- IEO, European Institute of Oncology IRCCS, Milan, Italy
In multicellular organisms, epithelial cells are key elements of tissue organization. In developing tissues, cellular proliferation and differentiation are under the tight regulation of morphogenetic programs, that ensure the correct organ formation and functioning. In these processes, mitotic rates and division orientation are crucial in regulating the velocity and the timing of the forming tissue. Division orientation, specified by mitotic spindle placement with respect to epithelial apico-basal polarity, controls not only the partitioning of cellular components but also the positioning of the daughter cells within the tissue, and hence the contacts that daughter cells retain with the surrounding microenvironment. Daughter cells positioning is important to determine signal sensing and fate, and therefore the final function of the developing organ. In this review, we will discuss recent discoveries regarding the mechanistics of planar divisions in mammalian epithelial cells, summarizing technologies and model systems used to study oriented cell divisions in vitro such as three-dimensional cysts of immortalized cells and intestinal organoids. We also highlight how misorientation is corrected in vivo and in vitro, and how it might contribute to the onset of pathological conditions.
Introduction
The mitotic spindle is a bipolar structure formed by microtubules (MTs) that in mitosis captures the duplicated chromosomes and segregates them equally between daughter cells. In unicellular and multicellular organisms the mitotic spindle can be regarded as a key player for the successful outcome of cell division (Pietro et al., 2016). In stem cells and progenitors, the mitotic spindle orientation contributes to define the fate choice of daughter cells and their positioning within the tissue, resulting in either symmetric or asymmetric division (Morin and Bellaïche, 2011). Oriented divisions have been extensively studied in invertebrate systems (Gönczy, 2008; Knoblich, 2008; Knoblich, 2010; Morin and Bellaïche, 2011; Pietro et al., 2016), however mechanistic insights into orientation mechanisms in vertebrates are still limited. Spindle positioning is known to impact on cell proliferation, cell fate and tissue development although a comprehensive understanding of the molecular details underlying these processes is just building (Pietro et al., 2016; Lechler and Mapelli, 2021). Timing and execution of spindle placement rely on intrinsic and extrinsic signals sensed by the dividing cell (Pietro et al., 2016).
In the epithelial tissues, contacts between the dividing cell and the adjacent ones are important factors determining the division orientation (Osswald and Morais-de-Sá, 2019). In polarized epithelial monolayers, cells divide by planar divisions with the mitotic spindle parallel to the epithelium, and the two daughter cells remain within the same monolayer, leading to tissue growth and expansion (Nakajima, 2018). Studies on division orientation in 3D culture, including organoids derived from various tissues, are just starting to reveal interesting differences between orientation mechanisms and misorientation correction compared to what observed in 2D and in invertebrate systems. In this review, we summarize what is known about mitotic spindle dynamics and oriented cell divisions in vertebrate 3D cysts and organoids. In the first section, we will present an overview of spindle orientation effectors. Then, we will describe mechanisms of oriented cell divisions in cysts grown from mammalian cell lines, while in the end of the review we will focus on more complex 3D cellular structures such as organoids. Finally, the potential role of mitotic spindle proteins in disease associated with defective epithelial morphogenesis and homeostasis will be discussed, with a few examples from Drosophila studies.
Mitotic Spindle Machinery: The Importance of the Gαi/LGN/NuMA Complex
Division orientation depends on mitotic spindle positioning, that is generally attained in metaphase and sometime corrected in telophase (Morin and Bellaïche, 2011; Lough et al., 2019). In many epithelial systems, division orientation follows the Hertwig’s rule, according to which the spindle aligns along the long axis of the dividing cell (Hertwig, 1884). To which extent spindle alignment to the long cell axis is guided by mechano-sensing pathways responding to compressional cues exerted by neighbouring cells, or it is contributed by cytoskeletal forces exerted by MT motors is still debated. Elegant studies in MDCK (Madin–Darby Canine Kidney) Extra-Cellular-Matrix-free (ECM-free) monolayers “in suspension” showed that the division orientation occurs along the longest cell axis and is instructed by the interphase geometry (Wyatt et al., 2015). In these cells, components of the force generators complexes including NuMA and Gαi accumulates at cortical polar sites. Consistently, studies in Xenopus epithelia indicate that cells divide according to interphase cellular shape that is defined by three-cell junction distribution, where LGN and E-cadherin accumulates (Nestor-Bergmann et al., 2019). Collectively, this evidence suggests that in mammalian epithelial cells interphase shape drives force generators distributions to orchestrate divisions along the longest cell axis. Notably, these findings in vertebrate cells are consistent with previous observations in Drosophila tissues (Bosveld et al., 2016), although do not seem to apply to the development of Drosophila follicular epithelium at early-stage egg chambers (Finegan et al., 2019).
Several studies elucidated the molecular mechanisms of orientation, in which a fundamental role is played by Gαi/LGN/NuMA proteins, an evolutionarily conserved ternary complex. Gαi is the subunit of heterotrimeric G-proteins that localizes at the plasma membrane, LGN acts as a molecular scaffold, and NuMA is the mitotic dynein-adaptor involved in MT-pulling force onset. The majority of studies addressing the mechanistics of orientation were conducted in adherent cells in isolation, such as HeLa cells (Du et al., 2001; Du and Macara, 2004; Zheng et al., 2010; Kiyomitsu and Cheeseman, 2012; Kotak et al., 2012; Gallini et al., 2016; Pirovano et al., 2019; Takayanagi et al., 2019), or in a monolayers of MDCK cells, in which the spindle axis aligns parallel to the substratum in an integrin-dependent manner (Reinsch and Karsenti, 1994; Tuncay et al., 2015; Chishiki et al., 2017; Lázaro-Diéguez and Müsch, 2017).
In metaphase, the Gαi/LGN/NuMA complex localizes at the plasma membrane above the spindle poles (Du et al., 2001; Du and Macara, 2004; Kotak et al., 2012; Gallini et al., 2016; Pirovano et al., 2019; Zheng et al., 2010; Machicoane et al., 2014) and recruits the MT motor dynein/dynactin (Kotak et al., 2012; Okumura et al., 2018; Woodard et al., 2010) (Figure 1A). Exploiting the minus-end directed movement of dynein, cortically localized dynein motors generate pulling forces on astral MTs branching from the spindle poles that in metaphase contribute to spindle placement (Théry et al., 2007; Kiyomitsu and Cheeseman, 2012; Kotak et al., 2012). Notably, ectopic recruitment of NuMA to the cell cortex by optogenetic techniques is necessary and sufficient to orient the spindle, while cortical targeting of dynein is not sufficient to generate enough pulling forces to place the spindle (Fielmich et al., 2018; Okumura et al., 2018), implying that the activity of MT motors requires a defined spatial cortical organization. In line with these findings, recent studies revealed that not only the levels of NuMA/dynein/dynactin motors present at the cortex, but also their spatial distribution plays a role in the onset of effective MT-pulling forces (Pirovano et al., 2019; Renna et al., 2020).
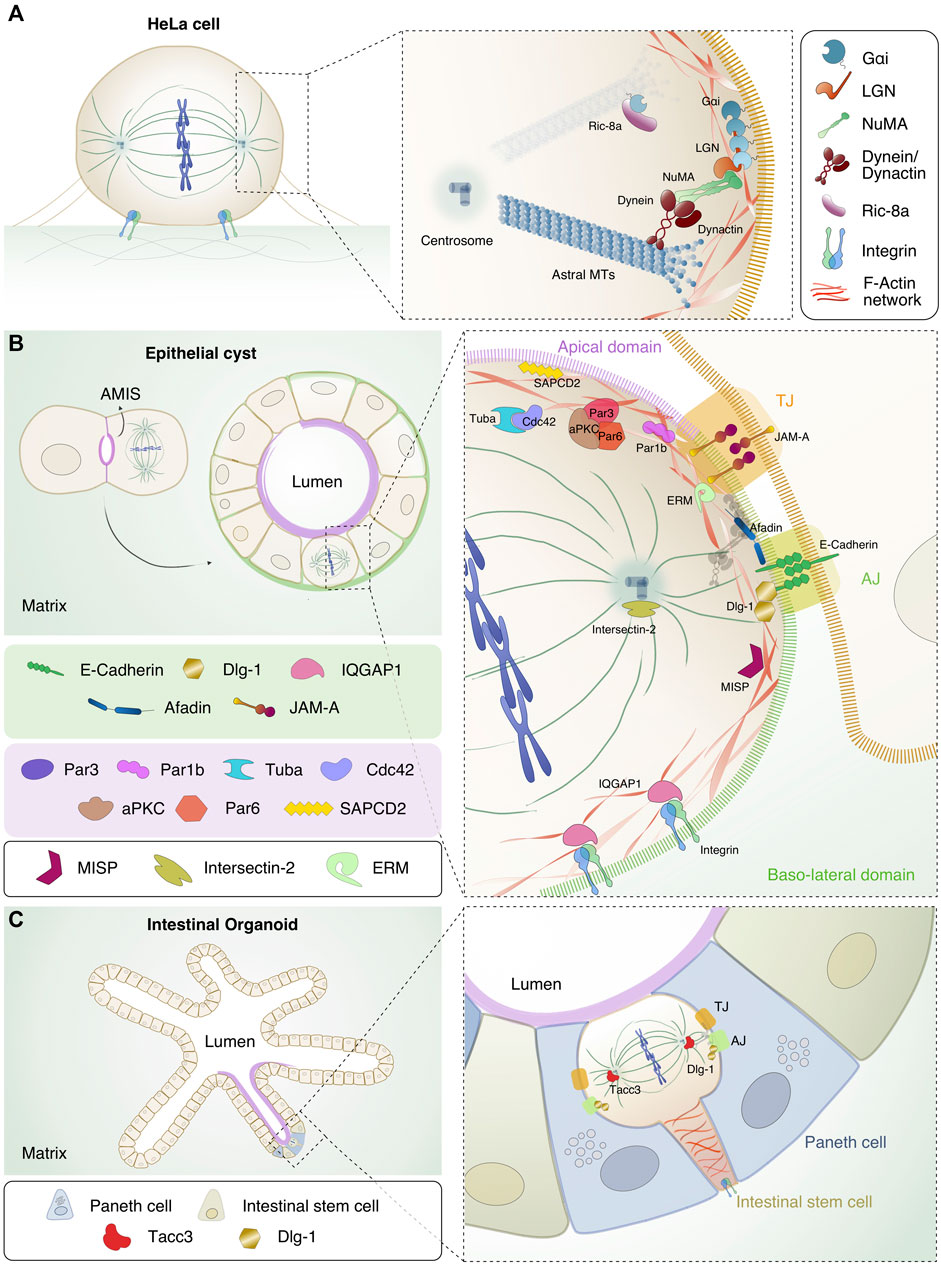
FIGURE 1. Localization and interaction of the spindle orientation and polarity proteins in different model systems. (A) HeLa cell in metaphase. Chromosomes (in blue) are aligned at the metaphase plate in the centre of the cell, MTs (in dark green) form the mitotic spindle and integrins important for adhesion of the mitotic cell to the substratum, are shown in light blue and green. In the inset, the details of the interaction interfaces between orientation proteins Gαi/LGN/NuMA, dynein/dynactin (in bordeaux) and astral MTs are shown. The Gαi/NuMA/LGN complex is recruited at the lateral sides above spindle poles. NuMA is in green, LGN in orange and Gαi in petrol blue. Ric8-A (in purple) is shown in the cytoplasm, close to the plasma membrane-bound Gαi. (B) Evolution from two-cell stage, in which the mitotic spindle orients parallel to the AMIS, to mature cyst with a single lumen. The diving cell in the mature cyst has the mitotic spindle parallel to the apical side. In the scheme, the apical domain is highlighted in purple while the basolateral side in green. In the close-up, the mitotic spindle proteins displayed in A are shown in relation with the polarity or junctional protein discussed in the text. At the level of cell-cell junctions, the tight junction (TJ, orange box) and the adherens junction (AJ, bright green box) are shown with key components highlighted. At the TJ, JAM-A (in rainbow orange), the polarity complex with Par3 (dark purple), Par6 (light red), aPKC (brown), Cdc42 (lilac) and Tuba (cyan) are depicted. Par1b (fuchsia) and SAPCD2 (yellow) are pictured at the apical side. At the AJ levels, E-cadherin (in green), Afadin (in blue) and Dlg-1 (in gold) are shown. At the basal side of the cell, IQGAP1 (in pink) and integrins are depicted. Intersectin-2 (in olive green) is present at the centrosomes. F-actin is shown in red, and the interacting proteins MISP (in purple wine) and ERM (in aqua green) connecting the mitotic cortex to the plasma membrane are indicated. (C) Left: intestinal organoids showing the crypt-villi structure that recapitulates the intestine architecture. The apical side of the organoids is shown in purple, the intestinal stem cells (in ocre) and the Paneth cells (in blue) are highlighted. In the inset on the right, mitotic ISC located apically in the monolayer is shown with the actin cable connecting the dividing cells to the basal membrane. Dlg-1 and Tacc3 (in tomato) are shown.
In mitosis Gαi proteins are uniformly enriched at the cell cortex, but only a GDP-loaded pool of Gαi (GαiGDP) accumulates above the spindle poles and is the one that selectively binds to LGN (Du et al., 2001; Willard et al., 2004; Mochizuki et al., 1996). The recruitment of LGN at the cortex by Gαi is controlled by GAPs (GTPase activating proteins) and GEFs (Guanine Exchange Factors) that tune the GTP-state of Gαi. An important Gαi GEF implicated in spindle placement is Ric-8A, which appears to play a key role in targeting LGN to the cortex (Chishiki et al., 2017; Woodard et al., 2010). In metaphase, LGN is spatially restricted to the cortical side facing the spindle poles by direct binding to Gαi (Zheng et al., 2010) (Figure 1A). Lateral recruitment of LGN and in turn NuMA/dynein motors promotes planar spindle orientation (Zheng et al., 2010). Notably, the conformation of LGN depends on its binding partners (Du and Macara, 2004; Pan et al., 2013): in the unliganded form LGN is kept in an inhibited conformation by intra-molecular interactions between the N-terminal TPR domain and the C-terminal GoLoco region. Cooperative binding of the four GoLoco motifs to cortical GαiGDP molecules recruits LGN to the cortex and induces a conformational change releasing the TPR domain that in turn associates with NuMA. These events result in the enrichment for NuMA/dynein/dynactin motors to specific cortical sites and onset of astral MT-pulling forces (Du and Macara, 2004; Pan et al., 2013) (Figure 1A). Notably, the TPR domain of LGN interacts not only with NuMA but also with Afadin and E-cadherin in a mutually exclusive manner, with functional implications that will be discussed below. NuMA shares the domain structures with other dynein-activator proteins (Kiyomitsu and Boerner, 2021), including a hook domain and a CC1-like box motifs, both at the N-terminus, responsible for the binding to dynein and dynactin. After a central 1500-residue long coiled-coil, NuMA codes for a C-terminal region binding to LGN, microtubules, as well as to the plasma membrane in anaphase (Kotak et al., 2013; Seldin et al., 2013; Carminati et al., 2016; Seldin et al., 2016; Pirovano et al., 2019; Renna et al., 2020). All these diverse functionalities of NuMA C-terminus contribute to spindle placement during mitotic progression, partly modulated by mitotic kinases’ phosphorylation (Lechler and Mapelli, 2021).
Polarity and Epithelial Junctions in Spindle Orientation in Polarized Monolayers and Cysts
In 2D systems such as polarized MDCK cells grown in monolayer, cells divide with the spindle axis aligned to the substratum by planar symmetric divisions that generate two daughter cells remaining in the same monolayer (Reinsch and Karsenti, 1994; Tuncay et al., 2015; Lázaro-Diéguez and Müsch, 2017). In this setting, spindle alignment is maintained by astral MTs captured by cortical cues localized at the lateral domains of the dividing cell, including cell-cell adhesion molecules (Gloerich et al., 2017; Lázaro-Diéguez and Müsch, 2017). Additional information has been obtained in more physiologically relevant 3D models, such as cysts.
The most common cells used to study oriented divisions in cysts are MDCK and Caco-2 (human colon adenocarcinoma) cells that, when plated on a substrate that mimics the ECM such as matrigel, grow as monolayered spheres by planar divisions occurring with the spindle axis perpendicular to the apico-basal polarity (Zegers et al., 2003; Jaffe et al., 2008). A cyst is characterized by a central lumen and a surrounding monolayer of polarized cells (Zegers et al., 2003) (Figure 1B). Notably, lumen formation in MDCK- or Caco-2-derived cysts relies on spindle orientation, as opposed to cysts obtained from MCF10A cells (human breast immortalized cells) where lumen forms by anoikis, i. e apoptosis of inner cells after a full sphere is formed (Debnath et al., 2002). After the first division, MDCK single cells have been shown to form an apical membrane initiation site (AMIS) between the two daughter cells, in the position where the midbody was located (Overeem et al., 2015), that will later become the lumen of the nascent cyst (Rodriguez-Boulan and Macara, 2014) (Figure 1B left). Cells composing the mature cyst have two types of domains: the apical side facing the central lumen where the PAR (partitioning defective) family proteins localize, and the baso-lateral domain where adhesion proteins such as integrins are in contact with the ECM, and where adjacent cells are in contact with each other by adherens (AJ) and tight junctions (TJ) (McCaffrey and Macara, 2011) (Figure 1B right). Importantly, each of these membrane domains is key for the localization of spindle orientation proteins instructing planar divisions including Gαi, NuMA and LGN (see below) (Overeem et al., 2015; Nakajima, 2018). In Table 1 we summarized the proteins involved in spindle orientation with their function, localization and defects occurring upon depletion.
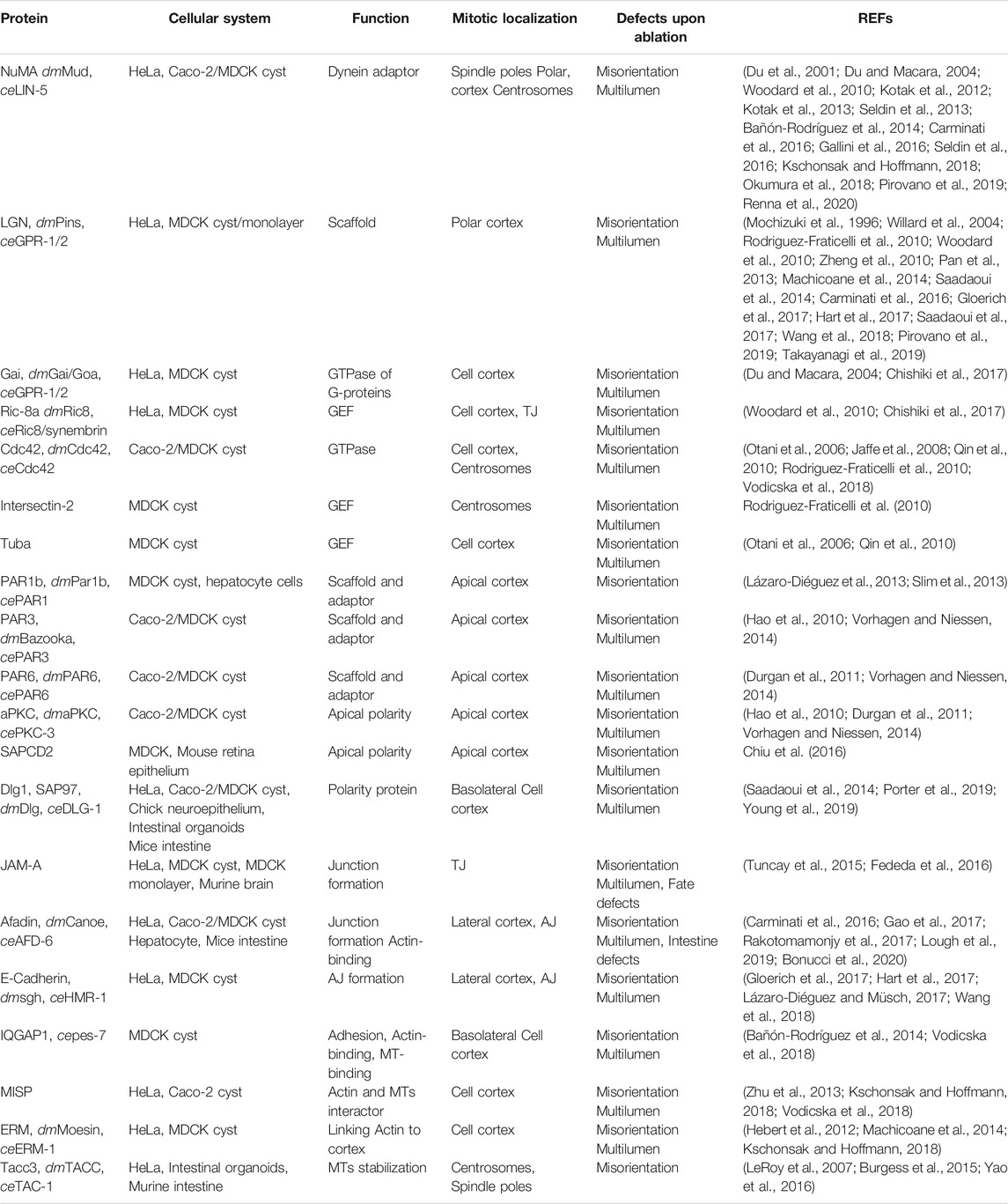
TABLE 1. Proteins involved in division orientation, and model systems in which they were studied (fly and worm orthologues are reported, when present).
One of the first proteins to be implicated in planar divisions in cysts was the GTPase Cdc42, whose depletion in Caco-2 cells results in multi-lumen cysts due to spindle misorientation (Jaffe et al., 2008). In MDCK cells, Cdc42 has been shown to be activated by the two GEFs Tuba, regulating cell-cell junctions and Cdc42 apical localization (Qin et al., 2010; Otani et al., 2006), and Intersectin-2, implicated in endocytosis and in the mitotic Cdc42 targeting at centrosomes (Rodriguez-Fraticelli et al., 2010; Okamoto et al., 1999; Hussain et al., 2001). Planar spindle orientation is also mediated by the apically-localized polarity complex composed by Par3, Par6 and the kinase aPKC (Figure 1B). Several studies in 3D systems have shown that depletion of Par3 leads to mislocalization of the kinase aPKC (Hao et al., 2010; Zheng et al., 2010; Durgan et al., 2011; Vorhagen and Niessen, 2014), which phosphorylates LGN on Ser401 to exclude it from the apical side ensuring its localization at the lateral cortex, possibly by direct association with the baso-lateral protein Dlg-1 (Saadaoui et al., 2014). An intriguing role has been described for the Par1b/MARK2 kinase that in MDCK cells monolayer with high Rho activity promotes LGN/NuMA recruitment at the lateral site and planar divisions with the spindle axis aligned to the substratum. Conversely, in hepatocytes, that in addition to apico-basal polarity also organize a lateral lumen for the development of bile canalicular networks and have reduced Rho activity, Par1b prevents NuMA/LGN lateral recruitment causing tilted spindles and asymmetric partitioning of the lateral lumen among daughter cells (Lázaro-Diéguez et al., 2013; Slim et al., 2013).
In addition to their cohesive role, also some junctional proteins have been shown to be involved in spindle orientation in cysts, including the Junctional adhesion molecule-A (JAM-A), Afadin (AF6), E-Cadherin and Dlg-1 (Discs large homolog 1) (Figure 1B). In MDCK cysts, JAM-A activates Cdc42 and PI(3)K (Phosphatidylinositol 3-kinases), generating a gradient of PtdIns(3,4,5)P3 enriched at the cortex area facing the spindle poles, which is required for correct localization of dynein/dynactin and for spindle orientation (Toyoshima et al., 2007; Tuncay et al., 2015). Consistently, JAM-A was shown to activate Cdc42 also in progenitors of the developing cerebral cortex this way contributing to spindle orientation (Fededa et al., 2016).
The actin-binding protein Afadin, localized at adherent junctions, mediates planar spindle orientation in Caco-2 cyst by recruiting LGN to the lateral cortex via direct interactions with the LGN-TPR domain (Carminati et al., 2016; Gao et al., 2017; Bonucci et al., 2020) (Figure 1B). Consistently, studies conducted in MDCK cysts (Gao et al., 2017), hepatocyte cells (Bonucci et al., 2020), and murine neuro glia (Rakotomamonjy et al., 2017) show that Afadin is crucial for spindle orientation as its depletion leads to an aberrant spindle placement (Lough et al., 2019; Carminati et al., 2016; Gao et al., 2017; Bonucci et al., 2020; Rakotomamonjy et al., 2017). In MDCK cysts, planar cell divisions also rely on the interaction between the intra-cellular domain of E-cadherin and LGN-TPR domain (Gloerich et al., 2017; Hart et al., 2017) (Figure 1B). As with Afadin, NuMA competes also with E-cadherin for LGN binding (Zhu et al., 2011; Carminati et al., 2016; Gloerich et al., 2017; Hart et al., 2017). This suggests that Afadin and E-cadherin might be needed for the initial LGN targeting at the cortex, when NuMA is still in the nucleus, and that these interactions dissociate later in mitosis. An alternative explanation envisions that the cortical GαiGDP-bound pool of LGN cycles between different mitotic binding partners associating with its TPR domain, including NuMA, Afadin and E-cadherin, in order to coordinate mechano-sensing junctional cues with spindle orientation and mitotic progression. Future live-imaging studies will clarify whether this is the case.
In addition to this role, E-cadherin was shown to be important for maintenance of cell polarity and spindle orientation in prostate epithelia by interacting with LGN, NuMA and Scrib at the lateral sites of mitotic cells, this way preserving correct apico-basal polarity, planar cell divisions and tissue integrity. Consistently, conditional loss of E-cadherin during murine prostate development leads to disorganized epithelia observed in early state prostate tumorigensis (Wang et al., 2018). Spindle orientation functions have been reported also for the baso-lateral polarity protein Dlg-1, that belongs to the membrane-associated guanylate kinase (MAGUK) family and is required for adherens junction formation and maintenance (Su et al., 2012) (Figure 1B). In HeLa cells, in MDCK cysts and in the chick neuroepithelium, Dlg-1 promotes spindle orientation by binding to the phosphorylated LGN protein (Saadaoui et al., 2014; Saadaoui et al., 2017; Porter et al., 2019), fully in line with was previously shown in Drosophila epithelial systems (Morin and Bellaïche, 2011; Pietro et al., 2016). In turn, the correct localization of Dlg-1 is influenced by other factors including Gαi (Saadaoui et al., 2014) and the tumor suppressor protein CASK (calcium/calmodulin-dependent serine protein kinase) (Porter et al., 2019). The binding of Dlg-1 to CASK and Gαi is key to direct LGN to restricted cortical regions before metaphase, and ultimately to target LGN and NuMA-dynein appropriately (Saadaoui et al., 2014; Saadaoui et al., 2017; Porter et al., 2019).
Another polarity protein affecting LGN cortical recruitment is the suppressor APC domain containing 2 (SAPCD2), that has been shown to interact with Gαi/LGN complexes to orchestrate mitotic spindle orientation in MDCK cyst and in mouse retina (Chiu et al., 2016). Specifically, SAPCD2 binding to the close conformation of LGN restricts LGN/NuMA accumulation at the lateral site providing a mechanism to balance the proportion of planar and vertical divisions, and hence the symmetric or asymmetric outcome of retinal progenitor mitosis (Chiu et al., 2016).
We already reported the relevance of the Gαi GEF Ric-8A for spindle orientation in HeLa cells (Woodard et al., 2010). Recent work highlighted a role for Ric-8A in tight junction formation in MDCK cysts and in LGN recruitment to the lateral cortex by generation of a localized Gαi-GDP pool promoting planar cell divisions (Chishiki et al., 2017) (Figure 1B).
Beside junctional and polarity proteins, the actin cytoskeleton, as well as actin and microtubule-binding proteins, contribute actively to spindle orientation (Pietro et al., 2016), as described in invertebrate systems such as Drosophila neuroblasts (Kunda and Baum, 2009) and HeLa cells (Pietro et al., 2016; Rizzelli et al., 2020). However, the role of actin in planar division and cystogenesis is less clear. In MDCK cysts, the microtubule-associated protein IQGAP1, localized at the basal site, participates to MTs dynamics and promotes planar spindle orientation by interacting with the MT plus-ends and by targeting NuMA laterally (Bañón-Rodríguez et al., 2014) (Figure 1B). Notably, in HeLa cells the interaction between IQGAP1 and Cdc42 has been shown to allow the binding of Cdc42 to the actin-binding protein MISP (Mitotic Interactor and Substrate of PLK1) implicated in spindle positioning (Zhu et al., 2013; Cadart et al., 2014; Vodicska et al., 2018). MISP associates to members of the ERM (Ezrin, Radixin and Moesin) protein family, that connects the mitotic acto-myosin cortex to the plasma membrane, in this way assisting the correct localization of NuMA at the cortex for correct spindle positioning (Hebert et al., 2012; Zhu et al., 2013; Machicoane et al., 2014; Kschonsak and Hoffmann, 2018) (Figure 1B).
Mitotic Spindle Orientation in Intestinal Organoids
Studies of oriented divisions in cysts provided great insights into the crosstalk between orientation pathways and epithelial polarity. However, cysts of immortalized cell lines do not entirely recapitulate the cell diversity and the signaling response of epithelial tissues in vivo (Lancaster and Knoblich, 2014; Clevers and Tuveson, 2019).
Tissue organoids, especially murine intestinal organoids, are becoming a relevant model to study division orientation in a more physiological setting. Organoids are model systems that recapitulate not only the morphology of the organ but also the cellular composition, from stem cells to differentiated lineages (Clevers, 2013; Sato and Clevers, 2013). Methods to grow, manipulate genetically and image intestinal organoids have been first established in the Clevers lab (Sato and Clevers, 2013; Sato et al., 2009), whose work revealed that the organoids grown from intestinal epithelial cells form crypt and villi-like domains mirroring the morphology of the intestinal epithelium, with an analogous composition and distribution of cell types (Sato and Clevers, 2013; Sato et al., 2009) (Figure 1C). These studies revealed that in intestinal organoids the proliferating cells reside at the bottom of the crypt, close to the stem cell niche compartment constituted by non-dividing Paneth cells, that generate a Wnt3 gradient decreasing along the crypt axis (Figure 1C). Intestinal stem cells (ISCs) divide symmetrically moving toward the apical side of the monolayer that faces the organoid lumen, with the metaphase plate perpendicular to the apical side (Figure 1C). These ISC apical mitosis retain a connection to the basal site, and hence to the ECM, through an actin cable (Carroll et al., 2017; McKinley et al., 2018) (Figure 1C) that is essential for daughter cells to move back to the basal side of the monolayer upon cytokinesis (Carroll et al., 2017). As a matter of fact, the use of intestinal organoids to study oriented division is still in its infancy, contributed mainly by descriptive imaging experiments and a few mechanistical studies investigating the molecular mechanisms of mitosis. Little is known on molecules executing oriented divisions in organoid, but it is plausible that the same set of polarity and junctional proteins important for correct cystogenesis is implicated in division orientation also in these systems, with molecular details that remain to be explored.
Ablation of Dlg-1 from the murine intestinal crypts has been shown to result in misoriented divisions of the intestinal stem cells with a consequent delay in cell migration from the crypts bottom to the villi that promotes tumorigenic events (Young et al., 2019). Similarly, depletion from the murine crypts of the protein Tacc3, which is involved in MT crosslinking and stabilization of the Aurora-A dependent kinetochore-microtubules attachment (LeRoy et al., 2007; Burgess et al., 2015), blocks proliferation (Yao et al., 2016). Interestingly, knock-out of Tacc3 from intestinal organoids derived from APC (Adenomatous polyposis coli) mutated mice, models for colorectal cancer (Merenda et al., 2020), increases chromosome misalignment and hypomorphic mitotic spindles, leading to prolonged mitosis or mitotic arrest (Yao et al., 2016), to a certain extent mimicking what observed in vivo. Both findings open the possibility to target specific mitotic spindle proteins for chemotherapeutic therapy. In conclusion, although organoids hold the potential to allow more insightful analyses on the orientation pathways and their relevance for morphogenesis and disease, more studies are required to elucidate the molecular mechanisms accounting for oriented divisions in these systems.
Spindle Misorientation: What Can Go Wrong and What Can be Done to Fix it
As discussed, oriented divisions are important for the regulation of epithelial morphogenesis and homeostasis. Consistently, their deregulation has been associated to several pathological conditions such as cancer, microcephaly, and developmental defects (Gillies and Cabernard, 2011; Nakajima, 2018; Lechler and Mapelli, 2021). However, not always the causal relationship between misorientation and diseases is clear. In vivo studies revealed that spindle misorientation is oftentimes corrected or is embryonic lethal (Nakajima, 2018; Lechler and Mapelli, 2021).
In murine hepatic epithelial cells in vivo, spindle misorientation leads to detachment of epithelial sheets from nephron epithelial tubules (Gao et al., 2017). Similarly, in stem cell systems, misorientation alters the balance between symmetric and asymmetric divisions resulting in defective changes in architecture and functioning. This has been documented for neuroepithelial progenitors during murine cortical development, in which misorientation leads to the expansion of the radial glial compartment with a delay in neurogenesis (Fededa et al., 2016).
Tissues have developed different mechanisms to rescue the damage that a misoriented spindle can cause, that have been first discovered in Drosophila and still await to be confirmed in mammalian tissues. The first mechanism impinges on the ability of epithelial tissue to reintegrate cells that after misoriented cytokinesis are misplaced above the epithelial layer (Bergstralh et al., 2015; Lough et al., 2019). As described for intestinal organoids (Carroll et al., 2017), in Drosophila imaginal disc the dividing cells have an actin protrusion that keeps them in connection to the basal side of the monolayer and assists the appropriate repositioning of daughters after cytokinesis (Nakajima et al., 2013). Parallel studies showed that also adhesive molecules, such as Fasciculin-2/3 and neuroglian, play a role in reintegrating in the epithelial layer the cells misplaced above the follicular epithelium due to orientation defects (Bergstralh et al., 2015; Cammarota et al., 2020). In Drosophila imaginal discs, evidence was provided that upon misorientation, one of the two daughter cells loses connection with the basal side and is displaced in the lumen (Nakajima et al., 2013). In the absence of re-integration, the misplaced cells can encounter two different fates: it either remains in the wrong position, where proliferation causes morphological defects (Dekanty et al., 2012; Nakajima et al., 2013; Poulton et al., 2014), or it undergoes apoptosis due to lack of survival signals (Nakajima et al., 2013; Poulton et al., 2014). Whether any of these mechanisms for misorientation correction is in place in vertebrate epithelial tissues remains an interesting open question.
Conclusion
Much is known about division orientation and how the spindle orientation components are recruited to the cortex in single cells in isolation and cysts. However, a clear picture of orientation mechanisms in more complex systems, such as organoids and tissues, is still missing. The complexity of cell-cell contacts and the presence of different cell populations in epithelial tissues contribute to determine the division orientation in ways that we do not fully grasp. We also still need to further understand the mechanisms that mammalian tissues have evolved to respond to misorientation in order to preserve tissue architecture. Some of the open questions that the field should address in the future are how the correction mechanisms work in mammalian systems and how we can leverage this knowledge to better understand physio-pathological processes associated with misoriented spindles in the presence or absence of other genetic lesions. We anticipate that the use organoids as model systems might be instrumental in these studies.
Author Contributions
FD and MM wrote the manuscript. SE illustrated the concepts covered by the review.
Funding
FD is founded from AIRC and from the European Union’s Horizon 2020 research and innovation program under the Marie Skłodowska-Curie grant agreement No. 800924. SE. is a PhD student within the European School of Molecular Medicine (SEMM). This work was supported by a grant to MM. from the Italian Association for Cancer Research (AIRC) (IG 2020 ID 25098) and partially supported by the Italian Ministry of Health with Ricerca Corrente and 5x1000 funds.
Conflict of Interest
The authors declare that the research was conducted in the absence of any commercial or financial relationships that could be construed as a potential conflict of interest.
Publisher’s Note
All claims expressed in this article are solely those of the authors and do not necessarily represent those of their affiliated organizations, or those of the publisher, the editors and the reviewers. Any product that may be evaluated in this article, or claim that may be made by its manufacturer, is not guaranteed or endorsed by the publisher.
References
Bañón-Rodríguez, I., Gálvez-Santisteban, M., Vergarajauregui, S., Bosch, M., Borreguero-Pascual, A., and Martín-Belmonte, F. (2014). EGFR Controls IQGAP Basolateral Membrane Localization and Mitotic Spindle Orientation during Epithelial Morphogenesis. Embo J. 33 (2), 129–145. doi:10.1002/embj.201385946
Bergstralh, D. T., Lovegrove, H. E., and St Johnston, D. (2015). Lateral Adhesion Drives Reintegration of Misplaced Cells into Epithelial Monolayers. Nat. Cel Biol 17 (11), 1497–1503. doi:10.1038/ncb3248
Bonucci, M., Kuperwasser, N., Barbe, S., Koka, V., de Villeneuve, D., Zhang, C., et al. (2020). mTOR and S6K1 Drive Polycystic Kidney by the Control of Afadin-dependent Oriented Cell Division. Nat. Commun. 11 (1), 3200. doi:10.1038/s41467-020-16978-z
Bosveld, F., Markova, O., Guirao, B., Martin, C., Wang, Z., Pierre, A., et al. (2016). Epithelial Tricellular Junctions Act as Interphase Cell Shape Sensors to orient Mitosis. Nature 530 (7591), 495–498. doi:10.1038/nature16970
Burgess, S. G., Peset, I., Joseph, N., Cavazza, T., Vernos, I., Pfuhl, M., et al. (2015). Aurora-A-Dependent Control of TACC3 Influences the Rate of Mitotic Spindle Assembly. Plos Genet. 11, e1005345. doi:10.1371/journal.pgen.1005345
Cadart, C., Zlotek-Zlotkiewicz, E., Le Berre, M., Piel, M., and Matthews, H. K. (2014). Exploring the Function of Cell Shape and Size during Mitosis. Dev. Cel 29 (2), 159–169. doi:10.1016/j.devcel.2014.04.009
Cammarota, C., Finegan, T. M., Wilson, T. J., Yang, S., and Bergstralh, D. T. (2020). An Axon-Pathfinding Mechanism Preserves Epithelial Tissue Integrity. Curr. Biol. 30 (24), 5049–5057. doi:10.1016/j.cub.2020.09.061
Carminati, M., Gallini, S., Pirovano, L., Alfieri, A., Bisi, S., and Mapelli, M. (2016). Concomitant Binding of Afadin to LGN and F-Actin Directs Planar Spindle Orientation. Nat. Struct. Mol. Biol. 23 (2), 155–163. doi:10.1038/nsmb.3152
Carroll, T. D., Langlands, A. J., Osborne, J. M., Newton, I. P., Appleton, P. L., and Näthke, I. (2017). Interkinetic Nuclear Migration and Basal Tethering Facilitates post-mitotic Daughter Separation in Intestinal Organoids. J. Cel Sci 130 (22), 3862–3877. doi:10.1242/jcs.211656
Chishiki, K., Kamakura, S., Hayase, J., and Sumimoto, H. (2017). Ric-8A, an Activator Protein of Gαi, Controls Mammalian Epithelial Cell Polarity for Tight junction Assembly and Cystogenesis. Genes Cells 22 (3), 293–309. doi:10.1111/gtc.12477
Chiu, C. W. N., Monat, C., Robitaille, M., Lacomme, M., Daulat, A. M., Macleod, G., et al. (2016). SAPCD2 Controls Spindle Orientation and Asymmetric Divisions by Negatively Regulating the Gαi-LGN-NuMA Ternary Complex. Dev. Cel 36 (1), 50–62. doi:10.1016/j.devcel.2015.12.016
Clevers, H. (2013). The Intestinal Crypt, A Prototype Stem Cell Compartment. Cell 154 (2), 274–284. doi:10.1016/j.cell.2013.07.004
Clevers, H., and Tuveson, D. A. (2019). Organoid Models for Cancer Research. Annu. Rev. Cancer Biol. 3 (1), 223–234. doi:10.1146/annurev-cancerbio-030518-055702
Debnath, J., Mills, K. R., Collins, N. L., Reginato, M. J., Muthuswamy, S. K., and Brugge, J. S. (2002). The Role of Apoptosis in Creating and Maintaining Luminal Space within normal and Oncogene-Expressing Mammary Acini. Cell 111 (1), 29–40. doi:10.1016/s0092-8674(02)01001-2
Dekanty, A., Barrio, L., Muzzopappa, M., Auer, H., and Milan, M. (2012). Aneuploidy-induced Delaminating Cells Drive Tumorigenesis in Drosophila Epithelia. Proc. Natl. Acad. Sci. 109 (50), 20549–20554. doi:10.1073/pnas.1206675109
Du, Q., and Macara, I. G. (2004). Mammalian Pins Is a Conformational Switch that Links NuMA to Heterotrimeric G Proteins. Cell 119 (4), 503–516. doi:10.1016/j.cell.2004.10.028
Du, Q., Stukenberg, P. T., and Macara, I. G. (2001). A Mammalian Partner of Inscuteable Binds NuMA and Regulates Mitotic Spindle Organization. Nat. Cel Biol 3 (12), 1069–1075. doi:10.1038/ncb1201-1069
Durgan, J., Kaji, N., Jin, D., and Hall, A. (2011). Par6B and Atypical PKC Regulate Mitotic Spindle Orientation during Epithelial Morphogenesis. J. Biol. Chem. 286 (14), 12461–12474. doi:10.1074/jbc.m110.174235
Fededa, J. P., Esk, C., Mierzwa, B., Stanyte, R., Yuan, S., Zheng, H., et al. (2016). Micro RNA ‐34/449 Controls Mitotic Spindle Orientation during Mammalian Cortex Development. Embo J. 35 (22), 2386–2398. doi:10.15252/embj.201694056
Fielmich, L. E., Schmidt, R., Dickinson, D. J., Goldstein, B., Akhmanova, A., and van den Heuvel, S. (2018). Optogenetic Dissection of Mitotic Spindle Positioning In Vivo. Elife 7, 7. doi:10.7554/eLife.38198
Finegan, T. M., Na, D., Cammarota, C., Skeeters, A. V., Nádasi, T. J., Dawney, N. S., et al. (2019). Tissue Tension and Not Interphase Cell Shape Determines Cell Division Orientation in the Drosophila Follicular Epithelium. EMBO J. 38, 38. doi:10.15252/embj.2018100072
Gallini, S., Carminati, M., De Mattia, F., Pirovano, L., Martini, E., Oldani, A., et al. (2016). NuMA Phosphorylation by Aurora-A Orchestrates Spindle Orientation. Curr. Biol. 26 (4), 458–469. doi:10.1016/j.cub.2015.12.051
Gao, L., Yang, Z., Hiremath, C., Zimmerman, S. E., Long, B., Brakeman, P. R., et al. (2017). Afadin Orients Cell Division to Position the Tubule Lumen in Developing Renal Tubules. Development 144 (19), 3511–3520. doi:10.1242/dev.148908
Gillies, T. E., and Cabernard, C. (2011). Cell Division Orientation in Animals. Curr. Biol. 21, R599–R609. doi:10.1016/j.cub.2011.06.055
Gloerich, M., Bianchini, J. M., Siemers, K. A., Cohen, D. J., and Nelson, W. J. (2017). Cell Division Orientation Is Coupled to Cell-Cell Adhesion by the E-Cadherin/LGN Complex. Nat. Commun. 8, 13996. doi:10.1038/ncomms13996
Gönczy, P. (2008). Mechanisms of Asymmetric Cell Division: Flies and Worms Pave the Way. Nat. Rev. Mol. Cel Biol 9 (5), 355–366. doi:10.1038/nrm2388
Hao, Y., Du, Q., Chen, X., Zheng, Z., Balsbaugh, J. L., Maitra, S., et al. (2010). Par3 Controls Epithelial Spindle Orientation by aPKC-Mediated Phosphorylation of Apical Pins. Curr. Biol. 20 (20), 1809–1818. doi:10.1016/j.cub.2010.09.032
Hart, K. C., Tan, J., Siemers, K. A., Sim, J. Y., Pruitt, B. L., Nelson, W. J., et al. (2017). E-cadherin and LGN Align Epithelial Cell Divisions with Tissue Tension Independently of Cell Shape. Proc. Natl. Acad. Sci. U S A. 114, E5845. doi:10.1073/pnas.1701703114
Hebert, A. M., DuBoff, B., Casaletto, J. B., Gladden, A. B., and McClatchey, A. I. (2012). Merlin/ERM Proteins Establish Cortical Asymmetry and Centrosome Position. Genes Dev. 26 (24), 2709–2723. doi:10.1101/gad.194027.112
Hussain, N. K., Jenna, S., Glogauer, M., Quinn, C. C., Wasiak, S., Guipponi, M., et al. (2001). Endocytic Protein Intersectin-L Regulates Actin Assembly via Cdc42 and N-WASP. Nat. Cel Biol 3 (10), 927–932. doi:10.1038/ncb1001-927
Jaffe, A. B., Kaji, N., Durgan, J., and Hall, A. (2008). Cdc42 Controls Spindle Orientation to Position the Apical Surface during Epithelial Morphogenesis. Biomed. Lit. 183 (4), 625–633. doi:10.1083/jcb.200807121
Kiyomitsu, T., and Boerner, S. (2021). The Nuclear Mitotic Apparatus (NuMA) Protein: A Key Player for Nuclear Formation, Spindle Assembly, and Spindle Positioning. Front. Cel Dev. Biol. 9, 653801. doi:10.3389/fcell.2021.653801
Kiyomitsu, T., and Cheeseman, I. M. (2012). Chromosome- and Spindle-Pole-Derived Signals Generate an Intrinsic Code for Spindle Position and Orientation. Nat. Cel Biol 14 (3), 311–317. doi:10.1038/ncb2440
Knoblich, J. A. (2010). Asymmetric Cell Division: Recent Developments and Their Implications for Tumour Biology. Nat. Rev. Mol. Cel Biol 11 (12), 849–860. doi:10.1038/nrm3010
Knoblich, J. A. (2008). Mechanisms of Asymmetric Stem Cell Division. Cell 132 (4), 583–597. doi:10.1016/j.cell.2008.02.007
Kotak, S., Busso, C., and Gönczy, P. (2012). Cortical Dynein Is Critical for Proper Spindle Positioning in Human Cells. J. Cel Biol 199 (1), 97–110. doi:10.1083/jcb.201203166
Kotak, S., Busso, C., and Gönczy, P. (2013). NuMA Phosphorylation by CDK1 Couples Mitotic Progression with Cortical Dynein Function. Embo J. 32 (18), 2517–2529. doi:10.1038/emboj.2013.172
Kschonsak, Y. T., and Hoffmann, I. (2018). Activated Ezrin Controls MISP Levels to Ensure Correct NuMA Polarization and Spindle Orientation. J. Cel Sci 131, 131. doi:10.1242/jcs.214544
Kunda, P., and Baum, B. (2009). The Actin Cytoskeleton in Spindle Assembly and Positioning. Trends Cel Biol. 19 (4), 174–179. doi:10.1016/j.tcb.2009.01.006
Lancaster, M. A., and Knoblich, J. A. (2014). Organogenesis in a Dish: Modeling Development and Disease Using Organoid Technologies. Science 345 (6194), 1247125. doi:10.1126/science.1247125
Lázaro-Diéguez, F., Cohen, D., Fernandez, D., Hodgson, L., van IJzendoorn, S. C. D., and Müsch, A. (2013). Par1b Links Lumen Polarity with LGN-NuMA Positioning for Distinct Epithelial Cell Division Phenotypes. J. Cel Biol. 203 (2), 251–264. doi:10.1083/jcb.201303013
Lázaro-Diéguez, F., and Müsch, A. (2017). Cell-cell Adhesion Accounts for the Different Orientation of Columnar and Hepatocytic Cell Divisions. J. Cel Biol 216 (11), 3847–3859. doi:10.1083/jcb.201608065
Lechler, T., and Mapelli, M. (2021). Spindle Positioning and its Impact on Vertebrate Tissue Architecture and Cell Fate. Nat. Rev. Mol. Cel Biol 22 (10), 691–708. doi:10.1038/s41580-021-00384-4
LeRoy, P. J., Hunter, J. J., Hoar, K. M., Burke, K. E., Shinde, V., Ruan, J., et al. (2007). Localization of Human TACC3 to Mitotic Spindles Is Mediated by Phosphorylation on Ser558by Aurora A: A Novel Pharmacodynamic Method for Measuring Aurora A Activity. Cancer Res. 67 (11), 5362–5370. doi:10.1158/0008-5472.can-07-0122
Lough, K. J., Byrd, K. M., Descovich, C. P., Spitzer, D. C., Bergman, A. J., Beaudoin, G. M., et al. (2019). Telophase Correction Refines Division Orientation in Stratified Epithelia. Elife 8, 8. doi:10.7554/eLife.49249
Machicoane, M., de Frutos, C. A., Fink, J., Rocancourt, M., Lombardi, Y., Garel, S., et al. (2014). SLK-dependent Activation of ERMs Controls LGN-NuMA Localization and Spindle Orientation. Fac. Opinions 205 (6), 791–799. doi:10.1083/jcb.201401049
McCaffrey, L. M., and Macara, I. G. (2011). Epithelial Organization, Cell Polarity and Tumorigenesis. Trends Cel Biol. 21 (12), 727–735. doi:10.1016/j.tcb.2011.06.005
McKinley, K. L., Stuurman, N., Royer, L. A., Schartner, C., Castillo-Azofeifa, D., Delling, M., et al. (2018). Cellular Aspect Ratio and Cell Division Mechanics Underlie the Patterning of Cell Progeny in Diverse Mammalian Epithelia. Elife 7, 7. doi:10.7554/eLife.36739
Merenda, A., Fenderico, N., and Maurice, M. M. (2020). Wnt Signaling in 3D: Recent Advances in the Applications of Intestinal Organoids. Trends Cel Biol. 30 (1), 60–73. doi:10.1016/j.tcb.2019.10.003
Mochizuki, N., Cho, G., Wen, B., and Insel, P. A. (1996). Identification and cDNA Cloning of a Novel Human Mosaic Protein, LGN, Based on Interaction with G Alpha I2. Gene 181 (1-2), 39–43. doi:10.1016/s0378-1119(96)00456-8
Morin, X., and Bellaïche, Y. (2011). Mitotic Spindle Orientation in Asymmetric and Symmetric Cell Divisions during Animal Development. Dev. Cel 21 (1), 102–119. doi:10.1016/j.devcel.2011.06.012
Nakajima, Y.-i., Meyer, E. J., Kroesen, A., McKinney, S. A., and Gibson, M. C. (2013). Epithelial Junctions Maintain Tissue Architecture by Directing Planar Spindle Orientation. Nature 500 (7462), 359–362. doi:10.1038/nature12335
Nakajima, Y.-i. (2018). Mitotic Spindle Orientation in Epithelial Homeostasis and Plasticity. J. Biochem. 164 (4), 277–284. doi:10.1093/jb/mvy064
Nestor-Bergmann, A., Stooke-Vaughan, G. A., Goddard, G. K., Starborg, T., Jensen, O. E., and Woolner, S. (2019). Decoupling the Roles of Cell Shape and Mechanical Stress in Orienting and Cueing Epithelial Mitosis. Cel Rep. 26 (8), 2088–2100. doi:10.1016/j.celrep.2019.01.102
Okamoto, M., Schoch, S., and Südhof, T. C. (1999). EHSH1/intersectin, a Protein that Contains EH and SH3 Domains and Binds to Dynamin and SNAP-25. J. Biol. Chem. 274 (26), 18446–18454. doi:10.1074/jbc.274.26.18446
Okumura, M., Natsume, T., Kanemaki, M. T., and Kiyomitsu, T. (2018). Dynein-Dynactin-NuMA Clusters Generate Cortical Spindle-Pulling Forces as a Multi-Arm Ensemble. Elife 7, 7. doi:10.7554/eLife.36559
Osswald, M., and Morais-de-Sá, E. (2019). Dealing with Apical-Basal Polarity and Intercellular Junctions: a Multidimensional challenge for Epithelial Cell Division. Curr. Opin. Cel Biol. 60, 75–83. doi:10.1016/j.ceb.2019.04.006
Otani, T., Ichii, T., Aono, S., and Takeichi, M. (2006). Cdc42 GEF Tuba Regulates the Junctional Configuration of Simple Epithelial Cells. Biomed. Lit. 175 (1), 135–146. doi:10.1083/jcb.200605012
Overeem, A. W., Bryant, D. M., and van IJzendoorn, S. C. D. (2015). Mechanisms of Apical-Basal axis Orientation and Epithelial Lumen Positioning. Trends Cel Biol. 25 (8), 476–485. doi:10.1016/j.tcb.2015.04.002
Pan, Z., Zhu, J., Shang, Y., Wei, Z., Jia, M., Xia, C., et al. (2013). An Autoinhibited Conformation of LGN Reveals a Distinct Interaction Mode between GoLoco Motifs and TPR Motifs. Structure 21 (6), 1007–1017. doi:10.1016/j.str.2013.04.005
Pietro, F., Echard, A., and Morin, X. (2016). Regulation of Mitotic Spindle Orientation: an Integrated View. EMBO Rep. 17 (8), 1106–1130. doi:10.15252/embr.201642292
Pirovano, L., Culurgioni, S., Carminati, M., Alfieri, A., Monzani, S., Cecatiello, V., et al. (2019). Hexameric NuMA:LGN Structures Promote Multivalent Interactions Required for Planar Epithelial Divisions. Nat. Commun. 10 (1), 2208. doi:10.1038/s41467-019-09999-w
Porter, A. P., White, G. R. M., Mack, N. A., and Malliri, A. (2019). The Interaction between CASK and the Tumour Suppressor Dlg1 Regulates Mitotic Spindle Orientation in Mammalian Epithelia. J. Cel Sci 132, 132. doi:10.1242/jcs.230086
Poulton, J. S., Cuningham, J. C., and Peifer, M. (2014). Acentrosomal Drosophila Epithelial Cells Exhibit Abnormal Cell Division, Leading to Cell Death and Compensatory Proliferation. Dev. Cel 30 (6), 731–745. doi:10.1016/j.devcel.2014.08.007
Qin, Y., Meisen, W. H., Hao, Y., and Macara, I. G. (2010). Tuba, a Cdc42 GEF, Is Required for Polarized Spindle Orientation during Epithelial Cyst Formation. J. Cel Biol. 189 (4), 661–669. doi:10.1083/jcb.201002097
Rakotomamonjy, J., Brunner, M., Jüschke, C., Zang, K., Huang, E. J., Reichardt, L. F., et al. (2017). Afadin Controls Cell Polarization and Mitotic Spindle Orientation in Developing Cortical Radial Glia. Neural Dev. 12 (1), 7. doi:10.1186/s13064-017-0085-2
Reinsch, S., and Karsenti, E. (1994). Orientation of Spindle axis and Distribution of Plasma Membrane Proteins during Cell Division in Polarized MDCKII Cells. J. Cel Biol. 126 (6), 1509–1526. doi:10.1083/jcb.126.6.1509
Renna, C., Rizzelli, F., Carminati, M., Gaddoni, C., Pirovano, L., Cecatiello, V., et al. (2020). Organizational Principles of the NuMA-Dynein Interaction Interface and Implications for Mitotic Spindle Functions. Structure 28 (7), 820–829. doi:10.1016/j.str.2020.04.017
Rizzelli, F., Malabarba, M. G., Sigismund, S., and Mapelli, M. (2020). The Crosstalk between Microtubules, Actin and Membranes Shapes Cell Division. Open Biol. 10 (3), 190314. doi:10.1098/rsob.190314
Rodriguez-Boulan, E., and Macara, I. G. (2014). Organization and Execution of the Epithelial Polarity Programme. Nat. Rev. Mol. Cel Biol 15 (4), 225–242. doi:10.1038/nrm3775
Rodriguez-Fraticelli, A. E., Vergarajauregui, S., Eastburn, D. J., Datta, A., Alonso, M. A., Mostov, K., et al. (2010). The Cdc42 GEF Intersectin 2 Controls Mitotic Spindle Orientation to Form the Lumen during Epithelial Morphogenesis. J. Cel Biol. 189 (4), 725–738. doi:10.1083/jcb.201002047
Saadaoui, M., Konno, D., Loulier, K., Goiame, R., Jadhav, V., Mapelli, M., et al. (2017). Loss of the Canonical Spindle Orientation Function in the Pins/LGN Homolog AGS 3. EMBO Rep. 18 (9), 1509–1520. doi:10.15252/embr.201643048
Saadaoui, M., Machicoane, M., di Pietro, F., Etoc, F., Echard, A., and Morin, X. (2014). Dlg1 Controls Planar Spindle Orientation in the Neuroepithelium through Direct Interaction with LGN. Biomed. Lit. 206 (6), 707–717. doi:10.1083/jcb.201405060
Sato, T., and Clevers, H. (2013). Growing Self-Organizing Mini-Guts from a Single Intestinal Stem Cell: Mechanism and Applications. Science 340 (6137), 1190–1194. doi:10.1126/science.1234852
Sato, T., Vries, R. G., Snippert, H. J., van de Wetering, M., Barker, N., Stange, D. E., et al. (2009). Single Lgr5 Stem Cells Build Crypt-Villus Structures In Vitro without a Mesenchymal Niche. Nature 459 (7244), 262–265. doi:10.1038/nature07935
Seldin, L., Muroyama, A., and Lechler, T. (2016). NuMA-microtubule Interactions Are Critical for Spindle Orientation and the Morphogenesis of Diverse Epidermal Structures. Elife 5, 5. doi:10.7554/eLife.12504
Seldin, L., Poulson, N. D., Foote, H. P., and Lechler, T. (2013). NuMA Localization, Stability, and Function in Spindle Orientation Involve 4.1 and Cdk1 Interactions. MBoC 24 (23), 3651–3662. doi:10.1091/mbc.e13-05-0277
Slim, C. L., Lázaro-Diéguez, F., Bijlard, M., Toussaint, M. J., de Bruin, A., Du, Q., et al. (2013). Par1b Induces Asymmetric Inheritance of Plasma Membrane Domains via LGN-dependent Mitotic Spindle Orientation in Proliferating Hepatocytes. Plos Biol. 11, e1001739. doi:10.1371/journal.pbio.1001739
Su, W. H., Mruk, D. D., Wong, E. W., Lui, W. Y., and Cheng, C. Y. (2012). Polarity Protein Complex Scribble/Lgl/Dlg and Epithelial Cell Barriers. Adv. Exp. Med. Biol. 763, 149–170. doi:10.1007/978-1-4614-4711-5_7
Takayanagi, H., Hayase, J., Kamakura, S., Miyano, K., Chishiki, K., Yuzawa, S., et al. (2019). Intramolecular Interaction in LGN, an Adaptor Protein that Regulates Mitotic Spindle Orientation. J. Biol. Chem. 294 (51), 19655–19666. doi:10.1074/jbc.ra119.011457
Théry, M., Jiménez-Dalmaroni, A., Racine, V., Bornens, M., and Jülicher, F. (2007). Experimental and Theoretical Study of Mitotic Spindle Orientation. Nature 447 (7143), 493–496. doi:10.1038/nature05786
Toyoshima, F., Matsumura, S., Morimoto, H., Mitsushima, M., and Nishida, E. (2007). PtdIns(3,4,5)P3 Regulates Spindle Orientation in Adherent Cells. Dev. Cel 13 (6), 796–811. doi:10.1016/j.devcel.2007.10.014
Tuncay, H., Brinkmann, B. F., Steinbacher, T., Schürmann, A., Gerke, V., Iden, S., et al. (2015). JAM-A Regulates Cortical Dynein Localization through Cdc42 to Control Planar Spindle Orientation during Mitosis. Nat. Commun. 6, 8128. doi:10.1038/ncomms9128
Vodicska, B., Cerikan, B., Schiebel, E., and Hoffmann, I. (2018). MISP Regulates the IQGAP1/Cdc42 Complex to Collectively Orchestrate Spindle Orientation and Mitotic Progression. Sci. Rep. 8 (1), 6330. doi:10.1038/s41598-018-24682-8
Vorhagen, S., and Niessen, C. M. (2014). Mammalian aPKC/Par Polarity Complex Mediated Regulation of Epithelial Division Orientation and Cell Fate. Exp. Cel Res. 328 (2), 296–302. doi:10.1016/j.yexcr.2014.08.008
Wang, X., Dong, B., Zhang, K., Ji, Z., Cheng, C., Zhao, H., et al. (2018). E-cadherin Bridges Cell Polarity and Spindle Orientation to Ensure Prostate Epithelial Integrity and Prevent Carcinogenesis In Vivo. Plos Genet. 14, e1007609. doi:10.1371/journal.pgen.1007609
Willard, F. S., Kimple, R. J., and Siderovski, D. P. (2004). Return of the GDI: the GoLoco Motif in Cell Division. Annu. Rev. Biochem. 73, 925–951. doi:10.1146/annurev.biochem.73.011303.073756
Woodard, G. E., Huang, N.-N., Cho, H., Miki, T., Tall, G. G., and Kehrl, J. H. (2010). Ric-8A and Giα Recruit LGN, NuMA, and Dynein to the Cell Cortex to Help Orient the Mitotic Spindle. Mol. Cel Biol 30 (14), 3519–3530. doi:10.1128/mcb.00394-10
Wyatt, T. P. J., Harris, A. R., Lam, M., Cheng, Q., Bellis, J., Dimitracopoulos, A., et al. (2015). Emergence of Homeostatic Epithelial Packing and Stress Dissipation through Divisions Oriented along the Long Cell axis. Proc. Natl. Acad. Sci. USA 112 (18), 5726–5731. doi:10.1073/pnas.1420585112
Yao, R., Oyanagi, J., Natsume, Y., Kusama, D., Kato, Y., Nagayama, S., et al. (2016). Suppression of Intestinal Tumors by Targeting the Mitotic Spindle of Intestinal Stem Cells. Oncogene 35 (47), 6109–6119. doi:10.1038/onc.2016.148
Young, M. A., May, S., Damo, A., Yoon, Y. S., Hur, M.-W., Swat, W., et al. (2019). Epigenetic Regulation of Dlg1, via Kaiso, Alters Mitotic Spindle Polarity and Promotes Intestinal Tumorigenesis. Mol. Cancer Res. 17 (3), 686–696. doi:10.1158/1541-7786.mcr-18-0280
Zegers, M. M. P., O'Brien, L. E., Yu, W., Datta, A., and Mostov, K. E. (2003). Epithelial Polarity and Tubulogenesis In Vitro. Trends Cel Biol. 13 (4), 169–176. doi:10.1016/s0962-8924(03)00036-9
Zheng, Z., Zhu, H., Wan, Q., Liu, J., Xiao, Z., Siderovski, D. P., et al. (2010). LGN Regulates Mitotic Spindle Orientation during Epithelial Morphogenesis. Fac. Opinions 189 (2), 275–288. doi:10.1083/jcb.200910021
Zhu, J., Wen, W., Zheng, Z., Shang, Y., Wei, Z., Xiao, Z., et al. (2011). LGN/mInsc and LGN/NuMA Complex Structures Suggest Distinct Functions in Asymmetric Cell Division for the Par3/mInsc/LGN and Gαi/LGN/NuMA Pathways. Mol. Cel 43 (3), 418–431. doi:10.1016/j.molcel.2011.07.011
Keywords: mitotic spindle orientation, epithelial polarity, cysts, organoids, planar divisions
Citation: Donà F, Eli S and Mapelli M (2022) Insights Into Mechanisms of Oriented Division From Studies in 3D Cellular Models. Front. Cell Dev. Biol. 10:847801. doi: 10.3389/fcell.2022.847801
Received: 03 January 2022; Accepted: 17 February 2022;
Published: 09 March 2022.
Edited by:
Jens Januschke, University of Dundee, United KingdomReviewed by:
Salah Elias, University of Southampton, United KingdomDan Bergstralh, University of Rochester, United States
Christopher A. Johnston, University of New Mexico, United States
Copyright © 2022 Donà, Eli and Mapelli. This is an open-access article distributed under the terms of the Creative Commons Attribution License (CC BY). The use, distribution or reproduction in other forums is permitted, provided the original author(s) and the copyright owner(s) are credited and that the original publication in this journal is cited, in accordance with accepted academic practice. No use, distribution or reproduction is permitted which does not comply with these terms.
*Correspondence: Marina Mapelli, bWFyaW5hLm1hcGVsbGlAaWVvLml0