- 1Senior Department of Ophthalmology, The Third Medical Center of Chinese PLA General Hospital, Beijing, China
- 2Department of Endocrinology, The Second Medical Center and National Clinical Research Center for Geriatric Diseases, The Chinese PLA General Hospital, Beijing, China
The lens is a relatively special and simple organ. It has become an ideal model to study the common developmental characteristics among different organic systems. Lens development is a complex process influenced by numerous factors, including signals from the intracellular and extracellular environment. Reactive oxygen species (ROS) are a group of highly reactive and oxygen-containing molecules that can cause endoplasmic reticulum stress in lens cells. As an adaptive response to ER stress, lens cells initiate the unfolded protein response (UPR) to maintain normal protein synthesis by selectively increasing/decreasing protein synthesis and increasing the degradation of misfolded proteins. Generally, the UPR signaling pathways have been well characterized in the context of many pathological conditions. However, recent studies have also confirmed that all three UPR signaling pathways participate in a variety of developmental processes, including those of the lens. In this review, we first briefly summarize the three stages of lens development and present the basic profiles of ROS and the UPR. We then discuss the interconnections between lens development and these two mechanisms. Additionally, the potential adoption of human pluripotent stem-cell-based lentoids in lens development research is proposed to provide a novel perspective on future developmental studies.
1 Introduction
The lens is a unique structure in the eyeball. With the main function of adjustably refracting light, the lens must be transparent, refractive, and elastic (Hejtmancik et al., 2015). To generate this sophisticated component, an organism needs an orchestrating embryonic developmental process that turns a mass of cells, namely, the lens placode, into what we know as the crystalline lens (Bassnett and Sikic, 2017). To some extent, lens development is simple. Only two kinds of cells are involved in this process: lens fiber cells (LFCs) and their antecedents, lens epithelial cells (LECs) (Bassnett and Sikic, 2017). This simplicity allows biologists and geneticists to use the lens as a perfect model to study complicated tissue formation and organization. However, lens development is also complex. Lens cell fate determination, precise cell migration, and timely organelle degradation all require a finely tuned gene regulatory network, which has attracted scientists interested in deciphering the underlying mechanism within this “black box.” In the past two decades, tremendous progress at the cellular and molecular levels has been made in understanding how lenses are formed. These studies have generated a complex yet incomplete picture describing factors that influence lens development from different perspectives. Signals from the extracellular microenvironment are among these factors. Human lens development involves the formation and degradation of the arteria hyaloidea, causing a microenvironmental shift favorable for lens formation (Ko et al., 1985). This process refers to the level change in an important factor that participates in the vast majority of physiological processes: oxygen. In 2019, three outstanding scientists were honored with the Nobel Prize in Physiology or Medicine for their contribution to cellular oxygen sensing, highlighting the significance of discovering the physiological and pathological influence of oxygen.
Oxygen not only participates in aerobic metabolism but also serves as the major coordinator in determining the cell fate (Mohyeldin et al., 2010). As the “byproduct” of oxygen, reactive oxygen species (ROS) are canonically regarded as the driving factors of many pathological conditions (Spector, 1995; Andersen, 2004). However, in the last two decades, growing evidence has clearly confirmed the important physiological mediating roles of ROS (Weidinger and Kozlov, 2015), including the regulation of normal development processes. Generally, low levels of ROS contribute to the maintenance of the stemness of stem cells, while relatively high levels contribute to the promotion of cell differentiation (Bigarella et al., 2014). ROS are also capable of causing endoplasmic reticulum stress, which initiates the unfolded protein response (UPR) (Koumenis et al., 2002; Romero-Ramirez et al., 2004; Koritzinsky et al., 2006; Zhang et al., 2019). The proapoptotic roles of the UPR in pathological processes have been widely studied. However, as an adaptive response, the UPR plays positive roles in physiological processes. Recent studies have also found evidence of its participation in normal organ development. Since human lens development may undergo a switch in microenvironmental oxygen concentration due to the formation and degradation of the arterial hyaloidea, we speculate that ROS/UPR may also participate in the cell fate determination of lens cells. Moreover, current studies were mainly founded on animal models that are profoundly different than humans. This review briefly summarizes the intrinsic characteristics of lens development and their possible relationship with the ROS/UPR and proposes possible future solutions to better understand the influence of the ROS/UPR during human lens development.
2 Major steps to form the lens and the corresponding molecular mechanism
Broadly speaking, lens development spans from the formation of zygotes until postnatal growth. Here, the review mainly discusses the development in the narrow sense, which is embryonic lens morphogenesis. The process comprises three general phases: the specification of lens progenitor cells and the formation of the lens placode, the invagination of the lens placode, and the differentiation of LFCs (Cvekl and Ashery-Padan, 2014).
2.1 Formation of lens placode
When a neural plate is formed, the anterior part of the neural plate “border,” which lies between the neural ectoderm and the nonneural ectoderm, is subsequently specified and known as the anterior preplacodal region (aPPR) (Litsiou et al., 2005; Grocott et al., 2012; Cvekl and Ashery-Padan, 2014). The aPPR is a sensory placode that contains a mixture of progenitor cells, including lens progenitor cells (Bailey and Streit, 2005; Sato et al., 2010). Growing evidence has shown that aPPR has a unique molecular signature (Sato et al., 2010). Upon specific signaling, progenitor cells within the aPPR migrate and converge into the adenohypophyseal, olfactory, and lens placodes (Cvekl and Ashery-Padan, 2014). Interestingly, all these placodes are initially specified as lenses, and only when the restriction of lens specification emerges can the formation of adenohypophyseal and olfactory placodes be promoted (Bailey et al., 2006). The molecular signals that drive the initial phase are combinatory actions driven by different pathways, mainly FGF, BMP, and Wnt. The FGF family plays a crucial role in the induction of the first PPRs (Ahrens and Schlosser, 2005; Litsiou et al., 2005). It was proposed that FGF family members promote the expression of “preneural” genes and regulate PPR-specific transcription factors (Grocott et al., 2012; Patthey and Gunhaga, 2014). During this stage, the expression level of BMP signaling first increases and then decreases (Kwon et al., 2010), but Wnt signaling remains consistently repressed (Litsiou et al., 2005; Patthey and Gunhaga, 2014). However, all progenitor cells within the aPPR have the ability to express PAX6. In a study of mice, deletion of Pax6 caused the failure of lens placode formation in mice embryos, indicating the critical status of PAX6 in subsequent lens placode formation (Huang et al., 2011). Similarly, FGF and BMP signaling is indispensable for specific PPR formation into the lens placode. Conditional deletion of Fgfr1 and Fgfr2 in mice resulted in the formation of small lenses, while conditional deletion of both Bmpr1a and Fgfr2 prevented lens formation (Garcia et al., 2011). More importantly, in another study of mice, deletion of Bmp4, a member of the BMP family, completely blocked the formation of lens placodes (Furuta and Hogan, 1998). Some other factors, including SIX3, OTX2, and PAR3, although not typically specific to this region, also participate in lens placode formation (Purcell et al., 2005; Ogino et al., 2008; Melo et al., 2017). However, during this stage, Wnt signaling can inhibit the establishment of aPPR and promote neural crest formation (Saint-Jeannet and Moody, 2014). Taken together, the specification of lens progenitor cells within the aPPR and the subsequent formation of lens placodes under specific signals and critical factors are the intrinsic properties of the initial phase of lens development.
2.2 Invagination of lens placodes
After the lens placode is formed, the invagination process is initiated and is synchronized with the invagination of the optic vesicle to form the optic cup. If induction of the lens cells is the principal event in the first phase, then lens cell proliferation and morphological construction are the core events in the second phase. The cells within the lens placode proliferate to increase its thickness and simultaneously accumulate extracellular matrix to confine the “area” of the lens (Cvekl and Ashery-Padan, 2014). When reaching a certain number, these columnar placodal cells elongate and transform into conical-shaped cells (Sawyer et al., 2010) and begin invaginating to form lens vesicles. This transformation process, also known as apical constriction or cell wedging, is an important process in epithelial invagination during organ development, including lens development (Chauhan et al., 2015). Studies of mice show that circumferential contraction of the actomyosin cytoskeleton in lens cells, which requires RhoA, Rho-kinase, Shroom3, and p120-catenin, ultimately decreases the apical bicellular junctional length and results in lens invagination (Plageman et al., 2010; Lang et al., 2014). Among these molecules, Shroom3 is necessary for the apical localization of F-actin and myosin Ⅱ, which are critical components for apical constriction. Additionally, the expression of Shroom3 is regulated by PAX6, the crucial transcription factor in lens induction, as mentioned earlier (Plageman et al., 2010), indicating the indispensable role of Shroom3 in the second phase of lens development. Recently, a study of mice showed that the formation of multicellular actomyosin cables is essential for the invagination of lens placodes (Houssin et al., 2020). These contractile factors converge into mechanical forces and promote the invaginating process (Hosseini and Taber, 2018). However, as invagination continues, a Rho GTPase, CDC42, antagonizes the contractile function that is promoted by Shroom3 and ensures the elongation of lens cells in a study of mice (Muccioli et al., 2016). Additionally, the core member of the Par complex, PAR3, was found to reduce the junctions of actomyosin contraction and to play an actomyosin-activation-regulation role in the lenses of mice (Houssin et al., 2020). In summary, the orchestration of these opposing processes under comprehensive cellular and molecular factors carefully pushes the invagination of the lens placode forward to form lens vesicles and guarantees its strict direction, which is the core event in the second phase of lens development.
2.3 Differentiation of LFCs
Characterized by both transparency and refractive power, the crystallin lens mainly consists of mature LFCs that contain a large amount of crystallin proteins and few organelles. Thus, once the invagination is complete, the lens pit closes to form the lens vesicle, which is a hollow structure containing lens progenitor cells. The anterior cells form LECs, and the LECs exit the cell cycle and undergo the initial LFC differentiation process toward the posterior part of the vesicle, which is the core event in the following developmental stage (Audette et al., 2017). RNA-sequence analysis revealed profound differences in gene expression in LECs and LFCs (Zhao Y. et al., 2018), indicating a complex transcriptional transition during LFC differentiation. Notably, there are two phenotypes but different LFC differentiation statuses. Primary LFC differentiation is undertaken by posterior embryonic lens progenitor cells, which later form the lens nucleus, and secondary LFC differentiation transpires with equatorial LECs, which later form the lens cortex (Cvekl and Zhang, 2017). Both LFC differentiation mechanisms contain three major processes: lens cell elongation (Rao and Maddala, 2006), crystallin protein production, intracellular organelle degradation (Bassnett, 2009), and cell-to-cell communication establishment (Martinez and de Iongh, 2010). A study of chicken embryos showed that microtubules play crucial roles in lens differentiation, mainly influencing elongation processes by interacting with actomyosin and N-cadherin junctions (Logan et al., 2018). (Please refer to the concise review (Audette et al., 2017).) FGF signaling also plays a very important role in LFC differentiation. It is widely accepted that a low dose of FGF mainly promotes the proliferation of LECs, but a high dose promotes LFC differentiation (Lovicu and McAvoy, 2005; Cvekl and Ashery-Padan, 2014; Audette et al., 2017; Cvekl and Zhang, 2017). Abundant studies of mice and rats have reported the specific mechanisms of FGF signaling. FGF facilitates LFC elongation through the CRK/FRS2/SHP2/GRB2 complex (Collins et al., 2018), Hippo–Yap signaling (Dawes et al., 2018), and the MAPK pathway (Lovicu and McAvoy, 2001; Upadhya et al., 2013). This effect can be antagonized by SPRY and SPREAD, negative regulators of the RTK-mediated MAPK pathway (Zhao G. et al., 2018). FGF signaling also promotes the synthesis of the crystallin protein (West-Mays et al., 2010). The proto-oncogene c-Maf binds to a distal enhancer of αA-crystallin (Cyraa), DCR1, and regulates the expression of CYRAA (Yang et al., 2006). Further research shows that FGF signaling can upregulate c-Maf through c-Jun and Etv5/ERM, an AP-1 factor and an Ets factor, respectively, in a study of embryonic chick lenses (Xie et al., 2016). Similarly, in a study of chicks, BMP participated in LFC differentiation, with two BMP family members, namely, BMP4 and BMP7, being particularly active and endogenously expressed by lens cells (Boswell et al., 2008). BMP signaling acts as the intermediate regulator of FGF and crystallin genes, as indicated by abolishing BMP2/4/7 signaling by noggin impeding FGF-induced crystallin gene expression (Boswell et al., 2008). In addition, since cellular organelles, including the endoplasmic reticulum, Golgi apparatus, mitochondria, and nuclei, can interfere with lens transparency, organelle degradation is necessary. This degradation process is synchronized with the production of large amounts of crystallin protein in LFCs, which indicates well-established coordination of these two contradictory procedures. Apoptosis, autophagy, nucleophagy, and mitophagy pathways are all involved in organelle degradation (Wride, 2011; Costello et al., 2013). Wnt signaling also participates in LFC differentiation; in contrast to its role in the first stage, Wnt activation favors the differentiation of LFCs from LECs in this stage (Stump et al., 2003; Cain et al., 2008), indicating the positive role of Wnt in LEC transdifferentiation. A recent study of mice showed that a protein that targets mitochondria for elimination during erythrocyte formation, called BCL2-interacting protein 3-like (BNIP3L/NIX), is indispensable and participates in the formation of the lens organelle–free zone (Brennan et al., 2018). In summary, LFC differentiation is an integrated process that involves different normal cellular and molecular functions to maintain ongoing development.
Lens development is a stepwise process influenced by ubiquitous but stage-specific cellular and molecular mechanisms. Although tremendous progress has been made in deciphering lens morphogenesis, the evidence is fragmented and disconnected. The remaining gaps need to be filled before the completed blueprint of lens development can be precisely constructed. Based on the knowledge discovered to date, studies now focus on the interactions between molecular pathways at the microlevel and the corresponding coordination among different organelles at the cellular level. For instance, the negative regulation of PTEN on FGFR signaling during lens development was recently confirmed in mice (Padula et al., 2020). It has been discovered that hedgehog signaling (Taira et al., 2020), the ETV family (Garg et al., 2020), the SPREAD family (Wazin and Lovicu, 2020), and heat shock factors (Evans et al., 2007; Cui et al., 2020) are involved in lens development. Notably, these results were obtained from models of avian embryos, CHO cells, mice, and embryonic zebrafish. Although direct molecular regulation during lens development is important, the effects of the developmental microenvironment are also drawing increasing attention. In the following sections, common chemicals produced during multiple biological activities, ROS, and their related cellular and molecular influence on lens development are discussed.
3 ROS and the UPR
ROS, including superoxide anion (O2−), hydrogen peroxide (H2O2), and hydroxyl radical (HO•), are mainly generated during mitochondrial oxidative metabolism (Murphy, 2009) and other cellular processes in response to exogenous substances (Ray et al., 2012). They participate in ubiquitous activities ranging from physiological to pathological conditions. The development of multicellular organisms is one of the processes involving ROS. The balance between the proliferation and differentiation of stem cells determines the direction of development and can be dramatically influenced by ROS (Schippers et al., 2012). The cellular ROS concentration is in dynamic equilibrium, which requires the harmonious cooperation of both ROS production and their consumption by antioxidants, resulting in a delicate balance between the proper redox state and oxidative stress.
3.1 Main sources of cellular ROS
Cellular ROS can be generated in both the cytosol and organelles (Figure 1). Several soluble components, including flavins, thiols, catecholamines, and hydroquinones, can produce ROS during redox reactions (Freeman and Crapo, 1982). Additionally, during their catalytic activities, several ROS-producing cytosolic enzymes, such as xanthine oxidase, produce ROS (McCord et al., 1985). However, a large proportion of intracellular ROS originate from organelles, including mitochondria, peroxisomes, and the endoplasmic reticulum (ER). Mitochondria serve not only as the major energy-producing “factories” in aerobic cells but also as continuous ROS-generating “machines.” The transport of electrons along the respiratory chain is accompanied by the production of ROS (Sena and Chandel, 2012). In addition, several oxidoreductases located in mitochondrial membranes participate in ROS generation (Andreyev et al., 2005). Peroxisomes are organelles involved in the cellular metabolism of H2O2, a species that can be generated by urate oxidase and xanthine oxidase within peroxisomes (Angermuller et al., 1987; Fritz et al., 2007). Another important ROS-generating organelle is the ER, which is involved in multiple functions, such as protein synthesis and protein folding, calcium storage, and lipid metabolism (Koch, 1990; Groenendyk and Michalak, 2005). The electron transport chain in the smooth ER is sustained by xenobiotic metabolism and unsaturated fatty acid production. ROS can be produced during these activities (Di Meo et al., 2016). Additionally, the ER stabilizes proteins through the oxidative protein folding (OPF) reaction, for which molecular oxygen is the source of the oxidizing equivalents necessary to form intramolecular disulfide bonds. OPF is the most common posttranslational modification undertaken in the ER and is a major source of H2O2 therein (Malhotra and Kaufman, 2007; Qin et al., 2015). It has been estimated that oxidative protein folding within the ER accounts for up to 25% of cellular ROS production (Tu and Weissman, 2004). Moreover, membrane contact sites (MCSs) between organelles contribute to ROS generation. In mammals, more than 90% of peroxisomes and 20% of mitochondria contact the ER (Valm et al., 2017). Vesicle-associated membrane protein (VAMP)-associated proteins (VAPs) are one kind of MCS between the ER and other organelles (Murphy and Levine, 2016). Studies have shown that there are close connections between VAPs and the UPR signaling pathways (Kamemura and Chihara, 2019). It has been demonstrated that ROS production occurs at ER–mitochondria contact sites, which are conducted by calcium channels, namely, inositol 1,4,5-trisphosphate (IP3) receptors (Booth et al., 2016). These results indicate that MCSs between the ER and mitochondria or peroxisomes can contribute to the interplay between the UPR and OS. Additionally, a feedback loop exists between ROS and the UPR. Excessive production of ROS can lead to the accumulation of misfolded proteins in the ER and subsequent UPR activation. Interestingly, prolonged UPR activation promotes the accumulation of ROS (Haynes et al., 2004; Malhotra and Kaufman, 2007). The results showed that UPR-deficient cells under sustained ER stress are unable to accumulate ROS; however, cells with a functional UPR accumulate ROS, resulting in cell death (Haynes et al., 2004). The generation of ROS induced by the UPR in age-related cataracts is reported to be related to ERO1-Lα, ERO1-Lβ, and protein disulfide isomerase (Periyasamy and Shinohara, 2017). Metabolic processes also generate ROS, and the primary function of NADPH oxidases is to produce ROS. NADPH oxidases are composed of membrane-bound subunits and catalyze molecular oxygen reduction. When NADPH oxidases are activated, NADPH transfers its electrons to flavin adenine nucleotides. Subsequently, the electrons are passed to two heme groups bound to the N-terminal domain, which finally pass the two electrons to two molecular oxygen atoms on the opposite side of the membrane, forming superoxide anions (Bedard and Krause, 2007; Brieger et al., 2012).
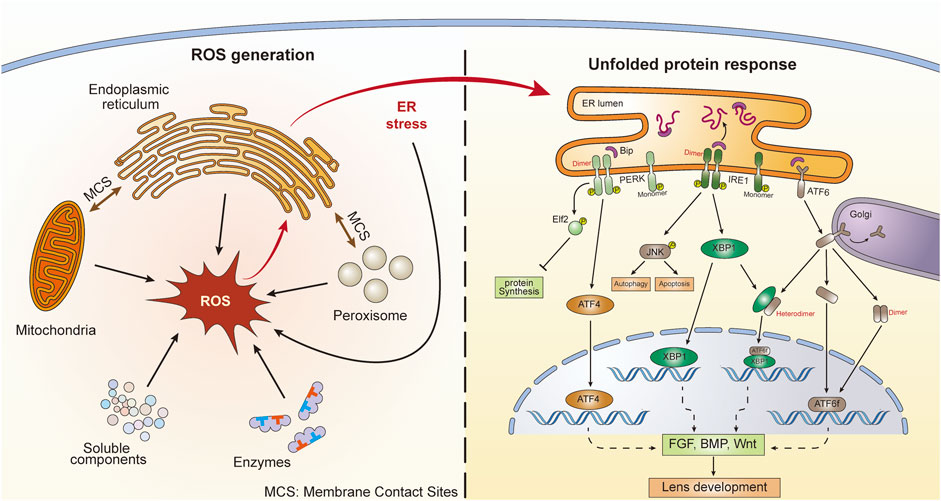
FIGURE 1. ROS generation and subsequent UPR activation in lens cells. There are five main ROS sources, including enzymes, soluble components, mitochondria, peroxisomes, and the endoplasmic reticulum (ER). ER stress and UPR can also generate ROS. There are membrane contact sites between ER and mitochondria as well as ER and peroxisomes. Three UPR signaling pathways are activated following ER stress, including ATF6, PERK, and IRE1. The dimerization and heterodimerization of key factors could lead to differential gene regulation. These UPR pathways might further influence lens development through action on the FGF, BMP, and Wnt pathways.
3.2 Roles of ROS in development
In addition to directly producing biological effects, generated ROS can serve as signaling molecules that trigger active cellular responses. These responses are involved in the development of primitive embryos and subsequent organs (Coffman and Su, 2019; Lin and Wang, 2020). A recent study showed that early cleavage of Xenopus embryos is promoted by an increase in mitochondrial ROS levels (Han Y. et al., 2018), indicating the early intervention of ROS during embryogenesis. Considerable evidence has established that ROS participate in many processes during neurogenesis (Borquez et al., 2016; Oswald et al., 2018). Promoting the differentiation of stem cells and maintaining the stemness of neural precursors are two important roles of ROS (Wang et al., 2013; Chaudhari et al., 2014). In addition, ROS can shape the polarity of neurons. A study has shown that the ROS level is increased in polarizing cerebellar granule neurons but is decreased under depolarizing conditions (Olguin-Albuerne and Moran, 2015). Cytoskeletal modifications have also been shown to be linked to ROS, as reflected by the influence of cysteine and methionine residues on β-actin upon redox signaling (Lassing et al., 2007). ROS can also alter the ubiquitination of Yap, which is closely related to organ size, during neurogenesis (Ji et al., 2017). All of these aspects, the differentiation of progenitor cells, the establishment of polarity, the modification of the cytoskeleton, and the expansion of cell volume, play roles not only in neurogenesis but also in lens development (Donaldson et al., 2009; Muccioli et al., 2016; Cheng et al., 2017). Although the regulatory effect of ROS on lens development is currently unknown, experience from the redox-regulating factor glutathione provides some evidence about the influence of ROS on lens development. A recent study confirmed that impairing the biosynthesis of glutathione can disrupt eye development, including lens development (Thompson et al., 2021). In this way, we hypothesize that ROS also play roles in lens development, the details of which require future studies to determine.
3.3 The link between ROS and UPR
The homeostasis state of ROS in the ER is vital. Although OPF requires an oxidative environment to function effectively, excessive accumulation of ROS or excessive oxidative stress can disrupt homeostasis and lead to the accumulation of misfolded proteins in the ER, which causes ER stress (Tu and Weissman, 2004; Malhotra and Kaufman, 2007). In turn, under conditions of ER stress, the induced activity of the ERO1 (ER oxidoreductase 1)-PDI (protein disulfide isomerase) system accelerates the production of ROS by transferring electrons to molecular oxygen (O2) and producing H2O2 (Cao and Kaufman, 2014; Zeeshan et al., 2016). Perturbation results in UPR induction and initiates the activation of a series of subsequent signaling pathways that restore ER equilibrium (Figure 1) (Gardner et al., 2013).
3.3.1 Initiation and modulation of the UPR
There are three UPR sensors distributed in the ER membrane, namely, activating transcription factor 6 (ATF6), protein kinase RNA-like endoplasmic reticulum kinase (PERK), and inositol-requiring protein 1 (IRE1) (Figure 1) (Zhang et al., 2019). ERO1 in the ER utilizes O2 to power the oxidization of PDI and generate H2O2, and this process forms a disulfide bond. However, when the concentration of H2O2 exceeds the threshold, redox homeostasis in the ER can be damaged. The OPF process is blocked and leads to the accumulation of unfolded proteins, which are seized by these three sensors to trigger different signaling pathways (Higa et al., 2014). Specifically, ATF6 is transported to the Golgi apparatus, where it is cleaved by two Golgi-resident proteases (Ye et al., 2000). Cleaved ATF6 (ATF6f) is further transported into the nucleus and acts as a transcription factor to promote the expression of activating transcription factor 4 (ATF4) and X-box-binding protein 1 (XBP1) (Yamamoto et al., 2007). PERK undergoes oligomerization and autophosphorylation when the UPR is initiated (McQuiston and Diehl, 2017) and then phosphorylates eukaryotic translation initiation factor 2α (eIF2α), which leads to selective expression of ATF4 (Rowlands et al., 1988). ATF4 is not only able to regulate adaptive genes that relieve ER stress but can also promote the expression of the CAAT/enhancer-binding protein homologous protein (CHOP), which participates in cell death induction (Rowlands et al., 1988; Han et al., 2013). After its autophosphorylation, IRE1α serves as an RNase and splices an intron of downstream XBP1 mRNA, which is then translated into the potent transcription factor XBP1 and initiates the transcription of ER-quality-regulation genes (Maurel et al., 2014). Activation of the UPR signaling pathway can restore ER viability and is vital for cell growth and survival (Bertolotti et al., 2000). Normally, no UPR activity is triggered until ER homeostasis is challenged by ER stress-causing chemicals, such as excessive ROS. However, these UPR-mediating genes also participate in developmental behaviors in a number of species (Mitra and Ryoo, 2019), suggesting that the UPR can also function in normal physiological processes. Under conditions of ER stress, the UPR induces the expression of these genes to restore ER equilibrium.
The over- or prolonged activation of the UPR can have a harmful effect. Proper modulation of the UPR helps to neutralize detrimental effects. For example, in neurodegenerative diseases, research has proven that dysregulated UPR signaling is a major pathogenic mechanism. However, genetic modulation of the PERK axis in animal models can prevent neurodegeneration (Hughes and Mallucci, 2019). Interestingly, both inhibition and activation of the PERK pathway were found to be beneficial in neurodegenerative disease models (Shacham et al., 2021). In diabetes mellitus, overactivation of IRE1α triggered the apoptosis and degeneration of islet β-cells. Several IRE1α-inhibiting small molecule compounds spared β-cells from death (Ghosh et al., 2019). These results indicate that modulating the activation status of the UPR through genetic interference or compound addition can have advantages for disease treatment. Moreover, modulation of the UPR can help elucidate the exact interaction between ROS and the UPR. In a study using human osteosarcoma cells, ROS generation was downregulated after adding the PERK inhibitor GSK2606414 (Wang et al., 2019), indicating a ROS generation-promotion effect of the UPR in this model. In contrast, by using a curcumin derivative to produce ROS in cultured human amniotic mesenchymal stem cells, researchers found that the ROS level was further elevated after adding the PERK inhibitor GSK2656157 (Wang et al., 2021), indicating an adaptive response from UPR activation.
3.3.2 Roles of ROS and the UPR in development
All three UPR branches are activated by the accumulation of misfolded proteins and/or the overload of unfolded proteins in the ER. The development of organisms is accompanied by an abundant synthesis of proteins, which requires the ER to operate under high loads. On the one hand, this load results in a dramatic increase in OPF, followed by the elevation of H2O2 levels. On the other hand, massive protein synthesis is more likely to lead to misfolded protein accumulation and the overload of unfolded proteins. Commonly, either situation can easily cause ER stress and UPR initiation signaling in developing cells. However, the roles of ROS and the UPR in development are far from clear. We currently know only the effect of core factors in the UPR signaling pathway.
There are two ATF6 species in vertebrate species, namely, ATF6α and ATF6β. Double knockout of Atf6α and Atf6β is lethal at the early embryonic stage, but single knockout of either type leads to more viable organisms (Yamamoto et al., 2007). Double-knockout mice present more severe malformations than mice with other types of UPR molecule knockouts, including Ire1α, Xbp1, Perk, or Atf4, indicating a broad role for the Atf6 gene in vertebrate development (Yoshida et al., 2000; Yamamoto et al., 2007). ATF6 participates in various developmental processes, including lens development (Firtina and Duncan, 2011; Clark et al., 2020), neurodevelopment (Saito et al., 2012; Naughton et al., 2015), mesoderm differentiation (Kroeger et al., 2018), osteogenesis and chondrogenesis (Maeda et al., 2015; Guo et al., 2016), adipogenesis and lipogenesis (Lowe et al., 2012), and the formation of the female reproductive system (Yang Y. et al., 2015; Xiong et al., 2015). Hyperactivation of ATF6 in human embryonic stem cells (hESCs) not only promotes the ER network but also facilitates mesodermal differentiation (Kroeger et al., 2018). In contrast, loss of ATF6 impedes the mesodermal fate of hESCs (Kroeger et al., 2018). In human eyes, mutation of ATF6 leads to defects in the macular central fovea, which results in achromatopsia, an ocular disease characterized by a lack of cone photoreceptor function (Ansar et al., 2015; Kohl et al., 2015). Additionally, ATF6 mutation can lead to cone-rod dystrophy. Patients suffering from this disease will gradually lose cone function followed by loss of rod function (Skorczyk-Werner et al., 2017). By using patient-specific iPSCs and retinal organoids, a recent study also showed that ATF6 is essential for the formation of human cone photoreceptors (Kroeger et al., 2021). All the evidence confirmed the important character of ATF6 during retinal development. Further studies confirmed that many different functions of ATF6, such as misfolded protein detection ability, were impaired upon ATF6 mutation (Chiang et al., 2017; Tam et al., 2018). ATF6 also participates in lens development, which is discussed in the following section. Nevertheless, how the ATF6 gene generally regulates the development of these ocular cells remains unclear.
Another UPR core organic macromolecule, PERK, also participates in the process of development. Loss-of-function mutation of PERK leads to a disease known as Walcott–Rallison syndrome, which is characterized by infantile-onset diabetes, exocrine pancreas dystrophy, and other problems in the bone, liver, and kidney (Durocher et al., 2006). Compared with that in adult tissues, the activation pattern of PERK/eIF2a is elevated in the brains of mouse embryos, indicating the participation of PERK during embryonic brain development (Zhang et al., 2007). PERK can affect miRNA networks associated with stemness and differentiation to determine the fate of myoblast differentiation. In one study of Perk knockdown in mice, myoblasts changed to stem-like cells with a marked transformation from a fusiform to a rounded morphology (Tan et al., 2021). A study in Perk-mutant β-islet cells showed that anterograde trafficking from the ER to the Golgi and retrotranslocation from the ER to the cytosol were impaired, as indicated by the reduced expression of ER chaperones (Gupta et al., 2010). In addition, a genome-wide association study (GWAS) revealed a set of human PERK variants in patients with progressive supranuclear palsy (PSP), which is characterized by abnormal tau deposits (Höglinger et al., 2011). However, the mechanism of PERK-mutant PSP is unclear because tau is not a secreted protein; thus, modification in the ER is unlikely. As the downstream factor of PERK, Atf4 deficiency can cause developmental defects, including pancreatic hypertrophy, in mice (Iida et al., 2007). Atf4-knockout mice also show defects in lens development. The details are discussed in the following section. However, the underlying mechanism by which PERK/ATF4 influences organ development remains unknown.
XBP1, generated by IRE1, is an important regulator during development. Mutation of Ire1 or Xbp1 in Drosophila is lethal at early larval stages. However, reintroduction of wild-type genes can reverse this effect (Huang et al., 2017). Xbp1-knockout (Xbp1−/−) mice die during embryogenesis because of liver dysfunction, indicating the requirement for XBP1 in liver development (Reimold et al., 2000). A study confirmed the requirement of spliced XBP1 (XBP1s) for the precise secretion of digestive enzymes in the Drosophila digestive tract (Huang et al., 2017). In fish, XBP1 is highly expressed in high-secretion cells, including those in the glands, notochord, and tail (Bennett et al., 2007; Ishikawa et al., 2017). XBP1s has been reported to be a differentiation factor required for hatching gland development (Bennett et al., 2007). In addition, XBP1s is the key factor for the growth and development of medaka fish because defects observed in XBP1-knockout or IRE1-knockout medaka are fully rescued by constitutive expression of XBP1s (Ishikawa et al., 2017). XBP1 also participates in the immune system response. Xbp1−/− mice fail to control infection with the B-cell-dependent polyoma virus because of the absence of plasma cells (Reimold et al., 2001). IRE1 shares some common features with XBP1 during development. Ire1α-knockout mice present with defects in the liver and pancreas and salivary gland cells, similar to Xbp1−/−mice (Iwawaki et al., 2010; Zhang et al., 2011). However, IRE1 also shows apparent differences with respect to XBP1. The development of the placenta in mice can be significantly influenced by IRE1α loss of function, while the developmental defects of the placenta in Xbp1−/− mice are moderate compared with those of Ire1α−/− mice (Iwawaki et al., 2009). This difference may result from the regulated IRE1-dependent decay (RIDD) of mRNA, which helps to maintain ER homeostasis by reducing the load of ER client proteins or inhibiting protein synthesis (Maurel et al., 2014). More importantly, IRE1 is active in early photoreceptors and is required for normal ER differentiation during the development of Drosophila photoreceptors (Xu et al., 2016). In addition, IRE1/XBP1 has been shown to be linked to retinitis pigmentosa (Chiang et al., 2015), indicating a connection between photoreceptors and IRE1 signaling. Since the generation of XBP1s can alleviate the protein-folding load in the ER, we hypothesize that the development-regulating effect of the IRE1–XBP1 axis might be part of a “production control” system. However, little is known about the underlying molecular mechanism.
In summary, the three UPR signaling pathways participate in developmental processes. Although some characteristics of different core factors in the UPR have been revealed, there is still a long way to go before the development-regulation blueprint of the UPR or the mutual relationship between the UPR and other canonical signaling pathways involved in development can be precisely outlined.
4 ROS and the UPR in lens development
As mentioned earlier, ROS play important roles in development, including the regulation of the proliferation and differentiation of stem cells (Holmstrom and Finkel, 2014). It has been shown that ROS are able to reduce the stemness of hESCs, promote their neural differentiation, and enhance the expression of PAX6 (Hu et al., 2018), an important factor that regulates lens development. ROS also directly influence the intracellular localization of PAX6. In corneal cells, PAX6 exhibits apparent nucleocytoplasmic shuttling following H2O2 treatment (Shukla and Mishra, 2018). Based on these results, we hypothesize that there might be a potential connection between ROS and lens development.
4.1 ROS and LECs
As an important type of lens cell, LECs may be closely related to ROS. Previous studies have shown that low levels of ROS can be generated following platelet-derived growth factor (PDGF) treatment of LECs (Chen et al., 2004; Wang and Lou, 2009). PDGF is a mitogenic factor that can promote the proliferation of LECs, in which ROS serve as signaling transducers to activate a series of downstream signaling pathways, including the MAPKs, ERK1/2, and JNK (Chen et al., 2004). It was confirmed that the upstream components of the PDGF receptor kinase, Src-family kinases, PI3K, RAC, RAS proteins, and arachidonic acid are required for ROS production and subsequent cell proliferation following PDGF treatment (Zhang et al., 2006; Chen et al., 2007). In addition, ROS are also involved in the cell-proliferation-promoting effect on other growth factors, including EGF and bFGF, in LECs (Ohguro et al., 1999). Importantly, these growth-factor-related proliferation-stimulating effects may be eradicated upon inhibition of ROS (both removal and blockade of ROS) (Zhang et al., 2008). Endogenous H2O2 is generated in LECs under basal conditions during lens development (Basu et al., 2014). We speculate that there is a basal developmental-regulatory role of ROS in LECs, which requires confirmation in future studies.
However, ROS can also contribute to cataract formation. We now understand that the homeostasis of the redox state in LECs is closely related to the transparency of the crystallin lens. The imbalance of free radical and antioxidant systems results in oxidative stress and subsequent cataracts (Wilhelm et al., 2007; Bai et al., 2013). Heme oxygenase-1 (HO-1) deficiency in LECs is an example of a stress-inducing condition. It has been shown that Ho-1 mutant transgenic mice display cataracts 12 weeks after birth, and this phenotype can be extended into adulthood (Zhou et al., 2011). Our previous work confirmed that HO-1 can reduce ROS levels and increase antioxidant levels in LECs, which inhibits the apoptosis of LECs (Ma et al., 2016). As the major byproduct of HO degradation, carbon monoxide (CO) confers a protective effect similar to that of HO-1 on LECs following ROS treatment, as indicated in our recent study (Huang et al., 2018a). Pretreatment with CO can be used to target mitochondria in LECs and promote the return of normal redox homeostasis (Huang et al., 2018b). The detailed molecular mechanism of the interaction between ROS and HO-1 in LECs remains unknown. However, the major regulator of HO-1, nuclear factor erythroid 2-like 2 (NRF2), attracted our attention. NRF2 is a transcription factor that is critical for the regulation of redox reactions and antioxidants in mammalian cells (Kensler et al., 2007). Under physiological conditions, NRF2 is sequestered by the inhibitor protein Kelch-like ECH-associated protein 1 (KEAP1). When oxidative stress occurs, NRF2 is released from KEAP1, translocates to the nucleus, and initiates the expression of a series of genes, including HO-1 (Baird and Dinkova-Kostova, 2011). Our recent research showed that the expression level of NRF2 in LECs increased significantly following ROS treatment, and transfecting LECs with the NRF2 gene enhanced the protective effect of NRF2 against oxidative damage (Ma et al., 2018). More importantly, NRF2 was found to interact with a UPR signaling pathway and the PERK pathway, by binding to the downstream factor ATF4. An increase in the NRF2-ATF4 complex level can be detected when the environmental H2O2 concentration increases, which facilitates NRF2-conferred protection (Ma et al., 2018). In summary, these results suggest a close relationship between ROS and LECs. Unfortunately, there is no direct research focusing on the potential effect of ROS on lens development, and future work is required to reveal the possible effect.
4.2 Cross-talk between components of the UPR and lens development
As an adaptive response to ROS, the UPR is established in the lens. The mechanism responsible for the activation of all three UPR pathways is highly conserved among vertebrates (Cvekl and Zhang, 2017), including mammals and fish (Ishikawa et al., 2011). These model animals are frequently used to study the influence of the UPR. There is considerable evidence showing UPR-related cataract formation (Torres-Bernal et al., 2014; Yang et al., 2015a; Yang et al., 2015b; Lyu et al., 2015) in these animals. However, in this situation, the UPR is highly activated, which induces apoptosis of both LECs and LFCs (Ikesugi et al., 2006). In contrast, moderate activation of the UPR was shown to influence normal lens development (Firtina et al., 2009). A study confirmed that all three UPR pathways are activated during mouse lens development but exhibit relatively different patterns (Firtina and Duncan, 2011).
ATF6, IRE1, and PERK are all expressed at low levels after the formation of lens vesicles (phase 2) in mice (Firtina and Duncan, 2011). As development continues, the ATF6 expression level increases sharply in LFCs and gradually diffuses in LECs. Through the end of lens development, the highest expression level of ATF6 is detected near the transition zone (Figures 2A1-A2) (Firtina and Duncan, 2011). To better investigate ATF6 activity during development, an ATF6-eGFP reporter was recently created for use in zebrafish (Clark et al., 2020). After injecting the reporter plasmid into zebrafish embryos, the highest eGFP expression was observed in the lens and skeletal muscle. The expression in skeletal muscle decreased as development continued, while the expression in lens remained relatively consistent (Clark et al., 2020). This exciting result indicates the full participation of ATF6 during lens development. However, current studies only provide us with correlative evidence. To further speculate on the underlying mechanism, we referred to the molecular function of ATF6. A previous study has shown that ATF6 is closely linked to tolerance to chronic stress (Wu et al., 2007). The subunit activity of ATF6 also plays important roles in maintaining tissue homeostasis (Hillary and FitzGerald, 2018). During the late stage of lens development, LFCs undertake extensive crystallin protein synthesis. Simultaneously, the elongation of LFCs requires multiple microtubules to promote dramatic deformation (Audette et al., 2017). The differentiation from LECs to LFCs at the transition zone is accompanied by a series of changes in gene expression patterns. As massive protein synthesis, microtubule movements, and changes in gene expression patterns might disturb homeostasis within lens cells, we hypothesize that the expression pattern of ATF6 during lens development could guarantee the relative stabilization of the internal microenvironment.
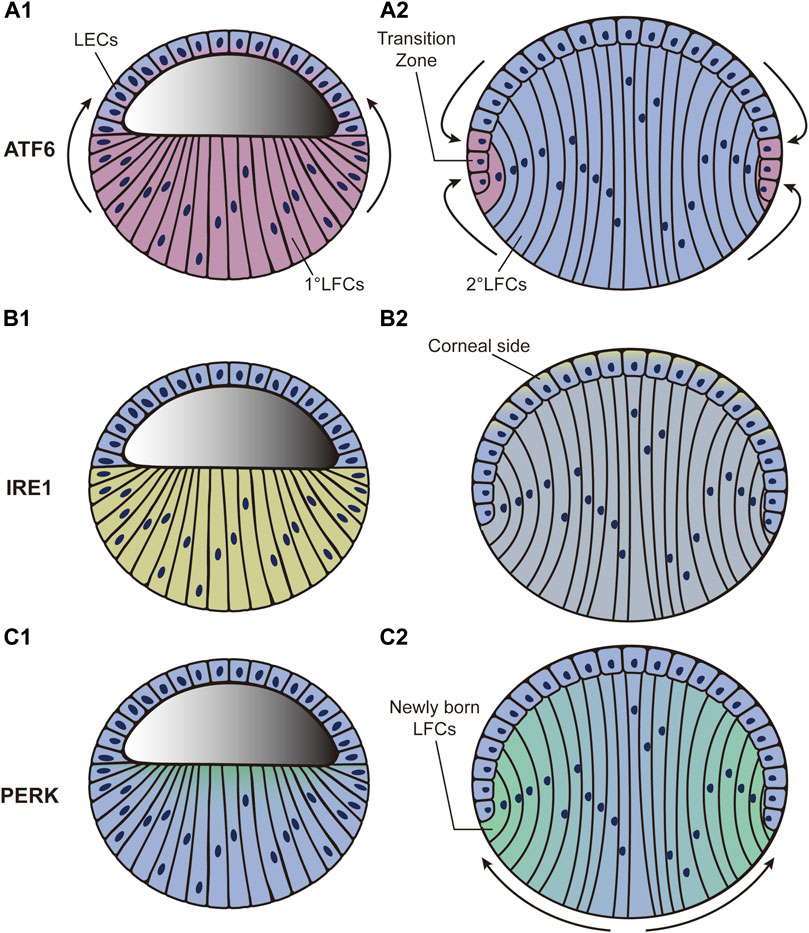
FIGURE 2. Dynamic distribution of three UPR pathways during the third phase of lens development. “Blue” refers to lens cells; “red,” “yellow,” and “green” in A, B, and C refer to ATF6, XBP1, and PERK expression, respectively. (A1–A2): ATF6 expression level increases sharply in LFCs and gradually diffuses to LECs. By the end of lens development, the highest expression level of ATF6 is detected around the transition zone. (B1–B2): XBP1 expression is initially increased in LFCs. It then decreases in LFCs but increases in LECs. By the end of development, the highest expression level of XBP1 is found in LECs adjacent to the corneal epithelium. (C1–C2): PERK expression level first appears at the apical tips of all LFCs and then accumulates predominantly in newly born LFCs while declining to nothing in central LFCs as development proceeds. LECs: lens epithelial cells; LFCs: lens fiber cells.
The expression level of XBP1, the downstream factor of IRE1, is also increased in LFCs during development (Firtina and Duncan, 2011). It then decreases in LFCs but increases in LECs during the middle stage (phase 3). By the end of development, the highest expression level of XBP1 is found in LECs adjacent to the corneal epithelium (Figures 2B1-B2) (Firtina and Duncan, 2011). Unfortunately, how the IRE1 branch actually influences lens development is far from clear. We presume that the role of IRE1 is linked to protective effects. Deletion of Xbp1 in epithelial cells dramatically inhibits cell proliferation and differentiation as well as characteristic protein synthesis in mice (Hasegawa et al., 2015), indicating the necessity of XBP1 in epithelial growth. In Drosophila, a study confirmed the physiological stress resolution characteristics of the IRE1–XBP1 axis during normal development (Huang et al., 2017). More specifically, IRE1–XBP1 was strongly activated in neuropil and peripheral glia in Drosophila and the counterparts of oligodendrocytes and Schwann cells in mammals, providing protective and homeostasis-maintaining effects to nervous systems (Sone et al., 2013). Additionally, activation of IRE1 was related to the activation of the proapoptotic JNK pathway, which can lead to apoptosis (Urano et al., 2000). As LECs are located at the frontal surface of the lens and directly face the aqueous humor and corneal epithelium, we hypothesize that the accumulation of IRE1 in LECs might protect cells against apoptosis and help maintain homeostasis in lens development.
The participation of PERK starts in the third phase of lens development. The PERK expression level first appears at the apical tips of all LFCs and then becomes predominant in new LFCs but is completely abolished in the central LFCs as development proceeds (Figures 2C1-C2) (Firtina and Duncan, 2011). As a downstream factor in the PERK pathway, ATF4 has also been shown to play an important role in lens development. Atf4-knockout mice display impaired secondary LFC formation, while their primary LFCs and normal retinal development seem to be unaffected (Tanaka et al., 1998). Histological analysis showed that LFCs undergo apoptosis as development proceeds. The failure of secondary LFC formation ultimately results in microphthalmia (Tanaka et al., 1998). To further determine whether Atf4 gene expression in the lens is essential for LFC differentiation, the Atf4 gene was transferred downstream of the αA-crystallin promoter in Atf4−/− mice. The microphthalmia defect was fully ameliorated in transgenic mice (Tanaka et al., 1998). In addition, a study based on transcriptomic analysis revealed the potential controlling role of eIF2 during lens development (Zhao Y. et al., 2018). These results are in agreement with the characteristics of the PERK activation pattern during lens development. In contrast to ATF6 and IRE1, which both induce a transcriptional response to relieve stress conditions, PERK mainly causes a rapid reduction in global rates of protein translation (Hughes and Mallucci, 2019). The conversion of LECs into LFCs is coupled with the elongation of the cell body together with the synthesis of crystallin protein. We hypothesize that PERK-ATF4 activation might help to stop irrelevant protein synthesis and minimize energy consumption.
Currently, there are no direct studies showing the influence of the UPR on signaling pathways that participate in lens development. However, the UPR is connected to these signaling pathways in other types of cells. As mentioned in the second section, FGF, BMP, and Wnt serve as core molecular regulators in three lens development stages. A study found that UPR activation is an inducer of FGF1 and FGF2 expressions in melanoma cells and is positively correlated with the activity of the ATF6 and PERK signaling pathways (Eigner et al., 2017). During angiogenesis, PERK kinase and its downstream factor ATF4 are drivers of FGF2 expression (Wang et al., 2012; Philippe et al., 2016). Additionally, moderate UPR activation can lead to the expression of FGF21 and its receptor FGFR1 in cardiomyocytes (Liang et al., 2017). The IRE1α–XBP1 pathway directly activates the transcriptional expression of Fgf21 (Jiang et al., 2014). However, in contrast to typical ROS-mediated UPR activation, hypoxia-induced UPR activation reduces FGF signaling in cardiac progenitor cells (Shi et al., 2016), indicating that hypoxia-induced UPR plays a different role than that of the ROS-mediated UPR. For BMP signals, an interconnected regulatory loop is established with XBP1 and BMP4 in Xenopus that synergistically participates in mesodermal and neural tissue development (Cao et al., 2006). However, studies are now focused more on BMP signaling as an upstream factor that initiates activation of the UPR during osteogenesis, not the downstream effector (Li et al., 2014; Tanaka et al., 2014). We speculate that BMP signals might also play a regulatory role through UPR signaling pathways during lens development, for which further studies are needed for verification. Wnt has also been linked to the UPR. In osteocytes, genome-wide RNA sequencing indicates a regulatory effect of upstream XBP1 on Wnt signaling (Zhou et al., 2018), and UPR activation may result in active Wnt signaling (Chan et al., 2017). In stem cell differentiation studies, UPR activation drives endodermal cell fate via the β-catenin signaling pathway (Xu et al., 2014). ATF4 and ATF6 were found to be linked with the β-catenin pathway during osteogenesis and adipogenesis, respectively (Nakamura et al., 2013; Yu et al., 2013). In some cancer cells, IRE1α–XBP1 plays a positive regulatory role in the Wnt signaling pathway (Hua et al., 2013; Rodvold et al., 2017). Based on the relevant evidence, we hypothesize that there might be a positive correlation between the UPR and Wnt signaling in lens development.
Taken together, the data indicate that the three branches of the UPR may participate in lens development and display potential interconnections with the signaling pathways involved. However, current evidence is still fragmented. Only several core molecules of the UPR have been demonstrated to participate in lens development. Since some factors, such as ATF4, are multifunctional, studies based on individual factors within the UPR cannot illustrate the precise mechanism by which the UPR influences lens development. Additionally, lens development is a continuous process that occurs in fetuses in the uterus. It is relatively difficult to acquire direct and timely morphological and molecular evidence to better understand developmental processes. Because of ethical problems, current knowledge is largely based on model animals that are profoundly different from humans. Although capturing the blueprint of the UPR molecular regulation pattern and finding direct evidence of human lens development are of great importance, selecting an appropriate model seems to have become a priority.
5 Future directions
Great progress has been made in recent regenerative medical studies. hESC- and human-induced pluripotent stem cell (hiPSC)-based 3D tissue generation has shed light on developmental biology. These tools not only provide new ways to elaborately study both morphological and molecular details during human organ formation but also serve as ideal models to study diseases and screen drugs (Rossi et al., 2018). Currently, using hESCs or hiPSCs, stem cell technology is applied to generate organ-like structures (organoids), including the optic cup, cerebrum, cerebellum, intestine, colon, liver, lung, and kidney (Eiraku et al., 2008; Spence et al., 2011; Nakano et al., 2012; Fordham et al., 2013; Takebe et al., 2013; Takasato et al., 2014; Muguruma et al., 2015; Dye et al., 2016). Cultivated tissues show organizational structures, cell composition, and functional characteristics similar to those of the original organs. Based on the 3D cultivation system, in our previous studies, we successfully analyzed the influence of microenvironmental changes during retinal development (Gao et al., 2016) and recently isolated a group of promising therapeutic cell sources to treat retinal degenerative diseases (Zou et al., 2019).
Due to the special construction of the lens, the ideal 3D cultivation method for generating intact and functional human lenses has not yet been developed. However, efforts are being made to form lens-like (lentoid) bodies or microlenses from hESCs and hiPSCs (Figure 3). Yang et al. (2010)first reported a three-stage system to differentiate hESCs into lens progenitor cells that subsequently formed small lentoid bodies (approximately 1,000 bodies per 30-mm well). Considering this result, Murphy et al. (2018)successfully generated microlenses with light-focusing ability, the characteristic function of the lens, from both hESCs and hiPSCs by aggregating ROR1+ LECs from induced lens progenitor cells. Later, the antibody isolation method was simplified through research and development of the cell strainer method (Dewi et al., 2020). The cells generated with this method showed RNA expression profiles similar to those of ROR1+ LECs and could also generate lentoid bodies with light-focusing ability (Dewi et al., 2020). A “fried egg” method was created by Fu et al., (2017a) in 2017. In this protocol, hiPSCs were induced into lens progenitor cells and lentoid bodies. This method significantly increased the induction efficiency and volume of the lentoid body (diameter approximately 3 mm). Further transcriptional analysis confirmed that the expression profiles of both the hESC- and hiPSC-derived lentoid bodies generated with the “fried egg” protocol were comparable (Ali et al., 2019) and mimicked the early stage of lens development (Ali et al., 2020). Nevertheless, the “fried egg” method may be regarded as an upgrade of the three-stage protocol, as the induction time window and induction supplement are relatively identical. In contrast, Nanog-negative peripheral cells were isolated at the end of the first step in the “fried egg” method. Both the ROR1+ cell aggregation and “fried egg” methods are enriched in LEC progenitor cells at second-stage induction, which contributes to lens fate establishment from ESCs and iPSCs.
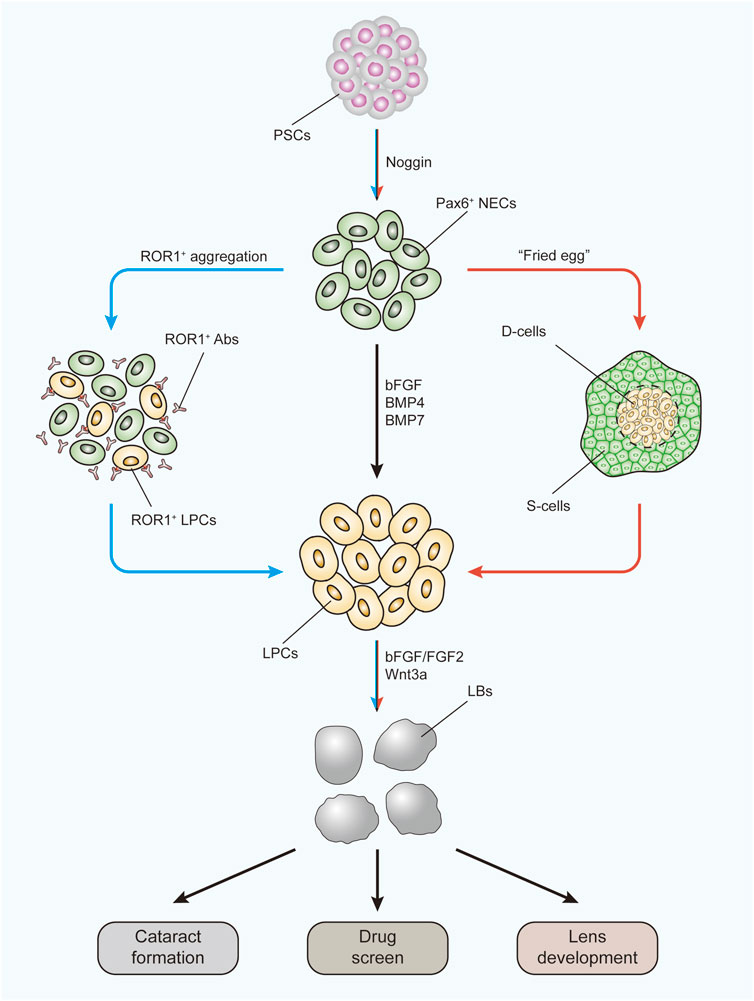
FIGURE 3. Three-stage protocol to form LBs from human PSCs using two improved methods. The black arrow indicates the main route of the three-stage protocol. The blue arrow indicates the ROR1+ LPC aggregation methods. The red arrow indicates the “fried egg” method. LBs generated under these protocols can be used to study the mechanism of cataract formation, to apply drug screens, and to investigate lens development. PSCs, pluripotent stem cells; NECs, neuroectodermal cells; Abs, antibodies; LPCs, lens progenitor cells; D-cells, differentiating cells; S-cells, supporting cells; LBs, lentoid bodies.
These models were proven to be excellent for exploring cataract formation mechanisms (Murphy et al., 2018; Qin et al., 2019) and studying autophagy during lens development (Fu et al., 2017b). More importantly, as they are more disposable and because the results are easier to monitor than in vivo models, these models are ideal by their nature for studying the influence of specific signaling pathways during human lens development (Figure 3). By using the “three-stage” protocol, a study confirmed the lens differentiation-promoting effect of the noncanonical Wnt pathway (Han C. et al., 2018). As the UPR is also involved in signaling pathway activation, these models can be similarly used to study the UPR influence. The pathological effects of the UPR have already been discovered using kidney, lung, and intestinal organoids (Dvela-Levitt et al., 2019; Bampi et al., 2020).
Additionally, progress in other technical areas also helps the study of lens development. Emerging high-throughput methods, including microarrays, RNA-sequencing, and tandem mass spectrometry, provide us with better approaches to understand the gene regulatory network and generate a global protein expression profile during lens development (Anand and Lachke, 2017; Aryal et al., 2020). Advances in single-cell transcriptomics further expand research tools. Using this technology, Dylan et al. discovered the temporospatial expression patterns of three crystallin proteins and examined the expression dynamics related to cytoskeletal, RNA-binding, membrane-associated, and transcription factor genes in the zebrafish lens (Farnsworth et al., 2021). With this technical progress, the technology of 3D tissue generation can be better used for future research on lens development.
In summary, it is foreseeable that lentoids derived from hESCs or hiPSCs will be able to provide a new and relatively direct understanding of the UPR in lens development and may become a momentous tool for development biology research.
6 Conclusion
Lens development and UPR signaling pathways have been elaborately investigated in recent decades. The former is usually considered a normal physiological process, while the latter is often studied under pathological conditions. However, growing evidence has demonstrated that some core factors of the UPR are also important during developmental processes, although errors in the UPR function can turn “physiological” into “pathological.” To date, there is still a large gap in understanding the effects of the UPR during lens development. Current studies only provide correlative evidence based on individual factors in the UPR. The detailed interrelations as well as the specific influence on each developmental stage will require extensive efforts to develop the precise blueprint. Indeed, the number of developing lens cells is small, in addition to unknown processes during lens development, which both become critical challenges faced by future research. As the novel 3D cultivation method continues to be developed, there is reason to believe that dramatic progress can be made with this technological advance. More importantly, the orchestrating but concise construction, simple cellular constitution, and easy monitoring methods make the lens a perfect model for deciphering developmental codes. Thus, determining the ROS and UPR mechanisms in lens development is of great value not only within the ophthalmological field but also for developmental research.
Author contributions
LG and ZY prepared the manuscript. LG, TM, and HL generated the figures. LG, NJ, YH, JD, and ZL revised the manuscript. NJ mainly contribute to the revision of the manuscript. All authors contributed to the article and approved the submitted version.
Funding
This study was supported by the National Nature Science Foundation of China (No. 82070937; No. 81870640; No. 82000923).
Conflict of interest
The authors declare that the research was conducted in the absence of any commercial or financial relationships that could be construed as a potential conflict of interest.
Publisher’s note
All claims expressed in this article are solely those of the authors and do not necessarily represent those of their affiliated organizations, or those of the publisher, the editors, and the reviewers. Any product that may be evaluated in this article, or claim that may be made by its manufacturer, is not guaranteed or endorsed by the publisher.
References
Ahrens, K., and Schlosser, G. (2005). Tissues and signals involved in the induction of placodal Six1 expression in Xenopus laevis. Dev. Biol. 288, 40–59. doi:10.1016/j.ydbio.2005.07.022
Ali, M., Kabir, F., Raskar, S., Renuse, S., Na, C. H., Delannoy, M., et al. fnm (2020). Generation and proteome profiling of PBMC-originated, iPSC-derived lentoid bodies. Stem Cell Res. 46, 101813. doi:10.1016/j.scr.2020.101813
Ali, M., Kabir, F., Thomson, J. J., Ma, Y., Qiu, C., Delannoy, M., et al. (2019). Comparative transcriptome analysis of hESC- and iPSC-derived lentoid bodies. Sci. Rep. 9, 18552. doi:10.1038/s41598-019-54258-z
Anand, D., and Lachke, S. A. (2017). Systems biology of lens development: A paradigm for disease gene discovery in the eye. Exp. Eye Res. 156, 22–33. doi:10.1016/j.exer.2016.03.010
Andersen, J. K. (2004). Oxidative stress in neurodegeneration: Cause or consequence? Nat. Med. 10 (1), S18–S25. doi:10.1038/nrn1434
Andreyev, A. Y., Kushnareva, Y. E., and Starkov, A. A. (2005). Mitochondrial metabolism of reactive oxygen species. Biochem. (Mosc) 70, 200–214. doi:10.1007/s10541-005-0102-7
Angermuller, S., Bruder, G., Volkl, A., Wesch, H., and Fahimi, H. D. (1987). Localization of xanthine oxidase in crystalline cores of peroxisomes. A cytochemical and biochemical study. Eur. J. Cell Biol. 45, 137–144.
Ansar, M., Santos-Cortez, R. L., Saqib, M. A., Zulfiqar, F., Lee, K., Ashraf, N. M., et al. (2015). Mutation of ATF6 causes autosomal recessive achromatopsia. Hum. Genet. 134, 941–950. doi:10.1007/s00439-015-1571-4
Aryal, S., Anand, D., Hernandez, F. G., Weatherbee, B. A. T., Huang, H., Reddy, A. P., et al. (2020). MS/MS in silico subtraction-based proteomic profiling as an approach to facilitate disease gene discovery: Application to lens development and cataract. Hum. Genet. 139, 151–184. doi:10.1007/s00439-019-02095-5
Audette, D. S., Scheiblin, D. A., and Duncan, M. K. (2017). The molecular mechanisms underlying lens fiber elongation. Exp. Eye Res. 156, 41–49. doi:10.1016/j.exer.2016.03.016
Bai, J., Dong, L., Song, Z., Ge, H., Cai, X., Wang, G., et al. (2013). The role of melatonin as an antioxidant in human lens epithelial cells. Free Radic. Res. 47, 635–642. doi:10.3109/10715762.2013.808743
Bailey, A. P., Bhattacharyya, S., Bronner-Fraser, M., and Streit, A. (2006). Lens specification is the ground state of all sensory placodes, from which FGF promotes olfactory identity. Dev. Cell 11, 505–517. doi:10.1016/j.devcel.2006.08.009
Bailey, A. P., and Streit, A. (2005). Sensory organs: Making and breaking the pre‐placodal region. Curr. Top. Dev. Biol. 72, 167–204. doi:10.1016/S0070-2153(05)72003-2
Baird, L., and Dinkova-Kostova, A. T. (2011). The cytoprotective role of the Keap1-Nrf2 pathway. Arch. Toxicol. 85, 241–272. doi:10.1007/s00204-011-0674-5
Bampi, G. B., Rauscher, R., Kirchner, S., Oliver, K. E., Bijvelds, M. J. C., Santos, L. A., et al. (2020). Global assessment of the integrated stress response in CF patient-derived airway and intestinal tissues. J. Cyst. Fibros. 19, 1021–1026. doi:10.1016/j.jcf.2020.04.005
Bassnett, S. (2009). On the mechanism of organelle degradation in the vertebrate lens. Exp. Eye Res. 88, 133–139. doi:10.1016/j.exer.2008.08.017
Bassnett, S., and Sikic, H. (2017). The lens growth process. Prog. Retin. Eye Res. 60, 181–200. doi:10.1016/j.preteyeres.2017.04.001
Basu, S., Rajakaruna, S., Dickinson, B. C., Chang, C. J., and Menko, A. S. (2014). Endogenous hydrogen peroxide production in the epithelium of the developing embryonic lens. Mol. Vis. 20, 458–467.
Bedard, K., and Krause, K. H. (2007). The NOX family of ROS-generating NADPH oxidases: Physiology and pathophysiology. Physiol. Rev. 87 (1), 245–313. doi:10.1152/physrev.00044.2005
Bennett, J. T., Joubin, K., Cheng, S., Aanstad, P., Herwig, R., Clark, M., et al. (2007). Nodal signaling activates differentiation genes during zebrafish gastrulation. Dev. Biol. 304, 525–540. doi:10.1016/j.ydbio.2007.01.012
Bertolotti, A., Zhang, Y., Hendershot, L. M., Harding, H. P., and Ron, D. (2000). Dynamic interaction of BiP and ER stress transducers in the unfolded-protein response. Nat. Cell Biol. 2, 326–332. doi:10.1038/35014014
Bigarella, C. L., Liang, R., and Ghaffari, S. (2014). Stem cells and the impact of ROS signaling. Development 141, 4206–4218. doi:10.1242/dev.107086
Booth, D. M., Enyedi, B., Geiszt, M., Varnai, P., and Hajnoczky, G. (2016). Redox nanodomains are induced by and control calcium signaling at the ER-mitochondrial interface. Mol. Cell 63, 240–248. doi:10.1016/j.molcel.2016.05.040
Borquez, D. A., Urrutia, P. J., Wilson, C., van Zundert, B., Nunez, M. T., and Gonzalez-Billault, C. (2016). Dissecting the role of redox signaling in neuronal development. J. Neurochem. 137, 506–517. doi:10.1111/jnc.13581
Boswell, B. A., Overbeek, P. A., and Musil, L. S. (2008). Essential role of BMPs in FGF-induced secondary lens fiber differentiation. Dev. Biol. 324, 202–212. doi:10.1016/j.ydbio.2008.09.003
Brennan, L. A., McGreal-Estrada, R., Logan, C. M., Cvekl, A., Menko, A. S., and Kantorow, M. (2018). BNIP3L/NIX is required for elimination of mitochondria, endoplasmic reticulum and Golgi apparatus during eye lens organelle-free zone formation. Exp. Eye Res. 174, 173–184. doi:10.1016/j.exer.2018.06.003
Brieger, K., Schiavone, S., Miller, F. J., and Krause, K. H. (2012). Reactive oxygen species: From health to disease. Swiss Med. Wkly. 142, w13659. doi:10.4414/smw.2012.13659
Cain, S., Martinez, G., Kokkinos, M. I., Turner, K., Richardson, R. J., Abud, H. E., et al. (2008). Differential requirement for beta-catenin in epithelial and fiber cells during lens development. Dev. Biol. 321, 420–433. doi:10.1016/j.ydbio.2008.07.002
Cao, S. S., and Kaufman, R. J. (2014). Endoplasmic reticulum stress and oxidative stress in cell fate decision and human disease. Antioxid. Redox Signal. 21 (3), 396–413. doi:10.1089/ars.2014.5851
Cao, Y., Knochel, S., Oswald, F., Donow, C., Zhao, H., and Knochel, W. (2006). XBP1 forms a regulatory loop with BMP-4 and suppresses mesodermal and neural differentiation in Xenopus embryos. Mech. Dev. 123, 84–96. doi:10.1016/j.mod.2005.09.003
Chan, W. C. W., Tsang, K. Y., Cheng, Y. W., Ng, V. C. W., Chik, H., Tan, Z. J., et al. (2017). Activating the unfolded protein response in osteocytes causes hyperostosis consistent with craniodiaphyseal dysplasia. Hum. Mol. Genet. 26, 4572–4587. doi:10.1093/hmg/ddx339
Chaudhari, P., Ye, Z., and Jang, Y. Y. (2014). Roles of reactive oxygen species in the fate of stem cells. Antioxid. Redox Signal. 20, 1881–1890. doi:10.1089/ars.2012.4963
Chauhan, B., Plageman, T., Lou, M., and Lang, R. (2015). Epithelial morphogenesis: The mouse eye as a model system. Curr. Top. Dev. Biol. 111, 375–399. doi:10.1016/bs.ctdb.2014.11.011
Chen, K. C., Zhou, Y., Xing, K., Krysan, K., and Lou, M. F. (2004). Platelet derived growth factor (PDGF)-induced reactive oxygen species in the lens epithelial cells: The redox signaling. Exp. Eye Res. 78, 1057–1067. doi:10.1016/j.exer.2004.02.004
Chen, K. C., Zhou, Y., Zhang, W., and Lou, M. F. (2007). Control of PDGF-induced reactive oxygen species (ROS) generation and signal transduction in human lens epithelial cells. Mol. Vis. 13, 374–387.
Cheng, C., Nowak, R. B., and Fowler, V. M. (2017). The lens actin filament cytoskeleton: Diverse structures for complex functions. Exp. Eye Res. 156, 58–71. doi:10.1016/j.exer.2016.03.005
Chiang, W. C., Chan, P., Wissinger, B., Vincent, A., Skorczyk-Werner, A., Krawczynski, M. R., et al. (2017). Achromatopsia mutations target sequential steps of ATF6 activation. Proc. Natl. Acad. Sci. U. S. A. 114, 400–405. doi:10.1073/pnas.1606387114
Chiang, W. C., Kroeger, H., Sakami, S., Messah, C., Yasumura, D., Matthes, M. T., et al. (2015). Robust endoplasmic reticulum-associated degradation of rhodopsin precedes retinal degeneration. Mol. Neurobiol. 52, 679–695. doi:10.1007/s12035-014-8881-8
Clark, E. M., Nonarath, H. J. T., Bostrom, J. R., and Link, B. A. (2020). Establishment and validation of an endoplasmic reticulum stress reporter to monitor zebrafish ATF6 activity in development and disease. Dis. Model. Mech. 13, dmm041426. doi:10.1242/dmm.041426
Coffman, J. A., and Su, Y. H. (2019). Redox regulation of development and regeneration. Curr. Opin. Genet. Dev. 57, 9–15. doi:10.1016/j.gde.2019.06.002
Collins, T. N., Mao, Y., Li, H., Bouaziz, M., Hong, A., Feng, G. S., et al. (2018). Crk proteins transduce FGF signaling to promote lens fiber cell elongation. Elife 7, e32586. doi:10.7554/eLife.32586
Costello, M. J., Brennan, L. A., Basu, S., Chauss, D., Mohamed, A., Gilliland, K. O., et al. (2013). Autophagy and mitophagy participate in ocular lens organelle degradation. Exp. Eye Res. 116, 141–150. doi:10.1016/j.exer.2013.08.017
Cui, X., Feng, R., Wang, J., Du, C., Pi, X., Chen, D., et al. (2020). Heat shock factor 4 regulates lysosome activity by modulating the alphaB-crystallin-ATP6V1A-mTOR complex in ocular lens. Biochim. Biophys. Acta Gen. Subj. 1864, 129496. doi:10.1016/j.bbagen.2019.129496
Cvekl, A., and Ashery-Padan, R. (2014). The cellular and molecular mechanisms of vertebrate lens development. Development 141, 4432–4447. doi:10.1242/dev.107953
Cvekl, A., and Zhang, X. (2017). Signaling and gene regulatory networks in mammalian lens development. Trends Genet. 33, 677–702. doi:10.1016/j.tig.2017.08.001
Dawes, L. J., Shelley, E. J., McAvoy, J. W., and Lovicu, F. J. (2018). A role for Hippo/YAP-signaling in FGF-induced lens epithelial cell proliferation and fibre differentiation. Exp. Eye Res. 169, 122–133. doi:10.1016/j.exer.2018.01.014
Dewi, C. U., Mason, M., Cohen-Hyams, T., Killingsworth, M. C., Harman, D. G., Gnanasambandapillai, V., et al. (2020). A simplified method for producing human lens epithelial cells and light-focusing micro-lenses from pluripotent stem cells. Exp. Eye Res. 202, 108317. doi:10.1016/j.exer.2020.108317
Di Meo, S., Reed, T. T., Venditti, P., and Victor, V. M. (2016). Role of ROS and RNS sources in ₹physiological and pathological conditions. Oxid. Med. Cell. Longev. 2016, 1245049. doi:10.1155/2016/1245049
Donaldson, P. J., Chee, K. S., Lim, J. C., and Webb, K. F. (2009). Regulation of lens volume: Implications for lens transparency. Exp. Eye Res. 88, 144–150. doi:10.1016/j.exer.2008.05.011
Durocher, F., Faure, R., Labrie, Y., Pelletier, L., Bouchard, I., and Laframboise, R. (2006). A novel mutation in the EIF2AK3 gene with variable expressivity in two patients with Wolcott-Rallison syndrome. Clin. Genet. 70, 34–38. doi:10.1111/j.1399-0004.2006.00632.x
Dvela-Levitt, M., Kost-Alimova, M., Emani, M., Kohnert, E., Thompson, R., Sidhom, E. H., et al. (2019). Small molecule targets TMED9 and promotes lysosomal degradation to reverse proteinopathy. Cell 178, 521–535. e523. doi:10.1016/j.cell.2019.07.002
Dye, B. R., Dedhia, P. H., Miller, A. J., Nagy, M. S., White, E. S., Shea, L. D., et al. (2016). A bioengineered niche promotes in vivo engraftment and maturation of pluripotent stem cell derived human lung organoids. Elife 5, e19732. doi:10.7554/eLife.19732
Eigner, K., Filik, Y., Mark, F., Schutz, B., Klambauer, G., Moriggl, R., et al. (2017). The unfolded protein response impacts melanoma progression by enhancing FGF expression and can be antagonized by a chemical chaperone. Sci. Rep. 7, 17498. doi:10.1038/s41598-017-17888-9
Eiraku, M., Watanabe, K., Matsuo-Takasaki, M., Kawada, M., Yonemura, S., Matsumura, M., et al. (2008). Self-organized formation of polarized cortical tissues from ESCs and its active manipulation by extrinsic signals. Cell Stem Cell 3, 519–532. doi:10.1016/j.stem.2008.09.002
Evans, T. G., Belak, Z., Ovsenek, N., and Krone, P. H. (2007). Heat shock factor 1 is required for constitutive Hsp70 expression and normal lens development in embryonic zebrafish. Comp. Biochem. Physiol. A Mol. Integr. Physiol. 146, 131–140. doi:10.1016/j.cbpa.2006.09.023
Farnsworth, D. R., Posner, M., and Miller, A. C. (2021). Single cell transcriptomics of the developing zebrafish lens and identification of putative controllers of lens development. Exp. Eye Res. 206, 108535. doi:10.1016/j.exer.2021.108535
Firtina, Z., Danysh, B. P., Bai, X., Gould, D. B., Kobayashi, T., and Duncan, M. K. (2009). Abnormal expression of collagen IV in lens activates unfolded protein response resulting in cataract. J. Biol. Chem. 284, 35872–35884. doi:10.1074/jbc.M109.060384
Firtina, Z., and Duncan, M. K. (2011). Unfolded Protein Response (UPR) is activated during normal lens development. Gene Expr. Patterns 11, 135–143. doi:10.1016/j.gep.2010.10.005
Fordham, R. P., Yui, S., Hannan, N. R., Soendergaard, C., Madgwick, A., Schweiger, P. J., et al. (2013). Transplantation of expanded fetal intestinal progenitors contributes to colon regeneration after injury. Cell Stem Cell 13, 734–744. doi:10.1016/j.stem.2013.09.015
Freeman, B. A., and Crapo, J. D. (1982). Biology of disease: Free radicals and tissue injury. Lab. Invest. 47, 412–426.
Fritz, R., Bol, J., Hebling, U., Angermuller, S., Volkl, A., Fahimi, H. D., et al. (2007). Compartment-dependent management of H(2)O(2) by peroxisomes. Free Radic. Biol. Med. 42, 1119–1129. doi:10.1016/j.freeradbiomed.2007.01.014
Fu, Q., Qin, Z., Jin, X., Zhang, L., Chen, Z., He, J., et al. (2017a). Generation of functional lentoid bodies from human induced pluripotent stem cells derived from urinary cells. Invest. Ophthalmol. Vis. Sci. 58, 517–527. doi:10.1167/iovs.16-20504
Fu, Q., Qin, Z., Zhang, L., Lyu, D., Tang, Q., Yin, H., et al. (2017b). A new long noncoding RNA ALB regulates autophagy by enhancing the transformation of LC3BI to LC3BII during human lens development. Mol. Ther. Nucleic. Acids 9, 207–217. doi:10.1016/j.omtn.2017.09.011
Furuta, Y., and Hogan, B. L. (1998). BMP4 is essential for lens induction in the mouse embryo. Genes Dev. 12, 3764–3775. doi:10.1101/gad.12.23.3764
Gao, L., Chen, X., Zeng, Y., Li, Q., Zou, T., Chen, S., et al. (2016). Intermittent high oxygen influences the formation of neural retinal tissue from human embryonic stem cells. Sci. Rep. 6, 29944. doi:10.1038/srep29944
Garcia, C. M., Huang, J., Madakashira, B. P., Liu, Y., Rajagopal, R., Dattilo, L., et al. (2011). The function of FGF signaling in the lens placode. Dev. Biol. 351, 176–185. doi:10.1016/j.ydbio.2011.01.001
Gardner, B. M., Pincus, D., Gotthardt, K., Gallagher, C. M., and Walter, P. (2013). Endoplasmic reticulum stress sensing in the unfolded protein response. Cold Spring Harb. Perspect. Biol. 5, a013169. doi:10.1101/cshperspect.a013169
Garg, A., Hannan, A., Wang, Q., Makrides, N., Zhong, J., Li, H., et al. (2020). Etv transcription factors functionally diverge from their upstream FGF signaling in lens development. Elife 9, e51915. doi:10.7554/eLife.51915
Ghosh, R., Colon-Negron, K., and Papa, F. R. (2019). Endoplasmic reticulum stress, degeneration of pancreatic islet beta-cells, and therapeutic modulation of the unfolded protein response in diabetes. Mol. Metab. 27S, S60–S68. doi:10.1016/j.molmet.2019.06.012
Grocott, T., Tambalo, M., and Streit, A. (2012). The peripheral sensory nervous system in the vertebrate head: A gene regulatory perspective. Dev. Biol. 370, 3–23. doi:10.1016/j.ydbio.2012.06.028
Groenendyk, J., and Michalak, M. (2005). Endoplasmic reticulum quality control and apoptosis. Acta Biochim. Pol. 52, 381–395. doi:10.18388/abp.2005_3451
Guo, F., Han, X., Wu, Z., Cheng, Z., Hu, Q., Zhao, Y., et al. (2016). ATF6a, a Runx2-activable transcription factor, is a new regulator of chondrocyte hypertrophy. J. Cell Sci. 129, 717–728. doi:10.1242/jcs.169623
Gupta, S., McGrath, B., and Cavener, D. R. (2010). PERK (EIF2AK3) regulates proinsulin trafficking and quality control in the secretory pathway. Diabetes 59, 1937–1947. doi:10.2337/db09-1064
Han, C., Li, J., Wang, C., Ouyang, H., Ding, X., Liu, Y., et al. (2018a). Wnt5a contributes to the differentiation of human embryonic stem cells into lentoid bodies through the noncanonical Wnt/JNK signaling pathway. Invest. Ophthalmol. Vis. Sci. 59, 3449–3460. doi:10.1167/iovs.18-23902
Han, J., Back, S. H., Hur, J., Lin, Y. H., Gildersleeve, R., Shan, J., et al. (2013). ER-stress-induced transcriptional regulation increases protein synthesis leading to cell death. Nat. Cell Biol. 15, 481–490. doi:10.1038/ncb2738
Han, Y., Ishibashi, S., Iglesias-Gonzalez, J., Chen, Y., Love, N. R., and Amaya, E. (2018b). Ca(2+)-Induced mitochondrial ROS regulate the early embryonic cell cycle. Cell Rep. 22, 218–231. doi:10.1016/j.celrep.2017.12.042
Hasegawa, D., Calvo, V., Avivar-Valderas, A., Lade, A., Chou, H. I., Lee, Y. A., et al. (2015). Epithelial Xbp1 is required for cellular proliferation and differentiation during mammary gland development. Mol. Cell Biol. 35, 1543–1556. doi:10.1128/MCB.00136-15
Haynes, C. M., Titus, E. A., and Cooper, A. A. (2004). Degradation of misfolded proteins prevents ER-derived oxidative stress and cell death. Mol. Cell 15, 767–776. doi:10.1016/j.molcel.2004.08.025
Hejtmancik, J. F., Riazuddin, S. A., McGreal, R., Liu, W., Cvekl, A., and Shiels, A. (2015). Lens biology and biochemistry. Prog. Mol. Biol. Transl. Sci. 134, 169–201. doi:10.1016/bs.pmbts.2015.04.007
Higa, A., Taouji, S., Lhomond, S., Jensen, D., Fernandez-Zapico, M. E., Simpson, J. C., et al. (2014). Endoplasmic reticulum stress-activated transcription factor ATF6alpha requires the disulfide isomerase PDIA5 to modulate chemoresistance. Mol. Cell. Biol. 34, 1839–1849. doi:10.1128/MCB.01484-13
Hillary, R. F., and FitzGerald, U. (2018). A lifetime of stress: ATF6 in development and homeostasis. J. Biomed. Sci. 25, 48. doi:10.1186/s12929-018-0453-1
Höglinger, G. U., Melhem, N. M., Dickson, D. W., Sleiman, P. M. A., Wang, L.-S., Klei, L., et al. (2011). Identification of common variants influencing risk of the tauopathy progressive supranuclear palsy. Nat. Genet. 43, 699–705. doi:10.1038/ng.859
Holmstrom, K. M., and Finkel, T. (2014). Cellular mechanisms and physiological consequences of redox-dependent signalling. Nat. Rev. Mol. Cell Biol. 15, 411–421. doi:10.1038/nrm3801
Hosseini, H. S., and Taber, L. A. (2018). How mechanical forces shape the developing eye. Prog. Biophys. Mol. Biol. 137, 25–36. doi:10.1016/j.pbiomolbio.2018.01.004
Houssin, N. S., Martin, J. B., Coppola, V., Yoon, S. O., and Plageman, T. F. (2020). Formation and contraction of multicellular actomyosin cables facilitate lens placode invagination. Dev. Biol. 462, 36–49. doi:10.1016/j.ydbio.2020.02.014
Hu, Q., Khanna, P., Ee Wong, B. S., Lin Heng, Z. S., Subhramanyam, C. S., Thanga, L. Z., et al. (2018). Oxidative stress promotes exit from the stem cell state and spontaneous neuronal differentiation. Oncotarget 9, 4223–4238. doi:10.18632/oncotarget.23786
Hua, Y., White-Gilbertson, S., Kellner, J., Rachidi, S., Usmani, S. Z., Chiosis, G., et al. (2013). Molecular chaperone gp96 is a novel therapeutic target of multiple myeloma. Clin. Cancer Res. 19, 6242–6251. doi:10.1158/1078-0432.CCR-13-2083
Huang, H. W., Zeng, X., Rhim, T., Ron, D., and Ryoo, H. D. (2017). The requirement of IRE1 and XBP1 in resolving physiological stress during Drosophila development. J. Cell Sci. 130, 3040–3049. doi:10.1242/jcs.203612
Huang, J., Rajagopal, R., Liu, Y., Dattilo, L. K., Shaham, O., Ashery-Padan, R., et al. (2011). The mechanism of lens placode formation: A case of matrix-mediated morphogenesis. Dev. Biol. 355, 32–42. doi:10.1016/j.ydbio.2011.04.008
Huang, Y., Ma, T., Ye, Z., Li, H., Zhao, Y., Chen, W., et al. (2018a). Carbon monoxide (CO) inhibits hydrogen peroxide (H2O2)-induced oxidative stress and the activation of NF-kappaB signaling in lens epithelial cells. Exp. Eye Res. 166, 29–39. doi:10.1016/j.exer.2017.08.016
Huang, Y., Ye, Z., Ma, T., Li, H., Zhao, Y., Chen, W., et al. (2018b). Carbon monoxide (CO) modulates hydrogen peroxide (H2O2)-mediated cellular dysfunction by targeting mitochondria in rabbit lens epithelial cells. Exp. Eye Res. 169, 68–78. doi:10.1016/j.exer.2018.01.023
Hughes, D., and Mallucci, G. R. (2019). The unfolded protein response in neurodegenerative disorders - therapeutic modulation of the PERK pathway. FEBS J. 286, 342–355. doi:10.1111/febs.14422
Iida, K., Li, Y., McGrath, B. C., Frank, A., and Cavener, D. R. (2007). PERK eIF2 alpha kinase is required to regulate the viability of the exocrine pancreas in mice. BMC Cell Biol. 8, 38. doi:10.1186/1471-2121-8-38
Ikesugi, K., Yamamoto, R., Mulhern, M. L., and Shinohara, T. (2006). Role of the unfolded protein response (UPR) in cataract formation. Exp. Eye Res. 83, 508–516. doi:10.1016/j.exer.2006.01.033
Ishikawa, T., Kashima, M., Nagano, A. J., Ishikawa-Fujiwara, T., Kamei, Y., Todo, T., et al. (2017). Unfolded protein response transducer IRE1-mediated signaling independent of XBP1 mRNA splicing is not required for growth and development of medaka fish. Elife 6, e26845. doi:10.7554/eLife.26845
Ishikawa, T., Taniguchi, Y., Okada, T., Takeda, S., and Mori, K. (2011). Vertebrate unfolded protein response: Mammalian signaling pathways are conserved in medaka fish. Cell Struct. Funct. 36, 247–259. doi:10.1247/csf.11036
Iwawaki, T., Akai, R., and Kohno, K. (2010). IRE1alpha disruption causes histological abnormality of exocrine tissues, increase of blood glucose level, and decrease of serum immunoglobulin level. PLoS One 5, e13052. doi:10.1371/journal.pone.0013052
Iwawaki, T., Akai, R., Yamanaka, S., and Kohno, K. (2009). Function of IRE1 alpha in the placenta is essential for placental development and embryonic viability. Proc. Natl. Acad. Sci. U. S. A. 106, 16657–16662. doi:10.1073/pnas.0903775106
Ji, F., Shen, T., Zou, W., and Jiao, J. (2017). UCP2 regulates embryonic neurogenesis via ROS-mediated Yap alternation in the developing neocortex. Stem Cells 35, 1479–1492. doi:10.1002/stem.2605
Jiang, S., Yan, C., Fang, Q. C., Shao, M. L., Zhang, Y. L., Liu, Y., et al. (2014). Fibroblast growth factor 21 is regulated by the IRE1alpha-XBP1 branch of the unfolded protein response and counteracts endoplasmic reticulum stress-induced hepatic steatosis. J. Biol. Chem. 289, 29751–29765. doi:10.1074/jbc.M114.565960
Kamemura, K., and Chihara, T. (2019). Multiple functions of the ER-resident VAP and its extracellular role in neural development and disease. J. Biochem. 165 (5), 391–400. doi:10.1093/jb/mvz011
Kensler, T. W., Wakabayashi, N., and Biswal, S. (2007). Cell survival responses to environmental stresses via the Keap1-Nrf2-ARE pathway. Annu. Rev. Pharmacol. Toxicol. 47, 89–116. doi:10.1146/annurev.pharmtox.46.120604.141046
Ko, M. K., Chi, J. G., and Chang, B. L. (1985). Hyaloid vascular pattern in the human fetus. J. Pediatr. Ophthalmol. Strabismus 22, 188–193. doi:10.3928/0191-3913-19850901-07
Koch, G. L. (1990). The endoplasmic reticulum and calcium storage. Bioessays 12, 527–531. doi:10.1002/bies.950121105
Kohl, S., Zobor, D., Chiang, W. C., Weisschuh, N., Staller, J., Gonzalez Menendez, I., et al. (2015). Mutations in the unfolded protein response regulator ATF6 cause the cone dysfunction disorder achromatopsia. Nat. Genet. 47, 757–765. doi:10.1038/ng.3319
Koritzinsky, M., Magagnin, M. G., van den Beucken, T., Seigneuric, R., Savelkouls, K., Dostie, J., et al. (2006). Gene expression during acute and prolonged hypoxia is regulated by distinct mechanisms of translational control. EMBO J. 25 (5), 1114–1125. doi:10.1038/sj.emboj.7600998
Koumenis, C., Naczki, C., Koritzinsky, M., Rastani, S., Diehl, A., Sonenberg, N., et al. (2002). Regulation of protein synthesis by hypoxia via activation of the endoplasmic reticulum kinase PERK and phosphorylation of the translation initiation factor eIF2alpha. Mol. Cell Biol. 22 (21), 7405–7416. doi:10.1128/MCB.22.21.7405-7416.2002
Kroeger, H., Grandjean, J. M. D., Chiang, W. J., Bindels, D. D., Mastey, R., Okalova, J., et al. (2021). ATF6 is essential for human cone photoreceptor development. Proc. Natl. Acad. Sci. U. S. A. 118 (39), e2103196118. doi:10.1073/pnas.2103196118
Kroeger, H., Grimsey, N., Paxman, R., Chiang, W. C., Plate, L., Jones, Y., et al. (2018). The unfolded protein response regulator ATF6 promotes mesodermal differentiation. Sci. Signal. 11, eaan5785. doi:10.1126/scisignal.aan5785
Kwon, H. J., Bhat, N., Sweet, E. M., Cornell, R. A., and Riley, B. B. (2010). Identification of early requirements for preplacodal ectoderm and sensory organ development. PLoS Genet. 6, e1001133. doi:10.1371/journal.pgen.1001133
Lang, R. A., Herman, K., Reynolds, A. B., Hildebrand, J. D., and Plageman, T. F. (2014). p120-catenin-dependent junctional recruitment of Shroom3 is required for apical constriction during lens pit morphogenesis. Development 141, 3177–3187. doi:10.1242/dev.107433
Lassing, I., Schmitzberger, F., Bjornstedt, M., Holmgren, A., Nordlund, P., Schutt, C. E., et al. (2007). Molecular and structural basis for redox regulation of beta-actin. J. Mol. Biol. 370, 331–348. doi:10.1016/j.jmb.2007.04.056
Li, T. F., Yukata, K., Yin, G., Sheu, T., Maruyama, T., Jonason, J. H., et al. (2014). BMP-2 induces ATF4 phosphorylation in chondrocytes through a COX-2/PGE2 dependent signaling pathway. Osteoarthr. Cartil. 22, 481–489. doi:10.1016/j.joca.2013.12.020
Liang, P., Zhong, L., Gong, L., Wang, J., Zhu, Y., Liu, W., et al. (2017). Fibroblast growth factor 21 protects rat cardiomyocytes from endoplasmic reticulum stress by promoting the fibroblast growth factor receptor 1-extracellular signalregulated kinase 1/2 signaling pathway. Int. J. Mol. Med. 40, 1477–1485. doi:10.3892/ijmm.2017.3140
Lin, J., and Wang, L. (2020). Oxidative stress in oocytes and embryo development: Implications for in vitro systems. Antioxid. Redox Signal 1, 1. doi:10.1089/ars.2020.8209
Litsiou, A., Hanson, S., and Streit, A. (2005). A balance of FGF, BMP and WNT signalling positions the future placode territory in the head. Development 132, 4051–4062. doi:10.1242/dev.01964
Logan, C. M., Bowen, C. J., and Menko, A. S. (2018). Functional role for stable microtubules in lens fiber cell elongation. Exp. Cell Res. 362, 477–488. doi:10.1016/j.yexcr.2017.12.012
Lovicu, F. J., and McAvoy, J. W. (2001). FGF-induced lens cell proliferation and differentiation is dependent on MAPK (ERK1/2) signalling. Development 128, 5075–5084. doi:10.1242/dev.128.24.5075
Lovicu, F. J., and McAvoy, J. W. (2005). Growth factor regulation of lens development. Dev. Biol. 280, 1–14. doi:10.1016/j.ydbio.2005.01.020
Lowe, C. E., Dennis, R. J., Obi, U., O'Rahilly, S., and Rochford, J. J. (2012). Investigating the involvement of the ATF6alpha pathway of the unfolded protein response in adipogenesis. Int. J. Obes. (Lond) 36, 1248–1251. doi:10.1038/ijo.2011.233
Lyu, L., Whitcomb, E. A., Jiang, S., Chang, M. L., Gu, Y., Duncan, M. K., et al. (2015). Unfolded‐protein response‐associated stabilization of p27(Cdkn1b) interferes with lens fiber cell denucleation, leading to cataract. FASEB J. 30, 1087–1095. doi:10.1096/fj.15-278036
Ma, T., Chen, T., Li, P., Ye, Z., Zhai, W., Jia, L., et al. (2016). Heme oxygenase-1 (HO-1) protects human lens epithelial cells (SRA01/04) against hydrogen peroxide (H2O2)-induced oxidative stress and apoptosis. Exp. Eye Res. 146, 318–329. doi:10.1016/j.exer.2016.02.013
Ma, T. J., Lan, D. H., He, S. Z., Ye, Z., Li, P., Zhai, W., et al. (2018). Nrf2 protects human lens epithelial cells against H2O2-induced oxidative and ER stress: The ATF4 may be involved. Exp. Eye Res. 169, 28–37. doi:10.1016/j.exer.2018.01.018
Maeda, T., Suzuki, A., Yuzawa, S., Baba, Y., Kimura, Y., and Kato, Y. (2015). Mineral trioxide aggregate induces osteoblastogenesis via Atf6. Bone Rep. 2, 36–43. doi:10.1016/j.bonr.2015.03.003
Malhotra, J. D., and Kaufman, R. J. (2007). Endoplasmic reticulum stress and oxidative stress: A vicious cycle or a double-edged sword? Antioxid. Redox Signal. 9, 2277–2293. doi:10.1089/ars.2007.1782
Martinez, G., and de Iongh, R. U. (2010). The lens epithelium in ocular health and disease. Int. J. Biochem. Cell Biol. 42, 1945–1963. doi:10.1016/j.biocel.2010.09.012
Maurel, M., Chevet, E., Tavernier, J., and Gerlo, S. (2014). Getting RIDD of RNA: IRE1 in cell fate regulation. Trends biochem. Sci. 39, 245–254. doi:10.1016/j.tibs.2014.02.008
McCord, J. M., Roy, R. S., and Schaffer, S. W. (1985). Free radicals and myocardial ischemia. The role of xanthine oxidase. Adv. Myocardiol. 5, 183–189. doi:10.1007/978-1-4757-1287-2_14
McQuiston, A., and Diehl, J. A. (2017). Recent insights into PERK-dependent signaling from the stressed endoplasmic reticulum. F1000Res. 6, 1897. doi:10.12688/f1000research.12138.1
Melo, M. O., Moraes Borges, R., and Yan, C. Y. I. (2017). Par3 in chick lens placode development. Genesis 55, 1. doi:10.1002/dvg.23032
Mitra, S., and Ryoo, H. D. (2019). The unfolded protein response in metazoan development. J. Cell. Sci. 132, jcs217216. doi:10.1242/jcs.217216
Mohyeldin, A., Garzon-Muvdi, T., and Quinones-Hinojosa, A. (2010). Oxygen in stem cell biology: A critical component of the stem cell niche. Cell Stem Cell 7, 150–161. doi:10.1016/j.stem.2010.07.007
Muccioli, M., Qaisi, D., Herman, K., and Plageman, T. F. (2016). Lens placode planar cell polarity is dependent on Cdc42-mediated junctional contraction inhibition. Dev. Biol. 412, 32–43. doi:10.1016/j.ydbio.2016.02.016
Muguruma, K., Nishiyama, A., Kawakami, H., Hashimoto, K., and Sasai, Y. (2015). Self-organization of polarized cerebellar tissue in 3D culture of human pluripotent stem cells. Cell Rep. 10, 537–550. doi:10.1016/j.celrep.2014.12.051
Murphy, M. P. (2009). How mitochondria produce reactive oxygen species. Biochem. J. 417, 1–13. doi:10.1042/BJ20081386
Murphy, S. E., and Levine, T. P. (2016). VAP, a Versatile Access Point for the Endoplasmic Reticulum: Review and analysis of FFAT-like motifs in the VAPome. Biochim. Biophys. Acta. 1861, 952–961. doi:10.1016/j.bbalip.2016.02.009
Murphy, P., Kabir, M. H., Srivastava, T., Mason, M. E., Dewi, C. U., Lim, S., et al. (2018). Light-focusing human micro-lenses generated from pluripotent stem cells model lens development and drug-induced cataract in vitro. Development 145, dev155838. doi:10.1242/dev.155838
Nakamura, Y., Hinoi, E., Iezaki, T., Takada, S., Hashizume, S., Takahata, Y., et al. (2013). Repression of adipogenesis through promotion of Wnt/beta-catenin signaling by TIS7 up-regulated in adipocytes under hypoxia. Biochim. Biophys. Acta 1832, 1117–1128. doi:10.1016/j.bbadis.2013.03.010
Nakano, T., Ando, S., Takata, N., Kawada, M., Muguruma, K., Sekiguchi, K., et al. (2012). Self-formation of optic cups and storable stratified neural retina from human ESCs. Cell Stem Cell 10, 771–785. doi:10.1016/j.stem.2012.05.009
Naughton, M. C., McMahon, J. M., and FitzGerald, U. (2015). Differential activation of ER stress pathways in myelinating cerebellar tracts. Int. J. Dev. Neurosci. 47, 347–360. doi:10.1016/j.ijdevneu.2015.08.002
Ogino, H., Fisher, M., and Grainger, R. M. (2008). Convergence of a head-field selector Otx2 and notch signaling: A mechanism for lens specification. Development 135, 249–258. doi:10.1242/dev.009548
Ohguro, N., Fukuda, M., Sasabe, T., and Tano, Y. (1999). Concentration dependent effects of hydrogen peroxide on lens epithelial cells. Br. J. Ophthalmol. 83, 1064–1068. doi:10.1136/bjo.83.9.1064
Olguin-Albuerne, M., and Moran, J. (2015). ROS produced by NOX2 control in vitro development of cerebellar granule neurons development. ASN Neuro 7, 1759091415578712. doi:10.1177/1759091415578712
Oswald, M. C. W., Garnham, N., Sweeney, S. T., and Landgraf, M. (2018). Regulation of neuronal development and function by ROS. FEBS Lett. 592, 679–691. doi:10.1002/1873-3468.12972
Padula, S. L., Sidler, E. P., Wagner, B. D., Manz, C. J., Lovicu, F. J., and Robinson, M. L. (2020). Lens fiber cell differentiation occurs independently of fibroblast growth factor receptor signaling in the absence of Pten. Dev. Biol. 467, 1–13. doi:10.1016/j.ydbio.2020.07.017
Patthey, C., and Gunhaga, L. (2014). Signaling pathways regulating ectodermal cell fate choices. Exp. Cell Res. 321, 11–16. doi:10.1016/j.yexcr.2013.08.002
Periyasamy, P., and Shinohara, T. (2017). Age-related cataracts: Role of unfolded protein response, Ca(2+) mobilization, epigenetic DNA modifications, and loss of Nrf2/Keap1 dependent cytoprotection. Prog. Retin. Eye Res. 60, 1–19. doi:10.1016/j.preteyeres.2017.08.003
Philippe, C., Dubrac, A., Quelen, C., Desquesnes, A., Van Den Berghe, L., Segura, C., et al. (2016). PERK mediates the IRES-dependent translational activation of mRNAs encoding angiogenic growth factors after ischemic stress. Sci. Signal. 9, ra44. doi:10.1126/scisignal.aaf2753
Plageman, T. F., Chung, M. I., Lou, M., Smith, A. N., Hildebrand, J. D., Wallingford, J. B., et al. (2010). Pax6-dependent Shroom3 expression regulates apical constriction during lens placode invagination. Development 137, 405–415. doi:10.1242/dev.045369
Purcell, P., Oliver, G., Mardon, G., Donner, A. L., and Maas, R. L. (2005). Pax6-dependence of Six3, Eya1 and Dach1 expression during lens and nasal placode induction. Gene Expr. Patterns 6, 110–118. doi:10.1016/j.modgep.2005.04.010
Qin, M., Wang, W., and Thirumalai, D. (2015). Protein folding guides disulfide bond formation. Proc. Natl. Acad. Sci. U. S. A. 112, 11241–11246. doi:10.1073/pnas.1503909112
Qin, Z., Zhang, L., Lyu, D., Li, J., Tang, Q., Yin, H., et al. (2019). Opacification of lentoid bodies derived from human induced pluripotent stem cells is accelerated by hydrogen peroxide and involves protein aggregation. J. Cell. Physiol. 234, 23750–23762. doi:10.1002/jcp.28943
Rao, P. V., and Maddala, R. (2006). The role of the lens actin cytoskeleton in fiber cell elongation and differentiation. Semin. Cell Dev. Biol. 17, 698–711. doi:10.1016/j.semcdb.2006.10.011
Ray, P. D., Huang, B. W., and Tsuji, Y. (2012). Reactive oxygen species (ROS) homeostasis and redox regulation in cellular signaling. Cell. Signal. 24, 981–990. doi:10.1016/j.cellsig.2012.01.008
Reimold, A. M., Etkin, A., Clauss, I., Perkins, A., Friend, D. S., Zhang, J., et al. (2000). An essential role in liver development for transcription factor XBP-1. Genes Dev. 14, 152–157. doi:10.1101/gad.14.2.152
Reimold, A. M., Iwakoshi, N. N., Manis, J., Vallabhajosyula, P., Szomolanyi-Tsuda, E., Gravallese, E. M., et al. (2001). Plasma cell differentiation requires the transcription factor XBP-1. Nature 412, 300–307. doi:10.1038/35085509
Rodvold, J. J., Chiu, K. T., Hiramatsu, N., Nussbacher, J. K., Galimberti, V., Mahadevan, N. R., et al. (2017). Intercellular transmission of the unfolded protein response promotes survival and drug resistance in cancer cells. Sci. Signal. 10, eaah7177. doi:10.1126/scisignal.aah7177
Romero-Ramirez, L., Cao, H., Nelson, D., Hammond, E., Lee, A. H., Yoshida, H., et al. (2004). XBP1 is essential for survival under hypoxic conditions and is required for tumor growth. Cancer Res. 64 (17), 5943–5947. doi:10.1158/0008-5472.CAN-04-1606
Rossi, G., Manfrin, A., and Lutolf, M. P. (2018). Progress and potential in organoid research. Nat. Rev. Genet. 19, 671–687. doi:10.1038/s41576-018-0051-9
Rowlands, A. G., Panniers, R., and Henshaw, E. C. (1988). The catalytic mechanism of guanine nucleotide exchange factor action and competitive inhibition by phosphorylated eukaryotic initiation factor 2. J. Biol. Chem. 263, 5526–5533. doi:10.1016/s0021-9258(18)60596-4
Saint-Jeannet, J. P., and Moody, S. A. (2014). Establishing the pre-placodal region and breaking it into placodes with distinct identities. Dev. Biol. 389, 13–27. doi:10.1016/j.ydbio.2014.02.011
Saito, A., Kanemoto, S., Kawasaki, N., Asada, R., Iwamoto, H., Oki, M., et al. (2012). Unfolded protein response, activated by OASIS family transcription factors, promotes astrocyte differentiation. Nat. Commun. 3, 967. doi:10.1038/ncomms1971
Sato, S., Ikeda, K., Shioi, G., Ochi, H., Ogino, H., Yajima, H., et al. (2010). Conserved expression of mouse Six1 in the pre-placodal region (PPR) and identification of an enhancer for the rostral PPR. Dev. Biol. 344, 158–171. doi:10.1016/j.ydbio.2010.04.029
Sawyer, J. M., Harrell, J. R., Shemer, G., Sullivan-Brown, J., Roh-Johnson, M., and Goldstein, B. (2010). Apical constriction: A cell shape change that can drive morphogenesis. Dev. Biol. 341, 5–19. doi:10.1016/j.ydbio.2009.09.009
Schippers, J. H., Nguyen, H. M., Lu, D., Schmidt, R., and Mueller-Roeber, B. (2012). ROS homeostasis during development: An evolutionary conserved strategy. Cell. Mol. Life Sci. 69, 3245–3257. doi:10.1007/s00018-012-1092-4
Sena, L. A., and Chandel, N. S. (2012). Physiological roles of mitochondrial reactive oxygen species. Mol. Cell 48, 158–167. doi:10.1016/j.molcel.2012.09.025
Shacham, T., Patel, C., and Lederkremer, G. Z. (2021). PERK pathway and neurodegenerative disease: To inhibit or to activate? Biomolecules 11, 354. doi:10.3390/biom11030354
Shi, H., O'Reilly, V. C., Moreau, J. L., Bewes, T. R., Yam, M. X., Chapman, B. E., et al. (2016). Gestational stress induces the unfolded protein response, resulting in heart defects. Development 143, 2561–2572. doi:10.1242/dev.136820
Shukla, S., and Mishra, R. (2018). Level of hydrogen peroxide affects expression and sub-cellular localization of Pax6. Mol. Biol. Rep. 45, 533–540. doi:10.1007/s11033-018-4190-z
Skorczyk-Werner, A., Chiang, W.-C., Wawrocka, A., Wicher, K., Jarmuż-Szymczak, M., Kostrzewska-Poczekaj, M., et al. (2017). Autosomal recessive cone-rod dystrophy can be caused by mutations in the ATF6 gene. Eur. J. Hum. Genet. 25, 1210–1216. doi:10.1038/ejhg.2017.131
Sone, M., Zeng, X., Larese, J., and Ryoo, H. D. (2013). A modified UPR stress sensing system reveals a novel tissue distribution of IRE1/XBP1 activity during normal Drosophila development. Cell Stress Chaperones 18, 307–319. doi:10.1007/s12192-012-0383-x
Spector, A. (1995). Oxidative stress-induced cataract: Mechanism of action. FASEB J. 9, 1173–1182. doi:10.1096/fasebj.9.12.7672510
Spence, J. R., Mayhew, C. N., Rankin, S. A., Kuhar, M. F., Vallance, J. E., Tolle, K., et al. (2011). Directed differentiation of human pluripotent stem cells into intestinal tissue in vitro. Nature 470, 105–109. doi:10.1038/nature09691
Stump, R. J., Ang, S., Chen, Y., von Bahr, T., Lovicu, F. J., Pinson, K., et al. (2003). A role for Wnt/beta-catenin signaling in lens epithelial differentiation. Dev. Biol. 259, 48–61. doi:10.1016/s0012-1606(03)00179-9
Taira, Y., Ikuta, Y., Inamori, S., Nunome, M., Nakano, M., Suzuki, T., et al. (2020). The formation of multiple pituitary pouches from the oral ectoderm causes ectopic lens development in hedgehog signaling-defective avian embryos. Dev. Dyn. 249, 1425–1439. doi:10.1002/dvdy.222
Takasato, M., Er, P. X., Becroft, M., Vanslambrouck, J. M., Stanley, E. G., Elefanty, A. G., et al. (2014). Directing human embryonic stem cell differentiation towards a renal lineage generates a self-organizing kidney. Nat. Cell Biol. 16, 118–126. doi:10.1038/ncb2894
Takebe, T., Sekine, K., Enomura, M., Koike, H., Kimura, M., Ogaeri, T., et al. (2013). Vascularized and functional human liver from an iPSC-derived organ bud transplant. Nature 499, 481–484. doi:10.1038/nature12271
Tam, A. B., Roberts, L. S., Chandra, V., Rivera, I. G., Nomura, D. K., Forbes, D. J., et al. (2018). The UPR activator ATF6 responds to proteotoxic and lipotoxic stress by distinct mechanisms. Dev. Cell 46, 327–343. e327. doi:10.1016/j.devcel.2018.04.023
Tan, Y. Y., Zhang, Y., Li, B., Ou, Y. W., Xie, S. J., Chen, P. P., et al. (2021). PERK signaling controls myoblast differentiation by regulating MicroRNA networks. Front. Cell Dev. Biol. 9, 670435. doi:10.3389/fcell.2021.670435
Tanaka, K., Kaji, H., Yamaguchi, T., Kanazawa, I., Canaff, L., Hendy, G. N., et al. (2014). Involvement of the osteoinductive factors, Tmem119 and BMP-2, and the ER stress response PERK-eIF2alpha-ATF4 pathway in the commitment of myoblastic into osteoblastic cells. Calcif. Tissue Int. 94, 454–464. doi:10.1007/s00223-013-9828-1
Tanaka, T., Tsujimura, T., Takeda, K., Sugihara, A., Maekawa, A., Terada, N., et al. (1998). Targeted disruption of ATF4 discloses its essential role in the formation of eye lens fibres. Genes cells. 3, 801–810. doi:10.1046/j.1365-2443.1998.00230.x
Thompson, B., Chen, Y., Davidson, E. A., Garcia-Milian, R., Golla, J. P., Apostolopoulos, N., et al. ₹(2021). Impaired GSH biosynthesis disrupts eye development, lens morphogenesis and PAX6 function. Ocul. Surf. 22, 190–203. doi:10.1016/j.jtos.2021.08.010
Torres-Bernal, B. E., Torres-Bernal, L. F., Gutierrez-Campos, R. R., Kershenobich Stalnikowitz, D. D., Barba-Gallardo, L. F., Chayet, A. A., et al. (2014). Unfolded protein response activation in cataracts. J. Cataract. Refract. Surg. 40, 1697–1705. doi:10.1016/j.jcrs.2014.02.038
Tu, B. P., and Weissman, J. S. (2004). Oxidative protein folding in eukaryotes: Mechanisms and consequences. J. Cell Biol. 164, 341–346. doi:10.1083/jcb.200311055
Upadhya, D., Ogata, M., and Reneker, L. W. (2013). MAPK1 is required for establishing the pattern of cell proliferation and for cell survival during lens development. Development 140, 1573–1582. doi:10.1242/dev.081042
Urano, F., Wang, X., Bertolotti, A., Zhang, Y., Chung, P., Harding, H. P., et al. (2000). Coupling of stress in the ER to activation of JNK protein kinases by transmembrane protein kinase IRE1. Science 287, 664–666. doi:10.1126/science.287.5453.664
Valm, A. M., Cohen, S., Legant, W. R., Melunis, J., Hershberg, U., Wait, E., et al. (2017). Applying systems-level spectral imaging and analysis to reveal the organelle interactome. Nature 546, 162–167. doi:10.1038/nature22369
Wang, H., Xu, Y., Sun, J., and Sui, Z. (2021). The novel curcumin derivative 1g induces mitochondrial and ER-stress-dependent apoptosis in colon cancer cells by induction of ROS production. Front. Oncol. 11, 644197. doi:10.3389/fonc.2021.644197
Wang, K., Zhang, T., Dong, Q., Nice, E. C., Huang, C., and Wei, Y. (2013). Redox homeostasis: The linchpin in stem cell self-renewal and differentiation. Cell Death Dis. 4, e537. doi:10.1038/cddis.2013.50
Wang, Y., Alam, G. N., Ning, Y., Visioli, F., Dong, Z., Nor, J. E., et al. (2012). The unfolded protein response induces the angiogenic switch in human tumor cells through the PERK/ATF4 pathway. Cancer Res. 72, 5396–5406. doi:10.1158/0008-5472.CAN-12-0474
Wang, Y., and Lou, M. F. (2009). The regulation of NADPH oxidase and its association with cell proliferation in human lens epithelial cells. Invest. Ophthalmol. Vis. Sci. 50, 2291–2300. doi:10.1167/iovs.08-2568
Wang, Z., Yin, F., Xu, J., Zhang, T., Wang, G., Mao, M., et al. (2019). CYT997(Lexibulin) induces apoptosis and autophagy through the activation of mutually reinforced ER stress and ROS in osteosarcoma. J. Exp. Clin. Cancer Res. 38, 44. doi:10.1186/s13046-019-1047-9
Wazin, F., and Lovicu, F. J. (2020). The negative regulatory Spred1 and Spred2 proteins are required for lens and eye morphogenesis. Exp. Eye Res. 191, 107917. doi:10.1016/j.exer.2020.107917
Weidinger, A., and Kozlov, A. V. (2015). Biological activities of reactive oxygen and nitrogen species: Oxidative stress versus signal transduction. Biomolecules 5, 472–484. doi:10.3390/biom5020472
West-Mays, J. A., Pino, G., and Lovicu, F. J. (2010). Development and use of the lens epithelial explant system to study lens differentiation and cataractogenesis. Prog. Retin. Eye Res. 29, 135–143. doi:10.1016/j.preteyeres.2009.12.001
Wilhelm, J., Smistik, Z., Mahelkova, G., and Vytasek, R. (2007). Redox regulation of proliferation of lens epithelial cells in culture. Cell biochem. Funct. 25, 317–321. doi:10.1002/cbf.1390
Wride, M. A. (2011). Lens fibre cell differentiation and organelle loss: Many paths lead to clarity. Philos. Trans. R. Soc. Lond B Biol. Sci. 366, 1219–1233. doi:10.1098/rstb.2010.0324
Wu, J., Rutkowski, D. T., Dubois, M., Swathirajan, J., Saunders, T., Wang, J., et al. (2007). ATF6alpha optimizes long-term endoplasmic reticulum function to protect cells from chronic stress. Dev. Cell 13, 351–364. doi:10.1016/j.devcel.2007.07.005
Xie, Q., McGreal, R., Harris, R., Gao, C. Y., Liu, W., Reneker, L. W., et al. (2016). Regulation of c-maf and alphaA-crystallin in ocular lens by fibroblast growth factor signaling. J. Biol. Chem. 291, 3947–3958. doi:10.1074/jbc.M115.705103
Xiong, Z., Jiang, R., Zhang, P., Han, X., and Guo, F. J. (2015). Transmission of ER stress response by ATF6 promotes endochondral bone growth. J. Orthop. Surg. Res. 10, 141. doi:10.1186/s13018-015-0284-7
Xu, H., Tsang, K. S., Wang, Y., Chan, J. C., Xu, G., and Gao, W. Q. (2014). Unfolded protein response is required for the definitive endodermal specification of mouse embryonic stem cells via Smad2 and beta-catenin signaling. J. Biol. Chem. 289, 26290–26301. doi:10.1074/jbc.M114.572560
Xu, Z., Chikka, M. R., Xia, H., and Ready, D. F. (2016). Ire1 supports normal ER differentiation in developing Drosophila photoreceptors. J. Cell Sci. 129 (5), 921–929. doi:10.1242/jcs.180406
Yamamoto, K., Sato, T., Matsui, T., Sato, M., Okada, T., Yoshida, H., et al. (2007). Transcriptional induction of mammalian ER quality control proteins is mediated by single or combined action of ATF6alpha and XBP1. Dev. Cell 13, 365–376. doi:10.1016/j.devcel.2007.07.018
Yang, C., Yang, Y., Brennan, L., Bouhassira, E. E., Kantorow, M., and Cvekl, A. (2010). Efficient generation of lens progenitor cells and lentoid bodies from human embryonic stem cells in chemically defined conditions. FASEB J. 24, 3274–3283. doi:10.1096/fj.10-157255
Yang, J., Zhou, S., Gu, J., Guo, M., Xia, H., and Liu, Y. (2015a). UPR activation and the down-regulation of alpha-crystallin in human high myopia-related cataract lens epithelium. PLoS One 10, e0137582. doi:10.1371/journal.pone.0137582
Yang, J., Zhou, S., Gu, J., Wang, Y., Guo, M., and Liu, Y. (2015b). Differences in unfolded protein response pathway activation in the lenses of three types of cataracts. PLoS One 10, e0130705. doi:10.1371/journal.pone.0130705
Yang, Y., Stopka, T., Golestaneh, N., Wang, Y., Wu, K., Li, A., et al. (2006). Regulation of alphaA-crystallin via Pax6, c-Maf, CREB and a broad domain of lens-specific chromatin. EMBO J. 25, 2107–2118. doi:10.1038/sj.emboj.7601114
Yang, Y., Sun, M., Shan, Y., Zheng, X., Ma, H., Ma, W., et al. (2015c). Endoplasmic reticulum stress-mediated apoptotic pathway is involved in corpus luteum regression in rats. Reprod. Sci. 22, 572–584. doi:10.1177/1933719114553445
Ye, J., Rawson, R. B., Komuro, R., Chen, X., Dave, U. P., Prywes, R., et al. (2000). ER stress induces cleavage of membrane-bound ATF6 by the same proteases that process SREBPs. Mol. Cell 6, 1355–1364. doi:10.1016/s1097-2765(00)00133-7
Yoshida, H., Okada, T., Haze, K., Yanagi, H., Yura, T., Negishi, M., et al. (2000). ATF6 activated by proteolysis binds in the presence of NF-Y (CBF) directly to the cis-acting element responsible for the mammalian unfolded protein response. Mol. Cell Biol. 20, 6755–6767. doi:10.1128/mcb.20.18.6755-6767.2000
Yu, S., Zhu, K., Lai, Y., Zhao, Z., Fan, J., Im, H. J., et al. (2013). atf4 promotes beta-catenin expression and osteoblastic differentiation of bone marrow mesenchymal stem cells. Int. J. Biol. Sci. 9, 256–266. doi:10.7150/ijbs.5898
Zeeshan, H. M., Lee, G. H., Kim, H. R., and Chae, H. J. (2016). Endoplasmic reticulum stress and associated ROS. Int. J. Mol. Sci. 17 (3), 327. doi:10.3390/ijms17030327
Zhang, K., Wang, S., Malhotra, J., Hassler, J. R., Back, S. H., Wang, G., et al. (2011). The unfolded protein response transducer IRE1alpha prevents ER stress-induced hepatic steatosis. EMBO J. 30, 1357–1375. doi:10.1038/emboj.2011.52
Zhang, L., Wang, N. L., Zhang, W., and Chang, Z. J. (2008). Suppression of cell proliferation by inhibitors to redox signaling in human lens epithelial cells. Zhonghua Yan Ke Za Zhi 44, 622–628.
Zhang, W., Wang, Y., Chen, C. W., Xing, K., Vivekanandan, S., and Lou, M. F. (2006). The positive feedback role of arachidonic acid in the platelet-derived growth factor-induced signaling in lens epithelial cells. Mol. Vis. 12, 821–831.
Zhang, X., Szabo, E., Michalak, M., and Opas, M. (2007). Endoplasmic reticulum stress during the embryonic development of the central nervous system in the mouse. Int. J. Dev. Neurosci. 25 (7), 455–463. doi:10.1016/j.ijdevneu.2007.08.007
Zhang, Z., Zhang, L., Zhou, L., Lei, Y., Zhang, Y., and Huang, C. (2019). Redox signaling and unfolded protein response coordinate cell fate decisions under ER stress. Redox. Biol. 25, 101047. doi:10.1016/j.redox.2018.11.005
Zhao, G., Bailey, C. G., Feng, Y., Rasko, J., and Lovicu, F. J. (2018a). Negative regulation of lens fiber cell differentiation by RTK antagonists Spry and Spred. Exp. Eye Res. 170, 148–159. doi:10.1016/j.exer.2018.02.025
Zhao, Y., Zheng, D., and Cvekl, A. (2018b). A comprehensive spatial-temporal transcriptomic analysis of differentiating nascent mouse lens epithelial and fiber cells. Exp. Eye Res. 175, 56–72. doi:10.1016/j.exer.2018.06.004
Zhou, L., Liu, Y., Zou, C., Ma, N., Hui, Y., Lv, G., et al. (2011). The effect of the Gly139His, Gly143His, and Ser142His mouse heme oxygenase-1 mutants on the HO reaction in vivo and in vitro. Anat. Rec. Hob. 294, 112–118. doi:10.1002/ar.21284
Zhou, Y., Chen, M., Ricupero, C. L., He, L., Wu, J., Chen, K., et al. (2018). Profiling of stem/progenitor cell regulatory genes of the synovial joint by genome-wide RNA-seq analysis. Biomed. Res. Int. 2018, 9327487. doi:10.1155/2018/9327487
Zou, T., Gao, L., Zeng, Y., Li, Q., Li, Y., Chen, S., et al. (2019). Organoid-derived C-Kit(+)/SSEA4(-) human retinal progenitor cells promote a protective retinal microenvironment during transplantation in rodents. Nat. Commun. 10, 1205. doi:10.1038/s41467-019-08961-0
Glossary
aPPR anterior pre-placodal region
ATF4 activating transcription factor 4
ATF6 activating transcription factor 6
BMP bone morphogenetic protein
BNIP3L BCL2-interacting protein 3-like
CHOP CAAT/enhancer-binding protein homologous protein
CO carbon monoxide
CRYAA αA-crystallin
DCR distal control region
eIF2α eukaryotic translation initiation factor 2α
ER endoplasmic reticulum
ERK1/2 extracellular signal-regulated kinases 1 and 2
ERO1 endoplasmic reticulum oxidoreductin 1
F-actin filamentous actin
FGF fibroblast growth factor
FSR2 fibroblast growth factor receptor substrate 2
GRB2 growth factor receptor-bound protein-2
GWAS genome-wide association study
hESC human embryonic stem cell
hiPSC human induced pluripotent stem cell
HO-1 heme oxygenase-1
IRE1 inositol-requiring protein 1
JNK c-Jun N-terminal kinase
Keap1 Kelch-like ECH-associated protein 1
LEC lens epithelial cell
LFC lens fiber cell
MAPK mitogen-activated protein kinase
MCS membrane contact site
NADPH nicotinamide adenine dinucleotide phosphate
NRF2 nuclear factor erythroid 2-like 2
OPF oxidative protein folding
OTX2 orthodenticle homeobox 2
PAR3 partitioning defective protein 3
PAX6 paired box protein 6
PDGF platelet-derived growth factor
PDI protein disulfide isomerase
PERK protein kinase RNA-like endoplasmic reticulum kinase
PI3K phosphoinositide 3-kinase
PSP progressive supranuclear palsy
PTEN Phosphatase and tensin homolog
RhoA Ras homolog family member A
RIDD regulated IRE1-dependent decay
ROR1 receptor tyrosine kinase-like orphan receptor 1
ROS reactive oxygen species
RTK receptor tyrosine kinase
SHP2 Src homology 2 domain-containing protein tyrosine phosphatase 2
UPR unfolded protein response
XBP1 X-box-binding protein 1
Yap Yes-associated protein.
Keywords: lens development, ROS, UPR, lentoid body, PSCs
Citation: Gao L, Jin N, Ye Z, Ma T, Huang Y, Li H, Du J and Li Z (2022) A possible connection between reactive oxygen species and the unfolded protein response in lens development: From insight to foresight. Front. Cell Dev. Biol. 10:820949. doi: 10.3389/fcell.2022.820949
Received: 23 November 2021; Accepted: 31 August 2022;
Published: 21 September 2022.
Edited by:
Moises Mallo, Gulbenkian Institute of Science (IGC), PortugalReviewed by:
Baris Uzilday, Ege University, TurkeyLisa M. Ryno, Oberlin College, United States
Paolo Remondelli, University of Salerno, Italy
Copyright © 2022 Gao, Jin, Ye, Ma, Huang, Li, Du and Li. This is an open-access article distributed under the terms of the Creative Commons Attribution License (CC BY). The use, distribution or reproduction in other forums is permitted, provided the original author(s) and the copyright owner(s) are credited and that the original publication in this journal is cited, in accordance with accepted academic practice. No use, distribution or reproduction is permitted which does not comply with these terms.
*Correspondence: Zhaohui Li, emhhb2h1aWxpNjUwQGhvdG1haWwuY29t