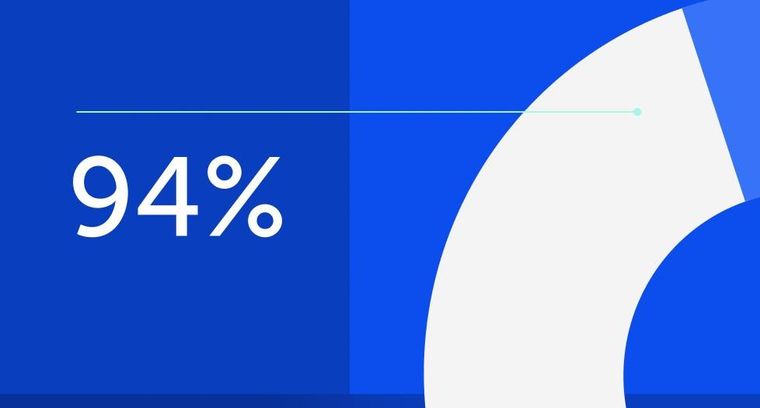
94% of researchers rate our articles as excellent or good
Learn more about the work of our research integrity team to safeguard the quality of each article we publish.
Find out more
REVIEW article
Front. Cell Dev. Biol., 28 April 2022
Sec. Cellular Biochemistry
Volume 10 - 2022 | https://doi.org/10.3389/fcell.2022.851542
This article is part of the Research TopicThe Isotypes of α, β and γ Tubulin: From Evolutionary Origins to Roles in Metazoan Development and Ligand Binding DifferencesView all 13 articles
Microtubule proteins form a dynamic component of the cytoskeleton, and play key roles in cellular processes, such as vesicular transport, cell motility and mitosis. Expression of microtubule proteins are often dysregulated in cancer. In particular, the microtubule protein βIII-tubulin, encoded by the TUBB3 gene, is aberrantly expressed in a range of epithelial tumours and is associated with drug resistance and aggressive disease. In normal cells, TUBB3 expression is tightly restricted, and is found almost exclusively in neuronal and testicular tissues. Understanding the mechanisms that control TUBB3 expression, both in cancer, mature and developing tissues will help to unravel the basic biology of the protein, its role in cancer, and may ultimately lead to the development of new therapeutic approaches to target this protein. This review is devoted to the transcriptional and posttranscriptional regulation of TUBB3 in normal and cancerous tissue.
Microtubules are one of the major constituents of the cell cytoskeleton and are made up of α- and β-tubulin heterodimers. Microtubules are highly dynamic filament structures that play critical roles in cellular processes, including vesicular transport, cell motility and mitosis. The α/β-tubulin heterodimers are made up of combinations of the different α- and β-tubulin isotypes (reviewed in Nogales 2000), of which in humans there are currently eight and seven different α- and β-tubulin isotypes, respectively (reviewed in Ludueña 2013). Each of the isotypes are encoded by a different gene and display different tissue and developmental stage expression (reviewed in Ludueña 1993; Verdier-Pinard et al., 2009). While each isotype shares high degrees of structural homology with each other, they have some differences at the peptide sequence level, specifically at their carboxy-terminal tails (Sullivan and Cleveland 1986). Sequence variations within the carboxy-terminal tails of various tubulin isotypes, have been demonstrated to regulate the dynamic assembly and disassembly of microtubule structures (Parker et al., 2018).
A strong interest exists for studying microtubules due to their importance as a target for anticancer therapies. Drugs targeting microtubules and microtubule dynamics are widely used in many cancer therapeutic regimens (reviewed in Jordan and Wilson 2004; La Regina et al., 2019). Clinically relevant Tubulin-Binding Agents (TBAs) such as the taxanes, vinca alkaloids, epothilones, and Eribulin, all bind to the β-tubulin subunits of the αβ-heterodimers (reviewed in Jordan and Wilson 2004; La Regina et al., 2019). These agents disrupt normal mitotic spindle function, block the metaphase to anaphase transition of the cell cycle, and induce mitotic arrest and cell death (reviewed in Jordan and Wilson 2004; La Regina et al., 2019). Despite the clinical success of TBAs and advances in chemotherapies, the persistent emergence of drug resistance largely hinders their clinical utility and is the primary cause of treatment failure for many cancers. Mechanisms mediating TBA resistance can occur at multiple levels (reviewed in Kavallaris, 2010; Katsetos and Draber, 2012; Parker et al., 2017). Previous studies have reported that altered expression of specific β-tubulin isotypes is strongly associated with resistance to TBAs (Kavallaris et al., 1997; Ranganathan et al., 1998a; Kavallaris et al., 1999). Of note, one particular isotype, βIII-tubulin, encoded by the TUBB3 gene, has demonstrated aberrant expression in the clinical setting, and has been identified as a marker of drug resistance and tumour aggressiveness in a sub-set of epithelial cancers (reviewed in Kavallaris, 2010; Karki et al., 2013; Mariani et al., 2015). In addition, there is clinical evidence in lung, ovarian, glioblastoma, and breast cancer, that patients with aberrant βIII-tubulin expression exhibit poorly differentiated tumour tissue, high grade malignancy, shorter disease progression, unfavourable prognosis and worse overall survival (reviewed in Seve and Dumontet 2008; Kavallaris 2010; Katsetos et al., 2011; Katsetos et al., 2015; Mariani et al., 2015; Kanakkanthara and Miller 2021). Post-translational modifications to tubulin proteins, including βIII-tubulin, are found in normal tissue and cancer cells, and have been well described elsewhere (Ludueña 1998; Wattanathamsan and Pongrakhananon 2021; Bär et al., 2022).
Interest in βIII-tubulin is not limited to its expression in cancer. Expression of βIII-tubulin is also observed in the early stages of neurogenesis of fetal development (Caccamo et al., 1989; Lee et al., 1990b; Jiang and Oblinger 1992; Linhartová et al., 1992; Easter et al., 1993; Hausrat et al., 2021). βIII-tubulin itself is primarily thought of as a neuronal protein, observed in neurons and involved with neurogenesis and axonal growth (Caccamo et al., 1989; Jiang and Oblinger 1992; Easter et al., 1993; Tischfield et al., 2010; Latremoliere et al., 2018; Hausrat et al., 2021). This notion has been strengthened by the identification of mutations in TUBB3, the gene that encodes for βIII-tubulin, resulting in nervous system disorders such as Congenital Fibrosis of the Extraocular Muscles type 3 (CFEOM3), which combines the weakening of the extraocular muscles with intellectual disability, as well as axonal abnormalities and disorganisation of cortical neurons (Poirier et al., 2010; Tischfield et al., 2010). Evidence also suggests βIII-tubulin has roles outside of neurogenesis, such as the formation of neural crest cell formation (Haendel et al., 1996; Chacon and Rogers 2019) and recently, in the mineralisation stages of tooth development (Oshima and Yawaka 2020). As such, despite the original neuronal findings, βIII-tubulin expression is increasingly observed outside of neuronal tissue, with reports of adult stem cells expressing βIII-tubulin, such as melanocytes (Locher et al., 2013) and spermatogenic cells (Person et al., 2017). Additionally, the expression of βIII-tubulin in induced pluripotent stem cells has also been observed (Daily et al., 2017; Kuang et al., 2019). However, the role of βIII-tubulin in these cells remains unclear.
Despite the relevance of βIII-tubulin protein in development and cancer, there is limited information on the precise elements that regulate the gene expression of TUBB3 in normal and cancerous human cells. This review will focus on the normal regulation of TUBB3 transcription, the role of this gene in neurogenesis and development, and on factors contributing to the dysregulation of TUBB3 expression in cancer and drivers of its aberrant expression. The review will present what is known and critically discuss gene regulatory elements including the drivers, or repressors, of TUBB3 gene expression.
Originally referred to as class III isotype β4, human βIII-tubulin was first identified in 1986 after being previously discovered in chickens a few years beforehand (Lopata et al., 1983; Sullivan and Cleveland 1986). At the time, the protein sequence of βIII-tubulin was found to be conserved across mammals, however, it was observed to be highly divergent from other β-tubulin isotypes in its carboxyl terminal region (Sullivan and Cleveland 1986). The sequence of the human βIII-tubulin gene TUBB3 was not identified until much later, with the sequence of the more common mRNA variant being determined in 1998 (Ranganathan et al., 1998b), and its genomic location confirmed in 2010 (Tischfield et al., 2010). The TUBB3 loci is present within the telomeric region of the long arm of chromosome 16 (Katsetos et al., 2002). Furthermore, up until 2010, TUBB3 was also referred to as CFEOM3 when Doherty et al. (1999) first described the gene in Extraocular Congenital Fibrosis Syndrome and identified the chromosomal location of the gene through linkage analysis of DNA microsatellite markers. It was Tischfield et al. (2010) who then identified that CFEOM3 and TUBB3 were one and the same after mapping eight different CFEOM3 causing mutations to TUBB3. TUBB3 mutations are also seen and have been reported in tumours, however the impact of these mutations are unknown (reviewed in Kanakkanthara and Miller 2021).
The human TUBB3 gene (NG_027810.1) is 21,089 bp in length and in a genomic context, the cytogenetic location of the gene is 16q24.3 on the plus strand (Figure 1). Within this locus, the Ensembl database reports that there are 15 unique TUBB3 transcripts (Table 1), however only 2, referred to as variants 1 and 2, have been studied. This could be due to these two having a higher abundance of mRNA than the other transcripts, with several transcripts being predicted to undergo nonsense mediated decay (Cunningham et al., 2018), or the other transcripts exist as mere sequencing artifacts. Given that TUBB3 is primarily expressed in neuronal tissue, it is possible that these alternative transcripts of TUBB3 represent further specialised neuronal forms of the transcript, as neuronal tissue is known to have an expanded repertoire of gene expression and alternative splicing (The GTEx Consortium, 2015; Melé et al., 2015). TUBB3 also has two pseudogenes, TUBB3P1 and TUBB3P2. TUBB3P1 the larger of the two is located on chromosome 6, while TUBB3P2 is located on chromosome 7. It is unknown if these pseudogenes possess any functional capacity.
FIGURE 1. Location of the human TUBB3 loci. Cytogenetic map of chromosome 16 showing all regions. TUBB3 is located within 16q24.3, located at the bottom of chromosome 16 (bolded).
From the transcripts identified for TUBB3, there are two main points of commonality between all of them. Firstly, the majority of the identified TUBB3 transcripts consist of four exons, and secondly, there are two exons that are present in the majority of transcripts (Table 1; Figure 2). In transcript variants 1 and 2, these two common exons are exons 2 and 3. These two particular exons also appear in the unusual read-through product of the upstream gene encoding for the G-coupled protein receptor melanocortin 1 receptor (MC1R), which results in the formation of an unusual chimeric MC1R protein featuring the βIII-tubulin carboxyl terminus that appears to attenuate MC1R signaling (Table 1; Figure 2) (Dalziel et al., 2011; Herraiz et al., 2015) (reviewed in Herraiz et al., 2017). Further investigation into the TUBB3 locus using alternative sequencing techniques may need to be performed to further validate these TUBB3 transcripts, and to better understand the prevalence of this MC1R-TUBB3 chimera. Because of this, only the two validated transcripts of TUBB3 will be referred to herein.
FIGURE 2. Structure of the human TUBB3 loci. A map of the human TUBB3 loci showing the main two transcripts of TUBB3 as well as several other genomic structures and DNA binding sites in the region. Gene loci’s (TUBB3, MC1R, retro-CYP2F1 and retro-TUBA3D) are represented by boxes with internal arrows showing sequence direction. Individual RNA transcripts are represented by a combination of thin and thick boxes for exons, and arrows for introns; thin boxes represent untranslated regions (5′ and 3′ UTRs), while thick boxes represent translated regions. TUBB3 transcript variants 1 and 2 are show in red and blue respectively, with addition observed TUBB3 exons presented in white boxes; additional information of TUBB3 transcripts is shown in Table 1. MC1R transcript is shown in green, the MC1R-TUBB3 chimera transcript is shown in purple, and the lncRNA AC092143.2 is shown in white. Known genomic binding sites are represented with black lines, with thickness corresponding to size; additional information on known genomic binding sites is shown in Table 2. CpG islands presented within the TUBB3 loci are represented by orange rectangles. Promoters/Enhancers are shown as white boxes with their name. Locations of TUBB3, MC1R and MC1R-TUBB3 transcripts, and CpG islands was extracted from the Ensembl database (Cunningham et al., 2018). Promoters/Enhancers sourced from Genehancer (Fishilevich et al., 2017). Location of retro-transposed genes and lncRNAs sourced from the UCSC genome browser (Haeussler et al., 2019). Positions based on human genome GCRh38/hg38 assembly.
Within the TUBB3 loci, there are several elements. The main TUBB3 promoter is the largest promoter found within the loci (consisting of the promoter GH16J089920 and the promoter/enhancer GH16J08995) (Fishilevich et al., 2017). Several CpG islands or regions of DNA methylation, are also observed within the loci. However, only one, which is located within the first intron of TUBB3, has been linked to modulating the expression of TUBB3 (Izutsu et al., 2008; Akasaka et al., 2009; Gao et al., 2012). Binding sites for several DNA binding proteins have also been identified in the human TUBB3 loci, the majority of which are observed on the plus strand. These include Retinoic Acid Response Elements, binding sites for AP1, a Ybox9 element and two Ybox4 elements, an RE1 site and overlapping Hypoxia Response Element (HRE) located in the 3′ region of the loci present on both the plus and minus strands (Figure 2; Table 2) (Saussede-Aim et al., 2009b; Shibazaki et al., 2012; Bordji et al., 2014; Raspaglio et al., 2014; Namekawa et al., 2020). The HRE element on the plus strand is also a canonical E-box motif, though it remains to be determined if other E-box binding proteins can bind to it. Studies performed in other animals also suggest that within the TUBB3 loci there is an Androgen Receptor Element (ARE), an additional E-box motif, and other elements listed in Table 3 (Dennis et al., 2002; De Gendt et al., 2011). The gene MC1R, whose loci is observed to be wholly within the TUBB3 loci, has a transcription region 2.5 kb upstream of the TUBB3 transcription start site (Figure 2). The MC1R promotor, GH16J089917, is also found within the 5’ region of the TUBB3 loci (Fishilevich et al., 2017).
TABLE 3. Transcription factors and Genomic elements associated with Tubb3 expression in Mice and Rats.
Outside of the above elements associated with the expression of TUBB3, two retro-transposed genes are located within the loci on the plus strand (Figure 2). The first retro-transposed gene is that of CYP2F1, located within the first intron of TUBB3, and the second are elements of several exons of TUBA3D (Baertsch et al., 2008). Finally, on the minus strand, a single lncRNA, known as AC092143.2 or lnc-CENPBD1-3:7 exists within the TUBB3 loci. Functionally, the potential role of these retro-transposed genes and lncRNA has yet to be investigated. In summary, the TUBB3 loci is complex, and contains many unexplored elements that may be involved with influencing its expression, and several elements associated with TUBB3 expression need to be mapped.
Traditionally, βIII-tubulin has been considered a neuronal specific protein, and has been primarily used as a marker for neurons (Caccamo et al., 1989). With the advancement of sequencing approaches, it has become evident that the gene encoding for βIII-tubulin, TUBB3, is expressed in a wide range of tissues across the body. TUBB3 expression is enriched in both the central and peripheral nervous systems, however, expression is also high in the testis (The GTEx Consortium, 2015). Recently, a large scale immuno-histological study was performed by Person et al. (2017) to gain a better understanding of βIII-tubulin expression across the human body in normal and cancerous tissues. Much like what was observed from sequencing studies, their work identified βIII-tubulin expression at varying amounts in the majority of human tissues, however, no comparison between expression in cancerous and normal tissues was performed (Person et al., 2017). Within the individual normal tissues, βIII-tubulin expression appeared predominantly in neurons, endothelial cells, fibroblasts and localized stem-like cells (Person et al., 2017).
As βIII-tubulin/TUBB3 displays differential expression across different cell types across the human body (The GTEx Consortium, 2015; Person et al., 2017), it suggests that there may be unique or tissue-specific transcriptional regulatory mechanisms for TUBB3 in different tissues. And indeed, several different mechanisms have been identified suggesting a complex nature to the regulation of TUBB3 in normal tissue. The presence of multiple different regulatory mechanisms does suggest however, that there are multiple routes that can lead to perturbed TUBB3 expression such as that observed in cancerous tissue. This section will focus on these mechanisms, by discussing what has been learnt about the normal regulation of TUBB3 in healthy tissues, after which the focus will shift to what has been uncovered from studies into dysregulated TUBB3 expression in cancer. Transcription factors with a mechanical link to the regulation of TUBB3 have been summarised in Table 4.
As mentioned, TUBB3 displays differential expression across the body, and factors driving its expression in different cell types in normal tissue have been proposed. To date, the primary focus into what drives TUBB3 expression in normal tissue has focused on its expression in both the central and peripheral nervous systems (CNS and PNS, respectively), and recently has been expanded to the roles TUBB3 may be playing in neural crest cell formation during development. Additionally, the field has made some headway in understanding why TUBB3 expression is predominantly repressed outside of neuronal tissues, and what mechanisms appear to be driving the observed TUBB3 enrichment in the testis.
βIII-tubulin has been considered as one of the earliest markers of neuronal differentiation of both the CNS and PNS where it is expressed either during, or prior to, terminal mitosis of the progenitor cells. This being either neuroepithelial cells for the CNS (Caccamo et al., 1989; Lee et al., 1990b; Linhartová et al., 1992; Easter et al., 1993), or neural crest cells for the PNS (Moody et al., 1989; Haendel et al., 1996). Indeed, cloning and in silico characterization of the 5’ flanking region of rat Tubb3 gene has revealed its minimal promoter region and several potential neuronal regulatory motifs (Dennis et al., 2002). This included putative binding sites for transcription factors Sp1, Ap2, Pea3, Pit1, and the C/EBP family, several E-box motifs, and a CNS enhancer motif (Table 3) (Dennis et al., 2002). There are differences in the expression of Tubb3 between the CNS and PNS. As shown in rats and mice, Tubb3 expression peaks during periods of axonal guidance and neuronal maturation, and then declines in the CNS with maturity while in the PNS continues to maintain high expression (Jiang and Oblinger 1992; Hausrat et al., 2021). This suggests that there may be specific regulatory mechanisms even within neuronal tissues. Indeed, several transcription factors have been identified in mouse and rat models that are involved with the expression of Tubb3 in neurogenesis in either the CNS or the PNS (Table 3).
A regulatory candidate in the CNS is Scratch1 (Scrt1), a Snail family zinc finger transcription factor that is specifically expressed in post-mitotic and newly differentiating neurons (Nakakura et al., 2001a). After initially identifying the co-expression of Scratch with βIII-tubulin by treating mouse P19 embryonal carcinoma cells with retinoic acid, Nakakura et al. (2001b) discovered that overexpressing Scratch1 by itself was sufficient to induce βIII-tubulin (Nakakura et al., 2001b). Two Retinoic Acid Response Elements have been recently identified within the human TUBB3 gene, and retinoic acid alone can induce TUBB3 expression (Namekawa et al., 2020). Another CNS transcription factor shown to stimulate βIII-tubulin expression during neuronal differentiation is the basic helix-loop-helix differentiation transcription factor Math2 (Uittenbogaard and Chiaramello 2002; Uittenbogaard and Chiaramello 2004). Although the binding sites of Scratch1 and Math2 in the Tubb3 loci have not been elucidated, both are known to bind to E-box motifs (Nakakura et al., 2001a; Uittenbogaard et al., 2003) and are potentially binding previously predicted sites (Dennis et al., 2002).
In addition to promoting gene expression, inhibitors of βIII-tubulin expression have also been identified in neuronal tissues. One of these inhibitors is ID2, which was originally speculated to be able to represses TUBB3 transcription (Katsetos et al., 2003). This was because elevated Id2 was shown to interfere with retinoblastoma tumour suppressor protein’s (pRb) capacity to bind to basic helix-loop-helix transcription factors, like the Tubb3 regulator Math2 (Uittenbogaard and Chiaramello 2002), and prevented the expression of neuronal specific genes in primary murine cortical progenitor cells (Toma et al., 2000). While alterations to Tubb3/βIII-tubulin expression were not examined by Toma et al. (2000), Id2 has since been demonstrated to indirectly impair Tubb3 transcription (Le Dréau et al., 2018). More recently, ID2 levels have been shown to influence neuronal differentiation of human glioblastoma stem cells, with elevated ID2 reducing the number of βIII-tubulin positive cells (Azzarelli et al., 2022). Another inhibitor of Tubb3 transcription is Pax3, which in rat neuronal stem cells was able to bind to the Tubb3 promoter regions and inhibit both transcription and translation (Cao et al., 2017). Subsequent work in mouse neuronal stem cells identified that during neurogenesis, Pax3 expression was reduced through elevated levels of miR-124, which resulted in increased Tubb3 expression and the development of neuronal phenotypes (Wei et al., 2018). In the same study, low levels of βIII-tubulin were present in these neuronal stem cells, suggesting that Pax3 partially suppresses Tubb3 expression (Wei et al., 2018).
Specific protein 1 (Sp1) is a transcription factor predicted to have many putative binding sites in the rat Tubb3 promoter region, suggesting it may have a potential role in the regulation of Tubb3 expression (Dennis et al., 2002). Sp1 is also predicted to have binding sites within the human TUBB3 promoter GH16J089920 (Fishilevich et al., 2017) (Figure 2). Sp1, a protein that is considered to be ubiquitously expressed in mammalian tissue, is known to function by binding GC-rich sequences and recruiting essential machineries to TATA boxes (one of which was also identified by Dennis et al., 2002) to initiate transcription of its target genes (Naar et al., 1998). The targeted inhibition of Sp1 activity in primary rat cortical neurons has been demonstrated to reduce the expression of Tubb3, alongside several other genes (Sleiman et al., 2011), suggesting that Sp1 is involved in Tubb3 transcription activation. Although Sp1 expression decreases after neuronal differentiation and is not detected in differentiated neurons (Mao et al., 2007; Mao et al., 2009). Tubb3 expression also declines in the CNS with neuronal maturity (Jiang and Oblinger 1992; Hausrat et al., 2021). Like Pax3, Sp1, is also a target of miR-124 (Mondanizadeh et al., 2015), although a link between Sp1, miR-124 and Tubb3 expression has not been reported.
While the factors involving Tubb3 expression described in the preceding section are associated with neuronal differentiation of the CNS, it is uncertain whether these shared by differentiating neurons of the PNS. During fetal development, however, βIII-tubulin has been shown to be expressed by neural crest cell progenitors (Pax7 positive cells), and by pre-migratory neural crest cells (Sox9 and Slug positive cells) prior to neurogenesis in the CNS (Chacon and Rogers 2019). Neural crest cells are considered to be multipotent progenitor cells able to give rise to various cells including neurons and melanocytes, and form the majority of the PNS (Acloque et al., 2008). This identification of βIII-tubulin expression in pre-migratory neural crest cells suggests that along with being involved with neurogenesis that βIII-tubulin has a separate role involved with neural crest cell development (Acloque et al., 2008). In this context, several factors linked with βIII-tubulin have been identified. For example, Ap2 has been linked to neural crest cell development (Mitchell et al., 1991) and identified to have several binding sites in the rat Tubb3 promoter (Dennis et al., 2002), however whether Ap2 can promote Tubb3 expression is yet to be determined.
The SRY-related HMG-box transcription factors of the SoxC gene family, Sox4, Sox11 and Sox12, are associated with the formation of neural crest cells (Uy et al., 2015). These transcription factors, which have been primarily linked with neuronal differentiation (Bergsland et al., 2006), are known to induce Tubb3 expression. Bergsland et al. (2006) first identified that the 5’ Untranslated Region (UTR) of the mouse Tubb3 gene contains three binding sites for either Sox4 or Sox11. Through increasing the expression of either Sox4 or Sox11 in developing murine embryos, Bergsland et al. (2006) observed an increase of Tubb3 and βIII-tubulin expression, and a reduction of both Tubb3 and βIII-tubulin when Sox4 or Sox11 was silenced through the use of siRNA. Subsequently Sox12 was also demonstrated to bind to the mouse Tubb3 promoter, and modulate βIII-tubulin as well (Hoser et al., 2008). Sox11 had the greatest impact on βIII-tubulin expression in neurogenesis (Bergsland et al., 2006; Hoser et al., 2008), and is required for binding to NeuroG1 in order to promote Tubb3 in early-born neurons, a process that can be inhibited through Bm2 (Chen et al., 2015). Since these three genes have been linked to neural crest formation (Uy et al., 2015), it is therefore plausible to think that the expression of Tubb3 observed by the neural crest progenitors (Chacon and Rogers 2019) could be driven by members of the SoxC family.
The animal studies discussed in the preceding section have been invaluable in deciphering the regulatory factors during neuronal development of βIII-tubulin. Equivalent studies examining TUBB3 expression in neuronal tissues and neural crest cells have yet to be validated in human cells, although genomic mapping has identified transcription factors that interact with the human and rat/mouse promotors. The validated human transcription factors involved with promoting TUBB3 expression in a neuronal setting are SOX11 and ZIC1 (Fu et al., 2019). While either SOX11 or ZIC1 promotes the expression of TUBB3/βIII-tubulin and induces a neuronal phenotype in U87 glioblastoma cells, the expression of ZIC1 greatly enhances the impact SOX11 has on TUBB3 expression and neuronal differentiation (Fu et al., 2019). SOX4 has also been shown to regulate TUBB3 expression cancer (Castillo et al., 2012), and this will be discussed in a subsequent section.
One potential reason the expression of TUBB3 is limited outside of neuronal tissue is due to the REST binding site (RE1) present within the first intronic region of TUBB3, which is located after the first exon of the first TUBB3 transcript variant (Figure 2; Table 2) (Shibazaki et al., 2012). An RE1 site is also present in the 5’ UTR of rat Tubb3 (Dennis et al., 2002). REST is a global transcriptional silencer that represses neuron-specific gene expression in non-neuronal cells (reviewed in Ooi and Wood 2007). Typically, REST forms complexes with chromatin-modifying enzymes, such as HDACs, coREST, mSin3a, MeCP2, and suppresses neuronal gene expression by epigenetic mechanisms (reviewed in Ooi and Wood 2007). Given the binding partners of REST, it is unsurprising that the human REST site located within the CpG island is found within the GH16J08995 “enhancer” (Figure 2). It is possible that in normal healthy tissue, as a result of REST binding, that this CpG island displays increased methylation, limiting the transcription of TUBB3 and potentially accounting for the reduced expression of TUBB3 observed in non-neuronal tissue. This needs to be further investigated as both TUBB3 and βIII-tubulin expression is observed in several non-neuronal tissues and cells including human fetal astrocytes, melanocytes, and spermatogenic cells (Dráberová et al., 2008; Leandro-García et al., 2010; Lehmann et al., 2017; Person et al., 2017).
Prior to identifying the REST binding site in TUBB3, Shibazaki et al. (2012) identified that TUBB3 expression in HEK293 and HeLa cells fluctuated with the cell cycle. TUBB3 expression increased throughout the S phase and βIII-tubulin expression peaked in the G2/M phase, where it appeared enriched around mitotic spindles (Shibazaki et al., 2012). Immunoprecipitation studies showed that REST was no longer bound to its RE1 site in TUBB3 during the G2/M phase, but rather rebound during the G1 phase, where TUBB3 expression was observed to decrease (Shibazaki et al., 2012). Knockdown studies also indicated that cell-cycle dependent TUBB3 expression is required for mitosis and normal cell growth in their cells (Shibazaki et al., 2012). This was further supported by studies that found that silencing TUBB3 expression sensitized cancer cell lines to epothilones, a TBA that causes cells to accumulate in G2M phase of the cell cycle (Gan et al., 2011; Narvi et al., 2013). This finding suggests that TUBB3 is not as neuronally specific as traditionally thought. Future studies are needed to better understand the role of REST in TUBB3 regulation.
An unexpected finding from a Lewis and Cowan 1988 study was the identification of βIII-tubulin expression in mouse testis (Lewis and Cowan 1988). This finding was initially dismissed, as the βIII-tubulin antibody that was used was also known to bind to βIVb-tubulin, which at the time was considered the only β-tubulin isotype to be expressed in the testis (Lewis and Cowan 1988). Lee et al., 1990a went on to validate βIII-tubulin expression in testis using a newly developed βIII-tubulin monoclonal antibody (TUJ1). Denolet et al. (2006) later identified the altered expression of Tubb3 in mouse Sertoli cells, “nurse” cells in the testes involved with spermatogenesis, in response to the loss of the androgen receptor. This work was then followed up by De Gendt et al. (2011) who identified several Androgen Response Elements (ARE) present in both mouse and rat Tubb3, and suggested that Tubb3 plays a critical role in spermatogenesis. The location of AREs in the human TUBB3 gene have not been reported and we cannot exclude the possibility that the association with the AR is indirect. Nevertheless, βIII-tubulin is expressed in human Sertoli cells (Person et al., 2017). Furthermore, testosterone has been shown to induce TUBB3/βIII-tubulin expression in human cell lines (Butler et al., 2001), suggesting that these elements potentially exist in the human TUBB3 gene. Person et al., 2017 also observed the strongest βIII-tubulin staining in testicular tissue in the spermatogenic cells, stem cells that give rise to sperm cells (Person et al., 2017), supporting the notion of TUBB3 being involved with spermatogenesis (De Gendt et al., 2011). In contrast, treating rat primary cortical neurons with supra-physiological doses of testosterone failed to elevate Tubb3 expression, despite the strong expression of the androgen receptor in the same cells (Zelleroth et al., 2021).
TUBB3 and βIII-tubulin expression has also been demonstrated to be controlled by the estrogen receptor (Saussede-Aim et al., 2009a), which could account for the observed expression in ovary tissue (Person et al., 2017). Though it is currently unclear if TUBB3 is expressed in oogonial stem cells in vivo, the female equivalent of spermatogenic cells, cultured murine oogonial stem cells have been shown to express βIII-tubulin (Cao et al., 2017). The location of the estrogen response element in the human TUBB3 loci is unknown, as it is not present in either the 5’ or 3’ UTR of TUBB3 (Saussede-Aim et al., 2009a).
In additional to studying the transcriptional regulation of TUBB3, there has also been investigations into the regulatory factors involved with the translation of, and the stability of the TUBB3 mRNA transcript. Work performed by Theodorakis and Cleveland (1992) demonstrated that increased cytosolic levels of β-tubulins results in a reduction to β-tubulin mRNA transcripts without impacting the level of α-tubulin transcripts. Their work suggested that there is an RNA binding agent that recognizes the first 13 coding nucleotides of the various β-tubulin transcripts that is involved with RNA stabilization, however as levels of β-tubulin protein increased, this unknown binding agent loses its affinity for the RNA resulting in destabilization of the mRNA (Theodorakis and Cleveland 1992). This was demonstrated by blocking the suspected binding site, which resulted in a loss in β-tubulin RNA (Theodorakis and Cleveland 1992). As this seminal work did not address the individual β-tubulin transcripts, how this relates to individual tubulin isotypes such as TUBB3/βIII-tubulin remains to be investigated.
In neurogenesis, translation of TUBB3 is also regulated in a neuronal specific manner. In mouse P19 and Neuro2a cells, RNA binding protein Tristetraprolin was shown to bind to Tubb3 and impair its translation (Dai et al., 2015). The authors identified many neuronal mRNAs to contain binding sites for Tristetraprolin (Dai et al., 2015). By initiating neuronal differentiation in these cells, they observed a reduction in Tristetraprolin levels followed by an increase in Tubb3 translation, a result they were able to mimic through Tristetraprolin knockdown studies as well (Dai et al., 2015). Human TUBB3 itself does contain a potential Tristetraprolin binding site, which appears to overlap with the binding site for the members of the miR-200 family, suggesting this mechanism of regulating TUBB3 transcription is likely to be active in the human developing nervous system as well.
Despite the well-established link between βIII-tubulin overexpression, drug resistance and poor clinical outcomes in patients, the regulation of TUBB3 expression in cancer cells remains poorly understood. It is becoming apparent that mechanisms driving aberrant TUBB3 expression in tumours are complex and may vary depending on cell type and gender. Indeed, the impact of aberrant TUBB3 expression impacts drug resistance in different types of cancer, as in ovarian and non-small cell lung cancer where elevated TUBB3 expression is associated with drug resistance (Kavallaris et al., 1997; Kavallaris et al., 1999), while increased TUBB3 expression in breast cancer and melanoma cells has been identified as a sign of increased drug sensitivity (Akasaka et al., 2009; Wang et al., 2013). Due to this perturbation of TUBB3 expression in cancer, several studies have investigated whether the altered expression of TUBB3 is a response to chemotherapeutic agents or as a result of gene dysregulation.
In cancers where TUBB3 is overexpressed, change in gene expression is often compared to the expression of total β-tubulin. For example, in neuronal tissues βIII-tubulin expression makes up approximately 25% of the β-tubulin pool, TUBB3 however only accounts for 4% of the total TUBB expression, with TUBB4 and TUBB2A making up at least 90% of the total TUBB expression (Cleveland et al., 1990; Leandro-García et al., 2010). This trend is seen in patient tumours, where TUBB3 only makes up a low to moderate proportion of the TUBB mRNA pool in ovarian, breast and lung cancer (with proportions ranging up to 7.5, 18, and 16% respectively) (Leandro-García et al., 2010). What makes this change aberrant though is that between normal and cancerous tissue, this change in TUBB3 expression accounts for a 71- and 43-fold increase in expression in lung and breast cancer respectively (Leandro-García et al., 2010).
Induction of TUBB3 expression has been widely reported in numerous cancer cell lines by both short term (Ranganathan et al., 1998b) and long term (Ranganathan et al., 1996; Kavallaris et al., 1997; Ranganathan et al., 1998a; Ranganathan et al., 1998b; Shalli et al., 2005) exposure to TBAs, a class of chemotherapeutics that target tubulin and microtubule dynamics (reviewed in Jordan and Wilson, 2004; La Regina et al., 2019). The factors responsible for this response may not be unique to βIII-tubulin as the levels of several other β-tubulin isotypes were also significantly increased (Ranganathan et al., 1996; Ranganathan et al., 1998a; Shalli et al., 2005). These results should be interpreted with caution though as very high doses of TBAs were used in some of the short-term studies. For example, in MCF7 cells, TUBB3 gene expression has been shown to be inducible following acute exposure to extremely high concentrations of vinorelbine, vinblastine or colchicine (1 μm), or paclitaxel (400 nm) (Saussede-Aim et al., 2009b; Lobert et al., 2011). Concentrations of vinblastine at 1 μm are known to completely depolymerise microtubules and increase microtubule polymer mass in vitro (Jordan et al., 1991; Toso et al., 1993). The concentration used is not clinically relevant and the Vinca alkaloid-induced TUBB3 expression is likely to be a compensatory response to microtubule depolymerisation, or an “off- target” effect on the transcriptional machinery or signalling pathways. Using this extreme dose of vinorelbine or vinblastine (1 μm) in mutagenesis studies, Saussede-Aim et al. (2009b) reported that Vinca alkaloid treatments were enhancing TUBB3 promoter activity via two AP1 binding sites located within the GH16J089920 promoter of the TUBB3 loci (Figure 2; Table 2) (Saussede-Aim et al., 2009b). However, using chromatin immunoprecipitation (ChIP) for canonical AP1 binding transcription factors failed to identify what was binding to the AP1 site in response to vinorelbine exposure, suggesting that there was a non-canonical AP1 binding protein inducing TUBB3 expression in response to vinorelbine (Saussede-Aim et al., 2009b). Future investigation using ChIP is required to identify transcription factors responsible for Vinca alkaloid-induced TUBB3 expression at clinically relevant doses.
Like Vinca alkaloids, Taxol has been reported to alter the level of TUBB3 expression in tumours. For example, Kavallaris et al. (1997) reported that, while the level of individual β-tubulin isotypes remained the same in normal ovary and primary untreated ovarian tumours, analysis of ovarian carcinoma specimens from the same patient before and after chemotherapy revealed that TUBB3 and TUBB2C gene expression increased significantly in Taxol-resistant tumours post-treatment (Kavallaris et al., 1997). As patients develop Taxol resistance after several cycles of Taxol/platinum combination therapy, it is difficult to differentiate whether the increased TUBB3 expression observed was a direct consequence of chemotherapy-induced changes, or as a result of selection of resistant cell populations where altered tubulin expression provided a survival advantage. Kavallaris et al. (1999) went on to show that Taxol resistant non-small cell lung cancer cells were overexpressing TUBB3 and βIII-tubulin, and that partial suppression of TUBB3 using antisense oligonucleotides sensitized cells to Taxol (Kavallaris et al., 1999), linking TUBB3/βIII-tubulin expression with Taxol sensitivity. Later, potent knockdown of TUBB3 using siRNA and shRNA confirmed a direct functional role for βIII-tubulin in mediating in vitro and in vivo sensitivity to broad classes of chemotherapy in non-small cell lung cancer, identifying βIII-tubulin as a survival factor in cancer cells (Gan et al., 2007; Gan et al., 2010b; McCarroll et al., 2010; Gan et al., 2011).
Several regulatory elements in addition to AP1 binding sites mentioned earlier, have been identified within the 5’ UTR of the TUBB3 loci, which includes two CpG islands. The shorter of the two (consisting of 38 CpGs) is located just upstream of the GH16J089920 promoter, while the second CpG island, and also the largest in the TUBB3 loci (132 CpGs), is located within the promoter and covering the first exon of the first TUBB3 transcript variant (Figure 2; Table 2). The larger CpG island has been identified as hypomethylated in several ovarian cancer cell lines, but not in non-cancerous ovarian tissues (Izutsu et al., 2008). Given the identification of multiple SP1 and AP2 binding sites within the rat genome around the first exon of Tubb3 (Dennis et al., 2002), Izutsu et al. (2008) suggested that these sites may be present at similar locations of the human TUBB3 loci too. Since SP1 and its DNA-binding activities are inducible under oxidative stress and DNA-damage (Ryu et al., 2003), and assuming there are SP1/AP2 binding sites within this region as suggested by Izutsu et al. (2008), it is possible that under chemotherapeutic insults, hypomethylated TUBB3 promoter regions with enhanced SP1 signalling may contribute to aberrant TUBB3 expression in ovarian cancer. Further studies are required to clarify whether SP1 and AP2 can directly bind to those hypomethylated regions and drive aberrant TUBB3 expression. Moreover, it will be important to determine if hypomethylation of TUBB3 occurs in patient samples with upregulated βIII-tubulin expression.
There are two Retinoic Acid Response Elements (RARE) upstream of the smaller CpG island, towards the extreme 5′ end of the TUBB3 loci and within the MC1R promoter region (Figure 2; Table 2). RAREs are bound to by the transcription factor Retinoic Acid Receptor α (RARα) in response to elevated levels of retinoic acid, resulting in gene expression. The two RAREs within the TUBB3 loci were recently discovered by Namekawa et al. while trying to improve the generation of long-term cultures of Patient Derived Cancer cells (PDCs) that were enriched for Cancer Stem-like Cells (CSCs) from surgically removed bladder tumours (Namekawa et al., 2020). CSCs are renewable cells that constitute a small population within a cancerous cell population, and are implicated in tumour drug resistance, as well as tumour recurrence and metastasis (reviewed in Clevers 2011; Diaz and Leon 2011). These PDCs were grown in a 3D spheroid culture to aid in CSC enrichment, and were observed to have elevated expression of ALDH1A1, a marker for CSCs and whose protein product RALDH1 oxidises retinaldehyde into retinoic acid (Namekawa et al., 2020). Knockdown studies of ALDH1A1 showed that its expression was required for the in vitro maintenance of the PDCs, and prevented spheroid formation, leading the authors to speculate that spheroid formation was occurring due to elevated levels of retinoic acid caused by elevated ALDH1A1 (Namekawa et al., 2020). After demonstrating that spheroid formation was reliant on retinoic acid levels independently of ALDH1A1 expression, Namekawa et al. (2020) proceeded to search for genes that were being upregulated by the retinoic acid response pathway. By performing ChIP for RARα, they identified two RAREs in the 5’ UTR of the TUBB3 loci, which were then confirmed to be able to promote the expression of TUBB3, and that TUBB3 expression was elevated by the PDCs too (Namekawa et al., 2020). Subsequent knockdown studies of TUBB3 in PDCs confirmed it as downstream to the elevation of ALDH1A1 expression, as TUBB3 expression was required for in vitro spheroid formation (Namekawa et al., 2020). This prompted the suggestion that TUBB3 expression may contribute to the maintenance of CSCs in bladder cancer (Namekawa et al., 2020), which could account for why elevated TUBB3 is observed with more aggressive subtypes of bladder cancer (Hinsch et al., 2017).
Recent work has identified that MZF1 is able to bind to the TUBB3 loci, with three potential sites predicted up to 600 base pairs upstream of the first exon of TUBB3 transcript variant 2 (Kanojia et al., 2020). The ability of MZF1 to bind to the TUBB3 loci was identified while looking for means to upregulate βIII-tubulin expression in HER2 positive breast cancer in an effort to induce sensitivity to the TBA, vinorelbine (Kanojia et al., 2020). Building on previous work that identified TUBB3 expression to be modulated by the members of the Bromdodmain and Extraterminal (BET) protein family (Piunti et al., 2017), Kanojia et al. (2020) identified increased TUBB3/βIII-tubulin expression in response to BET inhibition which led to increased sensitivity to Vinorelbine both in vitro and in vivo (Kanojia et al., 2020). Seeking a mechanism to account for why BET inhibition was promoting TUBB3 expression, the TUBB3 promoter (GH6J089920) was scanned and led to the identification of several potential binding sites for transcription factors (Kanojia et al., 2020). As MZF1 was associated with better survival in breast cancer patients, and because MZF1 expression decreased upon treatment with BET inhibitors, Kanojia et al. (2020) performed knockdown/overexpression studies and ChIP-qPCR, confirming that MZF1 could bind to the TUBB3 loci and repress TUBB3/βIII-tubulin expression.
Near the AP1 sites, exist two more transcription factor binding sites within the 5′ UTR of the first TUBB3 transcript variant, both of which are Ybox elements (Figure 2; Table 2). Ybox elements are canonically bound to by SRY-related HMG-box transcription factors, and as mentioned earlier several of these transcription factors have been linked to modulating TUBB3 expression in a neuronal setting. Two of these transcription factors have been linked to modulating TUBB3 expression in cancer, SOX4 and SOX9 (Castillo et al., 2012; Raspaglio et al., 2014). While both are linked to neurogenesis and neuronal crest cell formation (Bergsland et al., 2006; Martini et al., 2013; Uy et al., 2015), increased SOX4 expression is also commonly linked with several forms of cancer, in particular lung cancer, and has been suggested as a driver oncogene (Liu et al., 2006; Castillo et al., 2012). Despite its increase in expression, the impact of increased SOX4 expression on the genes it was upregulating was unknown. To address this issue, Castillo et al. (2012) investigated genes that were positively regulated by SOX4 expression in small cell lung cancer through knockdown studies. After identifying several potential genes downregulated upon knockdown of SOX4 expression, Castillo et al. (2012) screened these genes for potential SOX4 binding sites (Scharer et al., 2009), and subsequently confirmed SOX4 binding through ChIP and qPCR of the SOX4 bound sequences (Castillo et al., 2012). As Sox4 had been previously linked to regulating Tubb3/βIII-tubulin expression in neurogenesis (Bergsland et al., 2006), the authors used TUBB3 as a positive control for their assays as it was downregulated in the initial SOX4 knockdown microarray, and they went on to validate two SOX4 binding sites in the TUBB3 loci (Figure 2; Table 2) (Castillo et al., 2012). Of note, the Ybox4 element identified in the 5’ region was determined to be the more dominant (Figure 2) (Castillo et al., 2012). Thus Castillo et al. (2012) reported that the dysregulation of a direct factor associated with neurogenesis in cancer may be involved with promoting the aberrant expression of TUBB3 in some cancers. Due to its association with hypoxic stress response, the Ybox9 element and SOX9 will be discussed in a subsequent section.
Epigenetic dysregulation is a common feature of cancers (Hanahan 2022). Like many genes, TUBB3 can be epigenetically regulated. REST-mediated mechanisms and chromatin remodelling have been demonstrated to play an important role in TUBB3 regulation in several epithelial cancer cells (Izutsu et al., 2008; Akasaka et al., 2009; Gao et al., 2012; Shibazaki et al., 2012). For example, in ovarian cancer cells, DNA demethylation CpG island (containing 86 CpGs) within TUBB3 intron 1 has been shown to result in βIII-tubulin overexpression, with chromatin acetylation accelerating the process and increasing TUBB3 expression as well (Izutsu et al., 2008; Akasaka et al., 2009). Subsequently, Izutsu et al. (2008) performed in silico analysis’ within this region and identified the RE1 site, later validated by others (Shibazaki et al., 2012), suggesting REST may also be involved with the observed increase in TUBB3 expression. Follow-up investigations of this predicted RE1 site by Akasaka et al. (2009) identified that histone deacetylation of this RE1 motif partially contributes to TUBB3/βIII-tubulin overexpression in melanoma.
The loss of REST in a range of cancers has also been linked to the aberrant expression of neuronal genes in the clinic, including TUBB3. A negative correlation between REST and TUBB3 expression has been reported in skin, ovarian, and small cell lung cancer biopsy samples (Akasaka et al., 2009; Kreisler et al., 2010; Hatano et al., 2011; Gao et al., 2012), while in normal non-neoplastic tissues TUBB3 is barely detectable. Additionally, REST gene deletion and frame-shift mutations are frequently observed in colon and small cell lung cancers (Coulson et al., 2000; Westbrook et al., 2005). In mouse colonic crypts, targeted Rest genetic ablation has resulted in upregulation of Tubb3 expression (Hatano et al., 2011; Gao et al., 2012). Furthermore, TUBB3/βIII-tubulin expression can be independently induced upon REST siRNA treatment in cancer cells (Akasaka et al., 2009; Gao et al., 2012). Together, these findings suggest REST as a transcriptional silencer of TUBB3 and that dysfunctional REST, in conjunction with epigenetic modifications in TUBB3 intron 1, may be important mechanisms underlying aberrant TUBB3 expression in tumours of non-neuronal origin. Since other neuronal differentiation factors mentioned previously are also linked to this altered TUBB3 expression (Castillo et al., 2012; Raspaglio et al., 2014; Namekawa et al., 2020), it poses the question—are dysregulated processes associated with neuronal gene regulation the primary causes of aberrant TUBB3 expression in tumours of non-neuronal origin? Further research is required in order to better understand the role that these neuronal factors are playing in TUBB3 expression in cancer.
From the observed increases in TUBB3 expression in response to exposure to TBAs, (Ranganathan et al., 1996; Kavallaris et al., 1997; Ranganathan et al., 1998a; Ranganathan et al., 1998b; Shalli et al., 2005), one can speculate that the induction of βIII-tubulin could enable tumour cells to adapt and survive in a stressful microenvironment. Gan et al. (2007) provided the first evidence that expression of TUBB3/βIII-tubulin was a survival factor that when suppressed using gene silencing not only sensitized tumour cells to TBAs but also to broad classes of drugs including DNA-damaging agents and antimetabolites. A notion that is strengthened by the observation that the levels of TUBB3 were able to modulate the PTEN/AKT signaling axis (McCarroll et al., 2015a), a prosurvival pathway commonly perturbed in a range of tumours (reviewed in Song et al., 2012; Taddei et al., 2012). Indeed, growing evidence suggests that βIII-tubulin expression is a key adaptive response that is activated on cellular exposure to a stressful microenvironment, such as hypoxic conditions (Raspaglio et al., 2008; Forde et al., 2010; Danza et al., 2012; Bordji et al., 2014; Raspaglio et al., 2014) or glucose deprivation in cancers cells (Parker et al., 2016). In solid tumours, cells often grow within a hypoxic microenvironment, and cells with a highly efficient hypoxia-inducing factor orchestrated survival program possess an advantage to offset its selective pressure.
In tumours, the hypoxia-inducing factor HIF1α has been implicated in the transcriptional regulation of βIII-tubulin via the 3′UTR of the TUBB3 gene and is thought to protect tumours against hypoxic injury (Raspaglio et al., 2008; Forde et al., 2010; Danza et al., 2012; Bordji et al., 2014; Raspaglio et al., 2014). In A2780 ovarian cancer cells, hypoxia has been shown to strongly induce TUBB3 gene and βIII-tubulin protein expression and this phenotype was directly linked to cisplatin and paclitaxel resistance (Raspaglio et al., 2008; Raspaglio et al., 2014). This process was shown to be transcriptionally regulated through the binding of HIF1α to a hypoxia response element (HRE) within the 3′ UTR of TUBB3 (Raspaglio et al., 2008) (Figure 2; Table 2). An alternative transcriptional mechanism regulating TUBB3, involving HIF2α and the SoxC gene SOX9, has also been described (Raspaglio et al., 2014). In ovarian cancer specimens, high levels of TUBB3 mRNA and βIII-tubulin protein were significantly associated with increasing levels of SOX9 and HIF2α (Raspaglio et al., 2014). Silencing both SOX9 and HIF2α abrogated this hypoxia-activated TUBB3 expression, suggesting roles for SOX9 and HIF2α as positive TUBB3 regulators under hypoxic conditions. Subsequent in silico analysis and ChIP studies demonstrated the binding of SOX9 to a specific binding site (the Ybox9 element mentioned earlier) within the 5′ region of TUBB3 (Figure 2; Table 2), with gene-reporter and site-directed mutagenesis studies all supporting the involvement of SOX9 in TUBB3 regulation in hypoxia (Raspaglio et al., 2014).
HIF1α and HIF2α may potentially regulate TUBB3 expression in hypoxic conditions by mechanisms that differ in diverse cancer types. While both appear to have a positive impact on expression in ovarian cancer (Raspaglio et al., 2008; Raspaglio et al., 2014), HIF1α appears to play an inhibitory role on TUBB3/βIII-tubulin expression in glioblastoma cells (Bordji et al., 2014). In glioblastoma hypoxia reduced HIF1α expression, leading to HIF2α binding to the two overlapping HREs located in the 3′UTR of the gene (Bordji et al., 2014). Additionally, epigenetic regulation could account for this regulation in specific cancer cell lines, as hypomethylation of the HRE is required for TUBB3 expression in ovarian cancer cells, prostate cancer cells and prostate tumours (Raspaglio et al., 2008; Forde et al., 2010). This suggests that both HIF1α and HIF2α/SOX9 mediated TUBB3 regulation could be a cell-specific response, as it is not inducible upon hypoxia in some cell lines expressing high basal levels of βIII-tubulin (Raspaglio et al., 2008; Shen and Yu 2008; Danza et al., 2012; Levallet et al., 2012; Bordji et al., 2014; Raspaglio et al., 2014).
Another common mechanism used by cells as a means of translational regulation are microRNAs (miRNAs), small non-coding RNAs that can modulate the post-transcriptional regulation of gene expression through modulation of mRNA stability and translational efficiency through complementary base pair binding (Goodall et al., 2013; Maciotta et al., 2013). One particular family of miRNAs, the miR-200 family, has been linked to modulating the translation of TUBB3 in the context of cancer. The miR-200 family, consisting of miR-141, −200a, −200b, −200c, and −429, have an established role in cancer, with their downregulation being linked to angiogenesis, drug resistance and the epithelial-mesenchymal transition of cancer cells (Mongroo and Rustgi 2010; Pecot et al., 2013; Brozovic et al., 2015; Sulaiman et al., 2016). The expression of all five members of this miRNA family have been shown to inversely correlate with the levels of TUBB3 in ovarian cancer patients (Susanna et al., 2011), however only two of them, miR-200b and −200c, have been demonstrated to directly bind to TUBB3, while miR-429 is predicted to do so (Cochrane et al., 2009; Susanna et al., 2011; Wu et al., 2020). Given these miRNAs are from the same family, they all share a similar seed sequence and are able to bind to TUBB3 at the same location (Figure 3).
FIGURE 3. Structure of the common TUBB3 mRNA transcripts. Structure of the two common TUBB3 transcripts showing the validated binding sites of the HuR protein and the miR-200 family. Transcripts are represented by a combination of thin and thick boxes for exons, and arrows for introns; thin boxes represent untranslated regions (5′ and 3’ UTRs), while thick boxes represent translated regions. TUBB3 transcript variants 1 and 2 are show in red and blue respectively and have been aligned to show common regions. RNA binding sites represented by boxes under their approximate location, with thickness corresponding to size. HuR binding site validated by (Prislei et al., 2013); miR-200c binding confirmed by (Cochrane et al., 2009); miR-200b binding confirmed by (Wu et al., 2020); miR-429 binding predicted by (Susanna et al., 2011); miR-200c binding confirmed by (Cochrane et al., 2009); miR-200b binding confirmed by (Wu et al., 2020); miR-429 binding predicted by (Susanna et al., 2011).
The most well studied member of the miR-200 family in regards to TUBB3, is miR-200c, which has also been shown to have an interesting relationship with the RNA binding protein HuR in its modulation of TUBB3 translation (Cochrane et al., 2009; Cochrane et al., 2010; Raspaglio et al., 2010). Cochrane et al. (2009) and Cochrane et al. (2010) found that miR-200c binds to the TUBB3 3’ UTR (Figure 3) which results in a reduction of βIII-tubulin without impacting the expression of TUBB3 (Cochrane et al., 2009; Cochrane et al., 2010). In the context of cancer, identification of miR-200c regulating TUBB3 expression came from in vitro work examining reduced miR-200c in model breast, ovarian and endometrial cancer cell lines (Cochrane et al., 2009; Cochrane et al., 2010). Changes in miR-200c have also been reported in a number of cancer cell lines and clinical specimens. Specifically, several separate studies reported that low miR-200c expression is significantly associated with high βIII-tubulin protein levels, resistance to TBAs, high incidence of recurrence and poor survival in ovarian cancer patients (Susanna et al., 2011; Brozovic et al., 2015; Sulaiman et al., 2016). These findings suggest miR-200c negatively regulates TUBB3 expression and loss of miR-200c may result in βIII-tubulin overexpression in ovarian, breast and endometrial cancer. Additionally, recent work has demonstrated that intratumour delivery of miR-200c overexpressing exosomes can target TUBB3 in in vivo models of tongue squamous cell carcinoma and restore tumour chemosensitivity (Cui et al., 2020), suggesting miR-200c has potential as a therapeutic strategy to treat individuals with βIII-tubulin overexpressing tumours.
In contrast, another study examined miR-200c expression in patients with ovarian cancer and found no relation between elevated miR-200c, βIII-tubulin levels, or chemotherapy sensitivity, leading them to examine additional elements involved with βIII-tubulin translation (Prislei et al., 2013). One element Prislei et al. (2013) chose to focus on was the expression of the RNA binding protein HuR, that had been associated with promoting the translation of TUBB3 (Raspaglio et al., 2010). In ovarian cancer, Raspaglio et al. (2010) identified that while hypoglycaemic conditions caused an increase in TUBB3 expression, the expression of βIII-tubulin in these conditions was reliant on the stabilization of the TUBB3 transcript by cytosolic HuR binding to its 3’ UTR (Figure 3) (Raspaglio et al., 2010). The authors additionally identified that high cytosolic levels of HuR in tumours was associated with high βIII-tubulin expression and poor survival in ovarian cancer patients (Raspaglio et al., 2010). Building on this work, Prislei et al. (2013) divided up their patient cohort into those with high cytosolic or high nuclear HuR expression. They found that those with high cytosolic HuR expression with elevated miR-200c levels unexpectedly had elevated βIII-tubulin levels, exhibited chemotherapy resistance and poor patient outcomes (Prislei et al., 2013). In vitro work then identified that miR-200c was capable of recruiting cytosolic HuR to its binding site on the TUBB3 transcript (Figure 3), resulting in further stabilization of the TUBB3 transcript which potentially accounts for the higher expression of βIII-tubulin observed in patients (Prislei et al., 2013). How miR-200c impacts the recruitment of HuR to the TUBB3 transcript is unclear and understanding this relationship would be beneficial to unravelling how TUBB3 expression is modulated by miRNAs.
While the previous section highlighted elements that have been mapped to the TUBB3 loci, there are several elements that have been demonstrated to regulate TUBB3 expression but have no clear binding to the TUBB3 loci. Mentioned earlier, the gonadal steroids estrogen and testosterone have both been shown to induce TUBB3 expression and have emerged as potential drivers of TUBB3/βIII-tubulin expression in cancer (Butler et al., 2001; Saussede-Aim et al., 2009a; Mariani et al., 2012), however estrogen and androgen receptor elements (ERE and ARE respectively) in the TUBB3 loci not been identified. In breast cancer cells, Saussede-Aim et al. (2009a) described an estrogen-dependent TUBB3 regulatory pathway, where TUBB3/βIII-tubulin expression was inducible upon oestradiol exposure. While in silico analysis of the 5′ and 3’ UTRs of the TUBB3 loci failed to identify where the location of any EREs were, several binding sites for transcription factors known to be implicated in indirect estrogen-regulation such as AP1, NF-κB, and SP1 were identified in the first intron of TUBB3 (Saussede-Aim et al., 2009a). In the same study, oestradiol-induced TUBB3 expression could not be reproduced in estrogen receptor (ER) negative breast cancer cell lines, and was abrogated after exposure to the ER antagonists tamoxifen and fulvestrant in several ER-expressing breast cancer cell lines. These findings suggest that oestradiol-induced TUBB3 expression is ER-dependent. The authors proposed that ERs may regulate TUBB3 in an indirect manner, facilitating transcription factor binding to nearby corresponding sites in intron 1 and subsequent TUBB3 transcription activation. Conflicting results were reported in invasive breast cancer specimens, where high TUBB3 expression was identified in both ER positive and ER negative breast tumour specimens (Wang et al., 2013), raising the question as to whether ER is relevant to TUBB3 regulation in the clinic. This disparity could be explained by the different biology in cell models and clinical specimens. In the study by Wang et al. (2013), specimens were collected from patients with different pathological stages, with or without neoadjuvant chemotherapy, all of which could potentially contribute to high TUBB3 expression. In addition, patients in this study were not treated with estrogen and therefore further studies are required to assess the clinical value of ER in TUBB3 regulation in breast cancer.
In colorectal cancer, elevated TUBB3 expression is associated with invasive phenotypes in both genders (Portyanko et al., 2009; Zhao et al., 2016). In vitro analysis of 23 colorectal cancer cell lines suggested that TUBB3/βIII-tubulin is activated after exposure to androgens in males (Mariani et al., 2012), as seen with estrogens in breast cancer cells (Saussede-Aim et al., 2009a). In both male and female colorectal cancer cell lines, stable silencing of androgen receptors (AR) yielded significant downregulation of TUBB3/βIII-tubulin, raising the possibility that ARs play a significant role in driving TUBB3 expression. Importantly, in male colorectal cancer cells, the AR-dependent TUBB3 regulatory pathway is constitutively activated via testicular androgen, while in colorectal cancer cell lines derived from women TUBB3 is only inducible upon serum starvation (Mariani et al., 2012). This finding suggests that for males and females, there are differences in how the AR regulatory regions are impacted and are able to induce TUBB3 expression in response to external stimuli. While mapped in mice and rats (De Gendt et al., 2011), future mutagenesis and ChIP studies are required to identify AR binding regions within the human TUBB3 gene to understand this sex based expression pattern of TUBB3.
Other factors have also been proposed to play a role in TUBB3 regulation. For example, overexpression of Semaphorin-6A (SEMA6A) is correlated with TUBB3/βIII-tubulin upregulation in ovarian cancer cells, while the reverse is observed in SEMA6A knockdown cells (Prislei et al., 2008). Likewise, levels of the transcription factor ZEB1 have also been shown to influence TUBB3 expression in ovarian cancer in the same manner as Semaphorin-6A (Lobert et al., 2013). Additionally, Kanojia et al. (2020) identified a potential ZEB1 binding site within the TUBB3 5’ UTR, and their data supports that ZEB1 promotes TUBB3 expression, as increasing ZEB1 expression led to an elevation of TUBB3 expression. In contrast to Semaphorin-6A and ZEB1, the overexpression of the Snail family zinc finger transcription factor SLUG in non-small cell lung cancer cells suppressed expression of TUBB3/βIII-tubulin, as well as the β-tubulin isotype, TUBB4A/βIVa-tubulin (Tamura et al., 2013). This study then focused on the relationship between Slug and TUBB4A, and did not investigate the SLUG induced suppression of TUBB3 further (Tamura et al., 2013).
Slug is co-expressed with βIII-tubulin and Sox9 in pre-migratory avian neural crest cells (Chacon and Rogers 2019). Additionally, SLUG has been shown to directly interact with SOX9 to promote the formation of cancer stem-like cells in lung cancer (Luanpitpong et al., 2016), and there is the recent speculation that TUBB3 may be playing a role in the maintenance of cancer stem like cells (Namekawa et al., 2020). Though it does present as an oddity, these studies suggest that Slug may only be a TUBB3 repressor under certain conditions. Two other family members, Snail and Scratch1, are also involved in TUBB3 regulation. As previously mentioned, Scratch1 expression results in increased βIII-tubulin in a neuronal setting (Nakakura et al., 2001b). In contrast, Scratch1 may not be involved with TUBB3 regulation in a cancer setting due to its lack of expression in a wide range of patient samples obtained from different tumours (Bastid et al., 2010). The expression of the third family member, Snail/SNAI1 itself, has also been shown to correlate with the expression of TUBB3/βIII-tubulin in colon cancer cells (Sobierajska et al., 2016), however other than expression, no mechanistic study has been reported. Snail and Slug both present as interesting regulators of TUBB3, as they are both expressed in a large range of cancers (Bastid et al., 2010), and also because of their roles in the epithelial–mesenchymal transition in tumour cells, the process linked with metastasis (Thiery and Sleeman 2006). Additional studies are required to assess whether Snail or Slug can directly bind to the TUBB3 loci and regulate its expression, especially given βIII-tubulin’s roles in drug resistance and tumor aggressiveness.
Finally, K-Ras signalling has been associated with the regulation of βIII-tubulin translation in cancer (Levallet et al., 2012). While investigating K-Ras signalling in non-small cell lung cancer, Levallet et al. (2012) identified K-Ras mutations in clinical samples were strongly and frequently associated with positive βIII-tubulin expression. In immortalised human bronchial cells, expression of a K-Ras mutant protein was shown to significantly increase βIII-tubulin protein levels, while TUBB3 mRNA remained unchanged (Levallet et al., 2012). This observation raises the possibility that βIII-tubulin translation or turnover may be controlled by K-Ras-induced signalling cascades. In further support of this notion, siRNA knockdown of K-Ras and pharmacologic inhibition of K-Ras downstream effectors resulted in βIII-tubulin protein downregulation (Levallet et al., 2012). Additionally, overexpression of EGFR enhanced βIII-tubulin translation in both K-Ras wild type and mutant expressing cell lines, however non-small cell lung cancer associated EGFR mutations appeared to have no impact on βIII-tubulin translation (Levallet et al., 2012). Understanding, what is driving the increased translation of TUBB3 in this circumstance would greatly enhance our knowledge on βIII-tubulin translation and stability.
Due to the high degree of homology of βIII-tubulin with other β-tubulin isotypes, small molecule inhibitors against this protein are difficult to develop. Given the high expression of TUBB3/βIII-tubulin in epithelial cancers, strategies to silence TUBB3 have been explored. Cui et al. (2020) demonstrated that targeting the TUBB3 transcript directly was sufficient to restore tumour chemosensitivity. There is strong preclinical evidence that targeting the TUBB3 transcript through the use of transient or stable gene silencing can increase drug sensitivity, reduce tumour growth, and suppress metastasis in non-small cell lung cancer and pancreatic cancer (Kavallaris et al., 1999; Gan et al., 2007; Gan et al., 2010a; McCarroll et al., 2010; McCarroll et al., 2015b). Along with our colleagues, we have been exploring the development of therapeutic strategies to silence TUBB3, and hence βIII-tubulin, in tumors that overexpress this isotype. In pancreatic cancer, we developed polymeric star nanoparticles capable of delivering and potently silencing TUBB3 siRNA in a clinically relevant orthotopic model of pancreatic cancer and showed that this increased drug sensitivity and reduced metastasis (Teo et al., 2016; McCarroll et al., 2019; Conte et al., 2021). Recently we described the developed of nanoparticles loaded with docetaxel (DTX) and an siRNA against TUBB3, in order to have a synergistic effect in the treatment of lung cancer (Conte et al., 2021). In this study we showed that combining DTX/TUBB3-siRNA into nanoparticles led to a significant decrease in TUBB3 and cell viability of tumour cell spheroids compared to nanoparticles loaded with DTX alone—demonstrating the combined anticancer effects of βIII-tubulin reduction and increased drug sensitivity (Conte et al., 2021). Collectively, these studies highlight the potential of developing therapeutic strategies to target TUBB3 in cancer cells.
In both normal and cancerous tissue, it is clear the regulation of both TUBB3 expression and translation is controlled by a complex and multifaced system. This review highlights that a combination of transcriptional controls and altered epigenetic modifications, in conjunction with disrupted signalling pathways may all contribute to disrupted TUBB3 expression in cancers and subsequent response to therapy. Genomic advances such as single cell analysis and spatial transcriptomics may lead to improved identification of differences between cell-types, and the regulation of TUBB3 within the tumour microenvironment. Progressing our understanding of βIII-tubulin regulation is not only important in identifying how the nervous system develops but also in cancer, where it will aid in the identification of potential therapeutic targets and treatment strategies.
AD, FK and WT wrote the original draft. MK supervised and edited this work. All authors have read and agreed to the submitted version of this manuscript.
MK is supported by grants from the National Health and Medical Research (Program Grant APP1091261 and Principal Research Fellowship APP1119152), Cancer Institute New South Wales Program Grant (TPG 2037) and Cancer Australia grant (1141485). FK was supported by an Australian Postgraduate Award, Lung Foundation Australia Lung Cancer National Program Postgraduate Grant-in-Aid, and The LH Ainsworth Cancer Research Scholarship FK. This work was supported by the Children’s Cancer Institute, which is affiliated with the University of New South Wales (UNSW Sydney), and the Sydney Children’s Hospital Network.
The authors declare that the research was conducted in the absence of any commercial or financial relationships that could be construed as a potential conflict of interest.
All claims expressed in this article are solely those of the authors and do not necessarily represent those of their affiliated organizations, or those of the publisher, the editors and the reviewers. Any product that may be evaluated in this article, or claim that may be made by its manufacturer, is not guaranteed or endorsed by the publisher.
Acloque, H., Wilkinson, D. G., and Nieto, M. A. (2008). Chapter 9 In Situ Hybridization Analysis of Chick Embryos in Whole‐Mount and Tissue Sections. Methods Cel Biol. 87, 169–185. doi:10.1016/s0091-679x(08)00209-4
Akasaka, K., Maesawa, C., Shibazaki, M., Maeda, F., Takahashi, K., Akasaka, T., et al. (2009). Loss of Class III β-Tubulin Induced by Histone Deacetylation Is Associated with Chemosensitivity to Paclitaxel in Malignant Melanoma Cells. J. Invest. Dermatol. 129 (6), 1516–1526. doi:10.1038/jid.2008.406
Azzarelli, R., McNally, A., Dell’Amico, C., Onorati, M., Simons, B., and Philpott, A. (2022). ASCL1 Phosphorylation and ID2 Upregulation Are Roadblocks to Glioblastoma Stem Cell Differentiation. Sci. Rep. 12 (1), 2341. doi:10.1038/s41598-022-06248-x
Baertsch, R., Diekhans, M., Kent, W. J., Haussler, D., and Brosius, J. (2008). Retrocopy Contributions to the Evolution of the Human Genome. BMC Genomics 9 (1), 466. doi:10.1186/1471-2164-9-466
Bär, J., Popp, Y., Bucher, M., and Mikhaylova, M. (2022). Direct and Indirect Effects of Tubulin post-translational Modifications on Microtubule Stability: Insights and Regulations. Biochim. Biophys. Acta (Bba) - Mol. Cel Res. 1869 (6), 119241.
Bastid, J., Bouchet, B. P., Ciancia, C., Pourchet, J., Audoynaud, C., Grelier, G., et al. (2010). The SNAIL Family Member SCRATCH1 Is Not Expressed in Human Tumors. Oncol. Rep. 23 (2), 523–529.
Bergsland, M., Werme, M., Malewicz, M., Perlmann, T., and Muhr, J. (2006). The Establishment of Neuronal Properties Is Controlled by Sox4 and Sox11. Genes Dev. 20 (24), 3475–3486. doi:10.1101/gad.403406
Bordji, K., Grandval, A., Cuhna-Alves, L., Lechapt-Zalcman, E., and Bernaudin, M. (2014). Hypoxia-inducible Factor-2α (HIF-2α), but Not HIF-1α, Is Essential for Hypoxic Induction of Class III β-tubulin Expression in Human Glioblastoma Cells. FEBS J. 281 (23), 5220–5236. doi:10.1111/febs.13062
Brozovic, A., Duran, G. E., Wang, Y. C., Francisco, E. B., and Sikic, B. I. (2015). The miR-200 Family Differentially Regulates Sensitivity to Paclitaxel and Carboplatin in Human Ovarian Carcinoma OVCAR-3 and MES-OV Cells. Mol. Oncol. 9 (8), 1678–1693. doi:10.1016/j.molonc.2015.04.015
Butler, R., Leigh, P. N., and Gallo, J.-M. (2001). Androgen-induced Up-Regulation of Tubulin Isoforms in Neuroblastoma Cells. J. Neurochem. 78 (4), 854–861. doi:10.1046/j.1471-4159.2001.00475.x
Caccamo, D., Katsetos, C. D., Herman, M. M., Frankfurter, A., Collins, V. P., and Rubinstein, L. J. (1989). Immunohistochemistry of a Spontaneous Murine Ovarian Teratoma with Neuroepithelial Differentiation. Neuron-Associated β-Tubulin as a Marker for Primitive Neuroepithelium. Lab. Invest. 60 (3), 390–398.
Cao, S., Du, J., Lv, Y., Lin, H., Mao, Z., Xu, M., et al. (2017). PAX3 Inhibits β-Tubulin-III Expression and Neuronal Differentiation of Neural Stem Cell. Biochem. Biophysical Res. Commun. 485 (2), 307–311. doi:10.1016/j.bbrc.2017.02.086
Castillo, S. D., Matheu, A., Mariani, N., Carretero, J., Lopez-Rios, F., Lovell-Badge, R., et al. (2012). Novel Transcriptional Targets of the SRY-HMG Box Transcription Factor SOX4 Link its Expression to the Development of Small Cell Lung Cancer. Cancer Res. 72 (1), 176–186. doi:10.1158/0008-5472.can-11-3506
Chacon, J., and Rogers, C. D. (2019). Early Expression of Tubulin β-III in Avian Cranial Neural Crest Cells. Gene Expr. Patterns 34, 119067. doi:10.1016/j.gep.2019.119067
Chen, C., Lee, G. A., Pourmorady, A., Sock, E., and Donoghue, M. J. (2015). Orchestration of Neuronal Differentiation and Progenitor Pool Expansion in the Developing Cortex by SoxC Genes. J. Neurosci. 35 (29), 10629–10642. doi:10.1523/jneurosci.1663-15.2015
Cleveland, D. W., Joshi, H. C., and Murphy, D. B. (1990). Tubulin Site Interpretation. Nature 344 (6265), 389. doi:10.1038/344389a0
Clevers, H. (2011). The Cancer Stem Cell: Premises, Promises and Challenges. Nat. Med. 17 (3), 313–319. doi:10.1038/nm.2304
Cochrane, D. R., Howe, E. N., Spoelstra, N. S., and Richer, J. K. (2010). Loss of miR-200c: A Marker of Aggressiveness and Chemoresistance in Female Reproductive Cancers. J. Oncol. 2010, 821717. doi:10.1155/2010/821717
Cochrane, D. R., Spoelstra, N. S., Howe, E. N., Nordeen, S. K., and Richer, J. K. (2009). MicroRNA-200c Mitigates Invasiveness and Restores Sensitivity to Microtubule-Targeting Chemotherapeutic Agents. Mol. Cancer Ther. 8 (5), 1055–1066. doi:10.1158/1535-7163.mct-08-1046
Conte, C., Monteiro, P. F., Gurnani, P., Stolnik, S., Ungaro, F., Quaglia, F., et al. (2021). Multi-component Bioresponsive Nanoparticles for Synchronous Delivery of Docetaxel and TUBB3 siRNA to Lung Cancer Cells. Nanoscale 13 (26), 11414–11426. doi:10.1039/d1nr02179f
Coulson, J. M., Edgson, J. L., Woll, P. J., and Quinn, J. P. (2000). A Splice Variant of the Neuron-Restrictive Silencer Factor Repressor Is Expressed in Small Cell Lung Cancer: A Potential Role in Derepression of Neuroendocrine Genes and a Useful Clinical Marker. Cancer Res. 60 (7), 1840–1844.
Cui, J., Wang, H., Zhang, X., Sun, X., Zhang, J., and Ma, J. (2020). Exosomal miR-200c Suppresses Chemoresistance of Docetaxel in Tongue Squamous Cell Carcinoma by Suppressing TUBB3 and PPP2R1B. Aging 12 (8), 6756–6773. doi:10.18632/aging.103036
Cunningham, F., Achuthan, P., Akanni, W., Allen, J., Amode, M. R., Armean, I. M., et al. (2018). Ensembl 2019. Nucleic Acids Res. 47 (D1), D745–D751. doi:10.1093/nar/gky1113
Dai, W., Li, W., Hoque, M., Li, Z., Tian, B., and Makeyev, E. V. (2015). A post-transcriptional Mechanism Pacing Expression of Neural Genes with Precursor Cell Differentiation Status. Nat. Commun. 6 (1), 7576. doi:10.1038/ncomms8576
Daily, K., Ho Sui, S. J., Schriml, L. M., Dexheimer, P. J., Salomonis, N., Schroll, R., et al. (2017). Molecular, Phenotypic, and Sample-Associated Data to Describe Pluripotent Stem Cell Lines and Derivatives. Sci. Data 4, 170030. doi:10.1038/sdata.2017.30
Dalziel, M., Kolesnichenko, M., das Neves, R. P., Iborra, F., Goding, C., and Furger, A. (2011). α-MSH Regulates Intergenic Splicing of MC1R and TUBB3 in Human Melanocytes. Nucleic Acids Res. 39 (6), 2378–2392. doi:10.1093/nar/gkq1125
Danza, G., Di Serio, C., Rosati, F., Lonetto, G., Sturli, N., Kacer, D., et al. (2012). Notch Signaling Modulates Hypoxia-Induced Neuroendocrine Differentiation of Human Prostate Cancer Cells. Mol. Cancer Res. 10 (2), 230–238. doi:10.1158/1541-7786.mcr-11-0296
De Gendt, K., Denolet, E., Willems, A., Daniels, V. W., Clinckemalie, L., Denayer, S., et al. (2011). Expression of Tubb3, a β-Tubulin Isotype, Is Regulated by Androgens in Mouse and Rat Sertoli Cells1. Biol. Reprod. 85 (5), 934–945. doi:10.1095/biolreprod.110.090704
Dennis, K., Uittenbogaard, M., Chiaramello, A., and Moody, S. A. (2002). Cloning and Characterization of the 5'-flanking Region of the Rat Neuron-specific Class III β-Tubulin Gene. Gene 294 (1-2), 269–277. doi:10.1016/s0378-1119(02)00801-6
Denolet, E., De Gendt, K., Allemeersch, J., Engelen, K., Marchal, K., Van Hummelen, P., et al. (2006). The Effect of a Sertoli Cell-Selective Knockout of the Androgen Receptor on Testicular Gene Expression in Prepubertal Mice. Mol. Endocrinol. 20 (2), 321–334. doi:10.1210/me.2005-0113
Diaz, A., and Leon, K. (2011). Therapeutic Approaches to Target Cancer Stem Cells. Cancers 3 (3), 3331–3352. doi:10.3390/cancers3033331
D. Katsetos, C., Draber, P., and Kavallaris, M. (2011). Targeting βIII-Tubulin in Glioblastoma Multiforme: From Cell Biology and Histopathology to Cancer Therapeutics. Acamc 11 (8), 719–728. doi:10.2174/187152011797378760
D. Katsetos, C., and Draber, P. (2012). Tubulins as Therapeutic Targets in Cancer: from Bench to Bedside. Cpd 18 (19), 2778–2792. doi:10.2174/138161212800626193
Doherty, E. J., Macy, M. E., Wang, S. M., Dykeman, C. P., Melanson, M. T., and Engle, E. C. (1999). CFEOM3: a New Extraocular Congenital Fibrosis Syndrome that Maps to 16q24.2-q24.3. Invest. Ophthalmol. Vis. Sci. 40 (8), 1687–1694.
Dráberová, E., Del Valle, L., Gordon, J., Marková, V., Smejkalová, B., Bertrand, L., et al. (2008). Class III β-Tubulin Is Constitutively Coexpressed with Glial Fibrillary Acidic Protein and Nestin in Midgestational Human Fetal Astrocytes: Implications for Phenotypic Identity. J. Neuropathol. Exp. Neurol. 67 (4), 341–354.
Easter, S., Ross, L., and Frankfurter, A. (1993). Initial Tract Formation in the Mouse Brain. J. Neurosci. 13 (1), 285–299. doi:10.1523/jneurosci.13-01-00285.1993
Fishilevich, S., Nudel, R., Rappaport, N., Hadar, R., Plaschkes, I., Iny Stein, T., et al. (2017). GeneHancer: Genome-wide Integration of Enhancers and Target Genes in GeneCards. Database (Oxford) 2017, bax028. doi:10.1093/database/bax028
Forde, J. C., Perry, A. S., Brennan, K., Martin, L. M., Lawler, M. P., Lynch, T. H., et al. (2010). Docetaxel Maintains its Cytotoxic Activity under Hypoxic Conditions in Prostate Cancer Cells. Urol. Oncol. 30 (6), 912–919. doi:10.1016/j.urolonc.2010.08.015
Fu, J. Q., Chen, Z., Hu, Y. J., Fan, Z. H., Guo, Z. X., Liang, J. Y., et al. (2019). A Single Factor Induces Neuronal Differentiation to Suppress Glioma Cell Growth. CNS Neurosci. Ther. 25 (4), 486–495. doi:10.1111/cns.13066
Gan, P. P., McCarroll, J. A., Byrne, F. L., Garner, J., and Kavallaris, M. (2011). Specific β-Tubulin Isotypes Can Functionally Enhance or Diminish Epothilone B Sensitivity in Non-small Cell Lung Cancer Cells. PLoS ONE 6 (6), e21717. doi:10.1371/journal.pone.0021717
Gan, P. P., McCarroll, J. A., Po'uha, S. T., Kamath, K., Jordan, M. A., and Kavallaris, M. (2010a). Microtubule Dynamics, Mitotic Arrest, and Apoptosis: Drug-Induced Differential Effects of βIII-Tubulin. Mol. Cancer Ther. 9 (5), 1339–1348. doi:10.1158/1535-7163.mct-09-0679
Gan, P. P., McCarroll, J. A., Po'uha, S. T., Kamath, K., Jordan, M. A., and Kavallaris, M. (2010b). Microtubule Dynamics, Mitotic Arrest, and Apoptosis: Drug-Induced Differential Effects of βIII-Tubulin. Mol. Cancer Ther. 9 (5), 1339–1348. doi:10.1158/1535-7163.mct-09-0679
Gan, P. P., Pasquier, E., and Kavallaris, M. (2007). Class III β-Tubulin Mediates Sensitivity to Chemotherapeutic Drugs in Non-small Cell Lung Cancer. Cancer Res. 67 (19), 9356–9363. doi:10.1158/0008-5472.can-07-0509
Gao, S., Zhao, X., Lin, B., Hu, Z., Yan, L., and Gao, J. (2012). Clinical Implications of REST and TUBB3 in Ovarian Cancer and its Relationship to Paclitaxel Resistance. Tumor Biol. 33 (5), 1759–1765. doi:10.1007/s13277-012-0435-y
Goodall, E. F., Heath, P. R., Bandmann, O., Kirby, J., and Shaw, P. J. (2013). Neuronal Dark Matter: the Emerging Role of microRNAs in Neurodegeneration. Front. Cel. Neurosci. 7, 178. doi:10.3389/fncel.2013.00178
GTEx Consortium (2015). Human Genomics. The Genotype-Tissue Expression (GTEx) Pilot Analysis: Multitissue Gene Regulation in Humans. Science 348 (6235), 648–660. doi:10.1126/science.1262110
Haendel, M. A., Bollinger, K. E., and Baas, P. W. (1996). Cytoskeletal Changes during Neurogenesis in Cultures of Avain Neural Crest Cells. J. Neurocytol 25 (4), 289–301. doi:10.1007/BF02284803
Haeussler, M., Zweig, A. S., Tyner, C., Speir, M. L., Rosenbloom, K. R., Raney, B. J., et al. (2019). The UCSC Genome Browser Database: 2019 Update. Nucleic Acids Res. 47 (D1), D853–d858. doi:10.1093/nar/gky1095
Hanahan, D. (2022). Hallmarks of Cancer: New Dimensions. Cancer Discov. 12 (1), 31–46. doi:10.1158/2159-8290.cd-21-1059
Hatano, Y., Yamada, Y., Hata, K., Phutthaphadoong, S., Aoki, H., and Hara, A. (2011). Genetic Ablation of a Candidate Tumor Suppressor Gene, Rest, Does Not Promote Mouse colon Carcinogenesis. Cancer Sci. 102 (9), 1659–1664. doi:10.1111/j.1349-7006.2011.02006.x
Hausrat, T. J., Radwitz, J., Lombino, F. L., Breiden, P., and Kneussel, M. (2021). Alpha‐ and β‐tubulin Isotypes Are Differentially Expressed during Brain Development. Develop. Neurobiol. 81 (3), 333–350. doi:10.1002/dneu.22745
Herraiz, C., Garcia-Borron, J. C., Jiménez-Cervantes, C., and Olivares, C. (2017). MC1R Signaling. Intracellular Partners and Pathophysiological Implications. Biochim. Biophys. Acta (Bba) - Mol. Basis Dis. 1863 (10), 2448–2461. doi:10.1016/j.bbadis.2017.02.027
Herraiz, C., Olivares, C., Castejón-Griñán, M., Abrisqueta, M., Jiménez-Cervantes, C., and García-Borrón, J. C. (2015). Functional Characterization of MC1R-TUBB3 Intergenic Splice Variants of the Human Melanocortin 1 Receptor. PloS one 10 (12), e0144757. doi:10.1371/journal.pone.0144757
Hinsch, A., Chaker, A., Burdelski, C., Koop, C., Tsourlakis, M. C., Steurer, S., et al. (2017). β III-Tubulin Overexpression Is Linked to Aggressive Tumor Features and Genetic Instability in Urinary Bladder Cancer. Hum. Pathol. 61, 210–220. doi:10.1016/j.humpath.2016.11.005
Hoser, M., Potzner, M. R., Koch, J. M. C., Bösl, M. R., Wegner, M., and Sock, E. (2008). Sox12 Deletion in the Mouse Reveals Nonreciprocal Redundancy with the Related Sox4 and Sox11 Transcription Factors. Mol. Cel Biol 28 (15), 4675–4687. doi:10.1128/mcb.00338-08
Izutsu, N., Maesawa, C., Shibazaki, M., Oikawa, H., Shoji, T., Sugiyama, T., et al. (2008). Epigenetic Modification Is Involved in Aberrant Expression of Class III β-tubulin, TUBB3, in Ovarian Cancer Cells. Int. J. Oncol. 32 (6), 1227–1235. doi:10.3892/ijo.32.6.1227
Jiang, Y. Q., and Oblinger, M. M. (1992). Differential Regulation of β III and Other Tubulin Genes during Peripheral and central Neuron Development. J. Cel Sci 103 ( Pt 3) (Pt 3), 643–651. doi:10.1242/jcs.103.3.643
Jordan, M. A., Thrower, D., and Wilson, L. (1991). Mechanism of Inhibition of Cell Proliferation by Vinca Alkaloids. Cancer Res. 51 (8), 2212–2222.
Jordan, M. A., and Wilson, L. (2004). Microtubules as a Target for Anticancer Drugs. Nat. Rev. Cancer 4 (4), 253–265. doi:10.1038/nrc1317
Kanakkanthara, A., and Miller, J. H. (2021). Βiii-Tubulin Overexpression in Cancer: Causes, Consequences, and Potential Therapies. Biochim. Biophys. Acta (Bba) - Rev. Cancer 1876 (2), 188607. doi:10.1016/j.bbcan.2021.188607
Kanojia, D., Panek, W. K., Cordero, A., Fares, J., Xiao, A., Savchuk, S., et al. (2020). BET Inhibition Increases βIII-tubulin Expression and Sensitizes Metastatic Breast Cancer in the Brain to Vinorelbine. Sci. Transl Med. 12 (558). doi:10.1126/scitranslmed.aax2879
Karki, R., Mariani, M., Andreoli, M., He, S., Scambia, G., Shahabi, S., et al. (2013). Βiii-Tubulin: Biomarker of Taxane Resistance or Drug Target? Expert Opin. Ther. Targets 17 (4), 461–472. doi:10.1517/14728222.2013.766170
Katsetos, C. D., Del Valle, L., Geddes, J. F., Aldape, K., Boyd, J. C., Legido, A., et al. (2002). Localization of the Neuronal Class III β-Tubulin in Oligodendrogliomas: Comparison with Ki-67 Proliferative Index and 1p/19q Status. J. Neuropathol. Exp. Neurol. 61 (4), 307–320. doi:10.1093/jnen/61.4.307
Katsetos, C. D., Herman, M. M., and Mörk, S. J. (2003). Class III β-tubulin in Human Development and Cancer. Cell Motil. Cytoskeleton 55 (2), 77–96. doi:10.1002/cm.10116
Katsetos, C. D., Reginato, M. J., Baas, P. W., D’Agostino, L., Legido, A., Tuszyn´ski, J. A., et al. (2015). Emerging Microtubule Targets in Glioma Therapy. Semin. Pediatr. Neurol. 22 (1), 49–72. doi:10.1016/j.spen.2015.03.009
Kavallaris, M., Burkhart, C. A., and Horwitz, S. B. (1999). Antisense Oligonucleotides to Class III β-tubulin Sensitize Drug-Resistant Cells to Taxol. Br. J. Cancer 80 (7), 1020–1025. doi:10.1038/sj.bjc.6690507
Kavallaris, M., Kuo, D. Y., Burkhart, C. A., Regl, D. L., Norris, M. D., Haber, M., et al. (1997). Taxol-resistant Epithelial Ovarian Tumors Are Associated with Altered Expression of Specific β-Tubulin Isotypes. J. Clin. Invest. 100 (5), 1282–1293. doi:10.1172/jci119642
Kavallaris, M. (2010). Microtubules and Resistance to Tubulin-Binding Agents. Nat. Rev. Cancer 10 (3), 194–204. doi:10.1038/nrc2803
Kreisler, A., Strissel, P. L., Strick, R., Neumann, S. B., Schumacher, U., and Becker, C.-M. (2010). Regulation of the NRSF/REST Gene by Methylation and CREB Affects the Cellular Phenotype of Small-Cell Lung Cancer. Oncogene 29 (43), 5828–5838. doi:10.1038/onc.2010.321
Kuang, Y.-L., Munoz, A., Nalula, G., Santostefano, K. E., Sanghez, V., Sanchez, G., et al. (2019). Evaluation of Commonly Used Ectoderm Markers in iPSC Trilineage Differentiation. Stem Cel. Res. 37, 101434. doi:10.1016/j.scr.2019.101434
La Regina, G., Coluccia, A., Naccarato, V., and Silvestri, R. (2019). Towards Modern Anticancer Agents that Interact with Tubulin. Eur. J. Pharm. Sci. 131, 58–68. doi:10.1016/j.ejps.2019.01.028
Latremoliere, A., Cheng, L., DeLisle, M., Wu, C., Chew, S., Hutchinson, E. B., et al. (2018). Neuronal-Specific TUBB3 Is Not Required for Normal Neuronal Function but Is Essential for Timely Axon Regeneration. Cel Rep. 24 (7), 1865–1879. e1869. doi:10.1016/j.celrep.2018.07.029
Le Dréau, G., Escalona, R., Fueyo, R., Herrera, A., Martínez, J. D., Usieto, S., et al. (2018). E Proteins Sharpen Neurogenesis by Modulating Proneural bHLH Transcription Factors' Activity in an E-box-dependent Manner. Elife 7.
Leandro-García, L. J., Leskelä, S., Landa, I., Montero-Conde, C., López-Jiménez, E., Letón, R., et al. (2010). Tumoral and Tissue-specific Expression of the Major Human β-tubulin Isotypes. Cytoskeleton 67 (4), 214–223.
Lee, M. K., Rebhun, L. I., and Frankfurter, A. (1990a). Posttranslational Modification of Class III Beta-Tubulin. Proc. Natl. Acad. Sci. U.S.A. 87 (18), 7195–7199. doi:10.1073/pnas.87.18.7195
Lee, M. K., Tuttle, J. B., Rebhun, L. I., Cleveland, D. W., and Frankfurter, A. (1990b). The Expression and Posttranslational Modification of a Neuron-specific ?-tubulin Isotype during Chick Embryogenesis. Cel Motil. Cytoskeleton 17 (2), 118–132. doi:10.1002/cm.970170207
Lehmann, S. G., Bourgoin-Voillard, S., Seve, M., and Rachidi, W. (2017). Tubulin Beta-3 Chain as a New Candidate Protein Biomarker of Human Skin Aging: A Preliminary Study. Oxid Med. Cel Longev 2017, 5140360. doi:10.1155/2017/5140360
Levallet, G., Bergot, E., Antoine, M., Creveuil, C., Santos, A. O., Beau-Faller, M., et al. (2012). High TUBB3 Expression, an Independent Prognostic Marker in Patients with Early Non-small Cell Lung Cancer Treated by Preoperative Chemotherapy, Is Regulated by K-Ras Signaling Pathway. Mol. Cancer Ther. 11 (5), 1203–1213. doi:10.1158/1535-7163.mct-11-0899
Lewis, S. A., and Cowan, N. J. (1988). Complex Regulation and Functional Versatility of Mammalian Alpha- and Beta-Tubulin Isotypes during the Differentiation of Testis and Muscle Cells. J. Cel Biol 106 (6), 2023–2033. doi:10.1083/jcb.106.6.2023
Linhartová, I., Dráber, P., Dráberová, E., and Viklický, V. (1992). Immunological Discrimination of Beta-Tubulin Isoforms in Developing Mouse Brain. Post-translational Modification of Non-class-III Beta-Tubulins. Biochem. J. 288 (Pt 3Pt 3), 919–924.
Liu, L., Geisert, E. E., Frankfurter, A., Spano, A. J., Jiang, C. X., Yue, J., et al. (2007). A Transgenic Mouse Class-III β Tubulin Reporter Using Yellow Fluorescent Protein. genesis 45 (9), 560–569. doi:10.1002/dvg.20325
Liu, P., Ramachandran, S., Ali Seyed, M., Scharer, C. D., Laycock, N., Dalton, W. B., et al. (2006). Sex-Determining Region Y Box 4 Is a Transforming Oncogene in Human Prostate Cancer Cells. Cancer Res. 66 (8), 4011–4019. doi:10.1158/0008-5472.can-05-3055
Lobert, S., Graichen, M. E., and Morris, K. (2013). Coordinated Regulation of β-Tubulin Isotypes and Epithelial-To-Mesenchymal Transition Protein ZEB1 in Breast Cancer Cells. Biochemistry 52 (32), 5482–5490. doi:10.1021/bi400340g
Lobert, S., Jefferson, B., and Morris, K. (2011). Regulation of β-tubulin Isotypes by Micro-RNA 100 in MCF7 Breast Cancer Cells. Cytoskeleton 68 (6), 355–362. doi:10.1002/cm.20517
Locher, H., de Rooij, K. E., de Groot, J. C., van Doorn, R., Gruis, N. A., Löwik, C. W., et al. (2013). Class III β-tubulin, a Novel Biomarker in the Human Melanocyte Lineage. Differentiation 85 (4-5), 173–181. doi:10.1016/j.diff.2013.05.003
Lopata, M. A., Havercroft, J. C., Chow, L. T., and Cleveland, D. W. (1983). Four Unique Genes Required for β Tubulin Expression in Vertebrates. Cell 32 (3), 713–724. doi:10.1016/0092-8674(83)90057-0
Luanpitpong, S., Li, J., Manke, A., Brundage, K., Ellis, E., McLaughlin, S. L., et al. (2016). SLUG Is Required for SOX9 Stabilization and Functions to Promote Cancer Stem Cells and Metastasis in Human Lung Carcinoma. Oncogene 35 (22), 2824–2833. doi:10.1038/onc.2015.351
Ludueña, R. F. (2013). A Hypothesis on the Origin and Evolution of Tubulin. Int. Rev. Cel Mol Biol 302, 41–185.
Ludueña, R. F. (1993). Are Tubulin Isotypes Functionally Significant. Mol. Biol. Cel 4 (5), 445–457.
Ludueña, R. F. (1998). Multiple Forms of Tubulin: Different Gene Products and Covalent Modifications. Int. Rev. Cytol. 178, 207–275.
Maciotta, S., Meregalli, M., and Torrente, Y. (2013). The Involvement of microRNAs in Neurodegenerative Diseases. Front. Cel. Neurosci. 7, 265. doi:10.3389/fncel.2013.00265
Mao, X. R., Moerman-Herzog, A. M., Chen, Y., and Barger, S. W. (2009). Unique Aspects of Transcriptional Regulation in Neurons - Nuances in NFkappaB and Sp1-Related Factors. J. Neuroinflammation 6 (1), 16. doi:10.1186/1742-2094-6-16
Mao, X., Yang, S.-H., Simpkins, J. W., and Barger, S. W. (2007). Glutamate Receptor Activation Evokes Calpain-Mediated Degradation of Sp3 and Sp4, the Prominent Sp-Family Transcription Factors in Neurons. J. Neurochem. 100 (5), 1300–1314. doi:10.1111/j.1471-4159.2006.04297.x
Mariani, M., Karki, R., Spennato, M., Pandya, D., He, S., Andreoli, M., et al. (2015). Class III β-tubulin in normal and Cancer Tissues. Gene 563 (2), 109–114. doi:10.1016/j.gene.2015.03.061
Mariani, M., Zannoni, G. F., Sioletic, S., Sieber, S., Martino, C., Martinelli, E., et al. (2012). Gender Influences the Class III and V β-Tubulin Ability to Predict Poor Outcome in Colorectal Cancer. Clin. Cancer Res. 18 (10), 2964–2975. doi:10.1158/1078-0432.ccr-11-2318
Martini, S., Bernoth, K., Main, H., Ortega, G. D. C., Lendahl, U., Just, U., et al. (2013). A Critical Role for Sox9 in Notch-Induced Astrogliogenesis and Stem Cell Maintenance. STEM CELLS 31 (4), 741–751. doi:10.1002/stem.1320
McCarroll, J. A., Gan, P. P., Erlich, R. B., Liu, M., Dwarte, T., Sagnella, S. S., et al. (2015a). TUBB3/βIII-Tubulin Acts through the PTEN/AKT Signaling Axis to Promote Tumorigenesis and Anoikis Resistance in Non-small Cell Lung Cancer. Cancer Res. 75 (2), 415–425. doi:10.1158/0008-5472.can-14-2740
McCarroll, J. A., Gan, P. P., Liu, M., and Kavallaris, M. (2010). Βiii-Tubulin Is a Multifunctional Protein Involved in Drug Sensitivity and Tumorigenesis in Non-small Cell Lung Cancer. Cancer Res. 70 (12), 4995–5003. doi:10.1158/0008-5472.can-09-4487
McCarroll, J. A., Sharbeen, G., Kavallaris, M., and Phillips, P. A. (2019). The Use of Star Polymer Nanoparticles for the Delivery of siRNA to Mouse Orthotopic Pancreatic Tumor Models. Methods Mol. Biol., 329–353. doi:10.1007/978-1-4939-9220-1_23
McCarroll, J. A., Sharbeen, G., Liu, J., Youkhana, J., Goldstein, D., McCarthy, N., et al. (2015b). Βiii-Tubulin: A Novel Mediator of Chemoresistance and Metastases in Pancreatic Cancer. Oncotarget 6 (4), 2235–2249. doi:10.18632/oncotarget.2946
Melé, M., Ferreira, P. G., Reverter, F., DeLuca, D. S., Monlong, J., Sammeth, M., et al. (2015). The Human Transcriptome across Tissues and Individuals. Science 348 (6235), 660–665.
Mitchell, P. J., Timmons, P. M., Hébert, J. M., Rigby, P. W., and Tjian, R. (1991). Transcription Factor AP-2 Is Expressed in Neural Crest Cell Lineages during Mouse Embryogenesis. Genes Dev. 5 (1), 105–119. doi:10.1101/gad.5.1.105
Mondanizadeh, M., Arefian, E., Mosayebi, G., Saidijam, M., Khansarinejad, B., and Hashemi, S. M. (2015). MicroRNA‐124 Regulates Neuronal Differentiation of Mesenchymal Stem Cells by Targeting Sp1 mRNA. J. Cel. Biochem. 116 (6), 943–953. doi:10.1002/jcb.25045
Mongroo, P. S., and Rustgi, A. K. (2010). The Role of the miR-200 Family in Epithelial-Mesenchymal Transition. Cancer Biol. Ther. 10 (3), 219–222. doi:10.4161/cbt.10.3.12548
Moody, S. A., Quigg, M. S., and Frankfurter, A. (1989). Development of the Peripheral Trigeminal System in the Chick Revealed by an Isotype-specific Anti-beta-tubulin Monoclonal Antibody. J. Comp. Neurol. 279 (4), 567–580. doi:10.1002/cne.902790406
Näär, A. M., Beaurang, P. A., Robinson, K. M., Oliner, J. D., Avizonis, D., Scheek, S., et al. (1998). Chromatin, TAFs, and a Novel Multiprotein Coactivator Are Required for Synergistic Activation by Sp1 and SREBP-1a In Vitro. Genes Dev. 12 (19), 3020–3031. doi:10.1101/gad.12.19.3020
Nakakura, E. K., Watkins, D. N., Sriuranpong, V., Borges, M. W., Nelkin, B. D., and Ball, D. W. (2001b). Mammalian Scratch Participates in Neuronal Differentiation in P19 Embryonal Carcinoma Cells. Brain Res. Mol. Brain Res. 95 (1-2), 162–166. doi:10.1016/s0169-328x(01)00246-7
Nakakura, E. K., Watkins, D. N., Schuebel, K. E., Sriuranpong, V., Borges, M. W., Nelkin, B. D., et al. (2001a). Mammalian Scratch: a Neural-specific Snail Family Transcriptional Repressor. Proc. Natl. Acad. Sci. U.S.A. 98 (7), 4010–4015. doi:10.1073/pnas.051014098
Namekawa, T., Ikeda, K., Horie‐Inoue, K., Suzuki, T., Okamoto, K., Ichikawa, T., et al. (2020). ALDH1A1 in Patient‐derived Bladder Cancer Spheroids Activates Retinoic Acid Signaling Leading to TUBB3 Overexpression and Tumor Progression. Int. J. Cancer 146 (4), 1099–1113. doi:10.1002/ijc.32505
Narvi, E., Jaakkola, K., Winsel, S., Oetken-Lindholm, C., Halonen, P., Kallio, L., et al. (2013). Altered TUBB3 Expression Contributes to the Epothilone Response of Mitotic Cells. Br. J. Cancer 108 (1), 82–90. doi:10.1038/bjc.2012.553
Nogales, E. (2000). Structural Insights into Microtubule Function. Annu. Rev. Biochem. 69, 277–302. doi:10.1146/annurev.biochem.69.1.277
Ooi, L., and Wood, I. C. (2007). Chromatin Crosstalk in Development and Disease: Lessons from REST. Nat. Rev. Genet. 8 (7), 544–554. doi:10.1038/nrg2100
Oshima, S., and Yawaka, Y. (2020). Class III β-tubulin Expression during Hard Tissue Formation in Developing Mouse Teeth. Pediatr. Dental J. 30 (1), 9–16. doi:10.1016/j.pdj.2019.12.002
Parker, A. L., Teo, W. S., McCarroll, J. A., and Kavallaris, M. (2017). An Emerging Role for Tubulin Isotypes in Modulating Cancer Biology and Chemotherapy Resistance. Int. J. Mol. Sci. 18 (7). doi:10.3390/ijms18071434
Parker, A. L., Teo, W. S., Pandzic, E., Vicente, J. J., McCarroll, J. A., Wordeman, L., et al. (2018). β-Tubulin Carboxy-Terminal Tails Exhibit Isotype-specific Effects on Microtubule Dynamics in Human Gene-Edited Cells. Life Sci. Alliance 1 (2). doi:10.26508/lsa.201800059
Parker, A. L., Turner, N., McCarroll, J. A., and Kavallaris, M. (2016). Βiii-Tubulin Alters Glucose Metabolism and Stress Response Signaling to Promote Cell Survival and Proliferation in Glucose-Starved Non-small Cell Lung Cancer Cells. Carcin 37 (8), 787–798. doi:10.1093/carcin/bgw058
Pecot, C. V., Rupaimoole, R., Yang, D., Akbani, R., Ivan, C., Lu, C., et al. (2013). Tumour Angiogenesis Regulation by the miR-200 Family. Nat. Commun. 4 (1), 2427. doi:10.1038/ncomms3427
Person, F., Wilczak, W., Hube-Magg, C., Burdelski, C., Möller-Koop, C., Simon, R., et al. (2017). Prevalence of βIII-tubulin (TUBB3) Expression in Human normal Tissues and Cancers. Tumour Biol. 39 (10), 1010428317712166. doi:10.1177/1010428317712166
Piunti, A., Hashizume, R., Morgan, M. A., Bartom, E. T., Horbinski, C. M., Marshall, S. A., et al. (2017). Therapeutic Targeting of Polycomb and BET Bromodomain Proteins in Diffuse Intrinsic Pontine Gliomas. Nat. Med. 23 (4), 493–500. doi:10.1038/nm.4296
Poirier, K., Saillour, Y., Bahi-Buisson, N., Jaglin, X. H., Fallet-Bianco, C., Nabbout, R., et al. (2010). Mutations in the Neuronal β-tubulin Subunit TUBB3 Result in Malformation of Cortical Development and Neuronal Migration Defects. Hum. Mol. Genet. 19 (22), 4462–4473. doi:10.1093/hmg/ddq377
Portyanko, A., Kovalev, P., Gorgun, J., and Cherstvoy, E. (2009). Βiii-Tubulin at the Invasive Margin of Colorectal Cancer: Possible Link to Invasion. Virchows Arch. 454 (5), 541–548. doi:10.1007/s00428-009-0764-4
Prislei, S., Martinelli, E., Mariani, M., Raspaglio, G., Sieber, S., Ferrandina, G., et al. (2013). MiR-200c and HuR in Ovarian Cancer. BMC Cancer 13, 72. doi:10.1186/1471-2407-13-72
Prislei, S., Mozzetti, S., Filippetti, F., De Donato, M., Raspaglio, G., Cicchillitti, L., et al. (2008). From Plasma Membrane to Cytoskeleton: a Novel Function for Semaphorin 6A. Mol. Cancer Ther. 7 (1), 233–241. doi:10.1158/1535-7163.mct-07-0390
Ranganathan, S., Dexter, D. W., Benetatos, C. A., Chapman, A. E., Tew, K. D., and Hudes, G. R. (1996). Increase of Beta(III)- and beta(IVa)-tubulin Isotopes in Human Prostate Carcinoma Cells as a Result of Estramustine Resistance. Cancer Res. 56 (11), 2584–2589.
Ranganathan, S., Benetatos, C., Colarusso, P., Dexter, D., and Hudes, G. (1998a). Altered β-tubulin Isotype Expression in Paclitaxel-Resistant Human Prostate Carcinoma Cells. Br. J. Cancer 77 (4), 562–566. doi:10.1038/bjc.1998.91
Ranganathan, S., Dexter, D. W., Benetatos, C. A., and Hudes, G. R. (1998b). Cloning and Sequencing of Human βIII-tubulin cDNA: Induction of βIII Isotype in Human Prostate Carcinoma Cells by Acute Exposure to Antimicrotubule Agents. Biochim. Biophys. Acta (Bba) - Gene Struct. Expr. 1395 (2), 237–245. doi:10.1016/s0167-4781(97)00168-1
Raspaglio, G., Filippetti, F., Prislei, S., Penci, R., De Maria, I., Cicchillitti, L., et al. (2008). Hypoxia Induces Class III Beta-Tubulin Gene Expression by HIF-1alpha Binding to its 3' Flanking Region. Gene 409 (1-2), 100–108. doi:10.1016/j.gene.2007.11.015
Raspaglio, G., De Maria, I., Filippetti, F., Martinelli, E., Zannoni, G. F., Prislei, S., et al. (2010). HuR Regulates β-Tubulin Isotype Expression in Ovarian Cancer. Cancer Res. 70 (14), 5891–5900. doi:10.1158/0008-5472.can-09-4656
Raspaglio, G., Petrillo, M., Martinelli, E., Li Puma, D. D., Mariani, M., De Donato, M., et al. (2014). Sox9 and Hif-2α Regulate TUBB3 Gene Expression and Affect Ovarian Cancer Aggressiveness. Gene 542 (2), 173–181. doi:10.1016/j.gene.2014.03.037
Ryu, H., Lee, J., Zaman, K., Kubilis, J., Ferrante, R. J., Ross, B. D., et al. (2003). Sp1 and Sp3 Are Oxidative Stress-Inducible, Antideath Transcription Factors in Cortical Neurons. J. Neurosci. 23 (9), 3597–3606. doi:10.1523/jneurosci.23-09-03597.2003
Saussede-Aim, J., Matera, E. L., Herveau, S., Rouault, J. P., Ferlini, C., and Dumontet, C. (2009b). Vinorelbine Induces Beta3-Tubulin Gene Expression through an AP-1 Site. Anticancer Res. 29 (8), 3003–3009.
Saussede-Aim, J., Matera, E.-L., Ferlini, C., and Dumontet, C. (2009a). β3-Tubulin Is Induced by Estradiol in Human Breast Carcinoma Cells through an Estrogen-Receptor Dependent Pathway. Cel Motil. Cytoskeleton 66 (7), 378–388. doi:10.1002/cm.20377
Scharer, C. D., McCabe, C. D., Ali-Seyed, M., Berger, M. F., Bulyk, M. L., and Moreno, C. S. (2009). Genome-wide Promoter Analysis of the SOX4 Transcriptional Network in Prostate Cancer Cells. Cancer Res. 69 (2), 709–717. doi:10.1158/0008-5472.can-08-3415
Sève, P., and Dumontet, C. (2008). Is Class III β-tubulin a Predictive Factor in Patients Receiving Tubulin-Binding Agents? Lancet Oncol. 9 (2), 168–175. doi:10.1016/s1470-2045(08)70029-9
Shalli, K., Brown, I., Heys, S. D., and Schofield, C. A. (2005). Alterations of β‐tubulin Isotypes in Breast Cancer Cells Resistant to Docetaxel. FASEB j. 19 (10), 1299–1301. doi:10.1096/fj.04-3178fje
Shen, Y., and Yu, L.-C. (2008). Potential protection of Curcumin against Hypoxia-Induced Decreases in Beta-III Tubulin Content in Rat Prefrontal Cortical Neurons. Neurochem. Res. 33 (10), 2112–2117. doi:10.1007/s11064-008-9720-y
Shibazaki, M., Maesawa, C., Akasaka, K., Kasai, S., Yasuhira, S., Kanno, K., et al. (2012). Transcriptional and post-transcriptional Regulation of βIII-tubulin Protein Expression in Relation with Cell Cycle-dependent Regulation of Tumor Cells. Int. J. Oncol. 40 (3), 695–702. doi:10.3892/ijo.2011.1291
Sleiman, S. F., Langley, B. C., Basso, M., Berlin, J., Xia, L., Payappilly, J. B., et al. (2011). Mithramycin Is a Gene-Selective Sp1 Inhibitor that Identifies a Biological Intersection between Cancer and Neurodegeneration. J. Neurosci. 31 (18), 6858–6870. doi:10.1523/jneurosci.0710-11.2011
Sobierajska, K., Wieczorek, K., Ciszewski, W. M., Sacewicz-Hofman, I., Wawro, M. E., Wiktorska, M., et al. (2016). β-III Tubulin Modulates the Behavior of Snail Overexpressed during the Epithelial-To-Mesenchymal Transition in colon Cancer Cells. Biochim. Biophys. Acta (Bba) - Mol. Cel Res. 1863 (9), 2221–2233. doi:10.1016/j.bbamcr.2016.05.008
Song, M. S., Salmena, L., and Pandolfi, P. P. (2012). The Functions and Regulation of the PTEN Tumour Suppressor. Nat. Rev. Mol. Cel Biol 13 (5), 283–296. doi:10.1038/nrm3330
Sulaiman, S. A., Ab Mutalib, N. S., and Jamal, R. (2016). miR-200c Regulation of Metastases in Ovarian Cancer: Potential Role in Epithelial and Mesenchymal Transition. Front. Pharmacol. 7 (271), 271. doi:10.3389/fphar.2016.00271
Sullivan, K. F., and Cleveland, D. W. (1986). Identification of Conserved Isotype-Defining Variable Region Sequences for Four Vertebrate Beta Tubulin Polypeptide Classes. Proc. Natl. Acad. Sci. U.S.A. 83 (12), 4327–4331. doi:10.1073/pnas.83.12.4327
Susanna, L., Luis, J. L.-G., Marta, M., Jorge, B., Lucía, I.-P., Iván, M., et al. (2011). The miR-200 Family Controls β-tubulin III Expression and Is Associated with Paclitaxel-Based Treatment Response and Progression-free Survival in Ovarian Cancer Patients. Endocrine-Related Cancer 18 (1), 85–95.
Taddei, M., Giannoni, E., Fiaschi, T., and Chiarugi, P. (2012). Anoikis: an Emerging Hallmark in Health and Diseases. J. Pathol. 226 (2), 380–393. doi:10.1002/path.3000
Tamura, D., Arao, T., Nagai, T., Kaneda, H., Aomatsu, K., Fujita, Y., et al. (2013). Slug Increases Sensitivity to Tubulin‐binding Agents via the Downregulation of βIII and βIVa‐tubulin in Lung Cancer Cells. Cancer Med. 2 (2), 144–154. doi:10.1002/cam4.68
Teo, J., McCarroll, J. A., Boyer, C., Youkhana, J., Sagnella, S. M., Duong, H. T. T., et al. (2016). A Rationally Optimized Nanoparticle System for the Delivery of RNA Interference Therapeutics into Pancreatic Tumors In Vivo. Biomacromolecules 17 (7), 2337–2351. doi:10.1021/acs.biomac.6b00185
Theodorakis, N. G., and Cleveland, D. W. (1992). Physical Evidence for Cotranslational Regulation of Beta-Tubulin mRNA Degradation. Mol. Cel. Biol. 12 (2), 791–799. doi:10.1128/mcb.12.2.791
Thiery, J. P., and Sleeman, J. P. (2006). Complex Networks Orchestrate Epithelial-Mesenchymal Transitions. Nat. Rev. Mol. Cel Biol 7 (2), 131–142. doi:10.1038/nrm1835
Tischfield, M. A., Baris, H. N., Wu, C., Rudolph, G., Van Maldergem, L., He, W., et al. (2010). Human TUBB3 Mutations Perturb Microtubule Dynamics, Kinesin Interactions, and Axon Guidance. Cell 140 (1), 74–87. doi:10.1016/j.cell.2009.12.011
Toma, J. G., El-Bizri, H., Barnabé-Heider, F., Aloyz, R., and Miller, F. D. (2000). Evidence that helix-loop-helix Proteins Collaborate with Retinoblastoma Tumor Suppressor Protein to Regulate Cortical Neurogenesis. J. Neurosci. 20 (20), 7648–7656. doi:10.1523/jneurosci.20-20-07648.2000
Toso, R. J., Jordan, M. A., Farrell, K. W., Matsumoto, B., and Wilson, L. (1993). Kinetic Stabilization of Microtubule Dynamic Instability In Vitro by Vinblastine. Biochemistry 32 (5), 1285–1293. doi:10.1021/bi00056a013
Uittenbogaard, M., and Chiaramello, A. (2002). Constitutive Overexpression of the Basic helix-loop-helix Nex1/MATH-2 Transcription Factor Promotes Neuronal Differentiation of PC12 Cells and Neurite Regeneration. J. Neurosci. Res. 67 (2), 235–245. doi:10.1002/jnr.10119
Uittenbogaard, M., and Chiaramello, A. (2004). Expression Profiling upon Nex1/MATH-2-Mediated Neuritogenesis in PC12 Cells and its Implication in Regeneration. J. Neurochem. 91 (6), 1332–1343. doi:10.1111/j.1471-4159.2004.02814.x
Uittenbogaard, M., Martinka, D. L., and Chiaramello, A. (2003). The Basic helix-loop-helix Differentiation Factor Nex1/MATH-2 Functions as a Key Activator of the GAP-43 Gene. J. Neurochem. 84 (4), 678–688. doi:10.1046/j.1471-4159.2003.01572.x
Uy, B. R., Simoes-Costa, M., Koo, D. E. S., Sauka-Spengler, T., and Bronner, M. E. (2015). Evolutionarily Conserved Role for SoxC Genes in Neural Crest Specification and Neuronal Differentiation. Developmental Biol. 397 (2), 282–292. doi:10.1016/j.ydbio.2014.09.022
Verdier-Pinard, P., Pasquier, E., Xiao, H., Burd, B., Villard, C., Lafitte, D., et al. (2009). Tubulin Proteomics: towards Breaking the Code. Anal. Biochem. 384 (2), 197–206. doi:10.1016/j.ab.2008.09.020
Wang, Y., Sparano, J. A., Fineberg, S., Stead, L., Sunkara, J., Horwitz, S. B., et al. (2013). High Expression of Class III β-Tubulin Predicts Good Response to Neoadjuvant Taxane and Doxorubicin/Cyclophosphamide-Based Chemotherapy in Estrogen Receptor-Negative Breast Cancer. Clin. Breast Cancer 13 (2), 103–108. doi:10.1016/j.clbc.2012.11.003
Wattanathamsan, O., and Pongrakhananon, V. (2021). Post-translational Modifications of Tubulin: Their Role in Cancers and the Regulation of Signaling Molecules. Cancer Gene Ther. doi:10.1038/s41417-021-00396-4
Wei, C., Ren, L., Li, K., and Lu, Z. (2018). The Regulation of Survival and Differentiation of Neural Stem Cells by miR-124 via Modulating PAX3. Neurosci. Lett. 683, 19–26. doi:10.1016/j.neulet.2018.05.051
Westbrook, T. F., Martin, E. S., Schlabach, M. R., Leng, Y., Liang, A. C., Feng, B., et al. (2005). A Genetic Screen for Candidate Tumor Suppressors Identifies REST. Cell 121 (6), 837–848. doi:10.1016/j.cell.2005.03.033
Wu, Y. Z., Lin, H. Y., Zhang, Y., and Chen, W. F. (2020). miR-200b-3p Mitigates Oxaliplatin Resistance via Targeting TUBB3 in Colorectal Cancer. J. Gene Med., e3178.
Zelleroth, S., Nylander, E., Örtenblad, A., Stam, F., Nyberg, F., Grönbladh, A., et al. (2021). Structurally Different Anabolic Androgenic Steroids Reduce Neurite Outgrowth and Neuronal Viability in Primary Rat Cortical Cell Cultures. J. Steroid Biochem. Mol. Biol. 210, 105863. doi:10.1016/j.jsbmb.2021.105863
Keywords: TUBB3, βIII-tubulin, microtubule, gene regulation, cancer, neuronal tubulin, tubulin, human
Citation: Duly AMP, Kao FCL, Teo WS and Kavallaris M (2022) βIII-Tubulin Gene Regulation in Health and Disease. Front. Cell Dev. Biol. 10:851542. doi: 10.3389/fcell.2022.851542
Received: 10 January 2022; Accepted: 07 April 2022;
Published: 28 April 2022.
Edited by:
Jack Adam Tuszynski, University of Alberta, CanadaReviewed by:
Pavel Draber, Institute of Molecular Genetics (ASCR), CzechiaCopyright © 2022 Duly, Kao, Teo and Kavallaris. This is an open-access article distributed under the terms of the Creative Commons Attribution License (CC BY). The use, distribution or reproduction in other forums is permitted, provided the original author(s) and the copyright owner(s) are credited and that the original publication in this journal is cited, in accordance with accepted academic practice. No use, distribution or reproduction is permitted which does not comply with these terms.
*Correspondence: Maria Kavallaris, bS5rYXZhbGxhcmlzQGNjaWEudW5zdy5lZHUuYXU=
†These authors have contributed equally to this work
Disclaimer: All claims expressed in this article are solely those of the authors and do not necessarily represent those of their affiliated organizations, or those of the publisher, the editors and the reviewers. Any product that may be evaluated in this article or claim that may be made by its manufacturer is not guaranteed or endorsed by the publisher.
Research integrity at Frontiers
Learn more about the work of our research integrity team to safeguard the quality of each article we publish.