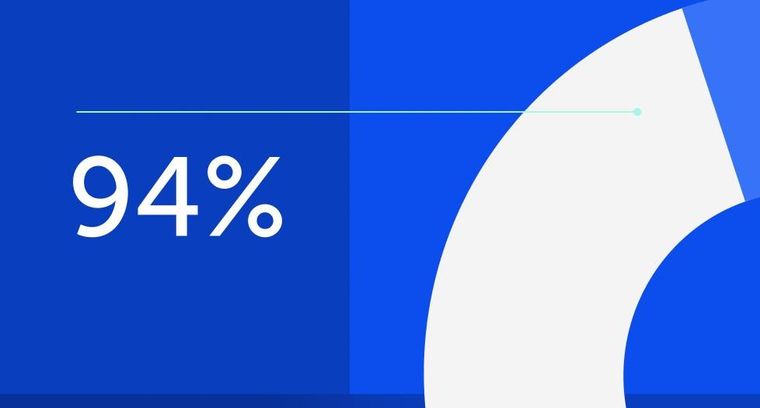
94% of researchers rate our articles as excellent or good
Learn more about the work of our research integrity team to safeguard the quality of each article we publish.
Find out more
REVIEW article
Front. Cell Dev. Biol., 24 March 2022
Sec. Stem Cell Research
Volume 10 - 2022 | https://doi.org/10.3389/fcell.2022.713934
This article is part of the Research TopicRejuvenation of Aging Adult Stem Cells to Improve their Regenerative PotentialView all 7 articles
Biomaterials and tissue regeneration represent two fields of intense research and rapid advancement. Their combination allowed the utilization of the different characteristics of biomaterials to enhance the expansion of stem cells or their differentiation into various lineages. Furthermore, the use of biomaterials in tissue regeneration would help in the creation of larger tissue constructs that can allow for significant clinical application. Several studies investigated the role of one or more biomaterial on stem cell characteristics or their differentiation potential into a certain target. In order to achieve real advancement in the field of stem cell-based tissue regeneration, a careful analysis of the currently published information is critically needed. This review describes the fundamental description of biomaterials as well as their classification according to their source, bioactivity and different biological effects. The effect of different biomaterials on stem cell expansion and differentiation into the primarily studied lineages was further discussed. In conclusion, biomaterials should be considered as an essential component of stem cell differentiation strategies. An intense investigation is still required. Establishing a consortium of stem cell biologists and biomaterial developers would help in a systematic development of this field.
Tissue engineering is an emerging field in medical care that links medicine to biology, engineering, physics and chemistry. The shortage of organ transplants as well as the limitations of artificial implants has enforced the research in this particular stream of regenerative medicine. The main goal of tissue engineering is the development of biological substitutes or functional constructs in order to restore or correct a defect in tissues or organs. Over the last few decades, human stem cells have become an attractive base for tissue engineering, with several case studies and proof of concept reports (Amini et al., 2012). Using stem cells in tissue engineering does not only open the potential up to produce specific tissues according to the patient’s need, but also reduces the risk of immune rejection. However, the full differentiation of stem cell populations into the desired target, is still an unmet challenge (El-Serafi, 2012). In-vivo, stem cells exist in an active and complex microenvironment; a fundamental factor of which is the extracellular matrix (ECM). The latter supports the cells and delivers physical and chemical clues that direct certain signaling pathways and affect the cell differentiation (Abagnale et al., 2015). Several biomaterials were designed to mimic the natural ECM effect in-vitro (Hellmund and Koksch, 2019). Furthermore, applying the biomaterials in 3D environment, can help in creating a human-based model that can reduce the use of animal in research (Nugud and El-Serafi, 2018).
In 2019, Williams define a biomaterial as “a material designed to take a form that can direct, through interactions with living systems, the course of any therapeutic or diagnostic procedure” (Williams, 2019). These materials need to be biocompatible in order to be applied in-vivo. Biocompatibility was defined earlier by Williams in 2008, as “the ability of a biomaterial to perform its desired function with respect to a medical therapy, without eliciting any undesirable local or systemic effects in the recipient or beneficiary of that therapy, but generating the most appropriate beneficial cellular or tissue response” (Williams, 2008). Biomaterials should be - at least - biotolerable; which is defined as the “ability of a material to reside in the body for long periods of time with only low degrees of inflammatory reaction” (Ratner, 2011). Ideally, biomaterials should be non-toxic, non-immunogenic, non-thrombogenic, non-carcinogenic and non-irritant. The early evaluation of biomaterials was restricted to the inert biocompatibility with the surrounding tissues or cells, although physical characteristics such as ultimate tensile strength, suture retention strength and stress–strain characteristics should also be considered. Metals were introduced to medical practice in bone fracture fixation around 1895. However, the use of metal in the field of biomaterials started to develop steadily when stainless steel was introduced for medical applications in early 1920s (Walker, 1912). Currently, there is a wide use of biomaterials in clinical practice, including hip prosthesis, steel rods for internal fixation, prosthetic heart valves, vascular stents, eye lens, contrast materials for magnetic resonance imaging as well as in dental materials and implants. The classical use of biomaterials in clinical practice are illustrated in Figure 1. Moreover, biocompatible polymers have also received special attention in the fields of biosensors as well as drug carrying and release (Bamford and Al-Lamee, 1992). Thus, it was not surprising for biomaterials to be involved in the tissue engineering technology (Williams, 2019). The ideal biomaterial should allow for functional replacement of tissue rather than providing simple physical support. Mostly are based on synthetic polymers, which should offer a combination of properties including good tensile strength and resemblance of the natural materials in the mechanical properties. Table 1 summarizes the most common application of biomaterials in clinical practice.
FIGURE 1. Applying a biomaterial in 3D configuration would enhance the cell-cell and cell-material interaction. The biomaterial surface charge, roughness, topography and chemical composition will affect the cells on the attachment boundary in case of 2D culture. Cells in 3D culture will receive the corresponding signals from the enclosing surroundings. The figure was created in BioRender.com.
The term “stem cells” is very widely used and includes different types of cells with diverse differentiation abilities. Embryonic Stem cells (ESC) are pluripotent cells that can differentiate into all cell types that are derived from the three embryonic layers i.e. ectoderm, mesoderm and endoderm. This differentiation potential is limited in adult stem cells (ASC). These cells can be found in all tissues and are responsible for tissue homeostasis. The most famous subgroup of ASC is the mesenchymal stem cells (MSC), which are capable to efficiently differentiate into all cell types derived from the mesoderm. These cells can be isolated, with relative ease, from bone marrow, adipose tissue, and umbilical cord blood (Wang and Yeung, 2017). Furthermore, MSC can differentiate into cells derived from other embryonic lineages, based on the culture conditions, with variable degrees of success. For example, MSC was shown to differentiate into several types of cells including hepatocytes, beta cell of the pancreas, neurons and neuroectodermal cells (Cooke M. J. et al., 2010; Elsharkawi et al., 2019; Mansour et al., 2019; Khan et al., 2020). In-vitro, the proliferation of ASC, is limited by Hayflick’s limit, associated with senescence and decreasing ability to differentiate following few passages in culture. On the other hand, undifferentiated ESC preserves pluripotency and can give rise to teratomas. For this reason, any stem cell therapeutic approach should have efficient and safe differentiation towards the target cell type, which could be directed by the administration of the appropriate differentiation factors, as well as the use of specific biomaterial scaffolds and (Gadalla et al., 2018; Nugud et al., 2018). Nevertheless, these cells can enhance the natural capacity of tissue repair through their local secretions and release of exosomes with their micro-RNA (miRNA) content (Gentile and Garcovich, 2019).
Induced pluripotent stem cells (IPCs) are somatic cells transfected with potency genes that can provide the cells with the differentiation potential of ESC. Researchers considered IPCs as an important tool for understanding normal development, as well as disease onset, progression and new drug testing. The latter would involve creating disease specific IPCs and differentiate them into specific targets, in the presence of an appropriate scaffold, in order to create a 3D in-vitro model of diseased organ (Nugud et al., 2018).
The main drive for in-vitro differentiation of stem cells is classically achieved via media supplementation with additives, such as growth and transcription factors. At the meantime, physical factors that exert regulatory effect on stem cells differentiation and proliferation remains to be clarified (Kshitiz et al., 2012). However, it is well-known from embryology that the morphogenetic cell movement and propagation result in the development of all body organs. Populations of progenitor cells undergo several processes whereby growing signals are gradually introduced, and they encounter a change in the cellular environment, resulting in cell division and expansion into tissues. In turn, some of these progenitors, along with stem cells, remain devoted to tissue turnover into organs. The control is based on cell-to-cell interactions and the molecular signals, adhesive signals and soluble matrix proteins present in the stem cell niche (Pennings et al., 2018). The interaction between ECM or biomaterials with stem cells revealed a potent effect on their differentiation, based on the adhesion and interaction between cells and underlying nanotopography. The latter is assembled at the scale of individual ECM molecules. The cells can also interact through contact guidance; a naturally occurring phenomena that regulate the cell orientation and movement based on the nature and shape of the surface. Similar effects can be obtained in biomaterials through designing grooves, pits, pores or other nanopatterns which can alter several cell characteristics, such as phenotype, survival, motility, proliferation, endocytic activity and gene regulation (Kshitiz et al., 2012; Driscoll et al., 2014). For example, Engler et al. (2006) showed that soft collagen coated with polysaccharides induced neuronal cell differentiation while the stiffer version induced osteogenic differentiation (Engler et al., 2006). The alterations of the cell behavior were explained by remodeling of the intranuclear factors that influence epigenetics (Kshitiz et al., 2012). Furthermore, several ECM proteins form large-scale structures (up to hundreds micrometers) can interact with multiple cells and organize a complex multicellular structure (Anderson et al., 2017).
Over the years, biomaterials have been classified according to different and overlapping approaches. Understanding these classifications could be crucial for the proper choice of a certain material for stem cell application.
Extracellular matrix components, such as fibronectin and collagen, represent natural resources for biomaterials that can be configured for medical and biological uses. Naturally, ECM provides a niche-like environment that accommodates and interacts with the surrounding cells. ECM components were shown to maintain cellular adhesion and promote cellular growth and differentiation of IPCs, in vitro (Dzhoyashvili et al., 2015). Natural biomaterials are known to be biodegradable and biocompatible, that give them an extra advantage. For example, decellularized extracellular matrix and lyophilized type I collagen were used for urinary bladder regeneration (Serrano-Aroca et al., 2018). Decellularization can be considered as a relatively safe procedure to produce a natural scaffold of the physiological nature of the original tissue (Gentile et al., 2020). Similarly, demineralized bone matrix allowed the integration into the bone defects and enhance endochondral ossification, thus, bone formation (Wang and Yeung, 2017).
Synthetic biomaterials can be made of different forms of organic and inorganic materials, as well as different combinations of them. The chemical and physical properties can be changed according to the manufacturing process. In comparison to natural biomaterials, synthetic biomaterials have many advantages including; the possibility of standardization, the control of mechanical and chemical properties, degradation rate and byproducts, as well as the relative long shelf life, flexibility in shaping, possibility of bulk production and cost effectiveness (Prasadh and Wong, 2018). Regulation of degradation rate offers an important advantage that can be harnessed in tissue engineering to control cellular differentiation. On the other hand, cell attachment to synthetic materials can be less than the natural counterpart. Moreover, some synthetic biomaterials can induce host immune reaction. Examples of synthetic biomaterials include; 1) polylactic acid (PLA), 2) polyglycolic acid (PGA), 3) polycaprolactone (PCL), 4) poly lactide- co-glycolic acid (PLGA) (Cooke M. J. et al., 2010).
Bioinert materials interact with surrounding microenvironment, but not to the extent causing change in cellular structure or material at the light microscopic level. They support the surrounding cells and tissues during the repair process without chemical interaction. Clinically applied examples include polytetrafluoroethylene (PTFE), polymethylmethacrylate (PMMA), ceramics such as alumina and zirconia, and metals such as the titanium and cobalt-chrome-molybdenum alloys. As these materials have considerable strength and high wear resistance, they are commonly used as joint prosthetics including acetabular cups, femoral heads and dental protheses. Recently, bioinert hydrogels were characterized for three-dimensional tissue engineering (Kieswetter et al., 1996; Marin et al., 2020; Unal and West, 2020).
Bioresorbable biomaterials are designed to provide temporary support, then get digested and absorbed through oxidation by free radicals, hydrolysis or through an enzymatic action, such as hydrolase, cholesterol esterase or phosphatases. Bioresorbable materials include sutures, stents and bone implants for the management of temporary clinical problems, such as narrowed arteries and fractured bones. These biomaterials are highly valuable in the field of regenerative medicine as stem cells should be able to differentiate and synthesis the natural extracellular matrix to replace the decaying biomaterial (Williams and Zhong, 1994; Ang et al., 2017; Cockerill et al., 2020).
Bioactive materials interact directly with the surrounding cells and tissues on chemical basis. These materials are more likely to be based on composites that can be metabolized by the body, such as collagen-derived products, polylactic and polyglycolic acid polymers and processed bone graft (Brown and Anseth, 2017). Bioactive materials are either derived from natural resources, such as replamineform-treated hydroxyapatite compounds or synthesized as bioglass and synthetic calcium phosphates (Nour et al., 2019). The physical properties of these materials , including the surface composition, surface topography and surface charge would confer to their effects (Schwartz and Boyan, 1994). Bioactive glass has been very attractive for research and therapeutics in the field of orthopedics due to several characteristics, including osteoconductivty, as bioglass initiates precipitation of hydroxyapatite matrix on their surfaces and fibular nature. In addition, bioactive glass is used as a defect filler as it integrates with bony tissues and induces stem cell differentiation into osteoblasts in-vivo (Zhang et al., 2002). Furthermore, bio-active glass can stimulate osteoid formation by MSC directly and through enhancing neovascularization (Westhauser et al., 2019).
Biomaterials surfaces are quickly coated with proteins in biological media. Adsorbed proteins could be related to the charge of the composite and the protein, material hydration at the interface and surface roughness of the biomaterials at the nanoscale. These factors contribute to a degree of selectivity for each material. As the protein surface integration happens, the molecular architecture of the surface change in correspondence of the orientation of ions, minerals, water and proteins. The expression of new cell adhesion molecules will result in change of the cell shape and behavior through the interaction with extracellular and intracellular signaling pathways (Scopelliti et al., 2010; Eliaz, 2019).
Cell adhesion results mainly from the interaction between the biomaterial surface and the cell surface ligands. The composition of the material itself plays an important role in cellular attachment. Hanein et al., have shown in several studies the difference in the ability of renal cell adherence to calcium crystals, based on the three-dimensional orientation. The crystals were chemically equivalent, but structurally different, that was reflected on the crystallographic surfaces, as well as the cellular adhesion characteristics (Hanein et al., 1993; Hanein et al., 1994; Hanein et al., 1996). Cell adhesion can be enhanced by nanograting which resembles in-vivo extracellular matrix proteins. For example, mimicking collagen would allow the attachment and adherence of cells along the longitudinal axis. On the other hand, the reduction of cell attachment and constant filopodia formation can be guided by nanopits and nanoposts (Maturavongsadit et al., 2016). Skeletal stem cell depends on the adhesion formation and cellular spreading for functional differentiation, and that processes - at least partially - dependent on nanotopographical cues (Hart et al., 2007).
The concept of cell migration is based on cell movement pattern influenced by the biomaterial. The adherence between the cells and biomaterial as well as the elasticity of the biomaterial and the extracellular microenvironment, influenced by the protein adsorption, would promote cell motility. Historical results of Abercrombie et al., 1972, indicated that renal cells interacted with any surface through ruffled membranes, focal adhesions, adhesion plaques and focal contacts, which consequently affect the amoebic movement of the cells (Abercrombie et al., 1972). Cell motility occurs through the intra cellular scaffolding composed of microtubules, as well as intermediate filaments, and actin filaments (Sanz-Herrera and Reina-Romo, 2011). Early studies of cellular migratory behavior and the motility of chick heart fibroblasts, in terms of a velocity and direction of movement, was thought to be random (Abercrombie et al., 1971). Gail et al., in 1970, suggested that when mouse fibroblasts cultured on glass, they move neither in a perfectly uniform nor in a perfectly random way. Their studies demonstrated an intermediate tendency to persist in their direction of motion, which was supported latter by Abercrombie et al., in 1972 (Gail and Boone, 1970; Abercrombie et al., 1972). Contact guidance considered as a fundamental factor that regulates cell migration, which is naturally controlled by proteins of ECM. Moreover, contact guidance is crucial for organelle formation, including growth cone motility and axonal guidance (Discher et al., 2005; Al-Haque et al., 2012). For example, epithelial cells were reported to migrate from smoother to stiffer regions on biomaterials, in a phenomena known as durotaxis (Sanz-Herrera and Reina-Romo, 2011). Furthermore, cell migration would be affected by the surrounding environment in-vivo. The inflammatory mediators, produced by tissue macrophages, are normally present around the scaffold. These mediators attract endothelial cells and enhance their migration through the pores of the scaffold. From a regenerative perspective, this can help in the vascularization of the scaffold and enhance the construct sustainability. On the other hand, extracellular matrix secretion by the implanted cells can be negatively affected (Li et al., 2017; Saleh and Bryant, 2018). However, the interaction between cell-laden scaffolds and the surrounding environment is complicated and the ultimate effect can be induced by the scaffold or the enclosed tissue. Studying the migration of cells to and from the scaffold is both interesting and challenging, being affected by the method of labelling the cells, the timepoints of the study and the cell proliferation and migration.
The interaction between the cell surface receptors and biomaterial surface can affect the cell proliferation. The chemistry-dependent interaction elicits a change in affinity specific integrin receptors, that affect gene expression via intracellular signaling pathways (Keselowsky et al., 2005; Lin et al., 2011).
The biomaterials are responsible for the formation of the stem cell microenvironment, where their physical, chemical and mechanical properties can influence cellular proliferation, integration and eventually differentiation. The use of biomaterials in stem cell differentiation has proved a great potential due to their specific properties that can guide differentiation and support the slowly forming tissue as well as the following biodegradability (Smith et al., 2010; Kumar et al., 2015). From a chemical perspective, the biomaterial important features consist of material composition, special ligands concentrations and possible biodegradability of the material. As for the physical properties, they involve the biomaterial mechanical properties, biomaterial topography, and morphology (Engler et al., 2006; Khetan et al., 2013).
Biomaterials presented topographical landscapes like grooves, pits or pillars that can influence the proliferation, differentiation of different types of stem cells (Kshitiz et al., 2012; Driscoll et al., 2014). The focal contacts are specialized microstructures anchored to cytoskeletal microfilaments which play a role in shaping the cytoplasmic membrane. Components of the microstructure traverse the cytoplasmic membrane, allowing the cells to bind to the extracellular matrix via integrins. While the basal lamina in some types of tissues have unique nanofibrous characteristics, their substrate topography encounter functionality that interact with the cells. Similarly, synthetic topography was able to induce different effects on cells through affecting cell adhesion, alignment, morphology, proliferation, migration and even cytoskeletal organization (Schwartz et al., 1993). The influence of material topography on morphogenesis depends on the cell type as well as cytoskeletal organization, cell adhesion and the interaction between cells. Moreover, triggers from the extracellular environment can be transferred to the cell as a consequence of changes in the interaction of integrins, resulting in the stimulation or inhibition of intracellular signaling cascades with possible induction of new gene(s) and the synthesis of new protein(s) (Maturavongsadit et al., 2016). Focal adhesion kinase (FAK) would be activated and regulate ERK signaling pathway, which can affect both transcription and post-translational modifications that can lead to the differentiation of the stem cells in a machano-sensory approach (Biggs et al., 2008; Hamilton and Brickman, 2014; Cembran et al., 2020).
Biomaterial surface chemistry can have a direct influence on stem cell differentiation. For example, side chains of polyacrylamide hydrogels would develop varying degrees of hydrophobicity without changing the mechanical properties of the gel. Such changes promoted -specifically - the differentiation of mesenchymal stem cells into osteogenic and myogenic lineages (Ayala et al., 2011). Similarly, high hydrophobic surfaces, such as octadecanethiol and polydimethylsiloxane, promoted differentiation of ESC toward the three germinal layers; endoderm, ectoderm, and mesoderm (Valamehr et al., 2008).
Implanted biomaterials can provoke an inflammatory response inside the body. This foreign body reaction could have deleterious effect on the chemical and biophysical properties of implanted biomaterials and alter its rate of biodegradability. This inflammatory response is considered as a major hurdle for the use of many biomaterials for in-vivo use (Sun et al., 2012). The description of host implant inflammatory response was introduced by Anderson et al., which included overlapping stages of injury, protein adsorption, acute inflammation, chronic inflammation, foreign body reaction, granulation tissue formation and encapsulation (Anderson, 1988). The acute phase of the reaction is dominated by neutrophils followed by macrophages within 48 h to in the latent phase of the reaction with the formation of foreign body giant cells (Henson, 1980). In fact, the host-macrophage response is an indispensable component of the constructive tissue remodeling process following the implantation of certain biologically derived scaffolds (Badylak et al., 2008; Valentin et al., 2009; Brown et al., 2012; Brown and Badylak, 2013). The type of adsorbed proteins onto the composite surface in addition to the surface chemistry of the material and the topographic features are all considered to be important and contributing factors in the severity of the host response (Anderson et al., 2008; Chen et al., 2010). Henceforth, the success of implanted biomaterials is dependent on evasion or control of inflammatory response either by implanting biocompatible materials or inhibiting the immune response (Ye et al., 2011).
The interaction between the host and biomaterial can affect the properties of the latter. For example, contact with body fluid can enhance the degradation rate which alter the material density as well as its volume and strength. This effect can be induced by the in-situ environment and the change in pH, the presence of certain enzymes and electrolytes as well as the mechanical forces around the biomaterial, such as the weight, muscle contraction, joint movement, and shear stresses (Wang et al., 2019).
Monolayer or two dimensional (2D) cell culture is the most convenient way of cultivating cells in vitro and it is carried out on flat surfaces with cells exposed to soluble elements in the media. This culture system is a simple method to dissect the role of individual components on stem cells differentiation (Lutolf et al., 2009). On the other hand, three dimensional (3D) microenvironment could provide more insight into an in-vivo like cellular behavior, including the cell shape and interactions, as well as enhancement of gene expression (Nugud and El-Serafi, 2018). For example, 3D culture allows cells to better communicate and migrate that can promote cellular differentiation (Figure 1) (Baharvand et al., 2006). Stem cells in particular are extremely sensitive to any change in the microenvironment. We have previously shown the difference in gene expression for stem cell differentiation into adipogenic lineage between monolayer and in a scaffold-free 3D culture. The latter method has clarified the upregulation of the relevant gene expression between the differentiated and control cells, which was not obvious in monolayer culture (El-Serafi et al., 2019). Stem cells cultured in 3D constructs, can differentiate and self-organize into a multi-type cell construct (El-Serafi et al., 2011; Sozen et al., 2018). Moreover, the presence of suitable biomaterials could enhance the cell “self-assembly” into larger and transferable constructs (Jean et al., 2011). Similarly, stem cells cultured on electrospun-nanofibrillar polyamide 3D scaffold had superior proliferation and migration potential when compared to a 2D model using the same biomaterial (Nur et al., 2006). Thus, the ideal scaffolds design should be complex enough to mimic native tissue architecture as well as allow cellular attachment, migration, proliferation and differentiation, taking in consideration the material physicochemical properties (Kloxin et al., 2009; El-Serafi et al., 2017; Nugud et al., 2018). Such approach will greatly help in the understanding of complex cellular pathways.
Native-like hydrogels, resembling in-vivo environment of the heart, were able to induce cardiac differentiation of MSC during a 2 weeks in-vitro culture. Around 80% of MSCs expressed cardiac myosin and troponin, which are important markers involved in cardiac cells contraction-relaxation process (Li et al., 2012). This method had a higher cardiac differentiation potential when compared to commonly used modalities such as co-culture with cardiac cells or using epigenetic modifiers such as 5-azacytidine (Pijnappels et al., 2008). In another model, human MSC were cultured as a multilayer and loaded on a porous acellular bovine scaffold and implanted in a rat myocardial infarction model. Native-like neo-connective tissue fibrils and neo-vascularization were developed and angiogenic cytokines (bFGF, vWF, and PDGF-B), cardiac markers (Nkx2.5 and MEF2D), and cardiac protective factors (IGF-1 and HGF) were expressed in the transplanted construct (Wei et al., 2008). The combination of stem cells and biomaterials would not only promote cardiac tissue repair and homeostasis, but also guide the differentiation of the 3 cell types in the heart wall; i.e., cardiomyocytes, smooth muscle, and endothelial cells. Different types of stem cells were involved in these studies, with particular success with cardiac progenitor cells, isolated from healthy cardiac tissue and identified with one of the cell surface markers c-Kit, Sca-1, CD31 and Flk-1. The biomaterials used are extracellular matrix protein-based, decellularized matrices and several polymer-based materials. These biomaterials can retain the intrinsic biotic activity to support cell adhesion, differentiation, and subsistence (Cutts et al., 2015). Furthermore, Young et al., aimed at mimicking cardiac development by creating a hydrogel that stiffens over a time period of 300 h after cell seeding. The authors reported an increase in functional cardiac muscle fibers by 60% and a three-fold increase in number of mature cardiac cell markers, indicating improvement of cardiomyocytes differentiation by the gradual stiffness of this biomaterial over time (Young and Engler, 2011).
MSCdifferentiation into cardiomyocytes could be influenced by biomaterial micro-topography. Coating PLGA material with ECM protein and human plasma derived fibronectin allows the formation of different topographies and forming spatially defined geometries. The latter promoted differentiation towards a cardiac muscle lineage, by induction of certain genes including; GATA4, MyoD1 which are early regulators of cardiomyogensis as well as β-MHC and cTnT genes (Tay et al., 2010). Hibino et al., in 2012, reported the differentiation of iPSCs to the vascular lineage on a flat non-woven porous PGA mesh and a co-polymer sealant solution of e-caprolactone and l -lactide scaffold. The differentiation was noted by expression of vascular and endothelial markers; VEGF, PECAM, and E-cadherin (Hibino et al., 2012). For blood vessel tissue engineering, murine stem cells were cultured on a macro-porous nanofibrous, polylactide scaffolds, in the presence of retinoid acid. The scaffold promoted stem cell differentiation into smooth muscle cells, by mimicking the architecture of vascular tissue, with expression of the early and late smooth muscle markers such as; α-SMA, MyoCD, smoothelin, SM22a, and SMMHC (Xie et al., 2011). In a pulsatile bioreactor, human iPSCs were seeded on PGA mesh over a silicone tube for 8 weeks. The cells expressed early stage smooth muscle markers including; α-SMA, SM22α and calponin, while the absence of the osteochondrogenic markers were absent. The extra cellular matrix was positive for the presence of collagen I, collagen II, and fibronectin. Interestingly, engineered vessel could withstand the pressure up to 700 mmHg, which is approximately half that of the normal veins pressure (Sundaram et al., 2014).
The progress in biomaterials development in relation to osteogenic differentiation is critical to the advancement of both dentistry and orthopedics. The use of proper biomaterial can help the healing process through the recruitment of MSCs and the stimulation of their differentiation (Logan and Brett, 2013; Lv et al., 2015). Materials used as a substitute or a fixative for bone should provide mechanical support and promote weight bearing as well as serving as a medium for osteo-regeneration. These biomaterials should be, 1) osteoconductive; i.e., able to support the attachment of osteoblasts and the promotion of bone formation on its surface, including neovasculogenesis, 2) osteoinductive; promote the expression of bone specific markers and osteogenic proteins in response to the biomaterial 3D configuration, chemical and physical composition, followed by mineralization and calcification of newly formed bony tissue; and 3) supports osteogenic differentiation of progenitor cells, osteoblasts, and bone marrow stromal cells into mature osteoblasts (Agrawal and Srivastava, 2020). Thus, an ideal bone biomaterial would be biocompatible, bioactive and biodegradable, such as bioceramics, polymers, and metals (Gao et al., 2017). In-vitro, the mechanical properties of a biomaterial can affect the osteogenic differentiation. For example, MSCs showed faster proliferation and osteogenic differentiation when cultured within 3D hydrogels with rapid relaxing rate; i.e. relaxing time of about 1 min (Chaudhuri et al., 2016). When MSC were cultured on stiff hydrogel before being transferred to soft hydrogel, the pre-osteogenic transcription factors (YAP, TAZ and Runx2) were permanently activated (Yang et al., 2014). Similarly, the physiochemical properties can affect the osteogenic differentiation. When the osteoblast cell line “MC3T3 -E1” were cultured on RGD-modified alginate hydrogel, both the proliferation rate and osteoblastic differentiation were enhanced, including a 4-fold increase in osteocalcin, a late stage osteoblastic differentiation marker (Lee et al., 2004). Micro and nano-topography, produced by electron beam lithography, colloidal lithography and polymer demixing techniques, were able to promote the differentiation of osteogenic of human MSCs. Nanograting can influence the polarization of MSCs by contact interaction and osteogenesis promotion. The symmetry and order of the nanopits enhanced the expression of bone specific ECM proteins, such as osteopontin and osteocalcin. When MSCs were cultured on completely random nanopits, no expression of both markers could be detected (Turner and Dalby, 2014; Aminuddin et al., 2016).
Micro aggregation, along with progenitor cells’ condensation, are the main events that create a specialized microenvironment drives chondrogenesis during bud development. Thus, the biomaterials involved in chondrogenesis should contain components that help to establish this microenvironment for the cells and hence promote their microaggregation and chondrogenesis (Leijten et al., 2016). Many natural and synthetic biomaterials have been investigated their chondrogenic differentiation potential, including synthetic and natural silk, cellulose, marine sponge fiber skeleton, hyaluronan and hyaluronic acid, in addition to a hybrid polymer of synthetic and natural material. The combination of PLGA, gelatin, chondroitin and hyaluronate provided a greater potential in repairing full thickness cartilage defects in rabbits when compared to PLGA biomaterial alone (Fan et al., 2006). Injectable polymers loaded with cells represent an intense area of research with potential for clinical application as it can be easily introduced to the joint space. In a preclinical trial, a thermosensitive hydrogel was loaded with human MSCs and injected into the bladder submucosal layer of rabbits. As the gel underwent structural changes by the effect of the body temperature, the new confirmation enhanced MSC chondrogenic differentiation and formed a tissue that resembled articular cartilage with a mixture of hyaline and fibrous cartilage (Cho et al., 2004). On the other hand, the material stiffness has been shown in another system to affect the chondrogenic differentiation. When MSC were cultured on a rigid surface-charged methyl acrylate/methyl methacrylate, the osteogenic differentiation prevails. Culturing the same cells on the lower rigidity substrate enhances chondrogenesis (Yoon et al., 2018). Other biomaterials, such as fibrin glue, type 1 collagen gel, peptide hydrogels, and Matrigel, have been also studied for in vitro chondrogenesis with MSCs and resulted in a cartilage-like transplants (Monaghan et al., 2016).
The role of biomaterials in peripheral nerve regeneration is crucial. In addition to enhancing the glial and tubular cell differentiation, the material physical conformation can guide the nerve growth direction. Several natural biomaterials have been tested for nerve tissue regeneration, including collagen, gelatin, chitin, elastin as well as hyaluronic acid. Synthetic biomaterials, such as poly-3-hydroxybutyrate, have been also used for neuronal repair, with the advantage of incorporating several neurotrophic factors during the manufacturing process (Mahoney and Saltzman, 2001; Seidlits et al., 2010; Wang et al., 2012; Samadian et al., 2020). Moreover, the neurogenic differentiation can be affected by the several physical and mechanical factors, based on the nature of the material and the surface topography. Additionally, stiffness of the biomaterial is a key influential factor for neurogenesis. MSC cultured on soft polyacrylamide hydrogel (∼0.5 kPa) were directed towards neurogenesis, while the stiffer substrate (∼40 kPa) would favour osteogenesis (Yoon et al., 2018). Wang et al. (2010) have studied the differentiation of neural stem cells (NSC) on chitosan on three different topologies; film, porous scaffold and multi-microtubule conduit. In the film topology and multi-microtubule conduit, cells were tightly adherent and elongated, exhibiting a star-like morphology with a network of inter-communicating processes that supported the differentiation to neuronal linage. On the other hand, porous scaffold did not drive NSC to differentiate. Gene expression study and immunostaining were performed for glial fibrillary acidic protein (GFAP; an astrocyte marker), b-tubulin III (an early neuronal marker), and O1 (an oligodendrocyte marker). The authors highlighted induction of GFAP, a mature astrocyte marker, in cells cultured on films, indicating a preference towards astrocytes differentiation. Cells seeded on porous scaffold and multi-microtubule conduit had an induction of b-tubulin III, suggesting differentiation toward neuronal lineage. However there were no significant differences in O1 gene expression, an oligodendrocyte marker, among the three experimental groups (Wang et al., 2010).
The combination of biomaterials allows further complexity of the constructs. For example, a double layered scaffold that is similar to white and gray matter of spinal cord, was seeded at the inner part with stem cells. The cells embedded in the biomaterial were transplanted into a hemisectiond spinal cord lesion in a rat model, which showed enhanced recovery of the lower limb neurological function in comparison to control subjects with cells or scaffold alone. The results were attributed to diminished glial scarring, reduction of tissue loss due to the injury, and the reestablishment of axonal connection. The same group reported later that an implanted glycolide scaffold seeded with stem cells was able to establish a two ways interaction between brain and viable areas in an ischemia-induced lesion model (Park et al., 2002; Teng et al., 2002).
The role of biomaterials in skin regeneration has been previously established in the clinic, as a temporary cover for extensive wounds, with improved re-epithelization, matrix formation and nerve growth. The list of examples includes dextran hydrogels, biodegradable polymers and bioactive glass microfibers (Martin et al., 2014; Shen et al., 2015; Zhou et al., 2016). Several biomaterials were acquired to the field of skin regeneration, using patient-derived keratinocytes or stem cells (El-Serafi et al., 2017). Skardal et al. (2017) designed a heparin-hyaluronic acid hydrogel, loaded with amniotic fluid-derived stem cells. This construct was introduced to a full thickness burn wound model and was associated with better production of extra-cellular matrix, increased rate of revascularization and eventually better rate of re-epithelialization. The authors relate these results to the ability of their hydrogel to sequester and release the paracrine secreted growth factors and cytokines, which prolonged their effect (Skardal et al., 2017). Various types of synthetic biomaterials are available as dermal substitute (DS) for clinical use. The main role of DS is to replace the lost skin until the patient is prepared for skin grafting, which fastens the wound healing and enhance the angiogenesis process (De Angelis et al., 2015; Gentile and Garcovich, 2021). Collagen-based DS showed efficiency also in skin ulcers of vasculogenic origin (De Angelis et al., 2019). Alternatively, animal derived, decellularized dermis can be used in severe cases, such as loss of a large surface area of skin (Gentile et al., 2013). In a different approach, autologous fat-derived scaffold can be considered as a clinical solution for wound and scar care with better skin quality and healing time. Fat tissue can be mechanically or enzymatically processed to provide adipogenic derived stem cells suspended in fatty tissue of the stromal vascular fraction, which contains other cell types such as endothelial cells, pericytes and immune cells (Gentile et al., 2017; Gentile et al., 2021). Similar approach was used in breast reconstruction following mastectomy instead of an implant with safe and effective clinical outcome (Gentile et al., 2019).
A major challenge with stem cell implementation for therapeutic approaches is to obtain enough yield of cells with a good quality, in an environment free from animal-derived products using reproducible technique. Multiple studies investigated the effect of biomaterials on stem cells, in terms of proliferation and preservation of their phenotype. For example, alkali treatment of PLA and PLGA on murine ESC increased the material hydrophilicity and the cell proliferation (Harrison et al., 2004). Furthermore, scaffold stiffness acted synergistically with fibronectin coating to enhance ESC differentiation compared to the less stiff matrigel scaffold which supported the growth of ESC (Levenberg et al., 2003). Similarly, vitronectin contained biomaterials provided a suitable matrix for the adherence and expansion of iPCS (Seale and Varghese, 2017). The same effect can be obtained for hematopoietic stem cells when cultured in a porous scaffold coated with tantalum that resembled the microarchitecture of bone marrow trabeculae without the need of any cytokines (Bagley et al., 1999; Ehring et al., 2003). The same type of stem cells showed enhanced colony formation and upregulation of growth factors-related genes when cultured on scaffold of collagen type 1 in the presence a cocktail of ligands (Oswald et al., 2006). Thus, each type of stem cell may require a special biomaterial to enhance its proliferation, without inducing differentiation.
Most of the body tissues consist of different types of cells that interact in a harmonized organization. Such interaction is not only responsible of the sustainability of the tissue, but also for the functionality. For example, bone and cartilage are present adjacent to each other in a well-defined configuration at the articular surface of the joints. This formation allows weight support as well as protection of the bone ends from erosion; thus a cartilage defect can be easily extended to the underlying bone. The 3D scaffolds, in this case, can be tailored to be a composite of two types of biomaterials which correspond to the two target tissues. Not only the material, but also the fabrication properties would differ according to the target tissue (Jia et al., 2018). Vascularization is another challenge for cell-biomaterial constructs for maintaining its viability in-vivo (Shahin et al., 2020). In classical scaffold-based 3D culture system, neovasculogenesis is dependent on the media supplement. Unfortunately, the osteogenic supplements in media do not support elongation of new vessels, while the angiogenic factors decrease the osteogenic differentiation ability (Schott et al., 2021). On the other hand, self-assembly bone construct can be associated with spontaneous chondrogenic matrix formation and the extension of capillary like structure. The biomaterial used in this case was glass, which served as a surface to allow folding of the monolayer in a 3D bony construct (Alghfeli et al., 2021).
Skin represents another example of complicated multilayer structure. Bioprinting and electrospinning provide a solution through the preparation of a multilayer scaffold that would support the attachment and survival of both keratinocytes and fibroblast to represent the epidermal and dermal components (Choi et al., 2021). The multilayer scaffold should take into consideration the physical properties of each biological layer. For example, stiffness gradient differs between the skin layers can be reflected on the amount of calcium ions at the corresponding level of the scaffold. Calcium is responsible for the activation of several intracellular signalling cascades and consequently can involve the determination of the cell fate (Chaudhari et al., 2016). In conclusion, combination of biomaterials or combination of different physicochemical properties of the same biomaterial may enhance the bioengineering of complex tissues.
The field of biomaterials has already proved its importance in the field of stem cell biology, as well as differentiation and potential medical applications. There are many technical challenges that yet to be solved, such as the adherence of seeded cells to the bio-scaffold and integration with host tissue, as well as cytotoxicity as a result of amplified immune response from immune system or inflammatory response. Accordingly, biomaterials should also provide a sort of isolation to the cells seeded in them. Establishing specific substrates for cell expansion or differentiation into various lineages will only not have a great biological benefit but also economical interest, which would result from avoiding the expenses of different peptides and growth factors. Another major challenge is the production of complex scaffolds that can co-culture multiple cell lines. There is vast number of studies that proved the biomaterial-stem cell interaction with or without fully explaining the underlying mechanisms. Protein adsorption, cell adhesion and sensing the underlying structure, signaling pathway(s) activation, gene response and differentiation are the generic processes. However, few studies compared the effect of various compositions, forms or nanostructure and topography of the same biomaterial on the proliferation versus the differentiation potential of stem cells into different lineages. A proper description of the biomaterials according to their chemical and physical properties in relation to their effect on stem cells will be a major addition to the regenerative medicine. Furthermore, a proper description of the role of these biomaterials as additive to the current differentiation protocols or as a replacement of one or of the differentiation components can have a great biological impact as well as medical and commercial applications. Thus, a consortium of stem cell biologists and biomaterial developers and producers is highly needed in order to investigate a matrix of available and in-development materials against various types of stem cell differentiation and proliferation. Such collaboration can enhance the progress of application of stem cell-based tissue regeneration in the clinic.
All authors listed have made a substantial, direct, and intellectual contribution to the work and approved it for publication.
The authors declare that the research was conducted in the absence of any commercial or financial relationships that could be construed as a potential conflict of interest.
All claims expressed in this article are solely those of the authors and do not necessarily represent those of their affiliated organizations, or those of the publisher, the editors and the reviewers. Any product that may be evaluated in this article, or claim that may be made by its manufacturer, is not guaranteed or endorsed by the publisher.
Abagnale, G., Steger, M., Nguyen, V. H., Hersch, N., Sechi, A., Joussen, S., et al. (2015). Surface Topography Enhances Differentiation of Mesenchymal Stem Cells towards Osteogenic and Adipogenic Lineages. Biomaterials 61, 316–326. doi:10.1016/j.biomaterials.2015.05.030
Abercrombie, M., Heaysman, J. E. M., and Pegrum, S. M. (1972). Locomotion of Fibroblasts in Culture. Exp. Cel Res. 73, 536–539. doi:10.1016/0014-4827(72)90090-0
Abercrombie, M., Heaysman, J. E. M., and Pegrum, S. M. (1971). The Locomotion of Fibroblasts in Culture. Exp. Cel Res. 67, 359–367. doi:10.1016/0014-4827(71)90420-4
Agrawal, S., and Srivastava, R. (2020). “Osteoinductive and Osteoconductive Biomaterials,” in Racing for the Surface: Antimicrobial and Interface Tissue Engineering. Editors B. Li, T. F. Moriarty, T. Webster, and M. Xing (Cham: Springer International Publishing), 355–395. doi:10.1007/978-3-030-34471-9_15
Al-Haque, S., Miklas, J. W., Feric, N., Chiu, L. L. Y., Chen, W. L. K., Simmons, C. A., et al. (2012). Hydrogel Substrate Stiffness and Topography Interact to Induce Contact Guidance in Cardiac Fibroblasts. Macromol. Biosci. 12, 1342–1353. doi:10.1002/mabi.201200042
Alghfeli, L., Parambath, D., Manzoor, S., Roach, H. I., Oreffo, R. O. C., and El-Serafi, A. T. (2021). Synthesis of Scaffold-free, Three Dimensional, Osteogenic Constructs Following Culture of Skeletal Osteoprogenitor Cells on Glass Surfaces. Bone Rep. 15, 101143. doi:10.1016/j.bonr.2021.101143
Amini, A. R., Laurencin, C. T., and Nukavarapu, S. P. (2012). Bone Tissue Engineering: Recent Advances and Challenges. Crit. Rev. Biomed. Eng. 40, 363–408. doi:10.1615/critrevbiomedeng.v40.i5.10
Aminuddin, N. I., Ahmad, R., Akbar, S. A., and Pingguan-Murphy, B. (2016). Osteoblast and Stem Cell Response to Nanoscale Topographies: a Review. Sci. Technology Adv. Mater. 17, 698–714. doi:10.1080/14686996.2016.1242999
Anderson, J. M. (1988). Inflammatory Response to Implants. ASAIO J. 34, 101–107. doi:10.1097/00002480-198804000-00005
Anderson, J. M., Rodriguez, A., and Chang, D. T. (2008). Foreign Body Reaction to Biomaterials. Semin. Immunol. 20, 86–100. doi:10.1016/j.smim.2007.11.004
Anderson, K. L., Page, C., Swift, M. F., Suraneni, P., Janssen, M. E. W., Pollard, T. D., et al. (2017). Nano-scale Actin-Network Characterization of Fibroblast Cells Lacking Functional Arp2/3 Complex. J. Struct. Biol. 197, 312–321. doi:10.1016/j.jsb.2016.12.010
Ang, H. Y., Bulluck, H., Wong, P., Venkatraman, S. S., Huang, Y., and Foin, N. (2017). Bioresorbable Stents: Current and Upcoming Bioresorbable Technologies. Int. J. Cardiol. 228, 931–939. doi:10.1016/j.ijcard.2016.11.258
Ayala, R., Zhang, C., Yang, D., Hwang, Y., Aung, A., Shroff, S. S., et al. (2011). Engineering the Cell-Material Interface for Controlling Stem Cell Adhesion, Migration, and Differentiation. Biomaterials 32, 3700–3711. doi:10.1016/j.biomaterials.2011.02.004
Badylak, S. F., Valentin, J. E., Ravindra, A. K., Mccabe, G. P., and Stewart-Akers, A. M. (2008). Macrophage Phenotype as a Determinant of Biologic Scaffold Remodeling. Tissue Eng. A 14, 1835–1842. doi:10.1089/ten.tea.2007.0264
Bagley, J., Rosenzweig, M., Marks, D. F., and Pykett, M. J. (1999). Extended Culture of Multipotent Hematopoietic Progenitors without Cytokine Augmentation in a Novel Three-Dimensional Device. Exp. Hematol. 27, 496–504. doi:10.1016/s0301-472x(98)00053-8
Baharvand, H., Hashemi, S. M., Kazemi Ashtiani, S., and Farrokhi, A. (2006). Differentiation of Human Embryonic Stem Cells into Hepatocytes in 2D and 3D Culture Systems In Vitro. Int. J. Dev. Biol. 50, 645–652. doi:10.1387/ijdb.052072hb
Bamford, C. H., and Al-Lamee, K. G. (1992). Chemical Methods for Improving the Haemocompatibility of Synthetic Polymers. Clin. Mater. 10, 243–261. doi:10.1016/0267-6605(92)90018-o
Biggs, M. J. P., Richards, R. G., Mcfarlane, S., Wilkinson, C. D. W., Oreffo, R. O. C., and Dalby, M. J. (2008). Adhesion Formation of Primary Human Osteoblasts and the Functional Response of Mesenchymal Stem Cells to 330 Nm Deep Microgrooves. J. R. Soc. Interf. 5, 1231–1242. doi:10.1098/rsif.2008.0035
Brown, B. N., and Badylak, S. F. (2013). Expanded Applications, Shifting Paradigms and an Improved Understanding of Host-Biomaterial Interactions. Acta Biomater. 9, 4948–4955. doi:10.1016/j.actbio.2012.10.025
Brown, B. N., Londono, R., Tottey, S., Zhang, L., Kukla, K. A., Wolf, M. T., et al. (2012). Macrophage Phenotype as a Predictor of Constructive Remodeling Following the Implantation of Biologically Derived Surgical Mesh Materials. Acta Biomater. 8, 978–987. doi:10.1016/j.actbio.2011.11.031
Brown, T. E., and Anseth, K. S. (2017). Spatiotemporal Hydrogel Biomaterials for Regenerative Medicine. Chem. Soc. Rev. 46, 6532–6552. doi:10.1039/c7cs00445a
Cembran, A., Bruggeman, K. F., Williams, R. J., Parish, C. L., and Nisbet, D. R. (2020). Biomimetic Materials and Their Utility in Modeling the 3-Dimensional Neural Environment. iScience 23, 100788. doi:10.1016/j.isci.2019.100788
Chaudhari, A. A., Vig, K., Baganizi, D. R., Sahu, R., Dixit, S., Dennis, V., et al. (2016). Future Prospects for Scaffolding Methods and Biomaterials in Skin Tissue Engineering: A Review. Int. J. Mol. Sci. 17. doi:10.3390/ijms17121974
Chaudhuri, O., Gu, L., Klumpers, D., Darnell, M., Bencherif, S. A., Weaver, J. C., et al. (2016). Hydrogels with Tunable Stress Relaxation Regulate Stem Cell Fate and Activity. Nat. Mater 15, 326–334. doi:10.1038/nmat4489
Chen, S., Jones, J. A., Xu, Y., Low, H.-Y., Anderson, J. M., and Leong, K. W. (2010). Characterization of Topographical Effects on Macrophage Behavior in a Foreign Body Response Model. Biomaterials 31, 3479–3491. doi:10.1016/j.biomaterials.2010.01.074
Cho, J. H., Kim, S.-H., Park, K. D., Jung, M. C., Yang, W. I., Han, S. W., et al. (2004). Chondrogenic Differentiation of Human Mesenchymal Stem Cells Using a Thermosensitive poly(N-Isopropylacrylamide) and Water-Soluble Chitosan Copolymer. Biomaterials 25, 5743–5751. doi:10.1016/j.biomaterials.2004.01.051
Choi, W. S., Kim, J. H., Ahn, C. B., Lee, J. H., Kim, Y. J., Son, K. H., et al. (2021). Development of a Multi-Layer Skin Substitute Using Human Hair Keratinic Extract-Based Hybrid 3D Printing. Polymers (Basel) 13. doi:10.3390/polym13162584
Cockerill, I., Su, Y., Lee, J. H., Berman, D., Young, M. L., Zheng, Y., et al. (2020). Micro-/Nanotopography on Bioresorbable Zinc Dictates Cytocompatibility, Bone Cell Differentiation, and Macrophage Polarization. Nano Lett. 20, 4594–4602. doi:10.1021/acs.nanolett.0c01448
Cooke, M. J., Vulic, K., and Shoichet, M. S. (2010a). Design of Biomaterials to Enhance Stem Cell Survival when Transplanted into the Damaged central Nervous System. Soft Matter 6, 4988–4998. doi:10.1039/c0sm00448k
Cooke, M. J., Vulic, K., and Shoichet, M. S. (2010b). Design of Biomaterials to Enhance Stem Cell Survival when Transplanted into the Damaged central Nervous System.
Cutts, J., Nikkhah, M., and Brafman, D. A. (2015). Biomaterial Approaches for Stem Cell-Based Myocardial Tissue Engineering. Biomark Insights 10, 77–90. doi:10.4137/BMI.S20313
De Angelis, B., Gentile, P., Tati, E., Bottini, D. J., Bocchini, I., Orlandi, F., et al. (2015). One-Stage Reconstruction of Scalp after Full-Thickness Oncologic Defects Using a Dermal Regeneration Template (Integra). Biomed. Res. Int. 2015, 698385. doi:10.1155/2015/698385
De Angelis, B., Orlandi, F., Morais D'autilio, M. F. L., Di Segni, C., Scioli, M. G., Orlandi, A., et al. (2019). Vasculogenic Chronic Ulcer: Tissue Regeneration with an Innovative Dermal Substitute. J. Clin. Med. 8. doi:10.3390/jcm8040525
Discher, D. E., Janmey, P., and Wang, Y.-l. (2005). Tissue Cells Feel and Respond to the Stiffness of Their Substrate. Science 310, 1139–1143. doi:10.1126/science.1116995
Driscoll, M. K., Sun, X., Guven, C., Fourkas, J. T., and Losert, W. (2014). Cellular Contact Guidance through Dynamic Sensing of Nanotopography. ACS Nano 8, 3546–3555. doi:10.1021/nn406637c
Dzhoyashvili, N. A., Shen, S., and Rochev, Y. A. (2015). Natural and Synthetic Materials for Self-Renewal, Long-Term Maintenance, and Differentiation of Induced Pluripotent Stem Cells. Adv. Healthc. Mater. 4, 2342–2359. doi:10.1002/adhm.201400798
Ehring, B., Biber, K., Upton, T. M., Plosky, D., Pykett, M., and Rosenzweig, M. (2003). Expansion of HPCs from Cord Blood in a Novel 3D Matrix. Cytotherapy 5, 490–499. doi:10.1080/14653240310003585
El-Serafi, A. T., El-Serafi, I. T., Elmasry, M., Steinvall, I., and Sjöberg, F. (2017). Skin Regeneration in Three Dimensions, Current Status, Challenges and Opportunities. Differentiation 96, 26–29. doi:10.1016/j.diff.2017.06.002
El-Serafi, A. T. (2012). “Epigenetic Modifiers and Stem Cell Differentiation,” in Stem Cells and Cancer Stem Cells. Editor M. Hayat (Dordrecht: Springer), 147–154. doi:10.1007/978-94-007-4798-2_14
El-Serafi, A. T., Sandeep, D., Abdallah, S., Lozansson, Y., Hamad, M., and Khan, A. A. (2019). Paradoxical Effects of the Epigenetic Modifiers 5-Aza-Deoxycytidine and Suberoylanilide Hydroxamic Acid on Adipogenesis. Differentiation 106, 1–8. doi:10.1016/j.diff.2019.02.003
El-Serafi, A., Wilson, D. I., Wilson, D., Roach, H., and Oreffo, R. (2011). Developmental Plasticity of Human Foetal Femur-Derived Cells in Pellet Culture: Self Assembly of an Osteoid Shell Around a Cartilaginous Core. eCM 21, 558–567. doi:10.22203/ecm.v021a42
Eliaz, N. (2019). Corrosion of Metallic Biomaterials: A Review. Materials (Basel) 12. doi:10.3390/ma12030407
Elsharkawi, I., Parambath, D., Saber-Ayad, M., Khan, A. A., and El-Serafi, A. T. (2019). Exploring the Effect of Epigenetic Modifiers on Developing Insulin-Secreting Cells. Hum. Cel 33 (1), 1–9. doi:10.1007/s13577-019-00292-y
Engler, A. J., Sen, S., Sweeney, H. L., and Discher, D. E. (2006). Matrix Elasticity Directs Stem Cell Lineage Specification. Cell 126, 677–689. doi:10.1016/j.cell.2006.06.044
Fan, H., Hu, Y., Zhang, C., Li, X., Lv, R., Qin, L., et al. (2006). Cartilage Regeneration Using Mesenchymal Stem Cells and a PLGA-Gelatin/chondroitin/hyaluronate Hybrid Scaffold. Biomaterials 27, 4573–4580. doi:10.1016/j.biomaterials.2006.04.013
Gadalla, S. M., Aubert, G., Wang, T., Haagenson, M., Spellman, S. R., Wang, L., et al. (2018). Donor Telomere Length and Causes of Death after Unrelated Hematopoietic Cell Transplantation in Patients with Marrow Failure. Blood 131, 2393–2398. doi:10.1182/blood-2017-10-812735
Gail, M. H., and Boone, C. W. (1970). The Locomotion of Mouse Fibroblasts in Tissue Culture. Biophysical J. 10, 980–993. doi:10.1016/s0006-3495(70)86347-0
Gao, C., Feng, P., Peng, S., and Shuai, C. (2017). Carbon Nanotube, Graphene and boron Nitride Nanotube Reinforced Bioactive Ceramics for Bone Repair. Acta Biomater. 61. 1–20. doi:10.1016/j.actbio.2017.05.020
Gentile, P., Casella, D., Palma, E., and Calabrese, C. (2019). Engineered Fat Graft Enhanced with Adipose-Derived Stromal Vascular Fraction Cells for Regenerative Medicine: Clinical, Histological and Instrumental Evaluation in Breast Reconstruction. J. Clin. Med. 8. doi:10.3390/jcm8040504
Gentile, P., and Garcovich, S. (2019). Concise Review: Adipose-Derived Stem Cells (ASCs) and Adipocyte-Secreted Exosomal microRNA (A-SE-miR) Modulate Cancer Growth and proMote Wound Repair. J. Clin. Med. 8. doi:10.3390/jcm8060855
Gentile, P., and Garcovich, S. (2021). Systematic Review: Adipose-Derived Mesenchymal Stem Cells, Platelet-Rich Plasma and Biomaterials as New Regenerative Strategies in Chronic Skin Wounds and Soft Tissue Defects. Int. J. Mol. Sci. 22. doi:10.3390/ijms22041538
Gentile, P., Sterodimas, A., Pizzicannella, J., Dionisi, L., De Fazio, D., Calabrese, C., et al. (2020). Systematic Review: Allogenic Use of Stromal Vascular Fraction (SVF) and Decellularized Extracellular Matrices (ECM) as Advanced Therapy Medicinal Products (ATMP) in Tissue Regeneration. Int. J. Mol. Sci. 21. doi:10.3390/ijms21144982
Gentile, P., Colicchia, G. M., Nicoli, F., Cervelli, G., Curcio, C. B., Brinci, L., et al. (2013). Complex Abdominal wall Repair Using a Porcine Dermal Matrix. Surg. Innov. 20, NP12–NP15. doi:10.1177/1553350611421022
Gentile, P., Scioli, M. G., Bielli, A., Orlandi, A., and Cervelli, V. (2017). Comparing Different Nanofat Procedures on Scars: Role of the Stromal Vascular Fraction and its Clinical Implications. Regenerative Med. 12, 939–952. doi:10.2217/rme-2017-0076
Gentile, P., Sterodimas, A., Calabrese, C., and Garcovich, S. (2021). Systematic Review: Advances of Fat Tissue Engineering as Bioactive Scaffold, Bioactive Material, and Source for Adipose-Derived Mesenchymal Stem Cells in Wound and Scar Treatment. Stem Cel Res Ther 12, 318. doi:10.1186/s13287-021-02397-4
Hamilton, W. B., and Brickman, J. M. (2014). Erk Signaling Suppresses Embryonic Stem Cell Self-Renewal to Specify Endoderm. Cel Rep. 9, 2056–2070. doi:10.1016/j.celrep.2014.11.032
Hanein, D., Sabanay, H., Addadi, L., and Geiger, B. (1993). Selective Interactions of Cells with crystal Surfaces. Implications for the Mechanism of Cell Adhesion. J. Cel Sci 104 ( Pt 2) (Pt 2), 275–288. doi:10.1242/jcs.104.2.275
Hanein, D., Geiger, B., and Addadi, L. (1994). Differential Adhesion of Cells to Enantiomorphous crystal Surfaces. Science 263, 1413–1416. doi:10.1126/science.8128221
Hanein, D., Yarden, A., Sabanay, H., Addadi, L., and Geiger, B. (1996). Cell-Adhesion to Crystal Surfaces: Adhesion-Induced Physiological Cell Death. Cel Adhes. Commun. 4, 341–353. doi:10.3109/15419069609010777
Harrison, J., Pattanawong, S., Forsythe, J. S., Gross, K. A., Nisbet, D. R., Beh, H., et al. (2004). Colonization and Maintenance of Murine Embryonic Stem Cells on Poly(α-Hydroxy Esters). Biomaterials 25, 4963–4970. doi:10.1016/j.biomaterials.2004.01.054
Hart, A., Gadegaard, N., Wilkinson, C. D. W., Oreffo, R. O. C., and Dalby, M. J. (2007). Osteoprogenitor Response to Low-Adhesion Nanotopographies Originally Fabricated by Electron Beam Lithography. J. Mater. Sci. Mater. Med. 18, 1211–1218. doi:10.1007/s10856-007-0157-7
Hellmund, K. S., and Koksch, B. (2019). Self-Assembling Peptides as Extracellular Matrix Mimics to Influence Stem Cell's Fate. Front. Chem. 7, 172. doi:10.3389/fchem.2019.00172
Henson, P. M. (1980). Mechanisms of Exocytosis in Phagocytic Inflammatory Cells. Parke-Davis Award Lecture. Am. J. Pathol. 101, 494–511.
Hibino, N., Duncan, D. R., Nalbandian, A., Yi, T., Qyang, Y., Shinoka, T., et al. (2012). Evaluation of the Use of an Induced Puripotent Stem Cell Sheet for the Construction of Tissue-Engineered Vascular Grafts. J. Thorac. Cardiovasc. Surg. 143, 696–703. doi:10.1016/j.jtcvs.2011.06.046
Jean, J., Bernard, G., Duque-Fernandez, A., Auger, F. A., and Pouliot, R. (2011). Effects of Serum-free Culture at the Air-Liquid Interface in a Human Tissue-Engineered Skin Substitute. Tissue Eng. Part A 17, 877–888. doi:10.1089/ten.tea.2010.0256
Jia, S., Wang, J., Zhang, T., Pan, W., Li, Z., He, X., et al. (2018). Multilayered Scaffold with a Compact Interfacial Layer Enhances Osteochondral Defect Repair. ACS Appl. Mater. Inter. 10, 20296–20305. doi:10.1021/acsami.8b03445
Keselowsky, B. G., Collard, D. M., and García, A. J. (2005). Integrin Binding Specificity Regulates Biomaterial Surface Chemistry Effects on Cell Differentiation. Proc. Natl. Acad. Sci. U.S.A. 102, 5953–5957. doi:10.1073/pnas.0407356102
Khan, A. A., Huat, T. J., Al Mutery, A., El-Serafi, A. T., Kacem, H. H., Abdallah, S. H., et al. (2020). Significant Transcriptomic Changes Are Associated with Differentiation of Bone Marrow-Derived Mesenchymal Stem Cells into Neural Progenitor-like Cells in the Presence of bFGF and EGF. Cell Biosci 10, 126. doi:10.1186/s13578-020-00487-z
Khetan, S., Guvendiren, M., Legant, W. R., Cohen, D. M., Chen, C. S., and Burdick, J. A. (2013). Degradation-mediated Cellular Traction Directs Stem Cell Fate in Covalently Crosslinked Three-Dimensional Hydrogels. Nat. Mater 12, 458–465. doi:10.1038/nmat3586
Kieswetter, K., Schwartz, Z., Dean, D. D., and Boyan, B. D. (1996). The Role of Implant Surface Characteristics in the Healing of Bone. Crit. Rev. Oral Biol. Med. 7, 329–345. doi:10.1177/10454411960070040301
Kloxin, A. M., Kasko, A. M., Salinas, C. N., and Anseth, K. S. (2009). Photodegradable Hydrogels for Dynamic Tuning of Physical and Chemical Properties. Science 324, 59–63. doi:10.1126/science.1169494
Kshitiz, , Park, J., Kim, P., Helen, W., Engler, A. J., Levchenko, A., et al. (2012). Control of Stem Cell Fate and Function by Engineering Physical Microenvironments. Integr. Biol. (Camb) 4, 1008–1018. doi:10.1039/c2ib20080e
Kumar, A., Young, C., Farina, J., Witzl, A., and Marks, E. D. (2015). Novel Nanocomposite Biomaterial to Differentiate Bone Marrow Mesenchymal Stem Cells to the Osteogenic Lineage for Bone Restoration. J. Orthopaedic Translation 3, 105–113. doi:10.1016/j.jot.2015.03.002
Lee, K. Y., Alsberg, E., Hsiong, S., Comisar, W., Linderman, J., Ziff, R., et al. (2004). Nanoscale Adhesion Ligand Organization Regulates Osteoblast Proliferation and Differentiation. Nano Lett. 4, 1501–1506. doi:10.1021/nl0493592
Leijten, J., Teixeira, L. S. M., Bolander, J., Ji, W., Vanspauwen, B., Lammertyn, J., et al. (2016). Bioinspired Seeding of Biomaterials Using Three Dimensional Microtissues Induces Chondrogenic Stem Cell Differentiation and Cartilage Formation under Growth Factor Free Conditions. Sci. Rep. 6, 36011. doi:10.1038/srep36011
Levenberg, S., Huang, N. F., Lavik, E., Rogers, A. B., Itskovitz-Eldor, J., and Langer, R. (2003). Differentiation of Human Embryonic Stem Cells on Three-Dimensional Polymer Scaffolds. Proc. Natl. Acad. Sci. U.S.A. 100, 12741–12746. doi:10.1073/pnas.1735463100
Li, X., Dai, Y., Shen, T., and Gao, C. (2017). Induced Migration of Endothelial Cells into 3D Scaffolds by Chemoattractants Secreted by Pro-inflammatory Macrophages In Situ. Regen. Biomater. 4, 139–148. doi:10.1093/rb/rbx005
Li, Z., Guo, X., Palmer, A. F., Das, H., and Guan, J. (2012). High-efficiency Matrix Modulus-Induced Cardiac Differentiation of Human Mesenchymal Stem Cells inside a Thermosensitive Hydrogel. Acta Biomater. 8, 3586–3595. doi:10.1016/j.actbio.2012.06.024
Lin, S., Sangaj, N., Razafiarison, T., Zhang, C., and Varghese, S. (2011). Influence of Physical Properties of Biomaterials on Cellular Behavior. Pharm. Res. 28, 1422–1430. doi:10.1007/s11095-011-0378-9
Logan, N., and Brett, P. (2013). The Control of Mesenchymal Stromal Cell Osteogenic Differentiation through Modified Surfaces. Stem Cell Int 2013, 361637. doi:10.1155/2013/361637
Lutolf, M. P., Gilbert, P. M., and Blau, H. M. (2009). Designing Materials to Direct Stem-Cell Fate. Nature 462, 433–441. doi:10.1038/nature08602
Lv, J., Xiu, P., Tan, J., Jia, Z., Cai, H., and Liu, Z. (2015). Enhanced Angiogenesis and Osteogenesis in Critical Bone Defects by the Controlled Release of BMP-2 and VEGF: Implantation of Electron Beam Melting-Fabricated Porous Ti 6 Al 4 V Scaffolds Incorporating Growth Factor-Doped Fibrin Glue. Biomed. Mater. 10, 035013. doi:10.1088/1748-6041/10/3/035013
Mahoney, M. J., and Saltzman, W. M. (2001). Transplantation of Brain Cells Assembled Around a Programmable Synthetic Microenvironment. Nat. Biotechnol. 19, 934–939. doi:10.1038/nbt1001-934
Mansour, M. F., Greish, S. M., El-Serafi, A. T., Abdelall, H., and El-Wazir, Y. M. (2019). Therapeutic Potential of Human Umbilical Cord Derived Mesenchymal Stem Cells on Rat Model of Liver Fibrosis. Am. J. Stem Cell 8, 7–18.
Marin, E., Boschetto, F., and Pezzotti, G. (2020). Biomaterials and Biocompatibility: An Historical Overview. J. Biomed. Mater. Res. 108, 1617–1633. doi:10.1002/jbm.a.36930
Martin, J. R., Gupta, M. K., Page, J. M., Yu, F., Davidson, J. M., Guelcher, S. A., et al. (2014). A Porous Tissue Engineering Scaffold Selectively Degraded by Cell-Generated Reactive Oxygen Species. Biomaterials 35, 3766–3776. doi:10.1016/j.biomaterials.2014.01.026
Maturavongsadit, P., Luckanagul, J. A., Metavarayuth, K., Zhao, X., Chen, L., Lin, Y., et al. (2016). Promotion of In Vitro Chondrogenesis of Mesenchymal Stem Cells Using In Situ Hyaluronic Hydrogel Functionalized with Rod-like Viral Nanoparticles. Biomacromolecules 17, 1930–1938. doi:10.1021/acs.biomac.5b01577
Monaghan, M. G., Linneweh, M., Liebscher, S., Van Handel, B., Layland, S. L., and Schenke-Layland, K. (2016). Endocardial-to-mesenchymal Transformation and Mesenchymal Cell Colonization at the Onset of Human Cardiac Valve Development. Development 143, 473–482. doi:10.1242/dev.133843
Nour, S., Baheiraei, N., Imani, R., Rabiee, N., Khodaei, M., Alizadeh, A., et al. (2019). Bioactive Materials: A Comprehensive Review on Interactions with Biological Microenvironment Based on the Immune Response. J. Bionic Eng. 16, 563–581. doi:10.1007/s42235-019-0046-z
Nugud, A. A. A., and El-Serafi, A. (2018). Three-dimensional Culturing Models for Bone Development. Adv. Tissue Eng. Regenerative Med. 4, 124–125.
Nugud, A., Sandeep, D., and El-Serafi, A. T. (2018). Two Faces of the coin: Minireview for Dissecting the Role of Reactive Oxygen Species in Stem Cell Potency and Lineage Commitment. J. Adv. Res. 14, 73–79. doi:10.1016/j.jare.2018.05.012
Nur-E-Kamal, A., Ahmed, I., Kamal, J., Schindler, M., and Meiners, S. (2006). Three-dimensional Nanofibrillar Surfaces Promote Self-Renewal in Mouse Embryonic Stem Cells. Stem Cells 24, 426–433. doi:10.1634/stemcells.2005-0170
Oswald, J., Steudel, C., Salchert, K., Joergensen, B., Thiede, C., Ehninger, G., et al. (2006). Gene-expression Profiling of CD34+ Hematopoietic Cells Expanded in a Collagen I Matrix. Stem Cells 24, 494–500. doi:10.1634/stemcells.2005-0276
Park, K. I., Teng, Y. D., and Snyder, E. Y. (2002). The Injured Brain Interacts Reciprocally with Neural Stem Cells Supported by Scaffolds to Reconstitute Lost Tissue. Nat. Biotechnol. 20, 1111–1117. doi:10.1038/nbt751
Pennings, S., Liu, K. J., and Qian, H. (2018). The Stem Cell Niche: Interactions between Stem Cells and Their Environment. Stem Cell Int 2018, 4879379. doi:10.1155/2018/4879379
Pijnappels, D. A., Schalij, M. J., Ramkisoensing, A. A., Van Tuyn, J., De Vries, A. A. F., Van Der Laarse, A., et al. (2008). Forced Alignment of Mesenchymal Stem Cells Undergoing Cardiomyogenic Differentiation Affects Functional Integration with Cardiomyocyte Cultures. Circ. Res. 103, 167–176. doi:10.1161/circresaha.108.176131
Prasadh, S., and Wong, R. C. W. (2018). Unraveling the Mechanical Strength of Biomaterials Used as a Bone Scaffold in Oral and Maxillofacial Defects. Oral Sci. Int. 15, 48–55. doi:10.1016/s1348-8643(18)30005-3
Ratner, B. D. (2011). The Biocompatibility Manifesto: Biocompatibility for the Twenty-First century. J. Cardiovasc. Trans. Res. 4, 523–527. doi:10.1007/s12265-011-9287-x
Saleh, L. S., and Bryant, S. J. (2018). The Host Response in Tissue Engineering: Crosstalk between Immune Cells and Cell-Laden Scaffolds. Curr. Opin. Biomed. Eng. 6, 58–65. doi:10.1016/j.cobme.2018.03.006
Samadian, H., Maleki, H., Fathollahi, A., Salehi, M., Gholizadeh, S., Derakhshankhah, H., et al. (2020). Naturally Occurring Biological Macromolecules-Based Hydrogels: Potential Biomaterials for Peripheral Nerve Regeneration. Int. J. Biol. Macromolecules 154, 795–817. doi:10.1016/j.ijbiomac.2020.03.155
Sanz-Herrera, J. A., and Reina-Romo, E. (2011). Cell-biomaterial Mechanical Interaction in the Framework of Tissue Engineering: Insights, Computational Modeling and Perspectives. Ijms 12, 8217–8244. doi:10.3390/ijms12118217
Schott, N. G., Friend, N. E., and Stegemann, J. P. (2021). Coupling Osteogenesis and Vasculogenesis in Engineered Orthopedic Tissues. Tissue Eng. B: Rev. 27, 199–214. doi:10.1089/ten.teb.2020.0132
Schwartz, Z., and Boyan, B. D. (1994). Underlying Mechanisms at the Bone-Biomaterial Interface. J. Cel. Biochem. 56, 340–347. doi:10.1002/jcb.240560310
Schwartz, Z., Braun, G., Kohavi, D., Brooks, B., Amir, D., Sela, J., et al. (1993). Effects of Hydroxyapatite Implants on Primary Mineralization during Rat Tibial Healing: Biochemical and Morphometric Analyses. J. Biomed. Mater. Res. 27, 1029–1038. doi:10.1002/jbm.820270808
Scopelliti, P. E., Borgonovo, A., Indrieri, M., Giorgetti, L., Bongiorno, G., Carbone, R., et al. (2010). The Effect of Surface Nanometre-Scale Morphology on Protein Adsorption. PLoS One 5, e11862. doi:10.1371/journal.pone.0011862
Seale, N. M., and Varghese, S. (2017). Biomaterials for Pluripotent Stem Cell Engineering: From Fate Determination to Vascularization.
Seidlits, S. K., Khaing, Z. Z., Petersen, R. R., Nickels, J. D., Vanscoy, J. E., Shear, J. B., et al. (2010). The Effects of Hyaluronic Acid Hydrogels with Tunable Mechanical Properties on Neural Progenitor Cell Differentiation. Biomaterials 31, 3930–3940. doi:10.1016/j.biomaterials.2010.01.125
Serrano-Aroca, Á., Vera-Donoso, C. D., and Moreno-Manzano, V. (2018). Bioengineering Approaches for Bladder Regeneration. Int. J. Mol. Sci. 19. doi:10.3390/ijms19061796
Shahin, H., Elmasry, M., Steinvall, I., Söberg, F., and El-Serafi, A. (2020). Vascularization Is the Next challenge for Skin Tissue Engineering as a Solution for Burn Management. Burns Trauma 8, tkaa022. doi:10.1093/burnst/tkaa022
Shen, Y.-I., Song, H.-H. G., Papa, A. E., Burke, J. A., Volk, S. W., and Gerecht, S. (2015). Acellular Hydrogels for Regenerative Burn Wound Healing: Translation from a Porcine Model. J. Invest. Dermatol. 135, 2519–2529. doi:10.1038/jid.2015.182
Skardal, A., Murphy, S. V., Crowell, K., Mack, D., Atala, A., and Soker, S. (2017). A Tunable Hydrogel System for Long‐term Release of Cell‐secreted Cytokines and Bioprinted In Situ Wound Cell Delivery. J. Biomed. Mater. Res. 105, 1986–2000. doi:10.1002/jbm.b.33736
Smith, L. A., Liu, X., Hu, J., and Ma, P. X. (2010). The Enhancement of Human Embryonic Stem Cell Osteogenic Differentiation with Nano-Fibrous Scaffolding. Biomaterials 31, 5526–5535. doi:10.1016/j.biomaterials.2010.03.065
Sozen, B., Amadei, G., Cox, A., Wang, R., Na, E., Czukiewska, S., et al. (2018). Self-assembly of Embryonic and Two Extra-embryonic Stem Cell Types into Gastrulating Embryo-like Structures. Nat. Cel Biol 20, 979–989. doi:10.1038/s41556-018-0147-7
Sun, H.-H., Qu, T.-J., Zhang, X.-H., Yu, Q., and Chen, F.-M. (2012). Designing Biomaterials for In Situ Periodontal Tissue Regeneration. Biotechnol. Prog. 28, 3–20. doi:10.1002/btpr.698
Sundaram, S., One, J., Siewert, J., Teodosescu, S., Zhao, L., Dimitrievska, S., et al. (2014). Tissue-engineered Vascular Grafts Created from Human Induced Pluripotent Stem Cells. Stem Cell Transl Med 3, 1535–1543. doi:10.5966/sctm.2014-0065
Tay, C. Y., Yu, H., Pal, M., Leong, W. S., Tan, N. S., Ng, K. W., et al. (2010). Micropatterned Matrix Directs Differentiation of Human Mesenchymal Stem Cells towards Myocardial Lineage. Exp. Cel Res. 316, 1159–1168. doi:10.1016/j.yexcr.2010.02.010
Teng, Y. D., Lavik, E. B., Qu, X., Park, K. I., Ourednik, J., Zurakowski, D., et al. (2002). Functional Recovery Following Traumatic Spinal Cord Injury Mediated by a Unique Polymer Scaffold Seeded with Neural Stem Cells. Proc. Natl. Acad. Sci. U.S.A. 99, 3024–3029. doi:10.1073/pnas.052678899
Turner, L.-A., and Dalby, M. J. (2014). Nanotopography - Potential Relevance in the Stem Cell Niche. Biomater. Sci. 2, 1574–1594. doi:10.1039/c4bm00155a
Unal, A., and West, J. L. (2020). Synthetic ECM: Bioactive Synthetic Hydrogels for 3D Tissue Engineering. Bioconjug. Chem. 31 (10), 2253–2271. doi:10.1021/acs.bioconjchem.0c00270
Valamehr, B., Jonas, S. J., Polleux, J., Qiao, R., Guo, S., Gschweng, E. H., et al. (2008). Hydrophobic Surfaces for Enhanced Differentiation of Embryonic Stem Cell-Derived Embryoid Bodies. Proc. Natl. Acad. Sci. U S A. 105, 14459–14464. doi:10.1073/pnas.0807235105
Valentin, J. E., Stewart-Akers, A. M., Gilbert, T. W., and Badylak, S. F. (2009). Macrophage Participation in the Degradation and Remodeling of Extracellular Matrix Scaffolds. Tissue Eng. Part. A. 15, 1687–1694. doi:10.1089/ten.tea.2008.0419
Walker, J. B. (1912). IV. Operative Treatment of Fractures. Ann. Surg. 56, 847–853. doi:10.1097/00000658-191212000-00004
Wang, G., Ao, Q., Gong, K., Wang, A., Zheng, L., Gong, Y., et al. (2010). Acta Biomaterialia the Effect of Topology of Chitosan Biomaterials on the Differentiation and Proliferation of Neural Stem Cells. Acta Biomater. 6, 3630–3639. doi:10.1016/j.actbio.2010.03.039
Wang, L., Wu, S., Cao, G., Fan, Y., Dunne, N., and Li, X. (2019). Biomechanical Studies on Biomaterial Degradation and Co-cultured Cells: Mechanisms, Potential Applications, Challenges and Prospects. J. Mater. Chem. B 7, 7439–7459. doi:10.1039/c9tb01539f
Wang, T. Y., Forsythe, J. S., Nisbet, D. R., and Parish, C. L. (2012). Promoting Engraftment of Transplanted Neural Stem Cells/progenitors Using Biofunctionalised Electrospun Scaffolds. Biomaterials 33, 9188–9197. doi:10.1016/j.biomaterials.2012.09.013
Wang, W., and Yeung, K. W. K. (2017). Bone Grafts and Biomaterials Substitutes for Bone Defect Repair: A Review. Bioact Mater. 2, 224–247. doi:10.1016/j.bioactmat.2017.05.007
Wei, H. J., Chen, C. H., Lee, W. Y., Chiu, I., Hwang, S. M., Lin, W. W., et al. (2008). Bioengineered Cardiac Patch Constructed from Multilayered Mesenchymal Stem Cells for Myocardial Repair. Biomaterials 29, 3547–3556. doi:10.1016/j.biomaterials.2008.05.009
Westhauser, F., Widholz, B., Nawaz, Q., Tsitlakidis, S., Hagmann, S., Moghaddam, A., et al. (2019). Favorable Angiogenic Properties of the Borosilicate Bioactive Glass 0106-B1 Result in Enhanced In Vivo Osteoid Formation Compared to 45S5 Bioglass. Biomater. Sci. 7, 5161–5176. doi:10.1039/c9bm01220f
Williams, D. F. (2008). On the Mechanisms of Biocompatibility. Biomaterials 29, 2941–2953. doi:10.1016/j.biomaterials.2008.04.023
Williams, D. F. (2019). Specifications for Innovative, Enabling Biomaterials Based on the Principles of Biocompatibility Mechanisms. Front. Bioeng. Biotechnol. 7, 255. doi:10.3389/fbioe.2019.00255
Williams, D. F., and Zhong, S. P. (1994). Biodeterioration Biodegradation of Polymeric Medical Devices In-Situ. Int. Biodeterioration Biodegradation 34, 95–130. doi:10.1016/0964-8305(94)90002-7
Xie, C., Hu, J., Ma, H., Zhang, J., Chang, L. J., Chen, Y. E., et al. (2011). Three-dimensional Growth of iPS Cell-Derived Smooth Muscle Cells on Nanofibrous Scaffolds. Biomaterials 32, 4369–4375. doi:10.1016/j.biomaterials.2011.02.049
Yang, C., Tibbitt, M. W., Basta, L., and Anseth, K. S. (2014). Mechanical Memory and Dosing Influence Stem Cell Fate. Nat. Mater. 13, 645–652. doi:10.1038/nmat3889
Ye, Q., Van Amerongen, M. J., Sandham, J. A., Bank, R. A., Van Luyn, M. J., and Harmsen, M. C. (2011). Site-specific Tissue Inhibitor of Metalloproteinase-1 Governs the Matrix Metalloproteinases-dependent Degradation of Crosslinked Collagen Scaffolds and Is Correlated with Interleukin-10. J. Tissue Eng. Regen. Med. 5, 264–274. doi:10.1002/term.311
Yoon, J. K., Kang, M. L., Park, J. H., Lee, K. M., Shin, Y. M., Lee, J. W., et al. (2018). Direct Control of Stem Cell Behavior Using Biomaterials and Genetic Factors. Stem Cell Int 2018, 8642989. doi:10.1155/2018/8642989
Young, J. L., and Engler, A. J. (2011). Hydrogels with Time-dependent Material Properties Enhance Cardiomyocyte Differentiation In Vitro. Biomaterials 32, 1002–1009. doi:10.1016/j.biomaterials.2010.10.020
Zhang, K., Ma, Y., and Francis, L. F. (2002). Porous Polymer/bioactive Glass Composites for Soft-To-Hard Tissue Interfaces. J. Biomed. Mater. Res. 61, 551–563. doi:10.1002/jbm.10227
Keywords: stem cells, biomaterials, tissue regeneration, topography, differentiation, bioengineering
Citation: Nugud A, Alghfeli L, Elmasry M, El-Serafi I and El-Serafi AT (2022) Biomaterials as a Vital Frontier for Stem Cell-Based Tissue Regeneration. Front. Cell Dev. Biol. 10:713934. doi: 10.3389/fcell.2022.713934
Received: 24 May 2021; Accepted: 11 March 2022;
Published: 24 March 2022.
Edited by:
Ming Pei, West Virginia University, United StatesReviewed by:
Janos Kanczler, University of Southampton, United KingdomCopyright © 2022 Nugud, Alghfeli, Elmasry, El-Serafi and El-Serafi. This is an open-access article distributed under the terms of the Creative Commons Attribution License (CC BY). The use, distribution or reproduction in other forums is permitted, provided the original author(s) and the copyright owner(s) are credited and that the original publication in this journal is cited, in accordance with accepted academic practice. No use, distribution or reproduction is permitted which does not comply with these terms.
*Correspondence: Ahmed T. El-Serafi, YWhtZWQuZWxzZXJhZnlAbGl1LnNl
Disclaimer: All claims expressed in this article are solely those of the authors and do not necessarily represent those of their affiliated organizations, or those of the publisher, the editors and the reviewers. Any product that may be evaluated in this article or claim that may be made by its manufacturer is not guaranteed or endorsed by the publisher.
Research integrity at Frontiers
Learn more about the work of our research integrity team to safeguard the quality of each article we publish.