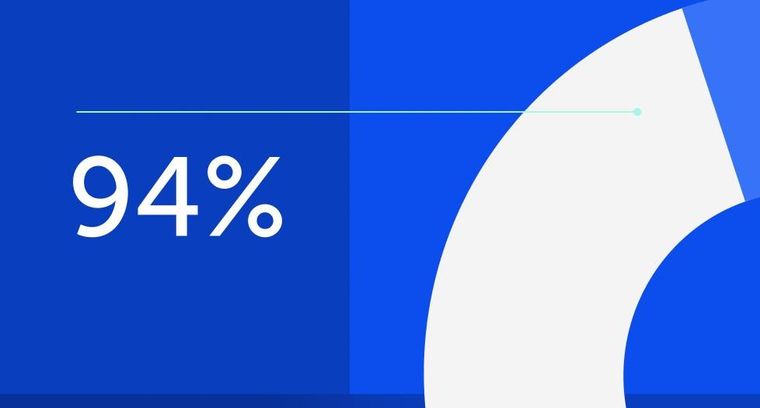
94% of researchers rate our articles as excellent or good
Learn more about the work of our research integrity team to safeguard the quality of each article we publish.
Find out more
REVIEW article
Front. Cell Dev. Biol., 03 March 2022
Sec. Stem Cell Research
Volume 10 - 2022 | https://doi.org/10.3389/fcell.2022.798826
This article is part of the Research TopicMicroenvironment-Derived Stem Cell Plasticity - Volume IIView all 5 articles
Stem cells, specifically embryonic stem cells (ESCs), mesenchymal stem cells (MSCs), induced pluripotent stem cells (IPSCs), and neural progenitor stem cells (NSCs), are a possible treatment for stroke, Parkinson’s disease (PD), and Huntington’s disease (HD). Current preclinical data suggest stem cell transplantation is a potential treatment for these chronic conditions that lack effective long-term treatment options. Finding treatments with a wider therapeutic window and harnessing a disease-modifying approach will likely improve clinical outcomes. The overarching concept of stem cell therapy entails the use of immature cells, while key in recapitulating brain development and presents the challenge of young grafted cells forming neural circuitry with the mature host brain cells. To this end, exploring strategies designed to nurture graft-host integration will likely enhance the reconstruction of the elusive neural circuitry. Enriched environment (EE) and exercise facilitate stem cell graft-host reconstruction of neural circuitry. It may involve at least a two-pronged mechanism whereby EE and exercise create a conducive microenvironment in the host brain, allowing the newly transplanted cells to survive, proliferate, and differentiate into neural cells; vice versa, EE and exercise may also train the transplanted immature cells to learn the neurochemical, physiological, and anatomical signals in the brain towards better functional graft-host connectivity.
Neurological diseases such as stroke, Parkinson’s disease (PD), and Huntington’s disease (HD) remain significant contributors to long-term disability and financial burden for patients worldwide. Despite decades of research into their underlying pathology and potential therapeutic targets, limited treatment options exist for these conditions. Regenerative medicine offers a promising intervention for these pathologies, but still faces significant hurdles to overcome. Of these issues, the ability to use stem cell-derived neural progenitors and integrate them fully into existing neural circuits to provide a more functional benefit while also enhancing the general outcome of transplantation has possible solutions; exercise and rehabilitation. This paper aims to discuss some of the current therapeutic uses of regenerative medicine and how rehabilitation strategies may increase the efficacy of stem cell grafts for long-term recovery. Stroke, PD, and HD were explicitly chosen due to the relative abundance of literature about exercise, EE, and stem cells (Table 1).
TABLE 1. The pathophysiology and common treatment options for ischemic stroke, Parkinson's disease, and Huntington's disease.
Regenerative medicine utilizes a multitude of progenitor cell lines that vary based on biological origin, differentiation, advantages, and disadvantages (Table 2). Embryonic Stem Cells (ESCs) are undifferentiated pluripotent cells derived from mammalian blastocysts and can differentiate into any cell of all three germ layers, but pose major ethical concerns and have potential for immune rejection (Niwa et al., 2000; Chew et al., 2005). Induced pluripotent stem cells (IPSCs) are generated from adult somatic cells and bypass the ethical and immunogenicity concerns seen in ESCs, but may be tumorigenic, and it can be challenging to produce specific neurons with high purity (Okita et al., 2007; Qin et al., 2013; Doi et al., 2014; Yu et al., 2014). Nonetheless, IPSCs are a popular cell line for studying neurodegenerative diseases such as PD and HD. IPSCs can be differentiated into disease-specific neurons (ex. dopaminergic neurons in PD) that reflect the donor’s genetic markers and provide insight into changes in neurite morphology during disease progression (Park et al., 2008; de Rus Jacquet, 2019; Simmnacher et al., 2020). Mesenchymal stem cells (MSCs) are multipotent cells that secrete therapeutic substances and may migrate to the site of injury and putatively differentiate into the neural lineage (Pittenger et al., 1999; Ries et al., 2007; Waterman et al., 2010). MSCs’ anti-inflammatory effects make this an effective cell type for transplantation in ischemic stroke; indeed, MSC transplantation improves neurologic function in stroke models (Lee et al., 2016; Stonesifer et al., 2017). However, human MSCs exhibit distinct stemness properties from murine MSCs; thereby, it is important to consider the species source of the cells (Bonab et al., 2006; Miura et al., 2006). MSCs are routinely harvested from the bone marrow, and referred to as bone-marrow-derived derived MSCs (BM-MSCs). Controversy exists on the migration of BM-MSCs across the BBB and thereafter differentiating into neural cells. While a few peripherally transplanted BM-MSCs may reach the brain, most of the grafted cells lodged into inflammation-riches peripheral organs, such as the spleen, thymus, and cervical lymph nodes. Accordingly, whereas a few BM-MSC may differentiate into neural lineage and may accompany functional recovery of transplant recipients, the most likely regenerative mechanism entails the bystander effects via secretion of growth factors and other therapeutic substances. Nonetheless, these multi-pronged regenerative processes, including cell replacement and bystander effects, may aid in the cerebrovascular restoration post-ischemia (Eckert et al., 2013; Chen et al., 2015; Shichinohe et al., 2015; Stonesifer et al., 2017; Li et al., 2021). Finally, neural stem cells (NSCs) are primarily located in the subventricular zone (SVZ) and subgranular zone (SGZ) zones of the brain and can be derived from adults (Eriksson et al., 1998; Doetsch et al., 1999). NSCs are utilized for studying stroke, neurodegenerative disease, and trauma and can be produced in vivo and in vitro. They bypass ethical and immunogenic concerns and secrete growth factors promoting neuronal survival, but may promote tumor growth and can be difficult to isolate (Laywell et al., 2007; Amariglio et al., 2009; Bacigaluppi et al., 2009). Recent advances in regenerative medicine also include organoids and directly induced neurons. Organoids are in vitro 3D models grown from either pluripotent embryonic stem cells or adult stem cells (Grassi et al., 2019). Such a 3D model reflects the complex tissue organization of the host tissue that a single cell layer culture cannot and can generate from all three germ layers if derived from ESCs or IPSCs. More specifically, brain tissue organoids are made from neural progenitor cells (NPCs), which can differentiate into neurons and astrocytes (Rossi et al., 2018; Grassi et al., 2019; Corrò et al., 2020). Directly induced neurons may be used for studying age-related neurodegenerative diseases, where transcription factors and chemical signals are used to convert terminally differentiated cells across stages of aging (Mertens et al., 2018).
TABLE 2. Common stem cells and neural-progenitor cell types used for the experimental treatment of ischemic stroke, Parkinson's disease, and Huntington's disease.
Environmental enrichment (EE) is the addition of physical, sensory, or social stimulation into an animal’s environment. In experimental models, EE exerts a positive role in promoting regeneration, neurogenesis, and CNS remodeling. Exposure prevents relapse, enhances attention performance, reduces anxiety levels during development, prevents DNA methylation changes brought by aging, and enhances neurogenesis by increasing NPCs (Korkhin et al., 2020; Zocher et al., 2021; Zorzin et al., 2021; Pintori et al., 2022). Similarly, exercise promotes positive effects on neurogenesis, trophic factor secretion, motor recovery following ischemia, cognitive and motor function, and neuroprotection (Pang et al., 2006; Mang et al., 2013; Tsai et al., 2019). Harnessing these beneficial effects of EE and exercise in combination with stem cell therapy holds significant promise for advancing treatment in stroke, PD, and HD, where therapeutic options remain limited.
Stroke is the second leading cause of death globally, costing patients in the United States nearly $103.5 billion in 2016 (Girotra et al., 2020). Approximately 87% of strokes are ischemic, where thrombotic or embolic events disrupt blood supply and deprive neurons of oxygen, leading to acute neuronal injury and death (Kuriakose and Xiao, 2020). The affected brain tissue with <20% of cerebral blood flow is defined as the ischemic core, where hypoxia results in irreversible damage and apoptosis within minutes to hours following the insult. Surrounding this core is the ischemic penumbra, where the neuronal function may still be recovered by restoring blood flow to the region (Anrather and Iadecola, 2016). Ischemic injury induces neuroinflammation, oxidative stress, and excitotoxicity, which contribute to underlying stroke pathology and impede healing (Kuriakose and Xiao, 2020; Anrather and Iadecola, 2016). Current reperfusion strategies utilize thrombolytic agents such as tissue plasminogen activator (tPA) or surgical intervention via mechanical thrombectomy (Table 1). The recent DAWN trial showed that the therapeutic window for mechanical thrombectomy could be prolonged to 24 h in select ischemic cases (Khaku and Tadi, 2021). Interestingly, after the critical period, reperfusion by mechanical thrombectomy or tPa amplifies existing neuronal injury due to reactive oxygen species (ROS) and subsequent neuroinflammation (Mandalaneni et al., 2021). While these techniques confer therapeutic advantages, they may also lead to hemorrhage following ischemic reperfusion (Nogueira et al., 2018). Additionally, tPA’s narrow therapeutic window, short half-life, and poor penetration of large clots warrant further investigation into effective therapies for stroke (Zamanlu et al., 2018).
PD is the second most common age-related neurodegenerative disease globally, characterized by the death of dopaminergic neurons in the substantia nigra. This complex disease process leads to motor and non-motor symptoms, with neurodegeneration implicating the central, autonomic, and enteric nervous systems (Table 1) (Reeve et al., 2014; Simon et al., 2020; Mak et al., 2017; Stoker and Barker, 2020). Classic motor manifestations include cogwheel rigidity, an asymmetric resting tremor, bradykinesia, and non-motor features such as depression, dementia, and rapid-eye-movement (REM) sleep disorders that precede motor symptoms and contribute to cognitive decline (Pantcheva et al., 2015; Hayes et al., 2019; Simon et al., 2020; Balestrino and Schapira, 2020). Current therapeutic approaches for PD focus on replenishing dopamine stores, as in the case of levodopa, which is considered the gold standard treatment for PD. Levodopa is the biological precursor to dopamine that improves motor function early in the disease process, but may cause dyskinesias and fluctuating periods of enhanced and impaired motor function due to alterations in extra-striatal dopamine (Stoker and Barker, 2020; Hayes et al., 2019). In patients with mid-stage and advanced-stage PD, deep brain stimulation (DBS) confers therapeutic benefit by alleviating dyskinesias and medication-refractory tremors. Surgically implanted electrodes stimulate the subthalamic nucleus and globus pallidus internus, and stimulation parameters are tailored to the patient’s clinical state (Figure 1). While DBS is a well-established treatment option for PD, adverse effects may include dyskinesias, imbalance, and dysarthria, which should be weighed against its therapeutic benefits (Habets et al., 2018; Hayes et al., 2019; Stoker & Barker 2020).
FIGURE 1. The disruption in the functional circuit in Parkinson’s disease. In PD, loss of the dopaminergic neurons of the substantia nigra pars compacta (SNc) leads to increased output by the indirect pathway and less motor output. The loss of dopaminergic neurons also impacts the direct pathway, increasing the circuit’s inhibition on the thalamus (VA/VL). Green arrows signify glutamatergic excitatory neurons and red arrows signify GABA expressing inhibitory neurons. enk, enkephalin; SP, Substance P; GPe, globus pallidus, external segment; GPi, globus pallidus, internal segment; SNc, substantia nigra pars compacta; SNr, substantia nigra pars reticulata; STN, subthalamic nucleus; VA/VL, ventral anterior/ventral lateral nucleus of the thalamus; D1r, D1 dopaminergic Gs coupled receptor; D2r dopaminergic Gi coupled receptor. Adapted from (McGregor and Nelson, 2019).
HD is a neurodegenerative disorder characterized by trinucleotide (CAG) repeat expansions in the huntingtin gene (HTT) on chromosome 4. It is inherited in an autosomal dominant manner, with genetic anticipation creating longer CAG expansions via paternal transmission. This mutation leads to a degeneration of GABAergic medium spiny neurons (MSNs) in the basal ganglia, disrupting neural circuitry and associated symptomology (Figure 2). Individuals with more repeats experience the disease earlier and progress more rapidly than those with fewer repeats (Wyant et al., 2017; Kim et al., 2021). HD’s molecular pathology is complex, involving aggregate formation, transcriptional dysregulation, altered synaptic plasticity, and glial dysfunction (Jimenez-Sanchez et al., 2017). Clinically, patients present with choreiform movements, which may progress to dystonia, rigidity, and ataxia as the disease progresses. Psychiatric conditions such as depression, anxiety, and suicidal ideations are also associated with HD. Currently, treatments for HD are primarily palliative (Table 1). Tetrabenazine (TBZ) is a monoamine transporter inhibitor that effectively reduces dopamine levels but has a black-box warning for increased risk of depression and suicidal ideation from concurrent depletion of serotonin and norepinephrine (Wyant et al., 2017; Kumar et al., 2020).
FIGURE 2. The disruption in the functional circuit in Huntington’s disease. Cerebral cortex atrophy and loss of the indirect pathway leads to more motor output and results in the choreiform movements seen in HD. Green arrows signify glutamatergic excitatory neurons and red arrows signify GABA expressing inhibitory neurons. enk, enkephalin; SP, Substance P; GPe, globus pallidus, external segment; GPi, globus pallidus, internal segment; SNc, substantia nigra pars compacta; SNr, substantia nigra pars reticulata; STN, subthalamic nucleus; VA/VL, ventral anterior/ventral lateral nucleus of the thalamus; D1r, D1 dopaminergic Gs coupled receptor; D2r dopaminergic Gi coupled receptor. Adapted from (Essa et al., 2019).
The pathophysiology and genetic mechanisms of stroke, PD, and HD have been well-studied, yet current therapeutic options leave much to be desired. tPA, the FDA-approved fibrinolytic agent, is commonly used to treat acute ischemic stroke, has a narrow therapeutic window and short half-life. A mechanical thrombectomy may be performed when patients do not meet tPA criteria. However, procedural and post-operative complications such as access-site vessel and nerve damage, intracerebral hemorrhage, and pseudoaneurysm may occur (Balami et al., 2018). Current strategies for PD and HD remain palliative and primarily focus on dopamine replacement and monoamine depletion. Finding targeted therapies that harness a disease-modifying approach for these conditions and a wider therapeutic window in the case of stroke will likely improve clinical outcomes for patients.
Regenerative medicine, specifically stem cell-derived neural progenitor therapy, offers promising therapeutic potential for treating these CNS disorders. These three pathologies exhibit cell death and prove to be good candidates for stem cell-derived neural progenitor therapy. This option provides cell regeneration, endogenous neural progenitor recruitment, anti-inflammatory properties, and circuitry reconstruction. While the exact mechanisms of stem cell-derived neural progenitors remain elusive, the therapeutic void for stroke persists and begs for new strategies for recovery.
In stroke, mass cell death presents unique complications as it is the death of neurons and the neurovascular unit as a whole within the ischemic core. The neurovascular unit comprises neurons, astrocytes, endothelial cells of the blood-brain barrier (BBB), myocytes, pericytes, and extracellular matrix components (Yasuhara et al., 2006; Muoio et al., 2014). The rescue of neurons and the neurovascular unit in the ischemic penumbra is feasible if acted upon promptly and thoughtfully using the suitable stem cells, dosage, and delivery route (Borlongan, 2019). In models of ischemic stroke, transplanted stem cell-derived neural progenitors not only replace infarcted tissue, but are neuroprotective, combat neuroinflammation, promote angiogenesis, reduce glial scar formation, and recruit endogenous neural progenitors to the area of injury (Doeppner and Hermann, 2010; Stonesifer et al., 2017; Borlongan, 2019; Saft et al., 2020). The discovery of endogenous neural stem cells (NSCs) in the human brain led to a new avenue of experiments with stem cell-derived neural progenitors and the eventual discovery of the biobridge (Renolds and Weiss, 1992; Ming and Song, 2011). The biobridge is the concept that transplanted neural progenitors not only replace dying cells and mitigate aberrant inflammation via the bystander effect but guide native neural progenitor cells from the host’s neurogenic niches to the area of infarction as well (Tajiri et al., 2013). The bystander effect is the process where transplanted stem cells secrete therapeutic substances rather than differentiating into neural cells, and may involve rapid attenuation of inflammation by reducing expression of proinflammatory factors, decreasing microglial activation, and promoting BBB repair. This anti-inflammatory effect was displayed when human-induced pluripotent stem cell-derived neural stem cells (hiPSC-NSCs) were transplanted into rodents 24 h after the onset of stroke. By the 48-h period, these transplanted cells migrated to the area of infarction and downregulated aggravating molecules such as tumor necrosis factor-α (TNF- α), IL-6, IL-1β, monocyte chemotactic protein 1 (MCP-1), macrophage inflammatory protein 1a (MIP-1a), intracellular adhesion molecule 1 (ICAM-1), and vascular cell adhesion molecule 1 (VCAM-1) (Eckert et al., 2015). In addition to the bystander effect, the endogenous NSCs from the neurogenic niche essentially replace transplanted stem cells and recapitulate the secretion of anti-inflammatory cytokines, proteomes, and neurotrophic factors to mitigate the harsh environment and facilitate functional recovery (Crowley and Tajiri, 2017; Liska et al., 2017). Additionally, these progenitor cells can differentiate into tissue-specific neurons, astrocytes, and oligodendrocytes to result in more favorable outcomes (Corey et al., 2019; Zhang et al., 2020). However, successful integration into fully functional neurons persists as a problem for both transplanted and endogenous neural progenitors.
Entry of peripherally administered cells across the BBB remains a controversial topic. Grafted BM-MSCs may use upregulated P- and E-selectins after stroke to adhere to endothelial cells in the cerebral vasculature (Huang et al., 2000; Yilmaz et al., 2011). Additionally, the upregulation of VCAM-1 after stroke aided NSC adhesion in another study, further highlighting the ability of these cells to use selectin mediated rolling and integrin associated adhesion to penetrate the BBB (Guzman et al., 2008). MSC adhesion to the cerebral endothelial cells led to secretion of CXCl-11 which binds CXCR-3 and increases BBB permeability through the ERK1/2 signaling pathway (Feng et al., 2014). Interestingly, MSCs have also shown to reduce BBB disruption by inhibiting matrix metallopeptidase 9 (MMP-9) and decreasing ICAM-1 (Cheng et al., 2018). This discrepancy may be due to the different stem cells used and the different stages of these stem cells, which needs to be further studied (Zhou et al., 2021). There seems to be a two-pronged mechanism where stem cells will use BBB permeability to extravasate and migrate to the injured area and then begin to secrete growth factors and therapeutic substances through the bystander effect (Ao et al., 2018).
Preclinical studies prove the viability of stem cell-derived neural progenitors in PD treatment. However, clinical trials display findings that show incongruities between preclinical and clinical results (Yasuhara et al., 2006; Kirkeby et al., 2017). Like in stroke, translating results from the preclinical to clinical settings poses barriers, including which type of stem cells to use, the route of administration, the timing, the induction of cell differentiation, and facilitating transplanted cell survival. Regardless, stem cells have proven to be an effective treatment in rodent models due to their ability to differentiate into tissue-specific cells, secrete neuroprotective factors, induce endogenous repair mechanisms, recruit endogenous neural progenitors, modulate immune processes, increase survival of existing cell populations, and lead to a more functional recovery (Yasuhara et al., 2017; Takahashi, 2018; Yasuhara et al., 2006; Tajiri, Yasuhara, Shingo et al., 2010). Current stem cell therapies utilize MSCs, NSCs, ESCs, and IPSCs (Table 2). ESCs are pluripotent, but create ethical concerns, may trigger tumorigenesis, and stand frequent immunological rejection (Bradley et al., 2002; Bieberich et al., 2004). Compared with other artificially-induced neural progenitors, IPSC cell lines can be differentiated into dopaminergic neurons in vitro before transplantation, genetically tailored to match the dysfunctional transcription factor responsible for the patient’s phenotype, and permit patient-specific human leukocyte antigen (HLA) matches to reduce immune incompatibility. The use of IPSC-derived neural progenitor cells additionally circumvents a crucial ethical dilemma in stem cell research, as embryos are not utilized in this technology. (Takahashi and Yamanaka, 2006; Morizane et al., 2017; Playne and Connor, 2017; Stoddard-Bennett and Pera, 2019).
After successful stem cell therapy in PD, an overarching issue still exists, specifically the full integration of the immature cells into the host’s neural circuitry (Weiss et al., 2006). In one preclinical study, human NSCs cloned by v-myc gene transfer (HB1.F3 cells), were transplanted into the 6-hydroxydopamine-lesioned striatum of rats. The treated rats showed significant recovery of parkinsonian symptoms in comparison to controls. The lesioned rats exhibited a nearly complete restoration of spontaneous motor activity after intracranial transplantation and a small fraction of the neurons were positive for tyrosine hydroxylase (TH) along the nigrostriatal pathway, indicating a successful integration into tissue-specific neurons. However, many of the neurons remained nestin-positive or lacked TH in the presence of MAP2, suggesting many of the grafted cells remained immature or mature with a lack of complete differentiation into dopaminergic neurons, respectively (Yasuhara et al., 2006). Despite lack of dopaminergic differentiation, the transplanted NSCs exhibited neuroprotective effects. Studies have also shown improved Parkinson’s symptomatology even with minimal dopaminergic differentiation, suggesting that the bystander effects may play a more substantial role as grafts improve functional recovery after the loss of dopaminergic neurons in the substantia nigra (Jung et al., 2004; Goldman, 2005; Rafuse et al., 2005; Yasuhara et al., 2006). In addition to increased secretion of neurotrophic factors such as human recombinant stem cell factor (SCF) in both the neural progenitor cells of the SVZ and the grafted HB1.F3 cells, the study also observed increases in endogenous neural stem cell production and preservation of existing neuronal circuitry and dopaminergic neurons (Yasuhara et al., 2006). This study suggest the efficacy of the stem cell treatment in early PD can ameliorate the functional loss associated with the disease pathology with minimal or even lack of neural differentiation and maturation. In another study by Kirkeby et al., human ESC derived neural progenitor cells were transplanted into the striatum of 6-hydroxydopamine (6-OHDA) lesioned rats to assess their ability to obtain regional specification and survive. Tumor-free grafts were able to proliferate and reach full maturity, displaying an affinity for axonal outgrowth (Kirkeby et al., 2012). Another issue that regenerative medicine faces is the threat of inflammation after injection of stem cells in PD models. One investigation administered ESC derived neuronal cells into the brains of rats that received surgery to cause brain injury. At the 7-week mark after implantation, histological analysis discovered extensive loss of the grafts at the ipsilateral site of only one animal. Further analysis revealed that macrophages infiltrated the graft site at a high concentration and activated astrogliosis despite the administration of immunosuppressants (Molcanyi et al., 2007).
With a compelling body of evidence, strategies adjunct to stem cell therapy have been designed to enhance neuronal survival, development, and differentiation into fully functional dopaminergic neurons capable of mitigating PD deficits. Two potential strategies to achieve this improved graft function involve exercise and EE, as discussed in section 3.2 below.
In a 2001 double-blind open clinical trial, embryonic dopamine neurons were surgically transplanted in 20 out of 39 patients with PD. Imaging and postmortem studies showed a significant increase in dopaminergic neurons and neuronal differentiation in 17 out of 20 patients. Only 15% of the patients reported a recurrence of dystonia and dyskinesias after a one-year follow-up. The results proved human embryonic dopamine neuron transplants survive and lead to functional recovery in younger patients compared to older (over 60). This difference between younger and older patient recovery is likely due to a lack of robust neural plasticity in the older patients (Freed et al., 2001). While the use of embryonic grafts remains controversial, these findings indicate a viable option in the treatment of early PD. However, the lack of neuroplasticity in the aged brain may be overcome by specific interventions such as exercise and enriched environments (EE) to reinforce the circuitry provided by cell transplantation. The TRANSEURO clinical trials are ongoing but have problems collecting the human fetal ventral mesencephalic tissue needed for transplantation and have subsequently stopped. This barrier, alongside ethical dilemmas, has led to researchers searching for an alternative tissue supply (Barker et al., 2017). In response to this need, momentum has continued after the trials by Freed et al. and TRANSEURO despite the ethical concerns concerning embryonic stem cell use with the modern use of pluripotent stem cell (PSC)-derived dopamine neurons in humans (Barker et al., 2017).
HD continues to be an incurable disease, and current therapeutic options primarily focus on symptomatic treatment. Significant barriers to HD therapy include limited knowledge on molecular mechanisms implicated by the Huntington protein (HTT), extra-striatal atrophy throughout disease progression, and its broad impact on multiple systems, thus necessitating a systemic approach to treatment. Fortunately, advances in cell reprogramming and gene therapy offer promising potential to regenerate the damaged circuitry in the striatum and improve motor outcomes (Zheng and Kozloski, 2017; Cho et al., 2019; Monk and Connor, 2020). Cho and colleagues utilized gene therapy and neural progenitor cell transplantation to improve the motor deficits seen in the HD phenotype. The authors genetically corrected a mutant HTT NPC line and produced a viable cell therapy product that, like the WT NPC line, improved motor performance and lifespan when grafted into the striatum of HD mice (Cho et al., 2019). However, it is worth noting that inducing genetic changes before transplantation may introduce genetic instability (i.e., malfunctions in DNA repair, spindle formation, and telomere abnormalities) that can collectively contribute to tumor formation (Ross et al., 2011). Nonetheless, stem cell-derived neural progenitor cell transplantation stands as a promising therapeutic tool for HD.
Altogether, the developments in stem cell research for these three pathologies offer an exciting new avenue of possible subacute and chronic treatments. However, one problem remains, specifically, the use of immature cells in a previously integrated neural network. The use of immature cells, while key in recapitulating brain development, presents the challenge of young grafted cells forming neural circuitry with the mature host brain cells. To this end, exploring strategies designed to nurture graft-host integration will likely enhance the reconstruction of the elusive neural circuitry. The subsequent section discusses the use of EE/exercise in stem cell therapy-induced repair of the neural circuitry after stroke, PD, and HD.
Environmental enrichment and exercise are validated animal models for rehabilitation that can be measured through behavioral assessments (Huo et al., 2021). Clinical rehabilitation strategies vary based on subjective criteria such as patient characteristics, clinician preferences, and preferred outcomes. This makes it hard to standardize and attribute preferable outcomes to specific strategies (Huo et al., 2021). However, it is well known that rehabilitation and exercise lead to better functional outcomes, but the mechanisms by which they accomplish this and the effective period for intervention can be elusive. Recent findings in understanding motor learning, neuroplasticity, and functional recovery have led researchers to dive deeper into possible mechanisms by which EE and exercise lead to better outcomes and how they may be used in conjunction with other treatments and interventions. Here we will evaluate the preclinical data for stroke, PD, and HD regarding EE and exercise and show possible mechanisms of recovery that are used in combination therapies and can be further used in translational studies.
EE and exercise have been well studied in the context of stroke. EE strategies lead to increased gross neuroglia, sensorimotor function, spatial learning, and memory. However, outcomes are primarily based on the animal’s activity (Zhan et al., 2020). A three-phase paradigm has been proposed for the evolution of the EE throughout the healing process in an ischemic stroke rat model. Rats were initially exposed to social interaction, voluntary exercise, and small cabins for the first 2–7 days. They were then moved to a two-layer cage with more environmental interaction such as tunnels, swing boards, ladders, and balance beams for days 8–14. The third and final phase consisted of a three-layered cage with increasing slopes of the ladders and balance beams as well as floating cabins for days 15–30. The study showed significant restorative benefits with increased survival of neurons in the striatum and cortex, improved cerebral blood flow, increased angiogenesis, increased endogenous progenitor cell proliferation, increased endogenous neuronal differentiation in the ischemic regions, increased axonal guidance proteins, and reduced ischemic BBB capillary damage compared to standard housing rats (Zhan et al., 2020). Many molecular mechanisms induced by EE supported these incredible findings. Angiogenesis and the rescue of the neurovascular unit were portrayed through signaling pathways such as phosphorylated PI3K, AKT, and GSK-3, but reduced phosphorylated β-catenin. In tandem with modified expressions of vascular endothelial growth factor (VEGF), Angiopoietin-1 (Ang-1), and Angiopoietin-2 (Ang-2), the benefit from a modified EE program highlights the importance of rehabilitation in the recovery from stroke (Zhan et al., 2020). It has been shown that neuronal and endothelial cell proliferation happen together in the context of the neurovascular unit. The effects of EE on adult neurogenesis are replicable and have been displayed in many studies. Spatial learning significantly increased when rats were subjected to EE, and the positive results diminished when adding temozolomide, a drug that decreases neurogenesis (Garthe et al., 2016).
In addition to the combined benefits on neuronal proliferation, endogenous repair mechanisms, and neurogenesis, EE has proven to effectively induce neuroplasticity, cell maturation, genetic changes, and neuronal integration in host tissue (Kempermann, 2020). The theory posits that the actual benefits of EE come from the physical activity, social interaction, and cognitive stimulation elicited by the environment and must be assessed together. When studying hippocampal-dependent memory in rats, spatial enrichment modified the PKA dependence of long-term potentiation (Duffy et al., 2001). The study of genetic modifications in response to EE began in 2000 and has progressed (Rampon, 2000; Zhang et al., 2018). Enrichment modifies DNA methylation, including a regulator of adult neurogenesis, NeuroD1 (Zhang et al., 2018). Along with the genetic effects, neuronal maturation and integration are essential for portraying the benefits of EE. The experience of the animal leads to differing synaptogenesis and dendritic spine formation in the dentate gyrus (DG) during neurogenesis (Zhao et al., 2015). New neurons need stimulation to mature into tissue-specific neurons that are capable of remaining viable. Yu et al. demonstrated the neurovascular and behavioral outcomes after rehabilitation in an ischemic stroke model. EE in the form of climbing platforms, plastic tubes and tunnels, chains, and small boxes elicited neuroprotective effects and accelerated motor coordination recovery, progenitor cell integration, memory performance and more after partial middle cerebral artery occlusion. In addition, higher levels of CD31 were detected in the ischemic penumbra, revealing an increase in vascular differentiation (Yu et al., 2014).
Immunological studies also highlight the ability of EE in the form of nest boxes, fabric tubes, running wheels, and swing to change genetic factors and T Cell phenotype, leading to increases in IL-10 and IL-17 with subsequent decreases in IFN-γ (Rattazzi et al., 2016). This resolution of inflammation points to a broader immunological role of behavior and recovery. A review paper published in 2014 revealed the immunological changes were largely based on the EE in which the animals were placed. Physical exercise (PE), a form of EE, led to downregulation of toll-like receptors on macrophages and monocytes, reduced secretion of adipokines, modulation of hippocampal T cells, upregulation of mitogen-activated protein kinase phosphatase-1, and decreases in IL-1β and TNF-α. These immunomodulatory effects last after the stimulus and point to a long-term positive effect of EE and exercise (Singhal et al., 2014). A novel form of apoptosis, named pyroptosis, is a mechanism of cell death through membrane pore formation in response to infection and danger signals (Kuang et al., 2017). An experiment by Liu et al. displayed that an EE with ladders, platforms, swings, colorful balls, different-shaped wooden blocks, plastic tunnels, and a running wheel led to neuronal anti-pyroptosis through inhibition of Nuclear Factor kappa B (NF-kB) p-65 signaling pathway resulting in decreased levels of the inflammasome NLRP1 and NLRP3 after ischemia. Downstream, this pathway led to decreased levels of IL-1B, IL-18, and pyroptosis in neurons showing EE’s anti-inflammatory effects (Liu et al., 2021).
Exercise after an ischemic event has been combined with stem cells transplants (Table 3). However, it should be noted that timing for exercise, especially intense exercise, after an ischemic event is a determining factor for rehabilitative efficacy. Very early exercise interventions have a negative overall effect on the outcome of recovery after traumatic brain injury and may follow a similar pattern in stroke.
TABLE 3. Experimental studies for stroke with stem cell transplantation, an enriched environment, exercise, and a combination of enriched environment or exercise with stem cells.
EE and exercise may offer a solution to the maintenance and protection of dopaminergic neurons, evidenced through rat models. A study was conducted to investigate the neuroprotective functions of exercise in rats that were treated with 6-OHDA to emulate PD. A baseline was taken, with rats having access to an exercise wheel 2 weeks before the 6-OHDA injection. Rats would travel 3,361 ± 932 m/day before treatment, and after the brain lesions were induced they traveled 1,292 ± 770 m/day (Tsai et al., 2019). The study also assessed gait patterns and akinesia and took histological samples before and after the brain lesion. The immunohistochemistry analysis revealed that rats in the exercise condition displayed a significantly higher amount of dopaminergic neurons than the control group. Ultimately, the study’s results support the long-term neuroprotective effects of exercise through immunehistological results and behavioral assessments (Tsai et al., 2019). This research is further supported by Rezaee et al., who also investigated the effects of exercise in rats treated with 6-OHDA. This experiment assessed the expression of various genes that play critical roles during neurodegeneration and regeneration such as Ampk, Sirt1, Pgc1a. The study found that treadmill exercise for rats with the 6-OHDA injection significantly increased TH expression and brain derived neurotrophic factor (BDNF). This also contributed to the amelioration of the behavioral abnormalities of the rats in exercises like the apomorphine-induced rotations (Rezaee et al., 2020). The increased expression of neurotrophic factors and TH levels supports that exercise has neuroprotective properties that can play a critical role in the treatment of PD. EEs are another tool that shows promise in the treatment of PD, but these settings are not standardized, which emphasizes the importance of descriptive details for each study (Table 4). Jadavji et al. found that rats with PD that were placed in an EE with ladders, multileveled cages, and toys saw significant improvements in motor deficits such as skilled reaching, walking, and apomorphine-induced rotation compared to rats with PD in a standard housing environment. Furthermore, a histological assay that measured the amount of TH-positive cells found that rats in the EE group had a significantly higher amount than the standard environment group (Jadavji et al., 2006). However, an important consideration to these results is that the rats were placed in an EE before 6-OHDA treatment as well. This timeline for exposure to EEs, particularly before the onset of PD has been a point of interest because a relationship may exist. Jungling et al. conducted another study where they placed rat pups in an EE with larger cages and were exposed to intensive complex stimuli for 5 weeks after birth and then were placed in a regular environment afterward. They then received 6-OHDA injections at 3 months old. The study results demonstrated that the rats with early exposure to an EE performed significantly better on motor function tests and had less dopaminergic neuron loss after 6-OHDA treatment (Jungling et al., 2017). However, in this study, the maximal lesion of dopaminergic neurons was only 24%. Regardless, this investigation still supported the use of EEs as a preventative tool against PD (Jungling et al., 2017). Other studies have extended these findings to measure the effects of a combined intervention utilizing both EEs and exercise. An investigation by Pradhan incorporated both treatments through exercise video games with human subjects. This study recruited patients with mild PD, and selected games that specifically target PD-induced deficits such as balance, reflex responses, and cognitive engagement (Pradhan, 2019). The specific games were tailored to each subject based on a 1–10 rating of difficulty each participant provided after trialing each game. Participants performed physical assessments before and after the intervention that assessed motor deficits associated with PD such as a functional reach test, single limb stance, and gait speed. These results ultimately displayed behavioral improvements from baseline to post-intervention for the assessments that the games had targeted (Pradhan, 2019). These studies show firm support for practical applications of EEs and exercise as a treatment regimen for alleviating the debilitating symptoms of PD. Increasing expression of neurotrophic factors and protecting dopaminergic neurons from decay allow for improvements in motor skill deficits that originate from PD.
TABLE 4. Experimental studies for Parkinson's disease with stem cell transplantation, an enriched environment, exercise, and a combination of enriched environment or exercise with stem cells.
The benefits of EE conditions for HD were first discovered in transgenic HD mice models during a landmark study in 2000. Van Dellen et al. found that HD mice exposed to stimulating environments demonstrate delayed cerebral atrophy and motor coordination compared to non-stimulated HD controls. Mice in the non-stimulated group also developed seizures, whereas the enriched group did not (van Dellen et al., 2000). Cognitive deficits in learning and memory, which typically precede motor manifestations in HD, were improved in the environmentally enriched group, specifically for task-specific flexibility and long-term spatial memory (Nithianantharajah et al., 2008). Furthermore, it is known that HD vastly affects intracellular signaling, transcriptional regulation, and protein expression, including downregulated BDNF expression (Cha, 2000; Spires, et al., 2004; Ferrer, et al., 2000). Environmental enrichment ameliorates the motor manifestations in HD and increases BDNF levels in the striatum and hippocampus, posing positive effects on neurogenesis and cell survival in this neurodegenerative disease (Spires et al., 2004; Lazic, et al., 2006). Like EE, PE demonstrates cognitive and motor benefits in rodent HD models (Pang et al., 2006). When spatial memory was assessed in HD mice by observing running alternations in a T maze, 45% of HD mice housed with running wheels alternated during the task, compared to only 29% of HD mice in standard housing. There was no significant difference in alternation rates between WT and standardized housing HD rodents, supporting that physical exercise can rescue cognitive function in HD. Decreased mRNA BDNF levels were also noted in the striatum, hippocampus, and anterior cortex of HD rodents, supporting dysregulated transcription. However, PE did not affect BDNF protein expression in either WT or HD groups. Running did increase striatal mRNA in this study, which the authors attribute to increased astrocytes secreting BDNF (Pang et al., 2006). Another study tested the long-term effects of PE on improving cognitive reserve in mice models, which interestingly revealed reduced escape latencies and longer survival in mice trained with cognitive stimulation compared with the only motor-trained group (Wood et al., 2011). Taken together, both environmental enrichment and physical exercise prove beneficial for ameliorating cognitive function, protein expression, and motor performance in HD (See Table 5).
TABLE 5. Experimental studies for Huntington's disease with stem cell transplantation, an enriched environment, exercise, and a combination of enriched environment or exercise with stem cells.
EE and exercise show distinct regenerative effects that may work synergistically with stem cell-derived neural progenitor therapy if used in combination and following certain guidelines with timing, dosage, and intensity. Altogether, the use of these rehabilitation strategies may produce the most impact by potentially training the immature transplanted cells to form functional circuitry with the host’s neural networks leading to improved neuroanatomical graft-host integration and behavioral outcomes.
Effective stem cell therapy not only hinges on cell replacement but necessitates appropriate functional integration, connectivity, and differentiation into the host environment. Utilizing environmental enrichment and exercise to facilitate this process is an active field of research of great therapeutic value for stroke, PD, and HD. This concept centers around stimulating the graft site through EEs to enhance neuroplasticity and recruit endogenous repair mechanisms that favor survival, growth, and functional integration for transplanted cells (Döbrössy and Dunnett, 2005; Dunnett, 2013; Clinch et al., 2017). However, in the case of PD, restoring dopamine levels alone is able to ameliorate parkinsonian symptoms. The positive benefits from both EE and stem cell transplantation can further be discussed through combination studies rather than the individual applications of both stem cells and EE (Figure 3).
FIGURE 3. Training the stem cell graft to integrate with the host brain. Stem cell transplantation offers many therapeutic benefits such as immune system modulation, dying cell rescue, neurogenesis, and angiogenesis. When combined with the benefits of EE and exercise, these outcomes synergize and lead to better outcomes.
Studies using a preclinical stroke model with a combination of stem cells and rehabilitation have consistently revealed the importance of using a combination therapy when assessing outcomes. BDNF is a neurotrophic factor correlated with neural plasticity that has been well studied in the context of motor rehabilitation and stem cell transplantation. It has been proposed that rehabilitation strategies that enhance BDNF also enhance motor recovery after ischemia (Mang et al., 2013). Because stem cells and rehabilitation have been shown to increase this neurotrophic factor, it can be theorized that the combination of both therapies will have an additive effect on functional outcome (Ploughman et al., 2009; Mang et al., 2013). In section 2, we assessed the current theories of stem cell mechanisms for brain repair. Indeed, stem cells lead to enhanced recovery through many means, but the biobridge paves a new avenue for understanding the amelioration of deficits after stroke. The increases in endogenous NPCs must be met with increased training to lead to functional integration of the grafts with the host tissue. Rehabilitation offers the means by which grafts mature and integrate effectively. However, the type of rehabilitation method is an important factor. Aerobic exercise has been shown to increase BDNF and endogenous neurogenesis (Mang et al., 2013). Forced low-intensity exercise increases NPC maturation and facilitates a more robust motor recovery (Morishita et al., 2020). Most importantly, both EE and stem cell transplantation increase adult neurogenesis which has been implicated as the main driving force behind recovery (Zhan et al., 2020; Garthe et al., 2016; Tajiri et al., 2103; Singhal et al., 2014; van Praag et al., 1999) The stem cells induce neuroprotection early and influence proliferation when the rodents are still in recovery. Once the rodents begin to display functional recovery, EE should be implemented quickly to increase neurogenesis and neuronal integration into the host tissue. The timing and implementation of exercise and EE remains questionable. Exercise, if implemented too early, results in increased thalamic atrophy and worse functional motor outcomes and should be further evaluated in combination studies (Kozlowski et al., 1996; Risedal et al., 1999). EE, byways of housing enrichment, is safe and effective when implemented early or late in the disease course and may not pose the same problems (Tang et al., 2019; Zhan et al., 2020).
The three-phase paradigm might combat this careful balancing effect between exploiting the positive results and mitigating adverse effects based on timing and the intervention needed (Zhan et al., 2020). Treadmill exercise enhances the therapeutic potency of MSCs by increasing the survival of existing neurons and transplanted neurons (Zhang et al., 2015). In addition, combined therapy led to reduced infarct volume, increased synaptogenesis, and overall improved behavioral outcomes compared to lesioned rats treated with either stem cells or rehabilitation (Akhoundzadeh et al., 2017). The timing for implementation between the stem cells and the rehabilitation strategy needs more data to find a balance accurately, as many studies show differences in timing and results. There is believed to be a defined plastic window in which neural plasticity can be exploited for better outcomes after a pathologic event or process (Hara, 2015; Zhan et al., 2020).
Exercise induces morphological changes in toxin-induced PD rat models. When dopaminergic grafts from the rat fetal ventral mesencephalon were transplanted into the striatum of 6-OHDA lesioned rats, rodents exposed to exercise had enhanced dopaminergic graft survival, maturation, and neurite extension into the dorsolateral striatum. These neurite extensions specifically grew in the same pathway taken by A9 dopaminergic neurons, which project into the striatum and are crucial for improved behavior (Figure 4). This is an important finding as A9 dopaminergic neurons preferentially degenerate in PD (Björklund and Dunnett, 2007; Torikoshi et al., 2020). Additionally, hemispheric dominance plays an influential role in dopaminergic neuroplasticity post-transplantation. Rats unilaterally lesioned with 6-OHDA were observed in skilled forelimb experiments pre- and post-DA graft placement. Rats that did not demonstrate a paw preference during the staircase test improved the most post-transplantation. Subjects with skilled movements contralateral to the lesion recovered moderately whereas those with ipsilateral lateralization recovered the least (Nikkah et al., 2001). Clinically, exercise-induced neuroplasticity plays a profound impact on long-term rehabilitation in PD patients. 8 weeks of balance training improved functional mobility and reduced falls in PD patients for up to 12 months following treatment, and Tai Chi training for 12–24 weeks reduced falls for up to 6 months post-training (Li et al., 2012; Gao et al., 2014; Shen and Mak, 2015; Wong-Yu and Mak, 2015; Mak et al., 2017). Furthermore, patients with early PD who practiced intensive treadmill exercise had improved postural control and increased striatal dopaminergic graft binding to the dopaminergic receptor, D2R, as confirmed by PET imaging. These changes were not observed in the non-exercise PD group (Fisher et al., 2013).
FIGURE 4. A diagram of the classical and simplified functional circuit of the basal ganglia. Dopaminergic neurons from the substantia nigra pars compacta (SNc) project to the medium spiny neurons (MSNs) of the striatum and modulate the direct and indirect pathway activity to modulate inhibition by the thalamus (VA/VL). Green arrows signify glutamatergic excitatory neurons and red arrows signify GABA expressing inhibitory neurons. enk, enkephalin; SP, Substance P; GPe, globus pallidus, external segment; GPi, globus pallidus, internal segment; SNc, substantia nigra pars compacta; SNr, substantia nigra pars reticulata; STN, subthalamic nucleus; VA/VL, ventral anterior/ventral lateral nucleus of the thalamus; D1r, D1 dopaminergic Gs coupled receptor; D2r dopaminergic Gi coupled receptor. Adapted from (McGregor and Nelson, 2019).
A seminal study by Brasted and colleagues revealed the importance of targeted training to recover lost motor function in HD rat models. These animals were specifically trained to perform a lateralized choice reaction task before receiving quinolinic acid injections into the neostriatum and retrained for 30 days to perform the same task with or without a striatal graft. After retraining post-recovery, only rats that received striatal grafts showed marked performance and eventually achieved similar results as non-HD controls. Additionally, motor training ipsilateral to the lesion did not confer additional benefits, whereas training the contralateral side resulted in marked recovery. Since ipsilateral lesions affect the contralateral side, this supports that rehabilitative training specific and targeted to the lesion is vital for functional recovery in HD (Brasted, et al., 1999). Further investigation into post-graft recovery with lateralized training showed that HD rats with striatal lesions trained to perform a food retrieval task with the ipsilateral paw performed similarly as non-HD controls, whereas those trained on the paw contralateral to the lesion showed motor deficits that recovered with graft transplantation (Döbrössy and Dunnett, 2006b). Döbrössy and Dunnett also investigated changes in BDNF levels, dendritic spinal densities, and cell volume in rodent HD models subjected to EE and exercise, post-striatal graft transplantation (Döbrössy and Dunnett, 2006a). The HD group exposed to EEs in the form of several cardboard tunnels, ladders and platforms revealed increased BDNF levels, spinal densities, and cell volume. In contrast, decreased spinal densities and BDNF were found in the exercise group, suggesting that surrounding environmental factors influence neural connectivity and plasticity post-transplantation (Döbrössy and Dunnett, 2006a).
Stem cell therapy not only relies on cell replacement, but also necessitates successful graft-host functional integration. Enhancing graft integration through rehabilitative strategies advances current treatment options for stroke and HD. This concept may not be as important for PD as replacement of dopaminergic neurons and the presence of dopamine ameliorates the symptoms. EE and exercise facilitate stem cell graft-host reconstruction of neural circuitry that may involve at least a two-pronged mechanism that creates a conducive microenvironment into the host brain, allowing the newly transplanted cells to survive, proliferate, and differentiate into neural cells. Both may additionally train the transplanted immature cells to learn the neurochemical, physiological, and anatomical signals in the brain towards better functional graft-host connectivity.
Limited therapeutic options exist for stroke, PD, and HD, prompting further research into their underlying pathology to improve functional recovery and slow disease progression. Regenerative medicine, specifically stem cell therapy, is a promising avenue that consistently improves outcomes in preclinical studies. This is an active field of research with underlying mechanisms that include cell replacement, endogenous stem cell recruitment, neurotrophic effects, and induction of anti-inflammatory properties. While replacing damaged neurons is the first step to functional recovery, grafts must integrate, differentiate, and enhance synaptic plasticity to maintain and promote healing. EE and exercise combined with stem cell therapy achieve this via enhanced synaptogenesis, neuronal survival, axonal regrowth, dendrite extension, and enhanced receptor binding. There is a continued need for clinical trials that demonstrate the rehabilitative effects of combined EE, exercise, and stem cell therapy. Further research is also required to elucidate appropriate timing between stem cell transplantation and rehabilitation. Ultimately, understanding how these rehabilitative strategies facilitate graft integration and neural repair encourages a team-based and multi-faceted approach to treating these diseases and is core to developing robust therapeutic options for patients across the globe.
RB, DC, DG-P, and CB conceptualized, wrote, revised, finalized, and approved the manuscript. CB provided supervision and overall guidance on the manuscript write-up.
The authors declare that the research was conducted in the absence of any commercial or financial relationships that could be construed as a potential conflict of interest.
All claims expressed in this article are solely those of the authors and do not necessarily represent those of their affiliated organizations, or those of the publisher, the editors and the reviewers. Any product that may be evaluated in this article, or claim that may be made by its manufacturer, is not guaranteed or endorsed by the publisher.
Akhoundzadeh, K., Vakili, A., Sameni, H. R., Vafaei, A. A., Rashidy-Pour, A., Safari, M., et al. (2017). Effects of the Combined Treatment of Bone Marrow Stromal Cells with Mild Exercise and Thyroid Hormone on Brain Damage and Apoptosis in a Mouse Focal Cerebral Ischemia Model. Metab. Brain Dis. 32 (4), 1267–1277. doi:10.1007/s11011-017-0034-0
Amariglio, N., Hirshberg, A., Scheithauer, B. W., Cohen, Y., Loewenthal, R., Trakhtenbrot, L., et al. (2009). Donor-derived Brain Tumor Following Neural Stem Cell Transplantation in an Ataxia Telangiectasia Patient. PLoS Med. 6 (2), e1000029. doi:10.1371/journal.pmed.1000029
Anrather, J., and Iadecola, C. (2016). Inflammation and Stroke: An Overview. Neurotherapeutics 13 (4), 661–670. doi:10.1007/s13311-016-0483-x
Ao, L. Y., Yan, Y. Y., Zhou, L., Li, C. Y., Li, W. T., and Fang, W. R. (2018). Immune Cells after Ischemic Stroke Onset: Roles, Migration, and Target Intervention. J. Mol. Neurosci. 66 (3), 342–355. doi:10.1007/s12031-018-1173-4
Armstrong, M. J., and Miyasaki, J. M.American Academy of Neurology (2012). Evidence-based Guideline: Pharmacologic Treatment of Chorea in Huntington Disease: Report of the Guideline Development Subcommittee of the American Academy of Neurology. Neurology 79 (6), 597–603. doi:10.1212/WNL.0b013e318263c443
Asgari Taei, A., Nasoohi, S., Hassanzadeh, G., Kadivar, M., Dargahi, L., and Farahmandfar, M. (2021). Enhancement of Angiogenesis and Neurogenesis by Intracerebroventricular Injection of Secretome from Human Embryonic Stem Cell-Derived Mesenchymal Stem Cells in Ischemic Stroke Model. Biomed. Pharmacother. = Biomedecine pharmacotherapie 140, 111709. doi:10.1016/j.biopha.2021.111709
Bacigaluppi, M., Pluchino, S., Peruzzotti-Jametti, L., Kilic, E., Kilic, U., Salani, G., et al. (2009). Delayed post-ischaemic Neuroprotection Following Systemic Neural Stem Cell Transplantation Involves Multiple Mechanisms. Brain. 132 (Pt 8), 2239–2251. doi:10.1093/brain/awp174
Balami, J. S., White, P. M., McMeekin, P. J., Ford, G. A., and Buchan, A. M. (2018). Complications of Endovascular Treatment for Acute Ischemic Stroke: Prevention and Management. Int. J. stroke : official J. Int. Stroke Soc. 13 (4), 348–361. doi:10.1177/1747493017743051
Balestrino, R., and Schapira, A. (2020). Parkinson Disease. Eur. J. Neurol. 27 (1), 27–42. doi:10.1111/ene.14108
Barker, R. A., Parmar, M., Studer, L., and Takahashi, J. (2017). Human Trials of Stem Cell-Derived Dopamine Neurons for Parkinson's Disease: Dawn of a New Era. Cell stem cell 21 (5), 569–573. doi:10.1016/j.stem.2017.09.014
Bergman, H., and Deuschl, G. (2002). Pathophysiology of Parkinson's Disease: from Clinical Neurology to Basic Neuroscience and Back. Mov. Disord. : official J. Mov. Disord. Soc. 17 (Suppl. 3), S28–S40. doi:10.1002/mds.10140
Bieberich, E., Silva, J., Wang, G., Krishnamurthy, K., and Condie, B. G. (2004). Selective Apoptosis of Pluripotent Mouse and Human Stem Cells by Novel Ceramide Analogues Prevents Teratoma Formation and Enriches for Neural Precursors in ES Cell-Derived Neural Transplants. J. Cel. Biol. 167 (4), 723–734. doi:10.1083/jcb.200405144
Biernaskie, J., and Corbett, D. (2001). Enriched Rehabilitative Training Promotes Improved Forelimb Motor Function and Enhanced Dendritic Growth after Focal Ischemic Injury. J. Neurosci. 21 (14), 5272–5280. doi:10.1523/JNEUROSCI.21-14-05272.2001
Björklund, A., and Dunnett, S. B. (2007). Dopamine Neuron Systems in the Brain: an Update. Trends Neurosciences 30 (5), 194–202. doi:10.1016/j.tins.2007.03.006
Bonab, M. M., Alimoghaddam, K., Talebian, F., Ghaffari, S. H., Ghavamzadeh, A., and Nikbin, B. (2006). Aging of Mesenchymal Stem Cell In Vitro. BMC Cel. Biol. 7, 14. doi:10.1186/1471-2121-7-14
Borlongan, C. V. (2019). Concise Review: Stem Cell Therapy for Stroke Patients: Are We There yet? Stem Cell translational Med. 8 (9), 983–988. doi:10.1002/sctm.19-0076
Bradley, J. A., Bolton, E. M., and Pedersen, R. A. (2002). Stem Cell Medicine Encounters the Immune System. Nat. Rev. Immunol. 2 (11), 859–871. doi:10.1038/nri934
Brasted, P. J., Watts, C., Robbins, T. W., and Dunnett, S. B. (1999). Associative Plasticity in Striatal Transplants. Proc. Natl. Acad. Sci. United States America 96 (18), 10524–10529. doi:10.1073/pnas.96.18.10524
Caplan, L. R. (2009). in Basic Pathology, Anatomy, and Pathophysiology of Stroke. Editors S. E. Kassner, and J. F. Dashe. 4th ed (Saunders Elsevier).
Cha, J. H. (2000). Transcriptional Dysregulation in Huntington's Disease. Trends Neurosciences 23 (9), 387–392. doi:10.1016/s0166-2236(00)01609-x
Chang, M. C., Park, C. R., Rhie, S. H., Shim, W. H., and Kim, D. Y. (2019). Early Treadmill Exercise Increases Macrophage Migration Inhibitory Factor Expression after Cerebral Ischemia/reperfusion. Neural Regen. Res. 14 (7), 1230–1236. doi:10.4103/1673-5374.251330
Chen, Q. H., Liu, A. R., Qiu, H. B., and Yang, Y. (2015). Interaction between Mesenchymal Stem Cells and Endothelial Cells Restores Endothelial Permeability via Paracrine Hepatocyte Growth Factor In Vitro. Stem Cel. Res. Ther. 6 (1), 44. doi:10.1186/s13287-015-0025-1
Cheng, Z., Wang, L., Qu, M., Liang, H., Li, W., Li, Y., et al. (2018). Mesenchymal Stem Cells Attenuate Blood-Brain Barrier Leakage after Cerebral Ischemia in Mice. J. Neuroinflammation 15 (1), 135. doi:10.1186/s12974-018-1153-1
Chew, J. L., Loh, Y. H., Zhang, W., Chen, X., Tam, W. L., Yeap, L. S., et al. (2005). Reciprocal Transcriptional Regulation of Pou5f1 and Sox2 via the Oct4/Sox2 Complex in Embryonic Stem Cells. Mol. Cell. Biol. 25 (14), 6031–6046. doi:10.1128/MCB.25.14.6031-6046.2005
Cho, I. K., Hunter, C. E., Ye, S., Pongos, A. L., and Chan, A. (2019). Combination of Stem Cell and Gene Therapy Ameliorates Symptoms in Huntington's Disease Mice. NPJ Regenerative Med. 4, 7. doi:10.1038/s41536-019-0066-7
Clinch, S., Busse, M., Döbrössy, M. D., and Dunnett, S. B. (2017). Rehabilitation Training in Neural Restitution. Prog. Brain Res. 230, 305–329. doi:10.1016/bs.pbr.2016.12.006
Connolly, B. S., and Lang, A. E. (2014). Pharmacological Treatment of Parkinson Disease: a Review. JAMA 311 (16), 1670–1683. doi:10.1001/jama.2014.3654
Corey, S., Bonsack, B., and Borlongan, C. V. (2019). Stem Cell-Based Regenerative Medicine for Neurological Disorders: A Special Tribute to Dr. Teng Ma. Brain Circ. 5 (3), 97–100. doi:10.4103/bc.bc_39_19
Corrò, C., Novellasdemunt, L., and Li, V. (2020). A Brief History of Organoids. Am. J. Physiol. Cel Physiol. 319 (1), C151–C165. doi:10.1152/ajpcell.00120.2020
Cova, L., Armentero, M. T., Zennaro, E., Calzarossa, C., Bossolasco, P., Busca, G., et al. (2010). Multiple Neurogenic and Neurorescue Effects of Human Mesenchymal Stem Cell after Transplantation in an Experimental Model of Parkinson's Disease. Brain Res. 1311, 12–27. doi:10.1016/j.brainres.2009.11.041
Crowley, M. G., and Tajiri, N. (2017). Exogenous Stem Cells pioneer a Biobridge to the Advantage of Host Brain Cells Following Stroke: New Insights for Clinical Applications. Brain Circ. 3 (3), 130–134. doi:10.4103/bc.bc_17_17
Cucarián, J. D., Berrío, J. P., Rodrigues, C., Zancan, M., Wink, M. R., and de Oliveira, A. (2019). Physical Exercise and Human Adipose-Derived Mesenchymal Stem Cells Ameliorate Motor Disturbances in a Male Rat Model of Parkinson's Disease. J. Neurosci. Res. 97 (9), 1095–1109. doi:10.1002/jnr.24442
Dayalu, P., and Albin, R. L. (2015). Huntington Disease: Pathogenesis and Treatment. Neurol. Clin. 33 (1), 101–114. doi:10.1016/j.ncl.2014.09.003
de Rus Jacquet, A. (2019). Preparation and Co-culture of iPSC-Derived Dopaminergic Neurons and Astrocytes. Curr. Protoc. Cel. Biol. 85 (1), e98. doi:10.1002/cpcb.98
Döbrössy, M. D., and Dunnett, S. B. (2006a). Morphological and Cellular Changes within Embryonic Striatal Grafts Associated with Enriched Environment and Involuntary Exercise. Eur. J. Neurosci. 24 (11), 3223–3233. doi:10.1111/j.1460-9568.2006.05182.x
Döbrössy, M. D., and Dunnett, S. B. (2005). Optimising Plasticity: Environmental and Training Associated Factors in Transplant-Mediated Brain Repair. Rev. neurosciences 16 (1), 1–21. doi:10.1515/revneuro.2005.16.1.1
Döbrössy, M. D., and Dunnett, S. B. (2006b). The Effects of Lateralized Training on Spontaneous Forelimb Preference, Lesion Deficits, and Graft-Mediated Functional Recovery after Unilateral Striatal Lesions in Rats. Exp. Neurol. 199 (2), 373–383. doi:10.1016/j.expneurol.2005.12.033
Doeppner, T. R., and Hermann, D. M. (2010). Mesenchymal Stem Cells in the Treatment of Ischemic Stroke: Progress and Possibilities. Stem Cell Cloning : Adv. Appl. 3, 157–163. doi:10.2147/SCCAA.S7820
Doetsch, F., Caillé, I., Lim, D. A., García-Verdugo, J. M., and Alvarez-Buylla, A. (1999). Subventricular Zone Astrocytes Are Neural Stem Cells in the Adult Mammalian Brain. Cell 97 (6), 703–716. doi:10.1016/s0092-8674(00)80783-7
Doi, D., Morizane, A., Kikuchi, T., Onoe, H., Hayashi, T., Kawasaki, T., et al. (2012). Prolonged Maturation Culture Favors a Reduction in the Tumorigenicity and the Dopaminergic Function of Human ESC-Derived Neural Cells in a Primate Model of Parkinson's Disease. Stem cells (Dayton, Ohio) 30 (5), 935–945. doi:10.1002/stem.1060
Doi, D., Samata, B., Katsukawa, M., Kikuchi, T., Morizane, A., Ono, Y., et al. (2014). Isolation of Human Induced Pluripotent Stem Cell-Derived Dopaminergic Progenitors by Cell Sorting for Successful Transplantation. Stem Cel. Rep. 2 (3), 337–350. doi:10.1016/j.stemcr.2014.01.013
Doyle, K. P., Simon, R. P., and Stenzel-Poore, M. P. (2008). Mechanisms of Ischemic Brain Damage. Neuropharmacology 55 (3), 310–318. doi:10.1016/j.neuropharm.2008.01.005
Duffy, S. N., Craddock, K. J., Abel, T., and Nguyen, P. V. (2001). Environmental Enrichment Modifies the PKA-Dependence of Hippocampal LTP and Improves Hippocampus-dependent Memory. Learn. Mem. (Cold Spring Harbor, N.Y.) 8 (1), 26–34. doi:10.1101/lm.36301
Dunnett, S. B. (2013). Neural Tissue Transplantation, Repair, and Rehabilitation. Handbook Clin. Neurol. 110, 43–59. doi:10.1016/B978-0-444-52901-5.00004-6
Eckert, A., Huang, L., Gonzalez, R., Kim, H. S., Hamblin, M. H., and Lee, J. P. (2015). Bystander Effect Fuels Human Induced Pluripotent Stem Cell-Derived Neural Stem Cells to Quickly Attenuate Early Stage Neurological Deficits after Stroke. Stem Cell translational Med. 4 (7), 841–851. doi:10.5966/sctm.2014-0184
Eckert, M. A., Vu, Q., Xie, K., Yu, J., Liao, W., Cramer, S. C., et al. (2013). Evidence for High Translational Potential of Mesenchymal Stromal Cell Therapy to Improve Recovery from Ischemic Stroke. J. Cereb. Blood flow Metab. : official J. Int. Soc. Cereb. Blood Flow Metab. 33 (9), 1322–1334. doi:10.1038/jcbfm.2013.91
Eriksson, P. S., Perfilieva, E., Björk-Eriksson, T., Alborn, A. M., Nordborg, C., Peterson, D. A., et al. (1998). Neurogenesis in the Adult Human hippocampus. Nat. Med. 4 (11), 1313–1317. doi:10.1038/3305
Essa, M. M., Moghadas, M., Ba-Omar, T., Walid Qoronfleh, M., Guillemin, G. J., Manivasagam, T., et al. (2019). Protective Effects of Antioxidants in Huntington's Disease: an Extensive Review. Neurotoxicity Res. 35 (3), 739–774. doi:10.1007/s12640-018-9989-9
Feng, Y., Yu, H. M., Shang, D. S., Fang, W. G., He, Z. Y., and Chen, Y. H. (2014). The Involvement of CXCL11 in Bone Marrow-Derived Mesenchymal Stem Cell Migration through Human Brain Microvascular Endothelial Cells. Neurochem. Res. 39 (4), 700–706. doi:10.1007/s11064-014-1257-7
Ferrer, I., Goutan, E., Marín, C., Rey, M. J., and Ribalta, T. (2000). Brain-derived Neurotrophic Factor in Huntington Disease. Brain Res. 866 (1-2), 257–261. doi:10.1016/s0006-8993(00)02237-x
Fisher, B. E., Li, Q., Nacca, A., Salem, G. J., Song, J., Yip, J., et al. (2013). Treadmill Exercise Elevates Striatal Dopamine D2 Receptor Binding Potential in Patients with Early Parkinson's Disease. Neuroreport 24 (10), 509–514. doi:10.1097/WNR.0b013e328361dc13
Freed, C. R., Greene, P. E., Breeze, R. E., Tsai, W. Y., DuMouchel, W., Kao, R., et al. (2001). Transplantation of Embryonic Dopamine Neurons for Severe Parkinson's Disease. New Engl. J. Med. 344 (10), 710–719. doi:10.1056/NEJM200103083441002
Gao, Q., Leung, A., Yang, Y., Wei, Q., Guan, M., Jia, C., et al. (2014). Effects of Tai Chi on Balance and Fall Prevention in Parkinson's Disease: a Randomized Controlled Trial. Clin. Rehabil. 28 (8), 748–753. doi:10.1177/0269215514521044
Garthe, A., Roeder, I., and Kempermann, G. (2016). Mice in an Enriched Environment Learn More Flexibly Because of Adult Hippocampal Neurogenesis. Hippocampus 26 (2), 261–271. doi:10.1002/hipo.22520
Gil, J. M., and Rego, A. C. (2008). Mechanisms of Neurodegeneration in Huntington's Disease. Eur. J. Neurosci. 27 (11), 2803–2820. doi:10.1111/j.1460-9568.2008.06310.x
Girotra, T., Lekoubou, A., Bishu, K. G., and Ovbiagele, B. (2020). A Contemporary and Comprehensive Analysis of the Costs of Stroke in the United States. J. Neurol. Sci. 410, 116643. doi:10.1016/j.jns.2019.116643
Goldberg, N. R., Haack, A. K., and Meshul, C. K. (2011). Enriched Environment Promotes Similar Neuronal and Behavioral Recovery in a Young and Aged Mouse Model of Parkinson's Disease. Neuroscience 172, 443–452. doi:10.1016/j.neuroscience.2010.09.062
Goldman, S. (2005). Stem and Progenitor Cell-Based Therapy of the Human central Nervous System. Nat. Biotechnol. 23 (7), 862–871. doi:10.1038/nbt1119
Grassi, L., Alfonsi, R., Francescangeli, F., Signore, M., De Angelis, M. L., Addario, A., et al. (2019). Organoids as a New Model for Improving Regenerative Medicine and Cancer Personalized Therapy in Renal Diseases. Cel Death Dis. 10 (3), 201. doi:10.1038/s41419-019-1453-0
Grealish, S., Diguet, E., Kirkeby, A., Mattsson, B., Heuer, A., Bramoulle, Y., et al. (2014). Human ESC-Derived Dopamine Neurons Show Similar Preclinical Efficacy and Potency to Fetal Neurons when Grafted in a Rat Model of Parkinson's Disease. Cell stem cell 15 (5), 653–665. doi:10.1016/j.stem.2014.09.017
Guzman, R., De Los Angeles, A., Cheshier, S., Choi, R., Hoang, S., Liauw, J., et al. (2008). Intracarotid Injection of Fluorescence Activated Cell-Sorted CD49d-Positive Neural Stem Cells Improves Targeted Cell Delivery and Behavior after Stroke in a Mouse Stroke Model. Stroke 39 (4), 1300–1306. doi:10.1161/STROKEAHA.107.500470
Habets, J., Heijmans, M., Kuijf, M. L., Janssen, M., Temel, Y., and Kubben, P. L. (2018). An Update on Adaptive Deep Brain Stimulation in Parkinson's Disease. Mov. Disord. : official J. Mov. Disord. Soc. 33 (12), 1834–1843. doi:10.1002/mds.115
Hallett, P. J., Deleidi, M., Astradsson, A., Smith, G. A., Cooper, O., Osborn, T. M., et al. (2015). Successful Function of Autologous iPSC-Derived Dopamine Neurons Following Transplantation in a Non-human Primate Model of Parkinson's Disease. Cell stem cell 16 (3), 269–274. doi:10.1016/j.stem.2015.01.018
Hara, Y. (2015). Brain Plasticity and Rehabilitation in Stroke Patients. J. Nippon Med. Sch. = Nippon Ika Daigaku zasshi 82 (1), 4–13. doi:10.1272/jnms.82.4
Hayes, M. W., Fung, V. S., Kimber, T. E., and O'Sullivan, J. D. (2019). Updates and Advances in the Treatment of Parkinson Disease. Med. J. Aust. 211 (6), 277–283. doi:10.5694/mja2.50224
Hicks, A. U., Hewlett, K., Windle, V., Chernenko, G., Ploughman, M., Jolkkonen, J., et al. (2007). Enriched Environment Enhances Transplanted Subventricular Zone Stem Cell Migration and Functional Recovery after Stroke. Neuroscience 146 (1), 31–40. doi:10.1016/j.neuroscience.2007.01.020
Huang, J., Choudhri, T. F., Winfree, C. J., McTaggart, R. A., Kiss, S., Mocco, J., et al. (2000). Postischemic Cerebrovascular E-Selectin Expression Mediates Tissue Injury in Murine Stroke. Stroke 31 (12), 3047–3053. doi:10.1161/01.str.31.12.3047
Huo, C. C., Zheng, Y., Lu, W. W., Zhang, T. Y., Wang, D. F., Xu, D. S., et al. (2021). Prospects for Intelligent Rehabilitation Techniques to Treat Motor Dysfunction. Neural Regen. Res. 16 (2), 264–269. doi:10.4103/1673-5374.290884
Ives, N. J., Stowe, R. L., Marro, J., Counsell, C., Macleod, A., Clarke, C. E., et al. (2004). Monoamine Oxidase Type B Inhibitors in Early Parkinson's Disease: Meta-Analysis of 17 Randomised Trials Involving 3525 Patients. BMJ (Clinical Res. ed.)AE 329 (7466), 593. doi:10.1136/bmj.38184.60616910.1136/bmj.38184.606169.ae
Jadavji, N. M., Kolb, B., and Metz, G. A. (2006). Enriched Environment Improves Motor Function in Intact and Unilateral Dopamine-Depleted Rats. Neuroscience 140 (4), 1127–1138. doi:10.1016/j.neuroscience.2006.03.027
Jimenez-Sanchez, M., Licitra, F., Underwood, B. R., and Rubinsztein, D. C. (2017). Huntington's Disease: Mechanisms of Pathogenesis and Therapeutic Strategies. Cold Spring Harbor Perspect. Med. 7 (7), a024240. doi:10.1101/cshperspect.a024240
Jung, C. G., Hida, H., Nakahira, K., Ikenaka, K., Kim, H. J., and Nishino, H. (2004). Pleiotrophin mRNA Is Highly Expressed in Neural Stem (Progenitor) Cells of Mouse Ventral Mesencephalon and the Product Promotes Production of Dopaminergic Neurons from Embryonic Stem Cell-Derived Nestin-Positive Cells. FASEB J. : official Publ. Fed. Am. Societies Exp. Biol. 18 (11), 1237–1239. doi:10.1096/fj.03-0927fje
Jungling, A., Reglodi, D., Karadi, Z. N., Horvath, G., Farkas, J., Gaszner, B., et al. (2017). Effects of Postnatal Enriched Environment in a Model of Parkinson's Disease in Adult Rats. Int. J. Mol. Sci. 18 (2), 406. doi:10.3390/ijms18020406
Jungling, A., Reglodi, D., Maasz, G., Zrinyi, Z., Schmidt, J., Rivnyak, A., et al. (2021). Alterations of Nigral Dopamine Levels in Parkinson's Disease after Environmental Enrichment and PACAP Treatment in Aging Rats. Life (Basel, Switzerland) 11 (1), 35. doi:10.3390/life11010035
Kempermann, G. (2020). Delayed Gratification in the Adult Brain. Elife 9, e59786. doi:10.7554/eLife.59786
Khaku, A. S., and Tadi, P. (2021). Cerebrovascular Disease. In: StatPearls [Internet] (Treasure Island (FL): StatPearls Publishing
Kikuchi, T., Morizane, A., Doi, D., Magotani, H., Onoe, H., Hayashi, T., et al. (2017). Human iPS Cell-Derived Dopaminergic Neurons Function in a Primate Parkinson's Disease Model. Nature 548 (7669), 592–596. doi:10.1038/nature23664
Kim, A., Lalonde, K., Truesdell, A., Gomes Welter, P., Brocardo, P. S., Rosenstock, T. R., et al. (2021). New Avenues for the Treatment of Huntington's Disease. Int. J. Mol. Sci. 22 (16), 8363. doi:10.3390/ijms22168363
Kirkeby, A., Grealish, S., Wolf, D. A., Nelander, J., Wood, J., Lundblad, M., et al. (2012). Generation of Regionally Specified Neural Progenitors and Functional Neurons from Human Embryonic Stem Cells under Defined Conditions. Cel Rep. 1 (6), 703–714. doi:10.1016/j.celrep.2012.04.009
Kirkeby, A., Parmar, M., and Barker, R. A. (2017). Strategies for Bringing Stem Cell-Derived Dopamine Neurons to the Clinic: A European Approach (STEM-PD). Prog. Brain Res. 230, 165–190. doi:10.1016/bs.pbr.2016.11.011
Klaissle, P., Lesemann, A., Huehnchen, P., Hermann, A., Storch, A., and Steiner, B. (2012). Physical Activity and Environmental Enrichment Regulate the Generation of Neural Precursors in the Adult Mouse Substantia Nigra in a Dopamine-dependent Manner. BMC Neurosci. 13, 132. doi:10.1186/1471-2202-13-132
Komitova, M., Zhao, L. R., Gidö, G., Johansson, B. B., and Eriksson, P. (2005). Postischemic Exercise Attenuates whereas Enriched Environment Has Certain Enhancing Effects on Lesion-Induced Subventricular Zone Activation in the Adult Rat. Eur. J. Neurosci. 21 (9), 2397–2405. doi:10.1111/j.1460-9568.2005.04072.x
Korkhin, A., Zubedat, S., Aga-Mizrachi, S., and Avital, A. (2020). Developmental Effects of Environmental Enrichment on Selective and Auditory Sustained Attention. Psychoneuroendocrinology 111, 104479. doi:10.1016/j.psyneuen.2019.104479
Kozlowski, D. A., James, D. C., and Schallert, T. (1996). Use-dependent Exaggeration of Neuronal Injury after Unilateral Sensorimotor Cortex Lesions. J. Neurosci. 16 (15), 4776–4786. doi:10.1523/JNEUROSCI.16-15-04776.1996
Kuang, S., Zheng, J., Yang, H., Li, S., Duan, S., Shen, Y., et al. (2017). Structure Insight of GSDMD Reveals the Basis of GSDMD Autoinhibition in Cell Pyroptosis. Proc. Natl. Acad. Sci. United States America 114 (40), 10642–10647. doi:10.1073/pnas.1708194114
Kumar, A., Kumar, V., Singh, K., Kumar, S., Kim, Y. S., Lee, Y. M., et al. (2020). Therapeutic Advances for Huntington's Disease. Brain Sci. 10 (1), 43. doi:10.3390/brainsci10010043
Kuriakose, D., and Xiao, Z. (2020). Pathophysiology and Treatment of Stroke: Present Status and Future Perspectives. Ijms 21 (20), 7609. doi:10.3390/ijms21207609
Laywell, E. D., Steindler, D. A., and Silver, D. J. (2007). Astrocytic Stem Cells in the Adult Brain. Neurosurg. Clin. North America 18 (1), 21–viii. doi:10.1016/j.nec.2006.10.003
Lazic, S. E., Grote, H. E., Blakemore, C., Hannan, A. J., van Dellen, A., Phillips, W., et al. (2006). Neurogenesis in the R6/1 Transgenic Mouse Model of Huntington's Disease: Effects of Environmental Enrichment. Eur. J. Neurosci. 23 (7), 1829–1838. doi:10.1111/j.1460-9568.2006.04715.x
Lee, J. Y., Kim, E., Choi, S. M., Kim, D. W., Kim, K. P., Lee, I., et al. (2016). Microvesicles from Brain-Extract-Treated Mesenchymal Stem Cells Improve Neurological Functions in a Rat Model of Ischemic Stroke. Scientific Rep. 6, 33038. doi:10.1038/srep33038
Lee, S. H., Kim, Y. H., Kim, Y. J., and Yoon, B. W. (2008). Enforced Physical Training Promotes Neurogenesis in the Subgranular Zone after Focal Cerebral Ischemia. J. Neurol. Sci. 269 (1-2), 54–61. doi:10.1016/j.jns.2007.12.028
Li, C., Ke, C., Su, Y., and Wan, C. (2021). Exercise Intervention Promotes the Growth of Synapses and Regulates Neuroplasticity in Rats with Ischemic Stroke through Exosomes. Front. Neurol. 12, 752595. doi:10.3389/fneur.2021.752595
Li, F., Harmer, P., Fitzgerald, K., Eckstrom, E., Stock, R., Galver, J., et al. (2012). Tai Chi and Postural Stability in Patients with Parkinson's Disease. New Engl. J. Med. 366 (6), 511–519. doi:10.1056/NEJMoa1107911
Liska, M. G., Crowley, M. G., and Borlongan, C. V. (2017). Regulated and Unregulated Clinical Trials of Stem Cell Therapies for Stroke. Translational stroke Res. 8 (2), 93–103. doi:10.1007/s12975-017-0522-x
Liu, J., Zheng, J., Xu, Y., Cao, W., Wang, J., Wang, B., et al. (2021). Enriched Environment Attenuates Pyroptosis to Improve Functional Recovery after Cerebral Ischemia/Reperfusion Injury. Front. Aging Neurosci. 13, 717644. doi:10.3389/fnagi.2021.717644
Luo, J., Hu, X., Zhang, L., Li, L., Zheng, H., Li, M., et al. (2014). Physical Exercise Regulates Neural Stem Cells Proliferation and Migration via SDF-1α/CXCR4 Pathway in Rats after Ischemic Stroke. Neurosci. Lett. 578, 203–208. doi:10.1016/j.neulet.2014.06.059
Mak, M. K., Wong-Yu, I. S., Shen, X., and Chung, C. L. (2017). Long-term Effects of Exercise and Physical Therapy in People with Parkinson Disease. Nat. Rev. Neurol. 13 (11), 689–703. doi:10.1038/nrneurol.2017.128
Mandalaneni, K., Rayi, A., and Jillella, D. V. (2021). “Stroke Reperfusion Injury,” in StatPearls (Treasure Island, FL: StatPearls Publishing).
Mang, C. S., Campbell, K. L., Ross, C. J., and Boyd, L. A. (2013). Promoting Neuroplasticity for Motor Rehabilitation after Stroke: Considering the Effects of Aerobic Exercise and Genetic Variation on Brain-Derived Neurotrophic Factor. Phys. Ther. 93 (12), 1707–1716. doi:10.2522/ptj.20130053
Markus, H. S. (2004). Cerebral Perfusion and Stroke. J. Neurol. Neurosurg. Psychiatry 75 (3), 353–361. doi:10.1136/jnnp.2003.025825
McGregor, M. M., and Nelson, A. B. (2019). Circuit Mechanisms of Parkinson's Disease. Neuron 101 (6), 1042–1056. doi:10.1016/j.neuron.2019.03.004
Mertens, J., Reid, D., Lau, S., Kim, Y., and Gage, F. H. (2018). Aging in a Dish: iPSC-Derived and Directly Induced Neurons for Studying Brain Aging and Age-Related Neurodegenerative Diseases. Annu. Rev. Genet. 52, 271–293. doi:10.1146/annurev-genet-120417-031534
Ming, G. L., and Song, H. (2011). Adult Neurogenesis in the Mammalian Brain: Significant Answers and Significant Questions. Neuron 70 (4), 687–702. doi:10.1016/j.neuron.2011.05.001
Miura, M., Miura, Y., Padilla-Nash, H. M., Molinolo, A. A., Fu, B., Patel, V., et al. (2006). Accumulated Chromosomal Instability in Murine Bone Marrow Mesenchymal Stem Cells Leads to Malignant Transformation. Stem cells (Dayton, Ohio) 24 (4), 1095–1103. doi:10.1634/stemcells.2005-0403
Molcanyi, M., Riess, P., Bentz, K., Maegele, M., Hescheler, J., Schäfke, B., et al. (2007). Trauma-associated Inflammatory Response Impairs Embryonic Stem Cell Survival and Integration after Implantation into Injured Rat Brain. J. neurotrauma 24 (4), 625–637. doi:10.1089/neu.2006.0180
Monk, R., and Connor, B. (2020). Cell Replacement Therapy for Huntington's Disease. Adv. Exp. Med. Biol. 1266, 57–69. doi:10.1007/978-981-15-4370-8_5
Moore, D. J., West, A. B., Dawson, V. L., and Dawson, T. M. (2005). Molecular Pathophysiology of Parkinson's Disease. Annu. Rev. Neurosci. 28, 57–87. doi:10.1146/annurev.neuro.28.061604.135718
Morishita, S., Hokamura, K., Yoshikawa, A., Agata, N., Tsutsui, Y., Umemura, K., et al. (2020). Different Exercises Can Modulate the Differentiation/maturation of Neural Stem/progenitor Cells after Photochemically Induced Focal Cerebral Infarction. Brain Behav. 10 (3), e01535. doi:10.1002/brb3.1535
Morizane, A., Kikuchi, T., Hayashi, T., Mizuma, H., Takara, S., Doi, H., et al. (2017). MHC Matching Improves Engraftment of iPSC-Derived Neurons in Non-human Primates. Nat. Commun. 8 (1), 385. doi:10.1038/s41467-017-00926-5
Muoio, V., Persson, P. B., and Sendeski, M. M. (2014). The Neurovascular Unit - Concept Review. Acta Physiol. (Oxford, England) 210 (4), 790–798. doi:10.1111/apha.12250
Nance, M., Paulsen, J. S., Rosenblatt, A., and Wheelock, V. (2011). in A Physician’s Guide to the Management of Huntington’s Disease. Editors D. Lovecky, and K. Tarapata. 3rd ed. (Kitchener, ON, Canada: Huntington’s disease Society of America).
Nikkhah, G., Falkenstein, G., and Rosenthal, C. (2001). Restorative Plasticity of Dopamine Neuronal Transplants Depends on the Degree of Hemispheric Dominance. J. Neurosci. 21 (16), 6252–6263. doi:10.1523/JNEUROSCI.21-16-06252.2001
Nithianantharajah, J., Barkus, C., Murphy, M., and Hannan, A. J. (2008). Gene-environment Interactions Modulating Cognitive Function and Molecular Correlates of Synaptic Plasticity in Huntington's Disease Transgenic Mice. Neurobiol. Dis. 29 (3), 490–504. doi:10.1016/j.nbd.2007.11.006
Niwa, H., Miyazaki, J., and Smith, A. G. (2000). Quantitative Expression of Oct-3/4 Defines Differentiation, Dedifferentiation or Self-Renewal of ES Cells. Nat. Genet. 24 (4), 372–376. doi:10.1038/74199
Nogueira, R. G., Jadhav, A. P., Haussen, D. C., Bonafe, A., Budzik, R. F., Bhuva, P., et al. DAWN Trial Investigators (2018). Thrombectomy 6 to 24 hours after Stroke with a Mismatch between Deficit and Infarct. New Engl. J. Med., 378(1), 11–21. doi:10.1056/NEJMoa1706442
Nygren, J., Wieloch, T., Pesic, J., Brundin, P., and Deierborg, T. (2006). Enriched Environment Attenuates Cell Genesis in Subventricular Zone after Focal Ischemia in Mice and Decreases Migration of Newborn Cells to the Striatum. Stroke 37 (11), 2824–2829. doi:10.1161/01.str.000024476910.1161/01.str.0000244769.39952.90
Okita, K., Ichisaka, T., and Yamanaka, S. (2007). Generation of Germline-Competent Induced Pluripotent Stem Cells. Nature 448 (7151), 313–317. doi:10.1038/nature05934
Pang, T., Stam, N. C., Nithianantharajah, J., Howard, M. L., and Hannan, A. J. (2006). Differential Effects of Voluntary Physical Exercise on Behavioral and Brain-Derived Neurotrophic Factor Expression Deficits in Huntington's Disease Transgenic Mice. Neuroscience 141 (2), 569–584. doi:10.1016/j.neuroscience.2006.04.013
Pantcheva, P., Reyes, S., Hoover, J., Kaelber, S., and Borlongan, C. V. (2015). Treating Non-motor Symptoms of Parkinson's Disease with Transplantation of Stem Cells. Expert Rev. neurotherapeutics 15 (10), 1231–1240. doi:10.1586/14737175.2015.1091727
Park, I. H., Arora, N., Huo, H., Maherali, N., Ahfeldt, T., Shimamura, A., et al. (2008). Disease-specific Induced Pluripotent Stem Cells. Cell 134 (5), 877–886. doi:10.1016/j.cell.2008.07.041
Pintori, N., Piva, A., Guardiani, V., Decimo, I., and Chiamulera, C. (2022). Brief Environmental Enrichment Exposure Enhances Contextual-Induced Sucrose-Seeking with and without Memory Reactivation in Rats. Behav. Brain Res. 416, 113556. doi:10.1016/j.bbr.2021.113556
Pittenger, M. F., Mackay, A. M., Beck, S. C., Jaiswal, R. K., Douglas, R., and Mosca, J. D. (1999). Multilineage Potential of Adult Human Mesenchymal Stem Cells. Science 284 (5411), 143–147. doi:10.1126/science.284.5411.143
Playne, R., and Connor, B. (2017). Understanding Parkinson's Disease through the Use of Cell Reprogramming. Stem Cel. Rev. Rep. 13 (2), 151–169. doi:10.1007/s12015-017-9717-5
Ploughman, M., Windle, V., MacLellan, C. L., White, N., Doré, J. J., and Corbett, D. (2009). Brain-derived Neurotrophic Factor Contributes to Recovery of Skilled Reaching after Focal Ischemia in Rats. Stroke 40 (4), 1490–1495. doi:10.1161/STROKEAHA.108.531806
Pradhan, S. (2019). The Use of Commercially Available Games for a Combined Physical and Cognitive challenge during Exercise for Individuals with Parkinson's Disease - a Case Series Report. Physiother. Theor. Pract. 35 (4), 355–362. doi:10.1080/09593985.2018.1444118
Qin, J., Song, B., Zhang, H., Wang, Y., Wang, N., Ji, Y., et al. (2013). Transplantation of Human Neuro-epithelial-like Stem Cells Derived from Induced Pluripotent Stem Cells Improves Neurological Function in Rats with Experimental Intracerebral Hemorrhage. Neurosci. Lett. 548, 95–100. doi:10.1016/j.neulet.2013.05.007
Rafuse, V. F., Soundararajan, P., Leopold, C., and Robertson, H. A. (2005). Neuroprotective Properties of Cultured Neural Progenitor Cells Are Associated with the Production of Sonic Hedgehog. Neuroscience 131 (4), 899–916. doi:10.1016/j.neuroscience.2004.11.048
Rampon, C., Jiang, C. H., Dong, H., Tang, Y. P., Lockhart, D. J., and Schultz, P. G. (2000). Effects of Environmental Enrichment on Gene Expression in the Brain. Proc Natl Acad Sci U S A 97 (23), 12880–12884. doi:10.1073/pnas.97.23.12880
Rattazzi, L., Piras, G., Brod, S., Smith, K., Ono, M., and D'Acquisto, F. (2016). Impact of Enriched Environment on Murine T Cell Differentiation and Gene Expression Profile. Front. Immunol. 7, 381. doi:10.3389/fimmu.2016.00381
Raymond, L. A., André, V. M., Cepeda, C., Gladding, C. M., Milnerwood, A. J., and Levine, M. S. (2011). Pathophysiology of Huntington's Disease: Time-dependent Alterations in Synaptic and Receptor Function. Neuroscience 198, 252–273. doi:10.1016/j.neuroscience.2011.08.052
Reeve, A., Simcox, E., and Turnbull, D. (2014). Ageing and Parkinson's Disease: Why Is Advancing Age the Biggest Risk Factor? Ageing Res. Rev. 14 (100), 19–30. doi:10.1016/j.arr.2014.01.004
Reynolds, B. A., and Weiss, S. (1992). Generation of Neurons and Astrocytes from Isolated Cells of the Adult Mammalian central Nervous System. Science (New York, N.Y.) 255 (5052), 1707–1710. doi:10.1126/science.1553558
Rezaee, Z., Marandi, S. M., Alaei, H., and Esfarjani, F. (2020). Exercise-Induced Neuroprotection in the 6-Hydroxydopamine Parkinson's Disease Model. Neurotoxicity Res. 38 (4), 850–858. doi:10.1007/s12640-020-00189-x
Ries, C., Egea, V., Karow, M., Kolb, H., Jochum, M., and Neth, P. (2007). MMP-2, MT1-MMP, and TIMP-2 Are Essential for the Invasive Capacity of Human Mesenchymal Stem Cells: Differential Regulation by Inflammatory Cytokines. Blood 109 (9), 4055–4063. doi:10.1182/blood-2006-10-051060
Risedal, A., Zeng, J., and Johansson, B. B. (1999). Early Training May Exacerbate Brain Damage after Focal Brain Ischemia in the Rat. J. Cereb. Blood flow Metab. : official J. Int. Soc. Cereb. Blood Flow Metab. 19 (9), 997–1003. doi:10.1097/00004647-199909000-00007
Rizek, P., Kumar, N., and Jog, M. S. (2016). An Update on the Diagnosis and Treatment of Parkinson Disease. CMAJ : Can. Med. Assoc. J. = J. de l'Association medicale canadienne 188 (16), 1157–1165. doi:10.1503/cmaj.151179
Ross, A. L., Leder, D. E., Weiss, J., Izakovic, J., and Grichnik, J. M. (2011). Genomic Instability in Cultured Stem Cells: Associated Risks and Underlying Mechanisms. Regenerative Med. 6 (5), 653–662. doi:10.2217/rme.11.44
Rossi, G., Manfrin, A., and Lutolf, M. P. (2018). Progress and Potential in Organoid Research. Nat. Rev. Genet. 19 (11), 671–687. doi:10.1038/s41576-018-0051-9
Roy, N. S., Cleren, C., Singh, S. K., Yang, L., Beal, M. F., and Goldman, S. A. (2006). Functional Engraftment of Human ES Cell-Derived Dopaminergic Neurons Enriched by Coculture with Telomerase-Immortalized Midbrain Astrocytes. Nat. Med. 12 (11), 1259–1268. doi:10.1038/nm1495
Saft, M., Gonzales-Portillo, B., Park, Y. J., Cozene, B., Sadanandan, N., Cho, J., et al. (2020). Stem Cell Repair of the Microvascular Damage in Stroke. Cells 9 (9), 2075. doi:10.3390/cells9092075
Sasaki, Y., Sasaki, M., Kataoka-Sasaki, Y., Nakazaki, M., Nagahama, H., Suzuki, J., et al. (2016). Synergic Effects of Rehabilitation and Intravenous Infusion of Mesenchymal Stem Cells after Stroke in Rats. Phys. Ther. 96 (11), 1791–1798. doi:10.2522/ptj.20150504
Schwab, R. S., Poskanzer, D. C., England, A. C., and Young, R. R. (1972). Amantadine in Parkinson's Disease. Review of More Than Two Years' Experience. JAMA 222 (7), 792–795. doi:10.1001/jama.222.7.79210.1001/jama.1972.03210070026008
Schweitzer, J. S., Song, B., Herrington, T. M., Park, T. Y., Lee, N., Ko, S., et al. (2020). Personalized iPSC-Derived Dopamine Progenitor Cells for Parkinson's Disease. New Engl. J. Med. 382 (20), 1926–1932. doi:10.1056/NEJMoa1915872
Shen, X., and Mak, M. K. (2015). Technology-assisted Balance and Gait Training Reduces Falls in Patients with Parkinson's Disease: a Randomized Controlled Trial with 12-month Follow-Up. Neurorehabil. Neural Repair 29 (2), 103–111. doi:10.1177/1545968314537559
Shichinohe, H., Ishihara, T., Takahashi, K., Tanaka, Y., Miyamoto, M., Yamauchi, T., et al. (2015). Bone Marrow Stromal Cells rescue Ischemic Brain by Trophic Effects and Phenotypic Change toward Neural Cells. Neurorehabil. Neural Repair 29 (1), 80–89. doi:10.1177/1545968314525856
Simmnacher, K., Lanfer, J., Rizo, T., Kaindl, J., and Winner, B. (2020). Modeling Cell-Cell Interactions in Parkinson's Disease Using Human Stem Cell-Based Models. Front. Cell. Neurosci. 13, 571. doi:10.3389/fncel.2019.00571
Simon, D. K., Tanner, C. M., and Brundin, P. (2020). Parkinson Disease Epidemiology, Pathology, Genetics, and Pathophysiology. Clin. Geriatr. Med. 36 (1), 1–12. doi:10.1016/j.cger.2019.08.002
Singhal, G., Jaehne, E. J., Corrigan, F., and Baune, B. T. (2014). Cellular and Molecular Mechanisms of Immunomodulation in the Brain through Environmental Enrichment. Front. Cell. Neurosci. 8, 97. doi:10.3389/fncel.2014.00097
Song, B., Cha, Y., Ko, S., Jeon, J., Lee, N., Seo, H., et al. (2020). Human Autologous iPSC-Derived Dopaminergic Progenitors Restore Motor Function in Parkinson's Disease Models. J. Clin. Invest. 130 (2), 904–920. doi:10.1172/JCI130767
Song, J. J., Oh, S. M., Kwon, O. C., Wulansari, N., Lee, H. S., Chang, M. Y., et al. (2018). Cografting Astrocytes Improves Cell Therapeutic Outcomes in a Parkinson's Disease Model. J. Clin. Invest. 128 (1), 463–482. doi:10.1172/JCI93924
Spires, T. L., Grote, H. E., Varshney, N. K., Cordery, P. M., van Dellen, A., Blakemore, C., et al. (2004). Environmental Enrichment Rescues Protein Deficits in a Mouse Model of Huntington's Disease, Indicating a Possible Disease Mechanism. J. Neurosci. 24 (9), 2270–2276. doi:10.1523/JNEUROSCI.1658-03.2004
Stoddard-Bennett, T., and Reijo Pera, R. (2019). Treatment of Parkinson's Disease through Personalized Medicine and Induced Pluripotent Stem Cells. Cells 8 (1), 26. doi:10.3390/cells8010026
Stoker, T. B., and Barker, R. A. (2020). Recent Developments in the Treatment of Parkinson's Disease. F1000Research 9, F1000. doi:10.12688/f1000research.25634
Stonesifer, C., Corey, S., Ghanekar, S., Diamandis, Z., Acosta, S. A., and Borlongan, C. V. (2017). Stem Cell Therapy for Abrogating Stroke-Induced Neuroinflammation and Relevant Secondary Cell Death Mechanisms. Prog. Neurobiol. 158, 94–131. doi:10.1016/j.pneurobio.2017.07.004
Tajiri, N., Acosta, S., Glover, L. E., Bickford, P. C., Jacotte Simancas, A., Yasuhara, T., et al. (2012). Intravenous Grafts of Amniotic Fluid-Derived Stem Cells Induce Endogenous Cell Proliferation and Attenuate Behavioral Deficits in Ischemic Stroke Rats. PloS one 7 (8), e43779. doi:10.1371/journal.pone.0043779
Tajiri, N., Kaneko, Y., Shinozuka, K., Ishikawa, H., Yankee, E., McGrogan, M., et al. (2013). Stem Cell Recruitment of Newly Formed Host Cells via a Successful Seduction? Filling the gap between Neurogenic Niche and Injured Brain Site. PloS one 8 (9), e748doi. doi:10.1371/journal.pone.0074857
Tajiri, N., Yasuhara, T., Shingo, T., Kondo, A., Yuan, W., Kadota, T., et al. (2010). Exercise Exerts Neuroprotective Effects on Parkinson's Disease Model of Rats. Brain Res. 1310, 200–207. doi:10.1016/j.brainres.2009.10.075
Takahashi, J. (2018). Stem Cells and Regenerative Medicine for Neural Repair. Curr. Opin. Biotechnol. 52, 102–108. doi:10.1016/j.copbio.2018.03.006
Takahashi, K., and Yamanaka, S. (2006). Induction of Pluripotent Stem Cells from Mouse Embryonic and Adult Fibroblast Cultures by Defined Factors. Cell 126 (4), 663–676. doi:10.1016/j.cell.2006.07.024
Tang, Y., Li, M. Y., Zhang, X., Jin, X., Liu, J., and Wei, P. H. (2019). Delayed Exposure to Environmental Enrichment Improves Functional Outcome after Stroke. J. Pharmacol. Sci. 140 (2), 137–143. doi:10.1016/j.jphs.2019.05.002
Tobin, M. K., Stephen, T., Lopez, K. L., Pergande, M. R., Bartholomew, A. M., Cologna, S. M., et al. (2020). Activated Mesenchymal Stem Cells Induce Recovery Following Stroke via Regulation of Inflammation and Oligodendrogenesis. J. Am. Heart Assoc. 9 (7), e013583. doi:10.1161/JAHA.119.013583
Torikoshi, S., Morizane, A., Shimogawa, T., Samata, B., Miyamoto, S., and Takahashi, J. (2020). Exercise Promotes Neurite Extensions from Grafted Dopaminergic Neurons in the Direction of the Dorsolateral Striatum in Parkinson's Disease Model Rats. J. Parkinson's Dis. 10 (2), 511–521. doi:10.3233/JPD-191755
Tsai, W. L., Chen, H. Y., Huang, Y. Z., Chen, Y. H., Kuo, C. W., Chen, K. Y., et al. (20192019). Long-Term Voluntary Physical Exercise Exerts Neuroprotective Effects and Motor Disturbance Alleviation in a Rat Model of Parkinson's Disease. Behav. Neurol., 4829572. doi:10.1155/2019/4829572
van Dellen, A., Blakemore, C., Deacon, R., York, D., and Hannan, A. J. (2000). Delaying the Onset of Huntington's in Mice. Nature 404 (6779), 721–722. doi:10.1038/35008142
van Praag, H., Kempermann, G., and Gage, F. H. (1999). Running Increases Cell Proliferation and Neurogenesis in the Adult Mouse Dentate Gyrus. Nat. Neurosci. 2 (3), 266–270. doi:10.1038/6368
Walker, F. O. (2007). Huntington's Disease. Lancet (London, England) 369 (9557), 218–228. doi:10.1016/S0140-6736(07)60111-1
Wang, F., Yasuhara, T., Shingo, T., Kameda, M., Tajiri, N., Yuan, W. J., et al. (2010). Intravenous Administration of Mesenchymal Stem Cells Exerts Therapeutic Effects on Parkinsonian Model of Rats: Focusing on Neuroprotective Effects of Stromal Cell-Derived Factor-1alpha. BMC Neurosci. 11, 52. doi:10.1186/1471-2202-11-52
Wang, Y. K., Zhu, W. W., Wu, M. H., Wu, Y. H., Liu, Z. X., Liang, L. M., et al. (2018). Human Clinical-Grade Parthenogenetic ESC-Derived Dopaminergic Neurons Recover Locomotive Defects of Nonhuman Primate Models of Parkinson's Disease. Stem Cel. Rep. 11 (1), 171–182. doi:10.1016/j.stemcr.2018.05.010
Wassouf, Z., Hentrich, T., Samer, S., Rotermund, C., Kahle, P. J., Ehrlich, I., et al. (2018). Environmental Enrichment Prevents Transcriptional Disturbances Induced by Alpha-Synuclein Overexpression. Front. Cell. Neurosci. 12, 112. doi:10.3389/fncel.2018.00112
Waterman, R. S., Tomchuck, S. L., Henkle, S. L., and Betancourt, A. M. (2010). A New Mesenchymal Stem Cell (MSC) Paradigm: Polarization into a Pro-Inflammatory MSC1 or an Immunosuppressive MSC2 Phenotype. PLoS One 5 (4), e10088. doi:10.1371/journal.pone.0010088
Weiss, M. L., Medicetty, S., Bledsoe, A. R., Rachakatla, R. S., Choi, M., Merchav, S., et al. (2006). Human Umbilical Cord Matrix Stem Cells: Preliminary Characterization and Effect of Transplantation in a Rodent Model of Parkinson's Disease. Stem cells (Dayton, Ohio) 24 (3), 781–792. doi:10.1634/stemcells.2005-0330
Wi, S., Lee, J. W., Kim, M., Park, C. H., and Cho, S. R. (2018). An Enriched Environment Ameliorates Oxidative Stress and Olfactory Dysfunction in Parkinson's Disease with α-Synucleinopathy. Cel Transplant. 27 (5), 831–839. doi:10.1177/0963689717742662
Wong-Yu, I. S., and Mak, M. K. (2015). Multi-dimensional Balance Training Programme Improves Balance and Gait Performance in People with Parkinson's Disease: A Pragmatic Randomized Controlled Trial with 12-month Follow-Up. Parkinsonism Relat. Disord. 21 (6), 615–621. doi:10.1016/j.parkreldis.2015.03.022
Wood, N. I., Glynn, D., and Morton, A. J. (2011). Brain Training" Improves Cognitive Performance and Survival in a Transgenic Mouse Model of Huntington's Disease. Neurobiol. Dis. 42 (3), 427–437. doi:10.1016/j.nbd.2011.02.005
Wyant, K. J., Ridder, A. J., and Dayalu, P. (2017). Huntington's Disease-Update on Treatments. Curr. Neurol. Neurosci. Rep. 17 (4), 33. doi:10.1007/s11910-017-0739-9
Xie, Q., Cheng, J., Pan, G., Wu, S., Hu, Q., Jiang, H., et al. (2019). Treadmill Exercise Ameliorates Focal Cerebral Ischemia/reperfusion-Induced Neurological Deficit by Promoting Dendritic Modification and Synaptic Plasticity via Upregulating Caveolin-1/VEGF Signaling Pathways. Exp. Neurol. 313, 60–78. doi:10.1016/j.expneurol.2018.12.005
Yang, L., Zhang, J., Deng, Y., and Zhang, P. (2017). The Effects of Early Exercise on Motor, Sense, and Memory Recovery in Rats with Stroke. Am. J. Phys. Med. Rehabil. 96 (3), e36–e43. doi:10.1097/PHM.0000000000000670
Yasuhara, T., Kameda, M., Sasaki, T., Tajiri, N., and Date, I. (2017). Cell Therapy for Parkinson's Disease. Cel Transplant. 26 (9), 1551–1559. doi:10.1177/0963689717735411
Yasuhara, T., Matsukawa, N., Hara, K., Yu, G., Xu, L., Maki, M., et al. (2006). Transplantation of Human Neural Stem Cells Exerts Neuroprotection in a Rat Model of Parkinson's Disease. J. Neurosci. 26 (48), 12497–12511. doi:10.1523/JNEUROSCI.3719-06.2006
Yilmaz, G., Vital, S., Yilmaz, C. E., Stokes, K. Y., Alexander, J. S., and Granger, D. N. (2011). Selectin-mediated Recruitment of Bone Marrow Stromal Cells in the Postischemic Cerebral Microvasculature. Stroke 42 (3), 806–811. doi:10.1161/STROKEAHA.110.597088
Yu, K., Wu, Y., Zhang, Q., Xie, H., Liu, G., Guo, Z., et al. (2014). Enriched Environment Induces Angiogenesis and Improves Neural Function Outcomes in Rat Stroke Model. J. Neurol. Sci. 347 (1-2), 275–280. doi:10.1016/j.jns.2014.10.022
Yuan, Z. Y., Yang, J., Ma, X. W., Wang, Y. Y., and Wang, M. W. (20181994). Enriched Environment Elevates Expression of Growth Associated Protein-43 in the Substantia Nigra of SAMP8 Mice. Neural Regen. Res. 13 (11), 1988. doi:10.4103/1673-5374.239447
Zamanlu, M., Farhoudi, M., Eskandani, M., Mahmoudi, J., Barar, J., Rafi, M., et al. (2018). Recent Advances in Targeted Delivery of Tissue Plasminogen Activator for Enhanced Thrombolysis in Ischaemic Stroke. J. Drug Target. 26 (2), 95–109. doi:10.1080/1061186X.2017.1365874
Zhan, Y., Li, M. Z., Yang, L., Feng, X. F., Lei, J. F., Zhang, N., et al. (2020). The Three-phase Enriched Environment Paradigm Promotes Neurovascular Restorative and Prevents Learning Impairment after Ischemic Stroke in Rats. Neurobiol. Dis. 146, 105091. doi:10.1016/j.nbd.2020.105091
Zhang, S., Lachance, B. B., Moiz, B., and Jia, X. (2020). Optimizing Stem Cell Therapy after Ischemic Brain Injury. J. stroke 22 (3), 286–305. doi:10.5853/jos.2019.03048
Zhang, T. Y., Keown, C. L., Wen, X., Li, J., Vousden, D. A., Anacker, C., et al. (2018). Environmental Enrichment Increases Transcriptional and Epigenetic Differentiation between Mouse Dorsal and Ventral Dentate Gyrus. Nat. Commun. 9 (1), 298. doi:10.1038/s41467-017-02748-x
Zhang, Y. X., Yuan, M. Z., Cheng, L., Lin, L. Z., Du, H. W., Chen, R. H., et al. (2015). Treadmill Exercise Enhances Therapeutic Potency of Transplanted Bone Mesenchymal Stem Cells in Cerebral Ischemic Rats via Anti-apoptotic Effects. BMC Neurosci. 16, 56. doi:10.1186/s12868-015-0196-9
Zhao, C., Jou, J., Wolff, L. J., Sun, H., and Gage, F. H. (2015). Spine Morphogenesis in Newborn Granule Cells Is Differentially Regulated in the Outer and Middle Molecular Layers. J. Comp. Neurol. 523 (10), 1588. doi:10.1002/cne.23800
Zheng, P., and Kozloski, J. (2017). Striatal Network Models of Huntington's Disease Dysfunction Phenotypes. Front. Comput. Neurosci. 11, 70. doi:10.3389/fncom.2017.00070
Zhou, G., Wang, Y., Gao, S., Fu, X., Cao, Y., and Peng, Y. (2021). Potential Mechanisms and Perspectives in Ischemic Stroke Treatment Using Stem Cell Therapies. Front Cel Dev Biol 9, 646927. doi:10.3389/fcell.2021.646927
Zocher, S., Overall, R. W., Lesche, M., Dahl, A., and Kempermann, G. (2021). Environmental Enrichment Preserves a Young DNA Methylation Landscape in the Aged Mouse hippocampus. Nat. Commun. 12 (1), 3892. doi:10.1038/s41467-021-23993-1
Keywords: stem cell, rehabiliatation, enriched enviroment, exercise, combination therapy, Parkinson’s disease, huntingtons’s disease, stroke
Citation: Berlet R, Galang Cabantan DA, Gonzales-Portillo D and Borlongan CV (2022) Enriched Environment and Exercise Enhance Stem Cell Therapy for Stroke, Parkinson’s Disease, and Huntington’s Disease. Front. Cell Dev. Biol. 10:798826. doi: 10.3389/fcell.2022.798826
Received: 20 October 2021; Accepted: 01 February 2022;
Published: 03 March 2022.
Edited by:
Marietta Herrmann, University Hospital Würzburg, GermanyReviewed by:
Julien Rossignol, Central Michigan University, United StatesCopyright © 2022 Berlet, Galang Cabantan, Gonzales-Portillo and Borlongan. This is an open-access article distributed under the terms of the Creative Commons Attribution License (CC BY). The use, distribution or reproduction in other forums is permitted, provided the original author(s) and the copyright owner(s) are credited and that the original publication in this journal is cited, in accordance with accepted academic practice. No use, distribution or reproduction is permitted which does not comply with these terms.
*Correspondence: Cesar V. Borlongan, Y2JvcmxvbmdAdXNmLmVkdQ==
†These authors have contributed equally to this work and share first authorship
Disclaimer: All claims expressed in this article are solely those of the authors and do not necessarily represent those of their affiliated organizations, or those of the publisher, the editors and the reviewers. Any product that may be evaluated in this article or claim that may be made by its manufacturer is not guaranteed or endorsed by the publisher.
Research integrity at Frontiers
Learn more about the work of our research integrity team to safeguard the quality of each article we publish.