- 1The First Clinical Medical College of Shandong University of Traditional Chinese Medicine, Jinan, China
- 2Affiliated Hospital of Shandong University of Traditional Chinese Medicine, Jinan, China
Extracellular vesicles (EVs) have become a research hotspot in recent years because they act as messengers between cells in the physiological and pathological processes of the human body. It can be produced by the follicle, prostate, embryo, uterus, and oviduct in the reproductive field and exists in the extracellular environment as follicular fluid, semen, uterine cavity fluid, and oviduct fluid. Because extracellular vesicles are more stable at transmitting information, it allows all cells involved in the physiological processes of embryo formation, development, and implantation to communicate with one another. Extracellular vesicles carried miRNAs and proteins as mail, and when the messenger delivers the mail to the recipient cell, the recipient cell undergoes a series of changes. Current research begins with intercepting and decoding the information carried by extracellular vesicles. This information may help us gain a better understanding of the secrets of reproduction, as well as assist reproductive technology as an emerging marker and treatment.
1 Introduction
In 1967, Peter Wolf discovered extracellular vesicles (EVs) (Wolf, 1967). It is a heterogeneous vesicle that is surrounded by lipid bilayers derived from endosomes or cell membranes. Exosomes, Microvesicles, and Apoptotic bodies can be classified based on vesicle diameter, with exosomes (EXOs) measuring 30–150 nm and Microvesicles (MVs) measuring 100 nm-1 μm. Apoptotic bodys (ABs) diameters range from 1 to 5 μm (Merchant et al., 2017). Small EVs have a diameter of less than 100 nm or 200 nm, while large EVs have a diameter greater than 200 nm (Théry et al., 2018). EXOs are the most well-defined EVs, with distinct markers such as CD81, CD89, and other molecules. EXO has a one-of-a-kind biological formation process. To begin, the cell’s plasma membrane shoots inward or outward to form polyvesicles. Vesicles are released after polyvesicles fuse with the plasma membrane to form EVs containing proteins, RNA, DNA, sugars, non-coding RNA, and lipids (Mishra et al., 2021). Through surface-related receptors and specific intercellular adhesion molecules, EVs can specifically recognize target cells. Then, signal transduction occurs via endocytosis, fusion, ligand receptor contact, or direct release of vesicle contents, and various target cell functions are regulated (Abels and Breakefield, 2016). Because EV’s structure is stable and resistant to proteases and nucleases in the extracellular environment, it can transmit information from the mother cell to multiple receptor cells throughout the body (Urbanelli et al., 2013). EVs can be produced directly by cells and released into the extracellular environment, or it can enter the extracellular environment via transcellular and biological barriers (Fuhrmann, 2020). For example, a large number of endogenous EVs are released from the yolk sac into the peripheral circulation during zebrafish embryogenesis (Verweij et al., 2019). Embryonic EVs can pass through the zona pellucida and enter the culture medium (Vyas et al., 2019). EVs transport also has certain tropism, which may be determined by the receptors it carries and the signals released by the receptor cells (Garofalo et al., 2019; Limongi et al., 2021).
When sperm and egg combine in the oviduct, capacitated sperm pass through the zona pellucida and into the secondary oocyte. Fertilization is completed when the egg pronucleus fuses with the sperm pronucleus. Following that, the fertilized egg develops until it reaches the blastocyst stage, at which point it will be implanted 6–8 days later. Endometrial epithelial cells form large smooth pinopodes in the mid-luteal phase, endometrial stromal cells proliferate and differentiate into decidual cells, and the endometrium enters the “window of implantation” for embryo implantation under the action of estrogen and progesterone. After hatching, the blastocyst enters the endometrium of the “window of implantation” and goes through three stages of positioning, adhesion, and invasion in preparation for implantation (Daikoku et al., 2011). EV’s involvement in vascular biology, immunology, neurology, and reproductive medicine has recently been widely reported. Ovum division, sperm capacitation, embryo development, and implantation all require interaction between the embryo and the mother via EVs (Saadeldin et al., 2014; Yáñez-Mó et al., 2015).
2 The role of EVs in gametes fertilization
2.1 The influence of EVs on oocyte development
Because follicular fluid is the initial environment for oocyte development and oviduct fluid is the final environment for oocyte maturation and fertilization, both follicular fluid and oviduct fluid are important for oocyte development (Da Broi et al., 2018; Saint-Dizier et al., 2019). Oocytes, granulosa cells, and cumulus cells can produce follicular fluid EVs (FF-EVs), which acted as a messenger to transmit information from each cell in the follicle and promote oocyte development (Andrade et al., 2017). When diseases occur in the organism, FF-EVs have a negative impact on oocytes. For example, miRNA-424-5p found in PCOS patients’ FF-EVs can inhibit cumulus cells, inhibit Rb/E2F1 signaling, and is not conducive to oocyte maturation (Yuan D et al., 2021). Of course, the contents of EVs change with menstrual hormone levels on a regular basis. Because the physiological state of follicles, fallopian tubes, and endometrium varies with hormone levels, so do the number of EVs and their contents (de Ávila et al., 2020; da Silveira et al., 2018; Almiñana et al., 2018; Vilella et al., 2015; Yi et al., 2022). The miRNAs contained in the equine FF-EVs differed depending on Deviation, mid-estrous period, and ovulation period, and the majority of these different mirnas were involved in growth and apoptosis pathways (da Silveira et al., 2015). The function of these various mirnas in oocyte development is still unknown. Given what is known, we can only speculate about their role. For example, miRNA-181a expression in FF-EVs is significantly reduced during ovulation in young horses. MiRNA-181 can activate activin receptor IIA, causing SMAD phosphorylation and inhibiting cell proliferation (Zhang et al., 2013). To some extent, the contents of FF-EVs can also reflect reproductive ability. TGF-β was the main targeted pathway of miRNA with differential expression when miRNAs in FF-EVs of young mares and old mares were measured, which was widely involved in cell proliferation, apoptosis, and differentiation (da Silveira et al., 2015; da Silveira et al., 2012). In FF-EVs of aged mares at mid-estrus, the expression of miRNA-23a and miRNA-132 was higher than in young horses, while the expression of miRNA-222 was lower. MiRNA-23a levels rise in patients with premature ovarian failure as FSH levels rise. Furthermore, before follicle maturation, miRNA-132 expression was higher in luteinized follicles than in dominant follicles (da Silveira et al., 2012). miRNA-222 has the ability to regulate the estrogen receptor and Cyclin-dependent kinase 1, as well as promote estrogen secretion (Sang et al., 2013). The connection between miRNA and ovarian function in these FF-EVs are still unknown. Existing studies, however, have revealed a correlation between these miRNAs and ovarian function, albeit without the involvement of EVs.
FF-EVs can regulate oocyte development in the early stages, possibly by regulating the level of cyclic adenosine monophosphate and blocking oocyte meiosis in the foaming stage (Pioltine et al., 2020). When bovine FF-EVs are used to culture bovine cumulus-oocyte complex, it can promote cumulus complex expansion, and larger FF-EVs can also inhibit granulosus cell apoptosis and promote estradiol secretion (Hung et al., 2015; Ying et al., 2023). This could be because miRNA-17b and miRNA-92a in FF-EVs can promote granulosa cell proliferation and cumulus expansion, which is linked to the activation of Src, PI3K/Akt, and MAPK signaling pathways (Hung et al., 2017; Inoue et al., 2020). Oocytes’ in vitro maturation rate can be increased when cultured with equine FF-EVs (Gabryś et al., 2022). Furthermore, FF-EVs can mitigate the negative effects of heat shock on oocytes (Rodrigues et al., 2019). In contrast, two studies have shown that the cumulus complex does not expand efficiently when cultured with FF-EVs. As a result, the effect of FF-EVs on the cumulus complex requires further investigation (Matsuno et al., 2017; Rodrigues et al., 2019). The rate of blastocyst formation, implantation, and abortion is determined by the quality of the oocytes. FF-EVs has been shown in studies to increase blastocyst rate while improving oocyte quality (da Silveira et al., 2017; Asaadi et al., 2021). However, the number of studies is small, and more research is needed.
When an egg develops in the oviduct, it is also exposed to oviduct EVs(O-EVs). O-EVs can promote oocyte maturation in vitro by upregulating the EGFR/MAPK pathway, reducing reactive oxygen species (ROS) accumulation, inhibiting cell apoptosis, promoting cumulus cell proliferation, and increasing the rate of oocyte maturation (Lee et al., 2020a; b). However, when the concentration of EVs are too high, the incidence of the second meiotic metaphase of egg cells is reduced (Lange-Consiglio et al., 2017). FF-EVs, of course, acts on the oviduct and causes changes in the transcriptome of oviduct epithelial cells. Treatment of oviduct epithelial cells with bovine FF-EVs, for example, increases signaling pathways such as oxidative phosphorylation, thermogenesis, and amino acid biosynthesis, all of which are linked to sperm swimming, fertilization, and embryo growth and development (Hasan et al., 2020). Wang et al. discovered that bovine FF-EVs could promote ovarian cortical stromal cell proliferation, inhibit apoptosis, and increase androstenedione and progesterone synthesis (Ying et al., 2021). MiRNA-31-5p in FF-EVs regulated the follicular growth suppressor gene SFRP4 and promoted ovarian granulosus cell proliferation and progesterone synthesis via the WNT/B-CATENIN pathway in another study of FF-EVs in pigs (Yuan C et al., 2021). It is also suggested that EVs acting as messengers may regulate the effects on hormones during oocyte development.
2.2 The influence of EVs on sperm fertilization
2.2.1 SP-EVs can both promote and protect sperm fertilization
Seminal plasma EVs (SP-EVs) may improve sperm fertilization ability. Seminal plasma contains EVs produced by the prostate, epididymis, and testis, and healthy EVs have been shown to promote sperm-egg binding. Cat SP-EVs have been shown to improve the fertilization ability and developmental potential of immature spermatozoa, as well as spermatozoa motility after freezing and thawing (Rowlison et al., 2021). SP-EVs have been shown to improve sperm motility after sperm culture, which may be due to the fact that SP-EVs contain many proteins that promote sperm survival and capacitance (Mahdavinezhad et al., 2022). Long-chain fatty acid transport protein 2 (LCFATP2), WAP four-disulfide core Domain protein 8 (WFDC8), and beta-Galactosidase-1-like Protein 4 (GLB1L4) are examples of proteins that can be transferred to sperm. WFDC8 protein, for example, is involved in male reproductive immunity and has anti-microbial properties. The GLB1L4 protein is associated with sperm capacitation, and when the GLB1L4 gene is knocked out, the sperm hyperactivation index and sperm protein phosphorylation level are significantly reduced (Dong et al., 2021; Barrachina et al., 2022). The acrosome reaction is essential for egg fertilization. Many enzymes involved in sperm acrosome reaction are found in SP-EVs, including arachidonic acid 15-lipoxygenase and extracellular diadenosine polyphosphate hydrolase. After fusion with spermatozoa, SP-EVs can stimulate acrosome reaction, making spermatozoa more sensitive to the effect of progesterone (Ronquist, 2012; Dong et al., 2021).
To some extent, SP-EVs can protect spermatozoa. Sperm and seminal plasma are foreign to the female body and be attacked by the reproductive system’s immune system. In the female reproductive system, anti-sperm antibodies recognize not only sperm membrane proteins but also SP-EVs (Allegrucci et al., 2001). Related research found that SP-EVs had an immune-protective effect on sperm. Natural killer cells play an important role in female reproduction. When SP-EVs are co-cultured with natural killer cells, the expression of the natural killer cell receptor 2B4 is reduced, as is the activity of the natural killer cells (Tarazona et al., 2011). Furthermore, high levels of ROS are the primary cause of idiopathic male infertility, and abnormally high levels of ROS are more likely to be found in infertile patients’ sperm samples. Sperm is vulnerable to oxidative stress and can be harmed by high levels of ROS. Currently, it is known that polymorphonuclear neutrophils are the primary producers of sperm ROS, and SP-EVs can reduce sperm ROS production while increasing sperm antioxidant capacity, which may be due to SP-EVs hardening the membrane of polymorphonuclear neutrophils. Glutathione S-transferase 2 regulation reduces the production of intrinsic ROS (Saez et al., 1998).
2.2.2 EVs found in the normal female reproductive system can aid in sperm fertilization
The semen transports the sperm into the woman’s vagina after it has been produced. The sperm then travels through the uterus, enters the fallopian tube, and combines with the egg cell to complete fertilization (Saint-Dizier et al., 2020). The environment in the uterus and fallopian tube is critical for sperm survival, transport, and capacitation. This decisive effect is most likely carried out via the oviduct and the EVs present in the uterus. Cat O-EVs can bind to spermatozoa at the acrosome and middle part, increasing sperm motility and maintaining acrosome integrity, as well as improving sperm fertilization ability. This could be due to the many proteins found in O-EVs that promote sperm motility and fertilization, such as oviduct-specific glycoprotein, T-complex protein 1 subunit gamma, and T-complex protein 1 subunit alpha (Ferraz et al., 2019). Plasma membrane calcium atpase 4(PMCA4) is a membrane protein that serves as the primary calcium ion discharge pump in sperm. When PMCA4 is missing, it causes severe hyperactivity and loss of sperm movement, which leads to infertility. Mice O-EVs can be absorbed by spermatozoa, transferring PMCA4 to them, promoting calcium outflow, and inducing sperm capacitation (Al-Dossary et al., 2013). Notably, almost all PMCA4a was found in uterine and oviduct fluid EVs.The content of PMCA4a increases by about threefold when cultured with EVs from uterine and oviduct fluid (Al-Dossary et al., 2013). The mechanism by which EVs transfer PMCA4 and other transmembrane proteins to the sperm plasma membrane may be related to the pro-integrinαVβ3 and Integrinα5β1 effects (Al-Dossary et al., 2015). When PMCA4 is absent in sperm, mice O-EVs can compensate by up-regulating Plasma membrane calcium atpase 1 (PMCA1), resulting in calcium ion efflux (Okunade et al., 2004). MiRNA-34c-5p can be transferred to the sperm head by O-EVs. Because the first cleavage of the zygote requires miRNA-34c-5p acting on the centromere, and miRNA-34c-5p can only be obtained from sperm, O-EVs can indirectly affect zygote development via sperm (Fereshteh et al., 2018).
Furthermore, intrauterine EVs(I-EVs)can deliver Hyaluronidase PH-20 (SPAM1) to the sperm membrane, and the uptake of SPAM1 by sperm in vitro is a sign of sperm maturation. SPAM1 protein can improve spermatozoa binding to hyaluronic acid, increase oocyte cumulus penetration, and induce acrosome reaction related to calcium signal to promote fertilization (Griffiths et al., 2008a). In comparison to the secreting stage, EVs secreted by endometrial cells in the proliferating stage can improve sperm capacitation and fertilization ability, which may be related to EV-induced protein tyrosine phosphorylation of sperm (Murdica et al., 2020). After entering the female reproductive system, sperm will first come into contact with the vaginal environment, and vaginal EVs (V-EVs) also has an effect on sperm. V-EVs contain proteins that inhibit premature sperm capacitation and acrosome reaction, which can increase the likelihood of progesterone-induced sperm acrosome reaction (Fereshteh et al., 2019). V-EVs also contains PMCA4a, which sperm can use (Al-Dossary et al., 2013). The exciting part is that eggs may be able to communicate with sperm via EVs before fertilization. According to Miyado et al.(2008) mature eggs can still deliver EVs to sperm. When spermatozoa penetrate the cumulus, they fuse, and this step is aided by the ovum’s secretion of EVs. Furthermore, after follicular fluid enters the fallopian tube following ovulation, FF-EVs may promote sperm motility and fertilization (Sysoeva et al., 2021). Surprisingly, oocytes and cumulus cells can take up SP-EVs, and rabbit SP-EVs can promote cumulus expansion and affect mRNA expression of cumulus cells and oocytes, including PTGS2 and NTRK, which are involved in oocyte development and maturation (Abumaghaid et al., 2022). SP-EVs can also be taken up by porcine cumulus cells, but not by oocytes. In cumulus cells, these EVs can regulate the expression of HAS2 and steroidogenesis associated with oocyte maturation, as well as CYP11A1 and HSD3B1 (Mateo-Otero et al., 2022). This raises the question of whether SP-EV plays a role in oocyte maturation after male and female life.
3 The role of EVs in embryonic development
3.1 Normal O-EVs may promote embryonic development
The sperm and egg combine to form a zygote, which develops into an early embryo over time. The oviduct is the early embryo’s growth and development environment, and oviduct fluid is an important medium of communication between the oviduct and the embryo (Hasan et al., 2020). O-EVs are an important messenger in this communication process because EVs can cross the zona pellucida. For example (Laezer et al., 2020), the volume of O-EVs change with hormonal levels during the menstrual cycle, and the volume of porcine O-EVs are smaller in the late ovulatory period than in the late oestrus and late oestrus. The protein content of O-EVs change over time. When compared to estrus, syntaxin-2 and syntaxin-binding protein 1 are upregulated during ovulation. These two proteins are involved in sperm acrosome reaction and sperm-oocyte membrane fusion. Embryo implantation alters the types and contents of RNA, protein, and miRNA in O-EVs. When the differences in miRNA expression in oviduct EVs between pregnant and non-pregnant cows were compared, it was discovered that eight miRNAs were down-regulated in pregnant cows, including miRNA-126-5p, miRNA-129, and miRNA-345-3p, while miRNA-331-5p was up-regulated in non-pregnant cows. PI3K/AKT, mTOR, and MAPK are among the signaling pathways regulated by these differentially expressed miRNAs (Mazzarella et al., 2021). The PI3K/AKT signal transduction pathway, for example, can regulate the growth and survival of embryonic cells prior to implantation, and the PI3K-Akt subunit in the pathway is expressed from the zygote to the blastocyst stage and is involved in blastomere proliferation (Fiorenza et al., 2020).
EVs produced by healthy fallopian tubes are beneficial to embryo development. In pigs, for example, O-EVs can promote embryonic development by increasing blastocyst formation rate and attachment ability (Fang et al., 2022a). The contents of O-EVs may be the cause of the positive effect, not only promoting early embryo development but also alleviating the embryo’s damage from adverse factors. ROS can harm cells, impair embryo development, and reduce embryo transfer success rates (Tsukazawa et al., 2021). 5-methylcytosine (5-MC) is a well-studied DNA modification base in prokaryotes and eukaryotes that is involved in nuclear reprogramming, embryonic development, and gene expression regulation (Kumar et al., 2018). When O-EVs are used to culture embryos, the blastocyst rate and hatching rate of embryos are significantly increased, the levels of ROS and 5-MC are decreased, the inner cell mass (ICM)/trophoblast cell (TE) index is increased, and the apoptosis rate is decreased, which may be related to the melatonin contained in O-EVs (Qu et al., 2020). During Apoptosis in mouse embryos cultured with O-EVs, Apoptosis Regulator Bcl-2 (Bcl-2) expression was increased while Apoptosis Regulator BAX (BAX) expression was decreased, which also promoted embryo differentiation and increased the birth rate after embryo transfer (Qu et al., 2019). Porcine O-EVs can decrease caspase-3 gene expression, decrease blastocyst cell apoptosis, and increase blastocyst formation rate. This could be caused by lowering the expression of the sXBP1, ATF4, and GRP78 genes in order to reduce embryonic ER stress (Fu et al., 2022). Furthermore, O-EVs have an energy regulation effect on embryo development. The addition of O-EVs to embryo culture medium has been shown to inhibit Pyruvate dehydrogenase kinase-mediated expression of isoform 4 and Sirtuin 4. Up-regulation of Pyruvate dehydrogenase and Glutamate dehydrogenase expression can increase Pyruvate flux in the tricarboxylic acid cycle, dynamically regulate embryo mitochondrial metabolism, and improve blastocyst quality and hatchability (Sidrat et al., 2020).
3.2 Normal I-EVs can promote embryonic development
The intrauterine fluid contains various enzymes, hormones, glucose, and amino acids required for embryonic development, and it serves as a conduit for communication between the mother and the embryo, determining the embryo’s development and survival (Silva et al., 2021). I-EVs are crucial in embryo implantation. When Lv et al. (2018). Cultured embryos with pregnant mouse uterus flushing solution, they discovered that the pregnant group’s EVs content were higher than the non-pregnant group’s. Furthermore, the I-EVs of pregnancy group had higher levels of miRNA-21 expression, which can promote embryonic development. The final culture results showed that I-EVs during pregnancy could increase the ICM/TE index, decrease BAX expression in embryos, and increase Bcl-2 and Octamer-binding protein 4 expression (OCT4). OCT4 controls gene expression in the early stages of embryo formation and plays a critical role in ensuring the number of pluripotent stem cells as a key protein to maintain the pluripotency of embryonic stem cells (Stirparo et al., 2021). However, the effects of EVs produced by the uterus in different states on embryo development vary. When EVs of endometritis cows were compared to that of healthy cows, 52 miRNAs were down-regulated and 66 miRNAs were up-regulated in the EVs of endometritis group, and I-EVs of endometritis could reduce embryo hatching rate (Wang et al., 2019). Similarly, when I-EVs from recurrent implantation failure (RIF) patients are used to incubate embryos, the total number of embryonic cells, hatching rate, and invasiveness are reduced when compared to I-EVs from healthy women (Liu et al., 2020a). Thus, the contents of EVs may play an important role in embryonic development.
EVs secreted by embryos may have an effect on embryo development in addition to the presence of EVs that can affect embryo development. Relevant research has shown that the presence of miRNA-378a-3p in EVs secreted from the blastocyst stage can increase the number of embryonic trophoblast cells and inner cells, as well as improve the hatching rate (Pavani et al., 2022). Surprisingly, SP-EVs may influence early embryo development. This does not necessarily imply that SP-EVs have the same effect in humans. The addition of SP-EVs to a medium containing mouse oocytes and sperm, on the other hand, can significantly increase the rate of blastocyst formation, reduce cell apoptosis, and increase ICM/TE in embryos (Ma et al., 2022). These findings also suggest that various seminal plasma components may have beneficial effects on embryo development and later embryo development when cultured in vitro.
4 The role of EVs in embryo implantation
4.1 The EVs are responsible for communicating between the embryo and the uterus
4.1.1 Luteal phase I-EVs can aid in embryo implantation
Because embryo implantation failure accounts for 75% of pregnancy failure in humans, embryo implantation is a critical step in achieving a successful pregnancy (Pavani et al., 2022). The requirements for embryo adhesion in this process are relatively stringent, requiring both sufficient implantation ability of the embryo and endometrium receptivity. Endometrium receptivity, in particular, requires an appropriate level of inflammation, which first ensures that the embryo is protected from the maternal immune system before implantation, and then requires a maternal endometrial inflammatory response after implantation to promote embryo invasion (Franasiak et al., 2021; Sehring et al., 2022). However, the embryo’s implantation ability and the endometrium’s receptivity must be obtained concurrently, which is more difficult. I-EVs can transmit embryo and endometrium information and is a messenger of signal communication between mother and embryo, which is critical in the embryo implantation process.
During pregnancy, I-EVs primarily consist of EVs produced by the endometrium and EVs produced by the embryo, but it is primarily produced by uterine gland epithelial cells (O'Neil et al., 2020; Hu et al., 2022). The I-EVs also varied with the mother’s estrous cycles, and the embryo can respond differently to endometrial EVs (EM-EVs) at different times to prepare for implantation. EVs secreted by the 13th estrous cycle in the sheep uterus can increase IFNT production of trophoectoderm cells and promote trophoectoderm cell proliferation (Ruiz-González et al., 2015). I-EVs at estrous cycle or day 9 of pregnancy can promote embryonic trophoblast cell proliferation and migration, whereas I-EVs at day 12 or 15 of pregnancy can inhibit embryonic trophoblast cell migration and proliferation (Hu et al., 2022). To simulate the endometrium in the luteal phase, treating human endometrium epithelial cells with estrogen and progesterone to produce EVs can increase trophoblast adhesion and invasion ability, which may be related to EM-EVs upregulating cell-cell adhesion and the cadherin-binding function in trophoblast cell spheroids (Evans et al., 2019). In this process, the “cargo” carried by the EVs can regulate the embryo. For example, miRNA-100-5p in EM-EVs activates FAK and C-jun N-terminal kinase (JNK), promoting trophoblast migration and invasion as well as angiogenesis (Tan et al., 2020a).
The different effects of I-EVs on embryos at different stages may be due to different hormone levels acting on the “cargo” carried by EM-EVs at different stages. Progesterone can not only increase the number of EVs produced by the endometrium, but it can also regulate the miRNA content of EVs. Progesterone-induced miRNA may promote embryo adhesion by regulating PI3K/AKT, BMP, and small RNA post-transcriptional silencing (Burns et al., 2018). Endometrial epithelial cells cultured with estrogen and progesterone produced EVs with higher levels of Laminin subunit alpha-5 (LAMA5) than endometrial epithelial cells cultured with estrogen alone. During embryonic development, LAMA5 mediates cell attachment, migration, and tissue entry. Endometrial epithelial cells cultured with estrogen and progesterone can enhance embryonic trophoblast cell adhesion and up-regulate the expression of FAK and FAK-TYR397. This could be mediated by EVs’ “command” via activation of the active adhesive FAK signal (Greening et al., 2016). Similarly, progesterone may affect the contents of EVs produced by embryos (EO-EVs). The EVs produced by trophoblast cells cultured with progesterone is more effective than that produced by trophoblast cells cultured without progesterone in improving IntegrinαV, Integrinβ3, and Protein WnT-7α and decreasing MUCin-1 of uterine epithelial cells (Su et al., 2022).
4.1.2 EVs produced by an abnormal uterus are not conducive to embryo implantation
I-EVs will not be conducive to embryo implantation if the uterus is abnormal. When I-EVs from RIF women were used for mouse embryo culture, but also weakened their invasion ability (Liu et al., 2020a). This could be because EM-EVs in RIF patients reduces trophoblast cell proliferation, migration, and invasion, resulting in implantation failure (Liu et al., 2021). Furthermore, abnormal cytokine and leukocyte expression in endometritis may impair the endometrium’s immune tolerance to the embryo, which is not conducive to the invasion of the embryo’s survival trophoblast, leading to infertility, Recurrent spontaneous abortion (RSA) and RIF (Buzzaccarini et al., 2020; Franasiak et al., 2021). The use of lipopolysaccharide to interfere with endometrial epithelium as a model for endometrial inflammation. EVs produced by it can inhibit the migration of bovine embryonic trophoblast cells and suppress the expression of CDX2, ER, and NANOG genes involved in bovine embryonic development (Wang et al., 2021a). Uterine fluid from a successful pregnancy benefits the embryo. ITGA5, CXCL10, and CXCL11 expression can be significantly up-regulated when cultured with I-EVs from successfully pregnant pigs, whereas ID2 expression can be significantly down-regulated. ITGA5 is a key gene in embryo implantation, and its high expression indicates endometrial receptivity (Ahmad et al., 2018). CXCL10 plays a role in the recruitment of immune cells to the endometrium during embryo implantation as well as the establishment of an immune tolerance environment in the embryo (Złotkowska and Andronowska, 2019). CXCL11 can cause trophoblast cells to migrate, endometrial stromal cells to proliferate, and endometrial epithelial cells to die (Hirota et al., 2006). ID2 is a transcription factor inhibitor that regulates cell differentiation, proliferation, and apoptosis (Xie et al., 2010).
4.2 EMT is closely related to the information carried by EVs
The epithelial-mesenchymal transition (EMT) is critical for embryo implantation. Through EMT, the trophoblast gains adhesion and invasion ability, whereas the epithelial-mesenchymal transition of endometrial epithelial cells causes the cell to lose polarity, recombines adhesion molecules on the cell membrane, and promotes endometrium-embryo adhesion (Uchida et al., 2007). As a result, an abnormal EMT between endometrial epithelial cells and trophoblast cells can cause embryo implantation failure (Gou et al., 2019). Communication between the embryo and the endometrium is required prior to implantation for synchronization. EVs are particularly important in the process of EMT. EVs produced by placental trophblast cells can also carry miRNA-1290, which can regulate the LHX6 gene in endometrial epithelial cells and suppress E-cadherin expression, promoting endometrial EMT (Shi et al., 2021). During embryo implantation, aldehyde reductase (AKR1B1) and macrophage-capping protein (CAPG) were up-regulated in the embryos, which also existed in EO-EVs. Both AKR1B1 and CAPG have the ability to promote the EMT process (Nakamura et al., 2016). EMT is the process by which an embryo invades the endometrium and is detected by maternal autoimmunity. Surprisingly, the number of M1 macrophages was increased in the decidua of RSA patients. However, EVs released by M1 macrophages can inhibit trophoblast EMT by promoting E-cadherin expression while inhibiting N-cadherin and vimentin expression (Ding et al., 2021). This process could be linked to TRAF6 downregulation by EVs containing miRNA-146a-5p and miRNA-146b-5p. In normal pregnancy, EVs released by decidual stromal cells increase the invasiveness of trophoblast cells after being ingested by trophoblast cells. This could be because EVs in decidual stromal cells promote EMT by upregulating the expression of phosphorylated Smad2, phosphorylated Smad3, and N-cadherin (Liu et al., 2020b).
4.3 EVs regulate the inflammatory and immune environments of embryo implantation
The inflammatory response of the endometrium prior to implantation is required for implantation. EVs produced by embryonic trophoblast cells recruit monocytes in a dose-dependent manner, increasing monocyte secretion of IL-1β, IL-6, and TNF-α to create an inflammatory environment in the uterus prior to pregnancy (Atay et al., 2011). Furthermore, EVs produced by placental trophoblast cells can significantly increase TNF-α, IL-6, and IL-8 expression in endometrial epithelial cells, resulting in an inflammatory uterine environment and increased angiogenesis to promote embryo implantation (Shi et al., 2021). Furthermore, mild endometrial inflammation may provide embryo protection. Mesenchymal stem cells are well known for their ability to regulate the immune microenvironment and influence the function of immune effector cells (Song et al., 2020). However, inflammation and implantation signals induce mesenchymal stem cells in peripheral blood under normal conditions (Calle et al., 2021a). Without recruiting embryonic stem cells, EVs secreted by embryonic trophoblast cells can recruit mesenchymal stem cells in the endometrium and peripheral blood. As a result, stem cells recruited by inflammatory factors produced by trophoblast EVs and trophoblast EVs can inhibit T lymphocyte proliferation, reducing maternal immune rejection of the embryo (Calle et al., 2021b). Despite the fact that embryo implantation necessitates an inflammatory response in the maternal endometrium, more does not appear to be better. For example, the previously mentioned endometrial inflammation is not conducive to embryo implantation. Other research has discovered that M1-M, which has an inflammatory promoting function, is significantly increased in the decidua tissue of RSA patients (Zhu et al., 2020). Furthermore, miRNA-196a-5p was found to be highly expressed in EVs produced by trophoblast cells in RSA patients’ villus tissue. EVs can transfer miRNA-196a-5p into macrophages, and the NF-κB pathway induces macrophage M1 polarization and secretes TNF-α to inhibit trophoblast development (Zhang et al., 2022). As a result, appropriate endometrial inflammation is critical to implantation success.
Seminal plasma not only protects sperm from maternal immune damage, but it also makes embryo implantation easier. This could be accomplished by inducing inflammation in order to improve endometrium receptivity and promote embryo implantation in the uterus. SP-EVs, for example, can promote decidualization of endometrial stromal cells in vitro, which may be due to the activation of the IL-11 signaling pathway by SP-EVs proteins (George et al., 2020). Although the mechanism of inflammation is unknown, endometrial epithelial cells treated with SP-EVs have significantly activated inflammatory and immune signaling pathways (Bai et al., 2018). It is also worth noting that the occurrence of inflammation may be related to endometrial stromal cell secretion of inflammatory factors or the transformation of macrophages to M1-M caused by EVs in seminal plasma (Paktinat et al., 2019; Paktinat et al., 2021). Seminal plasma, on the other hand, causes immune rejection or tolerance in the maternal endometrium, which is closely related to the balance of immune-related cells such as dendritic cells, macrophages, and neutrophils (Schjenken et al., 2015). Mouse SP-EVs can inhibit dendritic cell maturation in endometrial tissues, which may increase embryo implantation rates by establishing immune tolerance (Wang et al., 2021b). It should be noted that the extent to which SP-EVs inhibited maternal dendritic cell maturation varies with age. SP-EVs from old mice have weaker inhibition of dendritic cells in the endometrium, higher levels of IL-6, TNF-α, and IL-1β in the uterine cavity, lower levels of IL-10 and TGF-β, and a lower implantation rate of embryos (Wang et al., 2021b). This suggests that the pro-embryo transfer effect of SP-EVs may vary with age. Figure 1; Table 1 depicts a detailed description of the possible messenger roles of EVs at various stages of embryo formation, development, and implantation.
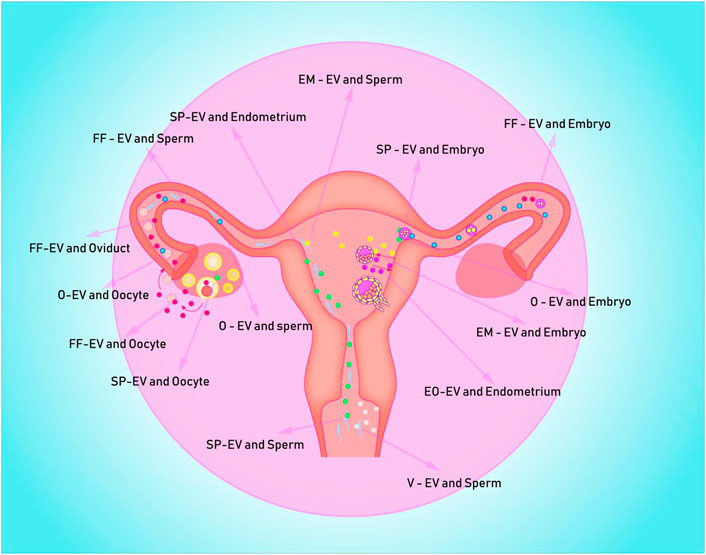
FIGURE 1. Depicts potential EVs messenger pathways in the human uterus for embryo formation, development, and implantation. EV is represented by the colored spheres. (A) The blue ball is the O-EVs, which transports information from the oviduct to the oocyte, sperm, and early embryo; (B) The red sphere represents FF-EVs, which can communicate information from the follicle to the fallopian tube, sperm, and early embryo; (C) The white ball represents the V-EVs, which can transmit vaginal information to the sperm; (D) The green sphere represents the SP-EVs, which is capable of transmitting seminal plasma information to sperm, endometrium, and follicle; (E) The purple spheres are mostly EO-EVs, which can send data from the embryo to the maternal endometrium; (F) The yellow spheres represent EM-EVs, which transmit information from the endometrium to sperm and embryos.
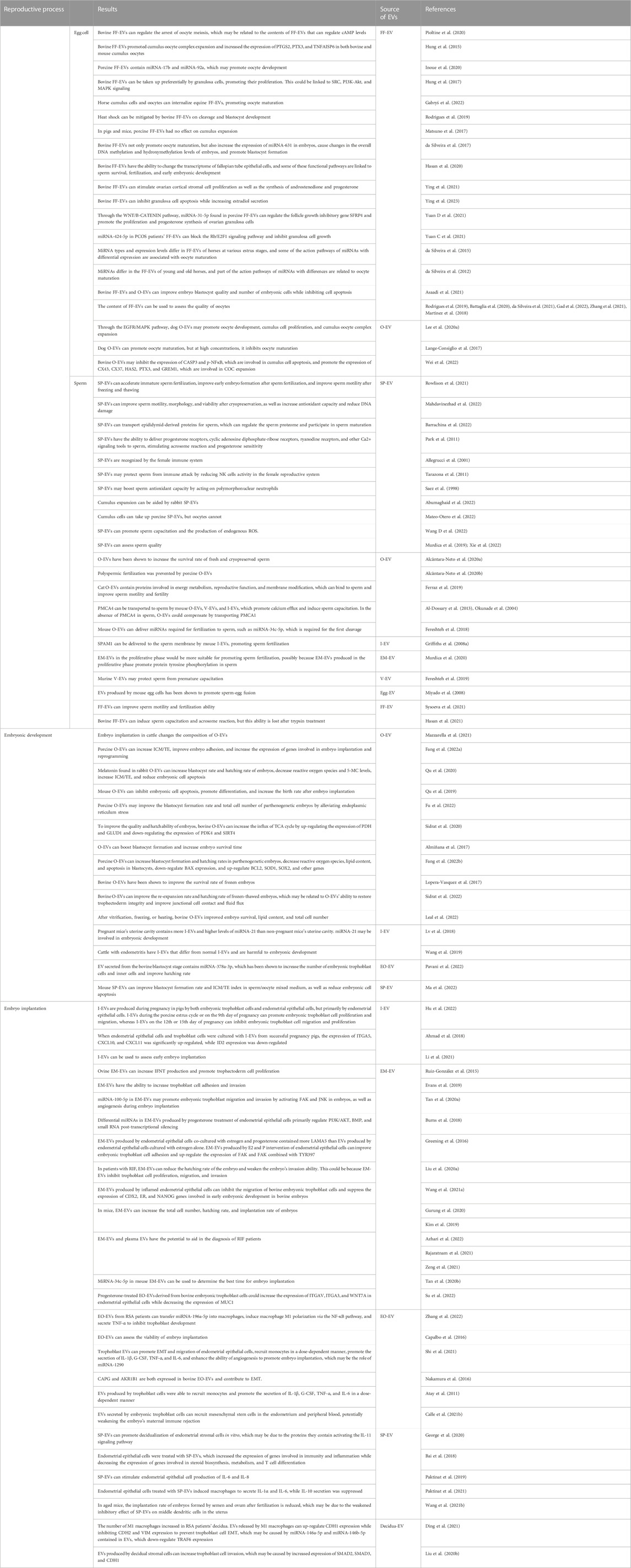
TABLE 1. EV’s potential role as a messenger in the human uterus during gametophyte fertilization, early embryonic development, and implantation.
5 Extracellular vesicles’ clinical utility in assisted reproduction
Assisted reproductive technology is a medical aid that is widely used in the clinical treatment of infertility. It includes artificial insemination and in vitro fertilization - embryo transfer (IVF-ET). Although assisted reproductive technology can help many infertility patients, its success rate is still low and it is costly. As a result, it is critical to raise the level and quality of assisted reproductive technology. The EVs described above are critical messengers for embryo formation, development, and implantation. However, most current studies are limited to animals, and human applications have not been thoroughly researched.
5.1 Potential application of EVs in the prediction and culture of egg cells
The ultimate success rate of assisted reproductive technology will be determined by the quality of oocytes. Cumulus cells, oocytes, and granulosa cells secrete follicular fluid. Cell communication in follicular fluid is critical for oocyte development, fertilization, and development (Yang et al., 2020; Zakerkish et al., 2020). As a messenger carrying vital components, EVs can facilitate cell communication in follicular fluid. As a result, FF-EVs can react and affect oocyte quality. FF-EVs primarily reflect oocyte quality through its content (Rodrigues et al., 2019; Battaglia et al., 2020). For example, because the amount and type of miRNA and lipid carried by FF-EVs differ between high and low quality follicles (da Silveira et al., 2021; Gad et al., 2022; Zhang et al., 2021), miRNA expression in FF-EVs differ between oocytes with and without successful fertilization (Martinez et al., 2018). Thus, we were able to assess oocyte developmental potential and fertilization ability using FF-EVs. Second, we can use extracellular vesicles’ ability to convey information to intervene in oocyte culture using EVs at the site of oocyte development. FF-EVs have been shown in previous studies to promote the expansion of cumulus cells (Hung et al., 2015), stimulate the proliferation of granulosa cells (Hung et al., 2017), and improve the developmental ability of oocytes (Asaadi et al., 2021). However, O-EVs can accelerate oocyte maturation and promote cumulus cell proliferation (Lange-Consiglio et al., 2017; Wei et al., 2022). Interestingly, O-EVs were also able to change gene expression in oviduct epithelial cells, which may represent FF-EV’s ability to improve sperm survival and fertilization (Hasan et al., 2020).
5.2 Potential application of EVs in culture and prediction of spermatozoa
Male sperm, like oocytes, is essential in artificial insemination and in vitro fertilization-embryo transfer. As a result, prior to using assisted reproductive technology, sperm must be optimized. EVs can have a significant impact on sperm survival and fertilization as a messenger in seminal plasma, oocyte transfusion fluid, and uterine fluid (Griffiths et al., 2008b; Ronquist, 2012; Al-Dossary et al., 2015). SP-EVs can protect sperm from maternal immune damage, increase sperm antioxidant capacity (Skibinski et al., 1992; Saez et al., 1998; Tarazona et al., 2011), improve sperm motility and fertilization capacity (Yang et al., 2017; Wang H et al., 2022), and improve sperm motility after cryopreservation (Rowlison et al., 2021). Furthermore, SP-EVs have some diagnostic value. SP-EV’s contents can reflect male infertility sperm status; for example, two tsRNA TRF-Val-AAC-010 and TRF-pro-AGG-003 in seminal plasma can be used to diagnose non-obstructive azoospermia (Han et al., 2022). SP-EVs, as messengers communicating with sperm in seminal plasma, can indicate the status of the sperm. Therefore, we can determine the quality of sperm using SP-EVs, making later use of sperm easier (Murdica et al., 2019). SP-EVs can help us understand the pathogenesis as well as evaluate the quality of the sperm. SP-EVs contain more miRNA-21-5p in incompetent pig sperm, which can inhibit sperm capacitation and provide new ideas for clinical treatment (Xie et al., 2022). O-EVs have been shown to improve sperm motility, promote sperm fertilization, extend sperm survival time (Ferraz et al., 2019; Alcântara-Neto et al., 2020a; Murdica et al., 2020), and reduce the occurrence of multiple fertilization (Alcântara-Neto et al., 2020b). I-EVs promoted sperm capacitation and acrosome reaction, whereas V-EVs inhibited sperm capacitation and acrosome reaction (Fereshteh et al., 2019). We wondered if the superposition effect of EVs in multiple reproductive anatomical parts on sperm would play a positive role in artificial insemination because of the effect of EVs in different parts of the female reproductive system. More intriguingly, FF-EVs promoted cumulus complex expansion and may induce oocyte maturation, whereas SP-EVs promoted sperm motility and spermatogenesis (Hasan et al., 2021; Abumaghaid et al., 2022). When FF-EVs are used to culture spermatozoa from asthenospermia patients, it can increase sperm motility and forward motility rate, improving spermatozoa fertilization ability (Abumaghaid et al., 2022).
5.3 Potential application of EVs in embryo culture and prediction
The oviduct is the site of zygote birth as well as preembryonic development. Current research indicates that O-EVs can improve embryo blastocyst formation rate, embryo survival rate, embryo hatching rate, and post-embryo transfer birth rate (Almiñana et al., 2017; Qu et al., 2019; Fang et al., 2022b; de Alcântara-Neto et al., 2022). Furthermore, O-EVs can improve embryo development after cryopreservation and survival rate after vitrification (Lopera-Vasquez et al., 2017; Leal et al., 2022; Sidrat et al., 2022). More than just O-EVs may influence embryo development in vivo. Other research has shown that SP-EVs and FF-EVs can also promote the development of in vitro embryos (da Silveira et al., 2017; Asaadi et al., 2021; Ma et al., 2022). This could pave the way for improved embryo culture and quality in the future. Another worthwhile application is that EO-EVs can promote embryo development when used to culture embryos (Qu et al., 2017; Pavani et al., 2022). Because both artificial insemination and in vitro fertilization embryo transfer rely on normal embryo development in the uterine cavity, the influence of the intrauterine environment on embryo growth cannot be overlooked. As previously stated, I-EVs from endometritis can inhibit embryonic development and have different contents than normal healthy I-EVs (Wang et al., 2019). As a result, we anticipate that we will be able to use the contents of I-EVs to evaluate the timing of the use of assisted reproductive technology.
5.4 Application and predictive potential of EVs in embryo implantation
Embryo implantation is a critical step in the completion of a pregnancy, which is unquestionably difficult for elderly women and patients with thin endometrium. After embryo transfer, embryo implantation failure will increase the psychological and financial burden on infertility patients. Of course, embryo implantation failure cannot be attributed solely to maternal factors. Poor embryo quality, poor developmental potential, and chromosomal abnormalities can all result in embryo implantation failure. Before implantation, the embryo will communicate with the mother’s uterus via EVs. However, there is no communication between the embryo and the mother prior to IVF-embryo transfer. We are unable to demonstrate that increasing the amount of time the embryo spends communicating with the mother increases the rate of implantation. However, recent research has shown that EVs produced by endometrial epithelial cells during the secretory phase can improve trophoblast cell adhesion (Greening et al., 2016; Evans et al., 2019) and increase embryo hatching and implantation rates (Kim et al., 2019; Gurung et al., 2020). This is because progesterone can increase the amount of EM-EVs and change its composition (Burns et al., 2018). Furthermore, EO-EVs can improve endometrial receptivity and regulate maternal immunity, facilitating implantation (Atay et al., 2011; Su et al., 2022). Although the role of seminal plasma in IVF is frequently overlooked, numerous studies have found that SP-EVs can regulate the maternal uterine immune system and create favorable conditions for embryo implantation (Paktinat et al., 2019; Wang et al., 2021b; Paktinat et al., 2021).
We strive to improve embryo quality and endometrial receptivity during IVF embryo transfer to ensure a successful pregnancy. The number of trophoblast cells, the number of inner cells, and the classification of cyst lumen are currently the main methods for screening high-quality embryos by embryo morphology, but embryo implantation potential is still unknown. Relevant studies have discovered that EO-EVs can alter the expression of specific transcripts in endometrial epithelial cells, such as the down-regulation of ZNF81 expression, but inferior embryos do not (Es-Haghi et al., 2019). Furthermore, miRNA-20a and miRNA-30c levels in EO-EVs were significantly higher than in unimplanted embryos, and both had some predictive value for embryo implantation (Capalbo et al., 2016). MiRNAs found in EVs in plasma and serum have been shown to have diagnostic value for embryo implantation in recent years (Rajaratnam et al., 2021; Zeng et al., 2021; Azhari et al., 2022). I-EVs can also indicate whether the endometrium is ready for embryo implantation. MiRNA-34c-5p, for example, is up-regulated in I-EVs during implantation (Tan et al., 2020b). In addition, miRNA-362-3p expression in I-EVs were significantly higher in non-pregnant patients following controlled ovarian stimulation-embryo transfer than in pregnant patients (Li et al., 2021). It can be concluded that during embryo transfer, we can perform noninvasive screening of embryos based on the signature content of EVs, and it can also be used to help with endometrial status assessment.
5.5 Through EVs, stem cells can regenerate reproductive capacity
EVs can act as messengers for stem cells, allowing them to repair and regenerate. The ability of stem cells to regenerate is primarily used in three areas: the restoration of female ovarian reproductive function, the restoration of male testicular reproductive ability, and the repair of female endometrial damage (Deng et al., 2019; Hong et al., 2022). Stem cell action pathways include homing, self-differentiation, paracrine, and immune regulation (Naji et al., 2017). EVs play a critical role in each process. For example, EVs can recruit stem cells (Liang et al., 2020; Wang D et al., 2022) and transmit stem cell information (Qu et al., 2022). Cells in the lesion site will release EVs in addition to recruitment factors to recruit surrounding tissues or peripheral stem cells (Chen et al., 2022). Stem cells can either release EVs to play a distant role or return to the lesion site to self-differentiate, replace damaged cells, and release EVs or other therapeutic factors (Fu et al., 2021). EVs released by stem cells have been shown to restore ovarian function (Liu et al., 2020c; Wang et al., 2021c), repair endometrial cells (Yao et al., 2019; Xin et al., 2020), improve testicular function (Deng et al., 2019). Despite extensive research in regenerative medicine, the mechanisms of action and clinical applications of stem cells in reproductive medicine remain unknown. Because EVs play an important role in the transmission of information between cells, they can assist us in deciphering the secret of stem cells for the restoration of reproductive ability.
6 Conclusion
EVs are shown in this review to play an important role in the reproductive system. The follicle, prostate, embryo, fallopian tube, uterus, and vagina all produce messengers that transport information to the follicular fluid, semen, fallopian tube fluid, uterine fluid, and vaginal secretions. The information carried by EVs are not constant; it can change depending on whether the reproductive system is healthy or sick. This information transfer can carry information not only from the sperm to the sperm, but also from the oocyte fluid to the sperm. Although current research on EVs in reproductive medicine is primarily focused on animals, the findings are expected to provide a new direction for the advancement of assisted reproductive technology. However, there is no denying that EVs raise many questions about how they reproduce. For example, What is the mechanism by which EVs are generated from cells? In terms of information transmission, how does it relate to the extracellular environment? Which receptors do target cells use to recognize EVs from different ligands? Is there a relationship between the EVs produced by different ligands and the EVs? These issues require further investigation in order to gain a better understanding of the physiological processes of sperm and egg cell development, embryo formation and development, embryo implantation, and so on.
Author contributions
WF conceived the article and wrote the full text. YQ is responsible for proofreading, writing and translating the full text. YW was responsible for the literature search and summary of embryo formation; HY was responsible for the literature search and summary of embryo implantation; XL was responsible for the literature search and summary of embryo development; YZ was responsible for article guidance and financial support.
Funding
This research was supported by the Natural Science Foundation of Shandong Province (ZR202103050651).
Conflict of interest
The authors declare that the research was conducted in the absence of any commercial or financial relationships that could be construed as a potential conflict of interest.
Publisher’s note
All claims expressed in this article are solely those of the authors and do not necessarily represent those of their affiliated organizations, or those of the publisher, the editors and the reviewers. Any product that may be evaluated in this article, or claim that may be made by its manufacturer, is not guaranteed or endorsed by the publisher.
References
Abels, E. R., and Breakefield, X. O. (2016). Introduction to extracellular vesicles: Biogenesis, RNA cargo selection, content, release, and uptake. Cell. Mol. Neurobiol. 36 (3), 301–312. doi:10.1007/s10571-016-0366-z
Abumaghaid, M. M., Abdelazim, A. M., Belali, T. M., Alhujaily, M., and Saadeldin, I. M. (2022). Shuttle transfer of mRNA transcripts via extracellular vesicles from male reproductive tract cells to the cumulus-oocyte complex in rabbits (Oryctolagus cuniculus). Front. Vet. Sci. 9, 816080. doi:10.3389/fvets.2022.816080
Ahmad, S. F., Brown, J. K., Campbell, L. L., Koscielniak, M., Oliver, C., Wheelhouse, N., et al. (2018). Pelvic chlamydial infection predisposes to ectopic pregnancy by upregulating integrin β1 to promote embryo-tubal attachment. EBioMedicine 29, 159–165. doi:10.1016/j.ebiom.2018.02.020
Al-Dossary, A. A., Bathala, P., Caplan, J. L., and Martin-DeLeon, P. A. (2015). Oviductosome-sperm membrane interaction in cargo delivery: Detection OF FUSION and underlying molecular players using three-dimensional super-resolution structured illumination microscopy (SR-SIM). J. Biol. Chem. 290 (29), 17710–17723. doi:10.1074/jbc.M114.633156
Al-Dossary, A. A., Strehler, E. E., and Martin-Deleon, P. A. (2013). Expression and secretion of plasma membrane Ca2+-ATPase 4a (PMCA4a) during murine estrus: Association with oviductal exosomes and uptake in sperm. PLoS One 8 (11), e80181. doi:10.1371/journal.pone.0080181
Alcântara-Neto, A. S., Fernandez-Rufete, M., Corbin, E., Tsikis, G., Uzbekov, R., Garanina, A. S., et al. (2020). Oviduct fluid extracellular vesicles regulate polyspermy during porcine in vitro fertilisation. Reprod. Fertil. Dev. 32 (4), 409–418. doi:10.1071/RD19058
Alcântara-Neto, A. S., Schmaltz, L., Caldas, E., Blache, M. C., Mermillod, P., and Almiñana, C. (2020). Porcine oviductal extracellular vesicles interact with gametes and regulate sperm motility and survival. Theriogenology 155, 240–255. doi:10.1016/j.theriogenology.2020.05.043
Allegrucci, C., Ronquist, G., Ove Nilsson, B., Carlsson, L., Lundqvist, M., Minelli, A., et al. (2001). Circulating human antisperm antibodies recognize prostasomes. Am. J. Reprod. Immunol. 46 (3), 211–219. doi:10.1034/j.1600-0897.2001.d01-4.x
Almiñana, C., Corbin, E., Tsikis, G., Alcântara-Neto, A. S., Labas, V., Reynaud, K., et al. (2017). Oviduct extracellular vesicles protein content and their role during oviduct-embryo cross-talk. Reproduction 154 (3), 153–168. doi:10.1530/REP-17-0054
Almiñana, C., Tsikis, G., Labas, V., Uzbekov, R., da Silveira, J. C., Bauersachs, S., et al. (2018). Deciphering the oviductal extracellular vesicles content across the estrous cycle: Implications for the gametes-oviduct interactions and the environment of the potential embryo. BMC Genomics 19 (1), 622. doi:10.1186/s12864-018-4982-5
Andrade, G. M., Meirelles, F. V., Perecin, F., and da Silveira, J. C. (2017). Cellular and extracellular vesicular origins of miRNAs within the bovine ovarian follicle. Reprod. Domest. Anim. 52 (6), 1036–1045. doi:10.1111/rda.13021
Asaadi, A., Dolatabad, N. A., Atashi, H., Raes, A., Van Damme, P., Hoelker, M., et al. (2021). Extracellular vesicles from follicular and ampullary fluid isolated by density gradient ultracentrifugation improve bovine embryo development and quality. Int. J. Mol. Sci. 22 (2), 578. doi:10.3390/ijms22020578
Atay, S., Gercel-Taylor, C., Suttles, J., Mor, G., and Taylor, D. D. (2011). Trophoblast-derived exosomes mediate monocyte recruitment and differentiation. Am. J. Reprod. Immunol. 65 (1), 65–77. doi:10.1111/j.1600-0897.2010.00880.x
Azhari, F., Pence, S., Hosseini, M. K., Balci, B. K., Cevik, N., Bastu, E., et al. (2022). The role of the serum exosomal and endometrial microRNAs in recurrent implantation failure. J. Matern. Fetal Neonatal Med. 35 (5), 815–825. doi:10.1080/14767058.2020.1849095
Bai, R., Latifi, Z., Kusama, K., Nakamura, K., Shimada, M., and Imakawa, K. (2018). Induction of immune-related gene expression by seminal exosomes in the porcine endometrium. Biochem. Biophys. Res. Commun. 495 (1), 1094–1101. doi:10.1016/j.bbrc.2017.11.100
Barrachina, F., Battistone, M. A., Castillo, J., Mallofré, C., Jodar, M., Breton, S., et al. (2022). Sperm acquire epididymis-derived proteins through epididymosomes. Hum. Reprod. 37 (4), 651–668. doi:10.1093/humrep/deac015
Battaglia, R., Musumeci, P., Ragusa, M., Barbagallo, D., Scalia, M., Zimbone, M., et al. (2020). Ovarian aging increases small extracellular vesicle CD81+ release in human follicular fluid and influences miRNA profiles. Aging (Albany NY) 12 (12), 12324–12341. doi:10.18632/aging.103441
Burns, G. W., Brooks, K. E., O'Neil, E. V., Hagen, D. E., Behura, S. K., and Spencer, T. E. (2018). Progesterone effects on extracellular vesicles in the sheep uterus. Biol. Reprod. 98 (5), 612–622. doi:10.1093/biolre/ioy011
Buzzaccarini, G., Vitagliano, A., Andrisani, A., Santarsiero, C. M., Cicinelli, R., Nardelli, C., et al. (2020). Chronic endometritis and altered embryo implantation: A unified pathophysiological theory from a literature systematic review. J. Assist. Reprod. Genet. 37 (12), 2897–2911. doi:10.1007/s10815-020-01955-8
Calle, A., Gutiérrez-Reinoso, M. Á., Re, M., Blanco, J., De la Fuente, J., Monguió-Tortajada, M., et al. (2021). Bovine peripheral blood MSCs chemotax towards inflammation and embryo implantation stimuli. J. Cell. Physiol. 236 (2), 1054–1067. doi:10.1002/jcp.29915
Calle, A., Toribio, V., Yáñez-Mó, M., and Ramírez, M. Á. (2021). Embryonic trophectoderm secretomics reveals chemotactic migration and intercellular communication of endometrial and circulating MSCs in embryonic implantation. Int. J. Mol. Sci. 22 (11), 5638. doi:10.3390/ijms22115638
Capalbo, A., Ubaldi, F. M., Cimadomo, D., Noli, L., Khalaf, Y., Farcomeni, A., et al. (2016). MicroRNAs in spent blastocyst culture medium are derived from trophectoderm cells and can be explored for human embryo reproductive competence assessment. Fertil. Steril. 105 (1), 225–235. e1-3. doi:10.1016/j.fertnstert.2015.09.014
Chen, Y., Ma, Y., Yang, X., Chen, J., Yang, B., and Tian, W. (2022). The application of pulp tissue derived-exosomes in pulp regeneration: A novel cell-homing approach. Int. J. Nanomedicine 17, 465–476. doi:10.2147/IJN.S342685
Da Broi, M. G., Giorgi, V. S. I., Wang, F., Keefe, D. L., Albertini, D., and Navarro, P. A. (2018). Influence of follicular fluid and cumulus cells on oocyte quality: Clinical implications. J. Assist. Reprod. Genet. 35 (5), 735–751. doi:10.1007/s10815-018-1143-3
da Silveira, J. C., Andrade, G. M., Del Collado, M., Sampaio, R. V., Sangalli, J. R., Silva, L. A., et al. (2017). Supplementation with small-extracellular vesicles from ovarian follicular fluid during in vitro production modulates bovine embryo development. PLoS One 12 (6), e0179451. doi:10.1371/journal.pone.0179451
da Silveira, J. C., Andrade, G. M., Simas, R. C., Martins-Júnior, H. A., Eberlin, M. N., Smith, L. C., et al. (2021). Lipid profile of extracellular vesicles and their relationship with bovine oocyte developmental competence: New players in intra follicular cell communication. Theriogenology 174, 1–8. doi:10.1016/j.theriogenology.2021.07.024
da Silveira, J. C., de Ávila, A. C. F. C. M., Garrett, H. L., Bruemmer, J. E., Winger, Q. A., and Bouma, G. J. (2018). Cell-secreted vesicles containing microRNAs as regulators of gamete maturation. J. Endocrinol. 236 (1), R15–R27. doi:10.1530/JOE-17-0200
da Silveira, J. C., Veeramachaneni, D. N., Winger, Q. A., Carnevale, E. M., and Bouma, G. J. (2012). Cell-secreted vesicles in equine ovarian follicular fluid contain miRNAs and proteins: A possible new form of cell communication within the ovarian follicle. Biol. Reprod. 86 (3), 71. doi:10.1095/biolreprod.111.093252
da Silveira, J. C., Winger, Q. A., Bouma, G. J., and Carnevale, E. M. (2015). Effects of age on follicular fluid exosomal microRNAs and granulosa cell transforming growth factor-β signalling during follicle development in the mare. Reprod. Fertil. Dev. 27 (6), 897–905. doi:10.1071/RD14452
Daikoku, T., Cha, J., Sun, X., Tranguch, S., Xie, H., Fujita, T., et al. (2011). Conditional deletion of Msx homeobox genes in the uterus inhibits blastocyst implantation by altering uterine receptivity. Dev. Cell. 21 (6), 1014–1025. doi:10.1016/j.devcel.2011.09.010
de Alcântara-Neto, A. S., Cuello, C., Uzbekov, R., Bauersachs, S., Mermillod, P., and Almiñana, C. (2022). Oviductal extracellular vesicles enhance porcine in vitro embryo development by modulating the embryonic transcriptome. Biomolecules 12 (9), 1300. doi:10.3390/biom12091300
de Ávila, A. C. F. C. M., Bridi, A., Andrade, G. M., Del Collado, M., Sangalli, J. R., Nociti, R. P., et al. (2020). Estrous cycle impacts microRNA content in extracellular vesicles that modulate bovine cumulus cell transcripts during in vitro maturation. Biol. Reprod. 102 (2), 362–375. doi:10.1093/biolre/ioz177
Deng, C., Xie, Y., Zhang, C., Ouyang, B., Chen, H., Lv, L., et al. (2019). Urine-derived stem cells facilitate endogenous spermatogenesis restoration of busulfan-induced nonobstructive azoospermic mice by paracrine exosomes. Stem Cells Dev. 28 (19), 1322–1333. doi:10.1089/scd.2019.0026
Ding, J., Zhang, Y., Cai, X., Zhang, Y., Yan, S., Wang, J., et al. (2021). Extracellular vesicles derived from M1 macrophages deliver miR-146a-5p and miR-146b-5p to suppress trophoblast migration and invasion by targeting TRAF6 in recurrent spontaneous abortion. Theranostics 11 (12), 5813–5830. doi:10.7150/thno.58731
Dong, D., Yang, J., Chen, Y., Peng, G., Cao, H., Gao, H., et al. (2021). Palmitoylated GLB1L4 transfers via exosomes to maintain sperm function in rat epididymis. Reproduction 161 (2), 159–172. doi:10.1530/REP-20-0423
Es-Haghi, M., Godakumara, K., Häling, A., Lättekivi, F., Lavrits, A., Viil, J., et al. (2019). Specific trophoblast transcripts transferred by extracellular vesicles affect gene expression in endometrial epithelial cells and may have a role in embryo-maternal crosstalk. Cell. Commun. Signal 17 (1), 146. doi:10.1186/s12964-019-0448-x
Evans, J., Rai, A., Nguyen, H. P. T., Poh, Q. H., Elglass, K., Simpson, R. J., et al. (2019). Human endometrial extracellular vesicles functionally prepare human trophectoderm model for implantation: Understanding bidirectional maternal-embryo communication. Proteomics 19 (23), e1800423. doi:10.1002/pmic.201800423
Fang, X., Tanga, B. M., Bang, S., Seo, C., Kim, H., Saadeldin, I. M., et al. (2022). Oviduct epithelial cell-derived extracellular vesicles improve porcine trophoblast outgrowth. Vet. Sci. 9 (11), 609. doi:10.3390/vetsci9110609
Fang, X., Tanga, B. M., Bang, S., Seong, G., Saadeldin, I. M., Lee, S., et al. (2022). Oviduct epithelial cells-derived extracellular vesicles improve preimplantation developmental competence of in vitro produced porcine parthenogenetic and cloned embryos. Mol. Reprod. Dev. 89 (1), 54–65. doi:10.1002/mrd.23550
Fereshteh, Z., Bathala, P., Galileo, D. S., and Martin-DeLeon, P. A. (2019). Detection of extracellular vesicles in the mouse vaginal fluid: Their delivery of sperm proteins that stimulate capacitation and modulate fertility. J. Cell. Physiol. 234 (8), 12745–12756. doi:10.1002/jcp.27894
Fereshteh, Z., Schmidt, S. A., Al-Dossary, A. A., Accerbi, M., Arighi, C., Cowart, J., et al. (2018). Murine Oviductosomes (OVS) microRNA profiling during the estrous cycle: Delivery of OVS-borne microRNAs to sperm where miR-34c-5p localizes at the centrosome. Sci. Rep. 8 (1), 16094. doi:10.1038/s41598-018-34409-4
Ferraz, M. A. M. M., Carothers, A., Dahal, R., Noonan, M. J., and Songsasen, N. (2019). Oviductal extracellular vesicles interact with the spermatozoon's head and mid-piece and improves its motility and fertilizing ability in the domestic cat. Sci. Rep. 9 (1), 9484. doi:10.1038/s41598-019-45857-x
Fiorenza, M. T., Russo, G., Narducci, M. G., Bresin, A., Mangia, F., and Bevilacqua, A. (2020). Protein kinase Akt2/PKBβ is involved in blastomere proliferation of preimplantation mouse embryos. J. Cell. Physiol. 235 (4), 3393–3401. doi:10.1002/jcp.29229
Franasiak, J. M., Alecsandru, D., Forman, E. J., Gemmell, L. C., Goldberg, J. M., Llarena, N., et al. (2021). A review of the pathophysiology of recurrent implantation failure. Fertil. Steril. 116 (6), 1436–1448. doi:10.1016/j.fertnstert.2021.09.014
Fu, B., Ma, H., Zhang, D. J., Wang, L., Li, Z. Q., Guo, Z. H., et al. (2022). Porcine oviductal extracellular vesicles facilitate early embryonic development via relief of endoplasmic reticulum stress. Cell. Biol. Int. 46 (2), 300–310. doi:10.1002/cbin.11730
Fu, Y. X., Ji, J., Shan, F., Li, J., and Hu, R. (2021). Human mesenchymal stem cell treatment of premature ovarian failure: New challenges and opportunities. Stem Cell. Res. Ther. 12 (1), 161. doi:10.1186/s13287-021-02212-0
Fuhrmann, G. (2020). Diffusion and transport of extracellular vesicles. Nat. Nanotechnol. 15 (3), 168–169. doi:10.1038/s41565-020-0651-3
Gabryś, J., Kij-Mitka, B., Sawicki, S., Kochan, J., Nowak, A., Łojko, J., et al. (2022). Extracellular vesicles from follicular fluid may improve the nuclear maturation rate of in vitro matured mare oocytes. Theriogenology 188, 116–124. doi:10.1016/j.theriogenology.2022.05.022
Gad, A., Murin, M., Bartkova, A., Kinterova, V., Marcollova, K., Laurincik, J., et al. (2022). Small-extracellular vesicles and their microRNA cargo from porcine follicular fluids: The potential association with oocyte quality. J. Anim. Sci. Biotechnol. 13 (1), 82. doi:10.1186/s40104-022-00723-1
Garofalo, M., Villa, A., Crescenti, D., Marzagalli, M., Kuryk, L., Limonta, P., et al. (2019). Heterologous and cross-species tropism of cancer-derived extracellular vesicles. Theranostics 9 (19), 5681–5693. doi:10.7150/thno.34824
George, A. F., Jang, K. S., Nyegaard, M., Neidleman, J., Spitzer, T. L., Xie, G., et al. (2020). Seminal plasma promotes decidualization of endometrial stromal fibroblasts in vitro from women with and without inflammatory disorders in a manner dependent on interleukin-11 signaling. Hum. Reprod. 35 (3), 617–640. doi:10.1093/humrep/deaa015
Gou, J., Hu, T., Li, L., Xue, L., Zhao, X., Yi, T., et al. (2019). Role of epithelial-mesenchymal transition regulated by twist basic helix-loop-helix transcription factor 2 (Twist2) in embryo implantation in mice. Reprod. Fertil. Dev. 31 (5), 932–940. doi:10.1071/RD18314
Greening, D. W., Nguyen, H. P., Elgass, K., Simpson, R. J., and Salamonsen, L. A. (2016). Human endometrial exosomes contain hormone-specific cargo modulating trophoblast adhesive capacity: Insights into endometrial-embryo interactions. Biol. Reprod. 94 (2), 38. doi:10.1095/biolreprod.115.134890
Griffiths, G. S., Galileo, D. S., Reese, K., and Martin-Deleon, P. A. (2008). Investigating the role of murine epididymosomes and uterosomes in GPI-linked protein transfer to sperm using SPAM1 as a model. Mol. Reprod. Dev. 75 (11), 1627–1636. doi:10.1002/mrd.20907
Griffiths, G. S., Miller, K. A., Galileo, D. S., and Martin-DeLeon, P. A. (2008). Murine SPAM1 is secreted by the estrous uterus and oviduct in a form that can bind to sperm during capacitation: Acquisition enhances hyaluronic acid-binding ability and cumulus dispersal efficiency. Reproduction 135 (3), 293–301. doi:10.1530/REP-07-0340
Gurung, S., Greening, D. W., Catt, S., Salamonsen, L., and Evans, J. (2020). Exosomes and soluble secretome from hormone-treated endometrial epithelial cells direct embryo implantation. Mol. Hum. Reprod. 26 (7), 510–520. doi:10.1093/molehr/gaaa034
Han, X., Hao, L., Shi, Z., Li, Y., Wang, L., Li, Z., et al. (2022). Seminal plasma extracellular vesicles tRF-Val-AAC-010 can serve as a predictive factor of successful microdissection testicular sperm extraction in patients with non-obstructive azoospermia. Reprod. Biol. Endocrinol. 20 (1), 106. doi:10.1186/s12958-022-00978-3
Hasan, M. M., Reshi, Q. U. A., Lättekivi, F., Viil, J., Godakumara, K., Dissanayake, K., et al. (2021). Bovine follicular fluid derived extracellular vesicles modulate the viability, capacitation and acrosome reaction of bull spermatozoa. Capacitation Acrosome React. Bull Spermatozoa. Biol. (Basel) 10 (11), 1154. doi:10.3390/biology10111154
Hasan, M. M., Viil, J., Lättekivi, F., Ord, J., Reshi, Q. U. A., Jääger, K., et al. (2020). Bovine follicular fluid and extracellular vesicles derived from follicular fluid alter the bovine oviductal epithelial cells transcriptome. Int. J. Mol. Sci. 21 (15), 5365. doi:10.3390/ijms21155365
Hirota, Y., Osuga, Y., Koga, K., Yoshino, O., Hirata, T., Morimoto, C., et al. (2006). The expression and possible roles of chemokine CXCL11 and its receptor CXCR3 in the human endometrium. J. Immunol. 177 (12), 8813–8821. doi:10.4049/jimmunol.177.12.8813
Hong, W., Wang, B., Zhu, Y., Wu, J., Qiu, L., Ling, S., et al. (2022). Female germline stem cells: Aging and anti-aging. J. Ovarian Res. 15 (1), 79. doi:10.1186/s13048-022-01011-2
Hu, Q., Zang, X., Ding, Y., Gu, T., Shi, J., Li, Z., et al. (2022). Porcine uterine luminal fluid-derived extracellular vesicles improve conceptus-endometrial interaction during implantation. Theriogenology 178, 8–17. doi:10.1016/j.theriogenology.2021.10.021
Hung, W. T., Hong, X., Christenson, L. K., and McGinnis, L. K. (2015). Extracellular vesicles from bovine follicular fluid support cumulus expansion. Biol. Reprod. 93 (5), 117. doi:10.1095/biolreprod.115.132977
Hung, W. T., Navakanitworakul, R., Khan, T., Zhang, P., Davis, J. S., McGinnis, L. K., et al. (2017). Stage-specific follicular extracellular vesicle uptake and regulation of bovine granulosa cell proliferation. Biol. Reprod. 97 (4), 644–655. doi:10.1093/biolre/iox106
Inoue, Y., Munakata, Y., Shinozawa, A., Kawahara-Miki, R., Shirasuna, K., and Iwata, H. (2020). Prediction of major microRNAs in follicular fluid regulating porcine oocyte development. J. Assist. Reprod. Genet. 37 (10), 2569–2579. doi:10.1007/s10815-020-01909-0
Kim, J., Lee, J., Lee, T. B., and Jun, J. H. (2019). Embryotrophic effects of extracellular vesicles derived from outgrowth embryos in pre- and peri-implantation embryonic development in mice. Mol. Reprod. Dev. 86 (2), 187–196. doi:10.1002/mrd.23093
Kumar, S., Chinnusamy, V., and Mohapatra, T. (2018). Epigenetics of modified DNA bases: 5-Methylcytosine and beyond. Front. Genet. 9, 640. doi:10.3389/fgene.2018.00640
Laezer, I., Palma-Vera, S. E., Liu, F., Frank, M., Trakooljul, N., Vernunft, A., et al. (2020). Dynamic profile of EVs in porcine oviductal fluid during the periovulatory period. Reproduction 159 (4), 371–382. doi:10.1530/REP-19-0219
Lange-Consiglio, A., Perrini, C., Albini, G., Modina, S., Lodde, V., Orsini, E., et al. (2017). Oviductal microvesicles and their effect on in vitro maturation of canine oocytes. Reproduction 154 (2), 167–180. doi:10.1530/REP-17-0117
Leal, C. L. V., Cañón-Beltrán, K., Cajas, Y. N., Hamdi, M., Yaryes, A., Millán de la Blanca, M. G., et al. (2022). Extracellular vesicles from oviductal and uterine fluids supplementation in sequential in vitro culture improves bovine embryo quality. J. Anim. Sci. Biotechnol. 13 (1), 116. doi:10.1186/s40104-022-00763-7
Lee, S. H., Oh, H. J., Kim, M. J., and Lee, B. C. (2020). Canine oviductal exosomes improve oocyte development via EGFR/MAPK signaling pathway. Reproduction 160 (4), 613–625. doi:10.1530/REP-19-0600
Lee, S. H., Oh, H. J., Kim, M. J., and Lee, B. C. (2020). Exosomes derived from oviduct cells mediate the EGFR/MAPK signaling pathway in cumulus cells. J. Cell. Physiol. 235 (2), 1386–1404. doi:10.1002/jcp.29058
Li, T., Greenblatt, E. M., Shin, M. E., Brown, T. J., and Chan, C. (2021). Cargo small non-coding RNAs of extracellular vesicles isolated from uterine fluid associate with endometrial receptivity and implantation success. Fertil. Steril. 115 (5), 1327–1336. doi:10.1016/j.fertnstert.2020.10.046
Liang, C., Huang, J., Luo, P., Wang, Z., He, J., Wu, S., et al. (2020). Platelet-derived microparticles mediate the intra-articular homing of mesenchymal stem cells in early-stage cartilage lesions. Stem Cells Dev. 29 (7), 414–424. doi:10.1089/scd.2019.0137
Limongi, T., Susa, F., Dumontel, B., Racca, L., Perrone Donnorso, M., Debellis, D., et al. (2021). Extracellular vesicles tropism: A comparative study between passive innate tropism and the active engineered targeting capability of lymphocyte-derived EVs. Membr. (Basel) 11 (11), 886. doi:10.3390/membranes11110886
Liu, C., Li, L., Wang, M., Shui, S., Yao, H., Sui, C., et al. (2021). Endometrial extracellular vesicles of recurrent implantation failure patients inhibit the proliferation, migration, and invasion of HTR8/SVneo cells. J. Assist. Reprod. Genet. 38 (4), 825–833. doi:10.1007/s10815-021-02093-5
Liu, C., Yao, W., Yao, J., Li, L., Yang, L., Zhang, H., et al. (2020). Endometrial extracellular vesicles from women with recurrent implantation failure attenuate the growth and invasion of embryos. Fertil. Steril. 114 (2), 416–425. doi:10.1016/j.fertnstert.2020.04.005
Liu, C., Yin, H., Jiang, H., Du, X., Wang, C., Liu, Y., et al. (2020). Extracellular vesicles derived from mesenchymal stem cells recover fertility of premature ovarian insufficiency mice and the effects on their offspring. Cell. Transpl. 29, 963689720923575. doi:10.1177/0963689720923575
Liu, M., Chen, X., Chang, Q. X., Hua, R., Wei, Y. X., Huang, L. P., et al. (2020). Decidual small extracellular vesicles induce trophoblast invasion by upregulating N-cadherin. Reproduction 159 (2), 171–180. doi:10.1530/REP-18-0616
Lopera-Vasquez, R., Hamdi, M., Maillo, V., Gutierrez-Adan, A., Bermejo-Alvarez, P., Ramírez, M. Á., et al. (2017). Effect of bovine oviductal extracellular vesicles on embryo development and quality in vitro. Reproduction 153 (4), 461–470. doi:10.1530/REP-16-0384
Lv, C., Yu, W. X., Wang, Y., Yi, D. J., Zeng, M. H., and Xiao, H. M. (2018). MiR-21 in extracellular vesicles contributes to the growth of fertilized eggs and embryo development in mice. Biosci. Rep. 38 (4), BSR20180036. doi:10.1042/BSR20180036
Ma, Y., Wang, J., Qiao, F., and Wang, Y. (2022). Extracellular vesicles from seminal plasma improved development of in vitro-fertilized mouse embryos. Zygote 30, 619–624. doi:10.1017/S0967199422000041
Mahdavinezhad, F., Gilani, M. A. S., Gharaei, R., Ashrafnezhad, Z., Valipour, J., Nashtaei, M. S., et al. (2022). Protective roles of seminal plasma exosomes and microvesicles during human sperm cryopreservation. Reprod. Biomed. Online 45 (2), 341–353. doi:10.1016/j.rbmo.2022.03.033
Martinez, R. M., Liang, L., Racowsky, C., Dioni, L., Mansur, A., Adir, M., et al. (2018). Extracellular microRNAs profile in human follicular fluid and IVF outcomes. Sci. Rep. 8 (1), 17036. doi:10.1038/s41598-018-35379-3
Mateo-Otero, Y., Yeste, M., Roca, J., Llavanera, M., Bucci, D., Galeati, G., et al. (2022). Seminal extracellular vesicles subsets modulate gene expression in cumulus cells of porcine in vitro matured oocytes. Sci. Rep. 12 (1), 19096. doi:10.1038/s41598-022-22004-7
Matsuno, Y., Onuma, A., Fujioka, Y. A., Yasuhara, K., Fujii, W., Naito, K., et al. (2017). Effects of exosome-like vesicles on cumulus expansion in pigs in vitro. J. Reprod. Dev. 63 (1), 51–58. doi:10.1262/jrd.2016-124
Mazzarella, R., Bastos, N. M., Bridi, A., Del Collado, M., Andrade, G. M., Pinzon, J., et al. (2021). Changes in oviductal cells and small extracellular vesicles miRNAs in pregnant cows. Front. Vet. Sci. 8, 639752. doi:10.3389/fvets.2021.639752
Merchant, M. L., Rood, I. M., Deegens, J. K. J., and Klein, J. B. (2017). Isolation and characterization of urinary extracellular vesicles: Implications for biomarker discovery. Nat. Rev. Nephrol. 13 (12), 731–749. doi:10.1038/nrneph.2017.148
Mishra, A., Ashary, N., Sharma, R., and Modi, D. (2021). Extracellular vesicles in embryo implantation and disorders of the endometrium. Am. J. Reprod. Immunol. 85 (2), e13360. doi:10.1111/aji.13360
Miyado, K., Yoshida, K., Yamagata, K., Sakakibara, K., Okabe, M., Wang, X., et al. (2008). The fusing ability of sperm is bestowed by CD9-containing vesicles released from eggs in mice. Proc. Natl. Acad. Sci. U. S. A. 105 (35), 12921–12926. doi:10.1073/pnas.0710608105
Murdica, V., Giacomini, E., Alteri, A., Bartolacci, A., Cermisoni, G. C., Zarovni, N., et al. (2019). Seminal plasma of men with severe asthenozoospermia contain exosomes that affect spermatozoa motility and capacitation. Fertil. Steril. 111 (5), 897–908. e2. doi:10.1016/j.fertnstert.2019.01.030
Murdica, V., Giacomini, E., Makieva, S., Zarovni, N., Candiani, M., Salonia, A., et al. (2020). In vitro cultured human endometrial cells release extracellular vesicles that can be uptaken by spermatozoa. Sci. Rep. 10 (1), 8856. doi:10.1038/s41598-020-65517-9
Naji, A., Suganuma, N., Espagnolle, N., Yagyu, K. I., Baba, N., Sensebé, L., et al. (2017). Rationale for determining the functional potency of mesenchymal stem cells in preventing regulated cell death for therapeutic use. Stem Cells Transl. Med. 6 (3), 713–719. doi:10.5966/sctm.2016-0289
Nakamura, K., Kusama, K., Bai, R., Sakurai, T., Isuzugawa, K., Godkin, J. D., et al. (2016). Induction of IFNT-stimulated genes by conceptus-derived exosomes during the attachment period. PLoS One 11 (6), e0158278. doi:10.1371/journal.pone.0158278
O'Neil, E. V., Burns, G. W., Ferreira, C. R., and Spencer, T. E. (2020). Characterization and regulation of extracellular vesicles in the lumen of the ovine uterus. Biol. Reprod. 102 (5), 1020–1032. doi:10.1093/biolre/ioaa019
Okunade, G. W., Miller, M. L., Pyne, G. J., Sutliff, R. L., O'Connor, K. T., Neumann, J. C., et al. (2004). Targeted ablation of plasma membrane Ca2+-ATPase (PMCA) 1 and 4 indicates a major housekeeping function for PMCA1 and a critical role in hyperactivated sperm motility and male fertility for PMCA4. J. Biol. Chem. 279 (32), 33742–33750. doi:10.1074/jbc.M404628200
Paktinat, S., Esfandyari, S., Karamian, A., Koochaki, A., Asadirad, A., Ghaffari Novin, M., et al. (2021). Conditioned medium derived from seminal extracellular vesicles-exposed endometrial stromal cells induces inflammatory cytokine secretion by macrophages. Eur. J. Obstet. Gynecol. Reprod. Biol. 262, 174–181. doi:10.1016/j.ejogrb.2021.05.019
Paktinat, S., Hashemi, S. M., Ghaffari Novin, M., Mohammadi-Yeganeh, S., Salehpour, S., Karamian, A., et al. (2019). Seminal exosomes induce interleukin-6 and interleukin-8 secretion by human endometrial stromal cells. Eur. J. Obstet. Gynecol. Reprod. Biol. 235, 71–76. doi:10.1016/j.ejogrb.2019.02.010
Park, K. H., Kim, B. J., Kang, J., Nam, T. S., Lim, J. M., Kim, H. T., et al. (2011). Ca2+ signaling tools acquired from prostasomes are required for progesterone-induced sperm motility. Sci. Signal 4 (173), ra31. doi:10.1126/scisignal.2001595
Pavani, K. C., Meese, T., Pascottini, O. B., Guan, X., Lin, X., Peelman, L., et al. (2022). Hatching is modulated by microRNA-378a-3p derived from extracellular vesicles secreted by blastocysts. Proc. Natl. Acad. Sci. U. S. A. 119 (12), e2122708119. doi:10.1073/pnas.2122708119
Pioltine, E. M., Machado, M. F., da Silveira, J. C., Fontes, P. K., Botigelli, R. C., Quaglio, A. E. V., et al. (2020). Can extracellular vesicles from bovine ovarian follicular fluid modulate the in-vitro oocyte meiosis progression similarly to the CNP-NPR2 system? Theriogenology 157, 210–217. doi:10.1016/j.theriogenology.2020.06.031
Qu, P., Luo, S., Du, Y., Zhang, Y., Song, X., Yuan, X., et al. (2020). Extracellular vesicles and melatonin benefit embryonic develop by regulating reactive oxygen species and 5-methylcytosine. J. Pineal Res. 68 (3), e12635. doi:10.1111/jpi.12635
Qu, P., Qing, S., Liu, R., Qin, H., Wang, W., Qiao, F., et al. (2017). Effects of embryo-derived exosomes on the development of bovine cloned embryos. PLoS One 12 (3), e0174535. doi:10.1371/journal.pone.0174535
Qu, P., Zhao, Y., Wang, R., Zhang, Y., Li, L., Fan, J., et al. (2019). Extracellular vesicles derived from donor oviduct fluid improved birth rates after embryo transfer in mice. Reprod. Fertil. Dev. 31 (2), 324–332. doi:10.1071/RD18203
Qu, Q., Liu, L., Cui, Y., Liu, H., Yi, J., Bing, W., et al. (2022). miR-126-3p containing exosomes derived from human umbilical cord mesenchymal stem cells promote angiogenesis and attenuate ovarian granulosa cell apoptosis in a preclinical rat model of premature ovarian failure. Stem Cell. Res. Ther. 13 (1), 352. doi:10.1186/s13287-022-03056-y
Rajaratnam, N., Ditlevsen, N. E., Sloth, J. K., Bæk, R., Jørgensen, M. M., and Christiansen, O. B. (2021). Extracellular vesicles: An important biomarker in recurrent pregnancy loss? J. Clin. Med. 10 (12), 2549. doi:10.3390/jcm10122549
Rodrigues, T. A., Tuna, K. M., Alli, A. A., Tribulo, P., Hansen, P. J., Koh, J., et al. (2019). Follicular fluid exosomes act on the bovine oocyte to improve oocyte competence to support development and survival to heat shock. Reprod. Fertil. Dev. 31 (5), 888–897. doi:10.1071/RD18450
Ronquist, G. (2012). Prostasomes are mediators of intercellular communication: From basic research to clinical implications. J. Intern Med. 271 (4), 400–413. doi:10.1111/j.1365-2796.2011.02487.x
Rowlison, T., Ottinger, M. A., and Comizzoli, P. (2021). Exposure to epididymal extracellular vesicles enhances immature sperm function and sustains vitality of cryopreserved spermatozoa in the domestic cat model. J. Assist. Reprod. Genet. 38 (8), 2061–2071. doi:10.1007/s10815-021-02214-0
Ruiz-González, I., Xu, J., Wang, X., Burghardt, R. C., Dunlap, K. A., and Bazer, F. W. (2015). Exosomes, endogenous retroviruses and toll-like receptors: Pregnancy recognition in ewes. Reproduction 149 (3), 281–291. doi:10.1530/REP-14-0538
Saadeldin, I. M., Kim, S. J., Choi, Y. B., and Lee, B. C. (2014). Improvement of cloned embryos development by co-culturing with parthenotes: A possible role of exosomes/microvesicles for embryos paracrine communication. Cell. Reprogr. 16 (3), 223–234. doi:10.1089/cell.2014.0003
Saez, F., Motta, C., Boucher, D., and Grizard, G. (1998). Antioxidant capacity of prostasomes in human semen. Mol. Hum. Reprod. 4 (7), 667–672. doi:10.1093/molehr/4.7.667
Saint-Dizier, M., Mahé, C., Reynaud, K., Tsikis, G., Mermillod, P., and Druart, X. (2020). Sperm interactions with the female reproductive tract: A key for successful fertilization in mammals. Mol. Cell. Endocrinol. 516, 110956. doi:10.1016/j.mce.2020.110956
Saint-Dizier, M., Schoen, J., Chen, S., Banliat, C., and Mermillod, P. (2019). Composing the early embryonic microenvironment: Physiology and regulation of oviductal secretions. Int. J. Mol. Sci. 21 (1), 223. doi:10.3390/ijms21010223
Sang, Q., Yao, Z., Wang, H., Feng, R., Wang, H., Zhao, X., et al. (2013). Identification of microRNAs in human follicular fluid: Characterization of microRNAs that govern steroidogenesis in vitro and are associated with polycystic ovary syndrome in vivo. J. Clin. Endocrinol. Metab. 98 (7), 3068–3079. doi:10.1210/jc.2013-1715
Schjenken, J. E., Glynn, D. J., Sharkey, D. J., and Robertson, S. A. (2015). TLR4 signaling is a major mediator of the female tract response to seminal fluid in mice. Biol. Reprod. 93 (3), 68. doi:10.1095/biolreprod.114.125740
Sehring, J., Beltsos, A., and Jeelani, R. (2022). Human implantation: The complex interplay between endometrial receptivity, inflammation, and the microbiome. Placenta 117, 179–186. doi:10.1016/j.placenta.2021.12.015
Shi, S., Tan, Q., Liang, J., Cao, D., Wang, S., Liang, J., et al. (2021). Placental trophoblast cell-derived exosomal microRNA-1290 promotes the interaction between endometrium and embryo by targeting LHX6. Mol. Ther. Nucleic Acids 26, 760–772. doi:10.1016/j.omtn.2021.09.009
Sidrat, T., Khan, A. A., Joo, M. D., Wei, Y., Lee, K. L., Xu, L., et al. (2020). Bovine oviduct epithelial cell-derived culture media and exosomes improve mitochondrial Health by restoring metabolic flux during pre-implantation development. Int. J. Mol. Sci. 21 (20), 7589. doi:10.3390/ijms21207589
Sidrat, T., Khan, A. A., Joo, M. D., Xu, L., El-Sheikh, M., Ko, J. H., et al. (2022). Extracellular vesicles improve embryo cryotolerance by maintaining the tight junction integrity during blastocoel re-expansion. Reproduction 163 (4), 219–232. doi:10.1530/REP-21-0320
Silva, F. A. C. C., da Silva, G. F., Vieira, B. S., Neto, A. L., Rocha, C. C., Lo Turco, E. G., et al. (2021). Peri-estrus ovarian, uterine, and hormonal variables determine the uterine luminal fluid metabolome in beef heifers. Biol. Reprod. 105 (5), 1140–1153. doi:10.1093/biolre/ioab149
Skibinski, G., Kelly, R. W., Harkiss, D., and James, K. (1992). Immunosuppression by human seminal plasma--extracellular organelles (prostasomes) modulate activity of phagocytic cells. Am. J. Reprod. Immunol. 28 (2), 97–103. doi:10.1111/j.1600-0897.1992.tb00767.x
Song, N., Scholtemeijer, M., and Shah, K. (2020). Mesenchymal stem cell immunomodulation: Mechanisms and therapeutic potential. Trends Pharmacol. Sci. 41 (9), 653–664. doi:10.1016/j.tips.2020.06.009
Stirparo, G. G., Kurowski, A., Yanagida, A., Bates, L. E., Strawbridge, S. E., Hladkou, S., et al. (2021). OCT4 induces embryonic pluripotency via STAT3 signaling and metabolic mechanisms. Proc. Natl. Acad. Sci. U. S. A. 118 (3), e2008890118. doi:10.1073/pnas.2008890118
Su, Y., Li, Q., Zhang, Q., Li, Z., Yao, X., Guo, Y., et al. (2022). Exosomes derived from placental trophoblast cells regulate endometrial epithelial receptivity in dairy cows during pregnancy. J. Reprod. Dev. 68 (1), 21–29. doi:10.1262/jrd.2021-077
Sysoeva, A. P., Makarova, N. P., Silachev, D. N., Lobanova, N. N., Shevtsova, Y. A., Bragina, E. E., et al. (2021). Influence of extracellular vesicles of the follicular fluid on morphofunctional characteristics of human sperm. Bull. Exp. Biol. Med. 172 (2), 254–262. doi:10.1007/s10517-021-05372-4
Tan, Q., Shi, S., Liang, J., Cao, D., Wang, S., and Wang, Z. (2020). Endometrial cell-derived small extracellular vesicle miR-100-5p promotes functions of trophoblast during embryo implantation. Mol. Ther. Nucleic Acids 23, 217–231. doi:10.1016/j.omtn.2020.10.043
Tan, Q., Shi, S., Liang, J., Zhang, X., Cao, D., and Wang, Z. (2020). MicroRNAs in small extracellular vesicles indicate successful embryo implantation during early pregnancy. Cells 9 (3), 645. doi:10.3390/cells9030645
Tarazona, R., Delgado, E., Guarnizo, M. C., Roncero, R. G., Morgado, S., Sánchez-Correa, B., et al. (2011). Human prostasomes express CD48 and interfere with NK cell function. Immunobiology 216 (1-2), 41–46. doi:10.1016/j.imbio.2010.03.002
Théry, C., Witwer, K. W., Aikawa, E., Alcaraz, M. J., Anderson, J. D., Andriantsitohaina, R., et al. (2018). Minimal information for studies of extracellular vesicles 2018 (MISEV2018): A position statement of the international society for extracellular vesicles and update of the MISEV2014 guidelines. J. Extracell. Vesicles 7 (1), 1535750. doi:10.1080/20013078.2018.1535750
Tsukazawa, K. S., Li, L., and Tse, W. K. F. (2021). 2, 4-dichlorophenol exposure induces lipid accumulation and reactive oxygen species formation in zebrafish embryos. Ecotoxicol. Environ. Saf. 230, 113133. doi:10.1016/j.ecoenv.2021.113133
Uchida, H., Maruyama, T., Ohta, K., Ono, M., Arase, T., Kagami, M., et al. (2007). Histone deacetylase inhibitor-induced glycodelin enhances the initial step of implantation. Hum. Reprod. 22 (10), 2615–2622. doi:10.1093/humrep/dem263
Urbanelli, L., Magini, A., Buratta, S., Brozzi, A., Sagini, K., Polchi, A., et al. (2013). Signaling pathways in exosomes biogenesis, secretion and fate. Genes (Basel) 4 (2), 152–170. doi:10.3390/genes4020152
Verweij, F. J., Revenu, C., Arras, G., Dingli, F., Loew, D., Pegtel, D. M., et al. (2019). Live tracking of inter-organ communication by endogenous exosomes in vivo. Dev. Cell. 48 (4), 573–589. e4. doi:10.1016/j.devcel.2019.01.004
Vilella, F., Moreno-Moya, J. M., Balaguer, N., Grasso, A., Herrero, M., Martínez, S., et al. (2015). Hsa-miR-30d, secreted by the human endometrium, is taken up by the pre-implantation embryo and might modify its transcriptome. Development 142 (18), 3210–3221. doi:10.1242/dev.124289
Vyas, P., Balakier, H., and Librach, C. L. (2019). Ultrastructural identification of CD9 positive extracellular vesicles released from human embryos and transported through the zona pellucida. Syst. Biol. Reprod. Med. 65 (4), 273–280. doi:10.1080/19396368.2019.1619858
Wang, D., Jueraitetibaike, K., Tang, T., Wang, Y., Jing, J., Xue, T., et al. (2021). Seminal plasma and seminal plasma exosomes of aged male mice affect early embryo implantation via immunomodulation. Front. Immunol. 12, 723409. doi:10.3389/fimmu.2021.723409
Wang, D., Lyu, Y., Yang, Y., Zhang, S., Chen, G., Pan, J., et al. (2022). Schwann cell-derived EVs facilitate dental pulp regeneration through endogenous stem cell recruitment via SDF-1/CXCR4 axis. Acta Biomater. 140, 610–624. doi:10.1016/j.actbio.2021.11.039
Wang, H., Zhu, Y., Tang, C., Zhou, Z., Wang, Z., Li, Z., et al. (2022). Reassessment of the proteomic composition and function of extracellular vesicles in the seminal plasma. Endocrinology 163 (1), bqab214. doi:10.1210/endocr/bqab214
Wang, X., Li, Q., Xie, T., Yuan, M., Sheng, X., Qi, X., et al. (2021). Exosomes from bovine endometrial epithelial cells ensure trophoblast cell development by miR-218 targeting secreted frizzled related protein 2. J. Cell. Physiol. 236 (6), 4565–4579. doi:10.1002/jcp.30180
Wang, X., Tian, F., Chen, C., Feng, Y., Sheng, X., Guo, Y., et al. (2019). Exosome-derived uterine microRNAs isolated from cows with endometritis impede blastocyst development. Reprod. Biol. 19 (2), 204–209. doi:10.1016/j.repbio.2019.06.003
Wang, X., Wu, P., Li, X., Zeng, C., Zhu, J., Zhou, Y., et al. (2021). Extracellular vesicles inhibit proliferation and invasion of ovarian endometrial stromal cells and their expression of SF-1, ERβ, and aromatase. Front. Endocrinol. (Lausanne) 12, 666195. doi:10.3389/fendo.2021.666195
Wei, Y., Idrees, M., Sidrat, T., Joo, M., Xu, L., Ko, J., et al. (2022). BOEC-exo addition promotes in vitro maturation of bovine oocyte and enhances the developmental competence of early embryos. Anim. (Basel) 12 (4), 424. doi:10.3390/ani12040424
Wolf, P. (1967). The nature and significance of platelet products in human plasma. Br. J. Haematol. 13 (3), 269–288. doi:10.1111/j.1365-2141.1967.tb08741.x
Xie, Y., Abdallah, M. E., Awonuga, A. O., Slater, J. A., Puscheck, E. E., and Rappolee, D. A. (2010). Benzo(a)pyrene causes PRKAA1/2-dependent ID2 loss in trophoblast stem cells. Mol. Reprod. Dev. 77 (6), 533–539. doi:10.1002/mrd.21178
Xie, Y., Xu, Z., Wu, C., Zhou, C., Zhang, X., Gu, T., et al. (2022). Extracellular vesicle-encapsulated miR-21-5p in seminal plasma prevents sperm capacitation via Vinculin inhibition. Theriogenology 193, 103–113. doi:10.1016/j.theriogenology.2022.09.014
Xin, L., Lin, X., Zhou, F., Li, C., Wang, X., Yu, H., et al. (2020). A scaffold laden with mesenchymal stem cell-derived exosomes for promoting endometrium regeneration and fertility restoration through macrophage immunomodulation. Acta Biomater. 113, 252–266. doi:10.1016/j.actbio.2020.06.029
Yáñez-Mó, M., Siljander, P. R., Andreu, Z., Zavec, A. B., Borràs, F. E., Buzas, E. I., et al. (2015). Biological properties of extracellular vesicles and their physiological functions. J. Extracell. Vesicles 4, 27066. doi:10.3402/jev.v4.27066
Yang, C., Guo, W. B., Zhang, W. S., Bian, J., Yang, J. K., Zhou, Q. Z., et al. (2017). Comprehensive proteomics analysis of exosomes derived from human seminal plasma. Andrology 5 (5), 1007–1015. doi:10.1111/andr.12412
Yang, J., Feng, T., Li, S., Zhang, X., and Qian, Y. (2020). Human follicular fluid shows diverse metabolic profiles at different follicle developmental stages. Reprod. Biol. Endocrinol. 18 (1), 74. doi:10.1186/s12958-020-00631-x
Yao, Y., Chen, R., Wang, G., Zhang, Y., and Liu, F. (2019). Exosomes derived from mesenchymal stem cells reverse EMT via TGF-β1/Smad pathway and promote repair of damaged endometrium. Stem Cell. Res. Ther. 10 (1), 225. doi:10.1186/s13287-019-1332-8
Yi, C., Ni, Y., Sun, P., Gao, T., and Li, K. (2022). Differential size distribution and estrogen receptor cargo of oviductal extracellular vesicles at various stages of estrous cycle in mice. Reprod. Sci. 29 (10), 2847–2858. doi:10.1007/s43032-022-00862-w
Ying, W., Hengqin, W., Xiaomei, W., Yunqi, Z., Yong, Z., and Fusheng, Q. (2021). Extracellular vesicles of bovine small follicular fluid promote ovarian cortical stromal cell proliferation and steroidogenesis. Reprod. Domest. Anim. 56 (11), 1425–1434. doi:10.1111/rda.14007
Ying, W., Yunqi, Z., Zimeng, L., Kangning, X., Deji, L., Chen, Q., et al. (2023). Large extracellular vesicles in bovine follicular fluid inhibit the apoptosis of granulosa cell and stimulate the production of steroid hormones. Theriogenology 195, 149–158. doi:10.1016/j.theriogenology.2022.10.027
Yuan, C., Li, Z., Zhao, Y., Wang, X., Chen, L., Zhao, Z., et al. (2021). Follicular fluid exosomes: Important modulator in proliferation and steroid synthesis of porcine granulosa cells. FASEB J. 35 (5), e21610. doi:10.1096/fj.202100030RR
Yuan, D., Luo, J., Sun, Y., Hao, L., Zheng, J., and Yang, Z. (2021). PCOS follicular fluid derived exosomal miR-424-5p induces granulosa cells senescence by targeting CDCA4 expression. Cell. Signal 85, 110030. doi:10.1016/j.cellsig.2021.110030
Zakerkish, F., Brännström, M., Carlsohn, E., Sihlbom, C., van der Post, S., and Thoroddsen, A. (2020). Proteomic analysis of follicular fluid during human ovulation. Acta Obstet. Gynecol. Scand. 99 (7), 917–924. doi:10.1111/aogs.13805
Zeng, H., Fu, Y., Shen, L., and Quan, S. (2021). MicroRNA signatures in plasma and plasma exosome during window of implantation for implantation failure following in-vitro fertilization and embryo transfer. Reprod. Biol. Endocrinol. 19 (1), 180. doi:10.1186/s12958-021-00855-5
Zhang, D., Lv, J., Tang, R., Feng, Y., Zhao, Y., Fei, X., et al. (2021). Association of exosomal microRNAs in human ovarian follicular fluid with oocyte quality. Biochem. Biophys. Res. Commun. 534, 468–473. doi:10.1016/j.bbrc.2020.11.058
Zhang, J., Tao, Y., Cai, R., and Wang, Y. (2022). miR-196a-5p-Rich extracellular vesicles from trophoblasts induce M1 polarization of macrophages in recurrent miscarriage. J. Immunol. Res. 2022, 6811632. doi:10.1155/2022/6811632
Zhang, Q., Sun, H., Jiang, Y., Ding, L., Wu, S., Fang, T., et al. (2013). MicroRNA-181a suppresses mouse granulosa cell proliferation by targeting activin receptor IIA. PLoS One 8 (3), e59667. doi:10.1371/journal.pone.0059667
Zhu, X., Liu, H., Zhang, Z., Wei, R., Zhou, X., Wang, Z., et al. (2020). MiR-103 protects from recurrent spontaneous abortion via inhibiting STAT1 mediated M1 macrophage polarization. Int. J. Biol. Sci. 16 (12), 2248–2264. doi:10.7150/ijbs.46144
Keywords: extracellular vesicles, embryo, development, implantation, the research progress
Citation: Fan W, Qi Y, Wang Y, Yan H, Li X and Zhang Y (2023) Messenger roles of extracellular vesicles during fertilization of gametes, development and implantation: Recent advances. Front. Cell Dev. Biol. 10:1079387. doi: 10.3389/fcell.2022.1079387
Received: 25 October 2022; Accepted: 16 December 2022;
Published: 05 January 2023.
Edited by:
Sofia Makieva, University Hospital Zürich, SwitzerlandReviewed by:
Kenji Miyado, National Center for Child Health and Development (NCCHD), JapanRenwu Hua, Shenzhen Hospital, The University of Hong Kong, China
Copyright © 2023 Fan, Qi, Wang, Yan, Li and Zhang. This is an open-access article distributed under the terms of the Creative Commons Attribution License (CC BY). The use, distribution or reproduction in other forums is permitted, provided the original author(s) and the copyright owner(s) are credited and that the original publication in this journal is cited, in accordance with accepted academic practice. No use, distribution or reproduction is permitted which does not comply with these terms.
*Correspondence: Yingjie Zhang, MjAyMTExMTAzMUBzZHV0Y20uZWR1LmNu
†These authors have contributed equally to this work