and eventually leads to β-cell growth arrest and apoptosis. Nuclear receptor subfamily 4 group A member 1 (NR4A1) is translocated to the nucleus in response to free fatty acids and plays a key role in lipotoxicity-induced β-cell apoptosis (Susini et al., 1998; Roche et al., 1999; Briand et al., 2012; Yu et al., 2015; Close et al., 2019). Mechanistically, NR4A1 binds to Foxo1 at the MafA promoter region and suppresses its expression to reduce insulin transcription (Briand et al., 2012). NR4A1 is required for Nkx6-1-mediated β-cell mass expansion (Tessem et al., 2014). Cholesterol sensor Liver X receptor (LXR; LXRα and LXRβ) stimulates GSIS and insulin biosynthesis (Efanov et al., 2004; Zitzer et al., 2006). LXRβKO mice exhibit a glucose-intolerant phenotype secondary to blunted GSIS in vivo (Gerin et al., 2005; Zitzer et al., 2006). Peroxisome proliferator-activated receptors (PPARs; PPARγ, PPARα, and PPARδ) broadly regulate fatty acid oxidation, thereby enhancing GSIS and reducing lipotoxic effects in β cells. Estrogen receptors (ERs; ERα and ERβ) also protect against lipotoxicity, especially in females. Testosterone-mediated andorogen receptor (AR) signaling enhances GSIS and glucagon-like peptide-1 (GLP-1)-mediated insulin secretion in males (Navarro et al., 2016). Vitamin D receptor (VDR) regulates SWI/SNF chromatin remodeling together with bromodomain-containing protein 7 (BRD7) and BRD9, and protection from cytokines induces dedifferentiation of β cells in rodents and hPSC-derived β-like cells (Wei et al., 2018). Estrogen-related receptor γ (ERRγ) regulates mitochondrial gene expression related to the TCA cycle and OxPhos (Yoshihara et al., 2016). Farnesoid X receptors (FXRs; FXRα and FXRβ) regulate Ins (Renga et al., 2010), adenylyl cyclase 8 (Adcy8) (Kong et al., 2019a), transient receptor potential ankyrin 1 (Trpa1) (Kong et al., 2019b), and GLP-1 receptor (Glp1r) (Kong et al., 2021) gene expression and protect from lipotoxicity (Popescu et al., 2010) in the rodents. Glucocorticoids and steroids are known to induce hyperglycemia, which may cause diabetes. Glucocorticoid receptor (GR) signaling through dexamethasone was initially believed to have a negative impact on islet physiology by reducing insulin synthesis and insulin secretion and inducing apoptosis in murine β cells (Philippe and Missotten, 1990; Goodman et al., 1996; Ranta et al., 2006; Reich et al., 2012; Aylward et al., 2021). In contrast, GR plays an essential role in human fetal and adult islet development by controlling β-cell transcriptomes, such as PDX-1, GCK, GLUT2, SIX2, and SIX3(Gesina et al., 2006; Matthews and Hanley, 2011; Ghazalli et al., 2018). Thyroid hormone receptors (TRs; TRα and TRβ) regulate MafA expression during postnatal rat β-cell maturation (Aguayo-Mazzucato et al., 2013). Hypothyroidism impairs hPSC-PP maturation in mice suggesting the importance of TRs signaling in vivo functional β-cell maturation (Bruin et al., 2016). Circadian rhythms regulate β-cell maturation and physiology (Marcheva et al., 2010; Perelis et al., 2015; Alvarez-Dominguez et al., 2020). The clock protein REV-ERVα regulates the circadian rhythms in β cells, and downregulation of REV-ERVα impairs time-dependent GSIS (Vieira et al., 2012). REV-ERVα may be an important physiological regulator of β cells in time-restricted feeding or preventing pathogenesis of T2D by modulating β-cell rhythmic transcriptional regulation by adapting to environmental changes over time (Petrenko et al., 2020; Brown et al., 2021). Hepatic nuclear receptor 4-α (HNF4α) is responsible for maturity-onset diabetes of the young 1 (MODY1) (Yamagata et al., 1996). Although NRs integrate gene regulation and cell function to adapt to environmental physiological stimulation, the contribution of NRs to functional maturation to adapt to environmental stimulation in islets is largely unknown. Understanding how these factors cooperatively regulate β-cell differentiation and physiological adaptation may improve generation of a physiological level of functional β cells.
I discussed the existing limitations of the technology to generate human islets from pluripotent stem cells. The current protocol is generated on the basis of lineage specification; however, sufficient number of integrated functional maturation pathways are not there. The challenge remains to create cells with protocols within a few weeks of additional maturation, which exhibit equal functionality with primary human islets in terms of 1) amount of insulin secretion, 2) speed and accuracy of response to glucose, 3) transcriptome, and 4) controlled differentiation to individual endocrine cell types by integrating the new pathways adapting to environmental physiological stimulation. The key challenge in the field is that maturation may not only be coordinated by single signaling but corporately regulates by multiple signaling as the environmental senor switch (Figure 1). Additionally, the required signaling may not be constitutively activated or suppressed; however, it is dynamically regulated by adapting to environmental physiological situations to work flexibly and properly in the spatiotemporal physiological situation. The future challenge of developing new technology should focus on dissecting many differential signaling and transcriptional networks that are involved in postnatal functional maturation and integrating how those signaling pathways synergistically regulate islet maturation. The robustness of scalability, consistency of high quality, and less batch-to-batch generation of different human islets in vitro should be continuously improved. One important notion for adapting to environmental signals is the duration and timing of the stimulation of those signals. The current protocol generates glucose-responsive hPSC-derived β-like cells or islets within 4–10 weeks of culturing in vitro. However, it takes approximately 40 weeks of differentiation and a few years of postnatal functional islet maturation in human body. This significant gap in the developmental time may need to be considered adapting to environmental cues in functional human islet organogenesis. A deeper understanding of islet differentiation and maturation is critical for generating fully functional human islets in vitro. Integrating the microenvironmental cues that mimic in vivo situations may help in further improving the protocol.
The original contributions presented in the study are included in the article/Supplementary Material, further inquiries can be directed to the corresponding author.
EY conceptualized and wrote the manuscript.
This work was supported by the California Institute for Regenerative Medicine (CIRM)-DISC2 discovery award and Allen Foundation. EY was supported by the JDRF (formerly known as Juvenile Diabetes Research Foundation) career development award, which was made possible through collaboration between JDRF International and the Leona M. and Harry B. Helmsley Charitable Trust.
EY is an inventor on licensed patents and patent applications related to the HILO technology described in this manuscript.
All claims expressed in this article are solely those of the authors and do not necessarily represent those of their affiliated organizations, or those of the publisher, the editors, and the reviewers. Any product that may be evaluated in this article, or claim that may be made by its manufacturer, is not guaranteed, or endorsed by the publisher.
The Supplementary Material for this article can be found online at: https://www.frontiersin.org/articles/10.3389/fcell.2022.854604/full#supplementary-material
Aguayo-Mazzucato, C., Koh, A., El Khattabi, I., Li, W.-C., Toschi, E., Jermendy, A., et al. (2011). Mafa Expression Enhances Glucose-Responsive Insulin Secretion in Neonatal Rat Beta Cells. Diabetologia 54, 583–593. doi:10.1007/s00125-010-2026-z
Aguayo-Mazzucato, C., Zavacki, A. M., Marinelarena, A., Hollister-Lock, J., El Khattabi, I., Marsili, A., et al. (2013). Thyroid Hormone Promotes Postnatal Rat Pancreatic β-Cell Development and Glucose-Responsive Insulin Secretion through MAFA. Diabetes 62, 1569–1580. doi:10.2337/db12-0849
Ahmadian, M., Liu, S., Reilly, S. M., Hah, N., Fan, W., Yoshihara, E., et al. (2018). ERRγ Preserves Brown Fat Innate Thermogenic Activity. Cel Rep. 22, 2849–2859. doi:10.1016/j.celrep.2018.02.061
Ahrén, B. (2000). Autonomic Regulation of Islet Hormone Secretion - Implications for Health and Disease. Diabetologia 43, 393–410. doi:10.1007/s001250051322
Alonso-Magdalena, P., Ropero, A. B., Carrera, M. P., Cederroth, C. R., Baquié, M., Gauthier, B. R., et al. (2008). Pancreatic Insulin Content Regulation by the Estrogen Receptor ERα. PLoS One 3, e2069. doi:10.1371/journal.pone.0002069
Alvarez-Dominguez, J. R., Donaghey, J., Rasouli, N., Kenty, J. H. R., Helman, A., Charlton, J., et al. (2020). Circadian Entrainment Triggers Maturation of Human In Vitro Islets. Cell Stem Cell 26, 108–122. e10. doi:10.1016/j.stem.2019.11.011
Alvarez-Dominguez, J. R., and Melton, D. A. (2021). Cell Maturation: Hallmarks, Triggers, and Manipulation. Cell 185, 235.
Arda, H. E., Li, L., Tsai, J., Torre, E. A., Rosli, Y., Peiris, H., et al. (2016). Age-Dependent Pancreatic Gene Regulation Reveals Mechanisms Governing Human β Cell Function. Cel Metab. 23, 909–920. doi:10.1016/j.cmet.2016.04.002
Artner, I., Hang, Y., Mazur, M., Yamamoto, T., Guo, M., Lindner, J., et al. (2010). MafA and MafB Regulate Genes Critical to β-Cells in a Unique Temporal Manner. Diabetes 59, 2530–2539. doi:10.2337/db10-0190
Assady, S., Maor, G., Amit, M., Itskovitz-Eldor, J., Skorecki, K. L., and Tzukerman, M. (2001). Insulin Production by Human Embyonic Stem Cells. Diabetes 50 (8), 1691–7. doi:10.2337/diabetes.50.8.1691
Augsornworawat, P., Maxwell, K. G., Velazco-Cruz, L., and Millman, J. R. (2020). Single-Cell Transcriptome Profiling Reveals β Cell Maturation in Stem Cell-Derived Islets after Transplantation. Cel Rep. 32, 108067. doi:10.1016/j.celrep.2020.108067
Augsornworawat, P., Maxwell, K. G., Velazco-Cruz, L., and Millman, J. R. (2021). Single-cell Transcriptome Profiling Reveals β Cell Maturation in Stem Cell-Derived Islets after Transplantation. Cel Rep. 34, 108850. doi:10.1016/j.celrep.2021.108850
Aylward, A., Okino, M.-L., Benaglio, P., Chiou, J., Beebe, E., Padilla, J. A., et al. (2021). Glucocorticoid Signaling in Pancreatic Islets Modulates Gene Regulatory Programs and Genetic Risk of Type 2 Diabetes. Plos Genet. 17, e1009531. doi:10.1371/journal.pgen.1009531
Bader, E., Migliorini, A., Gegg, M., Moruzzi, N., Gerdes, J., Roscioni, S. S., et al. (2016). Identification of Proliferative and Mature β-cells in the Islets of Langerhans. Nature 535, 430–434. doi:10.1038/nature18624
Balboa, D., Barsby, T., Lithovius, V., Saarimaki-Vire, J., Omar-Hmeadi, M., Dyachok, O., et al. (2022). Functional, Metabolic and Transcriptional Maturation of Human Pancreatic Islets Derived from Stem Cells. Nat. Biotechnol. doi:10.1038/s41587-022-01219-z
Baquié, M., St-Onge, L., Kerr-Conte, J., Cobo-Vuilleumier, N., Lorenzo, P. I., Jimenez Moreno, C. M., et al. (2018). The Liver Receptor Homolog-1 (LRH-1) Is Expressed in Human Islets and Protects β-cells against Stress-Induced Apoptosis. Hum. Mol. Genet. 27, 406. doi:10.1093/hmg/ddx402
Bardoux, P., Zhang, P., Flamez, D., Perilhou, A., Lavin, T. A., Tanti, J.-F., et al. (2005). Essential Role of Chicken Ovalbumin Upstream Promoter-Transcription Factor II in Insulin Secretion and Insulin Sensitivity Revealed by Conditional Gene Knockout. Diabetes 54, 1357–1363. doi:10.2337/diabetes.54.5.1357
Beckman, J. A., Paneni, F., Cosentino, F., and Creager, M. A. (2013). Diabetes and Vascular Disease: Pathophysiology, Clinical Consequences, and Medical Therapy: Part II. Eur. Heart J. 34, 2444–2452. doi:10.1093/eurheartj/eht142
Bevacqua, R. J., Lam, J. Y., Peiris, H., Whitener, R. L., Kim, S., Gu, X., et al. (2021). SIX2 and SIX3 Coordinately Regulate Functional Maturity and Fate of Human Pancreatic β Cells. Genes Dev. 35, 234–249. doi:10.1101/gad.342378.120
Boj, S. F., Párrizas, M., Maestro, M. A., and Ferrer, J. (2001). A Transcription Factor Regulatory Circuit in Differentiated Pancreatic Cells. Proc. Natl. Acad. Sci. U.S.A. 98, 14481–14486. doi:10.1073/pnas.241349398
Borden, P., Houtz, J., Leach, S. D., and Kuruvilla, R. (2013). Sympathetic Innervation during Development Is Necessary for Pancreatic Islet Architecture and Functional Maturation. Cel Rep. 4, 287–301. doi:10.1016/j.celrep.2013.06.019
Boutant, M., Ramos, O. H. P., Tourrel-Cuzin, C., Movassat, J., Ilias, A., Vallois, D., et al. (2012). COUP-TFII Controls Mouse Pancreatic β-Cell Mass through GLP-1-β-Catenin Signaling Pathways. PLoS One 7, e30847. doi:10.1371/journal.pone.0030847
Briand, O., Helleboid-Chapman, A., Ploton, M., Hennuyer, N., Carpentier, R., Pattou, F., et al. (2012). The Nuclear Orphan Receptor Nur77 Is a Lipotoxicity Sensor Regulating Glucose-Induced Insulin Secretion in Pancreatic β-Cells. Mol. Endocrinol. 26, 399–413. doi:10.1210/me.2011-1317
Brown, M. R., Sen, S. K., Mazzone, A., Her, T. K., Xiong, Y., Lee, J. H., et al. (2021). Time-restricted Feeding Prevents Deleterious Metabolic Effects of Circadian Disruption through Epigenetic Control of β Cell Function. Sci. Adv. 7, eabg6856. doi:10.1126/sciadv.abg6856
Bruin, J. E., Saber, N., O’Dwyer, S., Fox, J. K., Mojibian, M., Arora, P., et al. (2016). Hypothyroidism Impairs Human Stem Cell-Derived Pancreatic Progenitor Cell Maturation in Mice. Diabetes 65, 1297–1309. doi:10.2337/db15-1439
Brun, P. J., Grijalva, A., Rausch, R., Watson, E., Yuen, J. J., Das, B. C., et al. (2015). Retinoic Acid Receptor Signaling Is Required to Maintain Glucose‐stimulated Insulin Secretion and β‐cell Mass. FASEB j. 29, 671–683. doi:10.1096/fj.14-256743
Buijs, R. M., Chun, S. J., Niijima, A., Romijn, H. J., and Nagai, K. (2001). Parasympathetic and Sympathetic Control of the Pancreas: a Role for the Suprachiasmatic Nucleus and Other Hypothalamic Centers that Are Involved in the Regulation of Food Intake. J. Comp. Neurol. 431, 405–423. doi:10.1002/1096-9861(20010319)431:4<405::aid-cne1079>3.0.co;2-d
Burns, S. M., Vetere, A., Walpita, D., Dančík, V., Khodier, C., Perez, J., et al. (2015). High-throughput Luminescent Reporter of Insulin Secretion for Discovering Regulators of Pancreatic Beta-Cell Function. Cel Metab. 21, 126–137. doi:10.1016/j.cmet.2014.12.010
Cayabyab, F., Nih, L. R., and Yoshihara, E. (2021). Advances in Pancreatic Islet Transplantation Sites for the Treatment of Diabetes. Front. Endocrinol. 12, 732431. doi:10.3389/fendo.2021.732431
Cechin, S., Álvarez-Cubela, S., Giraldo, J. A., Molano, R. D., Villate, S., Ricordi, C., et al. (2014). Influence of In Vitro and In Vivo Oxygen Modulation on β Cell Differentiation from Human Embryonic Stem Cells. Stem Cell Transl Med 3, 277–289. doi:10.5966/sctm.2013-0160
Chandra, R., and Liddle, R. A. (2013). Modulation of Pancreatic Exocrine and Endocrine Secretion. Curr. Opin. Gastroenterol. 29, 517–522. doi:10.1097/mog.0b013e3283639326
Chiou, J., Geusz, R. J., Okino, M.-L., Han, J. Y., Miller, M., Melton, R., et al. (2021). Interpreting Type 1 Diabetes Risk with Genetics and Single-Cell Epigenomics. Nature 594, 398–402. doi:10.1038/s41586-021-03552-w
Cho, N. H., Shaw, J. E., Karuranga, S., Huang, Y., Da Rocha Fernandes, J. D., Ohlrogge, A. W., et al. (2018). IDF Diabetes Atlas: Global Estimates of Diabetes Prevalence for 2017 and Projections for 2045. Diabetes Res. Clin. Pract. 138, 271–281. doi:10.1016/j.diabres.2018.02.023
Chuang, J.-C., Cha, J.-Y., Garmey, J. C., Mirmira, R. G., and Joyce J., J. J. (2008). Research Resource: Nuclear Hormone Receptor Expression in the Endocrine Pancreas. Mol. Endocrinol. 22, 2353–2363. doi:10.1210/me.2007-0568
Clark, A. R., Stokes, Y. M., Lane, M., and Thompson, J. G. (2006). Mathematical Modelling of Oxygen Concentration in Bovine and Murine Cumulus-Oocyte Complexes. Reproduction 131, 999–1006. doi:10.1530/rep.1.00974
Close, A.-F., Dadheech, N., Villela, B. S., Rouillard, C., and Buteau, J. (2019). The Orphan Nuclear Receptor Nor1/Nr4a3 Is a Negative Regulator of β-cell Mass. J. Biol. Chem. 294, 4889–4897. doi:10.1074/jbc.ra118.005135
Cobo-Vuilleumier, N., Lorenzo, P. I., Rodríguez, N. G., Herrera Gómez, I. d. G., Fuente-Martin, E., López-Noriega, L., et al. (2018). LRH-1 Agonism Favours an Immune-Islet Dialogue Which Protects against Diabetes Mellitus. Nat. Commun. 9, 1488. doi:10.1038/s41467-018-03943-0
Creager, M. A., Lüscher, T. F., Cosentino, F., and Beckman, J. A. (2003). Diabetes and Vascular Disease. Circulation 108, 1527–1532. doi:10.1161/01.cir.0000091257.27563.32
D'Amour, K. A., Agulnick, A. D., Eliazer, S., Kelly, O. G., Kroon, E., and Baetge, E. E. (2005). Efficient Differentiation of Human Embryonic Stem Cells to Definitive Endoderm. Nat. Biotechnol. 23, 1534–1541. doi:10.1038/nbt1163
D'Amour, K. A., Bang, A. G., Eliazer, S., Kelly, O. G., Agulnick, A. D., Smart, N. G., et al. (2006). Production of Pancreatic Hormone-Expressing Endocrine Cells from Human Embryonic Stem Cells. Nat. Biotechnol. 24, 1392–1401. doi:10.1038/nbt1259
Davis, J. C., Alves, T. C., Helman, A., Chen, J. C., Kenty, J. H., Cardone, R. L., et al. (2020). Glucose Response by Stem Cell-Derived β Cells In Vitro Is Inhibited by a Bottleneck in Glycolysis. Cel Rep. 31, 107623. doi:10.1016/j.celrep.2020.107623
Dhawan, S., Tschen, S.-I., Zeng, C., Guo, T., Hebrok, M., Matveyenko, A., et al. (2015). DNA Methylation Directs Functional Maturation of Pancreatic β Cells. J. Clin. Invest. 125, 2851–2860. doi:10.1172/jci79956
Dubois, M., Pattou, F., Kerr-Conte, J., Gmyr, V., Vandewalle, B., Desreumaux, P., et al. (2000). Expression of Peroxisome Proliferator-Activated Receptor γ (PPARγ) in normal Human Pancreatic Islet Cells. Diabetologia 43, 1165–1169. doi:10.1007/s001250051508
Düfer, M., Hörth, K., Wagner, R., Schittenhelm, B., Prowald, S., Wagner, T. F. J., et al. (2012). Bile Acids Acutely Stimulate Insulin Secretion of Mouse β-Cells via Farnesoid X Receptor Activation and KATP Channel Inhibition. Diabetes 61, 1479–1489. doi:10.2337/db11-0815
Efanov, A. M., Sewing, S., Bokvist, K., and Gromada, J. (2004). Liver X Receptor Activation Stimulates Insulin Secretion via Modulation of Glucose and Lipid Metabolism in Pancreatic Beta-Cells. Diabetes 53 (Suppl. 3), S75–S78. doi:10.2337/diabetes.53.suppl_3.s75
El Ouaamari, A., Kawamori, D., Dirice, E., Liew, C. W., Shadrach, J. L., Hu, J., et al. (2013). Liver-derived Systemic Factors Drive β Cell Hyperplasia in Insulin-Resistant States. Cell Rep 3, 401–410. doi:10.1016/j.celrep.2013.01.007
Evans, R. M., and Mangelsdorf, D. J. (2014). Nuclear Receptors, RXR, and the Big Bang. Cell 157, 255–266. doi:10.1016/j.cell.2014.03.012
Fan, W., He, N., Lin, C. S., Wei, Z., Hah, N., Waizenegger, W., et al. (2018). ERRγ Promotes Angiogenesis, Mitochondrial Biogenesis, and Oxidative Remodeling in PGC1α/β-Deficient Muscle. Cel Rep. 22, 2521–2529. doi:10.1016/j.celrep.2018.02.047
Fedullo, A. L., Schiattarella, A., Morlando, M., Raguzzini, A., Toti, E., De Franciscis, P., et al. (2021). Mediterranean Diet for the Prevention of Gestational Diabetes in the Covid-19 Era: Implications of Il-6 in Diabesity. Int. J. Mol. Sci. 22. doi:10.3390/ijms22031213
Fraker, C. A., Álvarez, S., Papadopoulos, P., Giraldo, J., Gu, W., Ricordi, C., et al. (2007). Enhanced Oxygenation Promotes β-Cell Differentiation In Vitro. Stem Cells 25, 3155–3164. doi:10.1634/stemcells.2007-0445
Gerber, P. A., and Rutter, G. A. (2017). The Role of Oxidative Stress and Hypoxia in Pancreatic Beta-Cell Dysfunction in Diabetes Mellitus. Antioxid. Redox Signaling 26, 501–518. doi:10.1089/ars.2016.6755
Gerin, I., Dolinsky, V. W., Shackman, J. G., Kennedy, R. T., Chiang, S.-H., Burant, C. F., et al. (2005). LXRβ Is Required for Adipocyte Growth, Glucose Homeostasis, and β Cell Function. J. Biol. Chem. 280, 23024–23031. doi:10.1074/jbc.m412564200
Gesina, E., Blondeau, B., Milet, A., Le Nin, I., Duchene, B., Czernichow, P., et al. (2006). Glucocorticoid Signalling Affects Pancreatic Development through Both Direct and Indirect Effects. Diabetologia 49, 2939–2947. doi:10.1007/s00125-006-0449-3
Ghazalli, N., Wu, X., Walker, S., Trieu, N., Hsin, L.-Y., Choe, J., et al. (2018). Glucocorticoid Signaling Enhances Expression of Glucose-Sensing Molecules in Immature Pancreatic Beta-like Cells Derived from Murine Embryonic Stem Cells In Vitro. Stem Cell Develop. 27, 898–909. doi:10.1089/scd.2017.0160
Goodman, P. A., Medina-Martinez, O., and Fernandez-Mejia, C. (1996). Identification of the Human Insulin Negative Regulatory Element as a Negative Glucocorticoid Response Element. Mol. Cell Endocrinol. 120, 139–146. doi:10.1016/0303-7207(96)03830-0
Goto, R., Kondo, T., Ono, K., Kitano, S., Miyakawa, N., Watanabe, T., et al. (2019). Mineralocorticoid Receptor May Regulate Glucose Homeostasis through the Induction of Interleukin-6 and Glucagon-like Peptide-1 in Pancreatic Islets. J. Clin. Med. 8. doi:10.3390/jcm8050674
Hakim, F., Kaitsuka, T., Raeed, J. M., Wei, F.-Y., Shiraki, N., Akagi, T., et al. (2014). High Oxygen Condition Facilitates the Differentiation of Mouse and Human Pluripotent Stem Cells into Pancreatic Progenitors and Insulin-Producing Cells. J. Biol. Chem. 289, 9623–9638. doi:10.1074/jbc.m113.524363
Hale, M. A., Swift, G. H., Hoang, C. Q., Deering, T. G., Masui, T., Lee, Y.-K., et al. (2014). The Nuclear Hormone Receptor Family Member NR5A2 Controls Aspects of Multipotent Progenitor Cell Formation and Acinar Differentiation during Pancreatic Organogenesis. Development 141, 3123–3133. doi:10.1242/dev.109405
Hang, Y., and Stein, R. (2011). MafA and MafB Activity in Pancreatic β Cells. Trends Endocrinol. Metab. 22, 364–373. doi:10.1016/j.tem.2011.05.003
Harvey, A. J. (2007). The Role of Oxygen in Ruminant Preimplantation Embryo Development and Metabolism. Anim. Reprod. Sci. 98, 113–128. doi:10.1016/j.anireprosci.2006.10.008
Heinis, M., Simon, M.-T., Ilc, K., Mazure, N. M., Pouysségur, J., Scharfmann, R., et al. (2010). Oxygen Tension Regulates Pancreatic β-Cell Differentiation through Hypoxia-Inducible Factor 1α. Diabetes 59, 662–669. doi:10.2337/db09-0891
Hellerström, C., and Swenne, I. (1991). Functional Maturation and Proliferation of Fetal Pancreatic Beta-Cells. Diabetes 40 (Suppl. 2), 89–93. doi:10.2337/diab.40.2.s89
Helman, A., Cangelosi, A. L., Davis, J. C., Pham, Q., Rothman, A., Faust, A. L., et al. (2020). A Nutrient-Sensing Transition at Birth Triggers Glucose-Responsive Insulin Secretion. Cel Metab. 31, 1004–1016. e5. doi:10.1016/j.cmet.2020.04.004
Hogrebe, N. J., Augsornworawat, P., Maxwell, K. G., Velazco-Cruz, L., and Millman, J. R. (2020). Targeting the Cytoskeleton to Direct Pancreatic Differentiation of Human Pluripotent Stem Cells. Nat. Biotechnol. 38, 460–470. doi:10.1038/s41587-020-0430-6
Hrvatin, S., O’Donnell, C. W., Deng, F., Millman, J. R., Pagliuca, F. W., Diiorio, P., et al. (2014). Differentiated Human Stem Cells Resemble Fetal, Not Adult, β Cells. Proc. Natl. Acad. Sci. U.S.A. 111, 3038–3043. doi:10.1073/pnas.1400709111
Huang, Y., Karuranga, S., Malanda, B., and Williams, D. R. R. (2018). Call for Data Contribution to the IDF Diabetes Atlas 9th Edition 2019. Diabetes Res. Clin. Pract. 140, 351–352. doi:10.1016/j.diabres.2018.05.033
Huising, M. O. (2020). Paracrine Regulation of Insulin Secretion. Diabetologia 63, 2057–2063. doi:10.1007/s00125-020-05213-5
Jaafar, R., Tran, S., Shah, A. N., Sun, G., Valdearcos, M., Marchetti, P., et al. (2019). mTORC1 to AMPK Switching Underlies β-cell Metabolic Plasticity during Maturation and Diabetes. J. Clin. Invest. 129, 4124–4137. doi:10.1172/JCI127021
Jacovetti, C., Matkovich, S. J., Rodriguez-Trejo, A., Guay, C., and Regazzi, R. (2015). Postnatal β-cell Maturation Is Associated with Islet-specific microRNA Changes Induced by Nutrient Shifts at Weaning. Nat. Commun. 6, 8084. doi:10.1038/ncomms9084
Jansson, L., and Hellerström, C. (1983). Stimulation by Glucose of the Blood Flow to the Pancreatic Islets of the Rat. Diabetologia 25, 45–50. doi:10.1007/BF00251896
Jun, Y., Lee, J., Choi, S., Yang, J. H., Sander, M., Chung, S., et al. (2019). In Vivo-mimicking Microfluidic Perfusion Culture of Pancreatic Islet Spheroids. Sci. Adv. 5, eaax4520. doi:10.1126/sciadv.aax4520
Kadison, A., Kim, J., Maldonado, T., Crisera, C., Prasadan, K., Manna, P., et al. (2001). Retinoid Signaling Directs Secondary Lineage Selection in Pancreatic Organogenesis. J. Pediatr. Surg. 36, 1150–1156. doi:10.1053/jpsu.2001.25734
Kane, M. A., Folias, A. E., Pingitore, A., Perri, M., Obrochta, K. M., Krois, C. R., et al. (2010). Identification of 9- Cis -retinoic Acid as a Pancreas-specific Autacoid that Attenuates Glucose-Stimulated Insulin Secretion. Proc. Natl. Acad. Sci. U.S.A. 107, 21884–21889. doi:10.1073/pnas.1008859107
Komatsu, H., Cook, C., Wang, C.-H., Medrano, L., Lin, H., Kandeel, F., et al. (2017). Oxygen Environment and Islet Size Are the Primary Limiting Factors of Isolated Pancreatic Islet Survival. PLoS One 12, e0183780. doi:10.1371/journal.pone.0183780
Komatsu, H., Kang, D., Medrano, L., Barriga, A., Mendez, D., Rawson, J., et al. (2016). Isolated Human Islets Require Hyperoxia to Maintain Islet Mass, Metabolism, and Function. Biochem. Biophysical Res. Commun. 470, 534–538. doi:10.1016/j.bbrc.2016.01.110
Kong, X., Li, B., Deng, Y., and Ma, X. (2019a). FXR Mediates Adenylyl Cyclase 8 Expression in Pancreatic β-Cells. J. Diabetes Res. 2019, 8915818. doi:10.1155/2019/8915818
Kong, X., Feng, L., Yan, D., Li, B., Yang, Y., and Ma, X. (2021). FXR-mediated Epigenetic Regulation of GLP-1R Expression Contributes to Enhanced Incretin Effect in Diabetes after RYGB. J. Cel Mol Med.
Kong, X., Tu, Y., Li, B., Zhang, L., Feng, L., Wang, L., et al. (2019b). Roux-en-Y Gastric Bypass Enhances Insulin Secretion in Type 2 Diabetes via FXR-Mediated TRPA1 Expression. Mol. Metab. 29, 1–11. doi:10.1016/j.molmet.2019.08.009
Krivova, Y. S., Proshchina, A. E., Otlyga, D. A., Leonova, O. G., and Saveliev, S. V. (2022). Prenatal Development of Sympathetic Innervation of the Human Pancreas. Ann. Anat. 240, 151880. doi:10.1016/j.aanat.2021.151880
Kroon, E., Martinson, L. A., Kadoya, K., Bang, A. G., Kelly, O. G., Eliazer, S., et al. (2008). Pancreatic Endoderm Derived from Human Embryonic Stem Cells Generates Glucose-Responsive Insulin-Secreting Cells In Vivo. Nat. Biotechnol. 26, 443–452. doi:10.1038/nbt1393
Lalloyer, F., Vandewalle, B., Percevault, F., Torpier, G., Kerr-Conte, J., Oosterveer, M., et al. (2006). Peroxisome Proliferator-Activated Receptor α Improves Pancreatic Adaptation to Insulin Resistance in Obese Mice and Reduces Lipotoxicity in Human Islets. Diabetes 55, 1605–1613. doi:10.2337/db06-0016
Lees, J. G., Cliff, T. S., Gammilonghi, A., Ryall, J. G., Dalton, S., Gardner, D. K., et al. (2019). Oxygen Regulates Human Pluripotent Stem Cell Metabolic Flux. Stem Cell Int 2019, 8195614. doi:10.1155/2019/8195614
Lemos, J. R. N., Baidal, D. A., Ricordi, C., Fuenmayor, V., Alvarez, A., and Alejandro, R. (2021). Survival after Islet Transplantation in Subjects with Type 1 Diabetes: Twenty-Year Follow-Up. Diabetes Care 44, e67–e68. doi:10.2337/dc20-2458
Lin, E. E., Scott-Solomon, E., and Kuruvilla, R. (2021). Peripheral Innervation in the Regulation of Glucose Homeostasis. Trends Neurosciences 44, 189–202. doi:10.1016/j.tins.2020.10.015
Lumelsky, N., Blondel, O., Laeng, P., Velasco, I., Ravin, R., and McKay, R. (2001). Differentiation of Embryonic Stem Cells to Insulin-Secreting Structures Similar to Pancreatic Islets. Science 292 (5520), 1389–94. doi:10.1126/science.1058866
Lüscher, T. F., Creager, M. A., Beckman, J. A., and Cosentino, F. (2003). Diabetes and Vascular Disease: Pathophysiology, Clinical Consequences, and Medical Therapy: Part II. Circulation 108, 1655–1661. doi:10.1161/01.CIR.0000089189.70578.E2
Mamidi, A., Prawiro, C., Seymour, P. A., De Lichtenberg, K. H., Jackson, A., Serup, P., et al. (2018). Mechanosignalling via Integrins Directs Fate Decisions of Pancreatic Progenitors. Nature 564, 114–118. doi:10.1038/s41586-018-0762-2
Marcheva, B., Ramsey, K. M., Buhr, E. D., Kobayashi, Y., Su, H., Ko, C. H., et al. (2010). Disruption of the Clock Components CLOCK and BMAL1 Leads to Hypoinsulinaemia and Diabetes. Nature 466, 627–631. doi:10.1038/nature09253
Martin, C. C., Flemming, B. P., Wang, Y., Oeser, J. K., and O'Brien, R. M. (2008). Foxa2 and MafA Regulate Islet-specific Glucose-6-Phosphatase Catalytic Subunit-Related Protein Gene Expression. J. Mol. Endocrinol. 41, 315–328. doi:10.1677/jme-08-0062
Matthews, K. A., Rhoten, W. B., Driscoll, H. K., and Chertow, B. S. (2004). Vitamin A Deficiency Impairs Fetal Islet Development and Causes Subsequent Glucose Intolerance in Adult Rats. J. Nutr. 134, 1958–1963. doi:10.1093/jn/134.8.1958
Matthews, L. C., and Hanley, N. A. (2011). The Stress of Starvation: Glucocorticoid Restraint of Beta Cell Development. Diabetologia 54, 223–226. doi:10.1007/s00125-010-1963-x
Mühlbauer, E., Bazwinsky-Wutschke, I., Wolgast, S., Labucay, K., and Peschke, E. (2013). Differential and Day-Time Dependent Expression of Nuclear Receptors RORα, RORβ, RORγ and RXRα in the Rodent Pancreas and Islet. Mol. Cell Endocrinol. 365, 129–138. doi:10.1016/j.mce.2012.10.001
Nair, G. G., Liu, J. S., Russ, H. A., Tran, S., Saxton, M. S., Chen, R., et al. (2019). Recapitulating Endocrine Cell Clustering in Culture Promotes Maturation of Human Stem-Cell-Derived β Cells. Nat. Cel Biol 21, 263–274. doi:10.1038/s41556-018-0271-4
Navarro, G., Xu, W., Jacobson, D. A., Wicksteed, B., Allard, C., Zhang, G., et al. (2016). Extranuclear Actions of the Androgen Receptor Enhance Glucose-Stimulated Insulin Secretion in the Male. Cel Metab. 23, 837–851. doi:10.1016/j.cmet.2016.03.015
Ni, Q., Gu, Y., Xie, Y., Yin, Q., Zhang, H., Nie, A., et al. (2017). Raptor Regulates Functional Maturation of Murine Beta Cells. Nat. Commun. 8, 15755. doi:10.1038/ncomms15755
Noh, J.-R., Hwang, J. H., Kim, Y.-H., Kim, K.-S., Gang, G.-T., Kim, S.-W., et al. (2013). The Orphan Nuclear Receptor Small Heterodimer Partner Negatively Regulates Pancreatic Beta Cell Survival and Hyperglycemia in Multiple Low-Dose Streptozotocin-Induced Type 1 Diabetic Mice. Int. J. Biochem. Cel Biol. 45, 1538–1545. doi:10.1016/j.biocel.2013.05.004
Pagliuca, F. W., Millman, J. R., Gürtler, M., Segel, M., Van Dervort, A., Ryu, J. H., et al. (2014). Generation of Functional Human Pancreatic β Cells In Vitro. Cell 159, 428–439. doi:10.1016/j.cell.2014.09.040
Paneni, F., Beckman, J. A., Creager, M. A., and Cosentino, F. (2013). Diabetes and Vascular Disease: Pathophysiology, Clinical Consequences, and Medical Therapy: Part I. Eur. Heart J. 34, 2436–2443. doi:10.1093/eurheartj/eht149
Park, K.-G., Lee, K.-M., Seo, H.-Y., Suh, J.-H., Kim, H.-S., Wang, L., et al. (2007). Glucotoxicity in the INS-1 Rat Insulinoma Cell Line Is Mediated by the Orphan Nuclear Receptor Small Heterodimer Partner. Diabetes 56, 431–437. doi:10.2337/db06-0753
Perelis, M., Marcheva, B., Ramsey, K. M., Schipma, M. J., Hutchison, A. L., Taguchi, A., et al. (2015). Pancreatic β Cell Enhancers Regulate Rhythmic Transcription of Genes Controlling Insulin Secretion. Science 350, aac4250. doi:10.1126/science.aac4250
Pérez, R. J., Benoit, Y. D., and Gudas, L. J. (2013). Deletion of Retinoic Acid Receptor β (RARβ) Impairs Pancreatic Endocrine Differentiation. Exp. Cel Res. 319, 2196–2204. doi:10.1016/j.yexcr.2013.05.032
Perilhou, A., Tourrel-Cuzin, C., Zhang, P., Kharroubi, I., Wang, H., Fauveau, V., et al. (2008). The MODY1 Gene for Hepatocyte Nuclear Factor 4α and a Feedback Loop Control COUP-TFII Expression in Pancreatic Beta Cells. Mol. Cel Biol 28, 4588–4597. doi:10.1128/mcb.01191-07
Peterson, Q. P., Veres, A., Chen, L., Slama, M. Q., Kenty, J. H. R., Hassoun, S., et al. (2020). A Method for the Generation of Human Stem Cell-Derived Alpha Cells. Nat. Commun. 11, 2241. doi:10.1038/s41467-020-16049-3
Petrenko, V., Gandasi, N. R., Sage, D., Tengholm, A., Barg, S., and Dibner, C. (2020). In Pancreatic Islets from Type 2 Diabetes Patients, the Dampened Circadian Oscillators lead to Reduced Insulin and Glucagon Exocytosis. Proc. Natl. Acad. Sci. U.S.A. 117, 2484–2495. doi:10.1073/pnas.1916539117
Philippe, J., and Missotten, M. (1990). Dexamethasone Inhibits Insulin Biosynthesis by Destabilizing Insulin Messenger Ribonucleic Acid in Hamster Insulinoma Cells. Endocrinology 127, 1640–1645. doi:10.1210/endo-127-4-1640
Picard, F., Wanatabe, M., Schoonjans, K., Lydon, J., O'Malley, B. W., and Auwerx, J. (2002). Progesterone Receptor Knockout Mice Have an Improved Glucose Homeostasis Secondary to β-cell Proliferation. Proc. Natl. Acad. Sci. U.S.A. 99, 15644–15648. doi:10.1073/pnas.202612199
Popescu, I. R., Helleboid-Chapman, A., Lucas, A., Vandewalle, B., Dumont, J., Bouchaert, E., et al. (2010). The Nuclear Receptor FXR Is Expressed in Pancreatic β-cells and Protects Human Islets from Lipotoxicity. FEBS Lett. 584, 2845–2851. doi:10.1016/j.febslet.2010.04.068
Prentice, K. J., Saksi, J., Robertson, L. T., Lee, G. Y., Inouye, K. E., Eguchi, K., et al. (2021). A Hormone Complex of FABP4 and Nucleoside Kinases Regulates Islet Function. Nature 600, 720. doi:10.1038/s41586-021-04137-3
Pulimeno, P., Mannic, T., Sage, D., Giovannoni, L., Salmon, P., Lemeille, S., et al. (2013). Autonomous and Self-Sustained Circadian Oscillators Displayed in Human Islet Cells. Diabetologia 56, 497–507. doi:10.1007/s00125-012-2779-7
Qin, J., Chen, X., Yu-Lee, L.-y., Tsai, M.-J., and Tsai, S. Y. (2010). Nuclear Receptor COUP-TFII Controls Pancreatic Islet Tumor Angiogenesis by Regulating Vascular Endothelial Growth Factor/vascular Endothelial Growth Factor Receptor-2 Signaling. Cancer Res. 70, 8812–8821. doi:10.1158/0008-5472.can-10-0551
Ranta, F., Avram, D., Berchtold, S., Düfer, M., Drews, G., Lang, F., et al. (2006). Dexamethasone Induces Cell Death in Insulin-Secreting Cells, an Effect Reversed by Exendin-4. Diabetes 55, 1380–1390. doi:10.2337/db05-1220
Reich, E., Tamary, A., Sionov, R. V., and Melloul, D. (2012). Involvement of Thioredoxin-Interacting Protein (TXNIP) in Glucocorticoid-Mediated Beta Cell Death. Diabetologia 55, 1048–1057. doi:10.1007/s00125-011-2422-z
Renga, B., Mencarelli, A., Vavassori, P., Brancaleone, V., and Fiorucci, S. (2010). The Bile Acid Sensor FXR Regulates Insulin Transcription and Secretion. Biochim. Biophys. Acta (Bba) - Mol. Basis Dis. 1802, 363–372. doi:10.1016/j.bbadis.2010.01.002
Rezania, A., Bruin, J. E., Arora, P., Rubin, A., Batushansky, I., Asadi, A., et al. (2014). Reversal of Diabetes with Insulin-Producing Cells Derived In Vitro from Human Pluripotent Stem Cells. Nat. Biotechnol. 32, 1121–1133. doi:10.1038/nbt.3033
Rezania, A., Riedel, M. J., Wideman, R. D., Karanu, F., Ao, Z., Warnock, G. L., et al. (2011). Production of Functional Glucagon-Secreting α-Cells from Human Embryonic Stem Cells. Diabetes 60, 239–247. doi:10.2337/db10-0573
Rickels, M. R., Norris, A. W., and Hull, R. L. (2020). A Tale of Two Pancreases: Exocrine Pathology and Endocrine Dysfunction. Diabetologia 63, 2030–2039. doi:10.1007/s00125-020-05210-8
Robertson, R. P., Harmon, J., Tran, P. O., and Poitout, V. (2004). Beta-cell Glucose Toxicity, Lipotoxicity, and Chronic Oxidative Stress in Type 2 Diabetes. Diabetes 53 (Suppl. 1), S119–S124. doi:10.2337/diabetes.53.2007.s119
Roche, E., Buteau, J., Aniento, I., Reig, J. A., Soria, B., and Prentki, M. (1999). Palmitate and Oleate Induce the Immediate-Early Response Genes C-Fos and Nur-77 in the Pancreatic Beta-Cell Line INS-1. Diabetes 48, 2007–2014. doi:10.2337/diabetes.48.10.2007
Rodesch, F., Simon, P., Donner, C., and Jauniaux, E. (1992). Oxygen Measurements in Endometrial and Trophoblastic Tissues during Early Pregnancy. Obstet. Gynecol. 80, 283–285.
Rogers, M. A. M., and Kim, C. (2020). Congenital Infections as Contributors to the Onset of Diabetes in Children: A Longitudinal Study in the United States, 2001‐2017. Pediatr. Diabetes 21, 456–459. doi:10.1111/pedi.12957
Rogers, M. A. M., Wei, M. Y., Kim, C., and Lee, J. M. (2020). Sex Differences in Autoimmune Multimorbidity in Type 1 Diabetes Mellitus and the Risk of Cardiovascular and Renal Disease: A Longitudinal Study in the United States, 2001-2017. J. Women's Health 29, 511–519. doi:10.1089/jwh.2019.7935
Rorsman, P., Arkhammar, P., Bokvist, K., Hellerström, C., Nilsson, T., Welsh, M., et al. (1989). Failure of Glucose to Elicit a normal Secretory Response in Fetal Pancreatic Beta Cells Results from Glucose Insensitivity of the ATP-Regulated K+ Channels. Proc. Natl. Acad. Sci. U.S.A. 86, 4505–4509. doi:10.1073/pnas.86.12.4505
Rosario, W., Singh, I., Wautlet, A., Patterson, C., Flak, J., Becker, T. C., et al. (2016). The Brain-To-Pancreatic Islet Neuronal Map Reveals Differential Glucose Regulation from Distinct Hypothalamic Regions. Diabetes 65, 2711–2723. doi:10.2337/db15-0629
Rosen, E. D., Kulkarni, R. N., Sarraf, P., Ozcan, U., Okada, T., Hsu, C.-H., et al. (2003). Targeted Elimination of Peroxisome Proliferator-Activated Receptor γ in β Cells Leads to Abnormalities in Islet Mass without Compromising Glucose Homeostasis. Mol. Cel Biol 23, 7222–7229. doi:10.1128/mcb.23.20.7222-7229.2003
Russ, H. A., Parent, A. V., Ringler, J. J., Hennings, T. G., Nair, G. G., Shveygert, M., et al. (2015). Controlled Induction of Human Pancreatic Progenitors Produces Functional Beta‐like Cells In Vitro. EMBO J. 34, 1759–1772. doi:10.15252/embj.201591058
Russell, R., Carnese, P. P., Hennings, T. G., Walker, E. M., Russ, H. A., Liu, J. S., et al. (2020). Loss of the Transcription Factor MAFB Limits β-cell Derivation from Human PSCs. Nat. Commun. 11, 2742. doi:10.1038/s41467-020-16550-9
Saini, C., Petrenko, V., Pulimeno, P., Giovannoni, L., Berney, T., Hebrok, M., et al. (2016). A Functional Circadian Clock Is Required for Proper Insulin Secretion by Human Pancreatic Islet Cells. Diabetes Obes. Metab. 18, 355–365. doi:10.1111/dom.12616
Seo, H.-Y., Kim, Y. D., Lee, K.-M., Min, A.-K., Kim, M.-K., Kim, H.-S., et al. (2008). Endoplasmic Reticulum Stress-Induced Activation of Activating Transcription Factor 6 Decreases Insulin Gene Expression via Up-Regulation of Orphan Nuclear Receptor Small Heterodimer Partner. Endocrinology 149, 3832–3841. doi:10.1210/en.2008-0015
Shah, V. N., Grimsmann, J. M., Foster, N. C., Dost, A., Miller, K. M., Pavel, M., et al. (2020). Undertreatment of Cardiovascular Risk Factors in the Type 1 Diabetes Exchange Clinic Network ( United States ) and the Prospective Diabetes Follow‐up (Germany/Austria) Registries. Diabetes Obes. Metab. 22, 1577–1585. doi:10.1111/dom.14069
Shapiro, A. M. J., Lakey, J. R. T., Ryan, E. A., Korbutt, G. S., Toth, E., Warnock, G. L., et al. (2000). Islet Transplantation in Seven Patients with Type 1 Diabetes Mellitus Using a Glucocorticoid-free Immunosuppressive Regimen. N. Engl. J. Med. 343, 230–238. doi:10.1056/nejm200007273430401
Shi, X., Ma, D., Li, M., Zeng, L., Chen, J., and Yang, Y. (2019). Nuclear Receptor TLX Regulates Islet Beta Cell Proliferation via E2F6. Biochem. Biophysical Res. Commun. 513, 560–566. doi:10.1016/j.bbrc.2019.04.033
Shi, X., Xiong, X., Dai, Z., Deng, H., Sun, L., Hu, X., et al. (2015). Nuclear Orphan Receptor TLX Affects Gene Expression, Proliferation and Cell Apoptosis in Beta Cells. Biochem. Biophysical Res. Commun. 468, 387–393. doi:10.1016/j.bbrc.2015.10.042
Smalls, B. L., Ritchwood, T. D., Bishu, K. G., and Egede, L. E. (2020). Racial/Ethnic Differences in Glycemic Control in Older Adults with Type 2 Diabetes: United States 2003-2014. Int. J. Environ. Res. Public Health 17. doi:10.3390/ijerph17030950
Sneddon, J. B., Borowiak, M., and Melton, D. A. (2012). Self-renewal of Embryonic-Stem-Cell-Derived Progenitors by Organ-Matched Mesenchyme. Nature 491, 765–768. doi:10.1038/nature11463
Stafford, D., and Prince, V. E. (2002). Retinoic Acid Signaling Is Required for a Critical Early Step in Zebrafish Pancreatic Development. Curr. Biol. 12, 1215–1220. doi:10.1016/s0960-9822(02)00929-6
Stolovich-Rain, M., Enk, J., Vikesa, J., Nielsen, F. C., Saada, A., Glaser, B., et al. (2015). Weaning Triggers a Maturation Step of Pancreatic β Cells. Develop. Cel 32, 535–545. doi:10.1016/j.devcel.2015.01.002
Strowski, M. Z., Parmar, R. M., Blake, A. D., and Schaeffer, J. M. (2000). Somatostatin Inhibits Insulin and Glucagon Secretion via Two Receptor Subtypes: An In Vitro Study of Pancreatic Islets from Somatostatin Receptor 2 Knockout Mice*. Endocrinology 141, 111–117. doi:10.1210/endo.141.1.7263
Suh, Y.-H., Kim, S.-Y., Lee, H.-Y., Jang, B. C., Bae, J. H., Sohn, J.-N., et al. (2004). Overexpression of Short Heterodimer Partner Recovers Impaired Glucose-Stimulated Insulin Secretion of Pancreatic β-cells Overexpressing UCP2. J. Endocrinol. 183, 133–144. doi:10.1677/joe.1.05675
Susini, S., Roche, E., Prentki, M., and Schlegel, W. (1998). Glucose and Glucoincretin Peptides Synergize to Induce C‐ Fos , C‐ Jun , junB , Zif ‐268, and Nur‐ 77 Gene Expression in Pancreatic β(INS‐1) Cells. FASEB j. 12, 1173–1182. doi:10.1096/fasebj.12.12.1173
Tahbaz, M., and Yoshihara, E. (2021). Immune Protection of Stem Cell-Derived Islet Cell Therapy for Treating Diabetes. Front. Endocrinol. 12, 716625. doi:10.3389/fendo.2021.716625
Takahashi, K., Tanabe, K., Ohnuki, M., Narita, M., Ichisaka, T., Tomoda, K., et al. (2007). Induction of Pluripotent Stem Cells from Adult Human Fibroblasts by Defined Factors. Cell 131, 861–872. doi:10.1016/j.cell.2007.11.019
Tan, X., Lee, L. K., Huynh, S., Pawaskar, M., and Rajpathak, S. (2020). Sociodemographic Disparities in the Management of Type 2 Diabetes in the United States. Curr. Med. Res. Opin. 36, 967–976. doi:10.1080/03007995.2020.1756764
Taneera, J., Mohammed, A. K., Dhaiban, S., Hamad, M., Prasad, R. B., Sulaiman, N., et al. (2019). RORB and RORC Associate with Human Islet Dysfunction and Inhibit Insulin Secretion in INS-1 Cells. Islets 11, 10–20. doi:10.1080/19382014.2019.1566684
Tang, T., Abbott, M. J., Ahmadian, M., Lopes, A. B., Wang, Y., and Sul, H. S. (2013). Desnutrin/ATGL Activates PPARδ to Promote Mitochondrial Function for Insulin Secretion in Islet β Cells. Cel Metab. 18, 883–895. doi:10.1016/j.cmet.2013.10.012
Tessem, J. S., Moss, L. G., Chao, L. C., Arlotto, M., Lu, D., Jensen, M. V., et al. (2014). Nkx6.1 Regulates Islet β-cell Proliferation via Nr4a1 and Nr4a3 Nuclear Receptors. Proc. Natl. Acad. Sci. U.S.A. 111, 5242–5247. doi:10.1073/pnas.1320953111
Thomson, J. A., Itskovitz-Eldor, J., Shapiro, S. S., Waknitz, M. A., Swiergiel, J. J., Marshall, V. S., et al. (1998). Embryonic Stem Cell Lines Derived from Human Blastocysts. Science 282, 1145–1147. doi:10.1126/science.282.5391.1145
Tiano, J. P., Delghingaro-Augusto, V., Le May, C., Liu, S., Kaw, M. K., Khuder, S. S., et al. (2011). Estrogen Receptor Activation Reduces Lipid Synthesis in Pancreatic Islets and Prevents β Cell Failure in Rodent Models of Type 2 Diabetes. J. Clin. Invest. 121, 3331–3342. doi:10.1172/jci44564
van der Meulen, T., Donaldson, C. J., Cáceres, E., Hunter, A. E., Cowing-Zitron, C., Pound, L. D., et al. (2015). Urocortin3 Mediates Somatostatin-dependent Negative Feedback Control of Insulin Secretion. Nat. Med. 21, 769–776. doi:10.1038/nm.3872
Varum, S., Rodrigues, A. S., Moura, M. B., Momcilovic, O., Easley, C. A., Ramalho-Santos, J., et al. (2011). Energy Metabolism in Human Pluripotent Stem Cells and Their Differentiated Counterparts. PLoS One 6, e20914. doi:10.1371/journal.pone.0020914
Velazco-Cruz, L., Goedegebuure, M. M., Maxwell, K. G., Augsornworawat, P., Hogrebe, N. J., and Millman, J. R. (2020a). SIX2 Regulates Human β Cell Differentiation from Stem Cells and Functional Maturation In Vitro. Cel Rep. 31, 107687. doi:10.1016/j.celrep.2020.107687
Velazco-Cruz, L., Goedegebuure, M. M., and Millman, J. R. (2020b). Advances toward Engineering Functionally Mature Human Pluripotent Stem Cell-Derived β Cells. Front. Bioeng. Biotechnol. 8, 786. doi:10.3389/fbioe.2020.00786
Veres, A., Faust, A. L., Bushnell, H. L., Engquist, E. N., Kenty, J. H.-R., Harb, G., et al. (2019). Charting Cellular Identity during Human In Vitro β-cell Differentiation. Nature 569, 368–373. doi:10.1038/s41586-019-1168-5
Vergari, E., Knudsen, J. G., Ramracheya, R., Salehi, A., Zhang, Q., Adam, J., et al. (2019). Insulin Inhibits Glucagon Release by SGLT2-Induced Stimulation of Somatostatin Secretion. Nat. Commun. 10, 139. doi:10.1038/s41467-018-08193-8
Vieira, E., Marroquí, L., Batista, T. M., Caballero-Garrido, E., Carneiro, E. M., Boschero, A. C., et al. (2012). The Clock GeneRev-Erbα Regulates Pancreatic β-Cell Function: Modulation by Leptin and High-Fat Diet. Endocrinology 153, 592–601. doi:10.1210/en.2011-1595
Wei, Z., Yoshihara, E., He, N., Hah, N., Fan, W., Pinto, A. F. M., et al. (2018). Vitamin D Switches BAF Complexes to Protect β Cells. Cell 173, 1135–1149. doi:10.1016/j.cell.2018.04.013
Wong, W. P. S., Tiano, J. P., Liu, S., Hewitt, S. C., Le May, C., Dalle, S., et al. (2010). Extranuclear Estrogen Receptor-α Stimulates NeuroD1 Binding to the Insulin Promoter and Favors Insulin Synthesis. Proc. Natl. Acad. Sci. U.S.A. 107, 13057–13062. doi:10.1073/pnas.0914501107
Wortham, M., and Sander, M. (2021). Transcriptional Mechanisms of Pancreatic Beta-Cell Maturation and Functional Adaptation. Trends Endocrinol. Metab.
Xie, R., Everett, L. J., Lim, H.-W., Patel, N. A., Schug, J., Kroon, E., et al. (2013). Dynamic Chromatin Remodeling Mediated by Polycomb Proteins Orchestrates Pancreatic Differentiation of Human Embryonic Stem Cells. Cell Stem Cell 12, 224–237. doi:10.1016/j.stem.2012.11.023
Yamagata, K., Furuta, H., Oda, N., Kaisaki, P. J., Menzel, S., Cox, N. J., et al. (1996). Mutations in the Hepatocyte Nuclear Factor-4α Gene in Maturity-Onset Diabetes of the Young (MODY1). Nature 384, 458–460. doi:10.1038/384458a0
Yoshihara, E. (2020). TXNIP/TBP-2: A Master Regulator for Glucose Homeostasis. Antioxidants (Basel) 9. doi:10.3390/antiox9080765
Yoshihara, E., Chen, Z., Matsuo, Y., Masutani, H., and Yodoi, J. (2010a). Thiol Redox Transitions by Thioredoxin and Thioredoxin-Binding Protein-2 in Cell Signaling. Methods Enzymol. 474, 67–82. doi:10.1016/s0076-6879(10)74005-2
Yoshihara, E., Fujimoto, S., Inagaki, N., Okawa, K., Masaki, S., Yodoi, J., et al. (2010b). Disruption of TBP-2 Ameliorates Insulin Sensitivity and Secretion without Affecting Obesity. Nat. Commun. 1, 127. doi:10.1038/ncomms1127
Yoshihara, E., Masaki, S., Matsuo, Y., Chen, Z., Tian, H., and Yodoi, J. (2014). Thioredoxin/Txnip: Redoxisome, as a Redox Switch for the Pathogenesis of Diseases. Front. Immunol. 4, 514. doi:10.3389/fimmu.2013.00514
Yoshihara, E., O’Connor, C., Gasser, E., Wei, Z., Oh, T. G., Tseng, T. W., et al. (2020). Immune-evasive Human Islet-like Organoids Ameliorate Diabetes. Nature 586, 606–611. doi:10.1038/s41586-020-2631-z
Yoshihara, E., Wei, Z., Lin, C. S., Fang, S., Ahmadian, M., Kida, Y., et al. (2016). ERRγ Is Required for the Metabolic Maturation of Therapeutically Functional Glucose-Responsive β Cells. Cel Metab. 23, 622–634. doi:10.1016/j.cmet.2016.03.005
Yu, C., Cui, S., Zong, C., Gao, W., Xu, T., Gao, P., et al. (2015). The Orphan Nuclear Receptor NR4A1 Protects Pancreatic β-Cells from Endoplasmic Reticulum (ER) Stress-Mediated Apoptosis. J. Biol. Chem. 290, 20687–20699. doi:10.1074/jbc.m115.654863
Zhang, T., Li, X.-H., Zhang, D.-B., Liu, X.-Y., Zhao, F., Lin, X.-W., et al. (2017). Repression of COUP-TFI Improves Bone Marrow-Derived Mesenchymal Stem Cell Differentiation into Insulin-Producing Cells. Mol. Ther. - Nucleic Acids 8, 220–231. doi:10.1016/j.omtn.2017.06.016
Zhou, Y.-T., Shimabukuro, M., Wang, M.-Y., Lee, Y., Higa, M., Milburn, J. L., et al. (1998). Role of Peroxisome Proliferator-Activated Receptor α in Disease of Pancreatic β Cells. Proc. Natl. Acad. Sci. U.S.A. 95, 8898–8903. doi:10.1073/pnas.95.15.8898
Zitzer, H., Wente, W., Brenner, M. B., Sewing, S., Buschard, K., Gromada, J., et al. (2006). Sterol Regulatory Element-Binding Protein 1 Mediates Liver X Receptor-β-Induced Increases in Insulin Secretion and Insulin Messenger Ribonucleic Acid Levels. Endocrinology 147, 3898–3905. doi:10.1210/en.2005-1483
Keywords: human islet-like organoids, nuclear receptors, physiology, human pluripotent stem cells, diabetes
Citation: Yoshihara E (2022) Adapting Physiology in Functional Human Islet Organogenesis. Front. Cell Dev. Biol. 10:854604. doi: 10.3389/fcell.2022.854604
Received: 14 January 2022; Accepted: 22 March 2022;
Published: 26 April 2022.
Edited by:
Keiichiro Suzuki, Osaka University, JapanReviewed by:
Hirotake Komatsu, Beckman Research Institute, City of Hope, United StatesCopyright © 2022 Yoshihara. This is an open-access article distributed under the terms of the Creative Commons Attribution License (CC BY). The use, distribution or reproduction in other forums is permitted, provided the original author(s) and the copyright owner(s) are credited and that the original publication in this journal is cited, in accordance with accepted academic practice. No use, distribution or reproduction is permitted which does not comply with these terms.
*Correspondence: Eiji Yoshihara, ZWlqaS55b3NoaWhhcmFAbHVuZHF1aXN0Lm9yZw==
Disclaimer: All claims expressed in this article are solely those of the authors and do not necessarily represent those of their affiliated organizations, or those of the publisher, the editors and the reviewers. Any product that may be evaluated in this article or claim that may be made by its manufacturer is not guaranteed or endorsed by the publisher.
Research integrity at Frontiers
Learn more about the work of our research integrity team to safeguard the quality of each article we publish.