- Department of Instructive Biomaterial Engineering, MERLN Institute for Technology-Inspired Regenerative Medicine, Maastricht University, Maastricht, Netherlands
Advances in the field of stem cell-based models have in recent years lead to the development of blastocyst-like structures termed blastoids. Blastoids can be used to study key events in mammalian pre-implantation development, as they mimic the blastocyst morphologically and transcriptionally, can progress to the post-implantation stage and can be generated in large numbers. Blastoids were originally developed using mouse pluripotent stem cells, and since several groups have successfully generated blastocyst models of the human system. Here we provide a comparison of the mouse and human protocols with the aim of deriving the core requirements for blastoid formation, discuss the models’ current ability to mimic blastocysts and give an outlook on potential future applications.
Introduction
In the past few years, a set of novel stem cell-based embryo models named blastoids have seen the light that recapitulate the pre-implantation blastocyst-stage embryo. Blastoids are well-poised to complement research on natural embryos as they contain both the embryonic and extraembryonic cell types, can be formed in large numbers with similar genetic makeup, are amenable to new modes of experimental manipulation including genetic screens and show features of post-implantation morphogenesis when exposed to appropriate culture conditions. In particular, human blastoids may provide promising alternatives to the use of human embryos, which are scarce and come with substantial ethical concerns (Pereira Daoud et al., 2020).
Several days after conception the blastomeric cells of the mammalian embryo compact into a tight polarized cluster named the morula. This conserved morphological event initiates, in part via aPKC/Hippo-mediated signaling, the first lineage bifurcation into the embryonic inner cell mass (ICM) and the extra-embryonic trophectoderm (TE), that will form the fetal placenta (Cockburn and Rossant, 2010; Gerri et al., 2020a). The TE then forms an epithelium, starts pumping fluid inside and forms a cavitated sphere encasing the ICM on one side of the cavity, which is called the blastocyst (Molè et al., 2020). Interestingly, in human, commitment to either the ICM or TE does not occur until the start of cavitation (Meistermann et al., 2021). Around embryonic day 3.5 in mouse and day 5.0 in human, the ICM undergoes a second bifurcation and specifies into the epiblast (EPI), which forms the embryo proper, and primitive endoderm [PrE; named hypoblast in human (HYPO)], which gives rise to extra-embryonic endoderm tissues including parts of the yolk sac (Molè et al., 2020). In mouse, EPI and PrE cells are initially mixed in a salt/pepper-like distribution after which the PrE segregates and forms an epithelium on top of the EPI, facing the blastocyst cavity. There is evidence of HYPO formation and subsequent segregation taking place in human embryos as well, although it is not clear whether the initial distribution of HYPO cells in the ICM follows a similar salt and pepper-like pattern as observed in mouse (Roode et al., 2012). Meanwhile the TE differentiates and can be subdivided in the polar and mural TE. The polar TE refers to the TE directly in contact with the EPI and in mouse acts as a stem cell pool for the TE, whereas the mural TE is on the opposite side of the cavity. A striking difference between mouse and human development is that the mouse blastocyst attaches to the uterine wall with the mural side, while the human blastocyst is believed to attach with the polar side (Molè et al., 2020).
Thus, phenomenologically, pre-implantation development seems largely conserved between mouse and human, however the molecular underpinnings may differ. These differences become more pronounced in early post-implantation morphogenesis when also the embryo architecture and the maternal/fetal interface deviate from mouse [reviewed in Gerri et al. (2020b), Molè et al. (2020)]. As such, there is a clear need for a sophisticated model of the human pre- and peri-implantation embryo. In addition, the wide use of mouse as a model species and subsequent vast amounts of data available on mouse embryology and gene function form a solid foundation for generating and testing embryo models (Taft, 2008). Furthermore, in contrast to human, mouse embryo models may be amenable to model full organismal development.
For faithful recapitulation of blastocysts, it is essential that blastoids contain the EPI, HYPO/PrE and TE lineages. Embryonic stem cells (ESC) (Evans and Kaufman, 1981; Martin, 1981; Thomson et al., 1998), trophoblast stem cells (TSC) (Tanaka et al., 1998; Kubaczka et al., 2014; Okae et al., 2018; Frias-Aldeguer et al., 2020), and extra-embryonic endoderm cells (XEN) (Niakan et al., 2013; Anderson et al., 2017; Linneberg-Agerholm et al., 2019) form in vitro analogues of the EPI, TE and PrE/HYPO, respectively, and can generally be maintained as 2D monolayer cultures. More recently, chemically defined culture conditions have been identified that permit the expansion of multiple levels of pluripotent cells that reflect different developmental states of the pre-to early post-implantation EPI (Ying et al., 2008; Theunissen et al., 2014; Yang J. et al., 2017; Yang Y. et al., 2017; Guo et al., 2017; Gao et al., 2019; Bayerl et al., 2021; Shen et al., 2021). These 2D cultures form the basis of the more complex 3D models, such as blastoids, that are designed to capture both the specification of cell types and their spatiotemporal organization. As formation of 3D models relies heavily on self-organization, the starting conditions of the 2D cultures are critical for the successful generation of 3D models. On top of the stem cells themselves and the chemical cues that direct self-organization, the 3D culture platforms play an important role in providing the freedom to re-organize, as well as enabling in situ imaging-based readouts and enabling high-throughput screening assays.
The first report on the generation of blastoids recapitulated the mouse blastocyst formation by using a co-culture of mouse ESCs, which resemble the pre-implantation EPI (Evans and Kaufman, 1981; Martin, 1981), and mouse TSCs, which resemble the TE (Tanaka et al., 1998). When these types were combined through sequential seeding into microwells and exposed to a mixture of growth factors and small molecule signaling pathway modulators they formed blastocyst-like structures (Rivron et al., 2018). Following this report, several other labs have reported modified methods for blastoid generation that all recapitulate the blastocysts’ overall morphology and contain the three distinct cell lineages (Rivron et al., 2018; Kime et al., 2019; Li et al., 2019; Sozen et al., 2019; Zhang et al., 2019).
Although the culture of human ESCs and later TSCs has been possible for some time (Thomson et al., 1998; Okae et al., 2018), practical and ethical restrictions have impeded advances in human embryo models. Much of the work on human models consequently relies on preceding findings in mouse. However, this gap is closing with the recent accumulation of studies in both species, including the improved understanding of the similarities and differences in pluripotency (Brons et al., 2007; Tesar et al., 2007; Weinberger et al., 2016), methods to culture blastocysts towards the post-implantation stage in vitro (Bedzhov et al., 2014; Deglincerti et al., 2016; Shahbazi et al., 2016), advanced single cell omics studies to demarcate cell identities and regulatory networks that underlie EPI, PrE/HYPO and TE specification (Nakamura et al., 2015; Nakamura et al., 2016; Meistermann et al., 2021), and the in vitro differentiation of TE and its derivatives (Castel et al., 2020; Io et al., 2021). Through the use of human extended pluripotent/expanded potential stem cells (EPSCs), induced pluripotent stem cells (iPSCs) and naïve ESCs, several groups have reported their first successes with human blastoid generation (Fan et al., 2021; Kagawa et al., 2021; Liu et al., 2021; Sozen et al., 2021; Yanagida et al., 2021; Yu et al., 2021). The methods employed have yet some marked differences between the groups, underlining the different angles and controversies surrounding the cellular subtypes and signaling pathways involved in early human development.
In this review, we aim to give an overview of the current mouse and human blastoid protocols, compare mouse and human protocols to identify core requirements for blastoid formation and discuss to what extent current blastoids mimic blastocysts.
First, we will address several of the key components in the generation of mouse and human blastoids: the starting cell lines and their flavor of pluripotency, the soluble signaling pathways modulators used to steer differentiation of cells towards the blastocyst lineages and the culture platforms applied to facilitate 3D self-organization. Additionally, we will compare the timelines of the protocols and the extent of successful lineage specification. Next, we will delineate the capacity of blastoids to recapitulate principles of blastocyst development and the potential for post-implantation progression in vitro. Finally, we will provide an outlook on the future applications of both mouse and human blastoids.
Generation of Mouse and Human Blastocyst Models In Vitro
Cell Sources and Flavors of Pluripotency
As delineated above, the pre-implantation mammalian blastocyst is comprised of three lineages: the TE, the PrE/HYPO and the EPI. In mouse, stem cell analogues can be derived from all these three primary cell types and propagated as self-renewing stem cells, namely TSCs (Tanaka et al., 1998), XEN cells (Kunath et al., 2005; Anderson et al., 2017), and pluripotent ESCs (Evans and Kaufman, 1981; Martin, 1981), respectively. The term pluripotency describes the capacity of cells to form all cell types of the mature body in response to developmental cues (Silva and Smith, 2008). Leukemia inhibitory factor (LIF) was originally found to maintain pluripotency in mouse ESCs (Williams et al., 1988). Since, Ying et al., identified that the addition of the two inhibitors PD0325901 (ERK/MAPK inhibitor) and CHIR99021 (GSK3β inhibitor) can sustain mouse ESCs in a so-called pluripotent ground-state, which closely reflects the late blastocyst-stage epiblast and exhibits lower cell-to-cell variability and thereby manifests in more homogenous in vitro cultures (Ying et al., 2008). In the mouse embryo, progression of the EPI towards the post-implantation stage is characterized by a transiently evolving transcriptional and epigenetic signature of pluripotency, from which in vitro analogues can be captured in discrete states (Morgani et al., 2017; Neagu et al., 2020; Kinoshita et al., 2021), including the pluripotency endpoint (i.e., primed) post-implantation EPI cells named epiblast-derived stem cells (EpiSCs) (Brons et al., 2007; Tesar et al., 2007). In vitro at least, the pluripotency domain appears non-linear since additional states have been identified [reviewed in Morgani et al. (2017)], including so-called extended and expanded PSCs (EPSCs) (Yang J. et al., 2017; Yang Y. et al., 2017) that, reportedly, are able to also give rise to both the extraembryonic PrE and TE cell types. Although EPSCs do robustly form PrE, transcriptomic comparisons performed by Posfai et al. contested the claim of murine EPSC’ ability to form bona fide TE (Posfai et al., 2021). In fact, the data suggest that EPSC do not have an additional totipotent character relative to native ESC and cluster instead closer to early post-implantation pluripotent cells (Posfai et al., 2021).
In contrast to mouse, human blastocyst-derived ESCs were originally maintained in vitro in a so-called primed state that reflects more the post-implantation EPI (Thomson et al., 1998) rather than the blastocyst-stage EPI. Later, numerous regulatory cocktails have been developed that claim the expansion of human ESCs and iPSCs in a naïve state (Chan et al., 2013; Gafni et al., 2013; Takashima et al., 2014; Theunissen et al., 2014; Ware et al., 2014; Bredenkamp et al., 2019b; Bayerl et al., 2021) that shares features with mouse ground-state ESCs such as their dome-like colonies, predominant activation of the OCT4 distal enhancer, germline potency and pre-X-chromosome inactivation (Weinberger et al., 2016; Devika et al., 2019; Yilmaz and Benvenisty, 2019). Moreover, methods for culturing mouse EPSCs have been translated to human as well and termed human EPSCs (Gao et al., 2019). Nevertheless, establishing a consistent and pure human naïve line can be challenging as is evidenced by current efforts to optimize the combinations of factors that permit robust expansion of human naïve pluripotent PSCs from diverse genetic backgrounds (Bredenkamp et al., 2019a; Bayerl et al., 2021; Khan et al., 2021). The progress in the field of naïve pluripotency over the past decade has been covered extensively elsewhere and will therefore not be discussed in detail here (Weinberger et al., 2015; Smith, 2017; Yilmaz and Benvenisty, 2019; Taei et al., 2020). Important to note, whereas mouse PSC cannot readily differentiate into TE lineages, human ESCs appear to have a certain capacity to do so (Xu et al., 2002; Amita et al., 2013). Although there is no consensus yet about whether primed hPSC form bona fide trophoblast cells or something related (e.g., mesoderm or amnion), it appears that hPSCs maintained in EPSC and naïve conditions do form stable self-renewing lines reminiscent of early post-implantation trophoblast (Castel et al., 2020; Cinkornpumin et al., 2020; Guo et al., 2021; Io et al., 2021). Robust expansion cultures of pre-implantation TE have not been reported to date, however. Besides the differentiation to trophoblast, human naïve ESCs were reported capable of differentiating into HYPO-like cells (Linneberg-Agerholm et al., 2019).
Of note, it is important that, independent of the expansion protocol that is used, the maintenance of pure and uniform pluripotent cell populations is an essential starting point to form blastoids for both mouse and human.
For mouse, the first blastoids were formed using ESCs cultured in the 2i/LIF ground-state condition in order to form EPI and PrE, combined with multipotent TSCs to mimic the TE (Figure 1) (Rivron et al., 2018). In contrast to the protocols based on mouse ESCs, Li et al. reported blastoids based on murine EPSCs without addition of TSCs (EPSC-only blastoids; Table 1) (Rivron et al., 2018; Li et al., 2019; Sozen et al., 2019; Vrij et al., 2019). As mentioned before, while mouse ESCs are committed to the EPI lineage, mouse EPSCs are reportedly able to give rise to all cell types of the conceptus (Yang J. et al., 2017; Yang Y. et al., 2017). Although EPSCs do form PrE in blastocysts and in blastoids the claim of murine EPSC ability to form bona fide TE is contested (Posfai et al., 2021). Strikingly, although EPSC-only blastoids can form blastoids with fewer exogenous cues than the other models, the efficiency of blastoid generation appears higher when using TSCs for the TE compartment rather than TE induction from EPSCs alone (15 vs. 60% formation efficiency) (Li et al., 2019; Sozen et al., 2019). This is also supported by the findings of Kime et al. (2019). This group used their previously developed protocol for converting post-implantation mouse EPI cells to naïve mouse PSCs (Kime et al., 2016) and found that these converted cells could give rise to blastocyst-like structures on their own, albeit at a low efficiency (Kime et al., 2019).
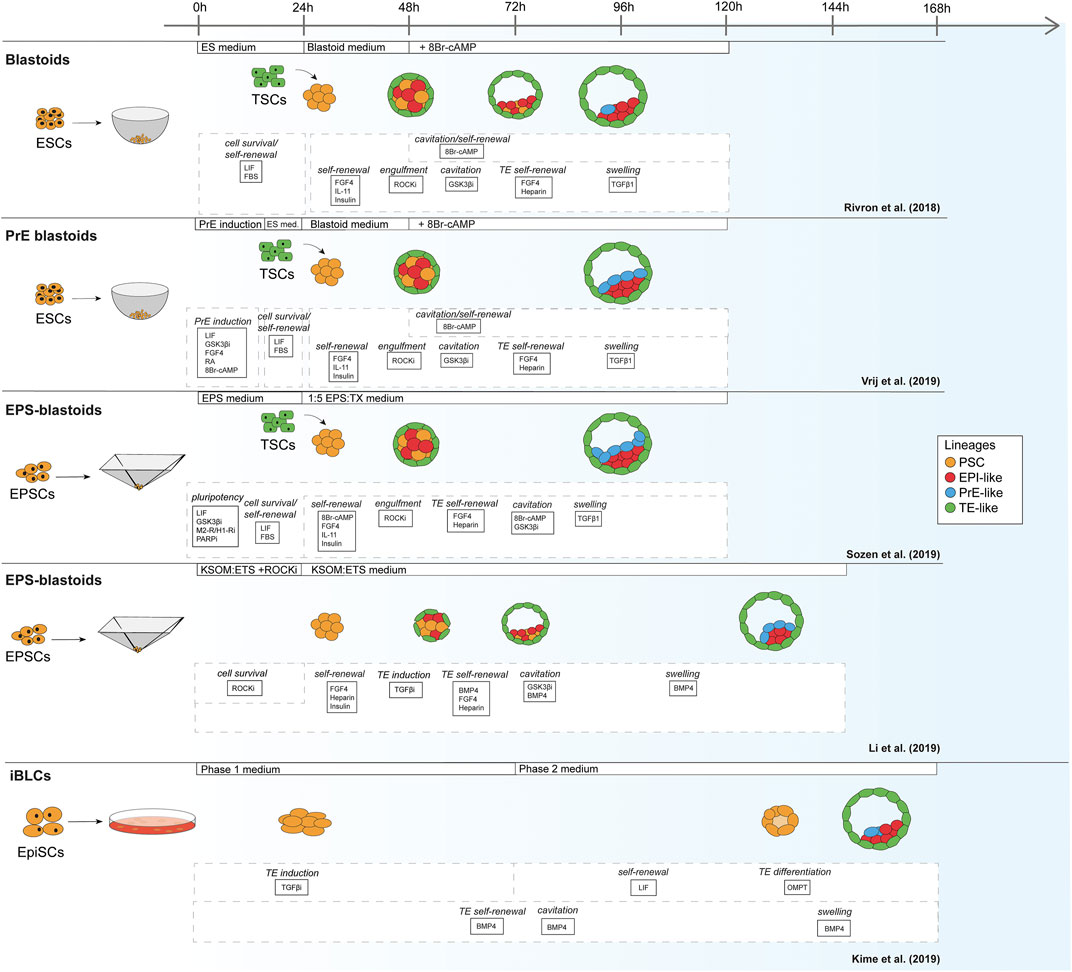
FIGURE 1. Schematic of mouse blastoid protocols. The names given are taken from the original publications.
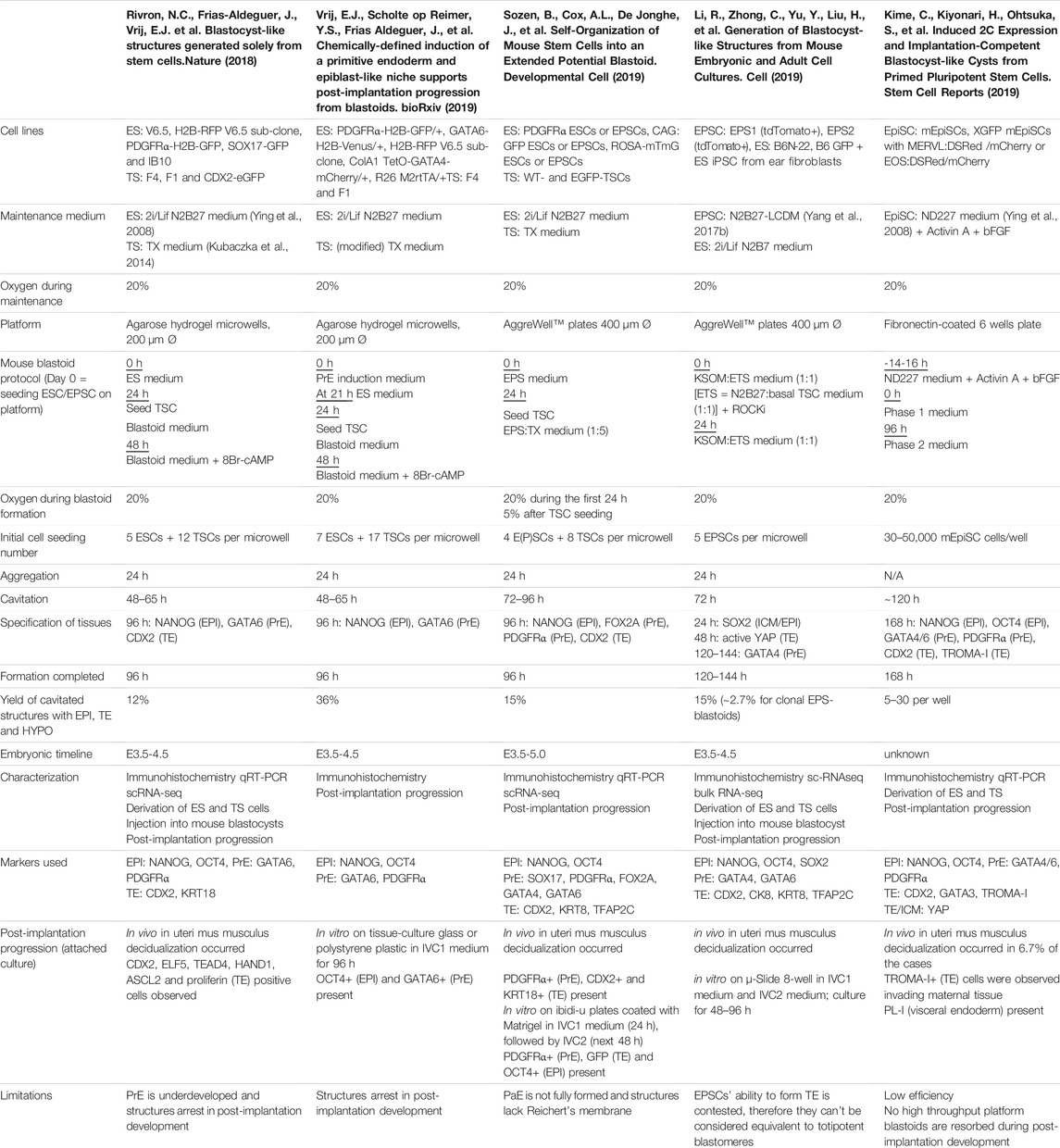
TABLE 1. Overview of mouse blastoid protocols, summarizing culture conditions, experimental time lines, blastocyst marker expression, characterization experiments performed, post-implantation progression and limitations of the individual protocols.
Similar to the mouse models, human blastoid models were created with various types of PSCs that are believed to confer different pluripotency states (Figure 2). While some labs use human ESCs and iPSCs grown in naïve conditions (Kagawa et al., 2021; Yanagida et al., 2021; Yu et al., 2021), others have used EPSC conditions (Fan et al., 2021; Sozen et al., 2021) and one group has used direct reprogramming of fibroblasts over a course of 21 days into cells that form blastoids (Liu et al., 2021). This diversity in starting material likely contributes to the variation between methods in blastoid generation efficiency and capacity for lineage specification (Table 2). Moreover, depending on the pluripotency state, triggers for directing the founding cells towards an organized blastocyst-like structure vary as well, as is also observed in the mouse protocols (Rivron et al., 2018; Li et al., 2019; Sozen et al., 2019; Vrij et al., 2019).
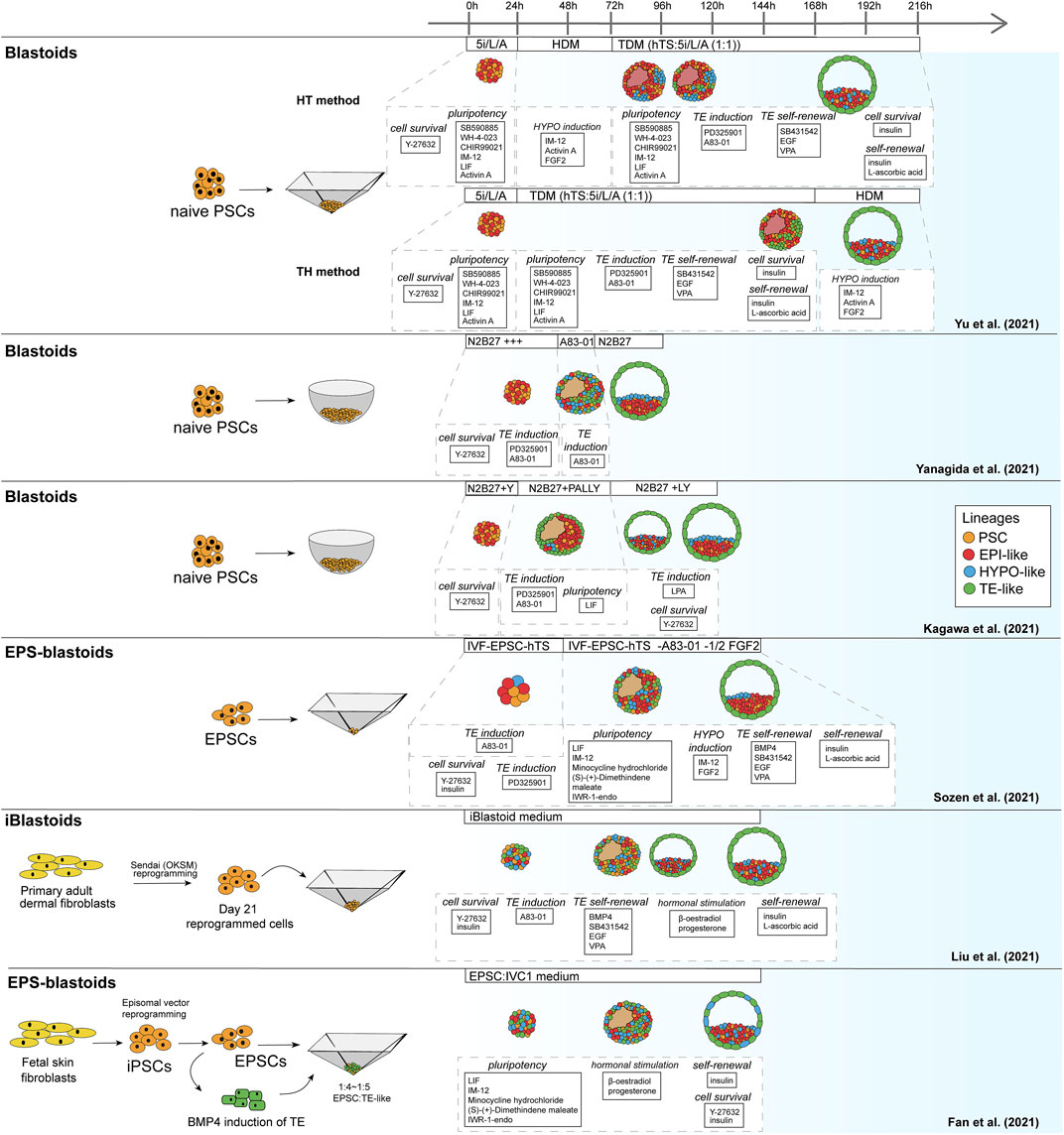
FIGURE 2. Schematic of human blastoid protocols. The names attributed to the models are taken from the original publications.
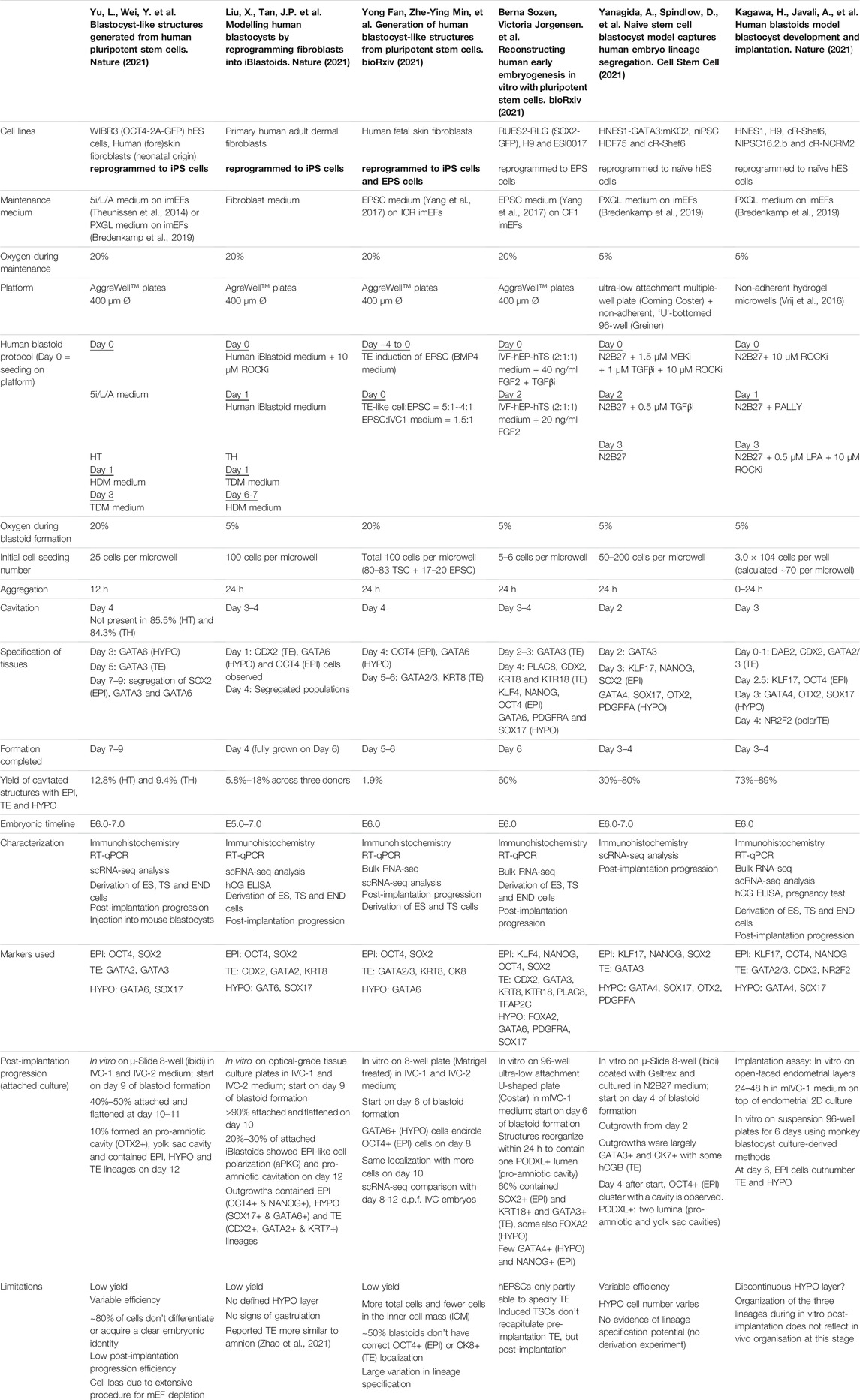
TABLE 2. Overview human blastoid protocols, summarizing culture conditions, experimental time lines, blastocyst marker expression.
As noted before, human PSCs appear more readily able to contribute to the TE lineage when they are in a naïve or EPSC conditions than mouse PSCs. This might explain why, contrary to murine blastoids, the majority of human blastoids have been formed from a single cell type and higher yields were reported in some of the single cell type approaches (Kagawa et al., 2021; Sozen et al., 2021; Yanagida et al., 2021) compared to the approach of Fan et al. that combines induced TE-like cells with EPSCs (Table 2) (Fan et al., 2021).
Blastoid Culture Conditions
In addition to 2D PSC cultures in the appropriate pluripotency state, several other conditions must be met to generate blastoids. The PSCs need to be exposed to the right signaling molecules at the right time to direct their differentiation towards the blastocyst lineages and they need the appropriate microenvironment to undergo their morphogenetic changes.
Signaling pathways that are involved in blastocyst formation have been thoroughly studied over the past decades in mouse [reviewed in Rossant and Tam (2009), Frum and Ralston (2015)]. While in mouse, in vitro work on the effects of pathway activation can be complemented with in vivo work, this is not the case for human due to ethical concerns. Much of what we know about signaling pathways in human development therefore stems from findings in the mouse and/or comparisons between mouse and human ESCs in vitro, substantiated with (single cell) omics approaches applied to human (Yan et al., 2013; Blakeley et al., 2015; Petropoulos et al., 2016) and mouse embryos in the recent years (Deng et al., 2014; Boroviak et al., 2015; Nakamura et al., 2016). Based on these studies, we are now able to attribute the activity of several pathways to the development of the blastocyst lineages in both mouse and human. Consequently, small molecules that regulate these pathways are applied in blastoid generation. Here we will discuss all pathway regulators that have reportedly been used so far to generate blastoids and explain their function(s) in blastoid formation (summarized in Tables 3, 4).
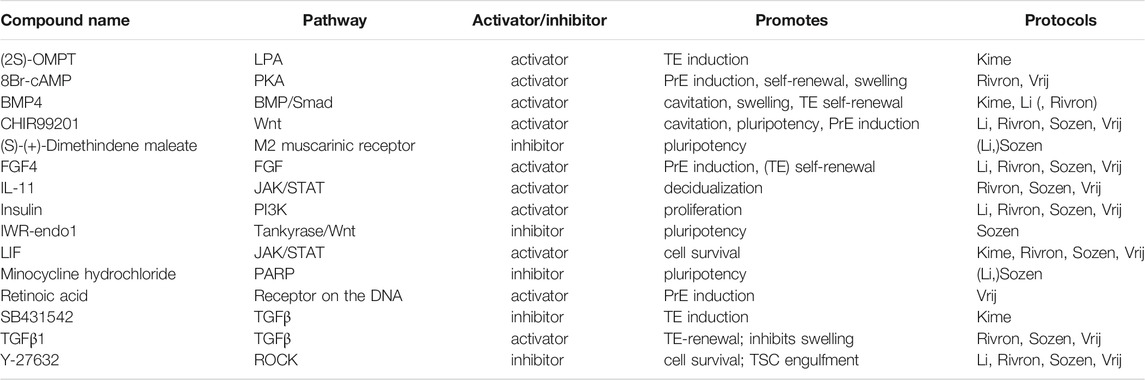
TABLE 3. Small molecules applied in mouse blastoid protocols. When a protocol is listed in brackets, the molecule is used in a screening or cell culture maintenance medium, but not included in the standard blastoid generation protocol.
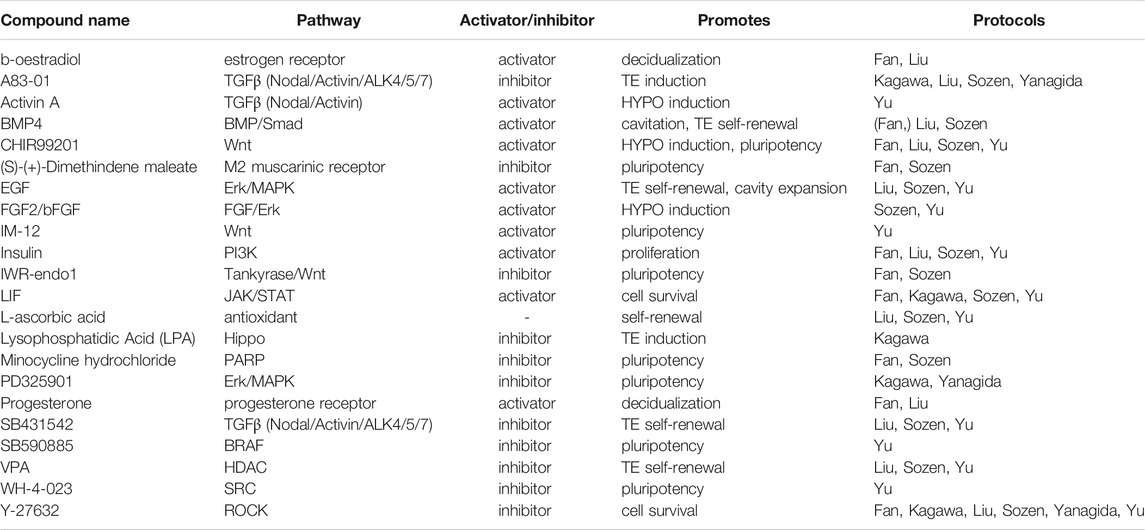
TABLE 4. Small molecules applied in human blastoid protocols. When a protocol is listed in brackets, the molecule is used in a screening or cell culture maintenance medium, but not included in the blastoid generation protocol.
Wnt Pathway Modulators
Wnt signaling is found to play an important role in both mouse and human development, although its role during pre-implantation remains controversial [reviewed in De Jaime-Soguero et al. (2018)]. Regardless of the disputes surrounding Wnt activity during pre-implantation stages, CHIR99201 (CHIR), a GSK3β inhibitor and subsequent canonical Wnt/β-catenin activator, is included in all mouse blastoid protocols except the one from Kime et al. Addition of CHIR significantly increased the yield of mouse blastoid formation (Rivron et al., 2018; Li et al., 2019) and Wnt inhibitors XAV939 and IWR-1-endo significantly inhibited blastoid formation (Rivron et al., 2018; Li et al., 2019). Additionally, it enhanced blastoid cavitation size (Rivron et al., 2018) and PrE induction (Rivron et al., 2018; Vrij et al., 2019).
CHIR is included in several of the human protocols (Fan et al., 2021; Sozen et al., 2021; Yu et al., 2021) and has similar functions as in mouse, namely, to maintain pluripotency of the human PSCs and support HYPO induction. Sozen et al. even found that human 3D cultures did not survive without CHIR (Sozen et al., 2021). However, CHIR is not the only Wnt regulator employed. The naïve pluripotency medium 5i/L/A that is used for blastoid generation by Yu et al. includes the selective GSK3β inhibitor IM-12 (Theunissen et al., 2014). In blastoid protocols based on human EPSCs, EPSC medium rather than naïve medium is used to maintain a pluripotent compartment. EPSC medium also contains CHIR and is paradoxically complemented with a canonical Wnt/β-catenin signaling inhibitor, IWR-endo-1 (Yang Y. et al., 2017; Fan et al., 2021; Sozen et al., 2021), that possibly mediates an effect by further increasing Axin2 levels (Kim et al., 2013).
ERK/MAPK Pathway Modulators
The ERK/MAPK pathway too is unanimously recognized as a key player. Downregulation of ERK/MAPK signaling was first described in murine ESC cultures to maintain naïve pluripotency alongside Wnt pathway activation (Ying et al., 2008). Through the inhibitor PD0325901, ERK/MAPK signaling is blocked, resulting in sustained pluripotency and self-renewal in both murine and human ESCs (Li et al., 2007; Ying et al., 2008; Nichols et al., 2009). As MEK inhibitors are suppressors of differentiation, they are omitted from mouse blastoid protocols to facilitate lineage specification.
In human however, maintaining ERK/MAPK inhibition in 3D culture supports the specification of the TE lineage and is therefore included in several protocols for human blastoids (Guo et al., 2021; Kagawa et al., 2021; Yanagida et al., 2021). Additionally, the previously mentioned human naïve pluripotency medium 5i/L/A contains both PD0325901 and a BRAF inhibitor, which is also linked to the inhibition of ERK/MAPK signaling (Theunissen et al., 2014).
TGFβ Pathway Modulators
Then, there are several members of the TGFβ-superfamily that are involved in the regulation of blastoid formation. Most mouse blastoid protocols apply low levels of TGFβ1 to maintain TE self-renewal and modulate swelling of the cavitated structures (Rivron et al., 2018; Sozen et al., 2019; Vrij et al., 2019). Notably, studies in mouse identified TGFβ1 as a co-regulator with FGF4 for TSC self-renewal (Erlebacher et al., 2004). Therefore it is striking that, when murine blastoids are generated from EPSCs alone, they are exposed to Activin/Nodal/ALK4/5/7 inhibitor A83-01 to induce the TE identity in EPSCs (Li et al., 2019). Similarly, Kime et al. apply Activin/Nodal/ALK4/5/7 inhibitor SB431542 in their blastoid protocol (Kime et al., 2019). Regarding the other branch of the TGFβ-superfamily, BMP4, is included in mouse blastoid cultures (Rivron et al., 2018; Kime et al., 2019; Li et al., 2019) to support cavitation, swelling and TE self-renewal (Coucouvanis and Martin, 1999; Hayashi et al., 2010).
Similar to aforementioned murine EPSC-only blastoids and EpiSC-based blastoids, human blastoids are formed in the presence of TGFβ inhibitors. In human naïve PSCs and EPSCs alike, A83-01 in combination with PD0325901 induces a TE identity, while the less potent inhibitor SB431542 is included to support TE self-renewal (Tojo et al., 2005; Okae et al., 2018; Guo et al., 2021). Through the other TGFβ signaling branch, BMP4 contributes to TE self-renewal as well (Xu et al., 2002; Amita et al., 2013; Okae et al., 2018). In one of the human protocols, TE induction before 3D culture is even performed with BMP4 alone (Fan et al., 2021). Whether it can give rise to pre-implantation TE-like cells is unclear, as most studies on BMP4-mediated induction observe trophoblast markers that are expressed by peri- and post-implantation trophoblast cell types (Li and Parast, 2014; Jain et al., 2017; Gao et al., 2019; Fan et al., 2021). Lastly, Activin A, an activator of Activin/Nodal/ALK4/5/7 signaling, is applied in human PSC culture to maintain naïve pluripotency (Theunissen et al., 2014; Bayerl et al., 2021). In combination with Wnt and FGF pathway regulators, Activin A is also used to induce HYPO differentiation (Linneberg-Agerholm et al., 2019; Yu et al., 2021).
FGF Pathway Modulators
In mice, FGF signaling is most notably involved in directing differentiation of ESCs during pre-implantation. FGF4 specifically is indispensable for mouse ESC differentiation towards the PrE lineage (Kunath et al., 2007; Nichols et al., 2009; Lanner and Rossant, 2010; Yamanaka et al., 2010; Krawchuk et al., 2013). Moreover, FGF4 does not only direct mouse ESCs towards the PrE, but also supports TE self-renewal (Tanaka et al., 1998; Goldin and Papaioannou, 2003). Overall, FGF4, including its chaperone heparin, is an all-round crucial factor for murine blastocyst formation (Allen and Rapraeger 2003; Furue et al., 2008) and therefore it is included in nearly all mouse blastoid models (Rivron et al., 2018; Li et al., 2019; Sozen et al., 2019; Vrij et al., 2019).
In human HYPO development on the other hand, FGF signaling is dispensable, showcasing a marked difference between mouse and human in the regulation of the second lineage bifurcation (Roode et al., 2012). While it might not be essential, FGF signaling through the protein FGF2/bFGF does support HYPO induction, alongside other pathways as mentioned above (Linneberg-Agerholm et al., 2019). Furthermore, it is well-established that FGF2/bFGF supports human ESC self-renewal in the conventional primed pluripotency state (Xu et al., 2005), indicating its importance for maintenance of the post-implantation epiblast.
JAK/STAT Pathway Modulators
One of the vital factors for mouse ESC proliferation and self-renewal is the JAK/STAT3 pathway activator LIF which is produced by the preimplantation TE and feeds the EPI (Williams et al., 1988). In addition to maintaining pluripotency and EPI identity, LIF also promotes PrE specification in blastocysts (Morgani and Brickman, 2015). A related activator of the JAK/STAT3 pathway, IL-11, complements FGF4 in the maintenance of TE self-renewal in blastoids (Rivron et al., 2018).
Several factors in the JAK/STAT3 pathway are applied in human blastoid protocols as well (Fan et al., 2021; Kagawa et al., 2021; Liu et al., 2021; Sozen et al., 2021; Yu et al., 2021). LIF is widely included to maintain the pluripotent EPI (Fan et al., 2021; Kagawa et al., 2021; Sozen et al., 2021; Yu et al., 2021), even though it appears less crucial for proliferation and self-renewal in human as it is in mouse (Humphrey et al., 2004; Dahéron et al., 2008). Meanwhile EGF is included in several blastoid protocols (Liu et al., 2021; Sozen et al., 2021; Yu et al., 2021) for its support of TE differentiation and self-renewal (Okae et al., 2018).
ROCK Inhibitor
One universally applied compound in blastoid protocols is the ROCK inhibitor Y-27632. In mouse blastoids, it was found to improve the engulfment of ESC aggregates by TSCs and was subsequently used in all protocols where TSCs are added separately (Rivron et al., 2018). Interestingly, studies in murine blastocysts indicate that ROCK inhibitor prevents cavitation in vivo and hampers TE induction through Hippo signaling (Kawagishi et al., 2004; Kono et al., 2014). Whether it similarly obstructs blastoid formation in some way in vitro is currently unknown.
For human PSC culture, ROCK inhibitor is a common addition to prevent apoptosis when human PSCs are reseeded as single cells (Watanabe et al., 2007). As such, it is included at least for the first 24 h in every human blastoid protocol to enhance single cell survival before aggregation.
Hippo Pathway Modulators
The mouse blastoid study by Kime et al. and the human blastoid study by Kagawa et al. show that Hippo signaling inhibition is key for TE specification in blastoids (Kime et al., 2019; Kagawa et al., 2021), similar to mouse (Yu et al., 2016) and human embryos (Gerri et al., 2020a). Kime et al. applied OMPT, an agonist of Hippo inhibitor Lysophosphatidic Acid (LPA) in their phase 2 medium (Kime et al., 2019), after they previously found that LPA promotes the conversion of EpiSCs to naïve PSCs (Kime et al., 2016). In the human blastoid system, Kagawa et al. added LPA and found that this significantly increased the blastoid formation efficiency (Kagawa et al., 2021).
cAMP Modulation in Mouse Blastoids
In most mouse blastoid models, 8Br-cAMP, a synthetic cyclic AMP analog, is applied in order to support both maintenance of the TE through upregulation of CDX2 expression, as well as to enhance cavitation of the blastoids (Rivron et al., 2018; Sozen et al., 2019; Vrij et al., 2019). This compound has however not been included in any of the human blastoid protocols.
Modulators Specific for Human Blastoids
Besides signaling pathway modulators with a clear connection to developmental processes, some of the human blastoid protocols include a number of small molecules that have no apparent strong connection to established developmental signaling pathways. Several of these, SRCi, M2-R/H1-Ri, and PARPi, are added to maintain the pluripotent compartment in the blastoids (Theunissen et al., 2014; Yang Y. et al., 2017). The histone deacetylase inhibitor valproic acid (VPA) is included as a component of the previously defined human trophoblast medium (Okae et al., 2018). Notably, the use of either VPA or a similar histone deacetylase inhibitor facilitates the induction of naïve pluripotency via resetting of the epigenome (Guo et al., 2017).
Hormones
Several hormones are also included in some of the models. Insulin has become a standard component of both mouse and human TSC medium compositions and as such is now also present in blastoid protocols of both species (Kubaczka et al., 2014; Okae et al., 2018). Insulin is a well-established activator of the PI3K pathway, through which it promotes cell survival and proliferation (Taniguchi et al., 2006). This support of cell survival and proliferation led to the inclusion of insulin in the media for human PSC cultures, alongside FGF2 and ascorbic acid (Chen et al., 2011). Correspondingly, insulin appears to give naïve human PSCs a growth advantage and thus curbs HYPO differentiation (Anderson et al., 2017). Finally, there are the two sex hormones, β-oestradiol and progesterone, which are known to increase endometrial receptivity in human and mice and upregulate cytokines that support implantation of the murine blastocyst (Norwitz et al., 2001; Basak et al., 2002; Young, 2013). Both hormones have been applied in human blastoid cultures, as components of a medium called “in vitro culture 1” (IVC1). This medium is developed for and typically used to permit in vitro post-implantation progression of blastocysts rather than for pre-implantation embryo culture (Morris et al., 2012; Bedzhov et al., 2014).
To summarize, there is evidently a myriad of components that have so far been included in blastoid protocols, especially in the human ones. This can be attributed to the fact that most of the currently published human protocols use mixes of pre-existing media to induce the blastocyst lineages in 3D cultures. Notably, the protocols developed by Yanagida et al. and Kagawa et al. only include a handful of pathway regulators and are therefore surprisingly minimalistic compared to the others. This begs the question which of the compounds applied so far are truly indispensable for human blastoid generation, particularly when using PSCs maintained in the naïve state.
Culture Platforms
Regulating the mechanical environment of stem cells to direct assembly, trigger differentiation and guide development has recently gained traction, since an increasing number of studies show a relation between physical properties of the microenvironment and stem cell behavior (Guilak et al., 2009; Han et al., 2014). For a review on the potential of in vitro models to study mechanical and geometrical cues in early mammalian embryogenesis, refer to (Vianello and Lutolf, 2019).
In the case of blastoids, the culture platforms need to facilitate several requirements. Firstly, after initial seeding, cells need to be in each other’s proximity to aggregate. Secondly, the cells/aggregates require the efficient exchange of nutrients, signaling molecules and growth factors to support their growth, differentiation and proliferation. Thirdly, it is important to take into account that mechanical cues also directly affect the signaling pathways involved in development (e.g., Hippo signaling) (Barzegari et al., 2020). As blastoids are a pre-implantation model, adherence to the culture platform should be prevented. Usually, this is established either through the use of a suitable, non-adherent material [e.g., agarose or polyethylene glycol (PEG) for hydrogel microwells], by pre-coating culture plates with an anti-adherence solution [e.g., polyethylene-oxide polypropylene-oxide (hydrophilic-hydrophobic)] block copolymers for Aggrewells or by using pre-treated plates (ultra-low attachment plates). Finally, through the use of microwells arrays, large quantities of blastoids can be produced for one experiment, facilitating high-throughput analysis.
For murine blastoids, either in-house produced agarose hydrogel microwells or commercial Aggrewell microwells have been used (Table 1). Both set-ups contain a large quantity of microwells (400–1200 per well of a well-plate) (Vrij et al., 2016). Advantages of agarose hydrogel microwells over hard-plastic Aggrewell microwells may be the aid in diffusion of nutrients and waste products throughout the gel and the circular geometry that is less obstructive compared to the inverted pyramidal-shape of Aggrewell microwells. Moreover, agarose hydrogel microwells are amenable to in situ bright-field and epi-fluorescence imaging and downstream analysis and therefore blastoids do not require transfer to other platforms prior to analysis. The disadvantage of agarose-hydrogel microwells however is that diffusion of soluble components into the gel hampers abrupt and complete switches in medium conditions. Additionally, transfer of blastoids is still required for high resolution (i.e., confocal-based) imaging.
Similar to mouse, most human blastoid protocols are developed using Aggrewell microwells. However, Kagawa et al. used the agarose hydrogel microwells and Yanagida et al. reported using ultra-low attachment multiwell plates and non-adherent, “U”-bottomed 96-wells plates (Table 2). Yanagida et al.’s method of manually transferring structures to new media conditions however is very laborious, time-consuming, likely leading to the loss of (developing) blastoids and not amenable to high-throughput screenings.
Although Aggrewell microwells are widely used and support the culture of more than 1000 structures per well, they too come with several disadvantages. While it may seem arbitrary, a technical issue due to their pyramidal shape is the difficulty of changing media without disturbing the forming structures. As such, switching media for sequential inductions of blastocyst lineages is practically hindered. In contrast, agarose hydrogel microwells are far less sensitive for flow disturbances due to their cylindrical geometry. Another disadvantage of Aggrewell microwells is that the inverted pyramidal shape significantly hinders the in situ imaging quality and thus image-based readouts of the structures. As a result, blastoids need to be manually transferred to different platforms for imaging and further analysis, which poses the same issues as listed above for the method employed by Yanagida et al. All in all, among the currently reported microwell platforms for blastoid generation, a platform in which blastoids can be imaged directly with high quality is still lacking.
Recapitulation of Development
The formation of blastoids consists of roughly the same steps in mouse and human protocols: aggregation, cavitation and maturation. First, upon seeding, the PSCs need to aggregate into spherical structures. This invariably occurs in the first 24 h with both mouse and human cells. Generally, media still mainly contain factors to support maintenance of pluripotency at this stage. Once the PSCs have clumped together, the medium composition is often changed to induce lineage specification and self-renewal of these lineages. For methods that require TSCs separately, TSCs are added after the first 24 h. Subsequently, the TSCs engulf the aggregated PSCs. From this stage onward, the forming blastoid structures self-organize and undergo cavitation, followed by a maturation process in which the blastoid increases in size, forms distinct EPI and PrE cells and occasionally organize themselves by sorting out a continuous epithelial layer of PrE cells overlying the EPI within the blastoid. Lineage specification takes place in parallel with these morphological changes. Thus, blastoids undergo embryonic events reminiscent of the pre-implantation embryo (Takaoka and Hamada, 2012).
In vivo, mouse and human development occur at a different pace. While the mouse embryo grows from a zygote to a blastocyst in approximately 3.5 days and implants around day 4.5–5, the human embryo reaches the blastocyst stage around day 5 and implants between day 7 and 8 (Molè et al., 2020). Interestingly, this difference in developmental pace between species can to some extent also be observed in vitro. Mouse blastoids generated with naïve ESCs have been reported to cavitate as early as 48 h after adding TSCs to ESC aggregates, while protocols using mouse EPSCs report cavitation around 72–96 h post TSC seeding. When using EpiSCs, cavitation may even occur as late as after 120 h, however this protocol is difficult to compare with the others, since the 3D structures arise from a converting 2D culture, rather than a 3D setup (Table 1). Similar to mouse EPSC-based blastoids, most human blastoids form a cavity between 72 and 96 h (Table 2). The blastoids from Kagawa et al. and Yanagida et al. based on naïve PSCs deviate from this timing. These blastoids were observed to have a cavity as early as at the 48-h time point (Yanagida et al.) or at the 60-h time point (Kagawa et al.).
When blastoids contain all three lineages and their morphology resembles the blastocyst, they are considered fully formed and their generation is complete. At what time point this stage is reached varies hugely between models. Mouse blastoids are generally fully formed at 96 h, except for the Li et al. EPSC-only blastoids, which are fully formed at 120–144 h, and the EpiSC-based blastoids from Kime et al., which may take up to 168 h. Completion of human blastoid generation on the other hand varies between 96 and 216 h. While naïve PSC-based blastoids by Yanagida et al. and Kagawa et al. are fully formed in 96–120 h (Kagawa et al., 2021; Yanagida et al., 2021), similar to the timeline for human morula to blastocyst development (Niakan et al., 2012; Rossant and Tam, 2017), Yu et al. report that their protocol, also based on naïve PSCs, takes 7–9 days (168–216 h) (Yu et al., 2021). The iBlastoids from Liu et al. and both EPSC-based blastoid models require approximately 144 h (Fan et al., 2021; Liu et al., 2021; Sozen et al., 2021). The difference in timing between mouse and human likely reflects the different rates of development also observed between the blastocysts of these species. The variation observed between models of the same species on the other hand may reflect the efficiency of PSC commitment to the blastocyst lineages and the efficiency of the subsequent interactions between lineages.
It is precisely the proper commitment of PSCs to the blastocyst lineages that is one of the general challenges in both mouse and human blastoid models. In mouse models, the initial naïve ESC-based blastoids often lack a well-defined PrE compartment (Rivron et al., 2018). Subsequent studies led to a modified protocol that specifically induces PrE formation in blastoids (Vrij et al., 2019). Notably, blastoids based on EPSCs form PrE more readily, which Sozen et al. linked to the enhanced pluripotency state of EPSCs compared to mouse ESCs (Sozen et al., 2019). Despite this enhanced potential, EPSC-only blastoids from Li et al. contain mislocalized EPI/PrE- or TE-like cells as well as significant cell populations that might consist of either uncommitted PSCs remaining in the EPSC state or intermediates between lineages (Li et al., 2019).
The presence of cell types in vitro that are not present in the natural blastocyst is usually ascribed to incomplete induction. These off-target cell types can either be entirely uncommitted to any lineage, intermediates between EPI, PrE/HYPO and/or TE, or they correspond to a later stage of development. For many of the current human blastoid models, either one or multiple types of off-target cells have been described. Further inspection of lineage markers in the iBlastoids led to the conclusion that the majority of the cells in these structures are not conclusively committed to a specific lineage, but express markers from at least two different lineages (Liu et al., 2021; Yanagida et al., 2021). Similar overlap in marker gene expression was observed by Fan et al. in their EPSC-based blastoids (Fan et al., 2021). The naïve cell-based blastoids from Yu et al. also contain a large fraction of off-target cells, but these seemingly remain in a naïve-like, uncommitted state (Yanagida et al., 2021; Yu et al., 2021). These uncommitted cells most resemble EPI and far outnumber the TE-like cells, while in the blastocyst TE is the most abundant of the three lineages (Yanagida et al., 2021). In contrast, Yanagida et al. showed that the majority of cells in their blastoids commit to the TE lineage. This is confirmed by the mutually exclusive expression of GATA2 in their TE compartment and expression of OCT4 and naïve marker KLF17 in the ICM compartment. In the same line, Kagawa et al. show that between 50 and 80% of the cells in their blastoids can be attributed to the TE lineage based on immunofluorescence staining. Additionally, they report that less than 3% of cells were transcriptionally similar to post-implantation tissues (amnion and extra-embryonic mesoderm), when comparing the single cell transcriptomes of blastoid cells to blastocysts, in vitro outgrowths of blastocysts and gastrulation-stage embryos (Petropoulos et al., 2016; Zhou et al., 2019; Tyser et al., 2020). All other cells matched pre-implantation blastocyst lineages.
While blastoids from Yu et al. contain cells stuck in a developmental stage preceding the blastocyst stage, the iBlastoids appear to contain cells that correspond to the post-implantation stage. When comparing their single cell transcriptomic data with post-implantation blastocysts, the cell population within iBlastoids that was identified as TE is found to more closely resemble amnion-like cells, rather than TE (Zhao et al., 2021). This could suggest that the cells used to make iBlastoids are not completely reprogrammed towards a state of pluripotency similar to naïve PSCs and therefore less able to give rise to the TE compartment (Zheng et al., 2019; Cinkornpumin et al., 2020; Guo et al., 2021). Sozen et al. found that their human EPSC-based blastoids too have only partially specified TE, as the TE compartment could not form a cohesive epithelium. Moreover, some of these EPSC -based blastoids contain multiple cavities (Sozen et al., 2021). Besides the specification of the TE, current human blastoid models also struggle with formation of the HYPO compartment. As was the case for the first mouse blastoids (Rivron et al., 2018), the human models often appear to lack the proper localization and/or HYPO cell numbers (Fan et al., 2021; Liu et al., 2021; Sozen et al., 2021; Yanagida et al., 2021; Yu et al., 2021).
Comparison of Blastoids With Blastocysts
In order to prove the functionality of blastoids as models of blastocysts, two experiments are generally conducted. First, following the identification and correct spatial allocation of the EPI, PrE/HYPO and TE lineages (e.g., through single cell RNAseq and immunohistochemistry), cell lines are derived from these cell types as a functional assay to verify their propagation potential in vitro and their downstream differentiation potential. The second experiment is to test the capacity of complete blastoids to undergo post-implantation progression.
Derivation of Stem Cell Lines
Originally developed for derivation from natural mouse blastocysts, the general approach to obtain stem cell lines from blastoids is to seed the blastoids on a culture plate with either ESC, TSC or PrE/hypoblast-like cell culture medium (Evans and Kaufman, 1981; Martin, 1981; Tanaka et al., 1998; Ying et al., 2008; Niakan et al., 2013; Okae et al., 2018; Linneberg-Agerholm et al., 2019) that selectively favors the desired cell type over several passages. The mouse naïve PSC-based blastoids of Rivron et al., the mouse EPSC-only blastoids of Li et al. and mouse EpiSC-based blastoids of Kime et al. all confirmed the presence of EPI and TE lineages in their blastoids with this approach (Rivron et al., 2018; Kime et al., 2019; Li et al., 2019). Additionally, blastocyst chimera assays confirmed the contribution of these cell lines to the expected compartments when grown out in utero. Moreover, besides the chimeric contribution of EPI and TE lineages, the PrE line derived from mouse EPSC-only blastoids contributed to the yolk sac.
As for the human blastoid models, derivation experiments were performed on the naïve PSC-based blastoids of Kagawa et al. and Yu et al., the EPSC-based blastoids of Fan et al. and the iBlastoids of Liu et al., though only Yu et al. included a culture selecting for HYPO-like cells (Linneberg-Agerholm et al., 2019; Yu et al., 2021). Remarkably, despite the low number of TE-like cells, Yu et al. was able to derive TSC cells from single plated blastoids, as well as naïve PSCs and naïve endoderm cells. The identity of these cell lines was confirmed with immunostaining, differentiation assays and injection of the derived cells into mouse blastocysts. Kagawa et al. were able to derive naïve PSCs and TSCs from their blastoid model. These derived TSCs differentiate towards syncytiotrophoblast and extravillous trophoblast cell fates in 3–6 days, as was confirmed through immunostaining and RT-qPCR of several markers. Strikingly, blastoid-derived naïve PSCs could be used to generate a second generation of blastoids. Also Liu et al. derived TSCs and naïve PSCs from their iBlastoids. The naïve PSCs were confirmed to be pluripotent through a tri-lineage differentiation assay and the TSCs were differentiated towards TE derivatives, with immunostainings supporting the presence of marker genes. Finally, Fan et al. derived PSCs and TSCs from their blastoids, which they confirmed with immunostainings of several lineage markers.
Though these derivations say little about the developmental potential of the blastoids as complete structures, they do indicate that blastoids contain PSCs or cells with similar potential capable of differentiating towards post-implantation cell types.
Post-Implantation Development In Vitro
Culture media that permit the in vitro post-implantation progression of blastocysts have in recent years contributed to embryo phenotyping and mechanistic understanding, particularly for human since this stage is non-accessible in vivo (Bedzhov et al., 2014; Deglincerti et al., 2016; Shahbazi et al., 2016; Xiang et al., 2020).
In vitro, blastocysts are transferred to a plastic or glass substrate and cultured in optimized in vitro culture (IVC) medium containing for the first 2 days serum, to promote growth and attachment of the TE cells, which is later substituted by knockout serum (Bedzhov et al., 2014). With this method, post-implantation morphological changes in the embryo, in particular the EPI transformation into the egg cylinder with concomitant visceral endoderm patterning, can easily be tracked for up to 5 days of development. Likewise, blastoids can be exposed to similar culture conditions to assess their potential for early post-implantation development. Naïve mouse ESC-based blastoids reportedly only continue development rarely in the in vitro system. The PrE-induced blastoids on the other hand manage to occasionally grow out into post-implantation epiblast-like structures encasing pro-amniotic-like cavities- and surrounded by a visceral endoderm-like epithelium (Vrij et al., 2019). Vrij et al. suggested this developmental potential is dependent on the abundance of PrE-like cells in the blastoids prior to post-implantation culture (Vrij et al., 2019). This is supported by the in vitro post-implantation assays performed with EPSC-based blastoids, which form PrE more robustly. In line with the development of blastocysts and post-implantation embryo models (Bedzhov et al., 2014), both EPSC-based mouse blastoid models could form egg cylinder-like structures (Li et al., 2019; Sozen et al., 2019). These structures contained cells similar to EPI, and post-implantation PrE and TE derivatives, visceral endoderm and extraembryonic ectoderm, respectively. Moreover, Li et al. observed that these structures recapitulated post-implantation polarization events on the molecular level (Li et al., 2019). Importantly, however, current 2D in vitro culture methods for post-implantation morphogenesis of blastocysts and blastoids do not readily permit the formation of mural trophectoderm, associated parietal endoderm and the ectoplacental cone [current gaps in embryo model development are reviewed in Shankar et al. (2021)].
The golden standard for mouse models however, is the injection of blastoids into the uteri of pseudo-pregnant mice to assess their capacity for full placentation and development beyond implantation. Rivron et al. reported the ability of naïve ESC-based blastoids to induce decidualization in utero (between 10 and 20% of injected blastoids implanted), but structures failed to develop further, similar to the in vitro findings. The EPSC-only blastoids of Li et al. resembled naïve ESC-based blastoids in that they could implant in the uterine wall and trigger decidualization (with ∼7% efficiency) but failed to continue development in this more stringent assay (Li et al., 2019). Sozen et al. reported similar findings for their EPSC-based blastoids. Upon closer inspection, they found that although the blastoids physically attached to the maternal tissue, they did not develop a Reichert’s membrane (Sozen et al., 2021). This membrane was found to play an important role in post-implantation morphogenesis (Ueda et al., 2020), therefore the lack of this structure in the post-implantation progression of blastoids may prevent further development.
Although it is possible to perform in vivo post-implantation assays for mouse blastoids, this is obviously unacceptable for the human equivalent. Therefore, human blastoids were only tested for their post-implantation developmental potential in vitro. Most groups use roughly the same attached culture method as described above for mouse blastoids and blastocysts (Bedzhov et al., 2014), adapted to support human blastocyst development in vitro (Deglincerti et al., 2016). Notably, all the groups that applied this method report a flattening of the blastoid structures upon attachment as is also observed in in vitro post-implantation blastocyst culture (Deglincerti et al., 2016; Shahbazi et al., 2016). Moreover, all confirm the presence of markers of at least two of the three blastocyst lineages and provide evidence of the onset of amniotic cavity formation. Additionally, Yu et al. and Yanagida et al. report indications of the onset of yolk sac formation. However, the success rates for the formation of post-implantation structures are quite low. Yu et al. obtained post-implantation-like structures from 10% of the blastoids, while 20–30% of the iBlastoids plated for attached culture formed these structures. Sozen et al. confirmed that ∼60% of their attached structures had clear EPI and TE compartments, but this rate is lower for structures that also contain a HYPO marker. What fraction of these post-implantation structures contains a pro-amniotic-like cavity is not quite clear. Meanwhile Fan et al. and Yanagida et al. reported post-implantation-like structures as well but did not include the formation efficiency.
Kawaga et al. had a slightly different approach for mimicking post-implantation progression. They applied a method similar to the common one described above, but theirs was adapted from a protocol designed for cynomulgus monkey embryos, rather than mouse (Ma et al., 2019). Using this attached culture method, they reported the differentiation and expansion of all three blastocyst lineages and the formation of an amniotic cavity in some attached blastoids. Their post-implantation culture could be maintained for 6 days, to a day 13 equivalent stage, however these cultures did not recapitulate the spatial organization of this developmental stage in vivo.
Taken together, the results of the assays discussed above indicate that both the current mouse and human blastoid models are capable of mimicking some of the morphogenetic events typical for the post-implantation blastocyst in vitro, albeit at a low efficiency. However, the more stringent in vivo experiments with mouse blastoids and the failure of human blastoids to comprehensively recapitulate spatial tissue organization of post-implantation development, underline that the developmental potential of current blastoid systems and/or post-implantation culture methods is still limited for both species. More sophisticated bioengineered 3D culture methods may be needed to overcome this barrier.
Modelling Processes of Blastocyst Development
Besides investigations of the potential of blastoid models to continue development to a post-implantation stage, some groups reported additional findings to showcase the capacity of their blastoid models to recapitulate essential processes during pre-implantation development.
In mouse blastoids, Rivron et al. found that the EPI maintains the self-renewal and epithelial identity in the adjacent polar TE (Rivron et al., 2018). This is in line with findings from Gardner et al. that trophoblast cells in contact with the ICM don’t terminally differentiate, but instead contribute to the proliferating polar TE-derived ectoplacental cone (Gardner and Johnson, 1972; Gardner et al., 1973).
Furthermore, Li et al. demonstrated that their EPSC-only blastoids accumulated ZO1 and E-Cadherin at cell-cell junctions during the aggregation of the EPSCs which resembles compaction in the 8-cell stage. They also provide evidence of apical enrichment of PARD6 expression during blastoid formation (Li et al., 2019), which is an indication of polarization (Chazaud and Yamanaka, 2016). Additionally, they show nuclear YAP expression in the outer cells and cytoplasmic YAP expression in the inner cells in about 60% of their EPSC-only blastoids on day 5 (Li et al., 2019). This intracellular localization pattern of YAP, part of the Hippo signaling, is proposed to play a role in TE specification in human and mouse during blastocyst formation (Gerri et al., 2020a).
Similar evidence of processes preceding blastocyst formation in vivo, such as compaction and polarization, was found in human blastoids as well. Sozen et al. observed the baso-lateral expression of E-cadherin and apical enrichment of F-actin and PARD6 (Sozen et al., 2021). In previous studies, the PLC-PKC pathway was suggested to play a role in cell polarization and TE specification in human embryos (Zhu et al., 2020). Using their blastoid system, Sozen et al. could observe that both PLC-specific inhibition and depletion reduced the expression of GATA3, one of the early TE markers, in outer cells of aggregates (Sozen et al., 2021). Simultaneously, the apical expression of PARD6, which marks polarization, was reduced too. Natural human embryos have a comparable response to in vitro PLC inhibition and depletion treatments (Zhu et al., 2020), supporting the capacity of the blastoid system to recapitulate pre-implantation processes.
Furthermore, Sozen et al. investigated the effect of WNT3A. In mouse blastoids, WNT3A supports cavitation and increased blastoid yield (Rivron et al., 2018). However, addition of WNT3A does not improve cavitation in human blastoid cultures. This may indicate a different role for WNT3A in human development (Sozen et al., 2021).
In line with the study of the PLC-PKC pathway by Sozen et al., Yu et al. more extensively investigated the presence of tight junctions in their blastoids and found that, similar to murine blastocysts (Eckert et al., 2004a; Eckert et al., 2004b), their blastoids required the activity of specific PKC for cavitation (Yu et al., 2021).
Kagawa et al. further complimented these findings by their study of Hippo signaling in their blastoids. They found that atypical PKC inhibition strongly reduced nuclear accumulation of YAP1. As aforementioned, as a downstream effector of Hippo signaling, YAP1 is widely established to be involved TE specification in both mouse and human in vivo (Gerri et al., 2020a). Kagawa et al. confirmed the same is true in their blastoids, as aPKC inhibition and reduced YAP1 nuclear accumulation also correlated with reduced number of GATA3-positive cells and overall failure of human PSC aggregates to form blastoids. In addition, they show that YAP1 overexpression accelerated cavitation of their blastoids (Kagawa et al., 2021). They further elucidated on the process of cavitation by demonstrating that, similar to the mouse blastocyst (Dumortier et al., 2019), the cavity of their blastoids forms through the merging of multiple fluid-filled cavities (Kagawa et al., 2021).
Beyond the processes of blastocyst formation prior to implantation, blastoids can be used to model the earliest maternal-embryo interaction. In fact, Kagawa et al. present an in vitro model of embryo adhesion, the first step in implantation, by combining their blastoid model with so-called open-faced endometrial layers (OFELs) that mimic the endometrium. Using this set-up, Kagawa et al. demonstrate that their blastoids are capable of attaching to receptive endometrium cells and repelling it. Moreover, they show blastoids specifically attach to OFELs with their polar TE region. This is the region of the TE that directly borders on the EPI cluster. Kagawa et al. generated several types of trophospheres, which are blastoids without an EPI compartment, and none of these trophospheres were unable to attach to receptive OFELs, neither did post-implantation stage TSCs and human PSC aggregates. Thus Kagawa et al. provide evidence that the TE receives cues from the EPI that enable it to interact with the endometrium. In silico ligand-receptor pair analysis performed by Kagawa et al. using single-cell transcriptomics data yielded a list of potential molecular interactions between TE and endometrium epithelium, which may provide clues for future studies on implantation.
All in all, both mouse and human blastoids, despite the abundance of off-target cell types detected, especially in some of the current human models, already show great potential for investigating pre-implantation processes, such as polarization and cavitation. Notably, Kagawa et al. demonstrated that human blastoids can be used to model implantation in vitro when combined with maternal endometrium epithelium cell types. Although it remains unknown whether blastoids fully mimic pre-implantation development while their lineage specification remains (partially) incomplete, current results indicate that some processes underlying blastocyst formation in vivo may be faithfully recapitulated in vitro.
Conclusion and Prospects
There can be little doubt that blastoids are a valuable addition to the collection of stem cell-based models. Compared to other stem cell-based embryo-like structures, including gastruloids, 2D micropatterned stem cells, post-implantation amniotic sac embryoids and embryonic-extraembryonic fusion embryoids [reviewed in Fu et al. (2021), Shankar et al. (2021)], blastoids have the unique potential to recapitulate the pre-implantation embryo and are therefore the singular model for studying this early stage of development in vitro.
The development of mouse blastoids several years ago has paved the way for the recent development of the human blastoid model. Although there are clear differences between mouse and human in terms of signaling pathway regulation, they share similar core signaling pathways that are tightly regulated during early embryogenesis. This knowledge has guided studies towards medium compositions that are now used to induce the three founding blastocyst lineages in the human blastoid. Moreover, microwell culture platforms first applied to mouse models, as well as several lineage markers originally identified in mice have been adopted for the human system as well. Improvements are still required for blastoids to more convincingly mimic peri- and post-implantation development, particularly regarding the extra-embryonic mesoderm, HYPO and TE compartments. Nevertheless, the first results are promising, as they show that the current models undergo morphological changes and upon attachment partly mimic the architecture of post-implantation embryonic and several extra-embryonic structures in vitro. More importantly, pre-implantation development is at least partially recapitulated and blastoids can be used to study cell-cell interactions typical for the blastocyst stage as well as molecular pathways involved in lineage specification.
The possibility of mimicking the pre-implantation embryo has huge implications for a plethora of studies, such as aneuploidy studies, toxicity screens, development of improved IVF compounds and studies on peri-implantation cell differentiation. Combined with more sophisticated, bioengineered in vitro models of the uterine wall, human blastoids may become an attractive tool for studying the process of implantation in humans. New insights in this area will indubitably deepen our understanding of key requirements for successful implantation and subsequently aid the development of new contraceptives and therapeutic routes towards treating implantation failure in the future.
The introduction of the human blastoids to the arena of stem cell-based embryo models does certainly not make the mouse models redundant, however. On the contrary, increasingly meaningful comparisons can be made as these models are further developed to more faithfully recapitulate early embryogenesis. Comparisons between mice and human embryogenesis are relevant from an evolutionary perspective, but may also serve the field of medicine, particularly when considering implantation-related complications, as mentioned above. The more extensively studied mouse model may provide more clues for specific pathways and mechanisms to probe in the human system, saving both time and human material. Moreover, while the first reports of human blastoids have sparked debates on the ethical restrictions on research performed with human embryo (-like) structures (Pereira Daoud et al., 2020; Clark et al., 2021), the mouse embryo models may remain the favored models for more ethically challenging experiments, e.g., involving gene-editing techniques. Additionally, mouse models may be able to help chart post-implantation to a stage that human models will not be allowed to reach for ethical and legal reasons, such as in vitro and in utero advanced organogenesis or organismal development. In conclusion, these two model systems will continue to develop alongside each other and together have broad future application, both for fundamental and clinical research.
Author Contributions
DL, SG, and EV wrote the manuscript. VS collected and processed the data for the different blastoid protocols. CB helped to direct the manuscript. All the authors discussed and corrected the manuscript. SG and EV contributed equally to the manuscript.
Funding
This research has received funding from the European Research Council (ERC) under the European Union’s Horizon 2020 Research and Innovation Programme grant agreement No 694801. The authors gratefully acknowledge the Gravitation Program “Materials Driven Regeneration”, funded by the Netherlands Organization for Scientific Research (024.003.013).
Conflict of Interest
The authors declare that the research was conducted in the absence of any commercial or financial relationships that could be construed as a potential conflict of interest.
Publisher’s Note
All claims expressed in this article are solely those of the authors and do not necessarily represent those of their affiliated organizations, or those of the publisher, the editors and the reviewers. Any product that may be evaluated in this article, or claim that may be made by its manufacturer, is not guaranteed or endorsed by the publisher.
Acknowledgments
The authors acknowledge financial support from Stichting De Weijerhorst and from the Dutch Province of Limburg (program “Limburg INvesteert in haar Kenniseconomie/LINK”).
References
Allen, B. L., and Rapraeger, A. C. (2003). Spatial and Temporal Expression of Heparan Sulfate in Mouse Development Regulates FGF and FGF Receptor Assembly. J. Cel Biol. 163, 637–648. doi:10.1083/jcb.200307053
Amita, M., Adachi, K., Alexenko, A. P., Sinha, S., Schust, D. J., Schulz, L. C., et al. (2013). Complete and Unidirectional Conversion of Human Embryonic Stem Cells to Trophoblast by BMP4. Proc. Natl. Acad. Sci. 110, E1212–E1221. doi:10.1073/pnas.1303094110
Anderson, K. G. V., Hamilton, W. B., Roske, F. V., Azad, A., Knudsen, T. E., Canham, M. A., et al. (2017). Insulin fine-tunes Self-Renewal Pathways Governing Naive Pluripotency and Extra-embryonic Endoderm. Nat. Cel Biol 19, 1164–1177. doi:10.1038/ncb3617
Barzegari, A., Gueguen, V., Omidi, Y., Ostadrahimi, A., Nouri, M., and Pavon‐Djavid, G. (2020). The Role of Hippo Signaling Pathway and Mechanotransduction in Tuning Embryoid Body Formation and Differentiation. J. Cel Physiol 235, 5072–5083. doi:10.1002/jcp.29455
Basak, S., Dubanchet, S., Zourbas, S., Chaouat, G., and Das, C. (2002). Expression of Pro-inflammatory Cytokines in Mouse Blastocysts during Implantation: Modulation by Steroid Hormones. Am. J. Reprod. Immunol. 47, 2–11. doi:10.1034/j.1600-0897.2002.1o047.x
Bayerl, J., Ayyash, M., Shani, T., Manor, Y. S., Gafni, O., Massarwa, R., et al. (2021). Principles of Signaling Pathway Modulation for Enhancing Human Naive Pluripotency Induction. Cell Stem Cell 28 (9), 1549–1565. e12. doi:10.1016/j.stem.2021.04.001
Bedzhov, I., Leung, C. Y., Bialecka, M., and Zernicka-Goetz, M. (2014). In Vitro culture of Mouse Blastocysts beyond the Implantation Stages. Nat. Protoc. 9, 2732–2739. doi:10.1038/nprot.2014.186
Blakeley, P., Fogarty, N. M., del Valle, I., Wamaitha, S. E., Hu, T. X., Elder, K., et al. (2015). Defining the Three Cell Lineages of the Human Blastocyst by Single-Cell RNA-Seq. Development 142, 3151–3165. doi:10.1242/dev.123547
Boroviak, T., Loos, R., Lombard, P., Okahara, J., Behr, R., Sasaki, E., et al. (2015). Lineage-Specific Profiling Delineates the Emergence and Progression of Naive Pluripotency in Mammalian Embryogenesis. Developmental Cel 35, 366–382. doi:10.1016/j.devcel.2015.10.011
Bredenkamp, N., Stirparo, G. G., Nichols, J., Smith, A., and Guo, G. (2019a). The Cell-Surface Marker Sushi Containing Domain 2 Facilitates Establishment of Human Naive Pluripotent Stem Cells. Stem Cel Rep. 12, 1212–1222. doi:10.1016/j.stemcr.2019.03.014
Bredenkamp, N., Yang, J., Clarke, J., Stirparo, G. G., von Meyenn, F., Dietmann, S., et al. (2019b). Wnt Inhibition Facilitates RNA-Mediated Reprogramming of Human Somatic Cells to Naive Pluripotency. Stem Cel Rep. 13, 1083–1098. doi:10.1016/j.stemcr.2019.10.009
Brons, I. G. M., Smithers, L. E., Trotter, M. W. B., Rugg-Gunn, P., Sun, B., Chuva de Sousa Lopes, S. M., et al. (2007). Derivation of Pluripotent Epiblast Stem Cells from Mammalian Embryos. Nature 448, 191–195. doi:10.1038/nature05950
Castel, G., Meistermann, D., Bretin, B., Firmin, J., Blin, J., Loubersac, S., et al. (2020). Induction of Human Trophoblast Stem Cells from Somatic Cells and Pluripotent Stem Cells. Cell Rep 33, 108419. doi:10.1016/j.celrep.2020.108419
Chan, Y.-S., Göke, J., Ng, J.-H., Lu, X., Gonzales, K. A. U., Tan, C.-P., et al. (2013). Induction of a Human Pluripotent State with Distinct Regulatory Circuitry that Resembles Preimplantation Epiblast. Cell Stem Cell 13, 663–675. doi:10.1016/j.stem.2013.11.015
Chazaud, C., and Yamanaka, Y. (2016). Lineage Specification in the Mouse Preimplantation Embryo. Development 143, 1063–1074. doi:10.1242/dev.128314
Chen, G., Gulbranson, D. R., Hou, Z., Bolin, J. M., Ruotti, V., Probasco, M. D., et al. (2011). Chemically Defined Conditions for Human iPSC Derivation and Culture. Nat. Methods 8, 424–429. doi:10.1038/nmeth.1593
Cinkornpumin, J. K., Kwon, S. Y., Guo, Y., Hossain, I., Sirois, J., Russett, C. S., et al. (2020). Naive Human Embryonic Stem Cells Can Give Rise to Cells with a Trophoblast-like Transcriptome and Methylome. Stem Cel Rep. 15, 198–213. doi:10.1016/j.stemcr.2020.06.003
Clark, A. T., Brivanlou, A., Fu, J., Kato, K., Mathews, D., Niakan, K. K., et al. (2021). Human Embryo Research, Stem Cell-Derived Embryo Models and In Vitro Gametogenesis: Considerations Leading to the Revised ISSCR Guidelines. Stem Cel Rep. 16, 1416–1424. doi:10.1016/j.stemcr.2021.05.008
Cockburn, K., and Rossant, J. (2010). Making the Blastocyst: Lessons from the Mouse. J. Clin. Invest. 120, 995–1003. doi:10.1172/jci41229
Coucouvanis, E., and Martin, G. R. (1999). BMP Signaling Plays a Role in Visceral Endoderm Differentiation and Cavitation in the Early Mouse Embryo. Development 126, 535–546. doi:10.1242/dev.126.3.535
Dahéron, L., Opitz, S. L., Zaehres, H., Lensch, W. M., Andrews, P. W., Itskovitz‐Eldor, J., et al. (2008). LIF/STAT3 Signaling Fails to Maintain Self‐Renewal of Human Embryonic Stem Cells. STEM CELLS 22, 770–778. doi:10.1634/stemcells.22-5-770
De Jaime-Soguero, A., Abreu de Oliveira, W., and Lluis, F. (2018). The Pleiotropic Effects of the Canonical Wnt Pathway in Early Development and Pluripotency. Genes 9, 93. doi:10.3390/genes9020093
Deglincerti, A., Croft, G. F., Pietila, L. N., Zernicka-Goetz, M., Siggia, E. D., and Brivanlou, A. H. (2016). Self-organization of the In Vitro Attached Human Embryo. Nature 533, 251–254. doi:10.1038/nature17948
Deng, Q., Ramsköld, D., Reinius, B., and Sandberg, R. (2014). Single-cell RNA-Seq Reveals Dynamic, Random Monoallelic Gene Expression in Mammalian Cells. Science 343, 193–196. doi:10.1126/science.1245316
Devika, A. S., Wruck, W., Adjaye, J., and Sudheer, S. (2019). The Quest for Pluripotency: a Comparative Analysis across Mammalian Species. Reproduction 158, R97–R111. doi:10.1530/REP-18-0083
Dumortier, J. G., Le Verge-Serandour, M., Tortorelli, A. F., Mielke, A., de Plater, L., Turlier, H., et al. (2019). Hydraulic Fracturing and Active Coarsening Position the Lumen of the Mouse Blastocyst. Science 365, 465–468. doi:10.1126/science.aaw7709
Eckert, J. J., McCallum, A., Mears, A., Rumsby, M. G., Cameron, I. T., and Fleming, T. P. (2004a). PKC Signalling Regulates Tight junction Membrane Assembly in the Pre-implantation Mouse Embryo. Reproduction 127, 653–667. doi:10.1530/rep.1.00150
Eckert, J. J., McCallum, A., Mears, A., Rumsby, M. G., Cameron, I. T., and Fleming, T. P. (2004b). Specific PKC Isoforms Regulate Blastocoel Formation during Mouse Preimplantation Development. Developmental Biol. 274, 384–401. doi:10.1016/j.ydbio.2004.07.027
Erlebacher, A., Price, K. A., and Glimcher, L. H. (2004). Maintenance of Mouse Trophoblast Stem Cell Proliferation by TGF-Β/activin. Developmental Biol. 275, 158–169. doi:10.1016/j.ydbio.2004.07.032
Evans, M. J., and Kaufman, M. H. (1981). Establishment in Culture of Pluripotential Cells from Mouse Embryos. Nature 292, 154–156. doi:10.1038/292154a0
Fan, Y., Min, Z., Alsolami, S., Ma, Z., Zhang, E., Chen, W., et al. (2021). Generation of Human Blastocyst-like Structures from Pluripotent Stem Cells. Cell Discov 7, 81. doi:10.1038/s41421-021-00316-8
Frias-Aldeguer, J., Kip, M., Vivié, J., Li, L., Alemany, A., Korving, J., et al. (2020). Embryonic Signals Perpetuate Polar-like Trophoblast Stem Cells and Pattern the Blastocyst axis. bioRxiv, 510362.
Frum, T., and Ralston, A. (2015). Cell Signaling and Transcription Factors Regulating Cell Fate during Formation of the Mouse Blastocyst. Trends Genet. 31, 402–410. doi:10.1016/j.tig.2015.04.002
Fu, J., Warmflash, A., and Lutolf, M. P. (2021). Stem-cell-based Embryo Models for Fundamental Research and Translation. Nat. Mater. 20, 132–144. doi:10.1038/s41563-020-00829-9
Furue, M. K., Na, J., Jackson, J. P., Okamoto, T., Jones, M., Baker, D., et al. (2008). Heparin Promotes the Growth of Human Embryonic Stem Cells in a Defined Serum-free Medium. Proc. Natl. Acad. Sci. 105, 13409–13414. doi:10.1073/pnas.0806136105
Gafni, O., Weinberger, L., Mansour, A. A., Manor, Y. S., Chomsky, E., Ben-Yosef, D., et al. (2013). Derivation of Novel Human Ground State Naive Pluripotent Stem Cells. Nature 504, 282–286. doi:10.1038/nature12745
Gao, X., Nowak-Imialek, M., Chen, X., Chen, D., Herrmann, D., Ruan, D., et al. (2019). Establishment of Porcine and Human Expanded Potential Stem Cells. Nat. Cel Biol 21, 687–699. doi:10.1038/s41556-019-0333-2
Gardner, R. L., and Johnson, M. H. (1972). An Investigation of Inner Cell Mass and Trophoblast Tissues Following Their Isolation from the Mouse Blastocyst. J. Embryol. Exp. Morphol. 28 (2), 279–312. doi:10.1242/dev.28.2.279
Gardner, R. L., Papaioannou, V. E., and Barton, S. C. (1973). Origin of the Ectoplacental Cone and Secondary Giant Cells in Mouse Blastocysts Reconstituted from Isolated Trophoblast and Inner Cell Mass. J. Embryol. Exp. Morphol. 30, 561–572. doi:10.1242/dev.30.3.561
Gerri, C., McCarthy, A., Alanis-Lobato, G., Demtschenko, A., Bruneau, A., Loubersac, S., et al. (2020a). Initiation of a Conserved Trophectoderm Program in Human, Cow and Mouse Embryos. Nature 587, 443–447. doi:10.1038/s41586-020-2759-x
Gerri, C., Menchero, S., Mahadevaiah, S. K., Turner, J. M. A., and Niakan, K. K. (2020b). Human Embryogenesis: A Comparative Perspective. Annu. Rev. Cel Dev. Biol. 36, 411–440. doi:10.1146/annurev-cellbio-022020-024900
Goldin, S. N., and Papaioannou, V. E. (2003). Paracrine Action of FGF4 during Periimplantation Development Maintains Trophectoderm and Primitive Endoderm. Genesis 36, 40–47. doi:10.1002/gene.10192
Guilak, F., Cohen, D. M., Estes, B. T., Gimble, J. M., Liedtke, W., and Chen, C. S. (2009). Control of Stem Cell Fate by Physical Interactions with the Extracellular Matrix. Cell Stem Cell 5, 17–26. doi:10.1016/j.stem.2009.06.016
Guo, G., Stirparo, G. G., Strawbridge, S. E., Spindlow, D., Yang, J., Clarke, J., et al. (2021). Human Naive Epiblast Cells Possess Unrestricted Lineage Potential. Cell Stem Cell 28, 1040–1056. e1046. doi:10.1016/j.stem.2021.02.025
Guo, G., von Meyenn, F., Rostovskaya, M., Clarke, J., Dietmann, S., Baker, D., et al. (2017). Epigenetic Resetting of Human Pluripotency. Development 144, 2748–2763. doi:10.1242/dev.146811
Han, Y. L., Wang, S., Zhang, X., Li, Y., Huang, G., Qi, H., et al. (2014). Engineering Physical Microenvironment for Stem Cell Based Regenerative Medicine. Drug Discov. Today 19, 763–773. doi:10.1016/j.drudis.2014.01.015
Hayashi, Y., Furue, M. K., Tanaka, S., Hirose, M., Wakisaka, N., Danno, H., et al. (2010). BMP4 Induction of Trophoblast from Mouse Embryonic Stem Cells in Defined Culture Conditions on Laminin. In Vitro Cell.Dev.Biol.-Animal 46, 416–430. doi:10.1007/s11626-009-9266-6
Humphrey, R. K., Beattie, G. M., Lopez, A. D., Bucay, N., King, C. C., Firpo, M. T., et al. (2004). Maintenance of Pluripotency in Human Embryonic Stem Cells Is STAT3 Independent. STEM CELLS 22, 522–530. doi:10.1634/stemcells.22-4-522
Io, S., Kabata, M., Iemura, Y., Semi, K., Morone, N., Minagawa, A., et al. (2021). Capturing Human Trophoblast Development with Naive Pluripotent Stem Cells In Vitro. Cell Stem Cell 28, 1023–1039. e1013. doi:10.1016/j.stem.2021.03.013
Jain, A., Ezashi, T., Roberts, R. M., and Tuteja, G. (2017). Deciphering Transcriptional Regulation in Human Embryonic Stem Cells Specified towards a Trophoblast Fate. Sci. Rep. 7, 17257. doi:10.1038/s41598-017-17614-5
Kagawa, H., Javali, A., Khoei, H. H., Sommer, T. M., Sestini, G., Novatchkova, M., et al. (2021). Human Blastoids Model Blastocyst Development and Implantation. Nature 601 (7894), 600–605. doi:10.1038/s41586-021-04267-8
Kawagishi, R., Tahara, M., Sawada, K., Ikebuchi, Y., Morishige, K., Sakata, M., et al. (2004). Rho-kinase Is Involved in Mouse Blastocyst Cavity Formation. Biochem. Biophysical Res. Commun. 319, 643–648. doi:10.1016/j.bbrc.2004.05.040
Khan, S. A., Park, K. M., Fischer, L. A., Dong, C., Lungjangwa, T., Jimenez, M., et al. (2021). Probing the Signaling Requirements for Naive Human Pluripotency by High-Throughput Chemical Screening. Cel Rep 35, 109233. doi:10.1016/j.celrep.2021.109233
Kim, H., Wu, J., Ye, S., Tai, C.-I., Zhou, X., Yan, H., et al. (2013). Modulation of β-catenin Function Maintains Mouse Epiblast Stem Cell and Human Embryonic Stem Cell Self-Renewal. Nat. Commun. 4, 2403. doi:10.1038/ncomms3403
Kime, C., Kiyonari, H., Ohtsuka, S., Kohbayashi, E., Asahi, M., Yamanaka, S., et al. (2019). Induced 2C Expression and Implantation-Competent Blastocyst-like Cysts from Primed Pluripotent Stem Cells. Stem Cel Rep. 13, 485–498. doi:10.1016/j.stemcr.2019.07.011
Kime, C., Sakaki-Yumoto, M., Goodrich, L., Hayashi, Y., Sami, S., Derynck, R., et al. (2016). Autotaxin-mediated Lipid Signaling Intersects with LIF and BMP Signaling to Promote the Naive Pluripotency Transcription Factor Program. Proc. Natl. Acad. Sci. USA 113, 12478–12483. doi:10.1073/pnas.1608564113
Kinoshita, M., Barber, M., Mansfield, W., Cui, Y., Spindlow, D., Stirparo, G. G., et al. (2021). Capture of Mouse and Human Stem Cells with Features of Formative Pluripotency. Cell Stem Cell 28, 453–471. e458. doi:10.1016/j.stem.2020.11.005
Kono, K., Tamashiro, D. A. A., and Alarcon, V. B. (2014). Inhibition of RHO-ROCK Signaling Enhances ICM and Suppresses TE Characteristics through Activation of Hippo Signaling in the Mouse Blastocyst. Developmental Biol. 394, 142–155. doi:10.1016/j.ydbio.2014.06.023
Krawchuk, D., Honma-Yamanaka, N., Anani, S., and Yamanaka, Y. (2013). FGF4 Is a Limiting Factor Controlling the Proportions of Primitive Endoderm and Epiblast in the ICM of the Mouse Blastocyst. Developmental Biol. 384, 65–71. doi:10.1016/j.ydbio.2013.09.023
Kubaczka, C., Senner, C., Araúzo-Bravo, M. J., Sharma, N., Kuckenberg, P., Becker, A., et al. (2014). Derivation and Maintenance of Murine Trophoblast Stem Cells under Defined Conditions. Stem Cel Rep. 2, 232–242. doi:10.1016/j.stemcr.2013.12.013
Kunath, T., Arnaud, D., Uy, G. D., Okamoto, I., Chureau, C., Yamanaka, Y., et al. (2005). Imprinted X-Inactivation in Extra-embryonic Endoderm Cell Lines from Mouse Blastocysts. Development 132, 1649–1661. doi:10.1242/dev.01715
Kunath, T., Saba-El-Leil, M. K., Almousailleakh, M., Wray, J., Meloche, S., and Smith, A. (2007). FGF Stimulation of the Erk1/2 Signalling cascade Triggers Transition of Pluripotent Embryonic Stem Cells from Self-Renewal to Lineage Commitment. Development 134, 2895–2902. doi:10.1242/dev.02880
Lanner, F., and Rossant, J. (2010). The Role of FGF/Erk Signaling in Pluripotent Cells. Development 137, 3351–3360. doi:10.1242/dev.050146
Li, J., Wang, G., Wang, C., Zhao, Y., Zhang, H., Tan, Z., et al. (2007). MEK/ERK Signaling Contributes to the Maintenance of Human Embryonic Stem Cell Self-Renewal. Differentiation 75, 299–307. doi:10.1111/j.1432-0436.2006.00143.x
Li, R., Zhong, C., Yu, Y., Liu, H., Sakurai, M., Yu, L., et al. (2019). Generation of Blastocyst-like Structures from Mouse Embryonic and Adult Cell Cultures. Cell 179, 687–702. e618. doi:10.1016/j.cell.2019.09.029
Li, Y., and Parast, M. M. (2014). BMP4 Regulation of Human Trophoblast Development. Int. J. Dev. Biol. 58, 239–246. doi:10.1387/ijdb.130341mp
Linneberg-Agerholm, M., Wong, Y. F., Romero Herrera, J. A., Monteiro, R. S., Anderson, K. G. V., and Brickman, J. M. (2019). Naïve Human Pluripotent Stem Cells Respond to Wnt, Nodal and LIF Signalling to Produce Expandable Naïve Extra-embryonic Endoderm. Development 146 (24), dev180620. doi:10.1242/dev.180620
Liu, X., Tan, J. P., Schröder, J., Aberkane, A., Ouyang, J. F., Mohenska, M., et al. (2021). Modelling Human Blastocysts by Reprogramming Fibroblasts into iBlastoids. Nature 591, 627–632. doi:10.1038/s41586-021-03372-y
Ma, H., Zhai, J., Wan, H., Jiang, X., Wang, X., Wang, L., et al. (2019). In Vitro culture of Cynomolgus Monkey Embryos beyond Early Gastrulation. Science 366, eaax7890. doi:10.1126/science.aax7890
Martin, G. R. (1981). Isolation of a Pluripotent Cell Line from Early Mouse Embryos Cultured in Medium Conditioned by Teratocarcinoma Stem Cells. Proc. Natl. Acad. Sci. 78, 7634–7638. doi:10.1073/pnas.78.12.7634
Meistermann, D., Bruneau, A., Loubersac, S., Reignier, A., Firmin, J., François-Campion, V., et al. (2021). Integrated Pseudotime Analysis of Human Pre-implantation Embryo Single-Cell Transcriptomes Reveals the Dynamics of Lineage Specification. Cell Stem Cell 28, 1625–1640. e1626. doi:10.1016/j.stem.2021.04.027
Molè, M. A., Weberling, A., and Zernicka-Goetz, M. (2020). “Comparative Analysis of Human and Mouse Development: From Zygote to Pre-gastrulation,” in Current Topics in Developmental Biology. Editor L. Solnica-Krezel (Academic Press), 113–138. doi:10.1016/bs.ctdb.2019.10.002
Morgani, S. M., and Brickman, J. M. (2015). LIF Supports Primitive Endoderm Expansion during Pre-implantation Development. Development 142, 3488–3499. doi:10.1242/dev.125021
Morgani, S., Nichols, J., and Hadjantonakis, A.-K. (2017). The many Faces of Pluripotency: In Vitro Adaptations of a Continuum of In Vivo States. BMC Dev. Biol. 17, 7. doi:10.1186/s12861-017-0150-4
Morris, S. A., Grewal, S., Barrios, F., Patankar, S. N., Strauss, B., Buttery, L., et al. (2012). Dynamics of Anterior-Posterior axis Formation in the Developing Mouse Embryo. Nat. Commun. 3, 673. doi:10.1038/ncomms1671
Nakamura, T., Okamoto, I., Sasaki, K., Yabuta, Y., Iwatani, C., Tsuchiya, H., et al. (2016). A Developmental Coordinate of Pluripotency Among Mice, Monkeys and Humans. Nature 537, 57–62. doi:10.1038/nature19096
Nakamura, T., Yabuta, Y., Okamoto, I., Aramaki, S., Yokobayashi, S., Kurimoto, K., et al. (2015). SC3-seq: a Method for Highly Parallel and Quantitative Measurement of Single-Cell Gene Expression. Nucleic Acids Res. 43, e60. doi:10.1093/nar/gkv134
Neagu, A., van Genderen, E., Escudero, I., Verwegen, L., Kurek, D., Lehmann, J., et al. (2020). In Vitro capture and Characterization of Embryonic Rosette-Stage Pluripotency between Naive and Primed States. Nat. Cel Biol 22, 534–545. doi:10.1038/s41556-020-0508-x
Niakan, K. K., Han, J., Pedersen, R. A., Simon, C., and Pera, R. A. R. (2012). Human Pre-implantation Embryo Development. Development 139, 829–841. doi:10.1242/dev.060426
Niakan, K. K., Schrode, N., Cho, L. T. Y., and Hadjantonakis, A.-K. (2013). Derivation of Extraembryonic Endoderm Stem (XEN) Cells from Mouse Embryos and Embryonic Stem Cells. Nat. Protoc. 8, 1028–1041. doi:10.1038/nprot.2013.049
Nichols, J., Silva, J., Roode, M., and Smith, A. (2009). Suppression of Erk Signalling Promotes Ground State Pluripotency in the Mouse Embryo. Development 136, 3215–3222. doi:10.1242/dev.038893
Norwitz, E. R., Schust, D. J., and Fisher, S. J. (2001). Implantation and the Survival of Early Pregnancy. N. Engl. J. Med. 345, 1400–1408. doi:10.1056/nejmra000763
Okae, H., Toh, H., Sato, T., Hiura, H., Takahashi, S., Shirane, K., et al. (2018). Derivation of Human Trophoblast Stem Cells. Cell Stem Cell 22, 50–63. e56. doi:10.1016/j.stem.2017.11.004
Pereira Daoud, A. M., Popovic, M., Dondorp, W. J., Trani Bustos, M., Bredenoord, A. L., Chuva de Sousa Lopes, S. M., et al. (2020). Modelling Human Embryogenesis: Embryo-like Structures Spark Ethical and Policy Debate. Hum. Reprod. Update 26, 779–798. doi:10.1093/humupd/dmaa027
Petropoulos, S., Edsgärd, D., Reinius, B., Deng, Q., Panula, S. P., Codeluppi, S., et al. (2016). Single-Cell RNA-Seq Reveals Lineage and X Chromosome Dynamics in Human Preimplantation Embryos. Cell 165, 1012–1026. doi:10.1016/j.cell.2016.03.023
Posfai, E., Schell, J. P., Janiszewski, A., Rovic, I., Murray, A., Bradshaw, B., et al. (2021). Evaluating Totipotency Using Criteria of Increasing Stringency. Nat. Cel Biol 23, 49–60. doi:10.1038/s41556-020-00609-2
Rivron, N. C., Frias-Aldeguer, J., Vrij, E. J., Boisset, J.-C., Korving, J., Vivié, J., et al. (2018). Blastocyst-like Structures Generated Solely from Stem Cells. Nature 557, 106–111. doi:10.1038/s41586-018-0051-0
Roode, M., Blair, K., Snell, P., Elder, K., Marchant, S., Smith, A., et al. (2012). Human Hypoblast Formation Is Not Dependent on FGF Signalling. Developmental Biol. 361, 358–363. doi:10.1016/j.ydbio.2011.10.030
Rossant, J., and Tam, P. P. L. (2009). Blastocyst Lineage Formation, Early Embryonic Asymmetries and axis Patterning in the Mouse. Development 136, 701–713. doi:10.1242/dev.017178
Rossant, J., and Tam, P. P. L. (2017). New Insights into Early Human Development: Lessons for Stem Cell Derivation and Differentiation. Cell Stem Cell 20, 18–28. doi:10.1016/j.stem.2016.12.004
Shahbazi, M. N., Jedrusik, A., Vuoristo, S., Recher, G., Hupalowska, A., Bolton, V., et al. (2016). Self-organization of the Human Embryo in the Absence of Maternal Tissues. Nat. Cel Biol 18, 700–708. doi:10.1038/ncb3347
Shankar, V., Blitterswijk, C., Vrij, E., and Giselbrecht, S. (2021). From Snapshots to Development: Identifying the Gaps in the Development of Stem Cell‐based Embryo Models along the Embryonic Timeline. Adv. Sci. 8, 2004250. doi:10.1002/advs.202004250
Shen, H., Yang, M., Li, S., Zhang, J., Peng, B., Wang, C., et al. (2021). Mouse Totipotent Stem Cells Captured and Maintained through Spliceosomal Repression. Cell 184, 2843–2859. e2820. doi:10.1016/j.cell.2021.04.020
Silva, J., and Smith, A. (2008). Capturing Pluripotency. Cell 132, 532–536. doi:10.1016/j.cell.2008.02.006
Smith, A. (2017). Formative Pluripotency: the Executive Phase in a Developmental Continuum. Development 144, 365–373. doi:10.1242/dev.142679
Sozen, B., Cox, A. L., De Jonghe, J., Bao, M., Hollfelder, F., Glover, D. M., et al. (2019). Self-Organization of Mouse Stem Cells into an Extended Potential Blastoid. Developmental Cel 51, 698–712. e698. doi:10.1016/j.devcel.2019.11.014
Sozen, B., Jorgensen, V., Weatherbee, B. A. T., Chen, S., Zhu, M., and Zernicka-Goetz, M. (2021). Reconstructing Aspects of Human Embryogenesis with Pluripotent Stem Cells. Nat. Commun. 12, 5550. doi:10.1038/s41467-021-25853-4
Taei, A., Rasooli, P., Braun, T., Hassani, S.-N., and Baharvand, H. (2020). Signal Regulators of Human Naïve Pluripotency. Exp. Cel Res. 389, 111924. doi:10.1016/j.yexcr.2020.111924
Taft, R. A. (2008). Virtues and Limitations of the Preimplantation Mouse Embryo as a Model System. Theriogenology 69, 10–16. doi:10.1016/j.theriogenology.2007.09.032
Takaoka, K., and Hamada, H. (2012). Cell Fate Decisions and axis Determination in the Early Mouse Embryo. Development 139, 3–14. doi:10.1242/dev.060095
Takashima, Y., Guo, G., Loos, R., Nichols, J., Ficz, G., Krueger, F., et al. (2014). Resetting Transcription Factor Control Circuitry toward Ground-State Pluripotency in Human. Cell 158, 1254–1269. doi:10.1016/j.cell.2014.08.029
Tanaka, S., Kunath, T., Hadjantonakis, A.-K., Nagy, A., and Rossant, J. (1998). Promotion of Trophoblast Stem Cell Proliferation by FGF4. Science 282, 2072–2075. doi:10.1126/science.282.5396.2072
Taniguchi, C. M., Emanuelli, B., and Kahn, C. R. (2006). Critical Nodes in Signalling Pathways: Insights into Insulin Action. Nat. Rev. Mol. Cel Biol 7, 85–96. doi:10.1038/nrm1837
Tesar, P. J., Chenoweth, J. G., Brook, F. A., Davies, T. J., Evans, E. P., Mack, D. L., et al. (2007). New Cell Lines from Mouse Epiblast Share Defining Features with Human Embryonic Stem Cells. Nature 448, 196–199. doi:10.1038/nature05972
Theunissen, T. W., Powell, B. E., Wang, H., Mitalipova, M., Faddah, D. A., Reddy, J., et al. (2014). Systematic Identification of Culture Conditions for Induction and Maintenance of Naive Human Pluripotency. Cell Stem Cell 15, 471–487. doi:10.1016/j.stem.2014.07.002
Thomson, J. A., Itskovitz-Eldor, J., Shapiro, S. S., Waknitz, M. A., Swiergiel, J. J., Marshall, V. S., et al. (1998). Embryonic Stem Cell Lines Derived from Human Blastocysts. Science 282, 1145–1147. doi:10.1126/science.282.5391.1145
Tojo, M., Hamashima, Y., Hanyu, A., Kajimoto, T., Saitoh, M., Miyazono, K., et al. (2005). The ALK-5 Inhibitor A-83-01 Inhibits Smad Signaling and Epithelial-To-Mesenchymal Transition by Transforming Growth Factor-Beta. Cancer Sci. 96, 791–800. doi:10.1111/j.1349-7006.2005.00103.x
Tyser, R. C. V., Mahammadov, E., Nakanoh, S., Vallier, L., Scialdone, A., and Srinivas, S. (20202020). A Spatially Resolved Single Cell Atlas of Human Gastrulation. bioRxiv 2007, 213512. doi:10.1101/2020.07.21.213512v1
Ueda, Y., Kimura-Yoshida, C., Mochida, K., Tsume, M., Kameo, Y., Adachi, T., et al. (2020). Intrauterine Pressures Adjusted by Reichert's Membrane Are Crucial for Early Mouse Morphogenesis. Cel Rep. 31, 107637. doi:10.1016/j.celrep.2020.107637
Vianello, S., and Lutolf, M. P. (2019). Understanding the Mechanobiology of Early Mammalian Development through Bioengineered Models. Developmental Cel 48, 751–763. doi:10.1016/j.devcel.2019.02.024
Vrij, E. J., Scholte op Reimer, Y. S., Frias Aldeguer, J., Misteli Guerreiro, I., Kind, J., Koo, B.-K., et al. (2019). Chemically-defined Induction of a Primitive Endoderm and Epiblast-like Niche Supports post-implantation Progression from Blastoids. bioRxiv 1, 510396. doi:10.1101/510396
Vrij, E., Rouwkema, J., LaPointe, V., van Blitterswijk, C., Truckenmüller, R., and Rivron, N. (2016). Directed Assembly and Development of Material-free Tissues with Complex Architectures. Adv. Mater. 28, 4032–4039. doi:10.1002/adma.201505723
Ware, C. B., Nelson, A. M., Mecham, B., Hesson, J., Zhou, W., Jonlin, E. C., et al. (2014). Derivation of Naive Human Embryonic Stem Cells. Proc. Natl. Acad. Sci. 111, 4484–4489. doi:10.1073/pnas.1319738111
Watanabe, K., Ueno, M., Kamiya, D., Nishiyama, A., Matsumura, M., Wataya, T., et al. (2007). A ROCK Inhibitor Permits Survival of Dissociated Human Embryonic Stem Cells. Nat. Biotechnol. 25, 681–686. doi:10.1038/nbt1310
Weinberger, L., Ayyash, M., Novershtern, N., and Hanna, J. H. (2016). Dynamic Stem Cell States: Naive to Primed Pluripotency in Rodents and Humans. Nat. Rev. Mol. Cel Biol 17, 155–169. doi:10.1038/nrm.2015.28
Weinberger, L., Ayyash, M., Novershtern, N., and Hanna, J. H. (2015). Understanding Stem Cell States: Naïve to Primed Pluripotency in Rodents and Humans. bioRxiv 1, 030676. doi:10.1101/030676
Williams, R. L., Hilton, D. J., Pease, S., Willson, T. A., Stewart, C. L., Gearing, D. P., et al. (1988). Myeloid Leukaemia Inhibitory Factor Maintains the Developmental Potential of Embryonic Stem Cells. Nature 336, 684–687. doi:10.1038/336684a0
Xiang, L., Yin, Y., Zheng, Y., Ma, Y., Li, Y., Zhao, Z., et al. (2020). A Developmental Landscape of 3D-Cultured Human Pre-gastrulation Embryos. Nature 577, 537–542. doi:10.1038/s41586-019-1875-y
Xu, R.-H., Chen, X., Li, D. S., Li, R., Addicks, G. C., Glennon, C., et al. (2002). BMP4 Initiates Human Embryonic Stem Cell Differentiation to Trophoblast. Nat. Biotechnol. 20, 1261–1264. doi:10.1038/nbt761
Xu, R.-H., Peck, R. M., Li, D. S., Feng, X., Ludwig, T., and Thomson, J. A. (2005). Basic FGF and Suppression of BMP Signaling Sustain Undifferentiated Proliferation of Human ES Cells. Nat. Methods 2, 185–190. doi:10.1038/nmeth744
Yamanaka, Y., Lanner, F., and Rossant, J. (2010). FGF Signal-dependent Segregation of Primitive Endoderm and Epiblast in the Mouse Blastocyst. Development 137, 715–724. doi:10.1242/dev.043471
Yan, L., Yang, M., Guo, H., Yang, L., Wu, J., Li, R., et al. (2013). Single-cell RNA-Seq Profiling of Human Preimplantation Embryos and Embryonic Stem Cells. Nat. Struct. Mol. Biol. 20, 1131–1139. doi:10.1038/nsmb.2660
Yanagida, A., Spindlow, D., Nichols, J., Dattani, A., Smith, A., and Guo, G. (2021). Naive Stem Cell Blastocyst Model Captures Human Embryo Lineage Segregation. Cell Stem Cell 28 (6), 1016–1022. e4. doi:10.1016/j.stem.2021.04.031
Yang, J., Ryan, D. J., Wang, W., Tsang, J. C.-H., Lan, G., Masaki, H., et al. (2017a). Establishment of Mouse Expanded Potential Stem Cells. Nature 550, 393–397. doi:10.1038/nature24052
Yang, Y., Liu, B., Xu, J., Wang, J., Wu, J., Shi, C., et al. (2017b). Derivation of Pluripotent Stem Cells with In Vivo Embryonic and Extraembryonic Potency. Cell 169, 243–257. e225. doi:10.1016/j.cell.2017.02.005
Yilmaz, A., and Benvenisty, N. (2019). Defining Human Pluripotency. Cell Stem Cell 25, 9–22. doi:10.1016/j.stem.2019.06.010
Ying, Q.-L., Wray, J., Nichols, J., Batlle-Morera, L., Doble, B., Woodgett, J., et al. (2008). The Ground State of Embryonic Stem Cell Self-Renewal. Nature 453, 519–523. doi:10.1038/nature06968
Young, S. L. (2013). Oestrogen and Progesterone Action on Endometrium: a Translational Approach to Understanding Endometrial Receptivity. Reprod. BioMedicine Online 27, 497–505. doi:10.1016/j.rbmo.2013.06.010
Yu, C., Ji, S.-Y., Dang, Y.-J., Sha, Q.-Q., Yuan, Y.-F., Zhou, J.-J., et al. (2016). Oocyte-expressed Yes-Associated Protein Is a Key Activator of the Early Zygotic Genome in Mouse. Cell Res 26, 275–287. doi:10.1038/cr.2016.20
Yu, L., Wei, Y., Duan, J., Schmitz, D. A., Sakurai, M., Wang, L., et al. (2021). Blastocyst-like Structures Generated from Human Pluripotent Stem Cells. Nature 591, 620–626. doi:10.1038/s41586-021-03356-y
Zhang, S., Chen, T., Chen, N., Gao, D., Shi, B., Kong, S., et al. (2019). Implantation Initiation of Self-Assembled Embryo-like Structures Generated Using Three Types of Mouse Blastocyst-Derived Stem Cells. Nat. Commun. 10, 496. doi:10.1038/s41467-019-08378-9
Zhao, C., Reyes, A. P., Schell, J. P., Weltner, J., Ortega, N., Zheng, Y., et al. (20212021). Reprogrammed iBlastoids Contain Amnion-like Cells but Not Trophectoderm. bioRxiv 2005, 442980. doi:10.1101/2021.05.07.442980
Zheng, Y., Xue, X., Shao, Y., Wang, S., Esfahani, S. N., Li, Z., et al. (2019). Controlled Modelling of Human Epiblast and Amnion Development Using Stem Cells. Nature 573, 421–425. doi:10.1038/s41586-019-1535-2
Zhou, F., Wang, R., Yuan, P., Ren, Y., Mao, Y., Li, R., et al. (2019). Reconstituting the Transcriptome and DNA Methylome Landscapes of Human Implantation. Nature 572, 660–664. doi:10.1038/s41586-019-1500-0
Keywords: blastoids, human blastocyst-like structures, embryonic development, pluripotent stem cells, implantation
Citation: Luijkx D, Shankar V, van Blitterswijk C, Giselbrecht S and Vrij E (2022) From Mice to Men: Generation of Human Blastocyst-Like Structures In Vitro. Front. Cell Dev. Biol. 10:838356. doi: 10.3389/fcell.2022.838356
Received: 17 December 2021; Accepted: 26 January 2022;
Published: 11 March 2022.
Edited by:
Ying Gu, Beijing Genomics Institute (BGI), ChinaReviewed by:
Yang Yu, Peking University Third Hospital, ChinaYong Fan, Guangzhou Medical University, China
Kiichiro Tomoda, Gladstone Institute of Cardiovascular Disease, United States
Copyright © 2022 Luijkx, Shankar, van Blitterswijk, Giselbrecht and Vrij. This is an open-access article distributed under the terms of the Creative Commons Attribution License (CC BY). The use, distribution or reproduction in other forums is permitted, provided the original author(s) and the copyright owner(s) are credited and that the original publication in this journal is cited, in accordance with accepted academic practice. No use, distribution or reproduction is permitted which does not comply with these terms.
*Correspondence: Erik Vrij, e.vrij@maastrichtuniversity.nl; Stefan Giselbrecht, s.giselbrecht@maastrichtuniversity.nl
†These authors have contributed equally to this work