- 1Red Sea Research Center (RSRC) and Computational Bioscience Research Center (CBRC), King Abdullah University of Science and Technology, Thuwal, Saudi Arabia
- 2Biological and Environmental Science and Engineering Division (BESE), King Abdullah University of Science and Technology, Thuwal, Saudi Arabia
The characterization, control, and reporting of environmental conditions in mammalian cell cultures is fundamental to ensure physiological relevance and reproducibility in basic and preclinical biomedical research. The potential issue of environment instability in routine cell cultures in affecting biomedical experiments was identified many decades ago. Despite existing evidence showing variable environmental conditions can affect a suite of cellular responses and key experimental readouts, the underreporting of critical parameters affecting cell culture environments in published experiments remains a serious problem. Here, we outline the main sources of potential problems, improved guidelines for reporting, and deliver recommendations to facilitate improved culture-system based research. Addressing the lack of attention paid to culture environments is critical to improve the reproducibility and translation of preclinical research, but constitutes only an initial step towards enhancing the relevance of in vitro cell cultures towards in vivo physiology.
Introduction
Mammalian cell cultures have been a foundational resource in almost every biomedical research program since the 1990s (Petricciani, 1995; Hu and Aunins, 1997; Merten, 2006). The use of mammalian cell cultures as preclinical models ranges from the characterization of in vivo physiological mechanisms and manipulation of disease-related pathways to the maintenance of stem cells for therapeutic purposes. Culture systems are used to maintain cells in a state that mimics in vivo physiological conditions (Papkovsky, 2004; Michl et al., 2019), ensuring the clinical compatibility of experimental findings. Physiological conditions in mammalian cell cultures typically aim to mimic conditions in extracellular fluids, including temperature, typically adjusted to 37°C, O2 to 18.6%–20.9%, CO2 to 5%, and pH adjusted to 7.4 units (Wenger et al., 2015). Maintaining relevant physiological conditions in cell cultures is of paramount importance to ensure the reproducibility of published findings and the translational relevance of experimental data to clinical applications. Yet, inadequate reproducibility of experimental findings in biomedical research is an increasingly well-recognized problem (Begley, 2013; Collins and Tabak, 2014), contributing to delays in drug discovery and therapies (Freedman et al., 2015).
Best-practice guidelines are used to ensure standards in biomedical research, encompassing multiple aspects of the research practice (Baust et al., 2017), but have not yet included comprehensive standards for the reporting or control of environmental conditions in cell-culture systems. The most common approach to in vitro cell culture is when cells are grown either in suspension or as adherent monolayers in standard media within tissue culture flasks (defined here as “standard batch culture”). This approach is most popular since it reliably induces the proliferation of cells, is affordable, and scalable in terms of the possible number of biological replicates and treatments. Although the standard batch culture of cells meets the critical need for continuous sources of biological material, most biomedical researchers using standard culture systems acknowledge that they are limited in their capacity to maintain cell homeostasis within the physiological limits experienced in vivo (Place et al., 2017; Al-Ani et al., 2018; Hirsch and Schildknecht, 2019). Despite this awareness and the scope for substantial environmental variability during the standard batch culture of cells (Michl et al., 2019), recent assessments show that the majority of research papers rely on nominal set points and fail to directly verify and report environmental parameters (Al-Ani et al., 2018; Michl et al., 2019; Klein et al., 2021b). Standard batch culture systems undergo substantial environmental changes owing to cell metabolic activity (Balin et al., 1976; Naciri et al., 2008; Vallejos et al., 2010; Pradhan et al., 2012; Al-Ani et al., 2018; Michl et al., 2019), with pH declines reaching 0.9 units, O2 levels declining down to 0.95%, and CO2 values reaching up to 10.45% (Klein et al., 2021b). In light of these reported changes, the apparent reliance on nominal set points to ensure physiological relevance and reproducibility in biomedical research requires urgent reconsideration. Although Eagle (1971) first highlighted environmental drift in standard batch cultures almost 50 years ago, we are aware of only three papers that collectively measured and reported O2 or CO2 regimes in in vitro cultures of mammalian cells (Balin et al., 1976; Naciri et al., 2008; Vallejos et al., 2010). Inadequate control and reporting of environmental conditions in cell cultures is, therefore, a candidate contributor to irreproducibility in basic and preclinical biomedical research.
Here, we provide guidelines for the reporting and control of environmental conditions in cell culture systems, with a focus on metabolic gases (O2 and CO2) and the associated acid-base balance driving pH. We (i) raise awareness of the imperative to control and monitor cell culture environments in biomedical research, (ii) propose short- and long-term standards for control, monitoring, and reporting with consideration of resource availability, and (iii) highlight the steps needed for these recommendations to be achieved. We outline the most common problems resulting in uncontrolled environmental conditions and associated confounding factors, and then provide a range of solutions. We also supply a reporting workflow that ensures improved standards for the reporting and control of culture environments to enhance reproducibility and progress in biomedical research.
The Problems
Environmental Instability in Cell Culture Media
Documented reports of deoxygenation and disruptions to acid-base stability in culture media caused by cellular metabolism equate to a median pH shift of 0.425 units and a median O2 shift of 10.6% from target (nominal) values (see, Klein et al., 2021b). Cells are capable of buffering against extracellular reductions in pH to maintain alkaline pH of the cytoplasm (Johnson et al., 1976; L’allemain et al., 1984; Lindström and Sehlin, 1984; Pouyssegur et al., 1985; McBrian et al., 2013). However, such mechanisms (e.g., Na+/H+ antiporters or histone deacetylation) consume cellular energy and can alter gene transcription and reduce cellular growth through changes in the acetylation state of chromatin (Boron and Russell, 1983; Bowen and Levinson, 1984; Boron, 2004). Changes in dissolved gases are also well known to substantially affect cellular physiology. Besides the role of O2 in affecting the most fundamental characteristics of in vitro cell cultures (Packer and Fuehr, 1977), including the dependence of cellular metabolism on O2 (Ast and Mootha, 2019), deoxygenation can also activate the hypoxia-inducible factor (HIF) transcription system, which triggers the expression of most genes responsible for cellular adaptation to varying O2 levels (Semenza et al., 1991; Wang et al., 1995; Semenza, 2012). Minor deviations in dCO2 can also induce a wide range of cellular responses (Bumke et al., 2003; Kikuchi et al., 2017; Kikuchi et al., 2019), affecting the function of biomolecules and the proteome (Duarte et al., 2020).
The impacts of compromised acid-base stability and O2 delivery on cellular responses during in vitro cell culture are not confined to theory (see syntheses by; Ast and Mootha, 2019; Keeley and Mann, 2019; Klein et al., 2021b). Briefly, for instance, Michl and others (2019) showed that cellular growth of three colorectal cell-lines (NCI-H747, DLD1, Caco2) was optimal at pH 7.4, but when medium pH deviated from 7.4 by > 0.3 units all three cell lines exhibited reduced rates of proliferation. Medium acidification during in vitro cell culture can also initiate pro-inflammatory signaling responses in human aortic smooth muscle cells (Tomura et al., 2005) and cells of the human nucleus pulposus (Gilbert et al., 2016). A transcriptomic study, focusing on human fibroblasts, revealed that reductions in medium pH (to pH 6.7) modulated the expression of 2,068 genes (out of 12,565) by more than two-fold after only 24 h of culture (Bumke et al., 2003). Constraining O2 availability during in vitro cell culture appears to be similarly crucial. For example, HepG2 cultures at confluence rapidly depleted O2 levels to <1% to self-inflict a switch from oxidative phosphorylation to glycolysis, despite being cultured in incubator conditions providing ambient atmospheric O2 levels (18.6%–20.9%) (Wolff et al., 1993). These findings are highly consistent with observations of other cell types, including human hepatocytes (Ng et al., 2014) and rat renal mesangial cells (Metzen et al., 1995), where perturbed O2 levels correlated with anomalous cellular responses (Keeley and Mann, 2019). Although limited, some evidence suggests that variable culture environments can also affect the reproducibility of cell culture experiments. Indeed, barcoding experiments showed that cancer cell-line evolution occurred from positive clonal selection that was highly sensitive to culture conditions (Ben-David et al., 2018). Further experiments testing the cell-line strains against anti-cancer compounds uncovered disparate drug responses, although the exact sources of instabilities in culture environments that promoted cell-line heterogeneity were not resolved (Ben-David et al., 2018).
Factors Contributing to Environmental Instability in Cell Culture Media
Most commercial media contain buffering systems that act only to regulate pH, whereas levels of dissolved O2 and CO2 are regulated by atmosphere re-equilibration. The initial stability of medium pH is typically achieved by mimicking the physiologically relevant CO2/HCO3− buffering system (Michl et al., 2019). Most media formulations contain a known concentration of HCO3−, which upon exposure to an incubator that nominally maintains a CO2-rich atmosphere (typically 5% CO2 in air), equilibrates to spontaneously produce H+ ions and stabilize pH (Supplementary Box S1). Although the CO2/HCO3− buffer system is indeed the primary physiological buffering system in mammalian fluids (Boron, 2004; Michl et al., 2019), standard cell cultures lack the regulatory systems (e.g., changes in respiratory rate, vascular remodeling, renal control of HCO3− and H+) present in the mammalian body. Such systems regulate for the changes in dissolved gases, waste products (e.g., lactic acid), and H+ ions involved in cellular metabolism, thereby achieving conditions that maintain homeostasis (Supplementary Box S1). Although consistent observations of pH instability in standard cell cultures prompted the use of additional exogenous buffers in media formulations to enhance medium buffering capacity (Eagle, 1971), such approaches can, in some cases, promote unpredictable changes in pH and introduce confounding artifacts (Michl et al., 2019, see below).
In the case of O2, medium deoxygenation is caused by the disparity between rates of O2 consumption via cellular metabolism and the replenishment of O2 at the air-medium interface (Place et al., 2017; Al-Ani et al., 2018). Specifically, O2 first dissolves at the air-medium interface and then diffuses through the liquid (at least several millimeters) to reach and oxygenate cell microenvironments (Place et al., 2017) even as cells undergo exponential growth. This is contrary to in vivo physiology, where most cells exist within 100 µm to the nearest capillaries that replenish O2 and act to remove excess CO2. Besides the role of CO2 in affecting medium acid-base chemistry, levels of CO2/HCO3− readily diffuse across cell membranes to moderate intracellular pH (Gutknecht et al., 1977), act as metabolic inhibitors, and may induce complex transcriptional responses (Cottier et al., 2012; Follonier et al., 2013), and signal other critical reactions (see, Blombach and Takors, 2015). In concert, these processes interact to create a changing environmental gradient from the surface of the medium down to the microenvironment of the cells (Place et al., 2017). The effect of unstirred medium layers also presumably determines the delivery of nutrients/growth factors and the removal of other metabolic waste products (e.g., lactic acid), which can also act to directly and indirectly moderate environmental variation (Michl et al., 2019).
Changes in the culture environment may also initiate complex feedback mechanisms, where cellular responses to variations in the culture environment could, in turn, inflict greater intrusions of environmental stability and promote unpredictable outcomes. For instance, perturbations to dissolved O2 levels in culture medium can induce cells to switch away from oxidative phosphorylation towards anaerobic glycolysis (Wolff et al., 1993), leading to large accumulations of lactic acid that force medium acidification (Michl et al., 2019). Another example lies in the role of carbonic anhydrases (CA), which catalyze the hydration of CO2. Švastová and others showed that medium deoxygenation in cell cultures of human cancer cell lines induced the expression and activity of carbonic anhydrases, which resulted in enhanced acidification of the culture medium (Švastová et al., 2004).
Lack of Detailed Methodological Reporting
The lack of monitoring and reporting of environmental conditions in cell culture-systems is a pervasive, but under-recognized problem (Hunter, 2017; Al-Ani et al., 2018; Michl et al., 2019; Klein et al., 2021a; Klein et al., 2021b). A recent synthesis examining this problem sub-sampled 688 papers published between 2014 and 2019 and found that most papers reported the medium manufacturer, but only one third reported the type of culture system utilized and 42% reported temperature and CO2 incubator settings (Klein et al., 2021b). Another post-publication analysis reported that less than half of studies published in Cancer Research and Nature in the third quarter of 2017 described the brand of medium, and only one-tenth declared the medium-buffering regime (Michl et al., 2019). Even when protocols are declared, there is an unfortunate prevalence of papers stating, “as previously described by ref. (x),” which often leads to a chain of citations that generate confusion as to the specific procedures, reagents, and materials involved (Freedman et al., 2015). In cases where environmental parameters are measured, these are often not reported. The apparent under appreciation of reporting measured environmental parameters is exemplified by published bioreactor experiments that report only the target levels of environmental parameters (e.g., Karst et al., 2016; Abecasis et al., 2017). Indeed, these systems, by design, typically require consistent monitoring of the controlled parameters via a feedback loop to achieve the desired control.
Failure to Monitor Mammalian Cell Culture Environments
A recent synthesis revealed that despite differences in cell type, medium formulation, and buffering components, all investigated standard batch cultures exhibited environmental drift after only a few days of culture (Klein et al., 2021b). Despite this, less than 0.05% of studies monitored pH, CO2, or O2 levels in cell cultures. Klein et al. (2021a) reported median declines in dissolved O2 down to 7.3%, and increases in dissolved CO2 to values ranging from 7.5% to 9.5%, compared to the nominal O2 and CO2 targets of 21% and 5%, respectively. The reported median decline in pH was 0.43 units, but in some particularly extreme cases, cell metabolic activity promoted pH reductions that approached one pH unit (Eagle, 1971) and dissolved O2 decreased down to 0.95% (Vallejos et al., 2010). In such extreme cases, variations in culture conditions may resemble levels consistent with hypercapnia and hypoxia rather than conditions typical of in vivo extracellular fluids, although in vivo environments vary considerably among selected tissues (Ast and Mootha, 2019). It is implicitly assumed that culture temperature is controlled at 37°C and thus, incubator temperatures were only reported in 42% of papers between 2014 and 2019 (Klein et al., 2021b). However, a number of studies used different culture temperatures (Brinkhof et al., 2015; Xu et al., 2017), which highlights the need to declare and monitor the incubation temperature.
Artefacts Introduced by Forcing of Non-Physiological Controls
Numerous approaches are used to control culture conditions, either to maintain physiological conditions or to test the effects of departures from those conditions (e.g., hypoxia; Grayson et al., 2006; acidosis; Kikuchi et al., 2017). However, some approaches misrepresent in vivo physiology and in doing so, inadvertently introduce artefacts and biases that could compromise reproducibility and relevance of the study to cellular function in the living organism. For instance, NVBs (e.g., HEPES, PIPES, or MES) are used to enhance acid-base stability of medium because the physiological HCO3−/CO2 buffering system can exhibit high volatility and a weak buffering capacity (Supplementary Box S1). However, it is vital to consider how NVBs introduce active molecules and acid-base reactions absent from mammalian fluids and existing evidence, although limited, indicates that NVBs could induce toxicity and anomalous cellular responses in particular cell types (Good et al., 1966; Lepe-Zuniga et al., 1987; Hanrahan and Tabcharani, 1990; Stea and Nurse, 1991). In particular, HEPES is commonly included in commercial media formulations, yet emerging reports demonstrate a range of possible side-effects. Briefly, HEPES activated lysosomal transcription factors in macrophages (Tol et al., 2018), inhibited the prion protein conversion in neural stem cells and affected their viability and differentiation (Delmouly et al., 2011). Another study showed cellular uptake of HEPES in human cell lines (MCF-7, U2OS, HeLa) that persisted for 48 h after cells were returned to HEPES-free media (Depping and Seeger, 2019).
Recent assessments also shows that NVB addition may not fully prevent pH declines in standard batch cultures and may lead to unexpected pH changes when interacting with the HCO3−/CO2 buffering system, although predictable pH levels can be obtained when appropriate protocols are used (see, Michl et al., 2019). Researchers often manipulate medium pH by titrating acids and bases to achieve a desired level. The titration of acids and bases (including HCl, NaOH, and NVBs) introduces osmolytes (Na+, Cl−) to cell medium and can result in substantial changes to medium osmolarity by > 10% (Michl et al., 2019). Supra-physiological osmolarity can directly affect cell membrane tension and volume (Pedersen et al., 2013), but can also moderate how cells respond to other environmental parameters (Dezengotita et al., 1998). For instance, hybridoma cells exposed to elevated CO2 conditions exhibited reduced growth rates when osmolarity was held constant at 361 mOsm kg−1, but cell growth rates further declined by 30% when medium osmolality was 415 mOsm kg−1 (Dezengotita et al., 1998).
The Solutions
Measure Environmental Parameters
Key environmental parameters (temperature, O2, CO2, and pH) should be accurately measured and reported. Researchers should also consider measurements of osmolarity and hydrostatic pressure (if experiments are not conducted at atmospheric pressure) because these variables are required for unit conversions of dissolved gases (Christmas and Bassingthwaighte, 2017), thereby facilitating accurate replication and comparisons of conditions among studies. Ideally, measurements of key parameters (O2, CO2, and pH) should be conducted to capture the variability that cell cultures experience, either continuously where logging systems can be used or via non-autonomous, regular measurements. A basic understanding of the expected variability for each of these parameters in specific experimental setups can be used to help guide the frequency of measurements required to capture the variability. As a minimum requirement in routine cell cultures, initial and final values are required for cases of linear drift characteristic of many batch culture experiments (Michl et al., 2019), whereas frequent recording (e.g., 1-min intervals) are likely required for advanced bioreactor systems involving gas and/or acid and base additions. Measuring these parameters at concurrent time points is critical to understand the interdependencies among parameters, and guide the explanation of their possible forcing on cellular responses (e.g., proliferation, metabolism, changes in gene transcription, epigenetic regulation). For instance, the solubility of dissolved gases, and thus the influence of CO2 on acid-base chemistry, is strongly dependent on temperature, osmolarity, humidity, and pressure (Christmas and Bassingthwaighte, 2017). Although thermal regimes of cell cultures may be reliably inferred from calibrated incubators, levels of dissolved O2 and CO2, as well as pH must be measured directly in the culture medium because cellular metabolism directly affects these parameters (Supplementary Table S1). Measuring systems capable of delivering the required precision and accuracy are available for all key environmental parameters. Such systems range in cost, from moderately priced sensors for temperature, pH, O2, and salinity to the more expensive sensing equipment required for monitoring dissolved CO2, which often require complex calibration protocols (Supplementary Table S1).
Levels of relative humidity and media evaporation are equally important considerations for the control of cell culture environments. Since variations in both factors result in changes to osmolarity as well as solute and gas concentrations, that in turn, affect diffusion. Unfortunately, variations in relative humidity even in sophisticated incubators are common (Triaud et al., 2003), but they should be recognized and remedied. Low-cost sensors are available to monitor relative humidity levels inside incubators (Supplementary Table S1, Rajan et al., 2017) and should also be reported alongside key environmental variables. For relative humidity and the minimization of medium evaporation, water baths or pans placed inside incubators provide a simple and cost-effect solution to maintaining adequate levels, although this approach is limited in its capacity for precise control and can elevate the risk of condensation and contamination. Watertight joints in culture incubators may also require frequent maintenance and replacement, where needed (Rajan et al., 2017). More sophisticated and costly options are available for the control of relative humidity (and minimization of medium desiccation), including direct steam humidification systems and incubators capable of two-sided controls (Supplementary Table S1).
Accordingly, we provide reporting guidelines (Figure 1, Supplementary Extended Materials S1) along with sample method descriptions (Supplementary Extended Materials S2), to guide practitioners into conducting and reporting characterizations of environmental regimes and promote a greater understanding of the factors that may affect precision and accuracy of experiments. Indeed, researchers could consider conducting pilot experiments to understand if variability in environmental factors significantly affects key experimental readouts.
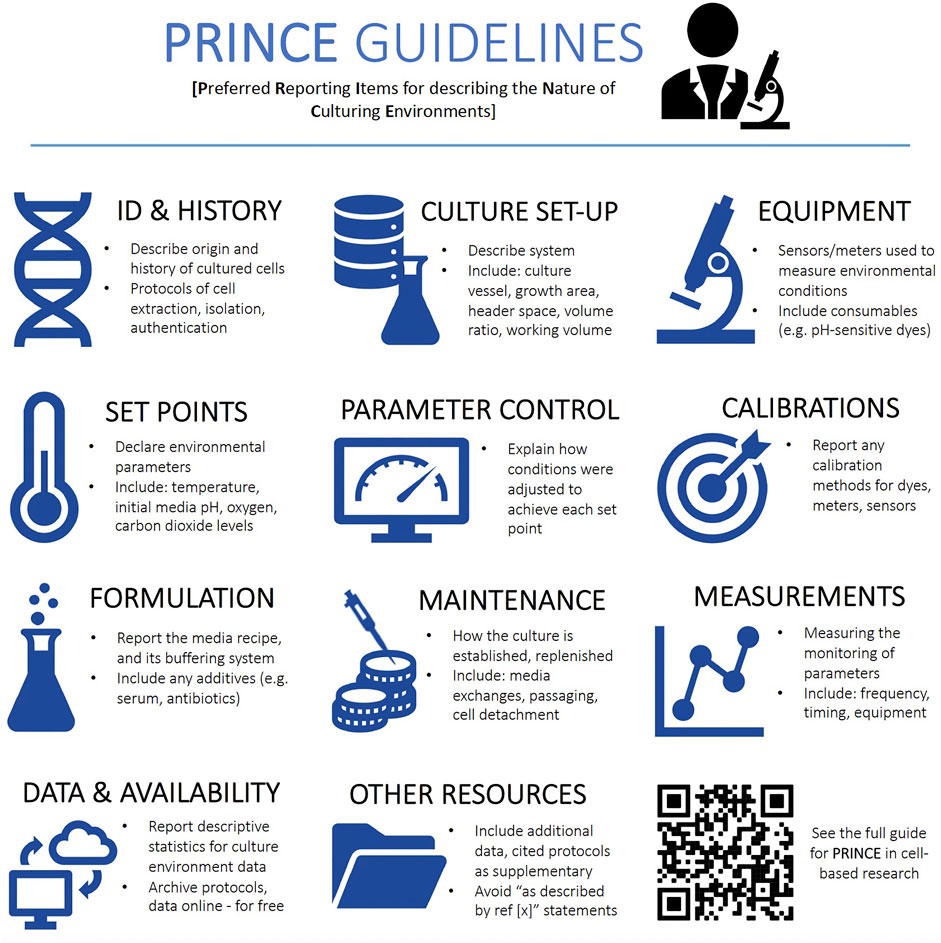
FIGURE 1. An overview of the PRINCE (Preferred Reporting Items for describing the Nature of Culturing Environments) guidelines. The QR provides access to the full PRINCE reporting checklist (also in Supplementary Extended Materials S1) containing an exhaustive list of reporting items.
Control Environmental Parameters
A reasonable degree of control over environmental conditions is achievable in routine culture systems, but requires consideration of workflow factors that can, directly and indirectly, promote environmental drift (Figure 2). Interpretation and reproducibility of biomedical experiments involving mammalian cell cultures mandate that environmental parameters (temperature, O2, CO2, and pH) be at least monitored and reported, and where possible environmental variation minimized and controlled. Environmental stability is most easily achieved in advanced bioreactor culture systems, whereas achieving stability in routine batch culture systems is most challenging, with perfusion systems (and chemostats) providing intermediate solutions (Table 1). Batch cultures are typically maintained in incubators that maintain temperature (typically 37°C) and guarantee a CO2 level (typically 5%) in the atmosphere. In batch culture set-ups, researchers often select culture medium that contains a pH indicator dye [e.g., Phenol Red (PhR)] to guide the renewal of cell medium, but medium color changes assessed “by eye” can lead to undetected pH declines (Michl et al., 2019). Indeed, batch cell cultures are the most popular, inexpensive and scalable culture system, in terms of the possible number of replicates and treatments, with <1% of the published literature utilizing cybernetic bioreactor or chemostat culture systems (2014–2019, Klein et al., 2021b). Chemostat and perfusion systems were first described in 1950 for use in bacterial cultures (Novick and Szilard, 1950a; b) and later adopted for mammalian cells in 1961 (Cohen and Eagle, 1961). Such systems can maintain environmental conditions and cell growth rates via a continuous dilution of the culture with fresh medium (Cohen and Eagle, 1961). More sophisticated bioreactor culture systems were first introduced in the 1970s to culture mammalian cells (Knazek et al., 1972; Fouron, 1987) and typically involve the automated control of temperature, gas addition (O2, CO2), and/or acids and bases to maintain set targets for temperature, dissolved gases and pH. Bioreactors provide the best capacity to control environmental conditions, but are most costly in the context of capital investment, maintenance, and operations. Importantly, many bioreactor systems lack flexibility in the number of biological replicates and the volume of culture media (often larger than that of batch culture) that can be manipulated, which translates into greater time and monetary costs. While traditional bioreactor systems are ideal for cells culture in suspension, attached cell monolayers require different solutions. For these cell types, advanced bench top culture systems providing convection of culture media (Supplementary Table S1), which are also expandable in terms of replication, will likely provide the best capacity for adherent cell types (see, Kreß et al., 2021). We provide considerations as well as suggestions for improved environmental control for culture systems ranging in complexity from batch cultures to bioreactors (Table 1 and Supplementary Table S2).
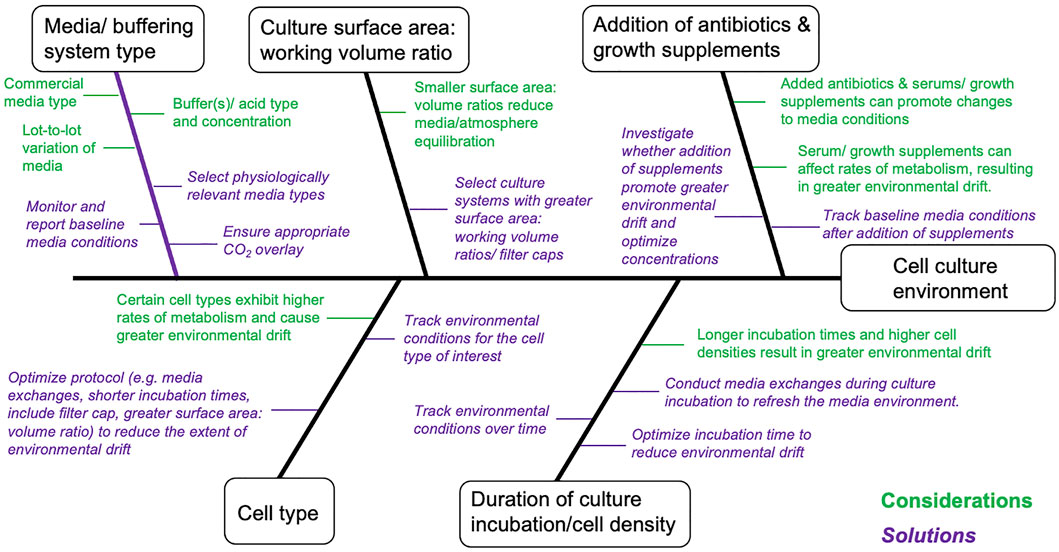
FIGURE 2. Cause-and-effect diagram summarizing considerations that can directly and indirectly affect environmental conditions in in vitro cell cultures. This diagram is non-exhaustive and should be further complemented/modified for specific cellular models and/or fields of research. Statements marked in green are technical considerations affecting in vitro cell cultures and statements marked in Purple (italicized) are proposed solutions.
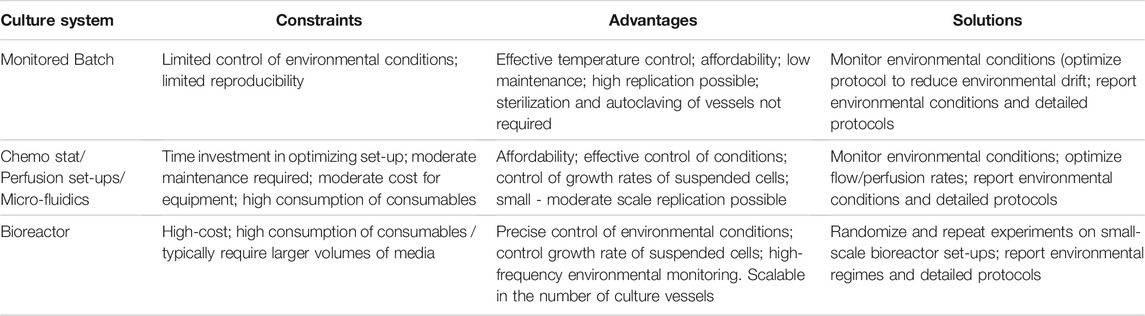
TABLE 1. Constraints, advantages, and solutions for improved environmental control and reproducibility for three major types of culture systems.
Report Procedures to Monitor and Control Environmental Data
Precise control and monitoring of environmental conditions for mammalian cell cultures need be accompanied by reporting of the procedures used—a requirement, that is, not yet sufficiently emphasized nor enforced by the majority of scientific journals. A survey assessing the reporting requirements of leading biomedical journals for publications involving mammalian cells (Cell, Nature, Science, etc.; cf. Supplementary Extended Materials S3) revealed that only Nature Research, Science, Cell, and EMBO press journals require a standardized declaration of reporting practices to be published as a form attached to the electronic version of the published papers (see Nature Reporting Standards and MDAR Reporting Standards). However, none of the information required in this form addresses the monitoring and control of critical environmental conditions for research conducted using mammalian cell cultures (hereafter referred to as “cell-based experiments”). Strengthening reporting requirements and standards will likely place greater emphasis on cell culturing environments and, in turn, likely enhance the reproducibility of cell-based experiments as well as their relevance to the in vivo environment. We address this gap by offering the PRINCE (Preferred Reporting Information on the Nature of Cell-culturing Environments) guidelines (Supplementary Extended Materials S1) as a checklist for the parsimonious reporting of the monitoring and control of critical environmental conditions in the experiments reported in the papers. The PRINCE reporting checklist is designed to be adopted by journals publishing cell-based experiments and be included as a required declaration at the time of submission, thereby available to be assessed by peer reviewers, to then become available as an appendix to the electronic version of the published papers (Supplementary Extended Materials S1). This will ensure much needed standardized reporting of cell culture conditions.
Report Resulting Environmental Data
Monitoring and controlling environmental conditions for cell-based experiments must be accompanied by reporting the data obtained as an essential step to identify possible environmental artifacts affecting the reproducibility of the findings and their comparisons among studies. The lack of detailed methodological and data reporting prevalent in studies published to date has been attributed to strict word and page limits enforced by publishers (Freedman et al., 2015). However, while many journals, dictate strict restrictions on the main body of the published text, most journals encourage providing all relevant details in extended materials, thereby extending the space available to accurately describe the procedures used and report additional data helping interpret the results presented. Online repositories are also available for more detailed reporting of protocols (e.g., Nature’s Protocol Exchange, Dryad) and datasets (e.g., Dryad, figshare and Zenodo). These data repositories were designed to meet journal and funder requirements for data availability and most of these offer data curation services that streamline the uploading process and ensure sustained access to the data.
Minimum reporting requirements should include the mean and a metric of dispersion (e.g., SD, SEM or range) for each of the monitored environmental parameters. In cases where environmental parameters display a monotonous trend over time, the slope, a metric of dispersion, as well as the probability of the slope being equal to zero may be reported to describe the change over time and can be fitted using simple linear regression analysis. Ideally, researchers would accompany such summary statistics with a supplementary figure displaying environmental regimes over time (Michl et al., 2019), so that the published findings can be interpreted alongside the nature of cell-culture conditions.
Recommendations
The task of enhancing standards for environmental control, monitoring, and reporting in biomedical research may initially seem overwhelming provided the current absence of a culture to this end (Collins and Tabak, 2014; Baker, 2016; Hunter, 2017). Enhancing standards is also hindered by the limited availability of affordable culture systems capable of advanced environmental monitoring and conrtol for a broad range of cell types. The lack of appropriate tools in turn contributes to the lack of awareness of the true extent of environmental instability. However, reporting only nominal set-points used in cell culture systems without verification cannot be a sustainable solution.
Resolving the issue without a systematic approach may risk putting more burden on researchers’ time, resources, and expertise. As an immediate requirement, initial and final values of key parameters should be measured and reported in the batch cultures of cell lines used in experiments. This requirement should capture existing environmental variation affecting published findings, ensuring accurate interpretation of the reported results and improved reproducibility. The provided PRINCE reporting checklist is designed to apply to a range of culture systems, from routine batch cultures to advanced culture systems (e.g., prefusion set-ups and bioreactors). Next, existing protocols must be optimized to minimize environmental variation in routine cultures (Supplementary Table S1). The third step is to build the capacity and infrastructure, supported by a sufficient understanding of the causes and consequences of variability in these conditions. Where needed, postgraduate biomedical programs may be revised to strengthen these competences. The next step, which requires significant investment over longer time frames (years to decades), involves the routine use of advanced cell culture technologies that allow precise and accurate control and monitoring of environmental conditions (Figure 3, Supplementary Table S1). Pending these advancements, reporting requirements should then extend to include proliferative, maintenance culture vessels, not only those dedicated to experimental assessments. Researchers should then consider the relevance in vitro culture environments to the levels under which particular cell types exist in vivo. For instance, O2 levels vary across human tissues and range from 13% O2 in the lung-pulmonary vein to 1–3% O2 in the uterus (Ast and Mootha, 2019). By considering how niche in vivo environments affect experimental outcomes, researchers could further increase the robustness of their experiments and increase the likelihood that findings have relevance to focal in vivo compartments (Ast and Mootha, 2019). Particular fields within biomedical science (e.g., 3D cultures and stem cell research) are already making great strides in this arena (Ryall et al., 2015; Shyh-Chang and Ng, 2017), although reliable reports of environmental parameters within selected human tissues are presently limited (Al-Ani et al., 2018; Ast and Mootha, 2019) and this research area warrants further attention.
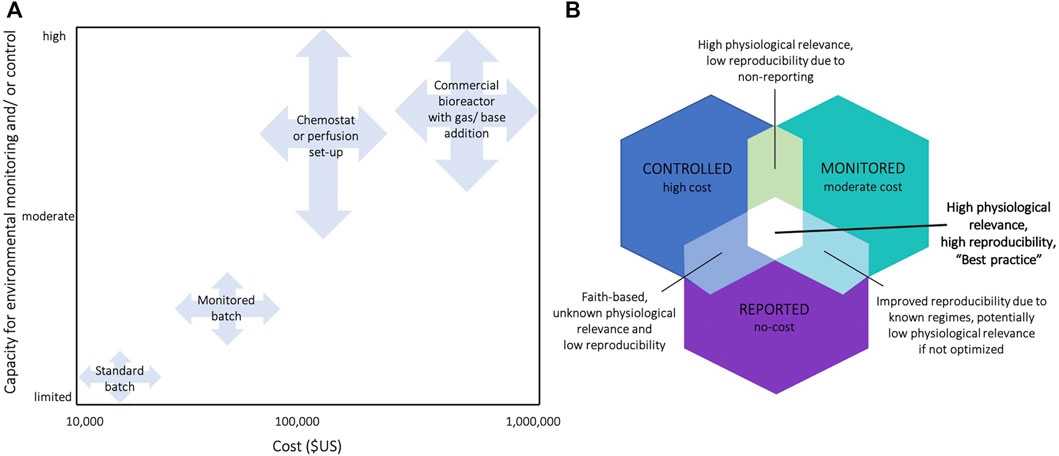
FIGURE 3. (A) Classification of cell culture systems by their capacity for environmental control/and or monitoring, and estimates of the required financial investments. Estimates for the required financial investment include the full costs associated with the required set-ups, including the key infrastructure required (see Supplementary Table S2 for prices of exemplary commercial systems) (B) Venn diagram summarizing the benefits and disadvantages of monitoring, controlling, and/or reporting cell culture environments in experiments. E.g., acquirement of a bioreactor is high in cost, but often only setpoints are reported without actually measuring environmental conditions in the medium (mean ± SE) leading to unknown physiological relevance and low reproducibility.
Ultimately, improved characterization and control over environmental conditions in cell cultures will enhance the reliability of experimental findings and the confidence in their translation to clinical applications, which should provide sufficient rationale for funding bodies and institutions to invest in the necessary infrastructure. Indeed, funding agencies could consider supporting research initiatives aiming to further investigate the effects on environmental factors on commonly studies biological responses (i.e., gene expression, histone modification, metabolic pathways) in model cell lines (e.g., Bumke et al., 2003; Ben-David et al., 2018; Muelas et al., 2018). This will provide a systematic understanding of the impacts of environmental control on cell culture experiments. Transitioning towards advanced culture systems capable of mimicking in vivo conditions not only requires consideration of environmental parameters, but also necessitates attention to other chemical and physical factors known to program cell fate. Such factors include, but are not limited to, the common usage non-physiological concentrations of growth factors (Rubin, 2007; Holohan et al., 2013; Langhans, 2018), and antibiotics (Ryu et al., 2017), as well as the physical structure of cell microenvironments, which can alter cell morphology and function (Darnell et al., 2018). For many of these factors, existing recommendations that aim to increase the relevance of in vitro cultures to in vivo physiology are available (Baker, 2016; Muelas et al., 2018; Hirsch and Schildknecht, 2019).
Author Contributions
CD, ML, and SK conceptualized the study. SK, AS and CD designed and wrote the first draft of the manuscript. SK, AS, AP, SMA and SA prepared the display figures and extended materials. All authors contributed to, and approved, the final version of the manuscript.
Funding
King Abdullah University of Science and Technology (KAUST) funded this research through baseline funding to CD and ML. (BAS/1/1080-01-01).
Conflict of Interest
The authors declare that the research was conducted in the absence of any commercial or financial relationships that could be construed as a potential conflict of interest.
Publisher’s Note
All claims expressed in this article are solely those of the authors and do not necessarily represent those of their affiliated organizations, or those of the publisher, the editors, and the reviewers. Any product that may be evaluated in this article, or claim that may be made by its manufacturer, is not guaranteed or endorsed by the publisher.
Acknowledgments
We thank J. E. Thomson for assistance with design of the display figures.
Supplementary Material
The Supplementary Material for this article can be found online at: https://www.frontiersin.org/articles/10.3389/fcell.2022.788808/full#supplementary-material
Reference
Abecasis, B., Aguiar, T., Arnault, É., Costa, R., Gomes-Alves, P., Aspegren, A., et al. (2017). Expansion of 3D Human Induced Pluripotent Stem Cell Aggregates in Bioreactors: Bioprocess Intensification and Scaling-Up Approaches. J. Biotechnol. 246, 81–93. doi:10.1016/j.jbiotec.2017.01.004
Al-Ani, A., Toms, D., Kondro, D., Thundathil, J., Yu, Y., and Ungrin, M. (2018). Oxygenation in Cell Culture: Critical Parameters for Reproducibility Are Routinely Not Reported. PLOS ONE 13, e0204269. doi:10.1371/journal.pone.0204269
Ast, T., and Mootha, V. K. (2019). Oxygen and Mammalian Cell Culture: Are We Repeating the experiment of Dr. Ox? Nat. Metab. 1, 858–860. doi:10.1038/s42255-019-0105-0
Balin, A. K., Goodman, D. B. P., Rasmussen, H., and Cristofalo, V. J. (1976). Atmospheric Stability in Cell Culture Vessels. In Vitro Cell.Dev.Biol.-Plant 12, 687–692. doi:10.1007/bf02797472
Baust, J. M., Buehring, G. C., Campbell, L., Elmore, E., Harbell, J. W., Nims, R. W., et al. (2017). Best Practices in Cell Culture: an Overview. In Vitro Cell.Dev.Biol.-Animal 53, 669–672. doi:10.1007/s11626-017-0177-7
Ben-David, U., Siranosian, B., Ha, G., Tang, H., Oren, Y., Hinohara, K., et al. (2018). Genetic and Transcriptional Evolution Alters Cancer Cell Line Drug Response. Nature 560, 325–330. doi:10.1038/s41586-018-0409-3
Blombach, B., and Takors, R. (2015). CO2–Intrinsicproduct ,essential Substrate ,and Regulatory Trigger of Microbial and Mammalian Production Processes. Front. Bioeng. Biotechnol. 3, a108. doi:10.3389/fbioe.2015.00108
Boron, W. F. (2004). Regulation of Intracellular pH. Adv. Physiol. Edu. 28, 160–179. doi:10.1152/advan.00045.2004
Boron, W. F., and Russell, J. M. (1983). Stoichiometry and Ion Dependencies of the Intracellular-pH-Regulating Mechanism in Squid Giant Axons. J. Gen. Physiol. 81, 373–399. doi:10.1085/jgp.81.3.373
Bowen, J. W., and Levinson, C. (1984). H+ Transport and the Regulation of Intracellular pH in Ehrlich Ascites Tumor Cells. J. Membrain Biol. 79, 7–18. doi:10.1007/bf01868522
Brinkhof, B., Van Tol, H. T., Groot Koerkamp, M. J., Riemers, F. M., Ijzer, S. G., Mashayekhi, K., et al. (2015). A mRNA Landscape of Bovine Embryos after Standard and MAPK-Inhibited Culture Conditions: a Comparative Analysis. BMC Genomics 16, 277. doi:10.1186/s12864-015-1448-x
Bumke, M. A., Neri, D., and Elia, G. (2003). Modulation of Gene Expression by Extracellular pH Variations in Human Fibroblasts: a Transcriptomic and Proteomic Study. Proteomics 3, 675–688. doi:10.1002/pmic.200300395
Christmas, K. M., and Bassingthwaighte, J. B. (2017). Equations for O2 and CO2 Solubilities in saline and Plasma: Combining Temperature and Density Dependences. J. Appl. Physiol. 122, 1313–1320. doi:10.1152/japplphysiol.01124.2016
Cohen, E. P., and Eagle, H. (1961). A Simplified Chemostat for the Growth of Mammalian Cells: Characteristics of Cell Growth in Continuous Culture. J. Exp. Med. 113, 467–474. doi:10.1084/jem.113.2.467
Collins, F. S., and Tabak, L. A. (2014). Policy: NIH Plans to Enhance Reproducibility. Nature 505, 612–613. doi:10.1038/505612a
Cottier, F., Raymond, M., Kurzai, O., Bolstad, M., Leewattanapasuk, W., Jiménez-López, C., et al. (2012). The bZIP Transcription Factor Rca1p Is a Central Regulator of a Novel CO2 Sensing Pathway in Yeast. Plos Pathog. 8, e1002485. doi:10.1371/journal.ppat.1002485
Darnell, M., O’Neil, A., Mao, A., Gu, L., Rubin, L. L., and Mooney, D. J. (2018). Material Microenvironmental Properties Couple to Induce Distinct Transcriptional Programs in Mammalian Stem Cells. Proc. Natl. Acad. Sci. USA 115, E8368–E8377. doi:10.1073/pnas.1802568115
Delmouly, K., Belondrade, M., Casanova, D., Milhavet, O., and Lehmann, S. (2011). HEPES Inhibits the Conversion of Prion Protein in Cell Culture. J. Gen. Virol. 92, 1244–1250. doi:10.1099/vir.0.027334-0
Depping, R., and Seeger, K. (2019). 1H-NMR Spectroscopy Shows Cellular Uptake of HEPES Buffer by Human Cell Lines-An Effect to Be Considered in Cell Culture Experiments. Anal. Bioanal. Chem. 411, 797–802. doi:10.1007/s00216-018-1518-4
Dezengotita, V. M., Kimura, R., and Miller, W. M. (1998). Effects of CO2 and Osmolality on Hybridoma Cells: Growth, Metabolism and Monoclonal Antibody Production. Cytotechnology 28, 213–227. doi:10.1007/978-94-011-4786-6_22
Duarte, C. M., Jaremko, Ł., and Jaremko, M. (2020). Hypothesis: Potentially Systemic Impacts of Elevated CO2 on the Human Proteome and Health. Front. Public Health 8, 543322. doi:10.3389/fpubh.2020.543322
Eagle, H. (1971). Buffer Combinations for Mammalian Cell Culture. Science 174, 500–503. doi:10.1126/science.174.4008.500
Follonier, S., Escapa, I. F., Fonseca, P. M., Henes, B., Panke, S., Zinn, M., et al. (2013). New Insights on the Reorganization of Gene Transcription in Pseudomonas Putida KT2440 at Elevated Pressure. Microb. Cel Fact 12, 30–19. doi:10.1186/1475-2859-12-30
Fouron, Y. (1987). Growing Cultures in Perfusion Bioreactors. Nature 327, 537–538. doi:10.1038/327537a0
Freedman, L. P., Gibson, M. C., Ethier, S. P., Soule, H. R., Neve, R. M., and Reid, Y. A. (2015). Reproducibility: Changing the Policies and Culture of Cell Line Authentication. Nat. Methods 12, 493–497. doi:10.1038/nmeth.3403
Gilbert, H. T. J., Hodson, N., Baird, P., Richardson, S. M., and Hoyland, J. A. (2016). Acidic pH Promotes Intervertebral Disc Degeneration: Acid-Sensing Ion Channel -3 as a Potential Therapeutic Target. Sci. Rep. 6, 37360. doi:10.1038/srep37360
Good, N. E., Winget, G. D., Winter, W., Connolly, T. N., Izawa, S., and Singh, R. M. M. (1966). Hydrogen Ion Buffers for Biological Research*. Biochemistry 5, 467–477. doi:10.1021/bi00866a011
Grayson, W. L., Zhao, F., Izadpanah, R., Bunnell, B., and Ma, T. (2006). Effects of Hypoxia on Human Mesenchymal Stem Cell Expansion and Plasticity in 3D Constructs. J. Cel. Physiol. 207, 331–339. doi:10.1002/jcp.20571
Gutknecht, J., Bisson, M. A., and Tosteson, F. C. (1977). Diffusion of Carbon Dioxide through Lipid Bilayer Membranes: Effects of Carbonic Anhydrase, Bicarbonate, and Unstirred Layers. J. Gen. Physiol. 69, 779–794. doi:10.1085/jgp.69.6.779
Hanrahan, J. W., and Tabcharani, J. A. (1990). Inhibition of an Outwardly Rectifying Anion Channel by HEPES and Related Buffers. J. Membrain Biol. 116, 65–77. doi:10.1007/bf01871673
Hirsch, C., and Schildknecht, S. (2019). In Vitro research Reproducibility: Keeping up High Standards. Front. Pharmacol. 10, 1484. doi:10.3389/fphar.2019.01484
Holohan, C., Van Schaeybroeck, S., Longley, D. B., and Johnston, P. G. (2013). Cancer Drug Resistance: an Evolving Paradigm. Nat. Rev. Cancer 13, 714–726. doi:10.1038/nrc3599
Hu, W.-S., and Aunins, J. G. (1997). Large-scale Mammalian Cell Culture. Curr. Opin. Biotechnol. 8, 148–153. doi:10.1016/s0958-1669(97)80093-6
Hunter, P. (2017). The Reproducibility "crisis". EMBO Rep. 18, 1493–1496. doi:10.15252/embr.201744876
Johnson, J. D., Epel, D., and Paul, M. (1976). Intracellular pH and Activation of Sea Urchin Eggs after Fertilisation. Nature 262, 661–664. doi:10.1038/262661a0
Karst, D. J., Serra, E., Villiger, T. K., Soos, M., and Morbidelli, M. (2016). Characterization and Comparison of ATF and TFF in Stirred Bioreactors for Continuous Mammalian Cell Culture Processes. Biochem. Eng. J. 110, 17–26. doi:10.1016/j.bej.2016.02.003
Keeley, T. P., and Mann, G. E. (2019). Defining Physiological Normoxia for Improved Translation of Cell Physiology to Animal Models and Humans. Physiol. Rev. 99, 161–234. doi:10.1152/physrev.00041.2017
Kikuchi, R., Iwai, Y., Tsuji, T., Watanabe, Y., Koyama, N., Yamaguchi, K., et al. (2019). Hypercapnic Tumor Microenvironment Confers Chemoresistance to Lung Cancer Cells by Reprogramming Mitochondrial Metabolism In Vitro. Free Radic. Biol. Med. 134, 200–214. doi:10.1016/j.freeradbiomed.2019.01.014
Kikuchi, R., Tsuji, T., Watanabe, O., Yamaguchi, K., Furukawa, K., Nakamura, H., et al. (2017). Hypercapnia Accelerates Adipogenesis: A Novel Role of High CO2in Exacerbating Obesity. Am. J. Respir. Cel Mol Biol 57, 570–580. doi:10.1165/rcmb.2016-0278oc
Klein, S. G., Alsolami, S. M., Arossa, S., Ramos Mandujano, G., Parry, A. J., Steckbauer, A., et al. (2021a). In Situ monitoring Reveals Cellular Environmental Instabilities in Human Pluripotent Stem Cell Culture. Commun. Biol. (in press). doi:10.1038/s42003-022-03065-w
Klein, S. G., Alsolami, S. M., Steckbauer, A., Arossa, S., Parry, A. J., Ramos Mandujano, G., et al. (2021b). A Prevalent Neglect of Environmental Control in Mammalian Cell Culture Calls for Best Practices. Nat. Biomed. Eng. 5, 787–792. doi:10.1038/s41551-021-00775-0
Knazek, R. A., Gullino, P. M., Kohler, P. O., and Dedrick, R. L. (1972). Cell Culture on Artificial Capillaries: an Approach to Tissue Growth In Vitro. Science 178, 65–67. doi:10.1126/science.178.4056.65
Kreß, S., Almeria, C., and Kasper, C. (2021). “Lab Equipment for 3D Cell Culture,” in Basic Concepts on 3D Cell Culture. Editors C. Kasper, D. Egger, and A. Lavrentieva (Cham: Springer International Publishing), 27–67.
L'allemain, G., Paris, S., and Pouysségur, J. (1984). Growth Factor Action and Intracellular pH Regulation in Fibroblasts. Evidence for a Major Role of the Na+/H+ Antiport. J. Biol. Chem. 259, 5809–5815. doi:10.1016/s0021-9258(18)91086-0
Langhans, S. A. (2018). Three-Dimensional In Vitro Cell Culture Models in Drug Discovery and Drug Repositioning. Front. Pharmacol. 9, 6. doi:10.3389/fphar.2018.00006
Lepe-Zuniga, J. L., Zigler, J. S., and Gery, I. (1987). Toxicity of Light-Exposed HEPES media. J. Immunological Methods 103, 145. doi:10.1016/0022-1759(87)90253-5
Lindström, P., and Sehlin, J. (1984). Effect of Glucose on the Intracellular pH of Pancreatic Islet Cells. Biochem. J. 218, 887–892.
Mcbrian, M. A., Behbahan, I. S., Ferrari, R., Su, T., Huang, T.-W., Li, K., et al. (2013). Histone Acetylation Regulates Intracellular pH. Mol. Cel 49, 310–321. doi:10.1016/j.molcel.2012.10.025
Merten, O.-W. (2006). Introduction to Animal Cell Culture Technology-Past, Present and Future. Cytotechnology 50, 1–7. doi:10.1007/s10616-006-9009-4
Metzen, E., Wolff, M., Fandrey, J., and Jelkmann, W. (1995). Pericellular PO2 and O2 Consumption in Monolayer Cell Cultures. Respiration Physiol. 100, 101–106. doi:10.1016/0034-5687(94)00125-j
Michl, J., Park, K. C., and Swietach, P. (2019). Evidence-based Guidelines for Controlling pH in Mammalian Live-Cell Culture Systems. Commun. Biol. 2, 144. doi:10.1038/s42003-019-0393-7
Naciri, M., Kuystermans, D., and Al-Rubeai, M. (2008). Monitoring pH and Dissolved Oxygen in Mammalian Cell Culture Using Optical Sensors. Cytotechnology 57, 245–250. doi:10.1007/s10616-008-9160-1
Ng, S., March, S., Galstian, A., Hanson, K., Carvalho, T., Mota, M. M., et al. (2014). Hypoxia Promotes Liver-Stage Malaria Infection in Primary Human Hepatocytes In Vitro. Dis. Model. Mech. 7, 215–224. doi:10.1242/dmm.013490
Novick, A., and Szilard, L. (1950a). Description of the Chemostat. Science 112, 715–716. doi:10.1126/science.112.2920.715
Novick, A., and Szilard, L. (1950b). Experiments with the Chemostat on Spontaneous Mutations of Bacteria. Proc. Natl. Acad. Sci. 36, 708–719. doi:10.1073/pnas.36.12.708
Packer, L., and Fuehr, K. (1977). Low Oxygen Concentration Extends the Lifespan of Cultured Human Diploid Cells. Nature 267, 423–425. doi:10.1038/267423a0
Papkovsky, D. B. (2004). Methods in Optical Oxygen Sensing: Protocols and Critical Analyses. Methods Enzymol. 381, 715–735. doi:10.1016/s0076-6879(04)81046-2
Pedersen, S. F., Hoffmann, E. K., and Novak, I. (2013). Cell Volume Regulation in Epithelial Physiology and Cancer. Front. Physiol. 4, 233. doi:10.3389/fphys.2013.00233
Petricciani, J. C. (1995). The Acceptability of Continuous Cell Lines: A Personal & Historical Perspective. Cytotechnology 18, 9–13. doi:10.1007/bf00744314
Place, T. L., Domann, F. E., and Case, A. J. (2017). Limitations of Oxygen Delivery to Cells in Culture: An Underappreciated Problem in Basic and Translational Research. Free Radic. Biol. Med. 113, 311–322. doi:10.1016/j.freeradbiomed.2017.10.003
Pouysségur, J., Franchi, A., L'allemain, G., and Paris, S. (1985). Cytoplasmic pH, a Key Determinant of Growth Factor-Induced DNA Synthesis in Quiescent Fibroblasts. FEBS Lett. 190, 115–119. doi:10.1016/0014-5793(85)80439-7
Pradhan, K., Pant, T., and Gadgil, M. (2012). In Situ pH Maintenance for Mammalian Cell Cultures in Shake Flasks and Tissue Culture Flasks. Biotechnol. Prog. 28, 1605–1610. doi:10.1002/btpr.1614
Rajan, D. K., Verho, J., Kreutzer, J., Välimäki, H., Ihalainen, H., Lekkala, J., et al. (2017). “Monitoring pH, Temperature and Humidity in Long-Term Stem Cell Culture in CO2 Incubator,” in 2017 IEEE International Symposium on Medical Measurements and Applications (MeMeA) (IEEE), 470–474.
Rubin, H. (2007). The Logic of the Membrane, Magnesium, Mitosis (MMM) Model for the Regulation of Animal Cell Proliferation. Arch. Biochem. Biophys. 458, 16–23. doi:10.1016/j.abb.2006.03.026
Ryall, J. G., Cliff, T., Dalton, S., and Sartorelli, V. (2015). Metabolic Reprogramming of Stem Cell Epigenetics. Cell stem cell 17, 651–662. doi:10.1016/j.stem.2015.11.012
Ryu, A. H., Eckalbar, W. L., Kreimer, A., Yosef, N., and Ahituv, N. (2017). Use Antibiotics in Cell Culture with Caution: Genome-wide Identification of Antibiotic-Induced Changes in Gene Expression and Regulation. Sci. Rep. 7, 7533. doi:10.1038/s41598-017-07757-w
Semenza, G. L. (2012). Hypoxia-inducible Factors in Physiology and Medicine. Cell 148, 399–408. doi:10.1016/j.cell.2012.01.021
Semenza, G. L., Nejfelt, M. K., Chi, S. M., and Antonarakis, S. E. (1991). Hypoxia-inducible Nuclear Factors Bind to an Enhancer Element Located 3' to the Human Erythropoietin Gene. Proc. Natl. Acad. Sci. 88, 5680–5684. doi:10.1073/pnas.88.13.5680
Shyh-Chang, N., and Ng, H.-H. (2017). The Metabolic Programming of Stem Cells. Genes Dev. 31, 336–346. doi:10.1101/gad.293167.116
Stea, A., and Nurse, C. A. (1991). Contrasting Effects of HEPES vs HCO3−-Buffered media on Whole-Cell Currents in Cultured Chemoreceptors of the Rat Carotid Body. Neurosci. Lett. 132, 239–242. doi:10.1016/0304-3940(91)90310-p
Švastová, E., Hulı́Ková, A., Rafajová, M., Zat'ovičová, M., Gibadulinová, A., Casini, A., et al. (2004). Hypoxia Activates the Capacity of Tumor-Associated Carbonic Anhydrase IX to Acidify Extracellular pH. FEBS Lett. 577, 439–445.
Tol, M. J., Van Der Lienden, M. J. C., Gabriel, T. L., Hagen, J. J., Scheij, S., Veenendaal, T., et al. (2018). HEPES Activates a MiT/TFE-dependent Lysosomal-Autophagic Gene Network in Cultured Cells: A Call for Caution. Autophagy 14, 437–449. doi:10.1080/15548627.2017.1419118
Tomura, H., Wang, J.-Q., Komachi, M., Damirin, A., Mogi, C., Tobo, M., et al. (2005). Prostaglandin I2 Production and cAMP Accumulation in Response to Acidic Extracellular pH through OGR1 in Human Aortic Smooth Muscle Cells. J. Biol. Chem. 280, 34458–34464. doi:10.1074/jbc.m505287200
Triaud, F., Clenet, D.-H., Cariou, Y., Le Neel, T., Morin, D., and Truchaud, A. (2003). Evaluation of Automated Cell Culture Incubators. JALA: J. Assoc. Lab. Automation 8, 82–86. doi:10.1016/s1535-5535(03)00018-2
Vallejos, J. R., Brorson, K. A., Moreira, A. R., and Rao, G. (2010). Dissolved Oxygen and pH Profile Evolution after Cryovial Thaw and Repeated Cell Passaging in a T-75 Flask. Biotechnol. Bioeng. 105, 1040–1047. doi:10.1002/bit.22649
Wang, G. L., Jiang, B. H., Rue, E. A., and Semenza, G. L. (1995). Hypoxia-inducible Factor 1 Is a basic-helix-loop-helix-PAS Heterodimer Regulated by Cellular O2 Tension. Proc. Natl. Acad. Sci. 92, 5510–5514. doi:10.1073/pnas.92.12.5510
Wenger, R., Kurtcuoglu, V., Scholz, C., Marti, H., and Hoogewijs, D. (2015). Frequently Asked Questions in Hypoxia Research. Hp 3, 35–43. doi:10.2147/HP.S92198
Wolff, M., Fandrey, J., and Jelkmann, W. (1993). Microelectrode Measurements of Pericellular PO2 in Erythropoietin-Producing Human Hepatoma Cell Cultures. Am. J. Physiology-Cell Physiol. 265, C1266–C1270. doi:10.1152/ajpcell.1993.265.5.c1266
Muelas, M. W., Ortega, F., Breitling, R., Bendtsen, C., and Westerhoff, H. V. (2018). Rational Cell Culture Optimization Enhances Experimental Reproducibility in Cancer Cells. Sci. Rep. 8, 3029. doi:10.1038/s41598-018-21050-4
Keywords: batch culture, reproducibility, pH, oxygen, carbon dioxide, stem cells, cancer cells
Citation: Klein SG, Steckbauer A, Alsolami SM, Arossa S, Parry AJ, Li M and Duarte CM (2022) Toward Best Practices for Controlling Mammalian Cell Culture Environments. Front. Cell Dev. Biol. 10:788808. doi: 10.3389/fcell.2022.788808
Received: 03 October 2021; Accepted: 26 January 2022;
Published: 21 February 2022.
Edited by:
Jangho Kim, Chonnam National University, South KoreaReviewed by:
Ignacio Gimenez, University of Zaragoza, SpainElisa Cimetta, University of Padua, Italy
Copyright © 2022 Klein, Steckbauer, Alsolami, Arossa, Parry, Li and Duarte. This is an open-access article distributed under the terms of the Creative Commons Attribution License (CC BY). The use, distribution or reproduction in other forums is permitted, provided the original author(s) and the copyright owner(s) are credited and that the original publication in this journal is cited, in accordance with accepted academic practice. No use, distribution or reproduction is permitted which does not comply with these terms.
*Correspondence: Mo Li, bW8ubGlAa2F1c3QuZWR1LnNh; Carlos M. Duarte, Y2FybG9zLmR1YXJ0ZUBrYXVzdC5lZHUuc2E=