- National Clinical Research Center for Child Health of the Children’s Hospital, Zhejiang University School of Medicine, Hangzhou, China
The Hedgehog (HH) signaling is one of the key agents that govern the precisely regulated developmental processes of multicellular organisms in vertebrates and invertebrates. The HH pathway in the receiving cell includes Patched1, a twelve-pass transmembrane receptor, and Smoothened, a seven-transmembrane G-protein coupled receptor (GPCR), and the downstream GLI family of three transcriptional factors (GLI1-GLI3). Mutations of HH gene and the main components in HH signaling are also associated with numerous types of diseases. Before secretion, the HH protein undergoes post-translational cholesterol modification to gain full activity, and cholesterol is believed to be essential for proper HH signaling transduction. In addition, results from recent studies show the reciprocal effect that HH signaling functions in cholesterol metabolism as well as in cholesterol homeostasis, which provides feedback to HH pathway. Here, we hope to provide new insights into HH signaling function by discussing the role of cholesterol in HH protein maturation, secretion and HH signaling transduction, and the potential role of HH in regulation of cholesterol as well.
Introduction
Members of Hedgehog (HH) family have been recognized as one of the major intercellular signalings involved in controlling cell growth, regulating cell survival, deciding cell fate, and patterning almost all aspects of the body plan during animal embryonic development, depending on the context. Along with establishing the patterns of cellular differentiation to direct complex organ formation, HH signaling also plays an important role in post-embryonic tissue regeneration and repair processes (Lee et al., 2016; Skoda et al., 2018; Chapouly et al., 2019; Kong et al., 2019; Salaritabar et al., 2019; Rallis et al., 2020). Dysregulation of HH signaling, including mutations, is at the root of several developmental defects, and can lead to tumorigenesis in adults as well, such as holoprosencephaly (HPE) and Gorlin’s syndrome (Abramyan, 2019; Onodera et al., 2020). The origin of the name Hedgehog comes from the phenotype of the HH gene mutant Drosophila larvae that is shorter and more spiked (Ingham, 1995). Further in 1980s, Nusslein-Volhard and Wieschaus identified mutations in the HH gene. Since the first isolation and cloning of HH gene in Drosophila in the early 1990s, studies have identified proteins of HH family of secreted signaling molecules in both vertebrates and invertebrates, as diverse as mammals, insects and echinoderms, although the Caenorhabditis elegans (round worm), one of the most famous model organisms, has no HH ortholog (Ingham, 1995; Kume et al., 2019; González-Méndez et al., 2020). The vertebrate homologs of the Drosophila HH gene can be categorized into three subgroups: Sonic Hedgehog (SHH), Indian Hedgehog (IHH), and Desert Hedgehog (DHH), among which SHH is the most broadly expressed and is thought to be responsible for the major effects on development of the craniofacial part, spinal cord, axial skeleton and limbs, while IHH is identified to play a major role in cartilage differentiation in the growth of long bones and affects the formation of midface and masticatory system, and DHH is mainly involved in the formation of mouse testis, ovary and development of nerves (Jung et al., 2015; Lopez-Rios, 2016; Liu et al., 2018; Sasai et al., 2019; Amano et al., 2020; Brooks et al., 2020; Hosoya et al., 2020; Moreau and Boucher, 2020; Ohba, 2020). In the past decades, by using Drosophila genetics, key components of the HH signaling pathway have been identified and a series of mechanisms have been clarified. Up to now accumulating evidence has suggested that cholesterol regulates this important signaling at many levels, without which will lead to congenital malformations and cause diseases in adults.
Processing and Maturation of Hedgehog Protein
All HH family members share the same tertiary structure and follow the same way of maturation in HH-producing cells (Valentini et al., 1997). After translation, initially synthesized HH pro-proteins undergo multiple post-translational processing modifications to obtain activated HH proteins and subsequently to be released from the producing cells (Banavali, 2020). As depicted in Figure 1, after the signal sequence is removed, the HH molecule (∼45 kDa) first undergoes a cleavage catalyzed by its own C-terminal domain that is between the conserved glycine and cysteine residues, generating two polypeptides including the N-terminal polypeptide (HH-Np) of ∼19 kDa that contains the whole functions of the signaling in both vertebrates and invertebrates, and the C-terminal polypeptide (HH-Cp) of ∼25 kDa that has no function other than catalyzing the autoproteolytic cleavage. Mutations that block this auto proteolysis will result in impairment of HH function. During the autoproteolytic cleavage, a hydroxyl-oxygen cholesterol moiety is covalently attached to the last amino acid of the N-terminal polypeptide (HH-Np). As a result, cholesterol modified HH-Np associates tightly with the membrane, and subsequently the HH-Cp diffuses away and modified by the endoplasmic reticulum (ER) transmembrane protein acyl-transferase Skinny HH (Ski, also known as sightless, rasp or central missing, and HHAT in humans), a palmitic acid moiety is attached to the first amino acid of the N-terminal polypeptide (HH-Np), which is also required for HH-N activity in vivo (Liang et al., 2019). HH cholesteroylation is not only dispensable in the early stages of HH production and maturation, but also essential for receiving cells to receive HH signals (Manikowski et al., 2021). Thus, HH-N of full activity is with cholesteroylation at its C-terminus and palmitoylation at its N-terminus (Porter et al., 1995).
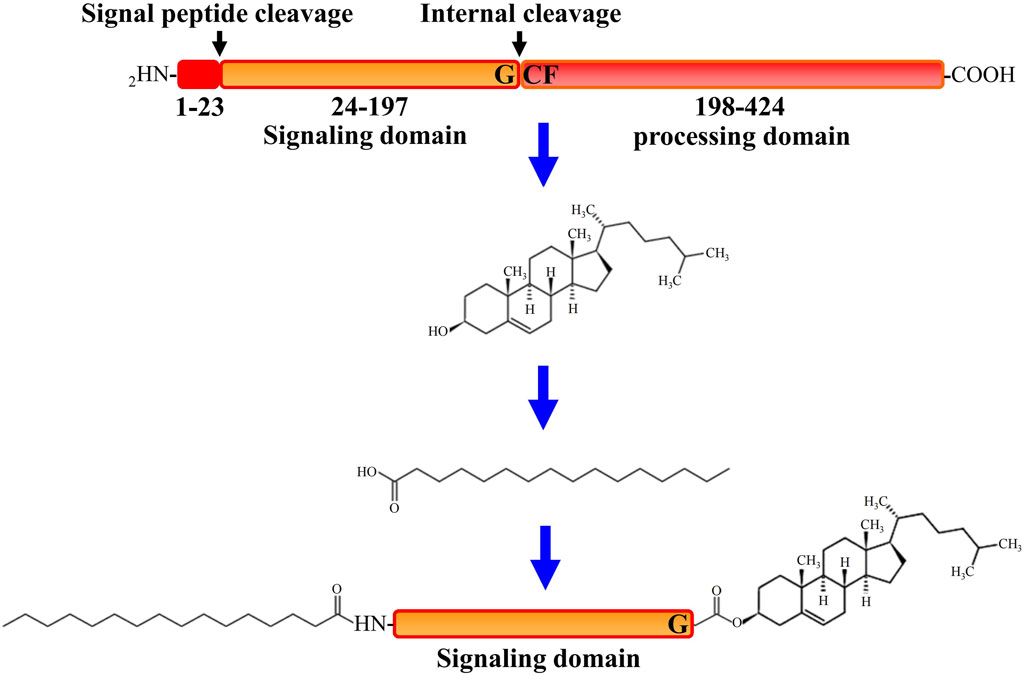
FIGURE 1. A schematic description of progress of Hedgehog protein maturation. In the first step of processing after Hedgehog (HH) protein translation, C-terminal initiates the catalytic action through its processing domain and leads to form an internal thioester between a cysteine and a glycine residue at the cleavage site. The C-terminal domain is then released by nucleophilic attack by the hydroxyl group of cholesterol, resulting in the covalent attachment of cholesterol to the C terminus of the N-terminal domain. Further more, the N terminus of the N-terminal domain is palmitoylated by the acyltransferase Skinny after signal peptide cleavage.
The Hedgehog Signaling Pathway
The major components of the HH pathway are identified to be conserved in D. melanogaster (Briscoe and Thérond, 2013). As the first HH receptor identified, PTCH1 binds to SMO to inhibit its activity in the absence of HH ligand. At this time, the transcription factor protein GLI is distributed at the tip of primary cilium and binds to the Suppressor of Fused (SuFu) protein to form a complex, and is phosphorylated by a series of protein kinases including protein Kinase A (PKA), glycogen synthesis kinase 3β (GSK-3β) and casein kinase 1 (CK1) to form a truncated repressor. However, binding of HH to PTCH1 relieved the inhibitory effect of PTCH1 on Smo. At this time, the activated SMO is translocated to the primary cilia, and finally the transcription factor GLI is depolymerized from the GLI-SuFu complex and enters the nucleus in the form of a transcription activator (GLIA) to activate the transcription of downstream target genes (Cannonier and Sterling, 2015) (Figure 2).
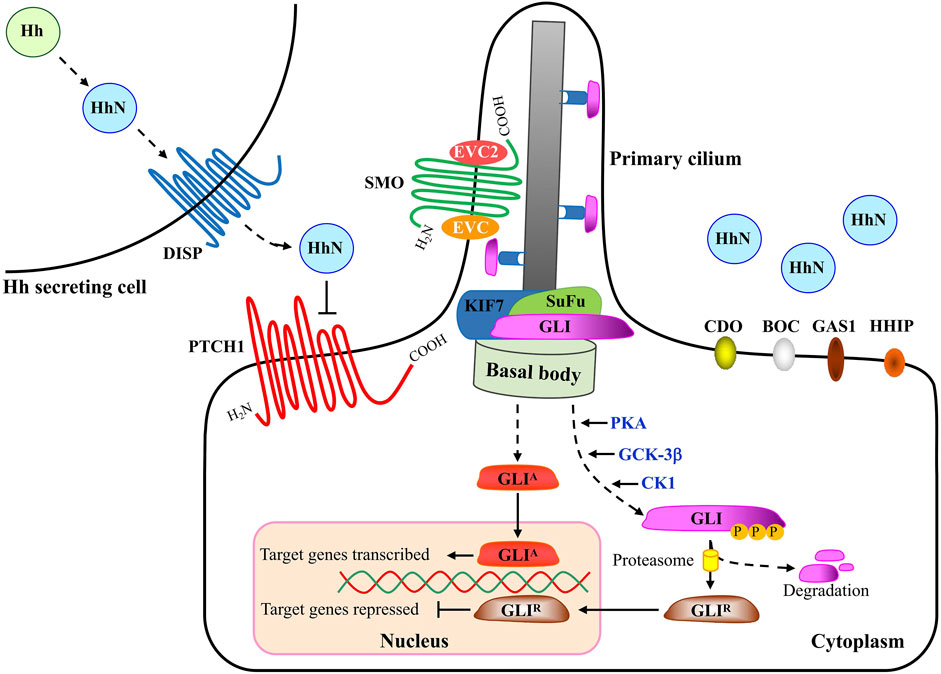
FIGURE 2. A schematic description of Hedgehog signaling transduction. When HH is absent, the 12-span-membrane receptor protein PTCH1 (Patched1) inhibits SMO (Smoothened) accumulation in the primary cilium and consequently SMO activity. SuFu can form complexes with GLI proteins and inhibit them from entering the nucleus and their transcriptional activity. Several kinases, including PKA, GSK3β and CK1 phosphorylate the C-terminal of GLIs, leading to the truncated form of full length GLI. C-terminal deleted-GLIs are thought to be strong transcriptional repressors (GLI-R) that go into the nucleus and inhibit the transcription of target genes. Upon HH ligand binding, the inhibition from PTCH1 is released and SMO is activated and translocates into the primary cilium. Within the primary cilium, SMO forms a complex with EVC and EVC2 to transduce the HH signaling response. Activated SMO leads to the release of GLIs from the SuFu complex and GLIs go into the nucleus as transcriptional activators (GLI-A) that activate the transcription of target genes. Movement of GLI proteins within the primary cilium occurs in conjunction with KIF7, a member of the kinesin family of anterograde motor proteins. Abbreviations: HH, Hedgehog; DISP, Dispatched; PTCH1, Patched1; CDO, Cell adhesion molecule-related/down-regulated by oncogenes; BOC, brother of Cdo/biregional Cdon-binding protein; Gas1, Growth arrest specific 1; HHIP, Hedgehog-interacting protein; SMO, Smoothened; EVC, Ellis van Creveld syndrome protein; EVC2, Ellis van Creveld syndrome protein 2; GLI, Glioma-associated oncogene; SuFu, Suppressor of Fused; KIF7, Kinesin family member 7.
In HH-responding cells, SMO is redistributed from unidentified intracellular compartments to the plasma membrane (Milenkovic et al., 2009). Activated SMO then transduces downstream signaling with the regulation of GLI transcriptional factors (Hu and Song, 2019). Three GLIs have been discovered in vertebrates, including GLI1, GLI2 and GLI3, which are in overlapping domains and are partially redundant. Similar to Ci (a 155 kDa cytoplasmic zinc finger protein) in Drosophila, GLI1 always acts as a transcriptional activator as it lacks the repressor domain and its expression is induced by HH ligands (Tang et al., 2015a; Infante et al., 2015; Doheny et al., 2020). Different from GLI2 that is essential for embryonic development, whose loss is embryonic lethal, GLI1 is dispensable for normal development, at least in the case of mice (Lee et al., 1997). Both GLI2 and GLI3 contain an amino-terminal repressor domain and a carboxy-terminal activator domain. In most cases GLI2 acts as a transcriptional activator (GLI-A) while GLI3 is thought to play a major role as a transcriptional repressor (GLI-R) that inhibits the expression of HH-downstream target genes. Ultimately, the balance between GLI-A and GLI-R influences HH pathway output through their accumulation and the subsequent transcription of target genes in nucleus.
In vertebrates, HH signaling is always present in primary cilia, the mechano-sensory organelles that respond to mechanical stimuli in the micro-environment. In the absence of HH signal, PTCH1 is found at the base of primary cilia, whereas GLI2 and GLI3 have been observed at the tips of the cilia and are retained in the cytoplasm in a protein complex associated with the inhibitory molecule SuFu that functions as a negative regulator in HH signaling network (Cannonier and Sterling, 2015). In addition to the well-known molecules, new members have been discovered, such as Ellis-van Creveld Syndrome (EVC) and Ellis-van Creveld Syndrome 2(EVC2), which interact with SMO and control HH signaling activity by regulating SuFu/GLI3 dissociation and GLI3 trafficking in primary cilia of chondrocytes and osteoblasts (Caparrós-Martín et al., 2013; Palencia-Campos et al., 2017). Protein kinases, including protein kinase A (PKA) and casein kinase 1 (CK1), phosphorylate SMO at several sites, leading to the increase of SMO cell-surface levels and signaling activity (Jia et al., 2004). Furthermore, a zipper-lock model was proposed in which the gradual phosphorylation at three clusters of serine residues causes a gradual conformational change in the cytoplasmic tail of Smo, thereby promoting the interaction between Smo and Costal2 in Drosophila (Fan et al., 2012). PKA, glycogen synthase kinase-3β (GSK-3β) and CK1 regulate GLI3 and a small fraction of GLI2 by phosphorylating their specific phosphor-sites in the region of C-terminus, after which, GLI3 and the small fraction of GLI2 enter the nucleus as truncated forms while most GLI2 is proteolytically degraded in the cytoplasm (Gomez et al., 2019; Yin et al., 2019). As a result, the truncated GLIs, also known as GLI repressors (GLI-R), function as repressors and suppress the transcription of HH signaling target genes. Once the canonical HH signaling is activated, GLI2 as well as GLI3 escapes from the proteolytic degradation and thereby goes into the nucleus as a full-length protein, and as transcriptional activators, the full length of GLIs activate transcription of target genes. GLIs play important roles in organ development during embryogenesis, including lungs, limbs, neural tube and craniofacial region, such as eyes, nose and teeth. Of note, during development of vertebrate neural tube, the absence of GLI2 disrupts the patterning of tissue types that are located closet to the origin of the HH signal, indicating activated GLI2 alone has the ability to induce the expression of downstream target genes of HH signaling (Minhas et al., 2015). In the nucleus, GLIs bind to the consensus and non-consensus binding sites to trans-activate or trans-inactivate their target genes. Despite the consensus GLI binding sequence “GACCACCCA”, some novel GLI binding sequences were confirmed in the past few years (Po et al., 2020), and up to now numerous target genes in HH signaling have been identified, including Bcl2, Myc, Wnt, Cyclin D, Cyclin E as well as PTCH1 and GLI1, two members in HH pathway, which provide negative and positive feedback loops to the pathway, respectively (Ma et al., 2015; Girardet et al., 2020; Tolosa et al., 2020).
Role of Cholesterol in Hedgehog Protein Transportation
Sterols, such as cholesterol, play a crucial role in the cell biology of all eukaryotes (Gelissen and Brown, 2017; Reboldi and Dang, 2018; Huang et al., 2020). Cellular cholesterol is derived from endogenous synthesis in the ER and from internalized lipoproteins. There is compelling evidence of the involvement of cholesterol in the proper HH signaling transduction that acts as a key regulator in both development and tumor progression (Niewiadomski et al., 2019). As a major developmental mediator in the morphogenetic field, the versatile HH molecule acts at both short and long ranges in a direct and concentration-dependent manner, which means HH family members can exert their effects not only on the cells nearby the source of the original signal but also over considerable distances (up to 30-cell diameters), at least acting as classic morphogens in some cases (Wu et al., 2019). Investigations of the past few years show that cholesterol modification affects the diffusion range of HH family members strikingly with the function to restrict the dilution and to alleviate the spread of HH ligand diffusion in the extracellular space (Dillon et al., 2003; Mateska et al., 2020; Gore et al., 2021). Although HH signaling is first thought to be a morphogen in the segmental patterning of the Drosophila larvae, from which a unique readout can be offered by the adult wing pattern, the importance of cholesterol modification has already been confirmed in the context of embryonic development (Purohit et al., 2020; Radhakrishnan et al., 2020). In Drosophila, cholesterol-unmodified HH form diffuses further away with an abnormally wide range, compared to the cholesterol-modified HH protein with a decreased affinity for plasma membrane by expressing a transgene that only encodes the HH N-terminal domain. Moreover, cholesterol-unmodified HH form does not interact properly with the Patched receptor protein, Shifted proteins and extracellular components such as heparan sulfate proteoglycan (HSPG) (The et al., 1999; Lewis et al., 2001; Dawber et al., 2005; Callejo et al., 2006; Kenwrick et al., 2019). However, recent studies indicate that cholesterol modification of HH-N is not necessary for HH-PTCH1 interaction, either functionally or structurally (Tukachinsky et al., 2016; Qi et al., 2018). In vertebrates, cholesterol modification of HH-N is essential for its function at long range, while cholesterol-unmodified HH-N only possesses the activity of short range, which was determined through a developmental ideal model system of limb bud pattern in embryonic mice. The discrepancy of conclusions between Drosophila and mouse possibly reflects the differences between tissues studied in each case (The et al., 1999).
Though the mechanism by which HH-Np is released from producing cells to exert long-range activity is not completely understood, one gene is identified to be involved in this process by genetic screens in Drosophila. This gene, Dispatched (Disp), which encodes the putative 12-pass transmembrane protein Dispatched (DISP) that contains a novel sterol-sensing domain (SSD), is found to be required for secretion of Hh-Np in Drosophila imaginal disc. SSD domain, first identified in proteins implicated in cholesterol homeostasis or trafficking, is required for the cholesterol-dependent regulation (Stewart et al., 2018; Cannac et al., 2020; Wierbowski et al., 2020). In Disp mutants, HH-Np can be hardly released to target cells or activate the target genes but accumulates in the producing cells. However, cholesterol-unmodified HH-N can be released and diffuse as it does not require DISP for secretion. Some studies have given the possible functions of DISP, that is, DISP is involved in the intracellular trafficking of cholesterol-modified HH-N in the secretory pathway, and once DISP reaches the cell surface it displaces cholesterol from the cell membrane (Wierbowski et al., 2020). Thus, it is likely that DISP is involved in packaging cholesterol-modified HH into freely diffusing aggregates.
In addition to the effect of cholesterol on HH protein, recent work further sheds light on another phenomenon that normal cholesterol concentrations are required for proper activation of SMO (Smoothened), the seven-transmembrane-span receptor-like protein in HH pathway, and that regulation of SMO by PTCH1 is thought to be dependent on small molecular intermediates, such as cholesterol (The et al., 1999; Callejo et al., 2006; Kenwrick et al., 2019; Dawber et al., 2005; Lewis et al., 2001; Tukachinsky et al., 2016; Qi et al., 2018; Stewart et al., 2018; Wierbowski et al., 2020; Cannac et al., 2020; Rohatgi et al., 2009; Byrne et al., 2016; Huang et al., 2016; Luchetti et al., 2016; Xiao et al., 2017; Kowatsch et al., 2019) (summarized in Table 1). The Asp 95 (D95) residue of SMO is proved to be covalently modified by cholesterol via an ester bond, which is enhanced by HH but inhibited by PTCH1(69). The D95N mutation of SMO is hardly modified by cholesterol and rarely localizes to the primary cilia, resulting in its failure in activation of downstream signaling transduction. Several possible mechanisms by which PTCH1 regulates SMO have been proposed, and these mechanisms are mainly focused on the primary cilium or the ciliary micro-domain: (Skoda et al., 2018) PTCH1 can regulate the overall membrane levels of cholesterol or distribution of cholesterol across the bilayer, thereby preventing cholesterol supply to the cysteine-rich domain (CRD) and/or transmembrane domain (TMD) of SMO (Zhang et al., 2018). (Kong et al., 2019) PTCH1 removes sterols from SMO CRD, thus inhibiting HH signaling (Salaritabar et al., 2019). As a sterol flippase, PTCH1 transfers sterols between the outer and the inner membrane leaflets (Deshpande et al., 2019; Kowatsch et al., 2019). More experiments are in need to address this issue.
Requirement for Cholesterol in Hedgehog Pathway Transduction
The HH signaling mainly comprises of four parts, as depicted in Figure 2, including HH ligands from the HH secreting cell, transmembrane receptors, the GLI (Glioma-associated oncogene) transcriptional factor family of zinc-finger DNA-binding proteins, and the downstream target genes (Carballo et al., 2018; Cortes et al., 2019). In the absence of HH ligand, PTCH1, a twelve-transmembrane-span receptor, inhibits the activity of SMO, a member of the Frizzled family of seven-pass transmembrane receptor-like proteins. Binding of HH to receptor PTCH1, which is promoted by the membrane co-receptor proteins CDO (CAM-related/down regulated by oncogenes), BOC (brother of CDO) and GAS1 (growth arrest-specific 1) (Kim H. et al., 2020; Echevarría-Andino and Allen, 2020; Kim Y. et al., 2020), leads to inactivation of PTCH1 and activation of SMO with its release from the inhibitory effect by PTCH1. Intriguingly, recent biochemical, structural and cell biological studies have demonstrated that cholesterol also acts as a second messenger to communicate HH signals between PTCH1 and SMO (Table 2). PTCH1 could move cholesterol from the outer to the inner leaflet of the membrane in exchange for potassium ion export (Kinnebrew et al., 2021). A study on the structural analysis of cholesterol-activated Xenopus laevis SMO (xSMO) indicated that cholesterol-mediated SMO activation is correlated with the rearrangement of CRD orientation and a cation-lock opening in the transmembrane domain (TMD) of SMO (Huang et al., 2018). A large number of signaling researches and molecular dynamics simulations analyze the structural basis for PTCH1-SMO regulation (Myers et al., 2017; Zhang et al., 2018; Kinnebrew et al., 2019; Radhakrishnan et al., 2020). For example, PTCH1 restricts the access of cholesterol to SMO by controlling the flipping of cholesterol in PM lipid bilayers. Specifically, PTCH1 limits the cholesterol content in the inner leaflet, which may affect cholesterol level in SMO-containing compartments (Zhang et al., 2018). Although the exact mechanisms remain largely vague, studies carried out in the past few years suggest that SMO exists in active and inactive conformational states and PTCH1 regulates the sub-cellular localization of SMO as well as several progressive phosphorylation sites in SMO (Byrne et al., 2018).
Insights From Diseases
As an essential sterol found in vertebrates, cholesterol plays a pivotal role in regulating normal functions of cells, tissues and organs, such as acting as a precursor for steroid hormone biosynthesis, regulating the fluidity and permeability of cell membranes and as well as playing roles as a covalently modifying protein (Porter et al., 1996; Miller, 2017; Xiao et al., 2017; Cole et al., 2019). During the early embryonic development, cholesterol plays an essential role in controlling the activity and function of HH signaling pathway, and without sufficient cholesterol, HH signaling works aberrantly, resulting in abnormal development and relevant syndromes. For example, Emopamil binding protein (EBP, also known as 3-beta-hydroxysteroid-Delta (Onodera et al., 2020), Delta(7)-isomerase) mutation-associated is caused by abnormal cholesterylation of SMO (Qiu et al., 2021). Patients with CDPX2 are characterized by cartilage abnormalities around vertebral column, pelvis and long bones (Qiu et al., 2021). Over the past 30 years, progresses in the field of etiology have emphasized that a large amount of developmental malformations of the syndromes caused by abnormal HH signaling transduction are due to the defects of cholesterol biosynthesis, such as holoprosencephaly, a severe brain malformation. Interestingly, it is recently found in mice that inactivation of SHH causes axial patterning defects with a phenotype of holoprosencephalic, which is similar to that found in the mice with deficiency of Megalin and in some of the human patients with Smith–Lemli–Opitz syndrome (SLOS) as well. In addition, by using chick embryos some studies also confirmed that treatment of cyclodextrin, a cyclic oligosaccharide that forms non-covalent complexes with sterols and can be thereby used to extract and deplete cholesterol from living cells, causes variable loss of the frontonasal process and other midline structures in chick embryos, and the spectrum of facial defects is similar to that resulted from the exposure to HH-pathway antagonist jervine (DeSesso, 2020). Likewise, mutation of SHH gene in human causes the similar phenotype as that has been shown in the patients of SLOS. It can be thereby confirmed that at least three ways in HH signaling transduction are associated with metabolism of cholesterol, including the covalent modification of HH in the process of HH maturation and secretion, the action of PTCH1 with putative SSD (Gelissen and Brown, 2017), and the cholesterylation of SMO.
Hedgehog Signaling Regulates Cholesterol
Since its discovery nearly 30 years ago, the essential role of HH signaling in embryonic development and cancer progress has been clarified, and several mechanisms by which cholesterol regulates HH signaling have been investigated, whereas there was no evidence until 2015 showing HH mutually affects cholesterol (Tang et al., 2015b). Hence, it is interesting to determine whether cholesterol is also regulated by HH signaling, which would further reflect the association of HH with process of other metabolic diseases. HH signaling plays a central role in the development of many steroidogenic tissues, such as the ovary, the adrenal cortex, the testis and the placenta, suggesting HH signaling is involved in the regulation of lipid metabolisms such as steroidogenesis (shown in Table 3) (Tang et al., 2015b; Finco et al., 2015; Matz-Soja et al., 2016; Zhu et al., 2016; Rennert et al., 2017; Albert et al., 2018; Finco et al., 2018; Takai et al., 2019; Luo et al., 2020). The abnormality of HH pathway is also identified to be involved in hepatic steatosis. A previous study utilizing transgenic mice with liver-specific deletion of SMO shows that inhibition of HH pathway in hepatocytes causes steatosis by altering the expression of transcription factors GLI1 and GLI3 (Matz-Soja et al., 2016). In contrast, activation of HH signaling reverses the GLI-code and alleviates hepatic steatosis. Additionally, Rennert et al. reported that female mice with downregulation of embryonic and hepatic HH signaling results in an increase of testosterone levels, and infertility characterized by reproductive organs and acyclicity, indicating the impaired hormonal balance in the liver via the abnormal induction of steroidogenesis (Rennert et al., 2017).
There are multiple steps in steroidogenesis from cholesterol with the first step of cellular allocation of cholesterol, and cholesterol is recognized to be the common original material for biosynthesis of all kinds of steroid hormones in cells (Luo et al., 2020; Xu et al., 2020; Schade et al., 2020; Guo et al., 2018). In cells many sources of cholesterol can be made available for steroidogenesis, including cholesterol that is delivered by serum low-density lipoprotein (LDL) and high-density lipoprotein (HDL), cholesterol that is internalized from the plasma membrane, hydrolysis of intracellular cholesterol esters (CE) stored in lipid droplets and as well as de novo synthesis (Figure 3A) (Luo et al., 2020). De novo synthesis of cholesterol in cells is from acetyl-CoA and acetoacetyl-CoA in the ER through several stepwise enzymatic reactions. First, in combination with acetoacetyl-CoA, acetyl-CoA is converted to 3-hydroxy-3-methylglutaryl-CoA (HMG-CoA) in the cytosol. Then, as the rate-limiting enzyme of the pathway, hydroxy-methylglutaryl-CoA reductase (HMG-CoAR) converts HMG-CoA to mevalonate. HMG-CoAR contains a conserved SSD, which is characterized by five membrane-spanning α-helices (Finco et al., 2015; Guo et al., 2018; Wangeline and Hampton, 2020) and is necessary for its attachment to the protein Insig on ER membrane as well as for the regulation of its degradation (Theesfeld and Hampton, 2013; Zhou et al., 2020). In the following steps, mevalonate is converted successively to isopentenyl pyrophosphate, squalene and lanosterol. Finally, lanosterol undergoes a series of 19-additional reactions to produce cholesterol. Some products from cholesterol have been discovered, such as oxysterol and cholesterol esters, and once cholesterol is successfully transferred into the mitochondria, it is catalyzed by P450 cholesterol side-chain cleavage enzyme (P450scc) to produce pregnenolone in mitochondria (Bose et al., 2021). Then pregnenolone moves from the mitochondria to the ER where following reactions happen with the results of production of different hormones (Finco et al., 2015).
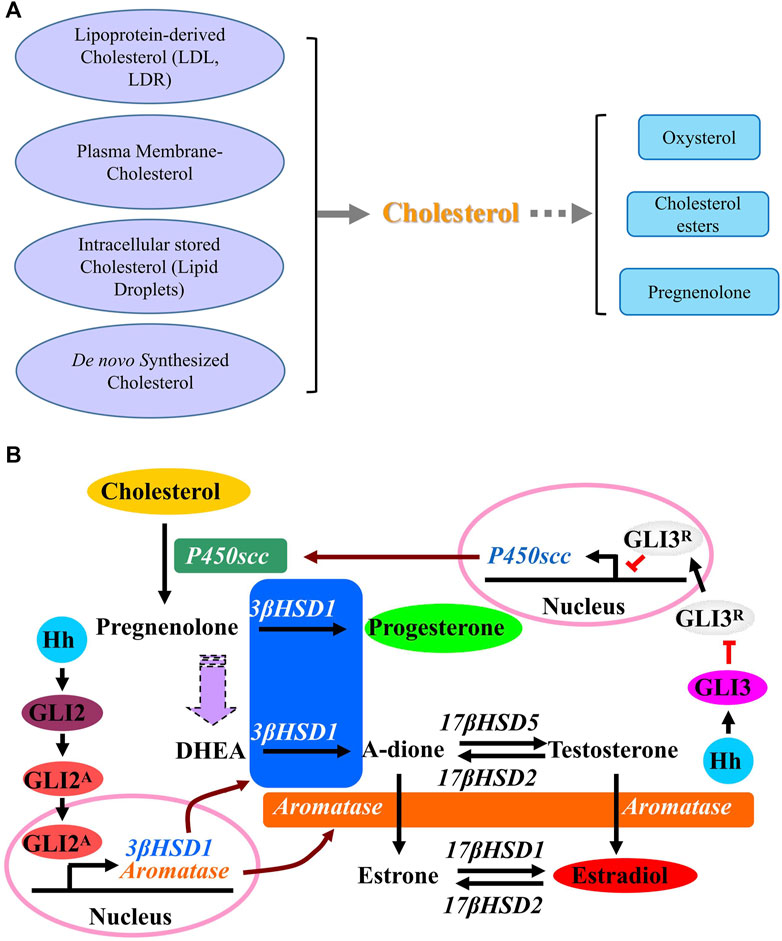
FIGURE 3. Cholesterol synthesis pathways. (A) A schematic description showing the sources of cholesterol and the products of cholesterol. (B) A schematic description of effect of Hedgehog signaling on metabolism of cholesterol. Activation of Hh signaling induces the conversion of cholesterol to progesterone (P4) and estradiol (E2) through up-regulating the expression of steroidogenic enzymes including P450scc, 3β-HSD1 and aromatase. GLI3 is required for Hh-induced P450scc expression, while GLI2 mediates the induction of 3β-HSD1and aromatase. Abbreviations: P450scc, P450 cholesterol side chain cleavage enzyme; 3β-HSD1, 3β-hydroxysteroid dehydrogenase type 1; 17β-HSD1, 17β-hydroxysteroid dehydrogenase type 1; 17β-HSD2, 17β-hydroxysteroid dehydrogenase type 2; 17β-HSD5, 17β-hydroxysteroid dehydrogenase type 5; DHEA, Dehydroepiandrosterone.
A previous report reveals the novel function of HH that HH acts as a regulator in stimulating the conversion of cholesterol to steroid. Activation of HH signaling induces the conversion of cholesterol to progesterone and estradiol through up-regulating the expression of steroidogenic enzymes including P450scc, 3β-hydroxysteroid dehydrogenase type 1 (3β-HSD1) and aromatase. As shown in Figure 3B, while GLI3 is required for HH-induced P450scc expression, GLI2 mediates the induction of 3β-HSD1 and aromatase. As a novel role of HH signaling in regulating the metabolism of cholesterol with up-regulated expression of genes P450scc, 3β-HSD1 and aromatase, which are essential for steroidogenesis during human pregnancy, it is likely that HH signaling is also essential for human pregnancy maintenance, since a successful pregnancy requires steroidogenesis from cholesterol in placenta. Later in 2016 and 2018, Zhu et al. and Raleigh et al. discovered that GLI up-regulates the expression of oxysterol synthase HSD11β2, and HSD11β2 generates oxysterols that are associated with the cellular cilia and bind to SMO, which leads to the activation of SMO and promotion of HH pathway activity (Zhu et al., 2016; Raleigh et al., 2018), suggesting that the inhibition of SMO-activating oxysterol production may be therapeutically useful for patients with HH-associated diseases.
Given that HH signaling regulates chondrocyte differentiation and is activated in human and murine osteoarthritis, recent study discovers that HH signaling regulates cholesterol homeostasis in osteoarthritis using human osteoarthritis samples by up-regulating genes that govern cholesterol homeostasis, which alters cholesterol accumulation in chondrocytes (Ali et al., 2016). Moreover, chondrocyte-specific cholesterol accumulation causes an osteoarthritis phenotype in genetically modified mice, while reducing cholesterol accumulation attenuates the severity of osteoarthritis in mice in vivo and decreases expression of proteases in human cartilage in vitro.
Perspective
The HH signaling that was first identified in Drosophila a few decades ago, has been clarified to be involved in the development of both vertebrates and invertebrates and in the progress of cancer. Owing to rapid progress over the last several years, we have a better understanding of the mechanisms by which HH signaling is regulated by cholesterol, and recent work further uncovers the mechanisms by which HH affects cholesterol regulation. Advances in our knowledge of the cross-talk between HH signaling and cholesterol provides a framework which can be used to illustrate unknown mechanisms of clinical diseases, and further fulfill the biological significance of HH signaling to the known mechanisms. Aberrant HH signaling can lead to abnormal lipid homeostasis, which affects atherosclerosis and hepatosteatosis. Further research, especially progress in metabolomics and genetic studies will help reveal novel and intricate details of the biochemical and molecular cellular biological aspects of HH signaling. Currently, there are still some challenges in this area. What is the specific mechanism by which sterol participates in the regulation of SMO by PTCH1? How exactly does cholesterol modification of SMO activate HH signaling and transduce downstream events? How aberrant cholesterol metabolism affects Hh signaling and leads to different clinical manifestations? Deeper investigations in this field will potentially benefit disease therapy, especially in understanding the relation between HH signaling and related sterol metabolisms, which will provide potential targets in developing novel drugs for relevant diseases in the future.
Author Contributions
SX wrote the manuscript. CT edited the manuscript.
Conflict of Interest
The authors declare that the research was conducted in the absence of any commercial or financial relationships that could be construed as a potential conflict of interest.
Publisher’s Note
All claims expressed in this article are solely those of the authors and do not necessarily represent those of their affiliated organizations, or those of the publisher, the editors and the reviewers. Any product that may be evaluated in this article, or claim that may be made by its manufacturer, is not guaranteed or endorsed by the publisher.
References
Abramyan, J. (2019). Hedgehog Signaling and Embryonic Craniofacial Disorders. J. Dev. Biol. 7 (2), 9. doi:10.3390/jdb7020009
Albert, E. A., Puretskaia, O. A., Terekhanova, N. V., Labudina, A., and Bökel, C. (2018). Direct Control of Somatic Stem Cell Proliferation Factors by the Drosophila Testis Stem Cell Niche. Development 145 (17), dev156315. doi:10.1242/dev.156315
Ali, S. A., Al‐Jazrawe, M., Ma, H., Whetstone, H., Poon, R., Farr, S., et al. (2016). Regulation of Cholesterol Homeostasis by Hedgehog Signaling in Osteoarthritic Cartilage. Arthritis Rheumatol. 68 (1), 127–137. doi:10.1002/art.39337
Amano, K., Okuzaki, D., Aikawa, T., and Kogo, M. (2020). Indian Hedgehog in Craniofacial Neural Crest Cells Links to Skeletal Malocclusion by Regulating Associated Cartilage Formation and Gene Expression. FASEB j. 34 (5), 6791–6807. doi:10.1096/fj.201903269r
Banavali, N. K. (2020). The Mechanism of Cholesterol Modification of Hedgehog Ligand. J. Comput. Chem. 41 (6), 520–527. doi:10.1002/jcc.26097
Bose, H. S., Whittal, R. M., Marshall, B., Rajapaksha, M., Wang, N. P., Bose, M., et al. (2021). A Novel Mitochondrial Complex of Aldosterone Synthase, Steroidogenic Acute Regulatory Protein, and Tom22 Synthesizes Aldosterone in the Rat Heart. J. Pharmacol. Exp. Ther. 377 (1), 108–120. doi:10.1124/jpet.120.000365
Briscoe, J., and Thérond, P. P. (2013). The Mechanisms of Hedgehog Signalling and its Roles in Development and Disease. Nat. Rev. Mol. Cel Biol 14 (7), 416–429. doi:10.1038/nrm3598
Brooks, E. R., Islam, M. T., Anderson, K. V., and Zallen, J. A. (2020). Sonic Hedgehog Signaling Directs Patterned Cell Remodeling during Cranial Neural Tube Closure. Elife 9, e60234. Published 2020 Oct 26. doi:10.7554/eLife.60234
Byrne, E. F., Luchetti, G., Rohatgi, R., and Siebold, C. (2018). Multiple Ligand Binding Sites Regulate the Hedgehog Signal Transducer Smoothened in Vertebrates. Curr. Opin. Cel Biol. 51, 81–88. doi:10.1016/j.ceb.2017.10.004
Byrne, E. F. X., Sircar, R., Miller, P. S., Hedger, G., Luchetti, G., Nachtergaele, S., et al. (2016). Structural Basis of Smoothened Regulation by its Extracellular Domains. Nature 535 (7613), 517–522. doi:10.1038/nature18934
Callejo, A., Torroja, C., Quijada, L., and Guerrero, I. (2006). Hedgehog Lipid Modifications Are Required for Hedgehog Stabilization in the Extracellular Matrix. Development 133 (3), 471–483. doi:10.1242/dev.02217
Cannac, F., Qi, C., Falschlunger, J., Hausmann, G., Basler, K., and Korkhov, V. M. (2020). Cryo-EM Structure of the Hedgehog Release Protein Dispatched. Sci. Adv. 6 (16), eaay7928. doi:10.1126/sciadv.aay7928
Cannonier, S. A., and Sterling, J. A. (2015). The Role of Hedgehog Signaling in Tumor Induced Bone Disease. Cancers (Basel) 7 (3), 1658–1683. doi:10.3390/cancers7030856
Caparrós-Martín, J. A., Valencia, M., Reytor, E., Pacheco, M., Fernandez, M., Perez-Aytes, A., et al. (2013). The Ciliary Evc/Evc2 Complex Interacts with Smo and Controls Hedgehog Pathway Activity in Chondrocytes by Regulating Sufu/Gli3 Dissociation and Gli3 Trafficking in Primary Cilia. Hum. Mol. Genet. 22 (1), 124–139. doi:10.1093/hmg/dds409
Carballo, G. B., Honorato, J. R., de Lopes, G. P. F., and Spohr, T. C. L. S. E. (2018). A Highlight on Sonic Hedgehog Pathway. Cell Commun Signal 16 (1), 11. doi:10.1186/s12964-018-0220-7
Chapouly, C., Guimbal, S., Hollier, P.-L., and Renault, M.-A. (2019). Role of Hedgehog Signaling in Vasculature Development, Differentiation, and Maintenance. Int. J. Mol. Sci. 20 (12), 3076. doi:10.3390/ijms20123076
Cole, T. J., Short, K. L., and Hooper, S. B. (2019). The Science of Steroids. Semin. Fetal Neonatal Med. 24 (3), 170–175. doi:10.1016/j.siny.2019.05.005
Cortes, J. E., Gutzmer, R., Kieran, M. W., and Solomon, J. A. (2019). Hedgehog Signaling Inhibitors in Solid and Hematological Cancers. Cancer Treat. Rev. 76, 41–50. doi:10.1016/j.ctrv.2019.04.005
Dawber, R. J., Hebbes, S., Herpers, B., Docquier, F., and van den Heuvel, M. (2005). Differential Range and Activity of Various Forms of the Hedgehog Protein. BMC Dev. Biol. 5, 21. doi:10.1186/1471-213x-5-21
DeSesso, J. M. (2020). Of Embryos and Tumors: Cyclopia and the Relevance of Mechanistic Teratology. Birth Defects Res. 112 (3), 219–233. doi:10.1002/bdr2.1636
Deshpande, I., Liang, J., Hedeen, D., Roberts, K. J., Zhang, Y., Ha, B., et al. (2019). Smoothened Stimulation by Membrane Sterols Drives Hedgehog Pathway Activity. Nature 571 (7764), 284–288. doi:10.1038/s41586-019-1355-4
Dillon, R., Gadgil, C., and Othmer, H. G. (2003). Short- and Long-Range Effects of Sonic Hedgehog in Limb Development. Proc. Natl. Acad. Sci. U.S.A. 100 (18), 10152–10157. doi:10.1073/pnas.1830500100
Doheny, D., Manore, S. G., Wong, G. L., and Lo, H.-W. (2020). Hedgehog Signaling and Truncated GLI1 in Cancer. Cells 9 (9), 2114. doi:10.3390/cells9092114
Echevarría-Andino, M. L., and Allen, B. L. (2020). The Hedgehog Co-receptor BOC Differentially Regulates SHH Signaling during Craniofacial Development. Development 147 (23), dev189076.
Fan, J., Liu, Y., and Jia, J. (2012). Hh-induced Smoothened Conformational Switch Is Mediated by Differential Phosphorylation at its C-Terminal Tail in a Dose- and Position-dependent Manner. Develop. Biol. 366 (2), 172–184. doi:10.1016/j.ydbio.2012.04.007
Finco, I., LaPensee, C. R., Krill, K. T., and Hammer, G. D. (2015). Hedgehog Signaling and Steroidogenesis. Annu. Rev. Physiol. 77, 105–129. doi:10.1146/annurev-physiol-061214-111754
Finco, I., Lerario, A. M., and Hammer, G. D. (2018). Sonic Hedgehog and WNT Signaling Promote Adrenal Gland Regeneration in Male Mice. Endocrinology 159 (2), 579–596. doi:10.1210/en.2017-03061
Gelissen, I. C., and Brown, A. J. (2017). An Overview of Cholesterol Homeostasis. Methods Mol. Biol. 1583, 1–6. doi:10.1007/978-1-4939-6875-6_1
Girardet, L., Bernet, A., Calvo, E., Soulet, D., Joly-Beauparlant, C., Droit, A., et al. (2020). Hedgehog Signaling Pathway Regulates Gene Expression Profile of Epididymal Principal Cells through the Primary Cilium. FASEB j. 34 (6), 7593–7609. doi:10.1096/fj.202000328r
Gomez, H. G., Noguchi, H., Castillo, J. G., Aguilar, D., Pleasure, S. J., and Yabut, O. R. (2019). Suppressor of Fused Regulates the Proliferation of Postnatal Neural Stem and Precursor Cells via a Gli3-dependent Mechanism. Biol. Open 8 (6), bio039248. doi:10.1242/bio.039248
González-Méndez, L., Gradilla, A. C., Sánchez-Hernández, D., González, E., Aguirre-Tamaral, A., Jiménez-Jiménez, C., et al. (2020). Polarized Sorting of Patched Enables Cytoneme-Mediated Hedgehog Reception in the Drosophila wing Disc. EMBO J. 39 (11), e103629. doi:10.15252/embj.2019103629
Gore, T., Matusek, T., D'Angelo, G., Giordano, C., Tognacci, T., Lavenant-Staccini, L., et al. (2021). The GTPase Rab8 Differentially Controls the Long- and Short-Range Activity of the Hedgehog Morphogen Gradient by Regulating Hedgehog Apico-Basal Distribution. Development 148 (5), dev191791. doi:10.1242/dev.191791
Guo, C., Chi, Z., Jiang, D., Xu, T., Yu, W., Wang, Z., et al. (2018). Cholesterol Homeostatic Regulator SCAP-SREBP2 Integrates NLRP3 Inflammasome Activation and Cholesterol Biosynthetic Signaling in Macrophages. Immunity 49 (5), 842–856. doi:10.1016/j.immuni.2018.08.021
Hosoya, A., Shalehin, N., Takebe, H., Shimo, T., and Irie, K. (2020). Sonic Hedgehog Signaling and Tooth Development. Int. J. Mol. Sci. 21 (5), 1587. doi:10.3390/ijms21051587
Hu, A., and Song, B.-L. (2019). The Interplay of Patched, Smoothened and Cholesterol in Hedgehog Signaling. Curr. Opin. Cel Biol. 61, 31–38. doi:10.1016/j.ceb.2019.06.008
Huang, B., Song, B.-l., and Xu, C. (2020). Cholesterol Metabolism in Cancer: Mechanisms and Therapeutic Opportunities. Nat. Metab. 2 (2), 132–141. doi:10.1038/s42255-020-0174-0
Huang, P., Nedelcu, D., Watanabe, M., Jao, C., Kim, Y., Liu, J., et al. (2016). Cellular Cholesterol Directly Activates Smoothened in Hedgehog Signaling. Cell 166 (5), 1176–1187. doi:10.1016/j.cell.2016.08.003
Huang, P., Zheng, S., Wierbowski, B. M., Kim, Y., Nedelcu, D., Aravena, L., et al. (2018). Structural Basis of Smoothened Activation in Hedgehog Signaling. Cell 175 (1), 295–297. doi:10.1016/j.cell.2018.09.003
Infante, P., Mori, M., Alfonsi, R., Ghirga, F., Aiello, F., Toscano, S., et al. (2015). Gli1/DNA Interaction Is a Druggable Target for Hedgehog‐dependent Tumors. Embo J. 34 (2), 200–217. doi:10.15252/embj.201489213
Ingham, P. W. (1995). Signalling by Hedgehog Family Proteins in Drosophila and Vertebrate Development. Curr. Opin. Genet. Dev. 5 (4), 492–498. doi:10.1016/0959-437x(95)90054-k
Jia, J., Tong, C., Wang, B., Luo, L., and Jiang, J. (2004). Hedgehog Signalling Activity of Smoothened Requires Phosphorylation by Protein Kinase A and Casein Kinase I. Nature 432 (7020), 1045–1050. doi:10.1038/nature03179
Jung, J., Frump, D., Su, J., Wang, W., Mozaffar, T., and Gupta, R. (2015). Desert Hedgehog Is a Mediator of Demyelination in Compression Neuropathies. Exp. Neurol. 271, 84–94. doi:10.1016/j.expneurol.2015.04.014
Kenwrick, K., Mukherjee, A., and Renault, A. D. (2019). Hmgcr Promotes a Long-Range Signal to Attract Drosophila Germ Cells Independently of Hedgehog. J. Cel Sci 132 (23), jcs232637. doi:10.1242/jcs.232637
Kim, H., Lee, S.-Y., Jeong, H.-J., Kang, J.-S., Cho, H., and Leem, Y.-E. (2020). Cdo Is Required for Efficient Motor Neuron Generation of Embryonic Stem Cells. Ijsc 13 (3), 342–352. doi:10.15283/ijsc20037
Kim, Y., Lee, J., Seppala, M., Cobourne, M. T., and Kim, S.-H. (2020). Ptch2/Gas1 and Ptch1/Boc Differentially Regulate Hedgehog Signalling in Murine Primordial Germ Cell Migration. Nat. Commun. 11 (1), 1994. [published correction appears in Nat Commun. 2020 May 5;11(1):2275]. doi:10.1038/s41467-020-16304-7
Kinnebrew, M., Iverson, E. J., Patel, B. B., Pusapati, G. V., Kong, J. H., Johnson, K. A., et al. (2019). Cholesterol Accessibility at the Ciliary Membrane Controls Hedgehog Signaling. Elife 8, e50051. doi:10.7554/eLife.50051
Kinnebrew, M., Luchetti, G., Sircar, R., Frigui, S., Viti, L. V., Naito, T., et al. (2021). Patched 1 Reduces the Accessibility of Cholesterol in the Outer Leaflet of Membranes. Elife 10, e70504. doi:10.7554/eLife.70504
Kong, J. H., Siebold, C., and Rohatgi, R. (2019). Biochemical Mechanisms of Vertebrate Hedgehog Signaling. Development 146 (10), dev166892. doi:10.1242/dev.166892
Kowatsch, C., Woolley, R. E., Kinnebrew, M., Rohatgi, R., and Siebold, C. (2019). Structures of Vertebrate Patched and Smoothened Reveal Intimate Links between Cholesterol and Hedgehog Signalling. Curr. Opin. Struct. Biol. 57, 204–214. doi:10.1016/j.sbi.2019.05.015
Kume, M., Chiyoda, H., Kontani, K., Katada, T., and Fukuyama, M. (2019). Hedgehog-Related Genes Regulate Reactivation of Quiescent Neural Progenitors in Caenorhabditis Elegans. Biochem. Biophysical Res. Commun. 520 (3), 532–537. doi:10.1016/j.bbrc.2019.10.045
Lee, J., Platt, K. A., Censullo, P., and Ruiz i Altaba, A. (1997). Gli1 Is a Target of Sonic Hedgehog that Induces Ventral Neural Tube Development. Development 124 (13), 2537–2552. doi:10.1242/dev.124.13.2537
Lee, R. T., Zhao, Z., and Ingham, P. W. (2016). Hedgehog Signalling. Development 143 (3), 367–372. doi:10.1242/dev.120154
Lewis, P. M., Dunn, M. P., McMahon, J. A., Logan, M., Martin, J. F., St-Jacques, B., et al. (2001). Cholesterol Modification of Sonic Hedgehog Is Required for Long-Range Signaling Activity and Effective Modulation of Signaling by Ptc1. Cell 105 (5), 599–612. doi:10.1016/s0092-8674(01)00369-5
Liang, R., Kagwiria, R., Zehender, A., Dees, C., Bergmann, C., Ramming, A., et al. (2019). Acyltransferase Skinny Hedgehog Regulates TGFβ-dependent Fibroblast Activation in SSc. Ann. Rheum. Dis. 78 (9), 1269–1273. doi:10.1136/annrheumdis-2019-215066
Liu, C., Rodriguez, K. F., Brown, P. R., and Yao, H. H.-C. (2018). Reproductive, Physiological, and Molecular Outcomes in Female Mice Deficient in Dhh and Ihh. Endocrinology 159 (7), 2563–2575. doi:10.1210/en.2018-00095
Lopez-Rios, J. (2016). The many Lives of SHH in Limb Development and Evolution. Semin. Cel Dev Biol 49, 116–124. doi:10.1016/j.semcdb.2015.12.018
Luchetti, G., Sircar, R., Kong, J. H., Nachtergaele, S., Sagner, A., Byrne, E. F., et al. (2016). Cholesterol Activates the G-Protein Coupled Receptor Smoothened to Promote Hedgehog Signaling. Elife 5, e20304. doi:10.7554/eLife.20304
Luo, J., Yang, H., and Song, B.-L. (2020). Mechanisms and Regulation of Cholesterol Homeostasis. Nat. Rev. Mol. Cel Biol 21 (4), 225–245. doi:10.1038/s41580-019-0190-7
Ma, J., Cheng, J., Gong, Y., Tian, L., and Huang, Q. (2015). Downregulation of Wnt Signaling by Sonic Hedgehog Activation Promotes Repopulation of Human Tumor Cell Lines. Dis. Model. Mech. 8 (4), 385–391. doi:10.1242/dmm.018887
Manikowski, D., Kastl, P., Schürmann, S., Ehring, K., Steffes, G., Jakobs, P., et al. (2021). C-terminal Peptide Modifications Reveal Direct and Indirect Roles of Hedgehog Morphogen Cholesteroylation. Front. Cel Dev. Biol. 8, 615698. doi:10.3389/fcell.2020.615698
Mateska, I., Nanda, K., Dye, N. A., Alexaki, V. I., and Eaton, S. (2020). Range of SHH Signaling in Adrenal Gland Is Limited by Membrane Contact to Cells with Primary Cilia. J. Cel Biol 219 (12), e201910087. doi:10.1083/jcb.201910087
Matz-Soja, M., Rennert, C., Schönefeld, K., Aleithe, S., Boettger, J., Schmidt-Heck, W., et al. (2016). Hedgehog Signaling Is a Potent Regulator of Liver Lipid Metabolism and Reveals a GLI-Code Associated with Steatosis. Elife 5, e13308. doi:10.7554/eLife.13308
Milenkovic, L., Scott, M. P., and Rohatgi, R. (2009). Lateral Transport of Smoothened from the Plasma Membrane to the Membrane of the Cilium. J. Cel Biol 187 (3), 365–374. doi:10.1083/jcb.200907126
Miller, W. L. (2017). Steroidogenesis: Unanswered Questions. Trends Endocrinol. Metab. 28 (11), 771–793. doi:10.1016/j.tem.2017.09.002
Minhas, R., Pauls, S., Ali, S., Doglio, L., Khan, M. R., Elgar, G., et al. (2015). Cis-regulatory Control of Human GLI2 Expression in the Developing Neural Tube and Limb Bud. Dev. Dyn. 244 (5), 681–692. doi:10.1002/dvdy.24266
Moreau, N., and Boucher, Y. (2020). Hedging against Neuropathic Pain: Role of Hedgehog Signaling in Pathological Nerve Healing. Int. J. Mol. Sci. 21 (23), 9115. doi:10.3390/ijms21239115
Myers, B. R., Neahring, L., Zhang, Y., Roberts, K. J., and Beachy, P. A. (2017). Rapid, Direct Activity Assays for Smoothened Reveal Hedgehog Pathway Regulation by Membrane Cholesterol and Extracellular Sodium. Proc. Natl. Acad. Sci. U S A. 114 (52), E11141–E11150. doi:10.1073/pnas.1717891115
Niewiadomski, P., Niedziółka, S. M., Markiewicz, Ł., Uśpieński, T., Baran, B., and Chojnowska, K. (2019). Gli Proteins: Regulation in Development and Cancer. Cells 8 (2), 147. doi:10.3390/cells8020147
Ohba, S. (2020). Hedgehog Signaling in Skeletal Development: Roles of Indian Hedgehog and the Mode of its Action. Int. J. Mol. Sci. 21 (18), 6665. doi:10.3390/ijms21186665
Onodera, S., Nakamura, Y., and Azuma, T. (2020). Gorlin Syndrome: Recent Advances in Genetic Testing and Molecular and Cellular Biological Research. Int. J. Mol. Sci. 21 (20), 7559. doi:10.3390/ijms21207559
Palencia-Campos, A., Ullah, A., Nevado, J., Yıldırım, R., Unal, E., Ciorraga, M., et al. (2017). GLI1 Inactivation Is Associated with Developmental Phenotypes Overlapping with Ellis-van Creveld Syndrome. Hum. Mol. Genet. 26 (23), 4556–4571. doi:10.1093/hmg/ddx335
Po, A., Citarella, A., Catanzaro, G., Besharat, Z. M., Trocchianesi, S., Gianno, F., et al. (2020). Hedgehog-GLI Signalling Promotes Chemoresistance through the Regulation of ABC Transporters in Colorectal Cancer Cells. Sci. Rep. 10 (1), 13988. doi:10.1038/s41598-020-70871-9
Porter, J. A., von Kessler, D. P., Ekker, S. C., Young, K. E., Lee, J. J., Moses, K., et al. (1995). The Product of Hedgehog Autoproteolytic Cleavage Active in Local and Long-Range Signalling. Nature 374 (6520), 363–366. doi:10.1038/374363a0
Porter, J. A., Young, K. E., and Beachy, P. A. (1996). Cholesterol Modification of Hedgehog Signaling Proteins in Animal Development. Science 274 (5285), 255–259. doi:10.1126/science.274.5285.255
Purohit, R., Peng, D. S., Vielmas, E., and Ondrus, A. E. (2020). Dual Roles of the Sterol Recognition Region in Hedgehog Protein Modification. Commun. Biol. 3 (1), 250. doi:10.1038/s42003-020-0977-2
Qi, X., Schmiege, P., Coutavas, E., Wang, J., and Li, X. (2018). Structures of Human Patched and its Complex with Native Palmitoylated Sonic Hedgehog. Nature 560 (7716), 128–132. doi:10.1038/s41586-018-0308-7
Qiu, Z.-P., Hu, A., and Song, B.-L. (2021). The 3-beta-hydroxysteroid-Delta(8), Delta(7)-isomerase EBP Inhibits Cholesterylation of Smoothened. Biochim. Biophys. Acta Mol. Cel Biol Lipids 1866 (12), 159041. doi:10.1016/j.bbalip.2021.159041
Radhakrishnan, A., Rohatgi, R., and Siebold, C. (2020). Cholesterol Access in Cellular Membranes Controls Hedgehog Signaling. Nat. Chem. Biol. 16 (12), 1303–1313. doi:10.1038/s41589-020-00678-2
Raleigh, D. R., Sever, N., Choksi, P. K., Sigg, M. A., Hines, K. M., Thompson, B. M., et al. (2018). Cilia-Associated Oxysterols Activate Smoothened. Mol. Cel 72 (2), 316–327. e5. doi:10.1016/j.molcel.2018.08.034
Rallis, A., Navarro, J. A., Rass, M., Hu, A., Birman, S., Schneuwly, S., et al. (2020). Hedgehog Signaling Modulates Glial Proteostasis and Lifespan. Cel Rep. 30 (8), 2627–2643. doi:10.1016/j.celrep.2020.02.006
Reboldi, A., and Dang, E. (2018). Cholesterol Metabolism in Innate and Adaptive Response. F1000Res 7, F1000. Faculty Rev-1647. doi:10.12688/f1000research.15500.1
Rennert, C., Eplinius, F., Hofmann, U., Johänning, J., Rolfs, F., Schmidt-Heck, W., et al. (2017). Conditional Loss of Hepatocellular Hedgehog Signaling in Female Mice Leads to the Persistence of Hepatic Steroidogenesis, Androgenization and Infertility. Arch. Toxicol. 91 (11), 3677–3687. doi:10.1007/s00204-017-1999-5
Rohatgi, R., Milenkovic, L., Corcoran, R. B., and Scott, M. P. (2009). Hedgehog Signal Transduction by Smoothened: Pharmacologic Evidence for a 2-step Activation Process. Proc. Natl. Acad. Sci. U.S.A. 106 (9), 3196–3201. doi:10.1073/pnas.0813373106
Salaritabar, A., Berindan-Neagoe, I., Darvish, B., Hadjiakhoondi, F., Manayi, A., Devi, K. P., et al. (2019). Targeting Hedgehog Signaling Pathway: Paving the Road for Cancer Therapy. Pharmacol. Res. 141, 466–480. doi:10.1016/j.phrs.2019.01.014
Sasai, N., Toriyama, M., and Kondo, T. (2019). Hedgehog Signal and Genetic Disorders. Front. Genet. 10, 1103. doi:10.3389/fgene.2019.01103
Schade, D. S., Shey, L., and Eaton, R. P. (2020). Cholesterol Review: A Metabolically Important Molecule. Endocr. Pract. 26 (12), 1514–1523. doi:10.4158/ep-2020-0347
Skoda, A. M., Simovic, D., Karin, V., Kardum, V., Vranic, S., and Serman, L. (2018). The Role of the Hedgehog Signaling Pathway in Cancer: A Comprehensive Review. Bosn J. Basic Med. Sci. 18 (1), 8–20. doi:10.17305/bjbms.2018.2756
Stewart, D. P., Marada, S., Bodeen, W. J., Truong, A., Sakurada, S. M., Pandit, T., et al. (2018). Cleavage Activates Dispatched for Sonic Hedgehog Ligand Release. Elife 7, e31678. doi:10.7554/eLife.31678
Takai, H., Kondoh, E., Mogami, H., Kawasaki, K., Chigusa, Y., Sato, M., et al. (2019). Placental Sonic Hedgehog Pathway Regulates Foetal Growth via Insulin-like Growth Factor axis in Preeclampsia. J. Clin. Endocrinol. Metab. 104, 4239–4252. doi:10.1210/jc.2019-00335
Tang, C., Mei, L., Pan, L., Xiong, W., Zhu, H., Ruan, H., et al. (2015a). Hedgehog Signaling through GLI1 and GLI2 Is Required for Epithelial-Mesenchymal Transition in Human Trophoblasts. Biochim. Biophys. Acta 1850 (7), 1438–1448. doi:10.1016/j.bbagen.2015.04.005
Tang, C., Pan, Y., Luo, H., Xiong, W., Zhu, H., Ruan, H., et al. (2015b). Hedgehog Signaling Stimulates the Conversion of Cholesterol to Steroids. Cell Signal. 27 (3), 487–497. doi:10.1016/j.cellsig.2015.01.004
The, I., Bellaiche, Y., and Perrimon, N. (1999). Hedgehog Movement Is Regulated through Tout Velu-dependent Synthesis of a Heparan Sulfate Proteoglycan. Mol. Cel. 4 (4), 633–639. doi:10.1016/s1097-2765(00)80214-2
Theesfeld, C. L., and Hampton, R. Y. (2013). Insulin-induced Gene Protein (INSIG)-dependent Sterol Regulation of Hmg2 Endoplasmic Reticulum-Associated Degradation (ERAD) in Yeast. J. Biol. Chem. 288 (12), 8519–8530. doi:10.1074/jbc.m112.404517
Tolosa, E. J., Fernandez-Barrena, M. G., Iguchi, E., McCleary-Wheeler, A. L., Carr, R. M., Almada, L. L., et al. (2020). GLI1/GLI2 Functional Interplay Is Required to Control Hedgehog/GLI Targets Gene Expression. Biochem. J. 477 (17), 3131–3145. doi:10.1042/bcj20200335
Tukachinsky, H., Petrov, K., Watanabe, M., and Salic, A. (2016). Mechanism of Inhibition of the Tumor Suppressor Patched by Sonic Hedgehog. Proc. Natl. Acad. Sci. U S A. 113 (40), E5866–E5875. doi:10.1073/pnas.1606719113
Valentini, R. P., Brookhiser, W. T., Park, J., Yang, T., Briggs, J., Dressler, G., et al. (1997). Post-translational Processing and Renal Expression of Mouse Indian Hedgehog. J. Biol. Chem. 272 (13), 8466–8473. doi:10.1074/jbc.272.13.8466
Wangeline, M. A., and Hampton, R. Y. (2020). An Autonomous, but INSIG-Modulated, Role for the Sterol Sensing Domain in Mallostery-Regulated ERAD of Yeast HMG-CoA Reductase. J. Biol. Chem. 296, 100063. doi:10.1074/jbc.RA120.015910
Wierbowski, B. M., Petrov, K., Aravena, L., Gu, G., Xu, Y., and Salic, A. (2020). Hedgehog Pathway Activation Requires Coreceptor-Catalyzed, Lipid-dependent Relay of the Sonic Hedgehog Ligand. Develop. Cel 55 (4), 450–467. doi:10.1016/j.devcel.2020.09.017
Wu, Z., Makihara, S., Yam, P. T., Teo, S., Renier, N., Balekoglu, N., et al. (2019). Long-Range Guidance of Spinal Commissural Axons by Netrin1 and Sonic Hedgehog from Midline Floor Plate Cells. Neuron 101 (4), 635–647. doi:10.1016/j.neuron.2018.12.025
Xiao, X., Tang, J.-J., Peng, C., Wang, Y., Fu, L., Qiu, Z.-P., et al. (2017). Cholesterol Modification of Smoothened Is Required for Hedgehog Signaling. Mol. Cel 66 (1), 154–162. doi:10.1016/j.molcel.2017.02.015
Xu, H., Zhou, S., Tang, Q., Xia, H., and Bi, F. (2020). Cholesterol Metabolism: New Functions and Therapeutic Approaches in Cancer. Biochim. Biophys. Acta (Bba) - Rev. Cancer 1874 (1), 188394. doi:10.1016/j.bbcan.2020.188394
Yin, W.-C., Satkunendran, T., Mo, R., Morrissy, S., Zhang, X., Huang, E. S., et al. (2019). Dual Regulatory Functions of SUFU and Targetome of GLI2 in SHH Subgroup Medulloblastoma. Develop. Cel 48 (2), 167–183. doi:10.1016/j.devcel.2018.11.015
Zhang, Y., Bulkley, D. P., Xin, Y., Roberts, K. J., Asarnow, D. E., Sharma, A., et al. (2018). Structural Basis for Cholesterol Transport-like Activity of the Hedgehog Receptor Patched. Cell 175 (5), 1352–1364. e14. doi:10.1016/j.cell.2018.10.026
Zhou, Z.-S., Li, M.-X., Liu, J., Jiao, H., Xia, J.-M., Shi, X.-J., et al. (2020). Competitive Oxidation and Ubiquitylation on the Evolutionarily Conserved Cysteine Confer Tissue-specific Stabilization of Insig-2. Nat. Commun. 11 (1), 379. doi:10.1038/s41467-019-14231-w
Keywords: Hedgehog, signaling transduction, GLI, cholesterol, modification
Citation: Xu S and Tang C (2022) Cholesterol and Hedgehog Signaling: Mutual Regulation and Beyond. Front. Cell Dev. Biol. 10:774291. doi: 10.3389/fcell.2022.774291
Received: 11 September 2021; Accepted: 06 April 2022;
Published: 27 April 2022.
Edited by:
Albena Todorova Dinkova-Kostova, University of Dundee, United KingdomReviewed by:
Anthony Ian Magee, Imperial College London, United KingdomMayumi Suzuki, Okinawa Institute of Science and Technology Graduate University, Japan
Rongrong Fan, Karolinska Institutet (KI), Sweden
Shiwen Luo, Nanchang University, China
Copyright © 2022 Xu and Tang. This is an open-access article distributed under the terms of the Creative Commons Attribution License (CC BY). The use, distribution or reproduction in other forums is permitted, provided the original author(s) and the copyright owner(s) are credited and that the original publication in this journal is cited, in accordance with accepted academic practice. No use, distribution or reproduction is permitted which does not comply with these terms.
*Correspondence: Chao Tang, Y2h0YW5nQHpqdS5lZHUuY24=