- 1Cellular Communication Laboratory, Program of Cellular & Molecular Biology, Center for Studies on Exercise, Metabolism and Cancer (CEMC), Instituto de Ciencias Biomédicas, Facultad de Medicina, Universidad de Chile, Santiago, Chile
- 2Advanced Center for Chronic Diseases (ACCDiS), Faculty of Chemical and Pharmaceutical Sciences, Universidad de Chile, Santiago, Chile
- 3Faculty of Medicine, Universidad de Chile, Santiago, Chile
- 4Division of Cardiology, Department of Medicine, Emory University, Atlanta, GA, United States
Acute skin wound healing is a multistage process consisting of a plethora of tightly regulated signaling events in specialized cells. The Thy-1 (CD90) glycoprotein interacts with integrins and the heparan sulfate proteoglycan syndecan 4, generating a trimolecular complex that triggers bi-directional signaling to regulate diverse aspects of the wound healing process. These proteins can act either as ligands or receptors, and they are critical for the successful progression of wound healing. The expression of Thy-1, integrins, and syndecan 4 is controlled during the healing process, and the lack of expression of any of these proteins results in delayed wound healing. Here, we review and discuss the roles and regulatory events along the stages of wound healing that support the relevance of Thy-1, integrins, and syndecan 4 as crucial regulators of skin wound healing.
1 Introduction
The skin is considered the largest human organ and functions as a natural barrier that protects the organs from environmental factors, such as light, heat, chemicals, dehydration, and infections (Yousef et al., 2020). After an acute skin injury, the body initiates a wound repair process, which is necessary to restore skin integrity and homeostasis. Wound repair consists of a plethora of tightly regulated biological and molecular processes that can be divided into four continuous and overlapping phases: hemostasis, inflammatory, proliferative, and remodeling. Hemostasis occurs immediately after an injury. Platelets aggregate and form a blood clot, which is mainly constituted by extracellular matrix (ECM) proteins forming the provisional matrix, which acts as a scaffold for cell migration (Chen and Lopez, 2005). The inflammatory phase involves the migration of cells, such as phagocytic neutrophils, macrophages, and leukocytes to the wound site. Subsequently, the phagocytic cells release cytokines and other soluble factors to induce fibroblast migration and proliferation (Martin and Leibovich, 2005; Nathan, 2006). During the proliferative phase, new blood vessels are formed (either by angiogenesis or neovascularization), giving rise to the synthesis of ECM components, as well as re-epithelialization. The final phase comprises collagen deposition and remodeling (Thiruvoth et al., 2015; Cañedo-Dorantes and Cañedo-Ayala, 2019).
These four phases can be arrested at any point, leading to the formation of a chronic non-healing wound. Alterations in any mediators, including soluble factors (e.g., inflammatory molecules and growth factors), proteases (e.g., matrix metalloproteinases), blood elements, the ECM, parenchymal and inflammatory cells, can also lead to impaired healing. Other comorbidities, such as diabetes, immunosuppression, renal failure, infection, and smoking, negatively affect the wound healing process.
Thy-1 (CD90), a glycosylphosphatidylinositol (GPI)-anchored protein, has been described as one of the cell receptors that participates in the acute wound healing process (Lee et al., 2013). Thy-1 is expressed in a variety of cells, including fibroblasts, neurons, endothelial, and hematopoietic cells. Thy-1 expression is tightly regulated during development, inflammation, and fibrosis. Moreover, Thy-1 is considered a cell marker for fibroblast and mesenchymal stem cells (MSCs), both of which play a transcendental role during wound repair. Most importantly, Thy-1 is the ligand/receptor for integrins and syndecan 4, and their interaction generates a trimolecular complex that triggers bi-directional signaling pathways to regulate several cellular processes, including cell adhesion, differentiation, migration, and proliferation (reviewed in Leyton et al., 2019).
Here, we review the direct role of Thy-1 and its co-receptors, integrins and syndecan 4, during the different phases of the acute skin wound healing process. Moreover, based on previously published functions related to other pathophysiological situations, we propose and critically discuss some new putative roles for the Thy-1/integrin/syndecan 4 trimolecular complex in the skin healing process, which could translate into potential therapeutics to improve clinical outcomes.
2 Thy-1, Integrins and Syndecan 4 Are Cell-Cell and Cell-Matrix Communication Molecules
Thy-1 is a small (25–37 kDa) glycoprotein that possesses two N-glycosylation sites in humans, and three in mice. Reports indicate differential Thy-1 expression between tissues and during development. In adults, Thy-1 protein is highly expressed in the brain, smooth muscle, kidney, and colon. In contrast, RNA studies have shown that Thy-1 is transcribed in many other tissues and cell types, such as the endometrium, adipose tissue, the urinary bladder, and T cells (Thul et al., 2017). During development, Thy-1 is undetectable in the neonatal and developing brain, compared to the higher levels observed in the adult brain. Thy-1 expression can also vary within the same cell type, defining cell subpopulations that possess different functions. For example, lung fibroblasts expressing higher levels of Thy-1 secrete ECM and inflammatory molecules different from those of fibroblasts expressing lower levels. Thy-1 presence in fibroblasts can also dictate if they differentiate into myofibroblasts or lipofibroblasts (Koumas et al., 2003).
Thy-1 localizes in lipid rafts at the cell membrane, but it can also be shed by specific phospholipases (PI-PLC or PLC-β) and other proteases not yet identified. Soluble Thy-1 (sThy-1) has been detected in serum, urine, wound fluid, and synovial fluid. In vitro, lung fibroblasts treated with IL-1β or TNFα shed Thy-1 into the culture media. Increased levels of sThy-1 exist in wound fluid from venous ulcers and synovial fluid from knee punction in patients with rheumatoid arthritis, as well as in serum from patients with systemic sclerosis and diabetic kidney disease, suggesting that sThy-1 exerts a role during inflammation and some pathological conditions (Freimuth et al., 1978; Saalbach et al., 1999; Kollert et al., 2013; Wu et al., 2021).
Thy-1 is a very versatile glycoprotein that can act as a receptor, a ligand, or a cell adhesion molecule. Thy-1 possesses an integrin-binding domain (RGD-like tripeptide: RLD) and a heparin-binding domain (HBD: REKRK, in mouse), which allow its interactions with several integrins and syndecan 4, respectively. The interaction of Thy-1 with its receptors occurs within the same cell (in Cis) or between cells (in Trans) to trigger diverse signaling pathways downstream of Thy-1, integrins, and/or syndecan 4 (reviewed in Herrera-Molina et al., 2013; Leyton et al., 2019).
Integrins are transmembrane receptors formed by α and β heterodimers that bind ECM proteins, cell surface molecules, and soluble ligands. At least 18 α and 8 β subunits have been described in humans, generating 24 different heterodimers, which recognize and interact with specific ligands. Integrins transduce signals from the ECM into the cell (outside-in), but the cell can also regulate the integrin affinity for its ligand (inside-out). To date, Thy-1 has been shown to interact with the αvβ3, αxβ2, αMβ2, α5β1, and αvβ5 integrins (Table 1) (Leyton et al., 2001; Wetzel et al., 2004; Saalbach et al., 2005; Hermosilla et al., 2008; Avalos et al., 2009; Zhou et al., 2010; Fiore et al., 2014).
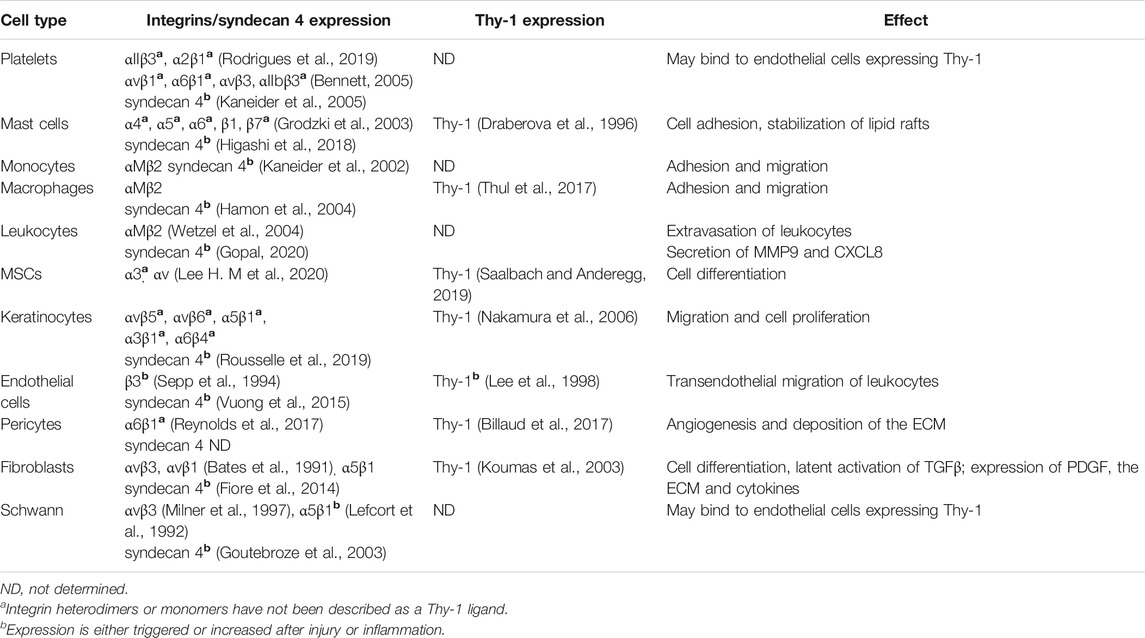
TABLE 1. Thy-1 and its integrins/syndecan 4 receptors are expressed in different cell types that participate in the wound healing process.
The syndecan family is comprised of four members (syndecan 1, 2, 3, and 4), which are timely and spatially expressed across every cell of the body. Syndecans are transmembrane heparan sulfate proteoglycans, composed of a divergent ectodomain, a conserved transmembrane region, and a short cytoplasmic tail. The ectodomain possesses glycosaminoglycan chains (GAGs) of heparan sulfate that interact with ECM proteins, cytokines, chemokines, growth factors, and its receptors. The ectodomain can also be shed from the cell surface to sequester soluble factors or compete for binding to the ECM. The cytoplasmic domain possesses two conserved regions (C1 and C2), flanking a variable (V) region unique to each syndecan, which triggers specific cell signaling pathways (Simons and Horowitz, 2001; Bertrand and Bollmann, 2019; Gondelaud and Ricard-Blum, 2019).
Of interest for this review, integrins act cooperatively with syndecan 4 to regulate focal adhesion (FA) and actin stress fiber formation in a RhoA-dependent manner (Avalos et al., 2009; Fiore et al., 2014). FA turnover is an important event during directional cell migration. Moreover, Thy-1 has been shown to directly interact with integrins and syndecan 4 to form a trimolecular complex that facilitates initial cell adhesion by enhancing FA formation and subsequently, promoting migration by regulating contractility and FA turnover (Avalos et al., 2004; Hermosilla et al., 2008; Avalos et al., 2009; Kong et al., 2013; Fiore et al., 2014; Fiore et al., 2015; Lagos-Cabre et al., 2017; Burgos-Bravo et al., 2020; Valdivia et al., 2020). Although the bidirectional signaling pathways triggered by Thy-1/integrin/syndecan 4 clustering and activation remain unexplored during skin injury, the biological significance of each of these molecules by themselves has been proven important for an efficient wound healing process.
Most of the cell types participating in the wound healing process express integrins, syndecan 4 and Thy-1 (Table 1). Furthermore, Thy-1, integrins and syndecan 4 are upregulated after skin injury and in response to inflammation (Table 1) (Lefcort et al., 1992; Sepp et al., 1994; Gallo et al., 1996; Lee et al., 1998; Goutebroze et al., 2003). The Thy-1 promoter becomes active right at the wound area and remains active up to 36 days after injury (Josvay et al., 2014). Similarly, transcriptome analysis has shown that human blood vessels in a wounded area express 24-fold higher Thy-1 levels than normal tissue vessels (Roy et al., 2007). Additionally, decreasing Thy-1 levels delays the wound healing process by generating abnormal re-epithelialization and TGFβ secretion (Lee et al., 2013).
Syndecan 4 is also upregulated in the epidermis after injury, and disruption of the syndecan 4 gene in mice delayed skin wound healing and impaired angiogenesis (Gallo et al., 1996; Echtermeyer et al., 2001). Syndecan 4 regulates wound healing in vitro by controlling the levels of integrins at the membrane to allow an efficient directional cell migration, and in vivo, by upregulating its levels within the granulation tissue, implying a role during wound-related angiogenesis (Fuster and Wang, 2010; Bass et al., 2011; Brooks et al., 2012; Vuong et al., 2015).
On the other hand, integrins have been proven transcendental for the recruitment of leukocytes, keratinocytes, and fibroblasts to the wound area, as well as for myofibroblast differentiation and blood vessel sprouting during angiogenesis (Columbo and Bochner, 2001; Annes et al., 2004; Asano et al., 2005; Asano et al., 2006; Carracedo et al., 2010; Zarbock et al., 2012; Darby et al., 2014; Koivisto et al., 2014; Kenny and Connelly, 2015; DiPersio et al., 2016; Lishko et al., 2018). Aberrant integrin signaling is associated with defective ECM deposition, which affects normal fibroblast differentiation and function, and also generates inefficient cell recruitment and insufficient angiogenesis, which cause a hypertrophic scar or chronic wounds (Koivisto et al., 2014).
Substantial evidence supports the importance of Thy-1 and its counteracting receptors during wound closure. In the next sections, we review the specific roles that Thy-1, integrins, and syndecan 4 play in each one of the phases of the acute skin wound healing process, with a special emphasis on yet unexplored functions, which may be relevant for future therapeutic development.
3 Hemostasis Phase
The immediate response after an acute wound is vasoconstriction and clot formation to prevent blood loss. Vasoconstriction is mediated by prostaglandins and endothelin released from circulating platelets and the damaged endothelial layer (Menter et al., 2017; Rodrigues et al., 2019). Moreover, circulating catecholamines and prostanoids, such as epinephrine, norepinephrine, and thrombocyclin can also generate vasoconstriction (Feletou et al., 2010). Platelet-derived growth factor (PDGF) activates smooth muscle cells in the vessel to cause contraction (Berk et al., 1986). After the initiation of the coagulation cascade, vasoconstriction resolves the bleeding through thromboxane A2, bradykinin, serotonin, and fibrinopeptide (Rodrigues et al., 2019). The clot formation process is initiated in response to the vascular wall damage, which exposes the subendothelial collagen to blood components (Chen and Lopez, 2005). Platelets adhere to collagen, become activated, and aggregate to form the initial hemostatic plug. At this point, the coagulation and complement cascades are activated, mediating the cleavage of prothrombin to thrombin, which subsequently cleaves fibrinogen to fibrin. Fibrin strands bind at the hemostatic plug along with platelets and erythrocytes to form an insoluble clot. Apart from the crosslinked fibrin strands, the clot also contains other ECM proteins, such as collagen type I, fibronectin, vitronectin, and thrombospondin (TSP), which create a provisional ECM that favors fibroblast and leukocyte migration. The active platelets in the clot will also undergo degranulation, and release soluble factors to mediate vasoconstriction, endothelial and fibroblast activators (e.g., TGFβ, VEGF, FGF2, and PDGF), and chemoattractant and inflammatory mediators [e.g., chemokine (C-X-C motif) ligand 4 (CXCL4 or PF4), chemokine (C-C motif) ligand 5 (CCL5), Interleukin 6 (IL-6), Interleukin 8 (IL-8), and Insulin growth factor 1 (IGF1)] (Martins-Green et al., 2013; Ridiandries et al., 2018; Cañedo-Dorantes and Cañedo-Ayala, 2019; Rodrigues et al., 2019).
Integrin αIIbβ3 is the main integrin on the surface of platelets and mediates adhesion to fibrinogen, fibronectin, vitronectin, and the Von Willebrand Factor (vWF). The function of αIIbβ3 is indispensable for platelet aggregation, clots retraction, and thrombus stability during wound healing. Platelets also express, to a lesser extent, α2β1, α5β1, α6β1, and αvβ3 integrins (Table 1) (Bennett, 2005; Bennett et al., 2009; Haling et al., 2011; Eisinger et al., 2018). Although, α2β1 mediates adhesion to collagen and αvβ3 to osteopontin, as well as to vitronectin during in vitro assays, it is uncertain whether they play a role during hemostasis or if they can interact with Thy-1 expressed on other cell types present in the wounded area (Bennett et al., 1997; Paul et al., 2003).
Syndecan 4 is ubiquitously expressed in adult human cells. Particularly in healthy skin, tissue analysis shows medium-to-high levels of syndecan 4 protein expression in keratinocytes, Langerhans (tissue-resident macrophages in epidermis), fibroblasts, and epidermal cells. Single-cell RNA analysis has also shown that skin endothelial cells, smooth muscle cells, melanocytes and T cells express syndecan 4 (Thul et al., 2017; Sole-Boldo et al., 2020; Karlsson et al., 2021; The Human Protein Atlas, 2021). Platelets themselves express syndecan 4, and clustering of syndecan 4 using antibodies increases platelet aggregation, though the role of syndecan 4 in platelet aggregation has not been described during skin hemostasis (Kaneider et al., 2005).
Single-cell RNA analysis of healthy skin shows that Thy-1 is normally expressed in fibroblasts and smooth muscle cells, while lower levels are observed in endothelial cells, keratinocytes, melanocytes, resident skin macrophages, and granulocytes (Thul et al., 2017; Sole-Boldo et al., 2020; Karlsson et al., 2021; The Human Protein Atlas, 2021). Even though Thy-1 is considered a dermal fibroblast and dermal mesenchymal stem cell marker, its expression levels in healthy skin are very low compared to other tissues, such as the cerebral cortex (<30-fold) (Thul et al., 2017; Sole-Boldo et al., 2020; Karlsson et al., 2021; The Human Protein Atlas, 2021). To date, it remains unknown whether Thy-1 basal levels play a relevant role in skin physiology or during the hemostasis phase. However, Thy-1 levels rapidly increase after skin injury (Figure 1). Indeed, using an ear injury model on transgenic mice expressing YFP under the control of the Thy-1 promoter, Jósvay et al., showed that a halo of fluorescence appears right at the wound edge, as soon as day 1 after injury. At day 3, the fluorescent area expands to the surrounding wounded area, and the fluorescence remains up to 2 weeks after the injury (Josvay et al., 2014). The fluorescent area around the wound starts decreasing at day 21 and disappears by day 36, indicating that the activity of the Thy-1 promoter is tightly controlled during the wound healing process (Freimuth et al., 1978). These changes in Thy-1 expression presumably occur in response to the inflammatory molecules and growth factors that are released in the wound area early during the hemostasis phase. Supporting this idea, cytokines such as IL-1β, IL-2, and TNFα, and growth factors such as VEGF can induce Thy-1 synthesis in other systems (Mason et al., 1996; Lee et al., 1998; Weston et al., 2002; Zhao et al., 2003; Wetzel et al., 2004). Notably, IL-1β, IL-6, and TNFα levels are elevated in the diabetic wound healing process. High levels of these chemokines during hemostasis activates the secretion of acute-phase proteins from the liver and recruit an excessive number of inflammatory cells to the wound site, thus affecting the healing process (Strang et al., 2020). Moreover, Thy-1 levels are also increased during diabetic foot ulcers (Januszyk et al., 2020). Therefore, we speculate that at least during diabetic wound healing these cytokines can induce Thy-1 overexpression to mediate the migration of inflammatory cells. Some of the mechanisms by which Thy-1 can stimulate cell migration on the surface of inflammatory cells will be discussed in detail in the description of the inflammatory phase (Section 4). Similarly, the expression of integrins and syndecan 4 is also regulated by inflammatory mediators (Herzberg et al., 1996; Werner and Grose, 2003; Okuyama et al., 2013; Lagos-Cabre et al., 2017; Schnittert et al., 2018; Gopal, 2020). It is noteworthy that CXCL4, the main chemokine secreted by platelets during the hemostasis phase, binds integrin αMβ2 (MAC-1) on leukocytes, as well as αvβ3 and α5β1 on endothelial cells (Figure 1) (Aidoudi et al., 2008; Lishko et al., 2018). Therefore, Thy-1 could potentially modulate the effects of CXCL4 by competing for its integrin receptor, although this idea needs to be evaluated in the context of skin wound healing.
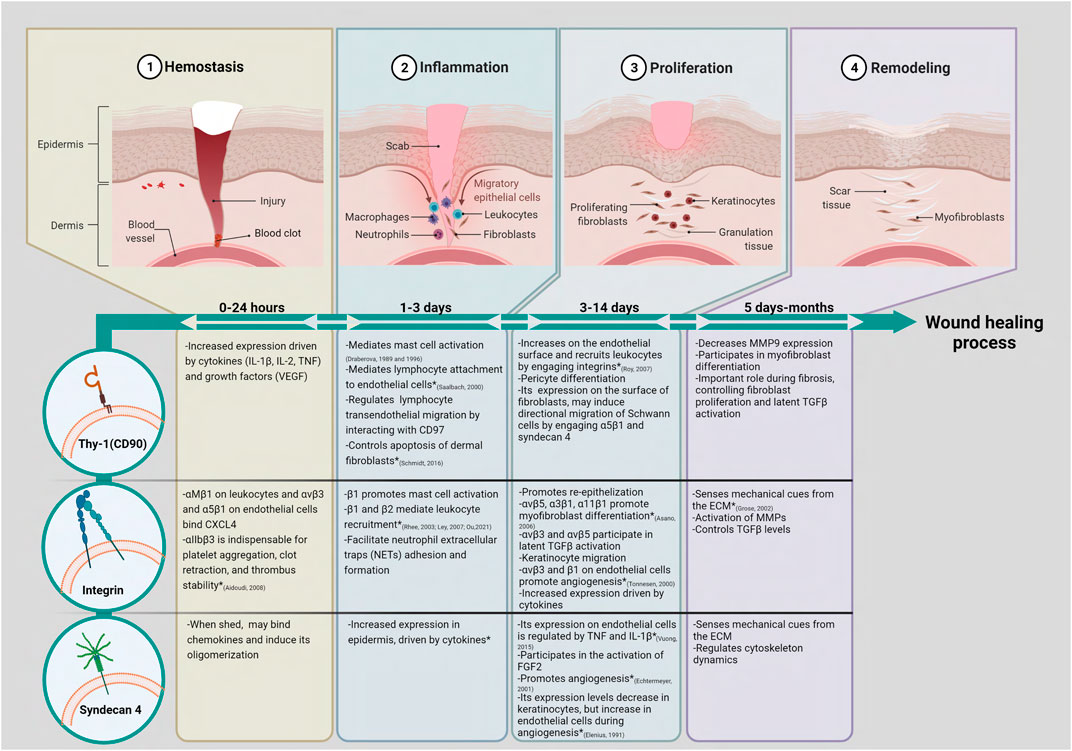
FIGURE 1. Thy-1 (CD90), integrins, and syndecan 4 could participate in multiple stages of skin wound healing. Based on data obtained in similar cell types from organs other than skin, we have proposed putative roles for Thy-1, integrins and syndecan 4 during the wound healing process. (*) Shows functions for these molecules that have already been studied in skin and/or skin wound models. In brief, the acute skin wound healing process consists of four phases that overlap and are tightly regulated. (1) The hemostasis phase starts immediately after injury. Platelets aggregate and form a blood clot containing ECM proteins and secrete soluble factors, such as CXCL4, which lead cell migration into the wound area; (2) The inflammatory phase involves the migration of neutrophils, macrophages, and leukocytes to the wound site. The inflammatory cells release cytokines, chemokines, and growth factors to recruit fibroblasts into the wound; (3) The proliferation phase is characterized by proliferation of fibroblasts, angiogenesis, formation of granulation tissue, peripheral nerve repair, recruitment of keratinocytes, and re-epithelization; (4) The remodeling phase is characterized by wound contraction and collagen remodeling. The roles for Thy-1 (CD90) and its co-receptors integrins and syndecan 4 are highlighted in each phase. Created with Biorender.com.
The role of syndecans in the activation of chemokines has been broadly studied. Chemokines such as CCL5 and CXCL4, which are secreted by activated platelets during the hemostasis phase, require both chemokine oligomerization and binding to GAGs to participate in cell recruitment. CCL5 induces syndecan 4 shedding from the surface of HeLa cells, and syndecan 4 can bind chemokines and induce chemokine oligomerization (Figure 1) (Charnaux et al., 2005; Dyer et al., 2016). Nonetheless, further research is necessary to establish a specific role of syndecan 4 in the oligomerization of chemokines during the hemostasis phase after acute skin injury.
Thy-1 can also be related to the secretion of ECM in other cell types not associated with skin. For instance, the induction of Thy-1 in human ovarian cancer cells enhances the expression of fibronectin and TSP1 (Figure 1). In addition, pulmonary Thy-1 (+) and Thy-1 (−) fibroblasts synthesize fibronectin, but the subpopulation of Thy-1 (+) fibroblasts produces two- to three-fold more collagen than the Thy-1 (−) cells (Derdak et al., 1992; Abeysinghe et al., 2005). Moreover, TSP1, one of the provisional ECM components, induces the disassembly of focal adhesions necessary to induce fibroblast migration in a Thy-1-dependent manner (Barker et al., 2004). However, it remains unknown whether Thy-1 can trigger ECM secretion or interact with TSP1 during skin wound healing.
Therefore, the expression of Thy-1, integrins, and syndecan 4 can potentially be modulated by soluble signals released during hemostasis, although more research is necessary to elucidate if Thy-1 and its receptors also exert a role in controlling key events for hemostasis, such as activation of chemokines, ECM secretion and early cell migration to the wounded area.
4 Inflammatory Phase
During the inflammatory phase, neutrophils, macrophages, and lymphocytes are recruited to the wound site. Bradykinin and anaphylatoxins (C3a and C5a), generated by the coagulation and complement cascades, respectively, disrupt cell-cell junctions of the endothelial cells and increase the permeability of the local vessels, facilitating the infiltration of inflammatory cells. Neutrophils are the first to arrive to the wound area, following a gradient of chemoattractant molecules composed of several growth factors and chemokines released by activated platelets in the blood clot, as well as N-formyl peptides released by bacteria and damaged cells (Mantovani et al., 2011). Within the wound, neutrophils will decontaminate the wound using diverse strategies, including phagocytosis, proteases, secretion of antimicrobial peptides, generation of reactive oxygen species (ROS), and neutrophil extracellular traps (NETs) to immobilize and kill microorganisms (Brinkmann et al., 2004; Nathan, 2006; Kolaczkowska and Kubes, 2013). Despite their relevant role in controlling infection, the absence of neutrophils does not impair the healing process. Indeed, wound repair can happen faster in animals deficient in neutrophils, suggesting that neutrophils can be inhibitory to some extent during the repair process (Simpson and Ross, 1972; Dovi et al., 2003; Martin and Leibovich, 2005). On the other hand, the sustained presence of neutrophils in the wound could be one of the causes of chronic non-healing wounds (Martin and Leibovich, 2005).
In addition to NET function as a host defense mechanism, NETs also participate in thrombus formation and metastatic dissemination of cancer cells. In this context, α9β1 integrin in the neutrophil plasma membrane promotes thrombosis and clot formation, and the adhesion of different tumor cells to NETs is facilitated by high expression of α5β1, αvβ3, and αvβ5 integrins (Martinod and Wagner, 2014; Monti et al., 2018). Similarly, integrins reportedly regulate neutrophil activation and NET formation (NETosis). In a mouse model of ventilator-induced lung injury, blocking integrin-mediated outside-in signaling decreases NET formation and lung injury (Rossaint et al., 2014). Alternatively, stimulation of neutrophils with phorbol 12-myristate 13-acetate (PMA), a potent protein kinase C (PKC) activator, induces complete NET formation independent of cell adhesion (Erpenbeck et al., 2019). Therefore, NET formation could occur in an integrin-dependent and -independent manner. However, the exact mechanism by which PMA induces NET formation remains unclear. Currently, there is no evidence that the Thy-1/integrin/syndecan 4 trimolecular complex can control NETosis, although, syndecan 4 can regulate PKC activity, and PMA can increase Thy-1 expression on endothelial cells to facilitate wound healing (Oh et al., 1997; Murakami et al., 2002; Wen et al., 2018). More importantly, increased NETosis is associated with delayed wound healing in diabetic skin wounds, which therefore posit NET formation mechanisms as an attractive subject of study (Wong et al., 2015; Fadini et al., 2016; Erpenbeck et al., 2019; Lee, Y. S. et al., 2020).
Following neutrophils, monocytes are recruited to the wound via C-C chemokines, such as CCL2. These chemokines are secreted initially by neutrophils and subsequently by keratinocytes and monocytes themselves (Gillitzer and Goebeler, 2001; Rees et al., 2015; Ridiandries et al., 2018). Within the wound, monocytes mature into macrophages, which initially acquire a pro-inflammatory phenotype (M1), and subsequently, as the wound heals, they transition to an anti-inflammatory and pro-regenerative phenotype (M2). As proposed by Krzyszczyk and other researchers, the M1/M2 definition is oversimplified, since in the wound bed, macrophages exhibit a different spectrum of M1- or M2-like characteristics (Martinez and Gordon, 2014; Krzyszczyk et al., 2018). M1-like macrophages remove dead cells, apoptotic neutrophils, bacteria, tissue debris, and foreign materials. They function as antigen-presenting cells, and secrete cytokines and growth factors, such as TGFα, TGFβ, bFGF, VEGF, and PDGF (Martins-Green et al., 2013; Krzyszczyk et al., 2018). These soluble factors attract and activate endothelial cells, fibroblasts, and keratinocytes. Subsequently, M2-like macrophages favor wound healing by inducing cell proliferation, angiogenesis, and ECM synthesis during the proliferative phase, and also by secreting matrix metalloproteinases (MMPs) in the remodeling phase, as discussed later (Sections 5 and 6). In a guinea pig wound model, macrophage depletion using antisera and steroids results in impaired disposal of damaged tissue and matrix, a decreased fibroblast count, and delayed wound healing (Leibovich and Ross, 1975; Martin and Leibovich, 2005). Surprisingly, antisera depletion of neutrophils does not affect the healing process (Simpson and Ross, 1972). More recent research using leukocyte-deficient mice has shown that not one of the inflammatory cell lineages is absolutely necessary to favor wound healing. Indeed, the absence of some leukocytes lineages can cause a faster wound repair and can also diminish scarring later during remodeling phase, suggesting that inflammatory cells can have an inhibitory effect during healing. In this context, neutrophil knockdown mice show faster wound repair than the control littermates, if the conditions are sterile (Dovi et al., 2003). Additionally, in PU.1 null mice, which lack macrophages and neutrophils, wound healing is not impaired; it follows a similar time course as in their WT littermates and healing occurs in the absence of fibrosis and scar formation, similarly to embryonic wound healing (Martin et al., 2003). Cytokines and growth factor levels are reduced in PU.1 null mice. However, they are not entirely absent, as observed in wounded embryonic tissue, since these soluble signals can still be produced in small amounts by keratinocytes and fibroblasts, suggesting that inflammation and macrophages are somehow not crucial for healing, although they may play an essential role in scar formation.
Evidence suggests that Thy-1 might mediate the recruitment of inflammatory cells to the wound site. As reported, Thy-1 expressed on endothelial cells mediates the binding of neutrophils and monocytes to activated microvascular endothelial cells (Figure 1) (Saalbach et al., 2000). Furthermore, αMβ2 integrin (Mac-1 or CD11b/CD18) expressed in the leukocyte membrane was identified as the counterreceptor for Thy-1 (Wetzel et al., 2004). Moreover, Thy-1 mediates the extravasation of monocytes and neutrophils in a thioglycollate-induced peritonitis model and the extravasation of eosinophils and monocytes in a lung inflammation model (Schubert et al., 2011). Additionally, the interaction between Thy-1 and αMβ2 integrin on neutrophils triggers effector functions in neutrophils, inducing the secretion of MMP9 and CXCL8 (Saalbach et al., 2008). Hence, by bringing together inflammatory cells to the wound bed, Thy-1 may contribute to modulate the inflammatory microenvironment.
The innate skin immune system, including neutrophils and monocyte/macrophages, provides a non-specific first-line response to pathogens, toxins, and foreign material. The innate response comprises toll-like receptors (TLRs) and receptor for advanced glycation end products (RAGE) receptors that recognize stress signals, such as lipopolysaccharide (LPS). These receptors then trigger signal transduction pathways that culminate with the release of TNF, IL-1β, IL-6, and NO. On the other hand, B- and T-lymphocytes complement the innate response more specifically: B-lymphocytes produce specific antibodies, and T-lymphocytes secrete cytokines and elicit cytolytic activity (Strbo et al., 2014). B-lymphocytes are present in the wound area from day 4 to day 17 after injury (Sirbulescu et al., 2017). In splenectomized nude mice, wound healing is delayed, and this effect is recovered after the addition of external antibody-producing B cells (Nishio et al., 2009). Similarly, topical treatment with B cells improves healing of acute wounds by 2–3 days in wild-type animals and 5–6 days in obese diabetic mice (Sirbulescu et al., 2017). Thy-1 is expressed on early B-cells in the thymus and has been related to the proliferation of B-cell lymphomas (Ritter et al., 1983; Ishiura et al., 2010). Nonetheless, Thy-1 is not expressed in mature B-cells. Relatedly, syndecan 4 blocking antibodies inhibit the directional migration of B-cells in an asthma model, and B-cells from syndecan 4−/− mice also show impaired directional cell migration in an arthritis model (Endo et al., 2015; Polte et al., 2015). Although these results suggest a possible role of syndecan 4 during B-cell migration, further experiments are necessary to elucidate its role in skin wound repair.
T-lymphocytes in the skin consist of regulatory cells, CD4+ helper cells, and CD8+ killer cells, which can be present in the circulation or be permanent residents of the skin. Regulatory CD4+ T cells migrate and accumulate in the skin, where they participate in skin homeostasis and tolerance to normal skin flora (Ali and Rosenblum, 2017). The role of regulatory T cells in skin injury was recently reviewed (Boothby et al., 2020). In brief, regulatory T cells control inflammation and reduce the number of macrophages and upregulate the expression of EGFR to favor re-epithelialization and wound closure. As proposed, skin regulatory T cells play an important role in preventing an immune response against self-antigens during cutaneous injury (Metzger and Anderson, 2011). On the other hand, helper CD4+ T cells contribute to the inflammatory response against pathogens by releasing cytokines that mediate the secretion of antimicrobial peptides. Several subsets of helper T cells (Th1, Th2, Th17, and Th22) secrete unique cytokines, which orchestrate defensins and antimicrobial peptides to protect the skin from infection. Skin resident dendritic cells participate in the phagocytosis of microorganisms and present the antigens to naïve CD8+ cells. Active CD8+ killer cells differentiate in homing effector memory (TEM) T cells and central memory (TCM) T cells. TEM cells migrate to the wound area and release proinflammatory cytokines to mediate pathogen clearance. After the infection is resolved, most of the TEM cells die by apoptosis, and the few remaining cells are known as tissue-resident memory (TRM) T cells. After reinfection, dendritic cells present the antigen to the TRM cells, which proliferate and recruit circulating TEM cells to mediate pathogen clearance. Human hypertrophic scars generated after a burn injury show a high infiltration of T cells, and murine models have shown that scar formation is mediated by cytokines secreted by Th2 helper T cells (Cañedo-Dorantes and Cañedo-Ayala, 2019; Rodrigues et al., 2019). Interferon γ secreted by Th1 cells can attenuate tissue fibrosis by downregulating collagen synthesis and decreasing fibroblast proliferation (Harrop et al., 1995; Wynn, 2004). Similarly, keloid fibroblasts synthetize less collagen when co-cultured with regulatory T cells (Murao et al., 2014; Chen et al., 2019). Therefore, regulatory T cells maintain immune homeostasis.
Thy-1 can also induce T lymphocyte activation. Thy-1 is abundantly expressed on CD4+ CD8+ double-positive thymocytes and, to a lesser extent, at the T cell surface (Haeryfar and Hoskin, 2004). Although early research showed that Thy-1 expression was restricted to mature mouse T cells and that mature human T cells did not express Thy-1, more recent reports have shown that Thy-1 is expressed in specific subsets of human T cells (Th17/Tc17) (Guillot-Delost et al., 2012). Differentiation into Th17/Tc17 is enhanced after tissue damage and the dysregulation of these cells can lead to skin inflammatory conditions, such as atopic dermatitis, psoriasis, and Lichen Planus (Baurecht et al., 2015; Brockmann et al., 2017; Linehan et al., 2018; Norsgaard et al., 2019; Solimani et al., 2019). Moreover, single-cell RNA data has shown that T cells express low Thy-1 levels in healthy human skin; nonetheless, the significance of this observation has not been studied in healthy skin nor in wound healing models (Thul et al., 2017; Sole-Boldo et al., 2020; Vorstandlechner et al., 2020; Karlsson et al., 2021; The Human Protein Atlas, 2021). The role of Thy-1 in T cell function has been studied primarily on T cells from rodent models. In this context, it is known that T cells from Thy1−/− mice show enhanced T cell antigen receptor (TCR) activity. Additionally, anti-Thy-1 antibodies activate mouse T cell proliferation and cytokine synthesis in the absence of an antigen-specific signal in the context of CD28 co-stimulation (Pont, 1987; Hueber et al., 1994) or a co-stimulatory signal from syngeneic bone marrow-derived dendritic cells (Haeryfar and Hoskin, 2001). Thus, Thy-1 can regulate the T cell response in the absence of TCR activation and enhance antigen-induced T cell responses in mouse cells. Noteworthy, Thy-1-regulated TCR activation has not been shown in human skin resident T cells during wound healing nor in the aforementioned skin conditions, where Th17/Tc17 cells are relevant.
The last leukocytes recruited to the wound area are the mast cells, which typically reside in the skin. They play an essential role in innate immunity during the healing process of infected wounds, where they release TNFα to chemoattract professional phagocytic cells, such as polymorphonuclear neutrophils (PMN). Knockout of mast cells in mice impairs wound healing and reduces the inflammatory response that diminishes fibrosis and scar formation (Szpaderska et al., 2003; Gallant-Behm et al., 2008; Shiota et al., 2010). In the activation of mast cells, Thy-1 membrane microdomains serve as a platform to aggregate Src-related proteins to induce activation of rat mast cells (Figure 1) (Draberova, 1989; Draberova et al., 1996). This activation also involves Thy-1 ligands, such as integrins, and in this context, the engagement of β1 integrin in mast cells increases their sensitivity to cellular activation (Ra et al., 1994). Mast cells can show an adherent or non-adherent behavior according to the integrin profile, which might include the expression of α4, α5, α6, β1, and β7 integrin subunits. The expression of α4 integrin is higher in adherent mast cells (Grodzki et al., 2003). β1 and αvβ3 integrin expression have also been related to mast cell adhesion in human skin (Columbo et al., 1995; Columbo and Bochner, 2001). Interestingly, α5β1 and αvβ3 integrins are reported Thy-1 receptors/ligands, and therefore, their interaction in this biological process could potentially contribute to the adhesive behavior of mast cells.
A role of Thy-1 in recruiting leukocyte cells and regulating inflammation has been sustained by studies that show that monocytes and neutrophils expressing αxβ2 and αMβ2 integrins use Thy-1 expressed on the surface of activated endothelial cells to migrate (Wetzel et al., 2004). The recruitment of leukocytes and the subsequent increase in cytokines can exacerbate the expression of Thy-1 in the endothelial cells at the wound site, as discussed during angiogenesis in the proliferative phase (Section 5). Thy-1 also interacts with CD97, an adhesion G-protein coupled receptor (GPCR) present at the adherent junctions formed between endothelial cells; such interaction probably mediates the transmigration of the leukocytes to the wound (Figure 1). Additionally, Thy-1 regulates the extravasation of leukocytes during acute lung inflammation, controlling the recruitment of different cells and preparing the inflammatory environment (Schubert et al., 2011).
Inflammatory cell recruitment and subsequent secretion of regenerative factors at the wound bed are tightly coordinated processes (Cañedo-Dorantes and Cañedo-Ayala, 2019). As discussed before, changes in the timing and inflammatory components can both positively and negatively affect the healing process. Another component reviewed in the literature is the heparan sulfate proteoglycan syndecan. Its role during inflammation depends on the degree of sulfation of the heparan sulfate chains, the rate of ectodomain shedding, the solubility of the ectodomains, and the expression levels of different syndecan family members (Gopal, 2020).
On the other hand, integrins mediate leukocyte accumulation at the sites of inflammation. Inflammatory mediators trigger integrin activation, and this is a crucial step to initiate leukocyte migration to inflamed tissues. Subsequently, integrin deactivation is indispensable for maintaining proper leukocyte migration (Zarbock et al., 2012; Park et al., 2015). Integrins are also important for leukocyte adhesion and transmigration through blood-vessel walls to get access to the site of inflammation, in neutrophil recruitment, lymphocyte recirculation, and monocyte trafficking. Specifically, β1 and β2 integrins have been addressed as central players in regulating leukocyte recruitment and vascular permeability during acute inflammation (Figure 1) (Rhee et al., 2003; Ley et al., 2007; Ou et al., 2021). Taken together, Thy-1 and its receptors integrins and syndecan 4 play a crucial role in recruiting leukocytes and restraining the immune response in the wound area. Therefore, further studies are necessary to evaluate if, for instance, the shedding of Thy-1 and syndecan 4 or changes in the expression of these receptors are important to clear up inflammation and lead to the remodeling phase of wound healing.
Lymphocyte-induced inflammation is cleared by a massive apoptosis process induced by interferon (INFc) and TNFα production at the wound site (Martins-Green et al., 2013). Liu et al., demonstrated that Bcl-2 and Bcl-xL synthesis was decreased in response to Thy-1 expression and could lead to apoptosis by activating the effector caspase-3 and poly ADP-ribose polymerase (PARP). In addition, they demonstrated that Thy-1 mediates apoptotic signaling via both caspase-9- and caspase-8-dependent pathways (Liu X. et al., 2017). Moreover, Thy-1/β3 integrin-induced apoptosis of dermal fibroblasts is mediated by upregulation of Fas ligand (FasL) expression (Figure 1) (Schmidt et al., 2016). FasL binds to and activates Fas, a cell-surface death receptor belonging to the TNF receptor superfamily, to induce apoptosis through the activation of caspase-8. Therefore, we hypothesize that Thy-1 and β3 integrin could play a vital role in terminating the inflammatory phase by inducing apoptosis of lymphocytes.
5 Proliferative Phase
During the proliferative phase, fibroblasts, keratinocytes, and endothelial cells divide, differentiate, and migrate to the wound area. This phase overlaps with the inflammatory phase, starts 2 days after injury with the degradation of the fibrin matrix and invasion of fibroblasts and endothelial cells, and lasts up to 3 weeks to completely repair the wounded area. The signature events during this phase include:
a) Fibroplasia, which consists in the formation of fibrous tissue to fill the wound area. During this phase, fibroblasts are stimulated by multiple cytokines and growth factors released by platelets, macrophages, keratinocytes, mast cells and endothelial cells. The fibroblasts become active, start to proliferate, and secrete MMPs and other proteases to allow cell migration through the provisional fibrin matrix. Active fibroblasts produce fibronectin, hyaluronic acid, proteoglycans, and collagen to form a new ECM that allows keratinocyte migration. The provisional fibrin matrix becomes the granulation tissue formed by a loose ECM, embedding blood vessels, macrophages, and fibroblasts. The fibroblasts differentiate in myofibroblasts, which contract to pull the wound together and decrease its area (Li and Wang, 2011; Darby et al., 2014). The myofibroblast differentiation process is mediated by TGFβ and CXCL8 and requires the interaction between fibronectin and αvβ5 integrin (Asano et al., 2006). Similarly, αvβ3 integrin upregulation contributes to establish an autocrine TGFβ loop in scleroderma fibroblasts (Asano et al., 2005). Interestingly, αvβ5 integrin is a reported Thy-1 ligand/receptor, and Thy-1 has been addressed as a negative regulator of latent TGFβ activation induced by fibroblast contraction, and as an inhibitor of myofibroblast differentiation through its interaction with αvβ5 integrin in lung cells (Zhou et al., 2010). TGFβ itself can also be regulated by mechanical strain. Within the wound, TGFβ is found inactive, and interacts with the latency-associated peptide (LAP) and the latent TGFβ binding proteins (LTBP). LTBPs (LTBP1, 3, and 4) link the TGFβ/LAP complex to the ECM, providing a dormant TGFβ pool that can be activated as wound healing progresses. The myofibroblasts express integrins that bind to the LAP, and mechanical force transduced by these integrins either from the ECM-bounded LTBP or from the myofibroblast contraction can release TGFβ by pulling LAP and allowing the TGFβ to bind its receptor (Munger et al., 1998; Annes et al., 2002; Annes et al., 2004; Wipff et al., 2007). The newly released TGFβ can increase the myofibroblast contractile phenotype and its ECM synthetic activity. Here, it is possible that Thy-1 in the same cell (Cis interaction) and through its RLD motif, compete with LAP and the ECM for integrin binding, maintaining the inactive state of the integrin and thus, allowing displacement of the other interactions without inducing myofibroblast differentiation (Zhou et al., 2010; Herrera-Molina et al., 2013). Blocking other integrins such as α3β1, α11β1, αvβ5 also inhibits myofibroblast development (Figure 1), highlighting the importance of integrin signaling during fibroplasia (Asano et al., 2006; Kim et al., 2009; Carracedo et al., 2010).
Thy-1 has a crucial role in suppressing proliferation and promoting differentiation of dermal fibroblasts. The lack of Thy-1 in dermal fibroblasts increases proliferation rates and reduces apoptosis by modulating β3 integrin function. Moreover, Thy-1−/− fibroblasts display reduced expression of myofibroblast differentiation markers, such as αSMA, fibronectin, collagen I and III, and a reduced amount of biologically active TGFβ (Schmidt et al., 2015). Consistently, increased fibroblast proliferation in the absence of Thy-1 expression has also been described in fibrotic foci in lungs of patients with pulmonary fibrosis and fibroblasts derived from patients with hypersensitivity pneumonitis, supporting the idea that Thy-1 expression is necessary to avoid fibrosis (Ramirez et al., 2011). Alternatively, syndecan 4 regulates fibroblast migration during wound healing (Bass et al., 2011; Brooks et al., 2012). Interestingly, Thy-1-mediated migration and focal adhesion dynamics in embryonic fibroblasts depend on the recruitment of Partitioning-defective 3 (PAR3) to the cytoplasmic tail of syndecan 4. Consequently, PAR3 silencing inhibits FA disassembly triggered by Thy-1 stimulation, favoring fibroblast adhesion instead of migration (Valdivia et al., 2020). Further research is necessary to determine if the Thy-1/syndecan 4 interaction can also modulate fibroblast proliferation, differentiation, or ECM secretion.
b) Re-epithelialization starts approximately 16–24 h after injury, and during this phase, keratinocytes and MSCs work together to resurface the skin wound with new epithelium. Growth factors, chemokines, and cytokines released in the wound bed activate the keratinocytes adjacent to the wound edge to allow migration and proliferation (Peplow and Chatterjee, 2013). The keratinocyte-activated phenotype is characterized by changes in the cytoskeleton and membrane receptors, allowing keratinocytes to migrate. Wound keratinocytes express at least seven different integrins, which cooperatively control essential cell functions to promote proper re-epithelialization, including adhesion, migration, proliferation, survival, and basal membrane assembly (DiPersio et al., 2016). Migrating keratinocytes exhibit upregulation of keratins (K6, K16, and K17), MMPs, ECM proteins (Laminin), integrins (αvβ5, αvβ6, α5β1), and proteoglycans (perlacan and syndecans) (Figure 1) (Rousselle et al., 2019). In a porcine wound model, TGFβ stimulates expression of keratinocyte integrins during re-epithelialization of cutaneous wounds, and increases mRNA levels of integrin subunits α5, αv, and β5, with little effect on β1 in human keratinocytes (Gailit et al., 1994). Integrins and proteoglycans are essential for the keratinocytes to interact with the fibronectin-rich provisional matrix and later, with the collagen-rich matrix (Rousselle et al., 2019). Indeed, keratinocyte-specific β1 integrin knockout mice show a severe defect in wound healing. Furthermore, α3β1 integrin has been related to inhibition of migration and wound re-epithelialization in the skin, where α3-deficient keratinocytes migrate with an increased velocity and persistence (Margadant et al., 2009). Furthermore, the role of α6β4 integrin was determined in a human three-dimensional wound healing model by blocking β4 integrin with antibodies, which delay keratinocyte re-epithelialization (Egles et al., 2010). Additionally, epithelial-mesenchymal interactions between keratinocytes and fibroblasts generate a bidirectional signaling, in which keratinocytes stimulate fibroblasts to release growth factors that, in return, stimulate keratinocyte proliferation (Werner et al., 2007). Syndecan expression is increased in proliferating and migrating keratinocytes at the periphery of wounds in the skin (Figure 1) (Elenius et al., 1991). Particularly, syndecan 4 protein expression is significantly increased during tissue repair in the mouse and human dermis and is localized at the site of injury. Importantly, mice deficient in syndecan 4 experience delayed healing of excisional dermal wounds (Gallo et al., 1996; Echtermeyer et al., 2001). In a mouse wound healing model, Gallo and co-workers studied the upregulation of syndecan 4 throughout the dermis at the injury site; however, they observed that expression of syndecan 4 returns to basal levels in the re-epithelialization stage (Gallo et al., 1996). In addition, dermal fibroblasts isolated from syndecan 4 null mice exhibit decreased cell migration in wound healing assays in vitro and the inability to contract three-dimensional fibrin/fibronectin matrices during in vitro wound closure experiments (Midwood et al., 2004). On the other hand, Thy-1 expression in keratinocytes has been reported as a helpful marker of human keratinocyte stem cells (Nakamura et al., 2006). However, a role for Thy-1 in keratinocyte function and re-epithelialization has not been investigated yet.
The ECM plays a transcendental role not only during re-epithelialization, but also during every phase of the wound healing process (reviewed in Olczyk et al., 2014; Maquart, 2015; Xue and Jackson, 2015; Tracy et al., 2016; Rousselle et al., 2019). The secretion of ECM components occurs in a coordinated fashion in response to specific inflammatory and growth factors. The sequential deposition of ECM, along with cell-specific expression of receptors that interact with the ECM, are responsible for the synchronized recruitment of specific cell types to the wound area. Additionally, the ECM retains and modulates the delivery of growth factors and inflammatory molecules. Thus, aberrant ECM leads to delayed wound healing and abnormal scarring. Therefore, both ECM proteins and their integrin receptors play a key role in the re-epithelialization phase.
c) Angiogenesis is the formation of new capillaries from pre-existent blood vessels. This process starts approximately 4 days after the injury and forms the microvascular network throughout the granulation tissue. The granulation tissue is a stroma composed of connective tissue containing ECM proteins, as well as the cells necessary to allow the sprouting of blood vessels and subsequent wound closure. The reduction in blood supply and the exacerbated metabolism of the cells actively working in the healing process cause hypoxia, a major angiogenesis stimulus (Semenza, 2007; Castilla et al., 2012; Kimmel et al., 2016). This hypoxic environment induces an increase in the levels of the hypoxia inducible factor 1 (Hif-1) in macrophages, endothelial cells, fibroblasts, and keratinocytes (Andrikopoulou et al., 2011). Hif-1 activates the transcription of the main angiogenic factors, including VEGF, angiopoietin 1, TSP, and CXCL8. The presence of VEGF and macrophages induce the differentiation of endothelial cells into three different phenotypes: tip cells that secrete proteases, which degrade the basement membrane to allow them to detach from the vessel wall and migrate; proliferative stalk cells that elongate the new capillary sprout; and the quiescent phalanx cells that form the vessel lining. After the new capillaries are formed, PDGF, along with TGFβ and angiopoietin 1, stimulate mesenchymal cells to differentiate into pericytes and recruit other pericytes into the wound area. The pericytes wrap around immature capillaries and contribute to vessel maturation (Gaengel et al., 2009).
Even though the Thy-1 promoter lacks hypoxia regulatory elements, Thy-1 expression can be enhanced by Hif-1 downstream targets, such as cytokines and growth factors, as observed in the hypoxic-ischemic brain after injury or stroke. In a model of choroidal neovascularization, Thy-1 expression increases in primary endothelial cells after VEGF or CCL11 treatment, and after laser-induced injury. Moreover, Thy-1 ablation or inhibition reduces VEGF-induced migration and proliferation of endothelial cells, as well as VEGFR2, Rac and β3 integrin activation (Wang et al., 2016). Noteworthy, CCL11—a CCR3 ligand—is expressed by fibroblasts after the initial recruitment of lymphocytes during the inflammatory phase (Section 4). CCL11 positively affects neovascularization and enhances wound repair in vitro by inducing the migration of human primary dermal microvascular endothelial cells, dermal fibroblasts, and epidermal keratinocytes into the wound area (Salcedo et al., 2001; Park et al., 2017; Bunemann et al., 2018). Additionally, CCR3 is markedly expressed in dermal fibroblasts, and it is upregulated after cutaneous injury (Bunemann et al., 2018). Altogether, these findings suggest that CCL11 and its receptor CCR3 play a crucial role during the wound healing process; however, further studies are necessary to relate these effects to CCL11-CCR3-induced Thy-1 expression enhancement and to integrin/syndecan 4 signaling during wound closure.
Lee et al., showed that Thy-1 is expressed in newly formed vessels in four different models of adult angiogenesis: balloon injury of the carotid, tumor implantation in the cornea, renal artery ligation, and uterine capillaries formed during pregnancy (Lee et al., 1998). Accordingly, since embryonic angiogenesis occurs by different mechanisms than those of adult angiogenesis, Thy-1 is not detected on blood vessels of rat embryos. Moreover, cytokines such as IL-1β and TNFα upregulate the expression of Thy-1 in endothelial cells, and after carotid damage, Thy-1 appears in the newly formed vessels adjacent to zones with macrophage infiltration, indicating a biological function of Thy-1 during inflammation (Lee et al., 1998; Wen et al., 2013). Similarly, Thy-1 is upregulated in the EA.hy926 endothelial cell line when treated with TNFα (Brenet et al., 2020). Thy-1 expression in glioblastoma vasculature is associated with endothelial, smooth muscle, and pericyte cells, supporting Thy-1 function during angiogenesis (Inoue et al., 2016). Further in vitro studies have shown that Thy-1 overexpression decreases migration and tube formation of endothelial cells (Wen et al., 2013). Although the effect on tube formation can sound contradictory for a successful angiogenesis process, these results cannot be easily extrapolated to in vivo angiogenesis since they were performed in cells overexpressing Thy-1 and in Matrigel containing high amounts of cytokines and growth factors that can overstimulate the endothelial cells. One possible interpretation of these results is that the role of Thy-1 is to stabilize the newly formed blood vessels.
Most importantly, transcriptome analysis of blood vessels from chronic wound edge tissue showed that Thy-1 expression is 24-fold higher than in vessels from healthy human skin (Figure 1) (Roy et al., 2007). The biological significance of Thy-1 expression on the surface of endothelial cells after a skin wound is still not fully understood. However, Thy-1 would exert a role in recruiting lymphocytes and allowing trans-epithelial migration, as discussed in detail above (Section 4).
One of the essential factors for wound angiogenesis is αvβ3 integrin, a receptor for provisional matrix proteins, including vitronectin, fibronectin, and fibrin, which is expressed on the tips of angiogenic capillary sprouts (Figure 1) (Tonnesen et al., 2000). Blockage of αvβ3 integrin using antibodies or specific cyclic peptides inhibits the formation of granulation tissue and wound healing (Clark et al., 1996). Provisional matrix proteins, such as fibronectin and fibrin, increase αvβ3 integrin expression in endothelial cells, while a collagen-rich matrix has the opposite effect (Tonnesen et al., 2000). Noteworthy, the provisional matrix deposited during the hemostasis phase is subsequently replaced by collagen type I during the remodeling phase, suggesting that the distribution of αvβ3 at the tip of the capillaries is spatially and temporally regulated by the ECM. On the other hand, β1 integrins are expressed along the full length of the blood vessels, supporting the growing and sprouting of the vasculature when the new capillaries are extending (Yamamoto et al., 2015). β1 integrin expression increases when endothelial cells are plated on collagen-rich coated surfaces, suggesting a role in stabilizing the newly formed blood vessels at the end of the wound healing process (Tonnesen et al., 2000). Likewise, syndecan 4 is upregulated throughout the granulation tissue in endothelial cells and fibroblasts after injury (Gallo et al., 1996). In vitro assays using endothelial cells have shown that syndecan 4 is regulated by inflammatory factors, such as TNFα and IL-1β, and that decreasing syndecan 4 expression in endothelial cells impairs tube formation (Figure 1) (Vuong et al., 2015). Accordingly, mice lacking syndecan 4 expression showed reduced angiogenesis and delayed wound healing (Echtermeyer et al., 2001). The syndecan 4 cytoplasmic domain has also been described to be important for the function of FGF2, a potent angiogenesis inducer and signaling molecule in endothelial cells. Cells transfected with syndecan 4 mutated in the cytoplasmic domain show decreased PKCα activation, which is associated with impaired migration, proliferation, and tube formation (Horowitz et al., 2002). Full activation of FGF2 requires not only its interaction with the FGF receptor, but also its internalization (Goldfarb, 2001). Interestingly, FGF2 binds to and activates syndecan 4 (Simons and Horowitz, 2001). At the same time, active syndecan 4 leads to FGF2 complete activation by triggering FGF2 internalization through macropinocytosis in endothelial cells (Tkachenko et al., 2004). Recent reports indicate that FGF2 also upregulates syndecan 4 in endothelial cells at a high cell density, an event which might help repair damaged vascular endothelial cell layer or that could be related to the regulation of angiogenesis (Hara et al., 2020).
Thy-1 is also found in pericytes and mesenchymal progenitor cells that can differentiate into pericytes. A recent study identified two different pericyte populations associated with brain vessels, based on Thy-1 expression levels. Pericytes expressing high levels of Thy-1 exhibit a blunted inflammatory response, produce less ECM, and express lower levels of pericyte markers (e.g., SMA and PDGFR-β), when compared with pericytes expressing lower Thy-1 levels (Park et al., 2016). Although such heterogenicity in pericytes has not been determined after skin injury, these results suggest that Thy-1 may play a role in microvessel maturation and ECM deposition to form the granulation tissue (Figure 1). Moreover, pericytes expressing Thy-1 appear to secrete vesicles that exhibit Thy-1 on their surface and localize in the intercellular area (Bukovsky et al., 2001). Although these Thy-1 (+) vesicles are present between the epithelial-mesenchymal interphase, their biological role remains uncertain.
d) Peripheral nerve repair occurs by collateral reinnervation and nerve regeneration. After an injury, the undamaged axons are stimulated to produce collateral sprouting to reinnervate the skin. Severed nerves of the peripheral nervous system (PNS) can regrow the tips of two myelinated axon stumps and reconnect them to restore their homeostatic function in the skin (Gaudet et al., 2011). During this process, Schwann cells (SCs) present at the distal degenerating stump lose their myelin sheath and dedifferentiate to a progenitor-like cell to promote axonal regrowth. SCs leave the damaged nerve stump by directly interacting with fibroblasts accumulated at the wound bed, migrate, align, and form columnar bands of Büngner, which provide a substrate for axonal regeneration. Simultaneously, macrophages help to clean myelin and axon debris. The hypoxic environment and chemoattractant molecules released by SCs and other cell types, recruit macrophages/monocytes, which contribute to angiogenesis, as discussed above. Subsequently, SCs use the newly formed vasculature as a scaffold to guide the regrowing axons. Once the axons reinnervate, SCs differentiate and remyelinate the axons (Chernousov and Carey, 2000; Gaudet et al., 2011).
SCs express integrins that bind laminin (α2β1, α6β1, α6β4), collagen (α1β1, α2β1), and fibronectin (αvβ3, α5β1). Following a peripheral nerve lesion, both α5β1 and syndecan 4 levels are strongly increased in SCs nearby the wounded nerve (Lefcort et al., 1992; Milner et al., 1997; Chernousov and Carey, 2000; Goutebroze et al., 2003). On the other hand, fibroblasts in the wound area overexpress Thy-1 on their membranes. Since fibroblasts are responsible for guiding the SCs to the lesion site, this close interaction likely occurs by engagement of α5β1 integrin and syndecan 4 on the surface of SCs, with Thy-1 present at the fibroblast membrane (Figure 1). Similarly, our group has described in other glial cells (astrocytes) expressing αvβ3 and syndecan 4, that binding to Thy-1 promotes astrocyte adhesion to the ECM and subsequent cell migration (Hermosilla et al., 2008; Avalos et al., 2009; Kong et al., 2013; Valdivia et al., 2020).
An early investigation showed that Thy-1 expression in the PNS is restricted to the nodes of Ranvier within the sciatic nerve and that it colocalizes with laminin at the SC membranes (Liesi et al., 1990). Further research using transgenic rodents expressing fluorescent proteins under the control of the Thy-1 promoter (Thy-1-YFP) revealed that most of the neurons in the PNS were fluorescent, suggesting that Thy-1 is broadly expressed in the axons of adult peripheral neurons (Morris et al., 1983). Noteworthy, this kind of evidence is not functional and does not discard the possibility of a differential Thy-1 distribution in microdomains within the axon. Perhaps the area closer to the wound has a different density or less active Thy-1 than the rest of the axon, in order to favor the extension of the axon during repair. Indeed, experiments using the saphenous nerve crush model in Thy-1-YFP mice, followed by transcutaneous imaging to evaluate nerve generation, implied that the Thy-1 promoter is timely and spatially regulated after nerve damage. YFP expression driven by the Thy-1 promoter decreased nearby the crush injury. After 7 days, a second crush injury prompted a decrease in YFP levels distal to the crush site, up to complete disappearance 24 h later. After 2 days, YFP expression started increasing proximal to the crush site, to fully recover 7 days post-injury. Moreover, YFP expression at the crush site is exceptionally high compared with distal areas (Yan et al., 2011). This study suggests that the Thy-1 promoter is active in healthy and fully regenerated axons and at the site of nerve injury. Even though these cell-specific fluorescent reporters are highly popular to study peripheral nerve repair, very few studies have focused on the role of Thy-1 during this type of nerve repair in wounded skin (Feng et al., 2000; Pan et al., 2003; Kemp et al., 2013). For example, Thy-1 expressed on the axons of PNS facilitates the regeneration of peripheral nerves, such as the sciatic nerve (Kemp et al., 2013).
6 Remodeling Phase
The remodeling phase, also known as the maturation phase, is the final and longest phase of the wound healing process. It can last from 21 days to 2 years and occurs concurrently with granulation tissue formation. During remodeling, all the tissues formed in the previous phases, including the epidermis, vasculature, nerves, and myofibers, are mature and functional. In parallel, there is a decreased tissue cellularity due to apoptosis of fibroblasts, myofibroblasts, endothelial cells, pericytes, and inflammatory cells present within the granulation tissue. Additionally, the number of proteoglycans and glycosaminoglycans diminished, reducing the amount of water in the wound. The remodeling phase is characterized by wound contraction and ECM turnover. Myofibroblasts direct wound contraction, in which wound tensile strength increases as the collagen fibers are cross-linked by lysyl oxidase. The scar tissue has a tensile strength that is never greater than 80% of the unwounded skin, but this can be improved using MMP inhibitors. Throughout matrix turnover, MMPs released by fibroblasts and macrophages break down collagen type III and replace it with collagen type I, which is organized into parallel fibers (Toriseva and Kahari, 2009). As the remodeling process progresses, the ECM is reorganized and later suffers degradation, which is controlled by the balance of MMPs and tissue inhibitors of MMPs (TIMPs).
Remodeling is also regulated by growth factors such as TGFβ, PDGF, and FGF. TGFβ can increase collagen deposition by enhancing the synthesis of TIMPs. In contrast, PDGF and FGF increase the expression of collagenase. The balance between these growth factors controls the rate of remodeling. Importantly, PDGF also mediates fibroblast differentiation into myofibroblasts, which participate in wound contraction (Jinnin et al., 2005; Barrientos et al., 2008).
Macrophages are also crucial during remodeling since they adopt a fibrolytic phenotype to help engulf the ECM and cell debris. The removal of fibrous tissue is an important step during remodeling. When myofibroblasts express the CD47 on their surface (a “don’t-eat-me-signal”), they are not phagocyted by macrophages, resulting in excessive matrix deposition and hypertrophic scarring (Lech and Anders, 2013). As proposed, activation of the fibrolytic phenotype on macrophages and blockade of CD47 are relevant factors in reversing tissue fibrosis (Wernig et al., 2017). Interestingly, CD47, integrins, and syndecan 4 can bind and activate TSP1 and TSP2. Although controversial, TSP can regulate tissue remodeling and act as a negative regulator of wound healing (Kyriakides et al., 1999; Streit et al., 2000; Kyriakides et al., 2001; Agah et al., 2002; Kyriakides and Maclauchlan, 2009; Soto-Pantoja et al., 2014). Indeed, blocking of CD47/TSP signaling is effective in promoting wound closure and preventing necrosis of skin graft (Isenberg et al., 2008; Soto-Pantoja et al., 2014; Kale et al., 2021). However, the role of Thy-1/integrin/syndecan 4 trimolecular complex has not been studied in the context of CD47/TSP signaling in wounded skin.
Scar formation is the final step of wound repair. Occasionally, an imbalance may occur during ECM turnover, resulting in abnormal scar formation, such as hypertrophic or keloid scarring. Keloid scars are characterized and differentiated from hypertrophic scars by an excess of connective tissue forming a firm and raised scar that extends beyond the original wound limits and does not regress over time. A TGFβ signaling dysregulation, in which overexpression of TGFβ1 and β2, and blunted expression of β3, is believed to cause hyperactivation of fibroblasts, leading to increased ECM production. Consequently, collagen production is increased 3-fold in hypertrophic scars and 20-fold in keloids (Carswell and Borger, 2021). Likewise, a change in the expression of pro-inflammatory (IL-6 and IL-8) and anti-inflammatory (IL-10) cytokines have been associated with increased incidence of hypertrophic and keloid scarring (Berman et al., 2017). Despite this knowledge, the etiology of hypertrophic and keloid scarring is not fully understood yet; however, mechanical tension seems to be relevant, among other pathological factors (Butler et al., 2008). Physical tension has been proposed as a guide in keloid growth patterns, and skin areas subject to stretching have an increased incidence of keloid formation due to high mechanical stimulation (Akaishi et al., 2008; Carswell and Borger, 2021). Therefore, mechanical tension could play an important role during wound remodeling.
Every phase of the wound healing process is influenced by mechanical forces (Agha et al., 2011). Mechanical strain can alter the microenvironment of a healing wound, causing changes in cellular function, motility, and signaling (Kuehlmann et al., 2020). Fibroblasts are essential in supporting wound healing, and the signaling between the ECM-integrin-cytoskeleton is the classical pathway of mechanotransduction that regulates fibroblast viability, collagen production, and myofibroblast transformation during the remodeling phase (Bainbridge, 2013; Sun et al., 2016; Tschumperlin et al., 2018). Integrins are the main mechanotransducers of bidirectional information between cells and the ECM, and fibroblasts transduce mechanical strain predominantly through β1 integrins (Table 1) (Katsumi et al., 2004). Other important cells participating in wound healing that are affected by mechanical forces are keratinocytes, endothelial cells, and adipocytes (Fu et al., 2021). Cultured human keratinocytes and fibroblasts on a collagen membrane in a tensile device show asymmetric keratinocyte migration regulated by growth factors secreted by fibroblasts (Lu et al., 2016). Keratinocyte proliferation is regulated by mechanical strain through matrix-integrin signaling, epithelial-mesenchymal interactions, and Ca2+ channels, which transduce mechanical signals to PKC, PLC, and mitogen-activated protein (MAP) kinase pathways (Reichelt, 2007; Fu et al., 2021). Additionally, keratinocyte migration requires integrins to facilitate wound closure, as previously described during re-epithelialization (Section 5, c) (Kenny and Connelly, 2015). Indeed, β1 integrin can sense collagen and fibronectin ECM stiffness, and β1-null keratinocytes show a severe defect in skin wound healing (Figure 1) (Grose et al., 2002; Reynolds et al., 2008). Moreover, keratinocytes and fibroblasts rearrange their cytoskeleton in response to matrix stiffness, and the scar hardness and elevation observed in hypertrophic/keloid scarring can be influenced by rigidity-induced collagen production and cell proliferation (Hossain et al., 2005; Solon et al., 2007; Hadjipanayi et al., 2009). Focal adhesion kinase (FAK) is the most studied signaling pathway in skin mechanotransduction. FAK is activated downstream of integrins in fibroblasts and keratinocytes, and mechanical strain during wound healing leads to FAK hyperactivation, which correlates with hypertrophic scarring and fibrosis (Aarabi et al., 2007; Kuehlmann et al., 2020). In contrast, blunted FAK activation has been related to nonhealing wounds and delayed healing (e.g., diabetic wounds) (Wong et al., 2014; Liu W. et al., 2017). These data support the idea that the cell-centric view of wound remodeling is incomplete and suggests that tissue stiffness and mechanical strain within the wound may significantly influence the healing process. More investigations are necessary to unravel the mechanotransducer proteins that communicate internal with external forces in cells and their microenvironment.
The role of Thy-1 in integrin-mediated mechanotransduction has been mainly studied in Trans interactions, and little is known about Cis interactions (Hu and Barker, 2019). Thy-1, as well as integrins and syndecan 4, have been described as mechanotransducer proteins (Figure 1). Barker and co-workers showed that Thy-1 regulates fibroblast mechanotransduction. Thy-1 binding to αvβ3 integrin modulates rigidity-dependent Rho signaling, cytoskeleton remodeling, focal adhesion formation, and substrate rigidity sensing (Fiore et al., 2015). Furthermore, syndecan 4 is also considered a mechanotransducer protein (Bellin et al., 2009; Ross et al., 2013). Remarkably, integrins and syndecan 4 form a trimolecular complex with Thy-1, where two triads have been reported: α5β1/Thy-1/syndecan 4 in melanoma/endothelial cells and αvβ3/Thy-1/syndecan 4 in a neuron/astrocyte model (Fiore et al., 2014; Burgos-Bravo et al., 2020). However, further investigation is necessary to assess the specific role of Thy-1 and its binding partners during force transduction in cells involved in every phase of the skin wound healing process.
Additionally, tissue stiffness has been posited to drive stromal cells to differentiate into active myofibroblasts, which remodel the tissue, leading to fibrosis progression. Interestingly, fibroblasts show reduced Thy-1 expression in areas of active fibrogenesis in human idiopathic pulmonary fibrosis, and in vitro experiments have shown that the lack of Thy-1 expression in fibroblasts is enough to induce myofibroblast differentiation on soft matrix substrates (Hagood et al., 2005; Fiore et al., 2015; Fiore et al., 2018). Thus, Thy-1 expression in fibroblasts may represent a physiological mechanism relevant to fibrosis.
7 Mesenchymal Stem Cells
MSCs are multipotent stromal cells with the potential to differentiate into a variety of cell types, including adipocytes, chondrocytes, osteoblasts, myocytes, and stromal cells. MSCs can promote angiogenesis, granulation tissue formation, and epithelialization, which result in accelerated wound healing (Hu et al., 2018). MSCs have an anti-inflammatory effect during the inflammatory phase and can also stimulate fibroblasts, keratinocytes, and endothelial cells during the proliferative phase of wound healing.
Thy-1 (CD90) is a marker of MSCs and has a role regulating the fate of cells during cell differentiation. For instance, Thy-1 positive-MSCs follow osteogenic differentiation, while MSCs expressing reduced Thy-1 levels can promote adipogenic differentiation in cells isolated from dental pulp, adipose tissue, and amniotic fluid (Moraes et al., 2016; Picke et al., 2018). Additionally, strong evidence indicates that resident MSCs are activated to differentiate into other cell types, such as pericytes, myofibroblasts, and endothelial cells during wound healing and fibrosis (Yamaguchi et al., 2005; Wu et al., 2007; Sasaki et al., 2008; Lozito et al., 2009; Jeon et al., 2010; Nie et al., 2011; Ojeh and Navsaria, 2014; Park et al., 2016; Michelis et al., 2018). However, it is still unknown whether Thy-1 participates in MSC differentiation into these cell types in the skin. In addition, the mechanisms by which Thy-1 promotes MSCs differentiation are still controversial and need further investigation (Yang et al., 2020).
8 Current Therapeutic Strategies Targeting Thy-1, Integrins and Syndecan 4
Different therapeutic approaches to promote wound healing have been reported. Among these, ECM-based skin substitutes and cell-based therapies, such as implantation of MSCs, hydrocolloid pads, and chitosan dressing have shown promising results (Dreifke et al., 2015). In view of the important role that integrins, syndecan 4 and Thy-1 play in the wound healing process, we summarize and discuss here some of the current therapeutic efforts to modify these molecules and their interactions in order to improve wound healing outcomes and design potential future applications.
Integrins have been related with important roles along most of the wound healing stages (Figure 1). Impaired wound healing by overhealing (hypertrophic scar) or failure to heal (chronic wound) can result from aberrant integrin signaling. Many clinical studies in cancer and fibrosis have used integrin antagonists, such as blocking peptides, antibodies, and small molecules, to inhibit angiogenesis, cell migration, and proliferation (Silva et al., 2008; Lasinska and Mackiewicz, 2019; Su et al., 2020; Zhang et al., 2021). However, less is known about the advantages of these therapeutical approaches during wound healing of the skin.
To date, several pre-clinical studies in skin models have shown that integrin antagonists can potentially improve wound healing. For example, the antibody P1F6 (against αvβ5) and LM609 (against αvβ3) are effective in decreasing myofibroblast differentiation markers and TGFβ-induced gel contractility in human dermal fibroblasts (Lygoe et al., 2004). Similarly, both anti αvβ5 antibodies and RGD synthetic peptides have been used for blocking the interaction of this integrin with LAP-β1 and small latent complex (SLC) binding during the activation of latent TGFβ in dermal fibroblasts (Asano et al., 2006). Furthermore, blockade of other integrins, such as α3β1 and α11β1, also inhibits myofibroblast development (Kim et al., 2009; Carracedo et al., 2010). Thus, integrins related to TGFβ activation are biological targets for preventing fibrosis (Nishimura, 2009).
Integrins can also be targeted by creating substrates that mimic ECM components or binding peptides. The conjugation of integrin-targeting peptides to biomaterials has been shown transcendental for therapeutic success and has been recently reviewed (Zhao et al., 2020). In this context, for example, a thermoresponsive hydrogel formulated with laminin-derived peptides (PPCN-A5G81) that activate α3β1 and α6β1 integrins, has been tested in a diabetic mouse model, showing significant acceleration of wound closure (Zhu et al., 2018). During in vitro assays, the PPCN-A5G81 hydrogel induces cell spreading and migration of keratinocytes and dermal fibroblasts and controls the integrin α3- and α6-dependent proliferation of human dermal fibroblasts. As found in in vivo assays, the PPCN-A5G81 hydrogel accelerates the closure of excisional splinted wounds in diabetic mice by enhancing the granulation tissue formation and re-epithelialization (Zhu et al., 2018). Similarly, a peptide derived from angiopoietin 1 (QHREDGS), delivered as a soluble peptide, or collagen-chitosan hydrogel, or covering nanosheets of silk fibroin, positively influences re-epithelialization, angiogenesis, and granulation tissue formation to accelerate wound healing by binding αvβ3 and α5β1 integrins (Miklas et al., 2013; Xiao et al., 2016; Zhao et al., 2020). Moreover, a clinical trial indicated that the topical application of RGD peptide matrix scaffold, on partial-thickness scald burns in pediatric patients accelerates wound healing (Hansbrough et al., 1995). Therefore, to positively influence wound healing, the efforts to develop therapeutic tools can be focused on the activation or inhibition of specific integrins.
Some yet unexplored roles of syndecan 4 during wound healing include its function in regulating oligomerization and activation of chemokines, shedding from the cell surface in response to inflammatory molecules, and acting as a co-receptor for growth factors to enhance the signaling downstream of the growth factor receptor. In diabetic and obese mouse models, proteoliposomes containing syndecan 4 and PDGF-BB enhance PDGF-BB activity, improving wound healing. These proteoliposomes increase re-epithelialization and angiogenesis, compared to the treatment with PDGF-BB alone. The proportion of M1/M2 macrophages also improves, suggesting that the syndecan 4 proteoliposomes improve PDGF-BB efficacy in wound healing (Das et al., 2016). Most of syndecan 4 functions are mediated by their extracellular HS moiety (Bernfield et al., 1999; Tumova et al., 2000). Alternatively, heparanase, an endoglycosidase that cleaves HS from the cell surface and ECM, leads to the release and activation of HS-bound growth factors and cytokines that participate in the wound healing process. Heparanase itself is expressed in the granulation tissue and transgenic mice overexpressing heparanase show increased wound angiogenesis (Zcharia et al., 2005). Remarkably, topical application of heparanase enhances wound healing, vessel maturation and skin survival, suggesting that syndecan and other HS-proteoglycans can be important therapeutic targets (Zcharia et al., 2005; Fuster and Wang, 2010).
Thus far, the potential of Thy-1 as a therapeutic target has not been studied in skin wound healing. Some pre-clinical studies have provided some clues about the delivery of recombinant Thy-1 being beneficial in reversing fibrosis in biological systems other than skin. For instance, during idiopathic pulmonary fibrosis, fibroblasts lacking Thy-1 have increased proliferation and decreased myofibroblast differentiation markers. Similarly, Thy-1 deficit in an in vivo lung fibrosis mouse model is restored by administrating soluble Thy-1, showing that treatment with soluble Thy-1 therapeutically inhibits integrin-mediated fibrosis (Tan et al., 2019). Even though fibrosis mechanisms are considered to be similar between different tissues, the fibroblast heterogenicity within and between tissues suggest that these observations need to be carefully evaluated in skin wound healing fibrosis models.
9 Concluding Remarks
Here, we have reviewed diverse instances where either Thy-1, integrins or syndecan 4 are biologically relevant during wound healing. Specifically, we described that each of these proteins are upregulated during wound healing and that wound closure is delayed after decreasing the expression of any of these proteins. Our revision also pointed out to some putative functions of Thy-1, integrins and syndecan 4, based in observations performed in similar cell types in different biological systems. However, the effect of the Thy-1/integrin/syndecan 4 trimolecular complex remains mostly unexplored during skin injury.
Integrin or syndecan 4 modulation by itself has been shown to be effective in improving wound outcomes during pre-clinical assays. However, most of the successful treatments currently used in the clinic consist of complex skin substitutes or allographs composed of numerous ECM proteins, GAGs, growth factors, and cytokines, suggesting that a simplistic approach of modulating only one molecule may not be ideal.
Much less is known regarding the role of Thy-1 during skin wound and its potential as a therapeutic agent. Nonetheless, the fact that Thy-1 can act as the ligand of several integrins and syndecan 4, expressed by diverse cell types, highlights the importance of this glycoprotein as a broad modulator of cell function, and posits Thy-1 as an adequate biological target to develop future therapeutics for wound healing.
Wound healing is a complex process that involves an orchestrated plethora of events and requires the participation of many molecules and cells. An altered balance of these events results in aberrant wound phenotypes, where too little (chronic wounds) or too much (scarring and fibrosis) leads to unwanted outcomes. The trilogy of Thy-1/integrin/syndecan 4 proteins and its interaction as a trimolecular complex, either in Cis or Trans, offers an interesting window of opportunity for the development of new combined therapies to control the process of wound healing.
Author Contributions
Conceptualization: AV; writing—original draft preparation: AV and LP; writing—review and editing: AV and LL; visualization: LP; funding acquisition: LL. All authors have read and agreed to the published version of the manuscript.
Funding
This work was funded by National Institute of Health grant HL095070 (AV), the Agencia Nacional de Investigación y Desarrollo (ANID); grants FONDECYT #1200836 (LL), ANID Ph.D. student fellowship (Beca Doctorado Nacional) #21181617 (LP), and CONICYT-FONDAP ACCDiS #15130011 (LL).
Conflict of Interest
The authors declare that the research was conducted in the absence of any commercial or financial relationships that could be construed as a potential conflict of interest.
Publisher’s Note
All claims expressed in this article are solely those of the authors and do not necessarily represent those of their affiliated organizations, or those of the publisher, the editors and the reviewers. Any product that may be evaluated in this article, or claim that may be made by its manufacturer, is not guaranteed or endorsed by the publisher.
Acknowledgments
The authors thank Dr. Ana María Avalos for her careful review of the English writing and style.
References
Aarabi, S., Bhatt, K. A., Shi, Y., Paterno, J., Chang, E. I., Loh, S. A., et al. (2007). Mechanical Load Initiates Hypertrophic Scar Formation through Decreased Cellular Apoptosis. FASEB j. 21 (12), 3250–3261. doi:10.1096/fj.07-8218com
Abeysinghe, H. R., Li, L. Q., Guckert, N. L., Reeder, J., and Wang, N. (2005). THY-1 Induction Is Associated with Up-Regulation of Fibronectin and Thrombospondin-1 in Human Ovarian Cancer. Cancer Genet. Cytogenet. 161 (2), 151–158. doi:10.1016/j.cancergencyto.2005.02.014
Agah, A., Kyriakides, T. R., Lawler, J., and Bornstein, P. (2002). The Lack of Thrombospondin-1 (TSP1) Dictates the Course of Wound Healing in Double-Tsp1/tsp2-Null Mice. Am. J. Pathol. 161 (3), 831–839. doi:10.1016/s0002-9440(10)64243-5
Agha, R., Ogawa, R., Pietramaggiori, G., and Orgill, D. P. (2011). A Review of the Role of Mechanical Forces in Cutaneous Wound Healing. J. Surg. Res. 171 (2), 700–708. doi:10.1016/j.jss.2011.07.007
Aidoudi, S., Bujakowska, K., Kieffer, N., and Bikfalvi, A. (2008). The CXC-Chemokine CXCL4 Interacts with Integrins Implicated in Angiogenesis. PLoS One 3 (7), e2657. doi:10.1371/journal.pone.0002657
Akaishi, S., Akimoto, M., Ogawa, R., and Hyakusoku, H. (2008). The Relationship between Keloid Growth Pattern and Stretching Tension. Ann. Plast. Surg. 60 (4), 445–451. doi:10.1097/sap.0b013e3181238dd7
Ali, N., and Rosenblum, M. D. (2017). Regulatory T Cells in Skin. Immunology 152 (3), 372–381. doi:10.1111/imm.12791
Andrikopoulou, E., Zhang, X., Sebastian, R., Marti, G., Liu, L., Milner, S. M., et al. (2011). Current Insights into the Role of HIF-1 in Cutaneous Wound Healing. Cmm 11 (3), 218–235. doi:10.2174/156652411795243414
Annes, J. P., Rifkin, D. B., and Munger, J. S. (2002). The Integrin alphaVbeta6 Binds and Activates Latent TGFbeta3. FEBS Lett. 511 (1-3), 65–68. doi:10.1016/s0014-5793(01)03280-x
Annes, J. P., Chen, Y., Munger, J. S., and Rifkin, D. B. (2004). Integrin αVβ6-mediated Activation of Latent TGF-β Requires the Latent TGF-β Binding Protein-1. J. Cel Biol 165 (5), 723–734. doi:10.1083/jcb.200312172
Asano, Y., Ihn, H., Yamane, K., Jinnin, M., Mimura, Y., and Tamaki, K. (2005). Increased Expression of Integrin αvβ3 Contributes to the Establishment of Autocrine TGF-β Signaling in Scleroderma Fibroblasts. J. Immunol. 175 (11), 7708–7718. doi:10.4049/jimmunol.175.11.7708
Asano, Y., Ihn, H., Yamane, K., Jinnin, M., and Tamaki, K. (2006). Increased Expression of Integrin αvβ5 Induces the Myofibroblastic Differentiation of Dermal Fibroblasts. Am. J. Pathol. 168 (2), 499–510. doi:10.2353/ajpath.2006.041306
Avalos, A. M., Valdivia, A. D., Muñoz, N., Herrera-Molina, R., Tapia, J. C., Lavandero, S., et al. (2009). Neuronal Thy-1 Induces Astrocyte Adhesion by Engaging Syndecan-4 in a Cooperative Interaction with Alphavbeta3 Integrin that Activates PKCalpha and RhoA. J. Cel Sci 122 (Pt 19), 3462–3471. doi:10.1242/jcs.034827
Avalos, A. M., Arthur, W. T., Schneider, P., Quest, A. F. G., Burridge, K., and Leyton, L. (2004). Aggregation of Integrins and RhoA Activation Are Required for Thy-1-Induced Morphological Changes in Astrocytes. J. Biol. Chem. 279 (37), 39139–39145. doi:10.1074/jbc.m403439200
Bainbridge, P. (2013). Wound Healing and the Role of Fibroblasts. J. Wound Care 22 (8), 407410–412412. doi:10.12968/jowc.2013.22.8.407
Barker, T. H., Pallero, M. A., MacEwen, M. W., Tilden, S. G., Woods, A., Murphy-Ullrich, J. E., et al. (2004). Thrombospondin-1-induced Focal Adhesion Disassembly in Fibroblasts Requires Thy-1 Surface Expression, Lipid Raft Integrity, and Src Activation. J. Biol. Chem. 279 (22), 23510–23516. doi:10.1074/jbc.m402169200
Barrientos, S., Stojadinovic, O., Golinko, M. S., Brem, H., and Tomic-Canic, M. (2008). PERSPECTIVE ARTICLE: Growth Factors and Cytokines in Wound Healing. Wound Repair Regen. 16 (5), 585–601. doi:10.1111/j.1524-475x.2008.00410.x
Bass, M. D., Williamson, R. C., Nunan, R. D., Humphries, J. D., Byron, A., Morgan, M. R., et al. (2011). A Syndecan-4 Hair Trigger Initiates Wound Healing through Caveolin- and RhoG-Regulated Integrin Endocytosis. Develop. Cel 21 (4), 681–693. doi:10.1016/j.devcel.2011.08.007
Bates, R. C., Rankin, L. M., Lucas, C. M., Scott, J. L., Krissansen, G. W., and Burns, G. F. (1991). Individual Embryonic Fibroblasts Express Multiple Beta Chains in Association with the Alpha V Integrin Subunit. Loss of Beta 3 Expression with Cell confluence. J. Biol. Chem. 266 (28), 18593–18599. doi:10.1016/s0021-9258(18)55104-8
Baurecht, H., Hotze, M., Brand, S., Büning, C., Cormican, P., Corvin, A., et al. (2015). Genome-wide Comparative Analysis of Atopic Dermatitis and Psoriasis Gives Insight into Opposing Genetic Mechanisms. Am. J. Hum. Genet. 96 (1), 104–120. doi:10.1016/j.ajhg.2014.12.004
Bellin, R. M., Kubicek, J. D., Frigault, M. J., Kamien, A. J., Steward, R. L., Barnes, H. M., et al. (2009). Defining the Role of Syndecan-4 in Mechanotransduction Using Surface-Modification Approaches. Pnas 106 (52), 22102–22107. doi:10.1073/pnas.0902639106
Bennett, J. S., Berger, B. W., and Billings, P. C. (2009). The Structure and Function of Platelet Integrins. J. Thromb. Haemost. 7 (Suppl. 1), 200–205. doi:10.1111/j.1538-7836.2009.03378.x
Bennett, J. S., Chan, C., Vilaire, G., Mousa, S. A., and DeGrado, W. F. (1997). Agonist-activated αvμ3 on Platelets and Lymphocytes Binds to the Matrix Protein Osteopontin. J. Biol. Chem. 272 (13), 8137–8140. doi:10.1074/jbc.272.13.8137
Bennett, J. S. (2005). Structure and Function of the Platelet Integrin IIb 3. J. Clin. Invest. 115 (12), 3363–3369. doi:10.1172/jci26989
Berk, B. C., Alexander, R. W., Brock, T. A., Gimbrone, M. A., and Webb, R. C. (1986). Vasoconstriction: a New Activity for Platelet-Derived Growth Factor. Science 232 (4746), 87–90. doi:10.1126/science.3485309
Berman, B., Maderal, A., and Raphael, B. (2017). Keloids and Hypertrophic Scars: Pathophysiology, Classification, and Treatment. Dermatol. Surg. 43 (Suppl. 1), S3–S18. doi:10.1097/DSS.0000000000000819
Bernfield, M., Götte, M., Park, P. W., Reizes, O., Fitzgerald, M. L., Lincecum, J., et al. (1999). Functions of Cell Surface Heparan Sulfate Proteoglycans. Annu. Rev. Biochem. 68, 729–777. doi:10.1146/annurev.biochem.68.1.729
Bertrand, J., and Bollmann, M. (2019). Soluble Syndecans: Biomarkers for Diseases and Therapeutic Options. Br. J. Pharmacol. 176 (1), 67–81. doi:10.1111/bph.14397
Billaud, M., Donnenberg, V. S., Ellis, B. W., Meyer, E. M., Donnenberg, A. D., Hill, J. C., et al. (2017). Classification and Functional Characterization of Vasa Vasorum-Associated Perivascular Progenitor Cells in Human Aorta. Stem Cel Rep. 9 (1), 292–303. doi:10.1016/j.stemcr.2017.04.028
Boothby, I. C., Cohen, J. N., and Rosenblum, M. D. (2020). Regulatory T Cells in Skin Injury: At the Crossroads of Tolerance and Tissue Repair. Sci. Immunol. 5 (47), eaaz9631. doi:10.1126/sciimmunol.aaz9631
Brenet, M., Martínez, S., Pérez-Nuñez, R., Pérez, L. A., Contreras, P., Díaz, J., et al. (2020). Thy-1 (CD90)-Induced Metastatic Cancer Cell Migration and Invasion Are β3 Integrin-dependent and Involve a Ca2+/P2X7 Receptor Signaling Axis. Front Cel Dev Biol 8, 592442. doi:10.3389/fcell.2020.592442
Brinkmann, V., Reichard, U., Goosmann, C., Fauler, B., Uhlemann, Y., Weiss, D. S., et al. (2004). Neutrophil Extracellular Traps Kill Bacteria. Science 303 (5663), 1532–1535. doi:10.1126/science.1092385
Brockmann, L., Giannou, A. D., Gagliani, N., and Huber, S. (2017). Regulation of TH17 Cells and Associated Cytokines in Wound Healing, Tissue Regeneration, and Carcinogenesis. Int. J. Mol. Sci. 18 (5). doi:10.3390/ijms18051033
Brooks, R., Williamson, R. C., and Bass, M. D. (2012). Syndecan-4 Independently Regulates Multiple Small GTPases to Promote Fibroblast Migration during Wound Healing. Small GTPases 3 (2), 73–79. doi:10.4161/sgtp.19301
Bukovsky, A., Caudle, M. R., Keenan, J. A., Upadhyaya, N. B., Van Meter, S. E., Wimalasena, J., et al. (2001). Association of Mesenchymal Cells and Immunoglobulins with Differentiating Epithelial Cells. BMC Dev. Biol. 1, 11. doi:10.1186/1471-213x-1-11
Bünemann, E., Hoff, N.-P., Buhren, B. A., Wiesner, U., Meller, S., Bölke, E., et al. (2018). Chemokine Ligand-Receptor Interactions Critically Regulate Cutaneous Wound Healing. Eur. J. Med. Res. 23 (1), 4. doi:10.1186/s40001-017-0299-0
Burgos-Bravo, F., Martínez-Meza, S., Quest, A. F. G., Wilson, C. A. M., and Leyton, L. (2020). Application of Force to a Syndecan-4 Containing Complex with Thy-1-Αvβ3 Integrin Accelerates Neurite Retraction. Front. Mol. Biosci. 7, 582257. doi:10.3389/fmolb.2020.582257
Butler, P. D., Longaker, M. T., and Yang, G. P. (2008). Current Progress in Keloid Research and Treatment. J. Am. Coll. Surgeons 206 (4), 731–741. doi:10.1016/j.jamcollsurg.2007.12.001
Cañedo-Dorantes, L., and Cañedo-Ayala, M. (2019). Skin Acute Wound Healing: A Comprehensive Review. Int. J. Inflamm. 2019, 3706315. doi:10.1155/2019/3706315
Carracedo, S., Lu, N., Popova, S. N., Jonsson, R., Eckes, B., and Gullberg, D. (2010). The Fibroblast Integrin α11β1 Is Induced in a Mechanosensitive Manner Involving Activin A and Regulates Myofibroblast Differentiation. J. Biol. Chem. 285 (14), 10434–10445. doi:10.1074/jbc.m109.078766
Carswell, L., and Borger, J. (2021). Hypertrophic Scarring Keloids. Treasure Island (FL: StatPearls.
Castilla, D. M., Liu, Z.-J., and Velazquez, O. C. (2012). Oxygen: Implications for Wound Healing. Adv. Wound Care 1 (6), 225–230. doi:10.1089/wound.2011.0319
Charnaux, N., Brule, S., Chaigneau, T., Saffar, L., Sutton, A., Hamon, M., et al. (2005). RANTES (CCL5) Induces a CCR5-dependent Accelerated Shedding of Syndecan-1 (CD138) and Syndecan-4 from HeLa Cells and Forms Complexes with the Shed Ectodomains of These Proteoglycans as Well as with Those of CD44. Glycobiology 15 (2), 119–130. doi:10.1093/glycob/cwh148
Chen, J., and López, J. A. (2005). Interactions of Platelets with Subendothelium and Endothelium. Microcirculation 12 (3), 235–246. doi:10.1080/10739680590925484
Chen, Y., Jin, Q., Fu, X., Qiao, J., and Niu, F. (2019). Connection between T Regulatory Cell Enrichment and Collagen Deposition in Keloid. Exp. Cel Res. 383 (2), 111549. doi:10.1016/j.yexcr.2019.111549
Chernousov, M. A., and Carey, D. J. (2000). Schwann Cell Extracellular Matrix Molecules and Their Receptors. Histol. Histopathol 15 (2), 593–601. doi:10.14670/HH-15.593
Clark, R. A., Tonnesen, M. G., Gailit, J., and Cheresh, D. A. (1996). Transient Functional Expression of alphaVbeta 3 on Vascular Cells during Wound Repair. Am. J. Pathol. 148 (5), 1407–1421.
Columbo, M., Bochner, B. S., and Marone, G. (1995). Human Skin Mast Cells Express Functional Beta 1 Integrins that Mediate Adhesion to Extracellular Matrix Proteins. J. Immunol. 154 (11), 6058–6064.
Columbo, M., and Bochner, B. S. (2001). Human Skin Mast Cells Adhere to Vitronectin via the αvβ3 Integrin Receptor (CD51/CD61). J. Allergy Clin. Immunol. 107 (3), 554. doi:10.1067/mai.2001.113238
Darby, I. A., Laverdet, B., Desmoulière, F. A., and Desmouliere, A. (2014). Fibroblasts and Myofibroblasts in Wound Healing. Clin. Cosmet. Investig. Dermatol. 7, 301–311. doi:10.2147/CCID.S50046
Das, S., Majid, M., and Baker, A. B. (2016). Syndecan-4 Enhances PDGF-BB Activity in Diabetic Wound Healing. Acta Biomater. 42, 56–65. doi:10.1016/j.actbio.2016.07.001
Derdak, S., Penney, D. P., Keng, P., Felch, M. E., Brown, D., and Phipps, R. P. (1992). Differential Collagen and Fibronectin Production by Thy 1+ and Thy 1- Lung Fibroblast Subpopulations. Am. J. Physiol. 263 (2 Pt 1), L283–L290. doi:10.1152/ajplung.1992.263.2.L283
DiPersio, C. M., Zheng, R., Kenney, J., and Van De Water, L. (2016). Integrin-mediated Regulation of Epidermal Wound Functions. Cell Tissue Res 365 (3), 467–482. doi:10.1007/s00441-016-2446-2
Dovi, J. V., He, L.-K., and DiPietro, L. A. (2003). Accelerated Wound Closure in Neutrophil-Depleted Mice. J. Leukoc. Biol. 73 (4), 448–455. doi:10.1189/jlb.0802406
Dráberová, L., Amoui, M., and Dráber, P. (1996). Thy-1-mediated Activation of Rat Mast Cells: the Role of Thy-1 Membrane Microdomains. Immunology 87 (1), 141–148.
Dráberová, Ľ. (1989). The Involvement of Thy-1 Antigen in the Activation of Rat Mast Cells. Eur. J. Immunol. 19 (9), 1715–1720. doi:10.1002/eji.1830190928
Dreifke, M. B., Jayasuriya, A. A., and Jayasuriya, A. C. (2015). Current Wound Healing Procedures and Potential Care. Mater. Sci. Eng. C 48, 651–662. doi:10.1016/j.msec.2014.12.068
Dyer, D. P., Salanga, C. L., Volkman, B. F., Kawamura, T., and Handel, T. M. (2016). The Dependence of Chemokine-Glycosaminoglycan Interactions on Chemokine Oligomerization. Glycobiology 26 (3), 312–326. doi:10.1093/glycob/cwv100
Echtermeyer, F., Streit, M., Wilcox-Adelman, S., Saoncella, S., Denhez, F., Detmar, M., et al. (2001). Delayed Wound Repair and Impaired Angiogenesis in Mice Lacking Syndecan-4. J. Clin. Invest. 107 (2), R9–R14. doi:10.1172/jci10559
Egles, C., Huet, H. A., Dogan, F., Cho, S., Dong, S., Smith, A., et al. (2010). Integrin-blocking Antibodies Delay Keratinocyte Re-epithelialization in a Human Three-Dimensional Wound Healing Model. PLoS One 5 (5), e10528. doi:10.1371/journal.pone.0010528
Eisinger, F., Patzelt, J., and Langer, H. F. (2018). The Platelet Response to Tissue Injury. Front. Med. 5, 317. doi:10.3389/fmed.2018.00317
Elenius, K., Vainio, S., Laato, M., Salmivirta, M., Thesleff, I., and Jalkanen, M. (1991). Induced Expression of Syndecan in Healing Wounds. J. Cel Biol 114 (3), 585–595. doi:10.1083/jcb.114.3.585
Endo, T., Ito, K., Morimoto, J., Kanayama, M., Ota, D., Ikesue, M., et al. (2015). Syndecan 4 Regulation of the Development of Autoimmune Arthritis in Mice by Modulating B Cell Migration and Germinal Center Formation. Arthritis Rheumatol. 67 (9), 2512–2522. doi:10.1002/art.39193
Erpenbeck, L., Gruhn, A. L., Kudryasheva, G., Günay, G., Meyer, D., Busse, J., et al. (2019). Effect of Adhesion and Substrate Elasticity on Neutrophil Extracellular Trap Formation. Front. Immunol. 10, 2320. doi:10.3389/fimmu.2019.02320
Fadini, G. P., Menegazzo, L., Rigato, M., Scattolini, V., Poncina, N., Bruttocao, A., et al. (2016). NETosis Delays Diabetic Wound Healing in Mice and Humans. Diabetes 65 (4), 1061–1071. doi:10.2337/db15-0863
Félétou, M., Huang, Y., and Vanhoutte, P. M. (2010). Vasoconstrictor Prostanoids. Pflugers Arch. - Eur. J. Physiol. 459 (6), 941–950. doi:10.1007/s00424-010-0812-6
Feng, G., Mellor, R. H., Bernstein, M., Keller-Peck, C., Nguyen, Q. T., Wallace, M., et al. (2000). Imaging Neuronal Subsets in Transgenic Mice Expressing Multiple Spectral Variants of GFP. Neuron 28 (1), 41–51. doi:10.1016/s0896-6273(00)00084-2
Fiore, V. F., Wong, S. S., Tran, C., Tan, C., Xu, W., Sulchek, T., et al. (2018). αvβ3 Integrin Drives Fibroblast Contraction and Strain Stiffening of Soft Provisional Matrix during Progressive Fibrosis. JCI Insight 3 (20), 97597. doi:10.1172/jci.insight.97597
Fiore, V. F., Ju, L., Chen, Y., Zhu, C., and Barker, T. H. (2014). Dynamic Catch of a Thy-1-Α5β1+syndecan-4 Trimolecular Complex. Nat. Commun. 5, 4886. doi:10.1038/ncomms5886
Fiore, V. F., Strane, P. W., Bryksin, A. V., White, E. S., Hagood, J. S., and Barker, T. H. (2015). Conformational Coupling of Integrin and Thy-1 Regulates Fyn Priming and Fibroblast Mechanotransduction. J. Cel Biol 211 (1), 173–190. doi:10.1083/jcb.201505007
Freimuth, W. W., Esselman, W. J., and Miller, H. C. (1978). Release of thy-1.2 and thy-1.1 from Lymphoblastoid Cells: Partial Characterization and Antigenicity of Shed Material. J. Immunol. 120 (5), 1651–1658.
Fu, S., Panayi, A., Fan, J., Mayer, H. F., Daya, M., Khouri, R. K., et al. (2021). Mechanotransduction in Wound Healing: From the Cellular and Molecular Level to the Clinic. Adv. Skin Wound Care 34 (2), 67–74. doi:10.1097/01.asw.0000725220.92976.a7
Fuster, M. M., and Wang, L. (2010). Endothelial Heparan Sulfate in Angiogenesis. Prog. Mol. Biol. Transl Sci. 93, 179–212. doi:10.1016/s1877-1173(10)93009-3
Gaengel, K., Genové, G., Armulik, A., and Betsholtz, C. (2009). Endothelial-mural Cell Signaling in Vascular Development and Angiogenesis. Atvb 29 (5), 630–638. doi:10.1161/atvbaha.107.161521
Gailit, J., Welch, R. A. F. M. P., and Clark, R. A. (1994). TGF-β1 Stimulates Expression of Keratinocyte Integrins during Re-epithelialization of Cutaneous Wounds. J. Invest. Dermatol. 103 (2), 221–227. doi:10.1111/1523-1747.ep12393176
Gallant-Behm, C. L., Hildebrand, K. A., and Hart, D. A. (2008). The Mast Cell Stabilizer Ketotifen Prevents Development of Excessive Skin Wound Contraction and Fibrosis in Red Duroc Pigs. Wound Repair Regen. 16 (2), 226–233. doi:10.1111/j.1524-475x.2008.00363.x
Gallo, R., Kim, C., Kokenyesi, R., Scott Adzick, N., and Bernfield, M. (1996). Syndecans-1 and -4 Are Induced during Wound Repair of Neonatal but Not Fetal Skin. J. Invest. Dermatol. 107 (5), 676–683. doi:10.1111/1523-1747.ep12365571
Gaudet, A. D., Popovich, P. G., and Ramer, M. S. (2011). Wallerian Degeneration: Gaining Perspective on Inflammatory Events after Peripheral Nerve Injury. J. Neuroinflammation 8, 110. doi:10.1186/1742-2094-8-110
Gillitzer, R., and Goebeler, M. (2001). Chemokines in Cutaneous Wound Healing. J. Leukoc. Biol. 69 (4), 513–521. doi:10.1189/jlb.69.4.513
Goldfarb, M. (2001). Signaling by Fibroblast Growth Factors: the inside story. Sci. STKE 2001 (106), pe37. doi:10.1126/stke.2001.106.pe37
Gondelaud, F., and Ricard‐Blum, S. (2019). Structures and Interactions of Syndecans. FEBS J. 286 (15), 2994–3007. doi:10.1111/febs.14828
Gopal, S. (2020). Syndecans in Inflammation at a Glance. Front. Immunol. 11, 227. doi:10.3389/fimmu.2020.00227
Goutebroze, L., Carnaud, M., Denisenko, N., Boutterin, M.-C., and Girault, J.-A. (2003). Syndecan-3 and Syndecan-4 Are Enriched in Schwann Cell Perinodal Processes. BMC Neurosci. 4, 29. doi:10.1186/1471-2202-4-29
Grodzki, A. C. G., Dávila Pástor, M. V., Sousa, J. F., Oliver, C., and Jamur, M. C. (2003). Differential Expression of Integrin Subunits on Adherent and Nonadherent Mast Cells. Braz. J. Med. Biol. Res. 36 (8), 1101–1109. doi:10.1590/s0100-879x2003000800017
Grose, R., Hutter, C., Bloch, W., Thorey, I., Watt, F. M., Fässler, R., et al. (2002). A Crucial Role of β1 Integrins for Keratinocyte Migration In Vitro and during Cutaneous Wound Repair. Development 129 (9), 2303–2315. doi:10.1242/dev.129.9.2303
Guillot-Delost, M., Le Gouvello, S., Mesel-Lemoine, M., Cheraï, M., Baillou, C., Simon, A., et al. (2012). Human CD90 Identifies Th17/Tc17 T Cell Subsets that Are Depleted in HIV-Infected Patients. J.I. 188 (3), 981–991. doi:10.4049/jimmunol.1101592
Hadjipanayi, E., Mudera, V., and Brown, R. A. (2009). Close Dependence of Fibroblast Proliferation on Collagen Scaffold Matrix Stiffness. J. Tissue Eng. Regen. Med. 3 (2), 77–84. doi:10.1002/term.136
Haeryfar, S. M. M., and Hoskin, D. W. (2001). Selective Pharmacological Inhibitors Reveal Differences between Thy-1- and T Cell Receptor-Mediated Signal Transduction in Mouse T Lymphocytes. Int. Immunopharmacology 1 (4), 689–698. doi:10.1016/s1567-5769(01)00002-9
Haeryfar, S. M. M., and Hoskin, D. W. (2004). Thy-1: More Than a Mouse Pan-T Cell Marker. J. Immunol. 173 (6), 3581–3588. doi:10.4049/jimmunol.173.6.3581
Hagood, J. S., Prabhakaran, P., Kumbla, P., Salazar, L., MacEwen, M. W., Barker, T. H., et al. (2005). Loss of Fibroblast Thy-1 Expression Correlates with Lung Fibrogenesis. Am. J. Pathol. 167 (2), 365–379. doi:10.1016/s0002-9440(10)62982-3
Haling, J. R., Monkley, S. J., Critchley, D. R., and Petrich, B. G. (2011). Talin-dependent Integrin Activation Is Required for Fibrin Clot Retraction by Platelets. Blood 117 (5), 1719–1722. doi:10.1182/blood-2010-09-305433
Hamon, M., Mbemba, E., Charnaux, N., Slimani, H., Brule, S., Saffar, L., et al. (2004). A syndecan-4/CXCR4 Complex Expressed on Human Primary Lymphocytes and Macrophages and HeLa Cell Line Binds the CXC Chemokine Stromal Cell-Derived Factor-1 (SDF-1). Glycobiology 14 (4), 311–323. doi:10.1093/glycob/cwh038
Hansbrough, J. F., Herndon, D. N., Heimbach, D. M., Solem, L. D., Gamelli, R. L., and Tompkins, R. G. (1995). Accelerated Healing and Reduced Need for Grafting in Pediatric Patients with Burns Treated with Arginine-Glycine-Aspartic Acid Peptide Matrix. J. Burn Care Rehabil. 16 (4), 377–387. doi:10.1097/00004630-199507000-00002
Hara, T., Yabushita, S., Yamamoto, C., and Kaji, T. (2020). Cell Density-dependent Fibroblast Growth Factor-2 Signaling Regulates Syndecan-4 Expression in Cultured Vascular Endothelial Cells. Int. J. Mol. Sci. 21 (10), 3698. doi:10.3390/ijms21103698
Harrop, A. R., Ghahary, A., Scott, P. G., Forsyth, N., Uji-Friedland, R. T. A., and Tredget, E. E. (1995). Regulation of Collagen Synthesis and mRNA Expression in Normal and Hypertrophic Scar Fibroblasts In Vitro by Interferon-γ. J. Surg. Res. 58 (5), 471–477. doi:10.1006/jsre.1995.1074
Hermosilla, T., Muñoz, D., Herrera-Molina, R., Valdivia, A., Muñoz, N., Nham, S.-U., et al. (2008). Direct Thy-1/αVβ3 Integrin Interaction Mediates Neuron to Astrocyte Communication. Biochim. Biophys. Acta (Bba) - Mol. Cel Res. 1783 (6), 1111–1120. doi:10.1016/j.bbamcr.2008.01.034
Herrera-Molina, R., Valdivia, A., Kong, M., Alvarez, A., Cárdenas, A., Quest, A. F. G., et al. (2013). Thy-1-interacting Molecules and Cellular Signaling in Cis and Trans. Int. Rev. Cel Mol Biol 305, 163–216. doi:10.1016/b978-0-12-407695-2.00004-4
Herzberg, F., Schöning, M., Schirner, M., Topp, M., Thiel, E., and Kreuser, E.-D. (1996). IL-4 and TNF-? Induce Changes in Integrin Expression and Adhesive Properties and Decrease the Lung-Colonizing Potential of HT-29 colon Carcinoma Cells. Clin. Exp. Metast 14 (2), 165–175. doi:10.1007/bf00121213
Higashi, N., Waki, M., Sudo, Y., Suzuki, S., Oku, T., Tsuiji, M., et al. (2018). Incorporation, Intracellular Trafficking and Processing of Extracellular Heparanase by Mast Cells: Involvement of Syndecan-4-dependent Pathway. Biochem. Biophysical Res. Commun. 503 (4), 3235–3241. doi:10.1016/j.bbrc.2018.08.132
Horowitz, A., Tkachenko, E., and Simons, M. (2002). Fibroblast Growth Factor-specific Modulation of Cellular Response by Syndecan-4. J. Cel Biol 157 (4), 715–725. doi:10.1083/jcb.200112145
Hossain, M. M., Crish, J. F., Eckert, R. L., Lin, J. J.-C., and Jin, J.-P. (2005). h2-Calponin Is Regulated by Mechanical Tension and Modifies the Function of Actin Cytoskeleton. J. Biol. Chem. 280 (51), 42442–42453. doi:10.1074/jbc.m509952200
Hu, M. S., Borrelli, M. R., Lorenz, H. P., Longaker, M. T., and Wan, D. C. (20182018). Mesenchymal Stromal Cells and Cutaneous Wound Healing: A Comprehensive Review of the Background, Role, and Therapeutic Potential. Stem Cell Int 2018, 6901983. doi:10.1155/2018/6901983
Hu, P., and Barker, T. H. (2019). Thy-1 in Integrin Mediated Mechanotransduction. Front. Cel Dev. Biol. 7, 22. doi:10.3389/fcell.2019.00022
Hueber, A. O., Pierres, M., and He, H. T. (1994). Thy-1 Functions as a Recognition/signaling Molecule during Mouse T Lymphocyte Development. Braz. J. Med. Biol. Res. 27 (2), 275–281.
Inoue, A., Tanaka, J., Takahashi, H., Kohno, S., Ohue, S., Umakoshi, A., et al. (2016). Blood Vessels Expressing CD90 in Human and Rat Brain Tumors. Neuropathology 36 (2), 168–180. doi:10.1111/neup.12244
Isenberg, J. S., Pappan, L. K., Romeo, M. J., Abu-Asab, M., Tsokos, M., Wink, D. A., et al. (2008). Blockade of Thrombospondin-1-CD47 Interactions Prevents Necrosis of Full Thickness Skin Grafts. Ann. Surg. 247, 180–190. doi:10.1097/sla.0b013e31815685dc
Ishiura, Y., Kotani, N., Yamashita, R., Yamamoto, H., Kozutsumi, Y., and Honke, K. (2010). Anomalous Expression of Thy1 (CD90) in B-Cell Lymphoma Cells and Proliferation Inhibition by Anti-thy1 Antibody Treatment. Biochem. Biophysical Res. Commun. 396 (2), 329–334. doi:10.1016/j.bbrc.2010.04.092
Januszyk, M., Chen, K., Henn, D., Foster, D. S., Borrelli, M. R., Bonham, C. A., et al. (2020). Characterization of Diabetic and Non-diabetic Foot Ulcers Using Single-Cell RNA-Sequencing. Micromachines (Basel) 11 (9), 815. doi:10.3390/mi11090815
Jeon, Y. K., Jang, Y. H., Yoo, D. R., Kim, S. N., Lee, S. K., and Nam, M. J. (2010). Mesenchymal Stem Cells' Interaction with Skin: Wound-Healing Effect on Fibroblast Cells and Skin Tissue. Wound Repair Regen. 18 (6), 655–661. doi:10.1111/j.1524-475x.2010.00636.x
Jinnin, M., Ihn, H., Mimura, Y., Asano, Y., Yamane, K., and Tamaki, K. (2005). Regulation of Fibrogenic/fibrolytic Genes by Platelet-Derived Growth Factor C, a Novel Growth Factor, in Human Dermal Fibroblasts. J. Cel. Physiol. 202 (2), 510–517. doi:10.1002/jcp.20154
Jósvay, K., Winter, Z., Katona, R. L., Pecze, L., Marton, A., Buhala, A., et al. (2014). Besides Neuro-Imaging, the Thy1-YFP Mouse Could Serve for Visualizing Experimental Tumours, Inflammation and Wound-Healing. Sci. Rep. 4, 6776. doi:10.1038/srep06776
Kale, A., Rogers, N. M., and Ghimire, K. (2021). Thrombospondin-1 CD47 Signalling: From Mechanisms to Medicine. Int. J. Mol. Sci. 22 (8), 4062. doi:10.3390/ijms22084062
Kaneider, N. C., Feistritzer, C., Gritti, D., Mosheimer, B. A., Ricevuti, G., Patsch, J. R., et al. (2005). Expression and Function of Syndecan-4 in Human Platelets. Thromb. Haemost. 93 (6), 1120–1127. doi:10.1160/TH04-11-0763
Kaneider, N. C., Reinisch, C. M., Dunzendorfer, S., Römisch, J. C. J., and Wiederman, C. J. (2002). Syndecan-4 Mediates Antithrombin-Induced Chemotaxis of Human Peripheral Blood Lymphocytes and Monocytes. J. Cel Sci 115 (Pt 1), 227–236. doi:10.1242/jcs.115.1.227
Karlsson, M., Zhang, C., Méar, L., Zhong, W., Digre, A., Katona, B., et al. (2021). A Single–Cell Type Transcriptomics Map of Human Tissues. Sci. Adv. 7, 1–9. doi:10.1126/sciadv.abh2169
Katsumi, A., Orr, A. W., Tzima, E., and Schwartz, M. A. (2004). Integrins in Mechanotransduction. J. Biol. Chem. 279 (13), 12001–12004. doi:10.1074/jbc.r300038200
Kemp, S. W. P., Phua, P. D., Stanoulis, K. N., Wood, M. D., Liu, E. H., Gordon, T., et al. (2013). Functional Recovery Following Peripheral Nerve Injury in the Transgenic Thy1 -GFP Rat. J. Peripher. Nerv Syst. 18 (3), 220–231. doi:10.1111/jns5.12035
Kenny, F. N., and Connelly, J. T. (2015). Integrin-mediated Adhesion and Mechano-Sensing in Cutaneous Wound Healing. Cel Tissue Res 360 (3), 571–582. doi:10.1007/s00441-014-2064-9
Kim, K. K., Wei, Y., Szekeres, C., Kugler, M. C., Wolters, P. J., Hill, M. L., et al. (2009). Epithelial Cell Alpha3beta1 Integrin Links Beta-Catenin and Smad Signaling to Promote Myofibroblast Formation and Pulmonary Fibrosis. J. Clin. Invest. 119 (1), 213–224. doi:10.1172/JCI36940
Kimmel, H. M., Grant, A., and Ditata, J. (2016). The Presence of Oxygen in Wound Healing. Wounds 28 (8), 264–270.
Koivisto, L., Heino, J., Häkkinen, L., and Larjava, H. (2014). Integrins in Wound Healing. Adv. Wound Care 3 (12), 762–783. doi:10.1089/wound.2013.0436
Kolaczkowska, E., and Kubes, P. (2013). Neutrophil Recruitment and Function in Health and Inflammation. Nat. Rev. Immunol. 13 (3), 159–175. doi:10.1038/nri3399
Kollert, F., Christoph, S., Probst, C., Budweiser, S., Bannert, B., Binder, M., et al. (2013). Soluble CD90 as a Potential Marker of Pulmonary Involvement in Systemic Sclerosis. Arthritis Care Res. 65 (2), 281–287. doi:10.1002/acr.21799
Kong, M., Muñoz, N., Valdivia, A., Alvarez, A., Herrera-Molina, R., Cárdenas, A., et al. (2013). Thy-1-mediated Cell-Cell Contact Induces Astrocyte Migration through the Engagement of αVβ3 Integrin and Syndecan-4. Biochim. Biophys. Acta (Bba) - Mol. Cel Res. 1833 (6), 1409–1420. doi:10.1016/j.bbamcr.2013.02.013
Koumas, L., Smith, T. J., Feldon, S., Blumberg, N., and Phipps, R. P. (2003). Thy-1 Expression in Human Fibroblast Subsets Defines Myofibroblastic or Lipofibroblastic Phenotypes. Am. J. Pathol. 163 (4), 1291–1300. doi:10.1016/s0002-9440(10)63488-8
Krzyszczyk, P., Schloss, R., Palmer, A., and Berthiaume, F. (2018). The Role of Macrophages in Acute and Chronic Wound Healing and Interventions to Promote Pro-wound Healing Phenotypes. Front. Physiol. 9, 419. doi:10.3389/fphys.2018.00419
Kuehlmann, B., Bonham, C. A., Zucal, I., Prantl, L., and Gurtner, G. C. (2020). Mechanotransduction in Wound Healing and Fibrosis. J. Clin. Med. 9 (5), 1423. doi:10.3390/jcm9051423
Kyriakides, T. R., and Maclauchlan, S. (2009). The Role of Thrombospondins in Wound Healing, Ischemia, and the Foreign Body Reaction. J. Cel Commun Signal 3 (3-4), 215–225. doi:10.1007/s12079-009-0077-z
Kyriakides, T. R., Tam, J. W. Y., and Bornstein, P. (1999). Accelerated Wound Healing in Mice with a Disruption of the Thrombospondin 2 Gene. J. Invest. Dermatol. 113 (5), 782–787. doi:10.1046/j.1523-1747.1999.00755.x
Kyriakides, T. R., Zhu, Y.-H., Yang, Z., Huynh, G., and Bornstein, P. (2001). Altered Extracellular Matrix Remodeling and Angiogenesis in Sponge Granulomas of Thrombospondin 2-Null Mice. Am. J. Pathol. 159 (4), 1255–1262. doi:10.1016/s0002-9440(10)62512-6
Lagos-Cabré, R., Alvarez, A., Kong, M., Burgos-Bravo, F., Cárdenas, A., Rojas-Mancilla, E., et al. (2017). αVβ3 Integrin Regulates Astrocyte Reactivity. J. Neuroinflammation 14 (1), 194. doi:10.1186/s12974-017-0968-5
Lasinska, I., and Mackiewicz, J. (2019). Integrins as A New Target for Cancer Treatment. Anticancer Agents Med. Chem. 19 (5), 580–586. doi:10.2174/1871520618666181119103413
Lech, M., and Anders, H.-J. (2013). Macrophages and Fibrosis: How Resident and Infiltrating Mononuclear Phagocytes Orchestrate All Phases of Tissue Injury and Repair. Biochim. Biophys. Acta (Bba) - Mol. Basis Dis. 1832 (7), 989–997. doi:10.1016/j.bbadis.2012.12.001
Lee, H. M., Seo, S.-R., Kim, J., Kim, M. K., Seo, H., Kim, K. S., et al. (2020). Expression Dynamics of Integrin α2, α3, and αV upon Osteogenic Differentiation of Human Mesenchymal Stem Cells. Stem Cel Res Ther 11 (1), 210. doi:10.1186/s13287-020-01714-7
Lee, M.-J., Shin, J.-O., and Jung, H.-S. (2013). Thy-1 Knockdown Retards Wound Repair in Mouse Skin. J. Dermatol. Sci. 69 (2), 95–104. doi:10.1016/j.jdermsci.2012.11.009
Lee, W.-S., Jain, M. K., Arkonac, B. M., Zhang, D., Shaw, S.-Y., Kashiki, S., et al. (1998). Thy-1, a Novel Marker for Angiogenesis Upregulated by Inflammatory Cytokines. Circ. Res. 82 (8), 845–851. doi:10.1161/01.res.82.8.845
Lee, Y. S., Kang, S. U., Lee, M.-H., Kim, H.-J., Han, C.-H., Won, H.-R., et al. (2020). GnRH Impairs Diabetic Wound Healing through Enhanced NETosis. Cell Mol Immunol 17 (8), 856–864. doi:10.1038/s41423-019-0252-y
Lefcort, F., Venstrom, K., McDonald, J. A., and Reichardt, L. F. (1992). Regulation of Expression of Fibronectin and its Receptor, Alpha 5 Beta 1, during Development and Regeneration of Peripheral Nerve. Development 116 (3), 767–782. doi:10.1242/dev.116.3.767
Leibovich, S. J., and Ross, R. (1975). The Role of the Macrophage in Wound Repair. A Study with Hydrocortisone and Antimacrophage Serum. Am. J. Pathol. 78 (1), 71–100.
Ley, K., Laudanna, C., Cybulsky, M. I., and Nourshargh, S. (2007). Getting to the Site of Inflammation: the Leukocyte Adhesion cascade Updated. Nat. Rev. Immunol. 7 (9), 678–689. doi:10.1038/nri2156
Leyton, L., Díaz, J., Martínez, S., Palacios, E., Pérez, L. A., and Perez, R. D. (2019). Thy-1/CD90 a Bidirectional and Lateral Signaling Scaffold. Front. Cel Dev. Biol. 7, 132. doi:10.3389/fcell.2019.00132
Leyton, L., Schneider, P., Labra, C. V., Rüegg, C., Hetz, C. A., Quest, A. F. G., et al. (2001). Thy-1 Binds to Integrin β3 on Astrocytes and Triggers Formation of Focal Contact Sites. Curr. Biol. 11 (13), 1028–1038. doi:10.1016/s0960-9822(01)00262-7
Li, B., and Wang, J. H.-C. (2011). Fibroblasts and Myofibroblasts in Wound Healing: Force Generation and Measurement. J. Tissue Viability 20 (4), 108–120. doi:10.1016/j.jtv.2009.11.004
Liësi, P., Salonen, E. M., Dahl, D., Vaheri, A., and Richards, S. J. (1990). Thy-1 Is a Neuronal and Glial Surface Antigen Which Interacts with Matrix Proteins and Plasminogen Activator. Exp. Brain Res. 79 (3), 642–650. doi:10.1007/BF00229332
Linehan, J. L., Harrison, O. J., Han, S.-J., Byrd, A. L., Vujkovic-Cvijin, I., Villarino, A. V., et al. (2018). Non-classical Immunity Controls Microbiota Impact on Skin Immunity and Tissue Repair. Cell 172 (4), 784–796. e718. doi:10.1016/j.cell.2017.12.033
Lishko, V. K., Yakubenko, V. P., Ugarova, T. P., and Podolnikova, N. P. (2018). Leukocyte Integrin Mac-1 (CD11b/CD18, αMβ2, CR3) Acts as a Functional Receptor for Platelet Factor 4. J. Biol. Chem. 293 (18), 6869–6882. doi:10.1074/jbc.ra117.000515
Liu, W., Ma, K., Kwon, S. H., Garg, R., Patta, Y. R., Fujiwara, T., et al. (2017). The Abnormal Architecture of Healed Diabetic Ulcers Is the Result of FAK Degradation by Calpain 1. J. Invest. Dermatol. 137 (5), 1155–1165. doi:10.1016/j.jid.2016.11.039
Liu, X., Wong, S. S., Taype, C. A., Kim, J., Shentu, T.-P., Espinoza, C. R., et al. (2017). Thy-1 Interaction with Fas in Lipid Rafts Regulates Fibroblast Apoptosis and Lung Injury Resolution. Lab. Invest. 97 (3), 256–267. doi:10.1038/labinvest.2016.145
Lozito, T. P., Kuo, C. K., Taboas, J. M., and Tuan, R. S. (2009). Human Mesenchymal Stem Cells Express Vascular Cell Phenotypes upon Interaction with Endothelial Cell Matrix. J. Cel. Biochem. 107 (4), 714–722. doi:10.1002/jcb.22167
Lü, D., Li, Z., Gao, Y., Luo, C., Zhang, F., Zheng, L., et al. (2016). β1 Integrin Signaling in Asymmetric Migration of Keratinocytes under Mechanical Stretch in a Co-cultured Wound Repair Model. Biomed. Eng. Online 15 (Suppl. 2), 130. doi:10.1186/s12938-016-0263-1
Lygoe, K. A., Norman, J. T., Marshall, J. F., and Lewis, M. P. (2004). AlphaV Integrins Play an Important Role in Myofibroblast Differentiation. Wound Repair Regen. 12 (4), 461–470. doi:10.1111/j.1067-1927.2004.12402.x
Mantovani, A., Cassatella, M. A., Costantini, C., and Jaillon, S. (2011). Neutrophils in the Activation and Regulation of Innate and Adaptive Immunity. Nat. Rev. Immunol. 11 (8), 519–531. doi:10.1038/nri3024
Maquart, F.-X. (2015). La matrice extracellulaire : un partenaire majeur de la cicatrisation des plaies. Bull. de l'Académie Nationale de Médecine 199 (7), 1199–1209. doi:10.1016/s0001-4079(19)30865-9
Margadant, C., Raymond, K., Kreft, M., Sachs, N., Janssen, H., and Sonnenberg, A. (2009). Integrin Alpha3beta1 Inhibits Directional Migration and Wound Re-epithelialization in the Skin. J. Cel Sci 122 (Pt 2), 278–288. doi:10.1242/jcs.029108
Martin, P., D'Souza, D., Martin, J., Grose, R., Cooper, L., Maki, R., et al. (2003). Wound Healing in the PU.1 Null Mouse-Tissue Repair Is Not Dependent on Inflammatory Cells. Curr. Biol. 13 (13), 1122–1128. doi:10.1016/s0960-9822(03)00396-8
Martin, P., and Leibovich, S. J. (2005). Inflammatory Cells during Wound Repair: the Good, the Bad and the Ugly. Trends Cel Biol. 15 (11), 599–607. doi:10.1016/j.tcb.2005.09.002
Martinez, F. O., and Gordon, S. (2014). The M1 and M2 Paradigm of Macrophage Activation: Time for Reassessment. F1000prime Rep. 6, 13. doi:10.12703/P6-13
Martinod, K., and Wagner, D. D. (2014). Thrombosis: Tangled up in NETs. Blood 123 (18), 2768–2776. doi:10.1182/blood-2013-10-463646
Martins-Green, M., Petreaca, M., and Wang, L. (2013). Chemokines and Their Receptors Are Key Players in the Orchestra that Regulates Wound Healing. Adv. Wound Care 2 (7), 327–347. doi:10.1089/wound.2012.0380
Mason, J. C., Yarwood, H., Tárnok, A., Sugars, K., Harrison, A. A., Robinson, P. J., et al. (1996). Human Thy-1 Is Cytokine-Inducible on Vascular Endothelial Cells and Is a Signaling Molecule Regulated by Protein Kinase C. J. Immunol. 157 (2), 874–883.
Menter, D. G., Kopetz, S., Hawk, E., Sood, A. K., Loree, J. M., Gresele, P., et al. (2017). Platelet "first Responders" in Wound Response, Cancer, and Metastasis. Cancer Metastasis Rev. 36 (2), 199–213. doi:10.1007/s10555-017-9682-0
Metzger, T. C., and Anderson, M. S. (2011). Control of central and Peripheral Tolerance by Aire. Immunol. Rev. 241 (1), 89–103. doi:10.1111/j.1600-065x.2011.01008.x
Michelis, K. C., Nomura-Kitabayashi, A., Lecce, L., Franzén, O., Koplev, S., Xu, Y., et al. (2018). CD90 Identifies Adventitial Mesenchymal Progenitor Cells in Adult Human Medium- and Large-Sized Arteries. Stem Cel Rep. 11 (1), 242–257. doi:10.1016/j.stemcr.2018.06.001
Midwood, K. S., Valenick, L. V., Hsia, H. C., and Schwarzbauer, J. E. (2004). Coregulation of Fibronectin Signaling and Matrix Contraction by Tenascin-C and Syndecan-4. MBoC 15 (12), 5670–5677. doi:10.1091/mbc.e04-08-0759
Miklas, J. W., Dallabrida, S. M., Reis, L. A., Ismail, N., Rupnick, M., and Radisic, M. (2013). QHREDGS Enhances Tube Formation, Metabolism and Survival of Endothelial Cells in Collagen-Chitosan Hydrogels. PLoS One 8 (8), e72956. doi:10.1371/journal.pone.0072956
Milner, R., Wilby, M., Nishimura, S., Boylen, K., Edwards, G., Fawcett, J., et al. (1997). Division of Labor of Schwann Cell Integrins during Migration on Peripheral Nerve Extracellular Matrix Ligands. Develop. Biol. 185 (2), 215–228. doi:10.1006/dbio.1997.8547
Monti, M., De Rosa, V., Iommelli, F., Carriero, M. V., Terlizzi, C., Camerlingo, R., et al. (2018). Neutrophil Extracellular Traps as an Adhesion Substrate for Different Tumor Cells Expressing RGD-Binding Integrins. Int. J. Mol. Sci. 19 (8), 2350. doi:10.3390/ijms19082350
Moraes, D. A., Sibov, T. T., Pavon, L. F., Alvim, P. Q., Bonadio, R. S., Da Silva, J. R., et al. (2016). A Reduction in CD90 (THY-1) Expression Results in Increased Differentiation of Mesenchymal Stromal Cells. Stem Cel Res Ther 7 (1), 97. doi:10.1186/s13287-016-0359-3
Morris, R. J., Barber, P. C., Beech, J., and Raisman, G. (1983). The Distribution of Thy-1 Antigen in the P.N.S. Of the Adult Rat. J. Neurocytol 12 (6), 1017–1039. doi:10.1007/bf01153348
Munger, J. S., Harpel, J. G., Giancotti, F. G., and Rifkin, D. B. (1998). Interactions between Growth Factors and Integrins: Latent Forms of Transforming Growth Factor-β Are Ligands for the Integrin αvβ1. MBoC 9 (9), 2627–2638. doi:10.1091/mbc.9.9.2627
Murakami, M., Horowitz, A., Tang, S., Ware, J. A., and Simons, M. (2002). Protein Kinase C (PKC) δ Regulates PKCα Activity in a Syndecan-4-dependent Manner. J. Biol. Chem. 277 (23), 20367–20371. doi:10.1074/jbc.m202501200
Murao, N., Seino, K.-i., Hayashi, T., Ikeda, M., Funayama, E., Furukawa, H., et al. (2014). Treg-enriched CD4+ T Cells Attenuate Collagen Synthesis in Keloid Fibroblasts. Exp. Dermatol. 23 (4), 266–271. doi:10.1111/exd.12368
Nakamura, Y., Muguruma, Y., Yahata, T., Miyatake, H., Sakai, D., Mochida, J., et al. (2006). Expression of CD90 on Keratinocyte Stem/progenitor Cells. Br. J. Dermatol. 154 (6), 1062–1070. doi:10.1111/j.1365-2133.2006.07209.x
Nathan, C. (2006). Neutrophils and Immunity: Challenges and Opportunities. Nat. Rev. Immunol. 6 (3), 173–182. doi:10.1038/nri1785
Nie, C., Yang, D., Xu, J., Si, Z., Jin, X., and Zhang, J. (2011). Locally Administered Adipose-Derived Stem Cells Accelerate Wound Healing through Differentiation and Vasculogenesis. Cel Transpl. 20 (2), 205–216. doi:10.3727/096368910x520065
Nishimura, S. L. (2009). Integrin-Mediated Transforming Growth Factor-β Activation, a Potential Therapeutic Target in Fibrogenic Disorders. Am. J. Pathol. 175 (4), 1362–1370. doi:10.2353/ajpath.2009.090393
Nishio, N., Ito, S., Suzuki, H., and Isobe, K.-I. (2009). Antibodies to Wounded Tissue Enhance Cutaneous Wound Healing. Immunology 128 (3), 369–380. doi:10.1111/j.1365-2567.2009.03119.x
Norsgaard, H., Lovato, P., Jiang, L., Hebsgaard, J., and Ewald, D. A. (2019). Improvements of Both Inflammation and Skin Barrier in a Human Th17 Skin Inflammation Model by Topical Treatment with Fixed-Dose Combination Calcipotriene/betamethasone Dipropionate Foam. JAAD 81 (4), AB79. doi:10.1016/j.jaad.2019.06.309
Oh, E.-S., Woods, A., and Couchman, J. R. (1997). Syndecan-4 Proteoglycan Regulates the Distribution and Activity of Protein Kinase C. J. Biol. Chem. 272 (13), 8133–8136. doi:10.1074/jbc.272.13.8133
Ojeh, N. O., and Navsaria, H. A. (2014). Anin Vitroskin Model to Study the Effect of Mesenchymal Stem Cells in Wound Healing and Epidermal Regeneration. J. Biomed. Mater. Res. 102 (8), 2785–2792. doi:10.1002/jbm.a.34950
Okuyama, E., Suzuki, A., Murata, M., Ando, Y., Kato, I., Takagi, Y., et al. (2013). Molecular Mechanisms of Syndecan-4 Upregulation by TNF- in the Endothelium-like EAhy926 Cells. J. Biochem. 154 (1), 41–50. doi:10.1093/jb/mvt024
Olczyk, P., Mencner, Ł., and Komosinska-Vassev, K. (20142014). The Role of the Extracellular Matrix Components in Cutaneous Wound Healing. Biomed. Res. Int. 2014, 747584. doi:10.1155/2014/747584
Ou, Z., Dolmatova, E., Lassègue, B., and Griendling, K. K. (2021). β1- and β2-integrins: central Players in Regulating Vascular Permeability and Leukocyte Recruitment during Acute Inflammation. Am. J. Physiology-Heart Circulatory Physiol. 320 (2), H734–H739. doi:10.1152/ajpheart.00518.2020
Pan, Y. A., Misgeld, T., Lichtman, J. W., and Sanes, J. R. (2003). Effects of Neurotoxic and Neuroprotective Agents on Peripheral Nerve Regeneration Assayed by Time-Lapse ImagingIn Vivo. J. Neurosci. 23 (36), 11479–11488. doi:10.1523/jneurosci.23-36-11479.2003
Park, E. J., Yuki, Y., Kiyono, H., and Shimaoka, M. (2015). Structural Basis of Blocking Integrin Activation and Deactivation for Anti-inflammation. J. Biomed. Sci. 22, 51. doi:10.1186/s12929-015-0159-6
Park, J. Y., Kang, Y. W., Choi, B. Y., Yang, Y. C., Cho, B. P., and Cho, W. G. (2017). CCL11 Promotes Angiogenic Activity by Activating the PI3K/Akt Pathway in HUVECs. J. Receptors Signal Transduction 37 (4), 416–421. doi:10.1080/10799893.2017.1298132
Park, T. I.-H., Feisst, V., Brooks, A. E. S., Rustenhoven, J., Monzo, H. J., Feng, S. X., et al. (2016). Cultured Pericytes from Human Brain Show Phenotypic and Functional Differences Associated with Differential CD90 Expression. Sci. Rep. 6, 26587. doi:10.1038/srep26587
Paul, B. Z. S., Vilaire, G., Kunapuli, S. P., and Bennett, J. S. (2003). Concurrent Signaling from Galphaq- and Galphai-Coupled Pathways Is Essential for Agonist-Induced Alphavbeta3 Activation on Human Platelets. J. Thromb. Haemost. 1, 814–820. doi:10.1046/j.1538-7836.2003.00099.x
Peplow, P. V., and Chatterjee, M. P. (2013). A Review of the Influence of Growth Factors and Cytokines in In Vitro Human Keratinocyte Migration. Cytokine 62 (1), 1–21. doi:10.1016/j.cyto.2013.02.015
Picke, A. K., Campbell, G. M., Blüher, M., Krügel, U., Schmidt, F. N., Tsourdi, E., et al. (2018). Thy-1 (CD90) Promotes Bone Formation and Protects against Obesity. Sci. Transl Med. 10 (453), eaao6806. doi:10.1126/scitranslmed.aao6806
Polte, T., Petzold, S., Bertrand, J., Schütze, N., Hinz, D., Simon, J. C., et al. (2015). Critical Role for Syndecan-4 in Dendritic Cell Migration during Development of Allergic Airway Inflammation. Nat. Commun. 6, 7554. doi:10.1038/ncomms8554
Pont, S. (1987). Thy-1: a Lymphoid Cell Subset Marker Capable of Delivering an Activation Signal to Mouse T Lymphocytes. Biochimie 69 (4), 315–320. doi:10.1016/0300-9084(87)90022-8
Ra, C., Yasuda, M., Yagita, H., and Okumura, K. (1994). Fibronectin Receptor Integrins Are Involved in Mast Cell Activation. J. Allergy Clin. Immunol. 94 (3 Pt 2), 625–628. doi:10.1016/0091-6749(94)90139-2
Ramírez, G., Hagood, J. S., Sanders, Y., Ramírez, R., Becerril, C., Segura, L., et al. (2011). Absence of Thy-1 Results in TGF-β Induced MMP-9 Expression and Confers a Profibrotic Phenotype to Human Lung Fibroblasts. Lab. Invest. 91 (8), 1206–1218. doi:10.1038/labinvest.2011.80
Rees, P. A., Greaves, N. S., Baguneid, M., and Bayat, A. (2015). Chemokines in Wound Healing and as Potential Therapeutic Targets for Reducing Cutaneous Scarring. Adv. Wound Care 4 (11), 687–703. doi:10.1089/wound.2014.0568
Reichelt, J. (2007). Mechanotransduction of Keratinocytes in Culture and in the Epidermis. Eur. J. Cel Biol 86 (11-12), 807–816. doi:10.1016/j.ejcb.2007.06.004
Reynolds, L. E., Conti, F. J., Silva, R., Robinson, S. D., Iyer, V., Rudling, R., et al. (2008). alpha3beta1 Integrin-Controlled Smad7 Regulates Reepithelialization during Wound Healing in Mice. J. Clin. Invest. 118 (3), 965–974. doi:10.1172/JCI33538
Reynolds, L. E., D'Amico, G., Lechertier, T., Papachristodoulou, A., Muñoz-Félix, J. M., De Arcangelis, A., et al. (2017). Dual Role of Pericyte α6β1-integrin in Tumour Blood Vessels. J. Cel Sci 130 (9), 1583–1595. doi:10.1242/jcs.197848
Rhee, J.-S., Santoso, S., Herrmann, M., Bierhaus, A., Kanse, S., May, A., et al. (2003). New Aspects of Integrin-Mediated Leukocyte Adhesion in Inflammation: Regulation by Haemostatic Factors and Bacterial Products. Cmm 3 (4), 387–392. doi:10.2174/1566524033479753
Ridiandries, A., Tan, J. T. M., and Bursill, C. A. (2018). The Role of Chemokines in Wound Healing. Int. J. Mol. Sci. 19 (10), 3217. doi:10.3390/ijms19103217
Ritter, M. A., Sauvage, C. A., and Delia, D. (1983). Human Thy-1 Antigen: Cell Surface Expression on Early T and B Lymphocytes. Immunology 49 (3), 555–564.
Rodrigues, M., Kosaric, N., Bonham, C. A., and Gurtner, G. C. (2019). Wound Healing: A Cellular Perspective. Physiol. Rev. 99 (1), 665–706. doi:10.1152/physrev.00067.2017
Ross, T. D., Coon, B. G., Yun, S., Baeyens, N., Tanaka, K., Ouyang, M., et al. (2013). Integrins in Mechanotransduction. Curr. Opin. Cel Biol. 25 (5), 613–618. doi:10.1016/j.ceb.2013.05.006
Rossaint, J., Herter, J. M., Van Aken, H., Napirei, M., Döring, Y., Weber, C., et al. (2014). Synchronized Integrin Engagement and Chemokine Activation Is Crucial in Neutrophil Extracellular Trap-Mediated Sterile Inflammation. Blood 123 (16), 2573–2584. doi:10.1182/blood-2013-07-516484
Rousselle, P., Montmasson, M., and Garnier, C. (2019). Extracellular Matrix Contribution to Skin Wound Re-epithelialization. Matrix Biol. 75-76, 12–26. doi:10.1016/j.matbio.2018.01.002
Roy, S., Patel, D., Khanna, S., Gordillo, G. M., Biswas, S., Friedman, A., et al. (2007). Transcriptome-wide Analysis of Blood Vessels Laser Captured from Human Skin and Chronic Wound-Edge Tissue. Proc. Natl. Acad. Sci. 104 (36), 14472–14477. doi:10.1073/pnas.0706793104
Saalbach, A., and Anderegg, U. (2019). Thy‐1: More Than a Marker for Mesenchymal Stromal Cells. FASEB j. 33 (6), 6689–6696. doi:10.1096/fj.201802224r
Saalbach, A., Arnhold, J., Lessig, J., Simon, J. C., and Anderegg, U. (2008). Human Thy-1 Induces Secretion of Matrix Metalloproteinase-9 and CXCL8 from Human Neutrophils. Eur. J. Immunol. 38 (5), 1391–1403. doi:10.1002/eji.200737901
Saalbach, A., Haustein, U. F., and Anderegg, U. (2000). A Ligand of Human Thy-1 Is Localized on Polymorphonuclear Leukocytes and Monocytes and Mediates the Binding to Activated Thy-1-Positive Microvascular Endothelial Cells and Fibroblasts. J. Invest. Dermatol. 115 (5), 882–888. doi:10.1046/j.1523-1747.2000.00104.x
Saalbach, A., Wetzel, A., Haustein, U.-F., Sticherling, M., Simon, J. C., and Anderegg, U. (2005). Interaction of Human Thy-1 (CD 90) with the Integrin αvβ3 (CD51/CD61): an Important Mechanism Mediating Melanoma Cell Adhesion to Activated Endothelium. Oncogene 24 (29), 4710–4720. doi:10.1038/sj.onc.1208559
Saalbach, A., Wetzig, T., Haustein, U. F., and Anderegg, U. (1999). Detection of Human Soluble Thy-1 in Serum by ELISA. Cel Tissue Res. 298 (2), 307–315. doi:10.1007/s004419900079
Salcedo, R., Young, H. A., Ponce, M. L., Ward, J. M., Kleinman, H. K., Murphy, W. J., et al. (2001). Eotaxin (CCL11) Induces In Vivo Angiogenic Responses by Human CCR3+Endothelial Cells. J. Immunol. 166 (12), 7571–7578. doi:10.4049/jimmunol.166.12.7571
Sasaki, M., Abe, R., Fujita, Y., Ando, S., Inokuma, D., and Shimizu, H. (2008). Mesenchymal Stem Cells Are Recruited into Wounded Skin and Contribute to Wound Repair by Transdifferentiation into Multiple Skin Cell Type. J. Immunol. 180 (4), 2581–2587. doi:10.4049/jimmunol.180.4.2581
Schmidt, M., Gutknecht, D., Anastassiadis, K., Eckes, B., Anderegg, U., and Saalbach, A. (2016). Thy-1/β3 Integrin Interaction-Induced Apoptosis of Dermal Fibroblasts Is Mediated by Up-Regulation of FasL Expression. J. Invest. Dermatol. 136 (2), 526–529. doi:10.1016/j.jid.2015.10.063
Schmidt, M., Gutknecht, D., Simon, J. C., Schulz, J.-N., Eckes, B., Anderegg, U., et al. (2015). Controlling the Balance of Fibroblast Proliferation and Differentiation: Impact of Thy-1. J. Invest. Dermatol. 135 (7), 1893–1902. doi:10.1038/jid.2015.86
Schnittert, J., Bansal, R., Storm, G., and Prakash, J. (2018). Integrins in Wound Healing, Fibrosis and Tumor Stroma: High Potential Targets for Therapeutics and Drug Delivery. Adv. Drug Deliv. Rev. 129, 37–53. doi:10.1016/j.addr.2018.01.020
Schubert, K., Polte, T., Bönisch, U., Schader, S., Holtappels, R., Hildebrandt, G., et al. (2011). Thy-1 (CD90) Regulates the Extravasation of Leukocytes during Inflammation. Eur. J. Immunol. 41 (3), 645–656. doi:10.1002/eji.201041117
Semenza, G. L. (2007). Vasculogenesis, Angiogenesis, and Arteriogenesis: Mechanisms of Blood Vessel Formation and Remodeling. J. Cel. Biochem. 102 (4), 840–847. doi:10.1002/jcb.21523
Sepp, N. T., Li, L.-J., Lee, K. H., Brown, E. J., Caughman, S. W., Lawley, T. J., et al. (1994). Basic Fibroblast Growth Factor Increases Expression of the αvβ3 Integrin Complex on Human Microvascular Endothelial Cells. J. Invest. Dermatol. 103 (3), 295–299. doi:10.1111/1523-1747.ep12394617
Shiota, N., Nishikori, Y., Kakizoe, E., Shimoura, K., Niibayashi, T., Shimbori, C., et al. (2010). Pathophysiological Role of Skin Mast Cells in Wound Healing after Scald Injury: Study with Mast Cell-Deficient W/WV Mice. Int. Arch. Allergy Immunol. 151 (1), 80–88. doi:10.1159/000232573
Silva, R., D'Amico, G., Hodivala-Dilke, K. M., and Reynolds, L. E. (2008). Integrins. Atvb 28 (10), 1703–1713. doi:10.1161/atvbaha.108.172015
Simons, M., and Horowitz, A. (2001). Syndecan-4-mediated Signalling. Cell Signal. 13 (12), 855–862. doi:10.1016/s0898-6568(01)00190-5
Simpson, D. M., and Ross, R. (1972). The Neutrophilic Leukocyte in Wound Repair. J. Clin. Invest. 51 (8), 2009–2023. doi:10.1172/jci107007
Sîrbulescu, R. F., Boehm, C. K., Soon, E., Wilks, M. Q., Ilieş, I., Yuan, H., et al. (2017). Mature B Cells Accelerate Wound Healing after Acute and Chronic Diabetic Skin Lesions. Wound Rep. Reg. 25 (5), 774–791. doi:10.1111/wrr.12584
Solé-Boldo, L., Raddatz, G., Schütz, S., Mallm, J.-P., Rippe, K., Lonsdorf, A. S., et al. (2020). Single-cell Transcriptomes of the Human Skin Reveal Age-Related Loss of Fibroblast Priming. Commun. Biol. 3 (1), 188. doi:10.1038/s42003-020-0922-4
Solimani, F., Pollmann, R., Schmidt, T., Schmidt, A., Zheng, X., Savai, R., et al. (2019). Therapeutic Targeting of Th17/Tc17 Cells Leads to Clinical Improvement of Lichen Planus. Front. Immunol. 10, 1808. doi:10.3389/fimmu.2019.01808
Solon, J., Levental, I., Sengupta, K., Georges, P. C., and Janmey, P. A. (2007). Fibroblast Adaptation and Stiffness Matching to Soft Elastic Substrates. Biophysical J. 93 (12), 4453–4461. doi:10.1529/biophysj.106.101386
Soto-Pantoja, D. R., Shih, H. B., Maxhimer, J. B., Cook, K. L., Ghosh, A., Isenberg, J. S., et al. (2014). Thrombospondin-1 and CD47 Signaling Regulate Healing of thermal Injury in Mice. Matrix Biol. 37, 25–34. doi:10.1016/j.matbio.2014.05.003
Strang, H., Kaul, A., Parikh, U., Masri, L., Saravanan, S., Li, H., et al. (2020). “Role of Cytokines and Chemokines in Wound Healing,” in Wound Healing, Tissue Repair, and Regeneration in Diabetes. Editors D. Bangchi, A. Das, and S. Roy (Cambridge, Massachusetts, United States: Academic Press), 197–235. doi:10.1016/b978-0-12-816413-6.00011-3
Strbo, N., Yin, N., and Stojadinovic, O. (2014). Innate and Adaptive Immune Responses in Wound Epithelialization. Adv. Wound Care 3 (7), 492–501. doi:10.1089/wound.2012.0435
Streit, M., Velasco, P., Riccardi, L., Spencer, L., Brown, L. F., Janes, L., et al. (2000). Thrombospondin-1 Suppresses Wound Healing and Granulation Tissue Formation in the Skin of Transgenic Mice. EMBO J. 19 (13), 3272–3282. doi:10.1093/emboj/19.13.3272
Su, C.-Y., Li, J.-Q., Zhang, L.-L., Wang, H., Wang, F.-H., Tao, Y.-W., et al. (2020). The Biological Functions and Clinical Applications of Integrins in Cancers. Front. Pharmacol. 11, 579068. doi:10.3389/fphar.2020.579068
Sun, Z., Guo, S. S., and Fässler, R. (2016). Integrin-mediated Mechanotransduction. J. Cel Biol 215 (4), 445–456. doi:10.1083/jcb.201609037
Szpaderska, A. M., Egozi, E. I., Gamelli, R. L., and DiPietro, L. A. (2003). The Effect of Thrombocytopenia on Dermal Wound Healing. J. Invest. Dermatol. 120 (6), 1130–1137. doi:10.1046/j.1523-1747.2003.12253.x
Tan, C., Jiang, M., Wong, S. S., Espinoza, C. R., Kim, C., Li, X., et al. (2019). Soluble Thy-1 Reverses Lung Fibrosis via its Integrin-Binding Motif. JCI Insight 4 (21), e131152. doi:10.1172/jci.insight.131152
The Human Protein Atlas (2021). Protein Atlas. Retrieved November, 2021, Available at: https://www.proteinatlas.org.
Thiruvoth, F., Mohapatra, D., Sivakumar, D., Chittoria, R., and Nandhagopal, V. (2015). Current Concepts in the Physiology of Adult Wound Healing. Plast. Aesthet. Res. 2, 250–256. doi:10.4103/2347-9264.158851
Thul, P. J., Åkesson, L., Wiking, M., Mahdessian, D., Geladaki, A., Ait Blal, H., et al. (2017). A Subcellular Map of the Human Proteome. Science 356 (6340), eaal3321. doi:10.1126/science.aal3321
Tkachenko, E., Lutgens, E., Stan, R. V., and Simons, M. (2004). Fibroblast Growth Factor 2 Endocytosis in Endothelial Cells Proceed via Syndecan-4-dependent Activation of Rac1 and a Cdc42-dependent Macropinocytic Pathway. J. Cel Sci 117 (Pt 15), 3189–3199. doi:10.1242/jcs.01190
Tonnesen, M. G., Feng, X., and Clark, R. A. F. (2000). Angiogenesis in Wound Healing. J. Invest. Dermatol. Symp. Proc. 5 (1), 40–46. doi:10.1046/j.1087-0024.2000.00014.x
Toriseva, M., and Kähäri, V.-M. (2009). Proteinases in Cutaneous Wound Healing. Cell. Mol. Life Sci. 66 (2), 203–224. doi:10.1007/s00018-008-8388-4
Tracy, L. E., Minasian, R. A., and Caterson, E. J. (2016). Extracellular Matrix and Dermal Fibroblast Function in the Healing Wound. Adv. Wound Care 5 (3), 119–136. doi:10.1089/wound.2014.0561
Tschumperlin, D. J., Ligresti, G., Hilscher, M. B., and Shah, V. H. (2018). Mechanosensing and Fibrosis. J. Clin. Invest. 128 (1), 74–84. doi:10.1172/jci93561
Tumova, S., Woods, A., and Couchman, J. R. (2000). Heparan Sulfate Proteoglycans on the Cell Surface: Versatile Coordinators of Cellular Functions. Int. J. Biochem. Cel Biol. 32 (3), 269–288. doi:10.1016/s1357-2725(99)00116-8
Valdivia, A., Cárdenas, A., Brenet, M., Maldonado, H., Kong, M., Díaz, J., et al. (2020). Syndecan-4/PAR-3 Signaling Regulates Focal Adhesion Dynamics in Mesenchymal Cells. Cell Commun Signal 18 (1), 129. doi:10.1186/s12964-020-00629-3
Vorstandlechner, V., Laggner, M., Kalinina, P., Haslik, W., Radtke, C., Shaw, L., et al. (2020). Deciphering the Functional Heterogeneity of Skin Fibroblasts Using Single‐cell RNA Sequencing. FASEB j. 34 (3), 3677–3692. doi:10.1096/fj.201902001rr
Vuong, T. T., Reine, T. M., Sudworth, A., Jenssen, T. G., and Kolset, S. O. (2015). Syndecan-4 Is a Major Syndecan in Primary Human Endothelial Cells In Vitro, Modulated by Inflammatory Stimuli and Involved in Wound Healing. J. Histochem. Cytochem. 63 (4), 280–292. doi:10.1369/0022155415568995
Wang, H., Han, X., Kunz, E., and Hartnett, M. E. (2016). Thy-1 Regulates VEGF-Mediated Choroidal Endothelial Cell Activation and Migration: Implications in Neovascular Age-Related Macular Degeneration. Invest. Ophthalmol. Vis. Sci. 57 (13), 5525–5534. doi:10.1167/iovs.16-19691
Wen, H.-C., Huo, Y. N., Chou, C.-M., and Lee, W.-S. (2018). PMA Inhibits Endothelial Cell Migration through Activating the PKC-δ/Syk/NF-Κb-Mediated Up-Regulation of Thy-1. Sci. Rep. 8 (1), 16247. doi:10.1038/s41598-018-34548-8
Wen, H.-C., Kao, C., Hsu, R.-C., Huo, Y.-N., Ting, P.-C., Chen, L.-C., et al. (2013). Thy-1-induced Migration Inhibition in Vascular Endothelial Cells through Reducing the RhoA Activity. PLoS One 8 (4), e61506. doi:10.1371/journal.pone.0061506
Werner, S., and Grose, R. (2003). Regulation of Wound Healing by Growth Factors and Cytokines. Physiol. Rev. 83 (3), 835–870. doi:10.1152/physrev.2003.83.3.835
Werner, S., Krieg, T., and Smola, H. (2007). Keratinocyte-fibroblast Interactions in Wound Healing. J. Invest. Dermatol. 127 (5), 998–1008. doi:10.1038/sj.jid.5700786
Wernig, G., Chen, S.-Y., Cui, L., Van Neste, C., Tsai, J. M., Kambham, N., et al. (2017). Unifying Mechanism for Different Fibrotic Diseases. Proc. Natl. Acad. Sci. USA 114 (18), 4757–4762. doi:10.1073/pnas.1621375114
Weston, G. C., Haviv, I., and Rogers, P. A. (2002). Microarray Analysis of VEGF-Responsive Genes in Myometrial Endothelial Cells. Mol. Hum. Reprod. 8 (9), 855–863. doi:10.1093/molehr/8.9.855
Wetzel, A., Chavakis, T., Preissner, K. T., Sticherling, M., Haustein, U.-F., Anderegg, U., et al. (2004). Human Thy-1 (CD90) on Activated Endothelial Cells Is a Counterreceptor for the Leukocyte Integrin Mac-1 (CD11b/CD18). J. Immunol. 172 (6), 3850–3859. doi:10.4049/jimmunol.172.6.3850
Wipff, P.-J., Rifkin, D. B., Meister, J.-J., and Hinz, B. (2007). Myofibroblast Contraction Activates Latent TGF-Β1 from the Extracellular Matrix. J. Cel Biol 179 (6), 1311–1323. doi:10.1083/jcb.200704042
Wong, S. L., Demers, M., Martinod, K., Gallant, M., Wang, Y., Goldfine, A. B., et al. (2015). Diabetes Primes Neutrophils to Undergo NETosis, Which Impairs Wound Healing. Nat. Med. 21, 815–819. doi:10.1038/nm.3887
Wong, V. W., Garg, R. K., Sorkin, M., Rustad, K. C., Akaishi, S., Levi, K., et al. (2014). Loss of Keratinocyte Focal Adhesion Kinase Stimulates Dermal Proteolysis through Upregulation of MMP9 in Wound Healing. Ann. Surg. 260 (6), 1138–1146. doi:10.1097/sla.0000000000000219
Wu, L., Chang, D.-Y., Zhang, L.-X., Chen, M., and Zhao, M.-H. (2021). Urinary Soluble CD90 Predicts Renal Prognosis in Patients with Diabetic Kidney Disease. Ann. Transl Med. 9 (4), 282. doi:10.21037/atm-20-6528
Wu, Y., Chen, L., Scott, P. G., and Tredget, E. E. (2007). Mesenchymal Stem Cells Enhance Wound Healing through Differentiation and Angiogenesis. Stem Cells 25 (10), 2648–2659. doi:10.1634/stemcells.2007-0226
Wynn, T. A. (2004). Fibrotic Disease and the TH1/TH2 Paradigm. Nat. Rev. Immunol. 4 (8), 583–594. doi:10.1038/nri1412
Xiao, Y., Reis, L. A., Feric, N., Knee, E. J., Gu, J., Cao, S., et al. (2016). Diabetic Wound Regeneration Using Peptide-Modified Hydrogels to Target Re-epithelialization. Proc. Natl. Acad. Sci. USA 113 (40), E5792–E5801. doi:10.1073/pnas.1612277113
Xue, M., and Jackson, C. J. (2015). Extracellular Matrix Reorganization during Wound Healing and its Impact on Abnormal Scarring. Adv. Wound Care 4 (3), 119–136. doi:10.1089/wound.2013.0485
Yamaguchi, Y., Kubo, T., Murakami, T., Takahashi, M., Hakamata, Y., Kobayashi, E., et al. (2005). Bone Marrow Cells Differentiate into Wound Myofibroblasts and Accelerate the Healing of Wounds with Exposed Bones when Combined with an Occlusive Dressing. Br. J. Dermatol. 152 (4), 616–622. doi:10.1111/j.1365-2133.2005.06402.x
Yamamoto, H., Ehling, M., Kato, K., Kanai, K., van Lessen, M., Frye, M., et al. (2015). Integrin β1 Controls VE-Cadherin Localization and Blood Vessel Stability. Nat. Commun. 6, 6429. doi:10.1038/ncomms7429
Yan, Y., Sun, H. H., Mackinnon, S. E., and Johnson, P. J. (2011). Evaluation of Peripheral Nerve Regeneration via In Vivo Serial Transcutaneous Imaging Using Transgenic Thy1-YFP Mice. Exp. Neurol. 232 (1), 7–14. doi:10.1016/j.expneurol.2011.06.013
Yang, J., Zhan, X.-Z., Malola, J., Li, Z.-Y., Pawar, J. S., Zhang, H.-T., et al. (2020). The Multiple Roles of Thy-1 in Cell Differentiation and Regeneration. Differentiation 113, 38–48. doi:10.1016/j.diff.2020.03.003
Yousef, H., Alhajj, M., and Sharma, S. (2020). Anatomy, Skin (Integument), Epidermis. Treasure Island (FL: StatPearls.
Zarbock, A., Kempf, T., Wollert, K. C., and Vestweber, D. (2012). Leukocyte Integrin Activation and Deactivation: Novel Mechanisms of Balancing Inflammation. J. Mol. Med. 90 (4), 353–359. doi:10.1007/s00109-011-0835-2
Zcharia, E., Zilka, R., Yaar, A., Yacoby-Zeevi, O., Zetser, A., Metzger, S., et al. (2005). Heparanase Accelerates Wound Angiogenesis and Wound Healing in Mouse and Rat Models. FASEB j. 19 (2), 211–221. doi:10.1096/fj.04-1970com
Zhang, J., Wang, T., Saigal, A., Johnson, J., Morrisson, J., Tabrizifard, S., et al. (2021). Discovery of a New Class of Integrin Antibodies for Fibrosis. Sci. Rep. 11 (1), 2118. doi:10.1038/s41598-021-81253-0
Zhao, J., Santino, F., Giacomini, D., and Gentilucci, L. (2020). Integrin-Targeting Peptides for the Design of Functional Cell-Responsive Biomaterials. Biomedicines 8 (9), 307. doi:10.3390/biomedicines8090307
Zhao, Y., Ohdan, H., Manilay, J. O., and Sykes, M. (2003). NK Cell Tolerance in Mixed Allogeneic Chimeras. J. Immunol. 170 (11), 5398–5405. doi:10.4049/jimmunol.170.11.5398
Zhou, Y., Hagood, J. S., Lu, B., Merryman, W. D., and Murphy-Ullrich, J. E. (2010). Thy-1-Integrin αvβ5 Interactions Inhibit Lung Fibroblast Contraction-Induced Latent Transforming Growth Factor-Β1 Activation and Myofibroblast Differentiation. J. Biol. Chem. 285 (29), 22382–22393. doi:10.1074/jbc.m110.126227
Zhu, Y., Cankova, Z., Iwanaszko, M., Lichtor, S., Mrksich, M., and Ameer, G. A. (2018). Potent Laminin-Inspired Antioxidant Regenerative Dressing Accelerates Wound Healing in Diabetes. Proc. Natl. Acad. Sci. USA 115 (26), 6816–6821. doi:10.1073/pnas.1804262115
Glossary
αSMA α smooth muscle actin
bFGF basic fibroblast growth factor
CD90 cluster of differentiation 90
ECM extracellular matrix
FA focal adhesion
FAK focal adhesion kinase
Fas TNF receptor superfamily, member 6
FasL Fas ligand
FGF2 fibroblast growth factor 2
GAGs glycosaminoglycans
GPCR G-protein coupled receptor
HBD heparin-binding domain
Hif-1 hypoxia inducible factor 1
IGF1 insulin growth factor 1
INFc interferon c
LAP latency-associated peptide
LPS lipopolysaccharide
LTBP latent TGF-β binding protein
MAPK mitogen-activated protein kinase
MMPs matrix metalloproteinases
MSCs mesenchymal stem cells
NETs neutrophil extracellular traps
NO nitric oxide
PARP poly ADP-ribose polymerase
PDGF platelet-derived growth factor
PI-PLC phosphoinositide-specific phospholipase C
PMA phorbol 12-myristate 13-acetate
PMN polymorphonuclear neutrophils
RAGE receptor for advanced glycation end products
ROS reactive oxygen species
SCs Schwann cells
TCR T-cell antigen receptor
TGFβ transforming growth factor subunit β
Thy-1 thymus cell antigen 1
TIMPs tissue inhibitors of matrix metalloproteinases
TLR toll-like receptor
VEGF vascular endothelial growth factor
YFP yellow fluorescent protein
Keywords: Thy-1, CD90, integrins, mesenchymal cells, wound healing, cell migration, angiogenesis, syndecan 4
Citation: Pérez LA, Leyton L and Valdivia A (2022) Thy-1 (CD90), Integrins and Syndecan 4 are Key Regulators of Skin Wound Healing. Front. Cell Dev. Biol. 10:810474. doi: 10.3389/fcell.2022.810474
Received: 07 November 2021; Accepted: 06 January 2022;
Published: 03 February 2022.
Edited by:
Andreja Ambriovic-Ristov, Rudjer Boskovic Institute, CroatiaReviewed by:
Ulf Anderegg, Leipzig University, GermanyDusko Ilic, King’s College London, United Kingdom
Copyright © 2022 Pérez, Leyton and Valdivia. This is an open-access article distributed under the terms of the Creative Commons Attribution License (CC BY). The use, distribution or reproduction in other forums is permitted, provided the original author(s) and the copyright owner(s) are credited and that the original publication in this journal is cited, in accordance with accepted academic practice. No use, distribution or reproduction is permitted which does not comply with these terms.
*Correspondence: Lisette Leyton, bGxleXRvbkB1Y2hpbGUuY2w=; Alejandra Valdivia, YWxlamFuZHJhLnZhbGRpdmlhQGVtb3J5LmVkdQ==